- 1State Key Laboratory of Medicinal Chemical Biology, College of Pharmacy and Tianjin Key Laboratory of Molecular Drug Research, Nankai University, Tianjin, China
- 2The National Institutes of Pharmaceutical R&D Co., Ltd., Beijing, China
- 3Tianjin Key Laboratory of Molecular Drug Research, Tianjin International Joint Academy of Biomedicine, Tianjin, China
Introduction: Deglycosylated azithromycin (Deg-AZM), a newly developed Class I drug with good therapeutic effects on slow transit constipation, is a small-molecule transgelin agonist that has been approved for clinical trials in 2024. The preclinical pharmacokinetic profile of Deg-AZM was investigated to support further development.
Methods: A LC-MS/MS method was established and validated to detected the concentration of Deg-AZM in various biological samples. In vivo tests such as pharmacokinetic studies in rats and dogs, tissue distribution studies in rats, and extraction studies in rats were conducted to investigated the preclinical pharmacokinetic behaviors of Deg-AZM comprehensively. The plasma protein rate of Deg-AZM was determined by rapid equilibrium dialysis method in vitro. The metabolic stability and metabolite profile of Deg-AZM was assessed using pooled mice, rats, dogs, monkeys and humans microsomes in vitro. The PK profiles of Deg-AZM in human was predicted based on physiologically based pharmacokinetic (PBPK) models.
Results: The plasma protein binding rates of Deg-AZM were lower in mice and rats, higher in dogs, and moderate in humans. The metabolic process of Deg-AZM was similar in rat and human liver microsomes. From Pharmacokinetic studies in rats and dogs, Deg-AZM was rapidly absorbed into the blood and then quickly eliminated. Plasma exposure of Deg-AZM was dose dependent with no accumulation after continuous gavage administration. In addition, there is no significant gender difference in the pharmacokinetic behavior of Deg-AZM. Deg-AZM was widely distributed in the tissues without obvious accumulation, and mainly excreted from the urinary excretion pathway. Furthermore, the pharmacokinetic profiles of Deg-AZM in humans showed dose dependency.
Conclusion: The pharmacokinetic profiles of Deg-AZM was fully explored, these results could provide valuable information to support the first-in-human dosage prediction and phase I clinical design.
1 Introduction
Slow transit constipation (STC), also known as colonic inertia, is a refractory constipation caused by weakened colonic transit function, leading to the retention of fecal matter in the colon (Rao et al., 2016; Bharucha and Wald, 2019). The main clinical manifestations of STC include reduced frequency of defecation, difficulty in defecation, dry and hard feces, abdominal distension, and a series of serious complications that severely affect people’s quality of life (Bharucha et al., 2013; Shah et al., 2015). Nowadays, the incidence of constipation is increasing year by year with the acceleration of people’s pace of life, changes in dietary structure and habits, and the influence of social and physiological factors (Bharucha and Lacy, 2020; Crosby and Husk, 2021). STC is a pathological process involving multiple pathogenic mechanisms with no unified treatment plan (Pannemans et al., 2020; Vriesman et al., 2020). The first-line treatment in clinical practice is conservative drug therapy and maintaining good lifestyle habits (Camilleri et al., 2016; Yamamoto et al., 2021). Surgery, such as colon segment resection, anastomosis, and ileostomy, may be necessary in clinical practice (Huang et al., 2019). However, surgical treatment often leads to serious complications, such as recurrent constipation, diarrhea, and obstruction (Knowles, 2015). Currently, there is no ideal method for effectively treating STC, and there is an urgent need for new therapies to accelerate colonic transit. It is necessary to develop efficient and low-toxicity drugs to treat chronic constipation.
Deglycosylated azithromycin (Deg-AZM), a new Class I drug with good therapeutic effects on slow transit constipation developed by our research group, is a small-molecule transgelin agonist that has been approved for clinical trials in 2024. Deg-AZM is a unique metabolite of azithromycin (AZM). AZM can be used to treat gastrointestinal motility disorders (Brown et al., 1997; Parnham et al., 2014; Zeng et al., 2020). However, AZM has a side effect of gastrointestinal irritation in clinic, which varies greatly among different people (Firth and Prathapan, 2020; Ong et al., 2022; Wang et al., 2022). In addition, as an antibiotic, AZM is not suitable for development as a constipation drug. Our previous study found that Deg-AZM was a positive intestinal agonist that can induce intestinal activity, consistent with the side effect of AZM (Zhong et al., 2020). As an orally bioavailable small-molecule transgelin agonist, Deg-AZM (Figure 1) has been developed to treat STC (Zhong et al., 2020) and obtained implied approval for clinical trials from the Center for Drug Evaluation of China (acceptance number CXHL2400005). Transgelin, a 22 kDa protein, is the actin-binding protein in the Calponin family first discovered in chicken gizzard smooth muscle and named for its ability to bind to actin (Lees-Miller et al., 1987; Shapland et al., 1993; Li et al., 2008). Deg-AZM can stimulate the expression of transgelin in intestinal smooth muscle cells, promote the polymerization of G-actin into F-actin, increase the formation of stress fiber bundles in intestinal smooth muscle cells, and promote intestinal peristalsis (Zhong et al., 2020). Remarkably, because no similar drug targets transgelin to treat STC, Deg-AZM could potentially be a new option for treating STC.
Clinical pharmacokinetic (PK) data could not be obtained in the early stage of drug development. First-in-human (FIH) dosage prediction based on preclinical data is an important reference for phase I clinical design, which can effectively reduce the risk of human toxicity (Heller et al., 2018; Davies et al., 2020). Physiologically based pharmacokinetic (PBPK) modeling is a simulation model based on knowledge of physiology, biochemistry, and anatomy that simulates the blood flow in the body’s circulatory system, interconnecting various tissues or organs within the body (Kuepfer et al., 2016). It follows the principle of mass balance to simulate the metabolic processes of drugs in the body. A PBPK model could predict the PK profiles of drugs in humans (Zhang et al., 2024). The purpose of the present study was to investigate the preclinical PK profile of Deg-AZM, including the absorption, distribution, metabolism, and excretion properties of Deg-AZM across various biological matrices in nonclinical species, with a primary focus on rats and dogs. Furthermore, the PK profiles of Deg-AZM in healthy Asian humans at various doses were simulated to support the single ascending dose (SAD) clinical trial.
2 Materials and methods
2.1 Chemicals, reagents, and materials
Deg-AZM was provided by Nankai University (Tianjin, China). Roxithromycin (internal standard, IS), propranolol hydrochloride, and diphenhydramine hydrochloride were provided by the National Institutes for Food and Drug Control (Beijing, China). Midazolam was obtained from Cerilliant Co. Ltd. (Texas, United States). All of the reference substances were 98% purity. HPLC-grade methanol and acetonitrile were obtained from Thermo Fisher Scientific India Pvt. Ltd. (Waltham, MA, United States). HPLC-grade ammonium acetate was purchased from Tianjin Guangfu Technology Development Co., Ltd. (Tianjin, China). Carboxymethylcellulose sodium (CMC-Na) was provided by Sinopharm Chemical Reagent Co. Ltd. (Shanghai, China). N,N-dimethylformamide (DMF) was obtained from Concord Technology Co. Ltd. (Tianjin, China). Magnesium chloride, disodium hydrogen phosphate, and sodium dihydrogen phosphate were purchased from Tianjin Guangfu Fine Chemical Research Institute (Tianjin, China). NADP and glucose-6-phosphate dehydrogenase (G-6-PD) were purchased from Sigma-Aldrich Pty Ltd. (City of Saint Louis, United States). Blank human plasma, and liver microsomes of human, dog, mouse, and monkey were purchased from the Research Institute for Liver Diseases Co. Ltd. (Shanghai, China). Blank mouse plasma, blank rat plasma, and blank dog plasma were prepared by Tiancheng New Drug Evaluation Co. Ltd. (Tianjin, China). Deionized water used throughout the study was provided by Wahaha Corporation (Hangzhou, China).
2.2 Animals
Sprague–Dawley (SD) rats were purchased from Beijing Vital River Laboratory Animal Technology Co., Ltd. During the experiment, the temperature of the animal feeding environment was 19.84–26.14°C, and the humidity was 36.05–60.50%, which met the temperature and humidity requirements of the feeding environment. The beagle dogs were provided by the Shanghai Xingang Experimental Animal Research Center. During the experiment, the temperature of the animal feeding environment was 21.81–25.28°C, and the humidity was 41.94–56.39%, which met the temperature and humidity requirements of the feeding environment.
The animal experiments in this study were conducted in animal experimental facilities certified by the International Laboratory Animal Evaluation and Certification Management Committee and approved by the Institutional Animal Care and Use Committee at Tianjin Tiancheng New Drug Evaluation Co., Ltd. (Permit No. SYXK 2016–0009).
2.3 LC-MS/MS conditions
The concentrations of Deg-AZM in biological samples processed by precipitation of protein were analyzed by LC-MS/MS. The mobile phases used were methanol (A) and water/methanol (100:5, v/v) with 5 mM ammonium acetate (B). The chromatographic separation was performed on an LC-20A HPLC system (Shimadzu, Japan) using Agilent ZORBAX Eclipse C18 (50 mm × 4.6 mm, 5 µm). The column temperature was 40°C, and the flow rate was 0.5 mL/min. The gradient started with 55% A, progressed to 95% A over 3 min, and was maintained for 1.5 min. The column was then re-equilibrated with 55% A for 1.5 min. The mass spectrometric detection was performed on an API 4000 QTRAP (AB SCIEX) under the positive electrospray ionization (ESI+) source condition. The multiple reaction monitoring (MRM) of Deg-AZM and IS were m/z 434.4→300.4 and m/z 837.5→679.5, respectively, and are presented in Table 1 and Figure 1.
2.4 Drug preparation before administration
An amount of Deg-AZM was weighed, dispersed in 0.5% carboxymethyl cellulose sodium solution, and then stirred evenly to prepare the formulation for oral administration to rats and dogs in in vivo pharmacokinetic studies, tissue distribution studies, and excretion studies. An amount of Deg-AZM was weighed and dissolved into N, N-dimethylformamide-5% glucose injection (7:3; v/v) solution to prepare the formulation for intravenous administration for rats and dogs in pharmacokinetic studies, tissue distribution studies, and excretion studies in vivo. All formulations were prepared freshly before dosing.
2.5 Biological sample preparation for LC-MS/MS analysis
2.5.1 Rat plasma sample preparation
Plasma samples were prepared by protein precipitation. Aliquots (30 μL of rat plasma, 50 µL of IS solution (250 ng/mL, dissolved in acetonitrile), and 300 µL acetonitrile) were added to EP tubes. The mixtures were vortexed for 1 min and centrifuged at 12,000 rpm, 4°C for 10 min, and 50 μL of the supernatant was taken and diluted with 100 µL of distilled water, vortexed for 1 min, and centrifuged at 12,000 rpm, 4°C for 5 min. Finally, the upper layer was analyzed by the LC-MS/MS system.
2.5.2 Dog plasma sample preparation
Plasma samples were prepared by protein precipitation. Aliquots (30 μL of dog plasma, 50 µL of IS solution (1 μg/mL, dissolved in acetonitrile), and 300 µL acetonitrile) were added to EP tubes. The mixtures were vortexed for 1 min and centrifuged at 12,000 rpm, 4°C for 10 min, and 50 μL of the supernatant was taken and diluted with 200 µL methanol-water (1:3, v/v), vortexed for 1 min, and centrifuged at 12,000 rpm, 4°C for 5 min. Finally, the upper layer was analyzed by the LC-MS/MS system.
2.5.3 Tissue sample preparation
Aliquots (30 µL of the tissue homogenate, 50 µL of IS solution (250 ng/mL, dissolved in acetonitrile), and 300 µL acetonitrile) were mixed and vortexed for 1 min. After centrifuging at 4°C, 12,000 rpm for 10 min, 50 µL of the supernatant was diluted with 100 µL methanol–water (1:4, v/v), vortexed for 1 min, and centrifuged at 12,000 rpm, 4°C for 5 min. Finally, the upper layer was analyzed using the LC-MS/MS system.
2.5.4 Urine sample preparation
Aliquots (30 µL of urine, 50 µL of 50% methanol aqueous solution, 50 µL of IS solution (1,500 ng/mL, dissolved in acetonitrile), and 300 µL of acetonitrile) were added to EP tubes. The mixtures were vortexed for 1 min, centrifuged at 12,000 rpm, 4°C for 10 min, and 50 μL of the supernatant was taken and diluted with 200 µL methanol–water (1:3, v/v), vortexed for 1 min, and centrifuged at 12,000 rpm, 4°C for 5 min. Finally, the upper layer was analyzed by the LC-MS/MS system.
2.5.5 Feces sample preparation
Aliquots (50 µL of feces homogenate, 50 µL of 50% methanol aqueous solution, 50 µL of IS solution (250 ng/mL, dissolved in acetonitrile), and 100 µL of acetonitrile) were mixed and vortexed for 1 min. After centrifuging at 4°C, 12,000 rpm for 10 min, 100 µL of the supernatant was diluted with 100 µL distilled water, vortexed for 1 min, and centrifuged at 12,000 rpm, 4°C for 5 min. Finally, the upper layer was analyzed using the LC-MS/MS system.
2.5.6 Bile sample preparation
A 50-µL aliquot of rat bile, 50 µL of 50% methanol aqueous solution, 50 µL of IS solution (250 ng/mL, dissolved in acetonitrile), and 1 mL of extraction solvent (ethyl acetate: isopropanol = 3:1, v/v) were added to tubes and vortexed for 1 min. After centrifuging at 4°C, 12,000 rpm for 10 min, 800 μL of the supernatant was dried with nitrogen in a 40°C water bath. The residues were reconstituted with 400 µL of 50% methanol aqueous solution, then vortexed for 1 min, and centrifuged at 12,000 rpm, 4°C for 5 min. Finally, the upper layer was analyzed using the LC-MS/MS system.
2.6 Method validation
The developed LC-MS/MS method for absolute quantification of Deg-AZM in biological samples was validated in accordance with the guidelines outlined in the Food and Drug Administration (FDA) Guidance for Industry on Bioanalytical Method Validation (FDA, 2018), addressing selectivity, crosstalk, carryover effect, matrix effect, recovery, linearity, lower limit of quantification (LLOQ), upper limit of quantification (ULOQ), accuracy, precision, and stability.
2.7 Pharmacokinetic studies in rats and dogs
Deg-AZM was administered to SD rats via a single intravenous bolus at the dose of 10 mg/kg or by single and multiple oral administration at the dose levels defined in each study. The dosages for a single oral administration were 10 mg/kg, 25 mg/kg, and 50 mg/kg, and the dose of repeated oral administration was 25 mg/kg. Six rats (half male and half female) were used for administration and sampling in each pharmacokinetic study at different doses. Blood samples (approximately 200 µL) were collected at pre-dose (0) and at 0.0333 h, 0.0833 h, 0.25 h, 0.5 h, 1 h, 2 h, 4 h, 6 h, 8 h, 10 h, and 12 h after intravenous administration. Blood samples were also collected at pre-dose (0) and at 0.0833 h, 0.25 h, 0.5 h, 1 h, 2 h, 4 h, 6 h, 8 h, 10 h, and 12 h after administration. Plasma was prepared by centrifugation at 12,000 rpm for 4 min and stored frozen at approximately −70°C until analysis.
Deg-AZM was administered to beagle dogs via a single intravenous bolus at a dose of 3 mg/kg or single and multiple oral administration at the dose levels defined in each study. The dosages for a single administration were 3 mg/kg, 10 mg/kg, and 30 mg/kg, and the dose of repeated administration by gavage administration was 10 mg/kg. Six dogs (half male and half female) were used for administration and sampling in each pharmacokinetic study at different doses. Approximately 0.5 of mL blood samples was collected at pre-dose (0) and at 0.0333 h, 0.0833 h, 0.25 h, 0.5 h, 1 h, 2 h, 4 h, 6 h, 8 h, 10 h, and 12 h post dose. Plasma was prepared by centrifugation at 12,000 rpm for 4 min and stored frozen at approximately −70°C until analysis.
Non-compartmental PK parameters were evaluated via the individual concentration–time data using WinNonlin 8.3.1 (Certara United States, Princeton, NJ), including time of maximum concentration (Tmax), maximum concentration (Cmax), area under the concentration versus time curve (AUC), mean residence time (MRT), volume of distribution (Vz), and terminal half-life (T1/2). Finally, the plasma kinetic characteristics of the drug in rats were analyzed, including exposure level, absorption characteristics, dose relationship, elimination rate, possible accumulation, and absolute bioavailability. The T-test was used to compare data between different groups, and P < 0.05 was considered statistically significant. The absolute bioavailability was calculated using the average AUC value without statistical analysis.
2.8 Tissue distribution studies in rats
SD rats were randomly divided into four groups (tissues were taken at 0.167 h, 0.5 h, 3 h, and 8 h post dose), with six rats in each group (half male and half female). The rats were fasted for 12 h and allowed ad libitum access to water before the experiment. After oral administration of 25 mg/kg of Deg-AZM, the rats were anesthetized at different time points, and the plasma was prepared by venous blood collected from the abdominal aorta. The rats were euthanized, and brain, muscles, fat, testes (ovaries), epididymis (uterus), bladder, spleen, kidneys, liver, heart, lungs, colon, stomach, and duodenum tissues were collected. Tissue homogenates were prepared using a 50% methanol aqueous solution in a ratio of 1:5 (g/mL). The above biological samples were stored at −70°C for determination.
2.9 Plasma protein binding
The rapid equilibrium dialysis method was used to determine the plasma protein rates of Deg-AZM in the plasma of mice, rats, dogs, and humans in vitro. Propranolol was selected as the positive control drug for this study. A 0.04-mL aliquot of Deg-AZM acetonitrile solution at concentrations of 50 μg/mL, 200 μg/mL, and 1,000 μg/mL was added into 1.96 mL blank plasma of mice, rats, dogs, and humans, respectively, then mixed evenly to prepare drug-containing plasma samples with final concentrations of 1 μg/mL, 4 μg/mL, and 20 μg/mL for Deg-AZM. Then, 0.024 mL of propranolol methanol solution (2.5 μg/mL) was added into 1.176 mL blank plasma of mice, rats, dogs, and humans, respectively, with mixing evenly to prepare drug-containing plasma samples with final concentrations of 50 ng/mL for propranolol.
In the balanced dialysis tube (n = 3), drug-containing plasma (300 µL) of Deg-AZM at concentrations of 1 μg/mL, 4 μg/mL, and 20 μg/mL or propranolol at concentrations of 50 ng/mL were added to side A, respectively, and 500 µL of phosphate-buffered saline (PBS) was added to side B. The dialysis devices were sealed with a sealing film and incubated in a 37°C water bath with gentle shaking at 100 rpm for 4 h. A 30-μL sample of plasma from side A and another 30-μL sample of the solution from side B were aspirated and analyzed to determine the concentration of Deg-AZM using LC-MS/MS. The protein binding rate was calculated according to the following formula:
Ctotal represents the drug concentration on the plasma side, and Cfree represents the drug concentration on the PBS side.
2.10 In vitro metabolic stability
The metabolic stability and metabolite profile of Deg-AZM (1 µM) were assessed using pooled mouse, rat, dog, monkey, and human microsomes (Research Institute for Liver Diseases Co. Ltd., Shanghai, China). Midazolam was employed as the positive control drug to monitor the activity of enzymes in the test. An inactivated rat liver microsome incubation system was set as the negative control group to investigate the self-stability of Deg-AZM in the incubation system.
The total incubation volume of 1 mL included 745 µL PBS (pH 7.4), 50 µL microsomal protein (final concentration at 1 mg/mL), NADPH regeneration system, and 5 µL Deg-AZM (final concentration at 1 µM). Incubation was carried out in a water bath at 37°C. At 0 min, 3 min, 6 min, 9 min, 15 min, and 30 min (for monkey microsomes) and 0 min, 6 min, 15 min, 30 min, 60 min, and 120 min (for the other four species and the negative control), 50 µL of the reaction mixture was withdrawn and added into an equal volume of cold acetonitrile to stop the reaction and precipitate proteins. A positive control group was set up to ensure the reliability of the study. At the end of the assay, the remaining content of Deg-AZM was determined by LC-MS/MS.
The elimination rate constant (−ke) was the slope of the linear regression of ln (% remaining content of Deg-AZM) with incubation time. T1/2, intrinsic clearance (CLint), hepatic clearance (CLh), and extraction ratio (ER) of Deg-AZM were calculated according to the following formula:
In the formula, Cprotein was 1 mg/mL. The scaling factor (SF) = microsomal weight per gram of liver (mg/g) × liver weight to body weight ratio (g/kg). The microsomal weights per gram of the livers of mice, rats, dogs, monkeys, and humans were 45 mg/g, 44.8 mg/g, 77.9 mg/g, 45 mg/g, and 48.8 mg/g, respectively (Yang et al., 2022). The liver weight to body weight ratios of mice, rats, dogs, monkeys, and humans were 87.5 g/kg, 40.0 g/kg, 32.0 g/kg, 32.5 g/kg, and 25.7 g/kg, respectively (Yang et al., 2022; Pearson and Wienkers, 2008). SF values in mice, rats, dogs, monkeys, and humans were 3937.5 mg/kg, 1792 mg/kg, 2492.8 mg/kg, 1462.5 mg/kg, and 1254.16 mg/kg, respectively. The liver blood flow rates (Q) in mice, rats, dogs, monkeys, and humans were 90 mL/min/kg, 55.2 mL/min/kg, 30.9 mL/min/kg, 44 mL/min/kg, and 20.7 mL/min/kg, respectively. fµb was approximately equal to 1.
2.11 Metabolite profiling
After incubating Deg-AZM with liver microsomes, the resulting samples from “2.10 In vitro metabolic stability” were analyzed by ultra-high performance liquid chromatography with quadrupole time-of-flight mass spectrometry (UPLC-QTOF-MS). UNIFI software was used to identify the metabolites of Deg-AZM. Potential metabolites were identified by combining standard metabolic reaction patterns. Based on the precise molecular weight and formula of each metabolite, fragment ions of the parent compound and metabolites were compared to elucidate the structures of the metabolites. The metabolic spectrum differences of Deg-AZM in liver microsomes of different species were analyzed by semi-quantitative peak area normalization.
2.12 Excretion studies in rats
Twelve SD rats (half male and half female) were used to collect urine, fecal, and bile samples after administration of Deg-AZM. Two rats of the same gender were placed in a metabolic cage. After oral administration of Deg-AZM at 25 mg/kg, urine samples were collected at 0–2 h, 2–4 h, 4–6 h, 6–8 h, 8–12 h, 12–24 h, 24–30 h, 30–48 h, 48–72 h, and 72–96 h post dose at room temperature. Fecal samples were collected at 0–6 h, 6–12 h, 12–24 h, 24–30 h, 30–48 h, 48–72 h, and 72–96 h post dose at room temperature. Six SD rats (half male and half female) were used to collect bile. Bile samples were collected from 0–2 h, 2–4 h, 4–6 h, 6–8 h, 8–12 h, 12–24 h, 24–30 h, 30–48 h, and 48–72 h post dose using bile duct intubation surgery. The volume of urine and bile and the weight of feces were measured. The above biological samples were stored at −70°C until further determination.
2.13 Prediction of PK profiles of Deg-AZM in healthy Asian humans based on PBPK models
2.13.1 Parameters employed for the development of PBPK
Parameters related to physiological and drug-specific were collected to establish the PBPK models of Deg-AZM, including molecular weight, solubility, LogP, pKa, fraction unbound in plasma (fup), apparent permeability coefficient (Papp), clearance in microsomes (CL), and T1/2. The solubility of Deg-AZM was determined in a phosphate buffer liquid system at pH 7.4. The logP of Deg-AZM was measured using a shake flask method with n-octanol/buffer. The pKa of Deg-AZM was determined by UV–visible spectrophotometry according to the Chinese Pharmacopoeia. The fup of Deg-AZM could be calculated from the “2.9. Plasma protein binding” experiment using the following formula: fup = 1 − (the plasma protein binding rates of Deg-AZM). The Papp of Deg-AZM was determined on Caco-2 cell monolayers. The CL and T1/2 of Deg-AZM were measured following the experiment described in “2.10. In vitro metabolic stability”.
2.13.2 Establishment and validation of PBPK models for Deg-AZM in rats
PBPK models of Deg-AZM in rats were developed using PK-Sim (Version 9.1.2, Bayer Technology Services, Leverkusen, Germany). The cellular permeability of the models was estimated using the PK-Sim standard method. The Rodgers and Rowland method was employed to optimize the distribution part of the models. The simulated PK parameters of Deg-AZM in rats were compared with the observed values from experiments to validate the PBPK models. The accepted criterion was that the ratio between the predicted and observed values should fall within the range of 0.5–2.0.
2.13.3 Prediction of PK profiles of Deg-AZM in healthy Asian humans
After successfully establishing and validating the PBPK models of Deg-AZM in rats, the PK profiles in healthy Asian humans of Deg-AZM at various doses were simulated to support the SAD clinical trial.
3 Results
3.1 Absorption
3.1.1 Deg-AZM plasma pharmacokinetics in rats
The plasma pharmacokinetics of Deg-AZM in male and female rats were estimated after a single intravenous dose or single and multiple oral administration. The PK parameters are listed in Table 2. The plasma concentration versus time curves are displayed in Figure 2. After a single intravenous injection of Deg-AZM at a dose of 10 mg/kg, Deg-AZM was eliminated quickly with a T1/2 of 1.05 h and 1.72 h for male and female rats, respectively. The apparent distribution volumes (Vd) for male and female rats were 4.85 L/kg and 6.82 L/kg, which is significantly greater than the total body fluid volume (0.6 L/kg), indicating that Deg-AZM was mainly distributed in tissues. The clearance (CL) values of Deg-AZM were 3.22 L/h/kg and 2.81 L/h/kg for male and female rats. The absolute bioavailabilities of Deg-AZM at the dosage of 10 mg/kg were 23.8% and 47.46% for male and female rats. The absolute bioavailability of Deg-AZM may increase with increased dose because the exposure increased more than the dose. There were no apparent sex differences in the PK parameters in rats. After single gavage of Deg-AZM at doses of 10 mg/kg, 25 mg/kg, and 50 mg/kg, Cmax values were 402 ng/mL, 2000 ng/mL, and 6,730 ng/mL for males and 1,062 ng/mL, 3,287 ng/mL, and 7,117 ng/mL for females. The AUC0–12 h values were 765 h⋅ng/mL, 3,367 h⋅ng/mL, and 11,633 h⋅ng/mL for males and 1927 h⋅ng/mL, 6,580 h⋅ng/mL, and 10,857 h⋅ng/mL for females, respectively. We found that Cmax and AUC0–12 h increased with increased dose, with ratios of 1:4.98:16.74 (male) and 1:3.1:6.76 (female) for Cmax and 1:4.4:15.21 (male) and 1:3.41:5.63 (female) for AUC0–12 h (Figure 2D). The Tmax in male and female rats was 0.278–0.917 h and 0.197–0.333 h, respectively, and the absorption rate of Deg-AZM were eliminated fast both in female and male rats. The T1/2 values in male and female rates were approximately 1.07–1.27 h and 1.13–1.18 h, respectively, with the fast elimination rate. Tmax and T1/2 did not change with dose. Compared with a single gavage administration, the Cmax ratios of the last dose to the first dose for male and female rats were 0.58 and 0.69, and the AUC0–12 h ratios were 0.98 (male) and 0.50 (female), respectively. The Tmax and T1/2 remained unchanged after multiple doses compared to those after a single dose. Therefore, there was no accumulation of the drug, and there was no change in the elimination behavior.
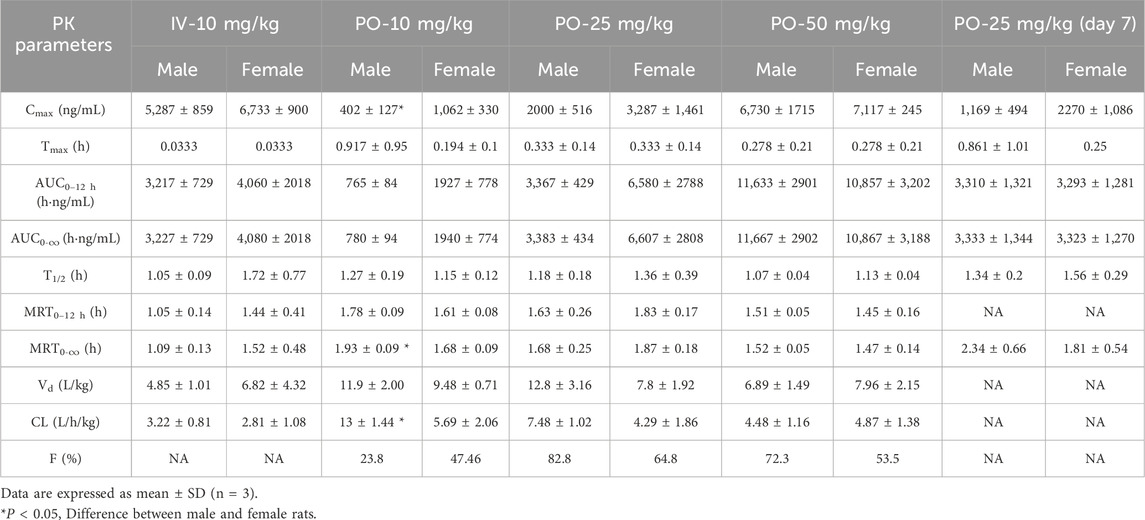
Table 2. Overall mean Deg-AZM plasma pharmacokinetic parameters in rats after intravenous bolus or oral administration.
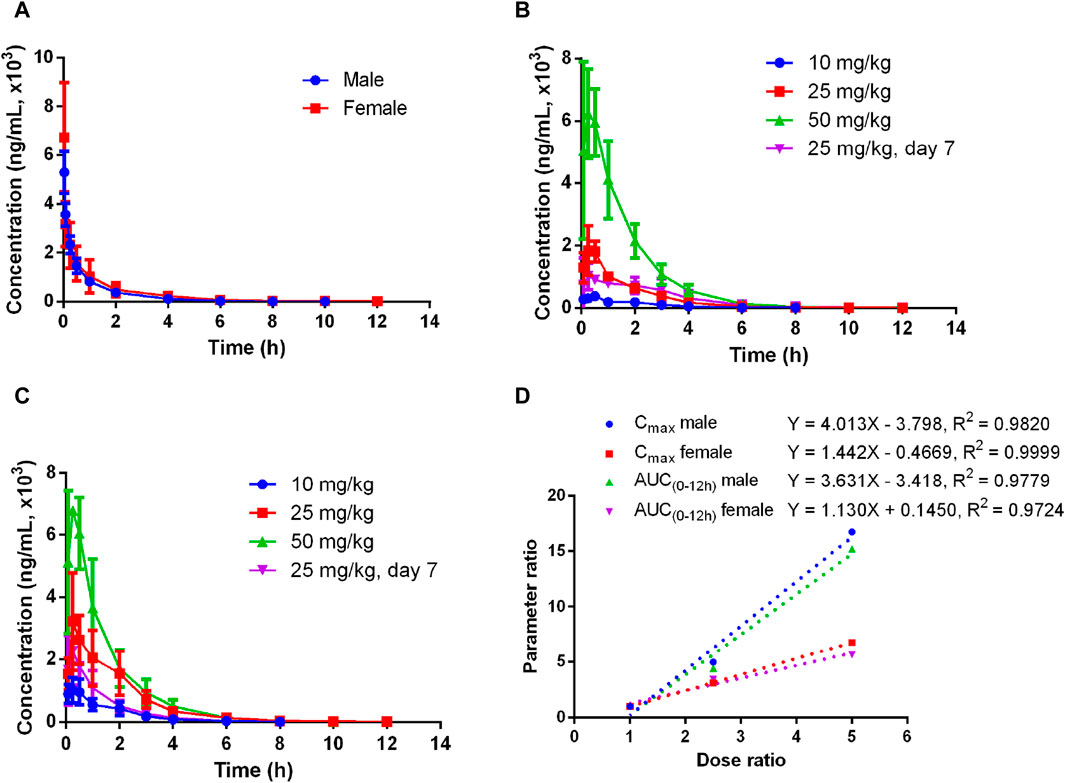
Figure 2. Mean plasma concentration–time curves of Deg-AZM in rats (A) after a single 10 mg/kg intravenous administration, (B) after single or multiple oral doses in male rats, (C) after single or multiple oral doses in female rats, and (D) exposure–dose relationship after single oral doses of 10 mg/kg, 25 mg/kg, and 50 mg/kg (n = 3).
3.1.2 Deg-AZM plasma pharmacokinetics in dogs
The plasma pharmacokinetics of Deg-AZM in male and female dogs were estimated after a single intravenous dose or single and multiple oral administration. The PK parameters are listed in Table 3. The plasma concentration versus time curves are displayed in Figure 3. After a single intravenous injection of Deg-AZM at a dose of 3 mg/kg in dogs, Deg-AZM was eliminated quickly with a T1/2 of 1.29 h and 1.36 h for male and female dogs. The absolute bioavailability of Deg-AZM at the dosage of 3 mg/kg was 32.8% and 57.4% for male and female dogs. The absolute bioavailability of Deg-AZM showed no significant change with increasing doses. There were no significant sex differences in the main PK parameters in dogs. After single gavage of Deg-AZM at doses of 3 mg/kg, 10 mg/kg, and 30 mg/kg in dogs, Cmax were 1,617 ng/mL, 5,830 ng/mL, and 10,973 ng/mL for male dogs and 1760 ng/mL, 4,603 ng/mL, and 11,117 ng/mL for female dogs. AUC0–24 h values were 3,627 h ng/mL, 14,300 h ng/mL, and 40,200 hng/mL for male dogs and 4,603 h ng/mL, 16,700 h ng/mL, and 34,700 hng/mL for female dogs, respectively. We found that Cmax and AUC0–24 h values increased with increasing dose, with a ratio of 1:3.61:6.79 (male) and 1:2.62:6.32 (female) for Cmax and 1:3.94:11.08 (male) and 1:3.63:7.52 (female) for AUC0–24 h (Figure 3D). The Tmax in male and female rats was 0.333–0.833 h and 0.25–1.5 h, respectively, and the absorption rate of Deg-AZM was relatively fast. The T1/2 values in male and female dogs were 1.21–3.41 h and 1.38–2.31 h, respectively, with a faster elimination rate. Tmax and T1/2 showed no significant change with increasing dose. Compared with a single gavage administration, the Cmax ratios of the last dose to the first dose for male and female dogs were 1.05 and 0.76, and the AUC0–24 h ratios were 0.96 (male) and 0.81 (female), respectively. The Tmax and T1/2 remained unchanged after multiple doses compared to those after a single dose. Therefore, there was no accumulation of drugs, and there was no change in the elimination behavior.
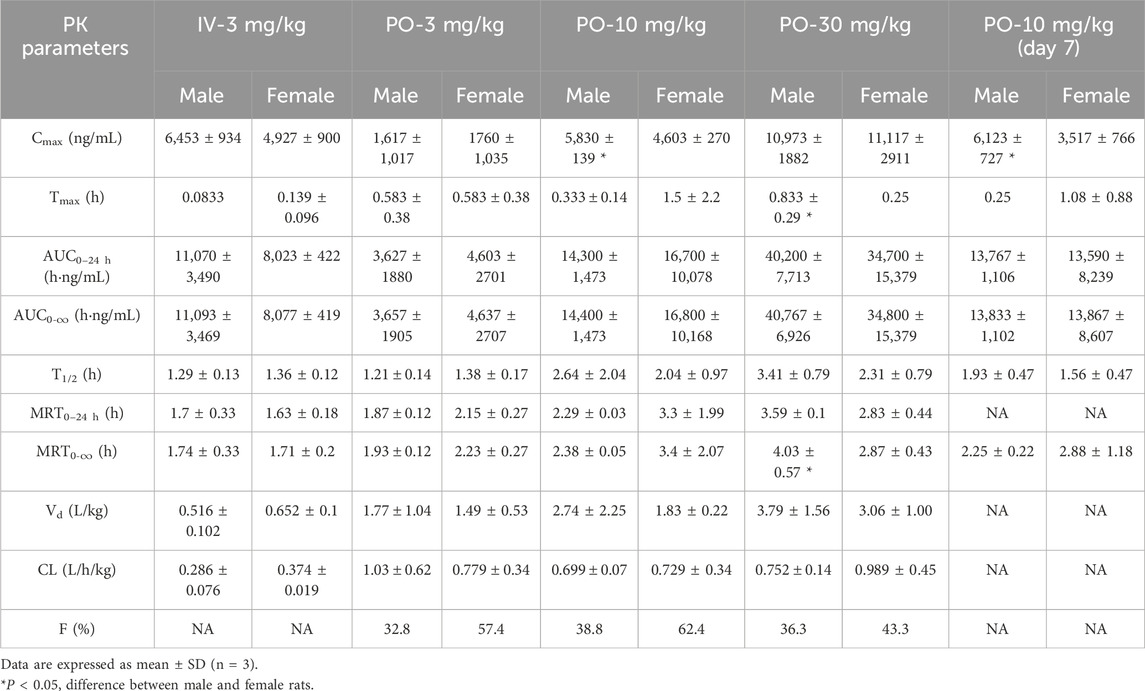
Table 3. Overall mean Deg-AZM plasma pharmacokinetic parameters in dogs after intravenous bolus or oral administration.
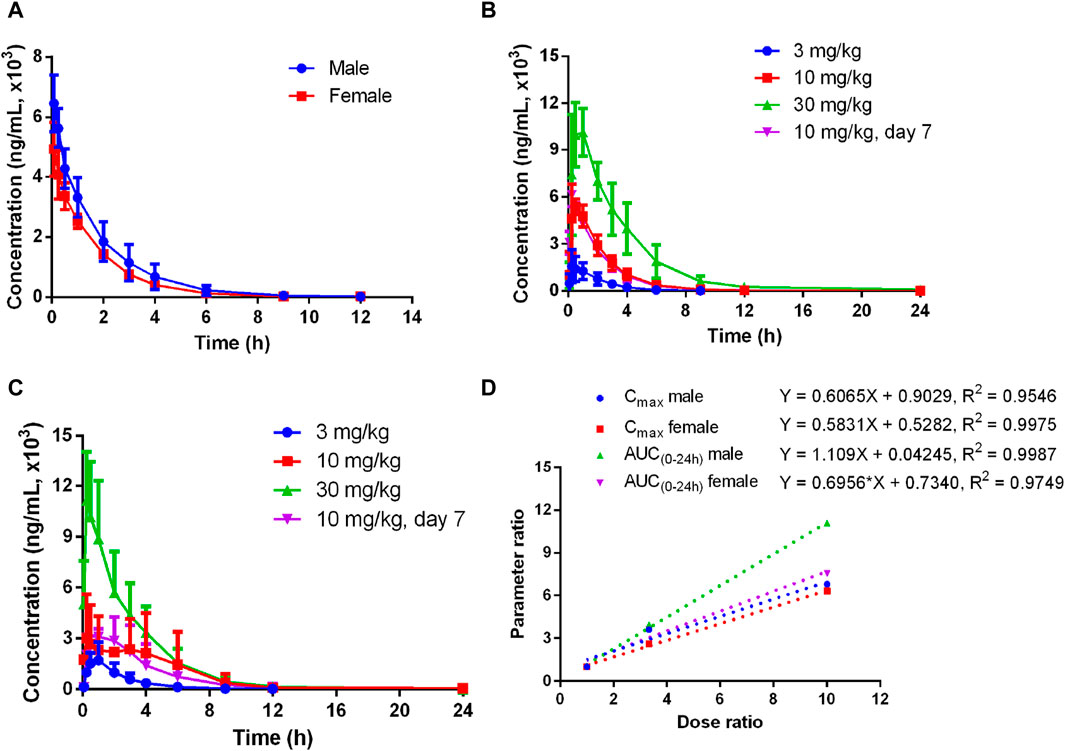
Figure 3. Mean plasma concentration–time curves of Deg-AZM in dogs (A) after a single 3 mg/kg intravenous administration, (B) after single or multiple oral doses in male dogs, (C) after single or multiple oral doses in female dogs, and (D) exposure–dose relationship after single oral doses of 3 mg/kg, 10 mg/kg, and 30 mg/kg (n = 3).
3.2 Distribution
3.2.1 Plasma protein binding
The binding rates of propranolol to plasma proteins of mice, rats, dogs, and humans were 92.7%, 90.5%, 97.0%, and 84.7%, respectively, which indicated that the experimental conditions were suitable. The in vitro binding rates of Deg-AZM to plasma proteins of mice, rats, dogs, and humans are shown in Table 4. The average binding rates of Deg-AZM to plasma proteins of mice, rats, dogs, and humans were 49.3%, 39.2%, 87.4%, and 73.3% in the concentration range of 1–20 μg/mL, respectively. This indicated that the plasma protein binding rate of Deg-AZM is lower in mice and rats, higher in dogs, and moderate in humans. In addition, the binding rate of Deg-AZM to human plasma proteins decreased with increasing concentration.
3.2.2 Tissue distribution in rats
The tissue distributions of Deg-AZM in male and female rats are shown in Figure 4. Deg-AZM could be detected in all tissues, and the exposure level in most tissues was higher than in plasma, indicating the widespread distribution of Deg-AZM in rat tissues. There was no significant gender difference in the exposure levels of Deg-AZM in tissues (Figures 4A,B). It was consistent with the high value of Vd (5.83 L/kg) for Deg-AZM. The exposure levels of Deg-AZM in rat tissues were ranked as follows: stomach (12.8 times that of plasma)>bladder, spleen, kidney, duodenum, liver (6–9 times that of plasma)>colon, lungs, ovaries, epididymis, muscles, testicle, heart, uterus (2–5 times that of plasma)>plasma > fat (35.7% that of plasma)>brain (8.36% that of plasma). Deg-AZM reached peak distribution at 0.167 h in the liver, stomach, intestine, and ovaries after administration, at 3 h in testes, and at 0.5 h in other tissues. At 8 h after administration, Deg-AZM in the testicle decreased to approximately one-third of its peak distribution, while in other tissues, Deg-AZM decreased to approximately 1/100–1/10 of the peak distribution, indicating no significant accumulation of Deg-AZM in these tissues of male and female rats.
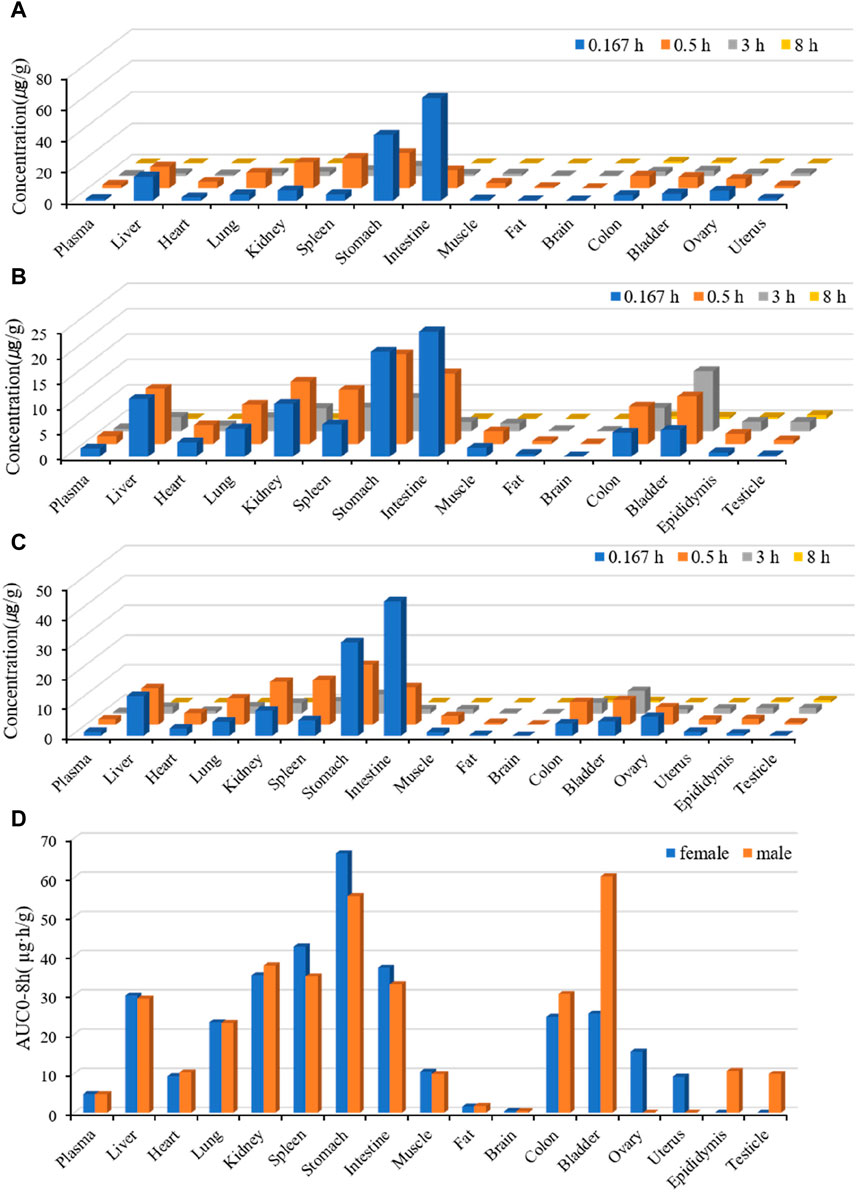
Figure 4. Tissue distribution of Deg-AZM at 0.167 h, 0.5 h, 3 h, and 8 h after a single 25 mg/kg oral dose. (A) The tissue concentration of Deg-AZM in female rats. (B) The tissue concentration of Deg-AZM in male rats. (C) The average tissue concentration of Deg-AZM in male and female rats. (D) The tissue AUC of Deg-AZM in male and female rats.
3.3 Metabolism
3.3.1 In Vitro metabolic stability in liver microsomes
After incubation for 30 min in the positive control group, the remaining proportion of midazolam was 0.00338%, indicating the effectiveness of the incubation system and reaction conditions. After incubation for 120 min in the negative control group, the remaining substrate proportion was about 108%, indicating that Deg-AZM did not exhibit self-degradation in this incubation system.
The residual substrate ratio time curves are shown in Figure 5. After incubating Deg-AZM with mouse, rat, dog, and human liver microsomes for 120 min, the average remaining substrate ratios were 62.7%, 12.9%, 81.9%, and 16.5%, respectively. After incubating Deg-AZM with the monkey liver microsome for 30 min, the average remaining substrate ratio was 7.12%. The metabolic rate of Deg-AZM in various types of liver microsomes was in the order of monkey > rat > human > mouse > dog.
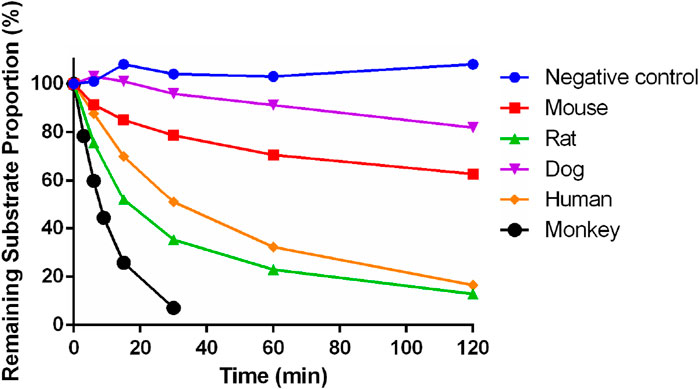
Figure 5. Metabolic stability of Deg-AZM in mouse, rat, dog, monkey, and human liver microsomes (n = 2).
The parameters of Deg-AZM in liver microsomes are shown in Table 5. T1/2 was greater than 120 min, and ER was less than 0.3 of Deg-AZM in mouse and dog liver microsomes. The T1/2 values in rat, monkey, and human liver microsomes were between 7 min and 50 min, and the ER values were 0.343, 0.747, and 0.474 in rat, monkey, and human liver microsomes, respectively. Deg-AZM had a higher liver extraction rate in monkey liver microsome, a moderate liver extraction rate in rat and human liver microsomes, and a lower liver extraction rate in mouse and dog liver microsomes, indicating that Deg-AZM was metabolized and eliminated most rapidly in monkey liver microsomes, while it exhibited a similar metabolic elimination profile in rat and human liver microsomes.
3.3.2 Metabolite profiling
In addition to the prototype of Deg-AZM, 8, 8, 9, 13, and 10 metabolites of Deg-AZM were identified from the liver microsomes of mice, rats, dogs, monkeys, and humans, respectively. The metabolic pathways include hydroxylation, methylation, and combinations of different metabolic pathways. Detailed information on each metabolite is listed Table 6. The metabolic pathway analysis is shown in Figure 6. The proportion of metabolites analyzed based on the mass spectrometry response peak areas is shown in Figure 7. In the human liver microsomes, Deg-AZM, M1-2 (hydroxylation of Deg-AZM), and M2 (demethylation of Deg-AZM) were the main forms of the drug, accounting for 34.4%, 30.0%, and 17.1% of the total related substances, respectively. In the rat liver microsomes, Deg-AZM, M1-2, and M2 were the main forms of the drug, accounting for 24.8%, 19.3%, and 44.6% of the total related substances, respectively. In the mouse liver microsomes, Deg-AZM accounted for 78.8% of the total related substances, and the proportion of each metabolite was less than 10%. In the dog liver microsomes, Deg-AZM accounted for 91.1% of the total related substances, and the proportion of each metabolite was less than 4%. In the monkey liver microsomes, M1-2 and M2 accounted for 31.4% and 38.5% of the total related substances; the proportion of each metabolite was less than 9%, and Deg-AZM was not detected.
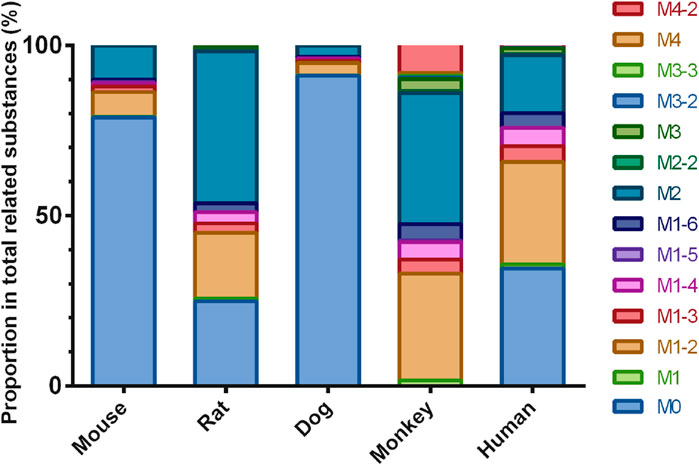
Figure 7. Comparison of metabolite profiles of Deg-AZM in liver microsome incubations of different species.
3.4 Excretion in rats
The excretion routes of Deg-AZM after oral administration of a dose of 25 mg/kg in both intact and bile duct-cannulated rats were determined. The mean cumulative excretion fraction curves of prototype Deg-AZM in the urine, bile, and feces of male and female rats are presented in Figure 8. At 96 h post-dosing, 20.022% (17.3% in urine, 0.752% in bile, and 1.97% in feces) and 14.763% (12.5% in urine, 0.623% in bile, and 1.64% in feces) of Deg-AZM were excreted from male and female rats, respectively. Deg-AZM was mainly excreted from the body through urine, with minimal excretion in its prototype form, indicating extensive metabolism within the body.
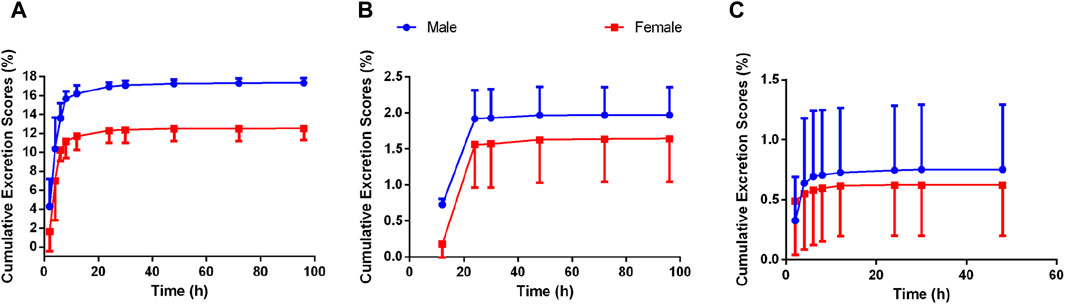
Figure 8. Mean cumulative excretion fraction curve of Deg-AZM in (A) urine, (B) feces, and (C) bile of rats after oral administration of Deg-AZM at 25 mg/kg (n = 3).
3.5 Establishment and validation of Deg-AZM PBPK models in rats
The parameters collected from preclinical studies were used to establish PBPK models of Deg-AZM (listed in Table 7), followed by validation using PK data of rats. Figure 9 showed the predicted/observed ratios of Cmax and AUC0–24 h in rats at different oral doses, with all values falling within the acceptable range of 0.5–2.0, indicating a well-established PBPK model.
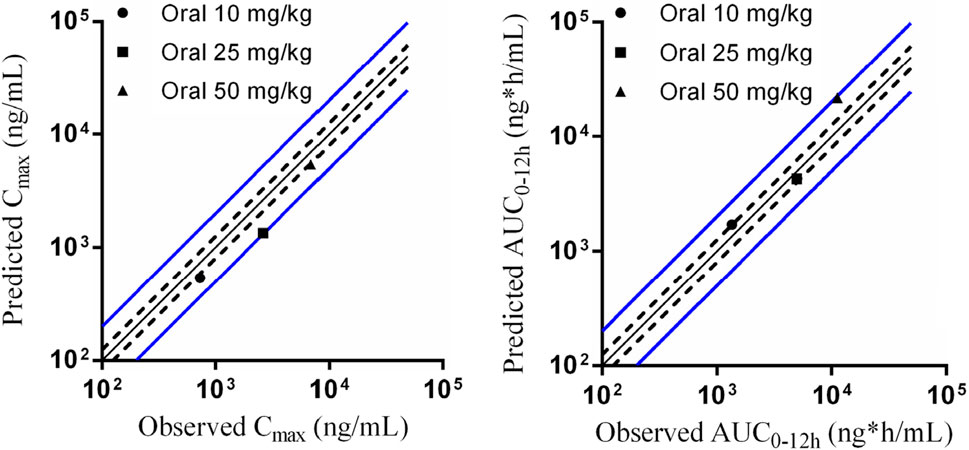
Figure 9. Predicted and validated PK parameters of Deg-AZM in SD rats. The solid black line represents the predicted values corresponding to the observed values. The dashed black line represents 0.8 and 1.25 times the observed values. The solid blue line represents 0.5 and 2 times the observed values.
3.6 Prediction of PK profiles for Deg-AZM in a healthy Asian population
Figure 10 showed the PK profiles of Deg-AZM in healthy Asian individuals at seven different doses, which were simulated based on the established PBPK model. The PK behavior of Deg-AZM showed dose dependency. These results could provide valuable information for SAD studies in a FIH clinical trial.
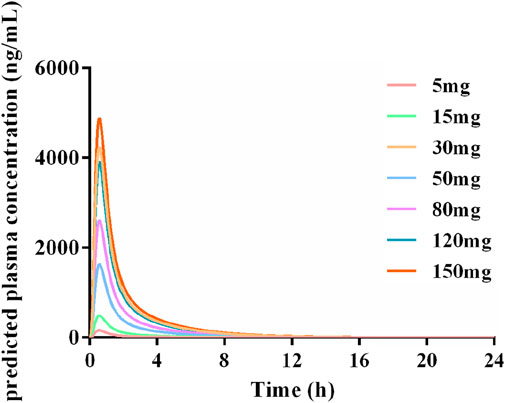
Figure 10. Simulated PK profiles of Deg-AZM in a healthy Asian population at doses of 5 mg, 15 mg, 30 mg, 50 mg, 80 mg, 120 mg, and 150 mg.
4 Discussion
Deg-AZM, a new Class I drug for clinical treatment of STC, was found to stimulate the expression of transgelin in intestinal smooth muscle cells, promote the polymerization of G-actin into F-actin, increase the formation of stress fiber bundles in intestinal smooth muscle cells, and promote intestinal peristalsis (Zhong et al., 2020). Because no similar drug targets transgelin to treat STC, Deg-AZM could potentially be a new option for treating STC.
In the early stage of drug development, comprehensively elucidating the ADME properties of the drug candidate in animals is a crucial prerequisite for understanding its efficacy and safety (Lucas et al., 2019). Predicting the first human dose based on preclinical data is a crucial reference for phase I clinical trial design, as it can effectively reduce the risk of human toxicity (Heller et al., 2018; Davies et al., 2020). In this study, an LC-MS/MS method was developed and validated to quantify the concentration of Deg-AZM in various biological matrices. The ADME profiles of Deg-AZM were investigated systematically in vivo and in vitro. PK studies conducted in rats or dogs indicated no significant gender differences in the PK behavior of Deg-AZM. Additionally, Deg-AZM was rapidly absorbed into the bloodstream and then rapidly cleared from the body. The plasma exposure of Deg-AZM was dose-dependent, and there was no accumulation after continuous oral administration. Results from plasma protein binding assays showed that the plasma protein binding rate of Deg-AZM was low in mice and rats, high in dogs, and moderate in humans. Metabolic stability studies in liver microsomes revealed that Deg-AZM was more easily metabolized and eliminated in monkey liver microsomes, with similar levels of metabolism and elimination in rat and human liver microsomes. Therefore, rats and dogs were chosen as animal species in preclinical experiments. Deg-AZM was widely distributed in the tissues of rats without significant accumulation. Deg-AZM was primarily excreted from the body of rats through the urinary tract. These studies provided comprehensive preclinical data for Deg-AZM, supporting initial human dose predictions and phase I clinical trials.
PBPK modeling based on computer simulation can simulate a holistic model by building on the physiological, biochemical, anatomical, pharmacological, and thermodynamic properties of the body. The PBPK model describes the behavior of drugs in vivo from a mechanistic perspective (Kostewicz et al., 2014; Kaur et al., 2018). In addition, the PBPK model can also simulate the effects of metabolism, enterohepatic circulation, physicochemical parameters, transport proteins, in vitro data, or the influence of ingestion (Pathak et al., 2019). In recent years, PBPK models have been widely applied in drug development, including dose selection for FIH (Damle et al., 2011), drug interaction studies (Dong et al., 2022; Ni et al., 2023), drug formulation studies (Wang et al., 2009), food effects studies (Arredondo et al., 1995), as well as the PK study of special populations (such as pregnant women, children, older people, and patients with liver and kidney dysfunction) (Chetty et al., 2018; Fan et al., 2022; Pillai et al., 2022; Chen et al., 2023). Various professional computer software programs are available for PBPK modeling, such as Simcyp Simulator, GastroPlus, and PK-Sim (Wang et al., 2023). In this study, PK-Sim software was selected to establish the PBPK models of Deg-AZ-M. By inputting PK parameters, physicochemical and biological properties obtained from preclinical studies, as well as anatomical, physiological, and biochemical parameters of the human body, PBPK models of Deg-AZM in rats and dogs were established and further validated using the observed PK real data of rats and dogs. All the predicted/observed ratios of Cmax and AUC0–24 h fell within the acceptable range of 0.5–2.0, indicating a well-established PBPK model. Animal PK data can be extrapolated to humans, which, to some extent, solves the problem of ethical limitations that make it difficult to obtain certain human data. The predicted PK profiles in humans indicated that the PK behavior of Deg-AZM was dose dependent.
5 Conclusion
This study revealed the preclinical PK profile of Deg-AZM, a new transgelin agonist at the clinical stage. No significant gender differences were noted in the PK behavior of Deg-AZM in rats and dogs. After oral administration, Deg-AZM was rapidly absorbed into the blood, widely distributed in the tissues without obvious accumulation, and quickly eliminated from the body. Repeated administration did not change the elimination behavior of Deg-AZM. Deg-AZM was mainly excreted from the body through the urinary excretion pathway. The predicted PK profiles in humans indicated that the PK behavior of Deg-AZM was dose dependent. Clinical PK data could not be obtained in the early stage of drug development, making this study an important reference for reducing the risk of human toxicity in phase I clinical design.
Data availability statement
The raw data supporting the conclusions of this article will be made available by the authors, without undue reservation.
Ethics statement
Ethical approval was not required for the studies on humans in accordance with the local legislation and institutional requirements because only commercially available established cell lines were used. The animal study was approved by the Experimental Animal Welfare Ethics Review Committee of Nankai University. The study was conducted in accordance with the local legislation and institutional requirements.
Author contributions
XA: conceptualization, supervision, writing–review and editing, and funding acquisition. XG: conceptualization, methodology, writing–original draft, data curation, formal analysis, funding acquisition, software, validation, and writing–review and editing. XL: methodology, validation, writing–original draft, and data curation. WT: data curation, writing–review and editing, formal analysis, and resources. CZ: writing–original draft, investigation, software, methodology, and validation. YC: writing–original draft, investigation, methodology, software, and validation. XX: writing–original draft, investigation, methodology, and software. CZ: writing–original draft, investigation, methodology, and software. HL: writing–original draft, software, investigation, and validation. YS: writing–original draft, validation, investigation, and software. ZL: writing–original draft, investigation, and software. SZ: writing–original draft, investigation, and software. HZ: writing–review and editing, conceptualization, project administration, resources, and supervision. CY: writing–review and editing, funding acquisition, project administration, resources, supervision, and conceptualization.
Funding
The author(s) declare that financial support was received for the research, authorship, and/or publication of this article. This research was supported by the National Natural Science Foundation of China (82304856), the Fundamental Research Funds for the Central Universities, Nankai University (63231203), the Fundamental Research Funds for the Central Universities, Nankai University (63231200), the Natural Science Foundation of Tianjin (22JCQNJC01610), the Natural Science Foundation of Tianjin (22JCQNJC00510), and the 111 Project B20016.
Conflict of interest
Author WT was employed by The National Institutes of Pharmaceutical R&D Co., Ltd.
The remaining authors declare that the research was conducted in the absence of any commercial or financial relationships that could be construed as a potential conflict of interest.
Publisher’s note
All claims expressed in this article are solely those of the authors and do not necessarily represent those of their affiliated organizations, or those of the publisher, the editors, and the reviewers. Any product that may be evaluated in this article, or claim that may be made by its manufacturer, is not guaranteed or endorsed by the publisher.
Abbreviations
AUC, area under the concentration versus time curve; AZM, azithromycin; CLh, hepatic clearance; CLint, intrinsic clearance; Cmax, maximum concentration; CMC-Na, carboxymethylcellulose sodium; Deg-AZM, deglycosylated azithromycin; DMF, N,N-dimethylformamide; ER, extraction ratio; ESI, electrospray ionization; glucose-6-phosphate dehydrogenase; IS, internal standard; ke, elimination rate constant; LC-MS/MS, liquid chromatography coupled with tandem mass spectroscopy; LLOQ, lower limit of quantification; m/z, mass-to-charge ratio; MF, matrix factor; MRM, multiple reaction monitoring; NA, not applicable; NADP, nicotinamide adenine dinucleotide phosphate; PBPK, physiologically based pharmacokinetic. PK, pharmacokinetics; QC, quality control; RE, relative error; RSD, relative standard deviation; STC, slow transit constipation; t1/2, elimination half-life; T1/2, terminal half-life; Tmax, time of maximum concentration; ULOQ, upper limit of quantification; Vd, apparent distribution volume.
References
Arredondo, G., Calvo, R., Marcos, F., Martínez-Jordá, R., and Suarez, E. (1995). Protein binding of itraconazole and fluconazole in patients with cancer. Int. J. Clin. Pharmacol. Ther. 33 (8), 449–452.
Bharucha, A. E., and Lacy, B. E. (2020). Mechanisms, evaluation, and management of chronic constipation. Gastroenterology 158 (5), 1232–1249.e3. doi:10.1053/j.gastro.2019.12.034
Bharucha, A. E., Pemberton, J. H., and Locke, G. R. (2013). American Gastroenterological Association technical review on constipation. Gastroenterology 144 (1), 218–238. doi:10.1053/j.gastro.2012.10.028
Bharucha, A. E., and Wald, A. (2019). Chronic constipation. Mayo Clin. Proc. 94 (11), 2340–2357. doi:10.1016/j.mayocp.2019.01.031
Brown, B. A., Griffith, D. E., Girard, W., Levin, J., and Wallace, R. J. (1997). Relationship of adverse events to serum drug levels in patients receiving high-dose azithromycin for mycobacterial lung disease. Clin. Infect. Dis. 24 (5), 958–964. doi:10.1093/clinids/24.5.958
Camilleri, M., Piessevaux, H., Yiannakou, Y., Tack, J., Kerstens, R., Quigley, E. M. M., et al. (2016). Efficacy and safety of prucalopride in chronic constipation: an integrated analysis of six randomized, controlled clinical trials. Dig. Dis. Sci. 61 (8), 2357–2372. doi:10.1007/s10620-016-4147-9
Chen, J., You, X., Wu, W., Guo, G., Lin, R., Ke, M., et al. (2023). Application of PBPK modeling in predicting maternal and fetal pharmacokinetics of levetiracetam during pregnancy. Eur. J. Pharm. Sci. 181, 106349. doi:10.1016/j.ejps.2022.106349
Chetty, M., Johnson, T. N., Polak, S., Salem, F., Doki, K., and Rostami-Hodjegan, A. (2018). Physiologically based pharmacokinetic modelling to guide drug delivery in older people. Adv. Drug Deliv. Rev. 135, 85–96. doi:10.1016/j.addr.2018.08.013
Crosby, E. C., and Husk, K. E. (2021). Defecatory dysfunction. Obstet. Gynecol. Clin. North Am. 48 (3), 653–663. doi:10.1016/j.ogc.2021.05.016
Damle, B., Varma, M. V., and Wood, N. (2011). Pharmacokinetics of voriconazole administered concomitantly with fluconazole and population-based simulation for sequential use. Antimicrob. Agents Chemother. 55 (11), 5172–5177. doi:10.1128/aac.00423-11
Davies, M., Jones, R. D. O., Grime, K., Jansson-Lofmark, R., Fretland, A. J., Winiwarter, S., et al. (2020). Improving the accuracy of predicted human pharmacokinetics: lessons learned from the AstraZeneca drug pipeline over two decades. Trends Pharmacol. Sci. 41 (6), 390–408. doi:10.1016/j.tips.2020.03.004
Dong, J., Liu, S. B., Rasheduzzaman, J. M., Huang, C. R., and Miao, L. Y. (2022). Development of physiology based pharmacokinetic model to predict the drug interactions of voriconazole and venetoclax. Pharm. Res. 39 (8), 1921–1933. doi:10.1007/s11095-022-03289-9
Fan, J., Yang, Y., Grimstein, M., Zhang, X., Kitabi, E., Earp, J. C., et al. (2022). Whole body PBPK modeling of remdesivir and its metabolites to aid in estimating active metabolite exposure in the lung and liver in patients with organ dysfunction. Clin. Pharmacol. Ther. 111 (3), 624–634. doi:10.1002/cpt.2445
FDA (2018). Bioanalytical method validation guidance for Industry. Available at: https://www.fda.gov/regulatory-information/search-fda-guidance-documents/bioanalytical-method-validation-guidance-industry (Accessed May 21, 2018).
Firth, A., and Prathapan, P. (2020). Azithromycin: the first broad-spectrum therapeutic. Eur. J. Med. Chem. 207, 112739. doi:10.1016/j.ejmech.2020.112739
Heller, A. A., Lockwood, S. Y., Janes, T. M., and Spence, D. M. (2018). Technologies for measuring pharmacokinetic profiles. Annu. Rev. Anal. Chem. (Palo Alto Calif) 11 (1), 79–100. doi:10.1146/annurev-anchem-061417-125611
Huang, X., Xiao, Z., and Huang, Z. (2019). Chronic abdominal pain and difficult defecation. Gastroenterology 157 (2), 306–308. doi:10.1053/j.gastro.2019.03.055
Kaur, N., Narang, A., and Bansal, A. K. (2018). Use of biorelevant dissolution and PBPK modeling to predict oral drug absorption. Eur. J. Pharm. Biopharm. 129, 222–246. doi:10.1016/j.ejpb.2018.05.024
Knowles, C. H. (2015). Defecation: colectomy for constipation-a time for renewed caution? Nat. Rev. Gastroenterol. Hepatol. 12 (12), 675–676. doi:10.1038/nrgastro.2015.191
Kostewicz, E. S., Aarons, L., Bergstrand, M., Bolger, M. B., Galetin, A., Hatley, O., et al. (2014). PBPK models for the prediction of in vivo performance of oral dosage forms. Eur. J. Pharm. Sci. 57, 300–321. doi:10.1016/j.ejps.2013.09.008
Kuepfer, L., Niederalt, C., Wendl, T., Schlender, J. F., Willmann, S., Lippert, J., et al. (2016). Applied concepts in PBPK modeling: how to build a PBPK/PD model. CPT Pharmacometrics Syst. Pharmacol. 5 (10), 516–531. doi:10.1002/psp4.12134
Lees-Miller, J. P., Heeley, D. H., Smillie, L. B., and Kay, C. M. (1987). Isolation and characterization of an abundant and novel 22-kDa protein (SM22) from chicken gizzard smooth muscle. J. Biol. Chem. 262 (7), 2988–2993. doi:10.1016/S0021-9258(18)61457-7
Li, M., Li, S., Lou, Z., Liao, X., Zhao, X., Meng, Z., et al. (2008). Crystal structure of human transgelin. J. Struct. Biol. 162 (2), 229–236. doi:10.1016/j.jsb.2008.01.005
Lucas, A. J., Sproston, J. L., Barton, P., and Riley, R. J. (2019). Estimating human ADME properties, pharmacokinetic parameters and likely clinical dose in drug discovery. Expert Opin. Drug Discov. 14 (12), 1313–1327. doi:10.1080/17460441.2019.1660642
Ni, L., Zheng, L., Liu, Y., Xu, W., Zhao, Y., Wang, L., et al. (2023). Physiologically based pharmacokinetic modeling to simulate CYP3A4-mediated drug-drug interactions for pyrotinib. Adv. Ther. 40 (10), 4310–4320. doi:10.1007/s12325-023-02602-1
Ong, J. J., Aguirre, I., Unemo, M., Kong, F. Y. S., Fairley, C. K., Hocking, J. S., et al. (2022). Comparison of gastrointestinal side effects from different doses of azithromycin for the treatment of gonorrhoea. J. Antimicrob. Chemother. 77 (7), 2011–2016. doi:10.1093/jac/dkac118
Pannemans, J., Masuy, I., and Tack, J. (2020). Functional constipation: individualising assessment and treatment. Drugs 80 (10), 947–963. doi:10.1007/s40265-020-01305-z
Parnham, M. J., Erakovic Haber, V., Giamarellos-Bourboulis, E. J., Perletti, G., Verleden, G. M., and Vos, R. (2014). Azithromycin: mechanisms of action and their relevance for clinical applications. Pharmacol. Ther. 143 (2), 225–245. doi:10.1016/j.pharmthera.2014.03.003
Pathak, S. M., Schaefer, K. J., Jamei, M., and Turner, D. B. (2019). Biopharmaceutic IVIVE-mechanistic modeling of single- and two-phase in vitro experiments to obtain drug-specific parameters for incorporation into PBPK models. J. Pharm. Sci. 108 (4), 1604–1618. doi:10.1016/j.xphs.2018.11.034
Pearson, P. G., and Wienkers, L. C. (2008). Handbook of drug metabolism. Second Edition. Boca Raton: CRC Press.
Pillai, V. C., Shah, M., Rytting, E., Nanovskaya, T. N., Wang, X., Clark, S. M., et al. (2022). Prediction of maternal and fetal pharmacokinetics of indomethacin in pregnancy. Br. J. Clin. Pharmacol. 88 (1), 271–281. doi:10.1111/bcp.14960
Rao, S. S., Rattanakovit, K., and Patcharatrakul, T. (2016). Diagnosis and management of chronic constipation in adults. Nat. Rev. Gastroenterol. Hepatol. 13 (5), 295–305. doi:10.1038/nrgastro.2016.53
Shah, B. J., Rughwani, N., and Rose, S. (2015). In the clinic. Constipation. Ann. Intern Med. 162 (7), ITC1. doi:10.7326/AITC201504070
Shapland, C., Hsuan, J. J., Totty, N. F., and Lawson, D. (1993). Purification and properties of transgelin: a transformation and shape change sensitive actin-gelling protein. J. Cell Biol. 121 (5), 1065–1073. doi:10.1083/jcb.121.5.1065
Vriesman, M. H., Koppen, I. J. N., Camilleri, M., Di Lorenzo, C., and Benninga, M. A. (2020). Management of functional constipation in children and adults. Nat. Rev. Gastroenterol. Hepatol. 17 (1), 21–39. doi:10.1038/s41575-019-0222-y
Wang, G., Lei, H. P., Li, Z., Tan, Z. R., Guo, D., Fan, L., et al. (2009). The CYP2C19 ultra-rapid metabolizer genotype influences the pharmacokinetics of voriconazole in healthy male volunteers. Eur. J. Clin. Pharmacol. 65 (3), 281–285. doi:10.1007/s00228-008-0574-7
Wang, H., Zhao, M., Liu, S., and Wang, X. (2022). Efficacy and safety of reduning injection combined with azithromycin in the treatment of mycoplasma pneumonia among children: a systematic review and meta-analysis. Phytomedicine 106, 154402. doi:10.1016/j.phymed.2022.154402
Wang, X., Chen, F., Guo, N., Gu, Z., Lin, H., Xiang, X., et al. (2023). Application of physiologically based pharmacokinetics modeling in the research of small-molecule targeted anti-cancer drugs. Cancer Chemother. Pharmacol. 92 (4), 253–270. doi:10.1007/s00280-023-04566-z
Yamamoto, S., Kawamura, Y., Yamamoto, K., Yamaguchi, Y., Tamura, Y., Izawa, S., et al. (2021). Internet survey of Japanese patients with chronic constipation: focus on correlations between sleep quality, symptom severity, and quality of life. J. Neurogastroenterol. Motil. 27 (4), 602–611. doi:10.5056/jnm20135
Yang, X., Liu, A., Yang, L., Wen, T., Wang, J., Shi, J., et al. (2022). Preclinical pharmacokinetics, tissue distribution and in vitro metabolism of FHND6091, a novel oral proteasome inhibitor. Drug Des. Devel Ther. 16, 3087–3107. doi:10.2147/DDDT.S371020
Zeng, L., Xu, P., Choonara, I., Bo, Z., Pan, X., Li, W., et al. (2020). Safety of azithromycin in pediatrics: a systematic review and meta-analysis. Eur. J. Clin. Pharmacol. 76 (12), 1709–1721. doi:10.1007/s00228-020-02956-3
Zhang, L., Feng, F., Wang, X., Liang, H., Yao, X., and Liu, D. (2024). Dose prediction and pharmacokinetic simulation of XZP-5610, a small molecule for NASH therapy, using allometric scaling and physiologically based pharmacokinetic models. Pharm. (Basel) 17 (3), 369. doi:10.3390/ph17030369
Keywords: Deg-AZM, nonclinical pharmacokinetics, absorption, distribution, metabolism, excretion
Citation: Gu X, Li X, Tian W, Zheng C, Cai Y, Xu X, Zhao C, Liu H, Sun Y, Luo Z, Zhu S, zhou H, Ai X and Yang C (2024) Preclinical pharmacokinetic studies and prediction of human PK profiles for Deg-AZM, a clinical-stage new transgelin agonist. Front. Pharmacol. 15:1423175. doi: 10.3389/fphar.2024.1423175
Received: 25 April 2024; Accepted: 30 July 2024;
Published: 26 August 2024.
Edited by:
Charles Oo, Morris Plains, United StatesReviewed by:
Jingwen Qiu, UMR8214 Institut des Sciences Moléculaires d'Orsay (ISMO), FranceSandhya Subash, Washington State University Health Sciences Spokane, United States
Copyright © 2024 Gu, Li, Tian, Zheng, Cai, Xu, Zhao, Liu, Sun, Luo, Zhu, zhou, Ai and Yang. This is an open-access article distributed under the terms of the Creative Commons Attribution License (CC BY). The use, distribution or reproduction in other forums is permitted, provided the original author(s) and the copyright owner(s) are credited and that the original publication in this journal is cited, in accordance with accepted academic practice. No use, distribution or reproduction is permitted which does not comply with these terms.
*Correspondence: Cheng Yang, eWFuZ2NoZW5nQG5hbmthaS5lZHUuY24=; Xiaoyu Ai, YWl4aWFveXVAbmFua2FpLmVkdS5jbg==; Honggang zhou, aG9uZ2dhbmcuemhvdUBuYW5rYWkuZWR1LiBjbg==
†These authors have contributed equally to this work