- 1Department of Experimental Medicine, University of Campania “Luigi Vanvitelli”, Naples, Italy
- 2Department of Biological and Environmental Sciences and Technologies, University of Salento, Lecce, Italy
- 3Department of Molecular Medicine and Medical Biotechnologies, University of Naples “Federico II”, Naples, Italy
- 4CEINGE-Advanced Biotechnologies, Naples, Italy
Heart failure and cognitive impairment emerge as public health problems that need to be addressed due to the aging global population. The conditions that often coexist are strongly related to advancing age and multimorbidity. Epidemiological evidence indicates that cardiovascular disease and neurodegenerative processes shares similar aspects, in term of prevalence, age distribution, and mortality. Type 2 diabetes increasingly represents a risk factor associated not only to cardiometabolic pathologies but also to neurological conditions. The pathophysiological features of type 2 diabetes and its metabolic complications (hyperglycemia, hyperinsulinemia, and insulin resistance) play a crucial role in the development and progression of both heart failure and cognitive dysfunction. This connection has opened to a potential new strategy, in which new classes of anti-diabetic medications, such as glucagon-like peptide-1 receptor (GLP-1R) agonists and sodium-glucose cotransporter 2 (SGLT2) inhibitors, are able to reduce the overall risk of cardiovascular events and neuronal damage, showing additional protective effects beyond glycemic control. The pleiotropic effects of GLP-1R agonists and SGLT2 inhibitors have been extensively investigated. They exert direct and indirect cardioprotective and neuroprotective actions, by reducing inflammation, oxidative stress, ions overload, and restoring insulin signaling. Nonetheless, the specificity of pathways and their contribution has not been fully elucidated, and this underlines the urgency for more comprehensive research.
1 Introduction
Heart failure (HF) and cognitive impairment are two common health conditions that often coexist in the general population (Virani et al., 2021). Both syndromes affecting cardiac and cognitive functions loom as public health problems in the coming decades due to the aging global population (Patnode et al., 2020; Díez-Villanueva et al., 2021).
HF is a clinical syndrome with symptoms and/or signs caused by a structural and/or functional cardiac abnormality affecting more than 60 million people worldwide (Groenewegen et al., 2020). Nearly half of the patients with HF are diagnosed with HF with preserved ejection fraction (HFpEF), a subclass of HF in which patients are more likely to be older and female, with multiple chronic comorbidities, such as hypertension, type 2 diabetes, and obesity (Owan et al., 2006; Dunlay et al., 2017). In addition to traditional comorbidities, cognitive impairment, which represents an independent risk factor for HF, significantly increases the hospitalization and mortality, and decreases quality of life of patients with HF (Dodson et al., 2013; Vellone et al., 2020).
Epidemiological evidence indicates that neurodegenerative processes share similar profiles with HF, in term of prevalence, age distribution, and mortality (Pappolla et al., 2003; Razay et al., 2007). Cognitive impairment encompasses a range of cognitive functions, including memory, attention, understanding, reasoning, problem-solving, decision-making, and language production (Murman, 2015). Interestingly, HF represents per se a risk factor for developing dementia (Qiu et al., 2006). Particularly prevalent in those over 70, where it reaches up to 10%, HF has long been recognized as a potential cause of cognitive dysfunction, sometimes referred to as cardiogenic dementia (Cardiogenic dementia, 1977; Liu et al., 2024). On the other hand, these syndromes can occur independently. The relationship between HF and cognitive impairment stems from partly common background. Age, metabolic disorders, genetic profile, or dysfunctional neuroendocrine systems are capable to affect both systems (Packer, 2018; Nguyen et al., 2020).
In this review, we will provide an overview of physiological connections between heart and brain. In particular, we will discuss the impact of metabolic disorders in affecting HF and cognitive function, and the implication of clinical treatments, focusing on paramount effects of new antidiabetic drugs.
2 A common milieu of metabolic disorders in heart failure and cognitive impairment
2.1 Heart failure
According to epidemiologic analyses, HF is highly prevalent in patients with type 2 diabetes, who are at a higher risk (up to 2-fold in men and 4-fold in women) of developing HF than patients without diabetes (Dunlay et al., 2019). However, it is important to note that type 2 diabetes, obesity, and metabolic dysfunction may specifically increase the likelihood of developing HFpEF more than HF with reduced ejection fraction (HFrEF) (Samson et al., 2016). Evidence from community-based cohorts has shown that higher body mass index and insulin resistance may be strongly associated with future HFpEF, especially among women (Savji et al., 2018). Furthermore, a sedentary lifestyle is associated with a greater risk of HFpEF compared with HFrEF (Pandey et al., 2016).
Several pathophysiological mechanisms linking type 2 diabetes and HF are recognized, with hyperglycemia, hyperinsulinemia, and insulin resistance known to initiate and perpetuate disease progression. Impairment in glucose metabolism, alterations of nitric oxide signaling, and reactive oxygen species (ROS) accumulation with mounting oxidative stress are among mechanisms underlying both micro- and macrovasculopathy, and diabetic cardiomyopathy with cardiac hypertrophy and fibrosis (Kolwicz and Tian, 2011; Piperi et al., 2015). Of note, insulin resistance is emerging as a major risk factor for the development of HF (Aroor et al., 2012a).
In this context, a prominent feature is functional alteration of mitochondria—the major source of intracellular ROS that compromise insulin signaling (Kim et al., 2008; Aroor et al., 2012b). In diabetic patients, ATP production relies even more on fatty acid oxidation rather than glycolysis (Lopaschuk, 2016). This process disrupts the oxidative phosphorylation and produces more ROS causing oxidative damage to cellular proteins and lipids (Gollmer et al., 2020). Changes in mitochondrial bioenergetics also affect myocardial ion handling resulting in cytosolic sodium and calcium overload (Trost et al., 2002; Bers, 2006). Abnormalities in the expression or activity of ryanodin receptor, sarcoplasmic endoplasmic reticulum Ca2+-ATPase, and sodium/calcium exchanger (NCX), reported in HF and diabetic cardiomyopathy, contribute to myocardial cell death, cardiac fibrosis, and diastolic dysfunction (Lebeche et al., 2008; Tuunanen and Knuuti, 2011).
In patients with type 2 diabetes, systemic chronic inflammation, caused by hyperglycemia and insulin resistance, participates in the development of vascular complications, and contributes to HF (Tsalamandris et al., 2019). Activation of nuclear factor-κB (NF-κB) results in downstream pro-inflammatory cytokine release, such as tumor necrosis factor-α (TNF-α), interleukin (IL)-6, and IL-1β, which amplify ROS-induced myocardial stress further promoting adverse remodeling, fibrosis, and myocardial dysfunction (Brasier, 2010; Jia et al., 2018).
The activation of renin-angiotensin-aldosterone system (RAAS) is associated with insulin resistance as well (Lastra et al., 2010). Upregulation of angiotensin II can activate NADPH oxidase enzyme complex in vascular smooth muscle cells and cardiomyocytes, further increasing the generation of ROS and participating in the progression of HF (Doughan et al., 2008). In addition, aldosterone, released upon the action of angiotensin II and sympathetic nervous system hyperactivation, reduces insulin sensitivity in several cell types triggering maladaptive responses in type 2 diabetes, hypertension, and HF (Aroor et al., 2012a). This is only a general outline, and excellent detailed reviews are available. However, the effective role of key pathways in the pathogenesis of cardiometabolic HF is still understudied and require further investigation. Understanding these mechanisms is crucial, as it can lead to the development of targeted interventions aimed at preserving cardiovascular health in individuals with type 2 diabetes.
2.2 Cognitive impairment
Cognitive impairment is generally referred to Alzheimer’s disease and vascular dementia, recognized as the two most prevalent causes of dementia that affects about 50 million people worldwide, with cases projected to triple by 2050 (GBD, 2016 Dementia Collaborators, 2019; Ou et al., 2024). Type 2 diabetes has increasingly represented a risk factor associated not only to cardiometabolic pathologies but also to neurological conditions, highlighting its negative impact on cognitive function (Banday et al., 2020; Tomic et al., 2022). Hyperglycemia in diabetic patients is a risk factor for Alzheimer’s disease that is also called “type 3 diabetes” (Barone et al., 2021).
The relationship between type 2 diabetes and cognitive impairment is multifaceted and intricate. Although exact mechanisms are still unknown, several potential pathways have emerged. The combination of vascular damage and neurodegeneration represents the main postulated mechanism underlying the development of cognitive impairment in patients with type 2 diabetes or HF (Dao et al., 2023). Chronic hyperglycemia and hyperinsulinemia drive a metabolic imbalance promoting oxidative stress, inflammation, and endothelial damage, responsible for non-enzymatic biomolecule glycation, accelerating cerebrovascular atherosclerosis and neurodegenerative damage that further promote cognitive dysfunction and dementia (Barone et al., 2021; Kopp et al., 2022). Under physiologic conditions, insulin promotes amyloid-β protein clearance thereby preventing its accumulation (Mullins et al., 2017). Alteration in insulin signaling may accelerate the onset of Alzheimer’s disease, by changing the intracellular concentration of neurotransmitters involved in memory formation and functioning, and influencing on amyloid-β-related processes and amyloid clearance (Craft, 2007; Spinelli et al., 2019; Rizzo et al., 2022). Additionally, insulin resistance increases the number of neuritic plaques by affecting tau phosphorylation, widely considered a pivotal pathological hallmark in Alzheimer’s disease (Wegmann et al., 2021).
Experimental studies provide strong evidence that insulin resistance-related neuroinflammation feeds cognitive impairment neuropathology, leading to a raised-up brain levels of pro-inflammatory cytokines (Mullins et al., 2017; Denver et al., 2018). Hyperphosphorylation of tau triggers pro-inflammatory signaling cascades, as a consequence of vascular inflammation and vasoconstriction (Paris et al., 2000). Activated microglia drive the immune response to the inflammatory state releasing immune signaling molecules (cytokines, and chemokines), and inducing neuronal cell death (Calsolaro and Edison, 2016).
These data underline the importance of optimal glycemic control and call for major pharmacological interventions to reduce the risk of cardiometabolic-related cognitive impairment.
The pathophysiological overlap between cardiometabolic HF and cognitive impairment opens to reasonable possibility that new successful pharmacological approaches used in type 2 diabetes may be beneficial for cardiovascular and neurological diseases. Indeed, there is growing interest in the potential of new classes of anti-diabetic medications such as glucagon-like peptide-1 receptor (GLP-1R) agonists and sodium-glucose cotransporter 2 (SGLT2) inhibitors, to reduce the risk of cardiovascular events and slow down the progression of neuronal damage. Notably, these drugs not only act on the glycemic control, but also show protective effects independently of their anti-diabetic effect (Table 1, Figure 1).
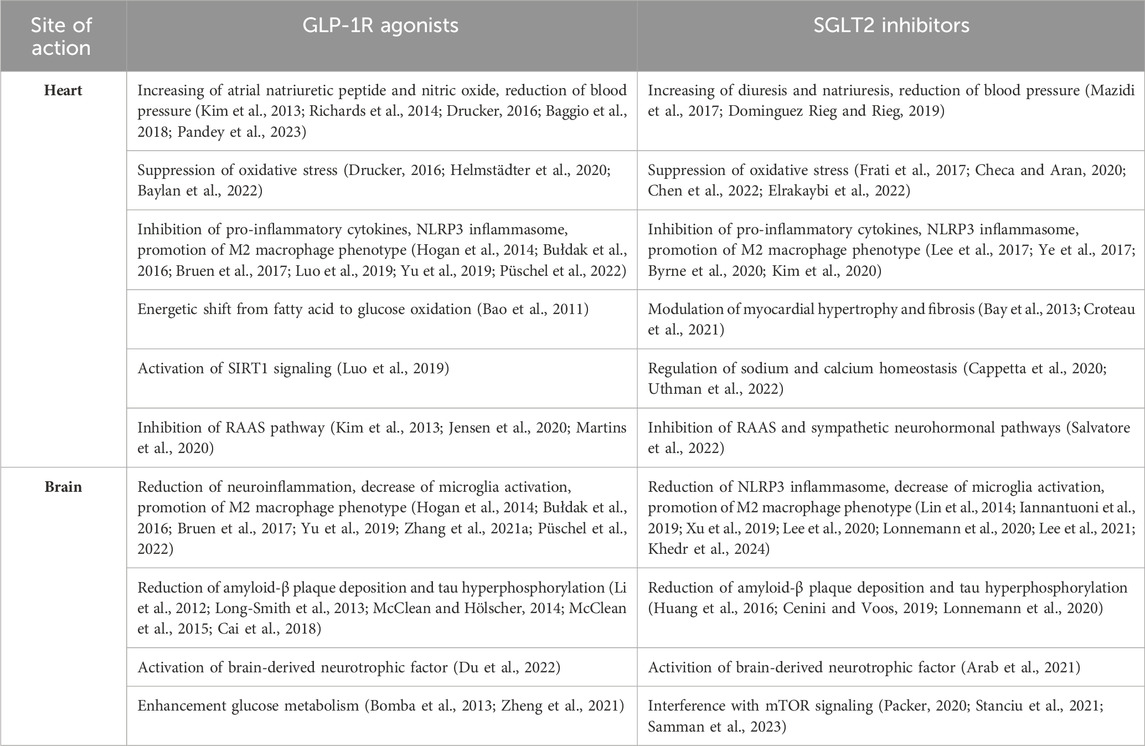
Table 1. Summary of cardioprotective and neuroprotective mechanisms by GLP-1R agonists and SGLT2 inhibitors.
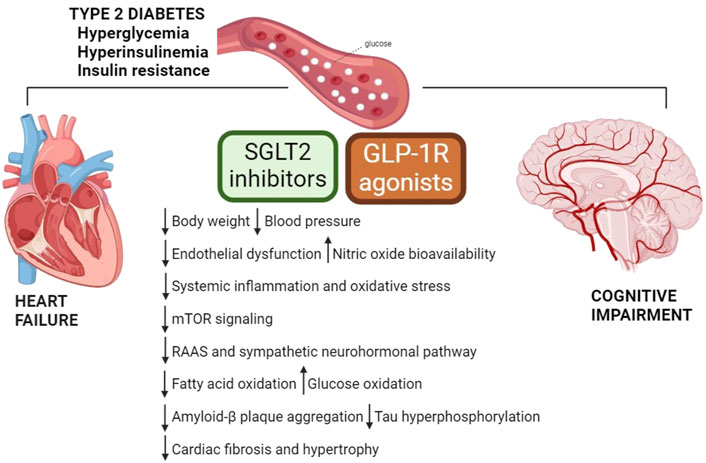
Figure 1. Mechanisms underlying favorable effects of GLP-1R agonists and SGLT2 inhibitors. Metabolic complications play a crucial role in the development and progression of HF and cognitive dysfunction. GLP-1R agonists and SGLT2 inhibitors exert direct and indirect cardioprotective and neuroprotective actions, and their benefits lay beyond glycemic control. GLP-1R, glucagon-like peptide-1 receptor; mTOR, mammalian target of rapamycin; RAAS, renin-angiotensin-aldosterone system; SGLT2, sodium-glucose cotransporter 2.
3 Glucagon-like peptide-1 receptor agonists
Glucagon-like peptide-1 (GLP-1) represents a particular incretin that has generated a lot of interest. Discovered for the first time in 1987, GLP-1 is an intestinal gut hormone that is secreted in response to food intake and potentiates the glucose-dependent insulin secretion from pancreatic β-cells (Drucker et al., 1987). It acts by binding to the G protein-coupled receptor GLP-1 receptor (GLP-1R). In addition to metabolic effects (promotion of insulin secretion, inhibition of appetite, reduction of gastric emptying, and increase of natriuretic and diuretic processes), experimental studies have pointed out the cardio- and neuroprotective effects of GLP-1 (During et al., 2003; Bendotti et al., 2022; Yang et al., 2022). GLP-1 is a labile peptide and is rapidly removed from circulation by enzymatic degradation by the serine protease dipeptidyl peptidase (DPP)-4. The therapeutic approach to target the incretin system for the treatment of type 2 diabetes involves the use of GLP-1 agonists or DPP-4 inhibitors to increase the levels of endogenous GLP-1 (Cosentino et al., 2020).
In addition to pancreatic localization, GLP-1R has been found in the gastrointestinal system, kidneys, central nervous system, and cardiovascular system (Pyke et al., 2014). The additional non-glycemic effect related to incretins may be linked to extra-pancreatic tissue localization of GLP-1R.
GLP-1R agonists are classified on their molecular backbone derived either from human GLP-1 (liraglutide, semaglutide, and dulaglutide) or from exendin-4 (exenatide and lixisenatide), a salivary gland hormone from the Gila monster lizard (Colin et al., 2023). In addition, a dual GLP-1/glucose-dependent insulinotropic polypeptide (GIP) co-agonist, tirzepatide, has been recently developed for the treatment of type 2 diabetes and obesity (Jakubowska et al., 2024). GIP is an incretin hormone secreted by small intestine in response to luminal presence of nutrients (Deacon and Ahrén, 2011). It stimulates insulin secretion, facilitates fat deposition, promotes bone formation and may be involved in memory formation and control of appetite (Seino et al., 2010). Changes in GIP secretion could contribute to the loss of incretin effect observed in patients with type 2 diabetes (Holst et al., 2011).
GLP-1R agonists are known to significantly decrease the cardiovascular risk of diabetic patients, while they are currently being investigated to determine their potential efficacy for neurodegenerative diseases associated to cardiometabolic pathology.
3.1 GLP-1R agonists in the cardiovascular system
GLP-1R is expressed in the heart and vasculature, raising the possibility that GLP-1 might have both direct and indirect actions on the cardiovascular system (Ussher and Drucker, 2023). GLP-1R expression has been found in both the atria and ventricles (Baggio et al., 2018). Its regulation contributes to the reduction of blood pressure through the secretion of atrial natriuretic peptide or by regulating the availability of nitric oxide (Pandey et al., 2023). GLP-1 and its receptor agonists have been shown to reduce endothelial inflammation and oxidative stress, through the activation of nitric oxide production, crucial for maintaining healthy vascular tone and function (Drucker, 2016).
Incretin-based therapies has evidenced a reduced cardiovascular risk in high-risk individuals with type 2 diabetes, independent of the need for additional glucose control. Among GLP-1R agonists, liraglutide, semaglutide, and dulaglutide are widely available with licensed indications for the prevention of cardiovascular disease (Marx et al., 2022).
However, understanding how activation of the GLP-1R contributes to myocardial protection and decreases cardiac injury is challenging.
GLP-1R activation reduces blood pressure, as shown in experimental models of hypertension (Hirata et al., 2009; Laugero et al., 2009). The anti-hypertensive effect of GLP-1R agonists has been observed with liraglutide that is able to mediate atrial natriuretic peptide secretion from atrial cardiomyocytes, thus lowering systolic and diastolic blood pressure increased by angiotensin II (Kim et al., 2013). In addition, it has been demonstrated the specific involvement of endothelial GLP-1R in contributing to the anti-hypertensive effects by controlling nitric oxide bioavailability (Richards et al., 2014; Baggio et al., 2018). Liraglutide shows efficacy in experimental arterial hypertension, independently of glycemic control. Mechanistically, liraglutide suppresses the angiotensin II-induced inflammatory cascade and oxidative stress in the vascular wall, leading to reduced expression of adhesion molecules at endothelial level. As a result, function of endothelial nitric oxide synthase is preserved, thus restoring nitric oxide level (Helmstädter et al., 2020; Baylan et al., 2022). Additionally, the relevant effect of GLP-1R signaling on blood pressure control may be attributable to the action of agonists on sodium reabsorption in the proximal tubule, and interference with intrarenal angiotensin II, thus augmenting renal blood flow and promoting natriuresis (Jensen et al., 2020; Martins et al., 2020).
Reduced cardiac and vascular inflammation has been well documented for GLP-1R agonists in experimental and human studies (Drucker, 2016). Liraglutide lowers vascular dysfunction and systemic inflammation in a lipopolysaccharide-induced sepsis model, by normalizing the expression of adhesion molecules and cytokines (Steven et al., 2015). Interestingly, in GLP-1R-deficient mice, liraglutide loses its beneficial effects (Steven et al., 2017). In regulating the response to inflammation of the endothelium, dulaglutide counteracts high glucose-induced activation of the NLR family pyrin domain containing 3 (NLRP3) inflammasome and suppresses maturation and release of IL-1β and IL-18; the lack of effects of dulaglutide on endothelial inflammation by SIRT1 siRNA evidences the involvement of SIRT1 pathway in this process (Luo et al., 2019). In human peripheral blood cells, liraglutide has been reported to decrease the production of pro-inflammatory cytokines (TNF-α, IL-1β, and IL-6), and activation of macrophages (Hogan et al., 2014; Bruen et al., 2017). Under pathological stress, the role of macrophages in inflammatory responses, which drive insulin resistance and diabetes, is significant (Püschel et al., 2022). In this regard, GLP-1R agonists (i.e., exenatide) improve insulin resistance suppressing pro-inflammatory phenotypes of monocytes/macrophages, and directly modulating NF-κB activation and IL-1β, IL-6, and TNF-α expression (Bułdak et al., 2016; Yu et al., 2019).
Data suggests that GLP-1R-dependent cardioprotection may also result from effects on cardiac energetic metabolism. In ischemia–reperfusion model, albiglutide promotes energetic shift from fatty acid to glucose oxidation, reducing myocardial infarct size and improving contractile function (Bao et al., 2011). Likewise, liraglutide increases myocardial glucose oxidation, and alleviates diastolic dysfunction in mice subjected to experimental diabetic cardiomyopathy (Almutairi et al., 2021).
Dual GLP-1R/GIP receptor agonists co-stimulate both GLP-1R and GIP receptor, and enhance insulinotropic and antihyperglycemic effects compared with selective GLP-1R agonists (Finan et al., 2013). Tirzepatide is the only approved incretin multi-agonist. It has been proven superior to single GLP-1R agonist or insulin analog treatments in reducing blood glucose, body mass index, and weight (Dutta et al., 2021). Tirzepatide has been associated with improvements in lipoprotein profiles, blood pressure, and inflammation, although favorable effects on cardiovascular risk factors have been reported in only a single clinical trial (Wilson et al., 2020; Del et al., 2021).
Triple agonists are unimolecular peptide drugs that target GLP-1R, GIP receptor, and glucagone receptor, developed primarily for treating metabolic diseases (Knerr et al., 2022). Preclinical data available so far have highlighted metabolic efficacy and its superior performance to dual agonists in reducing cardiovascular risk factors, such as plasma glucose levels, and body weight (Finan et al., 2015; Knerr et al., 2022).
The above findings underscore the potential of GLP-1R agonists as efficacious and safe drugs with pleiotropic activities on cardiovascular system that are independent of their glycemic control effects emphasizing cardiovascular outcomes as one of the primary goals in the management of type 2 diabetes.
3.2 GLP-1R agonists in the central nervous system
GLP-1R, expressed in endothelial cells, microglia, astrocytes, and neurons, is widely distributed in the hypothalamus, cortex, subventricular zone, and substantia nigra (Hamilton and Holscher, 2009; Cork et al., 2015; Reich and Hölscher, 2022). GLP-1R overexpression has been found in neurons, microglia, and endothelial cells, where is correlated to low level of inflammation and improved cognitive function (Du et al., 2022). GLP-1R activation, by stimulating adenylyl cyclase and consequently elevating cyclic adenosine monophosphate levels, enhance neuroprotective signaling through upregulation of anti-inflammatory and anti-apoptotic signaling (Holz, 2004; Delghandi et al., 2005; Robichaux and Cheng, 2018; Wicinski et al., 2019; Mehdi et al., 2023).
Pharmacological approaches able to promote healthy incretin and insulin signaling in the brain may represent a potential direction for neurodegenerative disease treatment. Clinical trials have pointed out a neuroprotective effect and an improvement of clinical outcome for some anti-diabetic treatments, translating them into new therapies against neurodegenerative conditions, such as Alzheimer’s disease, dementia, or Parkinson’s disease (Green et al., 2019; Kopp et al., 2022; Colin et al., 2023).
GLP-1R agonists cross the blood-brain barrier and have been widely tested in preclinical studies as therapeutic option for neuroinflammation and neurodegeneration. In an experimental model of Alzheimer’s disease, liraglutide exerts neuronal anti-inflammatory effects, decreasing the number of activated microglia cells in the hippocampus, and astrocyte in the cortex as well as reducing the expression of pro-inflammatory cytokines (IL-6, IL-12, and IL-1β). It also shows properties as growth and differentiation factor, increasing stem cell proliferation and differentiation into mature neurons (Parthsarathy and Holscher, 2013; Batista et al., 2018). In a model of cognitive impairment associated to neuropathic pain, the inhibition of GLP-1/GLP-R axis increases the expression of pro-inflammatory cytokines IL-1β and TNF-α, while exenatide treatment prevents the phosphorylation of NF-κB and decreases inflammatory burden (Zhang LQ. et al., 2021).
GLP-1 activation can reduce the pathological process of amyloid-β plaque aggregation/deposition and tau hyperphosphorylation enhanced by neuroinflammation, thus preventing age-related neurodegenerative changes, such as decline of learning and memory (Li et al., 2012). Lixisenatide is a long-lasting GLP-1R agonist that crosses blood-brain barrier at very low doses (Hunter and Holscher, 2012). In a mouse model of Alzheimer’s disease, it reduces amyloid-β plaques, tau neurofibrillary tangles, and neuroinflammation, by activating CREB pathway and inhibiting p38/MAPK signaling (Cai et al., 2018). A large body of evidence reports a beneficial effect of liraglutide on amyloid pathology. The drug, as prophylactic or long-term treatments, significantly reduces amyloid-β plaque size, number, and load (Long-Smith et al., 2013; McClean and Hölscher, 2014; McClean et al., 2015). Both liraglutide and dulaglutide ameliorate impaired learning and memory ability limiting tau hyperphosphorylation and neurofilament aggregates through the modulation of JNK or Akt activity (Qi et al., 2016; Chen et al., 2017; Zhou et al., 2019).
Neuronal apoptosis, induced by plaque deposition and stress, is a pathophysiological marker in cognitive impairment (Engidawork et al., 2001). In a mouse model of Parkinson’s disease, both lixisenatide and liraglitude are associated with neuroprotective benefits and reduced motor impairment by decreasing pro-apoptotic BAX signaling and increasing expression of anti-apoptotic Bcl-2 (Liu et al., 2015). Likewise, the neuroprotective effect of semaglutide involves modulation of apoptotic signaling (Chang et al., 2020).
GLP-1R agonists may also exert their neuroprotective action increasing level and activity of brain-derived neurotrophic factor, regulating calcium homeostasis, promoting glycolysis, and reducing vascular damage (Du et al., 2022). Of note, it has been shown that liraglutide improves learning and memory as well as cognitive function by regulating intracellular calcium homeostasis in cortical or hippocampal pyramidal cells (Wang et al., 2013). Moreover, both exenatide and liraglutide enhance glucose metabolism and aerobic glycolysis by increasing brain lactate dehydrogenase activity (Bomba et al., 2013; Zheng et al., 2021).
Dual GLP-1R/GIP receptor agonists exert anti-neuroinflammatory and anti-neurodegenerative benefits in preclinical studies and improve memory function, synaptic health, and neurogenesis (Pathak et al., 2018; Zhang et al., 2020). Interestingly, the dual agonist tirzepatide has shown efficacy in neuroprotection, by counteracting hyperglycemia and insulin resistance-related effects at the neuronal level (Fontanella et al., 2024).
Also triple agonists have demonstrated to possess anti-neurodegenerative properties, indicating a potential for treating Alzheimer’s disease by reducing neuroinflammation and oxidative stress, enhancing neuronal health and viability, and minimizing glutamate excitotoxicity (Kopp et al., 2022). In mouse models of Alzheimer’s disease, triple agonists play a neuroprotective role and ameliorate cognitive deficits by significantly reducing hippocampal amyloid-β, phosphorylated tau aggregates, and neuroinflammation, and stimulating neurogenesis and brain-derived neurotrophic factor expression (Li et al., 2018; Tai et al., 2018).
All these experimental data support the potential benefit of this drug class to translate neuroprotective properties from cellular and animal studies to humans. Although several clinical trials have been completed or are currently underway, repurposing GLP-1R agonists to treat cardiometabolic syndrome-related neurodegenerative diseases will require additional research to confirm safety and efficacy.
4 Sodium/glucose co-transporter 2 inhibitors
Sodium/glucose co-transporter 2 (SGLT2) inhibitors or gliflozins represent a new class of approved oral anti-hyperglycemic drugs widely used in clinical practice for the treatment of type 2 diabetes, alone or in association with metformin (Kalra, 2014; Nauck, 2014). Their main mechanism of action consists in the inhibition of SGLT receptors, of which two different isoforms are known, SGLT1 and SGLT2. SGLTs are membrane proteins that act as co-transporters of glucose and sodium in the cell, and their expression and function have been detected in many organs of the body (Pandey and Tamrakar, 2019; Koepsell, 2020). Selective SGLT2 inhibitors block glucose reabsorption in the proximal renal tubule, increasing glucose elimination and reducing hyperglycemia. They improve both insulin secretion by β-cells and peripheral insulin sensitivity (Ni et al., 2020).
Dapagliflozin, empagliflozin, and ertugliflozin are the most selective SGLT2 inhibitors (Ferrannini, 2017). Sotagliflozin, referred to as a dual SGLT1/2 inhibitor, has the highest affinity for SGLT1 (Markham and Keam, 2019), while canagliflozin can act as a dual SGLT1/2 inhibitor and is able to inhibit intestinal glucose absorption by blocking intestinal SGLT1 (Dominguez Rieg and Rieg, 2019). Although these drugs were initially developed for the treatment of type 2 diabetes, clinical studies have largely demonstrated their beneficial effects in metabolic, renal, and cardiovascular diseases that go beyond glucose control (Minze et al., 2018; Mascolo et al., 2022a; Urbanek et al., 2023).
Based on the results of clinical data, empagliflozin and dapagliflozin are now recommended for the treatment of HFpEF, as well as for HFrEF also in the absence of diabetes (McDonagh et al., 2021; McDonagh et al., 2023).
4.1 SGLT2 inhibitors in the cardiovascular system
Numerous studies have proved that SGLT2 inhibitors reduce the combined risk of cardiovascular death and hospitalization in HF patients with or without diabetes. The benefits include osmotic diuresis and natriuresis, weight loss and lipid metabolism shift, blood pressure reduction, decrease in uric acid, oxidative stress, and inflammation with a lower risk of hypoglycaemia or pancreatic β-cell overstimulation. They represent a new therapeutic approach able to act directly on the heart or kidneys, independently of insulin sensitivity (Lopaschuk and Verma, 2020; Goldberg, 2021). SGLT2 inhibitor effects have been established on cardiomyocytes, endothelial cells, fibroblasts, and smooth muscle cells (Uthman et al., 2018a; Cappetta et al., 2021).
Oxidative stress and inflammation significantly and interdependently contribute to the onset and progression of cardiovascular diseases (Frati et al., 2017). Inflammation can induce acute or chronic oxidative stress via cytosolic protein kinase C and calcium release; vice versa, oxidative stress is a positive modulator of the inflammatory response through NLRP3 inflammasome and NF-κB (Checa and Aran, 2020; Chen et al., 2022). SGLT2 inhibitors exhibit considerable potential as anti-inflammatory agents, through indirect mechanisms that involve metabolic improvement, oxidative stress reduction, as well as direct modulation of inflammatory pathways (Elrakaybi et al., 2022). In obese mice with diabetes, dapagliflozin reduces cardiac dysfunction interfering with activation of NLRP3 inflammasome and affects cardiac fibrosis by attenuating collagen 1 and collagen 3 deposition (Ye et al., 2017). Similarly, empagliflozin suppresses NLRP3 inflammasome activation in macrophages of diabetic patients (Kim et al., 2020). Furthermore, it has been shown that empagliflozin attenuates the decline of cardiac function in mice with HF in the absence of diabetes and that this is associated with reduced priming of NLRP3 inflammasome in the heart (Byrne et al., 2020). In post-ischemic rat hearts, treatment with dapagliflozin results in higher level of IL-10, a cytokine that facilitates the conversion of macrophages from a pro-inflammatory M1 to anti-inflammatory M2 phenotype, thereby inhibiting myofibroblast differentiation and extracellular matrix formation (Lee et al., 2017).
Cardiomyocytes and endothelial cells are major targets for SGLT2 inhibitors in the heart (Chen et al., 2022). Studies support that intracellular sodium and calcium overload induce signaling cascades that lead to dysregulation of mitochondrial homeostasis, with energy impairment and elevated free radical production, and consequent increase in cardiac hypertrophy and remodeling (Bay et al., 2013). In mice with diabetic cardiomyopathy, ertugliflozin prevents myocardial hypertrophy, fibrosis, and diastolic dysfunction by preserving mitochondrial function and improving myocardial energetics (Croteau et al., 2021). Activation of NCX and sodium/hydrogen exchanger-1 (NHE-1), at cardiac and vascular level, mediate the maladaptive neurohormonal stimulation (sympathetic nervous system, RAAS, and natriuretic peptide system) during the pathophysiological course toward HF (Baartscheer et al., 2003; Packer, 2017). SGLT2 inhibitors show a strict interaction with human and murine cardiomyocytes through direct inhibition of the myocardial isoform of NHE (Uthman et al., 2018b; Trum et al., 2020). Moreover, both dapagliflozin and empagliflozin have been shown to directly inhibit NHE-1 activity in human endothelial cells (Cappetta et al., 2020; Uthman et al., 2022). Another factor in the development of HF is the late component of the cardiac voltage-gated sodium current, termed late-INa (Horvath and Bers, 2014). In silico approach confirms a reduction of late-INa by empagliflozin demonstrating that SGLT2 inhibitors may be the ligands for the cardiac sodium channel Nav1.5 isoform that drives late-INa (Philippaert et al., 2021). Amelioration of dysfunctional sodium and calcium homeostasis in cardiomyocytes from diabetic hearts is able to reverse cardiac remodeling and counteract the development of diabetic cardiomyopathy (Lee et al., 2019; Philippaert et al., 2021). Moreover, the regulation of sodium and calcium homeostasis in cardiomyocytes and coronary endothelium is directly translated into improved contractility and relaxation of the heart (Cappetta et al., 2020).
Diuretic and natriuretic effects of SGLT2 inhibitors contribute to the reduction of intravascular volume and decrease in blood pressure, lowering afterload, improving cardiac efficiency, and reducing ventricular mass index (Mazidi et al., 2017; Dominguez Rieg and Rieg, 2019). SGLT2 inhibitors can reduce natriuretic peptides leading to a reduction in ventricular pressure and distention, and both pulmonary and systemic decongestion (Mascolo et al., 2022b). Moreover, the favorable hemodynamic effects seem to be related with an attenuated endothelial cell activation and improved vasorelaxation via voltage-gated potassium channels (Dimitriadis et al., 2023). Additionally, benefits include the capacity to decrease the RAAS and sympathetic neurohormonal pathways and a positive pharmacodynamic interaction with RAAS inhibitors (Salvatore et al., 2022).
The above cardiac and extracardiac effects outline the cardioprotective mechanisms of SGLT2 inhibitors that stay behind the success of these drugs in the cardiovascular field.
4.2 SGLT2 inhibitors in the central nervous system
SGLT1 is localized in the pyramidal cells of the brain cortex, Purkinje cerebellum cells, hippocampus pyramidal, and granular cells (Pawlos et al., 2021). SGLT2 brain expression is lower compared to SGLT1, and it is localized mainly in the microvessels of the blood-brain barrier, in the amygdala, hypothalamus, periaqueductal gray, and in the dorsomedial medulla (Enerson and Drewes, 2006). Both co-transporters are also described in the membrane of the capillary endothelium (Koepsell, 2020). Interestingly, the presence of SGLT1/2 co-transporters has been found mainly in areas involved in processes such as learning, food intake, energy and glucose homeostasis, and central cardiovascular and autonomic regulation (Wiciński et al., 2020).
Growing evidence suggests that SGLT2 inhibitors have a neuroprotective potential and may improve the impaired cognitive function of diabetic patients by exerting pleiotropic effects and modulating numerous molecular mechanisms.
Chronic inflammation negatively affects the blood-brain barrier and leads to a pro-inflammatory phenotype of astrocytes and microglia (Rochfort and Cummins, 2015). Pro-inflammatory M1 polarization associates with neurodegeneration and cognitive impairment (Zhang Z. et al., 2021). SGLT2 inhibitors (i.e., dapagliflozin) have been proven to strongly promote macrophage polarization towards a M2 phenotype, thus alleviating inflammation and atherosclerotic processes (Lee et al., 2020). Similarly, empagliflozin can mitigate inflammation and macrophage stimulation by downregulating JAK2/STAT1 pathway and optimize energy expenditure (Xu et al., 2019; Lee et al., 2021). SGLT2 inhibitors may also regulate macrophage activation by decreasing the generation of free radicals and enhancing the expression of antioxidant enzymes (Lin et al., 2014; Iannantuoni et al., 2019). In Alzheimer’s disease, NLRP3 inflammasome has emerged as a pathological component participating in the impaired removal of amyloid-β by microglia (Lonnemann et al., 2020). Notably, SGLT2 inhibitors empagliflozin and canagliflozin act on macrophages and attenuate NLRP3 inflammasome in atherosclerosis and cognitive impairment, respectively (Kim et al., 2020; Khedr et al., 2024).
The inflammatory state may contribute to the overproduction of ROS or the decrease in antioxidant defense by compromising mitochondrial function (Guo et al., 2013). Oxidative stress is associated with amyloid-β- or tau-induced neurotoxicity, which results in impaired synaptic plasticity, neurotransmitter imbalance, and neuronal loss, leading to cognitive impairment (Huang et al., 2016; Cenini and Voos, 2019). Interestingly, the administration of dapagliflozin in obese mice is associated with a significant improvement in brain mitochondrial function and decreased ROS production, preventing cognitive decline (Sa-Nguanmoo et al., 2017).
Another crucial target involved in metabolic diseases and cognitive impairment is the mammalian target of rapamycin (mTOR). Hyperglycemia-induced mTOR upregulation leads to blood-brain barrier disruption and endothelial dysfunction, and contributes to tau hyperphosphorylation and amyloid-β aggregation in Alzheimer’s disease (Van Skike and Galvan, 2018; Uddin et al., 2020). SGLT2 inhibitors contribute to switching energy metabolism and reinstate mTOR activity in cardiovascular system, and by restoring mTOR cycle may interfere with a cognitive impairment (Packer, 2020; Stanciu et al., 2021).
Brain insulin resistance is heavily implicated in the pathogenesis of Alzheimer’s disease, and takes part in amyloid-β- and tau phosphorylation-mediated neuronal damage (Mullins et al., 2017). In a murine model of Alzheimer’s disease and type 2 diabetes, empagliflozin has been shown to ameliorate metabolic alterations and glucose levels lowering senile plaque burden; these effects are accompanied by an improvement of cognitive deficits (Hierro-Bujalance et al., 2020). In an experimental model of Alzheimer’s disease induced by aluminum chloride, dapagliflozin shows neuroprotective effects by suppressing brain amyloid-β protein deposition; improved cognition is mediated through different mechanisms, such as attenuation of oxidative stress, enhancement of glucose metabolism, and regulation of mTOR signaling (Samman et al., 2023). Moreover, dapagliflozin markedly alleviates neuronal oxidative stress, tau phosphorylation and amyloid-β production due to the ability to counteract neuronal apoptosis and upregulating glial cell-derived neurotrophic factor (Arab et al., 2021).
Alzheimer’s disease is also characterized by a reduced level of neuronal acetylcholine and the first line pharmacological treatment is based on the use of acetylcholinesterase inhibitors (Ferreira-Vieira et al., 2016). In an experimental model of cognitive impairment induced by scopolamine, the beneficial effect of canagliflozin on cognitive function has been attributed to the capacity to inhibit acetylcholinesterase and increase acetylcholine M1 receptor expression (Arafa et al., 2017). These results have been confirmed by molecular docking studies evaluating the binding energy of dapagliflozin to the catalytic site of SGLT2 and acetylcholinesterase enzymes, thus suggesting that dapagliflozin might act as a dual inhibitor to treat diabetes-associated neurological disorders (Shaikh et al., 2016).
5 Future directions
The mechanisms underlying HF and cognitive impairment have been extensively investigated although studies to provide a comprehensive view of the pathophysiological aspects at stake are needed. At this regard, epigenetic signature, observed in type 2 diabetes as well as in chronic cardiovascular and neurological diseases, is emerging as an innovative research field and a potential pharmacological target. Future research should explores the capability of GLP-1R agonists and SGLT2 inhibitors to modulate epigenetic writers, readers and erasers in the context of cardiovascular and brain homeostasis (Scisciola et al., 2020; Donniacuo et al., 2023).
The effectiveness of these anti-diabetic drugs in HF patients has been clearly established. Instead, the use of GLP-1R agonists and SGLT2 inhibitors as a potential therapeutic option for cognitive dysfunction is a relatively new area of investigation, and clinical studies are still limited. Therefore, more research is needed to understand the underlying mechanisms and to determine long-term safety and efficacy in humans. Intriguingly, the association of these pharmacological classes may represent a promising therapeutic strategy, and some clinical studies have already shown adjunctive benefits of the combination therapy.
Given the impact of type 2 diabetes on cardiovascular and neurological diseases, GLP-1 agonists and SGLT2 inhibitors represent a valid pharmacological tool for improving cardiometabolic profile and diabetes-related cognitive impairment. Because the vast array of molecular and cellular mechanisms modulated by these two new drug classes often intercept in pathophysiological background of chronic cardiovascular and neurodegenerative diseases, it is possible that any future new anti-diabetic drug may be also relevant for cardiometabolic and cognitive decline, independently from the anti-diabetic action.
Author contributions
MR: Writing–original draft, Conceptualization. EM: Investigation, Writing–original draft. MD: Writing–original draft, Investigation. MT: Writing–original draft, Investigation. GB: Investigation, Writing–original draft. GC: Writing–review and editing, Supervision. FR: Writing–review and editing, Supervision. AD: Writing–review and editing, Supervision. DC: Writing–review and editing, Conceptualization, Writing–original draft. KU: Writing–review and editing, Funding acquisition. LB: Writing–review and editing, Supervision, Funding acquisition.
Funding
The author(s) declare that financial support was received for the research, authorship, and/or publication of this article. Work supported by #NEXTGENERATIONEU (NGEU) and funded by the Ministry of University and Research (MUR), National Recovery and Resilience Plan (NRRP), project MNESYS (PE0000006)—A Multiscale integrated approach to the study of the nervous system in health and disease (DN. 1553 11.10.2022).
Conflict of interest
Authors GC and KU were employed by CEINGE-Advanced Biotechnologies.
The remaining authors declare that the research was conducted in the absence of any commercial or financial relationships that could be construed as a potential conflict of interest.
The author(s) declared that they were an editorial board member of Frontiers, at the time of submission. This had no impact on the peer review process and the final decision.
Publisher’s note
All claims expressed in this article are solely those of the authors and do not necessarily represent those of their affiliated organizations, or those of the publisher, the editors and the reviewers. Any product that may be evaluated in this article, or claim that may be made by its manufacturer, is not guaranteed or endorsed by the publisher.
Abbreviations
DPP-4, protease dipeptidyl peptidase 4; GIP, glucose-dependent insulinotropic polypeptide; GLP-1, glucagon-like peptide-1; GLP-1R, glucagon-like peptide-1 receptor; HF, heart failure; HFpEF, heart failure with preserved ejection fraction; HFrEF, heart failure with reduced ejection fraction; IL, interleukin; INa, sodium current; mTOR, mammalian target of rapamycin; NCX, sodium/calcium exchanger; NF-κB, nuclear factor-κB; NHE-1, sodium/hydrogen exchanger-1; NLRP3, NLR family pyrin domain containing 3 inflammasome; RAAS, renin-angiotensin-aldosterone system; ROS, reactive oxygen species; SGLT2, sodium-glucose cotransporter 2; TNF-α, tumor necrosis factor-α.
References
Almutairi, M., Gopal, K., Greenwell, A. A., Young, A., Gill, R., Aburasayn, H., et al. (2021). The GLP-1 receptor agonist liraglutide increases myocardial glucose oxidation rates via indirect mechanisms and mitigates experimental diabetic cardiomyopathy. Can. J. Cardiol. 37 (1), 140–150. doi:10.1016/j.cjca.2020.02.098
Arab, H. H., Safar, M. M., and Shahin, N. N. (2021). Targeting ROS-dependent AKT/GSK-3β/NF-κB and DJ-1/nrf2 pathways by dapagliflozin attenuates neuronal injury and motor dysfunction in rotenone-induced Parkinson's disease rat model. ACS Chem. Neurosci. 12 (4), 689–703. doi:10.1021/acschemneuro.0c00722
Arafa, N. M. S., Ali, E. H. A., and Hassan, M. K. (2017). Canagliflozin prevents scopolamine-induced memory impairment in rats: comparison with galantamine hydrobromide action. Chem. Biol. Interact. 277, 195–203. doi:10.1016/j.cbi.2017.08.013
Aroor, A. R., Mandavia, C., Ren, J., Sowers, J. R., and Pulakat, L. (2012b). Mitochondria and oxidative stress in the cardiorenal metabolic syndrome. Cardiorenal Med. 2, 87–109. doi:10.1159/000335675
Aroor, A. R., Mandavia, C. H., and Sowers, J. R. (2012a). Insulin resistance and heart failure: molecular mechanisms. Heart Fail Clin. 8 (4), 609–617. doi:10.1016/j.hfc.2012.06.005
Baartscheer, A., Schumacher, C. A., van Borren, M. M., Belterman, C. N., Coronel, R., and Fiolet, J. W. (2003). Increased Na+/H+-exchange activity is the cause of increased [Na+]i and underlies disturbed calcium handling in the rabbit pressure and volume overload heart failure model. Cardiovasc Res. 57 (4), 1015–1024. doi:10.1016/s0008-6363(02)00809-x
Baggio, L. L., Yusta, B., Mulvihill, E. E., Cao, X., Streutker, C. J., Butany, J., et al. (2018). GLP-1 receptor expression within the human heart. Endocrinology 159 (4), 1570–1584. doi:10.1210/en.2018-00004
Banday, M. Z., Sameer, A. S., and Nissar, S. (2020). Pathophysiology of diabetes: an overview. Avicenna J. Med. 10, 174–188. doi:10.4103/ajm.ajm_53_20
Bao, W., Aravindhan, K., Alsaid, H., Chendrimada, T., Szapacs, M., Citerone, D. R., et al. (2011). Albiglutide, a long lasting glucagon-like peptide-1 analog, protects the rat heart against ischemia/reperfusion injury: evidence for improving cardiac metabolic efficiency. PLoS One 6 (8), e23570. doi:10.1371/journal.pone.0023570
Barone, E., Di Domenico, F., Perluigi, M., and Butterfield, D. A. (2021). The interplay among oxidative stress, brain insulin resistance and AMPK dysfunction contribute to neurodegeneration in type 2 diabetes and Alzheimer disease. Free Radic. Biol. Med. 176, 16–33. doi:10.1016/j.freeradbiomed.2021.09.006
Batista, A. F., Forny-Germano, L., Clarke, J. R., Lyra, E. S. N. M., Brito-Moreira, J., Boehnke, S. E., et al. (2018). The diabetes drug liraglutide reverses cognitive impairment in mice and attenuates insulin receptor and synaptic pathology in a non-human primate model of Alzheimer’s disease. J. Pathol. 245 (1), 85–100. doi:10.1002/path.5056
Bay, J., Kohlhaas, M., and Maack, C. (2013). Intracellular Na⁺ and cardiac metabolism. J. Mol. Cell Cardiol. 61, 20–27. doi:10.1016/j.yjmcc.2013.05.010
Baylan, U., Korn, A., Emmens, R. W., Schalkwijk, C. G., Niessen, H. W. M., Krijnen, P. A. J., et al. (2022). Liraglutide treatment attenuates inflammation markers in the cardiac, cerebral and renal microvasculature in streptozotocin-induced diabetic rats. Eur. J. Clin. Invest. 52 (9), e13807. doi:10.1111/eci.13807
Bendotti, G., Montefusco, L., Lunati, M. E., Usuelli, V., Pastore, I., Lazzaroni, E., et al. (2022). The anti-inflammatory and immunological properties of GLP-1 Receptor Agonists. Pharmacol. Res. 182, 106320. doi:10.1016/j.phrs.2022.106320
Bers, D. M. (2006). Altered cardiac myocyte Ca regulation in heart failure. Physiol. (Bethesda) 21, 380–387. doi:10.1152/physiol.00019.2006
Bomba, M., Ciavardelli, D., Silvestri, E., Canzoniero, L. M., Lattanzio, R., Chiappini, P., et al. (2013). Exenatide promotes cognitive enhancement and positive brain metabolic changes in PS1-KI mice but has no effects in 3xTg-AD animals. Cell Death Dis. 4, e612. doi:10.1038/cddis.2013.139
Brasier, A. R. (2010). The nuclear factor-kappaB-interleukin-6 signalling pathway mediating vascular inflammation. Cardiovasc Res. 86 (2), 211–218. doi:10.1093/cvr/cvq076
Bruen, R., Curley, S., Kajani, S., Crean, D., O'Reilly, M. E., Lucitt, M. B., et al. (2017). Liraglutide dictates macrophage phenotype in apolipoprotein E null mice during early atherosclerosis. Cardiovasc Diabetol. 16 (1), 143. doi:10.1186/s12933-017-0626-3
Bułdak, Ł., Machnik, G., Bułdak, R. J., Łabuzek, K., Bołdys, A., and Okopień, B. (2016). Exenatide and metformin express their anti-inflammatory effects on human monocytes/macrophages by the attenuation of MAPKs and NFκB signaling. Naunyn-Schmiedeberg's archives Pharmacol. 389 (10), 1103–1115. doi:10.1007/s00210-016-1277-8
Byrne, N. J., Matsumura, N., Maayah, Z. H., Ferdaoussi, M., Takahara, S., Darwesh, A. M., et al. (2020). Empagliflozin blunts worsening cardiac dysfunction associated with reduced NLRP3 (Nucleotide-Binding domain-like receptor protein 3) inflammasome activation in heart failure. Circ. Heart Fail 13 (1), e006277. doi:10.1161/CIRCHEARTFAILURE.119.006277
Cai, H. Y., Yang, J. T., Wang, Z. J., Zhang, J., Yang, W., Wu, M. N., et al. (2018). Lixisenatide reduces amyloid plaques, neurofibrillary tangles and neuroinflammation in an APP/PS1/tau mouse model of Alzheimer’s disease. Biochem. Biophys. Res. Commun. 495 (1), 1034–1040. doi:10.1016/j.bbrc.2017.11.114
Calsolaro, V., and Edison, P. (2016). Neuroinflammation in Alzheimer’s disease: current evidence and future directions. Alzheimers Dement. 12, 719–732. doi:10.1016/j.jalz.2016.02.010
Cappetta, D., De Angelis, A., Bellocchio, G., Telesca, M., Cianflone, E., Torella, D., et al. (2021). Sodium-glucose cotransporter 2 inhibitors and heart failure: a bedside-to-bench journey. Front. Cardiovasc Med. 8, 810791. doi:10.3389/fcvm.2021.810791
Cappetta, D., De Angelis, A., Ciuffreda, L. P., Coppini, R., Cozzolino, A., Miccichè, A., et al. (2020). Amelioration of diastolic dysfunction by dapagliflozin in a non-diabetic model involves coronary endothelium. Pharmacol. Res. 157, 104781. doi:10.1016/j.phrs.2020.104781
Cardiogenic dementia (1977). Cardiogenic dementia. Lancet. 1, 27–28. doi:10.1016/S0140-6736(77)91660-9
Cenini, G., and Voos, W. (2019). Mitochondria as potential targets in alzheimer disease therapy: an update. Front. Pharmacol. 10, 902. doi:10.3389/fphar.2019.00902
Chang, Y. F., Zhang, D., Hu, W. M., Liu, D. X., and Li, L. (2020). Semaglutide-mediated protection against Aβ correlated with enhancement of autophagy and inhibition of apotosis. J. Clin. Neurosci. 81, 234–239. doi:10.1016/j.jocn.2020.09.054
Checa, J., and Aran, J. M. (2020). Reactive oxygen species: drivers of physiological and pathological processes. J. Inflamm. Res. 13, 1057–1073. doi:10.2147/JIR.S275595
Chen, S., Coronel, R., Hollmann, M. W., Weber, N. C., and Zuurbier, C. J. (2022). Direct cardiac effects of SGLT2 inhibitors. Cardiovasc Diabetol. 21 (1), 45. doi:10.1186/s12933-022-01480-1
Chen, S., Sun, J., Zhao, G., Guo, A., Chen, Y., Fu, R., et al. (2017). Liraglutide improves water maze learning and memory performance while reduces hyperphosphorylation of tau and neurofilaments in APP/PS1/tau triple transgenic mice. Neurochem. Res. 42 (8), 2326–2335. doi:10.1007/s11064-017-2250-8
Colin, I. M., Szczepanski, L. W., Gérard, A. C., and Elosegi, J. A. (2023). Emerging evidence for the use of antidiabetic drugs, glucagon-like peptide 1 receptor agonists, for the treatment of alzheimer's disease. touchREV Endocrinol. 19 (1), 16–24. doi:10.17925/EE.2023.19.1.16
Cork, S. C., Richards, J. E., Holt, M. K., Gribble, F. M., Reimann, F., and Trapp, S. (2015). Distribution and characterisation of glucagon-like peptide-1 receptor expressing cells in the mouse brain. Mol. Metab. 4, 718–731. doi:10.1016/j.molmet.2015.07.008
Cosentino, F., Grant, P. J., Aboyans, V., Bailey, C. J., Ceriello, A., Delgado, V., et al. (2020). 2019 ESC Guidelines on diabetes, pre-diabetes, and cardiovascular diseases developed in collaboration with the EASD. Eur. Heart J. 41 (2), 255–323. doi:10.1093/eurheartj/ehz486
Craft, S. (2007). Insulin resistance and alzheimer's disease pathogenesis: potential mechanisms and implications for treatment. Curr. Alzheimer Res. 4, 147–152. doi:10.2174/156720507780362137
Croteau, D., Luptak, I., Chambers, J. M., Hobai, I., Panagia, M., Pimentel, D. R., et al. (2021). Effects of sodium-glucose linked transporter 2 inhibition with ertugliflozin on mitochondrial function, energetics, and metabolic gene expression in the presence and absence of diabetes mellitus in mice. J. Am. Heart Assoc. 10 (13), e019995. doi:10.1161/JAHA.120.019995
Dao, L., Choi, S., and Freeby, M. (2023). Type 2 diabetes mellitus and cognitive function: understanding the connections. Curr. Opin. Endocrinol. Diabetes Obes. 30 (1), 7–13. doi:10.1097/MED.0000000000000783
Deacon, C. F., and Ahrén, B. (2011). Physiology of incretins in health and disease. Rev. Diabet. Stud. 8 (3), 293–306. doi:10.1900/RDS.2011.8.293
Del, P. S., Kahn, S. E., Pavo, I., Weerakkody, G. J., Yang, Z., Doupis, J., et al. (2021). Tirzepatide versus insulin glargine in type 2 diabetes and increased cardiovascular risk (SURPASS-4): a randomised, open-label, parallel-group, multicentre, phase 3 trial. Lancet 398 (10313), 1811–1824. doi:10.1016/S0140-6736(21)02188-7
Delghandi, M. P., Johannessen, M., and Moens, U. (2005). The cAMP signalling pathway activates CREB through PKA, p38 and MSK1 in NIH 3T3 cells. Cell Signal 17 (11), 1343–1351. doi:10.1016/j.cellsig.2005.02.003
Denver, P., English, A., and McClean, P. L. (2018). Inflammation, insulin signaling and cognitive function in aged APP/PS1 mice. Brain Behav. Immun. 70, 423–434. doi:10.1016/j.bbi.2018.03.032
Díez-Villanueva, P., Jiménez-Méndez, C., and Alfonso, F. (2021). Heart failure in the elderly. J. Geriatr. Cardiol. 18 (3), 219–232. doi:10.11909/j.issn.1671-5411.2021.03.009
Dimitriadis, K., Adamopoulou, E., Pyrpyris, N., Sakalidis, A., Leontsinis, I., Manta, E., et al. (2023). The effect of SGLT2 inhibitors on the endothelium and the microcirculation: from bench to bedside and beyond. Eur. Heart J. Cardiovasc Pharmacother. 9 (8), 741–757. doi:10.1093/ehjcvp/pvad053
Dodson, J. A., Truong, T. T., Towle, V. R., Kerins, G., and Chaudhry, S. I. (2013). Cognitive impairment in older adults with heart failure: prevalence, documentation, and impact on outcomes. Am. J. Med. 126 (2), 120–126. doi:10.1016/j.amjmed.2012.05.029
Dominguez Rieg, J. A., and Rieg, T. (2019). What does sodium-glucose co-transporter 1 inhibition add: prospects for dual inhibition. Diabetes Obes. Metab. 21 (Suppl. 2), 43–52. doi:10.1111/dom.13630
Donniacuo, M., De Angelis, A., Telesca, M., Bellocchio, G., Riemma, M. A., Paolisso, P., et al. (2023). Atrial fibrillation: epigenetic aspects and role of sodium-glucose cotransporter 2 inhibitors. Pharmacol. Res. 188, 106591. doi:10.1016/j.phrs.2022.106591
Doughan, A. K., Harrison, D. G., and Dikalov, S. I. (2008). Molecular mechanisms of angiotensin II-mediated mitochondrial dysfunction: linking mitochondrial oxidative damage and vascular endothelial dysfunction. Circ. Res. 102 (4), 488–496. doi:10.1161/CIRCRESAHA.107.162800
Drucker, D. J. (2016). The cardiovascular biology of glucagon-like peptide-1. Cell Metab. 24 (1), 15–30. doi:10.1016/j.cmet.2016.06.009
Drucker, D. J., Philippe, J., Mojsov, S., Chick, W. L., and Habener, J. F. (1987). Glucagon-like peptide I stimulates insulin gene expression and increases cyclic AMP levels in a rat islet cell line. Proc. Natl. Acad. Sci. U. S. A. 84, 3434–3438. doi:10.1073/pnas.84.10.3434
Du, H., Meng, X., Yao, Y., and Xu, J. (2022). The mechanism and efficacy of GLP-1 receptor agonists in the treatment of Alzheimer's disease. Front. Endocrinol. (Lausanne) 13, 1033479. doi:10.3389/fendo.2022.1033479
Dunlay, S. M., Givertz, M. M., Aguilar, D., Allen, L. A., Chan, M., Desai, A. S., et al. (2019). Type 2 diabetes mellitus and heart failure: a scientific statement from the American heart association and the heart failure society of America: this statement does not represent an update of the 2017 ACC/AHA/HFSA heart failure guideline update. Circulation 140 (7), e294–e324. doi:10.1161/CIR.0000000000000691
Dunlay, S. M., Roger, V. L., and Redfield, M. M. (2017). Epidemiology of heart failure with preserved ejection fraction. Nat. Rev. Cardiol. 14 (10), 591–602. doi:10.1038/nrcardio.2017.65
During, M. J., Cao, L., Zuzga, D. S., Francis, J. S., Fitzsimons, H. L., Jiao, X. Y., et al. (2003). Glucagon-like peptide-1 receptor is involved in learning and neuroprotection. Nat. Med. 9, 1173–1179. doi:10.1038/nm919
Dutta, D., Surana, V., Singla, R., Aggarwal, S., and Sharma, M. (2021). Efficacy and safety of novel twincretin tirzepatide a dual GIP and GLP-1 receptor agonist in the management of type-2 diabetes: a Cochrane meta-analysis. Indian J. Endocrinol. Metab. 25 (6), 475–489. doi:10.4103/ijem.ijem_423_21
Elrakaybi, A., Laubner, K., Zhou, Q., Hug, M. J., and Seufert, J. (2022). Cardiovascular protection by SGLT2 inhibitors - do anti-inflammatory mechanisms play a role? Mol. Metab. 64, 101549. doi:10.1016/j.molmet.2022.101549
Enerson, B. E., and Drewes, L. R. (2006). The rat blood-brain barrier transcriptome. J. Cereb. Blood Flow. Metab. 26 (7), 959–973. doi:10.1038/sj.jcbfm.9600249
Engidawork, E., Gulesserian, T., Seidl, R., Cairns, N., and Lubec, G. (2001). Expression of apoptosis related proteins in brains of patients with Alzheimer's disease. Neurosci. Lett. 303 (2), 79–82. doi:10.1016/s0304-3940(01)01618-4
Ferrannini, E. (2017). Sodium-glucose Co-transporters and their inhibition: clinical physiology. Cell Metab. 26 (1), 27–38. doi:10.1016/j.cmet.2017.04.011
Ferreira-Vieira, T. H., Guimaraes, I. M., Silva, F. R., and Ribeiro, F. M. (2016). Alzheimer's disease: targeting the cholinergic system. Curr. Neuropharmacol. 14 (1), 101–115. doi:10.2174/1570159x13666150716165726
Finan, B., Ma, T., Ottaway, N., Muller, T. D., Habegger, K. M., Heppner, K. M., et al. (2013). Unimolecular dual incretins maximize metabolic benefits in rodents, monkeys, and humans. Sci. Transl. Med. 5, 209ra151. doi:10.1126/scitranslmed.3007218
Finan, B., Yang, B., Ottaway, N., Smiley, D. L., Ma, T., Clemmensen, C., et al. (2015). A rationally designed monomeric peptide triagonist corrects obesity and diabetes in rodents. Nat. Med. 21 (1), 27–36. doi:10.1038/nm.3761
Fontanella, R. A., Ghosh, P., Pesapane, A., Taktaz, F., Puocci, A., Franzese, M., et al. (2024). Tirzepatide prevents neurodegeneration through multiple molecular pathways. J. Transl. Med. 22 (1), 114. doi:10.1186/s12967-024-04927-z
Frati, G., Schirone, L., Chimenti, I., Yee, D., Biondi-Zoccai, G., Volpe, M., et al. (2017). An overview of the inflammatory signalling mechanisms in the myocardium underlying the development of diabetic cardiomyopathy. Cardiovasc Res. 113 (4), 378–388. doi:10.1093/cvr/cvx011
GBD 2016 Dementia Collaborators (2019). Global, regional, and national burden of Alzheimer's disease and other dementias, 1990-2016: a systematic analysis for the Global Burden of Disease Study 2016. Lancet Neurol. 18 (1), 88–106. doi:10.1016/S1474-4422(18)30403-4
Goldberg, L. R. (2021). The pleiotropic effects of SGLT2 inhibitors: remodeling the treatment of heart failure. J. Am. Coll. Cardiol. 77, 256–258. doi:10.1016/j.jacc.2020.11.029
Gollmer, J., Zirlik, A., and Bugger, H. (2020). Mitochondrial mechanisms in diabetic cardiomyopathy. Diabetes Metab. J. 44 (1), 33–53. doi:10.4093/dmj.2019.0185
Green, H., Tsitsi, P., Markaki, I., Aarsland, D., and Svenningsson, P. (2019). Novel treatment opportunities against cognitive impairment in Parkinson's disease with an emphasis on diabetes-related pathways. CNS Drugs 33, 143–160. doi:10.1007/s40263-018-0601-x
Groenewegen, A., Rutten, F. H., Mosterd, A., and Hoes, A. W. (2020). Epidemiology of heart failure. Eur. J. Heart Fail 22 (8), 1342–1356. doi:10.1002/ejhf.1858
Guo, C., Sun, L., Chen, X., and Zhang, D. (2013). Oxidative stress, mitochondrial damage and neurodegenerative diseases. Neural Regen. Res. 8 (21), 2003–2014. doi:10.3969/j.issn.1673-5374.2013.21.009
Hamilton, A., and Holscher, C. (2009). Receptors for the incretin glucagon-like peptide-1 are expressed on neurons in the central nervous system. Neuroreport 20, 1161–1166. doi:10.1097/WNR.0b013e32832fbf14
Helmstädter, J., Frenis, K., Filippou, K., Grill, A., Dib, M., Kalinovic, S., et al. (2020). Endothelial GLP-1 (Glucagon-Like peptide-1) receptor mediates cardiovascular protection by liraglutide in mice with experimental arterial hypertension. Arterioscler. Thromb. Vasc. Biol. 40 (1), 145–158. doi:10.1161/atv.0000615456.97862.30
Hierro-Bujalance, C., Infante-Garcia, C., Del Marco, A., Herrera, M., Carranza-Naval, M. J., Suarez, J., et al. (2020). Empagliflozin reduces vascular damage and cognitive impairment in a mixed murine model of Alzheimer's disease and type 2 diabetes. Alzheimers Res. Ther. 12 (1), 40. doi:10.1186/s13195-020-00607-4
Hirata, K., Kume, S., Araki, S., Sakaguchi, M., Chin-Kanasaki, M., Isshiki, K., et al. (2009). Exendin-4 has an anti-hypertensive effect in salt-sensitive mice model. Biochem. Biophys. Res. Commun. 380 (1), 44–49. doi:10.1016/j.bbrc.2009.01.003
Hogan, A. E., Gaoatswe, G., Lynch, L., Corrigan, M. A., Woods, C., O'Connell, J., et al. (2014). Glucagon-like peptide 1 analogue therapy directly modulates innate immune-mediated inflammation in individuals with type 2 diabetes mellitus. Diabetologia 57 (4), 781–784. doi:10.1007/s00125-013-3145-0
Holst, J. J., Knop, F. K., Vilsbøll, T., Krarup, T., and Madsbad, S. (2011). Loss of incretin effect is a specific, important, and early characteristic of type 2 diabetes. Diabetes Care 34 (Suppl. 2), S251–S257. doi:10.2337/dc11-s227
Holz, G. G. (2004). EPAC: a new cAMP-binding protein in support of glucagon-like peptide 1 receptor mediated signal transduction in the pancreatic beta-cell. Diabetes 53 (1), 5–13. doi:10.2337/diabetes.53.1.5
Horvath, B., and Bers, D. M. (2014). The late sodium current in heart failure: pathophysiology and clinical relevance. Esc. Heart Fail 1 (1), 26–40. doi:10.1002/ehf2.12003
Huang, W. J., Zhang, X., and Chen, W. W. (2016). Role of oxidative stress in Alzheimer's disease. Biomed. Rep. 4 (5), 519–522. doi:10.3892/br.2016.630
Hunter, K., and Holscher, C. (2012). Drugs developed to treat diabetes, liraglutide and lixisenatide, cross the blood brain barrier and enhance neurogenesis. BMC Neurosci. 13, 33. doi:10.1186/1471-2202-13-33
Iannantuoni, F., M de Marañon, A., Diaz-Morales, N., Falcon, R., Bañuls, C., Abad-Jimenez, Z., et al. (2019). The SGLT2 inhibitor empagliflozin ameliorates the inflammatory profile in type 2 diabetic patients and promotes an antioxidant response in leukocytes. J. Clin. Med. 8 (11), 1814. doi:10.3390/jcm8111814
Jakubowska, A., Roux, C. W. L., and Viljoen, A. (2024). The road towards triple agonists: glucagon-like peptide 1, glucose-dependent insulinotropic polypeptide and glucagon receptor - an update. Endocrinol. Metab. Seoul. 39 (1), 12–22. doi:10.3803/EnM.2024.1942
Jensen, E. P., Møller, S., Hviid, A. V., Veedfald, S., Holst, J. J., Pedersen, J., et al. (2020). GLP-1-induced renal vasodilation in rodents depends exclusively on the known GLP-1 receptor and is lost in prehypertensive rats. Am. J. Physiol. Ren. Physiol. 318 (6), F1409–F1417. doi:10.1152/ajprenal.00579.2019
Jia, G., Hill, M. A., and Sowers, J. R. (2018). Diabetic cardiomyopathy: an update of mechanisms contributing to this clinical entity. Circ. Res. 122, 624–638. doi:10.1161/CIRCRESAHA.117.311586
Kalra, S. (2014). Sodium glucose Co-Transporter-2 (SGLT2) inhibitors: a review of their basic and clinical Pharmacology. Diabetes Ther. 5 (2), 355–366. doi:10.1007/s13300-014-0089-4
Khedr, L. H., Rahmo, R. M., Eldemerdash, O. M., Helmy, E. M., Ramzy, F. A., Lotfy, G. H., et al. (2024). Implication of M2 macrophage on NLRP3 inflammasome signaling in mediating the neuroprotective effect of Canagliflozin against methotrexate-induced cognitive impairment. Int. Immunopharmacol. 130, 111709. doi:10.1016/j.intimp.2024.111709
Kim, J., Wei, Y., and Sowers, J. R. (2008). Role of mitochondrial dysfunction in insulin resistance. Circ. Res. 102, 401–414. doi:10.1161/CIRCRESAHA.107.165472
Kim, M., Platt, M. J., Shibasaki, T., Quaggin, S. E., Backx, P. H., Seino, S., et al. (2013). GLP-1 receptor activation and Epac2 link atrial natriuretic peptide secretion to control of blood pressure. Nat. Med. 19 (5), 567–575. doi:10.1038/nm.3128
Kim, S. R., Lee, S. G., Kim, S. H., Kim, J. H., Choi, E., Cho, W., et al. (2020). SGLT2 inhibition modulates NLRP3 inflammasome activity via ketones and insulin in diabetes with cardiovascular disease. Nat. Commun. 11 (1), 2127. doi:10.1038/s41467-020-15983-6
Knerr, P. J., Mowery, S. A., Douros, J. D., Premdjee, B., Hjollund, K. R., He, Y., et al. (2022). Next generation GLP-1/GIP/glucagon triple agonists normalize body weight in obese mice. Mol. Metab. 63, 101533. doi:10.1016/j.molmet.2022.101533
Koepsell, H. (2020). Glucose transporters in the small intestine in health and disease. Pflugers Arch. 472 (9), 1207–1248. doi:10.1007/s00424-020-02439-5
Kolwicz, S. C., and Tian, R. (2011). Glucose metabolism and cardiac hypertrophy. Cardiovasc Res. 90 (2), 194–201. doi:10.1093/cvr/cvr071
Kopp, K. O., Glotfelty, E. J., Li, Y., and Greig, N. H. (2022). Glucagon-like peptide-1 (GLP-1) receptor agonists and neuroinflammation: implications for neurodegenerative disease treatment. Pharmacol. Res. 186, 106550. doi:10.1016/j.phrs.2022.106550
Lastra, G., Dhuper, S., Johnson, M. S., and Sowers, J. R. (2010). Salt, aldosterone, and insulin resistance: impact on the cardiovascular system. Nat. Rev. Cardiol. 7, 577–584. doi:10.1038/nrcardio.2010.123
Laugero, K. D., Stonehouse, A. H., Guss, S., Landry, J., Vu, C., and Parkes, D. G. (2009). Exenatide improves hypertension in a rat model of the metabolic syndrome. Metab. Syndr. Relat. Disord. 7 (4), 327–334. doi:10.1089/met.2008.0095
Lebeche, D., Davidoff, A. J., and Hajjar, R. J. (2008). Interplay between impaired calcium regulation and insulin signaling abnormalities in diabetic cardiomyopathy. Nat. Clin. Pract. Cardiovasc Med. 5, 715–724. doi:10.1038/ncpcardio1347
Lee, N., Heo, Y. J., Choi, S. E., Jeon, J. Y., Han, S. J., Kim, D. J., et al. (2021). Anti-inflammatory effects of empagliflozin and gemigliptin on LPS-stimulated macrophage via the IKK/NF-κB, MKK7/JNK, and JAK2/STAT1 signalling pathways. J. Immunol. Res. 2021, 9944880. doi:10.1155/2021/9944880
Lee, S. G., Lee, S. J., Lee, J. J., Kim, J. S., Lee, O. H., Kim, C. K., et al. (2020). Anti-inflammatory effect for atherosclerosis progression by sodium-glucose cotransporter 2 (SGLT-2) inhibitor in a normoglycemic rabbit model. Korean Circ. J. 50 (5), 443–457. doi:10.4070/kcj.2019.0296
Lee, T. I., Chen, Y. C., Lin, Y. K., Chung, C. C., Lu, Y. Y., Kao, Y. H., et al. (2019). Empagliflozin attenuates myocardial sodium and calcium dysregulation and reverses cardiac remodeling in streptozotocin-induced diabetic rats. Int. J. Mol. Sci. 20 (7), 1680. doi:10.3390/ijms20071680
Lee, T. M., Chang, N. C., and Lin, S. Z. (2017). Dapagliflozin, a selective SGLT2 Inhibitor, attenuated cardiac fibrosis by regulating the macrophage polarization via STAT3 signaling in infarcted rat hearts. Free Radic. Biol. Med. 104, 298–310. doi:10.1016/j.freeradbiomed.2017.01.035
Li, L., Zhang, Z. F., Holscher, C., Gao, C., Jiang, Y. H., and Liu, Y. Z. (2012). (Val⁸) glucagon-like peptide-1 prevents tau hyperphosphorylation, impairment of spatial learning and ultra-structural cellular damage induced by streptozotocin in rat brains. Eur. J. Pharmacol. 674 (2-3), 280–286. doi:10.1016/j.ejphar.2011.11.005
Li, T., Jiao, J. J., Hölscher, C., Wu, M. N., Zhang, J., Tong, J. Q., et al. (2018). A novel GLP-1/GIP/Gcg triagonist reduces cognitive deficits and pathology in the 3xTg mouse model of Alzheimer's disease. Hippocampus 28 (5), 358–372. doi:10.1002/hipo.22837
Lin, B., Koibuchi, N., Hasegawa, Y., Sueta, D., Toyama, K., Uekawa, K., et al. (2014). Glycemic control with empagliflozin, a novel selective SGLT2 inhibitor, ameliorates cardiovascular injury and cognitive dysfunction in obese and type 2 diabetic mice. Cardiovasc Diabetol. 13, 148. doi:10.1186/s12933-014-0148-1
Liu, J., Xiao, G., Liang, Y., He, S., Lyu, M., and Zhu, Y. (2024). Heart-brain interaction in cardiogenic dementia: pathophysiology and therapeutic potential. Front. Cardiovasc Med. 11, 1304864. doi:10.3389/fcvm.2024.1304864
Liu, W., Jalewa, J., Sharma, M., Li, G., Li, L., and Holscher, C. (2015). Neuroprotective effects of lixisenatide and liraglutide in the 1-methyl-4-phenyl-1,2,3,6-tetrahydropyridine mouse model of Parkinson’s disease. Neuroscience 303, 42–50. doi:10.1016/j.neuroscience.2015.06.054
Long-Smith, C. M., Manning, S., McClean, P. L., Coakley, M. F., O'Halloran, D. J., Holscher, C., et al. (2013). The diabetes drug liraglutide ameliorates aberrant insulin receptor localisation and signalling in parallel with decreasing both amyloid-β plaque and glial pathology in a mouse model of Alzheimer's disease. Neuromolecular Med. 15 (1), 102–114. doi:10.1007/s12017-012-8199-5
Lonnemann, N., Hosseini, S., Marchetti, C., Skouras, D. B., Stefanoni, D., D'Alessandro, A., et al. (2020). The NLRP3 inflammasome inhibitor OLT1177 rescues cognitive impairment in a mouse model of Alzheimer's disease. Proc. Natl. Acad. Sci. U. S. A. 117 (50), 32145–32154. doi:10.1073/pnas.2009680117
Lopaschuk, G. D. (2016). Fatty acid oxidation and its relation with insulin resistance and associated disorders. Ann. Nutr. Metab. 68 (3), 15–20. doi:10.1159/000448357
Lopaschuk, G. D., and Verma, S. (2020). Mechanisms of cardiovascular benefits of sodium glucose Co-transporter 2 (SGLT2) inhibitors: a state-of-the-art review. JACC Basic Transl. Sci. 5 (6), 632–644. doi:10.1016/j.jacbts.2020.02.004
Luo, X., Hu, Y., He, S., Ye, Q., Lv, Z., Liu, J., et al. (2019). Dulaglutide inhibits high glucose-induced endothelial dysfunction and NLRP3 inflammasome activation. Arch. Biochem. Biophys. 671, 203–209. doi:10.1016/j.abb.2019.07.008
Markham, A., and Keam, S. J. (2019). Sotagliflozin: first global approval. Drugs 79 (9), 1023–1029. doi:10.1007/s40265-019-01146-5
Martins, F. L., Bailey, M. A., and Girardi, A. C. C. (2020). Endogenous activation of glucagon-like peptide-1 receptor contributes to blood pressure control: role of proximal tubule Na+/H+ exchanger isoform 3, renal angiotensin II, and insulin sensitivity. Hypertension 76 (3), 839–848. doi:10.1161/HYPERTENSIONAHA.120.14868
Marx, N., Husain, M., Lehrke, M., Verma, S., and Sattar, N. (2022). GLP-1 receptor agonists for the reduction of atherosclerotic cardiovascular risk in patients with type 2 diabetes. Circulation 146 (24), 1882–1894. doi:10.1161/CIRCULATIONAHA.122.059595
Mascolo, A., di Mauro, G., Cappetta, D., De Angelis, A., Torella, D., Urbanek, K., et al. (2022b). Current and future therapeutic perspective in chronic heart failure. Pharmacol. Res. 175, 106035. doi:10.1016/j.phrs.2021.106035
Mascolo, A., Di Napoli, R., Balzano, N., Cappetta, D., Urbanek, K., De Angelis, A., et al. (2022a). Safety profile of sodium glucose co-transporter 2 (SGLT2) inhibitors: a brief summary. Front. Cardiovasc Med. 9, 1010693. doi:10.3389/fcvm.2022.1010693
Mazidi, M., Rezaie, P., Gao, H. K., and Kengne, A. P. (2017). Effect of sodium-glucose cotransport-2 inhibitors on blood pressure in people with type 2 diabetes mellitus: a systematic review and meta-analysis of 43 randomized control trials with 22 528 patients. J. Am. Heart Assoc. 6 (6), e004007. doi:10.1161/JAHA.116.004007
McClean, P. L., and Hölscher, C. (2014). Liraglutide can reverse memory impairment, synaptic loss and reduce plaque load in aged APP/PS1 mice, a model of Alzheimer's disease. Neuropharmacology 76 Pt A, 57–67. doi:10.1016/j.neuropharm.2013.08.005
McClean, P. L., Jalewa, J., and Hölscher, C. (2015). Prophylactic liraglutide treatment prevents amyloid plaque deposition, chronic inflammation and memory impairment in APP/PS1 mice. Behav. Brain Res. 293, 96–106. doi:10.1016/j.bbr.2015.07.024
McDonagh, T. A., Metra, M., Adamo, M., Gardner, R. S., Baumbach, A., Böhm, M., et al. (2021). 2021 ESC Guidelines for the diagnosis and treatment of acute and chronic heart failure. Eur. Heart J. 42 (36), 3599–3726. doi:10.1093/eurheartj/ehab368
McDonagh, T. A., Metra, M., Adamo, M., Gardner, R. S., Baumbach, A., Böhm, M., et al. (2023). 2023 Focused Update of the 2021 ESC Guidelines for the diagnosis and treatment of acute and chronic heart failure. Eur. Heart J. 44 (37), 3627–3639. doi:10.1093/eurheartj/ehad195
Mehdi, S. F., Pusapati, S., Anwar, M. S., Lohana, D., Kumar, P., Nandula, S. A., et al. (2023). Glucagon-like peptide-1: a multi-faceted anti-inflammatory agent. Front. Immunol. 14, 1148209. doi:10.3389/fimmu.2023.1148209
Minze, M. G., Will, K. J., Terrell, B. T., Black, R. L., and Irons, B. K. (2018). Benefits of SGLT2 inhibitors beyond glycemic control - a focus on metabolic, cardiovascular and renal outcomes. Curr. Diabetes Rev. 14 (6), 509–517. doi:10.2174/1573399813666170816142351
Mullins, R. J., Diehl, T. C., Chia, C. W., and Kapogiannis, D. (2017). Insulin resistance as a link between amyloid-beta and Tau pathologies in Alzheimer’s disease. Front. Aging Neurosci. 9, 118. doi:10.3389/fnagi.2017.00118
Murman, D. L. (2015). The impact of age on cognition. Semin. Hear 36 (3), 111–121. doi:10.1055/s-0035-1555115
Nauck, M. A. (2014). Update on developments with SGLT2 inhibitors in the management of type 2 diabetes. Drug Des. Devel Ther. 8, 1335–1380. doi:10.2147/DDDT.S50773
Nguyen, T. T., Ta, Q. T. H., Nguyen, T. K. O., Nguyen, T. T. D., and Giau, V. V. (2020). Type 3 diabetes and its role implications in alzheimer's disease. Int. J. Mol. Sci. 21 (9), 3165. doi:10.3390/ijms21093165
Ni, L., Yuan, C., Chen, G., Zhang, C., and Wu, X. (2020). SGLT2i: beyond the glucose-lowering effect. Cardiovasc Diabetol. 19 (1), 98. doi:10.1186/s12933-020-01071-y
Ou, Y. N., Zhang, Y. B., Li, Y. Z., Huang, S. Y., Zhang, W., Deng, Y. T., et al. (2024). Socioeconomic status, lifestyle and risk of incident dementia: a prospective cohort study of 276730 participants. Geroscience 46 (2), 2265–2279. doi:10.1007/s11357-023-00994-0
Owan, T. E., Hodge, D. O., Herges, R. M., Jacobsen, S. J., Roger, V. L., and Redfield, M. M. (2006). Trends in prevalence and outcome of heart failure with preserved ejection fraction. N. Engl. J. Med. 355 (3), 251–259. doi:10.1056/NEJMoa052256
Packer, M. (2017). Activation and inhibition of sodium-hydrogen exchanger is a mechanism that links the pathophysiology and treatment of diabetes mellitus with that of heart failure. Circulation 136 (16), 1548–1559. doi:10.1161/CIRCULATIONAHA.117.030418
Packer, M. (2018). Heart failure: the most important, preventable, and treatable cardiovascular complication of type 2 diabetes. Diabetes Care 41 (1), 11–13. doi:10.2337/dci17-0052
Packer, M. (2020). SGLT2 inhibitors produce cardiorenal benefits by promoting adaptive cellular reprogramming to induce a state of fasting mimicry: a paradigm shift in understanding their mechanism of action. Diabetes Care 43 (3), 508–511. doi:10.2337/dci19-0074
Pandey, A., Salahuddin, U., Garg, S., Ayers, C., Kulinski, J., Anand, V., et al. (2016). Continuous dose-response association between sedentary time and risk for cardiovascular disease: a meta-analysis. JAMA Cardiol. 1 (5), 575–583. doi:10.1001/jamacardio.2016.1567
Pandey, J., and Tamrakar, A. K. (2019). SGLT2 inhibitors for the treatment of diabetes: a patent review (2013-2018). Expert Opin. Ther. Pat. 29 (5), 369–384. doi:10.1080/13543776.2019.1612879
Pandey, S., Mangmool, S., and Parichatikanond, W. (2023). Multifaceted roles of GLP-1 and its analogs: a review on molecular mechanisms with a cardiotherapeutic perspective. Pharm. (Basel) 16 (6), 836. doi:10.3390/ph16060836
Pappolla, M. A., Bryant-Thomas, T. K., Herbert, D., Pacheco, J., Fabra Garcia, M., Manjon, M., et al. (2003). Mild hypercholesterolemia is an early risk factor for the development of Alzheimer amyloid pathology. Neurology 61 (2), 199–205. doi:10.1212/01.wnl.0000070182.02537.84
Paris, D., Town, T., Mori, T., Parker, T. A., Humphrey, J., and Mullan, M. (2000). Soluble beta-amyloid peptides mediate vasoactivity via activation of a pro-inflammatory pathway. Neurobiol. Aging 21 (2), 183–197. doi:10.1016/s0197-4580(99)00111-6
Parthsarathy, V., and Holscher, C. (2013). The type 2 diabetes drug liraglutide reduces chronic inflammation induced by irradiation in the mouse brain. Eur. J. Pharmacol. 700 (1-3), 42–50. doi:10.1016/j.ejphar.2012.12.012
Pathak, N. M., Pathak, V., Gault, V. A., McClean, S., Irwin, N., and Flatt, P. R. (2018). Novel dual incretin agonist peptide with antidiabetic and neuroprotective potential. Biochem. Pharmacol. 155, 264–274. doi:10.1016/j.bcp.2018.07.021
Patnode, C. D., Perdue, L. A., Rossom, R. C., Rushkin, M. C., Redmond, N., Thomas, R. G., et al. (2020). Screening for cognitive impairment in older adults: updated evidence report and systematic review for the US preventive services task force. JAMA 323 (8), 764–785. doi:10.1001/jama.2019.22258
Pawlos, A., Broncel, M., Woźniak, E., and Gorzelak-Pabiś, P. (2021). Neuroprotective effect of SGLT2 inhibitors. Molecules 26 (23), 7213. doi:10.3390/molecules26237213
Philippaert, K., Kalyaanamoorthy, S., Fatehi, M., Long, W., Soni, S., Byrne, N. J., et al. (2021). Cardiac late sodium channel current is a molecular target for the sodium/glucose cotransporter 2 inhibitor empagliflozin. Circulation 143 (22), 2188–2204. doi:10.1161/CIRCULATIONAHA.121.053350
Piperi, C., Goumenos, A., Adamopoulos, C., and Papavassiliou, A. G. (2015). AGE/RAGE signalling regulation by miRNAs: associations with diabetic complications and therapeutic potential. Int. J. Biochem. Cell Biol. 60, 197–201. doi:10.1016/j.biocel.2015.01.009
Püschel, G. P., Klauder, J., and Henkel, J. (2022). Macrophages, low-grade inflammation, insulin resistance and hyperinsulinemia: a mutual ambiguous relationship in the development of metabolic diseases. J. Clin. Med. 11 (15), 4358. doi:10.3390/jcm11154358
Pyke, C., Heller, R. S., Kirk, R. K., Ørskov, C., Reedtz-Runge, S., Kaastrup, P., et al. (2014). GLP-1 receptor localization in monkey and human tissue: novel distribution revealed with extensively validated monoclonal antibody. Endocrinology 155 (4), 1280–1290. doi:10.1210/en.2013-1934
Qi, L., Ke, L., Liu, X., Liao, L., Ke, S., Liu, X., et al. (2016). Subcutaneous administration of liraglutide ameliorates learning and memory impairment by modulating tau hyperphosphorylation via the glycogen synthase kinase-3β pathway in an amyloid β protein induced alzheimer disease mouse model. Eur. J. Pharmacol. 783, 23–32. doi:10.1016/j.ejphar.2016.04.052
Qiu, C., Winblad, B., Marengoni, A., Klarin, I., Fastbom, J., and Fratiglioni, L. (2006). Heart failure and risk of dementia and Alzheimer disease: a population-based cohort study. Arch. Intern Med. 166 (9), 1003–1008. doi:10.1001/archinte.166.9.1003
Razay, G., Vreugdenhil, A., and Wilcock, G. (2007). The metabolic syndrome and Alzheimer disease. Arch. Neurol. 64 (1), 93–96. doi:10.1001/archneur.64.1.93
Reich, N., and Hölscher, C. (2022). The neuroprotective effects of glucagon-like peptide 1 in Alzheimer's and Parkinson's disease: an in-depth review. Front. Neurosci. 16, 970925. doi:10.3389/fnins.2022.970925
Richards, P., Parker, H. E., Adriaenssens, A. E., Hodgson, J. M., Cork, S. C., Trapp, S., et al. (2014). Identification and characterization of GLP-1 receptor-expressing cells using a new transgenic mouse model. Diabetes 63 (4), 1224–1233. doi:10.2337/db13-1440
Rizzo, M. R., Di Meo, I., Polito, R., Auriemma, M. C., Gambardella, A., di Mauro, G., et al. (2022). Cognitive impairment and type 2 diabetes mellitus: focus of SGLT2 inhibitors treatment. Pharmacol. Res. 176, 106062. doi:10.1016/j.phrs.2022.106062
Robichaux, W. G., and Cheng, X. (2018). Intracellular cAMP sensor EPAC: physiology, pathophysiology, and therapeutics development. Physiol. Rev. 98 (2), 919–1053. doi:10.1152/physrev.00025.2017
Rochfort, K. D., and Cummins, P. M. (2015). The blood-brain barrier endothelium: a target for pro-inflammatory cytokines. Biochem. Soc. Trans. 43 (4), 702–706. doi:10.1042/BST20140319
Salvatore, T., Galiero, R., Caturano, A., Rinaldi, L., Di Martino, A., Albanese, G., et al. (2022). An overview of the cardiorenal protective mechanisms of SGLT2 inhibitors. Int. J. Mol. Sci. 23 (7), 3651. doi:10.3390/ijms23073651
Samman, W. A., Selim, S. M., El Fayoumi, H. M., El-Sayed, N. M., Mehanna, E. T., and Hazem, R. M. (2023). Dapagliflozin ameliorates cognitive impairment in aluminum-chloride-induced alzheimer's disease via modulation of AMPK/mTOR, oxidative stress and glucose metabolism. Pharm. (Basel) 16 (5), 753. doi:10.3390/ph16050753
Samson, R., Jaiswal, A., Ennezat, P. V., Cassidy, M., and Le Jemtel, T. H. (2016). Clinical phenotypes in heart failure with preserved ejection fraction. J. Am. Heart Assoc. 5 (1), e002477. doi:10.1161/JAHA.115.002477
Sa-Nguanmoo, P., Tanajak, P., Kerdphoo, S., Jaiwongkam, T., Pratchayasakul, W., Chattipakorn, N., et al. (2017). SGLT2-inhibitor and DPP-4 inhibitor improve brain function via attenuating mitochondrial dysfunction, insulin resistance, inflammation, and apoptosis in HFD-induced obese rats. Toxicol. Appl. Pharmacol. 333, 43–50. doi:10.1016/j.taap.2017.08.005
Savji, N., Meijers, W. C., Bartz, T. M., Bhambhani, V., Cushman, M., Nayor, M., et al. (2018). The association of obesity and cardiometabolic traits with incident HFpEF and HFrEF. JACC Heart Fail 6 (8), 701–709. doi:10.1016/j.jchf.2018.05.018
Scisciola, L., Rizzo, M. R., Cataldo, V., Fontanella, R. A., Balestrieri, M. L., D'Onofrio, N., et al. (2020). Incretin drugs effect on epigenetic machinery: new potential therapeutic implications in preventing vascular diabetic complications. FASEB J. 34 (12), 16489–16503. doi:10.1096/fj.202000860RR
Seino, Y., Fukushima, M., and Yabe, D. (2010). GIP and GLP-1, the two incretin hormones: similarities and differences. J. Diabetes Investig. 1 (1-2), 8–23. doi:10.1111/j.2040-1124.2010.00022.x
Shaikh, S., Rizvi, S. M., Shakil, S., Riyaz, S., Biswas, D., and Jahan, R. (2016). Forxiga (dapagliflozin): plausible role in the treatment of diabetes-associated neurological disorders. Biotechnol. Appl. Biochem. 63 (1), 145–150. doi:10.1002/bab.1319
Spinelli, M., Fusco, S., and Grassi, C. (2019). Brain insulin resistance and hippocampal plasticity: mechanisms and biomarkers of cognitive decline. Front. Neurosci. 31, 788. doi:10.3389/fnins.2019.00788
Stanciu, G. D., Rusu, R. N., Bild, V., Filipiuc, L. E., Tamba, B. I., and Ababei, D. C. (2021). Systemic actions of SGLT2 inhibition on chronic mTOR activation as a shared pathogenic mechanism between alzheimer's disease and diabetes. Biomedicines 9 (5), 576. doi:10.3390/biomedicines9050576
Steven, S., Hausding, M., Kröller-Schön, S., Mader, M., Mikhed, Y., Stamm, P., et al. (2015). Gliptin and GLP-1 analog treatment improves survival and vascular inflammation/dysfunction in animals with lipopolysaccharide-induced endotoxemia. Basic Res. Cardiol. 110 (2), 6. doi:10.1007/s00395-015-0465-x
Steven, S., Jurk, K., Kopp, M., Kröller-Schön, S., Mikhed, Y., Schwierczek, K., et al. (2017). Glucagon-like peptide-1 receptor signalling reduces microvascular thrombosis, nitro-oxidative stress and platelet activation in endotoxaemic mice. Br. J. Pharmacol. 174 (12), 1620–1632. doi:10.1111/bph.13549
Tai, J., Liu, W., Li, Y., Li, L., and Holscher, C. (2018). Neuroprotective effects of a triple GLP-1/GIP/glucagon receptor agonist in the APP/PS1 transgenic mouse model of Alzheimer’s disease. Brain Res. 1678, 64–74. doi:10.1016/j.brainres.2017.10.012
Tomic, D., Shaw, J. E., and Magliano, D. J. (2022). The burden and risks of emerging complications of diabetes mellitus. Nat. Rev. Endocrinol. 18, 525–539. doi:10.1038/s41574-022-00690-7
Trost, S. U., Belke, D. D., Bluhm, W. F., Meyer, M., Swanson, E., and Dillmann, W. H. (2002). Overexpression of the sarcoplasmic reticulum Ca(2+)-ATPase improves myocardial contractility in diabetic cardiomyopathy. Diabetes 51, 1166–1171. doi:10.2337/diabetes.51.4.1166
Trum, M., Riechel, J., Lebek, S., Pabel, S., Sossalla, S. T., Hirt, S., et al. (2020). Empagliflozin inhibits Na+/H+ exchanger activity in human atrial cardiomyocytes. Esc. Heart Fail 7, 4429–4437. doi:10.1002/ehf2.13024
Tsalamandris, S., Antonopoulos, A. S., Oikonomou, E., Papamikroulis, G. A., Vogiatzi, G., Papaioannou, S., et al. (2019). The role of inflammation in diabetes: current concepts and future perspectives. Eur. Cardiol. Rev. 14 (1), 50–59. doi:10.15420/ecr.2018.33.1
Tuunanen, H., and Knuuti, J. (2011). Metabolic remodelling in human heart failure. Cardiovasc Res. 90, 251–257. doi:10.1093/cvr/cvr052
Uddin, M. S., Rahman, M. A., Kabir, M. T., Behl, T., Mathew, B., Perveen, A., et al. (2020). Multifarious roles of mTOR signaling in cognitive aging and cerebrovascular dysfunction of Alzheimer's disease. IUBMB Life 72 (9), 1843–1855. doi:10.1002/iub.2324
Urbanek, K., Cappetta, D., Bellocchio, G., Coppola, M. A., Imbrici, P., Telesca, M., et al. (2023). Dapagliflozin protects the kidney in a non-diabetic model of cardiorenal syndrome. Pharmacol. Res. 188, 106659. doi:10.1016/j.phrs.2023.106659
Ussher, J. R., and Drucker, D. J. (2023). Glucagon-like peptide 1 receptor agonists: cardiovascular benefits and mechanisms of action. Nat. Rev. Cardiol. 20 (7), 463–474. doi:10.1038/s41569-023-00849-3
Uthman, L., Baartscheer, A., Bleijlevens, B., Schumacher, C. A., Fiolet, J. W. T., Koeman, A., et al. (2018b). Class effects of SGLT2 inhibitors in mouse cardiomyocytes and hearts: inhibition of Na+/H+ exchanger, lowering of cytosolic Na+ and vasodilation. Diabetologia 61, 722–726. doi:10.1007/s00125-017-4509-7
Uthman, L., Baartscheer, A., Schumacher, C. A., Fiolet, J. W. T., Kuschma, M. C., Hollmann, M. W., et al. (2018a). Direct cardiac actions of sodium glucose cotransporter 2 inhibitors target pathogenic mechanisms underlying heart failure in diabetic patients. Front. Physiol. 9, 1575. doi:10.3389/fphys.2018.01575
Uthman, L., Li, X., Baartscheer, A., Schumacher, C. A., Baumgart, P., Hermanides, J., et al. (2022). Empagliflozin reduces oxidative stress through inhibition of the novel inflammation/NHE/[Na+]c/ROS-pathway in human endothelial cells. Biomed. Pharmacother. 146, 112515. doi:10.1016/j.biopha.2021.112515
Van Skike, C. E., and Galvan, V. (2018). A perfect sTORm: the role of the mammalian target of rapamycin (mTOR) in cerebrovascular dysfunction of alzheimer's disease: a mini-review. Gerontology 64 (3), 205–211. doi:10.1159/000485381
Vellone, E., Chialà, O., Boyne, J., Klompstra, L., Evangelista, L. S., Back, M., et al. (2020). Cognitive impairment in patients with heart failure: an international study. Esc. Heart Fail 7 (1), 46–53. doi:10.1002/ehf2.12542
Virani, S. S., Alonso, A., Aparicio, H. J., Benjamin, E. J., Bittencourt, M. S., Callaway, C. W., et al. (2021). Heart disease and stroke statistics-2021 update: a report from the American heart association. Circulation 143 (8), e254–e743. doi:10.1161/CIR.0000000000000950
Wang, X. H., Yang, W., Holscher, C., Wang, Z. J., Cai, H. Y., Li, Q. S., et al. (2013). Val⁸-GLP-1 remodels synaptic activity and intracellular calcium homeostasis impaired by amyloid β peptide in rats. J. Neurosci. Res. 91 (4), 568–577. doi:10.1002/jnr.23181
Wegmann, S., Biernat, J., and Mandelkow, E. (2021). A current view on Tau protein phosphorylation in Alzheimer's disease. Curr. Opin. Neurobiol. 69, 131–138. doi:10.1016/j.conb.2021.03.003
Wicinski, M., Socha, M., Malinowski, B., Wodkiewicz, E., Walczak, M., Gorski, K., et al. (2019). Liraglutide and its neuroprotective properties-focus on possible biochemical mechanisms in alzheimer’s disease and cerebral ischemic events. Int. J. Mol. Sci. 20 (5), 1050. doi:10.3390/ijms20051050
Wiciński, M., Wódkiewicz, E., Górski, K., Walczak, M., and Malinowski, B. (2020). Perspective of SGLT2 inhibition in treatment of conditions connected to neuronal loss: focus on alzheimer's disease and ischemia-related brain injury. Pharm. (Basel) 13 (11), 379. doi:10.3390/ph13110379
Wilson, J. M., Nikooienejad, A., Robins, D. A., Roell, W. C., Riesmeyer, J. S., Haupt, A., et al. (2020). The dual glucose-dependent insulinotropic peptide and glucagon-like peptide-1 receptor agonist, tirzepatide, improves lipoprotein biomarkers associated with insulin resistance and cardiovascular risk in patients with type 2 diabetes. Diabetes Obes. Metab. 22 (12), 2451–2459. doi:10.1111/dom.14174
Xu, L., Nagata, N., Chen, G., Nagashimada, M., Zhuge, F., Ni, Y., et al. (2019). Empagliflozin reverses obesity and insulin resistance through fat browning and alternative macrophage activation in mice fed a high-fat diet. BMJ Open Diabetes Res. Care 7 (1), e000783. doi:10.1136/bmjdrc-2019-000783
Yang, X., Qiang, Q., Li, N., Feng, P., Wei, W., and Hölscher, C. (2022). Neuroprotective mechanisms of glucagon-like peptide-1-based therapies in ischemic stroke: an update based on preclinical research. Front. Neurol. 13, 844697. doi:10.3389/fneur.2022.844697
Ye, Y., Bajaj, M., Yang, H. C., Perez-Polo, J. R., and Birnbaum, Y. (2017). SGLT-2 inhibition with dapagliflozin reduces the activation of the Nlrp3/ASC inflammasome and attenuates the development of diabetic cardiomyopathy in mice with type 2 diabetes. Further augmentation of the effects with saxagliptin, a DPP4 inhibitor. Cardiovasc Drugs Ther. 31 (2), 119–132. doi:10.1007/s10557-017-6725-2
Yu, H., Rimbert, A., Palmer, A. E., Toyohara, T., Xia, Y., Xia, F., et al. (2019). GPR146 deficiency protects against hypercholesterolemia and atherosclerosis. Cell 179 (6), 1276–1288.e14. doi:10.1016/j.cell.2019.10.034
Zhang, L. Q., Zhang, W., Li, T., Yang, T., Yuan, X., Zhou, Y., et al. (2021a). GLP-1R activation ameliorated novel-object recognition memory dysfunction via regulating hippocampal AMPK/NF-κB pathway in neuropathic pain mice. Neurobiol. Learn Mem. 182, 107463. doi:10.1016/j.nlm.2021.107463
Zhang, L. Y., Zhang, L. P., Li, Y. W., Li, L., Melchiorsen, J. U., Rosenkilde, M., et al. (2020). The novel dual GLP-1/GIP receptor agonist DA-CH5 is superior to single GLP-1 receptor agonists in the MPTP model of Parkinson?s disease. J. Park. Dis. 10 (2), 523–542. doi:10.3233/JPD-191768
Zhang, Z., Li, X. G., Wang, Z. H., Song, M., Yu, S. P., Kang, S. S., et al. (2021b). δ-Secretase-cleaved Tau stimulates Aβ production via upregulating STAT1-BACE1 signaling in Alzheimer's disease. Mol. Psychiatry 26 (2), 586–603. doi:10.1038/s41380-018-0286-z
Zheng, J., Xie, Y., Ren, L., Qi, L., Wu, L., Pan, X., et al. (2021). GLP-1 improves the supportive ability of astrocytes to neurons by promoting aerobic glycolysis in alzheimer’s disease. Mol. Metab. 47, 101180. doi:10.1016/j.molmet.2021.101180
Zhou, M., Chen, S., Peng, P., Gu, Z., Yu, J., Zhao, G., et al. (2019). Dulaglutide ameliorates STZ induced AD-like impairment of learning and memory ability by modulating hyperphosphorylation of tau and NFs through GSK3β. Biochem. Biophys. Res. Commun. 511 (1), 154–160. doi:10.1016/j.bbrc.2019.01.103
Keywords: heart failure, cognitive impairment, type 2 diabetes, glucagon-like peptide-1 receptor agonists, sodium-glucose cotransporter 2 inhibitors
Citation: Riemma MA, Mele E, Donniacuo M, Telesca M, Bellocchio G, Castaldo G, Rossi F, De Angelis A, Cappetta D, Urbanek K and Berrino L (2024) Glucagon-like peptide-1 receptor agonists and sodium-glucose cotransporter 2 inhibitors, anti-diabetic drugs in heart failure and cognitive impairment: potential mechanisms of the protective effects. Front. Pharmacol. 15:1422740. doi: 10.3389/fphar.2024.1422740
Received: 24 April 2024; Accepted: 21 May 2024;
Published: 14 June 2024.
Edited by:
Anca Hermenean, Vasile Goldis Western University of Arad, RomaniaReviewed by:
Danila Gurgone, University of Glasgow, United KingdomEra Gorica, University of Zurich, Switzerland
Copyright © 2024 Riemma, Mele, Donniacuo, Telesca, Bellocchio, Castaldo, Rossi, De Angelis, Cappetta, Urbanek and Berrino. This is an open-access article distributed under the terms of the Creative Commons Attribution License (CC BY). The use, distribution or reproduction in other forums is permitted, provided the original author(s) and the copyright owner(s) are credited and that the original publication in this journal is cited, in accordance with accepted academic practice. No use, distribution or reproduction is permitted which does not comply with these terms.
*Correspondence: Donato Cappetta, ZG9uYXRvLmNhcHBldHRhQHVuaXNhbGVudG8uaXQ=
†These authors have contributed equally to this work