- 1College of Animal Science and Technology, Yangzhou University, Yangzhou, China
- 2Department of Veterinary Biomedical Sciences, Botswana University of Agriculture and Agriculture and Natural Resources, Gaborone, Botswana
- 3Department of Animal Production, Jordan University of Science and Technology, Irbid, Jordan
- 4Jiangsu Key Laboratory of Animal Genetic Breeding and Molecular Design, College of Animal Science and Technology, Yangzhou University, Yangzhou, China
- 5International Livestock Research Institute, Addis Ababa, Ethiopia
- 6Department of Animal Resource and Science, Dankook University, Cheonan, Republic of Korea
Introduction: The gastrointestinal tract is integral to defending against external contaminants, featuring a complex array of immunological, physical, chemical, and microbial barriers. Mycotoxins, which are toxic metabolites from fungi, are pervasive in both animal feed and human food, presenting substantial health risks.
Methods: This review examines the pharmacological, toxicological, and microbiological impacts of natural products on mycotoxicosis, with a particular focus on the gut-x axis. The analysis synthesizes current understanding and explores the role of natural products rich in polysaccharides, polyphenols, flavonoids, and saponins.
Results: The review highlights that mycotoxins can disrupt intestinal integrity, alter inflammatory responses, damage the mucus layer, and disturb the bacterial balance. The toxins' effects are extensive, potentially harming the immune system, liver, kidneys, and skin, and are associated with serious conditions such as cancer, hormonal changes, genetic mutations, bleeding, birth defects, and neurological issues. Natural products have shown potential anticancer, anti-tumor, antioxidant, immunomodulatory, and antitoxic properties.
Discussion: The review underscores the emerging therapeutic strategy of targeting gut microbial modulation. It identifies knowledge gaps and suggests future research directions to deepen our understanding of natural products' role in gut-x axis health and to mitigate the global health impact of mycotoxin-induced diseases.
1 Introduction
Mycotoxins, which are low molecular weight (MW around 700 Da) secondary metabolites primarily produced by Aspergillus, Penicillium, and Fusarium, are highly toxic to humans and animals (Liew and Mohd-Redzwan, 2018). Their impact on health is influenced by the type of mycotoxin, exposure levels and duration, physiological and nutritional status, and potential interactions with other substances (Gajęcka et al., 2013). Genetic variability means some individuals are more susceptible to mycotoxicosis due to gene mutations in the cytochrome P450 (CYP450) gene, affecting mycotoxin metabolism (Sun et al., 2016). The FAO estimates that mycotoxins contaminate about 25% of global agricultural production, leading to an annual loss of 50 million tons of food (Iheshiulor et al., 2011). However, the actual prevalence may be as high as 60%–80% (Eskola et al., 2020). Mycotoxins can cause a range of severe health effects, including immunotoxicity, organ damage, cancer promotion, and neurological harm (Romina and Romina Alina, 2022).
Mycotoxins can cause a range of severe health effects, including immunotoxicity, organ damage, cancer promotion, and neurological harm (Rajakovich and Balskus, 2019). Disruptions in gut microbiota, or dysbiosis, have been linked to various diseases (Fung et al., 2017). Many human and animal studies have shown that dysbiosis of gut microbiota is linked with diseases as asthma, autism, colon cancer, Crohn’s disease, cardiovascular disease, diabetes, food allergies, eczema, irritable bowel syndrome, hepatic encephalopathy, obesity, and mental disorders (Korem et al., 2015). External factors like toxins, diet, and medication can alter gut microbiota composition (Izco et al., 2021). Some bacteria can metabolize or bind to mycotoxins, protecting against their harmful effects, but mycotoxins can also have adverse effects on the gut microbiota, such as affecting the composition at various levels of the community, threatening the colonization of beneficial bacteria, and promoting the growth of harmful bacteria (Izco et al., 2021). However, not much research has been conducted on how mycotoxins affect the gut microbiome (Robert et al., 2017; Liew and Mohd-Redzwan, 2018). The gut microbiota axis (GMA) is a vital communication network between the gut and other organs (Sampson and Mazmanian Sarkis, 2015). Studies on the microbiome have shed light on the interactions between the gut and other systems like the liver, brain, and lungs (Shi et al., 2023).
Chinese herbal medicine (CHM), known for its antioxidant, immunomodulatory, anti-tumor, and antifungal properties, is widely used to prevent and treat metabolic diseases (Ding et al., 2023). Common natural antioxidants mostly contain flavonoids, polyphenols, polysaccharides, and saponins as their active metabolites, which work as antioxidants by inhibiting the synthesis of lipid reactive oxygen species (ROS) and simultaneously turning H2O2 into H2O (Huang et al., 2017; Wang et al., 2022). These extracts contain natural antioxidants that are safer and more environmentally friendly than synthetic ones (Ates and Ortatatli, 2021). Antioxidant botanical drugs such as curcumin, lycopene, fucoidan, and artemisinin have been used widely in recent years to prevent mycotoxin exposure and disorders related to ferroptosis (Li et al., 2021; Wu et al., 2022). Some CHM preparations are widely used to treat cancer due to their properties of invigorating vital energy and anti-cancer characteristics, primarily by resisting inflammatory responses and enhancing immune function (Wang et al., 2020). The aim of this study was to discuss the pharmacological, toxicological and microbiological effects of natural products on gut-x axis in mycotoxin-caused diseases to provide novel insight into future therapies.
2 Gut health, microbiota and mycotoxins
In general, the GI tract’s intestinal barrier serves as a filter for eliminating harmful mycotoxins. Nevertheless, it has been found that a number of mycotoxins cause harm to the gastrointestinal tract. For example, mycotoxins may cause disruption with the normal functioning of the intestinal barrier and absorption of nutrients. Certain mycotoxins also affect the histomorphology of intestine (Liew and Mohd-Redzwan, 2018). However, the liver and the digestive tract are both sites of mycotoxin metabolism. Liver is responsible for detoxification that following mycotoxin exposure, as the central metabolic site (Zong et al., 2022). The adverse effects of mycotoxins within the GIT may be restricted by intestinal metabolism, which can take place in the gut epithelium or by gut microbes. This is particularly true for ruminants, who may convert a variety of mycotoxins into metabolites that are non-toxic. It has been suggested that the microbial population in the rumen has a detoxifying role in ruminant resistance to some mycotoxins. Many compounds, like ZEA, DON, or OTA, are efficiently made non-toxic by the microorganisms of the rumen prior to absorption (Cavret and Lecoeur, 2006).
2.1 Aflatoxin (AFB)
The animal mostly gets into contact with AFB through ingestion, and the gastrointestinal tract’s microbiota is first exposed (Huang et al., 2023). The small intestine is the main site of AFB absorption, with the duodenum being the intestinal location with the highest absorption efficiency (Sayin et al., 2013). AFB1 exposure resulted in intestinal diseases such as villi degeneration and the formation of sub-epithelial space in the duodenum and ileum. Exposure to AFB1 can have negative effects on the gut, such as immune system problems, intestinal barrier degradation, cell proliferation, and apoptosis. Compared to the other mycotoxins, AFB1 is the most life-threatening mycotoxin affected the gut (Liew and Mohd-Redzwan, 2018). The impact of AFB1 on the gut microbiota was evaluated by extracting the contents of the broiler chickens’ duodenum, following AFB1 exposure and using 16S rRNA sequencing to analyze the changes in abundance and diversity of the gut microbiota (Huang et al., 2023). AFB1 at a dosage level of 1 ppm significantly (p < 0.05) decreased total LAB in the broiler, according to a recent study. On the other hand, the broiler group offered 1.5 and 2 ppm of AFB1 showed a significant (p < 0.05) increase in the total number of Gram-negative bacteria and LAB. Additionally, it has been observed that broilers exposed to 2.5 ppm of AFB1 produced more total volatile fatty acids, indicating an association between higher AFB1 dose and a higher incidence of LAB in the gut (Galarza-Seeber et al., 2016).
2.2 Fusarium graminearum (T-2, DON)
Fusarium graminearum is the main fungi species that produces tricothecenes and Deoxynivalenol (DON). DON’s effect is often dependent on dose- and/or exposure time, and can cause severe damage to the gastrointestinal tract, liver, and other metabolic and immunity-related organs (Pestka, 2010). Furthermore, the histological analysis provided features such as nuclear vacuolation, neutrophil infiltration, and dilated and congested blood vessels, confirming liver damage (Wang et al., 2023). Oxidative stress has been a serious issue involved in DON-induced hepatotoxicity that leads to overproduce of ROS, and extreme ROS could trigger lipid peroxidation (LPO) and damage cellular macromolecule function (Wang et al., 2023). Using porcine epithelial cell, trichothecenes improved intestinal permeability by the downregulation of tight junction protein expression (Osselaere et al., 2013). Furthermore, in animal treated with trichothecenes, previous studies showed a significant (p < 0.05) decrease in the number of goblet cells secreting mucin. Mucin is mainly involved in the gut barrier function. Furthermore, trichothecenes have been linked to a reduced intestinal level of IL-8, which is in charge of eliminating pathogens (Kadota et al., 2013). Generally, trichothecenes exert negative impacts on GI tracts specifically on the gut absorption, immunity, and integrity (Liew and Mohd-Redzwan, 2018). It was demonstrated that giving T-2 toxin to pigs for 1 week was enough to substantially increase the number of aerobic bacteria in their intestines (Verbrugghe et al., 2012). Trichothecene has been demonstrated to have an important effect on bacterial populations; however, the exact mechanism causing this alteration is still not completely understood.
2.3 Zearalenone (ZEA)
Zearalenone (ZEA) was shown to accelerate cell migration, increase colony formation, and enhance cell proliferation in a colon cancer cell line HC-116 (Abassi et al., 2016). Additionally, ZEA has been demonstrated to downregulate the expression of tumor-suppressor genes particularly (PCDH11X, DKK1, and TC5313860) in intestinal cells (Taranu et al., 2015). In fact, the carcinogenic effects of ZEA were caused by the modification of gene expression. An in vitro study revealed that the human gut bacteria transformed zearalenone molecules into unknown metabolites (Gratz et al., 2017). The alterations in the gut microbiota were assessed using the Biolog-Eco Plate method, which allows only the quantification of culturable bacteria. After 6 weeks of ZEA application, the findings demonstrated a significant (p < 0.05) decrease in the concentrations of E. coli, Enterobacteriaceae, and Clostridium perfringens. (Piotrowska et al., 2014).
2.4 Fumonisins (FB)
By using intestinal cell lines (IPEC-1, Caco-2, and HT29), it was discovered that FB1 reduced cell viability and proliferation in a concentration-dependent manner (Minervini et al., 2014). A potential mechanism has been proposed through the accumulation of sphinganine by FB1. Sphinganine accumulation in intestinal epithelial cells inhibited the G0/G1 phase of the cell, resulting in to growth inhibition and apoptosis (Angius et al., 2015). Furthermore, FB1 altered the integrity of the intestinal barrier by decreasing the expression of the tight junction (TJ) protein (Romero et al., 2016). Besides, elevated FB1 levels also caused growth in the intestinal goblet cells of pigs and broilers. Mucin secretion is known to be elevated in goblet-cell hyperplasia. On the other hand, continuous mucin hypersecretion may reduce the quantity of goblet cells, which would destroy the mucus barrier (Johansson and Hansson, 2016). Upon LPS exposure to the FB1-treated cell line, a decrease in IL-8 synthesis has been detected (Brazil et al., 2013). FB1 often resulted in immune dysfunction, reduced intestinal barrier function, and increased intestinal cell death in the gut. By using capillary electrophoresis single-stranded conformation polymorphism (CE-SSCP), it is demonstrated that fumonisins reduced the fecal microbiota SSCP-profiles similarity of the fumonisins treated swine, in comparison to the untreated control group. The findings indicated that the variety of the microbiota is increasing (Burel et al., 2013).
2.5 Ochratoxin (OTA)
Studies conducted in vitro demonstrated that OTA reduced the SGLT1 transporter’s ability to absorb glucose (Peraica et al., 2011). In addition, compared to control animals, OTA-treated animals developed more quickly and harmful parasite infections in chicks and turkeys (which is caused by Eimeria acervulina and E. adenoeides). The finding revealed that animals fed with OTA had developed lesion and oocyst indexes in the intestine and more damage at mucosa (Manafi et al., 2011). It has been found that the oxidative stress caused by OTA is related to the intestine IPEC-J2 cells’ apoptosis (Wang H. et al., 2017). Inflammation pathway in the intestine was also affected by OTA. Piglets exposed to the toxin revealed a significant (p < 0.05) decrease in the expression of inflammation-related cytokines, including IL-8, IL-6, IL-17A, IL-12, and IL-18, in their intestines (Marin et al., 2017). OTA affected the gut through decreasing the absorption of nutrients, disrupting the permeability of the intestinal lining, causing cell apoptosis, and modifying the immune system (Liew and Mohd-Redzwan, 2018). An in vivo study on rats showed that the gut microbiota’s diversity was reduced by OTA treatment. Moreover, at the genus level the OTA increases the quantity of Lactobacillus and decreased the populations of Bacteroides, Dorea, Escherichia, Oribacterium, Ruminococcus, and Syntrophococcus. The findings showed that lactobacillus was more resistant to OTA and that it might be involved in the process of OTA detoxification. Additionally, it was reported that the OTA treatment has positive effects on facultative anaerobes (Guo et al., 2014). This may indicate that the OTA could alter the gut microbiota in a way that is harmful to the host’s health.
3 Gut-liver axis and mycotoxin
There exists a delicate connection between gut and liver for their functional and anatomical familiarity (Luo and Zhou, 2021). Gut dysbiosis has been related with the pathogenesis of a wide spectrum of liver diseases including autoimmune hepatitis, autoimmune cholangiopathies, alcoholic liver disease, non-alcoholic liver disease (NAFLD), hepatocellular carcinoma, acute liver injury, and liver fibrosis/cirrhosis (Hartmann et al., 2019). Aflatoxin B1, DON, fumonisin B1, ochratoxin A, and T2 are among the mycotoxins that have been shown in multiple studies to increase the permeability of the intestinal epithelium in many animals (Alassane-Kpembi et al., 2019). This is mainly the consequence of protein synthesis being inhibited. The passage of antigens into the bloodstream increases as a result. This makes the animal more susceptible to infectious intestinal diseases. Moreover, mycotoxins are absorbed more quickly due to the damage they cause to the intestinal barrier. Aflatoxin toxicity is mostly aimed towards the liver through entero-hepatic circulation after absorption. For example, AFB1 can induce inflammatory damage, apoptosis, oxidative stress, and autophagy to cause liver function damage (Zhang et al., 2021; Lin et al., 2022) (Figure 1).
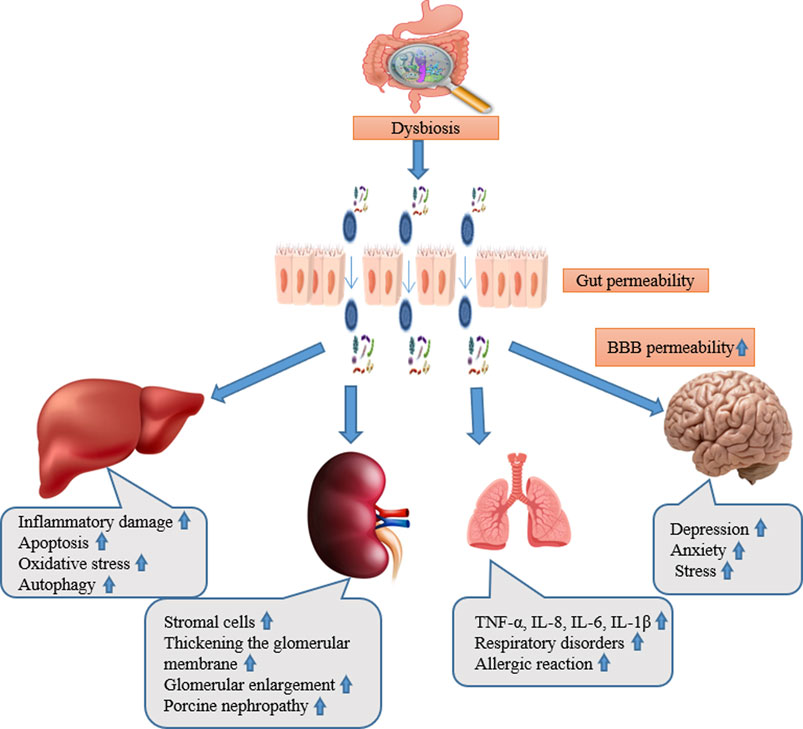
Figure 1. The abstract of gut liver, kidney, lung, and brain axis and their main pathological alteration during mycotoxicosis.
4 Gut-lung axis and mycotoxin
Both the gastrointestinal and respiratory systems are physically close to one another, and they also have similar embryonic origins and structures, which raises the possibility that their interactions are multifaceted. (Haldar et al., 2023). Aspiration may allow the microbiota from the gut to enter the lungs, exposing the respiratory tract’s epithelial surface to a variety of microorganisms (Hartman et al., 2010). The lung is a functional organ for gas exchange, the interaction between the gut microbiota and nutrition is associated with respiratory disorders, such as asthma, chronic obstructive pulmonary disease (COPD), and allergies (Segal et al., 2016). The lungs’ inflammatory response is impeded by short chain fatty acids generated from gut bacteria (Barcik et al., 2020). In addition, gut bacteria have the ability to produce anti-inflammatory active metabolites such biogenic amines, that are represented by histamine (Pugin et al., 2017) and oxylipins (Levan et al., 2019). Research indicates that various microbes, viruses, changes in the microbiome, stress, exposure to the environment, and dietary modifications can all have an effect on the lungs (Mims, 2015), and they are risk factors for such diseases. The majority of experimental studies on lung disorders has only discussed how the gut microbiota contributes to the development of lung disorders. Longitudinal studies are required to correlate the severity of chronic lung diseases with changes in the gut microbiome (Shi et al., 2023). DON has been demonstrated to have a distinct cytotoxic effect on human primary cells. Collagens I, III, and IV, fibronectin secretion, apoptotic and necrotic cell death, and cell viability were all evaluated. A decrease in viability can be observed in 2 cell types, with fibroblasts reacting more sensitive than epithelial cells. Additionally, fibroblasts treated with DON mostly experienced apoptotic cell death (Königs et al., 2007) (Figure 1).
5 Gut-brain axis and mycotoxin
Maintaining the host’s healthy mental state, particularly brain function, depends on the mutualistic relationship that exists between the gut microbiota and the brain (Mohajeri et al., 2018; Ganci et al., 2019). In fact, the microbiota-gut-brain axis permits bilateral interaction between the gut microbiota and the central nervous system (CNS) (Cryan et al., 2019). This communication is based on multiple complex pathways, most of which transfer sensory information from the gastrointestinal (GI) tract and then convert it into signals related to hormones, brain activity, and immunity (Pferschy-Wenzig et al., 2022). These signals supplementary transfer information to the central nervous system (CNS) whichever individually or cooperatively (Lach et al., 2018). Three main pathways are thought to be involved in the interactions between the gut microbiota and the brain: 1) direct and indirect signaling through chemical transmitters, such as hormones, neurotransmitters, or microbial metabolites (e.g., short-chain fatty acids, or SCFAs), that can be directly synthesized by the gut microbiota or have their levels modulated by gut microbiota; 2) neural pathways, such as modulation of vagus nerve activity; and 3) immune system signaling, such as microglia-mediated effects or effects of circulating cytokines that can modify the activity of the hypothalamic–pituitary–adrenal (HPA) axis (Cenit et al., 2017; Farzi et al., 2018; Cryan et al., 2019).
Once the mycotoxin passes through the intestinal wall and reaches the plasma, some of them (FUM, DON) may alter the brain functions. Data demonstrates that the neurologic effects of DON are partially dependent on DON’s direct action on brain cells, even though some of these modifications may be related to peripheral effects (Maresca, 2013). This needs the passage of the blood-brain barrier (BBB) by the toxin. Endothelial and glial cells adhere closely to create the blood-brain barrier (BBB) that acts as a selective barrier to restrict the passage of chemicals from the plasma into the cerebrospinal fluid (CSF) (Nico and Ribatti, 2012). The capability of DON to cross the BBB depends on the animal species. In pigs, 25%–30% of the plasmatic DON is found in the CSF, the toxin remaining detectable in the fluid 20 h after ingestion and having a half-life in the CSF similar to its plasmatic one (Maresca, 2013). Nothing is known regarding the mechanism(s) permitting DON to be transported (absorbed and excreted) across the BBB. In conclusion, mycotoxins can cause changes in the gut microbiota, intestinal permeability, neuroinflammation, oxidative stress, and consequent neurological and behavioral symptoms. All of these may disrupt the delicate balance of the gut-brain axis. To improve animals’ general health and wellbeing,such as improving animal immunity to viruses and developing ways to dispose of toxin residues from within. It is still necessary to investigate how these toxins impact the gut-brain axis and how to reduce their effects (Figure 1).
6 Gut-kidney axis and mycotoxin
The kidney is considered the metabolic organ of the body, with its main function of removing metabolic waste products and maintaining the equilibrium of the body (Sutherland, 2020). The gut-kidney axis (GKA) is linked with a diversity of kidney diseases, such as kidney stones (Ticinesi et al., 2019), chronic kidney disease (Ramezani et al., 2016), and diabetes (Sircana et al., 2019). The intestinal microflora will change when the alterations in renal function result in excessive ammonia in blood and increased intestinal pH (Shi et al., 2023). These alterations motivate the intestine to reduce mucus manufacture (Vaziri et al., 2012), destructive the intestinal epithelium (Hobby et al., 2019), disturbing the integrity of the intestinal barrier (Plata et al., 2019), changing intestinal permeability (Meijers et al., 2018), and permitting the microflora to be transported to the systemic circulation through the blood to act on the kidneys (Shi et al., 2014). The microbiota in the gut breaks down macromolecules to produce neurotransmitters that have an impact on the kidney (Santisteban et al., 2017). In addition, the gut microbiota can produce neurotransmitters such as acetylcholine, norepinephrine, and gamma-aminobutyric acid (Lyte, 2014), which connect with the metabolically dependent immune pathways through the sympathetic nervous system (SNS) to control the function of the kidney (Al Khodor and Shatat, 2017). The effects of dietary mycotoxin on broiler kidneys include increased stromal cells and thickened of the glomerular basement membrane, glomerular enlargement, cytoplasmic vacuolation of tubular epithelial cells, collapse of the renal glomerulus, and structural damage (Śliżewska et al., 2019; Saleemi et al., 2020), poultry ochratoxicosis, porcine nephropathy, human endemic nephropathies, and urinary tract tumors (Heussner and Bingle, 2015) (Figure 1).
7 Effect of natural products on gut-x axis (pharmacology, toxicology and microbiology) during mycotoxicosis
The pharmacological effects of natural metabolites have drawn an enormous amount more attention in recent years (Pan et al., 2019). Clinical data and in vitro studies have shown the beneficial effects of natural products on a wide range of diseases (Ennab et al., 2019; Mustafa et al., 2019; Ullah et al., 2020; Ennab et al., 2023). These changes in the gut microbiota caused by natural substances demonstrate that natural products have a preventive effect on the development of inflammation into cancer. Natural products have the ability to control the Firmicutes/Bacteroidetes ratio, and they also increase the number of potentially helpful microorganisms in feces and decrease the relative abundance of potentially pathogenic microbes (Lu et al., 2022). For instance, in HFD-induced obese mice, the ratio of Firmicutes/Bacteroidetes (F/B) reduced to normal levels after receiving a high dose of 300 mg/kg of MDG-1, which was extracted from the root of Ophiopogon japonicas, for a duration of 12 weeks (Shi et al., 2015), declined the number of pathogenic bacteria (Streptococcus and Escherichia coli) (Wang et al., 2011). Feeding mice 0.5% curcumin for 14 weeks can significantly prevent age-related decreases in alpha diversity, increase bacterial richness, increase the relative abundance of Lactobacillales, and decrease the relative plenty of members of the Coriobacterales order (McFadden et al., 2015), that can utilize strong anti-inflammatory, antioxidative and antiproliferative effects (McFadden et al., 2015). Microbes are vital contributors to the host metabolism, considering that numerous important components, such as vitamin K, indoles, gamma amino butyric acid, folate, and short-chained fatty acids (SCFAs), are produced by microbiota (Brown and Hazen, 2018). These metabolites are generally implicated in many physiological and pathological processes, which may be related to a number of diseases. Natural products may control the microbiome’s metabolism by changing the bacterial structure, which is important in preserving intestinal homeostasis (Ma et al., 2018). Some microbial population (specially the gut bacteria) have a significant detoxification role in the gut-liver cycle during mycotoxin injury (Zhang et al., 2022); therefore, natural products may enhance the capability of the microbial induced mycotoxin resistance.
Mycotoxins can overwhelm the immune system, leading to increased susceptibility to infections. Several natural products have demonstrated immunomodulatory properties, such as echinacea (Echinacea purpurea), ginger (Zingiber officinale), and garlic (Allium sativum), that may increase resistance to mycotoxicosis and enhance the immune response. Natural products modulate the immunological status by modifying the concentration of immune components like IL-22, activating T reg cells, or preventing the development of Th17 cells (Pan et al., 2019). Some natural products can help the body eliminate mycotoxins more effectively since they possess detoxifying properties. For example, it's been discovered that activated charcoal binds to mycotoxins in the gastrointestinal tract, blocking their absorption and promoting their elimination. In addition of the forementioned activities of the natural product, there are many other activities has been demonstrated in previous studies, to reduce mycotoxin toxicity in vivo and vitro (Table 1). It is worth noting that, as shown in the table, due to differences in toxin types, species, and methods of administration, there may be significant variation in the effective dosage of natural products. Additionally, the manner in which natural products are administered (gavage, oral intake, injection) to achieve the optimal effect must also be taken into consideration. A considerable amount of experimentation is still required to determine the appropriate intake methods and dosages for practical application in production.
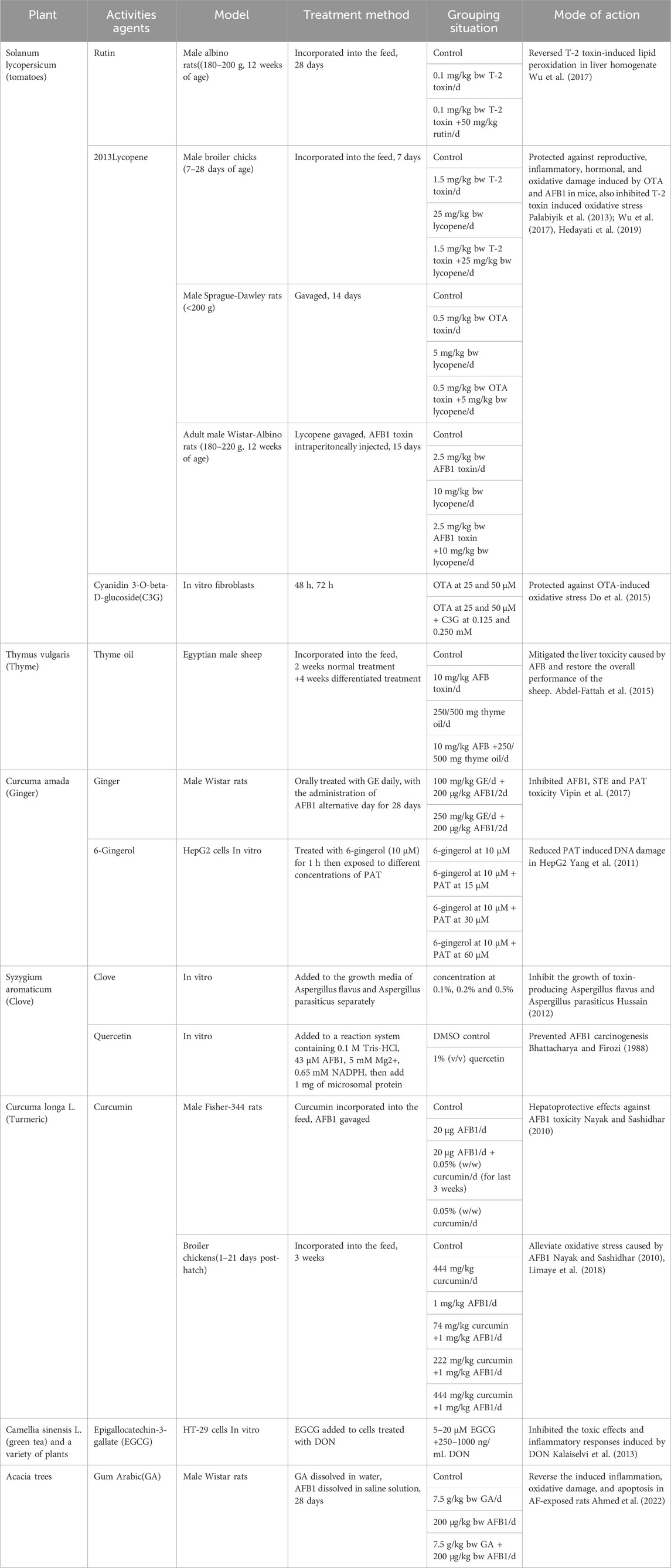
Table 1. Analysis on the impact of plant extracts and their metabolites on the toxicity caused by mycotoxin.
The toxicity of medicinal plants and natural products is strictly associated to the presence of bioactive metabolites in the plant substantial and their toxic potential (Woo et al., 2012). The issue becomes considerably more intricate when considering complex, heterogeneous botanical drugs mix which can cause unpredictable effects (Efferth et al., 2012). Many natural products have hepatotoxic, carcinogenic, cytotoxic, genotoxic, phototoxic, possible pulmonary toxicity, embryotoxic, and neurotoxic effects (Wang et al., 2021). Utilizing natural products during mycotoxicosis may have a taxological effect; however, the precise effect will differ based on the natural product used, the dosage, the individual’s susceptibility, and any interactions with other medications or substances.
8 Natural products, gut tight junction and regulating of intestinal microbes
Tight junction often serves as a barrier to protect intestinal epithelial cells from bacterial endotoxins (Lu et al., 2022). A high-fat diet (HFD) can increase the gut microbiota-induced production of lipophilic acid synthase (LPS), and this in turn may disrupt the expression of tight junction proteins and ultimately enhance intestinal permeability (Cani et al., 2008). Flos Lonicera, is one of the best-known traditional Chinese medicines, has the ability to modify tight junctions at the cellular level by reversing the negative effects of lipopolysaccharides (LPS) and increasing certain microbiota. These actions had a positive impact on preserving the integrity of the intestinal barrier (Wang et al., 2014). Berberine pretreatment has the potential to decrease intestinal permeability and enhance the LPS-induced redistribution of the tight junction-related proteins Claudin-1 and Claudin-4 (Gu et al., 2011). In a previous study, Curcumin has been demonstrated to be able to reduce the disruption of intestinal epithelial barrier functions when its effects were investigated on Caco-2 and HT-29 cells. Curcumin inhibited the release of LPS-secreted IL-1β, stimulated macrophages and IEC, and prevented the disintegration of tight junction proteins, such as ZO-1, Claudin-1, Claudin-7, and actin filaments (Wang J. et al., 2017). All of the aforementioned results demonstrated that tight junction proteins, inflammation, and probiotic abundance can all be positively impacted by natural products on the intestinal barrier (Lu et al., 2022).
9 Discussion
Natural products hold significant promise for mitigating mycotoxin-related issues and preserving gut-x axis health. They have exhibited beneficial pharmacological, toxicological, and microbiological properties that can counteract the negative impacts of mycotoxins along the entire gut-x axis. These substances can help balance gut microbiota, regulate gut permeability, boost immunity, alleviate inflammation, and the detoxification of mycotoxins in both animals and humans. Given the complexity of gut-x axis dynamics and host-mycotoxin interactions, in-depth research is essential to establish an effective intervention model including changes in gut microbiota, alterations in metabolites and key targets. This includes exploring cellular processes and molecular mechanisms that enable natural products to enhance gut health and facilitate mycotoxin removal. A thorough toxicological evaluation of these products is necessary to establish their safety profiles, especially regarding potential medication interactions and long-term or high-dose use side effects. Additionally, the economic feasibility of incorporating natural products into the diet, food products, or supplements should also be taken into consideration.
The potential of natural products in treating mycotoxin-induced diseases should be further explored, with a focus on their incorporation into dietary feeds, food products, and supplements. Effective collaboration between researchers and the food industry is crucial for the successful implementation of these natural solutions. By addressing current research gaps and adopting a multidisciplinary approach, our knowledge of how natural products can support gut-x axis health amidst mycotoxin challenges can be significantly enhanced.
Author contributions
KL: Resources, Supervision, Writing–review and editing. SW: Investigation, Supervision, Writing–review and editing. WQ: Supervision, Writing–review and editing. AA: Supervision, Writing–original draft. WE: Resources, Writing–review and editing. MO: Supervision, Writing–review and editing. H-YL: Supervision, Writing–review and editing. TD Alemayehu: Methodology, Writing–review and editing. IK: Writing–review and editing. SA: Conceptualization, Methodology, Resources, Writing–original draft, Writing–review and editing. DC: Conceptualization, Funding acquisition, Methodology, Resources, Supervision, Writing–original draft, Writing–review and editing.
Funding
The author(s) declare that financial support was received for the research, authorship, and/or publication of this article. This work was funded by the Jiangsu Agricultural Science and Technology Innovation Fund [CX (21) 2014], Natural Science Foundation of Jiangsu Province (BK20220582 and BK20210812), the Priority Academic Program Development of Jiangsu Higher Education Institutions (PAPD).
Conflict of interest
The authors declare that the research was conducted in the absence of any commercial or financial relationships that could be construed as a potential conflict of interest.
The author(s) declared that they were an editorial board member of Frontiers, at the time of submission. This had no impact on the peer review process and the final decision.
Publisher’s note
All claims expressed in this article are solely those of the authors and do not necessarily represent those of their affiliated organizations, or those of the publisher, the editors and the reviewers. Any product that may be evaluated in this article, or claim that may be made by its manufacturer, is not guaranteed or endorsed by the publisher.
Supplementary material
The Supplementary Material for this article can be found online at: https://www.frontiersin.org/articles/10.3389/fphar.2024.1419844/full#supplementary-material
References
Abassi, H., Ayed-Boussema, I., Shirley, S., Abid, S., Bacha, H., and Micheau, O. (2016). The mycotoxin zearalenone enhances cell proliferation, colony formation and promotes cell migration in the human colon carcinoma cell line HCT116. Toxicol. Lett. 254, 1–7. doi:10.1016/j.toxlet.2016.04.012
Abdel-Fattah, S., Abosrea, S. Y. H., Flourage, F. E., and Helal, M. R. (2015). The efficacy of thyme oil as antitoxicant of aflatoxin(s) toxicity in sheep. J. Am. Sci. doi:10.13140/RG.2.1.2947.8485
Ahmed, N., El-Rayes, S. M., Khalil, W. F., Abdeen, A., Abdelkader, A., Youssef, M., et al. (2022). Arabic gum could alleviate the aflatoxin B1-provoked hepatic injury in rat: the involvement of oxidative stress, inflammatory, and apoptotic pathways. Toxins 14 (9), 605. doi:10.3390/toxins14090605
Alassane-Kpembi, I., Pinton, P., and Oswald, I. P. (2019). Effects of mycotoxins on the intestine. Toxins 11 (3), 159. doi:10.3390/toxins11030159
Al Khodor, S., and Shatat, I. F. (2017). Gut microbiome and kidney disease: a bidirectional relationship. Pediatr. Nephrol. Berl. Ger. 32 (6), 921–931. doi:10.1007/s00467-016-3392-7
Angius, F., Spolitu, S., Uda, S., Deligia, S., Frau, A., Banni, S., et al. (2015). High-density lipoprotein contribute to G0-G1/S transition in Swiss NIH/3T3 fibroblasts. Sci. Rep. 5 (1), 17812. doi:10.1038/srep17812
Ates, M. B., and Ortatatli, M. (2021). The effects of Nigella sativa seeds and thymoquinone on aflatoxin phase-2 detoxification through glutathione and glutathione-S-transferase alpha-3, and the relationship between aflatoxin B1-DNA adducts in broilers. Toxicon 193, 86–92. doi:10.1016/j.toxicon.2021.01.020
Barcik, W., Boutin, R. C. T., Sokolowska, M., and Finlay, B. B. (2020). The role of lung and gut microbiota in the pathology of asthma. Immunity 52 (2), 241–255. doi:10.1016/j.immuni.2020.01.007
Bhattacharya, R. K., and Firozi, P. F. (1988). Effect of plant flavonoids on microsome catalyzed reactions of aflatoxin B1 leading to activation and DNA adduct formation. Cancer Lett. 39 (1), 85–91. doi:10.1016/0304-3835(88)90043-2
Brazil, J. C., Louis, N. A., and Parkos, C. A. (2013). The role of polymorphonuclear leukocyte trafficking in the perpetuation of inflammation during inflammatory bowel disease. Inflamm. Bowel Dis. 19 (7), 1556–1565. doi:10.1097/MIB.0b013e318281f54e
Brown, J. M., and Hazen, S. L. (2018). Microbial modulation of cardiovascular disease. Nat. Rev. Microbiol. 16 (3), 171–181. doi:10.1038/nrmicro.2017.149
Burel, C., Tanguy, M., Guerre, P., Boilletot, E., Cariolet, R., Queguiner, M., et al. (2013). Effect of low dose of fumonisins on pig health: immune status, intestinal microbiota and sensitivity to Salmonella. Toxins 5 (4), 841–864. doi:10.3390/toxins5040841
Cani, P. D., Bibiloni, R., Knauf, C., Waget, A., Neyrinck, A. M., Delzenne, N. M., et al. (2008). Changes in gut microbiota control metabolic endotoxemia-induced inflammation in high-fat diet-induced obesity and diabetes in mice. Diabetes 57 (6), 1470–1481. doi:10.2337/db07-1403
Cavret, S., and Lecoeur, S. (2006). Fusariotoxin transfer in animal. Food Chem. Toxicol. Int. J. Publ. Br. Industrial Biol. Res. Assoc. 44 (3), 444–453. doi:10.1016/j.fct.2005.08.021
Cenit, M. C., Sanz, Y., and Codoñer-Franch, P. (2017). Influence of gut microbiota on neuropsychiatric disorders. World J. gastroenterology 23 (30), 5486–5498. doi:10.3748/wjg.v23.i30.5486
Cryan, J. F., O'Riordan, K. J., Cowan, C. S. M., Sandhu, K. V., Bastiaanssen, T. F. S., Boehme, M., et al. (2019). The microbiota-gut-brain Axis. Physiol. Rev. 99 (4), 1877–2013. doi:10.1152/physrev.00018.2018
Ding, W., Lin, L., Yue, K., He, Y., Xu, B., Shaukat, A., et al. (2023). Ferroptosis as a potential therapeutic target of traditional Chinese medicine for mycotoxicosis: a review. Toxics 11 (4), 395. doi:10.3390/toxics11040395
Do, K. H., An, T. J., Oh, S.-K., and Moon, Y. (2015). Nation-based occurrence and endogenous biological reduction of mycotoxins in medicinal herbs and spices. Toxins 7 (10), 4111–4130. doi:10.3390/toxins7104111
Efferth, T., and Greten, H. J. (2012). “Chapter 9 - potential of ‘omics’ technologies for implementation in research on phytotherapeutical toxicology,” in Advances in botanical research. Editors L.-F. Shyur, and A. S. Y. Lau (Massachusetts, United States: Academic Press), 343–363.
Ennab, W., Mustafa, S., Wei, Q., Lv, Z., Kavita, N. M., Ullah, S., et al. (2019). Resveratrol protects against restraint stress effects on stomach and spleen in adult male mice. Animals 9 (10), 736. doi:10.3390/ani9100736
Ennab, W., Ye, N., Wu, H., Ullah, S., Hadi, T., Bassey, A. P., et al. (2023). The synergistic effects of the combination of L-carnitine and lycopene on the lycopene bioavailability and duodenal health of roosters. Animals 13 (8), 1274. doi:10.3390/ani13081274
Eskola, M., Kos, G., Elliott, C. T., Hajšlová, J., Mayar, S., and Krska, R. (2020). Worldwide contamination of food-crops with mycotoxins: validity of the widely cited ‘FAO estimate’ of 25. Crit. Rev. Food Sci. Nutr. 60 (16), 2773–2789. doi:10.1080/10408398.2019.1658570
Farzi, A., Fröhlich, E. E., and Holzer, P. (2018). Gut microbiota and the neuroendocrine system. Neurotherapeutics 15 (1), 5–22. doi:10.1007/s13311-017-0600-5
Fung, T. C., Olson, C. A., and Hsiao, E. Y. (2017). Interactions between the microbiota, immune and nervous systems in health and disease. Nat. Neurosci. 20 (2), 145–155. doi:10.1038/nn.4476
Gajęcka, M., Stopa, E., Tarasiuk, M., Zielonka, Ł., and Gajęcki, M. (2013). The expression of type-1 and type-2 nitric oxide synthase in selected tissues of the gastrointestinal tract during mixed mycotoxicosis. Toxins 5 (11), 2281–2292. doi:10.3390/toxins5112281
Galarza-Seeber, R., Latorre, J. D., Bielke, L. R., Kuttappan, V. A., Wolfenden, A. D., Hernandez-Velasco, X., et al. (2016). Leaky gut and mycotoxins: aflatoxin B1 does not increase gut permeability in broiler chickens. Front. veterinary Sci. 3, 10. doi:10.3389/fvets.2016.00010
Ganci, M., Suleyman, E., Butt, H., and Ball, M. (2019). The role of the brain–gut–microbiota axis in psychology: the importance of considering gut microbiota in the development, perpetuation, and treatment of psychological disorders. Brain Behav. 9, e01408. doi:10.1002/brb3.1408
Gratz, S. W., Dinesh, R., Yoshinari, T., Holtrop, G., Richardson, A. J., Duncan, G., et al. (2017). Masked trichothecene and zearalenone mycotoxins withstand digestion and absorption in the upper GI tract but are efficiently hydrolyzed by human gut microbiota in vitro. Mol. Nutr. Food Res. 61 (4), 1600680. doi:10.1002/mnfr.201600680
Gu, L., Li, N., Gong, J., Li, Q., Zhu, W., and Li, J. (2011). Berberine ameliorates intestinal epithelial tight-junction damage and down-regulates myosin light chain kinase pathways in a mouse model of endotoxinemia. J. Infect. Dis. 203 (11), 1602–1612. doi:10.1093/infdis/jir147
Guo, M., Huang, K., Chen, S., Qi, X., He, X., Cheng, W.-H., et al. (2014). Combination of metagenomics and culture-based methods to study the interaction between ochratoxin A and gut microbiota. Toxicol. Sci. 141 (1), 314–323. doi:10.1093/toxsci/kfu128
Haldar, S., Jadhav, S. R., Gulati, V., Beale, D. J., Balkrishna, A., Varshney, A., et al. (2023). Unravelling the gut-lung axis: insights into microbiome interactions and Traditional Indian Medicine's perspective on optimal health. FEMS Microbiol. Ecol. 99 (10), fiad103. doi:10.1093/femsec/fiad103
Hartman, T. J., Albert, P. S., Zhang, Z., Bagshaw, D., Kris-Etherton, P. M., Ulbrecht, J., et al. (2010). Consumption of a legume-enriched, low-glycemic index diet is associated with biomarkers of insulin resistance and inflammation among men at risk for colorectal cancer. J. Nutr. 140 (1), 60–67. doi:10.3945/jn.109.114249
Hartmann, P., Chu, H., Duan, Y., and Schnabl, B. (2019). Gut microbiota in liver disease: too much is harmful, nothing at all is not helpful either. Am. J. physiology Gastrointest. liver physiology 316 (5), G563–G73. doi:10.1152/ajpgi.00370.2018
Hedayati, N., Naeini, M. B., Nezami, A., Hosseinzadeh, H., Wallace Hayes, A., Hosseini, S., et al. (2019). Protective effect of lycopene against chemical and natural toxins: a review. BioFactors 45 (1), 5–23. doi:10.1002/biof.1458
Heussner, A. H., and Bingle, L. E. H. (2015). Comparative ochratoxin toxicity: a review of the available data. Toxins 7 (10), 4253–4282. doi:10.3390/toxins7104253
Hobby, G. P., Karaduta, O., Dusio, G. F., Singh, M., Zybailov, B. L., and Arthur, J. M. (2019). Chronic kidney disease and the gut microbiome. Am. J. Physiology-Renal Physiology 316 (6), F1211–F7. doi:10.1152/ajprenal.00298.2018
Huang, G., Mei, X., and Hu, J. (2017). The antioxidant activities of natural polysaccharides. Curr. drug targets 18 (11), 1296–1300. doi:10.2174/1389450118666170123145357
Huang, S., Lin, L., Wang, S., Ding, W., Zhang, C., Shaukat, A., et al. (2023). Total flavonoids of rhizoma drynariae mitigates aflatoxin B1-induced liver toxicity in chickens via microbiota-gut-liver Axis interaction mechanisms. Antioxidants Basel, Switz. 12 (4), 819. doi:10.3390/antiox12040819
Hussain, A. (2012). Inhibition of aflatoxin producing fungus growth using chemical, herbal compoundsspices and plants. Pure Appl. Biol. 1, 8–13. doi:10.19045/bspab.2012.11002
Iheshiulor, O., Esonu, B. O., Chuwuka, O. K., Omede, A., Ifeanyi Charles, O., and Princewill, O. (2011). Effects of mycotoxins in animal nutrition: a review. Asian J. Animal Sci. 5, 19–33. doi:10.3923/ajas.2011.19.33
Izco, M., Vettorazzi, A., de Toro, M., Sáenz, Y., and Alvarez-Erviti, L. (2021). Oral sub-chronic ochratoxin A exposure induces gut microbiota alterations in mice. Toxins 13 (2), 106. doi:10.3390/toxins13020106
Johansson, M. E. V., and Hansson, G. C. (2016). Immunological aspects of intestinal mucus and mucins. Nat. Rev. Immunol. 16 (10), 639–649. doi:10.1038/nri.2016.88
Kadota, T., Furusawa, H., Hirano, S., Tajima, O., Kamata, Y., and Sugita-Konishi, Y. (2013). Comparative study of deoxynivalenol, 3-acetyldeoxynivalenol, and 15-acetyldeoxynivalenol on intestinal transport and IL-8 secretion in the human cell line Caco-2. Toxicol. Vitro 27 (6), 1888–1895. doi:10.1016/j.tiv.2013.06.003
Kalaiselvi, P., Rajashree, K., Priya, L. B., and Padma, V. V. (2013). Cytoprotective effect of epigallocatechin-3-gallate against deoxynivalenol-induced toxicity through anti-oxidative and anti-inflammatory mechanisms in HT-29 cells. Food Chem. Toxicol. 56, 110–118. doi:10.1016/j.fct.2013.01.042
Königs, M., Lenczyk, M., Schwerdt, G., Holzinger, H., Gekle, M., and Humpf, H.-U. (2007). Cytotoxicity, metabolism and cellular uptake of the mycotoxin deoxynivalenol in human proximal tubule cells and lung fibroblasts in primary culture. Toxicology 240 (1), 48–59. doi:10.1016/j.tox.2007.07.016
Korem, T., Zeevi, D., Suez, J., Weinberger, A., Avnit-Sagi, T., Pompan-Lotan, M., et al. (2015). Growth dynamics of gut microbiota in health and disease inferred from single metagenomic samples. Science 349 (6252), 1101–1106. doi:10.1126/science.aac4812
Lach, G., Schellekens, H., Dinan, T. G., and Cryan, J. F. (2018). Anxiety, depression, and the microbiome: a role for gut peptides. Neurotherapeutics 15 (1), 36–59. doi:10.1007/s13311-017-0585-0
Levan, S. R., Stamnes, K. A., Lin, D. L., Panzer, A. R., Fukui, E., McCauley, K., et al. (2019). Elevated faecal 12,13-diHOME concentration in neonates at high risk for asthma is produced by gut bacteria and impedes immune tolerance. Nat. Microbiol. 4 (11), 1851–1861. doi:10.1038/s41564-019-0498-2
Li, S., Liu, R., Wei, G., Guo, G., Yu, H., Zhang, Y., et al. (2021). Curcumin protects against Aflatoxin B1-induced liver injury in broilers via the modulation of long non-coding RNA expression. Ecotoxicol. Environ. Saf. 208, 111725. doi:10.1016/j.ecoenv.2020.111725
Liew, W. P., and Mohd-Redzwan, S. (2018). Mycotoxin: its impact on gut health and microbiota. Front. Cell. Infect. Microbiol. 8, 60. doi:10.3389/fcimb.2018.00060
Limaye, A., Yu, R.-C., Chou, C.-C., Liu, J.-R., and Cheng, K.-C. (2018). Protective and detoxifying effects conferred by dietary selenium and curcumin against AFB1-mediated toxicity in livestock: a review. Toxins 10 (1), 25. doi:10.3390/toxins10010025
Lin, L., Fu, P., Chen, N., Gao, N., Cao, Q., Yue, K., et al. (2022). Total flavonoids of Rhizoma Drynariae protect hepatocytes against aflatoxin B1-induced oxidative stress and apoptosis in broiler chickens. Ecotoxicol. Environ. Saf. 230, 113148. doi:10.1016/j.ecoenv.2021.113148
Lu, L., Dong, J., Liu, Y., Qian, Y., Zhang, G., Zhou, W., et al. (2022). New insights into natural products that target the gut microbiota: effects on the prevention and treatment of colorectal cancer. Front. Pharmacol. 13, 964793. doi:10.3389/fphar.2022.964793
Luo, Y., and Zhou, T. (2021). Connecting the dots: targeting the microbiome in drug toxicity. Med. Res. Rev. 42, 83–111. doi:10.1002/med.21805
Lyte, M. (2014). Microbial endocrinology and the microbiota-gut-brain axis. Adv. Exp. Med. Biol. 817, 3–24. doi:10.1007/978-1-4939-0897-4_1
Ma, C., Han, M., Heinrich, B., Fu, Q., Zhang, Q., Sandhu, M., et al. (2018). Gut microbiome-mediated bile acid metabolism regulates liver cancer via NKT cells. Science 360 (6391), eaan5931. doi:10.1126/science.aan5931
Manafi, M., Mohan, K., and Ali, M. N. (2011). Effect of ochratoxin A on coccidiosis-challenged broiler chicks. World Mycotoxin J. 4 (2), 177–181. doi:10.3920/wmj2010.1234
Maresca, M. (2013). From the gut to the brain: journey and pathophysiological effects of the food-associated trichothecene mycotoxin deoxynivalenol. Toxins 5 (4), 784–820. doi:10.3390/toxins5040784
Marin, D. E., Pistol, G. C., Gras, M. A., Palade, M. L., and Taranu, I. (2017). Comparative effect of ochratoxin A on inflammation and oxidative stress parameters in gut and kidney of piglets. Regul. Toxicol. Pharmacol. 89, 224–231. doi:10.1016/j.yrtph.2017.07.031
McFadden, R.-M. T., Larmonier, C. B., Shehab, K. W., Midura-Kiela, M., Ramalingam, R., Harrison, C. A., et al. (2015). The role of curcumin in modulating colonic microbiota during colitis and colon cancer prevention. Inflamm. Bowel Dis. 21 (11), 2483–2494. doi:10.1097/MIB.0000000000000522
Meijers, B., Farré, R., Dejongh, S., Vicario, M., and Evenepoel, P. (2018). Intestinal barrier function in chronic kidney disease. Toxins 10 (7), 298. doi:10.3390/toxins10070298
Mims, J. W. (2015). Asthma: definitions and pathophysiology. Int. Forum Allergy & Rhinology 5 (S1), S2–S6. doi:10.1002/alr.21609
Minervini, F., Garbetta, A., D’Antuono, I., Cardinali, A., Martino, N. A., Debellis, L., et al. (2014). Toxic mechanisms induced by fumonisin B1 mycotoxin on human intestinal cell line. Archives Environ. Contam. Toxicol. 67 (1), 115–123. doi:10.1007/s00244-014-0004-z
Mohajeri, M. H., La Fata, G., Steinert, R. E., and Weber, P. (2018). Relationship between the gut microbiome and brain function. Nutr. Rev. 76 (7), 481–496. doi:10.1093/nutrit/nuy009
Mustafa, S., Wei, Q., Ennab, W., Lv, Z., Nazar, K., Siyal, F. A., et al. (2019). Resveratrol ameliorates testicular histopathology of mice exposed to restraint stress. Animals 9 (10), 743. doi:10.3390/ani9100743
Nayak, S., and Sashidhar, R. B. (2010). Metabolic intervention of aflatoxin B1 toxicity by curcumin. J. Ethnopharmacol. 127 (3), 641–644. doi:10.1016/j.jep.2009.12.010
Nico, B., and Ribatti, D. (2012). Morphofunctional aspects of the blood-brain barrier. Curr. drug Metab. 13 (1), 50–60. doi:10.2174/138920012798356970
Osselaere, A., Santos, R., Hautekiet, V., De Backer, P., Chiers, K., Ducatelle, R., et al. (2013). Deoxynivalenol impairs hepatic and intestinal gene expression of selected oxidative stress, tight junction and inflammation proteins in broiler chickens, but addition of an adsorbing agent shifts the effects to the distal parts of the small intestine. PLOS ONE 8 (7), e69014. doi:10.1371/journal.pone.0069014
Palabiyik, S. S., Erkekoglu, P., Zeybek, N. D., Kizilgun, M., Baydar, D. E., Sahin, G., et al. (2013). Protective effect of lycopene against ochratoxin A induced renal oxidative stress and apoptosis in rats. Exp. Toxicol. Pathology 65 (6), 853–861. doi:10.1016/j.etp.2012.12.004
Pan, C., Guo, Q., and Lu, N. (2019). Role of gut microbiota in the pharmacological effects of natural products. Evidence-based complementary Altern. Med. eCAM. 2019, 2682748. doi:10.1155/2019/2682748
Peraica, M., Flajs, D., Mladinic, M., Zeljezic, D., Eror, D. B., Koepsell, H., et al. (2011). Oxidative stress and Na+-glucose cotransporters Sglt1 and Sglt2 in kidneys of ochratoxin A-treated rats. Toxicol. Lett. 205, S275. doi:10.1016/j.toxlet.2011.05.933
Pestka, J. J. (2010). Deoxynivalenol: mechanisms of action, human exposure, and toxicological relevance. Archives Toxicol. 84 (9), 663–679. doi:10.1007/s00204-010-0579-8
Pferschy-Wenzig, E. M., Pausan, M. R., Ardjomand-Woelkart, K., Röck, S., Ammar, R. M., Kelber, O., et al. (2022). Medicinal plants and their impact on the gut microbiome in mental health: a systematic review. Nutrients 14 (10), 2111. doi:10.3390/nu14102111
Piotrowska, M., Śliżewska, K., Nowak, A., Zielonka, Ł., Żakowska, Z., Gajęcka, M., et al. (2014). The effect of experimental Fusarium mycotoxicosis on microbiota diversity in porcine ascending colon contents. Toxins 6 (7), 2064–2081. doi:10.3390/toxins6072064
Plata, C., Cruz, C., Cervantes, L. G., and Ramírez, V. (2019). The gut microbiota and its relationship with chronic kidney disease. Int. Urology Nephrol. 51 (12), 2209–2226. doi:10.1007/s11255-019-02291-2
Pugin, B., Barcik, W., Westermann, P., Heider, A., Wawrzyniak, M., Hellings, P., et al. (2017). A wide diversity of bacteria from the human gut produces and degrades biogenic amines. Microb. Ecol. Health Dis. 28 (1), 1353881. doi:10.1080/16512235.2017.1353881
Rajakovich, L. J., and Balskus, E. P. (2019). Metabolic functions of the human gut microbiota: the role of metalloenzymes. Nat. Product. Rep. 36 (4), 593–625. doi:10.1039/c8np00074c
Ramezani, A., Massy, Z. A., Meijers, B., Evenepoel, P., Vanholder, R., and Raj, D. S. (2016). Role of the gut microbiome in uremia: a potential therapeutic target. Am. J. Kidney Dis. 67 (3), 483–498. doi:10.1053/j.ajkd.2015.09.027
Robert, H., Payros, D., Pinton, P., Théodorou, V., Mercier-Bonin, M., and Oswald, I. P. (2017). Impact of mycotoxins on the intestine: are mucus and microbiota new targets? J. Toxicol. Environ. Health, Part B 20 (5), 249–275. doi:10.1080/10937404.2017.1326071
Romero, A., Ares, I., Ramos, E., Castellano, V., Martínez, M., Martínez-Larrañaga, M.-R., et al. (2016). Mycotoxins modify the barrier function of Caco-2 cells through differential gene expression of specific claudin isoforms: protective effect of illite mineral clay. Toxicology 353-354, 21–33. doi:10.1016/j.tox.2016.05.003
Romina, A. M. (2022). “Implications of mycotoxins in food safety,” in Mycotoxins and food safety. Editor M. Romina Alina (Rijeka: IntechOpen). Ch. 1.
Saleemi, M. K., Ashraf, K., Gul, S. T., Naseem, M. N., Sajid, M. S., Mohsin, M., et al. (2020). Toxicopathological effects of feeding aflatoxins B1 in broilers and its ameliosration with indigenous mycotoxin binder. Ecotoxicol. Environ. Saf. 187, 109712. doi:10.1016/j.ecoenv.2019.109712
Sampson, T. R., and Mazmanian Sarkis, K. (2015). Control of brain development, function, and behavior by the microbiome. Cell Host Microbe 17 (5), 565–576. doi:10.1016/j.chom.2015.04.011
Santisteban, M. M., Qi, Y., Zubcevic, J., Kim, S., Yang, T., Shenoy, V., et al. (2017). Hypertension-linked pathophysiological alterations in the gut. Circulation Res. 120 (2), 312–323. doi:10.1161/CIRCRESAHA.116.309006
Sayin, S. I., Wahlström, A., Felin, J., Jäntti, S., Marschall, H.-U., Bamberg, K., et al. (2013). Gut microbiota regulates bile acid metabolism by reducing the levels of tauro-beta-muricholic acid, a naturally occurring FXR antagonist. Cell Metab. 17 (2), 225–235. doi:10.1016/j.cmet.2013.01.003
Segal, L. N., Clemente, J. C., Tsay, J. C., Koralov, S. B., Keller, B. C., Wu, B. G., et al. (2016). Enrichment of the lung microbiome with oral taxa is associated with lung inflammation of a Th17 phenotype. Nat. Microbiol. 1, 16031. doi:10.1038/nmicrobiol.2016.31
Shi, K., Wang, F., Jiang, H., Liu, H., Wei, M., Wang, Z., et al. (2014). Gut bacterial translocation may aggravate microinflammation in hemodialysis patients. Dig. Dis. Sci. 59 (9), 2109–2117. doi:10.1007/s10620-014-3202-7
Shi, L. L., Li, Y., Wang, Y., and Feng, Y. (2015). MDG-1, an Ophiopogon polysaccharide, regulate gut microbiota in high-fat diet-induced obese C57BL/6 mice. Int. J. Biol. Macromol. 81, 576–583. doi:10.1016/j.ijbiomac.2015.08.057
Shi, R., Huang, C., Gao, Y., Li, X., Zhang, C., and Li, M. (2023). Gut microbiota axis: potential target of phytochemicals from plant-based foods. Food Sci. Hum. Wellness 12 (5), 1409–1426. doi:10.1016/j.fshw.2023.02.001
Sircana, A., De Michieli, F., Parente, R., Framarin, L., Leone, N., Berrutti, M., et al. (2019). Gut microbiota, hypertension and chronic kidney disease: recent advances. Pharmacol. Res. 144, 390–408. doi:10.1016/j.phrs.2018.01.013
Śliżewska, K., Cukrowska, B., Smulikowska, S., and Cielecka-Kuszyk, J. (2019). The effect of probiotic supplementation on performance and the histopathological changes in liver and kidneys in broiler chickens fed diets with aflatoxin B₁. Toxins 11 (2), 112. doi:10.3390/toxins11020112
Sun, L.-H., Zhang, N.-Y., Zhu, M.-K., Zhao, L., Zhou, J.-C., and Qi, D.-S. (2016). Prevention of aflatoxin B1 hepatoxicity by dietary selenium is associated with inhibition of cytochrome P450 isozymes and up-regulation of 6 selenoprotein genes in chick liver. J. Nutr. 146 (4), 655–661. doi:10.3945/jn.115.224626
Sutherland, M. R. (2020). Introduction to a special issue on kidney development and disease. Anat. Rec. Hob. N. J. 2007 303 (10), 2507–2510. doi:10.1002/ar.24467
Taranu, I., Braicu, C., Marin, D. E., Pistol, G. C., Motiu, M., Balacescu, L., et al. (2015). Exposure to zearalenone mycotoxin alters in vitro porcine intestinal epithelial cells by differential gene expression. Toxicol. Lett. 232 (1), 310–325. doi:10.1016/j.toxlet.2014.10.022
Ticinesi, A., Nouvenne, A., and Meschi, T. (2019). Gut microbiome and kidney stone disease: not just an Oxalobacter story. Kidney Int. 96 (1), 25–27. doi:10.1016/j.kint.2019.03.020
Ullah, S., Mustafa, S., Ennab, W., Muhammad, J., Shafiq, M., Kavita, N. M., et al. (2020). A protective role of resveratrol against the effects of immobilization stress in corpora lutea of mice in early pregnancy. J. Integr. Agric. 19 (7), 1857–1866. doi:10.1016/s2095-3119(19)62856-x
Vaziri, N. D., Yuan, J., and Norris, K. (2012). Role of urea in intestinal barrier dysfunction and disruption of epithelial tight junction in chronic kidney disease. Am. J. Nephrol. 37 (1), 1–6. doi:10.1159/000345969
Verbrugghe, E., Vandenbroucke, V., Dhaenens, M., Shearer, N., Goossens, J., De Saeger, S., et al. (2012). T-2 toxin induced Salmonella Typhimurium intoxication results in decreased Salmonella numbers in the cecum contents of pigs, despite marked effects on Salmonella-host cell interactions. Veterinary Res. 43 (1), 22. doi:10.1186/1297-9716-43-22
Vipin, A. V., Raksha, R. K., Kurrey, N. K., Anu Appaiah, A. K., and Venkateshwaran, G. (2017). Protective effects of phenolics rich extract of ginger against Aflatoxin B1-induced oxidative stress and hepatotoxicity. Biomed. Pharmacother. 91, 415–424. doi:10.1016/j.biopha.2017.04.107
Wang, H., Chen, Y., Zhai, N., Chen, X., Gan, F., Li, H., et al. (2017a). Ochratoxin A-induced apoptosis of IPEC-J2 cells through ROS-mediated mitochondrial permeability transition pore opening pathway. J. Agric. food Chem. 65 (48), 10630–10637. doi:10.1021/acs.jafc.7b04434
Wang, J., Ghosh, S. S., and Ghosh, S. (2017b). Curcumin improves intestinal barrier function: modulation of intracellular signaling, and organization of tight junctions. Am. J. physiology Cell physiology 312 (4), C438–C45. doi:10.1152/ajpcell.00235.2016
Wang, J.-H., Bose, S., Kim, G.-C., Hong, S.-U., Kim, J.-H., Kim, J.-E., et al. (2014). Flos Lonicera ameliorates obesity and associated endotoxemia in rats through modulation of gut permeability and intestinal microbiota. PloS one 9, e86117. doi:10.1371/journal.pone.0086117
Wang, L. Y., Wang, S., Wang, Y., Ruan, K.-F., and Feng, Y. (2011). Effect of MDG-1 on oral glucose tolerance and intestinal microecological balance in diabetic mice. World Chin. J. Dig. 19, 2058–2062. doi:10.11569/wcjd.v19.i19.2058
Wang, S. M., Long, S. Q., Deng, Z. Y., and Wu, W. Y. (2020). Positive role of Chinese herbal medicine in cancer immune regulation. Am. J. Chin. Med. 48 (7), 1577–1592. doi:10.1142/S0192415X20500780
Wang, Y. H., Wang, M., Chen, J. X., Li, Y., Kuang, Z., Dende, C., et al. (2023). The gut microbiota reprograms intestinal lipid metabolism through long noncoding RNA. Science 381 (6660), 851–856.
Wang, Y. J., Li, Y. X., Li, S., He, W., Wang, Z. R., Zhan, T. P., et al. (2022). Progress in traditional Chinese medicine and natural extracts for the treatment of lupus nephritis. Biomed. Pharmacother. = Biomedecine Pharmacother. 149, 112799. doi:10.1016/j.biopha.2022.112799
Wang, Y. K., Li, W. Q., Xia, S., Guo, L., Miao, Y., and Zhang, B. K. (2021). Metabolic activation of the toxic natural products from herbal and dietary supplements leading to toxicities. Front. Pharmacol. 12, 758468. doi:10.3389/fphar.2021.758468
Woo, C. S. J., Lau, J. S. H., and El-Nezami, H. (2012). “Chapter 10 - herbal medicine: toxicity and recent trends in assessing their potential toxic effects,” in Advances in botanical research. Editors L.-F. Shyur, and A. S. Y. Lau (Massachusetts, United States: Academic Press), 365–384.
Wu, Q., Wang, X., Nepovimova, E., Wang, Y., Yang, H., Li, L., et al. (2017). Antioxidant agents against trichothecenes: new hints for oxidative stress treatment. Oncotarget 8 (66), 110708–110726. doi:10.18632/oncotarget.22800
Wu, Z., Zhong, M., Liu, Y., Xiong, Y., Gao, Z., Ma, J., et al. (2022). Application of natural products for inducing ferroptosis in tumor cells. Biotechnol. Appl. Biochem. 69 (1), 190–197. doi:10.1002/bab.2096
Yang, G., Zhong, L., Jiang, L., Geng, C., Cao, J., Sun, X., et al. (2011). 6-Gingerol prevents patulin-induced genotoxicity in HepG2 cells. Phytotherapy Res. 25 (10), 1480–1485. doi:10.1002/ptr.3446
Zhang, Q., Feng, Z., Lu, J., Lu, J., Guan, S., and Chen, Y. (2021). Aflatoxin B1 inhibited autophagy flux by inducing lysosomal alkalinization in HepG2 cells. Toxicol. Mech. Methods 31 (6), 450–456. doi:10.1080/15376516.2021.1909196
Zhang, X., Liu, H., Hashimoto, K., Yuan, S. Y., and Zhang, J. C. (2022). The gut-liver axis in sepsis: interaction mechanisms and therapeutic potential. Crit. Care 26 (1), 213. doi:10.1186/s13054-022-04090-1
Keywords: mycotoxin, mycotoxin-induced diseases, natural products, gut-x axis, gut microbial modulation
Citation: Li K, Wang S, Qu W, Ahmed AA, Enneb W, Obeidat MD, Liu H-Y, Dessie T, Kim IH, Adam SY and Cai D (2024) Natural products for Gut-X axis: pharmacology, toxicology and microbiology in mycotoxin-caused diseases. Front. Pharmacol. 15:1419844. doi: 10.3389/fphar.2024.1419844
Received: 19 April 2024; Accepted: 29 May 2024;
Published: 19 June 2024.
Edited by:
Yi Wu, Nanjing Agricultural University, ChinaReviewed by:
Lei Li, China Agricultural University, ChinaAdelijiang Wusiman, Xinjiang Agricultural University, China
Wenjing Sun, Yulin Normal University, China
Weijie Lv, South China Agricultural University, China
Copyright © 2024 Li, Wang, Qu, Ahmed, Enneb, Obeidat, Liu, Dessie, Kim, Adam and Cai. This is an open-access article distributed under the terms of the Creative Commons Attribution License (CC BY). The use, distribution or reproduction in other forums is permitted, provided the original author(s) and the copyright owner(s) are credited and that the original publication in this journal is cited, in accordance with accepted academic practice. No use, distribution or reproduction is permitted which does not comply with these terms.
*Correspondence: Saber Y. Adam, saaber5757@gmail.com; Demin Cai, demincai@yzu.edu.cn
†These authors have contributed equally to this work