- 1Cancept Therapeutics Laboratory, Department of Pharmacy, Indira Gandhi National Tribal University, Lalpur, India
- 2Chhattrapati Shivaji Institute of Pharmacy, Durg, Chhattisgarh, India
- 3Department of Pharmaceutical Sciences, College of Pharmacy, St. John’s University, Queens, NY, United States
- 4Department of Pharmacology and Experimental Therapeutics, College of Pharmacy and Pharmaceutical Sciences, University of Toledo, Toledo, OH, United States
- 5Department of Nutrition, State College, Pennsylvania State University, University Park, PA, United States
- 6Department of Drug Discovery and Development, Harrison School of Pharmacy, Auburn University, Auburn, AL, United States
- 7Department of Pharmaceutical Sciences, College of Pharmacy, University of Arkansas for Medical Sciences, Little Rock, AR, United States
Multiwalled carbon nanotubes (MWCNTs) are at the forefront of nanotechnology-based advancements in cancer therapy, particularly in the field of targeted drug delivery. The nanotubes are characterized by their concentric graphene layers, which give them outstanding structural strength. They can deliver substantial doses of therapeutic agents, potentially reducing treatment frequency and improving patient compliance. MWCNTs’ diminutive size and modifiable surface enable them to have a high drug loading capacity and penetrate biological barriers. As a result of the extensive research on these nanomaterials, they have been studied extensively as synthetic and chemically functionalized molecules, which can be combined with various ligands (such as folic acid, antibodies, peptides, mannose, galactose, polymers) and linkers, and to deliver anticancer drugs, including but not limited to paclitaxel, docetaxel, cisplatin, doxorubicin, tamoxifen, methotrexate, quercetin and others, to cancer cells. This functionalization facilitates selective targeting of cancer cells, as these ligands bind to specific receptors overexpressed in tumor cells. By sparing non-cancerous cells and delivering the therapeutic payload precisely to cancer cells, this therapeutic payload delivery ability reduces chemotherapy systemic toxicity. There is great potential for MWCNTs to be used as targeted delivery systems for drugs. In this review, we discuss techniques for functionalizing and conjugating MWCNTs to drugs using natural and biomacromolecular linkers, which can bind to the cancer cells’ receptors/biomolecules. Using MWCNTs to administer cancer drugs is a transformative approach to cancer treatment that combines nanotechnology and pharmacotherapy. It is an exciting and rich field of research to explore and optimize MWCNTs for drug delivery purposes, which could result in significant benefits for cancer patients.
1 Introduction
Cancer continues to be a major global health concern, with 20 million new cases and 9.7 million deaths in 2022. This situation is not expected to improve, as projected statistics highlight a significant rise in the number of new cancer cases (29.9 million) and mortality (15.3 million) by the year 2040 (Bray et al., 2024).
Chemotherapeutic agents, e.g., Cisplatin, Docetaxel, Doxorubicin, Fluorouracil, Gemcitabine, Methotrexate, and Paclitaxel are the mainstay of therapeutic regimens for several types of cancer (Raza et al., 2016; Thakur et al., 2016). Still, dose-dependent adverse and toxic effects, low aqueous solubility, poor tissue specificity, low stability, short half-lives, and low tissue penetration seriously limit their clinical use (Dy and Adjei, 2013; Tran et al., 2019; Rele et al., 2024). Nanocarrier-based drug delivery systems such as liposomes (e.g., polymeric liposomes and magneto-liposomes), nanoparticles (e.g., magnetic nanoparticles and polymeric nanoparticles), and advanced technology polymers (e.g., dendrimers and carbon nanotubes) can overcome these limitations by entrapping and delivering the drug in the cancer tissue (Mainarden et al., 2004; Yun et al., 2015; Reza et al., 2018).
Among the different classes of nanocarrier-based drug delivery systems, carbon nanotubes (CNTs) represent a novel class of nanocarriers that offers numerous advantages such as a high drug loading capacity, large surface area, sustained drug release, and selective targeting potential (Pate et al., 2019; Thakur et al., 2023).
Currently, CNTs, with unidimensional and graphitic hexagonal structure, containing horizontally arranged benzene rings, are being developed (Kesharwani et al., 2012; Mehra et al., 2015). Clinically, CNTs have been used based on their high aspect ratios, diminutively minuscule sizes, significant voluminous surface areas, facile perforate membrane and their conjugation or encapsulation with numerous therapeutic molecules (Patole et al., 2008; Kesharwani et al., 2012; Rele et al., 2024). Overall, CNTs represent an important biomaterial that can be used for the distribution of biomolecules and drugs, that can be generally classified into the following groups, according to their sizes and construction or arrangement, as single walled, double walled or multiwalled carbon nanotubes (MWCNTs), as shown in Figure 1.
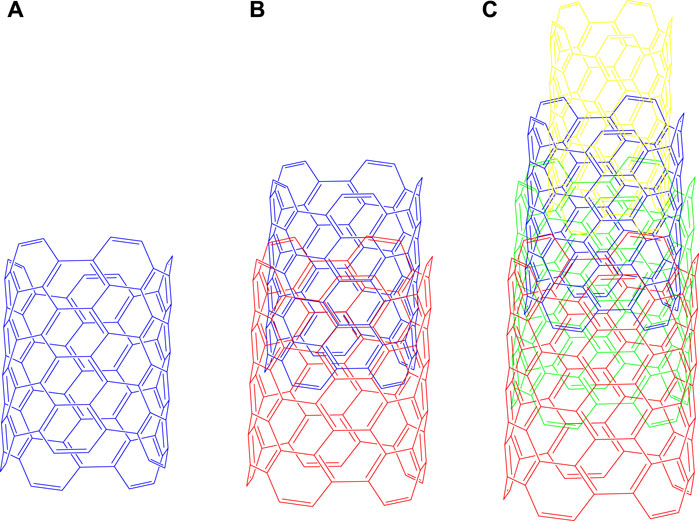
Figure 1. Types of Carbon Nanotubes (A) Single - walled, (B) Double - walled, (C) Multi - walled carbon nanotubes. Single-walled carbon nanotubes consist of only one layer of graphene, which forms six-atom carbon rings in a hexagonal shape, whereas the multilayer nanotubes consist of concentric layers of graphene in cylindrical shape.
1.1 Single-walled carbon nanotubes (SWCNTs)
MWCNTs consist of a graphene unilayer sheet that is wrapped into a cylindrical tube. MWCNTs can be further classified as zigzag, armchair and chiral (Cherukuri et al., 2006). Its diameter is approximately 1 nm and the length of tube is long because of the geometric arrangement of the nanotubes (Murakami et al., 2004; Jha et al., 2020).
1.2 Double - walled carbon nanotubes (DWCNTs)
DWCNTs consist of two layers of a cylindrically arranged graphite sheet. The structure and properties of DWCNTs are comparable to MWCNTs; however, the outer wall of the DWCNTs is a coaxial nanostructure that contains a two dimensional graphene sheet rolled into a cylindrical form (Jha et al., 2020). The cylindrical tubes that are less than 1 nm in diameter are not considered to be DWCNTs (Tison et al., 2008).
1.3 Multiwalled - carbon nanotubes (MWCNTs)
Currently, MWCNTs are nanotubes that are being used to develop effective nanomedicines (Srivastava et al., 2011; Garcia et al., 2015; Singh et al., 2016; Martins et al., 2023). MWCNTs offer several advantages over SWCNTs and DWCNTs, including higher drug loading capacity, larger surface area, and multiple sites for ligand, linker, and drug conjugation. MWCNTs provide a sustained release and a greater selective targeting potential that decreases toxicity and increases metabolic stability, with a more rigid and higher aspect ratio 1:1000 diameter/length ratio and a decrease the leakage of loaded drugs due to the presence of multiple layers nanomedicines (Tison et al., 2008; Kayat et al., 2011; Prasek et al., 2011; Mehra et al., 2016). Furthermore, fabricated/modified/conjugated MWCNTs have been used as biomarkers in cancer cells and as imaging and cancer diagnostic compounds nanomedicines (Ji et al., 2010; Wang et al., 2015). Many reviews published in this area predominantly focus on the use of carbon nanotubes for drug-delivery nanomedicines (Ethissi et al., 2012; Kushwaha et al., 2013; Son et al., 2016; Sanginario et al., 2017). In this review, we focus on the functionalization and ligand conjugation strategies of MWCNTs concerning their applications in the delivery of drugs to cancer cells.
1.3.1 The structure of MWCNTs
MWCNTs consist of 3–10 layers of concentric tubes of graphene that interact with one another by van der Waals (vdW) forces (Figure 1), with each layer being separated from one another by approximately 0.34 nm, a distance that is greater than that of the inner layer sheets nanomedicines (Kulkarni et al., 2010; Lehman et al., 2011; Sahebian et al., 2016; Martins et al., 2023). The structures of the MWCNTs have been explained by the Russian doll model (RDM) and parchment model (PM) nanomedicines (Das et al., 2015a). In the RDM, the graphite sheets are arranged in cylinders, whereas in the PM, the graphite sheets are rolled into one sheet in the region, similar to a newspaper (Yang et al., 2007). MWCNTs have been reported to target, release and distribute drugs in various tissues, including cancer cells (Das et al., 2015b). Consequently, MWCNTS are used as nanocarriers for anticancer drug delivery and therapy to a targeted site in a sustained manner (Froy et al., 1999; Raza et al., 2016). The pristine MWCNTs are not appropriate for interaction with biomolecules or bioactive compounds, as they typically form bundles or aggregates that poorly disperse in aqueous solution (Yang et al., 2007; Sahoo et al., 2011). This problem could be circumvented by functionalization, which is one of the methods used to improve the dispersion and solubility of MWCNTs. For example, the modification of the surface of MWCNTs with various chemical groups decreases the likelihood of aggregation, which can decrease toxicity (Froy et al., 1999; Raza et al., 2016; Sheikhpour et al., 2017).
1.3.2 Advances in the functionalization of MWCNTs
Activated MWCNTs are novel nanocarriers that can be modified by different types of surfactants (including but not limited to, phosphoric acid esters, sodium lauryl sulfate, carboxylic acid salts and alkylbenzene sulfonates) and biopolymers (e.g., peptide, chitosan, nucleic acid) (Table 1). The introduction of several functional groups on the surface can be used to disperse the carbon nanocomposite material (Sun et al., 2002; Thakur et al., 2022). These functionalized MWCNTs have good biocompatibility, decreased toxicity and the capacity to cross cell membranes, which is important for targeted drug distribution (Vardharajula et al., 2012; Rele et al., 2024). Chemical methods, such as exohedral functionalization (noncovalent and covalent) and endohedral functionalization, are used to functionalize MWCNTs (Figure 2) (Jeon et al., 2011; Pandurangappa et al., 2011; Thakur et al., 2022). Figure 3 illustrates the common methodologies used to functionalize MWCNTs, which includes the covalent carboxylation method, using oxidizing compounds, such as sulphuric acid and nitric acid (Hirsch et al., 2005). These carboxylic groups allow for further covalent and noncovalent chemical reactions of the ligand and the linker (Menezes et al., 2019).
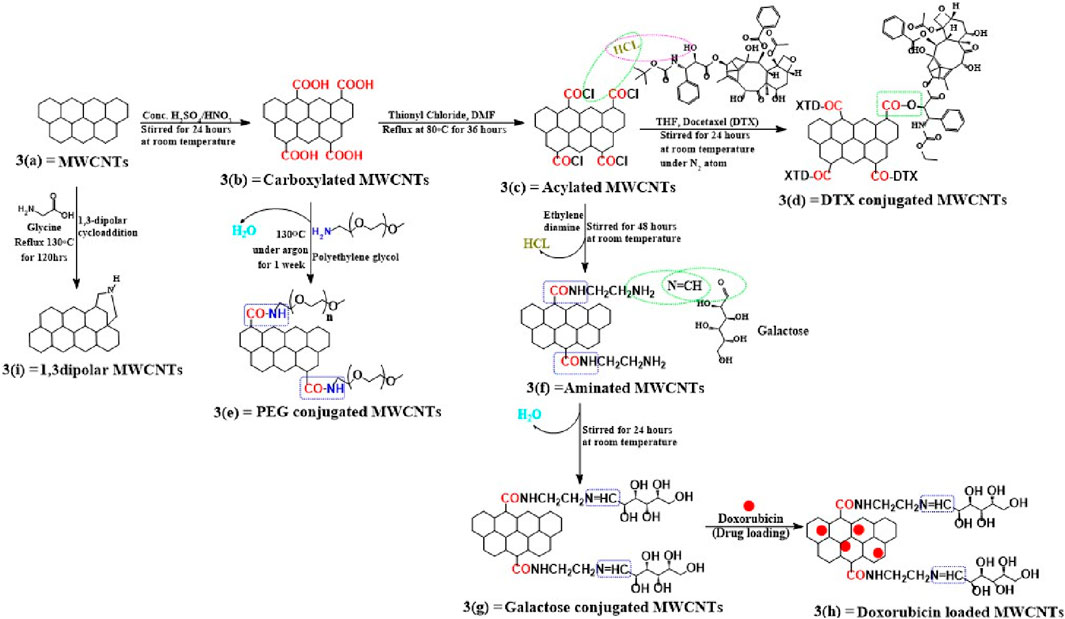
Figure 3. Mechanism for functionalizing pristine MWCNTs. Using this scheme, we show the different ways of covalently functionalizing (a) pristine MWCNTs and (b) describe how pristine MWCNTs are oxidized by strong acidic solvents and how carboxyl functionalized MWCNTs have been further covalently modified, whether through conversion to acylated form (c) or direct conjugation with polymers, such as PEG (e). A variety of methods have been used to conjugate acylated MWCNTs, including conjugation with linkers (f) or ligands (g), followed by drug loading, as shown in figure (h), or for the direct conjugation of docetaxel with acylated MWCNTs. (d) A second method is shown in this (i) that explains the cycloaddition reaction using glycine and this also directly modifies pristine MWCNTs without carboxylation or acylation.
1.3.2.1 Exohedral functionalization
This process involves the grafting of molecules on the outer surface of the nanotubes with surfactants, using direct functionalization, covalent functionalization and non-covalent functionalization (Pandurangappa et al., 2011; Menezes et al., 2019). Exohedral functionalization can be classified according to the nature of interactions between the surface of MWCNTs and the functional groups or polymer chain, as shown in Figure 4. These interactions occur due to the formation of covalent or non-covalent bonds (Melchionna et al., 2013).
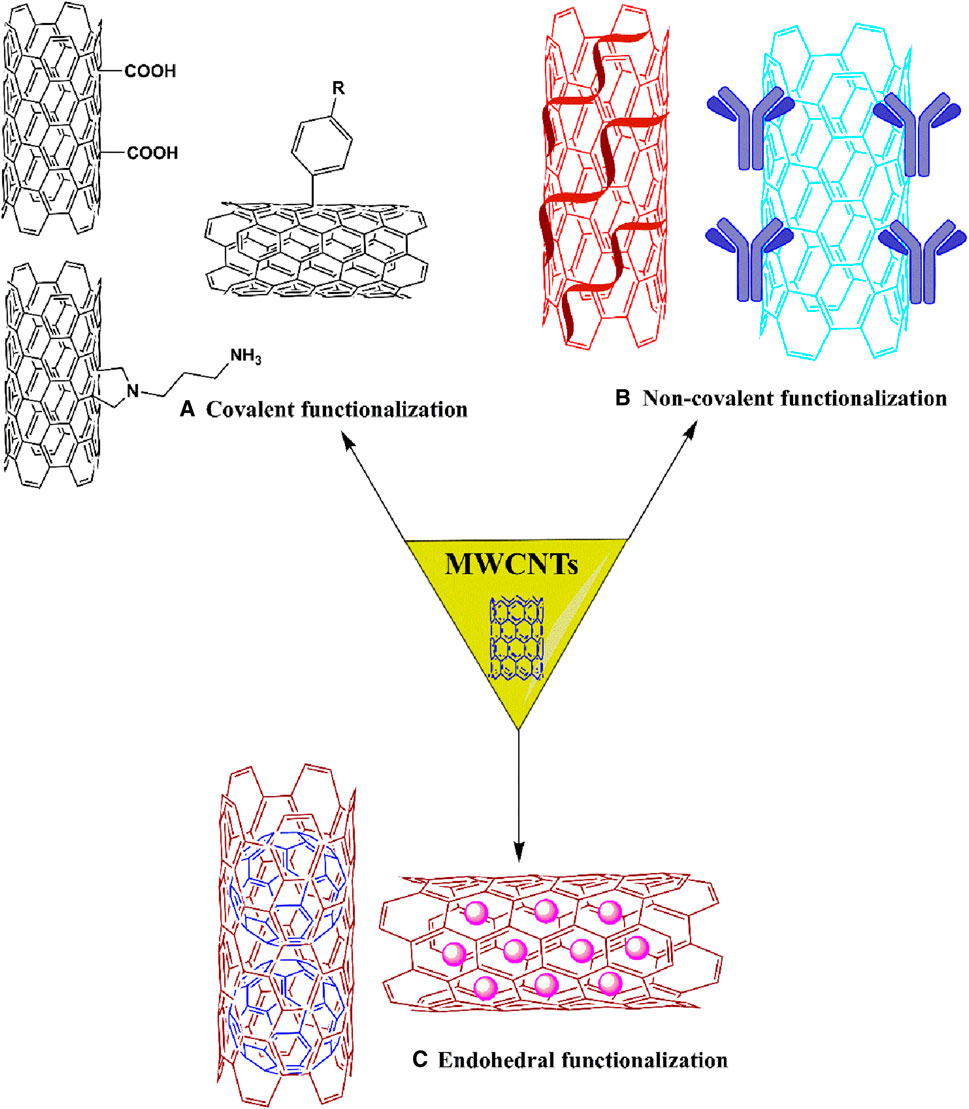
Figure 4. Schematic representation of exohedral [covalent (A) and non-covalent (B)] and endohedral (C) functionalization.
1.3.2.1.1 Covalent functionalization
This method involves covalent interactions between the functional groups and the carbon skeleton of the nanotubes. The functional groups are formed by the incorporation of various chemical moieties or organic particles at different tips or in the sidewall of the MWCNTs. Covalent functionalization has been categorized as direct or indirect functionalization (Meng et al., 2009; Jeon et al., 2011). Direct sidewall covalent functionalization occurs by sp2 to sp3 hybridization and synchronization by removing the conjugated ligand (Sahoo et al., 2010). In contrast, indirect or direct covalent functionalization involves the chemical transformation of the open ends and sidewalls of holes, such as carboxylic groups, pentagon and hexagon graphene scaffolds, among others (Jeon et al., 2011).
1.3.2.1.2 Noncovalent functionalization
Noncovalent modifications occur via supramolecular complexation formed by numerous noncovalent interactions, such as hydrogen bonds, π-π stacking interactions, vdW interactions and electrostatic interactions. Generally, surfactants, polymers and biopolymers are used for the dispersion of nanotubes, using self-assembling methods to produce hydrophobicity (Meng et al., 2009; Raza et al., 2016). The advantage of noncovalent functionalization is that it does not destroy the conjugated system of the MWCNTs sidewalls and thus, the structural properties of the final molecules are not significantly affected (Karousis et al., 2010). Furthermore, noncovalent functionalization is an alternative method for modulating the interfacial properties of the nanotubes (Meng et al., 2009).
1.3.2.2 Endohedral functionalization
Nanotubes can be modified by bioactive molecules and nanoparticles that increase the hydrophilicity of the nanotubes, thus increasing their solubility in hydrophilic solvents. This process is illustrated in Figure 4 by the encapsulation of fullerene molecules and bioactive molecules inside MWCNTs (Delgado et al., 2008; Marega et al., 2014; Iglesias et al., 2019).
1.3.3 The application of MWCNTs
At the beginning of the 21st century, MWCNTs have been used by academic and industrial scientists to develop targeted drug delivery systems (Bianco et al., 2005; Bakry et al., 2007). Currently, the majority of MWCNTs - based research involves the formulation of drug delivery systems for various drugs and for gene therapy, diagnostic detection and drug vectors, among others (Hirlekar et al., 2009; Thakur et al., 2022). As a results of their significant chemical stability, large surface area and a high level of electronic aromatic structure, MWCNTs can be readily conjugated and interact with various ligands, enzymes, proteins, RNA and DNA, to delivery these molecules to the targeted sites (Lamberti et al., 2014).
2 MWCNTs as a conjugated ligand-based drug delivery system
A receptor is a biomacromolecule that recognizes and binds to signaling molecules and drugs (i.e., ligands) to produce a response that alters cellular physiology. For the targeted delivery of drugs, the drug-receptor interaction plays a critical role in mediating the efficacy of a drug. Consequently, there are numerous drugs that can be delivered to cells in a tissue and interact with a specific receptor to produce a specific response (Allen, 2002; Mehra et al., 2013).
Target - based drug delivery system conjugate drugs and ligands with MWCNTs to deliver the drugs to the target. The internalization of the MWCNTs nanocarrier by the cells can occur by endocytosis, a process that involves the engulfment of the material by the cell membrane where it forms an endosome (Yaron et al., 2011; Maruyama et al., 2015) (see Figure 5). Once internalized, the conjugated MWCNT undergo biodegradation and release the drugs (Lamberti et al., 2014). This drug delivery method was first used to conjugate antibiotic and anticancer compounds with MWCNTs nanocarriers to treat certain types of microbial infections and cancers. Indeed, previous studies have reported that anticancer drugs, such as paclitaxel, docetaxel, cisplatin, doxorubicin, epirubicin, tamoxifen, methotrexate and quercetin, could be conjugated or loaded with the modified MWCNTs and their efficacy was validated by in vitro and in vivo experiments (Mehra et al., 2014; Raza et al., 2016). In order to deliver the drugs efficiently, various types of ligands, including but not limited to, folic acid, mannose, galactose and polymers, have been conjugated with MWCNTs (Figure 6) (Singh et al., 2016; Thakur et al., 2022).
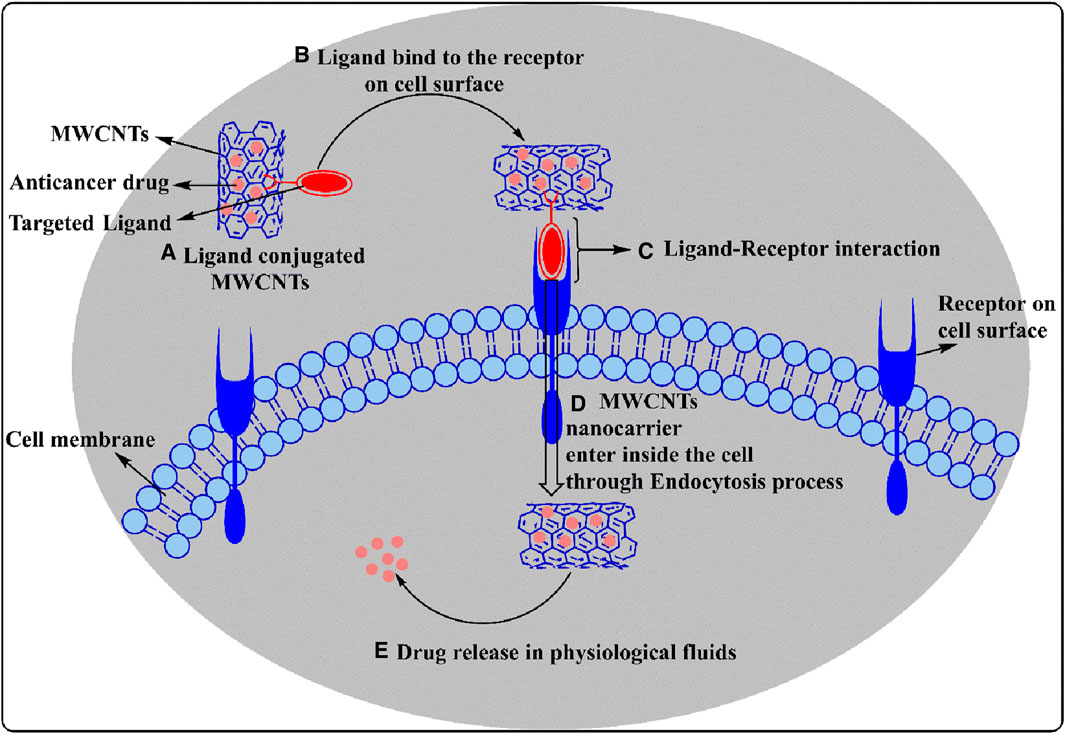
Figure 5. A diagrammatic representation of ligand conjugated MWCNT endocytosis mechanisms shows how drug-loaded ligand modified MWCNTs interact with receptors and enter the cells, and under which conditions the drug is released.
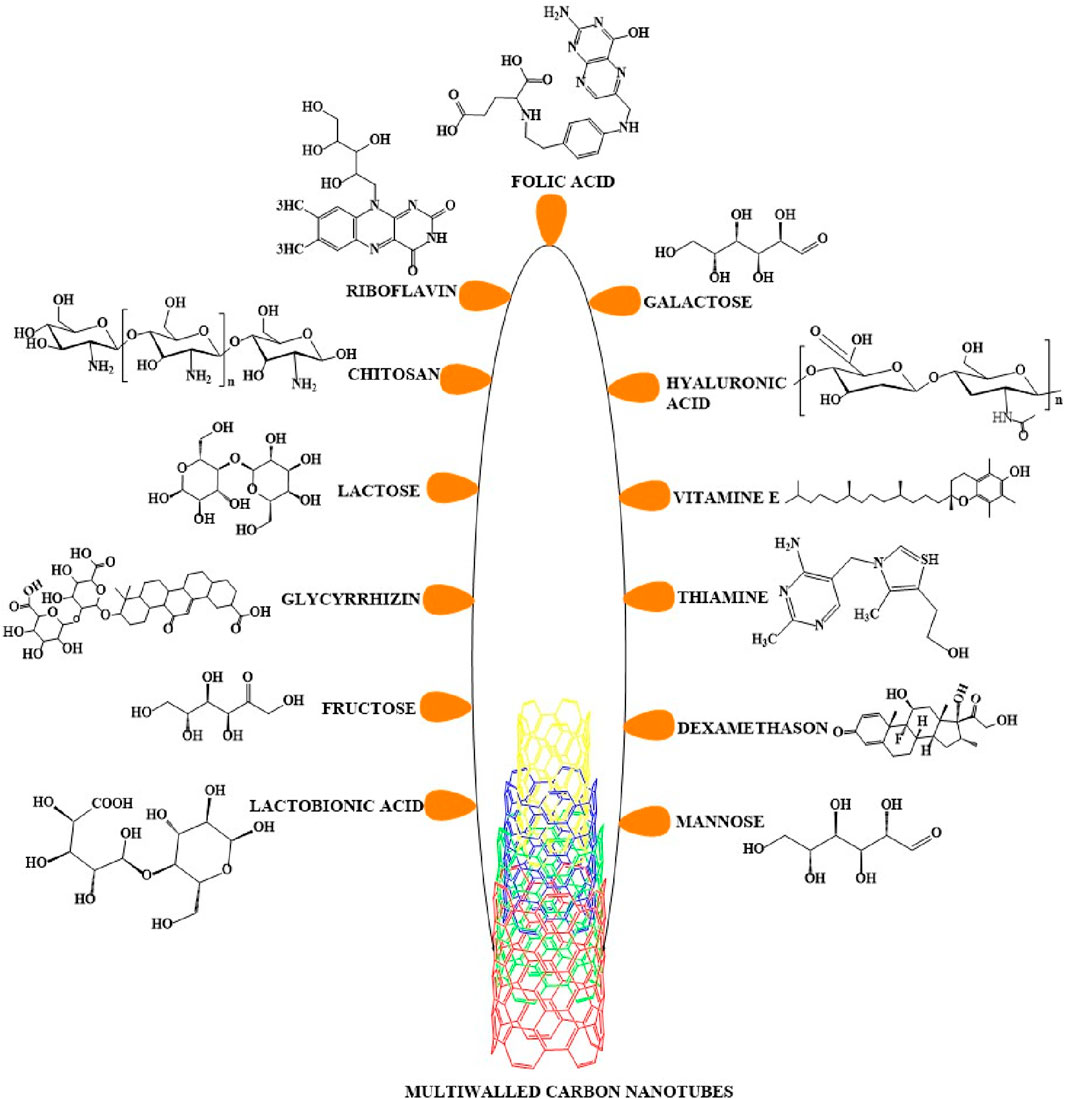
Figure 6. Multiwalled carbon nanotubes conjugated with various ligands. A variety of ligands can be covalently or noncovalently conjugated with MWCNTs to increase their recognition and binding to cellular receptors. The figure here includes all types of ligands that have been conjugated with MWCNTs for use in the treatment of cancer.
2.1 Vitamins conjugated with MWCNTs
A variety of vitamins can be delivered to a specific target by conjugation with MWCNTs (Gao et al., 2018). In this section, we will discuss the targeted delivery of different vitamins-MWCNTs.
2.1.1 Folic Acid
Numerous studies have reported that folic acid receptors are overexpressed in lung tumors, ovarian carcinomas, breast carcinomas, meningiomas, ependymal encephalon tumors, osteosarcomas, non-Hodgkin’s lymphomas, colon tumors, renal carcinomas and uterine sarcomas (Veetil et al., 2009; Fraczyk et al., 2017). Consequently, this led to the development of MWCNTs conjugated with folate that also contained anticancer drugs, thereby increasing the selectivity of drug delivery to the types of cancer.
Recently, Fraczyk et al. (Fraczyk et al., 2017) formulated oxidized MWCNTs, using ethylenediamine in the presence of 4-(4,6-dimethoxy-1,3,5 triazin-2-yl)-4-methyl morpholinium tetrafluoroborate (DMTMM), to link a hydrophilic peptide linker (6-aminohexanoic acid and derivative of β-alanine) that was subsequently conjugated with folic acid (using a folate targeted ligand conjugation mechanism, as shown in Figure 7A. These nanomolecules had proteolytic efficacy in HT-29 colon cancer cells for at least 7 days. Furthermore, the conjugated MWCNTs did not significantly alter the viability of normal colonocyte cells, CCD 841 CoN. The metabolic stability of the nano-molecules was determined under physiological condition (37°C, pH.7.4, in PBS) in human heparinized plasma 7 days later. The results indicated that functionalized MWCNTs were stable and resistant to proteolytic degradation. Furthermore, the cellular uptake of folic acid functionalized MWCNTs was determined in Saos-2 cells (human osteosarcoma cell line), by measuring the fluorescence of fluorescein isothiocyanate (FITC), using a fluorescence microscope. The results indicated a high level of the functionalized folic acid inside the Saos-2 cells However, MWCNTs that were not functionalized with folic acid did not significantly increase the fluorescence intensity in the Saos-2 cells, compared to cells incubated with the folic acids functionalized MWCNTs. Zhang et al. (Zhang et al., 2018) reported the synthesis of multi-functionalized magneto-fluorescent - based carbon nanotubes (Figure 7A, B). In these molecules, amide bonds were formed between the free amino group of polyethyleneimine and the free carboxylic group of folic acid, yielding a folate-carbon nanotubes carrier for the delivery of doxorubicin cancer cells. Furthermore, the MWCNTs had the properties of magnetic resonance imaging/double modular fluorescence that could be used as a chemo-photothermal synergistic therapy that could decrease the growth of certain tumors. Wang et al. (Wang et al., 2017) reported the surface modification of MWCNTs, using poly (N-vinyl pyrrole) and conjugation with functionalized folic acid, using a thiolenated polyethylene glycol (Figure 7A, C), which increased the time in the circulation and the drug loading capacity. The loading ratio of doxorubicin was 453% and 365% of the drug loading ratio in MWCNTs coated with poly (N-vinyl pyrrole)-folic acid and poly (N-vinyl pyrrole), respectively. Moreover, in an in vitro drug release study in 10 mM of phosphate buffer at pH 5.5 and 7.4, the MWCNTs coated with poly (N-vinyl pyrrole)-folic acid released a significantly greater amount of doxorubicin at pH 5.5, compared to pH 7.4, indicating a pH responsive drug release that allows for the targeting of the acidic tumor microenvironment. The in vitro incubation of Hela cells with Dox - loaded MWCNTs formulation and pure MWCNTs formulation produced as 40% and 80% cell viability, respectively, results indicated pure MWCNTs formulations are nontoxic and better carrier for delivery of Dox. Yang et al. (Yang et al., 2017) reported the potential use of CNTs as a dual drug delivery system (DDDS), by incorporating cisplatin and doxorubicin (DOX) in MWCNTs, using a wet-chemical approach with folic acid and polyethylene glycol. DOX was attached to the external surface by non-covalent interactions, whereas cisplatin was encapsulated inside the nanotube. The antitumor efficacy of DDDS in MCF7 breast cancer cells was determined at pH 6.4 and 7.4. Based on the percent viable MCF7 cells, DOX produced significant antitumor efficacy at pH 6.5, compared to pH 7.4, after 72h of incubation. It has been reported that cisplatin can be encapsulated inside nano-vector MWCNTs, with external surface attachment of doxorubicin (Figure 7A, D). In addition, folic acid and polyethylene glycol were used to increase the drug loading capacity. The % DOX loading efficiency was increased in the folic acid modified MWCNTs, compared to non-modified MWCNTs was 192.67% and 174.07% of DOX loaded folic acid modified MWCNTs and DOX - loaded pristine MWCNTs, respectively. Furthermore, cisplatin was shown to be loaded in the pristine MWCNTs (92.80%) as compared to folic acid modified MWCNTs (84.56%). The cumulative release of the DOX-loaded folic acid MWCNTs and cisplatin - loaded folic acid MWCNTs was determined using a UV spectroscopy method at pH 7.4 and pH 6.5. At pH 6.5, the release of DOX and cisplatin was 22% and 26% respectively, which was significantly greater than that obtained at pH 7.4 (8% DOX release and 13% cisplatin release) after 72 h of incubation. The cytotoxicity experiments indicated that in MCF-7 cancer cells, the antitumor efficacy of DDDS was significantly greater at pH 6.5, compared to pH 7.4, as the acidic pH increases the release of DOX and cisplatin from the folic acid conjugated MWCNTs (Fraczyk et al., 2017).
5-Flurouracil (5-FU) was functionalized on the surface of MWCNTs, using folic acid and PEG for targeted delivery by Kaur et al. (Kaur et al., 2017). The sequestration of 5-FU in functionalized MWCNTs (using the carboxylic group of folates that were conjugated with the amino group of PEGylation bis - amine) (Figure 7A, E) was synthesized to target MCF-7 breast cancer cells. In vitro, 5-FU - loaded FA-PEG modified MWCNTs (20% cell viability) more inhibited the MCF-7 cells as compared to pure 5-FU (60% cell viability) at concentration 10 μg/mL. Following the i. v administration (3 mg/kg) of free 5-FU and 5-FU functionalized MWCNTs, in Wistar rats, the elimination half-lives (t1/2) were 5.62 and 16.48 h, respectively, These clearly indicated that the 5-FU loaded FA-PEG modified MWCNTs was more efficacious than free 5-FU in decreasing the proliferation of MCF7 cells. Mehra and Jain (Mehra et al., 2013) developed a novel, site effective delivery system by loading doxorubicin (DOX) PEGylated MWCNTs conjugated with folic acid (Figure 7A, F). The IC50 of DOX functionalized MWCNTs was 15 μg/mL, which was significantly lower than that for free DOX (50 μg/mL), due to its targeted interaction with the folic acid receptors on the cancer cells. The bio-distribution profile in mice indicated the accumulation of DOX in the liver, tumors and kidneys, after the i. v. administration of 5 mg/kg of the DOX functionalized MWCNTs. The elimination half-lives for the DOX functionalized MWCNTs and free DOX formulations were 14.96 h and 1.89 h, respectively. Thus, compare to free Dox, the levels of the DOX functionalized MWNCTs were greater in the tumors and its clearance time was greater than that for free DOX. The novel ethylenedioxy)-bis-ethyl ammonium (EDBE) modified MWCNTs (Figure 7A, G) were synthesized, using theranostic prodrug conjugation with methotrexate, folic acid (the target ligand), a radiotracer (Technitium-99m) and a fluorochrome (Alexa-fluor, AF488/647) (Das et al., 2013a). The in vitro stability indicated that the non-modified MWCNTs compounds had a stability of 91%–94% after 24 h of incubation in PBS at pH 7.4. The folic acid conjugated MWCNTs accumulated in MCF-7 and A549 cancer cells, as these cancer cells overexpress folic acid receptors (Kaittanis et al., 2009; Santra et al., 2009). In contrast, in Neuro 2A cells, which do not express folic acid receptors, there was no accumulation of the folic acid conjugated MWCNTs. In vitro, methotrexate and FA-modified MWCNTs-methotrexate inhibited the proliferation of MCF-7 cells (IC50 values of 7.36 and 1.95 µM, respectively) and A549 cell (7.26 and 2.13 µM, respectively). Thus, in vitro, the modified MWCNTs were more efficacious than free methotrexate in inhibiting the proliferation of MCF7 and A549 cancer cells. Das et al. (2013a) reported that 24 h after the i. v. administration of 5 mg/kg of free methotrexate and MWCNTs-conjugated methotrexate in 7, 12- dimethylbenzα anthracene (DMBA) carcinogen induced female Sprague-Dawley rats, the tumor growth inhibition of free methotrexate and MWCNTs-conjugated methotrexate was 25.37% and 38.37% after 24 h respectively. The inhibition of tumor growth, 1 week after 2 i. v. treatments with free methotrexate and MWCNTs-conjugated methotrexate was 80% and 50%. Thus, the MWCNTs-conjugated methotrexate formulation was significantly more efficacious than free methotrexate. Twenty-four hours after the i. v. administration of free methotrexate high levels was present in the stomach, intestine and heart. In contrast, the amount of methotrexate loaded MWNCTs in the stomach, intestine and heart was 0.04 ± 0.01, 0.07 ± 0.01, 0.05% ± 0.01% injected dose (ID)/g, respectively. According to these finding MWCNTs-conjugated methotrexate formulation are more effective in tumor and less accumulated in organs as compared to free methotrexate (Das et al., 2013a; Das et al., 2013b).
Das et al. (2013b) designed a bioactive functionalized MWCNTs (see Figure 7A, H) linked to estradiol (EA), folic acid (FA), hyaluronic acid (HA), a modified polymer (polyethyleneglycol), fluorophores (AF-647), and the anticancer drugs, methotrexate (MTX), doxorubicin (Dox) and paclitaxel (PTX). These multifunctionalized MWCNTs formulations were individually loaded with MTX, Dox or PTX. The % drug loading efficacy of PTX (91.4% ± 2.6%) was less than that for MWCNTs-FA-MTX (97.8% ± 2.1%) and MWCNTs-FA-Dox (97.8% ± 2.4%). The IC50 value of compounds in A549 cells, HeLa cells and MCF-7 cells is shown in Table 1, Based on the IC50 values, the MWCNTs-FA loaded with anticancer drugs were more efficacious than the pure anticancer drug alone.
The conjugation of folic acid to MWCNTs containing gemcitabine (Figure 7B, I), was developed to target human breast cancer cells (MCF-7) (Singh et al., 2013). The % drug loading efficiency of gemcitabine was greater in the modified MWCNTs (79.60 ± 0.45), compared to the non-modified MWCNTs (71.60 ± 0.25). In vitro, the incubation of MCF-7 cells with 80 μg/mL of MWNCTs loaded with gemcitabine produced a 40% decrease in cell viability, compared to 20% for free gemcitabine. Bio-distribution experiments in Sprague-Dawley rats indicated that 1 h after the i. v. administration of 1 mg/kg of gemcitabine formulated MWCNTs, the levels of gemcitabine were significantly lower in the liver, speen, stomach, kidney, compared to 1 mg/kg i. v. of pure gemcitabine. In vivo pharmacokinetic data indicated that the bioavailability and plasma levels of the MWCNTs formulated with gemcitabine was significantly greater than that of pure gemcitabine. Finally, the incubation of human red blood cells with conc. 500 μL of effect of MWCNTs formulated with gemcitabine or pure gemcitabine produced a level of hemolysis of 8.23% ± 0.65% and 17.34% ± 0.56%, respectively, indicating the gemcitabine formulated MWNCTs were less toxic than pure gemcitabine.
Lu et al. (Lu et al., 2012) designed MWCNTs noncovalently grafted with poly (acrylic acid), iron-oxide magnetic nanovectors and a folate ligand (Figure 7A, J), and the nanotubes were loaded with doxorubicin for targeted drug delivery. The drug loading capacity of the formulation was increased, as was the cytotoxic efficacy. The loading capacity of the MWCNTs for Dox was 96% when the Dox concentration was 0.5 mg, whereas the loading capacity for Dox was 92%, when the Dox concentration was 1 mg. The percentage cumulative release of Dox at pH 5.3 and 7.4, after 192 h of incubation, was 71% and 14%, respectively. The proliferation of U87 glioblastoma cells was inhibited by pure Dox (IC50 = 50 μg/mL) and Dox-loaded MWCNTs (IC50 = 15 μg/mL). The conjugation of glycine to MWCNTs/C60 fullerene, using the 1,3-dipolar cycloaddition method, was used to increase the targeted delivery of methotrexate (MTX) (Joshi et al., 2017). The MTX loading efficiency was greater in the C60 fullerene (79.98% ± 3.21%) formulation, as compared to the MWCNT formulation (75.47% ± 1.79%). The in vitro incubation of MDA-MB-231 breast cancer cells with the C60 fullerene-MTX compound produced a 2.6-folds greater decrease in cell viability, compared to pure MTX. The i. v administration of 2.5 mg/kg of MTX equivalent (either as pure MTX, C60 fullerene and MWCNTs formulation) to Wistar rats indicated that the C60 fullerene and MWCNTs formulations significantly increased the half-life (6.84 and 8.85 h, respectively) of MTX, compared to the pure MTX formulation (2.49 h). Furthermore, the clearance of MTX was significantly lower for the C60 fullerene and MWCNTs formulations (0.0047 mL/min and 0.0034 mL/min, respectively), compared to pure MTX (0.019 mL/min). The AUC for the C60 fullerene and MWCNTs formulations was greater (129.08 μg/mL/h and 179.92 μg/mL/h, respectively), compared to pure MTX (30.96 μg/mL/h).
Li et al. (Li et al., 2011) reported the synthesis of MWCNTs conjugated with folate and iron nanoparticles for the dual-targeted delivery of doxorubicin to HeLa cells. Initially, the folic acid and FITC were individually conjugated with hexamethylene diamine, then bonded with the carboxylic acid group of MWCNTs and encapsulated with iron nanoparticles or Dox (Figure 7B, K). The loading efficiency of Dox in the Dox - loaded MWCNTs nanocarrier and Dox loaded iron nanocarrier was 32 μg/mg and 24 μg/mg, respectively. The % cell viability of pure Dox and the Dox-loaded MWNCTs was 80% and 20% at 40 μg/mL concentration, results finding indicated Dox loaded MWCNTs are more effective in Hela cell as compared to pure Dox. MWCNTs-folic acid modified dendrimers (PAMAM) were synthesized to target KB-HFAR and KB-LFAR human mouth epidermal cancer cells (Shi et al., 2009). The aminated dendrimers (De) were noncovalently bonded to folic acid (FA) and FITC and subsequently linked with carboxylated MWCNTs (Figure 7B, L). The FA/FI/De modified MWCNTs compound produced a concentration-dependent inhibition of the proliferation of KB-HFAR cells due to the presence of the folic acid ligand that interacts with folic acid receptors. However, the FI/De modified MWCNTs nanocarriers did not interact with KB-HFAR cells as this formulation lacked a folic acid ligand. KB-LFAR cells did not interact significantly with either FA/FI/De or FI/De modified MWCNTs nanocarriers due to absence of folic acid receptor on KB-LFAR cancer cells. The proliferation the KB-HFAR and KB-LFAR cells was not significantly altered by incubation with FA/FI/De or FI/De modified MWCNTs nanocarriers (1–100 μg/mL). Overall, these data suggest that the MWCNTs formulation in this study were biocompatible and safe for targeted drug delivery. Wang et al. (Wang et al., 2019) designed a novel MWCNTs-folic acid-protohemin modified/encapsulated compound that was covalently conjugated with oridonin (Figure 7B, M), an anticancer drug, to target its delivery to human HepG2 cancer cells. In vitro, 12 μg/mL of oridonin loaded liposome or the folic acid modified oridonin MWCNTs formulation inhibited the proliferation of HepG2 cells by 42.3% ± 2.9% and 95.4% ± 5.9%, respectively. The folic acid modified MWCNTs had the highest uptake into HepG2 cells due to the presence of folic acid receptors on their surface. In BALB/c male mice bearing HepG2 cells, the i. v. administration of 0.01 g/kg/day of either oridonin loaded liposome or folic acid modified oridonin MWCNTs for 10 days decreased tumor growth by 32.5% and 90.0%, respectively.
A multifunctional drug delivery nanocarrier of doxorubicin - loaded MWCNTs, with covalently adhered polyethyleneimine, FITC, polyethylene glycol and folic acid (Figure 7B, N), was synthesized to target Hela cancer cells. In vitro, the proliferation of HeLa cells was inhibited by pure Dox (IC50 = 3.45 mg/L) and Dox - loaded folate conjugated MWCNTs (3.53 mg/L). In HeLa-HFAR (high folic acid receptors) and HeLa-LFAR (low folic acid receptor) cells, incubation with the Dox - loaded folate conjugated MWCNTs inhibited their proliferation by 84.5% and 54.2%, respectively. These results indicate that a low level of folic acid receptors decreases the efficacy of the MWCNTs conjugated with folic acid. An intravenous injection of 5 mg/kg equivalent of Dox, Dox + folate conjugated MWNCTs or MWNCTs alone produced a 42.5, 73.3% and 9.1% increase, respectively in the apoptosis of tumors cells in male BALB/c nude mice xenografted with Hela tumors. Twelve hours after the i. v. administration of 5 mg/kg of Dox-loaded folate conjugated MWCNTs in male BALB/c nude mice, Dox was present in the heart, kidney, liver and spleen, at 5.99, 1.50, 20.15% and 7.49%, respectively, of the injected dose per Gram of tissue (% ID/g) (Yan et al., 2018).
Docetaxel or coumarin-6 encapsulated with chitosan-folic acid modified MWCNTs, was reported to increase the delivery of docetaxel and coumarin-6 to A549 cancer cells, which overexpress folic acid receptor, thereby increasing the drug loading capacity. Chitosan links folic acid and MWCNTs via covalent bonds between the carboxylic group of the folates and the amino group of chitosan (forming an amide bond formation), whereas the hydroxy group of chitosan interacts with the carboxylic groups of MWCNTs, forming an ester bond (Figure 7B, O). The drug loading capacity of docetaxel and coumarin-6 were increased in functionalized MWCNTs (79.29% ± 1.3% and 83.62% ± 1.2%, respectively), compared to non-functionalized MWCNTs (59.72% ± 2.3% and 63.08% ± 3.2%, respectively). The proliferation of A549 cells was inhibited by docetaxel formulated in MWNCTs (IC50 = 0.56 μg/mL) and docetaxel (IC50 = 50.19 ± 2.5 μg/mL). The intracellular levels of docetaxel formulated in MWNCTs was increased in the A549 cells, due to the presence of chitosan and folic acid in the formulation (Singh et al., 2017).
Wang et al. (Wang et al., 2017) synthesized a compound where folic acid was noncovalently adhered to gold nanoparticles with polypyrrole that was grafted layer by layer with MWCNTs that contained doxorubicin bound by π-π stretching interactions, for cancer therapy (Figure 7B, P). In vitro, the release of docetaxel at pH 5.5 and 7.4 was 25% and 5%, respectively, after 72 h of incubation. This is important as the pH of cancer cells is typically acidic (Logozzi et al., 2018; Lyra et al., 2021) and thus, more drugs should be released at the tumor site. The in vitro cytotoxicity studies indicated that the docetaxel - loaded functionalized MWCNTs, at 50 μg/mL highest conc. used, inhibited the proliferation of Hela cells and H9C2 cardiomyoblast cells, by 70% and 65%, respectively. In contrast, the functionalized MWCNTs (maximal conc. 5 μg/mL–100 μg/mL) without docetaxel, decreased HeLa and H9C2 cardiomyoblast cell proliferation by 30% and 26%, respectively.
Ozgen et al. (Ozgen et al., 2020), synthesized a novel glycol-block copolymer conjugated MWCNTs for dual targeting delivery in breast cancer. For the preparation of glycol-block copolymer using poly (1-O-methacryloyl-b-D- fructo-pyranose-b-(2-methacryloxyethoxy)) benzaldehyde in which Dox was attached then these copolymer noncovalently coupled with MWCNTs further conjugated with folic acid for dual targeting of folate receptor as well as glucose transporter protein in MDAMB231 and MCF7 breast carcinoma cells. Developed copolymer and folic acid conjugated MWCNTs formulation showed higher dispersibility in aqueous medium as compared to pristine MWCNTs. Cell viability and Apoptosis studies were performed in MDAMB231 and MCF7 breast carcinoma cells and results were found as copolymer and folic acid conjugated MWCNTs formulation showed higher cell viability or apoptosis (96.58% in MDAMB231 or 87.46% in MCF7) as compared to pure Dox (72.80% in MDAMB231 or 42.68% in MCF7) & without folic acid conjugated copolymer MWCNTs formulation (81.24% in MDAMB231 or 85.00% in MCF7) after 24 h s of incubations, these finding data demonstrated developed formulations enhanced the dispersibility, apoptosis and provided dual targeted effect in breast cancer cells.
Zhou and coworker (Zhou et al., 2022), developed a novel nanocarrier functionalized carboxylated MWCNTs with polyethylene glycol, hyperbranched poly-L-lysine cross linked with adipic acid and finally conjugated with folic acid ligand, this functionalized MWCNTs nanocarrier loaded with Dox for targeted delivery of cancer cells like HepG2 (human liver cancer) and HEK293 (human embryonic kidney). Cell viability and Apoptosis studies were performed in HepG2 and HEK293 cancer cell and results was found as MWCNTs nanocarrier loaded with Dox showed higher cell viability (>20%) as compared to pure Dox (>40%) and pure MWCNTs nanocarrier showed less cytotoxicity in both the cell line (>90%). Prepared formulations are biocompatible and safe for delivery of anticancer drug.
2.1.2 Riboflavin and thiamine
Singh et al. (Singh et al., 2016 (a)) synthesized novel forms of the ligands, riboflavin (Figure 8B) and thiamine (Figure 8C), for the targeted delivery of paclitaxel to the breast cancer cell line, MCF-7. Paclitaxel - loaded MWCNTs were conjugated with the receptor targeted ligands, thiamine or riboflavin, and their levels were determined in the MCF-7 cells. In this formulation, the pristine MWCNTs were chemically modified by carboxylation, acylation and amination, and the ligand was ultimately conjugated with amine groups in the MWCNTs to form an amide bond, using an ethylenediamine linker. The in vitro incubation of MCF-7 cells with conc. (10–80 μg/mL) of pure paclitaxel or the MWNCTs-paclitaxel formulation decreased their viability by 40% or 20%, respectively. The MWNCTs-paclitaxel formulation was more efficacious than pure paclitaxel due to their nanosize, which increased their penetration across the tumor cell membranes, increasing the magnitude of tumor cell apoptosis. Following the i. v. administration of 10 mg/kg of pure paclitaxel to Sprague Dawley rats, the plasma conc. of paclitaxel was less than 5 μg/mL after 12 h, result indicated pure paclitaxel showed rapid clearance from blood plasma. In contrast, following the i. v. administration of 10 mg/kg i. v. of the paclitaxel loaded MWCNTs formulations, the plasma conc. of paclitaxel was 5 μg/mL at 36 h, showed the presence in blood for long duration as compared to pure paclitaxel. The % of human RBC hemolyzed after incubation of human RBC with conc. 0.5 mL of PTX functionalized in MWCNTs containing thiamine or 0.5 mL conc. of riboflavin, was 14.6 ± 0.84 and 11.17 ± 0.77, respectively, which was lower than that for 0.5 mL conc. of pure paclitaxel (20.49 ± 0.97) and 0.5 mL conc. of PTX functionalized in MWCNTs (37.39% ± 0.78%).
2.1.3 D-α-tocopheryl polyethylene glycol 1000 succinate
Vitamin E (also known as D-α-tocopherol) conjugated with polyethylene glycol 1000 produced the compound, D-α-tocopheryl polyethylene glycol 1000 succinate (TPGS) (Pawar et al., 2016). It was a water-soluble non-ionic compound that increased the solubility, absorption, bioavailability and efficacy of camptothecin, taxanes, docetaxel, coumarin-6, paclitaxel, emodin, gambogic acid, doxorubicin, cisplatin, quercetin and toptecan. Furthermore, TPGS loaded with plumbagin/gambogic acid circumvents multidrug resistance by inhibiting P-glycoprotein in MCF-7 breast cancer cells (Dou et al., 2014; Singh et al., 2016).
The covalent conjugation of TPGS on the noncovalent surface of the MWCNTs (Figure 9) was synthesized and loaded with docetaxel/coumarin 6 to increase the therapeutic efficacy of these drugs for A549 lung cancer cells (Singh et al., 2016 (b)). Cellular uptake assays indicated a higher concentration of the docetaxel-loaded TPGS-MWCNT compounds, compared to pure docetaxel, in the A549 cells. The loading efficiency of docetaxel in the TPGS coated MWCNTs and the non TPGS coated MWCNTs was 71.44% ± 1.8% and 76.53% ± 1.3%, respectively, whereas the loading efficiency of coumarin-6 in noncoated TPGS-MWCNTs was 62.61% ± 2.2% and 60.04% ± 2.8%, respectively. In vitro, A549 cells incubated with 5 μg/mL concentration of coumarin 6 loaded with TPGS coated MWCNTs, noncoated TPGS-MWCNTs and free coumarin 6. The results clearly indicated that a significantly greater amount of the MWCNTs loaded with coumarin 6 accumulated in the cytoplasm of the A549 cancer cells, compared to free coumarin 6. In vitro, pure docetaxel, docetaxel loaded TPGS coated MWCNTs and noncoated TPGS-MWCNTs inhibited the proliferation of A549 cells (IC50 values of 45.98 ± 1.7, 0.57 ± 0.02 and 1.49 ± 0.21 μg/mL, respectively). These results indicated that docetaxel - loaded TPGS coated MWCNTs were more efficacious than free docetaxel and noncoated TPGS-MWCNTs in inhibiting the proliferation of A549 cells.
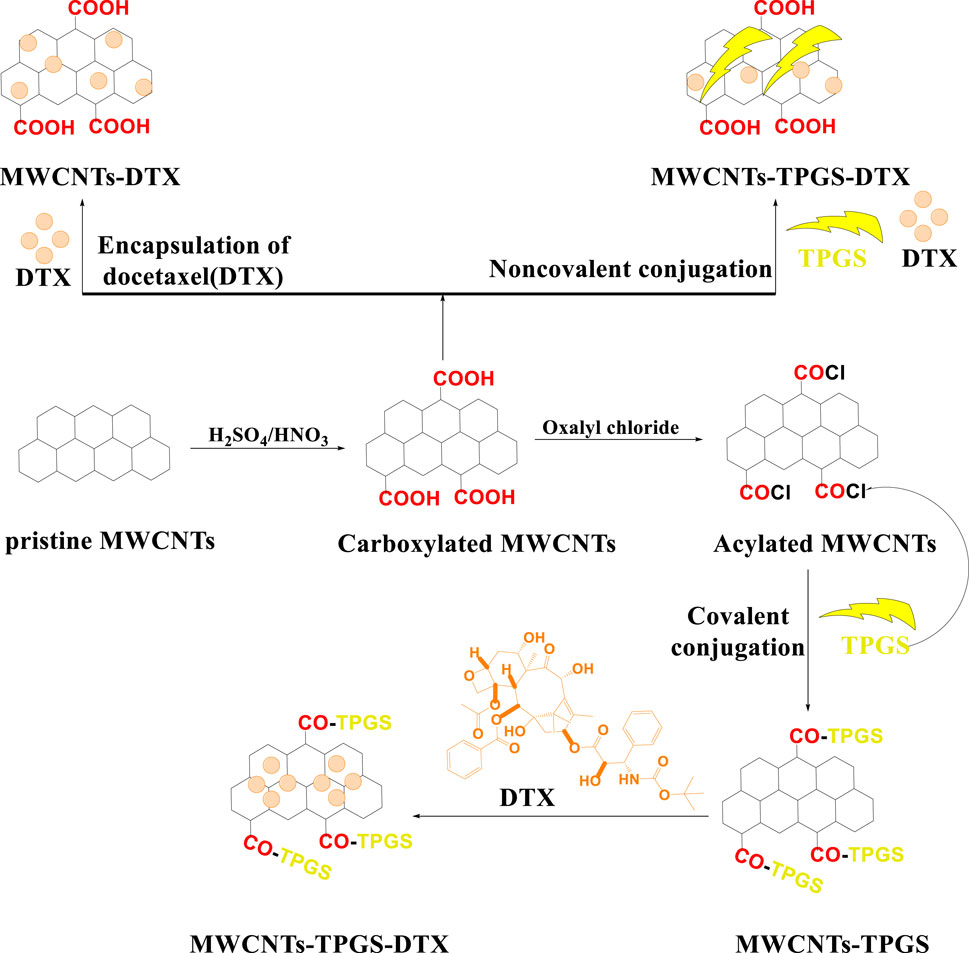
Figure 9. A schematic representation of MWCNTs covalently and noncovalently conjugated with the ligand, D-α-tocopheryl polyethylene glycol 1000 succinate (TPGS).
Singhai et al. (2020) developed effective novel functionalized MWCNTs using α-tocopheryl succinate and chondroitin sulphate for improving therapeutic effect of Dox. Functionalized MWCNTs showed high cellular uptake in MDA-MB-231 breast cells as compared to pure Dox and which showed high apoptosis rate (53.40% ± 3.32%) compared to pure Dox (17.20% ± 1.22%) respectively. This functionalized MWCNTs has also enhanced the Dox loading capacity and provided pH dependent release, that is beneficial for cancer therapy.
2.2 Gonadotrophin releasing hormone (GnRH)
Studies have shown that the receptor for the protein, GnRH, is overexpressed in many types of cancers, particularly in the plasma membrane (Chen et al., 2002). Based on this, Moretti et al. covalently bonded GnRH to oxidized MWCNTs to target DU145 prostate cancer cells as they have been reported to overexpress GnRH receptors (Moretti et al., 2014). The GnRH - modified MWCNTs had a high magnitude of interaction with the cytoplasm of DU145 cells, compared to non-modified pristine MWCNTs. These results indicate that GnRH - modified MWCNTs can bind to the GnRH receptor and induce DU145 cell death. In addition, the GnRH - modified MWCNTs interacted with HeLa cells but not L929 cells, as these cells express low levels of GnRH. In vitro, the GnRH modified MWCNTs (0.010–0.050 mg/mL) decreased the proliferation of DU145 and HeLa cells by 85% and 35%, respectively, but it did not significantly alter the proliferation of L929 cells. The pristine MWCNTs (0.010–0.050 mg/mL) decreased the proliferation of HeLa, DU145 and L929 cells and GnRH (0.010–0.050 mg/mL) also decreased the proliferation of HeLa, DU145 and L929 cells, by above 80% cell viability in all cells respectively. The results of this study indicate that GnRH - modified MWCNTs have a high selectivity for detecting cancer cells expressing GnRH receptors.
2.3 Polymers
Hyaluronic acid is a glycosaminoglycan polysaccharide that consists of glucuronic acid and N-acetylglucosamine (Smith et al., 2016). Hyaluronic acid can be used as a ligand to target the hyaluronic receptor in cancer cells and to increase the selectivity and therapeutic efficacy of the anticancer drug, doxorubicin (Dox) (Datir et al., 2012; Lokeshwar et al., 2014), because hyaluronic acid delivers drugs to targeted sites (Chadar et al., 2021). Datir et al. (Datir et al., 2012) covalently modified MWCNTs, using ethylenedioxy-bis-ethylammonium and then incorporated doxorubicin conjugated with hyaluronic acid, (Figure 10B), to target hyaluronan receptors in A549 lung cancer cells. In the lung cancer cells, the optimized MWCNT nanoformulation was 3.2-fold more efficacious in inhibiting the proliferation and 5-fold more efficacious in inducing apoptosis, compared to a solution of only doxorubicin.
Cao et al. (2015) developed an innovative nanocarrier for specific targeted drug delivery by conjugating hyaluronic acid and fluorescein isothiocyanate with modified MWCNTs using polyethyleneimine (10A). In this formulation, Dox was incorporated for targeted delivery to L929 (a mouse fibroblast cell) and HeLa cells which express CD44 receptors (Wang et al., 2016; Liu et al., 2018). The Dox loading capacity, using a UV spectroscopy method, was 72.0% in multifunctionalized MWCNTs. Dox release experiments indicated that a greater % of Dox was released at pH 5.8 (54.6%), compared to pH 7.4 (40.8%). This is important as the pH of the tumor microenvironment is typically acidic and thus, more Dox will be available to interact with the tumor cells. The cytotoxicity experiments indicated that the Dox - loaded multifunctionalized MWCNT and pure Dox decreased HeLa cell proliferation by 40% and 60%, respectively. The non-Dox multifunctionalized MWCNTs did not significantly alter the proliferation of the HeLa cells, suggesting that multifunctionalized MWCNTs should be safe and biocompatible. Using flow cytometry, it was shown that L929 cells expressed a low level of CD44, whereas HeLa cells expressed a high level of CD44. These results explain why multifunctionalized MWCNTs uptake in HeLa cell was seven times greater than that of L929 cells. Thus, the formulation reported in this study selectively targets cancer cells that overexpress CD44.
Singhai et al. (2020) synthesized MWCNTs using α-Tocopheryl succinate and hyaluronic acid, then loaded with Dox to develop a novel and effective nanocarrier for targeting the CD44 receptor which is overexpressed in MDA-MB-231 breast cancer. The cytotoxicity results indicated that functionalized MWCNTs showed high growth inhibition as compared to pure Dox with GI50 values of 0.810 ± 0.017 and 2.621 ± 0.153 μg/mL respectively. This finding revealed that hyaluronic acid is used to target the CD44 receptor for breast cancer therapy.
Dong et al. (2017) formulated doxorubicin encapsulated with a chitosan modified MWCNTs (Figure 11A), where the acidic groups of the carboxylated MWCNTs were covalently conjugated with the free amino group of chitosan to form an amide bridge, and doxorubicin was encapsulated inside these modified nanotubes. In vitro, the percent loading capacity for MWCNTs: Dox, at ratios of 1:1, 2:1 and 1:3 were 24%, 33%, and 50%, respectively. The release of Dox from the MWCNT chitosan formulation was significantly greater at pH 5.8 (more than 75% Dox release) compared to pH 7.4 (less than 20% Dox release) after 72h of incubation. The uptake of pure Dox was higher than that of Dox - loaded chitosan MWCNTs, after 4 h of incubation with BEL-7402 cancer cells. However, the uptake of the Dox - loaded chitosan MWCNTs by BEL-7402 cells was greater than that of pure Dox, most likely due to the prolonged release of Dox from the nanocarrier system. Pure Dox, chitosan modified MWCNT and Dox - loaded chitosan MWCNTs produced a 10%, 75% and 9% decrease, respectively, in the proliferation of BEL-7402 cancer cells. PEG containing MWCNTs were synthesized by Lay et al., (2010) for the targeted delivery of paclitaxel to MCF-7 and HeLa cancer cells, using monomethyl polyethylene glycol (PEG) grafted MWCNTs (Figure 11B) formed by an endohedral process. The percentage of paclitaxel loaded by the PEG modified MWCNTs was 45% and the release of paclitaxel from this formulation at pH 5.0, 7.0 was 42% and 38%, respectively, compared to only 11% for paclitaxel as free paclitaxel had a lower aqueous solubility compared to paclitaxel loaded in the MWCNTs and the slower rate of release free paclitaxel. However, the PEG - modified MWCNTs provided a sustained release of paclitaxel over a period of 40 days at pH 5 and 7. Pure paclitaxel inhibited the proliferation of MCF7 and HeLa cells (IC50 values of 0.10 μg/mL and 0.021 μg/mL respectively), as did the paclitaxel loaded MWCNTs in MCF7 (IC50 value of 0.080 μg/mL) and HeLa cells (IC50 value of 0.010 μg/mL).
A multifunctionalized magnetically modified nanocarrier for targeted drug delivery to cancer cells was reported by Rezaei et al., (2018) Pristine side - walled MWCNTs were modified with cis-Pt (1,7-phenanthroline), ferric oxide, PEG, and poly (citric acid), followed by encapsulation of cisplatin (Figure 11C). The cisplatin loading capacity of the multifunctionalized MWCNTs was 86% and 76.6% of the cisplatin was released at pH 5.6, compared to 16.5% at pH 7.4, for up to 50 h. In vitro, the multifunctionalized MWCNTs loaded with cis-platin (concentration - 25, 50, 100 μg mL−1), inhibited the proliferation of MDA-MB-231 and HeLa cancer cells (less than 20% viability for these cell lines). In contrast, non-drug loaded multifunctionalized MWCNTs decreased the viability of MDA-MB-231 and HeLa cells 4% and 2%, respectively Thus, in vitro, the carrier alone was nontoxic, suggesting that it would be safe carrier for drug delivery.
The compound, curcumin (which has anticancer efficacy; (Allegra et al., 2017; Hindumathi et al., 2018; Rodrigue et al., 2019)) was incorporated into a biocompatible polyethylene glycol functionalized MWCNTs (Figure 11D) nanocarrier to target C6 cancer brain cells (Hindumathi et al., 2018). This formulation, compared to curcumin alone: 1) increased the drug loading of curcumin by 30%; 2) increased the uptake in the C6 brain cancer cells and 3) increased the solubility of curcumin in the aqueous medium. However, curcumin alone was not present in the C6 brain cancer cells as it did not cross cell membrane. The curcumin loaded MWNCTs, at 50 μg/mL, produced as 95% inhibition of the proliferation of C6 brain cancer.
The covalent conjugation of a poly (acrylic acid)-PEG copolymer with MWCNTs was synthesized to increase the delivery and efficacy of cyclophosphamide (Figure 11E) and methotrexate (Figure 11F) (Azghandi et al., 2017). The acylated MWCNTs reacted with the carboxylic acid moieties of PEG to form an ester link grafted with polymer polyacrylic acid to form a novel polymeric nanocarrier. The drugs, cyclophosphamide and methotrexate were loaded on to the MWCNTs and their % release in the buffer media at pH 4, was >80% for cyclophosphamide and >80% for methotrexate, after 1 h of incubation and at pH 7.4, 80% of both drugs (cyclophosphamide and methotrexate) was released after 3 h of incubation in PBS. Both drugs were released in a sustained pattern at pH 4 and their release was faster at pH 7.4.
Raza et al. (2016) designed a carboxylated MWCNTs containing piperine and covalent side walls modified with docetaxel (Figure 11G) for the targeted delivery of docetaxel in MCF-7 and MDA-MB-231 triple-negative breast cancer cells. In vitro experiments indicated that pure docetaxel, piperine and modified MWCNTs inhibited the proliferation of MCF-7 (IC50 values of 25, 121 and 8 μg/mL, respectively) and MDA-MB-231 (IC50 values of 15, 72.6 and 6 μg/mL, respectively) cells. The incubation of rat red blood cells with 2% (w/v) of the docetaxel conjugated piperine - modified MWCNTs for 1 h at 37°C, lysed <5% of the cells. In vivo pharmacokinetic experiments in male Wistar rats indicated that following the i. v. administration of 5 mg/kg equivalent of docetaxel (pure docetaxel and the Dox MWCNT formulation), there was a 50% decrease in the clearance and a 6.4-fold greater AUC for the Dox MWCNT formulation, compared to pure docetaxel.
Polymeric nanocarriers, built on chitosan covalently attached to MWCNTs, were loaded with the anticancer drug, 5-fluorouracil (Figure 11H) (Nivethaa et al., 2016). The 5-fluorouracil loading efficiency was 97% for the chitosan modified MWCNTs. At pH 5.0, 71.2% of the loaded 5-fluorouracil was released after 72 h in the release medium. The proliferation of MCF7 cells was decreased by 50%, after incubation with 100 μg/mL) of the 5-fluorouracil - loaded formulation.
Pistone et al. (2016) developed a drug delivery carrier, where doxorubicin was encapsulated with PEG and polylactic acid (PLA) modified MWCNTs (Figure 11I), for the targeted release of doxorubicin to HepG2, SH-SY5Y and HT-29 cancer cells. The loading capacity of the Dox - loaded functionalized MWCNTs was 25.4 wt%. The incubation of HepG2, SH-SY5Y and HT-29 cancer cells with 1–10 μg/mL of the Dox - loaded MWCNTs or Dox alone, decreased cell proliferation by <20%. The modified MWCNTs without Dox did not significantly alter the proliferation of HepG2, SH-SY5Y and HT-29 cancer cells, compared to Dox - loaded MWCNTs and Dox alone. The results indicated that pure MWCNTs modified formulation are nontoxic and a safe carrier for the delivery of Dox.
Selim et al., (2023) was formulated biotinylated chitosan noncovalently functionalized with MWCNTs for breast carcinoma treatment with using neratinib as a model drug which is having capacity to inhibit the tyrosine kinase. Firstly, MWCNTs was modified to carboxylated MWCNTs then noncovalently attached with biotinylated chitosan and finally loaded neratinib. Then prepared formulation was characterized by different spectroscopy technology and determine drug loading efficiency was 95.6%. Invitro release results of developed formulations shown in acidic condition showed high release upto 90% drug release at 72 h s but in physiological condition drug release below 40% at 72 h s, this results clearly showed developed formulation provided pH depended on release or it is essential for cancer treatments. SkBr3 cell line (human breast cancer cell line) was used to perform the cell cytotoxicity of free neratinib and neratinib loaded formulations, finding results was observed that free neratinib (IC50 value - 548.43 mg/mL) showed low cytotoxicity as compared to neratinib loaded formulations (IC50 value - 319.55 mg/mL & 257.75 mg/mL) respectively. Biotinylated chitosan noncovalently functionalized with MWCNTs formulations showed higher cell cytotoxicity as compared to without biotinylated chitosan functionalized MWCNTs formulations. Finding results clearly indicated biotinylated chitosan functionalized MWCNTs formulations enhanced the cytotoxicity as well as provided pH depended on releases.
Lyra et al., (2021) were formulated novel and effective guanidinylated dendritic conjugated MWCNTs for cancer targeting and Dox was loaded into modified formulations. Guanidinylated dendritic conjugated MWCNTs formulations showed higher drug loading efficiency as 99.5% as compared to unmodified MWCNTs as 78.7% respectively. Guanidinylated dendritic conjugated MWCNTs formulations enhanced the dispersibility in aqueous medium compared to pure MWCNTs, it is also enhanced the apoptosis and cell viability compared than pristine MWCNTs, Guanidinylated dendritic conjugated MWCNTs formulations showed higher cell viability in PC3 and DU145 human prostate carcinoma cells upto 40% and in normal cells HEK293 showed negligible cytotoxicity more than 90%. Finding results clearly shown prepared formulation enhanced the cell cytotoxicity, dispersibility as well as loading capacity.
2.4 Carbohydrate
The carbohydrates, galactose, lactose, mannose and fucose, among others, can be conjugated or modified to MWCNTs to form glyconanotubes, to increase the cellular uptake and efficacy of certain anticancer drugs (Chen et al., 2013).
2.4.1 Galactose
Qi et al. Qi et al. (2015) designed doxorubicin-loaded MWCNTs conjugated with galactosylated chitosan to target the liver cancer cell line (HepG2). Initially, the pristine MWCNTs undergo oxidation in the presence of sulphuric and nitric acid. Subsequently, they are non-covalently conjugated with galactosylated chitosan and finally, doxorubicin is loaded into this nanoformulation, as shown in Figure 12. The loading ratio of the Dox-loaded galactosylated MWCNTs was 25% ± 2%. The cumulative percentage release of Dox from the loaded galactosylated MWCNTs, at pH 7.4, 6.5 and 5.5 was 30%, 35% and 55%, respectively, after 24 h of incubation in a phosphate buffer saline media. % decrease in cell viability in HepG2 cells after incubation with Dox-loaded galactosylated MWCNTs (0.04–40.00 μg/mL), pure Dox (0.04–40.00 μg/mL) and pure galactosylated MWCNTs (0.04–40.00 μg/mL) was 9%, 10% and 80% respectively. These results indicate that pure galactosylated MWCNTs are nontoxic and effective carrier for delivery of anticancer drugs.
Jain et al. (2009), synthesized covalently modified MWCNTs galactose to increase the dispersibility of MWCNTs in aqueous medium. The carboxylated MWCNTs were converted to acylated MWCNTs by chemical modification, which formed covalent bonds with the amino group of ethylenediamine and the free amino group was conjugated with the aldehyde group of galactoses. Galactosylated MWCNTs (Figure 8D). The dispersion of pristine, acylated and carboxylated MWCNTs was determined using the visualization technique, The pristine MWCNTs and acylated MWCNTs were poorly dispersed in solutions at pH 4, 7 and 9. The carboxylated MWCNTs had a high degree of dispersion at pH 9 due to the greater ionization of the carboxylic acid residues. The aminated MWCNTs dispersion was greater at pH 4, compared to pH 7 and 9, because of the greater extent of protonation and ionization of the aminated groups at pH 4, compared to pH 7 and 9. The dispersion of the galactosylated MWCNTs was highest at pH 9, compared to pH 4 and 7, as the pKa value of galactose is approximately 12.35, which is similar to pH 9.
Thakur et al. (2022) was reported Carboxylated MWCNTs functionalized with lysine further conjugated with three different carbohydrate ligands such as galactose, mannose, lactose, and Dox used as model drug for targeting the MDAMB231 & MCF7 breast cancer cell lines. These functionalized MWCNTs were characterized with different spectroscopy techniques. Galactose, Mannose, Lactose functionalized MWCNTs showed higher drug loading capacity as compared to lysine functionalized MWCNTs as well as carboxylated MWCNTs as 96.78%, 97.29%, 95.56%, 93.30% and 90.83% respectively and these prepared formulations also provided pH depended on release. Dox loaded ligand (galactose & mannose) conjugated MWCNTs showed higher percentage of cell viability in both breast cancer cell line (MDAMB231 & MCF7) compared to pure Dox at higher concentration more than 12.5 μg/mL but lactose functionalized MWCNTs showed minimal cytotoxicity with pure Dox and without Dox loaded MWCNTs formulations showed very less cytotoxicity more than 90% in both breast cancer cells, It means pure formulations does not causes any cytotoxicity with cancer cells is safe, biocompatible and provided effective targeted delivery of drug to the targeted sites.
2.4.2 Lactose
Multifunctional MWCNTs, combined with iron oxide - based superparamagnetic nanoparticles and lactose-glycine (Figure 13), coated with poly-diallyl-dimethyl-ammonium-chloride (PDDA), were developed by Liu et al. (2014). The % cell viability of HEK293 and Huh7 cells following incubation with 150 ug/mL of multifunctionalized MWCNTs was >25% and <90%, respectively. There was a significantly greater accumulation of the multifunctionalized MWCNTs in liver tumors in Harlan BALBc mice, compared to normal liver tissue (2.77-fold), after the i. v. administration of 10 mg/kg of multifunctionalized MWCNTs.
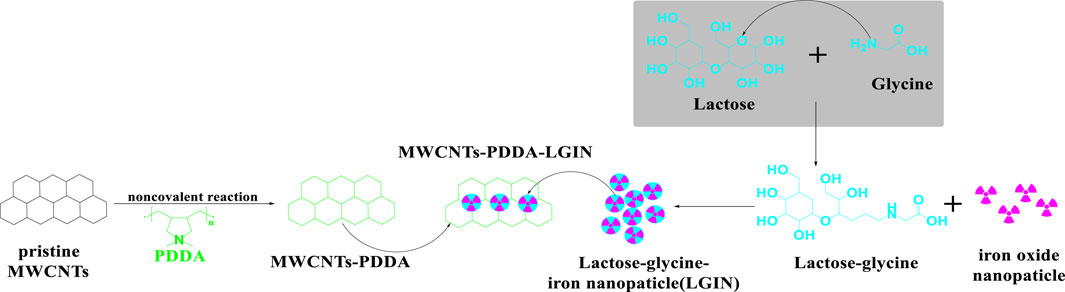
Figure 13. Conjugation sequences for the modification of MWCNTS-PDDA and lactose-glycine functionalized iron nanoparticles.
2.4.3 Fucose
Gupta et al. (2014) synthesized a MWCNT with a fucose sugar moiety that incorporated sulfasalazine (Figure 8F), for targeted delivery to J774 liver cancer cells. The loading capacity of sulfasalazine into the fucosylated MWCNTs was 87.77% ± 0.11%. In vitro, 100 µM of fucosylated MWCNTs loaded with sulfasalazine produced a 25% decrease in the viability of J774 cells (a macrophage cell line isolated from humans). However, unloaded fucosylated MWCNTs and pure sulfasalazine, at 100 μM, only decreased J774 cell viability by 95% and 40%, respectively, indicating that the pure formulation is non-toxic and fucosylated MWCNTs loaded with sulfasalazine are more effective in J774 cells than pure sulfasalazine. The in vivo pharmacokinetic experiments indicated that after the i. v. administration of either 20 mg/kg of pure sulfasalazine, MWCNTs loaded sulfasalazine or fucosylated MWCNTs - loaded sulfasalazine, in male Sprague-Dawley rats, the 1) rates of clearance were 168.01 ± 0.93, 155.62 ± 0.61 and 124.80 ± 0.74 μg/mL, respectively; 2) half-lives were 5.06, 6.33, 9.92 h, respectively and 3) AUCs were 1004.55, 1262.47, 1646.99 μg h/mL, respectively. Overall, these data clearly indicated that the fucosylated MWCNTs - loaded sulfasalazine had a longer half-life, a greater AUC, and a lower clearance, compared to the other formulations.
2.5 Dexamethasone
Dexamethasone is a synthetic corticosteroid that is used in the treatment of numerous diseases, including certain types of solid and non-solid cancers (Ruy et al., 2003; Park et al., 2006). It was reported that dexamethasone can be conjugated on the surface of MWCNTs using an ethylenediamine linker, to form aminated MWCNTs, in the presence of 2-iminothiolane (Figure 8E). Doxorubicin (Dox) was incorporated into the functionalized MWCNTs using a nano extraction method, where doxorubicin was dissolved in acetone and triethylamine and added to functionalized MWCNTs and stirred for 24h at room temperature (Lodhi et al., 2013). In vitro, the incubation of A549 cells with 1–10 μg/mL of formulated Dox and pure Dox decreased their viability by 40% and 45%, respectively. The % hemolysis of human red blood cells by Dox alone (17.1% ± 0.2%) was significantly greater than that of the Dox - loaded dexamethasone-MWCNTs (9.5% ± 0.6%).
2.6 Glycyrrhizin
Glycyrrhizin is a compound present in the plant, glycyrrhiza glabra, and studies suggest that it has in vitro and in vivo anticancer efficacy (Wang et al., 2019; Thirugnanam et al., 2008; Cai et al., 2017). Chopdey et al. (2015) developed MWCNTs conjugated with glycyrrhizin and encapsulated with doxorubicin, for targeted delivery to HepG2 cancer cells. Glycyrrhizinated MWCNTs (Figure 8G) were developed by modifying the pristine MWCNTs to carboxylated MWCNTs, which were aminated with ethylenediamine and the N-terminal of the aminated MWCNTs was conjugated with carboxylic group of the glycyrrhizins to form amide bonds. The Dox loading efficacy was greater for Dox-Glycyrrhizinated MWCNTs (87.26 ± 0.57%), compared to Dox-MWCNTs (77.08 ± 0.62%). 1 mg/ml of Dox-Glycyrrhizinated MWCNTs (9.82 ± 0.67) produced a lower % hemolysis of human red blood cells than Dox-MWCNTs (18.36 ± 0.33%). Dox alone, Dox-MWCNTs and Dox-Glycyrrhizinated MWCNTs significantly decreased the proliferation of HepG2 cells (IC50 values of 4.19 ± 0.08, 4.15 ± 0.01 2.7 ± 0.03 µM, respectively).
3 Biological disposition and toxicity of MWCNTs in cancer drug delivery
MWCNTs have several benefits for use in cancer drug delivery (Panigrahi et al., 2020). These have been shown to have good biocompatibility and slow biodegradability and can undergo enzymatic fragmentation in the body over time, which can reduce the risk of long-term toxicity and make them a safer option for drug delivery use (Cao et al., 2019; Aoki et al., 2020). MWCNTs have the versatility of easy detection by a different imaging technique, which makes them useful for tracking their movement in the tumor tissue. Moreover, the excellent electrical, and thermal characteristics enable MWCNTs serve as cargos for cancer drugs and deliver them directly to tumor cells, and they can be used to heat up tumors during thermotherapy to kill cancer cells (Masoudi et al., 2023).
The use of MWCNTs as biomaterials has seen a significant rise due to their demonstrated efficacy as drug delivery systems and their biological safety. While it cannot be conclusively claimed that MWCNTs are completely safe biological systems, extensive studies have shown no evidence of biological risk, leading most researchers to deem them safe for use as long as the appropriate delivery method and site are used (Ciofani et al., 2010; Saito et al., 2014). Studies have shown that the toxicity of CNTs is related to their physicochemical properties, such as dimensions and functionalization, as well as their method of entry and site of injection (Shvedova et al., 2005; Liu et al., 2008; Chall et al., 2021). Studies have shown that the toxicity of MWCNTs is largely dependent on their length, diameter, and surface chemistry. For instance, longer MWCNTs have been found to be more toxic than shorter ones, while smaller diameter MWCNTs are more toxic than larger ones. When longer MWCNTs (20 μm) are injected, it causes more serious toxicity like aggregation because phages are not able to engulf the micro size fibers. 825 nm longer MWCNTs causes inflammation because microphages could not envelop more than 220 nm, while 50 nm diameter of MWCNTs showed cytotoxicity or inflammogenic due to high crystallin nature, but 150 nm–220 nm of MWCNTs showed less cytotoxicity and inflammogenic (Liu et al., 2013; Kobayashi et al., 2017; Ali, 2023). Moreover, the surface chemistry of MWCNTs can influence their toxicity as it affects their interactions with biological systems. Managing these properties can be a useful approach in minimizing the toxicity of MWCNTs (Yan et al., 2011; Bussy et al., 2012; Louro, 2018; Ali, 2023). Extensive biological studies and regulatory standards must be established to validate the safety of MWCNTs. Extreme caution is necessary when taking the risky step towards clinical application involving the entry of CNTs into the circulation to ensure safe use (Van et al., 2011).
The presence of metallic impurities together with hydrophobic accumulation contributes significantly to the toxicity of MWCNTs, which can be reduced by the functionalization. Several studies or being conducted to develop safe and effective CNT nanocarriers by purifying metallic impurities (Mehra et al., 2015; Francis et al., 2017). Functionalized CNTs are considered as promising nanocarrier for biomedical applications. Modifying the surface of MWCNTs with targeting ligands, biodegradable and biocompatible polymers can improve biocompatibility and reduce toxicity. In addition, the utilization of specific sizes and shapes of MWCNTs has been shown to improve biodistribution and decrease toxicity (Liu et al., 2013; Francis et al., 2017).
4 Distribution and elimination of MWCNTs
The subcutaneously injected MWCNTs into mice showed that MWCNTs did not accumulate in organs except for lymph nodes, and there was no observed injury. The study demonstrates that subcutaneous injection of MWCNTs induces short-term immunological reactions that can be eliminated over time and SC route of administration was believed to be safer than systemic administration (Meng et al., 2011). In a recent study, the biodistribution of chitosan-MWCNT, and chitosan crosslinked MWCNT loaded with anti-gastritis drug, HEP (hericium erinaceus polysaccharide) was studied in mice following intraperitoneal injection. Real-time fluorescence imaging results indicated that CS-MWCNT-HEP had higher accumulation in liver and spleen. Authors claimed that the accumulation could enhance the body’s immunity and metabolism, but it is not clear how the emptied MWCNT will be cleared from the body (Ren et al., 2020).
Systemic administration via intravenous injection and distribution in the bloodstream has the potential to accumulate in various organs and tissues. The exact distribution pattern may depend on factors such as the size (length and diameter), shape, surface properties, and functionalization of the MWCNTs, as well as the dose and injection site (Jacobsen et al., 2017). Studies have shown that after injection, MWCNTs can accumulate in organs such as the liver, spleen, lungs, kidneys, and brain (Cherukuri et al., 2006; Deng et al., 2007; Liu et al., 2007; Georgin et al., 2009; Krug et al., 2011). For example, smaller MWCNTs have been found to distribute more widely throughout the body compared to larger ones (Shvedova et al., 2012). It has been reported that the intravenous administration of MWCNTs was safe with very low toxicity. Yang et al. investigated the long-term effects of intravenously administered MWCNTs in mice and found that the nanotubes accumulated in the lungs, liver, and spleen, with minimal inflammatory cell infiltration in the lungs (Yang et al., 2008). Similarly, Schipper et al. conducted a pilot study in mice and reported no observable toxicity when MWCNTs were administered intravenously. These findings suggest that intravenous administration of MWCNTs is relatively safe and may be a promising method for drug delivery and other applications (Schipper et al., 2008).
The biodistribution of MWCNTs in mice was determined by using the skeleton 13C-enriched SWNTs and isotope ratio mass spectroscopy. The study found that there were no acute toxic effects observed in animals exposed to high doses of 13C-SWNTs, and no animals died during the 4-week test period. However, the clearance rate of the nanotubes from most organs was slow, with significant accumulations in the liver, lungs, and spleen (Yang et al., 2007). In another study, the long-term toxicity of MWCNTs in mice after intravenous exposure was investigated. It was found that the MWCNTs accumulated in various organs, including the liver, spleen, and lungs, for up to 3 months post-exposure (Deng et al., 2008). Similarly, the biodistribution of radio labelled MWCNTs in mice using in vivo positron emission tomography was investigated. The study found that MWCNTs functionalized with phospholipids bearing PEG were stable in vivo, and PEG chain length affected their biodistribution and circulation. MWCNTs coated with PEG chains linked to RGD peptide efficiently targeted integrin-positive tumors in mice, exhibiting high tumor accumulation due to the multivalent effect of the MWCNTs. The MWCNTs were largely localized in the liver and lesser localized in spleen and kidney. The PEG caused a slower blood clearance and lower concentration in the liver (Liu et al., 2007).
The distribution of 14C-taurine-MWCNT after intravenous injection was investigated in mice. Results showed that 75% of the dose accumulated in the liver after 1 month but decreased to 20% after 90 days. MWCNT were observed in Kupffer cells via TEM imaging, with <5% found in the spleen and lungs which are almost eliminated in 90 days (Deng et al., 2007). The same group of researchers tested biodistribution of two types of functionalized MWCNTs, 125I-Taurine-MWCNTs, and 125I-Tween-80-MWCNTs by intravenous administration into mice. About 75% of 125I-tau-MWCNT was in the liver up to 6 h, very small amount was seen in other organs such as spleen lungs. 125I-Tween-MWCNT was found to be distributed in many organs, liver, spleen and lung, stomach, kidney, large and small intestine, probably due to amphiphilic properties of Tween 80 (Deng et al., 2008). The biodistribution of 14C-MWCNT suspension (in rat serum) after intravenous administration was followed in rats. The MWCNTs were cleared from the bloodstream and were predominantly distributed to the liver. The lungs, spleen, and kidneys also had lower amounts, whereas no radioactivity was found in the brain, heart, bones, stomach, and muscles. Dark clusters were observed in the lungs and liver via optical microscopy, which coincided with radioactive hotspots. Throughout the study, radioactivity in all organs decreased (Deng et al., 2008; Mortensen et al., 2022). The impact of MWCNTs of two different diameters on the biodistribution was followed. The MWCNTs were functionalized and conjugated with radionuclide chelating moieties and IgG antibodies and a radioactive tracer. The results showed that the modification did not affect the distribution but the narrow MWCNTs (9 nm) had less tissue affinity than wider MWCNTs (40 nm) suggesting their suitability of biological application (Singh et al., 2006).
In a recent study, the influence of the diameter and length MWCNTs on biodistribution was investigated. Three different sized carboxylated MWCNTs were administered as a single intravenous dose of 1 mg/kg MWCNTs. Biodistribution was evaluated in liver, lung, spleen, and lymph nodes using microscopy and hyperspectral imaging. The results showed that the observed tissue location of MWCNTs is size dependent. Overlaps in the perturbation of endogenous metabolite profiles were found regardless of their size. The tissue distribution and persistence of MWCNTs in liver, spleen, lymph node, and lung are influenced by their size. The metabolomics findings suggest that the liver is impacted by the MWCNTs observed in the tissue. Water-soluble MWCNTs functionalized with DTPA and labeled with indium were used for urine excretion studies in rats. Imaging showed that within a minute, the CNT began to accumulate in the kidneys and bladder. At 30 min, most of the detected activity was in the kidneys/bladder. At 6 h, almost all CNT eliminated via renal excretion route. Urinary excretion of the vast majority of radiolabeling (nanotubes) was confirmed at 24 h, where it was shown that 11.5% of the dose/g tissue was in the urine, whereas the liver, spleen, bladder, and kidneys all had content below 1% (Singh et al., 2006; Deng et al., 2008; Mortensen et al., 2022). While the biodistribution of CNTs and qualitative assessments of CNT deposition in tissues have been well reported in the literature, the quantification of CNTs at this point is technically demanding.
Factors affecting the distribution and elimination of MWCNTs:
MWCNTs are distributed throughout the body and eliminated. Various factors, including size, shape, and surface functionalization have been shown influence the absorption, distribution, metabolism, and excretion of MWCNTs, potentially impacting their pharmacokinetics and toxicity (Liu et al., 2013; Mehra et al., 2015; Mortensen et al., 2022).
• MWCNTs that are smaller in size have the potential to penetrate tissue more deeply and may be eliminated more effectively by renal filtration. Larger MWCNTs on the other hand accumulate in the spleen and liver, among other organs.
• Variations in the shapes of MWCNTs, such as straight, curved, or tangled forms, can affect cellular uptake and their interaction with biological membranes.
• Surface functionalization of MWCNTs influences circulation time, biodistribution, and clearance routes by altering their interaction with bio molecules and cells.
• The surface charge of MWCNTs influences how they interact with proteins, cells, and tissues, which in turn impacts their biodistribution and clearance from the body.
5 Conclusion
Ligand-functionalized MWCNTs have emerged as effective nanocarriers for anticancer drug delivery, owing to their biocompatibility and low toxicity to healthy cells and tissues. A variety of ligand molecules (Table 2) that bind to specific receptors found on cancer cells can be attached to MWCNTs via chemical/biochemical linker moieties, affording significant opportunities for the targeted delivery of anticancer therapeutics in different cancers. Ligand-functionalized MWCNTs have demonstrated increased aqueous dispersibility, cellular uptake, bioavailability, and drug loading capacity, making them useful for tumor-targeted delivery of anticancer drugs with poor pharmacokinetic properties, and poor cell permeability. Furthermore, ligand-functionalized MWCNTs display relatively less toxicity and greater penetrability through biological membranes making them ideal for biological applications. Overall, we conclude that functionalized MWCNTS are unique, multifunctional nanocarriers that hold considerable promise for cancer-targeted drug delivery. The rapid advancement in MWCNT functionalization strategies together with the availability of a wider array of cancer cell targeting ligands would pave the way for the translational development of these novel nanoplatforms for the diagnosis and treatment of cancer.
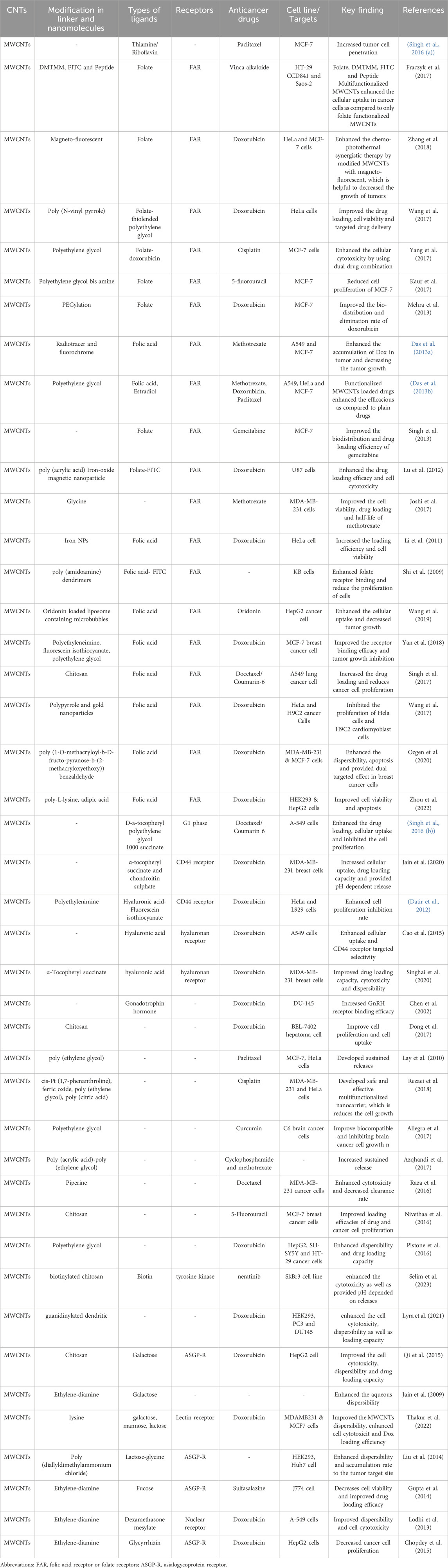
Table 2. A summary of the in vitro and in vivo effect of bioactive targeting ligands conjugated with MWCNTs on cancer cells and tumors.
Author contributions
CT: Conceptualization, Writing–original draft. CK: Supervision, Writing–review and editing, Conceptualization. CA: Writing–review and editing, Formal Analysis. RN: Formal Analysis, Writing–review and editing. VS: Formal Analysis, Writing–review and editing. RB: Conceptualization, Formal Analysis, Writing–review and editing. NN: Formal Analysis, Supervision, Writing–review and editing. AT: Formal Analysis, Supervision, Writing–review and editing.
Funding
The author(s) declare that financial support was received for the research, authorship, and/or publication of this article. AT is supported by VCRI-Arkansas Bioscience Institute funds (GR020025/FD224).
Acknowledgments
The author CT is thankful to Ministry of Tribal Affairs, Govt. of India for providing National Fellowship for Higher Education of ST Students (201819-NFST-CHH-00112) to carry out this research work.
Conflict of interest
The authors declare that the research was conducted in the absence of any commercial or financial relationships that could be construed as a potential conflict of interest.
The author(s) declared that they were an editorial board member of Frontiers, at the time of submission. This had no impact on the peer review process and the final decision.
Publisher’s note
All claims expressed in this article are solely those of the authors and do not necessarily represent those of their affiliated organizations, or those of the publisher, the editors and the reviewers. Any product that may be evaluated in this article, or claim that may be made by its manufacturer, is not guaranteed or endorsed by the publisher.
References
Ali, M. (2023). What function of nanoparticles is the primary factor for their hyper-toxicity? Adv. Colloid Interface Sci. 314, 102881. doi:10.1016/j.cis.2023.102881
Allegra, A., Innao, V., Russo, S., Gerace, D., Alonci, A., and Musolino, C. J. C. (2017). Anticancer activity of curcumin and its analogues: preclinical and clinical studies, Cancer Investig. 35, 1–22. doi:10.1080/07357907.2016.1247166
Allen, T. M. (2002). Ligand-targeted therapeutics in anticancer therapy. Nat. Rev. Cancer 2, 750–763. doi:10.1038/nrc903
Aoki, K., and Saito, N. (2020). Biocompatibility and carcinogenicity of carbon nanotubes as biomaterials. Nanomaterials 10, 264. doi:10.3390/nano10020264
Azqhandi, M. H. A., Farahani, B. V., and Dehghani, N. (2017). Encapsulation of methotrexate and cyclophosphamide in interpolymer complexes formed between poly acrylic acid and poly ethylene glycol on multi-walled carbon nanotubes as drug delivery systems. Mater. Sci. Eng. C 79, 841–847. doi:10.1016/j.msec.2017.05.089
Bakry, R., Vallant, R. M., Najam-ul-Haq, M., Rainer, M., Szabo, Z., Huck, C. W., et al. (2007). Medicinal applications of fullerenes. Int. J. nanomedicine 2, 639–649. doi:10.2147/IJN.S2.4.639
Bianco, A., Kostarelos, K., Partidos, C. D., and Prato, M. (2005). Biomedical applications of functionalised carbon nanotubes. Chem. Commun., 571–577. doi:10.1039/b410943k
Bray, F., Laversanne, M., Sung, H., Ferlay, J., Siegel, R. L., Soerjomataram, I., et al. (2024). Global cancer statistics 2022: GLOBOCAN estimates of incidence and mortality worldwide for 36 cancers in 185 countries. CA Cancer J. Clin. 74, 229–263. doi:10.3322/caac.21834
Bussy, C., Pinault, M., Cambedouzou, J., Landry, M. J., Jegou, P., Mayne-L'Hermite, M., et al. (2012). Critical role of surface chemical modifications induced by length shortening on multi-walled carbon nanotubes-induced toxicity. Part. fibre Toxicol. 9, 46–15. doi:10.1186/1743-8977-9-46
Cai, Y., Zhao, B., Liang, Q., Zhang, Y., Cai, J., and Li, G. (2017). The selective effect of glycyrrhizin and glycyrrhetinic acid on topoisomerase IIα and apoptosis in combination with etoposide on triple negative breast cancer MDA-MB-231 cells. Eur. J. Pharmacol. 809, 87–97. doi:10.1016/j.ejphar.2017.05.026
Cao, X., Tao, L., Wen, S., Hou, W., and Shi, X. (2015). Hyaluronic acid-modified multiwalled carbon nanotubes for targeted delivery of doxorubicin into cancer cells. Carbohydr. Res. 405, 70–77. doi:10.1016/j.carres.2014.06.030
Cao, Y., and Luo, Y. (2019). Pharmacological and toxicological aspects of carbon nanotubes (CNTs) to vascular system: a review. Toxicol. Appl. Pharmacol. 385, 114801. doi:10.1016/j.taap.2019.114801
Chadar, R., Afzal, O., Alqahtani, S. M., and Kesharwani, P. (2021). Carbon nanotubes as an emerging nanocarrier for the delivery of doxorubicin for improved chemotherapy. Colloids Surf. B Biointerfaces 208, 112044. doi:10.1016/j.colsurfb.2021.112044
Chall, A., Stagg, J., Mixson, A., Gato, E., Quirino, R. L., and Sittaramane, V. (2021). Ablation of cells in mice using antibody-functionalized multiwalled carbon nanotubes (Ab-MWCNTs) in combination with microwaves. Nanotechnology 32, 195102. doi:10.1088/1361-6528/abe32a
Chen, A., Kaganovsky, E., Rahimipour, S., Ben-Aroya, N., Okon, E., and Koch, Y. (2002). Two forms of gonadotropin-releasing hormone (GnRH) are expressed in human breast tissue and overexpressed in breast cancer: a putative mechanism for the antiproliferative effect of GnRH by down-regulation of acidic ribosomal phosphoproteins P1 and P2. Cancer Res. 62, 1036–1044.
Chen, Y., Star, A., and Vidal, S. (2013). Sweet carbon nanostructures: carbohydrate conjugates with carbon nanotubes and graphene and their applications. Chem. Soc. Rev. 42, 4532–4542. doi:10.1039/c2cs35396b
Cherukuri, P., Gannon, C. J., Leeuw, T. K., Schmidt, H. K., Smalley, R. E., Curley, S. A., et al. (2006). Mammalian pharmacokinetics of carbon nanotubes using intrinsic near-infrared fluorescence. Proc. Natl. Acad. Sci. 103, 18882–18886. doi:10.1073/pnas.0609265103
Chopdey, P. K., Tekade, R. K., Mehra, N. K., Mody, N., and Jain, N. K. (2015). Glycyrrhizin conjugated dendrimer and multi-walled carbon nanotubes for liver specific delivery of doxorubicin. J. Nanosci. Nanotechnol. 15 (2), 1088–1100. doi:10.1166/jnn.2015.9039
Ciofani, G., Raffa, V., Vittorio, O., Cuschieri, A., Pizzorusso, T., Costa, M., et al. (2010). In vitro and in vivo biocompatibility testing of functionalized carbon nanotubes. Carbon Nanotub. Methods Protoc. 625, 67–83. doi:10.1007/978-1-60761-579-8_7
Das, M., Datir, S. R., Singh, R. P., and Jain, S. (2013a). Augmented anticancer activity of a targeted, intracellularly activatable, theranostic nanomedicine based on fluorescent and radiolabeled, methotrexate-folic acid-multiwalled carbon nanotube conjugate. Mol. Pharm. 10 (7), 2543–2557. doi:10.1021/mp300701e
Das, M., Singh, R. P., Datir, S. R., and Jain, S. (2013b). Surface chemistry dependent “switch” regulates the trafficking and therapeutic performance of drug-loaded carbon nanotubes. Bioconjugate Chem. 24, 626–639. doi:10.1021/bc300598z
Das, R., Bee Abd Hamid, S., Eaqub Ali, M., Ramakrishna, S., and Yongzhi, W. (2015a). Carbon nanotubes characterization by X-ray powder diffraction–a review. Curr. Nanosci. 11, 23–35. doi:10.2174/1573413710666140818210043
Das, R., Hamid, S. B. A., Ali, M., Annuar, M., Samsudin, E. M. B., and Bagheri, S. (2015b). Covalent functionalization schemes for tailoring solubility of multi-walled carbon nanotubes in water and acetone solvents. Sci. Adv. Mater. 7, 2726–2737. doi:10.1166/sam.2015.2694
Datir, S. R., Das, M., Singh, R. P., and Jain, S. (2012). Hyaluronate tethered,“smart” multiwalled carbon nanotubes for tumor-targeted delivery of doxorubicin. Bioconjugate Chem. 23, 2201–2213. doi:10.1021/bc300248t
Delgado, J. L., Herranz, M. Á., and Martin, N. (2008). The nano-forms of carbon. J. Mater. Chem. 18, 1417–1426. doi:10.1039/b717218d
Deng, X., Jia, G., Wang, H., Sun, H., Wang, X., Yang, S., et al. (2007). Translocation and fate of multi-walled carbon nanotubes in vivo. Carbon 45, 1419–1424. doi:10.1016/j.carbon.2007.03.035
Deng, X., Yang, S., Nie, H., Wang, H., and Liu, Y. (2008). A generally adoptable radiotracing method for tracking carbon nanotubes in animals. Nanotechnology 19, 075101. doi:10.1088/0957-4484/19/7/075101
Dong, X., Sun, Z., Wang, X., Zhu, D., Liu, L., and Leng, X. (2017). Simultaneous monitoring of the drug release and antitumor effect of a novel drug delivery system-MWCNTs/DOX/TC. Drug Deliv. 24, 143–151. doi:10.1080/10717544.2016.1233592
Dou, J., Zhang, H., Liu, X., Zhang, M., and Zhai, G. (2014). Preparation and evaluation in vitro and in vivo of docetaxel loaded mixed micelles for oral administration. Colloids Surfaces B Biointerfaces 114, 20–27. doi:10.1016/j.colsurfb.2013.09.010
Dy, G. K., and Adjei, A. A. (2013). Understanding, recognizing, and managing toxicities of targeted anticancer therapies. CA a cancer J. Clin. 63, 249–279. doi:10.3322/caac.21184
Elhissi, A., Ahmed, W., Hassan, I. U., Dhanak, V., and D'Emanuele, A. (2012). Carbon nanotubes in cancer therapy and drug delivery. J. drug Deliv. 2012, 837327. doi:10.1155/2012/837327
Fraczyk, J., Walczak, M., Szymanski, L., Kolacinski, Z., Wrzosek, H., Majsterek, I., et al. (2017). Carbon nanotubes functionalized with folic acid attached via biomimetic peptide linker. Nanomedicine 12, 2161–2182. doi:10.2217/nnm-2017-0120
Fraczyk, J., Walczak, M., Szymanski, L., Kolacinski, Z., Wrzosek, H., Majsterek, I., et al. (2017). Carbon nanotubes functionalized with folic acid attached via biomimetic peptide linker. Nanomedicine 12 (18), 2161–2182. doi:10.2217/nnm-2017-0120
Francis, A. P., and Devasena, T. (2017). Toxicity of carbon nanotubes: a review. Toxicol. Industrial Health 1, 1–10. doi:10.1177/0748233717747472
Froy, O., Sagiv, T., Poreh, M., Urbach, D., Zilberberg, N., and Gurevitz, M. (1999). Dynamic diversification from a putative common ancestor of scorpion toxins affecting sodium, potassium, and chloride channels. J. Mol. Evol. 48, 187–196. doi:10.1007/pl00006457
Gao, X., Yue, H., Huang, S., Lin, X., Gao, X. P., Wang, B., et al. (2018). Synthesis of graphene/ZnO nanowire arrays/graphene foam and its application for determination of folic acid. J. Electroanal. Chem. 808, 189–194. doi:10.1016/j.jelechem.2017.12.017
García-Hevia, L., Valiente, R., González, J., Luis Fernandez-Luna, J., C Villegas, J., and L Fanarraga, M. (2015). Anti-cancer cytotoxic effects of multiwalled carbon nanotubes. Curr. Pharm. Des. 21, 1920–1929. doi:10.2174/1381612821666150302144101
Georgin, D., Czarny, B., Botquin, M., Mayne-L’Hermite, M., Pinault, M., Bouchet-Fabre, B., et al. (2009). Preparation of 14C-labeled multiwalled carbon nanotubes for biodistribution investigations. J. Am. Chem. Soc. 131, 14658–14659. doi:10.1021/ja906319z
Gupta, R., Mehra, N. K., and Jain, N. K. (2014). Fucosylated multiwalled carbon nanotubes for Kupffer cells targeting for the treatment of cytokine-induced liver damage. Pharm. Res. 31, 322–334. doi:10.1007/s11095-013-1162-9
Hindumathi, R., Jagannatham, M., Haridoss, P., and Sharma, C. P. (2018). Novel nano-cocoon like structures of polyethylene glycol–multiwalled carbon nanotubes for biomedical applications. Nano-Structures Nano-Objects 13, 30–35. doi:10.1016/j.nanoso.2017.11.001
Hindumathi, R., Jagannatham, M., Haridoss, P., and Sharma, C. P. (2018). Novel nano-cocoon like structures of polyethylene glycol–multiwalled carbon nanotubes for biomedical applications. Nano-Structures Nano-Objects 13, 30–35. doi:10.1016/j.nanoso.2017.11.001
Hirlekar, R., Yamagar, M., Garse, H., Vij, M., and Kadam, V. (2009). Carbon nanotubes and its applications: a review. Asian J. Pharm. Clin. Res. 2, 17–27.
Hirsch, A., and Vostrowsky, O. (2005). “Functionalization of carbon nanotubes,” in Functional molecular nanostructures, 193–237.
Iglesias, D., and Melchionna, M. (2019). Enter the tubes: carbon nanotube endohedral catalysis. Catalysts 9, 128. doi:10.3390/catal9020128
Jacobsen, N. R., Møller, P., Clausen, P. A., Saber, A. T., Micheletti, C., Jensen, K. A., et al. (2017). Biodistribution of carbon nanotubes in animal models. Basic & Clin. Pharmacol. Toxicol. 121, 30–43. doi:10.1111/bcpt.12705
Jain, A. K., Dubey, V., Mehra, N. K., Lodhi, N., Nahar, M., Mishra, D. K., et al. (2009). Carbohydrate-conjugated multiwalled carbon nanotubes: development and characterization. Nanomedicine Nanotechnol. Biol. Med. 5, 432–442. doi:10.1016/j.nano.2009.03.001
Jain, K., Kumar Mehra, N., and K Jain, N. (2015). Nanotechnology in drug delivery: safety and toxicity issues. Curr. Pharm. Des. 21, 4252–4261. doi:10.2174/1381612821666150901103208
Jeon, I.-Y., Chang, D. W., Kumar, N. A., and Baek, J.-B. (2011). Functionalization of carbon nanotubes. Carbon nanotubes-polymer nanocomposites 6, 91–110.
Jha, R., Singh, A., Sharma, P., and Fuloria, N. K. (2020). Smart carbon nanotubes for drug delivery system: a comprehensive study. J. Drug Deliv. Sci. Technol. 58, 101811. doi:10.1016/j.jddst.2020.101811
Ji, S.-r., Liu, C., Zhang, B., Yang, F., Xu, J., Long, J., et al. (2010). Carbon nanotubes in cancer diagnosis and therapy. Biochimica Biophysica Acta (BBA)-Reviews Cancer 1806, 29–35. doi:10.1016/j.bbcan.2010.02.004
Joshi, M., Kumar, P., Kumar, R., Sharma, G., Singh, B., Katare, O. P., et al. (2017). Aminated carbon-based “cargo vehicles” for improved delivery of methotrexate to breast cancer cells. Mater. Sci. Eng. C 75, 1376–1388. doi:10.1016/j.msec.2017.03.057
Kaittanis, C., Santra, S., and Perez, J. M. (2009). Role of nanoparticle valency in the nondestructive magnetic-relaxation-mediated detection and magnetic isolation of cells in complex media. J. Am. Chem. Soc. 131, 12780–12791. doi:10.1021/ja9041077
Karousis, N., Tagmatarchis, N., and Tasis, D. (2010). Current progress on the chemical modification of carbon nanotubes. Chem. Rev. 110, 5366–5397. doi:10.1021/cr100018g
Kaur, J., Gill, G. S., and Jeet, K. (2019). “Applications of carbon nanotubes in drug delivery: a comprehensive review,” in Characterization and biology of nanomaterials for drug delivery (Elsevier), 113–135.
Kaur, S., Mehra, N. K., Jain, K., and Jain, N. K. (2017). Development and evaluation of targeting ligand-anchored CNTs as prospective targeted drug delivery system. Artif. cells, nanomedicine, Biotechnol. 45, 242–250. doi:10.3109/21691401.2016.1146728
Kayat, J., Gajbhiye, V., Tekade, R. K., and Jain, N. K. (2011). Pulmonary toxicity of carbon nanotubes: a systematic report. Nanomedicine Nanotechnol. Biol. Med. 7, 40–49. doi:10.1016/j.nano.2010.06.008
Kesharwani, P., Ghanghoria, R., and Jain, N. K. (2012). Carbon nanotube exploration in cancer cell lines. Drug Discov. Today 17, 1023–1030. doi:10.1016/j.drudis.2012.05.003
Kobayashi, N., Izumi, H., and Morimoto, Y. (2017). Review of toxicity studies of carbon nanotubes. J. Occup. Health 59, 394–407. doi:10.1539/joh.17-0089-RA
Krug, H. F., and Wick, P. (2011). Nanotoxicology: an interdisciplinary challenge. Angew. Chem. Int. Ed. 50, 1260–1278. doi:10.1002/anie.201001037
Kulkarni, C., and Khot, A. (2010). Carbon nanotubes as interconnects. Indian J. Pure Appl. Phys. 120, 1–20.
Kushwaha, S. K. S., Ghoshal, S., Rai, A. K., and Singh, S. (2013). Carbon nanotubes as a novel drug delivery system for anticancer therapy: a review. Braz. J. Pharm. Sci. 49, 629–643. doi:10.1590/s1984-82502013000400002
Lamberti, M., Pedata, P., Sannolo, N., Porto, S., De Rosa, A., and Caraglia, M. (2015). Carbon nanotubes: properties, biomedical applications, advantages and risks in patients and occupationally-exposed workers. Int. J. Immunopathol. Pharmacol. 28, 4–13. doi:10.1177/0394632015572559
Lamberti, M., Zappavigna, S., Sannolo, N., Porto, S., and Caraglia, M. (2014). Advantages and risks of nanotechnologies in cancer patients and occupationally exposed workers. Expert Opin. drug Deliv. 11, 1087–1101. doi:10.1517/17425247.2014.913568
Lay, C. L., Liu, H. Q., Tan, H. R., and Liu, Y. (2010). Delivery of paclitaxel by physically loading onto poly (ethylene glycol)(PEG)-graftcarbon nanotubes for potent cancer therapeutics. Nanotechnology 21, 065101. doi:10.1088/0957-4484/21/6/065101
Lehman, J. H., Terrones, M., Mansfield, E., Hurst, K. E., and Meunier, V. (2011). Evaluating the characteristics of multiwall carbon nanotubes. Carbon 49, 2581–2602. doi:10.1016/j.carbon.2011.03.028
Li, R., Wu, R. a., Zhao, L., Hu, Z., Guo, S., Pan, X., et al. (2011). Folate and iron difunctionalized multiwall carbon nanotubes as dual-targeted drug nanocarrier to cancer cells. Carbon 49, 1797–1805. doi:10.1016/j.carbon.2011.01.003
Liu, M., Xu, Y., Huang, C., Jia, T., Zhang, X., Yang, D.-P., et al. (2018). Hyaluronic acid-grafted three-dimensional MWCNT array as biosensing interface for chronocoulometric detection and fluorometric imaging of CD44-overexpressing cancer cells. Microchim. Acta 185, 338–8. doi:10.1007/s00604-018-2861-z
Liu, Y., Hughes, T. C., Muir, B. W., Waddington, L. J., Gengenbach, T. R., Easton, C. D., et al. (2014). Water-dispersible magnetic carbon nanotubes as T2-weighted MRI contrast agents. Biomaterials 35, 378–386. doi:10.1016/j.biomaterials.2013.09.079
Liu, Y., Zhao, Y., Sun, B., and Chen, C. (2013). Understanding the toxicity of carbon nanotubes. Accounts Chem. Res. 46, 702–713. doi:10.1021/ar300028m
Liu, Z., Cai, W., He, L., Nakayama, N., Chen, K., Sun, X., et al. (2007). In vivo biodistribution and highly efficient tumour targeting of carbon nanotubes in mice. Nat. Nanotechnol. 2, 47–52. doi:10.1038/nnano.2006.170
Liu, Z., Davis, C., Cai, W., He, L., Chen, X., and Dai, H. (2008). Circulation and long-term fate of functionalized, biocompatible single-walled carbon nanotubes in mice probed by Raman spectroscopy. Proc. Natl. Acad. Sci. 105, 1410–1415. doi:10.1073/pnas.0707654105
Lodhi, N., Mehra, N. K., and Jain, N. K. (2013). Development and characterization of dexamethasone mesylate anchored on multi walled carbon nanotubes. J. drug Target. 21, 67–76. doi:10.3109/1061186X.2012.729213
Logozzi, M., Mizzoni, D., Angelini, D. F., Di Raimo, R., Falchi, M., Battistini, L., et al. (2018). Microenvironmental pH and exosome levels interplay in human cancer cell lines of different histotypes. Cancers 10, 370. doi:10.3390/cancers10100370
Lokeshwar, V. B., Mirza, S., and Jordan, A. (2014). Targeting hyaluronic acid family for cancer chemoprevention and therapy. Adv. cancer Res. 123, 35–65. doi:10.1016/B978-0-12-800092-2.00002-2
Louro, H. (2018). Relevance of physicochemical characterization of nanomaterials for understanding nano-cellular interactions. Cell. Mol. Toxicol. Nanoparticles 1048, 123–142. doi:10.1007/978-3-319-72041-8_8
Lu, Y.-J., Wei, K.-C., Ma, C.-C. M., Yang, S.-Y., and Chen, J.-P. (2012). Dual targeted delivery of doxorubicin to cancer cells using folate-conjugated magnetic multi-walled carbon nanotubes. Colloids Surfaces B Biointerfaces 89, 1–9. doi:10.1016/j.colsurfb.2011.08.001
Lyra, K., Kaminari, A., Panagiotaki, K. N., Spyrou, K., Papageorgiou, S., Sakellis, E., et al. (2021). Multi-walled carbon nanotubes decorated with guanidinylated dendritic molecular transporters: an efficient platform for the selective anticancer activity of doxorubicin. Pharmaceutics 13, 858. doi:10.3390/pharmaceutics13060858
Lyra, K.-M., Kaminari, A., Panagiotaki, K. N., Spyrou, K., Papageorgiou, S., Sakellis, E., et al. (2021). Multi-walled carbon nanotubes decorated with guanidinylated dendritic molecular transporters: an efficient platform for the selective anticancer activity of doxorubicin. Pharmaceutics 13, 1–28. doi:10.3390/pharmaceutics13060858
Mainardes, R. M., and Silva, L. P. (2004). Drug delivery systems: past, present, and future. Curr. drug targets 5, 449–455. doi:10.2174/1389450043345407
Marega, R., and Bonifazi, D. (2014). Filling carbon nanotubes for nanobiotechnological applications. New J. Chem. 38, 22–27. doi:10.1039/c3nj01008b
Martins, F. G., Thakur, C. K., Karthikeyan, C., Narayana, N. S. H., and Sousa, S. F. (2023). Use of lysinated multiwalled carbon nanotubes with carbohydrate ligands as a doxorubicin nanocarrier: a molecular dynamics analysis. Carbon Trends 12, 100280. doi:10.1016/j.cartre.2023.100280
Maruyama, K., Haniu, H., Saito, N., Matsuda, Y., Tsukahara, T., Kobayashi, S., et al. (2015). Endocytosis of multiwalled carbon nanotubes in bronchial epithelial and mesothelial cells. BioMed Res. Int. 2015, 793186. doi:10.1155/2015/793186
Masoudi Asil, S., Guerrero, E. D., Bugarini, G., Cayme, J., De Avila, N., Garcia, J., et al. (2023). Theranostic applications of multifunctional carbon nanomaterials.
Mehra, N. K., Jain, K., and Jain, N. K. (2015). Pharmaceutical and biomedical applications of surface engineered carbon nanotubes. Drug Discov. Today 20, 750–759. doi:10.1016/j.drudis.2015.01.006
Mehra, N. K., and Jain, N. (2013). Development, characterization and cancer targeting potential of surface engineered carbon nanotubes. J. Drug Target. 21, 745–758. doi:10.3109/1061186X.2013.813028
Mehra, N. K., and Jain, N. K. (2015). Functioalized carbon nanotubes and their drug delivery applications. Nanostructured Drug Deliv. 4, 328–335.
Mehra, N. K., Mishra, V., and Jain, N. (2014). A review of ligand tethered surface engineered carbon nanotubes. Biomaterials 35, 1267–1283. doi:10.1016/j.biomaterials.2013.10.032
Mehra, N. K., Mishra, V., and Jain, N. K. (2013). Receptor-based targeting of therapeutics. Ther. Deliv. 4, 369–394. doi:10.4155/tde.13.6
Mehra, N. K., and Palakurthi, S. (2016). Interactions between carbon nanotubes and bioactives: a drug delivery perspective. Drug Discov. Today 21, 585–597. doi:10.1016/j.drudis.2015.11.011
Melchionna, M., and Prato, M. (2013). Functionalizing carbon nanotubes: an indispensable step towards applications. ECS J. Solid State Sci. Technol. 2, M3040–M3045. doi:10.1149/2.008310jss
Menezes, B. R. C., Rodrigues, K. F., da Silva Fonseca, B. C., Ribas, R. G., do Amaral Montanheiro, T. L., and Thim, G. P. (2019). Recent advances in the use of carbon nanotubes as smart biomaterials. J. Mater. Chem. B 7, 1343–1360. doi:10.1039/c8tb02419g
Meng, J., Yang, M., Jia, F., Xu, Z., Kong, H., and Xu, H. (2011). Immune responses of BALB/c mice to subcutaneously injected multi-walled carbon nanotubes. Nanotoxicology 5, 583–591. doi:10.3109/17435390.2010.523483
Meng, L., Fu, C., and Lu, Q. (2009). Advanced technology for functionalization of carbon nanotubes. Prog. Nat. Sci. 19, 801–810. doi:10.1016/j.pnsc.2008.08.011
Moretti, R. M., Marelli, M. M., Taylor, D. M., Martini, P. G., Marzagalli, M., and Limonta, P. (2014). Gonadotropin-releasing hormone agonists sensitize, and resensitize, prostate cancer cells to docetaxel in a p53-dependent manner. PLoS One 9, e93713. doi:10.1371/journal.pone.0093713
Mortensen, N. P., Snyder, R. W., Pathmasiri, W., Moreno Caffaro, M., Sumner, S. J., and Fennell, T. R. (2022). Intravenous administration of three multiwalled carbon nanotubes to female rats and their effect on urinary biochemical profile. J. Appl. Toxicol. 42, 409–422. doi:10.1002/jat.4226
Murakami, T., Ajima, K., Miyawaki, J., Yudasaka, M., Iijima, S., and Shiba, K. (2004). Drug-loaded carbon nanohorns: adsorption and release of dexamethasone in vitro. Mol. Pharm. 1, 399–405. doi:10.1021/mp049928e
Nivethaa, E., Dhanavel, S., Narayanan, V., and Stephen, A. (2016). Fabrication of chitosan/MWCNT nanocomposite as a carrier for 5-fluorouracil and a study of the cytotoxicity of 5-fluorouracil encapsulated nanocomposite towards MCF-7. Polym. Bull. 73, 3221–3236. doi:10.1007/s00289-016-1651-1
Ozgen, O., Sinem, P., Atasoy, S., Kurt, B. Z., Durmus, Z., Yigit, G., et al. (2020). Glycopolymer decorated multiwalled carbon nanotubes for dual targeted breast cancer therapy. J. Mater Chem. B 8, 3123–3137. doi:10.1039/c9tb02711d
Pandurangappa, M., and Raghu, G. K. (2011). “Chemically modified carbon nanotubes: derivatization and their applications,” in Carbon nanotubes applications on electron devices (London, United Kingdom: IntechOpen).
Panigrahi, B. K., and Nayak, A. K. (2020). Carbon nanotubes: an emerging drug delivery carrier in cancer therapeutics. Curr. Drug Deliv. 17, 558–576. doi:10.2174/1567201817999200508092821
Park, T. G., and Yoo, H. S. (2006). Dexamethasone nano-aggregates composed of PEG–PLA–PEG triblock copolymers for anti-proliferation of smooth muscle cells. Int. J. Pharm. 326, 169–173. doi:10.1016/j.ijpharm.2006.06.041
Patel, K. D., Singh, R. K., and Kim, H.-W. (2019). Carbon-based nanomaterials as an emerging platform for theranostics. Mater. Horizons 6, 434–469. doi:10.1039/c8mh00966j
Patel, K. D., Singh, R. K., and Kim, H.-W. (2019). Carbon-based nanomaterials as an emerging platform for theranostics. Mater. Horizons 6 (3), 434–469. doi:10.1039/c8mh00966j
Patole, S., Alegaonkar, P., Lee, H.-C., and Yoo, J.-B. (2008). Optimization of water assisted chemical vapor deposition parameters for super growth of carbon nanotubes. Carbon 46, 1987–1993. doi:10.1016/j.carbon.2008.08.009
Pawar, A., Patel, R., Arulmozhi, S., and Bothiraja, C. (2016). D-α-Tocopheryl polyethylene glycol 1000 succinate conjugated folic acid nanomicelles: towards enhanced bioavailability, stability, safety, prolonged drug release and synergized anticancer effect of plumbagin. RSC Adv. 6, 78106–78121. doi:10.1039/c6ra12714b
Pistone, A., Iannazzo, D., Ansari, S., Milone, C., Salamò, M., Galvagno, S., et al. (2016). Tunable doxorubicin release from polymer-gated multiwalled carbon nanotubes. Int. J. Pharm. 515, 30–36. doi:10.1016/j.ijpharm.2016.10.010
Prasek, J., Drbohlavova, J., Chomoucka, J., Hubalek, J., Jasek, O., Adam, V., et al. (2011). Methods for carbon nanotubes synthesis—review. J. Mater. Chem. 21, 15872–15884. doi:10.1039/c1jm12254a
Qi, X., Rui, Y., Fan, Y., Chen, H., Ma, N., and Wu, Z. (2015). Galactosylated chitosan-grafted multiwall carbon nanotubes for pH-dependent sustained release and hepatic tumor-targeted delivery of doxorubicin in vivo. Colloids Surfaces B Biointerfaces 133, 314–322. doi:10.1016/j.colsurfb.2015.06.003
Raza, K., Kumar, D., Kiran, C., Kumar, M., Guru, S. K., Kumar, P., et al. (2016). Conjugation of docetaxel with multiwalled carbon nanotubes and codelivery with piperine: implications on pharmacokinetic profile and anticancer activity. Mol. Pharm. 13, 2423–2432. doi:10.1021/acs.molpharmaceut.6b00183
Rele, S., Thakur, C. K., Khan, F., Baral, B., Saini, V., Karthikeyan, C., et al. (2024). Curcumin coating: a novel solution to mitigate inherent carbon nanotube toxicity. J. material Sci. Mater. Med. 35, 24–10. doi:10.1007/s10856-024-06789-9
Ren, Z., Luo, Y., Liu, X., Zhang, J., Chen, S., Yu, R., et al. (2020). Preparation, characterization and controlled-release property of CS crosslinked MWCNT based on Hericium erinaceus polysaccharides. Int. J. Biol. Macromol. 153, 1310–1318. doi:10.1016/j.ijbiomac.2019.10.266
Rezaei, S. J., Hesami, A., Khorramabadi, H., Amani, V., Malekzadeh, A. M., Ramazani, A., et al. (2018). Pt (II) complexes immobilized on polymer-modified magnetic carbon nanotubes as a new platinum drug delivery system. Appl. Organomet. Chem. 32, e4401. doi:10.1002/aoc.4401
Reza Rezaie, H., Esnaashary, M., Reza Rezaie, H., Esnaashary, M., Aref arjmand, A., and Öchsner, A. (2018). The history of drug delivery systems. A Rev. Biomaterials Their Appl. Drug Deliv., 1–8. doi:10.1007/978-981-10-0503-9_1
Rodrigues, F. C., Kumar, N. A., and Thakur, G. (2019). Developments in the anticancer activity of structurally modified curcumin: an up-to-date review. Eur. J. Med. Chem. 177, 76–104. doi:10.1016/j.ejmech.2019.04.058
Ruy, C., Silvia, S., Rodrigo, J. F., Cecília, B. M., Barcellos, I., and Funck, J. (2003). Nanoparticles containing dexamethasone: physicochemical properties and antiinflammatory activity. Acta Farm Bonaer. 22, 11–15.
Sahebian, S., Zebarjad, S., Vahdati Khaki, J., and Lazzeri, A. (2016). The decoration of multi-walled carbon nanotubes with nickel oxide nanoparticles using chemical method. Int. Nano Lett. 6, 183–190. doi:10.1007/s40089-016-0185-8
Sahoo, N. G., Bao, H., Pan, Y., Pal, M., Kakran, M., Cheng, H. K. F., et al. (2011). Functionalized carbon nanomaterials as nanocarriers for loading and delivery of a poorly water-soluble anticancer drug: a comparative study. Chem. Commun. 47, 5235–5237. doi:10.1039/c1cc00075f
Sahoo, N. G., Rana, S., Cho, J. W., Li, L., and Chan, S. H. (2010). Polymer nanocomposites based on functionalized carbon nanotubes. Prog. Polym. Sci. 35, 837–867. doi:10.1016/j.progpolymsci.2010.03.002
Saito, N., Haniu, H., Usui, Y., Aoki, K., Hara, K., Takanashi, S., et al. (2014). Safe clinical use of carbon nanotubes as innovative biomaterials. Chem. Rev. 114, 6040–6079. doi:10.1021/cr400341h
Sanginario, A., Miccoli, B., and Demarchi, D. (2017). Carbon nanotubes as an effective opportunity for cancer diagnosis and treatment. Biosensors 7, 9. doi:10.3390/bios7010009
Santra, S., Kaittanis, C., Grimm, J., and Perez, J. M. (2009). Drug/dye-loaded, multifunctional iron oxide nanoparticles for combined targeted cancer therapy and dual optical/magnetic resonance imaging. small 5, 1862–1868. doi:10.1002/smll.200900389
Schipper, M. L., Nakayama-Ratchford, N., Davis, C. R., Kam, N. W. S., Chu, P., Liu, Z., et al. (2008). A pilot toxicology study of single-walled carbon nanotubes in a small sample of mice. Nat. Nanotechnol. 3, 216–221. doi:10.1038/nnano.2008.68
Selim, A., Lila, A., Bhattacharya, R., Moin, A., Hagbani, A., Danish Rizvi, S. M., et al. (2023). Dual targeting multiwalled carbon nanotubes for improved neratinib delivery in breast cancer. RSC Adv. 13, 24309–24318. doi:10.1039/d3ra04732f
Sheikhpour, M., Golbabaie, A., and Kasaeian, A. (2017). Carbon nanotubes: a review of novel strategies for cancer diagnosis and treatment. Mater. Sci. Eng. C 76, 1289–1304. doi:10.1016/j.msec.2017.02.132
Shi, X., Wang, S. H., Shen, M., Antwerp, M. E., Chen, X., Li, C., et al. (2009). Multifunctional dendrimer-modified multiwalled carbon nanotubes: synthesis, characterization, and in vitro cancer cell targeting and imaging. Biomacromolecules 10, 1744–1750. doi:10.1021/bm9001624
Shvedova, A. A., Kapralov, A. A., Feng, W. H., Kisin, E. R., Murray, A. R., Mercer, R. R., et al. (2012). Impaired clearance and enhanced pulmonary inflammatory/fibrotic response to carbon nanotubes in myeloperoxidase-deficient mice. PLoS one 7, e30923. doi:10.1371/journal.pone.0030923
Shvedova, A. A., Kisin, E. R., Mercer, R., Murray, A. R., Johnson, V. J., Potapovich, A. I., et al. (2005). Unusual inflammatory and fibrogenic pulmonary responses to single-walled carbon nanotubes in mice. Am. J. Physiology-Lung Cell. Mol. Physiology 289, L698–L708. doi:10.1152/ajplung.00084.2005
Singh, R., Mehra, N. K., Jain, V., and Jain, N. K. (2013). Gemcitabine-loaded smart carbon nanotubes for effective targeting to cancer cells. J. drug Target. 21, 581–592. doi:10.3109/1061186X.2013.778264
Singh, R., Pantarotto, D., Lacerda, L., Pastorin, G., Klumpp, C., Prato, M., et al. (2006). Tissue biodistribution and blood clearance rates of intravenously administered carbon nanotube radiotracers, in Proceedings of the national academy of Sciences, 3357–3362. doi:10.1073/pnas.0509009103
Singh, R. P., Sharma, G., Singh, S., Bharti, S., Pandey, B. L., Koch, B., et al. (2017). Chitosan-folate decorated carbon nanotubes for site specific lung cancer delivery. Mater. Sci. Eng. C 77, 446–458. doi:10.1016/j.msec.2017.03.225
Singh, R. P., Sharma, G., Singh, S., Kumar, M., Pandey, B. L., Koch, B., et al. (2016a). Vitamin E TPGS conjugated carbon nanotubes improved efficacy of docetaxel with safety for lung cancer treatment. Colloids surfaces B Biointerfaces 141, 429–442. doi:10.1016/j.colsurfb.2016.02.011
Singh, R. P., Sharma, G., Singh, S., Patne, S. C., Pandey, B. L., Koch, B., et al. (2016b). Effects of transferrin conjugated multi-walled carbon nanotubes in lung cancer delivery. Mater. Sci. Eng. C 67, 313–325. doi:10.1016/j.msec.2016.05.013
Singh, S., Mehra, N. K., and Jain, N. (2016c). Development and characterization of the paclitaxel loaded riboflavin and thiamine conjugated carbon nanotubes for cancer treatment. Pharm. Res. 33, 1769–1781. doi:10.1007/s11095-016-1916-2
Singhai, N. J., Maheshwari, R., Jain, N. K., and Ramteke, S. (2020). Chondroitin sulphate and α -tocopheryl succinate tethered multiwalled carbon nanotubes for dual-action therapy of triple-negative breast cancer. J. Drug Deliv. Sci. Technol. 60, 102080. doi:10.1016/j.jddst.2020.102080
Singhai, N. J., Maheshwari, R., and Ramteke, S. (2020). CD44 receptor targeted ‘smart’multi-walled carbon nanotubes for synergistic therapy of triple-negative breast cancer. Colloid Interface Sci. Commun. 35, 100235. doi:10.1016/j.colcom.2020.100235
Smith, A., Moxon, S., and Morris, G. (2016). Biopolymers as wound healing materials Wound Heal. Biomater 2, 261–287.
Son, K. H., Hong, J. H., and Lee, J. W. (2016). Carbon nanotubes as cancer therapeutic carriers and mediators. Int. J. nanomedicine 11, 5163–5185. doi:10.2147/IJN.S112660
Srivastava, R. K., Pant, A. B., Kashyap, M. P., Kumar, V., Lohani, M., Jonas, L., et al. (2011). Multi-walled carbon nanotubes induce oxidative stress and apoptosis in human lung cancer cell line-A549. Nanotoxicology 5, 195–207. doi:10.3109/17435390.2010.503944
Sun, Y.-P., Fu, K., Lin, Y., and Huang, W. (2002). Functionalized carbon nanotubes: properties and applications. Accounts Chem. Res. 35, 1096–1104. doi:10.1021/ar010160v
Thakur, C. K., Karthikeyan, C., Abou-dahech, M. S., Altabakha, M. M. A. M., Jamal, M., A, S., et al. (2023). Microwave-Assisted functionalization of multi-walled carbon nanotubes for biosensor and drug delivery applications. Pharmaceutics 15, 1–30. doi:10.3390/pharmaceutics15020335
Thakur, C. K., Neupane, R., Karthikeyan, C., Ashby, C. R., Babu, R. J., Boddu, S. H. S., et al. (2022). Lysinated multiwalled carbon nanotubes with carbohydrate ligands as an effective nanocarrier for targeted doxorubicin delivery to breast cancer cells. Molecules 27, 1–20. doi:10.3390/molecules27217461
Thakur, C. K., Thotakura, N., Kumar, R., Kumar, P., Singh, B., Chitkara, D., et al. (2016). Chitosan-modified PLGA polymeric nanocarriers with better delivery potential for tamoxifen. Int. J. Biol. Macromol. 93, 381–389. doi:10.1016/j.ijbiomac.2016.08.080
Thirugnanam, S., Xu, L., Ramaswamy, K., and Gnanasekar, M. (2008). Glycyrrhizin induces apoptosis in prostate cancer cell lines DU-145 and LNCaP. Oncol. Rep. 20, 1387–1392.
Tison, Y., Giusca, C. E., Stolojan, V., Hayashi, Y., and Silva, S. R. P. (2008). The inner shell influence on the electronic structure of double-walled carbon nanotubes. Adv. Mater. 20, 189–194. doi:10.1002/adma.200700399
Tran, P., Pyo, Y.-C., Kim, D.-H., Lee, S.-E., Kim, J.-K., and Park, J.-S. (2019). Overview of the manufacturing methods of solid dispersion technology for improving the solubility of poorly water-soluble drugs and application to anticancer drugs. Pharmaceutics 11, 132. doi:10.3390/pharmaceutics11030132
Van der Zande, M., Junker, R., Walboomers, X. F., and Jansen, J. A. (2011). Carbon nanotubes in animal models: a systematic review on toxic potential. Tissue Eng. Part B Rev. 17, 57–69. doi:10.1089/ten.TEB.2010.0472
Vardharajula, S., Ali, S. Z., Tiwari, P. M., Eroğlu, E., Vig, K., Dennis, V. A., et al. (2012). Functionalized carbon nanotubes: biomedical applications. Int. J. nanomedicine 7, 5361–5374. doi:10.2147/IJN.S35832
Veetil, J. V., and Ye, K. (2009). Tailored carbon nanotubes for tissue engineering applications. Biotechnol. Prog. 25, 709–721. doi:10.1002/btpr.165
Wang, C.-J., Wang, H.-Z., and Li, W. (2019). A novel conjunction of folate-targeted carbon nanotubes containing protohemin and oridonin-liposome loaded microbubbles for cancer chemo-sonodynamic therapy. J. Drug Target. 27, 1076–1083. doi:10.1080/1061186X.2019.1591422
Wang, D., Hou, C., Meng, L., Long, J., Jing, J., Dang, D., et al. (2017). Stepwise growth of gold coated cancer targeting carbon nanotubes for the precise delivery of doxorubicin combined with photothermal therapy. J. Mater. Chem. B 5, 1380–1387. doi:10.1039/c6tb02755e
Wang, D., Ren, Y., Shao, Y., Yu, D., and Meng, L. (2017). Facile preparation of doxorubicin-loaded and folic acid-conjugated carbon nanotubes@ poly (N-vinyl pyrrole) for targeted synergistic chemo–Photothermal Cancer treatment. Bioconjugate Chem. 28, 2815–2822. doi:10.1021/acs.bioconjchem.7b00515
Wang, L., Shi, J., Hao, Y., Zhang, P., Zhao, Y., Meng, D., et al. (2015). Magnetic multi-walled carbon nanotubes for tumor theranostics. J. Biomed. Nanotechnol. 11, 1653–1661. doi:10.1166/jbn.2015.2103
Wang, Q.-S., Gao, L.-N., Zhu, X.-N., Zhang, Y., Zhang, C.-N., Xu, D., et al. (2019). Co-delivery of glycyrrhizin and doxorubicin by alginate nanogel particles attenuates the activation of macrophage and enhances the therapeutic efficacy for hepatocellular carcinoma. Theranostics 9, 6239–6255. doi:10.7150/thno.35972
Wang, Z., Tian, Y., Zhang, H., Qin, Y., Li, D., Gan, L., et al. (2016). Using hyaluronic acid-functionalized pH stimuli-responsive mesoporous silica nanoparticles for targeted delivery to CD44-overexpressing cancer cells. Int. J. Nanomedicine 11, 6485–6497. doi:10.2147/IJN.S117184
Xu, Y., Wu, H., Huang, C., Hao, C., Wu, B., Miao, C., et al. (2015). Sensitive detection of tumor cells by a new cytosensor with 3D-MWCNTs array based on vicinal-dithiol-containing proteins (VDPs). Biosens. Bioelectron. 66, 321–326. doi:10.1016/j.bios.2014.11.008
Yan, L., Zhao, F., Li, S., Hu, Z., and Zhao, Y. (2011). Low-toxic and safe nanomaterials by surface-chemical design, carbon nanotubes, fullerenes, metallofullerenes, and graphenes. Nanoscale 3, 362–382. doi:10.1039/c0nr00647e
Yan, Y., Wang, R., Hu, Y., Sun, R., Song, T., Shi, X., et al. (2018). Stacking of doxorubicin on folic acid-targeted multiwalled carbon nanotubes for in vivo chemotherapy of tumors. Drug Deliv. 25, 1607–1616. doi:10.1080/10717544.2018.1501120
Yang, M., Gao, Y., Li, H., and Adronov, A. (2007a). Functionalization of multiwalled carbon nanotubes with polyamide 6 by anionic ring-opening polymerization. Carbon 45, 2327–2333. doi:10.1016/j.carbon.2007.07.021
Yang, S., Guo, W., Lin, Y., Deng, X.-y., Wang, H.-f., Sun, H.-f., et al. (2007b). Biodistribution of pristine single-walled carbon nanotubes in vivo. J. Phys. Chem. C 111, 17761–17764. doi:10.1021/jp070712c
Yang, S.-T., Wang, X., Jia, G., Gu, Y., Wang, T., Nie, H., et al. (2008). Long-term accumulation and low toxicity of single-walled carbon nanotubes in intravenously exposed mice. Toxicol. Lett. 181, 182–189. doi:10.1016/j.toxlet.2008.07.020
Yang, T., Wu, Z., Wang, P., Mu, T., Qin, H., Zhu, Z., et al. (2017). A large-inner-diameter multi-walled carbon nanotube-based dual-drug delivery system with pH-sensitive release properties. J. Mater. Sci. Mater. Med. 28, 110–113. doi:10.1007/s10856-017-5920-9
Yaron, P. N., Holt, B. D., Short, P. A., Lösche, M., Islam, M. F., and Dahl, K. N. (2011). Single wall carbon nanotubes enter cells by endocytosis and not membrane penetration. J. nanobiotechnology 9, 45–15. doi:10.1186/1477-3155-9-45
Yu, B.-Z., Yang, J.-S., and Li, W.-X. (2007). In vitro capability of multi-walled carbon nanotubes modified with gonadotrophin releasing hormone on killing cancer cells. Carbon 45, 1921–1927. doi:10.1016/j.carbon.2007.06.015
Yun, Y. H., Lee, B. K., and Park, K. (2015). Controlled drug delivery: historical perspective for the next generation. J. Control. Release 219, 2–7. doi:10.1016/j.jconrel.2015.10.005
Zhang, M., Wang, W., Cui, Y., Zhou, N., and Shen, J. (2018). Magnetofluorescent carbon quantum dot decorated multiwalled carbon nanotubes for dual-modal targeted imaging in chemo-photothermal synergistic therapy. ACS Biomaterials Sci. Eng. 4, 151–162. doi:10.1021/acsbiomaterials.7b00531
Keywords: multiwalled carbon nanotubes, cancer, vitamins, targeted drug delivery, anticancer drugs, cytotoxicity
Citation: Thakur CK, Karthikeyan C, Ashby CR Jr., Neupane R, Singh V, Babu RJ, Narayana Moorthy NSH and Tiwari AK (2024) Ligand-conjugated multiwalled carbon nanotubes for cancer targeted drug delivery. Front. Pharmacol. 15:1417399. doi: 10.3389/fphar.2024.1417399
Received: 14 April 2024; Accepted: 24 June 2024;
Published: 25 July 2024.
Edited by:
Chao Zhao, University of Alabama, United StatesReviewed by:
Qi Li, University of Alabama, United StatesKandasamy Vinothini, Mohamed Sathak Engineering College (MSEC), India
Preeti Kush, Shobhit University, India
Copyright © 2024 Thakur, Karthikeyan, Ashby, Neupane, Singh, Babu, Narayana Moorthy and Tiwari. This is an open-access article distributed under the terms of the Creative Commons Attribution License (CC BY). The use, distribution or reproduction in other forums is permitted, provided the original author(s) and the copyright owner(s) are credited and that the original publication in this journal is cited, in accordance with accepted academic practice. No use, distribution or reproduction is permitted which does not comply with these terms.
*Correspondence: R. Jayachandra Babu, ramapjb@auburn.edu; N. S. Hari Narayana Moorthy, nsharinarayana.moorthy@igntu.ac.in; Amit K. Tiwari, atiwari@uams.edu