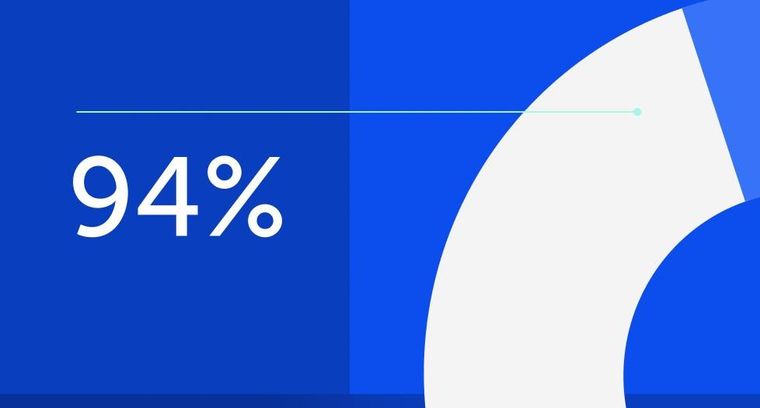
94% of researchers rate our articles as excellent or good
Learn more about the work of our research integrity team to safeguard the quality of each article we publish.
Find out more
REVIEW article
Front. Pharmacol., 19 June 2024
Sec. Pharmacology of Anti-Cancer Drugs
Volume 15 - 2024 | https://doi.org/10.3389/fphar.2024.1416382
This article is part of the Research TopicNatural Products and Nanoparticles in Cancer Treatment: Is There a Light at the End of the Tunnel?View all 5 articles
Ferroptosis is a form of regulated cell death (RCD) characterized by iron-dependent lipid peroxidation. Ferroptosis is currently proposed as one of the most promising means of combating tumor resistance. Nevertheless, the problem of ferroptosis resistance in certain cancer cells has been identified. This review first, investigates the mechanisms of ferroptosis induction in cancer cells. Next, the problem of cancer cell resistance to ferroptosis, as well as the underlying mechanisms is discussed. Recently discovered ferroptosis-suppressing biomarkers have been described. The various types of nanoparticles that can induce ferroptosis are also discussed. Given the ability of nanoparticles to combine multiple agents, this review proposes nanoparticle-based ferroptosis cell death as a viable method of circumventing this resistance. This review suggests combining ferroptosis with other forms of cell death, such as apoptosis, cuproptosis and autophagy. It also suggests combining ferroptosis with immunotherapy.
Cancer remains one of the world’s biggest killers, despite considerable progress in diagnostic and therapeutic techniques (Siegel et al., 2021; Sung et al., 2021). The challenges of cancer therapy are manifold. They include issues such as drug resistance, tumor heterogeneity and deleterious side effects on normal tissues (Alfarouk et al., 2015; Kudelova et al., 2022; Romani, 2022). These obstacles call for the development of innovative therapeutic strategies capable of targeting cancer cells more effectively, while circumventing the problem of resistance.
One such emerging strategy is the induction of ferroptosis, a form of regulated cell death (RCD). Ferroptosis is characterized by the accumulation of iron-dependent lipid peroxidation. Unlike other RCDs such as apoptosis and necrosis, ferroptosis targets the vulnerability of cancer cells to iron overload and oxidative stress, offering a unique angle for tackling drug-resistant and difficult-to-treat cancers (Wang Huan et al., 2023). The significance of ferroptosis in cancer treatment lies not only in its potential to eliminate cancer cells but also in its capacity to induce a form of cell death that is fundamentally different from other RCD, thereby potentially overcoming resistance mechanisms. Ferroptosis is characterized by a complex interplay of processes leading to cell death due to reactive oxygen species (ROS) attacking membrane lipids. In particular, it involves the disintegration of the cell membrane, mitochondria or various organelles (Do et al., 2023; Krusenstiern et al., 2023; Lyamzaev et al., 2023). This event is a consequence of multiple changes at the cellular level (Logie et al., 2021; Ma et al., 2022). These transformations are attributed to increased lipid peroxidation, which disrupts membrane integrity, leading to a loss of normal membrane permeability (Mortensen et al., 2023). This intensification of lipid peroxidation plays a central role in the ferroptosis process, effectively serving as a death signal for the cell (Mortensen et al., 2023; Usukhbayar et al., 2023). Notably, ferroptosis is driven by four interdependent mechanisms: iron accumulation, reactive oxygen species (ROS) generation, lipid peroxidation, and glutathione depletion. These interconnected pathways act synergistically to induce this unique form of cell death, offering a nuanced understanding of the ferroptosis process and its impact on cancer therapy (Xu et al., 2023).
Nanotechnology offers a cutting-edge platform for inducing ferroptosis in a targeted manner. Nanoparticles can be engineered to induce ferroptosis or deliver ferroptosis-inducing agents specifically to cancer cells, thereby reducing off-target effects (Cai et al., 2022; Abu-Serie, 2023; Zhang D. et al., 2023). Moreover, the physicochemical properties of nanoparticles, such as size, shape, and surface characteristics, can be precisely controlled to optimize their delivery and therapeutic efficiency (Tian et al., 2022; Wang Bingqing et al., 2023). Nanoparticles also offer the advantage of combining multiple therapeutic agents, enabling a synergistic approach to induce ferroptosis at the same time as other forms of cell death, thus widening the therapeutic window (Zhang J. et al., 2023). This review explores the versatile role of nanoparticles that trigger ferroptosis to treat cancer. These findings also suggest how these nanoparticles could be combined with other treatments for even better results.
Iron is involved in several major biological processes. It includes cellular respiration, heme production, enzyme activity, DNA and RNA synthesis, immune function and metabolism (Winter et al., 2014). Normal and cancer cells depend on iron for survival and growth (Lee and Roh, 2023a; Crescenzi et al., 2023). The dysregulation of iron balance can lead to pathological conditions such as tumor initiation, proliferation, and metastasis (Liu J. et al., 2022; Crescenzi et al., 2023). However, excessive production of iron can have adverse effects on cellular components, leading to cell death. Understanding the mechanisms involved in iron uptake, storage, and release within cells is essential for developing effective strategies to target cancer cells (Crescenzi et al., 2023).
Iron can enter the cell through different routes depending on its source (Ma S. et al., 2023). In the ferric form (Fe3+), iron from dietary sources is converted to ferrous iron (Fe2+) by the action of duodenal cytochrome B (DyctB) and then transported into the cell via the divalent metal transporter 1 (DMT1) (Ma X. et al., 2023; Crescenzi et al., 2023). In the bloodstream, Fe3+ is taken up by the cell through the transferrin-transferrin receptor 1 (TF-TFR1) system (Akashi et al., 2021; Qu et al., 2022). Once inside the endosome, Fe3+ is converted to Fe2+ by the six-transmembrane epithelial antigen of the prostate 3 (STEAP3) and then transported into the cytoplasm via endosomal DMT1, contributing to the formation of the labile iron pool (LIP). Excess Fe2+ is exported by the transmembrane exporter ferroportin (FPN) and converted to Fe3+(Yanatori et al., 2010; Velkova et al., 2023).
Other transporters are involved in the transport of iron into the cell cytoplasm or mitochondria. Heme, a circulating form of iron, can enter the cell by endocytosis and then be degraded to Fe2+ by the action of heme oxygenase 1 (HMOX1) (Yanatori et al., 2010; Wang Luping et al., 2023). Multiple divalent metal transporters, such as ZRT/IRT-like protein 14 (ZIP14) or ZIP8, can carry Fe2+ into the cell cytoplasm (Lee and Roh, 2023b). Fe2+ can enter mitochondria through the complex mitoferrin 1/2 (MFRN 1/2)/ATP-binding cassette subfamily B member 10 (ABCB 10) enabling the synthesis of heme and iron-sulfur (Fe-S) clusters. The Fe-S clusters are involved in the generation of reactive oxygen species (ROS), which ultimately contribute to lipid peroxidation and the induction of ferroptosis (Lee and Roh, 2023b).
Regulation of the labile iron pool (LIP) in the cell is tightly controlled by iron transporters involved in iron entry and exit. Iron regulatory proteins (IRPs), notably IRP1 and IRP2, play an important role in this mechanism. Under conditions of iron deficiency, IRPs bind to the 5′untranslated region (UTR) of FPN, reducing iron export and storage. Similarly, IRPs bind to the 3′UTR of the TFR, preventing iron degradation and increasing iron uptake. Conversely, during iron replenishment, the binding of IRP1 to the Fe-S cluster induces a conformational change, inhibiting its binding activity to ferritin or FPN. IRP2, regulated by the iron sensor F-box and leucine-rich repeat protein 5 (FBXL5), undergoes ubiquitination and degradation, preventing its activity (Zhou and Tan, 2017; Cardona and Montgomery, 2023).
In addition to regulating iron transport and storage, cells can also release iron through ferritinophagy. Nuclear receptor coactivator 4 (NCOA4) facilitates the proteolysis of lysosomes, releasing iron from ferritin (Mancias et al., 2014). Nuclear factor erythroid 2-related factor 2 (NRF2), which is controlled by Kelch-like ECH-associated protein 1 (Keap1) also plays an important role in iron metabolism by regulating the activity of NCOA4 and HMOX1 (Fan et al., 2017; Lee and Roh, 2023c).
Reactive oxygen species (ROS), which are part of the reactive species family, are highly reactive oxidants derived from molecular oxygen (O2) (Sies et al., 2022). ROS can be generated enzymatically or nonenzymatically within cells and play an essential role in various cellular functions (Sies et al., 2022). Several studies have demonstrated the involvement of ROS in several cell death processes, including apoptosis, autophagy and ferroptosis (Wang Ling et al., 2023).
Excessive accumulation of Fe2+ in the cell in the form of LIP is one of the mechanisms by which ROS can be generated (Huang J. et al., 2023). When it accumulates excessively, Fe2+ can trigger the Fenton reaction, oxidizing to Fe3+. Electron transfer occurs in the presence of hydrogen peroxide (H2O2), resulting in the formation of the hydroxyl radicals (HO•), a highly reactive type of ROS. (38). The mitochondria and enzymes of the nicotinamide adenine dinucleotide phosphate (NADPH) oxidase (NOX) family also play important roles in ROS generation (Tang and Kroemer, 2020; Zeng K. et al., 2023).
Lipid peroxidation is a complex process that occurs when reactive oxygen species (ROS), such as HO•, damage unsaturated fatty acids in cell membranes. Lipid peroxidation occurs via two mechanisms: enzymatic and nonenzymatic (Ayala et al., 2014).
Nonenzymatic lipid peroxidation is triggered by the Fenton reaction, where an excess of intracellular Fe2+ reacts with H2O2 to produce HO•. HO• then initiates the oxidation of polyunsaturated fatty acyl chains (PUFAs) by extracting hydrogen from them, forming carbon-centered phospholipid radicals that react with oxygen to form lipid peroxide radicals (PLOO•). These PLOO• radicals propagate the chain reaction by extracting hydrogen from adjacent PUFAs, resulting in the formation of lipid hydroperoxides (PLOOH) and new lipid free radicals. This process triggers further lipid peroxidation. The process is terminated by radical-trapping antioxidants or by reduction catalyzed by glutathione peroxidase. This termination process forms alkoxy lipid radicals (PLO•), which react with PUFAs from another phospholipid (PL), producing PL alcohol (PLOH) and a new PL•. This perpetuates the lipid radical chain reaction (Mortensen et al., 2023).
Several key enzymes, including lipoxygenase (LOX), cytochrome P450 oxidoreductase (POR) and cyclooxygenases (COXs), control enzymatic lipid peroxidation. PUFAs can be synthesized through the action of acyl-CoA synthetase long-chain family member 4 (ACSL4). ACSL4 binds PUFAs to coenzyme A (CoA) to produce PUFA-CoA. Subsequently, lysophosphatidylcholine acyltransferase 3 (LPCAT3) re-esters PUFA-CoA into phospholipids. The most abundant PUFAs in cells, linoleic acid, and arachidonic acid, can be oxidized into PLOOH by the action of LOX, a nonheme iron dioxygenase. LOX catalyzes the dioxygenation of free and esterified PUFAs, generating various PLOOH. In the presence of ferrous iron, PLOOH can breakdown into alkoxy PLO• which further propagates lipid peroxidation or is converted into 4-hydroxynonenal (4-HNE) and malondialdehyde (MDA). These compounds can lead to the formation of protein adducts and disrupt protein structure and function. This in-depth exploration offers valuable insights into the complex mechanisms and regulation of lipid peroxidation, highlighting its critical role as a hallmark of ferroptosis (Bayır et al., 2020; Endale et al., 2023).
Glutamate synthesis and its regulation play a crucial role in the induction of ferroptosis (Yao et al., 2021). This synthesis is dependent on the proper functioning of the system xc-, which comprises the light chain SLC7A11 and the heavy chain SLC3A2. System xc-operates as a cystine/glutamate antiporter and is responsible for importing cystine into the cell and exporting glutamate. Once inside the cell, cystine is reduced to cysteine, which serves as a key element in the synthesis of reduced glutathione. (GSH) (Li B. et al., 2022).
Glutathione peroxidase 4 (GPX4) is a critical regulator of lipid peroxidation, acting to reduce PLOOH to PLOH, thereby preventing the damaging spread of lipid peroxidation (Ursini and Maiorino, 2020; Xu et al., 2021).
Significantly, GSH acts as a vital cofactor for GPX4’s lipid peroxide reductase activity. GPX4 utilizes its catalytic selenocysteine residue and relies on the two electrons donated by GSH, low-molecular thiols, or protein thiols to efficiently counteract lipid peroxidation (Ursini and Maiorino, 2020).
A number of advances have been made in the search for a ferroptosis treatments for cancer. Several molecules have been demonstrated to be able to effectively induce cancer cell death via ferroptosis. These include erastin, RSL3, sorafenib, cisplatin and others. Like all therapeutic approaches, ferroptosis induction faces several challenges. Resistance is one such challenge. Several biomarkers whose expression in cancer cells renders ferroptosis-based treatment ineffective have been discovered. The FerrDb database is a free and open resource for identifying ferroptosis-suppressor genes. (http://www.zhounan.org/ferrdb/) (Zhou H. et al., 2023). Several other ferroptosis suppressors have recently been discovered. Table 1 summarizes these ferroptosis suppressors and their mechanisms of action.
GPX4 expression, as previously stated, inhibits lipid peroxidation. Several studies have revalated proteins that can upregulate GPX4. Most ferroptosis-inducing drugs become ineffective in these situations. Protein disulfide isomerase A member 4 (PDIA4) suppresses ferroptosis via endoplasmic reticulum stress. SLC7A11 is targeted by the latter via the PERK/ATF4 pathway. Salinomycin has recently been shown to suppress PDIA4 expression, thereby increasing ferroptosis (Kang et al., 2023). SLC12A5 is an ion transporter whose expression is increased in hepatocellular carcinoma. It focuses on the xCt system, increasing its activity. It increases GPX4 expression while decreasing ACSL4, resulting in ferroptosis resistance (Tong et al., 2023). The Cystatin SN, encoded by the CST1 gene, has been linked to gastric cancer metastasis. It also suppresses ferroptosis. It was discovered that the CST1 protein and GPX4 have a strong interaction. This interaction stabilizes the GPX4 protein and increases its expression when CST1 levels increase. CST1 relieves GPX4 ubiquitination via the deubiquitinase OTUB1 (Li D. et al., 2023).
Forkhead box transcription factor A2 (FOXA2) is a biomarker capable of increasing GPX4 activity in colorectal cancer (CRC) (Liu J. et al., 2022). Another GPX4 promoter is creatatine kinase B. By phosphorylating GPX4, creatine kinase B can suppress ferroptosis (Wu et al., 2023).
As lipid peroxidation is the key component of ferroptosis, its inhibition is an obstacle to ferroptosis-based therapy. Several studies have identified biomarkers capable of directly inhibiting lipid peroxidation. TXNDC12 (thioredoxin domain-containing protein 12) is a protein found primarily in the endoplasmic reticulum. When expressed, it inhibits lipid peroxidation independently of the GPX4 pathway. Its upregulation has been shown to be mediated by the expression of the transcription factor ATF4 (Tang J. et al., 2023). ASCL4 plays an important role in lipid peroxidation. When it is inhibited, lipid peroxidation is also inhibited, rendering cancer cells resistant to ferroptosis. Cytochrome P450 1B1 (CYP1B1), promotes ACLS4 ubiquitination and degradation in colorectal cancer (Chen C. et al., 2023).
Ferroptosis suppressor protein 1 (FSP1) is one of the main regulators of ferroptosis. FSP1 converts CoQ10 to CoQ10H2 by consuming NADH/NADPH. CoQ10H2 acts as an antioxidant, preventing lipid peroxidation. FSP1 upregulation is thus a factor in resistance to ferroptosis (Li H. et al., 2023). ACSL1 has been shown to upregulate FSP1 in ovarian cancer (Zhang Q. et al., 2023). FSP1 can convert vitamin K (VK) to VKH2. VKH2 then acts as an antioxidant by scavenging free radicals and thus inhibiting lipid peroxidation (Mishima et al., 2022; Jin D.-Y. et al., 2023). The tripartite motif family member TRIM21 can ubiquitinating the FSP1 protein. Overexpression of TRIM21 promotes FSP1 translocation into the plasma membrane and causes ferroptosis resistance (Gong et al., 2023). CoQ10 synthesis requires the mevalonate pathway (Zheng and Conrad, 2020). Certain enzymes can cause ferroptosis resistance by acting on this pathway. This is the case for P4HA1, a subtype of the prolyl 4-hydroxylase (P4H) family. In fact, P4HA1 can upregulate HMGCS1, a key enzyme in the mevalonate pathway, and thus induce ferroptosis resistance (Zhou N. et al., 2023).
Dihydroorotate dehydrogenase (DHODH) is a mitochondrial enzyme that, like FSP1, inhibits lipid peroxidation (Wang Qin et al., 2023). It catalyzes the conversion of dihydroorotate (DHO) to orotate (OA), as well as the reduction of CoQ to CoQH2. CoQH2 prevents lipid peroxidation. Lysyl oxidase-like 3 (LOXL3) in mitochondria induces ferroptosis resistance by stabilizing DHODH. In effect, LOXL3 prevents DHODH-K344 ubiquitination, which promotes CoQ reduction to CoQH2 in mitochondria (Zhan et al., 2023).
Guanosine 5′-triphosphate cyclohydrolase 1 (GCH1) is an enzyme that regulates ferroptosis. It catalyzes the reaction that produces tetrahydrobiopterin (BH4) from GTP. When GCH1 is overexpressed, BH4 biosynthesis increases, preventing lipid peroxidation. Recently, it was discovered that SR-rich splicing factor 1 (SRSF1) can increase GCH1 expression by preventing its ubiquitination. First, SRSF1 binds to circSEPT9, increasing its expression. In turn, circSEPT9 binds to GCH1, preventing its ubiquitination (Song et al., 2024).
Cholesterol biosynthesis is governed by the mevalonate pathway. Isopentenyl pyrophosphate (IPP) is one of the byproducts of cholesterol synthesis. IPP stimulates the production of GPX4 (Zheng and Conrad, 2020). Cholesterol has also been shown to increase ferroptosis resistance via the SLC38A9-mTOR axis by upregulating GPX4. Furthermore, cholesterol accumulation inhibits ferritinophagy in long term hematopoietic stem cells via the SLC38A9-mTOR axis (Liu C. et al., 2023). B7H3 is a ferroptosis inhibitor that interacts with cholesterol biosynthesis. B7H3 downregulates sterol regulatory element binding protein 2 (SREBP2), a transcription factor that plays an important role in regulating cholesterol metabolism by activating the ATK pathway (Jin H. et al., 2023). 7-Hydroxycholesterol (27HC), a common circulating cholesterol metabolite, is a regulator of cholesterol biosynthesis (Liu W. et al., 2021). 27HC increases GPX4 expression and inhibits lipid peroxidation. According to one study, TMEM147 may increase 27HC activity by activating the enzyme 7-dehydrocholesterol reductase (DHCR7) (J. Huang L. et al., 2023).
Recently, two genes whose expression is controlled by sex hormone were shown to cause ferroptosis resistance. Indeed, two Membrane Bound O-Acyltransferase Domain Containing (MBOAT1/2) genes have been implicated in ferroptosis resistance. Compared with GPX4 and FSP1, MBOAT1 and MBOAT2 induce resistance to ferroptosis via a different. They are controlled by two sex hormone receptors, the estrogen receptor and the androgen receptor. MBOAT1 and MBOAT2 are indeed upregulated by these two receptors. (Liang D. et al., 2023).
The wnt/ß-catenin pathway plays an important role in the regulation of the body’s homeostasis (Liu S. et al., 2022). It has been found that its abnormal activation is involved in the progression of several types of tumors. Several studies have shown that beta-catenin plays a role in cancer cell resistance to ferroptosis. By binding to a transcription factor, ß-catenin can promote GPX4 activity and thus induce resistance to ferroptosis. The transcription factor TCF4, by binding to beta-catenin, induces resistance to ferroptosis. TCF4 has been identified with upregulated expression in gastric cancer. One study showed that it targets GPX4 by upregulating its expression, rendering cancer cells resistant to ferroptosis (Wang Jingmei et al., 2022). Another involves the deubiquitinating enzyme USP8. USP8 has been shown to deubiquitinate ß-catenin by removing the K48-linked ubiquitin chain. By doing so, USP8 stabilizes ß-catenin, resulting in ferroptosis resistance (Tang L. et al., 2023).
The mitochondrial tricarboxylic acid (TCA) cycle is necessary for ROS production. The TCA cycle is fed by glutamine metabolism (glutaminolysis). Glutaminolysis via the enzyme glutaminase (GLS2) is therefore necessary for ferroptosis induced by cysteine deprivation (Gao et al., 2019). Clearly, glutaminolysis inhibition is an important factor in ferroptosis resistance. ASS1, a key enzyme in the urea cycle, is important for ferroptosis resistance. ASS1 interferes with the tricarboxylic acid oxidative cycle from glutamine anaplerosis and promotes the reductive carboxylation of cytosolic glutamine, leading to a reduction in ROS production by mitochondria (Hu et al., 2023).
One of the most effective methods for inducing ferroptosis is the use of nanotherapy. It enables the combination of multiple inducers targeting different pathways. As a result, it is the most effective method for dealing with resistance.
Emerging evidence has highlighted the involvement of specific nanoparticles (NPs) in the modulation and initiation of ferroptosis (Acosta et al., 2022; Sun et al., 2022) (Figure 1).
Figure 1. Nanoparticles targeting major metabolic pathways involved in ferroptosis. (A) NPs can promote ferroptosis by increasing the LIP (e.g., Iron NPs) either through an Fe2+/Fe3+ ion supply, HMOX1-mediated heme degradation (e.g., FPBC@SN) or ferritinophagy (e.g., PHPF NMs). Excess Fe2+ from LIP as well as metal ions (e.g., Cu2+; Mn2+; Au (I)) generated by gold nanoparticles can trigger the fenton reaction generating excess ROS (HO•). (B) Certain NPs are able to inhibit the activity of DHODH (e.g., siR/IONs@LDH) and FSP1 (e.g., Cu-SF(RSV) NPs), thus reducing the production of CoQH2, an inhibitor of lipid peroxidation. The Fe2+ ions supplied by iron NPS can also enter mitochondria and promote ROS production. (C) Some NPs can increase lipid peroxidation either by inhibiting the Xc system (e.g., FGSTR NPs) or GPX4 (e.g., CBD NPs). On the other hand, other compounds can increase ACSL4 activity, thereby promoting lipid peroxidation (e.g., ADM/Fe3O4-MS).
Iron nanoparticles stand out as prime candidates that induce ferroptosis by enhancing the cell’s LIP through the release of iron ions (Zhu G. et al., 2022). In addition to iron nanoparticles, certain NPs have the potential to fast-track ferritinophagy. NPs based on Polyherbal formulations and the combined use of doxorubicin with gold nanoparticles have been shown to accelerate ferritinophagy, leading to the degradation of ferritin and consequently increasing the LIP (Chittineedi et al., 2023). Additionally, certain nanoparticles (e.g., FPBC@SN) have been noted to upregulate NCOA4 activity, revealing yet another mechanism through which nanoparticles affect ferritinophagy (Zuo et al., 2021). In addition to catalyzing ferritinophagy, certain NPs can amplify the activity of HMOX1, as observed with PHPF NMs (Zhang R. et al., 2023). Furthermore, some nanoparticles, such as iCoDMSN, have been shown to modulate the interactions between NRF2 and KEAP1 (Zhao et al., 2023a).
Certain nanoparticles can enhance ferroptosis by inducing substantial amounts of ROS. A prime example includes metal NPs and NPs specifically designed for therapeutic modalities such as phototherapy, radiotherapy, and sonotherapy (e.g., BDPB NPs) (Zheng G. et al., 2023; Chen D. et al., 2023).
The xc-system, which plays a central role in glutathione synthesis, is a possible target for ferroptosis-inducing nanoparticles. By acting on this system (inhibiting SLC7A11), nanoparticles such as SOR@TF-Fe3+ NVs and Fe3O4-SAS@PLT have shown potential for ferroptosis induction (Xiao et al., 2023). In addition, nanoparticles such as MFC-Gem NPs and dGPX4@401-TK-12 can either inhibit the synthesis of GPX4 or reduce GSH, both of which are essential components in the regulation of lipid peroxidation (Luo et al., 2022; Zhang et al., 2022).
The upregulation of lipid peroxidation is crucial to ferroptosis (Krusenstiern et al., 2023). Some nanoparticles, such as ADM/Fe3O4-MS, have been found to increase lipid peroxidation by upregulating ACSL4 (Chen M. et al., 2022). Others, such as siR/IONs@LDH NPs, inhibit DHODH, while Cu-SF(SRV) NPs inhibit FSP1, contributing to the intricate nanoparticle-mediated modulation of lipid peroxidation and ferroptosis (Yang et al., 2022; Chen S. et al., 2023).
Metal nanoparticles have shown significant promise in cancer therapeutics due to their unique physicochemical properties, particularly their ability to deliver metal ions to cancer cells, stimulating ferroptosis (Dong et al., 2022; Chen X. et al., 2023; Zhong et al., 2023). (Figure 2) This programmed cell death, triggered by metal ion-dependent lipid peroxidation, represents a promising new approach for cancer treatment.
Figure 2. Metal nanoparticles trigger ferroptosis. Iron NPs release Fe3+/Fe2+ ions into the cell via endocytosis. Fe3+ is reduced to Fe2+ by STEAP3 and then completes the LIP. Fe2+, on the other hand, can be added directly to LIP. In addition, LIP releases Fe2+ to trigger the Fenton reaction, or to penetrate mitochondria to generate ROS. ROS attack cell and mitochondrial membrane lipids, inducing their degradation (ferroptosis). Other metallic NPs can also increase ROS generation by releasing ions that trigger Fenton reactivation.
In addition to delivering metal ions, metal nanoparticles can be combined with established ferroptosis-inducing agents including drugs (e.g., sorafenib, dihydroxyartemisinin, RSL3) and noncoding RNAs (miRNAs, siRNA) (Guan et al., 2020; Wang Jianxin et al., 2022; Guo et al., 2022; Huang et al., 2022).
Ferrous nanoparticles designed to deliver ferrous ions have been identified as effective agents for inducing ferroptosis in cancer cells. The delivered ions generate reactive oxygen species (ROS) through the Fenton reaction, leading to lipid peroxidation and, subsequently, ferroptosis (Chi et al., 2022).
Iron oxide nanoparticles (IONs), a subtype of ferrous nanoparticles, have gained increased research attention due to their ability to induce ferroptosis across various types of cancers both in vitro and in vivo (Chen Z. et al., 2022; Chen Y. et al., 2022; Chin et al., 2022). One specific example includes a type of ION synthesized by Huang et al., which successfully induced ferroptosis in diffuse large-cell B lymphoma by introducing iron ions into target cells. This promoted the generation of ROS through the Fenton reaction, leading to lipid peroxidation and cell membrane destruction. Additionally, these IONs influence the regulation of iron metabolism-related proteins, such as ferroportin (FPN) and transferrin receptor (TFR), thus increasing the intracellular labile iron pool (LIP) (Q.-T. Huang P. et al., 2023).
Moreover, IONs can serve as a core for creating multifaceted nanoparticles that combine various components (biopolymers, ferroptosis-inducing agents, peptides), enhancing their therapeutic effect (Lin et al., 2022; Liang Z. et al., 2023). For example, the Fe3O4-PEI@HA-RSL3 nanoparticle developed by Liang et al., has demonstrated great potential for inducing ferroptosis in hepatocellular Carcinoma (HCC) by enhancing ROS production and suppressing several ferroptosis-related genes (Han et al., 2022). Another complex nanoparticle is MNP@BQR@ANG-EXO-siGPX4 which was designed by Boyan Li et al., can target glioblastoma cells via its CD36 antibody and effectively release Fe2+ ions (Li F.-J. et al., 2022).
Another strategy involves the integration of iron ions (Fe2+ or Fe3+) into the nanoparticle system through chelation. These ions can be derived from FeCl2 or FeCl3 and can be added to other nanoparticles through chelation (UPDA-PEG@Fe2+/3+) (Chen et al., 2019a). Fe (acac)3 and FeSO4 can also serve as sources of Fe3+ and Fe2+ respectively when incorporated into nanoparticles (Zhang S. et al., 2023; Zhong et al., 2023).
The use of ferrocene, a biocompatible, hydrophobic, low-toxicity, and redox-friendly organometallic compound, has been effective in designing ferroptosis inducing nanoparticles (Wang Yingjie et al., 2022). For instance, Lin et al. created a nanoparticle (mPEG-b-PPLGFc@Dox) by assembling ferrocene with methoxypolyethylene glycol-b-poly (γ-3-azidopropanyl-L-glutamate) (mPEGb-PAPLG) and doxorubicin, achieving therapeutic effectiveness in prostate cancer while limiting collateral damage to nontarget cells (Lin et al., 2023).
Moreover, the inclusion of hemin, a potent source of Fe2+ and enhancer of HMOX1 activity, is another strategy for designing ferroptosis-inducing nanoparticles (Su I.-C. et al., 2023). Hemin can also consume glutathione (GSH), inhibiting the activity of GPX4, thereby promoting lipid peroxidation (Xiao K. et al., 2022). Zhang et al. exploited the synergistic potential of hemin and ferrocene to design a PHPF nanomotor, achieving significant antitumor therapeutic effects via ferroptosis (Zhang X. et al., 2023).
The ability of Cu2+ ions to catalyze the Fenton reaction and produce reactive oxygen species (ROS) has sparked interest in developing copper-based nanoparticles for ferroptosis induction. Several innovative copper-based nanosystems have been designed that capitalize on the redox transformation between Cu2+ and Cu + to generate cytotoxic hydroxyl radicals (OH•) that promote ferroptosis (Yuan et al., 2022).
For instance, the PAA-Cu3(PO4)2-DOX-CMCS nanoparticle, as designed by Sheng Zhao et al., efficiently delivers doxorubicin and Cu2+ ions into 4T1 cells. The released Cu2+ ions undergo a reduction to Cu + by GSH, promoting the Fenton reaction and the subsequent generation of ROS (Zhao et al., 2022). Similarly, the Cu-SF(RSV) NPs, developed by Jie Yang et al., leverage copper ions and silk fibroin (SF) to trigger substantial ROS production, which in tandem with the action of rosuvastatin (RSV), induces lipid peroxidation and eventual ferroptosis (Yang et al., 2022).
Nickel-based nanoparticles are also under scrutiny for their potential to generate ROS and induce ferroptosis (Yang Qunfang et al., 2023; Wang Weikai et al., 2023). The NiB@IrOx nanoparticles developed by Qin Wang et al. represent an innovation in this field. This nanoparticle effectively induces ROS production and subsequent ferroptosis through the catalytic action of IrOx and nickel (Ni2+/Ni0) in Hepa1-6 tumors in female BALB/c nude mice (Wang et al., 2023g).
Cobalt-based nanoparticles, due to their capacity to generate ROS, have shown potential in cancer therapy (Alarifi et al., 2013; Huang et al., 2021). iCoDMSNs, nanoparticles designed by Jianqi Zhao et al., combine cobalt oxide nanodots with iRGD peptides and dendritic mesoporous silica nanoparticles. This complex of nanoparticles selectively accumulates in tumor sites, triggering ferroptosis by inducing ROS production and regulating the expression of KEAP1, NRF2, and HMOX1, which in turn elevates the labile iron pool in 4T1 tumor-bearing BALB/c model mice (Zhao et al., 2023b).
Manganese ions (Mn2+) can induce ferroptosis in cancer cells by catalyzing the Fenton reaction and generating ROS (Dong et al., 2022). In the quest for novel cancer therapeutics, researchers have utilized this property to develop manganese-based nanoparticles such as PEG-PDA@Mn NPs, which have shown efficacy in gastric cancer therapy (Chen and Wen, 2022).
Zinc’s role in ferroptosis has been demonstrated in various studies, with zinc oxide nanoparticles (ZONPs) showing potential in cervical and breast cancer treatment (Chen J. et al., 2021; Li Q. et al., 2023). In cervical cancer, ZONPs not only induce lipid ROS and malondialdehyde (MDA) production but also suppress CD164 by enhancing miR-505-3p levels (Lei et al., 2022). In breast cancer, ZONPs have been shown to counteract resistance to doxorubicin by inhibiting ABCC9 and inducing ferroptosis (Li Y. et al., 2022).
The unique ability of gold to induce ferroptosis is being leveraged in the development of gold-based anticancer drugs (Zou et al., 2015; Xiao X. et al., 2022). The released Au(I) ions can inhibit the activity of thioredoxin reductase (TrxR), leading to an increase in ROS levels, rapid lipid peroxidation, and the subsequent induction of ferroptosis (Xiao K. et al., 2022). A notable example is the Au(I)-based NIR-II ferroptosis nanoparticles (TBTP-Au NPs) created by Jing Zhang et al. for glioblastoma treatment. These nanoparticles exploit the high ROS gradient in the glioma microenvironment to trigger the selective binding of Au(I) to overexpressed TrxR, activating HMOX1-regulated noncanonical ferroptosis in glioma cells and leading to tumor suppression (Zhang Xiaoqian et al., 2023).
Cerium oxide nanoparticles, also known as CeO2 NPs, are another type of NP that can induce ferroptosis (Wang et al., 2022d, p. 53). These nanoparticles work by triggering the Fenton reaction (Gao et al., 2023). Researchers like Xuan Gao and his team have shown that when these CeO2 NPs enter a cell, they can transform H2O2 into HO•. The nanoparticles release ions called Ce(III) when they enter the cell. These ions interact with H2O2 present in the cell to create another form of cerium ion, Ce(IV) and HO•. HO• then induces lipid peroxidation, ultimately leading to cell death through ferroptosis (Gao et al., 2023).
Silver nanoparticles are another type of metal NP that can trigger ferroptosis. These NPs achieve this by ROS (G. Zheng S. et al., 2023). Christina M. Snyder and her team have shown that silver nanoparticles can be particularly effective against triple-negative breast cancer. They do this by causing a lipid peroxidation (Snyder et al., 2021).
Although the use of metal-based nanoparticles in ferroptosis has been explored extensively, the emergence of metal-free nanoplatforms has opened new avenues in cancer therapeutics. Given the high iron content in cancer cells, ferroptosis induction using iron-based materials might lead to iron intoxication after the cancer cells burst and release their contents. Therefore, the development of nonferric, or even better, nonmetallic nanoparticles, provides an alternative approach to induce ferroptosis without this risk.
Cannabinoids (CBDs), have long been implicated in various cell death mechanisms critical to cancer therapy (Salazar et al., 2009; Erzurumlu et al., 2023; Whynot et al., 2023). Recently, studies by researchers such as Qunfang Yang et al. have revealed its potential in stimulating ferroptosis. They found that CBD not only was capable of inducing ROS production but also exhibited regulatory effects on GPX4, SLC7A11 and FTH1 by downregulating them and upregulating ACSL4. Researchers have proceeded to design a nanoparticle named CP NPs, which couples CBD to the Toll-like receptor three agonist, poly (I:C). This ingenious combination facilitated the delivery of CBD into murine melanoma cells, generating a ferroptosis response alongside an enhanced immune reaction induced by poly (I:C) (Yang Qing et al., 2023).
In addition to these strategies, ferroptosis-inducing metal-free nanoparticles can be fashioned by integrating ferroptosis drugs or molecules into nanoparticles. For instance, GPX4 inhibitors such as FIN56 or even GPX4 degraders have been used to develop such nanoparticles (Luo et al., 2022; Sun et al., 2023). Expanding on this concept, Xinxin Sun et al. created a nanoparticle by amalgamating FIN56 with arachidonic acid (AA). A small amount of lipid-PEG containing a disulfide bond (DSPE-SS-PEG2K) was then added to this pair, resulting in redox-sensitive lipid peroxidation nanoamplifiers (FAS NPs). This 'drug-deliver-drug’ nanoparticle strategy leveraged the inherent tendency of AA to augment lipid peroxidation, thereby boosting the ferroptotic response (Sun et al., 2023).
Another intriguing development in this field revolves around a pentadecapeptide derivative of cleaved high molecular weight kininogen (HKa), HKN15. Because of its strong binding affinity for ferritin, HKN15 has been used by Luwen Zhu et al. to create a modified nanoparticle, Ce6-PEG-HKN15 NPs. Upon HKN15-mediated uptake, these nanoparticles were found to congregate around ferritin, triggering its destruction and subsequent release of iron into the cytoplasm. This liberated iron, in turn, catalyzed the production of ROS through the Fenton reaction, successfully driving the cells toward ferroptosis (Zhu L. et al., 2022).
Traditional therapies such as phototherapy, sonotherapy, microwave-assisted thermal therapy, and radiotherapy have been found to potentiate ferroptosis by generating ROS. Nanoparticles can be engineered to carry agents receptive to each therapy type and can be activated by corresponding stimuli including light, ultrasound, microwaves, or ionizing radiation (Lang et al., 2019; Liu X. et al., 2021; Feng S. et al., 2023; Liu S. et al., 2023).
In the domain of phototherapy, light radiation (spanning near-infrared (NIR), X-ray, or UV light) is used to deliver tumor-inhibiting or tumor-destroying agents. NIR-based therapy is especially beneficial for localized cancers due to its selectivity, low-toxicity, resistance-limiting properties and minimal collateral damage. Moreover, NIR can amplify the ferroptosis response by boosting the Fenton reaction, a process that produces harmful hydroxyl radicals from iron and hydrogen peroxide (Chen Z. et al., 2022; Yu et al., 2022; Zhang et al., 2023h). Researchers, such as Lu Chen et al. and Hongli Yu et al. have developed PDA-based nanoparticles that can chelate with ferroptosis-inducing agents such as iron ions (Chen et al., 2019b; Yu et al., 2023). Particularly notable is the work of Hongli Yu et al. where Fe3+ ion-chelated PDA nanoparticles, covered with a red blood cell membrane, showed significant tumor inhibition under NIR irradiation, which was linked to increased ROS production and reduced GPX4 expression (Yu et al., 2023).
Microwave-assisted thermal therapy, which utilizes microwaves to induce hyperthermia and damage cancer cells, has also been explored in the context of ferroptosis (Chen Y. et al., 2022). Hui Zhou et al.'s work with CuCy nanoparticles exemplifies this approach, demonstrating increased ROS generation and lipid peroxidation, coupled with a reduction in GPX4 expression when subjected to microwaves (Zhou et al., 2023c).
Radiotherapy, a cornerstone of cancer treatment, not only fractures the DNA double bonds but also generates ROS through ionizing radiation (Gong et al., 2021). This ROS-generating property can be harnessed to enhance ferroptosis using nanoparticles sensitive to radiotherapy. For instance, Shuting Zheng et al.'s study presented a nanoparticle design, (HMON)-GOx@MnO2 NPs, which exhibited a pronounced ferroptosis effect following exposure to ionizing radiation (Zheng G. et al., 2023).
Sonodynamic therapy (SDT), similar to radiotherapy, promotes ROS production and can thereby boost the effect of ferroptosis when combined with a ferroptosis inducer (Pan et al., 2020; Zhang et al., 2023i). Recently Jianxin Wang et al. developed nanoparticles (IRP NPs) that amalgamated the sonosensitizer IR780 and a ferroptosis inducer RSL-3, enveloped in biocompatible poly-(lactic-co-glycolic acid) (PLGA). These nanoparticles, which leverage the mitochondrial targeting capabilities of RSL-3, successfully induced ferroptosis by impairing the mitochondria of TNBC cells (Wang Yue et al., 2022).
The innovative domain of immunotherapy has joined forces with conventional cancer treatments to create a potent therapeutic approach (J. Chen P.-H. et al., 2021; Formenti et al., 2018; Twyman-Saint Victor et al., 2015). Importantly, the ferroptosis mechanism has been found to enhance these immunological responses, specifically by inducing immune cell death (ICD) (Zhou et al., 2023d).
It begins with the aftermath of ferroptosis: the deceased cancer cells release damage-associated molecular patterns (DAMPs), effectively initiating the immune response. Research has revealed that ferroptosis cells expel DAMPs, specifically high mobility group box 1 (HMGB1), into the tumor microenvironment. These DAMPs, in turn, promote dendritic cell (DC) maturation and lead to the recruitment of a broader range of immune cells, thereby amplifying ICD (Hsieh et al., 2021; Zhou H. et al., 2023).
In another application, nanoparticles capable of inducing ferroptosis have emerged as potential immunostimulants. These nanoparticles help mature dendritic cells through antigens produced during cell death. Once they mature, these dendritic cells recruit T cells to enter the tumor microenvironment and kill more cancer cells. Additionally, the release of DAMPs (e.g., HMGB1 and KRAS) can repolarize M2 macrophages, which usually help tumors grow into M1 macrophages, which actively fight against the tumors (Dai et al., 2020b; 2020a; Jiang et al., 2020; Lei et al., 2023) (Figure 3).
Figure 3. Nanoparticles induce ferroptosis and immunotherapy. Antigens released during NP-induced ferroptosis can activate DC maturation and repolarization of M2 to M1. Mature DCs in turn activate T cells to attack and kill cancer cells.
This was aptly demonstrated in a study by the research group, Hsieh et al., 2021. They used zero-valent iron nanoparticles (ZVI-NPs) to stimulate ferroptosis in cancer cells, which not only initiated cell death but also spurred a significant immune response. ZVI-NPs transformed protumor M2 macrophages into antitumor M1 macrophages, reduced the population of regulatory T cells, and downregulated PD-1 and CTLA4 in CD8+ T cells to boost their cancer cell cytolytic activity. Notably, they also managed to curtail PD-L1 expression in cancer cells (Hsieh et al., 2021).
Similarly, in 2023, Zhou et al., in 2023, worked with FeGd-HN@Sorafenib@TGF-β-antibody@RGD2 NPs (FG-STR NPs) to treat MC38 tumor-bearing C57BL/6 mice. Treatment results in the infiltration of various immune cells, including memory B cells, eosinophils, lymphoid cells, mast cells, NK cells, monocytes, M1 macrophages, and CD8+ T cells, into the tumor microenvironment. This led to the production of IFN-γ+, provoking antitumor cytotoxicity. Moreover, increased expression of markers such as CD11c+, CD80+, and MHC-II + confirmed the maturation of DCs (Zhou et al., 2023c).
Building on these findings, specific components can be integrated into the nanosystems to further augment immune cell activation. A key example includes the indoleamine-2,3-dioxygenase (IDO) inhibitors (e.g., NLG919), which are used in the creation of FPBC@SN nanoparticles, as detailed in the work of Zuo et al. This is particularly relevant because an increase in IDO expression can increase tryptophan degradation, which is crucial for T cell activation and proliferation (Zuo et al., 2021).
Another intricate nanoformulation is CP nanoparticles, which are composed of CBD nanoparticles and poly (I:C). As illustrated by Kim et al., these nanoparticles not only inhibit the increase in PD-L1 expression on tumor cells but also enhance tumor sensitivity to T-cell-mediated destruction. This prevents tumor escape from immunosurveillance, thereby improving the overall efficacy of immunotherapy (Yang Qunfang et al., 2023).
The potential of nanoparticles to promote ferroptosis, has led to promising developments in cancer therapeutics. However, these findings have shed light on an even more intriguing capability: their role in concurrently inducing apoptosis in cancer cells. This dual function serves as a multifaceted attack on cancer cells, opening up the possibility of exploiting multiple cell death pathways for a more comprehensive therapeutic strategy (Zhong et al., 2023).
The common denominator in both ferroptosis and apoptosis induction is the generation of reactive oxygen species (ROS). NPs prompt ROS production, leading to lipid peroxidation, a critical factor in initiating both ferroptosis and apoptosis. The products of lipid peroxidation interact with membrane receptors, transcription factors, or inhibitors, triggering apoptosis signaling. In parallel, ROS can breach the mitochondrial membrane integrity, releasing cytochrome c into the cytoplasm, an event that initiates caspase three activation and thus initiates apoptosis (Su et al., 2019; Wang Yaxuan et al., 2023).
This interplay between ferroptosis and apoptosis induced by nanoparticles has been highlighted in numerous studies. For instance, Yuan et al.'s work with CuCP Lipo nanoparticles demonstrated their dual ability to induce ferroptosis and apoptosis in cancer cells. The nanoparticles initiated lipid peroxidation and triggered the upregulation of the p53 protein, a well-known regulator of cell cycle arrest, senescence, apoptosis, and ferroptosis (Yuan et al., 2022).
A similar pattern was observed in the research conducted by Zhou et al. where HMPB/ML210@TA-BLM-Fe3+ nanoparticles were used to treat breast cancer in a mouse model. Their results reveal elevated caspase three expression in the treated cancer cells, indicating the nanoparticles tended to induce apoptosis and ferroptosis (Zhou et al., 2021).
Another noteworthy aspect of using nanoparticles in cancer therapy is their ability to carry other therapeutic agents, thereby amplifying their overall impact. This was exemplified by the research led by Jiaxin Zhu et al. who developed a nonmetallic nanoparticle known as an ASP NPs. In combination with aurantiamide acetate, scutebarbatine A, and palmitin, these nanoparticles not only induced ferroptosis but also induced apoptosis in breast cancer cells. They increase the expression of apoptosis-related proteins, including cytochrome C, caspase 3, caspase-9, and Bax, while reducing the expression of the antiapoptotic protein, Bcl-2 (Zhu et al., 2023).
Autophagy-dependent cell death is a crucial regulatory mechanism essential for maintaining cellular equilibrium. It is initiated by a myriad of triggers, including peptide accumulation, endoplasmic reticulum (ER) stress, and nutrient deprivation (Shen et al., 2023). Core to the autophagy machinery is signaling cascades involving the mammalian target of rapamycin (mTOR) and the mitogen-activated protein kinase (AMPK). These factors, in turn, modulate key autophagy-related proteins such as the Unc-51 like autophagy activating kinase 1 (ULK1) complex (Inoki et al., 2012; Wang et al., 2023i).
Interestingly, lipid peroxidation, a marker of cellular oxidative stress, can induce ER stress, subsequently driving autophagy through the PKR-like ER kinase (PERK) pathway. This often leads to the activation of c-Jun-N-terminal kinase (JNK). Integral to this cascade are processes such as the lipidation of microtubule-associated light chain-3 (LC3-I) to form LC3-II and the liberation of Beclin one from its inhibitory association with BCL2, both of which are hallmarks of autophagosome formation and autophagic progression (Wang Zuo et al., 2023).
Reactive oxygen species (ROS), omnipresent in cellular processes, have their own intricate link to autophagy. They can stimulate the adenosine 5′-monophosphate (AMP)-activated protein kinase (AMPK), driving autophagy either through mTOR inhibition or by influencing the ULK complex to release Beclin one from BCL2 (Egan et al., 2015; Zhang Z. et al., 2023; Wang Zhangjie et al., 2023).
Within the realm of cancer research, certain nanoparticles have shown their ability to induce both autophagy and ferroptosis. A prime example is ultrasmall iron oxide nanoparticles (USIONPs). When applied to human glioblastoma cells, these nanoparticles exhibited an increased expression the of autophagic markers Beclin 1/ATG5, alongside markers indicative of ferroptosis (Ren et al., 2019; Wen et al., 2021). Similarly, IONP@PTX NPs mirrored the autophagy and apoptosis induced by USIONPs, as indicated by increases in Beclin 1, LC3 II, LC3 I levels and a concurrent decrease in P62 (Chen and Wen, 2022). Further confirming the dual-role of these nanoparticles, the introduction of Fe2O3@Co-PEG to fibrosarcoma cells, led to autophagy-dependent ferroptosis, as reported by Thanpisit Lomphithak et al. (Lomphithak et al., 2023).
The association of copper with cell death can be traced back to the 1980s. Its involvement was initially thought to be intertwined with reactive oxygen species (ROS) mechanisms, drawing it closer to well-charted cell death processes such as apoptosis, ferroptosis, autophagy, and necroptosis (Halliwell and Gutteridge, 1984). This framework, however, was challenged and redefined in 2022 by Tsvetkov et al. who shed light on “cuproptosis”. In contrast to previous assumptions, this pathway deviates from ROS and focuses on the tricarboxylic acid (TCA) cycle in mitochondria (Tsvetkov et al., 2022).
Lipoylation, a highly conserved posttranslational modification of lysine, has been observed in a select group of enzymes: pyruvate dehydrogenase (PDH), alpha-ketoglutarate dehydrogenase (KDH), branched-chain alpha-ketoacid dehydrogenase (BCKDH), and the glycine cleavage system (GCV). When copper binds to these lipoylated enzymes, particularly within the TCA cycle, it leads to their aggregation, culminating in proteotoxic stress and subsequent cell death (Tsvetkov et al., 2022).
Essential participants in this process include ferrodoxin-1 (FDX1) (a reductase that reduces Cu2+ to Cu+), the lipoic acid enzymes such as lipolytransferase 1 (LIPT1), lipoyl synthase (LIAS), and dihydrolipoamide dehydrogenase (DLD), and key components of the PDH complex and complex I genes. The PDH complex includes pyruvate dehydrogenase E1 subunit alpha 1 (PDHA1), pyruvate dehydrogenase E1 subunit beta (PDHB), and dihydrolipoamide S-acetyltransferase (DLAT) (Wang Zhenjie et al., 2022; Tsvetkov et al., 2022).
A therapeutic breakthrough has emerged in the form of the chemotherapy agent, Elesclomol (ES). When paired with copper, ES has emerged as a promising initiator of curroptosis, signifying its ability to target mitochondria and induce this specialized form of cell death (Hasinoff et al., 2014; Li Yongyun et al., 2022; Liu T. et al., 2023).
In addition to inducing ferroptosis, copper nanoparticles (Cu NPs), play a role in inducing cuproptosis, making them particularly useful for treating cancer. For example, a study by Boda Guo’s team developed a specific copper nanoparticle called NP@ESCu that successfully induced cuproptosis in mice with bladder cancer. These nanoparticles successfully targeted mitochondria using ES. NP@ESCu not only delivers copper ions into the cells but also generates reactive oxygen species (ROS) and initiates an immune response (Guo B. et al., 2023).
When Cu NPs penetrate cells, they release copper ions (Cu2+; Cu+). Cu2+ then interacts with GSH, leading to the increased production of ROS. This influx of ROS helps induce another forms of cell death (apoptosis, ferroptosis and autophagy) and contributes to the rapid accumulation of Cu+ in the cell (Halliwell and Gutteridge, 1984; Zhao et al., 2023a). This excess Cu + can bind to a protein named DLAT, causing it to clump together. This clumping, also known as agglomeration, creates a type of harmful stress in the cell that activates cuproptosis. Additionally, the copper ions are capable of inhibiting proteins that contain iron-sulfur groups, which also leads to harmful stress in the cell and triggers cuproptosis (Zhao et al., 2023b).
One research direction involves increasing the level of copper inside cells by blocking GSH (Tsvetkov et al., 2022). This technique can be particularly effective when used alongside copper nanoparticles (Wang Bingqing et al., 2023). Cu-GA NPs, which are copper nanoparticles combined with a chemotherapy drug called galic acid, have shown promise in triggering cuproptosis, while also increasing the production of ROS and inhibiting GSH (Zhao J. et al., 2023).
Furthermore, modulating the copper transporter ATP7B offers a method to optimize intracellular copper concentrations, potentially supercharging therapeutic interventions against cancer (Polishchuk et al., 2014). Fan Zhao et al. have found a way to improve the effectiveness of cuproptosis by inhibiting the copper-transporting protein, ATP7B. They created a unique copper nanoparticle, Cu2(PO4) (OH) NPs, that block the activity of ATP7B, thereby optimizing the intracellular copper concentration and increasing the efficiency of the cuproptosis process (Zhao Y. et al., 2023).
Table 2 lists some recently developed ferroptosis-inducing nanoparticles for combination therapy.
Ferroptosis, a form of iron-dependent cell death, has been increasingly recognized for its transformative potential in the landscape of cancer treatment. With distinct mechanisms that differ from those of regular cell death pathways, ferroptosis presents a compelling avenue for circumventing resistance to existing therapies. Recent advancements in nanoparticle-based approaches have further catalyzed this promise, demonstrating multifaceted capabilities, ranging from inducing reactive oxygen species (ROS) to activating other cellular death pathways (Figure 4).
Figure 4. Copper nanoparticles induce cuproptosis and other forms of cell death. Cu NPs can release copper ions into the cell. Cu + can be oxidized to Cu2+, generating ROS (HO). ROS can thus induce lipid peroxidation leading to ferroptosis, apoptosis or autophagy. ROS can itself induce apoptosis by inducing the release of CytC from mitochondria, which in turn activates the casp3 pathway. ROS can also induce autophagy via the AMPK/ULK complex pathway, leading to the release of BECN1. On the other hand, Cu2+ can be reduced to Cu + by the action of FDX1 or by reducing GSH to GSSG. Cu + can thus enter mitochondria, bind to DALT and form a DALT-Cu aggregate under the action of FDX1 and LIAS. This aggregation and the inhibition of sulfide-containing proteins (loss of the Fe-S protein) are the causes of cuproptosis.
The synergistic benefits of ferroptosis-inducing nanoparticles are particularly salient. These nanoparticles not only cause cell death but they also stimulate an immune response. The DAMPs generated by cell death results in the maturation of dendritic cells (DCs), the recruitment of T lymphocytes and the polarization of M2 macrophages into M1 macrophages. This immunogenicity has proven invaluable in the treatment of cancer in animal models by combining ferroptosis and vaccines (Ma S. et al., 2023; Fang et al., 2023; Shi et al., 2023). Further research is required before these formulations can be tested on humans. The ferroptosis and nanovaccine combination tested today has always been a single nanoparticle formulation. Combining two nanoparticle formulations, one for the ferroptosis and one for the vaccination, could improve the efficacy of the combinatorial effect.
Despite the advantages of ferroptosis-inducing nanoparticles, it is crucial to acknowledge the potential hazards associated with the use of metal nanoparticles and the excessive ROS generation (Makhdoumi et al., 2020). These include toxicity to nearby normal cells and unknown long-term health effects, warranting rigorous in vivo evaluations for safety and efficacy. There is an imperative need to develop nanoparticles that are highly selective for cancer cells while sparing normal tissues, in order to minimize unintended consequences. Manufacturing nanoparticles that can target cancer cells exclusively or primarily through their specific receptors may reduce the toxicity to nearby normal cells. The therapeutic combinations used to induce ferroptosis must also be thoroughly researched. The type of cancer as well as the resistance mechanisms associated with that type of cancer must be considered.
KA: Conceptualization, Visualization, Writing–original draft. WZ: Writing–review and editing. XH: Writing–review and editing. WS: Funding acquisition, Supervision, Writing–review and editing.
The author(s) declare that financial support was received for the research, authorship, and/or publication of this article. This work is financially supported by the National Natural Science Foundation of China (NSFC31770999), and Beijing Municipal Natural Science Foundation (7202002).
The authors declare that the research was conducted in the absence of any commercial or financial relationships that could be construed as a potential conflict of interest.
All claims expressed in this article are solely those of the authors and do not necessarily represent those of their affiliated organizations, or those of the publisher, the editors and the reviewers. Any product that may be evaluated in this article, or claim that may be made by its manufacturer, is not guaranteed or endorsed by the publisher.
Abu-Serie, M. M. (2023). Targeted ferroptotic potency of ferrous oxide nanoparticles-diethyldithiocarbamate nanocomplex on the metastatic liver cancer. Front. Pharmacol. 13, 1089667. doi:10.3389/fphar.2022.1089667
Acosta, R., Iriarte-Mesa, C., Alvarez-Alminaque, D., Hassannia, B., Wiernicki, B., Díaz-García, A., et al. (2022). Novel iron oxide nanoparticles induce ferroptosis in a panel of cancer cell lines. Molecules 27, 3970. doi:10.3390/molecules27133970
Akashi, K., Nagashima, Y., Tabata, T., and Oda, H. (2021). Immunochemical analysis of iron transporters and M2 macrophages in ovarian endometrioma and clear cell adenocarcinoma. Mol. Clin. Oncol. 15, 159–168. doi:10.3892/mco.2021.2321
Alarifi, S., Ali, D., Y, A. O. S., Ahamed, M., Siddiqui, M. A., and Al-Khedhairy, A. A. (2013). Oxidative stress contributes to cobalt oxide nanoparticles-induced cytotoxicity and DNA damage in human hepatocarcinoma cells. Int. J. Nanomedicine 8, 189–199. doi:10.2147/IJN.S37924
Alfarouk, K. O., Stock, C.-M., Taylor, S., Walsh, M., Muddathir, A. K., Verduzco, D., et al. (2015). Resistance to cancer chemotherapy: failure in drug response from ADME to P-gp. Cancer Cell Int. 15, 71. doi:10.1186/s12935-015-0221-1
Ayala, A., Muñoz, M. F., and Argüelles, S. (2014). Lipid peroxidation: production, metabolism, and signaling mechanisms of malondialdehyde and 4-hydroxy-2-nonenal. Oxid. Med. Cell. Longev. 2014, e360438. doi:10.1155/2014/360438
Bayır, H., Anthonymuthu, T. S., Tyurina, Y. Y., Patel, S. J., Amoscato, A. A., Lamade, A. M., et al. (2020). Achieving life through death: redox biology of lipid peroxidation in ferroptosis. Cell Chem. Biol. 27, 387–408. doi:10.1016/j.chembiol.2020.03.014
Bi, F., Qiu, Y., Wu, Z., Liu, S., Zuo, D., Huang, Z., et al. (2023). METTL9-SLC7A11 axis promotes hepatocellular carcinoma progression through ferroptosis inhibition. Cell Death Discov. 9, 428–511. doi:10.1038/s41420-023-01723-4
Cai, X., Hua, S., Deng, J., Du, Z., Zhang, D., Liu, Z., et al. (2022). Astaxanthin activated the Nrf2/HO-1 pathway to enhance autophagy and inhibit ferroptosis, ameliorating acetaminophen-induced liver injury. ACS Appl. Mat. Interfaces 14, 42887–42903. doi:10.1021/acsami.2c10506
Cardona, C., and Montgomery, M. (2023). Iron regulatory proteins: players or pawns in ferroptosis and cancer? Front. Mol. Biosci. 10, 1229710. doi:10.3389/fmolb.2023.1229710
Chen, C., Yang, Y., Guo, Y., He, J., Chen, Z., Qiu, S., et al. (2023a). CYP1B1 inhibits ferroptosis and induces anti-PD-1 resistance by degrading ACSL4 in colorectal cancer. Cell Death Dis. 14, 271–279. doi:10.1038/s41419-023-05803-2
Chen, D., Liang, C., Qu, X., Zhang, T., Mou, X., Cai, Y., et al. (2023b). Metal-free polymer nano-photosensitizer actuates ferroptosis in starved cancer. Biomaterials 292, 121944. doi:10.1016/j.biomaterials.2022.121944
Chen, H., and Wen, J. (2022). Iron oxide nanoparticles loaded with paclitaxel inhibits glioblastoma by enhancing autophagy-dependent ferroptosis pathway. Eur. J. Pharmacol. 921, 174860. doi:10.1016/j.ejphar.2022.174860
Chen, J., Qiu, M., Ye, Z., Nyalile, T., Li, Y., Glass, Z., et al. (2021a). In situ cancer vaccination using lipidoid nanoparticles. Sci. Adv. 7, eabf1244. doi:10.1126/sciadv.abf1244
Chen, L., Lin, Z., Liu, L., Zhang, X., Shi, W., Ge, D., et al. (2019a). Fe2+/Fe3+ ions chelated with ultrasmall polydopamine nanoparticles induce ferroptosis for cancer therapy. ACS Biomater. Sci. Eng. 5, 4861–4869. doi:10.1021/acsbiomaterials.9b00461
Chen, L., Lin, Z., Liu, L., Zhang, X., Shi, W., Ge, D., et al. (2019b). Fe2+/Fe3+ ions chelated with ultrasmall polydopamine nanoparticles induce ferroptosis for cancer therapy. ACS Biomater. Sci. Eng. 5, 4861–4869. doi:10.1021/acsbiomaterials.9b00461
Chen, M., Li, J., Shu, G., Shen, L., Qiao, E., Zhang, N., et al. (2022a). Homogenous multifunctional microspheres induce ferroptosis to promote the anti-hepatocarcinoma effect of chemoembolization. J. Nanobiotechnology 20, 179. doi:10.1186/s12951-022-01385-x
Chen, P.-H., Wu, J., Xu, Y., Ding, C.-K. C., Mestre, A. A., Lin, C.-C., et al. (2021b). Zinc transporter ZIP7 is a novel determinant of ferroptosis. Cell Death Dis. 12, 198–212. doi:10.1038/s41419-021-03482-5
Chen, S., Yang, J., Liang, Z., Li, Z., Xiong, W., Fan, Q., et al. (2023c). Synergistic functional nanomedicine enhances ferroptosis therapy for breast tumors by a blocking defensive redox system. ACS Appl. Mat. Interfaces 15, 2705–2713. doi:10.1021/acsami.2c19585
Chen, S.-J., Zhang, J., Zhou, T., Rao, S.-S., Li, Q., Xiao, L.-Y., et al. (2024). Epigenetically upregulated NSUN2 confers ferroptosis resistance in endometrial cancer via m5C modification of SLC7A11 mRNA. Redox Biol. 69, 102975. doi:10.1016/j.redox.2023.102975
Chen, X., Wang, H., Shi, J., Chen, Z., Wang, Y., Gu, S., et al. (2023d). An injectable and active hydrogel induces mutually enhanced mild magnetic hyperthermia and ferroptosis. Biomaterials 298, 122139. doi:10.1016/j.biomaterials.2023.122139
Chen, Y., Su, M., Jia, L., and Zhang, Z. (2022c). Synergistic chemo-photothermal and ferroptosis therapy of polydopamine nanoparticles for esophageal cancer. Nanomed 17, 1115–1130. doi:10.2217/nnm-2022-0064
Chen, Z., Li, Z., Li, C., Huang, H., Ren, Y., Li, Z., et al. (2022b). Manganese-containing polydopamine nanoparticles as theranostic agents for magnetic resonance imaging and photothermal/chemodynamic combined ferroptosis therapy treating gastric cancer. Drug Deliv. 29, 1201–1211. doi:10.1080/10717544.2022.2059124
Chi, H., Zhu, G., Yin, Y., Diao, H., Liu, Z., Sun, S., et al. (2022). Dual-Responsive multifunctional “core–shell” magnetic nanoparticles promoting Fenton reaction for tumor ferroptosis therapy. Int. J. Pharm. 622, 121898. doi:10.1016/j.ijpharm.2022.121898
Chin, Y.-C., Yang, L.-X., Hsu, F.-T., Hsu, C.-W., Chang, T.-W., Chen, H.-Y., et al. (2022). Iron oxide@chlorophyll clustered nanoparticles eliminate bladder cancer by photodynamic immunotherapy-initiated ferroptosis and immunostimulation. J. Nanobiotechnology 20, 373. doi:10.1186/s12951-022-01575-7
Chittineedi, P., Mohammed, A., Abdul Razab, M. K. A., Mat Nawi, N., and Pandrangi, S. (2023). Polyherbal formulation conjugated to gold nanoparticles induced ferroptosis in drug-resistant breast cancer stem cells through ferritin degradation. Front. Pharmacol. 14, 1134758. doi:10.3389/fphar.2023.1134758
Crescenzi, E., Leonardi, A., and Pacifico, F. (2023). Iron metabolism in cancer and senescence: a cellular perspective. Biology 12, 989. doi:10.3390/biology12070989
Dai, E., Han, L., Liu, J., Xie, Y., Kroemer, G., Klionsky, D. J., et al. (2020a). Autophagy-dependent ferroptosis drives tumor-associated macrophage polarization via release and uptake of oncogenic KRAS protein. Autophagy 16, 2069–2083. doi:10.1080/15548627.2020.1714209
Dai, E., Han, L., Liu, J., Xie, Y., Zeh, H. J., Kang, R., et al. (2020b). Ferroptotic damage promotes pancreatic tumorigenesis through a TMEM173/STING-dependent DNA sensor pathway. Nat. Commun. 11, 6339. doi:10.1038/s41467-020-20154-8
Do, Q., Zhang, R., Hooper, G., and Xu, L. (2023). Differential contributions of distinct free radical peroxidation mechanisms to the induction of ferroptosis. JACS Au 3, 1100–1117. doi:10.1021/jacsau.2c00681
Dong, Z., Liang, P., Guan, G., Yin, B., Wang, Y., Yue, R., et al. (2022). Overcoming hypoxia-induced ferroptosis resistance via a 19F/1H-mri traceable core-shell nanostructure. Angew. Chem. Int. Ed. 61, e202206074. doi:10.1002/anie.202206074
Egan, D. F., Chun, M. G. H., Vamos, M., Zou, H., Rong, J., Miller, C. J., et al. (2015). Small molecule inhibition of the autophagy kinase ULK1 and identification of ULK1 substrates. Mol. Cell 59, 285–297. doi:10.1016/j.molcel.2015.05.031
Endale, H. T., Tesfaye, W., and Mengstie, T. A. (2023). ROS induced lipid peroxidation and their role in ferroptosis. Front. Cell Dev. Biol. 11, 1226044. doi:10.3389/fcell.2023.1226044
Erzurumlu, Y., Catakli, D., and Sezer, S. (2023). Cannabidiol negatively regulates androgenic signal in prostate cancer cells and fine-tunes the tumorigenesis by modulating endoplasmic reticulum-associated degradation, unfolded protein response, and autophagy. Rev. Bras. Farmacogn. 33, 316–325. doi:10.1007/s43450-023-00360-3
Fan, Z., Wirth, A.-K., Chen, D., Wruck, C. J., Rauh, M., Buchfelder, M., et al. (2017). Nrf2-Keap1 pathway promotes cell proliferation and diminishes ferroptosis. Oncogenesis 6, e371. doi:10.1038/oncsis.2017.65
Fang, T., Ma, S., Wei, Y., Yang, J., Zhang, J., and Shen, Q. (2023). Catalytic immunotherapy-photothermal therapy combination for melanoma by ferroptosis-activating vaccine based on artificial nanoenzyme. Mat. Today Chem. 27, 101308. doi:10.1016/j.mtchem.2022.101308
Feng, S., Rao, Z., Zhang, J., She, X., Chen, Y., Wan, K., et al. (2023a). Inhibition of CARM1-mediated methylation of ACSL4 promotes ferroptosis in colorectal cancer. Adv. Sci. 10, 2303484. doi:10.1002/advs.202303484
Feng, Y., Chen, Q., Jin, C., Ruan, Y., Chen, Qi, Lin, W., et al. (2023b). Microwave-activated Cu-doped zirconium metal-organic framework for a highly effective combination of microwave dynamic and thermal therapy. J. Control. Release 361, 102–114. doi:10.1016/j.jconrel.2023.07.046
Formenti, S. C., Rudqvist, N.-P., Golden, E., Cooper, B., Wennerberg, E., Lhuillier, C., et al. (2018). Radiotherapy induces responses of lung cancer to CTLA-4 blockade. Nat. Med. 24, 1845–1851. doi:10.1038/s41591-018-0232-2
Gao, M., Yi, J., Zhu, J., Minikes, A. M., Monian, P., Thompson, C. B., et al. (2019). Role of mitochondria in ferroptosis. Mol. Cell 73, 354–363. doi:10.1016/j.molcel.2018.10.042
Gao, X., Feng, J., Lv, K., Zhou, Y., Zhang, R., Song, S., et al. (2023). Engineering CeO2/CuO heterostructure anchored on upconversion nanoparticles with boosting ROS generation-primed apoptosis-ferroptosis for cancer dynamic therapy. Nano Res. 16, 5322–5334. doi:10.1007/s12274-022-5223-4
Gong, J., Liu, Y., Wang, W., He, R., Xia, Q., Chen, L., et al. (2023). TRIM21-Promoted FSP1 plasma membrane translocation confers ferroptosis resistance in human cancers. Adv. Sci. 10, 2302318. doi:10.1002/advs.202302318
Gong, L., Zhang, Y., Liu, C., Zhang, M., and Han, S. (2021). Application of radiosensitizers in cancer radiotherapy. Int. J. Nanomedicine 16, 1083–1102. doi:10.2147/IJN.S290438
Guan, Q., Guo, R., Huang, S., Zhang, F., Liu, J., Wang, Z., et al. (2020). Mesoporous polydopamine carrying sorafenib and SPIO nanoparticles for MRI-guided ferroptosis cancer therapy. J. Control. Release 320, 392–403. doi:10.1016/j.jconrel.2020.01.048
Guo, B., Yang, F., Zhang, L., Zhao, Q., Wang, W., Yin, L., et al. (2023a). Cuproptosis induced by ROS responsive nanoparticles with elesclomol and copper combined with αpd-L1 for enhanced cancer immunotherapy. Adv. Mat. 35, 2212267. doi:10.1002/adma.202212267
Guo, J., Huang, M., Deng, S., Wang, H., Wang, Z., and Yan, B. (2023b). Highly expressed RPLP2 inhibits ferroptosis to promote hepatocellular carcinoma progression and predicts poor prognosis. Cancer Cell Int. 23, 278. doi:10.1186/s12935-023-03140-0
Guo, W., Wu, Z., Chen, J., Guo, S., You, W., Wang, S., et al. (2022). Nanoparticle delivery of miR-21-3p sensitizes melanoma to anti-PD-1 immunotherapy by promoting ferroptosis. J. Immunother. Cancer 10, e004381. doi:10.1136/jitc-2021-004381
Halliwell, B., and Gutteridge, J. M. (1984). Oxygen toxicity, oxygen radicals, transition metals and disease. Biochem. J. 219, 1–14. doi:10.1042/bj2190001
Han, Y., Dong, Z., Wang, C., Li, Q., Hao, Y., Yang, Z., et al. (2022). Ferrous ions doped calcium carbonate nanoparticles potentiate chemotherapy by inducing ferroptosis. J. Control. Release 348, 346–356. doi:10.1016/j.jconrel.2022.06.002
Hasinoff, B. B., Yadav, A. A., Patel, D., and Wu, X. (2014). The cytotoxicity of the anticancer drug elesclomol is due to oxidative stress indirectly mediated through its complex with Cu(II). J. Inorg. Biochem. 137, 22–30. doi:10.1016/j.jinorgbio.2014.04.004
Heng, J., Li, Z., Liu, L., Zheng, Z., Zheng, Y., Xu, X., et al. (2023). Acetyl-CoA acetyltransferase 2 confers radioresistance by inhibiting ferroptosis in esophageal squamous cell carcinoma. Int. J. Radiat. Oncol. Biol. Phys. 117, 966–978. doi:10.1016/j.ijrobp.2023.05.031
Hsieh, C.-H., Hsieh, H.-C., Shih, F.-H., Wang, P.-W., Yang, L.-X., Shieh, D.-B., et al. (2021). An innovative NRF2 nano-modulator induces lung cancer ferroptosis and elicits an immunostimulatory tumor microenvironment. Theranostics 11, 7072–7091. doi:10.7150/thno.57803
Hsu, T.-W., Su, Y.-H., Chen, H.-A., Liao, P.-H., Shen, S. C., Tsai, K.-Y., et al. (2023). Galectin-1-mediated MET/AXL signaling enhances sorafenib resistance in hepatocellular carcinoma by escaping ferroptosis. Aging 15, 6503–6525. doi:10.18632/aging.204867
Hu, J., Hu, H., Liu, Q., Feng, B., Lu, Y., and Chen, K. (2024). Inhibition of Apoc1 reverses resistance of sorafenib by promoting ferroptosis in esophageal cancers. Gene 892, 147874. doi:10.1016/j.gene.2023.147874
Hu, Q., Dai, J., Zhang, Z., Yu, H., Zhang, J., Zhu, X., et al. (2023). ASS1-Mediated reductive carboxylation of cytosolic glutamine confers ferroptosis resistance in cancer cells. Cancer Res. 83, 1646–1665. doi:10.1158/0008-5472.CAN-22-1999
Huang, J., Pan, H., Sun, J., Wu, J., Xuan, Q., Wang, J., et al. (2023a). TMEM147 aggravates the progression of HCC by modulating cholesterol homeostasis, suppressing ferroptosis, and promoting the M2 polarization of tumor-associated macrophages. J. Exp. Clin. Cancer Res. 42, 286. doi:10.1186/s13046-023-02865-0
Huang, L., Feng, J., Zhu, J., Yang, J., Xiong, W., Lu, X., et al. (2023b). A strategy of fenton reaction cycloacceleration for high-performance ferroptosis therapy initiated by tumor microenvironment remodeling. Adv. Healthc. Mat. 12, 2203362. doi:10.1002/adhm.202203362
Huang, P., Duan, W., Ruan, C., Wang, L., Hosea, R., Wu, Z., et al. (2023c). NeuroD1-GPX4 signaling leads to ferroptosis resistance in hepatocellular carcinoma. PLOS Genet. 19, e1011098. doi:10.1371/journal.pgen.1011098
Huang, Q.-T., Hu, Q.-Q., Wen, Z.-F., and Li, Y.-L. (2023d). Iron oxide nanoparticles inhibit tumor growth by ferroptosis in diffuse large B-cell lymphoma. Am. J. Cancer Res. 13, 498–508.
Huang, S., Le, H., Hong, G., Chen, G., Zhang, F., Lu, L., et al. (2022). An all-in-one biomimetic iron-small interfering RNA nanoplatform induces ferroptosis for cancer therapy. Acta Biomater. 148, 244–257. doi:10.1016/j.actbio.2022.06.017
Huang, X., Cai, H., Zhou, H., Li, T., Jin, H., Evans, C. E., et al. (2021). Cobalt oxide nanoparticle-synergized protein degradation and phototherapy for enhanced anticancer therapeutics. Acta Biomater. 121, 605–620. doi:10.1016/j.actbio.2020.11.036
Huang, X., Wu, J., Wang, Y., Xian, Z., Li, J., Qiu, N., et al. (2023e). FOXQ1 inhibits breast cancer ferroptosis and progression via the circ_0000643/miR-153/SLC7A11 axis. Exp. Cell Res. 431, 113737. doi:10.1016/j.yexcr.2023.113737
Inoki, K., Kim, J., and Guan, K.-L. (2012). AMPK and mTOR in cellular energy homeostasis and drug targets. Annu. Rev. Pharmacol. Toxicol. 52, 381–400. doi:10.1146/annurev-pharmtox-010611-134537
Jian, H., Chen, Z.-Q., Du, H., Liao, T., Sun, Y.-C., Ke, D., et al. (2024). Inhibition of ferroptosis by POLE2 in gastric cancer cells involves the activation of NRF2/GPX4 pathway. J. Cell. Mol. Med. 28, e17983. doi:10.1111/jcmm.17983
Jiang, F., Jia, K., Chen, Y., Ji, C., Chong, X., Li, Z., et al. (2023). ANO1-Mediated inhibition of cancer ferroptosis confers immunotherapeutic resistance through recruiting cancer-associated fibroblasts. Adv. Sci. 10, 2300881. doi:10.1002/advs.202300881
Jiang, Q., Wang, K., Zhang, X., Ouyang, B., Liu, H., Pang, Z., et al. (2020). Platelet membrane-camouflaged magnetic nanoparticles for ferroptosis-enhanced cancer immunotherapy. Small 16, 2001704. doi:10.1002/smll.202001704
Jin, D.-Y., Chen, X., Liu, Y., Williams, C. M., Pedersen, L. C., Stafford, D. W., et al. (2023a). A genome-wide CRISPR-Cas9 knockout screen identifies FSP1 as the warfarin-resistant vitamin K reductase. Nat. Commun. 14, 828. doi:10.1038/s41467-023-36446-8
Jin, H., Zhu, M., Zhang, D., Liu, X., Guo, Y., Xia, L., et al. (2023b). B7H3 increases ferroptosis resistance by inhibiting cholesterol metabolism in colorectal cancer. Cancer Sci. 114, 4225–4236. doi:10.1111/cas.15944
Kang, L., Wang, D., Shen, T., Liu, X., Dai, B., Zhou, D., et al. (2023). PDIA4 confers resistance to ferroptosis via induction of ATF4/SLC7A11 in renal cell carcinoma. Cell Death Dis. 14, 193–212. doi:10.1038/s41419-023-05719-x
Krusenstiern, A., Robson, R., Qian, N., Qiu, B., Hu, F., Reznik, E., et al. (2023). Identification of essential sites of lipid peroxidation in ferroptosis. Nat. Chem. Biol. 19, 1–12. doi:10.1038/s41589-022-01249-3
Kudelova, E., Smolar, M., Holubekova, V., Hornakova, A., Dvorska, D., Lucansky, V., et al. (2022). Genetic heterogeneity, tumor microenvironment and immunotherapy in triple-negative breast cancer. Int. J. Mol. Sci. 23, 14937. doi:10.3390/ijms232314937
Lang, X., Green, M. D., Wang, W., Yu, J., Choi, J. E., Jiang, L., et al. (2019). Radiotherapy and immunotherapy promote tumoral lipid oxidation and ferroptosis via synergistic repression of SLC7A11. Cancer Discov. 9, 1673–1685. doi:10.1158/2159-8290.CD-19-0338
Lee, J., and Roh, J.-L. (2023a). Altered iron metabolism as a target for ferroptosis induction in head and neck cancer. Cell. Oncol. 46, 801–810. doi:10.1007/s13402-023-00784-y
Lee, J., and Roh, J.-L. (2023b). Targeting iron-sulfur clusters in cancer: opportunities and challenges for ferroptosis-based therapy. Cancers 15, 2694. doi:10.3390/cancers15102694
Lee, J., and Roh, J.-L. (2023c). Targeting Nrf2 for ferroptosis-based therapy: implications for overcoming ferroptosis evasion and therapy resistance in cancer. Biochim. Biophys. Acta BBA - Mol. Basis Dis. 1869, 166788. doi:10.1016/j.bbadis.2023.166788
Lei, H., Li, Q., Pei, Z., Liu, L., Yang, N., and Cheng, L. (2023). Nonferrous ferroptosis inducer manganese molybdate nanoparticles to enhance tumor immunotherapy. Small n/a 19, 2303438. doi:10.1002/smll.202303438
Lei, J.-Y., Li, S.-X., Li, F., Li, H., and Lei, Y.-S. (2022). Zinc oxide nanoparticle regulates the ferroptosis, proliferation, invasion and steaminess of cervical cancer by miR-506-3p/CD164 signaling. Cancer Nanotechnol. 13, 33. doi:10.1186/s12645-022-00134-x
Li, B., Chen, X., Qiu, W., Zhao, R., Duan, J., Zhang, S., et al. (2022a). Synchronous disintegration of ferroptosis defense Axis via engineered exosome-conjugated magnetic nanoparticles for glioblastoma therapy. Adv. Sci. 9, e2105451. doi:10.1002/advs.202105451
Li, D., Wang, Y., Dong, C., Chen, T., Dong, A., Ren, J., et al. (2023a). CST1 inhibits ferroptosis and promotes gastric cancer metastasis by regulating GPX4 protein stability via OTUB1. Oncogene 42, 83–98. doi:10.1038/s41388-022-02537-x
Li, F.-J., Long, H.-Z., Zhou, Z.-W., Luo, H.-Y., Xu, S.-G., and Gao, L.-C. (2022b). System Xc−/GSH/GPX4 axis: an important antioxidant system for the ferroptosis in drug-resistant solid tumor therapy. Front. Pharmacol. 13, 910292. doi:10.3389/fphar.2022.910292
Li, H., Lan, H., Zhang, M., Zhao, F., An, N., and Yi, C. (2023b). TEA domain transcription factor 1 inhibits ferroptosis and sorafenib sensitivity of hepatocellular carcinoma cells. Dig. Dis. Sci. 68, 3070–3082. doi:10.1007/s10620-023-07824-5
Li, Q., Yang, Q., Guo, P., Feng, Y., Wang, S., Guo, J., et al. (2023c). Mitophagy contributes to zinc-induced ferroptosis in porcine testis cells. Food Chem. Toxicol. 179, 113950. doi:10.1016/j.fct.2023.113950
Li, W., Liang, L., Liu, S., Yi, H., and Zhou, Y. (2023d). FSP1: a key regulator of ferroptosis. Trends Mol. Med. 29, 753–764. doi:10.1016/j.molmed.2023.05.013
Li, Y., Jiang, C., Zhang, X., Liao, Z., Chen, L., Li, S., et al. (2022c). Inhibition of ABCC9 by zinc oxide nanoparticles induces ferroptosis and inhibits progression, attenuates doxorubicin resistance in breast cancer. Cancer Nanotechnol. 13, 3. doi:10.1186/s12645-021-00109-4
Li, Y., Yang, J., Zhang, Q., Xu, S., Sun, W., Ge, S., et al. (2022d). Copper ionophore elesclomol selectively targets GNAQ/11-mutant uveal melanoma. Oncogene 41, 3539–3553. doi:10.1038/s41388-022-02364-0
Liang, D., Feng, Y., Zandkarimi, F., Wang, H., Zhang, Z., Kim, J., et al. (2023a). Ferroptosis surveillance independent of GPX4 and differentially regulated by sex hormones. Cell 186, 2748–2764.e22. doi:10.1016/j.cell.2023.05.003
Liang, Z., Wang, Y., Wang, J., Xu, T., Ma, S., Liu, Q., et al. (2023b). Multifunctional Fe3O4-PEI@HA nanoparticles in the ferroptosis treatment of hepatocellular carcinoma through modulating reactive oxygen species. Colloids Surf. B Biointerfaces 227, 113358. doi:10.1016/j.colsurfb.2023.113358
Lin, J., Yang, H., Zhang, Y., Zou, F., He, H., Xie, W., et al. (2023). Ferrocene-based polymeric nanoparticles carrying doxorubicin for oncotherapeutic combination of chemotherapy and ferroptosis. Small 19, 2205024. doi:10.1002/smll.202205024
Lin, J., Zhang, J., Wang, K., Guo, S., and Yang, W. (2022). Zwitterionic polymer coated sorafenib-loaded Fe3O4 composite nanoparticles induced ferroptosis for cancer therapy. J. Mat. Chem. B 10, 5784–5795. doi:10.1039/D2TB01242A
Liu, C., Liao, W., Chen, J., Yu, K., Wu, Y., Zhang, S., et al. (2023a). Cholesterol confers ferroptosis resistance onto myeloid-biased hematopoietic stem cells and prevents irradiation-induced myelosuppression. Redox Biol. 62, 102661. doi:10.1016/j.redox.2023.102661
Liu, J., Xiao, Q., Xiao, J., Niu, C., Li, Y., Zhang, X., et al. (2022a). Wnt/β-catenin signalling: function, biological mechanisms, and therapeutic opportunities. Signal Transduct. Target. Ther. 7, 3–23. doi:10.1038/s41392-021-00762-6
Liu, S., Cao, X., Wang, D., and Zhu, H. (2022b). Iron metabolism: state of the art in hypoxic cancer cell biology. Arch. Biochem. Biophys. 723, 109199. doi:10.1016/j.abb.2022.109199
Liu, S., Li, W., Ding, H., Tian, B., Fang, L., Zhao, X., et al. (2023b). Biomineralized RuO2 nanozyme with multi-enzyme activity for ultrasound-triggered peroxynitrite-boosted ferroptosis. Small n/a 19, 2303057. doi:10.1002/smll.202303057
Liu, T., Dahle, M. A., Lystad, M. H., Marignol, L., Karlsen, M., and Redalen, K. R. (2023c). In vitro and in vivo characterization of [64Cu] [Cu(elesclomol)] as a novel theranostic agent for hypoxic solid tumors. Eur. J. Nucl. Med. Mol. Imaging. 50, 3576–3588. doi:10.1007/s00259-023-06310-4
Liu, W., Chakraborty, B., Safi, R., Kazmin, D., Chang, C., and McDonnell, D. P. (2021a). Dysregulated cholesterol homeostasis results in resistance to ferroptosis increasing tumorigenicity and metastasis in cancer. Nat. Commun. 12, 5103. doi:10.1038/s41467-021-25354-4
Liu, X., Sui, B., Camargo, P. H. C., Wang, J., and Sun, J. (2021b). Tuning band gap of MnO2 nanoflowers by Alkali metal doping for enhanced Ferroptosis/phototherapy synergism in Cancer. Appl. Mat. Today 23, 101027. doi:10.1016/j.apmt.2021.101027
Liu, X., Yan, C., Chang, C., Meng, F., Shen, W., Wang, S., et al. (2023d). FOXA2 suppression by TRIM36 exerts anti-tumor role in colorectal cancer via inducing NRF2/GPX4-regulated ferroptosis. Adv. Sci. n/a 10, 2304521. doi:10.1002/advs.202304521
Logie, E., Van Puyvelde, B., Cuypers, B., Schepers, A., Berghmans, H., Verdonck, J., et al. (2021). Ferroptosis induction in multiple myeloma cells triggers DNA methylation and histone modification changes associated with cellular senescence. Int. J. Mol. Sci. 22, 12234. doi:10.3390/ijms222212234
Lomphithak, T., Helvacioglu, S., Armenia, I., Keshavan, S., G Ovejero, J., Baldi, G., et al. (2023). High-dose exposure to polymer-coated iron oxide nanoparticles elicits autophagy-dependent ferroptosis in susceptible cancer cells. Nanomaterials 13, 1719. doi:10.3390/nano13111719
Luo, T., Zheng, Q., Shao, L., Ma, T., Mao, L., and Wang, M. (2022). Intracellular delivery of glutathione peroxidase degrader induces ferroptosis in vivo. Angew. Chem. Int. Ed. 61, e202206277. doi:10.1002/anie.202206277
Lyamzaev, K. G., Panteleeva, A. A., Simonyan, R. A., Avetisyan, A. V., and Chernyak, B. V. (2023). Mitochondrial lipid peroxidation is responsible for ferroptosis. Cells 12, 611. doi:10.3390/cells12040611
Ma, S., Adzavon, Y. M., Wen, X., Zhao, P., Xie, F., Liu, M., et al. (2022). Novel insights in the regulatory mechanisms of ferroptosis in hepatocellular carcinoma. Front. Cell Dev. Biol. 10, 873029. doi:10.3389/fcell.2022.873029
Ma, S., Liang, X., Yang, N., Yang, J., Zhang, J., Pan, X., et al. (2023a). Boosting cancer immunotherapy by biomineralized nanovaccine with ferroptosis-inducing and photothermal properties. Biomater. Sci. 11, 518–532. doi:10.1039/D2BM01126C
Ma, X., Zhao, J., and Feng, H. (2023b). Targeting iron metabolism in osteosarcoma. Discov. Oncol. 14, 31. doi:10.1007/s12672-023-00637-y
Makhdoumi, P., Karimi, H., and Khazaei, M. (2020). Review on metal-based nanoparticles: role of reactive oxygen species in renal toxicity. Chem. Res. Toxicol. 33, 2503–2514. doi:10.1021/acs.chemrestox.9b00438
Mancias, J. D., Wang, X., Gygi, S. P., Harper, J. W., and Kimmelman, A. C. (2014). Quantitative proteomics identifies NCOA4 as the cargo receptor mediating ferritinophagy. Nature 509, 105–109. doi:10.1038/nature13148
Mishima, E., Ito, J., Wu, Z., Nakamura, T., Wahida, A., Doll, S., et al. (2022). A non-canonical vitamin K cycle is a potent ferroptosis suppressor. Nature 608, 778–783. doi:10.1038/s41586-022-05022-3
Mortensen, M., Ruiz, J., and Watts, J. (2023). Polyunsaturated fatty acids drive lipid peroxidation during ferroptosis. Cells 12, 804. doi:10.3390/cells12050804
Pan, X., Wang, W., Huang, Z., Liu, S., Guo, J., Zhang, F., et al. (2020). MOF-derived double-layer hollow nanoparticles with oxygen generation ability for multimodal imaging-guided sonodynamic therapy. Angew. Chem. 132, 13659–13663. doi:10.1002/ange.202004894
Polishchuk, E. V., Concilli, M., Iacobacci, S., Chesi, G., Pastore, N., Piccolo, P., et al. (2014). Wilson disease protein ATP7B utilizes lysosomal exocytosis to maintain copper homeostasis. Dev. Cell 29, 686–700. doi:10.1016/j.devcel.2014.04.033
Qiu, S., Zhong, X., Meng, X., Li, S., Qian, X., Lu, H., et al. (2023). Mitochondria-localized cGAS suppresses ferroptosis to promote cancer progression. Cell Res. 33, 299–311. doi:10.1038/s41422-023-00788-1
Qu, L., He, X., Tang, Q., Fan, X., Liu, J., and Lin, A. (2022). Iron metabolism, ferroptosis, and lncRNA in cancer: knowns and unknowns. J. Zhejiang Univ.-Sci. B 23, 844–862. doi:10.1631/jzus.B2200194
Ren, Z., Liang, J., Zhang, P., Chen, J., and Wen, J. (2019). Inhibition of human glioblastoma cell invasion involves PION@E6 mediated autophagy process. Cancer Manag. Res. 11, 2643–2652. doi:10.2147/CMAR.S200151
Romani, A. M. P. (2022). Cisplatin in cancer treatment. Biochem. Pharmacol. 206, 115323. doi:10.1016/j.bcp.2022.115323
Salazar, M., Carracedo, A., Salanueva, Í. J., Hernández-Tiedra, S., Lorente, M., Egia, A., et al. (2009). Cannabinoid action induces autophagy-mediated cell death through stimulation of ER stress in human glioma cells. J. Clin. Invest. 119, 1359–1372. doi:10.1172/JCI37948
Shen, X., Deng, Y., Chen, L., Liu, C., Li, L., and Huang, Y. (2023). Modulation of autophagy direction to enhance antitumor effect of endoplasmic-reticulum-targeted therapy: left or right? Adv. Sci. 2301434, e2301434. doi:10.1002/advs.202301434
Shi, W., Feng, W., Li, S., Cui, Y., Liu, S., Jiang, H., et al. (2023). Ferroptosis and necroptosis produced autologous tumor cell lysates Co-delivering with combined immnoadjuvants as personalized in situ nanovaccines for antitumor immunity. ACS Nano 17, 14475–14493. doi:10.1021/acsnano.3c00901
Siegel, R. L., Miller, K. D., Fuchs, H. E., and Jemal, A. (2021). Cancer statistics, 2021. Ca. Cancer J. Clin. 71, 7–33. doi:10.3322/caac.21654
Sies, H., Belousov, V. V., Chandel, N. S., Davies, M. J., Jones, D. P., Mann, G. E., et al. (2022). Defining roles of specific reactive oxygen species (ROS) in cell biology and physiology. Nat. Rev. Mol. Cell Biol. 23, 499–515. doi:10.1038/s41580-022-00456-z
Snyder, C. M., Rohde, M. M., Fahrenholtz, C. D., Swanner, J., Sloop, J., Donati, G. L., et al. (2021). Low doses of silver nanoparticles selectively induce lipid peroxidation and proteotoxic stress in mesenchymal subtypes of triple-negative breast cancer. Cancers 13, 4217. doi:10.3390/cancers13164217
Song, X., Wang, X., Chen, X., Yu, Z., and Zhou, Y. (2024). SRSF1 inhibits ferroptosis and reduces cisplatin chemosensitivity of triple-negative breast cancer cells through the circSEPT9/GCH1 axis. J. Proteomics 292, 105055. doi:10.1016/j.jprot.2023.105055
Su, I.-C., Su, Y.-K., Setiawan, S. A., Yadav, V. K., Fong, I.-H., Yeh, C.-T., et al. (2023a). NADPH oxidase subunit CYBB confers chemotherapy and ferroptosis resistance in mesenchymal glioblastoma via nrf2/SOD2 modulation. Int. J. Mol. Sci. 24, 7706. doi:10.3390/ijms24097706
Su, L.-J., Zhang, J.-H., Gomez, H., Murugan, R., Hong, X., Xu, D., et al. (2019). Reactive oxygen species-induced lipid peroxidation in apoptosis, autophagy, and ferroptosis. Oxid. Med. Cell. Longev. 2019, e5080843. doi:10.1155/2019/5080843
Su, Y., Zhang, Z., Lee, L. T. O., Peng, L., Lu, L., He, X., et al. (2023b). Amphiphilic dendrimer doping enhanced pH-sensitivity of liposomal vesicle for effective Co-delivery toward synergistic ferroptosis–apoptosis therapy of hepatocellular carcinoma. Adv. Healthc. Mat. 12, 2202663. doi:10.1002/adhm.202202663
Sun, H., Cai, H., Xu, C., Zhai, H., Lux, F., Xie, Y., et al. (2022). AGuIX nanoparticles enhance ionizing radiation-induced ferroptosis on tumor cells by targeting the NRF2-GPX4 signaling pathway. J. Nanobiotechnology 20, 449. doi:10.1186/s12951-022-01654-9
Sun, X., Yang, X., Wang, J., Shang, Y., Wang, P., Sheng, X., et al. (2023). Self-engineered lipid peroxidation nano-amplifier for ferroptosis-driven antitumor therapy. Chem. Eng. J. 451, 138991. doi:10.1016/j.cej.2022.138991
Sung, H., Ferlay, J., Siegel, R. L., Laversanne, M., Soerjomataram, I., Jemal, A., et al. (2021). Global cancer statistics 2020: GLOBOCAN estimates of incidence and mortality worldwide for 36 cancers in 185 countries. Ca. Cancer J. Clin. 71, 209–249. doi:10.3322/caac.21660
Tang, D., and Kroemer, G. (2020). Ferroptosis. Curr. Biol. 30, R1292–R1297. doi:10.1016/j.cub.2020.09.068
Tang, J., Long, G., Xiao, L., and Zhou, L. (2023a). USP8 positively regulates hepatocellular carcinoma tumorigenesis and confers ferroptosis resistance through β-catenin stabilization. Cell Death Dis. 14, 360–413. doi:10.1038/s41419-023-05747-7
Tang, L., Yu, Y., Deng, W., Liu, J., Wang, Y., Ye, F., et al. (2023b). TXNDC12 inhibits lipid peroxidation and ferroptosis. iScience 26, 108393. doi:10.1016/j.isci.2023.108393
Tian, H., Zhang, T., Qin, S., Huang, Z., Zhou, L., Shi, J., et al. (2022). Enhancing the therapeutic efficacy of nanoparticles for cancer treatment using versatile targeted strategies. J. Hematol. Oncol.J Hematol. Oncol. 15, 132. doi:10.1186/s13045-022-01320-5
Tong, Q., Qin, W., Li, Z.-H., Liu, C., Wang, Z.-C., Chu, Y., et al. (2023). SLC12A5 promotes hepatocellular carcinoma growth and ferroptosis resistance by inducing ER stress and cystine transport changes. Cancer Med. 12, 8526–8541. doi:10.1002/cam4.5605
Trombetti, S., Iaccarino, N., Riccio, P., Sessa, R., Catapano, R., Salvatore, M., et al. (2023). Over-expressed GATA-1S, the short isoform of the hematopoietic transcriptional factor GATA-1, inhibits ferroptosis in K562 myeloid leukemia cells by preventing lipid peroxidation. Antioxidants 12, 537. doi:10.3390/antiox12030537
Tsvetkov, P., Coy, S., Petrova, B., Dreishpoon, M., Verma, A., Abdusamad, M., et al. (2022). Copper induces cell death by targeting lipoylated TCA cycle proteins. Science 375, 1254–1261. doi:10.1126/science.abf0529
Twyman-Saint Victor, C., Rech, A. J., Maity, A., Rengan, R., Pauken, K. E., Stelekati, E., et al. (2015). Radiation and dual checkpoint blockade activate non-redundant immune mechanisms in cancer. Nature 520, 373–377. doi:10.1038/nature14292
Ursini, F., and Maiorino, M. (2020). Lipid peroxidation and ferroptosis: the role of GSH and GPx4. Free Radic. Biol. Med. 152, 175–185. doi:10.1016/j.freeradbiomed.2020.02.027
Usukhbayar, N., Takano, Y., Uesugi, S., Muroi, M., Osada, H., and Kimura, K. (2023). 3,6-Epidioxy-1,10-bisaboladiene induces ferroptosis-like cell death through lipid peroxidation. Free Radic. Res. 57, 208–222. doi:10.1080/10715762.2023.2229005
Velkova, I., Pasino, M., Khalid, Z., Menichini, P., Martorana, E., Izzotti, A., et al. (2023). Modulation of ferroptosis by microRNAs in human cancer. Cancer. J. Pers. Med. 13, 719. doi:10.3390/jpm13050719
Wang, B., Wang, Y., Zhang, J., Hu, C., Jiang, J., Li, Y., et al. (2023a). ROS-induced lipid peroxidation modulates cell death outcome: mechanisms behind apoptosis, autophagy, and ferroptosis. Arch. Toxicol. 97, 1439–1451. doi:10.1007/s00204-023-03476-6
Wang, H., Cheng, Q., Bao, L., Li, M., Chang, K., and Yi, X. (2023b). Cytoprotective role of heme oxygenase-1 in cancer chemoresistance: focus on antioxidant, antiapoptotic, and pro-autophagy properties. Antioxidants 12, 1217. doi:10.3390/antiox12061217
Wang, J., Yang, W., He, X., Zhang, Z., and Zheng, X. (2022a). Assembling p53 activating peptide with CeO2 nanoparticle to construct a metallo-organic supermolecule toward the synergistic ferroptosis of tumor. Front. Bioeng. Biotechnol. 10, 929536. doi:10.3389/fbioe.2022.929536
Wang, J., Zhao, Z., Liu, Y., Cao, X., Li, F., Ran, H., et al. (2022b). Mito-Bomb’: a novel mitochondria-targeting nanosystem for ferroptosis-boosted sonodynamic antitumor therapy. Drug Deliv. 29, 3111–3122. doi:10.1080/10717544.2022.2126027
Wang, L., Han, H., Feng, Y., Ma, J., Han, Z., Li, R., et al. (2023c). Capilliposide B inhibits the migration of prostate cancer by inducing autophagy through the ROS/AMPK/mTOR pathway. Phytother. Res. 37, 2902–2914. doi:10.1002/ptr.7785
Wang, L., Wang, J., and Chen, L. (2023d). TIMP1 represses sorafenib-triggered ferroptosis in colorectal cancer cells by activating the PI3K/Akt signaling pathway. Immunopharmacol. Immunotoxicol. 45, 419–425. doi:10.1080/08923973.2022.2160731
Wang, Q., Shaik, F., Lu, X., Zhang, W., Wu, Y., Qian, H., et al. (2023e). Amorphous NiB@IrOx nanozymes trigger efficient apoptosis-ferroptosis hybrid therapy. Acta Biomater. 155, 575–587. doi:10.1016/j.actbio.2022.10.048
Wang, W., Lu, K., Jiang, X., Wei, Q., Zhu, L., Wang, X., et al. (2023f). Ferroptosis inducers enhanced cuproptosis induced by copper ionophores in primary liver cancer. J. Exp. Clin. Cancer Res. 42, 142. doi:10.1186/s13046-023-02720-2
Wang, Y., Chen, F., Zhou, H., Huang, L., Ye, J., Liu, X., et al. (2022c). Redox dyshomeostasis with dual stimuli-activatable dihydroartemisinin nanoparticles to potentiate ferroptotic therapy of pancreatic cancer. Small Methods 7, 2200888. doi:10.1002/smtd.202200888
Wang, Y., Hu, J., Wu, S., Fleishman, J. S., Li, Y., Xu, Y., et al. (2023g). Targeting epigenetic and posttranslational modifications regulating ferroptosis for the treatment of diseases. Signal Transduct. Target. Ther. 8, 449–545. doi:10.1038/s41392-023-01720-0
Wang, Y., Wu, X., Bao, X., and Mou, X. (2023h). Progress in the mechanism of the effect of Fe3O4 nanomaterials on ferroptosis in tumor cells. Molecules 28, 4562. doi:10.3390/molecules28114562
Wang, Y., Wu, X., Ren, Z., Li, Y., Zou, W., Chen, J., et al. (2023i). Overcoming cancer chemotherapy resistance by the induction of ferroptosis. Drug resist. updat. 66, 100916. doi:10.1016/j.drup.2022.100916
Wang, Y., Zhang, L., and Zhou, F. (2022d). Cuproptosis: a new form of programmed cell death. Cell. Mol. Immunol. 19, 867–868. doi:10.1038/s41423-022-00866-1
Wang, Y., Zheng, L., Shang, W., Yang, Z., Li, T., Liu, F., et al. (2022e). Wnt/beta-catenin signaling confers ferroptosis resistance by targeting GPX4 in gastric cancer. Cell Death Differ. 29, 2190–2202. doi:10.1038/s41418-022-01008-w
Wang, Z., Li, K., Xu, Y., Song, Z., Lan, X., Pan, C., et al. (2023j). Ferroptosis contributes to nickel-induced developmental neurotoxicity in zebrafish. Sci. Total Environ. 858, 160078. doi:10.1016/j.scitotenv.2022.160078
Wang, Z., Wang, Y., Gao, H., Tang, C., Feng, Z., Lin, L., et al. (2022f). Phototheranostic nanoparticles with aggregation-induced emission as a four-modal imaging platform for image-guided photothermal therapy and ferroptosis of tumor cells. Biomaterials 289, 121779. doi:10.1016/j.biomaterials.2022.121779
Wang, Z., Xia, Y., Wang, Y., Zhu, R., Li, H., Liu, Y., et al. (2023k). The E3 ligase TRIM26 suppresses ferroptosis through catalyzing K63-linked ubiquitination of GPX4 in glioma. Cell Death Dis. 14, 695–713. doi:10.1038/s41419-023-06222-z
Wen, J., Chen, H., Ren, Z., Zhang, P., Chen, J., and Jiang, S. (2021). Ultrasmall iron oxide nanoparticles induced ferroptosis via Beclin1/ATG5-dependent autophagy pathway. Nano Converg. 8, 10. doi:10.1186/s40580-021-00260-z
Whynot, E. G., Tomko, A. M., and Dupré, D. J. (2023). Anticancer properties of cannabidiol and Δ9-tetrahydrocannabinol and synergistic effects with gemcitabine and cisplatin in bladder cancer cell lines. J. Cannabis Res. 5, 7. doi:10.1186/s42238-023-00174-z
Winter, W. E., Bazydlo, L. A. L., and Harris, N. S. (2014). The molecular biology of human iron metabolism. Lab. Med. 45, 92–102. doi:10.1309/LMF28S2GIMXNWHMM
Wu, K., Yan, M., Liu, T., Wang, Z., Duan, Y., Xia, Y., et al. (2023). Creatine kinase B suppresses ferroptosis by phosphorylating GPX4 through a moonlighting function. Nat. Cell Biol. 25, 714–725. doi:10.1038/s41556-023-01133-9
Xiao, K., Zhang, N., Li, F., Hou, D., Zhai, X., Xu, W., et al. (2022a). Pro-oxidant response and accelerated ferroptosis caused by synergetic Au(I) release in hypercarbon-centered gold(I) cluster prodrugs. Nat. Commun. 13, 4669. doi:10.1038/s41467-022-32474-y
Xiao, X., Chen, M., Zhang, Y., Li, L., Peng, Y., Li, J., et al. (2022b). Hemin-incorporating DNA nanozyme enabling catalytic oxygenation and GSH depletion for enhanced photodynamic therapy and synergistic tumor ferroptosis. J. Nanobiotechnology 20, 410. doi:10.1186/s12951-022-01617-0
Xiao, Y., Xu, Z., Cheng, Y., Huang, R., Xie, Y., Tsai, H.-I., et al. (2023). Fe3+-binding transferrin nanovesicles encapsulating sorafenib induce ferroptosis in hepatocellular carcinoma. Biomater. Res. 27, 63. doi:10.1186/s40824-023-00401-x
Xie, J., Lan, T., Zheng, D.-L., Ding, L.-C., and Lu, Y.-G. (2023). CDH4 inhibits ferroptosis in oral squamous cell carcinoma cells. BMC Oral Health 23, 329. doi:10.1186/s12903-023-03046-3
Xu, C., Sun, S., Johnson, T., Qi, R., Zhang, S., Zhang, J., et al. (2021). The glutathione peroxidase Gpx4 prevents lipid peroxidation and ferroptosis to sustain Treg cell activation and suppression of antitumor immunity. Cell Rep. 35, 109235. doi:10.1016/j.celrep.2021.109235
Xu, R., Wang, W., and Zhang, W. (2023). Ferroptosis and the bidirectional regulatory factor p53. Cell Death Discov. 9, 197–198. doi:10.1038/s41420-023-01517-8
Yanatori, I., Tabuchi, M., Kawai, Y., Yasui, Y., Akagi, R., and Kishi, F. (2010). Heme and non-heme iron transporters in non-polarized and polarized cells. BMC Cell Biol. 11, 39. doi:10.1186/1471-2121-11-39
Yang, J., Jia, Z., Zhang, J., Pan, X., Wei, Y., Ma, S., et al. (2022). Metabolic intervention nanoparticles for triple-negative breast cancer therapy via overcoming FSP1-mediated ferroptosis resistance. Adv. Healthc. Mat. 11, 2102799. doi:10.1002/adhm.202102799
Yang, Q., Liu, T., Zheng, H., Zhou, Z., Huang, Y., Jia, H., et al. (2023a). A nanoformulation for immunosuppression reversal and broad-spectrum self-amplifying antitumor ferroptosis-immunotherapy. Biomaterials 292, 121936. doi:10.1016/j.biomaterials.2022.121936
Yang, Q., Zuo, Z., Zeng, Y., Ouyang, Y., Cui, H., Deng, H., et al. (2023b). Autophagy-mediated ferroptosis involved in nickel-induced nephrotoxicity in the mice. Ecotoxicol. Environ. Saf. 259, 115049. doi:10.1016/j.ecoenv.2023.115049
Yao, Y., Chen, Z., Zhang, H., Chen, C., Zeng, M., Yunis, J., et al. (2021). Selenium–GPX4 axis protects follicular helper T cells from ferroptosis. Nat. Immunol. 22, 1127–1139. doi:10.1038/s41590-021-00996-0
Ye, F., Wu, J., and Zhang, F. (2023). METTL16 epigenetically enhances GPX4 expression via m6A modification to promote breast cancer progression by inhibiting ferroptosis. Biochem. Biophys. Res. Commun. 638, 1–6. doi:10.1016/j.bbrc.2022.10.065
Yu, H., Yan, J., Li, Z., Song, T., Ning, F., Tan, J., et al. (2023). Enhanced photothermal-ferroptosis effects based on RBCm-coated PDA nanoparticles for effective cancer therapy. J. Mat. Chem. B 11, 415–429. doi:10.1039/D2TB02329F
Yu, Z., Tong, S., Wang, C., Wu, Z., Ye, Y., Wang, S., et al. (2022). PPy@Fe3O4 nanoparticles inhibit the proliferation and metastasis of CRC via suppressing the NF-κB signaling pathway and promoting ferroptosis. Front. Bioeng. Biotechnol. 10, 1001994. doi:10.3389/fbioe.2022.1001994
Yuan, H., Xia, P., Sun, X., Ma, J., Xu, X., Fu, C., et al. (2022). Photothermal nanozymatic nanoparticles induce ferroptosis and apoptosis through tumor microenvironment manipulation for cancer therapy. Small 18, 2202161. doi:10.1002/smll.202202161
Zeng, K., Li, W., Wang, Y., Zhang, Z., Zhang, L., Zhang, W., et al. (2023a). Inhibition of CDK1 overcomes oxaliplatin resistance by regulating ACSL4-mediated ferroptosis in colorectal cancer. Adv. Sci. 10, 2301088. doi:10.1002/advs.202301088
Zeng, Z., Luo, Y., Xu, X., Shan, T., Chen, M., Huang, Z., et al. (2023b). A mitochondria-targeting ROS-activated nanoprodrug for self-augmented antitumor oxidation therapy. J. Control. Release 359, 415–427. doi:10.1016/j.jconrel.2023.06.004
Zhan, M., Ding, Y., Huang, S., Liu, Y., Xiao, J., Yu, H., et al. (2023). Lysyl oxidase-like 3 restrains mitochondrial ferroptosis to promote liver cancer chemoresistance by stabilizing dihydroorotate dehydrogenase. Nat. Commun. 14, 3123. doi:10.1038/s41467-023-38753-6
Zhang, D., Man, D., Lu, J., Jiang, Y., Ding, B., Su, R., et al. (2023a). Mitochondrial TSPO promotes hepatocellular carcinoma progression through ferroptosis inhibition and immune evasion. Adv. Sci. 10, 2206669. doi:10.1002/advs.202206669
Zhang, G., Li, N., Qi, Y., Zhao, Q., Zhan, J., and Yu, D. (2022). Synergistic ferroptosis-gemcitabine chemotherapy of the gemcitabine loaded carbonaceous nanozymes to enhance the treatment and magnetic resonance imaging monitoring of pancreatic cancer. Acta Biomater. 142, 284–297. doi:10.1016/j.actbio.2022.02.006
Zhang, J., Han, L., Haigang, W., Zhong, Y., Shangguan, P., Liu, Y., et al. (2023b). A brain-targeting NIR-II ferroptosis system: effective visualization and oncotherapy for orthotopic glioblastoma. Adv. Sci. 10, e2206333. doi:10.1002/advs.202206333
Zhang, Q., Li, N., Deng, L., Jiang, X., Zhang, Y., Lee, L. T. O., et al. (2023c). ACSL1-induced ferroptosis and platinum resistance in ovarian cancer by increasing FSP1 N-myristylation and stability. Cell Death Discov. 9, 83–12. doi:10.1038/s41420-023-01385-2
Zhang, R., Xu, S., Yuan, M., Guo, L., Xie, L., Liao, Y., et al. (2023d). An ultrasmall PVP–Fe–Cu–Ni–S nano-agent for synergistic cancer therapy through triggering ferroptosis and autophagy. Nanoscale 15, 12598–12611. doi:10.1039/D3NR02708B
Zhang, S., Xia, S., Chen, L., Chen, Y., and Zhou, J. (2023e). Covalent organic framework nanobowls as activatable nanosensitizers for tumor-specific and ferroptosis-augmented sonodynamic therapy. Adv. Sci. 10, 2206009. doi:10.1002/advs.202206009
Zhang, X., Gao, H., Wei, D., Pei, X., Zhang, Y., Wang, J., et al. (2023f). ROS responsive nanoparticles encapsulated with natural medicine remodel autophagy homeostasis in breast cancer. ACS Appl. Mat. Interfaces 15, 29827–29840. doi:10.1021/acsami.3c03068
Zhang, X., Zheng, X., Ying, X., Xie, W., Yin, Y., and Wang, X. (2023g). CEBPG suppresses ferroptosis through transcriptional control of SLC7A11 in ovarian cancer. J. Transl. Med. 21, 334. doi:10.1186/s12967-023-04136-0
Zhang, Y., Dong, P., Liu, N., Yang, J.-Y., Wang, H.-M., and Geng, Q. (2023h). TRIM6 reduces ferroptosis and chemosensitivity by targeting SLC1A5 in lung cancer. Oxid. Med. Cell. Longev. 2023, e9808100. doi:10.1155/2023/9808100
Zhang, Y., Zhang, K., Yang, H., Hao, Y., Zhang, J., Zhao, W., et al. (2023i). Highly penetrable drug-loaded nanomotors for photothermal-enhanced ferroptosis treatment of tumor. ACS Appl. Mat. Interfaces 15, 14099–14110. doi:10.1021/acsami.3c00297
Zhang, Z., Lo, H., Zhao, X., Li, W., Wu, K., Fanchu, Z., et al. (2023j). Mild photothermal/radiation therapy potentiates ferroptosis effect for ablation of breast cancer via MRI/PA imaging guided all-in-one strategy. J. Nanobiotechnology 21, 150. doi:10.1186/s12951-023-01910-6
Zhao, F., Liang, L., Wang, H., Wang, C., Su, D., Ying, Y., et al. (2023a). H2S-Activated ion-interference therapy: a novel tumor targeted therapy based on copper-overload-mediated cuproptosis and pyroptosis. Adv. Funct. Mater 33, 2300941. doi:10.1002/adfm.202300941
Zhao, F., Yu, H., Liang, L., Wang, C., Shi, D., Zhang, X., et al. (2023b). Redox homeostasis disruptors based on metal-phenolic network nanoparticles for chemo/chemodynamic synergistic tumor therapy through activating apoptosis and cuproptosis. Adv. Healthc. Mater 12, 2301346. doi:10.1002/adhm.202301346
Zhao, J., Chen, Y., Xiong, T., Han, S., Li, C., He, Y., et al. (2023c). Clustered cobalt nanodots initiate ferroptosis by upregulating heme oxygenase 1 for radiotherapy sensitization. Small 19, 2206415. doi:10.1002/smll.202206415
Zhao, S., He, L., Sun, Y., Xu, T., Chen, C., Ouyang, Y., et al. (2022). Acid-responsive drug-loaded copper phosphate nanoparticles for tumor cell therapy through synergistic apoptosis and ferroptosis strategy. J. Nanoparticle Res. 25, 7. doi:10.1007/s11051-022-05655-5
Zhao, Y., Liu, Z., Liu, G., Zhang, Y., Liu, S., Gan, D., et al. (2023d). Neutrophils resist ferroptosis and promote breast cancer metastasis through aconitate decarboxylase 1. Cell Metab. 35, 1688–1703.e10. doi:10.1016/j.cmet.2023.09.004
Zheng, G., Zhang, J., Zhang, X., Zhang, Z., Liu, S., Zhang, S., et al. (2023a). Implications of ferroptosis in silver nanoparticle-induced cytotoxicity of macrophages. Ecotoxicol. Environ. Saf. 259, 115057. doi:10.1016/j.ecoenv.2023.115057
Zheng, J., and Conrad, M. (2020). The metabolic underpinnings of ferroptosis. Cell Metab. 32, 920–937. doi:10.1016/j.cmet.2020.10.011
Zheng, S., Hu, H., Hou, M., Zhu, K., Wu, Z., Qi, L., et al. (2023b). Proton pump inhibitor-enhanced nanocatalytic ferroptosis induction for stimuli-responsive dual-modal molecular imaging guided cancer radiosensitization. Acta Biomater. 162, 72–84. doi:10.1016/j.actbio.2023.03.011
Zhong, Y., Peng, Z., Peng, Y., Li, B., Pan, Y., Ouyang, Q., et al. (2023). Construction of Fe-doped ZIF-8/DOX nanocomposites for ferroptosis strategy in the treatment of breast cancer. J. Mat. Chem. B 11, 6335–6345. doi:10.1039/D3TB00749A
Zhou, H., Liu, Z., Zhang, Z., Pandey, N., Amador, E., Nguyen, W., et al. (2023a). Copper-cysteamine nanoparticle-mediated microwave dynamic therapy improves cancer treatment with induction of ferroptosis. Bioact. Mat. 24, 322–330. doi:10.1016/j.bioactmat.2022.12.023
Zhou, L., Chen, J., Li, R., Wei, L., Xiong, H., Wang, C., et al. (2021). Metal-polyphenol-network coated prussian blue nanoparticles for synergistic ferroptosis and apoptosis via triggered GPX4 inhibition and concurrent in situ bleomycin toxification. Small 17, 2103919. doi:10.1002/smll.202103919
Zhou, N., Yuan, X., Du, Q., Zhang, Z., Shi, X., Bao, J., et al. (2023b). FerrDb V2: update of the manually curated database of ferroptosis regulators and ferroptosis-disease associations. Nucleic Acids Res. 51, D571–D582. doi:10.1093/nar/gkac935
Zhou, R., Liu, Y., Wang, Z., Lv, J., Liao, W., Shen, Z., et al. (2023c). Nanoparticle-based MRI-guided tumor microenvironment heating via the synergistic effect of ferroptosis and inhibition of TGF-β signaling. Adv. Healthc. Mat. n/a 12, 2300176. doi:10.1002/adhm.202300176
Zhou, R., Qiu, L., Zhou, L., Geng, R., Yang, S., and Wu, J. (2023d). P4HA1 activates HMGCS1 to promote nasopharyngeal carcinoma ferroptosis resistance and progression. Cell. Signal. 105, 110609. doi:10.1016/j.cellsig.2023.110609
Zhou, Y., Chen, K., Lin, W. K., Liu, J., Kang, W., Zhang, Y., et al. (2023e). Photo-enhanced synergistic induction of ferroptosis for anti-cancer immunotherapy. Adv. Healthc. Mat. n/a 12, 2300994. doi:10.1002/adhm.202300994
Zhou, Z. D., and Tan, E.-K. (2017). Iron regulatory protein (IRP)-iron responsive element (IRE) signaling pathway in human neurodegenerative diseases. Mol. Neurodegener. 12, 75. doi:10.1186/s13024-017-0218-4
Zhu, G., Chi, H., Liu, M., Yin, Y., Diao, H., Liu, Z., et al. (2022a). Multifunctional “ball-rod” Janus nanoparticles boosting Fenton reaction for ferroptosis therapy of non-small cell lung cancer. J. Colloid Interface Sci. 621, 12–23. doi:10.1016/j.jcis.2022.04.021
Zhu, J., Zhang, K., Zhou, Y., Wang, R., Gong, L., Wang, C., et al. (2023). A carrier-free nanomedicine enables apoptosis-ferroptosis synergistic breast cancer therapy by targeting subcellular organelles. ACS Appl. Mat. Interfaces 15, 22403–22414. doi:10.1021/acsami.3c01350
Zhu, L., You, Y., Zhu, M., Song, Y., Zhang, J., Hu, J., et al. (2022b). Ferritin-hijacking nanoparticles spatiotemporally directing endogenous ferroptosis for synergistic anticancer therapy. Adv. Mat. Deerf. Beach Fla 34, e2207174. doi:10.1002/adma.202207174
Zou, T., Lum, C. T., Lok, C.-N., Zhang, J.-J., and Che, C.-M. (2015). Chemical biology of anticancer gold(III) and gold(I) complexes. Chem. Soc. Rev. 44, 8786–8801. doi:10.1039/c5cs00132c
Keywords: ferroptosis, ferroptosis resistance, nanoparticles, combinatory therapy, cancer therapy
Citation: Adzavon KP, Zhao W, He X and Sheng W (2024) Ferroptosis resistance in cancer cells: nanoparticles for combination therapy as a solution. Front. Pharmacol. 15:1416382. doi: 10.3389/fphar.2024.1416382
Received: 12 April 2024; Accepted: 20 May 2024;
Published: 19 June 2024.
Edited by:
Zdeněk Kejík, Charles University, CzechiaReviewed by:
Dalin Zhang, Stanford University, United StatesCopyright © 2024 Adzavon, Zhao, He and Sheng. This is an open-access article distributed under the terms of the Creative Commons Attribution License (CC BY). The use, distribution or reproduction in other forums is permitted, provided the original author(s) and the copyright owner(s) are credited and that the original publication in this journal is cited, in accordance with accepted academic practice. No use, distribution or reproduction is permitted which does not comply with these terms.
*Correspondence: Wang Sheng, c2hlbmd3YW5nQGJqdXQuZWR1LmNu
Disclaimer: All claims expressed in this article are solely those of the authors and do not necessarily represent those of their affiliated organizations, or those of the publisher, the editors and the reviewers. Any product that may be evaluated in this article or claim that may be made by its manufacturer is not guaranteed or endorsed by the publisher.
Research integrity at Frontiers
Learn more about the work of our research integrity team to safeguard the quality of each article we publish.