- 1Unit of Pharmacology and Pharmacovigilance, Department of Clinical and Experimental Medicine, University of Pisa, Pisa, Italy
- 2Unit of Neurology, Department of Clinical and Experimental Medicine, University of Pisa, Pisa, Italy
- 3Department of Physiology and Pharmacology “V.Erspamer”, Sapienza University of Rome, Rome, Italy
- 4Unit of Gastroenterology, Department of Translational Research and New Technologies in Medicine and Surgery, University of Pisa, Pisa, Italy
- 5Unit of Histology and Medical Embryology, Department of Clinical and Experimental Medicine, University of Pisa, Pisa, Italy
Parkinson’s disease (PD) is a common and slow-progressing neurodegenerative disorder characterized by motor and non-motor symptoms, including gastrointestinal (GI) dysfunctions. Over the last years, the microbiota-gut-brain (MGB) axis is emerging as a bacterial-neuro-immune ascending pathway that contributes to the progression of PD. Indeed, PD patients are characterized by changes in gut microbiota composition, alterations of intestinal epithelial barrier (IEB) and enteric neurogenic/inflammatory responses that, besides determining intestinal disturbances, contribute to brain pathology. In this context, despite the causal relationship between gut dysbiosis, impaired MGB axis and PD remains to be elucidated, emerging evidence shows that MGB axis modulation can represent a suitable therapeutical strategy for the treatment of PD. This review provides an overview of the available knowledge about the beneficial effects of gut-directed therapies, including dietary interventions, prebiotics, probiotics, synbiotics and fecal microbiota transplantation (FMT), in both PD patients and animal models. In this context, particular attention has been devoted to the mechanisms by which the modulation of MGB axis could halt or slow down PD pathology and, most importantly, how these approaches can be included in the clinical practice.
1 Introduction
Parkinson’s disease (PD) is a frequent, slow-progressing neurodegenerative disease that affects over six million individuals worldwide with a growing economic impact on society (Ray Dorsey et al., 2018). Common symptoms of PD involve progressive motor dysfunctions, with tremor, bradykinesia, postural instability, and stiffness, caused by the degeneration of dopaminergic (DA) neurons in the substantia nigra pars compacta (SNpc) (Kalia and Lang, 2015). Along with the classical PD manifestations (motor symptoms), other symptoms, that can be detected years before central manifestations, include non-motor alterations, such as gastrointestinal (GI) disturbances, rapid eye movement (REM) sleep behavior disorder (RBD), hyposmia, asymmetric nonspecific shoulder pain, depression, and others (Chaudhuri and Schapira, 2009; Gaenslen et al., 2011; Al-Wardat et al., 2024). Nowadays, available therapies might alleviate these symptoms, but does not exist treatment that can halt the disease’s onset and progression (Armstrong and Okun, 2020). Indeed, it is critical to individuate disease-modifying therapies because of PD progression variability and patients’ clinical heterogeneity and so, nowadays, pharmacological treatments are only intended to treat PD symptoms, both motor and non-motor (Jankovic and Tan, 2020). Among the options for the treatment of motor-symptoms, levodopa (L-dopa), the precursor of dopamine, is the most used and effective one almost always combined with carbidopa or benserazide or catechol-O-methyl transferase (COMT) inhibitors, which prevent its peripheral metabolism and increasing its brain bioavailability. Unfortunately, L-dopa showed several side effects, including the worsening of GI symptoms and the appearance of bradykinesia (Djaldetti et al., 1996; Jankovic and Tan, 2020). Monoamine oxidase inhibitors (selegiline, rasagiline), dopamine agonists, anti-cholinergic and anti-glutamatergic are also included in the therapeutic toolkit for PD treatment (Jankovic and Tan, 2020). In the other hand, PD non-motor symptoms are treated with cholinesterase inhibitors (donepezil and rivastigmine) and NMDA receptor antagonists (memantine) that exert beneficial effects in PD-associated dementia and cognitive dysfunctions (Jankovic and Tan, 2020). Unfortunately, many patients with moderate to advanced disease experienced drugs fluctuating response, annoying dyskinesia, and L-dopa-unresponsiveness, which extremely reduce their quality of life (Jankovic and Tan, 2020). In this context, recently device-assisted therapies (DATs), such as deep brain stimulation (DBS) (Okun, 2012), levodopa-carbidopa intestinal gel infusion (LCIG) (Nyholm et al., 2012) and subcutaneous apomorphine injections (Pollak et al., 1989), have been explored and recommended to patients with tremors inadequately controlled by medications. However, research aimed at identifying new therapeutical strategies are imperative to improve quality of life and extend patients lifespan. In the last decade, scientists have pointed out a strict relationship between the brain and the gut microbiota, known as the microbiota-gut-brain (MGB) axis. Indeed, MGB axis alterations are known to affect both intestinal epithelial barrier (IEB) and blood-brain barrier (BBB) integrity, triggering an immune reaction, and potentially increasing brain inflammation and oxidative stress (Tillisch, 2014; Parashar and Udayabanu, 2017; Grillo et al., 2022; Bellini et al., 2023). Recognizing the diverse functions of gut microbiota within the GI tract and the host, we will delve into existing evidence exploring the possibility of targeting the gut microbiota as an innovative avenue for potential disease-modifying therapy in PD. In this review we will provide a comprehensive overview on recent studies suggesting that acting on the gut microenvironment may contribute to ameliorate PD condition, especially focusing on the molecular mechanisms mediated by the several gut-directed therapies and trying to individuate the most appropriate and beneficial one in the different phases of PD.
1.1 Pathophysiology of PD
PD is characterized by the aggregation of α-synuclein in Lewy bodies and Lewy neurites in the central nervous system (CNS). Interestingly, similar alterations can be observed in numerous organs, ranging from the skin, salivary glands, and colon, implying that PD could be considered a multiorgan pathology (Chahine et al., 2020). The relationship between early manifestations in the GI tract and later neurodegeneration in the CNS remains unknown. Constipation can appear several years before motor symptoms (Postuma et al., 2019), in accordance with the Braak hypothesis (the veracity of which is debatable) that suppose a primary deposition of α-synuclein within the gastrointestinal system (and in the olfactory bulb) spreading than to the brain through the vagal nerve (Braak et al., 2003a). In this regard, α-synuclein aggregates have been discovered in the dorsal motor nucleus of the vagus nerve (DMV), the locus coeruleus, the olfactory bulb, the submandibular glands, and the enteric nervous system (ENS) in addition to the SNpc (Klingelhoefer and Reichmann, 2015). Misfolded α-synuclein has been reported in enteric neurons of untreated Parkinson’s disease patients even in the early stages of the disease (Cersosimo and Benarroch, 2012). These findings led Braak and his colleagues to suggest that α-synucleinopathy in the ENS might be the earliest PD occurrence, with misfolded α-synuclein spreading to the DMV and then to higher CNS areas via retrograde vagal transmission, contributing to the origins of some cases of PD (Braak et al., 2003b). According to recent research, subdiaphragmatic vagotomy (a surgical method which injures the dorsal and ventral subdiaphragmatic vagus nerve and eliminates vagal afferent (sensory) and efferent (motor) signaling below the diaphragm (Suarez et al., 2018)) can stop misfolded α-synuclein from spreading from the gut to SNpc, which may additionally prevent or delay parkinsonism, confirming the key role of the vagus nerve in the cross talk between the brain and gut (Pan-Montojo et al., 2012; Svensson et al., 2015; Zhang et al., 2020).
Of note, astrocytes and microglial cells could be triggered by misfolded α-synuclein to produce pro-inflammatory cytokines, such as interleukin (IL)-1β and IL-6, contributing to the PD-associated neuroinflammation. Pathological α-synuclein depositions can also cause significant cellular stress, including mitochondrial, lysosomal, and endoplasmic reticulum dysfunctions (Feng et al., 2021). Noteworthy, α-synuclein aggregates could also trigger intestinal and circulating inflammatory/immune cells, leading to the release of pro-inflammatory cytokines, that can infiltrate to the CNS promoting and sustaining the neuroinflammation (De Virgilio et al., 2016; Brown, 2019; Pellegrini et al., 2020). In summary, current research supports a top-down (which begins in the SNpc) and a bottom-up (which begins in the GI tract) origin for PD genesis. Recent research supported this viewpoint by hypothesizing that PD may be separated into two main subtypes: peripheral nervous system (PNS)-first and CNS-first (Miraglia et al., 2015; Horsager et al., 2020). The PNS phenotype, also called body-first, is related with RBD during the prodromal stage and can be recognized by significant autonomic damage as well as digestive symptoms that arise earlier than central dopaminergic system involvement. The CNS-first PD, also known as the brain-first phenotype, is generally RBD-negative during the prodromal phase and is defined by nigrostriatal dopaminergic impairment, which begins without autonomic PNS involvement (Miraglia et al., 2015; Horsager et al., 2020). Despite the remarkable advancement in knowledge relating to the pathophysiology of PD, further investigations are needed to better understand the underlying pathophysiology of the PD subtypes, thus allowing to discover new targets and to develop subtype specific therapies.
1.2 The role of the microbiota-gut-brain axis in PD
The GI tract comprises numerous organs that extends from the mouth to the anus and is essential for a variety of activities such as digesting, absorption, and defense against external agents (Yoo and Mazmanian, 2017). In addition, an increasing number of research demonstrates the importance of gut microbiota in preserving brain homeostasis through different mechanisms of interconnection (Powell et al., 2017; Cryan et al., 2019). In particular, MGB communications mostly depend on the gut microbiota’s interactions with the immune system, intestinal barrier, and/or ENS-vagus nerve pathways. Especially, enteric bacteria and their metabolites, such as short chain fatty acids (SCFAs), can directly stimulate enterochromaffin cells to produce various neurotransmitters, such as serotonin, or neuropeptides, such as peptide YY, neuropeptide Y, cholecystokinin, glucagon-like peptide-1 and -2 (GLP-1 and 2), and substance P (Stasi et al., 2017). These substances can then spread into the bloodstream, reach the brain, and directly influence CNS functions (Liu et al., 2020). In addition, specific bacterial products (e.g., SCFAs, vitamins or neurotransmitters, such as acetylcholine, dopamine, noradrenaline, gamma-aminobutyric acid or serotonin) can translocate in the bloodstream through the IEB, reaching the CNS (Matsumoto et al., 2013; Gao et al., 2020; Wenzel et al., 2020). Thus, it seems that neuropeptides, neurotransmitters, and metabolites generated from the circulating microbiota can penetrate the brain and directly impact its neurobiology (Stasi et al., 2012). On the other hand, the CNS alters the intestinal microenvironment by modulating gut motility and neuroendocrine pathways (Gallo and Hooper, 2012; Varatharaj and Galea, 2017; Stasi et al., 2019).
The immune system may be involved in communications across the MGB axis, as intestinal bacteria and their byproducts can activate circulating and intestinal innate and adaptive immune cells. These cells then migrate to the CNS and impact brain functions through the brain lymphatic network (Gorjifard and Goldszmid, 2016). Further research is required as the processes by which immune cells regulate the gut-brain axis are still not widely understood. Notably, it has been discovered that the gut bacteria interact with the ENS-vagus nerve pathways. For instance, altered gut microbiota may stimulate peripheral immunological signals, such as increased levels of pro-inflammatory cytokines (tumor necrosis factor (TNF), IL-6, IL-1, and interferon-γ (IFN- γ)) and reduced anti-inflammatory cytokines (IL-4 and IL-10), which can be detected by the vagus nerve (Cawthon and de La Serre, 2018; Liu et al., 2020).
Changes in the communication processes between the intestinal microbiota and the CNS appear linked to alterations in the composition of the intestinal microbiota. In this regard, variety of research has inquired into shifts in the gut microbiota in PD patients compared to healthy controls (Cirstea et al., 2021; Romano et al., 2021; Toh et al., 2022). According to meta-analyses, the relative abundance of numerous phyla of anti-inflammatory and SCFA-producing bacteria, which includes Roseburia, Lachnospira, Blautia, and Faecalibacterium, is lower in PD patients compared to controls. Notably, PD is associated with an increased abundance in opportunistic pathogens and pro-inflammatory bacteria, including Bacteroides and Escherichia (Toh et al., 2022). Faecalibacterium can maintain gut barrier function by producing the SCFA butyrate and secreting anti-inflammatory mediators (Lenoir et al., 2020). Lower levels of butyrate have been associated to postural instability, gait abnormalities, Movement Disorder Society-Sponsored Revised Unified Parkinson’s Disease Rating Scale (MDS-UPDRS) part III motor scores, and depression in PD patients (Li et al., 2017; Xie et al., 2022). Surprisingly, Lactobacillus, Bifidobacterium, and Akkermansia levels are greater in PD patients (Nishiwaki et al., 2020), despite these bacteria are widely recognized as “beneficial”, and often included in probiotic preparations (Tan et al., 2020). The causes for their higher abundance in PD are unknown, although they may be connected to their better adaptation to live in a changed gut environment. Notably, higher Akkermansia abundance has been linked to reduced intestinal transit time and low body weight, common features of PD (Fasano et al., 2015; Yong et al., 2020). In PD patients, a decrease in SCFA-producing bacteria and an increase in pro-inflammatory microorganisms is associated with motor and cognitive symptoms (Nishiwaki et al., 2020; Ren et al., 2020) and also with greater fecal calprotectin levels, which is an established clinical biomarker for the evaluation of enteric inflammation (Weis et al., 2019). The increase in Bacteroides and Bifidobacterium has also been related to increased expression of serum and fecal inflammatory parameters such as IFN-γ or TNF (Lin et al., 2019; Aho et al., 2021). These results highlight the relevant role of gut microbiota in the bidirectional communication between the gut and the brain making itself as a promising target to slow down the progression of PD.
2 Therapeutical gut-directed approaches in PD
The involvement of MGB axis in the pathophysiology of PD is pushing the research on the potential therapeutical benefits, in terms of anti-inflammatory and neuroprotective activity, resulting from gut-directed therapies. At present, the most of available studies have investigated the effects of gut microbiota modulation in several experimental models of PD, characterized by similar features to those observed in PD-patients, including GI disturbances (Rota et al., 2019; Pellegrini and Travagli, 2024) and CNS dysfunctions (summarized in Table 1). In addition, several gut-directed therapeutics (Schmidt et al., 2018; Sanders et al., 2019; Zmora et al., 2019) have been found to exert beneficial effects also in PD patients, in terms of improvement of motor and non-motor GI symptoms (Harkins et al., 2020). Accordingly, the following sections and Tables 2, 3 summarize preclinical and clinical data regarding gut-directed therapies in different animal models of PD as well as in patients.
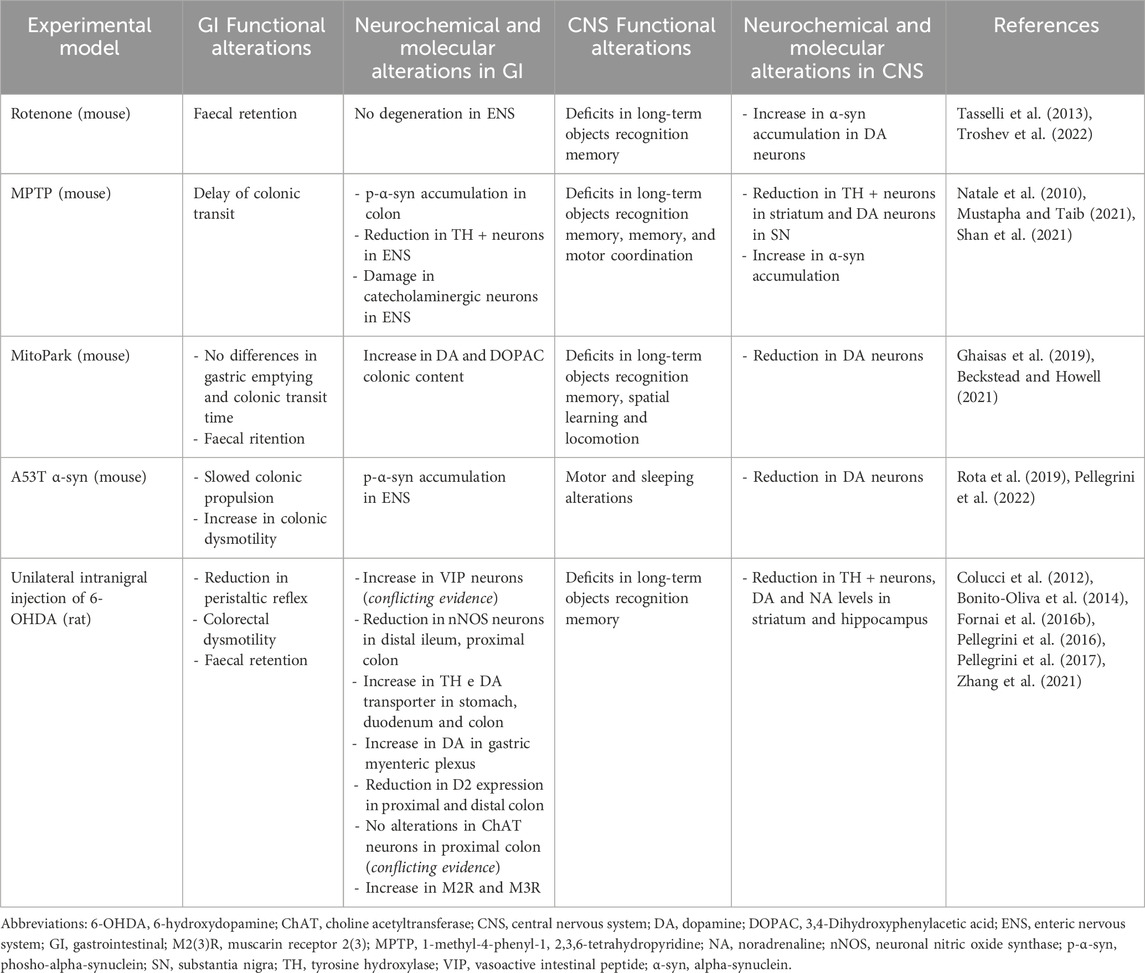
Table 1. Summary of the main functional, neurochemical, and molecular alterations in GI tract and CNS in different animal models of PD employed to evaluate the effect of gut-directed therapies.
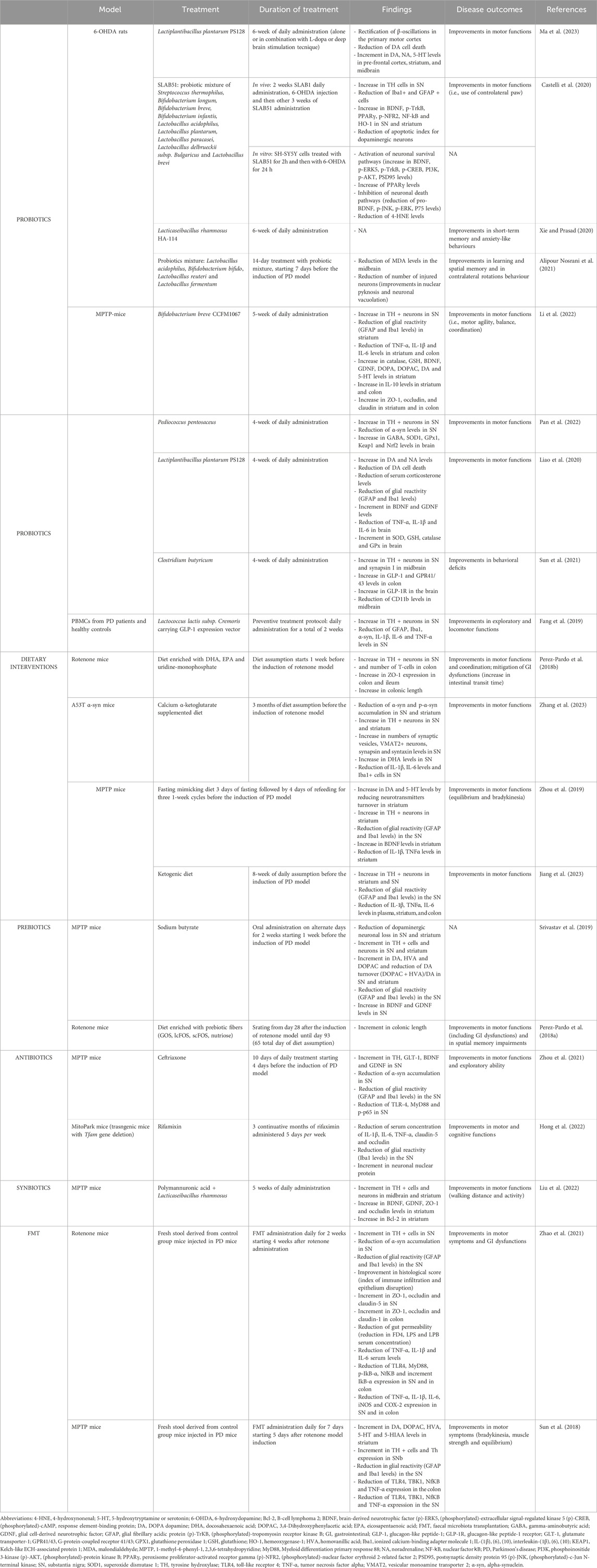
Table 2. Preclinical studies investigating the effect of gut-directed therapies in different animal model of Parkinson’s disease.
2.1 Preclinical evidence
2.1.1 Antibiotics
Antibiotics are drugs regarded to influence gut microbiota composition by affecting both pathogenetic and beneficial microbes’ population (Fornai et al., 2016a; Becattini et al., 2016). In addition, besides targeting gut microbiota, antibiotics are also endowed with additional properties, such as anti-inflammatory and neuroprotective activities (Stoilova et al., 2013; Sultan et al., 2013; Fornai et al., 2016a; Santa-Cecília et al., 2019). In this context, a previous study by Zhou et al. showed how gut microbiota modulation via ceftriaxone, a third-generation cephalosporin active against both Gram-negative and positive bacteria, exerted beneficial effects in 1-Methyl-4-phenyl-1,2,3,6-tetrahydropyridine (MPTP)-induced PD in mice. Authors demonstrated that the administration of ceftriaxone in MPTP mice improved motor dysfunctions and maintained neuronal integrity by restoring brain-derived neurotrophic factor (BDNF) and glial derived neurotrophic factor (GDNF) expression levels in CNS. Such effects were associated with an anti-inflammatory activity, exerted by the downregulation of TLR4 and MyD88, locally restricted to the colon (Zhou et al., 2021). However, more investigations are needed to clarify if such effects depend only on the direct modulation of gut microbiota or also on systemic anti-inflammatory and neuroprotective effects induced by the antibiotic. Notably, a recent and pioneering study has tested antibiotic rifaximin, a poorly absorbed antibiotic with a wide spectrum of antibacterial activity, in PD animals at the early phases of the disease. In particular, Hong and colleagues reported that a 3-month rifaximin treatment protected MitoPark PD mice against neuronal loss and attenuated systemic inflammation, preventing BBB breakdown (Hong et al., 2022). Overall, these findings suggest that a gut-directed therapy aimed at modulating microbiota composition via particular antibiotics can halt disease progression and symptoms, representing a suitable therapeutic option for the management of this neurodegenerative disorder, but more researches are needed to identify the specific activity of any antibiotics and the more effective one.
2.1.2 Dietary interventions
Growing evidence highlights how dietary interventions can influence gut microbiota composition and, in turn, impact on neurological disorders, including PD (Larroya-García et al., 2019). Healthy diet habits have been found to exert neuroprotective effects, decreasing neuroinflammatory responses, oxidative stress, and mitochondrial dysfunctions in the brain (Muth and Park, 2021). Several studies have reported the beneficial effects mediated by different dietary interventions in PD animals. Among all the preclinical models, the rotenone model (which consists in the intravenous, oral, subcutaneous, or intraperitoneal administration of rotenone toxin) represents the most used one because it depicts as fully as possible all the PD features, including GI dysfunctions, olfactory deficits, dopaminergic cell death, neuronal oxidative stress and α-synuclein phosphorylation and aggregation (Radad et al., 2019) (see Table 1 for more detailed information about all the PD preclinical models). Indeed, Perez-Pardo et al. investigated the effect of a dietary intervention combining uridine and docosahexaenoic acid (DHA) in rotenone-induced PD mice (Perez-Pardo et al., 2018b). In particular, authors observed that the diet ameliorated motor coordination and exerted a neuroprotective effect, increasing the survival of DA neurons. Moreover, the enriched dietary intervention mitigated GI dysfunctions, α-synuclein accumulation and enhanced zonulin-1 expression in colonic tissues from rotenone-treated mice (Perez-Pardo et al., 2018b). Such effects were attributed both to uridine, a phospholipid precursor, able to increase synaptogenesis and to DHA, regarded to exert anti-inflammatory and antioxidant effects (Perez-Pardo et al., 2018b). Likewise, Zhang and colleagues proved that the dietary intake of α-ketoglutarate ameliorated central α-synuclein pathology, rescued DA neuron degeneration, and suppressed pro-inflammatory mediators such as IL-6, IL-1β and TNF in transgenic A53T α-syn mice via enhancing nigral DHA levels (Zhang et al., 2023). Nevertheless, more studies are needed to better clarify whether the effects resulting from uridine and DHA depend on a gut locally activity or on a direct effect in the brain, since uridine can be transported across cellular membranes in all tissues, including the CNS (Wurtman et al., 2010). Zhou et al. showed that a fasting mimicking diet, consisting in 3 days of fasting followed by 4 days of refeeding in MPTP PD mice, reshaped gut microbiota composition and attenuated neuroinflammation by decreasing IL-1β levels and increasing BDNF levels in the striatum, thus offering innovative perspectives for the treatment of PD (Zhou et al., 2019). Similar results were obtained in a very recent study by Jiang et al. in which the administration of a ketogenic diet, characterized by low carbohydrate, high fat, and moderate-protein levels, restructured gut microbiota composition and ameliorated motor dysfunctions in MPTP PD mice (Jiang et al., 2023). Overall, these findings suggest that dietary interventions ameliorate PD and related motor and GI symptoms by exerting anti-inflammatory and neuroprotective effects. However, the exact mechanisms by which such interventions may influence gut microbiota composition, and, in turn, PD progression remain still unclear.
2.1.3 Prebiotics
Prebiotics are primarily indigestible dietary fibers that improve host’s health by selectively promoting the development and/or function of some bacteria in the gut (Davani-Davari et al., 2019). Soybeans, raw oats, unprocessed grains such as barley and wheat, indigestible sugars and oligosaccharides are the most prevalent sources of prebiotics (Markowiak and Ślizewska, 2017). The indigestible fibers, taken with the diet, reach the colon and are locally fermented by several bacteria strains, including Bifidobacteria (Hutkins et al., 2016). The final products of the fermentation include SCFAs (butyrate, acetate, and propionate), which promote beneficial activities to the host, such as inhibition of pro-inflammatory genes transcription (i.e., NF-kB, MAPK/ERK) and maintenance of gut barrier function and homeostasis (Blaak et al., 2020). Indeed, Srivastav et al. showed that the administration of sodium butyrate counteracted neurotoxicity, mitigated gliosis, and elevated neurotrophic factors levels in brain tissues from MPTP mice, confirming SCFAs as potent neuroprotective agents (Srivastav et al., 2019). Of note, sodium butyrate is in large part absorbed in the upper parts of GI tract and consequently its beneficial effects could be mainly addressed to a direct influence on the brain and, only in a lesser extent, to a gut-directed activity (Pellegrini et al., 2018). On these bases, prebiotic fibers targeting butyrogenic bacteria, such as chitin-glucan and β-glucan, able to promote butyrate production locally in the gut, are emerging for the treatment of PD (Cantu-Jungles et al., 2019). In this context, a recent study showed that the administration of four different monomers of chitosan oligosaccharide protected MPTP mice from DA neuron loss (Wang et al., 2022). Accordingly, several studies in PD mice have shown that non-digestible oligosaccharides such as galacto- and fructo-oligosaccharides (GOS and FOS, respectively) normalized motor and non-motor symptoms, including GI dysfunctions (Perez-Pardo et al., 2018a). The mechanisms underlying GOS and FOS effects resulted from a polarization of microbiota towards beneficial bacteria strain proliferation along with an improvement of synaptic functions in the ENS (Perez-Pardo et al., 2018a). Therefore, GOS and FOS supplementation might confer clinical benefits on PD patients, although more studies are essential to provide further insights on how FOS and GOS beneficially affect PD motor and non-motor symptoms.
2.1.4 Probiotics
Probiotics are live microorganisms able to induce benefits in the host and there are lot of evidence regarding their use in neurodegenerative disorders, including PD (Zommiti et al., 2022). Their beneficial activity results from different mechanisms, such as preservation of barrier integrity through the modulation of tight junctions’ expression (i.e., zonulin-1, occludin and claudin-1) and mucus production as well as reduction of enteric and systemic immune/inflammatory responses (Klaenhammer et al., 2012; Toscano et al., 2017; Sanders et al., 2019). Several efforts have been made to investigate the effects of probiotics and their metabolites in PD animal models, focusing the attention on their effects in halting or slowing down disease progression. Sun et al. showed that treatment with Clostridium butyricum (Cb) to MPTP mice reshaped gut microbiota composition, augmenting the relative abundance of SCFAs-producing bacteria such as Akkermansia, along with a normalization of SCFAs receptor expression, including GPR41/43, as well as an increase of GLP-1 in colonic tissues (Sun et al., 2021). Of note, GLP-1 can cross the BBB and, through the interaction with its receptor (GLP-1R) located with high density on SNpc neurons, astrocytes, and microglia, can, in turn, promote neurogenesis and reverse neuronal loss (Sun et al., 2021). In agreement with the previous results, Fang et al. showed how an engineered probiotic, carrying GLP-1 expression vector, ameliorated locomotor impairment, increased tyrosine hydroxylase levels in neurons and counteracted inflammation in MPTP mice, opening the way to implement genetically modified bacteria for the treatment of brain diseases (Fang et al., 2019). Other studies have evaluated the effects of Bifidobacterium and Lactobacillus in PD animals (Liao et al., 2020; Wang et al., 2022; Li et al., 2022). In particular, Li et al. showed that a 5-week supplementation with Bifidobacterium breve CCFM1067 suppressed pathogenic bacteria strains (e.g., Escherichia-Shigella), increased beneficial bacteria growth (e.g., Bifidobacterium and Akkermansia) and enhanced the expression of tight junction proteins, along with a decrease in pro-inflammatory cytokines levels in colon and brain tissues from MPTP mice (T. Li et al., 2022). Accordingly, a plethora of studies conducted in different animal model of CNS disorders showed that the assumption of probiotics increased intestinal tight junctions’ expression, restored colonic mucus cells loss, that, in turn, can reduce immune/inflammatory responses and alleviate central symptoms (Pellegrini et al., 2023). Another study by Ma and colleagues showed that Lactiplantibacillus plantarum PS128, administrated to rats with nigrostriatal dopaminergic neurodegeneration induced by 6-hydroxydopamine (6-OHDA), increased DA content in the striatum and attenuated dopaminergic neuronal loss (Ma et al., 2023), highlighting the neuroprotective effects of Lactobacillus supplementation. Interestingly, the neurotrophic effects of Lactobacillus and Bifidobacterium supplementation were tested in a study conducted by Castelli et al. in which SLAB51 (a probiotic mixture of Streptococcus thermophilus, Bifidobacterium longum, B. breve, Bifidobacterium infantis, Lactobacillus acidophilus, Lactobacillus plantarum, Lactobacillus paracasei, Lactobacillus delbrueckii subsp. Bulgaricus and Lactobacillus brevis) counteracted dopaminergic neuronal loss and modulated BDNF expression levels in the substantia nigra and in the striatum from 6-OHDA injected mice, by increasing peroxisome proliferator-activated receptor gamma (PPAR-γ) expression levels (Castelli et al., 2020). These findings suggest that the assumption of probiotics containing Bifidobacterium and Lactobacillus can represent a suitable therapeutical tool to manage PD via decreasing pro-inflammatory responses, counteracting oxidative stress and exerting neuroprotective effects (Magistrelli et al., 2019; Castelli et al., 2020; Xie and Prasad, 2020; Alipour Nosrani et al., 2021). Of interest, some probiotics displayed their potential to release neurotransmitters, including GABA, serotonin, acetylcholine, and noradrenaline that, successively, can influence brain functions. Accordingly, Pan et al. showed the ability of Pediococcus pentosaceus to counteract α-synuclein accumulation in SNpc, to maintain intracellular redox homeostasis and protect neurons from oxidative damage, through the increment of GABA levels in brain tissues from MPTP mice (Pan et al., 2022). However, whether P. pentosaceus-released GABA can influence indirectly brain functions through the maintenance of intestinal homeostasis, or rather it can migrate in the bloodstream and cross the BBB influencing the CNS, remain to be clarified.
2.1.5 Synbiotics
In recent years, researchers are investigating the effects of “synbiotics”, the synergistic combination of pro- and prebiotics, in neurodegenerative disorders (Lye et al., 2018; Ton et al., 2020). In this context, Liu et al. tested the administration of Lacticaseibacillus rhamnosus GG (LGG) and polymannuronic acid (PM) either alone or in combination (PM + LGG) in MPTP mice (Liu et al., 2022). The results showed that all treatments protect MPTP mice from dopaminergic neuronal loss and neuroinflammation, with a more pronounced beneficial activity in those receiving PM + LGG. Interestingly, the underlying mechanisms of PM, LGG or PM + LGG seem to be different in protecting dopaminergic neurons. In particular, PM displayed an anti-inflammation activity in brain tissues via microbiota derived SCFAs, whereas LGG improved GDNF striatal expressions levels, emphasizing the hypothesis that the synergistic effects of synbiotics are closely dependent on the combination of a pro- and a prebiotic. However, the available preclinical data in PD models about the effectiveness of synbiotics are limited, and more studies are needed to define the most effective combination in slowing down the progression of PD.
2.1.6 Fecal microbiota transplantation (FMT)
Fecal microbiota transplantation (FMT) is a procedure that consists in the transplantation of a gut microbiota extract, obtained from the feces of a healthy donor, into the GI tract of a recipient (Bakken et al., 2013). FMT has been tested in different pathological settings (e.g., Clostridium difficile infection, ulcerative colitis, and others) because of its capacity to thoroughly and extensively regenerate gut microbiota, modifying intestinal microbial diversity and restoring atypical intestinal flora (Li et al., 2019; Kim et al., 2021). Several preclinical studies have explored the effect of FMT as a potential therapeutical strategy for the treatment of CNS disorders, including PD. In this regard, Zhao et al. showed that FTM improved both intestinal and motor dysfunctions in rotenone-treated mice and elicited a remarkable reduction of lipopolysaccharide levels in serum, colon, and CNS tissues, underlying how the reshaping of gut microbiota can ameliorate IEB integrity and, successively, reduce immune/inflammatory processes (Zhao et al., 2021). Moreover, based on another study by Sun et al., FMT significantly mitigated gut dysbiosis and reduced microglia and astrocyte activation, thus suggesting a significant neuroprotective effect (Sun et al., 2018). Overall, the above findings shed light on the possibility to take advantages through the modulation of MGB axis using FMT practice, although further investigations are necessary to better understand how identify healthy donors, long-term safety, delivery methods, and clinical benefits of FMT.
2.2 Clinical evidence
2.2.1 Dietary interventions
Among the numerous dietary regimens, the mediterranean diet (MD) is recognized as a dietary model able to improve health in several disorders, including cardiovascular diseases, cancer, and metabolic syndrome in both Mediterranean and non-Mediterranean populations (Sánchez-Sánchez et al., 2020). In particular, the beneficial effects mediated by the MD seem to be addressed to its content in anti-inflammatory and antioxidant compounds (such as β-carotene and ascorbic acid) and to its ability to control glycemic levels and improve endothelial functions, thus resulting in a normalization of blood pressure levels (Martín-Peláez et al., 2020). Moreover, it is well accepted that a diet intake is responsible for gut microbiota shaping, in terms of composition and function. In this regard, the MD has been observed to positively influence gut microbiota, thanks to its enrichment in dietary fibers and complex carbohydrates which, once fermented by gut microorganisms, lead to the consequent production of SCFAs, capable of regulating glucose and lipid metabolism in several tissues (Martín-Peláez et al., 2020). Recently, a new field of research has showed that a higher adherence to the MD is associated with a lower risk of mental and brain disorders (Aridi et al., 2017). Indeed, case-control studies reported that MD assumption was associated with a decreased risk to develop PD (Alcalay et al., 2012; Maraki et al., 2019). A recent randomized clinical trial examined the impact of the MD on cognitive performance in PD participants. In this study, authors observed that the diet improved cognitive and executive functions, as well as attention, memory, and language (Paknahad et al., 2020). The major source of fat in the MD is olive (Olea europaea L.) oil, containing monounsaturated fatty acids, which possesses antioxidant activity and can reduce α-synuclein aggregation (Mohammad-Beigi et al., 2019). Furthermore, the dietary vitamin E and carotene intake from MD were associated with a lower risk of PD, likely minimizing oxidative damage by neutralizing the effect of oxygen free radicals (Yang et al., 2017). Moreover, the ketogenic diet can activate dopaminergic nuclei of the CNS (Naneix et al., 2017) through the increased utilization of ketone bodies by the brain. After 12 weeks, the above effects ameliorate cognitive functions and alleviate both motor and non-motor symptoms in PD patients (Phillips et al., 2018), and improve the feeling of anxiety, described as a persistent condition of worry not connected to stressful events (Tidman et al., 2022). Overall, these findings underscore the potential of tailored nutritional strategies in mitigating the symptoms of PD (Baroni et al., 2011). Further research and understanding of the intricate relationship between diet and PD may pave the way for innovative approaches in the management of this neurodegenerative disorder.
2.2.2 Prebiotics
The use of prebiotics in PD patients has been demonstrated to improve GI peristalsis, enhance immunological function, and alleviate constipation. In one small open-label investigation, a diet high in insoluble fibers (a typical prebiotic food source) ameliorated constipation, motor capacities and systemic L-dopa bioavailability (Astarloa et al., 1992). Furthermore, in an open-label trial enrolling 87 participants, the authors found that individuals with PD receiving resistant starch improved non-motor symptom ratings and had also substantially incremented fecal butyrate levels, as well as decreased fecal calprotectin levels, compared to patients that received dietary recommendations only (Becker et al., 2022). In another open-label study, the administration of a prebiotic mixture to PD patients determined the decrease in total UPRDS score and a reduction in both intestinal inflammation and circulating zonulin levels, a marker of IEB dysfunction (Hall et al., 2023). Therefore, prebiotics could have beneficial effect on PD by stimulating the production of SCFA-producing bacteria and their metabolites. However, there is currently scarce clinical data on prebiotics in PD patients, hence more trials are needed to adequately study the process.
2.2.3 Probiotics
Many studies evaluated the effect of multistrain probiotics in the treatment of PD constipation (Georgescu et al., 2016; Ibrahim et al., 2020; Tan et al., 2021; Du et al. 2022). Tan and colleagues observed a significant increase in bowel movements and constipation symptoms in PD patients treated with probiotic capsules containing strains of Lactobacillus, Bifidobacterium, and Enterococcus (Tan et al., 2021). In another study with a different formulation containing Lactobacillus, Bifidobacterium, and Enterococcus the researchers also found a significant reduction in Bristol stool scale (BSS) score, patient assessment of constipation quality of life questionnaire (PAC -QOL) score and degree of defecation effort score, associated with positive changes in the gut microbiota composition of PD patients (n = 46) (Du et al., 2022). Other studies have evaluated the effect of probiotics in motor and non-motor symptoms (Tamtaji et al., 2019; Yang et al., 2023). Tamtaji et al. in their randomized, double-blind trial (n = 60) observed that a 12-week treatment with probiotic capsules containing L. acidophilus, Bifidobacterium bifidum, Lactobacillus reuteri, and Lactobacillus fermentum determined a significant decrease in MDS-UPRDS, other than reducing inflammatory parameters and oxidative stress. In a recent research, involving 128 PD patients, a fermented milk containing Lacticaseibacillus paracasei strain Shirota was able to improve constipation-related symptoms but also ameliorate other non-motor symptoms (i.e., depression and anxiety) (Yang et al., 2023). Overall, probiotics are likely to represent a promising therapeutic strategy for the treatment of PD-related GI symptoms, but their safety needs to be further evaluated, as Enterococcus spp., often incorporated in probiotics, has shown significant levodopa breakdown capability (Rekdal et al., 2019), stressing the need for caution during its utilization.
2.2.4 Fecal microbiota transplantation
Considering the association between GI symptoms and PD, FMT might represent a possible therapeutical strategy. Several trials (NCT04854291, NCT05204641 and NCT04837313 on ClinicalTrials.gov) are at present being conducted to explore the impact of FMT on both motor and non-motor symptoms in PD. In 2019, the first human case report including the employment of FMT in PD was published (Huang et al., 2019). FMT was performed in a 71-year-old man who had a 7-year history of PD. The patient got nearly immediate alleviation from constipation, and his tremor in his lower extremities was also significantly decreased and remained reduced at the 3-month follow-up (Huang et al., 2019). In a more recent study, Segal and colleagues examined a group of six PD patients who received FMT (Segal et al., 2021). Four weeks after FMT, most of patients showed improvements in their PD-related constipation and an amelioration in UPDRS-III and Non-Motor Symptoms Scale scores, with few adverse effects reported (Segal et al., 2021). FMT from healthy donors, administered via a nasoduodenal tube, has also demonstrated to elicit significant benefits for both motor and non-motor PD symptoms (Kuai et al., 2021). In another study conducted on 15 patients, at a 1-month follow-up, it was observed that FMT was linked with better sleep and overall quality of life, in addition to decreases in motor symptoms, anxiety, and depression scores (Xue et al., 2020). A 3-month follow-up of the same population revealed long-term gains among the 12 participants who remained in the trial. Interestingly, ten of the fifteen patients in the trial received colonic FMT, while the remaining five received FMT through a nasoduodenal tube. The latter group showed major improvements on several motor and non-motor PD-related evaluations, as compared with patients receiving FMT via the colonic method, indicating that method of delivery may be a significant variable in FMT success (Xue et al., 2020). The adverse effects observed in the previous studies were minor to mild and of short duration (Huang et al., 2019; Xue et al., 2020; Kuai et al., 2021; Segal et al., 2021). Overall, this research shows that FMT could represent a feasible treatment for PD. Still, given the different rates of response in relation to FMT delivery, more research is needed to determine the appropriate route of administration, the choice of proper endpoints, and the rate of administration required to sustain desired beneficial effects.
2.2.5 Postbiotics, antibiotics, small molecules and biological drugs
Among the antibiotics, rifaximin, has been used to treat small intestinal bacterial overgrowth (SIBO) also in PD. In a recent study, involving 33 PD patients, SIBO eradication with rifaximin resulted in lower GI motor fluctuations, but without ameliorating L-DOPA bioavailability (Fasano et al., 2013). Additional microbial-directed therapies having the potential to assist PD patients, such as postbiotics, small-molecules and biological drugs, have yet to be fully investigated in the clinical context. SCFAs administration and selective blocking of levodopa-metabolizing bacterial enzymes appear to offer special promises, although more testing in human interventional research is required. Other immunomodulatory and IEB-restoring therapies need more consideration. Anti-TNF treatment, which has been linked to a lower PD incidence in IBD patients (Peter et al., 2018), has been shown to change fecal microbiota composition, with fecal amounts of butyrate and SCFAs in general being linked to clinical remission (Aden et al., 2019). Another new frontiers could be targeting PD-related molecular pathogenesis (for example, involving α-synuclein or glucocerebrosidase) in the ENS. In an open label trial, orally administered squalamine (that seems to relocate α-synuclein aggregates from ENS neurons) was safe and significantly reduced the symptoms of constipation in patients with PD (Hauser et al., 2019).
3 Future challenges and perspectives
Despite the promising results of gut-directed therapies in PD, certainly, several questions remain open. For instance, the introduction of probiotics in the clinical practice (i.e., combo-therapy bacteria-drugs) needs furthers investigations. Indeed, some bacteria species, endowed with a specific enzymatic activity, such as Enterococcus faecalis, Lactobacillus fermentum and Lactobacillus plantarum were found to lower L-dopa bioavailability, converting L-dopa in dopamine in the intestine, even in the presence of human peripheral decarboxylase inhibitors (i.e., carbidopa). Such an activity reduces L-dopa brain levels, thus decreasing drug beneficial outcomes (Cirstea et al., 2023). In parallel, L-dopa treated patients showed an increment in Peptoniphilus, Finegoldia and Enterococcus relative abundance, along with a decrease in Faecalibacterium, Blautia and Lachnospirae relative abundance in comparison with L-dopa-untreated-PD patients, demonstrating that L-dopa, itself can influence gut microbiota composition (Weis et al., 2019). Accordingly, L-dopa assumption induces gut dysbiosis, that, in turn, can contribute to GI symptoms observed in L-dopa treated patients (Chen et al., 2021). Likewise, the utilization of DATs for the treatment of long-term PD has a notable impact on gut bacteria species (Melis et al., 2021; Lubomski et al., 2022), which were found altered following DATs initiation in comparison with PD-control patients, but clinical trials are scarce and further investigations are necessary in this field (Hey et al., 2023). However, it is necessary to better clarify the pharmacokinetic interactions between PD drugs and gut-directed therapies and individuate the better combo bacteria-drugs regimen with the aim to creating a boosted beneficial impact on PD symptoms and progression. Indeed, certainly, Lactobacillus can reduce L-dopa bioavailability, but at the same time have demonstrated the capacity to slow down PD progression and counteract PD symptoms. Therefore, Lactobacillus could represent a suitable gut-directed therapy in the pre-motor phases of PD before the beginning of L-DOPA treatment. In addition, other limitations regard the difference therapy response in male and female, since gut microbiota is influenced (and, in turn, can influence) by sex hormone levels, especially estrogens (Rizzetto et al., 2018). In this context, further clinical studies should be performed in sex-oriented groups.
4 Conclusion
In conclusion, the present review provide evidence about gut-directed microbial therapies in the context of PD and how they represent a promising frontier in our understanding and potential treatment of this complex neurodegenerative disorders. The intricate interplay between the gut microbiota and the CNS is increasingly recognized as a significant factor in the development and progression of PD. While the precise mechanisms underlying these interactions are still being elucidated, the potential therapeutic implications are substantial. Still, a lot of questions remain regarding how MGB influences the onset and the progression of PD and the potential involvement of ENS together with how to identify the optimal probiotics strains to employ, the choice of one-single strain or multi-strains, the inter-subject’s variability, the appropriate administration schedule, the safety of probiotics and the lasting of their effects. Furthermore, for FMT, while case studies are useful as inspiration for future research, at present clinical trials are limited and lack in standardization (Seguella et al., 2023). However, the concept of modulating the gut microbiota through microbial therapies, such as probiotics, prebiotics, or FMT, holds promise as a novel approach to manage or even mitigate the symptoms of PD (Figure 1). These therapies have the potential to restore microbial balance, enhance the production of beneficial metabolites and exert a positive influence on immune function, thereby offering hope for beneficial outcomes not only in PD, but also in other brain disorders characterized by intestinal symptoms, gut dysbiosis, enteric barrier alterations and activation of inflammatory pathways such as Alzheimer’s disease, depression, and autism (Pellegrini et al., 2018). However, several limitations in the translational from preclinical model to clinical practice are still present. For instance, preclinical models, though reflect the main neuropathological and clinical features of PD, do not completely reproduce the pathophysiology seen in humans. Indeed, environmental factors and genetic susceptibility play a role in the onset and progression of PD in humans. Moreover, it is fundamental to set up specific studies on PD patients selected on the disease phase to better identify the most effective timing for gut-directed therapies administration and their integration with the commonly used pharmacological treatments (in Figure 2, a diagram for the potential application of gut-directed therapies based on PD stages is proposed). Based on the results discussed in the present review, we can speculate that the gut-directed therapies could be used both as disease-modifying therapies as well as symptomatic therapies. Indeed, they have displayed their capacity to reduce PD symptoms (i.e., motor and intestinal symptoms) in several clinical studies, but only preclinical evidence is available about their effect in reducing dopaminergic cell death or decreasing the accumulation of α-syn. In this context, more clinical studies are imperative to confirm the above evidence also in PD patients. However, it is essential to acknowledge that further research is needed to fully understand the complexities of this relationship, to develop safe and effective therapies tailored to unique needs of PD patients. In summary, the emerging field of gut-directed microbial therapies in PD holds significant promise for enhancing our therapeutic arsenal against this debilitating condition. While challenges and questions remain, the potential benefits for patients and their quality of life make this an area of continued exploration and innovation in the quest to conquer PD.
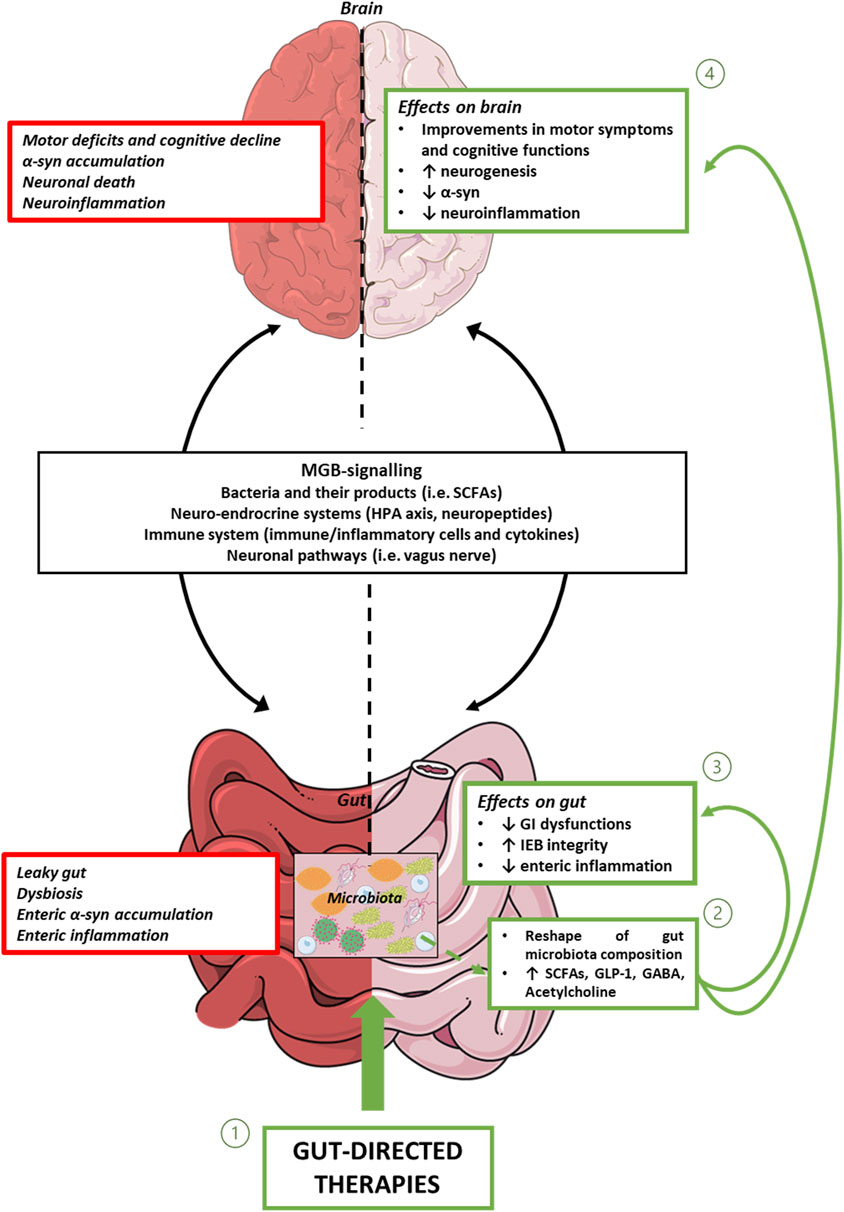
Figure 1. Representative diagram showing MGB signalling, and intestinal and brain alterations associated with PD. In this context, gut-directed therapies (1), through the reshaping of gut microbiota composition and the consequent release of beneficial products, including bacterial metabolites and neurotransmitters (2), besides counteracting enteric inflammation, reinforcing gut barrier integrity, and improving intestinal symptoms (3), exert beneficial effects on CNS alterations. Abbreviations: α-syn: α-synuclein; CNS: central nervous system; GABA: γ-Aminobutyric acid; GI: gastrointestinal; GLP-1: glucagon-like peptide-1; IEB: intestinal epithelial barrier; HPA axis: hypothalamic–pituitary–adrenal axis; MGB: microbiota gut-brain axis; PD: Parkinson’s disease; SCFAs: Short-chain fatty acids.
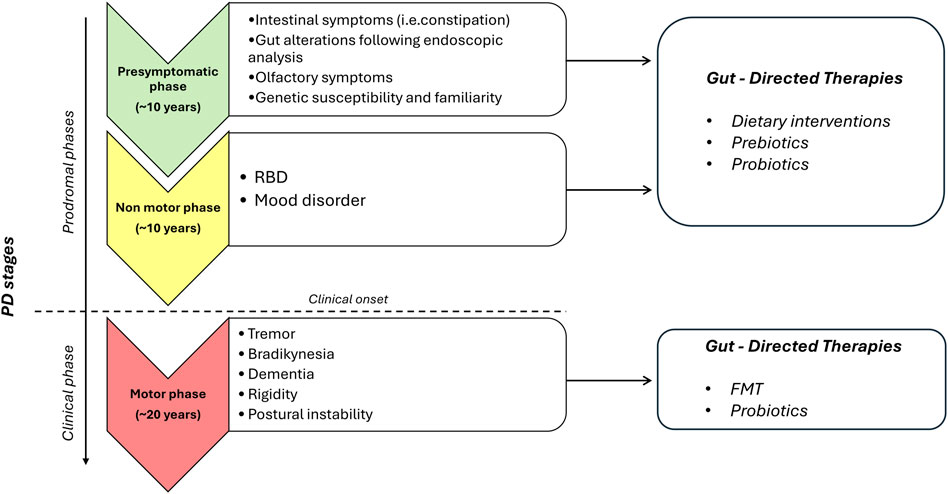
Figure 2. Proposed diagram for the potential application of gut-directed therapies based on PD stages. The present diagram is based on clinical and preclinical studies, so it should always be interpreted in conjunction with patient clinical history, clinical examinations, and PD drugs assumption. For patients in the prodromal stages of PD with intestinal symptoms and gut alterations following endoscopic analysis and subjects with RBD and/or mood disorder, gut-directed therapies, including dietary interventions, prebiotics, and probiotics, can be proposed. For patients in the clinical phase of PD, additional gut directed therapies, including FMT and probiotics, can be added to validated PD drugs. Abbreviations: PD, Parkinson’s disease; RBD, rapid eye movement (REM) sleep behavior disorder.
Author contributions
LB: Writing–original draft, Writing–review and editing. CD: Writing–original draft, Writing–review and editing. GB: Writing–review and editing. LS: Writing–original draft. FR: Writing–review and editing. GE: Conceptualization, Writing–review and editing. LA: Writing–original draft. RC: Conceptualization, Writing–review and editing. NB: Supervision, Writing–review and editing. CP: Conceptualization, Writing–review and editing. MF: Conceptualization, Writing–review and editing.
Funding
The author(s) declare that financial support was received for the research, authorship, and/or publication of this article. This work was supported by research grant from MIUR (PRIN 2022_20229WP2JJ).
Acknowledgments
Figure 1 was partly generated using Servier Medical Art (SMART - Servier Medical ART), provided by Servier, licensed under a Creative Commons Attribution 4.0 unported license.
Conflict of interest
The authors declare that the research was conducted in the absence of any commercial or financial relationships that could be construed as a potential conflict of interest.
The author(s) declared that they were an editorial board member of Frontiers, at the time of submission. This had no impact on the peer review process and the final decision.
Publisher’s note
All claims expressed in this article are solely those of the authors and do not necessarily represent those of their affiliated organizations, or those of the publisher, the editors and the reviewers. Any product that may be evaluated in this article, or claim that may be made by its manufacturer, is not guaranteed or endorsed by the publisher.
References
Aden, K., Rehman, A., Waschina, S., Pan, W. H., Walker, A., Lucio, M., et al. (2019). Metabolic functions of gut microbes associate with efficacy of tumor necrosis factor antagonists in patients with inflammatory bowel diseases. Gastroenterology 157, 1279–1292. doi:10.1053/J.GASTRO.2019.07.025
Aho, V. T. E., Houser, M. C., Pereira, P. A. B., Chang, J., Rudi, K., Paulin, L., et al. (2021). Relationships of gut microbiota, short-chain fatty acids, inflammation, and the gut barrier in Parkinson’s disease. Mol. Neurodegener. 16, 6. doi:10.1186/S13024-021-00427-6
Alcalay, R. N., Gu, Y., Mejia-Santana, H., Cote, L., Marder, K. S., and Scarmeas, N. (2012). The association between Mediterranean diet adherence and Parkinson’s disease. Mov. Disord. 27, 771–774. doi:10.1002/MDS.24918
Alipour Nosrani, E., Tamtaji, O. R., Alibolandi, Z., Sarkar, P., Ghazanfari, M., Azami Tameh, A., et al. (2021). Neuroprotective effects of probiotics bacteria on animal model of Parkinson’s disease induced by 6-hydroxydopamine: a behavioral, biochemical, and histological study. J. Immunoass. Immunochem. 42, 106–120. doi:10.1080/15321819.2020.1833917
Al-Wardat, M., Grillo, P., Schirinzi, T., Pavese, C., Salimei, C., Pisani, A., et al. (2024). Constipation and pain in Parkinson’s disease: a clinical analysis. J. Neural Transm. (Vienna) 131, 165–172. doi:10.1007/S00702-023-02696-5
Aridi, Y. S., Walker, J. L., and Wright, O. R. L. (2017). The association between the mediterranean dietary pattern and cognitive health: a systematic review. Nutrients 9, 674. doi:10.3390/NU9070674
Armstrong, M. J., and Okun, M. S. (2020). Diagnosis and treatment of Parkinson disease: a review. JAMA 323, 548–560. doi:10.1001/JAMA.2019.22360
Astarloa, R., Mena, M. A., Sanchez, V., De la Vega, L., and De Yebenes, J. G. (1992). Clinical and pharmacokinetic effects of a diet rich in insoluble fiber on Parkinson disease. Clin. Neuropharmacol. 15, 375–380. doi:10.1097/00002826-199210000-00004
Bakken, J. S., Polgreen, P. M., Beekmann, S. E., Riedo, F. X., and Streit, J. A. (2013). Treatment approaches including fecal microbiota transplantation for recurrent Clostridium difficile infection (RCDI) among infectious disease physicians. Anaerobe 24, 20–24. doi:10.1016/J.ANAEROBE.2013.08.007
Baroni, L., Bonetto, C., Tessan, F., Goldin, D., Cenci, L., Magnanini, P., et al. (2011). Pilot dietary study with normoproteic protein-redistributed plant-food diet and motor performance in patients with Parkinson’s disease. Nutr. Neurosci. 14, 1–9. doi:10.1179/174313211X12966635733231
Becattini, S., Taur, Y., and Pamer, E. G. (2016). Antibiotic-induced changes in the intestinal microbiota and disease. Trends Mol. Med. 22, 458–478. doi:10.1016/J.MOLMED.2016.04.003
Becker, A., Schmartz, G. P., Gröger, L., Grammes, N., Galata, V., Philippeit, H., et al. (2022). Effects of resistant starch on symptoms, fecal markers, and gut microbiota in Parkinson’s disease - the RESISTA-PD trial. Genomics Proteomics Bioinforma. 20, 274–287. doi:10.1016/J.GPB.2021.08.009
Beckstead, M. J., and Howell, R. D. (2021). Progressive parkinsonism due to mitochondrial impairment: lessons from the MitoPark mouse model. Exp. Neurol. 341, 113707. doi:10.1016/J.EXPNEUROL.2021.113707
Bellini, G., Benvenuti, L., Ippolito, C., Frosini, D., Segnani, C., Rettura, F., et al. (2023). Intestinal histomorphological and molecular alterations in patients with Parkinson’s disease. Eur. J. Neurol. 30, 3440–3450. doi:10.1111/ENE.15607
Blaak, E. E., Canfora, E. E., Theis, S., Frost, G., Groen, A. K., Mithieux, G., et al. (2020). Short chain fatty acids in human gut and metabolic health. Benef. Microbes 11, 411–455. doi:10.3920/BM2020.0057
Bonito-Oliva, A., Pignatelli, M., Spigolon, G., Yoshitake, T., Seiler, S., Longo, F., et al. (2014). Cognitive impairment and dentate gyrus synaptic dysfunction in experimental parkinsonism. Biol. Psychiatry 75, 701–710. doi:10.1016/J.BIOPSYCH.2013.02.015
Braak, H., Del Tredici, K., Rüb, U., De Vos, R. A. I., Jansen Steur, E. N. H., and Braak, E. (2003a). Staging of brain pathology related to sporadic Parkinson’s disease. Neurobiol. Aging 24, 197–211. doi:10.1016/S0197-4580(02)00065-9
Braak, H., Rüb, U., Gai, W. P., and Del Tredici, K. (2003b). Idiopathic Parkinson’s disease: possible routes by which vulnerable neuronal types may be subject to neuroinvasion by an unknown pathogen. J. Neural Transm. (Vienna) 110, 517–536. doi:10.1007/S00702-002-0808-2
Brown, G. C. (2019). The endotoxin hypothesis of neurodegeneration. J. Neuroinflammation 16, 180. doi:10.1186/S12974-019-1564-7
Cantu-Jungles, T. M., Rasmussen, H. E., and Hamaker, B. R. (2019). Potential of prebiotic butyrogenic fibers in Parkinson’s disease. Front. Neurol. 10, 663. doi:10.3389/FNEUR.2019.00663
Castelli, V., d’Angelol, M., Lombardi, F., Alfonsetti, M., Antonosante, A., Catanesi, M., et al. (2020). Effects of the probiotic formulation SLAB51 in in vitro and in vivo Parkinson’s disease models. Aging 12, 4641–4659. doi:10.18632/AGING.102927
Cawthon, C. R., and de La Serre, C. B. (2018). Gut bacteria interaction with vagal afferents. Brain Res. 1693, 134–139. doi:10.1016/J.BRAINRES.2018.01.012
Cersosimo, M. G., and Benarroch, E. E. (2012). Autonomic involvement in Parkinson’s disease: pathology, pathophysiology, clinical features and possible peripheral biomarkers. J. Neurol. Sci. 313, 57–63. doi:10.1016/J.JNS.2011.09.030
Chahine, L. M., Beach, T. G., Brumm, M. C., Adler, C. H., Coffey, C. S., Mosovsky, S., et al. (2020). In vivo distribution of α-synuclein in multiple tissues and biofluids in Parkinson disease. Neurology 95, E1267–E1284. doi:10.1212/WNL.0000000000010404
Chaudhuri, K. R., and Schapira, A. H. (2009). Non-motor symptoms of Parkinson’s disease: dopaminergic pathophysiology and treatment. Lancet Neurol. 8, 464–474. doi:10.1016/S1474-4422(09)70068-7
Chen, Z. J., Liang, C. Y., Yang, L. Q., Ren, S. M., Xia, Y. M., Cui, L., et al. (2021). Association of Parkinson’s disease with microbes and microbiological therapy. Front. Cell Infect. Microbiol. 11, 619354. doi:10.3389/FCIMB.2021.619354
Cirstea, M. S., Creus-Cuadros, A., Lo, C., Yu, A. C., Serapio-Palacios, A., Neilson, S., et al. (2023). A novel pathway of levodopa metabolism by commensal Bifidobacteria. Sci. Rep. 13, 19155. doi:10.1038/S41598-023-45953-Z
Cirstea, M. S., Sundvick, K., Golz, E., Yu, A. C., Boutin, R. C. T., Kliger, D., et al. (2021). The gut mycobiome in Parkinson’s disease. J. Park. Dis. 11, 153–158. doi:10.3233/JPD-202237
Colucci, M., Cervio, M., Faniglione, M., De Angelis, S., Pajoro, M., Levandis, G., et al. (2012). Intestinal dysmotility and enteric neurochemical changes in a Parkinson’s disease rat model. Auton. Neurosci. 169, 77–86. doi:10.1016/J.AUTNEU.2012.04.005
Cryan, J. F., O’riordan, K. J., Cowan, C. S. M., Sandhu, K. V., Bastiaanssen, T. F. S., Boehme, M., et al. (2019). The microbiota-gut-brain Axis. Physiol. Rev. 99, 1877–2013. doi:10.1152/PHYSREV.00018.2018
Davani-Davari, D., Negahdaripour, M., Karimzadeh, I., Seifan, M., Mohkam, M., Masoumi, S. J., et al. (2019). Prebiotics: definition, types, sources, mechanisms, and clinical applications. Foods 8, 92. doi:10.3390/FOODS8030092
De Virgilio, A., Greco, A., Fabbrini, G., Inghilleri, M., Rizzo, M. I., Gallo, A., et al. (2016). Parkinson’s disease: autoimmunity and neuroinflammation. Autoimmun. Rev. 15, 1005–1011. doi:10.1016/j.autrev.2016.07.022
Djaldetti, R., Baron, J., Ziv, I., and Melamed, E. (1996). Gastric emptying in Parkinson’s disease: patients with and without response fluctuations. Neurology 46, 1051–1054. doi:10.1212/WNL.46.4.1051
Du, Y., Li, Y., Xu, X., Li, R., Zhang, M., Cui, Y., et al. (2022). Probiotics for constipation and gut microbiota in Parkinson’s disease. Park. Relat. Disord. 103, 92–97. doi:10.1016/J.PARKRELDIS.2022.08.022
Fang, X., Tian, P., Zhao, X., Jiang, C., and Chen, T. (2019). Neuroprotective effects of an engineered commensal bacterium in the 1-methyl-4-phenyl-1, 2, 3, 6-tetrahydropyridine Parkinson disease mouse model via producing glucagon-like peptide-1. J. Neurochem. 150, 441–452. doi:10.1111/JNC.14694
Fasano, A., Bove, F., Gabrielli, M., Petracca, M., Zocco, M. A., Ragazzoni, E., et al. (2013). The role of small intestinal bacterial overgrowth in Parkinson’s disease. Mov. Disord. 28, 1241–1249. doi:10.1002/MDS.25522
Fasano, A., Visanji, N. P., Liu, L. W. C., Lang, A. E., and Pfeiffer, R. F. (2015). Gastrointestinal dysfunction in Parkinson’s disease. Lancet Neurol. 14, 625–639. doi:10.1016/S1474-4422(15)00007-1
Feng, S. T., Wang, Z. Z., Yuan, Y. H., Sun, H. M., Chen, N. H., and Zhang, Y. (2021). Update on the association between alpha-synuclein and tau with mitochondrial dysfunction: implications for Parkinson’s disease. Eur. J. Neurosci. 53, 2946–2959. doi:10.1111/EJN.14699
Fornai, M., Antonioli, L., Pellegrini, C., Colucci, R., Sacco, D., Tirotta, E., et al. (2016a). Small bowel protection against NSAID-injury in rats: effect of rifaximin, a poorly absorbed, GI targeted, antibiotic. Pharmacol. Res. 104, 186–196. doi:10.1016/J.PHRS.2015.12.031
Fornai, M., Pellegrini, C., Antonioli, L., Segnani, C., Ippolito, C., Barocelli, E., et al. (2016b). Enteric dysfunctions in experimental Parkinson’s disease: alterations of excitatory cholinergic neurotransmission regulating colonic motility in rats. J. Pharmacol. Exp. Ther. 356, 434–444. doi:10.1124/JPET.115.228510
Gaenslen, A., Swid, I., Liepelt-Scarfone, I., Godau, J., and Berg, D. (2011). The patients’ perception of prodromal symptoms before the initial diagnosis of Parkinson’s disease. Mov. Disord. 26, 653–658. doi:10.1002/MDS.23499
Gallo, R. L., and Hooper, L. V. (2012). Epithelial antimicrobial defence of the skin and intestine. Nat. Rev. Immunol. 12, 503–516. doi:10.1038/NRI3228
Gao, K., Mu, C. L., Farzi, A., and Zhu, W. Y. (2020). Tryptophan metabolism: a link between the gut microbiota and brain. Adv. Nutr. 11, 709–723. doi:10.1093/ADVANCES/NMZ127
Georgescu, D., Ancusa, O. E., Georgescu, L. A., Ionita, I., and Reisz, D. (2016). Nonmotor gastrointestinal disorders in older patients with Parkinson’s disease: is there hope? Clin. Interv. Aging 11, 1601–1608. doi:10.2147/CIA.S106284
Ghaisas, S., Langley, M. R., Palanisamy, B. N., Dutta, S., Narayanaswamy, K., Plummer, P. J., et al. (2019). MitoPark transgenic mouse model recapitulates the gastrointestinal dysfunction and gut-microbiome changes of Parkinson’s disease. Neurotoxicology 75, 186–199. doi:10.1016/J.NEURO.2019.09.004
Gorjifard, S., and Goldszmid, R. S. (2016). Microbiota-myeloid cell crosstalk beyond the gut. J. Leukoc. Biol. 100, 865–879. doi:10.1189/JLB.3RI0516-222R
Grillo, P., Sancesario, G. M., Mascioli, D., Geusa, L., Zenuni, H., Giannella, E., et al. (2022). Constipation distinguishes different clinical-biochemical patterns in de novo Parkinson’s disease. Park. Relat. Disord. 102, 64–67. doi:10.1016/J.PARKRELDIS.2022.08.001
Hall, D. A., Voigt, R. M., Cantu-Jungles, T. M., Hamaker, B., Engen, P. A., Shaikh, M., et al. (2023). An open label, non-randomized study assessing a prebiotic fiber intervention in a small cohort of Parkinson’s disease participants. Nat. Commun. 14, 926. doi:10.1038/S41467-023-36497-X
Harkins, C. P., Kong, H. H., and Segre, J. A. (2020). Manipulating the human microbiome to manage disease. JAMA 323, 303–304. doi:10.1001/JAMA.2019.19602
Hauser, R. A., Sutherland, D., Madrid, J. A., Rol, M. A., Frucht, S., Isaacson, S., et al. (2019). Targeting neurons in the gastrointestinal tract to treat Parkinson’s disease. Clin. Park Relat. Disord. 1, 2–7. doi:10.1016/J.PRDOA.2019.06.001
Hey, G., Nair, N., Klann, E., Gurrala, A., Safarpour, D., Mai, V., et al. (2023). Therapies for Parkinson’s disease and the gut microbiome: evidence for bidirectional connection. Front. Aging Neurosci. 15, 1151850. doi:10.3389/FNAGI.2023.1151850
Hong, C. T., Chan, L., Chen, K. Y., Lee, H. H., Huang, L. K., Yang, Y. C. S. H., et al. (2022). Rifaximin modifies gut microbiota and attenuates inflammation in Parkinson’s disease: preclinical and clinical studies. Cells 11, 3468. doi:10.3390/CELLS11213468
Horsager, J., Andersen, K. B., Knudsen, K., Skjærbæk, C., Fedorova, T. D., Okkels, N., et al. (2020). Brain-first versus body-first Parkinson’s disease: a multimodal imaging case-control study. Brain 143, 3077–3088. doi:10.1093/BRAIN/AWAA238
Huang, H., Xu, H., Luo, Q., He, J., Li, M., Chen, H., et al. (2019). Fecal microbiota transplantation to treat Parkinson’s disease with constipation: a case report. Medicine 98, e16163. doi:10.1097/MD.0000000000016163
Hutkins, R. W., Krumbeck, J. A., Bindels, L. B., Cani, P. D., Fahey, G., Goh, Y. J., et al. (2016). Prebiotics: why definitions matter. Curr. Opin. Biotechnol. 37, 1–7. doi:10.1016/J.COPBIO.2015.09.001
Ibrahim, A., Raja Ali, R. A., Abdul Manaf, M. R., Ahmad, N., Tajurruddin, F. W., Qin, W. Z., et al. (2020). Multi-strain probiotics (Hexbio) containing MCP BCMC strains improved constipation and gut motility in Parkinson’s disease: a randomised controlled trial. PLoS One 15, e0244680. doi:10.1371/JOURNAL.PONE.0244680
Jankovic, J., and Tan, E. K. (2020). Parkinson’s disease: etiopathogenesis and treatment. J. Neurol. Neurosurg. Psychiatry 91, 795–808. doi:10.1136/JNNP-2019-322338
Jiang, Z., Wang, X., Zhang, H., Yin, J., Zhao, P., Yin, Q., et al. (2023). Ketogenic diet protects MPTP-induced mouse model of Parkinson’s disease via altering gut microbiota and metabolites. MedComm (Beijing) 4, e268. doi:10.1002/MCO2.268
Kalia, L. V., and Lang, A. E. (2015). Parkinson’s disease. Lancet 386, 896–912. doi:10.1016/S0140-6736(14)61393-3
Kim, N., Jeon, S. H., Ju, I. G., Gee, M. S., Do, J., Oh, M. S., et al. (2021). Transplantation of gut microbiota derived from Alzheimer’s disease mouse model impairs memory function and neurogenesis in C57BL/6 mice. Brain Behav. Immun. 98, 357–365. doi:10.1016/J.BBI.2021.09.002
Klaenhammer, T. R., Kleerebezem, M., Kopp, M. V., and Rescigno, M. (2012). The impact of probiotics and prebiotics on the immune system. Nat. Rev. Immunol. 12, 728–734. doi:10.1038/NRI3312
Klingelhoefer, L., and Reichmann, H. (2015). Pathogenesis of Parkinson disease—the gut–brain axis and environmental factors. Nat. Rev. Neurol. 2015 11, 625–636. doi:10.1038/nrneurol.2015.197
Kuai, X. Y., Yao, X. H., Xu, L. J., Zhou, Y. Q., Zhang, L. P., Liu, Y., et al. (2021). Evaluation of fecal microbiota transplantation in Parkinson’s disease patients with constipation. Microb. Cell Fact. 20. 98. doi:10.1186/s12934-021-01589-0
Larroya-García, A., Navas-Carrillo, D., and Orenes-Piñero, E. (2019). Impact of gut microbiota on neurological diseases: diet composition and novel treatments. Crit. Rev. Food Sci. Nutr. 59, 3102–3116. doi:10.1080/10408398.2018.1484340
Lenoir, M., Martín, R., Torres-Maravilla, E., Chadi, S., González-Dávila, P., Sokol, H., et al. (2020). Butyrate mediates anti-inflammatory effects of Faecalibacterium prausnitzii in intestinal epithelial cells through Dact3. Gut Microbes 12, 1–16. doi:10.1080/19490976.2020.1826748
Li, N., Wang, Q., Wang, Y., Sun, A., Lin, Y., Jin, Y., et al. (2019). Fecal microbiota transplantation from chronic unpredictable mild stress mice donors affects anxiety-like and depression-like behavior in recipient mice via the gut microbiota-inflammation-brain axis. Stress 22, 592–602. doi:10.1080/10253890.2019.1617267
Li, T., Chu, C., Yu, L., Zhai, Q., Wang, S., Zhao, J., et al. (2022). Neuroprotective effects of Bifidobacterium breve CCFM1067 in MPTP-induced mouse models of Parkinson’s disease. Nutrients 14, 4678. doi:10.3390/NU14214678
Li, W., Wu, X., Hu, X., Wang, T., Liang, S., Duan, Y., et al. (2017). Structural changes of gut microbiota in Parkinson’s disease and its correlation with clinical features. Sci. China Life Sci. 60, 1223–1233. doi:10.1007/S11427-016-9001-4
Liao, J. F., Cheng, Y. F., You, S. T., Kuo, W. C., Huang, C. W., Chiou, J. J., et al. (2020). Lactobacillus plantarum PS128 alleviates neurodegenerative progression in 1-methyl-4-phenyl-1,2,3,6-tetrahydropyridine-induced mouse models of Parkinson’s disease. Brain Behav. Immun. 90, 26–46. doi:10.1016/J.BBI.2020.07.036
Lin, C. H., Chen, C. C., Chiang, H. L., Liou, J. M., Chang, C. M., Lu, T. P., et al. (2019). Altered gut microbiota and inflammatory cytokine responses in patients with Parkinson’s disease. J. Neuroinflammation 16, 129. doi:10.1186/S12974-019-1528-Y
Liu, S., Guo, R., Liu, F., Yuan, Q., Yu, Y., and Ren, F. (2020). Gut microbiota regulates depression-like behavior in rats through the neuroendocrine-immune-mitochondrial pathway. Neuropsychiatr. Dis. Treat. 16, 859–869. doi:10.2147/NDT.S243551
Liu, X., Du, Z. R., Wang, X., Sun, X. R., Zhao, Q., Zhao, F., et al. (2022). Polymannuronic acid prebiotic plus Lacticaseibacillus rhamnosus GG probiotic as a novel synbiotic promoted their separate neuroprotection against Parkinson’s disease. Food Res. Int. 155, 111067. doi:10.1016/J.FOODRES.2022.111067
Lubomski, M., Xu, X., Holmes, A. J., Yang, J. Y. H., Sue, C. M., and Davis, R. L. (2022). The impact of device-assisted therapies on the gut microbiome in Parkinson’s disease. J. Neurol. 269, 780–795. doi:10.1007/S00415-021-10657-9
Lye, H. S., Lee, Y. T., Ooi, S. Y., Teh, L. K., Lim, L. N., and Wei, L. K. (2018). Modifying progression of aging and reducing the risk of neurodegenerative diseases by probiotics and synbiotics. Front. Biosci. (Elite Ed.) 10, 344–351. doi:10.2741/E826
Ma, Y. F., Lin, Y. A., Huang, C. L., Hsu, C. C., Wang, S., Yeh, S. R., et al. (2023). Lactiplantibacillus plantarum PS128 alleviates exaggerated cortical beta oscillations and motor deficits in the 6-hydroxydopamine rat model of Parkinson’s disease. Probiotics Antimicrob. Proteins 15, 312–325. doi:10.1007/S12602-021-09828-X
Magistrelli, L., Amoruso, A., Mogna, L., Graziano, T., Cantello, R., Pane, M., et al. (2019). Probiotics may have beneficial effects in Parkinson’s disease: in vitro evidence. Front. Immunol. 10, 969. doi:10.3389/FIMMU.2019.00969
Maraki, M. I., Yannakoulia, M., Stamelou, M., Stefanis, L., Xiromerisiou, G., Kosmidis, M. H., et al. (2019). Mediterranean diet adherence is related to reduced probability of prodromal Parkinson’s disease. Mov. Disord. 34, 48–57. doi:10.1002/MDS.27489
Markowiak, P., and Ślizewska, K. (2017). Effects of probiotics, prebiotics, and synbiotics on human health. Nutrients 9, 1021. doi:10.3390/NU9091021
Martín-Peláez, S., Fito, M., and Castaner, O. (2020). Mediterranean diet effects on type 2 diabetes prevention, disease progression, and related mechanisms. A review. Nutrients 12, 2236–2315. doi:10.3390/NU12082236
Matsumoto, M., Kibe, R., Ooga, T., Aiba, Y., Sawaki, E., Koga, Y., et al. (2013). Cerebral low-molecular metabolites influenced by intestinal microbiota: a pilot study. Front. Syst. Neurosci. 7, 9. doi:10.3389/FNSYS.2013.00009
Melis, M., Vascellari, S., Santoru, M. L., Oppo, V., Fabbri, M., Sarchioto, M., et al. (2021). Gut microbiota and metabolome distinctive features in Parkinson disease: focus on levodopa and levodopa-carbidopa intrajejunal gel. Eur. J. Neurol. 28, 1198–1209. doi:10.1111/ENE.14644
Miraglia, F., Betti, L., Palego, L., and Giannaccini, G. (2015). Parkinson’s disease and alpha-synucleinopathies: from arising pathways to therapeutic challenge. Cent. Nerv. Syst. Agents Med. Chem. 15, 109–116. doi:10.2174/1871524915666150421114338
Mohammad-Beigi, H., Aliakbari, F., Sahin, C., Lomax, C., Tawfike, A., Schafer, N. P., et al. (2019). Oleuropein derivatives from olive fruit extracts reduce α-synuclein fibrillation and oligomer toxicity. J. Biol. Chem. 294, 4215–4232. doi:10.1074/JBC.RA118.005723
Mustapha, M., and Taib, C. N. M. (2021). MPTP-induced mouse model of Parkinson’s disease: a promising direction of therapeutic strategies. Bosn. J. Basic Med. Sci. 21, 422–433. doi:10.17305/BJBMS.2020.5181
Muth, A. K., and Park, S. Q. (2021). The impact of dietary macronutrient intake on cognitive function and the brain. Clin. Nutr. 40, 3999–4010. doi:10.1016/J.CLNU.2021.04.043
Naneix, F., Tantot, F., Glangetas, C., Kaufling, J., Janthakhin, Y., Boitard, C., et al. (2017). Impact of early consumption of high-fat diet on the mesolimbic dopaminergic system. eNeuro 4, ENEURO.0120–17.2017. doi:10.1523/ENEURO.0120-17.2017
Natale, G., Kastsiushenka, O., Fulceri, F., Ruggieri, S., Paparelli, A., and Fornai, F. (2010). MPTP-induced parkinsonism extends to a subclass of TH-positive neurons in the gut. Brain Res. 1355, 195–206. doi:10.1016/J.BRAINRES.2010.07.076
Nishiwaki, H., Ito, M., Ishida, T., Hamaguchi, T., Maeda, T., Kashihara, K., et al. (2020). Meta-analysis of gut dysbiosis in Parkinson’s disease. Mov. Disord. 35, 1626–1635. doi:10.1002/MDS.28119
Nyholm, D., Klangemo, K., and Johansson, A. (2012). Levodopa/carbidopa intestinal gel infusion long-term therapy in advanced Parkinson’s disease. Eur. J. Neurol. 19, 1079–1085. doi:10.1111/J.1468-1331.2012.03679.X
Okun, M. S. (2012). Deep-brain stimulation for Parkinson’s disease. N. Engl. J. Med. 367, 1529–1538. doi:10.1056/NEJMCT1208070
Paknahad, Z., Sheklabadi, E., Derakhshan, Y., Bagherniya, M., and Chitsaz, A. (2020). The effect of the Mediterranean diet on cognitive function in patients with Parkinson’s disease: a randomized clinical controlled trial. Complement. Ther. Med. 50, 102366. doi:10.1016/J.CTIM.2020.102366
Paknahad, Z., Sheklabadi, E., Moravejolahkami, A. R., Chitsaz, A., and Hassanzadeh, A. (2022). The effects of Mediterranean diet on severity of disease and serum Total Antioxidant Capacity (TAC) in patients with Parkinson’s disease: a single center, randomized controlled trial. Nutr. Neurosci. 25, 313–320. doi:10.1080/1028415X.2020.1751509
Pan, S., Wei, H., Yuan, S., Kong, Y., Yang, H., Zhang, Y., et al. (2022). Probiotic Pediococcus pentosaceus ameliorates MPTP-induced oxidative stress via regulating the gut microbiota-gut-brain axis. Front. Cell Infect. Microbiol. 12, 1022879. doi:10.3389/FCIMB.2022.1022879
Pan-Montojo, F., Schwarz, M., Winkler, C., Arnhold, M., O’Sullivan, G. A., Pal, A., et al. (2012). Environmental toxins trigger PD-like progression via increased alpha-synuclein release from enteric neurons in mice. Sci. Rep. 2, 898. doi:10.1038/SREP00898
Parashar, A., and Udayabanu, M. (2017). Gut microbiota: implications in Parkinson’s disease. Park. Relat. Disord. 38, 1–7. doi:10.1016/J.PARKRELDIS.2017.02.002
Pellegrini, C., Antonioli, L., Calderone, V., Colucci, R., Fornai, M., and Blandizzi, C. (2020). Microbiota-gut-brain axis in health and disease: is NLRP3 inflammasome at the crossroads of microbiota-gut-brain communications? Prog. Neurobiol. 191, 101806. doi:10.1016/J.PNEUROBIO.2020.101806
Pellegrini, C., Antonioli, L., Colucci, R., Blandizzi, C., and Fornai, M. (2018). Interplay among gut microbiota, intestinal mucosal barrier and enteric neuro-immune system: a common path to neurodegenerative diseases? Acta Neuropathol. 136, 345–361. doi:10.1007/S00401-018-1856-5
Pellegrini, C., Antonioli, L., Colucci, R., Tirotta, E., Gentile, D., Ippolito, C., et al. (2017). Effects of L-DOPA/benserazide co-treatment on colonic excitatory cholinergic motility and enteric inflammation following dopaminergic nigrostriatal neurodegeneration. Neuropharmacology 123, 22–33. doi:10.1016/J.NEUROPHARM.2017.05.016
Pellegrini, C., D’Antongiovanni, V., Miraglia, F., Rota, L., Benvenuti, L., Di Salvo, C., et al. (2022). Enteric α-synuclein impairs intestinal epithelial barrier through caspase-1-inflammasome signaling in Parkinson’s disease before brain pathology. NPJ Park. Dis. 8, 9. doi:10.1038/S41531-021-00263-X
Pellegrini, C., Fornai, M., Colucci, R., Tirotta, E., Blandini, F., Levandis, G., et al. (2016). Alteration of colonic excitatory tachykininergic motility and enteric inflammation following dopaminergic nigrostriatal neurodegeneration. J. Neuroinflammation 13, 146. doi:10.1186/S12974-016-0608-5
Pellegrini, C., Fornai, M., D’Antongiovanni, V., Antonioli, L., Bernardini, N., and Derkinderen, P. (2023). The intestinal barrier in disorders of the central nervous system. Lancet Gastroenterol. Hepatol. 8, 66–80. doi:10.1016/S2468-1253(22)00241-2
Pellegrini, C., and Travagli, R. A. (2024). Gastrointestinal dysmotility in rodent models of Parkinson’s disease. Am. J. Physiol. Gastrointest. Liver Physiol. 326, G345–G359. doi:10.1152/AJPGI.00225.2023
Perez-Pardo, P., Broersen, L. M., Kliest, T., van Wijk, N., Attali, A., Garssen, J., et al. (2018a). Additive effects of levodopa and a neurorestorative diet in a mouse model of Parkinson’s disease. Front. Aging Neurosci. 10, 237. doi:10.3389/FNAGI.2018.00237
Perez-Pardo, P., Dodiya, H. B., Broersen, L. M., Douna, H., van Wijk, N., Lopes da Silva, S., et al. (2018b). Gut-brain and brain-gut axis in Parkinson’s disease models: effects of a uridine and fish oil diet. Nutr. Neurosci. 21, 391–402. doi:10.1080/1028415X.2017.1294555
Peter, I., Dubinsky, M., Bressman, S., Park, A., Lu, C., Chen, N., et al. (2018). Anti-tumor necrosis factor therapy and incidence of Parkinson disease among patients with inflammatory bowel disease. JAMA Neurol. 75, 939–946. doi:10.1001/JAMANEUROL.2018.0605
Phillips, M. C. L., Murtagh, D. K. J., Gilbertson, L. J., Asztely, F. J. S., and Lynch, C. D. P. (2018). Low-fat versus ketogenic diet in Parkinson’s disease: a pilot randomized controlled trial. Mov. Disord. 33, 1306–1314. doi:10.1002/MDS.27390
Pollak, P., Champay, A. S., Hommel, M., Perret, J. E., Benabid, A. L., and Tirelli, U. (1989). Lethal neurotoxicity associated to azidothymidine therapy. J. Neurol. Neurosurg. Psychiatry 52, 544–545. doi:10.1136/jnnp.52.4.544-a
Postuma, R. B., Iranzo, A., Hu, M., Högl, B., Boeve, B. F., Manni, R., et al. (2019). Risk and predictors of dementia and parkinsonism in idiopathic REM sleep behaviour disorder: a multicentre study. Brain 142, 744–759. doi:10.1093/BRAIN/AWZ030
Powell, N., Walker, M. M., and Talley, N. J. (2017). The mucosal immune system: master regulator of bidirectional gut-brain communications. Nat. Rev. Gastroenterology Hepatology 14, 143–159. doi:10.1038/nrgastro.2016.191
Radad, K., Al-Shraim, M., Al-Emam, A., Wang, F., Kranner, B., Rausch, W. D., et al. (2019). Rotenone: from modelling to implication in Parkinson’s disease. Folia Neuropathol. 57, 317–326. doi:10.5114/FN.2019.89857
Ray Dorsey, E., Elbaz, A., Nichols, E., Abd-Allah, F., Abdelalim, A., Adsuar, J. C., et al. (2018). Global, regional, and national burden of Parkinson’s disease, 1990-2016: a systematic analysis for the Global Burden of Disease Study 2016. Lancet Neurol. 17, 939–953. doi:10.1016/S1474-4422(18)30295-3
Rekdal, V. M., Bess, E. N., Bisanz, J. E., Turnbaugh, P. J., and Balskus, E. P. (2019). Discovery and inhibition of an interspecies gut bacterial pathway for Levodopa metabolism. Science 364, eaau6323. doi:10.1126/SCIENCE.AAU6323
Ren, T., Gao, Y., Qiu, Y., Jiang, S., Zhang, Q., Zhang, J., et al. (2020). Gut microbiota altered in mild cognitive impairment compared with normal cognition in sporadic Parkinson’s disease. Front. Neurol. 11, 137. doi:10.3389/FNEUR.2020.00137
Rizzetto, L., Fava, F., Tuohy, K. M., and Selmi, C. (2018). Connecting the immune system, systemic chronic inflammation and the gut microbiome: the role of sex. J. Autoimmun. 92, 12–34. doi:10.1016/J.JAUT.2018.05.008
Romano, S., Savva, G. M., Bedarf, J. R., Charles, I. G., Hildebrand, F., and Narbad, A. (2021). Meta-analysis of the Parkinson’s disease gut microbiome suggests alterations linked to intestinal inflammation. NPJ Park. Dis. 7, 27. doi:10.1038/S41531-021-00156-Z
Rota, L., Pellegrini, C., Benvenuti, L., Antonioli, L., Fornai, M., Blandizzi, C., et al. (2019). Constipation, deficit in colon contractions and alpha-synuclein inclusions within the colon precede motor abnormalities and neurodegeneration in the central nervous system in a mouse model of alpha-synucleinopathy. Transl. Neurodegener. 8, 5. doi:10.1186/S40035-019-0146-Z
Rusch, C., Beke, M., Tucciarone, L., Dixon, K., Nieves, C., Mai, V., et al. (2021). Effect of a Mediterranean diet intervention on gastrointestinal function in Parkinson’s disease (the MEDI-PD study): study protocol for a randomised controlled trial. BMJ Open 11, e053336. doi:10.1136/BMJOPEN-2021-053336
Sánchez-Sánchez, M. L., García-Vigara, A., Hidalgo-Mora, J. J., García-Pérez, M. Á., Tarín, J., and Cano, A. (2020). Mediterranean diet and health: a systematic review of epidemiological studies and intervention trials. Maturitas 136, 25–37. doi:10.1016/J.MATURITAS.2020.03.008
Sanders, M. E., Merenstein, D. J., Reid, G., Gibson, G. R., and Rastall, R. A. (2019). Probiotics and prebiotics in intestinal health and disease: from biology to the clinic. Nat. Rev. Gastroenterol. Hepatol. 16, 605–616. doi:10.1038/S41575-019-0173-3
Santa-Cecília, F. V., Leite, C. A., Del-Bel, E., and Raisman-Vozari, R. (2019). The neuroprotective effect of doxycycline on neurodegenerative diseases. Neurotox. Res. 35, 981–986. doi:10.1007/S12640-019-00015-Z
Schmidt, T. S. B., Raes, J., and Bork, P. (2018). The human gut microbiome: from association to modulation. Cell 172, 1198–1215. doi:10.1016/J.CELL.2018.02.044
Segal, A., Zlotnik, Y., Moyal-Atias, K., Abuhasira, R., and Ifergane, G. (2021). Fecal microbiota transplant as a potential treatment for Parkinson’s disease - a case series. Clin. Neurol. Neurosurg. 207, 106791. doi:10.1016/J.CLINEURO.2021.106791
Seguella, L., Palenca, I., Franzin, S. B., Zilli, A., and Esposito, G. (2023). Mini-review: interaction between intestinal microbes and enteric glia in health and disease. Neurosci. Lett. 806, 137221. doi:10.1016/J.NEULET.2023.137221
Shan, J., Qu, Y., Wang, S., Wei, Y., Chang, L., Ma, L., et al. (2021). Regulation of neurotoxicity in the striatum and colon of MPTP-induced Parkinson’s disease mice by gut microbiome. Brain Res. Bull. 177, 103–110. doi:10.1016/J.BRAINRESBULL.2021.09.009
Srivastav, S., Neupane, S., Bhurtel, S., Katila, N., Maharjan, S., Choi, H., et al. (2019). Probiotics mixture increases butyrate, and subsequently rescues the nigral dopaminergic neurons from MPTP and rotenone-induced neurotoxicity. J. Nutr. Biochem. 69, 73–86. doi:10.1016/J.JNUTBIO.2019.03.021
Stasi, C., Bellini, M., Gambaccini, D., Duranti, E., de Bortoli, N., Fani, B., et al. (2017). Neuroendocrine dysregulation in irritable bowel syndrome patients: a pilot study. J. Neurogastroenterol. Motil. 23, 428–434. doi:10.5056/JNM16155
Stasi, C., Caserta, A., Nisita, C., Cortopassi, S., Fani, B., Salvadori, S., et al. (2019). The complex interplay between gastrointestinal and psychiatric symptoms in irritable bowel syndrome: a longitudinal assessment. J. Gastroenterol. Hepatol. 34, 713–719. doi:10.1111/JGH.14375
Stasi, C., Rosselli, M., Bellini, M., Laffi, G., and Milani, S. (2012). Altered neuro-endocrine-immune pathways in the irritable bowel syndrome: the top-down and the bottom-up model. J. Gastroenterol. 47, 1177–1185. doi:10.1007/S00535-012-0627-7
Stoilova, T., Colombo, L., Forloni, G., Tagliavini, F., and Salmona, M. (2013). A new face for old antibiotics: tetracyclines in treatment of amyloidoses. J. Med. Chem. 56, 5987–6006. doi:10.1021/JM400161P
Suarez, A. N., Hsu, T. M., Liu, C. M., Noble, E. E., Cortella, A. M., Nakamoto, E. M., et al. (2018). Gut vagal sensory signaling regulates hippocampus function through multi-order pathways. Nat. Commun. 9, 2181. doi:10.1038/S41467-018-04639-1
Sultan, S., Gebara, E., and Toni, N. (2013). Doxycycline increases neurogenesis and reduces microglia in the adult hippocampus. Front. Neurosci. 7, 131. doi:10.3389/FNINS.2013.00131
Sun, J., Li, H., Jin, Y., Yu, J., Mao, S., Su, K. P., et al. (2021). Probiotic Clostridium butyricum ameliorated motor deficits in a mouse model of Parkinson’s disease via gut microbiota-GLP-1 pathway. Brain Behav. Immun. 91, 703–715. doi:10.1016/J.BBI.2020.10.014
Sun, M. F., Zhu, Y. L., Zhou, Z. L., Jia, X. B., Xu, Y.Da, Yang, Q., et al. (2018). Neuroprotective effects of fecal microbiota transplantation on MPTP-induced Parkinson’s disease mice: gut microbiota, glial reaction and TLR4/TNF-α signaling pathway. Brain Behav. Immun. 70, 48–60. doi:10.1016/J.BBI.2018.02.005
Svensson, E., Horváth-Puhó, E., Thomsen, R. W., Djurhuus, J. C., Pedersen, L., Borghammer, P., et al. (2015). Vagotomy and subsequent risk of Parkinson’s disease. Ann. Neurol. 78, 522–529. doi:10.1002/ANA.24448
Tamtaji, O. R., Taghizadeh, M., Daneshvar Kakhaki, R., Kouchaki, E., Bahmani, F., Borzabadi, S., et al. (2019). Clinical and metabolic response to probiotic administration in people with Parkinson’s disease: a randomized, double-blind, placebo-controlled trial. Clin. Nutr. 38, 1031–1035. doi:10.1016/J.CLNU.2018.05.018
Tan, A. H., Hor, J. W., Chong, C. W., and Lim, S. Y. (2020). Probiotics for Parkinson’s disease: current evidence and future directions. JGH Open 5, 414–419. doi:10.1002/JGH3.12450
Tan, A. H., Lim, S. Y., Chong, K. K., A Manap, M. A. A., Hor, J. W., Lim, J. L., et al. (2021). Probiotics for constipation in Parkinson disease: a randomized placebo-controlled study. Neurology 96, e772–e782. doi:10.1212/WNL.0000000000010998
Tasselli, M., Chaumette, T., Paillusson, S., Monnet, Y., Lafoux, A., Huchet-Cadiou, C., et al. (2013). Effects of oral administration of rotenone on gastrointestinal functions in mice. Neurogastroenterol. Motil. 25, e183–e193. doi:10.1111/NMO.12070
Tidman, M. M., White, D., and White, T. (2022). Effects of an low carbohydrate/healthy fat/ketogenic diet on biomarkers of health and symptoms, anxiety and depression in Parkinson’s disease: a pilot study. Neurodegener. Dis. Manag. 12, 57–66. doi:10.2217/NMT-2021-0033
Tillisch, K. (2014). The effects of gut microbiota on CNS function in humans. Gut Microbes 5, 404–410. doi:10.4161/GMIC.29232
Toh, T. S., Chong, C. W., Lim, S. Y., Bowman, J., Cirstea, M., Lin, C. H., et al. (2022). Gut microbiome in Parkinson’s disease: new insights from meta-analysis. Park. Relat. Disord. 94, 1–9. doi:10.1016/J.PARKRELDIS.2021.11.017
Ton, A. M. M., Campagnaro, B. P., Alves, G. A., Aires, R., Côco, L. Z., Arpini, C. M., et al. (2020). Oxidative stress and dementia in Alzheimer’s patients: effects of synbiotic supplementation. Oxid. Med. Cell Longev. 2020, 2638703. doi:10.1155/2020/2638703
Toscano, M., De Grandi, R., Pastorelli, L., Vecchi, M., and Drago, L. (2017). A consumer’s guide for probiotics: 10 golden rules for a correct use. Dig. Liver Dis. 49, 1177–1184. doi:10.1016/J.DLD.2017.07.011
Troshev, D., Voronkov, D., Pavlova, A., Abaimov, D., Latanov, A., Fedorova, T., et al. (2022). Time course of neurobehavioral disruptions and regional brain metabolism changes in the rotenone mice model of Parkinson’s disease. Biomedicines 10, 466. doi:10.3390/BIOMEDICINES10020466
Varatharaj, A., and Galea, I. (2017). The blood-brain barrier in systemic inflammation. Brain Behav. Immun. 60, 1–12. doi:10.1016/J.BBI.2016.03.010
Wang, B., Wang, L., Qu, Y., Lu, J., and Xia, W. (2022a). Chitosan oligosaccharides exert neuroprotective effects via modulating the PI3K/Akt/Bcl-2 pathway in a Parkinsonian model. Food Funct. 13, 5838–5853. doi:10.1039/D1FO04374A
Wang, L., Zhao, Z., Zhao, L., Zhao, Y., Yang, G., Wang, C., et al. (2022b). Lactobacillus plantarum DP189 reduces α-SYN aggravation in MPTP-induced Parkinson’s disease mice via regulating oxidative damage, inflammation, and gut microbiota disorder. J. Agric. Food Chem. 70, 1163–1173. doi:10.1021/acs.jafc.1c07711
Weis, S., Schwiertz, A., Unger, M. M., Becker, A., Faßbender, K., Ratering, S., et al. (2019). Effect of Parkinson’s disease and related medications on the composition of the fecal bacterial microbiota. NPJ Park. Dis. 5, 28. doi:10.1038/S41531-019-0100-X
Wenzel, T. J., Gates, E. J., Ranger, A. L., and Klegeris, A. (2020). Short-chain fatty acids (SCFAs) alone or in combination regulate select immune functions of microglia-like cells. Mol. Cell Neurosci. 105, 103493. doi:10.1016/J.MCN.2020.103493
Wurtman, R. J., Cansev, M., Sakamoto, T., and Ulus, I. (2010). Nutritional modifiers of aging brain function: use of uridine and other phosphatide precursors to increase formation of brain synapses. Nutr. Rev. 68 (Suppl. 2), S88–S101. doi:10.1111/J.1753-4887.2010.00344.X
Xie, A., Ensink, E., Li, P., Gordevičius, J., Marshall, L. L., George, S., et al. (2022). Bacterial butyrate in Parkinson’s disease is linked to epigenetic changes and depressive symptoms. Mov. Disord. 37, 1644–1653. doi:10.1002/MDS.29128
Xie, C., and Prasad, A. A. (2020). Probiotics treatment improves hippocampal dependent cognition in a rodent model of Parkinson’s disease. Microorganisms 8, 1661–1713. doi:10.3390/MICROORGANISMS8111661
Xue, L. J., Yang, X. Z., Tong, Q., Shen, P., Ma, S. J., Wu, S. N., et al. (2020). Fecal microbiota transplantation therapy for Parkinson’s disease: a preliminary study. Medicine 99, E22035. doi:10.1097/MD.0000000000022035
Yang, F., Wolk, A., Håkansson, N., Pedersen, N. L., and Wirdefeldt, K. (2017). Dietary antioxidants and risk of Parkinson’s disease in two population-based cohorts. Mov. Disord. 32, 1631–1636. doi:10.1002/MDS.27120
Yang, X., He, X., Xu, S., Zhang, Y., Mo, C., Lai, Y., et al. (2023). Effect of Lacticaseibacillus paracasei strain Shirota supplementation on clinical responses and gut microbiome in Parkinson’s disease. Food Funct. 14, 6828–6839. doi:10.1039/D3FO00728F
Yong, V. W., Tan, Y. J., Ng, Y.De, Choo, X. Y., Sugumaran, K., Chinna, K., et al. (2020). Progressive and accelerated weight and body fat loss in Parkinson’s disease: a three-year prospective longitudinal study. Park. Relat. Disord. 77, 28–35. doi:10.1016/J.PARKRELDIS.2020.06.015
Yoo, B. B., and Mazmanian, S. K. (2017). The enteric network: interactions between the immune and nervous systems of the gut. Immunity 46, 910–926. doi:10.1016/J.IMMUNI.2017.05.011
Zhang, J., Ma, L., Chang, L., Pu, Y., Qu, Y., and Hashimoto, K. (2020). A key role of the subdiaphragmatic vagus nerve in the depression-like phenotype and abnormal composition of gut microbiota in mice after lipopolysaccharide administration. Transl. Psychiatry 10, 186. doi:10.1038/S41398-020-00878-3
Zhang, W., Ding, L., Zhang, M., Zheng, S., Ma, R., Gong, J., et al. (2023). Dietary intake of α-ketoglutarate ameliorates α-synuclein pathology in mouse models of Parkinson’s disease. Cell Mol. Life Sci. 80, 155. doi:10.1007/S00018-023-04807-7
Zhang, X. L., Zhang, X. H., Yu, X., Zheng, L. F., Feng, X. Y., Liu, C. Z., et al. (2021). Enhanced contractive tension and upregulated muscarinic receptor 2/3 in colorectum contribute to constipation in 6-hydroxydopamine-induced Parkinson’s disease rats. Front. Aging Neurosci. 13, 770841. doi:10.3389/FNAGI.2021.770841
Zhao, Z., Ning, J., Bao, X., Shang, M., Ma, J., Li, G., et al. (2021). Fecal microbiota transplantation protects rotenone-induced Parkinson’s disease mice via suppressing inflammation mediated by the lipopolysaccharide-TLR4 signaling pathway through the microbiota-gut-brain axis. Microbiome 9, 226. doi:10.1186/S40168-021-01107-9
Zhou, X., Lu, J., Wei, K., Wei, J., Tian, P., Yue, M., et al. (2021). Neuroprotective effect of ceftriaxone on MPTP-induced Parkinson’s disease mouse model by regulating inflammation and intestinal microbiota. Oxid. Med. Cell Longev. 2021, 9424582. doi:10.1155/2021/9424582
Zhou, Z. L., Jia, X. B., Sun, M. F., Zhu, Y. L., Qiao, C. M., Zhang, B. P., et al. (2019). Neuroprotection of fasting mimicking diet on MPTP-induced Parkinson’s disease mice via gut microbiota and metabolites. Neurotherapeutics 16, 741–760. doi:10.1007/S13311-019-00719-2
Zmora, N., Soffer, E., and Elinav, E. (2019). Transforming medicine with the microbiome. Sci. Transl. Med. 11, eaaw1815. doi:10.1126/SCITRANSLMED.AAW1815
Keywords: Parkinson’s disease, microbiota-gut-brain axis, enteric nervous system, enteric inflammation, prebiotics, probiotics, fecal microbiota transplantation
Citation: Benvenuti L, Di Salvo C, Bellini G, Seguella L, Rettura F, Esposito G, Antonioli L, Ceravolo R, Bernardini N, Pellegrini C and Fornai M (2024) Gut-directed therapy in Parkinson’s disease. Front. Pharmacol. 15:1407925. doi: 10.3389/fphar.2024.1407925
Received: 27 March 2024; Accepted: 17 May 2024;
Published: 21 June 2024.
Edited by:
Cinzia Parolini, University of Milan, ItalyReviewed by:
Parisa Gazerani, Oslo Metropolitan University, NorwayGiovanni Albani, Italian Auxological Institute (IRCCS), Italy
Carsten Theiss, Ruhr University Bochum, Germany
Copyright © 2024 Benvenuti, Di Salvo, Bellini, Seguella, Rettura, Esposito, Antonioli, Ceravolo, Bernardini, Pellegrini and Fornai. This is an open-access article distributed under the terms of the Creative Commons Attribution License (CC BY). The use, distribution or reproduction in other forums is permitted, provided the original author(s) and the copyright owner(s) are credited and that the original publication in this journal is cited, in accordance with accepted academic practice. No use, distribution or reproduction is permitted which does not comply with these terms.
*Correspondence: Carolina Pellegrini, carolina.pellegrini@unipi.it, carolina.pellegrini87@gmail.com
†These authors have contributed equally to this work and share first authorship