- 1Department of Pediatrics, Case Western Reserve University, Cleveland, OH, United States
- 2Department of Pediatrics, University of Virginia, Charlottesville, VA, United States
- 3Galleon Pharmaceuticals, Inc., Horsham, PA, United States
- 4Department of Anesthesiology, University of Iowa Hospitals and Clinics Iowa, Iowa City, IA, United States
- 5Division of Pulmonary, Critical Care and Sleep Medicine, University Hospitals Case Medical Center, Case Western Reserve University, Cleveland, OH, United States
- 6Department of Pharmacology, Case Western Reserve University, Cleveland, OH, United States
- 7Functional Electrical Stimulation Center, Case Western Reserve University, Cleveland, OH, United States
Our lab is investigating the efficacy profiles of tropine analogs against opioid-induced respiratory depression. The companion manuscript reports that the cell-permeant tropeine, tropine ester (Ibutropin), produces a rapid and sustained reversal of the deleterious actions of fentanyl on breathing, alveolar-arterial (A-a) gradient (i.e., index of alveolar gas exchange), and arterial blood-gas (ABG) chemistry in freely-moving male Sprague Dawley rats, while not compromising fentanyl analgesia. We report here that in contrast to Ibutropin, the injection of the parent molecule, tropine (200 μmol/kg, IV), worsens the adverse actions of fentanyl (75 μg/kg, IV) on ventilatory parameters (e.g., frequency of breathing, tidal volume, minute ventilation, peak inspiratory and expiratory flows, and inspiratory and expiratory drives), A-a gradient, ABG chemistry (e.g., pH, pCO2, pO2, and sO2), and sedation (i.e., the righting reflex), while not affecting fentanyl antinociception (i.e., the tail-flick latency) in freely-moving male Sprague Dawley rats. These data suggest that tropine augments opioid receptor-induced signaling events that mediate the actions of fentanyl on breathing and alveolar gas exchange. The opposite effects of Ibutropin and tropine may result from the ability of Ibutropin to readily enter peripheral and central cells. Of direct relevance is that tropine, resulting from the hydrolysis of Ibutropin, would combat the Ibutropin-induced reversal of the adverse effects of fentanyl. Because numerous drug classes, such as cocaine, atropine, and neuromuscular blocking drugs contain a tropine moiety, it is possible that their hydrolysis to tropine has unexpected/unintended consequences. Indeed, others have found that tropine exerts the same behavioral profile as cocaine upon central administration. Together, these data add valuable information about the pharmacological properties of tropine.
Introduction
Tropine ring structures (Trautner and McCallum, 1950; Zeile and Heusner, 1959; Issekutz, 1963) are vital components of many bioactive drugs, such as cocaine, atropine, and a variety of neuromuscular blockers, such as decamethylene bis N,N′ atropinium and bis [N (3,4-diacetoxybenzyl) tropaninium (alpha-yl) glutarate] dibromide (Gyermek, 2002; Kohnen-Johannsen and Kayser, 2019). Our companion manuscript (Getsy et al., 2024) details evidence that intravenous injection of the cell-permeant tropeine, tropine ester (Ibutropin), also known as isobutyric tropine ester; butropine; tropine isobutyrate, iisobutyroyl tropine, elicits a rapid and sustained reversal of the adverse actions of fentanyl on breathing, alveolar-arterial (A-a) gradient (i.e., index of alveolar gas exchange), and arterial blood-gas (ABG) chemistry (i.e., pH, pCO2, pO2, and sO2), while not reducing fentanyl-induced analgesia in unanesthetized male Sprague Dawley rats. Although we do not have direct information about Ibutropin, we expect that it will be highly cell-penetrant like other tropeines (Gyermek, 2002; Kohnen-Johannsen and Kayser, 2019). The actions of Ibutropin are likely to involve effects on functional proteins in the plasma membrane (e.g., ion-channels and receptors) and functional proteins within cells. Previous research has shown that tropeines exert direct effects on serotonin, acetylcholine, and histamine receptor subtypes (Gyermek, 1953a; b, Gyermek, 1953, 2002; Zaĭtseva and Gerchikov, 1969; Mashkovskiĭ and Shvarts, 1979; Gyermek and Lee, 2009a; b), and allosterically regulate glycine ion-channel receptor activity (Macksay et al., 2004; 2008; 2009a; b; San Martin et al., 2019) along with the activity of other ion channels, such as Na+ and K+-channels (Friess et al., 1961a; b; 1963; 1964a; b, 1965a; b; 1966; 1968; 1969; Blaustein, 1968; Thron et al., 1963). All the receptors and ion-channels mentioned above have important roles in the central regulation of breathing (Richter et al., 2003; Shao and Feldman, 2009; Manzke et al., 2011). Nonetheless, to date, no information has been reported as to whether tropine has any effects on ventilatory control systems.
At present, there is little direct information about the actual metabolites of Ibutropin or their pharmacological actions in vivo. However, the potential desterification of Ibutropin to tropine (Supplementary Figure S1) by nonspecific carboxyesterases within blood plasma (Butterworth et al., 1993; Nishida et al., 1996; Hemmings and Egan, 2018) may be a factor in the ability of Ibutropin to exert its potent effects against opioid-induced respiratory depression (OIRD) (Getsy et al., 2024). This is especially possible because tropine exerts the same behavioral profile as cocaine upon central administration to rats (Zakusov et al., 1978). As such, the objectives of this study were to determine the effects an injection of tropine (200 μmol/kg, IV) has on the deleterious actions of fentanyl (75 μg/kg, IV) on breathing by looking at changes in ventilatory parameters, A-a gradient, ABG chemistry, antinociception and sedation (i.e., righting reflex) in freely-moving (unanesthetized) adult male Sprague Dawley rats. The data collected in this study provides evidence that in contrast to Ibutropin, the administration of tropine largely exacerbates the opioid receptor signaling events that mediate the effects of fentanyl on breathing, alveolar gas exchange, and sedation, while not affecting fentanyl-induced antinociception. Therefore tropine, when liberated by the hydrolysis of Ibutropin, appears to combat the Ibutropin-induced reversal of the adverse effects of fentanyl on breathing. Whether the ability of tropine to exacerbate the effects of fentanyl is due to allosteric modulation of opioid receptors and/or modulation of intracellular cascades elicited by fentanyl will be the focus of future studies.
Material and methods
Permissions, rats, and surgical procedures
All studies were carried out in accordance with the NIH Guide for Care and Use of Laboratory Animals (NIH Publication No. 80-23) revised in 2011, and in compliance with the ARRIVE (Animal Research: Reporting of In Vivo Experiments) guidelines (https://arriveguidelines.org/). All protocols involving rats were approved by the Animal Care and Use Committees of Galleon Pharmaceuticals, Case Western Reserve University, and the University of Virginia. Adult male Sprague Dawley rats were purchased from Harlan Industries (Madison, WI, United States). After 4 days of recovery from transportation, rats were implanted with a jugular vein catheter only or with a jugular vein catheter and a femoral artery catheter under 2%–3% isoflurane anesthesia (Henderson et al., 2014; Gaston et al., 2021). The rats were given 4 days to recover from surgery before use in any experiment. All catheters were flushed with a heparin solution (50 units of heparin in 0.1 M, pH 7.4 phosphate-buffered saline) immediately after surgery and again 8 h later. The catheters were also flushed twice-daily at 8 a.m. and 4 p.m. on recovery days 2–4 and again 3–4 h before starting a study on post-surgery day 5 (therefore, nine flushes in total). Injectable (liquid) fentanyl citrate and tropine powder were obtained from Sigma-Aldrich (St. Louis, MO, United states). The pH of all stock solutions of vehicle and tropine was adjusted to 7.0 with 0.25 M NaOH. All studies were performed in a quiet room with a relative humidity of 49% ± 2% and room temperature of 21.3°C ± 0.2°C. The ABG chemistry and antinociception studies were done in separate groups of rats so as not to compromise the ventilatory recording studies. The plethysmography, antinociception recording sessions and arterial blood sampling studies (used for ABG measurements) were performed by an investigator who was blinded to the study protocol and thus used syringes with the opioid, vehicle or test drugs that were prepared by another investigator who was not involved in the study protocol. In every case, the data files resulting from each study were collated and analyzed by yet another investigator in the group who did not make up the syringes or perform the study protocol.
Whole body plethysmography measurement of ventilatory parameters
Ventilatory parameters were recorded continuously in unrestrained, freely-moving rats by whole body plethysmography (PLY3223; Data Sciences International, St. Paul, MN), as detailed previously (Getsy et al., 2022a; b). The directly recorded and calculated (derived) parameters are defined in Supplementary Table S1. The ventilatory parameters and abbreviations are frequency of breathing (Freq), tidal volume (TV), minute ventilation (MV), inspiratory time (Ti), expiratory time (Te), Ti/Te, end inspiratory pause (EIP), end expiratory pause (EEP), peak inspiratory flow (PIF), peak expiratory flow (PEF), PIF/PEF, expiratory flow at 50% expired TV (EF50), relaxation time (RT), inspiratory drive (TV/Ti), expiratory drive (TV/Te), expiratory delay (Te-RT), non-eupneic breathing index (NEBI), and NEBI corrected for Freq (NEBI/Freq). A diagram, adapted from Lomask (2006), showing relationships between some directly recorded parameters is shown in Supplementary Figure S2. On the day of the study, each rat was placed in an individual plethysmography chamber and allowed at least 60 min to acclimatize so that resting (baseline, Pre) ventilatory parameter values could be accurately defined. Two groups of rats (see Supplementary Table S2 for numbers, ages, weights, and baseline ventilatory parameters) received an injection of fentanyl (75 μg/kg, IV). After 5 min, one group was injected with vehicle (saline, 100 μL/100 g body weight, IV), and the other group was injected with tropine (200 μmol/kg, IV). Ventilatory parameters were monitored for 60 min after these injections. The body weights of both groups were similar to one another (p > 0.05), therefore, ventilatory parameters related to volumes (e.g., TV, PIF, and PEF) are presented without body weight corrections. The FinePointe (DSI) software constantly corrected digitized ventilatory values originating from actual waveforms for alterations in chamber humidity and chamber temperature. Pressure changes associated with respiratory waveforms were converted to volumes (e.g., TV, PIF, and PEF) using the algorithms of Epstein and Epstein (1978) and Epstein et al. (1980). Factoring in the chamber humidity and temperature, cycle analyzers filtered the acquired signals, and FinePointe algorithms generated an array of box flow data that identified a waveform segment as an acceptable breath. From this array, minimum and maximum box flow values were obtained and multiplied by a compensation factor provided by the selected algorithm, thereby producing TV, PIF, and PEF values used to determine non-eupneic breathing events expressed as non-eupneic breathing index (NEBI, % of non-eupneic breathing events per epoch) (Getsy et al., 2014). Apneic pause was calculated by the formula (Expiratory time/Relaxation time) − 1 (Gaston et al., 2021).
Protocols for blood-gas measurements and determination of arterial-alveolar gradient
Changes in ABG chemistry values (pH, pCO2, pO2, and sO2) and A-a gradients were determined as detailed previously (Getsy et al., 2022e; f). The A-a gradient defines differences between alveolar and arterial blood O2 concentrations (Stein et al., 1995; Story, 1996). For example, a decrease in PaO2 without a concomitant alteration in the A-a gradient is the result of hypoventilation, whereas a decrease in PaO2 with a concomitant increase in A-a gradient indicates an ongoing mismatch in ventilation–perfusion in alveoli. A-a gradient = PAO2 – PaO2, where PAO2 is the partial pressure (p) of alveolar O2, and PaO2 is pO2 in sampled arterial blood. PAO2 = [(FiO2 × (Patm – PH2O) − (PaCO2/respiratory quotient)], where FiO2 is the fraction of O2 in inspired air; Patm is atmospheric pressure; PH2O is the partial pressure of H2O in inspired air; PaCO2 is the pCO2 in arterial blood; and respiratory quotient (RQ) is the ratio of (CO2 eliminated)/(O2 consumed). We took FiO2 of room-air to be 21% = 0.21, Patm to be 760 mmHg, and PH2O to be 47 mmHg (Gaston et al., 2021). We took the RQ value of our adult male rats to be 0.9 (Stengel et al., 2010; Chapman et al., 2012). Briefly, on the day of the study, an arterial blood sample (100 μL) was taken from two groups of rats to determine pre-drug (baseline) ABG and A-a gradient values. Thirty minutes later, both groups received a bolus injection of fentanyl (75 μg/kg, IV), and an arterial blood sample (100 μL) was taken post 5 min. Immediately afterward, one group (83.7 ± 0.7 days of age; 339 ± 2 g body weight) received a bolus injection of vehicle, and the other group (83.3 ± 0.9 days of age; 336 ± 2 g body weight) received a bolus injection of tropine (200 μmol/kg, IV). Arterial blood samples were taken from both groups 5 min, 10 min, and 15 min later for determination of ABG values. All ABG chemistry values were determined by a radiometer blood-gas analyzer (ABL800 FLEX).
Antinociception assessment by tail-flick latency assay
The antinociceptive actions of fentanyl and tropine were determined by tail-flick latencies (TFL) via the use of a Tail-Flick Analgesia Meter (IITC Life Science Inc., United States) as detailed previously (Lewis et al., 1991; Meller et al., 1991; Getsy et al., 2022a; g). This involved a minor degree of manual restraint while positioning the tail to apply a thermal beam sufficient to induce a latency of tail withdrawal of approximately 2.5 s. Baseline TFL were tested in all rats 30–60 min prior to drug injection. Next, all rats received an injection of fentanyl (75 μg/kg, IV), and TFL were recorded at 5 min. One group of rats (83.3 ± 0.9 days of age; 336 ± 1 g body weight, n = 6) then received an injection of vehicle (saline, 100 μL/100 g body weight, IV), and the second group (83.0 ± 0.8 days of age; 335 ± 2 g body weight, n = 6) received a bolus injection of tropine (200 μmol/kg, IV). TFL were then recorded 10 min, 25 min, 40 min, and 55 min after these injections.
Sedation—righting reflex
Separate groups of rats were used to evaluate the effects of tropine (200 μmol/kg, IV) on the duration of fentanyl (75 μg/kg, IV) impairment of the righting reflex (i.e., the inability to stand on all four legs) as described previously (Jenkins et al., 2021). Each rat was placed in an open plastic chamber to allow the duration of loss of righting reflex to be accurately assessed. The time when the rat spontaneously stood on all four paws and remained so for at least 10 s was taken as the point of recovery of the righting reflex (Ren et al., 2015; 2020; Yu et al., 2018). One group of rats (80.0 ± 0.5 days of age; 333 ± 1 g, n = 9) received an injection of fentanyl and, after 5 min, an injection of vehicle. A second group of rats (79.7 ± 0.4 days of age; 332 ± 1 g, n = 9) received an injection of fentanyl and, after 5 min, an injection of tropine. The duration of the effect of fentanyl was defined as the time interval from the time of injection of fentanyl administration to the recovery of the righting reflex.
Data analyses
All data are presented as mean ± SEM and were analyzed by one-way and two-way ANOVA with Bonferroni corrections for multiple comparisons between means using the error mean square terms from the ANOVAs (Getsy et al., 2022a—c). A p < 0.05 value was taken as the initial significance level and was modified by the number of between-mean comparisons. The modified t-statistic for two groups, for instance, is t = (mean group 1 − mean group 2)/[s × (1/n1 + 1/n2)1/2], where s2 = mean square within groups term from the ANOVA analysis, and n1 and n2 are the number of rats in each group. Statistical analyses were done with GraphPad Prism software (GraphPad Software, Inc., La Jolla, CA).
Results
Ventilatory parameters
The ages, body weights, and baseline ventilatory parameters of the two groups of rats used in the ventilatory studies are provided in Supplementary Table S2. There were no between-group differences for any of the ventilatory parameters (p > 0.05 for all comparisons). As detailed below, two groups of rats received an injection of fentanyl (75 μg/kg, IV), and, after 5 min, one group received an injection of vehicle (VEH), and the other group received an injection of tropine (200 μmol/kg, IV). Summaries of frequency of breathing (Freq), tidal volume (TV), and minute ventilation (MV) before (Pre), after injection of fentanyl (75 μg/kg, IV), and after subsequent injection of vehicle or tropine (200 μmol/kg, IV) are given in Figure 1. Fentanyl elicited pronounced decreases in Freq (Panel A), TV (Panel B), and, consequently, MV (Panel C) in both groups of rats. Tropine elicited a prompt worsening of Freq, TV, and MV that remained for about 20 min post injection. Values for inspiratory time (Ti), expiratory time (Te), and Ti/Te before (Pre), following injection of fentanyl (75 μg/kg, IV), and after subsequent injection of vehicle or tropine (200 μmol/kg, IV) are summarized in Figure 2. The injection of fentanyl elicited pronounced increases in Ti (Panel A) and Te (Panel B), with the relative changes resulting in brief increases in Ti/Te (Panel C) in both groups. The injection of tropine did not affect Ti, whereas it elicited a prompt increase in Te of about 10 min in duration before declining to similar levels observed in the vehicle-injected rats. As such, tropine markedly diminished the fentanyl-induced increases in Ti/Te. Values for end-inspiratory pause (EIP) and end-expiratory pause (EEP) before (Pre), following the injection of fentanyl (75 μg/kg, IV), and after subsequent injection of vehicle or tropine (200 μmol/kg, IV) are shown in Figure 3. The injection of fentanyl elicited pronounced increases in EIP (Panel A) and EEP (Panel B) in both groups of rats. EIP remained elevated for about 30 min after the injection of vehicle, whereas EEP fell back to baseline within 5 min. The injection of tropine elicited a prompt and relatively sustained decrease in EIP, whereas it lengthened the time of increase in EEP compared to vehicle-injected rats.
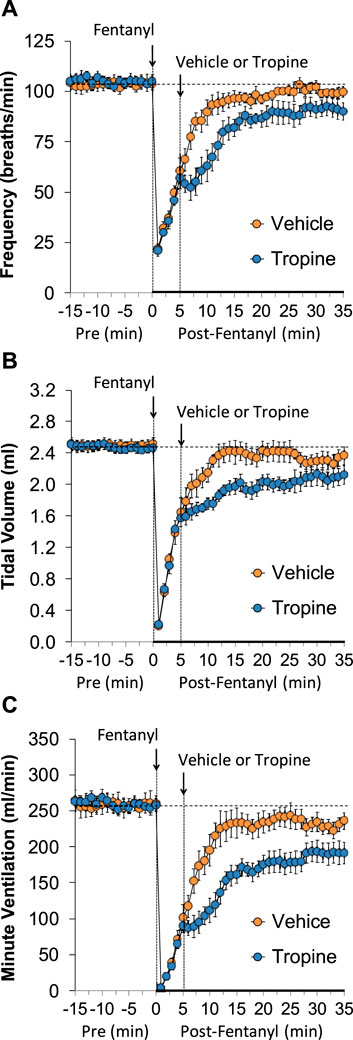
Figure 1. A summary of the values for frequency of breathing (A), tidal volume (B), and minute ventilation (C) before (Pre), following injection of fentanyl (75 μg/kg, IV), and subsequent injection of vehicle or tropine (200 μmol/kg, IV) in freely-moving adult male rats. The data are presented as mean ± SEM. There were six rats in each group.
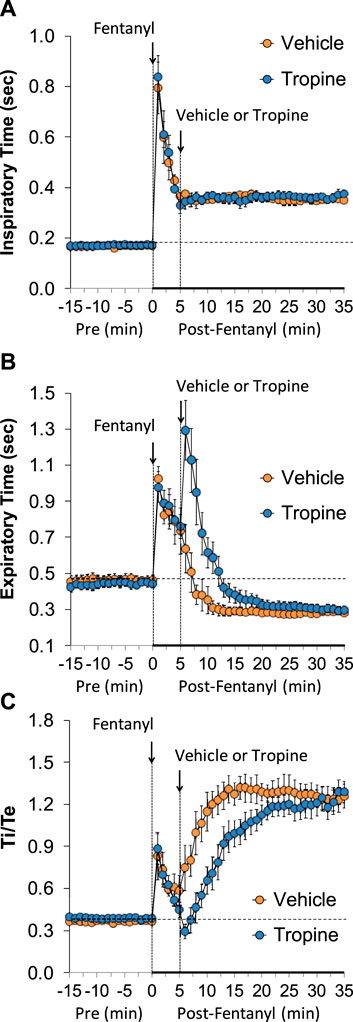
Figure 2. A summary of the values for inspiratory time (A), expiratory time (B), and inspiratory time/expiratory time (Ti/Te) (C) before (Pre), following injection of fentanyl (75 μg/kg, IV), and subsequent injection of vehicle or tropine (200 μmol/kg, IV) in freely-moving adult male rats. The data are presented as mean ± SEM. There were six rats in each group.
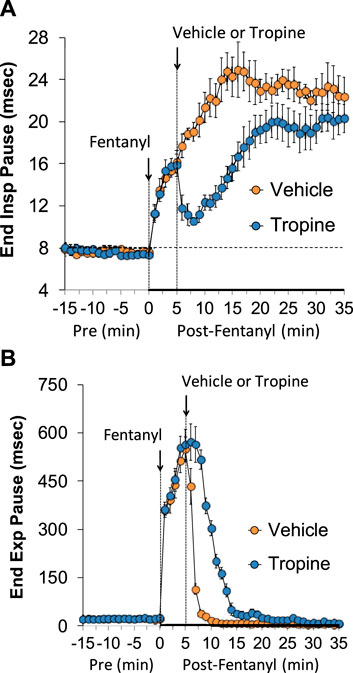
Figure 3. A summary of the values for end-inspiratory pause (A) and end-expiratory pause (B) before (Pre), following injection of fentanyl (75 μg/kg, IV), and subsequent injection of vehicle or tropine (200 μmol/kg, IV) in freely-moving adult male rats. The data are presented as mean ± SEM. There were six rats in each group.
The values for peak inspiratory flow (PIF), peak expiratory flow (PEF), and PIF/PEF before (Pre), following the injection of fentanyl (75 μg/kg, IV), and after subsequent injection of vehicle or tropine (200 μmol/kg, IV) are summarized in Figure 4. Fentanyl elicited a pronounced and sustained decrease in PIF (Panel A) and a pronounced decrease in PEF (Panel B). PEF levels gradually returned to baseline and then rose above pre-fentanyl values with injection of vehicle (Panel B). The injection of tropine further diminished the fentanyl-induced decrease in PIF and prevented the baseline overshoot in PEF. Taken together, these changes in PIF and PEF resulted in sustained decreases in PIF/PEF that were similar in both groups of rats (Panel C). The values for relaxation time (RT) and expiratory delay (Te-RT) before (Pre), following the injection of fentanyl (75 μg/kg, IV), and after subsequent injection of vehicle or tropine (200 μmol/kg, IV) are summarized in Figure 5. The injection of fentanyl elicited transient increases and then sustained decreases in RT in both groups of rats that were minimally affected by the injection of tropine (Panel A). Fentanyl elicited pronounced increases in expiratory delay that gradually fell to below pre-injection levels in the rats that received an injection of vehicle (Panel B). The injection of tropine caused an immediate and relatively sustained increase in expiratory delay before the values fell to values equal to those of the vehicle-injected rats. The values for inspiratory drive (TV/Ti) and expiratory drive (TV/Te) before (Pre), following the injection of fentanyl (75 μg/kg, IV), and after subsequent injection of vehicle or tropine (200 μmol/kg, IV) are shown in Figure 6. Fentanyl elicited a pronounced and sustained decrease in inspiratory drive (Panel A), and a pronounced decrease in expiratory drive (Panel B) that gradually rose to values above pre-fentanyl injection in vehicle-injected rats. The injection of tropine minimally affected inspiratory drive, but augmented the fentanyl-induced decrease in expiratory drive and thus delayed the subsequent overshoot to above pre-fentanyl levels as seen in the vehicle-injected group. The values for NEBI and NEBI corrected for frequency of breathing (NEBI/Freq) before (Pre), after injection of fentanyl (75 μg/kg, IV), and subsequent injection of vehicle or tropine (200 μmol/kg, IV) are shown in Figure 7. Fentanyl elicited a substantial increase in NEBI (Panel A) and NEBI/Freq (Panel B) that eventually returned to and remained below pre-fentanyl injection values in vehicle-injected rats. The injection of tropine did not affect either parameter.
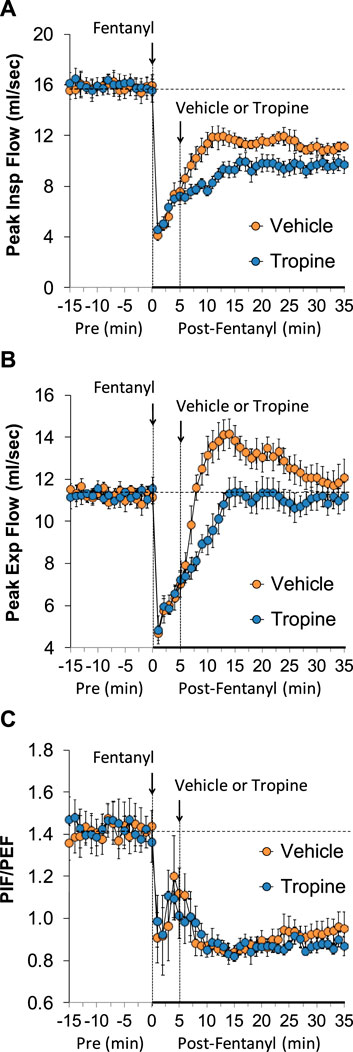
Figure 4. A summary of the values for peak inspiratory flow (A), peak expiratory flow (B), and peak inspiratory flow/peak expiratory flow (PIF/PEF) (C) before (Pre), following injection of fentanyl (75 μg/kg, IV), and subsequent injection of vehicle or tropine (200 μmol/kg, IV) in freely-moving adult male rats. The data are presented as mean ± SEM. There were six rats in each group.
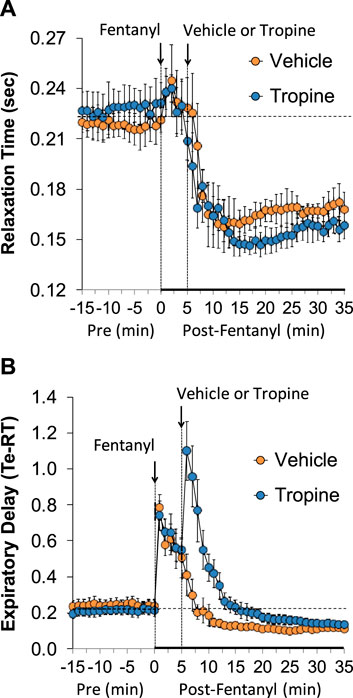
Figure 5. A summary of the values for relaxation time (A) and expiratory delay (expiratory time–relaxation time, Te-RT) (B) before (Pre), following injection of fentanyl (75 μg/kg, IV), and subsequent injection of vehicle or tropine (200 μmol/kg, IV) in freely-moving adult male rats. The data are presented as mean ± SEM. There were six rats in each group.
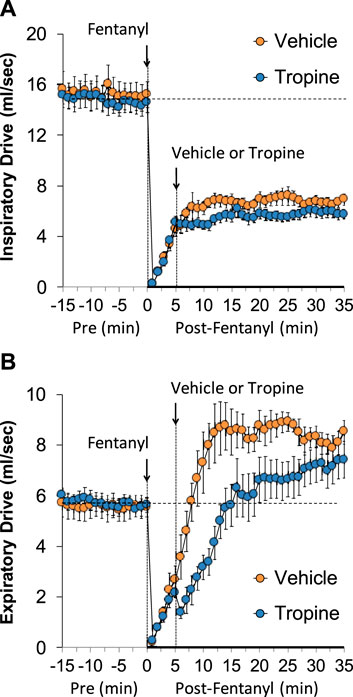
Figure 6. A summary of the values for inspiratory drive (A) and expiratory drive (B) before (Pre), after injection of fentanyl (75 μg/kg, IV), and subsequent injection of vehicle or tropine (200 μmol/kg, IV) in freely-moving adult male rats. The data are presented as mean ± SEM. There were six rats in each group.
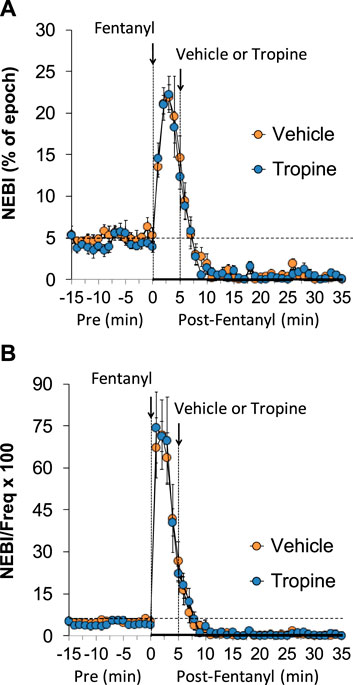
Figure 7. A summary of the values for non-eupneic breathing index (NEBI) (A) and NEBI corrected for frequency of breathing (NEBI/Freq) (B) before (Pre), following injection of fentanyl (75 μg/kg, IV), and subsequent injection of vehicle or tropine (200 μmol/kg, IV) in freely-moving adult male rats. The data are presented as mean ± SEM. There were six rats in each group.
The total (cumulative) changes in ventilatory parameters elicited by fentanyl (75 μg/kg, IV) over the 0 to 5 min period prior to injection of vehicle or tropine are summarized in Supplementary Figure S3. Fentanyl elicited significant decreases in Freq, TV, and MV that were associated with increases in Ti, Te, Ti/Te, and EIP (Panel A). Fentanyl elicited substantial decreases in PIF, PEF, PIF/PEF, inspiratory drive (TV/Ti), and expiratory drive (TV/Te), with a substantial increase in expiratory delay (Te-RT) (Panel B). Fentanyl also elicited substantial increases in EEP, NEBI, and NEBI/Freq (Panel C). The total (cumulative) changes in ventilatory parameters over the 0 to 5 min period following injection of vehicle or tropine are summarized in Figure 8. As seen in Panel A, fentanyl elicited decreases in Freq, TV, and MV in vehicle-injected rats that were associated with increases in Ti, Te, Ti/Te, and EIP. The decreases in Freq and MV were greater in tropine-treated rats, whereas the increases in Ti/Te and EIP were diminished in the tropine-treated rats. Additionally, the increases in Te were greater in the tropine-treated rats compared to the vehicle-treated rats. As seen in Panel B, fentanyl elicited substantial decreases in PIF, PIF/PEF, and TV/Ti, but not PEF, RT, or TV/Te in vehicle-treated rats. Tropine-treated rats displayed exaggerated decreases in PIF, PEF, and TV/Te. As seen in Panel C, fentanyl elicited marked increases in EEP, but minimal changes in Te-RT, NEBI, or NEBI/Freq in vehicle-treated rats. The fentanyl-induced increases in EEP and Te-RT were markedly greater in the tropine-treated rats compared to the vehicle-treated rats. The total (cumulative) changes in ventilatory parameters over 6 to 10 min period after the injection of vehicle or tropine are shown in Figure 9. The injection of tropine prevented the effects of fentanyl from fully resolving and instead enhanced the adverse effects of fentanyl on Freq, TV, MV, and Te, while reducing the effects of fentanyl on Ti/Te and EIP (Panel A). Tropine enhanced the adverse effects of fentanyl on PIF, PEF, and TV/Te (Panel B), and EEP and Te-RT (Panel C). The total (overall cumulative) changes in ventilatory parameters over the 0 to 30 min period following the injection of vehicle or tropine are summarized in Figure 10. The effects of fentanyl on Freq, TV, MV, Te, Ti/Te, and EIP (Panel C), TV/Te (Panel B), and EEP and Te-RT (Panel C) were markedly affected by the administration of tropine.
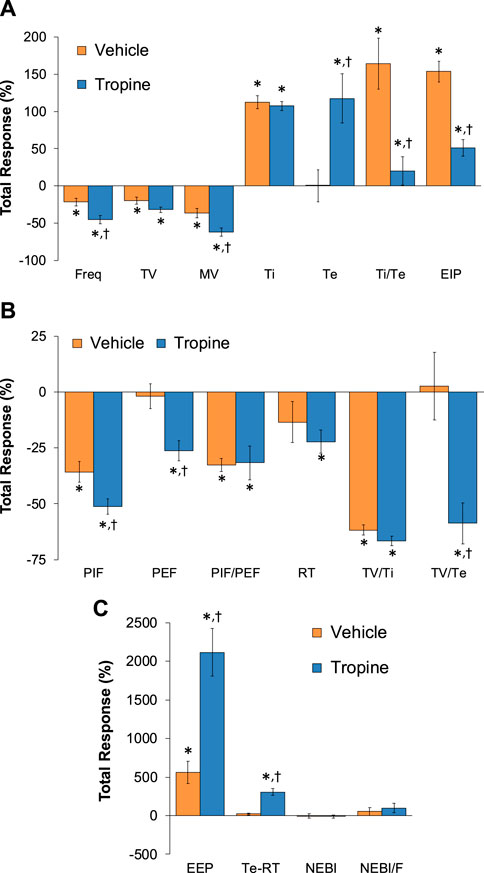
Figure 8. Total (cumulative) changes in ventilatory parameters elicited by fentanyl (75 μg/kg, IV) over the 0–5 min period following the injection of vehicle or tropine (200 μmol/kg, IV). (A) Frequency of breathing (Freq), tidal volume (TV), minute ventilation (MV), inspiratory time (Ti), expiratory time (Te), Ti/Te, and end-inspiratory pause (EIP). (B) Peak inspiratory flow (PIF), peak expiratory flow (PEF), PIF/PEF, relaxation time (RT), inspiratory drive (TV/Ti), and expiratory drive (TV/Te). (C) End-expiratory pause (EEP), expiratory delay (Te-RT), non-eupneic breathing index (NEBI), and NEBI/Freq (NEBI/F). The data are presented as mean ± SEM. There were six rats in each group. *p < 0.05 indicates a significant response from Pre-values. †p < 0.05 for tropine versus vehicle.
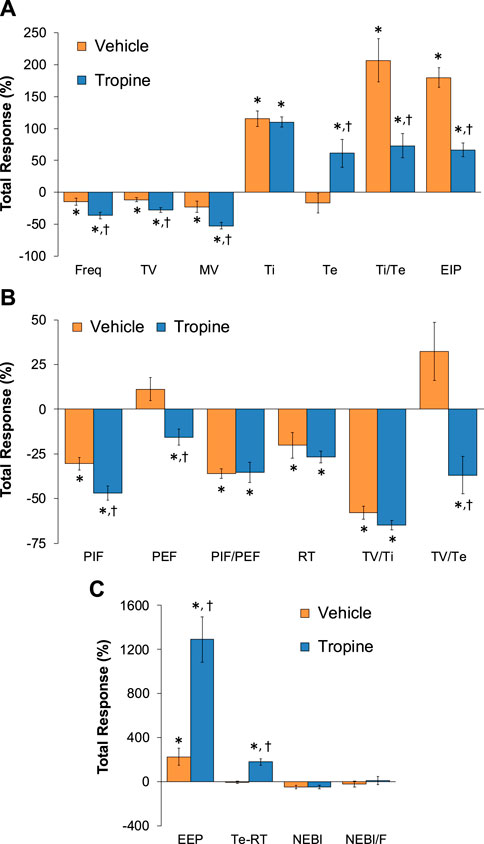
Figure 9. Total (cumulative) changes in ventilatory parameters elicited by fentanyl (75 μg/kg, IV) over the 6–10 min period following injection of vehicle or tropine (200 μmol/kg, IV). (A) Frequency of breathing (Freq), tidal volume (TV), minute ventilation (MV), inspiratory time (Ti), expiratory time (Te), Ti/Te, and end-inspiratory pause (EIP). (B) Peak inspiratory flow (PIF), peak expiratory flow (PEF), PIF/PEF, relaxation time (RT), inspiratory drive (TV/Ti), and expiratory drive (TV/Te). (C) End-expiratory pause (EEP), expiratory delay (Te-RT), non-eupneic breathing index (NEBI), and NEBI/Freq (NEBI/F). Data are presented as mean ± SEM. There were six rats in each group. *p < 0.05 indicates a significant response from Pre-values. †p < 0.05 for tropine versus vehicle.
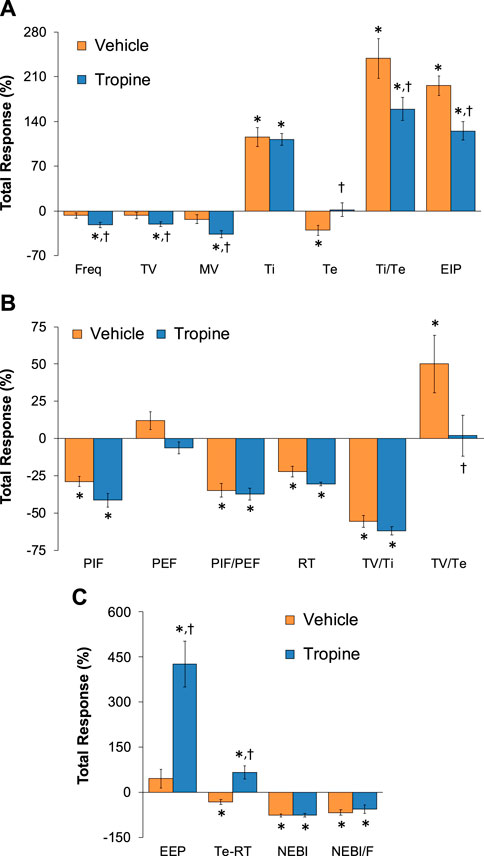
Figure 10. Total (cumulative) changes in ventilatory parameters elicited by fentanyl (75 μg/kg, IV) over the 0–30 min period following injection of vehicle or tropine (200 μmol/kg, IV). (A) Frequency of breathing (Freq), tidal volume (TV), minute ventilation (MV), inspiratory time (Ti), expiratory time (Te), Ti/Te, and end-inspiratory pause (EIP). (B) Peak inspiratory flow (PIF), peak expiratory flow (PEF), PIF/PEF, relaxation time (RT), inspiratory drive (TV/Ti), and expiratory drive (TV/Te). (C) End-expiratory pause (EEP), expiratory delay (Te-RT), non-eupneic breathing index (NEBI), and NEBI/Freq (NEBI/F). Data are shown as mean ± SEM. There were six rats in each group. *p < 0.05 indicates a significant response from Pre-values. †p < 0.05 for tropine versus vehicle.
ABG chemistry and A-a gradient
As summarized in Figure 11, the injection of fentanyl (75 μg/kg, IV) elicited substantial decreases in arterial blood pH, pO2, and sO2 and substantial increases in pCO2 and A-a gradient in both groups of rats. All of these deleterious fentanyl responses, except for the increases in A-a gradient, were augmented following the injection of tropine (200 μmol/kg, IV).
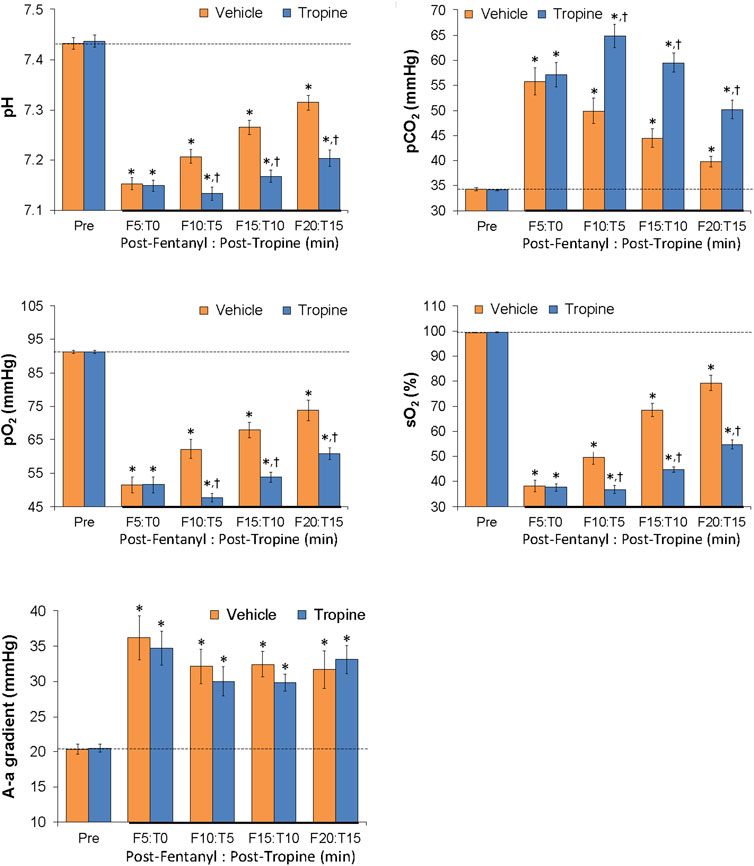
Figure 11. Tropine exacerbates the effects of fentanyl on ABG chemistry and A-a gradient. Values of pH, pCO2, pO2, sO2, and A-a gradient before (Pre) and after injection of fentanyl (75 μg/kg, IV) and then an injection of tropine (200 μmol/kg, IV) given 5 min after fentanyl in freely-moving rats. The data are shown as mean ± SEM. There were six rats in each group. *p < 0.05 indicates a significant change from Pre-values. †p < 0.05 indicates a significant difference from vehicle.
Tail-flick latencies
As shown in Figure 12, the injection of fentanyl (75 μg/kg, IV) elicited a pronounced antinociception (i.e., increase in tail-flick latencies) that was maintained during the 60-min recording period in rats that received the vehicle injection. Moreover, these antinociceptive effects of fentanyl were identical in magnitude and duration to the vehicle-treated rats in the rats that received tropine (200 μmol/kg, IV).
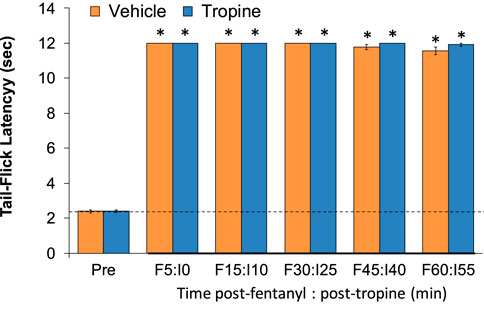
Figure 12. Tropine does not diminish the antinociceptive actions of fentanyl. Tail-flick latency values before (Pre) and after injection of fentanyl (75 μg/kg, IV) and subsequent injections of vehicle or tropine (200 μmol/kg, IV) given 5 min afterward in freely-moving rats. There were six rats in each group. *p < 0.05 indicates a significant change from Pre-values.
Sedation—righting reflex
The injection of fentanyl (75 μg/kg, IV) caused rapid sedative effects in all of the rats studied. More specifically, the rats become immobile with evident chest-wall rigidity. They usually remained on their side with their eyes closed. Full return of the righting reflex (i.e., the ability to stand on four legs) in the tropine (200 μmol/kg, IV)-treated rats (80.1. ± 7.0 min) occurred more slowly than in vehicle-treated rats (57.1 ± 5.6 min) (p > 0.05). The injection of tropine did not induce any obvious behavioral differences in the fentanyl-treated rats, except that the fentanyl-induced rigidity appeared to be enhanced, both in strength and duration, in the tropine-treated rats. Upon establishing steady positioning on all four paws, no differences in the normal behaviors of the rats, such as exploring, sniffing, rearing, or grooming, were noted between the vehicle-treated or tropine-treated rats.
Discussion
This study confirms that the intravenous injection of fentanyl elicits a series of rapid and deleterious effects on ventilatory parameters in unrestrained adult male Sprague Dawley rats (Henderson et al., 2014; Jenkins et al., 2021; Seckler et al., 2022; Getsy et al., 2022a; b). These responses are summarized in Table 1 and described in detail in our companion manuscript (Getsy et al., 2024). These responses included (a) reductions in Freq associated with a sustained elevation of Ti and EIP accompanied by a transient elevation of Te and EEP followed by a sustained reduction in Te and EEP, (b) sustained reductions in TV, MV, and PIF, and more transient decreases in PEF, (c) sustained decreases in RT associated with an initial increase followed by a sustained decrease in expiratory delay (Te-RT), (d) large and sustained decreases in inspiratory drive (TV/Ti) and equally pronounced but more transient decreases in expiratory drive (TV/Te), and (e) substantial increases in NEBI and NEBI/Freq. The ventilatory effects of fentanyl in these rats, including the destabilization of breathing (i.e., increase in NEBI), were expressed when they were heavily sedated and non-moving. As such, it appears that all of these effects of fentanyl were a result of intrinsic mechanisms of action, rather than being caused indirectly by fentanyl-induced behaviors. Several structurally different opioid analgesics, such as fentanyl, morphine oxymorphone, butorphanol, buprenorphine, and methadone, elevate A-a gradients in rats (May et al., 2013a; b; Henderson et al., 2014; Getsy et al., 2022a; b,c; d; e; f; g), goats (Meyer et al., 2006), dogs (Jacobson et al., 1994), rabbits (Shafford and Schadt, 2008), impala (Meyer et al., 2010), and humans (Goetz et al., 1994; Teichtahl et al., 2004; Wang et al., 2005). As was expected, fentanyl elevated the A-a gradient by direct mechanisms involving impairment of alveolar gas exchange or by indirect mechanisms, such as atelectasis (Henderson et al., 2014; Jenkins et al., 2021; Seckler et al., 2022; Getsy et al., 2022a; b). The fentanyl-induced impairment in gas exchange, together with reduced ventilation, resulted in decreases in pH, pO2, and sO2, and an increase in pCO2. The mechanisms responsible for the effects of fentanyl on breathing, alveolar gas exchange, and ABG chemistry involve central and peripheral mechanisms (Henderson et al., 2014). As expected, fentanyl caused robust and long-lasting antinociception and sedation in these rats that has been thoroughly documented and explored (Henderson et al., 2014; Jenkins et al., 2021; Getsy et al., 2022a; b).
This study shows that the injection of tropine augmented the adverse effects of fentanyl on ventilatory parameters in male Sprague Dawley rats and augmented the deleterious changes in A-a gradient and ABG chemistry produced by the synthetic opioid. In addition, tropine increased the duration of fentanyl-induced sedation, but did not alter fentanyl-induced analgesia. The question arises as to where and how tropine exerts these effects on fentanyl. The effects of fentanyl on ventilatory parameters, A-a gradient, ABG chemistry, and analgesia are mediated by central and peripheral opioid receptors (Henderson et al., 2014). As such, the ability of tropine to worsen the effects of fentanyl on ventilation and ABG chemistry may be due to actions in the brain, spinal cord, and peripheral sites (e.g., carotid bodies and chest-wall/diaphragmatic structures). The absence of the effect of tropine on the adverse effects of fentanyl on alveolar gas exchange (A-a gradient) argues against a peripheral site of action of tropine within the lungs. Opioids elevate A-a gradients by impairing ventilation–perfusion ratios in the lungs, known as ventilation–perfusion mismatch (Meyer et al., 2006; Henderson et al., 2014). Opioids diminish pulmonary perfusion via hypoxemia-mediated pulmonary vasoconstriction (Nun, 1993) and by pulmonary vasoconstriction (Santiago and Edelman, 1985; Hakim et al., 1992) via central activation of the sympathetic drive into lung structures (Roquebert and Delgoulet, 1988) and by histamine release in the lungs (Hakim et al., 1992; Mather, 1994). As will be discussed below, Ibutropin may reverse fentanyl-induced increases in A-a gradient via an increase in TV (thereby preventing atelectasis) and/or by modulating the other mechanisms detailed above. The lack of effect of tropine on the A-a gradient suggests that it cannot interact with peripheral and/or central mechanisms by which Ibutropin elicits its effects. In contrast, the ability of tropine to enhance the duration of fentanyl sedation also points to the likelihood that tropine enters and acts within the brain (e.g., cortical) sites responsible for the sedative actions of opioids (Young-McCaughan and Miaskowski, 2001a; b). Importantly, the apparent lack of effect of tropine on the antinociceptive actions of fentanyl suggests that the tropine does not directly block central or peripheral opioid receptors or their cell-signaling pathways that engender analgesia (Henderson et al., 2014; Jenkins et al., 2021). These results suggest that tropine modulates several of the pharmacological effects of fentanyl by actions within the central nervous system and periphery.
As mentioned, our findings with tropine contrast starkly with those obtained with tropine ester, Ibutropin (Getsy et al., 2024). As summarized in Table 1, Ibutropin beneficially reversed and tropine worsened the adverse effects of fentanyl on ventilatory parameters, A-a gradient, and ABG chemistry while not affecting the antinociceptive actions of the opioid. Ibutropin shortened and tropine lengthened the duration of fentanyl-induced sedation. Although sedation is known to be a common effect of opioid analgesics, the mechanisms and behavioral characteristics of sedation are poorly understood (Young-McCaughan and Miaskowski, 2001a; b). However, on the basis of our findings here and in Getsy et al. (2024), we assume that tropine and Ibutropin enter the central nervous system, but exert opposite effects on opioid receptor signaling events. We hypothesize that the effects of Ibutropin involve its ready entry into cells, whereas the effects of tropine may be restricted to modulating functional proteins (e.g., receptors, ion-channels, and enzymes) on the extracellular surface of plasma membranes. Moreover, regarding the different molecular mechanisms of opioid effects on ventilation and antinociception, it has been established that the tropeine-mediated glycine receptor α3-5-HT1A–receptor complex initiates subcellular events that overcome fentanyl-induced respiratory depression but not fentanyl-induced analgesia (Manzke et al., 2011). As such, one possibility is that tropine inhibits, whereas Ibutropin activates this receptor complex. Although we do not know whether tropine or Ibutropin differentially modulate the glycine receptor α3-5-HT1A-receptor complex or down-stream signaling pathways as do other tropeines (Macksay et al., 2004; 2008; 2009a; b; San Martin et al., 2019; Gallagher et al., 2022), it appears that activation of discrete signaling pathways differentially modulates the ventilatory and analgesic actions of opioids (Manzke et al., 2011). An important difference between Ibutropin and tropine was that Ibutropin, but not tropine, immediately diminished the fentanyl-induced elevation of both NEBI and NEBI/Freq. Considering the evidence as to how opioids affect ventilatory patterns, most non-eupneic breathing events are likely to be apneas, abnormal breaths (mismatch between inspiratory and expiratory phases), and, less often, type 1 and type 2 sighs (Zutler, 2011; Nagappa et al., 2017). The ability of fentanyl to elicit apneas in humans and experimental animals is well established (Willette et al., 1987; Yeadon and Kitchen, 1990; Ren et al., 2009; Zhang et al., 2012a; b; Zhuang et al., 2012; Haouzi et al., 2020; Saunders and Levitt, 2020). The mechanisms by which opioids induce apneas involve opioid receptor-induced signaling events in the Kölliker–Fuse-parabrachial nucleus complex (Saunders and Levitt, 2020), ventrolateral medulla (Willette et al., 1987), and nucleus tractus solitarius (Zhang et al., 2012a; Zhuang et al., 2012). In addition, it has been established that opioids suppress ventilatory responses to hypoxic, hypercapnic, and hypoxic–hypercapnic challenges (Berkenbosch et al., 1997; May et al., 2013a, b). As such, the abilities of fentanyl to depress breathing and elicit apneas may also involve depression of breathing responses to fentanyl-induced alterations in ABG chemistry. The precise sites and mechanisms by which Ibutropin improves the fentanyl-induced increases in NEBI and NEBI/Freq have not been established, but it is evident that tropine cannot mimic the actions of the tropeine. In summary, the processes by which tropine modulates the effects of fentanyl may be multi-factorial and may involve altering the opioid receptor signaling transduction processes triggered by fentanyl acting as a biased-ligand at opioid receptors (Grim et al., 2020a; b). Excitatory–inhibitory interactions between neurons within brainstem respiratory networks provide the basis for steady rhythmic breathing. The rhythmic activity of these networks is maintained by tonic 5HT1-receptor-mediated signal transduction processes that maintain synaptic glycine α3 receptors in the active/dephosphorylated state (Manzke et al., 2010). Accordingly, reduced inhibitory glycinergic cell-signaling negatively impacts breathing rhythms (Schmid et al., 1991; Pierrefiche et al., 1998; Busselberg et al., 2001), which may lead to immediate fatalities (Busselberg et al., 2001; Markstahler et al., 2002; Harvey et al., 2008). Moreover, drugs that selectively activate glycine a3 receptors may be beneficial for ventilatory disorders, including those caused by opioids (Lynch et al., 2016). As such, a tropine-mediated inhibition of glycine a3 receptors or their signaling events may be an important factor in the ability of tropine to worsen fentanyl-induced suppression of breathing.
Study limitations
An important limitation is that we have not performed dose–response relationships with tropine against lower and higher doses of fentanyl in order to maximize our understanding of the efficacy profile of tropine. Additionally, because the effects of opioids can often be quantitatively and qualitatively different in female compared to male rats (Dahan et al., 1998; Sarton et al., 1998; Hosseini et al., 2011), it is imperative to determine the effects of tropine on the pharmacological actions of fentanyl in female rats. It is also imperative to determine whether tropine can prevent the latent deleterious actions of opioids on ventilatory responses elicited by hypoxic–hypercapnic stimuli. Another key limitation of our work is the lack of knowledge about the cellular and molecular mechanisms by which tropine modulates the effects of fentanyl. Based on previous research, we are currently performing receptor binding experiments to establish if tropine directly binds to glycine receptors as do parent tropeine molecules (Macksay et al., 2004; 2008; 2009a; b; Manzke et al., 2010; 2011; San Martin et al., 2019; Gallagher et al., 2022). Finally, we must gather information about the pharmacokinetic profiles of tropine and its potential metabolites in order to better understand the sites of action of these compounds. We are presently modifying our liquid chromatography-mass spectrometry method (Altawallbeh et al., 2019) to establish the distribution of tropine in rats that received the vehicle and those that received fentanyl.
Conclusion
This study demonstrates that the injection of tropine causes an immediate worsening of the adverse effects produced by fentanyl on ventilatory parameters, alveolar gas exchange (A-a gradient), and ABG chemistry in male Sprague Dawley rats. Additionally, although tropine did not affect fentanyl-induced antinociception, it did lengthen the duration of fentanyl-induced sedation, as assessed by the righting reflex. Taken together, it appears that tropine may not directly block opioid receptors, but rather may modulate the opioid receptor-induced signaling events triggered by fentanyl. Because tropine and Ibutropin have opposing effects on fentanyl-induced changes in ventilatory parameters and ABG chemistry (Getsy et al., 2024), it is possible that the actions of tropine involve interactions with the extracellular domains of functional proteins in plasma membranes, whereas those of Ibutropin may involve interactions with intracellular signaling cascades. The opposing effects of tropine and Ibutropin on the sedative effects of fentanyl suggest that both compounds enter the central nervous system but that the sites and/or mechanisms of action are clearly different. As such, the conversion of Ibutropin to tropine in the body would lead to diminished efficacy of the tropeine. If conversion of Ibutropin to tropine occurs via the hydrolytic actions of blood plasma carboxylesterases, then preventing this hydrolysis by inhibitors, such as bis(4-nitrophenyl) phosphate (Boyce et al., 1976; Butterworth et al., 1993; Nishida et al., 1996), may indeed augment the potency of Ibutropin.
Data availability statement
The raw data supporting the conclusion of this article will be made available by the authors, without undue reservation.
Ethics statement
The animal study was approved by and all studies were carried out in accordance with the NIH Guide for Care and Use of Laboratory Animals (NIH Publication No. 80-23) revised in 2011 and in compliance with the ARRIVE (Animal Research: Reporting of In Vivo Experiments) guidelines (https://arriveguidelines.org/). All protocols involving rats were approved by the Animal Care and Use Committees of Galleon Pharmaceuticals, Case Western Reserve University, and the University of Virginia. The study was conducted in accordance with the local legislation and institutional requirements.
Author contributions
PG: writing–review and editing, writing–original draft, formal analysis, data curation, and conceptualization. WM: writing–review and editing, writing–original draft, data curation, conceptualization. AY: writing–review and editing, writing–original draft, data curation, and conceptualization. SB: writing–review and editing, writing–original draft, data curation, and conceptualization. GC: writing–review and editing, writing–original draft, formal analysis, data curation, and conceptualization. JB: writing–review and editing, writing–original draft, data curation, and conceptualization. Y-HH: writing–review and editing, writing–original draft, data curation, and conceptualization. SL: writing–review and editing, writing–original draft, visualization, validation, supervision, software, resources, project administration, methodology, investigation, funding acquisition, formal analysis, data curation, and conceptualization.
Funding
The author(s) declare that financial support was received for the research, authorship, and/or publication of this article. These studies were supported in part by grants from Galleon Pharmaceuticals, Inc. (to SL) and from NIH/NIDA U01DA051373 (to SL). The authors declare that this study received funding from Galleon Pharmaceuticals, Inc. The funder was not involved in the study design, collection, analysis, interpretation of data, the writing of this article, or the decision to submit it for publication.
Acknowledgments
The authors wish to sincerely thank the staff at the Animal Care Facilities at Case Western Reserve University, the University of Virginia, and Galleon Pharmaceuticals, Inc., for their expert assistance with the care of the rats. The authors also wish to acknowledge David Kalergis (CEO, Atelerix Life Sciences) for his assistance in detailing the clinical significance of our findings.
Conflict of interest
Author SB was employed by Galleon Pharmaceuticals, Inc.
The remaining authors declare that the research was conducted in the absence of any commercial or financial relationships that could be construed as a potential conflict of interest.
The author(s) declared that they were an editorial board member of Frontiers at the time of submission. This had no impact on the peer review process and the final decision.
Publisher’s note
All claims expressed in this article are solely those of the authors and do not necessarily represent those of their affiliated organizations, or those of the publisher, the editors, and the reviewers. Any product that may be evaluated in this article, or claim that may be made by its manufacturer, is not guaranteed or endorsed by the publisher.
Supplementary material
The Supplementary Material for this article can be found online at: https://www.frontiersin.org/articles/10.3389/fphar.2024.1405461/full#supplementary-material
References
Algera, M. H., Kamp, J., van der Schrier, R., van Velzen, M., Niesters, M., Aarts, L., et al. (2019). Opioid-induced respiratory depression in humans: a review of pharmacokinetic-pharmacodynamic modelling of reversal. Br. J. Anaesth. 122, e168–e179. doi:10.1016/j.bja.2018.12.023
Altawallbeh, G., Smith, L., Lewis, S. J., Authier, S., Bujold, K., Gaston, B., et al. (2019). Pharmacokinetic study of Sudaxine in dog plasma using novel LC-MS/MS method. Drug Test. Anal. 11, 403–410. doi:10.1002/dta.2507
Arendt, F. (2021). The opioid-overdose crisis and fentanyl: the role of online information seeking via internet search engines. Health Commun. 36, 1148–1154. doi:10.1080/10410236.2020.1748820
Armenian, P., Vo, K. T., Barr-Walker, J., and Lynch, K. L. (2018). Fentanyl, fentanyl analogs and novel synthetic opioids: a comprehensive review. Neuropharmacology 134, 121–132. doi:10.1016/j.neuropharm.2017.10.016
Baby, S., Gruber, R., Discala, J., Puskovic, V., Jose, N., Cheng, F., et al. (2021a). Systemic administration of tempol attenuates the cardiorespiratory depressant effects of fentanyl. Front. Pharmacol. 12, 690407. doi:10.3389/fphar.2021.690407
Baby, S. M., Discala, J. F., Gruber, R., Getsy, P. M., Cheng, F., Damron, D. S., et al. (2021b). Tempol reverses the negative effects of morphine on arterial blood-gas chemistry and tissue oxygen saturation in freely-moving rats. Front. Pharmacol. 12, 749084. doi:10.3389/fphar.2021.749084
Benda, L., and Kraupp, O. (1954). Pharmacological properties and clinical effects of the hydrochloride of the benzilic acid ester of tropine. Wien Klin. Wochenschr 66, 445–448.
Berkenbosch, A., Teppema, L. J., Olievier, C. N., and Dahan, A. (1997). Influences of morphine on the ventilatory response to isocapnic hypoxia. Anesthesiology 86, 1342–1349. doi:10.1097/00000542-199706000-00016
Blaustein, M. P. (1968). Action of certain tropine esters on voltage-clamped lobster axon. J. Gen. Physiol. 51, 309–319. doi:10.1085/jgp.51.3.309
Boyce, J. R., Wright, F. J., Cervenko, F. W., Pietak, S. P., and Faulkner, S. (1976). Prolongation of anesthetic action by BNPP (bis-[p-nitrophenyl] phosphate). Anesthesiology 45, 629–634. doi:10.1097/00000542-197612000-00006
Büsselberg, D., Bischoff, A. M., Becker, K., Becker, C. M., and Richter, D. W. (2001). The respiratory rhythm in mutant oscillator mice. Neurosci. Lett. 316, 99–102. doi:10.1016/s0304-3940(01)02382-5
Butterworth, M., Upshall, D. G., and Cohen, G. M. (1993). A novel role for carboxylesterase in the elevation of cellular cysteine by esters of cysteine. Biochem. Pharmacol. 46, 1131–1137. doi:10.1016/0006-2952(93)90460-e
Chapman, C. D., Dono, L. M., French, M. C., Weinberg, Z. Y., Schuette, L. M., and Currie, P. J. (2012). Paraventricular nucleus anandamide signaling alters eating and substrate oxidation. Neuroreport 23, 425–429. doi:10.1097/WNR.0b013e32835271d1
Comer, S. D., and Cahill, C. M. (2019). Fentanyl: receptor pharmacology, abuse potential, and implications for treatment. Neurosci. Biobehav Rev. 106, 49–57. doi:10.1016/j.neubiorev.2018.12.005
Dahan, A., Sarton, E., Teppema, L., and Olievier, C. (1998). Sex-related differences in the influence of morphine on ventilatory control in humans. Anesthesiology 88, 903–913. doi:10.1097/00000542-199804000-00009
Dahan, A., van der Schrier, R., Smith, T., Aarts, L., van Velzen, M., and Niesters, M. (2018). Averting opioid-induced respiratory depression without affecting analgesia. Anesthesiology 128, 1027–1037. doi:10.1097/ALN.0000000000002184
Decsi, L., Varszegi, M. K., and Nador, K. (1963). Effect of some quaternary tropine esters on the gastrointestinal system. Arzneimittelforschung 13, 776–779.
Deo, V. S., Gilson, T. P., Kaspar, C., and Singer, M. E. (2021). The fentanyl phase of the opioid epidemic in Cuyahoga County, Ohio, United States. J. Forensic Sci. 66, 926–933. doi:10.1111/1556-4029.14665
Epstein, M. A., and Epstein, R. A. (1978). A theoretical analysis of the barometric method for measurement of tidal volume. Respir. Physiol. 32, 105–120. doi:10.1016/0034-5687(78)90103-2
Epstein, R. A., Epstein, M. A., Haddad, G. G., and Mellins, R. B. (1980). Practical implementation of the barometric method for measurement of tidal volume. J. Appl. Physiol. 49, 1107–1115. doi:10.1152/jappl.1980.49.6.1107
Friess, S. L., Baldridge, H. D., Durant, R. C., and Reber, L. J. (1965b). Some toxic properties of 2-alpha-tropine aryl esters. Toxicol. Appl. Pharmacol. 7, 794–803. doi:10.1016/0041-008x(65)90004-9
Friess, S. L., Baldridge, H. D., Durant, R. C., Reber, L. J., and Allen, C. H. (1964a). Structure vs. toxicologic parameters in new esters of tropine and psi-tropine. VI. Toxicol. Appl. Pharmacol. 6, 459–475. doi:10.1016/s0041-008x(64)80013-2
Friess, S. L., and Durant, R. C. (1963). Interference patterns in twitch potentiation of the rat phrenic nerve-diaphragm by aryl esters of tropine and psi-tropine. IV. Toxicol. Appl. Pharmacol. 5, 358–370. doi:10.1016/0041-008x(63)90095-4
Friess, S. L., Durant, R. C., and Martin, H. L. (1966). Potency convergence and crossover in tertiary vs. quaternary tropine amino esters. Toxicol. Appl. Pharmacol. 9, 240–256. doi:10.1016/0041-008x(66)90119-0
Friess, S. L., Durant, R. C., Reber, L. J., and Thommesen, W. C. (1961b). Further toxicologic properties of aromatic esters in the tropine and psi-tropine series. Toxicol. Appl. Pharmacol. 3, 224–235. doi:10.1016/s0041-008x(61)80008-2
Friess, S. L., and Fash, F. J. (1968). Substituent effects on ester carbonyl absorption and chemoreceptor responses initiated by tropanyl phenylacetates. J. Med. Chem. 11, 175–176. doi:10.1021/jm00307a047
Friess, S. L., Hudak, W. V., and Weems, H. (1969). Blockade of superior cervical ganglionic transmission by tropine-p-tolylacetate and its quaternary methiodide derivative. Toxicol. Appl. Pharmacol. 15, 540–551. doi:10.1016/0041-008x(69)90056-8
Friess, S. L., and Minzghor, K. R. (1965a). Blockade of excitable nodes of Ranvier by stereoisomeric phenylacetate esters in the tropine and psi-tropine series. 3. Arch. Biochem. Biophys. 111, 61–66. doi:10.1016/0003-9861(65)90322-x
Friess, S. L., ReberDurant, LJRC, Baldridge, H. D. J., and Allen, C. H. (1964b). Interactions of aryl esters in the tropine and psi-tropine series with tissue chemoreceptors. the ortho effect. 7. Toxicol. Appl. Pharmacol. 6, 676–689. doi:10.1016/0041-008x(64)90118-8
Friess, S. L., Whitcomb, E. R., Thron, C. D., Durant, R. C., Reber, L. J., and Patterson, R. N. (1961a). Studies on blockade of single nodes of Ranvier and of the acetylcholinesterase system by cyclic aromatic esters. II. Arch. Biochem. Biophys. 95, 85–92. doi:10.1016/0003-9861(61)90112-6
Gallagher, C. I., Ha, D. A., Harvey, R. J., and Vandenberg, R. J. (2022). Positive allosteric modulators of Glycine receptors and their potential use in pain therapies. Pharmacol. Rev. 74, 933–961. doi:10.1124/pharmrev.122.000583
Gaston, B., Baby, S. M., May, W. J., Young, A. P., Grossfield, A., Bates, J. N., et al. (2021). D-Cystine di(m)ethyl ester reverses the deleterious effects of morphine on ventilation and arterial blood gas chemistry while promoting antinociception. Sci. Rep. 11, 10038. doi:10.1038/s41598-021-89455-2
Getsy, P. M., Baby, S. M., Gruber, R. B., Gaston, B., Lewis, T. H. J., Grossfield, A., et al. (2022a). S-Nitroso-L-Cysteine stereoselectively blunts the deleterious effects of fentanyl on breathing while augmenting antinociception in freely-moving rats. Front. Pharmacol. 13, 892307. doi:10.3389/fphar.2022.892307
Getsy, P. M., Baby, S. M., May, W. J., Bates, J. N., Ellis, C. R., Feasel, M. G., et al. (2022c). L-cysteine methyl ester overcomes the deleterious effects of morphine on ventilatory parameters and arterial blood-gas chemistry in unanesthetized rats. Front. Pharmacol. 13, 968378. doi:10.3389/fphar.2022.968378
Getsy, P. M., Baby, S. M., May, W. J., Bates, J. N., Ellis, C. R., Feasel, M. G., et al. (2022e). L-cysteine methyl ester overcomes the deleterious effects of morphine on ventilatory parameters and arterial blood-gas chemistry in unanesthetized rats. Front. Pharmacol. 13, 968378. doi:10.3389/fphar.2022.968378
Getsy, P. M., Baby, S. M., May, W. J., Lewis, T. H. J., Bates, J. N., Hsieh, Y. H., et al. (2022b). L-NAC reverses of the adverse effects of fentanyl infusion on ventilation and blood-gas chemistry. Biomed. Pharmacother. 153, 113277. doi:10.1016/j.biopha.2022.113277
Getsy, P. M., Baby, S. M., May, W. J., Young, A. P., Gaston, B., Hodges, M. R., et al. (2022f). D-cysteine ethyl ester reverses the deleterious effects of morphine on breathing and arterial blood-gas chemistry in freely-moving rats. Front. Pharmacol. 13, 883329. doi:10.3389/fphar.2022.883329
Getsy, P. M., Davis, J., Coffee, G. A., May, W. J., Palmer, L. A., Strohl, K. P., et al. (2014). Enhanced non-eupneic breathing following hypoxic, hypercapnic or hypoxic-hypercapnic gas challenges in conscious mice. Respi. Physiol. Neurobiol. 204, 147–159. doi:10.1016/j.resp.2014.09.006
Getsy, P. M., May, W. J., Young, A. P., Baby, S. M., Lewis, T. H. J., McShine, A., et al. (2024). Isobutyric tropine ester (Ibutropin) overcomes fentanyl-induced respiratory depression in unanesthetized rats without compromising analgesia. Front. Pharmacol.
Getsy, P. M., Young, A. P., Bates, J. N., Baby, S. M., Seckler, J. M., Grossfield, A., et al. (2022d). S-nitroso-L-cysteine stereoselectively blunts the adverse effects of morphine on breathing and arterial blood gas chemistry while promoting analgesia. Biomed. Pharmacother. 153, 113436. doi:10.1016/j.biopha.2022.113436
Getsy, P. M., Young, A. P., Grossfield, A., Seckler, J. M., Wilson, C. G., Gaston, B., et al. (2022g). D-cysteine ethyl ester and D-cystine dimethyl ester reverse the deleterious effects of morphine on arterial blood-gas chemistry and Alveolar-arterial gradient in anesthetized rats. Resp. Physiol. Neurobiol. 302, 103912. doi:10.1016/j.resp.2022.103912
Goetz, A. M., Rogers, P. L., Schlichtig, R., Muder, R. R., Diven, W. F., and Prior, R. B. (1994). Adult respiratory distress syndrome associated with epidural fentanyl infusion. Crit. Care Med. 22, 1579–1583. doi:10.1097/00003246-199410000-00012
Grim, T. W., Acevedo-Canabal, A., and Bohn, L. M. (2020a). Toward directing opioid receptor signaling to refine opioid therapeutics. Biol. Psychiatry 87, 15–21. doi:10.1016/j.biopsych.2019.10.020
Grim, T. W., Schmid, C. L., Stahl, E. L., Pantouli, F., Ho, J. H., Acevedo-Canabal, A., et al. (2020b). A G protein signaling-biased agonist at the μ-opioid receptor reverses morphine tolerance while preventing morphine withdrawal. Neuropsychopharmacology 45, 416–425. doi:10.1038/s41386-019-0491-8
Grynkiewicz, G., and Gadzikowska, M. (2008). Tropane alkaloids as medicinally useful natural products and their synthetic derivatives as new drugs. Pharmacol. Rep. 60, 439–463.
Guyenet, P. G., Stornetta, R. L., and Bayliss, D. A. (2010). Central respiratory chemoreception. J. Comp. Neurol. 518, 3883–3906. doi:10.1002/cne.22435
Gyermek, L. (1953a). Quaternary derivatives of benzoyltropine and benzoyl-psi-tropine with anticholinergic and local anaesthetic properties. Nature 171, 788. doi:10.1038/171788a0
Gyermek, L. (1953b). Studies on cholinergic blocking substances. 4. Correlation between structure and effect of quaternary derivatives of benzoyltropine and benzoyl psi tropine compounds. Acta Physiol. Acad. Sci. Hung 4, 333–340.
Gyermek, L. (2002). Structure-activity relationships among derivatives of dicarboxylic acid esters of tropine. Pharmacol. Ther. 96, 1–21. doi:10.1016/s0163-7258(02)00296-6
Gyermek, L., and Lee, C. (2009a). The development of ultrashort acting neuromuscular relaxant tropane derivatives. J. Crit. Care 24, 58–65. doi:10.1016/j.jcrc.2008.08.005
Gyermek, L., and Lee, C. (2009b). The development of ultrashort acting neuromuscular relaxant tropane derivatives. J. Crit. Care 24, 58–65. doi:10.1016/j.jcrc.2008.08.005
György, L., Doda, M., and Nador, K. (1960). Pharmacological studies on new tertiary tropine esters. Acta Physiol. Acad. Sci. Hung 17, 473–478.
György, L., Doda, M., and Nador, K. (1965). Some pharmacological effects of N-alkyl- and N-aralkyl-nor-tropine esters. Acta Physiol. Acad. Sci. Hung 26, 369–376.
György, L., Pfeifer, A. K., Dóda, M., Galambos, E., Molnár, J., Kraiss, G., et al. (1968). Pharmacology of new tropine ethers with central activity. Arzneimittelforschung 18, 517–524.
Hakim, T. S., Grunstein, M. M., and Michel, R. P. (1992). Opiate action in the pulmonary circulation. Pulm. Pharmacol. 5, 159–165. doi:10.1016/0952-0600(92)90036-g
Han, Y., Yan, W., Zheng, Y., Khan, M. Z., Yuan, K., and Lu, L. (2019). The rising crisis of illicit fentanyl use, overdose, and potential therapeutic strategies. Transl. Psychiatry 9, 282. doi:10.1038/s41398-019-0625-0
Haouzi, P., Mellen, N., McCann, M., Sternick, M., Guck, D., and Tubbs, N. (2020). Evidence for the emergence of an opioid-resistant respiratory rhythm following fentanyl overdose. Respir. Physiol. Neurobiol. 277, 103428. doi:10.1016/j.resp.2020.103428
Harvey, R. J., Topf, M., Harvey, K., and Rees, M. I. (2008). The genetics of hyperekplexia: more than startle. Trends Genet. 24, 439–447. doi:10.1016/j.tig.2008.06.005
Hemmings, H. C., and Egan, T. D. (2019). Neuromuscular blockers and reversal drugs: foundations and clinical application. Pharmacol. Physiology Anesth., 428–454.
Henderson, F., May, W. J., Gruber, R. B., Discala, J. F., Puskovic, V., Young, A. P., et al. (2014). Role of central and peripheral opiate receptors in the effects of fentanyl on analgesia, ventilation and arterial blood-gas chemistry in conscious rats. Respir. Physiol. Neurobiol. 191, 95–105. doi:10.1016/j.resp.2013.11.005
Hosseini, M., Taiarani, Z., Hadjzadeh, M. A., Salehabadi, S., Tehranipour, M., and Alaei, H. A. (2011). Different responses of nitric oxide synthase inhibition on morphine-induced antinociception in male and female rats. Pathophysiology 18, 143–149. doi:10.1016/j.pathophys.2010.05.004
Issekutz, B. Sr (1963). Spatial configuration and activity of tropine derivatives. Pol. Tyg. Lek. 18, 670–674.
Jacobson, J. D., McGrath, C. J., and Smith, E. P. (1994). Cardiorespiratory effects of induction and maintenance of anesthesia with ketamine-midazolam combination, with and without prior administration of butorphanol or oxymorphone. Am. J. Vet. Res. 55, 543–550. doi:10.2460/ajvr.1994.55.04.543
Jenkins, M. W., Khalid, F., Baby, S. M., May, W. J., Young, A. P., Bates, J. N., et al. (2021). Glutathione ethyl ester reverses the deleterious effects of fentanyl on ventilation and arterial blood-gas chemistry while prolonging fentanyl-induced analgesia. Sci. Rep. 11, 6985. doi:10.1038/s41598-021-86458-x
Kohnen-Johannsen, K. L., and Kayser, O. (2019). Tropane alkaloids: chemistry, pharmacology, biosynthesis and production. Molecules 24, 796. doi:10.3390/molecules24040796
Lewis, S. J., Meller, S. T., Brody, M. J., and Gebhart, G. F. (1991). Reduced nociceptive effects of intravenous serotonin (5-HT) in the spontaneously hypertensive rat. Clin. Exp. Hypertens. A 13, 849–857. doi:10.3109/10641969109042089
Lewis, T. H. J., May, W. J., Young, A. P., Bates, J. N., Baby, S. M., Getsy, P. M., et al. (2022). The ventilatory depressant actions but not the antinociceptive effects of morphine are blunted in rats receiving intravenous infusion of L-cysteine ethyl ester. Biomed. Pharmacother. 156, 113939. doi:10.1016/j.biopha.2022.113939
Lomask, M. (2006). Further exploration of the Penh parameter. Exp. Toxicol. Pathol. 57 (Suppl. 2), 13–20. doi:10.1016/j.etp.2006.02.014
Lynch, J. W., Zhang, Y., Talwar, S., and Estrada-Mondragon, A. (2017). Glycine receptor drug Discovery. Adv. Pharmacol. 79, 225–253. doi:10.1016/bs.apha.2017.01.003
Maksay, G., Laube, B., Schemm, R., Grudzinska, J., Drwal, M., and Betz, H. (2009a). Different binding modes of tropeines mediating inhibition and potentiation of alpha1 glycine receptors. J. Neurochem. 109, 1725–1732. doi:10.1111/j.1471-4159.2009.06083.x
Maksay, G., Nemes, P., and Bíró, T. (2004). Synthesis of tropeines and allosteric modulation of ionotropic glycine receptors. J. Med. Chem. 47, 6384–6391. doi:10.1021/jm040814g
Maksay, G., Nemes, P., Vincze, Z., and Bíró, T. (2008). Synthesis of (nor)tropeine (di)esters and allosteric modulation of glycine receptor binding. Bioorg Med. Chem. 16, 2086–2092. doi:10.1016/j.bmc.2007.10.097
Maksay, G., Vincze, Z., and Nemes, P. (2009b). Synthesis of heteroaromatic tropeines and heterogeneous binding to glycine receptors. Bioorg Med. Chem. 17, 6872–6878. doi:10.1016/j.bmc.2009.08.029
Manzke, T., Niebert, M., Koch, U. R., Caley, A., Vogelgesang, S., Bischoff, A. M., et al. (2011). Serotonin receptor 1A-modulated dephosphorylation of glycine receptor α3: a new molecular mechanism of breathing control for compensation of opioid-induced respiratory depression without loss of analgesia. Schmerz 25, 272–281. doi:10.1007/s00482-011-1044-1
Manzke, T., Niebert, M., Koch, U. R., Caley, A., Vogelgesang, S., Hülsmann, S., et al. (2010). Serotonin receptor 1A-modulated phosphorylation of glycine receptor α3 controls breathing in mice. J. Clin. Invest. 120, 4118–4128. doi:10.1172/JCI43029
Markstahler, U., Kremer, E., Kimmina, S., Becker, K., and Richter, D. W. (2002). Effects of functional knock-out of alpha 1 glycine-receptors on breathing movements in oscillator mice. Respir. Physiol. Neurobiol. 130, 33–42. doi:10.1016/s0034-5687(01)00334-6
Mashkovskiĭ, M. D., and Shvarts, G. I. (1979). Antihistaminic and antiserotonin properties of new complex tropine esters. Farmakol. Toksikol. 42, 3–7.
Mather, L. (1994). “Opioid analgesic drugs,” in Anaesthesia (St Hoboken, NJ, USA: Blackwell Scientific).
May, W. J., Gruber, R. B., Discala, J. F., Puskovic, V., Henderson, F., Palmer, L. A., et al. (2013b). Morphine has latent deleterious effects on the ventilatory responses to a hypoxic challenge. Open J. Mol. Integr. Physiol. 3, 166–180. doi:10.4236/ojmip.2013.34022
May, W. J., Henderson, F., Gruber, R. B., Discala, J. F., Young, A. P., Bates, J. N., et al. (2013a). Morphine has latent deleterious effects on the ventilatory responses to a hypoxic-hypercapnic challenge. Open J. Mol. Integr. Physiol. 3, 134–145. doi:10.4236/ojmip.2013.33019
Meller, S. T., Lewis, S. J., Brody, M. J., and Gebhart, G. F. (1991). The peripheral nociceptive actions of intravenously administered 5-HT in the rat requires dual activation of both 5-HT2 and 5-HT3 receptor subtypes. Brain Res. 561, 61–68. doi:10.1016/0006-8993(91)90749-l
Meyer, L. C., Fuller, A., and Mitchell, D. (2006). Zacopride and 8-OH-DPAT reverse opioid-induced respiratory depression and hypoxia but not catatonic immobilization in goats. Am. J. Physiol. Regul. Integr. Comp. Physiol. 290, R405–R413. doi:10.1152/ajpregu.00440.2005
Meyer, L. C., Hetem, R. S., Fick, L. G., Mitchell, D., and Fuller, A. (2010). Effects of serotonin agonists and doxapram on respiratory depression and hypoxemia in etorphine-immobilized impala (Aepyceros melampus). J. Wildl. Dis. 46, 514–524. doi:10.7589/0090-3558-46.2.514
Nádor, K., and Scheiber, P. (1972). Conformation analysis of tropines: conformation of benzoyl tropine and benzoyl-psi-tropine (tropacocaine) and their cholinolytic actions. Arzneimittelforschung 22, 459–462.
Nagappa, M., Weingarten, T. N., Montandon, G., Sprung, J., and Chung, F. (2017). Opioids, respiratory depression, and sleep-disordered breathing. Best. Pract. Res. Clin. Anaesthesiol. 31, 469–485. doi:10.1016/j.bpa.2017.05.004
Nishida, K., Ohta, Y., Ito, M., Nagamura, Y., Kitahara, S., Fujii, K., et al. (1996). Conversion of gamma-glutamylcysteinylethyl ester to glutathione in rat hepatocytes. Biochim. Biophys. Acta 1313, 47–53. doi:10.1016/0167-4889(96)00054-7
Nunn, J. F. (1993). Nunn’s applied respiratory physiology. Oxford, United Kingdom: Butterworth Leinemann.
Patel, J. M., and Lemberger, A. P. (1963). Comparative hydrolytic rates of some tropine esters. J. Pharm. Sci. 52, 1129–1133. doi:10.1002/jps.2600521206
Pierrefiche, O., Schwarzacher, S. W., Bischoff, A. M., and Richter, D. W. (1998). Blockade of synaptic inhibition within the pre-Bötzinger complex in the cat suppresses respiratory rhythm generation in vivo. J. Physiol. 509, 245–254. doi:10.1111/j.1469-7793.1998.245bo.x
Reber, L. J., Allen, C. H., Durant, R. C., and Friess, S. L. (1963). Structure vs. Toxicity Relationships in aryl esters of tropine and pseudotropine. V. Toxicol. Appl. Pharmacol. 5, 625–636. doi:10.1016/0041-008x(63)90007-3
Ren, J., Ding, X., Funk, G. D., and Greer, J. J. (2009). Ampakine CX717 protects against fentanyl-induced respiratory depression and lethal apnea in rats. Anesthesiology 110, 1364–1370. doi:10.1097/ALN.0b013e31819faa2a
Ren, J., Ding, X., and Greer, J. J. (2015). 5-HT1A receptor agonist Befiradol reduces fentanyl-induced respiratory depression, analgesia, and sedation in rats. Anesthesiology 122, 424–434. doi:10.1097/ALN.0000000000000490
Ren, J., Ding, X., and Greer, J. J. (2020). Countering opioid-induced respiratory depression in male rats with nicotinic acetylcholine receptor partial agonists varenicline and ABT 594. Anesthesiology 132, 1197–1211. doi:10.1097/ALN.0000000000003128
Richter, D. W., Manzke, T., Wilken, B., and Ponimaskin, E. (2003). Serotonin receptors: guardians of stable breathing. Trends Mol. Med. 9, 542–548. doi:10.1016/j.molmed.2003.10.010
Roquebert, J., and Delgoulet, C. (1988). Cardiovascular effects of etorphine in rats. J. Auton. Pharmacol. 8, 39–43. doi:10.1111/j.1474-8673.1988.tb00167.x
San Martín, V. P., Burgos, C. F., Marileo, A. M., Lara, C. O., Sazo, A., Fuentealba, J., et al. (2019). Inhibitory actions of tropeines on the α3 Glycine receptor function. Front. Pharmacol. 10, 331. doi:10.3389/fphar.2019.00331
Santiago, T. V., and Edelman, N. H. (1985). Opioids and breathing. J. Appl. Physiol. 59, 1675–1685. doi:10.1152/jappl.1985.59.6.1675
Sarton, E., Dahan, A., and Teppema, L. (1998). Do sex-related differences exist in the respiratory pharmacology of opioids? Adv. Exp. Med. Biol. 450, 141–145. doi:10.1007/978-1-4757-9077-1_23
Saunders, S. E., and Levitt, E. S. (2020). Kölliker-fuse/parabrachial complex mu opioid receptors contribute to fentanyl-induced apnea and respiratory rate depression. Respir. Physiol. Neurobiol. 275, 103388. doi:10.1016/j.resp.2020.103388
Schmid, K., Böhmer, G., and Gebauer, K. (1991). Glycine receptor-mediated fast synaptic inhibition in the brainstem respiratory system. Respir. Physiol. 84, 351–361. doi:10.1016/0034-5687(91)90129-7
Seckler, J. M., Grossfield, A., May, W. J., Getsy, P. M., and Lewis, S. J. (2022). Nitrosyl factors play a vital role in the ventilatory depressant effects of fentanyl in unanesthetized rats. Biomed. Pharmacother. 146, 112571. doi:10.1016/j.biopha.2021.112571
Shafford, H. L., and Schadt, J. C. (2008). Respiratory and cardiovascular effects of buprenorphine in conscious rabbits. Vet. Anaesth. Analg. 35, 326–332. doi:10.1111/j.1467-2995.2007.00383.x
Shao, X. M., and Feldman, J. L. (2009). Central cholinergic regulation of respiration: nicotinic receptors. Acta Pharmacol. Sin. 30, 761–770. doi:10.1038/aps.2009.88
Stein, P. D., Goldhaber, S. Z., and Henry, J. W. (1995). Alveolar-arterial oxygen gradient in the assessment of acute pulmonary embolism. Chest 107, 139–143. doi:10.1378/chest.107.1.139
Stengel, A., Coskun, T., Goebel, M., Wang, L., Craft, L., Alsina-Fernandez, J., et al. (2010). Central injection of the stable somatostatin analog ODT8-SST induces a somatostatin2 receptor-mediated orexigenic effect: role of neuropeptide Y and opioid signaling pathways in rats. Endocrinology 151, 4224–4235. doi:10.1210/en.2010-0195
Story, D. A. (1996). Alveolar oxygen partial pressure, alveolar carbon dioxide partial pressure, and the alveolar gas equation. Anesthesiology 84, 1011. doi:10.1097/00000542-199604000-00036
Teichtahl, H., Wang, D., Cunnington, D., Kronborg, I., Goodman, C., Prodromidis, A., et al. (2004). Cardiorespiratory function in stable methadone maintenance treatment (MMT) patients. Addict. Biol. 9, 247–253. doi:10.1080/13556210412331292578
Thron, C. D., Durant, R. C., Patterson, R. N., Reber, L. J., and Friess, S. L. (1963). Further elements of structural specificity in potentiation and blockade of excitable tissue preparations by aryl esters of tropine and pseudotropine. III. Toxicol. Appl. Pharmacol. 5, 79–104. doi:10.1016/0041-008x(63)90084-x
Trautner, E. M., and McCallum, I. A. (1950). The action of tropine and heliotridine-alkaloids on excitation, propagation and recovery in muscle. Aust. J. Exp. Biol. Med. Sci. 28, 343–360. doi:10.1038/icb.1950.35
Wang, D., Teichtahl, H., Drummer, O., Goodman, C., Cherry, G., Cunnington, D., et al. (2005). Central sleep apnea in stable methadone maintenance treatment patients. Chest 128, 1348–1356. doi:10.1378/chest.128.3.1348
Willette, R. N., Doorley, B. M., and Sapru, H. N. (1987). Activation of cholinergic mechanisms in the medulla oblongata reverse intravenous opioid-induced respiratory depression. J. Pharmacol. Exp. Ther. 240, 352–358.
Yeadon, M., and Kitchen, I. (1990). Multiple opioid receptors mediate the respiratory depressant effects of fentanyl-like drugs in the rat. Gen. Pharmacol. 21, 655–664. doi:10.1016/0306-3623(90)91013-h
Young, A. P., Gruber, R. B., Discala, J. F., May, W. J., Palmer, L. A., Lewis, S. J., et al. (2013). Co-activation of μ- and δ-opioid receptors elicits tolerance to morphine-induced ventilatory depression via generation of peroxynitrite. Resp. Physiol. Neurobiol. 186, 255–264. doi:10.1016/j.resp.2013.02.028
Young-McCaughan, S., and Miaskowski, C. (2001a). Measurement of opioid-induced sedation. Pain Manag. Nurs. 2 (4), 132–149. doi:10.1053/jpmn.2001.25169
Young-McCaughan, S., and Miaskowski, C. (2001b). Definition of and mechanism for opioid-induced sedation. Pain Manag. Nurs. 2, 84–97. doi:10.1053/jpmn.2001.25012
Yu, C., Yuan, M., Yang, H., Zhuang, X., and Li, H. (2018). P-glycoprotein on blood-brain barrier plays a vital role in fentanyl brain exposure and respiratory toxicity in rats. Toxicol. Sci. 164, 353–362. doi:10.1093/toxsci/kfy093
Zaĭtseva, K. A., and Gerchikov, L. N. (1969). Comparative pharmacological activity of some esters of tropine, piperidol and oxymethylpyrrolidine. Farmakol. Toksikol. 32, 53–58.
Zakusov, V. V., Kostochka, L. M., and Skoldinov, A. P. (1978). Effect of cocaine molecule fragments on the central nervous system. Biull Eksp. Biol. Med. 86, 435–438.
Zeile, K., and Heusner, A. (1959). Synthesis and pharmacology of various tropine and speudotropine esters with substituted pyrrolidine ring. Arch. Pharm. Ber. Dtsch. Pharm. Ges. 292 (/64), 238–255. doi:10.1002/ardp.19592920505
Zhang, Z., Zhang, C., Zhou, M., and Xu, F. (2012b). Activation of opioid μ-receptors, but not δ- or κ-receptors, switches pulmonary C-Fiber-Mediated rapid shallow breathing into an apnea in anesthetized rats. Respir. Physiol. Neurobiol. 183, 211–217. doi:10.1016/j.resp.2012.06.032
Zhang, Z., Zhang, C., Zhuang, J., and Xu, F. (2012a). Contribution of central μ-receptors to switching pulmonary C-Fibers-Mediated rapid shallow breathing into an apnea by fentanyl in anesthetized rats. Brain Res. 1469, 73–81. doi:10.1016/j.brainres.2012.06.028
Zhuang, J., Zhang, Z., Zhang, C., and Xu, F. (2012). 8-OH-DPAT abolishes the pulmonary C-Fiber-Mediated apneic response to fentanyl largely via acting on 5HT1A receptors in the nucleus tractus solitarius. Am. J. Physiol. Regul. Integr. Comp. Physiol. 303, R449–R458. doi:10.1152/ajpregu.00016.2012
Keywords: tropine, fentanyl, ventilatory depression, arterial blood-gas chemistry, analgesia, male Sprague Dawley rats
Citation: Getsy PM, May WJ, Young AP, Baby SM, Coffee GA, Bates JN, Hsieh Y-H and Lewis SJ (2024) Tropine exacerbates the ventilatory depressant actions of fentanyl in freely-moving rats. Front. Pharmacol. 15:1405461. doi: 10.3389/fphar.2024.1405461
Received: 22 March 2024; Accepted: 15 May 2024;
Published: 24 June 2024.
Edited by:
Mariana Spetea, University of Innsbruck, AustriaReviewed by:
Kendall Francis Morris, University of South Florida, United StatesPatricio Ernesto Iturriaga-Vasquez, University of La Frontera, Chile
Katarzyna Kaczynska, Mossakowski Medical Research Institute, Polish Academy of Sciences, Poland
Copyright © 2024 Getsy, May, Young, Baby, Coffee, Bates, Hsieh and Lewis. This is an open-access article distributed under the terms of the Creative Commons Attribution License (CC BY). The use, distribution or reproduction in other forums is permitted, provided the original author(s) and the copyright owner(s) are credited and that the original publication in this journal is cited, in accordance with accepted academic practice. No use, distribution or reproduction is permitted which does not comply with these terms.
*Correspondence: Stephen J. Lewis, sjl78@case.edu
†Present addresses: Santhosh M. Baby,Translational Sciences Treatment Discovery, Galvani Bioelectronics, Collegeville, PA, United States
James N. Bates, Atelerix Life Sciences Inc., Charlottesville, VA, United States.