- 1Department of Pharmacy, Taizhou Second People’s Hospital (Mental Health Center affiliated to Taizhou University School of Medicine), Taizhou University, Taizhou, Zhejiang, China
- 2Department of Pharmacology, Shenyang Pharmaceutical University, Shenyang, China
- 3Department of Pharmacology, Taizhou University, Taizhou, Zhejiang, China
Tumor-associated macrophages (TAMs), fundamental constituents of the tumor microenvironment (TME), significantly influence cancer development, primarily by promoting epithelial-mesenchymal transition (EMT). EMT endows cancer cells with increased motility, invasiveness, and resistance to therapies, marking a pivotal juncture in cancer progression. The review begins with a detailed exposition on the origins of TAMs and their functional heterogeneity, providing a foundational understanding of TAM characteristics. Next, it delves into the specific molecular mechanisms through which TAMs induce EMT, including cytokines, chemokines and stromal cross-talking. Following this, the review explores TAM-induced EMT features in select cancer types with notable EMT characteristics, highlighting recent insights and the impact of TAMs on cancer progression. Finally, the review concludes with a discussion of potential therapeutic targets and strategies aimed at mitigating TAM infiltration and disrupting the EMT signaling network, thereby underscoring the potential of emerging treatments to combat TAM-mediated EMT in cancer. This comprehensive analysis reaffirms the necessity for continued exploration into TAMs’ regulatory roles within cancer biology to refine therapeutic approaches and improve patient outcomes.
1 TAMs at the crossroads of cancer: a dual-edged sword within the TME
Cancer, a multifaceted pathological entity, is increasingly understood through its interactions within the tumor microenvironment (TME), a diverse ecosystem comprising stromal cells, immune cells, and the extracellular matrix (ECM) (Hawkins et al., 2016). Among the immune cells, tumor-associated macrophages (TAMs) are of particular interest due to their significant, yet paradoxical roles in cancer progression (Iriondo et al., 2018).
TAMs represent a unique subset of myeloid cells within the TME, known to be associated with adverse clinical outcomes in a variety of cancers such as breast, lung, and pancreatic cancers (Akimoto et al., 2016). Their functionalities extend from phagocytosis and cytokine secretion to the remodeling of the ECM (Kitamura et al., 2015; Colwell et al., 2017). A significant aspect of TAMs’ influence is their facilitation of epithelial-mesenchymal transition (EMT) (Tekpli et al., 2019), a process enabling epithelial cells to acquire mesenchymal traits, thereby enhancing their migratory and invasive capacities (Oh et al., 2018). This phenotypic shift, marked by the downregulation of epithelial markers such as E-cadherin and the upregulation of mesenchymal markers including N-cadherin, Vimentin, and Fibronectin, is instrumental in the metastatic cascade. It enables malignant cells to detach from the primary tumor, intravasate into the bloodstream or lymphatic system, and subsequently establish secondary tumors in distant organs (Urbas et al., 2016).
Emerging research delineates the multifaceted roles of TAMs in orchestrating the EMT. TAMs exert their influence through the secretion of an array of bioactive molecules, including cytokines such as IL-6 and TGF-β, and various growth factors that synergistically activate EMT pathways in cancer cells (Kartikasari et al., 2021). This complex cocktail not only modulates gene expression to favor a mesenchymal phenotype but also disrupts cell-cell adhesion, enhancing migratory and invasive properties of cancer cells (Huang et al., 2020; Zhang et al., 2021).
TAMs play a pivotal role in orchestrating the TME, extending beyond their secretory roles to foster a pro-metastatic niche in collaboration with cancer-associated fibroblasts (CAFs) and other stromal cells. In this complex interplay, TAMs and CAFs collectively contribute to the creation of a pro-inflammatory milieu, characterized by the release of inflammatory mediators that sustain chronic inflammation and enhance mesenchymal phenotype (Habanjar et al., 2023; Nakamura et al., 2022). Moreover, TAMs express matrix metalloproteinases (MMPs) like MMP-9 (Niland et al., 2021), while CAFs contribute to ECM deposition through the production of fibronectin and collagen (Belhabib et al., 2021), leading to increased tissue stiffness and the creation of conduits that facilitate cancer cell invasion. The interaction between TAMs and CAFs in ECM remodeling is further facilitated by integrins and discoidin domain receptors (DDRs) on their surfaces, which mediate cell-ECM interactions and signal transduction involved in cancer cell migration and invasion (Lee et al., 2021; Gunaydin, 2021a).
Understanding the intricate relationship between TAMs and EMT is paramount for the development of innovative therapeutic strategies. This review commences with a detailed examination of the diversity and plasticity of macrophages, highlighting their aberrant functions within the TME. We then dissect the current knowledge on the role of TAMs in EMT induction and subsequent cancer cell metastasis. Given the heterogeneity of both tumors and TAMs, we underscore the variable impact of TAM-mediated EMT four cancer types. This review endeavors to elucidate the integral role of TAMs in cancer progression within the TME that could potentially impact patient outcomes.
2 Deciphering the complex nature of TAMs: Origins, classification, and the role in cancer dynamics
Macrophages are pivotal in tumor immune surveillance, capable of recognizing and eradicating malignant cells. However, in the context of cancer, these cells often undergo functional reprogramming, leading to the emergence of TAMs that can paradoxically support tumor growth and metastasis (Ratnam et al., 2019).
2.1 Lineage tracing and phenotype of TAMs
Within the TME, TAMs exemplify cellular adaptability, performing roles that transition from anti-tumorigenic to pro-tumorigenic in response to TME cues. TAM ontogeny is predominantly attributed to two lineages: the MerTK + CX3CR1+ resident tissue macrophages (RTMs) and the Ly6C + CCR2+ bone marrow-derived macrophages (BMDMs). The expression and kinase activity of MerTK are crucial for efferocytosis in human RTMs (Wanke et al., 2021), while CX3CR1 originates from embryonic CX3CR1+ precursors that migrate into tissues during embryogenesis and differentiate into various RTMs (Ensan et al., 2016). Ly6C is a classical surface marker highly expressed on inflammatory monocytes, facilitating their migration from the bone marrow to peripheral tissues (Miyake et al., 2024). CCR2, a chemokine receptor, is essential for guiding these monocytes during their transit to sites of inflammation, where they then differentiate into macrophages and partake in the immune response (Chen et al., 2023a).
RTMs, the custodians of tissue homeostasis, are established during embryogenesis, deriving from yolk sac and fetal liver progenitors. These cells disperse to various organs, where they acquire specialized functions unique to each tissue (Wu and Hirschi, 2019). For example, embryonically derived resident CD163+ Tim4+ omental macrophages have been shown to facilitate the metastatic progression of ovarian cancer (Etzerodt et al., 2020). RTMs are initially found in close proximity to tumor cells, where they contribute to the process of EMT and enhance tumor invasiveness. With tumor progression, however, RTMs are relegated to the periphery of the TME, making way for an influx of BMDMs that become predominant in the TME of both mouse models and human cases of NSCLC (Casanova-Acebes et al., 2021). Intriguingly, in breast cancer (BC), RTMs defy the anticipated dominance of BMDMs, playing an essential role in sculpting the tumor milieu (Hirano et al., 2023a). This finding underscores the importance of considering the origin of TAMs when studying their roles in tumor biology.
Conversely, BMDMs originate from a lineage of CD34+CD38−CD90+ hematopoietic stem cells within the bone marrow. These cells are lured into the tumultuous environment of the TME by a myriad of chemokines and growth factors. Monocytes characterized by Ly6C + CCR2+ surface markers can evolve into CD68+ macrophages, influenced by local mediators such as macrophage colony-stimulating factor (M-CSF) and granulocyte-macrophage colony-stimulating factor (GM-CSF) (Wu et al., 2020). The recruitment and functional roles of BMDMs are highly dynamic, often initiated by inflammatory signals, making them integral to the TME’s immunological landscape. Their propensity for rapid infiltration and response to inflammatory stimuli renders BMDMs pivotal in the later stages of tumor evolution and metastatic dissemination (Kielbassa et al., 2022). In melanoma tumors, F4/80+ TAM subsets have been primarily traced back to bone marrow progenitors, challenging the conventional belief of their derivation from skin-resident macrophages (Pizzurro et al., 2023). The findings accentuate the contributions of both RTMs and BMDMs to the intricacies of the TME. The distinct origins and developmental trajectories of these macrophages engender a diversity of roles within the TME, underscored by their context-dependent functions in cancer progression.
The phenotypic spectrum of TAMs is a complex mosaic, intricately crafted from the TME diverse array of cytokines, chemokines, growth factors, and metabolic by-products. This spectrum transcends the traditional binary categorization into CD80+CD86+ M1-like (anti-tumorigenic) and CD163+CD206+ M2-like (pro-tumorigenic) phenotypes, revealing a much broader continuum of TAM states (Fernandez et al., 2017; Pe et al., 2022). This continuum reflects the dynamic nature of the TME, with TAMs adopting hybrid phenotypes (Boutilier and Elsawa, 2021).
The advent of single-cell analytic technologies has marked a new epoch in TAM characterization, enabling a more nuanced understanding of their roles within the TME. For instance, in BC, such analyses have distinguished TAM subpopulations like TREM2+ TAMs, characterized by the expression of TREM2 and SPP1, and FOLR2+ TAMs, identifiable by FOLR2 and CD206 markers. These refined subdivisions underscore the potential for developing targeted therapies aimed at modulating TAM-driven oncogenic processes, opening up new avenues for cancer treatment (Nalio Ramos et al., 2022).
Furthermore, the characterization of TAMs varies across different models and tumor types, reflecting the adaptability and diversity of these cells in response to unique TME. For instance, a study utilizing a larval Drosophila model, which harbors a dominant-active version of the Ras oncogene (RasV12), shed light on dysplastic growth during early tumor progression. Analysis of hemocytes in this model revealed five distinct clusters, each exhibiting unique gene expression profiles. This suggests that, even in Drosophila, TAMs can be categorized into multiple subtypes based on their molecular signatures, hinting at the complex roles these cells play in tumor biology (Khalili et al., 2023).
In human glioblastoma, research has unveiled a broad spectrum of TAM subtypes that extends well beyond the conventional M1/M2 dichotomy. This includes as many as 11 distinct subtypes, encompassing hypoxic, proliferating, and chemokine-producing TAMs, among others. Such findings underscore the heterogeneity and complexity of TAM populations within tumors, challenging the traditional binary classification and highlighting the nuanced roles these cells play in tumorigenesis (Anand et al., 2022).
2.2 Heterogeneity and plasticity of TAMs
The remarkable heterogeneity and plasticity of TAMs within the TME are shaped by a complex web of signals, steering them towards roles that either support or counteract tumor growth. M1 polarization is incited by pro-inflammatory mediators such as interferon gamma (IFN-γ), intratumoral bacterial lipopolysaccharide (LPS), and tumor necrosis factor alpha (TNF-α), orchestrating the activation of transcription factors including STAT1, interferon regulatory factor 5 (IRF5), and NF-κB (Chin et al., 2021). Conversely, M2 polarization is fostered by anti-inflammatory cues, notably IL-4, IL-13, and IL-10, which mobilize transcription factors like STAT6, IRF4, and PPAR-γ (Ren et al., 2021).
The conventional dichotomy of TAMs into M1 and M2 macrophages has undergone significant scrutiny with the emergence of scRNA-seq technologies. These analyses have revealed that TAMs often exhibit a blend of M1 and M2 characteristics at the single-cell level, demonstrating a more intricate spectrum of TAM phenotypes within the TME. This methodological advancement has also enabled the identification of multiple TAM subsets across different cancer types, each characterized by distinct gene expression profiles and functional roles. For instance, in pancreatic ductal adenocarcinoma (PDAC), scRNA-seq has delineated TAMs into seven distinct clusters, with the CCL2+CCL3 + cluster1 and TOP2A + cluster3 being particularly relevant to PDAC pathology. These findings highlight the exceptional diversity and context-specific nature of TAMs in various tumor settings, prompting a reconsideration of the simplistic M1/M2 paradigm and encouraging a deeper exploration of TAM functions in cancer (Yang et al., 2023a).
In addition to cytokine engagement, the metabolic landscape of the TME plays a critical role in modulating TAM polarization, with factors like lactate accumulation and the presence of lipid-rich apoptotic debris through efferocytosis significantly influencing TAM behavior, potentially even surpassing the impact of canonical cytokine signaling. The TME’s enriched metabolic milieu, characterized by these components, profoundly dictates TAM functionality (Zhang et al., 2022; Cheng et al., 2020; Okikawa et al., 2022; Kloosterman and Akkari, 2023). The accumulation of lipid droplets, in particular, has been shown to drive TAMs towards an M2-like phenotype, conducive to tumor progression. This process involves the hydrolysis of stored triglycerides into free fatty acids (FFAs) by enzymes such as adipose triglyceride lipase (ATGL) and hormone-sensitive lipase (HSL), underscoring the link between lipid metabolism and TAM-mediated tumor support mechanisms (Wu et al., 2019). TAMs exhibit remarkable metabolic flexibility, adapting their metabolic pathways to the diverse and fluctuating demands of the TME. This adaptation enables TAMs to efficiently utilize glucose, amino acids, and lipids, tailoring their energy production and biosynthetic activities to optimally support tumor growth and survival (Xiao et al., 2023).
Epigenetic mechanisms further refine the functional spectrum of TAMs. In M2-like TAMs, DNA methyltransferase 3B (DNMT3B) modulates the arginase 1 (ARG1) gene, integral to the M2 phenotype, where DNMT3B deficiency augments ARG1 expression in BMDMs (Qin et al., 2022). Similarly, the inhibition of histone deacetylase 6 (HDAC6) has been shown to reduce M2 polarization, emphasizing the role of histone modifications in determining TAM functionality (Shi et al., 2022). The overexpression of HDAC2 in M2-like TAMs has been associated with adverse outcomes for lung cancer patients. Targeting the HDAC2-SP1 axis may disrupt the pro-tumorigenic activities of M2-like TAMs, thereby impairing their support for tumor progression (Zheng et al., 2023).
The metabolic dichotomy between M1 and M2 macrophages is underscored by their respective reliance on glycolysis showing interruptions in the tricarboxylic acid TCA cycle, which leads to the stabilization of hypoxia-inducible factor 1-alpha (HIF1-α) and fatty acid synthesis for pro-inflammatory activities (Qian et al., 2024), and oxidative phosphorylation (OXPHOS) alongside fatty acid oxidation for anti-inflammatory and tissue repair functions, but the glycometabolism of TAMs within the TME becomes more complex and diverse (Zhang et al., 2022; Kes et al., 2020), reflecting their adaptation to the diverse conditions of the TME (Wculek et al., 2022; Elia and Haigis, 2021).
TAMs display remarkable metabolic flexibility, oscillating between glycolysis and OXPHOS in response to the dynamic oxygen and nutrient availability within the TME. This metabolic plasticity is highlighted by the altered expression of key metabolic components in TAMs, including an upsurge in glucose transporters like GLUT1 and glycolytic enzymes such as hexokinase 2, signifying a propensity towards aerobic glycolysis (Shin and Koo, 2021). Furthermore, modifications in lipid metabolism are apparent through the enhanced expression of enzymes involved in fatty acid synthesis, like fatty acid synthase, alongside shifts in cholesterol metabolism, which may contribute to the production of pro-inflammatory and immunosuppressive lipid mediator (Li et al., 2022).
The process of efferocytosis, the engulfment of apoptotic cells, further influences TAM metabolism by inducing fatty acid oxidation, which not only supports TAM survival in the nutrient-depleted TME but may also enhance the stability of the TME (Kloosterman and Akkari, 2023). Consequently, targeting TAM lipid metabolism presents a promising strategy in cancer therapy. For example, arenobufagin has been shown to affect macrophage polarization by modulating PCSK9-mediated cholesterol metabolism. By inhibiting the PCSK9/LDL-R pathway, arenobufagin promotes M1-type polarization, illustrating the therapeutic potential of manipulating TAM metabolic pathways (Figure 1) (Li et al., 2024).
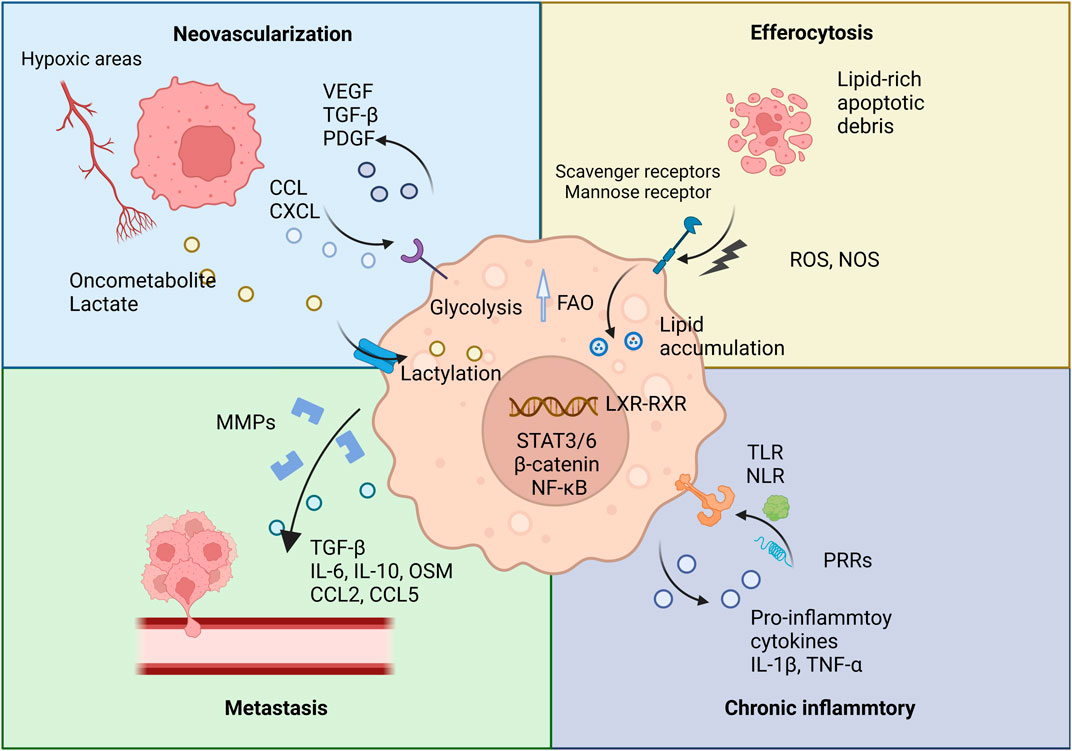
Figure 1. TAMs and their pro-tumorigenic functions. This figure illustrates the various pro-tumorigenic roles of TAMs within the TME. TAMs contribute to several key processes that promote tumor progression.
2.3 Dual role of TAMs in tumor progression and suppression
The dual role of TAMs is highlighted by their controversial relationship with cancer prognosis. Some studies associate TAMs with poor prognosis, while others report better outcomes in different cancer types (Liu et al., 2021).
Theoretically, the TME significantly influences TAMs, often impairing their antigen-presenting capabilities and steering them towards an M2-like phenotype, linked with tumor-promoting activities such as immunosuppression, angiogenesis, and tissue remodeling (Cheng et al., 2020; Wischhusen et al., 2020). Yet, the adaptability and diversity of TAMs are remarkable, with their functions being profoundly context-specific. While M1-like TAMs are generally associated with anti-tumorigenic properties, certain TME conditions can paradoxically lead them to contribute to tumor dissemination (You et al., 2022). In lung cancer, CXCL9+ M1 TAMs recruit tissue-resident T cells and facilitate fatty acid uptake, enhancing their metabolic fitness and anti-tumor effects (Eva et al., 2020).
The activation of TAMs using toll-like receptor ligands, for example, can induce a transition towards a pro-inflammatory phenotype. This phenotypic shift not only elevates their antigen-presentation capabilities but also amplifies cytokine secretion, ultimately bolstering the activation of antitumor T cells (Zhang et al., 2021b). TAMs also facilitate antibody-dependent cellular cytotoxicity (ADCC) and phagocytosis, contributing to vascular disruption and tumor necrosis, thereby destabilizing the TME and impeding cancer progression (Wettersten et al., 2019; Mantovani et al., 2017). Current research explores therapeutic targeting of macrophages, including chimeric antigen receptor macrophages (CAR-M), highlighting their potential in cancer treatment (Klichinsky et al., 2020).
3 The role of TAMs in facilitating EMT during cancer progression
TAMs are central to the orchestration of EMT, a crucial process in cancer metastasis. Through the secretion of signaling molecules, direct interactions with cancer cells, and modulation of immune responses, TAMs conduct the complex orchestra that regulates EMT. Understanding this interplay between TAMs and cancer cells not only elucidates the mechanisms of EMT but also reveals potential therapeutic targets.
3.1 TAM-secreted signaling molecules: Key conductors in the EMT orchestra
TAMs significantly influence the TME by secreting a diverse array of signaling molecules that drive the EMT process. This section explores the interactions between TAM-derived factors, such as growth factors, cytokines, and chemokines—including TGF-β, IL-6, and oncostatin M (OSM)—and their roles in inducing EMT.
3.1.1 TGF-β signaling
TGF-β, abundantly secreted by TAMs, emerges as a pivotal orchestrator of the EMT. The multifaceted roles of TGF-β in EMT modulation, highlighting its ability to actuate downstream transcriptional effectors, particularly Smad2/3, thereby influencing the expression of EMT-associated transcription factors (EMT-TFs) (Cai et al., 2019).
3.1.1.1 Direct promotion of EMT
In lung squamous cell carcinoma, increased secretion of TGF-β1 from TAMs has been confirmed and promotes EMT by the role of TGF-β in the Smad/zinc finger e-box binding homeobox (ZEB) pathway (Sumitomo et al., 2023). Addressing this mechanism, innovative approaches like macrophage membrane-coated nanoparticles loaded with SD-208 (Mφ-SDNP), a TGF-βR1 kinase inhibitor, aim to directly inhibit the TGF-β signaling pathway, thereby preventing the initiation of EMT in cancer cells. SD-208 within the Mφ-SDNP inhibits the TGF-β receptor’s kinase activity, blocking the downstream signaling pathways that lead to EMT. In addition to targeting cancer cells, Mφ-SDNP also prevents the polarization of macrophages to the M2 type. Treatment with Mφ-SDNP results in a significant decrease in mRNA and protein levels of EMT markers. Furthermore, it increases the population of cytotoxic T lymphocytes within the tumor, thereby enhancing the effectiveness of immune checkpoint inhibitors (Kim et al., 2023).
In colorectal cancer (CRC), TAM-derived TGF-β is known to escalate EMT by enhancing phosphorylated SMAD2/3 and SMAD4 levels, exemplifying the crucial role of TAMs in this regulatory cascade (Cai et al., 2019). Notably, it also has been implicated in augmenting HIF-1α levels, leading to the upregulation of tribbles pseudokinase 3 (TRIB3), which in turn activates the Wingless/Integrated (Wnt)/β-catenin pathway, thus propelling EMT and lung metastasis (Liu C. et al., 2021). Besides, collagen triple helix repeat containing 1 (CTHRC1), a protein highly expressed in CRC, can upregulate chemokine (C-C motif) ligand 15 (CCL15) via the TGF-β/Smad signaling pathway, enhancing TAM infiltration and creating a feedback loop that further propels the disease progression (Liu et al., 2024). In cholangiocarcinoma, TAM-secreted TGF-β has been shown to activate Gli2, intensifying EMT processes and maintaining endoplasmic reticulum (ER) homeostasis (Chen Z. et al., 2023). The anticancer agent emodin demonstrates potential in curtailing TGF-β secretion by TAMs, consequently mitigating TAM-induced EMT and cellular stemness (Liu et al., 2020a).
3.1.1.2 Chemotherapy resistance
TAM-secreted TGF-β fosters chemotherapy resistance, with prolonged exposure linked to elevated EMT, stemness, and drug resistance (Katsuno et al., 2019). For instance, in esophageal squamous cell carcinoma (ESCC), TAM-derived TGF-β has been associated with cisplatin resistance through the enhancement of cancer stem cell (CSC) properties (Yang et al., 2023b). Conversely, there is evidence that certain pharmaceutical agents can modulate TAM behavior to counteract these effects. Simvastatin, a cardiovascular drug, shows promise in re-polarizing TAMs towards an anti-tumorigenic phenotype and reducing TGF-β secretion, decreasing EMT and increasing lung cancer cell sensitivity to paclitaxel (Jin et al., 2019).
3.1.1.3 Feedback loop and regulation mechanisms
TAMs reinforce TGF-β feedback loops within cancer and stromal cells, amplifying EMT and cancer progression. For instance, the E3 ubiquitin ligase RAD18 has been shown to strengthen a positive feedback loop between triple-negative breast cancer (TNBC) cells and macrophages mediated by TGF-β, thereby increasing cellular stemness (Yan et al., 2022). TGF-β also prompts fibroblast growth factor 2 (FGF2) secretion in CAFs, which subsequently influences TAM polarization in gastric cancer (GC), significantly propelling migration, invasion and CSC traits (Li et al., 2023a). In bladder cancer, cancer cells prompt TAMs to secrete TGF-β, facilitated by the HIF-1α signaling pathway—a response linked to aerobic glycolysis within the tumor milieu. In a reciprocal interaction, TGF-β released by TAMs fosters glycolysis in bladder cancer cells via the Smad2/3 signaling pathways. This interaction not only amplifies the CSCs but also promotes EMT process (Shen et al., 2023).
3.1.1.4 Receptor modulation
Additionally, TAMs alter the ratio of TGF-β receptors on neoplastic cells, modulating EMT induction. In PDAC, for instance, TAMs have been observed to downregulate the expression of TGF-β receptor 3 (TGFBR3) while concurrently upregulating TGFBR1 and TGFBR2. This modulation in receptor expression enhances the sensitivity of PDAC cells to TGF-β, thereby facilitating EMT through increased SMAD3 signaling and the subsequent upregulation of EMT-TFs (Yin et al., 2019).
3.1.1.5 ECM stiffening
In BC, macrophage-derived TGF-β is a key player in stimulating the transdifferentiation of fibroblasts and inducing the expression of collagen crosslinking enzymes such as lysyl oxidase (LOX) and lysyl hydroxylase 2 (LH2). The activity of these enzymes not only increases the tensile strength and structural integrity of the collagen network but also contributes to the pathological stiffening of the tumor stroma. A positive correlation exists between the TAM marker CD163 and collagen crosslinking enzymes LOX and PLOD2, but not LOXL2, suggesting a regulatory relationship between TAMs and ECM stiffening. This relationship underscores the critical role of macrophage-secreted TGF-β in shaping the extracellular matrix and influencing tumor metastasis in BC (Maller et al., 2021).
3.1.1.6 Stromal cell modulation
TAMs also augment TGF-β production and modulate the functions of other stromal cells to advance tumor progression. Studies have highlighted that TAMs secrete TGF-β1, which plays a pivotal role in the differentiation of CAFs from mesenchymal stromal cells (MSCs), as well as in enhancing their synthesis of ECM proteins. These CAFs, in turn, secrete TGF-β1, contributing to the induction of EMT and the development of drug resistance in neuroblastoma cells (Louault et al., 2022a). Targeting the TGF-β1/IL-6 pathway has been shown to reduce tumor burden and metastasis in vivo while enhancing neuroblastoma cell sensitivity to chemotherapy, underscoring the therapeutic potential of interrupting this pathway (Louault et al., 2022b).
3.1.2 IL-6 family signaling
IL-6, secreted by TAMs, is recognized for its pro-inflammatory role within TME, particularly under hypoxic conditions and in response to inflammatory stimuli. The importance of IL-6 as a biomarker in various cancers is increasingly recognized, highlighting its role in oncogenesis and tumor progression (Zhang et al., 2020; Unver and McAllister, 2018), with TAM-derived IL-6 pivotal in activating the STAT3 cascade, thereby upregulating EMT-TFs and propelling tumor progression (Siersbaek et al., 2020; Huang et al., 2022).
3.1.2.1 Mechanisms of IL-6 elevation
3.1.2.1.1 YY1 transcriptional complex
For example, in M2-like macrophages, the yin yang 1 (YY1) transcriptional complex amplifies IL-6 expression through long-range chromatin interactions between an M2-specific IL-6 enhancer and the IL-6 promoter. Regulation of YY1 by the IL-4/STAT6 pathway, along with the phase separation of the YY1 complex during M2 macrophage polarization, are key mechanisms in the elevation of IL-6 expression (Chen et al., 2023c).
3.1.2.1.2 Metabolic influence
In oral squamous cell carcinoma (OSCC), the glycolytic enzyme alpha-enolase (ENO1) is released into the TME, eliciting an IL-6 surge in TAMs that bolsters ENO1 expression and propels the EMT process (Lin Y. et al., 2023). Additionally, lactate, a prevalent metabolic byproduct in the TME, induces lactylation of retinoic acid receptor gamma (RARγ) in macrophages, leading to increased IL-6 secretion and activation of the oncogenic STAT3 signaling pathway (Li X.-M. et al., 2024).
3.1.2.2 Pro-inflammatory role of IL-6
The IL-6 family, known for its pro-inflammatory properties, empowers M1 phenotype TAMs to drive tumor progression. In OSCC, M1-like TAMs are characterized by a significant surge in IL-6 secretion, which enhances EMT and fosters cellular stemness. This effect is mediated through the IL-6/STAT3/thrombospondin 1 (THBS1) signaling pathway, leading to an upregulation of MMP14 expression (You et al., 2022). Additionally, the upregulation of high mobility group box 1 (HMGB1) in OSCC has been observed to induce M1 polarization of macrophages. This polarization leads to an augmented secretion of IL-6, which furthers migration and invasion capabilities of OSCC cells via the activation of the NF-κB/IL-6 signaling axis, highlighting a critical mechanism through which pro-inflammatory M1 TAM signals contribute to cancer aggressiveness (Jiang et al., 2024).
3.1.2.3 Feedback loop and regulation mechanisms
In CRC, exosomes from tumor cells, rich in circular RNAs, activate TAMs’ NF-κB pathway, leading to IL-6 secretion and triggering tumor cells’ Janus kinase 2 (Jak2)/STAT3 pathway. This enhances chemokine CCL2 levels, contributing to EMT and macrophage recruitment (Zhou et al., 2023). Likewise, TAMs conditioned by CRC notably elevate IL-6 secretion, activating the Jak2/STAT3 pathway and indirectly boosting forkhead box Q1 (FoxQ1) expression in cancer, thus reinforcing EMT and cancer stem cell attributes (Wei et al., 2019a). Intriguingly, in CRC, macrophages amplify IL-6 production from cancer cells themselves, suggesting that a substantial portion of IL-6 present in CRC’s TME might primarily be derived from the tumor cells rather than the macrophages (Gao et al., 2018). Besides, IL-6 is capable of inducing its own expression in both macrophages and gastric epithelial cells, creating an autocrine and paracrine positive feedback loop. This loop is further potentiated by H. pylori stimulation, which may initially induce an M1 polarization characterized by an inflammatory phenotype. However, chronic infection with Helicobacter pylori can lead to a shift towards M2 polarization, driven by continuous IL-6 production, highlighting the dynamic nature of TAM polarization and its impact on cancer progression (Yu et al., 2024).
3.1.2.4 Other pathways
The DNMT family, especially DNMT1, influences TAM polarization, with TAM-induced IL-6/STAT3/ZEB1 signaling promoting DNMT1 expression in BC, correlated with elevated DNMT1, CD163, and ZEB1 expression in patients’ breast tissues (Li Z. et al., 2022). Besides, BC cells release exosomal circular RNA cSERPINE2, which is taken up by TAMs. This interaction leads to an increase in MALT1 (mucosa-associated lymphoid tissue lymphoma translocation protein 1) levels within TAMs, subsequently activating the NF-κB pathway. Activation of this pathway triggers the secretion of IL-6 by TAMs, which then enhances the levels of eukaryotic initiation factor 4A3 (EIF4A3) and the chemokine CCL2 in tumor cells. This cascade establishes a positive feedback loop that not only increases the biogenesis of cSERPINE2 within tumor cells but also promotes further recruitment of TAMs and facilitates the invasion capabilities of BC cells (Boxuan Zhou et al., 2023).
In lung cancer, IL-6 mediates interactions between cancer cells and microglia via Jak2/STAT3 signaling, aiding brain metastasis, a significant mortality driver. Brain-metastatic lung cancer cells secrete IL-6, prompting microglia’s M2 polarization, which facilitates metastatic colonization (Jin et al., 2022). TAMs secrete IL-6, which activates the JAK2/STAT3 pathway through autocrine secretion in TAMs. This activation leads to the stimulation of CCAAT/enhancer-binding protein β (C/EBPβ), which in turn promotes the transcription and further expression of IL-6, thus creating an IL-6-STAT3-C/EBPβ-IL-6 positive feedback loop. This loop is significant in perpetuating the inflammatory and tumor-promoting environment within the TME (Hu et al., 2024). Targeting IL-6 signaling, particularly through dual STAT3 and IL-6R inhibition, emerges as a viable strategy to restrict migration and metastasis (Hu et al., 2024; Méndez-Clemente et al., 2022).
3.1.2.5 OSM-another key IL-6 family member in tumor progression
OSM, characterized by its pro-inflammatory properties, plays a pivotal role in the dynamics between TAMs and tumor cells (Masjedi et al., 2021). OSM is abundantly expressed in M2-polarized THP-1 derived macrophages, triggering the STAT3 signaling pathway to initiate EMT in BC (Guo et al., 2013). In glioblastoma, OSM released by TAMs is linked to the enhancement of mesenchymal-like (MES-like) cellular states, correlating with increased tumor aggression and poorer clinical outcomes (Weidner et al., 2023). In early-onset tongue cancer, areas that exhibit vascular mimicry show a high prevalence of macrophage markers, including OSM, suggesting its role in supporting tumor growth and enhancing metastatic potential (Marina et al., 2023).
In BC, Gr1+CD11b+ cells, educated by the tumor, secrete OSM and IL6, significantly expanding the metastatic SCA1+ cell population within the cancer (Peyvandi et al., 2024). Mechanistically, OSM has been shown to induce a mesenchymal phenotype in PDAC cells through the activation of the mitogen-activated protein kinase (MAPK) signaling pathway. This activation is pivotal for the stemness traits induced by OSM, as evidenced by comprehensive transcriptomic analyses. The perpetuation of this effect involves a feed-forward loop, where OSM stimulates transcriptional upregulation of the OSM receptor (OSMR), thus reinforcing the mesenchymal phenotype and stemness characteristics in PDAC cells (Polak et al., 2023).
Recent studies have explored the interplay mediated by OSM between TAMs and other stromal cells, such as CAFs. OSM secreted by TAMs has been found to stimulate the expression of inflammatory genes in CAFs, supporting tumor survival and promoting metastasis. In PDAC mouse models, the absence of Osm significantly reduces the metastatic spread of the tumor, underlining the critical role of OSM in tumor progression (Lee et al., 2021). In BC, OSM produced by myeloid cells, including TAMs, activates OSMR on CAFs, inducing secretion of chemokines such as CXCL1 and CXCL16, which attract more TAMs to the tumor site, reinforcing a pro-inflammatory feedback loop that facilitates lung metastasis (Araujo et al., 2022). Recent reports highlight the significance of heterocellular OSM-OSMR signaling in promoting tumor metastasis through TAMs, tumor cells, and other stromal cells intercellular communication (Figure 2).
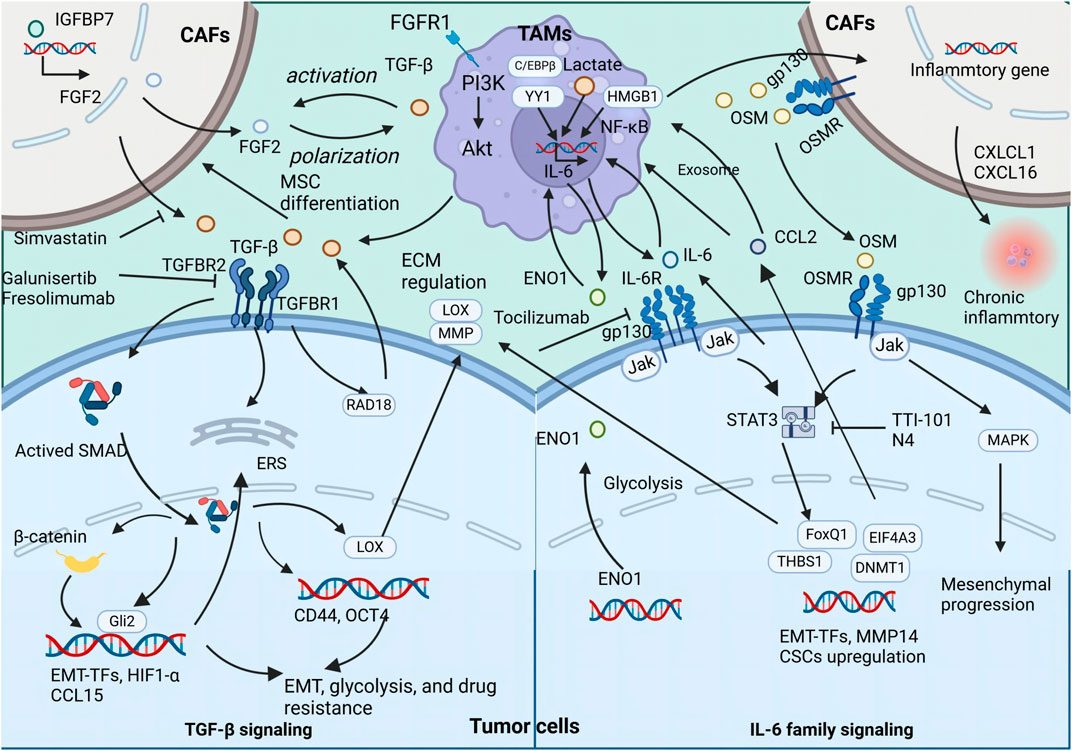
Figure 2. The secretion of TGFβ, IL-6, and OSM by TAMs exert both direct and indirect influences on the promotion of tumor metastasis and CSC properties The illustration depicts the signaling cascade of TAM-secreted TGFβ, IL-6, and OSM that promotes EMT and augments the stemness of neoplastic cells.
3.1.3 Chemokine signaling
Chemokines derived from TAMs are increasingly recognized as critical facilitators of EMT in malignant cells, drawing significant attention for their potential as targets in novel therapeutic strategies aimed at halting tumor progression. These chemokines, emanating from diverse cellular subsets within the TME—including immune, endothelial, and stromal cells (Ozga et al., 2021) (Kumari and Choi, 2022).
3.1.3.1 CCL18
3.1.3.1.1 Receptor and mechanism
Phosphatidylinositol transfer protein, membrane-associated 3 (PITPNM3), has been identified as the receptor for CCL18, a chemokine linked with EMT induction. Subsequent investigations have unveiled the role of CCL18-PITPNM3 signaling in promoting extracellular matrix adherence and cellular migration in BC (Chen et al., 2011). Recent findings indicate that CCL18, upregulated in M2 macrophages, facilitates metastasis in squamous cell carcinoma of the head and neck (SCCHN) (She et al., 2018). The underlying mechanisms of CCL18-induced EMT in SCCHN involve activation of the metadherin (MTDH)/NF-κB cascade (Qin et al., 2019). Moreover, TAM-derived CCL18 can stimulate Annexin A2 (AnxA2), which then activates the EMT through the PI3K/Akt/GSK3β/Snail pathway in BC, and similar activation of PI3K/Akt in gallbladder cancer (GBC) promotes cell migration (Zhao et al., 2019; Zhou et al., 2018).
In ESCC, advanced analyses integrating single-cell transcriptomic sequencing with bulk microarray data have uncovered that CCL18, released by TAMs, drives tumor cell proliferation via the JAK2/STAT3 signaling pathway, correlating with a poorer prognosis in ESCC (Sui et al., 2023). Further investigations have identified the immune responsive gene 1 (IRG1) as a regulator of CCL18 secretion by TAMs. It has been found that the overexpression of IRG1 in macrophages leads to a reduction in CCL18 levels. This decrease in CCL18 secretion results in the suppression of malignant behaviors in intrahepatic cholangiocarcinoma (ICC) cells, suggesting a promising therapeutic avenue (Zhou et al., 2024).
3.1.3.1.2 Feedback loop and regulation mechanisms
In HNSCC, TAMs expressing SPP1 give rise to a pro-angiogenic SPP1+CCL18+ TAM subset, implicated in tumor growth and metastasis. These SPP1+CCL18+ TAMs are associated with increased tumor growth and metastasis, primarily due to their high expression levels in metastasis-associated and EMT pathways (Wu et al., 2024). Furthermore, in the context of perineural invasion (PNI) in PDAC, elevated CCL18 levels in TAMs engage in paracrine interactions with Schwann cells, integral to the neural microenvironment, thereby facilitating PDAC progression (Zhang et al., 2024).
A notable feedback loop involving CCL18 in ovarian cancer has been shown to increase metastasis through a cascade beginning with CCL18-induced ZEB1 upregulation in cancer cells, which in turn leads to increased secretion of M-CSF. The heightened levels of M-CSF contribute to an enhanced TAM phenotype, fostering a TME conducive to cancer metastasis. The interplay among CCL18, ZEB1, and M-CSF accelerates the metastatic process (Long et al., 2021). CCL18 also mediates communication between TAMs and CAFs in BC, fostering chemoresistance. CCL18 promotes the transition of CAFs towards a CD10+ GPR77+ phenotype, a change associated with the acquisition of stemness properties and chemoresistance (Zeng et al., 2022).
3.1.3.2 CCL2
The role of CCL2 varies depending on the tumor stage. It can promote or suppress tumors based on the immune cells it recruits, such as TAMs, NK cells, and T cells (Archer et al., 2023).
3.1.3.2.1 Mesenchymal promotion mechanisms
In TNBC, CCL2 is known to activate Akt signaling, leading to β-catenin nuclear translocation and promoting both stemness and EMT through the CCL2/Akt/β-catenin pathway (Chen X. et al., 2022). The impact of this signaling pathway is further magnified by the direct interaction between β-catenin and the CCL2 promoter, which amplifies the characteristics of breast cancer stem cells, thus potentiating the aggressive nature of TNBC (Zhang F. et al., 2021; Tigue et al., 2023). Moreover, CCL2 secreted by TAMs has been linked to the induction of tamoxifen resistance in breast cancer cells through the PI3K/Akt/mammalian target of rapamycin (mTOR) pathway (Li et al., 2020).
In renal cell carcinoma, CCL2 secreted by M2 macrophages increases muscleblind like splicing regulator 2 (MBNL2) expression, which in turn stabilizes B-cell lymphoma 2 (Bcl-2) mRNA, leading to the inhibition of Beclin 1-dependent autophagy and endowing RCC cells with invasion properties (He et al., 2023).
3.1.3.2.2 Feedback loop and regulation mechanisms
CCL2 also serves as a chemokine for TAMs, enhancing their pro-tumoral functions and infiltration. For instance, Wnt5a + TAMs activate the Wnt5a-CaMKⅡ-ERK cascade, increasing CCL2 production and enhancing TAMs and CRC cells migration in vitro. (Liu et al., 2020b). In NSCLC, a feedback loop involving circHSPB6, let-7a-2-3p, and CCL2 promotes TAM polarization to the M2 phenotype, supporting infiltration and fostering EMT (Li et al., 2023b). In ovarian cancer, MYBL2 activates CCL2 transcription, recruiting macrophages and promoting their M2 polarization (Pan et al., 2023).
3.1.3.2.3 Therapeutic potential
Given these dynamics, combination therapy involving CCR2 antagonists and anti-PD-1 antibodies has shown greater efficacy in suppressing tumor growth and lung metastasis in solid cancer compared to control or monotherapy, highlighting the potential for targeted intervention in CCL2-mediated pathways to enhance cancer treatment outcomes (Weng et al., 2024; Lin et al., 2024).
3.1.3.3 CCL22
3.1.3.3.1 Mesenchymal promotion mechanisms
Recent studies have illuminated the role of CCL22, particularly its elevated expression in TAMs, in activating diverse signaling pathways that enhance the metastatic capabilities of tumor cells.
In ESCC, for instance, TAM-derived CCL22 can hyperactivate focal adhesion kinase (FAK) in ESCC cells by promoting the formation of a complex with diacylglycerol kinase alpha (DGKα). This activation leads to increased tumor cell migration and invasion, which can be mitigated by pharmacological inhibition of FAK or by employing anti-CCL22 treatments (Chen J. et al., 2022). Furthermore, CCL22 has been found to stimulate DGKα activity in tumor cells, suppressing NADPH oxidase 4 (NOX4) and averting cisplatin-induced ROS overproduction. This mechanism is closely tied to the development of chemoresistance in ESCC (Chen et al., 2024). Additionally, the activation of RSK4 in ESCC cells can elevate CCL22 expression, enhancing the release of soluble ICAM-1 and indirectly influencing STAT3 phosphorylation. Experiments with recombinant CCL22 and neutralizing antibodies have demonstrated significant alterations in the expression of EMT markers and tumor cell invasion (He et al., 2024). Moreover, TAM-secreted CCL22 can activate FAK, which subsequently mediates the phosphorylation of Gli1 at specific residues, boosting Gli1’s transcriptional activity and propelling ESCC progression. TAMs positive for CCL22 markedly enhance the invasive capacity and anchorage-independent growth of ESCC cells (Chen J. et al., 2023). Pexidartinib targets the M-CSFR to inhibit TAMs, leading to a reduction in CCL22 production. This mechanism underpins the rationale for clinical trials exploring the use of pexidartinib (Zhang et al., 2023a).
3.1.3.3.2 Regulation mechanisms
Hypoxia increases CCL22 expression in TAMs, which significantly boosts the migration and lung metastasis of TNBC cells via the CCL22/CCR4 axis (Zhao et al., 2022). CCL22 expression by macrophages is modulated through the IL-4/STAT6 signaling pathway, which is pivotal for T cell differentiation into Th2 cells and crucial for CCL22 and VEGF-C expression in tongue squamous cell carcinoma, contributing to lymph node metastasis (Kimura et al., 2021). In osteosarcoma, M2 macrophages promote cell migration and EMT, with CCL22 expression being notably high in LINC00662 exosome-treated M2 macrophages. The neutralization of CCL22 using a specific antibody has been shown to reverse these pro-tumorigenic effects, suggesting a therapeutic approach to mitigating cancer progression by targeting CCL22 (Zhang Y. et al., 2023). Additionally, TAM-secreted TGF-β induces CCL22 expression via c-Fos, facilitating the recruitment of regulatory T cells and establishing a feedback loop with IL-8 and TGF-β that enhances CCL22 secretion in TAMs (Wang et al., 2019).
3.1.3.3.3 Therapeutic potential
CCL22 also plays a crucial role in CRC chemoresistance by activating the PI3K/Akt pathway, thereby reducing the effectiveness of 5-fluorouracil in curbing tumor growth (Wei et al., 2019b).
3.2 Interaction with CAFs
CAFs, a distinct group within the tumor stroma, play a crucial role in facilitating EMT and the metastatic spread of cancer (Yang et al., 2022). TAMs interact with CAFs in numerous ways, significantly influencing tumor behavior and treatment response (Gunaydin, 2021b). A noteworthy interaction involves TAMs secreting CCL18, which engages CAFs expressing CD10 and GPR77. This interaction is associated with the promotion of CSC characteristics and the development of chemotherapy resistance in BC (Zeng et al., 2022).
3.2.1 OSM-OSMR signaling
OSM-OSMR signaling pathway is crucial in the reprogramming of CAFs, thereby significantly impacting tumor metastasis, particularly in PDAC. OSM, produced by macrophages, induces CAFs to secrete IL-6 and CCL2. These cytokines then activate key signaling pathways, specifically the PI3K/Akt and Jak/STAT pathways, which play a critical role in promoting EMT (Lee et al., 2021). Furthermore, CAFs mitigate the pro-inflammatory effects of the chemotherapy regimen FOLFIRINOX on CD200R+CD209+ macrophages, limiting cell death and reinforcing the M2 phenotype of TAMs (Hussain et al., 2024).
3.2.2 Reciprocal interactions and recruitment
The interplay between TAMs and CAFs is reciprocal, with the influence of CAFs on TAMs becoming increasingly apparent. CAFs actively recruit macrophages to the TME by secreting a variety of chemokines, leading to a significant co-infiltration of CAFs and TAMs, which is often correlated with tumor progression. In OSCC, for instance, CAFs have been observed to recruit monocytes via the CXCL12/CXCR4 axis (Li et al., 2019). Additionally, CAFs can induce an M2-like polarization in TAMs, with both tumor-conditioned and CAF-conditioned mediums synergistically enhancing the expression of CD206 and IL-10 in TAMs. This synergistic effect is largely attributed to factors secreted by CAFs, notably GM-CSF and IL-6 (Cho et al., 2018). Moreover, CAFs also have the capability to elevate ROS levels in TAMs, further promoting their polarization toward an M2 phenotype (Pakravan et al., 2022). Furthermore, TAMs establish crucial interactions with CAFs through the CD74/macrophage migration inhibitory factor (MIF) pathway. This interaction is pivotal for the recruitment and polarization of TAMs within the TME. In addition, TAMs plays a significant role in attracting regulatory B cells (Bregs) that express PD-L1, utilizing the CXCL12/CXCR4 axis (Lian et al., 2024).
3.2.3 Transformation of noncancerous fibroblasts
Mixed-polarized macrophages, expressing both M1 and M2 markers, activate noncancerous fibroblasts (NFs) to transform into cancer-associated fibroblast-like (CAF-like) cells, which in turn promote the malignant phenotype of diffuse-type gastric cancer (DGC) cells. IL-1β, released by these mixed-polarized macrophages, triggers NF-kB signaling in NFs, catalyzing their activation and subsequent transdifferentiation into CAF-like cells. This process is evidenced by the nuclear translocation of p65 and a surge in the expression of fibroblast activation protein alpha (FAP) (Zhang et al., 2023c).
3.2.4 TAM-endothelial cell (EC) interaction
Moreover, the reciprocal interaction between TAMs and ECs is critical within the TME, especially in facilitating intratumoral angiogenesis. Recent advancements in biomimetic 3D models have shown that TAM-EC communication relies on direct contact, with M2-like TAMs increasing the secretion of anti-inflammatory cytokines, thereby fostering invasion in glioblastoma (Figure 3) (Cui et al., 2018).
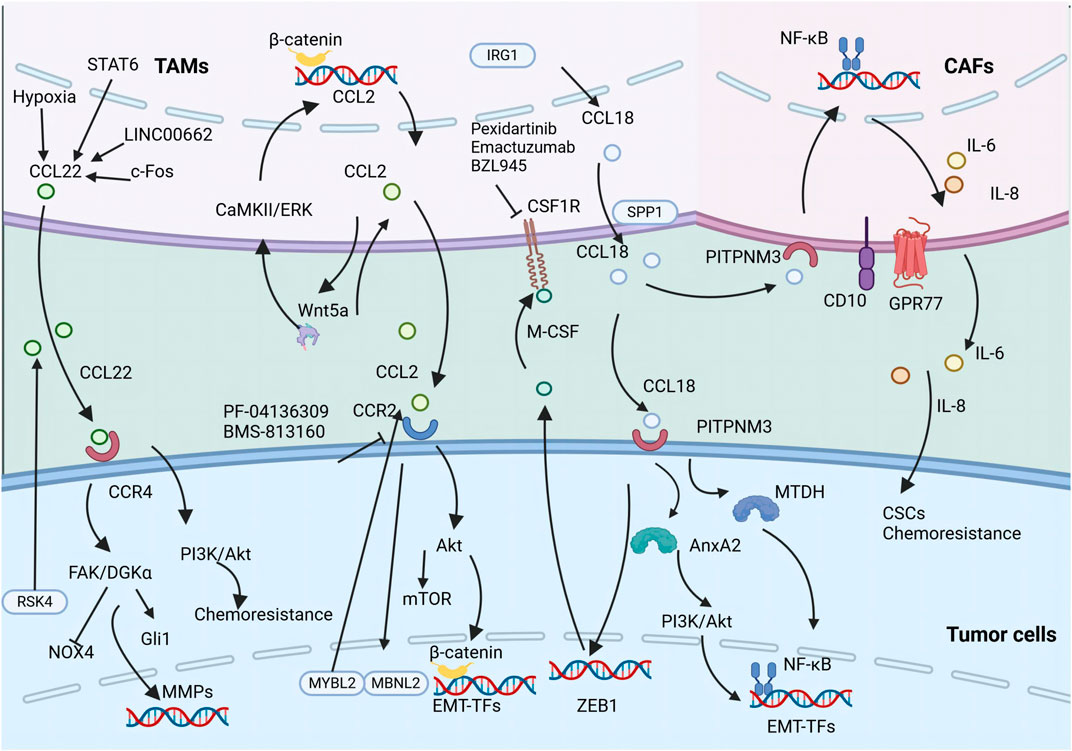
Figure 3. The secretion of various chemokines including CCL18, CCL2, and CCL22 by TAMs promotes tumor metastasis and CSC properties. The illustration depicts the signaling cascade of TAM-secreted CCL18, CCL2, and CCL22 that promotes EMT and augments the stemness of neoplastic cells.
4 TAM-induced EMT: Shaping cancer diversity
The intersection of TAMs with the EMT process underscores a fundamental aspect of tumor biology, revealing a vast heterogeneity across various cancers (Feng et al., 2022). In this analysis, we aim to dissect the complex relationships between TAMs and EMT within four specific cancer contexts, each chosen for its unique clinical challenges and the significant role TAMs play in their progression.
1. TNBC is characterized by its aggressive nature and a conspicuous lack of targeted therapies. This section will elucidate the contribution of TAMs to TNBC progression, with a particular focus on their involvement in EMT mechanisms.
2. The resistance to EGFR-TKI in lung cancer represents a significant clinical hurdle. Our discussion will center on how TAMs influence this resistance, potentially through EMT modulation.
3. The fibrotic microenvironment, a hallmark of pancreatic cancer, is significantly shaped by pro-fibrotic cytokines such as TGF-β and OSM, which are instrumental in the pathology of this malignancy. We aim to explore the impact of TAMs within this context, specifically their influence on EMT processes.
4. Androgen deprivation therapy (ADT) is a fundamental component of prostate cancer treatment, yet its long-term efficacy remains limited. This investigation will focus on how TAMs interact with the prostate cancer microenvironment in the context of ADT, particularly regarding their influence on EMT and disease progression.
4.1 Breast cancer——Entry point for TNBC
TNBC is a particularly aggressive subtype of BC, characterized by the absence of estrogen receptors, progesterone receptors, and the human epidermal growth factor receptor 2 (HER2). This lack of receptors renders TNBC without specific targets for established therapies, significantly complicating treatment efforts. Consequently, there has been a heightened focus on exploring the interactions between TAMs and TNBC cells to identify new therapeutic avenues. Given the critical role TAMs play in TNBC progression, macrophage-targeting immunotherapy emerges as a promising strategy (Fernandez et al., 2023).
4.1.1 Epigenetic reprogramming of TAMs
In TNBC, TAMs compared to BMDMs and MGMs showed distinct DNA methylation patterns. These methylation patterns retain imprints from their monocytic origins but undergo cancer-specific epigenetic changes. Key transcription factors such as FOSL2, STAT1, and RUNX3 are involved in this epigenetic reprogramming, which correlates with more severe tumor grades and poorer prognoses in breast cancer patients (Hey et al., 2023). Additionally, a significant negative correlation exists between the TAM marker CD163 and E-cadherin expression in TNBC, indicating that higher TAM presence is associated with worse patient outcomes (Zhang et al., 2018).
4.1.2 Chemokine-mediated EMT
TAM-secreted chemokines, particularly within the CXCL8/CXCR2 axis, play a crucial role in facilitating EMT processes in TNBC. The antagonism of CXCR2, for example, with Danirixin, presents a potential therapeutic strategy to counteract this mechanism (Nie et al., 2021). Additionally, Additionally, CCL2 released by M2 macrophages activates β-catenin, enhancing cancer stemness and promoting the EMT process in TNBC (Chen X. et al., 2022). This CCL2/β-catenin signaling loop highlights the complex interplay between TAM signaling and TNBC progression (Zhang F. et al., 2021). Furthermore, the endogenous protein visfatin, produced by TNBC cells, recruits and polarizes macrophages. These TAMs subsequently augment tumor cell mobility and self-renewal capacity through the secretion of CXCL1 (Wang et al., 2020).
4.1.3 VEGFA and cancer stemness
TAMs contribute significantly to the complexity of the TME in TNBC by secreting VEGFA. This secretion interacts with TNBC cells through the neuropilin-1 (NRP-1) receptor, activating the GAPVD1/Wnt/β-catenin signaling pathway, thereby enhancing the cancer stemness of TNBC cells. This process fuels tumor progression and metastasis while also promoting the further polarization of macrophages towards the M2 pro-tumorigenic phenotype. The release of VEGFA by M2-like TAMs and its subsequent actions establish a feedback loop that exacerbates the metastatic capabilities of TNBC (Wang L. et al., 2024).
4.1.4 Hippo-YAP pathway
The Hippo signaling pathway, particularly the YAP, is aberrantly expressed in both TAMs and TNBC cells, emerging as a pivotal player (Xu et al., 2022; Yang et al., 2020). Research reveals that TNBC cells can upregulate YAP expression in TAMs through otu deubiquitinase 5 (OTUD5)-dependent deubiquitination and stabilization of YAP. This overexpression leads to M2 macrophage polarization and enhances metastatic capabilities via the CCL2/CCR2 pathway in TNBC (Zhang et al., 2021c). Furthermore, the activation of the Hippo-YAP pathway in TNBC cells, driven by RAD18, stimulates cancer cell proliferation and invasion. This pathway activation also correlates with increased expression of the M2 macrophage marker CD163 in TAMs, further underlining the contribution of the Hippo-YAP axis to TNBC progression (Yang et al., 2020). In a reciprocal manner, M2-polarized TAMs secrete TGF-β to activate RAD18, thus promoting cancer stemness and establishing a feedback loop. Disrupting this loop can suppress cancer stemness, highlighting the critical interplay between RAD18, YAP, and TGF-β in TNBC CSC characteristic (Yan et al., 2022).
4.1.5 GLUT3 and glycolytic activity
Recent studies have underscored the significant role of the GLUT3 in TNBC metastasis. The endogenous expression of GLUT3 directly promotes the EMT process. When GLUT3 expression is upregulated, it enhances the glycolytic activity of TNBC cells, leading to increased lactate production. This lactate stimulates TNBC cells to secrete CXCL8, inducing a phenotype in macrophages that resembles M1-like pro-inflammatory macrophages. Once activated, these M1-like macrophages contribute to further upregulation of GLUT3 expression, creating a feedback loop that sustains high glycolytic activity and EMT in TNBC cells (Tsai et al., 2021).
4.1.6 Targeted therapy innovations
The mannose receptor serves as a crucial target for directing therapies towards TAMs in TNBC. Innovative theranostic nanoformulations leverage the interactions between mannose and its receptor to specifically target TAMs in the acidic environment characteristic of TNBC tumors. These nanoformulations are engineered with an acid-sensitive polyethylene glycol (PEG) layer that sheds to reveal mannose ligands upon exposure to the acidic TME. One such application involves DOX (doxorubicin)-loaded nanovehicles, which have shown significant promise in targeting TAMs and improving therapeutic outcomes (Scialla et al., 2023). Additionally, cutting-edge nanocomplexes designed to react to the acidic and oxidative tumor environment can facilitate the release of miR-155. This release encourages a shift towards an M1-like phenotype in TAMs and fosters the maturation of tumor-infiltrating dendritic cells (TIDCs), thereby amplifying the anti-tumor immune responses in TNBC (Jing et al., 2023).
4.1.7 Role of MGRTMs in early TNBC
Notably, research has identified that in the early stages of TNBC, the pro-tumoral macrophages are predominantly mammary gland tissue-resident macrophages (MGRTMs), rather than monocyte-derived TAMs. MGRTMs, characterized as F4/80+FOLR2+CD206+CADM1-, stimulate in vitro proliferation and enhance in vivo angiogenesis. Local depletion of MGRTMs has been shown to reduce metastatic potential (Hirano et al., 2023b).
4.1.8 Inflammatory breast cancer (IBC)
IBC, an exceptionally malignant form of BC, is characterized by its aggressive nature, stem cell-like traits, and high propensity for metastasis, distinguishing it from other subtypes (Lim et al., 2018). Co-cultivation of IBC cells with monocytes significantly enhances CSC characteristics. IBC cells attract and differentiate monocytes into M2 TAMs, which release large amounts of IL-8 and growth-related oncogene (GRO). This release activates the STAT cascade in IBC cells, further exacerbating the malignant phenotype (Valeta-Magara et al., 2019).
4.2 Lung cancer——EGFR-TKIs and oncometabolites
4.2.1 Role of TAMs in EGFR-TKI resistance
In NSCLC, particularly in adenocarcinoma subtypes common among non-smokers or light smokers, mutations in the epidermal growth factor receptor (EGFR) are a primary oncogenic driver (Vyse and Huang, 2019). Emerging evidence implicates TAMs in facilitating the EMT process in NSCLC, thereby contributing to resistance against EGFR-TKIs. Specifically, in the context of EGFR-TKI resistance, TAMs exhibit M2-like reprogramming and reduced phagocytosis. This reprogramming correlates with the resistance observed in gefitinib-resistant lung cancer cells and tumor xenografts (Lu et al., 2023).
4.2.2 Activation and recruitment of TAMs
The activation of EGFR signaling in tumor cells initiates a cascade that leads to the recruitment of TAMs, which subsequently promote the upregulation of EMT-TFs in NSCLC (Ravi et al., 2016; Zhu et al., 2019). An intriguing finding relates to the dual effect produced by the activation of cannabinoid receptor 2 (CB2) through the compound JWH-015. This activation appears to counteract the pro-tumorigenic signaling mediated by M2-like TAMs, specifically inhibiting EGFR/ERK/STAT3 signaling and N-cadherin expression, both promoted by M2-like TAMs. Moreover, JWH-015 reduces ARG1 expression in TAMs (Ravi et al., 2016).
A recent study has highlighted the role of TAM-derived IL-6, particularly in EGFR mutant NSCLC, revealing that IL-6 can augment the immunotherapy resistance of tumor cells with EMT-associated resistance to EGFR-TKI (Wang et al., 2023). Significantly, TAMs have been identified as the primary source of IL-6, underscoring their crucial role in the resistance mechanism and highlighting potential therapeutic targets to overcome resistance in EGFR-TKI therapy.
4.2.3 Phagocytic checkpoints
The Mer receptor tyrosine kinase (MERTK) present on macrophages acts as a phagocytic checkpoint and is associated with EGFR-TKI resistance in NSCLC. Elevated MERTK expression in both tumor cells and macrophages contributes to TKI resistance by promoting cell polarity and stemness in tumor cells while sending 'do not eat me’ signals that help maintain an immunosuppressive TME (Chen and Liu, 2021). Another critical phagocytic checkpoint is SIRPα. TAMs express high levels of SIRPα, which is positively correlated with IL-6 expression. The SIRPα-IL-6 axis forms a self-reinforcing feedback loop, where each component upregulates the other through STAT3 signaling in macrophages (Wang et al., 2023).
4.2.4 Resistance to osimertinib
Resistance to the third-generation EGFR-TKI, osimertinib, presents a formidable challenge in treating certain cancers. Research has highlighted the roles of IL-6, the EMT process, and TAM activity in contributing to this resistance. Studies utilizing RNA sequencing and immune infiltration analysis have revealed an association between increased macrophage infiltration, particularly M0 and M2 macrophages, and resistance to osimertinib in patients (Han et al., 2023). The EMT process in tumor cells has been identified as a key mechanism behind osimertinib resistance. The involvement of TAMs in inducing EMT underscores a potential therapeutic target; by mitigating TAM-induced EMT, it may be possible to overcome resistance to osimertinib and potentially other TKIs (Jiang et al., 2022; Yan et al., 2023).
4.2.5 Innovative approaches
One mechanism by which tumor cells develop TKI resistance involves the upregulation of CD47, helping them evade macrophage-mediated phagocytosis—a key way macrophages can contribute to overcoming drug resistance (Lu et al., 2023). Innovative approaches, including the use of STING agonists and anti-CD47 monoclonal antibodies, are being explored to reprogram TAMs to combat this resistance effectively (Lu et al., 2023). TAMs can suppress IFNγ and Granzyme B production in CD8 T cells. MSA-2, a STING agonist, triggers IFNβ production in TAMs, further emphasizing the importance of macrophages in the STING-mediated antitumor response (Lin Z. et al., 2023).
4.2.6 Metabolic interplay and resistance
The metabolic interplay between TAMs and tumor cells significantly influences osimertinib resistance. Succinate, an intermediate in the TCA cycle, has been highlighted for its role in TAM polarization and EMT induction in lung cancer through activation of the succinate receptor 1 (SUCNR1) and subsequent triggering of the PI3K/HIF-1α pathway. This promotes M2 polarization of TAMs and EMT in tumor cells (Wu J.-Y. et al., 2020). Additionally, targeting lipid metabolism with simvastatin has emerged as a potential therapeutic approach. By disrupting critical pathways involved in TAM polarization and EMT, simvastatin can restore sensitivity to chemotherapy and offer a new strategy to combat resistance in EGFR-TKI-treated NSCLC (Jin et al., 2019).
4.3 Pancreatic cancer——Profibrotic cytokines
Pancreatic cancer, known for its aggressive behavior and dire prognosis, is characterized by a distinct microenvironment heavily marked by fibrosis (Pratt et al., 2021). This fibrotic milieu is largely governed by key profibrotic cytokines such as TGF-β and OSM, which are central to the disease’s pathology. Within this fibrotic landscape, TAMs stand out as a predominant cell type, exerting a significant influence on cancer progression through their interaction with these cytokines and their downstream signaling pathways (Stawski and Trojanowska, 2019; Maria et al., 2022).
4.3.1 TAM modulation of TGF-β signaling
TAMs are recognized for their role in modulating the TGF-β signaling pathway, particularly through the production of miR-501-3p. This microRNA regulates TGF-β signaling by inhibiting TGFBR3 and upregulating TGFBR1, TGFBR2, and phosphorylated SMAD3, thereby amplifying TGF-β signaling and promoting EMT (Yin et al., 2019). Research indicated that treatment with TAM-CM can escalate the expression of EMT markers such as Snail, Vimentin, and N-cadherin in PDAC cells, while reducing E-cadherin expression and augmenting SMAD3 phosphorylation. The effectiveness of TGF-β neutralizing antibodies in inhibiting TAM-induced EMT further underscores the pivotal role of TAM-derived TGF-β in PDAC progression (Xiong et al., 2021).
The TGFβ vaccine has demonstrated efficacy in PDAC by decreasing the proportion of M2-like TAMs and influencing the polarization of CAFs away from the myofibroblast-like phenotype. This phenotype is associated with the development of a rigid extracellular matrix that impedes T cell infiltration into tumors. T cells specific to TGF-β, stimulated by the vaccine, can alter fibroblast phenotypes, reducing the expression of myofibroblast-associated markers such as alpha-smooth muscle actin (αSMA) and transgelin (TAGLN). By targeting and modifying TAMs and CAFs, the TGF-β vaccine aims to alleviate immunosuppression and circumvent immune exclusion within pancreatic tumors. This strategic modulation of the TME seeks to make PDAC more responsive to immune-based therapies, potentially enhancing the effectiveness of such treatments (Perez-Penco et al., 2024).
Additionally, TAMs characterized by CD51 expression, indicative of M2 polarization, have been found to promote CSC characteristics in PDAC by releasing TGF-β1. This cytokine activates SMAD2/3 signaling in cancer cells, leading to an upregulation of stemness markers like Nanog, Sox2, and Oct3/4, thereby contributing to the complexity of PDAC progression (Zhang et al., 2019).
4.3.2 OSM-OSMR signaling in PDAC
OSM plays a crucial role in TAM-tumor interactions by binding to its receptor, OSMR, on tumor and stromal cells, activating various signaling pathways, including Jak-STAT (West, 2019). Elevated OSMR expression in PDAC cells is associated with poor patient outcomes (Smigiel et al., 2017).
TAMs are capable of secreting OSM, which can activate the OSM-OSMR signaling pathway in neighboring mesenchymal cells, specifically CAFs. This cascade promotes the expression of proinflammatory cytokines in CAFs, establishing a feedback loop with TAMs and tumor cells that facilitates tumor metastasis (Lee et al., 2021).
Recent studies using patient-derived xenograft models of PDAC have shown that TAM-secreted OSM can enhance EMT. This is evidenced by increased expression of LOXL2 and Snail, with LOXL2 reduction linked to decreased metastasis and CSC traits in PDAC (Alonso-Nocelo et al., 2022). Targeting the OSM-LOXL2 signaling pathway with LOXL2 inhibitors, possibly in combination with TAM-targeting therapies, may offer a new strategy to curb PDAC metastasis (Alonso-Nocelo et al., 2022).
4.3.3 IL-1β+ TAMs and inflammatory loop
IL-1β+ TAMs play a pivotal role in the progression of PDAC by participating in a reciprocal inflammatory loop with PDAC cells. Exposure to pro-inflammatory stimuli like PGE2 and TNF induces these TAMs to polarization, which not only reprograms adjacent PDAC cells but also boosts the production of PGE2, TNF, and other factors, sustaining the IL-1β TAM phenotype. IL-1β+ TAMs are crucial in PDAC progression, forming a reciprocal inflammatory loop with PDAC cells (Caronni et al., 2023).
4.3.4 Gemcitabine resistance
Besides, TAMs are involved in gemcitabine resistance. Tissue-resident macrophages within PDAC endure chemotherapy by increasing deoxycytidine (dC) production while reducing deoxycytidine kinases (dCKs) levels, thereby decreasing gemcitabine uptake (Zhang et al., 2023d). Nonetheless, targeting the myeloid spleen tyrosine kinase (Syk) in macrophages can induce a shift towards an immunostimulatory phenotype. The use of the FDA-approved Syk inhibitor R788 has been shown to transform the tumor immune microenvironment, reprogramming pro-tumorigenic macrophages into an immunostimulatory state. This reprogramming significantly bolsters CD8+ T-cell activity, thereby enhancing the therapeutic efficacy of gemcitabine (Rohila et al., 2023).
4.4 Prostate cancer——Androgen deprivation therapy
Prostate cancer, a prevalent malignancy among men, is closely associated with the inflammatory TME in terms of its development and progression. A notable aspect of prostate cancer treatment involves ADT, which, despite its initial effectiveness, often leads to resistance and disease progression. This section explores the role of TAMs in this context, particularly their influence on the EMT process and therapeutic resistance.
4.4.1 TAM-induced chemokines secretion
In 2013, a key discovery revealed that TAMs can stimulate the secretion of CCL4 through the activation of the macrophage androgen receptor (AR). This activation triggers STAT3 and initiates EMT in prostate tumor cells, downregulating tumor suppressors like P53/PTEN and raising levels of EMT markers such as Snail and MMP9. The utilization of anti-CCL4 neutralizing antibodies presents a potential strategy to counteract TAM-mediated EMT and metastasis (Fang et al., 2013).
Further, TAM-derived CCL5 contributes significantly to metastasis and chemotherapy resistance. Elevated CCL5 expression in prostate cancer enhances tumor cell migration and invasion, upregulating N-cadherin, MMP2, MMP9, and the CD44+CD133+ cell subpopulation. Neutralizing CCL5 can inhibit these effects, suggesting a potential intervention strategy (Huang et al., 2020). Furthermore, CCL5 derived from TAMs can induce STAT3 activation, facilitating the EMT process and CSC phenotype. Inhibiting STAT3 signaling can attenuate chemoresistance and inhibit lung metastasis in prostate cancer (Ma et al., 2021).
4.4.2 ADT resistance
ADT is an established first-line therapy for managing advanced prostate cancer (Cooperberg et al., 2003). However, suppression of AR function has been linked to increased TAM infiltration, fostering EMT and metastasis (Cooperberg et al., 2003). Recent studies highlight the critical role of macrophages in the bone-metastatic prostate cancer microenvironment. Macrophages promote resistance to ADT through the cytokine activin A, which triggers the fibronectin (FN1)-integrin alpha 5 (ITGA5)-tyrosine kinase Src (SRC) cascade. Genetic models show that both bone-resident and monocyte-derived macrophages are essential for enzalutamide resistance, a common anti-androgen therapy (Li X. F. et al., 2023).
Moreover, cathepsin K (CTSK) expression, linked to M2 TAM infiltration, is upregulated in castration-resistant prostate cancer (CRPC) and may activate IL-17 signaling, promoting metastasis (Wu N. et al., 2022). ADT treatment also inhibits the SAM-pointed domain-containing ETS transcription factor (SPDEF), increasing CCL2 expression and leading to EMT and ADT resistance (Tsai et al., 2018). Inhibition of AR function significantly promotes CCL2 expression and secretion in TAMs, inducing EMT. Neutralizing CCL2 attenuates STAT3 cascades and inhibits the EMT process. Moreover, inhibiting both AR and CCL2/CCR2 signaling could reduce tumor progression in vivo (Izumi et al., 2013). These findings highlight the significant role of CCL2-mediated TAM-tumor interactions in promoting EMT and resistance to therapeutic interventions in the context of ADT for prostate cancer.
4.4.3 NOTCH signaling
Direct contact between macrophages and tumor cells in prostate cancer promotes the EMT process. The NOTCH signaling pathway, which relies on the physical interaction between a receptor and its ligand, is pivotal in this context (Siebel and Lendahl, 2017). When TAMs interact directly with prostate cancer cells, downstream factors associated with NOTCH1 signaling are significantly upregulated. This contact enhances the expression of genes related to EMT. Inhibiting TAMs phenotype or NOTCH1 signaling could be a viable strategy to impede prostate cancer progression (Shi F. et al., 2022).
5 Clinical implications
5.1 Targeting TAM infiltration
Therapeutic strategies aimed at curbing TAM infiltration have garnered significant interest as a potential avenue for cancer treatment. The focus on pharmacological agents that can selectively target TAMs has led to the development and clinical evaluation of various compounds, with some showing promising results in reducing TAM-mediated pro-tumorigenic functions.
5.1.1 M-CSF/CSF-1R
M-CSF, a crucial cytokine in macrophage regulation, plays a vital role in the differentiation, survival, and functional activity of macrophages. Its signaling pathway is implicated in TAM recruitment and the promotion of their pro-tumorigenic activities. Targeting M-CSF or its receptor CSF-1R has emerged as a promising strategy (Mun et al., 2020).
Pexidartinib (PLX3397), a small molecule inhibitor of CSF-1R, has shown efficacy in both preclinical studies and clinical trials. PLX3397 induces cell death in tenosynovial giant cell tumors (TGCT), which are highly infiltrated by M-CSF-dependent macrophages (Thongchot et al., 2023). Additionally, PLX3397 treatment reduces M2 polarization and TAM proliferation in sarcomas, blocks tumor metastasis, and improves lymphocyte infiltration in an orthotopic osteosarcoma mouse model (Fujiwara et al., 2021). Mechanistically, PLX3397 disrupts TAM recruitment and inhibits CCL22 release, thereby impairing TAM-mediated immune suppression and metastasis promotion (Zhang et al., 2023a). Notably, this drug has also been shown to inhibit the infiltration of microglia in the brain while sparing peripheral macrophages, highlighting its potential for targeting central nervous system (CNS) tumors without significantly impacting peripheral immune functions (Okojie et al., 2023).
A phase 3 clinical trial evaluating the efficacy of PLX3397 in the treatment of TGCT demonstrated promising results in terms of ameliorating patient symptoms and improving overall outcomes in adults with TGCT (NCT02371369). Nonetheless, careful attention must be paid to the potential hepatotoxicity associated with this therapeutic agent (Tap et al., 2019). Currently, the further safety and efficacy assessment of PLX3397 remains ongoing (NCT04635111, NCT04488822, NCT04635111). Recently, there has also been attention focused on the targeted delivery and synergistic therapy of PLX3397 (Liang et al., 2023; Zhang et al., 2023e).
Emactuzumab (RG7155), an anti-CSF1R monoclonal antibody, reduces the quantity and functional activity of TAMs in various cancer types and promotes CD8+ T cell infiltration. Additionally, RG7155 significantly decreases the population of CSF-1R+CD163+ TAMs in patients with diffuse-type giant cell tumors (Ries et al., 2014). However, under certain circumstances, TAMs may evade RG7155 therapy by relying on alternative factors, such as IL-4 (Pradel et al., 2016a). Therefore, combining a CSF-1R inhibitor with IL-4 signaling blockade could be a promising therapeutic approach. RG7155 has undergone phase 1 clinical evaluation in advanced solid tumor patients. The trial, involving 217 patients, assessed the safety, tolerability, pharmacokinetics, pharmacodynamics, and preliminary efficacy of RG7155. Results indicated that RG7155 has a favorable tolerability profile and clinical efficacy in inhibiting M2 TAMs (NCT01494688) (Gomez-Roca et al., 2019). Additionally, combining RG7155 with the CD40 agonist selicrelumab significantly improves the immune microenvironment in solid tumors, notably increasing CD8+ T cell infiltration and showing effectiveness when used in combination with atezolizumab (Machiels et al., 2020; Gomez-Roca et al., 2022).
BLZ945, a small inhibitor of CSF-1R, has shown promise in research studies. It can inhibit tumor progression by reducing TAM and MDSC infiltration in HCC (He et al., 2021). Additionally, BLZ945 impedes the M2 polarization of TAMs and reduces the tumor burden in OSCC (Guo et al., 2020). A phase I clinical trial evaluating BLZ945 alone or with PDR001 for advanced solid tumors demonstrated favorable safety and tolerability profiles (NCT02829723). Recent study has shown that BLZ945 exerts immunomodulatory effects both in the periphery and within the TME of solid tumors, including inhibiting monocyte infiltration and promoting T cell infiltration (Alcazar et al., 2024).
CSF-1R inhibitors like PLX3397 and RG7155 offer several advantages and disadvantages in cancer treatment. These inhibitors are known for their target specificity, allowing them to specifically target macrophages while reducing off-target effects and sparing other immune cells, thereby minimizing unintended side effects. Additionally, they have significant combination potential, as they can be used with other therapies, such as chemotherapy and checkpoint inhibitors, to enhance overall treatment efficacy (Gomez-Roca et al., 2019). By reducing TAM populations, these inhibitors can modulate the immune system, potentially reversing immunosuppression within the tumor microenvironment and enhancing anti-tumor immune responses.
However, there are notable disadvantages associated with CSF-1R inhibitors. Toxicity, particularly hepatotoxicity, is a concern, necessitating careful monitoring and dose adjustments during treatment (Lewis et al., 2021; Viganò et al., 2023). Tumors may also develop resistance mechanisms by upregulating alternative pathways, such as IL-4 (Pradel et al., 2016a), to maintain TAM populations, which can limit the long-term efficacy of these treatments. Furthermore, while preclinical data is promising, more extensive clinical trials are required to fully establish the safety and efficacy profiles of CSF-1R inhibitors.
5.1.2 CCL2/CCR2
The CCL2/CCR2 signaling pathway represents a pivotal mechanism that governs the recruitment and activation of TAMs within the TME. Interventions that selectively target this pathway have demonstrated considerable promise in impeding the process of EMT mediated by TAMs.
PF-04136309, a specific CCR2 inhibitor, significantly reduces inflammatory TAM infiltration and inhibits tumor metastasis in preclinical PDAC models. It effectively suppresses macrophage infiltration in both primary tumors and liver metastases (Yang and Zhang, 2017). Combining PF-04136309 with a CXCR inhibitor suppresses TAM and TAN infiltration in PDAC, enhancing T cell-mediated anti-tumor responses. (Nywening et al., 2018). Additionally, PF-04136309 inhibits GOLM1-mediated MDSC infiltration, reducing CRC metastasis (Dang et al., 2021).
A phase 1b study evaluated PF-04136309 combined with FOLFIRINOX chemotherapy, it shows a higher rate of tumor response and local tumor control compared to FOLFIRINOX alone, although it is associated with significant adverse events, particularly neutropenia and hypokalemia (Wang-Gillam et al., 2015; Nywening T. et al., 2016). Another phase 1b clinical study established the safety and feasibility of combining PF-04136309 with nab-paclitaxel and gemcitabine for treating metastatic PDAC. Importantly, the treatment led to a decrease in CD14+ CCR2+ inflammatory monocytes in the peripheral blood, although these cells did not accumulate in the bone marrow (Noel et al., 2020a).
BMS-813160, a recently developed small molecule, impedes the binding of chemokines CCL2 and CCL5 to their receptors, CCR2 and CCR5. It is highly potent and selective, with IC50 values of 6.2 and 3.6 nM, respectively. BMS-813160 also has favorable pharmacokinetic properties, including good permeability, stability, oral bioavailability, and low clearance in animal models (Tu et al., 2020). An ongoing phase 1b/2 clinical trial is investigating BMS-813160 combined with chemotherapy or nivolumab for treating metastatic colorectal or pancreatic cancers (NCT03184870). And BMS-813160 reduces the recruitment and activity of MDSCs and regulatory T cells, thereby diminishing the immunosuppressive milieu and potentially enhancing antitumor immune responses (Le et al., 2018). Besides, a phase IIa trial indicates that while BMS-813160 and BMS-986253 (anti-IL8) show biological activity by affecting chemokine levels in NSCLC and HCC, reducing the recruitment of circulating monocytes to the TME (Venturini et al., 2023).
Despite the promising results from preclinical and early-phase clinical studies, there are still limitations. More extensive trials are needed to fully establish the long-term safety and efficacy of these therapies. Regarding the adverse events associated with PF-04136309, a significant number of patients experienced grade 3 or higher neutropenia when combined with FOLFIRINOX. This condition involves a low count of neutrophils, which are essential for fighting infections (Nywening T. M. et al., 2016). Additionally, when PF-04136309 was used in combination with nab-paclitaxel and gemcitabine, there was a high incidence (24%) of pulmonary toxicity. This raises concerns about the safety profile of PF-04136309 in such combinations (Noel et al., 2020a).
In addition to BMS-813160 and PF-04136309, several other CCR2 inhibitors have shown promising potential in cancer therapy. CCX872-B has demonstrated efficacy in combination with chemotherapy for PDAC by reducing immunosuppressive myeloid cell recruitment (Noel et al., 2017). MLN1202, a monoclonal antibody targeting CCR2, has been explored in bone metastasis of cancer and inflammatory conditions, effectively blocking the migration of CCR2+ cells into the TME (Yumimoto et al., 2019). RS504393, a non-peptide CCR2 antagonist, has shown preclinical success in inhibiting myeloid cell recruitment (Mu et al., 2019). These compounds highlight the ongoing advancements in targeting CCR2 to enhance cancer treatment outcomes.
5.2 Targeting cytokine and growth factor signaling
TAMs secrete a diverse array of cytokines and growth factors that facilitate the progression of tumor growth, invasion, and metastasis. Interfering with these signaling molecules has demonstrated considerable potential in restraining TAM-mediated EMT, thereby serving as a propitious therapeutic approach.
5.2.1 TGF-β
TGF-β promotes tumor growth and metastasis by inducing EMT. Several drugs have been developed to inhibit TGF-β signaling and suppress TAM-induced EMT.
Galunisertib, a selective TGFBR1 inhibitor, has shown promising preclinical results, combining galunisertib with PD-L1 blockade enhanced tumor growth inhibition (Holmgaard R. et al., 2018). It inhibits macrophage infiltration and M2 polarization in vivo in prostate cancer models and attenuates TAMs-derived CXCL5 mediated EMT process (Wu et al., 2022b). Galunisertib is currently under evaluation in clinical trials as a monotherapy or in combination with chemotherapy or immunotherapy for treating advanced solid tumors, including HCC (Kelley et al., 2019; Faivre et al., 2019), metastatic PDAC (Melisi et al., 2021a) and locally advanced rectal cancer (Yamazaki et al., 2022). However, in a trial involving 32 patients with metastatic pancreatic cancer, only one patient experienced a partial response, and the disease control rate was 25.0%, highlighting limited clinical activity (NCT02734160) (Melisi et al., 2021b).
Fresolimumab, a human monoclonal antibody that neutralizes all three isoforms of TGF-β, has yet to undergo comprehensive preclinical investigation regarding its association with TAMs (Peng et al., 2022). Higher dose group exhibited a stronger systemic immune response, evidenced by increased peripheral blood mononuclear cells and an expansion of the CD8+ T cell central memory pool (Formenti et al., 2018). It has been examined in both phase 1 and 2 clinical trials. A phase 1 trial in patients with malignant melanoma and renal cell carcinoma showed that fresolimumab had acceptable safety profiles and demonstrated antitumor efficacy, though some adverse events were reported (Morris et al., 2014). A phase 2 trial in patients with NSCLC is currently ongoing (NCT02581787). Additionally, there have been instances where fresolimumab treatment led to the development of skin lesions resembling benign keratoacanthomas, and in one patient with a history of skin cancer, there was a potential link to the development of squamous cell carcinoma (Arjaans et al., 2012).
5.2.2 IL-6
IL-6 plays a vital role in orchestrating EMT, facilitating neoplastic progression and promoting immune-related adverse events (irAEs). Multiple pharmacological agents have been evaluated for their efficacy in blocking IL-6 or its downstream signaling cascades to impede TAM-induced EMT and suppress malignant growth.
Tocilizumab, a monoclonal antibody targeting the IL-6 receptor, has been extensively investigated. Many preclinical studies show its potential in inhibiting TAM-induced EMT and mitigating cancer progression. In 2014, it was found that tocilizumab inhibits CSC characteristics induced by TAMs by blocking IL-6/STAT3 signaling in HCC. In vitro and in vivo studies showed that tocilizumab significantly reduced the CD44+ subset (Wan et al., 2014a). In BC, tocilizumab attenuated the MCT-1/IL-6/IL-6R signaling between tumor cells and TAMs, inhibiting stemness (Weng Y. et al., 2019).
IL-6, a pro-inflammatory cytokine, has recently gained attention for its role in reducing immunotherapy toxicity when combined with immune checkpoint blockade (ICB) therapy. In patients and mice receiving ICB therapy, IL-6 expression, neutrophil infiltration, and chemotactic markers increased in inflamed tissues (Holmstroem et al., 2022). An open-label study showed that tocilizumab effectively treated ICB-induced colitis and arthritis with manageable safety profiles (Holmstroem et al., 2022). Tocilizumab treatment reduced the severity and frequency of irAEs in patients and mice receiving ICB therapy without compromising the anti-tumor immune response. This shift was due to blocking IL-6, changing the immune balance in the TME from a Th17-dominated response to a Th1- and CD8+ T cell-dominated response (Hailemichael et al., 2022). Clinical trials are assessing the safety and tolerability of tocilizumab with PD-1 and CTLA-4 targeting drugs (NCT04940299).
TTI-101, the latest STAT3 inhibitor, has received orphan drug designation by the FDA for HCC treatment. It has been indicated that TTI-101 could inhibit neuropathic pain caused by cancer treatment (Kasembeli et al., 2021). Additionally, TTI-101 exhibited antitumor activity and clinical benefits in patients with solid tumors (NCT03195699). N4, another well-studied and novel STAT3 inhibitor, specifically binds to STAT3 and prevents the phosphorylation of tyrosine 705. N4 inhibits the proliferation and viability of pancreatic cancer cells in a dose-dependent manner and also inhibits migration and invasion in vitro. Importantly, in mouse models, N4 demonstrates a significant inhibitory effect on the growth and metastasis of pancreatic tumors. Additionally, N4 treatment inhibits TAM infiltration and the EMT process (Chen et al., 2021). However, N4 is still in the preclinical stage of development (Table 1).
6 Controversies and gaps
While the role of TAMs in driving EMT is well-documented, the literature presents several conflicting findings that necessitate a more nuanced discussion. For instance, the dual role of TAMs in cancer progression and suppression remains a contentious area (Aras and Zaidi, 2017).
For instance, TAMs have been shown to enhance EMT and metastasis by secreting factors, which activate signaling pathways in tumor cells leading to increased invasiveness. This pro-tumorigenic role is evident in GC, where high levels of TAM infiltration correlate with increased EMT marker expression and poorer patient outcomes (Zhang et al., 2016). Conversely, research in CRC presents a different perspective. In a CRC model, TAMs were found to be pro-inflammatory and inhibited the proliferation of tumor cells. Additionally, these TAMs produced chemokines that attract T cells, stimulated the proliferation of allogeneic T cells, and activated type-1 T cells associated with anti-tumor immune responses (Ong et al., 2012).
Interestingly, the phenomenon cannot be solely attributed to the “pro-inflammatory” nature. Recent studies have shown that pro-inflammatory macrophages with high expression of IL-1β can promote the progression of PDAC (Caronni et al., 2023). Despite all being digestive tract tumors, the differences are still significant. Furthermore, numerous reports indicate that even M1 macrophages, typically associated with anti-tumor activity, can support tumor progression (You et al., 2022; Tsai et al., 2021). This dichotomy underscores the complexity of TAM behavior within the TME.
In addition, the differences in some research results are likely due to the choice of TAM model. Different experimental systems, including THP-1 derived macrophages, human peripheral blood mononuclear cells (PBMCs), murine BMDMs, and macrophages isolated directly from tumors, each have unique advantages and limitations that can affect study outcomes. THP-1 derived macrophages, originating from a human monocytic cell line, are popular due to their ease of use and consistency. However, they often fail to fully recapitulate the complexity and heterogeneity of primary TAMs. Human PBMCs, isolated from blood and differentiated into macrophages in vitro, offer a more physiologically relevant source. However, donor variability and the influence of ex vivo differentiation conditions can result in significant heterogeneity. Murine BMDMs are widely used in preclinical research due to their ease of obtaining large numbers of cells and genetic manipulation. Nonetheless, murine models may not always accurately reflect human macrophage biology. Macrophages isolated directly from tumors represent the most relevant model for studying TAM function within the TME. These cells have been exposed to the unique biochemical milieu of the tumor, including hypoxia, metabolic byproducts, and tumor-derived signals. However, the isolation process can be technically challenging and may alter macrophage phenotypes. Moreover, transitioning TAMs from a 3D tumor environment to 2D culture conditions can significantly impact their behavior. The current priority is to find the most suitable and universal TAM model for research. For example, a 2019 study provided a detailed comparison of different in vitro induction methods to obtain the most realistic TAM phenotypes (Benner et al., 2019).
7 Conclusion and future direction
TAMs, integral to the TME, play a pivotal role in cancer progression, influencing tumor growth, invasion, and metastasis. Their capacity to induce EMT stands as a key mechanism propelling cancer metastasis. This review has sought to collate and synthesize recent advancements in understanding the modalities through which TAMs orchestrate EMT and the potential therapeutic interventions aimed at curtailing TAM-mediated EMT.
TAMs expedite the EMT process via the secretion of an array of cytokines, growth factors, and chemokines, culminating in the upregulation of EMT-inducing transcription factors within tumor cells. These transcription factors subsequently downregulate epithelial markers and upregulate mesenchymal markers, endowing cancer cells with enhanced invasive and metastatic capabilities. Furthermore, TAMs, as predominant immune cells within solid tumors, contribute significantly to the EMT process by fostering an inflammatory milieu, enabling tumor immune evasion, and facilitating interactions with other stromal cells. To elucidate the complex signaling pathways, the specific EMT signals occurring within tumors are detailed in Figure 4.
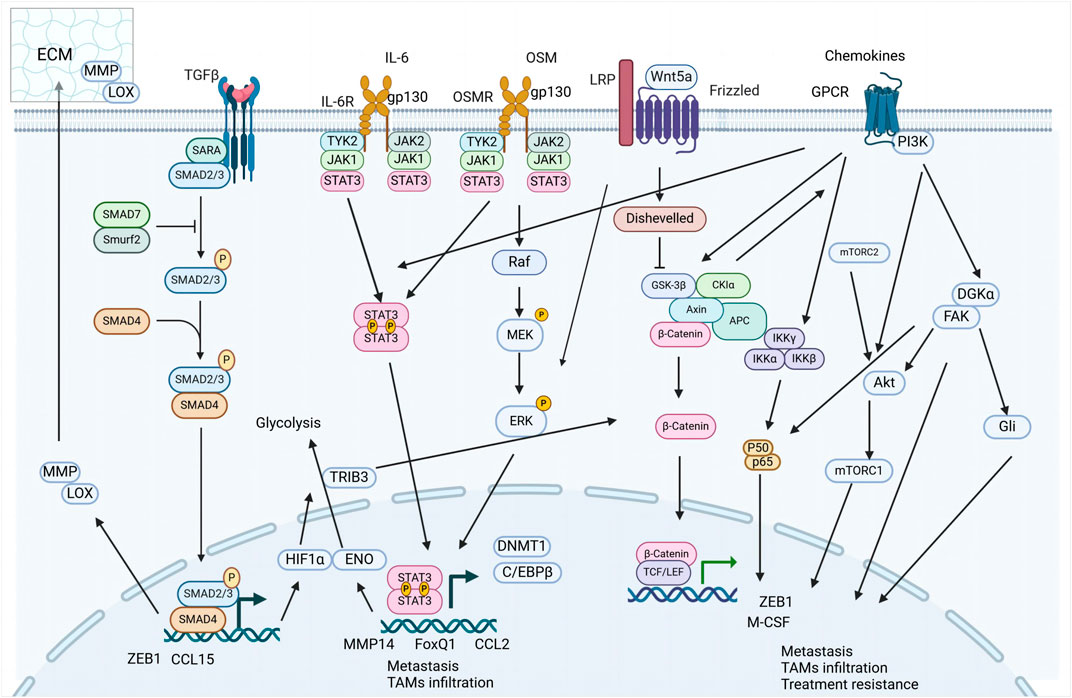
Figure 4. Key signaling pathways involved in tumor mesenchymal progression. This figure presents an overview of critical signaling pathways implicated in tumor mesenchymal progression, highlighting the complex network of interactions that contribute to various aspects of tumor biology, including metastasis, immune cell infiltration, and treatment resistance.
Various therapeutic approaches are being explored to disrupt TAM-induced EMT, including strategies aimed at reducing TAM recruitment, inhibiting TAM-secreted factors, and blocking the signaling pathways involved in TAM-driven EMT. Notably, interventions targeting the CSF/CSFR and CCL2/CCR2 signaling axes have shown promise in curtailing TAM recruitment, while inhibitors of key TAM-derived cytokines such as TGF-β and IL-6 are being investigated to hinder the pro-metastatic activities of TAMs. The ultimate goal of these strategies is to disrupt the interactions between TAMs and cancer cells, thereby preventing the EMT and acquisition of stemness traits in tumor cells.
However, it is crucial to acknowledge that TAMs are implicated in adverse clinical outcomes across a broader spectrum of cancers, including colorectal (Hou et al., 2024), ovarian (Schweer et al., 2022), and gastric cancers (Cao et al., 2023), among others. Due to space constraints, this review has focused on specific cancers such as breast cancer (including TNBC and IBC), lung cancer (particularly NSCLC), pancreatic cancer, and prostate cancer, which offer unique insights into TAM-mediated EMT and therapeutic interventions. Future research should aim to explore and summarize the role of TAMs in these additional cancer types, providing a more comprehensive understanding of their impact on cancer progression and therapeutic resistance.
Despite the promising developments in TAMs, several challenges persist in this field. The heterogeneity and plasticity of TAMs complicate efforts to selectively target them without affecting the normal functions of macrophages (Wang S. et al., 2024). Moreover, the intricate dynamics of TAM-tumor interactions and their variable impact on cancer progression across different contexts and cancer stages necessitate further investigation. The precise molecular signals and pathways driving the polarization of macrophages towards a pro-tumor phenotype in the TME remain inadequately understood (Vadevoo et al., 2021; Zhang A. et al., 2021). Recent studies have reported that lactate in the TME can promote the M2-like TAM phenotype, making lactylation in TAMs a promising direction for future research (Su et al., 2023). Additionally, the activation of β-catenin also facilitates the M2-like TAM phenotype (Tigue et al., 2023). These findings suggest potential targets for therapeutic intervention. Strategies to inhibit these pathways could help in reprogramming TAMs towards a more anti-tumor phenotype. However, these approaches need to be further explored and validated in clinical settings.
The role of TAMs in cancer metastasis requires further elucidation. Specifically, understanding how TAMs contribute to the metastatic spread of cancer cells and identifying the key factors involved in this process are critical areas of ongoing research. Developing effective strategies to therapeutically target TAMs without compromising normal immune functions remains a crucial objective. Furthermore, the interaction between TAMs and CSCs is another area requiring investigation. TAMs can support CSC maintenance and promote their stemness properties, which are associated with increased resistance to therapies and metastatic potential. Targeting this interaction could be a promising strategy to prevent cancer recurrence and metastasis. Lastly, identifying reliable biomarkers for monitoring TAM activity and therapeutic responses in cancer patients is essential. Biomarkers would enable the assessment of treatment efficacy and guide therapeutic decisions, ensuring more personalized and effective cancer treatment approaches.
As research in this area progresses, it is hoped that a deeper understanding of TAM-mediated mechanisms in cancer will lead to more effective and targeted therapeutic strategies, ultimately improving outcomes for patients with metastatic cancers.
Author contributions
YZ: Conceptualization, Data curation, Formal Analysis, Funding acquisition, Investigation, Methodology, Project administration, Resources, Software, Supervision, Validation, Visualization, Writing–original draft, Writing–review and editing. XD: Investigation, Writing–review and editing. XZ: Investigation, Writing–review and editing. YL: Investigation, Writing–review and editing. RX: Investigation, Writing–review and editing. H-JL: Investigation, Writing–review and editing. DZ: Conceptualization, Data curation, Formal Analysis, Funding acquisition, Investigation, Methodology, Project administration, Resources, Software, Supervision, Validation, Visualization, Writing–review and editing. GC: Conceptualization, Data curation, Formal Analysis, Funding acquisition, Investigation, Methodology, Project administration, Resources, Software, Supervision, Validation, Visualization, Writing–review and editing.
Funding
The author(s) declare that financial support was received for the research, authorship, and/or publication of this article. This research was supported by the Natural Science Foundation of Liaoning Province of China (Grant No. 2020-MS-187). The Foundation provided generous financial support for the research activities. Specifically, they supported the experimental design and data collection phases of the study. We gratefully acknowledge the Natural Science Foundation of Liaoning Province of China (2020-MS-187) for the generous financial support. Additionally, this work was supported by the Zhejiang Provincial Natural Science Foundation of China under Grant Nos. LHDMY22H310001, LGD22H160002, LBY23H200004, LTGD24H160006, LTGD23H160001, LTGY23H010007, LBY23H200004, and LTGD24H160006.
Conflict of interest
The authors declare that the research was conducted in the absence of any commercial or financial relationships that could be construed as a potential conflict of interest.
Publisher’s note
All claims expressed in this article are solely those of the authors and do not necessarily represent those of their affiliated organizations, or those of the publisher, the editors and the reviewers. Any product that may be evaluated in this article, or claim that may be made by its manufacturer, is not guaranteed or endorsed by the publisher.
References
Akimoto, M., Maruyama, R., Takamaru, H., Ochiya, T., and Takenaga, K. (2016). Soluble IL-33 receptor sST2 inhibits colorectal cancer malignant growth by modifying the tumour microenvironment. Nat. Commun. 7, 13589. doi:10.1038/ncomms13589
Alcazar, C. R. G. D., Xu, V., Lin, C.-c., Gil-Martin, M., Naing, A., Fan, L., et al. (2024). Abstract CT094: targeting CSF1R with BLZ945 results in effective peripheral and tumor immune microenvironment modulation in advanced solid tumors and may be associated with limited efficacy in recurrent non-mesenchymal glioblastoma. Cancer Res. 84, CT094. doi:10.1158/1538-7445.am2024-ct094
Alonso-Nocelo, M., Ruiz-Canas, L., Sancho, P., Gorgulu, K., Alcala, S., Pedrero, C., et al. (2022). Macrophages direct cancer cells through a LOXL2-mediated metastatic cascade in pancreatic ductal adenocarcinoma. Gut 72, 345–359. doi:10.1136/gutjnl-2021-325564
Anand, A., Olsen, R. F. S., Burton, M., Harwood, D. S. L., Poulsen, F. R., Halle, B., et al. (2022). TMIC-24. SINGLE-CELL ANALYSIS OF TUMOR-ASSOCIATED MICROGLIA AND MACROPHAGES FROM HUMAN GLIOBLASTOMA. Neuro Oncol. 24, vii276. doi:10.1093/neuonc/noac209.1068
Aras, S., and Zaidi, M. R. (2017). TAMeless traitors: macrophages in cancer progression and metastasis. Br. J. Cancer 117, 1583–1591. doi:10.1038/bjc.2017.356
Araujo, A. M., Abaurrea, A., Azcoaga, P., Lopez-Velazco, J. I., Manzano, S., Rodriguez, J., et al. (2022). Stromal oncostatin M cytokine promotes breast cancer progression by reprogramming the tumor microenvironment. J. Clin. Invest. 132, e165107. doi:10.1172/JCI165107
Archer, M., Bernhardt, S. M., Hodson, L. J., Woolford, L., Van der Hoek, M., Dasari, P., et al. (2023). CCL2-Mediated stromal interactions drive macrophage polarization to increase breast tumorigenesis. Int. J. Mol. Sci. 24, 7385. doi:10.3390/ijms24087385
Arjaans, M., Oude Munnink, T. H., Timmer-Bosscha, H., Reiss, M., Walenkamp, A. M. E., Lub-de Hooge, M. N., et al. (2012). Transforming growth factor (TGF)-β expression and activation mechanisms as potential targets for anti-tumor therapy and tumor imaging. Pharmacol. Ther. 135, 123–132. doi:10.1016/j.pharmthera.2012.05.001
Belhabib, I., Zaghdoudi, S., Lac, C., Bousquet, C., and Jean, C. (2021). Extracellular matrices and cancer-associated fibroblasts: targets for cancer diagnosis and therapy? Cancers (Basel) 13, 3466. doi:10.3390/cancers13143466
Benner, B., Scarberry, L., Suarez-Kelly, L. P., Duggan, M. C., Campbell, A. R., Smith, E., et al. (2019). Generation of monocyte-derived tumor-associated macrophages using tumor-conditioned media provides a novel method to study tumor-associated macrophages in vitro. J. Immunother. Cancer 7, 140. doi:10.1186/s40425-019-0622-0
Boutilier, A. J., and Elsawa, S. F. (2021). Macrophage polarization states in the tumor microenvironment. Int. J. Mol. Sci. 22, 6995. doi:10.3390/ijms22136995
Boxuan Zhou, Z. M., Lai, G., Chen, X., Li, R., Wu, R., Jia, Z., et al. (2023). Targeting tumor exosomal circular RNA cSERPINE2 suppresses breast cancer progression by modulating MALT1-NF-kB-IL-6 axis of tumor-associated macrophages, J. Exp. Clin. Cancer Res. 42, 48. doi:10.1186/s13046-023-02620-5
Cai, J., Xia, L., Li, J., Ni, S., Song, H., and Wu, X. (2019). Tumor-associated macrophages derived TGF-β‒induced epithelial to mesenchymal transition in colorectal cancer cells through smad2,3-4/snail signaling pathway. Cancer Res. Treat. 51, 252–266. doi:10.4143/crt.2017.613
Cao, F., Liu, Y., Cheng, Y., Wang, Y., He, Y., and Xu, Y. (2023). Multi-omics characteristics of tumor-associated macrophages in the tumor microenvironment of gastric cancer and their exploration of immunotherapy potential. Sci. Rep. 13, 18265. doi:10.1038/s41598-023-38822-2
Caronni, N., La Terza, F., Vittoria, F. M., Barbiera, G., Mezzanzanica, L., Cuzzola, V., et al. (2023). IL-1β+ macrophages fuel pathogenic inflammation in pancreatic cancer. Nature 623, 415–422. doi:10.1038/s41586-023-06685-2
Casanova-Acebes, M., Dalla, E., Leader, A. M., LeBerichel, J., Nikolic, J., Morales, B. M., et al. (2021). Tissue-resident macrophages provide a pro-tumorigenic niche to early NSCLC cells. Nature 595, 578–584. doi:10.1038/s41586-021-03651-8
Chen, C. J., and Liu, Y. P. (2021). MERTK inhibition: potential as a treatment strategy in EGFR tyrosine kinase inhibitor-resistant non-small cell lung cancer. Pharm. (Basel) 14, 130. doi:10.3390/ph14020130
Chen, H., Bian, A., Yang, L. F., Yin, X., Wang, J., Ti, C., et al. (2021). Targeting STAT3 by a small molecule suppresses pancreatic cancer progression. Oncogene 40, 1440–1457. doi:10.1038/s41388-020-01626-z
Chen, J., Yao, Y., Gong, C., Yu, F., Su, S., Chen, J., et al. (2011). CCL18 from tumor-associated macrophages promotes breast cancer metastasis via PITPNM3. Cancer Cell 19, 541–555. doi:10.1016/j.ccr.2011.02.006
Chen, J., Zhao, D., Zhang, L., Zhang, J., Xiao, Y., Wu, Q., et al. (2022b). Tumor-associated macrophage (TAM)-derived CCL22 induces FAK addiction in esophageal squamous cell carcinoma (ESCC). Cell. Mol. Immunol. 19, 1054–1066. doi:10.1038/s41423-022-00903-z
Chen, J., Zhao, D., Zhang, L., Zhang, J., Xiao, Y., Wu, Q., et al. (2024). Tumor-associated macrophage (TAM)-secreted CCL22 confers cisplatin resistance of esophageal squamous cell carcinoma (ESCC) cells via regulating the activity of diacylglycerol kinase α (DGKα)/NOX4 axis. Drug Resist. Updat. 73, 101055. doi:10.1016/j.drup.2024.101055
Chen, J., Zhu, Y., Zhao, D., Zhang, L., Zhang, J., Xiao, Y., et al. (2023d). Co-targeting FAK and Gli1 inhibits the tumor-associated macrophages-released CCL22-mediated esophageal squamous cell carcinoma malignancy. MedComm 4, e381. doi:10.1002/mco2.381
Chen, S., Lu, K., Hou, Y., You, Z., Shu, C., Wei, X., et al. (2023c). YY1 complex in M2 macrophage promotes prostate cancer progression by upregulating IL-6. J. Immunother. Cancer 11, e006020. doi:10.1136/jitc-2022-006020
Chen, S., Saeed, A. F. U. H., Liu, Q., Jiang, Q., Xu, H., Xiao, G. G., et al. (2023a). Macrophages in immunoregulation and therapeutics. Signal Transduct. Target. Ther. 8, 207. doi:10.1038/s41392-023-01452-1
Chen, X., Yang, M., Yin, J., Li, P., Zeng, S., Zheng, G., et al. (2022a). Tumor-associated macrophages promote epithelial-mesenchymal transition and the cancer stem cell properties in triple-negative breast cancer through CCL2/AKT/β-catenin signaling. Cell Commun. Signal 20, 92. doi:10.1186/s12964-022-00888-2
Chen, Z., Li, H., Li, Z., Chen, S., Huang, X., Zheng, Z., et al. (2023b). SHH/GLI2-TGF-β1 feedback loop between cancer cells and tumor-associated macrophages maintains epithelial-mesenchymal transition and endoplasmic reticulum homeostasis in cholangiocarcinoma. Pharmacol. Res. 187, 106564. doi:10.1016/j.phrs.2022.106564
Cheng, L. C., Chao, Y. J., Wang, C. Y., Phan, N. N., Chen, Y. L., Wang, T. W., et al. (2020). Cancer-derived transforming growth factor-β modulates tumor-associated macrophages in ampullary cancer. Onco Targets Ther. 13, 7503–7516. doi:10.2147/OTT.S246714
Chin, W. Y., He, C. Y., Chow, T. W., Yu, Q. Y., Lai, L. C., and Miaw, S. C. (2021). Adenylate kinase 4 promotes inflammatory gene expression via Hif1α and AMPK in macrophages. Front. Immunol. 12, 630318. doi:10.3389/fimmu.2021.630318
Cho, H., Seo, Y., Loke, K. M., Kim, S. W., Oh, S. M., Kim, J. H., et al. (2018). Cancer-stimulated CAFs enhance monocyte differentiation and protumoral TAM activation via IL6 and GM-CSF secretion. Clin. Cancer Res. 24, 5407–5421. doi:10.1158/1078-0432.CCR-18-0125
Colwell, N., Larion, M., Giles, A. J., Seldomridge, A. N., Sizdahkhani, S., Gilbert, M. R., et al. (2017). Hypoxia in the glioblastoma microenvironment: shaping the phenotype of cancer stem-like cells. Neuro Oncol. 19, 887–896. doi:10.1093/neuonc/now258
Cooperberg, M., Small, E., D'Amico, A., and Carroll, P. (2003). The evolving role of androgen deprivation therapy in the management of prostate cancer. Minerva urologica e nefrologica = Italian J. urology Nephrol. 55, 219–238.
Cui, X., Morales, R.-T. T., Qian, W., Wang, H., Gagner, J.-P., Dolgalev, I., et al. (2018). Hacking macrophage-associated immunosuppression for regulating glioblastoma angiogenesis. Biomaterials 161, 164–178. doi:10.1016/j.biomaterials.2018.01.053
Dang, Y., Yu, J., Zhao, S., Jin, L., Cao, X., and Wang, Q. (2021). GOLM1 drives colorectal cancer metastasis by regulating myeloid-derived suppressor cells. J. Cancer 12, 7158–7166. doi:10.7150/jca.61567
Elia, I., and Haigis, M. C. (2021). Metabolites and the tumour microenvironment: from cellular mechanisms to systemic metabolism. Nat. Metab. 3, 21–32. doi:10.1038/s42255-020-00317-z
Ensan, S., Li, A., Besla, R., Degousee, N., Cosme, J., Roufaiel, M., et al. (2016). Self-renewing resident arterial macrophages arise from embryonic CX3CR1+ precursors and circulating monocytes immediately after birth. Nat. Immunol. 17, 159–168. doi:10.1038/ni.3343
Etzerodt, A., Moulin, M., Doktor, T. K., Delfini, M., Mossadegh-Keller, N., Bajenoff, M., et al. (2020). Tissue-resident macrophages in omentum promote metastatic spread of ovarian cancer. J. Exp. Med. 217, e20191869. doi:10.1084/jem.20191869
Eva, M.G.-M., Toby, W. P. M., James, C., Anusha-Preethi, G., Oliver, W., Angelica, C., et al. (2020). M1 hot tumor-associated macrophages boost tissue-resident memory T cells infiltration and survival in human lung cancer. J. Immunother. Cancer 8, e000778. doi:10.1136/jitc-2020-000778
Faivre, S., Santoro, A., Kelley, R. K., Gane, E., Costentin, C. E., Gueorguieva, I., et al. (2019). Novel transforming growth factor beta receptor I kinase inhibitor galunisertib (LY2157299) in advanced hepatocellular carcinoma. Liver Int. 39, 1468–1477. doi:10.1111/liv.14113
Fang, L. Y., Izumi, K., Lai, K. P., Liang, L., Li, L., Miyamoto, H., et al. (2013). Infiltrating macrophages promote prostate tumorigenesis via modulating androgen receptor-mediated CCL4-STAT3 signaling. Cancer Res. 73, 5633–5646. doi:10.1158/0008-5472.CAN-12-3228
Feng, Y., Ye, Z., Song, F., He, Y., and Liu, J. (2022). The role of TAMs in tumor microenvironment and new research progress. Stem Cells Int. 2022, 5775696. doi:10.1155/2022/5775696
Fernandez, A., Vermeren, M., Humphries, D., Subiros-Funosas, R., Barth, N., Campana, L., et al. (2017). Chemical modulation of in vivo macrophage function with subpopulation-specific fluorescent prodrug conjugates. ACS Cent. Sci. 3, 995–1005. doi:10.1021/acscentsci.7b00262
Fernandez, M. E., Fines, C. B., Subul, F. S., Alhudaithi, S. S., Bear, H. D., Sweet, D. H., et al. (2023). Abstract 2328: macrophage-targeting immunotherapy for triple negative breast cancer. Cancer Res. 83, 2328. doi:10.1158/1538-7445.am2023-2328
Formenti, S. C., Lee, P., Adams, S., Goldberg, J. D., Li, X., Xie, M. W., et al. (2018). Focal irradiation and systemic TGFβ blockade in metastatic breast cancer. Clin. Cancer Res. 24, 2493–2504. doi:10.1158/1078-0432.CCR-17-3322
Fujiwara, T., Yakoub, M. A., Chandler, A., Christ, A. B., Yang, G., Ouerfelli, O., et al. (2021). CSF1/CSF1R signaling inhibitor pexidartinib (PLX3397) reprograms tumor-associated macrophages and stimulates T-cell infiltration in the sarcoma microenvironment. Mol. Cancer Ther. 20, 1388–1399. doi:10.1158/1535-7163.MCT-20-0591
Gao, S., Hu, J., Wu, X., and Liang, Z. (2018). PMA treated THP-1-derived-IL-6 promotes EMT of SW48 through STAT3/ERK-dependent activation of Wnt/β-catenin signaling pathway. Biomed. Pharmacother. 108, 618–624. doi:10.1016/j.biopha.2018.09.067
Gomez-Roca, C., Cassier, P., Zamarin, D., Machiels, J. P., Perez Gracia, J. L., Stephen Hodi, F., et al. (2022). Anti-CSF-1R emactuzumab in combination with anti-PD-L1 atezolizumab in advanced solid tumor patients naïve or experienced for immune checkpoint blockade. J. Immunother. Cancer 10, e004076. doi:10.1136/jitc-2021-004076
Gomez-Roca, C. A., Italiano, A., Le Tourneau, C., Cassier, P. A., Toulmonde, M., D'Angelo, S. P., et al. (2019). Phase I study of emactuzumab single agent or in combination with paclitaxel in patients with advanced/metastatic solid tumors reveals depletion of immunosuppressive M2-like macrophages. Ann. Oncol. 30, 1381–1392. doi:10.1093/annonc/mdz163
Gunaydin, G. (2021a). CAFs interacting with TAMs in tumor microenvironment to enhance tumorigenesis and immune evasion. Front. Oncol. 11, 668349. doi:10.3389/fonc.2021.668349
Gunaydin, G. (2021b). CAFs interacting with TAMs in tumor microenvironment to enhance tumorigenesis and immune evasion. Front. Oncol. 11, 668349. doi:10.3389/fonc.2021.668349
Guo, L., Chen, C., Shi, M., Wang, F., Chen, X., Diao, D., et al. (2013). Stat3-coordinated Lin-28-let-7-HMGA2 and miR-200-ZEB1 circuits initiate and maintain oncostatin M-driven epithelial-mesenchymal transition. Oncogene 32, 5272–5282. doi:10.1038/onc.2012.573
Guo, X., Zhang, J., Shi, X., Wang, Q., Shen, W., Zhu, W., et al. (2020). Upregulation of CSF-1 is correlated with elevated TAM infiltration and poor prognosis in oral squamous cell carcinoma. Am. J. Transl. Res. 12, 6235–6249.
Habanjar, O., Bingula, R., Decombat, C., Diab-Assaf, M., Caldefie-Chezet, F., and Delort, L. (2023). Crosstalk of inflammatory cytokines within the breast tumor microenvironment. Int. J. Mol. Sci. 24, 4002. doi:10.3390/ijms24044002
Hailemichael, Y., Johnson, D., Abdel-Wahab, N., Foo, W., Bentebibel, S., Daher, M., et al. (2022). Interleukin-6 blockade abrogates immunotherapy toxicity and promotes tumor immunity. Cancer Cell 40, 509–523.e6. doi:10.1016/j.ccell.2022.04.004
Han, R., Guo, H., Shi, J., Wang, H., Zhao, S., Jia, Y., et al. (2023). Tumour microenvironment changes after osimertinib treatment resistance in non-small cell lung cancer. Eur. J. Cancer 189, 112919. doi:10.1016/j.ejca.2023.05.007
Hawkins, E. D., Duarte, D., Akinduro, O., Khorshed, R. A., Passaro, D., Nowicka, M., et al. (2016). T-cell acute leukaemia exhibits dynamic interactions with bone marrow microenvironments. Nature 538, 518–522. doi:10.1038/nature19801
He, C., Li, Y., Chen, Z.-Y., and Huang, C.-K. (2023). Crosstalk of renal cell carcinoma cells and tumor-associated macrophages aggravates tumor progression by modulating muscleblind-like protein 2/B-cell lymphoma 2/beclin 1-mediated autophagy. Cytotherapy 25, 298–309. doi:10.1016/j.jcyt.2022.09.001
He, Q., Liu, M., Huang, W., Chen, X., Zhang, B., Zhang, T., et al. (2021). IL-1β-Induced elevation of solute carrier family 7 member 11 promotes hepatocellular carcinoma metastasis through up-regulating programmed death ligand 1 and colony-stimulating factor 1. Hepatology 74, 3174–3193. doi:10.1002/hep.32062
He, S., Lu, M., Zhang, L., and Wang, Z. (2024). RSK4 promotes the macrophage recruitment and M2 polarization in esophageal squamous cell carcinoma. Biochimica Biophysica Acta (BBA) - Mol. Basis Dis. 1870, 166996. doi:10.1016/j.bbadis.2023.166996
Hey, J., Halperin, C., Hartmann, M., Mayer, S., Schönung, M., Lipka, D. B., et al. (2023). DNA methylation landscape of tumor-associated macrophages reveals pathways, transcription factors and prognostic value relevant to triple-negative breast cancer patients. Int. J. Cancer 152, 1226–1242. doi:10.1002/ijc.34364
Hirano, R., Okamoto, K., Shinke, M., Sato, M., Watanabe, S., Watanabe, H., et al. (2023a). Tissue-resident macrophages are major tumor-associated macrophage resources, contributing to early TNBC development, recurrence, and metastases. Commun. Biol. 6, 144. doi:10.1038/s42003-023-04525-7
Hirano, R., Okamoto, K., Shinke, M., Sato, M., Watanabe, S., Watanabe, H., et al. (2023b). Tissue-resident macrophages are major tumor-associated macrophage resources, contributing to early TNBC development, recurrence, and metastases. Commun. Biol. 6, 144. doi:10.1038/s42003-023-04525-7
Holmgaard, R., Schaer, D., Li, Y., Castaneda, S., Murphy, M., Xu, X., et al. (2018a). Targeting the TGFβ pathway with galunisertib, a TGFβRI small molecule inhibitor, promotes anti-tumor immunity leading to durable, complete responses, as monotherapy and in combination with checkpoint blockade. J. Immunother. Cancer 6, 47. doi:10.1186/s40425-018-0356-4
Holmgaard, R. B., Schaer, D. A., Li, Y., Castaneda, S. P., Murphy, M. Y., Xu, X., et al. (2018b). Targeting the TGFβ pathway with galunisertib, a TGFβRI small molecule inhibitor, promotes anti-tumor immunity leading to durable, complete responses, as monotherapy and in combination with checkpoint blockade. J. Immunother. Cancer 6, 47. doi:10.1186/s40425-018-0356-4
Holmstroem, R. B., Nielsen, O. H., Jacobsen, S., Riis, L. B., Theile, S., Bjerrum, J. T., et al. (2022). COLAR: open-label clinical study of IL-6 blockade with tocilizumab for the treatment of immune checkpoint inhibitor-induced colitis and arthritis. J. Immunother. Cancer 10, e005111. doi:10.1136/jitc-2022-005111
Hou, S., Zhao, Y., Chen, J., Lin, Y., and Qi, X. (2024). Tumor-associated macrophages in colorectal cancer metastasis: molecular insights and translational perspectives. J. Transl. Med. 22, 62. doi:10.1186/s12967-024-04856-x
Hu, Z., Sui, Q., Jin, X., Shan, G., Huang, Y., Yi, Y., et al. (2024). IL6-STAT3-C/EBPβ-IL6 positive feedback loop in tumor-associated macrophages promotes the EMT and metastasis of lung adenocarcinoma. J. Exp. Clin. Cancer Res. 43, 63. doi:10.1186/s13046-024-02989-x
Huang, B., Lang, X., and Li, X. (2022). The role of IL-6/JAK2/STAT3 signaling pathway in cancers. Front. Oncol. 12, 1023177. doi:10.3389/fonc.2022.1023177
Huang, R., Wang, S., Wang, N., Zheng, Y., Zhou, J., Yang, B., et al. (2020). CCL5 derived from tumor-associated macrophages promotes prostate cancer stem cells and metastasis via activating β-catenin/STAT3 signaling. Cell Death Dis. 11, 234. doi:10.1038/s41419-020-2435-y
Hussain, Z., Bertran, T., Finetti, P., Lohmann, E., Mamessier, E., Bidaut, G., et al. (2024). Macrophages reprogramming driven by cancer-associated fibroblasts under FOLFIRINOX treatment correlates with shorter survival in pancreatic cancer. Cell Commun. Signal. 22, 1. doi:10.1186/s12964-023-01388-7
Iriondo, O., Liu, Y., Lee, G., Elhodaky, M., Jimenez, C., Li, L., et al. (2018). TAK1 mediates microenvironment-triggered autocrine signals and promotes triple-negative breast cancer lung metastasis. Nat. Commun. 9, 1994. doi:10.1038/s41467-018-04460-w
Izumi, K., Fang, L. Y., Mizokami, A., Namiki, M., Li, L., Lin, W. J., et al. (2013). Targeting the androgen receptor with siRNA promotes prostate cancer metastasis through enhanced macrophage recruitment via CCL2/CCR2-induced STAT3 activation. EMBO Mol. Med. 5, 1383–1401. doi:10.1002/emmm.201202367
Jiang, M., Liu, L., Huang, W., Qi, Y., Li, Y., and Li, B. (2024). HMGB1-activated tumor-associated macrophages promote migration and invasion via NF-κB/IL-6 signaling in oral squamous cell carcinoma. Int. Immunopharmacol. 126, 111200. doi:10.1016/j.intimp.2023.111200
Jiang, Y., Zhuo, X., Wu, Y., Fu, X., and Mao, C. (2022). PAR2 blockade reverses osimertinib resistance in non-small-cell lung cancer cells via attenuating ERK-mediated EMT and PD-L1 expression. Biochimica Biophysica Acta (BBA) - Mol. Cell Res. 1869, 119144. doi:10.1016/j.bbamcr.2021.119144
Jin, H., He, Y., Zhao, P., Hu, Y., Tao, J., Chen, J., et al. (2019). Targeting lipid metabolism to overcome EMT-associated drug resistance via integrin β3/FAK pathway and tumor-associated macrophage repolarization using legumain-activatable delivery. Theranostics 9, 265–278. doi:10.7150/thno.27246
Jin, Y., Kang, Y., Wang, M., Wu, B., Su, B., Yin, H., et al. (2022). Targeting polarized phenotype of microglia via IL6/JAK2/STAT3 signaling to reduce NSCLC brain metastasis. Signal Transduct. Target Ther. 7, 52. doi:10.1038/s41392-022-00872-9
Jing, Z., Li, Y., Song, J., and Zang, X. (2023). Efficient TNBC immunotherapy by dual reprogramming tumor-infiltrating dendritic cells and tumor-associated macrophages with stimulus-responsive miR155 nanocomplexes. Int. J. Biol. Macromol. 253, 126912. doi:10.1016/j.ijbiomac.2023.126912
Kartikasari, A. E. R., Huertas, C. S., Mitchell, A., and Plebanski, M. (2021). Tumor-induced inflammatory cytokines and the emerging diagnostic devices for cancer detection and prognosis. Front. Oncol. 11, 692142. doi:10.3389/fonc.2021.692142
Kasembeli, M. M., Singhmar, P., Ma, J., Edralin, J., Tang, Y., Adams, C., et al. (2021). TTI-101: a competitive inhibitor of STAT3 that spares oxidative phosphorylation and reverses mechanical allodynia in mouse models of neuropathic pain. Biochem. Pharmacol. 192, 114688. doi:10.1016/j.bcp.2021.114688
Katsuno, Y., Meyer, D. S., Zhang, Z., Shokat, K. M., Akhurst, R. J., Miyazono, K., et al. (2019). Chronic TGF-β exposure drives stabilized EMT, tumor stemness, and cancer drug resistance with vulnerability to bitopic mTOR inhibition. Sci. Signal. 12, eaau8544. doi:10.1126/scisignal.aau8544
Kelley, R. K., Gane, E., Assenat, E., Siebler, J., Galle, P. R., Merle, P., et al. (2019). A phase 2 study of galunisertib (TGF-β1 receptor type I inhibitor) and sorafenib in patients with advanced hepatocellular carcinoma. Clin. Transl. Gastroenterol. 10, e00056. doi:10.14309/ctg.0000000000000056
Kes, M. M. G., Van den Bossche, J., Griffioen, A. W., and Huijbers, E. J. M. (2020). Oncometabolites lactate and succinate drive pro-angiogenic macrophage response in tumors. Biochim. Biophys. Acta Rev. Cancer 1874, 188427. doi:10.1016/j.bbcan.2020.188427
Khalili, D., Mohammed, M., Kunc, M., Sindlerova, M., Ankarklev, J., and Theopold, U. (2023). Single-cell sequencing of tumor-associated macrophages in a Drosophila model. Front. Immunol. 14, 1243797. doi:10.3389/fimmu.2023.1243797
Kielbassa, K., Vegna, S., Ramirez, C., and Akkari, L. (2022). Understanding the origin and diversity of macrophages to tailor their targeting in solid cancers.
Kim, J., Kim, M., Yong, S.-B., Han, H., Kang, S., Lahiji, S. F., et al. (2023). Engineering TGF-β inhibitor-encapsulated macrophage-inspired multi-functional nanoparticles for combination cancer immunotherapy. Biomaterials Res. 27, 136. doi:10.1186/s40824-023-00470-y
Kimura, S., Noguchi, H., Nanbu, U., and Nakayama, T. (2021). Macrophage CCL22 expression promotes lymphangiogenesis in patients with tongue squamous cell carcinoma via IL-4/STAT6 in the tumor microenvironment. Oncol. Lett. 21, 383. doi:10.3892/ol.2021.12644
Kitamura, T., Qian, B. Z., and Pollard, J. W. (2015). Immune cell promotion of metastasis. Nat. Rev. Immunol. 15, 73–86. doi:10.1038/nri3789
Klichinsky, M., Ruella, M., Shestova, O., Lu, X. M., Best, A., Zeeman, M., et al. (2020). Human chimeric antigen receptor macrophages for cancer immunotherapy. Nat. Biotechnol. 38, 947–953. doi:10.1038/s41587-020-0462-y
Kloosterman, D. J., and Akkari, L. (2023). Mapping the uncharted territories of human brain malignancies. Cell 181, 1454–1457. doi:10.1016/j.cell.2020.06.003
Kumari, N., and Choi, S. H. (2022). Tumor-associated macrophages in cancer: recent advancements in cancer nanoimmunotherapies. J. Exp. Clin. Cancer Res. 41, 68. doi:10.1186/s13046-022-02272-x
Le, D., Gutierrez, M. E., Saleh, M., Chen, E., Mallick, A. B., Pishvaian, M. J., et al. (2018). Abstract CT124: a phase Ib/II study of BMS-813160, a CC chemokine receptor (CCR) 2/5 dual antagonist, in combination with chemotherapy or nivolumab in patients (pts) with advanced pancreatic or colorectal cancer. Cancer Res. 78, CT124. doi:10.1158/1538-7445.am2018-ct124
Lee, B. Y., Hogg, E. K. J., Below, C. R., Kononov, A., Blanco-Gomez, A., Heider, F., et al. (2021). Heterocellular OSM-OSMR signalling reprograms fibroblasts to promote pancreatic cancer growth and metastasis. Nat. Commun. 12, 7336. doi:10.1038/s41467-021-27607-8
Lewis, J. H., Gelderblom, H., van de Sande, M., Stacchiotti, S., Healey, J. H., Tap, W. D., et al. (2021). Pexidartinib long-term hepatic safety profile in patients with tenosynovial giant cell tumors. Oncol. 26, e863–e873. doi:10.1002/onco.13629
Li, D., Du, F., Jiao, H., Zhang, F., Wang, X., and Zhang, S. (2023b). CircHSPB6 promotes tumor-associated macrophages M2 polarization and infiltration to accelerate cell malignant properties in lung adenocarcinoma by CCL2. Biochem. Genet. 62, 1379–1395. doi:10.1007/s10528-023-10482-x
Li, D., Ji, H., Niu, X., Yin, L., Wang, Y., Gu, Y., et al. (2020). Tumor-associated macrophages secrete CC-chemokine ligand 2 and induce tamoxifen resistance by activating PI3K/Akt/mTOR in breast cancer. Cancer Sci. 111, 47–58. doi:10.1111/cas.14230
Li, D., Xia, L., Huang, P., Wang, Z., Guo, Q., Huang, C., et al. (2023a). Cancer-associated fibroblast-secreted IGFBP7 promotes gastric cancer by enhancing tumor associated macrophage infiltration via FGF2/FGFR1/PI3K/AKT axis. Cell Death Discov. 9, 17. doi:10.1038/s41420-023-01336-x
Li, J., DeNicola, G. M., and Ruffell, B. (2022a). Metabolism in tumor-associated macrophages. Int. Rev. Cell Mol. Biol. 367, 65–100. doi:10.1016/bs.ircmb.2022.01.004
Li, X., Bu, W., Meng, L., Liu, X., Wang, S., Jiang, L., et al. (2019). CXCL12/CXCR4 pathway orchestrates CSC-like properties by CAF recruited tumor associated macrophage in OSCC. Exp. Cell Res. 378, 131–138. doi:10.1016/j.yexcr.2019.03.013
Li, X. F., Selli, C., Zhou, H. L., Cao, J., Wu, S., Ma, R. Y., et al. (2023c). Macrophages promote anti-androgen resistance in prostate cancer bone disease. J. Exp. Med. 220, e20221007. doi:10.1084/jem.20221007
Li, X.-M., Yang, Y., Jiang, F.-Q., Hu, G., Wan, S., Yan, W.-Y., et al. (2024b). Histone lactylation inhibits RARγ expression in macrophages to promote colorectal tumorigenesis through activation of TRAF6-IL-6-STAT3 signaling. Cell Rep. 43, 113688. doi:10.1016/j.celrep.2024.113688
Li, Y., Chen, Y., Zhao, C., Yang, Y., Zhang, M., Cheng, H., et al. (2024a). Arenobufagin modulation of PCSK9-mediated cholesterol metabolism induces tumor-associated macrophages polarisation to inhibit hepatocellular carcinoma progression. Phytomedicine 128, 155532. doi:10.1016/j.phymed.2024.155532
Li, Z., Wang, P., Cui, W., Yong, H., Wang, D., Zhao, T., et al. (2022b). Tumour-associated macrophages enhance breast cancer malignancy via inducing ZEB1-mediated DNMT1 transcriptional activation. Cell Biosci. 12, 176. doi:10.1186/s13578-022-00913-4
Lian, S. L., Lu, Y. T., Lu, Y. J., Yao, Y. L., Wang, X. L., and Jiang, R. Q. (2024). Tumor-associated macrophages promoting PD-L1 expression in infiltrating B cells through the CXCL12/CXCR4 axis in human hepatocellular carcinoma. Am. J. Cancer Res. 14, 832–853. doi:10.62347/ZIAX8828
Liang, D.-S., You, W.-P., Zhu, F.-F., Wang, J.-H., Guo, F., Xu, J.-J., et al. (2023). Targeted delivery of pexidartinib to tumor-associated macrophages via legumain-sensitive dual-coating nanoparticles for cancer immunotherapy. Colloids Surfaces B Biointerfaces 226, 113283. doi:10.1016/j.colsurfb.2023.113283
Lim, B., Woodward, W., Wang, X., Reuben, J., and Ueno, N. (2018). Inflammatory breast cancer biology: the tumour microenvironment is key. Nat. Rev. Cancer 18, 485–499. doi:10.1038/s41568-018-0010-y
Lin, H., Fu, L., Zhou, X., Yu, A., Chen, Y., Liao, W., et al. (2024). LRP1 induces anti-PD-1 resistance by modulating the DLL4-NOTCH2-CCL2 axis and redirecting M2-like macrophage polarisation in bladder cancer. Cancer Lett. 593, 216807. doi:10.1016/j.canlet.2024.216807
Lin, Y., Zhang, W., Liu, L., Li, W., Li, Y., and Li, B. (2023a). ENO1 promotes OSCC migration and invasion by orchestrating IL-6 secretion from macrophages via a positive feedback loop. Int. J. Mol. Sci. 24, 737. doi:10.3390/ijms24010737
Lin, Z., Wang, Q., Jiang, T., Wang, W., and Zhao, J. J. (2023b). Targeting tumor-associated macrophages with STING agonism improves the antitumor efficacy of osimertinib in a mouse model of EGFR-mutant lung cancer. Front. Immunol. 14. doi:10.3389/fimmu.2023.1077203
Liu, C., Zhang, W., Wang, J., Si, T., and Xing, W. (2021b). Tumor-associated macrophage-derived transforming growth factor-β promotes colorectal cancer progression through HIF1-TRIB3 signaling. Cancer Sci. 112, 4198–4207. doi:10.1111/cas.15101
Liu, M., Zhang, L., Zhou, Q., Wang, Y., Sun, Q., and Ren, X. (2021a). The distinct impact of TAM infiltration on the prognosis of patients with cardia and non-cardia gastric cancer and its association with H. pylori infection. Front. Oncol. 11, 737061. doi:10.3389/fonc.2021.737061
Liu, Q., Hodge, J., Wang, J., Wang, Y., Wang, L., Singh, U., et al. (2020a). Emodin reduces breast cancer lung metastasis by suppressing macrophage-induced breast cancer cell epithelial-mesenchymal transition and cancer stem cell formation. Theranostics 10, 8365–8381. doi:10.7150/thno.45395
Liu, Q., Song, J., Pan, Y., Shi, D., Yang, C., Wang, S., et al. (2020b). Wnt5a/CaMKII/ERK/CCL2 axis is required for tumor-associated macrophages to promote colorectal cancer progression. Int. J. Biol. Sci. 16, 1023–1034. doi:10.7150/ijbs.40535
Liu, Y., Chen, X., Xu, Y., Yang, T., Wang, H., Wang, Z., et al. (2024). CTHRC1 promotes colorectal cancer progression by recruiting tumor-associated macrophages via up-regulation of CCL15. J. Mol. Med. 102, 81–94. doi:10.1007/s00109-023-02399-0
Long, L., Hu, Y., Long, T., Lu, X., Tuo, Y., Li, Y., et al. (2021). Tumor-associated macrophages induced spheroid formation by CCL18-ZEB1-M-CSF feedback loop to promote transcoelomic metastasis of ovarian cancer. J. Immunother. Cancer 9, e003973. doi:10.1136/jitc-2021-003973
Louault, K., Porras, T., Lee, M. H., Muthugounder, S., Kennedy, R. J., Blavier, L., et al. (2022a). Fibroblasts and macrophages cooperate to create a pro-tumorigenic and immune resistant environment via activation of TGF-β/IL-6 pathway in neuroblastoma. Oncoimmunology 11, 2146860. doi:10.1080/2162402x.2022.2146860
Louault, K., Porras, T., Lee, M. H., Muthugounder, S., Kennedy, R. J., Blavier, L., et al. (2022b). Fibroblasts and macrophages cooperate to create a pro-tumorigenic and immune resistant environment via activation of TGF-β/IL-6 pathway in neuroblastoma. Oncoimmunology 11, 2146860. doi:10.1080/2162402X.2022.2146860
Lu, J., Li, J., Lin, Z., Li, H., Lou, L., Ding, W., et al. (2023). Reprogramming of TAMs via the STAT3/CD47-SIRPα axis promotes acquired resistance to EGFR-TKIs in lung cancer. Cancer Lett. 564, 216205. doi:10.1016/j.canlet.2023.216205
Ma, J., Shayiti, F., Ma, J., Wei, M., Hua, T., Zhang, R., et al. (2021). Tumor-associated macrophage-derived CCL5 promotes chemotherapy resistance and metastasis in prostatic cancer. Cell Biol. Int. 45, 2054–2062. doi:10.1002/cbin.11630
Machiels, J. P., Gomez-Roca, C., Michot, J. M., Zamarin, D., Mitchell, T., Catala, G., et al. (2020). Phase Ib study of anti-CSF-1R antibody emactuzumab in combination with CD40 agonist selicrelumab in advanced solid tumor patients. J. Immunother. Cancer 8, e001153. doi:10.1136/jitc-2020-001153
Maller, O., Drain, A. P., Barrett, A. S., Borgquist, S., Ruffell, B., Zakharevich, I., et al. (2021). Tumour-associated macrophages drive stromal cell-dependent collagen crosslinking and stiffening to promote breast cancer aggression. Nat. Mater. 20, 548–559. doi:10.1038/s41563-020-00849-5
Mantovani, A., Marchesi, F., Malesci, A., Laghi, L., and Allavena, P. (2017). Tumour-associated macrophages as treatment targets in oncology. Nat. Rev. Clin. Oncol. 14, 399–416. doi:10.1038/nrclinonc.2016.217
Maria, P.-P., Stine Emilie, W.-B., Aimilia, S., Majken, S., Mie Linder, H., Mia Aaboe, J., et al. (2022). TGFβ-derived immune modulatory vaccine: targeting the immunosuppressive and fibrotic tumor microenvironment in a murine model of pancreatic cancer. J. Immunother. Cancer 10, e005491. doi:10.1136/jitc-2022-005491
Marina, R. P., Elena, S. K., Anna, A. K., Elizaveta, A. P., Maxim, E. M., Irina, V. L., et al. (2023). Revealing molecular mechanisms of early-onset tongue cancer by spatial transcriptomics. bioRxiv. doi:10.1101/2023.10.12.562054
Masjedi, A., Hajizadeh, F., Beigi Dargani, F., Beyzai, B., Aksoun, M., Hojjat-Farsangi, M., et al. (2021). Oncostatin M: a mysterious cytokine in cancers. Int. Immunopharmacol. 90, 107158. doi:10.1016/j.intimp.2020.107158
Melisi, D., Oh, D. Y., Hollebecque, A., Calvo, E., Varghese, A., Borazanci, E., et al. (2021a). Safety and activity of the TGFβ receptor I kinase inhibitor galunisertib plus the anti-PD-L1 antibody durvalumab in metastatic pancreatic cancer. J. Immunother. Cancer 9, e002068. doi:10.1136/jitc-2020-002068
Melisi, D., Oh, D.-Y., Hollebecque, A., Calvo, E., Varghese, A., Borazanci, E., et al. (2021b). Safety and activity of the TGFβ receptor I kinase inhibitor galunisertib plus the anti-PD-L1 antibody durvalumab in metastatic pancreatic cancer. J. Immunother. Cancer 9, e002068. doi:10.1136/jitc-2020-002068
Méndez-Clemente, A., Bravo-Cuellar, A., González-Ochoa, S., Santiago-Mercado, M., Palafox-Mariscal, L., Jave-Suárez, L., et al. (2022). Dual STAT-3 and IL-6R inhibition with stattic and tocilizumab decreases migration, invasion and proliferation of prostate cancer cells by targeting the IL-6/IL-6R/STAT-3 axis. Oncol. Rep. 48, 138. doi:10.3892/or.2022.8349
Miyake, K., Ito, J., Takahashi, K., Nakabayashi, J., Brombacher, F., Shichino, S., et al. (2024). Single-cell transcriptomics identifies the differentiation trajectory from inflammatory monocytes to pro-resolving macrophages in a mouse skin allergy model. Nat. Commun. 15, 1666. doi:10.1038/s41467-024-46148-4
Morris, J. C., Tan, A. R., Olencki, T. E., Shapiro, G. I., Dezube, B. J., Reiss, M., et al. (2014). Phase I study of GC1008 (fresolimumab): a human anti-transforming growth factor-beta (TGFβ) monoclonal antibody in patients with advanced malignant melanoma or renal cell carcinoma. PLoS One 9, e90353. doi:10.1371/journal.pone.0090353
Mu, X.-Y., Wang, R.-J., Yao, Z.-X., Zheng, Z., Jiang, J.-T., Tan, M.-Y., et al. (2019). RS 504393 inhibits M-MDSCs recruiting in immune microenvironment of bladder cancer after gemcitabine treatment. Mol. Immunol. 109, 140–148. doi:10.1016/j.molimm.2019.02.014
Mun, S., Park, P., and Park-Min, K. (2020). The M-CSF receptor in osteoclasts and beyond. Exp. Mol. Med. 52, 1239–1254. doi:10.1038/s12276-020-0484-z
Nakamura, Y., Kinoshita, J., Yamaguchi, T., Aoki, T., Saito, H., Hamabe-Horiike, T., et al. (2022). Crosstalk between cancer-associated fibroblasts and immune cells in peritoneal metastasis: inhibition in the migration of M2 macrophages and mast cells by Tranilast. Gastric Cancer 25, 515–526. doi:10.1007/s10120-021-01275-5
Nalio Ramos, R., Missolo-Koussou, Y., Gerber-Ferder, Y., Bromley, C. P., Bugatti, M., Núñez, N. G., et al. (2022). Tissue-resident FOLR2+ macrophages associate with CD8+ T cell infiltration in human breast cancer. Cell 185, 1189–1207.e25. doi:10.1016/j.cell.2022.02.021
Nie, G., Cao, X., Mao, Y., Lv, Z., Lv, M., Wang, Y., et al. (2021). Tumor-associated macrophages-mediated CXCL8 infiltration enhances breast cancer metastasis: suppression by Danirixin. Int. Immunopharmacol. 95, 107153. doi:10.1016/j.intimp.2020.107153
Niland, S., Riscanevo, A. X., and Eble, J. A. (2021). Matrix metalloproteinases shape the tumor microenvironment in cancer progression. Int. J. Mol. Sci. 23, 146. doi:10.3390/ijms23010146
Noel, M., O'Reilly, E. M., Wolpin, B. M., Ryan, D. P., Bullock, A. J., Britten, C. D., et al. (2020a). Phase 1b study of a small molecule antagonist of human chemokine (C-C motif) receptor 2 (PF-04136309) in combination with nab-paclitaxel/gemcitabine in first-line treatment of metastatic pancreatic ductal adenocarcinoma. Invest. New Drugs 38, 800–811. doi:10.1007/s10637-019-00830-3
Noel, M., O’Reilly, E. M., Wolpin, B. M., Ryan, D. P., Bullock, A. J., Britten, C. D., et al. (2020b). Phase 1b study of a small molecule antagonist of human chemokine (C-C motif) receptor 2 (PF-04136309) in combination with nab-paclitaxel/gemcitabine in first-line treatment of metastatic pancreatic ductal adenocarcinoma. Invest. New Drugs 38, 800–811. doi:10.1007/s10637-019-00830-3
Noel, M. S., Hezel, A. F., Linehan, D., Wang-Gillam, A., Eskens, F., Sleijfer, S., et al. (2017). Orally administered CCR2 selective inhibitor CCX872-b clinical trial in pancreatic cancer. J. Clin. Oncol. 35, 276. doi:10.1200/jco.2017.35.4_suppl.276
Nywening, T., Wang-Gillam, A., Sanford, D., Belt, B., Panni, R., Cusworth, B., et al. (2016a). Targeting tumour-associated macrophages with CCR2 inhibition in combination with FOLFIRINOX in patients with borderline resectable and locally advanced pancreatic cancer: a single-centre, open-label, dose-finding, non-randomised, phase 1b trial. Lancet. Oncol. 17, 651–662. doi:10.1016/S1470-2045(16)00078-4
Nywening, T. M., Belt, B. A., Cullinan, D. R., Panni, R. Z., Han, B. J., Sanford, D. E., et al. (2018). Targeting both tumour-associated CXCR2+ neutrophils and CCR2+ macrophages disrupts myeloid recruitment and improves chemotherapeutic responses in pancreatic ductal adenocarcinoma. Gut 67, 1112–1123. doi:10.1136/gutjnl-2017-313738
Nywening, T. M., Wang-Gillam, A., Sanford, D. E., Belt, B. A., Panni, R. Z., Cusworth, B. M., et al. (2016b). Targeting tumour-associated macrophages with CCR2 inhibition in combination with FOLFIRINOX in patients with borderline resectable and locally advanced pancreatic cancer: a single-centre, open-label, dose-finding, non-randomised, phase 1b trial. Lancet Oncol. 17, 651–662. doi:10.1016/S1470-2045(16)00078-4
Oh, S. C., Sohn, B. H., Cheong, J. H., Kim, S. B., Lee, J. E., Park, K. C., et al. (2018). Clinical and genomic landscape of gastric cancer with a mesenchymal phenotype. Nat. Commun. 9, 1777. doi:10.1038/s41467-018-04179-8
Okikawa, S., Morine, Y., Saito, Y., Yamada, S., Tokuda, K., Teraoku, H., et al. (2022). Inhibition of the VEGF signaling pathway attenuates tumor-associated macrophage activity in liver cancer, Oncol. Rep., 47. doi:10.3892/or.2022.8282
Okojie, A. K., Uweru, J. O., Coburn, M. A., Li, S., Cao-Dao, V. D., and Eyo, U. B. (2023). Distinguishing the effects of systemic CSF1R inhibition by PLX3397 on microglia and peripheral immune cells. J. Neuroinflammation 20, 242. doi:10.1186/s12974-023-02924-5
Ong, S. M., Tan, Y. C., Beretta, O., Jiang, D., Yeap, W. H., Tai, J. J., et al. (2012). Macrophages in human colorectal cancer are pro-inflammatory and prime T cells towards an anti-tumour type-1 inflammatory response. Eur. J. Immunol. 42, 89–100. doi:10.1002/eji.201141825
Ozga, A., Chow, M., and Luster, A. (2021). Chemokines and the immune response to cancer. Immunity 54, 859–874. doi:10.1016/j.immuni.2021.01.012
Pakravan, K., Mossahebi-Mohammadi, M., Ghazimoradi, M. H., Cho, W. C., Sadeghizadeh, M., and Babashah, S. (2022). Monocytes educated by cancer-associated fibroblasts secrete exosomal miR-181a to activate AKT signaling in breast cancer cells. J. Transl. Med. 20, 559. doi:10.1186/s12967-022-03780-2
Pan, B., Wan, T., Zhou, Y., Huang, S., Yuan, L., Jiang, Y., et al. (2023). The MYBL2–CCL2 axis promotes tumor progression and resistance to anti-PD-1 therapy in ovarian cancer by inducing immunosuppressive macrophages. Cancer Cell Int. 23, 248. doi:10.1186/s12935-023-03079-2
Patwardhan, P. P., Surriga, O., Beckman, M. J., de Stanchina, E., Dematteo, R. P., Tap, W. D., et al. (2014). Sustained inhibition of receptor tyrosine kinases and macrophage depletion by PLX3397 and rapamycin as a potential new approach for the treatment of MPNSTs. Clin. Cancer Res. 20, 3146–3158. doi:10.1158/1078-0432.CCR-13-2576
Pe, K. C. S., Saetung, R., Yodsurang, V., Chaotham, C., Suppipat, K., Chanvorachote, P., et al. (2022). Triple-negative breast cancer influences a mixed M1/M2 macrophage phenotype associated with tumor aggressiveness. PLoS One 17, e0273044. doi:10.1371/journal.pone.0273044
Peng, D., Fu, M., Wang, M., Wei, Y., and Wei, X. (2022). Targeting TGF-β signal transduction for fibrosis and cancer therapy. Mol. Cancer 21, 104. doi:10.1186/s12943-022-01569-x
Perez-Penco, M., Lara de la Torre, L., Lecoq, I., Martinenaite, E., and Andersen, M. H. (2024). TGFβ-specific T cells induced by a TGFβ-derived immune modulatory vaccine both directly and indirectly modulate the phenotype of tumor-associated macrophages and fibroblasts. J. Immunother. Cancer 12, e008405. doi:10.1136/jitc-2023-008405
Peyvandi, S., Bulliard, M., Yilmaz, A., Kauzlaric, A., Marcone, R., Haerri, L., et al. (2024). Tumor-educated Gr1+CD11b+ cells drive breast cancer metastasis via OSM/IL6-JAK-induced cancer cell plasticity. J. Clin. Investig. 134, e166847. doi:10.1172/JCI166847
Pizzurro, G. A., Bridges, K., Jiang, X., Vidyarthi, A., Miller-Jensen, K., and Colegio, O. R. (2023). Functionally and metabolically divergent melanoma-associated macrophages originate from common bone-marrow precursors. Cancers (Basel) 15, 3330. doi:10.3390/cancers15133330
Polak, K. L., Tamagno, I., Parameswaran, N., Smigiel, J., Chan, E. R., Yuan, X., et al. (2023). Oncostatin-M and OSM-receptor feed-forward activation of MAPK induces separable stem-like and mesenchymal programs. Mol. Cancer Res. 21, 975–990. doi:10.1158/1541-7786.MCR-22-0715
Pradel, L. P., Ooi, C. H., Romagnoli, S., Cannarile, M. A., Sade, H., Ruttinger, D., et al. (2016a). Macrophage susceptibility to emactuzumab (RG7155) treatment. Mol. Cancer Ther. 15, 3077–3086. doi:10.1158/1535-7163.MCT-16-0157
Pradel, L. P., Ooi, C.-H., Romagnoli, S., Cannarile, M. A., Sade, H., Rüttinger, D., et al. (2016b). Macrophage susceptibility to emactuzumab (RG7155) treatment. Mol. Cancer Ther. 15, 3077–3086. doi:10.1158/1535-7163.MCT-16-0157
Pratt, H., Steinberger, K., Mihalik, N., Ott, S., Whalley, T., Szomolay, B., et al. (2021). Macrophage and neutrophil interactions in the pancreatic tumor microenvironment drive the pathogenesis of pancreatic cancer. Cancers (Basel) 14, 194. doi:10.3390/cancers14010194
Qian, Y., Yin, Y., Zheng, X., Liu, Z., and Wang, X. (2024). Metabolic regulation of tumor-associated macrophage heterogeneity: insights into the tumor microenvironment and immunotherapeutic opportunities. Biomark. Res. 12, 1. doi:10.1186/s40364-023-00549-7
Qin, W., Spek, C. A., Scicluna, B. P., van der Poll, T., and Duitman, J. (2022). Myeloid DNA methyltransferase3b deficiency aggravates pulmonary fibrosis by enhancing profibrotic macrophage activation. Respir. Res. 23, 162. doi:10.1186/s12931-022-02088-5
Qin, Y., Wang, J., Zhu, G., Li, G., Tan, H., Chen, C., et al. (2019). CCL18 promotes the metastasis of squamous cell carcinoma of the head and neck through MTDH-NF-κB signalling pathway. J. Cell. Mol. Med. 23, 2689–2701. doi:10.1111/jcmm.14168
Ratnam, N. M., Gilbert, M. R., and Giles, A. J. (2019). Immunotherapy in CNS cancers: the role of immune cell trafficking. Neuro Oncol. 21, 37–46. doi:10.1093/neuonc/noy084
Ravi, J., Elbaz, M., Wani, N. A., Nasser, M. W., and Ganju, R. K. (2016). Cannabinoid receptor-2 agonist inhibits macrophage induced EMT in non-small cell lung cancer by downregulation of EGFR pathway. Mol. Carcinog. 55, 2063–2076. doi:10.1002/mc.22451
Ren, J., Han, X., Lohner, H., Liang, R., Liang, S., and Wang, H. (2021). Serum- and glucocorticoid-inducible kinase 1 promotes alternative macrophage polarization and restrains inflammation through FoxO1 and STAT3 signaling. J. Immunol. 207, 268–280. doi:10.4049/jimmunol.2001455
Ries, C. H., Cannarile, M. A., Hoves, S., Benz, J., Wartha, K., Runza, V., et al. (2014). Targeting tumor-associated macrophages with anti-CSF-1R antibody reveals a strategy for cancer therapy. Cancer Cell 25, 846–859. doi:10.1016/j.ccr.2014.05.016
Rohila, D., Park, I. H., Pham, T. V., Weitz, J., Hurtado de Mendoza, T., Madheswaran, S., et al. (2023). Syk inhibition reprograms tumor-associated macrophages and overcomes gemcitabine-induced immunosuppression in pancreatic ductal adenocarcinoma. Cancer Res. 83, 2675–2689. doi:10.1158/0008-5472.CAN-22-3645
Sanford, D. E., Belt, B. A., Panni, R. Z., Mayer, A., Deshpande, A. D., Carpenter, D., et al. (2013). Inflammatory monocyte mobilization decreases patient survival in pancreatic cancer: a role for targeting the CCL2/CCR2 Axis. Clin. Cancer Res. 19, 3404–3415. doi:10.1158/1078-0432.CCR-13-0525
Schweer, D., McAtee, A., Neupane, K., Richards, C., Ueland, F., and Kolesar, J. (2022). Tumor-associated macrophages and ovarian cancer: implications for therapy. Cancers (Basel) 14, 2220. doi:10.3390/cancers14092220
Scialla, S., Hanafy, M. S., Wang, J.-L., Genicio, N., Costa Da Silva, M., Costa, M., et al. (2023). Targeted treatment of triple-negative-breast cancer through pH-triggered tumour associated macrophages using smart theranostic nanoformulations. Int. J. Pharm. 632, 122575. doi:10.1016/j.ijpharm.2022.122575
She, L., Qin, Y., Wang, J., Liu, C., Zhu, G., Li, G., et al. (2018). Tumor-associated macrophages derived CCL18 promotes metastasis in squamous cell carcinoma of the head and neck. Cancer Cell Int. 18, 120. doi:10.1186/s12935-018-0620-1
Shen, C., Liu, J., Jiao, W., Zhang, X., Zhao, X., Yang, X., et al. (2023). A feed-forward loop based on aerobic glycolysis and TGF-β between tumor-associated macrophages and bladder cancer cells promoted malignant progression and immune escape. J. Cancer Res. Clin. Oncol. 149, 12867–12880. doi:10.1007/s00432-023-05164-5
Shi, F., Sun, M. H., Zhou, Z., Wu, L., Zhu, Z., Xia, S. J., et al. (2022b). Tumor-associated macrophages in direct contact with prostate cancer cells promote malignant proliferation and metastasis through NOTCH1 pathway. Int. J. Biol. Sci. 18, 5994–6007. doi:10.7150/ijbs.73141
Shi, Y., Li, J., Chen, H., Hu, Y., Tang, L., Zhou, X., et al. (2022a). Pharmacologic inhibition of histone deacetylase 6 prevents the progression of chlorhexidine gluconate-induced peritoneal fibrosis by blockade of M2 macrophage polarization. Front. Immunol. 13, 899140. doi:10.3389/fimmu.2022.899140
Shin, E., and Koo, J. S. (2021). Glucose metabolism and glucose transporters in breast cancer. Front. Cell Dev. Biol. 9, 728759. doi:10.3389/fcell.2021.728759
Siebel, C., and Lendahl, U. (2017). Notch signaling in development, tissue homeostasis, and disease. Physiol. Rev. 97, 1235–1294. doi:10.1152/physrev.00005.2017
Siersbaek, R., Scabia, V., Nagarajan, S., Chernukhin, I., Papachristou, E. K., Broome, R., et al. (2020). IL6/STAT3 signaling hijacks estrogen receptor alpha enhancers to drive breast cancer metastasis. Cancer Cell 38, 412–423 e419. doi:10.1016/j.ccell.2020.06.007
Smeester, B. A., Slipek, N. J., Pomeroy, E. J., Laoharawee, K., Osum, S. H., Larsson, A. T., et al. (2020). PLX3397 treatment inhibits constitutive CSF1R-induced oncogenic ERK signaling, reduces tumor growth, and metastatic burden in osteosarcoma. Bone 136, 115353. doi:10.1016/j.bone.2020.115353
Smigiel, J. M., Parameswaran, N., and Jackson, M. W. (2017). Potent EMT and CSC phenotypes are induced by oncostatin-M in pancreatic cancer. Mol. Cancer Res. 15, 478–488. doi:10.1158/1541-7786.MCR-16-0337
Su, J., Zheng, Z., Bian, C., Chang, S., Bao, J., Yu, H., et al. (2023). Functions and mechanisms of lactylation in carcinogenesis and immunosuppression. Front. Immunol. 14, 1253064. doi:10.3389/fimmu.2023.1253064
Sui, X., Chen, C., Zhou, X., Wen, X., Shi, C., Chen, G., et al. (2023). Integrative analysis of bulk and single-cell gene expression profiles to identify tumor-associated macrophage-derived CCL18 as a therapeutic target of esophageal squamous cell carcinoma. J. Exp. Clin. Cancer Res. 42, 51. doi:10.1186/s13046-023-02612-5
Sumitomo, R., Menju, T., Shimazu, Y., Toyazaki, T., Chiba, N., Miyamoto, H., et al. (2023). M2-like tumor-associated macrophages promote epithelial–mesenchymal transition through the transforming growth factor β/Smad/zinc finger e-box binding homeobox pathway with increased metastatic potential and tumor cell proliferation in lung squamous cell carcinoma. Cancer Sci. 114, 4521–4534. doi:10.1111/cas.15987
Tap, W. D., Gelderblom, H., Palmerini, E., Desai, J., Bauer, S., Blay, J. Y., et al. (2019). Pexidartinib versus placebo for advanced tenosynovial giant cell tumour (ENLIVEN): a randomised phase 3 trial. Lancet 394, 478–487. doi:10.1016/S0140-6736(19)30764-0
Tekpli, X., Lien, T., Rossevold, A. H., Nebdal, D., Borgen, E., Ohnstad, H. O., et al. (2019). An independent poor-prognosis subtype of breast cancer defined by a distinct tumor immune microenvironment. Nat. Commun. 10, 5499. doi:10.1038/s41467-019-13329-5
Thongchot, S., Duangkaew, S., Yotchai, W., Maungsomboon, S., Phimolsarnti, R., Asavamongkolkul, A., et al. (2023). Novel CSF1R-positive tenosynovial giant cell tumor cell lines and their pexidartinib (PLX3397) and sotuletinib (BLZ945)-induced apoptosis. Hum. Cell 36, 456–467. doi:10.1007/s13577-022-00823-0
Tigue, M., Loberg, M., Goettel, J., Weiss, W., Lee, E., and Weiss, V. (2023). Wnt signaling in the phenotype and function of tumor-associated macrophages. Cancer Res. 83, 3–11. doi:10.1158/0008-5472.CAN-22-1403
Tsai, T. H., Yang, C. C., Kou, T. C., Yang, C. E., Dai, J. Z., Chen, C. L., et al. (2021). Overexpression of GLUT3 promotes metastasis of triple-negative breast cancer by modulating the inflammatory tumor microenvironment. J. Cell. Physiol. 236, 4669–4680. doi:10.1002/jcp.30189
Tsai, Y. C., Chen, W. Y., Abou-Kheir, W., Zeng, T., Yin, J. J., Bahmad, H., et al. (2018). Androgen deprivation therapy-induced epithelial-mesenchymal transition of prostate cancer through downregulating SPDEF and activating CCL2. Biochim. Biophys. Acta Mol. Basis Dis. 1864, 1717–1727. doi:10.1016/j.bbadis.2018.02.016
Tsimberidou, A. M., Achaval, S. d., Alibhai, I., and Kaseb, A. O. (2021). First-in-man phase I clinical trial evaluating TTI-101, an orally bioavailable, small molecule inhibitor of STAT3, in patients with advanced solid tumors. J. Clin. Oncol. 39, TPS3158. doi:10.1200/jco.2021.39.15_suppl.tps3158
Tu, M. M., Abdel-Hafiz, H. A., Jones, R. T., Jean, A., Hoff, K. J., Duex, J. E., et al. (2020). Inhibition of the CCL2 receptor, CCR2, enhances tumor response to immune checkpoint therapy. Commun. Biol. 3, 720. doi:10.1038/s42003-020-01441-y
Unver, N., and McAllister, F. (2018). IL-6 family cytokines: key inflammatory mediators as biomarkers and potential therapeutic targets. Cytokine Growth Factor Rev. 41, 10–17. doi:10.1016/j.cytogfr.2018.04.004
Urbas, R., Mayr, C., Klieser, E., Fuereder, J., Bach, D., Stattner, S., et al. (2016). Relevance of MicroRNA200 family and MicroRNA205 for epithelial to mesenchymal transition and clinical outcome in biliary tract cancer patients. Int. J. Mol. Sci. 17, 2053. doi:10.3390/ijms17122053
Vadevoo, S. M. P., Gunassekaran, G. R., Lee, C., Lee, N., Lee, J., Chae, S., et al. (2021). The macrophage odorant receptor Olfr78 mediates the lactate-induced M2 phenotype of tumor-associated macrophages. Proc. Natl. Acad. Sci. U. S. A. 118, e2102434118. doi:10.1073/pnas.2102434118
Valeta-Magara, A., Gadi, A., Volta, V., Walters, B., Arju, R., Giashuddin, S., et al. (2019). Inflammatory breast cancer promotes development of M2 tumor-associated macrophages and cancer mesenchymal cells through a complex chemokine network. Cancer Res. 79, 3360–3371. doi:10.1158/0008-5472.CAN-17-2158
Venturini, N., Hamon, P., Ward, S., Fiel, M., Beasley, M., Kim, E., et al. (2023). 170P Targeting myeloid cells in non-small cell lung cancer and hepatocellular carcinoma: a window-of-opportunity trial of nivolumab with BMS-813160 (CCR2/5i) or BMS-986253 (anti-IL8). Immuno-Oncology Technol. 20, 100629. doi:10.1016/j.iotech.2023.100629
Viganò, M., La Milia, M., Grassini, M. V., Pugliese, N., De Giorgio, M., and Fagiuoli, S. (2023). Hepatotoxicity of small molecule protein kinase inhibitors for cancer. Cancers (Basel), 15. doi:10.3390/cancers15061766
Vyse, S., and Huang, P. H. (2019). Targeting EGFR exon 20 insertion mutations in non-small cell lung cancer. Signal Transduct. Target. Ther. 4, 5. doi:10.1038/s41392-019-0038-9
Wan, S., Zhao, E., Kryczek, I., Vatan, L., Sadovskaya, A., Ludema, G., et al. (2014a). Tumor-associated macrophages produce interleukin 6 and signal via STAT3 to promote expansion of human hepatocellular carcinoma stem cells. Gastroenterology 147, 1393–1404. doi:10.1053/j.gastro.2014.08.039
Wan, S., Zhao, E., Kryczek, I., Vatan, L., Sadovskaya, A., Ludema, G., et al. (2014b). Tumor-associated macrophages produce interleukin 6 and signal via STAT3 to promote expansion of human hepatocellular carcinoma stem cells. Gastroenterology 147, 1393–1404. doi:10.1053/j.gastro.2014.08.039
Wang, B., Pan, L., Chen, M., Ma, Y., Gao, J., Tang, D., et al. (2023). SIRP-alpha-IL-6 axis induces immunosuppressive macrophages in non-small-cell lung cancer. Biochem. Biophys. Res. Commun. 682, 386–396. doi:10.1016/j.bbrc.2023.10.035
Wang, D., Yang, L., Yue, D., Cao, L., Li, L., Wang, D., et al. (2019). Macrophage-derived CCL22 promotes an immunosuppressive tumor microenvironment via IL-8 in malignant pleural effusion. Cancer Lett. 452, 244–253. doi:10.1016/j.canlet.2019.03.040
Wang, L., Zhang, L., Zhao, L., Shao, S., Ning, Q., Jing, X., et al. (2024a). VEGFA/NRP-1/GAPVD1 axis promotes progression and cancer stemness of triple-negative breast cancer by enhancing tumor cell-macrophage crosstalk. Int. J. Biol. Sci. 20, 446–463. doi:10.7150/ijbs.86085
Wang, S., Wang, J., Chen, Z., Luo, J., Guo, W., Sun, L., et al. (2024b). Targeting M2-like tumor-associated macrophages is a potential therapeutic approach to overcome antitumor drug resistance. npj Precis. Oncol. 8, 31. doi:10.1038/s41698-024-00522-z
Wang, Y. Y., Chen, H. D., Lo, S., Chen, Y. K., Huang, Y. C., Hu, S. C., et al. (2020). Visfatin enhances breast cancer progression through CXCL1 induction in tumor-associated macrophages. Cancers (Basel) 12, 3526. doi:10.3390/cancers12123526
Wang-Gillam, A., Nywening, T. M., Sanford, D. E., Lockhart, A. C., Suresh, R., Tan, B. R., et al. (2015). Phase IB study of FOLFIRINOX plus PF-04136309 in patients with borderline resectable and locally advanced pancreatic adenocarcinoma (PC). J. Clin. Oncol. 33, 338. doi:10.1200/jco.2015.33.3_suppl.338
Wanke, F., Gutbier, S., Rümmelin, A., Steinberg, M., Hughes, L. D., Koenen, M., et al. (2021). Ligand-dependent kinase activity of MERTK drives efferocytosis in human iPSC-derived macrophages. Cell Death Dis. 12, 538. doi:10.1038/s41419-021-03770-0
Wculek, S. K., Dunphy, G., Heras-Murillo, I., Mastrangelo, A., and Sancho, D. (2022). Metabolism of tissue macrophages in homeostasis and pathology. Cell. Mol. Immunol. 19, 384–408. doi:10.1038/s41423-021-00791-9
Wei, C., Yang, C., Wang, S., Shi, D., Zhang, C., Lin, X., et al. (2019a). Crosstalk between cancer cells and tumor associated macrophages is required for mesenchymal circulating tumor cell-mediated colorectal cancer metastasis. Mol. Cancer 18, 64. doi:10.1186/s12943-019-0976-4
Wei, C., Yang, C., Wang, S., Shi, D., Zhang, C., Lin, X., et al. (2019b). M2 macrophages confer resistance to 5-fluorouracil in colorectal cancer through the activation of CCL22/PI3K/AKT signaling. Onco Targets Ther. 12, 3051–3063. doi:10.2147/OTT.S198126
Weidner, L., Lorenz, J., Quach, S., Braun, F. K., Rothhammer-Hampl, T., Ammer, L.-M., et al. (2023). Translocator protein (18kDA) (TSPO) marks mesenchymal glioblastoma cell populations characterized by elevated numbers of tumor-associated macrophages. Acta Neuropathol. Commun. 11, 147. doi:10.1186/s40478-023-01651-5
Weng, J., Wang, Z., Hu, Z., Xu, W., Sun, J.-L., Wang, F., et al. (2024). Repolarization of immunosuppressive macrophages by targeting SLAMF7-regulated CCL2 signaling sensitizes hepatocellular carcinoma to immunotherapy. Cancer Res. 84, 1817–1833. doi:10.1158/0008-5472.CAN-23-3106
Weng, Y., Tseng, H., Chen, Y., Shen, P., Al Haq, A., Chen, L., et al. (2019a). MCT-1/miR-34a/IL-6/IL-6R signaling axis promotes EMT progression, cancer stemness and M2 macrophage polarization in triple-negative breast cancer. Mol. Cancer 18, 42. doi:10.1186/s12943-019-0988-0
Weng, Y.-S., Tseng, H.-Y., Chen, Y.-A., Shen, P.-C., Al Haq, A. T., Chen, L.-M., et al. (2019b). MCT-1/miR-34a/IL-6/IL-6R signaling axis promotes EMT progression, cancer stemness and M2 macrophage polarization in triple-negative breast cancer. Mol. Cancer 18, 42. doi:10.1186/s12943-019-0988-0
West, N. R. (2019). Coordination of immune-stroma crosstalk by IL-6 family cytokines. Front. Immunol. 10, 1093. doi:10.3389/fimmu.2019.01093
Wettersten, H. I., Weis, S. M., Pathria, P., Von Schalscha, T., Minami, T., Varner, J. A., et al. (2019). Arming tumor-associated macrophages to reverse epithelial cancer progression. Cancer Res. 79, 5048–5059. doi:10.1158/0008-5472.CAN-19-1246
Wischhusen, J. C., Chowdhury, S. M., Lee, T., Wang, H., Bachawal, S., Devulapally, R., et al. (2020). Ultrasound-mediated delivery of miRNA-122 and anti-miRNA-21 therapeutically immunomodulates murine hepatocellular carcinoma in vivo. J. Control. Release 321, 272–284. doi:10.1016/j.jconrel.2020.01.051
Wu, H., Han, Y., Rodriguez Sillke, Y., Deng, H., Siddiqui, S., Treese, C., et al. (2019). Lipid droplet-dependent fatty acid metabolism controls the immune suppressive phenotype of tumor-associated macrophages. EMBO Mol. Med. 11, e10698. doi:10.15252/emmm.201910698
Wu, J., Shen, Y., Zeng, G., Liang, Y., and Liao, G. (2024). SPP1+ TAM subpopulations in tumor microenvironment promote intravasation and metastasis of head and neck squamous cell carcinoma. Cancer Gene Ther. 31, 311–321. doi:10.1038/s41417-023-00704-0
Wu, J.-Y., Huang, T.-W., Hsieh, Y.-T., Wang, Y.-F., Yen, C.-C., Lee, G.-L., et al. (2020b). Cancer-derived succinate promotes macrophage polarization and cancer metastasis via succinate receptor. Mol. Cell 77, 213–227. doi:10.1016/j.molcel.2019.10.023
Wu, K., Lin, K., Li, X., Yuan, X., Xu, P., Ni, P., et al. (2020a). Redefining tumor-associated macrophage subpopulations and functions in the tumor microenvironment. Front. Immunol. 11, 1731. doi:10.3389/fimmu.2020.01731
Wu, N., Wang, Y., Wang, K., Zhong, B., Liao, Y., Liang, J., et al. (2022a). Cathepsin K regulates the tumor growth and metastasis by IL-17/CTSK/EMT axis and mediates M2 macrophage polarization in castration-resistant prostate cancer. Cell Death Dis. 13, 813. doi:10.1038/s41419-022-05215-8
Wu, T., Wang, W., Shi, G., Hao, M., Wang, Y., Yao, M., et al. (2022b). Targeting HIC1/TGF-β axis-shaped prostate cancer microenvironment restrains its progression. Cell Death Dis. 13, 624. doi:10.1038/s41419-022-05086-z
Wu, T., Wang, W., Shi, G., Hao, M., Wang, Y., Yao, M., et al. (2022c). Targeting HIC1/TGF-β axis-shaped prostate cancer microenvironment restrains its progression. Cell Death Dis. 13, 624. doi:10.1038/s41419-022-05086-z
Xiao, L., Wang, Q., and Peng, H. (2023). Tumor-associated macrophages: new insights on their metabolic regulation and their influence in cancer immunotherapy. Front. Immunol. 14, 1157291. doi:10.3389/fimmu.2023.1157291
Xiong, C., Zhu, Y., Xue, M., Jiang, Y., Zhong, Y., Jiang, L., et al. (2021). Tumor-associated macrophages promote pancreatic ductal adenocarcinoma progression by inducing epithelial-to-mesenchymal transition. Aging 13, 3386–3404. doi:10.18632/aging.202264
Xu, J., Liu, X.-Y., Zhang, Q., Liu, H., Zhang, P., Tian, Z.-B., et al. (2022). Crosstalk among YAP, LncRNA, and tumor-associated macrophages in tumorigenesis development. Front. Oncol. 11, 810893. doi:10.3389/fonc.2021.810893
Yamazaki, T., Gunderson, A., Gilchrist, M., Whiteford, M., Kiely, M., Hayman, A., et al. (2022). Galunisertib plus neoadjuvant chemoradiotherapy in patients with locally advanced rectal cancer: a single-arm, phase 2 trial. Lancet. Oncol. 23, 1189–1200. doi:10.1016/S1470-2045(22)00446-6
Yan, R., Huang, X., Liu, H., Xiao, Z., Liu, J., An, G., et al. (2023). DCLK1 drives EGFR-TKI-acquired resistance in lung adenocarcinoma by remodeling the epithelial–mesenchymal transition status. Biomedicines 11, 1490. doi:10.3390/biomedicines11051490
Yan, X., He, Y., Yang, S., Zeng, T., Hua, Y., Bao, S., et al. (2022). A positive feedback loop: RAD18-YAP-TGF-β between triple-negative breast cancer and macrophages regulates cancer stemness and progression. Cell Death Discov. 8, 196. doi:10.1038/s41420-022-00968-9
Yang, K., Wang, Y., Zhang, F., Li, Q., Luo, B., Feng, D., et al. (2022). CAF-derived midkine promotes EMT and cisplatin resistance by upregulating lncRNA ST7-AS1 in gastric cancer. Mol. Cell. Biochem. 477, 2493–2505. doi:10.1007/s11010-022-04436-x
Yang, K., Xie, Y., Xue, L., Li, F., Luo, C., Liang, W., et al. (2023b). M2 tumor-associated macrophage mediates the maintenance of stemness to promote cisplatin resistance by secreting TGF-β1 in esophageal squamous cell carcinoma. J. Transl. Med. 21, 26. doi:10.1186/s12967-022-03863-0
Yang, K., Yang, T., Yu, J., Li, F., and Zhao, X. (2023a). Integrated transcriptional analysis reveals macrophage heterogeneity and macrophage-tumor cell interactions in the progression of pancreatic ductal adenocarcinoma. BMC Cancer 23, 199. doi:10.1186/s12885-023-10675-y
Yang, L., and Zhang, Y. (2017). Tumor-associated macrophages, potential targets for cancer treatment. Biomark. Res. 5, 25. doi:10.1186/s40364-017-0106-7
Yang, W., Yang, S., Zhang, F., Cheng, F., Wang, X., and Rao, J. (2020). Influence of the Hippo-YAP signalling pathway on tumor associated macrophages (TAMs) and its implications on cancer immunosuppressive microenvironment. Ann. Transl. Med. 8, 399. doi:10.21037/atm.2020.02.11
Yin, Z., Ma, T., Huang, B., Lin, L., Zhou, Y., Yan, J., et al. (2019). Macrophage-derived exosomal microRNA-501-3p promotes progression of pancreatic ductal adenocarcinoma through the TGFBR3-mediated TGF-β signaling pathway. J. Exp. Clin. Cancer Res. 38, 310. doi:10.1186/s13046-019-1313-x
You, Y., Tian, Z., Du, Z., Wu, K., Xu, G., Dai, M., et al. (2022). M1-like tumor-associated macrophages cascade a mesenchymal/stem-like phenotype of oral squamous cell carcinoma via the IL6/Stat3/THBS1 feedback loop. J. Exp. Clin. Cancer Res. 41, 10. doi:10.1186/s13046-021-02222-z
Yu, B., de Vos, D., Guo, X., Peng, S., Xie, W., Peppelenbosch, M. P., et al. (2024). IL-6 facilitates cross-talk between epithelial cells and tumor-associated macrophages in Helicobacter pylori-linked gastric carcinogenesis. Neoplasia 50, 100981. doi:10.1016/j.neo.2024.100981
Yumimoto, K., Sugiyama, S., Mimori, K., and Nakayama, K. I. (2019). Potentials of C-C motif chemokine 2–C-C chemokine receptor type 2 blockers including propagermanium as anticancer agents. Cancer Sci. 110, 2090–2099. doi:10.1111/cas.14075
Zeng, W., Xiong, L., Wu, W., Li, S., Liu, J., Yang, L., et al. (2022). CCL18 signaling from tumor-associated macrophages activates fibroblasts to adopt a chemoresistance-inducing phenotype. Oncogene 42, 224–237. doi:10.1038/s41388-022-02540-2
Zhang, A., Xu, Y., Xu, H., Ren, J., Meng, T., Ni, Y., et al. (2021d). Lactate-induced M2 polarization of tumor-associated macrophages promotes the invasion of pituitary adenoma by secreting CCL17. Theranostics 11, 3839–3852. doi:10.7150/thno.53749
Zhang, B., Guo, X., Huang, L., Zhang, Y., Li, Z., Su, D., et al. (2024). Tumour-associated macrophages and Schwann cells promote perineural invasion via paracrine loop in pancreatic ductal adenocarcinoma. Br. J. Cancer 130, 542–554. doi:10.1038/s41416-023-02539-w
Zhang, B., Ye, H., Ren, X., Zheng, S., Zhou, Q., Chen, C., et al. (2019). Macrophage-expressed CD51 promotes cancer stem cell properties via the TGF-β1/smad2/3 axis in pancreatic cancer. Cancer Lett. 459, 204–215. doi:10.1016/j.canlet.2019.06.005
Zhang, F., Li, P., Liu, S., Yang, M., Zeng, S., Deng, J., et al. (2021a). β-Catenin-CCL2 feedback loop mediates crosstalk between cancer cells and macrophages that regulates breast cancer stem cells. Oncogene 40, 5854–5865. doi:10.1038/s41388-021-01986-0
Zhang, J., Fu, L., Yasuda-Yoshihara, N., Yonemura, A., Wei, F., Bu, L., et al. (2023c). IL-1β derived from mixed-polarized macrophages activates fibroblasts and synergistically forms a cancer-promoting microenvironment. Gastric Cancer 26, 187–202. doi:10.1007/s10120-022-01352-3
Zhang, J., Song, J., Tang, S., Zhao, Y., Wang, L., Luo, Y., et al. (2023d). Multi-omics analysis reveals the chemoresistance mechanism of proliferating tissue-resident macrophages in PDAC via metabolic adaptation. Cell Rep. 42, 112620. doi:10.1016/j.celrep.2023.112620
Zhang, J., Yan, Y., Yang, Y., Wang, L., Li, M., Wang, J., et al. (2016). High infiltration of tumor-associated macrophages influences poor prognosis in human gastric cancer patients, associates with the phenomenon of EMT. Med. Baltim. 95, e2636. doi:10.1097/MD.0000000000002636
Zhang, W., Jiang, X., Zou, Y., Yuan, L., and Wang, X. (2023a). Pexidartinib synergize PD-1 antibody through inhibiting treg infiltration by reducing TAM-derived CCL22 in lung adenocarcinoma. Front. Pharmacol. 14, 1092767. doi:10.3389/fphar.2023.1092767
Zhang, W., Jiang, X., Zou, Y., Yuan, L., and Wang, X. (2023e). Pexidartinib synergize PD-1 antibody through inhibiting treg infiltration by reducing TAM-derived CCL22 in lung adenocarcinoma. Front. Pharmacol. 14, 1092767. doi:10.3389/fphar.2023.1092767
Zhang, W., Liu, Y., Yan, Z., Yang, H., Sun, W., Yao, Y., et al. (2020). IL-6 promotes PD-L1 expression in monocytes and macrophages by decreasing protein tyrosine phosphatase receptor type O expression in human hepatocellular carcinoma. J. Immunother. Cancer 8, e000285. doi:10.1136/jitc-2019-000285
Zhang, W. J., Wang, X. H., Gao, S. T., Chen, C., Xu, X. Y., Sun, Q., et al. (2018). Tumor-associated macrophages correlate with phenomenon of epithelial-mesenchymal transition and contribute to poor prognosis in triple-negative breast cancer patients. J. Surg. Res. 222, 93–101. doi:10.1016/j.jss.2017.09.035
Zhang, Y., Chen, Y., Chen, C., Guo, H., Zhou, C., Wang, H., et al. (2023b). PITX1 suppresses osteosarcoma metastasis through exosomal LINC00662-mediated M2 macrophage polarization. Clin. Exp. Metastasis 40, 79–93. doi:10.1007/s10585-022-10192-5
Zhang, Y., Chen, Y., Li, J., Zhu, X., Liu, Y., Wang, X., et al. (2021b). Development of toll-like receptor agonist-loaded nanoparticles as precision immunotherapy for reprogramming tumor-associated macrophages. ACS Appl. Mater. and Interfaces 13, 24442–24452. doi:10.1021/acsami.1c01453
Zhang, Y., Fan, Y., Jing, X., Zhao, L., Liu, T., Wang, L., et al. (2021c). OTUD5-mediated deubiquitination of YAP in macrophage promotes M2 phenotype polarization and favors triple-negative breast cancer progression. Cancer Lett. 504, 104–115. doi:10.1016/j.canlet.2021.02.003
Zhang, Y., Zhang, X., Meng, Y., Xu, X., and Zuo, D. (2022). The role of glycolysis and lactate in the induction of tumor-associated macrophages immunosuppressive phenotype. Int. Immunopharmacol. 110, 108994. doi:10.1016/j.intimp.2022.108994
Zhao, C., Zheng, S., Yan, Z., Deng, Z., Wang, R., and Zhang, B. (2019). CCL18 promotes the invasion and metastasis of breast cancer through Annexin A2. Oncol. Rep. 43, 571–580. doi:10.3892/or.2019.7426
Zhao, W., Zhou, B., Sun, J., Qiao, H., Li, J., Ma, Q., et al. (2022). Macrophage CCL22 secretion under hypoxic conditions promotes the metastasis of triple-negative breast cancer. Proc. Anticancer Res. 6, 8–14. doi:10.26689/par.v6i6.4493
Zheng, X., Sarode, P., Weigert, A., Turkowski, K., Chelladurai, P., Günther, S., et al. (2023). The HDAC2-SP1 axis orchestrates protumor macrophage polarization. Cancer Res. 83, 2345–2357. doi:10.1158/0008-5472.CAN-22-1270
Zhou, B., Mo, Z., Lai, G., Chen, X., Li, R., Wu, R., et al. (2023). Targeting tumor exosomal circular RNA cSERPINE2 suppresses breast cancer progression by modulating MALT1-NF-?B-IL-6 axis of tumor-associated macrophages. J. Exp. and Clin. cancer Res. CR 42, 48. doi:10.1186/s13046-023-02620-5
Zhou, M., Yu, H., Bai, M., Lu, S., Wang, C., Ke, S., et al. (2024). IRG1 restrains M2 macrophage polarization and suppresses intrahepatic cholangiocarcinoma progression via the CCL18/STAT3 pathway. Cancer Sci. 115, 777–790. doi:10.1111/cas.16068
Zhou, Z., Peng, Y., Wu, X., Meng, S., Yu, W., Zhao, J., et al. (2018). CCL18 secreted from M2 macrophages promotes migration and invasion via the PI3K/Akt pathway in gallbladder cancer. Cell. Oncol. 42, 81–92. doi:10.1007/s13402-018-0410-8
Zhu, X., Chen, L., Liu, L., and Niu, X. (2019). EMT-mediated acquired EGFR-TKI resistance in NSCLC: mechanisms and strategies. Front. Oncol. 9, 1044. doi:10.3389/fonc.2019.01044
Glossary
Keywords: TAM, tumor associated macrophage, TME, tumor microenvironment, EMT, epithelialmesenchymal transition, ECM, extracellular matrix, CSF1R, colony-stimulating factor 1 receptor
Citation: Zhang Y, Ding X, Zhang X, Li Y, Xu R, Li H-J, Zuo D and Chen G (2024) Unveiling the contribution of tumor-associated macrophages in driving epithelial-mesenchymal transition: a review of mechanisms and therapeutic Strategies. Front. Pharmacol. 15:1404687. doi: 10.3389/fphar.2024.1404687
Received: 21 March 2024; Accepted: 15 August 2024;
Published: 02 September 2024.
Edited by:
Lu Xie, Shanghai Institute for Biomedical and Pharmaceutical Technologies, ChinaCopyright © 2024 Zhang, Ding, Zhang, Li, Xu, Li, Zuo and Chen. This is an open-access article distributed under the terms of the Creative Commons Attribution License (CC BY). The use, distribution or reproduction in other forums is permitted, provided the original author(s) and the copyright owner(s) are credited and that the original publication in this journal is cited, in accordance with accepted academic practice. No use, distribution or reproduction is permitted which does not comply with these terms.
*Correspondence: Guang Chen, Z2NoZW5AdHpjLmVkdS5jbg==; Daiying Zuo, enVvZGFpeWluZ0AxNjMuY29t; Hai-Jun Li, bGhqeXlAMTI2LmNvbQ==