- 1Faculty of Health Sciences, Institute of Medical, Pharmaceutical and Health Sciences, Kanazawa University, Kanazawa, Japan
- 2Department of Radioisotope Medicine, Atomic Bomb Disease Institute, Nagasaki University, Nagasaki, Japan
- 3Division of Cancer Genome and Pharmacotherapy, Department of Clinical Pharmacy, Showa University School of Pharmacy, Tokyo, Japan
- 4Department of Radiologic Technology, National Cancer Center Hospital East, Kashiwa, Japan
- 5Department of Radiological Technology, Faculty of Health Science, Juntendo University, Tokyo, Japan
- 6Division of Health Sciences, Graduate School of Medical Sciences, Kanazawa University, Kanazawa, Japan
- 7Faculty of Pharmaceutical Sciences, Matsuyama University, Matsuyama, Japan
- 8Preeminent Medical Photonics Education and Research Center, Hamamatsu University School of Medicine, Hamamatsu, Japan
- 9Department of Integrated Health Sciences, Graduate School of Medicine, Nagoya University, Nagoya, Japan
- 10Department of Radiological Sciences, Ibaraki Prefectural University of Health Sciences, Ibaraki, Japan
- 11Department of Psychiatry, Faculty of Medicine, University of Miyazaki, Miyazaki, Japan
- 12Faculty of Pharmaceutical Sciences, Kobe Gakuin University, Kobe, Japan
- 13Division of Medical Technology and Science, Department of Medical Physics and Engineering, Course of Health Science, Graduate School of Medicine, Osaka University, Osaka, Japan
- 14Biomedical Imaging Research Center, University of Fukui, Fukui, Japan
Background: As drug-metabolizing enzyme activities are affected by a variety of factors, such as drug-drug interactions, a method to evaluate drug-metabolizing enzyme activities in real time is needed. In this study, we developed a novel SPECT imaging probe for evaluation of hepatic CYP2D activity.
Methods: Iodine-123- and 125-labeled 4-iodobenzylmequitazine (123/125I-BMQ) was synthesized with high labeling and purity. CYP isozymes involved in the metabolism of 125I-BMQ in mouse liver microsomes were evaluated, and the utility of 123/125I-was assessed from biological distribution and SPECT imaging evaluation in normal and CYP2D-inhibited mice.
Results: In vitro metabolite analysis using mouse liver microsomes showed that 125I-BMQ is specifically metabolized by CYP2D. Biological distribution and SPECT imaging of 123/125I-BMQ in normal mice showed that injection 123/125I-BMQ accumulated early in the liver and was excreted into the gallbladder and intestines. In CYP2D-inhibited mice, accumulation in the liver was increased, but accumulation in the gallbladder and intestines, the excretory organ, was delayed. Since only metabolites of 125I-BMQ are detected in bile, visualization and measuring of the accumulation of metabolites over time in the intestine, where bile is excreted, could predict the amount of metabolites produced in the body and evaluate CYP2D activity, which would be useful in determining the dosage of various drugs metabolized by CYP2D.
Conclusion: 123/125I-BMQ is useful as a SPECT imaging probe for comprehensive and direct assessment of hepatic CYP2D activity in a minimally invasive and simple approach.
1 Introduction
After ingestion, drugs are converted to a hydrophilic form by cytochrome P450 (CYP) and other drug-metabolizing enzymes to facilitate excretion from the body (Ingelman-Sundberg et al., 1999; Rogers et al., 2002; Manikandan and Nagini, 2018). As the activity of drug-metabolizing enzymes varies between individuals, the effects of administered drugs can vary greatly as well (Singh et al., 2011; Reed and Backes, 2012; Ross et al., 2012). Individual-specific responses to drug administration are affected by many multifaceted and complex factors, such as the genotype with regard to drug-metabolizing enzymes, physiological factors (age, gender, body size, ethnicity), environmental factors (toxin exposure, diet, smoking), and even pathological conditions (liver and kidney dysfunction, diabetes, obesity, drug interactions), which can act alone or in combination to influence drug responses (Almazroo et al., 2017). Measuring the activity and capacity of drug-metabolizing enzymes in order to predict drug effects in individuals could be useful for selecting optimal drugs and determining optimal doses in personalized medicine, as well as for real-time measurement of individual drug responses. Although genotyping of CYPs is generally well established, the analyses have not been comprehensive, only evaluating genetic polymorphisms. A variety of approaches, such as serial blood, urine and breath sampling, have been used to comprehensively evaluate CYP activity (Michael et al., 2012; Matthaei et al., 2020). However, these approaches require specialized chemical analysers such as liquid chromatographs and mass spectrometers, which are not generally available in clinics and hospitals. A method for evaluating drug-metabolizing enzyme activities that is simple and applicable to many facilities is needed, and we have previously reported that SPECT imaging could be used to analyze drug metabolizing enzyme activities in mice and to evaluate the activities of hepatic carboxylesterase (Mizutani et al., 2018), CYP3A4 and 2D6 (Mizutani et al., 2022) by measuring the amounts of various radioactive metabolites accumulated in the gallbladder. The following conditions are considered important for SPECT imaging probes to evaluate drug-metabolising enzyme activity in the liver: 1) the SPECT imaging probe accumulate in metabolizing organs; 2) the SPECT imaging probe is metabolized by specific drug-metabolizing enzymes, and the metabolites are radioactive; 3) The radioactive metabolites are selectively transported from the metabolic organs to the excretory organs; and 4) the radioactive metabolites accumulate in excretory organs can be visualized and measured. In previous studies, 123/125I-O-desmethylvenlafaxine was a SPECT imaging probe with the potential to directly and comprehensively detect and measure hepatic CYP3A4 and 2D6 activity (Mizutani et al., 2022). The new SPECT imaging probe in this study is based on the same concept as 123/125I-O-desmethylvenlafaxine, but aims to specifically assess CYP2D6 activity only. Of the CYP isozymes, CYP2D6 contributes to the metabolism of approximately 13% of all prescription drugs (Williams et al., 2004). As many psychiatric prescription drugs, such as antidepressants and antipsychotics, are metabolized by CYP2D6, methods that allow estimation of CYP2D6 activity in each individual are very important an evidence-based medicine perspective. The purpose of this study was to evaluate hepatic CYP2D activity in mice as an initial study for future human application.
2 Materials and methods
2.1 Materials
Dichloromethane, acetate, sodium thiosulfate, chloroform, acetonitrile, potassium dihydrogen phosphate, dipotassium hydrogen phosphate, ethylene diamide tetra acetic acid (EDTA), and glucose-6-phosphate (G6P) were purchased from Nacalai Tesque (Kyoto, Japan). β-Nicotinamide-adenine dinucleotide phosphate (β-NADP+) and isoflurane were purchased from Wako Pure Chemical Industries (Osaka, Japan), and glucose-6-phosphate dehydrogenase (G6PD) was purchased from Oriental Yeast (Osaka, Japan). tert-Butyl hydroperoxide (t-BuOOH) was purchased from Tokyo Chemical Industry (Tokyo, Japan). 125I-NaI was purchased from American Radiolabeled Chemicals (St. Louis, United States). 123I-NaI was purchased from FUJIFILM Toyama Chemical (Tokyo, Japan). Mequitazine was provided by Sumitomo Chemical (Tokyo, Japan). All other reagents and chemicals were of analytical or high-performance liquid chromatography (HPLC) grade.
2.2 Labeling of 125I-4-iodobenzylmequitazine
Mequitazine {10-[(3RS)-1-azabicyclo [2.2.2] oct-3-ylmethyl]-10H-phenothiazine} is a long-acting and selective histamine H1–receptor antagonist used clinically. To develop a SPECT imaging probe using mequitazine, 4-(tributylstannyl) benzyl bromide was synthesized as a precursor. Mequitazine was labeled with radioiodine in two steps (Figure 1). In the first step, 1.0 μL of 4-(tributylstannyl) benzyl bromide was diluted by adding 50 μL of dichloromethane. This solution was mixed with 20 μL of acetic acid (70%), 33 μL of t-BuOOH, and 3.7 MBq of 125I-NaI. The reaction mixture was then incubated at room temperature for 5 min. The oxidation reaction was stopped by the addition of saturated sodium chloride solution and heated to reflux under N2. The resultant product was 125I-4-iodobenzyl bromide. In the second step, 125I-4-iodobenzyl bromide was diluted by adding 1 mL of acetonitrile. This solution was mixed with 32 mg of mequitazine and incubated at room temperature for 5 min. The resultant product was 125I-4-iodobenzylmequitazine (this new SPECT imaging probe was designated 125I-BMQ). Separation and purification of 125I-BMQ was carried out on an HPLC system consisting of a pump (model L-7100, Hitachi, Japan), UV detector (Chromaster 5410, Hitachi, Japan), and γ-ray detector (model RLC-701, Hitachi-Aloka Medical, Japan) equipped with a 5C18 AR-II-column (4.6 mm × 250 mm; 5 μm, Nacalai Tesque, Kyoto, Japan). PowerChrom (ver. 2.3.3, eDAQ, Japan) was used for data processing. The mobile phase consisted of acetonitrile (solvent A) and 20 mM phosphate buffer (pH 2.3) (solvent B). Separation was carried out as follows: 35% solvent A; 65% solvent B, 0–2 min; 35%–50% linear gradient, 2–9.5 min; 50%–80% linear gradient, 9.5–16 min; flow rate 1.0 mL/min. The elution of 125I-BMQ was monitored at 250 nm UV. For UV chromatograms, mequitazine and 127I-BMQ (cold-labeling) were mixed for comparison of radioisotope (RI) chromatograms (hot 125I-labeling). The structure of 127I-BMQ was confirmed by proton nuclear magnetic resonance spectroscopy (1H-NMR, JEOL JNM-ECS400; Jeol Resonance Inc., Tokyo, Japan). 1H-NMR data were as follows: (400 MHz, CDCl3/TMS) δ: 7.66 (d, J = 8.2 Hz, 2H), 7.34 (d, J = 8.7 Hz, 2H), 7.17–7.15 (m, 4H), 6.95 (t, J = 7.8 Hz, 4H), 5.00 (d, J = 12.8 Hz, 1H), 4.96 (d, J = 12.8 Hz, 1H), 4.13 (dd, J = 14.2, 9.6 Hz, 1H), 3.98 (dd, J = 14.2, 6.0 Hz, 1H), 3.77–3.72 (m, 4H), 3.57–3.46 (m, 2H), 2.66 (br s, 1H), 2.28–2.27 (m, 1H), 1.96–1.81 (m, 4H). 13C-NMR data were as follows: (100 MHz, CDCl3) δ: 144.56, 138.31, 134.87, 127.79, 127.71, 126.34, 126.11, 123.26, 116.10, 97.49, 65.96, 57.27, 54.13, 53.92, 48.29, 31.98, 24.86, 21.87, 19.54.
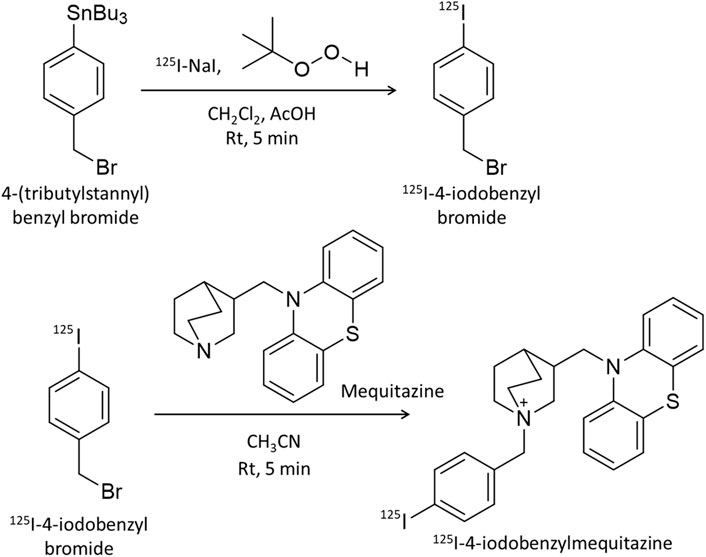
Figure 1. Scheme for 125I-labeling of mequitazine in two steps. In the first step, 4-(tributylstannyl) benzyl bromide was labeled with 125I-NaI. In the second step, 125I-4-iodobenzyl bromide was synthesized with mequitazine to give 125I-4-iodobenzyl mequitazine (125I-BMQ).
2.3 Metabolism of 125I-BMQ in vitro
Animal studies were approved by the Animal Care Committee at Kanazawa University (AP-173851) and conducted in accordance with international standards for animal welfare and institutional guidelines. For preparation of pooled mouse liver microsomes (MLMs), three 6-week-old male ddY mice (Japan SLC, Tokyo, Japan) were euthanized under anesthesia using isoflurane. The liver of each mouse was removed and weighed. After adding 3 mL of Krebs-Ringer’s phosphate buffer (pH 7.4) per gram of liver, the livers were pooled and homogenized using an ultrasonic homogenizer (SONIFIER250, Branson, MO, United States). The homogenate was centrifuged for 20 min at 9,000 g, and the supernatant was centrifuged for 60 min at 100,500 g. The resulting precipitate was suspended with Krebs-Ringer phosphate buffer and centrifuged for 60 min at 10,500 g. The resulting precipitate was obtained as microsomes, and the protein content was determined according to the bicinchoninic acid method (Smith et al., 1985). The pooled MLMs were stored at −80°C.
To analyze CYP-mediated metabolism of 125I-BMQ, nicotinamide adenine dinucleotide phosphate (NADPH) was used as an energy source for CYP. The NADPH-generating system created a CYP-activated state for 125I-BMQ metabolism. Metabolism of 125I-BMQ via CYP was carried out using the NADPH-generating system (0.5 mM β-NADP+, 5 mM MgCl2, 5 mM G6P, 1 U/mL G6PD), 100 mM sodium potassium phosphate buffer (pH 7.4), 50 μM EDTA disodium salt, and 1,000 μg protein/20 μL pooled MLMs in a final volume of 250 μL (NADPH [+]). The same mixture without the NADPH-generating system was used as control (NADPH [−]). Samples were incubated at 37°C for 60 min with gentle shaking, and the reaction was stopped by adding 100 µL of ethanol. The samples were then centrifuged at 18,000 g for 5 min, and the supernatant of each sample was analyzed by thin layer chromatography (TLC). The supernatant was spotted onto a silica gel TLC plate 60 F254 (Merck, Darmstadt, Germany), which was developed using methanol/acetic acid in a 100:1 ratio. After development and complete drying, the TLC plates were cut into 21 fractions, and the radioactivity associated with each fraction was measured using a γ-ray counter (AccuFLEX γ 7000, Aloka, Tokyo, Japan). The fractionation ratio of 125I-BMQ, 125I-BMQ, and other metabolites was calculated by dividing the radioactivity count for each fraction by the total radioactivity count.
In order to analyze the inhibition of specific 125I-BMQ-metabolizing CYP isoenzymes, specific inhibitors of the human CYP isoenzymes employed in this study were used, including α-naphthoflavone (inhibitor of CYP1A1 and 1A2) (Kim et al., 2005; Dinger et al., 2014; Valicherla et al., 2019), sulfaphenazole (inhibitor of CYP2C9) (Kim et al., 2005; Turpeinen et al., 2005; Wang et al., 2010; Dinger et al., 2014; Valicherla et al., 2019), fluconazole (inhibitor of CYP2C19) (Dinger et al., 2014; Valicherla et al., 2019), paroxetine and quinidine (inhibitors of CYP2D6) (Kim et al., 2005; Turpeinen et al., 2005; Wang et al., 2010; Dinger et al., 2014; Valicherla et al., 2019), 4-methylpyrazole (inhibitor of CYP2E1) (Wang et al., 2010; Valicherla et al., 2019), and ketoconazole (inhibitor of CYP3A4) (Kim et al., 2005; Turpeinen et al., 2005; Valicherla et al., 2019). These specific inhibitors were used to identify the CYPs involved in the biotransformation of 125I-BMQ. The inhibitors and mequitazine (self-inhibitor) were dissolved at a concentration of 10 μM (0.8% [v/v] final concentration DMSO).
A mixture consisting of 50 µL of NADPH-generating system (NADPH [+]), 50 µM disodium EDTA salt in 100 mM sodium potassium phosphate buffer (pH 7.4), 1,000 µg protein/20 µL pooled MLMs, and 25 µL of inhibitor in a final volume of 250 µL was used to examine the inhibition of 125I-BMQ metabolism. Samples were incubated at 37°C for 15 min with gentle shaking, and the reaction was stopped by addition of 100 µL of ethanol and centrifugation at 18,000 g for 5 min. The final supernatant was analyzed by TLC using methanol/acetic acid at a 100:1 ratio. The percentages of 125I-BMQ metabolites in each sample were then calculated (n = 3).
2.4 Biological distribution of 125I-BMQ in normal mice
125I-BMQ was prepared to a specific radioactivity of 185 kBq/mL by adding saline. A total of 20 fasting 6-week-old male ddY mice were injected with 125I-BMQ into the tail vein (18.5 kBq/100 µL/mouse), and after 2, 10, 30, 60, and 120 min, four mice each were euthanized under isoflurane, and blood, brain, thyroid, lung, heart, liver, gallbladder, stomach, pancreas, spleen, intestine, kidney, and urine tissue were collected. The tissue was weighted and the radioactivity was measured using a γ-ray counter to calculate the injected dose percent (%ID) or injected dose percent per gram of tissue (%ID/g).
2.5 Metabolism of 125I-BMQ in vivo
125I-BMQ was prepared to a specific radioactivity of 10 MBq/mL by adding saline and injected via tail vein into three mice (2.0 MBq/200 µL/mouse). After 30 min, each of the three mice was euthanized under isoflurane, and the gallbladder was removed. Radioactive products in the bile were analyzed by TLC using chloroform/acetic acid/H2O at a ratio of 60:40:1. Bile was spotted directly onto the TLC plate.
2.6 Whole-body imaging of 123I-BMQ in normal mice and CYP2D-inhibited mice
123I-BMQ was labeled and purified according to the same method used for 125I-BMQ. Whole-body SPECT imaging of normal mice was performed using a U-SPECT-II/CT system (MILabs, Utrecht, Netherlands). Two normal mice were injected with 14.5 MBq of 123I-BMQ via the tail vein. Whole-body SPECT images were acquired under 2.0% isoflurane anesthesia every 10 min from 5 to 115 min after injection. The images were reconstructed using the filter-backed projection method, with 16 subsets and six iterations. The voxel size was set to 0.8 mm × 0.8 mm × 0.8 mm. Neither attenuation nor scatter correction was performed. Post-reconstruction smoothing filtering was applied using a Gaussian smooth 3D filter at 1.2 mm. Images were displayed using PMOD (ver. 3.7). On SPECT images, volumes of interest were drawn for the liver, intestines (duodenum, small and large intestines), and kidney. Respective %ID values were calculated over time.
For whole-body SPECT imaging in CYP2D-inhibited mice, two mice were injected intraperitoneally with 30 mg/kg of paroxetine (MacLeod et al., 2017). At 60 min after paroxetine injection, 14.5 MBq of 123I-BMQ was injected via the tail vein. Whole-body SPECT images were acquired under 2.0% isoflurane anesthesia every 10 min from 5 to 115 min after 123I-BMQ injection. SPECT images of CYP2D-inhibited mice were analyzed according to the same method used for control mice.
2.7 Statistical analysis
All results represent the mean of at least three experiments, and data are expressed as the mean ± standard deviation. Data were analyzed using the F-test and Student’s t-test, and p < 0.01 or <0.05 was considered statistically significant.
3 Results
3.1 Labeling of 125I-BMQ
To identify 125I-BMQ, 127I-BMQ (cold-label) was used as a reference standard. Figure 2 shows HPLC chromatograms of mequitazine, 127I-BMQ (cold-label), and 125I-BMQ. The UV chromatogram confirmed the separation of mequitazine (retention time: 8 min) and 127I-BMQ (retention time: 13.5 min), and the RI chromatogram showed that the main peak (retention time: 13.5 min) had the same retention time as 127I-BMQ (In the HPLC system used in this study, the RI detector is located after the UV detector. The slight difference in retention times of the 125/127I-BMQ peaks is due to the longer tube between the RI and UV detectors.) This confirmed that two-step 125I labeling of mequitazine provided 125I-BMQ with a labeling index of approximately 88%–91% and radiochemical purity >98%.
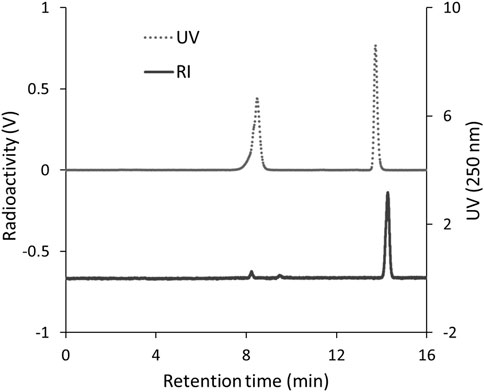
Figure 2. HPLC analysis of 125I-BMQ. Labeled 125I-BMQ was detected separately from the raw material mequitazine.
3.2 Metabolism of 125I-BMQ in vitro
In this study, 125I-BMQ and its metabolites were separated by TLC. The rate of flow (Rf) values in this analytical condition ranged from 0.15–0.30 for 125I-BMQ and 0.65–0.75 for 125I-NaI. In the metabolic reaction without NADPH (NADPH [−]), 125I− was not detected and only 125I-BMQ was detected. On the other hand, in the NADPH-mediated metabolic reaction (NADPH [+]), as in NADPH [−], 125I− was not detected, 125I-BMQ was detected, and other radioactive metabolites (Rf value: 0.05–0.10) were detected (Figure 3). With NADPH [+] conditions, the percentage of radioactive metabolite significantly increased from approximately 3%–25% with reaction time, and the percentage of 125I-BMQ significantly decreased from approximately 93%–76% with reaction time. These results showed that the radioactive metabolite was an NADPH-mediated metabolite of 125I-BMQ.
Figure 4 shows the percentages of radioactive metabolite produced from 125I-BMQ. Under the condition with NADPH [−], paroxetine, quinidine, and mequitazine, the percentage of metabolites were 2.64%, 12.5%, 4.47%, and 1.34%, respectively significantly lower than under NADPH [+]. Under the condition with 4-methylpyrazole and ketoconazole, the percentage of 125I-BMQ was slightly lower, but not significantly.
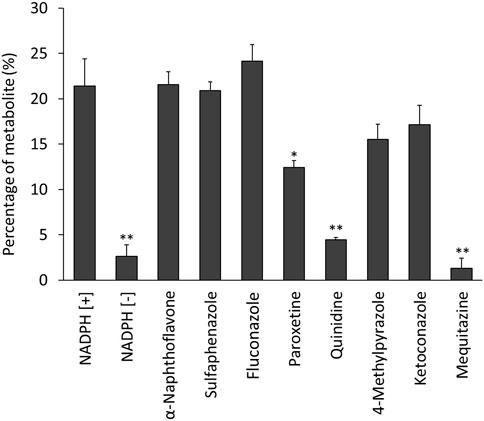
Figure 4. Effect of CYP inhibitors and mequitazine (a substrate of CYP2D6) on the metabolism of 125I-BMQ. Under NADPH [−], paroxetine, quinidine and mequitazine conditions, the percentage of radioactive metabolite was significantly lower than under NADPH [+].
3.3 Biological distribution of 125I-BMQ in normal mice
Table 1 shows the biodistribution of 125I-BMQ in normal mice. The injected 125I-BMQ was rapidly distributed to tissues throughout the body. In the thyroid and stomach, radioactivity was consistently low. Radioactivity in the liver increased immediately after injection and then decreased gradually. In the gallbladder, intestine, and kidney, radioactivity increased gradually after injection.
3.4 Metabolism of 125I-BMQ in vivo
The percentage of radioactive metabolites of 125I-BMQ in mouse bile was analyzed by in vitro TLC. In this analytical conditions, the Rf values of 125I− and 125I-BMQ in bile were 0.45–0.55 and 0.65–0.75, respectively. In the bile of mice injected with 125I-BMQ, no 125I-BMQ was detected, only 125I− and radioactive metabolite of 125I-BMQ (Rf values; 0.00–0.35). The percentage of metabolites of 125I-BMQ was >90% (Figure 5).
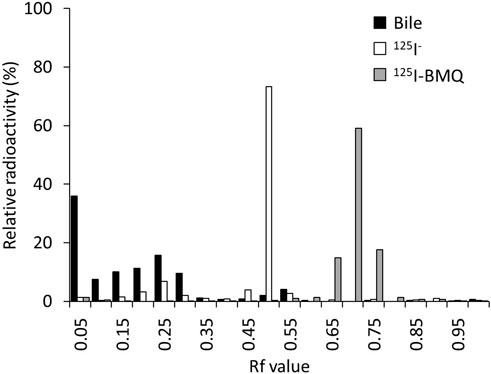
Figure 5. TLC analysis of radioactive metabolites in bile collected from mice after 125I-BMQ injection. The bars indicate 125I-NaI (white), 125I-BMQ (gray) and bile content (black), respectively. 125I-BMQ was not detected in the bile and more than 90% of the radioactivity was metabolites of 125I-BMQ.
3.5 Whole-body imaging of 123I-BMQ in normal mice and CYP2D-inhibited mice
Figure 6 shows SPECT images of 123I-BMQ in normal mice and CYP2D-inhibited mice. In normal mice, radioactivity was found in the liver and intestine (Figure 6A, frame 1). Radioactivity in the liver gradually decreased and that in the intestine gradually increased. Radioactivity excreted in the small intestine translocated to the large intestine (Figure 6A, frame 10). In CYP2D-inhibited mice, radioactivity was detected in the liver but not clearly in the intestine (Figure 6B, frame 1). The radioactivity in the intestine gradually increased, but remained on the duodenal side and did not translocated to the large intestine side (Figure 6B, frame 10).
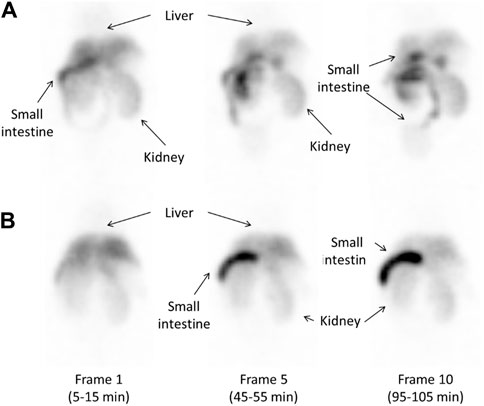
Figure 6. Whole-body images obtained approximately 10, 50, and 100 min after injection of 14.5 MBq of 123I-BMQ in normal mice (A) and CYP2D-inhibited mice (B). 123I-BMQ accumulated in the liver, gallbladder, small intestines, and kidney.
Figure 7 shows the time-activity curves of 123I-BMQ in the liver and intestines in SPECT images of normal and CYP2D-inhibited mice. CYP2D-inhibited mice accumulated more in the liver than control mice. Accumulation in the intestines was low in the early stages of 123I-BMQ injection, but gradually accumulated to the same level as in control mice.
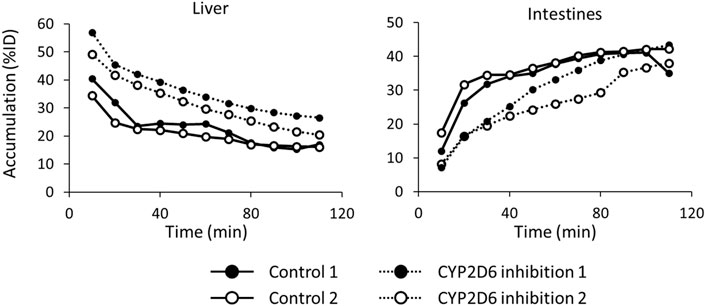
Figure 7. The time-activity curves of 123I-BMQ in the liver and intestines in SPECT images of normal and CYP2D-inhibited mice. Mice were scanned from 5 to 115 min after 123I-BMQ injection. Accumulation in the liver was increased and accumulation in the intestines was decreased in CYP2D-inhibited mice.
4 Discussion
In this study, we attempted to evaluate hepatic CYP2D activity by SPECT imaging using 123I-BMQ. In general, SPECT imaging probe used in nuclear medicine are intended to evaluate their function in vivo, and the SPECT imaging probe themselves are not metabolized. Our previous study showed that 123I-iomazenil was metabolized by hepatic carboxylesterase, after which almost all of the radioactive metabolites were translocated to the gallbladder and urinary bladder. Thus, hepatic carboxylesterase activity could be measured by detecting and evaluating the radioactive metabolites of 123I-iomazenil accumulated in the gallbladder and/or urinary bladder (Mizutani et al., 2018). Using the same technique, we also reported that the newly developed 123/125I-O-desmethylvenlafaxine is useful for direct and comprehensive detection and measurement of hepatic CYP3A4 and 2D6 in a simple and minimally invasive approach (Mizutani et al., 2022). However, since 123/125I-O-desmethylvenlafaxine can only evaluate the total activity of CYP3A4 and 2D6, we tried to develop a new SEPCT imaging probe that can evaluate only CYP2D6 activity. It is very difficult to prescribe psychiatric pharmacotherapy probe in clinical practice due to significant variability between individuals. CYP2D6 contributes to the clearance of many psychiatric drugs, and thus, the ability to accurately evaluate CYP2D6 activity in a minimally invasive and simple manner is critical for proper prescribing of medications. The metabolic pathway of mequitazine has already been reported and primarily involves conversion to S-oxidized and hydroxylated compounds (Figure 8). Hydroxylation is the major metabolic fate of mequitazine, and this conversion primarily involves CYP2D6 (Nakamura et al., 1998). Thus, mequitazine was selected as a substrate for CYP2D6 and used as a raw material for a new SPECT imaging probe that can measure hepatic CYP2D6 activity. A stable radioiodine labelling method for mequitazine was established in this study and 123/125I-BMQ showed very high labelling yield and radiochemical purity.
To confirm the involvement of CYP in the in vitro metabolism of 125I-BMQ, the effect of an NADPH-generating system was examined. Under the condition of NADPH [+], 125I−, 125I-BMQ, and a radioactive metabolite of 125I-BMQ were detected. In contrast, only 125I− and 125I-BMQ were detected under the condition of NADPH [−]. Thus, the radioactive metabolite of 125I-BMQ was only produced under condition of NADPH-generating system supplied energy to CYP, indicating the involvement of CYP in the metabolism of 125I-BMQ. To further identify the CYP isozymes involved in 125I-BMQ metabolites, the effects of inhibitors specific for each CYP were examined in an in vitro 125I-BMQ metabolism inhibition study. Paroxetine, quinidine, and mequitazine significantly inhibited the metabolism of 125I-BMQ (42%, 80%, and 94% inhibition, respectively). Therefore, 125I-BMQ is mainly metabolized by CYP2D. Not only the raw material mequitazine, but also 125I-BMQ, was shown to be specifically metabolised by CYP2D, making 123I-BMQ a candidate for a new SPECT imaging probe to evaluate mouse hepatic CYP2D and human hepatic CYP2D6 activity.
To directly detect and evaluate the activity of drug-metabolizing enzymes in metabolic drug clearance, it is necessary to image and measure the accumulation in the excretory organs. The biodistribution of 125I-BMQ in mice showed high radioactivity in the liver, gall bladder, and kidney immediately after injection. The radioactivity in the metabolic organ (liver) decreased with time, whereas the radioactivity in the excretory organs (gallbladder, intestines, and kidney) increased early. Furthermore, the low accumulation in the thyroid and stomach indicated that 125I-BMQ is rarely metabolized to 125I− and that the majority of the radioactivity detected in vivo in mice is 125I-BMQ or a radioactive metabolite of 125I-BMQ. To determine whether the radioactivity in the bile was 125I-BMQ or a radioactive metabolite of 125I-BMQ, the gallbladder was removed 60 min after the mice were injected with 125I-BMQ. As a result, more than 90% of the radioactivity in the bile was radioactive metabolite, and 125I− or 125I-BMQ was not detected. Therefore, it is suggested that the radioactivity in the bile is the radioactive metabolite of 125I-BMQ and that the amount of radioactivity in the bile increases or decreases according to the amount of radioactive metabolites produced. With regard to radioactive metabolite, no chemical structure was identified in this study, but TLC analysis confirmed that was clearly radioactive metabolite of CYP2D rather than 125I− or 125I-BMQ. In the concept of this study, the chemical structure of the radioactive metabolite is not important, but the selective excretion of CYP2D-specific radioactive metabolite, and 125I-BMQ fulfilled this requirement.
SPECT imaging of 123I-BMQ was performed to visualize the accumulation of radioactive metabolite for the purpose of assessing CYP2D activity. Images of normal mice injected with 123I-BMQ showed a decrease in accumulation in the liver over time, but increased accumulation in the intestines over time. In CYP2D-inhibited mice, accumulation in the liver was initially higher than in control mice, but accumulation in intestines was lower than in control mice. Loading paroxetine, a CYP2D6 inhibitor, inhibited or delayed the metabolism of 123I-BMQ. Inhibition of CYP2D resulted in 123I-BMQ being retained unmetabolized, accumulation in the liver was less likely to decrease, while accumulation in the intestines decreased due to reduced production of metabolite. Accumulation in the intestine remained in the duodenal region and did not migrate to the lower part of the intestine. Paroxetine, used as an inhibitor of CYP2D6, has gastrointestinal symptoms as a side effect, which may have affected the results. In a preliminary study, the effect of intraperitoneal administration of paroxetine on hepatobiliary excretion was investigated. After the hepatobiliary probe 99mTc-N-pyridoxyl-5-methyltryptophan (99mTc-PMT) was administered to normal mice and biliary excretion function was obtained by SPECT dynamic imaging, the same mice were treated with paroxetine as CYP2D inhibitors and similar SPECT dynamic imaging was performed. The results showed that hepatobiliary excretion and excretion rate of 99mTc-PMT were similar in normal and CYP2D-inhibited mice. This result showed that the use of paroxetine in CYP2D-inhibited mice did not affect the biliary excretory function itself. Therefore, the observed changes in distribution in this study reflected reduced metabolite production and delayed metabolic responses associated with decreased CYP2D activity, and CYP2D activity can be evaluated by imaging intestinal accumulation over time after 123I-BMQ injection.
These results indicated that 123/125I-BMQ meets four conditions: 1) 123/125I-BMQ accumulated in the liver as the metabolic organ; 2) 123/125I-BMQ was metabolized specifically by CYP2D, and the metabolite is radioactive; 3) the radioactive metabolite of 123/125I-BMQ selectively translocated from the liver to the gallbladder and intestines; and 4) the excretory organs in which radioactive metabolite selectively accumulate could be visualized and measured.
5 Conclusion
By visualizing and measuring the accumulation over time in the intestine, where bile containing only 123/125I-BMQ metabolites is excreted, it is possible to evaluate CYP2D activity from the decrease or delay in accumulation. Therefore, 123/125I-BMQ is useful as a SPECT imaging probe for comprehensive and direct assessment of hepatic CYP2D activity in a minimally invasive and simple approach.
Data availability statement
The original contributions presented in the study are included in the article/Supplementary Material, further inquiries can be directed to the corresponding author.
Ethics statement
The animal study was approved by the Animal Care Committee at Kanazawa University. The study was conducted in accordance with the local legislation and institutional requirements.
Author contributions
AM: Conceptualization, Funding acquisition, Investigation, Visualization, Writing–original draft. MKo: Conceptualization, Writing–review and editing. KN: Methodology, Writing–review and editing. KeF: Methodology, Writing–review and editing. KT: Investigation, Writing–review and editing. YuM: Investigation, Writing–review and editing. KS: Investigation, Writing–review and editing. MKi: Resources, Writing–review and editing. CS: Data curation, Writing–review and editing. RN: Writing–review and editing. NS: Writing–review and editing. YaM: Data curation, Supervision, Writing–review and editing. YI: Supervision, Writing–review and editing. MKu: Resources, Supervision, Writing–review and editing. KaF: Supervision, Writing–review and editing. KK: Conceptualization, Funding acquisition, Methodology, Project administration, Supervision, Writing–review and editing.
Funding
The author(s) declare that financial support was received for the research, authorship, and/or publication of this article. This study was funded in part by Grants-in-Aid for Scientific Research from the Japan Society for the Promotion of Science (No. 18K19508 and 19K17194).
Acknowledgments
We thank laboratory staff of Kanazawa University.
Conflict of interest
The authors declare that the research was conducted in the absence of any commercial or financial relationships that could be construed as a potential conflict of interest.
Publisher’s note
All claims expressed in this article are solely those of the authors and do not necessarily represent those of their affiliated organizations, or those of the publisher, the editors and the reviewers. Any product that may be evaluated in this article, or claim that may be made by its manufacturer, is not guaranteed or endorsed by the publisher.
References
Almazroo, O. A., Miah, M. K., and Venkataramanan, R. (2017). Drug metabolism in the liver. Clin. Liver Dis. 21 (1), 1–20. doi:10.1016/j.cld.2016.08.001
Dinger, J., Meyer, M. R., and Maurer, H. H. (2014). Development of an in vitro cytochrome P450 cocktail inhibition assay for assessing the inhibition risk of drugs of abuse. Toxicol. Lett. 230, 28–35. doi:10.1016/j.toxlet.2014.08.004
Ingelman-Sundberg, M., Oscarson, M., and McLellan, R. A. (1999). Polymorphic human cytochrome P450 enzymes: an opportunity for individualized drug treatment. Trends Pharmacol. Sci. 20, 342–349. doi:10.1016/s0165-6147(99)01363-2
Kim, M. J., Kim, H., Cha, I. J., Park, J. S., Shon, J. H., Liu, K. H., et al. (2005). High-throughput screening of inhibitory potential of nine cytochrome P450 enzymes in vitro using liquid chromatography/tandem mass spectrometry. Rapid Commun. Mass Spectrom. 19, 2651–2658. doi:10.1002/rcm.2110
MacLeod, A. K., McLaughlin, L. A., Henderson, C. J., and Wolf, C. R. (2017). Application of mice humanized for CYP2D6 to the study of tamoxifen metabolism and drug-drug interaction with antidepressants. Drug Metab. Dispos. 45, 17–22. doi:10.1124/dmd.116.073437
Manikandan, P., and Nagini, S. (2018). Cytochrome P450 structure, function and clinical significance: a review. Curr. Drug Targets 19 (1), 38–54. doi:10.2174/1389450118666170125144557
Matthaei, J., Bonat, W. H., Kerb, R., Tzvetkov, M. V., Strube, J., Brunke, S., et al. (2020). Inherited and acquired determinants of hepatic CYP3A activity in Humans. Front. Genet. 11, 944. doi:10.3389/fgene.2020.00944
Michael, M., Cullinane, C., Hatzimihalis, A., O'Kane, C., Milner, A., Booth, R., et al. (2012). Docetaxel pharmacokinetics and its correlation with two in vivo probes for cytochrome P450 enzymes: the C(14)-erythromycin breath test and the antipyrine clearance test. Cancer Chemother. Pharmacol. 69 (1), 125–135. doi:10.1007/s00280-011-1676-y
Mizutani, A., Kobayashi, M., Aibe, R., Muranaka, Y., Nishi, K., Kitamura, M., et al. (2022). Measurement of hepatic CYP3A4 and 2D6 activity using radioiodine-labeled O-desmethylvenlafaxine. Int. J. Mol. Sci. 23 (19), 11458. doi:10.3390/ijms231911458
Mizutani, A., Kobayashi, M., Fujita, K., Takahashi, K., Hokama, T., Takasu, H., et al. (2018). 123I-iomazenil whole-body imaging to detect hepatic carboxylesterase drug-metabolizing enzyme activity. Nucl. Med. Commun. 39, 825–833. doi:10.1097/MNM.0000000000000875
Nakamura, K., Yokoi, T., Kodama, T., Inoue, K., Nagashima, K., Shimada, N., et al. (1998). Oxidation of histamine H1 antagonist mequitazine is catalyzed by cytochrome P450 2D6 in human liver microsomes. J. Pharmacol. Exp. Ther. 284, 437–442.
Reed, J. R., and Backes, W. L. (2012). Formation of P450 · P450 complexes and their effect on P450 function. Pharmacol. Ther. 133, 299–310. doi:10.1016/j.pharmthera.2011.11.009
Rogers, J. F., Nafziger, A. N., and Bertino, J. S. (2002). Pharmacogenetics affects dosing, efficacy, and toxicity of cytochrome P450-metabolized drugs. Am. J. Med. 113, 746–750. doi:10.1016/s0002-9343(02)01363-3
Ross, M. K., Borazjani, A., Wang, R., Crow, J. A., and Xie, S. (2012). Examination of the carboxylesterase phenotype in human liver. Arch. Biochem. Biophys. 522, 44–56. doi:10.1016/j.abb.2012.04.010
Singh, D., Kashyap, A., Pandey, R. V., and Saini, K. S. (2011). Novel advances in cytochrome P450 research. Drug Discov. Today 16, 793–799. doi:10.1016/j.drudis.2011.08.003
Smith, P. K., Krohn, R. I., Hermanson, G. T., Mallia, A. K., Gartner, F. H., Provenzano, M. D., et al. (1985). Measurement of protein using bicinchoninic acid. Anal. Biochem. 150, 76–85. doi:10.1016/0003-2697(85)90442-7
Turpeinen, M., Uusitalo, J., Jalonen, J., Pelkonen, O., and Jorma, J. (2005). Multiple P450 substrates in a single run: rapid and comprehensive in vitro interaction assay. Eur. J. Pharm. Sci. 24, 123–132. doi:10.1016/j.ejps.2004.10.006
Valicherla, G. R., Mishra, A., Lenkalapelly, S., Jillela, B., Francis, F. M., Rajagopalan, L., et al. (2019). Investigation of the inhibition of eight major human cytochrome P450 isozymes by a probe substrate cocktail in vitro with emphasis on CYP2E1. Xenobiotica 49, 1396–1402. doi:10.1080/00498254.2019.1581301
Wang, L., Zhang, D., Raghavan, N., Yao, M., Ma, L., Frost, C. E., et al. (2010). In vitro assessment of metabolic drug-drug interaction potential of apixaban through cytochrome P450 phenotyping, inhibition, and induction studies. Drug Metab. Dispos. 40, 448–458. doi:10.1124/dmd.109.029694
Williams, J. A., Hyland, R., Jones, B. C., Smith, D. A., Hurst, S., Goosen, T. C., et al. (2004). Drug-drug interactions for UDP-glucuronosyltransferase substrates: a pharmacokinetic explanation for typically observed low exposure (AUCi/AUC) ratios. Drug Metab. Dispos. 32, 1201–1208. doi:10.1124/dmd.104.000794
Keywords: mequitazine, whole-body imaging, drug-metabolizing enzyme, CYP2D, individualized medicine
Citation: Mizutani A, Kobayashi M, Nishi K, Fujita K-i, Takahashi K, Muranaka Y, Sato K, Kitamura M, Suzuki C, Nishii R, Shikano N, Magata Y, Ishida Y, Kunishima M, Fukuchi K and Kawai K (2024) Development of radioiodine-labeled mequitazine for evaluation of hepatic CYP2D activity. Front. Pharmacol. 15:1397288. doi: 10.3389/fphar.2024.1397288
Received: 07 March 2024; Accepted: 31 May 2024;
Published: 19 June 2024.
Edited by:
Yurong Lai, Gilead, United StatesReviewed by:
Kazuya Maeda, Kitasato University, JapanPatrick Dansette, UMR8601 Laboratoire de Chimie et Biochimie Pharmacologiques et Toxicologiques, France
Copyright © 2024 Mizutani, Kobayashi, Nishi, Fujita, Takahashi, Muranaka, Sato, Kitamura, Suzuki, Nishii, Shikano, Magata, Ishida, Kunishima, Fukuchi and Kawai. This is an open-access article distributed under the terms of the Creative Commons Attribution License (CC BY). The use, distribution or reproduction in other forums is permitted, provided the original author(s) and the copyright owner(s) are credited and that the original publication in this journal is cited, in accordance with accepted academic practice. No use, distribution or reproduction is permitted which does not comply with these terms.
*Correspondence: Keiichi Kawai, a2VpQG1ocy5tcC5rYW5hemF3YS11LmFjLmpw