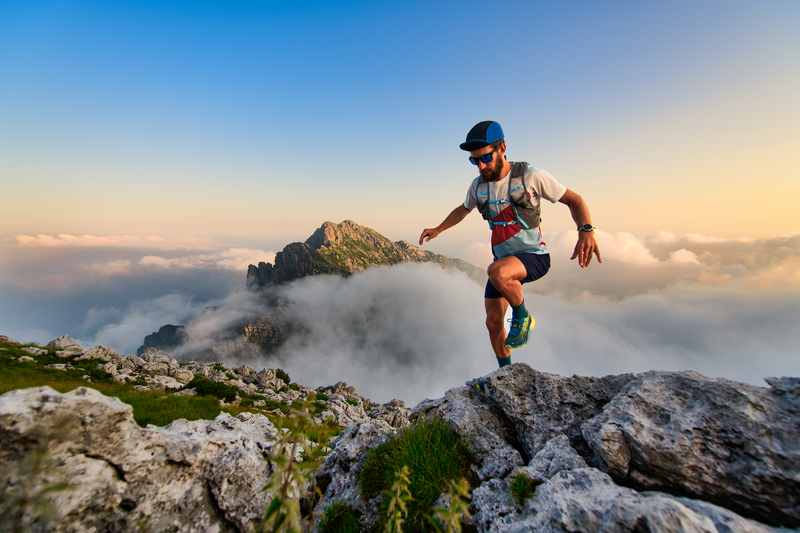
95% of researchers rate our articles as excellent or good
Learn more about the work of our research integrity team to safeguard the quality of each article we publish.
Find out more
REVIEW article
Front. Pharmacol. , 26 June 2024
Sec. Ethnopharmacology
Volume 15 - 2024 | https://doi.org/10.3389/fphar.2024.1396292
This article is part of the Research Topic Natural Sources as Potential Therapeutic Agents against Obesity and Type-II Diabetes View all 10 articles
Plant extracts are increasingly recognized for their potential in modulating (postprandial) blood glucose levels. In this context, root extracts are of particular interest due to their high concentrations and often unique spectrum of plant bioactives. To identify new plant species with potential glucose-lowering activity, simple and robust methodologies are often required. For this narrative review, literature was sourced from scientific databases (primarily PubMed) in the period from June 2022 to January 2024. The regulatory targets of glucose homeostasis that could be modulated by bioactive plant compounds were used as search terms, either alone or in combination with the keyword “root extract”. As a result, we present a comprehensive methodological toolbox for studying the glucose homeostasis modulating properties of plant extracts and its constituents. The described assays encompass in-vitro investigations involving enzyme inhibition (α-amylase, α-glucosidase, dipeptidyl peptidase 4), assessment of sodium-dependent glucose transporter 1 activity, and evaluation of glucose transporter 4 translocation. Furthermore, we describe a patch-clamp technique to assess the impact of extracts on KATP channels. While validating in-vitro findings in living organisms is imperative, we introduce two screenable in-vivo models (the hen’s egg test and Drosophila melanogaster). Given that evaluation of the bioactivity of plant extracts in rodents and humans represents the current gold standard, we include approaches addressing this aspect. In summary, this review offers a systematic guide for screening plant extracts regarding their influence on key regulatory elements of glucose homeostasis, culminating in the assessment of their potential efficacy in-vivo. Moreover, application of the presented toolbox might contribute to further close the knowledge gap on the precise mechanisms of action of plant-derived compounds.
Insufficient reduction of (postprandial) blood glucose levels is strongly associated with the pathogenesis of obesity and chronic metabolic disorders such as type 2 diabetes mellitus and cardiovascular diseases (Blaak et al., 2012). High postprandial glucose levels are known to cause microvascular dysfunction (Aroda and Eckel, 2022), and are also a prevailing risk factor for cardiovascular mortality even in the healthy population (European Diabetes Epidemiology Group, 1999). This may in part be due to the enhanced postprandial insulin levels in response to impaired glucose tolerance (Pyörälä et al., 1998; Shen et al., 2022), emphasising the importance of preventing increased postprandial glucose levels and subsequent progressive development of prediabetic phenotypes. Interestingly, lowering postprandial glucose peaks seems to be the more relevant target than lowering average blood glucose levels/HbA1c with regard to cardiovascular disease prevention (Hanssen et al., 2020).
The blood glucose concentration is dependent on the postprandial absorption rate of glucose, the endogenous production of glucose, the uptake of glucose by the liver and peripheral tissues as well as the disposal of glucose (Wu et al., 2020). Among these, gastrointestinal responses to meal ingestion (i.e., gastric emptying, intestinal digestion and absorption, and the secretion of gastrointestinal hormones) are key determinants of postprandial glucose levels. In order to improve glycemic control, different strategies can be employed. These comprise lifestyle interventions such as the modulation of physical activity and diet, but also the prescription of (oral) hypoglycemic medications (Pasmans et al., 2022). Plant-based medications which are assumed to have benefits in availability, side effects, and financial issues over conventional (oral) antihyperglycemic drugs (Salehi et al., 2019), might be a promising and effective strategy as well. In this context, it should be emphasized that plant extracts are a complex mixture of putative bioactive compounds, that have the potential to act at multiple targets simultaneously, including various enzymes or transporters involved in the regulation of glucose homeostasis. Figure 1 summarizes the most important mechanisms by which plant extracts or plant-derived molecules may affect postprandial blood glucose levels.
Figure 1. Overview of key regulatory elements of (postprandial) blood glucose regulation and their possible modulation by bioactive compounds from plant extracts. Sodium-dependent glucose transporter (SGLT); Glucose transporter 4 (GLUT4); Dipeptidyl peptidase 4 (DPP4).
Among the most frequently studied putative antihyperglycemic plant species are Morus alba L., Cinnamomum zeylanicum J. Presl, Trigonella foenum-graecum L., Phaseolus vulgaris L., Zingiber officinale Rosc., and Panax ginseng C.A.Meyer as recently summarized. On the one hand, this is due to the fact that the bioactive lead substances are identified here, such as phaseolamine from P. vulgaris, ginsenosides from P. ginseng or shogaol and gingerol from Z. officinale. On the other hand, these plant species are readily available, low cost and generally considered safe (Przeor, 2022). Interestingly, a combined formulation of M. alba, P. vulgaris and green coffee extract showed high potential to lower postprandial blood glucose and insulin concentrations in randomized, double-blind, placebo-controlled, cross-over experiments, which is attributed to the impact of bioactive compounds in the extracts on different regulatory pathways of glucose homeostasis (Adamska-Patruno et al., 2018). Figure 2 gives the structure of selected plant-derived compounds that are highly recognized for their putative antihyperglycemic effects, namely, 6-shogaol (Yi et al., 2019), berberine (Utami et al., 2023), eugenol (Srinivasan et al., 2014), 1-deoxynojirimycin (Ramappa et al., 2020), myricetin (Imran et al., 2021), and chlorogenic acid (Zuñiga et al., 2018).
Figure 2. Chemical structure of selected plant-derived compounds with putative antihyperglycemic activity. The structures of 6-shogaol (PubChem CID: 5281794), berberine (PubChem CID: 2353), eugenol (PubChem CID: 3314), 1-deoxynojirimycin (PubChem CID: 29435), myricetin (PubChem CID: 5281672) and chlorogenic acid (PubChem CID: 1794427) were taken from PubChem (Kim et al., 2023)
However, there is a great effort in research to discover novel antihyperglycemic plants and their related bioactive compounds and to decipher their mechanisms of action, with the overall intention to identify even more potent plant-based compounds or combination of these. Collections of plant extracts, commonly established by biotechnology companies and academic research institutes, are a tremendous help when a large number of plant extracts should be screened (Onur et al., 2013; Potterat and Hamburger, 2014; Wilson et al., 2020). For example, the screening library established by the US National Cancer Institute is one of the most relevant with over 230,000 extracts (McCloud, 2010; Thornburg et al., 2018). The largest French extract library of the Institut de Chimie des Substances Naturelles contains approximately 14,000 extracts (Marinetti, 2018). Extracts from 4,000 different plant species can be purchased from the company PhytoPharmacon (PhytoPharmacon, n. d.). The German “Plant Extract Collection Kiel in Schleswig-Holstein” (PECKISH) is a screening library with over 4,500 extracts, which is distinguished by its open accessibility (Onur et al., 2013). In this context, well documented, extensive plant extract libraries can be used to generate tailored sub-libraries selecting extracts with specific properties, e.g., extracts based on plants from the same region, plant family or plant part. In this regard, the targeted use of plant root extracts derived from such libraries might be of particular interest. In general, the extensive use of plant roots and rhizomes is frequently described in the traditional medicine of various cultures worldwide (Bais et al., 2001), as for example, by traditional healers (called “root doctors”) in Angola (Novotna et al., 2020). Despite this, as indicated by reviews concerning antihyperglycemic activity of medicinal plant extracts, underground plant material is not as widely studied compared to the aerial parts (Bailey and Day, 1989; Arumugam et al., 2013; Mamun-or-Rashid et al., 2014; Ardalani et al., 2021; Kifle et al., 2022). At this point, it should be noted that the occurrence and concentration of bioactive compounds within a plant species can vary greatly depending on the plant part. For example, leaves apparently have the highest content of flavonoids (Hao et al., 2023) although these are also present in the roots. However, additional bioactive compounds, such as phenols, alkaloids, phytosteroids, saponins, tannins, terpenoids, anthraquinones, and cardiac glycosides, which are abundant in roots, make these plant parts particularly interesting (Ardalani et al., 2021). Moreover, roots are one of the most important plant organs for accumulating these active components (Li et al., 2020). A root-specific metabolism leads to uniquely synthesized compounds in the root system, which are generally considered to have great potential for pharmacological applications, such as forskolin (diterpenoid) or the shikonins (group of naphthoquinones) (Bais et al., 2001; Ardalani et al., 2021).
However, easy-to-use methods are crucial enabling plant (root derived) extracts to be screened for multiple targets. In addition, alternative in-vivo models capable of replacing classical rodent studies, while being without ethical concerns and less time-consuming, are becoming increasingly important.
In the first part of this review, major regulatory elements of glucose homeostasis that might be modulated by plant extracts are outlined. The currently used antihyperglycemic drugs are considered in context of these molecular targets. In the second part, a comprehensive methodological toolbox including in-vitro and in-vivo assays related to the regulatory elements is presented that enables screening and verification of plant (root) extracts and bioactive compounds with respect to their antihyperglycemic properties. The assays described herein cover well known standard methods (e.g., spectrophotometric determination of α-amylase and α-glucosidase activity) but also less common methods such as patch-clamp measurements for KATP channels. Likewise, the in-vivo assays presented, using hen’s eggs and Drosophila melanogaster (D. melanogaster) as model organisms, are not yet widely used in the context of blood glucose regulation. Advantages and limitations of the different methods are discussed. In particular, this extensive collection of assays could facilitate the systematic investigation of novel plant material for future research and further decipher their mechanisms of action. Finally, root extracts (which could be particularly interesting for research in the future, but have not yet received widespread attention) targeting the aforementioned glucose homeostasis pathways are summarized in particular.
The diet usually contains a mixture of mono-, di- and polysaccharides such as glucose, sucrose and starch. Within the digestive tract, complex carbohydrates are initially converted into monosaccharides before they are absorbed and lead to a postprandial elevation of blood glucose level. The first step of dietary starch breakdown is accomplished by the enzyme α-amylase, which is predominantly secreted by salivary glands and the pancreas (Zakowski and Bruns, 1985). As a member of endoenzymes, α-amylase degrades complex starch molecules into oligosaccharides of 6 - 8 glucose units by catalysing the hydrolysis of internal α-1,4 glycosidic bonds (Sales et al., 2012). Since neither terminal glucose groups nor α-1,6 glycosidic bonds can be cleaved by this enzyme (Whitcomb and Lowe, 2007), both linear and branched products are generated that have to be broken down by further degrading enzymes. α-Glucosidases, located on the brush border membrane of the small intestine, catalyses the hydrolysis of α-1,4 and α-1,6 glycosidic bonds of α-amylase degradation products and also of dietary disaccharides via an exo-mechanism thereby releasing α-glucose from the non-reducing end of the substrate which can be absorbed by enterocytes (Chiba, 1997) (Figure 3). Interestingly, about 80% of starch can be broken down into glucose by α-glucosidase without the involvement of α-amylase (Lin et al., 2010; Lin et al., 2012). As these, enzyme actions, which can be considered as a rate-limiting step in carbohydrate digestion, are relatively easy to access pharmacologically due to their location within the gastrointestinal tract, both α-amylase and α-glucosidase are favoured targets of antihyperglycemic medications.
Figure 3. Schematic overview of glucose release and transport. In the oral cavity and the gut, the enzyme α-amylase, secreted by salivary glands and the pancreas, catalyses the hydrolysis of internal α-1,4-glycosidic bonds in dietary starch molecules. In the intestinal lumen, the resulting oligosaccharides are further broken down by the membrane-bound α-glucosidase. The α-glucosidase catalyses the hydrolysis of terminal α-glycosidic bonds thus releases glucose. At the apical membrane of enterocytes, one molecule glucose and two sodium ions are co-transported into the cell via the sodium-dependent glucose transporter 1 (SGLT1). Glucose is transported into the circulation on the basolateral side via passive diffusion through the Glucose transporter 2 (GLUT2). The driving force of the intestinal glucose uptake is a sodium gradient established under energy consumption by the Na+/K+-ATPase, which transported sodium basolaterally out of the cell (modified from (Koepsell, 2020; Barber et al., 2021). Used under CC BY 4.0: https://creativecommons.org/licenses/by/4.0/.
Acarbose, a pseudo-oligosaccharide of microbial origin derived from cultures of the soil bacteria Actinoplanes sp., is a well-known α-amylase and α-glucosidase inhibitor (Wehmeier and Piepersberg, 2004). Acarbose is composed of an unsaturated cyclitol, an amino-deoxyhexose and a maltose moiety and is recognized as a substrate by hydrolase enzymes involved in di- and polysaccharide digestion (Wehmeier, 2003; Tsunoda et al., 2022). However, the nitrogen linkage between the cyclitol (non-reducing end) and the deoxyhexose cannot be cleaved by these enzymes (Li et al., 2005; Tsunoda et al., 2022). Moreover, acarbose has an increased binding affinity for α-glucosidase compared to dietary saccharides (Rosak and Mertes, 2012). Acarbose is reported as a competitive inhibitor for α-glucosidase and a mixed non-competitive inhibitor for α-amylase (Kim et al., 1999). Other α-glucosidase inhibitors to be named are miglitol and voglibose. However, all these inhibitors are limited by their relatively high production cost, for example, due to complex biosynthesis for acarbose (Zhao Q. et al., 2017), and gastrointestinal (GI) adverse effects such as flatulence, abdominal discomfort and diarrhea caused by fermentation of carbohydrates by gut bacteria (Krentz and Bailey, 2005).
Cellular uptake of glucose from diet or blood is mediated by integral transport proteins. Depending on the transport mechanism, glucose transporters are classified into two families: (i) the facilitative glucose transporters (GLUTs) using a diffusion gradient and (ii) the sodium-dependent glucose co-transporters (SGLTs) through which glucose is actively transported by a Na+-electrochemical gradient (Bell et al., 1990; Wood and Trayhurn, 2003). Various isoforms of GLUTs and SGLTs have been identified showing different tissue distribution patterns (Wright et al., 2011; Mueckler and Thorens, 2013). The predominant glucose transporters in the intestine enabling dietary glucose (and galactose) uptake into circulation are SGLT1 and GLUT2 (Gorboulev et al., 2012). In Figure 3, the generally accepted mechanism of intestinal glucose absorption is depicted. SGLT1 is localized in the brush-border membrane of enterocytes, with its expression upregulated by dietary sugar (Margolskee et al., 2007; Song et al., 2016). Along with sodium, glucose is taken up into epithelial cells via SGLT1, whereas GLUT2 mediates its release into circulation on the basolateral side following the concentration gradient. Apparently, suppression of the SGLT1 transport protein can attenuate postprandial glucose peaks.
Much attention is currently also being given to another isoform of the SGLT family, namely, SGLT2 (Song et al., 2016). This low-affinity, high-capacity glucose transporter accounts for approximately 90% of renal glucose reabsorption, while the remaining 10% are reabsorbed by the high-affinity, low-capacity transporter SGLT1 (Kanai et al., 1994). SGLT2 inhibitors (e.g., gliflozins) have the potential not only to improve glycemic control by increasing glucose excretion in the urine (Chao and Henry, 2010; Scheen, 2015), but are also reported to have cardiovascular benefits (Zelniker et al., 2019). However, even if SGLT2 is completely blocked, glucose reabsorption in the kidney is partially maintained by SGLT1, indicating the prospective potential of dual SGLT1/2 inhibitors (Powell et al., 2013).
Phlorizin, a glycoside of the dihydrochalcone phloretin, was first isolated from the bark of the apple tree in 1835. This natural compound turned out to be the first non-selective inhibitor of both SGLT isoforms with a competitive mode of action (Ehrenkranz et al., 2005). However, phlorizin entails disadvantages e.g., poor water solubility, low oral bioavailability (Crespy et al., 2001) and rapid degradation (Tian et al., 2021), but the structure of phlorizin has been served as a template for (synthetic) SGLT inhibitors, which comprise individual SGLT2 and SGLT1 inhibitors as well as dual SGLT1/2 blockers (Dominguez Rieg and Rieg, 2019). Nevertheless, the discovery of novel natural SGLT inhibitors is becoming increasingly important (Oranje et al., 2019).
The presence of dietary carbohydrates triggers a group of intestinal hormones known as the incretin system. An important step in the discovery of the incretin system was made in 1964 when it was shown for the first time that oral glucose provided a more potent insulin response compared with intravenous glucose stimulus (Elrick et al., 1964; McIntyre et al., 1964; Rehfeld, 2018). Nowadays it is known that this phenomenon is mainly due to two gut-derived hormones: glucose-dependent insulinotropic polypeptide (GIP) and glucagon-like peptide-1 (GLP-1) (Drucker and Nauck, 2006; Rehfeld, 2018). While the plasma level of incretin hormones is low in the fasting state, it increases within minutes after meal ingestion (Drucker and Nauck, 2006). Subsequently, GIP and GLP-1 stimulate insulin secretion form the pancreas by binding to their receptors GIPR and GLP-1R (Mayendraraj et al., 2022). Thus, incretin hormones play a particularly important role in postprandial glucose regulation being responsible for approximately 70% of insulin secretion after eating (Gallwitz, 2019). Other incretin effects have also been described, such as decelerated gastric emptying or reduced appetite (Drucker and Nauck, 2006). Interestingly, modulation of the incretin effect has proven to be a remarkably effective strategy for the management of obesity, as recently demonstrated by the therapeutic use of semaglutide (Chao et al., 2023).
The incretin system represents an excellent target for the modulation of glucose homeostasis (Holst et al., 2009) via two different strategies. On the one hand, GLP-1 receptor agonists bind to the GLP-1R stimulating pancreatic insulin secretion, thereby improving postprandial glucose levels (Meier, 2012; Gilbert and Pratley, 2020). On the other hand, GIP and GLP-1 are substrates for the enzyme dipeptidyl peptidase 4 (DPP4), whose cleavage activity leads to their rapid degradation (Figure 4) (Mentlein et al., 1993). Hence, inhibitors of regulatory DPP4 can be used to prolong the circulating half-life of incretin hormones (Gilbert and Pratley, 2020).
Figure 4. GLP-1 function and its interaction with DPP4. The incretin hormone glucagon-like peptide-1 (GLP-1) is released from intestinal cells as a response to nutrient intake. Subsequently, GLP-1 elicits pleiotropic effects on the target organs such as the pancreas (Mojsov et al., 1987; Ramracheya et al., 2018), brain (Flint et al., 1998; Perry et al., 2002), gastrointestinal (GI) tract (Tolessa et al., 1998; Chelikani et al., 2005), or heart (Barragán et al., 1994; Read et al., 2012; McCormick et al., 2015). Rapid inactivation of GLP-1 occurs by cleavage of a terminal dipeptide by the enzyme dipeptidyl peptidase 4 (DPP4) (modified from (Saraiva and Sposito, 2014; Razavi et al., 2022)). Used under CC BY 4.0: https://creativecommons.org/licenses/by/4.0/.
Several GLP-1 receptor agonists e.g., exenatide, lixisenatide, liraglutide or albiglutide as well as DPP4 inhibitors, known as gliptins such as sitagliptin, vildagliptin, linagliptin, saxagliptin or allogliptin are established as a class of oral hypoglycemic medications (Meier, 2012; Gallwitz, 2019; Gilbert and Pratley, 2020). Since there is evidence that SGLT1 inhibition increases plasma GLP-1 concentrations (Powell et al., 2013; Oguma et al., 2015; Io et al., 2019), combined use of SGLT1 and DPP4 inhibitors may be a particularly potent strategy for improved glycemic control (Zambrowicz et al., 2013). However, both GLP-1 receptor agonists and DPP4 inhibitors are associated with adverse effects. While DPP4 inhibitors are mainly associated with headache, nasal pharyngitis, and upper respiratory tract infections, GLP-1 receptor agonists are reported to contribute to gastrointestinal complaints (Gilbert and Pratley, 2020).
Insulin is considered the major blood glucose-lowering hormone. It is produced and stored in the β-cells of the pancreas until its release, which is in part triggered by glucose entry into the cells and subsequent alterations in the activity of ion channels (Ashcroft et al., 1984; Ashcroft and Rorsman, 1989), with the inwardly rectifying K+ channel Kir6.2 (KCNJ11) and its accessory subunit SUR1 (ABCC8) playing a particularly central role (Gloyn et al., 2004; Hattersley and Ashcroft, 2005; Flanagan et al., 2009; Rubio-Cabezas et al., 2012). Together, Kir6.2 and SUR1 form in a 4:4 stoichiometry the KATP channel in pancreatic β-cells, which functions as a metabolic sensor (Ashcroft and Rorsman, 2013). At elevated blood glucose levels, the increasing glucose uptake by β-cells results in a high intracellular ATP:ADP ratio. This leads to an inhibition of the ATP-sensitive KATP channels, which in turn provokes a membrane depolarization and a subsequent opening of voltage-gated Ca2+ channels. The accompanying increase of intracellular Ca2+ levels then elicits increased insulin secretion (Figure 5).
Figure 5. Schematic illustration of glucose-stimulated insulin secretion. At elevated blood glucose levels, more glucose is transported via glucose transporter 2 (GLUT2) into pancreatic β-cells. Subsequent glucose metabolism results in an augmented generation of ATP, which closes the ATP-sensitive KATP channel Kir6.2. This leads to a depolarization of the cell membrane and an opening of voltage-gated Ca2+ channels, followed by an influx of calcium into the cell. The increase of intracellular Ca2+ levels then triggers the exocytosis of insulin granules.
Because of its prominent role in insulin secretion, the pancreatic β-cell KATP channel is a suitable target for modulating blood glucose regulation. Sulfonylureas such as glibenclamide and tolbutamide, for example, bind to SUR1 and inhibit the KATP channels, thereby increasing insulin secretion. Due to its opposite effect, the KATP channel activator diazoxide is used to counteract hypoglycemia. In general, sulfonylureas can be grouped into two generations of oral blood glucose-lowering medication. As the first-generation sulfonylureas are associated with a considerably higher risk of hypoglycemia and cardiovascular issues, these drugs have been replaced by improved (prolonged-release) second-generation sulfonylureas, which are associated with a lower risk of these adverse effects (Sola et al., 2015).
Insulin acts on three key target tissues to reduce blood glucose: liver, muscle and adipose tissue (Wilcox, 2005; Petersen and Shulman, 2018). While it suppresses endogenous glucose production and concurrently stimulates glycogen synthesis in the liver, insulin signaling promotes glucose uptake and metabolism in muscle and adipose tissue (Stadlbauer et al., 2020). Approximately 80% of postprandial rise in blood glucose levels is decreased by muscle tissue (Merz and Thurmond, 2020). The principal transporter responsible for glucose absorption by peripheral tissues is GLUT4, thus a key protein in whole-body glucose regulation (Huang and Czech, 2007). Since GLUT4 belongs to the facilitative glucose transporters, glucose is taken up into skeletal myocytes and adipocytes by passive diffusion following a concentration gradient (Satoh, 2014). The regulation of GLUT4-mediated glucose transport occurs primarily via its intracellular localization (Govers, 2014). In unstimulated (basal) cells, GLUT4 is retained in intracellular storage vesicles, while an increase of blood glucose level and subsequent insulin secretion triggers the translocation of GLUT4 to the plasma membrane (Govers, 2014; Lanzerstorfer et al., 2014). This process is mediated by the insulin signalling cascade (Kanai et al., 1993) illustrated in Figure 6. In brief, upon insulin binding, the tyrosine kinase activity of the insulin receptor phosphorylates the insulin receptor substrate protein (IRS), which in turn triggers the activation of phosphoinositide 3-kinase (PI3K) and the subsequent formation of phosphatidylinositol (3,4,5)-trisphosphate (PIP3) in the plasma membrane. PIP3 then recruits the protein kinase B (Akt) to the cell membrane, where Akt is phosphorylated and thereby activated by phosphoinositide-dependent kinase (PDK) 1/2 (Alessi et al., 1997; Stokoe et al., 1997; Dangelmaier et al., 2014). Active Akt suppresses the Akt substrate 160 (AS160) via phosphorylation, resulting in GTP loading of Ras-associated binding (Rab) proteins responsible for the initiation of GLUT4 translocation (Mîinea et al., 2005; Tan et al., 2012). Although PI3K/Akt is the generally recognized major signalling pathway leading to GLUT4 translocation, PI3K independent signals are also known to stimulate this trafficking event. In particular, the activation of the Rho family member GTPase TC10 via Cbl associated protein (CAP)/Cbl pathway, turned out to be a relevant factor for insulin-stimulated translocation of GLUT4 (Chiang et al., 2001; Watson et al., 2001; Chang et al., 2007).
Figure 6. Insulin-stimulated GLUT4 translocation via the PI3K/Akt signalling cascade. Upon binding, insulin activates its receptor, which then phosphorylates and binds the insulin receptor substrate (IRS) protein. IRS binding recruits phosphoinositide 3-kinase (PI3K) to the cell membrane. PI3K phosphorylates membrane-bound phosphatidylinositol (4,5)-bisphosphate (PIP2) leading to the generation of phosphatidylinositol (3,4,5)-triphosphate (PIP3). This results in the recruitment of protein kinase B (Akt) to the membrane of the cell and the activation of phosphoinositide-dependent kinase (PDK) 1/2, which in turn phosphorylates Akt. Activated Akt subsequently phosphorylates the Akt substrate of 160 kDa (AS160), which thereby loses its GTPase activity. Consequently, Ras-associated binding (Rab) protein is activated as the hydrolysis of its bound GTP is prevented. Rab activation is responsible for the fusion of GLUT4 storage vesicles (GSV) with the cell membrane and thus for GLUT4 translocation. When integrated into the plasma membrane, GLUT4 finally enables the uptake of glucose into the cell (modified from (Thorn et al., 2013; Lankatillake et al., 2019; Heckmann et al., 2022). Used under CC BY 4.0: https://creativecommons.org/licenses/by/4.0/.
The enzyme activity of α-amylase is mainly determined in two ways: with a disc diffusion method and spectrophotometrically. The disc assay is a method used for over 70 years to determine α-amylase activity (Goodman, 1950). It is based on α-amylase diffusing from a filter paper (disc) into a starch-containing agar gel, where it degrades the polysaccharide. Accordingly, a clear zone appears around the filter disc indicating the diffusion radius and the activity of the α-amylase when the undegraded starch in the plate is stained with iodine. The clear zone diameter can be determined, with the size of the diameter depending on the α-amylase concentration/activity (Briggs, 1962) (Figure 7). Thus, the disc assay is also applicable to study inhibition of the enzyme, e.g., by plant extracts. To this end, the α-amylase solution is mixed with the extract (preferably in various concentrations) before the filter discs are soaked (Correia et al., 2004). By comparing the diameter of the clear zone of a control sample (only enzyme solution) with those of the clear zone where the extract was added, the inhibition of α-amylase can be calculated. The assay is simple to perform and does not require expensive materials or equipment. In order to save material, downscaling of the protocol might be advantageous for screening approaches, thereby using 4 filter discs per agar-starch plate. A major advantage of this method is that the usually intense staining of plant extracts, which often interferes with the photometer-based assays discussed below, has no influence. However, due to its rather high inaccuracy, the disc assay is only suitable for general assessment of α-amylase inhibition by plant extracts.
Figure 7. The disc assay for measuring inhibition of α-amylase activity. (A) Four “discs” made of filter paper with a diameter of 0.5 cm are placed on a Petri dish filled with medium (composed of 1% agar-agar and 1% starch). First, α-amylase (sourced from porcine pancreas) is added to solutions containing decreasing concentrations of an extract and pre-incubated, before the filter discs are soaked with the reaction mixture. The Petri plate is incubated at 37 °C for at least 8 h until the filter discs are removed. (B) Iodide-staining enables the calculation of the inhibition of enzyme activity. For this, the clear zone diameter of the control filter disc soaked with α-amylase alone (a) is compared with clear zone diameters of filter discs soaked with a solution of the extract and α-amylase (b, c and d). The stronger the inhibition of enzyme activity by the extract, the smaller the clear zone diameter.
A comprehensive protocol for spectrophotometrically determining α-amylase inhibition by plant extracts was developed by Apostolidis et al., 2006. After a porcine pancreatic α-amylase solution is pre-incubated with the plant extract, a solution of soluble starch is added as a substrate. The reaction incubated at 25 °C is stopped after 10 min by the addition of a colour reagent consisting of 3,5-dinitrosalicylic acid and sodium potassium tartrate and a 5 min incubation at 100 °C. The aromatic substance dinitrosalicylic acid reacts with the reducing sugars produced during starch degradation (Keharom et al., 2016). After cooling to room temperature, the absorbance of the reaction mixture, which reflects the α-amylase activity, can be measured at a wavelength of 540 nm (Apostolidis et al., 2006).
To determine the inhibition of α-glucosidase by plant extracts, a spectrophotometric assay is commonly used (Lankatillake et al., 2021). For this purpose, the enzyme is pre-incubated with the extract, followed by the addition of the chromogenic substrate p-nitrophenyl-α-D-glucopyranoside. The reaction incubated at 37 °C is terminated by the addition of a basic Na2CO3 stop solution, which also intensifies the yellow colour of the product p-nitrophenol. The α-glucosidase activity is proportional to the amount of p-nitrophenol released by the enzymatic reaction, the absorbance of which can be recorded spectrophotometrically at 405 nm (Lankatillake et al., 2021). For screening purposes, this assay can be easily performed in 96-well plate format, enabling a large number of samples to be run simultaneously.
Commercially available DPP4 inhibitor screening kits are suitable for high-throughput screening of putative DPP4 inhibitors. It can be performed in black 96-well microtiter plates according to the manufacturer’s instructions. The measurement is based on the cleavage of the substrate Gly-Pro-7-amido-4-methylcoumarin hydrobromide with yield of the fluorescence product 7-amido-4-methylcoumarin by active DPP4 enzyme (Kęska and Stadnik, 2022). The fluorescence signal (excitation/emission: 360/465 nm), which is proportional to the enzymatic activity present, can be measured over time.
For all assays, the percentage inhibition of the enzyme by an extract compared to a control can be determined. When performing the assay with suitable ascending extract concentrations, the half-maximal inhibitory concentration (IC50) (Aykul and Martinez-Hackert, 2016), can be calculated e.g., by using online IC50 calculator tools.
The activity of the SGLT1 transporter can be determined in cellular monolayers by employing the Ussing chamber. The Ussing chamber, developed by Hans Ussing in the 1950s (Larsen, 2002; Thomson et al., 2019), is a laboratory device that enables the measurement of substance fluxes such as ions, nutrients or drugs through epithelial tissues. The device (Figure 8) consists of two liquid-filled half-chambers separated by an epithelial tissue into an apical (mucosal) and basolateral (serosal) side, with substance flow between the two compartments via the epithelium. The liquid, a physiological buffer, is kept at a constant temperature of 37 °C and is continuously oxygenated with carbogen, a 95/5 (v/v) mixture of O2 and CO2. Moreover, by using identical electrolyte solutions of the same volume on both sides, there are no osmotic and electrochemical gradients either (Clarke, 2009). An inner pair of electrodes enables the measurement of the transepithelial potential difference between the apical and basolateral sides. Via an outer pair of electrodes, a short-circuit current (ISC) can be applied, which clamps this potential difference to 0 mV and reflects the actual active transepithelial ion transport (Clarke, 2009; He et al., 2013). This ISC is recorded over time to monitor changes in transepithelial ion transport.
Figure 8. The Ussing chamber system. One Ussing chamber unit consists of two half-chambers, representing the apical and basolateral side, separated by a slider mounting an epithelial tissue or a cell monolayer. Both half-chambers are filled with equal volumes of the same 37 °C heated physiological salt solution. The solutions are oxygenated by carbogen gas while the temperature is maintained constant, so each side faces the same conditions. The transepithelial potential difference is continuously monitored through voltage electrodes (inner black electrodes). By using an automatic voltage clamp, the short-circuit current (ISC) is measured through current electrodes (outer white electrodes).
Different sliders available for an Ussing chamber system enables the variable use of epithelial tissues as well as Snapwell cell culture inserts comprising a permeable membrane supported by a detachable ring (Yeste et al., 2018). The intestine is one of the most intensively studied tissues in Ussing chambers in terms of gastrointestinal barrier function, permeability and nutrient absorption (He et al., 2013). Particularly, the active sodium and glucose co-transport via SGLT1 has mostly been studied by using animal tissue (Clarke, 2009; Schloesser et al., 2017; Klinger and Breves, 2018; Pallauf et al., 2019; Klinger, 2020). However, mounting Caco-2 (a human colorectal adenocarcinoma cell line) monolayers in Ussing chambers (Zheng et al., 2012; Steffansen et al., 2017) represent an intriguing alternative (Darling et al., 2020) to study plant extracts or compounds targeting the SGLT1-mediated glucose transport. Based on its properties, Caco-2 cells are a suitable in-vitro model for transport studies. Initiated by confluence, Caco-2 cells are starting to polarize and differentiate while developing an enterocyte-like phenotype, e.g., by forming a brush border and expressing specific brush border membrane enzymes (Hidalgo et al., 1989) and transporters for e.g., bile acids, amino acids and sugars including SGLT1 (van Breemen and Li, 2005). It should be pointed out that different Caco-2 clones exist distinguished by drastic differences in the expression of SGLT1. The highest SGLT1 expression is reported to be exhibited by the PD7 clone (Mahraoui et al., 1994).
For transport studies, Caco-2 cells are typically cultured for 21 days prior to use, as they have usually reached a fully differentiated state at this point (Darling et al., 2020). It has been proven effective to use the mucosal medium without serum supplementation after 7 days of cultivation, thereby simulating a more physiological condition and promoting the polarization process (Ferruzza et al., 2012). However, measurement of the transepithelial electrical resistance (TEER) as a marker of barrier tightness of a cellular monolayer is a suitable method to confirm the differentiation state of Caco-2 cells (Grasset et al., 1984).
Prior to Ussing chamber experiments the chambers are sealed with a blank slide and filled with the physiological buffer which is heated and oxygenated. Subsequently, adjustments are made to correct the offset potential between voltage-sensing electrodes and the fluid resistance. To start Ussing chamber experiments, the Snapwell inserts with the differentiated cell monolayer are transferred to Ussing chamber slides, before they are mounted into the prepared Ussing chamber. After refilling both half-chambers with the 37 °C physiological buffer, 10 mM glucose is added basolaterally as an energy substrate while 10 mM mannitol is added apically for osmotic reasons. The transepithelial potential difference is continuously recorded via Ag-AgCl electrodes and salt bridges (consisting of agar melted in the buffer used) (Clarke, 2009). Subsequently, the ISC is measured via automatic voltage clamp (Clarke, 2009; Schloesser et al., 2017; Pallauf et al., 2019). By adding glucose to the mucosal side of the chamber (with simultaneous addition of mannitol to the serosal side) the sodium-coupled glucose transport is stimulated as indicated by elevated ISC values. To determine SGLT1 inhibitory activity of plant extracts, they are added to both sides of the Ussing chamber and the reduction of the glucose-induced ISC value is determined.
In general, it should be kept in mind that, although Ussing chamber studies provide an excellent opportunity to make accurate and rapid measurements of substance fluxes in a physiological context, the complexity of the physiological system is still not being mimicked to its full extent (He et al., 2013).
The potassium transport activity of the inwardly rectifying K+ channel Kir6.2, which is crucial for insulin secretion by pancreatic β-cells, can be determined by the patch-clamp technique. In the early 1950s, the concept of ion channels with conductances selective for different ions was proven by the fundamental work of Alan Hodgkin and Andrew Huxley (Hodgkin and Huxley, 1952b; 1952a). Thirty years later, the first genes coding for ion channels were identified and since then more than 400 ion channel genes have been cloned (Giraudat et al., 1982; Noda et al., 1982; Imbrici et al., 2016). In parallel, the patch-clamp technique has been developed (Hamill et al., 1981), which made it possible to examine the properties of the various ion channels after heterologous expression in a feasible expression system and to uncover their influence on cell physiology and thus also on physiology of the body.
Figure 9A depicts a typical set-up used for patch-clamp measurements. It is basically built around an inverse microscope placed on an anti-vibration table and surrounded by a Faraday cage. The heart of the setup is a preamplifier (probe or headstage) on which the patch pipette is mounted. The technique is based on the formation of a very tight contact of a glass pipette with the cell membrane (giga seal; Figure 9B). After the formation of the giga seal, the cell-attached configuration is achieved (Figure 9C), allowing the ion channels inside the patch to be measured without disturbing the intracellular milieu. Accordingly, the modulation of the channels by intracellular signal cascades can be evaluated. Starting from the cell-attached configuration, there are two possibilities to conduct measurements. One is to remove the membrane patch under the pipette by gentle suction obtaining the whole cell configuration. Here, all channels of the cytoplasma membrane contribute to the measurement, resulting in the largest currents being recorded. The cell is now filled with the defined pipette solution and the extracellular condition can be changed simply e.g., by adding drugs such as ion channel modulators and ligands of membrane receptors. The whole cell configuration is the standard configuration used when measuring the influence of modulators such as plant extracts on ion channel function. The second possibility is to withdraw the pipette from the cell-attached configuration. This leads to the inside-out configuration, where free access to the inside of the membrane is obtained. Consequently, the influence of pharmaka, changes in intracellular pH, fatty acids etc. can be measured in this configuration. From the whole cell configuration, you can also reach the outside-out configuration to investigate the channel without the intracellular milieu.
Figure 9. The patch-clamp technique. (A) Setup used for the patch-clamp measurements (1: headstage; 2: micromanipulator; 3: bath chamber; 4: microscope; 5: anti-vibration table; 6: Faraday cage; 7: application system; 8: software). (B) Electric circuit of the patch-clamp technique. (C) Different configurations of the patch-clamp technique.
The patch-clamp technique can be used in two different modes: In the current clamp mode, the actual current seen by the cell can be either clamped to a certain value or no current at all is injected to the cell. In this mode, the potential between the intra- and extracellular milieu is measured. If the modulation of a channel leads to its opening or closing, this can be recorded as a change in the membrane potential or, in the case of excitable cells, as the generation of an action potential. In the current clamp mode, for example, the inhibition of inwardly rectifying K+ channels is noticeable as depolarization of the membrane, resembling the inhibition of the KATP channel in pancreatic β-cells after ATP elevation (Ashcroft and Rorsman, 2013). The second mode is the voltage-clamp mode. Here, the voltage is clamped to a certain value and the ionic current flowing through the membrane is measured as the counter current required to maintain the set voltage. Using the patch-clamp technique in combination with the preparation of slices of the organ of interest, every cell type in the body is available for electrophysiological measurements. Pharmacological investigations of ion channels are mainly done in the voltage clamp mode.
Most commonly used for the heterologous expression of ion channels are oocytes from Xenopus laevis, HEK293 cells, CHO cells or COS7 cells. These cell types have in common a relatively low background expression of endogenous ion channel genes. This allows the measurement of the respective channels without interfering endogenous currents. Following standard transfection protocols, plasmids for co-expression of Kir6.2 and SUR1 (e.g., available from Addgene; www.addgene.org/) are introduced into the cells and K+ channel function can be examined after 24 h already. The sulfonylurea drug glibenclamide is a suitable positive control having an IC50 value of about 1 µM (Houtman et al., 2021).
The determination of GLUT4 translocation by total internal reflection fluorescence (TIRF) microscopy has been established as an excellent method for identifying drugs and plant extracts that can enhance GLUT4 translocation independently of insulin (Lanzerstorfer et al., 2014; Stadlbauer et al., 2016; Stadlbauer et al., 2020) (Figure 10). TIRF microscopy enables detection of fluorophores such as green fluorescent protein (GFP) located close to the interface between two media such as cover glass and sample and is characterized by extremely high sensitivity (Stadlbauer et al., 2020). Moreover, TIRF microscopy has been proven in several studies to be a suitable technique for screening approaches (Lanzerstorfer et al., 2014; Stadlbauer et al., 2016; Haselgrübler et al., 2018a; Stadlbauer et al., 2021). Nevertheless, it should be recognized that there are additional suitable methods for quantifying GLUT4 translocation, including microscopy-based approaches as well as biochemical and spectrometric approaches, as recently comprehensively reviewed (Heckmann et al., 2022).
Figure 10. Schematic overview of TIRF microscopy for the quantification of GLUT4 translocation. The total internal reflection fluorescence (TIRF) microscopy is used to visualize fluorescent molecules located in an evanescent field very close to the interface between cover glass and water/specimen. This allows detection of membrane-associated proteins. The evanescent field is generated by a laser beam which is totally reflected at the interface. In unstimulated cells, stably expressing a glucose transporter 4 (GLUT4)-myc-green fluorescent fusion protein (GFP), GLUT4:GFP remains within the intracellular storage vesicles leading to the absence of fluorophores in the evanescent field. Insulin (or insulin activity mimicking plant extract/test compound) stimulation of cells results in the translocation and insertion of GLUT4-myc-GFP to the cell membrane. Consequently, the GFP signal intensity recoded by TIRF microscopy increases in the evanescent field (modified from (Lanzerstorfer et al., 2014; Stadlbauer et al., 2020)). Used under CC BY 4.0: https://creativecommons.org/licenses/by/4.0/.
To determine GLUT4 translocation modulating properties of substances or plant extracts, cell lines stably expressing a GLUT4-myc-GFP fusion protein are generated. Next to CHO-K1, HeLa turned out to be a suitable cell line, since no differentiation is required and the cells are characterized by good adhesion properties on various surfaces (Stadlbauer et al., 2020). In general, GLUT4-GFP expressing cells are grown in 96-well imaging plates, starved for at least 3 h and then treated with insulin (positive control), buffer (control) or test samples. Images can be taken at different time points before and after stimulation of the cells, using a microscope in TIRF configuration where the GFP fluorescence signal intensity correlates with GLUT4 accumulation at the cell membrane (Lanzerstorfer et al., 2014; Stadlbauer et al., 2016; Haselgrübler et al., 2018a; Stadlbauer et al., 2020; Stadlbauer et al., 2021). The analysis tool Spotty, which can be retrieved online (Research Group Bioinformatics Hagenberg, n. d.), provides e.g., an automated detection of fluorescent cells, the quantification of fluorescence signals or an automated calculation of signal changes (Lanzerstorfer et al., 2014), enabling increased throughput due to simplified data evaluation.
Spectrophotometric and fluorometric assays are traditionally employed to quantify the inhibition potency of plant extracts against enzymes (Lankatillake et al., 2021). They are usually simple low cost assays suitable for high-throughput approaches. However, protocols often differ in some aspects e.g., enzyme concentration, incubation times or volumes used. Therefore, standardization of these assays should be attempted in order to improve comparability of results. To this end, the toolbox presented in this review might offer a valuable contribution. In general, limitations in terms of spectral interference from extract colour and turbidity should be noted. Therefore, blank-correction in spectrophotometric- and fluorometric-based enzyme assays is mandatory (Lankatillake et al., 2021). Considering that some plant extracts might exhibit autofluorescence, results should be interpreted critically with respect to possible false-positive hits (Stadlbauer et al., 2020). Moreover, the general use of enzymes of non-mammalian origin (Miller and Joubert, 2022) (e.g., α-glucosidase from Saccharomyces cerevisiae or porcine pancreatic α-amylase) and of non-natural substrates in enzyme screening assays may be additional pitfalls to be aware of.
Although the combination of Ussing chamber with Caco-2 cell culture has rarely been used to examine SGLT1-mediated glucose transport (Yin et al., 2014), recent publications show that this technique is excellent for studying the impact of extracts/substances on this target (Günther et al., 2021; Bauer et al., 2023; Lüersen et al., 2023). A particularly important parameter might be using the PD7 clone of the Caco-2 cell line (Mahraoui et al., 1994). However, it should be noted that the use of Caco-2 in monocultures has its limitations, including the lack of mucus, making co-cultures with mucin-secreting HT29 cells increasingly important (Béduneau et al., 2014). Another major disadvantage is that cytochrome P450 isozymes are poorly expressed in Caco-2 cells, resulting in a lack of metabolism of compounds during absorption process (van Breemen and Li, 2005). In this sense, it should be pointed out that in-vitro assays per se do not cover crucial aspects of pharmacology (Croston, 2017; Günther et al., 2021). Especially for target molecules located within the body such as Kir6.1/Sur2, Glut4 or DPP4, it should be borne in mind that high concentrations of plant extract-derived active ingredients may not be achieved in-vivo. On the other hand, the lack of biotransformation and the absence of a microbiota metabolism in in-vitro assays may prevent the formation of certain metabolites with putative bioactivity from plant extract ingredients.
The patch-clamp technique is ideally suited to characterize biophysical and pharmacological properties of certain ion channels after heterologous expression in the above-mentioned expression systems. If endogenous ion currents (for example, KATP channels in pancreatic β-cells) are to be measured, it is necessary to separate these currents from other endogenous ion currents. This can only be done successfully if specific inhibitors are available or if the biophysical properties can be clearly assigned to the channel under investigation. Furthermore, it is necessary to weigh up the patch-clamp-configuration used for the measurements: in cell-attached, inside-out and outside-out mode the currents are often very small and it must be ensured that the channels to be examined are in the patch; in whole-cell mode you have larger currents, but the intracellular solution is replaced by the pipette solution, so that intracellular signal molecules are washed out.
In general, when studying the bioactivity of plant extracts, one should be bear in mind that the solvent used for extraction can have a significant influence on the efficacy against individual targets (Jaradat et al., 2021), which is due to the fact that individual bioactive compounds dissolve differently depending on the solvent. However, even minor variations in the structure of putative bioactive compounds might significantly modify the effectiveness against a target. For example, studies postulate that structural characteristics such as the presence of hydroxyl groups in the B-ring and the C2-C3 double bond in the C-ring of flavonoids might be essential for α-glucosidase and DPP4 inhibition (Sarian et al., 2017; Li et al., 2018), while an additional Cl ion at 3-position of the C-ring might be particularly important for effective suppression of α-amylase (Proença et al., 2019). Moreover, Oranje et al. (2019) demonstrated that a substitution at the 9’ and 10’ C-atom of an angular pyranocoumarin backbone with an alkyl or alkene ester of 2–5 C-atoms was pivotal for SGLT1 inhibition. However, one should be aware that the effectiveness might not be necessarily due to a single compound and that synergistic modes of action can even be assumed as frequently discussed (Jaradat et al., 2024).
Blood glucose-reducing properties of synthetic compounds and plant extracts at an organismic level can be identified by a modified HET-CAM assay, the Gluc-HET test (Figure 11) (Haselgrübler et al., 2017; 2018b). The hen’s egg test (HET) is a well-established method for studying embryonic development, embryotoxicity, systemic toxicity, immunopathology and metabolic pathways (Luepke and Kemper, 1986). In general, the HET method offers advantages such as simple handling, acceptable costs and sufficient throughput rates (Haselgrübler et al., 2017; 2018b; Sandner et al., 2022, p. 20). An extended version of this test, the HET-chorioallantoic membrane (HET-CAM) test was developed as an alternative to the Draize rabbit eye test which is widely used in industry for evaluating membrane irritating properties of chemicals (Luepke and Kemper, 1986; Steiling et al., 1999; Haselgrübler et al., 2017). The CAM represents a highly vascularized extraembryonic membrane involved in gas exchange, acid-base homeostasis, calcium transport and reabsorption processes in the chicken embryo (Gabrielli and Accili, 2010). In brief, the HET-CAM assay is based on direct application of test chemicals onto the CAM of fertilized eggs and subsequent observation of adverse changes (Spielmann, 1995).
Figure 11. Schematic overview of the Gluc-HET experiment. (A) The location of the air bladder of a fertilized hen’s egg (11 days old) is marked and test substance-containing solution is applied into the air compartment. (B) The chorioallantoic membrane of the embryo is incubated with the solution. Afterwards, the eggshell is removed and the eggshell membrane is equilibrated. (C) After removal of the eggshell membrane, the chorioallantoic membrane is cut. (D) A main blood vessel is prepared on a pH-strip and (E) blood is collected from the vessel. (F) The blood glucose level is determined by using a blood glucose meter (modified from (Stadlbauer et al., 2021)). Used under CC BY 4.0: https://creativecommons.org/licenses/by/4.0/.
A similar strategy is applied in the Gluc-HET assay, where plant extracts or compounds diluted in buffer are injected into the air compartment of hen’s eggs and which are subsequently incubated for a defined time. This is followed by the preparation of the eggs for blood collection. To this end, the eggshell and, after an equilibration step, the eggshell membrane are removed, before the CAM can be uncovered to prepare a blood vessel on a pH-strip. Finally, blood collected from the vessel can be used to determine blood glucose levels (Haselgrübler et al., 2018b).
For this purpose, fertilized 10 or 11 day old eggs are used, with chicken embryos aged 11 days proving most suitable (Haselgrübler et al., 2017). This age provides several advantages. (i) Although not fully differentiated, the growth of the CAM during embryonic development is completed (Nowak-Sliwinska et al., 2014). (ii) Embryos at this age have high glucose levels (Haselgrübler et al., 2017). (iii) Insulin is produced by the embryos from day four or five, but the concentration of serum insulin is negligible until day 12, reducing the potential for interference between endogenously produced insulin and test substances. (iv) Insulin sensitivity is nevertheless already present at this stage (Yoshiyama et al., 2005; Haselgrübler et al., 2017). (v) The experiments are conducted at a stage in embryonic development when they are not considered as animal experiments (Haselgrübler et al., 2017; Sandner et al., 2022). These characteristics may make hen’s eggs a more attractive vertebrate model for determining the blood glucose-reducing properties of plant extracts than other vertebrate models such as the zebrafish, which is also frequently used as a model organism in the context of glucose homeostasis (Eames et al., 2010; Kakouri et al., 2020).
The fruit fly D. melanogaster has proven to be a valuable model organism in nutrition research in recent decades (Staats et al., 2018; Lüersen et al., 2019; Eickelberg et al., 2022), especially due to the partly considerable similarity between human and Drosophila metabolism (e.g., approximately 65% of human disease-related genes having functional homologs in the fly) (Ugur et al., 2016). This also applies to the pathways of energy metabolism and glucose homeostasis in particular (Graham and Pick, 2017; Biglou et al., 2021; Chatterjee and Perrimon, 2021). Corresponding to the situation in humans, α-amylases and α-glucosidases are responsible for the intestinal degradation of poly- and disaccharides and GLUT transporters convey cellular glucose uptake. A sugar-responsive hormone neuropeptide F (NPF) acts similar to mammalian incretin-like hormones, and it is suggested that glucose is taken up via a sodium-dependent transporter (SLC5A5) in the midgut region (Li Y. et al., 2021). Moreover, Drosophila insulin-like peptides (Dilp), whose release from insulin producing cells (IPC) in response to rising glucose levels is mediated by a Kir6.1/SUR1-dependent mechanism similar to that in mammalian β-cells, counteract hyperglycaemia. Accordingly, the fruit fly has been found to be a suitable in-vivo model that can be employed not only to study and decipher molecular mechanisms of glucose homeostasis and related metabolic dysfunctions (Graham and Pick, 2017; Biglou et al., 2021; Chatterjee and Perrimon, 2021) but also to evaluate or verify modulating properties of plant extracts in this context (Bai et al., 2018; Günther et al., 2021; Nakitto et al., 2021; Ollinger et al., 2022; Bauer et al., 2023; Lüersen et al., 2023; Omoboyowa et al., 2023). This is underlined by the fact that established oral hypoglycemic agents such as acarbose (Günther et al., 2021), metformin (Abrat et al., 2018) and glibenclamide (Kréneisz et al., 2010) have also been found to be effective in D. melanogaster.
Being a genetic model in the first place, several Drosophila mutants and transgenes related to glucose homeostasis are available (Murillo-Maldonado and Riesgo-Escovar, 2017). Among these models, the Ilp21gd2HF strain has been established expressing a double-tagged insulin-like peptide 2 (Ilp2HF), which allows quantification of circulating insulin in the fruit fly by an enzyme-linked immunosorbent assay (ELISA) (Park et al., 2014). Of the Drosophila Ilps, Ilp2 is essential for the maintenance of normoglycemia in flies (Grönke et al., 2010). The transgenetic flies were employed to monitor oral glucose stimulated insulin secretion in a fasting and re-feeding assay (Park et al., 2014). As recently demonstrated by Jans et al. (2024), the strain has also been proven to evaluate the impact of dietary supplements on levels of circulating postprandial Ilp2 in fruit flies. Further studies will reveal whether this transgene can also be used to screen plant extracts and bioactives with insulin secretion promoting properties.
Next to the genetic models, hyperglycemia and insulin resistance can be obtained through dietary approaches, feeding a high-sugar (HSD) or a high-fat diet (HFD) (Birse et al., 2010; Musselman et al., 2011; Morris et al., 2012). Similar to humans, this usually induces an obese phenotype associated with elevated triacylglyceride (TAG) levels. Notably, HSD feeding and a manifested diabetes-like status in the fruit fly have been shown to go along with long-term complications known from humans such as cardiopathy (Na et al., 2013) and nephropathy (dysfunction of the Malphigian tubules, the fruit fly kidney analog) (Na et al., 2015). Considering the conserved intestinal carbohydrate digestion enzymes, a dietary starch-induced obesity model was established to verify putative beneficial effects of plant extracts on glucose metabolism in a living organism (Figure 12). To this end, synchronized eggs are given onto a starch-based diet consisting of 10% soluble starch, 4% yeast and 1% agar according to Abrat et al. (2018). This diet is used either without supplement (control diet) or with acarbose given at 1.8 μg/mL (positive control) and plant extract (experimental diet), respectively. To avoid putative adverse developmental effects of the plant extracts, the treatment can be alternatively started in the adult stage on day 3 after eclosion. On day 10 after eclosion various readouts such as body weight and body composition can be determined (Eickelberg et al., 2022). These analyses are carried out on a sex-specific basis. For each group, the average body weight per fly is calculated by weighing 10–20 animals with a precision scale. For further analysis of TAG and protein content, a fly homogenate is made by grinding the flies in a buffer solution and subsequent centrifugation. Commercial kits are available for both parameters, which can be used according to the manufacturer’s instructions. If a reduction in the TAG level occurs in the treatment groups, this indicates that the glucose metabolism is targeted by the plant extract or compound.
Figure 12. Schematic overview of the feeding experiment used in the Drosophila melanogaster model for dietary starch-induced obesity. Synchronized eggs are given in vials with a starch-based diet (control) or diets that were additionally supplemented with acarbose (positive control) or an extract (experimental diets). After eclosion, male and female flies are mated for 2 days. On day three, only mated females are transferred to fresh vials containing the respective diets. On day 10, the female flies are harvested. Subsequently, analyses are performed to determine the body weight and body composition of the flies (modified from (Eickelberg et al., 2022). Used under CC BY 4.0: https://creativecommons.org/licenses/by/4.0/.
Compared to rodents, this invertebrate model organism has numerous advantages. Due to an affordable maintenance, a high reproduction rate and a relatively short lifespan of D. melanogaster, high-throughput analyses are possible (Staats et al., 2018; Jans et al., 2021). Moreover, as the hen’s egg test, experiments can be carried out without serious ethical concerns and regulatory frameworks. Supplementation studies with D. melanogaster do not only cover most of the key features present in human carbohydrate metabolism but also many pharmacological aspects (e.g., bioavailability, biotransformation, role of gut microbiota, toxicity) (Günther et al., 2021; Lüersen et al., 2024). Despite this, physiological differences between the fruit fly and humans should be mentioned. In contrast to humans with one insulin hormone, the fruit fly possesses at least three Dilps involved in glucose homeostasis, which are expressed and secreted by neuroendocrine cells of the brain, as fruit flies lack a pancreas-like organ. Furthermore, trehalose rather than glucose is the predominant circulating sugar (Graham and Pick, 2017).
The evaluation of potential bioactive compounds or plant extracts in rodents and humans still represents the current gold standard, however obviously this is significantly more complex in terms of approval, time and ethical issues. There are numerous rodent models with impaired glycemic control, which are referred to as diabetes models. These are mainly mouse and rat models, which are thoroughly described in the literature (Chen and Wang, 2005, p. 200; Rees and Alcolado, 2005; Cefalu, 2006; Neubauer and Kulkarni, 2006; King, 2012; Kleinert et al., 2018; Kottaisamy et al., 2021), but none of these models reflect the pathological aspects of this disease entirely (Cefalu, 2006). In general, a phenotype with impaired glucose tolerance in rodents can be induced by chemical substances, such as alloxan (Dunn and McLetchie, 1943) and streptozotocin (Junod et al., 1969), or results from genetic alterations concerning, for example, leptin (ob/ob mice (Coleman, 1978)) or the leptin receptor (db/db mice (Coleman, 1978) and Zucker diabetic fatty rats (Peterson et al., 1990)). A high-fat diet represents an alternative to induce impaired glucose tolerance in these animals (Winzell and Ahrén, 2004; King, 2012; Calligaris et al., 2013).
Besides the determination of basal glucose and insulin levels, the glucose tolerance test, which monitors the change in blood glucose levels after glucose administration over time, is considered the standard method for assessing the impact of substances or plant extracts on glucose tolerance in rodents. The test can be varied considerably e.g., with respect to the applied glucose dosage, the route of its administration (oral, intraperitoneal injection or intravenous injection) or the expression of the results (absolute values vs. area under the curve) (Andrikopoulos et al., 2008; Bowe et al., 2014). However, the protocol as described here has proven useful in recent years to study the impact of dietary supplements in mice on glucose tolerance. After several weeks on an experimental diet (containing a supplement/plant extract), mice are fasted for 5–6 h prior to the glucose tolerance test. For this purpose, 2 g glucose/kg body weight is administered orally by gavage. Glucose levels are determined in blood taken from the tail tip by using a glucometer. Measurements are conducted prior to glucose administration and at several time points (ranging from 30 to 120 min) after administration (Schloesser et al., 2017; Fischer et al., 2020; Wüpper et al., 2020).
Similar to the glucose tolerance test, an insulin tolerance test, as indicator of insulin sensitivity, can be performed (Bowe et al., 2014). Moreover, a meal tolerance test is a suitable alternative in rodents (Manzano et al., 2016), which are thought to be more in a physiological context (Brodovicz et al., 2011). Despite the comparatively high effort (Muniyappa et al., 2008), some rodent studies using the glucose clamp technique to evaluate the antihyperglycemic activity of plant extracts can also be found (Manzano et al., 2016; Eddouks et al., 2017).
These methods are not only applied in animal models, but also find their implementation in human studies (Rijkelijkhuizen et al., 2009; Ajala et al., 2012; Besser et al., 2012; Lian et al., 2014; Vitetta et al., 2020). Clinical trials with plant extracts indicate that a variety of different parameters may reflect an antihyperglycemic activity in humans including HbA1c (glycated haemoglobin A1c) values, postprandial levels of glucose, insulin or C-peptide or the HOMA-IR (homeostasis model assessment of insulin resistance) index (Ahmad R. et al., 2021; Lim et al., 2021). Since its development in 1979 (DeFronzo et al., 1979), the glucose clamp technique has emerged as the most appropriate method to evaluate potential antidiabetic agents in humans (Tam et al., 2012; Pelegrin et al., 2019). Depending on the specific issue, either the hyperglycemic clamp technique, as an indicator of islet cell functionality, or the euglycemic insulin clamp technique, as an indicator of insulin sensitivity, can be employed. Both methods rely on the use of variable glucose infusion rates administered to probands to maintain (clamp) blood glucose at a constant level following an initial glucose bolus (hyperglycemic clamp) or a continuous insulin infusion (euglycemic insulin clamp) (DeFronzo et al., 1979).
Based on their traditional use against various metabolic diseases such as diabetes, the in-vivo blood glucose-lowering potential of medicinal plants and plant extracts is widely reported for rodent models or humans (Bailey and Day, 1989; Eddouks et al., 2017). As discussed above, the use of root extracts, which have received little attention so far, is of particular interest. In this context, Ardalani et al. (2021) recently summarized hyperglycemia-reducing properties of root and rhizome extracts derived from 104 plant species in rodent models, but the mechanism of action commonly remains unclear. However, although mechanistic investigations concerning the impact of root and rhizome extracts on key regulatory elements of glucose homeostasis are rare, some studies address this particular issue. They are summarized in Table 1. For this purpose, studies which are published after 2002, were searched via scientific databases using the targets of glucose homeostasis as search terms in combination with the keyword “root extract(s).” Studies were excluded when extracts without a defined solvent were used.
Table 1. Overview of root and rhizome extracts evaluated for their impact on key regulatory elements of glucose homeostasis.
Almost all of the plant species listed in Table 1 share a significant ethnopharmacological background, which is also referred to in the table. For example, with plant species such as Hedychium coronarium J. Koenig (Panigrahy et al., 2020), Pueraria tuberosa (Roxb. Ex Willd.) DC. (Srivastava et al., 2015), Salacia oblonga Wall. ex Wight & Arn (Deepak et al., 2020). and Withania somnifera (L.) Duna (Kempegowda et al., 2018), reference is made to their intensive use in traditional Indian medicine/Ayurveda. In African folk medicine Annona stenophylla Engl. & Diels (Taderera et al., 2019), Aristolochia ringens Vahl (Sulymean et al., 2016), Cissus cornifolia (Baker) Planch. (Chipiti et al., 2017), Myrica salicifolia Hochst. Ex A. Rich. (Emiru et al., 2020), Solanum incanum L. (Andargie et al., 2022) or Vernonia amygdalina Delile (Medjiofack Djeujo et al., 2021) are employed for the treatment of various health problems while in the Chinese Pharmacopoeia the use of plant species such as Dioscorea batatas Decne. (Go et al., 2015), M. alba L. (Zhao et al., 2018), Polygonum viviparum L. (Li H. et al., 2021) or Rehmannia glutinosa Libosch (Jeong et al., 2013) is prominent. Basically, the studies with the plant root extracts listed were set up for various reasons. (i) The studies attempt to find a scientific rationale for the traditional use of the plant species studied in the treatment of diabetes. (ii) The studies are intended to elucidate the underlying molecular mechanisms responsible for the demonstration of antihyperglycemic effects of the plant roots in an in-vivo rodent model. (iii) A screening approach, for example, using a selection of Mongolian plants (Kobayashi et al., 2003) or using a plant extract library (Günther et al., 2021), was carried out with respect to one of the targets of glucose homeostasis to identify new bioactive plant material.
Approximately 50 plant root extracts have been described with respect to their activity toward α-amylase, α-glucosidase, SGLT1, GLP-1, DPP4 and GLUT4. The most abundant targets evaluated in these studies are the carbohydrate-hydrolysing enzymes α-amylase and α-glucosidase followed by GLUT4. Interestingly, a comparison of IC50 values indicated that an ethyl acetate extract of M. alba L. was the most potent inhibitor of both α-amylase (IC50 value of 5.16 μg/mL) and α-glucosidase (IC50 value of 1.70 μg/mL), while, for example, root extracts from various Limonium species were found to specifically inhibit the α-amylase (Senizza et al., 2021) and root extracts from different Moltkia species or from Geum urbanum L. were found to specifically inhibit the α-glucosidase (Günther et al., 2021; Orhan et al., 2021). There are only few studies investigating the modulating activity of plant root derived extracts on the incretin system (DPP4, GLP-1) and on SGLT1. However, concerning the incretin system, the aqueous extract of roots from P. tuberosa (Roxb. Ex Willd.) DC. might be of interest as a modulation of DPP4 and GLP-1 was demonstrated both in-vitro and in-vivo (Srivastava et al., 2015).
Besides conventional targets (α-amylase, α-glucosidase, SGLT1, GLP-1, DPP4 and GLUT4), whose modulation by root extracts has been investigated so far, inhibition of KATP channels could be an emerging focus. Modulation of pancreatic insulin secretion by plant extracts and their phytochemicals has already been demonstrated and might be relevant for future (Govindappa, 2015; Hager et al., 2021). For example, a high-content screen of aqueous plant extracts led to the identification of several potential modulators of glucose-stimulated insulin secretion in living MIN6 β-cells by using an insulin-Gaussia luciferase biosensor (Hager et al., 2021). In this context, it could be particularly interesting to examine the influence of root extracts on pancreatic β-cell KATP channels via patch-clamp technique.
Recent results of studies that have systematically investigated several of the above-mentioned targets indicate that plant (root) extracts indeed have the potential to influence more than one of the key regulatory elements of glucose homeostasis simultaneously, as demonstrated by the example of a G. urbanum L. root extract (Günther et al., 2021), a Bistorta officinalis Delarbre root extract (Bauer et al., 2023) and a Morus mesozygia Stapf. root bark extract (Olufolabo et al., 2024). In this context, we are convinced that the systematic investigation of the key elements of glucose homeostasis could also be of further interest for the other promising root extracts listed in Table 1 and that the toolbox presented here could considerably facilitates this approach. Moreover, it should be noted that it might be highly beneficial to use a combination of two or more plant roots (extracts), as this combination could be significantly more effective by modulating different mechanisms of glucose homeostasis simultaneously. Fundamental scientific findings in this context could also be beneficial to traditional medicines. Modern biomedical science continues to explore the ethnobotanical value of roots to validate traditional knowledge and discover novel applications. Thus, integrating traditional knowledge with modern scientific research technologies holds great promise for discovering new therapeutic agents and improving public health, as exemplified by metformin, which originated from the traditional used Galega officinalis L. (Bailey, 2017). Accordingly, ethnobotanical research often focuses on isolating active compounds, understanding their underlying cellular and molecular mechanisms of action, and conducting clinical trials to confirm their efficacy and safety in humans.
Natural substances, and plant extracts in particular, already play an important role in the control of glucose homeostasis. It is therefore promising to expand our portfolio of natural substances in this field and to find even more effective drugs with fewer side effects. This also applies in particular to compounds from plant roots, which have been little investigated to date. The growing importance of medicinal plant extracts as a source of blood glucose-regulating medications increases the demand for a systematic experimental approach to screen larger amount of plant extracts, such as those generated e.g., when employing extract libraries and sub-libraries, against multiple targets of glucose homeostasis and to evaluate promising extracts in appropriate in-vivo models and humans. The corresponding workflow proposed in this review (Figure 13) is intended to represent such a roadmap starting with enzyme inhibition assays suitable for (high-throughput) screening-approaches, through in-vivo models suitable for intermediate screenings such as the hen’s egg assay or the D. melanogaster models, up to complex experiments concerning the blood glucose-lowering potential of plant extracts in rodents and humans.
Figure 13. Schematic overview of a methodological approach to systematically study glucose homeostasis modulating properties of plant extracts. This approach comprises in-vitro studies in terms of α-amylase inhibition, α-glucosidase inhibition, dipeptidyl-peptidase 4 (DPP4) inhibition, sodium-dependent glucose transporter 1 (SGLT1) inhibition, KATP channel inhibition and glucose transporter 4 translocation. Also included are in-vivo studies in model organisms, namely, chicken embryos, Drosophila melanogaster and rodents, and also in humans.
From the previous studies on plant (root derived) extracts with blood glucose-lowering activity, two principal weaknesses can often be deduced. First, based on their traditional use, the blood glucose-lowering potential of medicinal plants and plant extracts is directly demonstrated in-vivo in diabetic rodents or humans, but the mechanism of action usually remains unclear. Second, when plant extracts were tested in in-vitro assays, only selected targets of glucose homeostasis are commonly studied, rather than systematically examining a broader range of key regulatory elements of glucose homeostasis. With respect to plant extracts, this is particularly important, as a pleiotropic effect can certainly be assumed. Thus, the application of the presented toolbox allows to cover many important steps of glucose homeostasis in a rational way and may complement promising study results available so far on root extracts as listed in Table 1. Our own studies in recent years, in which the majority of the assays included in this toolbox were systematically applied, revealed that plant extracts have the potential to influence several key elements of glucose homeostasis simultaneously and demonstrated the relevance of combining in-vitro assays and (relatively simple) in-vivo models when evaluating the putative bioactivity of plant extracts in this context (Günther et al., 2021; Bauer et al., 2023). Subsequent steps could focus on counter-current fractionation and bioassay-guided separation to identify the bioactive compounds.
However, further expansion of the toolbox may be worthwhile, for example, the investigation of the impact of plant extracts on incretin hormones such as GLP-1, by determining GLP-1 secretion in cultured enteroendocrine GLUTag cells (Esatbeyoglu et al., 2016), thereby complementing DPP4 data. Moreover, GLUT2 represents a promising target, since it was shown that GLUT2 is translocated to the apical membrane of enterocytes under high-glucose concentrations (Zheng et al., 2012; Müller et al., 2018). Likewise, inhibition of the enzyme protein tyrosine phosphatase 1B (PTP1B) by plant extracts is getting increasingly more attention (Zhao Y. et al., 2017; 2018; Sun et al., 2019). As a negative regulator of insulin receptor signaling, PTP1B inhibition may lead to improved insulin sensitivity and/or increased glucose uptake (Elchebly et al., 1999; Klaman et al., 2000; Nandi and Saxena, 2020). A recently published review provides a comprehensive overview of additional molecular targets and in-vitro methods (Zhang et al., 2022). However, greater emphasis should be placed on validating in-vitro results in-vivo. In this context, continued Drosophila experiments could be implemented. Studies on glucose transport inhibition in this in-vivo model would be of great interest, since the first Drosophila glucose transporter was recently discovered in the midgut (Li Y. et al., 2021).
In summary, we suggest that the comprehensive screening toolbox proposed here could facilitate the identification of new plant-derived bioactive compounds with glucose homeostasis modulating properties.
IB: Conceptualization, Writing–original draft. GR: Conceptualization, Supervision, Writing–review and editing. SC: Methodology, Writing–original draft. AB-W: Writing–original draft. JW: Methodology, Writing–review and editing. II: Methodology, Writing–review and editing. KL: Conceptualization, Methodology, Supervision, Writing–original draft.
The author(s) declare that financial support was received for the research, authorship, and/or publication of this article. We acknowledge financial support by the German Research Foundation (DFG) within the funding programme Open Access-Publication Costs. This research was funded by the Christian Doppler Forschungsgesellschaft (Josef Ressel Center for Phytogenic Drug Research). Additionally, this study was funded by a research project of the Austrian Competence Centre for Feed and Food Quality, Safety and Innovation (FFoQSI). The COMET-K1 Competence Centre FFoQSI is funded by the Austrian ministries BMVIT, BMDW and the Austrian provinces Lower Austria, Upper Austria and Vienna within the scope of COMET—Competence Centers for Excellent Technologies. The program COMET is handled by the Austrian Research Promotion Agency FFG.
The authors declare that the research was conducted in the absence of any commercial or financial relationships that could be construed as a potential conflict of interest.
The author(s) declared that they were an editorial board member of Frontiers, at the time of submission. This had no impact on the peer review process and the final decision.
All claims expressed in this article are solely those of the authors and do not necessarily represent those of their affiliated organizations, or those of the publisher, the editors and the reviewers. Any product that may be evaluated in this article, or claim that may be made by its manufacturer, is not guaranteed or endorsed by the publisher.
Akt, protein kinase B; Dilp, Drosophila insulin-like peptides; D. melanogaster, Drosophila melanogaster; DPP4, dipeptidyl peptidase 4; GI, gastrointestinal; GIP, glucose-dependent insulinotropic polypeptide; GLP1, glucagon-like peptide-1; GLUT, glucose transporter; HET-CAM, hen’s egg test - chorioallantoic membrane; HFD, high-fat diet; HSD, high-sugar diet; IGT, impaired glucose tolerance; ISC, short-circuit current; PECKISH, Plant Extract Collection Kiel in Schleswig-Holstein; PI3K, phosphoinositide 3-kinase; PTP1B, protein tyrosine phosphatase 1B; SGLT1, sodium-dependent glucose cotransporter; TAG, triacylglyceride; TIRF, total internal reflection fluorescence.
Abdel-Sattar, E., Shams, M. M., Abd-Rabo, M. M., Mahmoud, N., and Mahrous, E. A. (2021). Chemical and biological investigations of Limonium axillare reveal mechanistic evidence for its antidiabetic activity. PLOS ONE 16, e0255904. doi:10.1371/journal.pone.0255904
Abrat, O. B., Storey, J. M., Storey, K. B., and Lushchak, V. I. (2018). High amylose starch consumption induces obesity in Drosophila melanogaster and metformin partially prevents accumulation of storage lipids and shortens lifespan of the insects. Comp. Biochem. Physiol. Part A Mol. Integr. Physiol. 215, 55–62. doi:10.1016/j.cbpa.2017.10.011
Adamska-Patruno, E., Billing-Marczak, K., Orlowski, M., Gorska, M., Krotkiewski, M., and Kretowski, A. (2018). A synergistic formulation of plant extracts decreases postprandial glucose and insulin peaks: results from two randomized, controlled, cross-over studies using real-world meals. Nutrients 10, 956. doi:10.3390/nu10080956
Ahmad, J. B., Ajani, E. O., and Sabiu, S. (2021a). Chemical group profiling, in vitro and in silico evaluation of Aristolochia ringens on α-amylase and α-glucosidase activity. Evidence-Based Complementary Altern. Med. 2021, e6679185. doi:10.1155/2021/6679185
Ahmad, R., AlLehaibi, L. H., AlSuwaidan, H. N., Alghiryafi, A. F., Almubarak, L. S., AlKhalifah, K. N., et al. (2021b). Evaluation of clinical trials for natural products used in diabetes: an evidence-based systemic literature review. Med. Baltim. 100, e25641. doi:10.1097/MD.0000000000025641
Ajala, O., Lockett, H., Twine, G., and Flanagan, D. E. (2012). Depth and duration of hypoglycaemia achieved during the insulin tolerance test. Eur. J. Endocrinol. 167, 59–65. doi:10.1530/EJE-12-0068
Alessi, D. R., James, S. R., Downes, C. P., Holmes, A. B., Gaffney, P. R. J., Reese, C. B., et al. (1997). Characterization of a 3-phosphoinositide-dependent protein kinase which phosphorylates and activates protein kinase Balpha. Curr. Biol. 7, 261–269. doi:10.1016/S0960-9822(06)00122-9
Andargie, Y., Sisay, W., Molla, M., and Tessema, G. (2022). Evaluation of antidiabetic and antihyperlipidemic activity of 80% methanolic extract of the root of Solanum incanum linnaeus (solanaceae) in mice. Evid. Based Complement. Altern. Med. 2022, 4454881. doi:10.1155/2022/4454881
Andrikopoulos, S., Blair, A. R., Deluca, N., Fam, B. C., and Proietto, J. (2008). Evaluating the glucose tolerance test in mice. Am. J. Physiol. Endocrinol. Metab. 295, E1323–E1332. doi:10.1152/ajpendo.90617.2008
Apostolidis, E., Kwon, Y.-I., and Shetty, K. (2006). Potential of cranberry-based herbal synergies for diabetes and hypertension management. Asia Pac J. Clin. Nutr. 15, 433–441.
Ardalani, H., Hejazi Amiri, F., Hadipanah, A., and Kongstad, K. T. (2021). Potential antidiabetic phytochemicals in plant roots: a review of in vivo studies. J. Diabetes Metab. Disord. 20, 1837–1854. doi:10.1007/s40200-021-00853-9
Aroda, V. R., and Eckel, R. H. (2022). Reconsidering the role of glycaemic control in cardiovascular disease risk in type 2 diabetes: a 21st century assessment. Diabetes Obes. Metab. 24, 2297–2308. doi:10.1111/dom.14830
Arumugam, G., Manjula, P., and Paari, N. (2013). A review: anti diabetic medicinal plants used for diabetes mellitus. J. Acute Dis. 2, 196–200. doi:10.1016/S2221-6189(13)60126-2
Ashcroft, F. M., Harrison, D. E., and Ashcroft, S. J. (1984). Glucose induces closure of single potassium channels in isolated rat pancreatic beta-cells. Nature 312, 446–448. doi:10.1038/312446a0
Ashcroft, F. M., and Rorsman, P. (1989). Electrophysiology of the pancreatic beta-cell. Prog. Biophysics Mol. Biol. 54, 87–143. doi:10.1016/0079-6107(89)90013-8
Ashcroft, F. M., and Rorsman, P. (2013). K(ATP) channels and islet hormone secretion: new insights and controversies. Nat. Rev. Endocrinol. 9, 660–669. doi:10.1038/nrendo.2013.166
Aykul, S., and Martinez-Hackert, E. (2016). Determination of half-maximal inhibitory concentration using biosensor-based protein interaction analysis. Anal. Biochem. 508, 97–103. doi:10.1016/j.ab.2016.06.025
Bai, Y., Li, K., Shao, J., Luo, Q., and Jin, L. H. (2018). Flos Chrysanthemi Indici extract improves a high-sucrose diet-induced metabolic disorder in Drosophila. Exp. Ther. Med. 16, 2564–2572. doi:10.3892/etm.2018.6470
Bailey, C. J. (2017). Metformin: historical overview. Diabetologia 60, 1566–1576. doi:10.1007/s00125-017-4318-z
Bailey, C. J., and Day, C. (1989). Traditional plant medicines as treatments for diabetes. Diabetes Care 12, 553–564. doi:10.2337/diacare.12.8.553
Bais, H. P., Loyola-Vargas, V. M., Flores, H. E., and Vivanco, J. M. (2001). Root-specific metabolism: the biology and biochemistry of underground organs. Vitro Cell.Dev.Biol.-Plant 37, 730–741. doi:10.1007/s11627-001-0122-y
Barber, E., Houghton, M. J., and Williamson, G. (2021). Flavonoids as human intestinal α-glucosidase inhibitors. Foods 10, 1939. doi:10.3390/foods10081939
Barragán, J. M., Rodríguez, R. E., and Blázquez, E. (1994). Changes in arterial blood pressure and heart rate induced by glucagon-like peptide-1-(7-36) amide in rats. Am. J. Physiol. 266, E459–E466. doi:10.1152/ajpendo.1994.266.3.E459
Bauer, I., Rimbach, G., Nevermann, S., Neuhauser, C., Schwarzinger, B., Schwarzinger, C., et al. (2023). In-vitro antidiabetic activity of a Bistorta officinalis Delarbre root extract can not be confirmed in the in-vivo models hen’s egg test and Drosophila melanogaster. J. Physiol. Pharmacol. 74. doi:10.26402/jpp.2023.1.04
Béduneau, A., Tempesta, C., Fimbel, S., Pellequer, Y., Jannin, V., Demarne, F., et al. (2014). A tunable Caco-2/HT29-MTX co-culture model mimicking variable permeabilities of the human intestine obtained by an original seeding procedure. Eur. J. Pharm. Biopharm. 87, 290–298. doi:10.1016/j.ejpb.2014.03.017
Bell, G. I., Kayano, T., Buse, J. B., Burant, C. F., Takeda, J., Lin, D., et al. (1990). Molecular biology of mammalian glucose transporters. Diabetes Care 13, 198–208. doi:10.2337/diacare.13.3.198
Berrani, A., Marmouzi, I., Bouyahya, A., Kharbach, M., El Hamdani, M., El Jemli, M., et al. (2021). Phenolic compound analysis and pharmacological screening of vitex agnus-castus functional parts. Biomed. Res. Int. 2021, 6695311. doi:10.1155/2021/6695311
Besser, R. E. J., Jones, A. G., McDonald, T. J., Shields, B. M., Knight, B. A., and Hattersley, A. T. (2012). The impact of insulin administration during the mixed meal tolerance test. Diabet. Med. 29, 1279–1284. doi:10.1111/j.1464-5491.2012.03649.x
Biglou, S. G., Bendena, W. G., and Chin-Sang, I. (2021). An overview of the insulin signaling pathway in model organisms Drosophila melanogaster and Caenorhabditis elegans. Peptides 145, 170640. doi:10.1016/j.peptides.2021.170640
Birse, R. T., Choi, J., Reardon, K., Rodriguez, J., Graham, S., Diop, S., et al. (2010). High-fat-diet-induced obesity and heart dysfunction are regulated by the TOR pathway in Drosophila. Cell. Metab. 12, 533–544. doi:10.1016/j.cmet.2010.09.014
Blaak, E. E., Antoine, J.-M., Benton, D., Björck, I., Bozzetto, L., Brouns, F., et al. (2012). Impact of postprandial glycaemia on health and prevention of disease. Obes. Rev. 13, 923–984. doi:10.1111/j.1467-789X.2012.01011.x
Bowe, J. E., Franklin, Z. J., Hauge-Evans, A. C., King, A. J., Persaud, S. J., and Jones, P. M. (2014). Metabolic phenotyping guidelines: assessing glucose homeostasis in rodent models. J. Endocrinol. 222, G13–G25. doi:10.1530/JOE-14-0182
Briggs, D. E. (1962). Gel-diffusion method for the assay of α-amylase. J. Inst. Brew. 68, 27–32. doi:10.1002/j.2050-0416.1962.tb01844.x
Brodovicz, K. G., Girman, C. J., Simonis-Bik, A. M. C., Rijkelijkhuizen, J. M., Zelis, M., Bunck, M. C., et al. (2011). Postprandial metabolic responses to mixed versus liquid meal tests in healthy men and men with type 2 diabetes. Diabetes Res. Clin. Pract. 94, 449–455. doi:10.1016/j.diabres.2011.09.002
Calligaris, S. D., Lecanda, M., Solis, F., Ezquer, M., Gutiérrez, J., Brandan, E., et al. (2013). Mice long-term high-fat diet feeding recapitulates human cardiovascular alterations: an animal model to study the early phases of diabetic cardiomyopathy. PLOS ONE 8, e60931. doi:10.1371/journal.pone.0060931
Cefalu, W. T. (2006). Animal models of type 2 diabetes: clinical presentation and pathophysiological relevance to the human condition. ILAR J. 47, 186–198. doi:10.1093/ilar.47.3.186
Chang, L., Chiang, S.-H., and Saltiel, A. R. (2007). TC10alpha is required for insulin-stimulated glucose uptake in adipocytes. Endocrinology 148, 27–33. doi:10.1210/en.2006-1167
Chao, A. M., Tronieri, J. S., Amaro, A., and Wadden, T. A. (2023). Semaglutide for the treatment of obesity. Trends Cardiovasc Med. 33, 159–166. doi:10.1016/j.tcm.2021.12.008
Chao, E. C., and Henry, R. R. (2010). SGLT2 inhibition--a novel strategy for diabetes treatment. Nat. Rev. Drug Discov. 9, 551–559. doi:10.1038/nrd3180
Chatterjee, N., and Perrimon, N. (2021). What fuels the fly: energy metabolism in Drosophila and its application to the study of obesity and diabetes. Sci. Adv. 7, eabg4336. doi:10.1126/sciadv.abg4336
Chelikani, P. K., Haver, A. C., and Reidelberger, R. D. (2005). Intravenous infusion of glucagon-like peptide-1 potently inhibits food intake, sham feeding, and gastric emptying in rats. Am. J. Physiol. Regul. Integr. Comp. Physiol. 288, R1695–R1706. doi:10.1152/ajpregu.00870.2004
Chen, D., and Wang, M.-W. (2005). Development and application of rodent models for type 2 diabetes. Diabetes, Obes. Metabolism 7, 307–317. doi:10.1111/j.1463-1326.2004.00392.x
Chiang, S. H., Baumann, C. A., Kanzaki, M., Thurmond, D. C., Watson, R. T., Neudauer, C. L., et al. (2001). Insulin-stimulated GLUT4 translocation requires the CAP-dependent activation of TC10. Nature 410, 944–948. doi:10.1038/35073608
Chiba, S. (1997). Molecular mechanism in alpha-glucosidase and glucoamylase. Biosci. Biotechnol. Biochem. 61, 1233–1239. doi:10.1271/bbb.61.1233
Chipiti, T., Ibrahim, M. A., Singh, M., and Islam, M. S. (2017). In vitro α-amylase and α-glucosidase inhibitory and cytotoxic activities of extracts from Cissus cornifolia Planch parts. Pharmacogn. Mag. 13, S329-S333–S333. doi:10.4103/pm.pm_223_16
Clarke, L. L. (2009). A guide to Ussing chamber studies of mouse intestine. Am. J. Physiol. Gastrointest. Liver Physiol. 296, G1151–G1166. doi:10.1152/ajpgi.90649.2008
Coleman, D. L. (1978). Obese and diabetes: two mutant genes causing diabetes-obesity syndromes in mice. Diabetologia 14, 141–148. doi:10.1007/BF00429772
Correia, R. T. P., Mccue, P., Vattem, D. A., Magalhães, M. M. A., Macêdo, G. R., and Shetty, K. (2004). Amylase and Helicobacter pylori inhibition by phenolic extracts of pineapple wastes bioprocessed by rhizopus oligosporus. J. Food Biochem. 28, 419–434. doi:10.1111/j.1745-4514.2004.06003.x
Crespy, V., Aprikian, O., Morand, C., Besson, C., Manach, C., Demigné, C., et al. (2001). Bioavailability of phloretin and phloridzin in rats. J. Nutr. 131, 3227–3230. doi:10.1093/jn/131.12.3227
Croston, G. E. (2017). The utility of target-based discovery. Expert Opin. Drug Discov. 12, 427–429. doi:10.1080/17460441.2017.1308351
Dangelmaier, C., Manne, B. K., Liverani, E., Jin, J., Bray, P., and Kunapuli, S. P. (2014). PDK1 selectively phosphorylates Thr(308) on Akt and contributes to human platelet functional responses. Thromb. Haemost. 111, 508–517. doi:10.1160/TH13-06-0484
Darling, N. J., Mobbs, C. L., González-Hau, A. L., Freer, M., and Przyborski, S. (2020). Bioengineering novel in vitro Co-culture models that represent the human intestinal mucosa with improved caco-2 structure and barrier function. Front. Bioeng. Biotechnol. 8, 992. doi:10.3389/fbioe.2020.00992
Deepak, K. G. K., Challa, S., Suhasin, G., Nagesewara Rao Reddy, N., Elansary, H. O., and El-Ansary, D. O. (2020). Antidiabetic and antilipidemic activity of root extracts of Salacia oblonga against streptozotocin-induced diabetes in wistar rats. Processes 8, 301. doi:10.3390/pr8030301
DeFronzo, R. A., Tobin, J. D., and Andres, R. (1979). Glucose clamp technique: a method for quantifying insulin secretion and resistance. Am. J. Physiol. 237, E214–E223. doi:10.1152/ajpendo.1979.237.3.E214
Dominguez Rieg, J. A., and Rieg, T. (2019). What does sodium-glucose co-transporter 1 inhibition add: prospects for dual inhibition. Diabetes Obes. Metab. 21, 43–52. doi:10.1111/dom.13630
Drucker, D. J., and Nauck, M. A. (2006). The incretin system: glucagon-like peptide-1 receptor agonists and dipeptidyl peptidase-4 inhibitors in type 2 diabetes. Lancet 368, 1696–1705. doi:10.1016/S0140-6736(06)69705-5
Dunn, J. S., and McLetchie, N. G. B. (1943). Experimental alloxan diabetes in the rat. Lancet 242, 384–387. doi:10.1016/S0140-6736(00)87397-3
Eames, S. C., Philipson, L. H., Prince, V. E., and Kinkel, M. D. (2010). Blood sugar measurement in zebrafish reveals dynamics of glucose homeostasis. Zebrafish 7, 205–213. doi:10.1089/zeb.2009.0640
Eddouks, M., Lemhadri, A., Hebi, M., el Hidani, A., Zeggwagh, N. A., el Bouhali, B., et al. (2017). Capparis spinosa L. aqueous extract evokes antidiabetic effect in streptozotocin-induced diabetic mice. Avicenna J. Phytomed 7, 191–198.
Ehrenkranz, J. R. L., Lewis, N. G., Kahn, C. R., and Roth, J. (2005). Phlorizin: a review. Diabetes/Metabolism Res. Rev. 21, 31–38. doi:10.1002/dmrr.532
Eickelberg, V., Lüersen, K., Staats, S., and Rimbach, G. (2022). Phenotyping of Drosophila melanogaster—a nutritional perspective. Biomolecules 12, 221. doi:10.3390/biom12020221
Elchebly, M., Payette, P., Michaliszyn, E., Cromlish, W., Collins, S., Loy, A. L., et al. (1999). Increased insulin sensitivity and obesity resistance in mice lacking the protein tyrosine phosphatase-1B gene. Science 283, 1544–1548. doi:10.1126/science.283.5407.1544
Elrick, H., Stimmler, L., Hlad, C. J., and Arai, Y. (1964). Plasma insulin response to oral and intravenous glucose administration. J. Clin. Endocrinol. Metab. 24, 1076–1082. doi:10.1210/jcem-24-10-1076
Emiru, Y. K., Periasamy, G., Karim, A., Ur Rehman, N., and Ansari, M. N. (2020). Evaluation of in vitro α-amylase inhibitory activity and antidiabetic effect of Myrica salicifolia in streptozotocin-induced diabetic mice. Pak J. Pharm. Sci. 33, 1917–1926. doi:10.36721/PJPS.2020.33.4.SUP.1917-1926.1
Emordi, J. E., Agbaje, E. O., Oreagba, I. A., and Iribhogbe, O. I. (2018). Antidiabetic effects of the ethanolic root extract of uvaria chamae P. Beauv (annonaceae) in alloxan-induced diabetic rats: a potential alternative treatment for diabetes mellitus. Adv. Pharmacol. Sci. 2018, 1314941. doi:10.1155/2018/1314941
Esatbeyoglu, T., Rodríguez-Werner, M., Schlösser, A., Liehr, M., Ipharraguerre, I., Winterhalter, P., et al. (2016). Fractionation of plant bioactives from black carrots (daucus carota subspecies sativus varietas atrorubens alef.) by adsorptive membrane chromatography and analysis of their potential anti-diabetic activity. J. Agric. Food Chem. 64, 5901–5908. doi:10.1021/acs.jafc.6b02292
European Diabetes Epidemiology Group (1999). Glucose tolerance and mortality: comparison of WHO and American diabetes association diagnostic criteria. The DECODE study group. European diabetes Epidemiology group. Diabetes Epidemiology: collaborative analysis of diagnostic criteria in europe. Lancet 354, 617–621. doi:10.1016/S0140-6736(98)12131-1
Fageyinbo, M. S., Akindele, A. J., Adenekan, S. O., and Agbaje, E. O. (2019). Evaluation of in-vitro and in-vivo antidiabetic, antilipidemic and antioxidant potentials of aqueous root extract of Strophanthus hispidus DC (Apocynaceae). J. Complement. Integr. Med. 16, 20180055. doi:10.1515/jcim-2018-0055
Ferruzza, S., Rossi, C., Scarino, M. L., and Sambuy, Y. (2012). A protocol for differentiation of human intestinal Caco-2 cells in asymmetric serum-containing medium. Toxicol Vitro 26, 1252–1255. doi:10.1016/j.tiv.2012.01.008
Fischer, A., Lüersen, K., Schultheiß, G., de Pascual-Teresa, S., Mereu, A., Ipharraguerre, I. R., et al. (2020). Supplementation with nitrate only modestly affects lipid and glucose metabolism in genetic and dietary-induced murine models of obesity. J. Clin. Biochem. Nutr. 66, 24–35. doi:10.3164/jcbn.19-43
Flanagan, S. E., Clauin, S., Bellanné-Chantelot, C., de Lonlay, P., Harries, L. W., Gloyn, A. L., et al. (2009). Update of mutations in the genes encoding the pancreatic beta-cell K(ATP) channel subunits Kir6.2 (KCNJ11) and sulfonylurea receptor 1 (ABCC8) in diabetes mellitus and hyperinsulinism. Hum. Mutat. 30, 170–180. doi:10.1002/humu.20838
Flint, A., Raben, A., Astrup, A., and Holst, J. J. (1998). Glucagon-like peptide 1 promotes satiety and suppresses energy intake in humans. J. Clin. Investig. 101, 515–520. doi:10.1172/JCI990
Funke, I., and Melzig, M. F. (2006). Traditionally used plants in diabetes therapy: phytotherapeutics as inhibitors of alpha-amylase activity. Rev. Bras. Farmacogn. 16, 1–5. doi:10.1590/S0102-695X2006000100002
Gabrielli, M. G., and Accili, D. (2010). The chick chorioallantoic membrane: a model of molecular, structural, and functional adaptation to transepithelial ion transport and barrier function during embryonic development. J. Biomed. Biotechnol. 2010, 940741. doi:10.1155/2010/940741
Gallwitz, B. (2019). Clinical use of DPP-4 inhibitors. Front. Endocrinol. 10, 389. doi:10.3389/fendo.2019.00389
Gilbert, M. P., and Pratley, R. E. (2020). GLP-1 analogs and DPP-4 inhibitors in type 2 diabetes therapy: review of head-to-head clinical trials. Front. Endocrinol. (Lausanne) 11, 178. doi:10.3389/fendo.2020.00178
Giraudat, J., Devillers-Thiery, A., Auffray, C., Rougeon, F., and Changeux, J. P. (1982). Identification of a cDNA clone coding for the acetylcholine binding subunit of Torpedo marmorata acetylcholine receptor. EMBO J. 1, 713–717. doi:10.1002/j.1460-2075.1982.tb01235.x
Gloyn, A. L., Pearson, E. R., Antcliff, J. F., Proks, P., Bruining, G. J., Slingerland, A. S., et al. (2004). Activating mutations in the gene encoding the ATP-sensitive potassium-channel subunit Kir6.2 and permanent neonatal diabetes. N. Engl. J. Med. 350, 1838–1849. doi:10.1056/NEJMoa032922
Go, H. K., Rahman, M. M., Kim, G. B., Na, C. S., Song, C.-H., Kim, J. S., et al. (2015). Antidiabetic effects of yam (Dioscorea batatas) and its active constituent, allantoin, in a rat model of streptozotocin-induced diabetes. Nutrients 7, 8532–8544. doi:10.3390/nu7105411
Goodman, J. J. (1950). Adaptive production of amylase and lipase by three species of fungi. Science 112, 176–179. doi:10.1126/science.112.2902.176
Gorboulev, V., Schürmann, A., Vallon, V., Kipp, H., Jaschke, A., Klessen, D., et al. (2012). Na(+)-D-glucose cotransporter SGLT1 is pivotal for intestinal glucose absorption and glucose-dependent incretin secretion. Diabetes 61, 187–196. doi:10.2337/db11-1029
Govers, R. (2014). Molecular mechanisms of GLUT4 regulation in adipocytes. Diabetes & Metabolism 40, 400–410. doi:10.1016/j.diabet.2014.01.005
Graham, P., and Pick, L. (2017). Drosophila as a model for diabetes and diseases of insulin resistance. Curr. Top. Dev. Biol. 121, 397–419. doi:10.1016/bs.ctdb.2016.07.011
Grasset, E., Pinto, M., Dussaulx, E., Zweibaum, A., and Desjeux, J. F. (1984). Epithelial properties of human colonic carcinoma cell line Caco-2: electrical parameters. Am. J. Physiol. 247, C260–C267. doi:10.1152/ajpcell.1984.247.3.C260
Grönke, S., Clarke, D.-F., Broughton, S., Andrews, T. D., and Partridge, L. (2010). Molecular evolution and functional characterization of Drosophila insulin-like peptides. PLoS Genet. 6, e1000857. doi:10.1371/journal.pgen.1000857
Günther, I., Rimbach, G., Nevermann, S., Neuhauser, C., Stadlbauer, V., Schwarzinger, B., et al. (2021). Avens root (Geum urbanum L.) extract discovered by target-based screening exhibits antidiabetic activity in the hen’s egg test model and Drosophila melanogaster. Front. Pharmacol. 12, 794404. doi:10.3389/fphar.2021.794404
Hamill, O. P., Marty, A., Neher, E., Sakmann, B., and Sigworth, F. J. (1981). Improved patch-clamp techniques for high-resolution current recording from cells and cell-free membrane patches. Pflugers Arch. 391, 85–100. doi:10.1007/BF00656997
Han, Y., Jung, H. W., and Park, Y. K. (2012). The roots of Atractylodes japonica Koidzumi promote adipogenic differentiation via activation of the insulin signaling pathway in 3T3-L1 cells. BMC Complementary Altern. Med. 12, 154. doi:10.1186/1472-6882-12-154
Hanssen, N. M. J., Kraakman, M. J., Flynn, M. C., Nagareddy, P. R., Schalkwijk, C. G., and Murphy, A. J. (2020). Postprandial glucose spikes, an important contributor to cardiovascular disease in diabetes? Front. Cardiovasc Med. 7, 570553. doi:10.3389/fcvm.2020.570553
Hao, J., Na, R., Sun, L., Jia, Y., Han, F., Fu, Z., et al. (2023). Chemical profile and quantitative comparison of constituents in different medicinal parts of Lactuca indica during varied harvest periods using UPLC-MS/MS method. Food Chem. X 20, 101031. doi:10.1016/j.fochx.2023.101031
Haselgrübler, R., Stadlbauer, V., Stübl, F., Schwarzinger, B., Rudzionyte, I., Himmelsbach, M., et al. (2018a). Insulin mimetic properties of extracts prepared from bellis perennis. Molecules 23, 2605. doi:10.3390/molecules23102605
Haselgrübler, R., Stübl, F., Essl, K., Iken, M., Schröder, K., and Weghuber, J. (2017). Gluc-HET, a complementary chick embryo model for the characterization of antidiabetic compounds. PLOS ONE 12, e0182788. doi:10.1371/journal.pone.0182788
Haselgrübler, R., Stübl, F., Stadlbauer, V., Lanzerstorfer, P., and Weghuber, J. (2018b). An in ovo model for testing insulin-mimetic compounds. J. Vis. Exp., e57237. doi:10.3791/57237
Hattersley, A. T., and Ashcroft, F. M. (2005). Activating mutations in Kir6.2 and neonatal diabetes: new clinical syndromes, new scientific insights, and new therapy. Diabetes 54, 2503–2513. doi:10.2337/diabetes.54.9.2503
He, L., Yin, Y., Li, T., Huang, R., Xie, M., Wu, Z., et al. (2013). Use of the Ussing chamber technique to study nutrient transport by epithelial tissues. Front. Biosci. Landmark Ed. 18, 1266–1274. doi:10.2741/4178
Heckmann, M., Klanert, G., Sandner, G., Lanzerstorfer, P., Auer, M., and Weghuber, J. (2022). Fluorescence microscopy-based quantitation of GLUT4 translocation. Methods Appl. Fluoresc. 10, 022001. doi:10.1088/2050-6120/ac4998
Hidalgo, I. J., Raub, T. J., and Borchardt, R. T. (1989). Characterization of the human colon carcinoma cell line (Caco-2) as a model system for intestinal epithelial permeability. Gastroenterology 96, 736–749. doi:10.1016/0016-5085(89)90897-4
Hodgkin, A. L., and Huxley, A. F. (1952a). A quantitative description of membrane current and its application to conduction and excitation in nerve. J. Physiol. 117, 500–544. doi:10.1113/jphysiol.1952.sp004764
Hodgkin, A. L., and Huxley, A. F. (1952b). Currents carried by sodium and potassium ions through the membrane of the giant axon of Loligo. J. Physiol. 116, 449–472. doi:10.1113/jphysiol.1952.sp004717
Holst, J. J., Vilsbøll, T., and Deacon, C. F. (2009). The incretin system and its role in type 2 diabetes mellitus. Mol. Cell. Endocrinol. 297, 127–136. doi:10.1016/j.mce.2008.08.012
Houtman, M. J. C., Friesacher, T., Chen, X., Zangerl-Plessl, E.-M., van der Heyden, M. A. G., and Stary-Weinzinger, A. (2021). Development of IKATP ion channel blockers targeting sulfonylurea resistant mutant KIR6.2 based channels for treating DEND syndrome. Front. Pharmacol. 12, 814066. doi:10.3389/fphar.2021.814066
Huang, S., and Czech, M. P. (2007). The GLUT4 glucose transporter. Cell. Metab. 5, 237–252. doi:10.1016/j.cmet.2007.03.006
Husain, G. M., Rai, R., Rai, G., Singh, H. B., Thakur, A. K., and Kumar, V. (2014). Potential mechanism of anti-diabetic activity of Picrorhiza kurroa. TANG 4, 27.1–27.5. doi:10.5667/tang.2014.0013
Igbinidu, G., and Nimenibo Uadia, R. (2019). GC-MS analysis, phytochemical screening and in vitro alpha amylase and alpha glucosidase inhibitory activities of Vernonia amygdalina root extract and fractions. J. Pharmacogn. Phytochem. 8, 2125–2131.
Imbrici, P., Liantonio, A., Camerino, G. M., De Bellis, M., Camerino, C., Mele, A., et al. (2016). Therapeutic approaches to genetic ion channelopathies and perspectives in drug discovery. Front. Pharmacol. 7, 121. doi:10.3389/fphar.2016.00121
Imran, M., Saeed, F., Hussain, G., Imran, A., Mehmood, Z., Gondal, T. A., et al. (2021). Myricetin: a comprehensive review on its biological potentials. Food Sci. Nutr. 9, 5854–5868. doi:10.1002/fsn3.2513
Inbaraj, S., and Muniappan, M. (2020). Correlation between the in-vitro and in-vivo antihyperglycemic effect of ocimum sanctum, Trigonella foenum graecum and curcuma longa. PJ 12, 369–376. doi:10.5530/pj.2020.12.58
Io, F., Gunji, E., Koretsune, H., Kato, K., Sugisaki-Kitano, M., Okumura-Kitajima, L., et al. (2019). SGL5213, a novel and potent intestinal SGLT1 inhibitor, suppresses intestinal glucose absorption and enhances plasma GLP-1 and GLP-2 secretion in rats. Eur. J. Pharmacol. 853, 136–144. doi:10.1016/j.ejphar.2019.03.023
Jans, K., Lüersen, K., and Rimbach, G. (2021). Drosophila melanogaster as a model organism to study lithium and boron bioactivity. Int. J. Mol. Sci. 22, 11710. doi:10.3390/ijms222111710
Jaradat, N., Dwikat, M., Amer, J., Ghanim, M., Hawash, M., Hussein, F., et al. (2024). Total phenolic contents, cytotoxic, free radicals, porcine pancreatic α-amylase, and lipase suppressant activities of Artemisia dracunculus plant from Palestine. Front. Pharmacol. 15, 1351743. doi:10.3389/fphar.2024.1351743
Jaradat, N., Qadi, M., Ali, I., Hussein, F., Issa, L., Rashdan, D., et al. (2021). Phytochemical screening, antiobesity, antidiabetic and antimicrobial assessments of Orobanche aegyptiaca from Palestine. BMC Complement. Med. Ther. 21, 256. doi:10.1186/s12906-021-03431-x
Jeong, H. J., Kim, J. S., Hyun, T. K., Yang, J., Kang, H.-H., Cho, J. C., et al. (2013). In vitro antioxidant and antidiabetic activities of Rehmannia glutinosa tuberous root extracts. ScienceAsia 39, 605–609. doi:10.2306/scienceasia1513-1874.2013.39.605
Junod, A., Lambert, A. E., Stauffacher, W., and Renold, A. E. (1969). Diabetogenic action of streptozotocin: relationship of dose to metabolic response. J. Clin. Investig. 48, 2129–2139. doi:10.1172/JCI106180
Kakouri, E., Agalou, A., Kanakis, C., Beis, D., and Tarantilis, P. A. (2020). Crocins from crocus sativus L. In the management of hyperglycemia. in vivo evidence from zebrafish. Molecules 25, 5223. doi:10.3390/molecules25225223
Kanai, F., Nishioka, Y., Hayashi, H., Kamohara, S., Todaka, M., and Ebina, Y. (1993). Direct demonstration of insulin-induced GLUT4 translocation to the surface of intact cells by insertion of a c-myc epitope into an exofacial GLUT4 domain. J. Biol. Chem. 268, 14523–14526. doi:10.1016/s0021-9258(19)85269-9
Kanai, Y., Lee, W. S., You, G., Brown, D., and Hediger, M. A. (1994). The human kidney low affinity Na+/glucose cotransporter SGLT2. Delineation of the major renal reabsorptive mechanism for D-glucose. J. Clin. Investig. 93, 397–404. doi:10.1172/JCI116972
Keharom, S., Mahachai, R., and Chanthai, S. (2016). The optimization study of α-amylase activity based on central composite design-response surface methodology by dinitrosalicylic acid method. Int. Food Res. J. 23, 10–17.
Kempegowda, P. K., Zameer, F., Narasimashetty, C., Kollur, S., and Murari, S. (2018). Inhibitory potency of Withania somnifera extracts against DPP-4: an in vitro evaluation. Afr. J. Traditional, Complementary Altern. Med. 15, 11–25. doi:10.21010/ajtcam.vi15.1.2
Kęska, P., and Stadnik, J. (2022). Dipeptidyl peptidase IV inhibitory peptides generated in dry-cured pork loin during aging and gastrointestinal digestion. Nutrients 14, 770. doi:10.3390/nu14040770
Kifle, Z. D., Abdelwuhab, M., Melak, A. D., Genet, G., Meseret, T., and Adugna, M. (2022). Pharmacological evaluation of medicinal plants with antidiabetic activities in Ethiopia: a review. Metabol. Open 13, 100174. doi:10.1016/j.metop.2022.100174
Kim, M. J., Lee, S. B., Lee, H. S., Lee, S. Y., Baek, J. S., Kim, D., et al. (1999). Comparative study of the inhibition of alpha-glucosidase, alpha-amylase, and cyclomaltodextrin glucanosyltransferase by acarbose, isoacarbose, and acarviosine-glucose. Arch. Biochem. Biophys. 371, 277–283. doi:10.1006/abbi.1999.1423
Kim, S., Chen, J., Cheng, T., Gindulyte, A., He, J., He, S., et al. (2023). PubChem 2023 update. Nucleic Acids Res. 51, D1373–D1380. doi:10.1093/nar/gkac956
King, A. J. (2012). The use of animal models in diabetes research. Br. J. Pharmacol. 166, 877–894. doi:10.1111/j.1476-5381.2012.01911.x
Kingsley, B., Arun, K. P., Selvaraj, S., and Brindha, P. (2014). Stereospermum tetragonam as an antidiabetic agent by activating PPARγ and GLUT4. Bangladesh J. Pharmacol. 9, 250–256. doi:10.3329/bjp.v9i2.18488
Klaman, L. D., Boss, O., Peroni, O. D., Kim, J. K., Martino, J. L., Zabolotny, J. M., et al. (2000). Increased energy expenditure, decreased adiposity, and tissue-specific insulin sensitivity in protein-tyrosine phosphatase 1B-deficient mice. Mol. Cell. Biol. 20, 5479–5489. doi:10.1128/MCB.20.15.5479-5489.2000
Kleinert, M., Clemmensen, C., Hofmann, S. M., Moore, M. C., Renner, S., Woods, S. C., et al. (2018). Animal models of obesity and diabetes mellitus. Nat. Rev. Endocrinol. 14, 140–162. doi:10.1038/nrendo.2017.161
Klinger, S. (2020). Segment-specific effects of resveratrol on porcine small intestinal dipeptide absorption depend on the mucosal pH and are due to different mechanisms: potential roles of different transport proteins and protein kinases. J. Nutr. Biochem. 85, 108467. doi:10.1016/j.jnutbio.2020.108467
Klinger, S., and Breves, G. (2018). Resveratrol inhibits porcine intestinal glucose and alanine transport: potential roles of Na+/K+-ATPase activity, protein kinase A, AMP-activated protein kinase and the association of selected nutrient transport proteins with detergent resistant membranes. Nutrients 10, 302. doi:10.3390/nu10030302
Kobayashi, K., Baba, E., Fushiya, S., Takano, F., Batkhuu, J., Dash, T., et al. (2003). Screening of Mongolian plants for influence on amylase activity in mouse plasma and gastrointestinal tube. Biol. Pharm. Bull. 26, 1045–1048. doi:10.1248/bpb.26.1045
Koepsell, H. (2020). Glucose transporters in the small intestine in health and disease. Pflugers Arch. 472, 1207–1248. doi:10.1007/s00424-020-02439-5
Kottaisamy, C. P. D., Raj, D. S., Prasanth Kumar, V., and Sankaran, U. (2021). Experimental animal models for diabetes and its related complications—a review. Lab. Anim. Res. 37, 23. doi:10.1186/s42826-021-00101-4
Kréneisz, O., Chen, X., Fridell, Y.-W. C., and Mulkey, D. K. (2010). Glucose increases activity and Ca2+ in insulin-producing cells of adult Drosophila. Neuroreport 21, 1116–1120. doi:10.1097/WNR.0b013e3283409200
Krentz, A. J., and Bailey, C. J. (2005). Oral antidiabetic agents: current role in type 2 diabetes mellitus. Drugs 65, 385–411. doi:10.2165/00003495-200565030-00005
Lankatillake, C., Huynh, T., and Dias, D. A. (2019). Understanding glycaemic control and current approaches for screening antidiabetic natural products from evidence-based medicinal plants. Plant Methods 15, 105. doi:10.1186/s13007-019-0487-8
Lankatillake, C., Luo, S., Flavel, M., Lenon, G. B., Gill, H., Huynh, T., et al. (2021). Screening natural product extracts for potential enzyme inhibitors: protocols, and the standardisation of the usage of blanks in α-amylase, α-glucosidase and lipase assays. Plant Methods 17, 3. doi:10.1186/s13007-020-00702-5
Lanzerstorfer, P., Stadlbauer, V., Chtcheglova, L. A., Haselgrübler, R., Borgmann, D., Wruss, J., et al. (2014). Identification of novel insulin mimetic drugs by quantitative total internal reflection fluorescence (TIRF) microscopy. Br. J. Pharmacol. 171, 5237–5251. doi:10.1111/bph.12845
Larsen, E. H. (2002). Hans H. Ussing—scientific work: contemporary significance and perspectives. Biochimica Biophysica Acta (BBA) - Biomembr. 1566, 2–15. doi:10.1016/S0005-2736(02)00592-8
Li, C., Begum, A., Numao, S., Park, K. H., Withers, S. G., and Brayer, G. D. (2005). Acarbose rearrangement mechanism implied by the kinetic and structural analysis of human pancreatic alpha-amylase in complex with analogues and their elongated counterparts. Biochemistry 44, 3347–3357. doi:10.1021/bi048334e
Li, H., He, Z., Shen, Q., Fan, W., Tan, G., Zou, Y., et al. (2021a). Rapid screening alpha-glucosidase inhibitors from polygoni vivipari rhizoma by multi-step matrix solid-phase dispersion, ultrafiltration and HPLC. Molecules 26, 6111. doi:10.3390/molecules26206111
Li, J., Luo, J., Chai, Y., Guo, Y., Tianzhi, Y., and Bao, Y. (2021b). Hypoglycemic effect of Taraxacum officinale root extract and its synergism with Radix Astragali extract. Food Sci. Nutr. 9, 2075–2085. doi:10.1002/fsn3.2176
Li, K., Yao, F., Xue, Q., Fan, H., Yang, L., Li, X., et al. (2018). Inhibitory effects against α-glucosidase and α-amylase of the flavonoids-rich extract from Scutellaria baicalensis shoots and interpretation of structure–activity relationship of its eight flavonoids by a refined assign-score method. Chem. Central J. 12, 82. doi:10.1186/s13065-018-0445-y
Li, Y., Kong, D., Fu, Y., Sussman, M. R., and Wu, H. (2020). The effect of developmental and environmental factors on secondary metabolites in medicinal plants. Plant Physiology Biochem. 148, 80–89. doi:10.1016/j.plaphy.2020.01.006
Li, Y., Wang, W., and Lim, H.-Y. (2021c). Drosophila solute carrier 5A5 regulates systemic glucose homeostasis by mediating glucose absorption in the midgut. Int. J. Mol. Sci. 22, 12424. doi:10.3390/ijms222212424
Lian, F., Li, G., Chen, X., Wang, X., Piao, C., Wang, J., et al. (2014). Chinese herbal medicine tianqi reduces progression from impaired glucose tolerance to diabetes: a double-blind, randomized, placebo-controlled, multicenter trial. J. Clin. Endocrinol. Metabolism 99, 648–655. doi:10.1210/jc.2013-3276
Liang, J. H., Lin, H. R., Yang, C. S., Liaw, C. C., Wang, I. C., and Chen, J. J. (2022). Bioactive components from ampelopsis japonica with antioxidant, anti-α-glucosidase, and antiacetylcholinesterase activities. Antioxidants (Basel) 11, 1228. doi:10.3390/antiox11071228
Lim, W. X. J., Gammon, C. S., von Hurst, P., Chepulis, L., and Page, R. A. (2021). A narrative review of human clinical trials on the impact of phenolic-rich plant extracts on prediabetes and its subgroups. Nutrients 13, 3733. doi:10.3390/nu13113733
Lin, A. H., Nichols, B. L., Quezada-Calvillo, R., Rose, D. R., Sim, L., and Hamaker, B. R. (2010). Specific starch digestion of maize alpha-limit dextrins by recombinant mucosal glucosidase enzymes. FASEB J. 24, 231–236. doi:10.1096/fasebj.24.1_supplement.231.6
Lin, A. H. M., Nichols, B. L., Quezada-Calvillo, R., Avery, S. E., Sim, L., Rose, D. R., et al. (2012). Unexpected high digestion rate of cooked starch by the ct-maltase-glucoamylase small intestine mucosal α-glucosidase subunit. PLOS ONE 7, e35473. doi:10.1371/journal.pone.0035473
Luepke, N. P., and Kemper, F. H. (1986). The HET-CAM test: an alternative to the draize eye test. Food Chem. Toxicol. 24, 495–496. doi:10.1016/0278-6915(86)90099-2
Lüersen, K., Fischer, A., Bauer, I., Huebbe, P., Uekaji, Y., Chikamoto, K., et al. (2023). Soy extract, rich in hydroxylated isoflavones, exhibits antidiabetic properties in vitro and in Drosophila melanogaster in vivo. Nutrients 15, 1392. doi:10.3390/nu15061392
Lüersen, K., Jöckel, T., Chin, D., Demetrowitsch, T., Schwarz, K., and Rimbach, G. (2024). Reduced iron and cobalt levels in response to curcumin supplementation are not responsible for the prolonged larval development and do not affect the oxidative stress tolerance and polyamine status of D. melanogaster. BioFactors 50, 161–180. doi:10.1002/biof.2000
Lüersen, K., Roeder, T., and Rimbach, G. (2019). Drosophila melanogaster in nutrition research - the importance of standardizing experimental diets. Genes. & Nutr. 14, 3. doi:10.1186/s12263-019-0627-9
Mahmood, R., Kayani, W. K., Ahmed, T., Malik, F., Hussain, S., Ashfaq, M., et al. (2020). Assessment of antidiabetic potential and phytochemical profiling of Rhazya stricta root extracts. BMC Complement. Med. Ther. 20, 293. doi:10.1186/s12906-020-03035-x
Mahraoui, L., Rodolosse, A., Barbat, A., Dussaulx, E., Zweibaum, A., Rousset, M., et al. (1994). Presence and differential expression of SGLT1, GLUT1, GLUT2, GLUT3 and GLUT5 hexose-transporter mRNAs in Caco-2 cell clones in relation to cell growth and glucose consumption. Biochem. J. 298 (Pt 3), 629–633. doi:10.1042/bj2980629
Mamun-or-Rashid, A. N. M., Hossain, M. S., Hassan, N., Dash, B. K., Sapon, M. A., and Sen, M. K. (2014). A review on medicinal plants with antidiabetic activity. J. Pharmacogn. Phytochem. 3, 149–159.
Manzano, M., Giron, M. D., Vilchez, J. D., Sevillano, N., El-Azem, N., Rueda, R., et al. (2016). Apple polyphenol extract improves insulin sensitivity in vitro and in vivo in animal models of insulin resistance. Nutr. Metabolism 13, 32. doi:10.1186/s12986-016-0088-8
Margolskee, R. F., Dyer, J., Kokrashvili, Z., Salmon, K. S. H., Ilegems, E., Daly, K., et al. (2007). T1R3 and gustducin in gut sense sugars to regulate expression of Na+-glucose cotransporter 1. Proc. Natl. Acad. Sci. U. S. A. 104, 15075–15080. doi:10.1073/pnas.0706678104
Marinetti, A. (2018). The Institut de Chimie des Substances Naturelles (ICSN): Past and Present. Eur. J. Org. Chem. 2018, 5774–5776. doi:10.1002/ejoc.201801558
Mayendraraj, A., Rosenkilde, M. M., and Gasbjerg, L. S. (2022). GLP-1 and GIP receptor signaling in beta cells – a review of receptor interactions and co-stimulation. Peptides 151, 170749. doi:10.1016/j.peptides.2022.170749
McCloud, T. G. (2010). High throughput extraction of plant, marine and fungal specimens for preservation of biologically active molecules. Molecules 15, 4526–4563. doi:10.3390/molecules15074526
McCormick, L. M., Heck, P. M., Ring, L. S., Kydd, A. C., Clarke, S. J., Hoole, S. P., et al. (2015). Glucagon-like peptide-1 protects against ischemic left ventricular dysfunction during hyperglycemia in patients with coronary artery disease and type 2 diabetes mellitus. Cardiovasc Diabetol. 14, 102. doi:10.1186/s12933-015-0259-3
McIntyre, N., Holdsworth, C. D., and Turner, D. S. (1964). New interpretation of oral glucose tolerance. Lancet 2, 20–21. doi:10.1016/s0140-6736(64)90011-x
Medjiofack Djeujo, F., Cusinato, F., Ragazzi, E., and Froldi, G. (2021). α-Glucosidase and advanced glycation end products inhibition with Vernonia amygdalina root and leaf extracts: new data supporting the antidiabetic properties. J. Pharm. Pharmacol. 73, 1240–1249. doi:10.1093/jpp/rgab057
Meier, J. J. (2012). GLP-1 receptor agonists for individualized treatment of type 2 diabetes mellitus. Nat. Rev. Endocrinol. 8, 728–742. doi:10.1038/nrendo.2012.140
Mentlein, R., Gallwitz, B., and Schmidt, W. E. (1993). Dipeptidyl-peptidase IV hydrolyses gastric inhibitory polypeptide, glucagon-like peptide-1(7-36)amide, peptide histidine methionine and is responsible for their degradation in human serum. Eur. J. Biochem. 214, 829–835. doi:10.1111/j.1432-1033.1993.tb17986.x
Merz, K. E., and Thurmond, D. C. (2020). Role of skeletal muscle in insulin resistance and glucose uptake. Compr. Physiol. 10, 785–809. doi:10.1002/cphy.c190029
Mîinea, C. P., Sano, H., Kane, S., Sano, E., Fukuda, M., Peränen, J., et al. (2005). AS160, the Akt substrate regulating GLUT4 translocation, has a functional Rab GTPase-activating protein domain. Biochem. J. 391, 87–93. doi:10.1042/BJ20050887
Miller, N., and Joubert, E. (2022). Critical assessment of in vitro screening of α-glucosidase inhibitors from plants with acarbose as a reference standard. Planta Med. 88, 1078–1091. doi:10.1055/a-1557-7379
Mojsov, S., Weir, G. C., and Habener, J. F. (1987). Insulinotropin: glucagon-like peptide I (7-37) co-encoded in the glucagon gene is a potent stimulator of insulin release in the perfused rat pancreas. J. Clin. Investig. 79, 616–619. doi:10.1172/JCI112855
Morris, S. N. S., Coogan, C., Chamseddin, K., Fernandez-Kim, S. O., Kolli, S., Keller, J. N., et al. (2012). Development of diet-induced insulin resistance in adult Drosophila melanogaster. Biochim. Biophys. Acta 1822, 1230–1237. doi:10.1016/j.bbadis.2012.04.012
Mueckler, M., and Thorens, B. (2013). The SLC2 (GLUT) family of membrane transporters. Mol. Asp. Med. 34, 121–138. doi:10.1016/j.mam.2012.07.001
Müller, U., Stübl, F., Schwarzinger, B., Sandner, G., Iken, M., Himmelsbach, M., et al. (2018). In vitro and in vivo inhibition of intestinal glucose transport by guava (psidium guajava) extracts. Mol. Nutr. Food Res. 62, e1701012. doi:10.1002/mnfr.201701012
Muniyappa, R., Lee, S., Chen, H., and Quon, M. J. (2008). Current approaches for assessing insulin sensitivity and resistance in vivo: advantages, limitations, and appropriate usage. Am. J. Physiology. Endocrinol. Metabolism 294, E15–E26. doi:10.1152/ajpendo.00645.2007
Murillo-Maldonado, J. M., and Riesgo-Escovar, J. R. (2017). Development and diabetes on the fly. Mech. Dev. 144, 150–155. doi:10.1016/j.mod.2016.09.004
Musselman, L. P., Fink, J. L., Narzinski, K., Ramachandran, P. V., Hathiramani, S. S., Cagan, R. L., et al. (2011). A high-sugar diet produces obesity and insulin resistance in wild-type Drosophila. Dis. Models Mech. 4, 842–849. doi:10.1242/dmm.007948
Na, J., Musselman, L. P., Pendse, J., Baranski, T. J., Bodmer, R., Ocorr, K., et al. (2013). A Drosophila model of high sugar diet-induced cardiomyopathy. PLoS Genet. 9, e1003175. doi:10.1371/journal.pgen.1003175
Na, J., Sweetwyne, M. T., Park, A. S. D., Susztak, K., and Cagan, R. L. (2015). Diet-induced podocyte dysfunction in Drosophila and mammals. Cell. Rep. 12, 636–647. doi:10.1016/j.celrep.2015.06.056
Nakitto, A. M. S., Rudloff, S., Borsch, C., and Wagner, A. E. (2021). Solanum anguivi Lam. fruit preparations counteract the negative effects of a high-sugar diet on the glucose metabolism in Drosophila melanogaster. Food Funct. 12, 9238–9247. doi:10.1039/D1FO01363G
Nandi, S., and Saxena, M. (2020). Potential inhibitors of protein tyrosine phosphatase (PTP1B) enzyme: promising target for type-II diabetes mellitus. Curr. Top. Med. Chem. 20, 2692–2707. doi:10.2174/1568026620999200904121432
Neubauer, N., and Kulkarni, R. N. (2006). Molecular approaches to study control of glucose homeostasis. ILAR J. 47, 199–211. doi:10.1093/ilar.47.3.199
Niu, C. S., Chen, L. J., and Niu, H. S. (2014). Antihyperglycemic action of rhodiola-aqeous extract in type1-like diabetic rats. BMC Complement. Altern. Med. 14, 20. doi:10.1186/1472-6882-14-20
Noda, M., Takahashi, H., Tanabe, T., Toyosato, M., Furutani, Y., Hirose, T., et al. (1982). Primary structure of alpha-subunit precursor of Torpedo californica acetylcholine receptor deduced from cDNA sequence. Nature 299, 793–797. doi:10.1038/299793a0
Novotna, B., Polesny, Z., Pinto-Basto, M. F., Van Damme, P., Pudil, P., Mazancova, J., et al. (2020). Medicinal plants used by ‘root doctors’, local traditional healers in Bié province, Angola. J. Ethnopharmacol. 260, 112662. doi:10.1016/j.jep.2020.112662
Nowak-Sliwinska, P., Segura, T., and Iruela-Arispe, M. L. (2014). The chicken chorioallantoic membrane model in biology, medicine and bioengineering. Angiogenesis 17, 779–804. doi:10.1007/s10456-014-9440-7
Oguma, T., Nakayama, K., Kuriyama, C., Matsushita, Y., Yoshida, K., Hikida, K., et al. (2015). Intestinal sodium glucose cotransporter 1 inhibition enhances glucagon-like peptide-1 secretion in normal and diabetic rodents. J. Pharmacol. Exp. Ther. 354, 279–289. doi:10.1124/jpet.115.225508
Ollinger, N., Neuhauser, C., Schwarzinger, B., Wallner, M., Schwarzinger, C., Blank-Landeshammer, B., et al. (2022). Anti-hyperglycemic effects of oils and extracts derived from sea buckthorn – a comprehensive analysis utilizing in vitro and in vivo models. Mol. Nutr. Food Res. 66, 2101133. doi:10.1002/mnfr.202101133
Olufolabo, K. O., Lüersen, K., Oguntimehin, S. A., Nchiozem-Ngnitedem, V.-A., Agbebi, E. A., Faloye, K. O., et al. (2024). In vitro and in silico studies reveal antidiabetic properties of arylbenzofurans from the root bark of Morus mesozygia Stapf. Front. Pharmacol. 15, 1338333. doi:10.3389/fphar.2024.1338333
Omoboyowa, D. A., Agoi, M. D., Shodehinde, S. A., Saibu, O. A., and Saliu, J. A. (2023). Antidiabetes study of Spondias mombin (Linn) stem bark fractions in high-sucrose diet-induced diabetes in Drosophila melanogaster. J. Taibah Univ. Med. Sci. 18, 663–675. doi:10.1016/j.jtumed.2023.01.011
Onur, S., Stöckmann, H., Zenthoefer, M., Piker, L., and Döring, F. (2013). The plant extract collection Kiel in schleswig-holstein (PECKISH) is an open access screening library. J. Food Res. 2, 101–106. doi:10.5539/jfr.v2n4p101
Ooi, D. J., Azmi, N. H., Imam, M. U., Alitheen, N. B., and Ismail, M. (2018). Curculigoside and polyphenol-rich ethyl acetate fraction of Molineria latifolia rhizome improved glucose uptake via potential mTOR/AKT activated GLUT4 translocation. J. Food Drug Analysis 26, 1253–1264. doi:10.1016/j.jfda.2018.03.003
Oranje, P., Gouka, R., Burggraaff, L., Vermeer, M., Chalet, C., Duchateau, G., et al. (2019). Novel natural and synthetic inhibitors of solute carriers SGLT1 and SGLT2. Pharmacol. Res. Perspect. 7, e00504. doi:10.1002/prp2.504
Orhan, N., Gökbulut, A., and Deliorman Orhan, D. (2021). In vitro enzyme inhibitory properties, antioxidant activities, and phytochemical profiles of Moltkia aurea and Moltkia coerulea. Turk J. Pharm. Sci. 18, 204–212. doi:10.4274/tjps.galenos.2020.12258
Orumwense, G. E., Osagie, A. M., Omage, S. O., Omage, K., and Azeke, M. A. (2022). Synclisia scabrida protects against oxidative stress, hepatotoxicity and hyperglycaemia in alloxan-induced diabetic rats. J. Diabetes Metab. Disord. 21, 669–680. doi:10.1007/s40200-022-01029-9
Pallauf, K., Chin, D., Günther, I., Birringer, M., Lüersen, K., Schultheiß, G., et al. (2019). Resveratrol, lunularin and dihydroresveratrol do not act as caloric restriction mimetics when administered intraperitoneally in mice. Sci. Rep. 9, 4445. doi:10.1038/s41598-019-41050-2
Panigrahy, S. K., Kumar, A., and Bhatt, R. (2020). Hedychium coronarium rhizomes: promising antidiabetic and natural inhibitor of α-amylase and α-glucosidase. J. Diet. Suppl. 17, 81–87. doi:10.1080/19390211.2018.1483462
Park, S., Alfa, R. W., Topper, S. M., Kim, G. E. S., Kockel, L., and Kim, S. K. (2014). A genetic strategy to measure circulating Drosophila insulin reveals genes regulating insulin production and secretion. PLoS Genet. 10, e1004555. doi:10.1371/journal.pgen.1004555
Pasmans, K., Meex, R. C. R., van Loon, L. J. C., and Blaak, E. E. (2022). Nutritional strategies to attenuate postprandial glycemic response. Obes. Rev. 23, e13486. doi:10.1111/obr.13486
Pelegrin, S., Galtier, F., Chalançon, A., Gagnol, J. P., Barbanel, A. M., Pélissier, Y., et al. (2019). Effects of Nigella sativa seeds (black cumin) on insulin secretion and lipid profile: a pilot study in healthy volunteers. Br. J. Clin. Pharmacol. 85, 1607–1611. doi:10.1111/bcp.13922
Pérez-Nájera, V. C., Gutiérrez-Uribe, J. A., Antunes-Ricardo, M., Hidalgo-Figueroa, S., Del-Toro-Sánchez, C. L., Salazar-Olivo, L. A., et al. (2018). Smilax aristolochiifolia root extract and its compounds chlorogenic acid and astilbin inhibit the activity of α-amylase and α-glucosidase enzymes. Evid. Based Complement. Altern. Med. 2018, 6247306. doi:10.1155/2018/6247306
Perry, T., Haughey, N. J., Mattson, M. P., Egan, J. M., and Greig, N. H. (2002). Protection and reversal of excitotoxic neuronal damage by glucagon-like peptide-1 and exendin-4. J. Pharmacol. Exp. Ther. 302, 881–888. doi:10.1124/jpet.102.037481
Petersen, M. C., and Shulman, G. I. (2018). Mechanisms of insulin action and insulin resistance. Physiol. Rev. 98, 2133–2223. doi:10.1152/physrev.00063.2017
Peterson, R. G., Shaw, W. N., Neel, M.-A., Little, L. A., and Eichberg, J. (1990). Zucker diabetic fatty rat as a model for non-insulin-dependent diabetes mellitus. ILAR J. 32, 16–19. doi:10.1093/ilar.32.3.16
PhytoPharmacon (2023). Natural product library. Available at: https://phytopharmacon.com/natural-product-library.html (Accessed February 4, 2023).
Potterat, O., and Hamburger, M. (2014). Combined use of extract libraries and HPLC-based activity profiling for lead discovery: potential, challenges, and practical considerations. Planta Med. 80, 1171–1181. doi:10.1055/s-0034-1382900
Powell, D. R., DaCosta, C. M., Gay, J., Ding, Z.-M., Smith, M., Greer, J., et al. (2013). Improved glycemic control in mice lacking Sglt1 and Sglt2. Am. J. Physiol. Endocrinol. Metab. 304, E117–E130. doi:10.1152/ajpendo.00439.2012
Proença, C., Freitas, M., Ribeiro, D., Tomé, S. M., Oliveira, E. F. T., Viegas, M. F., et al. (2019). Evaluation of a flavonoids library for inhibition of pancreatic α-amylase towards a structure–activity relationship. J. Enzyme Inhibition Med. Chem. 34, 577–588. doi:10.1080/14756366.2018.1558221
Przeor, M. (2022). Some common medicinal plants with antidiabetic activity, known and available in europe (A mini-review). Pharmaceuticals 15, 65. doi:10.3390/ph15010065
Pujimulyani, D., Yulianto, W. A., Setyowati, A., Arumwardana, S., Sari Widya Kusuma, H., Adhani Sholihah, I., et al. (2020). Hypoglycemic activity of curcuma mangga val. Extract via modulation of GLUT4 and PPAR-γ mRNA expression in 3T3-L1 adipocytes. J. Exp. Pharmacol. 12, 363–369. doi:10.2147/JEP.S267912
Purintrapiban, J., Suttajit, M., and Forsberg, N. E. (2006). Differential activation of glucose transport in cultured muscle cells by polyphenolic compounds from Canna indica L. Root. Biol. Pharm. Bull. 29, 1995–1998. doi:10.1248/bpb.29.1995
Pyörälä, M., Miettinen, H., Laakso, M., and Pyörälä, K. (1998). Hyperinsulinemia predicts coronary heart disease risk in healthy middle-aged men: the 22-year follow-up results of the Helsinki Policemen Study. Circulation 98, 398–404. doi:10.1161/01.cir.98.5.398
Ramappa, V. K., Srivastava, D., Singh, P., Kumar, U., and Singh, V. (2020). Mulberry 1-deoxynojirimycin (DNJ): an exemplary compound for therapeutics. J. Hortic. Sci. Biotechnol. 95, 679–686. doi:10.1080/14620316.2020.1760738
Ramracheya, R., Chapman, C., Chibalina, M., Dou, H., Miranda, C., González, A., et al. (2018). GLP-1 suppresses glucagon secretion in human pancreatic alpha-cells by inhibition of P/Q-type Ca2+ channels. Physiol. Rep. 6, e13852. doi:10.14814/phy2.13852
Rani, M. P., Krishna, M. S., Padmakumari, K. P., Raghu, K. G., and Sundaresan, A. (2012). Zingiber officinale extract exhibits antidiabetic potential via modulating glucose uptake, protein glycation and inhibiting adipocyte differentiation: an in vitro study. J. Sci. Food Agric. 92, 1948–1955. doi:10.1002/jsfa.5567
Razavi, M., Wei, Y.-Y., Rao, X.-Q., and Zhong, J.-X. (2022). DPP-4 inhibitors and GLP-1RAs: cardiovascular safety and benefits. Mil. Med. Res. 9, 45. doi:10.1186/s40779-022-00410-2
Read, P. A., Khan, F. Z., and Dutka, D. P. (2012). Cardioprotection against ischaemia induced by dobutamine stress using glucagon-like peptide-1 in patients with coronary artery disease. Heart 98, 408–413. doi:10.1136/hrt.2010.219345
Rees, D. A., and Alcolado, J. C. (2005). Animal models of diabetes mellitus. Diabet. Med. 22, 359–370. doi:10.1111/j.1464-5491.2005.01499.x
Rehfeld, J. F. (2018). The origin and understanding of the incretin concept. Front. Endocrinol. (Lausanne) 9, 387. doi:10.3389/fendo.2018.00387
Research Group Bioinformatics Hagenberg (2021). Spotty. Available at: https://bioinformatics.fh-hagenberg.at/site/fileadmin/user_upload/img_upload/projects/spotty.html (Accessed September 7, 2021).
Rijkelijkhuizen, J. M., Girman, C. J., Mari, A., Alssema, M., Rhodes, T., Nijpels, G., et al. (2009). Classical and model-based estimates of beta-cell function during a mixed meal vs. an OGTT in a population-based cohort. Diabetes Res. Clin. Pract. 83, 280–288. doi:10.1016/j.diabres.2008.11.017
Rosak, C., and Mertes, G. (2012). Critical evaluation of the role of acarbose in the treatment of diabetes: patient considerations. Diabetes Metab. Syndr. Obes. 5, 357–367. doi:10.2147/DMSO.S28340
Rubio-Cabezas, O., Flanagan, S. E., Damhuis, A., Hattersley, A. T., and Ellard, S. (2012). KATP channel mutations in infants with permanent diabetes diagnosed after 6 months of life. Pediatr. Diabetes 13, 322–325. doi:10.1111/j.1399-5448.2011.00824.x
Sabir, S. M., Zeb, A., Mahmood, M., Abbas, S. R., Ahmad, Z., and Iqbal, N. (2021). Phytochemical analysis and biological activities of ethanolic extract of Curcuma longa rhizome. Braz J. Biol. 81, 737–740. doi:10.1590/1519-6984.230628
Saleem, H., Khurshid, U., Sarfraz, M., Ahmad, I., Alamri, A., Anwar, S., et al. (2021). Investigation into the biological properties, secondary metabolites composition, and toxicity of aerial and root parts of Capparis spinosa L.: an important medicinal food plant. Food Chem. Toxicol. 155, 112404. doi:10.1016/j.fct.2021.112404
Salehi, B., Ata, A., V. Anil Kumar, N., Sharopov, F., Ramírez-Alarcón, K., Ruiz-Ortega, A., et al. (2019). Antidiabetic potential of medicinal plants and their active components. Biomolecules 9, 551. doi:10.3390/biom9100551
Sales, P. M., Souza, P. M., Simeoni, L. A., and Silveira, D. (2012). α-Amylase inhibitors: a review of raw material and isolated compounds from plant source. J. Pharm. Pharm. Sci. 15, 141–183. doi:10.18433/j35s3k
Sandner, G., König, A., Wallner, M., and Weghuber, J. (2022). Alternative model organisms for toxicological fingerprinting of relevant parameters in food and nutrition. Crit. Rev. Food Sci. Nutr. 62, 5965–5982. doi:10.1080/10408398.2021.1895060
Saraiva, F. K., and Sposito, A. C. (2014). Cardiovascular effects of Glucagon-like peptide 1 (GLP-1) receptor agonists. Cardiovasc Diabetol. 13, 142. doi:10.1186/s12933-014-0142-7
Sarian, M. N., Ahmed, Q. U., Mat So’ad, S. Z., Alhassan, A. M., Murugesu, S., Perumal, V., et al. (2017). Antioxidant and antidiabetic effects of flavonoids: a structure-activity relationship based study. Biomed. Res. Int. 2017, 8386065. doi:10.1155/2017/8386065
Satoh, T. (2014). Molecular mechanisms for the regulation of insulin-stimulated glucose uptake by small guanosine triphosphatases in skeletal muscle and adipocytes. Int. J. Mol. Sci. 15, 18677–18692. doi:10.3390/ijms151018677
Scheen, A. J. (2015). Pharmacodynamics, efficacy and safety of sodium-glucose co-transporter type 2 (SGLT2) inhibitors for the treatment of type 2 diabetes mellitus. Drugs 75, 33–59. doi:10.1007/s40265-014-0337-y
Schloesser, A., Esatbeyoglu, T., Schultheiß, G., Vollert, H., Lüersen, K., Fischer, A., et al. (2017). Antidiabetic properties of an apple/kale extract in vitro, in situ, and in mice fed a western-type diet. J. Med. Food 20, 846–854. doi:10.1089/jmf.2017.0019
Senizza, B., Zhang, L., Rocchetti, G., Zengin, G., Ak, G., Yıldıztugay, E., et al. (2021). Metabolomic profiling and biological properties of six Limonium species: novel perspectives for nutraceutical purposes. Food Funct. 12, 3443–3454. doi:10.1039/D0FO02968H
Shen, X., He, S., Wang, J., Qian, X., Wang, H., Zhang, B., et al. (2022). Phenotype of higher post-load insulin response as a predictor of all-cause mortality and cardiovascular mortality in the Chinese non-diabetic population. Diabetol. Metab. Syndr. 14, 19. doi:10.1186/s13098-022-00786-0
Sola, D., Rossi, L., Schianca, G. P. C., Maffioli, P., Bigliocca, M., Mella, R., et al. (2015). Sulfonylureas and their use in clinical practice. Arch. Med. Sci. 11, 840–848. doi:10.5114/aoms.2015.53304
Song, P., Onishi, A., Koepsell, H., and Vallon, V. (2016). Sodium glucose cotransporter SGLT1 as a therapeutic target in diabetes mellitus. Expert Opin. Ther. Targets 20, 1109–1125. doi:10.1517/14728222.2016.1168808
Srinivasan, S., Sathish, G., Jayanthi, M., Muthukumaran, J., Muruganathan, U., and Ramachandran, V. (2014). Ameliorating effect of eugenol on hyperglycemia by attenuating the key enzymes of glucose metabolism in streptozotocin-induced diabetic rats. Mol. Cell. Biochem. 385, 159–168. doi:10.1007/s11010-013-1824-2
Srivastava, S., Koley, T., Singh, S., and Tripathi, Y. B. (2015). The tuber extract of pueraria tuberosa Linn. competitively inhibits DPP-IV activity in normoglycemic rats. Int. J. Pharm. Pharm. Sci. 7, 227–231.
Srivastava, S., Shree, P., Pandey, H., and Tripathi, Y. B. (2018). Incretin hormones receptor signaling plays the key role in antidiabetic potential of PTY-2 against STZ-induced pancreatitis. Biomed. Pharmacother. 97, 330–338. doi:10.1016/j.biopha.2017.10.071
Staats, S., Lüersen, K., Wagner, A., and Rimbach, G. (2018). Drosophila melanogaster as a versatile model organism in food and nutrition research. J. Agric. Food Chem. 66, 3737–3753. doi:10.1021/acs.jafc.7b05900
Stadlbauer, V., Haselgrübler, R., Lanzerstorfer, P., Plochberger, B., Borgmann, D., Jacak, J., et al. (2016). Biomolecular characterization of putative antidiabetic herbal extracts. PLOS ONE 11, e0148109. doi:10.1371/journal.pone.0148109
Stadlbauer, V., Lanzerstorfer, P., Neuhauser, C., Weber, F., Stübl, F., Weber, P., et al. (2020). Fluorescence microscopy-based quantitation of GLUT4 translocation: high throughput or high content? Int. J. Mol. Sci. 21, 7964. doi:10.3390/ijms21217964
Stadlbauer, V., Neuhauser, C., Aumiller, T., Stallinger, A., Iken, M., and Weghuber, J. (2021). Identification of insulin-mimetic plant extracts: from an in vitro high-content screen to blood glucose reduction in live animals. Molecules 26, 4346. doi:10.3390/molecules26144346
Steffansen, B., Pedersen, M. D. L., Laghmoch, A. M., and Nielsen, C. U. (2017). SGLT1-Mediated transport in caco-2 cells is highly dependent on cell bank origin. J. Pharm. Sci. 106, 2664–2670. doi:10.1016/j.xphs.2017.04.033
Steiling, W., Bracher, M., Courtellemont, P., and de Silva, O. (1999). The HET–CAM, a useful in vitro assay for assessing the eye irritation properties of cosmetic formulations and ingredients. Toxicol. Vitro 13, 375–384. doi:10.1016/S0887-2333(98)00091-5
Stokoe, D., Stephens, L. R., Copeland, T., Gaffney, P. R., Reese, C. B., Painter, G. F., et al. (1997). Dual role of phosphatidylinositol-3,4,5-trisphosphate in the activation of protein kinase B. Science 277, 567–570. doi:10.1126/science.277.5325.567
Suh, H. W., Lee, K. B., Kim, K. S., Yang, H. J., Choi, E.-K., Shin, M. H., et al. (2015). A bitter herbal medicine Gentiana scabra root extract stimulates glucagon-like peptide-1 secretion and regulates blood glucose in db/db mouse. J. Ethnopharmacol. 172, 219–226. doi:10.1016/j.jep.2015.06.042
Sulyman, A. O., Akolade, J. O., Sabiu, S. A., Aladodo, R. A., and Muritala, H. F. (2016). Antidiabetic potentials of ethanolic extract of Aristolochia ringens (Vahl.) roots. J. Ethnopharmacol. 182, 122–128. doi:10.1016/j.jep.2016.02.002
Sun, R., Deng, X., Zhang, D., Xie, F., Wang, D., Wang, J., et al. (2019). Anti-diabetic potential of Pueraria lobata root extract through promoting insulin signaling by PTP1B inhibition. Bioorg. Chem. 87, 12–15. doi:10.1016/j.bioorg.2019.02.046
Taderera, T., Chagonda, L. S., Gomo, E., Katerere, D., and Shai, L. J. (2019). Annona stenophylla aqueous extract stimulate glucose uptake in established C2Cl2 muscle cell lines. Afr. Health Sci. 19, 2219–2229. doi:10.4314/ahs.v19i2.47
Tam, C. S., Xie, W., Johnson, W. D., Cefalu, W. T., Redman, L. M., and Ravussin, E. (2012). Defining insulin resistance from hyperinsulinemic-euglycemic clamps. Diabetes Care 35, 1605–1610. doi:10.2337/dc11-2339
Tan, S.-X., Ng, Y., Burchfield, J. G., Ramm, G., Lambright, D. G., Stöckli, J., et al. (2012). The rab GTPase-activating protein tbc1d4/as160 contains an atypical phosphotyrosine-binding domain that interacts with plasma membrane phospholipids to facilitate GLUT4 trafficking in adipocytes. Mol. Cell. Biol. 32, 4946–4959. doi:10.1128/MCB.00761-12
Thomson, A., Smart, K., Somerville, M. S., Lauder, S. N., Appanna, G., Horwood, J., et al. (2019). The Ussing chamber system for measuring intestinal permeability in health and disease. BMC Gastroenterol. 19, 98. doi:10.1186/s12876-019-1002-4
Thorn, S. L., Gollob, M. H., Harper, M.-E., Beanlands, R. S., deKemp, R. A., and DaSilva, J. N. (2013). Chronic AMPK activity dysregulation produces myocardial insulin resistance in the human Arg302Gln-PRKAG2 glycogen storage disease mouse model. EJNMMI Res. 3, 48. doi:10.1186/2191-219X-3-48
Thornburg, C. C., Britt, J. R., Evans, J. R., Akee, R. K., Whitt, J. A., Trinh, S. K., et al. (2018). NCI program for natural product discovery: a publicly-accessible library of natural product fractions for high-throughput screening. ACS Chem. Biol. 13, 2484–2497. doi:10.1021/acschembio.8b00389
Tian, L., Cao, J., Zhao, T., Liu, Y., Khan, A., and Cheng, G. (2021). The bioavailability, extraction, biosynthesis and distribution of natural dihydrochalcone: phloridzin. Int. J. Mol. Sci. 22, 962. doi:10.3390/ijms22020962
Tian, L.-L., Bi, Y.-X., Wang, C., Zhu, K., Xu, D.-F., and Zhang, H. (2024). Bioassay-guided discovery and identification of new potent α-glucosidase inhibitors from Morus alba L. and the interaction mechanism. J. Ethnopharmacol. 322, 117645. doi:10.1016/j.jep.2023.117645
Tolessa, T., Gutniak, M., Holst, J. J., Efendic, S., and Hellström, P. M. (1998). Inhibitory effect of glucagon-like peptide-1 on small bowel motility. Fasting but not fed motility inhibited via nitric oxide independently of insulin and somatostatin. J. Clin. Investig. 102, 764–774. doi:10.1172/JCI942
Tsunoda, T., Samadi, A., Burade, S., and Mahmud, T. (2022). Complete biosynthetic pathway to the antidiabetic drug acarbose. Nat. Commun. 13, 3455. doi:10.1038/s41467-022-31232-4
Ugur, B., Chen, K., and Bellen, H. J. (2016). Drosophila tools and assays for the study of human diseases. Dis. Model. Mech. 9, 235–244. doi:10.1242/dmm.023762
Utami, A. R., Maksum, I. P., and Deawati, Y. (2023). Berberine and its study as an antidiabetic compound. Biol. (Basel) 12, 973. doi:10.3390/biology12070973
Uysal, S., Zengin, G., Sinan, K. I., Ak, G., Ceylan, R., Mahomoodally, M. F., et al. (2021). Chemical characterization, cytotoxic, antioxidant, antimicrobial, and enzyme inhibitory effects of different extracts from one sage (Salvia ceratophylla L.) from Turkey: open a new window on industrial purposes. RSC Adv. 11, 5295–5310. doi:10.1039/d0ra10044g
van Breemen, R. B., and Li, Y. (2005). Caco-2 cell permeability assays to measure drug absorption. Expert Opin. Drug Metabolism Toxicol. 1, 175–185. doi:10.1517/17425255.1.2.175
Vitetta, L., Butcher, B., Dal Forno, S., Vitetta, G., Nikov, T., Hall, S., et al. (2020). A double-blind randomized placebo-controlled study assessing the safety, tolerability and efficacy of a herbal medicine containing pycnogenol combined with papain and aloe vera in the prevention and management of pre-diabetes. Medicines 7, 22. doi:10.3390/medicines7040022
Watson, R. T., Shigematsu, S., Chiang, S.-H., Mora, S., Kanzaki, M., Macara, I. G., et al. (2001). Lipid raft microdomain compartmentalization of TC10 is required for insulin signaling and GLUT4 translocation. J. Cell. Biol. 154, 829–840. doi:10.1083/jcb.200102078
Wehmeier, U. F. (2003). The biosynthesis and metabolism of acarbose in Actinoplanes sp. se 50/110: a progress report. Biocatal. Biotransformation 21, 279–284. doi:10.1080/10242420310001614388
Wehmeier, U. F., and Piepersberg, W. (2004). Biotechnology and molecular biology of the alpha-glucosidase inhibitor acarbose. Appl. Microbiol. Biotechnol. 63, 613–625. doi:10.1007/s00253-003-1477-2
Whitcomb, D. C., and Lowe, M. E. (2007). Human pancreatic digestive enzymes. Dig. Dis. Sci. 52, 1–17. doi:10.1007/s10620-006-9589-z
Wilson, B. A. P., Thornburg, C. C., Henrich, C. J., Grkovic, T., and O’Keefe, B. R. (2020). Creating and screening natural product libraries. Nat. Prod. Rep. 37, 893–918. doi:10.1039/c9np00068b
Winzell, M. S., and Ahrén, B. (2004). The high-fat diet-fed mouse: a model for studying mechanisms and treatment of impaired glucose tolerance and type 2 diabetes. Diabetes 53 (Suppl. 3), S215–S219. doi:10.2337/diabetes.53.suppl_3.s215
Wood, I. S., and Trayhurn, P. (2003). Glucose transporters (GLUT and SGLT): expanded families of sugar transport proteins. Br. J. Nutr. 89, 3–9. doi:10.1079/BJN2002763
Wright, E. M., Loo, D. D. F., and Hirayama, B. A. (2011). Biology of human sodium glucose transporters. Physiol. Rev. 91, 733–794. doi:10.1152/physrev.00055.2009
Wu, T., Rayner, C. K., Jones, K. L., Xie, C., Marathe, C., and Horowitz, M. (2020). Role of intestinal glucose absorption in glucose tolerance. Curr. Opin. Pharmacol. 55, 116–124. doi:10.1016/j.coph.2020.10.017
Wüpper, S., Fischer, A., Luersen, K., Ipharraguerre, I. R., Chikamoto, K., Furune, T., et al. (2020). Effects of dietary gamma-cyclodextrin on voluntary activity and muscle strength in mice. J. Physiol. Pharmacol. 71. doi:10.26402/jpp.2020.3.08
Yang, H. S., Choi, Y. J., Jin, H. Y., Lee, S. C., and Huh, C. K. (2016). Effects of Allium hookeri root water extracts on inhibition of adipogenesis and GLUT-4 expression in 3T3-L1 adipocytes. Food Sci. Biotechnol. 25, 615–621. doi:10.1007/s10068-016-0086-7
Yang, S. E., Lin, Y. F., Liao, J. W., Chen, J. T., Chen, C. L., Chen, C. I., et al. (2022). Insulin sensitizer and antihyperlipidemic effects of Cajanus cajan (L.) millsp. root in methylglyoxal-induced diabetic rats. Chin. J. Physiology 65, 125–135. doi:10.4103/cjp.cjp_88_21
Yeste, J., Illa, X., Alvarez, M., and Villa, R. (2018). Engineering and monitoring cellular barrier models. J. Biol. Eng. 12, 18. doi:10.1186/s13036-018-0108-5
Yi, J.-K., Ryoo, Z.-Y., Ha, J.-J., Oh, D.-Y., Kim, M.-O., and Kim, S.-H. (2019). Beneficial effects of 6-shogaol on hyperglycemia, islet morphology and apoptosis in some tissues of streptozotocin-induced diabetic mice. Diabetol. Metab. Syndr. 11, 15. doi:10.1186/s13098-019-0407-0
Yin, L., Vijaygopal, P., MacGregor, G. G., Menon, R., Ranganathan, P., Prabhakaran, S., et al. (2014). Glucose stimulates calcium-activated chloride secretion in small intestinal cells. Am. J. Physiology. Cell. Physiology 306, C687–C696. doi:10.1152/ajpcell.00174.2013
Yoshiyama, Y., Sugiyama, T., and Kanke, M. (2005). Experimental diabetes model in chick embryos treated with streptozotocin. Biol. Pharm. Bull. 28, 1986–1988. doi:10.1248/bpb.28.1986
Yue, H., Wang, L., Jiang, S., Banma, C., Jia, W., Tao, Y., et al. (2022). Hypoglycemic effects of Rhodiola crenulata (HK. f. et. Thoms) H. Ohba in vitro and in vivo and its ingredient identification by UPLC-triple-TOF/MS. Food Funct. 13, 1659–1667. doi:10.1039/D1FO03436G
Zabidi, N. A., Ishak, N. A., Hamid, M., Ashari, S. E., and Mohammad Latif, M. A. (2021). Inhibitory evaluation of Curculigo latifolia on α-glucosidase, DPP (IV) and in vitro studies in antidiabetic with molecular docking relevance to type 2 diabetes mellitus. J. Enzyme Inhib. Med. Chem. 36, 109–121. doi:10.1080/14756366.2020.1844680
Zakowski, J. J., and Bruns, D. E. (1985). Biochemistry of human alpha amylase isoenzymes. Crit. Rev. Clin. Laboratory Sci. 21, 283–322. doi:10.3109/10408368509165786
Zambrowicz, B., Ding, Z.-M., Ogbaa, I., Frazier, K., Banks, P., Turnage, A., et al. (2013). Effects of LX4211, a dual SGLT1/SGLT2 inhibitor, plus sitagliptin on postprandial active GLP-1 and glycemic control in type 2 diabetes. Clin. Ther. 35, 273–285. doi:10.1016/j.clinthera.2013.01.010
Zelniker, T. A., Wiviott, S. D., Raz, I., Im, K., Goodrich, E. L., Bonaca, M. P., et al. (2019). SGLT2 inhibitors for primary and secondary prevention of cardiovascular and renal outcomes in type 2 diabetes: a systematic review and meta-analysis of cardiovascular outcome trials. Lancet 393, 31–39. doi:10.1016/S0140-6736(18)32590-X
Zhang, X., Kupczyk, E., Schmitt-Kopplin, P., and Mueller, C. (2022). Current and future approaches for in vitro hit discovery in diabetes mellitus. Drug Discov. Today 27, 103331. doi:10.1016/j.drudis.2022.07.016
Zhao, Q., Xie, H., Peng, Y., Wang, X., and Bai, L. (2017a). Improving acarbose production and eliminating the by-product component C with an efficient genetic manipulation system of Actinoplanes sp. SE50/110. Synth. Syst. Biotechnol. 2, 302–309. doi:10.1016/j.synbio.2017.11.005
Zhao, Y., Chen, M. X., Kongstad, K. T., Jäger, A. K., and Staerk, D. (2017b). Potential of Polygonum cuspidatum root as an antidiabetic food: dual high-resolution α-glucosidase and PTP1B inhibition profiling combined with HPLC-HRMS and NMR for identification of antidiabetic constituents. J. Agric. Food Chem. 65, 4421–4427. doi:10.1021/acs.jafc.7b01353
Zhao, Y., Kongstad, K. T., Jäger, A. K., Nielsen, J., and Staerk, D. (2018). Quadruple high-resolution α-glucosidase/α-amylase/PTP1B/radical scavenging profiling combined with high-performance liquid chromatography–high-resolution mass spectrometry–solid-phase extraction–nuclear magnetic resonance spectroscopy for identification of antidiabetic constituents in crude root bark of Morus alba L. J. Chromatogr. A 1556, 55–63. doi:10.1016/j.chroma.2018.04.041
Zheng, Y., Scow, J. S., Duenes, J. A., and Sarr, M. G. (2012). Mechanisms of glucose uptake in intestinal cell lines: role of GLUT2. Surgery 151, 13–25. doi:10.1016/j.surg.2011.07.010
Zuñiga, L. Y., Aceves-de la Mora, M. C. A., González-Ortiz, M., Ramos-Núñez, J. L., and Martínez-Abundis, E. (2018). Effect of chlorogenic acid administration on glycemic control, insulin secretion, and insulin sensitivity in patients with impaired glucose tolerance. J. Med. Food 21, 469–473. doi:10.1089/jmf.2017.0110
Keywords: carbohydrate-hydrolysing enzymes, ussing chamber, sodium-dependent glucose transporter 1, dipeptidyl-peptidase 4, glucose transporter 4, KATP channel, Drosophila melanogaster, hen’s egg test
Citation: Bauer I, Rimbach G, Cordeiro S, Bosy-Westphal A, Weghuber J, Ipharraguerre IR and Lüersen K (2024) A comprehensive in-vitro/in-vivo screening toolbox for the elucidation of glucose homeostasis modulating properties of plant extracts (from roots) and its bioactives. Front. Pharmacol. 15:1396292. doi: 10.3389/fphar.2024.1396292
Received: 05 March 2024; Accepted: 10 June 2024;
Published: 26 June 2024.
Edited by:
Adolfo Andrade-Cetto, National Autonomous University of Mexico, MexicoReviewed by:
Nidal Jaradat, An-Najah National University, PalestineCopyright © 2024 Bauer, Rimbach, Cordeiro, Bosy-Westphal, Weghuber, Ipharraguerre and Lüersen. This is an open-access article distributed under the terms of the Creative Commons Attribution License (CC BY). The use, distribution or reproduction in other forums is permitted, provided the original author(s) and the copyright owner(s) are credited and that the original publication in this journal is cited, in accordance with accepted academic practice. No use, distribution or reproduction is permitted which does not comply with these terms.
*Correspondence: Ilka Bauer, YmF1ZXJAZm9vZHNjaS51bmkta2llbC5kZQ==
Disclaimer: All claims expressed in this article are solely those of the authors and do not necessarily represent those of their affiliated organizations, or those of the publisher, the editors and the reviewers. Any product that may be evaluated in this article or claim that may be made by its manufacturer is not guaranteed or endorsed by the publisher.
Research integrity at Frontiers
Learn more about the work of our research integrity team to safeguard the quality of each article we publish.