- Liaoning Provincial Key Laboratory of Follicular Development and Reproductive Health, Jinzhou Medical University, Jinzhou, Liaoning, China
Group B streptococcal (GBS) is a Gram-positive bacterium that is commonly found in the gastrointestinal tract and urogenital tract. GBS infestation during pregnancy is a significant contributor to maternal and neonatal morbidity and mortality globally. This article aims to discuss the infectious diseases caused by GBS in the field of obstetrics and gynecology, as well as the challenges associated with the detection, treatment, and prevention of GBS.
1 Introduction of GBS
1.1 Microbiology
Group B streptococcal (GBS) is a Gram-positive, beta-hemolytic bacterium that appears as round or elliptical chains of cocci, usually in pairs or short chains, with a cell diameter of approximately 0.5–1.5 μm (Burcham et al., 2019). GBS is mainly classified based on its polysaccharide antigens, with at least ten different types of polysaccharide antigens identified (Tiruvayipati et al., 2021). The most common classification method is based on the capsular polysaccharide (CPS), which divides GBS into types Ia, Ib, II, III, IV, and others (Bianchi-Jassir et al., 2020). GBS is commonly found in the human digestive and reproductive tracts and typically ferments carbohydrates to produce lactic acid and carbon dioxide gas during the fermentation process (Goel et al., 2020). GBS requires a culture medium rich in blood components for growth and thrives in an acidic environment with a pH range of 5–6.5 (Bnfaga et al., 2023). GBS is sensitive to multiple antibiotics but may also exhibit some resistance (Koide et al., 2019). The genome size of GBS is approximately 2-3 Mbp. Its genome is a circular chromosome containing numerous coding and non-coding sequences. The structure and arrangement of the genome may vary among different strains. GBS exhibits genetic diversity, meaning that different strains may have distinct genome compositions and variations (Liu et al., 2023). The gene expression of GBS is influenced by complex regulatory networks, including transcription factors and other regulatory proteins, which help the bacterium adapt to and survive in different environments (Erickson Keesha et al., 2017). The GBS genome encodes many factors (Spencer et al., 2019) associated with pathogenicity, such as capsule polysaccharides, surface proteins, hemolysins, and enterotoxins. These factors play important roles in pathogenicity and the interaction with the host (Rajagopal, 2009).
Genomic analysis plays an important role in studying the genetic characteristics and pathogenic mechanisms of GBS (Schindler et al., 2023). Through sequencing technology (Preenanka and Safeena, 2023), the complete genome sequence of GBS can be obtained, which can then be used to study aspects such as genome structure, gene coding, and function. By comparing and analyzing the genome sequences of different strains, differences between different strains can be revealed, such as genome rearrangements and single nucleotide polymorphism (SNP) variations, and further research can be conducted on their relationship with pathogenicity. Transcriptome analysis techniques can be used to study changes in gene expression of GBS under different environmental conditions (Sitkiewicz et al., 2009), revealing its adaptability and biological characteristics. The pathogenic mechanisms of GBS include several aspects (Zadoks et al., 2011): the polysaccharide capsule of GBS is one of its main pathogenic factors. The capsule polysaccharide helps bacteria evade attacks from the host immune system and enhances their resistance to phagocytic cells, thereby increasing the chances of infection (Wang et al., 2022a). Surface proteins of GBS are also an important part of its pathogenic mechanisms (Xu et al., 2022). Surface proteins can bind to receptors on host cell surfaces, promoting bacterial adhesion and invasion. Some surface proteins also exhibit variability, making it more difficult for bacteria to be recognized and eliminated by the immune system. GBS produces hemolysins (Rosa-Fraile et al., 2014), which can destroy the membranes of host cells, leading to cell lysis and further promoting bacterial invasion and spread. GBS also causes inflammation through cell infiltration (Kuperwaser et al., 2023). It can stimulate host immune cells to release inflammatory mediators such as cytokines and chemokines, leading to tissue inflammation and damage. When infected with GBS, the host immune system produces specific antibodies and cellular immune responses. However, bacteria can interfere with host immune responses through various mechanisms, such as inhibiting cytokine production, evading phagocytosis by immune cells, and developing resistance, thereby enhancing their survival and reproduction. GBS is one of the main pathogens causing preterm birth and neonatal death (Le Gallou et al., 2023). GBS infections have certain epidemiological characteristics worldwide (Shabayek et al., 2018), influenced by factors such as geographic location, population demographics, and healthcare practices (Sidky and Thomas, 2002). The distribution and prevalence of GBS infections can differ significantly across various parts of the world, often due to environmental factors, climate, and the presence of specific GBS strains, which can affect local population susceptibility and the effectiveness of regional health strategies. Age distribution, genetic predispositions among certain populations, and socio-economic factors can influence the rate of GBS colonization and infection, leading to variations in disease incidence and outcomes among different demographic groups (Alizzi et al., 2022). The availability and implementation of screening and prevention measures, such as intrapartum antibiotic prophylaxis for GBS-positive pregnant women, greatly influence the incidence of neonatal GBS infections, with variations in healthcare quality and policies impacting overall disease management and outcomes (Schuchat, 1995). GBS is one of the main causes of preterm birth and neonatal death (Zhu and Lin, 2021). The main mode of transmission of GBS is vertical transmission (Mei et al., 2023), that is, transmission from an infected individual to a newborn or uninfected pregnant woman. Other modes of transmission include close contact transmission and healthcare-associated infections, but they are relatively rare. Under normal circumstances, the human immune system has a certain degree of protection against GBS (Korir et al., 2017). However, newborns and immunocompromised individuals are susceptible to infection. To prevent GBS infection, many countries and regions have implemented a series of preventive strategies, such as prenatal screening and prophylactic administration of antibiotics (Vieira et al., 2019).
The diagnostic methods of GBS are commonly used techniques in research and clinical practice. For the diagnosis of maternal infection, amniotic fluid samples can be cultured to detect the growth of GBS (Sayres et al., 2023). For screening of maternal infection, commonly used methods involve collecting vaginal and/or rectal samples for culture (Pierański et al., 2023). Screening before delivery is an important preventive strategy, especially for the diagnosis of neonatal infection, which can be detected through blood culture to determine the presence of GBS infection. Molecular biology techniques such as polymerase chain reaction (PCR) can detect the nucleic acid of GBS with high sensitivity and specificity (d'Otreppe et al., 2023). Understanding the susceptibility of GBS to antibiotics can guide the selection of clinical treatment (Husen et al., 2023). Commonly used antibiotic susceptibility testing methods include: disc diffusion method (Totadhri et al., 2022), where paper discs containing different antibiotics are placed on a culture medium to observe the relationship between bacterial growth and inhibition zones; broth dilution method (Stepanović et al., 2003), which gradually dilutes different concentrations of antibiotics in a culture medium to observe the minimum inhibitory concentration; E-test (Persson et al., 2008), which uses a gradient concentration of antibiotics on a strip to observe the relative position between bacterial growth and inhibition zones. These methods can be used to determine the susceptibility of GBS to a specific antibiotic, helping doctors choose appropriate drugs for treatment.
Preventing and controlling GBS infections is crucial for high-risk populations such as newborns and pregnant women. It is recommended to screen pregnant women for GBS colonization in the vagina and rectum, typically during late pregnancy (around 35–37 weeks). This can help detect the presence of GBS carriage and take appropriate preventive measures. For pregnant women who test positive for GBS carriage, it is advised to receive intravenous antibiotic prophylaxis during labor to reduce the risk of neonatal infection (Gurudas et al., 2022). Commonly used antibiotics include penicillin and ceftriaxone (Ali et al., 2022a), with specific antibiotic choices based on local treatment guidelines. If the mother is at risk of GBS infection, the newborn usually undergoes special observation and monitoring after birth. For high-risk newborns, antibiotic treatment may be needed to prevent infection. Necessary isolation and protective measures should be implemented in neonatal intensive care units or other settings prone to infection outbreaks to minimize the risk of pathogen transmission. Education and awareness campaigns about GBS infection should be conducted for healthcare workers, pregnant women, and families to enhance understanding and consciousness of prevention and control measures. Strengthening surveillance and reporting mechanisms, tracking the epidemiological characteristics of infection cases, and promptly implementing public health interventions are essential to reduce the spread and occurrence of GBS infections.
2 Virulence Factors
GBS commonly colonizes the human genital tract and is one of the major pathogens during the perinatal period (Armistead et al., 2019). It can cause infections in pregnant women and, in severe cases, even jeopardize the lives of newborns. GBS possesses multiple virulence factors that are associated with bacterial adhesion, immune evasion, and invasive damage. These virulence factors enable the bacteria to persist within the human body, increasing the likelihood of transmission and worsening the infection, thereby affecting patient prognosis. GBS virulence factors elucidated in this review are shown in Figure 1.
2.1 Adherence-associated virulence factors
Fibrinogen-binding proteins (Fbs) are crucial proteins found on GBS (Buscetta et al., 2014). Three types of Fbs proteins have been identified: FbsA, FbsB, and FbsC. These proteins adhere to human skin cells to facilitate the colonization of GBS in the vaginal area. FbsA promotes bacterial adhesion to mucosal surfaces and increases their sensitivity to phagocytosis. FbsB is involved in the formation of bacterial biofilms and facilitates the invasion of lung epithelial cells by interacting with fibrinogen. Conversely, the loss of FbsC significantly impairs the adhesion, invasion, and biofilm formation abilities of the bacteria. FbsC, a pivotal factor in the brain colonization process by GBS, is notably absent in the notably aggressive ST17 strains, which are a sequence type known for their heightened virulence and strong association with serious neonatal infections, such as meningitis (Kardos et al., 2019; Yao et al., 2020). These findings are of significant importance in understanding the adhesion, invasion, and colonization mechanisms of GBS (Liu et al., 2022).
Serine-rich repeat proteins (Srr), which are rich in serine and characterized by amino acid sequence variations, can be divided into two subtypes, Srr1 and Srr2, in GBS (Chan et al., 2020). These proteins not only mediate invasion of endothelial cells by the bacteria but also assist in bacterial adherence by locking onto docking mechanisms. The process referred to as “locking onto docking mechanisms” implies the precise attachment or binding of these proteins to specific structures or receptors on the surface of host cells. This binding can be likened to inserting a key into a lock, where the Srr proteins (the “key”) have a specific molecular structure that allows them to securely attach to certain cell surface receptors or structures (the “dock”). This interaction facilitates bacterial adherence and invasion into host cells, thereby aiding the infection process. Through this precise docking mechanism, Srr proteins help to solidify the initial contact between GBS and the cells, further facilitating bacterial invasion and colonization. This mechanism is crucial not only for the pathogen’s adherence phase but also plays a role in its subsequent penetration through cellular barriers and dissemination within the host. Understanding this mechanism is therefore of significant importance for developing new strategies to combat pathogens that employ such mechanisms for infection. Most strains of GBS express Srr1, which promotes better adherence to the vaginal epithelium through its binding to human fibrinogen. Additionally, Srr1 enhances stability by inhibiting proteolytic activities through glycosylation, thereby prolonging bacterial adhesion and persistence. The stability enhanced by Srr1 refers to the structural and functional stability of the Srr1 protein itself on the surface of GBS bacteria. This stability is crucial for the prolonged adhesion and persistence of the bacteria on host tissues, such as the vaginal epithelium. Glycosylation of Srr1, a biochemical process in which a carbohydrate is covalently attached to the protein, plays a key role in this context. This glycosylation process can protect Srr1 from being degraded by proteolytic enzymes present in the host environment. Proteolytic enzymes are capable of breaking down proteins into peptides or amino acids, which could potentially disrupt the adherence mechanism of the bacteria to host cells. Therefore, by inhibiting proteolytic activities through glycosylation, Srr1 maintains its integrity and functionality longer, promoting a more stable bacterial adherence to host tissues. On the other hand, Srr2, a homologue of Srr1, is associated with the highly virulent clonal complex CC17. It exhibits stronger binding to human fibrinogen than Srr1 and strains expressing Srr2 are more pathogenic compared to those lacking Srr2. While Srr1 is expressed more abundantly in GBS, it cannot bind to plasminogen and plasmin, whereas Srr2 effectively interacts with them to enhance adherence strength. The interaction between bacterial surface proteins and host proteins plays a crucial role in the virulence of pathogens. In the case of GBS, the glycoproteins Srr1 and Srr2 have been identified as key players in adherence strength. While Srr1 is the most dominant glycoprotein, it is unable to bind to plasminogen and plasmin (Liu et al., 2022). On the other hand, Srr2 effectively interacts with plasminogen and plasmin, enhancing adherence strength (Liu et al., 2022). This difference in binding capabilities between Srr1 and Srr2 highlights the importance of specific protein interactions in bacterial pathogenicity. In a similar context, Staphylococcus aureus has been shown to utilize adhesive virulence factors to resist host defenses. The staphylokinase (SAK) protein interacts with the serine protease domain of plasmin, enhancing resistance to digestion (Risser et al., 2022). This interaction with plasmin is crucial for the pathogen’s ability to evade host immune responses. Additionally, the molecular interactions of human plasminogen with fibronectin-binding proteins further emphasize the significance of protein-protein interactions in bacterial adherence and virulence (Risser et al., 2022). Overall, the ability of bacterial surface proteins to interact with host proteins such as plasminogen and plasmin is a key determinant of pathogenicity. While some proteins like Srr1 lack the ability to bind to these host proteins, others like Srr2 can effectively interact with them to enhance adherence strength. The structures of Srr1 and Srr2 are highly conserved in GBS, and vaccination with the corresponding “latch peptide” has been shown to provide serotype-independent protection against relevant infections in mice (Lin et al., 2017).
The laminin-binding protein (Lmb) (Spellerberg et al., 1999) in GBS, encoded by the lmb gene, facilitates adherence of the bacteria to extracellular matrix molecules in the human body and binds to the major component of the basement membrane, laminin. Lmb participates in the regulation of intracellular metal homeostasis by coordinating zinc ions with histidine residues to form a tetrahedral structure. This enables the control of zinc influx and efflux in bacterial cells, thereby prolonging survival in the human body and promoting pathogenicity. Bacteria lacking Lmb not only exhibit reduced invasiveness towards human brain microvascular endothelial cells and impaired neurotropism but also display decreased resistance to zinc ions. Lmb mediates the attachment of GBS to human laminin, facilitating bacterial colonization and invasion (Liu et al., 2022). The lmb gene encodes Lmb, which plays a crucial role in binding to laminin, a component of host cells, thereby increasing GBS’s pathogenic potential (Armistead et al., 2019). Additionally, Lmb promotes GBS adherence to host tissues, reflecting changes in GBS pathogenicity (Upadhyay et al., 2022). Studies have shown that Lmb, along with other virulence factors such as hypervirulent GBS adhesin (HvgA), contributes to the high pathogenicity of certain GBS strains (Shimizu et al., 2020; Kamińska et al., 2024). Furthermore, Lmb is identified as an immunogenic protein of GBS, interacting with host immune cells and potentially modulating host immune responses (Dobrut and Brzychczy-Włoch, 2022). The crystal structure of Lmb has been elucidated, providing insights into its function and potential as a target for therapeutic interventions (Ragunathan et al., 2013). Overall, the laminin-binding protein Lmb is a critical virulence factor in GBS pathogenicity, highlighting its importance in the colonization and invasion processes of this pathogen (Liu et al., 2019; Lacasse et al., 2022).
The immunogenic bacterial adhesin (BibA) (Santi et al., 2007) is a cell wall-anchored protein produced by GBS that promotes bacterial adherence to the surface of human cervical and lung epithelial cells. This protein can also interfere with the host’s antimicrobial defense mechanisms, such as phagocytosis by white blood cells, by regulating the interaction between the bacteria and complement C4-binding protein, thereby aiding the survival of GBS in the bloodstream. A report suggest that BibA is a strong and specific vaccine target. It demonstrated in a mouse model that a vaccine formulation containing BibA induced the production of protective antibodies against GBS, which could help prevent vaginal colonization and invasive infections caused by this bacterium (Dos Santos et al., 2020).
The hypervirulent GBS adhesin (HvgA) is a cell wall-anchored protein specific to the highly pathogenic clone CC17 of GBS (Li et al., 2019). It is closely associated with the development of late-onset diseases (LOD), such as neonatal meningitis (Pietrocola et al., 2018). Enhanced expression of HvgA facilitates bacterial adherence to intestinal epithelial cells, choroid plexus epithelial cells, and microvascular endothelial cells that constitute the blood-brain barrier (BBB). In a mouse experiment, HvgA-expressing GBS showed greater ability to colonize and penetrate the blood-brain barrier compared to strains lacking HvgA, leading to severe consequences. This suggests that GBS, under the mediation of HvgA, can breach the blood-brain barrier and cause central nervous system infections (Kekic et al., 2021).
The pili (PI) of GBS are considered essential structures for promoting bacterial colonization, biofilm formation, and central nervous system invasion (Danne and Dramsi, 2012). The genes encoding these pili are categorized into two types: Pili-1 (PI-1) and Pili-2 (PI-2). Among them, PI-2 is further divided into two subtypes, PI-2a and PI-2b. While the genes for pili may be present in varying degrees in the bacterial genome, a single strain of GBS may express only one type of pili. The GBS pili consist of three structural protein subunits: pili associated adhesin (PilA) at the tip, pili shaft backbone protein (PilB), and pili anchor (PilC) at the base. Research has shown that PilA enhances bacterial adherence to vaginal and cervical epithelial cells (Pezzicoli et al., 2008), while the biofilm synthesized by PilB is involved in bacterial invasion and resistance to phagocytosis (Maeda et al., 2021). One study has found that since almost all GBS strains possess pili, a vaccine containing conserved components of the pili island would provide high-level protection against the majority of GBS strains (Margarit et al., 2009).
2.2 Bacterial immune evasion related virulence factors
The capsular polysaccharide (CPS) of GBS aids in bacterial colonization and survival in the human body. CPS is an important virulence factor that mediates immune evasion. Its specificity is determined by the specific arrangement of sugars within each polysaccharide repeat unit. GBS can be classified into 10 CPS serotypes (Ia, Ib, II-IX). All 10 serotypes can cause disease, although the types and rates of disease vary among different serotypes (Lin E. et al., 2021). The distribution of CPS serotypes is influenced by factors such as geographic region and ethnicity (Wu et al., 2019). CPS not only resists phagocytosis by immune cells but also inhibits the activation of neutrophils and macrophages, thereby helping the bacteria evade the immune defenses of the host. It also promotes biofilm formation and interferes with complement defense, playing an important role in the infection process. GBS CPS contains α2,3-linked sialic acid residues (Sia), which effectively inhibit platelet-mediated killing of GBS, counteract antibacterial components produced by platelets, and can bind to the Siglec-9 receptor on the surface of platelets, thus inhibiting platelet activation (Armistead et al., 2019). CPS is an important target for vaccine development. Monovalent vaccines designed based on common CPS serotypes (Ia, Ib, II, III, and V) have entered phase I clinical trials (Baker et al., 1999; Baker et al., 2000; Baker et al., 2003; Baker et al., 2004). Trivalent vaccines targeting serotypes Ia, Ib, and III have shown high levels of specificity, safety, and tolerance in infants (Madhi et al., 2017; Swamy et al., 2020). A hexavalent vaccine containing serotypes Ia, Ib, II, III, IV, and V has been developed by BUURMAN et al. and it is the most comprehensive vaccine to date, including the largest number of serotypes (Buurman et al., 2019). Animal experimental results have shown that this hexavalent vaccine has a good immunogenicity and is expected to apply for clinical trials.
ALP family proteins are commonly expressed virulence factors in GBS that are also associated with immune evasion (Paoletti and Kasper, 2019; Lin L. et al., 2021). This family of proteins includes ALP-C, ALP-1, ALP-2, ALP-3, ALP-4, and Rib, encoded by the genes bca, alp1, alp2, alp3, alp4, and rib, respectively, and their amino acid sequences exhibit homology (Furfaro et al., 2018). A study has found that antibodies designed against ALP family proteins in mouse models attenuate infections caused by homologous GBS strains, indicating that loss of the repetitive gene sequences in this protein family is a mechanism by which bacteria interact with and evade the human immune system (Paoletti and Kasper, 2019). Beta-C protein, which is similar to ALP-C and encoded by the bac gene, can bind to IgA antibodies and inhibit complement-mediated phagocytosis. Immunizing pregnant mice with this protein immunogen protects newborn mice from invasive GBS infection, possibly by accelerating the phagocytosis of bacteria by white blood cells (Zastempowska et al., 2022). On the other hand, since over 90% of GBS strains express one or more proteins from this family, the ALP protein family is a highly specific vaccine target (Gabrielsen et al., 2017). Vaccines based on the highly immunogenic N-terminal domain of ALP-C and Rib (GBS-NN) have completed phase I clinical trials, resulting in over 30 times increase in GBS-specific antibodies in the sera of 240 female participants (Lin et al., 2018).
Streptococcal C5a peptidase from GBS is encoded by the scpB gene and is a serine protease. It can cleave the neutrophil chemoattractant C5a, thereby interrupting complement activation. It also functions as an allergenic toxin involved in the invasion of epithelial cells (Shabayek et al., 2018), inhibits neutrophil recruitment (Tulyaprawat et al., 2021), and aids in GBS binding to fibronectin, facilitating the invasion of human epithelial cells (Liu et al., 2022). Bone marrow-derived mast cells (BMMC) contain abundant factor XIIIA (FXIIIA), which has been recently demonstrated to crosslink fibrinogen through the contribution of scpB gene, increasing the capture probability of GBS within fibrin thrombi and assisting in host defense against GBS infection (Piliponsky et al., 2022). C5a peptidase is highly conserved and widely expressed in GBS. Researchers further evaluated the potential as a vaccine antigen by using a mouse model. They encapsulated C5a peptidase in microspheres and inoculated mice, finding that mice immunized with C5a peptidase encapsulated microspheres exhibited a high immune response against GBS, and the mortality rate was significantly reduced compared to mice not receiving C5a peptidase encapsulated microspheres (Santillan et al., 2008; Santillan et al., 2011).
2.3 Bacterial invasion associated virulence factors
GBS belonging to beta-hemolytic streptococci produces β-hemolysin encoded by the cyl E gene and the CAMP factor encoded by the cfb gene, which cause various tissue damage by lysing human cells (Liu et al., 2022). β-hemolysin itself possesses lytic properties, disrupting cell membrane structure and function, leading to cytolysis and cell death. Transcription of the cyl E gene and production of hemolysin are negatively regulated by the CovR/S two-component system, promoting the release of inflammatory factors by host cells to enhance bacterial damage to the host (Koo et al., 2019). The CAMP factor aggregates on the cell membrane surface, forming dispersed pores that induce cell lysis. A crucial phenotypic test used in clinical laboratories to identify GBS is the CAMP test, which is based on the synergy between the CAMP factor and β-hemolysin from Staphylococcus aureus, resulting in the lysis of blood cells and the formation of a characteristic arrowhead-shaped hemolytic zone (Liu et al., 2022).
Streptococcal fibronectin-binding protein A (SfbA) is highly conserved in GBS and facilitates the invasion of GBS into human vaginal and cervical cells, brain microvascular endothelial cells, and astrocytes, but it does not enhance GBS adhesion to host cells (Shabayek et al., 2018). Additionally, SfbA plays a crucial role in the interaction between GBS and the blood-brain barrier and in the pathogenesis of GBS meningitis. Immunization targeting SfbA can help prevent neonatal GBS meningitis infection (Mu et al., 2014). The fibronectin-binding protein encoded by the pavA gene is an extracellular surface protein of GBS that is involved in GBS colonization. This protein, along with SfbA, contributes to GBS colonization and establishment of the ecological niche in the vagina (Gendrin et al., 2018; Yoshida et al., 2021). The pathogenesis of streptococcal infections is a complex process involving various virulence factors and regulatory mechanisms. One such factor is the fibronectin-binding protein A gene, which plays a crucial role in the adherence of streptococci to host cells. A study has shown that disruption of genes encoding fibronectin-binding proteins can reduce bacterial adherence to human endothelial cells (Deng et al., 2019). Additionally, fibronectin-binding proteins have been implicated in promoting inflammation during the pathogenesis of meningitis caused by streptococci (Deng et al., 2019).
Hyaluronidase, an extracellular enzyme released by GBS, is encoded by the hylB gene. This enzyme degrades hyaluronic acid polymers, which are present in the extracellular matrix of human cells, into disaccharide units, disrupting cellular signaling and promoting the expression of inflammatory mediators. It has the capability to break down hyaluronic acid in the connective tissue matrix, disintegrate proteoglycans in connective tissues, and regulate the immune response during colonization and invasion by the bacteria, suppressing the production of reactive oxygen species (ROS) and resisting the action of neutrophils (Coleman et al., 2021; Kurian and Modi, 2022). Most importantly, hyaluronidase can breach the barrier between mother and fetus, allowing GBS to ascend from the vagina to the fetus, leading to fatal infections in the fetus (Liu et al., 2022). GBS virulence factors with their specific targets and mechanisms are shown in Table 1.
3 Antibiotic resistance in GBS
GBS poses considerable risks for both expectant mothers and their babies. In pregnant women, it can lead to serious infections such as sepsis, inflammation of the fetal membranes known as chorioamnionitis, and postpartum endometritis. Additionally, GBS can cause adverse pregnancy outcomes, including premature rupture of membranes, miscarriage, preterm delivery, and intrauterine growth restriction. Late-pregnancy colonization by GBS stands as a major threat for neonatal infection (Chattopadhyay et al., 2011), with approximately 1%–2% of newborns from GBS colonization-positive mothers contracting invasive infections (Yu et al., 2011). These newborns primarily suffer from sepsis and meningitis that are both aggressive and life-threatening. Consequently, such infections have high mortality and disability rates, jeopardizing the health and wellbeing of the affected neonates.
The implementation of intrapartum antibiotic prophylaxis (IAP) strategies has significantly reduced the incidence and adverse impacts of perinatal GBS infections in European and American countries (MMWR, 1997). However, the overuse of antibiotics in recent years has led to growing concerns about the emergence of antibiotic-resistant GBS strains on a global scale. Understanding the resistance patterns of GBS is of critical importance for guiding the rational use of antibiotics in clinical settings.
3.1 Penicillin resistance in GBS and its underlying mechanisms
Compared to the high resistance rates observed with erythromycin and clindamycin, numerous studies have confirmed that GBS retains high sensitivity towards penicillin, which remains the preferred drug for the prophylactic treatment of GBS infections (Verani et al., 2010). However, with the rising use of antibiotics, a change in sensitivity has been detected. Since 1994, reports of GBS strains with reduced penicillin susceptibility (PRGBS) have emerged sporadically. Since 2008, there has been evidence suggesting an increasing trend in the minimum inhibitory concentration (MIC) values of penicillin against GBS, indicating a tendency towards resistance (Kimura et al., 2008; Nagano et al., 2012). PRGBS resistant to beta-lactam antibiotics is an important emerging problem. Cases of PRGBS have been reported in regions such as Hong Kong (Chu et al., 2007), the United States (Dahesh et al., 2008), Canada (Longtin et al., 2011), and Japan (Kimura et al., 2008), with MIC values reaching from 0.25 to 1.00 mg/L. In Japan, studies have shown a high isolation rate of multidrug-resistant (MDR) group B streptococci with reduced penicillin susceptibility in Japan, indicating a growing issue with antibiotic resistance in this region (Ali et al., 2022b; Koide et al., 2022; Khan et al., 2023; Verma et al., 2023). Currently, the mechanisms underlying the reduced sensitivity of GBS to penicillin are not fully understood. Japanese researchers have attributed the decrease in penicillin susceptibility to mutations in the genes encoding penicillin-binding proteins (PBPs), specifically PBP1A, PBP2B, and PBP2X genes [101–103]. Notably, amino acid substitutions V405A and Q557E in PBP2X gene have been found to form unstable proteins, leading to a reduction and weakened affinity of the associated penicillin-binding proteins, which is a major mechanism of decreased penicillin sensitivity in GBS (Uruén et al., 2022). Moreover, multiple amino acid substitutions in PBPs 2X, 2B, and 1A have been discovered (Kimura et al., 2008; Nagano et al., 2012). Research in Canada on PRGBS identified amino acid substitutions in multiple PBPs but did not find the V405A and Q557E substitutions in PBP2X gene (V405A refers to a substitution where valine (V) at position 405 in the protein sequence is replaced by alanine (A), and Q557E refers to a substitution where glutamine (Q) at position 557 is replaced by glutamic acid (E)) (Longtin et al., 2011).
3.2 Resistance to erythromycin and clindamycin in GBS and its underlying mechanisms
Penicillin is the front-line treatment for both prevention and management of GBS infections. For those allergic to penicillin, clindamycin and erythromycin serve as the primary alternatives and are used by approximately 20% of GBS carriers. With the increasing use of these drugs, there has been a global rise in resistance to erythromycin and clindamycin (Savoia et al., 2008; Sadowy et al., 2010).
The resistance rate of GBS among pregnant women has been a growing concern in recent years. Studies from various regions have reported high rates of antimicrobial resistance in GBS isolates. Du et al. (Du et al., 2021) found that in Vietnamese pregnant women, the multidrug-resistance rate was 59.19%, with 8.46% of isolates resistant to six to seven antibiotics. Similarly, Bae et al. (Bae et al., 2022) reported a nationwide GBS colonization rate of 10.6% in pregnant Korean women. Furthermore, Du et al. (Van Du et al., 2021) highlighted the importance of considering the high rates of erythromycin, clindamycin, and multidrug resistance in GBS as a risk factor for neonates. This is supported by Hsu et al. (Hsu et al., 2023), who found that serotype Ib GBS strains had significantly higher rates of resistance to erythromycin and clindamycin compared to other serotypes. Moreover, Wang et al. (Wang et al., 2023) conducted a systematic review and meta-analysis in China, indicating a concerning emergence of penicillin resistance among GBS strains. This aligns with the findings of Verma et al. (Verma et al., 2023), who reported the highest resistance rate for penicillin among all tested antibiotics in GBS isolates of Indian origin.
GBS exhibits resistance to macrolide(M), clindamycin(L), and Streptogramin B(SB), together classified as the MLS group, encompassing three distinct yet functionally related types of antibiotics. There are three predominant mechanisms of GBS resistance to macrolide antibiotics:
M Phenotype Resistance: The resistance mechanism involves active efflux, where efflux pumps extrude the antibiotic out of the cell, leading to resistance. The efflux pump-related proteins are encoded by mef genes, which confer resistance to 14- and 15-membered ring macrolides but sensitivity to 16-membered macrolides, clindamycin, and Streptogramin B. This typically results in moderate-level resistance, with erythromycin MIC ranging from 1 to 32 mg/L. The mefA gene, one of two subtypes of the mef gene, is located on the Tn1207.1 transposon in pyogenic streptococci (Bacciaglia et al., 2007).
MLSB Phenotype Resistance: The mechanism involves an alteration in ribosomal target sites, primarily mediated by erm genes encoding ribosomal methylases that methylate a single adenine residue in 23SrRNA. This methylation reduces the affinity of the macrolide antibiotics to the ribosomal binding sites (Lopardo et al., 2005). erm gene-mediated macrolide resistance is generally of a high level, with erythromycin MIC values exceeding 256 mg/L, and cross-resistance occurs with clindamycin and Streptogramin B. The MLSB phenotype is divided into constitutive (cMLSB) and inducible (iMLSB) types. cMLSB occurs when erm genes are stably expressed, which results in resistance to erythromycin, clindamycin, and other MLS group members. iMLSB relates to scenarios where the erm genes require inducers to express resistance to clindamycin; erythromycin can act as such an inducer. Otherwise, clindamycin sensitivity might appear in vitro tests (Shen et al., 2005).
Both the M phenotype resistance and iMLSB resistance appear with erythromycin resistance but clindamycin sensitivity (Akdoğan Kittana et al., 2019). The presence of erythromycin ribosome methylase (erm) genes has been linked to the expression of inducible clindamycin resistance in Staphylococcus aureus (Heyar et al., 2020). However, the prevalence of iMLSB phenotype may vary depending on the study population, with lower rates observed in rural areas where antimicrobial exposure is limited (Heyar et al., 2020). In clinical settings, it is crucial to accurately identify clindamycin resistance, as studies have shown that a significant proportion of Staphylococcus aureus isolates can exhibit inducible clindamycin resistance, which may be misidentified as clindamycin susceptible using standard methods (Padekar et al., 2020). The cMLSB phenotype has been reported as the predominant form of resistance, followed by the iMLSB phenotype in some studies (Kumar Chaudhary and Piya, 2021). To differentiate these two phenotypes, the National Committee for Clinical Laboratory Standards (NCCLS) in the United States recommended the D-test in 2004. This involves placing a clindamycin disk (2 μg/disk) 20 mm away from an erythromycin disk (15 μg/disk), incubating at 35°C for 16–18 h. A “D” shape flattening or blunting of the inhibition zone adjacent to the erythromycin disc indicates a positive D-test, suggesting inducible clindamycin resistance (iMLSB type); otherwise, the test is negative (M type resistance) (Back et al., 2012). The D-test distinguishes iMLSB resistance and corrects clindamycin sensitivity results, aiding in rational pharmacotherapy.
L Phenotype Resistance: This resistance is due to adenylation. Enzymes encoded by the linB and lnu genes mediate the inactivation of lincosamide antibiotics (de Azavedo et al., 2001; Faccone et al., 2010; Seo et al., 2010). It is characterized by sensitivity to erythromycin and resistance to clindamycin. Studies such as by Lu et al. indicated that 4.5% of GBS strains are L phenotype resistant (Lu et al., 2014), with the prevalence of the linB gene being significantly lower than reported in Korea, suggesting geographical variation in L phenotype resistance and linB gene carriage. The linB gene, which is linked to clindamycin resistance, can lead to an L phenotype, conferring resistance to lincosamides only (Santana et al., 2020). The presence of antibiotic resistance genes, such as linB, in GBS strains highlights the importance of monitoring and understanding geographical variation in resistance patterns to inform treatment strategies and vaccine design (Santana et al., 2020; Barros, 2021; Mudzana et al., 2021). The inactivation of lincosamide antibiotics mediated by the lnu gene was first reported in Enterococcus faecium HM1025 (Bozdogan et al., 1999). L phenotype resistance regulated by the lnuB gene has been documented in various regions, including Latin America, Canada, Korea, and Spain (Arana et al., 2014).
3.3 Mechanisms of GBS resistance to telithromycin
A study from the United States between 2001 and 2004 indicated a 53.5% resistance rate to erythromycin in GBS, while the non-susceptibility rate for tetracycline was only 1.5% (DiPersio and DiPersio, 2006). Research in China has shown that among pregnant women colonized with GBS, non-susceptibility rates for erythromycin, clarithromycin, and azithromycin all exceeded 85.0%, while for tetracycline it was only 31.0%. This suggests a sensitivity to tetracycline despite resistance to other macrolides (Wang et al., 2015).
Telithromycin, the first ketolide and a 14-membered ring macrolide, demonstrates a strong affinity towards bacterial ribosomes, enabling it to counteract common macrolide antibiotic resistance mechanisms. These mechanisms include methyltransferase enzymatic activity encoded by the ermB gene, which results in the dimethylation of an adenine residue at the N-6 position on the 23SrRNA, and ribosomal protein variations that interfere with the binding of macrolides to bacteria. Telithromycin has been proven to exhibit greater antimicrobial activity against erythromycin-resistant strains, as confirmed in Streptococcus pneumoniae (Farrell and Felmingham, 2004; Takaya et al., 2010).
3.4 Resistance to fluoroquinolone antibiotics in GBS and the mechanisms involved
In 2003, Japan first reported the isolation of fluoroquinolone-resistant GBS strains, although the initial rate was low. Then several countries and regions have reported the emergence of GBS isolates resistant to fluoroquinolones (Ali et al., 2020; Hayes et al., 2020). In Taiwan the resistance rate to quinolones ranges between 0.3% and 5.0%, and all GBS strains resistant to levofloxacin also exhibited higher MIC values for ciprofloxacin, gatifloxacin, moxifloxacin, and gemifloxacin (Back et al., 2012). In 2014, Italy first reported the presence of levofloxacin-resistant GBS strains with a resistance rate of 1.4% (Piccinelli et al., 2015a), and another study in the same year reported a resistance rate of 3.4% (Piccinelli et al., 2015b). Mutations in the quinolone resistance-determining regions (QRDRs) of genes encoding the topoisomerase IV subunit C (ParC) and the DNA gyrase subunit A (GyrA) have been closely associated with GBS resistance to fluoroquinolone antibiotics (Wehbeh et al., 2005; Murayama et al., 2009). Double mutations in GyrA Ser-81 to Leu and in ParC Ser-79 to Phe or Tyr are associated with high-level resistance to levofloxacin. Additional mutations have been discovered in ParC, such as Asp-83 to Tyr and Asp-83 to Asn. Similar mutations have also been found in GyrB, but their significance is yet to be clarified (Piccinelli et al., 2015b). Clinical isolates of GBS resistant to levofloxacin have been reported to belong predominantly to clonal complex III/ST19. Wang et al. found that the resistance rate of III/ST19 GBS strains to levofloxacin reached 92.9%, with 75% of the levofloxacin-resistant strains belonging to CC19, whereas all III/ST17 type GBS strains were sensitive to levofloxacin (Wang et al., 2013). Research in Italy indicated that the majority of levofloxacin-resistant GBS strains were of the Ib/ST19 type, also within the CC19 (Piccinelli et al., 2015b). It is speculated that the sensitivity of levofloxacin in bacteria may be related to their molecular biological characteristics and serotype, suggesting possible clonal spread.
3.5 Resistance to tetracycline in GBS and the underlying mechanisms
Research both nationally and internationally has consistently shown high rates of resistance to tetracycline in GBS. Resistance rates reported include 62% in Canada, 80% in Italy, 97% in Brazil, and 98% in Egypt (Shabayek and Abdalla, 2014; Piccinelli et al., 2015b). The tetracycline resistance gene primarily involves tetM (Granlund et al., 2010), which encodes ribosomal protection proteins. In China, tetM and tetO genes are the main tetracycline resistance genes found in GBS, with the detection of tetK and tetL genes also reported (Huiling et al., 2010; Jia-de, 2010). In Brazil, resistance is predominantly due to the tetM gene (99.3%), with a 1.8% carriage rate for tetO (Dutra et al., 2014). In Egypt, tetM is also the main resistance gene, with an individual carriage rate of 83.7%, and the presence of tetL, tetK, and tetO genes has been detected (Shabayek and Abdalla, 2014). Tetracyclines are known to affect the development of teeth and bones in children, and due to concerns about severe hepatorenal toxicity reactions, its use has been largely discontinued in pediatric clinical practice for many years. However, the problem of tetracycline resistance remains very serious in China (Liu et al., 2021). This issue may be related to the overuse of these antibiotics in agriculture and food animals, as well as the stable resistance of bacteria to this class of antibiotics. Further investigation is warranted into this matter.
3.6 Vancomycin resistance in GBS
Due to rising resistance rates to erythromycin and clindamycin, vancomycin is sometimes necessary for the prevention and treatment of GBS infections in patients allergic to penicillin. Park et al. explored two laboratory-confirmed cases of invasive GBS strains resistant to vancomycin (Park et al., 2014). This study employed PCR amplification with primers, EG1 and, EG2 to produce a sequence similar to the vanG (941bp) of Enterococci and confirmed that the strains contained sequences corresponding to vanW, vanG and vanXY, with sequence similarities of 89.8%, 91.0%, and 95.7%, respectively. One of the isolates had a 2658bp tandem repeat sequence completely identical to the vanG of Enterococcus faecalis. Since there was no epidemiological link between the strains, it is conjectured that independent mechanisms of resistance acquisition exist. Further research is needed, in conjunction with clinical outcomes, to investigate their origins and patterns of spread.
3.7 Multidrug resistance in GBS
In recent years, the problem of drug resistance in GBS has become increasingly serious globally, with reports emerging of multidrug-resistant GBS strains (Talebi Bezmin Abadi et al., 2019). Additionally, a study revealed an increasing trend in macrolide-resistant GBS isolates (Khan et al., 2023). PRGBS is capable of surviving and spreading in hospital settings, leading to nosocomial infections. There is a potential risk of global transmission and epidemic spread in the future.
GBS colonization is a significant risk factor for various adverse outcomes in pregnant women and neonates. Studies have shown that GBS colonization in the vaginal tract is associated with preterm birth (Tano et al., 2021) and neonatal GBS early-onset disease (Zhu and Lin, 2021). The prevalence of GBS colonization varies depending on the detection method used, with enrichment media improving the detection rate (Song et al., 2022). In the context of GBS colonization and infection, alternative antimicrobials such as cefazolin have been explored as prophylactic regimens, especially in situations where penicillins are contraindicated or unavailable (Antonello et al., 2020). Additionally, the relationship between the gut microbiota composition in pregnant women colonized with GBS and maternal blood routine as well as neonatal blood-gas analysis has been investigated to understand the interplay between GBS colonization and adverse birth outcomes (Wang et al., 2022b). Furthermore, the prevalence and clinical relevance of colonization with methicillin-resistant Staphylococcus aureus (MRSA) in the obstetric population have been studied to assess the potential impact on both mother and child (Bauters et al., 2022). Maternal GBS colonization has been identified as a major risk factor for neonatal GBS infection, emphasizing the importance of understanding and addressing GBS colonization in pregnant women (Jung et al., 2021).
The epidemiology of multidrug-resistant GBS remains a significant concern globally, with studies focusing on different aspects of this pathogen. Huang et al. (Huang et al., 2019) reviewed data from China to determine the maternal GBS colonization rate, incidence of invasive GBS disease in infants, and associated clinical outcomes. The systematic literature review reveals that in mainland China, the maternal GBS colonization rate varies from 3.7% to 14.52%, and the incidence of invasive GBS disease in infants is 0.55–1.79 per 1000 live births, indicating a significant health concern with Serotype III being the most prevalent. The available data in China suggest that specific GBS serotypes are predominant in causing disease. This comprehensive analysis highlights the varied prevalence of GBS colonization among pregnant women in China and the consequent risks of invasive GBS diseases in infants, with relatively high fatality rates. Furthermore, the study underscores the potential of immunization strategies targeting pregnant women, focusing on vaccines covering the major serotypes (Ia, Ib, II, III, and V) identified, to significantly mitigate the burden of GBS infections. Kao et al. (Kao et al., 2019) focused on the clinical characteristics and impacts of emerging serotype III sequence type 17 GBS invasive infections in infants in Taiwan. The study aimed to determine serotype distribution, antimicrobial resistance, clinical features, and molecular characteristics of invasive GBS isolates. The study identifies significant variations in serotype distribution, antimicrobial resistance profiles, clinical manifestations, and molecular characteristics of invasive GBS isolates from Taiwanese infants. This research highlights the diversity of GBS serotypes affecting Taiwanese infants, each with distinct antimicrobial resistance and clinical characteristics, emphasizing the need for tailored healthcare strategies. It also suggests the importance of continued surveillance and molecular epidemiological studies to better understand and combat GBS infection in this vulnerable population. Slotved et al. (Slotved and Hoffmann, 2020) analyzed the epidemiology of invasive GBS infections in Denmark from 2005 to 2018, presenting data on serotype distribution and antibiotic susceptibility in all age groups. The study reveals a significant increase in the incidence of invasive GBS infections among the elderly in Denmark from 2005 to 2018, alongside a rise in resistance to erythromycin and clindamycin. While the incidence of early-onset and late-onset GBS disease in newborns remained stable and low, there was a notable rise in GBS infections in older adults, particularly in those aged 65 and above. Additionally, the study observed an increasing trend in antibiotic resistance among GBS isolates, underscoring the need for ongoing surveillance and tailored antibiotic stewardship programs. In contrast, Choi et al. (Choi et al., 2021) discussed recent epidemiological changes in GBS among pregnant Korean women, highlighting the evolving nature of GBS epidemiology. The study indicates an increase in GBS colonization rates among pregnant Korean women to levels comparable with those in Western countries, along with notable antimicrobial resistance. The colonization rate of GBS in pregnant Korean women is 19.8%, showing an upward trend and aligning with rates in Western countries. Additionally, there is a significant presence of antimicrobial resistance, particularly to clindamycin, erythromycin, and tetracycline, underscoring the importance of periodic and comprehensive epidemiological studies to guide prevention and treatment strategies. Additionally, Zhang et al. (Zhang et al., 2021) conducted a retrospective study in Shanxi, China, focusing on the molecular characterization of pathogenic GBS strains, with a high incidence of sequence type 10 strains in infants and pregnant women. A high prevalence of ST10 was found in both pregnant women (44.4%) and infants (72.2%) with GBS, highlighting its significant role in regional infections. The majority of GBS isolates harbored the pilus island combinations PI-1+PI-2a, indicating its potential importance in the pathogenesis and transmission of GBS, thus suggesting targets for future interventions and vaccine development.
The treatment of infections caused by MDR pathogens poses a significant challenge in clinical practice. Various studies have explored different therapeutic interventions and their outcomes in combating MDR infections. Nørgaard et al. (Nørgaard et al., 2019) conducted a systematic review to identify current antimicrobial treatment options for infections with MDR Gram-negative bacteria. The study found that monotherapy and colistin combination therapy showed clinical and microbiological success rates ranging from 70% to 100%, depending on the infection site and severity. In the context of specific bacterial infections, Liu et al. (Liu et al., 2020) investigated the influence of Autoinducer-2 (AI-2) on tetracycline resistance in Streptococcus suis. The study demonstrated that the addition of exogenous AI-2 led to an increase in MIC compared to the wild type strain, highlighting the importance of exploring new approaches to combating antimicrobial resistance. Furthermore, Xiangru et al. (Xiangru et al., 2023) evaluated the clinical efficacy of Buzhong Yiqi decoction (BZYQ) in the treatment of hospital-acquired pneumonia (HAP) with multi-drug-resistant bacteria (MDRB). The study reported a higher clinical success rate and pathogen eradication rate in the intervention group compared to the control group, indicating the potential of BZYQ as a treatment option for MDRB infections.
Therefore, epidemiological surveillance of GBS, assessment of PRGBS, and evaluation of multidrug-resistant genotypes are of crucial importance (Nagano et al., 2012). Antibiotic resistance in GBS with their specific targets and mechanisms are shown in Table 2.
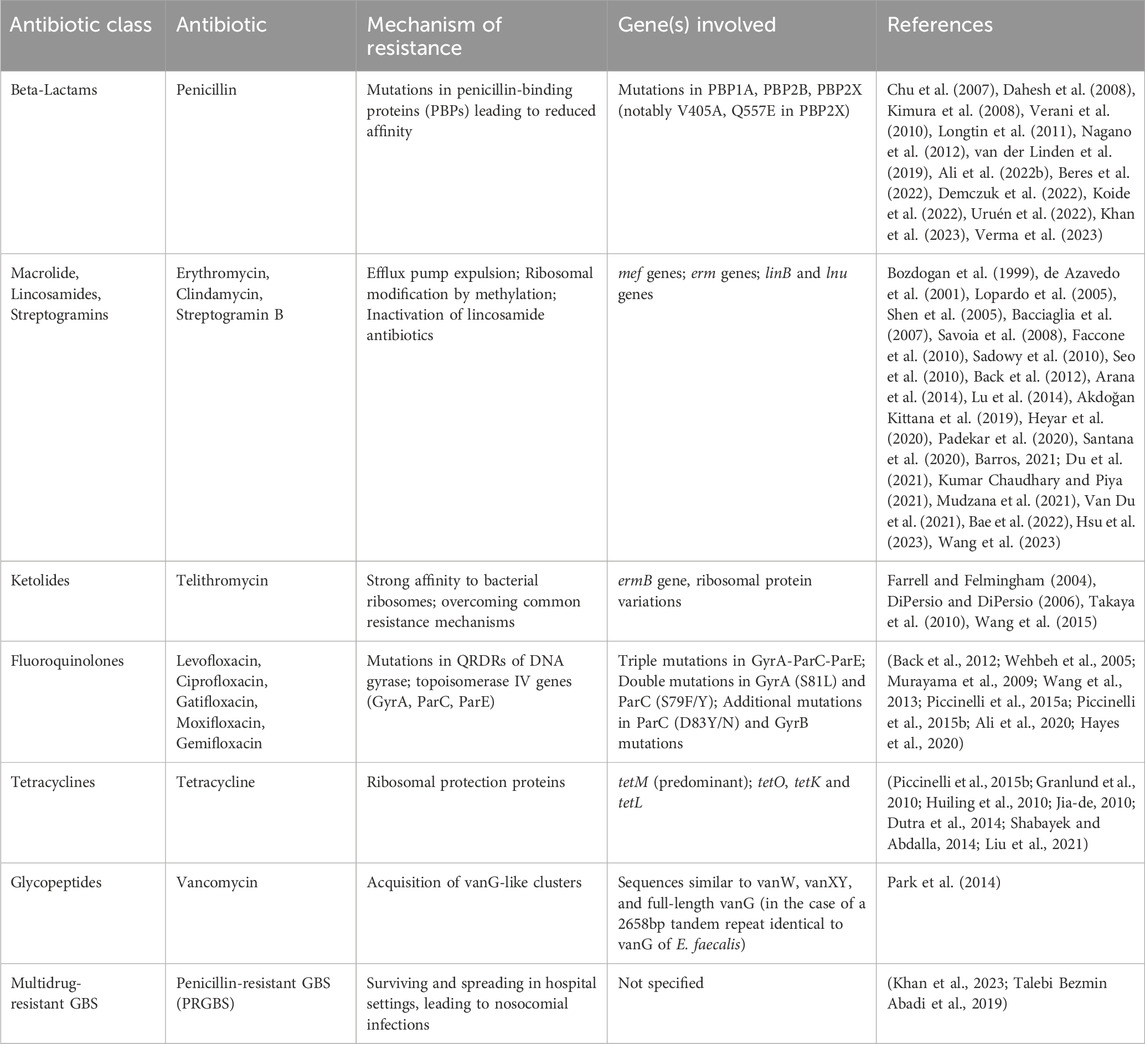
Table 2. Summary of Antibiotic resistance in Group B Streptococcus with their specific targets, mechanisms and references.
4 GBS related clinical diseases in obstetrics and gynecology
4.1 GBS infection in non-pregnant women
The incidence of GBS disease is increasing in non-pregnant adults or adults with impaired immune function, especially among those with underlying conditions. Approximately 20%–70% of infections are nosocomial (Miselli et al., 2022). Several clinical diseases have been confirmed to be caused by GBS infection. The most common diseases are skin and soft tissue infections (Akbari et al., 2023), and GBS can also cause vaginal infections in non-pregnant women. This infection can cause symptoms such as vaginal inflammation (Tano et al., 2021), abnormal vaginal discharge (Dilrukshi et al., 2021), and itching (Tano et al., 2021). GBS is also one of the common pathogens that cause urinary tract infections in non-pregnant women (Balasubramanian et al., 2023). Urinary tract infections can cause symptoms such as frequent urination, urgency, and pain during urination. Although GBS infection is not usually considered a sexually transmitted disease, it can be transmitted to non-pregnant women through sexual contact (El Beitune et al., 2006). In this case, the infection may affect areas such as the vagina, cervix, and urethra. Non-pregnant individuals with compromised immune function (Bebien et al., 2012), such as those receiving immunosuppressive therapy, with chronic diseases, or undergoing chemotherapy, may be more susceptible to GBS infection. For GBS infection in non-pregnant women, the general approach includes diagnosis and laboratory testing by a healthcare professional to determine the presence of GBS infection. If the infection is confirmed, appropriate antibiotic treatment such as penicillin or other antibiotics may be prescribed to eliminate bacterial infection. Symptomatic measures such as pain relief and anti-itch treatment can also be taken.
4.2 GBS infection in pregnant women
GBS infection in pregnant women can manifest as asymptomatic clinical infection or progress to sepsis. GBS infection can cause maternal bacterial urinary tract infections, pyelonephritis, postpartum mastitis, and endometritis (Sundin et al., 2021). Among systemic GBS infections in mothers, serotypes Ia, III, and VI account for the majority (Barro et al., 2023). GBS infection is also associated with premature birth, premature rupture of membranes, chorioamnionitis, fetal infection, and stillbirth. Approximately 1%–3% of infected newborns will develop early-onset disease within 7 days after birth (Preventing neonatal group B streptococcal infection, 2011). The main causes of early-onset neonatal infection are vertical transmission from the mother and GBS infection of the amniotic membranes. Over 95% of early-onset infections are related to GBS serotypes Ia, Ib, II, III, IV, and V. Among newborns with early-onset infection, 80%–85% will develop sepsis (Simonsen et al., 2014), 10% will develop pneumonia (Finsterer, 2022), and 5%–10% will develop meningitis (Manzanares et al., 2023). Meningitis is a late-onset disease that occurs between 6 days and more than 90 days after birth. Currently, there is limited understanding of the pathogenesis of late-onset GBS infection (Delara et al., 2023), which may be related to vertical transmission, nosocomial infection, or community-acquired infection. Serotype III GBS is highly associated with meningitis (Hsu et al., 2021). Recent studies have shown that preterm birth is a major risk factor for late-onset GBS infection (Gonçalves et al., 2022; Choi et al., 2023; Paul et al., 2023). In addition to meningitis, clinical manifestations of late-onset infection also include bacteremia and osteoarticular infections (Raabe et al., 2019). Currently, there are no effective preventive measures for GBS infection in pregnant women. Extremely late-onset GBS infection refers to GBS infection in infants older than 3 months. The risk factors for extremely late-onset GBS infection are similar to those for late-onset infection. However, most cases of extremely late-onset GBS infection occur in preterm or extremely low birth weight infants (Liu and Tong, 2019; Suffolk et al., 2019). Infants with extremely late-onset GBS infection are more susceptible to immunodeficiency disorders. The most common clinical manifestations of extremely late-onset GBS infection are bacteremia and meningitis.
In infants infected with GBS, the mortality rate of early-onset infection is about 2%–3%, while the mortality rate of late-onset infection is about 1%–3% (Stephens et al., 2023). In premature infants, the mortality rate of early-onset GBS infection is approximately 20%–30%, and the mortality rate of late-onset infection is about 5%–8%. Although infants infected with GBS can survive, their 10-year survival rate is very low (Kalliola et al., 1999), and they often require multiple hospitalizations within the first 5 years of life. Research has found that children with GBS infection are three times more likely to die or be hospitalized within 11 years after birth (Platt and Gilson, 1994). GBS infection can increase the risk of permanent neurological disabilities such as cerebral palsy and epilepsy. 51% of infants with GBS meningitis can grow up, while 25% of infants with GBS meningitis have mild to moderate neurological disabilities, and the remaining infants with GBS meningitis will develop severe neurological or functional impairments. Therefore, early detection and prevention of GBS infection in newborns and infants is crucial.
According to the recommendation of CDC, GBS screening should be performed in pregnant women between 36 weeks 0/7 days and 37 weeks 6/7 days (approximately 5 weeks before delivery) (Verani et al., 2010). If the screening result is positive for GBS, antibiotics should be given during delivery to prevent infection. If GBS is found in the vaginal flora of pregnant women at any time, regardless of the concentration, it indicates an overgrowth of GBS. If the concentration of bacteria in the urine is higher than 105 CFU/mL at any time during pregnancy, antibiotic treatment should be given to the pregnant woman before delivery, and intrauterine injection should be performed during delivery. If the concentration of bacteria in the urine is lower than 105 CFU/mL, antibiotic treatment before delivery is not necessary, but antibiotic prophylaxis during delivery is still necessary. For pregnant women who have already given birth or have premature rupture of membranes before 36 weeks of pregnancy, antibiotic prophylaxis will be continued until the baby is born. GBS screening in pregnant women significantly reduces the incidence of early-onset GBS infection in newborns, reducing it by nearly 85% compared to no screening (Hanson et al., 2022). However, late-onset infection is not prevented.
Penicillin G is the preferred drug for prophylaxis against GBS infection due to its low cost, low toxicity, and narrow spectrum of antibacterial activity (Ikebe et al., 2023). According to the guidelines of the American Academy of Pediatrics, antibiotics should be administered at least 4 h before delivery to ensure that the concentration of Penicillin G in the amniotic fluid and placental circulation reaches a sufficient level, thereby reducing the transmission of GBS from mother to baby. If a pregnant woman is allergic to beta-lactam antibiotics, cefazolin should be used instead. Pregnant women who are sensitive to clindamycin should receive clindamycin treatment, while those who are resistant to clindamycin should receive vancomycin treatment (Duffy et al., 2022). Unlike conventional antibiotic treatment, antibiotic prophylaxis is used only as “local antibiotic treatment”. “Comprehensive antibiotic treatment” is a method used to eradicate Helicobacter pylori (Luo et al., 2023), which has been classified as a class I human carcinogen by the World Health Organization’s International Agency for Research on Cancer. This high-dose antibiotic therapy can eradicate Helicobacter pylori colonization and treat gastric cancer. However, in the field of obstetrics, this “comprehensive antibiotic treatment” cannot be implemented because it may cause serious harm to the health of both the mother and the fetus, including fatal diseases or chronic disabilities.
However, there are also certain limitations to prophylactic antibiotic treatment. Due to the risk of allergic reactions, the necessity of conducting antimicrobial sensitivity tests on pregnant women is increasingly being emphasized. Some research reports indicate that the rate at which maternal antibodies are transferred to newborns is approximately 0.5–0.7, indicating a relatively poor effectiveness of prophylactic antibiotic treatment (Dad et al., 2021).
4.3 GBS infection and the microbiota of pregnant women
GBS infection is closely related to the microecology of pregnant women. Microecology refers to the balance between beneficial bacteria (such as Lactobacillus) and other microorganisms in the human body (Mejia et al., 2023). Under normal circumstances, the vagina and intestines of healthy pregnant women may carry a certain amount of GBS, but it maintains a balance with other beneficial bacteria. However, certain factors may lead to an imbalance in microecology, causing GBS to overgrow and cause infection.
GBS primarily colonizes in the vagina, where there is a normal presence of Lactobacillus and other beneficial bacteria that maintain an acidic environment by producing substances such as lactic acid (Kling et al., 2009), inhibiting the growth of pathogens. When the number or balance of Lactobacillus in the vagina decreases or becomes imbalanced, the proliferation and risk of GBS infection increase. In addition, the gut microbiota is closely related to the microecology of other parts of the body. Imbalances in the gut microbiota can affect overall immune system function and the colonization and infection process of GBS.
During pregnancy, the immune system undergoes a series of regulatory changes to tolerate the fetus (Sweeney et al., 2020). These changes may affect the immune response to GBS infection. When immune system regulation becomes imbalanced, the risk of GBS infection may increase. Imbalances in vaginal microecology usually involve a lack of Lactobacillus and excessive growth of other pathogenic microorganisms (Mei and Li, 2022). Bacterial vaginosis is a mixed infection caused by an imbalance in normal vaginal flora, where lactobacillus is reduced and other bacteria multiply, mostly anaerobic bacteria (Kamga et al., 2019). Bacterial vaginosis and aerobic vaginitis are considered to be associated with various severe obstetric complications, such as preterm birth, miscarriage, premature rupture of membranes, fetal infection, and low birth weight infants (Choi et al., 2022). Abnormal vaginal flora can lead to cervical shortening, resulting in preterm birth. Bacterial vaginosis often accompanies an increase in GBS infection (Xiao et al., 2023). Lactobacillus count significantly decreases and streptococcus count increases in pregnant women with bacterial vaginosis (Mohammed et al., 2020). Therefore, the balance of microecology in pregnant women is crucial for preventing GBS infection.
There are some strategies that can help maintain or improve the balance of microecology in pregnant women, such as consuming foods rich in lactobacillus and probiotics, such as yogurt and fermented foods, which help maintain gut health (Sroka-Oleksiak et al., 2020). Excessive or inappropriate use of antibiotics can disrupt beneficial bacteria and lead to an imbalance in microecology (Pulingam et al., 2022). Antibiotics should be used under the guidance of a doctor. Long-term exposure to high-stress environments can also affect the balance of microecology. Pregnant women can reduce stress through appropriate rest, relaxation techniques, and stress management.
5 Prevention, detection, and treatment of GBS
5.1 Intrapartum antibiotic prophylaxis
Intrapartum antibiotic chemoprophylaxis (IAP) is an effective strategy for preventing early-onset neonatal GBS disease by inhibiting or reducing the colonization of GBS within the maternal genitourinary and gastrointestinal tracts, which in turn reduces the vertical transmission of GBS to the newborn (Le Doare et al., 2017). In the 1990s, the incidence of GBS-EOD (early-onset disease) in live births in the United States was 1.80 per 1,000. However, following the widespread implementation of the IAP policy, the incidence dropped significantly to 0.23 per 1,000 in 2015, representing an 80% decrease (Nanduri et al., 2019). IAP has been proven to be an effective means for preventing early-onset GBS disease in newborns. The approach to IAP currently represents the most widely used strategy to prevent GBS infections in pregnant women in many developed countries (Nuccitelli et al., 2015). The criteria for administering IAP are primarily based on the colonization status of GBS in the pregnant woman and/or an assessment of perinatal clinical risk factors. The basis for these assessments may vary among different countries or regions (Tsega et al., 2015; Wang et al., 2021).
In 1996, the American College of Obstetricians and Gynecologists (ACOG) recommended in its guidelines on preventing neonatal GBS-EOD that IAP should be determined by a combination of microbiological screening and assessment of risk factors (ACOG committee opinion, 1996). However, the revised guidelines from the U.S. Centers for Disease Control and Prevention (CDC) in 2002 emphasized the greater efficacy of microbiological screening, and recommended IAP for women with positive GBS bacteriuria, those with a history of neonatal GBS infection, or those with unknown GBS status but presenting labor risk factors (Schrag et al., 2002). Countries such as the United Kingdom and the Netherlands do not advocate for microbiological screening of pregnant women around the time of delivery, opting instead to rely on an assessment of clinical risk factors to determine whether to administer IAP (ACOG committee opinion, 1996). The decision to use risk factor assessments over microbiological screening is informed by the cost of screening tests and a desire to prevent the overuse of antibiotics (Le Doare et al., 2017).
A systematic review in 2017, which included IAP policies from 60 countries, identified the following major risk factors for prioritizing IAP: 1) Preterm birth (<37 weeks); 2) Premature rupture of membranes; 3) Prolonged duration of membrane rupture; 4) Positive GBS bacteriuria; 5) History of neonatal GBS infection; 6) Maternal fever (temperature >38°C); 7) Intra-amniotic infection. Of the 60 countries, 25 implemented an IAP policy based on clinical risk factors, and all (60/60) countries recommended IAP for women with a history of neonatal GBS infection. Most countries (23/25) recommended IAP for cases with prolonged duration after membrane rupture, premature rupture of membranes for >18 h (PROM), or maternal GBS bacteriuria (Le Doare et al., 2017).
β-Lactam antibiotics exhibit high sensitivity against GBS and have always been the drugs of choice for the prevention or treatment of GBS infections. Nevertheless, drug sensitivity monitoring data indicate that in recent years, there has been a reduction in GBS sensitivity to β-lactam antibiotics, including penicillin (Dahesh et al., 2008; Longtin et al., 2011; Metcalf et al., 2017; Yi et al., 2019), and high levels of resistance to secondary antibiotics such as erythromycin and clindamycin (Lamagni et al., 2013). Additionally, resistance to other antibiotics, like fluoroquinolones and tetracyclines, is also on the rise (Nagano et al., 2012; Wang et al., 2015).
Intrapartum intravenous administration of penicillin is the preferred IAP treatment protocol due to its efficacy (Prevention of Group B Streptococcal Early-Onset Disease in Newborns: ACOG Committee Opinion, 2020). In case of penicillin allergy, clindamycin is used. Six countries recommend cephalosporins instead of penicillin, and four South American countries and two Asian countries, concerned about the risk of clindamycin-resistant strains in penicillin-allergic patients, suggest adding vancomycin as an alternative (Le Doare et al., 2017). In the 2020 guidelines, ACOG also recommends intravenous penicillin or ampicillin as the first-line treatment. For pregnant women with a low-risk penicillin allergy or uncertain severity of allergy, cefazolin is recommended. For those with a high-risk allergic response, clindamycin treatment can be considered after confirming the GBS strain’s sensitivity to this antibiotic (Prevention of Group B Streptococcal Early-Onset Disease in Newborns: ACOG Committee Opinion, 2020).
5.2 Various detection methods for GBS
In the detection of tumors caused by GBS, a combined screening and diagnostic method for GBS infection and gynecological malignancies is usually used (Achten et al., 2020). The doctor will ask the patient if there are any symptoms of infection or any previous infection records. Physical examinations may include vaginal examinations, cervical smears, and endometrial biopsies to check for any abnormal signs. Cervical smears are a commonly used screening method that involves collecting cervical cells and observing them under a microscope to look for abnormal cells or lesions (Kamal, 2022). This method can help detect cervical cancer and other early abnormalities. Human papillomavirus (HPV) is closely associated with the development of cervical cancer (Sravani et al., 2023). HPV virus screening can detect HPV infections, including high-risk types of the virus (Gavinski and DiNardo, 2023). This screening method can help detect infections early and take appropriate further actions. In some cases, specific biomarkers or blood tests can be used to assess the risk or diagnose malignancies. For example, in the screening and diagnosis of endometrial cancer, the levels of CA-125 (Pourmadadi et al., 2023) and other related markers in the blood can be measured to assess the level of risk.
5.3 Microbial therapy
The current administration methods of antibiotics have low cost and wide applicability, making them a better method for preventing GBS infections, especially in countries with low socioeconomic status or limited resources. However, the use of antibiotics still has limitations due to the increased risk of allergic reactions and serious risks to newborns. In this regard, microbiota therapy can serve as an alternative treatment method (Nader-Macías et al., 2021). Microbiota therapy has become a hot topic in obstetrics, gynecology, and translational research fields. Some studies have reported on the treatment of gut microbiota, such as fecal microbiota transplantation (FMT) (Cheng and Fischer, 2023), for cancer treatment. In addition, a research report has shown that the composition of gut microbiota can regulate immune response mechanisms (de Vos et al., 2022), such as anti-tumor activity, thereby producing interactions between microbiota and tumors. This microbiota regulation mechanism may be direct, but its specific downstream pathways still need to be elucidated. For general microbiota therapy, known biomarkers are used as diagnostic tools to screen and monitor patients. Microbiota-based treatment methods are used to treat various diseases and are applied in different ways (Elinav et al., 2019), including dietary interventions, probiotics, prebiotics, postbiotics, bacteriophage therapy, and fecal microbiota transplantation. Each method has its advantages and disadvantages. Probiotics are considered relatively safe (Fugaban et al., 2021). However, they do not target specific diseases and only provide a temporary therapeutic response. In addition, the effectiveness of probiotic therapy depends on specific microbial colonies and the gut microenvironment (Zhao et al., 2023). Bacteriophage therapy is a highly specific targeted treatment method (Cold et al., 2020). However, an important limitation of bacteriophage therapy is its narrow host range, where a bacteriophage can only kill certain strains of the same bacteria species and cannot kill multiple strains or different bacteria species (Azam and Tanji, 2019).
These different microbiota-based treatment methods can also be applied as new approaches to treat patients with GBS infection in the vaginal microbiota. As mentioned earlier, poor vaginal microbiota is closely associated with gynecologic malignancies and adverse obstetric outcomes, and adjusting the vaginal microbiota may potentially alter the incidence of GBS infection in pregnant women. Microbiota-based treatment methods can be similar to those used for the gut microbiota. Probiotics can be used to rebalance the vaginal flora, mainly by increasing the number of lactobacilli. Synbiotics, which combine probiotics and prebiotics, aim to overcome the limitations of probiotics, specifically their dependence on lactobacilli (Calder et al., 2022). However, symbiosis may require a specific environment. Bacteriophages bind to specific receptors on bacterial cell walls and deliver engineered therapeutic materials into host cells, resulting in promising effects. Biofilm disruptors are another treatment option (Reza et al., 2019). Polymicrobial infections produce biofilms on the vaginal epithelium and generate short-chain fatty acids, ultimately increasing the vaginal environment’s pH and leading to vaginal inflammation. A report has indicated that using antibiotics alone can reduce microbial diversity and restore populations of lactobacilli but cannot completely destroy biofilms (Nitzan et al., 2016). Therefore, antibiotic therapy combined with biofilm disruptor adjuvants would be a more comprehensive treatment approach.
Finally, vaginal microbiota transplantation is another microbial therapy for treating vaginal diseases (Wei and Chen, 2021). In this treatment method, volunteers are recruited and undergo medical evaluations. Their vaginal microbiota is assessed through microscopic evaluations. After screening, the best vaginal microbiota is transplanted into the recipient’s vagina. Vaginal microbiota transplantation significantly alleviates patients’ symptoms and successfully restores the composition of vaginal microbiota, including increased lactobacillus count. However, this relatively new method still remains controversial. Therefore, full supervision should be implemented throughout the screening process to minimize the risk of potential disease transmission, especially those that may lead to antibiotic resistance in microbes.
6 GBS Vaccine
While GBS remains highly sensitive to first-line β-lactam antibiotics, the widespread implementation of IAP comes with an extensive use of antibiotics, which may enhance the resistance of GBS. It can also disrupt the body’s microecology, leading to an imbalance of microbial communities. Moreover, the transfer of resistance genes can result in a greater prevalence of antibiotic-resistant pathogens across humans, animals, and the environment (McGee et al., 2021). Therefore, the development of alternative interventions to replace intrapartum antibiotic treatment has become an area of keen interest. The research and application of GBS vaccines have emerged as a promising solution. Currently, there are three main types of vaccines under investigation: capsular polysaccharide vaccines, conjugate vaccine, and protein-based vaccines.
6.1 Preventive vaccination with GBS vaccine
In order to reduce the global incidence and mortality rate of neonatal infections related to GBS, it is crucial to develop a vaccine against GBS (Madhi et al., 2023). It is estimated that vaccinating 70% of pregnant women with a GBS vaccine could prevent nearly 50,000 deaths related to GBS infections and 170,000 cases of preterm birth each year. However, there is currently no licensed vaccine available for preventing GBS. In 2016, the World Health Organization held consultations specifically on the development of maternal immunization vaccines and declared an urgent need for a vaccine to prevent mother-to-child transmission of GBS in order to protect the health and lives of infants worldwide (Kobayashi et al., 2016). It also proposed a strategic goal of developing a safe, effective, and affordable GBS vaccine for pregnant women to prevent neonatal deaths, stillbirths, and GBS-related diseases. Currently, two GBS vaccines have entered Phase II or III clinical trials. The first is a multivalent conjugate vaccine aimed at targeting the majority of pathogenic serotypes, while the other is a protein subunit vaccine (Duke et al., 2021). The multivalent conjugate vaccine has the potential to prevent 95% of GBS infections in pregnant women, 99% of stillbirths, and 99% of neonatal GBS infections by targeting the majority of pathogenic serotypes. The protein-based vaccine approach provides broader protection against all GBS serotypes (Dominguez and Randis, 2022). Pharmaceutical companies such as Pfizer and MinervaX have been working on developing GBS vaccines. Pfizer recently announced that the U.S. Food and Drug Administration has designated their investigational GBS vaccine (Absalon et al., 2022), Bacterial GBS 6 (PF-06760805), for prevention of the six most prominent GBS serotypes that account for 98% of GBS disease cases. MinervaX is developing a GBS candidate vaccine based on traditional multivalent conjugate technology and is preparing for Phase III clinical trials (Pawlowski et al., 2022). In low- and middle-income countries, the vaccine will greatly improve the occurrence of GBS infectious diseases and make it possible to prevent the majority of GBS-related diseases (Procter et al., 2023). Despite the advantages of GBS vaccines, their limitations include high cost, lack of coverage for all GBS strains, and the possibility of resistance. Therefore, some researchers believe it is important to detect GBS before infection progresses or develops into a severe condition.
6.2 Capsular polysaccharide vaccines
Capsular polysaccharide (CPS) is one of the virulence factors of GBS, which enables the bacteria to evade the host’s immune response. GBS uses its capsular polysaccharide to inhibit complement deposition and resist phagocytosis by immune cells. Additionally, CPS promotes the formation of biofilms and hampers the binding of antimicrobial peptides and Neutrophil Extracellular Traps (NETs), thereby enhancing the invasive capability of GBS. Based on the antigenic components of GBS capsular polysaccharides, GBS can be classified into ten serotypes: Ia, Ib, II, III, IV, V, VI, VII, VIII, and IX (Carreras-Abad et al., 2020).
CPS vaccines refer to vaccines developed by targeting the highly expressed CPS on the surface of GBS as the antigen and conducting research on CPS-specific antibodies. Currently, the phase I and phase II clinical trials for CPS vaccines have preliminarily confirmed their safety and efficacy. However, the immunogenicity and reactogenicity of CPS vaccines are generally low. Additionally, the IgM produced does not cross the placenta, providing only short-term protection to the fetus and no significant protection to neonates. Moreover, due to considerable structural differences between the CPS of different serotypes and the absence of cross-protective effects, the immunological protection range of monovalent vaccines is limited. Consequently, CPS vaccines have not yet been adopted for clinical use (Mettu et al., 2020).
6.3 Conjugate vaccine
Conjugate CPS vaccines aim to enhance immunogenicity through the covalent bonding of GBS’s own capsular polysaccharide with carrier proteins, thereby inducing the production of IgG and the memory of T-cells and B-cells (Aceil et al., 2022). The early development of CPS conjugate vaccines involved the covalent attachment of tetanus toxoid (TT) to type III CPS to form a monovalent conjugate vaccine (III-TT). Currently, monovalent, bivalent, and trivalent vaccines targeting GBS serotypes Ia, Ib, II, III, and V have been researched in non-pregnant and pregnant women, demonstrating safety and efficacy in phase I and phase II clinical trials (Baker et al., 2003; Madhi et al., 2016). In 2021, Absalon et al. evaluated the safety and immunogenicity of a novel hexavalent vaccine (GBS6) for serotypes Ia, Ib, II, III, IV, and V, which proved to be safe and effective in healthy, non-pregnant adults through phase I and II clinical trials (Absalon et al., 2021). Future research will further investigate the vaccine’s effects in varying populations and its capacity to transfer antibodies to newborns.
6.4 Protein-based vaccine
CPS vaccines offer protection limited to specific serotypes, presenting significant constraints. On the other hand, protein vaccines are created from proteins common to all serotypes of CPS, providing a broader protective range. Moreover, protein vaccines may prevent serotype replacement or switching problems that might arise with the use of CPS vaccines (Dominguez and Randis, 2022).
Current research has been focusing extensively on protein vaccines made by fusing the N-terminus of GBS surface Alpha C (αC) protein and Rib protein to produce a vaccine (GBS-NN). In 2021, Fischer et al. published the results of a phase I clinical trial for the GBS-NN vaccine, confirming its safety and immunogenicity in healthy women (Fischer et al., 2021). Building upon this in 2022, Pawlowski et al. demonstrated that the vaccine consisting of αC-N and Rib-N induced strong and persistent IgG and IgA responses against the homotypic αC-N (Pawlowski et al., 2022). It also elicited variable immune responses to heterotypic Alpha-like proteins (Alp1∼3). The study further confirmed that the IgG elicited by the GBS-NN vaccine was predominantly IgG1, which is an effective antibody subtype transferred to the fetus during the later stages of pregnancy through the placenta. Researchers are now developing additional GBS protein vaccines based on the different structural domains of the N-terminus of Alpha-like proteins.
7 Conclusion
The presence of GBS implies that infants and newborns may experience severe clinical outcomes. However, for elderly individuals with GBS infection, the lethality of the infection itself is relatively low. Considering the potential role of GBS in the development of gynecologic malignancies, although GBS may not be the sole major cause, it is a key factor leading to adverse outcomes. GBS may play a crucial role in the development of severe clinical symptoms, but its detection becomes challenging due to interference from many other factors. GBS can even act as a powerful dormant pathogen, manipulating and regulating other bacteria, thereby resulting in serious clinical consequences. Therefore, it is crucial to study the interaction and impact mechanisms between GBS and bacteria and the host environment. More research is needed in the future to examine the pathogenesis and mechanisms of action of GBS, such as high-throughput sequencing technologies like RNA-seq, metagenomics, and metabolomics. In addition, professional discussions and collaborative research should be encouraged to develop better management strategies for GBS, aiming to control and reduce the maternal and infant mortality and morbidity caused by GBS infections. This comprehensive approach not only allows for better understanding of GBS but also contributes to the health of pregnant women and newborns nationwide and even globally.
Author contributions
YL: Writing–original draft. HA: Writing–review and editing.
Funding
The author(s) declare that financial support was received for the research, authorship, and/or publication of this article. This study was provided financial support from the following projects: Liaoning Province Science and Technology Program Joint Program Fund Project (grant no. 2023-MSLH-059), Postgraduate Education Teaching Research and Reform Project of Jinzhou Medical University (grant no. YJ2023-018), Jie Bang Gua Shuai Project of Science & Technology Department of Liaoning Province (grant no. 2022JH1/10800070), Basic Scientific Research Project of Colleges and Universities of Education Department of Liaoning Province (Key project) (grant no. 1821240403), 2023 Jinzhou Medical University first-class discipline construction project.
Conflict of interest
The authors declare that the research was conducted in the absence of any commercial or financial relationships that could be construed as a potential conflict of interest.
Publisher’s note
All claims expressed in this article are solely those of the authors and do not necessarily represent those of their affiliated organizations, or those of the publisher, the editors and the reviewers. Any product that may be evaluated in this article, or claim that may be made by its manufacturer, is not guaranteed or endorsed by the publisher.
References
Absalon, J., Segall, N., Block, S. L., Center, K. J., Scully, I. L., Giardina, P. C., et al. (2021). Safety and immunogenicity of a novel hexavalent group B streptococcus conjugate vaccine in healthy, non-pregnant adults: a phase 1/2, randomised, placebo-controlled, observer-blinded, dose-escalation trial. Lancet Infect. Dis. 21 (2), 263–274. doi:10.1016/S1473-3099(20)30478-3
Absalon, J., Simon, R., Radley, D., Giardina, P. C., Koury, K., Jansen, K. U., et al. (2022). Advances towards licensure of a maternal vaccine for the prevention of invasive group B streptococcus disease in infants: a discussion of different approaches. Hum. vaccines Immunother. 18 (1), 2037350. doi:10.1080/21645515.2022.2037350
Aceil, J., Paschall, A. V., Knoot, C. J., Robinson, L. S., Scott, N. E., Feldman, M. F., et al. (2022). Immunogenicity and protective efficacy of a prototype pneumococcal bioconjugate vaccine. Vaccine 40 (42), 6107–6113. doi:10.1016/j.vaccine.2022.09.018
Achten, N. B., Dorigo-Zetsma, J. W., van Rossum, A. M. C., Oostenbrink, R., and Plötz, F. B. (2020). Risk-based maternal group B Streptococcus screening strategy is compatible with the implementation of neonatal early-onset sepsis calculator. Clin. Exp. Pediatr. 63 (10), 406–410. doi:10.3345/cep.2020.00094
ACOG committee opinion (1996). ACOG committee opinion. Prevention of early-onset group B streptococcal disease in newborns. Number 173--June 1996. Committee on Obstetric Practice. American College of Obstetrics and Gynecologists. Int. J. Gynaecol. obstetrics official organ Int. Fed. Gynaecol. Obstetrics 54 (2), 197–205. doi:10.1016/S0020-7292(96)90083-1
Akbari, M. S., Keogh, R. A., Radin, J. N., Sanchez-Rosario, Y., Johnson, M. D. L., Horswill, A. R., et al. (2023). The impact of nutritional immunity on Group B streptococcal pathogenesis during wound infection. mBio 14, e0030423. doi:10.1128/mbio.00304-23
Akdoğan Kittana, F. N., Mustak, I. B., Hascelik, G., Saricam, S., Gurler, N., and Diker, K. S. (2019). Erythromycin-resistant Streptococcus pneumoniae: phenotypes, genotypes, transposons and pneumococcal vaccine coverage rates. J. Med. Microbiol. 68 (6), 874–881. doi:10.1099/jmm.0.000995
Ali, M., Alamin, M. A., Ali G, A., Alzubaidi, K., Ali, B., Ismail, A., et al. (2022b). Microbiological and clinical characteristics of invasive Group B Streptococcal blood stream infections in children and adults from Qatar. BMC Infect. Dis. 22 (1), 881. doi:10.1186/s12879-022-07801-9
Ali, M., Alamin, M. A., G, A. A., Alzubaidi, K., Ali, B., Ismail, A., et al. (2022a). Microbiological and clinical characteristics of invasive Group B Streptococcal blood stream infections in children and adults from Qatar. BMC Infect. Dis. 22 (1), 881. doi:10.1186/s12879-022-07801-9
Ali, M. M., Woldeamanuel, Y., Asrat, D., Fenta, D. A., Beall, B., Schrag, S., et al. (2020). Features of Streptococcus agalactiae strains recovered from pregnant women and newborns attending different hospitals in Ethiopia. BMC Infect. Dis. 20 (1), 848. doi:10.1186/s12879-020-05581-8
Alizzi, M., Rathnayake, R., Sivabalan, P., Emeto, T. I., and Norton, R. (2022). Group B streptococcal bacteraemia: changing trends in a tropical region of Australia. Intern. Med. J. 52 (5), 800–807. doi:10.1111/imj.15164
Antonello, V. S., Dallé, J., Dall'Oglio, E., Ramos, S., Bassols, F., and Jimenez, M. F. (2020). Alternative antimicrobials for prophylaxis of the Group B Streptococcus maternal-fetal disease. J. Infect. Dev. Ctries. 14 (6), 664–668. doi:10.3855/jidc.12180
Arana, D. M., Rojo-Bezares, B., Torres, C., and Alós, J. I. (2014). First clinical isolate in Europe of clindamycin-resistant group B Streptococcus mediated by the lnu(B) gene. Rev. espanola Quimioter. publicacion Of. Soc. Espanola Quimioter. 27 (2), 106–109.
Armistead, B., Oler, E., Adams Waldorf, K., and Rajagopal, L. (2019). The double life of group B Streptococcus: asymptomatic colonizer and potent pathogen. J. Mol. Biol. 431 (16), 2914–2931. doi:10.1016/j.jmb.2019.01.035
Azam, A. H., and Tanji, Y. (2019). Peculiarities of Staphylococcus aureus phages and their possible application in phage therapy. Appl. Microbiol. Biotechnol. 103 (11), 4279–4289. doi:10.1007/s00253-019-09810-2
Bacciaglia, A., Brenciani, A., Varaldo, P. E., and Giovanetti, E. (2007). SmaI typeability and tetracycline susceptibility and resistance in Streptococcus pyogenes isolates with efflux-mediated erythromycin resistance. Antimicrob. agents Chemother. 51 (8), 3042–3043. doi:10.1128/AAC.00249-07
Back, E. E., O'Grady, E. J., and Back, J. D. (2012). High rates of perinatal group B Streptococcus clindamycin and erythromycin resistance in an upstate New York hospital. Antimicrob. agents Chemother. 56 (2), 739–742. doi:10.1128/AAC.05794-11
Bae, H. G., Hong, J., Kim, Y. J., Lee, K. R., Lee, K., Choi, S. J., et al. (2022). A retrospective national study on colonization rate and antimicrobial susceptibility of Streptococcus agalactiae in pregnant Korean women, 2018-2020. Yonsei Med. J. 63 (8), 717–723. doi:10.3349/ymj.2022.63.8.717
Baker, C. J., Paoletti, L. C., Rench, M. A., Guttormsen, H.-K., Carey, V. J., Hickman, M. E., et al. (2000). Use of capsular polysaccharide—tetanus toxoid conjugate vaccine for type II group B Streptococcus in healthy women. J. Infect. Dis. 182 (4), 1129–1138. doi:10.1086/315839
Baker, C. J., Paoletti, L. C., Rench, M. A., Guttormsen, H. K., Edwards, M. S., and Kasper, D. L. (2004). Immune response of healthy women to 2 different group B streptococcal type V capsular polysaccharide-protein conjugate vaccines. J. Infect. Dis. 189 (6), 1103–1112. doi:10.1086/382193
Baker, C. J., Paoletti, L. C., Wessels, M. R., Guttormsen, H.-K., Rench, M. A., Hickman, M. E., et al. (1999). Safety and immunogenicity of capsular polysaccharide—tetanus toxoid conjugate vaccines for group B streptococcal types Ia and Ib. J. Infect. Dis. 179 (1), 142–150. doi:10.1086/314574
Baker, C. J., Rench, M. A., and McInnes, P. (2003). Immunization of pregnant women with group B streptococcal type III capsular polysaccharide-tetanus toxoid conjugate vaccine. Vaccine 21 (24), 3468–3472. doi:10.1016/s0264-410x(03)00353-0
Balasubramanian, N., Pounpandi, P., Varatharaju, G., Shanmugaiah, V., Balakrishnan, K., and Thirunarayan, M. A. (2023). Distribution of virulence genes and biofilm characterization of human isolates of Streptococcus agalactiae: a pilot study. Colloids surfaces B, Biointerfaces 223, 113151. doi:10.1016/j.colsurfb.2023.113151
Barro, C., Salloum, M., Lim, S., Delputte, P., and Le Doare, K. (2023). Simultaneous carriage of multiple serotypes of Group B Streptococcus: systematic review and meta-analysis. Vaccine 41 (1), 15–22. doi:10.1016/j.vaccine.2022.11.024
Barros, R. R. (2021). Antimicrobial resistance among beta-hemolytic Streptococcus in Brazil: an overview. Antibiotics 10 (8), 973. doi:10.3390/antibiotics10080973
Bauters, E., Jonckheere, S., Dehaene, I., Vandecandelaere, P., Argudín, M. A., and Page, G. (2022). Prevalence and clinical relevance of colonization with methicillin-resistant Staphylococcus aureus in the obstetric population. J. maternal-fetal neonatal Med. official J. Eur. Assoc. Perinat. Med. Fed. Asia Ocean. Perinat. Soc. Int. Soc. Perinat. Obstet 35 (25), 8186–8191. doi:10.1080/14767058.2021.1966411
Bebien, M., Hensler, M. E., Davanture, S., Hsu, L. C., Karin, M., Park, J. M., et al. (2012). The pore-forming toxin β hemolysin/cytolysin triggers p38 MAPK-dependent IL-10 production in macrophages and inhibits innate immunity. PLoS Pathog. 8 (7), e1002812. doi:10.1371/journal.ppat.1002812
Beres, S. B., Zhu, L., Pruitt, L., Olsen, R. J., Faili, A., Kayal, S., et al. (2022). Integrative reverse genetic analysis identifies polymorphisms contributing to decreased antimicrobial agent susceptibility in Streptococcus pyogenes. mBio 13 (1), e0361821. doi:10.1128/mbio.03618-21
Bianchi-Jassir, F., Paul, P., To, K. N., Carreras-Abad, C., Seale, A. C., Jauneikaite, E., et al. (2020). Systematic review of Group B Streptococcal capsular types, sequence types and surface proteins as potential vaccine candidates. Vaccine 38 (43), 6682–6694. doi:10.1016/j.vaccine.2020.08.052
Bnfaga, A. A., Lee, K. W., Than, L. T. L., and Amin-Nordin, S. (2023). Antimicrobial and immunoregulatory effects of Lactobacillus delbrueckii 45E against genitourinary pathogens. J. Biomed. Sci. 30 (1), 19. doi:10.1186/s12929-023-00913-7
Bozdogan, B., Berrezouga, L., Kuo, M. S., Yurek, D. A., Farley, K. A., Stockman, B. J., et al. (1999). A new resistance gene, linB, conferring resistance to lincosamides by nucleotidylation in Enterococcus faecium HM1025. Antimicrob. agents Chemother. 43 (4), 925–929. doi:10.1128/AAC.43.4.925
Burcham, L. R., Spencer, B. L., Keeler, L. R., Runft, D. L., Patras, K. A., Neely, M. N., et al. (2019). Determinants of Group B streptococcal virulence potential amongst vaginal clinical isolates from pregnant women. PloS one 14 (12), e0226699. doi:10.1371/journal.pone.0226699
Buscetta, M., Papasergi, S., Firon, A., Pietrocola, G., Biondo, C., Mancuso, G., et al. (2014). FbsC, a novel fibrinogen-binding protein, promotes Streptococcus agalactiae-host cell interactions. J. Biol. Chem. 289 (30), 21003–21015. doi:10.1074/jbc.M114.553073
Buurman, E. T., Timofeyeva, Y., Gu, J., Kim, J. H., Kodali, S., Liu, Y., et al. (2019). A novel hexavalent capsular polysaccharide conjugate vaccine (GBS6) for the prevention of neonatal group B streptococcal infections by maternal immunization. J. Infect. Dis. 220 (1), 105–115. doi:10.1093/infdis/jiz062
Calder, P. C., Ortega, E. F., Meydani, S. N., Adkins, Y., Stephensen, C. B., Thompson, B., et al. (2022). Nutrition, immunosenescence, and infectious disease: an overview of the scientific evidence on micronutrients and on modulation of the gut microbiota. Adv. Nutr. (Bethesda, Md) 13 (5), S1–s26. doi:10.1093/advances/nmac052
Carreras-Abad, C., Ramkhelawon, L., Heath, P. T., and Le Doare, K. (2020). A vaccine against group B Streptococcus: recent advances. Infect. drug Resist. 13, 1263–1272. doi:10.2147/IDR.S203454
Chan, J. M., Gori, A., Nobbs, A. H., and Heyderman, R. S. (2020). Streptococcal serine-rich repeat proteins in colonization and disease. Front. Microbiol. 11, 593356. doi:10.3389/fmicb.2020.593356
Chattopadhyay, D., Carey, A. J., Caliot, E., Webb, R. I., Layton, J. R., Wang, Y., et al. (2011). Phylogenetic lineage and pilus protein Spb1/SAN1518 affect opsonin-independent phagocytosis and intracellular survival of Group B Streptococcus. Microbes Infect. 13 (4), 369–382. doi:10.1016/j.micinf.2010.12.009
Cheng, Y. W., and Fischer, M. (2023). Fecal microbiota transplantation. Clin. colon rectal Surg. 36 (2), 151–156. doi:10.1055/s-0043-1760865
Choi, E. K., Kim, H. J., Je, B. K., Choi, B. M., and Kim, S. D. (2023). Morbidity and mortality trends in preterm infants of <32 Weeks gestational age with severe intraventricular hemorrhage: a 14-year single-center retrospective study. J. Korean Neurosurg. Soc. 66 (3), 316–323. doi:10.3340/jkns.2022.0264
Choi, S. J., Kang, J., and Uh, Y. (2021). Recent epidemiological changes in group B Streptococcus among pregnant Korean women. Ann. laboratory Med. 41 (4), 380–385. doi:10.3343/alm.2021.41.4.380
Choi, Y., Han, H. S., Chong, G. O., Le, T. M., Nguyen, H. D. T., Lee, O. E., et al. (2022). Updates on group B Streptococcus infection in the field of obstetrics and gynecology. Microorganisms 10 (12), 2398. doi:10.3390/microorganisms10122398
Chu, Y. W., Tse, C., Tsang, G. K., So, D. K., Fung, J. T., and Lo, J. Y. (2007). Invasive group B Streptococcus isolates showing reduced susceptibility to penicillin in Hong Kong. J. Antimicrob. Chemother. 60 (6), 1407–1409. doi:10.1093/jac/dkm390
Cold, F., Olsen, N. S., Djurhuus, A., and Hansen, L. H. (2020). Bacteriophage therapy. Ugeskrift laeger 182 (27), V01200041. [Bacteriophage therapy].
Coleman, M., Armistead, B., Orvis, A., Quach, P., Brokaw, A., Gendrin, C., et al. (2021). Hyaluronidase impairs neutrophil function and promotes group B Streptococcus invasion and preterm labor in nonhuman primates. mBio 12 (1), e03115. doi:10.1128/mBio.03115-20
Dad, N., Buhmaid, S., and Mulik, V. (2021). Vaccination in pregnancy - the when, what and how? Eur. J. obstetrics, Gynecol. reproductive Biol. 265, 1–6. doi:10.1016/j.ejogrb.2021.08.009
Dahesh, S., Hensler, M. E., Van Sorge, N. M., Gertz, R. E., Schrag, S., Nizet, V., et al. (2008). Point mutation in the group B streptococcal pbp2x gene conferring decreased susceptibility to beta-lactam antibiotics. Antimicrob. agents Chemother. 52 (8), 2915–2918. doi:10.1128/AAC.00461-08
Danne, C., and Dramsi, S. (2012). Pili of Gram-positive bacteria: roles in host colonization. Res. Microbiol. 163 (9), 645–658. doi:10.1016/j.resmic.2012.10.012
de Azavedo, J. C., McGavin, M., Duncan, C., Low, D. E., and McGeer, A. (2001). Prevalence and mechanisms of macrolide resistance in invasive and noninvasive group B streptococcus isolates from Ontario, Canada. Antimicrob. agents Chemother. 45 (12), 3504–3508. doi:10.1128/AAC.45.12.3504-3508.2001
Delara, M., Vadlamudi, N. K., and Sadarangani, M. (2023). Strategies to prevent early and late-onset group B streptococcal infection via interventions in pregnancy. Pathog. Basel, Switz. 12 (2), 229. doi:10.3390/pathogens12020229
Demczuk, W., Martin, I., Griffith, A., Lefebvre, B., McGeer, A., Tyrrell, G. J., et al. (2022). Linear regression equations to predict β-lactam, macrolide, lincosamide, and fluoroquinolone MICs from molecular antimicrobial resistance determinants in Streptococcus pneumoniae. Antimicrob. agents Chemother. 66 (1), 01370211–e201321. doi:10.1128/AAC.01370-21
Deng, L., Spencer, B. L., Holmes, J. A., Mu, R., Rego, S., Weston, T. A., et al. (2019). The Group B Streptococcal surface antigen I/II protein, BspC, interacts with host vimentin to promote adherence to brain endothelium and inflammation during the pathogenesis of meningitis. PLoS Pathog. 15 (6), e1007848. doi:10.1371/journal.ppat.1007848
de Vos, W. M., Tilg, H., Van Hul, M., and Cani, P. D. (2022). Gut microbiome and health: mechanistic insights. Gut 71 (5), 1020–1032. doi:10.1136/gutjnl-2021-326789
Dilrukshi, G. N., Kottahachchi, J., Dissanayake, D., Pathiraja, R. P., Karunasingha, J., Sampath, M. K. A., et al. (2021). Group B Streptococcus colonisation and their antimicrobial susceptibility among pregnant women attending antenatal clinics in tertiary care hospitals in the Western Province of Sri Lanka. J. obstetrics Gynaecol. J. Inst. Obstetrics Gynaecol. 41 (1), 1–6. doi:10.1080/01443615.2020.1716313
DiPersio, L. P., and DiPersio, J. R. (2006). High rates of erythromycin and clindamycin resistance among OBGYN isolates of group B Streptococcus. Diagnostic Microbiol. Infect. Dis. 54 (1), 79–82. doi:10.1016/j.diagmicrobio.2005.07.003
Dobrut, A., and Brzychczy-Włoch, M. (2022). Immunogenic proteins of group B Streptococcus—potential antigens in immunodiagnostic assay for GBS detection. Pathog. Basel, Switz. 11 (1), 43. doi:10.3390/pathogens11010043
Dominguez, K., and Randis, T. M. (2022). Toward the development of a protein-based group B Streptococcus vaccine. Cell. Rep. Med. 3 (2), 100536. doi:10.1016/j.xcrm.2022.100536
Dos Santos, N. F. B., da Silva, L. R., Costa, F., de Mattos, D. M., de Carvalho, E., Ferreira, L. C. S., et al. (2020). Immunization with a recombinant BibA surface protein confers immunity and protects mice against group B Streptococcus (GBS) vaginal colonization. Vaccine 38 (33), 5286–5296. doi:10.1016/j.vaccine.2020.05.076
dOtreppe, S., Lefèvre, P., Meex, C., Devey, A., Sacheli, R., Gerard, M., et al. (2023). Multicenter performance evaluation of the Revogene(®) GBS DS real-time PCR assay for group B Streptococcus detection during labor. Mol. diagnosis Ther. 27 (5), 611–620. doi:10.1007/s40291-023-00660-3
Du, Vu, Thai Dung, P., and Toan, N. L. (2021). al. e: High Rates of Streptococcus Agalactiae Clindamycin and Erythromycin Resistance in Vietnamese Pregnant Women. Res. Square. doi:10.21203/rs.21203.rs-196314/v196311
Duffy, C. R., Huang, Y., Andrikopoulou, M., Stern-Ascher, C. N., Wright, J. D., D'Alton, M. E., et al. (2022). Vancomycin during delivery hospitalizations for women with group B streptococcus. J. maternal-fetal neonatal Med. official J. Eur. Assoc. Perinat. Med. Fed. Asia Ocean. Perinat. Soc. Int. Soc. Perinat. Obstet 35 (5), 898–906. doi:10.1080/14767058.2020.1733520
Duke, J. A., Paschall, A. V., Robinson, L. S., Knoot, C. J., Vinogradov, E., Scott, N. E., et al. (2021). Development and immunogenicity of a prototype multivalent group B Streptococcus bioconjugate vaccine. ACS Infect. Dis. 7 (11), 3111–3123. doi:10.1021/acsinfecdis.1c00415
Dutra, V. G., Alves, V. M., Olendzki, A. N., Dias, C. A., de Bastos, A. F., Santos, G. O., et al. (2014). Streptococcus agalactiae in Brazil: serotype distribution, virulence determinants and antimicrobial susceptibility. BMC Infect. Dis. 14, 323. doi:10.1186/1471-2334-14-323
El Beitune, P., Duarte, G., Maffei, C. M., Quintana, S. M., De Sá Rosa, ESAC, and Nogueira, A. A. (2006). Group B Streptococcus carriers among HIV-1 infected pregnant women: prevalence and risk factors. Eur. J. obstetrics, Gynecol. reproductive Biol. 128 (1-2), 54–58. doi:10.1016/j.ejogrb.2006.02.017
Elinav, E., Garrett, W. S., Trinchieri, G., and Wargo, J. (2019). The cancer microbiome. Nat. Rev. Cancer 19 (7), 371–376. doi:10.1038/s41568-019-0155-3
Erickson Keesha, E., Otoupal Peter, B., and Chatterjee, A. (2017). Transcriptome-level signatures in gene expression and gene expression variability during bacterial adaptive evolution. mSphere 2 (1), e00009. doi:10.1128/mSphere.00009-17
Faccone, D., Lalonardi, F., Abel, S., Machain, M., Errecalde, L., Littvik, A., et al. (2010). Multiple-Clones of Streptococcus agalactiae harbouring lnuB gene. J. Infect. Dev. Ctries. 4 (9), 580–582. doi:10.3855/jidc.941
Farrell, D. J., and Felmingham, D. (2004). Activities of telithromycin against 13,874 Streptococcus pneumoniae isolates collected between 1999 and 2003. Antimicrob. agents Chemother. 48 (5), 1882–1884. doi:10.1128/aac.48.5.1882-1884.2004
Finsterer, J. (2022). Triggers of guillain-barré syndrome: Campylobacter jejuni predominates. Int. J. Mol. Sci. 23 (22), 14222. doi:10.3390/ijms232214222
Fischer, P., Pawlowski, A., Cao, D., Bell, D., Kitson, G., Darsley, M., et al. (2021). Safety and immunogenicity of a prototype recombinant alpha-like protein subunit vaccine (GBS-NN) against Group B Streptococcus in a randomised placebo-controlled double-blind phase 1 trial in healthy adult women. Vaccine 39 (32), 4489–4499. doi:10.1016/j.vaccine.2021.06.046
Fugaban, J. I. I., Holzapfel, W. H., and Todorov, S. D. (2021). Probiotic potential and safety assessment of bacteriocinogenic Enterococcus faecium strains with antibacterial activity against Listeria and vancomycin-resistant enterococci. Curr. Res. Microb. Sci. 2, 100070. doi:10.1016/j.crmicr.2021.100070
Furfaro, L. L., Chang, B. J., and Payne, M. S. (2018). Perinatal Streptococcus agalactiae epidemiology and surveillance targets. Clin. Microbiol. Rev. 31 (4), e00049. doi:10.1128/CMR.00049-18
Gabrielsen, C., Mæland, J. A., Lyng, R. V., Radtke, A., and Afset, J. E. (2017). Molecular characteristics of Streptococcus agalactiae strains deficient in alpha-like protein encoding genes. J. Med. Microbiol. 66 (1), 26–33. doi:10.1099/jmm.0.000412
Gavinski, K., and DiNardo, D. (2023). Cervical cancer screening. Med. Clin. N. Am. 107 (2), 259–269. doi:10.1016/j.mcna.2022.10.006
Gendrin, C., Shubin, N. J., Boldenow, E., Merillat, S., Clauson, M., Power, D., et al. (2018). Mast cell chymase decreases the severity of group B Streptococcus infections. J. allergy Clin. Immunol. 142 (1), 120–129. doi:10.1016/j.jaci.2017.07.042
Goel, N., Wattal, C., Gujral, K., Dhaduk, N., Mansukhani, C., Garg, P., et al. (2020). Group B Streptococcus in Indian pregnant women: its prevalence and risk factors. Indian J. Med. Microbiol. 38 (3 and 4), 357–361. doi:10.4103/ijmm.IJMM_20_333
Gonçalves, B. P., Procter, S. R., Paul, P., Chandna, J., Lewin, A., Seedat, F., et al. (2022). Group B streptococcus infection during pregnancy and infancy: estimates of regional and global burden. Lancet Glob. health 10 (6), e807–e819. doi:10.1016/S2214-109X(22)00093-6
Granlund, M., Axemo, P., Bremme, K., Bryngelsson, A. L., Carlsson Wallin, M., Ekström, C. M., et al. (2010). Antimicrobial resistance in colonizing group B Streptococci before the implementation of a Swedish intrapartum antibiotic prophylaxis program. Eur. J. Clin. Microbiol. Infect. Dis. 29 (10), 1195–1201. doi:10.1007/s10096-010-0877-3
Gurudas, G., Arjun, R., Jain, N., Ranganayaki, V., Sasikumar, C., Mohan, V., et al. (2022). Prevalence of Group B Streptococcus in pregnant women in Kerala and relation to neonatal outcomes: a prospective cross-sectional study. J. Trop. Pediatr. 68 (6), fmac092. doi:10.1093/tropej/fmac092
Hanson, S., Nelson, G., Preszler, M., Laible, B., Nazir, J., and Siewert, A. (2022). Antibiotic prescribing practices in group B Streptococcus positive obstetric patients with penicillin allergy. S. D. Med. J. S. D. State Med. Assoc. 75 (10), 462–468.
Hayes, K., O'Halloran, F., and Cotter, L. (2020). A review of antibiotic resistance in Group B Streptococcus: the story so far. Crit. Rev. Microbiol. 46 (3), 253–269. doi:10.1080/1040841X.2020.1758626
Heyar, A. K., Kaur, K., Gill, A. K., and Gill, P. K. (2020). Induction of clindamycin resistance in clinical isolates of staphylococcus aureus from a tertiary care hospital. Int. J. Med. Biomed. Stud. 4 (12). doi:10.32553/ijmbs.v4i12.1566
Hsu, J. F., Chen, Y. N., Chu, S. M., Lee, W. J., Huang, H. R., Chiang, M. C., et al. (2023). Clonal complex 12 serotype Ib Streptococcus agalactiae strain causing complicated sepsis in neonates: clinical features and genetic characteristics. Microbiol. Spectr. 11 (1), e0377822. doi:10.1128/spectrum.03778-22
Hsu, J. F., Lu, J. J., Lin, C., Chu, S. M., Lin, L. C., Lai, M. Y., et al. (2021). Clustered regularly interspaced short palindromic repeat analysis of clonal complex 17 serotype III group B Streptococcus strains causing neonatal invasive diseases. Int. J. Mol. Sci. 22 (21), 11626. doi:10.3390/ijms222111626
Huang, J., Lin, X.-Z., Zhu, Y., and Chen, C. (2019). Epidemiology of group B streptococcal infection in pregnant women and diseased infants in mainland China. Pediatr. Neonatol. 60 (5), 487–495. doi:10.1016/j.pedneo.2019.07.001
Huiling, C., Jia-de, D., Hui-fen, Y., YouMin, L., Yingzi, C., and Xiao-mian, Z. (2010). Detection of erythromycin and tetracycline resistance-related genes in invasive infections due to group B streptococci. Chin. J. Nosocomiology 20, 1354–1357.
Husen, O., Kannaiyan Abbai, M., Aliyo, A., Daka, D., Gemechu, T., Tilahun, D., et al. (2023). Prevalence, antimicrobial susceptibility pattern and associated factors of group B Streptococcus among pregnant women attending antenatal care at bule hora university teaching hospital, southern Ethiopia. Infect. drug Resist. 16, 4421–4433. doi:10.2147/IDR.S415414
Ikebe, T., Okuno, R., Uchitani, Y., Takano, M., Yamaguchi, T., Otsuka, H., et al. (2023). Serotype distribution and antimicrobial resistance of Streptococcus agalactiae isolates in nonpregnant adults with streptococcal toxic shock syndrome in Japan in 2014 to 2021. Microbiol. Spectr. 11 (2), e0498722. doi:10.1128/spectrum.04987-22
Jia-de, D. (2010) Detection of erythromycin and tetracycline resistant-related genes in skin and soft tissue infection due to group B streptococci. Int. J. Lab. Med. 31 (10), 1057–1058. doi:10.3969/j.issn.1673-4130.2010.10.001
Jung, Y. J., Huynh, B. T., Seck, A., Bercion, R., Sarr, F. D., Herindrainy, P., et al. (2021). Prevalence and factors associated with maternal group B Streptococcus colonization in Madagascar and Senegal. Am. J. Trop. Med. Hyg. 105 (5), 1339–1346. doi:10.4269/ajtmh.21-0113
Kalliola, S., Vuopio-Varkila, J., Takala, A. K., and Eskola, J. (1999). Neonatal group B streptococcal disease in Finland: a ten-year nationwide study. Pediatr. Infect. Dis. J. 18 (9), 806–810. doi:10.1097/00006454-199909000-00012
Kamal, M. (2022). Pap smear collection and preparation: key points. CytoJournal 19, 24. doi:10.25259/CMAS_03_05_2021
Kamga, Y. M., Ngunde, J. P., and Akoachere, J. K. T. (2019). Prevalence of bacterial vaginosis and associated risk factors in pregnant women receiving antenatal care at the Kumba Health District (KHD), Cameroon. BMC pregnancy childbirth 19 (1), 166. doi:10.1186/s12884-019-2312-9
Kamińska, D., Ratajczak, M., Nowak-Malczewska, D. M., Karolak, J. A., Kwaśniewski, M., Szumala-Kakol, A., et al. (2024). Macrolide and lincosamide resistance of Streptococcus agalactiae in pregnant women in Poland. Sci. Rep. 14 (1), 3877. doi:10.1038/s41598-024-54521-y
Kao, Y., Tsai, M.-H., Lai, M.-Y., Chu, S.-M., Huang, H.-R., Chiang, M.-C., et al. (2019). Emerging serotype III sequence type 17 group B streptococcus invasive infection in infants: the clinical characteristics and impacts on outcomes. BMC Infect. Dis. 19 (1), 538. doi:10.1186/s12879-019-4177-y
Kardos, S., Tóthpál, A., Laub, K., Kristóf, K., Ostorházi, E., Rozgonyi, F., et al. (2019). High prevalence of group B streptococcus ST17 hypervirulent clone among non-pregnant patients from a Hungarian venereology clinic. BMC Infect. Dis. 19 (1), 1009. doi:10.1186/s12879-019-4626-7
Kekic, D., Gajic, I., Opavski, N., Kojic, M., Vukotic, G., Smitran, A., et al. (2021). Trends in molecular characteristics and antimicrobial resistance of group B streptococci: a multicenter study in Serbia, 2015-2020. Sci. Rep. 11 (1), 540. doi:10.1038/s41598-020-79354-3
Khan, U. B., Portal, E. A. R., Sands, K., Lo, S., Chalker, V. J., Jauneikaite, E., et al. (2023). Genomic analysis reveals new integrative conjugal elements and transposons in GBS conferring antimicrobial resistance. Antibiotics 12 (3), 544. doi:10.3390/antibiotics12030544
Kimura, K., Suzuki, S., Wachino, J., Kurokawa, H., Yamane, K., Shibata, N., et al. (2008). First molecular characterization of group B streptococci with reduced penicillin susceptibility. Antimicrob. agents Chemother. 52 (8), 2890–2897. doi:10.1128/AAC.00185-08
Kling, D. E., Cavicchio, A. J., Sollinger, C. A., Madoff, L. C., Schnitzer, J. J., and Kinane, T. B. (2009). Lactic acid is a potential virulence factor for group B Streptococcus. Microb. Pathog. 46 (1), 43–52. doi:10.1016/j.micpath.2008.10.009
Kobayashi, M., Vekemans, J., Baker, C. J., Ratner, A. J., Le Doare, K., Schrag, S. J., et al. (2016). Group B Streptococcus vaccine development: present status and future considerations, with emphasis on perspectives for low and middle income countries. F1000Research 5, 2355. doi:10.12688/f1000research.9363.1
Koide, S., Hayashi, W., Taniguchi, Y., Tanaka, H., Kimura, K., Nagano, Y., et al. (2019). Potential effect of selective pressure with different β-lactam molecules on the emergence of reduced susceptibility to β-lactams in group B Streptococci. Microbiol. Immunol. 63 (2), 65–76. doi:10.1111/1348-0421.12667
Koide, S., Nagano, Y., Takizawa, S., Sakaguchi, K., Soga, E., Hayashi, W., et al. (2022). Genomic traits associated with virulence and antimicrobial resistance of invasive group B Streptococcus isolates with reduced penicillin susceptibility from elderly adults. Microbiol. Spectr. 10 (3), 00568222–e100522. doi:10.1128/spectrum.00568-22
Koo, J., Escajadillo, T., Zhang, L., Nizet, V., and Lawrence, S. M. (2019). Erythrocyte-coated nanoparticles block cytotoxic effects of group B Streptococcus β-hemolysin/cytolysin. Front. Pediatr. 7, 410. doi:10.3389/fped.2019.00410
Korir, M. L., Manning, S. D., and Davies, H. D. (2017). Intrinsic maturational neonatal immune deficiencies and susceptibility to group B Streptococcus infection. Clin. Microbiol. Rev. 30 (4), 973–989. doi:10.1128/CMR.00019-17
Kumar Chaudhary, N., and Piya, R. (2021). Macrolide-lincosamide-streptogramin B resistance among staphylococcus aureus in chitwan medical college teaching hospital, Nepal. Asian J. Pharm. Clin. Res., 61–65. doi:10.22159/ajpcr.2021.v14i5.41012
Kuperwaser, F., Avital, G., Vaz, M. J., Noble, K. N., Dammann, A. N., Randis, T. M., et al. (2023). Host inflammatory dynamics reveal placental immune modulation by Group B Streptococcus during pregnancy. Mol. Syst. Biol. 19 (3), e11021. doi:10.15252/msb.202211021
Kurian, N. K., and Modi, D. (2022). Mechanisms of group B Streptococcus-mediated preterm birth: lessons learnt from animal models. Reproduction Fertil. 3 (3), R109–r120. doi:10.1530/RAF-21-0105
Lacasse, M., Valentin, A.-S., Corvec, S., Bémer, P., Jolivet-Gougeon, A., Plouzeau, C., et al. (2022). Genotypic characterization and biofilm production of group B Streptococcus strains isolated from bone and Joint infections. Microbiol. Spectr. 10 (2), 02329211–e302321. doi:10.1128/spectrum.02329-21
Lamagni, T. L., Keshishian, C., Efstratiou, A., Guy, R., Henderson, K. L., Broughton, K., et al. (2013). Emerging trends in the epidemiology of invasive group B streptococcal disease in England and Wales, 1991-2010. Clin. Infect. Dis. official Publ. Infect. Dis. Soc. Am. 57 (5), 682–688. doi:10.1093/cid/cit337
Le Doare, K., O'Driscoll, M., Turner, K., Seedat, F., Russell, N. J., Seale, A. C., et al. (2017). Intrapartum antibiotic chemoprophylaxis policies for the prevention of group B streptococcal disease worldwide: systematic review. Clin. Infect. Dis. official Publ. Infect. Dis. Soc. Am. 65 (Suppl. l_2), S143–s151. doi:10.1093/cid/cix654
Le Gallou, B., Pastuszka, A., Lemaire, C., Mereghetti, L., and Lanotte, P. (2023). Group B Streptococcus CRISPR1 typing of maternal, fetal, and neonatal infectious disease isolates highlights the importance of CC1 in in utero fetal death. Microbiol. Spectr. 11 (4), e0522122. doi:10.1128/spectrum.05221-22
Li, J., Ji, W., Gao, K., Zhou, H., Zhang, L., Mu, X., et al. (2019). Molecular characteristics of group B Streptococcus isolates from infants in southern mainland China. BMC Infect. Dis. 19 (1), 812. doi:10.1186/s12879-019-4434-0
Lin, E., Zou, S., Wang, Y., Lee, C. C., Chiu, C. H., and Feng, Y. (2021a). Phylogeny, recombination, and invasiveness of group B Streptococcus revealed by genomic comparisons of its global strains. Eur. J. Clin. Microbiol. Infect. Dis. official Publ. Eur. Soc. Clin. Microbiol. 40 (3), 581–590. doi:10.1007/s10096-020-04067-4
Lin, L., Huang, X., Yang, H., He, Y., He, X., Huang, J., et al. (2021b). Molecular epidemiology, antimicrobial activity, and virulence gene clustering of Streptococcus agalactiae isolated from dairy cattle with mastitis in China. J. dairy Sci. 104 (4), 4893–4903. doi:10.3168/jds.2020-19139
Lin, S. M., Jang, A. Y., Zhi, Y., Gao, S., Lim, S., Lim, J. H., et al. (2017). Vaccination with a latch peptide provides serotype-independent protection against group B Streptococcus infection in mice. J. Infect. Dis. 217 (1), 93–102. doi:10.1093/infdis/jix565
Lin, S. M., Zhi, Y., Ahn, K. B., Lim, S., and Seo, H. S. (2018). Status of group B streptococcal vaccine development. Clin. Exp. vaccine Res. 7 (1), 76–81. doi:10.7774/cevr.2018.7.1.76
Liu, B., Yi, L., Li, J., Wang, Y., Mao, C., and Wang, Y. (2020). Autoinducer-2 influences tetracycline resistance in Streptococcus suis by regulating the tet(M) gene via transposon Tn916. Res. veterinary Sci. 128, 269–274. doi:10.1016/j.rvsc.2019.12.007
Liu, D., Tan, W., Wang, H., Li, W., Fu, J., Li, J., et al. (2023). Genetic diversity and genome-wide association study of 13 agronomic traits in 977 Beta vulgaris L. germplasms. BMC genomics 24 (1), 413. doi:10.1186/s12864-023-09522-y
Liu, H. Q., and Tong, X. M. (2019). A clinical analysis of late-onset sepsis in very low birth weight and extremely low birth weight infants. Zhongguo dang dai er ke za zhi = Chin. J. Contemp. Pediatr. 21 (10), 1038–1043. doi:10.7499/j.issn.1008-8830.2019.10.016
Liu, Y., Li, L., Huang, T., Wu, W., Liang, W., and Chen, M. (2019). The interaction between phagocytes and Streptococcus agalactiae (GBS) mediated by the activated complement system is the key to GBS inducing acute bacterial meningitis of Tilapia. Animals 9 (10), 818. doi:10.3390/ani9100818
Liu, Y., Liu, J., and Group, B. (2022). Group B Streptococcus: virulence factors and pathogenic mechanism. Microorganisms 10 (12), 2483. doi:10.3390/microorganisms10122483
Liu, Y., Zheng, X., Xu, L., Tong, P., Zhu, M., Peng, B., et al. (2021). Prevalence, antimicrobial resistance, and molecular characterization of Staphylococcus aureus isolated from animals, meats, and market environments in xinjiang, China. Foodborne pathogens Dis. 18 (10), 718–726. doi:10.1089/fpd.2020.2863
Longtin, J., Vermeiren, C., Shahinas, D., Tamber, G. S., McGeer, A., Low, D. E., et al. (2011). Novel mutations in a patient isolate of Streptococcus agalactiae with reduced penicillin susceptibility emerging after long-term oral suppressive therapy. Antimicrob. agents Chemother. 55 (6), 2983–2985. doi:10.1128/AAC.01243-10
Lopardo, H. A., Vidal, P., Sparo, M., Jeric, P., Centron, D., Facklam, R. R., et al. (2005). Six-month multicenter study on invasive infections due to Streptococcus pyogenes and Streptococcus dysgalactiae subsp. equisimilis in Argentina. J. Clin. Microbiol. 43 (2), 802–807. doi:10.1128/JCM.43.2.802-807.2005
Lu, B., Li, D., Cui, Y., Sui, W., Huang, L., and Lu, X. (2014). Epidemiology of Group B streptococcus isolated from pregnant women in Beijing, China. Clin. Microbiol. Infect. 20 (6), O370–O373. doi:10.1111/1469-0691.12416
Luo, Q., Liu, N., Pu, S., Zhuang, Z., Gong, H., and Zhang, D. (2023). A review on the research progress on non-pharmacological therapy of Helicobacter pylori. Front. Microbiol. 14, 1134254. doi:10.3389/fmicb.2023.1134254
Madhi, S. A., Anderson, A. S., Absalon, J., Radley, D., Simon, R., Jongihlati, B., et al. (2023). Potential for maternally administered vaccine for infant group B Streptococcus. N. Engl. J. Med. 389 (3), 215–227. doi:10.1056/NEJMoa2116045
Madhi, S. A., Cutland, C. L., Jose, L., Koen, A., Govender, N., Wittke, F., et al. (2016). Safety and immunogenicity of an investigational maternal trivalent group B streptococcus vaccine in healthy women and their infants: a randomised phase 1b/2 trial. Lancet Infect. Dis. 16 (8), 923–934. doi:10.1016/S1473-3099(16)00152-3
Madhi, S. A., Koen, A., Cutland, C. L., Jose, L., Govender, N., Wittke, F., et al. (2017). Antibody kinetics and response to routine vaccinations in infants born to women who received an investigational trivalent group B Streptococcus polysaccharide crm197-conjugate vaccine during pregnancy. Clin. Infect. Dis. official Publ. Infect. Dis. Soc. Am. 65 (11), 1897–1904. doi:10.1093/cid/cix666
Maeda, T., Fukushima, Y., Yoshida, H., Goto, M., Fujita, T., Tsuyuki, Y., et al. (2021). Biofilm production ability and associated characteristics of Streptococcus agalactiae isolates from companion animals and humans. J. Infect. Chemother. official J. Jpn. Soc. Chemother. 27 (11), 1571–1577. doi:10.1016/j.jiac.2021.06.018
Manzanares, Á., Prieto-Tato, L. M., Escosa-García, L., Navarro, M., Guillén, S., Penin, M., et al. (2023). Increased risk of group B streptococcal sepsis and meningitis in HIV-exposed uninfected infants in a high-income country. Eur. J. Pediatr. 182 (2), 575–579. doi:10.1007/s00431-022-04710-6
Margarit, I., Rinaudo, C. D., Galeotti, C. L., Maione, D., Ghezzo, C., Buttazzoni, E., et al. (2009). Preventing bacterial infections with pilus-based vaccines: the group B streptococcus paradigm. J. Infect. Dis. 199 (1), 108–115. doi:10.1086/595564
McGee, L., Chochua, S., Li, Z., Mathis, S., Rivers, J., Metcalf, B., et al. (2021). Multistate, population-based distributions of candidate vaccine targets, clonal complexes, and resistance features of invasive Group B Streptococci within the US: 2015-2017. Clin. Infect. Dis. official Publ. Infect. Dis. Soc. Am. 72 (6), 1004–1013. doi:10.1093/cid/ciaa151
Mei, J. Y., Silverman, N. S., and Group, B. (2023). Group B Streptococcus in pregnancy. Obstetrics Gynecol. Clin. N. Am. 50 (2), 375–387. doi:10.1016/j.ogc.2023.02.009
Mei, Z., and Li, D. (2022). The role of probiotics in vaginal health. Front. Cell. Infect. Microbiol. 12, 963868. doi:10.3389/fcimb.2022.963868
Mejia, M. E., Mercado-Evans, V., Zulk, J. J., Ottinger, S., Ruiz, K., Ballard, M. B., et al. (2023). Vaginal microbial dynamics and pathogen colonization in a humanized microbiota mouse model. bioRxiv Prepr. Serv. Biol., 527909. doi:10.1101/2023.02.09.527909
Metcalf, B. J., Chochua, S., Gertz, R. E., Hawkins, P. A., Ricaldi, J., Li, Z., et al. (2017). Short-read whole genome sequencing for determination of antimicrobial resistance mechanisms and capsular serotypes of current invasive Streptococcus agalactiae recovered in the USA. Clin. Microbiol. Infect. 23 (8), 574.e7–574.e14. doi:10.1016/j.cmi.2017.02.021
Mettu, R., Chen, C. Y., and Wu, C. Y. (2020). Synthetic carbohydrate-based vaccines: challenges and opportunities. J. Biomed. Sci. 27 (1), 9. doi:10.1186/s12929-019-0591-0
Miselli, F., Frabboni, I., Di Martino, M., Zinani, I., Buttera, M., Insalaco, A., et al. (2022). Transmission of Group B Streptococcus in late-onset neonatal disease: a narrative review of current evidence. Ther. Adv. Infect. Dis. 9, 20499361221142732. doi:10.1177/20499361221142732
MMWR (1997). Decreasing incidence of perinatal Group B streptococcal disease--United States, 1993-1995. MMWR Morb. Mortal. Wkly. Rep. 46 (21), 473–477.
Mohammed, L., Javed, M., Althwanay, A., Ahsan, F., Oliveri, F., Goud, H. K., et al. (2020). Live bacteria supplementation as probiotic for managing fishy, odorous vaginal discharge disease of bacterial vaginosis: an alternative treatment option? Cureus 12 (12), e12362. doi:10.7759/cureus.12362
Mu, R., Kim, B. J., Paco, C., Del Rosario, Y., Courtney, H. S., and Doran, K. S. (2014). Identification of a group B streptococcal fibronectin binding protein, SfbA, that contributes to invasion of brain endothelium and development of meningitis. Infect. Immun. 82 (6), 2276–2286. doi:10.1128/IAI.01559-13
Mudzana, R., Mavenyengwa, R. T., and Gudza-Mugabe, M. (2021). Analysis of virulence factors and antibiotic resistance genes in group B streptococcus from clinical samples. BMC Infect. Dis. 21 (1), 125. doi:10.1186/s12879-021-05820-6
Murayama, S. Y., Seki, C., Sakata, H., Sunaoshi, K., Nakayama, E., Iwata, S., et al. (2009). Capsular type and antibiotic resistance in Streptococcus agalactiae isolates from patients, ranging from newborns to the elderly, with invasive infections. Antimicrob. agents Chemother. 53 (6), 2650–2653. doi:10.1128/AAC.01716-08
Nader-Macías, M. E. F., De Gregorio, P. R., and Silva, J. A. (2021). Probiotic lactobacilli in formulas and hygiene products for the health of the urogenital tract. Pharmacol. Res. Perspect. 9 (5), e00787. doi:10.1002/prp2.787
Nagano, N., Nagano, Y., Toyama, M., Kimura, K., Tamura, T., Shibayama, K., et al. (2012). Nosocomial spread of multidrug-resistant group B streptococci with reduced penicillin susceptibility belonging to clonal complex 1. J. Antimicrob. Chemother. 67 (4), 849–856. doi:10.1093/jac/dkr546
Nanduri, S. A., Petit, S., Smelser, C., Apostol, M., Alden, N. B., Harrison, L. H., et al. (2019). Epidemiology of invasive early-onset and late-onset group B streptococcal disease in the United States, 2006 to 2015: multistate laboratory and population-based surveillance. JAMA Pediatr. 173 (3), 224–233. doi:10.1001/jamapediatrics.2018.4826
Nitzan, O., Elias, M., Peretz, A., and Saliba, W. (2016). Role of antibiotics for treatment of inflammatory bowel disease. World J. gastroenterology 22 (3), 1078–1087. doi:10.3748/wjg.v22.i3.1078
Nørgaard, S. M., Jensen, C. S., Aalestrup, J., Vandenbroucke-Grauls, C., de Boer, M. G. J., and Pedersen, A. B. (2019). Choice of therapeutic interventions and outcomes for the treatment of infections caused by multidrug-resistant gram-negative pathogens: a systematic review. Antimicrob. Resist. Infect. control 8, 170. doi:10.1186/s13756-019-0624-1
Nuccitelli, A., Rinaudo, C. D., and Maione, D. (2015). Group B Streptococcus vaccine: state of the art. Ther. Adv. vaccines 3 (3), 76–90. doi:10.1177/2051013615579869
Padekar, H., Samal, B., Dash, L., and J, S. (2020). Prevalence of inducible clindamycin resistance among Staphylococcus aureus isolates from a tertiary care hospital. Ip. Int. J. Med. Microbiol. Trop. Dis. 6 (3), 161–164. doi:10.18231/j.ijmmtd.2020.036
Paoletti, L. C., and Kasper, D. L. (2019). Surface structures of group B Streptococcus important in human immunity. Microbiol. Spectr. 7 (2). doi:10.1128/microbiolspec.GPP3-0001-2017
Park, C., Nichols, M., and Schrag, S. J. (2014). Two cases of invasive vancomycin-resistant group B streptococcus infection. N. Engl. J. Med. 370 (9), 885–886. doi:10.1056/NEJMc1308504
Paul, P., Gonçalves, B. P., Le Doare, K., and Lawn, J. E. (2023). 20 million pregnant women with group B streptococcus carriage: consequences, challenges, and opportunities for prevention. Curr. Opin. Pediatr. 35 (2), 223–230. doi:10.1097/MOP.0000000000001223
Pawlowski, A., Lannergård, J., Gonzalez-Miro, M., Cao, D., Larsson, S., Persson, J. J., et al. (2022). A group B Streptococcus alpha-like protein subunit vaccine induces functionally active antibodies in humans targeting homotypic and heterotypic strains. Cell. Rep. Med. 3 (2), 100511. doi:10.1016/j.xcrm.2022.100511
Persson, E., Berg, S., Bergseng, H., Bergh, K., Valsö-Lyng, R., and Trollfors, B. (2008). Antimicrobial susceptibility of invasive group B streptococcal isolates from south-west Sweden 1988-2001. Scand. J. Infect. Dis. 40 (4), 308–313. doi:10.1080/00365540701678702
Pezzicoli, A., Santi, I., Lauer, P., Rosini, R., Rinaudo, D., Grandi, G., et al. (2008). Pilus backbone contributes to group B Streptococcus paracellular translocation through epithelial cells. J. Infect. Dis. 198 (6), 890–898. doi:10.1086/591182
Piccinelli, G., Biscaro, V., Gargiulo, F., Caruso, A., and De Francesco, M. A. (2015b). Characterization and antibiotic susceptibility of Streptococcus agalactiae isolates causing urinary tract infections. Infect. Genet. Evol. J. Mol. Epidemiol. Evol. Genet. Infect. Dis. 34, 1–6. doi:10.1016/j.meegid.2015.07.001
Piccinelli, G., Gargiulo, F., Corbellini, S., Ravizzola, G., Bonfanti, C., Caruso, A., et al. (2015a). Emergence of the first levofloxacin-resistant strains of Streptococcus agalactiae isolated in Italy. Antimicrob. agents Chemother. 59 (4), 2466–2469. doi:10.1128/AAC.05127-14
Pierański, M. K., Kosiński, J. G., Szymczak, K., Sadowski, P., and Grinholc, M. (2023). Antimicrobial photodynamic inactivation: an alternative for group B Streptococcus vaginal colonization in a murine experimental model. Antioxidants Basel, Switz. 12 (4), 847. doi:10.3390/antiox12040847
Pietrocola, G., Arciola, C. R., Rindi, S., Montanaro, L., and Speziale, P. (2018). Streptococcus agalactiae non-pilus, cell wall-anchored proteins: involvement in colonization and pathogenesis and potential as vaccine candidates. Front. Immunol. 9, 602. doi:10.3389/fimmu.2018.00602
Piliponsky, A. M., Sharma, K., Quach, P., Brokaw, A., Nguyen, S., Orvis, A., et al. (2022). Mast cell-derived factor XIIIA contributes to sexual dimorphic defense against group B streptococcal infections. J. Clin. investigation 132 (20), e157999. doi:10.1172/JCI157999
Platt, M. W., and Gilson, G. J. (1994). Group B streptococcal disease in the perinatal period. Am. Fam. physician 49 (2), 434–442.
Pourmadadi, M., Moammeri, A., Shamsabadipour, A., Moghaddam, Y. F., Rahdar, A., and Pandey, S. (2023). Application of various optical and electrochemical nanobiosensors for detecting cancer antigen 125 (CA-125): a review. Biosensors 13 (1), 99. doi:10.3390/bios13010099
Preenanka, R., and Safeena, M. P. (2023). Morphological, biological and genomic characterization of lytic phages against Streptococcus agalactiae causing streptococcosis in tilapia. Microb. Pathog. 174, 105919. doi:10.1016/j.micpath.2022.105919
Preventing neonatal group B streptococcal infection (2011). Preventing neonatal group B streptococcal infection. Intrapartum antibiotic prophylaxis in some high-risk situations. Prescrire Int. 20 (114), 72–77.
Prevention of Group B Streptococcal Early-Onset Disease in Newborns: ACOG Committee Opinion (2020). Prevention of group B streptococcal early-onset disease in newborns: ACOG committee opinion, number 797. Obstetrics Gynecol. 135 (2), e51–e72. doi:10.1097/AOG.0000000000003668
Procter, S. R., Gonçalves, B. P., Paul, P., Chandna, J., Seedat, F., Koukounari, A., et al. (2023). Maternal immunisation against Group B Streptococcus: a global analysis of health impact and cost-effectiveness. PLoS Med. 20 (3), e1004068. doi:10.1371/journal.pmed.1004068
Pulingam, T., Parumasivam, T., Gazzali, A. M., Sulaiman, A. M., Chee, J. Y., Lakshmanan, M., et al. (2022). Antimicrobial resistance: prevalence, economic burden, mechanisms of resistance and strategies to overcome. Eur. J. Pharm. Sci. official J. Eur. Fed. Pharm. Sci. 170, 106103. doi:10.1016/j.ejps.2021.106103
Raabe, V. N., Shane, A. L., and Group, B. (2019). Group B Streptococcus (Streptococcus agalactiae). Microbiol. Spectr. 7 (2). doi:10.1128/microbiolspec.GPP3-0007-2018
Ragunathan, P., Sridaran, D., Weigel, A., Shabayek, S., Spellerberg, B., and Ponnuraj, K. (2013). Metal binding is critical for the folding and function of laminin binding protein, lmb of Streptococcus agalactiae. PloS one 8, e67517. doi:10.1371/journal.pone.0067517
Rajagopal, L. (2009). Understanding the regulation of Group B Streptococcal virulence factors. Future Microbiol. 4 (2), 201–221. doi:10.2217/17460913.4.2.201
Reza, A., Sutton, J. M., and Rahman, K. M. (2019). Effectiveness of efflux pump inhibitors as biofilm disruptors and resistance breakers in gram-negative (ESKAPEE) bacteria. Antibiot. Basel, Switz. 8 (4), 229. doi:10.3390/antibiotics8040229
Risser, F., López-Morales, J., and Nash, M. A. (2022). Adhesive virulence factors of Staphylococcus aureus resist digestion by coagulation proteases thrombin and plasmin. ACS Bio Med Chem Au 2 (6), 586–599. doi:10.1021/acsbiomedchemau.2c00042
Rosa-Fraile, M., Dramsi, S., and Spellerberg, B. (2014). Group B streptococcal haemolysin and pigment, a tale of twins. FEMS Microbiol. Rev. 38 (5), 932–946. doi:10.1111/1574-6976.12071
Sadowy, E., Matynia, B., and Hryniewicz, W. (2010). Population structure, virulence factors and resistance determinants of invasive, non-invasive and colonizing Streptococcus agalactiae in Poland. J. Antimicrob. Chemother. 65 (9), 1907–1914. doi:10.1093/jac/dkq230
Santana, F. A. F., de Oliveira, T. V. L., Filho, M. B. S., da Silva, L. S. C., de Brito, B. B., de Melo, F. F., et al. (2020). Streptococcus agalactiae: identification methods, antimicrobial susceptibility, and resistance genes in pregnant women. World J. Clin. cases 8 (18), 3988–3998. doi:10.12998/wjcc.v8.i18.3988
Santi, I., Scarselli, M., Mariani, M., Pezzicoli, A., Masignani, V., Taddei, A., et al. (2007). BibA: a novel immunogenic bacterial adhesin contributing to group B Streptococcus survival in human blood. Mol. Microbiol. 63 (3), 754–767. doi:10.1111/j.1365-2958.2006.05555.x
Santillan, D. A., Andracki, M. E., and Hunter, S. K. (2008). Protective immunization in mice against group B streptococci using encapsulated C5a peptidase. Am. J. obstetrics Gynecol. 198 (1), 114.e111–e6. doi:10.1016/j.ajog.2007.06.003
Santillan, D. A., Rai, K. K., Santillan, M. K., Krishnamachari, Y., Salem, A. K., and Hunter, S. K. (2011). Efficacy of polymeric encapsulated C5a peptidase–based group B streptococcus vaccines in a murine model. Am. J. obstetrics Gynecol. 205 (3), 249.e241–e8. doi:10.1016/j.ajog.2011.06.024
Savoia, D., Gottimer, C., Crocilla, C., and Zucca, M. (2008). Streptococcus agalactiae in pregnant women: phenotypic and genotypic characters. J. Infect. 56 (2), 120–125. doi:10.1016/j.jinf.2007.11.007
Sayres, L. C., Younge, N. E., Rikard, B., Corcoran, D. L., Modliszewski, J. L., and Hughes, B. L. (2023). The gestational membrane microbiome in the presence or absence of intraamniotic infection. Am. J. obstetrics Gynecol. MFM 5 (3), 100837. doi:10.1016/j.ajogmf.2022.100837
Schindler, Y., Rahav, G., Nissan, I., Treygerman, O., Prajgrod, G., Attia, B. Z., et al. (2023). Group B streptococcus virulence factors associated with different clinical syndromes: asymptomatic carriage in pregnant women and early-onset disease in the newborn. Front. Microbiol. 14, 1093288. doi:10.3389/fmicb.2023.1093288
Schrag, S., Gorwitz, R., Fultz-Butts, K., and Schuchat, A. (2002). Prevention of perinatal group B streptococcal disease. Revised guidelines from CDC. MMWR Recomm. Rep. Morb. Mortal. Wkly. Rep. Recomm. Rep. 51 (Rr-11), 1–22.
Schuchat, A. (1995). Group B streptococcal disease in newborns: a global perspective on prevention. Biomed. Pharmacother. = Biomedecine Pharmacother. 49 (1), 19–25. doi:10.1016/0753-3322(96)82573-X
Seo, Y. S., Srinivasan, U., Oh, K. Y., Shin, J. H., Chae, J. D., Kim, M. Y., et al. (2010). Changing molecular epidemiology of group B streptococcus in Korea. J. Korean Med. Sci. 25 (6), 817–823. doi:10.3346/jkms.2010.25.6.817
Shabayek, S., and Abdalla, S. (2014). Macrolide- and tetracycline-resistance determinants of colonizing group B streptococcus in women in Egypt. J. Med. Microbiol. 63 (Pt 10), 1324–1327. doi:10.1099/jmm.0.077057-0
Shabayek, S., Spellerberg, B., and Group, B. (2018). Group B streptococcal colonization, molecular characteristics, and epidemiology. Front. Microbiol. 9, 437. doi:10.3389/fmicb.2018.00437
Shen, A. D., Zhang, G. R., Wang, Y. H., and Yang, Y. H. (2005). Susceptibility patterns and mechanisms of macrolide resistance in group B streptococcus isolates. Zhonghua er ke za zhi = Chin. J. Pediatr. 43 (9), 661–664.
Shimizu, A., Tsukagoshi, H., Sekizuka, T., Kuroda, M., Koizumi, A., Fujita, M., et al. (2020). Meningitis and bacteremia by nonhemolytic Group B Streptococcus strain: a whole genome analysis. Microbiol. Immunol. 64 (9), 630–634. doi:10.1111/1348-0421.12826
Sidky, I., and Thomas, M. (2002). Prevalence of Group B streptococcal infection colonisation in pregnant women and their offspring in the Middle East. J. Obstetrics Gynaecol. 22 (2), 179–180. doi:10.1080/01443610120113364
Simonsen, K. A., Anderson-Berry, A. L., Delair, S. F., and Davies, H. D. (2014). Early-onset neonatal sepsis. Clin. Microbiol. Rev. 27 (1), 21–47. doi:10.1128/CMR.00031-13
Sitkiewicz, I., Green, N. M., Guo, N., Bongiovanni, A. M., Witkin, S. S., and Musser, J. M. (2009). Transcriptome adaptation of group B Streptococcus to growth in human amniotic fluid. PloS one 4 (7), e6114. doi:10.1371/journal.pone.0006114
Slotved, H. C., and Hoffmann, S. (2020). The epidemiology of invasive group B Streptococcus in Denmark from 2005 to 2018. Front. public health 8, 40. doi:10.3389/fpubh.2020.00040
Song, K. E., Hwang, N., Ham, J. Y., Cha, H. H., Chong, G. O., and Lee, N. Y. (2022). Prevalence of group B Streptococcus colonization in pregnant women at a university hospital in Korea. Clin. Lab. 68 (8). doi:10.7754/Clin.Lab.2021.211126
Spellerberg, B., Rozdzinski, E., Martin, S., Weber-Heynemann, J., Schnitzler, N., Lütticken, R., et al. (1999). Lmb, a protein with similarities to the LraI adhesin family, mediates attachment of Streptococcus agalactiae to human laminin. Infect. Immun. 67 (2), 871–878. doi:10.1128/IAI.67.2.871-878.1999
Spencer, B. L., Deng, L., Patras, K. A., Burcham, Z. M., Sanches, G. F., Nagao, P. E., et al. (2019). Cas9 contributes to group B streptococcal colonization and disease. Front. Microbiol. 10, 1930. doi:10.3389/fmicb.2019.01930
Sravani, A. B., Ghate, V., and Lewis, S. (2023). Human papillomavirus infection, cervical cancer and the less explored role of trace elements. Biol. trace Elem. Res. 201 (3), 1026–1050. doi:10.1007/s12011-022-03226-2
Sroka-Oleksiak, A., Gosiewski, T., Pabian, W., Gurgul, A., Kapusta, P., Ludwig-Słomczyńska, A. H., et al. (2020). Next-generation sequencing as a tool to detect vaginal microbiota disturbances during pregnancy. Microorganisms 8 (11), 1813. doi:10.3390/microorganisms8111813
Stepanović, S., Djukić, S., Veljković, M., Arsić, B., Garalejić, E., and Ranin, L. (2003). Antimicrobial activity of human follicular fluids. Gynecol. obstetric investigation 56 (3), 173–178. doi:10.1159/000074103
Stephens, K., Charnock-Jones, D. S., and Smith, G. C. S. (2023). Group B Streptococcus and the risk of perinatal morbidity and mortality following term labor. Am. J. obstetrics Gynecol. 228 (5s), S1305–s1312. doi:10.1016/j.ajog.2022.07.051
Suffolk, R., Agertoft, L., Johansen, M., and Zachariassen, G. (2019). Late-onset group B streptococcus infections and severe bronchopulmonary dysplasia in an extremely preterm born infant. BMJ case Rep. 12 (7), e229255. doi:10.1136/bcr-2019-229255
Sundin, C. S., Rigg, K., and Ellis, K. K. (2021). Maternal sepsis: presentation, course, treatment, and outcomes. MCN Am. J. maternal child Nurs. 46, 155–160. doi:10.1097/NMC.0000000000000712
Swamy, G. K., Metz, T. D., Edwards, K. M., Soper, D. E., Beigi, R. H., Campbell, J. D., et al. (2020). Safety and immunogenicity of an investigational maternal trivalent group B streptococcus vaccine in pregnant women and their infants: results from a randomized placebo-controlled phase II trial. Vaccine 38 (44), 6930–6940. doi:10.1016/j.vaccine.2020.08.056
Sweeney, E. L., Gardiner, S., Tickner, J., Trim, L., Beagley, K. W., Carey, A. J., et al. (2020). Group B Streptococcus serotypes Ia and V induce differential vaginal immune responses that may contribute to long term colonization of the female reproductive tract. Am. J. reproductive Immunol. 83 (1), e13199. doi:10.1111/aji.13199
Takaya, A., Kitagawa, N., Kuroe, Y., Endo, K., Okazaki, M., Yokoyama, E., et al. (2010). Mutational analysis of reduced telithromycin susceptibility of Streptococcus pneumoniae isolated clinically in Japan. FEMS Microbiol. Lett. 307 (1), 87–93. doi:10.1111/j.1574-6968.2010.01962.x
Talebi Bezmin Abadi, A., Rizvanov, A. A., Haertlé, T., and Blatt, N. L. (2019). World health organization report: current crisis of antibiotic resistance. BioNanoScience 9 (4), 778–788. doi:10.1007/s12668-019-00658-4
Tano, S., Ueno, T., Mayama, M., Yamada, T., Takeda, T., Uno, K., et al. (2021). Relationship between vaginal group B streptococcus colonization in the early stage of pregnancy and preterm birth: a retrospective cohort study. BMC pregnancy childbirth 21 (1), 141. doi:10.1186/s12884-021-03624-9
Tiruvayipati, S., Tang, W. Y., Barkham, T. M. S., and Chen, S. L. (2021). GBS-SBG - GBS serotyping by genome sequencing. Microb. genomics 7 (12), 000688. doi:10.1099/mgen.0.000688
Totadhri, M., Lakshmanan, A., Saraswathy, M. P., and Mane, M. S. (2022). Asymptomatic bacteriuria of pregnant women in a tertiary care centre. J. Educ. health Promot. 11, 249. doi:10.4103/jehp.jehp_1752_21
Tsega, K. G., Tamrat Abebe, Z., Adane, M., and Mulugeta Desta, T. (2015). Prevalence and antibiotic susceptibility pattern of Streptococcus agalactiae among pregnant women at adigrat zonal hospital and adigrat health center, tigray, Ethiopia. J. Gynecol. Obstetrics 3 (2), 29–35. doi:10.11648/j.jgo.20150302.13
Tulyaprawat, O., Pharkjaksu, S., Shrestha, R. K., and Ngamskulrungroj, P. (2021). Emergence of multi-drug resistance and its association with uncommon serotypes of Streptococcus agalactiae isolated from non-neonatal patients in Thailand. Front. Microbiol. 12, 719353. doi:10.3389/fmicb.2021.719353
Upadhyay, K., Talati, A., and Group, B. (2022). Group B streptococcal infections in neonates. Newborn 1 (1), 109–119. doi:10.5005/jp-journals-11002-0022
Uruén, C., García, C., Fraile, L., Tommassen, J., and Arenas, J. (2022). How Streptococcus suis escapes antibiotic treatments. Veterinary Res. 53 (1), 91. doi:10.1186/s13567-022-01111-3
van der Linden, M., Mamede, R., Levina, N., Helwig, P., Vila-Cerqueira, P., Carriço, J. A., et al. (2019). Heterogeneity of penicillin-non-susceptible group B streptococci isolated from a single patient in Germany. J. Antimicrob. Chemother. 75 (2), 296–299. doi:10.1093/jac/dkz465
Van Du, V., Dung, P. T., Toan, N. L., Van Mao, C., Bac, N. T., Van Tong, H., et al. (2021). Antimicrobial resistance in colonizing group B Streptococcus among pregnant women from a hospital in Vietnam. Sci. Rep. 11 (1), 20845. doi:10.1038/s41598-021-00468-3
Verani, J. R., McGee, L., and Schrag, S. J. (2010). Prevention of perinatal group B streptococcal disease--revised guidelines from CDC. MMWR Recomm. Rep. Morb. Mortal. Wkly. Rep. Recomm. Rep. 59 (10), 1–36.
Verma, S., Kumari, M., Pathak, A., Yadav, V., Johri, A. K., and Yadav, P. (2023). Antibiotic resistance, biofilm formation, and virulence genes of Streptococcus agalactiae serotypes of Indian origin. BMC Microbiol. 23 (1), 176. doi:10.1186/s12866-023-02877-y
Vieira, L. L., Perez, A. V., Machado, M. M., Kayser, M. L., Vettori, D. V., Alegretti, A. P., et al. (2019). Group B Streptococcus detection in pregnant women: comparison of qPCR assay, culture, and the Xpert GBS rapid test. BMC pregnancy childbirth 19 (1), 532. doi:10.1186/s12884-019-2681-0
Wang, H., Zhao, C., He, W., Zhang, F., Zhang, L., Cao, B., et al. (2013). High prevalence of fluoroquinolone-resistant group B streptococci among clinical isolates in China and predominance of sequence type 19 with serotype III. Antimicrob. agents Chemother. 57 (3), 1538–1541. doi:10.1128/AAC.02317-12
Wang, J., Zhang, Y., Lin, M., Bao, J., Wang, G., Dong, R., et al. (2023). Maternal colonization with group B Streptococcus and antibiotic resistance in China: systematic review and meta-analyses. Ann. Clin. Microbiol. Antimicrob. 22 (1), 5. doi:10.1186/s12941-023-00553-7
Wang, P., Tong, J. J., Ma, X. H., Song, F. L., Fan, L., Guo, C. M., et al. (2015). Serotypes, antibiotic susceptibilities, and multi-locus sequence type profiles of Streptococcus agalactiae isolates circulating in Beijing, China. PloS one 10 (3), e0120035. doi:10.1371/journal.pone.0120035
Wang, Y., Zhao, Y., Zou, L., Qiao, J., and Benitz, W. E. (2021). Regional variation of early-onset neonatal group B streptococcal disease prevention strategies in mainland China. Pediatr. Infect. Dis. J. 40 (7), 663–668. doi:10.1097/INF.0000000000003089
Wang, Z., Enotarpi, J., Buffi, G., Pezzicoli, A., Gstöttner, C. J., Nicolardi, S., et al. (2022a). Chemical synthesis and immunological evaluation of fragments of the multiantennary group-specific polysaccharide of group B Streptococcus. JACS Au 2 (7), 1724–1735. doi:10.1021/jacsau.2c00302
Wang, Z., Pu, W., Liu, Q., Zhu, M., Chen, Q., Xu, Y., et al. (2022b). Association of gut microbiota composition in pregnant women colonized with group B Streptococcus with maternal blood routine and neonatal blood-gas analysis. Pathog. Basel, Switz. 11 (11), 1297. doi:10.3390/pathogens11111297
Wehbeh, W., Rojas-Diaz, R., Li, X., Mariano, N., Grenner, L., Segal-Maurer, S., et al. (2005). Fluoroquinolone-resistant Streptococcus agalactiae: epidemiology and mechanism of resistance. Antimicrob. agents Chemother. 49 (6), 2495–2497. doi:10.1128/AAC.49.6.2495-2497.2005
Wei, K., and Chen, T. (2021). Vaginal microbiota transplantation for treatment of bacterial vaginosis: a review. Sheng wu gong cheng xue bao = Chin. J. Biotechnol. 37 (11), 3820–3827. doi:10.13345/j.cjb.210163
Wu, B., Su, J., Li, L., Wu, W., Wu, J., Lu, Y., et al. (2019). Phenotypic and genetic differences among group B Streptococcus recovered from neonates and pregnant women in Shenzhen, China: 8-year study. BMC Microbiol. 19 (1), 185. doi:10.1186/s12866-019-1551-2
Xiangru, X. U., Yi, Z., Gang, C., Ming, L., Wen, Z., Xinxin, W. U., et al. (2023). Clinical efficacy of Buzhong Yiqi decoction in the treatment of hospital-acquired pneumonia with multi-drug resistant bacteria: a prospective, randomized, multicenter controlled trial. J. traditional Chin. Med. = Chung i tsa chih ying wen pan 43 (5), 1010–1018. doi:10.19852/j.cnki.jtcm.20230713.002
Xiao, X., Zheng, Z., and Sun, H. (2023). Study on the correlation between genital tract microenvironment and GBS carrier rate of late-stage pregnant women in dongguan. Clin. Lab. 69 (4). doi:10.7754/Clin.Lab.2022.220742
Xu, X., Lewis Marffy, A. L., Keightley, A., McCarthy, A. J., and Geisbrecht, B. V. (2022). Group B Streptococcus surface protein β: structural characterization of a complement factor H-binding motif and its contribution to immune evasion. J. Immunol. 208 (5), 1232–1247. doi:10.4049/jimmunol.2101078
Yao, Z., Jiayin, W., Xinyi, Z., Ling, C., Mingyuan, H., Simin, M., et al. (2020). Identification of group B Streptococcus serotypes and genotypes in late pregnant women and neonates that are associated with neonatal early-onset infection in a south China population. Front. Pediatr. 8, 265. doi:10.3389/fped.2020.00265
Yi, A., Kim, C. K., Kimura, K., Arakawa, Y., Hur, M., Yun, Y. M., et al. (2019). First case in Korea of group B Streptococcus with reduced penicillin susceptibility harboring amino acid substitutions in penicillin-binding protein 2X. Ann. laboratory Med. 39 (4), 414–416. doi:10.3343/alm.2019.39.4.414
Yoshida, H., Goto, M., Takahiro, M., Fukushima, Y., Fujita, T., Tsuyuki, Y., et al. (2021). Intracellular invasion ability of Streptococcus agalactiae among non-invasive isolates from human adults and companion animals in Japan. J. Infect. Chemother. official J. Jpn. Soc. Chemother. 27 (7), 999–1004. doi:10.1016/j.jiac.2021.02.017
Yu, H. W., Lin, H. C., Yang, P. H., Hsu, C. H., Hsieh, W. S., Tsao, L. Y., et al. (2011). Group B streptococcal infection in Taiwan: maternal colonization and neonatal infection. Pediatr. Neonatol. 52 (4), 190–195. doi:10.1016/j.pedneo.2011.05.008
Zadoks, R. N., Middleton, J. R., McDougall, S., Katholm, J., and Schukken, Y. H. (2011). Molecular epidemiology of mastitis pathogens of dairy cattle and comparative relevance to humans. J. mammary Gl. Biol. neoplasia 16 (4), 357–372. doi:10.1007/s10911-011-9236-y
Zastempowska, E., Twarużek, M., Grajewski, J., and Lassa, H. (2022). Virulence factor genes and cytotoxicity of Streptococcus agalactiae isolated from bovine mastitis in Poland. Microbiol. Spectr. 10 (3), e0222421. doi:10.1128/spectrum.02224-21
Zhang, L., Ma, L., Zhu, L., Zhou, X.-H., Xu, L.-J., Guo, C., et al. (2021). Molecular characterization of pathogenic group B streptococcus from a tertiary hospital in Shanxi, China: high incidence of sequence type 10 strains in infants/pregnant women. J. Microbiol. Immunol. Infect. 54 (6), 1094–1100. doi:10.1016/j.jmii.2020.07.018
Zhao, L.-Y., Mei, J.-X., Yu, G., Lei, L., Zhang, W.-H., Liu, K., et al. (2023). Role of the gut microbiota in anticancer therapy: from molecular mechanisms to clinical applications. Signal Transduct. Target. Ther. 8 (1), 201. doi:10.1038/s41392-023-01406-7
Keywords: group B streptococcal, obstetrics and gynecology, antibiotic prophylaxis, group B streptococcal vaccine, microbial therapy
Citation: Liu Y and Ai H (2024) Current research update on group B streptococcal infection related to obstetrics and gynecology. Front. Pharmacol. 15:1395673. doi: 10.3389/fphar.2024.1395673
Received: 04 March 2024; Accepted: 31 May 2024;
Published: 17 June 2024.
Edited by:
Adrian Oo, National University of Singapore, SingaporeReviewed by:
Zhi Xian Kong, University of Malaya, MalaysiaAdzzie Shazleen Azman, Monash University Malaysia, Malaysia
Copyright © 2024 Liu and Ai. This is an open-access article distributed under the terms of the Creative Commons Attribution License (CC BY). The use, distribution or reproduction in other forums is permitted, provided the original author(s) and the copyright owner(s) are credited and that the original publication in this journal is cited, in accordance with accepted academic practice. No use, distribution or reproduction is permitted which does not comply with these terms.
*Correspondence: Hao Ai, bWlyYWNsZXBla2luZzIwMTBAMTYzLmNvbQ==