Introduction
Bevacizumab, a recombinant humanized monoclonal antibody targeting vascular endothelial growth factor A (VEGFA), is pivotal in treating various malignancies (Zondor and Medina, 2004; Pavlidis and Pavlidis, 2013; Kim et al., 2018; Garcia et al., 2020). It primarily neutralizes VEGFA, inhibiting new blood vessel formation necessary for sustained tumor growth and metastasis (Claesson-Welsh and Welsh, 2013; Peach et al., 2018; Apte et al., 2019; Pérez-Gutiérrez and Ferrara, 2023). This anti-angiogenic approach targets the lifeline of the tumor—its vascular systems, focusing on endothelial cells. However, it is widely acknowledged that the role of VEGFA in cancer extends beyond angiogenesis. VEGFA influences the function of stromal and immune cells within the tumor microenvironment and directly modulates the functionality of tumor cells (Goel and Mercurio, 2013; Pérez-Gutiérrez and Ferrara, 2023), particularly in promoting their survival and proliferation. Therefore, it leads to an intriguing paradox in the therapeutic role of bevacizumab. Many tumor cells express high levels of VEGFA and its receptors/co-receptors (Lalla et al., 2003; Donnem et al., 2009; Capp et al., 2010; Goel and Mercurio, 2013; Nordby et al., 2015; Morimoto et al., 2019); thus, bevacizumab can theoretically exert direct cytotoxic effects on these cells by blocking VEGFA. However, tumor cells have evaded the direct cytotoxic effect of bevacizumab, and the underlying reasons remain unexplained.
For years, bevacizumab’s purported lack of direct inhibitory effects on tumor cells was primarily based on early in vitro experiments, which failed to demonstrate significant direct cytotoxic effects (Naumov et al., 2009; Hasan et al., 2011; Hein and Graver, 2013; Mesti et al., 2014). However, the tide of evidence is shifting. Increasingly, studies suggest that bevacizumab does exert direct cytotoxic effects on encountered tumor cells (Miranda-Goncalves et al., 2017; Zhao et al., 2018; Suo et al., 2020; Alonso-Diez et al., 2021; Fan et al., 2022; Wei et al., 2023), with even bevacizumab-induced cell death observed in certain transplant tumor models (Ramezani et al., 2017; Zhao et al., 2018; Ramezani et al., 2019; Xiang et al., 2022). These emerging findings challenge the notion that bevacizumab lacks direct anti-tumor activity, prompting a need for rigorous scrutiny and scientific exploration.
In this opinion article, we re-examined bevacizumab’s anti-tumor mechanism. A comprehensive understanding of its therapeutic potential necessitates considering its direct cytotoxic effects on tumor cells alongside its anti-angiogenic action. Our goal is to emphasize bevacizumab’s direct anti-tumor action and elucidate why its historical oversight of direct cytotoxic effects on tumor cells have historically been overlooked. Incorporating bevacizumab’s direct cytotoxic effects on tumor cells into its mechanism of action represents not only an academic advancement, but also carries significant implications for its clinical application.
Traditional mechanism of action of bevacizumab and its challenges
Bevacizumab’s antitumor activity traditionally stems from its suppression of angiogenesis. This mechanism relies on the premise that tumor growth and metastasis necessitate new blood vessel formation to ensure oxygen and nutrient supply (Sherwood et al., 1971; Bergers and Benjamin, 2003). Pro-angiogenic factors perpetuate tumor blood vessel formation, with VEGFA being the most critical (Claesson-Welsh and Welsh, 2013; Apte et al., 2019). VEGFA binds to VEGFRs on endothelial cells, triggering downstream signaling pathways that promote endothelial cell proliferation, migration, and new blood vessel formation (Peach et al., 2018; Pérez-Gutiérrez and Ferrara, 2023). Bevacizumab binds to circulating VEGFA, hindering its interaction with VEGFRs on endothelial cell surfaces, thereby impeding crucial steps in tumor blood vessel formation. This inhibition not only halts new blood vessel formation but also impairs the functionality of existing tumor blood vessels. Consequently, by inhibiting this process, bevacizumab effectively starves the tumor of vital nutrition and oxygen, thus stunting its growth. Moreover, anti-VEGFA therapy has a significant impact on the tumor microenvironment (Finley and Popel, 2013; Tu et al., 2023), which has promoted the development of combined treatment strategies of anti-VEGFA with other tumor immunotherapies such as immune checkpoint inhibitors (Hack et al., 2020; Chambers et al., 2021; Zheng et al., 2022; Tu et al., 2023). New blood vessels typically have structural abnormalities and have increased permeability, crucial for tumor spread and metastasis. Bevacizumab indirectly suppresses tumor cells’ invasive and metastatic potential by improving the quality of these vessels and reducing their number. Additionally, it recalibrates abnormal tumor blood vessels, causing a brief “normalization” period (Goel et al., 2011; Chen et al., 2016; Zhang et al., 2021; Li et al., 2022). During this window, previously tortuous and highly permeable tumor blood vessels become more organized and leak less, improving tissue perfusion and drug delivery. This phenomenon offers another therapeutic angle: enhancing the efficacy of combined chemotherapy drugs and radiotherapy (Becker et al., 2015; Kulinich et al., 2021; Socinski et al., 2021; Hardesty et al., 2022).
While bevacizumab is effective in treating tumors, certain clinical challenges have highlighted its complex mechanism of action. For instance, despite high expression levels of VEGFA and its receptors in patients, responses to bevacizumab vary significantly. Some patients respond well, while other patients exhibit resistance (Giantonio et al., 2007; Haunschild and Tewari, 2020; Dai et al., 2022). VEGFA-independent angiogenesis is considered the primary reason for bevacizumab resistance; however, numerous attempts to inhibit VEGFA-independent angiogenesis have failed to reverse this resistance. These issues pose serious challenges to traditional anti-angiogenic mechanisms involving bevacizumab. As such, research should also explore its effects in addition to its anti-angiogenic properties. With research progress, studies have focused on the direct cytotoxic effects of bevacizumab on tumor cells.
Beyond angiogenesis: direct cytotoxic effects of bevacizumab on tumor cells
The in vivo tumor microenvironment complexity has led to a reliance on in vitro cell cytotoxicity assays to demonstrate the direct antitumor efficacy of drugs. Early in vitro studies significantly influenced our comprehension of bevacizumab’s antitumor activity, predominantly indicating that its direct inhibition of tumor cells might be inconsequential (Naumov et al., 2009; Hasan et al., 2011; Hein and Graver, 2013; Mesti et al., 2014). This prompts the question: why do tumor cells, bathed in high VEGFA concentrations and theoretically susceptible to bevacizumab, seemingly resist its direct cytotoxic effects? Despite acknowledging the multifaceted role of the VEGFA signaling axis in tumors, extending beyond angiogenesis regulation alone, this puzzle persists. As our understanding of bevacizumab’s pharmacological characteristics deepens, numerous findings challenge longstanding beliefs. This is evident in bevacizumab’s capacity to induce direct cytotoxic effects in various tumor cells expressing high VEGFA levels (Miranda-Goncalves et al., 2017; Zhao et al., 2018; Suo et al., 2020; Alonso-Diez et al., 2021; Fan et al., 2022; Wei et al., 2023). At the molecular level, studies proposed mechanisms through which bevacizumab induces tumor cell death. One mechanism involves blocking VEGFA-mediated survival pathways, increasing apoptosis. Another potential pathway is the alteration of intracellular signaling cascades crucial for tumor cell homeostasis. Bevacizumab may disrupt these pathways’ stability, resulting in direct cytotoxic effects. In vivo models further support the direct cytotoxic hypothesis, showing bevacizumab’s ability to induce cell death within tumor masses in xenograft models, evident from increased apoptosis and morphological changes in tumor cells (Ramezani et al., 2017; Zhao et al., 2018; Ramezani et al., 2019; Xiang et al., 2022). Traditional metrics like tumor volume and microvascular density may not fully capture these changes, suggesting additional mechanisms at play and reinforcing the concept of a multipronged attack on cancer cells.
Table 1 summarizes the inconsistent results of studies on the direct cytotoxic effects of bevacizumab on various VEGFA-expressing tumor cells. For instance, studies on the same tumor cell line A549 at similar concentration ranges (0.0001–0.2 mg/L) and identical time points (24–72 h) have shown conflicting results; that is, some studies have reported that bevacizumab elicits no direct cytotoxic effects (Naumov et al., 2009; Liu et al., 2022), while other studies have revealed significant findings (Wang et al., 2015; Xiao et al., 2016; Wei et al., 2023). On examining these literatures, it appears that studies confirming bevacizumab’s direct cytotoxic effects often employ a variety of methods to assess its direct cytotoxicity. Thus, the choice of evaluation method might have been a crucial factor previously overlooked in recognizing the direct cytotoxic effects of bevacizumab.
Limitations of in vitro experimental methodologies
When assessing bevacizumab’s direct cytotoxic effects, it is crucial to recognize the limitations inherent in traditional in vitro evaluations. Standard anti-tumor assays expose tumor cells to bevacizumab for 24–72 h, relying on end-point measurements for direct cytotoxic effects determination. These methods often focus on a single biomarker, such as cell membrane integrity or metabolic activity, disregarding the multifactorial and multi-stage nature of cell death and survival, leading to oversimplified conclusions about cellular states (Adan et al., 2016; Parboosing et al., 2016; Halim, 2020).
For example, colorimetric assays like 3-(4,5-Dimethylthiazol-2-yl)-2,5- diphenyl tetrazolium bromide (MTT) and water soluble tetrazolium (WST) assays, widely used for quantifying viable cells in vitro (Berridge et al., 2005; Lutter et al., 2017; Präbst et al., 2017; Stockert et al., 2018), are common for assessing bevacizumab’s direct cytotoxic effects (Naumov et al., 2009; Hasan et al., 2011; Hein and Graver, 2013; Mesti et al., 2014). These assays operate on the principle of converting soluble tetrazolium salts into insoluble formazan by living cells, measurable through absorbance changes upon dissolution in a solvent. However, research suggests that tetrazolium salt reduction is significantly influenced by various factors, notably changes in cellular metabolism—a process that bevacizumab has been shown to induce through metabolic reprogramming in tumor cells (Zulato et al., 2012; Miranda-Goncalves et al., 2017; Yue and Lu, 2023). Thus, in our previous research, we identified the use of tetrazolium-based colorimetric assays as a methodological oversight in directly assessing bevacizumab’s direct cytotoxic effects on tumor cells (Wei et al., 2023). Specifically, MTT or WTS assays have shown that 0.2 mg/L bevacizumab does not significantly exhibit direct cytotoxic effects on tumor cells. Conversely, precise counting has revealed that bevacizumab inhibits tumor cell growth by 40%–47%. This discrepancy occurs because bevacizumab enhances mitochondrial metabolism in tumor cells. As a result, it increases the production of succinate dehydrogenase that consequently reduces more tetrazolium salts to formazan, indicating an increased number of living cells.
Furthermore, the end-point nature of such analyses obscures the dynamic morphological and biochemical changes that occur during exposure to bevacizumab. Given the tumor cells’ heterogeneity, it is plausible that while some succumb to bevacizumab’s effects and undergo cell death, others adapt and proliferate, possibly through metabolic shifts or alterations in their secretory profiles. Consequently, the ultimate measurement might reflect an apparent equilibrium between cell death and compensatory proliferation, masking bevacizumab’s true direct cytotoxic effects. For example, El-Hajjar et al. (2019) found that the number of tumor cells doubled after 24 h of treatment with 0.05 mg/mL bevacizumab compared with that of the control group. This increase could be a result of compensatory proliferation exceeding the number of dead cells.
In summary, these methodological subtleties could inadvertently mask the direct cytotoxic effects, presenting an incomplete view of bevacizumab’s capabilities. Consequently, we propose a redefined framework to comprehend bevacizumab’s role in cancer therapy, one that incorporates both its direct anti-tumor and anti-angiogenic properties.
Redefining the antitumor mechanism of bevacizumab
Here, as depicted in Figure 1, we propose an expanded mechanistic framework that involves the direct cytotoxic effects of bevacizumab on tumor cells and thus emphasizes its ability to target both the tumor vasculature and the tumor itself. Specifically, bevacizumab weakens angiogenesis by inhibiting VEGFA signaling; as a consequence, tumors are deprived of critical nutrients and oxygen. This antibody also directly binds to VEGFA-expressing tumor cells, thereby triggering apoptosis pathways and inhibiting proliferation and survival signaling.
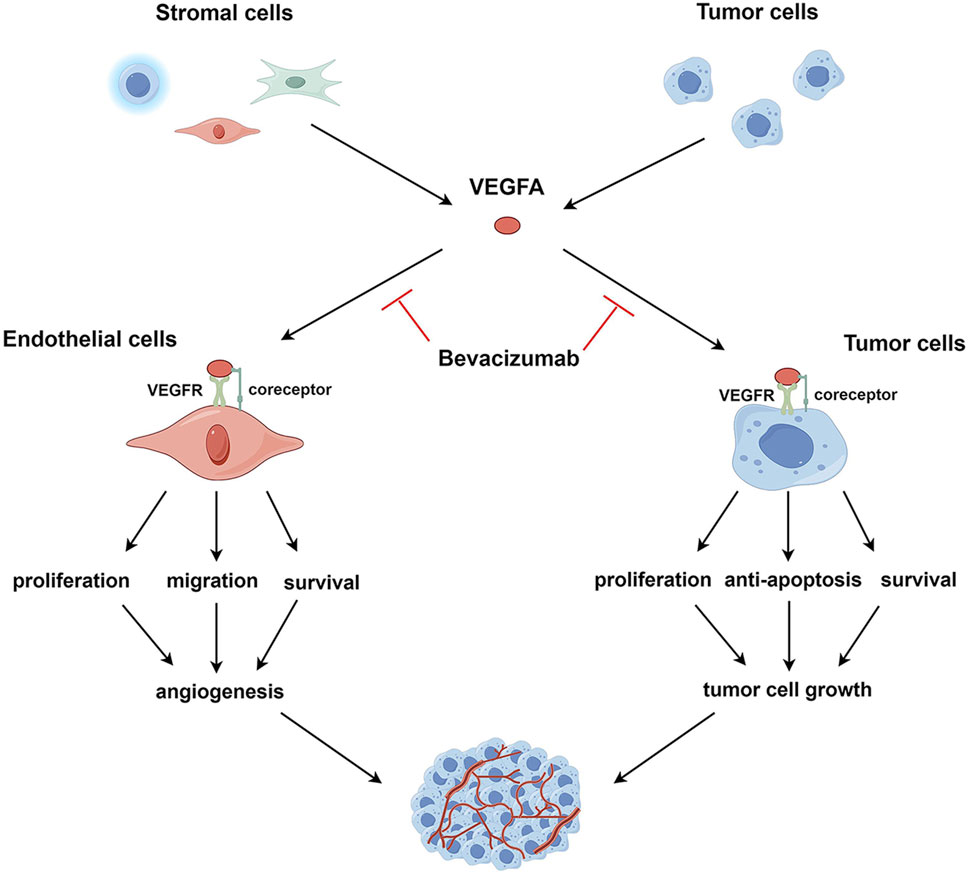
Figure 1. VEGFA signaling in endothelial and tumor cells. VEGFA, secreted by tumor and stromal cells, such as endothelial cells, immune cells, and fibroblasts, binds to VEGFR and co-receptors on the surface of endothelial or tumor cells, activating intracellular VEGFA signaling pathways. In endothelial cells, activated VEGFA signaling promotes cell survival, proliferation, and migration, leading to the induction of tumor angiogenesis. In tumor cells, the activation of the VEGFA pathway enhances cell survival, proliferation, and resistance to apoptosis, facilitating tumor cell growth. VEGFA targets both endothelial and tumor cells; correspondingly, bevacizumab exerts anti-angiogenic and direct antitumor effects by neutralizing VEGFA.
Recognizing this composite mechanism of action—targeting angiogenesis and tumor cells alike—has profound implications for oncological treatment strategies. Traditionally, bevacizumab’s impact was understood mainly in terms of its effect on the tumor’s blood supply through VEGFA inhibition, but this does not fully explain the lack of sensitivity observed in certain highly vascularized tumors among some patients. If bevacizumab exerts direct cytotoxic effects on VEGFA-expressing tumor cells, individual variances in VEGFA expression levels might be a key determinant in the variability of therapeutic responses. Furthermore, when devising treatment regimens, clinicians might tailor therapies based on the VEGFA expression levels in a patient’s tumor cells. For instance, identifying markers indicative of a direct tumor cell response will become as critical as those suggesting angiogenesis when predicting treatment outcomes. Additionally, understanding the temporal patterns of tumor response and resistance to bevacizumab could offer avenues to circumvent the emergence of resistant cancer cell populations. Once bevacizumab suppresses VEGFA within the tumor microenvironment, it affects the tumor’s ability to access oxygen and nutrients, not only through reduced vasculature but potentially by exerting direct survival pressures on the tumor cells. The cells must then adapt to these new conditions to sustain growth, a process that may involve activating alternative growth factor pathways, enhancing intracellular signaling to compensate for growth factor deprivation, or modulating apoptosis mechanisms to evade death signals. Elucidating these critical aspects will aid in preventing or overcoming resistance to bevacizumab.
Finally, incorporating bevacizumab’s direct cytotoxic effect enriches our understanding of its mechanism, fostering a comprehensive view of the tumor microenvironment—a complex ecosystem encompassing tumor cells, vessels, immune cells, and diverse cellular types and molecular signals. Following bevacizumab treatment, the tumor microenvironment may undergo significant changes such as cytokine and growth factor profile shifts, immune cell infiltration alterations, and extracellular matrix component modifications. These multifactorial effects may arise not only from anti-angiogenic actions but also from bevacizumab’s direct pressure on tumor cell survival.
In summary, under the new mechanistic framework, the therapeutic effects of bevacizumab are possibly attributed to a combination of its anti-angiogenic and direct cytotoxic effects. As such, drug administration protocols, therapeutic combinations, and predictive biomarkers should be reassessed to optimize the therapeutic outcomes of bevacizumab.
Further research is required to validate the direct cytotoxic effects of bevacizumab
A multifaceted experimental approach should be used to address the gaps in our understanding of the cytotoxic effects of bevacizumab on tumor cells. This strategy should initially commence with in vitro experiments to verify the direct cytotoxic effects of bevacizumab on cancer cells. With limitations inherent to endpoint assays, advanced imaging technologies should be adopted to monitor cellular responses in real time. In this way, cell death and potential compensatory proliferation, which can obscure the interpretation of the efficacy of bevacizumab, can be detected. Fluorescent markers specifically targeting cell death indicators, such as caspases, can be used to intricately analyze drug-induced cell death; routine medium replacement can prevent the confounding effects of proliferative cytokines that accumulate in a culture environment. Moreover, appropriate control cell lines should be chosen to elucidate the specificity of the cytotoxic effects of bevacizumab. Cells devoid of VEGFA expression and its receptors are key controls to definitively attribute the observed cytotoxicity to the disruption of the VEGFA signaling pathway. Furthermore, the effect of bevacizumab on normal cells expressing VEGFA and its receptors should be assessed to predict potential adverse effects accurately. Additionally, current research findings indicate that the direct cytotoxic effects of bevacizumab are not cell type-specific, as it exhibits significant cytotoxicity across 26 cell types from up to 8 different tumor classes. These findings also suggest that the direct cytotoxic effects of bevacizumab generally increases with concentration and time. However, it has been noted that these studies do not clearly define the relative expression levels of VEGFA and its receptors/co-receptors in different cells, nor do they test each cell’s sensitivity to a unit concentration of VEGFA. Therefore, it is imperative to re-evaluate the direct cytotoxic effects of bevacizumab on various tumor cells, upon clarifying the relative expression levels of VEGFA and its receptors/co-receptors, as well as the sensitivity of different cells to unit concentrations of VEGFA. This will aid in further elucidating the mechanisms through which bevacizumab exerts its direct cytotoxic effects. Subsequently, once the specific cytotoxic effects of bevacizumab against tumor cells are validated, the potential development of tumor cell resistance to this direct action should be explored. Such insights remarkably help reveal resistance mechanisms and reaffirm the potency of bevacizumab in inducing cell death. Furthermore, considering the standard incorporation of bevacizumab with chemotherapeutic agents in clinical settings, researchers should assess the in vitro synergistic cytotoxic effects of bevacizumab and various chemotherapeutics by investigating how tumor cells may adapt to resist this combination therapy. Thus, valuable guidance for clinical applications can be provided. Finally, in vitro findings should be translated into in vivo contexts to verify the observed mechanisms and effects. However, sophisticated experimental designs are necessary to distinguish between the direct cytotoxic effects of bevacizumab and those mediated indirectly through immune modulation. They may involve comparing outcomes between immunocompromised and immunocompetent animal models to elucidate the contribution of immune-mediated effects to the overall antitumor activity of bevacizumab.
Challenges and limitations in the clinical translation of bevacizumab’s direct cytotoxic effects
Although the direct cytotoxic effects of bevacizumab on tumor cells in vitro show potential for cancer therapy, translating these findings into clinical practice is impeded by limitations. First, tumors have inherent heterogeneity. The genetic and phenotypic diversity within and among tumors can considerably influence the efficacy of bevacizumab; as such, predicting responses based solely on in vitro data becomes difficult. Because of this variability, personalized approaches should be implemented to select patients who will likely benefit from bevacizumab treatment, emphasizing the importance of identifying response-predictive biomarkers. Second, the optimal concentration of bevacizumab that reflects the effective in vitro doses cannot be easily determined because of factors such as physiological barriers to drug delivery, systemic toxicity, and varying pharmacokinetics across patients. For this reason, dosing regimens should be cautiously optimized to maximize efficacy while minimizing adverse effects; thus, extensive clinical trials should be performed to establish safe yet effective dosage guidelines. Furthermore, the direct cytotoxic effects observed in isolated cell environments do not account for the dynamic interplay with the patient’s immune system. In vivo, the interaction of bevacizumab with immune mechanisms influences its effectiveness, but such interaction complicates the direct translation of its cytotoxic effects. Therefore, as a multidirectional avenue for future research, understanding how bevacizumab modulates immune system responses against tumors is crucial for achieving its full therapeutic potential. Moreover, the combination of bevacizumab with chemotherapeutic or targeted agents is a routine clinical strategy; therefore, incorporating the direct cytotoxic effects into the mechanism of action of bevacizumab undoubtedly further expands its potential for combination therapy. This potential not only lies in the “vascular normalization” induced by bevacizumab, making it easier for drugs to enter the tumor, but also in the added direct cytotoxic effects on tumor cells when used in conjunction with chemotherapeutic or targeted agents. Considering the important role of VEGFA in chemotherapy or targeted drug resistance, especially in regulating cancer stem cells (Goel and Mercurio, 2013; Mercurio, 2019), the combination of bevacizumab is also very attractive for preventing the development of drug resistance. However, just as a coin has two sides, if drug resistance occurs during the combination treatment, it also means the formation of more complex drug resistance mechanisms, making it more challenging to overcome resistance. Ultimately, bevacizumab should be integrated into cancer treatment regimens to improve patient outcomes, including extending survival times and enhancing the quality of life. However, the straightforward relationship between in vitro cytotoxicity and patient-centric endpoints is complicated by biological, environmental, and treatment-related factors. Therefore, a holistic approach should be implemented in clinical trials to evaluate not only the efficacy of bevacizumab but also its effect on overall patient wellbeing.
Although transitioning from the controlled environment of a laboratory to the complex realities of patient treatment has numerous challenges, this process is necessary to achieve the potential of bevacizumab for cancer therapy. By recognizing these obstacles and investing in interdisciplinary collaborative research efforts, we can narrow the gap between in vitro findings and clinical applications; thus, we can advance the development of targeted and effective cancer treatments.
Conclusion
This opinion article revisits bevacizumab’s antitumor activity mechanisms, underscoring the necessity to consider its direct cytotoxic effects on tumor cells alongside traditional angiogenesis inhibition views. Moreover, it also examines endpoint measurement technique limitations in evaluating bevacizumab’s cytotoxic impact, highlighting methodological oversights in previous studies that ignored its direct cytotoxic efficacy. Expanding our understanding of bevacizumab’s mechanism not only enriches comprehension of its therapeutic modality but also offers new perspectives for devising and refining cancer treatment strategies. However, fully grasping bevacizumab’s anticancer potential and optimal clinical application requires further investigation. Future research should focus on deeper exploration of bevacizumab’s direct mechanisms and translating these insights into clinical practices to enhance therapeutic outcomes for cancer patients.
Author contributions
ZW: Writing–original draft. JL: Writing–review and editing. JG: Writing–review and editing. PW: Writing–review and editing.
Funding
The author(s) declare that financial support was received for the research, authorship, and/or publication of this article. This work was supported by the grants from the National Natural Science Foundation of China (No. 81503104), the Guizhou Province Science and Technology Foundation [(2021)564], and the Scientific Research Foundation of Zunyi Medical University (Nos F955 and FZH004).
Acknowledgments
The authors would like to express their gratitude to EditSprings (https://www.editsprings.cn) for the expert linguistic services provided. We also thank Figdraw (www.figdraw.com) developed by HOME for Researchers (www.home-for-researchers.com) for the assistance for the VEGFA signaling illustration (ID No. WYYWPf3aaf).
Conflict of interest
The authors declare that the research was conducted in the absence of any commercial or financial relationships that could be construed as a potential conflict of interest.
Publisher’s note
All claims expressed in this article are solely those of the authors and do not necessarily represent those of their affiliated organizations, or those of the publisher, the editors and the reviewers. Any product that may be evaluated in this article, or claim that may be made by its manufacturer, is not guaranteed or endorsed by the publisher.
References
Adan, A., Kiraz, Y., and Baran, Y. (2016). Cell proliferation and cytotoxicity assays. Curr. Pharm. Biotechnol. 17 (14), 1213–1221. doi:10.2174/1389201017666160808160513
Alonso-Diez, Á., Cáceres, S., Peña, L., Crespo, B., and Illera, J. C. (2021). Anti-angiogenic treatments interact with steroid secretion in inflammatory breast cancer triple negative cell lines. Cancers 13 (15), 3668. doi:10.3390/cancers13153668
Apte, R. S., Chen, D. S., and Ferrara, N. (2019). VEGF in signaling and disease: beyond discovery and development. Cell 176 (6), 1248–1264. doi:10.1016/j.cell.2019.01.021
Becker, S., Bohn, P., Bouyeure-Petit, A. C., Modzelewski, R., Gensanne, D., Picquenot, J. M., et al. (2015). Bevacizumab enhances efficiency of radiotherapy in a lung adenocarcinoma rodent model: role of αvβ3 imaging in determining optimal window. Nucl. Med. Biol. 42 (12), 923–930. doi:10.1016/j.nucmedbio.2015.08.002
Bergers, G., and Benjamin, L. E. (2003). Tumorigenesis and the angiogenic switch. Nat. Rev. Cancer. 3 (6), 401–410. doi:10.1038/nrc1093
Berridge, M. V., Herst, P. M., and Tan, A. S. (2005). Tetrazolium dyes as tools in cell biology: new insights into their cellular reduction. Biotechnol. Annu. Rev. 11, 127–152. doi:10.1016/S1387-2656(05)11004-7
Capp, C., Wajner, S. M., Siqueira, D. R., Brasil, B. A., Meurer, L., and Maia, A. L. (2010). Increased expression of vascular endothelial growth factor and its receptors, VEGFR-1 and VEGFR-2, in medullary thyroid carcinoma. Thyroid 20 (8), 863–871. doi:10.1089/thy.2009.0417
Chambers, A., Kundranda, M., Rao, S., Mahmoud, F., and Niu, J. (2021). Anti-angiogenesis revisited: combination with immunotherapy in solid tumors. Curr. Oncol. Rep. 23 (9), 100. doi:10.1007/s11912-021-01099-7
Chen, B. B., Lu, Y. S., Lin, C. H., Chen, W. W., Wu, P. F., Hsu, C. Y., et al. (2016). A pilot study to determine the timing and effect of bevacizumab on vascular normalization of metastatic brain tumors in breast cancer. Bmc. Cancer. 16, 466. doi:10.1186/s12885-016-2494-8
Claesson-Welsh, L., and Welsh, M. (2013). VEGFA and tumour angiogenesis. J. Intern. Med. 273 (2), 114–127. doi:10.1111/joim.12019
Dai, M., Hua, C., Wang, M., Gao, L., Jiang, L., and Liu, Y. (2022). Targeting regulation of VEGF by BPTF in non-small cell lung cancer and its potential clinical significance. Eur. J. Med. Res. 27 (1), 299. doi:10.1186/s40001-022-00935-1
Donnem, T., Al-Shibli, K., Al-Saad, S., Delghandi, M. P., Busund, L. T., and Bremnes, R. M. (2009). VEGF-A and VEGFR-3 correlate with nodal status in operable non-small cell lung cancer: inverse correlation between expression in tumor and stromal cells. Lung. Cancer 63 (2), 277–283. doi:10.1016/j.lungcan.2008.05.022
El-Hajjar, L., Jalaleddine, N., Shaito, A., Zibara, K., Kazan, J. M., El-Saghir, J., et al. (2019). Bevacizumab induces inflammation in MDA-MB-231 breast cancer cell line and in a mouse model. Cell. Signal. 53, 400–412. doi:10.1016/j.cellsig.2018.11.007
Emlet, D. R., Brown, K. A., Kociban, D. L., Pollice, A. A., Smith, C. A., Ong, B. B., et al. (2007). Response to trastuzumab, erlotinib, and bevacizumab, alone and in combination, is correlated with the level of human epidermal growth factor receptor-2 expression in human breast cancer cell lines. Mol. Cancer. Ther. 6 (10), 2664–2674. doi:10.1158/1535-7163.MCT-07-0079
Fan, Y., Cheng, H., Liu, Y., Liu, S., Lowe, S., Li, Y., et al. (2022). Metformin anticancer: reverses tumor hypoxia induced by bevacizumab and reduces the expression of cancer stem cell markers CD44/CD117 in human ovarian cancer SKOV3 cells. Front. Pharmacol. 13, 955984. doi:10.3389/fphar.2022.955984
Filali el, M., Ly, L. V., Luyten, G. P., Versluis, M., Grossniklaus, H. E., van der Velden, P. A., et al. (2012). Bevacizumab and intraocular tumors: an intriguing paradox. Mol. Vis. 18, 2454–2467.
Finley, S. D., and Popel, A. S. (2013). Effect of tumor microenvironment on tumor VEGF during anti-VEGF treatment: systems biology predictions. J. Natl. Cancer. Inst. 105 (11), 802–811. doi:10.1093/jnci/djt093
Garcia, J., Hurwitz, H. I., Sandler, A. B., Miles, D., Coleman, R. L., Deurloo, R., et al. (2020). Bevacizumab (Avastin®) in cancer treatment: a review of 15 years of clinical experience and future outlook. Cancer. Treat. Rev. 86, 102017. doi:10.1016/j.ctrv.2020.102017
Giantonio, B. J., Catalano, P. J., Meropol, N. J., O'Dwyer, P. J., Mitchell, E. P., Alberts, S. R., et al. (2007). Bevacizumab in combination with oxaliplatin, fluorouracil, and leucovorin (FOLFOX4) for previously treated metastatic colorectal cancer: results from the Eastern Cooperative Oncology Group Study E3200. J. Clin. Oncol. 25 (12), 1539–1544. doi:10.1200/JCO.2006.09.6305
Goel, H. L., and Mercurio, A. M. (2013). VEGF targets the tumour cell. Nat. Rev. Cancer. 13 (12), 871–882. doi:10.1038/nrc3627
Goel, S., Duda, D. G., Xu, L., Munn, L. L., Boucher, Y., Fukumura, D., et al. (2011). Normalization of the vasculature for treatment of cancer and other diseases. Physiol. Rev. 91 (3), 1071–1121. doi:10.1152/physrev.00038.2010
Hack, S. P., Zhu, A. X., and Wang, Y. (2020). Augmenting anticancer immunity through combined targeting of angiogenic and PD-1/PD-L1 pathways: challenges and opportunities. Front. Immunol. 11, 598877. doi:10.3389/fimmu.2020.598877
Halim, A. B. (2020). Do We have a satisfactory cell viability assay? review of the currently commercially-available assays. Curr. Drug. Discov. Technol. 17 (1), 2–22. doi:10.2174/1570163815666180925095433
Hardesty, M. M., Krivak, T. C., Wright, G. S., Hamilton, E., Fleming, E. L., Belotte, J., et al. (2022). OVARIO phase II trial of combination niraparib plus bevacizumab maintenance therapy in advanced ovarian cancer following first-line platinum-based chemotherapy with bevacizumab. Gynecol. Oncol. 166 (2), 219–229. doi:10.1016/j.ygyno.2022.05.020
Hasan, M. R., Ho, S. H., Owen, D. A., and Tai, I. T. (2011). Inhibition of VEGF induces cellular senescence in colorectal cancer cells. Int. J. Cancer. 129 (9), 2115–2123. doi:10.1002/ijc.26179
Haunschild, C. E., and Tewari, K. S. (2020). Bevacizumab use in the frontline, maintenance and recurrent settings for ovarian cancer. Future. Oncol. 16 (7), 225–246. doi:10.2217/fon-2019-0042
Hein, M., and Graver, S. (2013). Tumor cell response to bevacizumab single agent therapy in vitro. Cancer. Cell. Int. 13 (1), 94. doi:10.1186/1475-2867-13-94
Heo, J. W., Kim, J. H., Cho, C. S., Jun, H. O., Kim, D. H., Yu, Y. S., et al. (2012). Inhibitory activity of bevacizumab to differentiation of retinoblastoma cells. PLoS. One. 7 (3), e33456. doi:10.1371/journal.pone.0033456
Huang, H., Song, J., Liu, Z., Pan, L., and Xu, G. (2018). Autophagy activation promotes bevacizumab resistance in glioblastoma by suppressing AKT/mTOR signaling pathway. Oncol. Lett. 15 (2), 1487–1494. doi:10.3892/ol.2017.7446
Itashiki, Y., Harada, K., Takenawa, T., Ferdous, T., Ueyama, Y., and Mishima, K. (2021). Antitumor effects of bevacizumab in combination with fluoropyrimidine drugs on human oral squamous cell carcinoma. Oncol. Lett. 22 (4), 730. doi:10.3892/ol.2021.12991
Kim, M. M., Umemura, Y., and Leung, D. (2018). Bevacizumab and glioblastoma: past, present, and future directions. Cancer. J. 24 (4), 180–186. doi:10.1097/PPO.0000000000000326
Kulinich, D. P., Sheppard, J. P., Nguyen, T., Kondajji, A. M., Unterberger, A., Duong, C., et al. (2021). Radiotherapy versus combination radiotherapy-bevacizumab for the treatment of recurrent high-grade glioma: a systematic review. Acta. Neurochir. 163 (7), 1921–1934. doi:10.1007/s00701-021-04794-3
Lalla, R. V., Boisoneau, D. S., Spiro, J. D., and Kreutzer, D. L. (2003). Expression of vascular endothelial growth factor receptors on tumor cells in head and neck squamous cell carcinoma. Arch. Otolaryngol. Head. Neck. Surg. 129 (8), 882–888. doi:10.1001/archotol.129.8.882
Li, B., Xu, D., Zhou, J., Wang, S. C., Cai, Y. X., Li, H., et al. (2022). Monitoring bevacizumab-induced tumor vascular normalization by intravoxel incoherent motion diffusion-weighted MRI. J. Magn. Reson. Imaging. 56 (2), 427–439. doi:10.1002/jmri.28012
Liu, Z., Qin, T., Yuan, X., Yang, J., Shi, W., Zhang, X., et al. (2022). Anlotinib downregulates RGC32 which provoked by bevacizumab. Front. Oncol. 12, 875888. doi:10.3389/fonc.2022.875888
Lutter, A. H., Scholka, J., Richter, H., and Anderer, U. (2017). Applying XTT, WST-1, and WST-8 to human chondrocytes: a comparison of membrane-impermeable tetrazolium salts in 2D and 3D cultures. Clin. Hemorheol. Microcirc. 67 (3-4), 327–342. doi:10.3233/CH-179213
Mercurio, A. M. (2019). VEGF/Neuropilin signaling in cancer stem cells. Int. J. Mol. Sci. 20 (3), 490. doi:10.3390/ijms20030490
Mesti, T., Bouchemal, N., Banissi, C., Triba, M. N., Marbeuf-Gueye, C., Cemazar, M., et al. (2018). Nuclear magnetic resonance metabolic fingerprint of bevacizumab in mutant IDH1 glioma cells. Radiol. Oncol. 52 (4), 392–398. doi:10.2478/raon-2018-0046
Mesti, T., Savarin, P., Triba, M. N., Le Moyec, L., Ocvirk, J., Banissi, C., et al. (2014). Metabolic impact of anti-angiogenic agents on U87 glioma cells. PLoS. One. 9 (6), e99198. doi:10.1371/journal.pone.0099198
Miranda-Goncalves, V., Cardoso-Carneiro, D., Valbom, I., Cury, F. P., Silva, V. A., Granja, S., et al. (2017). Metabolic alterations underlying bevacizumab therapy in glioblastoma cells. Oncotarget 8, 103657–103670. doi:10.18632/oncotarget.21761
Molhoek, K. R., Griesemann, H., Shu, J., Gershenwald, J. E., Brautigan, D. L., and Slingluff, C. L. (2008). Human melanoma cytolysis by combined inhibition of mammalian target of rapamycin and vascular endothelial growth factor/vascular endothelial growth factor receptor-2. Cancer. Res. 68 (11), 4392–4397. doi:10.1158/0008-5472.CAN-07-5844
Morimoto, Y., Tamura, R., Ohara, K., Kosugi, K., Oishi, Y., Kuranari, Y., et al. (2019). Prognostic significance of VEGF receptors expression on the tumor cells in skull base chordoma. J. Neurooncol. 144 (1), 65–77. doi:10.1007/s11060-019-03221-z
Naumov, G. N., Nilsson, M. B., Cascone, T., Briggs, A., Straume, O., Akslen, L. A., et al. (2009). Combined vascular endothelial growth factor receptor and epidermal growth factor receptor (EGFR) blockade inhibits tumor growth in xenograft models of EGFR inhibitor resistance. Clin. Cancer. Res. 15 (10), 3484–3494. doi:10.1158/1078-0432.CCR-08-2904
Nordby, Y., Andersen, S., Richardsen, E., Ness, N., Al-Saad, S., Melbø-Jørgensen, C., et al. (2015). Stromal expression of VEGF-A and VEGFR-2 in prostate tissue is associated with biochemical and clinical recurrence after radical prostatectomy. Prostate 75 (15), 1682–1693. doi:10.1002/pros.23048
Parboosing, R., Mzobe, G., Chonco, L., and Moodley, I. (2016). Cell-based assays for assessing toxicity: a basic guide. Med. Chem. 13 (1), 13–21. doi:10.2174/1573406412666160229150803
Pavlidis, E. T., and Pavlidis, T. E. (2013). Role of bevacizumab in colorectal cancer growth and its adverse effects: a review. World. J. Gastroenterol. 19 (31), 5051–5060. doi:10.3748/wjg.v19.i31.5051
Peach, C. J., Mignone, V. W., Arruda, M. A., Alcobia, D. C., Hill, S. J., Kilpatrick, L. E., et al. (2018). Molecular pharmacology of VEGF-A isoforms: binding and signalling at VEGFR2. Int. J. Mol. Sci. 19 (4), 1264. doi:10.3390/ijms19041264
Pérez-Gutiérrez, L., and Ferrara, N. (2023). Biology and therapeutic targeting of vascular endothelial growth factor A. Nat. Rev. Mol. Cell. Biol. 24 (11), 816–834. doi:10.1038/s41580-023-00631-w
Präbst, K., Engelhardt, H., Ringgeler, S., and Hübner, H. (2017). Basic colorimetric proliferation assays: MTT, WST, and Resazurin. Methods. Mol. Biol. 1601, 1–17. doi:10.1007/978-1-4939-6960-9_1
Ramezani, S., Vousooghi, N., Ramezani Kapourchali, F., and Joghataei, M. T. (2017). Perifosine enhances bevacizumab-induced apoptosis and therapeutic efficacy by targeting PI3K/AKT pathway in a glioblastoma heterotopic model. Apoptosis 22 (8), 1025–1034. doi:10.1007/s10495-017-1382-2
Ramezani, S., Vousooghi, N., Ramezani Kapourchali, F., Yousefzadeh-Chabok, S., Reihanian, Z., Alizadeh, A. M., et al. (2019). Rolipram optimizes therapeutic effect of bevacizumab by enhancing proapoptotic, antiproliferative signals in a glioblastoma heterotopic model. Life. Sci. 239, 116880. doi:10.1016/j.lfs.2019.116880
Sherwood, L. M., Parris, E. E., and Folkman, J. (1971). Tumor angiogenesis: therapeutic implications. N. Engl. J. Med. 285 (21), 1182–1186. doi:10.1056/NEJM197111182852108
Socinski, M. A., Nishio, M., Jotte, R. M., Cappuzzo, F., Orlandi, F., Stroyakovskiy, D., et al. (2021). IMpower150 final overall survival analyses for atezolizumab plus bevacizumab and chemotherapy in first-line metastatic nonsquamous NSCLC. J. Thorac. Oncol. 16 (11), 1909–1924. doi:10.1016/j.jtho.2021.07.009
Stockert, J. C., Horobin, R. W., Colombo, L. L., and Blázquez-Castro, A. (2018). Tetrazolium salts and formazan products in cell biology: viability assessment, fluorescence imaging, and labeling perspectives. Acta. Histochem. 120 (3), 159–167. doi:10.1016/j.acthis.2018.02.005
Suo, B., Wu, C., and Mei, F. (2020). Effect of bevacizumab on expression level of GLI1 and ING4 in colon cancer animal model. Oncol. Lett. 20 (2), 1263–1269. doi:10.3892/ol.2020.11677
Taha, A. M., Aboulwafa, M. M., Zedan, H., and Helmy, O. M. (2022). Ramucirumab combination with sorafenib enhances the inhibitory effect of sorafenib on HepG2 cancer cells. Sci. Rep. 12 (1), 17889. doi:10.1038/s41598-022-21582-w
Tu, J., Liang, H., Li, C., Huang, Y., Wang, Z., Chen, X., et al. (2023). The application and research progress of anti-angiogenesis therapy in tumor immunotherapy. Front. Immunol. 14, 1198972. doi:10.3389/fimmu.2023.1198972
Tura, A., Pawlik, V. E., Rudolf, M., Ernesti, J. S., Stutzer, J. N., Grisanti, S., et al. (2019). Uptake of ranibizumab but not bevacizumab into uveal melanoma cells correlates with a sustained decline in VEGF-A levels and metastatic activities. Cancers 11 (6), 868. doi:10.3390/cancers11060868
Wang, C., Lv, Y., Sha, Z., Zhang, J., Wu, J., Qi, Y., et al. (2021). Dicer enhances bevacizumab-related inhibition of hepatocellular carcinoma via blocking the vascular endothelial growth factor pathway. J. Hepatocell. Carcinoma. 8, 1643–1653. doi:10.2147/JHC.S327258
Wang, L. L., Hu, R. C., Dai, A. G., and Tan, S. X. (2015). Bevacizumab induces A549 cell apoptosis through the mechanism of endoplasmic reticulum stress in vitro. Int. J. Clin. Exp. Pathol. 8 (5), 5291–5299.
Wei, P., Wang, M., Lin, M., and Wang, Z. Y. (2023). Tetrazolium-based colorimetric assays underestimat the direct antitumor effects of anti-VEGF agent bevacizumab. Toxicol. Vitro. 91, 105631. doi:10.1016/j.tiv.2023.105631
Xiang, Z., Deng, X., He, W., Yang, Q., Ni, L., Dehghan Shasaltaneh, M., et al. (2022). Treatment of malignant pleural effusion in non-small cell lung cancer with VEGF-directed therapy. Ann. Med. 54 (1), 1357–1371. doi:10.1080/07853890.2022.2071977
Xiao, J., Xu, X., Li, X., Li, Y., Liu, G., Tan, H., et al. (2016). Re-188 enhances the inhibitory effect of bevacizumab in non-small-cell lung cancer. Molecules 21 (10), 1308. doi:10.3390/molecules21101308
Xu, C. S., Wang, Z. F., Dai, L. M., Chu, S. H., Gong, L. L., Yang, M. H., et al. (2014). Induction of proline-rich tyrosine kinase 2 activation-mediated C6 glioma cell invasion after anti-vascular endothelial growth factor therapy. J. Transl. Med. 12, 148. doi:10.1186/1479-5876-12-148
Yang, L., You, S., Kumar, V., Zhang, C., and Cao, Y. (2012). In vitro the behaviors of metastasis with suppression of VEGF in human bone metastatic LNCaP-derivative C4-2B prostate cancer cell line. J. Exp. Clin. Cancer. Res. 31 (1), 40. doi:10.1186/1756-9966-31-40
Yue, H., and Lu, X. (2023). Metabolic reprogramming of the ovarian cancer microenvironment in the development of antiangiogenic resistance. Acta. Biochim. Biophys. Sin. 55 (6), 938–947. doi:10.3724/abbs.2023046
Zhang, H., Cao, G., Ren, W., Gu, J., Ji, W., Zhu, D., et al. (2021). Vascular normalization therapy with targeted localized vessel bevacizumab infusion in hepatocellular carcinoma after transarterial chemoembolization failure. Ann. Palliat. Med. 10 (8), 9149–9156. doi:10.21037/apm-21-2123
Zhang, L., Chen, Y., Li, F., Bao, L., and Liu, W. (2019). Atezolizumab and bevacizumab attenuate cisplatin resistant ovarian cancer cells progression synergistically via suppressing epithelial-mesenchymal transition. Front. Immunol. 10, 867. doi:10.3389/fimmu.2019.00867
Zhang, L., and Zou, W. (2015). Inhibition of integrin β1 decreases the malignancy of ovarian cancer cells and potentiates anticancer therapy via the FAK/STAT1 signaling pathway. Mol. Med. Rep. 12 (6), 7869–7876. doi:10.3892/mmr.2015.4443
Zhao, Z., Xia, G., Li, N., Su, R., Chen, X., and Zhong, L. (2018). Autophagy inhibition promotes bevacizumab-induced apoptosis and proliferation inhibition in colorectal cancer cells. J. Cancer 9 (18), 3407–3416. doi:10.7150/jca.24201
Zheng, W., Qian, C., Tang, Y., Yang, C., Zhou, Y., Shen, P., et al. (2022). Manipulation of the crosstalk between tumor angiogenesis and immunosuppression in the tumor microenvironment: insight into the combination therapy of anti-angiogenesis and immune checkpoint blockade. Front. Immunol. 13, 1035323. doi:10.3389/fimmu.2022.1035323
Zondor, S. D., and Medina, P. J. (2004). Bevacizumab: an angiogenesis inhibitor with efficacy in colorectal and other malignancies. Ann. Pharmacother. 38 (7), 1258–1264. doi:10.1345/aph.1D470
Keywords: vascular endothelial growth factor A, bevacizumab, antiangiogenesis, direct antitumor activity, tumor cells
Citation: Wang Z, Li J, Guo J and Wei P (2024) Direct antitumor activity of bevacizumab: an overlooked mechanism?. Front. Pharmacol. 15:1394878. doi: 10.3389/fphar.2024.1394878
Received: 02 March 2024; Accepted: 08 April 2024;
Published: 23 April 2024.
Edited by:
Hira Lal Goel, UMass Chan Medical School, United StatesReviewed by:
Fei Chai, University of Massachusetts Medical School, United StatesDivya Nair, University of Massachusetts Medical School, United States
Copyright © 2024 Wang, Li, Guo and Wei. This is an open-access article distributed under the terms of the Creative Commons Attribution License (CC BY). The use, distribution or reproduction in other forums is permitted, provided the original author(s) and the copyright owner(s) are credited and that the original publication in this journal is cited, in accordance with accepted academic practice. No use, distribution or reproduction is permitted which does not comply with these terms.
*Correspondence: Pei Wei, d2VpcGVpQHptdS5lZHUuY24=