- 1Hospital of Chengdu University of Traditional Chinese Medicine, Chengdu, China
- 2Hainan Medical University, Haikou, Hainan, China
Diabetic nephropathy (DN) constitutes a major microvascular complication of diabetes and is a primary cause of mortality in diabetic individuals. With the global rise in diabetes, DN has become an urgent health issue. Currently, there is no definitive cure for DN. Alpinia oxyphylla, a Chinese herbal medicine traditionally used, exhibits a wide range of pharmacological effects and is frequently used in the prevention and management of DN. This paper offers an extensive review of the biological mechanisms by which A. oxyphylla delivers therapeutic advantages in DN management. These mechanisms include activating podocyte autophagy, regulating non-coding RNA, modulating gut microbiota, alleviating lipotoxicity, counteracting oxidative stress, and diminishing inflammatory responses, underscoring the therapeutic potential of A. oxyphylla in DN treatment.
1 Introduction
Diabetic nephropathy (DN) constitutes a significant microvascular complication among diabetes patients, with 30%–40% estimated to develop DN over their lifetimes (Deshpande, Harris-Hayes, and Schootman, 2008). It is the leading cause of end-stage renal disease (Gupta, Dominguez, and Golestaneh, 2023), thus generating a considerable global economic impact. DN is clinically characterized by progressive proteinuria and a reduction in glomerular filtration rate (GFR) (Pelle et al., 2022). Present treatments primarily target modulating risk factors, such as blood pressure and glucose levels, and mitigating proteinuria. However, these therapies, when applied long-term, can lead to various side effects, including persistent dry cough and angioedema (Shen et al., 2017), and frequently prove ineffective in significantly halting DN progression. This underscores an urgent need to explore and validate effective treatments. Recent studies highlight the promising role of Chinese herbal medicine in DN management due to its unique advantages (Yang et al., 2020; Liu et al., 2022; Wang et al., 2023).
Alpinia oxyphylla, commonly known as Yizhi, has been esteemed for its medicinal properties since its initial mention in the “Kaibao Bencao.” In 1998, the Chinese Ministry of Health designated it as a plant with dual purposes: medicinal and edible (Kai et al., 2021a). Current studies reveal that A. oxyphylla primarily contains diarylheptanoids, flavonoids, sesquiterpenes, and glycosides (Wu et al., 2019). Pharmacological research has identified several properties of this plant, such as anti-inflammatory, anti-aging, antioxidant, antidiuretic, immunostimulatory, and neuroprotective activities (Wang et al., 2018; Yu et al., 2020; Zhang et al., 2020; Park et al., 2022; Wang et al., 2023). Its renal protective effects have gained considerable attention, with studies highlighting the efficacy of A. oxyphylla extract in combating DN. Notably, the petroleum ether extract appears to be the most effective (Xie et al., 2015). Early intervention with A. oxyphylla and its extracts can decelerate DN progression, as shown by the alleviation of polyuria, reduction in 24-h urine protein levels, blood urea nitrogen (BUN), serum creatinine (Scr), and improvement in glomerular hypertrophy, mesangial expansion, and basement membrane thickening in DN mice (Xie et al., 2015; Chen et al., 2016). The critical aspect is that Suoquan Yishen Fang, primarily consisting of A. oxyphylla, is a traditional Chinese medicine compound that has been granted a national patent (patent number: ZL 2016 1 0423677.5) for treating DN. Studies have indicated that the Suoquan Yishen Fang can mitigate the clinical symptoms of DN and decrease urea nitrogen and urine protein levels in patients (Yin et al., 2019). This article examines the molecular mechanism of action of A. oxyphylla in DN, exploring potential strategies for mitigating and reversing renal injury, thereby informing future research and product development efforts in the treatment of DN with A. oxyphylla.
2 Roles of Alpinia oxyphylla on DN
A plethora of evidence indicates that mechanisms such as autophagy, non-coding RNA, the gut microbiome, lipotoxicity, oxidative stress, and inflammatory responses contribute to DN (Cui et al., 2020; Opazo-Ríos et al., 2020; Jin et al., 2023; Szostak et al., 2023; Zhao et al., 2023). Recent pharmacological studies have revealed that A. oxyphylla possesses therapeutic capabilities, including the activation of autophagy, regulation of non-coding RNA, modulation of intestinal microbiota, attenuation of lipotoxicity, reduction of oxidative damage, and mitigation of inflammatory responses (Du et al., 2017; Xie et al., 2017; Xie et al., 2018; Wang et al., 2022; Wang et al., 2023c; Ni et al., 2023; Yin et al., 2023). Such findings underscore A. oxyphylla, whether as a single agent, an extract, or in combination, as a promising multipronged intervention for DN, targeting various underlying mechanisms (Figure 1) (Table 1).
2.1 Activating podocyte autophagy
Autophagy, a self-degradative process in eukaryotic cells, involves lysosomal elimination of cytoplasmic proteins and impaired organelles, regulated by autophagy-related genes (Atg) (Lahiri et al., 2019). This mechanism operates continuously under normal physiological conditions—known as basal autophagy—and intensifies in response to cellular stress (Galluzzi and Green, 2019). As a protective cellular mechanism, autophagy contributes to cell growth and resilience, shielding cells from metabolic strain and oxidative harm, thereby playing a pivotal role in sustaining intracellular equilibrium as well as the synthesis, degradation, and recycling of cellular constituents (Lahiri et al., 2019). However, excessive activation of autophagy may lead to metabolic imbalances, unnecessary degradation of cellular structures, and potentially result in cell death (Xu and Hu, 2022).
Research has demonstrated that autophagy plays a crucial role in DN by maintaining glomerular and tubular homeostasis (Liu et al., 2022; Liu et al., 2022; Su et al., 2022). Unlike other glomerular cells, podocytes cannot remove harmful substances through cellular division due to their terminally differentiated state, necessitating increased basal autophagy for their elimination (Sun et al., 2021). As a result, these cells exhibit enhanced basal autophagic activity. However, chronic exposure to high glucose conditions leads to a reduced autophagic response in podocytes, significantly compromising their protective mechanism and aggravating cellular injury (Xu et al., 2021). Notably, such damage to podocytes is a critical factor in the progression of proteinuria and DN (Barutta et al., 2022; Li et al., 2023).
Recent research indicates that the phosphatidylinositol-3-kinase/protein kinase B/mammalian target of rapamycin (PI3K/Akt/mTOR) signaling pathway plays a crucial role in podocytes, primarily by downregulating autophagy (Yang X et al., 2020; Lai et al., 2023). Following DN, elevated levels of inflammatory factors and reactive oxygen species (ROS) can activate cell surface receptors, initiating the PI3K activation process. This activation of PI3K leads to downstream activation of Akt and mTOR (Miricescu et al., 2021). Moreover, mTOR phosphorylates several autophagy-related proteins, such as UNC-51 like kinase 1 (ULK1), inhibiting critical autophagy complexes and thereby impeding autophagosome formation and affecting podocyte autophagy (Al-Bari and Xu, 2020; Yang et al., 2023). A study by Yin Dehui et al. on DN mice revealed that A. oxyphylla suppresses the activation of the PI3K/Akt/mTOR pathway, enhances podocyte autophagy, mitigates podocyte damage (Yin et al., 2023), and may provide therapeutic benefits for DN (Figure 2).
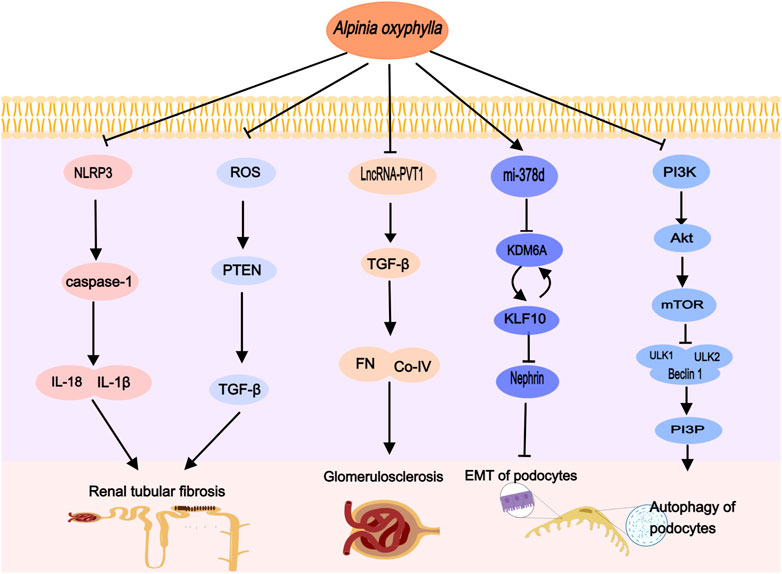
Figure 2. Alpinia oxyphylla treats DN by regulating the above signaling pathways, activating podocyte autophagy, inhibiting EMT, improving glomerulosclerosis and renal tubular fibrosis.
2.2 Regulating non-coding RNA
Noncoding RNAs (ncRNAs) constitute a broad category of RNAs that do not encode proteins (Liu et al., 2021). This group includes diverse types of ncRNAs, with microRNA (miRNA), long noncoding RNA (lncRNA), and circular RNA (circRNA) as the primary functional classes. These have been shown to influence the pathogenesis of DN by regulating gene expression (Lv et al., 2020). This review will focus on the regulatory roles of miRNAs and lncRNAs in DN and investigate the therapeutic potential of A. oxyphylla in modulating these ncRNAs to treat DN.
2.2.1 MicroRNA
MiRNAs, encompassing 19–22 base pairs, are single-stranded and function by downregulating gene expression through the targeted degradation of specific messenger RNAs (mRNAs) (Mauceri, 2022). Studies have demonstrated variations in miRNA expression levels when comparing kidney tissue samples from healthy individuals to those from patients with DN (Li et al., 2021). Similarly, research indicates differences in miRNA profiles within the kidneys of normal, db/db mice, and DN mice (Du et al., 2017; Yang, 2018; Zhu. et al., 2019). Notably, miRNA-378, which is under-expressed in the kidneys of db/db and DN mice compared to normal mice, is closely linked with insulin resistance and has the potential to modulate inflammatory stress alongside insulin resistance (Wang et al., 2021). Consequentl, miRNA-378 represents a promising target for the treatment of metabolic disorders (Machado et al., 2020).
It has been documented that A. oxyphylla is capable of restoring miR-378d expression in the kidneys of DN mice (Du et al., 2017). Concurrently, Suoquan Yishen Fang, which primarily contains A. oxyphylla, has been demonstrated to attenuate DN damage by upregulating miR-378d expression in the kidneys of db/db mice. This upregulation inhibits the histone demethylating enzyme KDM6A and the transcription factor KLF10, disrupting the epithelial-mesenchymal transition of podocytes (Wang, 2023). Additionally, A. oxyphylla has been recognized for enhancing the expression of six other microRNAs: let-7k, miR-129-1-3p, miR-21a-5p, miR-29c-3p, miR-203-3p, and miR-7a-5p (Du et al., 2017). Importantly, miR-21a-5p, miR-29c-3p, and miR-203-3p are implicated in podocyte damage and renal interstitial fibrosis, functioning through diverse signaling pathways (Long et al., 2011; Chen et al., 2018; Ji et al., 2019). This evidence suggests that A. oxyphylla may exert therapeutic effects on DN through the modulation of miRNA expression.
2.2.2 Long noncoding RNA
lncRNAs, defined as ncRNAs longer than 200 nucleotides, play a pivotal role in gene regulation through interactions with proteins, DNA, and RNA (Yao et al., 2019; Ruffo et al., 2023). Extensive research has highlighted the significance of lncRNAs in the pathophysiological mechanisms underlying DN, involving glomerular and renal tubular injury, microvascular lesions, endoplasmic reticulum stress, inflammation, autophagy, apoptosis, and cell proliferation (Yi et al., 2017; Ebadi et al., 2019; Leung et al., 2019). Certain lncRNAs have been identified as prospective diagnostic markers and therapeutic targets for DN (Xu et al., 2022). Notably, Plasmacytoma Variant Translocation 1 (PVT1) was the first lncRNA associated with kidney disease and is characterized by increased expression in various kidney cell types (Hanson et al., 2007). Elevated PVT1 levels can enhance cellular proliferation and extracellular matrix (ECM) accrual, consequently accelerating DN progression (Qin and Cao, 2021). Glomerular mesangial cells (GMCs) are primary ECM secretors, proliferating and secreting components such as collagen type IV (Col-IV), fibronectin (FN), and laminin in response to high glucose, lipids, and filtration pressure (Alvarez et al., 2016). Transforming growth factor-β1 (TGF-β1) is recognized as a significant fibrogenic factor and a central pathway in renal fibrosis, critically involved in ECM buildup, renal fibrosis exacerbation, and DN progression (Lovisa et al., 2015). Elevated PVT1 expression in human mesangial cells (HMCs) under high glucose conditions, along with significant increases in FN1, COL4α1, TGF-β1, and plasminogen activator inhibitor-1 (PAI-1) levels, has been documented (Mok et al., 2022). Silencing PVT1 partially reverses high glucose-induced mesangial cell proliferation and fibrosis (Zhong et al., 2020), suggesting PVT1 as a promising target for DN treatment. Importantly, Suoquan Yishen Fang, primarily comprised of A. oxyphylla, reduces lncRNA-PVT1 and suppresses TGF-β1 and ECM components FN and Col-IV protein expression, thereby mitigating glomerular basement membrane thickening, reducing ECM deposition, and ameliorating kidney tissue damage in DN mice (Jiaxin, 2021).
2.3 Modulating gut microbiota
The concept of the gut-kidney axis introduces a new paradigm in understanding the interplay between gut microbiota and various kidney diseases. It underscores the significant influence of gut microbiota and their metabolites on the pathophysiology of DN through this axis (Zhao et al., 2023). This involves bidirectional communication between the intestines and kidneys, wherein pathological and physiological changes in either organ can mutually influence and precipitate lesions in the other (Lobel et al., 2020).
A study revealed a markedly lower abundance and diversity of intestinal flora in DN patients compared to healthy individuals. Patients with DN displayed increased levels of Actinobacteria, Bacilli, Coriobacteriia, and Negativicutes, whereas Alphaproteobacteria and Clostridia were found in reduced quantities (Du et al., 2021). Tao et al. (Tao et al., 2019) identified Escherichia-Shigella and Prevotella as potential microbial markers for distinguishing DN from DM. In db/db mice, an increase in the Firmicutes/Bacteroides ratio was observed (Singh et al., 2020), alongside decreased levels of Akkermansia and Blautia (Cani and de Vos, 2017; Liu et al., 2021). These changes might originate from renal dysfunction in DN, which hampers the elimination of purine metabolism by-products such as urea, uric acid, and oxalate, leading to the accumulation of uremic toxins and disruption of the intestinal flora balance (Vaziri et al., 2013). Moreover, DN increases the intestinal pH through ammonia production, causing dysbiosis and an imbalance in intestinal homeostasis, which further affects mucosal stimulation and alters the structure of the intestinal barrier (Richardson et al., 2013; Ramezani and Raj, 2014). Importantly, the intestinal flora and its metabolites play a significant role in the progression of DN (Zhu et al., 2023). Lipopolysaccharide (LPS), an essential component of the outer membrane of Gram-negative bacteria, triggers an inflammatory response and is pivotal in DN development (Salguero et al., 2019). In DN patients with intestinal flora disorder, an overgrowth of Gram-negative bacteria and elevated LPS levels occur, leading to inflammatory responses through the activation of Toll-like receptors 2 (TLR2) and 4 (TLR4) and the nuclear factor kappa B (NF-κB) signaling pathway. This results in the production of inflammatory cytokines like tumor necrosis factor alpha (TNF-α), transforming growth factor beta (TGF-β), and interleukins (IL)-1 and IL-6, contributing to glomerulosclerosis and tubulointerstitial fibrosis, thus exacerbating kidney damage (Griffin et al., 2015; Wu et al., 2019; Mercer et al., 2020). Consequently, treatments targeting gut microbiota modulation have shown potential in restoring kidney function in chronic kidney disease (CKD) (Mao et al., 2023), highlighting therapeutic strategies that involve increasing beneficial bacterial phyla such as Bacteroidetes (Albuquerque et al., 2019) and Akkermansia (Luo et al., 2022) to counter DN progression.
Recent research has demonstrated that A. oxyphylla exhibits a promising therapeutic effect on kidney diseases. Compound preparations, individual drugs, and extracts of this plant have been shown to lower blood sugar levels and ameliorate DN by correcting dysbiosis of the gut microbiota. In DN mice, Suoquan Yishen Fang, which primarily features A. oxyphylla, modulated the Firmicutes-to-Bacteroides ratio, enhance the presence of Cyanobacteria and Akkermansia, and subsequently reduce blood glucose while improving renal function (Ni. 2019). Comparable benefits were observed in DN rats treated with raw or salt-processed forms of A. oxyphylla; these preparations decreased the species abundance of Proteobacteria and Enterobacter while fostering an increase in Prevotella and Rombutella, leading to improvements in blood glucose, body weight, and renal function parameters (Wu, 2021). Moreover, in T2DM mice, A. oxyphylla succeeded in diminishing blood glucose concentrations and significantly mitigating renal pathological alterations by promoting Bacteroides, inhibiting Firmicella and Helicobacter pylori, and boosting the presence of Akkermansia (Xie et al., 2018). Consequently, it is suggested that A. oxyphylla is instrumental in DN protection through the modulation of gut microbiome composition, the enhancement of beneficial bacterial populations, the suppression of detrimental bacterial communities, and involvement in blood glucose regulation (Figure 3).
2.4 Ameliorating lipotoxicity
Lipotoxicity is characterized by the abnormal accumulation of lipids within non-adipose tissues, notably in organs like the kidney that are rich in mitochondria and, consequently, have high energy demands. Lipids, being a primary energy source, tend to accumulate in the kidney. This accumulation particularly affects podocytes and tubular cells within the renal tissue (Mitrofanova et al., 2023). Recent evidence suggests that lipids play a role in causing renal damage through various mechanisms, including damage to podocytes, impairment of tubular function, proliferation of mesangial cells, and activation of endothelial cells. These pathophysiological alterations are thought to be driven by inflammation, oxidative stress, mitochondrial dysfunction, and cell death (Opazo-Ríos et al., 2020; Mitrofanova et al., 2023).
In DN mice, disturbances in lipid metabolism were closely linked to the sphingolipid and glycerophospholipid metabolic pathways, with sphingomyelin, phosphatidylcholine, lysophosphatidylcholine, and phosphatidylethanolamine identified as key metabolites (Ni et al., 2023). Notably, sphingomyelin and phosphatidylcholine have been recognized as predictive biomarkers for DN (Tofte et al., 2019). Elevated serum sphingomyelin levels in DN significantly correlate with adverse clinical outcomes, including a reduced estimated glomerular filtration rate (eGFR), progression to end-stage renal disease (ESRD), and the transition from microalbuminuria to macroalbuminuria (Pongrac Barlovic et al., 2020). Increased phosphatidylcholine levels are implicated in the development of insulin resistance (Kumar et al., 2021) and its diacyl form has been linked to renal dysfunction in individuals with prediabetes (Huang et al., 2021). Lysophosphatidylcholine, a derivative of phosphatidylcholine, detrimentally affects various cell types through G protein-coupled receptor signaling, leading to inflammation, apoptosis, and insulin resistance (Liu et al., 2020). It may also trigger lipid droplet accumulation by overactivating peroxisome proliferator-activated receptor δ (PPAR-δ), resulting in increased expression of the lipid droplet-coating protein Perilipin 2, decreased mitochondrial autophagic flux, and subsequent stress and apoptosis in proximal tubular organelles, ultimately causing a fast deterioration in renal function in DN (Yoshioka et al., 2022). Moreover, phosphatidylethanolamine, a lipid metabolite, is implicated in the early stages of diabetic kidney damage, with considerably higher levels observed in the glomeruli and renal tubules of diabetic patients compared to non-diabetic controls (Wiggenhauser et al., 2022).
Recent research indicates that A. oxyphylla can regulate lipid metabolism, aiding in the treatment of DN. Studies have shown that Alpinia oxyphylla can reduce levels of sphingomyelin, phosphatidylcholine, lysophosphatidylcholine, and phosphatidylethanolamine in DN mice. Furthermore, it can enhance the excretion of urinary protein, creatinine, and urea nitrogen within a 24-h urine collection period, and reduce glomerular mesangial cell proliferation and mesangial expansion in these mice (Ni et al., 2023). These findings suggest that A. oxyphylla may offer a protective effect for DN by impacting molecular mechanisms associated with lipotoxicity (Figure 3).
2.5 Combating oxidative stress
Oxidative stress is instrumental in the development and progression of DN. Chronic hyperglycemia triggers oxidative stress by increasing the production of reactive oxygen species (ROS), reducing antioxidant defenses, causing oxidative damage to DNA and proteins, and prompting the release of inflammatory mediators and cytokines. These mechanisms adversely affect the structure and function of glomerular capillaries and tubules, thereby intensifying renal and systemic damage (Jin et al., 2023). Normally, antioxidants such as reduced glutathione (GSH), vitamin C, and vitamin E, along with antioxidant enzymes including superoxide dismutase (SOD), peroxidase (POD), and catalase, maintain ROS levels essential for cellular health. However, in Type 2 Diabetes Mellitus (T2DM), endogenous antioxidant responses are overwhelmed, leading to ROS accumulation. Compared to control mice, the db-/db mice exhibit higher levels of malondialdehyde (MDA), lower GSH levels, and diminished activities of POD and SOD (Xie et al., 2017). Reactive oxygen species also affect the protein phosphatase and tensin homolog (PTEN), an inhibitor of insulin signaling (Hao et al., 2023). An increase in PTEN expression, potentially initiating or worsening DN, is associated with chronic oxidative stress, and inhibition of PTEN can reduce ROS-induced insulin resistance (Butler et al., 2002). DN is marked by tubulointerstitial fibrosis (TIF) and epithelial-mesenchymal transition (EMT), processes in which PTEN plays a vital regulatory role (Khokhar et al., 2020). Furthermore, PTEN contributes to DN pathogenesis by facilitating partial EMT through the action of transforming growth factor beta 1 (TGF-β1) (Li et al., 2019).
It is critical to acknowledge that a Phase 3 international study investigating the treatment of stage G4 DN patients with Bardoxolone Methyl was prematurely halted due to an elevated risk of heart failure (de Zeeuw et al., 2013). Bardoxolone Methyl, which activates the transcription factor Nrf2 to regulate antioxidant genes (Liby and Sporn, 2012), experienced a clinical trial failure. However, this outcome does not necessarily disqualify antioxidant therapy as a controversial approach in diabetes nephropathy management. The trial’s failure may result from the drug’s toxic side effects, dosage concerns, or patients’ pre-existing health conditions, such as high levels of B-type natriuretic peptide or prior hospitalizations for heart failure (Chin et al., 2014). Importantly, subsequent research suggests that excluding high-risk patients allows Bardoxolone Methyl to potentially delay the progression to End-Stage Kidney Disease (ESKD) in Chronic Kidney Disease (CKD) patients (Chin et al., 2018), indicating its potential as a novel treatment for CKD, including DN (Kanda and Yamawaki, 2020). Current studies have determined that oxidative stress contributes to the accumulation of extracellular matrix, endothelial dysfunction, podocyte injury, and DN inflammation, thus exacerbating kidney damage and expediting the progression of DN. This underscores its pivotal role in the advancement of DN (Samsu, 2021).
Alpinia oxyphylla, rich in compounds like sesquiterpenes, diterpenes, flavonoids, and diarylheptanes, exhibits strong antioxidant properties (Miao et al., 2015). Research indicates that the extract of A. oxyphylla has a concentration-dependent antioxidant effect (Mo et al., 2016). In DN mice, A. oxyphylla intake mitigates oxidative stress and reduces PTEN protein expression. It also lowers blood glucose levels, as well as urinary albumin, creatinine, and urea nitrogen concentrations (Xie et al., 2017) (Figure 2).
2.6 Mitigating inflammatory responses
Mounting epidemiological and preclinical evidence strongly suggests a link between inflammatory response and the onset and progression of DN. Elevated inflammatory markers, including tumor necrosis factor-alpha (TNF-α), interleukin-1 beta (IL-1β), interleukin-6 (IL-6), and NOD-like receptor protein 3 (NLRP3), have been observed in the serum of DN rats (Fu et al., 2017; Ou et al., 2021). Moreover, patients with type 2 diabetes nephropathy exhibit increased levels of interleukin-18 (IL-18) and IL-1β (Buraczynska et al., 2019; Guo et al., 2023). Importantly, Duan et al. identified inflammation as an independent risk factor for DN progression through renal biopsies (Duan et al., 2021). Experimental models have shown that various anti-inflammatory strategies targeting mediators such as cell adhesion molecules, chemokines, cytokines, and intracellular signaling pathways effectively reduce proteinuria and renal pathology (Rayego-Mateos et al., 2020).
IL-18, a cytokine in the Interleukin-1 (IL-1) family, functions as a predictive marker for diabetic nephropathy (DN) and shows a positive correlation with albuminuria and the progression of renal damage (Fujita et al., 2012; Sueud et al., 2019). Elevated IL-18 levels have been observed in the proximal tubular epithelial cells in renal biopsies from diabetic patients, likely due to the activation of MAPK signaling pathways by diabetic tubular epithelial cells (Miyauchi et al., 2009). IL-1β, a significant innate immune molecule predominantly produced by macrophages, induces the production of other proinflammatory mediators by renal cells (Sims and Smith, 2010) and promotes tubulointerstitial fibrosis, further impairing glycolysis and matrix production (Lemos et al., 2018). The NLRP3 inflammasome, an integral multiprotein complex in chronic inflammation, activates in response to cellular damage (Williams et al., 2022) and plays a vital role in the persistent inflammatory response associated with DN (Wen, Ting, and O'Neill, 2012). Research indicates that hyperglycemia increases NLRP3 expression and activates caspase-1, leading to the release of IL-1β and IL-18 (Wang et al., 2017; Ram et al., 2020), positioning the NLRP3 inflammasome–caspase-1–IL-1β/IL-18 pathway as crucial in the initiation and progression of DN. Notably, Li Kai et al. demonstrated that A. oxyphylla suppresses the activation of the NLRP3 inflammasome, caspase-1, and proinflammatory factors such as IL-18 and IL-1β, thereby reducing inflammation and enhancing the survival of human kidney-2 cells (HK-2) (Wang et al., 2022) (Figure 2).
3 Conclusion and prospects
As modern society progresses, the incidence of DN continues to increase. The complex pathogenesis of DN makes current medical treatments less effective. Notably, A. oxyphylla and its extracts offer significant advantages in managing DN. These natural treatments focus on regulating non-coding RNAs, modulating autophagy and gut microbiota, and reducing lipotoxicity, oxidative stress, and inflammation. Together, these mechanisms alleviate DN symptoms and slow the disease’s progression.
The therapeutic potential of A. oxyphylla in DN has been preliminarily validated through animal and cellular studies. Future research should focus on clinical trials to confirm the efficacy of A. oxyphylla and its extracts in treating DN patients.
Moreover, the complex interactions between autophagy, noncoding RNAs, the gut microbiome, lipotoxicity, oxidative stress, and inflammation may collectively influence key mechanisms underlying DN. Specifically, reducing NLRP3 inflammasome expression can alleviate podocyte damage not only by decreasing lipid accumulation and reducing lipotoxic effects but also by modulating podocyte autophagy. The PI3K/Akt/mTOR signaling pathway plays a crucial role in important processes such as inflammation, oxidative stress, autophagy, and apoptosis in DN. Future investigations may reveal the effects of A. oxyphylla and its compounds on the crosstalk between these cellular pathways in DN from various angles. In the subsequent phase, we will use network pharmacology to pinpoint the advantageous targets of A. oxyphylla in DN treatment and proceed with experimental confirmation. This strategy is designed to identify the most efficacious mechanism by which A. oxyphylla ameliorates DN.
Crucially, the molecular intricacies of A. oxyphylla have not been thoroughly examined, especially concerning its monomeric constituents. Future research should focus on identifying these monomeric components to unveil the essential elements responsible for its therapeutic efficacy in treating DN.
Author contributions
JW: Conceptualization, Investigation, Visualization, Writing–original draft. XW: Investigation, Writing–review and editing. TM: Investigation, Visualization, Writing–review and editing. YX: Funding acquisition, Supervision, Writing–review and editing.
Funding
The author(s) declare that financial support was received for the research, authorship, and/or publication of this article. This work was supported by the National Natural Science Foundation of China (82174334), and Potential Talents of “Hundred Talents Program” for Enhancement of Scientific Research Ability in Affiliated Hospital of Chengdu University of Traditional Chinese Medicine (No. 22-Q36).
Conflict of interest
The authors declare that the research was conducted in the absence of any commercial or financial relationships that could be construed as a potential conflict of interest.
Publisher’s note
All claims expressed in this article are solely those of the authors and do not necessarily represent those of their affiliated organizations, or those of the publisher, the editors and the reviewers. Any product that may be evaluated in this article, or claim that may be made by its manufacturer, is not guaranteed or endorsed by the publisher.
Abbreviations
Akt, protein kinase B; Atg5, autophagy protein5; Becn1, beclin1; BUN, blood urea nitrogen; COI-IV, collagen type IV; CAT, Catalase; DN, diabetic nephropathy; FN, fibronectin; GBM, glomerular basement membrane; GSH, glutathione; GMCs, glomerular mesangial cells; Glu, blood glucose; GM, glomerular mesangial; GS, glomerulosclerosis; GPCR, G protein-coupled receptor; HK-2, human Renal Cortex Proximal Tubule Epithelial Cells; KDM6A, lysine-specific demethylase 6A; KLF10, kruppel-like factor 10; LC3, Microtuble-associated protein light chain 3; LN, Laminin; LncRNA-PVT1, long noncoding RNA -plasmacytoma variant translocation 1; LPC, lysophosphatidylcholine; LPS, lipopolysaccharide; IL-1, interleukins-1; IL-6, interleukins-6; IL-18, interleukin-16; IL-1β, interleukin-1β; IMD, intestinal microflora diversity; INS, insulin; mTOR, mammalian target of rapamycin; MDA, malondialdehyde; MMP-9, Matrixmetalloproteinase-9; NLRP3, the NOD like receptor protein3; NF-κB, nuclear factor kappa B; PC, phosphatidylcholine; PE, phosphatidylethanolamine; POD, peroxidase; P62, sequestosome1; PI3K, phosphatidylinositol-3-kinase; PI3P, phosphatidylinositol-3-phosphate; PTEN, phosphatase and tension protein homolog; PPAR-δ, peroxisome proliferator-activated receptor δ; ROS, reactive oxygen species; SM, sphingomyelin; SOD, superoxide dismutase; Scr, serum creatinine; TC, total cholesterol; TG, triglycerides; TIMP-1, Tissue Inhibitors of Metalloproteinase-1; TGF-β1, Transforming Growth Factor-beta 1; TNF-α, tumor necrosis factor α; TLR, toll-like receptors; UAER, urinary albumin excretion rate; UACR, urine albumin to creatinine ratio; UAE, urinary albumin excretion; UCr, urea creatinine; ULK1, UNC-51 like kinase 1; ULK2, UNC-51 like kinase 2; α-SMA, α-Smooth muscle actin.
References
Al-Bari, M. A. A., and Xu, P. (2020). Molecular regulation of autophagy machinery by mTOR-dependent and -independent pathways. Ann. N. Y. Acad. Sci. 1467 (1), 3–20. doi:10.1111/nyas.14305
Albuquerque, R., Brandão, A. B. P., De Abreu, I., Ferreira, F. G., Santos, L. B., Moreira, L. N., et al. (2019). Saccharomyces boulardii Tht 500101 changes gut microbiota and ameliorates hyperglycaemia, dyslipidaemia, and liver inflammation in streptozotocin-diabetic mice. Benef. Microbes 10 (8), 901–912. doi:10.3920/bm2019.0056
Alvarez, M. L., Khosroheidari, M., Eddy, E., Kiefer, J., and DiStefano, J. K. (2016). Correction: role of MicroRNA 1207-5P and its host gene, the long non-coding RNA Pvt1, as mediators of extracellular matrix accumulation in the kidney: implications for diabetic nephropathy. PLoS One 11 (12), e0168353. doi:10.1371/journal.pone.0168353
Barutta, F., Bellini, S., and Gruden, G. (2022). Mechanisms of podocyte injury and implications for diabetic nephropathy. Clin. Sci. (Lond) 136 (7), 493–520. doi:10.1042/cs20210625
Buraczynska, M., Ksiazek, K., Wacinski, P., and Zaluska, W. (2019). Interleukin-1β gene (IL1B) polymorphism and risk of developing diabetic nephropathy. Immunol. Invest. 48 (6), 577–584. doi:10.1080/08820139.2019.1595642
Butler, M., McKay, R. A., Popoff, I. J., Gaarde, W. A., Witchell, D., Murray, S. F., et al. (2002). Specific inhibition of PTEN expression reverses hyperglycemia in diabetic mice. Diabetes 51 (4), 1028–1034. doi:10.2337/diabetes.51.4.1028
Cani, P. D., and de Vos, W. M. (2017). Next-generation beneficial microbes: the case of Akkermansia muciniphila. Front. Microbiol. 8, 1765. doi:10.3389/fmicb.2017.01765
Chen, F., Wei, Y., and Xie, Y. (2016). The effects of alpinia oxyphylla extracts on the reducing urination and nourishing kidney in rats with kidney -Yang deficiency. Chin. Archives Traditional Chin. Med. 34 (10), 2346–2347. doi:10.13193/j.issn.1673-7717.2016.10.011
Chen, X., Zhao, L., Xing, Y., and Lin, B. (2018). Down-regulation of microRNA-21 reduces inflammation and podocyte apoptosis in diabetic nephropathy by relieving the repression of TIMP3 expression. Biomed. Pharmacother. 108, 7–14. doi:10.1016/j.biopha.2018.09.007
Chin, M. P., Bakris, G. L., Block, G. A., Chertow, G. M., Goldsberry, A., Inker, L. A., et al. (2018). Bardoxolone methyl improves kidney function in patients with chronic kidney disease stage 4 and type 2 diabetes: post-hoc analyses from bardoxolone methyl evaluation in patients with chronic kidney disease and type 2 diabetes study. Am. J. Nephrol. 47 (1), 40–47. doi:10.1159/000486398
Chin, M. P., Wrolstad, D., Bakris, G. L., Chertow, G. M., de Zeeuw, D., Goldsberry, A., et al. (2014). Risk factors for heart failure in patients with type 2 diabetes mellitus and stage 4 chronic kidney disease treated with bardoxolone methyl. J. Card. Fail 20 (12), 953–958. doi:10.1016/j.cardfail.2014.10.001
Cui, J., Bai, X., and Chen, X. (2020). Autophagy and diabetic nephropathy. Adv. Exp. Med. Biol. 1207, 487–494. doi:10.1007/978-981-15-4272-5_36
Deshpande, A. D., Harris-Hayes, M., and Schootman, M. (2008). Epidemiology of diabetes and diabetes-related complications. Phys. Ther. 88 (11), 1254–1264. doi:10.2522/ptj.20080020
de Zeeuw, D., Akizawa, T., Audhya, P., Bakris, G. L., Chin, M., Christ-Schmidt, H., et al. (2013). Bardoxolone methyl in type 2 diabetes and stage 4 chronic kidney disease. N. Engl. J. Med. 369 (26), 2492–2503. doi:10.1056/NEJMoa1306033
Du, G., Xiao, M., Zhang, X., Wen, M., Pang, C., Jiang, S., et al. (2017). Alpinia oxyphylla Miq. extract changes miRNA expression profiles in db-/db-mouse kidney. Biol. Res. 50 (1), 9. doi:10.1186/s40659-017-0111-1
Du, X., Liu, J., Xue, Y., Kong, X., Lv, C., Li, Z., et al. (2021). Alteration of gut microbial profile in patients with diabetic nephropathy. Endocrine 73 (1), 71–84. doi:10.1007/s12020-021-02721-1
Duan, S., Sun, L., Zhang, C., Wu, L., Nie, G., Huang, Z., et al. (2021). Association of platelet-to-lymphocyte ratio with kidney clinicopathologic features and renal outcomes in patients with diabetic kidney disease. Int. Immunopharmacol. 93, 107413. doi:10.1016/j.intimp.2021.107413
Ebadi, Z., Moradi, N., Kazemi Fard, T., Balochnejadmojarrad, T., Chamani, E., Fadaei, R., et al. (2019). Captopril and spironolactone can attenuate diabetic nephropathy in wistar rats by targeting microRNA-192 and microRNA-29a/b/c. DNA Cell Biol. 38 (10), 1134–1142. doi:10.1089/dna.2019.4732
Fu, Y., Wu, N., and Zhao, D. (2017). Function of NLRP3 in the pathogenesis and development of diabetic nephropathy. Med. Sci. Monit. 23, 3878–3884. doi:10.12659/msm.903269
Fujita, T., Ogihara, N., Kamura, Y., Satomura, A., Fuke, Y., Shimizu, C., et al. (2012). Interleukin-18 contributes more closely to the progression of diabetic nephropathy than other diabetic complications. Acta Diabetol. 49 (2), 111–117. doi:10.1007/s00592-010-0178-4
Galluzzi, L., and Green, D. R. (2019). Autophagy-independent functions of the autophagy machinery. Cell 177 (7), 1682–1699. doi:10.1016/j.cell.2019.05.026
Griffin, J. L., Wang, X., and Stanley, E. (2015). Does our gut microbiome predict cardiovascular risk? A review of the evidence from metabolomics. Circ. Cardiovasc Genet. 8 (1), 187–191. doi:10.1161/circgenetics.114.000219
Guo, X., Gan, M., Zhou, L., Wei, Q., Li, Z., Luo, Z., et al. (2023). Correlation between plasma long non-coding RNA MEG-3 and inflammatory cytokines in patients with diabetic nephropathy. Clin. Nephrol. 100 (2), 67–73. doi:10.5414/cn110996
Gupta, S., Dominguez, M., and Golestaneh, L. (2023). Diabetic kidney disease: an update. Med. Clin. North Am. 107 (4), 689–705. doi:10.1016/j.mcna.2023.03.004
Hanson, R. L., Craig, D. W., Millis, M. P., Yeatts, K. A., Kobes, S., Pearson, J. V., et al. (2007). Identification of PVT1 as a candidate gene for end-stage renal disease in type 2 diabetes using a pooling-based genome-wide single nucleotide polymorphism association study. Diabetes 56 (4), 975–983. doi:10.2337/db06-1072
Hao, Z., Huajun, S., Zhen, G., Yu, X., Qian, L., Ziling, C., et al. (2023). AQP8 promotes glioma proliferation and growth, possibly through the ROS/PTEN/AKT signaling pathway. BMC Cancer 23 (1), 516. doi:10.1186/s12885-023-11025-8
Huang, J., Covic, M., Huth, C., Rommel, M., Adam, J., Zukunft, S., et al. (2021). Validation of candidate phospholipid biomarkers of chronic kidney disease in hyperglycemic individuals and their organ-specific exploration in leptin receptor-deficient db/db mouse. Metabolites 11 (2), 89. doi:10.3390/metabo11020089
Ji, T. T., Wang, Y. K., Zhu, Y. C., Gao, C. P., Li, X. Y., Li, J., et al. (2019). Long noncoding RNA Gm6135 functions as a competitive endogenous RNA to regulate toll-like receptor 4 expression by sponging miR-203-3p in diabetic nephropathy. J. Cell Physiol. 234 (5), 6633–6641. doi:10.1002/jcp.27412
Jiaxin, L. (2021) Study on protective effect of suo quan pill on renal function of diabetic nephropathy db/db mice. Chengdu University of Traditional Chinese Medicine. doi:10.26988/d.cnki.gcdzu.2021.000651
Jin, Q., Liu, T., Qiao, Y., Liu, D., Yang, L., Mao, H., et al. (2023). Oxidative stress and inflammation in diabetic nephropathy: role of polyphenols. Front. Immunol. 14, 1185317. doi:10.3389/fimmu.2023.1185317
Kai, L., Tianpeng, M., Niu, K., Xie, Y., and Mi, L. (2021b). A review of the related studys of the prevention and treatment of diabetic nephropathy by the alpinia oxyphylla. Chin. Med. Mod. Distance Educ. China 19 (03), 192–195.
Kai, L., Xie, Y., Chen, G., Qian, L., Mao, X., Yali, N., et al. (2021a). Study on the mechanism of early application of alpinia oxyphyllae in prevention and treatment of diabetic nephropathy through NLRP3 inflammatory body pathway based on the thought of "synopsis of golden chamber. Available at: https://kns.cnki.net/kcms2/article/abstract?v=1ya23wS0yuABz5Hq0l6DDKKJnmzU_KUlGmr30D1EYLFTELXdofIOSj4QXTAYJWHIOo09YAPGQVTqI2Yx18KKOnHQW8WHUlKe3vzRiS_qv7Vrbhvr2wG46bhiBbVqd9ChYmhHS8d_f5Q72PxNRfRw==uniplatform=NZKPTlanguage=CHS.
Kanda, H., and Yamawaki, K. (2020). Bardoxolone methyl: drug development for diabetic kidney disease. Clin. Exp. Nephrol. 24 (10), 857–864. doi:10.1007/s10157-020-01917-5
Khokhar, M., Roy, D., Modi, A., Agarwal, R., Yadav, D., Purohit, P., et al. (2020). Perspectives on the role of PTEN in diabetic nephropathy: an update. Crit. Rev. Clin. Lab. Sci. 57 (7), 470–483. doi:10.1080/10408363.2020.1746735
Kumar, A., Sundaram, K., Mu, J., Dryden, G. W., Sriwastva, M. K., Lei, C., et al. (2021). High-fat diet-induced upregulation of exosomal phosphatidylcholine contributes to insulin resistance. Nat. Commun. 12 (1), 213. doi:10.1038/s41467-020-20500-w
Lahiri, V., Hawkins, W. D., and Klionsky, D. J. (2019). Watch what you (self-) eat: autophagic mechanisms that modulate metabolism. Cell Metab. 29 (4), 803–826. doi:10.1016/j.cmet.2019.03.003
Lai, W., Luo, D., Li, Y., Li, Y., Wang, Q., Hu, Z., et al. (2023). Irisin ameliorates diabetic kidney disease by restoring autophagy in podocytes. Faseb J. 37 (10), e23175. doi:10.1096/fj.202300420R
Lemos, D. R., McMurdo, M., Karaca, G., Wilflingseder, J., Leaf, I. A., Gupta, N., et al. (2018). Interleukin-1β activates a MYC-dependent metabolic switch in kidney stromal cells necessary for progressive tubulointerstitial fibrosis. J. Am. Soc. Nephrol. 29 (6), 1690–1705. doi:10.1681/asn.2017121283
Leung, A., Amaram, V., and Natarajan, R. (2019). Linking diabetic vascular complications with LncRNAs. Vasc. Pharmacol. 114, 139–144. doi:10.1016/j.vph.2018.01.007
Li, X., Zhang, Y., Xing, X., Li, M., Liu, Y., Xu, A., et al. (2023). Podocyte injury of diabetic nephropathy: novel mechanism discovery and therapeutic prospects. Biomed. Pharmacother. 168, 115670. doi:10.1016/j.biopha.2023.115670
Li, Y., Hu, Q., Li, C., Liang, K., Xiang, Y., Hsiao, H., et al. (2019). PTEN-induced partial epithelial-mesenchymal transition drives diabetic kidney disease. J. Clin. Invest. 129 (3), 1129–1151. doi:10.1172/jci121987
Li, Y., Xu, Y., Hou, Y., and Li, R. (2021). Construction and bioinformatics analysis of the miRNA-mRNA regulatory network in diabetic nephropathy. J. Healthc. Eng. 2021, 8161701. doi:10.1155/2021/8161701
Liby, K. T., and Sporn, M. B. (2012). Synthetic oleanane triterpenoids: multifunctional drugs with a broad range of applications for prevention and treatment of chronic disease. Pharmacol. Rev. 64 (4), 972–1003. doi:10.1124/pr.111.004846
Liu, L., Bai, F., Song, H., Xiao, R., Wang, Y., Yang, H., et al. (2022c). Upregulation of TIPE1 in tubular epithelial cell aggravates diabetic nephropathy by disrupting PHB2 mediated mitophagy. Redox Biol. 50, 102260. doi:10.1016/j.redox.2022.102260
Liu, P., Zhu, W., Chen, C., Yan, B., Zhu, L., Chen, X., et al. (2020). The mechanisms of lysophosphatidylcholine in the development of diseases. Life Sci. 247, 117443. doi:10.1016/j.lfs.2020.117443
Liu, T., Yang, L., Mao, H., Ma, F., Wang, Y., Li, S., et al. (2022a). Sirtuins as novel pharmacological targets in podocyte injury and related glomerular diseases. Biomed. Pharmacother. 155, 113620. doi:10.1016/j.biopha.2022.113620
Liu, X., Mao, B., Gu, J., Wu, J., Cui, S., Wang, G., et al. (2021a). Blautia-a new functional genus with potential probiotic properties? Gut Microbes 13 (1), 1–21. doi:10.1080/19490976.2021.1875796
Liu, X. J., Hu, X. K., Yang, H., Gui, L. M., Cai, Z. X., Qi, M. S., et al. (2022b). A review of traditional Chinese medicine on treatment of diabetic nephropathy and the involved mechanisms. Am. J. Chin. Med. 50 (7), 1739–1779. doi:10.1142/s0192415x22500744
Liu, Y., Liu, X., Lin, C., Jia, X., Zhu, H., Song, J., et al. (2021b). Noncoding RNAs regulate alternative splicing in Cancer. J. Exp. Clin. Cancer Res. 40 (1), 11. doi:10.1186/s13046-020-01798-2
Lobel, L., Cao, Y. G., Fenn, K., Glickman, J. N., and Garrett, W. S. (2020). Diet posttranslationally modifies the mouse gut microbial proteome to modulate renal function. Science 369 (6510), 1518–1524. doi:10.1126/science.abb3763
Long, J., Wang, Y., Wang, W., Chang, B. H., and Danesh, F. R. (2011). MicroRNA-29c is a signature microRNA under high glucose conditions that targets Sprouty homolog 1, and its in vivo knockdown prevents progression of diabetic nephropathy. J. Biol. Chem. 286 (13), 11837–11848. doi:10.1074/jbc.M110.194969
Lovisa, S., LeBleu, V. S., Tampe, B., Sugimoto, H., Vadnagara, K., Carstens, J. L., et al. (2015). Epithelial-to-mesenchymal transition induces cell cycle arrest and parenchymal damage in renal fibrosis. Nat. Med. 21 (9), 998–1009. doi:10.1038/nm.3902
Luo, L., Luo, J., Cai, Y., Fu, M., Li, W., Shi, L., et al. (2022). Inulin-type fructans change the gut microbiota and prevent the development of diabetic nephropathy. Pharmacol. Res. 183, 106367. doi:10.1016/j.phrs.2022.106367
Lv, J., Wu, Y., Mai, Y., and Bu, S. (2020). Noncoding RNAs in diabetic nephropathy: pathogenesis, biomarkers, and therapy. J. Diabetes Res. 2020, 3960857. doi:10.1155/2020/3960857
Machado, I. F., Teodoro, J. S., Palmeira, C. M., and Rolo, A. P. (2020). miR-378a: a new emerging microRNA in metabolism. Cell Mol. Life Sci. 77 (10), 1947–1958. doi:10.1007/s00018-019-03375-z
Mao, L., Huang, L., Xiao, M., Du, G., Yao, Y., Li, X., et al. (2018). Inhibitory effect of Yizhiren combined with Obtusiloba on proliferation of high glucose-cultured mesangial cells. Shandong Med. J. 58 (33), 32–35. doi:10.3969/j.issn.1002-266X.2018.33.009
Mao, Z. H., Gao, Z. X., Liu, D. W., Liu, Z. S., and Wu, P. (2023). Gut microbiota and its metabolites - molecular mechanisms and management strategies in diabetic kidney disease. Front. Immunol. 14, 1124704. doi:10.3389/fimmu.2023.1124704
Mauceri, D. (2022). Role of epigenetic mechanisms in chronic pain. Cells 11 (16), 2613. doi:10.3390/cells11162613
Mercer, K. E., Yeruva, L., Pack, L., Graham, J. L., Stanhope, K. L., Chintapalli, S. V., et al. (2020). Xenometabolite signatures in the UC Davis type 2 diabetes mellitus rat model revealed using a metabolomics platform enriched with microbe-derived metabolites. Am. J. Physiol. Gastrointest. Liver Physiol. 319 (2), G157–g169. doi:10.1152/ajpgi.00105.2020
Miao, Q., Kong, W., Zhao, X., Yang, S., and Yang, M. (2015). GC-FID coupled with chemometrics for quantitative and chemical fingerprinting analysis of Alpinia oxyphylla oil. J. Pharm. Biomed. Anal. 102, 436–442. doi:10.1016/j.jpba.2014.10.014
Miricescu, D., Balan, D. G., Tulin, A., Stiru, O., Vacaroiu, I. A., Mihai, D. A., et al. (2021). PI3K/AKT/mTOR signalling pathway involvement in renal cell carcinoma pathogenesis (Review). Exp. Ther. Med. 21 (5), 540. doi:10.3892/etm.2021.9972
Mitrofanova, A., Merscher, S., and Fornoni, A. (2023). Kidney lipid dysmetabolism and lipid droplet accumulation in chronic kidney disease. Nat. Rev. Nephrol. 19 (10), 629–645. doi:10.1038/s41581-023-00741-w
Miyauchi, K., Takiyama, Y., Honjyo, J., Tateno, M., and Haneda, M. (2009). Upregulated IL-18 expression in type 2 diabetic subjects with nephropathy: TGF-beta1 enhanced IL-18 expression in human renal proximal tubular epithelial cells. Diabetes Res. Clin. Pract. 83 (2), 190–199. doi:10.1016/j.diabres.2008.11.018
Mo, J., Liu, S., and Xie, Y. (2016). Effect of Alpiniae Oxyphyllae Fractus ether extract on oxidative stress in mice with diabetic nephropathy. China Trop. Med. 16 (05), 463–465. doi:10.13604/j.cnki.46-1064/r.2016.05.15
Mok, H., Al-Jumaily, A., and Lu, J. (2022). Plasmacytoma variant translocation 1 (PVT1) gene as a potential novel target for the treatment of diabetic nephropathy. Biomedicines 10 (11), 2711. doi:10.3390/biomedicines10112711
Ni, Y. (2019). Studies on the mechanism of YiZhiRen in the treatment of diabetic nephropathy based on gut microbiota and metabonomics analysis. Master, First Affil. Hosp. Hainan Med. Univ., Cnki. doi:10.27952/d.cnki.ghnyx.2019.000002
Ni, Y., Yao, Y., Can, T., Huang, X., and Xie, Y. (2021). Study on the difference of effect of Sou-quan-yi-shen prescription and Yizhiren on the efficacy and gut microbiome of DKD mice. J. Hainan Med. Univ. 27 (11), 820–826. doi:10.13210/j.cnki.jhmu.20210104.002
Ni, Y., Yao, Y., Wu, S., and Xie, Y. (2023). Study on the protective mechanism of Yizhiren regulating lipid metabolism in mice with diabetic nephropathy. J. Hainan Med. Univ. 29 (11), 801–807. doi:10.13210/j.cnki.jhmu.20230329.001
Opazo-Ríos, L., Mas, S., Marín-Royo, G., Mezzano, S., Gómez-Guerrero, C., Moreno, J. A., et al. (2020). Lipotoxicity and diabetic nephropathy: novel mechanistic insights and therapeutic opportunities. Int. J. Mol. Sci. 21 (7), 2632. doi:10.3390/ijms21072632
Ou, Y., Zhang, W., Chen, S., and Deng, H. (2021). Baicalin improves podocyte injury in rats with diabetic nephropathy by inhibiting PI3K/Akt/mTOR signaling pathway. Open Med. (Wars) 16 (1), 1286–1298. doi:10.1515/med-2021-0335
Park, C. L., Kim, J. H., Jeon, J. S., Lee, J. H., Zhang, K., Guo, S., et al. (2022). Protective effect of alpinia oxyphylla fruit against tert-butyl hydroperoxide-induced toxicity in HepG2 cells via Nrf2 activation and free radical scavenging and its active molecules. Antioxidants (Basel) 11 (5), 1032. doi:10.3390/antiox11051032
Pelle, M. C., Provenzano, M., Busutti, M., Porcu, C. V., Zaffina, I., Stanga, L., et al. (2022). Up-date on diabetic nephropathy. Life (Basel) 12 (8), 1202. doi:10.3390/life12081202
Pongrac Barlovic, D., Harjutsalo, V., Sandholm, N., Forsblom, C., and Groop, P. H.FinnDiane Study Group (2020). Sphingomyelin and progression of renal and coronary heart disease in individuals with type 1 diabetes. Diabetologia 63 (9), 1847–1856. doi:10.1007/s00125-020-05201-9
Qin, B., and Cao, X. (2021). LncRNA PVT1 regulates high glucose-induced viability, oxidative stress, fibrosis, and inflammation in diabetic nephropathy via miR-325-3p/snail1 Axis. Diabetes Metab. Syndr. Obes. 14, 1741–1750. doi:10.2147/dmso.S303151
Ram, C., Jha, A. K., Ghosh, A., Gairola, S., Syed, A. M., Murty, U. S., et al. (2020). Targeting NLRP3 inflammasome as a promising approach for treatment of diabetic nephropathy: preclinical evidences with therapeutic approaches. Eur. J. Pharmacol. 885, 173503. doi:10.1016/j.ejphar.2020.173503
Ramezani, A., and Raj, D. S. (2014). The gut microbiome, kidney disease, and targeted interventions. J. Am. Soc. Nephrol. 25 (4), 657–670. doi:10.1681/asn.2013080905
Rayego-Mateos, S., Morgado-Pascual, J. L., Opazo-Ríos, L., Guerrero-Hue, M., García-Caballero, C., Vázquez-Carballo, C., et al. (2020). Pathogenic pathways and therapeutic approaches targeting inflammation in diabetic nephropathy. Int. J. Mol. Sci. 21 (11), 3798. doi:10.3390/ijms21113798
Richardson, A. J., McKain, N., and Wallace, R. J. (2013). Ammonia production by human faecal bacteria, and the enumeration, isolation and characterization of bacteria capable of growth on peptides and amino acids. BMC Microbiol. 13, 6. doi:10.1186/1471-2180-13-6
Ruffo, P., De Amicis, F., Giardina, E., and Conforti, F. L. (2023). Long-noncoding RNAs as epigenetic regulators in neurodegenerative diseases. Neural Regen. Res. 18 (6), 1243–1248. doi:10.4103/1673-5374.358615
Salguero, M. V., Al-Obaide, M. A. I., Singh, R., Siepmann, T., and Vasylyeva, T. L. (2019). Dysbiosis of Gram-negative gut microbiota and the associated serum lipopolysaccharide exacerbates inflammation in type 2 diabetic patients with chronic kidney disease. Exp. Ther. Med. 18 (5), 3461–3469. doi:10.3892/etm.2019.7943
Samsu, N. (2021). Diabetic nephropathy: challenges in pathogenesis, diagnosis, and treatment. Biomed. Res. Int. 2021, 1497449. doi:10.1155/2021/1497449
Shen, Z., Fang, Y., Xing, T., and Wang, F. (2017). Diabetic nephropathy: from pathophysiology to treatment. J. Diabetes Res. 2017, 2379432. doi:10.1155/2017/2379432
Sims, J. E., and Smith, D. E. (2010). The IL-1 family: regulators of immunity. Nat. Rev. Immunol. 10 (2), 89–102. doi:10.1038/nri2691
Singh, H., Miyamoto, S., Darshi, M., Torralba, M. G., Kwon, K., Sharma, K., et al. (2020). Gut microbial changes in diabetic db/db mice and recovery of microbial diversity upon pirfenidone treatment. Microorganisms 8 (9), 1347. doi:10.3390/microorganisms8091347
Su, P. P., Liu, D. W., Zhou, S. J., Chen, H., Wu, X. M., and Liu, Z. S. (2022). Down-regulation of Risa improves podocyte injury by enhancing autophagy in diabetic nephropathy. Mil. Med. Res. 9 (1), 23. doi:10.1186/s40779-022-00385-0
Sueud, T., Hadi, N. R., Abdulameer, R., Jamil, D. A., and Al-Aubaidy, H. A. (2019). Assessing urinary levels of IL-18, NGAL and albumin creatinine ratio in patients with diabetic nephropathy. Diabetes Metab. Syndr. 13 (1), 564–568. doi:10.1016/j.dsx.2018.11.022
Sun, B., Zhai, S., Zhang, L., and Sun, G. (2021). The role of extracellular vesicles in podocyte autophagy in kidney disease. J. Cell Commun. Signal 15 (3), 299–316. doi:10.1007/s12079-020-00594-z
Szostak, J., Gorący, A., Durys, D., Dec, P., Modrzejewski, A., and Pawlik, A. (2023). The role of MicroRNA in the pathogenesis of diabetic nephropathy. Int. J. Mol. Sci. 24 (7), 6214. doi:10.3390/ijms24076214
Tao, S., Li, L., Li, L., Liu, Y., Ren, Q., Shi, M., et al. (2019). Understanding the gut-kidney axis among biopsy-proven diabetic nephropathy, type 2 diabetes mellitus and healthy controls: an analysis of the gut microbiota composition. Acta Diabetol. 56 (5), 581–592. doi:10.1007/s00592-019-01316-7
Tofte, N., Suvitaival, T., Ahonen, L., Winther, S. A., Theilade, S., Frimodt-Møller, M., et al. (2019). Lipidomic analysis reveals sphingomyelin and phosphatidylcholine species associated with renal impairment and all-cause mortality in type 1 diabetes. Sci. Rep. 9 (1), 16398. doi:10.1038/s41598-019-52916-w
Vaziri, N. D., Wong, J., Pahl, M., Piceno, Y. M., Yuan, J., DeSantis, T. Z., et al. (2013). Chronic kidney disease alters intestinal microbial flora. Kidney Int. 83 (2), 308–315. doi:10.1038/ki.2012.345
Wang, B., Jin, Z., Ren, X., and Yinfeng, T. (2023a). Research progress on chemical components and pharmacological action of Alpiniae oxyphyllae Fructus in the past five years. J. Guangdong Pharm. Univ. 39 (04), 120–127. doi:10.16809/j.cnki.2096-3653.2023041101
Wang, F. (2023). Study on the mechanism of Suoquan Yishen Formula regulating KDM6A/KLF10 signal axis through miR-378d on EMT of diabetic nephropathy. Master, First Affil. Hosp. Hainan Med. Univ., Cnki. doi:10.27952/d.cnki.ghnyx.2023.000201
Wang, F., Xiao, M., Chen, B., Huang, M., Ma, Y., Liu, S., et al. (2023c). Regulation of epithelial-mesenchymal transition of glomerular podocytes by suoquan yishen formula containing serum through inhibition of KDM6A. Pharmacol. Clin. Chin. Materia Medica, 1–14. doi:10.13412/j.cnki.zyyl.20231208.001
Wang, H., Song, Y., Wu, Y., Kumar, V., Mahato, R. I., and Su, Q. (2021). Activation of dsRNA-dependent protein kinase R by MicroRNA-378 sustains metabolic inflammation in hepatic insulin resistance. Diabetes, db200181. doi:10.2337/20-0181
Wang, S., Qin, S., Cai, B., Zhan, J., and Chen, Q. (2023b). Promising therapeutic mechanism for Chinese herbal medicine in ameliorating renal fibrosis in diabetic nephropathy. Front. Endocrinol. (Lausanne) 14, 932649. doi:10.3389/fendo.2023.932649
Wang, S., Zhao, X., Yang, S., Chen, B., and Shi, J. (2017). Salidroside alleviates high glucose-induced oxidative stress and extracellular matrix accumulation in rat glomerular mesangial cells by the TXNIP-NLRP3 inflammasome pathway. Chem. Biol. Interact. 278, 48–53. doi:10.1016/j.cbi.2017.10.012
Wang, X., Jiang, H., Wang, F., Chang, H., Rui, K., Ma, T., et al. (2022). A study on the effect of yizhiren fangfeng drug pairs and their decomposed formulas on high glucose induced HK-2 cell survival and inflammatory factors based on kidney tonifying and wind dispelling methods. Lishizhen Med. Materia Medica Res. 33 (01), 68–71. doi:10.3969/j.issn.1008-0805.2022.01.16
Wang, Y., Wang, M., Fan, K., Li, T., Yan, T., Wu, B., et al. (2018). Protective effects of Alpinae Oxyphyllae Fructus extracts on lipopolysaccharide-induced animal model of Alzheimer's disease. J. Ethnopharmacol. 217, 98–106. doi:10.1016/j.jep.2018.02.015
Wen, H., Ting, J. P., and O'Neill, L. A. (2012). A role for the NLRP3 inflammasome in metabolic diseases--did Warburg miss inflammation? Nat. Immunol. 13 (4), 352–357. doi:10.1038/ni.2228
Wiggenhauser, L. M., Metzger, L., Bennewitz, K., Soleymani, S., Boger, M., Tabler, C. T., et al. (2022). pdx1 knockout leads to a diabetic nephropathy- like phenotype in zebrafish and identifies phosphatidylethanolamine as metabolite promoting early diabetic kidney damage. Diabetes 71 (5), 1073–1080. doi:10.2337/db21-0645
Williams, B. M., Cliff, C. L., Lee, K., Squires, P. E., and Hills, C. E. (2022). The role of the NLRP3 inflammasome in mediating glomerular and tubular injury in diabetic nephropathy. Front. Physiol. 13, 907504. doi:10.3389/fphys.2022.907504
Wu, J. (2021). Comparison of chemical composition content and diabetic nephropathy efficacy of non-processed or SaltProcessed alpinia oxyphylla. Master, First Affil. Hosp. Hainan Med. Univ. Cnki. doi:10.27952/d.cnki.ghnyx.2021.000034
Wu, X. Y., Yu, J., and Tian, H. M. (2019). Effect of SOCS1 on diabetic renal injury through regulating TLR signaling pathway. Eur. Rev. Med. Pharmacol. Sci. 23 (18), 8068–8074. doi:10.26355/eurrev_201909_19023
Xie, Y., Liu, Q., Wei, Y., Xing, M., Yin, D., and Zhu, Y. (2015). Screening of effective fraction for anti-diabetic nephropathy by alpiniae oxyphyllae fructus extract. Chin. J. Exp. Traditional Med. Formulae 21 (03), 114–117. doi:10.13422/j.cnki.syfjx.2015030114
Xie, Y., Xiao, M., Li, D., Liu, H., Yun, F., Wei, Y., et al. (2017). Anti-diabetic effect of Alpinia oxyphylla extract on 57BL/KsJ db-/db-mice. Exp. Ther. Med. 13 (4), 1321–1328. doi:10.3892/etm.2017.4152
Xie, Y., Xiao, M., Ni, Y., Jiang, S., Feng, G., Sang, S., et al. (2018). Alpinia oxyphylla miq. Extract prevents diabetes in mice by modulating gut microbiota. J. Diabetes Res. 2018, 4230590. doi:10.1155/2018/4230590
Xu, H. M., and Hu, F. (2022). The role of autophagy and mitophagy in cancers. Arch. Physiol. Biochem. 128 (2), 281–289. doi:10.1080/13813455.2019.1675714
Xu, J., Kitada, M., Ogura, Y., Liu, H., and Koya, D. (2021). Dapagliflozin restores impaired autophagy and suppresses inflammation in high glucose-treated HK-2 cells. Cells 10 (6), 1457. doi:10.3390/cells10061457
Xu, Y. X., Pu, S. D., Li, X., Yu, Z. W., Zhang, Y. T., Tong, X. W., et al. (2022). Exosomal ncRNAs: novel therapeutic target and biomarker for diabetic complications. Pharmacol. Res. 178, 106135. doi:10.1016/j.phrs.2022.106135
Yang, C., Zhang, Z., Liu, J., Chen, P., Li, J., Shu, H., et al. (2023). Research progress on multiple cell death pathways of podocytes in diabetic kidney disease. Mol. Med. 29 (1), 135. doi:10.1186/s10020-023-00732-4
Yang, F., Qu, Q., Zhao, C., Liu, X., Yang, P., Li, Z., et al. (2020a). Paecilomyces cicadae-fermented Radix astragali activates podocyte autophagy by attenuating PI3K/AKT/mTOR pathways to protect against diabetic nephropathy in mice. Biomed. Pharmacother. 129, 110479. doi:10.1016/j.biopha.2020.110479
Yang, X., Hu, C., Wang, S., and Chen, Q. (2020b). Clinical efficacy and safety of Chinese herbal medicine for the treatment of patients with early diabetic nephropathy: a protocol for systematic review and meta-analysis. Med. Baltim. 99 (29), e20678. doi:10.1097/md.0000000000020678
Yang, Y. (2018). Anti-diabetic mechanism of AOE through regulating miRNA expression down-regulates PTEN. Master, First Affil. Hosp. Hainan Med. Univ. Available at: https://kns.cnki.net/kcms2/article/abstract?v=1ya23wS0yuB6cswntBScXQXQLDvKaPcI86QH5IX7uw277JXNLvtkD6QbKV2Kp_9pC3061Ys5yiIhd2YyRWg1I877LXuDe4D9nrcnPSrt8J2fvPR47NiZqhcgL1ZX3c211f36i7jek=&uniplatform=NZKPT&language=CHS.
Yao, R. W., Wang, Y., and Chen, L. L. (2019). Cellular functions of long noncoding RNAs. Nat. Cell Biol. 21 (5), 542–551. doi:10.1038/s41556-019-0311-8
Yi, H., Peng, R., Zhang, L. Y., Sun, Y., Peng, H. M., Liu, H. D., et al. (2017). LincRNA-Gm4419 knockdown ameliorates NF-κB/NLRP3 inflammasome-mediated inflammation in diabetic nephropathy. Cell Death Dis. 8 (2), e2583. doi:10.1038/cddis.2016.451
Yin, D., Li, S., Xie, Y., Ye, Z., and Zhu, W. (2021). Study on the protective effect of the compatibility of Yizhiren and Wuyao on podocytes in diabetes nephropathy mice by regulating autophagy. Lishizhen Med. Materia Medica Res. 32 (09):2088–2090. doi:10.3969/j.issn.1008-0805.2021.09.11
Yin, D., Tang, S., Wu, Z., Chen, Y., and Zhu, Y. (2023). Study on the mechanism of the Yizhiren-Wuyao pair in regulating PI3K/Akt/mTOR pathway-mediated cellular autophagy to protect glomerular foot cells. Chin. Archives Traditional Chin. Med., 1–16. doi:10.13193/j.issn.1673-7717.2024.01.006
Yin, D., Zhu, Y., Ni, Y., Yao, Y., and Xie, Y. (2019). Clinical effect of Suoquan Yishen Fang on type 2 diabetic nephropathy. Chin. J. Gerontology 39 (09), 2091–2092. doi:10.3969/j.issn.1005-9202.2019.09.014
Yoshioka, K., Hirakawa, Y., Kurano, M., Ube, Y., Ono, Y., Kojima, K., et al. (2022). Lysophosphatidylcholine mediates fast decline in kidney function in diabetic kidney disease. Kidney Int. 101 (3), 510–526. doi:10.1016/j.kint.2021.10.039
Yu, S. H., Kim, H. J., Jeon, S. Y., Kim, M. R., Lee, B. S., Lee, J. J., et al. (2020). Anti-inflammatory and anti-nociceptive activities of Alpinia Oxyphylla Miquel extracts in animal models. J. Ethnopharmacol. 260, 112985. doi:10.1016/j.jep.2020.112985
Zhang, T., Qiu, J., Wu, X., Huang, S., Yuan, H., and Park, S. (2020). Schizonepeta tenuifolia with alpinia oxyphylla alleviates atopic dermatitis and improves the gut microbiome in nc/nga mice. Pharmaceutics 12 (8), 722. doi:10.3390/pharmaceutics12080722
Zhao, H., Yang, C. E., Liu, T., Zhang, M. X., Niu, Y., Wang, M., et al. (2023). The roles of gut microbiota and its metabolites in diabetic nephropathy. Front. Microbiol. 14, 1207132. doi:10.3389/fmicb.2023.1207132
Zhong, W., Zeng, J., Xue, J., Du, A., and Xu, Y. (2020). Knockdown of lncRNA PVT1 alleviates high glucose-induced proliferation and fibrosis in human mesangial cells by miR-23b-3p/WT1 axis. Diabetol. Metab. Syndr. 12, 33. doi:10.1186/s13098-020-00539-x
Zhu, G., Wang, X., Qi, C., and Luan, J. (2019). The regulatory effect of Astragalus total flavonoids on high glucoseinduced inflammatory factors in HK-2 cells through miR-378. Immunol. J. 35 (08), 653–658. doi:10.13431/j.cnki.immunol.j.20190102
Keywords: diabetic nephropathy, Alpinia oxyphylla, Chinese herbal medicine, mechanism, research progress
Citation: Wang J, Wang X, Ma T and Xie Y (2024) Research progress on Alpinia oxyphylla in the treatment of diabetic nephropathy. Front. Pharmacol. 15:1390672. doi: 10.3389/fphar.2024.1390672
Received: 23 February 2024; Accepted: 13 May 2024;
Published: 14 June 2024.
Edited by:
Somasundaram Arumugam, National Institute of Pharmaceutical Education and Research, IndiaReviewed by:
Song Yi, First Affiliated Hospital of Zhengzhou University, ChinaVivian Soetikno, University of Indonesia, Indonesia
Shiladitya Chattopadhyay, National Institute of Pharmaceutical Education and Research, India
Copyright © 2024 Wang, Wang, Ma and Xie. This is an open-access article distributed under the terms of the Creative Commons Attribution License (CC BY). The use, distribution or reproduction in other forums is permitted, provided the original author(s) and the copyright owner(s) are credited and that the original publication in this journal is cited, in accordance with accepted academic practice. No use, distribution or reproduction is permitted which does not comply with these terms.
*Correspondence: Yiqiang Xie, mrxieyiqiang@163.com