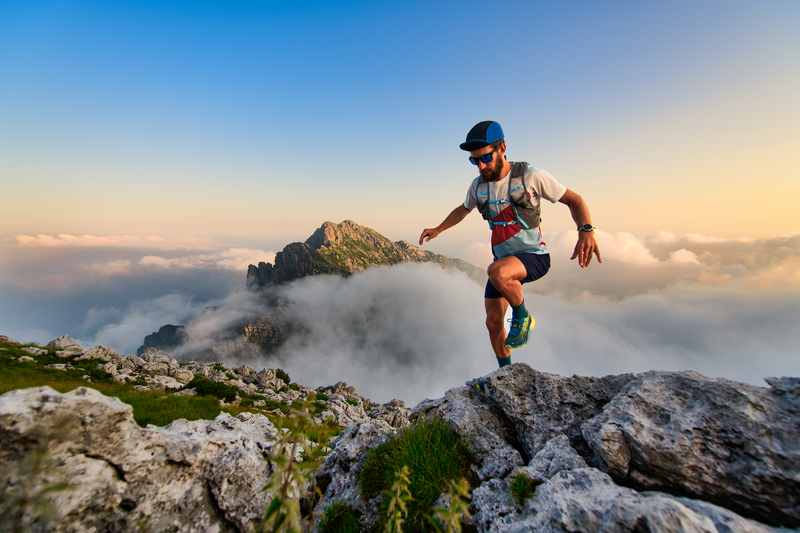
94% of researchers rate our articles as excellent or good
Learn more about the work of our research integrity team to safeguard the quality of each article we publish.
Find out more
REVIEW article
Front. Pharmacol. , 23 May 2024
Sec. Ethnopharmacology
Volume 15 - 2024 | https://doi.org/10.3389/fphar.2024.1389179
Background: Quercetin, a widespread polyphenolic flavonoid, is known for its extensive health benefits and is commonly found in the plant kingdom. The natural occurrence and extraction methods of quercetin are crucial due to its bioactive potential.
Purpose: This review aims to comprehensively cover the natural sources of quercetin, its extraction methods, bioavailability, pharmacokinetics, and its role in various cell death pathways and liver fibrosis.
Methods: A comprehensive literature search was performed across several electronic databases, including PubMed, Embase, CNKI, Wanfang database, and ClinicalTrials.gov, up to 10 February 2024. The search terms employed were “quercetin”, “natural sources of quercetin”, “quercetin extraction methods”, “bioavailability of quercetin”, “pharmacokinetics of quercetin”, “cell death pathways”, “apoptosis”, “autophagy”, “pyroptosis”, “necroptosis”, “ferroptosis”, “cuproptosis”, “liver fibrosis”, and “hepatic stellate cells”. These keywords were interconnected using AND/OR as necessary. The search focused on studies that detailed the bioavailability and pharmacokinetics of quercetin, its role in different cell death pathways, and its effects on liver fibrosis.
Results: This review details quercetin’s involvement in various cell death pathways, including apoptosis, autophagy, pyroptosis, necroptosis, ferroptosis, and cuproptosis, with particular attention to its regulatory influence on apoptosis and autophagy. It dissects the mechanisms through which quercetin affects these pathways across different cell types and dosages. Moreover, the paper delves into quercetin’s effects on liver fibrosis, its interactions with hepatic stellate cells, and its modulation of pertinent signaling cascades. Additionally, it articulates from a physical organic chemistry standpoint the uniqueness of quercetin’s structure and its potential for specific actions in the liver.
Conclusion: The paper provides a detailed analysis of quercetin, suggesting its significant role in modulating cell death mechanisms and mitigating liver fibrosis, underscoring its therapeutic potential.
Quercetin is a plant-derived flavonol, belonging to the broad category of polyphenolic substances and is ubiquitous in nature (Balestrini et al., 2021). Its etymology traces back to 1857, derived from the Latin “Quercus,” meaning “oak forest” or an area abundant with oaks (Batiha et al., 2020). These metabolites, characterized by a basic C6-C3-C6 diphenylpropane structure, are prevalent in the plant kingdom. Among them, quercetin stands out due to its widespread presence across various plant species. It often forms glycosides when bonded with sugar groups, which endows it with water solubility for easier absorption while maintaining the hydrophobic properties of phenolic substances, although it also exists in its aglycone form, such as in quercetin and kaempferol (García Díaz et al., 2022). As a fundamental flavonoid compound, quercetin forms the structural basis of several other flavonoid compounds like rutin, hesperidin, and naringenin (Rahvar et al., 2018), all sharing a core chemical framework with only minor variations in substituents. From a nomenclature perspective, quercetin is named 2-(3,4-dihydroxyphenyl)-3,5,7-trihydroxy-4H-chromen-4-one, with the chemical formula C15H10O7, accurately depicting its molecular structure (Li et al., 2016) (see Figure 1). Due to the unique chemical characteristics of the benzene ring and the balance between hydrophobicity and hydrophilicity brought by hydroxyl groups and sugar bindings, it plays a significant role in regulating cell death pathways and improving liver fibrosis. Quercetin has been found to participate in various cell death pathways such as apoptosis, autophagy, necrosis, and more, particularly playing a critical role in regulating apoptosis and autophagy processes. Additionally, quercetin has shown significant efficacy in treating liver fibrosis, especially impacting hepatic stellate cells. Moreover, the bioavailability and pharmacokinetics of quercetin are discussed in detail, including its sources and extraction methods. In summary, this article elaborates on the origins, extraction methods, and bioavailability of quercetin, emphasizing its specific mechanisms in intervening various cell death modalities (apoptosis, autophagy, necrosis, pyroptosis, ferroptosis, cuproptosis) and explores its specificity in action mechanisms from a physical organic chemistry perspective, especially concerning the liver. Further discussions include the impact of quercetin on liver fibrosis.
To ensure a comprehensive and systematic review of the existing literature on quercetin, a meticulous search strategy was implemented. Several electronic databases were queried for relevant publications, including PubMed, Embase, CNKI, Wanfang database, and ClinicalTrials.gov. The time frame for the literature search was up to and including 10 February 2024, to capture the most recent studies available.
The search terms were carefully selected to encompass the broad spectrum of research areas relevant to quercetin. Keywords included “quercetin”, “natural sources of quercetin”, “quercetin extraction methods”, “bioavailability of quercetin”, “pharmacokinetics of quercetin”, “cell death pathways”, “apoptosis”, “autophagy”, “pyroptosis”, “necroptosis”, “ferroptosis”, “cuproptosis”, “liver fibrosis”, and “hepatic stellate cells”. Boolean operators (AND/OR) were employed to combine these terms effectively and to ensure the search was both comprehensive and precise.
The inclusion criteria for the studies were as follows: 1) studies that report on the bioavailability and pharmacokinetics of quercetin, 2) research investigating the role of quercetin in various cell death pathways, and 3) studies focusing on the effects of quercetin on liver fibrosis. Studies were excluded if they did not directly pertain to these areas or if they were not in the English language. The search was not restricted by study design to capture a wide range of methodologies, from in vitro experiments to clinical trials. Upon retrieval, records were screened based on titles and abstracts to identify those that met the inclusion criteria. Full-text articles were then obtained and further assessed for relevance. Data extraction focused on the methods of quercetin extraction, its pharmacokinetic profile, and detailed accounts of its role in cellular pathways leading to cell death, with a special focus on liver fibrosis. The extracted data was then synthesized and prepared for comprehensive analysis within the review.
Quercetin is a polyphenolic flavonoid produced by plants through the phenylpropanoid pathway, a complex process initiated from cinnamic acid that encompasses eight distinct steps (Nabavi et al., 2020). This metabolites, serving as a secondary metabolite, enjoys a broad distribution across the plant world, predominantly in the form of glycosides, which are quercetin molecules bound to sugar molecules (Morimoto et al., 2023). A wide array of foodstuffs features quercetin, making it easily obtainable from dietary sources. Notable examples include onions, capers, various berries, apples, grapes, vegetables of the Brassica genus, tea, shallots, tomatoes, and an assortment of seeds, nuts, barks, flowers, and leaves. Medicinal botanical drugs such as Hypericum perforatum (St. John’s Wort), Ginkgo biloba, and Sambucus canadensis (Elderberry) also contain quercetin (Wiczkowski et al., 2008; Li et al., 2016).
The quercetin content significantly differs among foods, with raw capers being particularly rich in quercetin, boasting 234 mg per 100 g of the edible part. Conversely, teas like black or green tea from Camellia sinensis are on the lower end, with a mere 2 mg per 100 g (Bhagwat et al., 2011). The extraction of quercetin from plant sources has evolved, moving from traditional methods such as Soxhlet extraction, which is known for its solvent-heavy, time-consuming, and less efficient yield process, to more modern techniques (M et al., 2023; Gligor et al., 2023). Techniques such as microwave-assisted extraction (MAE) and supercritical fluid extraction (SFE) are now preferred for their efficiency and rapid processing times (Belwal et al., 2018).
As a metabolites with a vast pharmacological profile, quercetin is often incorporated into dietary supplements, available in powder and capsule forms. Consumption levels of quercetin vary globally, influenced by the intake of fruits, vegetables, and tea, with daily intakes estimated between 50 and 800 mg. For example, average quercetin consumption has been reported at 18 mg in China, 16.2 mg in Japan, 18.48 mg in Spain, and 9.75 mg in the United States (Li et al., 2016). In summary, quercetin’s widespread presence in various plant-based foods, coupled with advancements in extraction methodologies, has enhanced its accessibility and utility. Its varied dietary sources and established health benefits position quercetin as a valuable nutrient within the realms of nutrition and healthcare.
The burgeoning interest in quercetin’s health benefits has catalyzed the production of nutraceuticals containing high doses of this metabolites (Naghibi et al., 2013). Nonetheless, the challenge of quercetin’s low oral bioavailability, particularly after a single dose due to macronutrient absorption barriers, remains significant (Rich et al., 2017). Human pharmacokinetic studies have pinpointed the oral bioavailability of quercetin at a mere 2% following a single dose (Li et al., 2016), a limitation often ascribed to its slow absorption, quick metabolism, and swift bodily elimination. The bioavailability variance of quercetin metabolites is notably influenced by their sugar moiety (Graefe et al., 2001), with quercetin glycosides exhibiting more efficient human absorption than the aglycone form (Wagner et al., 2006). The absorption efficiency also varies with the type of sugar attached to the quercetin molecule (Scholz and Williamson, 2007), further moderated by the dietary matrix and presence of other dietary factors like fiber and fat (Guo et al., 2013).
In plant sources, quercetin primarily exists as hydrophilic glycosides, which can be converted into the aglycone form by β-glucosidases in the small intestine, facilitating passive diffusion through the intestinal epithelium (Ader et al., 2000). This process is possibly aided by the intestinal sodium/glucose cotransporter-1, allowing direct bloodstream entry of quercetin glycosides (Murota and Terao, 2003). Inside the body, quercetin may be oxidized, forming derivatives such as quercetin-quinone and quercetin-quinone methides (Rietjens et al., 2005; van der Woude et al., 2005). Unabsorbed quercetin undergoes colonic microbiota degradation, producing phenolic acids that are absorbed and transported to the liver for further conjugation (Manach et al., 2005; Thilakarathna and Rupasinghe, 2013).
Post-absorption, quercetin is metabolized in enterocytes and the liver through glucuronidation, sulfation, and methylation, generating a spectrum of metabolites (van der Woude et al., 2004; Harwood et al., 2007). The primary metabolites include isorhamnetin and glucoside acid-sulfated derivatives, constituting 91.5% of all quercetin metabolites, alongside glucuronoside and methylated variants (Morand et al., 1998). The primary metabolites include isorhamnetin and glucoside acid-sulfated derivatives, constituting 91.5% of all quercetin metabolites, alongside glucuronoside and methylated variants (de Boer et al., 2005), with notable deconjugated quercetin presence in certain tissues, albeit with variations in free quercetin proportions across different organs (Bieger et al., 2008). The distribution of quercetin aglycone in tissues, however, remains uncertain due to potential postmortem deconjugation variances (de Boer et al., 2005). Some studies propose mitochondrial quercetin accumulation, though evidence is still emerging (Moon et al., 2008; Konrad and Nieman, 2015).
Quercetin’s urinary concentration escalates with intake dosage and time post-consumption, highlighting the kidneys’ role in its excretion (Young et al., 1999; Mullen et al., 2006). Metabolic by-products include 3-hydroxyphenylacetic acid, hippuric acid, and benzoic acid (Guo and Bruno, 2015), with liver-produced metabolites potentially being expelled via the biliary tract through MRP2 (Arts et al., 2004; Guo and Bruno, 2015) and from the lungs in high quantities (Li et al., 2016). Quercetin elimination is protracted, with half-lives ranging between 11 and 28 h, and an average terminal half-life of approximately 3.5 h (Konrad and Nieman, 2015). Research by Ferry et al. on intravenous quercetin in cancer patients identified a safe dosage at 945 mg/m^2, contrasting with higher doses that induced adverse effects such as emesis and nephrotoxicity (Hollman and Katan, 1998). Graefe et al.'s study maintained a dosage up to 200 mg, observing a Cmax of 2.3 ± 1.5 μg/mL and a Tmax of 0.7 ± 0.3 h [39], underscoring the metabolite pharmacokinetic complexity.
Cell death occurs through various pathways, including apoptosis, autophagy, necroptosis, pyroptosis, ferroptosis, and cuproptosis, each with distinct triggers and processes (refer to Table 1). Quercetin, a versatile flavonoid, has shown efficacy in influencing these diverse cell death pathways. (Table 2 provides detailed listings of the dosages, models, and treatment durations related to quercetin mentioned in the cited literature).
Apoptosis is a form of programmed cell death characterized by specific morphological and biochemical changes such as cell shrinkage, membrane blebbing, chromatin condensation, DNA fragmentation, and caspase activation (Shi, 2004). It is driven by two main pathways: the intrinsic (mitochondrial) and the extrinsic (death receptor) pathways (Harada, 2003). The intrinsic pathway is activated by internal stimuli including DNA damage, oxidative stress, and mitochondrial dysfunction, leading to the release of cytochrome c from mitochondria. This release triggers a cascade that activates caspase-9, followed by caspase-3, culminating in cell death (Wei et al., 2001; Meyer et al., 2005; Dewson and Kluck, 2009; Gupta et al., 2009; Parrish et al., 2013). The extrinsic pathway, on the other hand, begins when external ligands bind to death receptors on the cell surface, activating caspase-8, which then activates caspase-3, leading to apoptosis (Bodmer et al., 1997; Stennicke HR et al., 1998; Guicciardi and Gores, 2009).
Quercetin has been identified as a potent inducer of apoptosis in various cancer cell types including breast, prostate, lung, colon, and leukemia cells, as evidenced by its ability to disrupt the balance between pro-apoptotic and anti-apoptotic proteins (see Figure 2). In an experiment conducted by Cheng et al., mice were administered quercetin intraperitoneally at doses of 0, 20, and 40 mg/kg body weight daily for 15 consecutive days. Researchers observed that in leukemia cells treated with quercetin, the expression of the anti-apoptotic protein Mcl-1 was downregulated, while the pro-apoptotic protein Bax was activated and translocated to the mitochondrial membrane. Furthermore, the extent of these effects was found to increase with the dosage of quercetin, indicating a dose-dependent relationship (Cheng et al., 2010). Studies have observed that quercetin concentrations above 20 μM significantly inhibit the proliferation of hepatic stellate cells (HSCs) with an IC50 of 27.2 μM, but it only affects liver cell growth at concentrations up to 80 μM (IC50 of 68.5 μM). Quercetin stimulates apoptosis in HSCs, with an apoptosis rate reaching 40% at a concentration of 40 μM (EC50 of 51.6 μM). Quercetin reduces the expression of Bcl-2, increases the expression of Bax, and leads to the release of cytochrome C within cells. Additionally, quercetin also enhances the mRNA and protein expression of calnexin and CHOP in HSCs, but does not affect liver cells. Moreover, quercetin increases the phosphorylation of PERK and IRE1 and the cleavage of ATF6. However, the ER stress inhibitor alubrinal significantly reduces the apoptotic effect of quercetin induced in HSCs (He et al., 2016).
Figure 2. Quercetin-Induced Apoptotic Pathways in Cellular Regulation. The dual apoptotic pathways are within cells: the intrinsic and extrinsic pathways. The intrinsic pathway, activated by internal signals such as DNA damage or mitochondrial disruptions, leads to the activation of caspase-9 and caspase-3. Conversely, the extrinsic pathway is initiated by the external binding of death ligands to cellular death receptors, catalyzing the activation of caspase-8. Quercetin’s role in inducing apoptosis is highlighted through its capacity to disrupt the balance between anti-apoptotic and pro-apoptotic proteins. Specifically, quercetin downregulates the anti-apoptotic protein Mcl-1 and activates the pro-apoptotic protein Bax, while also inhibiting the activity of survival proteins Bcl-2 and Bcl-xL. Moreover, quercetin’s induction of reactive oxygen species (ROS) precipitates the release of cytochrome c and diminishes mitochondrial membrane potential, paving the way for apoptosis. Additionally, quercetin triggers endoplasmic reticulum stress and obstructs the PI3K/Akt signaling pathway, further augmenting apoptotic processes.
Quercetin’s apoptotic effect in A549 lung cancer cells includes increasing the levels of Bax and Bad while decreasing Bcl-2, with the rate of apoptosis rising in correlation with the concentration of quercetin. The study employed four different concentrations of quercetin: 14.5, 29.0, 43.5, and 58.0 μM, with treatment durations of 24 and 48 h. The results indicate that the apoptosis rate increases as the concentration of quercetin increases (Nguyen et al., 2004). Quercetin triggers the production of reactive oxygen species (ROS), mitochondrial membrane depolarization, and the release of cytochrome c, demonstrating its capability to initiate apoptosis through mitochondrial pathways. The concentrations of quercetin used in this study ranged from 30 to 90 μM (Bishayee et al., 2013). Quercetin induces apoptosis in fetal rat hepatocytes by enhancing ROS production, leading to the loss of mitochondrial transmembrane potential, cytochrome c release, and caspase-3 activation. The study found that an 18-h treatment with quercetin can induce apoptosis in HepG2 cells, while a shorter treatment duration (4 h) has no effect on cell viability. Moreover, the inducing effect of quercetin is dose-dependent. Therefore, the specific dosage of quercetin needs to be determined based on the circumstances (Granado-Serrano AB et al., 2006).
Furthermore, quercetin induces endoplasmic reticulum (ER) stress by increasing UPR-related gene expression and promoting PERK and IRE1 phosphorylation, thereby activating apoptosis in hepatic stellate cells (He et al., 2016). It also inhibits the PI3K/Akt signaling pathway, reducing Akt phosphorylation and consequently inactivating procaspase-9, which plays a critical role in apoptosis regulation (Gamet-Payrastre et al., 1999; Granado-Serrano AB et al., 2006). Additionally, quercetin activates the ERK pathway in a dose- and time-dependent manner, influencing apoptosis by potentially promoting caspase-3 activation (Nguyen et al., 2004). Recent studies have shown that quercetin at concentrations of 10, 20, or 30 µM can significantly reduce the viability of HepG2 cells, especially with the treatment of 30 µM quercetin. This treatment also significantly lowers the mitochondrial membrane potential, demonstrating quercetin’s ability to induce apoptosis in HepG2 cells. Flow cytometry experiments have proven that quercetin induces apoptosis in a dose-dependent manner. This effect occurs through the direct interaction of quercetin with YY1, disrupting the YY1-p53 interaction, promoting p53 activation, and leading to an increased Bax/Bcl-2 ratio (Guan et al., 2023). Quercetin also has great potential in the prevention and treatment of liver cancer. By regulating multiple signaling pathways, including upregulating Bax, caspase-3, and p21, and downregulating Akt, PLK-1, cyclin-B1, cyclin-A, CDC-2, CDK-2, and Bcl-2, quercetin has been shown to induce apoptotic cell death in liver cancer cells (Sethi et al., 2023). In summary, quercetin modulates cell death by altering the equilibrium between pro- and anti-apoptotic proteins, generating oxidative stress, disrupting mitochondrial function, and inducing ER stress. It also affects key signaling pathways like PI3K/Akt and MAPK/ERK, contributing to its apoptotic effects. This evidence underscores quercetin’s potential as an anti-cancer agent, with future research needed to further elucidate its mechanisms and optimize its therapeutic applications.
Autophagy, a critical cellular mechanism for the degradation and recycling of cytoplasmic components, plays a pivotal role in maintaining cellular equilibrium, adapting to stress, and ensuring cellular vitality (Takeshige K et al., 1992). This process manifests in three primary forms: macroautophagy, microautophagy, and chaperone-mediated autophagy (CMA), each tailored to specific cellular needs and degradation targets (Abdrakhmanov A and Zhivotovsky, 2020) (Figures 3, 4).
Figure 3. Quercetin’s Enhancement of Macroautophagy for Cellular Regulation. The quercetin’s dynamic role in bolstering macroautophagy is a critical cellular process for maintaining homeostasis through the degradation and recycling of proteins and organelles. Quercetin modulates the mechanistic target of rapamycin (mTOR) pathway and activates AMP-activated protein kinase (AMPK), leading to decreased mTOR activity and the initiation of autophagy.
Figure 4. Regulation of CMA and Macroautophagy by Quercetin. Quercetin notably promotes lysosomal biogenesis, crucial for producing lysosomes that contain the enzymes needed for breaking down cellular metabolite s. Quercetin triggers the nuclear translocation of Transcription Factor EB (TFEB), a key regulator of lysosomal biogenesis and autophagy genes, thus amplifying autophagic activity for improved cellular clearance. Additionally, quercetin modulates the mechanistic target of rapamycin (mTOR) pathway and activates AMP-activated protein kinase (AMPK), leading to decreased mTOR activity and the initiation of autophagy. Through these actions, quercetin serves as a potent facilitator of cellular maintenance and health, highlighting its significant impact on enhancing autophagy for cellular equilibrium and stress management.
Macroautophagy, the most studied form, involves the creation of autophagosomes, a process orchestrated by the ULK1 complex, which comprises ULK1, FIP200, ATG13, and ATG101 (Ganley et al., 2009) (Figure 3). Activation of this complex is modulated by AMPK in response to nutrient scarcity or energy stress, and by mTORC1, a nutrient-sensitive regulator (Egan et al., 2011; Egan et al., 2011). Following activation, the ULK1 complex initiates autophagosome formation by phosphorylating key proteins like Beclin-1, VPS34, and ATG14 (Nazarko and Zhong, 2013; Russell et al., 2013; Wold et al., 2016). PI3K activity at the ER generates PI3P, facilitating the recruitment of DFCP1 to form the omegasome, which aids in membrane formation (Dooley et al., 2014; Nascimbeni et al., 2017; Birgisdottir Å et al., 2019). WIPI and ATG2A/B play roles in sourcing membranes via ATG9A for the burgeoning autophagosome (Lystad et al., 2019). Two ubiquitin-like systems, the ATG12-ATG5-ATG16L1 complex and LC3-PE conjugation, govern membrane expansion and closure (Tanida et al., 2004; Bento et al., 2016). LC3 processing involves ATG4, converting LC3-I to its membrane-bound form, LC3-II (Maruyama and Noda, 2017). Fusion of autophagosomes with lysosomes, a step facilitated by Rab7, SNAREs, and LAMP2, completes the degradation process (Bucci et al., 2000; Liu et al., 2020; Tian et al., 2021). CMA targets specific proteins for lysosomal degradation, identified by a KFERQ motif and mediated by Hsc70, leading to their translocation across the LAMP-2A receptor (Majeski and Dice, 2004) (Figure 4). Microautophagy involves direct engulfment of cargo by lysosomes, with the ESCRT machinery playing a role in cargo selection and membrane invagination (Frankel et al., 2017; Avalos-Padilla et al., 2021) (Figure 4).
Quercetin has been observed to stimulate autophagy in various models and conditions, including diabetic nephropathy (Lu et al., 2015), intervertebral disc degeneration (Wang et al., 2020), and retinal pigment epithelial cells under oxidative stress (Li et al., 2021). It exerts effects through modulation of the mTORC1 pathway, promoting autophagy as a protective mechanism against cellular stress and damage.
In studies, quercetin administration showed dose-dependent effects on autophagy induction. For diabetic nephropathy, high doses (90 mg/kg/d) significantly reduced renal fibrosis, mediated through the mTORC1/p70S6K pathway affecting epithelial-mesenchymal transition in tubular epithelial cells (Lu et al., 2015). In retinal cells, quercetin alleviated oxidative stress, promoting the accumulation of the tight junction protein ZO-1 and upregulating autophagy markers such as Beclin-1 and LC3-II. Experiments showed that in ARPE-19 cells, quercetin concentrations of 5 μM and 80 μM were not non-toxic, whereas concentrations of 10 μM, 20 μM, 40 μM, and 60 μM were found to enhance cell viability. Although the clinical application of quercetin is still limited by its low water solubility, poor bioavailability, and extensive first-pass metabolism, new formulations with clinical potential have been proposed. Further research is needed to explore the autophagy-inducing process of quercetin and confirm its protective effects in animal models (Li et al., 2021). This suggests quercetin’s potential to protect against oxidative damage by promoting autophagic pathways.
Quercetin’s ability to directly inhibit mTORC1 enhances its properties as an inducer of autophagy. Experimental evidence shows that quercetin promotes the nuclear translocation of TFEB, a master regulator of lysosomal biogenesis and autophagy, suggesting its role in enhancing cellular degradation mechanisms. The study also investigated the optimal dosage of quercetin for augmenting cellular degradation pathways. Cells treated with varying concentrations of quercetin ranging from 0.5 to 20 μM showed no significant differences in activity whether sourced from Sigma-Aldrich or USANA Health Sciences. Therefore, it is recommended that future studies consider using a dosage range of 0.5–20 μM for quercetin as an enhancer of cellular degradation pathways (Huang et al., 2018). Additionally, quercetin has been shown to activate AMPK, further influencing the mTOR pathway and autophagy induction (Xiao et al., 2022). This activation, along with mitochondrial membrane potential changes, suggests a complex interplay between quercetin, energy sensing, and autophagic regulation (Viollet et al., 2006; Dhanya et al., 2017). Research continues to uncover the breadth of quercetin’s impact on autophagy across various cell types and conditions. Its ability to modulate key signaling pathways like mTORC1 and AMPK underscores its therapeutic potential. However, the intricate mechanisms of quercetin’s action on autophagy demand further exploration to fully understand its benefits and applications in medical and clinical settings.
Necroptosis, an inflammatory form of cell death, diverges from the orderly process of apoptosis, unfolding in response to extreme cellular distress caused by factors like trauma, infection, or toxic exposure (Zhang et al., 2018). This cell death pathway is marked by the uncontrolled rupture of the plasma membrane, leading to the release of cellular contents that can exacerbate inflammation (Golstein and Kroemer, 2007). Activation of necroptosis is mediated through specific receptors such as TLR-4, TLR3, TNFR, and Fas. These receptors, upon engagement, recruit adaptor proteins including FADD, TRADD, and TRIF, setting the stage for downstream signaling events culminating in necroptosis (Laster et al., 1988; Holler et al., 2000; Kaiser et al., 2013). Central to this pathway is RIPK1, which, under normal circumstances, is kept in check by ubiquitination. However, upon receiving death signals, RIPK1 is deubiquitinated, turning active and capable of initiating cell death (Feoktistova et al., 2011; Tenev et al., 2011). RIPK1, once activated, collaborates with RIPK3 to form the ripoptosome, a crucial assembly in determining cell fate towards necroptosis (Cho et al., 2009; Li et al., 2012; Wegner et al., 2017). The interaction between RIPK3 and MLKL leads to the formation of the necrosome, which migrates to the plasma membrane to form disruptive pores, furthering the necroptotic process (Cai et al., 2014; Wang et al., 2014; Dovey et al., 2018). Furthermore, cytosolic nucleic acid sensors like RIG-I and cGAS/STING, upon detecting foreign or abnormal nucleic acids, can trigger immune responses that inadvertently promote necroptosis, adding layers to the cell’s defensive mechanisms (Schock et al., 2017; Chen et al., 2018). Caspase-8, generally associated with apoptosis, plays a dual role by cleaving cytokine blockers like N4BP1 post-TNFR or TLR activation, thus facilitating cytokine release (Yang et al., 2018). Quercetin, known for its anti-inflammatory properties, has shown potential in modulating necroptosis by influencing the RIPK1 pathway. Studies indicate that quercetin can prevent the formation of necrosome complexes, thereby averting necroptosis and its associated inflammatory responses (Yu et al., 2022). By administering doses ranging from 50 to 200 μM, quercetin demonstrated an inhibitory effect on LPS-induced RIPK1 expression in chicken embryos, suggesting a protective role against necroptotic damage (Yu et al., 2022).
Moreover, quercetin can dampen the activation and nuclear translocation of NF-κB, a key regulator of inflammation, by targeting proteins in the NF-κB signaling pathway (Martínez-Flórez et al., 2005; Gao et al., 2020). Research by Susana Martínez-Flórez et al. demonstrated that quercetin could significantly reduce NO production in IL-1 stimulated rat liver cells by suppressing the expression of iNOS, likely through the inhibition of NF-κB activation, highlighting its therapeutic potential in inflammatory conditions. The study employed primary cultures of rat liver cells stimulated with IL-1 and treated with concentrations of quercetin ranging from 5 to 100 μmol/L. NO production was measured through the generation of reactive oxygen species and the release of nitrates into the culture medium. The researchers also analyzed the protein and mRNA levels of iNOS using Western blotting and reverse transcription-polymerase chain reaction, respectively. Furthermore, the activation of nuclear factor (NF)-κB and the levels of its inhibitors were assessed using electrophoretic mobility shift assay and Western blotting. The results suggest that quercetin suppresses NO production in IL-1 stimulated liver cells by inhibiting the expression of iNOS, likely through the suppression of IL-1 induced NF-κB activation (Martínez-Flórez et al., 2005). Recent research has discovered that quercetin supplementation in weaned pigs and the porcine intestinal epithelial cell line (IPEC-1) improved villus height in pigs following exposure to DON, enhanced intestinal barrier function, and inhibited necrotic signaling pathways. The study found that quercetin treatment, by suppressing the necrotic signal transduction pathway, offers potential protective effects against damage caused by exposure to deoxynivalenol (Liu et al., 2023). In essence, quercetin exhibits a protective mechanism against necroptosis by interfering with key signaling pathways and receptors involved in this cell death process. Its ability to inhibit RIPK1 activity, prevent necrosome formation, and reduce inflammatory responses positions quercetin as a promising natural metabolite for mitigating necroptotic cell death and inflammation in various pathological contexts.
Pyroptosis, a lytic and pro-inflammatory form of cell death, is essential for combating infections and managing cellular stress, differentiating itself from other cell death mechanisms by its ability to invoke an inflammatory response (Jorgensen and Miao, 2015; Rathinam et al., 2019; Yu et al., 2021). It operates through two principal pathways: the canonical pathway, driven by caspase-1, and the non-canonical pathway, involving human caspase-4/5 or mouse caspase-11, both of which lead to the expulsion of inflammatory cytokines (Yu et al., 2021).
The initiation of pyroptosis via the canonical pathway starts with the detection of pathogenic or stress signals by pattern recognition receptors (PRRs), which sense pathogen-associated molecular patterns (PAMPs) or danger-associated molecular patterns (DAMPs). This detection triggers the assembly of inflammasomes, multiprotein complexes crucial for activating caspase-1 (Strowig et al., 2012; Liston and Masters, 2017). Various PRRs, such as NLRP1, NLRP3, NLRC4, AIM2, and pyrin, upon activation, collaborate with pro-caspase-1 and the adaptor protein ASC to form inflammasomes, which are instrumental in setting off pyroptosis (Fleshner, 2013; Sagulenko et al., 2013; Yang et al., 2019; Saadi et al., 2020). In this cascade, the activation of caspase-1 within the inflammasome complex leads to the cleavage of gasdermin-D (GSDMD) and the precursors of interleukin-1β (IL-1β) and interleukin-18 (IL-18), marking the cell for pyroptotic death (Martinon et al., 2002; Bergsbaken et al., 2009; Hornung et al., 2009; Sahoo et al., 2011; Zhao et al., 2018). The non-canonical pathway is characterized by the direct recognition of cytosolic lipopolysaccharide (LPS) by caspase-4/5 in humans or caspase-11 in mice, which also culminates in GSDMD cleavage and pyroptosis (Shi et al., 2014). Quercetin, a flavonoid with notable anti-inflammatory properties, has been identified as a potent modulator of pyroptosis. Studies have illustrated quercetin’s ability to inhibit the NLRP3 inflammasome pathway in THP-1 macrophages, reducing the maturation of N-terminal gasdermin-D (N-GSDMD) and IL-1β in a concentration-dependent manner (Luo et al., 2022). This inhibition is thought to occur through the suppression of P65 nuclear translocation and phosphorylation, alongside a reduction in reactive oxygen species (ROS) production (Luo et al., 2022). In experiments with LPS-primed mouse bone marrow-derived macrophages, quercetin administration resulted in a dose-dependent attenuation of NLRP3 and AIM2 inflammasome activation, highlighting its potential in reducing inflammatory responses (Domiciano et al., 2017). Additionally, quercetin’s influence extends to IFN-γ-primed keratinocytes, where it was found to suppress poly (dA:dT)-induced IL-18 secretion by downregulating the expressions of AIM2 and pro-caspase-1. This effect is attributed to the inhibition of JAK2 and STAT1 phosphorylation and their nuclear translocation, although high concentrations of quercetin were noted to negatively impact cell viability. The study recommends further research to determine the optimal dosage of quercetin, as the concentrations used in the study (5 and 10 mM) are relatively high (Lee et al., 2018). Quercetin’s effectiveness in controlling pyroptosis underscores its therapeutic potential in addressing inflammatory diseases and enhancing overall health. By targeting crucial components of the pyroptosis pathway, quercetin presents a promising natural solution for mitigating inflammation and preventing inflammatory cell death. However, further studies are essential to optimize quercetin’s dosage for maximal therapeutic benefits without adverse effects.
Ferroptosis is a distinct, iron-dependent form of cell death, marked by excessive lipid peroxidation and oxidative stress, diverging from the genetically programmed nature of apoptosis. This cell demise mechanism is fueled by iron and entails the degradation of cellular lipids, leading to significant oxidative damage and metabolic disturbances (Dixon et al., 2012). Central to ferroptosis are the intricate processes of lipid, antioxidant, and iron metabolisms, each playing a pivotal role in the regulation and execution of this cell death pathway (Xiong et al., 2022; Zhou et al., 2022).
In the realm of lipid metabolism, the breakdown of lipid droplets through lipophagy releases polyunsaturated fatty acids (PUFAs), which are then activated by acyl-CoA synthetase long-chain family member 4 (ACSL4) to form PUFA-CoAs (Das, 2019). These fatty acids are further processed by lysophosphatidylcholine acetyltransferase 3 (LPCAT3), which incorporates PUFA-CoAs into phospholipids, contributing to the cellular lipid composition and influencing membrane dynamics and signaling (Liang et al., 2022). The oxidative stress facilitated by iron catalysis leads to the peroxidation of these PUFA-containing phospholipids, significantly compromising cell membrane integrity and ion homeostasis (Stockwell, 2022). Conversely, replacing PUFA-phospholipids with monounsaturated fatty acid (MUFA) counterparts serves as a protective mechanism against oxidative damage, stabilizing cell membranes (Magtanong et al., 2019).
Antioxidant metabolism plays a critical role in countering ferroptosis through the action of glutathione peroxidase 4 (GPX4), which utilizes glutathione (GSH) and selenium to detoxify lipid peroxides (Ursini and Maiorino, 2020; Miao et al., 2022). The System Xc-transporter, comprising subunits SLC7A11 and SLC3A2, is crucial for maintaining intracellular GSH levels by facilitating the exchange of extracellular cystine for intracellular glutamate (Li et al., 2022). In addition to the GPX4 pathway, alternative mechanisms such as FSP1-CoQ10 and GCH1-BH4 provide additional defense against lipid peroxidation, underscoring the multi-faceted nature of cellular protection against ferroptosis (Friedmann Angeli et al., 2019; Li et al., 2020).
Iron metabolism within cells involves the regulated uptake and storage of iron, with proteins such as transferrin, STEAP3, DMT1, and ferritin ensuring iron is appropriately managed to prevent oxidative damage. Excess ferrous iron (Fe2+) can catalyze the Fenton reaction, generating hydroxyl radicals that exacerbate lipid peroxidation, a hallmark of ferroptosis (Cheng et al., 2004; Sendamarai et al., 2008; Frazer and Anderson, 2014; Ji and Kosman, 2015).
Quercetin, a potent flavonoid, has emerged as a significant modulator of ferroptosis by influencing key aspects of this cell death pathway. It has been shown to mitigate the activation of lipid peroxidation and iron accumulation, thereby reducing the susceptibility of cells to ferroptosis-induced damage. Through its antioxidant properties, quercetin enhances the cellular defense against oxidative stress, potentially by stabilizing lipid membranes, regulating iron metabolism, and boosting the antioxidant capacity of cells (Shen et al., 2018; Henning et al., 2022). The intricate interplay between lipid oxidation, iron catalysis, and antioxidant defenses in ferroptosis underscores the potential of quercetin as a therapeutic agent. By targeting the molecular underpinnings of ferroptosis, quercetin offers a promising avenue for mitigating oxidative damage and preserving cellular integrity in conditions predisposed to iron-induced oxidative stress. Further research into the precise mechanisms by which quercetin influences ferroptosis will be crucial for harnessing its full therapeutic potential in combating diseases associated with oxidative damage and iron dysregulation.
Quercetin, a flavonoid with a notable affinity for iron, demonstrates significant efficacy in mitigating ferroptosis. Quercetin achieves this through its iron-chelating capabilities, particularly via its B ring catechol, which significantly reduces hydroxyl radical formation from metal-catalyzed reactions. In research by Evangelia Vlachodimitropoulou et al., it was highlighted that increasing quercetin concentration from 0 to 0.1 μM can increase the rate of cytosolic iron efflux by 2.5 times in control cells, and by 3.2 times following pre-loading with 10 μM Fe(II/III). Increasing the quercetin concentration to 1 or 5 μM significantly reduces the rate of cellular iron loss. Furthermore, the intake of flavonoids, including quercetin, may vary depending on dietary habits (Vlachodimitropoulou et al., 2011; Wang et al., 2021). Evangelia Vlachodimitropoulou’s research underscores quercetin’s dynamic role in modulating iron homeostasis. The study observed a 2.5- to 3.2-fold increase in cytosolic iron efflux in cells treated with increasing quercetin concentrations, highlighting its potential to alleviate iron overload (Vlachodimitropoulou et al., 2011).
Animal studies further corroborate quercetin’s protective effects against iron-induced oxidative damage and lipid peroxidation, showcasing its ability to safeguard organ function (Kim and Choe, 2018; Gholampour and Saki, 2019; Li et al., 2020). Dan Li et al.'s investigation into type 2 diabetes mellitus (T2DM) mouse models revealed quercetin’s capacity to restore glucose homeostasis and pancreatic β-cell integrity, attributing these effects to quercetin’s inhibition of pancreatic iron deposition and ferroptosis (Li et al., 2020).
Quercetin has also been credited with DNA protection in human colon carcinoma cells, illustrating its broader protective scope against iron overload (Glei et al., 2002). Moreover, it modulates the activity of the iron transporter ferroportin, possibly through microRNA interactions, suggesting a nuanced approach to iron regulation in the body. Marija Lesjak et al. provided insights into quercetin’s influence on intestinal iron absorption and ferroportin expression, demonstrating its dual role in modulating iron uptake and efflux. In the study, rats were treated with a dose of 50 mg/kg, and when treating Caco-2 cells, various concentrations of quercetin ranging from 0.1 to 10 mmol/L were used (Lesjak et al., 2014).
Quercetin’s antioxidant properties extend to the inhibition of lipoxygenase activity, reducing the uptake of oxygen and the peroxidation of linoleic acid, thereby curbing the generation of lipid peroxides. Wang et al.’s study further illustrates quercetin’s role in repressing ferroptosis in acute kidney injury scenarios by modulating ATF3 expression, system Xc-activity, GPX4 levels, and enhancing glutathione availability (Wang et al., 2021). Quercetin emerges as a multifaceted agent against ferroptosis, acting through iron chelation, antioxidant defense enhancement, and modulation of critical ferroptosis-related pathways. Its broad spectrum of activity not only highlights its potential in mitigating oxidative stress and lipid peroxidation but also underscores its therapeutic promise in conditions marked by iron overload and ferroptosis. Further exploration into quercetin’s mechanisms of action and optimal dosing will be vital for harnessing its full potential in preventing and treating ferroptosis-related diseases.
Cuproptosis, a novel type of cell death driven by copper accumulation, is emerging as a significant area of study within cellular biology. Unlike other forms of cell death, cuproptosis is distinguished by its reliance on copper, an essential yet potentially toxic trace element in cellular functions (Chen et al., 2022; Tsvetkov et al., 2022). The intricacies of cuproptosis involve the interaction of copper with cellular proteins, particularly through a process known as protein lipoylation. This post-translational modification, crucial for the activity of certain enzymes within the tricarboxylic acid (TCA) cycle, becomes a focal point for copper toxicity when excessive copper binds to the lipoyl groups, disrupting normal cellular processes (Solmonson and DeBerardinis, 2018).
Research by Tsvetkov et al. has underscored the vulnerability of cells with active TCA cycles and abundant lipoylated enzymes to copper-induced toxicity. The binding of copper to these enzymes precipitates protein aggregation, destabilizes iron-sulfur cluster proteins, and prompts a stress response, marking cells for cuproptosis (Tsvetkov et al., 2022; Zheng et al., 2022).
Quercetin, known for its antioxidant and metal-chelating properties, is under investigation for its potential to mitigate copper-induced cellular damage. Although detailed mechanisms by which quercetin affects cuproptosis remain to be fully elucidated, evidence suggests its capability to chelate copper ions, thereby reducing oxidative stress induced by copper overload (Lomozová et al., 2021). Studies exploring the interactions between quercetin metabolites and copper reveal that these metabolite can form complexes with copper, potentially attenuating copper-induced hemolysis, with isorhamnetin showing particular efficacy in this regard.
Moreover, quercetin has been shown to modulate copper’s effect on metallothionein, a protein involved in metal detoxification, in a dose-dependent manner. This modulation suggests quercetin’s role in enhancing the cellular response to copper toxicity, offering insights into its therapeutic potential for managing copper-related disorders. The research found that quercetin enhances the induction of metallothionein by copper in a dose-dependent manner and produces a cumulative effect after repeated exposures. Compared to a single treatment, the effective concentration of quercetin remains lower after repeated treatments. Just 10 µM of quercetin is sufficient to reduce metallothionein expression stimulated by zinc, and quercetin has no effect on cadmium-induced metallothionein (Kuo et al., 2001). The metabolite anti-inflammatory effects, possibly through the inhibition of NF-κB/IκBα and p38 MAPK signaling pathways, further contribute to its protective actions against copper-induced cellular stress (Wang et al., 2017). The therapeutic implications of quercetin in cuproptosis and copper toxicity are promising, yet necessitate further investigation to determine optimal dosages and to fully understand its mechanisms of action. The exploration of quercetin as a natural metabolite for counteracting copper-related cellular damage opens new avenues for the development of treatments for diseases characterized by copper dysregulation. With ongoing research, quercetin could potentially become a valuable tool in the modulation of cuproptosis and the management of copper-induced pathologies.
Liver fibrosis, marked by the excessive buildup of fibrous tissue, arises as a consequence of chronic liver damage, often stemming from factors like viral hepatitis, alcohol abuse, non-alcoholic fatty liver disease (NAFLD), and autoimmune disorders (Poynard et al., 2003; Kondili et al., 2005; Yönem et al., 2006; Pais et al., 2013; Wu et al., 2014; Okura et al., 2015; Wu et al., 2019; Wang et al., 2020). This condition, characterized by a progressive alteration in liver architecture, can escalate to cirrhosis, liver failure, or even hepatocellular carcinoma if not adequately addressed (Roche et al., 2008; Sakurai and Kudo, 2013; Zhou et al., 2014). Understanding the pathogenesis of liver fibrosis is essential for developing effective treatments aimed at mitigating or reversing this fibrotic process.
The pivotal role in liver fibrosis development is attributed to hepatic stellate cells (HSCs), which reside in the liver sinusoids. These cells, upon activation by liver injury or inflammation, transform into myofibroblast-like cells that produce excessive amounts of extracellular matrix (ECM), leading to the fibrotic scarring of the liver (Brenner et al., 2000; Bekki et al., 2017; Zhang et al., 2017; Cai et al., 2018; Kiagiadaki et al., 2018). HSC activation is driven by both intracellular signals, such as the TGFβ/Smad, Notch, and Wnt/β-catenin pathways, and extracellular stimuli from other liver cells or external factors like cytokines and viral infections (Yan et al., 2021). This dual pathway of activation underscores the complex regulatory environment that promotes liver fibrosis.
Activated HSCs and fibroblasts are central to the fibrosis process, contributing to the pathological accumulation of collagen types I and III in the liver. This overproduction of collagen, a key component of the ECM, disrupts liver tissue architecture, impairs organ function, and obstructs blood flow (Musso et al., 1998; Du et al., 1999; Higashi et al., 2017; Ortiz et al., 2021). Quercetin, a flavonoid present in various TCM formulations, has demonstrated potential in addressing liver fibrosis. Through its multifaceted actions, quercetin targets the underlying mechanisms of fibrosis, offering a promising therapeutic avenue. Its potential to modulate HSC activity, reduce oxidative stress, and inhibit the excessive deposition of collagen marks quercetin as a significant metabolite in the fight against liver fibrosis.
While phenolic chemicals, as characteristic chemical structures of quercetin, are also widely present in other plants, and from the perspective of organic chemistry electronic effects, the benzene ring inherently involves the overlap of sp2 orbitals between carbon atoms when they come close to each other. Due to the close proximity of the carbon atoms, this further leads to the formation of side-by-side chemical bonds by the carbon atoms’ p orbitals vertical to the sp2 orbitals, resulting in a unique cyclic large pi-bond structure with 6 carbon atoms sharing 6 electrons. Therefore, according to the Huckel 4n+2 rule, this will possess aromaticity. This leads to benzene derivatives exhibiting unusual antioxidative properties, creating a paradox where other phenolic plants with benzene ring structures also possess similar chemical characteristics, suggesting that similar extracts could achieve similar effects. However, if we revisit the chemical formula of quercetin (Figure 1), we can see that while aromaticity brings hydrophobic interactions, a major driving force in chemical biology for protein folding and protein-ligand binding, the same hydrophobicity means that extracts with this structure cannot be absorbed through the small intestine and portal system. Considering most microbes reside in the large intestine, this implies that most phenolic plants are unlikely to be decomposed and absorbed by microbes. The presence of numerous hydroxyl functional groups in the chemical structure of quercetin means that although water molecules have a strong tendency to form hydrogen bonds, they cannot do so with the cyclic large pi-bond structure of the benzene ring, and one of the water molecule’s four charges will always face the benzene ring. Thus, to form as many hydrogen bonds as possible, water molecules will choose to rearrange, enveloping the entire benzene ring structure to form hydrophobic interactions, but the hydroxyl functional groups, which readily form hydrogen bonds, effectively break or alter the local arrangement of water molecules, thus forming hydrophilicity, making it easier to be absorbed through the small intestine. Moreover, in plants, quercetin’s hydroxyl functional groups are usually substituted by sugars, ethers, and phenolic acids (Materska, 2008), making quercetin with glycosides better at hydrophilizing, thereby increasing the likelihood of absorption by the body. Since liver cells require sugars for various biochemical reactions, when the sugar ligands are removed, the original hydrophobicity is restored. This clever structure gives quercetin characteristics different from other phenolic plants, and this property is likely specifically targeted towards the liver. Therefore, research into quercetin’s effects on liver fibrosis highlights its role in traditional Chinese medicine as a natural remedy with hepatoprotective properties. By interfering with the pathways that lead to HSC activation and collagen overproduction, quercetin contributes to the preservation of liver function and the prevention of fibrosis progression (Figure 5). Further exploration into quercetin’s therapeutic potential, within the context of TCM and beyond, could pave the way for novel interventions in liver fibrosis management, emphasizing the importance of early detection and treatment in curtailing the adverse outcomes associated with this condition.
Figure 5. Quercetin’s Mechanisms in Mitigating Liver Fibrosis. This figure illustrates quercetin’s multifaceted role in combating liver fibrosis, a condition exacerbated by factors like viral hepatitis, alcoholic and non-alcoholic fatty liver disease (NAFLD), through the activation of hepatic stellate cells (HSCs). Central to fibrosis progression, HSCs, upon activation, produce an excess of extracellular matrix metabolites, including collagen, fostering fibrous scar tissue formation within the liver. Quercetin intervenes by inducing apoptosis in activated HSCs, thus diminishing their numbers and fibrogenic output. It notably inhibits the TGF-β1/Smads and PI3K/Akt pathways, crucial for HSC activation and collagen synthesis, and associated with cell survival and proliferation, respectively. Through these actions, quercetin effectively reduces HSC activation and their fibrogenic activity, offering a promising approach to slowing liver fibrosis progression.
Liver fibrosis emerges from an intricate web of signaling pathways and molecular interactions, underlining the complexity of its pathogenesis. A deep understanding of these mechanisms is pivotal for devising effective strategies for its prevention and treatment. Among the myriad pathways implicated, the Notch and JAK/STAT signaling pathways are of particular interest due to their significant roles in the progression of liver fibrosis. The Notch signaling pathway, integral to cell communication and various biological functions such as cell fate determination and differentiation, is implicated in liver fibrosis through its influence on hepatic stellate cells (HSCs) and collagen synthesis (Meliou et al., 2011; Espinoza et al., 2013). This pathway involves four receptors (Notch1-4) and five ligands (Dll1, Dll3, Dll4, Jagged1, and Jagged2), which orchestrate the cellular response during fibrosis (Shimizu et al., 2000; Briot and Iruela-Arispe, 2015). Notably, increased activity of Notch3 has been observed in fibrotic liver, promoting HSC activation and exacerbating fibrosis (Chen et al., 2012; Ni et al., 2018). The pathway also affects macrophage polarization, skewing the balance towards a pro-fibrotic environment (Bansal et al., 2015; Aimaiti et al., 2019).
Concurrently, the JAK/STAT signaling pathway, known for regulating cell growth and immune responses, has been identified as a key player in liver repair and fibrosis. It is activated by cytokines and growth factors like IL-6, IFN-γ, and TGF-β, directly influencing HSC transformation and fibrosis severity (Klein et al., 2005; Kong et al., 2012; Kagan et al., 2017; Tang et al., 2017; Xin et al., 2020). IL-6, for example, can induce HSCs to transform into myofibroblast-like cells, highlighting a potential target for therapeutic intervention.
Quercetin, a flavonoid present in various Traditional Chinese Medicine (TCM) formulations, has shown promise in targeting HSCs through these pathways, suggesting its potential in reversing liver fibrosis. Studies have demonstrated quercetin’s ability to inhibit HSC proliferation, induce apoptosis, and modulate significant pathways involved in fibrosis, such as TGF-β1/Smads and PI3K/Akt (He et al., 2016; Wu et al., 2017).
Research within the realm of TCM, particularly in China, has explored quercetin-containing decoctions for their anti-fibrotic effects. Formulations like Qiwei Qinggan Powder, Yinchenhao Decoction, and Fuzheng Huayu Recipe have been studied for their regulatory effects on key targets and signaling pathways, showing efficacy in reducing fibrosis and promoting liver health (Cai et al., 2019; Zhang et al., 2021; Hong et al., 2022; Huang et al., 2022; Sun et al., 2022; Zhang et al., 2022; Jiang et al., 2023).
While clinical trials specifically targeting liver fibrosis with TCM formulations containing quercetin are yet to be conducted, preliminary studies on quercetin’s impact on hepatocyte injury are promising. A randomized, placebo-controlled trial among Japanese subjects suggested improvements in liver function with quercetin-rich onion consumption (Nishimura et al., 2019). Furthermore, a phase I study indicated quercetin’s safety and potential antiviral activity in chronic hepatitis C patients (Lu et al., 2016) (Table 2 provides detailed listings of the dosages, duration of action, models, and other specific details of quercetin interventions in liver fibrosis mentioned in this paper).
Quercetin, a polyphenolic flavonoid ubiquitously found in a variety of foods, offers a spectrum of health benefits, serving as the focus of this detailed exploration. Originating as a plant secondary metabolite, quercetin predominantly exists in glycosylated forms across numerous food sources, with onions, apples, berries, and leafy greens being particularly rich in this metabolite. Advances in extraction technologies, such as microwave-assisted and supercritical fluid extraction, have significantly surpassed traditional methods in efficiency and effectiveness, presenting a leap forward in quercetin utilization.
Despite its promising attributes, quercetin’s clinical application is hindered by its low oral bioavailability, attributed to challenges in absorption and rapid metabolic clearance. Innovations in dietary strategies and formulation technologies aim to enhance its bioavailability, ensuring a broader realization of quercetin’s health-promoting potential. The endeavor to amplify quercetin’s systemic availability is supported by extensive research, aiming to fully harness its therapeutic prospects.
Quercetin’s role extends to the modulation of various cellular death pathways, including but not limited to apoptosis, autophagy, necroptosis, pyroptosis, ferroptosis, and cuproptosis. It has shown efficacy in inducing apoptosis across a range of cancer cell lines, disrupting the balance between cell survival and death signals. The evidence presented herein underscores quercetin’s capacity to engage with multiple molecular mechanisms underlying cell death, supported by a robust body of scientific research.
The interaction between quercetin and liver fibrosis unveils another layer of its therapeutic potential. Exhibiting antioxidant, anti-inflammatory, and antitumor properties, quercetin emerges as a valuable agent in combating liver fibrosis. It achieves this through the regulation of critical signaling pathways, including NF-κB/IκBα, p38 MAPK, Bcl-2/Bax, TGF-β1/Smads, and PI3K/Akt. Moreover, the efficacy of Traditional Chinese Medicine (TCM) formulations containing quercetin against liver fibrosis has been highlighted, revealing promising outcomes in research studies.
This article serves as a detailed guide to quercetin, elucidating its sources, extraction methodologies, bioavailability challenges, and therapeutic roles in modulating cell death and combating liver fibrosis. Incorporating a wealth of research findings, this comprehensive overview not only illuminates the extensive benefits of quercetin but also emphasizes the ongoing efforts to enhance its bioavailability and therapeutic efficacy.
In summary, quercetin has been extensively validated in cellular and animal experiments, and future work should further explore the possibility of clinical trials to identify safe and effective dosages. Particularly, conducting more stringent, rigorous, and objective randomized double-blind controlled trials specifically targeting liver fibrosis will be a crucial step in the potential application of quercetin in the treatment of liver fibrosis. Overall, quercetin holds significant potential in the treatment of liver fibrosis and is worthy of further investigation.
FX: Writing–original draft. YZ: Writing–original draft. TL: Writing–original draft. Yi-ping YT: Writing–original draft. S-YS: Conceptualization, Investigation, Writing–review and editing. QZ: Funding acquisition, Writing–review and editing. YW: Conceptualization, Funding acquisition, Investigation, Project administration, Supervision, Validation, Writing–review and editing.
The author(s) declare that financial support was received for the research, authorship, and/or publication of this article. This research was supported by the National Natural Science Foundation of China (81802504), grants from the Sichuan Science and Technology Department (2023YFH0010 and 2021YFS0380).
The authors declare that the research was conducted in the absence of any commercial or financial relationships that could be construed as a potential conflict of interest.
All claims expressed in this article are solely those of the authors and do not necessarily represent those of their affiliated organizations, or those of the publisher, the editors and the reviewers. Any product that may be evaluated in this article, or claim that may be made by its manufacturer, is not guaranteed or endorsed by the publisher.
Abdrakhmanov A, G. V., and Zhivotovsky, B. (2020). To eat or to die: deciphering selective forms of autophagy. Trends Biochem. Sci. 45 (4), 347–364. doi:10.1016/j.tibs.2019.11.006
Ader, P., Wessmann, A., and Wolffram, S. (2000). Bioavailability and metabolism of the flavonol quercetin in the pig. Free Radic. Biol. Med. 28, 1056–1067. doi:10.1016/s0891-5849(00)00195-7
Aimaiti, Y., Yusufukadier, M., Li, W., Tuerhongjiang, T., Shadike, A., Meiheriayi, A., et al. (2019). TGF-β1 signaling activates hepatic stellate cells through Notch pathway. Cytotechnology 71, 881–891. doi:10.1007/s10616-019-00329-y
Arts, I. C., Sesink, A. L., Faassen-Peters, M., and Hollman, P. C. (2004). The type of sugar moiety is a major determinant of the small intestinal uptake and subsequent biliary excretion of dietary quercetin glycosides. Br. J. Nutr. 91, 841–847. doi:10.1079/bjn20041123
Avalos-Padilla, Y., Georgiev, V. N., and Dimova, R. (2021). ESCRT-III induces phase separation in model membranes prior to budding and causes invagination of the liquid-ordered phase. Biochimica biophysica acta. Biomembr. 1863, 183689. doi:10.1016/j.bbamem.2021.183689
Balestrini, R., Brunetti, C., Cammareri, M., Caretto, S., Cavallaro, V., Cominelli, E., et al. (2021). Strategies to modulate specialized metabolism in mediterranean crops: from molecular aspects to field. Int. J. Mol. Sci. 22, 2887. doi:10.3390/ijms22062887
Bansal, R., van Baarlen, J., Storm, G., and Prakash, J. (2015). The interplay of the Notch signaling in hepatic stellate cells and macrophages determines the fate of liver fibrogenesis. Sci. Rep. 5, 18272. doi:10.1038/srep18272
Batiha, G. E.-S., Beshbishy, A. M., Ikram, M., Mulla, Z. S., El-Hack, M. E. A., Taha, A. E., et al. (2020). The pharmacological activity, biochemical properties, and pharmacokinetics of the major natural polyphenolic flavonoid: quercetin. Foods 9, 374. doi:10.3390/foods9030374
Bekki, Y., Yoshizumi, T., Shimoda, S., Itoh, S., Harimoto, N., Ikegami, T., et al. (2017). Hepatic stellate cells secreting WFA(+) -M2BP: its role in biological interactions with Kupffer cells. J. gastroenterology hepatology 32, 1387–1393. doi:10.1111/jgh.13708
Belwal, T., Ezzat, S. M., Rastrelli, L., Bhatt, I. D., Daglia, M., Baldi, A., et al. (2018). A critical analysis of extraction techniques used for botanicals: trends, priorities, industrial uses and optimization strategies. TrAC Trends Anal. Chem. 100, 82–102. doi:10.1016/j.trac.2017.12.018
Bento, C. F., Renna, M., Ghislat, G., Puri, C., Ashkenazi, A., Vicinanza, M., et al. (2016). Mammalian autophagy: how does it work? Annu. Rev. Biochem. 85, 685–713. doi:10.1146/annurev-biochem-060815-014556
Bergsbaken, T., Fink, S. L., and Cookson, B. T. (2009). Pyroptosis: host cell death and inflammation. Nat. Rev. Microbiol. 7, 99–109. doi:10.1038/nrmicro2070
Bhagwat, S., Haytowitz, D. B., and Holden, J. (2011) USDA database for the flavonoid content of selected foods, release 3.
Bieger, J., Cermak, R., Blank, R., de Boer, V. C. J., Hollman, P. C. H., Kamphues, J., et al. (2008). Tissue distribution of quercetin in pigs after long-term dietary supplementation. J. Nutr. 138, 1417–1420. doi:10.1093/jn/138.8.1417
Birgisdottir Å, B., Mouilleron, S., Bhujabal, Z., Wirth, M., Sjøttem, E., Evjen, G., et al. (2019). Members of the autophagy class III phosphatidylinositol 3-kinase complex I interact with GABARAP and GABARAPL1 via LIR motifs. Autophagy 15, 1333–1355. doi:10.1080/15548627.2019.1581009
Bishayee, K., Ghosh, S., Mukherjee, A., Sadhukhan, R., Mondal, J., and Khuda-Bukhsh, A. R. (2013). Quercetin induces cytochrome-c release and ROS accumulation to promote apoptosis and arrest the cell cycle in G2/M, in cervical carcinoma: signal cascade and drug-DNA interaction. Cell Prolif. 46, 153–163. doi:10.1111/cpr.12017
Bodmer, J. L. B. K., Schneider, P., Hofmann, K., Steiner, V., Thome, M., Bornand, T., et al. (1997). TRAMP, a novel apoptosis-mediating receptor with sequence homology to tumor necrosis factor receptor 1 and Fas(Apo-1/CD95). Immunity 6 (1), 79–88. doi:10.1016/s1074-7613(00)80244-7
Brenner, D. A., Waterboer, T., Choi, S. K., Lindquist, J. N., Stefanovic, B., Burchardt, E., et al. (2000). New aspects of hepatic fibrosis. J. hepatology 32, 32–38. doi:10.1016/s0168-8278(00)80413-4
Briot, A., and Iruela-Arispe, M. L. (2015). Blockade of specific NOTCH ligands: a new promising approach in cancer therapy. Cancer Discov. 5, 112–114. doi:10.1158/2159-8290.Cd-14-1501
Bucci, C., Thomsen, P., Nicoziani, P., McCarthy, J., and van Deurs, B. (2000). Rab7: a key to lysosome biogenesis. Mol. Biol. Cell 11, 467–480. doi:10.1091/mbc.11.2.467
Cai, F. F., Bian, Y. Q., Wu, R., Sun, Y., Chen, X. L., Yang, M. D., et al. (2019). Yinchenhao decoction suppresses rat liver fibrosis involved in an apoptosis regulation mechanism based on network pharmacology and transcriptomic analysis. Biomed. Pharmacother. = Biomedecine Pharmacother. 114, 108863. doi:10.1016/j.biopha.2019.108863
Cai, X., Li, Z., Zhang, Q., Qu, Y., Xu, M., Wan, X., et al. (2018). CXCL6-EGFR-induced Kupffer cells secrete TGF-β1 promoting hepatic stellate cell activation via the SMAD2/BRD4/C-MYC/EZH2 pathway in liver fibrosis. J. Cell. Mol. Med. 22, 5050–5061. doi:10.1111/jcmm.13787
Cai, Z., Jitkaew, S., Zhao, J., Chiang, H. C., Choksi, S., Liu, J., et al. (2014). Plasma membrane translocation of trimerized MLKL protein is required for TNF-induced necroptosis. Nat. Cell Biol. 16, 55–65. doi:10.1038/ncb2883
Chen, D., Tong, J., Yang, L., Wei, L., Stolz, D. B., Yu, J., et al. (2018). PUMA amplifies necroptosis signaling by activating cytosolic DNA sensors. Proc. Natl. Acad. Sci. U. S. A. 115, 3930–3935. doi:10.1073/pnas.1717190115
Chen, L., Min, J., and Wang, F. (2022). Copper homeostasis and cuproptosis in health and disease. Signal Transduct. Target. Ther. 7, 378. doi:10.1038/s41392-022-01229-y
Chen, Y. X., Weng, Z. H., and Zhang, S. L. (2012). Notch3 regulates the activation of hepatic stellate cells. World J. gastroenterology 18, 1397–1403. doi:10.3748/wjg.v18.i12.1397
Cheng, S., Gao, N., Zhang, Z., Budhraja, A., and Ke, Z. (2010). Quercetin induces tumor-selective apoptosis through downregulation of Mcl-1 and activation of Bax. Clin. cancer Res. official J. Am. Assoc. Cancer Res. 16, 5679–5691. doi:10.1158/1078-0432.Ccr-10-1565
Cheng, Y., Zak, O., Aisen, P., Harrison, S. C., and Walz, T. (2004). Structure of the human transferrin receptor-transferrin complex. Cell 116, 565–576. doi:10.1016/s0092-8674(04)00130-8
Cho, Y. S., Challa, S., Moquin, D., Genga, R., Ray, T. D., Guildford, M., et al. (2009). Phosphorylation-driven assembly of the RIP1-RIP3 complex regulates programmed necrosis and virus-induced inflammation. Cell 137, 1112–1123. doi:10.1016/j.cell.2009.05.037
Das, U. N. (2019). Saturated fatty acids, MUFAs and PUFAs regulate ferroptosis. Cell Chem. Biol. 26, 309–311. doi:10.1016/j.chembiol.2019.03.001
de Boer, V. C., Dihal, A. A., van der Woude, H., Arts, I. C. W., Wolffram, S., Alink, G. M., et al. (2005). Tissue distribution of quercetin in rats and pigs. J. Nutr. 135, 1718–1725. doi:10.1093/jn/135.7.1718
Dewson, G., and Kluck, R. M. (2009). Mechanisms by which Bak and Bax permeabilise mitochondria during apoptosis. J. Cell Sci. 122, 2801–2808. doi:10.1242/jcs.038166
Dhanya, R. A. A., Nisha, P., and Jayamurthy, P. (2017). Quercetin, a lead compound against type 2 diabetes ameliorates glucose uptake via AMPK pathway in skeletal muscle cell line. Front. Pharmacol. 8, 336. doi:10.3389/fphar.2017.00336
Dixon, S. J., Lemberg, K. M., Lamprecht, M. R., Skouta, R., Zaitsev, E. M., Gleason, C. E., et al. (2012). Ferroptosis: an iron-dependent form of nonapoptotic cell death. Cell 149, 1060–1072. doi:10.1016/j.cell.2012.03.042
Domiciano, T. P., Wakita, D., Jones, H. D., Crother, T. R., Verri, W. A., Arditi, M., et al. (2017). Quercetin inhibits inflammasome activation by interfering with ASC oligomerization and prevents interleukin-1 mediated mouse vasculitis. Sci. Rep. 7, 41539. doi:10.1038/srep41539
Dooley, H. C., Razi, M., Polson, H. E. J., Girardin, S. E., Wilson, M. I., and Tooze, S. A. (2014). WIPI2 links LC3 conjugation with PI3P, autophagosome formation, and pathogen clearance by recruiting Atg12-5-16L1. Mol. Cell 55, 238–252. doi:10.1016/j.molcel.2014.05.021
Dovey, C. M., Diep, J., Clarke, B. P., Hale, A. T., McNamara, D. E., Guo, H., et al. (2018). MLKL requires the inositol phosphate code to execute necroptosis. Mol. Cell 70, 936–948. doi:10.1016/j.molcel.2018.05.010
Du, W. D., Zhang, Y. E., Zhai, W. R., and Zhou, X. M. (1999). Dynamic changes of type I,III and IV collagen synthesis and distribution of collagen-producing cells in carbon tetrachloride-induced rat liver fibrosis. World J. gastroenterology 5, 397–403. doi:10.3748/wjg.v5.i5.397
Egan, D., Kim, J., Shaw, R. J., and Guan, K. L. (2011b). The autophagy initiating kinase ULK1 is regulated via opposing phosphorylation by AMPK and mTOR. Autophagy 7, 643–644. doi:10.4161/auto.7.6.15123
Egan, D. F., Shackelford, D. B., Mihaylova, M. M., Gelino, S., Kohnz, R. A., Mair, W., et al. (2011a). Phosphorylation of ULK1 (hATG1) by AMP-activated protein kinase connects energy sensing to mitophagy. Sci. (New York, N.Y.) 331, 456–461. doi:10.1126/science.1196371
Espinoza, I., Pochampally, R., Xing, F., Watabe, K., and Miele, L. (2013). Notch signaling: targeting cancer stem cells and epithelial-to-mesenchymal transition. OncoTargets Ther. 6, 1249–1259. doi:10.2147/ott.S36162
Feoktistova, M., Geserick, P., Kellert, B., Dimitrova, D. P., Langlais, C., Hupe, M., et al. (2011). cIAPs block Ripoptosome formation, a RIP1/caspase-8 containing intracellular cell death complex differentially regulated by cFLIP isoforms. Mol. Cell 43, 449–463. doi:10.1016/j.molcel.2011.06.011
Fleshner, M. (2013). Stress-evoked sterile inflammation, danger associated molecular patterns (DAMPs), microbial associated molecular patterns (MAMPs) and the inflammasome. Brain, Behav. Immun. 27, 1–7. doi:10.1016/j.bbi.2012.08.012
Frankel, E. B., Shankar, R., Moresco, J. J., Yates, J. R., Volkmann, N., and Audhya, A. (2017). Ist1 regulates ESCRT-III assembly and function during multivesicular endosome biogenesis in Caenorhabditis elegans embryos. Nat. Commun. 8, 1439. doi:10.1038/s41467-017-01636-8
Frazer, D. M., and Anderson, G. J. (2014). The regulation of iron transport. BioFactors Oxf. Engl. 40, 206–214. doi:10.1002/biof.1148
Friedmann Angeli, J. P., Miyamoto, S., and Schulze, A. (2019). Ferroptosis: the greasy side of cell death. Chem. Res. Toxicol. 32, 362–369. doi:10.1021/acs.chemrestox.8b00349
Gamet-Payrastre, L., Manenti, S., Gratacap, M. P., Tulliez, J., Chap, H., and Payrastre, B. (1999). Flavonoids and the inhibition of PKC and PI 3-kinase. General Pharmacol. 32, 279–286. doi:10.1016/s0306-3623(98)00220-1
Ganley, I. G., Lam, D. H., Wang, J., Ding, X., Chen, S., and Jiang, X. (2009). ULK1.ATG13.FIP200 complex mediates mTOR signaling and is essential for autophagy. J. Biol. Chem. 284, 12297–12305. doi:10.1074/jbc.M900573200
Gao, C., Liu, Y., Jiang, C., Liu, L., Li, J., Li, D., et al. (2020). Intensive running enhances NF-κB activity in the mice liver and the intervention effects of quercetin. Nutrients 12, 2770. doi:10.3390/nu12092770
García Díaz, J., González Fernández, R., Escalona Arranz, J. C., Llauradó Maury, G., Méndez Rodríguez, D., De Vooght, L., et al. (2022). Inhibitory effect on nitric oxide release in LPS-stimulated macrophages and free radical scavenging activity of Croton linearis jacq. Leaves. Antioxidants (Basel, Switz.) 11, 1915. doi:10.3390/antiox11101915
Gholampour, F., and Saki, N. (2019). Hepatic and renal protective effects of quercetin in ferrous sulfateinduced toxicity. General physiology biophysics 38, 27–38. doi:10.4149/gpb_2018038
Glei, M., Latunde-Dada, G. O., Klinder, A., Becker, T. W., Hermann, U., Voigt, K., et al. (2002). Iron-overload induces oxidative DNA damage in the human colon carcinoma cell line HT29 clone 19A. Mutat. Res. 519, 151–161. doi:10.1016/s1383-5718(02)00135-3
Gligor, O., Clichici, S., Moldovan, R., Muntean, D., Vlase, A. M., Nadăș, G. C., et al. (2023). The effect of extraction methods on phytochemicals and biological activities of green coffee beans extracts. Plants (Basel, Switz.) 12, 712. doi:10.3390/plants12040712
Golstein, P., and Kroemer, G. (2007). Cell death by necrosis: towards a molecular definition. Trends Biochem. Sci. 32, 37–43. doi:10.1016/j.tibs.2006.11.001
Graefe, E. U., Wittig, J., Mueller, S., Riethling, A. K., Uehleke, B., Drewelow, B., et al. (2001). Pharmacokinetics and bioavailability of quercetin glycosides in humans. J. Clin. Pharmacol. 41, 492–499. doi:10.1177/00912700122010366
Granado-Serrano Ab, M. M., Bravo, L., Goya, L., and Ramos, S. (2006). Quercetin induces apoptosis via caspase activation, regulation of Bcl-2, and inhibition of PI-3-kinase/Akt and ERK pathways in a human hepatoma cell line (HepG2). J. Nutr. 136 (11), 2715–2721. doi:10.1093/jn/136.11.2715
Guan, H., Zhang, W., Liu, H., Jiang, Y., Li, F., Wu, M., et al. (2023). Quercetin induces apoptosis in HepG2 cells via directly interacting with YY1 to disrupt YY1-p53 interaction. Metabolites 13, 229. doi:10.3390/metabo13020229
Guicciardi, M. E., and Gores, G. J. (2009). Life and death by death receptors. FASEB J. official Publ. Fed. Am. Soc. Exp. Biol. 23, 1625–1637. doi:10.1096/fj.08-111005
Guo, Y., and Bruno, R. S. (2015). Endogenous and exogenous mediators of quercetin bioavailability. J. Nutr. Biochem. 26, 201–210. doi:10.1016/j.jnutbio.2014.10.008
Guo, Y., Mah, E., Davis, C. G., Jalili, T., Ferruzzi, M. G., Chun, O. K., et al. (2013). Dietary fat increases quercetin bioavailability in overweight adults. Mol. Nutr. food Res. 57, 896–905. doi:10.1002/mnfr.201200619
Gupta, S. K. G., Szegezdi, E., and Joseph, B. (2009). The mitochondrial death pathway: a promising therapeutic target in diseases. J. Cell Mol. Med. 13 (6), 1004–1033. doi:10.1111/j.1582-4934.2009.00697.x
Harwood, M., Danielewska-Nikiel, B., Borzelleca, J. F., Flamm, G. W., Williams, G. M., and Lines, T. C. (2007). A critical review of the data related to the safety of quercetin and lack of evidence of in vivo toxicity, including lack of genotoxic/carcinogenic properties. Food Chem. Toxicol. Int. J. Publ. Br. Industrial Biol. Res. Assoc. 45, 2179–2205. doi:10.1016/j.fct.2007.05.015
He, L., Hou, X., Fan, F., and Wu, H. (2016). Quercetin stimulates mitochondrial apoptosis dependent on activation of endoplasmic reticulum stress in hepatic stellate cells. Pharm. Biol. 54, 3237–3243. doi:10.1080/13880209.2016.1223143
Henning, Y., Blind, U. S., Larafa, S., Matschke, J., and Fandrey, J. (2022). Hypoxia aggravates ferroptosis in RPE cells by promoting the Fenton reaction. Cell death Dis. 13, 662. doi:10.1038/s41419-022-05121-z
Higashi, T., Friedman, S. L., and Hoshida, Y. (2017). Hepatic stellate cells as key target in liver fibrosis. Adv. drug Deliv. Rev. 121, 27–42. doi:10.1016/j.addr.2017.05.007
Holler, N., Zaru, R., Micheau, O., Thome, M., Attinger, A., Valitutti, S., et al. (2000). Fas triggers an alternative, caspase-8-independent cell death pathway using the kinase RIP as effector molecule. Nat. Immunol. 1, 489–495. doi:10.1038/82732
Hollman, P. C., and Katan, M. B. (1998). Bioavailability and health effects of dietary flavonols in man. Archives Toxicol. Suppl. = Archiv fur Toxikologie. Suppl. 20, 237–248. doi:10.1007/978-3-642-46856-8_21
Hong, B., Wang, Y., Hou, Y., Liu, R., and Li, W. (2022). Study on the mechanism of anti-hepatic fibrosis of Glycyrrhiza Uralensis-Salvia miltiorrhiza prescription based on serum and urine metabolomics and network pharmacology. J. Chromatogr. B, Anal. Technol. Biomed. life Sci. 1209, 123416. doi:10.1016/j.jchromb.2022.123416
Hornung, V., Ablasser, A., Charrel-Dennis, M., Bauernfeind, F., Horvath, G., Caffrey, D. R., et al. (2009). AIM2 recognizes cytosolic dsDNA and forms a caspase-1-activating inflammasome with ASC. Nature 458, 514–518. doi:10.1038/nature07725
Huang, S. P., Chen, S., Ma, Y. Z., Zhou, A., Jiang, H., and Wu, P. (2022). Evaluation of the mechanism of jiedu huazhuo quyu formula in treating wilson's disease-associated liver fibrosis by network pharmacology analysis and molecular dynamics simulation. Evidence-based complementary Altern. Med. eCAM 2022, 9363131. doi:10.1155/2022/9363131
Huang, Y., Chen, Y., Shaw, A. M., Goldfine, H., Tian, J., and Cai, J. (2018). Enhancing TFEB-mediated cellular degradation pathways by the mTORC1 inhibitor quercetin. Oxidative Med. Cell. Longev. 2018, 5073420. doi:10.1155/2018/5073420
Ji, C., and Kosman, D. J. (2015). Molecular mechanisms of non-transferrin-bound and transferring-bound iron uptake in primary hippocampal neurons. J. Neurochem. 133, 668–683. doi:10.1111/jnc.13040
Jiang, M., Huang, C., Wu, Q., Su, Y., Wang, X., Xuan, Z., et al. (2023). Sini San ameliorates CCl4-induced liver fibrosis in mice by inhibiting AKT-mediated hepatocyte apoptosis. J. Ethnopharmacol. 303, 115965. doi:10.1016/j.jep.2022.115965
Jorgensen, I., and Miao, E. A. (2015). Pyroptotic cell death defends against intracellular pathogens. Immunol. Rev. 265, 130–142. doi:10.1111/imr.12287
Kagan, P., Sultan, M., Tachlytski, I., Safran, M., and Ben-Ari, Z. (2017). Both MAPK and STAT3 signal transduction pathways are necessary for IL-6-dependent hepatic stellate cells activation. PloS one 12, e0176173. doi:10.1371/journal.pone.0176173
Kaiser, W. J., Sridharan, H., Huang, C., Mandal, P., Upton, J. W., Gough, P. J., et al. (2013). Toll-like receptor 3-mediated necrosis via TRIF, RIP3, and MLKL. J. Biol. Chem. 288, 31268–31279. doi:10.1074/jbc.M113.462341
Kiagiadaki, F., Kampa, M., Voumvouraki, A., Castanas, E., Kouroumalis, E., and Notas, G. (2018). Activin-A causes Hepatic stellate cell activation via the induction of TNFα and TGFβ in Kupffer cells. Biochimica biophysica acta. Mol. basis Dis. 1864, 891–899. doi:10.1016/j.bbadis.2017.12.031
Kim, J., and Choe, E. (2018). Effect of the pH on the lipid oxidation and polyphenols of soybean oil-in-water emulsion with added peppermint (Mentha piperita) extract in the presence and absence of iron. Food Sci. Biotechnol. 27, 1285–1292. doi:10.1007/s10068-018-0324-2
Klein, C., Wüstefeld, T., Assmus, U., Roskams, T., Rose-John, S., Müller, M., et al. (2005). The IL-6-gp130-STAT3 pathway in hepatocytes triggers liver protection in T cell-mediated liver injury. J. Clin. investigation 115, 860–869. doi:10.1172/jci23640
Kondili, L. A., Taliani, G., Cerga, G., Tosti, M. E., Babameto, A., and Resuli, B. (2005). Correlation of alcohol consumption with liver histological features in non-cirrhotic patients. Eur. J. gastroenterology hepatology 17, 155–159. doi:10.1097/00042737-200502000-00005
Kong, X., Horiguchi, N., Mori, M., and Gao, B. (2012). Cytokines and STATs in liver fibrosis. Front. physiology 3, 69. doi:10.3389/fphys.2012.00069
Konrad, M., and Nieman, D. C. J. S. N. (2015). Evaluation of quercetin as a countermeasure to exercise-induced physiological stress in Antioxidants in sport nutrition (Taylor and Francis: CRC Press).
Kuo, S. M., Huang, C. T., Blum, P., and Chang, C. (2001). Quercetin cumulatively enhances copper induction of metallothionein in intestinal cells. Biol. trace Elem. Res. 84, 1–10. doi:10.1385/bter:84:1-3:001
Laster, S. M., Wood, J. G., and Gooding, L. R. (1988). Tumor necrosis factor can induce both apoptic and necrotic forms of cell lysis. J. Immunol. 141, 2629–2634. doi:10.4049/jimmunol.141.8.2629
Lee, K. M., Kang, J. H., Yun, M., and Lee, S. B. (2018). Quercetin inhibits the poly(dA:dT)-induced secretion of IL-18 via down-regulation of the expressions of AIM2 and pro-caspase-1 by inhibiting the JAK2/STAT1 pathway in IFN-γ-primed human keratinocytes. Biochem. biophysical Res. Commun. 503, 116–122. doi:10.1016/j.bbrc.2018.05.191
Lesjak, M., Hoque, R., Balesaria, S., Skinner, V., Debnam, E. S., Srai, S. K. S., et al. (2014). Quercetin inhibits intestinal iron absorption and ferroportin transporter expression in vivo and in vitro. PloS one 9, e102900. doi:10.1371/journal.pone.0102900
Li, C., Zhang, W. J., Choi, J., and Frei, B. (2016b). Quercetin affects glutathione levels and redox ratio in human aortic endothelial cells not through oxidation but formation and cellular export of quercetin-glutathione conjugates and upregulation of glutamate-cysteine ligase. Redox Biol. 9, 220–228. doi:10.1016/j.redox.2016.08.012
Li, D., Jiang, C., Mei, G., Zhao, Y., Chen, L., Liu, J., et al. (2020b). Quercetin alleviates ferroptosis of pancreatic β cells in type 2 diabetes. Nutrients 12, 2954. doi:10.3390/nu12102954
Li, D. L., Mao, L., Gu, Q., Wei, F., and Gong, Y. Y. (2021). Quercetin protects retina external barrier from oxidative stress injury by promoting autophagy. Cutan. ocular Toxicol. 40, 7–13. doi:10.1080/15569527.2020.1860082
Li, F. J., Long, H. Z., Zhou, Z. W., Luo, H. Y., Xu, S. G., and Gao, L. C. (2022). System X(c) (-)/GSH/GPX4 axis: an important antioxidant system for the ferroptosis in drug-resistant solid tumor therapy. Front. Pharmacol. 13, 910292. doi:10.3389/fphar.2022.910292
Li, J., Cao, F., Yin, H. L., Huang, Z. J., Lin, Z. T., Mao, N., et al. (2020a). Ferroptosis: past, present and future. Cell death Dis. 11, 88. doi:10.1038/s41419-020-2298-2
Li, J., McQuade, T., Siemer, A. B., Napetschnig, J., Moriwaki, K., Hsiao, Y. S., et al. (2012). The RIP1/RIP3 necrosome forms a functional amyloid signaling complex required for programmed necrosis. Cell 150, 339–350. doi:10.1016/j.cell.2012.06.019
Li, Y., Yao, J., Han, C., Yang, J., Chaudhry, M. T., Wang, S., et al. (2016a). Quercetin, inflammation and immunity. Nutrients 8, 167. doi:10.3390/nu8030167
Liang, D., Minikes, A. M., and Jiang, X. (2022). Ferroptosis at the intersection of lipid metabolism and cellular signaling. Mol. Cell 82, 2215–2227. doi:10.1016/j.molcel.2022.03.022
Liston, A., and Masters, S. L. (2017). Homeostasis-altering molecular processes as mechanisms of inflammasome activation. Nat. Rev. Immunol. 17, 208–214. doi:10.1038/nri.2016.151
Liu, J., Zhou, M., Xu, Q., Lv, Q., Guo, J., Qin, X., et al. (2023). Quercetin ameliorates deoxynivalenol-induced intestinal injury and barrier dysfunction associated with inhibiting necroptosis signaling pathway in weaned pigs. Int. J. Mol. Sci. 24, 15172. doi:10.3390/ijms242015172
Liu, X., Liao, X., Rao, X., Wang, B., Zhang, J., Xu, G., et al. (2020). The lysosomal membrane protein LAMP-2 is dispensable for PINK1/Parkin-mediated mitophagy. FEBS Lett. 594, 823–840. doi:10.1002/1873-3468.13663
Lomozová, Z., Catapano, M. C., Hrubša, M., Karlíčková, J., Macáková, K., Kučera, R., et al. (2021). Chelation of iron and copper by quercetin B-ring methyl metabolites, isorhamnetin and tamarixetin, and their effect on metal-based Fenton chemistry. J. Agric. food Chem. 69, 5926–5937. doi:10.1021/acs.jafc.1c01729
Lu, N. T., Crespi, C. M., Liu, N. M., Vu, J. Q., Ahmadieh, Y., Wu, S., et al. (2016). A phase I dose escalation study demonstrates quercetin safety and explores potential for bioflavonoid antivirals in patients with chronic hepatitis C. Phytotherapy Res. PTR 30, 160–168. doi:10.1002/ptr.5518
Lu, Q., Ji, X. J., Zhou, Y. X., Yao, X. Q., Liu, Y. Q., Zhang, F., et al. (2015). Quercetin inhibits the mTORC1/p70S6K signaling-mediated renal tubular epithelial-mesenchymal transition and renal fibrosis in diabetic nephropathy. Pharmacol. Res. 99, 237–247. doi:10.1016/j.phrs.2015.06.006
Luo, X., Bao, X., Weng, X., Bai, X., Feng, Y., Huang, J., et al. (2022). The protective effect of quercetin on macrophage pyroptosis via TLR2/Myd88/NF-κB and ROS/AMPK pathway. Life Sci. 291, 120064. doi:10.1016/j.lfs.2021.120064
Lystad, A. H., Carlsson, S. R., and Simonsen, A. (2019). Toward the function of mammalian ATG12-ATG5-ATG16L1 complex in autophagy and related processes. Autophagy 15, 1485–1486. doi:10.1080/15548627.2019.1618100
M, M. A.-A., Al-Humaid, L., Aldawsari, M., Abid, I. F., Jhanani, G. K., and Shanmuganathan, R. (2023). Quercetin extraction from small onion skin (Allium cepa L. var. aggregatum Don.) and its antioxidant activity. Environ. Res. 224, 115497. doi:10.1016/j.envres.2023.115497
Magtanong, L., Ko, P. J., To, M., Cao, J. Y., Forcina, G. C., Tarangelo, A., et al. (2019). Exogenous monounsaturated fatty acids promote a ferroptosis-resistant cell state. Cell Chem. Biol. 26, 420–432. doi:10.1016/j.chembiol.2018.11.016
Majeski, A. E., and Dice, J. F. (2004). Mechanisms of chaperone-mediated autophagy. Int. J. Biochem. Cell Biol. 36, 2435–2444. doi:10.1016/j.biocel.2004.02.013
Manach, C., Williamson, G., Morand, C., Scalbert, A., and Rémésy, C. (2005). Bioavailability and bioefficacy of polyphenols in humans. I. Review of 97 bioavailability studies. Am. J. Clin. Nutr. 81, 230S–242s. doi:10.1093/ajcn/81.1.230S
Martínez-Flórez, S., Sánchez-Campos, S., González-Gallego, J., Tuñón, M. J., and Tuñón, M. J. (2005). Quercetin attenuates nuclear factor-kappaB activation and nitric oxide production in interleukin-1beta-activated rat hepatocytes. J. Nutr. 135 (6), 1359–1365. doi:10.1093/jn/135.6.1359
Martinon, F., Burns, K., and Tschopp, J. (2002). The inflammasome: a molecular platform triggering activation of inflammatory caspases and processing of proIL-beta. Mol. Cell 10, 417–426. doi:10.1016/s1097-2765(02)00599-3
Maruyama, T., and Noda, N. N. (2017). Autophagy-regulating protease Atg4: structure, function, regulation and inhibition. J. antibiotics 71, 72–78. doi:10.1038/ja.2017.104
Materska, M. (2008). Quercetin and its derivatives: chemical structure and bioactivity-a review. Pol. J. food Nutr. Sci. 58.
Meliou, E., Kerezoudis, N., Tosios, K., Lafkas, D., and Kiaris, H. (2011). Immunohistochemical expression of Notch signaling in the lining epithelium of periapical cysts. J. Endod. 37, 176–180. doi:10.1016/j.joen.2010.10.007
Meyer, K., Basu, A., Saito, K., Ray, R. B., and Ray, R. (2005). Inhibition of hepatitis C virus core protein expression in immortalized human hepatocytes induces cytochrome c-independent increase in Apaf-1 and caspase-9 activation for cell death. Virology 336, 198–207. doi:10.1016/j.virol.2005.03.016
Miao, Y., Chen, Y., Xue, F., Liu, K., Zhu, B., Gao, J., et al. (2022). Contribution of ferroptosis and GPX4's dual functions to osteoarthritis progression. EBioMedicine 76, 103847. doi:10.1016/j.ebiom.2022.103847
Moon, Y. J., Wang, L., DiCenzo, R., and Morris, M. E. (2008). Quercetin pharmacokinetics in humans. Biopharm. drug Dispos. 29, 205–217. doi:10.1002/bdd.605
Morand, C., Crespy, V., Manach, C., Besson, C., Demigné, C., and Rémésy, C. (1998). Plasma metabolites of quercetin and their antioxidant properties. Am. J. physiology 275, R212–R219. doi:10.1152/ajpregu.1998.275.1.R212
Morimoto, Y., Aiba, Y., Miyanaga, K., Hishinuma, T., Cui, L., Baba, T., et al. (2023). CID12261165, a flavonoid compound as antibacterial agents against quinolone-resistant Staphylococcus aureus. Sci. Rep. 13, 1725. doi:10.1038/s41598-023-28859-8
Mullen, W., Edwards, C. A., and Crozier, A. (2006). Absorption, excretion and metabolite profiling of methyl-glucuronyl-glucosyl- and sulpho-conjugates of quercetin in human plasma and urine after ingestion of onions. Br. J. Nutr. 96, 107–116. doi:10.1079/bjn20061809
Murota, K., and Terao, J. J. A. (2003). Antioxidative flavonoid quercetin: implication of its intestinal absorption and metabolism. Arch. Biochem. Biophys. 417, 12–17. doi:10.1016/s0003-9861(03)00284-4
Musso, O., Rehn, M., Saarela, J., Théret, N., Liétard, J., Hintikka, , et al. (1998). Collagen XVIII is localized in sinusoids and basement membrane zones and expressed by hepatocytes and activated stellate cells in fibrotic human liver. Hepatology 28, 98–107. doi:10.1002/hep.510280115
Nabavi, S. M., Šamec, D., Tomczyk, M., Milella, L., Russo, D., Habtemariam, S., et al. (2020). Flavonoid biosynthetic pathways in plants: versatile targets for metabolic engineering. Biotechnol. Adv. 38, 107316. doi:10.1016/j.biotechadv.2018.11.005
Naghibi, F., Esmaeili, S., Abdullah, N. R., Nateghpour, M., Taghvai, M., Kamkar, S., et al. (2013). In vitro and in vivo antimalarial evaluations of myrtle extract, a plant traditionally used for treatment of parasitic disorders. BioMed Res. Int. 2013, 316185. doi:10.1155/2013/316185
Nascimbeni, A. C., Codogno, P., and Morel, E. (2017). Phosphatidylinositol-3-phosphate in the regulation of autophagy membrane dynamics. FEBS J. 284, 1267–1278. doi:10.1111/febs.13987
Nazarko, V. Y., and Zhong, Q. (2013). ULK1 targets Beclin-1 in autophagy. Nat. Cell Biol. 15, 727–728. doi:10.1038/ncb2797
Nguyen, T. T., Tran, E., Do, P. T., Huynh, T. H., and Huynh, H. (2004). The role of activated MEK-ERK pathway in quercetin-induced growth inhibition and apoptosis in A549 lung cancer cells. Carcinogenesis 25, 647–659. doi:10.1093/carcin/bgh052
Ni, M. M., Wang, Y. R., Wu, W. W., Xia, C. C., Zhang, Y. H., Xu, J., et al. (2018). Novel Insights on Notch signaling pathways in liver fibrosis. Eur. J. Pharmacol. 826, 66–74. doi:10.1016/j.ejphar.2018.02.051
Nishimura, M., Muro, T., Kobori, M., and Nishihira, J. (2019). Effect of daily ingestion of quercetin-rich onion powder for 12 Weeks on visceral fat: a randomised, double-blind, placebo-controlled, parallel-group study. Nutrients 12, 91. doi:10.3390/nu12010091
Okura, H., Soeda, M., Morita, M., Fujita, M., Naba, K., Ito, C., et al. (2015). Therapeutic potential of human adipose tissue-derived multi-lineage progenitor cells in liver fibrosis. Biochem. biophysical Res. Commun. 456, 860–865. doi:10.1016/j.bbrc.2014.11.122
Ortiz, C., Schierwagen, R., Schaefer, L., Klein, S., Trepat, X., and Trebicka, J. (2021). Extracellular matrix remodeling in chronic liver disease. Curr. tissue Microenviron. Rep. 2, 41–52. doi:10.1007/s43152-021-00030-3
Pais, R., Charlotte, F., Fedchuk, L., Bedossa, P., Lebray, P., Poynard, T., et al. (2013). A systematic review of follow-up biopsies reveals disease progression in patients with non-alcoholic fatty liver. J. hepatology 59, 550–556. doi:10.1016/j.jhep.2013.04.027
Parrish, A. B., Freel, C. D., and Kornbluth, S. (2013). Cellular mechanisms controlling caspase activation and function. Cold Spring Harb. Perspect. Biol. 5, a008672. doi:10.1101/cshperspect.a008672
Poynard, T., Mathurin, P., Lai, C. L., Guyader, D., Poupon, R., Tainturier, M. H., et al. (2003). A comparison of fibrosis progression in chronic liver diseases. J. hepatology 38, 257–265. doi:10.1016/s0168-8278(02)00413-0
Rahvar, M., Owji, A. A., and Mashayekhi, F. J. (2018). Effect of quercetin on the brain-derived neurotrophic factor gene expression in the rat brain. Bratisl. Lek. listy 119, 28–31. doi:10.4149/bll_2018_006
Rathinam, V. A. K., Zhao, Y., and Shao, F. (2019). Innate immunity to intracellular LPS. Nat. Immunol. 20, 527–533. doi:10.1038/s41590-019-0368-3
Rich, G. T., Buchweitz, M., Winterbone, M. S., Kroon, P. A., and Wilde, P. J. (2017). Towards an understanding of the low bioavailability of quercetin: a study of its interaction with intestinal lipids. Nutrients 9, 111. doi:10.3390/nu9020111
Rietjens, I. M., Boersma, M. G., van der Woude, H., Jeurissen, S. M. F., Schutte, M. E., and Alink, G. M. (2005). Flavonoids and alkenylbenzenes: mechanisms of mutagenic action and carcinogenic risk. Mutat. Res. 574, 124–138. doi:10.1016/j.mrfmmm.2005.01.028
Roche, B., Sebagh, M., Canfora, M. L., Antonini, T., Roque-Afonso, A. M., Delvart, V., et al. (2008). Hepatitis C virus therapy in liver transplant recipients: response predictors, effect on fibrosis progression, and importance of the initial stage of fibrosis. Liver Transplant. 14, 1766–1777. doi:10.1002/lt.21635
Russell, R. C., Tian, Y., Yuan, H., Park, H. W., Chang, Y. Y., Kim, J., et al. (2013). ULK1 induces autophagy by phosphorylating Beclin-1 and activating VPS34 lipid kinase. Nat. Cell Biol. 15, 741–750. doi:10.1038/ncb2757
Saadi, M., Karkhah, A., Pourabdolhossein, F., Ataie, A., Monif, M., and Nouri, H. R. (2020). Involvement of NLRC4 inflammasome through caspase-1 and IL-1β augments neuroinflammation and contributes to memory impairment in an experimental model of Alzheimer's like disease. Brain Res. Bull. 154, 81–90. doi:10.1016/j.brainresbull.2019.10.010
Sagulenko, V., Thygesen, S. J., Sester, D. P., Idris, A., Cridland, J. A., Vajjhala, P. R., et al. (2013). AIM2 and NLRP3 inflammasomes activate both apoptotic and pyroptotic death pathways via ASC. Cell death Differ. 20, 1149–1160. doi:10.1038/cdd.2013.37
Sahoo, M., Ceballos-Olvera, I., del Barrio, L., and Re, F. (2011). Role of the inflammasome, IL-1β, and IL-18 in bacterial infections. TheScientificWorldJournal 11, 2037–2050. doi:10.1100/2011/212680
Sakurai, T., and Kudo, M. (2013). Molecular link between liver fibrosis and hepatocellular carcinoma. Liver cancer 2, 365–366. doi:10.1159/000343851
Schock, S. N., Chandra, N. V., Sun, Y., Irie, T., Kitagawa, Y., Gotoh, B., et al. (2017). Induction of necroptotic cell death by viral activation of the RIG-I or STING pathway. Cell death Differ. 24, 615–625. doi:10.1038/cdd.2016.153
Scholz, S., and Williamson, G. (2007). Interactions affecting the bioavailability of dietary polyphenols in vivo. Int. J. Vitam. Nutr. Res. Int. Zeitschrift fur Vitamin- und Ernahrungsforschung. J. Int. de vitaminologie de Nutr. 77, 224–235. doi:10.1024/0300-9831.77.3.224
Sendamarai, A. K., Ohgami, R. S., Fleming, M. D., and Lawrence, C. M. (2008). Structure of the membrane proximal oxidoreductase domain of human Steap3, the dominant ferrireductase of the erythroid transferrin cycle. Proc. Natl. Acad. Sci. U. S. A. 105, 7410–7415. doi:10.1073/pnas.0801318105
Sethi, G., Rath, P., Chauhan, A., Ranjan, A., Choudhary, R., Ramniwas, S., et al. (2023). Apoptotic mechanisms of quercetin in liver cancer: recent trends and advancements. Pharmaceutics 15, 712. doi:10.3390/pharmaceutics15020712
Shen, Z., Liu, T., Li, Y., Lau, J., Yang, Z., Fan, W., et al. (2018). Fenton-reaction-acceleratable magnetic nanoparticles for ferroptosis therapy of orthotopic brain tumors. ACS Nano 12, 11355–11365. doi:10.1021/acsnano.8b06201
Shi, J., Zhao, Y., Wang, Y., Gao, W., Ding, J., Li, P., et al. (2014). Inflammatory caspases are innate immune receptors for intracellular LPS. Nature 514, 187–192. doi:10.1038/nature13683
Shi, Y. (2004). Caspase activation, inhibition, and reactivation: a mechanistic view. Protein Sci. a Publ. Protein Soc. 13, 1979–1987. doi:10.1110/ps.04789804
Shimizu, K., Chiba, S., Saito, T., Kumano, K., and Hirai, H. (2000). Physical interaction of Delta1, Jagged1, and Jagged2 with Notch1 and Notch3 receptors. Biochem. biophysical Res. Commun. 276, 385–389. doi:10.1006/bbrc.2000.3469
Solmonson, A., and DeBerardinis, R. J. (2018). Lipoic acid metabolism and mitochondrial redox regulation. J. Biol. Chem. 293, 7522–7530. doi:10.1074/jbc.TM117.000259
Stennicke Hr, J. J., Shin, H., Deveraux, Q., Wolf, B. B., Yang, X., Zhou, Q., et al. (1998). Pro-caspase-3 is a major physiologic target of caspase-8. J. Biol. Chem. 273 (42), 27084–27090. doi:10.1074/jbc.273.42.27084
Stockwell, B. R. (2022). Ferroptosis turns 10: emerging mechanisms, physiological functions, and therapeutic applications. Cell 185, 2401–2421. doi:10.1016/j.cell.2022.06.003
Strowig, T., Henao-Mejia, J., Elinav, E., and Flavell, R. (2012). Inflammasomes in health and disease. Nature 481, 278–286. doi:10.1038/nature10759
Sun, X., Tan, Y., Lyu, J., Liu, H. L., Zhao, Z. M., and Liu, C. H. (2022). Active components formulation developed from Fuzheng Huayu Recipe for anti-liver fibrosis. Chin. J. Integr. Med. 28, 538–544. doi:10.1007/s11655-021-3293-x
Takeshige K, B. M., Tsuboi, S., Noda, T., and Ohsumi, Y. (1992). Autophagy in yeast demonstrated with proteinase-deficient mutants and conditions for its induction. J. Cell Biol. 119 (2), 301–311. doi:10.1083/jcb.119.2.301
Tang, L. Y., Heller, M., Meng, Z., Yu, L. R., Tang, Y., Zhou, M., et al. (2017). Transforming growth factor-β (TGF-β) directly activates the JAK1-STAT3 Axis to induce hepatic fibrosis in coordination with the SMAD pathway. J. Biol. Chem. 292, 4302–4312. doi:10.1074/jbc.M116.773085
Tanida, I., Ueno, T., and Kominami, E. (2004). LC3 conjugation system in mammalian autophagy. Int. J. Biochem. Cell Biol. 36, 2503–2518. doi:10.1016/j.biocel.2004.05.009
Tenev, T., Bianchi, K., Darding, M., Broemer, M., Langlais, C., Wallberg, F., et al. (2011). The Ripoptosome, a signaling platform that assembles in response to genotoxic stress and loss of IAPs. Mol. Cell 43, 432–448. doi:10.1016/j.molcel.2011.06.006
Thilakarathna, S. H., and Rupasinghe, H. P. (2013). Flavonoid bioavailability and attempts for bioavailability enhancement. Nutrients 5, 3367–3387. doi:10.3390/nu5093367
Tian, X., Teng, J., and Chen, J. (2021). New insights regarding SNARE proteins in autophagosome-lysosome fusion. Autophagy 17, 2680–2688. doi:10.1080/15548627.2020.1823124
Tsvetkov, P., Coy, S., Petrova, B., Dreishpoon, M., Verma, A., Abdusamad, M., et al. (2022). Copper induces cell death by targeting lipoylated TCA cycle proteins. Sci. (New York, N.Y.) 375, 1254–1261. doi:10.1126/science.abf0529
Ursini, F., and Maiorino, M. (2020). Lipid peroxidation and ferroptosis: the role of GSH and GPx4. Free Radic. Biol. Med. 152, 175–185. doi:10.1016/j.freeradbiomed.2020.02.027
van der Woude, H., Alink, G. M., van Rossum, B. E. J., Walle, K., van Steeg, H., Walle, T., et al. (2005). Formation of transient covalent protein and DNA adducts by quercetin in cells with and without oxidative enzyme activity. Chem. Res. Toxicol. 18, 1907–1916. doi:10.1021/tx050201m
van der Woude, H., Boersma, M. G., Vervoort, J., and Rietjens, I. M. (2004). Identification of 14 quercetin phase II mono- and mixed conjugates and their formation by rat and human phase II in vitro model systems. Chem. Res. Toxicol. 17, 1520–1530. doi:10.1021/tx049826v
Viollet, B. F. M., Guigas, B., Horman, S., Dentin, R., Bertrand, L., Hue, L., et al. (2006). Activation of AMP-activated protein kinase in the liver: a new strategy for the management of metabolic hepatic disorders. J. Physiol. 574 (Pt 1), 41–53. doi:10.1113/jphysiol.2006.108506
Vlachodimitropoulou, E., Sharp, P. A., and Naftalin, R. J. (2011). Quercetin-iron chelates are transported via glucose transporters. Free Radic. Biol. Med. 50, 934–944. doi:10.1016/j.freeradbiomed.2011.01.005
Wagner, C., Fachinetto, R., Dalla Corte, C. L., Brito, V. B., Severo, D., de Oliveira Costa Dias, G., et al. (2006). Quercitrin, a glycoside form of quercetin, prevents lipid peroxidation in vitro. Brain Res. 1107, 192–198. doi:10.1016/j.brainres.2006.05.084
Wang, D., He, X., Peng, P., Xu, X., and Gao, B. (2020a). Quercetin suppresses apoptosis and attenuates intervertebral disc degeneration via the SIRT1-autophagy pathway. Front. Cell Dev. Biol. 8, 613006. doi:10.3389/fcell.2020.613006
Wang, H., Sun, L., Su, L., Rizo, J., Liu, L., Wang, L. F., et al. (2014). Mixed lineage kinase domain-like protein MLKL causes necrotic membrane disruption upon phosphorylation by RIP3. Mol. Cell 54, 133–146. doi:10.1016/j.molcel.2014.03.003
Wang, L., Liao, Y., Yang, R., Yu, Z., Zhang, L., Zhu, Z., et al. (2020b). Sja-miR-71a in Schistosome egg-derived extracellular vesicles suppresses liver fibrosis caused by schistosomiasis via targeting semaphorin 4D. J. Extracell. vesicles 9, 1785738. doi:10.1080/20013078.2020.1785738
Wang, R., Zhang, H., Wang, Y., Song, F., and Yuan, Y. (2017). Inhibitory effects of quercetin on the progression of liver fibrosis through the regulation of NF-кB/IкBα, p38 MAPK, and Bcl-2/Bax signaling. Int. Immunopharmacol. 47, 126–133. doi:10.1016/j.intimp.2017.03.029
Wang, X., Li, Y., Han, L., Li, J., Liu, C., and Sun, C. (2021a). Role of flavonoids in the treatment of iron overload. Front. Cell Dev. Biol. 9, 685364. doi:10.3389/fcell.2021.685364
Wang, Y., Quan, F., Cao, Q., Lin, Y., Yue, C., Bi, R., et al. (2021b). Quercetin alleviates acute kidney injury by inhibiting ferroptosis. J. Adv. Res. 28, 231–243. doi:10.1016/j.jare.2020.07.007
Wegner, K. W., Saleh, D., and Degterev, A. (2017). Complex pathologic roles of RIPK1 and RIPK3: moving beyond necroptosis. Trends Pharmacol. Sci. 38, 202–225. doi:10.1016/j.tips.2016.12.005
Wei, M. C., Zong, W. X., Cheng, E. H., Lindsten, T., Panoutsakopoulou, V., Ross, A. J., et al. (2001). Proapoptotic BAX and BAK: a requisite gateway to mitochondrial dysfunction and death. Sci. (New York, N.Y.) 292, 727–730. doi:10.1126/science.1059108
Wiczkowski, W., Romaszko, J., Bucinski, A., Szawara-Nowak, D., Honke, J., Zielinski, H., et al. (2008). Quercetin from shallots (Allium cepa L. var. aggregatum) is more bioavailable than its glucosides. J. Nutr. 138, 885–888. doi:10.1093/jn/138.5.885
Wold, M. S., Lim, J., Lachance, V., Deng, Z., and Yue, Z. (2016). ULK1-mediated phosphorylation of ATG14 promotes autophagy and is impaired in Huntington's disease models. Mol. Neurodegener. 11, 76. doi:10.1186/s13024-016-0141-0
Wu, L., Zhang, Q., Mo, W., Feng, J., Li, S., Li, J., et al. (2017). Quercetin prevents hepatic fibrosis by inhibiting hepatic stellate cell activation and reducing autophagy via the TGF-β1/Smads and PI3K/Akt pathways. Sci. Rep. 7, 9289. doi:10.1038/s41598-017-09673-5
Wu, S., Yang, Z., Zhou, J., Zeng, N., He, Z., Zhan, S., et al. (2019). Systematic review: diagnostic accuracy of non-invasive tests for staging liver fibrosis in autoimmune hepatitis. Hepatol. Int. 13, 91–101. doi:10.1007/s12072-018-9907-5
Wu, Y. J., Xu, M. Y., and Lu, L. G. (2014). Clinical advances in fibrosis progression of chronic hepatitis B and C. J. Clin. Transl. hepatology 2, 222–227. doi:10.14218/jcth.2014.00029
Xiao, J., Zhang, B., Yin, S., Xie, S., Huang, K., Wang, J., et al. (2022). Quercetin induces autophagy-associated death in HL-60 cells through CaMKKβ/AMPK/mTOR signal pathway. Acta biochimica biophysica Sinica 54, 1244–1256. doi:10.3724/abbs.2022117
Xin, P., Xu, X., Deng, C., Liu, S., Wang, Y., Zhou, X., et al. (2020). The role of JAK/STAT signaling pathway and its inhibitors in diseases. Int. Immunopharmacol. 80, 106210. doi:10.1016/j.intimp.2020.106210
Xiong, F., Zhou, Q., Huang, X., Cao, P., and Wang, Y. (2022). Ferroptosis plays a novel role in nonalcoholic steatohepatitis pathogenesis. Front. Pharmacol. 13, 1055793. doi:10.3389/fphar.2022.1055793
Yan, Y., Zeng, J., Xing, L., and Li, C. (2021). Extra- and intra-cellular mechanisms of hepatic stellate cell activation. Biomedicines 9, 1014. doi:10.3390/biomedicines9081014
Yang, Y., Wang, H., Kouadir, M., Song, H., and Shi, F. (2019). Recent advances in the mechanisms of NLRP3 inflammasome activation and its inhibitors. Cell death Dis. 10, 128. doi:10.1038/s41419-019-1413-8
Yang, Z., Wang, Y., Zhang, Y., He, X., Zhong, C. Q., Ni, H., et al. (2018). RIP3 targets pyruvate dehydrogenase complex to increase aerobic respiration in TNF-induced necroptosis. Nat. Cell Biol. 20, 186–197. doi:10.1038/s41556-017-0022-y
Yönem, O., Ozkayar, N., Balkanci, F., Harmanci, O., Sökmensüer, C., Ersoy, O., et al. (2006). Is congenital hepatic fibrosis a pure liver disease? Am. J. gastroenterology 101, 1253–1259. doi:10.1111/j.1572-0241.2006.00642.x
Young, J. F., Nielsen, S. E., Haraldsdóttir, J., Daneshvar, B., Lauridsen, S. T., Knuthsen, P., et al. (1999). Effect of fruit juice intake on urinary quercetin excretion and biomarkers of antioxidative status. Am. J. Clin. Nutr. 69, 87–94. doi:10.1093/ajcn/69.1.87
Yu, J., Hu, G., Cao, H., and Guo, X. (2022). Quercetin ameliorates lipopolysaccharide-induced duodenal inflammation through modulating autophagy, programmed cell death and intestinal mucosal barrier function in chicken embryos. Animals open access J. MDPI 12, 3524. doi:10.3390/ani12243524
Yu, P., Zhang, X., Liu, N., Tang, L., Peng, C., and Chen, X. (2021). Pyroptosis: mechanisms and diseases. Signal Transduct. Target. Ther. 6, 128. doi:10.1038/s41392-021-00507-5
Zhang, C., Yan, Y., Gao, X., and Ma, Y. (2021). Therapeutic mechanism of the Mongolian medicine Qiwei Qinggan Powder against liver fibrosis based on UHPLC-TOF-MS combined with network pharmacological methods. Nan fang yi ke da xue xue bao = J. South. Med. Univ. 41, 1131–1141. doi:10.12122/j.issn.1673-4254.2021.08.02
Zhang, J. B., Jin, H. L., Feng, X. Y., Feng, S. L., Zhu, W. T., Nan, H. M., et al. (2022). The combination of Lonicerae Japonicae Flos and Forsythiae Fructus herb-pair alleviated inflammation in liver fibrosis. Front. Pharmacol. 13, 984611. doi:10.3389/fphar.2022.984611
Zhang, K., Han, X., Zhang, Z., Zheng, L., Hu, Z., Yao, Q., et al. (2017). The liver-enriched lnc-LFAR1 promotes liver fibrosis by activating TGFβ and Notch pathways. Nat. Commun. 8, 144. doi:10.1038/s41467-017-00204-4
Zhang, Y., Chen, X., Gueydan, C., and Han, J. (2018). Plasma membrane changes during programmed cell deaths. Cell Res. 28, 9–21. doi:10.1038/cr.2017.133
Zhao, Y., Shi, J., and Shao, F. (2018). Inflammatory caspases: activation and cleavage of gasdermin-D in vitro and during pyroptosis. Methods Mol. Biol. Clift. N.J. 1714, 131–148. doi:10.1007/978-1-4939-7519-8_9
Zheng, P., Zhou, C., Lu, L., Liu, B., and Ding, Y. (2022). Elesclomol: a copper ionophore targeting mitochondrial metabolism for cancer therapy. J. Exp. Clin. cancer Res. CR 41, 271. doi:10.1186/s13046-022-02485-0
Zhou, Q., Yang, L., Li, T., Wang, K., Huang, X., Shi, J., et al. (2022). Mechanisms and inhibitors of ferroptosis in psoriasis. Front. Mol. Biosci. 9, 1019447. doi:10.3389/fmolb.2022.1019447
Zhou, W. C., Zhang, Q. B., and Qiao, L. (2014). Pathogenesis of liver cirrhosis. World J. gastroenterology 20, 7312–7324. doi:10.3748/wjg.v20.i23.7312
Keywords: quercetin, cuproptosis, liver fibrosis, apoptosis, necroptosis, autophagy, pyroptosis, ferroptosis
Citation: Xiong F, Zhang Y, Li T, Tang Y, Song S-Y, Zhou Q and Wang Y (2024) A detailed overview of quercetin: implications for cell death and liver fibrosis mechanisms. Front. Pharmacol. 15:1389179. doi: 10.3389/fphar.2024.1389179
Received: 21 February 2024; Accepted: 29 April 2024;
Published: 23 May 2024.
Edited by:
Mohammad Reza Khazdair, Birjand University of Medical Sciences, IranReviewed by:
Ravindra Babu Pingili, SVKM’s Narsee Moonjee Institute of Management and Studies (NMIMS), IndiaCopyright © 2024 Xiong, Zhang, Li, Tang, Song, Zhou and Wang. This is an open-access article distributed under the terms of the Creative Commons Attribution License (CC BY). The use, distribution or reproduction in other forums is permitted, provided the original author(s) and the copyright owner(s) are credited and that the original publication in this journal is cited, in accordance with accepted academic practice. No use, distribution or reproduction is permitted which does not comply with these terms.
*Correspondence: Yi Wang, d195aTIwMjJAMTYzLmNvbQ==; Si-Yuan Song, c2kteXVhbi5zb25nQGJjbS5lZHU=; Qiao Zhou, MjI5MjI5MzRAcXEuY29t
†These authors have contributed equally to this work
Disclaimer: All claims expressed in this article are solely those of the authors and do not necessarily represent those of their affiliated organizations, or those of the publisher, the editors and the reviewers. Any product that may be evaluated in this article or claim that may be made by its manufacturer is not guaranteed or endorsed by the publisher.
Research integrity at Frontiers
Learn more about the work of our research integrity team to safeguard the quality of each article we publish.