- Department of Pharmacy, Daping Hospital, Army Medical University, Chongqing, China
Hepatocellular carcinoma (HCC) is one of the cancers that seriously threaten human health. Immunotherapy serves as the mainstay of treatment for HCC patients by targeting the programmed cell death protein 1/programmed cell death 1 ligand 1 (PD-1/PD-L1) axis. However, the effectiveness of anti-PD-1/PD-L1 treatment is limited when HCC becomes drug-resistant. Tumor-associated macrophages (TAMs) are an important factor in the negative regulation of PD-1 antibody targeted therapy in the tumor microenvironment (TME). Therefore, as an emerging direction in cancer immunotherapy research for the treatment of HCC, it is crucial to elucidate the correlations and mechanisms between TAMs and PD-1/PD-L1-mediated immune tolerance. This paper summarizes the effects of TAMs on the pathogenesis and progression of HCC and their impact on HCC anti-PD-1/PD-L1 immunotherapy, and further explores current potential therapeutic strategies that target TAMs in HCC, including eliminating TAMs in the TME, inhibiting TAMs recruitment to tumors and functionally repolarizing M2-TAMs (tumor-supportive) to M1-TAMs (antitumor type).
1 Introduction
Hepatocellular carcinoma (HCC) is a primary liver malignancy clinically characterized by a relatively high mortality, insidious onset, rapid progression, early recurrence, and poor prognosis. Since it is technically challenging to diagnose HCC at a very early stage, most patients present with late-stage HCC at initial diagnosis and thus miss the ideal time for hepatectomy or radiofrequency ablation (Bray et al., 2018). In addition, HCC is not sensitive to chemotherapy and has fewer targeted benefits, which makes immunotherapy a source of hope for long-term survival. Current immunotherapies mainly focus on HCC treatments with proved anticancer efficacy, primarily including immune checkpoint inhibition, vaccination, and adoptive T-cell therapy. Particularly, the immunotherapy targeting programmed cell death protein 1/programmed cell death 1 ligand 1 (PD-1/PD-L1) is the latest treatment available for HCC patients (Balar and Weber, 2017). Anti-PD-1/PD-L1 immunotherapy is a life-changing regimen for HCC patients as it substantially improves the overall survival (OS) and progression-free survival (PFS) (Hamanishi et al., 2016). However, clinical data demonstrate that the treatment effect of solid tumors treated with anti-PD-1/PD-L1 monoclonal antibodies is far from satisfactory, with only a few patients benefiting from anti-PD-1 therapy, and most of them experience drug resistance and disease progression in the process of anti-PD-1/PD-L1 immunotherapy. The efficacy of anti-PD-1/PD-L1 immunotherapy is associated with many factors, including (i) insufficient T cell infiltration into tumor tissue, (ii) the immunosuppressive tumor microenvironment (TME) consisting of bone marrow-derived immunosuppressive cells and regulatory T cells (Treg), (iii) physiological barriers such as vascular malformations, high interstitial fluid hydrostatic pressure, and dense extracellular matrix (ECM) that impede effective accumulation and penetration of anti-PD-1 (aPD-1) antibodies into tumor tissue, and (iv) immunosuppressive activities of tumor-associated macrophages (TAMs) (Maute et al., 2015; Pitt Jonathan et al., 2016; Sharma et al., 2017; Binnewies et al., 2018; Yang and Hu, 2019).
Particularly, TAMs represent about 40% of tumor-infiltrating inflammatory cells, serving as key players in the development and progression of HCC, and are associated with a poorer prognosis for HCC. TAMs are infiltrating macrophages in tumor tissue, which are mainly differentiated from monocytes. Chemokines such as CSF1 and CCL2, secreted by tumor cells, can recruit monocytes from peripheral blood to the TME, and then monocytes differentiate into macrophages. TAMs are a heterogeneous population which varies temporarily and spatially with the progression of the disease. They are the most plasticity and the highest proportion of immune cells in TME, and depending on the microenvironmental cues in the TME, they can undergo significant changes in their function. TAMs were generally divided into two polarization states: classically activated M1 and alternatively activated M2 subtypes. The M1/M2 macrophage paradigm plays a key role in tumor progression. M1 macrophages are historically regarded as anti-tumor, while M2-polarized macrophages contribute to many pro-tumorigenic outcomes in cancer through angiogenic and lymphangiogenic regulation, immune suppression, hypoxia induction, tumor cell proliferation, and metastasis. Abundant evidence suggests that TAMs are involved in anticancer signaling pathways, immunosuppressive, proangiogenic activities, and epithelial-to-mesenchymal transition (EMT) (Zhang et al., 2019a; DeNardo and Ruffell, 2019). It has recently been revealed that TAMs play an important role in the development of drug resistance in patients receiving anti-PD-1/PD-L1 therapy (Gordon et al., 2017). TAMs are plastic cells that adopt either anti-tumor (M1) or pro-tumor (M2) features to maintain homeostasis. M1-polarized TAMs are often detected in patients with early-stage cancers and are mediated by human interferons and endogenous/exogenous tumor necrosis factors (TNFs) to play a role in chronic inflammatory responses (Li et al., 2019a). In the TME, macrophages are always induced and transformed into the M2 phenotype, which is regarded as an important trigger of the immune escape mechanism as it supports the release of pro-tumor cytokines, promotes the formation of hypoxic TME, mediates EMT, and promotes cancer cell stemness and drug resistance, thereby contributing to tumor growth and progression (Vitale et al., 2019). As a new target, researchers are becoming increasingly interested in modulating TAMs for therapeutic purposes. Therefore it is crucial to clarify the relationship between TAMs and resistance to anti-PD-1/PD-L1 immunotherapy and elucidate the underlying molecular mechanism.
2 Effects of TAMS on HCC development
2.1 TAMs and tumorigenic factors
As a key player in anti-PD-1/PD-L1 immunotherapy, TAMs interact with tumorigenic factors and are involved in the development and progression of HCC. The majority of TAMs exhibit an M2 phenotype and is correlated with poor prognosis in a number of malignancies (Sica and Mantovani, 2012; Xu F. et al., 2020). Polarization of M2 macrophages is regulated by multiple cytokines in the TME. For example, overexpression of oxidored-nitro domain-containing protein 1 (NOR1) in TAMs is found to accelerate HCC progression by stimulating M2 polarization and TAMs-mediated inflammation (Chen et al., 2018). Colony-stimulating factor 1 (CSF1) secreted by HCC cells promotes overexpression of allograft inflammatory factor 1 (AIF1) in macrophages and induces a transformation of macrophages into the M2 phenotype, resulting in enhanced migration of tumor cells (Cai et al., 2017). TH2 cells secrete IL-4 and IL-13, cytokines that drive the polarization of the M2 phenotype (Cohen and Mosser, 2013). Malignant cells can secrete M2-like cytokines including IL-10, CCL2/3/4/5/7/8, CXCL12, VEGF and platelet-derived growth factor (PDGF), in an attempt to recruit a greater number of monocytes and M0 macrophages to the region and differentiate them into the M2 phenotype (Zhang et al., 2014). Hypoxia may be a key driver of macrophage recruitment and polarization in the TME. The high levels of chemokine, hypoxia inducible factor (HIF)-1/2, and endothelin-2 secreted by hypoxic tissues attract macrophages to areas of hypoxia (Murdoch et al., 2005; McGettrick and O’Neill, 2020). Damage associated molecular pattern (DAMP), specifically the high mobility group box 1 protein (HMGB1) is most commonly associated with hypoxia-induced macrophage polarization. It has been shown that HMGB1 is overexpressed in many solid tumors and correlates with the development of HCC (Liu Y. et al., 2015). Tumor cell-derived exosomes can also promote macrophage polarization toward M2 phenotype. For example, Wu et al. found that exosomes mediated transfer of functional CD11b/CD18 integrin (protein) from TAMs to tumor cells could potentially promote the migratory capability of HCC cells (Wu et al., 2021).
TAMs, which are a major component of the TME, can also promote tumor growth by secreting a variety of cytokines that induce immune suppression (Table 1). M2 TAMs play an important immunosuppressive role and have been found to secrete immunosuppressive molecules into the TME, including IL-10, transforming growth factor-β (TGF-β), Arg-1 and human leukocyte antigen G (HLA-G) (Komohara et al., 2016; Petty and Yang, 2017). Among them, TGF-β is noteworthy due to its strong immunosuppressive effect and close correlation with TAMs. TGF-β has been shown to affect anti-PD-1/PD-L1 immunotherapy by inhibiting the activation of T-cell and by inducing PD-L1 upregulation in tumor cells and tumor-associated angiogenesis. In HCC, TGF-β stimulates immune escape and tumor progression through TAMs recruitment (Mao J. et al., 2016). TGF-β has been demonstrated to promote the polarization of M1/M2 macrophages and plays a crucial role in neutrophil migration and cytotoxicity (Flavell et al., 2010; Santarpia et al., 2015). This suggests a potential therapeutic approach for HCC, which involves inhibiting the expression of TGF-β in TAMs. For instance, Sorafenib has been found to exhibit anti-tumor activities by reducing the level of TGF-β secreted by TAMs, thereby inhibiting liver cancer growth, metastasis, and EMT (epithelial-mesenchymal transition) (Fridlender et al., 2009; Wei et al., 2015). Furthermore, M2-type cells directly interact with myeloid-derived suppressor cells (MDSC) and actively suppress antitumor responses mediated by T cells (Parker et al., 2014). Tumor growth is driven directly by M2-like macrophages through the release of IL-1β, IL-6, IL-10, CCL18 and CCL20, as well as indirectly through the suppression of cytotoxic cell populations, including CD8⁺ T cells and NK cells (Arvanitakis et al., 2022). Guo et al. also provided evidence that infiltrating M2-TAMs were markedly elevated in the HCC TME, producing IL-17, a pro-inflammatory cytokine, and were augmented upon oxaliplatin treatment (Guo et al., 2017). TAMs containing a high level of CXCL8 can also promote cancer cell proliferation and metastasis (Yin et al., 2017). Recent publications have indicated that miRNAs derived from TAMs also confer drug resistance and immune escape in various cancers (Binenbaum et al., 2018; Sahraei et al., 2019; Ma et al., 2021). Therefore, a macrophage-targeted precision therapy should be considered, which may be the next step in reversing the drug resistance to PD-1/PD-L1 treatment.
2.2 TAMs and related signaling pathways
TAMs are involved in many signaling pathways contributing to HCC development, and the link between TAMs and tumor initiation has been extensively investigated in a variety of clinical samples and preclinical models (Figure 1) (Sica et al., 2008; Szebeni et al., 2017). For instance, active Wnt/β-catenin signaling in macrophages may promote the growth of tumor progenitor cells, thereby increasing the risk of HCC (Boulter et al., 2015; Debebe et al., 2017). Moreover, TGF-β/SMAD signaling pathway also plays an important role in immunosuppression, which can promote tumor cell escape through modulating the activity of immune cells, including macrophages and effector T cells (Batlle and Massagué, 2019). It is demonstrated that M2 macrophages promote the development and progression of HCC through the IL-6/STAT3 signaling pathway. Concurrently, the Toll-like receptor 4 (TLR4)/STAT3 pathway is also implicated, and this pathway can be activated by various hormones and growth factors via Janus kinase (JAK) activation. These activations lead to the phosphorylation and dimerization of STAT3, mediating its nuclear translocation and DNA binding, ultimately resulting in cell proliferation and survival (Wan et al., 2014; Yao et al., 2018; Li et al., 2019a). Activated STAT-3 in immunocytes features immunosuppressive activities, and STAT-3 at a high level is associated with a poor prognosis (Lu et al., 2018; Durham et al., 2019; Huang et al., 2019; Ren et al., 2019; Witt et al., 2021). TAMs-mediated upregulation of B7-H1 expression in HCC cells can be blocked by inhibiting the STAT-3 signaling pathway (Chen J. et al., 2012). It has been reported that overexpression of NOR1 in TAMs can accelerate diethyl nitrosamine (DEN)-induced HCC progression (Li et al., 2016; Chen et al., 2018). CCL17 expression in M2 macrophages can activate Wnt/β-catenin signaling and promote EMT of tumor cells, while tumor cells in turn activate TAMs through the TLR4/TRIF/NF-κB signaling pathway and increase IL-1β production mediated by HIF1α to trigger EMT (Zhu et al., 2016). Zhang et al. discovered that, under conditions of moderate hypoxia, M2 macrophages promote the secretion of IL-1β in HCC via the HIF-1α/IL-1β signaling loop, thereby enhancing tumor metastasis and contributing to the poor prognosis observed in HCC patients (Zhang J. et al., 2018). Recent studies have discovered that TAMs produce a large amount of ROS in response to massive upstream activation signals and mediate immunosuppression via the NF-κB pathway. It is inferred that the NF-κB pathway can modulate signal activation in cancer cells and tumor-infiltrating leukocytes to stimulate inflammation in the TME. Therefore, TNF-α blockage by anti-TNF-α antibodies is regarded as supportive of HCC treatment (Singh et al., 2019). The HIF1α signaling pathway plays a crucial role in the adaptation and progression of tumor cells in a hypoxic environment. It is demonstrated that in a hypoxic environment, the NF-κB pathway in macrophages is activated, leading to increased HIF1α expression (Cramer et al., 2003; Bandarra et al., 2015). Absence of HIF-1α can effectively inhibit macrophage motility and ECM migration (Krieg et al., 2000; Li et al., 2019b). Yue S et al. found that hepatocyte phosphatase and tensin homolog (PTEN) is quantitatively dominant and regulate liver immune response. Mice with a myeloid-specific PTEN knockout, which promotes liver PI3K/Akt/Foxo1 signaling, have been shown to exhibit increased levels of M2 macrophages and demonstrate lower TNF-α responses and higher IL-10 levels in response to TLR ligands, suggesting that PTEN plays a key role in macrophage differentiation (Yue et al., 2014).
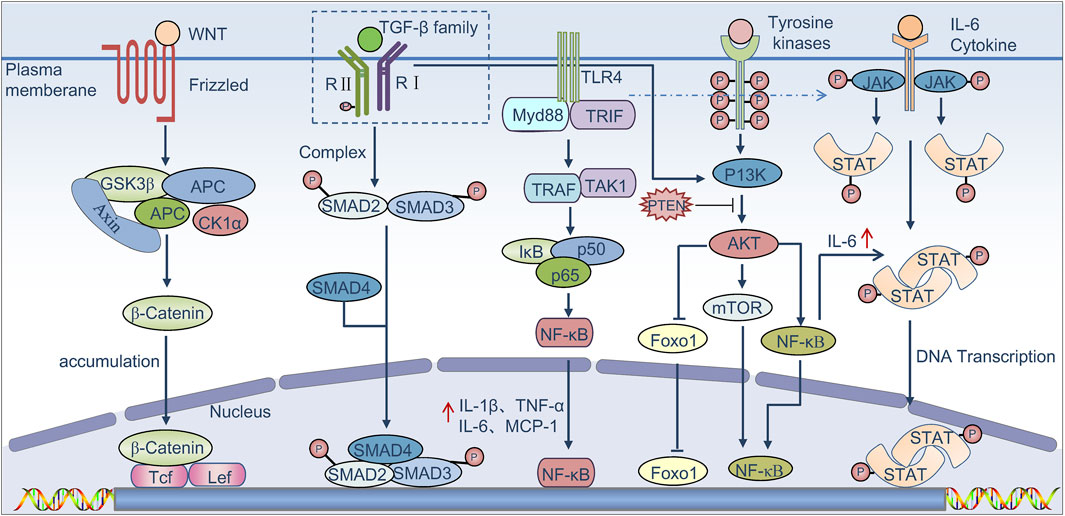
Figure 1. Signaling pathways that regulate TAMs to promote tumor progression. Binding of canonical WNT ligands to the Frizzled family of membrane receptors results in activation of the canonical WNT pathway. β-Catenin then translocates to the nucleus, resulting in the release of β-catenin from the GSK3β complex (glycogen synthase kinase-3β)–AXIN complex (axis inhibition protein)–APC complex (adenomatous polyposis coli protein), where it is known to bind to the transcription factors Tcf (T cell factor) and Lef (lymphoid enhancer-binding factor) in order to activate genes that promote the growth of tumor progenitor cells. TGF-β proteins bind to the TGF-β family of receptors, leading to phosphorylation of the receptors and activation of SMAD complexes, which induce immune suppression. The TGF-β pathway is also known to collaborate with the PI3K–AKT pathway, which in turn triggers activation of the mTOR complex and the nuclear factor-κB signaling axis (NF-κB). TLR4 binds to its ligand and can phosphorylate the IkB protein via TRIF, dissociate NF-κB from the trimer, promotes its nuclear transfer, and then promotes the release of factors such as IL-1β, TNF-α, and IL-6, which are known to promote tumor growth and induce immune suppression. In addition, the binding of several cytokines such as IL-6 to their receptors triggers the phosphorylation and activation of Janus kinases (JAKs) as well as the signal transducer and activator of transcription proteins (STATs); STAT dimers promote the proliferation and survival of tumor cells and induce immune suppression.
2.3 TAMs and immunosuppressive TME
Immunosuppression is the key feature of TAMs biology. Infiltrating TAMs were found to be markedly elevated in HCC TME (Guo et al., 2017; Arvanitakis et al., 2022). The TAMs-induced TME mainly consists of immunocytes and mesenchymal stem cells (MSCs) that exhibit immunosuppressive properties and accelerate HCC progression (Li et al., 2019a). In the TME, M2-polarized TAMs are associated with tissue remodeling and inflammation inhibition, suggesting involvement of TAMs in HCC and their complex interaction with other immunocytes and MSCs. In HCC, high TAMs infiltration is positively correlated with T cells regulated by CD4, CD25, and FoxP3 and negatively correlated with CD8⁺ T cells. It is reported that blockade of colony stimulating factor 1 receptor (GSF1R) in TAMs can enhance CD8⁺ T cell infiltration while also reducing CD4⁺T cell infiltration (Ao et al., 2017). In addition to the effects on the densities of CD4⁺and CD8⁺ T cells, TAMs upregulate B7-H1 (PD-L1, CD274) expression in HCC cells, thereby suppressing the function of CD8⁺ T cells (Zhao et al., 2011; Chen J. et al., 2012). In the HCC TME, PD-L1 expression is mainly upregulated in Kupffer cells (KCs) instead of other antigen presenting cells (APCs) or tumor cells (Zhu et al., 2019). CD69⁺ T cells induce TAMs to produce indoleamine 2, 3-dioxygenase (IDO) and exert pro-tumor effects by releasing IFN-γ (Zhao et al., 2012). Additionally, decreases in T cell counts are associated with the FASL/FAS pathway (Muraoka et al., 2019; Cersosimo et al., 2020). In nonalcoholic steatohepatitis (NASH), IFN-γ is strongly correlated with inflammation, which imposes a risk of developing HCC; NKp46⁺NK cells can stimulate M1 polarization of TAMs. Therefore, NKp46⁺NK cells deficiency may twist the polarization of TAMs toward the M2-like phenotype and accelerate hepatic fibrosis progression (Tosello-Trampont et al., 2016). However, high infiltration of TAMs in HCC tissue is associated with reduced NK cell activity. TAMs are thought to induce early NK cell activation and subsequent dysfunction mediated by CD48/2B4 interactions (Wu et al., 2013). Sorafenib has been demonstrated to support the anti-tumor activities of NK cells by modulating the crosstalk between TAMs and NK cells (Sprinzl et al., 2013). T cells and NK cells are both crucial to tumor cell eradication, whereas other immune-related clusters (e.g., interaction between B cells and TAMs) play a modulating role in this process, which is infrequently addressed in related studies. Currently, there is merely one published study exploring the correlations of CXCR3⁺ B cells with IL-17-mediated inflammation and the polarization of pro-tumor macrophages in HCC (Liu R-X. et al., 2015).
2.4 TAMs promote angiogenesis and lymphangiogenesis in HCC
TAMs are a major driving force in tumor angiogenesis and lymphangiogenesis. Tumor angiogenesis is supported by TAMs primarily through the production of factors such as VEGFA, PDGF, PIGF, FGF, and TGF-β (Dhanasekaran et al., 2016; Llovet et al., 2016; Lai et al., 2019). Increased TAMs around TME are closely associated with poor prognosis in cancer patients, and M2 TAMs promote tumor growth and tumor metastasis by stimulating angiogenesis and lymphangiogenesis. Given the highly active metabolism in tumor cells and the metastatic properties of advanced tumors, angiogenesis and lymphangiogenesis are induced frequently for survival of the tumor cells. TAMs are essential to angiogenesis and lymphangiogenesis in HCC. Patients with HCC are shown to have a relatively high serum level of IL-23 secreted by inflammatory macrophages, which promotes macrophage-mediated angiogenesis by positively regulating the expression of IL-23 receptors on macrophages and accelerates HCC progression resulting from chronic hepatitis virus infection (Zang et al., 2018). Meng et al. discovered that macrophages accelerate the process of angiogenesis by modulating vascular CXCR4 expression via the ERK pathway (Meng et al., 2017). Peng et al. found that enrichment of TAMs in tumor cells is associated with microvascular density (MVD) in HCC (Peng et al., 2005). TAMs also secrete enzymes including CathepsinB, iNOS, COX2 and MMPs, which enhance angiogenesis via degradation of the extracellular matrix and invasion of endothelial cells (Ruffell and Coussens, 2015; Kumari and Choi, 2022). For example, TAMs can sense hypoxia in tumors, which can stimulate the chemotaxis of macrophages and increase the expression of MMP9 in TAMs, and finally promote the angiogenesis (Wu et al., 2021). Besides pro-tumor angiogenesis, TAMs are associated with lymphangiogenesis. Alishekevitz et al. found that TAMs promote lymphangiogenesis through the VEGF-C/VEGFR-3 pathway (Alishekevitz et al., 2016). Recently, Hwang et al. demonstrated that TAMs stimulate VEGF-A and VEGF-C in tumor cells, thereby promoting angiogenesis and lymphangiogenesis at tumor site (Hwang et al., 2020). It is demonstrated that TAMs promote lymphangiogenesis through transdifferentiation, direct penetration into the endothelial layer, and local stimulation of existing lymphatic endothelial cells (Iurca et al., 2020). The number of TAMs in the lymph nodes affected by tumor metastasis is shown to have a negative correlation with the prognosis of cancer patients (Oosterling et al., 2005). Macrophages can promote lymphangiogenesis through the secretion of growth factors and proteases that lead to the formation of lymphatic vessel (Gordon et al., 2010). Recent research found that IL-10 produced by hypoxic TAMs adjacent to lymphatic vessels was essential for the formation of lymphatic vessels by inducing the upregulation of Sp1 (specific β1 glycoprotein) in lymphatic endothelial cells (Chen et al., 2021). TIE-2 (Tyrosine kinase receptor with immunoglobulin and EGF homology domains-2) expressing TAMs are highly angiogenic and lymphangiogenic cells that exhibit immune-suppressive activity through secreting IL-10 and VEGF (Guex et al., 2015; Bron et al., 2016). Lymphangiogenesis leads to tumor metastasis, so the role of TAMs in regulation is important to understand and further investigate (Wang X. et al., 2021).
2.5 TAMs and EMT
EMT is an evolutionarily conserved developmental program. TAMs can promote EMT by secreting different cytokines and chemokines. This promotion mechanism has been extensively studied (Fan et al., 2014). The long-term interaction with macrophages results in poor migration and infiltration of CD8⁺ T cells within the tumor island, which makes PD-1/PD-L1 treatment resistant. TAMs secrete TGF-β in a manner similar to TGF-β secreted by cancer-associated fibroblasts (CAFs) and induce EMT (Fan et al., 2014). In addition, studies in xenograft nude mouse models of HCC indicate that M2 TAMs release TGF-β, mediate Smad2/3 binding to the miR-362-3p promoter, lead to miR-362-3p overexpression, and promotes EMT processes that significantly contribute to proliferation, invasion, and metastasis of HCC cells (Zhang et al., 2019b). EMT inducers such as TGF-β and receptor tyrosine kinase (RTK) ligands bring about changes in gene expression through an intricate signaling network, resulting in upregulated expression of C2H2 zinc finger (ZnF) proteins, zinc finger E-box binding homeobox 1 and 2 (ZEB1 and ZEB2/SIP1), and fundamental helix-loop-helix (bHLH) factors like TWIST and E47 (Peinado et al., 2007; Roy et al., 2021). These proteins bind to the E-box element situated in the promoter region of E-cadherin and recruit histone deacetylases (HDACs) and other factors to promote chromatin condensation and subsequent inhibition of E-cadherin transcription. Reduced E-cadherin expression can induce disruption of adherent junctions and other signaling events, such as functional regulation of Rho GTPases, eventually leading to loss of cell polarity (Cai et al., 2018). A normal EMT process is strictly dependent on gene expression, while tumor cells are prone to EMT for invasion and migration. TAMs are shown to play a role in the EMT process in tumor cells. CCL17 expression in M2 macrophages can induce EMT and activate the Wnt/β-catenin signaling pathway in tumor cells (Liu R-X. et al., 2015; Elia and Fallahi, 2017). Additionally, tumor cells activate TAMs through the TLR4/TRIF/NF-κB signaling pathway and upregulate HIF1α-mediated IL-1β production to stimulate EMT of tumor cells (Li et al., 2019a). Zhang et al. discovered that the HIF-1α/IL-1β feedback loop between tumor cells and TAMs contributes to EMT and in vitro metastasis of cancer cells in the hypoxic TME (Zhao et al., 2011). Recent studies have demonstrated that mesenchymal carcinoma cells induce TAMs formation via granulocyte-macrophage colony stimulating factor (GM-CSF) secretion. Once TAMs are formed, CCL18 is secreted, which induces EMT and promotes cancer metastasis (Su et al., 2014). TAMs can also secrete IL-6 which activates cyclooxygenase 2 (COX2), prostaglandin E2 (PGE2) and β-catenin signaling pathways, ultimately leading to EMT and cell invasion (Lu et al., 2014; Che et al., 2017). Thus, macrophage depletion could reactivate CD8⁺ T cells to migrate and invade tumor islands, and enhance the therapeutic effect of anti-PD-1 therapy.
3 The interaction between TAMs and the immune system in HCC
3.1 Interaction between TAMs and CD4⁺T lymphocytes promotes hepatocarcinogenesis
In addition to the major role in adaptive immune responses, CD4⁺ T cells are critical in modulating host immunity in response to different classes of pathogens. Upon activation, naïve CD4⁺ T cells differentiate into T helper (Th) effector cells, including Th1, Th2, Th17 and Tregs, and different subsets are shown to have distinct effects on cancer growth (Jia et al., 2015). Th1 cells are primarily characterized by their anti-tumor activity, whereas Th2 cells are associated with tumor-promoting effects. Treg cells suppress anti-tumor immune response by inhibiting the functions of multiple immune cells, including Th1 cells, CD8⁺ T cells, NK cells, and tumor-infiltrating DC cells. These cells affect the tumor TME by altering the direction of macrophage polarization. Th1 cells secrete IFN-γ to induce M1 polarization, and Th2 cells secrete IL-4, IL-5, and IL-10 to promote M2 macrophage production (Mills et al., 2017). TAMs, induced by tumor activated HIF1α, could secrete IL-23, thereby promoting the proliferation of Tregs and the expression of IL-10 and TGF-β. Additionally, they could inhibit cytotoxic lymphocytes from killing tumor cells (Fu et al., 2019). Additionally, TAMs overexpress CCL1, the ligand of CCR8 expressed on Tregs, to attract Tregs into the tumor area, inhibiting T cell immunity and promoting tumor growth. CD4⁺T cells at a reduced level are shown to promote HCC development (Brown et al., 2018). Moreover, Th17 and Treg cells are two subsets of CD4⁺T cells. Th17 cells are considered pathogenic effectors that contribute to disease exacerbation, while Treg cells suppress liver protection (Zhang H. et al., 2019). These findings indicate the critical role of CD4⁺ T cells in immune surveillance against HCC. Early studies have established a strong correlation between HCC development and chronic inflammation, highlighting the crucial role of TAMs in the pathogenesis of chronic liver disease due to their M1/M2 phenotype shifts. A study proposed that monocyte-derived TAMs exert an inhibitory influence on liver cancer progression by aiding CD4⁺ T cells in eliminating senescent liver cells (Kang et al., 2011). Conversely, another study revealed a shift in TAMs from anti-tumorigenic into pro-tumorigenic phenotype preceding the emergence of HCC. This shift triggers the production of chemokines (e.g., CCL17 and CCL22) and recruits of CD4⁺ Treg cells, ultimately leading to HCC development (Hefetz-Sela et al., 2014). Overall, in a chronic inflammatory environment, TAMs are susceptible to transformation into a pro-tumorigenic M2 phenotype, which promotes HCC development through the recruitment of CD4⁺ Treg cells.
TAMs promote HCC development and progress through recruitment of CD4⁺ T cells. In turn, CD4⁺ T cells accelerate tumor growth by releasing cytokines which in turn affect TAMs. For example, Katharina et al. discovered that CD4⁺CD25⁺Treg cells had direct inhibitory effects on the activation and functions of human monocytes/macrophages, which consequently impeded their functions as innate and adaptive immune effector cells (Giesbrecht et al., 2019). Wei et al. pointed out that activated CD4⁺ T cells in liver tissue stimulated CXCL10 production by macrophages to generate IgG-expressing plasma cells through the interaction of CXCL10 with CXCR3 on B cells (Wei et al., 2019). IgG is capable of activating the Fc receptor on macrophages and thereby inhibiting cytokine production during anti-tumor immune response. In the mouse model with HCC, the activation of macrophages was suppressed by the depletion of B cells, which enhanced the anti-tumor T cell response and inhibited HCC growth. In addition, DG Denardo et al. showed that CD4⁺ T cells amplified the pro-tumorigenic properties of macrophages by responding to IL-4 to modulate breast cancer metastasis to the lungs (DeNardo et al., 2009). In conclusion, TAMs promote tumorigenesis by interacting with CD4⁺ T cells, while CD4⁺T cells in turn act on TAMs to further enhance tumor immune escape.
3.2 Interaction between TAMs and CD8⁺ T lymphocytes promotes hepatocarcinogenesis
M2-like macrophages directly or indirectly promote tumor growth by inhibiting cytotoxic cells, including CD8⁺ T cells. CD8⁺ T cells are shown to produce opposite effects in a chronic pro-inflammatory microenvironment and exert antitumor surveillance. CD8⁺ cytotoxic T lymphocytes (CTLs) achieve target cell killing through direct cell-to-cell contact and interaction with the Fas receptor and its ligand FasL on the surface of cells as well as through the secretion of signals mediated by the synthesis of perforins and granzymes A and B (Ringelhan et al., 2018). However, there is a highly limited number of CD8⁺ T cells in the TME, which is correlated with activation of immune checkpoints such as PD-1 and CTLA-4. TAMs are shown to suppress the antitumor effects of CD8⁺ T cells by expressing ligands such as PD-L1 and CD80. Stress in the endoplasmic reticulum has been reported to trigger HCC cells to positively regulate PD-L1 expression in macrophages through exosome production and to inhibit T cell functions via the miR-23a-PTEN-AKT pathway (Liu J. et al., 2019). CC Chemokine Receptor 2 (CCR2) antagonists enhance the generation of CD8⁺ T cells by suppressing the immunosuppressive effect of TAMs in HCC (Yao et al., 2017). Besides immune checkpoints, TAMs release cytokines to inhibit CD8⁺ T cells proliferation and function. For instance, CCL2 released by HCC cells increases the number of M2 macrophages that inhibit CD8⁺ T cell proliferation by producing cytokines such as IL-6 and MIP-2 and thereby accelerate HCC progression (Li et al., 2017) (Figure 2A).
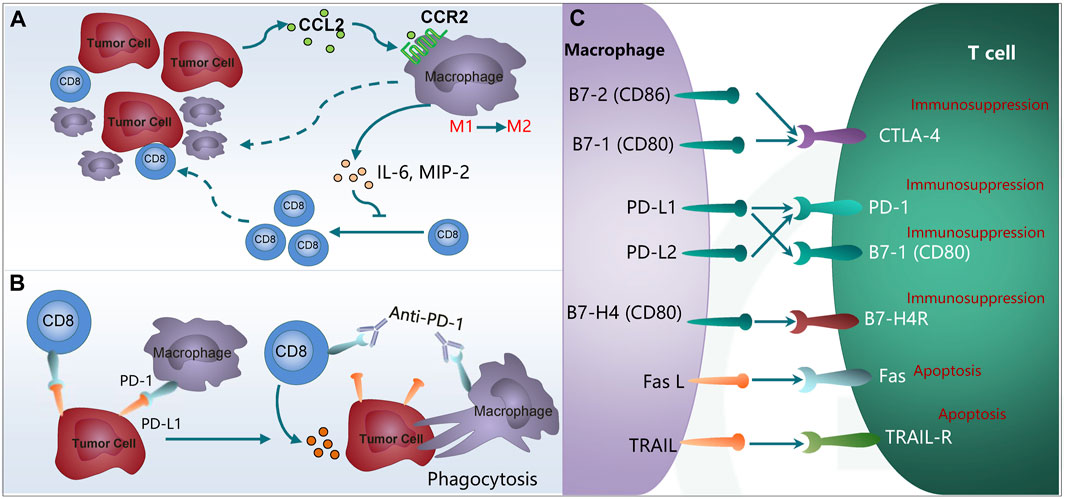
Figure 2. TAMs inhibit the immunotherapy through interaction with T cells. (A) High CCL2 expression correlates with more tumor-infiltrated TAMs and fewer CD8+ T lymphocytes. (B) Expression of PD-1 on the TAMs inhibits macrophages on phagocytosis and anti-tumor immunity. (C) Expression of PD-L1/L2, Fas L and TRAIL in M2 macrophages promotes T cell anergy and apoptosis. TAMs, tumor-associated macrophages; CCL2, chemokine (C-C motif) ligand 2; CCR2, chemokine (C-C motif) receptor 2; IL-6, Interleukin-6; MIP-2, macrophage inflammatory protein 2; PD-1, programmed cell death protein 1; PD-L1/L2, programmed cell death 1 ligand 1/ligand 2; CTLA4, cytotoxic T-lymphocyte-associated protein 4; Fas L, Fas ligand; TRAIL, TNF related apoptosis inducing ligand.
3.3 Interaction between TAMs and regulatory T cells promotes hepatocarcinogenesis
Regulatory T cells are a major immunosuppressive cell population and exerting various effects on tumor progression. A recent study utilizing the CIBERSORT algorithm assessed the relative abundances of 22 subsets of immune cells infiltrating tumors in 1,090 HCC instances, unveiling four distinct tumor clusters. Notably, tumors characterized by augmented regulatory T cell counts and depleted M1 macrophages were associated with a more dire prognosis (Yu et al., 2020). Interestingly, it was observed that M2 TAMs, rather than M1 macrophages, tended to foster the recruitment of regulatory T cells to the tumor microenvironment. Specifically, the infiltration of TREM-1+ TAMs within the hypoxic HCC microenvironment plays a pivotal role in conferring resistance to anti-PD-L1 therapy, accomplished through the recruitment of CCR6+ Foxp3+ regulatory T cells through CCL20 secretion (Wu et al., 2019a). These recruited or induced regulatory T cells can further potentiate the immunosuppressive capabilities of TAMs. Regulatory T cells facilitate the sterol regulatory element-binding protein 1 (SREBP1)-driven metabolic processes of M2 TAMs by suppressing the production of IFN-γ by CD8+ T cells (Liu C. et al., 2019). Consequently, regulatory T cells indirectly sustain the survival of M2 TAMs, thus establishing a positive feedback pool (Liu C. et al., 2019).
3.4 Interaction between TAMs and NK cells promotes hepatocarcinogenesis
In the TME of HCC, natural killer (NK) cells are less prevalent and functionally impaired (Park et al., 2020). A key regulatory mechanism that affects NK cell function is the intricate crosstalk that occurs between diverse immune cell types, including tumor-associated macrophages (TAMs), within the HCC TME (Sung and Jang, 2018). TAMs indirectly suppress NK cells through the release of cytokines, including interleukin-10 (IL-10) and transforming growth factor-β (TGF-β) (Sung and Jang, 2018). Furthermore, TAMs express CD48 in the HCC TME and may engage directly with the 2B4 receptor on NK cells, leading to dysfunction of these immune effectors (Wu et al., 2013). In contrast, M1 macrophages have the potential to enhance the overall count of activated intrahepatic NK cells in chronic liver diseases (Ma et al., 2017). This implies that by inducing the repolarization of TAMs to the M1 phenotype, they might stimulate NK cells to eliminate tumor cells.
4 The role of TAMs in resistance to anti-PD-1/PD-L1 immunotherapy in HCC
Effective therapy that blocks PD-1/PD-L1 interactions relies heavily on the function of T cells. Inhibitors targeting these checkpoints aim to disrupt the interaction between PD-1 and PD-L1, thereby preserving the anti-tumor properties of T cells, reversing immune evasion, and restoring their capacity to induce tumor cell death. However, any disruption in the immune functions of T cells can lead to the failure of PD-1/PD-L1 blockade therapy. Additionally, the activation of T cells is yet another pivotal process for the success of PD-1/PD-L1 blockade therapy. Despite blocking PD-1/PD-L1, tumor cells can still counter the immune checkpoints’ activity by activating additional inhibitory pathways. This occurs through the expression of other immune checkpoints and their ligands within the tumor’s immune microenvironment (Kwantwi, 2023). Given that TAMs are an important component in the TME, and there is intricate relationship between TAMs and the PD-1/PD-L1 axis, TAMs will play a pivotal role in the resistance to anti-PD-1/PD-L1. TAMs contribute to T cell dysfunction and exhaustion through the expression of various immunosuppressive factors, such as PD1 and PD-L1 expression, Fas L and TRAIL, FcγRI, and so forth (Morrissey et al., 2021; Pu et al., 2021).
4.1 TAMs contribute to PD-1/PD-L1 blockade resistance by inhibiting the migration of T cells activated by anti-PD-1 antibodies to the tumor islets
The primary purpose of anti-PD-1/PD-L1 immunotherapy is to restore T cell function (Chen and Mellman, 2017). However, there has been a lack of research focusing on promoting the migration of T cells to tumor nests. The extracellular stroma plays a crucial role in tumor growth and progression, with its main components including neovasculature, fibroblasts, and TAMs. Tumor extracellular stroma has been observed to inhibit the infiltration and activation of T cells (Cai et al., 2019). Numerous studies have confirmed that the exclusion of CD8+ T cells from tumor nests is consistently associated with a poor clinical response. TAMs have been shown to promote the formation of extracellular matrix (Afik et al., 2016), and the poor migration and infiltration of CD8+ T cells into tumor islets is a result of prolonged interaction with TAMs, which can trap lymphocytes (Peranzoni et al., 2018). TAMs control the recruitment of T cells and induce their exclusion within the tumor, thereby contributing to anti-PD-1/PD-L1 resistance (Kwantwi, 2023). Quaranta et al. have identified granulin as potentially involved in the specific mechanism of inhibiting the migration of CD8+ T cells to tumor nests mediated by TAMs (Quaranta et al., 2018). Moreover, TAMs can secrete granulin to exclude CD8+ T cells from metastatic livers, thereby promoting tumor progression. Genetic depletion of granulin has been found to reduce fibrotic stroma formation, thereby allowing T cells to migrate to metastatic sites and exert an anti-tumor effect (Nielsen et al., 2016). Consequently, the depletion of macrophages may reactivate the migration of CD8+ T cells and their invasion of tumor islets, ultimately improving the therapeutic effect of anti-PD-1 treatment.
4.2 TAMs contribute to PD-1/PD-L1 blockade resistance by inhibiting phagocytosis and anti-tumor immunity through expression of PD-1
PD-1 was first identified as an immune checkpoint receptor present on activated T cells, and its key role in suppressing T-cell activation upon binding with PD-L1 has been extensively established (Parry et al., 2005). However, it is noteworthy that PD-1 expression is not confined to T cells alone; it has also been reported to be expressed on other immune cells, including B cells (Sun et al., 2022) and NK cells (Hsu et al., 2018). Amongst these cells, TAMs constitute the predominant immune cells found within tumor islets. Recently, there has been a surge in research investigating the role of PD-1-expressing TAMs in tumor immune evasion. After interacting with its ligand PD-L1, it plays cardinal role in inducing immune evasion of cancer cells. Mouse and human M2 macrophages are both shown to express PD-1, with a later stage of cancer indicating a higher level of PD-1 expression and weaker phagocytic function of M2 macrophages (Gordon et al., 2017). PD-1/PD-L1 blockage in vivo can effectively enhance phagocytosis of macrophages, suppress tumor growth and achieve prolonged survival in tumor-bearing mouse models; moreover, a greater number of tumor-infiltrating macrophages in HCC tissue are indicative of longer survival. Gordon et al. found that the phagocytic potency of PD-1⁺ macrophages is rescued by the inhibition of PD-1/PD-L1 activity, which prolongs survival in preclinical colon cancer models in a macrophage dependent manner (Gordon et al., 2017; Li CW. et al., 2018). The researchers further suggested that the functions of PD-1+ TAMs are significantly compromised, and an increased proportion of PD-1+ macrophages aggravates the prognosis of patients (Li W. et al., 2022). This demonstrates that anti-PD-1/PD-L1 immunotherapy produces anti-tumor effects by acting on macrophages (Gordon et al., 2017). Besides, it is reported that the PD-1 expression level in M2 macrophages is negatively correlated with the anticancer activity, while PD-1/PD-L1 blockage in vivo is shown to enhance macrophage phagocytic capacity, suppressing tumor growth and prolonging the survival period of tumor bearing mice (Gordon et al., 2017; Hutchinson, 2017). M2 macrophages are suggested to inhibit T cell functions by releasing IL-17 to interact with PD-L1 in HCC (Chen J. et al., 2012). Ruan et al. also found that TAMs with high PD-1 expression tended to polarize toward M2-like macrophages, which may enhance the immune escape of tumor cells and may predict a poor prognosis (Ruan et al., 2021). The PD-1 overexpression significantly inhibited M1 macrophage polarization and phagocytosis as well as secreting more IL-10, which is a typical M2-related cytokine as an immunosuppressive cytokine. IL-10 has been shown to play an important role in promoting tumor immune evasion by decreasing the antitumor immune response in the tumor microenvironment (Mannino et al., 2015; Ham et al., 2017). These changes may enhance the pro-tumor effects of tumor microenvironment. In addition, anti-PD-1 therapy enhances the phagocytic ability of macrophages and the ability of macrophages to promote T cell activation by releasing immune activating factor, enhancing the anti-tumor immune response (Li CW. et al., 2018) (Figure 2B). On this basis, it is inferred that anti-PD-1 therapy may enhance the anti-tumor immune response of TAMs in HCC models by TAMs phagocytosis, which provides a new method for treating HCC of anti-PD-1 resistance.
4.3 TAMs contribute to PD-1/PD-L1 blockade resistance by promoting T cell anergy and apoptosis through the expression of PD-L1/L2
Besides PD-1, the expression of PD-L1 (B7-H1, CD274)/L2 (B7-DC, CD273) is detected in TAMs, which induce CD8⁺ T cells anergy and apoptosis through PD-1 binding on the surface of CD8⁺ T cells to inhibit anti-tumor immune response (Figure 2C). Furthermore, the expression of PD-L1was more frequently detected on immune cells, particularly TAMs, compared to malignant cells in HCC. Recent studies have also demonstrated that PD-L1 expression on TAMs is more closely related to clinical response to anti-PD-L1 treatment than PD-L1 expression on tumor cells (Kuang et al., 2009; Liu C. et al., 2019). In this condition, treatment with anti-PD-1/PD-L1 will be less effective. Kuang et al. were the first to show that macrophages are the predominant immune cells that express PD-L1 in HCC (Kuang et al., 2009; Wu et al., 2009). Recently study demonstrated that PD-L1 was preferentially expressed on CD68⁺ macrophages in the TME of HCC (Park et al., 2021). These PD-L1⁺TAMs, activated by tumor-derived IL-10, have been proposed to mediate CD8⁺ T cell dysfunction through the PD-1/PD-L1 interaction (Kuang et al., 2009; Wu et al., 2009). Reportedly, PD-1/PD-L1 protein-protein interaction mediates CD8⁺ T cell inhibition by resident hepatic macrophages (KCs) in HCC (Moreno-Cubero and Larrubia, 2016). Interestingly, PD-L1 overexpression in HCC is found to have correlations with TAMs (Chen J. et al., 2012). B7-1/CD80 and B7-2/CD86 in TAMs are shown to inhibit T cell activation by binding with T cell co-inhibitory receptor CTLA-4, which exhibits a higher affinity than the T cell co-stimulatory receptor CD28 (Wong et al., 2005). The above studies demonstrated that PD-L1 expression on TAMs plays a more critical role in suppressing CD8+ T cell effector function than tumor-derived PD-L1. PD-L1 and PD-L2 overexpression is observed in the TAMs of many cancer tissues. These ligands are shown to inhibit TCR (T cell receptor) signal transduction and promote T cell anergy and apoptosis by binding with PD-1 on CD4⁺ and CD8⁺ T cells (Lu et al., 2019). Additionally, B7-H4, another member of the B7 family, is also found in TAMs, which binds with the receptors on activated T cells to inhibit T cell proliferation, cytotoxicity, cell cycle progression and cytokine production (Kryczek et al., 2007). In addition to causing T-cell dysfunction, researchers also found that TAMs attenuated PD-L1 expression in tumors and inhibited T cell infiltration (Jeong et al., 2019). CD4 and CD8α T cell infiltration was found to be significantly increased after TAMs were depleted from tumors (Bloch et al., 2013). Numerous factors in the TME have also been shown to affect PD-L1 expression on TAMs, such as the IL-27/STAT3 axis induces PD-L1/2 overexpression in TAMs (Horlad et al., 2016). Progranulin could upregulate PD-L1 on TAMs through STAT3 pathway to promote T-cell exhaustion and immune evasion (Fang et al., 2021). TAMs also can induce PD-L1 expression by the secretion of IFN-γ through the JAK/STAT3 and PI3K/AKT/mTOR signaling pathways (Zhang et al., 2017). Furthermore, PD-L1-expressing TAMs are immunosuppressive and able to reduce the number of activated T cells through apoptosis (Prima et al., 2017). Taken together, these studies suggest that TAMs-derived PD-L1 in the TME of HCC is a major and important source for PD-L1/PD-1 axis–mediated suppression on intratumor CD8+ T cells, which further induce resistance to anti-PD-1/PD-L1 therapy. TAMs seem to be a novel target to resolve TAMs-related PD-1/PD-L1 blockade resistance (Li W. et al., 2022). In this case, if TAMs can be prevented from expressing PD-L1/L2, it will benefit the therapeutic effect of ICIs.
4.4 TAMs contribute to PD-1/PD-L1 blockade resistance by promoting T cell apoptosis through the expression of FasL and TRAIL
Both FasL (Fas ligand) and TRAIL (TNF-related apoptosis-inducing ligand) belong to the death ligand family. They are capable of binding to death receptors on the cell surface, such as the Fas receptor and TRAIL receptor, thereby initiating apoptotic signal transduction pathways. In M2 macrophages, when the expression of FasL and TRAIL increases, they can bind to the corresponding receptors on the surface of T cells. By inducing T cell apoptosis, they further suppress the activity of T cells, enhancing the immune escape capability of tumor cells and subsequently resulting in resistance to anti-PD-1/PD-L1 treatment. TRAIL demonstrates selective cytotoxic activities and induces apoptosis of tumor cells, transformants or virus infected cells without affecting normal cells (Huang et al., 2005). Therefore, TRAIL is regarded as a new path to anti-tumor therapies. However, recent studies have confirmed that M2 macrophages are shown to directly induce T cell apoptosis by expressing Fas L and TRAIL to demonstrate their immunosuppressive properties (Figure 2C). For example, Ritsuko et al. revealed that FasL expression and phagocytosis of M2 macrophages were modulated by enhanced NF-κB activation to accelerate T cell apoptosis, denoting the essential role of FasL expression in M2 macrophages to maintain T cell homeostasis in peripheral immune systems (Oura et al., 2013). Brown et al. demonstrated that induced TAMs can express FasL and release active soluble FasL in order to induce apoptosis of the Fas⁺lymphocyte (Brown and Savill, 1999), while CAFs (cancer associated fibroblasts) can upregulate FasL and PD-L2 via the cross-presentation of MHC-1 (major histocompatibility complex1) antigen and inhibit CD8⁺ T cells activity, thus elicit an immunosuppressive TME (De Jaeghere et al., 2019). Tsai et al. showed that TAMs expressed TRAIL to bind with corresponding receptors and mediate T cell apoptosis (Tsai et al., 2004). T cell activation is a critical process for PD-1/PD-L1 blockade therapy. Effective PD-1/PD-L1 blockade therapy relies on the T cell function, and any disruption in the processes of T cell immune function will result in the failure of PD-1/PD-L1 blockade therapy (Sun et al., 2020; Toor et al., 2020). In general, TAMs can directly promote T-cell apoptosis by expressing Fas L and TRAIL to further inhibit the activity of T cells, thereby enhancing the immune escape ability of tumor cells and leading to resistance to PD-1/PD-L1 therapy. Therefore, targeting Fas L and TRAIL expression in M2 TAMs may be a potential strategy to overcome anti-PD-1/PD-L1 resistance in cancer treatment. This is one of their important mechanisms for regulating immune response and affecting tumor development. This mechanism has important guiding significance for understanding the function of TAMs in the TME and developing tumor treatment methods targeting TAMs.
4.5 TAMs contribute to PD-1/PD-L1 blockade resistance by rapidly capturing anti-PD-1 from T cells and inducing an M2 phenotype through expressing FcγRI
Unrelated to the previously mentioned mechanisms, it has recently been suggested that TAMs contribute to resistance against anti-PD-1 strategies through another mechanism that relies on Fc receptors and the elimination of monoclonal antibodies from T cells. Fcγ receptors (FcγRs) are a class of glycoproteins situated in the Fc domain of IgG and expressed on the membrane of immune cells, which link humoral and cell-mediated immunity by interaction with specific antibodies and effector cells. The Fc domain of PD-1 inhibitors is capable of binding with Type I FcR (FcγRI/CD64)-expressing cells, which appears to affect the efficacy of PD-1 inhibitors (Zhang T. et al., 2018). There are three reasons. First, the TME contains a high density of FcγRI⁺M2 macrophages, and aPD-1 antibodies bind with FcγRIs on the surface of M2 macrophages to induce antibody-dependent cellular cytotoxicity (ADCC), antibody-dependent cellular phagocytosis (ADCP), and complement-dependent cytotoxicity (CDC), resulting in T cell exhaustion, non-combat loss of T lymphocytes and eventually a significant reduction in-the anti-tumor activities induced by aPD-1 antibodies (Dahan et al., 2015). In vitro studies demonstrate that in FcγR-knockout mouse models, aPD-1 antibodies exhibit anti-tumor activities significantly higher than those without FcγR knockout, suggesting the inhibitory effects of FcγR on anti-tumor activities by binding with the Fc domain (Dahan et al., 2015; Chen et al., 2019). Small animal imaging results showed that in tumor-bearing mouse models injected with labeled aPD-1 antibodies, PD-1 effectively bound with tumor-infiltrating PD-1⁺CD8⁺T cells early after injection; however, TAMs subsequently scavenged the aPD-1 antibodies from T cells in a FcγRI-dependent manner to deactivate the antibodies (Arlauckas et al., 2017). Besides, T cells were preincubated with stained aPD-1 antibodies for 30 min and subsequently co-cultured with macrophages, following which a massive number of aPD-1 antibodies were detected in the macrophages; anti-PD-1 antibody transfer from T cells to macrophages was prevented by pre-addition of FcγR inhibitor (Arlauckas et al., 2017). Second, aPD-1 antibodies are identified to induce macrophage infiltration by binding with FcγR, which entails a risk of cancer hyperprogression. Existing studies show that accelerated tumor growth is observed in some patients who receive treatment with immune checkpoint inhibitors (Lo Russo et al., 2019). Further, a blockade of Fcγ receptors before PD-1 antibody treatment showed prolonged binding of anti-PD-1 antibodies and CD8+ T cells infiltration (Lo Russo et al., 2019). Third, FcγRI exhibiting high affinity for IgG4 is highly expressed in M2 macrophages. As a class of IgG4 antibodies, PD-1 inhibitors are shown to perpetuate the transformation of TAMs into the immunosuppressive M2 phenotype by interacting with FcγRI (Swisher et al., 2014). In addition, aPD-1 IgG4S228P antibody stimulates macrophage-induced phagocytosis of PD-1⁺ T cells by mediating cross-linking between PD-1⁺ T cells and FcγRI⁺ macrophages, which indicates a shift from PD-1 blockage to the activation and induction of IL-10 gene expression, thereby suppressing T cell-mediated immune response (Zhang T. et al., 2018). In short, aPD-1 immunotherapies can achieve better efficacy with reduced side effects by inhibiting the expression of FcγRI on the surface of TAMs, which demonstrates significant clinical value and attracting prospects (Figure 3).
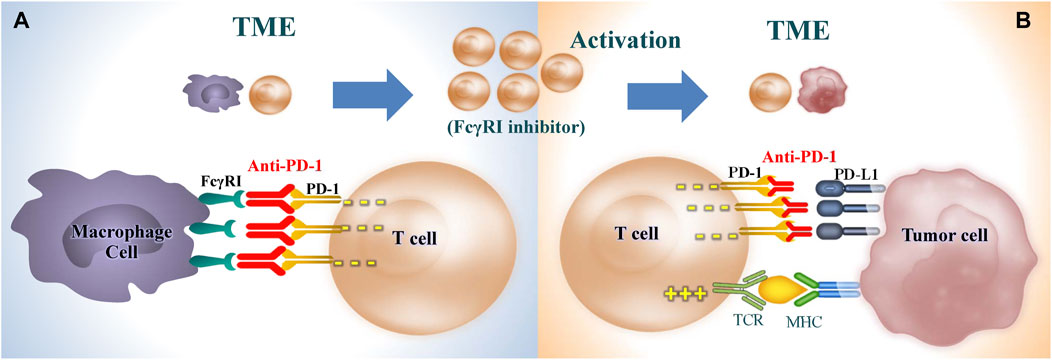
Figure 3. Restoration of T cell immunity by inhibiting FcγRI receptor on TAMs. (A) Inhibition of TAMs capturing anti-PD-1 from T cells in a FcγRI-dependent manner. (B) Restore the killing effect of T cells on tumor cells and enhance the efficacy of anti-PD-1. TME, tumor microenvironment; PD-1, programmed cell death protein 1; PD-L1, programmed cell death 1 ligand 1; FcγRI, CD64, Receptor I for the Fc Region of Immunoglobulin G; TCR, T cell receptor; MHC, major histocompatibility complex.
4.6 TAMs contribute to PD-1/PD-L1 blockade resistance by secreting IDO to inhibit T-cell functions
Indoleamine 2,3-dioxygenase (IDO) is renowned for its negative immunomodulatory properties, including the degradation of tryptophan and the generation of immune-modulating tryptophan metabolites. This enzymatic activity suppresses T-cell proliferation, ultimately leading to immunosuppression (Jürgens et al., 2009). Research conducted by Toulmonde et al. revealed that the majority of soft-tissue sarcomas (STS) and gastrointestinal stromal tumors (GIST) resistant to PD-1 blockade exhibited a substantial infiltration of M2 TAMs, which were found to express IDO (Toulmonde and Italiano, 2018). This suggests that the presence of these macrophages and their secretion of IDO may contribute significantly to the resistance observed in these tumor types. Furthermore, prior studies have illuminated the mechanisms underlying the immunosuppressive effects of IDO in the tumor microenvironment. Firstly, IDO expressed by APCs in tumor-draining lymph nodes can deactivate effective T cells, thereby reducing their antitumor activity (Cai et al., 2019). Secondly, the expression of IDO by tumor cells themselves suppresses the anti-tumor immune response, enabling immune evasion (Brandacher et al., 2006). Meanwhile, IDO has the ability to trigger macrophages to polarize towards the M2 phenotype. In essence, the secretion of IDO by macrophages can contribute to the creation of an immunosuppressive microenvironment, representing a pivotal mechanism in resistance to PD-1 blockade. Recently, a promising strategy for enhancing the effectiveness of tumor immunotherapy has emerged through the combined application of IDO inhibition and immune checkpoint blockade, as reported in studies (Zamarin and Postow, 2015; Tierney et al., 2020). Additionally, it has been observed that the secretion of IDO by M2 TAMs can lead to the polarization of M1 TAMs towards the immunosuppressive M2 subtype, thereby leading to resistance to PD-1/PD-L1 blockade (Wang et al., 2014). In a phase 2 clinical trial conducted by Toulmonde et al. (2018), a significant infiltration of M2 TAMs expressing IDO1 within tumor sites was demonstrated. Notably, the IDO1/kynurenine pathway plays a pivotal role in promoting the immunosuppressive phenotype of M2 TAMs, contributing to their resistance to PD-1 inhibition (Toulmonde et al., 2018). However, the mechanisms underlying PD-1/PD-L1 blockade resistance mediated by IDO derived from M2 TAMs remain incompletely understood in this study. Taken together, the secretion of IDO by M2 TAMs creates an immunosuppressive tumor microenvironment, serving as a crucial mechanism underlying resistance to PD-1/PD-L1 blockade. This understanding may pave the way for the development of novel therapeutic strategies that target IDO and its associated immunosuppressive pathways, ultimately enhancing the effectiveness of immunotherapy in cancer treatment (Toulmonde and Italiano, 2018).
4.7 The role of TAMs in anti-PD-1/PD-L1 immunotherapy for HCC caused by different etiology
HCC accounts for 90% of the incidence of liver cancer. Chronic hepatitis caused by hepatitis B virus (HBV) and hepatitis C virus (HCV) infection is a major risk factor for HCC. Much attention has been paid to the efficacy and safety of ICIs immunotherapy in HCC patients with chronic viral hepatitis. As a cornerstone, CheckMate-040 studies have shown that the objective remission rate (ORR) of Nivolumab (anti-PD-1) is 20% (El-Khoueiry et al., 2017). A systematic review study showed that the effective rate of ICIs treatment in the HCC cohort with HBV/HCV infection was 18.6%, which was similar to the CheckMate-040 study (Pu et al., 2020). In addition, efficacy survival indicators for the HBV/HCV infected cohort were not statistically significant compared to the non-HBV/HCV infected cohort in the subgroup of Asian patients with CheckMate-040 (Yau et al., 2019). Therefore, HCC patients may benefit from ICIs regardless of whether or not they are associated with HBV/HCV infection (Pan et al., 2022).
Other non-viral etiologies include chronic alcohol consumption and non-alcoholic fatty liver disease (NAFLD/MASH). Although the incidence of hepatitis virus-related HCC has been reduced in recent years by the HBV vaccine and HCV antiviral therapy, the incidence of HCC has been increasing, mainly due to strong links with alcohol abuse, obesity and diabetes. NAFLD has developed into one of the leading causes of HCC, and has become the fastest growing cause of HCC patients in the world (Anstee et al., 2019). The disease spectrum of NAFLD includes simple fatty liver, NASH (non-alcoholic hepatitis), liver fibrosis, cirrhosis and HCC. Compared with viral hepatitis-related HCC, NAFLD-HCC has a more insidious course, often with larger tumors and poorer prognosis (Leung et al., 2015). A recent study published in Nature involving 1,656 patients with advanced HCC showed that NASH-HCC patients did not benefit from ICIs treatment, possibly due to impaired immune surveillance of CD8⁺ T cells in NASH patients, resulting in poor response to ICIs and even induce MASH-HCC (Pfister et al., 2021). At the same time, the study found that patients with MASH-HCC who received ICIs had lower survival (11 cases, 8.8 months survival) than HCC patients with other causes (107 cases, 17.7 months survival) (p = 0.034). In response to the above problems, the researchers found that macrophage polarization is involved in the pathogenesis and progression of NASH. Macrophages in the liver (Kupffer cells) promote HCC initiation and progression under the long-term chronic inflammatory infiltration (Zhang P. et al., 2022). Using single cell transcriptome analysis, they identified two notable features of the hepatic immune microenvironment during the pathogenesis of NASH: macrophage expansion with molecular features of TAMs and induction of CD8⁺ T cell exhaustion. The induction of T-cell exhaustion may serve as a protective mechanism to limit further hepatic damage caused by the function of cytotoxic T cells (Dudek et al., 2021; Pfister et al., 2021). ICIs aggravate liver injury in diet-induced NASH mice, thus limiting immunotherapies targeting HCC. Unlike T-cells, TAMs undergo massive expansion during diet induced NASH. TAMs have been implicated in the context of liver tumorigenesis by orchestrating an immunosuppressive and tumor promoting microenvironment that facilitates the development of HCC. In summary, due to the impairment of T-cell function, PD-1 inhibition is less effective in patients with HCC complicated by NAFLD/NASH than in patients with viral hepatitis. The treatment based on TAMs is of great significance in the anti-PD-1/PDL1 treatment of NAFLD-HCC.
5 Strategies for overcoming resistance to anti-PD-1/PD-L1 blockade mediated by TAMs
Given that the complex biology of TAMs and their involved role in tumor proliferation, angiogenesis, EMT, metastasis, immune suppression, TAMs have a multichannel inhibitory effect on anti-PD-1/PD-L1 immunotherapy (Weiskopf and Weissman, 2015; Villanueva, 2017), so that a single ICI (Immune checkpoint inhibitor) cannot effectively activate the immune response. Therefore, targeting TAMs is of great significance to improve the efficacy of anti-PD-1/PD-L1 immunotherapy. There are several therapies available to recover the anticancer efficacy of PD-1/PD-L1 inhibitors by targeting TAMs, including eliminate TAMs already present in the TME, suppress macrophages recruitment, or reprogramming TAMs from the M1 to M2 phenotype (Table 2) (Kumari and Choi, 2022).
5.1 Eliminate TAMs already present in the TME
Given that the infiltration of TAMs restricts the clinically significant immune response of anti-PD1/PD-L1, eliminating them appears to be an appealing strategy. In early studies, clodronate-loaded liposomes (clodrolip) were often used to deplete liver macrophages (Van Rooijen et al., 1990). After injection, liposomes are artificially prepared vesicles that undergo phagocytosis by macrophages, and then intracellular delivery and accumulation of clodronate can induce macrophage apoptosis (Van Rooijen and Sanders, 1994). Li et al. demonstrated that nanoliposome C6-ceramide (LipC6) could enhance the anti-tumor immune response and hinder HCC growth in mice according to reducing the number of TAMs and their ability to suppress the anti-tumor immune response (Li G. et al., 2018). Xie et al. demonstrated that administration of clodronate liposomes partially depleted TAMs, resulting in reduced tumor growth and metastasis in a murine PLC/PRF/5, MHCC97H, H22 and Hepa1-6 cell- orthotopic tumor models (Xie et al., 2023). Similarly, Zhang et al. also found that compared with rats treated with Doxorubicin-liposome alone, macrophages depletion with clodronate-liposome (CL-LIP) in combination with Doxorubicin-liposome (Doxil) could significantly induce tumor cell apoptosis and thereby inhibit the progression of HCC (Zhang H. et al., 2022).
In recent years, researchers have found that Colony-stimulating factor-1 receptor (CSF-1R) blockers are the main way to deplete M2-TAMs (Gomez-Roca et al., 2019). When CSF-1 binds to its receptor, it initiates autophosphorylation, a vital process for macrophage proliferation, differentiation, and sustenance (Peranzoni et al., 2018). Therefore, employing a CSF-1R inhibitor (CSF-1Ri) may amplify the therapeutic potential of anti-PD-1/PD-L1 immunotherapy. PLX3397, a kinase inhibitor specifically tailored for CSF-1R, synergizes with anti-PD-1 therapy to enhance the infiltration and antitumor activities of CD8+ T cells within tumor tissues (Shi et al., 2019). Furthermore, it effectively alleviates tumor neovascularization and ascites formation (Dammeijer et al., 2017). Another notable CSF-1Ri, BLZ945, when combined with a PD-1/PD-L1 blocking antibody, also exhibits promising results in controlling tumor growth (Mao Y. et al., 2016). These existing antitumor drugs targeting CSF-1R present a novel avenue for integrated immunotherapy strategies. Some researchers have used CSF1R inhibitors to eliminate TAMs and redirect anti-PD-1 to CD8+ T cells, aiming to enhance the efficacy of combined therapy (Beider et al., 2014). A recent study explored the utilization of alginate-based hydrogel loaded with Pexidartinib (PLX)-encapsulated nanoparticles. These nanoparticles were designed to release PLX specifically at the tumor site, effectively interfering with the infiltration of TAMs. As reported by Li et al., regulating TAMs infiltration facilitated the infiltration of T cells, ultimately enhancing the local and systemic delivery of anti-PD-1 antibody-conjugated platelets. This approach aimed to prevent recurrence following surgical intervention (Li Z. et al., 2022). However, despite these preclinical successes, the persistence of the response to TAMs depletion is still lower than expected. In addition, compared to TAMs-targeted methods such as inhibiting TAMs recruitment or reprogramming, it has been found that TAMs depletion is less effective in preventing cancer growth.
5.2 Suppresses macrophages recruitment
Termination of macrophages recruitment is another treatment to combat the multi-faceted functions of TAMs in tumor progression and resistance. Zhou et al. demonstrated that ZA treatment enhanced the effects of hepatic transcatheter arterial chemoembolization (TACE) through inhibiting TAMs infiltration and tumor angiogenesis in rat models of HCC (Zhou et al., 2017). CCL2/CCR2 signaling plays a central regulatory role in circulatory monocytes and their infiltration into the TME. Teng et al. found that CCL2 is strongly upregulated in patients with HCC, and that gene silencing and administration of a neutralizing antibody to CCL2 or a CCR2 antagonist reduced recruitment of circulating monocytes, then decreased TAMs number, and downregulated M2 secretion function as TAMs. Finally, the liver damage, HCC incidence and tumor burden were significantly reduced (Teng et al., 2017; Yao et al., 2017). Li et al. found that blockade of the CCL2/CCR2 axis inhibits the recruitment of M2-TAMs, leading to a reversal in the status of immunosuppression in HCC (Li et al., 2017). A CCR2 antagonist, naturally occurring in Abies georgei and designated as 747, exhibited antitumor activity in mouse models of HCC. This was evident through a reduction in TAM levels and a concurrent increase in CD8 T-cells within the tumor microenvironment (Yao et al., 2017). Furthermore, studies revealed that concurrent administration of anti-CCL2 antibodies improved the effectiveness of chemotherapy and checkpoint inhibitors (Brana et al., 2015). Additionally, combining the CCR2 antagonist, RS504393, with anti-PD-1 therapy resulted in a superior tumor response compared to anti-PD-1 monotherapy in various murine tumor models. This combination therapy achieved an enhanced antitumor response by simultaneously boosting CD8+ T-cell recruitment and activation while reducing CD4+ regulatory T-cells. Chen et al. demonstrated that high-mobility group A1 (HMGA1) as a crucial regulator of macrophage recruitment through the activation of NF-κB-CCL2 signaling in HCC (Chen et al., 2022). Another key pathway in macrophages recruitment is the CXCL12/CXCR4 interaction. The upregulation of stromal cell derived factor 1alpha (SDF-1α/CXCL12) induced by hypoxia also contributes to the recruitment of suppressive M2 macrophages (Chen et al., 2014). Using the CXCR4 antagonist AMD3100, Chen et al. found that inhibition of the SDF-1α receptor (CXCR4) relieved regional immunosuppression and facilitated treatment with anti-PD-1 antibodies in a model of HCC resistant to sorafenib (Chen et al., 2015).
5.3 Reprogram TAMs from M1 to M2 phenotype
Recent findings suggest that re-education of M2 TAMs, rather than their depletion, could be a more efficient strategy. Previous research has indicated that the protumor M2 phenotype can be successfully re-educated to the tumoricidal M1 phenotype, effectively inhibiting the supportive role of TAMs in tumors. This approach may enhance the response to anti-PD-1 treatment (Kwantwi, 2023). As CSF-1R has been observed to be expressed in a restrictive manner by TAMs and monocytes that prefer to locate at the boundary of HCC (Jiang et al., 2020). CSF-1 and its receptor, CSF-1R, have been shown to regulate macrophage differentiation and function and to play an important role in macrophage infiltration in HCC (Ao et al., 2017). Targeting CSF1/CSF1R signaling in protumoral TAMs represents an attractive strategy for eliminating CSF1R-dependent or reprogramming M2-like TAMs in HCC (Pyonteck et al., 2013; Cannarile et al., 2017). Ao et al. found that treatment with the CSF-1R inhibitor PLX3397 resulted in delayed tumor growth in both xenograft and allograft models in HCC, and this delay was probably mediated by the transition from M2 to M1 macrophages in a population of TAMs induced by a block in the CSF1/CSF-1R signaling pathway (Ao et al., 2017). Xu et al. demonstrated that the Lmdd-MPFG (LM) vaccine activates the NF-κB pathway in TAMs through the TLR2-MyD88 pathways (Chen Y. et al., 2012; Xu G. et al., 2020), which skewed the TAMs from M2 into M1, and skewed the TME cytokine profile of the TME toward an anti-tumor profile, this change restored the T cell reactivity to anti-PD-1 blockade (Xu G. et al., 2020). Wu et al. demonstrated that HIF-1α induced increased expression of the triggering receptor expressed on myeloid cells-1 (TREM-1) in TAMs, leading to immunosuppression. Blocking TREM-1 treatment with the GF9 inhibitor reversed immunosuppression and resistance to anti-PD-L1 in HCC (Wu et al., 2019b). In addition, a study by Yang et al. identified that 17β-estradiol (E2) could attenuate the progression of HCC through the regulation of macrophage polarization, as E2 rechallenge reduced tumor growth in both orthotopic and ectopic mouse models of HCC, which functions as an inhibitor of alternative macrophage activation and tumor progression by keeping the estrogen receptor-β away from interacting with ATP5J and by interfering with the JAK1-STAT6 signaling pathway (Yang et al., 2012). Ubiquitin-specific protease 7 (USP7), an enzyme responsible for deubiquitination, holds significant promise as a therapeutic target due to its regulatory function in DNA damage repair and epigenetic inheritance (Wang et al., 2019). USP7 has been recognized as a gene that is highly expressed in M2 TAMs. Notably, specific inhibition of USP7 has the potential to reprogram M2 TAMs into M1 phenotype via the P38 MAPK pathway (Dai et al., 2020). Furthermore, targeting USP7 has been demonstrated to enhance the infiltration and cytotoxic activity of CD8+ T cells within the TME, while concurrently suppressing the expression of PD-L1 in tumor cells, thereby enhancing the response to anti-PD-1/PD-L1 therapy (Dai et al., 2020; Wang Z. et al., 2021).
Emerging studies have used nanoparticles to enhance drug delivery into TAMs to facilitate polarization to M1 phenotype. For example, Systemic targeting of TAMs with nanomedicines is an attractive approach because TAMs are ideal therapeutic targets due to their considerable propensity for phagocytose of nanoparticles (Han et al., 2021; Kumari and Choi, 2022). Recent studies have used nanoparticles containing TLR agonists or tumor peptides to promote TAMs reprogramming, utilizing nanoparticles to simultaneously target TAMs and promote anti-tumor immunity. For example, Rodell et al. found that nanoparticles loaded with the TLR7/TLR8 agonist R848 preferentially accumulated in TAMs in mouse models and promoted the polarization of cells towards M1 macrophage re-education (Rodell et al., 2018). Clinical trials are also underway for TLR agonists to treat solid tumors. TLR9 agonists, such as lefitolimod, effectively modulate the TME and induce anti-tumor responses by promoting the infiltration of CD8 T-cells and reprogramming TAMs in the TME (Kapp et al., 2019). In addition, the nanoparticle-based delivery of TLR agonists, bisphosphonates, DNA, mRNA, and miRNA repolarizes TAMs more effectively. Wang et al. encapsulated mRNA which can bispecifically bind and neutralize CCL2 and CCL5 (BisCCL2/5i) in a clinically approved lipid nanoparticle platform. The nanoplatform of This BisCCL2/5i mRNA significantly induced TAMs polarization from M2 to M1 and reduced the immunosuppression of the TME. Morever, BisCCL2/5i combination with anti-PD-L1 resulted in long term survival in mouse HCC models (Wang Y. et al., 2021). Chang et al. designed a NanoMnto tumor-targeted and biodegradable nano-delivery system to deliver sorafenib and MnO2. NanoMnSor reprograms the immunosuppressive TME by reducing the hypoxia-induced tumor TAMs infiltration, promote macrophage polarization to the M1 immunostimulatory phenotype, and increase the number of CD8⁺ cytotoxic T-cell numbers in tumors, thereby increasing the efficacy of the anti-PD-1 antibody, synergistically inhibited the progression of both primary HCC as well as distant metastases (Chang et al., 2020). Another recent study, selenium nanoparticles (SeNPs) were combined with cytokine induced killer cells (CIK), which effectively enhanced natural killer cell infiltration into tumors and reprogrammed tumor promoting M2 macrophages into anti-tumor M1 macrophages, stimulating a robust anti-tumor response to combat progression (Liu et al., 2020). Targeting TAMs offers a new approach to improving immunotherapy for HCC by harnessing the immune system. However, to obtain accurate therapeutic targets, further understanding of the molecular mechanisms controlling phenotypic changes in different processes of liver cancer is still needed.
6 Conclusion
HCC is a major public health threat that responds well to immunotherapy as an emerging treatment option. However, the evolution of drug resistance and the presence of immunosuppressive cells in the TME impose a challenge to sustain the efficacy of anti-PD-1/PD-L1 immunotherapy for HCC. M2 macrophages play a major role in achieving immunosuppression in the TME of HCC. Through interaction with different tumor-associated factors and cells, M2 macrophages can promote tumor angiogenesis and lymphangiogenesis, EMT, and drug resistance, revealing the mechanism responsible for the negative regulation of immunotherapy for HCC. TAMs affect anti-PD-1/PD-L1 immunotherapy for HCC in many ways. To be specific, TAMs interact with T cells, suppress phagocytosis and anti-tumor immunity through PD-1 expression, amplify T cell anergy and apoptosis through PD-L1/L2 expression, mediate T cell apoptosis through FasL and TRAIL expression, scavenge aPD-1 antibodies from T cells and transform into M2 phenotype polarization through FcγRI expression. Therefore, personalized immunotherapy for HCC can be developed by reference to the mechanisms responsible for the immunosuppressive activities of TAMs and related factors, such as blockage of PD-1, PD-L1/L2, Fas L and TRAIL, and FcγRI expression in TAMs, in order to enhance the efficacy of anti-PD-1/PD-L1 immunotherapy and improve the survival rate. Increasing evidence suggest that potential mechanisms of TAMs mediated tumor immune escape imply interpretation and breakthrough to bottleneck of current tumor immunotherapy (Qiu et al., 2021). TAMs appear to be an important potential target for cancer therapy, also new possibilities for improving the efficacy of anti-PD-1 monoclonal antibodies (Bouhlel et al., 2007; Orecchioni et al., 2019; Wang C. et al., 2021). Despite all that, the immunosuppressive effects of TAMs in the TME and the related mechanisms are not yet fully understood, and thus further explorations and discussions are needed to confirm the synergistic effect of TAMs targeted drugs and anti-PD-1 monoclonal antibody, and develop potential new strategies to overcome TAMs related immune tolerance. Furthermore, the differences among TAMs function in different tumors are primarily attributed to variations in their origins, proportions, and anatomic locales. Therefore, future investigations related to the development of TAMs-targeted therapies should be narrowed down to a specific tumor type, such as HCC. In the future, the customization of TAMs-targeted treatments in combination with anti-PD-1/PDL1 therapeutic approaches would be a promising alternative treatment strategy for HCC patients.
Author contributions
ZL: Conceptualization, Investigation, Writing–original draft, Writing–review and editing. DD: Writing–original draft. LL: Investigation, Visualization, Writing–review and editing. DP: Investigation, Visualization, Writing–review and editing. YM: Investigation, Visualization, Writing–review and editing. RN: Data curation, Writing–review and editing. YL: Funding acquisition, Supervision, Writing–review and editing.
Funding
The author(s) declare financial support was received for the research, authorship, and/or publication of this article. This work was funded by the Traditional Chinese Medicine Key Project of Chongqing Science and Health Joint (Grant No. 2023ZDXM032), the Innovative ability enhancement program for medical staff of the Army Medical Center (Grant No. 130333), Chongqing Clinical Pharmacy Key Specialties Construction Project, and the general program of Development Center for Medical Science and Technology, National Health Commission of the People’s Republic of China (Grant No. WKZX2023CX210006).
Conflict of interest
The authors declare that the research was conducted in the absence of any commercial or financial relationships that could be construed as a potential conflict of interest.
Publisher’s note
All claims expressed in this article are solely those of the authors and do not necessarily represent those of their affiliated organizations, or those of the publisher, the editors and the reviewers. Any product that may be evaluated in this article, or claim that may be made by its manufacturer, is not guaranteed or endorsed by the publisher.
References
Afik, R., Zigmond, E., Vugman, M., Klepfish, M., Shimshoni, E., Pasmanik Chor, M., et al. (2016). Tumor macrophages are pivotal constructors of tumor collagenous matrix. J. Exp. Med. 213 (11), 2315–2331. doi:10.1084/jem.20151193
Alishekevitz, D., Gingis-Velitski, S., Kaidar-Person, O., Gutter-Kapon, L., Scherer, S. D., Raviv, Z., et al. (2016). Macrophage-induced lymphangiogenesis and metastasis following paclitaxel chemotherapy is regulated by VEGFR3. Cell Rep. 17 (5), 1344–1356. doi:10.1016/j.celrep.2016.09.083
Anstee, Q. M., Reeves, H. L., Kotsiliti, E., Govaere, O., and Heikenwalder, M. (2019). From NASH to HCC: current concepts and future challenges. Nat. Rev. Gastroenterol. Hepatol. 16 (7), 411–428. doi:10.1038/s41575-019-0145-7
Ao, J.-Y., Zhu, X.-D., Chai, Z.-T., Cai, H., Zhang, Y.-Y., Zhang, K.-Z., et al. (2017). Colony-stimulating factor 1 receptor blockade inhibits tumor growth by altering the polarization of tumor-associated macrophages in hepatocellular carcinoma. Mol. Cancer Ther. 16 (8), 1544–1554. doi:10.1158/1535-7163.mct-16-0866
Arlauckas, S. P., Garris, C. S., Kohler, R. H., Kitaoka, M., Cuccarese, M. F., Yang, K. S., et al. (2017). In vivo imaging reveals a tumor-associated macrophage-mediated resistance pathway in anti-PD-1 therapy. Sci. Transl. Med. 9 (389), eaal3604. doi:10.1126/scitranslmed.aal3604
Arvanitakis, K., Koletsa, T., Mitroulis, I., and Germanidis, G. (2022). Tumor-associated macrophages in hepatocellular carcinoma pathogenesis, prognosis and therapy. Cancers (Basel) 14 (1), 226. doi:10.3390/cancers14010226
Balar, A. V., and Weber, J. S. (2017). PD-1 and PD-L1 antibodies in cancer: current status and future directions. Cancer Immunol. Immun. 66 (5), 551–564. doi:10.1007/s00262-017-1954-6
Bandarra, D., Biddlestone, J., Mudie, S., Müller, H. A. J., and Rocha, S. (2015). HIF-1α restricts NF-κB-dependent gene expression to control innate immunity signals. Dis. Model Mech. 8 (2), 169–181. doi:10.1242/dmm.017285
Batlle, E., and Massagué, J. (2019). Transforming growth factor-β signaling in immunity and cancer. Immunity 50 (4), 924–940. doi:10.1016/j.immuni.2019.03.024
Beider, K., Bitner, H., Leiba, M., Gutwein, O., Koren-Michowitz, M., Ostrovsky, O., et al. (2014). Multiple myeloma cells recruit tumor-supportive macrophages through the CXCR4/CXCL12 Axis and promote their polarization toward the M2 phenotype. Oncotarget 5, 11283–11296. doi:10.18632/oncotarget.2207
Binenbaum, Y., Fridman, E., Yaari, Z., Milman, N., Schroeder, A., Ben, D. G., et al. (2018). Transfer of miRNA in macrophage-derived exosomes induces drug resistance in pancreatic adenocarcinoma. Cancer Res. 78 (18), 5287–5299. doi:10.1158/0008-5472.can-18-0124
Binnewies, M., Roberts, E. W., Kersten, K., Chan, V., Fearon, D. F., Merad, M., et al. (2018). Understanding the tumor immune microenvironment (TIME) for effective therapy. Nat. Med. 24 (5), 541–550. doi:10.1038/s41591-018-0014-x
Bloch, O., Crane, C. A., Kaur, R., Safaee, M., Rutkowski, M. J., and Parsa, A. T. (2013). Gliomas promote immunosuppression through induction of B7-H1 expression in tumor-associated macrophages. Clin. Cancer Res. 19 (12), 3165–3175. doi:10.1158/1078-0432.Ccr-12-3314
Bouhlel, M. A., Derudas, B., Rigamonti, E., Dièvart, R., Brozek, J., Haulon, S., et al. (2007). PPARgamma activation primes human monocytes into alternative M2 macrophages with anti-inflammatory properties. Cell Metab. 6 (2), 137–143. doi:10.1016/j.cmet.2007.06.010
Boulter, L., Guest, R. V., Kendall, T. J., Wilson, D. H., Wojtacha, D., Robson, A. J., et al. (2015). WNT signaling drives cholangiocarcinoma growth and can be pharmacologically inhibited. J. Clin. Invest. 125 (3), 1269–1285. doi:10.1172/jci76452
Brana, I., Calles, A., LoRusso, P. M., Yee, L. K., Puchalski, T. A., Seetharam, S., et al. (2015). Carlumab, an anti-C-C chemokine ligand 2 monoclonal antibody, in combination with four chemotherapy regimens for the treatment of patients with solid tumors: an open-label, multicenter phase 1b study. Target Oncol. 10 (1), 111–123. doi:10.1007/s11523-014-0320-2
Brandacher, G., Perathoner, A., Ladurner, R., Schneeberger, S., Obrist, P., Winkler, C., et al. (2006). Prognostic value of indoleamine 2,3-dioxygenase expression in colorectal cancer: effect on tumor-infiltrating T cells. Clin. Cancer Res. 12 (4), 1144–1151. doi:10.1158/1078-0432.CCR-05-1966
Bray, F., Ferlay, J., Soerjomataram, I., Siegel, R. L., Torre, L. A., and Jemal, A. (2018). Global cancer statistics 2018: GLOBOCAN estimates of incidence and mortality worldwide for 36 cancers in 185 countries. CA-Cancer J. Clin. 68 (6), 394–424. doi:10.3322/caac.21492
Bron, S., Henry, L., Faes-Van't Hull, E., Turrini, R., Vanhecke, D., Guex, N., et al. (2016). TIE-2-expressing monocytes are lymphangiogenic and associate specifically with lymphatics of human breast cancer. Oncoimmunology 5 (2), e1073882. doi:10.1080/2162402x.2015.1073882
Brown, S. B., and Savill, J. (1999). Phagocytosis triggers macrophage release of Fas ligand and induces apoptosis of bystander leukocytes. J. Immunol. 162 (1), 480–485. doi:10.4049/jimmunol.162.1.480
Brown, Z. J., Fu, Q., Ma, C., Kruhlak, M., Zhang, H., Luo, J., et al. (2018). Carnitine palmitoyltransferase gene upregulation by linoleic acid induces CD4(+) T cell apoptosis promoting HCC development. Cell Death Dis. 9 (6), 620. doi:10.1038/s41419-018-0687-6
Cai, H., Zhu, X.-D., Ao, J.-Y., Ye, B.-G., Zhang, Y.-Y., Chai, Z.-T., et al. (2017). Colony-stimulating factor-1-induced AIF1 expression in tumor-associated macrophages enhances the progression of hepatocellular carcinoma. Oncoimmunology 6 (9), e1333213–e. doi:10.1080/2162402X.2017.1333213
Cai, J., Qi, Q., Qian, X., Han, J., Zhu, X., Zhang, Q., et al. (2019). The role of PD-1/PD-L1 axis and macrophage in the progression and treatment of cancer. J. Cancer Res. Clin. Oncol. 145 (6), 1377–1385. doi:10.1007/s00432-019-02879-2
Cai, Z., Cao, Y., Luo, Y., Hu, H., and Ling, H. (2018). Signalling mechanism(s) of epithelial–mesenchymal transition and cancer stem cells in tumour therapeutic resistance. Clin. Chim. Acta 483, 156–163. doi:10.1016/j.cca.2018.04.033
Cannarile, M. A., Weisser, M., Jacob, W., Jegg, A. M., Ries, C. H., and Rüttinger, D. (2017). Colony-stimulating factor 1 receptor (CSF1R) inhibitors in cancer therapy. J. Immunother. Cancer 5 (1), 53. doi:10.1186/s40425-017-0257-y
Cersosimo, F., Lonardi, S., Bernardini, G., Telfer, B., Mandelli, G. E., Santucci, A., et al. (2020). Tumor-associated macrophages in osteosarcoma: from mechanisms to therapy. Int. J. Mol. Sci. 21 (15), 5207. doi:10.3390/ijms21155207
Chang, C. C., Dinh, T. K., Lee, Y. A., Wang, F. N., Sung, Y. C., Yu, P. L., et al. (2020). Nanoparticle delivery of MnO(2) and antiangiogenic therapy to overcome hypoxia-driven tumor escape and suppress hepatocellular carcinoma. ACS Appl. Mater Interfaces 12 (40), 44407–44419. doi:10.1021/acsami.0c08473
Che, D., Zhang, S., Jing, Z., Shang, L., Jin, S., Liu, F., et al. (2017). Macrophages induce EMT to promote invasion of lung cancer cells through the IL-6-mediated COX-2/PGE2/β-catenin signalling pathway. Mol. Immunol. 90, 197–210. doi:10.1016/j.molimm.2017.06.018
Chen, D. S., and Mellman, I. (2017). Elements of cancer immunity and the cancer-immune set point. Nature 541 (7637), 321–330. doi:10.1038/nature21349
Chen, J., Ji, K., Gu, L., Fang, Y., Pan, M., and Tian, S. (2022). HMGA1 promotes macrophage recruitment via activation of NF-κB-CCL2 signaling in hepatocellular carcinoma. J. Immunol. Res. 2022, 4727198. doi:10.1155/2022/4727198
Chen, J., Li, G., Meng, H., Fan, Y., Song, Y., Wang, S., et al. (2012a). Upregulation of B7-H1 expression is associated with macrophage infiltration in hepatocellular carcinomas. Cancer Immunol. Immun. 61 (1), 101–108. doi:10.1007/s00262-011-1094-3
Chen, S., Zheng, P., Wang, W., Yi, M., Chen, P., Cai, J., et al. (2018). Abberent expression of NOR1 protein in tumor associated macrophages contributes to the development of DEN-induced hepatocellular carcinoma. J. Cell Physiol. 233 (6), 5002–5013. doi:10.1002/jcp.26349
Chen, X., Song, X., Li, K., and Zhang, T. (2019). FcγR-binding is an important functional attribute for immune checkpoint antibodies in cancer immunotherapy. Front. Immunol. 10, 292. doi:10.3389/fimmu.2019.00292
Chen, X. J., Wei, W. F., Wang, Z. C., Wang, N., Guo, C. H., Zhou, C. F., et al. (2021). A novel lymphatic pattern promotes metastasis of cervical cancer in a hypoxic tumour-associated macrophage-dependent manner. Angiogenesis 24 (3), 549–565. doi:10.1007/s10456-020-09766-2
Chen, Y., Huang, Y., Reiberger, T., Duyverman, A. M., Huang, P., Samuel, R., et al. (2014). Differential effects of sorafenib on liver versus tumor fibrosis mediated by stromal-derived factor 1 alpha/C-X-C receptor type 4 axis and myeloid differentiation antigen-positive myeloid cell infiltration in mice. Hepatology 59 (4), 1435–1447. doi:10.1002/hep.26790
Chen, Y., Ramjiawan, R. R., Reiberger, T., Ng, M. R., Hato, T., Huang, Y., et al. (2015). CXCR4 inhibition in tumor microenvironment facilitates anti-programmed death receptor-1 immunotherapy in sorafenib-treated hepatocellular carcinoma in mice. Hepatology 61 (5), 1591–1602. doi:10.1002/hep.27665
Chen, Y., Yang, D., Li, S., Gao, Y., Jiang, R., Deng, L., et al. (2012b). Development of a Listeria monocytogenes-based vaccine against hepatocellular carcinoma. Oncogene 31 (17), 2140–2152. doi:10.1038/onc.2011.395
Cohen, H. B., and Mosser, D. M. (2013). Extrinsic and intrinsic control of macrophage inflammatory responses. J. Leukoc. Biol. 94 (5), 913–919. doi:10.1189/jlb.0413236
Cramer, T., Yamanishi, Y., Clausen, B. E., Förster, I., Pawlinski, R., Mackman, N., et al. (2003). HIF-1alpha is essential for myeloid cell-mediated inflammation. Cell 112 (5), 645–657. doi:10.1016/s0092-8674(03)00154-5
Dahan, R., Sega, E., Engelhardt, J., Selby, M., Korman Alan, J., and Ravetch Jeffrey, V. (2015). FcγRs modulate the anti-tumor activity of antibodies targeting the PD-1/PD-L1 Axis. Cancer Cell 28 (3), 285–295. doi:10.1016/j.ccell.2015.08.004
Dai, X., Lu, L., Deng, S., Meng, J., Wan, C., Huang, J., et al. (2020). USP7 targeting modulates anti-tumor immune response by reprogramming tumor associated macrophages in lung cancer. Theranostics 10, 9332–9347. doi:10.7150/thno.47137
Dammeijer, F., Lievense, L. A., Kaijen-Lambers, M. E., van Nimwegen, M., Bezemer, K., Hegmans, J. P., et al. (2017). Depletion of tumor-associated macrophages with aCSF-1r kinase inhibitor enhances antitumor immunity and SurvivalInduced by DC immunotherapy. Cancer Immunol. Res. 5, 535–546. doi:10.1158/2326-6066.CIR-16-0309
Debebe, A., Medina, V., Chen, C. Y., Mahajan, I. M., Jia, C., Fu, D., et al. (2017). Wnt/β-catenin activation and macrophage induction during liver cancer development following steatosis. Oncogene 36 (43), 6020–6029. doi:10.1038/onc.2017.207
De Jaeghere, E. A., Denys, H. G., and De Wever, O. (2019). Fibroblasts fuel immune escape in the tumor microenvironment. Trends Cancer 5 (11), 704–723. doi:10.1016/j.trecan.2019.09.009
DeNardo, D. G., Barreto, J. B., Andreu, P., Vasquez, L., Tawfik, D., Kolhatkar, N., et al. (2009). CD4(+) T cells regulate pulmonary metastasis of mammary carcinomas by enhancing protumor properties of macrophages. Cancer Cell 16 (2), 91–102. doi:10.1016/j.ccr.2009.06.018
DeNardo, D. G., and Ruffell, B. (2019). Macrophages as regulators of tumour immunity and immunotherapy. Nat. Rev. Immunol. 19 (6), 369–382. doi:10.1038/s41577-019-0127-6
Dhanasekaran, R., Venkatesh, S. K., Torbenson, M. S., and Roberts, L. R. (2016). Clinical implications of basic research in hepatocellular carcinoma. J. Hepatol. 64 (3), 736–745. doi:10.1016/j.jhep.2015.09.008
Dudek, M., Pfister, D., Donakonda, S., Filpe, P., Schneider, A., Laschinger, M., et al. (2021). Auto-aggressive CXCR6+ CD8 T cells cause liver immune pathology in NASH. Nature 592 (7854), 444–449. doi:10.1038/s41586-021-03233-8
Durham, G. A., Williams, J. J. L., Nasim, M. T., and Palmer, T. M. (2019). Targeting SOCS proteins to control JAK-STAT signalling in disease. Trends Pharmacol. Sci. 40 (5), 298–308. doi:10.1016/j.tips.2019.03.001
Elia, G., and Fallahi, P. (2017). Hepatocellular carcinoma and CXCR3 chemokines: a narrative review. La Clin. Ter. 168 (1), e37–e41. doi:10.7417/ct.2017.1980
El-Khoueiry, A. B., Sangro, B., Yau, T., Crocenzi, T. S., Kudo, M., Hsu, C., et al. (2017). Nivolumab in patients with advanced hepatocellular carcinoma (CheckMate 040): an open-label, non-comparative, phase 1/2 dose escalation and expansion trial. Lancet 389 (10088), 2492–2502. doi:10.1016/S0140-6736(17)31046-2
Fan, Q. M., Jing, Y. Y., Yu, G. F., Kou, X. R., Ye, F., Gao, L., et al. (2014). Tumor-associated macrophages promote cancer stem cell-like properties via transforming growth factor-beta1-induced epithelial-mesenchymal transition in hepatocellular carcinoma. Cancer Lett. 352 (2), 160–168. doi:10.1016/j.canlet.2014.05.008
Fang, W., Zhou, T., Shi, H., Yao, M., Zhang, D., Qian, H., et al. (2021). Progranulin induces immune escape in breast cancer via up-regulating PD-L1 expression on tumor-associated macrophages (TAMs) and promoting CD8(+) T cell exclusion. J. Exp. Clin. Cancer Res. 40 (1), 4. doi:10.1186/s13046-020-01786-6
Flavell, R. A., Sanjabi, S., Wrzesinski, S. H., and Licona-Limón, P. (2010). The polarization of immune cells in the tumour environment by TGFbeta. Nat. Rev. Immunol. 10 (8), 554–567. doi:10.1038/nri2808
Fridlender, Z. G., Sun, J., Kim, S., Kapoor, V., Cheng, G., Ling, L., et al. (2009). Polarization of tumor-associated neutrophil phenotype by TGF-beta: "N1" versus "N2" TAN. Cancer Cell 16 (3), 183–194. doi:10.1016/j.ccr.2009.06.017
Fu, Q., Xu, L., Wang, Y., Jiang, Q., Liu, Z., Zhang, J., et al. (2019). Tumor-associated macrophage-derived interleukin-23 interlinks kidney cancer glutamine addiction with immune evasion. Eur. Urol. 75 (5), 752–763. doi:10.1016/j.eururo.2018.09.030
Giesbrecht, K., Förmer, S., Sähr, A., Heeg, K., and Hildebrand, D. (2019). Streptococcal pyrogenic exotoxin A-stimulated monocytes mediate regulatory T-cell accumulation through PD-L1 and kynurenine. Int. J. Mol. Sci. 20 (16), 3933. doi:10.3390/ijms20163933
Gomez-Roca, C. A., Italiano, A., Le Tourneau, C., Cassier, P. A., Toulmonde, M., D'Angelo, S. P., et al. (2019). Phase I study of emactuzumab single agent or in combination with paclitaxel in patients with advanced/metastatic solid tumors reveals depletion of immunosuppressive M2-like macrophages. Ann. Oncol. 30, 1381–1392. doi:10.1093/annonc/mdz163
Gordon, E. J., Rao, S., Pollard, J. W., Nutt, S. L., Lang, R. A., and Harvey, N. L. (2010). Macrophages define dermal lymphatic vessel calibre during development by regulating lymphatic endothelial cell proliferation. Development 137 (22), 3899–3910. doi:10.1242/dev.050021
Gordon, S. R., Maute, R. L., Dulken, B. W., Hutter, G., George, B. M., McCracken, M. N., et al. (2017). PD-1 expression by tumour-associated macrophages inhibits phagocytosis and tumour immunity. Nature 545 (7655), 495–499. doi:10.1038/nature22396
Guex, N., Crespo, I., Bron, S., Ifticene-Treboux, A., Faes-Van't Hull, E., Kharoubi, S., et al. (2015). Angiogenic activity of breast cancer patients' monocytes reverted by combined use of systems modeling and experimental approaches. PLoS Comput. Biol. 11 (3), e1004050. doi:10.1371/journal.pcbi.1004050
Guo, B., Li, L., Guo, J., Liu, A., Wu, J., Wang, H., et al. (2017). M2 tumor-associated macrophages produce interleukin-17 to suppress oxaliplatin-induced apoptosis in hepatocellular carcinoma. Oncotarget 8 (27), 44465–44476. doi:10.18632/oncotarget.17973
Ham, J. S., Park, H. Y., Ryu, K. J., Ko, Y. H., Kim, W. S., and Kim, S. J. (2017). Elevated serum interleukin-10 level and M2 macrophage infiltration are associated with poor survival in angioimmunoblastic T-cell lymphoma. Oncotarget 8 (44), 76231–76240. doi:10.18632/oncotarget.19301
Hamanishi, J., Mandai, M., Matsumura, N., Abiko, K., Baba, T., and Konishi, I. (2016). PD-1/PD-L1 blockade in cancer treatment: perspectives and issues. Int. J. Clin. Oncol. 21 (3), 462–473. doi:10.1007/s10147-016-0959-z
Han, S., Wang, W., Wang, S., Yang, T., Zhang, G., Wang, D., et al. (2021). Tumor microenvironment remodeling and tumor therapy based on M2-like tumor associated macrophage-targeting nano-complexes. Theranostics 11 (6), 2892–2916. doi:10.7150/thno.50928
Hefetz-Sela, S., Stein, I., Klieger, Y., Porat, R., Sade-Feldman, M., Zreik, F., et al. (2014). Acquisition of an immunosuppressive protumorigenic macrophage phenotype depending on c-Jun phosphorylation. Proc. Natl. Acad. Sci. U. S. A. 111 (49), 17582–17587. doi:10.1073/pnas.1409700111
Horlad, H., Ma, C., Yano, H., Pan, C., Ohnishi, K., Fujiwara, Y., et al. (2016). An IL-27/Stat3 axis induces expression of programmed cell death 1 ligands (PD-L1/2) on infiltrating macrophages in lymphoma. Cancer Sci. 107 (11), 1696–1704. doi:10.1111/cas.13065
Hsu, J., Hodgins, J. J., Marathe, M., Nicolai, C. J., Bourgeois-Daigneault, M. C., Trevino, T. N., et al. (2018). Contribution of NK cells to immunotherapy mediated by PD-1/PD-L1 blockade. J. Clin. Invest. 128 (10), 4654–4668. doi:10.1172/JCI99317
Huang, S., Zhou, D., Li, Y.-X., Ming, Z.-Y., Li, K.-Z., Wu, G.-B., et al. (2019). Retracted: in vivo and in vitro effects of microRNA-221 on hepatocellular carcinoma development and progression through the JAK–STAT3 signaling pathway by targeting SOCS3. J. Cell Physiol. 234 (4), 3500–3514. doi:10.1002/jcp.26863
Huang, Y., Erdmann, N., Zhao, J., and Zheng, J. (2005). The signaling and apoptotic effects of TNF-related apoptosis-inducing ligand in HIV-1 associated dementia. Neurotox. Res. 8 (1), 135–148. doi:10.1007/BF03033825
Hutchinson, L. (2017). Exploiting PD-1 on TAMs for tumour cell kill. Nat. Rev. Clin. Oncol. 14 (7), 393. doi:10.1038/nrclinonc.2017.87
Hwang, I., Kim, J. W., Ylaya, K., Chung, E. J., Kitano, H., Perry, C., et al. (2020). Tumor-associated macrophage, angiogenesis and lymphangiogenesis markers predict prognosis of non-small cell lung cancer patients. J. Transl. Med. 18 (1), 443. doi:10.1186/s12967-020-02618-z
Iurca, I., Tirpe, A., Zimta, A.-A., Moldovan, C., Gulei, D., Slabý, O., et al. (2020). Macrophages interaction and MicroRNA interplay in the modulation of cancer development and metastasis. Front. Immunol. 11, 870. doi:10.3389/fimmu.2020.00870
Jeong, H., Kim, S., Hong, B. J., Lee, C. J., Kim, Y. E., Bok, S., et al. (2019). Tumor-associated macrophages enhance tumor hypoxia and aerobic glycolysis. Cancer Res. 79 (4), 795–806. doi:10.1158/0008-5472.Can-18-2545
Jia, Y., Zeng, Z., Li, Y., Li, Z., Jin, L., Zhang, Z., et al. (2015). Impaired function of CD4+ T follicular helper (Tfh) cells associated with hepatocellular carcinoma progression. PLoS One 10 (2), e0117458–e. doi:10.1371/journal.pone.0117458
Jiang, Q., Zeng, Y., Xu, Y., Xiao, X., Liu, H., Zhou, B., et al. (2020). Ultrasound molecular imaging as a potential non-invasive diagnosis to detect the margin of hepatocarcinoma via CSF-1R targeting. Front. Bioeng. Biotechnol. 8, 783. doi:10.3389/fbioe.2020.00783
Jürgens, B., Hainz, U., Fuchs, D., Felzmann, T., and Heitger, A. (2009). Interferon-gamma-triggered indoleamine 2,3-dioxygenase competence in human monocyte-derived dendritic cells induces regulatory activity in allogeneic T cells. Blood 114 (15), 3235–3243. doi:10.1182/blood-2008-12-195073
Kang, T.-W., Yevsa, T., Woller, N., Hoenicke, L., Wuestefeld, T., Dauch, D., et al. (2011). Senescence surveillance of pre-malignant hepatocytes limits liver cancer development. Nature 479 (7374), 547–551. doi:10.1038/nature10599
Kapp, K., Volz, B., Oswald, D., Wittig, B., Baumann, M., and Schmidt, M. (2019). Beneficial modulation of the tumor microenvironment and generation of anti-tumor responses by TLR9 agonist lefitolimod alone and in combination with checkpoint inhibitors. Oncoimmunology 8 (12), e1659096. doi:10.1080/2162402X.2019.1659096
Komohara, Y., Fujiwara, Y., Ohnishi, K., and Takeya, M. (2016). Tumor-associated macrophages: potential therapeutic targets for anti-cancer therapy. Adv. Drug Deliv. Rev. 99 (Pt B), 180–185. doi:10.1016/j.addr.2015.11.009
Krieg, M., Haas, R., Brauch, H., Acker, T., Flamme, I., and Plate, K. H. (2000). Up-regulation of hypoxia-inducible factors HIF-1alpha and HIF-2alpha under normoxic conditions in renal carcinoma cells by von Hippel-Lindau tumor suppressor gene loss of function. Oncogene 19 (48), 5435–5443. doi:10.1038/sj.onc.1203938
Kryczek, I., Wei, S., Zhu, G., Myers, L., Mottram, P., Cheng, P., et al. (2007). Relationship between B7-H4, regulatory T cells, and patient outcome in human ovarian carcinoma. Cancer Res. 67 (18), 8900–8905. doi:10.1158/0008-5472.CAN-07-1866
Kuang, D. M., Zhao, Q., Peng, C., Xu, J., Zhang, J. P., Wu, C., et al. (2009). Activated monocytes in peritumoral stroma of hepatocellular carcinoma foster immune privilege and disease progression through PD-L1. J. Exp. Med. 206 (6), 1327–1337. doi:10.1084/jem.20082173
Kumari, N., and Choi, S. H. (2022). Tumor-associated macrophages in cancer: recent advancements in cancer nanoimmunotherapies. J. Exp. Clin. Cancer Res. 41 (1), 68. doi:10.1186/s13046-022-02272-x
Kwantwi, L. B. (2023). Overcoming anti-PD-1/PD-L1 immune checkpoint blockade resistance: the role of macrophage, neutrophils and mast cells in the tumor microenvironment. Clin. Exp. Med. 23 (7), 3077–3091. doi:10.1007/s10238-023-01059-4
Lai, Q., Vitale, A., Manzia, T. M., Foschi, F. G., Levi Sandri, G. B., Gambato, M., et al. (2019). Platelets and hepatocellular cancer: bridging the bench to the clinics. Cancers (Basel) 11 (10), 1568. doi:10.3390/cancers11101568
Leung, C., Yeoh, S. W., Patrick, D., Ket, S., Marion, K., Gow, P., et al. (2015). Characteristics of hepatocellular carcinoma in cirrhotic and non-cirrhotic non-alcoholic fatty liver disease. World J. Gastroenterol. 21 (4), 1189–1196. doi:10.3748/wjg.v21.i4.1189
Li, C. W., Lai, Y. J., Hsu, J. L., and Hung, M. C. (2018a). Activation of phagocytosis by immune checkpoint blockade. Front. Med. 12 (4), 473–480. doi:10.1007/s11684-018-0657-5
Li, D.-Q., Qiu, M., Nie, X.-M., Gui, R., and Huang, M.-Z. (2016). Oxidored-nitro domain-containing protein 1 expression is associated with the progression of hepatocellular carcinoma. Oncol. Lett. 11 (5), 3003–3008. doi:10.3892/ol.2016.4362
Li, G., Liu, D., Kimchi, E. T., Kaifi, J. T., Qi, X., Manjunath, Y., et al. (2018b). Nanoliposome C6-ceramide increases the anti-tumor immune response and slows growth of liver tumors in mice. Gastroenterology 154 (4), 1024–1036. doi:10.1053/j.gastro.2017.10.050
Li, W., Wu, F., Zhao, S., Shi, P., Wang, S., and Cui, D. (2022a). Correlation between PD-1/PD-L1 expression and polarization in tumor-associated macrophages: a key player in tumor immunotherapy. Cytokine Growth Factor Rev. 67, 49–57. doi:10.1016/j.cytogfr.2022.07.004
Li, X., Yao, W., Yuan, Y., Chen, P., Li, B., Li, J., et al. (2017). Targeting of tumour-infiltrating macrophages via CCL2/CCR2 signalling as a therapeutic strategy against hepatocellular carcinoma. Gut 66 (1), 157–167. doi:10.1136/gutjnl-2015-310514
Li, Z., Ding, Y., Liu, J., Wang, J., Mo, F., Wang, Y., et al. (2022b). Depletion of tumor associated macrophages enhances local and systemic platelet-mediated anti-PD-1 delivery for post-surgery tumor recurrence treatment. Nat. Commun. 13 (1), 1845. doi:10.1038/s41467-022-29388-0
Li, Z., Li, H., Zhao, Z. B., Zhu, W., Feng, P. P., Zhu, X. W., et al. (2019b). SIRT4 silencing in tumor-associated macrophages promotes HCC development via PPARδ signalling-mediated alternative activation of macrophages. J. Exp. Clin. Cancer Res. 38 (1), 469. doi:10.1186/s13046-019-1456-9
Li, Z., Wu, T., Zheng, B., and Chen, L. (2019a). Individualized precision treatment: targeting TAM in HCC. Cancer Lett. 458, 86–91. doi:10.1016/j.canlet.2019.05.019
Liu, C., Chikina, M., Deshpande, R., Menk, A. V., Wang, T., Tabib, T., et al. (2019b). Treg cells promote the SREBP1-dependent metabolic fitness of tumor-promoting macrophages via repression of CD8+ T cellderived interferon-γ. Immunity 51, 381–397. doi:10.1016/j.immuni.2019.06.017
Liu, J., Fan, L., Yu, H., Zhang, J., He, Y., Feng, D., et al. (2019a). Endoplasmic reticulum stress causes liver cancer cells to release exosomal miR-23a-3p and up-regulate programmed death ligand 1 expression in macrophages. Hepatol. Baltim. Md 70 (1), 241–258. doi:10.1002/hep.30607
Liu, R.-X., Wei, Y., Zeng, Q.-H., Chan, K.-W., Xiao, X., Zhao, X.-Y., et al. (2015b). Chemokine (C-X-C motif) receptor 3–positive B cells link interleukin-17 inflammation to protumorigenic macrophage polarization in human hepatocellular carcinoma. Hepatology 62 (6), 1779–1790. doi:10.1002/hep.28020
Liu, T., Xu, L., He, L., Zhao, J., Chen, T., Chen, Q., et al. (2020). Selenium nanoparticles regulates selenoprotein to boost cytokine-induced killer cells-based cancer immunotherapy. Nano Today 35, 100975. doi:10.1016/j.nantod.2020.100975
Liu, Y., Yan, W., Tohme, S., Chen, M., Fu, Y., Tian, D., et al. (2015a). Hypoxia induced HMGB1 and mitochondrial DNA interactions mediate tumor growth in hepatocellular carcinoma through Toll-like receptor 9. J. Hepatol. 63 (1), 114–121. doi:10.1016/j.jhep.2015.02.009
Llovet, J. M., Zucman-Rossi, J., Pikarsky, E., Sangro, B., Schwartz, M., Sherman, M., et al. (2016). Hepatocellular carcinoma. Nat. Rev. Dis. Prim. 2, 16018. doi:10.1038/nrdp.2016.18
Lo Russo, G., Moro, M., Sommariva, M., Cancila, V., Boeri, M., Centonze, G., et al. (2019). Antibody–fc/FcR interaction on macrophages as a mechanism for hyperprogressive disease in non–small cell lung cancer subsequent to PD-1/PD-L1 blockade. Clin. Cancer Res. 25 (3), 989–999. doi:10.1158/1078-0432.CCR-18-1390
Lu, H., Clauser, K. R., Tam, W. L., Fröse, J., Ye, X., Eaton, E. N., et al. (2014). A breast cancer stem cell niche supported by juxtacrine signalling from monocytes and macrophages. Nat. Cell Biol. 16 (11), 1105–1117. doi:10.1038/ncb3041
Lu, J., Xu, Y., Wu, Y., Huang, X.-Y., Xie, J.-W., Wang, J.-B., et al. (2019). Tumor-infiltrating CD8+ T cells combined with tumor-associated CD68+ macrophages predict postoperative prognosis and adjuvant chemotherapy benefit in resected gastric cancer. BMC Cancer 19 (1), 920. doi:10.1186/s12885-019-6089-z
Lu, X., Wo, G., Li, B., Xu, C., Wu, J., Jiang, C., et al. (2018). The anti-inflammatory NHE-06 restores antitumor immunity by targeting NF-κB/IL-6/STAT3 signaling in hepatocellular carcinoma. Biomed. Pharmacother. 102, 420–427. doi:10.1016/j.biopha.2018.03.099
Ma, P. F., Gao, C. C., Yi, J., Zhao, J. L., Liang, S. Q., Zhao, Y., et al. (2017). Cytotherapy with M1-polarized macrophages ameliorates liver fibrosis by modulating immune microenvironment in mice. J. Hepatol. 67, 770–779. doi:10.1016/j.jhep.2017.05.022
Ma, Y. S., Wu, T. M., Ling, C. C., Yu, F., Zhang, J., Cao, P. S., et al. (2021). M2 macrophage-derived exosomal microRNA-155-5p promotes the immune escape of colon cancer by downregulating ZC3H12B. Mol. Ther. oncolytics 20, 484–498. doi:10.1016/j.omto.2021.02.005
Mannino, M. H., Zhu, Z., Xiao, H., Bai, Q., Wakefield, M. R., and Fang, Y. (2015). The paradoxical role of IL-10 in immunity and cancer. Cancer Lett. 367 (2), 103–107. doi:10.1016/j.canlet.2015.07.009
Mao, J., Wang, D., Wang, Z., Tian, W., Li, X., Duan, J., et al. (2016a). Combretastatin A-1 phosphate, a microtubule inhibitor, acts on both hepatocellular carcinoma cells and tumor-associated macrophages by inhibiting the Wnt/β-catenin pathway. Cancer Lett. 380 (1), 134–143. doi:10.1016/j.canlet.2016.06.020
Mao, Y., Eissler, N., Blanc, K. L., Johnsen, J. I., Kogner, P., and Kiessling, R. (2016b). Targeting suppressive myeloid cells potentiates checkpoint inhibitors to ControlSpontaneous neuroblastoma. Clin. Cancer Res. 22, 3849–3859. doi:10.1158/1078-0432.CCR-15-1912
Maute, R. L., Gordon, S. R., Mayer, A. T., McCracken, M. N., Natarajan, A., Ring, N. G., et al. (2015). Engineering high-affinity PD-1 variants for optimized immunotherapy and immuno-PET imaging. Proc. Natl. Acad. Sci. U. S. A. 112 (47), E6506–E6514. doi:10.1073/pnas.1519623112
McGettrick, A. F., and O’Neill, L. A. J. (2020). The role of HIF in immunity and inflammation. Cell Metab. 32 (4), 524–536. doi:10.1016/j.cmet.2020.08.002
Meng, Y.-M., Liang, J., Wu, C., Xu, J., Zeng, D.-N., Yu, X.-J., et al. (2017). Monocytes/Macrophages promote vascular CXCR4 expression via the ERK pathway in hepatocellular carcinoma. Oncoimmunology 7 (3), e1408745. doi:10.1080/2162402X.2017.1408745
Mills, C. D., Kincaid, K., Alt, J. M., Heilman, M. J., and Hill, A. M. (2017). Pillars article: M-1/M-2 macrophages and the Th1/Th2 paradigm. J. Immunol. 2000. 164: 6166-6173. J. Immunol. 199 (7), 2194–2201. doi:10.4049/jimmunol.1701141
Moreno-Cubero, E., and Larrubia, J.-R. (2016). Specific CD8(+) T cell response immunotherapy for hepatocellular carcinoma and viral hepatitis. World J. Gastroenterol. 22 (28), 6469–6483. doi:10.3748/wjg.v22.i28.6469
Morrissey, S. M., Zhang, F., Ding, C., Montoya-Durango, D. E., Hu, X., Yang, C., et al. (2021). Tumor-derived exosomes drive immunosuppressive macrophages in a pre-metastatic niche through glycolytic dominant metabolic reprogramming. Cell Metab. 33 (10), 2040–2058.e10. doi:10.1016/j.cmet.2021.09.002
Muraoka, D., Seo, N., Hayashi, T., Tahara, Y., Fujii, K., Tawara, I., et al. (2019). Antigen delivery targeted to tumor-associated macrophages overcomes tumor immune resistance. J. Clin. Invest. 129 (3), 1278–1294. doi:10.1172/JCI97642
Murdoch, C., Muthana, M., and Lewis, C. E. (2005). Hypoxia regulates macrophage functions in inflammation. J. Immunol. 175 (10), 6257–6263. doi:10.4049/jimmunol.175.10.6257
Nielsen, S. R., Quaranta, V., Linford, A., Emeagi, P., Rainer, C., Santos, A., et al. (2016). Macrophage-secreted granulin supports pancreatic cancer metastasis by inducing liver fibrosis. Nat. Cell Biol. 18 (5), 549–560. doi:10.1038/ncb3340
Oosterling, S. J., van der Bij, G. J., Meijer, G. A., Tuk, C. W., van Garderen, E., van Rooijen, N., et al. (2005). Macrophages direct tumour histology and clinical outcome in a colon cancer model. J. Pathol. 207 (2), 147–155. doi:10.1002/path.1830
Orecchioni, M., Ghosheh, Y., Pramod, A. B., and Ley, K. (2019). Macrophage polarization: different gene signatures in M1(LPS+) vs. Classically and M2(LPS-) vs. Alternatively activated macrophages. Front. Immunol. 10, 1084. doi:10.3389/fimmu.2019.01084
Oura, R., Arakaki, R., Yamada, A., Kudo, Y., Tanaka, E., Hayashi, Y., et al. (2013). Induction of rapid T cell death and phagocytic activity by Fas-deficient lpr macrophages. J. Immunol. 190 (2), 578–585. doi:10.4049/jimmunol.1103794
Pan, S., Yu, Y., Wang, S., Tu, B., Shen, Y., Qiu, Q., et al. (2022). Correlation of HBV DNA and hepatitis B surface antigen levels with tumor response, liver function and immunological indicators in liver cancer patients with HBV infection undergoing PD-1 inhibition combinational therapy. Front. Immunol. 13, 892618. doi:10.3389/fimmu.2022.892618
Park, D. J., Sung, P. S., Kim, J. H., Lee, G. W., Jang, J. W., Jung, E. S., et al. (2020). EpCAM-high liver cancer stem cells resist natural killer cellmediated cytotoxicity by upregulating CEACAM1. J. Immunother. Cancer 8, e000301. doi:10.1136/jitc-2019-000301
Park, D. J., Sung, P. S., Lee, G. W., Cho, S., Kim, S. M., Kang, B. Y., et al. (2021). Preferential expression of programmed death ligand 1 protein in tumor-associated macrophages and its potential role in immunotherapy for hepatocellular carcinoma. Int. J. Mol. Sci. 22 (9), 4710. doi:10.3390/ijms22094710
Parker, K. H., Sinha, P., Horn, L. A., Clements, V. K., Yang, H., Li, J., et al. (2014). HMGB1 enhances immune suppression by facilitating the differentiation and suppressive activity of myeloid-derived suppressor cells. Cancer Res. 74 (20), 5723–5733. doi:10.1158/0008-5472.Can-13-2347
Parry, R. V., Chemnitz, J. M., Frauwirth, K. A., Lanfranco, A. R., Braunstein, I., Kobayashi, S. V., et al. (2005). CTLA-4 and PD-1 receptors inhibit T-cell activation by distinct mechanisms. Mol. Cell Biol. 25 (21), 9543–9553. doi:10.1128/MCB.25.21.9543-9553.2005
Peinado, H., Olmeda, D., and Snail, C. A. (2007). Zeb and bHLH factors in tumour progression: an alliance against the epithelial phenotype? Nat. Rev. Cancer 7 (6), 415–428. doi:10.1038/nrc2131
Peng, S.-H., Deng, H., Yang, J.-F., Xie, P.-P., Li, C., Li, H., et al. (2005). Significance and relationship between infiltrating inflammatory cell and tumor angiogenesis in hepatocellular carcinoma tissues. World J. Gastroenterol. 11 (41), 6521–6524. doi:10.3748/wjg.v11.i41.6521
Peranzoni, E., Lemoine, J., Vimeux, L., Feuillet, V., Barrin, S., Kantari Mimoun, C., et al. (2018). Macrophages impede CD8 T cells from reaching tumor cells and limit the efficacy of anti-PD-1 treatment. Proc. Natl. Acad. Sci. U. S. A. 115 (17), E4041–E4050. doi:10.1073/pnas.1720948115
Petty, A. J., and Yang, Y. (2017). Tumor-associated macrophages: implications in cancer immunotherapy. Immunotherapy 9 (3), 289–302. doi:10.2217/imt-2016-0135
Pfister, D., Nunez, N. G., Pinyol, R., Govaere, O., Pinter, M., Szydlowska, M., et al. (2021). NASH limits anti-tumour surveillance in immunotherapy-treated HCC. Nature 592 (7854), 450–456. doi:10.1038/s41586-021-03362-0
Pitt Jonathan, M., Vétizou, M., Daillère, R., Roberti María, P., Yamazaki, T., Routy, B., et al. (2016). Resistance mechanisms to immune-checkpoint blockade in cancer: tumor-intrinsic and -extrinsic factors. Immunity 44 (6), 1255–1269. doi:10.1016/j.immuni.2016.06.001
Prima, V., Kaliberova, L. N., Kaliberov, S., Curiel, D. T., and Kusmartsev, S. (2017). COX2/mPGES1/PGE2 pathway regulates PD-L1 expression in tumor-associated macrophages and myeloid-derived suppressor cells. Proc. Natl. Acad. Sci. U. S. A. 114 (5), 1117–1122. doi:10.1073/pnas.1612920114
Pu, D., Yin, L., Zhou, Y., Li, W., Huang, L., Cai, L., et al. (2020). Safety and efficacy of immune checkpoint inhibitors in patients with HBV/HCV infection and advanced-stage cancer: a systematic review. Med. Baltim. 99 (5), e19013. doi:10.1097/MD.0000000000019013
Pu, J., Xu, Z., Nian, J., Fang, Q., Yang, M., Huang, Y., et al. (2021). M2 macrophage-derived extracellular vesicles facilitate CD8+T cell exhaustion in hepatocellular carcinoma via the miR-21–5p/YOD1/YAP/β-catenin pathway. Cell Death Discov. 7 (1), 182. doi:10.1038/s41420-021-00556-3
Pyonteck, S. M., Akkari, L., Schuhmacher, A. J., Bowman, R. L., Sevenich, L., Quail, D. F., et al. (2013). CSF-1R inhibition alters macrophage polarization and blocks glioma progression. Nat. Med. 19 (10), 1264–1272. doi:10.1038/nm.3337
Qiu, Y., Chen, T., Hu, R., Zhu, R., Li, C., Ruan, Y., et al. (2021). Next frontier in tumor immunotherapy: macrophage-mediated immune evasion. Biomark. Res. 9 (1), 72. doi:10.1186/s40364-021-00327-3
Quaranta, V., Rainer, C., Nielsen, S. R., Raymant, M. L., Ahmed, M. S., Engle, D. D., et al. (2018). Macrophage-derived granulin drives resistance to immune checkpoint inhibition in metastatic pancreatic cancer. Cancer Res. 78 (15), 4253–4269. doi:10.1158/0008-5472.CAN-17-3876
Ren, W., Wu, S., Wu, Y., Liu, T., Zhao, X., and Li, Y. (2019). MicroRNA-196a/-196b regulate the progression of hepatocellular carcinoma through modulating the JAK/STAT pathway via targeting SOCS2. Cell Death Dis. 10 (5), 333. doi:10.1038/s41419-019-1530-4
Ringelhan, M., Pfister, D., O’Connor, T., Pikarsky, E., and Heikenwalder, M. (2018). The immunology of hepatocellular carcinoma. Nat. Immunol. 19 (3), 222–232. doi:10.1038/s41590-018-0044-z
Rodell, C. B., Arlauckas, S. P., Cuccarese, M. F., Garris, C. S., Li, R., Ahmed, M. S., et al. (2018). TLR7/8-agonist-loaded nanoparticles promote the polarization of tumour-associated macrophages to enhance cancer immunotherapy. Nat. Biomed. Eng. 2 (8), 578–588. doi:10.1038/s41551-018-0236-8
Roy, S., Sunkara, R. R., Parmar, M. Y., Shaikh, S., and Waghmare, S. K. (2021). EMT imparts cancer stemness and plasticity: new perspectives and therapeutic potential. Front. Biosci. 26 (2), 238–265. doi:10.2741/4893
Ruan, J., Ouyang, M., Zhang, W., Luo, Y., and Zhou, D. (2021). The effect of PD-1 expression on tumor-associated macrophage in T cell lymphoma. Clin. Transl. Oncol. 23 (6), 1134–1141. doi:10.1007/s12094-020-02499-0
Ruffell, B., and Coussens, L. M. (2015). Macrophages and therapeutic resistance in cancer. Cancer Cell 27 (4), 462–472. doi:10.1016/j.ccell.2015.02.015
Sahraei, M., Chaube, B., Liu, Y., Sun, J., Kaplan, A., Price, N. L., et al. (2019). Suppressing miR-21 activity in tumor-associated macrophages promotes an antitumor immune response. J. Clin. investigation 129 (12), 5518–5536. doi:10.1172/jci127125
Santarpia, M., González-Cao, M., Viteri, S., Karachaliou, N., Altavilla, G., and Rosell, R. (2015). Programmed cell death protein-1/programmed cell death ligand-1 pathway inhibition and predictive biomarkers: understanding transforming growth factor-beta role. Transl. Lung Cancer Res. 4 (6), 728–742. doi:10.3978/j.issn.2218-6751.2015.12.04
Sharma, P., Hu-Lieskovan, S., Wargo, J. A., and Ribas, A. (2017). Primary, adaptive, and acquired resistance to cancer immunotherapy. Cell 168 (4), 707–723. doi:10.1016/j.cell.2017.01.017
Shi, G., Yang, Q., Zhang, Y., Jiang, Q., Lin, Y., Yang, S., et al. (2019). Modulating the tumor microenvironment via oncolytic viruses and CSF-1r inhibition synergistically enhances anti-PD-1 immunotherapy. Mol. Ther. 27, 244–260. doi:10.1016/j.ymthe.2018.11.010
Sica, A., Allavena, P., and Mantovani, A. (2008). Cancer related inflammation: the macrophage connection. Cancer Lett. 267 (2), 204–215. doi:10.1016/j.canlet.2008.03.028
Sica, A., and Mantovani, A. (2012). Macrophage plasticity and polarization: in vivo veritas. J. Clin. Invest. 122 (3), 787–795. doi:10.1172/jci59643
Singh, N., Baby, D., Rajguru, J. P., Patil, P. B., Thakkannavar, S. S., and Pujari, V. B. (2019). Inflammation and cancer. Ann. Afr. Med. 18 (3), 121–126. doi:10.4103/aam.aam_56_18
Sprinzl, M. F., Reisinger, F., Puschnik, A., Ringelhan, M., Ackermann, K., Hartmann, D., et al. (2013). Sorafenib perpetuates cellular anticancer effector functions by modulating the crosstalk between macrophages and natural killer cells. Hepatology 57 (6), 2358–2368. doi:10.1002/hep.26328
Su, S., Liu, Q., Chen, J., Chen, J., Chen, F., He, C., et al. (2014). A positive feedback loop between mesenchymal-like cancer cells and macrophages is essential to breast cancer metastasis. Cancer Cell 25 (5), 605–620. doi:10.1016/j.ccr.2014.03.021
Sun, J. Y., Zhang, D., Wu, S., Xu, M., Zhou, X., Lu, X. J., et al. (2020). Resistance to PD-1/PD-L1 blockade cancer immunotherapy: mechanisms, predictive factors, and future perspectives. Biomark. Res. 8, 35. doi:10.1186/s40364-020-00212-5
Sun, X., Zhang, T., Li, M., Yin, L., and Xue, J. (2022). Immunosuppressive B cells expressing PD-1/PD-L1 in solid tumors: a mini review. QJM 115 (8), 507–512. doi:10.1093/qjmed/hcz162
Sung, P. S., and Jang, J. W. (2018). Natural killer cell dysfunction in hepatocellular carcinoma: pathogenesis and clinical implications. Int. J. Mol. Sci. 19, 3648. doi:10.3390/ijms19113648
Swisher, J. F. A., Haddad, D. A., McGrath, A. G., Boekhoudt, G. H., and Feldman, G. M. (2014). IgG4 can induce an M2-like phenotype in human monocyte-derived macrophages through FcγRI. MAbs 6 (6), 1377–1384. doi:10.4161/19420862.2014.975657
Szebeni, G. J., Vizler, C., Kitajka, K., and Puskas, L. G. (2017). Inflammation and cancer: extra- and intracellular determinants of tumor-associated macrophages as tumor promoters. Mediat. Inflamm. 2017, 9294018. doi:10.1155/2017/9294018
Teng, K. Y., Han, J., Zhang, X., Hsu, S. H., He, S., Wani, N. A., et al. (2017). Blocking the CCL2-CCR2 Axis using CCL2-neutralizing antibody is an effective therapy for hepatocellular cancer in a mouse model. Mol. Cancer Ther. 16 (2), 312–322. doi:10.1158/1535-7163.Mct-16-0124
Tierney, J. F., Vogle, A., Finnerty, B., Zarnegar, R., Ghai, R., Gattuso, P., et al. (2020). Indoleamine 2,3-dioxygenase-1 expression in adrenocortical carcinoma. J. Surg. Res. 256, 90–95. doi:10.1016/j.jss.2020.06.016
Toor, S. M., Sasidharan, N. V., Decock, J., and Elkord, E. (2020). Immune checkpoints in the tumor microenvironment. Semin. Cancer Biol. 65, 1–12. doi:10.1016/j.semcancer.2019.06.021
Tosello-Trampont, A.-C., Krueger, P., Narayanan, S., Landes, S. G., Leitinger, N., and Hahn, Y. S. (2016). NKp46(+) natural killer cells attenuate metabolism-induced hepatic fibrosis by regulating macrophage activation in mice. Hepatol. Baltim. Md 63 (3), 799–812. doi:10.1002/hep.28389
Toulmonde, M., and Italiano, A. (2018). PD-1 inhibition in sarcoma still needs investigation. Lancet Oncol. 19 (1), e6. doi:10.1016/S1470-2045(17)30921-X
Toulmonde, M., Penel, N., Adam, J., Chevreau, C., Blay, J. Y., Le Cesne, A., et al. (2018). Use of PD-1 targeting, macrophage infiltration, and Ido pathway activation in sarcomas: a phase 2 clinical trial. JAMA Oncol. 4 (1), 93–97. doi:10.1001/jamaoncol.2017.1617
Tsai, H.-F., Lai, J.-J., Chou, A.-H., Wang, T.-F., Wu, C.-S., and Hsu, P.-N. (2004). Induction of costimulation of human CD4 T cells by tumor necrosis factor–related apoptosis-inducing ligand: possible role in T cell activation in systemic lupus erythematosus. Arthritis Rheum. 50 (2), 629–639. doi:10.1002/art.20038
Van Rooijen, N., Kors, N., vd Ende, M., and Dijkstra, C. D. (1990). Depletion and repopulation of macrophages in spleen and liver of rat after intravenous treatment with liposome-encapsulated dichloromethylene diphosphonate. Cell Tissue Res. 260 (2), 215–222. doi:10.1007/bf00318625
Van Rooijen, N., and Sanders, A. (1994). Liposome mediated depletion of macrophages: mechanism of action, preparation of liposomes and applications. J. Immunol. Methods 174 (1-2), 83–93. doi:10.1016/0022-1759(94)90012-4
Villanueva, M. T. (2017). Immunotherapy: macrophages steal the show. Nat. Rev. Cancer 17 (7), 396–397. doi:10.1038/nrc.2017.47
Vitale, I., Manic, G., Coussens, L. M., Kroemer, G., and Galluzzi, L. (2019). Macrophages and metabolism in the tumor microenvironment. Cell Metab. 30 (1), 36–50. doi:10.1016/j.cmet.2019.06.001
Wan, S., Zhao, E., Kryczek, I., Vatan, L., Sadovskaya, A., Ludema, G., et al. (2014). Tumor-associated macrophages produce interleukin 6 and signal via STAT3 to promote expansion of human hepatocellular carcinoma stem cells. Gastroenterology 147 (6), 1393–1404. doi:10.1053/j.gastro.2014.08.039
Wang, C., Ma, C., Gong, L., Guo, Y., Fu, K., Zhang, Y., et al. (2021d). Macrophage polarization and its role in liver disease. Front. Immunol. 12, 803037. doi:10.3389/fimmu.2021.803037
Wang, X., Liu, Z., Sun, J., Song, X., Bian, M., Wang, F., et al. (2021a). Inhibition of NADPH oxidase 4 attenuates lymphangiogenesis and tumor metastasis in breast cancer. Faseb J. 35 (4), e21531. doi:10.1096/fj.202002533R
Wang, X. F., Wang, H. S., Wang, H., Zhang, F., Wang, K. F., Guo, Q., et al. (2014). The role of indoleamine 2,3-dioxygenase (Ido) in immune tolerance: focus on macrophage polarization of THP-1 cells. Cell Immunol. 289 (1-2), 42–48. doi:10.1016/j.cellimm.2014.02.005
Wang, Y., Tiruthani, K., Li, S., Hu, M., Zhong, G., Tang, Y., et al. (2021c). mRNA delivery of a bispecific single-domain antibody to polarize tumor-associated macrophages and synergize immunotherapy against liver malignancies. Adv. Mater 33 (23), e2007603. doi:10.1002/adma.202007603
Wang, Z., Kang, W., Li, O., Qi, F., Wang, J., You, Y., et al. (2021b). Abrogation of USP7 is an alternative strategy to downregulate PD-L1 and sensitize gastric CancerCells to T cells killing. Acta Pharm. Sin. B 11, 694–707. doi:10.1016/j.apsb.2020.11.005
Wang, Z., Kang, W., You, Y., Pang, J., Ren, H., Suo, Z., et al. (2019). USP7: novel DrugTarget in cancer therapy. Front. Pharmacol. 10, 427. doi:10.3389/fphar.2019.00427
Wei, X., Tang, C., Lu, X., Liu, R., Zhou, M., He, D., et al. (2015). MiR-101 targets DUSP1 to regulate the TGF-β secretion in sorafenib inhibits macrophage-induced growth of hepatocarcinoma. Oncotarget 6 (21), 18389–18405. doi:10.18632/oncotarget.4089
Wei, Y., Lao, X.-M., Xiao, X., Wang, X.-Y., Wu, Z.-J., Zeng, Q.-H., et al. (2019). Plasma cell polarization to the immunoglobulin G phenotype in hepatocellular carcinomas involves epigenetic alterations and promotes hepatoma progression in mice. Gastroenterology 156 (6), 1890–1904. doi:10.1053/j.gastro.2019.01.250
Weiskopf, K., and Weissman, I. L. (2015). Macrophages are critical effectors of antibody therapies for cancer. MAbs 7 (2), 303–310. doi:10.1080/19420862.2015.1011450
Witt, K., Evans-Axelsson, S., Lundqvist, A., Johansson, M., Bjartell, A., and Hellsten, R. (2021). Inhibition of STAT3 augments antitumor efficacy of anti-CTLA-4 treatment against prostate cancer. Cancer Immunol. Immun. 70, 3155–3166. doi:10.1007/s00262-021-02915-6
Wong, C. K., Lit, L. C. W., Tam, L. S., Li, E. K., and Lam, C. W. K. (2005). Aberrant production of soluble costimulatory molecules CTLA-4, CD28, CD80 and CD86 in patients with systemic lupus erythematosus. Rheumatology 44 (8), 989–994. doi:10.1093/rheumatology/keh663
Wu, J., Gao, W., Tang, Q., Yu, Y., You, W., Wu, Z., et al. (2021). M2 macrophage-derived exosomes facilitate HCC metastasis by transferring αM β2 integrin to tumor cells. Hepatology 73 (4), 1365–1380. doi:10.1002/hep.31432
Wu, K., Kryczek, I., Chen, L., Zou, W., and Welling, T. H. (2009). Kupffer cell suppression of CD8+ T cells in human hepatocellular carcinoma is mediated by B7-H1/programmed death-1 interactions. Cancer Res. 69 (20), 8067–8075. doi:10.1158/0008-5472.Can-09-0901
Wu, Q., Zhou, W., Yin, S., Zhou, Y., Chen, T., Qian, J., et al. (2019a). Blocking triggering receptor expressed on myeloid cells-1-positive tumor-associated macrophages induced by hypoxia reverses immunosuppression and anti-programmed cell death ligand 1 resistance in liver cancer. Hepatology 70, 198–214. doi:10.1002/hep.30593
Wu, Q., Zhou, W., Yin, S., Zhou, Y., Chen, T., Qian, J., et al. (2019b). Blocking triggering receptor expressed on myeloid cells-1-positive tumor-associated macrophages induced by hypoxia reverses immunosuppression and anti-programmed cell death ligand 1 resistance in liver cancer. Hepatology 70 (1), 198–214. doi:10.1002/hep.30593
Wu, Y., Kuang, D.-M., Pan, W.-D., Wan, Y.-L., Lao, X.-M., Wang, D., et al. (2013). Monocyte/macrophage-elicited natural killer cell dysfunction in hepatocellular carcinoma is mediated by CD48/2B4 interactions. Hepatology 57 (3), 1107–1116. doi:10.1002/hep.26192
Xie, M., Lin, Z., Ji, X., Luo, X., Zhang, Z., Sun, M., et al. (2023). FGF19/FGFR4-mediated elevation of ETV4 facilitates hepatocellular carcinoma metastasis by upregulating PD-L1 and CCL2. J. Hepatol. 79 (1), 109–125. doi:10.1016/j.jhep.2023.02.036
Xu, F., Wei, Y., Tang, Z., Liu, B., and Dong, J. (2020a). Tumor-associated macrophages in lung cancer: friend or foe? (Review). Mol. Med. Rep. 22 (5), 4107–4115. doi:10.3892/mmr.2020.11518
Xu, G., Feng, D., Yao, Y., Li, P., Sun, H., Yang, H., et al. (2020b). Listeria-based hepatocellular carcinoma vaccine facilitates anti-PD-1 therapy by regulating macrophage polarization. Oncogene 39 (7), 1429–1444. doi:10.1038/s41388-019-1072-3
Yang, J., and Hu, L. (2019). Immunomodulators targeting the PD-1/PD-L1 protein-protein interaction: from antibodies to small molecules. Med. Res. Rev. 39 (1), 265–301. doi:10.1002/med.21530
Yang, W., Lu, Y., Xu, Y., Xu, L., Zheng, W., Wu, Y., et al. (2012). Estrogen represses hepatocellular carcinoma (HCC) growth via inhibiting alternative activation of tumor-associated macrophages (TAMs). J. Biol. Chem. 287 (48), 40140–40149. doi:10.1074/jbc.M112.348763
Yao, R.-R., Li, J.-H., Zhang, R., Chen, R.-X., and Wang, Y.-H. (2018). M2-polarized tumor-associated macrophages facilitated migration and epithelial-mesenchymal transition of HCC cells via the TLR4/STAT3 signaling pathway. World J. Surg. Oncol. 16 (1), 9. doi:10.1186/s12957-018-1312-y
Yao, W., Ba, Q., Li, X., Li, H., Zhang, S., Yuan, Y., et al. (2017). A natural CCR2 antagonist relieves tumor-associated macrophage-mediated immunosuppression to produce a therapeutic effect for liver cancer. EBioMedicine 22, 58–67. doi:10.1016/j.ebiom.2017.07.014
Yau, T., Hsu, C., Kim, T. Y., Choo, S. P., Kang, Y. K., Hou, M. M., et al. (2019). Nivolumab in advanced hepatocellular carcinoma: sorafenib-experienced Asian cohort analysis. J. Hepatol. 71 (3), 543–552. doi:10.1016/j.jhep.2019.05.014
Yin, Z., Huang, J., Ma, T., Li, D., Wu, Z., Hou, B., et al. (2017). Macrophages activating chemokine (C-X-C motif) ligand 8/miR-17 cluster modulate hepatocellular carcinoma cell growth and metastasis. Am. J. Transl. Res. 9 (5), 2403–2411.
Yu, S., Wang, Y., Hou, J., Li, W., Wang, X., Xiang, L., et al. (2020). Tumor-infiltrating immune cells in hepatocellular carcinoma: Tregs is correlated with poor overall survival. PLoS One 15, e0231003. doi:10.1371/journal.pone.0231003
Yue, S., Rao, J., Zhu, J., Busuttil, R. W., Kupiec-Weglinski, J. W., Lu, L., et al. (2014). Myeloid PTEN deficiency protects livers from ischemia reperfusion injury by facilitating M2 macrophage differentiation. J. Immunol. 192 (11), 5343–5353. doi:10.4049/jimmunol.1400280
Zamarin, D., and Postow, M. A. (2015). Immune checkpoint modulation: rational design of combination strategies. Pharmacol. Ther. 150, 23–32. doi:10.1016/j.pharmthera.2015.01.003
Zang, M., Li, Y., He, H., Ding, H., Chen, K., Du, J., et al. (2018). IL-23 production of liver inflammatory macrophages to damaged hepatocytes promotes hepatocellular carcinoma development after chronic hepatitis B virus infection. BBA-Mol Basis Dis. 1864 (12), 3759–3770. doi:10.1016/j.bbadis.2018.10.004
Zhang, H., Jiang, Z., and Zhang, L. (2019c). Dual effect of T helper cell 17 (Th17) and regulatory T cell (Treg) in liver pathological process: from occurrence to end stage of disease. Int. Immunopharmacol. 69, 50–59. doi:10.1016/j.intimp.2019.01.005
Zhang, H., Sheng, D., Han, Z., Zhang, L., Sun, G., Yang, X., et al. (2022b). Doxorubicin-liposome combined with clodronate-liposome inhibits hepatocellular carcinoma through the depletion of macrophages and tumor cells. Int. J. Pharm. 629, 122346. doi:10.1016/j.ijpharm.2022.122346
Zhang, J., Zhang, Q., Lou, Y., Fu, Q., Chen, Q., Wei, T., et al. (2018a). Hypoxia-inducible factor-1α/interleukin-1β signaling enhances hepatoma epithelial-mesenchymal transition through macrophages in a hypoxic-inflammatory microenvironment. Hepatology 67 (5), 1872–1889. doi:10.1002/hep.29681
Zhang, M., He, Y., Sun, X., Li, Q., Wang, W., Zhao, A., et al. (2014). A high M1/M2 ratio of tumor-associated macrophages is associated with extended survival in ovarian cancer patients. J. Ovarian Res. 7, 19. doi:10.1186/1757-2215-7-19
Zhang, P., Chen, Z., Kuang, H., Liu, T., Zhu, J., Zhou, L., et al. (2022a). Neuregulin 4 suppresses NASH-HCC development by restraining tumor-prone liver microenvironment. Cell Metab. 34 (9), 1359–1376.e7. doi:10.1016/j.cmet.2022.07.010
Zhang, Q., He, Y., Luo, N., Patel, S. J., Han, Y., Gao, R., et al. (2019a). Landscape and dynamics of single immune cells in hepatocellular carcinoma. Cell 179 (4), 829–845. doi:10.1016/j.cell.2019.10.003
Zhang, Q., Huang, F., Yao, Y., Wang, J., Wei, J., Wu, Q., et al. (2019b). Interaction of transforming growth factor-β-Smads/microRNA-362-3p/CD82 mediated by M2 macrophages promotes the process of epithelial-mesenchymal transition in hepatocellular carcinoma cells. Cancer Sci. 110 (8), 2507–2519. doi:10.1111/cas.14101
Zhang, T., Song, X., Xu, L., Ma, J., Zhang, Y., Gong, W., et al. (2018b). The binding of an anti-PD-1 antibody to FcγRΙ has a profound impact on its biological functions. Cancer Immunol. Immun. 67 (7), 1079–1090. doi:10.1007/s00262-018-2160-x
Zhang, X., Zeng, Y., Qu, Q., Zhu, J., Liu, Z., Ning, W., et al. (2017). PD-L1 induced by IFN-γ from tumor-associated macrophages via the JAK/STAT3 and PI3K/AKT signaling pathways promoted progression of lung cancer. Int. J. Clin. Oncol. 22 (6), 1026–1033. doi:10.1007/s10147-017-1161-7
Zhao, Q., Kuang, D.-M., Wu, Y., Xiao, X., Li, X.-F., Li, T.-J., et al. (2012). Activated CD69+ T cells foster immune privilege by regulating Ido expression in tumor-associated macrophages. J. Immunol. 188 (3), 1117–1124. doi:10.4049/jimmunol.1100164
Zhao, Q., Xiao, X., Wu, Y., Wei, Y., Zhu, L.-Y., Zhou, J., et al. (2011). Interleukin-17-educated monocytes suppress cytotoxic T-cell function through B7-H1 in hepatocellular carcinoma patients. Eur J Immunol 41 (8), 2314–2322. doi:10.1002/eji.201041282
Zhou, D. Y., Qin, J., Huang, J., Wang, F., Xu, G. P., Lv, Y. T., et al. (2017). Zoledronic acid inhibits infiltration of tumor-associated macrophages and angiogenesis following transcatheter arterial chemoembolization in rat hepatocellular carcinoma models. Oncol. Lett. 14 (4), 4078–4084. doi:10.3892/ol.2017.6717
Zhu, F., Li, X., Chen, S., Zeng, Q., Zhao, Y., and Luo, F. (2016). Tumor-associated macrophage or chemokine ligand CCL17 positively regulates the tumorigenesis of hepatocellular carcinoma. Med. Oncol. 32 (2), 17. doi:10.1007/s12032-016-0729-9
Zhu, Y., Yang, J., Xu, D., Gao, X.-M., Zhang, Z., Hsu, J. L., et al. (2019). Disruption of tumour-associated macrophage trafficking by the osteopontin-induced colony-stimulating factor-1 signalling sensitises hepatocellular carcinoma to anti-PD-L1 blockade. Gut 68 (9), 1653–1666. doi:10.1136/gutjnl-2019-318419
Keywords: tumor-associated macrophages (TAMs), hepatocellular carcinoma (HCC), PD-1/PD-L1, T cell, immunotherapy
Citation: Li Z, Duan D, Li L, Peng D, Ming Y, Ni R and Liu Y (2024) Tumor-associated macrophages in anti-PD-1/PD-L1 immunotherapy for hepatocellular carcinoma: recent research progress. Front. Pharmacol. 15:1382256. doi: 10.3389/fphar.2024.1382256
Received: 05 February 2024; Accepted: 22 May 2024;
Published: 18 June 2024.
Edited by:
Claudia Moscheni, University of Milan, ItalyReviewed by:
Emma Assi, University of Milan, ItalyHaochen Yao, First Affiliated Hospital of Jilin University, China
Copyright © 2024 Li, Duan, Li, Peng, Ming, Ni and Liu. This is an open-access article distributed under the terms of the Creative Commons Attribution License (CC BY). The use, distribution or reproduction in other forums is permitted, provided the original author(s) and the copyright owner(s) are credited and that the original publication in this journal is cited, in accordance with accepted academic practice. No use, distribution or reproduction is permitted which does not comply with these terms.
*Correspondence: Rui Ni, ODUzMzU5NTY1QHFxLmNvbQ==; Yao Liu, c3dobGl1eWFvQDE2My5jb20=
†These authors have contributed equally to this work and share first authorship