- 1Department of Molecular Medicine and Medical Biotechnology, University of Naples Federico II, Naples, Italy
- 2Department of Medicine and Surgery, LUM University Giuseppe Degennaro, Bari, Italy
Cancer stem cells (CSC) are the leading cause of the failure of anti-tumor treatments. These aggressive cancer cells are preserved and sustained by adjacent cells forming a specialized microenvironment, termed niche, among which tumor-associated macrophages (TAMs) are critical players. The cycle of tricarboxylic acids, fatty acid oxidation path, and electron transport chain have been proven to play central roles in the development and maintenance of CSCs and TAMs. By improving their oxidative metabolism, cancer cells are able to extract more energy from nutrients, which allows them to survive in nutritionally defective environments. Because mitochondria are crucial bioenergetic hubs and sites of these metabolic pathways, major hopes are posed for drugs targeting mitochondria. A wide range of medications targeting mitochondria, electron transport chain complexes, or oxidative enzymes are currently investigated in phase 1 and phase 2 clinical trials against hard-to-treat tumors. This review article aims to highlight recent literature on the metabolic adaptations of CSCs and their supporting macrophages. A focus is provided on the resistance and dormancy behaviors that give CSCs a selection advantage and quiescence capacity in particularly hostile microenvironments and the role of TAMs in supporting these attitudes. The article also describes medicaments that have demonstrated a robust ability to disrupt core oxidative metabolism in preclinical cancer studies and are currently being tested in clinical trials.
Introduction
There is a consensus that conventional cancer treatments fail due to the failure to eliminate tumor stem cells (CSCs), i.e., the stem, regenerative, and undifferentiated component of the tumor. Tumor cells that survive treatment are more difficult to eradicate, are aggressive, are responsible for relapses, and possess stem-like properties overall (Baccelli and Trump, 2012; Fan et al., 2023). The expression of surface stemness markers (e.g., CD44, CD133, CD25, ABC transporters), stemness genes (e.g., OCT4, SOX2, NANOG) and aldehyde dehydrogenase 1-ADH1; the capacity for tumorigenicity when transplanted into mice even at low clonal density and the ability to grow in culture in non-adherent conditions forming spheres are classically used to identify CSCs. Although it is still under debate whether tumor-initiating cell originates from the transformation of normal stem cells or the clonal evolution of genetically unstable cells with a capacity for the interconversion of different cellular states (Jordan, 2004; Sell, 2010), the concept of plasticity is central in CSC biology (Plaks et al., 2015). The plasticity of CSCs enables them to adapt and survive throughout biological stresses caused by the treatment and the continuous TME changes during tumor evolution, allowing dynamic and reversible transitions between quiescent and proliferative states, epithelial and mesenchymal states, differentiation, and metastasis (Plaks et al., 2015; Agliano et al., 2017; Müller et al., 2020; Romano et al., 2020). Under a persistently hostile environment that can develop at either the primary or metastatic tumor site, CSCs exploit evolutionarily conserved adaptation mechanisms and become dormant (Garimella et al., 2023), leading to a type of clinical remission, in which cancer cells are occult, undetectable, and asymptomatic for a variably protracted period, after which the tumor can recur in primary or distant sites (Enderling et al., 2013). The extreme variability and plasticity of CSCs due to genetic and epigenetic remodeling (Garimella et al., 2023) make their targeting challenging.
The maintenance of CSCs is ensured by adjacent cells in the TME, in particular by tumor-associated macrophages (TAMs) that form a specialized microenvironment that supports their survival against stress and injury and exerts a central role in maintaining their self-renewal and resistance characteristics. Tumor adaptation with the TME and interactions with TAMs throughout cancer progression can also lead differentiated tumor cells to take on CSC characteristics. (Ayob and Ramasamy, 2018).
Over the past decade, thanks to a deeper understanding of the CSC biology of resistant tumors, numerous efforts have focused on designing tailored therapies to target CSCs towards personalized medicine (Khan et al., 2015). However, the results obtained to date are far from conclusive and therapies against cancer stem cells remain an unmet goal (Cole et al., 2020).
In recent years, our understanding of cancer metabolic adaptations in a stressful TME has placed new hopes in modern cancer chemotherapy that can hinder CSCs with their dynamic cellular states by targeting the cornerstone of energy metabolism (Ayob and Ramasamy, 2018). Metabolic adaptations of CSCs and their supporting TAMs actually represent a demanding field of investigation. Our article deals with such an urgent field of investigation that may give new directions to cancer treatment. We review the latest studies that converge on the perception that mitochondrial function and OXPHOS metabolism meet the requirements of CSCs and their supporting TAMs from different tumor types. We offer an overview of therapies that disrupt the core of oxidative metabolism and, having shown a robust ability against CSCs in preclinical cancer studies, are currently studied in phase 1 and phase 2 clinical trials in their aspects of pharmacodynamics, pharmacokinetics, bioavailability, toxicity, together to efficacy on refractory and resistant tumors.
CSCs and the mitochondrial respiratory machinery
The discovery that cancer has metabolic alterations dates back to the early 1920s when the biochemist Otto Warburg first proved that, oppositely to healthy cells, the metabolism of cancer cells mainly relies on glycolysis, uncoupled to OXPHOS, even under normal oxygen concentrations and fully functioning mitochondria. Tumor cells encompass hypoxia and re-oxygenation (Belisario et al., 2020), continuing their growth notwithstanding mutable environmental conditions. High lactate levels in the TME favor tumor acidosis and adaptation of cancer cells to hypoxia (Bononi et al., 2022). Hypoxia exerts a selection pressure that leads to the survival of subpopulations with the genetic machinery for malignant progression induced by HIF-1α and HIF-2α (Allavena et al., 2021). Lactate generated by glycolytic tumor cells is secreted outside the cell through the monocarboxylate transporter (MCT)4 and can be metabolized by adjacent cells (Martinez-Outschoorn et al., 2017). In oxygenated areas, lactate enters the tumor cell through MCT1 transporters and, upon conversion into pyruvate, produces the so-called “reverse Warburg phenotype” (Marchiq and Pouysségur, 2016). Pyruvate fuels the tricarboxylic acid (TCA) cycle and mitochondrial respiratory chain, increasing the NADH/NAD + ratio and mitochondrial biogenesis. In a physiological system of mouse adipocytes, Yang et al. showed that increased NADH/NAD + ratio induces Sirtuin 1 (SIRT1)-mediated deacetylation of the peroxisome proliferator-activated receptor gamma coactivator-1α (PGC-1α), leading to activation of such a pivotal promoter of mitochondrial biogenesis (Yang et al., 2020).
Several studies highlight expression of MCT trasporters in different cancer settings. Using varied tumor mouse models (colorectal adenocarcinoma, human cervix squamous cell carcinoma, hepatocarcinoma, lung adenocarcinoma), Sonveaux et al. found MCT1 expressed on a subset of resistant cancer stem-like cells and its targeting had clinical antitumor potential (Sonveaux et al., 2008). They demonstrated that MCT1 inhibition induced a switch from lactate-fueled respiration to glycolysis, which overcame cancer resistance and induced sensitivity to ionizing radiation (Sonveaux et al., 2008). Curry et al. interrogated head and neck cancer (HNSCC) tissues to assess metabolic compartmentation in primary tumors typically composed in upper layer of differentiating squamous carcinoma cells and a basal stem cell layer that regenerates the tumor. The basal layer was mitochondrial-rich and specialized for the use of mitochondrial fuels, such as L-lactate and ketone bodies and expressed high levels of MCT1. Conversely, the majority of well-differentiated carcinoma cells and cancer-associated fibroblasts (CAFs) showed strong MCT4 immunoreactivity (Curry et al., 2013).
Pancreatic ductal adenocarcinoma (PDAC) cells do express MCT1 and MCT4 (Kong et al., 2016). Through immunohistochemistry of PDAC tissues, Sandforth et al. demonstrated a co-localization of MCT1 with KLF4 (Sandforth et al., 2020). Moreover, they demonstrated that MCT1 expression on PDAC cell lines conferred greater potential of clonal growth, along with drug resistance and elevated expression of the stemness marker nestin and reprogramming factors (OCT4, KLF4, NANOG). These effects on stemness properties were abrogated by targeting of MCT1 (Sandforth et al., 2020). Pancreatic CSCs, defined using spheres and enriched through CD133 marker, were also shown to express increased levels of PGC-1α, demonstrated to be a relevant determinant of their OXPHOS dependency (Sancho et al., 2015). PGC-1α forced expression in CD133 pancreatic cancer cells accelerated OXPHOS metabolism and enabled their self-renewal and tumorigenic capacity (Valle et al., 2020).
MCT1 and MCT4 are expressed in glioblastoma tumors (Park et al., 2018). Takada et al. measured an upregulation of MCT1 along with stem cell markers (Nestin, NANOG, CD133, SOX-2, and OCT-4) in sphere-forming glioblastoma cells compared with adherent, non-sphere forming cells. Inhibition of MCT1 decreased the viability of glioblastoma CSCs compared with that of non-CSCs (Takada et al., 2016). Mudassar et al. showed MCT1 transporters were associated with high mitochondrial abundance in high grade glioma cells (Mudassar et al., 2020). PGC-1α suppression hampered spheroid formation of glioblastoma cells in vitro and their capability to form in vivo tumors (Bruns et al., 2019). Other studies, associate PGC-1α with cancer metastasis and resistance (Vazquez et al., 2013; LeBleu et al., 2014). PGC-1α expression was co-induced with EMT genetic program in breast cancer patients with distant metastasis and poor outcome (LeBleu et al., 2014). Also, PGC-1α supports high bioenergetic and ROS detoxification capacities of resistant melanoma tumors with higher rates of survival under oxidative stress compared to PGC-1α-negative melanomas (Vazquez et al., 2013). Mitochondrial biogenesis is essential for the anchorage-independent survival and propagation of stem-like cancer cells (De Luca et al., 2015). For a review of MCT transporters in cancer and the potential of new selective MCT1 and/or MCT4 inhibitors in cancer therapeutics, we refer to Singh et al. (Singh et al., 2023).
Evidence that oxidative phosphorylation is upregulated in CSCs is increasingly emerging (Abdullah and Chow, 2013; Sancho et al., 2016; Li et al., 2020; Karp and Lyakhovich, 2022). Studying one of the most aggressive and resistant cancers, i.e., pancreatic ductal adenocarcinoma, Viale et al. found that a subpopulation of dormant tumor cells responsible for tumor relapse relied on oxidative phosphorylation for survival and had features of cancer stem cells (CD133+CD44high cells with spherogenic and tumorigenic capabilities) (Viale et al., 2014). Valle et al. by changing the carbon source from glucose to galactose in vitro, induced a forced oxidative metabolism in pancreatic cancer cells (Valle et al., 2020). Such a metabolic switch produced enrichment in typical pancreatic CSC biomarkers (Hermann et al., 2007) including pluripotency gene expression, tumorigenic potential, upregulated immune evasion properties and acquisition of plastic features such as a reversible quiescence-like state (Valle et al., 2020). Dependency on mitochondrial metabolism has been demonstrated in CSCs from ovarian cancer, identified through coexpression of CD44 and CD117 and tumor-initiating capacity (Pastò et al., 2014). In ovarian cancer patients, comparative transcriptome analyses from ascites-derived tumor cell spheroids versus tumor samples revealed upregulation of genes involved in oxidative phosphorylation process along with those of chemoresistance, cell adhesion and cell-barrier integrity (Ding et al., 2021).
In small cell lung cancer, resistant CSC-like cells, identified based on selective expression of urokinase-type plasminogen activator receptor (uPAR+), showed higher dependency on oxidative phosphorylation than non-CSCs (uPAR−) (Gao et al., 2016). The glycosylphosphatidylinositol (GPI)-anchored protein uPAR is associated with multidrug resistance and with high clonogenic activity (Gao et al., 2016). Vlashi et al. showed that stem/progenitor cells from neurospheres depended on oxidative phosphorylation and higher ATP content compared with differentiated glioblastoma cells derived from culture in monolayers (Vlashi et al., 2011). They also show that such a OXPHOS dependence is lost during differentiation and accompanied with a switch to aerobic glycolysis (Vlashi et al., 2011). Evidence that mitochondrium is a relevant target to overcome resistance of colorectal CSCs are reviewed by Rainho et al. (Rainho et al., 2023). Following metabolic profiling of primary chronic myeloid leukemia (CML) cells, Kuntz et al. found a three-fold increase in the rate of mitochondrial oxygen consumption along with a pattern of metabolites indicating increased lipolysis and fatty acid oxidation in the stem cell-enriched population (CD34+CD38−), compared to differentiated CML cells (CD34−) (Kuntz et al., 2017). Inhibition of oxidative phosphorylation by tigecycline, an anti-bacterial FDA-approved antibiotic, produced a selective cytotoxic effect on CSC at clinically administrable doses (Kuntz et al., 2017). This study highlights that although the nature of CSCs differs and different origins of CSCs are postulated between hematological and solid tumors (Bonnet and Dick, 1997; Jordan, 2004), the requirements of CSCs appear to be met by oxidative metabolism across different tumors.
The concept of metabolic symbiosis between hypoxic/glycolytic- and OXPHOS-tumor cells that favors rapid adaptation of cancer to changing environmental oxygen conditions (Nakajima and Van Houten, 2013) can virtually unravel an interplay between non-CSCs and CSCs in which differentiated tumor cells provide glycolysis products that fuel oxidative metabolism of the stem, regenerative and resistant cellular component of the tumor. (Figure 1).
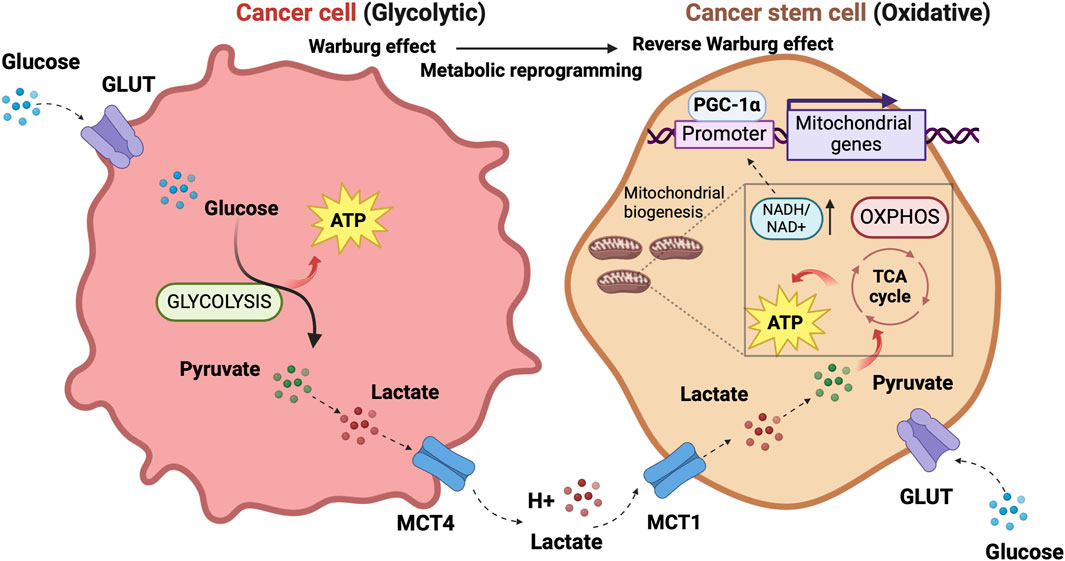
Figure 1. The prevalent metabolism adaptations in differentiated tumor cells compared to stem cell-like tumor cells support a metabolic symbiosis between the different cellular states. The illustration was started from scratch, created with BioRender.com original design.
OXPHOS and multidrug resistance
Increased activity of ATP binding cassette (ABC) transporter family members involved in multidrug resistance is a common feature of CSCs (Begicevic and Falasca, 2017). There are several efforts focused on creating druggable molecules to inhibit these transporters. Five-cyano-6-phenylpyrimidin derivatives containing an acylurea moiety demonstrated efficacy in inhibiting P-glycoprotein ABCB1, a leading member of ABC transport proteins found to be widely overexpressed in human solid tumors and hematologic malignancies (Wang et al., 2018a; Wang et al., 2018b). ABC transporters are highly dependent on ATP since they use the energy from ATP hydrolysis to pump substrates out of cells (Linton and Higgings, 2007). ATP generated by the respiration of mitochondria in the proximity of the plasma membrane and transported from the mitochondrial matrix to the cytosol nearby plasma membrane produces a local rise of ATP level for the active transporter’s need (Linton and Higgings, 2007), thus explaining why mitochondrial and not glycolytic ATP preferentially fuels ABC transporter activity in chemoresistant cancer cells (Linton and Higgings, 2007). In a model of chemoresistant cancer cells, Giddings et al. found that methylation-controlled J protein (MCJ) affected ABC transporter function through regulation of mitochondrial respiration (Giddings et al., 2021). MCJ localizes on the inner membrane of mitochondria and negatively regulates Complex I thus acting as an endogenous brake on mitochondrial respiration (Hatle et al., 2013) As MCJ is often downregulated in the tumors, the authors generated MCJ mimetics and investigated their capability to inhibit ABC transporter function and therapeutic efficacy in combination with doxorubicin, using ovarian and mammary cancer cells and an in vivo mouse model of mammary tumor (Giddings et al., 2021). MCJ mimetics attenuated mitochondrial respiration in chemoresistant cells and reversed cancer chemoresistance in vivo tumor model MCJ-KO. The tumors of mice treated with a combination of MCJ mimetics and doxorubicin showed a prominent size reduction compared to those treated with doxorubicin alone. There was no evidence of liver and heart toxicity by MCJ mimetics nor effect on mouse body weight (Giddings et al., 2021). Although not selectively involving CSCs, the study by Giddings et al. sheds light on the aspect of chemoresistance closely linked to the stem-cell-like concept.
OXPHOS and tumor dormancy
Dormancy is a strategy adopted by a tumor cell placed in a persistently hostile environment that exploits evolutionarily conserved adaptation mechanisms to succeed in tumor progression (Merlo et al., 2006). Proliferation arrest, metabolic quiescence, and immune occultation are the main features of tumor dormancy (Enderling et al., 2013). Dormant cancer cells can reawaken in response to signals which are not yet fully understood, resulting in recurrence and metastasis (Gao et al., 2016; Park and Nam, 2020). Adapting newly arrived cancer cells to the microenvironment of distal organs is a stringent rate-limiting step in metastasis, and the probability of completing this step varies widely depending on the tumor type and the target organ. A study of the metabolic signature associated with disseminated cancer cells suggested an activation of mitochondrial bioenergetic pathways (TCA cycle and OXPHOS) and the pentose-phosphate pathway (Dudgeon et al., 2020) upon seeding. Newly seeded cancer cells slow down bioenergetics and become dormant to survive in secondary sites (Ganguly and Kimmelman, 2023). Although how and when dormant tumor cells become reactivated after inactivity remains not well understood, a role for lipid metabolism in reawakening is emerging (Luo et al., 2017; Watt et al., 2019). Pascual et al. (Pascual et al., 2017) found a subpopulation of CD44bright slow-cycling cells in human oral carcinomas with a unique ability to initiate metastasis that expressed high levels of the fatty acid receptor CD36 and lipid metabolism genes (Pascual et al., 2017). Using neutralizing antibodies for CD36 blockade, they were able to inhibit metastasis formation in orthotopic mouse models of human oral cancer. Conversely, palmitic acid or a high-fat diet increased the metastatic potential of CD36+ cancer cells (Pascual et al., 2017). Ladanyi et al. demonstrated a role for adipocytes in the stimulation of CD36 and Fatty acid transport protein 1 (FATP1) in ovarian cancer cells (Ladanyi et al., 2018) suggesting a significant role for cancer-associated adipocytes in tumor growth and metastasis through favoring lipid utilization and uptake and metabolic reprogramming (Yao and He, 2021). Intriguingly, the oxidation of Cys272 and Cys333 promoted the activation of CD36, suggesting a regulatory effect of the redox signaling in the reactivation of dormant cancer cells (Wang et al., 2019). Also, oxidative stress enabled P450 epoxygenases to synthesize epoxyeicosatrienoic acids, metabolites of arachidonic acid, with a vasodilation effect facilitating exit from the dormant state (Borin et al., 2017).
The CSC niche and tumor associated macrophages
Adjacent cells to CSC form a specialized microenvironment, termed niche, essential for preserving and sustaining CSC against stress and injuries with growth factors, cytokines, and extracellular matrix compounds (Allavena et al., 2021). In analogy to the physiological stem cell niche, this specialized tissue structure allows CSCs to survive and remain quiescent and also provides cues for reactivation of proliferation, differentiation, and migration. (Allavena et al., 2021).
CSCs niche dynamics vary between leukemia and solid tumors. Tracing the cellular origins of human cancers has long been a complex and contentious area in cancer research. Pioneering work by John Dick and colleagues in the 1990s introduced the hierarchical model in acute myeloid leukemia (AML), proposing that a primitive stem or early progenitor cell serves as the cell of origin for malignant transformation in AML (Lapidot et al., 1994; Bonnet and Dick, 1997). This model delineates a rare population of leukemic stem cells (LSCs) with high self-renewal potential and immunophenotypic resemblance to healthy hematopoietic stem cells (HSCs), which are exclusively capable of reinitiating leukemia in immunodeficient mice (Lapidot et al., 1994; Bonnet and Dick, 1997). In leukemia, the bone marrow serves as primary niche, populated by healthy stem cells with which CSCs compete (Marchand and Pinho, 2021). The leukemic niche is populated by different cell types, such as mesenchymal stem cells (MSCs), endothelial cells, megakaryocytes, macrophages, osteoblasts, and nerve cells (Schepers et al., 2015). Bidirectional interactions between leukemic cells and the bone marrow microenvironment promote leukemic progression at the expense of healthy hematopoiesis, implicating bone marrow mesenchymal stem cells in the predisposition, manifestation, and evolution of hematological malignancies (Korn and Méndez-Ferrer, 2017).
In contrast, solid tumors exhibit phenotypic plasticity, where tumor cells can can interconvert between differentiated and stem-like states across a continuum of cell fate specification (Quail et al., 2012). Moreover, despite the presence of founder mutations within the parental clones, a large number of additional mutations between primitive and metastatic tumor implicate the concept of clonal evolution in CSC development (Campbell et al., 2010; Kreso and Dick, 2014). The fact that melanoma, breast, prostate, ovarian, and lung cancer cells are all able to alter their gene expression to resemble cell types that are not part of their original lineage (Quail et al., 2012) exemplifies cancer cell plasticity that enables cancer cells to gain/lose stem cell properties (Passalidou et al., 2002; Shirakawa et al., 2002; Lim et al., 2009). Solid tumors contain non-tumor stromal cells supporting CSCs including CAFs, MSCs, TAMs and other immune cells, and extracellular matrix proteins (Mancini et al., 2021). The niche is characterized by conditions of hypoxia, acidity, and low glucose levels (Olivares-Urbano et al., 2020). The niche concept extends to specialized pre-metastatic microenvironments that play a crucial role in the colonization of disseminated tumor cells at secondary sites, with organ-specific exosomes derived from primary tumors facilitating colonization (Fong et al., 2015; Hoshino al.al 2015). Once the pre-metastatic niche has finished priming, the metastatic niche generates a microenvironment that sustains metastatic cancer stem cells, providing physical anchorage, survival, immune surveillance protection, and metabolic requirements for CSCs in distant metastatic sites (Joseph et al., 2023).
TAMs are the leading players in the CSC niche, they physically interact with CSCs and secrete a variety of soluble factors to protect them from environmental damage (Jinushi et al., 2011; Fan et al., 2014; Zhou et al., 2015; Oshimori, 2020). Notably, similarities exist between TAMs from leukemias and solid tumors within their respective niches. Such similarities consist in abundant localization of TAMs in both leukemic and solid tumor niches that positively correlate with CSC distribution (Wang and Zheng, 2019; Basak et al., 2023) and accumulation within hypoxic tumor regions, where CSCs are also prevalent (Wang and Zheng, 2019; Basak et al., 2023). CSCs exert significant influence over TME by recruiting and polarizing macrophages toward a pro-tumor M2 phenotype. In turn, M2-TAMs actively support CSC maintenance, thus promoting a symbiotic relationship between these cellular populations (Wang and Zheng, 2019; Basak et al., 2023).
TAMs are able to activate signaling pathways essential to CSCs, including those driven by Sonic Hedgehog (SHH), Neurogenic locus notch homolog protein (NOTCH), STAT3, PI3k/Akt, Wingless integrated (WNT)/b-catenin and NANOG, through soluble factors or direct physical interaction with CSC (Allavena et al., 2021). Tumor cells produce chemotactic factors (Allavena et al., 2021), exosomes (Su et al., 2021) and metabolites (Diskin et al., 2021) to recruit circulating monocytes and tissue-resident macrophages and induce their polarization towards anti-inflammatory, angiogenic and protumor (M2) phenotype typical of TAMs. TAMs initiate reciprocal crosstalk with CSC to exert their trophic action in the niche (Allavena et al., 2021). Transcription factors involved in maintaining the pluripotency and self-renewal characteristics of CSCs are highly expressed by TAMs (Shang et al., 2023). The CSC’s role in modulating the TME and driving the recruitment and alternative polarization of macrophages and crosstalk between CSCs and TAMs have been extensively reviewed in several articles (Sainz et al., 2016; Muller et al., 2020; Allavena et al., 2021; Chae et al., 2023).
The primary tumor secretome influences the immune milieu at distant organs, thus preparing the permissive soil for colonization of disseminated cancer cells by re-educating the metabolic and epigenetic state of resident cells in the host organs (Ganguly and Kimmelman, 2023). Macrophages play a special role in priming and disseminating tumor cells for dormancy and stemness (Borriello et al., 2022). Using a technique termed Window for High-Resolution Imaging of the Lung (WHRIL) (Entenberg et al., 2018), Borriello et al. quantitatively measured, in real-time, spontaneously disseminating tumor cells during the process of metastasis to the lung, in a breast cancer mouse model (Borriello et al., 2022). They found a subset of macrophages within the primary tumor that caused activation of genetic programs related to dissemination, dormancy, and stemness in tumor cells approaching the intravasation site. Upon tumor cell contact with macrophages, tumor cell expresses high levels of the actin-regulatory protein MenaINV (Roussos et al., 2011). This actin isoform plays an active role in tumor cell migration during intravasation within the primary tumor (Pignatelli et al., 2016). Moreover, contact with macrophages activated in tumor cells expression of stem-like SOX-9 phenotype and Nuclear Receptor Subfamily 2 Group F Member 1 (NR2F1), the orphan nuclear receptor and one of the best molecular markers of dormancy that regulates expression of pluripotency genes (Sosa et al., 2015). The depletion of macrophages significantly reduced NR2F1 levels in the tumor cells and prevented dormancy (Borriello et al., 2022). Before disseminating, tumor cells establish microenvironmental niches incorporating macrophages in the primary tumor that enable them to acquire a pro-dissemination, stem-like dormancy phenotype that is carried to the secondary site and is lost during metastatic growth (Borriello et al., 2022). Dormancy represents a typical risk for long-term breast cancer survivors. Dormant breast cancer cells preferentially reside in the bone marrow. A study by Walker et al. in a breast cancer mouse model showed that bone marrow M2 macrophages supported tumor dormancy. Upon the M2 to M1 switch through activation of TLR4 with LPS, M1 macrophages reversed dormancy and induced sensitivity to carboplatin of breast cancer cells (Walker et al., 2019). The authors demonstrated that M1-derived exosomes produced clinical evidence of metastasis due to the activation of NF-κB in quiescent breast cancer cells to reverse non-cycling to cycling cells (Walker et al., 2019). Crosstalk between macrophages and dormant cancer cells has been extensively reviewed by Batoon and McCauley (Batoon et al., 2021). Figure 2 illustrates the interplay between dormant cell and macrophages.
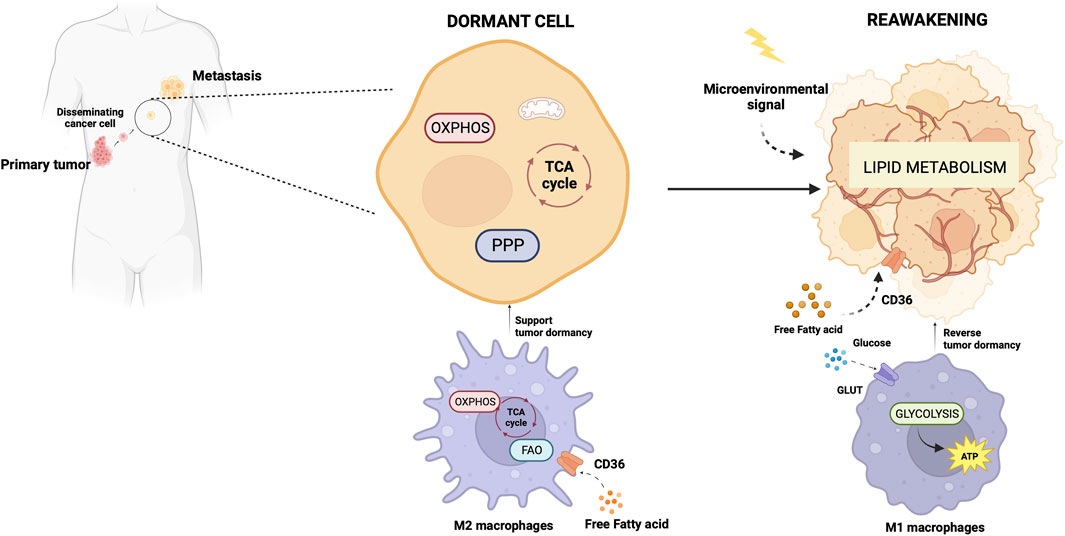
Figure 2. Metabolic aspects of TAMs in tumor dormancy and reawakening. The illustration was started from scratch, created with BioRender.com original design.
The mitochondrial respiratory machinery is a significant driving force in TAM polarization
Phenotype, function, and metabolic state are closely interconnected aspects in macrophages and coordinated with each other (Minhas et al., 2019; Emtenani et al., 2022; Gonzalez et al., 2023). Through single-cell transcriptomic profiling of macrophages phagocytosing neoplastic cells, Gonzales et al. demonstrated a strict linkage between phagocytosis, immune-suppressive phenotype, and gene expression changes toward OXPHOS, ribosomal, and other metabolic genes (Gonzalez et al., 2023). The correlation of the metabolic gene signature with worse clinical outcomes was validated in human lung cancer (Gonzalez et al., 2023). Consistent with the findings by Gonzales et al., Minhas et al. showed that genetic or pharmacological blockade of de novo NAD + synthesis, suppressed mitochondrial NAD + -dependent signaling and respiration, and impaired phagocytosis and resolution of inflammation due to changes in macrophage polarization state. (Minhas et al., 2019). Emtenani et al. (Emtenani et al., 2022), investigating gene expression in macrophages during the first migratory stages of the tissue invasion, found a metabolic reprogramming towards OXPHOS and ribosome biogenesis of migrating macrophages. In this cell model, the authors identified Atossa, a transcriptional regulator inducing expression of an RNA helicase termed Porthos. This factor increased the translation efficiency of short 5′UTR mRNAs that included a subset of mitochondrial OXPHOS genes of the respiratory complexes (Emtenani et al., 2022).
Like CSCs, M2 macrophages can resist and remain functional in adverse environmental conditions such as low nutrients, low pH, hypoxia, and oncometabolite abundance (Liu et al., 2021). Like CSCs, M2 macrophage metabolism exploits the mitochondrial respiratory machinery that is a significant driving force in alternative macrophage polarization (O'Neill et al., 2016; Liu et al., 2021). While aerobic glycolysis produces most of the ATP and intermediates for biosynthetic pathways required for effector (microbicidal and antitumor) functions of M1 macrophages (Warburg and Minami, 1923; Altenberg and Greulich, 2004; Tannahill et al., 2013), to sustain their activities, M2 macrophages use the TCA cycle to obtain reducing equivalents, assuring constant energy production in concert with mitochondrial OXPHOS (Figure 3). Acetyl-CoA oxidized in the TCA cycle mainly derives from fatty acid oxidation (Odegaard and Chawla, 2011; O'Neill et al., 2016). M2 macrophages actively extract fatty acids from circulating lipoproteins internalized through CD36 (Evans et al., 1993) and endocytosis (Huang et al., 2014). The pivotal role of fatty acids oxidation in alternative macrophage polarization is underscored by the observation that blocking palmitate entry into the mitochondrial matrix hampers IL-4-induced M2 polarization (Malandrino et al., 2015). Enhanced fatty acid oxidation in palmitate-incubated macrophages reduced the inflammatory profile (Malandrino et al., 2015). Proliferator-activated receptors of peroxisomes, organules involved in the oxidation of long-chain fatty acids and eicosanoid-CoA esters (Reddy and Hashimoto, 2001) were shown to regulate the transcription of M2 genes, (Odegaard et al., 2008; Chawla, 2010; Nelson et al., 2018).
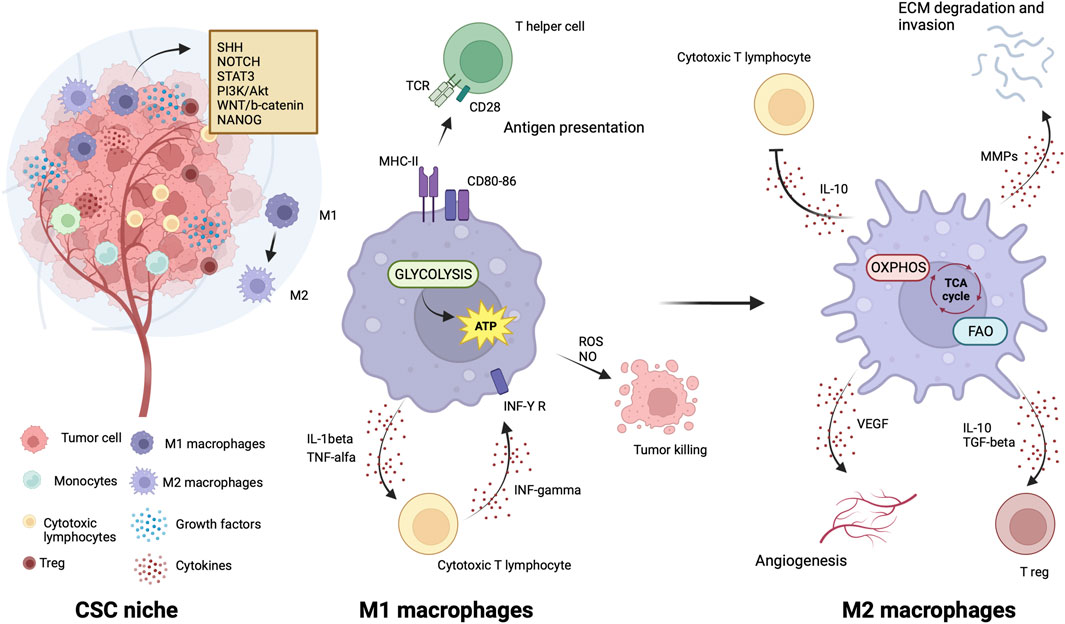
Figure 3. Metabolic features of TAMs in CSC niche. The illustration was started from scratch, created with BioRender.com original design.
OXPHOS-targeted drugs in clinical trials
Efforts in recent years led to design of numerous clinical trials for the assessment of the anticancer effectiveness of drugs targeting mitochondrial metabolism with the aim of hindering CSCs and microenvironmental signaling together.
The electron transport chain has indepth been explored for development of inhibitors for each complex (Sainero-Alcolado et al., 2022). However, these compounds can exhibit remarkable toxicity that prevents their use in clinical practice (Sainero-Alcolado et al., 2022). The antidiabetic agent metformin (Watanabe, 1918) counts more than 400 registered clinical trials in the last 5 years as a cancer chemopreventive or therapeutic agent, alone or in combination with neoadjuvant chemo-radiation therapy (https://www.clinicaltrials.gov). The master pathway of metformin anticancer activity is the activation of the adenosine monophosphate-activated protein kinase (AMPK) that inhibits mammalian target of rapamycin (mTOR) (Zhou et al., 2001) pathway triggered by inhibition of complex I through drug binding in the quinone channel (Owen et al., 2000). Recently, metformin was found to cause a mitochondrial effect independent of inhibition of complex1 by direct molecular targeting PEN2, a subunit of γ-secretase (Ma et al., 2022). PEN2 binds to ATPase H+transporting accessory protein 1, inhibits the activity of ATPase without increasing AMP or ADP, and then activates the lysosomal AMP-independent AMPK pathway (Ma et al., 2022). Still, other mechanisms concur to its anticancer activity that are still not well understood. Metformin reduces cancer risk, decreases cancer-related mortality in patients with diabetes (Decensi et al., 2010), and has excellent performance in preclinical studies. Particularly, a preclinical study shows that metformin selectively targets cancer stem cells and acts together with chemotherapy to block tumor growth and prolong remission (Hirsch et al., 2009). A prospective phase I clinical trial (NCT01442870) assessing the safety of metformin in combination with chemotherapy in patients with solid tumors suggests that metformin can be given safely with chemotherapy (Saif et al., 2019). Brown et al. evaluated the impact of metformin on CSC number and clinical outcomes in nondiabetic patients with advanced-stage epithelial ovarian cancer. Metformin decreased by 2.4-folds the number of ALDH+CD133+ CSCs and increased sensitivity to cisplatin ex vivo. Translational studies confirm an impact of metformin on ovarian cancer CSCs and suggest epigenetic change in the tumor stroma, specifically MSCs, may drive the platinum sensitivity ex vivo. Metformin treatment was associated with increased overall survival, supporting the use of metformin in phase III studies (Brown et al., 2020). However, benefits in cancer treatment are often quite vague in clinical trials; thus, there are challenges in the clinical translation of metformin. In a very recent review, Hua et al. (Hua et al., 2023) point out that the mechanisms of action of metformin must be seen in the context of cancer hallmarks, the well-standardized set of crucial functional capabilities for malignant transformation (Hanahan, 2022). In their article, after summarizing the current knowledge on the antitumor action of metformin, the authors elaborate the underlying mechanisms in terms of cancer hallmarks and propose new perspectives of metformin use potentially applicable to cancer treatment (Hanahan, 2022).
IACS-010759, an inhibitor of complex I, was found to reduce mitochondrial function of enriched tumor cell spheroids from the ascites of high-grade serous ovarian cancer patients. Also, IACS-010759 treatment reduced the fraction of CD34+ progenitor AML cells in a dose-dependent manner (Molina et al., 2018). Current clinical trials with IACS-010759 involve advanced tumors (phase 1, NCT03291938) and AML (phase 1, NCT02882321). Tamoxifen was found to interact with the flavin mononucleotide site of complex I leading to mitochondrial failure (Moreira et al., 2006). It is investigated in cancers other than breast, and genito-urinary tract, as intraocular melanoma, in combination with cisplatin (phase 2, NCT00489944); high risk stage III melanoma in combination with sorafenib (phase 2, NCT00492505); oesophageal cancer (phase 1, NCT02513849); osteosarcoma (phase 1, NCT00001436). Pyrvinium pamoate is a lipophilic cation belonging to the cyanine dye family, inhibiting complex I. (Schultz and Nevler, 2022). It has been used in the clinic as a safe and effective anthelminthic for over 70 years (Schultz and Nevler, 2022) and currently is investigated in pancreatic cancer to determine its safety and tolerability (phase 1, NCT05055323). Atovaquone, with a structure similar to protozoan ubiquinone, is an inhibitor of complex III (Mather et al., 2005) approved by the US Food and Drug Administration against plasmodium falciparum. Atovaquone reduced the tumorsphere formation and invasion ability of EpCAM+CD44+ CSCs isolated from HCT-116 colon carcinoma cell lines (Fu et al., 2020). It was found to inhibit proliferation and induce apoptosis of CSCs (CD44+CD24Low− and ALDH+) derived from the mammary breast cancer cell line MCF7 (Fiorillo et al., 2016b) and of ALDH+CD133+ cancer stem-like cells from two high-grade serous ovarian cancer patients (Kapur et al., 2022). Atovaquone anti-cancer efficacy has been assessed in varied mouse cancer models (Rodriguez-Berriguette et al., 2024) and is currently investigated in NSCLC (phase 1, NCT04648033), ovarian cancer (phase 2, NCT05998135), AML (phase 1, NCT03568994). Niclosamide is an uncoupler of electron transport chain (Chen et al., 2018). Jin et al., showed that niclosamide is a potent inhibitor of the NF-κB pathway and exerts a synergism with Ara-C or VP-16 against primary AML cells. They also suggested that this drug has the potential to eradicate AML blasts since they demonstrated that niclosamide kills AML CD34+CD38−stem-cells, while sparing normal bone marrow progenitors (Jin et al., 2010). Niclosamide efficiently decreased therapy resistance in colorectal cancers by reducing CSC populations and their self-renewal activity, thereby attenuating the survival potential of CSCs following chemoradiation (Park et al., 2019). Clinical trials with niclosamide involved treatment of refractory AML (phase 1, NCT05188170), colorectal cancer (phase 1, NCT02687009; phase 2, NCT02519582), and castration resistant prostate cancer (phase 1, NCT03123978; phase1, NCT02532114; phase 2, NCT02807805). Table 1 lists active or recently completed clinical trials investigating outcomes with respiratory-complex inhibitors in refractory tumors, ONC201 and ONC206 are imidazo-pyrido-pyrimidine derivatives that bind to the mitochondrial serine protease termed caseinolytic protease proteolytic subunit (ClpP) with the ability to reduce mitochondrial oxidative phosphorylation, oxygen consumption rate, ATP production and increase mitochondrial generation of reactive oxygen species (Przystal et al., 2022). They were found for the first time to affect mitochondrial activity in diffuse midline glioma cells in children and young adults and considered two promising agents against Histone three lysine27-to-methionine (H3.3K27M)-mutated gliomas. Treatment with ONC201 reduced self-renewal, clonogenicity and cell viability of GBM cells (He et al., 2021). Similar results of inhibition of tumorsphere formation, CSC genes NANOG and SOX2, and CSC frequency were obtained by Jeon et al., due to selective antagonism of dopamine receptor (Jeon et al., 2023). Moreover, ONC201 targets chemotherapy-resistant colorectal cancer stem-like cells (Prabhu et al., 2015) and significantly decreased CSC frequency and tumor initiation capability in a breast cancer mouse model (Greer et al., 2022). In chemo-refractory AML patient samples, ONC201 induced apoptosis in leukemia stem/progenitor cells (CD34+/CD38-) to an extent that was equivalently observed in non-CSCs (Ishizawa et al., 2016) Especially ONC201 is an investigational agent that has shown a favorable safety profile in phase 1 and phase 2 clinical trials in advanced cancers. Several clinical trials have been designed to assess efficacy of ONC201 and ONC206, alone or in combination with chemo or immunotherapy, against several cancer types, including colorectal cancer, pediatric H3. K27M-mutant gliomas, adults with recurrent H3.K27M-mutant gliomas, recurrent gliomas, rare primary central nervous system neoplasms, neuroendocrine tumors, multiple myeloma, endometrial cancer, advanced solid tumors, metastatic breast cancer, relapsed/refractory non-Hodgkin’s lymphoma, relapsed or refractory acute leukemias, oral cancer (Table 2). Two completed clinical trials (NCT02250781, NCT02324621) evaluated the safety, pharmacokinetics, and pharmacodynamics of ONC201 in patients with advanced solid tumor that is refractory to standard treatment, or for which no standard therapy is available. Results from these studies indicated that oral ONC201 is well-tolerated and had immunostimulatory activity. Patients treated with ONC201, who experienced at least stable disease by RECIST for 12 or more weeks, broad induction of immune cytokines and effector molecules was observed (Stein et al., 2019). Also, increased intratumoral infiltration of cytotoxic NK cells and granzyme B was observed in a metastatic prostate cancer patient in response to ONC201.
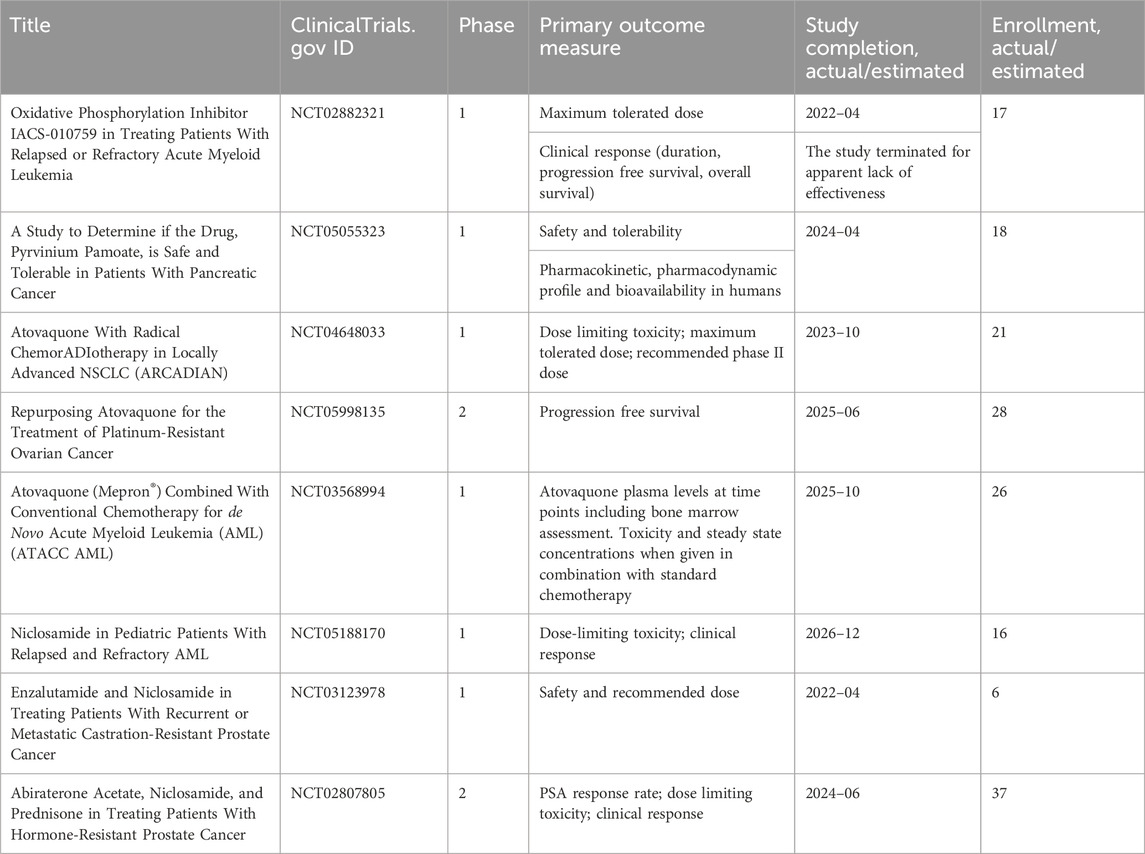
Table 1. Clinical trials investigating the outcomes of the treatments of refractory tumors with inhibitors of mitochondrial respiratory complexes.
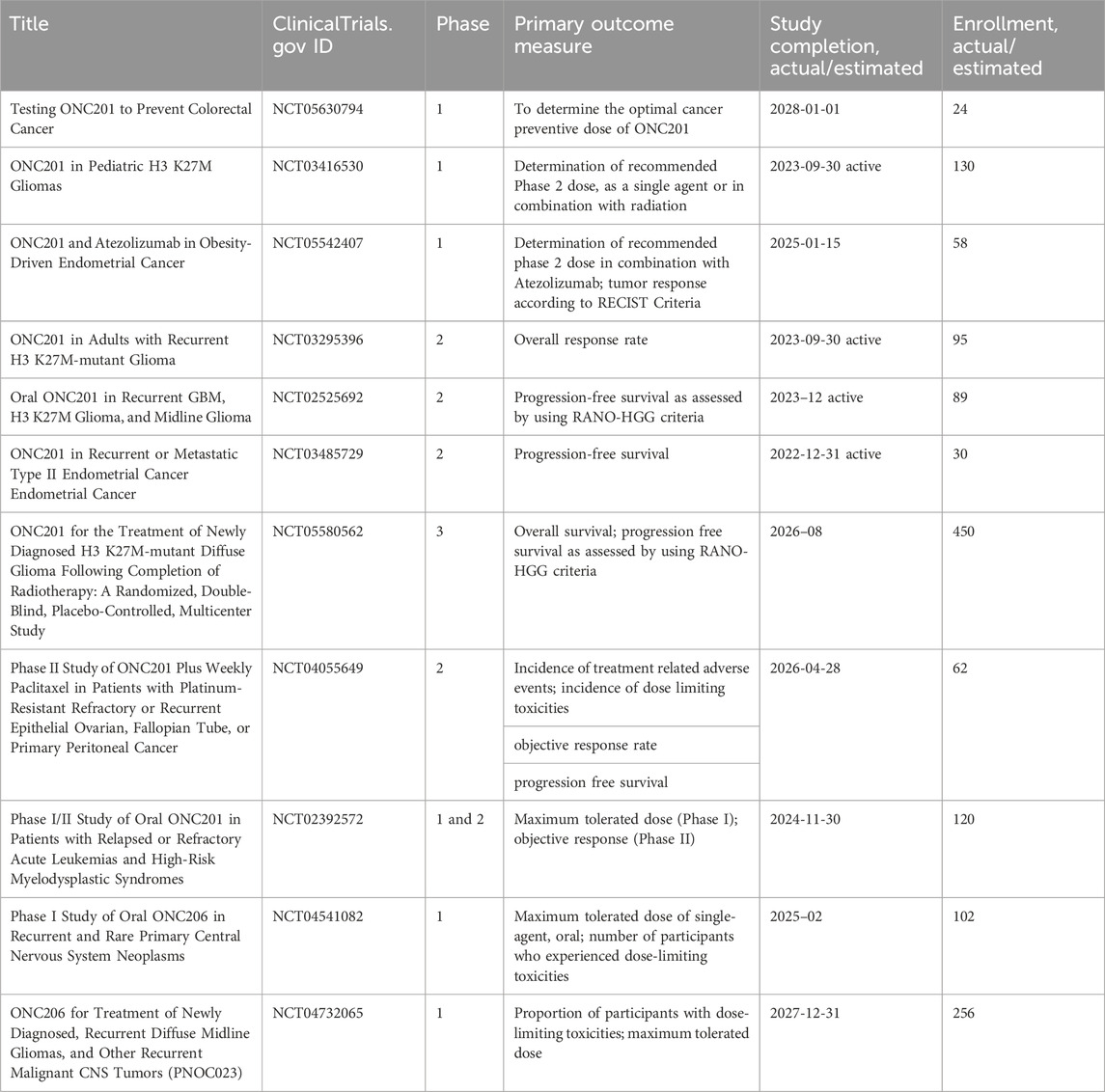
Table 2. Clinical trials investigating the outcomes of tumor treatments with ONC2091 and ONC206 (Imipridones).
In a recent review article, Karp and Lyakhovich outlined antibiotics that, by inducing mitochondrial dysfunction, hinder OXPHOS and the rate of oxygen consumption, reduce ATP and ΔΨm levels, and increase ROS (Karp and Lyakhovich, 2022). Antibiotics act on the prokaryotic ribosomal complex by binding to the bacterial 30S ribosomal subunit, thus preventing association with aminoacyl transfer RNAs (tRNAs) and counteract translation with a consequent bacteriostatic effect (Luger et al., 2018). Translation of mitochondria-encoded proteins occurs within the mitoribosome organelle and produces 13 proteins that are components of respiratory complexes (Luger et al., 2018). The evolutionary conserved link between mitochondria and bacteria supports the use of these drugs to target CSC metabolism (Luger et al., 2018; Karp and Lyakhovich, 2022). Bedaquiline is an anti-microbial agent that is approved by the FDA for the treatment of resistant tuberculosis. It significantly blocks the expansion CSCs generated by breast cancer MCF7 cell line, as determined by reduced expression of CD44 and ALDH1, under anchorage-independent growth conditions and the mammosphere assay (Fiorillo et al., 2016a). Several preclinical studies support efficacy of doxycycline against CSCs (Lamb et al., 2015; Yang et al., 2015; Liu et al., 2022). Lamb et al. found that doxycycline was effective against tumor-sphere formation across different cancer types including breast, ovarian, prostate, lung, pancreatic cancers, melanoma, and glioblastoma (Lamb et al., 2015). In early breast cancer patients, Scatena et al. conducted a clinical pilot study with doxycycline finding a significant decrease in cancer tissues of two CSC markers, namely, CD44 and ALDH1 (Scatena et al., 2018). Yang et al. show that doxycycline severely affected colony formation and viability of human cervical carcinoma stem cells (He-La CSCs), decreased expression of SOX-2 and surface markers CD133 and CD49f. Moreover, upon injection into NOD-SCID mice the doxycycline pretreated HeLa-CSCs had drastically reduced capacity of tumor growth (Yang et al., 2015). Liu et al. showed that the drug significantly inhibited the CSC-like properties of pancreatic cancer cells, namely, mammosphere formation and CD133 expression (Liu et al., 2022). Treatment of Panc-1 with doxycycline significantly enhanced the effect of chemotherapy drugs (i.e., cisplatin, oxaliplatin, 5-FU, sorafenib, and gemcitabine) in comparison with the results obtained when only chemotherapy drugs were used. Among the antibiotics with preclinical evidence of efficacy to suppress CSCs, for which we refer ad hoc review articles (Karp and Lyakhovich, 2022; Garimella et al., 2023), doxycycline, a tetracycline derivative is the most investigated in clinical trials. Clinical trials investigating the drug alone or in combination with standard therapy involve varied tumors, among which: pancreatic cancer (phase 2, NCT02775695); pleural neoplasm (observational, NCT03465774; interventional, NCT02583282; phase 2, NCT01411202); cutaneous T-cell lymphoma (phase 2, NCT02341209); advanced melanoma, in association with temozolomide and ipilimumab (phase 1, NCT01590082); relapsed NHL (phase 2, NCT02086591); bone metastatic breast cancer, in association with bisphosphonates (NCT01847976); in localized breast cancer and uterine cancer (phase2, NCT02874430) or head and neck cancer (phase 2, NCT03076281), in association with metformin. Tigecycline, a glycylcycline designed to overcome tetracycline resistance was shown to interfere with the generation of CSCs (LGR5⁺CD44⁺) in a colon adenocarcinoma murine model (Ruiz-Malagón et al., 2023). Moreover, tigecycline impacted tumorsphere formation in a number of cancer cell lines, including ER (−) breast, ovarian, lung, prostate, and pancreatic cancers and melanoma (Lamb et al., 2015). Currently, tigecycline is investigated in acute and chronic myeloid leukemia (phase 1, NCT01332786; observational, NCT02883036). The macrolide azithromycin exerted a very significant inhibitory effect on mammosphere formation when combined with doxycycline (Fiorillo et al., 2019). It is investigated in Familial Adenomatous Polyposis (FAP) carrying premature nonsense mutations (phase 4, NCT04454151). Table 3 resumes active or recently completed clinical trials investigating outcomes of antibiotics in refractory tumors.
CPI-613 (devimistat) is a nonredox active lipoate analog developed by Cornerstone Pharmaceuticals. CPI-613 mimics the cofactor of the E2 catalytic subunit of pyruvate dehydrogenase and ketoglutarate dehydrogenase (Stuart et al., 2014), inhibiting the enzymatic activity of these complexes operating on the TCA cycle (Stuart et al., 2014) and impairs ATP synthesis (Anderson et al., 2022). Also, TCA cycle inhibition leads to increased mitochondrial turnover due to mitophagy (Anderson et al., 2022).
In ovarian cancer, CPI-613 treatment was found to negatively impact CSC-rich spheres and resulted in a decrease in tumorigenicity in vivo. Moreover, CPI-613 treatment induced a decrease in CD133+ and CD117+ cell frequency in vitro and in vivo (Bellio et al., 2019). In early clinical trials in pancreatic cancer patients, devimistat produced impressive response rates (Alistar et al., 2017) leading to a phase 3 clinical trial (Philip et al., 2019). Moreover, in preclinical models, devimistat sensitized AML cells to chemotherapy and decreased mitochondrial respiration, leading to a phase I study in relapsed and refractory AML patients (Pardee et al., 2018). However, devimistat did not improve overall survival in the multi-center phase 3 randomized clinical trial (NCT03504423) where 528 patients with metastatic pancreatic adenocarcinoma were randomized to receive either devimistat in combination with modified Folfirinox or Folfirinox (Rafael Pharmaceuticals, Inc., 2021). Similarly, the phase 3 study ARMADA 2000 (NCT03504410) was not completed due to a lack of efficacy in patients with relapsed or refractory AML (Anderson et al., 2022). Despite preliminary unsuccessful results, investigations in the clinics continue with the aim of assessing with more precision devimistat capabilities against difficult-to-treat tumors and defining the best condition for devimistat use. Table 4 lists active clinical trials evaluating this drug in the treatment of advanced/refractory tumors. Figure 4 illustrates the mechanism of action of anti-mitochondrial drugs used in clinical trials.
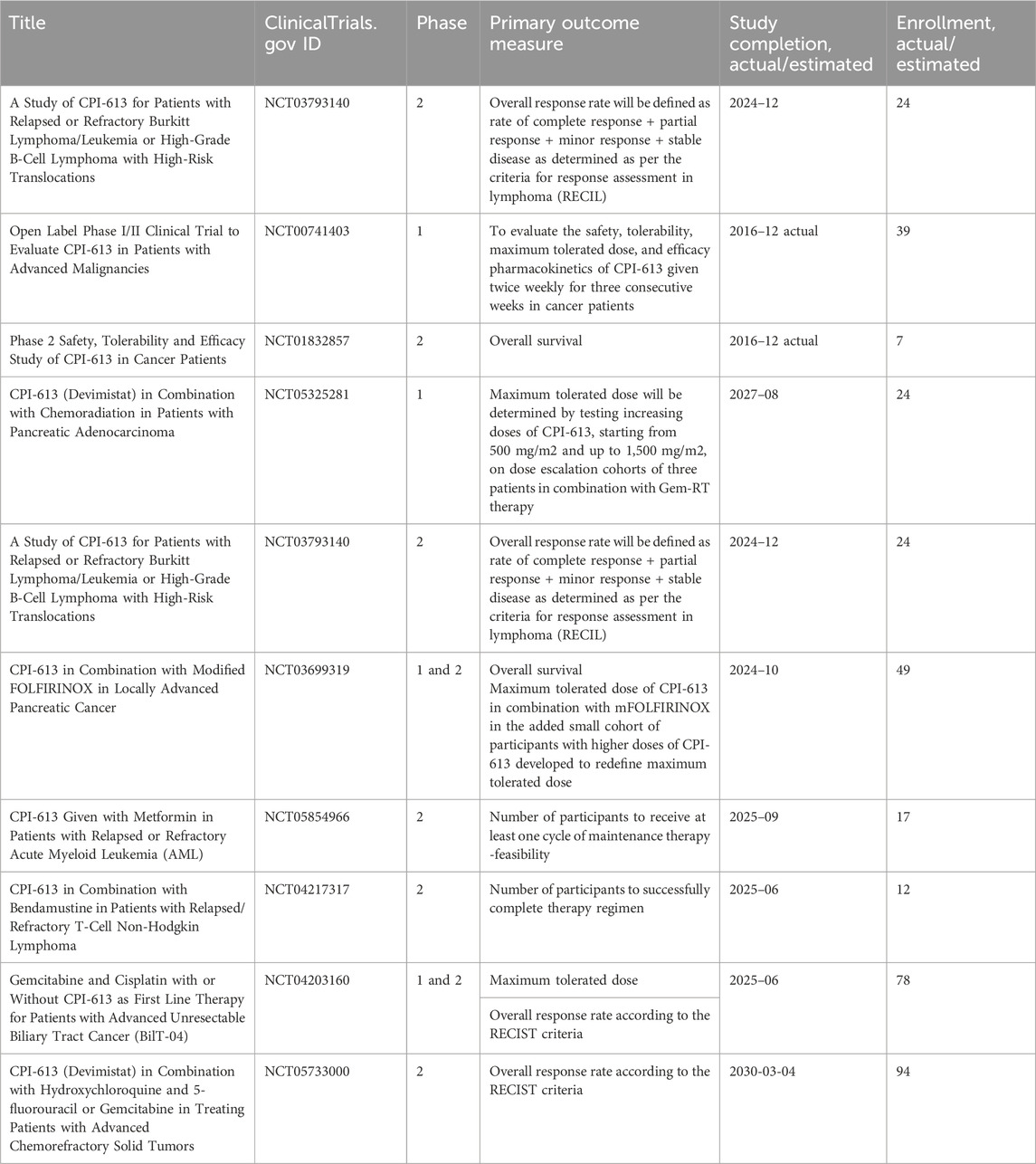
Table 4. Clinical trials investigating the outcomes of the treatments of refractory tumors with CPI-613 (Devimistat).
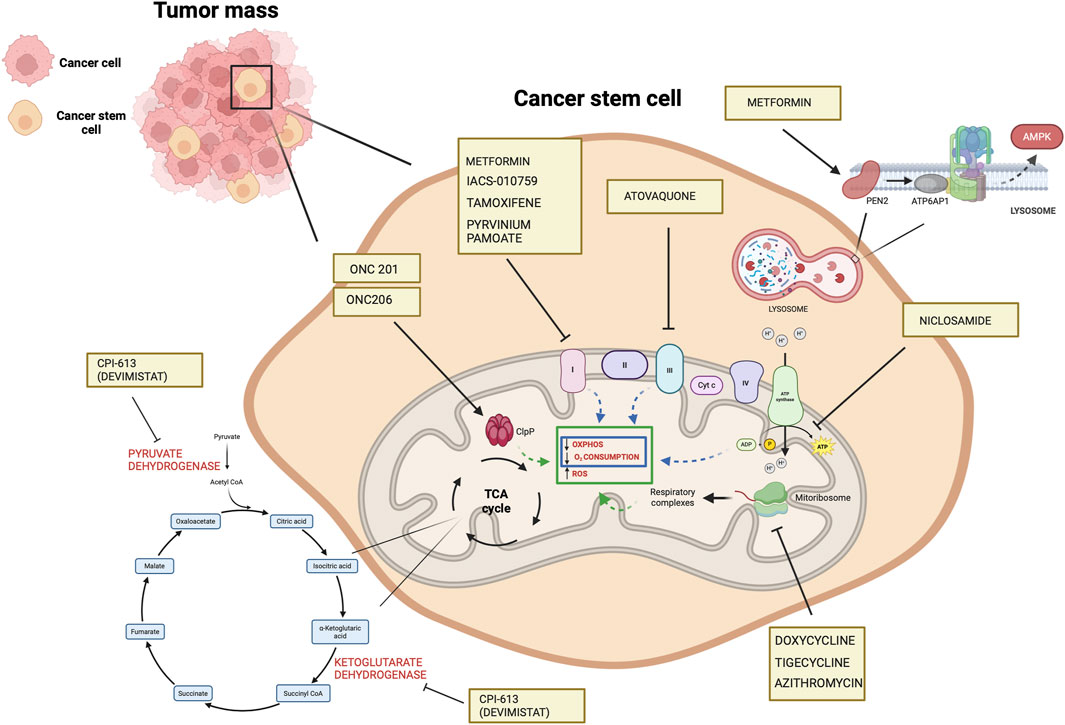
Figure 4. Mechanisms of the mitochondrial drugs used in clinical trials that have been shown to target CSCs in preclinical setting (https://www.clinicaltrials.gov). The illustration was started from scratch, created with BioRender.com original design.
OXPHOS-targeted drugs affect TAMs
Several studies suggest that the effects of pharmacological agents inhibiting mitochondrial metabolism, well reported for bulk tumor cells and cancer stem cells, extend beyond tumor cells and apply also to TAMs, which can contribute to their efficacy. Metformin has the potential to shift the balance of TAMs from an immunosuppressive M2 phenotype to an antitumor M1 phenotype (Wu et al., 2022; Abdelmoneim et al., 2023). A plethora of studies on different cancer models report its efficacy against TAMs (Liu et al., 2018; Wang et al., 2020; Munoz et al., 2021; Wei et al., 2021; Kang et al., 2022; Taylor et al., 2022; Cao et al., 2023). In mice-bearing prostate tumors, metformin remarkably suppressed the infiltration of TAMs mechanistically by inhibiting the cyclooxygenase-COX-2/prostaglandin-PGE2 axis in tumors. The reduction of TAMs following administration of metformin was responsible for the suppression of tumor growth and metastasis (Liu et al., 2018). Evaluating matched pre- and post-treatment tumor specimens from esophageal cancer patients in a phase II clinical trial of low-dose metformin treatment found significant changes in the TME. Precisely, metformin produced a decrease in tumor-promoting CD163+ macrophages and an increase in tumor-suppressive CD11c+ macrophages, in CD8+ cytotoxic T lymphocytes and CD20+ B lymphocytes. Also, metformin augmented macrophage-mediated phagocytosis of esophageal cancer cells in vitro. Similar results of TME reprogramming were obtained with short-term metformin treatment of an esophagus cancer mouse model together with inhibition of tumor growth. (Wang et al., 2020). Employing microparticles loading metformin, Wei et al. showed their efficacy in repolarizing M2-like TAMs to into M1-like phenotype and remodeling TME by increasing the recruitment of CD8+ T cells into tumor tissues and decreasing immunosuppressive infiltration of myeloid-derived suppressor cells and regulatory T cells (Wei et al., 2021). Metformin combined with a tumor vaccine significantly increased the expression of M1 markers CD86 and MHC-II in TME, reduced tumor growth and inhibited lung metastasis in select tumor models (Munoz et al., 2021). A study on epithelial ovarian cancer patients showed that metformin combined with platinum, in comparison with platinum alone, significantly reduced CD68+ macrophages and cancer-associated MSCs in TME of 38 cancer samples (Taylor et al., 2022). Two studies of colorectal cancer TME showed that metformin decreases CD206+ and CD163+ M2 macrophages in an AMPK-dependent manner (Kang et al., 2022) and promotes the polarization of TAMs to M1 through inhibition of HIF-1α and mTOR signal (Cao et al., 2023). A role for tamoxifen in TAM reprogramming to the M1 phenotype has been demonstrated in pituitary adenoma, resulting in inhibition of the migration of cancer cells. Mechanistically, such reprogramming was mediated by STAT6 inactivation and inhibition of the macrophage-specific protein tyrosine phosphatase SHP (Lv et al., 2022). Tamoxifen in combination with clodronate caused TAM depletion in castration-resistant ER-positive subtype of prostate cancer tumors (Semenas et al., 2021). It should be however noted that an expansion of an M2 population in the TME connoted tamoxifen resistance in the postmenopausal breast cancer (Xuan et al., 2014). Atovaquone, used within a stabilizer drug delivery platform composed by protoporphyrin IX nanoparticles, induced M2-type TAMs polarization toward M1-type TAMs, transforming “cold tumor” into “hot tumor” and synergized with anti-PD-L1 immunotherapy in a murine model of colon carcinoma (Feng et al., 2023). ONC201 affects macrophage immunometabolism and leads to a pro-inflammatory TME in glioblastoma (Geiß et al., 2021). Doxycycline inhibits M2-type polarization of human and bone marrow-derived mouse macrophages in a dose-dependent manner and in vivo M2-mediated neovascularization in a laser injury model of choroidal neovascularization (He and Marneros, 2014). In pulmonary metastases of osteosarcoma, doxycycline affects macrophage polarization by skewing the tumor induced M2-like TAMs to anti-tumor M1-like subsets, through this mechanism it prevents the progress of pulmonary micro-metastases to macro-metastases at early-stage disease. (Hadjimichael et al., 2022). Table 5 illustrates studies investigating the effects of OXPHOS-targeted drugs on TAMs.
Non-pharmacological disruption of energy metabolism
Studies conducted on triple-negative breast cancer (TNBC) suggest that starvation could have a therapeutic value in cancer. Specifically, Salvadori et al., showed that fasting mimicking (FMD) starvation produced a significant impairment of CSCs (CD44+CD24−) compared with non-CSC in TNBC murine model and decreased mammosphere generation and volume. FMD delayed tumor progression in a syngeneic TNBC mouse model. Moreover, combining FMD cycles with PI3K/AKT/mTOR inhibitors resulted in long-term animal survival and reduced the treatment-induced side effects (Salvadori et al., 2021). The authors suggest that FMD-induced depletion of TNBC CSCs when tumors are in a less advanced stage could enormously enhance the efficacy of subsequent treatments targeting both CSCs (such as the FMD) and more differentiated cancer cells (such as PI3K/AKT/mTORC1 inhibitors) in late-stage cancers (Salvadori et al., 2021).
Similarly, Pateras et al. showed that short-term starvation increased sensitivity to DNA-damaging chemotherapeutic agents (doxorubicin or cisplatin) and inhibited oxidative stress-induced DNA damage repair in TBNC cells. Mechanistically, the combination of STS and chemotherapy-induced an increase of ROS production in such cancer cells through a collapse of mitochondrial respiration and an altered ATP production. In contrast, in normal, non-transformed cells, this combination has a protective effect (Pateras et al., 2023). The reasons for the differential response of normal versus cancer cells to dietary restriction remain unknown. More insights into starvation-induced mechanisms may lead to safe and effective anti-cancer treatments and help to overcome the chemotherapy resistance of cancer. Future ad hoc designed clinical trials are needed to assess dietary recommendations as an adjunct to chemotherapy for TNBC treatment and to confirm the efficacy of the combined approach.
Concluding remarks
In various tumors, CSCs and their supporting macrophages have been shown to be highly dependent on mitochondrial function and OXPHOS metabolism. Such a metabolic dependency of CSCs has stimulated modern chemotherapy targeting mitochondria/OXPHOS for cancer cure. To date, numerous clinical trials are underway across a wide range of advanced, resistant, and refractory tumors with a wide range of anti-mitochondrial and anti-metabolic agents. Numerous further agents are the subject of preclinical investigations linking laboratory drug discovery to the initiation of human clinical trials. However, the clinical use of pharmacological agents targeting such metabolic vulnerabilities of CSCs presents numerous challenges. There are issues related to the toxicity of antimitochondrial drugs; also, results so far obtained with clinical trials are sometimes vague or unflattering. More studies are needed to codify and quantify drug effects on healthy cells and find a therapeutic window and valuable tools that assist personalized therapies for precise administration indication. Despite these issues, awareness of the metabolic plasticity of CSCs supports perseverance in the anti-mitochondrial therapeutic approach. An increasing number of investigations of anti-mitochondrial medications in clinical trials are underway to hinder hard-to-treat tumors. The precise definitions of the therapeutic window and dose of the drug, mode of administration, optimization strategies for selective delivery to tumor cells, and combination with distinct targeted agents are currently being investigated in an attempt to guarantee a safety profile and at the same time undermine CSCs and their selection advantage that causes relapse. Moreover, the implementation of ad hoc phase 1 and 2 studies could accelerate the combined use of drugs potentially active against CSCs with those of standard cancer protocols, thus improving helpful information to adopt the use of such combination as the first line of intervention against tumors with a high frequency of recurrence.
Limitation
We have not described the influence of all the components of the niches that vary between solid tumor, and leukemia, as well as primary and metastatic tumor. Still, we focused on TAMs because the study of the niche components would have opened up very broad scenarios that deserve to be treated and explored in depth in a separate article. Furthermore, the studies we have presented often extend to the concept of stem cell-like cells and refer to specific tumors and experimental contexts that are not generalizable to different CSCs from all kinds of tumors.
Author contributions
LM: Conceptualization, Writing–original draft, Writing–review and editing, Data curation, Resources, Software. SR: Conceptualization, Data curation, Resources, Writing–original draft, Visualization, Writing–review and editing. CM: Data curation, Visualization, Writing–original draft, Resources. VD: Data curation, Resources, Visualization, Software, Writing–original draft. AC: Data curation, Resources, Visualization, Writing–original draft. RA: Data curation, Resources, Visualization, Writing–original draft. MV: Data curation, Resources, Visualization, Writing–original draft. DF: Data curation, Visualization, Writing–original draft. MR: Visualization, Conceptualization, Funding acquisition, Supervision, Validation, Writing–original draft, Writing–review and editing.
Funding
The author(s) declare financial support was received for the research, authorship, and/or publication of this article. This work was funded by National Center for Gene Therapy and Drugs based on RNA Technology MUR-CN3 CUP E63C22000940007.
Conflict of interest
The authors declare that the research was conducted in the absence of any commercial or financial relationships that could be construed as a potential conflict of interest.
Publisher’s note
All claims expressed in this article are solely those of the authors and do not necessarily represent those of their affiliated organizations, or those of the publisher, the editors and the reviewers. Any product that may be evaluated in this article, or claim that may be made by its manufacturer, is not guaranteed or endorsed by the publisher.
References
Abdelmoneim, M., Aboalela, M. A., Naoe, Y., Matsumura, S., Eissa, R. I., Bustos-Villalobos, I., et al. (2023). The impact of metformin on tumor-infiltrated immune cells: preclinical and clinical studies. Int. J. Mol. Sci. 24 (17), 13353. doi:10.3390/ijms241713353
Abdullah, L. N., and Chow, E. K. (2013). Mechanisms of chemoresistance in cancer stem cells. Clin. Transl. Med. 2 (1), 3. doi:10.1186/2001-1326-2-3
Agliano, A., Calvo, A., and Box, C. (2017). The challenge of targeting cancer stem cells to halt metastasis. Semin. Cancer Biol. 44, 25–42. doi:10.1016/j.semcancer.2017.03.003
Alistar, A., Morris, B. B., Desnoyer, R., Klepin, H. D., Hosseinzadeh, K., Clark, C., et al. (2017). Safety and tolerability of the first-in-class agent CPI-613 in combination with modified FOLFIRINOX in patients with metastatic pancreatic cancer: a single-centre, open-label, dose-escalation, phase 1 trial. Lancet Oncol. 18 (6), 770–778. doi:10.1016/S1470-2045(17)30314-5
Allavena, P., Digifico, E., and Belgiovine, C. (2021). Macrophages and cancer stem cells: a malevolent alliance. Mol. Med. 27 (1), 121. doi:10.1186/s10020-021-00383-3
Altenberg, B., and Greulich, K. O. (2004). Genes of glycolysis are ubiquitously overexpressed in 24 cancer classes. Genomics 84 (6), 1014–1020. doi:10.1016/j.ygeno.2004.08.010
Anderson, R., Miller, L. D., Isom, S., Chou, J. W., Pladna, K. M., Schramm, N. J., et al. (2022). Phase II trial of cytarabine and mitoxantrone with devimistat in acute myeloid leukemia. Nat. Commun. 13 (1), 1673. doi:10.1038/s41467-022-29039-4
Ayob, A. Z., and Ramasamy, T. S. (2018). Cancer stem cells as key drivers of tumour progression. J. Biomed. Sci. 25 (1), 20. doi:10.1186/s12929-018-0426-4
Baccelli, I, , and Trumpp, A., (2012). The evolving concept of cancer and metastasis stem cells. J. Cell Biol. 198 (3), 281–293. doi:10.1083/jcb.201202014
Basak, U., Sarkar, T., Mukherjee, S., Chakraborty, S., Dutta, A., Dutta, S., et al. (2023). Tumor-associated macrophages: an effective player of the tumor microenvironment. Front. Immunol. 14, 1295257. doi:10.3389/fimmu.2023.1295257
Batoon, L., and McCauley, L. K. (2021). Cross talk between macrophages and cancer cells in the bone metastatic environment. Front. Endocrinol. (Lausanne) 12, 763846. doi:10.3389/fendo.2021.763846
Begicevic, R. R., and Falasca, M. (2017). ABC transporters in cancer stem cells: beyond chemoresistance. Int. J. Mol. Sci. 18 (11), 2362. doi:10.3390/ijms18112362
Belisario, D. C., Kopecka, J., Pasino, M., Akman, M., De Smaele, E., Donadelli, M., et al. (2020). Hypoxia dictates metabolic rewiring of tumors: implications for chemoresistance. Cells 9 (12), 2598. doi:10.3390/cells9122598
Bellio, C., DiGloria, C., Spriggs, D. R., Foster, R., Growdon, W. B., and Rueda, B. R. (2019). The metabolic inhibitor CPI-613 negates treatment enrichment of ovarian cancer stem cells. Cancers (Basel) 11 (11), 1678. doi:10.3390/cancers11111678
Bonnet, D., and Dick, J. E. (1997). Human acute myeloid leukemia is organized as a hierarchy that originates from a primitive hematopoietic cell. Nat. Med. 3 (7), 730–737. doi:10.1038/nm0797-730
Bononi, G., Masoni, S., Di Bussolo, V., Tuccinardi, T., Granchi, C., and Minutolo, F. (2022). Historical perspective of tumor glycolysis: a century with Otto Warburg. Semin. Cancer Biol. 86 (Pt 2), 325–333. doi:10.1016/j.semcancer.2022.07.003
Borin, T. F., Angara, K., Rashid, M. H., Achyut, B. R., and Arbab, A. S. (2017). Arachidonic acid metabolite as a novel therapeutic target in breast cancer metastasis. Int. J. Mol. Sci. 18 (12), 2661. doi:10.3390/ijms18122661
Borriello, L., Coste, A., Traub, B., Sharma, V. P., Karagiannis, G. S., Lin, Y., et al. (2022). Primary tumor associated macrophages activate programs of invasion and dormancy in disseminating tumor cells. Nat. Commun. 13 (1), 626. doi:10.1038/s41467-022-28076-3
Brown, J. R., Chan, D. K., Shank, J. J., Griffith, K. A., Fan, H., Szulawski, R., et al. (2020). Phase II clinical trial of metformin as a cancer stem cell-targeting agent in ovarian cancer. JCI Insight 5 (11), e133247. doi:10.1172/jci.insight.133247
Bruns, I., Sauer, B., Burger, M. C., Eriksson, J., Hofmann, U., Braun, Y., et al. (2019). Disruption of peroxisome proliferator-activated receptor γ coactivator (PGC)-1α reverts key features of the neoplastic phenotype of glioma cells. J. Biol. Chem. 294 (9), 3037–3050. doi:10.1074/jbc.RA118.006993
Campbell, P. J., Yachida, S., Mudie, L. J., Stephens, P. J., Pleasance, E. D., Stebbings, L. A., et al. (2010). The patterns and dynamics of genomic instability in metastatic pancreatic cancer. Nature 467 (7319), 1109–1113. doi:10.1038/nature09460
Cao, Y., Wo, M., Xu, C., Fei, X., Jin, J., and Shan, Z. (2023). An AMPK agonist suppresses the progress of colorectal cancer by regulating the polarization of TAM to M1 through inhibition of HIF-1α and mTOR signal pathway. J. Cancer Res. Ther. 19 (6), 1560–1567. doi:10.4103/jcrt.jcrt_2670_22
Chae, C. W., Choi, G., Kim, Y. J., Cho, M., Kwon, Y. W., and Kim, H. S. (2023). The maintenance mechanism of hematopoietic stem cell dormancy: role for a subset of macrophages. BMB Rep. 56 (9), 482–487. doi:10.5483/BMBRep.2023-0092
Chawla, A. (2010). Control of macrophage activation and function by PPARs. Circ. Res. 106 (10), 1559–1569. doi:10.1161/CIRCRESAHA.110.216523
Chen, W., Mook, R. A., Premont, R. T., and Wang, J. (2018). Niclosamide: beyond an antihelminthic drug. Cell Signal 41, 89–96. doi:10.1016/j.cellsig.2017.04.001
Cole, A. J., Fayomi, A. P., Anyaeche, V. I., Bai, S., and Buckanovich, R. J. (2020). An evolving paradigm of cancer stem cell hierarchies: therapeutic implications. Theranostics 10 (7), 3083–3098. doi:10.7150/thno.41647
Curry, J. M., Tuluc, M., Whitaker-Menezes, D., Ames, J. A., Anantharaman, A., Butera, A., et al. (2013). Cancer metabolism, stemness and tumor recurrence: MCT1 and MCT4 are functional biomarkers of metabolic symbiosis in head and neck cancer. Cell Cycle 12 (9), 1371–1384. doi:10.4161/cc.24092
Decensi, A., Puntoni, M., Goodwin, P., Cazzaniga, M., Gennari, A., Bonanni, B., et al. (2010). Metformin and cancer risk in diabetic patients: a systematic review and meta-analysis. Cancer Prev. Res. (Phila) 3 (11), 1451–1461. doi:10.1158/1940-6207.CAPR-10-0157
De Luca, A., Fiorillo, M., Peiris-Pagès, M., Ozsvari, B., Smith, D. L., Sanchez-Alvarez, R., et al. (2015). Mitochondrial biogenesis is required for the anchorage-independent survival and propagation of stem-like cancer cells. Oncotarget 6 (17), 14777–14795. doi:10.18632/oncotarget.4401
Ding, Y., Labitzky, V., Legler, K., Qi, M., Schumacher, U., Schmalfeldt, B., et al. (2021). Molecular characteristics and tumorigenicity of ascites-derived tumor cells: mitochondrial oxidative phosphorylation as a novel therapy target in ovarian cancer. Mol. Oncol. 15 (12), 3578–3595. doi:10.1002/1878-0261.13028
Diskin, C., Ryan, T. A. J., and O'Neill, L. A. J. (2021). Modification of proteins by metabolites in immunity. Immunity 54 (1), 19–31. doi:10.1016/j.immuni.2020.09.014
Dudgeon, C., Harris, C. R., Chen, Y., Ghaddar, B., Sharma, A., and Shah, M. M., (2020), A novel model of pancreatic cancer dormancy reveals mechanistic insights and a dormancy gene signature with human relevance, bioRxiv 2020.04.13.037374. doi:10.1101/2020.04.13.037374
Emtenani, S., Martin, E. T., Gyoergy, A., Bicher, J., Genger, J. W., Köcher, T., et al. (2022). Macrophage mitochondrial bioenergetics and tissue invasion are boosted by an Atossa-Porthos axis in Drosophila. EMBO J. 41 (12), e109049. doi:10.15252/embj.2021109049
Enderling, H., Almog, N., and Hlatky, L. (2013). Systems biology of tumor dormancy. New York, NY, USA: Springer. doi:10.1007/978-1-4614-1445-2
Entenberg, D., Voiculescu, S., Guo, P., Borriello, L., Wang, Y., Karagiannis, G. S., et al. (2018). A permanent window for the murine lung enables high-resolution imaging of cancer metastasis. Nat. Methods 15 (1), 73–80. doi:10.1038/nmeth.4511
Evans, A. J., Sawyez, C. G., Wolfe, B. M., Connelly, P. W., Maguire, G. F., and Huff, M. W. (1993). Evidence that cholesteryl ester and triglyceride accumulation in J774 macrophages induced by very low-density lipoprotein subfractions occurs by different mechanisms. J. Lipid Res. 34 (5), 703–717. doi:10.1016/s0022-2275(20)39692-9
Fan, M., Shi, Y., Zhao, J., and Li, L. (2023). Cancer stem cell fate determination: mito-nuclear communication. Cell Commun. Signal 21 (1), 159. doi:10.1186/s12964-023-01160-x
Fan, Q. M., Jing, Y. Y., Yu, G. F., Kou, X. R., Ye, F., Gao, L., et al. (2014). Tumor-associated macrophages promote cancer stem cell-like properties via transforming growth factor-beta1-induced epithelial–mesenchymal transition in hepatocellular carcinoma. Cancer Lett. 352 (2), 160–168. doi:10.1016/j.canlet.2014.05.008
Feng, X., Chen, Z., Liu, Z., Fu, X., Song, H., and Zhang, Q. (2023). Self-delivery photodynamic-hypoxia alleviating nanomedicine synergizes with anti-PD-L1 for cancer immunotherapy. Int. J. Pharm. 639, 122970. doi:10.1016/j.ijpharm.2023.122970
Fiorillo, M., Lamb, R., Tanowitz, H. B., Cappello, A. R., Martinez-Outschoorn, U. E., Sotgia, F., et al. (2016a). Bedaquiline, an FDA-approved antibiotic, inhibits mitochondrial function and potently blocks the proliferative expansion of stem-like cancer cells (CSCs). Aging 8, 1593–1607. doi:10.18632/aging.100983
Fiorillo, M., Lamb, R., Tanowitz, H. B., Mutti, L., Krstic-Demonacos, M., Cappello, A. R., et al. (2016b). Repurposing atovaquone: targeting mitochondrial complex III and OXPHOS to eradicate cancer stem cells. Oncotarget 7 (23), 34084–34099. doi:10.18632/oncotarget.9122
Fiorillo, M., Tóth, F., Sotgia, F., and Lisanti, M. P. (2019). Doxycycline, Azithromycin and Vitamin C (DAV): a potent combination therapy for targeting mitochondria and eradicating cancer stem cells (CSCs). Aging (Albany NY) 11 (8), 2202–2216. doi:10.18632/aging.101905
Fong, M. Y., Zhou, W., Liu, L., Alontaga, A. Y., Chandra, M., Ashby, J., et al. (2015). Breast-cancer-secreted miR-122 reprograms glucose metabolism in premetastatic niche to promote metastasis. Nat. Cell Biol. 17 (2), 183–194. doi:10.1038/ncb3094
Fu, C., Xiao, X., Xu, H., Lu, W., and Wang, Y. (2020). Efficacy of atovaquone on EpCAM+CD44+ HCT-116 human colon cancer stem cells under hypoxia. Exp. Ther. Med. 20 (6), 286. doi:10.3892/etm.2020.9416
Ganguly, K., and Kimmelman, A. C. (2023). Reprogramming of tissue metabolism during cancer metastasis. Trends Cancer 9 (6), 461–471. doi:10.1016/j.trecan.2023.02.005
Gao, C., Shen, Y., Jin, F., Miao, Y., and Qiu, X. (2016). Cancer stem cells in small cell lung cancer cell line H446: higher dependency on oxidative phosphorylation and mitochondrial substrate-level phosphorylation than non-stem cancer cells. PLoS One 11 (5), e0154576. doi:10.1371/journal.pone.0154576
Gao, H., Chakraborty, G., Zhang, Z., Akalay, I., Gadiya, M., Gao, Y., et al. (2016). Multi-organ site metastatic reactivation mediated by non-canonical discoidin domain receptor 1 signaling. Cell 166 (1), 47–62. doi:10.1016/j.cell.2016.06.009
Garimella, S. V., Gampa, S. C., and Chaturvedi, P. (2023). Mitochondria in cancer stem cells: from an innocent bystander to a central player in therapy resistance. Stem Cells Cloning 16, 19–41. doi:10.2147/SCCAA.S417842
Geiß, C., Witzler, C., Poschet, G., Ruf, W., and Régnier-Vigouroux, A. (2022). Metabolic and inflammatory reprogramming of macrophages by ONC201 translates in a pro-inflammatory environment even in presence of glioblastoma cells. Eur. J. Immunol. 2021 (5), 1246–1261. doi:10.1002/eji.202048957
Giddings, E. L., Champagne, D. P., Wu, M. H., Laffin, J. M., Thornton, T. M., Valenca-Pereira, F., et al. (2021). Mitochondrial ATP fuels ABC transporter-mediated drug efflux in cancer chemoresistance. Nat. Commun. 12 (1), 2804. doi:10.1038/s41467-021-23071-6
Gonzalez, M. A., Lu, D. R., Yousefi, M., Kroll, A., Lo, C. H., Briseño, C. G., et al. (2023). Phagocytosis increases an oxidative metabolic and immune suppressive signature in tumor macrophages. J. Exp. Med. 220 (6), e20221472. doi:10.1084/jem.20221472
Greer, Y. E., Hernandez, L., Fennell, E. M. J., Kundu, M., Voeller, D., Chari, R., et al. (2022). Mitochondrial matrix protease ClpP agonists inhibit cancer stem cell function in breast cancer cells by disrupting mitochondrial homeostasis. Cancer Res. Commun. 2 (10), 1144–1161. doi:10.1158/2767-9764.CRC-22-0142
Hadjimichael, A. C., Foukas, A. F., Papadimitriou, E., Kaspiris, A., Peristiani, C., Chaniotakis, I., et al. (2022). Doxycycline inhibits the progression of metastases in early-stage osteosarcoma by downregulating the expression of MMPs, VEGF and ezrin at primary sites. Cancer Treat. Res. Commun. 32, 100617. doi:10.1016/j.ctarc.2022.100617
Hanahan, D. (2022). Hallmarks of cancer: new dimensions. Cancer Discov. 12 (1), 31–46. doi:10.1158/2159-8290.CD-21-1059
Hatle, K. M., Gummadidala, P., Navasa, N., Bernardo, E., Dodge, J., Silverstrim, B., et al. (2013). MCJ/DnaJC15, an endogenous mitochondrial repressor of the respiratory chain that controls metabolic alterations. Mol. Cell Biol. 33 (11), 2302–2314. doi:10.1128/MCB.00189-13
He, L., Bhat, K., Ioannidis, A., Zhang, L., Nguyen, N. T., Allen, J. E., et al. (2021). Effects of the DRD2/3 antagonist ONC201 and radiation in glioblastoma. Radiother. Oncol. 161, 140–147. doi:10.1016/j.radonc.2021.05.027
He, L., and Marneros, A. G. (2014). Doxycycline inhibits polarization of macrophages to the proangiogenic M2-type and subsequent neovascularization. J. Biol. Chem. 289 (12), 8019–8028. doi:10.1074/jbc.M113.535765
Hermann, P. C., Huber, S. L., Herrler, T., Aicher, A., Ellwart, J. W., Guba, M., et al. (2007). Distinct populations of cancer stem cells determine tumor growth and metastatic activity in human pancreatic cancer. Cell Stem. 1 (3), 313–323. doi:10.1016/j.stem.2007.06.002
Hirsch, H. A., Iliopoulos, D., Tsichlis, P. N., and Struhl, K. (2009). Metformin selectively targets cancer stem cells, and acts together with chemotherapy to block tumor growth and prolong remission. Cancer Res. 69 (19), 7507–7511. doi:10.1158/0008-5472.CAN-09-2994
Hoshino, A., Costa-Silva, B., Shen, T. L., Rodrigues, G., Hashimoto, A., Tesic Mark, M., et al. (2015). Tumour exosome integrins determine organotropic metastasis. Nature 527 (7578), 329–335. doi:10.1038/nature15756
Hua, Y., Zheng, Y., Yao, Y., Jia, R., Ge, S., and Zhuang, A. (2023). Metformin and cancer hallmarks: shedding new lights on therapeutic repurposing. J. Transl. Med. 21 (1), 403. doi:10.1186/s12967-023-04263-8
Huang, S. C., Everts, B., Ivanova, Y., O'Sullivan, D., Nascimento, M., Smith, A. M., et al. (2014). Cell-intrinsic lysosomal lipolysis is essential for alternative activation of macrophages. Nat. Immunol. 15 (9), 846–855. doi:10.1038/ni.2956
Ishizawa, J., Kojima, K., Chachad, D., Ruvolo, P., Ruvolo, V., Jacamo, R. O., et al. (2016). ATF4 induction through an atypical integrated stress response to ONC201 triggers p53-independent apoptosis in hematological malignancies. Sci. Signal 9 (415), ra17. doi:10.1126/scisignal.aac4380
Jeon, H. M., Oh, Y. T., Shin, Y. J., Chang, N., Kim, D., Woo, D., et al. (2023). Dopamine receptor D2 regulates glioblastoma survival and death through MET and death receptor 4/5. Neoplasia 39, 100894. doi:10.1016/j.neo.2023.100894
Jin, Y., Lu, Z., Ding, K., Li, J., Du, X., Chen, C., et al. (2010). Antineoplastic mechanisms of niclosamide in acute myelogenous leukemia stem cells: inactivation of the NF-kappaB pathway and generation of reactive oxygen species. Cancer Res. 70 (6), 2516–2527. doi:10.1158/0008-5472.CAN-09-3950
Jinushi, M., Chiba, S., Yoshiyama, H., Masutomi, K., Kinoshita, I., Dosaka-Akita, H., et al. (2011). Tumor-associated macrophages regulate tumorigenicity and anticancer drug responses of cancer stem/initiating cells. Proc. Natl. Acad. Sci. U. S. A. 108 (30), 12425–12430. doi:10.1073/pnas.1106645108
Jones, C. L., Inguva, A., and Jordan, C. T. (2021). Targeting energy metabolism in cancer stem cells: progress and challenges in leukemia and solid tumors. Cell Stem Cell 28 (3), 378–393. doi:10.1016/j.stem.2021.02.013
Jordan, C. T. (2004). Cancer stem cell biology: from leukemia to solid tumors. Curr. Opin. Cell Biol. 16 (6), 708–712. doi:10.1016/j.ceb.2004.09.002
Joseph, S. S., Tran, D. H. V., Islam, F., and Gopalan, V. (2023). “Cancer stem cells and metastasis,” in Cancer stem cells: basic concept and therapeutic implications. Editors F. Islam,, and A. K. Lam (Singapore: Springer). doi:10.1007/978-981-99-3185-9_8
Kang, J., Lee, D., Lee, K. J., Yoon, J. E., Kwon, J. H., Seo, Y., et al. (2022). Tumor-suppressive effect of metformin via the regulation of M2 macrophages and myeloid-derived suppressor cells in the tumor microenvironment of colorectal cancer. Cancers (Basel) 14 (12), 2881. doi:10.3390/cancers14122881
Kapur, A., Mehta, P., Simmons, A. D., Ericksen, S. S., Mehta, G., Palecek, S. P., et al. (2022). Atovaquone: an inhibitor of oxidative phosphorylation as studied in gynecologic cancers. Cancers (Basel) 14 (9), 2297. doi:10.3390/cancers14092297
Karp, I., and Lyakhovich, A. (2022). Targeting cancer stem cells with antibiotics inducing mitochondrial dysfunction as an alternative anticancer therapy. Biochem. Pharmacol. 198, 114966. doi:10.1016/j.bcp.2022.114966
Khan, I. N., Al-Karim, S., Bora, R. S., Chaudhary, A. G., and Saini, K. S. (2015). Cancer stem cells: a challenging paradigm for designing targeted drug therapies. Drug Discov. Today 20 (10), 1205–1216. doi:10.1016/j.drudis.2015.06.013
Kong, S. C., Nøhr-Nielsen, A., Zeeberg, K., Reshkin, S. J., Hoffmann, E. K., Novak, I., et al. (2016). Monocarboxylate transporters MCT1 and MCT4 regulate migration and invasion of pancreatic ductal adenocarcinoma cells. Pancreas 45 (7), 1036–1047. doi:10.1097/MPA.0000000000000571
Korn, C., and Méndez-Ferrer, S. (2017). Myeloid malignancies and the microenvironment. Blood 129 (7), 811–822. doi:10.1182/blood-2016-09-670224
Kreso, A., and Dick, J. E. (2014). Evolution of the cancer stem cell model. Cell Stem Cell 14 (3), 275–291. doi:10.1016/j.stem.2014.02.006
Kuntz, E. M., Baquero, P., Michie, A. M., Dunn, K., Tardito, S., Holyoake, T. L., et al. (2017). Targeting mitochondrial oxidative phosphorylation eradicates therapy-resistant chronic myeloid leukemia stem cells. Nat. Med. 23 (10), 1234–1240. doi:10.1038/nm.4399
Ladanyi, A., Mukherjee, A., Kenny, H. A., Johnson, A., Mitra, A. K., Sundaresan, S., et al. (2018). Adipocyte-induced CD36 expression drives ovarian cancer progression and metastasis. Oncogene 37 (17), 2285–2301. doi:10.1038/s41388-017-0093-z
Lamb, R., Ozsvari, B., Lisanti, C. L., Tanowitz, H. B., Howell, A., Martinez-Outschoorn, U. E., et al. (2015). Antibiotics that target mitochondria effectively eradicate cancer stem cells, across multiple tumor types: treating cancer like an infectious disease. Oncotarget 6 (7), 4569–4584. doi:10.18632/oncotarget.3174
Lapidot, T., Sirard, C., Vormoor, J., Murdoch, B., Hoang, T., Caceres-Cortes, J., et al. (1994). A cell initiating human acute myeloid leukaemia after transplantation into SCID mice. Nature 367 (6464), 645–648. doi:10.1038/367645a0
LeBleu, V. S., O'Connell, J. T., Gonzalez Herrera, K. N., Wikman, H., Pantel, K., Haigis, M. C., et al. (2014). PGC-1α mediates mitochondrial biogenesis and oxidative phosphorylation in cancer cells to promote metastasis. Nat. Cell Biol. 16 (10), 992–1003. doi:10.1038/ncb3039
Li, H., Feng, Z., and He, M. L. (2020). Lipid metabolism alteration contributes to and maintains the properties of cancer stem cells. Theranostics 10 (16), 7053–7069. doi:10.7150/thno.41388
Lim, E., Vaillant, F., Wu, D., Forrest, N. C., Pal, B., Hart, A. H., et al. (2009). Aberrant luminal progenitors as the candidate target population for basal tumor development in BRCA1 mutation carriers. Nat. Med. 15 (8), 907–913. doi:10.1038/nm.2000
Linton, K. J., and Higgins, C. F. (2007). Structure and function of ABC transporters: the ATP switch provides flexible control. Pflugers Arch. 453 (5), 555–567. doi:10.1007/s00424-006-0126-x
Liu, H., Tao, H., Wang, H., Yang, Y., Yang, R., Dai, X., et al. (2022). Corrigendum: doxycycline inhibits cancer stem cell-like properties via PAR1/FAK/PI3K/AKT pathway in pancreatic cancer. Front. Oncol. 12, 830506. doi:10.3389/fonc.2022.830506
Liu, Q., Tong, D., Liu, G., Gao, J., Wang, L. A., Xu, J., et al. (2018). Metformin inhibits prostate cancer progression by targeting tumor-associated inflammatory infiltration. Clin. Cancer Res. 24 (22), 5622–5634. doi:10.1158/1078-0432.CCR-18-0420
Liu, Y., Xu, R., Gu, H., Zhang, E., Qu, J., Cao, W., et al. (2021). Metabolic reprogramming in macrophage responses. Biomark. Res. 9 (1), 1. doi:10.1186/s40364-020-00251-y
Luger, A. L., Sauer, B., Lorenz, N. I., Engel, A. L., Braun, Y., Voss, M., et al. (2018). Doxycycline impairs mitochondrial function and protects human glioma cells from hypoxia-induced cell death: implications of using tet-inducible systems. Int. J. Mol. Sci. 19 (5), 1504. doi:10.3390/ijms19051504
Luo, X., Cheng, C., Tan, Z., Li, N., Tang, M., Yang, L., et al. (2017). Emerging roles of lipid metabolism in cancer metastasis. Mol. Cancer 16 (1), 76. doi:10.1186/s12943-017-0646-3
Lv, T., Zhang, Z., Yu, H., Ren, S., Wang, J., Li, S., et al. (2022). Tamoxifen exerts anticancer effects on pituitary adenoma progression via inducing cell apoptosis and inhibiting cell migration. Int. J. Mol. Sci. 23 (5), 2664. doi:10.3390/ijms23052664
Ma, T., Tian, X., Zhang, B., Li, M., Wang, Y., Yang, C., et al. (2022). Low-dose metformin targets the lysosomal AMPK pathway through PEN2. Nature 603 (7899), 159–165. doi:10.1038/s41586-022-04431-8
Malandrino, M. I., Fucho, R., Weber, M., Calderon-Dominguez, M., Mir, J. F., Valcarcel, L., et al. (2015). Enhanced fatty acid oxidation in adipocytes and macrophages reduces lipid-induced triglyceride accumulation and inflammation. Am. J. Physiol. Endocrinol. Metab. 308 (9), E756–E769. doi:10.1152/ajpendo.00362.2014
Mancini, S. J. C., Balabanian, K., Corre, I., Gavard, J., Lazennec, G., Le Bousse-Kerdilès, M. C., et al. (2021). Deciphering tumor niches: lessons from solid and hematological malignancies. Front. Immunol. 12, 766275. doi:10.3389/fimmu.2021.766275
Marchand, T., and Pinho, S. (2021). Leukemic stem cells: from leukemic niche biology to treatment opportunities. Front. Immunol. 12, 775128. doi:10.3389/fimmu.2021.775128
Marchiq, I., and Pouysségur, J. (2016). Hypoxia, cancer metabolism and the therapeutic benefit of targeting lactate/H+ symporters. J. Mol. Med. 94, 155–171. doi:10.1007/s00109-015-1307-x
Martinez-Outschoorn, U. E., Peiris-Pagés, M., Pestell, R. G., Sotgia, F., and Lisanti, M. P. (2017). Cancer metabolism: a therapeutic perspective. Nat. Rev. Clin. Oncol. 14 (1), 11–31. doi:10.1038/nrclinonc.2016.60
Mather, M. W., Darrouzet, E., Valkova-Valchanova, M., Cooley, J. W., McIntosh, M. T., Daldal, F., et al. (2005). Uncovering the molecular mode of action of the antimalarial drug atovaquone using a bacterial system. J. Biol. Chem. 280 (29), 27458–27465. doi:10.1074/jbc.M502319200
Merlo, L. M., Pepper, J. W., Reid, B. J., and Maley, C. C. (2006). Cancer as an evolutionary and ecological process. Nat. Rev. Cancer 6 (12), 924–935. doi:10.1038/nrc2013
Minhas, P. S., Liu, L., Moon, P. K., Joshi, A. U., Dove, C., Mhatre, S., et al. (2019). Macrophage de novo NAD+ synthesis specifies immune function in aging and inflammation. Nat. Immunol. 20 (1), 50–63. doi:10.1038/s41590-018-0255-3
Molina, J. R., Sun, Y., Protopopova, M., Gera, S., Bandi, M., Bristow, C., et al. (2018). An inhibitor of oxidative phosphorylation exploits cancer vulnerability. Nat. Med. 24 (7), 1036–1046. doi:10.1038/s41591-018-0052-4
Moreira, P. I., Custódio, J., Moreno, A., Oliveira, C. R., and Santos, M. S. (2006). Tamoxifen and estradiol interact with the flavin mononucleotide site of complex I leading to mitochondrial failure. J. Biol. Chem. 281 (15), 10143–10152. doi:10.1074/jbc.M510249200
Mudassar, F., Shen, H., O'Neill, G., and Hau, E. (2020). Targeting tumor hypoxia and mitochondrial metabolism with anti-parasitic drugs to improve radiation response in high-grade gliomas. J. Exp. Clin. Cancer Res. 39 (1), 208. doi:10.1186/s13046-020-01724-6
Müller, L., Tunger, A., Plesca, I., Wehner, R., Temme, A., Westphal, D., et al. (2020). Bidirectional crosstalk between cancer stem cells and immune cell subsets. Front. Immunol. 11, 140. doi:10.3389/fimmu.2020.00140
Munoz, L. E., Huang, L., Bommireddy, R., Sharma, R., Monterroza, L., Guin, R. N., et al. (2021). Metformin reduces PD-L1 on tumor cells and enhances the anti-tumor immune response generated by vaccine immunotherapy. J. Immunother. Cancer 9 (11), e002614. doi:10.1136/jitc-2021-002614
Nakajima, E. C., and Van Houten, B. (2013). Metabolic symbiosis in cancer: refocusing the Warburg lens. Mol. Carcinog. 52 (5), 329–337. doi:10.1002/mc.21863
Nelson, V. L., Nguyen, H. C. B., Garcìa-Cañaveras, J. C., Briggs, E. R., Ho, W. Y., DiSpirito, J. R., et al. (2018). PPARγ is a nexus controlling alternative activation of macrophages via glutamine metabolism. Genes Dev. 32 (15-16), 1035–1044. doi:10.1101/gad.312355.118
Odegaard, J. I., and Chawla, A. (2011). Alternative macrophage activation and metabolism. Annu. Rev. Pathol. 6, 275–297. doi:10.1146/annurev-pathol-011110-130138
Odegaard, J. I., Ricardo-Gonzalez, R. R., Red Eagle, A., Vats, D., Morel, C. R., Goforth, M. H., et al. (2008). Alternative M2 activation of Kupffer cells by PPARdelta ameliorates obesity-induced insulin resistance. Cell Metab. 7 (6), 496–507. doi:10.1016/j.cmet.2008.04.003
Olivares-Urbano, M. A., Griñán-Lisón, C., Marchal, J. A., and Núñez, M. I. (2020). CSC radioresistance: a therapeutic challenge to improve radiotherapy effectiveness in cancer. Cells 9 (7), 1651. doi:10.3390/cells9071651
O'Neill, L. A., Kishton, R. J., and Rathmell, J. (2016). A guide to immunometabolism for immunologists. Nat. Rev. Immunol. 16 (9), 553–565. doi:10.1038/nri.2016.70
Oshimori, N. (2020). Cancer stem cells and their niche in the progression of squamous cell carcinoma. Cancer Sci. 111 (11), 3985–3992. doi:10.1111/cas.14639
Owen, M. R., Doran, E., and Halestrap, A. P. (2000). Evidence that metformin exerts its anti-diabetic effects through inhibition of complex 1 of the mitochondrial respiratory chain. Biochem. J. 348 (Pt 3), 607–614. doi:10.1042/bj3480607
Pardee, T. S., Anderson, R. G., Pladna, K. M., Isom, S., Ghiraldeli, L. P., Miller, L. D., et al. (2018). A phase I study of CPI-613 in combination with high-dose cytarabine and mitoxantrone for relapsed or refractory acute myeloid leukemia. Clin. Cancer Res. 24 (9), 2060–2073. doi:10.1158/1078-0432.CCR-17-2282
Park, S. J., Smith, C. P., Wilbur, R. R., Cain, C. P., Kallu, S. R., Valasapalli, S., et al. (2018). An overview of MCT1 and MCT4 in GBM: small molecule transporters with large implications. Am. J. Cancer Res. 8 (10), 1967–1976.
Park, S. Y., Kim, J. Y., Choi, J. H., Kim, J. H., Lee, C. J., Singh, P., et al. (2019). Inhibition of LEF1-mediated DCLK1 by niclosamide attenuates colorectal cancer stemness. Clin. Cancer Res. 25 (4), 1415–1429. doi:10.1158/1078-0432.CCR-18-1232
Park, S. Y., and Nam, J. S. (2020). The force awakens: metastatic dormant cancer cells. Exp. Mol. Med. 52 (4), 569–581. doi:10.1038/s12276-020-0423-z
Pascual, G., Avgustinova, A., Mejetta, S., Martín, M., Castellanos, A., Attolini, C. S., et al. (2017). Targeting metastasis-initiating cells through the fatty acid receptor CD36. Nature 541 (7635), 41–45. doi:10.1038/nature20791
Passalidou, E., Trivella, M., Singh, N., Ferguson, M., Hu, J., Cesario, A., et al. (2002). Vascular phenotype in angiogenic and non-angiogenic lung non-small cell carcinomas. Br. J. Cancer 86 (2), 244–249. doi:10.1038/sj.bjc.6600015
Pastò, A., Bellio, C., Pilotto, G., Ciminale, V., Silic-Benussi, M., Guzzo, G., et al. (2014). Cancer stem cells from epithelial ovarian cancer patients privilege oxidative phosphorylation, and resist glucose deprivation. Oncotarget 5 (12), 4305–4319. doi:10.18632/oncotarget.2010
Pateras, I. S., Williams, C., Gianniou, D. D., Margetis, A. T., Avgeris, M., Rousakis, P., et al. (2023). Short term starvation potentiates the efficacy of chemotherapy in triple negative breast cancer via metabolic reprogramming. J. Transl. Med. 21 (1), 169. doi:10.1186/s12967-023-03935-9
Philip, P. A., Buyse, M. E., Alistar, A. T., Rocha Lima, C. M., Luther, S., Pardee, T. S., et al. (2019). A Phase III open-label trial to evaluate efficacy and safety of CPI-613 plus modified FOLFIRINOX (mFFX) versus FOLFIRINOX (FFX) in patients with metastatic adenocarcinoma of the pancreas. Future Oncol. 15 (28), 3189–3196. doi:10.2217/fon-2019-0209
Pierce, S. R., Fang, Z., Yin, Y., West, L., Asher, M., Hao, T., et al. (2021). Targeting dopamine receptor D2 as a novel therapeutic strategy in endometrial cancer. J. Exp. Clin. Cancer Res. 40 (1), 61. doi:10.1186/s13046-021-01842-9
Pignatelli, J., Bravo-Cordero, J. J., Roh-Johnson, M., Gandhi, S. J., Wang, Y., Chen, X., et al. (2016). Macrophage-dependent tumor cell transendothelial migration is mediated by Notch1/MenaINV-initiated invadopodium formation. Sci. Rep. 6 37874. doi:10.1038/srep37874
Plaks, V., Kong, N., and Werb, Z. (2015). The cancer stem cell niche: how essential is the niche in regulating stemness of tumor cells? Cell Stem Cell 16 (3), 225–238. doi:10.1016/j.stem.2015.02.015
Prabhu, V. V., Allen, J. E., Dicker, D. T., and El-Deiry, W. S. (2015). Small-Molecule ONC201/TIC10 targets chemotherapy-resistant colorectal cancer stem-like cells in an akt/foxo3a/TRAIL-dependent manner. Cancer Res. 75 (7), 1423–1432. doi:10.1158/0008-5472.CAN-13-3451
Przystal, J. M., Cianciolo Cosentino, C., Yadavilli, S., Zhang, J., Laternser, S., Bonner, E. R., et al. (2022). Imipridones affect tumor bioenergetics and promote cell lineage differentiation in diffuse midline gliomas. Neuro Oncol. 24 (9), 1438–1451. doi:10.1093/neuonc/noac041
Quail, D. F., Taylor, M. J., and Postovit, L. M. (2012). Microenvironmental regulation of cancer stem cell phenotypes. Curr. Stem Cell Res. Ther. 7 (3), 197–216. doi:10.2174/157488812799859838
Rainho, M. A., Siqueira, P. B., de Amorim, Í. S. S., Mencalha, A. L., and Thole, A. A. (2023). Mitochondria in colorectal cancer stem cells - a target in drug resistance. Cancer Drug Resist 6 (2), 273–283. doi:10.20517/cdr.2022.116
Reddy, J. K., and Hashimoto, T. (2001). Peroxisomal beta-oxidation and peroxisome proliferator-activated receptor alpha: an adaptive metabolic system. Annu. Rev. Nutr. 21, 193–230. doi:10.1146/annurev.nutr.21.1.193
Rodriguez-Berriguete, G., Puliyadi, R., Machado, N., Barberis, A., Prevo, R., McLaughlin, M., et al. (2024). Antitumour effect of the mitochondrial complex III inhibitor Atovaquone in combination with anti-PD-L1 therapy in mouse cancer models. Cell Death Dis. 15 (1), 32. doi:10.1038/s41419-023-06405-8
Romano, S., Tufano, M., D'Arrigo, P., Vigorito, V., Russo, S., and Romano, M. F. (2020). Cell stemness, epithelial-to-mesenchymal transition, and immunoevasion: intertwined aspects in cancer metastasis. Semin. Cancer Biol. 60, 181–190. doi:10.1016/j.semcancer.2019.08.015
Roussos, E. T., Balsamo, M., Alford, S. K., Wyckoff, J. B., Gligorijevic, B., Wang, Y., et al. (2011). Mena invasive (MenaINV) promotes multicellular streaming motility and transendothelial migration in a mouse model of breast cancer. J. Cell Sci. 124 (Pt 13), 2120–2131. doi:10.1242/jcs.086231
Ruiz-Malagón, A. J., Hidalgo-García, L., Rodríguez-Sojo, M. J., Molina-Tijeras, J. A., García, F., Diez-Echave, P., et al. (2023). Tigecycline reduces tumorigenesis in colorectal cancer via inhibition of cell proliferation and modulation of immune response. Biomed. Pharmacother. 163, 114760. doi:10.1016/j.biopha.2023.114760
Saif, M. W., Rajagopal, S., Caplain, J., Grimm, E., Serebrennikova, O., Das, M., et al. (2019). A phase I delayed-start, randomized and pharmacodynamic study of metformin and chemotherapy in patients with solid tumors. Cancer Chemother. Pharmacol. 84 (6), 1323–1331. doi:10.1007/s00280-019-03967-3
Sainero-Alcolado, L., Liaño-Pons, J., Ruiz-Pérez, M. V., and Arsenian-Henriksson, M. (2022). Targeting mitochondrial metabolism for precision medicine in cancer. Cell Death Differ. 29 (7), 1304–1317. doi:10.1038/s41418-022-01022-y
Sainz, B., Carron, E., Vallespinós, M., and Machado, H. L. (2016). Cancer stem cells and macrophages: implications in tumor biology and therapeutic strategies. Mediat. Inflamm. 2016, 9012369. doi:10.1155/2016/9012369
Salvadori, G., Zanardi, F., Iannelli, F., Lobefaro, R., Vernieri, C., and Longo, V. D. (2021). Fasting-mimicking diet blocks triple-negative breast cancer and cancer stem cell escape. Cell Metab. 33 (11), 2247–2259.e6. doi:10.1016/j.cmet.2021.10.008
Sancho, P., Barneda, D., and Heeschen, C. (2016). Hallmarks of cancer stem cell metabolism. Br. J. Cancer 114 (12), 1305–1312. doi:10.1038/bjc.2016.152
Sancho, P., Burgos-Ramos, E., Tavera, A., Bou Kheir, T., Jagust, P., Schoenhals, M., et al. (2015). MYC/PGC-1α balance determines the metabolic phenotype and plasticity of pancreatic cancer stem cells. Cell Metabol. 22, 590–605. doi:10.1016/j.cmet.2015.08.015
Sandforth, L., Ammar, N., Dinges, L. A., Röcken, C., Arlt, A., Sebens, S., et al. (2020). Impact of the monocarboxylate transporter-1 (MCT1)-Mediated cellular import of lactate on stemness properties of human pancreatic adenocarcinoma cells. Cancers (Basel) 12 (3), 581. doi:10.3390/cancers12030581
Scatena, C., Roncella, M., Di Paolo, A., Aretini, P., Menicagli, M., Fanelli, G., et al. (2018). Doxycycline, an inhibitor of mitochondrial biogenesis, effectively reduces cancer stem cells (CSCs) in early breast cancer patients: a clinical pilot study. Front. Oncol. 8, 452. doi:10.3389/fonc.2018.00452
Schepers, K., Campbell, T. B., and Passegué, E. (2015). Normal and leukemic stem cell niches: insights and therapeutic opportunities. Cell Stem Cell 16 (3), 254–267. doi:10.1016/j.stem.2015.02.014
Schultz, C. W., and Nevler, A. (2022). Pyrvinium pamoate: past, present, and future as an anti-cancer drug. Biomedicines 10 (12), 3249. doi:10.3390/biomedicines10123249
Sell, S. (2010). On the stem cell origin of cancer. Am. J. Pathol. 176 (6), 2584–3494. doi:10.2353/ajpath.2010.091064
Semenas, J., Wang, T., Sajid Syed Khaja, A., Firoj Mahmud, A., Simoulis, A., Grundström, T., et al. (2020). Targeted inhibition of ERα signaling and PIP5K1α/Akt pathways in castration-resistant prostate cancer. Mol. Oncol. 15 (4), 968–986. doi:10.1002/1878-0261.12873
Shang, S., Yang, C., Chen, F., Xiang, R. S., Zhang, H., Dai, S. Y., et al. (2023). ID1 expressing macrophages support cancer cell stemness and limit CD8+ T cell infiltration in colorectal cancer. Nat. Commun. 14 (1), 7661. doi:10.1038/s41467-023-43548-w
Shirakawa, K., Kobayashi, H., Heike, Y., Kawamoto, S., Brechbiel, M. W., Kasumi, F., et al. (2002). Hemodynamics in vasculogenic mimicry and angiogenesis of inflammatory breast cancer xenograft. Cancer Res. 62 (2), 560–566.
Singh, M., Afonso, J., Sharma, D., Gupta, R., Kumar, V., Rani, R., et al. (2023). Targeting monocarboxylate transporters (MCTs) in cancer: how close are we to the clinics? Semin. Cancer Biol. 90, 1–14. doi:10.1016/j.semcancer.2023.01.007
Sonveaux, P., Végran, F., Schroeder, T., Wergin, M. C., Verrax, J., Rabbani, Z. N., et al. (2008). Targeting lactate-fueled respiration selectively kills hypoxic tumor cells in mice. J. Clin. Invest. 118 (12), 3930–3942. doi:10.1172/JCI36843
Sosa, M. S., Parikh, F., Maia, A. G., Estrada, Y., Bosch, A., Bragado, P., et al. (2015). NR2F1 controls tumour cell dormancy via SOX9-and RARβ-driven quiescence programmes. Nat. Commun. 6, 6170. doi:10.1038/ncomms7170
Stein, M. N., Malhotra, J., Tarapore, R. S., Malhotra, U., Silk, A. W., Chan, N., et al. (2019). Safety and enhanced immunostimulatory activity of the DRD2 antagonist ONC201 in advanced solid tumor patients with weekly oral administration. J. Immunother. Cancer 7 (1), 136. doi:10.1186/s40425-019-0599-8
Stuart, S. D., Schauble, A., Gupta, S., Kennedy, A. D., Keppler, B. R., Bingham, P. M., et al. (2014). A strategically designed small molecule attacks alpha-ketoglutarate dehydrogenase in tumor cells through a redox process. Cancer Metab. 2 (1), 4. doi:10.1186/2049-3002-2-4
Su, C., Zhang, J., Yarden, Y., and Fu, L. (2021). The key roles of cancer stem cell-derived extracellular vesicles. Signal Transduct. Target Ther. 6 (1), 109. doi:10.1038/s41392-021-00499-2
Takada, T., Takata, K., and Ashihara, E. (2016). Inhibition of monocarboxylate transporter 1 suppresses the proliferation of glioblastoma stem cells. J. Physiol. Sci. 66, 387–396. doi:10.1007/s12576-016-0435-6
Tannahill, G. M., Curtis, A. M., Adamik, J., Palsson-McDermott, E. M., McGettrick, A. F., Goel, G., et al. (2013). Succinate is an inflammatory signal that induces IL-1β through HIF-1α. Nature 496 (7444), 238–242. doi:10.1038/nature11986
Taylor, S. E., Chan, D. K., Yang, D., Bruno, T., Lieberman, R., Siddiqui, J., et al. (2022). Shifting the soil: metformin treatment decreases the protumorigenic tumor microenvironment in epithelial ovarian cancer. Cancers (Basel) 14 (9), 2298. doi:10.3390/cancers14092298
Valle, S., Alcalá, S., Martin-Hijano, L., Cabezas-Sáinz, P., Navarro, D., Muñoz, E. R., et al. (2020). Exploiting oxidative phosphorylation to promote the stem and immunoevasive properties of pancreatic cancer stem cells. Nat. Commun. 11 (1), 5265. doi:10.1038/s41467-020-18954-z
Vazquez, F., Lim, J. H., Chim, H., Bhalla, K., Girnun, G., Pierce, K., et al. (2013). PGC1α expression defines a subset of human melanoma tumors with increased mitochondrial capacity and resistance to oxidative stress. Cancer Cell 23 (3), 287–301. doi:10.1016/j.ccr.2012.11.020
Viale, A., Pettazzoni, P., Lyssiotis, C. A., Ying, H., Sánchez, N., Marchesini, M., et al. (2014). Oncogene ablation-resistant pancreatic cancer cells depend on mitochondrial function. Nature 514 (7524), 628–632. doi:10.1038/nature13611
Vlashi, E., Lagadec, C., Vergnes, L., Matsutani, T., Masui, K., Poulou, M., et al. (2011). Metabolic state of glioma stem cells and nontumorigenic cells. Proc. Natl. Acad. Sci. U. S. A. 108 (38), 16062–16067. doi:10.1073/pnas.1106704108
Walker, N. D., Elias, M., Guiro, K., Bhatia, R., Greco, S. J., Bryan, M., et al. (2019). Exosomes from differentially activated macrophages influence dormancy or resurgence of breast cancer cells within bone marrow stroma. Cell Death Dis. 10 (2), 59. doi:10.1038/s41419-019-1304-z
Wang, B., Ma, L. Y., Wang, J. Q., Lei, Z. N., Gupta, P., Zhao, Y. D., et al. (2018a). Discovery of 5-Cyano-6-phenylpyrimidin derivatives containing an acylurea moiety as orally bioavailable reversal agents against P-Glycoprotein-Mediated mutidrug resistance. J. Med. Chem. 61 (14), 5988–6001. doi:10.1021/acs.jmedchem.8b00335
Wang, B., Zhao, B., Chen, Z. S., Pang, L. P., Zhao, Y. D., Guo, Q., et al. (2018b). Exploration of 1,2,3-triazole-pyrimidine hybrids as potent reversal agents against ABCB1-mediated multidrug resistance. Eur. J. Med. Chem. 143, 1535–1542. doi:10.1016/j.ejmech.2017.10.041
Wang, L., and Zheng, G. (2019). Macrophages in leukemia microenvironment. Blood Sci. 1 (1), 29–33. doi:10.1097/BS9.0000000000000014
Wang, R., Tao, B., Fan, Q., Wang, S., Chen, L., Zhang, J., et al. (2019). Fatty-acid receptor CD36 functions as a hydrogen sulfide-targeted receptor with its Cys333-Cys272 disulfide bond serving as a specific molecular switch to accelerate gastric cancer metastasis. EBioMedicine 45, 108–123. doi:10.1016/j.ebiom.2019.06.037
Wang, S., Lin, Y., Xiong, X., Wang, L., Guo, Y., Chen, Y., et al. (2020). Low-dose metformin reprograms the tumor immune microenvironment in human esophageal cancer: results of a phase II clinical trial. Clin. Cancer Res. 26 (18), 4921–4932. doi:10.1158/1078-0432.CCR-20-0113
Warburg, O., and Minami, S. (1923). Versuche an überlebendem carcinom-gewebe. Klin. Wochenschr 2, 776–777. doi:10.1007/BF01712130
Watanabe, C. K. (1918). Studies in the metabolism changes induced by administration of guanidine bases: i. Influence of injected guanidine hydrochloride upon blood sugar content. J. Biol. Chem. 33 (2), 253–265. doi:10.1016/S0021-9258(18)86579-6
Watt, M. J., Clark, A. K., Selth, L. A., Haynes, V. R., Lister, N., Rebello, R., et al. (2019). Suppressing fatty acid uptake has therapeutic effects in preclinical models of prostate cancer. Sci. Transl. Med. 11 (478), eaau5758. doi:10.1126/scitranslmed.aau5758
Wei, Z., Zhang, X., Yong, T., Bie, N., Zhan, G., Li, X., et al. (2021). Boosting anti-PD-1 therapy with metformin-loaded macrophage-derived microparticles. Nat. Commun. 12 (1), 440. doi:10.1038/s41467-020-20723-x
Wu, Z., Zhang, C., and Najafi, M. (2022). Targeting of the tumor immune microenvironment by metformin. J. Cell Commun. Signal 16 (3), 333–348. doi:10.1007/s12079-021-00648-w
Xuan, Q. J., Wang, J. X., Nanding, A., Wang, Z. P., Liu, H., Lian, X., et al. (2014). Tumor-associated macrophages are correlated with tamoxifen resistance in the postmenopausal breast cancer patients. Pathol. Oncol. Res. 20 (3), 619–624. doi:10.1007/s12253-013-9740-z
Yang, B., Lu, Y., Zhang, A., Zhou, A., Zhang, L., Zhang, L., et al. (2015). Doxycycline induces apoptosis and inhibits proliferation and invasion of human cervical carcinoma stem cells. PLoS One 10 (6), e0129138. doi:10.1371/journal.pone.0129138
Yang, X., Liu, Q., Li, Y., Tang, Q., Wu, T., Chen, L., et al. (2020). The diabetes medication canagliflozin promotes mitochondrial remodelling of adipocyte via the AMPK-Sirt1-Pgc-1α signalling pathway. Adipocyte 9 (1), 484–494. doi:10.1080/21623945.2020.1807850
Yao, H., and He, S. (2021). Multi-faceted role of cancer-associated adipocytes in the tumor microenvironment (Review). Mol. Med. Rep. 24 (6), 866. doi:10.3892/mmr.2021.12506
Zhou, G., Myers, R., Li, Y., Chen, Y., Shen, X., Fenyk-Melody, J., et al. (2001). Role of AMP-activated protein kinase in mechanism of metformin action. J. Clin. Invest. 108 (8), 1167–1174. doi:10.1172/JCI13505
Keywords: cancer stem cells, tumor dormancy, tumor associated macrophages, oxidative metabolism, anti-mitochondrial drugs in clinical trials
Citation: Marrone L, Romano S, Malasomma C, Di Giacomo V, Cerullo A, Abate R, Vecchione MA, Fratantonio D and Romano MF (2024) Metabolic vulnerability of cancer stem cells and their niche. Front. Pharmacol. 15:1375993. doi: 10.3389/fphar.2024.1375993
Received: 24 January 2024; Accepted: 25 March 2024;
Published: 10 April 2024.
Edited by:
Bo Wang, Zhengzhou University, ChinaReviewed by:
Marco A. Velasco-Velazquez, National Autonomous University of Mexico, MexicoIoannis S. Pateras, National and Kapodistrian University of Athens, Greece
Copyright © 2024 Marrone, Romano, Malasomma, Di Giacomo, Cerullo, Abate, Vecchione, Fratantonio and Romano. This is an open-access article distributed under the terms of the Creative Commons Attribution License (CC BY). The use, distribution or reproduction in other forums is permitted, provided the original author(s) and the copyright owner(s) are credited and that the original publication in this journal is cited, in accordance with accepted academic practice. No use, distribution or reproduction is permitted which does not comply with these terms.
*Correspondence: Maria Fiammetta Romano, bWFyaWFmaWFtbWV0dGEucm9tYW5vQHVuaW5hLml0; Simona Romano, c2ltb25hLnJvbWFub0B1bmluYS5pdA==