- 1College of First Clinical Medicine, Shandong University of Traditional Chinese Medicine, Jinan, China
- 2College of Nursing, Shandong University of Traditional Chinese Medicine, Jinan, China
- 3Affiliated Hospital of Shandong University of Traditional Chinese Medicine, Jinan, China
Cardiovascular disease (CVD) is a serious public health problem, and among non-communicable diseases, CVD is now the leading cause of mortality and morbidity worldwide. CVD involves multiple organs throughout the body, especially the intestinal tract is the first to be involved. The impairment of the intestinal mucosal barrier is considered a significant pathological alteration in CVD and also contributes to the accelerated progression of the disease, thereby offering novel insights for CVD prevention and treatment. The treatment of Chinese medicine is characterized by multi-metabolites, multi-pathways, and multi-targets. In recent years, the studies of Traditional Chinese Medicine (TCM) in treating CVD by repairing the intestinal mucosal barrier have gradually increased, showing great therapeutic potential. This review summarizes the studies related to the treatment of CVD by TCM (metabolites of Chinese botanical drugs, TCM formulas, and Chinese patent medicine) targeting the repair of the intestinal mucosal barrier, as well as the potential mechanisms. We have observed that TCM exerts regulatory effects on the structure and metabolites of gut microbiota, enhances intestinal tight junctions, improves intestinal dyskinesia, repairs intestinal tissue morphology, and preserves the integrity of the intestinal vascular barrier through its anti-inflammatory, antioxidant, and anti-apoptotic properties. These multifaceted attributes position TCM as a pivotal modulator of inhibiting myocardial fibrosis, and hypertrophy, and promoting vascular repairment. Moreover, there exists a close association between cardiovascular risk factors such as hyperlipidemia, obesity, and diabetes mellitus with CVD. We also explore the mechanisms through which Chinese botanical drugs impact the intestinal mucosal barrier and regulate glucose and lipid metabolism. Consequently, these findings present novel insights and methodologies for treating CVD.
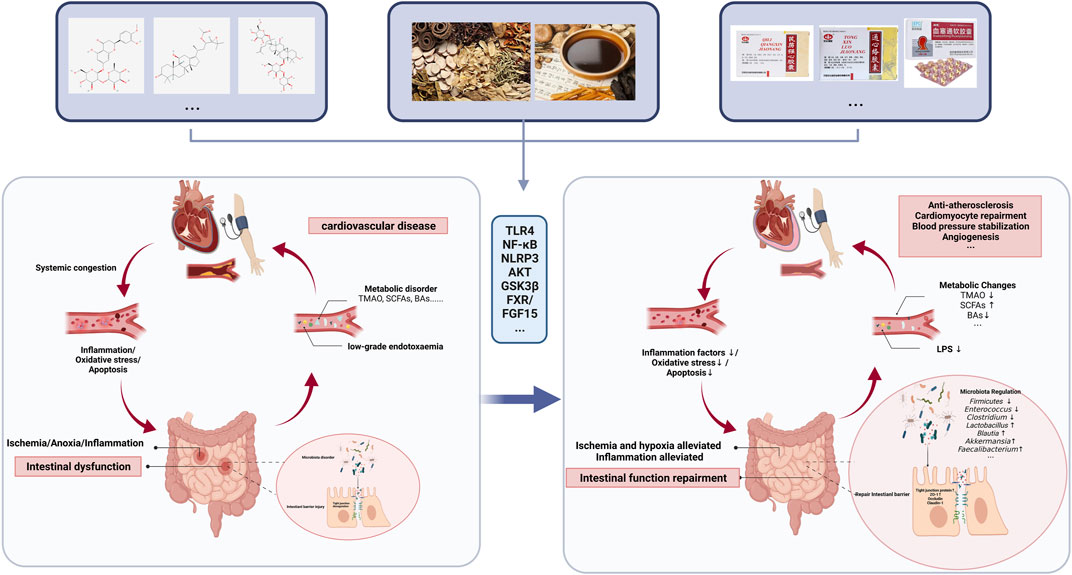
GRAPHICAL ABSTRACT | This review presents the progress of traditional Chinese medicines in treating CVD by repairing the intestinal mucosal barrier
Introduction
CVD poses a significant global health threat, affecting over 23 million individuals worldwide (Bui et al., 2011). In China, the incidence of CVD is steadily increasing each year, making it the leading cause of hospitalization and contributing to the overall disease burden (Wang et al., 2021a; Ren et al., 2021). CVD is a systemic ailment that impacts multiple organ systems beyond just the cardiovascular system, including the intestinal tract which often experiences initial manifestations (Krack et al., 2005; Keeter et al., 2022). Medications used for treating CVD such as nonsteroidal anti-inflammatory drugs (NSAIDs) and antithrombotic drugs can potentially compromise the integrity of the gastrointestinal mucosal barrier and lead to drug-induced gastrointestinal ulcers (US Preventive Services Task Force et al., 2022). Severe mucosal damage may result in intestinal dysfunction or even failure (Arutyunov et al., 2008; Krack et al., 2005). Experimental studies have demonstrated that impairment of the intestinal mucosal barrier, particularly alterations in gut microbiota and its metabolites, are closely associated with pathological mechanisms like inflammation, ischemia, and hypoxia, as well as metabolic disorders (Wang and Zhao, 2018). Targeting intestinal barrier dysfunction could potentially serve as a therapeutic approach for managing CVD (Lewis and Taylor, 2020).
However, drug studies targeting the intestinal mucosal barrier for CVD treatment in medicine are scattered across clinical and experimental reports. Recent research has demonstrated that candesartan cilexetil, a renin-angiotensin system blocker, not only reduces blood pressure and improves cardiac function but also repairs damage to the intestinal barrier (Wu et al., 2019a). Lubiprostone, a ClC-2 chloride channel activator utilized for constipation treatment, has demonstrated efficacy in reducing the lactulose/mannitol ratio in patients with NSAID-induced intestinal barrier dysfunction (Kato et al., 2017). Additionally, it has been found to restore the intestinal barrier and ameliorate inflammation in Western diet-induced Apolipoprotein E-deficient (ApoE−/−) mice by attenuating lipids, thereby mitigating atherosclerosis (AS) (Arakawa et al., 2019). Probiotics and prebiotics have demonstrated efficacy in clinical trials (Jin et al., 2019). However, the research and development of these therapeutic agents are still ongoing. The application of TCM formulas for CVD is extensively utilized in clinical settings (Chen et, al., 2023a). Hao et al. (Hao et al., 2017) systematically evaluated the efficacy and safety of TCM for CVD in conjunction with the potential pharmacological mechanisms of the active ingredients. The results suggest that TCM can be used as a complementary and alternative approach to the prevention and treatment of CVD. Many botanical drugs and their metabolites have been shown to repair intestinal barrier damage and improve the intestinal microbiota in CVD therapy (Li et al., 2017; Lyu et al., 2017). This article discusses the potential mechanisms by which botanical metabolites, botanical drugs, and proprietary Chinese medicines act on CVD from the perspective of the intestinal mucosal barrier and gut microbiota.
Relationship between the intestinal mucosal barrier and CVD
Functions of the intestinal mucosal barrier
The intestinal tract is the largest immune organ in the human body, and the intestinal mucosal barrier is an extremely important part of the intestinal tract that prevents the invasion of pathogens (Camilleri, 2019). Digestive juices and commensal bacteria in the gut degrade bacteria and antigens. Paneth cells secrete antimicrobial peptides that prevent bacterial colonization (Wang et al., 2018), and cuprocytes secrete a mucin barrier that prevents pathogen attachment (Johansson and Hansson, 2016). Tight junction proteins, including the occludin, claudin, zonula occludens, and junctional adhesion molecule families, prevent pathogen translocation (Odenwald and Turner, 2017). The lamina propria secretes IgA, cytokines, chemokines, and immune cells to play an immune role (Johansson and Hansson, 2016; Camilleri, 2019). In addition, the gut vascular barrier (GVB) is the last line of defense of the intestinal mucosal barrier and recognizes translocated microorganisms and antigens in the bloodstream (Spadoni et al., 2015).
Intestinal dysfunction in cardiovascular disease
Cardiovascular systems, such as heart, cardiac macro vessels, and capillaries are related to the circulation of the entire organism (Rakusan et al., 1992), and the gastrointestinal system contains abundant blood vessels, receiving about ¼ of the blood supply of the heart and is sensitive to ischemia and hypoxia (Krack et al., 2005). The presence of systemic inflammation and inadequate perfusion caused by cardiovascular disease results in a reduction in intestinal peristalsis, absorption, and the development of edema in the intestinal wall. This leads to disruption of the mucus layer, disarray within the bacterial flora, and loss of tight junctions, ultimately resulting in increased permeability commonly referred to as “leaky gut” (Usuda et al., 2021). Sandek et al. (2014) found that intestinal blood flow is reduced, and gastrointestinal symptoms are increased in patients with chronic heart failure (CHF). The study conducted by Drapala et al. (2020) demonstrated that intestinal blood barrier disturbances, such as decreased intestinal blood flow, reduced colonic mucosal thickness, and alterations in tight junctions, were observed in spontaneously hypertensive HF rats. Chronic inflammatory states induced by AS and dyslipidemia are involved in vascular injury and microvascular remodeling, increasing intestinal vascular permeability (Witjes et al., 2015). The combination of congestive heart failure, atrial fibrillation (AF), and other factors leads to intestinal hypoperfusion, while a hypercoagulable state of blood and blood stasis contribute to the development of intestinal ischemia (Mosinska and Fichna, 2015; Wang et al., 2016). Thus, both CVD itself and CVD-related risk factors (obesity, high-fat diet, diabetes, etc.) affect the function and structure of the intestinal barrier (Zhang et al., 2020a; Ding et al., 2020). The internal environment, such as inflammation and hypoxia, plays an important role (Kalogeris et al., 2016; Yuzefpolskaya et al., 2020). In a rat model of myocardial infarction, serum and intestinal levels of TNF-α and IL-6 were significantly increased, and IL-10 levels were significantly decreased, accompanied by increased tissue levels of cyclooxygenase-2 (COX-2) and positively correlated with matrix metalloproteinase-2 (MMP-2) activity (Chen et al., 2021).
Intestinal permeability and cardiovascular risk
After leaky gut, pathogens in the intestine as well as pathogen-associated molecular patterns (PAMPs), such as the harmful metabolite lipopolysaccharide (LPS), can readily leak from the damaged barrier into the bloodstream, causing systemic disease (Tripathi et al., 2018). Among them, LPS, a major component of the outer membrane of Gram-negative bacteria, translocates into the systemic circulation to develop low-grade endotoxaemia (Violi et al., 2023). Low-grade endotoxemia induces arterial inflammation, plaque instability, and thrombosis, which may lead to atherosclerosis and has been associated with cardiovascular events in patients at risk for or suffering from serious cardiovascular disease associated with cardiovascular events in patients at risk or with severe cardiovascular disease (Violi et al., 2023). Serum LPS levels are elevated in STEMI patients and correlate with levels of zonulin, a marker of intestinal permeability, and LPS is localized in coronary thrombi in STEMI patients (Carnevale et al., 2020). Zhang et al. (2022) found that intestinal barrier dysfunction in rats with a dramatic increase in LPS and glucose levels led to upregulated expression of NOD-like receptor protein-3 (NLRP-3) inflammasome, which promoted the development of AF. This may be one of the important reasons why intestinal disease induces the development of CVD and accelerates the development of CVD.
The role of gut microbiota in CVD
Gut microbiota is a very important part of the intestinal barrier, and intestinal commensal bacteria maintain intestinal homeostasis (Wells et al., 2017). The gut microbiota influences host immunity, metabolism, and development (Rosell-Mases et al., 2023). Structural disruption of gut microbiota or small intestinal bacterial overgrowth (SIBO) leads to impairment of intestinal barrier function, which directly or indirectly causes diseases, including CVD (Brown and Hazen, 2015; Adkins and Rezaie, 2018). Zhang et al. (2022) found that fecal Firmicutes transplantation (FMT) of feces from aged rats with high susceptibility to AF to young healthy rats increased AF susceptibility in young rats, whereas FMT of long-term healthy young rats to older rats prevented AF in older rats. Increased Firmicutes to Bacteroidetes ratios were found in the feces of hypertensive models and patients, whereas Coprococcus Pseudobutyrivibrio and Bifidobacterium, which produce acetate, butyrate, and lactate, were decreased (Yang et al., 2015).
The gut microbiota generally affects CVD through two pathways. One way is through changes in metabolites such as trimethylamine N-oxide (TMAO), bile acids (BAs), short-chain fatty acids (SCFAs), and tryptophan derivatives (Liu et al., 2021a). The gut microbiota can convert various dietary nutrients into trimethylamine (TMA). Most TMA enters the circulation and is subsequently oxidized to TMAO by heparin-containing monooxygenase (FMO) (Bennett et al., 2013). Studies have shown that TMAO is associated with strong pro-AS activity and myocardial remodeling, leading to a higher risk of adverse cardiovascular events (Tang et al., 2013; Organ et al., 2016; Zhu et al., 2016). The gut microbiota modifies primary BA through products such as 7α-dehydroxylase, and bile salt hydrolase (BSH), to produce secondary BA (Walker and Gilliland, 1993; Ridlon et al., 2006), which is associated with farnesoid X receptor (FXR), liver X receptor (LXR), pregnancy X receptor (PXR), and G protein-coupled receptor (GPCR) and interacts with various host nuclear receptors, causing lipid metabolism disorders and cardiovascular metabolic diseases such as AS (Hanniman et al., 2005; Hylemon et al., 2009; Pols et al., 2011; Li et al., 2013).SCFA is recognized as a beneficial factor for CVD (Hu et al., 2022). Cohort studies have shown that changes in fecal SCFAs, including acetate, propionate, and butyrate, are significantly associated with 24-h mean blood pressure in hypertensive patients (Huart et al., 2021). Intestinal butyrate-producing bacteria are enriched in the intestines of patients with STSMI infarction and rhesus monkeys undergoing ischemia-reperfusion, and supplementation with butyrate and colonization with butyrate-producing bacteria improves cardiac function and myocardial injury after myocardial injury (Chen et al., 2023a). Propionate was found to attenuate myocardial hypertrophy, myocardial fibrosis, vascular dysfunction, and hypertension in Ang II-induced wild-type NMRI mice or ApoE−/− mice (Bartolomaeus et al., 2019).
Another way is for the gut microbiota to induce changes in the immune pathways of the host. LPS can be sensed by Toll-like receptor 4 (TLR-4), which activates macrophages to take up oxidized low-density lipoproteins (ox-LDL) to form foam cells, triggering atherosclerotic progression (Caesar et al., 2010). A reduction in the relative abundance of butyrate-producing Roseburia and Faecalibacterium, as well as an increase in the TNFα: IFN-γ ratio and the production of TNFα and IL-6 in isolated peripheral blood mononuclear cells, can be observed in hypertensive patients (Kim et al., 2014). Butyrate has also been found to modulate CD4+ T helper cell differentiation, exerting an anti-inflammatory effect and thus influencing CVD (Hu et al., 2022).
Potential mechanisms of metabolites of Chinese botanical drugs affecting intestinal mucosal barrier for the treatment of CVD
Phenols
Resveratrol (RSV), is a plant polyphenol phytoalexin (Baur and Sinclair, 2006), which is mainly found in Chinese botanical drugs such as Smilax glabra Roxb. (Smilacaceae; Smilacis glabrae rhizoma), Veratrum album L. (Melanthiaceae; Veratrum album) and other traditional Chinese medicines. RSV could decrease TMAO levels and increase the abundance of BSH-active bacteria such as Lactobacillus and Bifidobacterium, which reduces BA degradation and fecal excretion. In contrast, RSV was found to neither reduce TMAO levels nor increase hepatic BA synthesis in antibiotic-treated sham-naive mice, suggesting that RSV acts by remodeling the gut microbiota. The FXR/FGF15 axis was inhibited in this process, and cholesterol 7a-hydroxylase (CYP7A1) expression was increased, exerting an anti-AS effect (Chen et al., 2016). This suggests that the beneficial cardiovascular effects of RV are related to the composition of the gut microbiota. According to Chen et al., (Chen et al., 2019), RV also prevents programmed hypertension associated with an increased abundance of the abundances of phylum Verrucomicrobia and genus Akkermansia Muciniphila (Chen et al., 2019).
Quercetin, a plant-derived polyphenol, found in Glehnia littoralis (A.Gray) F.Schmidt ex Miq. (Apiaceae; glehniae radix), Eucommia ulmoides Oliv. (Eucommiaceae; Eucommiae cortex) and many other botanical drugs. Quercetin treatment effectively attenuated weight gain and mitigated the extent of atherosclerotic lesions in the aortic sinus. The observed reduction in malondialdehyde levels and elevation of IL-6 levels further substantiated the protective effects of quercetin on immune/inflammatory responses and oxidative stress (Nie et al., 2019). Moreover, quercetin supplementation led to decreased intestinal cholesterol, lysophosphatidic acids, and atherogenic lysophosphatidylcholine (LPC 18:1) levels, while promoting an increased level of coprostanol (Nie et al., 2019). Microbiological analyses unveiled that quercetin treatment significantly enhanced Actinobacteria and Bacteroidetes populations, whereas it markedly reduced Firmicutes abundance (Nie et al., 2019).
Baicalin is found in botanical drugs, such as Scutellaria baicalensis Georgi (Lamiaceae; Scutellariae radixp). Wu et al. found in SHRs that baicalin attenuated hypertension-associated intestinal hyperpermeability and reduced serum high-sensitivity C-reactive protein (hs-CRP), inflammatory markers such as IL-1β and IL-6 (Wu et al., 2019a). And Baicalin treatment increased the abundance of SCFA-producing fecal flora, such as Streptococcus, Akkermansia, Allobaculum, Bifidobacterium, Lachnospiraceae_NK4B4_group, and Roseburia, as well as increased levels of SCFA (Wu et al., 2019b).
Naringin is a flavanone-7-O-glycoside between the flavanone Naringenin and the disaccharide neohesperidose. Naringin could promote the growth of 7α-dehydroxylase-producing bacteria, such as Eubacterium coprostanoligenes and Eubacterium brachy, and inhibit BSH-producing bacteria, such as Clostridium, Enterococcus, and Bacteroides, which may be important in alleviating AS by increasing bile acid synthesis through activation of the FXR/FXR/Fibroblast Growth Factor 15 (FGF15)-Cytochrome P450 7A1 (CYP7A1) pathway, thereby lowering cholesterol levels (Wang et, al., 2021a). Furthermore, the integrity of the GVB represents the ultimate defense mechanism for controlling bacterial translocation, with particular emphasis on the pivotal role played by the vascular endothelium within this context (Spadoni et al., 2016). The TNF-α-induced injury model of rat intestinal microvascular endothelial cells (RIMVEC) serves as a valuable tool to mimic inflammation-induced damage to GVB. Notably, intervention with Naringin effectively ameliorated tight junction proteins in RIMVEC, including Zonula Occludens-1 (ZO-1), occludin, and claudin-1, thereby preventing apoptosis and inhibiting cell migration while mitigating GVB disruption (Liu et al., 2020).
Naringenin is found in botanical drugs, such as Pericarpium Citri Reticulatae (Rutaceae; Citrus reticulata Blanco), and Paeonia lactiflora Pall. (Paeoniaceae; Paeoniae Radix Rubra). Naringenin has also been demonstrated to effectively mitigate TNF-α-induced disruption of the RIMVECs, as evidenced by the restoration of occludin and claudin-1 expression levels. This effect is partly attributed to the inhibition of nuclear factor kappa-B (NF-κB)-mediated activation of the Myosin Light Chain Kinases (MLCK)/p-MLC and TLR4/NF-κB/NLRP3 pathways (Zhong et al., 2021). Intestinal barrier dysfunction, including gastrointestinal motility disturbances, is associated with aberrant smooth muscle function and structure. Naringenin has been shown to hyperpolarize colonic smooth muscle cells (SMCs) through selective activation of large-conductance calcium-activated K+ (BKCa) channels, thereby reducing Ca2+ influx via voltage-dependent calcium channels (VDCC) and promoting SMCs relaxation for treating dyskinesia in rat colon (Yang et al., 2014). The activating effect of Naringenin on BKCa channels is also observed in vascular smooth muscle. In rat tail artery myocytes, (+/−)-Naringenin concentration-dependently increased BKCa currents. Additionally, (+/−)-Naringenin exerted a concentration-dependent relaxing effect on endothelium-denuded rat aortic rings precontracted with 20 mM KCl or norepinephrine (Saponara et, al., 2006). Naringenin not only regulates gastrointestinal motility disorders, but also exerts vasodilatory effects, but is not found in the same model, and its intrinsic mechanism of action needs to be investigated.
Terpenoids
Notoginsenoside R1 (NGR1) is a metabolite from Panax notoginseng (Burkill) F.H.Chen (Araliaceae; Notoginseng radix et rhizoma)NGR1 significantly reduced myocardial infarct size, alleviated cardiomyocyte injury, decreased cardiomyocyte apoptosis, and improved cardiac function in mice with myocardial ischemia/reperfusion (MI/R) by inhibiting transforming growth factor β-activated protein kinase 1 (TAK1)/c-Jun amino-terminal kinase (JNK). Pretreatment with NG-R1 (25 μM) significantly inhibited apoptosis in murine neonatal cardiomyocytes (CMs) induced by hypoxia/reoxygenation (H/R) (Zeng et al., 2023). Low-grade endotoxemia, resulting from increased intestinal permeability, stimulates leukocytes, platelets, and endothelial cells. This leads to inflammation and a hypercoagulable state, which in turn causes plaque instability and thrombosis (Violi et al., 2023). NGR1 inhibited the LPS-induced degradation of IκB-α (inhibitor of NF-κB) and increased synthesis of IκB-α protein in monocytic cell line THP-1. Simultaneously, it prevented LPS-induced upregulation of plasminogen activator inhibitor-1 (PAI-1) antigen and tissue factor in endothelial cells, improved LPS-induced PAI-1 in mice, and exerted anti-inflammatory and anti-thrombotic effects (Zhang et al., 1997). Interestingly, NGR1 also plays a protective role in intestinal ischemia-reperfusion (I/R). NGR1 attenuated intestinal I/R-induced microvascular hyperpermeability, reduced inflammatory cytokine production such as jejunal TNF-α, IL-1β, and IL-6, activated the NF-κB pathway and increased tight junction proteins, and improved energy metabolism during intestinal I/R (Li et al., 2014).
Ginsenoside Rc (GRc) is Panax ginseng C.A.Mey. (Araliaceae; Ginseng radix et rhizoma), and many drugs for the treatment of CVD contain ginsenoside, such as Shexiang Baoxin Pill, Tongxinluo, etc. Xie et al. (2022) found that GRc significantly attenuated AS injury in high-fat diet (HFD)-fed ApoE−/− mice by lowering blood lipids, IL-6, and IL-1β serum levels. And they also found that GRc reversed AS-induced changes in the abundance of Bacteroidetes and Firmicutes, Muribaculaceae, Lactobacillus, Ileibacterium, Bifidobacterium, Faecalibaculum, Oscillibacter, Blautia, and Eubacterium_Coprostanoligenes_group and other flora (Xie et al., 2022). Fecal metabolomics revealed that GRc treatment significantly reduced adenine and uric acid and was positively correlated with serum levels of TG, IL-6, IL-1β, and TNF-α (Xie et al., 2022). Oxidative stress, vascular injury, and systemic inflammation due to elevated uric acid have deleterious effects on CVD (Ndrepepa, 2018). GRc treatment also elevates levels of cholic acid (CA), chenodeoxycholic acid (CDCA), isolithocholic acid (isoLCA), taurochenodeoxycholic acid (TCDCCA), and tricarboxylic acid (TCA) (Xie et al., 2022). Primary bile acids, such as CA and CDCA, as well as secondary bile acids can activate FXR and TGR5 and reduce AS formation (Miyazaki-Anzai et al., 2018; Schoeler and Caesar, 2019). This may be one of the mechanisms by which GRc mitigates AS damage.
Alkaloids
Berberine (BBR) was revealed to inhibit deoxynivalenol-induced intestinal injury by increasing the expression of serum antioxidant enzymes and T-cell surface antigens and by decreasing the release of pro-inflammatory cytokines in the small intestine. BBR significantly increased ileal and jejunal mucosal ZO-1 by decreasing the expression of deoxynivalenol (DON)-induced genes and proteins in the jejunum and ileum in the presence of extracellular regulated protein kinases (ERK), JNK, and NF-κB, occludin and claudin-1 protein expression levels, and improved jejunal morphology. BBR is also known to improve jejunal morphology (Tang et, al., 2021). BBR treatment significantly reduced AS area and lipid levels decreased pro-inflammatory cytokines TNF-α, IL-1β, IL-6, and increased anti-inflammatory IL-10 and lipocalin levels in HFD-fed ApoE−/− mice (Wu et al., 2020). In this study, 16S rRNA sequencing and macrogenic findings revealed that BBR altered the abundance of Roseburia, Blautia, Allobaculum, Alistipes, Turicibacter, and Bilophila. These colonies showed beneficial anti-inflammatory effects, modulation of glycolipid metabolism, increased SCFAs, and decreased TMAO potential (Wu et al., 2020). Furthermore, BBR has been utilized for treating atherosclerotic patients (0.5 g, bid). However, oral administration of 5 g bid of BBR for 4 months resulted in an increased susceptibility to plaque formation among these patients. Notably, there was a significant decrease observed in the abundance of TMA-producing potential gut microbiota species, including Eubacterium_hallii_group, Anaerostipes, Faecalibacterium, Dialister, Eubacterium_coprostanoligenes_group, Coprococcus_3, Butyricoccus, and Clostridium_sensu_strito_1. Additionally, fecal and stool levels of both TMA and TMAO were significantly reduced along with a notable decrease in plaque score (Ma et al., 2022). Another study showed that BBR protects against NSAID-induced intestinal mucosal damage by upregulating the expression of Protein gene product 9.5 (PGP9.5), glial fibrillary acidic protein (GFAP), and Glial-cell-line-derived neurotrophic factor (GDNF) with the repair of enteric nervous system (Chao et al., 2020).
Leonurine is present in Leonurus japonicus Houtt. (Lamiaceae; Leonuri herba). Leonurine has been shown to attenuate Ang II-induced cardiomyocyte hypertrophy, fibrosis, and inflammation, and to preserve cardiac function (Shen et al., 2023) In the clinic, the intervention of 36 subjects using different doses of Leonurine revealed an increase in the relative abundance of Ruminococcus, Streptococcaceae, etc., and a relative abundance of Myobacterium, Veillonella, Lachnospiraceae, and Weissella was downward (Liao et al., 2021). Accumulating evidence suggests that high homocysteine and low methionine levels are associated with AS and myocardial infarction (Verhoef et al., 1996; Homocysteine Studies Collaboration, 2002; McCully, 2015). In the Liao et al. (2021) study, metabolomic analysis showed that Leonurine can affect homocysteine-methionine metabolism, increasing methionine levels.
Potential mechanisms of metabolites of Chinese botanical drugs affecting the intestinal mucosal barrier for the treatment of CVD are shown in Table 1.
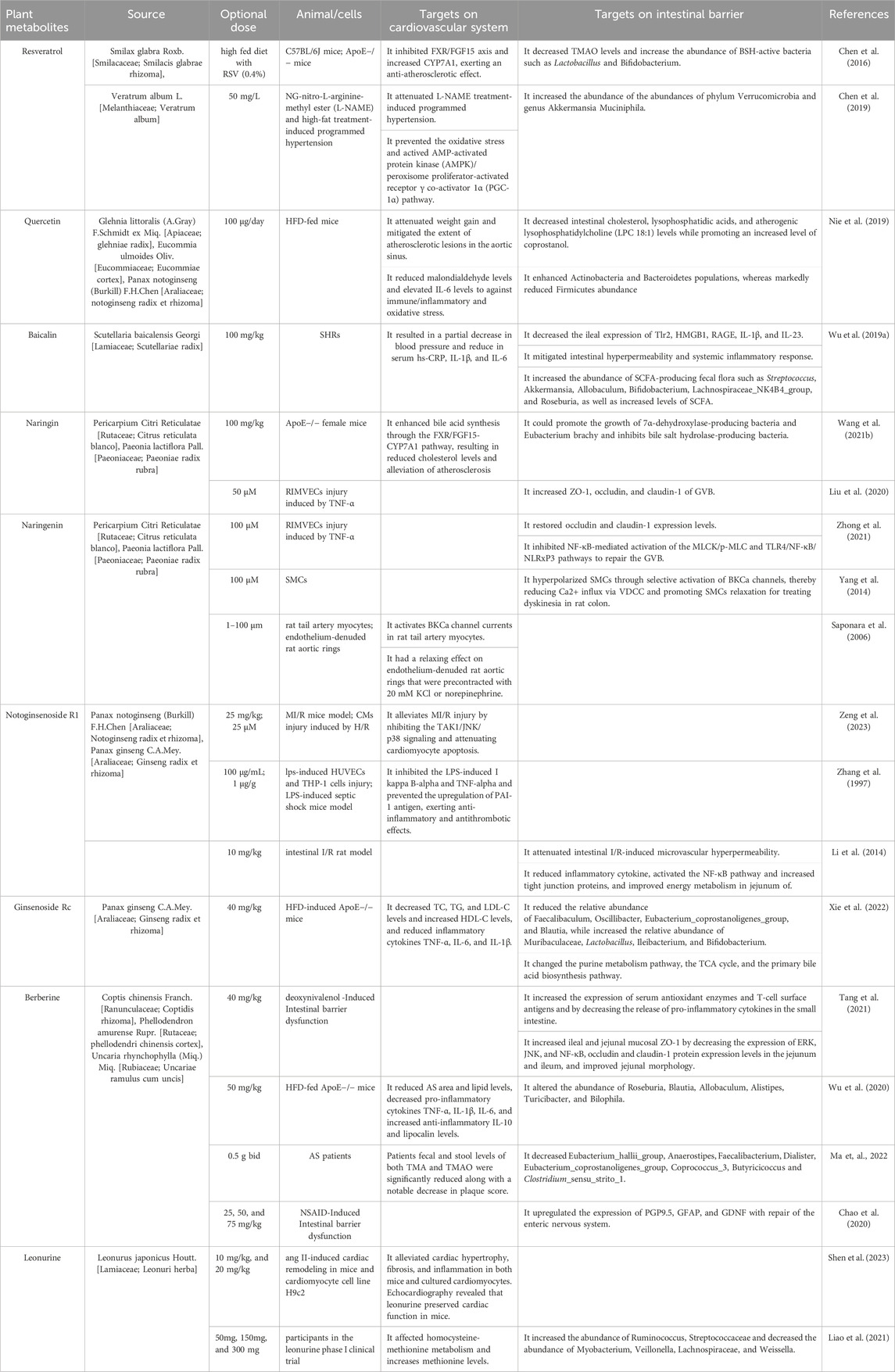
TABLE 1. Lists of metabolites of Chinese botanical drugs acting on intestinal barrier damage in the treatment of CVD.
Potential mechanisms of Chinese medicine formulas affecting intestinal mucosal barrier for the treatment of CVD
Shen-Fu Decoction (SFD), a classic traditional Chinese formula, is composed of Panax ginseng C.A.Mey. (Araliaceae; Ginseng radix et rhizoma), Aconitum carmichaelii Debeaux (Ranunculaceae; Aconiti lateralis radix praeparata). SFD significantly reduced the mortality rate of sepsis model induced by cecal ligation and puncture (CLP), prevented intestinal and liver injury, alleviated increased intestinal permeability and inflammation, and impaired intestinal barrier by regulating the expression of ZO-1, occludin, claudin-1, and Phosphorylated Vasodilator Stimulated Phosphoprotein (p-VASP) (Liu et al., 2021b). Yan et al. (2018) demonstrated that the regulation of Fas, Fas-L, Bcl-2, and Bax proteins in the apoptotic pathway by SFD and microRNAs played a pivotal role in ameliorating cardiac function and hemodynamic indices in rats with HF. Interestingly, another formula containing Ren Shen and Fu Zi, Fuzi decoction (FZD), has also been shown to have therapeutic effects on CHF. Gao et al. (Gao et al., 2022) found that FZD improved cardiac function and reduced the effects of pyruvate and lactate. Recent studies have shown that the pyruvate-lactate axis is critical for cardiac homeostasis (Cluntun et al., 2021) Gao et al. (2023) also found that FZD increased the Firmicutes-Bacteroidetes ratio and the abundance of Lactobacillus, and affected the β-diversity of the intestinal microbiota in rats with CHF. FZD increased the Firmicutes-Bacteroidetes ratio and Lactobacillus abundance and affected the β-diversity of the gut microbiota in rats with CHF (Gao et al., 2023). Non-targeted metabolomics analysis showed that FZD affected the biosynthesis of valine, leucine, and isoleucine. It increased the levels of acetic acid, propionic acid, butyric acid, and isovaleric acid in the feces of CHF rats. FMT demonstrates once again that the mechanism of action of FZD in the treatment of CHF may be related to the improvement of the gut microbiota, the elevation of SCFA content, and the realization of the amino acid pathway (Gao et al., 2023).
Lingguizhugan decoction (LGZGD), an ancient TCM formula from the Treatise on Cold Pathogenic and Miscellaneous Diseases, was prepared from Poria cocos (Schw.) Wolf (Polyporaceae; Poria), Neolitsea cassia (L.) Kosterm. (Lauraceae; Cinnamomi ramulus), Atractylodes macrocephala Koidz. (Asteraceae; Atractylodis macrocephalae rhizoma), Glycyrrhiza uralensis Fisch. ex DC. (Fabaceae; Glycyrrhizae radix et rhizoma). In DOX-induced myocardial injury in rats, LGZGD was found to ameliorate cardiac dysfunction and attenuate myocardial morphological injury and mitochondrial damage. LGZGD downregulated miR-24 expression, upregulated junctophilin-2 (JP-2) expression, and antagonized T-tubule-sarcoplasmic reticulum (TT-SR) microstructural remodeling, thereby improving cyclic Ca2+ transients and cell contraction (Li et al., 2019). In transverse aortic constriction (TAC)-induced HF mice, LGZGD significantly ameliorated cardiac dysfunction by downregulating p38 and ERK and bi-directionally regulating the expression of cardiac hypertrophy-related genes and proteins such as protein kinase B (AKT)- Glycosynthase kinase 3β (GSK3β)/mammalian target of rapamycin (mTOR)/P70S6K in TAC mice (Chen et al., 2022).
Buyang Huanwu Decoction (BYHWD) is composed of Astragalus mongholicus Bunge [Fabaceae; Astragali radix], Angelica sinensis (Oliv.) Diels [Apiaceae; Angelicae sinensis radix], Radix Paeonia lactiflora Pall. [Paeoniaceae; Paeoniae radix rubra], Pheretima Aspergillum (E.Perrier) [Megascolecidae; Pheretima], Rhizoma Ligustici Chuanxiong [Apiaceae; Chuanxiong rhizoma], Carthamus tinctorius L. [Asteraceae; Carthami Flos], Prunus persica (L.) Batsch [Rosaceae; Persicae semen] (Chen et al., 2020). Clinical studies have shown that BYHWD reduced levels of hs-CRP, TNF-α, IL-18, and TMAO, increased the abundance of Lactobacillus and Bifidobacterium, and reduced the abundance of Escherichia coli and fungi in patients with stable coronary artery disease (Liang et al., 2023). In vitro assays showed that BYHWD reduced the expression of IL-6, IL-1β, and matrix metalloproteinase 9 (MMP9), increased cell migration, decreased the proportion of RCFs in the S + G2 phase, and inhibited cell division and proliferation, thereby inhibiting myocardial fibrosis (Wang et al., 2022). The animal experiments demonstrated that BYHWD improved myocardial and cardiac function in HF rats, while also reducing serum d-lactate and TMAO levels. Furthermore, it increased the expression of occludin and claudin-1. Additionally, the taxonomic composition of gut microbiota was altered, with an observed increase in Lactobacillus abundance and a concurrent decrease in Romboutsia abundance following treatment with BYHWD in HF rats. These findings suggest that BYHWD has potential therapeutic implications for heart failure through its ability to restore intestinal mucosal barrier integrity (Weng et al., 2023).
Taohong Siwu Decoction (THSWD) consists of Rehmannia glutinosa (Gaertn.) DC. [Orobanchaceae, Rehmanniae radix praeparata], Angelica sinensis (Oliv.) Diels [Apiaceae; Angelicae sinensis radix], Radix Paeonia lactiflora Pall. [Paeoniaceae; Paeoniae radix rubra], Rhizoma Ligustici Chuanxiong [Apiaceae; Chuanxiong rhizoma], Carthamus tinctorius L. [Asteraceae; Carthami Flos], Prunus persica (L.) Batsch [Rosaceae; Persicae semen]. It is widely used in blood stasis type CVD (Liu et, al., 2022a; Luo et, al., 2019; Xia et, al., 2021). As found in a rat model of MI, THSWD administration improved cardiac function in MI rats. This study suggests that THSWD significantly increased the expression of basic bFGF, insulin-like growth factor-1 (IGF-1), reduced collagen deposition, promoted angiogenesis, reduced apoptosis, and activated the phosphatidylinositol 3-kinase (PI3K)/AKT signaling pathway. Notably, THSWD significantly reduced mitochondrial ROS production and inhibited excessive mitochondrial fission. Beneficial changes in the fecal microbiota of rats also occurred after THSWD intervention, as evidenced by increased Firmicutes/Bacteroidetes (F/B) value and increased Lactobacillus.
Potential mechanisms of Chinese medicine formulas affecting intestinal mucosal barrier for the treatment of CVD are shown in Table 2.
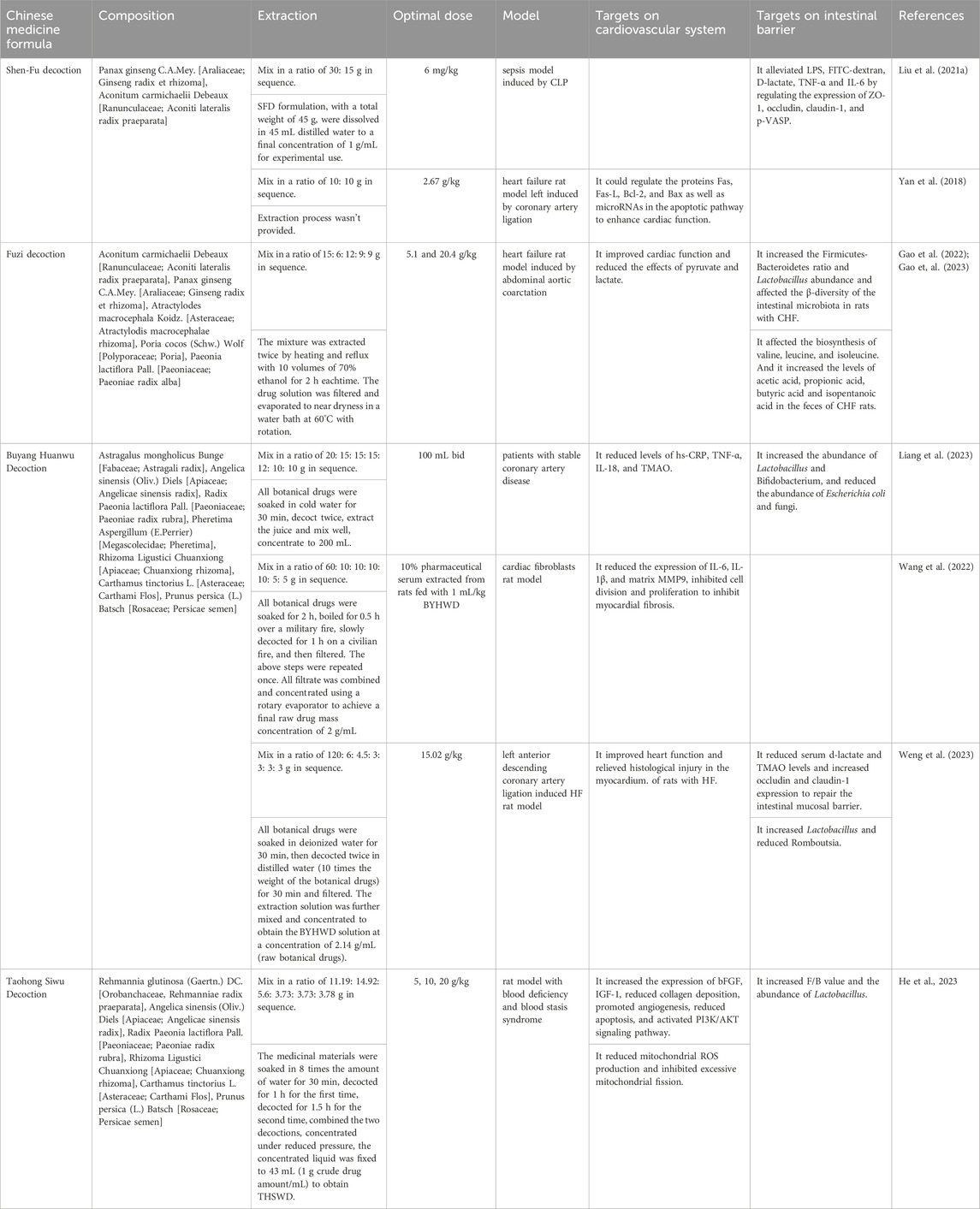
TABLE 2. Lists of Chinese medicine formulas acting on intestinal barrier damage in the treatment of CVD.
Potential mechanisms of Chinese patent medicine affecting intestinal mucosal barrier for the treatment of CVD
CPM has been extensively utilized in the secondary prevention and treatment of CVD (Chen et al., 2023a; Shang et al., 2009). Tongxinluo (TXL), is an approved CPM by the State Food and Drug Administration of China for angina pectoris and ischemic stroke (Qi et al., 2022). Qi et al. (2022) found TXL could enhance atherosclerotic plaque stability, suppress NLRP3, caspase-1, IL-1β, and IL-18 inflammatory pathways expression as well as increase the relative abundance of F/B ratio, Alipipes, Campylobacter, Rikenella, indistinctus, viscericola, nordii, subantarcticus, and other bacteria. The fecal metabolites erucic acid, N-acetylneuraminate, chenodeoxycholate, and 6-tuliposide B in the TXL intervention group were significantly reduced, while hydroxyphenyllactic acid, trans-ferulic acid (TFA), and others were significantly increased. This study found that some metabolites were significantly associated with some bacteria and some inflammatory factors. In particular, the metabolite trans-ferulic acid transcarboxylate inhibits the NLRP3 inflammatory pathway (Qi et al., 2022). Trans-ferulic acid, the major active form of ferulic acid (Cai et al., 2006), has been shown to reduce oxidative stress, apoptosis, and necrosis in peripheral monocytes (Perez-Ternero et al., 2017), and is involved in the activation of classical inflammatory pathways such as PPARγ and NF-κB//NLRP3 (Mahmoud et al., 2019). This evidence suggests that TXL may achieve improved plaque stability and inflammation in rabbits with atherosclerotic AS by regulating gut microbiota and metabolism (Qi et al., 2022). Another study demonstrated that TXL treatment attenuated intestinal I/R-induced mast cell activation, restrained overexpression of TLR4, NF-κB, TNF-α, platelet endothelial cell adhesion molecular (PECAM), High Mobility Group Protein 1 (HMGB1) expression, and protected VE-cadherin and Angiopoietin Like protein 4 (ANGPTL4) proteins to repair microvascular dysfunctionand endothelial injury (Zhang et al., 2020a; Zhang et al., 2018).
Qiliqiangxin (QL) capsules contain Astragalus mongholicus Bunge [Fabaceae, Astragali radix], Panax ginseng C.A.Mey. [Araliaceae; Ginseng radix et rhizoma], Aconitum carmichaelii Debeaux [Ranunculaceae; Aconiti lateralis radix praeparata], Salvia miltiorrhiza Bunge [Lamiaceae; Salvia miltiorrhizae radix et rhizoma], Descurainia sophia (L.) Webb ex Prantl [Brassicaceae, Descurainiae semen lepidii semen], etc. QL, also a CPM for the treatment of HF, reduced protein expression levels of myocardial NF-κB, NLRP3, ASC, caspase-1, and cleaved-IL-1β, preserved cardiac function, and significantly improved LV remodeling. In addition, QL treatment of HF rats resulted in increased Lactobacillus, improved intestinal villus edema, breakage or absence, and gaps between epithelial cells (Lu et al., 2022). In addition, Qiliqiangxin therapeutically protects the heart from ventricular remodeling and HF by modulating the microbiota of Paraprevotella, Phascolarctobacterium, and Intestinimonas and repairing the intestinal mucosal barrier (Lu et al., 2022).
Xuesaitong (XST) primarily consists of Panax notoginseng saponin (PNS), which exhibits dual effects of hemostasis and angiogenesis, making it extensively utilized for the treatment of cardiovascular and cerebrovascular diseases (Liao et al., 2023). Angiogenesis plays a crucial role in the reparative process following MI injury (Wu et al., 2021). In a mice model of MI, XST was demonstrated to enhance myocardial angiogenesis and mitigate myocardial fibrosis by specifically targeting nuclear hormone receptor77(Nur77) to suppress miR-3158-3p (Liao et al., 2023). Additionally, XST exhibited the ability to upregulate the expression of the VEGF signaling pathway, thereby promoting angiogenesis (Wang et al., 2012). Proteomic and cellular investigations unveiled that XST-induced alterations in protein levels of myocardial pyruvate dehydrogenase E1 alpha (PDHA1), hydroxyacyl-coenzyme A dehydrogenase (HADHA), peroxiredoxin 3 (PRX3), gamma-enolase, acetyl-coenzyme A acyltransferase 2 (ACAA2). In addition, XST promoted the activity of PDH, a crucial enzyme associated with the tricarboxylic acid (TCA) cycle, and enhanced intracellular levels of acetyl coenzyme A and ATP effectively mitigated malondialdehyde (MDA) release in cardiomyocytes (Zhao et al., 2017). Xu et al. investigated the impact of XST on intestinal barrier dysfunction and its underlying mechanisms in a rat model of intestinal I/R injury. XST demonstrated significant efficacy in alleviating I/R-induced intestinal barrier dysfunction by suppressing TNF-α expression, upregulating Bcl-2, downregulating caspase-3, and promoting intestinal peristalsis (Xu et al., 2015).
Zheng et al. (2023) conducted a randomized controlled trial in patients with acute ST-segment elevation myocardial infarction after percutaneous coronary intervention (PCI) and found that Tongguan capsules were able to improve the ventricular remodeling of patients. In addition, Tongguan capsules adjust the abundance of relevant genera of intestinal Prevotella, Agaricus, Microbacterium, and Enterococci, which increases the beneficial bacterial colonization and bacterial diversity, as well as adjust the structure of gut microbiota.
Shenfu injection, comprising ginsenosides and aconite alkaloid, exhibited enhanced hemodynamics and sustained intragastric pH in a myocardial I/R model, thereby preventing inadequate perfusion of the gastrointestinal microcirculation following myocardial I/R (Zhang et al., 2006). In children undergoing heart surgery for congenital heart defects, Shenfu injection significantly reduced the decrease in gastric mucosal pH during surgery. It also decreased plasma levels of diamine oxidase (DAO), MDA, LPS, IL-6, and creatine kinase isoenzyme MB. Additionally, Shenfu injection reduced postoperative utilization of positive inotropic drugs and length of stay in the care unit. Therefore, Shenfu injection attenuated gastrointestinal tract injury and suppressed inflammatory response after extracorporeal circulation in patients with congenital heart disease (Xia et al., 2005).
Potential mechanisms of Chinese patent medicine affecting intestinal mucosal barrier for the treatment of CVD are shown in Table 3.
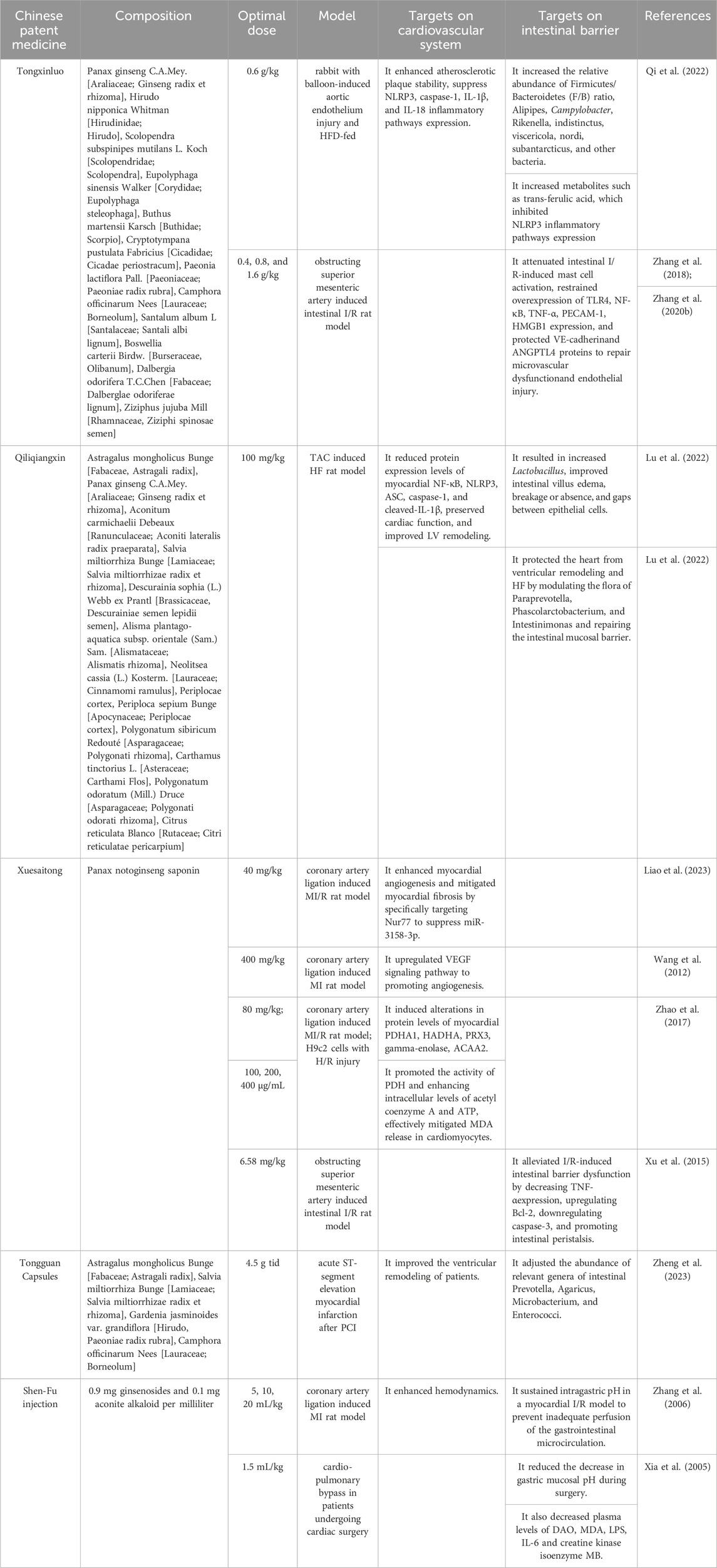
TABLE 3. Lists of Chinese patent medicine acting on intestinal barrier damage in the treatment of CVD.
Potential mechanisms of TCM affecting intestine to treat CVD risk factors
Fasting hyperglycemia, hypertriglyceridemia, low HDL cholesterol, hypertension, and/or metabolic disorders such as abdominal obesity and nonalcoholic fatty liver disease are risk factors for the development of coronary heart disease (CHD) and cardiovascular events (Lakka et al., 2002). RV intake decreased the relative abundance of Turicibacteraceae, Moryella, Lachnospiraceae, and Akkermansia and increased the relative abundance of Bacteroides and Parabacteroides in obese mice. And fecal FMT of healthy resveratrol-fed mice improved glucose homeostasis in obese mice (Sung et al., 2016).RV may indirectly treat CVD by modulating the microbial structure and improving lipid and glucose metabolism.
Porras et al. (Porras et al., 2017) identified the ability of quercetin to regulate lipid metabolism genes such as LXRα, sterol regulatory element binding protein (SREBP)-1c, fatty acid synthase (FAS), fatty acid binding protein (FABP)1 and FAT/CD36 and other lipid metabolism genes expression to regulate cytochrome P450 2E1 (CYP2E1)-dependent lipid peroxidation and related lipotoxicity, thereby reducing intrahepatic lipid accumulation and hence insulin resistance. Quercetin restored gut microbiota imbalance, SCFAs in HFD-fed mice, occludin, claudin 1, and intestinal alkaline phosphatase expression (Porras et, al., 2017). In addition, the associated endotoxemia-mediated TLR-4 pathway was also inhibited by quercetin, which subsequently inhibited inflammasome response and reticular stress pathway activation and blocked lipid metabolism gene expression (Porras et al., 2017).
Lu et al. (2020) demonstrated that Neohesperidin (Neo) attenuated the levels of inflammatory factors, including TNF-α, monocyte chemoattractant protein-1 (MCP-1), and IL-1β while modulating the expression of lipid transporter genes, adipogenic genes, and fatty acid oxidation in epididymal white adipose tissue. Additionally, Neo improved intestinal barrier integrity and reduced serum metabolic LPS levels in obese mice. However, eWAT was shown to be an exosome-dependent promoter of Ang II-induced cardiac fibrosis and subsequent cardiac dysfunction (Su et al., 2022). The study by Lu et al. also found that Neo neo-administration induced significant alterations in the composition of gut microbiota in high-fat diet-fed mice, characterized by a decrease in Faecalibaculum and an increase in Blautia, Mucispirillum, Lachnospiraceae_ UCG-006, Streptococcus, Enterorhabdus, and Bacteroides. Furthermore, FMT with Neo-treated samples effectively ameliorated obesity and metabolic disorders observed in HFD-fed mice (Lu et al., 2020).
Ginsenoside Rb1 (Rb1) oral supplementation ameliorated dyslipidemia, improved insulin sensitivity in HFD-induced obese mice, and reversed the expression of uncoupling protein 2 (UCP2), Nuclear receptor subfamily one group H member 4 (Nr1h4), and Fiaf. Rb1 altered gut microbiota composition and increased Akkermansia spp. abundance, and maintains amino acid metabolic homeostasis, particularly leucine (Leu), isoleucine (Iso), tryptophan (Trp), and alanine (Ala). Correlation analyses revealed that Akkermansia spp. alteration of the Ala gut microbiota and regulation of amino acid metabolism may be the mechanism by which Rb1 acts (Yang et al., 2021).
BYHWD was able to inhibit body fat accumulation and blood triglyceride levels in HFD rats significantly increase Bacteroidetes and dramatically decrease Firmicutes at the phyla level, and the remarkable increase in the abundance of Lactobacillus and Blautia (Liu et al., 2022b). Glycoside metabolites (astragaloside IV, paeoniflorin, and amygdalin) in BYHWD were shown to alleviate atherosclerotic inflammation. These glycosides were able to reduce pro-inflammatory factors and adhesion molecules, decrease the number of foam cells and intracellular lipid content, and prevent macrophage inflammation, all by inhibiting the activation of the Janus Kinase (JAK)/signal transducer and activator of transcription (STAT) pathway (Fu et al., 2022).
Lingguizhugan decoction (LGZGD) is an ancient Chinese herbal formula from the Treatise on Cold Pathogenic and Miscellaneous Diseases, consisting of Poria cocos (Fu Ling), Ramulus Cinnamomi (Gui Zhi), Atractylodis Macrocephalae Rhizoma (Bai Zhu), and Radix Glycyrrhizae (Gan Cao). It was found in the HFD rat model that LGZGD treatment not only reduced plasma insulin concentration, glucose tolerance, and lipids but also restored small intestinal villus morphology and upregulated occludin levels. This may be achieved by upregulating the relative abundance of Akkermansia, Faecalibacterium, and Phascolarctobacterium (Ning et al., 2022).
Potential mechanisms of TCM affecting intestine to treat CVD risk factors are shown in Table 4.
Summarize
In conclusion, TCM can regulate intestinal tight junctions, intestinal smooth muscle motility, intestinal mucus barrier, and gut microbiota, improve intestinal hemodynamics, and alleviate systemic or general inflammatory damage and oxidative stress injury, thus exerting potential therapeutic effects on CVD. In particular, the metabolites of the gut microbiota are closely related to the development of cardiovascular diseases. By intervening in the metabolism of gut microbiota, botanical drugs reduce the level of intestinal endotoxins and attenuate the inflammatory response. It is worth noting that the effect of some of these botanical drugs on altering the body’s glycolipid metabolism and CVD risk factors is also related to the intestinal mucosal barrier. Therefore, we hypothesized that the repair of intestinal mucosal barrier damage by botanical therapy is one of the mechanisms to protect the cardiovascular system.
However, the current understanding of the relationship and mechanisms between the cardiovascular system and the intestinal mucosal barrier remains limited in contemporary research. The pharmacological and toxicological mechanisms as well as the bioavailability of Chinese medicines to the organism and the gut microbiota also need to be continuously investigated. Chinese medicines emphasize individualized treatment, giving different prescriptions according to the different stages of the disease, and most of the studies are on simple disease models, such as simple hypertension, simple heart attack, and other animal models, which do not completely simulate the real clinical situation, especially when there are multiple confounding factors such as hyperlipidemia, hyperglycemia, or renal disease in the clinical patients, which needs to be further demonstrated with a combination of disease-evidence-based modeling and a high-quality clinical randomized controlled study or a real-world studies to further prove the scientific validity. Although the TCM formula and metabolites mentioned in the article were shown to treat CVD and repair the intestinal mucosal barrier, respectively, they were not in the same study and the same model. More importantly, most of the studies only demonstrate that botanical drugs can treat both CVD and repair the intestinal mucosal barrier, but these studies are not sufficient to confirm that botanical drugs can target the intestinal tract to treat CVD, direct evidence is still scarce, and there is a need for continued research on the intermediary mediators and pathways mediated by botanical drugs and on how certain flora specifically can affect CVD.
Author contributions
JL: Writing–original draft, Investigation, Writing–review and editing. XW: Writing–review and editing. TW: Writing–review and editing. MZ: Writing–review and editing. YG: Writing–review and editing. YC: Supervision, Writing–review and editing. LC: Supervision, Writing–review and editing.
Funding
The author(s) declare financial support was received for the research, authorship, and/or publication of this article. This work was supported by the Jinan Science and Technology Program Clinical (Grant No. 202134024), Qilu Traditional Chinese Medicine Advantage Specialty Cluster- Chest Pain Alliance (Grant No. 2021-02), Young Scientific Research Innovation Team of Affiliated Hospital of Shandong University of Traditional Chinese Medicine.
Conflict of interest
The authors declare that the research was conducted in the absence of any commercial or financial relationships that could be construed as a potential conflict of interest.
Publisher’s note
All claims expressed in this article are solely those of the authors and do not necessarily represent those of their affiliated organizations, or those of the publisher, the editors and the reviewers. Any product that may be evaluated in this article, or claim that may be made by its manufacturer, is not guaranteed or endorsed by the publisher.
References
Adak, A., and Khan, M. R. (2019). An insight into gut microbiota and its functionalities. Cell. Mol. Life Sci. CMLS 76 (3), 473–493. doi:10.1007/s00018-018-2943-4
Adkins, C., and Rezaie, A. (2018). Small intestinal bacterial overgrowth and coronary artery disease: what is in the CArDs? Dig. Dis. Sci. 63 (2), 271–272. doi:10.1007/s10620-017-4904-4
Arakawa, K., Ishigami, T., Nakai-Sugiyama, M., Chen, L., Doi, H., Kino, T., et al. (2019). Lubiprostone as a potential therapeutic agent to improve intestinal permeability and prevent the development of atherosclerosis in apolipoprotein E-deficient mice. PLoS ONE 14 (6), e0218096. doi:10.1371/journal.pone.0218096
Arutyunov, G. P., Kostyukevich, O. I., Serov, R. A., Rylova, N. V., and Bylova, N. A. (2008). Collagen accumulation and dysfunctional mucosal barrier of the small intestine in patients with chronic heart failure. Int. J. Cardiol. 125 (2), 240–245. doi:10.1016/j.ijcard.2007.11.103
Bartolomaeus, H., Balogh, A., Yakoub, M., Homann, S., Markó, L., Höges, S., et al. (2019). Short-chain fatty acid propionate protects from hypertensive cardiovascular damage. Circulation 139 (11), 1407–1421. doi:10.1161/CIRCULATIONAHA.118.036652
Baur, J. A., and Sinclair, D. A. (2006). Therapeutic potential of resveratrol: the in vivo evidence. Nat. Rev. Drug Discov. 5 (6), 493–506. doi:10.1038/nrd2060
Brown, J. M., and Hazen, S. L. (2015). The gut microbial endocrine organ: bacterially-derived signals driving cardiometabolic diseases. Annu. Rev. Med. 66, 343–359. doi:10.1146/annurev-med-060513-093205
Bui, A. L., Horwich, T. B., and Fonarow, G. C. (2011). Epidemiology and risk profile of heart failure. Nat. Rev. Cardiol. 8 (1), 30–41. doi:10.1038/nrcardio.2010.165
Caesar, R., Fåk, F., and Bäckhed, F. (2010). Effects of gut microbiota on obesity and atherosclerosis via modulation of inflammation and lipid metabolism. J. Intern. Med. 268 (4), 320–328. doi:10.1111/j.1365-2796.2010.02270.x
Cai, Y.-Z., Sun, M., Xing, J., Luo, Q., and Corke, H. (2006). Structure-radical scavenging activity relationships of phenolic compounds from traditional Chinese medicinal plants. Life Sci. 78 (25), 2872–2888. doi:10.1016/j.lfs.2005.11.004
Camilleri, M. (2019). Leaky gut: mechanisms, measurement and clinical implications in humans. Gut 68 (8), 1516–1526. doi:10.1136/gutjnl-2019-318427
Carnevale, R., Sciarretta, S., Valenti, V., di Nonno, F., Calvieri, C., Nocella, C., et al. (2020). Low-grade endotoxaemia enhances artery thrombus growth via Toll-like receptor 4: implication for myocardial infarction. Eur. Heart J. 41 (33), 3156–3165. doi:10.1093/eurheartj/ehz893
Chao, G., Ye, F., Yuan, Y., and Zhang, S. (2020). Berberine ameliorates non-steroidal anti-inflammatory drugs-induced intestinal injury by the repair of enteric nervous system. Fundam. Clin. Pharmacol. 34 (2), 238–248. doi:10.1111/fcp.12509
Chen, H.-C., Liu, Y.-W., Chang, K.-C., Wu, Y.-W., Chen, Y.-M., Chao, Y.-K., et al. (2023a). Gut butyrate-producers confer post-infarction cardiac protection. Nat. Commun. 14 (1), 7249. doi:10.1038/s41467-023-43167-5
Chen, H.-E., Lin, Y.-J., Lin, I.-C., Yu, H.-R., Sheen, J.-M., Tsai, C.-C., et al. (2019). Resveratrol prevents combined prenatal NG-nitro-L-arginine-methyl ester (L-NAME) treatment plus postnatal high-fat diet induced programmed hypertension in adult rat offspring: interplay between nutrient-sensing signals, oxidative stress and gut microbiota. J. Nutr. Biochem. 70, 28–37. doi:10.1016/j.jnutbio.2019.04.002
Chen, J., Wei, X., Zhang, Q., Wu, Y., Xia, G., Xia, H., et al. (2023b). The traditional Chinese medicines treat chronic heart failure and their main bioactive constituents and mechanisms. Acta Pharm. Sin. B 13 (5), 1919–1955. doi:10.1016/j.apsb.2023.02.005
Chen, M., Yi, L., Zhang, Y., Zhou, X., Ran, L., Yang, J., et al. (2016). Resveratrol attenuates trimethylamine-N-oxide (TMAO)-Induced atherosclerosis by regulating TMAO synthesis and bile acid metabolism via remodeling of the gut microbiota. mBio 7 (2), e02210–e02215. doi:10.1128/mBio.02210-15
Chen, R., Li, W., Qiu, Z., Zhou, Q., Zhang, Y., Li, W., et al. (2021). Ischemic postconditioning-mediated DJ-1 activation mitigate intestinal mucosa injury induced by myocardial ischemia reperfusion in rats through keap1/nrf2 pathway. Front. Mol. Biosci. 8, 655619. doi:10.3389/fmolb.2021.655619
Chen, X., Chen, H., He, Y., Fu, S., Liu, H., Wang, Q., et al. (2020). Proteomics-guided study on buyang Huanwu decoction for its neuroprotective and neurogenic mechanisms for transient ischemic stroke: involvements of EGFR/PI3K/Akt/Bad/14-3-3 and jak2/stat3/cyclin D1 signaling cascades. Mol. Neurobiol. 57 (10), 4305–4321. doi:10.1007/s12035-020-02016-y
Chen, Y., Li, L., Hu, C., Zhao, X., Zhang, P., Chang, Y., et al. (2022). Lingguizhugan decoction dynamically regulates MAPKs and AKT signaling pathways to retrogress the pathological progression of cardiac hypertrophy to heart failure. Phytomedicine 98, 153951. doi:10.1016/j.phymed.2022.153951
Cluntun, A. A., Badolia, R., Lettlova, S., Parnell, K. M., Shankar, T. S., Diakos, N. A., et al. (2021). The pyruvate-lactate axis modulates cardiac hypertrophy and heart failure. Cell Metab. 33 (3), 629–648.e10. doi:10.1016/j.cmet.2020.12.003
Ding, Q.-Y., Tian, J.-X., Li, M., Lian, F.-M., Zhao, L.-H., Wei, X.-X., et al. (2020). Interactions between therapeutics for metabolic disease, cardiovascular risk factors, and gut microbiota. Front. Cell. Infect. Microbiol. 10, 530160. doi:10.3389/fcimb.2020.530160
Drapala, A., Szudzik, M., Chabowski, D., Mogilnicka, I., Jaworska, K., Kraszewska, K., et al. (2020). Heart failure disturbs gut-blood barrier and increases plasma trimethylamine, a toxic bacterial metabolite. Int. J. Mol. Sci. 21 (17), 6161. doi:10.3390/ijms21176161
Fu, X., Sun, Z., Long, Q., Tan, W., Ding, H., Liu, X., et al. (2022). Glycosides from Buyang Huanwu Decoction inhibit atherosclerotic inflammation via JAK/STAT signaling pathway. Phytomedicine 105, 154385. doi:10.1016/j.phymed.2022.154385
Gao, T., Wang, R., Zhang, H., and Zhao, F. (2022). Network pharmacology combined with metabolomics reveals the mechanism of Fuzi decoction against chronic heart failure in rats. J. Chromatogr. B 1210, 123435. doi:10.1016/j.jchromb.2022.123435
Gao, T., Zhang, H., Li, Q., Zhao, F., Wang, N., He, W., et al. (2023). Fuzi decoction treats chronic heart failure by regulating the gut microbiota, increasing the short-chain fatty acid levels and improving metabolic disorders. J. Pharm. Biomed. Analysis 236, 115693. doi:10.1016/j.jpba.2023.115693
Hanniman, E. A., Lambert, G., McCarthy, T. C., and Sinal, C. J. (2005). Loss of functional farnesoid X receptor increases atherosclerotic lesions in apolipoprotein E-deficient mice. J. Lipid Res. 46 (12), 2595–2604. doi:10.1194/jlr.M500390-JLR200
Hao, P., Jiang, F., Cheng, J., Ma, L., Zhang, Y., and Zhao, Y. (2017). Traditional Chinese medicine for cardiovascular disease: evidence and potential mechanisms. J. Am. Coll. Cardiol. 69 (24), 2952–2966. doi:10.1016/j.jacc.2017.04.041
Homocysteine Studies Collaboration (2002). Homocysteine and risk of ischemic heart disease and stroke: a meta-analysis. JAMA 288 (16), 2015–2022. doi:10.1001/jama.288.16.2015
Hu, T., Wu, Q., Yao, Q., Jiang, K., Yu, J., and Tang, Q. (2022). Short-chain fatty acid metabolism and multiple effects on cardiovascular diseases. Ageing Res. Rev. 81, 101706. doi:10.1016/j.arr.2022.101706
Huart, J., Cirillo, A., Taminiau, B., Descy, J., Saint-Remy, A., Daube, G., et al. (2021). Human stool metabolome differs upon 24 h blood pressure levels and blood pressure dipping status: a prospective longitudinal study. Metabolites 11 (5), 282. doi:10.3390/metabo11050282
Hylemon, P. B., Zhou, H., Pandak, W. M., Ren, S., Gil, G., and Dent, P. (2009). Bile acids as regulatory molecules. J. Lipid Res. 50 (8), 1509–1520. doi:10.1194/jlr.R900007-JLR200
Jin, M., Qian, Z., Yin, J., Xu, W., and Zhou, X. (2019). The role of intestinal microbiota in cardiovascular disease. J. Cell. Mol. Med. 23 (4), 2343–2350. doi:10.1111/jcmm.14195
Johansson, M. E. V., and Hansson, G. C. (2016). Immunological aspects of intestinal mucus and mucins. Nat. Rev. Immunol. 16 (10), 639–649. doi:10.1038/nri.2016.88
Kalogeris, T., Baines, C. P., Krenz, M., and Korthuis, R. J. (2016). “Ischemia/reperfusion,” in Comprehensive physiology. Editor R. Terjung (Hoboken, New Jersey, United States: Wiley), 113–170. doi:10.1002/cphy.c160006
Kato, T., Honda, Y., Kurita, Y., Iwasaki, A., Sato, T., Kessoku, T., et al. (2017). Lubiprostone improves intestinal permeability in humans, a novel therapy for the leaky gut: a prospective randomized pilot study in healthy volunteers. PLoS ONE 12 (4), e0175626. doi:10.1371/journal.pone.0175626
Keeter, W. C., Ma, S., Stahr, N., Moriarty, A. K., and Galkina, E. V. (2022). Atherosclerosis and multi-organ-associated pathologies. Seminars Immunopathol. 44 (3), 363–374. doi:10.1007/s00281-022-00914-y
Kim, C. H., Park, J., and Kim, M. (2014). Gut microbiota-derived short-chain fatty acids, T cells, and inflammation. Immune Netw. 14 (6), 277–288. doi:10.4110/in.2014.14.6.277
Krack, A., Sharma, R., Figulla, H. R., and Anker, S. D. (2005). The importance of the gastrointestinal system in the pathogenesis of heart failure. Eur. Heart J. 26 (22), 2368–2374. doi:10.1093/eurheartj/ehi389
Lakka, H.-M., Laaksonen, D. E., Lakka, T. A., Niskanen, L. K., Kumpusalo, E., Tuomilehto, J., et al. (2002). The metabolic syndrome and total and cardiovascular disease mortality in middle-aged men. JAMA 288 (21), 2709–2716. doi:10.1001/jama.288.21.2709
Lewis, C. V., and Taylor, W. R. (2020). Intestinal barrier dysfunction as a therapeutic target for cardiovascular disease. Am. J. Physiology. Heart Circulatory Physiology 319 (6), H1227–H1233. doi:10.1152/ajpheart.00612.2020
Li, C., Li, Q., Liu, Y.-Y., Wang, M.-X., Pan, C.-S., Yan, L., et al. (2014). Protective effects of Notoginsenoside R1 on intestinal ischemia-reperfusion injury in rats. Am. J. Physiology. Gastrointest. Liver Physiology 306 (2), G111–G122. doi:10.1152/ajpgi.00123.2013
Li, F., Jiang, C., Krausz, K. W., Li, Y., Albert, I., Hao, H., et al. (2013). Microbiome remodelling leads to inhibition of intestinal farnesoid X receptor signalling and decreased obesity. Nat. Commun. 4 (1), 2384. doi:10.1038/ncomms3384
Li, X., Xu, G., Wei, S., Zhang, B., Yao, H., Chen, Y., et al. (2019). Lingguizhugan decoction attenuates doxorubicin-induced heart failure in rats by improving TT-SR microstructural remodeling. BMC Complementary Altern. Med. 19 (1), 360. doi:10.1186/s12906-019-2771-6
Li, Y., Wang, X., and Shen, Z. (2017). Traditional Chinese medicine for lipid metabolism disorders. Am. J. Transl. Res. 9 (5), 2038–2049.
Liang, C., Cai, D., Zhang, C., Qiu, Y., and Yu, Y. (2023). Effect of Buyang Huanwu decoction on inflammatory factors and intestinal flora in patients with stable coronary heart disease. Liaoning J. Traditional Chin. Med., 1–7.
Liao, J., Shao, M., Wang, Y., Yang, P., Fu, D., Liu, M., et al. (2023). Xuesaitong promotes myocardial angiogenesis in myocardial infarction mice by inhibiting MiR-3158-3p targeting Nur77. Aging (Albany NY) 15 (10), 4084–4095. doi:10.18632/aging.204671
Liao, J., Suguro, R., Zhao, X., Yu, Y., Cui, Y., and Zhu, Y. Z. (2021). Leonurine affected homocysteine-methionine metabolism based on metabolomics and gut microbiota studies of clinical trial samples. Clin. Transl. Med. 11 (10), e535. doi:10.1002/ctm2.535
Liu, F., Liu, J., Liu, Y., Zhang, Y., and Ding, X. (2021a). Shen-Fu Decoction could ameliorate intestinal permeability by regulating the intestinal expression of tight junction proteins and p-VASP in septic rats. J. Ethnopharmacol. 268, 113562. doi:10.1016/j.jep.2020.113562
Liu, J.-R., Miao, H., Deng, D.-Q., Vaziri, N. D., Li, P., and Zhao, Y.-Y. (2021b). Gut microbiota-derived tryptophan metabolism mediates renal fibrosis by aryl hydrocarbon receptor signaling activation. Cell. Mol. Life Sci. CMLS 78 (3), 909–922. doi:10.1007/s00018-020-03645-1
Liu, M., Yuan, G., Luo, G., Guo, X., Chen, M., Yang, H., et al. (2022a). Network pharmacology analysis and experimental verification strategies reveal the action mechanism of danshen decoction in treating ischemic cardiomyopathy. Evidence-Based Complementary Altern. Med. eCAM 2022, 7578055. doi:10.1155/2022/7578055
Liu, M., Zhao, Q., Liu, J., Huang, A., and Xia, X. (2022b). Buyang Huanwu decoction affects gut microbiota and lipid metabolism in a ZDF rat model of co-morbid type 2 diabetes mellitus and obesity: an integrated metabolomics analysis. Front. Chem. 10, 1036380. doi:10.3389/fchem.2022.1036380
Liu, P., Bian, Y., Fan, Y., Zhong, J., and Liu, Z. (2020). Protective effect of Naringin on in vitro gut-vascular barrier disruption of intestinal microvascular endothelial cells induced by TNF-α. J. Agric. Food Chem. 68 (1), 168–175. doi:10.1021/acs.jafc.9b06347
Lu, J. F., Zhu, M. Q., Zhang, H., Liu, H., Xia, B., Wang, Y. L., et al. (2020). Neohesperidin attenuates obesity by altering the composition of the gut microbiota in high-fat diet-fed mice. FASEB J. 34 (9), 12053–12071. doi:10.1096/fj.201903102RR
Lu, Y., Xiang, M., Xin, L., Zhang, Y., Wang, Y., Shen, Z., et al. (2022). Qiliqiangxin modulates the gut microbiota and NLRP3 inflammasome to protect against ventricular remodeling in heart failure. Front. Pharmacol. 13, 905424. doi:10.3389/fphar.2022.905424
Luo, Z., Li, H., Xiao, Z., Shao, S., Zhao, T., Zhao, Y., et al. (2019). Taohong Siwu decoction exerts a beneficial effect on cardiac function by possibly improving the microenvironment and decreasing mitochondrial fission after myocardial infarction. Cardiol. Res. Pract. 2019, 5198278. doi:10.1155/2019/5198278
Lyu, M., Wang, Y.-F., Fan, G.-W., Wang, X.-Y., Xu, S.-Y., and Zhu, Y. (2017). Balancing herbal medicine and functional Food for prevention and treatment of cardiometabolic diseases through modulating gut microbiota. Front. Microbiol. 8, 2146. doi:10.3389/fmicb.2017.02146
Ma, S.-R., Tong, Q., Lin, Y., Pan, L.-B., Fu, J., Peng, R., et al. (2022). Berberine treats atherosclerosis via a vitamine-like effect down-regulating Choline-TMA-TMAO production pathway in gut microbiota. Signal Transduct. Target. Ther. 7, 207. doi:10.1038/s41392-022-01027-6
Mahmoud, A. M., Hussein, O. E., Abd El-Twab, S. M., and Hozayen, W. G. (2019). Ferulic acid protects against methotrexate nephrotoxicity via activation of Nrf2/ARE/HO-1 signaling and PPARγ, and suppression of NF-κB/NLRP3 inflammasome axis. Food & Funct. 10 (8), 4593–4607. doi:10.1039/c9fo00114j
McCully, K. S. (2015). Homocysteine and the pathogenesis of atherosclerosis. Expert Rev. Clin. Pharmacol. 8 (2), 211–219. doi:10.1586/17512433.2015.1010516
Miyazaki-Anzai, S., Masuda, M., Kohno, S., Levi, M., Shiozaki, Y., Keenan, A. L., et al. (2018). Simultaneous inhibition of FXR and TGR5 exacerbates atherosclerotic formation. J. Lipid Res. 59 (9), 1709–1713. doi:10.1194/jlr.M087239
Mosinska, P., and Fichna, J. (2015). Ischemic colitis: current diagnosis and treatment. Curr. Drug Targets 16 (3), 209–218. doi:10.2174/1389450116666150113120549
Mouries, J., Brescia, P., Silvestri, A., Spadoni, I., Sorribas, M., Wiest, R., et al. (2019). Microbiota-driven gut vascular barrier disruption is a prerequisite for non-alcoholic steatohepatitis development. J. Hepatology 71 (6), 1216–1228. doi:10.1016/j.jhep.2019.08.005
Ndrepepa, G. (2018). Uric acid and cardiovascular disease. Clin. Chimica Acta; Int. J. Clin. Chem. 484, 150–163. doi:10.1016/j.cca.2018.05.046
Nie, J., Zhang, L., Zhao, G., and Du, X. (2019). Quercetin reduces atherosclerotic lesions by altering the gut microbiota and reducing atherogenic lipid metabolites. J. Appl. Microbiol. 127 (6), 1824–1834. doi:10.1111/jam.14441
Ning, Y., Gong, Y., Zheng, T., Xie, Y., Yuan, S., and Ding, W. (2022). Lingguizhugan decoction targets intestinal microbiota and metabolites to reduce insulin resistance in high-fat diet rats. Diabetes, Metabolic Syndrome Obes. Targets Ther. 15, 2427–2442. doi:10.2147/DMSO.S370492
Odenwald, M. A., and Turner, J. R. (2017). The intestinal epithelial barrier: a therapeutic target? Nat. Rev. Gastroenterology Hepatology 14 (1), 9–21. doi:10.1038/nrgastro.2016.169
Organ, C. L., Otsuka, H., Bhushan, S., Wang, Z., Bradley, J., Trivedi, R., et al. (2016). Choline diet and its gut microbe-derived metabolite, trimethylamine N-oxide, exacerbate pressure overload-induced heart failure. Circ. Heart Fail. 9 (1), e002314. doi:10.1161/CIRCHEARTFAILURE.115.002314
Perez-Ternero, C., Werner, C. M., Nickel, A. G., Herrera, M. D., Motilva, M.-J., Böhm, M., et al. (2017). Ferulic acid, a bioactive component of rice bran, improves oxidative stress and mitochondrial biogenesis and dynamics in mice and in human mononuclear cells. J. Nutr. Biochem. 48, 51–61. doi:10.1016/j.jnutbio.2017.06.011
Pols, T. W. H., Nomura, M., Harach, T., Sasso, G. L., Oosterveer, M. H., Thomas, C., et al. (2011). TGR5 activation inhibits atherosclerosis by reducing macrophage inflammation and lipid loading. Cell Metab. 14 (6), 747–757. doi:10.1016/j.cmet.2011.11.006
Porras, D., Nistal, E., Martínez-Flórez, S., Pisonero-Vaquero, S., Olcoz, J. L., Jover, R., et al. (2017). Protective effect of quercetin on high-fat diet-induced non-alcoholic fatty liver disease in mice is mediated by modulating intestinal microbiota imbalance and related gut-liver axis activation. Free Radic. Biol. Med. 102, 188–202. doi:10.1016/j.freeradbiomed.2016.11.037
Qi, Y., Liu, W., Yan, X., Zhang, C., Zhang, C., Liu, L., et al. (2022). Tongxinluo may alleviate inflammation and improve the stability of atherosclerotic plaques by changing the intestinal flora. Front. Pharmacol. 13, 805266. doi:10.3389/fphar.2022.805266
Rakusan, K., Flanagan, M. F., Geva, T., Southern, J., and Van Praagh, R. (1992). Morphometry of human coronary capillaries during normal growth and the effect of age in left ventricular pressure-overload hypertrophy. Circulation 86 (1), 38–46. doi:10.1161/01.cir.86.1.38
Ren, J., Wu, N. N., Wang, S., Sowers, J. R., and Zhang, Y. (2021). Obesity cardiomyopathy: evidence, mechanisms, and therapeutic implications. Physiol. Rev. 101 (4), 1745–1807. doi:10.1152/physrev.00030.2020
Ridlon, J. M., Kang, D.-J., and Hylemon, P. B. (2006). Bile salt biotransformations by human intestinal bacteria. J. Lipid Res. 47 (2), 241–259. doi:10.1194/jlr.R500013-JLR200
Rosell-Mases, E., Santiago, A., Corral-Pujol, M., Yáñez, F., Varela, E., Egia-Mendikute, L., et al. (2023). Mutual modulation of gut microbiota and the immune system in type 1 diabetes models. Nat. Commun. 14, 7770. doi:10.1038/s41467-023-43652-x
Sandek, A., Swidsinski, A., Schroedl, W., Watson, A., Valentova, M., Herrmann, R., et al. (2014). Intestinal blood flow in patients with chronic heart failure: a link with bacterial growth, gastrointestinal symptoms, and cachexia. J. Am. Coll. Cardiol. 64 (11), 1092–1102. doi:10.1016/j.jacc.2014.06.1179
Saponara, S., Testai, L., Iozzi, D., Martinotti, E., Martelli, A., Chericoni, S., et al. (2006). (+/−)-Naringenin as large conductance Ca2+-activated K+ (BKCa) channel opener in vascular smooth muscle cells. Br. J. Pharmacol. 149 (8), 1013–1021. doi:10.1038/sj.bjp.0706951
Schoeler, M., and Caesar, R. (2019). Dietary lipids, gut microbiota and lipid metabolism. Rev. Endocr. Metabolic Disord. 20 (4), 461–472. doi:10.1007/s11154-019-09512-0
Shang, H., Chen, J., Zhang, J., Xiang, Y., Cao, H., Ren, M., et al. (2009). Three therapeutic tendencies for secondary prevention of myocardial infarction and possible role of Chinese traditional patent medicine: viewpoint of evidence-based medicine. J. Evidence-Based Med. 2 (2), 84–91. doi:10.1111/j.1756-5391.2009.01004.x
Shen, S., Wu, G., Luo, W., Li, W., Li, X., Dai, C., et al. (2023). Leonurine attenuates angiotensin II-induced cardiac injury and dysfunction via inhibiting MAPK and NF-κB pathway. Phytomedicine Int. J. Phytotherapy Phytopharm. 108, 154519. doi:10.1016/j.phymed.2022.154519
Spadoni, I., Pietrelli, A., Pesole, G., and Rescigno, M. (2016). Gene expression profile of endothelial cells during perturbation of the gut vascular barrier. Gut Microbes 7 (6), 540–548. doi:10.1080/19490976.2016.1239681
Spadoni, I., Zagato, E., Bertocchi, A., Paolinelli, R., Hot, E., Sabatino, A. D., et al. (2015). A gut-vascular barrier controls the systemic dissemination of bacteria.
Su, M., Li, W., Yuan, Y., Liu, S., Liang, C., Liu, H. E., et al. (2022). Epididymal white adipose tissue promotes angiotensin II-induced cardiac fibrosis in an exosome-dependent manner. Transl. Res. J. Laboratory Clin. Med. 248, 51–67. doi:10.1016/j.trsl.2022.05.004
Sung, M. M., Kim, T. T., Denou, E., Soltys, C.-L. M., Hamza, S. M., Byrne, N. J., et al. (2016). Improved glucose homeostasis in obese mice treated with resveratrol is associated with alterations in the gut microbiome. Diabetes 66 (2), 418–425. doi:10.2337/db16-0680
Tang, M., Yuan, D., and Liao, P. (2021). Berberine improves intestinal barrier function and reduces inflammation, immunosuppression, and oxidative stress by regulating the NF-κB/MAPK signaling pathway in deoxynivalenol-challenged piglets. Environ. Pollut. 289, 117865. doi:10.1016/j.envpol.2021.117865
Tang, W. H. W., Wang, Z., Levison, B. S., Koeth, R. A., Britt, E. B., Fu, X., et al. (2013). Intestinal microbial metabolism of phosphatidylcholine and cardiovascular risk. N. Engl. J. Med. 368 (17), 1575–1584. doi:10.1056/NEJMoa1109400
Tripathi, A., Debelius, J., Brenner, D. A., Karin, M., Loomba, R., Schnabl, B., et al. (2018). The gut-liver axis and the intersection with the microbiome. Nat. Rev. Gastroenterology Hepatology 15 (7), 397–411. doi:10.1038/s41575-018-0011-z
US Preventive Services Task Force Davidson, K. W., Barry, M. J., Mangione, C. M., Cabana, M., Chelmow, D., Coker, T. R., et al. (2022). Aspirin use to prevent cardiovascular disease: US preventive Services Task Force recommendation statement. JAMA 327 (16), 1577–1584. doi:10.1001/jama.2022.4983
Usuda, H., Okamoto, T., and Wada, K. (2021). Leaky gut: effect of dietary fiber and fats on microbiome and intestinal barrier. Int. J. Mol. Sci. 22 (14), 7613. doi:10.3390/ijms22147613
Verhoef, P., Stampfer, M. J., Buring, J. E., Gaziano, J. M., Allen, R. H., Stabler, S. P., et al. (1996). Homocysteine metabolism and risk of myocardial infarction: relation with vitamins B6, B12, and folate. Am. J. Epidemiol. 143 (9), 845–859. doi:10.1093/oxfordjournals.aje.a008828
Violi, F., Cammisotto, V., Bartimoccia, S., Pignatelli, P., Carnevale, R., and Nocella, C. (2023). Gut-derived low-grade endotoxaemia, atherothrombosis and cardiovascular disease. Nat. Rev. Cardiol. 20 (1), 24–37. doi:10.1038/s41569-022-00737-2
Walker, D. K., and Gilliland, S. E. (1993). Relationship among bile tolerance, bile salt deconjugation, and assimilation of cholesterol by Lactobacillus acidophilus. J. Dairy Sci. 76 (4), 956–961. doi:10.3168/jds.s0022-0302(93)77422-6
Wang, C. C. L., Hess, C. N., Hiatt, W. R., and Goldfine, A. B. (2016). Clinical update: cardiovascular disease in diabetes mellitus: atherosclerotic cardiovascular disease and heart failure in type 2 diabetes mellitus - mechanisms, management, and clinical considerations. Circulation 133 (24), 2459–2502. doi:10.1161/CIRCULATIONAHA.116.022194
Wang, F., Zhao, C., Yang, M., Zhang, L., Wei, R., Meng, K., et al. (2021a). Four Citrus flavanones exert atherosclerosis alleviation effects in ApoE –/– mice via different metabolic and signaling pathways. J. Agric. Food Chem. 69 (17), 5226–5237. doi:10.1021/acs.jafc.1c01463
Wang, H., Chai, K., Du, M., Wang, S., Cai, J.-P., Li, Y., et al. (2021b). Prevalence and incidence of heart failure among urban patients in China: a national population-based analysis. Circ. Heart Fail. 14 (10), e008406. doi:10.1161/CIRCHEARTFAILURE.121.008406
Wang, S.-L., Shao, B.-Z., Zhao, S.-B., Fang, J., Gu, L., Miao, C.-Y., et al. (2018). Impact of paneth cell autophagy on inflammatory bowel disease. Front. Immunol. 9, 693. doi:10.3389/fimmu.2018.00693
Wang, T., Jiang, X., Ruan, Y., Zhuang, J., and Yin, Y. (2022). Based on network pharmacology and in vitro experiments to prove the effective inhibition of myocardial fibrosis by Buyang Huanwu decoction. Bioengineered 13 (5), 13767–13783. doi:10.1080/21655979.2022.2084253
Wang, Z., and Zhao, Y. (2018). Gut microbiota derived metabolites in cardiovascular health and disease. Protein & Cell 9 (5), 416–431. doi:10.1007/s13238-018-0549-0
Wang, Z.-T., Zhang, S.-J., Han, L.-H., and Chai, S.-B. (2012). Effects of xuesetong soft capsules on angiogenesis and VEGF mRNA expression in ischemic myocardium in rats with myocardial infarction. J. Traditional Chin. Med. = Chung I Tsa Chih Ying Wen Pan 32 (1), 71–74. doi:10.1016/s0254-6272(12)60035-1
Wells, J. M., Brummer, R. J., Derrien, M., MacDonald, T. T., Troost, F., Cani, P. D., et al. (2017). Homeostasis of the gut barrier and potential biomarkers. Am. J. Physiology - Gastrointest. Liver Physiology 312 (3), G171–G193. doi:10.1152/ajpgi.00048.2015
Weng, J., Li, J., Yuan, M., Yao, T., Zhang, J., Zeng, Y., et al. (2023). Effects of buyang Huanwu decoction on intestinal barrier, intestinal flora, and trimethylamine oxide in rats with heart failure. Chin. J. Integr. Med. 29 (2), 155–161. doi:10.1007/s11655-022-2898-z
Witjes, J. J., van Raalte, D. H., and Nieuwdorp, M. (2015). About the gut microbiome as a pharmacological target in atherosclerosis. Eur. J. Pharmacol. 763 (Pt A), 75–78. doi:10.1016/j.ejphar.2015.06.023
Wu, D., Ding, L., Tang, X., Wang, W., Chen, Y., and Zhang, T. (2019a). Baicalin protects against hypertension-associated intestinal barrier impairment in Part Through enhanced microbial production of short-chain fatty acids. Front. Pharmacol. 10, 1271. doi:10.3389/fphar.2019.01271
Wu, D., Tang, X., Ding, L., Cui, J., Wang, P., Du, X., et al. (2019b). Candesartan attenuates hypertension-associated pathophysiological alterations in the gut. Biomed. Pharmacother. = Biomedecine Pharmacother. 116, 109040. doi:10.1016/j.biopha.2019.109040
Wu, M., Yang, S., Wang, S., Cao, Y., Zhao, R., Li, X., et al. (2020). Effect of berberine on atherosclerosis and gut microbiota modulation and their correlation in high-fat diet-fed ApoE−/− mice. Front. Pharmacol. 11, 223. doi:10.3389/fphar.2020.00223
Wu, X., Reboll, M. R., Korf-Klingebiel, M., and Wollert, K. C. (2021). Angiogenesis after acute myocardial infarction. Cardiovasc. Res. 117 (5), 1257–1273. doi:10.1093/cvr/cvaa287
Xia, W., Hu, S., Wang, M., Xu, F., Han, L., and Peng, D. (2021). Exploration of the potential mechanism of the Tao Hong Si Wu Decoction for the treatment of postpartum blood stasis based on network pharmacology and in vivo experimental verification. J. Ethnopharmacol. 268, 113641. doi:10.1016/j.jep.2020.113641
Xia, Z., Liu, X.-Y., Zhan, L., He, Y., Luo, T., and Xia, Z. (2005). Ginsenosides compound (shen-fu) attenuates gastrointestinal injury and inhibits inflammatory response after cardiopulmonary bypass in patients with congenital heart disease. J. Thorac. Cardiovasc. Surg. 130 (2), 258–264. doi:10.1016/j.jtcvs.2005.02.046
Xie, B., Zu, X., Wang, Z., Xu, X., Liu, G., and Liu, R. (2022). Ginsenoside Rc ameliorated atherosclerosis via regulating gut microbiota and fecal metabolites. Front. Pharmacol. 13, 990476. doi:10.3389/fphar.2022.990476
Xu, X., Li, D., Gao, H., Gao, Y., Zhang, L., Du, Y., et al. (2015). Protective effect of the traditional Chinese medicine xuesaitong on intestinal ischemia-reperfusion injury in rats. Int. J. Clin. Exp. Med. 8 (2), 1768–1779.
Yan, X., Wu, H., Ren, J., Liu, Y., Wang, S., Yang, J., et al. (2018). Shenfu Formula reduces cardiomyocyte apoptosis in heart failure rats by regulating microRNAs. J. Ethnopharmacol. 227, 105–112. doi:10.1016/j.jep.2018.05.006
Yang, T., Santisteban, M. M., Rodriguez, V., Li, E., Ahmari, N., Carvajal, J. M., et al. (2015). Gut dysbiosis is linked to hypertension. Hypertension 65 (6), 1331–1340. doi:10.1161/HYPERTENSIONAHA.115.05315
Yang, X., Dong, B., An, L., Zhang, Q., Chen, Y., Wang, H., et al. (2021). Ginsenoside Rb1 ameliorates glycemic disorder in mice with high fat diet-induced obesity via regulating gut microbiota and amino acid metabolism. Front. Pharmacol. 12, 756491. doi:10.3389/fphar.2021.756491
Yang, Z., Pan, A., Zuo, W., Guo, J., and Zhou, W. (2014). Relaxant effect of flavonoid naringenin on contractile activity of rat colonic smooth muscle. J. Ethnopharmacol. 155 (2), 1177–1183. doi:10.1016/j.jep.2014.06.053
Yuzefpolskaya, M., Bohn, B., Nasiri, M., Zuver, A. M., Onat, D. D., Royzman, E. A., et al. (2020). Gut microbiota, endotoxemia, inflammation, and oxidative stress in patients with heart failure, left ventricular assist device, and transplant. J. Heart Lung Transplant. Official Publ. Int. Soc. Heart Transplant. 39 (9), 880–890. doi:10.1016/j.healun.2020.02.004
Zeng, J.-J., Shi, H.-Q., Ren, F.-F., Zhao, X.-S., Chen, Q.-Y., Wang, D.-J., et al. (2023). Notoginsenoside R1 protects against myocardial ischemia/reperfusion injury in mice via suppressing TAK1-JNK/p38 signaling. Acta Pharmacol. Sin. 44 (7), 1366–1379. doi:10.1038/s41401-023-01057-y
Zhang, J., Feng, Y., Yanyan, H., Yin, Z., Yi, L., Minghui, Y., et al. (2020a). Mast cell activation, TLR4-NF-κB/TNF-α pathway variation in rats’ intestinal ischemia-reperfusion injury and Tongxinluo’s therapeutic effect. Pak. J. Pharm. Sci. 33 (4), 1599–1608.
Zhang, J.-X., Li, S.-D., Liu, Y., and Yang, M.-H. (2018). Preventive effect of Tongxinluo on endothelial survival and vascular integrity, together with inhibition of inflammatory reaction in rats model of intestine ischemia/reperfusion injury. Pak. J. Pharm. Sci. 31 (6), 2403–2410.
Zhang, W.-J., Wojta, J., and Binder, B. R. (1997). Notoginsenoside R1 counteracts endotoxin-induced activation of endothelial cells in vitro and endotoxin-induced lethality in mice in vivo. Arteriosclerosis, Thrombosis, Vasc. Biol. 17 (3), 465–474. doi:10.1161/01.ATV.17.3.465
Zhang, X.-J., Song, L., Zhou, Z.-G., and Wang, X.-M. (2006). Effect of shenfu injection on gastrointestinal microcirculation in rabbits after myocardial ischemia-reperfusion injury. World J. Gastroenterology WJG 12 (27), 4389–4391. doi:10.3748/wjg.v12.i27.4389
Zhang, X.-Y., Chen, J., Yi, K., Peng, L., Xie, J., Gou, X., et al. (2020b). Phlorizin ameliorates obesity-associated endotoxemia and insulin resistance in high-fat diet-fed mice by targeting the gut microbiota and intestinal barrier integrity. Gut Microbes 12 (1), 1–18. doi:10.1080/19490976.2020.1842990
Zhang, Y., Zhang, S., Li, B., Luo, Y., Gong, Y., Jin, X., et al. (2022). Gut microbiota dysbiosis promotes age-related atrial fibrillation by lipopolysaccharide and glucose-induced activation of NLRP3-inflammasome. Cardiovasc. Res. 118 (3), 785–797. doi:10.1093/cvr/cvab114
Zhao, X., Zhang, F., and Wang, Y. (2017). Proteomic analysis reveals Xuesaitong injection attenuates myocardial ischemia/reperfusion injury by elevating pyruvate dehydrogenase-mediated aerobic metabolism. Mol. Biosyst. 13 (8), 1504–1511. doi:10.1039/C7MB00140A
Zheng, S., Yu, L., Zhao, X., Guo, L., Zhang, M., and Mao, S. (2023). Effects of tongguan capsules on left ventricular remodeling and gut microbiota in patients with acute ST-segment elevation myocardial infarction: a randomized controlled clinical study. J. Traditional Chin. Med. 64 (20), 2090–2100. doi:10.13288/j.11-2166/r.2023.20.009
Zhong, J., Yu, R., Zhou, Q., Liu, P., Liu, Z., and Bian, Y. (2021). Naringenin prevents TNF-α-induced gut-vascular barrier disruption associated with inhibiting the NF-κB-mediated MLCK/p-MLC and NLRP3 pathways. Food & Funct. 12 (6), 2715–2725. doi:10.1039/D1FO00155H
Zhu, W., Gregory, J. C., Org, E., Buffa, J. A., Gupta, N., Wang, Z., et al. (2016). Gut microbial metabolite TMAO enhances platelet hyperreactivity and thrombosis risk. Cell 165 (1), 111–124. doi:10.1016/j.cell.2016.02.011
Glossary
Keywords: metabolites of Chinese botanical drugs, traditional Chinese medicine formulas, Chinese patent medicine, cardiovascular disease, intestinal mucosal barrier, gut microbiota
Citation: Liu J, Wei X, Wang T, Zhang M, Gao Y, Cheng Y and Chi L (2024) Intestinal mucosal barrier: a potential target for traditional Chinese medicine in the treatment of cardiovascular diseases. Front. Pharmacol. 15:1372766. doi: 10.3389/fphar.2024.1372766
Received: 18 January 2024; Accepted: 12 February 2024;
Published: 26 February 2024.
Edited by:
Yi Wu, Nanjing Agricultural University, ChinaReviewed by:
Cui Liu, South China Agricultural University, ChinaLiwei Guo, Yangtze University, China
Yansheng Guo, Ningxia University, China
Copyright © 2024 Liu, Wei, Wang, Zhang, Gao, Cheng and Chi. This is an open-access article distributed under the terms of the Creative Commons Attribution License (CC BY). The use, distribution or reproduction in other forums is permitted, provided the original author(s) and the copyright owner(s) are credited and that the original publication in this journal is cited, in accordance with accepted academic practice. No use, distribution or reproduction is permitted which does not comply with these terms.
*Correspondence: Yan Cheng, ZG9jdG9yY2hlbmd5YW4yMDIzQDE2My5jb20=; Lili Chi, Y2hpbGlsaXlsQDEyNi5jb20=