- 1Department of Gastroenterology, Sir Run Run Shaw Hospital, College of Medicine, Zhejiang University, Hangzhou, China
- 2Inflammatory Bowel Disease Center, Sir Run Run Shaw Hospital, College of Medicine, Zhejiang University, Hangzhou, China
- 3Institute of Gastroenterology, Zhejiang University, Hangzhou, China
Non-alcoholic fatty liver disease (NAFLD) is the most common chronic liver disease, resulting in a huge medical burden worldwide. Accumulating evidence suggests that the gut microbiome and bile acids play pivotal roles during the development of NAFLD. Patients with NAFLD exhibit unique signatures of the intestinal microbiome marked by the priority of Gram-negative bacteria, decreased ratio of Firmicutes/Bacteroidetes (F/B), and increased Prevotella and Lachnospiraceae. The intestinal microbiota is involved in the metabolism of bile acids. Ursodeoxycholic acid (UDCA) is a key determinant in maintaining the dynamic communication between the host and gut microbiota. It generally shows surprising therapeutic potential in NAFLD with several mechanisms, such as improving cellular autophagy, apoptosis, and mitochondrial functions. This action is based on its direct or indirect effect, targeting the farnesoid X receptor (FXR) and various other nuclear receptors. This review aims to discuss the current studies on the involvement of the microbiome–UDCA interface in NAFLD therapy and provide prospective insights into future preventative and therapeutic approaches for NAFLD.
1 Introduction
Non-alcoholic fatty liver disease (NAFLD) is defined as the presence of ≥5% hepatic steatosis, which is not secondary to specific reasons such as significant alcohol consumption and drug damage. NAFLD is caused by metabolic dysfunction, being overweight or obese, and type 2 diabetes mellitus. Encompassing a broad spectrum of clinical phenotypes, NAFLD ranges from simple steatosis to non-alcoholic steatohepatitis (NASH), advanced liver fibrosis, cirrhosis, and hepatocellular carcinoma (Chalasani et al., 2018). The prevalence of NAFLD is estimated to be 20%–30% worldwide and is constantly increasing due to its association with obesity, diabetes, and metabolic syndrome (Younossi et al., 2016). Currently considered the second leading indication for liver transplantation, with numbers further increasing, NAFLD has become a heavy global burden that requires immediate attention (Younossi et al., 2018). The etiology of NAFLD is complex, including dietary and environmental factors that potentially lead to apoptosis, metabolism abnormalities, oxidative stress, and inflammation in the liver. In addition, the effect of patatin-like phospholipase domain-containing 3 (PNPLA3), transmembrane 6 superfamily member 2 protein (TM6SF2), and membrane-bound O-acyltransferase domain-containing 7 (MBOAT7) gene polymorphism provides convincing evidence for elucidating the genetic susceptibility to NAFLD (Xia et al., 2022). The application of Mendelian randomization has helped researchers discover more gene targets, like lipoprotein lipase (LPL) (Li et al., 2023). Recently, a novel hypothesis of “Multiple parallel hits” has been proposed, which suggests that gene polymorphism, lipotoxicity, and intestinal microbiome together contribute to the development of NAFLD (Tilg et al., 2021). However, the precise molecular network underlying NAFLD remains unclear, which is crucial for identifying novel and effective therapeutic targets to prevent or at least reduce the growing burden of liver transplantation.
Many studies have shed light on the indispensable role of the gut microbiome in the acceleration of NAFLD progression (Abenavoli et al., 2022). Mechanisms regarding the gut microbiome in NAFLD involve increased permeability of the intestinal barrier and perturbation of various bacterial metabolites, like bile acids and ethanol (Jiao et al., 2018; He et al., 2022). The gut–liver axis also occupies an important position in both mechanisms by providing a shortcut (Milosevic et al., 2019). Furthermore, microbiome features have been used to develop a new non-invasive risk assessment tool with a random forest machine learning model in NAFLD patients (Elsayed et al., 2022; Leung et al., 2022). It can provide potential new therapeutic strategies like probiotics and fecal microbiota transplantation (FMT) to attenuate chronic liver diseases (Scorletti et al., 2020; Xue et al., 2022). Of note, bile acids play a critical role in regulating metabolism and hepatic pathophysiology. They are initially synthesized as primary bile acids (PBAs) in the liver and then transformed into secondary bile acids (SBAs) by the gut microbiome. Although the microbiome is a prerequisite for SBA production, specific bile acids possess the capability to reciprocally modulate gut microbiome growth (Winston et al., 2020). In addition, it has been suggested that NAFLD is characterized by augmented synthesis of bile acids independent of downregulated bile acid signaling (Jiao et al., 2018). The modulation of bile acid profiles also represents a key step in ameliorating NAFLD (Jiao et al., 2018; Sun et al., 2019; Juarez-Fernandez et al., 2021). Indeed, modifications to the bile acid pool may serve as a new interventional target for NAFLD, as certified in both animal and human experiments (Kim et al., 2019; Gillard et al., 2022).
Ursodeoxycholic acid (UDCA) a secondary bile acid with the highest hydrophilicity and lowest toxicity. With anti-inflammatory, antioxidant, and anti-fibrotic properties, UDCA has been approved as the first-line drug for treating cholestatic liver diseases, such as primary biliary cholangitis. However, the evidence on its efficacy for NAFLD therapy is controversial. Studies have shown that UDCA alleviated hepatic steatosis and modulated inflammation and fibrosis (Marchiano et al., 2022; Xie et al., 2022; Marchianò et al., 2023). Despite its limited presence in the bile pool (only 1%–3%), UDCA interacts closely with the gut microbiome and can therefore directly or indirectly influence the progression of NAFLD. It warrants greater attention for its potential as a therapy for NAFLD.
In this review, we summarized the interaction between the gut microbiome, UDCA, and NAFLD, as well as the underlying mechanisms as revealed by recent research studies (Figure 1). The role of the gut microbiome and UDCA in liver immune response and inflammation will be discussed, with implications for development of preventive strategies, new therapeutics, and potential diagnostic tools for clinical applications in NAFLD.
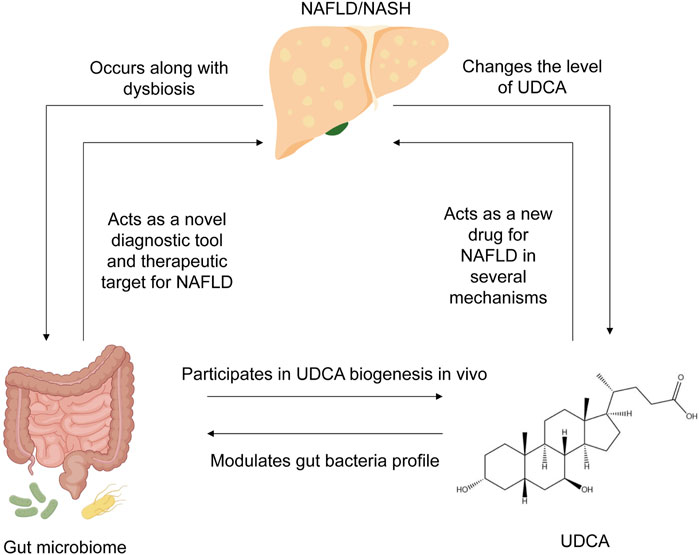
Figure 1. Summary of the interplay between NAFLD, UDCA, and gut microbiome. NAFLD occurs along with dysbiosis in the human gut, changes the level of UDCA, and alters bile acid composition. In turn, the gut microbiome has the potential to act as a novel diagnostic tool for NAFLD diagnosis and evaluation, as well as an effective therapeutic target for NAFLD therapy. UDCA, produced by the gut microbiome in vivo, can also remodel the bacterial community in the human gut. NAFLD, non-alcoholic fatty liver disease; UDCA, ursodeoxycholic acid.
2 Gut microbiome signatures and NAFLD
It has been clearly indicated that NAFLD patients harbored an obviously lower diversity and richness of the gut microbiome than healthy controls (Caussy et al., 2019; Schwimmer et al., 2019; Hullar et al., 2021). Gram-negative (G−) bacteria are prevalent in both obese and non-obese NAFLD patients (Wang et al., 2016; Schwimmer et al., 2019), which is represented by the phylum Proteobacteria as well as the genera Prevotella and Bacteroides. Critically, the genus Escherichia Shigella is sharply increased in the NAFLD group, especially in patients with significant fibrosis (Shen et al., 2017). Alternatively, two pro-inflammatory responses associated with G− bacteria, including genes for lipopolysaccharide biosynthesis in NASH and genes for flagellar assembly in moderate-to-severe fibrosis, are enriched in the NAFLD metagenome (Schwimmer et al., 2019). Collectively, dysbiosis that might contribute to pathogenesis of NAFLD is normally presented as endotoxemia, gut barrier leakiness, and subsequent inflammatory gene overexpression in the liver.
At the phylum level, Bacteroidetes and Firmicutes are the two dominant groups in the microbial community, with the prevalence accounting for over 90% in the human gut (Nkosi et al., 2022). Changes in the ratio of Firmicutes/Bacteroidetes (F/B) are considered an index of dysbiosis, whereas research studies regarding the changes in the F/B ratio had inconsistent conclusions. Firmicutes and Bacteroidetes were previously proposed as “fatty bacteria” and “lean bacteria,” respectively (Zhao, 2013); therefore, it could be speculated that high-fat diet-induced NAFLD theoretically results in the increased proportion of F/B (Carino et al., 2019). In most animal experiments, the F/B ratio followed the predicted tendency in NAFLD models and was restored along with the improvement in steatosis indicators (Porras et al., 2017; Gao et al., 2021; Yu et al., 2021; Romualdo et al., 2022). Both high-fat diet and Western diet-induced mouse NAFLD models showed similar results in the gut microbiome at the phylum level (the detailed information is displayed in Table 1). Interestingly, NAFLD and NASH patients showed different results, such as a higher abundance of Bacteroides and a lower abundance of Firmicutes (Wang et al., 2016), or no significant difference was observed in the F/B ratio between patients and controls (Tsai et al., 2020). The F/B ratio had contradictory results as it was highly affected by different methods of sequencing (different primer sets, shotgun vs. 16S, etc.), so the overall application, therefore, needed more validation. As predominant members of Firmicutes, the two families Lachnospiraceae and Ruminococcaceae are known as beneficial bacteria that promote human health. Ruminococcaceae exerts a protective influence on hepatic histopathology by producing SCFA and 7-α dehydrogenation. The Ruminococcus family is less abundant in NAFLD patients than in healthy samples (Tsai et al., 2020; Juárez-Fernández et al., 2023). On the contrary, however, some researchers previously reported that a high abundance of Ruminococcus was associated with advanced fibrosis (Boursier et al., 2016). The enrichment of Lachnospiraceae and Blautia demonstrated a growing tendency in the NAFLD rodent model and NASH patients. This may be attributed to the neutralization of impairment caused by pathogens and the effort to recover the subtle homeostasis during NAFLD progression (Shen et al., 2017). Another suggestion is that Lachnospiraceae expresses key bile acid-metabolizing enzymes required for deoxycholic acid (DCA) and downstream metabolite synthesis, while patients with significant liver fibrosis tend to have higher serum DCA levels (Smirnova et al., 2022).
Studies demonstrated that patients with different stages of NAFLD had their own gut microbial characteristics, which are presented in Table 2. Patients with early-stage NAFLD exhibited a growing abundance of Firmicutes; however, patients with severe NAFLD lesions tended to have a lower abundance of Firmicutes (Loomba et al., 2017). Although belonging to Firmicutes, Streptoccocus was found to be enriched in NAFLD with cirrhosis and hepatocellular carcinoma (Caussy et al., 2019; Ponziani et al., 2019). The abundance of G− bacteria, including Enterobacteriaceae, Proteobacteria, and Lachnospiraceae families, represented an increasing trend from simple hepatic steatosis progressing to fibrosis (Loomba et al., 2017; Shen et al., 2017; Caussy et al., 2019; Schwimmer et al., 2019). These bacteria profiles are detrimental to liver health, and the abundance increases as NAFLD progresses, thereby establishing a vicious circle. To assess the severity of NAFLD more accurately, NAFLD patients can be stratified based on multivariate analysis according to the abundance of Bacteroides and Ruminococcus (Boursier et al., 2016). However, further research is needed to quantify microbiome features as a model using a larger amount of data, which could be a novel method for diagnosing and predicting the prognosis of NAFLD.
3 Influence of UDCA on the gut microbiome in NAFLD
3.1 UDCA biosynthesis
Bile acids, amphipathic biological detergents, serve a wide range of regulatory functions in humans. PBAs, mainly including chenodeoxycholic acid (CDCA) and cholic acid (CA), are de novo synthesized in the liver by regulation of two main enzymes, sterol 27-hydroxylase (Cyp27a1) and cholesterol 7 alpha-hydroxylase (Cyp7a1). After being initially synthesized from cholesterol in the liver, bile acids undergo biotransformation to SBAs, secreted into bile, conjugated with either taurine or glycine, and then deconjugated and dehydrogenized by the microbiome residing in the gut. UDCA is produced as a collaborative effort by the production of primary bile acid in the host and the gut microbiome. The predominant secondary bile acids include DCA and lithocholic acid, formed from CA and CDCA, respectively (Sinha et al., 2020). Found in small quantities in the total bile acid (BA) pool, UDCA is a kind of SBA with beneficial effects on humans due to its hydrophilicity. In humans, the conversion of chenodeoxycholic acid to UDCA is facilitated by 7α- and 7β-hydroxysteroid dehydrogenases through epimerization of the 7-hydroxy group. Furthermore, this biotransformation of UDCA has been applied in artificial large-scale production and could be applied with a rational design of the bacterial consortium (Zhang et al., 2019; Zhou et al., 2023). Specific gut microbiomes are also essential in UDCA biosynthesis. There is a positive correlation between UDCA and the gut microbiome with aforementioned biocatalysts, including Ruminococcus, Peptococcaceae, Roseburia, and Faecalibacterium prausnitzii (Dempsey et al., 2019; Huang et al., 2019; Pearson et al., 2019).
3.2 Changed gut microbiome profile modulated by UDCA
UDCA and its conjugated forms have been proven to be effective regulators of the gut microbial community structure (Van den Bossche et al., 2017; Tang et al., 2018; Pearson et al., 2019) (Figure 2). In the colitis mouse model, tauroursodeoxycholic acid (TUDCA) and UDCA could normalize the Firmicutes/Bacteroidetes ratio and elevate the abundance of Akkermansia and Prevotellaceae (Van den Bossche et al., 2017). UDCA therapy also resulted in attenuation of pathogenesis of infectious intestinal disorders, like Clostridioides difficile infection, due to modulation in colonization resistance against pathogenic bacteria and immune response (Winston et al., 2020). Recent studies highlighted the role of Lachnospiraceae family in regulating microbial community structure through UDCA, a beneficial taxon known to participate in the production of SCFAs and conversion of SBAs (Sorbara et al., 2020; He et al., 2022). With elevated oral gavage of UDCA, the abundance of Lachnospiraceae in the rodent intestine increased in a dose-dependent manner (Wilson et al., 2021). Furthermore, the relative abundance of Lachnospiraceae remained significantly positively correlated with fecal levels of conjugated forms of UDCA (Huang et al., 2019; Kimmel et al., 2022) when treating with a prevalent traditional Chinese medicine named Gracilaria lemaneiformis. Additionally, genera Bifidobacterium and Prevotella were also reported to be related to elevated UDCA (Ghaffarzadegan et al., 2019). As facilitators and maintainers of human intestinal health, Alistipes was known to produce acetate to suppress the inflammatory response and tended to increase after UDCA treatment in NAFLD (Li et al., 2021). However, the relative abundance of the Faecalibaculum genus, that was generally considered beneficial, tended to be elevated in NASH mice and decreased with UDCA treatment (Li et al., 2021; He et al., 2022). Totally, numerous studies demonstrated an increasing trend for a beneficial microbiome with UDCA therapy, whereas a few studies exhibited the opposite results. The potential putative cause is that specific bacterial taxa play a disparate role in the pathogenesis of chronic liver diseases.
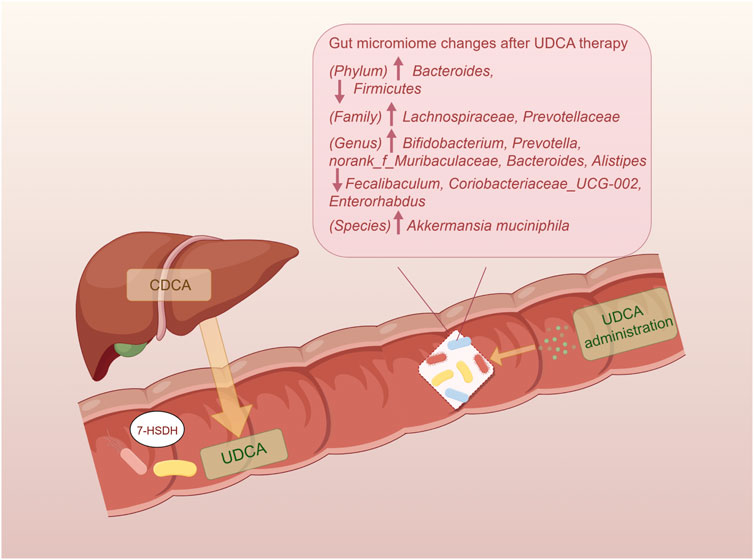
Figure 2. Impact of UDCA on the gut microbiome. The endogenous UDCA is produced by the gut microbiome with their 7-HSDH, which converts CDCA into UDCA. The administration of UDCA has the ability to regulate the intestinal bacteria and restore homeostasis. UDCA, ursodeoxycholic acid; 7-HSDH, 7-hydroxysteroid dehydrogenase; CDCA, chenodeoxycholic acid.
A novel concept of the gut microbiome–UDCA-host axis has been proposed in latest research studies, elucidating one of the mechanisms by which the microbiome modulates host metabolisms. The gut microbiome occupies a significant position in the synthesis of UDCA. UDCA subsequently alters the bile acid pool through affecting BA-producing bacteria and changes farnesoid X receptor (FXR) and Takeda G protein-coupled receptor 5 (TGR5) signaling ways in the host. However, the difference in affinity and agonist ability toward FXR and TGR5 of different bile acids complicates the axis, such that UDCA has no ability to activate FXR but is an agonist for TGR5, whereas TUDCA activates both of these receptors (Wilson et al., 2021). According to this axis, UDCA treatment results in alterations in the levels of bacteria associated with bile acid metabolism, ultimately leading to a reduction in unconjugated BAs and an increase in conjugated BAs (Chen et al., 2020).
4 Possible mechanisms of UDCA in NAFLD
Clinical studies comparing NAFLD and controls reported higher levels of total serum bile acids, secondary bile acids, deoxycholic acids, and chenodeoxycholyl-conjugates (Jiao et al., 2018; Caussy et al., 2019), while the relative abundance of unconjugated acids and UDCA tended to be decreased (Tang et al., 2019). UDCA, with hepatoprotective effects, is a gut microbiome-producing metabolite that significantly contributes to treating primary biliary cholangitis; however, it currently remains unknown if and how UDCA therapy confers protection against NAFLD. There are several possible mechanisms underlying the protective effect of UDCA on liver function, as shown in Figure 3. Although some of them have not been directly evidenced in NAFLD, it provides comprehensive possibilities for UDCA to modulate host metabolism and benefit liver functions.
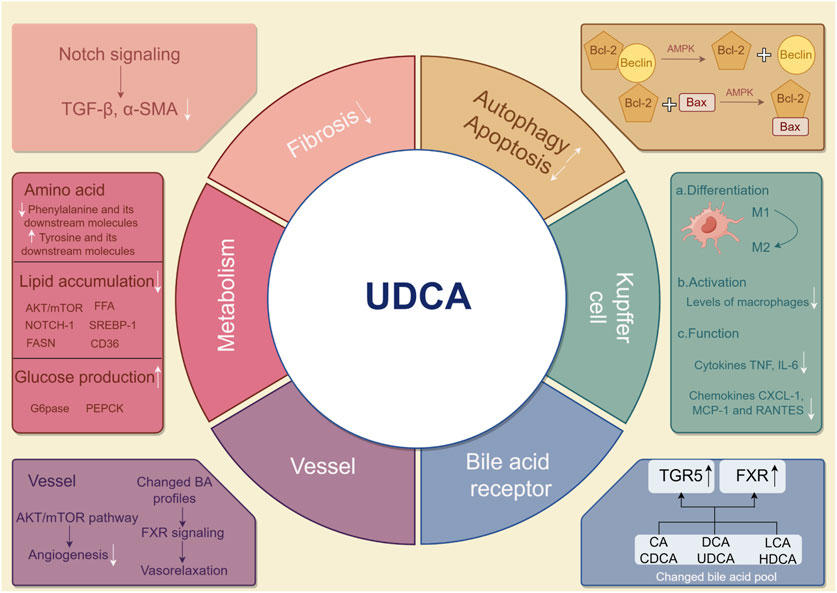
Figure 3. Possible mechanisms by which UDCA mitigates NAFLD. UDCA may treat NAFLD in the following ways. In hepatic cells, induced autophagy and alleviated apoptosis are found after UDCA therapy. Fibrosis and major metabolisms can be effectively modulated by UDCA. In Kupffer cells in the liver, UDCA attenuates the pro-inflammatory response. Angiogenesis is diminished, and vasorelaxation is found in vessels around the liver. By modulating the bile acid pool, UDCA indirectly and directly influences TGR5 and FXR. UDCA, ursodeoxycholic acid; NAFLD, non-alcoholic fatty liver disease; UDCA, ursodeoxycholic acid; TGR5, Takeda G protein-coupled receptor 5; FXR, farnesoid X receptor.
4.1 Modulation in host metabolism (amino acid, lipid, and glucose)
Amino acid metabolism mainly occurs in the liver, and metabolome analysis can be potentially applied in characterizing NAFLD. Patients generally exhibited liver dysfunction accompanied by instability of amino acid profiles. The ratio of branched-chain amino acids (BCAA) to aromatic amino acids (AAA) has been known as a diagnostic biomarker for assessing the severity of liver diseases (Middendorf et al., 2019; Devriendt et al., 2021). A quantitative model with superior predictive power was constructed base on amino acid profiles to identify NASH patients (Yamakado et al., 2017). Unlike antioxidants vitamins E and C, improving the amino acid metabolism dysfunction is a unique mechanism of UDCA in relieving hepatic steatosis. The metabolomic pathway of biosynthesis of phenylalanine, tyrosine, and tryptophan, was filtered out after UDCA administration in patients with choledocholithiasis (Guan et al., 2022). In addition, the level of L-phenylalanine and its downstream molecules was significantly decreased, while the level of N-acetyltryptophan, a tryptophan metabolite, increased with UDCA treatment in patients with liver dysfunction (Kim et al., 2018). In livers of mice, FXR activation could be a choice to modulate amino acid degradation via Hal and Prodh and ureagenesis via Cps1, Ass1, and Arg1 (Massafra et al., 2017). Further studies involving how UDCA influences amino acid levels in hepatic steatosis are required.
Hepatic lipid dysfunction serves as one of the most important mechanisms in NAFLD. The inhibitory effect of UDCA on adipogenic genes was confirmed, especially on the sterol-regulatory element-binding protein-1 (Srebp-1) family and its downstream enzymes such as Fasn and CD36. As a key molecule in adipogenesis and fat accumulation in the liver, Srebp-1 was upregulated in NAFLD according to most studies and downregulated upon treating with UDCA and its derivative norursodeoxycholic acid (norUDCA) (Chen et al., 2021; Marchiano et al., 2022). The downregulation of Srebp-1 was mediated by various pathways, such as the AKT/mTOR/Srebp-1 pathway. Activated by AKT, mTOR upregulated the CRTC2 complex and then promoted the activity of Srebp-1 (Hu et al., 2019). UDCA also repressed Srebp-1 via free fatty acid receptor 4 (FFA4)-dependent and Notch1 signaling transduction pathways (Xu et al., 2022). In addition to Srebp-1, Notch1 signaling repression simultaneously inhibited the expression of CD36 (Gheibi et al., 2019). CD36, namely, fatty acid translocase, formed a complex with insulin-induced gene-2 (INSIG-2) and activated Srebp-1 and adipogenesis (Zeng et al., 2022). However, the direct association between CD36 and UDCA remains unclear.
As a metabolic disease involving multiple systems, NAFLD is closely related to insulin resistance and dysregulated glucose homeostasis. UDCA significantly diminished gluconeogenesis and Notch1 signaling in the liver, evidenced by reduced protein levels and mRNA expression of glucose-6-phosphatase (G6pase) and PEPCK in leptin deficiency obese mice (Chen et al., 2019). Nevertheless, no significant changes were observed in levels of gluconeogenic genes after UDCA administration (Marchiano et al., 2022). Moreover, molecules related to cellular apoptosis were also found to be involved in glucose metabolism. The pro-apoptosis biomarker p53 weakens the pentose phosphate pathway glucose flux and intracytoplasmic carbohydrate storage (Jiang et al., 2011). Similarly, miR34a in the apoptosis pathway also dephosphorylates HMG reductase, influencing glucose biosynthesis (Castro et al., 2013). Both miR34a and p53 could be regulated by UDCA, indicating its potential mechanisms in glucose metabolism.
4.2 Restoration of apoptosis and oxidative stress
In NAFLD, autophagy is impaired while apoptosis increases, and UDCA treatment can reverse this alteration (Panzitt et al., 2021). The binding of Bcl-2 to Beclin inhibited autophagy induced by Beclin while maintaining the anti-apoptosis function of Bcl-2 at a high level. Hence, disrupting the Bcl-2–Beclin complex generally prevented premature aging (Fernandez et al., 2018; Papini et al., 2023). Unlike the Bcl-2–Beclin complex, the Bcl-2–Bax complex is proven to be highly effective in preventing apoptosis in vitro (Lu et al., 2017). UDCA exerted a favorable influence on the liver via apoptosis suppression and autophagy enhancement by promoting dissociation of the Bcl-2/Beclin complex and inhibiting dissociation of the Bcl-2/Bax complex through AMP-associated protein kinase activation (Wu et al., 2020). UDCA suppressed a pro-apoptosis pathway of microRNA-34a/Sirtuin 1/p53 and subsequent cellular apoptosis in the rat liver and primary rat hepatocytes (Castro et al., 2013). However, UDCA was reported to only reduce miR-34a in the vesicle-free fraction of serum and did not have a similar effect on the liver. Additionally, the potential of UDCA in enhancing the expression of the endoplasmic reticulum (ER) stress markers CHOP and Gpr78 and inducing apoptosis was also reported (Mueller et al., 2018). The following may explain for this contradiction: the FXR inhibition led by UDCA resulted in high cholesterol storage in the liver and the initiation of unfolding protein response, which was an attempt to restore the ER homeostasis but finally a promotor of apoptosis. Notably, while UDCA induces endoplasmic reticulum stress, apoptotic indicators like caspase-3 had no significant changes since apoptotic threshold and cytoprotective ability were enhanced (Mueller et al., 2018; Ali et al., 2020).
Reactive oxygen species (ROS), mainly induced when an electron escapes during ATP synthesis, causes an imbalance between oxidants and antioxidants, leading to mitochondrial dysfunction, excessive β-oxidation, and oxidative stress. ROS inflicts fatal damage to hepatocytes and also activates the inflammation of hepatic stellate cells, which further promoted the progress from NAFLD to liver cirrhosis (Borrelli et al., 2018; Shum et al., 2021). Furthermore, the superior clinical effect of antioxidants versus UDCA in NAFLD has been determined, including vitamins E and C (Borrelli et al., 2018; Fouda et al., 2021). Administration of UDCA notably altered the bile acid profile and subsequent hepatic steatosis. Bile acids with amphiphilicity modulated the activity of the electron transfer chain, membrane permeability, and biological synthesis to improve mitochondrial functions. UDCA therapy was revealed to improve hepatocyte mitochondrial function in rodent models (Chen et al., 2019; Pérez et al., 2021). Despite preclinical research studies, clinical evidence that UDCA depends on the mitochondria to treat NAFLD was still lacking. The combinational therapy of UDCA and antioxidants exhibited a remarkable effect with improved malondialdehyde and glutathione for NAFLD in animal experiments (Gheibi et al., 2019). Paradoxically, a few recent clinical studies failed to prove the capability of UDCA to change the oxidative status, and more studies are necessary to be designed to affirm the true role played by UDCA in oxidative stress.
4.3 Vasorelaxation and angiogenesis inhibition
The progression of hepatic steatosis to cirrhosis resulted in increased portal blood flow and splanchnic vasodilation due to enhanced circulating endogenous vasodilators and inhibited response to vasoconstrictors. Flow-mediated dilatation notably decreased in the brachial artery in NASH patients, indicating impaired endothelial function (Al-Hamoudi et al., 2020). Finally, the portal hypertension emerged as a consequence of the abovementioned process and increased intrahepatic vascular resistance (Chalasani et al., 2020; Sauerbruch et al., 2021). Bile acid, especially UDCA, is a crucial modulator in enterohepatic circulation. Elevated levels of hepatic angiogenesis markers were induced by liver inflammation and oxidative stress, while UDCA showed the ability to attenuate dysbiosis (Chen et al., 2019). Moreover, human M1 macrophages, the secretor of angiogenic stimulator VEGF, is inhibited in NAFLD and restored with administration of UDCA (Li et al., 2018; Chen et al., 2019). Hydrophilic UDCA had no direct evidence of a vasoactive effect but influenced angiogenesis (Jung and Hwang, 2021); however, UDCA might affect the vascular tone by altering bile acid profiles.
4.4 Regulation of inflammatory signaling pathways and liver fibrosis
Kupffer cells (KCs) are intrahepatic macrophages that represent the core immune cells during the pathogenesis of NAFLD. UDCA could modulate KCs and alleviate hepatic pathology via three ways: 1) differentiation: two subtypes of macrophages M1 and M2 represented pro-inflammatory and anti-inflammatory response, respectively. UDCA activated macrophage M2 (Chalasani et al., 2020) and modulated polarization between M1 and M2 via Notch1 signaling (Chen et al., 2019). 2) Activation: UDCA treatment resulted in lower levels of activated macrophages marked by soluble CD163 (Bossen et al., 2023). 3) Function: macrophage functions modulated by UDCA via secreting cytokines TNF and IL-6, as well as chemokines CXCL-1, MCP-1, and RANTES (Ludwig et al., 2018; Labiano et al., 2022; Marchiano et al., 2022). Interestingly, UDCA–lysophosphatidylethanolamide contributed to lower pro-inflammatory TNF and MCP-1 via KCs. It also activated the PI3K/AKT pathway to compensate for depressed hepatocyte proliferation due to TNF decline (Ludwig et al., 2018).
Signals including transforming growth factor-β (TGF-β), osteopontin, α-smooth muscle actin (α-SMA), and TAZ suggested the activation of the hepatic stellate cells (HSCs) (Kuchay et al., 2020; Dong et al., 2021). Bone morphogenetic protein 8B, found in TGF-β/BMP superfamily and absent in healthy livers, induced the proinflammatory phenotype of HSCs (Vacca et al., 2020). A recent research indicated the ability of UDCA to degrade TGF-β and of further enhancing antitumor immunity (Shen et al., 2022). Treating a rodent model of hepatic steatosis with UDCA and norUDCA significantly reduced fibrosis biomarkers TGF-β and α-SMA at the genetic level (Marchiano et al., 2022). Downregulation of Notch signaling by UDCA resulted in release of the signals that participated in the activation of resident HSCs synchronously (Zhu et al., 2018). Furthermore, liver regeneration significantly contributes to resistance of fibrosis, resulting in decreased macrophage infiltration and collagen deposition, which is promoted by UDCA via inhibitor of the DNA binding 1-dependent pathway (Dong et al., 2021). Even if potential protection of UDCA against hepatic fibrosis is nearly confirmed, the optimal dose and duration needs further exploration before becoming one of the first-line anti-fibrotic therapies for NAFLD (Ratziu et al., 2011; Parikh et al., 2016; Nadinskaia et al., 2021).
As a liver health promoter, UDCA targets various mechanisms corresponding with the pathophysiological process of NAFLD and NASH, incorporating effect of the glucose, lipid, and amino acid metabolism; cellular-level apoptosis; autophagy and oxidative stress; liver tissue-level blood vessels and inflammatory responses; and fibrosis. The effect of UDCA has been determined clearly in animal and cell experiments; however, the real clinical application needs explorations in future.
4.5 Bile acid receptors
FXR is widely expressed in the liver and ileum, functioning as the regulator of bile acid, lipid, and glucose metabolism. Clinical and preclinical studies confirmed that activators of FXR, OCA, and DCA showed a protective effect against liver steatosis (Younossi et al., 2019; Huang et al., 2021; Gillard et al., 2022; Rinella et al., 2022; Zhuge et al., 2023). Nevertheless, there is a consensus that UDCA is a weak ligand with little activation of FXR in humans, and it even exerts FXR-antagonistic effects (Li et al., 2021; Marchiano et al., 2022; Brevini et al., 2023; Wang et al., 2023). In current studies, UDCA is found to potentially interfere in the FXR pathway indirectly, mainly via gut microbiome remodeling and bile acid profile alteration. Patients receiving FXR-antagonistic UDCA surprisingly exhibited an activated effect of FXR with increased FGF19 (a FXR target gene), majorly due to the remodeled gut microbiome and induced high bile acid-deconjugating enzymes by UDCA. It subsequently enabled secondary modification and higher activity for endogenous FXR agonists (Ovadia et al., 2020). Without a notable activation effect on FXR in vitro, UDCA restored the expression and transduction of the FXR pathway in vivo with lower expression of Cyp7a1 (Marchiano et al., 2022). The efficacy of UDCA was contingent upon the presence of endogenous FXR ligands, as evidenced by its failure to increase FGF15 (a FXR target gene) in animals with biliary obstruction (Zaufel et al., 2021). It suggested that FXR-agonistic bile acids played a contributory role in mechanisms of UDCA. The evidence also suggested a possible role of reduced β-muricholic acids (MCAs), another FXR antagonist, in the reduction of hepatic lipid contents by UDCA, which could not be explained in humans in the absence of MCA (Fujita et al., 2017).
TGR5, also known as GPBAR1, is widely distributed in the skeletal muscle, white and brown adipocytes, ileum, and entero-hepatic tissues except hepatic parenchymal cells. TGR5-dependent pathways are crucial for protecting hepatocytes from injuries, involving improvement of glucose homeostasis, gallbladder dilatation, hepatic inflammation, and energy expenditure (Carino et al., 2017; Ginos et al., 2018; Iracheta-Vellve et al., 2018; Bidault-Jourdainne et al., 2021; Wang et al., 2022). In addition, diminished TGR5 signals were found to be correlated with downregulated secondary bile acids, attributed to alterations in the abundance of bacteria involved in bile acid transformation (Spatz et al., 2021). UDCA possessed the potential to improve histology for NASH and NAFLD as a treatment with TGR5 activation (Carino et al., 2017; Finn et al., 2019). The activated pathway of TGR5 signaling by UDCA has been certified to attenuate primary sclerosing cholangitis (PSC) and inhibit the proliferation of colorectal cancer cells and Escherichia coli infection (Reich et al., 2021; Zhang et al., 2021; He et al., 2022). However, the evidence on mechanisms of UDCA treatment for NAFLD via the TGR5 pathway is limited, which is possibly ascribed to the less abundance of TGR5 on hepatic parenchymal cells. One of possible mechanisms was stimulation of GLP-1 (a TGR5 target gene) release from intestinal L cells to protect hepatocytes against the inflammatory response (Carino et al., 2019; Marchiano et al., 2022). In addition to GLP-1, mechanisms of the UDCA-inducing TGR5 pathway need more explorations of the involved molecules to better illustrate the effect brought about by this pathway.
5 Therapeutic target of the UDCA–gut microbiome axis for NAFLD
5.1 Evidence on UDCA application in NAFLD
In previous clinical trials, UDCA administration had a beneficial effect on NAFLD and NASH patients with effectively improved liver function biomarkers (Dufour et al., 2006; Leuschner et al., 2010; Ratziu et al., 2011; Nadinskaia et al., 2021). Even the efficacy and safety of high-dose UDCA were already certified. However, significant differences in liver histology could not be detected using either invasive liver fibrosis tests or a second biopsy in humans, which was inconsistent with animal studies. In a rodent model of NASH, UDCA significantly attenuated hepatic inflammation histologically (Li et al., 2021). Since no evidence of significant histological improvement in large-scale clinical trials was demonstrated presently, UDCA could only be used as an adjunct to attenuate NAFLD and prevent it from progressing to severe fibrosis.
Interestingly, UDCA could partially restore intestinal dysbiosis induced by NAFLD and repair gut barrier integrity with increased expression of claudin-1 and ZO-1. At the phylum level, NAFLD mice treated with UDCA exhibited a decreased relative abundance of Firmicutes and increased relative abundance of Bacteroidetes. At the genus level, NAFLD mice treated by UDCA exhibited a lower abundance of Fecalibaculum, Coriobacteriaceae_UCG-002, and Enterorhabdus and higher abundance of norank_f_Muribaculaceae, Bacteroides, and Alistipes (Li et al., 2021). TUDCA, a conjugated bile acid derivative undergoing higher hydrophilicity as a candidate drug, attenuated hepatic steatosis and inflammation in the NAFLD mouse model (Wang et al., 2018). In the TUDCA-treated group, the changes in Proteobacteria, Paraprevotella, and Dehalobacterium tended to be partially reversed (Wang et al., 2018). Hence, these TUDCA-regulating microbiomes in the animal intestine might mediate the improvement of hepatic steatosis by gut–liver crosstalk. A side chain-shortened homolog of UDCA, norUDCA, also resulted in amelioration of NAFLD with improved liver function, yet without evidence that norUDCA remodeled the intestine microbiome (Traussnigg et al., 2019; Marchiano et al., 2022).
5.2 Dietary therapy
Currently, a balanced diet and healthy lifestyle are considered the best strategies for NAFLD patients. Notably, the Japanese diet pattern and Mediterranean diet were found to be effective in lowering the severity of liver fat accumulation and fibrosis in clinical studies (Montemayor et al., 2022; Matsumoto et al., 2023). These two diet patterns are characterized by a high intake of soybeans and soybean foods, vegetables, fruits, and seafood. In dietary therapy for hepatic steatosis, it was commonly observed that specific food remodeled the intestine microbial community. Changes in the bacteria profile led to comprehensive biochemical activities represented by alterations in bile acid metabolism, ultimately improving hepatic steatosis and altering the relative abundance of UDCA. Gracilaria lemaneiformis (GLP) increased the abundance of UDCA and TUDCA via elevating the abundance of Lachnospiraceae_NK4A136_group and Roseburia in the mouse intestine, which possibly explained for GLP protecting the liver from damage caused by a high-fat diet (Huang et al., 2019). Therefore, GLP could be used as a functional food to diminish NAFLD. Apple polyphenol extract (APE) significantly reduces the relative abundance of Lactobacillus and increases the relative abundance of Akkermansia, leading to reduced fecal UDCA in the NAFLD mouse model (Li et al., 2021). Interestingly, in another study, enriched Lactobacillus in both the grass carp and chicken groups was positively related to UDCA (Li et al., 2022). Collectively, increased UDCA tended to cause liver homeostasis in spite of different abundances of Lactobacillus. Meanwhile, more cautions should be taken in the prevention of NAFLD by dietary therapy, involving GLP, APE, and dietary white meat, before the efficiency is confirmed after robust clinical trials.
5.3 Probiotics and prebiotics
Nutritional interventions have been the first approach toward a healthy lifestyle as the main strategy to manage NAFLD. The synbiotic led to a significant steatosis remission in an in vivo rat model (Juarez-Fernandez et al., 2021). A variety of synbiotics and probiotics were suggested to be effective in changing gut dysbiosis in order to have a beneficial effect on NAFLD, as observed in numerous clinical studies (Ahn et al., 2019; Behrouz et al., 2020; Scorletti et al., 2020; Mohamad Nor et al., 2021) and rodent experiments (Wang et al., 2020). In animal studies, the intervention with the synbiotic of Akkermansia muciniphila and quercetin combination resulted in improvement of steatosis. This therapeutic capacity was shown to be driven by increased levels of hydrophilic bile acid UDCA along with the altered gut microbiome, in which a higher abundance of Cyanobacteria and Oscillospira as well as lower levels of Actinobacteria, Lactococcus, Lactobacillus, and Roseburia were observed (Juarez-Fernandez et al., 2021). Further investigations regarding the mechanisms of gut microbiome modulation and the shift in UDCA underlying beneficial effects of synbiotics and probiotics need to be conducted in clinical patients.
5.4 Fecal microbiota transplantation
Increasing evidence indicated that FMT is a novel approach for improving the manifestations of NAFLD by reconstructing the intestinal microecological balance and diversity. A randomized control trial reported that FMT has the potential to reduce gut permeability in NAFLD patients (Craven et al., 2020). Another clinical trial demonstrated that FMT affected hepatic DNA methylation and levels of phenylacetate- and choline-derived metabolites in individuals with NAFLD. As for gut microbial composition changes, Blautia wexlerae, a potential anti-obesogenic probiotic, was increased upon allogenic FMT compared with autologous FMT (Stols-Gonçalves et al., 2023). The proportions of beneficial bacteria Bacteroidetes, Christensenellaceae, and Lactobacillus were also increased by FMT intervention. Contrastingly, the proportions of Escherichia–Shigella, Odoribacter, and Oscillibacter decreased in patients with NAFLD after FMT (Zhou et al., 2017; Xue et al., 2022). These results showed that NAFLD-associated gut microbiome disturbance was, at least partially, corrected after FMT. While changes in the microbial metabolites, such as butyrate concentrations of the cecal content (Zhou et al., 2017), plasma phenylacetylcarnitine, and phenylacetylglutamine (Stols-Gonçalves et al., 2023), as well as gut microbiome structure were indicated, it would be highly interesting to explore the correlation between another microbial metabolite, bile acids, especially UDCA, and FMT intervention in NAFLD.
6 Discussion
Although NAFLD has become a pandemic attributed mainly to Western diet, the current methods of treatment are still limited. Accumulating evidence has recently shown the interactions between bile acids and gut microbiome and their roles in NAFLD. Additionally, a growing number of studies have indicated that certain bile acids, such as UDCA, exhibit beneficial effects on NAFLD through multiple mechanisms. In this study, the possible functional roles of UDCA and the gut microbiome in NAFLD were discussed.
However, there are still many limitations in this regard regarding existing research. First, there are many factors that affect the microbial community, and different research studies yielded inconsistent conclusions with different detection and modeling methods. Second, the significant therapeutic efficacy of UDCA has been clearly confirmed in animal models, whereas the improvement of liver biochemistry, not histological changes, is recognized in clinical patients. The reason for this inconsistency deserves further study and discussion so as to clarify the actual effect of UDCA in the treatment of NAFLD. Third, extensive animal experiments were analyzed when elucidating the interplay between the NAFLD, UDCA, and gut microbiome. It is well-recognized that lifestyle, including eating habits and exercise, is closely related to the progression of NAFLD. However, there are significant differences in lifestyle between humans and animals, hindering the representativeness of animal experimental results. Furthermore, because the bile acid composition in animals is substantially different from that in humans, changes in bile acid pool and host metabolism in humans and animal models of NAFLD must be interpreted cautiously. For instance, muricholic acid in the bile acid pool of mice is almost undetectable in humans.
Hence, the evidence from current studies is inadequate. The recovery of bacterial function may be an important direction of treatment of NAFLD, including further research on direct UDCA application, dietary therapy, probiotics, and standardization of FMT. In the future, more clinical and animal studies are necessary to explore the clinical efficacy and specific mechanisms between UCDA and the gut microbiome in NAFLD.
Author contributions
QM: writing–review and editing, writing–original draft, visualization, formal analysis, and conceptualization. BL: writing–review and editing, writing–original draft, visualization, and formal analysis. WZ: writing–original draft and visualization. YZ (4th author): writing–original draft and formal analysis. YZ (5th author): writing–review and editing and funding acquisition. QC: writing–review and editing, writing–original draft, validation, supervision, and project administration. MX: writing–review and editing, writing–original draft, validation, supervision, project administration, and conceptualization.
Funding
The author(s) declare financial support was received for the research, authorship, and/or publication of this article. This work was supported by the Medical Health Technology Project of Zhejiang, China under grant no. 2021KY749, 2023KY804, and 2024ZL101 to MX) and the Natural Science Foundation of Zhejiang, China under grant LQ21H030010 to YZ (5th author) and LY24H030002 to MX. All authors are indebted to the Medical Health Technology Project of Zhejiang, China and Natural Science Foundation of Zhejiang, China for secretarial support.
Acknowledgments
All authors are indebted to the Medical Health Technology Project of Zhejiang, China, and the Natural Science Foundation for secretarial support. All Figures were created by Figdraw (www.figdraw.com)
Conflict of interest
The authors declare that the research was conducted in the absence of any commercial or financial relationships that could be construed as a potential conflict of interest.
Publisher’s note
All claims expressed in this article are solely those of the authors and do not necessarily represent those of their affiliated organizations, or those of the publisher, the editors, and the reviewers. Any product that may be evaluated in this article, or claim that may be made by its manufacturer, is not guaranteed or endorsed by the publisher.
References
Abenavoli, L., Giubilei, L., Procopio, A. C., Spagnuolo, R., Luzza, F., Boccuto, L., et al. (2022). Gut microbiota in non-alcoholic fatty liver disease patients with inflammatory bowel diseases: a complex interplay. Nutrients 14 (24), 5323. doi:10.3390/nu14245323
Ahn, S. B., Jun, D. W., Kang, B. K., Lim, J. H., Lim, S., and Chung, M. J. (2019). Randomized, double-blind, placebo-controlled study of a multispecies probiotic mixture in nonalcoholic fatty liver disease. Sci. Rep. 9 (1), 5688. doi:10.1038/s41598-019-42059-3
Al-Hamoudi, W., Alsadoon, A., Hassanian, M., Alkhalidi, H., Abdo, A., Nour, M., et al. (2020). Endothelial dysfunction in nonalcoholic steatohepatitis with low cardiac disease risk. Sci. Rep. 10 (1), 8825. doi:10.1038/s41598-020-65835-y
Ali, F. E. M., Hassanein, E. H. M., Bakr, A. G., El-Shoura, E. A. M., El-Gamal, D. A., Mahmoud, A. R., et al. (2020). Ursodeoxycholic acid abrogates gentamicin-induced hepatotoxicity in rats: role of NF-κB-p65/TNF-α, Bax/Bcl-xl/Caspase-3, and eNOS/iNOS pathways. Life Sci. 254, 117760. doi:10.1016/j.lfs.2020.117760
Behrouz, V., Aryaeian, N., Zahedi, M. J., and Jazayeri, S. (2020). Effects of probiotic and prebiotic supplementation on metabolic parameters, liver aminotransferases, and systemic inflammation in nonalcoholic fatty liver disease: a randomized clinical trial. J. Food Sci. 85 (10), 3611–3617. doi:10.1111/1750-3841.15367
Bidault-Jourdainne, V., Merlen, G., Glenisson, M., Doignon, I., Garcin, I., Pean, N., et al. (2021). TGR5 controls bile acid composition and gallbladder function to protect the liver from bile acid overload. JHEP Rep. 3 (2), 100214. doi:10.1016/j.jhepr.2020.100214
Borrelli, A., Bonelli, P., Tuccillo, F. M., Goldfine, I. D., Evans, J. L., Buonaguro, F. M., et al. (2018). Role of gut microbiota and oxidative stress in the progression of non-alcoholic fatty liver disease to hepatocarcinoma: current and innovative therapeutic approaches. Redox Biol. 15, 467–479. doi:10.1016/j.redox.2018.01.009
Bossen, L., Lau, T. S., Nielsen, M. B., Nielsen, M. C., Andersen, A. H., Ott, P., et al. (2023). The association between soluble CD163, disease severity, and ursodiol treatment in patients with primary biliary cholangitis. Hepatol. Commun. 7 (4), e0068. doi:10.1097/hc9.0000000000000068
Boursier, J., Mueller, O., Barret, M., Machado, M., Fizanne, L., Araujo-Perez, F., et al. (2016). The severity of nonalcoholic fatty liver disease is associated with gut dysbiosis and shift in the metabolic function of the gut microbiota. Hepatology 63 (3), 764–775. doi:10.1002/hep.28356
Brevini, T., Maes, M., Webb, G. J., John, B. V., Fuchs, C. D., Buescher, G., et al. (2023). FXR inhibition may protect from SARS-CoV-2 infection by reducing ACE2. Nature 615 (7950), 134–142. doi:10.1038/s41586-022-05594-0
Carino, A., Biagioli, M., Marchiano, S., Fiorucci, C., Zampella, A., Monti, M. C., et al. (2019). Ursodeoxycholic acid is a GPBAR1 agonist and resets liver/intestinal FXR signaling in a model of diet-induced dysbiosis and NASH. Biochim. Biophys. Acta Mol. Cell Biol. Lipids 1864 (10), 1422–1437. doi:10.1016/j.bbalip.2019.07.006
Carino, A., Cipriani, S., Marchiano, S., Biagioli, M., Scarpelli, P., Zampella, A., et al. (2017). Gpbar1 agonism promotes a Pgc-1α-dependent browning of white adipose tissue and energy expenditure and reverses diet-induced steatohepatitis in mice. Sci. Rep. 7 (1), 13689. doi:10.1038/s41598-017-13102-y
Castro, R. E., Ferreira, D. M., Afonso, M. B., Borralho, P. M., Machado, M. V., Cortez-Pinto, H., et al. (2013). miR-34a/SIRT1/p53 is suppressed by ursodeoxycholic acid in the rat liver and activated by disease severity in human non-alcoholic fatty liver disease. J. Hepatol. 58 (1), 119–125. doi:10.1016/j.jhep.2012.08.008
Caussy, C., Tripathi, A., Humphrey, G., Bassirian, S., Singh, S., Faulkner, C., et al. (2019). A gut microbiome signature for cirrhosis due to nonalcoholic fatty liver disease. Nat. Commun. 10 (1), 1406. doi:10.1038/s41467-019-09455-9
Chalasani, N., Abdelmalek, M. F., Garcia-Tsao, G., Vuppalanchi, R., Alkhouri, N., Rinella, M., et al. (2020). Effects of belapectin, an inhibitor of galectin-3, in patients with nonalcoholic steatohepatitis with cirrhosis and portal hypertension. Gastroenterology 158 (5), 1334–1345.e5. doi:10.1053/j.gastro.2019.11.296
Chalasani, N., Younossi, Z., Lavine, J. E., Charlton, M., Cusi, K., Rinella, M., et al. (2018). The diagnosis and management of nonalcoholic fatty liver disease: practice guidance from the American Association for the Study of Liver Diseases. Hepatology 67 (1), 328–357. doi:10.1002/hep.29367
Chen, H.-T., Huang, H.-L., Li, Y.-Q., Xu, H.-M., and Zhou, Y.-J. (2020). Therapeutic advances in non-alcoholic fatty liver disease: a microbiota-centered view. World J. Gastroenterology 26 (16), 1901–1911. doi:10.3748/wjg.v26.i16.1901
Chen, J., Ding, C., Chen, Y., Hu, W., Yu, C., Peng, C., et al. (2021). ACSL4 reprograms fatty acid metabolism in hepatocellular carcinoma via c-Myc/SREBP1 pathway. Cancer Lett. 502, 154–165. doi:10.1016/j.canlet.2020.12.019
Chen, Y. S., Liu, H. M., and Lee, T. Y. (2019). Ursodeoxycholic acid regulates hepatic energy homeostasis and white adipose tissue macrophages polarization in leptin-deficiency obese mice. Cells 8 (3), 253. doi:10.3390/cells8030253
Craven, L., Rahman, A., Nair Parvathy, S., Beaton, M., Silverman, J., Qumosani, K., et al. (2020). Allogenic fecal microbiota transplantation in patients with nonalcoholic fatty liver disease improves abnormal small intestinal permeability: a randomized control trial. Am. J. Gastroenterol. 115 (7), 1055–1065. doi:10.14309/ajg.0000000000000661
Demir, M., Lang, S., Hartmann, P., Duan, Y., Martin, A., Miyamoto, Y., et al. (2022). The fecal mycobiome in non-alcoholic fatty liver disease. J. Hepatol. 76 (4), 788–799. doi:10.1016/j.jhep.2021.11.029
Dempsey, J. L., Wang, D., Siginir, G., Fei, Q., Raftery, D., Gu, H., et al. (2019). Pharmacological activation of PXR and CAR downregulates distinct bile acid-metabolizing intestinal bacteria and alters bile acid homeostasis. Toxicol. Sci. 168 (1), 40–60. doi:10.1093/toxsci/kfy271
Devriendt, N., Paepe, D., Serrano, G., Vandenabeele, S., Stock, E., Van Acker, L., et al. (2021). Plasma amino acid profiles in dogs with closed extrahepatic portosystemic shunts are only partially improved 3 months after successful gradual attenuation. J. Vet. Intern Med. 35 (3), 1347–1354. doi:10.1111/jvim.16135
Dong, X., Luo, Y., Lu, S., Ma, H., Zhang, W., Zhu, Y., et al. (2021). Ursodesoxycholic acid alleviates liver fibrosis via proregeneration by activation of the ID1-WNT2/HGF signaling pathway. Clin. Transl. Med. 11 (2), e296. doi:10.1002/ctm2.296
Dufour, J. F., Oneta, C. M., Gonvers, J. J., Bihl, F., Cerny, A., Cereda, J. M., et al. (2006). Randomized placebo-controlled trial of ursodeoxycholic acid with vitamin e in nonalcoholic steatohepatitis. Clin. Gastroenterol. Hepatol. 4 (12), 1537–1543. doi:10.1016/j.cgh.2006.09.025
Elsayed, A., Ismaiel, A., Procopio, A. C., Luzza, F., Abenavoli, L., and Dumitrascu, D. L. (2022). Noninvasive biochemical markers and surrogate scores in evaluating nonalcoholic steatohepatitis. Minerva Med. 113 (5), 864–874. doi:10.23736/s0026-4806.22.08185-x
Fernandez, A. F., Sebti, S., Wei, Y., Zou, Z., Shi, M., McMillan, K. L., et al. (2018). Disruption of the beclin 1-BCL2 autophagy regulatory complex promotes longevity in mice. Nature 558 (7708), 136–140. doi:10.1038/s41586-018-0162-7
Finn, P. D., Rodriguez, D., Kohler, J., Jiang, Z., Wan, S., Blanco, E., et al. (2019). Intestinal TGR5 agonism improves hepatic steatosis and insulin sensitivity in Western diet-fed mice. Am. J. Physiol. Gastrointest. Liver Physiol. 316 (3), G412–g424. doi:10.1152/ajpgi.00300.2018
Fouda, A., Abdelaziz, A. E., Hussien, M., Ali, A. A., Abdelkawy, K. S., and Elbarbry, F. (2021). A randomized controlled trial comparing the effects of Vitamin E, Ursodeoxycholic acid and Pentoxifylline on Egyptian non-alcoholic steatohepatitis patients. Eur. Rev. Med. Pharmacol. Sci. 25 (23), 7449–7459. doi:10.26355/eurrev_202112_27442
Fujita, K., Iguchi, Y., Une, M., and Watanabe, S. (2017). Ursodeoxycholic acid suppresses lipogenesis in mouse liver: possible role of the decrease in β-muricholic acid, a farnesoid X receptor antagonist. Lipids 52 (4), 335–344. doi:10.1007/s11745-017-4242-5
Gao, L. L., Ma, J. M., Fan, Y. N., Zhang, Y. N., Ge, R., Tao, X. J., et al. (2021). Lycium barbarum polysaccharide combined with aerobic exercise ameliorated nonalcoholic fatty liver disease through restoring gut microbiota, intestinal barrier and inhibiting hepatic inflammation. Int. J. Biol. Macromol. 183, 1379–1392. doi:10.1016/j.ijbiomac.2021.05.066
Ghaffarzadegan, T., Essen, S., Verbrugghe, P., Marungruang, N., Hallenius, F. F., Nyman, M., et al. (2019). Determination of free and conjugated bile acids in serum of Apoe(-/-) mice fed different lingonberry fractions by UHPLC-MS. Sci. Rep. 9 (1), 3800. doi:10.1038/s41598-019-40272-8
Gheibi, S., Gouvarchin Ghaleh, H. E., Motlagh, B. M., Azarbayjani, A. F., and Zarei, L. (2019). Therapeutic effects of curcumin and ursodexycholic acid on non-alcoholic fatty liver disease. Biomed. Pharmacother. 115, 108938. doi:10.1016/j.biopha.2019.108938
Gillard, J., Clerbaux, L. A., Nachit, M., Sempoux, C., Staels, B., Bindels, L. B., et al. (2022). Bile acids contribute to the development of non-alcoholic steatohepatitis in mice. JHEP Rep. 4 (1), 100387. doi:10.1016/j.jhepr.2021.100387
Ginos, B. N. R., Navarro, S. L., Schwarz, Y., Gu, H., Wang, D., Randolph, T. W., et al. (2018). Circulating bile acids in healthy adults respond differently to a dietary pattern characterized by whole grains, legumes and fruits and vegetables compared to a diet high in refined grains and added sugars: a randomized, controlled, crossover feeding study. Metabolism 83, 197–204. doi:10.1016/j.metabol.2018.02.006
Guan, Y., Xu, F., Zhang, X., Fu, X., Wang, J., Song, S., et al. (2022). Roles of ursodeoxycholic acid in the bile biochemistry and metabolomics in patients with choledocholithiasis: a prospective study. Metabolomics 18 (7), 46. doi:10.1007/s11306-022-01906-7
He, Q., Zhang, Y., Ma, D., Zhang, W., and Zhang, H. (2022a). Lactobacillus casei Zhang exerts anti-obesity effect to obese glut1 and gut-specific-glut1 knockout mice via gut microbiota modulation mediated different metagenomic pathways. Eur. J. Nutr. 61 (4), 2003–2014. doi:10.1007/s00394-021-02764-0
He, S., Cui, S., Song, W., Jiang, Y., Chen, H., Liao, D., et al. (2022b). Interleukin-17 weakens the NAFLD/NASH process by facilitating intestinal barrier restoration depending on the gut microbiota. mBio 13 (2), e0368821. doi:10.1128/mbio.03688-21
He, Z., Ma, Y., Yang, S., Zhang, S., Liu, S., Xiao, J., et al. (2022c). Gut microbiota-derived ursodeoxycholic acid from neonatal dairy calves improves intestinal homeostasis and colitis to attenuate extended-spectrum β-lactamase-producing enteroaggregative Escherichia coli infection. Microbiome 10 (1), 79. doi:10.1186/s40168-022-01269-0
Hu, J., Hong, W., Yao, K. N., Zhu, X. H., Chen, Z. Y., and Ye, L. (2019). Ursodeoxycholic acid ameliorates hepatic lipid metabolism in LO2 cells by regulating the AKT/mTOR/SREBP-1 signaling pathway. World J. Gastroenterol. 25 (12), 1492–1501. doi:10.3748/wjg.v25.i12.1492
Huang, S., Pang, D., Li, X., You, L., Zhao, Z., Cheung, P. C., et al. (2019). A sulfated polysaccharide from Gracilaria Lemaneiformis regulates cholesterol and bile acid metabolism in high-fat diet mice. Food Funct. 10 (6), 3224–3236. doi:10.1039/c9fo00263d
Huang, S., Wu, Y., Zhao, Z., Wu, B., Sun, K., Wang, H., et al. (2021). A new mechanism of obeticholic acid on NASH treatment by inhibiting NLRP3 inflammasome activation in macrophage. Metabolism 120, 154797. doi:10.1016/j.metabol.2021.154797
Hullar, M. A. J., Jenkins, I. C., Randolph, T. W., Curtis, K. R., Monroe, K. R., Ernst, T., et al. (2021). Associations of the gut microbiome with hepatic adiposity in the multiethnic cohort adiposity phenotype study. Gut Microbes 13 (1), 1965463. doi:10.1080/19490976.2021.1965463
Iracheta-Vellve, A., Calenda, C. D., Petrasek, J., Ambade, A., Kodys, K., Adorini, L., et al. (2018). FXR and TGR5 agonists ameliorate liver injury, steatosis, and inflammation after binge or prolonged alcohol feeding in mice. Hepatol. Commun. 2 (11), 1379–1391. doi:10.1002/hep4.1256
Jiang, P., Du, W., Wang, X., Mancuso, A., Gao, X., Wu, M., et al. (2011). p53 regulates biosynthesis through direct inactivation of glucose-6-phosphate dehydrogenase. Nat. Cell Biol. 13 (3), 310–316. doi:10.1038/ncb2172
Jiao, N., Baker, S. S., Chapa-Rodriguez, A., Liu, W., Nugent, C. A., Tsompana, M., et al. (2018). Suppressed hepatic bile acid signalling despite elevated production of primary and secondary bile acids in NAFLD. Gut 67 (10), 1881–1891. doi:10.1136/gutjnl-2017-314307
Juárez-Fernández, M., Goikoetxea-Usandizaga, N., Porras, D., García-Mediavilla, M. V., Bravo, M., Serrano-Maciá, M., et al. (2023). Enhanced mitochondrial activity reshapes a gut microbiota profile that delays NASH progression. Hepatology 77 (5), 1654–1669. doi:10.1002/hep.32705
Juarez-Fernandez, M., Porras, D., Petrov, P., Roman-Saguillo, S., Garcia-Mediavilla, M. V., Soluyanova, P., et al. (2021). The synbiotic combination of Akkermansia muciniphila and quercetin ameliorates early obesity and NAFLD through gut microbiota reshaping and bile acid metabolism modulation. Antioxidants (Basel) 10 (12), 2001. doi:10.3390/antiox10122001
Jung, H. W., and Hwang, J. H. (2021). Anticancer effects of ursi fel Extract and its active compound, ursodeoxycholic acid, in FRO anaplastic thyroid cancer cells. Molecules 26 (17), 5309. doi:10.3390/molecules26175309
Kim, D. J., Chung, H., Ji, S. C., Lee, S., Yu, K. S., Jang, I. J., et al. (2019). Ursodeoxycholic acid exerts hepatoprotective effects by regulating amino acid, flavonoid, and fatty acid metabolic pathways. Metabolomics 15 (3), 30. doi:10.1007/s11306-019-1494-5
Kim, D. J., Yoon, S., Ji, S. C., Yang, J., Kim, Y. K., Lee, S., et al. (2018). Ursodeoxycholic acid improves liver function via phenylalanine/tyrosine pathway and microbiome remodelling in patients with liver dysfunction. Sci. Rep. 8 (1), 11874. doi:10.1038/s41598-018-30349-1
Kimmel, M., Jin, W., Xia, K., Lun, K., Azcarate-Peril, A., Plantinga, A., et al. (2022). Metabolite trajectories across the perinatal period and mental health: a preliminary study of tryptophan-related metabolites, bile acids and microbial composition. Behav. Brain Res. 418, 113635. doi:10.1016/j.bbr.2021.113635
Kuchay, M. S., Choudhary, N. S., and Mishra, S. K. (2020). Pathophysiological mechanisms underlying MAFLD. Diabetes Metab. Syndr. 14 (6), 1875–1887. doi:10.1016/j.dsx.2020.09.026
Labiano, I., Agirre-Lizaso, A., Olaizola, P., Echebarria, A., Huici-Izagirre, M., Olaizola, I., et al. (2022). TREM-2 plays a protective role in cholestasis by acting as a negative regulator of inflammation. J. Hepatol. 77 (4), 991–1004. doi:10.1016/j.jhep.2022.05.044
Lee, G., You, H. J., Bajaj, J. S., Joo, S. K., Yu, J., Park, S., et al. (2020). Distinct signatures of gut microbiome and metabolites associated with significant fibrosis in non-obese NAFLD. Nat. Commun. 11 (1), 4982. doi:10.1038/s41467-020-18754-5
Leung, H., Long, X., Ni, Y., Qian, L., Nychas, E., Siliceo, S. L., et al. (2022). Risk assessment with gut microbiome and metabolite markers in NAFLD development. Sci. Transl. Med. 14 (648), eabk0855. doi:10.1126/scitranslmed.abk0855
Leuschner, U. F. H., Lindenthal, B., Herrmann, G., Arnold, J. C., Rössle, M., Cordes, H.-J., et al. (2010). High-dose ursodeoxycholic acid therapy for nonalcoholic steatohepatitis: a double-blind, randomized, placebo-controlled trial. Hepatology 52 (2), 472–479. doi:10.1002/hep.23727
Li, D., Cui, Y., Wang, X., Liu, F., and Li, X. (2021a). Apple polyphenol Extract improves high-fat diet-induced hepatic steatosis by regulating bile acid synthesis and gut microbiota in C57bl/6 male mice. J. Agric. Food Chem. 69 (24), 6829–6841. doi:10.1021/acs.jafc.1c02532
Li, H., Wang, Q., Chen, P., Zhou, C., Zhang, X., and Chen, L. (2021b). Ursodeoxycholic acid treatment restores gut microbiota and alleviates liver inflammation in non-alcoholic steatohepatitic mouse model. Front. Pharmacol. 12, 788558. doi:10.3389/fphar.2021.788558
Li, J., Li, Y., Feng, S., He, K., Guo, L., Chen, W., et al. (2022). Differential effects of dietary white meat and red meat on NAFLD progression by modulating gut microbiota and metabolites in rats. Oxid. Med. Cell Longev. 2022, 6908934. doi:10.1155/2022/6908934
Li, T., Peng, M., Yang, Z., Zhou, X., Deng, Y., Jiang, C., et al. (2018). 3D-printed IFN-γ-loading calcium silicate-β-tricalcium phosphate scaffold sequentially activates M1 and M2 polarization of macrophages to promote vascularization of tissue engineering bone. Acta Biomater. 71, 96–107. doi:10.1016/j.actbio.2018.03.012
Li, Z., Zhang, B., Liu, Q., Tao, Z., Ding, L., Guo, B., et al. (2023). Genetic association of lipids and lipid-lowering drug target genes with non-alcoholic fatty liver disease. EBioMedicine 90, 104543. doi:10.1016/j.ebiom.2023.104543
Loomba, R., Seguritan, V., Li, W., Long, T., Klitgord, N., Bhatt, A., et al. (2017). Gut microbiome-based metagenomic signature for non-invasive detection of advanced fibrosis in human nonalcoholic fatty liver disease. Cell Metab. 25 (5), 1054–1062. doi:10.1016/j.cmet.2017.04.001
Lu, Z., Miao, Y., Muhammad, I., Tian, E., Hu, W., Wang, J., et al. (2017). Colistin-induced autophagy and apoptosis involves the JNK-Bcl2-Bax signaling pathway and JNK-p53-ROS positive feedback loop in PC-12 cells. Chem. Biol. Interact. 277, 62–73. doi:10.1016/j.cbi.2017.08.011
Ludwig, J. M., Zhang, Y., Chamulitrat, W., Stremmel, W., and Pathil, A. (2018). Anti-inflammatory properties of ursodeoxycholyl lysophosphatidylethanolamide in endotoxin-mediated inflammatory liver injury. PLoS One 13 (5), e0197836. doi:10.1371/journal.pone.0197836
Marchianò, S., Biagioli, M., Morretta, E., Di Giorgio, C., Roselli, R., Bordoni, M., et al. (2023). Combinatorial therapy with BAR502 and UDCA resets FXR and GPBAR1 signaling and reverses liver histopathology in a model of NASH. Sci. Rep. 13 (1), 1602. doi:10.1038/s41598-023-28647-4
Marchiano, S., Biagioli, M., Roselli, R., Zampella, A., Di Giorgio, C., Bordoni, M., et al. (2022). Beneficial effects of UDCA and norUDCA in a rodent model of steatosis are linked to modulation of GPBAR1/FXR signaling. Biochim. Biophys. Acta Mol. Cell Biol. Lipids 1867 (11), 159218. doi:10.1016/j.bbalip.2022.159218
Massafra, V., Milona, A., Vos, H. R., Ramos, R. J. J., Gerrits, J., Willemsen, E. C. L., et al. (2017). Farnesoid X receptor activation promotes hepatic amino acid catabolism and ammonium clearance in mice. Gastroenterology 152 (6), 1462–1476. doi:10.1053/j.gastro.2017.01.014
Matsumoto, Y., Fujii, H., Harima, M., Okamura, H., Yukawa-Muto, Y., Odagiri, N., et al. (2023). Severity of liver fibrosis is associated with the Japanese diet pattern and skeletal muscle mass in patients with nonalcoholic fatty liver disease. Nutrients 15 (5), 1175. doi:10.3390/nu15051175
Middendorf, L., Radko, D., Düngelhoef, K., Sieverding, E., Windhaus, H., Mischok, D., et al. (2019). Amino acid pattern in the liver and blood of fattening turkeys suffering from hepatic lipidosis. Poult. Sci. 98 (9), 3950–3962. doi:10.3382/ps/pez131
Milosevic, I., Vujovic, A., Barac, A., Djelic, M., Korac, M., Radovanovic Spurnic, A., et al. (2019). Gut-liver Axis, gut microbiota, and its modulation in the management of liver diseases: a review of the literature. Int. J. Mol. Sci. 20 (2), 395. doi:10.3390/ijms20020395
Mohamad Nor, M. H., Ayob, N., Mokhtar, N. M., Raja Ali, R. A., Tan, G. C., Wong, Z., et al. (2021). The effect of probiotics (MCP® BCMC® strains) on hepatic steatosis, small intestinal mucosal immune function, and intestinal barrier in patients with non-alcoholic fatty liver disease. Nutrients 13 (9), 3192. doi:10.3390/nu13093192
Montemayor, S., Mascaró, C. M., Ugarriza, L., Casares, M., Llompart, I., Abete, I., et al. (2022). Adherence to mediterranean diet and NAFLD in patients with metabolic syndrome: the FLIPAN study. Nutrients 14 (15), 3186. doi:10.3390/nu14153186
Mueller, M., Castro, R. E., Thorell, A., Marschall, H. U., Auer, N., Herac, M., et al. (2018). Ursodeoxycholic acid: effects on hepatic unfolded protein response, apoptosis and oxidative stress in morbidly obese patients. Liver Int. 38 (3), 523–531. doi:10.1111/liv.13562
Nadinskaia, M., Maevskaya, M., Ivashkin, V., Kodzoeva, K., Pirogova, I., Chesnokov, E., et al. (2021). Ursodeoxycholic acid as a means of preventing atherosclerosis, steatosis and liver fibrosis in patients with nonalcoholic fatty liver disease. World J. Gastroenterol. 27 (10), 959–975. doi:10.3748/wjg.v27.i10.959
Nkosi, B. V. Z., Padayachee, T., Gront, D., Nelson, D. R., and Syed, K. (2022). Contrasting health effects of Bacteroidetes and Firmicutes lies in their genomes: analysis of P450s, ferredoxins, and secondary metabolite clusters. Int. J. Mol. Sci. 23 (9), 5057. doi:10.3390/ijms23095057
Ovadia, C., Perdones-Montero, A., Fan, H. M., Mullish, B. H., McDonald, J. A. K., Papacleovoulou, G., et al. (2020). Ursodeoxycholic acid enriches intestinal bile salt hydrolase-expressing Bacteroidetes in cholestatic pregnancy. Sci. Rep. 10 (1), 3895. doi:10.1038/s41598-020-60821-w
Panzitt, K., Fickert, P., and Wagner, M. (2021). Regulation of autophagy by bile acids and in cholestasis - CholestoPHAGY or CholeSTOPagy. Biochim. Biophys. Acta Mol. Basis Dis. 1867 (2), 166017. doi:10.1016/j.bbadis.2020.166017
Papini, N., Todisco, R., Giussani, P., Dei Cas, M., Paroni, R., Giallanza, C., et al. (2023). Impaired autophagy in krabbe disease: the role of BCL2 and beclin-1 phosphorylation. Int. J. Mol. Sci. 24 (6), 5984. doi:10.3390/ijms24065984
Parikh, P., Ingle, M., Patel, J., Bhate, P., Pandey, V., and Sawant, P. (2016). An open-label randomized control study to compare the efficacy of vitamin e versus ursodeoxycholic acid in nondiabetic and noncirrhotic Indian NAFLD patients. Saudi J. Gastroenterol. 22 (3), 192–197. doi:10.4103/1319-3767.182451
Pearson, T., Caporaso, J. G., Yellowhair, M., Bokulich, N. A., Padi, M., Roe, D. J., et al. (2019). Effects of ursodeoxycholic acid on the gut microbiome and colorectal adenoma development. Cancer Med. 8 (2), 617–628. doi:10.1002/cam4.1965
Pérez, A., Rivoira, M. A., Rodríguez, V., Marchionatti, A., and Tolosa de Talamoni, N. (2021). Role of mitochondria in the differential action of sodium deoxycholate and ursodeoxycholic acid on rat duodenum. Can. J. Physiol. Pharmacol. 99 (3), 270–277. doi:10.1139/cjpp-2019-0561
Ponziani, F. R., Bhoori, S., Castelli, C., Putignani, L., Rivoltini, L., Del Chierico, F., et al. (2019). Hepatocellular carcinoma is associated with gut microbiota profile and inflammation in nonalcoholic fatty liver disease. Hepatology 69 (1), 107–120. doi:10.1002/hep.30036
Porras, D., Nistal, E., Martinez-Florez, S., Pisonero-Vaquero, S., Olcoz, J. L., Jover, R., et al. (2017). Protective effect of quercetin on high-fat diet-induced non-alcoholic fatty liver disease in mice is mediated by modulating intestinal microbiota imbalance and related gut-liver axis activation. Free Radic. Biol. Med. 102, 188–202. doi:10.1016/j.freeradbiomed.2016.11.037
Ratziu, V., de Ledinghen, V., Oberti, F., Mathurin, P., Wartelle-Bladou, C., Renou, C., et al. (2011). A randomized controlled trial of high-dose ursodesoxycholic acid for nonalcoholic steatohepatitis. J. Hepatol. 54 (5), 1011–1019. doi:10.1016/j.jhep.2010.08.030
Rau, M., Rehman, A., Dittrich, M., Groen, A. K., Hermanns, H. M., Seyfried, F., et al. (2018). Fecal SCFAs and SCFA-producing bacteria in gut microbiome of human NAFLD as a putative link to systemic T-cell activation and advanced disease. United Eur. Gastroenterol. J. 6 (10), 1496–1507. doi:10.1177/2050640618804444
Reich, M., Spomer, L., Klindt, C., Fuchs, K., Stindt, J., Deutschmann, K., et al. (2021). Downregulation of TGR5 (GPBAR1) in biliary epithelial cells contributes to the pathogenesis of sclerosing cholangitis. J. Hepatol. 75 (3), 634–646. doi:10.1016/j.jhep.2021.03.029
Rinella, M. E., Dufour, J. F., Anstee, Q. M., Goodman, Z., Younossi, Z., Harrison, S. A., et al. (2022). Non-invasive evaluation of response to obeticholic acid in patients with NASH: results from the REGENERATE study. J. Hepatol. 76 (3), 536–548. doi:10.1016/j.jhep.2021.10.029
Romualdo, G. R., Valente, L. C., Sprocatti, A. C., Bacil, G. P., de Souza, I. P., Rodrigues, J., et al. (2022). Western diet-induced mouse model of non-alcoholic fatty liver disease associated with metabolic outcomes: features of gut microbiome-liver-adipose tissue axis. Nutrition 103-104, 111836. doi:10.1016/j.nut.2022.111836
Sauerbruch, T., Hennenberg, M., Trebicka, J., and Beuers, U. (2021). Bile acids, liver cirrhosis, and extrahepatic vascular dysfunction. Front. Physiology 12, 718783. doi:10.3389/fphys.2021.718783
Schwimmer, J. B., Johnson, J. S., Angeles, J. E., Behling, C., Belt, P. H., Borecki, I., et al. (2019). Microbiome signatures associated with steatohepatitis and moderate to severe fibrosis in children with nonalcoholic fatty liver disease. Gastroenterology 157 (4), 1109–1122. doi:10.1053/j.gastro.2019.06.028
Scorletti, E., Afolabi, P. R., Miles, E. A., Smith, D. E., Almehmadi, A., Alshathry, A., et al. (2020). Synbiotics alter fecal microbiomes, but not liver fat or fibrosis, in a randomized trial of patients with nonalcoholic fatty liver disease. Gastroenterology 158 (6), 1597–1610. doi:10.1053/j.gastro.2020.01.031
Shen, F., Zheng, R. D., Sun, X. Q., Ding, W. J., Wang, X. Y., and Fan, J. G. (2017). Gut microbiota dysbiosis in patients with non-alcoholic fatty liver disease. Hepatobiliary Pancreat. Dis. Int. 16 (4), 375–381. doi:10.1016/S1499-3872(17)60019-5
Shen, Y., Lu, C., Song, Z., Qiao, C., Wang, J., Chen, J., et al. (2022). Ursodeoxycholic acid reduces antitumor immunosuppression by inducing CHIP-mediated TGF-β degradation. Nat. Commun. 13 (1), 3419. doi:10.1038/s41467-022-31141-6
Shum, M., Ngo, J., Shirihai, O. S., and Liesa, M. (2021). Mitochondrial oxidative function in NAFLD: friend or foe? Mol. Metab. 50, 101134. doi:10.1016/j.molmet.2020.101134
Sinha, S. R., Haileselassie, Y., Nguyen, L. P., Tropini, C., Wang, M., Becker, L. S., et al. (2020). Dysbiosis-induced secondary bile acid deficiency promotes intestinal inflammation. Cell Host Microbe 27 (4), 659–670. doi:10.1016/j.chom.2020.01.021
Smirnova, E., Muthiah, M. D., Narayan, N., Siddiqui, M. S., Puri, P., Luketic, V. A., et al. (2022). Metabolic reprogramming of the intestinal microbiome with functional bile acid changes underlie the development of NAFLD. Hepatology 76 (6), 1811–1824. doi:10.1002/hep.32568
Sorbara, M. T., Littmann, E. R., Fontana, E., Moody, T. U., Kohout, C. E., Gjonbalaj, M., et al. (2020). Functional and genomic variation between human-derived isolates of Lachnospiraceae reveals inter- and intra-species diversity. Cell Host Microbe 28 (1), 134–146. doi:10.1016/j.chom.2020.05.005
Spatz, M., Ciocan, D., Merlen, G., Rainteau, D., Humbert, L., Gomes-Rochette, N., et al. (2021). Bile acid-receptor TGR5 deficiency worsens liver injury in alcohol-fed mice by inducing intestinal microbiota dysbiosis. JHEP Rep. 3 (2), 100230. doi:10.1016/j.jhepr.2021.100230
Stols-Gonçalves, D., Mak, A. L., Madsen, M. S., van der Vossen, E. W. J., Bruinstroop, E., Henneman, P., et al. (2023). Faecal Microbiota transplantation affects liver DNA methylation in Non-alcoholic fatty liver disease: a multi-omics approach. Gut Microbes 15 (1), 2223330. doi:10.1080/19490976.2023.2223330
Sun, L., Pang, Y., Wang, X., Wu, Q., Liu, H., Liu, B., et al. (2019). Ablation of gut microbiota alleviates obesity-induced hepatic steatosis and glucose intolerance by modulating bile acid metabolism in hamsters. Acta Pharm. Sin. B 9 (4), 702–710. doi:10.1016/j.apsb.2019.02.004
Tang, R., Wei, Y., Li, Y., Chen, W., Chen, H., Wang, Q., et al. (2018). Gut microbial profile is altered in primary biliary cholangitis and partially restored after UDCA therapy. Gut 67 (3), 534–541. doi:10.1136/gutjnl-2016-313332
Tang, Y., Zhang, J., Li, J., Lei, X., Xu, D., Wang, Y., et al. (2019). Turnover of bile acids in liver, serum and caecal content by high-fat diet feeding affects hepatic steatosis in rats. Biochim. Biophys. Acta Mol. Cell Biol. Lipids 1864 (10), 1293–1304. doi:10.1016/j.bbalip.2019.05.016
Tilg, H., Adolph, T. E., and Moschen, A. R. (2021). Multiple parallel hits hypothesis in nonalcoholic fatty liver disease: revisited after a decade. Hepatology 73 (2), 833–842. doi:10.1002/hep.31518
Traussnigg, S., Schattenberg, J. M., Demir, M., Wiegand, J., Geier, A., Teuber, G., et al. (2019). Norursodeoxycholic acid versus placebo in the treatment of non-alcoholic fatty liver disease: a double-blind, randomised, placebo-controlled, phase 2 dose-finding trial. Lancet Gastroenterol. Hepatol. 4 (10), 781–793. doi:10.1016/s2468-1253(19)30184-0
Tsai, M. C., Liu, Y. Y., Lin, C. C., Wang, C. C., Wu, Y. J., Yong, C. C., et al. (2020). Gut microbiota dysbiosis in patients with biopsy-proven nonalcoholic fatty liver disease: a cross-sectional study in taiwan. Nutrients 12 (3), 820. doi:10.3390/nu12030820
Vacca, M., Leslie, J., Virtue, S., Lam, B. Y. H., Govaere, O., Tiniakos, D., et al. (2020). Bone morphogenetic protein 8B promotes the progression of non-alcoholic steatohepatitis. Nat. Metab. 2 (6), 514–531. doi:10.1038/s42255-020-0214-9
Van den Bossche, L., Hindryckx, P., Devisscher, L., Devriese, S., Van Welden, S., Holvoet, T., et al. (2017). Ursodeoxycholic acid and its taurine- or glycine-conjugated species reduce colitogenic dysbiosis and equally suppress experimental colitis in mice. Appl. Environ. Microbiol. 83 (7), e02766-16. doi:10.1128/AEM.02766-16
Wang, B., Jiang, X., Cao, M., Ge, J., Bao, Q., Tang, L., et al. (2016). Altered fecal microbiota correlates with liver biochemistry in nonobese patients with non-alcoholic fatty liver disease. Sci. Rep. 6, 32002. doi:10.1038/srep32002
Wang, L., Rui, X., He, H. W., Zhou, X., and Long, Y. (2023). Ursodeoxycholic acid (UDCA) promotes lactate metabolism in mouse hepatocytes through cholic acid (CA) - farnesoid X receptor (FXR) pathway. Curr. Mol. Med. 23 (6), 661–666. doi:10.2174/1566524020666200123161340
Wang, W., Xu, A. L., Li, Z. C., Li, Y., Xu, S. F., Sang, H. C., et al. (2020). Combination of probiotics and salvia miltiorrhiza polysaccharide alleviates hepatic steatosis via gut microbiota modulation and insulin resistance improvement in high fat-induced NAFLD mice. Diabetes Metab. J. 44 (2), 336–348. doi:10.4093/dmj.2019.0042
Wang, W., Zhao, J., Gui, W., Sun, D., Dai, H., Xiao, L., et al. (2018). Tauroursodeoxycholic acid inhibits intestinal inflammation and barrier disruption in mice with non-alcoholic fatty liver disease. Br. J. Pharmacol. 175 (3), 469–484. doi:10.1111/bph.14095
Wang, X. X., Xie, C., Libby, A. E., Ranjit, S., Levi, J., Myakala, K., et al. (2022). The role of FXR and TGR5 in reversing and preventing progression of Western diet-induced hepatic steatosis, inflammation, and fibrosis in mice. J. Biol. Chem. 298 (11), 102530. doi:10.1016/j.jbc.2022.102530
Wilson, B. A., Winston, J. A., Rivera, A., Cai, J., Patterson, A. D., and Theriot, C. M. (2021). Secondary bile acid ursodeoxycholic acid alters weight, the gut microbiota, and the bile acid pool in conventional mice. Plos One 16 (2), e0246161. doi:10.1371/journal.pone.0246161
Winston, J. A., Rivera, A. J., Cai, J., Thanissery, R., Montgomery, S. A., Patterson, A. D., et al. (2020). Ursodeoxycholic acid (UDCA) mitigates the host inflammatory response during Clostridioides difficile infection by altering gut bile acids. Infect. Immun. 88 (6), e00045-20. doi:10.1128/iai.00045-20
Wu, P., Zhao, J., Guo, Y., Yu, Y., Wu, X., and Xiao, H. (2020). Ursodeoxycholic acid alleviates nonalcoholic fatty liver disease by inhibiting apoptosis and improving autophagy via activating AMPK. Biochem. Biophys. Res. Commun. 529 (3), 834–838. doi:10.1016/j.bbrc.2020.05.128
Xia, M., Ma, S., Huang, Q., Zeng, H., Ge, J., Xu, W., et al. (2022). NAFLD-related gene polymorphisms and all-cause and cause-specific mortality in an Asian population: the Shanghai Changfeng Study. Aliment. Pharmacol. Ther. 55 (6), 705–721. doi:10.1111/apt.16772
Xie, P., Peng, Y., and Qiu, L. (2022). Responsive oligochitosan nano-vesicles with ursodeoxycholic acid and exenatide for NAFLD synergistic therapy via SIRT1. Carbohydr. Polym. 288, 119388. doi:10.1016/j.carbpol.2022.119388
Xu, F., Wang, J., Wang, P., Hou, T., Zhou, H., Zhao, Y., et al. (2022). Ursodesoxycholic acid is an FFA4 agonist and reduces hepatic steatosis via FFA4 signaling. Eur. J. Pharmacol. 917, 174760. doi:10.1016/j.ejphar.2022.174760
Xue, L., Deng, Z., Luo, W., He, X., and Chen, Y. (2022). Effect of fecal microbiota transplantation on non-alcoholic fatty liver disease: a randomized clinical trial. Front. Cell Infect. Microbiol. 12, 759306. doi:10.3389/fcimb.2022.759306
Yamakado, M., Tanaka, T., Nagao, K., Imaizumi, A., Komatsu, M., Daimon, T., et al. (2017). Plasma amino acid profile associated with fatty liver disease and co-occurrence of metabolic risk factors. Sci. Rep. 7 (1), 14485. doi:10.1038/s41598-017-14974-w
Younossi, Z., Anstee, Q. M., Marietti, M., Hardy, T., Henry, L., Eslam, M., et al. (2018). Global burden of NAFLD and NASH: trends, predictions, risk factors and prevention. Nat. Rev. Gastroenterol. Hepatol. 15 (1), 11–20. doi:10.1038/nrgastro.2017.109
Younossi, Z. M., Koenig, A. B., Abdelatif, D., Fazel, Y., Henry, L., and Wymer, M. (2016). Global epidemiology of nonalcoholic fatty liver disease-Meta-analytic assessment of prevalence, incidence, and outcomes. Hepatology 64 (1), 73–84. doi:10.1002/hep.28431
Younossi, Z. M., Ratziu, V., Loomba, R., Rinella, M., Anstee, Q. M., Goodman, Z., et al. (2019). Obeticholic acid for the treatment of non-alcoholic steatohepatitis: interim analysis from a multicentre, randomised, placebo-controlled phase 3 trial. Lancet 394 (10215), 2184–2196. doi:10.1016/S0140-6736(19)33041-7
Yu, J. S., Youn, G. S., Choi, J., Kim, C. H., Kim, B. Y., Yang, S. J., et al. (2021). Lactobacillus lactis and Pediococcus pentosaceus-driven reprogramming of gut microbiome and metabolome ameliorates the progression of non-alcoholic fatty liver disease. Clin. Transl. Med. 11 (12), e634. doi:10.1002/ctm2.634
Zaufel, A., van de Wiel, S. M. W., Yin, L., Fauler, G., Chien, D., Dong, X., et al. (2021). Secondary (iso)BAs cooperate with endogenous ligands to activate FXR under physiological and pathological conditions. Biochim. Biophys. Acta Mol. Basis Dis. 1867 (8), 166153. doi:10.1016/j.bbadis.2021.166153
Zeng, H., Qin, H., Liao, M., Zheng, E., Luo, X., Xiao, A., et al. (2022). CD36 promotes de novo lipogenesis in hepatocytes through INSIG2-dependent SREBP1 processing. Mol. Metab. 57, 101428. doi:10.1016/j.molmet.2021.101428
Zhang, H., Xu, H., Zhang, C., Tang, Q., and Bi, F. (2021). Ursodeoxycholic acid suppresses the malignant progression of colorectal cancer through TGR5-YAP axis. Cell Death Discov. 7 (1), 207. doi:10.1038/s41420-021-00589-8
Zhang, X., Fan, D., Hua, X., and Zhang, T. (2019). Large-scale production of ursodeoxycholic acid from chenodeoxycholic acid by engineering 7α- and 7β-hydroxysteroid dehydrogenase. Bioprocess Biosyst. Eng. 42 (9), 1537–1545. doi:10.1007/s00449-019-02151-4
Zhao, L. (2013). The gut microbiota and obesity: from correlation to causality. Nat. Rev. Microbiol. 11 (9), 639–647. doi:10.1038/nrmicro3089
Zhou, C., Wang, Y., Li, C., Xie, Z., and Dai, L. (2023). Amelioration of colitis by a gut bacterial consortium producing anti-inflammatory secondary bile acids. Microbiol. Spectr. 11 (2), e0333022. doi:10.1128/spectrum.03330-22
Zhou, D., Pan, Q., Shen, F., Cao, H. X., Ding, W. J., Chen, Y. W., et al. (2017). Total fecal microbiota transplantation alleviates high-fat diet-induced steatohepatitis in mice via beneficial regulation of gut microbiota. Sci. Rep. 7 (1), 1529. doi:10.1038/s41598-017-01751-y
Zhu, C., Kim, K., Wang, X., Bartolome, A., Salomao, M., Dongiovanni, P., et al. (2018). Hepatocyte Notch activation induces liver fibrosis in nonalcoholic steatohepatitis. Sci. Transl. Med. 10 (468), eaat0344. doi:10.1126/scitranslmed.aat0344
Zhuge, A., Li, S., Yuan, Y., Han, S., Xia, J., Wang, Q., et al. (2023). Microbiota-induced lipid peroxidation impairs obeticholic acid-mediated antifibrotic effect towards nonalcoholic steatohepatitis in mice. Redox Biol. 59, 102582. doi:10.1016/j.redox.2022.102582
Glossary
Keywords: ursodeoxycholic acid, gut microbiome, bile acid receptors, non-alcoholic fatty liver disease, bile acid–gut microbiome axis
Citation: Mao Q, Lin B, Zhang W, Zhang Y, Zhang Y, Cao Q and Xu M (2024) Understanding the role of ursodeoxycholic acid and gut microbiome in non-alcoholic fatty liver disease: current evidence and perspectives. Front. Pharmacol. 15:1371574. doi: 10.3389/fphar.2024.1371574
Received: 23 January 2024; Accepted: 05 March 2024;
Published: 21 March 2024.
Edited by:
Ludovico Abenavoli, Magna Græcia University, ItalyReviewed by:
Wei Guo, University of North Carolina at Greensboro, United StatesAnna Caterina Procopio, Magna Græcia University, Italy
Copyright © 2024 Mao, Lin, Zhang, Zhang, Zhang, Cao and Xu. This is an open-access article distributed under the terms of the Creative Commons Attribution License (CC BY). The use, distribution or reproduction in other forums is permitted, provided the original author(s) and the copyright owner(s) are credited and that the original publication in this journal is cited, in accordance with accepted academic practice. No use, distribution or reproduction is permitted which does not comply with these terms.
*Correspondence: Mengque Xu, eHVtZW5ncXVlQHpqdS5lZHUuY24=; Qian Cao, Y2FvcUB6anUuZWR1LmNu
†These authors have contributed equally to this work and shared first authorship.