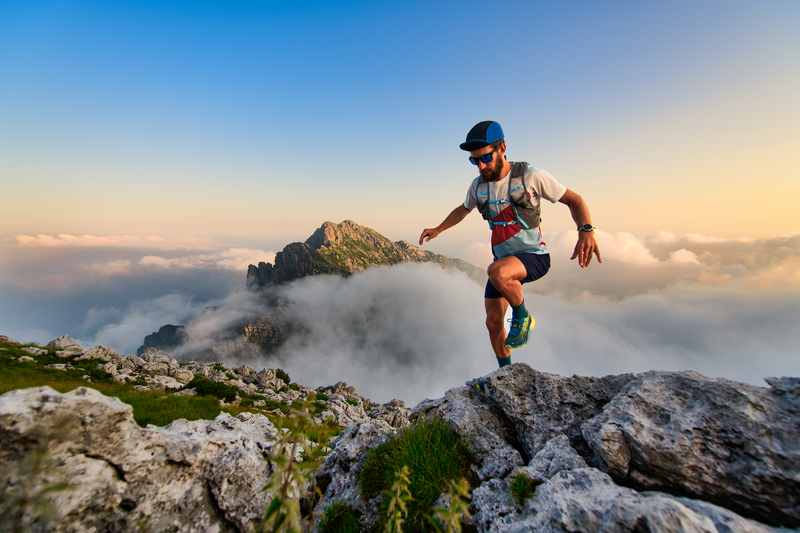
94% of researchers rate our articles as excellent or good
Learn more about the work of our research integrity team to safeguard the quality of each article we publish.
Find out more
REVIEW article
Front. Pharmacol. , 18 June 2024
Sec. Ethnopharmacology
Volume 15 - 2024 | https://doi.org/10.3389/fphar.2024.1368950
Background: Metabolic imbalance is the common basis of many diseases. As natural isoquinoline alkaloid, berberine (BBR) has shown great promise in regulating glucose and lipids metabolism and treating metabolic disorders. However, the related mechanism still lacks systematic research.
Aim: To discuss the role of BBR in the whole body’s systemic metabolic regulation and further explore its therapeutic potential and targets.
Method: Based on animal and cell experiments, the mechanism of BBR regulating systemic metabolic processes is reviewed. Potential metabolism-related targets were summarized using Therapeutic Target Database (TTD), DrugBank, GeneCards, and cutting-edge literature. Molecular modeling was applied to explore BBR binding to the potential targets.
Results: BBR regulates the whole-body metabolic response including digestive, circulatory, immune, endocrine, and motor systems through adenosine 5’-monophosphate (AMP)-activated protein kinase (AMPK)/mammalian target of rapamycin (mTOR), sirtuin (SIRT)1/forkhead box O (FOXO)1/sterol regulatory element-binding protein (SREBP)2, nuclear factor erythroid 2-related factor (Nrf) 2/heme oxygenase (HO)-1, and other signaling pathways. Through these reactions, BBR exerts hypoglycemic, lipid-regulating, anti-inflammatory, anti-oxidation, and immune regulation. Molecular docking results showed that BBR could regulate metabolism targeting FOXO3, Nrf2, NAD(P)H quinone oxidoreductase 1 (NQO1), glutathione peroxidase (Gpx) 4 and phosphatidylinositol-4,5-bisphosphate 3-kinase catalytic subunit alpha (PIK3CA). Evaluating the target clinical effects, we found that BBR has the therapeutic potential of anti-aging, anti-cancer, relieving kidney disease, regulating the nervous system, and alleviating other chronic diseases.
Conclusion: This review elucidates the interaction between potential targets and small molecular metabolites by exploring the mechanism of BBR regulating metabolism. That will help pharmacologists to identify new promising metabolites interacting with these targets.
The life of the human body depends on the normal operation of metabolism. If metabolism cannot function normally, it can lead to different degrees of physiological abnormalities and to diseases. Metabolic diseases, such as type 2 diabetes, obesity, and non-alcoholic fatty liver disease, have become increasingly serious global health challenges due to the sharp increase in their incidence (Ling et al., 2023). As of 2021, the estimated prevalence of diabetes among adults aged 20–79 worldwide is 536.6 million, of which 90% are type 2 diabetes. Long-term hyperglycemia can lead to multiple complications, including vision loss, kidney disease and neuropathy, affecting patients’ quality of life (Sun et al., 2022). About one-third of the world’s population suffers from non-alcoholic fatty liver disease, and some non-alcoholic fatty liver disease patients will further develop liver cirrhosis or hepatocellular carcinoma, threatening their lives (Wong et al., 2023). Obesity increases the risk of patients suffering from heart disease, diabetes, sleep apnea, joint disease and certain types of cancer. In addition, obesity can also have an impact on mental health, leading to depression and low self-esteem (Jin et al., 2023). The occurrence of these diseases is related to various factors, including genetics, environment, and lifestyle. However, effective treatment methods are still limited, especially for improving the fundamental causes of metabolic diseases. How to restore the relative balance of metabolism in the body, explore metabolic regulation strategies, and analyze the information exchange mechanisms in these regulatory processes are urgent problems to be solved.
More and more researchers are attempting to find effective drugs for metabolic diseases from natural products. Berberine (BBR) is an isoquinoline alkaloid found in the plant families Ranunculaceae, Berberidaceae, and Papaveraceae, particularly in the Coptis genus (Wong et al., 2020). Medicinal plants containing BBR have been used for more than 3,000 years. In clinical practice, BBR was originally used primarily for the treatment of digestive tract diseases, owing to its antibacterial and anti-inflammatory properties (Song et al., 2020). In recent years, BBR has attracted great interest of researchers due to its extensive pharmacological activities, especially its potential to regulate metabolism. More and more studies have shown that BBR plays a role in body metabolism, by regulating lipid and glucose metabolism, inhibiting liver fat accumulation, and alleviating vascular endothelial injury (Xu et al., 2021). In addition, BBR can also regulate intestinal microbiota, further affecting host metabolism. Therefore, BBR is considered a promising agent for the treatment of metabolism-related diseases. The therapeutic effect of BBR is based on the regulation of various metabolic aspects and pathophysiological processes, involving multiple cellular signaling pathways and action targets. For example, BBR enhances the cell’s utilization of glucose and fat by activating AMP-activated protein kinase (AMPK), thus improving insulin sensitivity and lipid metabolism (Chi et al., 2014). BBR can upregulate the expression of low-density lipoprotein receptors on the surface of liver cells, thereby increasing the clearance of the low-density lipoprotein (LDL) receptor (LDL-R) and reducing the level of LDL cholesterol (LDL-C) in the blood (Kong et al., 2004). However, metabolic diseases can involve multiple systems and organs, including the endocrine system, digestive system, and circulatory system. Although the metabolic regulatory function of BBR has been supported by many studies, further studies are still needed to understand the specific mechanism of how BBR regulates body metabolism and its potential clinical application.
This paper systematically reviews the recent research progress on the regulation of body’s metabolism by BBR, summarizing its mechanism and key targets in the system. It has been found that BBR has definite and extensive effects in regulating blood glucose, blood lipids, insulin resistance, and anti-inflammation. The targets involved in these effects mainly include glucagon-like peptide-1 (GLP1), glucose transporter (GLUT) 2 and mitochondrial pyruvate carrier (MPC) 1. In addition, there are far more pathways and targets for BBR to regulate metabolism. We summarize potential metabolism-related targets using TTD, DrugBank, GeneCards, and cutting-edge literature. Through the molecular docking of BBR and disease targets, more potential metabolic targets have been identified and may play a role in therapeutic effects of BBR, such as forkhead box O (FOXO) 3, nuclear factor erythroid 2-related factor (Nrf) 2, NAD (P) H quinone oxidoreductase 1 (NQO1), glutathione peroxidase (GPx) 4 and phosphatidylinositol-4,5-bisphosphate 3-kinase catalytic subunit alpha (PIK3CA). We thus explored the therapeutic potential of BBR in diseases and found that it has therapeutic effects on aging, cancer and hyperuricemia in addition to metabolism-related diseases.
As an important system for digestion and absorption of nutrients, the digestive system can be regarded as the “big chemical factory” of body’s metabolism, which maintains the source of energy for life activities. In particular, the liver has a strong metabolic capacity, and hepatocytes participate in various metabolic processes such as glucose metabolism, fat metabolism, and protein metabolism. Besides the function of digestion and absorption, the differentiated intestinal cells also play a balanced metabolic role through endocrine regulation and barrier protection.
The intestine is an important site for nutrient absorption, as well as an important metabolic and immune organ. Intestinal cell metabolism is key to regulating intestinal homeostasis (Litvak et al., 2018). Among them, enteroendocrine cells secrete intestinal hormones that regulate metabolism by intervening in digestion and absorption, insulin secretion, and controlling appetite. GLP-1 is mainly produced by enteroendocrine L cells, and its release not only stimulates insulin secretion in pancreatic islets, but also indirectly inhibits gluconeogenesis in the liver. BBR can promote the secretion of GLP-1 in experimental diabetic rats, which may be related to the promotion of the proliferation of intestinal L cells in rats. BBR increases the messenger ribonucleic acid (mRNA) level of proglucagon and prohormone convertase 3 which control GLP-1 biosynthesis in intestinal endocrine cell line NCI-H716, thereby promoting GLP-1 biosynthesis. This process is associated with dependency protein kinase C pathway (Yu et al., 2010). In addition, BBR promotes intestinal GLP-1 secretion by targeting the activation of the bitter receptor taste receptor 2 member 38 expression in the intestinal mucosa (Yu et al., 2015). The activation of bitter receptors by BBR may be a potential target for the treatment of type 2 diabetes.
One of the main functions of intestinal cells is to absorb glucose from food and then transport it into the blood. This process is mediated by specific glucose transporters. BBR reduces intestinal glucose absorption by reducing glucose transport in intestinal epithelial cells-6. This process is mainly through inhibiting the insulin-like growth factor 1-phospholipase C (PLC)-β2-GLUT 2 signaling pathway, reducing localization of PLC-β2 in the membrane, and thereby reducing GLUT2 translocation (Zhang et al., 2021). The expression of sodium-dependent glucose cotransporter, another glucose transporter protein in intestinal epithelial cells, is also inhibited by BBR to some extent, but whether it can exert glucose-lowering effect at high glucose concentrations remains to be verified (Zhang et al., 2021) (Table 1)
In addition, some studies have shown that intestinal permeability is associated with certain metabolic diseases in humans (Gummesson et al., 2011; Teixeira et al., 2012). When intestinal mucosal barrier is damaged, bacterial components such as endotoxin and bacterial metabolites can enter blood circulation, triggering certain inflammatory signals in the body, and then leading to metabolic diseases. This process is associated with Toll-like receptor (TLR) transduction and proinflammatory cytokines expression (Massier et al., 2021). BBR can protect intestinal mucosal barrier injury and inhibit inflammatory response in early septic rats by targeting TLR signaling pathway (Li et al., 2015; Chen et al., 2018). In addition, BBR can also improve pro-inflammatory factor-induced tight junction injury in intestinal epithelial cell lines (Li et al., 2010), restore tight junction protein zone 1 levels in diabetes rats (Shan et al., 2013), and repair intestinal barrier. Some studies have supported the hypothesis that BBR alleviates diabetes and regulates intestinal function. It is believed that the hypoglycemic effect of BBR on type 2 diabetes rats may play a role partly by improving intestinal mucosal barrier, but further experiments are needed to confirm the link (Shan et al., 2013).
The intestinal flora is regarded as a large metabolic ‘organ’ in the body that plays an important role in metabolic disorders. Intestinal microorganisms are involved in important physiological processes, such as nutrient absorption, substance metabolism, and immune defense, which affect host metabolism at many levels. Although the intestine is the main site of action of BBR, its oral bioavailability is poor and the mechanisms by which BBR regulates metabolic disorders are not yet fully understood. BBR has been shown to exert a metabolic protective effect by regulating the intestinal microbiota (Zhang et al., 2020; Wang et al., 2021); this effect can be attributed to an enhanced abundance of beneficial bacteria and a reduced abundance of harmful bacteria in the body. BBR also improves glucose metabolism in Zucker diabetic fatty rats by remodeling the intestinal flora, specifically reducing the abundance of pathogenic Desulfovibrionaceae and Proteobacteria (Li et al., 2021). In addition, BBR can improve obesity and insulin resistance in high-fat diet (HFD) rats, at least in part, by modulating the structure of the gut microbiota (Zhang et al., 2012). After BBR treatment, the improvement in insulin resistance in Zucker diabetic fatty rats was consistent with a decrease in the abundance of Prevotella copri, which is a bacterium that can directly affect host metabolism and induce obesity when combined with a high-fat diet. In addition, Akkermansia is a beneficial gut microbiota species that exerts a positive regulatory effect against obesity and metabolic disorders (Everard et al., 2013; Dao et al., 2016). BBR reportedly alleviates atherosclerosis in HFD mice by increasing the abundance of intestinal Akkermansia muciniphila species. BBR can impact certain short-chain fatty acid (SCFA) producers, which are known intestinal metabolites closely related to glucose and lipid metabolism (Wang et al., 2021). Treatment with BBR can increase the number of SCFA-producing bacteria in diabetes db/db mice, aid in restoring the intestinal SCFA content, and modify the structure of intestinal flora, thereby improving blood glucose levels and reducing diabetic complications (Zhang et al., 2019). Bile acid is an important metabolic signaling molecule closely related to lipid regulation (Fiorucci et al., 2010). BBR exerts a lipid-lowering role by activating the intestinal farnesoid X receptor signaling pathway and microbial metabolism of taurocholic acid in high-fat obese mice (Sun et al., 2017).
Furthermore, intestinal bacteria have been shown to metabolize oral BBR. It was found that the gut microbiota can use their nitroreductases to reduce BBR to an absorbable form known as dihydroberberine (dhBBR), which can then enter the bloodstream (Feng et al., 2015). In this process, the chemical structure and function of BBR is altered by intestinal microorganisms. This solves the problem of poor solubility and doubles BBR intestinal absorption rate, which is of great importance in drug research.
The liver is the primary source of endogenous glucose production: under basal conditions, about 80% of endogenous glucose production comes from hepatic glycogenolysis and gluconeogenesis (Klein et al., 2022). In lean individuals, both glycogenolysis and gluconeogenesis raise blood glucose, but in obese or type 2 diabetes patients, the contribution of gluconeogenesis is much higher. So suppressing hepatic gluconeogenesis is an effective way to control fasting blood glucose in diabetes (Gastaldelli et al., 2000). BBR has the therapeutic potential to lower blood glucose level by inhibiting hepatic gluconeogenesis. The MPC is composed of MPC1 and MPC2, and the input of pyruvate into the mitochondria is mediated by MPC. Once pyruvate enters the mitochondria, it is guided by the enzyme pyruvate carboxylase to undergo gluconeogenesis; the entry of pyruvate into the mitochondria through MPC is considered a pivotal step in hepatic gluconeogenesis. BBR inhibits SIRT-3 to protect the acetylation of MPC1 and disrupts the stability of the MPC1 protein through proteolysis, thereby limiting the supply of mitochondrial pyruvate for gluconeogenesis and reducing hepatic gluconeogenesis (Li et al., 2018). Glucagon physiologically induces hepatic gluconeogenesis. BBR reduces glucagon-stimulated cyclic adenosine 3'-5' monophosphate (cAMP) levels by activating phosphodiesterase, thereby downregulating the phosphorylation of the cAMP response element binding, and ultimately downregulating gluconeogenesis genes in liver cells, including phosphoenolpyruvate carboxykinase, glucose 6-phosphatase, and peroxisome proliferator-activated receptor-gamma coactivator 1-alpha (PGC-1α), thereby reducing hepatic gluconeogenesis (Zhong et al., 2020). Another study also showed that BBR can inhibit cAMP response element-binding protein phosphorylation and downregulate glucose 6-phosphatase and phosphoenolpyruvate carboxykinase, thereby inhibiting hepatic gluconeogenesis. This effect is related to the BBR-activated AKT-dependent mitogen-activated protein kinase (MAPK) pathway (Lu et al., 2023).
The liver is the main site for lipid metabolism. Digestion, absorption, transport, catabolism, and anabolism of lipids are closely linked to liver metabolism. Excessive fat intake leads to excess fat accumulation in liver cells in the form of lipid droplets, and intrahepatic fat accumulation may lead to hepatic fat metabolism disorders. Once liver fat metabolism disorder occurs, it not only causes liver disease but may also aggravate metabolic disorders in the body. BBR has good efficacy and safety in the treatment of patients with hyperlipidemia without the adverse reactions seen for statins (Wang et al., 2022), and is a promising lipid-lowering drug. BBR regulates lipid metabolism by acting on hepatocytes, and proprotein convertase subtilisin/kexin type 9 (PCSK9) is an important target. PCSK9 is a serine protease that is mainly synthesized and secreted by the liver. It mainly binds to LDL receptor (LDL-R) to promote its degradation, thereby reducing the clearance of LDL cholesterol (LDL-C). LDL-C binds to LDL-R on the membrane surface of hepatocytes and enters hepatocytes via endocytosis, and a decrease in intracellular pH dissociates LDL-C from LDL-R. After dissociation, LDL-C is degraded in lysosomes, whereas LDL-R returns to the hepatocyte membrane surface and continues to bind to the remaining LDL-C. PCSK9 binds to LDLR to form PCSK9–LDL-R-LDL complexes, which together enter lysosomes for degradation, leading to a decrease in LDL-R on the surface of hepatocytes and a corresponding decrease in the degradation of LDL-C (Liu et al., 2022). BBR, a natural cholesterol-lowering metabolite, has been found to be a promising PCSK9 inhibitor (Momtazi et al., 2017). It has been demonstrated that PCSK9 is upregulated by hepatic nuclear factor 1 alpha (HNF1α) and sterol regulatory element-binding protein (SREBP)-2 transcription factors, and BBR inhibits HNF1α-mediated PCSK9 transcription by reducing hepatic HNF1α protein content without affecting its mRNA levels (Dong et al., 2015), thereby reducing PCSK9 mRNA and protein levels (Cameron et al., 2008). BBR also increased LDL-C uptake by enhancing the stability of LDL-R mRNA in hepatocytes and increasing LDL-R density in hepatocytes (Kong et al., 2004). In animal models of dyslipidemia, BBR had no effect on the mRNA or protein expression of SREBP-2 when it reduced PCSK9 expression (Dong et al., 2015; Liu, D.-l. et al., 2015). Many current lipid-lowering drugs upregulate LDL-R by stimulating SREBP-2, leading to PCSK9-mediated drug resistance in dyslipidemia, which can be regarded as a useful adjunct to statin therapy (Figure 1).
Figure 1. BBR regulates lipid metabolism by reducing PCSK9 levels: 1) BBR inhibits the formation of PCSK9–LDL-R–LDL complexes and reduces the degradation of LDL-R by lysosomes, 2) HNF1α mediates the transcription of PCSK9, and BBR can reduce PCSK9 mRNA and protein levels by decreasing the content of HNF1α protein in liver, 3) BBR can increase the uptake of LDL-C by enhancing the stability of LDL-R mRNA and increasing LDL-R density in hepatocytes.
Hepatic steatosis is a common consequence of metabolic or toxic stress, and long-term hepatocyte degeneration can lead to hepatocyte damage. This in turn can lead to liver fibrosis and cirrhosis (Koyama and Brenner, 2017); therefore, early intervention is very important. The liver is a central metabolic organ that regulates lipid homeostasis, and reducing lipid production can effectively improve hepatic steatosis. BBR reduces liver triglyceride (TG) synthesis and alleviates hepatic steatosis by activating the AMPK-SREBP-1c-stearoyl-coenzyme A desaturase 1 (SCD1) pathway. Specifically, increased phosphorylation of AMPK promotes the phosphorylation of SREBP-1c and inhibits its cleavage and nuclear translocation. The binding of SREBP-1c to the sterol regulatory element motif on the promoter of SCD1 is reduced, resulting in the decreased expression of SCD1, thus alleviating hepatocyte steatosis (Zhu et al., 2019). BBR may reduce cholesterol synthesis and alleviate non-alcoholic hepatic steatosis by downregulating the SIRT1-forkhead box O (FOXO)1-SREBP2 signaling pathway in HepG2 cells. This is evidenced by SIRT1 and FOXO1 inhibitors blocking the effects of BBR treatment (Shan et al., 2021). Lipoautophagy regulates intracellular lipid homeostasis in response to various nutritional signals, and impaired lipoautophagy leads to excessive lipid accumulation in the liver (Liu and Czaja, 2013). BBR alleviates hepatic steatosis and controls energy balance in mice by inducing autophagy and fibroblast growth factor (FGF)-21, where SIRT1 is critical to this process (Sun et al., 2018). BBR stimulates SIRT1 deacetylation and induces autophagy in hepatocytes in an autophagy protein 5-dependent manner. In addition, BBR induced the expression of micro-RNA (miR)-373 in non-tumor hepatic MIHA cells. Upregulation of miR-373 depletes the levels of mRNA of target gene serine/threonine-protein kinase 1 (AKT1), and depletion of AKT1 results in decreased AKT1-mammalian target of rapamycin (mTOR) pathway activity. This is followed by inhibition of phosphorylated S6 kinase levels in hepatocytes, thereby attenuating S6K-mediated hepatic steatosis (Li, C.H. et al., 2018).
Metabolic intermediates such as sugars, fats, and proteins are transported through the circulatory system to ensure normal cellular metabolism. When important cells in the circulatory system are damaged, it can lead to changes in the composition and properties of substances in the blood, resulting in the accumulation of local metabolites or inflammatory products, causing circulation and metabolic disorders. Among them, endothelial cells and macrophages have a significant influence.
Endothelial cells have important endocrine functions, regulating vascular function through the secretion of vasoactive substances. Abnormal endothelial cell metabolism can lead to various metabolic diseases, which are related to endothelial dysfunction, inflammation, and oxidative stress (Deanfield et al., 2007). When endothelial cells are damaged by pathological stimuli, other cells in the blood (such as smooth muscle cells) aggregate and form a fibrin network with fibrin to repair the damage to endothelial cells. However, when the vascular endothelium is severely damaged, endothelial cells will secrete a large number of growth factors and active substances, attract too many monocytes to adhere to them, and migrate to deposit in the intima of blood vessels. Monocytes differentiate into macrophages and then absorb oxidatively modified LDLs in the blood, forming foam cells, which become potential risk factors for atherosclerosis (Rui et al., 2021). In response to this, BBR inhibits certain cell factors or proteins that stimulate endothelial cell damage. Adiponectin, with its pro-inflammatory effect, induction of cholesterol accumulation, and regulation of scavenger receptors (SR)-A and CD36 expression, has a harmful effect in atherosclerosis. BBR improves mouse endothelial function and alleviates atherosclerosis by inhibiting p38 MAPK and c-Jun N-terminal kinase (JNK) signaling pathways and downregulating adiponectin expression (Wan et al., 2018). Another study showed that BBR exhibited strong antioxidant capacity against LDL oxidation and had a protective effect against oxidized (ox) LDL-induced endothelial cell toxicity. In this process, BBR primarily inhibited oxLDL-induced reactive oxygen species (ROS) production, mitochondrial membrane damage, and cell apoptosis, including the inhibition of cytochrome C release and cysteine-aspartic protease-3 activation (Hsieh et al., 2007).
Vascular inflammation is one of the main causes of vascular remodeling (Hansson, 2005), especially high oxidative stress and low nitric oxide utilization. BBR improves the bioavailability of nitric oxide by reducing the protein expression of nicotinamide adenine dinucleotide phosphate oxidase (NOX) 4 in endothelial cells, thereby downregulating the expression of NOX. This is the main source of ROS production by endothelial cells, and ultimately improves endothelial function of diabetes rats (Wang et al., 2009). Endothelial nitric oxide synthase (eNOS) acts as a key enzyme for nitric oxide production in endothelial cells and its upstream kinases include AMPK, Akt, and protein kinase A (Mount et al., 2007). Among them, AMPK plays a central role in BBR-induced endothelial nitric oxide production and vasodilation, which promotes the binding of eNOS to heat shock protein 90 and thus maximizes eNOS activation and NO release, protecting against endothelial cell damage caused by high glucose. When AMPK is inhibited, BBR-mediated eNOS phosphorylation, NO production, and endothelium-dependent vasodilation are all eliminated (Wang et al., 2009). Endoplasmic reticulum (ER) stress in endothelial cells exacerbates oxidative stress. BBR may attenuate endothelial-dependent vasoconstriction by activating AMPK phosphorylation in the carotid arteries of spontaneously hypertensive rats, thereby inhibiting ER stress and protecting endothelial function. In this process, BBR inhibits the phosphorylation of eukaryotic translation initiation factor 2A and attenuates the expression of activating transcription factor-3, activating transcription factor-6, and X-box binding protein-1 (Liu et al., 2015). These three proteins mediate ER stress and are involved in complex downstream signaling pathways (Yang et al., 2010).
In addition, BBR participates in the regulation of lipid metabolism, thereby improving endothelial function. Treatment with BBR reversed and upregulated paraoxonase-1 (PON1) and apolipoprotein A1 (APOA1) expression and downregulated glycerol-3-phosphate dehydrogenase 2 (GPD2) expression in apolipoprotein E-deficient mice (Tan et al., 2020). These three proteins are strongly associated with atherosclerosis and lipid metabolism. As an antioxidant, paraoxonase-1 can hydrolyze lipid hydroperoxides and prevent oxidation of LDL (Aviram et al., 2005). GPD2 can regulate the transition of the inflammatory response and inhibit the oxidative metabolic response (Langston et al., 2019). APOA1 is the main protein component of HDL in the plasma and promotes the outflow of cholesterol from tissues. Studies have shown that regulating APOA1 transcription and increasing APOA1 levels in mice can reduce atherosclerosis (Guo et al., 2015). Thus, APOA1 may be a potential target for BBR to exert endothelial protection.
Atherosclerosis is the most important pathological process in the development of cardiovascular diseases. Dysregulation of lipid metabolism and abnormal inflammatory responses are considered major risk factors for atherosclerosis. BBR is a candidate for the treatment of atherosclerosis due to its pleiotropic antidyslipidemic and anti-inflammatory effects. Macrophage-derived foam cells play a key role in both the early and late stages of atherosclerosis (Chinetti-Gbaguidi et al., 2015); BBR can reduce foam cell formation. The process of macrophages taking up oxLDL to become foam cells depends on SRs, such as SR-A type I, cluster of differentiation (CD)36, and lectin-like oxLDL receptor (LOX)-1. The adenosine triphosphate (ATP)-binding cassette transporter G1 (ABCG1) and SR-B type I in macrophages participate in reverse cholesterol transport, and the upregulation of these proteins can weaken the formation of foam cells. BBR can reduce oxLDL uptake by inhibiting scavenger receptors CD36 and LOX-1, and increase cholesterol efflux by inhibiting the expression of adipocyte enhancer-binding protein 1 in macrophages (Huang et al., 2012). Another study showed that BBR can inhibit the influence of oxLDL on the foam cell formation from macrophages by inhibiting LOX-1 and upregulating SR-B type I expression (Guan et al., 2010). ABCG1 plays an important role in cholesterol efflux from foam cells. ABCG1 can directly mediate the efflux of cholesterol from the cells (Rosenson et al., 2016), and BBR can upregulate ABCG1 through nuclear factor, erythroid 2-related factor 2 (Nrf2)/HO-1 signaling in human macrophages, thereby regulating lipid homeostasis and inhibiting macrophage foam cell formation (Yang et al., 2020). BBR inhibits foam cell formation by activating the AMPK-SIRT1-proliferator-activated receptor γ (PPAR-γ) pathway and reducing oxLDL uptake. Compared with atorvastatin alone, the use of BBR in combination with atorvastatin can more effectively prevent the process of atherosclerosis (Chi et al., 2014).
Macrophages are prominent inflammatory cells in atherosclerotic lesions and produce a series of inflammatory factors, such as interleukin (IL)-1β, tumor necrosis factor (TNF)-α, IL-6, IL-8, monocyte chemoattractant protein-1 (MCP-1), triggering inflammation-induced atherosclerosis. BBR reduces inflammatory responses by activating AMPK to downregulate the secretion of various inflammatory factors in macrophages (Jeong et al., 2009). Additionally, BBR inhibits the secretion of inflammatory factors in oxLDL-induced J774A.1 cells by inducing autophagy, a process related to the activation of the AMPK/mTOR signaling pathway (Fan et al., 2015). In the treatment of atherosclerosis, it is of great significance to prevent atherosclerotic plaque rupture, which can cause thrombosis, leading to myocardial infarction, stroke, and death. Plaques with large lipid cores and covered by thin fibrous caps have a higher risk of rupture (Schaar et al., 2004). Macrophages and foam cells produce matrix metalloproteinases and extracellular matrix metalloproteinase inducers, which digest extracellular matrix proteins and weaken the fibrous cap, thereby reducing the stability of atherosclerotic plaques. BBR activates the p38 pathway to reduce the expression of matrix metalloproteinase-9 and extracellular matrix metalloproteinase inducer in macrophages to effectively stabilize the plaque (Huang et al., 2011). Another study also demonstrated the role of BBR in stabilizing plaques. In oxLDL-induced macrophages, BBR significantly increased miR-150-5p, reduced P2X7R expression, and downregulated the expression of matrix metallopeptidase 9 and EMMPRIN (Lu et al., 2021). In conclusion, BBR has the potential to prevent or treat atherosclerotic plaque formation and progression.
The endocrine system is closely related to metabolism, with endocrine cells synthesizing and secreting various hormones to distribute and transfer information between cells. Insulin secreted by islet cells binds to specific receptors on other target cells, producing a regulatory effect on the cells, such as adipocytes. Insulin resistance is often the core mechanism of dysregulated sugar and fat metabolism, and adipocyte metabolism is an important determinant of systemic insulin sensitivity.
Type II diabetes mellitus is a growing epidemic characterized by insulin resistance, relative insulin deficiency, and islet β-cell failure. BBR can reduce HbA1c and fasting glucose levels in type II diabetes patients, and its efficacy and safety have been demonstrated in clinical trials (Yin et al., 2008). As an effective oral hypoglycemic agent, BBR can regulate glucose metabolism in vitro and in vivo, and its hypoglycemic effect is closely related to the regulation of insulin secretion and improvement of islet β cell function.
BBR, the main active metabolite of Rhizoma coptifolia rhizoma, has been used in traditional Chinese medicine to treat diabetes for thousands of years. The hypoglycemic effect of BBR has been verified in a variety of animal experiments and human clinical trials, and this may be achieved by stimulating islet β-cells to secrete insulin. SIRT1 is involved in the regulation of various physiological activities of islet β-cells, and the activation of SIRT1 has a beneficial effect on β cell function (Kitada et al., 2019). BBR enhanced insulin secretion through the miR-204/SIRT1 signaling pathway. MiR-204 reduces insulin production and SIRT1 expression in mouse INsulinoma 6 cells, but BBR blocks this effect, instead promoting insulin secretion in islet tissues of diabetic mice (Lv et al., 2021). Insulin secretion is also regulated by ATP-sensitive K+ channels, which have been used as targets of insulin secretagogues. The potassium voltage-gated channel subfamily H member 6 (KCNH6) is a voltage-dependent K+ channel. BBR directly binds to the KCNH6 channel and accelerates channel closure, blocking outward Kv channel currents and prolonging the duration of action potential in islet β-cells. This causes Ca2+ influx into cells via voltage-gated calcium channels, and intracellular Ca2+ concentration triggers increased insulin secretion. Clinical trials have shown that BBR significantly promotes insulin secretion in the hyperglycemic state without affecting human basal insulin secretion; therefore, some researchers believe that BBR is an insulin secretagogue, and is different from other insulin secretagogues, because BBR only effectively reduces blood glucose levels under hyperglycemic conditions without causing hypoglycemia targeting KCNH6 channels (Zhao et al., 2021). However, it has been reported that BBR can reduce insulin secretion and inhibit the insulin gene promoter by activating AMPK in mice. This may be due to AMPK activation in the muscle and liver improving insulin resistance, reducing insulin demand, and reducing the synthetic burden on β-cells (Shen et al., 2012).
BBR not only stimulates the secretion of insulin but also protects islet β-cells. The inflammatory response can change the normal structure of β-cells, induce insulin resistance, and reduce insulin secretion. Reducing the inflammatory response improves β-cell function. BBR can inhibit the lipopolysaccharide-induced inflammatory response in pancreatic β-cells by interfering with the TLR 4/JNK/nuclear factor kappa-light-chain-enhancer of activated B cells (NF-κB) pathway. The lipopolysaccharide can increase the levels of inflammatory cytokines such as MCP-1, IL-6, and TNF-α. BBR may alleviate inflammatory injury by blocking early intracellular events in TLR4 signaling (Wang, 2013). BBR can inhibit β-cell apoptosis by upregulating calcium-independent phospholipase A2β (iPLA2β), cardiolipin (CL), and optic atrophy 1 (Opa1). The activation of iPLA2β is necessary for islet β-cells to secrete glucose to stimulate insulin. In iPLA2β silenced cells, the insulinogenic effect of BBR was weakened. In addition, CL/Opa1 expression was upregulated and decreased (Li et al., 2019). The occurrence and development of oxidative stress can lead to cardiovascular disease in diabetes. BBR inhibits the expression of miR-106b and upregulates SIRT1 to alleviate oxidative stress in islets of diabetic mice (Chen and Yang, 2017). Thus, BBR may reduce the incidence of diabetic cardiovascular diseases. Ferroptosis, a type of non-apoptotic cell death, has been linked to the pathogenesis of type I diabetes. BBR stimulation of GPx4 expression reduces Fe2+ and ROS, thereby inhibiting ferroptosis in islet β-cells. Its function is similar to that of the ferroptosis inhibitor ferrostatin-1 (Bao et al., 2022), which provides a new approach for improving the function of islet β-cells (Figure 2).
Figure 2. BBR can regulate insulin secretion and improve islet β cell function: 1) BBR inhibits the TLR4/JNK pathway and reduces MCP-6, IL-1, and TNF-α to alleviate cellular inflammation. 2) BBR stimulates GPX4 expression to reduce Fe2+ and ROS, thereby inhibiting ferroptosis in islet β-cells. 3) miR-204 decreases insulin production and SIRT1 expression in MIN6 cells, which was improved by BBR treatment. BBR inhibited miR-106b expression, upregulated SIRT1 expression, and weakened cell apoptosis. 4) BBR binds directly to KCNH6 channels, blocking outward Kv channel current and increasing intracellular Ca2+ concentration, thereby triggering more insulin secretion. 5) BBR attenuates β cell apoptosis by enhancing the iPLA2β/CL/Opa1 signaling pathway.
Obesity is defined as the accumulation of excess adipose tissue caused by various metabolic disorders. The development of adipocyte dysfunction is closely related to the development of obesity and insulin resistance. BBR can reduce body weight in overweight individuals and improve metabolic disorders in polycystic ovary syndrome, and its main effect may be related to changes in body composition in patients with obesity and dyslipidemia (Wei et al., 2012; Qiu et al., 2022). BBR has shown significant anti-obesity and functional improvement effects on adipocytes both in vivo and in vitro, partly owing to its action in adipocytes.
Obesity is a most common result of chronic energy metabolism imbalance and is considered an important risk factor for the development of type II diabetes and other metabolic diseases. Accumulation of fat is the direct cause of obesity, and many studies have shown that BBR can directly act on adipose tissue to achieve anti-obesity effects. Brown adipocytes are potential therapeutic targets for obesity-related metabolic diseases and stimulate FGF21 expression by regulating the molecular clock brain and muscle Arnt-like 1 in brown adipocytes. FGF21 secretion may act in a paracrine manner to promote thermogenic gene expression in brown adipocytes, thus alleviating diet-induced obesity (Hirai et al., 2019). Adipocyte differentiation and proliferation are regulated by a series of factors or molecules, among which galectin-3 (Gal-3) stimulates adipocyte differentiation and proliferation and is positively correlated with obesity. BBR downregulates Gal-3 by affecting its transcription, thereby inhibiting the differentiation and proliferation of preadipocytes isolated from epididymal white adipose tissue (WAT) used for fat storage, which contributes to its anti-obesity effect. Along with the downregulation of Gal-3 in differentiated adipocytes, the expression levels of adipogenic genes, such as PPARγ, were also reduced by BBR (Wang et al., 2019). The accumulation of TG in adipocytes contributes to obesity, therefore, reducing TG content in adipocytes is important to combat obesity. Adipose TG lipase is the rate-limiting enzyme that regulates the lipolysis of TG in adipocytes and mainly catalyzes the hydrolysis of TG to diacylglycerol. BBR directly increased the expression of adipose TG lipase in 3T3-L1 adipocytes and decreases TG levels in adipocytes (Jiang et al., 2016). In adipose tissue, increased autophagy may contribute to the pathogenesis of obesity (Kovsan et al., 2011) and plays an important role in regulating cellular metabolism and energy homeostasis. Beclin 1 (BECN1) is an essential protein for autophagy in differentiated 3T3-L1 adipocytes. BBR inhibits basal autophagy in mature adipocytes of fed mice by downregulating BECN1 expression by reducing the stability of BECN1 mRNA through the microRNA 30 family (Deng et al., 2014).
Brown adipocytes play a key role in the burning of glucose and lipids to maintain brown adipose tissue thermogenesis. In response to cold stimulation or β3-adrenergic receptor agonists, white adipocytes turn into brown-like adipocytes, a process known as the browning of white adipose tissue. Beige adipocytes are white or brown adipocytes. Uncoupling protein-1 (UCP1) is a hallmark of brown adipocytes. UCP1 can uncouple cellular respiration and ATP synthesis, contribute to heat production, and prevent obesity, and an increase in UCP1 content in WAT leads to a significant increase in whole-body energy expenditure. BBR can attract PGC-1α to UCP1 promoters by activating AMPK and inducing the expression of thermogenic genes, such as UCP1. Therefore, BBR can significantly induce the development of brown-like adipocytes in the groin (Zhang et al., 2014). PPARγ also promotes the expression of UCP-1 specific gene in brown adipocytes, inhibits the expression of specific genes in white adipocytes, and induces a brown-like phenotype in WAT. Selective deacetylation of PPARγ may be a regulatory switch in WAT conversion to brown adipose tissue. BBR activates the AMPK/SIRT1 axis to increase the level of PPARγ deacetylation, thereby regulating browning of adipose tissue and increasing the expression of the thermogenic protein UCP-1 (Xu et al., 2021). BBR can act as a selective PPARγ activator to promote adipose tissue remodeling and thermogenesis. BBR promotes white adipose tissue browning not only by regulating the expression of UCP-1 but also by affecting the genes related to brown adipogenesis. BBR treatment reduces the activity of the c13orf25 promoter, which drives the transcription of miR-92a. Reduction in miR-92a leads to an increase in the RNA-binding motif protein 4a protein, which activates gene expression and splicing networks involved in brown adipogenesis (Lin et al., 2019). However, individual studies have shown that BBR selectively promotes brown adipogenesis through AMPK activation but has no significant effect on beige adipogenesis (Han et al., 2020). Further studies are needed to investigate the mechanism of BBR-promoted browning of white adipose tissue.
Insulin resistance is a feature of metabolic diseases, such as obesity and type II diabetes. Insulin resistance is associated with the adipose tissue and chronic low-grade inflammation in adipose tissue is essential for its development. BBR upregulates the expression of SIRT1 in 3T3L-1 adipocytes, thereby inhibiting obesity-related inflammatory responses and improving local and systemic insulin resistance (Shan et al., 2020). BBR may also exert anti-inflammatory effects and ameliorate insulin resistance by inhibiting the phosphorylation of inhibitor kappa B kinase β Ser (Yi et al., 2008). In addition to low-grade inflammation in adipose tissue, adipose tissue fibrosis also contributes to obesity-related insulin resistance. BBR can significantly improve glucose tolerance in HFD-fed mice by inhibiting the abnormal deposition of a extracellular matrix in white adipose tissue and inhibiting the upregulation of fibrosis genes related to transforming growth factor-β1 signaling (Wang et al., 2018). BBR also regulates the cytokines secreted by adipocytes, such as retinol-binding protein 4. Retinol binding protein 4 is negatively correlated with the expression of GLUT4 during insulin resistance and BBR can significantly reduce the serum retinol binding protein 4 levels and upregulate the expression of GLUT4 in T2DM rats (Zhang et al., 2008). In addition, methylation of hypoxia-inducible factor-3α has previously been shown to be highly correlated with insulin resistance in patients with gestational diabetes and is considered a promising therapeutic target. BBR has been shown to be effective in improving insulin sensitivity in adipocyte models of insulin resistance, which may depend on reduced methylation and increased expression of hypoxia-inducible factor-3α (Wang et al., 2019).
The skeletal muscle is the main organ of the movement system. The normal life activities of skeletal muscle cells also require the supply of various substances and energy. Skeletal muscle cells are the main target cells for human glucose uptake, metabolism, and utilization mediated by insulin. BBR can enhance the sensitivity of skeletal muscle to insulin, increase the uptake and metabolism of glucose by skeletal muscle, thereby regulating glucose metabolism. The Insulin Receptor (InsR) protein is a complete cell membrane glycoprotein and is crucial for the binding of insulin to target cells. Insulin-resistant individuals lack or reduce the expression of InsR in peripheral tissues. In in vitro models, BBR increases the expression of InsR in L6 skeletal muscle cells and glucose consumption by 97% in L6 skeletal muscle cells in the presence of insulin (Kong et al., 2009). Insulin signal transduction is defective in metabolic diseases. Under insulin resistance, BBR treatment improves insulin-mediated glucose transport in muscle tubes and GLUT4 translocation, promoting glucose uptake. In L6myc cells, BBR improves insulin-induced tyrosine phosphorylation of insulin receptor substrate 1 and the recruitment of p85 to insulin receptor substrate 1. These results show that BBR improves insulin signal transduction in muscle cells and may overcome insulin resistance by regulating key molecules in the insulin signaling pathway (Liu et al., 2010). Excessive glucocorticoids can induce insulin resistance and obesity through intracellular glucocorticoid receptors. Excessive glucocorticoid receptors in skeletal muscle may play an important role in the occurrence and development of type 2 diabetes. In vivo and in vitro experiments have shown that BBR preparations with high bioavailability can reduce glucocorticoid receptor-mediated skeletal muscle insulin resistance. This mechanism is related to its reduction of the binding of glucocorticoid receptors to phosphatidylinositol-3-kinase (Meng et al., 2020). Oxidative stress is closely related to the occurrence and development of insulin resistance. BBR has a protective effect on fructose-induced rat insulin resistance. BBR treatment significantly upregulates Nrf2 and HO-1 protein expression in rat skeletal muscle, and can inhibit malondialdehyde levels, alleviate oxidative stress by increasing superoxide dismutase, catalase and glutathione levels, and at least partially improve insulin resistance through the Nrf2/HO-1 pathway (Cheng et al., 2023). Although the pathogenesis of insulin resistance is still unclear, elevated intralipid can induce insulin resistance (IR). The expression of cyclophilin D (CypD) is closely related to IR. Compared to high-fat diet mice, CypD-deficient mice have significantly reduced high-fat diet-induced IR (Tavecchio et al., 2015). BBR relieves intralipid-induced insulin resistance in skeletal muscle by inhibiting the expression of CypD, without affecting blood glucose (Dong et al., 2021). Therefore, BBR clinical intervention for IR before the onset of diabetes may be a very promising strategy for diabetes prevention.
Intracellular lipid accumulation in muscle cells may exacerbate metabolic disorders, higher intramuscular lipid content is observed in insulin-resistant subjects (Kim et al., 2000). BBR is thought to be able to regulate skeletal muscle lipid metabolism and prevent lipid deposition in skeletal muscle. BBR can improve the quantity and function of skeletal muscle mitochondria, which may be the target of BBR to improve metabolic disorders in patients with type 2 diabetes. BBR promotes the expression of the key transcriptional coactivator PGC-1α through the AMPK pathway. PGC-1α has been proven to be a major regulator of mitochondrial biosynthesis and function, able to increase the number and function of mitochondria, and prevent ectopic lipid deposition in skeletal muscle (Yao et al., 2020) (Figure 3).
In addition to metabolic mechanisms associated with various systems and those typically observed in cells, BBR exerts metabolic regulatory effects on specific cell types associated with other diseases. Fibroblast-like synoviocytes (FLS) are the main cell type in the synovial membrane of joints, and in rheumatoid arthritis, these cells cause joint destruction by invading cartilage. In the diseased state, FLS proliferate excessively and become abnormally activated, interacting with migrating immune cells to secrete large amounts of inflammatory factors. Under the induction of IL-21 and adjuvants, FLS undergo uncontrolled proliferation (Noack and Miossec, 2017). BBR was found to suppress this excessive proliferation by increasing BAX expression and decreasing B cell lymphoma-2 (Bcl-2) levels (Dinesh and Rasool, 2019). During autophagy, which is an intracellular catabolic mechanism, BBR reportedly inhibits LC3-I/II autophagy factors and activates CHOP to suppress AA-FLS autophagy response (Dinesh and Rasool, 2019). Moreover, BBR may exert anti-arthritic effects by regulating the balance between Treg and Th17 cells (Wang et al., 2017). BBR suppressed the proliferation and differentiation of Th17 cells by downregulating RORγ-t and CD196 and induced Foxp3 expression by activating the aryl hydrocarbon receptor, thereby promoting Treg cell differentiation and ultimately regulating the imbalance of Th17/Treg (Dinesh and Rasool, 2019). Furthermore, BBR was shown to regulate the proliferation and adhesion of RA-FLS cells through the RAS/MAPK/FOXO/HIF-1 signaling pathway, as well as reduce the expression of matrix metalloproteinase (MMP)-1, MMP-3, RANKL, and TNF-α, thereby combating rheumatoid arthriti (Li et al., 2023). The anti-arthritic action of BBR may be related to AMPK activation. By activating AMPK, BBR downregulated the expression of mTORC1/HIF-1α, thereby regulating the polarization of macrophages from the pro-inflammatory M1 type to the anti-inflammatory M2 type. This suppressed the glycolytic capacity of M1 macrophages, thus exerting an anti-arthritic effect (Cheng et al., 2023). Notably, in rheumatoid arthritis, macrophages and T cells jointly mediate the inflammatory cascade response. Exosomes derived from M1 macrophages can deliver miR-155 to CD4+ T cells to mediate immune metabolic disorder, and BBR reportedly inhibits this delivery process, thereby regulating the immune metabolism of CD4+ T cells (Cai et al., 2024).
BBR can also impact the metabolic mechanisms of certain oral and dental diseases. BBR was found to play a role in suppressing the growth of Porphyromonas gingivalis and the activity of gingival proteases. Osteoblasts and osteoclasts, as the main functional cells involved in bone formation, work in conjunction to regulate bone metabolism. BBR reportedly promotes osteogenic differentiation through the Wnt/β-catenin signaling pathway and enhances the expression of osteogenic-related genes in bone mesenchymal stem cells, thereby demonstrating its therapeutic potential to promote periodontal tissue regeneration (Zhang et al., 2019). The Wnt/β-catenin signaling pathway, as a classic route for BBR-stimulated osteogenic differentiation, promotes the differentiation of bone marrow mesenchymal stem cells into the osteogenic lineage (Tao et al., 2016) and regulates the development of dental tissues and the self-renewal of stem cells (Song et al., 2017; Wang et al., 2018). BBR also promotes odontogenic cell differentiation through the Wnt/β-catenin signaling pathway, where odontogenic cells, derived from dental pulp stem cells, are crucial in repairing dentin and protecting the deeper dental pulp in tooth lesions. This evidence supports the potential application of BBR in treating dental caries. Additionally, treatment with BBR was shown to repair bone loss in rats with apical periodontitis by restoring the balance between bone resorption and formation while also reducing the activity and quantity of osteoclasts and enhancing osteoblast differentiation. More importantly, BBR increased the expression of lysyl oxidase and decreased the levels of MMPs, which play important roles in extracellular matrix biosynthesis, thus promoting the stability of the extracellular matrix in periapical periodontitis (Cui et al., 2023), which is crucial for bone homeostasis. Collectively, these results indicate that BBR regulates bone metabolism in periodontitis and apical periodontitis, repairs bone damage by altering gingiva and extracellular matrix enzyme activity, and promotes odontogenic cell differentiation and bone homeostasis.
In previous explanations of animal and cell studies that have been validated, it has been partially demonstrated that BBR plays a metabolic role and mechanism in systemic regulation. It has been found that BBR exerts metabolic regulatory effects by targeting certain cells or regulatory protein targets. We further searched metabolism-related targets through TTD, DrugBank, and GeneCards, and included cutting-edge literature research to summarize potential metabolic targets. In addition, the binding of BBR to these targets was evaluated by molecular docking. Among them, 26 targets capable of docking with BBR were retrieved from three databases (Figure 4). PPAR-γ was identified as a common target in the TTD and GeneCards databases. Based on docking and metabolic correlation, we selected the top 20 targets with the highest affinity scores (Table 2), and BBR interacted with these macromolecular proteins (Figure 5) (Supplementary Figure S1). Although certain important signaling molecules, such as NFKB, LDLR, and APOA1, were detected in only one database and were not identified as common targets, they were still considered to have important metabolic effects and mechanisms in the above-mentioned animal and cell studies exploring BBR intervention. We further merged and compared the top 20 scoring molecules with the targets mentioned earlier, revealing 5 targets that were predicted by molecular docking and validated by experiments, namely, Nrf2, INS, PPAR-γ, LDLR, and NF-KB. Further validation confirmed the regulatory effects of these targets in the treatment of metabolic diseases such as diabetes, obesity, and hyperlipidemia by BBR.
Table 2. Binding energy of the top 20 docking scores of BBR and the amino acid residues to the metabolism-related targets.
At present, the therapeutic effect of BBR on metabolic disorders in clinical practice has been well described. The relatively clear effects include reducing blood glucose level, alleviating lipid disorders, improving insulin resistance, alleviating inflammation and oxidative stress. It has been shown in the previous discussion that BBR stimulates insulin secretion, reduces glucose transport, and inhibits hepatic gluconeogenesis by acting on GLP1, GLUT2, MPC1, TAS2R38, SIRT1, and other targets, which partially reveals the possible mechanism of BBR in treating diabetes. There are clinical trial reports that BBR can significantly improve serum fasting blood glucose and HbA1c levels in T2DM patients, and help restore pancreatic island function. In addition, BBR also inhibits the formation of foam cells, regulates lipid metabolism, and inhibits atherosclerosis related inflammatory reactions by regulating the levels of visfatin, oxLDL, PON1, APOA1, GPD2, ABCG1, and AMPK, which may have good clinical value for the treatment of cardiovascular diseases. A randomized, placebo-controlled, multicenter clinical trial showed that BBR could improve postprandial total cholesterol and low-density lipoprotein cholesterol levels in type 2 diabetes patients, thereby reducing the risk of cardiovascular disease in dyslipidemic patients with type 2 diabetes (Wang et al., 2022). This further confirmed from a clinical perspective that BBR can improve metabolic abnormalities such as blood glucose, blood lipid, and insulin resistance in T2DM patients, and its therapeutic applications cover type 2 diabetes and cardiovascular diseases. BBR also regulates lipid metabolism and steatosis of hepatocytes by regulating PCSK9, LDL-R, SIRT1, and SREBP2 levels, which suggests that BBR may have potential in the clinical application of non-alcoholic fatty liver disease and hyperlipidemia. A randomized controlled trial showed that BBR can reduce liver fat content, improve blood lipid levels, and reduce body weight in nonalcoholic fatty liver disease (NAFLD) patients (Yan et al., 2015). In conclusion, BBR has shown a good clinical potential to alleviate diabetes, cardiovascular disease, NAFLD, and obesity in clinical trials, which is related to reducing blood glucose, alleviating lipid disorders, improving insulin resistance, and alleviating inflammation. However, its exact mechanism needs to be further confirmed by experiments.
Based on metabolic related disease target database and molecular docking results mentioned above, the clinical therapeutic potential of BBR on potential targets was further predicted. We found that FOXO3, Nrf2, NQO1, Gpx4 and PIK3CA are not only related to regulating metabolic diseases, but also may have therapeutic effects on aging, cancer, or hyperuricemia (Figure 6). As a mediator of antioxidant responses and DNA damage repair, FOXO 3 is thought to have the potential to combat aging and stimulate tissue regeneration (de Keizer, 2017). BBR may play a role in preventing and treating aging by regulating FOXO3. Activation of AMPK can increase FOXO3 transcriptional activity. Notably, BBR can activate AMPK (Xu et al., 2017). Based on this, we suggest that BBR has a great possibility of inhibiting aging by regulating FOXO3 activity. Similarly, Nrf2 and NQO1 are also associated with antioxidant and aging control. Nrf2 promotes NQO1 transcription and expression by combining to NQO1 gene promoter regions. As an important oxidoreductase, NQO1 can reduce quinone to hydroquinone and reduce harmful oxidation of quinone (Chen et al., 2000). NQO1 is positively correlated with life span extension in animal models and may contribute to caloric restriction and control aging. Whether the anti-aging effect of BBR is related to NQO1 regulation, deserves further research. New research suggests a link between cancer and metabolic disorders (Glover and Glick, 1987). In cancer therapy, promoting ferroptosis of drug-resistant cancer cells by inhibiting GPX4 is seen as a potential therapeutic strategy. It was found that BBR has a high affinity to Gpx4 through molecular docking. As a key regulator of ferroptosis, Gpx4 may be a potential target for BBR to promote ferroptosis and exert anti-cancer effects. PIK3CA gene mutation and its resulting protein dysfunction can trigger dysregulation of PI3K/Akt signaling pathway, thereby promoting tumor formation and development. BBR has been shown to regulate the PI3K/Akt signaling pathway to promote apoptosis in thyroid cancer cells. Therefore, whether BBR can affect the activation of PI3K and Akt in cancer cells with PIK3CA mutations and inhibit PI3K phosphorylation is worthy of further research. In a mouse model of hyperuricemia after drug intervention, it was found that reducing uric acid was accompanied by upregulation of FOXO3 protein expression and changes of glutathione peroxidase levels. It is suggested that the regulation of FOXO3 and GPx4 may be related to the therapeutic mechanism of improving hyperuricemia, but there is no clinical evidence to prove it (An et al., 2023).
In summary, BBR has a positive therapeutic effect and broad application prospects in the pathological changes of human-related metabolic diseases and chronic diseases by participating in the metabolic activities of various systems. Many signaling pathways mediated by target receptors play a crucial role in regulating system metabolism and target cell fate. Targeting these pathways in metabolic regulation may bring new strategies for the treatment of metabolic diseases. Our molecular docking study further predicts that BBR may act on specific potential targets, such as FOXO3, Nrf2, NQO1, Gpx4, PIK3CA, and GPx, which are promising molecular targets for treating aging, cancer, or hyperuricemia. However, the limitations of this study need to be addressed. As BBR itself is a pan-assay interfering metabolite, it may possess the capacity to bind to multiple proteins. There is a lack of correlation between the binding states predicted by molecular docking and the actual biological effects generated by molecules. The metabolic mechanism of BBR involves several broad-spectrum signaling pathways, several of which are yet to be comprehensively elucidated, and research on related signal transduction pathways remains poorly clarified, necessitating further animal and cell experiments. Currently, there is a lack of research on the therapeutic mechanisms of BBR at the molecular and cellular levels in humans, and it is necessary to fully utilize metabolomics, transcriptomics, or proteomics technologies for further elucidation. Furthermore, the appropriate BBR dose remains unclear, and the effects of different dosages on systemic metabolism remain poorly explored. In the future, additional high-quality basic and clinical trials are needed to establish the dose-response effects of BBR, which will also facilitate the development of BBR and related products. In future research endeavors, key priorities include in-depth investigations into BBR-mediated molecular mechanisms that regulate metabolism through potential targets, the use of novel research techniques to identify scientific evidence of ligand-receptor interactions of potential targets, and the development of new biomarkers to explore the possibility and specificity of BBR in treating chronic diseases, as well as for specific targeting strategies.
AS: Conceptualization, Investigation, Writing–original draft, Writing–review and editing. HY: Conceptualization, Investigation, Writing–original draft. TL: Writing–review and editing. JL: Writing–review and editing. LZ: Writing–review and editing. RC: Writing–review and editing. LH: Conceptualization, Project administration, Resources, Writing–review and editing. YL: Conceptualization, Project administration, Writing–review and editing.
The author(s) declare that financial support was received for the research, authorship, and/or publication of this article. This work was supported by the National Natural Science Foundation of China (No. 82104840) and Scientific and technological innovation project of China Academy of Chinese Medical Sciences (No. CI 2021A01615).
Figures 1–3 were created by Figdraw (www.figdraw.com).
Author JL was employed by China Traditional Chinese Medicine Holdings Co. Limited, Guangdong e-fong Pharmaceutical Co., Ltd.
The remaining authors declare that the research was conducted in the absence of any commercial or financial relationships that could be construed as a potential conflict of interest.
All claims expressed in this article are solely those of the authors and do not necessarily represent those of their affiliated organizations, or those of the publisher, the editors and the reviewers. Any product that may be evaluated in this article, or claim that may be made by its manufacturer, is not guaranteed or endorsed by the publisher.
The Supplementary Material for this article can be found online at: https://www.frontiersin.org/articles/10.3389/fphar.2024.1368950/full#supplementary-material
An, M.-F., Shen, C., Zhang, S.-S., Wang, M.-Y., Sun, Z.-R., Fan, M.-S., et al. (2023). Anti-hyperuricemia effect of hesperetin is mediated by inhibiting the activity of xanthine oxidase and promoting excretion of uric acid. Front. Pharmacol. 14, 1128699. doi:10.3389/fphar.2023.1128699
Aviram, M., Kaplan, M., Rosenblat, M., and Fuhrman, B. (2005). Dietary antioxidants and paraoxonases against LDL oxidation and atherosclerosis development. Handb. Exp. Pharmacol. 170, 263–300. doi:10.1007/3-540-27661-0_9
Bao, L., Jin, Y., Han, J., Wang, W., Qian, L., and Wu, W. (2022). Berberine regulates GPX4 to inhibit ferroptosis of islet β cells. Planta Med. 89, 254–261. doi:10.1055/a-1939-7417
Cai, W. W., Gao, Y., Cheng, J. W., Yu, Y., Zong, S. Y., Li, Y. H., et al. (2024). Berberine modulates the immunometabolism and differentiation of CD4(+) T cells alleviating experimental arthritis by suppression of M1-exo-miR155. Phytomedicine 124, 155255. doi:10.1016/j.phymed.2023.155255
Cameron, J., Ranheim, T., Kulseth, M. A., Leren, T. P., and Berge, K. E. (2008). Berberine decreases PCSK9 expression in HepG2 cells. Atherosclerosis 201 (2), 266–273. doi:10.1016/j.atherosclerosis.2008.02.004
Chen, C. Y., Kao, C. L., and Liu, C. M. (2018). The cancer prevention, anti-inflammatory and anti-oxidation of bioactive phytochemicals targeting the TLR4 signaling pathway. Int. J. Mol. Sci. 19 (9), 2729. doi:10.3390/ijms19092729
Chen, D.-L., and Yang, K.-Y. (2017). Berberine alleviates oxidative stress in islets of diabetic mice by inhibiting miR-106b expression and up-regulating SIRT1. J. Cell Biochem. 118 (12), 4349–4357. doi:10.1002/jcb.26089
Chen, S., Wu, K., and Knox, R. (2000). Structure-function studies of DT-diaphorase (NQO1) and NRH: quinone oxidoreductase (NQO2). Free Radic. Biol. Med. 29 (3-4), 276–284. doi:10.1016/s0891-5849(00)00308-7
Cheng, J., Ma, X., Yan, G., Yu, Q., Huang, Z., Lin, G., et al. (2023). High fructose-induced skeletal muscle insulin resistance could be alleviated by berberine via AMPD1 and ADSL. Food Chem. Toxicol. 175, 113731. doi:10.1016/j.fct.2023.113731
Cheng, J. W., Yu, Y., Zong, S. Y., Cai, W. W., Wang, Y., Song, Y. N., et al. (2023). Berberine ameliorates collagen-induced arthritis in mice by restoring macrophage polarization via AMPK/mTORC1 pathway switching glycolytic reprogramming. Int. Immunopharmacol. 124 (Pt B), 111024. doi:10.1016/j.intimp.2023.111024
Chi, L., Peng, L., Pan, N., Hu, X., and Zhang, Y. (2014). The anti-atherogenic effects of berberine on foam cell formation are mediated through the upregulation of sirtuin 1. Int. J. Mol. Med. 34 (4), 1087–1093. doi:10.3892/ijmm.2014.1868
Chinetti-Gbaguidi, G., Colin, S., and Staels, B. (2015). Macrophage subsets in atherosclerosis. Nat. Rev. Cardiol. 12 (1), 10–17. doi:10.1038/nrcardio.2014.173
Cui, Y., Xie, J., Cai, L., Zhang, D., Sun, J., and Zhou, X. (2023). Berberine regulates bone metabolism in apical periodontitis by remodelling the extracellular matrix. Oral Dis. 29 (3), 1184–1196. doi:10.1111/odi.14094
Dao, M. C., Everard, A., Aron-Wisnewsky, J., Sokolovska, N., Prifti, E., Verger, E. O., et al. (2016). Akkermansia muciniphila and improved metabolic health during a dietary intervention in obesity: relationship with gut microbiome richness and ecology. Gut 65 (3), 426–436. doi:10.1136/gutjnl-2014-308778
Deanfield, J. E., Halcox, J. P., and Rabelink, T. J. (2007). Endothelial function and dysfunction: testing and clinical relevance. Circulation 115 (10), 1285–1295. doi:10.1161/CIRCULATIONAHA.106.652859
de Keizer, P. L. J. (2017). The fountain of youth by targeting senescent cells? Trends Mol. Med. 23 (1), 6–17. doi:10.1016/j.molmed.2016.11.006
Deng, Y., Xu, J., Zhang, X., Yang, J., Zhang, D., Huang, J., et al. (2014). Berberine attenuates autophagy in adipocytes by targeting BECN1. Autophagy 10 (10), 1776–1786. doi:10.4161/auto.29746
Dinesh, P., and Rasool, M. (2019). Berberine mitigates IL-21/IL-21R mediated autophagic influx in fibroblast-like synoviocytes and regulates Th17/Treg imbalance in rheumatoid arthritis. Apoptosis 24 (7-8), 644–661. doi:10.1007/s10495-019-01548-6
Dong, B., Li, H., Singh, A. B., Cao, A., and Liu, J. (2015). Inhibition of PCSK9 transcription by berberine involves down-regulation of hepatic HNF1α protein expression through the ubiquitin-proteasome degradation pathway. J. Biol. Chem. 290 (7), 4047–4058. doi:10.1074/jbc.M114.597229
Dong, Z.-H., Lin, H.-Y., Chen, F.-L., Che, X.-Q., Bi, W.-K., Shi, S.-L., et al. (2021). Berberine improves intralipid-induced insulin resistance in murine. Acta Pharmacol. Sin. 42 (5), 735–743. doi:10.1038/s41401-020-0493-4
Everard, A., Belzer, C., Geurts, L., Ouwerkerk, J. P., Druart, C., Bindels, L. B., et al. (2013). Cross-talk between Akkermansia muciniphila and intestinal epithelium controls diet-induced obesity. Proc. Natl. Acad. Sci. U. S. A. 110 (22), 9066–9071. doi:10.1073/pnas.1219451110
Fan, X., Wang, J., Hou, J., Lin, C., Bensoussan, A., Chang, D., et al. (2015). Berberine alleviates ox-LDL induced inflammatory factors by up-regulation of autophagy via AMPK/mTOR signaling pathway. J. Transl. Med. 13, 92. doi:10.1186/s12967-015-0450-z
Feng, R., Shou, J. W., Zhao, Z. X., He, C. Y., Ma, C., Huang, M., et al. (2015). Transforming berberine into its intestine-absorbable form by the gut microbiota. Sci. Rep. 15 (5), 12155. doi:10.1038/srep12155
Fiorucci, S., Cipriani, S., Mencarelli, A., Renga, B., Distrutti, E., and Baldelli, F. (2010). Counter-regulatory role of bile acid activated receptors in immunity and inflammation. Curr. Mol. Med. 10 (6), 579–595. doi:10.2174/1566524011009060579
Gastaldelli, A., Baldi, S., Pettiti, M., Toschi, E., Camastra, S., Natali, A., et al. (2000). Influence of obesity and type 2 diabetes on gluconeogenesis and glucose output in humans: a quantitative study. Diabetes 49 (8), 1367–1373. doi:10.2337/diabetes.49.8.1367
Glover, D. J., and Glick, J. H. (1987). Metabolic oncologic emergencies. CA Cancer J. Clin. 37 (5), 302–320. doi:10.3322/canjclin.37.5.302
Guan, S., Wang, B., Li, W., Guan, J., and Fang, X. (2010). Effects of berberine on expression of LOX-1 and SR-BI in human macrophage-derived foam cells induced by ox-LDL. Am. J. Chin. Med. 38 (6), 1161–1169. doi:10.1142/S0192415X10008548
Gummesson, A., Carlsson, L. M., Storlien, L. H., Bäckhed, F., Lundin, P., Löfgren, L., et al. (2011). Intestinal permeability is associated with visceral adiposity in healthy women. Obes. (Silver Spring) 19 (11), 2280–2282. doi:10.1038/oby.2011.251
Guo, Y., Fan, Y., Zhang, J., Lomberk, G. A., Zhou, Z., Sun, L., et al. (2015). Perhexiline activates KLF14 and reduces atherosclerosis by modulating ApoA-I production. J. Clin. Invest. 125 (10), 3819–3830. doi:10.1172/JCI79048
Han, Y.-B., Tian, M., Wang, X.-X., Fan, D.-H., Li, W.-Z., Wu, F., et al. (2020). Berberine ameliorates obesity-induced chronic inflammation through suppression of ER stress and promotion of macrophage M2 polarization at least partly via downregulating lncRNA Gomafu. Int. Immunopharmacol. 86, 106741. doi:10.1016/j.intimp.2020.106741
Hansson, G. K. (2005). Inflammation, atherosclerosis, and coronary artery disease. N. Engl. J. Med. 352 (16), 1685–1695. doi:10.1056/NEJMra043430
Hirai, T., Mitani, Y., Kurumisawa, K., Nomura, K., Wang, W., Nakashima, K.-I., et al. (2019). Berberine stimulates fibroblast growth factor 21 by modulating the molecular clock component brain and muscle Arnt-like 1 in brown adipose tissue. Biochem. Pharmacol. 164, 165–176. doi:10.1016/j.bcp.2019.04.017
Hsieh, Y. S., Kuo, W. H., Lin, T. W., Chang, H. R., Lin, T. H., Chen, P. N., et al. (2007). Protective effects of berberine against low-density lipoprotein (LDL) oxidation and oxidized LDL-induced cytotoxicity on endothelial cells. J. Agric. Food Chem. 55 (25), 10437–10445. doi:10.1021/jf071868c
Huang, Z., Dong, F., Li, S., Chu, M., Zhou, H., Lu, Z., et al. (2012). Berberine-induced inhibition of adipocyte enhancer-binding protein 1 attenuates oxidized low-density lipoprotein accumulation and foam cell formation in phorbol 12-myristate 13-acetate-induced macrophages. Eur. J. Pharmacol. 690 (1-3), 164–169. doi:10.1016/j.ejphar.2012.07.009
Huang, Z., Wang, L., Meng, S., Wang, Y., Chen, T., and Wang, C. (2011). Berberine reduces both MMP-9 and EMMPRIN expression through prevention of p38 pathway activation in PMA-induced macrophages. Int. J. Cardiol. 146 (2), 153–158. doi:10.1016/j.ijcard.2009.06.023
Jeong, H. W., Hsu, K. C., Lee, J.-W., Ham, M., Huh, J. Y., Shin, H. J., et al. (2009). Berberine suppresses proinflammatory responses through AMPK activation in macrophages. Am. J. Physiology. Endocrinol. Metabolism 296 (4), E955–E964. doi:10.1152/ajpendo.90599.2008
Jiang, D., Wang, D., Zhuang, X., Wang, Z., Ni, Y., Chen, S., et al. (2016). Berberine increases adipose triglyceride lipase in 3T3-L1 adipocytes through the AMPK pathway. Lipids Health Dis. 15 (1), 214. doi:10.1186/s12944-016-0383-4
Jin, X., Qiu, T., Li, L., Yu, R., Chen, X., Li, C., et al. (2023). Pathophysiology of obesity and its associated diseases. Acta Pharm. Sin. B 13 (6), 2403–2424. doi:10.1016/j.apsb.2023.01.012
Kim, J. Y., Hickner, R. C., Cortright, R. L., Dohm, G. L., and Houmard, J. A. (2000). Lipid oxidation is reduced in obese human skeletal muscle. Am. J. Physiology. Endocrinol. Metabolism 279 (5), E1039–E1044. doi:10.1152/ajpendo.2000.279.5.E1039
Kitada, M., Ogura, Y., Monno, I., and Koya, D. (2019). Sirtuins and type 2 diabetes: role in inflammation, oxidative stress, and mitochondrial function. Front. Endocrinol. (Lausanne) 10, 187. doi:10.3389/fendo.2019.00187
Klein, S., Gastaldelli, A., Yki-Järvinen, H., and Scherer, P. E. (2022). Why does obesity cause diabetes? Cell Metab. 34 (1), 11–20. doi:10.1016/j.cmet.2021.12.012
Kong, W., Wei, J., Abidi, P., Lin, M., Inaba, S., Li, C., et al. (2004). Berberine is a novel cholesterol-lowering drug working through a unique mechanism distinct from statins. Nat. Med. 10 (12), 1344–1351. doi:10.1038/nm1135
Kong, W.-J., Zhang, H., Song, D.-Q., Xue, R., Zhao, W., Wei, J., et al. (2009). Berberine reduces insulin resistance through protein kinase C-dependent up-regulation of insulin receptor expression. Metabolism Clin. Exp. 58 (1), 109–119. doi:10.1016/j.metabol.2008.08.013
Kovsan, J., Blüher, M., Tarnovscki, T., Klöting, N., Kirshtein, B., Madar, L., et al. (2011). Altered autophagy in human adipose tissues in obesity. J. Clin. Endocrinol. Metab. 96 (2), E268–E277. doi:10.1210/jc.2010-1681
Koyama, Y., and Brenner, D. A. (2017). Liver inflammation and fibrosis. J. Clin. Invest. 127 (1), 55–64. doi:10.1172/JCI88881
Langston, P. K., Nambu, A., Jung, J., Shibata, M., Aksoylar, H. I., Lei, J., et al. (2019). Glycerol phosphate shuttle enzyme GPD2 regulates macrophage inflammatory responses. Nat. Immunol. 20 (9), 1186–1195. doi:10.1038/s41590-019-0453-7
Li, A., Liu, Q., Li, Q., Liu, B., Yang, Y., and Zhang, N. (2018). Berberine reduces pyruvate-driven hepatic glucose production by limiting mitochondrial import of pyruvate through mitochondrial pyruvate carrier 1. EBioMedicine 34, 243–255. doi:10.1016/j.ebiom.2018.07.039
Li, C., Cao, H., Huan, Y., Ji, W., Liu, S., Sun, S., et al. (2021). Berberine combined with stachyose improves glycometabolism and gut microbiota through regulating colonic microRNA and gene expression in diabetic rats. Life Sci. 284, 119928. doi:10.1016/j.lfs.2021.119928
Li, C. H., Tang, S. C., Wong, C. H., Wang, Y., Jiang, J.-D., and Chen, Y. (2018). Berberine induces miR-373 expression in hepatocytes to inactivate hepatic steatosis associated AKT-S6 kinase pathway. Eur. J. Pharmacol. 825, 107–118. doi:10.1016/j.ejphar.2018.02.035
Li, G. X., Wang, X. M., Jiang, T., Gong, J. F., Niu, L. Y., and Li, N. (2015). Berberine prevents intestinal mucosal barrier damage during early phase of sepsis in rat through the toll-like receptors signaling pathway. Korean J. Physiol. Pharmacol. 19 (1), 1–7. doi:10.4196/kjpp.2015.19.1.1
Li, J., Du, H., Zhang, M., Zhang, Z., Teng, F., Zhao, Y., et al. (2019). Amorphous solid dispersion of Berberine mitigates apoptosis via iPLA2β/Cardiolipin/Opa1 pathway in db/db mice and in Palmitate-treated MIN6 β-cells. Int. J. Biol. Sci. 15 (7), 1533–1545. doi:10.7150/ijbs.32020
Li, N., Gu, L., Qu, L., Gong, J., Li, Q., Zhu, W., et al. (2010). Berberine attenuates pro-inflammatory cytokine-induced tight junction disruption in an in vitro model of intestinal epithelial cells. Eur. J. Pharm. Sci. 40 (1), 1–8. doi:10.1016/j.ejps.2010.02.001
Li, Z., Chen, M., Wang, Z., Fan, Q., Lin, Z., Tao, X., et al. (2023). Berberine inhibits RA-FLS cell proliferation and adhesion by regulating RAS/MAPK/FOXO/HIF-1 signal pathway in the treatment of rheumatoid arthritis. Bone Jt. Res. 12 (2), 91–102. doi:10.1302/2046-3758.122.BJR-2022-0269.R1
Lin, Y.-C., Lee, Y.-C., Lin, Y.-J., and Lin, J.-C. (2019). Berberine promotes beige adipogenic signatures of 3T3-L1 cells by regulating post-transcriptional events. Cells 8 (6), 632. doi:10.3390/cells8060632
Ling, Z. N., Jiang, Y. F., Ru, J. N., Lu, J. H., Ding, B., and Wu, J. (2023). Amino acid metabolism in health and disease. Signal Transduct. Target Ther. 8 (1), 345. doi:10.1038/s41392-023-01569-3
Litvak, Y., Byndloss, M. X., and Bäumler, A. J. (2018). Colonocyte metabolism shapes the gut microbiota. Science 362 (6418), eaat9076. doi:10.1126/science.aat9076
Liu, C., Chen, J., Chen, H., Zhang, T., He, D., Luo, Q., et al. (2022). PCSK9 inhibition: from current advances to evolving future. Cells 11 (19), 2972. doi:10.3390/cells11192972
Liu, D.-l., Xu, L.-j., Dong, H., Chen, G., Huang, Z.-y., Zou, X., et al. (2015). Inhibition of proprotein convertase subtilisin/kexin type 9: a novel mechanism of berberine and 8-hydroxy dihydroberberine against hyperlipidemia. Chin. J. Integr. Med. 21 (2), 132–138. doi:10.1007/s11655-014-1775-1
Liu, K., and Czaja, M. J. (2013). Regulation of lipid stores and metabolism by lipophagy. Cell Death Differ. 20 (1), 3–11. doi:10.1038/cdd.2012.63
Liu, L., Liu, J., Huang, Z., Yu, X., Zhang, X., Dou, D., et al. (2015). Berberine improves endothelial function by inhibiting endoplasmic reticulum stress in the carotid arteries of spontaneously hypertensive rats. Biochem. Biophys. Res. Commun. 458 (4), 796–801. doi:10.1016/j.bbrc.2015.02.028
Liu, L.-Z., Cheung, S. C. K., Lan, L.-L., Ho, S. K. S., Xu, H.-X., Chan, J. C. N., et al. (2010). Berberine modulates insulin signaling transduction in insulin-resistant cells. Mol. Cell. Endocrinol. 317 (1-2), 148–153. doi:10.1016/j.mce.2009.12.027
Lu, L., Huang, J., Xue, X., Wang, T., Huang, Z., and Li, J. (2021). Berberine regulated miR150-5p to inhibit P2X7 receptor, EMMPRIN and MMP-9 expression in oxLDL induced macrophages. Front. Pharmacol. 12, 639558. doi:10.3389/fphar.2021.639558
Lu, M., Wang, Y., Jiang, Y., Zhang, C., Wang, H., Sha, W., et al. (2023). Berberine inhibits gluconeogenesis in spontaneous diabetic rats by regulating the AKT/MAPK/NO/cGMP/PKG signaling pathway. Mol. Cell. Biochem. 478, 2013–2027. doi:10.1007/s11010-022-04604-z
Lv, X., Zhao, Y., Yang, X., Han, H., Ge, Y., Zhang, M., et al. (2021). Berberine potentiates insulin secretion and prevents β-cell dysfunction through the miR-204/SIRT1 signaling pathway. Front. Pharmacol. 12, 720866. doi:10.3389/fphar.2021.720866
Massier, L., Blüher, M., Kovacs, P., and Chakaroun, R. M. (2021). Impaired intestinal barrier and tissue bacteria: pathomechanisms for metabolic diseases. Front. Endocrinol. (Lausanne) 12, 616506. doi:10.3389/fendo.2021.616506
Meng, Z., Yu, Y., Zhang, Y., Yang, X., Lv, X., Guan, F., et al. (2020). Highly bioavailable berberine formulation improves glucocorticoid receptor-mediated insulin resistance via reduction in association of the glucocorticoid receptor with phosphatidylinositol-3-kinase. Int. J. Biol. Sci. 16 (14), 2527–2541. doi:10.7150/ijbs.39508
Momtazi, A. A., Banach, M., Pirro, M., Katsiki, N., and Sahebkar, A. (2017). Regulation of PCSK9 by nutraceuticals. Pharmacol. Res. 120, 157–169. doi:10.1016/j.phrs.2017.03.023
Mount, P. F., Kemp, B. E., and Power, D. A. (2007). Regulation of endothelial and myocardial NO synthesis by multi-site eNOS phosphorylation. J. Mol. Cell Cardiol. 42 (2), 271–279. doi:10.1016/j.yjmcc.2006.05.023
Noack, M., and Miossec, P. (2017). Selected cytokine pathways in rheumatoid arthritis. Semin. Immunopathol. 39 (4), 365–383. doi:10.1007/s00281-017-0619-z
Qiu, Y., Li, M., Zhang, Y., Liu, Y., Zhao, Y., Zhang, J., et al. (2022). Berberine treatment for weight gain in patients with schizophrenia by regulating leptin rather than adiponectin. Asian J. Psychiatr. 67, 102896. doi:10.1016/j.ajp.2021.102896
Rosenson, R. S., Brewer, H. B., Ansell, B. J., Barter, P., Chapman, M. J., Heinecke, J. W., et al. (2016). Dysfunctional HDL and atherosclerotic cardiovascular disease. Nat. Rev. Cardiol. 13 (1), 48–60. doi:10.1038/nrcardio.2015.124
Rui, R., Yang, H., Liu, Y., Zhou, Y., Xu, X., Li, C., et al. (2021). Effects of berberine on atherosclerosis. Front. Pharmacol. 12, 764175. doi:10.3389/fphar.2021.764175
Schaar, J. A., Muller, J. E., Falk, E., Virmani, R., Fuster, V., Serruys, P. W., et al. (2004). Terminology for high-risk and vulnerable coronary artery plaques. Report of a meeting on the vulnerable plaque, June 17 and 18, 2003, Santorini, Greece. Eur. Heart J. 25 (12), 1077–1082. doi:10.1016/j.ehj.2004.01.002
Shan, C. Y., Yang, J. H., Kong, Y., Wang, X. Y., Zheng, M. Y., Xu, Y. G., et al. (2013). Alteration of the intestinal barrier and GLP2 secretion in Berberine-treated type 2 diabetic rats. J. Endocrinol. 218 (3), 255–262. doi:10.1530/JOE-13-0184
Shan, M.-Y., Dai, Y., Ren, X.-D., Zheng, J., Zhang, K.-B., Chen, B., et al. (2021). Berberine mitigates nonalcoholic hepatic steatosis by downregulating SIRT1-FoxO1-SREBP2 pathway for cholesterol synthesis. J. Integr. Med. 19 (6), 545–554. doi:10.1016/j.joim.2021.09.003
Shan, Y., Zhang, S., Gao, B., Liang, S., Zhang, H., Yu, X., et al. (2020). Adipose tissue SIRT1 regulates insulin sensitizing and anti-inflammatory effects of berberine. Front. Pharmacol. 11, 591227. doi:10.3389/fphar.2020.591227
Shen, N., Huan, Y., and Shen, Z.-f. (2012). Berberine inhibits mouse insulin gene promoter through activation of AMP activated protein kinase and may exert beneficial effect on pancreatic β-cell. Eur. J. Pharmacol. 694 (1-3), 120–126. doi:10.1016/j.ejphar.2012.07.052
Song, D., Hao, J., and Fan, D. (2020). Biological properties and clinical applications of berberine. Front. Med. 14 (5), 564–582. doi:10.1007/s11684-019-0724-6
Song, Y., Liu, X., Feng, X., Gu, Z., Gu, Y., Lian, M., et al. (2017). NRP1 accelerates odontoblast differentiation of dental pulp stem cells through classical wnt/β-catenin signaling. Cell Reprogr. 19 (5), 324–330. doi:10.1089/cell.2017.0020
Sun, H., Saeedi, P., Karuranga, S., Pinkepank, M., Ogurtsova, K., Duncan, B. B., et al. (2022). IDF Diabetes Atlas: global, regional and country-level diabetes prevalence estimates for 2021 and projections for 2045. Diabetes Res. Clin. Pract. 183, 109119. doi:10.1016/j.diabres.2021.109119
Sun, R., Yang, N., Kong, B., Cao, B., Feng, D., Yu, X., et al. (2017). Orally administered berberine modulates hepatic lipid metabolism by altering microbial bile acid metabolism and the intestinal FXR signaling pathway. Mol. Pharmacol. 91 (2), 110–122. doi:10.1124/mol.116.106617
Sun, Y., Xia, M., Yan, H., Han, Y., Zhang, F., Hu, Z., et al. (2018). Berberine attenuates hepatic steatosis and enhances energy expenditure in mice by inducing autophagy and fibroblast growth factor 21. Br. J. Pharmacol. 175 (2), 374–387. doi:10.1111/bph.14079
Tan, W., Wang, Y., Wang, K., Wang, S., Liu, J., Qin, X., et al. (2020). Improvement of endothelial dysfunction of berberine in atherosclerotic mice and mechanism exploring through TMT-based proteomics. Oxid. Med. Cell Longev. 2020, 8683404. doi:10.1155/2020/8683404
Tao, K., Xiao, D., Weng, J., Xiong, A., Kang, B., and Zeng, H. (2016). Berberine promotes bone marrow-derived mesenchymal stem cells osteogenic differentiation via canonical Wnt/β-catenin signaling pathway. Toxicol. Lett. 240 (1), 68–80. doi:10.1016/j.toxlet.2015.10.007
Tavecchio, M., Lisanti, S., Bennett, M. J., Languino, L. R., and Altieri, D. C. (2015). Deletion of cyclophilin D impairs β-oxidation and promotes glucose metabolism. Sci. Rep. 5, 15981. doi:10.1038/srep15981
Teixeira, T. F., Souza, N. C., Chiarello, P. G., Franceschini, S. C., Bressan, J., Ferreira, C. L., et al. (2012). Intestinal permeability parameters in obese patients are correlated with metabolic syndrome risk factors. Clin. Nutr. 31 (5), 735–740. doi:10.1016/j.clnu.2012.02.009
Wan, Q., Liu, Z., Yang, Y., and Cui, X. (2018). Suppressive effects of berberine on atherosclerosis via downregulating visfatin expression and attenuating visfatin-induced endothelial dysfunction. Int. J. Mol. Med. 41 (4), 1939–1948. doi:10.3892/ijmm.2018.3440
Wang, C., Li, J., Lv, X., Zhang, M., Song, Y., Chen, L., et al. (2009). Ameliorative effect of berberine on endothelial dysfunction in diabetic rats induced by high-fat diet and streptozotocin. Eur. J. Pharmacol. 620 (1-3), 131–137. doi:10.1016/j.ejphar.2009.07.027
Wang, C., Song, Y., Gu, Z., Lian, M., Huang, D., Lu, X., et al. (2018). Wedelolactone enhances odontoblast differentiation by promoting wnt/β-catenin signaling pathway and suppressing NF-κB signaling pathway. Cell Reprogr. 20 (4), 236–244. doi:10.1089/cell.2018.0004
Wang, C., Wang, Y., Ma, S.-R., Zuo, Z.-Y., Wu, Y.-B., Kong, W.-J., et al. (2019). Berberine inhibits adipocyte differentiation, proliferation and adiposity through down-regulating galectin-3. Sci. Rep. 9 (1), 13415. doi:10.1038/s41598-019-50103-5
Wang, L., Ye, X., Hua, Y., and Song, Y. (2018). Berberine alleviates adipose tissue fibrosis by inducing AMP-activated kinase signaling in high-fat diet-induced obese mice. Biomed. Pharmacother. 105, 121–129. doi:10.1016/j.biopha.2018.05.110
Wang, S., Ren, H., Zhong, H., Zhao, X., Li, C., Ma, J., et al. (2022). Combined berberine and probiotic treatment as an effective regimen for improving postprandial hyperlipidemia in type 2 diabetes patients: a double blinded placebo controlled randomized study. Gut Microbes 14 (1), 2003176. doi:10.1080/19490976.2021.2003176
Wang, X., He, X., Zhang, C. F., Guo, C. R., Wang, C. Z., and Yuan, C. S. (2017). Anti-arthritic effect of berberine on adjuvant-induced rheumatoid arthritis in rats. Biomed. Pharmacother. 89, 887–893. doi:10.1016/j.biopha.2017.02.099
Wang, Y. (2013). Attenuation of berberine on lipopolysaccharide-induced inflammatory and apoptosis responses in β-cells via TLR4-independent JNK/NF-κB pathway. Pharm. Biol. 52, 532–538. doi:10.3109/13880209.2013.840851
Wang, Y., Gong, W., Lv, S., Qu, H., and He, Y. (2019). Berberine improves insulin resistance in adipocyte models by regulating the methylation of hypoxia-inducible factor-3α. Biosci. Rep. 39 (10), BSR20192059. doi:10.1042/BSR20192059
Wang, Y., Huang, Y., Lam, K. S., Li, Y., Wong, W. T., Ye, H., et al. (2009). Berberine prevents hyperglycemia-induced endothelial injury and enhances vasodilatation via adenosine monophosphate-activated protein kinase and endothelial nitric oxide synthase. Cardiovasc Res. 82 (3), 484–492. doi:10.1093/cvr/cvp078
Wang, Y., Liu, H., Zheng, M., Yang, Y., Ren, H., Kong, Y., et al. (2021). Berberine slows the progression of prediabetes to diabetes in Zucker diabetic fatty rats by enhancing intestinal secretion of glucagon-like peptide-2 and improving the gut microbiota. Front. Endocrinol. (Lausanne) 12, 609134. doi:10.3389/fendo.2021.609134
Wei, W., Zhao, H., Wang, A., Sui, M., Liang, K., Deng, H., et al. (2012). A clinical study on the short-term effect of berberine in comparison to metformin on the metabolic characteristics of women with polycystic ovary syndrome. Eur. J. Endocrinol. 166 (1), 99–105. doi:10.1530/EJE-11-0616
Wong, S. K., Chin, K.-Y., and Ima-Nirwana, S. (2020). Berberine and musculoskeletal disorders: the therapeutic potential and underlying molecular mechanisms. Phytomedicine 73, 152892. doi:10.1016/j.phymed.2019.152892
Wong, V.W.-S., Ekstedt, M., Wong, G.L.-H., and Hagström, H. (2023). Changing epidemiology, global trends and implications for outcomes of NAFLD. J. Hepatol. 79 (3), 842–852. doi:10.1016/j.jhep.2023.04.036
Xu, X., Yi, H., Wu, J., Kuang, T., Zhang, J., Li, Q., et al. (2021). Therapeutic effect of berberine on metabolic diseases: both pharmacological data and clinical evidence. Biomed. Pharmacother. 133, 110984. doi:10.1016/j.biopha.2020.110984
Xu, Y., Yu, T., Ma, G., Zheng, L., Jiang, X., Yang, F., et al. (2021). Berberine modulates deacetylation of PPARγ to promote adipose tissue remodeling and thermogenesis via AMPK/SIRT1 pathway. Int. J. Biol. Sci. 17 (12), 3173–3187. doi:10.7150/ijbs.62556
Xu, Z., Feng, W., Shen, Q., Yu, N., Yu, K., Wang, S., et al. (2017). Rhizoma coptidis and berberine as a natural drug to combat aging and aging-related diseases via anti-oxidation and AMPK activation. Aging Dis. 8 (6), 760–777. doi:10.14336/AD.2016.0620
Yan, H.-M., Xia, M.-F., Wang, Y., Chang, X.-X., Yao, X.-Z., Rao, S.-X., et al. (2015). Efficacy of berberine in patients with non-alcoholic fatty liver disease. PloS One 10 (8), e0134172. doi:10.1371/journal.pone.0134172
Yang, L., Li, P., Fu, S., Calay, E. S., and Hotamisligil, G. S. (2010). Defective hepatic autophagy in obesity promotes ER stress and causes insulin resistance. Cell Metab. 11 (6), 467–478. doi:10.1016/j.cmet.2010.04.005
Yang, X.-J., Liu, F., Feng, N., Ding, X.-S., Chen, Y., Zhu, S.-X., et al. (2020). Berberine attenuates cholesterol accumulation in macrophage foam cells by suppressing AP-1 activity and activation of the Nrf2/HO-1 pathway. J. Cardiovasc Pharmacol. 75 (1), 45–53. doi:10.1097/FJC.0000000000000769
Yao, S., Yuan, Y., Zhang, H., Meng, X., Jin, L., Yang, J., et al. (2020). Berberine attenuates the abnormal ectopic lipid deposition in skeletal muscle. Free Radic. Biol. Med. 159, 66–75. doi:10.1016/j.freeradbiomed.2020.07.028
Yi, P., Lu, F.-E., Xu, L.-J., Chen, G., Dong, H., and Wang, K.-F. (2008). Berberine reverses free-fatty-acid-induced insulin resistance in 3T3-L1 adipocytes through targeting IKKbeta. World J. Gastroenterol. 14 (6), 876–883. doi:10.3748/wjg.14.876
Yin, J., Xing, H., and Ye, J. (2008). Efficacy of berberine in patients with type 2 diabetes mellitus. Metabolism Clin. Exp. 57 (5), 712–717. doi:10.1016/j.metabol.2008.01.013
Yu, Y., Hao, G., Zhang, Q., Hua, W., Wang, M., Zhou, W., et al. (2015). Berberine induces GLP-1 secretion through activation of bitter taste receptor pathways. Biochem. Pharmacol. 97 (2), 173–177. doi:10.1016/j.bcp.2015.07.012
Yu, Y., Liu, L., Wang, X., Liu, X., Liu, X., Xie, L., et al. (2010). Modulation of glucagon-like peptide-1 release by berberine: in vivo and in vitro studies. Biochem. Pharmacol. 79 (7), 1000–1006. doi:10.1016/j.bcp.2009.11.017
Zhang, L., Wu, X., Yang, R., Chen, F., Liao, Y., Zhu, Z., et al. (2020). Effects of berberine on the gastrointestinal microbiota. Front. Cell Infect. Microbiol. 10, 588517. doi:10.3389/fcimb.2020.588517
Zhang, M., Yang, H., Yang, E., Li, J., and Dong, L. (2021). Berberine decreases intestinal GLUT2 translocation and reduces intestinal glucose absorption in mice. Int. J. Mol. Sci. 23 (1), 327. doi:10.3390/ijms23010327
Zhang, M., Yang, H., Yang, E., Li, J., and Dong, L. (2021). Berberine decreases intestinal GLUT2 translocation and reduces intestinal glucose absorption in mice. Int. J. Mol. Sci. 23 (1), 327. doi:10.3390/ijms23010327
Zhang, R., Yang, J., Wu, J., Xiao, L., Miao, L., Qi, X., et al. (2019). Berberine promotes osteogenic differentiation of mesenchymal stem cells with therapeutic potential in periodontal regeneration. Eur. J. Pharmacol. 851, 144–150. doi:10.1016/j.ejphar.2019.02.026
Zhang, W., Xu, J. H., Yu, T., and Chen, Q. K. (2019). Effects of berberine and metformin on intestinal inflammation and gut microbiome composition in db/db mice. Biomed. Pharmacother. 118, 109131. doi:10.1016/j.biopha.2019.109131
Zhang, W., Xu, Y.-c., Guo, F.-j., Meng, Y., and Li, M.-l. (2008). Anti-diabetic effects of cinnamaldehyde and berberine and their impacts on retinol-binding protein 4 expression in rats with type 2 diabetes mellitus. Chin. Med. J. Engl. 121 (21), 2124–2128. doi:10.1097/00029330-200811010-00003
Zhang, X., Zhao, Y., Zhang, M., Pang, X., Xu, J., Kang, C., et al. (2012). Structural changes of gut microbiota during berberine-mediated prevention of obesity and insulin resistance in high-fat diet-fed rats. PLoS One 7 (8), e42529. doi:10.1371/journal.pone.0042529
Zhang, Z., Zhang, H., Li, B., Meng, X., Wang, J., Zhang, Y., et al. (2014). Berberine activates thermogenesis in white and brown adipose tissue. Nat. Commun. 5, 5493. doi:10.1038/ncomms6493
Zhao, M.-M., Lu, J., Li, S., Wang, H., Cao, X., Li, Q., et al. (2021). Berberine is an insulin secretagogue targeting the KCNH6 potassium channel. Nat. Commun. 12 (1), 5616. doi:10.1038/s41467-021-25952-2
Zhong, Y., Jin, J., Liu, P., Song, Y., Zhang, H., Sheng, L., et al. (2020). Berberine attenuates hyperglycemia by inhibiting the hepatic glucagon pathway in diabetic mice. Oxidative Med. Cell. Longev. 2020, 6210526. doi:10.1155/2020/6210526
Zhu, X., Bian, H., Wang, L., Sun, X., Xu, X., Yan, H., et al. (2019). Berberine attenuates nonalcoholic hepatic steatosis through the AMPK-SREBP-1c-SCD1 pathway. Free Radic. Biol. Med. 141, 192–204. doi:10.1016/j.freeradbiomed.2019.06.019
Keywords: berberine, metabolism, potential targets, signaling pathway, molecular docking
Citation: Sun A, Yang H, Li T, Luo J, Zhou L, Chen R, Han L and Lin Y (2024) Molecular mechanisms, targets and clinical potential of berberine in regulating metabolism: a review focussing on databases and molecular docking studies. Front. Pharmacol. 15:1368950. doi: 10.3389/fphar.2024.1368950
Received: 11 January 2024; Accepted: 29 May 2024;
Published: 18 June 2024.
Edited by:
Sonia Piacente, University of Salerno, ItalyReviewed by:
Fang Zhong, Icahn School of Medicine at Mount Sinai, United StatesCopyright © 2024 Sun, Yang, Li, Luo, Zhou, Chen, Han and Lin. This is an open-access article distributed under the terms of the Creative Commons Attribution License (CC BY). The use, distribution or reproduction in other forums is permitted, provided the original author(s) and the copyright owner(s) are credited and that the original publication in this journal is cited, in accordance with accepted academic practice. No use, distribution or reproduction is permitted which does not comply with these terms.
*Correspondence: Lin Han, aGFubGluX21lZEAxNjMuY29t; Yiqun Lin, ODQyODk5NTlAcXEuY29t
†These authors have contributed equally to this work
Disclaimer: All claims expressed in this article are solely those of the authors and do not necessarily represent those of their affiliated organizations, or those of the publisher, the editors and the reviewers. Any product that may be evaluated in this article or claim that may be made by its manufacturer is not guaranteed or endorsed by the publisher.
Research integrity at Frontiers
Learn more about the work of our research integrity team to safeguard the quality of each article we publish.