- 1Department of Experimental Research, Guangxi Medical University Cancer Hospital, Nanning, China
- 2Department of Gastrointestinal Surgery, Guangxi Clinical Research Center for Colorectal Cancer, Guangxi Medical University Cancer Hospital, Nanning, China
Acting as a cysteine protease, small ubiquitin-like modifier (SUMO)/sentrin-specific protease1 (SENP1) involved in multiple physiological and pathological processes through processing the precursor SUMO protein into mature form and deSUMOylating target protein. It has been reported that SENP1 is highly expressed and plays a carcinogenic role in various cancers. In this paper, we mainly explore the function and mechanism of SENP1 in tumor cell proliferation, apoptosis, invasion, metastasis, stemness, angiogenesis, metabolism and drug resistance. Furthermore, the research progress of SENP1 inhibitors for cancer treatment is introduced. This study aims to provide theoretical references for cancer therapy by targeting SENP1.
Introduction
SUMO, discovered in 1996, is widely expressed in eukaryote to regulate target protein localization, activity and the interaction with other biomacromolecule through covalently modifying substrate proteins (Chang and Yeh, 2020). There are five different SUMO proteins encoding by human genome including SUMO1, SUMO2, SUMO3, SUMO4 and SUMO5. SUMO1, SUMO2 and SUMO3 are the main SUMO proteins while the expression of SUMO4 and SUMO5 is restricted to specific tissues (Kukkula et al., 2021). The amino acid sequence between SUMO2 and SUMO3 is 97% homologous, while they share only 50% homology with SUMO1 (Gareau and Lima, 2010). Since SUMO2 and SUMO3 cannot be distinguished by antibody. These two isoforms are collectively referred as SUMO2/3 (Hickey et al., 2012). Different amino acid sequence leads to that SUMO1 and SUMO2/3 modifies different substrates (Shen et al., 2006).
As a crucial protein post-translational modification (PTM), SUMOylation participates in various biological processes including gene expression, DNA replication/repair, RNA processing, and nucleocytoplasmic transport. SUMOylation is a dynamic and reversible enzymatic cascade reaction process which is catalyzed by SUMO-specific activating enzyme (E1; SAE1 and SAE2), conjugating enzyme (E2; Ubc9) and ligating enzyme (E3) (Zhao, 2018). The SUMOylation process includes four phases: maturation, activation, conjugation and ligation (Ryu et al., 2020). The first step in the SUMO conjugation pathway is cleaving their COOH termini via hydrolyzing ATP to expose the diglycine (GG) residues required for conjugation. Second, the mature SUMO protein is activated by binding to activating enzyme E1. And then, the SUMO protein is transferred to conjugating enzyme E2. Finally, SUMO forms isopeptide bonds with the specific lysine residues (K) on substrate with the assistance of ligase E3 (Figure 1). The classic motif of SUMO modification site on target protein is ΨKXE (Ψ is an amino acid whose side chain is hydrophobic and X is any amino acid) (Tatham et al., 2001; Vertegaal, 2022).
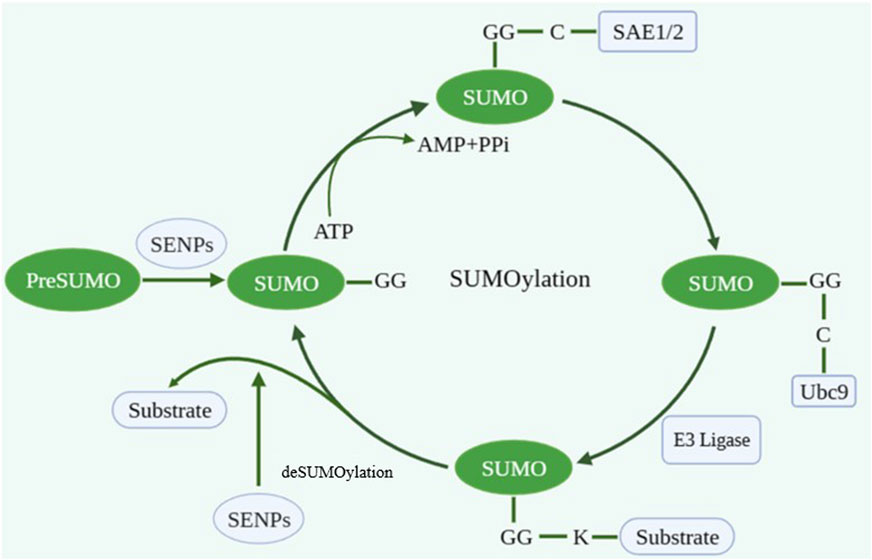
FIGURE 1. The process of SUMOylation. Created with BioRender.com.
The SUMOylation cycle is a dynamic and reversible process. The removal of SUMO protein from substrate protein is known as deSUMOylation, which is mediated by SUMO/sentrin-specific proteases (SENPs). The SENPs family is a class of cysteine proteases, including six members (SENP1, SENP2, SENP3, SENP5, SENP6, and SENP7) (Tokarz and Wozniak, 2021). They all have a conserved protease catalytic domain at the C-terminal while the N-terminal sequence and structure are different (Nayak and Müller, 2014). According to their sequence homology, cellular location and substrate specificity, they are divided into three subfamilies. SENP1 and SENP2, which are capable to deconjugate SUMO1 and SUMO2/3 modification, belong to the first subfamily while the second and the third subfamily of SENPs prefer SUMO2/3 as their substrates. SENP1 is the first mammalian enzyme to be reported and is primarily localized in the nucleus (Bailey and O'Hare, 2004). The homo sapiens SENP1 gene locates on chromosome 12q13.11 (Gong et al., 2000). SENP1 contains triad characteristics of cysteine proteases, consisting of Cys603, His533 and Asp550. It has been reported that SENP1 can process SUMO precursor into mature form and release SUMO protein from target protein. The mutation of the catalytic triad residues can abolish SENP1 functional activity (Liu W. et al., 2023). In recent years, overexpression of SENP1 has been reported in different types of cancers. Imbalance of target protein SUMOylation homeostasis mediated by SENP1 is closely associated with tumor development (Tokarz and Wozniak, 2021). The article will comprehensively summarize the regulatory mechanism of SENP1 and discuss the research progress of its inhibitors, aiming to provide new ideas for cancer therapy.
The expression of SENP1 in cancers
SENP1 was overexpressed in breast cancer cell lines compared to immortalized mammary epithelia MCF10A cells. The expression of SENP1 in breast primary tumor tissues was significantly higher than that in normal tissues (Sun et al., 2018). Data from the Cancer Genome Atlas (TCGA) showed that SENP1 expression was significantly increased in breast tumors compared to adjacent normal tissues. SENP1 high signal is detected in the majority of triple-negative breast cancer (TNBC) clinical samples (Gao et al., 2022). Moreover, SENP1 expression in TNBC tissues is markedly higher than that in non-TNBC tissues (Wang Z. et al., 2016). For lung cancer, the mRNA and protein levels of SENP1 both are upregulated in cancer tissues (Wang RT. et al., 2013). In prostate cancer, SENP1 is overexpressed in prostatic intraepithelial neoplasia and prostate cancer lesion compared to normal prostate epithelia (Bawa-Khalfe et al., 2007). Another research also detected the overexpression of SENP1 in prostate intraepithelial neoplasia lesions and prostate cancer tissues from patients (Wang Q. et al., 2013). For liver cancer, the expression level of SENP1 is obviously higher in tumor tissues than para-carcinoma tissues (Tao et al., 2020). In pancreatic cancer, the SENP1 marker is expressed at high level (Ma et al., 2014). It was also observed that SENP1 was upregulated in colorectal cancer cell lines and clinical samples (Xu et al., 2011; Chen et al., 2021). The integration of GSE12452 and GSE53819 datasets identified eleven SUMOylation regulators whose mRNA expression upregulated in nasopharyngeal carcinoma, including SENP1. SENP1 also expresses highly in hematologic neoplasms. Overexpressed SENP1 is found in leukemia, multiple myeloma (MM) and mantle cell lymphoma (Ohbayashi et al., 2008; Xu et al., 2015; Wang FF. et al., 2016; Zhang et al., 2021; Niu et al., 2022; Liu J. et al., 2023). In conclusion, SENP1 might play an oncogenic role not only in solid tumor, but also in malignant tumor, which deserves further exploring about the specific mechanism.
SENP1 in tumor cell proliferation
Cancer is characterized by the process of normal cell evolving into tumor cell to acquire special abilities, including the ability of tumor cells to proliferate indefinitely due to the severe dysregulation of cell cycle. Therefore, studying the mechanism of unlimited proliferation of tumor cells will help to find new treatment options and strategies. In nasopharyngeal carcinoma, SENP1 overexpression enhances cell viability, cell proliferation rate and cell clonality (Zhang et al., 2023). A study of Wilms tumor showed that overexpressed SENP1 dramatically increased cell proliferate capacity through stimulating Cyclin E1 expression. SENP1 silence diminished the expression of proliferation marker Ki67 and proliferating cell nuclear antigen (PCNA). Flow cytometry proved that SENP1 silence promoted G0/G1 phase arrest and decreased the S phase cell proportion (Zhu et al., 2021). In mantle cell lymphoma, SENP1 inhibits cell proliferation while has no significant effect on cell cycle distribution (Zhang et al., 2021). MicroRNA-186 overexpression directly downregulates SENP1 protein expression and inhibits cell proliferation in renal cell carcinoma (Jiao et al., 2018). The study in hepatocellular carcinoma demonstrated that SENP1 deletion inhibited proliferation and induced cell cycle dysregulation through the deSUMOylation of ubiquitin conjugating enzyme E2 T (UBE2T) (Tao et al., 2020). Overexpressed SENP1 promotes androgen receptor-dependent cell proliferation in prostate cancer cells (Bawa-Khalfe et al., 2007). Knockdown SENP1 significantly attenuates colony formation ability in prostate cancer (Wang Q. et al., 2013). Additionally, microRNA-145 mediates proliferation arrest through suppressing SENP1 activity in prostate cancer (Wang C. et al., 2015). In colorectal cancer, microRNA-133α-3p targets SENP1 to block cell cycle (Zhou GQ. et al., 2018). SENP1 silence upregulates cyclin dependent kinase inhibitors such as p16, p19, p21 and p27, resulting in colorectal cancer cell cycle arrest (Xu et al., 2011). In summary, these results illustrate that SENP1 promotes tumor cell proliferation through various mechanisms.
SENP1 in tumor cell apoptosis
Apoptosis, considered as an important mechanism to prevent tumor progression is an autonomous and orderly form of cell death controlled by specific genes. The resistance of tumor cells to apoptosis is a significant factor in cancer treatment failure (Schmitt, 2003; Hanahan and Weinberg, 2011). Downregulation of SENP1 increases the apoptosis rate of human glioma cells (Zhang QS. et al., 2016). Another research of astroglioma cells indicated that SENP1 knockdown downregulated anti-apoptotic protein B-cell lymphoma-2 (Bcl-2) through blocking nuclear factor kappa-B (NF-кB) signal activation, which promotes cell apoptosis (Xia et al., 2016). Similarly, SENP1 deletion inhibits NF-кB signaling pathway, resulting in tumor cell apoptosis in multiple myeloma (Xu et al., 2015). In osteosarcoma, overexpressed SENP1 decreases the expression of apoptotic protein BCL2-Associated X (BAX) while upregulates Bcl-2, consequently preventing cell apoptosis (Wang et al., 2018). In mantle cell lymphoma (MCL), SENP1 knockdown increases apoptosis by suppressing JAK-STAT5 signal transduction and increasing the expression of tumor suppressor cytokine signaling 2 (SOCS2) (Zhang et al., 2021). Conclusively, these studies suggest that SENP1 leads to apoptosis resistant and promotes cell survival.
SENP1 in tumor invasion and metastasis
Tumor invasion and metastasis are key factors leading to poor prognosis and death of cancer patients. Deeply exploring the specific regulatory mechanism of SENP1 in tumor invasion and metastasis is helpful to limit tumor progression. The biological process by which epithelial cells transform into cells with a mesenchymal phenotype is called epithelial-mesenchymal transition (EMT), which is considered as an important pathway for tumor invasion and metastasis (Pastushenko and Blanpain, 2019). EMT is characterized by downregulation of epithelial markers (cytokeratin and E-cadherin) and upregulation of mesenchymal markers (N-cadherin, vimentin, and fibronectin) (Mittal, 2018). SENP1 has been reported to promote tumor invasion and metastasis in various cancers. In breast cancer, SENP1 inhibition prevents the deSUMOylation of hypoxia-inducible factor 1α (HIF-1α), ultimately leading to HIF-1α degradation and cancer metastasis suppression (Jia et al., 2020). SENP1 directly contributes to the bone metastasis of prostate cancer cells. Mechanistically, SENP1 increases the expression of bone remodeling proteins such as matrix metalloproteinases 2 (MMP2) and matrix metalloproteinases 9 (MMP9) through activating HIF-1α signaling pathway (Wang Q. et al., 2013). Matrix metalloproteinases (MMPs) can facilitate the degradation of basement membrane and promote cell invasion. Studies in neuroblastoma demonstrated that SENP1 silence suppressed tumor invasion and metastasis via downregulating MMP2 and MMP9 levels (Xiang-Ming et al., 2016). The function of SENP1 in invasion and metastasis was also proved in several other cancers including TNBC and pancreatic cancer (Ma et al., 2014; Wang Z. et al., 2016). In TNBC, SENP1 deSUMOylates GATA binding protein 1 (GATA1) at lysine residue K137 and promotes the binding of GATA1 and COP9 signalosome complex subunit 5 (CSN5) promoters. CSN5 is a deubiquitinate ligase of Zinc finger E-box-binding homeobox 1 (ZEB1). Thus, activated CSN5 prevents ZEB1 degradation and maintains its protein stability, resulting in EMT and tumor metastasis (Gao et al., 2022). In prostate cancer, SENP1 silence enhances E-cadherin expression while inhibits vimentin expression (Zhang et al., 2017). In hepatocellular carcinoma, SENP1 knockdown suppresses EMT by increasing the expression of E-cadherin and zonula occludens-1 (ZO-1) while decreasing fibronectin and N-cadherin (Zhang W. et al., 2016). Researchers also found that SENP1 knockdown suppressed the invasive ability of osteosarcoma cells through modulating EMT marked genes (Wang et al., 2018). In nasopharyngeal carcinoma, SENP1 increases STAT1 protein level and promotes its nuclear translocation by inhibiting STAT1 SUMOylation, resulting in cancer invasion and metastasis (Zhang et al., 2023). Additionally, SENP1 facilitates the lung metastasis of colorectal cancer cells (Zhou et al., 2021). For upstream of SENP1, microRNA-1236 abolishes hypoxia-induced EMT through suppressing SENP1 (Chen et al., 2016). Summarily, SENP1 promotes cancer invasion and metastasis mainly through regulating EMT as well as other signaling pathway.
SENP1 in tumor stemness
Cancer stem cells (CSCS) are a group of tumor cells with the ability of self-renewal, which is a crucial factor of cell carcinogenesis and cancer progression. Multiple studies have identified that SENP1 plays an important role in cancer stemness. In hepatocellular carcinoma cells, SENP1 induces stemness-related genes expression such as Oct3/4, Nanog, NOTCH1 and BMI-1 through enhancing the stability and transcriptional activity of HIF-1α, ultimately leading to cancer cell self-renewal (Cui et al., 2017). In renal cell carcinoma cells, overexpressed SENP1 enhances the transcriptional activity of hypoxia-inducible factor 2α (HIF-2α) through deSUMOylation, resulting in stemness-related genes expression increasing (Lee et al., 2022). SENP1 increases the sphere formation ability of hepatocellular carcinoma cells through deSUMOylating HIF-1α (Sun et al., 2023). CD24 is a known marker of liver cancer stem cells. SENP1 overexpression increases CD24+ cell population and upregulates stemness-related genes expression in liver cancer cells (Dai et al., 2023). In glioblastoma, the study observed that SENP1-mediated deSUMOylation of methyltransferase like 3 (METTL3) promoted MYC protein expression, thereby accelerating self-renewal of tumor cells (You et al., 2022). A recent study showed that ubiquitin-specific protease 51 (USP51) could directly bind to Elongin C (ELOC) and form a functional complex with VHL E3 ligase to suppress ubiquitin-dependent proteasomal degradation of HIF-1α. The deSUMOylation of ELOC induced by SENP1 promoted USP51 bind to ELOC and facilitated the deubiquitylation and stabilization of HIF-1α, consequently enhancing colorectal cancer cells stemness (Mu et al., 2023). In summary, SENP1 plays an important role in tumor stemness and malignant development. Targeting SENP1 might be a potential therapeutic target.
SENP1 in tumor angiogenesis
Tumor angiogenesis is a hallmark of malignant transformation of cancer (Hanahan, 2022). Tumor growth is largely dependent on growth of blood vessels and formation of new blood vessels to provide nutrients and oxygen for tumor metabolism as well as remove metabolism waste (Hanahan and Weinberg, 2011). Vascular endothelial growth factors (VEGFs) are critical regulators that stimulate endothelial cells to proliferation, migration, and forming new vessels (Viallard and Larrivée, 2017). SENP1-deficient endothelial cells show increased SUMOylation of VEGFR2 through which impaired VEGFR2 signaling pathway. Mechanistically, SUMOylation retains VEGFR2 in Golgi and reduces its membrane surface distribution, thereby reducing angiogenesis in endothelial cells. However, VEGFR2 will be deconjugated rapidly and transported to plasma membrane for strong angiogenesis response when SENP1 expression exists (Zhou HJ. et al., 2018). In addition, SENP1 can increase the expression and secretion of VEGFs under hypoxic condition (Xu et al., 2010; Wang et al., 2018; Zhou and Sun, 2020). Similarly, SENP1 allows HIF-1α to escape degradation and maintain stability, subsequently facilitating the transcription of downstream gene VEGF under hypoxia through which further induces angiogenesis of adjacent glomerular endothelial cells via binding VEGFR2 (Wang L. et al., 2015). Furthermore, overexpression SENP1 in a mouse model enhances HIF-1α protein stability, which increases VEGF production and angiogenesis (Bawa-Khalfe et al., 2010). Fibroblast growth factor (FGF2) is recognized as the first discovered proangiogenic molecule, which facilitates angiogenesis through activating FGF receptor 1 (FGFR1) signal in endothelial cells (Lee et al., 2023). Researchers demonstrated that SENP1-induced deSUMOylation of FGFR1 acted as a crucial mechanism in response to proangiogenic stimuli (Zhu et al., 2022). NOTCH pathway is a prominent negative regulator of endothelial sprouting and vascular growth (Benedito et al., 2009). SENP1 deletion promotes NOTCH1 to cleavage and stabilize NOTCH1 intracellular domain (N1ICD) for translocation to nucleus through enhancing the SUMOylation of NOTCH1, consequently activating NOTCH signal and resulting in less angiogenesis (Zhu et al., 2017). In conclusion, SENP1 plays an important role in endothelia angiogenesis through various pathway. However, the direct evidence of SENP1 function in tumor angiogenesis requires further investigation.
SENP1 in tumor metabolism
Tumor growth mainly obtains energy through glycolytic pathway. Studies revealed that SENP1 played an essential role in Warburg effect. SENP1 and HIF-1α interacts with each other to regulate tumorigenesis through enhancing glycolysis in prostatic carcinoma cells (Wang et al., 2019). Another research also implicated that SENP1 contributed to tumor metabolism in prostate cancer cells. Hexokinase 2 (HK2) is a crucial molecule that activates the glycolysis pathway of tumor cells. The study found that SENP1 induced HK2 deSUMOylation and facilitated HK2 to combine with mitochondria. This process greatly consumes glucose and produces lactic acid, thereby supporting cancer cell proliferation (Shangguan et al., 2021). In renal clear cell carcinoma, there is a positive connection between SENP1 and glycolysis level. SENP1 enhances HIF-1α protein stability, which further promotes the expression of key glycolysis enzymes and increases glycolysis flux (Dong et al., 2016). Conclusively, SENP1 participates in tumor metabolism mainly through regulating glycolytic pathway.
SENP1 in tumor drug resistance
At present, drug resistance is a great challenge affecting therapy efficacy and prognosis of cancer patients. SENP1 has been reported as a promising therapeutic target to overcome drug resistance in a variety of cancers. In colorectal cancer, SENP1 drives chemotherapy resistance through reducing RNF168 SUMOylation. On the contrary, disrupting SENP1 enhances sensitivity to chemotherapeutic drugs (Wei et al., 2023). Overexpressed SENP1 facilitates JAK2 to accumulate in cytoplasm through deSUMOylating JAK2, thereby activating JAK2/STAT3 signaling transduction and leading to platinum therapy resistance (Li et al., 2021). The deSUMOylation of HK2 mediated by SENP1 desensitizes chemotherapy response in prostate cancer cells with docetaxel treatment (Shangguan et al., 2021). SENP1 activates sirtuin 3 (SIRT3) by preventing its proteasome degradation through which exacerbates resistance against chemotherapy in acute myeloid leukemia (AML) cells (Zhang et al., 2022). In MM cells, SENP1 is regarded as a key modifier of steroid receptor coactivator-3 (SRC-3) stability. SENP1-mediated SRC-3 deSUMOylation attenuates its K11-linked polyubiquitination and thus SENP1 knockdown accelerates the degradation of SRC-3 and remarkably overcomes resistance to proteasome inhibitors (Guo et al., 2022). Furthermore, SENP1 was identified as a desensitization factor for cisplatin treatment in ovarian cancer (Ao et al., 2015). Targeting SENP1 can significantly reduce irinotecan resistance in colorectal cancer patients (Chen et al., 2021). Non-small cell lung cancer (NSCLC) patients with higher SENP1 expression show lower rates of complete response, higher partial and non-response rate to chemoradiotherapy (Liu et al., 2018). The expression of SENP1 is dramatically increases in multidrug-resistant hepatocellular carcinoma cells (Qin et al., 2014). MicroRNA-122 targets SENP1 to decrease drug-resistance protein levels (p-glycoprotein and multidrug resistance protein), thereby attenuating chemoresistance (Dai et al., 2023). In summary, SENP1 promotes drug resistance in various cancers through different molecule mechanism.
The prognostic value of SENP1 in cancer
Many researches have showed that SENP1 has prognostic value in various cancers. In Wilms tumor, the overall survival (OS) and disease-free survival (DFS) of patients with high SENP1 expression are dramatically shorter than those with low SENP1 expression (Zhu et al., 2021). High level of SENP1 predicts poor prognosis in prostate cancer patients (Shangguan et al., 2021). The positive expression of SENP1 in prostate cancer patients is significantly associated with poor survival (Li et al., 2013). Plasma exosome-derived SENP1 is a potential prognostic biomarker in osteosarcoma. Patients with higher SENP1 content have worse prognosis and lower survival rate (Wang et al., 2021). Similarly, melanoma patients with high plasma exosome-derived SENP1 level have shorter OS and DFS. SENP1 high expression represents larger tumor volume, deeper invasion extent, later pathological stage, and distant metastasis (Hu et al., 2021). In bladder cancer, the level of SENP1 in urine can detect tumor recurrence (Brems-Eskildsen et al., 2010). In AML, SENP1 decreases after induction therapy and its reduction predicts low disease risk, favorable treatment response, and long survival (Liu J. et al., 2023). Multivariate Cox regression analysis showed that SENP1 was an independent prognostic factors for the survival of NSCLC patients (Mu et al., 2014). Also, SENP1 is able to be used as a prognostic biomarker for glioblastoma (Li and Meng, 2021). In addition, high expression of SENP1 was strongly related to poor prognosis in renal cell carcinoma, nasopharyngeal carcinoma and colorectal cancer (Lee et al., 2022; Wei et al., 2023; Zhang et al., 2023). Collectively, these findings clarify that SENP1 is of great value in cancers prognosis.
Inhibitors of SENP1
SENP1 plays a crucial role in tumor progression and is expected to be a critical target for cancer therapy (Figure 2). Therefore, the development of SENP1 inhibitors might bring new hope for cancer treatment. In recent years, tremendous efforts have been spent in SENP1 inhibitors study and development. Next, we will summarize the progress of SENP1 inhibitors in this paper, aiming to explore beneficial treatments for cancers (Table 1).
Momordin Ιc (Mc), a natural pentacyclic triterpenoid, presents a good inhibitory activity of SENP1 with an IC50 value of 15.37 μM. The cellular thermal shift assay (CETSA) and the drug affinity responsive target stability (DARTS) assay show that Mc directly interacts with SENP1 and inhibits its activity. Mc inhibits proliferation and promotes apoptosis in prostate cancer cells. However, SENP1 overexpression reversals Mc-induced proliferation inhibition and apoptosis. In nude mouse xenograft model, Mc significantly attenuates tumor growth (Wu et al., 2016). Furthermore, Mc treatment dramatically overcomes platinum-resistance in ovarian cancer (Li et al., 2021). In colorectal cancer, Mc induces cell cycle arrest and apoptosis through inhibiting SENP1-mediated c-Myc deSUMOylation (Xianjun et al., 2021).
Triptolide, an active component extracted from the Chinese herb Tripterygium wilfordii Hook F, has been reported to exhibit antitumor effects in various cancers. In prostate cancer, Triptolide decreases SENP1 mRNA and protein levels in both dose-dependent and time-dependent manner, resulting in cellular SUMOylation level increasing. In vitro, triptolide inhibits cell proliferation and induces cell apoptosis. And in vivo, triptolide decreases the weight and volume of xenograft tumors, which indicates that Triptolide has potent anti-tumor effect through suppressing SENP1 (Huang et al., 2012).
Hinokiflavone belongs to a plant flavonoid family, which has antitumor effects. Studies found that Hinokiflavone induced cell cycle arrest and cell death in both cervical cancer and acute promyelocytic leukemia. Hinokiflavone can inhibit target protein deSUMOylation through directly binding to SENP1 and inhibiting its activity (Pawellek et al., 2017).
Bethanidine, a strong adrenergic neuron blocking factor, is commonly used to treat heart failure in clinical practice. Through performing molecular docking and molecular dynamics simulation of Bethanidine with SENP1, researchers found that Betanidine constituted a stable complex with SENP1 to inhibit its activity. Although the specific mechanism needs to be further studied, these data provide the possibility of Bethanidine targeting SENP1 for cancer therapy (Taghvaei et al., 2022).
Vialinin A and thelephantin G are para-terphenyl compounds isolated from the edible Chinese mushroom Thelephora vialis. Both of them can inhibit the enzymatic activity of SENP1. Vialinin A and thelephantin G inhibit full-length recombinant human SENP1 (rhSENP1) activity with IC50 values of 1.64 ± 0.23 μM and 2.48 ± 0.02 μM. The IC50 values of Vialinin A and thelephantin G inhibit catalytic domain human SENP1 (cSENP1) are 1.89 ± 0.04 μM and 1.52 ± 0.06 μM, respectively. The structure-activity relationship (SAR) implicates that two benzyl acetyl moieties and two phenyl acetyl moieties of vialinin A and thelephantin G play an important role in inhibiting SENP1 activity. Moreover, the inhibition of SENP1 activity requires the 2-hydroxymoiety of p-terphenyl skeleton. The catechol moiety of center benzyl ring of p-terphenyl skeleton is also critical (Yoshioka et al., 2016).
Streptonigrin (SN) is a natural product isolated from Streptomyces flocculus. Recently, SN was identified as a small molecule inhibitor of SENP1. The study tested the SENPs−SUMO inhibition potency of SN by using different combinations of SENPs and AMC-tagged fluorogenic SUMO substrates. When SUMO1 acted as a substrate, SN preferred to inhibit SENP1 activity (IC50 = 0.518 ± 0.100 μM) rather than SENP2 activity (IC50 = 6.919 ± 0.676 μM). In order to investigate the specificity of SN, they tested SENP6 activity through using SUMO2−AMC as a substrate. The result proved that the inhibitory effect of SN on SENP6 was similar to that on SENP2 (IC50 = 5.205 ± 0.853 μM). These results indicate that SN has higher SENP1 inhibitory effect compared with other SENPs (Ambaye et al., 2018).
Gallic acid (GA), an endogenous plant polyphenol, exists abundantly in tea and fruits. This compound exerts antitumor effects through regulating cell cycle, cell apoptosis, angiogenesis, invasion and metastasis. By performing molecular docking and molecular dynamics simulation, studies found that GA suppressed SENP1 catalytic activity through directly interacting with its active site. GA shows high stability, high hydrogen bonds, high binding energy and the highest intermolecular bonds with SENP1. Moreover, Gallic acid has lower toxicity than Mc, indicating that Gallic acid is an ideal SENP1 inhibitor in cancer treatment. However, these data were obtained by computational analysis and still required experimental verification (Taghvaei et al., 2021).
Camptothecin is a pentacyclic monoterpene alkaloid isolated from the Chinese tree Camptotheca acuminate. Topotecan and irinotecan, two camptothecin derivatives, are currently used as anti-tumor drugs in clinic. Topotecan is mainly used in ovarian and lung cancer. And Irinotecan is commonly used in colorectal cancer therapy. In acute lymphoblastic leukemia (ALL), Topotecan was found to inhibit cells proliferation through SENP1 reduction. 7-ethylcamptothecin (7E-CPT), another camptothecin derivative, can downregulate SENP1 mRNA and protein levels, which suppresses ALL cells proliferation and induced apoptosis (Niu et al., 2022).
Ursolic acid (UA) is a natural pentacyclic triterpene which has been identified as an effective SENP1 inhibitor with an IC50 value of 2.58 μM. One study examined the SENP1 inhibitory efficiency of 29 commercially available natural ursane-type aglycones. Pomolic acid and tormentic acid are identified as effective SENP1 inhibitors with IC50 values of 5.1 μM and 4.3 μM. They can reverse cisplatin resistance greatly in ovarian cancer cells. Compared with cisplatin (IC50 = 28.23 μM) only, the IC50 values of cisplatin decreased to 3.69 μM (with pomolic acid) and 2.40 μM (with tormentic acid), which indicates the synergy effect between cisplatin and pomolic acid or tormentic acid (Wei et al., 2022a). However, some medicinal defects such as poor water solubility, rapid metabolism, and low bioavailability limit their clinical application. Another research designed a series of pentacyclic triterpene derivatives based on the structure of UA. Introducing hydrophilic or basic side-chain moieties is beneficial to improve the pharmaceutical properties of UA, and it is a rational drug design strategy in structure modifications based on pentacyclic triterpenes. The molecular docking experiment showed that the 3-hydroxyl group was the key structure for the interaction between UA and SENP1, and the 28-carboxyl group pointed to the solvent region. Therefore, they retained the 3-hydroxyl group and modified the 28-carboxyl group with hydrophilic amide to synthesize compound 1 which could inhibit SENP1 in a dose-dependent manner with an IC50 value of 0.51 μM. Researchers also demonstrated that UA and its derivatives could increase the radiosensitivity of HeLa cells. There is a dramatic positive correlation between SENP1 inhibitory activity and the sensitivity enhancement ratio (SER). Compound 1 presents the best radiosensitive activity with a SER value of 1.45. This is the first time to develop small molecule SENP1 inhibitors as radiosensitizers, which is of great significance for its application in tumor radiotherapy (Wei et al., 2022b).
Another research reported the design, synthesis and biological evaluation of SENP1 inhibitors based on benzodiazepines. In the crystal structure of SENP1 complexed with immature SUMO1, the catalytic Cys603 is located in a cleft. Once substrate binds, the cleft will be closed to form a channel-like structure. The cleft is occupied by the terminal peptide tail of precursor SUMO, leading to numerous hydrogen bonds formation. They observed that the benzodiazepine core structure could attach into the catalytic cleft and mimic the conformation of substrate peptides. The formyl group binds Cys603 and forms a covalent bond. Also, the substitution at the C–NH2 position might have an orientation similar to that of substrate peptides. Thus, they synthesized a series of carboxamides with extended substituents on the phenyl. Compound 2 was synthesized by installing NHCbz at the C (4′) position of the phenyl. They found its activity improved obviously with an IC50 value of 15.5 μM. The research further synthesized compound 3 through transferring the NHCbz group to the C (3’) position. Compound 3 showed higher activity (IC50 = 9.2 μM). In order to evaluate the inhibitory effect of compound 3, they subjected it to the SUMO-ΔRanGAP cleavage assay and found that compound 3 attenuated the enzymatic hydrolysis of SUMO-ΔRanGAP through SENP1 inhibition. In addition, they detected the inhibitory effect of them in prostate cancer cells. The IC50 values of compound 2 and compound 3 against prostate cancer cells were calculated to be 13.0 μM and 35.7 μM. This is the first report about designing and synthesizing SENP1 inhibitors, which opens the way for further research of SENP1 inhibitors based on benzodiazepines (Qiao et al., 2011).
Studies have identified eleven SENP1 inhibitors with various scaffolds through silico screening. Based on their structure, a series of new SENP1 inhibitors were designed and synthesized. Compound 4 (IC50 = 3.5 μM) has the highest SENP1 inhibitory effect (Zhao et al., 2016). They also developed many benzothiophene-2-carboxamide inhibitors based on protein structures of SENP1, SENP2, and SENP5. Compound 5 presented the best inhibitory potency with IC50 values of 1.3 μM (SENP1), 0.7 μM (SENP2), and 22.7 μM (SENP5) (Wang et al., 2020).
Masaharu et al. developed 1-[4-(N-benzylamino)phenyl]-3-phenylurea derivatives based on the HIF-1α inhibitor (GN6797) as SENP1 inhibitors. GN6797 was found to interact SENP1 directly by pull-down assay. In order to detect the effect on SENP1 enzyme activity, they combined GN6767 with SENP1 catalytic domain and incubated with fluorogenic SUMO-1-AMC (7-amino-4-methylcoumarin). GN6767 showed 40% inhibition of SENP1 endopeptidase activity at 100 μM concentration. However, this inhibition disappeared at 50 μM concentration. They found that the methyl substituent could increase the inhibitory potency of SENP1. GN6860 and GN6958 inhibit SENP1 protease activity in a concentration-dependent manner. GN6860 showed 74% inhibition of SENP1 endopeptidase activity at 50 μM concentration. GN6958 exhibited 97% inhibition at the same concentration. Their IC50 values are 39.5 ± 0.8 μM and 29.6 ± 0.5 μM, respectively. The study further demonstrated that GN6958 displayed no significant inhibition toward other proteases in the range of 10–100 μM concentrations such as trypsin, chymotripsin and thermolysin. These results suggest that GN6958 is a selective inhibitor of SENP1 enzymatic activity (Uno et al., 2012).
Chen et al. screened 38 compounds from SPECS library by using virtual screening and docking methods. The study identified compound 6 as the highest inhibitory potency of SENP1 with an IC50 value of 2.4 μM. Compound 6 can embed well in the binding site composed with Trp465, Asp468, Phe496, His529, Val532, Gln597 and Cys603. The amide nitrogen and carbonyl oxygen of it bonds with the side chain nitrogen of Gln597 and epsilon 2 nitrogen of His529, respectively, which stabilizes its extended binding, resulting in SENP1 inhibition. Thus, the research further designed and synthesized a series of 2-(4-Chlorophenyl)-2-oxoethyl 4-benzamidobenzoate derivatives based on compound 6. Considering the perpendicular π-π interaction between meta benzene-ring substitute and the phenyl ring of Phe496, they designed compound 7 through replacing the meta methoxy with a benzoxy, which showed efficient inhibition effect of SENP1 (IC50 = 1.080 ± 0.010 μM). Compound 8, 9, 10 were obtained by replacing the meta methoxy with a nitro, a fluorine and a bromine, respectively. These compounds all exhibited enhanced inhibitory potency of SNEP1 with IC50 values of 1.856 ± 0.205 μM, 1.735 ± 0.020 μM and 1.175 ± 0.033 μM, respectively (Chen et al., 2012).
Madu et al. screened 250,000 compound libraries and found the most potent compounds against SENP1 contained sulfonyl-benzene group. They found the inhibitory potency of these compounds depended on both enzyme and substrate. When SUMO-1 precursor acted as a substrate, four compounds (SPI-01 to SPI-04) inhibited SNEP2 more effectively than SENP1. However, for the cleavage of SUMO-2 precursor, they displayed similar inhibitory effect toward SENP1 and SENP2. They further tested SUMOylated proteins in Hela cells with SPI-01 treatment and performed heat-shock experiments. The result showed that SPI-01 had the inhibition of intracellular SENPs. The nuclear magnetic resonance (NMR) and quantitative enzyme kinetic analysis implicated that the inhibition mechanism was mainly non-competitive manner. These findings provide the possibility of designing substrate specific SENPs inhibitors (Madu et al., 2013).
Another study designed a new class of non-covalent SENP1 inhibitors based on pyridone scaffold via virtual design strategy. When SUMO1-AMC acted as a substrate, compound 11 showed an IC50 of 22 μM (with 0.1% BSA) and 3.7 μM (with 0.01% CHAPS) against SENP1. Compound 12 was obtained by replacing methyl amide with nitrile group. Compound 12 showed a larger difference in IC50 values when measured with different buffer additives. Compound 13 was obtained through replacing the central ester with an amide bond based on compound 11. To investigate the substrate specificity of SENP1 inhibitors, they detected the inhibitory concentrations when SUMO1, SUMO2 and SUMO3 acted as substrates, respectively. Compound 12 showed no selectivity on different SUMO substrates. Compound 11 and 13 exhibited 10-fold and 3-fold inhibitory activities on SUMO1 than on SUMO2 and SUMO3, respectively (Lindenmann et al., 2020).
Conclusion and future perspectives
In this review, we summarized the function and mechanism of SENP1 in tumor proliferation, apoptosis, invasion, metastasis, stemness, angiogenesis, metabolism and drug resistance. Studies demonstrated that SENP1 was significantly overexpressed in many malignant tumors. Moreover, SENP1 acts as a promotive role in the occurrence and development of cancers through the deSUMOylation of target proteins. Previous researches have found that there is an interaction between ubiquitination and SUMOylation, which is critical for protein stability and activity. However, it is still unclear whether SUMOylation and other post-translational modifications exist similar interactions. The relation between SENP1-mediated deSUMOylation and protein translocation or distribution need more effort to investigate. In terms of angiogenesis, several studies have demonstrated the significant role of SENP1 in blood vessel growth and new neovascularization. However, the direct function of SENP1 in tumor angiogenesis has not been elucidated. These might be important directions for future research in cancer treatment targeting angiogenesis. Studies showed that SENP1 could be used as a novel prognostic marker and a potential therapeutic target for cancers. Thus, we should be committed to understand the action mechanism of SENP1 in cancers, which will help the development of antitumor drugs targeting SENP1. In recent years, many SENP1 inhibitors have been identified, designed and synthesized. Most of them have no entered clinical trials. SENP1 inhibitors are mainly natural products and synthetic compounds. Their species and quantity are very rich and have broad application prospects. Meanwhile, natural inhibitors have advantages of high activity, low toxicity, wide plant sources and low price as anti-cancer drugs. However, the application of SENP1 natural inhibitors faces many challenges and difficulties. For example, they have disadvantages such as poor water solubility, fast metabolism and low bioavailability. These defects limit them from becoming practical clinical drugs. Therefore, how to improve drug property through rational structural modification is a crucial problem. To date, many small molecule SENP1 inhibitors have been designed and synthesized based on the structure of natural products. Most of them are obtained by computational analysis and screening of compound libraries. Furthermore, the inhibitory potency of them is usually detected by molecular docking, molecular dynamics simulation or examination in vitro. However, they lack validation in cell experiments and animal models, which is a long way from practical application. In summary, directly targeting SENP1 promises to be a constructive anticancer strategy. Deeply exploring the action mechanism of its inhibitors and optimizing drug efficacy will provide more effective treatment options for tumorigenesis. Developing effective inhibitors targeting SENP1 and applying them to clinical practice are directions for future efforts in cancer therapy.
Author contributions
ML: Writing–original draft, Writing–review and editing. MZ: Visualization, Writing–review and editing. BY: Visualization, Writing–review and editing. JC: Writing–review and editing. SW: Writing–review and editing. RC: Writing–review and editing. TC: Writing–review and editing. ZL: Conceptualization, Funding acquisition, Project administration, Supervision, Writing–review and editing.
Funding
The author(s) declare financial support was received for the research, authorship, and/or publication of this article. This work is supported by the National Natural Science Foundation of China (82170421 and82360464), Guangxi Science and Technology Base and Talent Special Project (2021AC19098), Youth Project of Guangxi Natural Science Foundation (2022JJB140663) and Outstanding Young Talent Training Program of Guangxi Medical University.
Conflict of interest
The authors declare that the research was conducted in the absence of any commercial or financial relationships that could be construed as a potential conflict of interest.
Publisher’s note
All claims expressed in this article are solely those of the authors and do not necessarily represent those of their affiliated organizations, or those of the publisher, the editors and the reviewers. Any product that may be evaluated in this article, or claim that may be made by its manufacturer, is not guaranteed or endorsed by the publisher.
References
Ambaye, N., Chen, C. H., Khanna, S., Li, Y. J., and Chen, Y. (2018). Streptonigrin inhibits SENP1 and reduces the protein level of hypoxia-inducible factor 1α (HIF1α) in cells. Biochemistry 57 (11), 1807–1813. doi:10.1021/acs.biochem.7b00947
Ao, Q., Su, W., Guo, S., Cai, L., and Huang, L. (2015). SENP1 desensitizes hypoxic ovarian cancer cells to cisplatin by up-regulating HIF-1α. Sci. Rep. 5, 16396. doi:10.1038/srep16396
Bailey, D., and O'Hare, P. (2004). Characterization of the localization and proteolytic activity of the SUMO-specific protease, SENP1. SENP1. J. Biol. Chem. 279 (1), 692–703. doi:10.1074/jbc.M306195200
Bawa-Khalfe, T., Cheng, J., Lin, S. H., Ittmann, M. M., and Yeh, E. T. (2010). SENP1 induces prostatic intraepithelial neoplasia through multiple mechanisms. J. Biol. Chem. 285 (33), 25859–25866. doi:10.1074/jbc.M110.134874
Bawa-Khalfe, T., Cheng, J., Wang, Z., and Yeh, E. T. H. (2007). Induction of the SUMO-specific protease 1 transcription by the androgen receptor in prostate cancer cells. J. Biol. Chem. 282 (52), 37341–37349. doi:10.1074/jbc.M706978200
Benedito, R., Roca, C., Sörensen, I., Adams, S., Gossler, A., Fruttiger, M., et al. (2009). The notch ligands Dll4 and Jagged1 have opposing effects on angiogenesis. Cell 137 (6), 1124–1135. doi:10.1016/j.cell.2009.03.025
Brems-Eskildsen, A. S., Zieger, K., Toldbod, H., Holcomb, C., Higuchi, R., Mansilla, F., et al. (2010). Prediction and diagnosis of bladder cancer recurrence based on urinary content of hTERT, SENP1, PPP1CA, and MCM5 transcripts. BMC Cancer 10, 646. doi:10.1186/1471-2407-10-646
Chang, H. M., and Yeh, E. T. H. (2020). SUMO: from bench to bedside. Physiol. Rev. 100 (4), 1599–1619. doi:10.1152/physrev.00025.2019
Chen, M. C., Nhan, D. C., Hsu, C. H., Wang, T. F., Li, C. C., Ho, T. J., et al. (2021). SENP1 participates in Irinotecan resistance in human colon cancer cells. J. Cell Biochem. 122 (10), 1277–1294. doi:10.1002/jcb.29946
Chen, S. Y., Teng, S. C., Cheng, T. H., and Wu, K. J. (2016). miR-1236 regulates hypoxia-induced epithelial-mesenchymal transition and cell migration/invasion through repressing SENP1 and HDAC3. Cancer Lett. 378 (1), 59–67. doi:10.1016/j.canlet.2016.05.006
Chen, Y., Wen, D., Huang, Z., Huang, M., Luo, Y., Liu, B., et al. (2012). 2-(4-Chlorophenyl)-2-oxoethyl 4-benzamidobenzoate derivatives, a novel class of SENP1 inhibitors: virtual screening, synthesis and biological evaluation. Bioorg. Med. Chem. Lett. 22 (22), 6867–6870. doi:10.1016/j.bmcl.2012.09.037
Cui, C. P., Wong, C. C., Kai, A. K., Ho, D. W., Lau, E. Y., Tsui, Y. M., et al. (2017). SENP1 promotes hypoxia-induced cancer stemness by HIF-1α deSUMOylation and SENP1/HIF-1α positive feedback loop. Gut 66 (12), 2149–2159. doi:10.1136/gutjnl-2016-313264
Dai, J., Hao, Y., Chen, X., Yu, Q., and Wang, B. (2023). miR-122/SENP1 axis confers stemness and chemoresistance to liver cancer through Wnt/β-catenin signaling. Oncol. Lett. 26 (3), 390. doi:10.3892/ol.2023.13976
Dong, B., Gao, Y., Kang, X., Gao, H., Zhang, J., Guo, H., et al. (2016). SENP1 promotes proliferation of clear cell renal cell carcinoma through activation of glycolysis. Oncotarget 7 (49), 80435–80449. doi:10.18632/oncotarget.12606
Gao, Y., Wang, R., Liu, J., Zhao, K., Qian, X., He, X., et al. (2022). SENP1 promotes triple-negative breast cancer invasion and metastasis via enhancing CSN5 transcription mediated by GATA1 deSUMOylation. Int. J. Biol. Sci. 18 (5), 2186–2201. doi:10.7150/ijbs.60594
Gareau, J. R., and Lima, C. D. (2010). The SUMO pathway: emerging mechanisms that shape specificity, conjugation and recognition. Nat. Rev. Mol. Cell Biol. 11 (12), 861–871. doi:10.1038/nrm3011
Gong, L., Millas, S., Maul, G. G., and Yeh, E. T. (2000). Differential regulation of sentrinized proteins by a novel sentrin-specific protease. J. Biol. Chem. 275 (5), 3355–3359. doi:10.1074/jbc.275.5.3355
Guo, J., Lv, Y., Wang, S., Peng, Z., Xie, Y., Wang, Y., et al. (2022). Hypoxia induces chemoresistance to proteasome inhibitors through orchestrating deSUMOylation and ubiquitination of SRC-3 in multiple myeloma. Oncogene 41 (45), 4971–4979. doi:10.1038/s41388-022-02494-5
Hanahan, D. (2022). Hallmarks of cancer: new dimensions. Cancer Discov. 12 (1), 31–46. doi:10.1158/2159-8290.CD-21-1059
Hanahan, D., and Weinberg, R. A. (2011). Hallmarks of cancer: the next generation. Cell 144 (5), 646–674. doi:10.1016/j.cell.2011.02.013
Hickey, C. M., Wilson, N. R., and Hochstrasser, M. (2012). Function and regulation of SUMO proteases. Nat. Rev. Mol. Cell Biol. 13 (12), 755–766. doi:10.1038/nrm3478
Hu, H., Ling, B., Shi, Y., Wu, H., Zhu, B., Meng, Y., et al. (2021). Plasma exosome-derived SENP1 may Be a potential prognostic predictor for melanoma. Front. Oncol. 11, 685009. doi:10.3389/fonc.2021.685009
Huang, W., He, T., Chai, C., Yang, Y., Zheng, Y., Zhou, P., et al. (2012). Triptolide inhibits the proliferation of prostate cancer cells and down-regulates SUMO-specific protease 1 expression. PLoS One 7 (5), e37693. doi:10.1371/journal.pone.0037693
Jia, Y., Guo, Y., Jin, Q., Qu, H., Qi, D., Song, P., et al. (2020). A SUMOylation-dependent HIF-1α/CLDN6 negative feedback mitigates hypoxia-induced breast cancer metastasis. J. Exp. Clin. Cancer Res. 39 (1), 42. doi:10.1186/s13046-020-01547-5
Jiao, D., Wu, M., Ji, L., Liu, F., and Liu, Y. (2018). MicroRNA-186 suppresses cell proliferation and metastasis through targeting sentrin-specific protease 1 in renal cell carcinoma. Oncol. Res. 26 (2), 249–259. doi:10.3727/096504017X14953948675430
Kukkula, A., Ojala, V. K., Mendez, L. M., Sistonen, L., Elenius, K., and Sundvall, M. (2021). Therapeutic potential of targeting the SUMO pathway in cancer. Cancers (Basel). 13 (17), 4402. doi:10.3390/cancers13174402
Lee, C., Chen, R., Sun, G., Liu, X., Lin, X., He, C., et al. (2023). VEGF-B prevents excessive angiogenesis by inhibiting FGF2/FGFR1 pathway. Signal Transduct. Target. Ther. 8 (1), 305. doi:10.1038/s41392-023-01539-9
Lee, M. H., Sung, K., Beebe, D., Huang, W., Shapiro, D., Miyamoto, S., et al. (2022). The SUMO protease SENP1 promotes aggressive behaviors of high HIF2α expressing renal cell carcinoma cells. Oncogenesis 11 (1), 65. doi:10.1038/s41389-022-00440-4
Li, J., Wu, R., Yung, M. M. H., Sun, J., Li, Z., Yang, H., et al. (2021). SENP1-mediated deSUMOylation of JAK2 regulates its kinase activity and platinum drug resistance. Cell Death Dis. 12 (4), 341. doi:10.1038/s41419-021-03635-6
Li, T., Huang, S., Dong, M., Gui, Y., and Wu, D. (2013). Prognostic impact of SUMO-specific protease 1 (SENP1) in prostate cancer patients undergoing radical prostatectomy. Urol. Oncol. 31 (8), 1539–1545. doi:10.1016/j.urolonc.2012.03.007
Li, X., and Meng, Y. (2021). SUMOylation regulator-related molecules can Be used as prognostic biomarkers for glioblastoma. Front. Cell Dev. Biol. 9, 658856. doi:10.3389/fcell.2021.658856
Lindenmann, U., Brand, M., Gall, F., Frasson, D., Hunziker, L., Kroslakova, I., et al. (2020). Discovery of a class of potent and selective non-competitive sentrin-specific protease 1 inhibitors. ChemMedChem 15 (8), 675–679. doi:10.1002/cmdc.202000067
Liu, J., Li, Y., and Zhang, G. (2023b). SUMO specific peptidase 1 decreases after induction treatment, and its reduction predicts lower disease risk, better treatment response, longer survival of acute myeloid leukemia. Scand. J. Clin. Lab. Invest. 83 (5), 283–289. doi:10.1080/00365513.2023.2175237
Liu, K., Zhang, J., and Wang, H. (2018). Small ubiquitin-like modifier/sentrin-specific peptidase 1 associates with chemotherapy and is a risk factor for poor prognosis of non-small cell lung cancer. J. Clin. Lab. Anal. 32 (9), e22611. doi:10.1002/jcla.22611
Liu, W., Wang, Y., Bozi, L. H. M., Fischer, P. D., Jedrychowski, M. P., Xiao, H., et al. (2023a). Lactate regulates cell cycle by remodelling the anaphase promoting complex. Nature 616 (7958), 790–797. doi:10.1038/s41586-023-05939-3
Ma, C., Wu, B., Huang, X., Yuan, Z., Nong, K., Dong, B., et al. (2014). SUMO-specific protease 1 regulates pancreatic cancer cell proliferation and invasion by targeting MMP-9. Tumor Biol. 35 (12), 12729–12735. doi:10.1007/s13277-014-2598-1
Madu, I. G., Namanja, A. T., Su, Y., Wong, S., Li, Y.-J., and Chen, Y. (2013). Identification and characterization of a new chemotype of noncovalent SENP inhibitors. ACS Chem. Biol. 8 (7), 1435–1441. doi:10.1021/cb400177q
Mittal, V. (2018). Epithelial mesenchymal transition in tumor metastasis. Annu. Rev. Pathol. 13, 395–412. doi:10.1146/annurev-pathol-020117-043854
Mu, J., Zuo, Y., Yang, W., Chen, Z., Liu, Z., Tu, J., et al. (2014). Over-expression of small ubiquitin-like modifier proteases 1 predicts chemo-sensitivity and poor survival in non-small cell lung cancer. Chin. Med. J. Engl. 127 (23), 4060–4065. doi:10.3760/cma.j.issn.0366-6999.20141013
Mu, M., Zhang, Q., Li, J., Zhao, C., Li, X., Chen, Z., et al. (2023). USP51 facilitates colorectal cancer stemness and chemoresistance by forming a positive feed-forward loop with HIF1A. Cell Death Differ. 30, 2393–2407. doi:10.1038/s41418-023-01228-8
Nayak, A., and Müller, S. (2014). SUMO-specific proteases/isopeptidases: SENPs and beyond. Genome Biol. 15 (7), 422. doi:10.1186/s13059-014-0422-2
Niu, Q., Hou, W., Yan, Y., Sun, S., Lin, Y., Fang, H., et al. (2022). Antileukemic effects of topoisomerase I inhibitors mediated by de-SUMOylase SENP1. Biochimica Biophysica Acta (BBA) - Mol. Basis Dis. 1868 (12), 166492. doi:10.1016/j.bbadis.2022.166492
Ohbayashi, N., Kawakami, S., Muromoto, R., Togi, S., Ikeda, O., Kamitani, S., et al. (2008). The IL-6 family of cytokines modulates STAT3 activation by desumoylation of PML through SENP1 induction. Biochem. Biophysical Res. Commun. 371 (4), 823–828. doi:10.1016/j.bbrc.2008.04.179
Pastushenko, I., and Blanpain, C. (2019). EMT transition States during tumor progression and metastasis. Trends Cell Biol. 29 (3), 212–226. doi:10.1016/j.tcb.2018.12.001
Pawellek, A., Ryder, U., Tammsalu, T., King, L. J., Kreinin, H., Ly, T., et al. (2017). Characterisation of the biflavonoid hinokiflavone as a pre-mRNA splicing modulator that inhibits SENP. Elife 6, e27402. doi:10.7554/eLife.27402
Qiao, Z., Wang, W., Wang, L., Wen, D., Zhao, Y., Wang, Q., et al. (2011). Design, synthesis, and biological evaluation of benzodiazepine-based SUMO-specific protease 1 inhibitors. Bioorg. Med. Chem. Lett. 21 (21), 6389–6392. doi:10.1016/j.bmcl.2011.08.101
Qin, Y., Bao, H., Pan, Y., Yin, M., Liu, Y., Wu, S., et al. (2014). SUMOylation alterations are associated with multidrug resistance in hepatocellular carcinoma. Mol. Med. Rep. 9 (3), 877–881. doi:10.3892/mmr.2014.1882
Ryu, H.-Y., Ahn, S. H., and Hochstrasser, M. (2020). SUMO and cellular adaptive mechanisms. Exp. Mol. Med. 52 (6), 931–939. doi:10.1038/s12276-020-0457-2
Schmitt, C. A. (2003). Senescence, apoptosis and therapy — cutting the lifelines of cancer. Nat. Rev. Cancer 3 (4), 286–295. doi:10.1038/nrc1044
Shangguan, X., He, J., Ma, Z., Zhang, W., Ji, Y., Shen, K., et al. (2021). SUMOylation controls the binding of hexokinase 2 to mitochondria and protects against prostate cancer tumorigenesis. Nat. Commun. 12 (1), 1812. doi:10.1038/s41467-021-22163-7
Shen, L., Tatham, M. H., Dong, C., Zagórska, A., Naismith, J. H., and Hay, R. T. (2006). SUMO protease SENP1 induces isomerization of the scissile peptide bond. Nat. Struct. Mol. Biol. 13 (12), 1069–1077. doi:10.1038/nsmb1172
Sun, W., Lei, X., Lu, Q., Wu, Q., Ma, Q., Huang, D., et al. (2023). LncRNA FRMD6-AS1 promotes hepatocellular carcinoma cell migration and stemness by regulating SENP1/HIF-1α axis. Pathol. Res. Pract. 243, 154377. doi:10.1016/j.prp.2023.154377
Sun, X. X., Chen, Y., Su, Y., Wang, X., Chauhan, K. M., Liang, J., et al. (2018). SUMO protease SENP1 deSUMOylates and stabilizes c-Myc. Proc. Natl. Acad. Sci. U. S. A. 115 (43), 10983–10988. doi:10.1073/pnas.1802932115
Taghvaei, S., Minuchehr, Z., and Sabouni, F. (2022). Computational drug repurposing of bethanidine for SENP1 inhibition in cardiovascular diseases treatment. Life Sci. 292, 120122. doi:10.1016/j.lfs.2021.120122
Taghvaei, S., Sabouni, F., Minuchehr, Z., and Taghvaei, A. (2021). Identification of novel anti-cancer agents, applying in silico method for SENP1 protease inhibition. J. Biomol. Struct. Dyn. 40 (14), 6228–6242. doi:10.1080/07391102.2021.1880480
Tao, Y., Li, R., Shen, C., Li, J., Zhang, Q., Ma, Z., et al. (2020). SENP1 is a crucial promotor for hepatocellular carcinoma through deSUMOylation of UBE2T. Aging (Albany NY) 12 (2), 1563–1576. doi:10.18632/aging.102700
Tatham, M. H., Jaffray, E., Vaughan, O. A., Desterro, J. M. P., Botting, C. H., Naismith, J. H., et al. (2001). Polymeric chains of SUMO-2 and SUMO-3 are conjugated to protein substrates by SAE1/SAE2 and Ubc9. J. Biol. Chem. 276 (38), 35368–35374. doi:10.1074/jbc.M104214200
Tokarz, P., and Wozniak, K. (2021). SENP proteases as potential targets for cancer therapy. Cancers (Basel) 13 (9), 2059. doi:10.3390/cancers13092059
Uno, M., Koma, Y., Ban, H. S., and Nakamura, H. (2012). Discovery of 1-[4-(N-benzylamino)phenyl]-3-phenylurea derivatives as non-peptidic selective SUMO-sentrin specific protease (SENP)1 inhibitors. Bioorg. Med. Chem. Lett. 22 (16), 5169–5173. doi:10.1016/j.bmcl.2012.06.084
Vertegaal, A. C. O. (2022). Signalling mechanisms and cellular functions of SUMO. Nat. Rev. Mol. Cell Biol. 23 (11), 715–731. doi:10.1038/s41580-022-00500-y
Viallard, C., and Larrivée, B. (2017). Tumor angiogenesis and vascular normalization: alternative therapeutic targets. Angiogenesis 20 (4), 409–426. doi:10.1007/s10456-017-9562-9
Wang, C., Tao, W., Ni, S., and Chen, Q. (2019). SENP1 interacts with HIF1α to regulate glycolysis of prostatic carcinoma cells. Int. J. Biol. Sci. 15 (2), 395–403. doi:10.7150/ijbs.27256
Wang, C., Tao, W., Ni, S., Chen, Q., Zhao, Z., Ma, L., et al. (2015a). Tumor-suppressive microRNA-145 induces growth arrest by targeting SENP1 in human prostate cancer cells. Cancer Sci. 106 (4), 375–382. doi:10.1111/cas.12626
Wang, F. F., Liu, M. Z., Sui, Y., Cao, Q., Yan, B., Jin, M. L., et al. (2016b). Deficiency of SUMO-specific protease 1 induces arsenic trioxide-mediated apoptosis by regulating XBP1 activity in human acute promyelocytic leukemia. Oncol. Lett. 12 (5), 3755–3762. doi:10.3892/ol.2016.5162
Wang, L., Wu, J., Song, S., Chen, H., Hu, Y., Xu, B., et al. (2021). Plasma exosome-derived sentrin SUMO-specific protease 1: a prognostic biomarker in patients with osteosarcoma. Front. Oncol. 11, 625109. doi:10.3389/fonc.2021.625109
Wang, L., Zhang, T., Fang, M., Shen, N., Wang, D., Teng, J., et al. (2015b). Podocytes protect glomerular endothelial cells from hypoxic injury via deSUMOylation of HIF-1α signaling. Int. J. Biochem. Cell Biol. 58, 17–27. doi:10.1016/j.biocel.2014.10.030
Wang, Q., Xia, N., Li, T., Xu, Y., Zou, Y., Zuo, Y., et al. (2013b). SUMO-specific protease 1 promotes prostate cancer progression and metastasis. Oncogene 32 (19), 2493–2498. doi:10.1038/onc.2012.250
Wang, R. T., Zhi, X. Y., Zhang, Y., and Zhang, J. (2013a). Inhibition of SENP1 induces radiosensitization in lung cancer cells. Exp. Ther. Med. 6 (4), 1054–1058. doi:10.3892/etm.2013.1259
Wang, X., Liang, X., Liang, H., and Wang, B. (2018). SENP1/HIF-1α feedback loop modulates hypoxia-induced cell proliferation, invasion, and EMT in human osteosarcoma cells. J. Cell Biochem. 119 (2), 1819–1826. doi:10.1002/jcb.26342
Wang, Z., Jin, J., Zhang, J., Wang, L., and Cao, J. (2016a). Depletion of SENP1 suppresses the proliferation and invasion of triple-negative breast cancer cells. Oncol. Rep. 36 (4), 2071–2078. doi:10.3892/or.2016.5036
Wang, Z., Liu, Y., Zhang, J., Ullah, S., Kang, N., Zhao, Y., et al. (2020). Benzothiophene-2-carboxamide derivatives as SENPs inhibitors with selectivity within SENPs family. Eur. J. Med. Chem. 204, 113918. doi:10.1016/j.ejmech.2021.113918
Wei, H., Guo, J., Sun, X., Gou, W., Ning, H., Fang, Z., et al. (2022b). Discovery and radiosensitization research of ursolic acid derivatives as SENP1 inhibitors. Eur. J. Med. Chem. 227, 113918. doi:10.1016/j.ejmech.2021.113918
Wei, H., Guo, J., Sun, X., Gou, W., Ning, H., Shang, H., et al. (2022a). Discovery of natural ursane-type SENP1 inhibitors and the platinum resistance reversal activity against human ovarian cancer cells: a structure–activity relationship study. J. Nat. Prod. 85 (5), 1248–1255. doi:10.1021/acs.jnatprod.1c01166
Wei, M., Huang, X., Liao, L., Tian, Y., and Zheng, X. (2023). SENP1 decreases RNF168 phase separation to promote DNA damage repair and drug resistance in colon cancer. Cancer Res. 83 (17), 2908–2923. doi:10.1158/0008-5472.CAN-22-4017
Wu, J., Lei, H., Zhang, J., Chen, X., Tang, C., Wang, W., et al. (2016). Momordin Ic, a new natural SENP1 inhibitor, inhibits prostate cancer cell proliferation. Oncotarget 7 (37), 58995–59005. doi:10.18632/oncotarget.10636
Xia, W., Tian, H., Cai, X., Kong, H., Fu, W., Xing, W., et al. (2016). Inhibition of SUMO-specific protease 1 induces apoptosis of astroglioma cells by regulating NF-κB/Akt pathways. Gene 595 (2), 175–179. doi:10.1016/j.gene.2016.09.040
Xiang-Ming, Y., Zhi-Qiang, X., Ting, Z., Jian, W., Jian, P., Li-Qun, Y., et al. (2016). SENP1 regulates cell migration and invasion in neuroblastoma. Biotechnol. Appl. Biochem. 63 (3), 435–440. doi:10.1002/bab.1375
Xianjun, F., Xirui, X., Jie, T., Huiwen, M., Shaojun, Z., Qiaoyun, L., et al. (2021). Momordin Ic induces G0/1 phase arrest and apoptosis in colon cancer cells by suppressing SENP1/c-MYC signaling pathway. J. Pharmacol. Sci. 146 (4), 249–258. doi:10.1016/j.jphs.2021.04.007
Xu, J., Sun, H. Y., Xiao, F. J., Wang, H., Yang, Y., Wang, L., et al. (2015). SENP1 inhibition induces apoptosis and growth arrest of multiple myeloma cells through modulation of NF-κB signaling. Biochem. Biophys. Res. Commun. 460 (2), 409–415. doi:10.1016/j.bbrc.2015.03.047
Xu, Y., Li, J., Zuo, Y., Deng, J., Wang, L.-S., and Chen, G.-Q. (2011). SUMO-specific protease 1 regulates the in vitro and in vivo growth of colon cancer cells with the upregulated expression of CDK inhibitors. Cancer Lett. 309 (1), 78–84. doi:10.1016/j.canlet.2011.05.019
Xu, Y., Zuo, Y., Zhang, H., Kang, X., Yue, F., Yi, Z., et al. (2010). Induction of SENP1 in endothelial cells contributes to hypoxia-driven VEGF expression and angiogenesis. J. Biol. Chem. 285 (47), 36682–36688. doi:10.1074/jbc.M110.164236
Yoshioka, Y., Namiki, D., Makiuchi, M., Sugaya, K., Onose, J.-i., Ashida, H., et al. (2016). Vialinin A and thelephantin G, potent inhibitors of tumor necrosis factor-α production, inhibit sentrin/SUMO-specific protease 1 enzymatic activity. Bioorg. Med. Chem. Lett. 26 (17), 4237–4240. doi:10.1016/j.bmcl.2016.07.051
You, J., Tao, B., Peng, L., Peng, T., He, H., Zeng, S., et al. (2022). Transcription factor YY1 mediates self-renewal of glioblastoma stem cells through regulation of the SENP1/METTL3/MYC axis. Cancer Gene Ther. 30 (5), 683–693. doi:10.1038/s41417-022-00580-0
Zhang, J., Tan, G.-l., Jiang, M., Wang, T.-s., Liu, G.-h., Xiong, S.-s., et al. (2023). Effects of protease in soybean meal-reduced diets on growth performance, nutrient digestibility, and intestinal health of weaned piglets. Cell. Signal. 14, 101. doi:10.3390/ani14010101
Zhang, Q. S., Zhang, M., Huang, X. J., Liu, X. J., and Li, W. P. (2016a). Downregulation of SENP1 inhibits cell proliferation, migration and promotes apoptosis in human glioma cells. Oncol. Lett. 12 (1), 217–221. doi:10.3892/ol.2016.4558
Zhang, W., Sun, H., Shi, X., Wang, H., Cui, C., Xiao, F., et al. (2016b). SENP1 regulates hepatocyte growth factor-induced migration and epithelial-mesenchymal transition of hepatocellular carcinoma. Tumour Biol. 37 (6), 7741–7748. doi:10.1007/s13277-015-4406-y
Zhang, X., Wang, H., Wang, H., Xiao, F., Seth, P., Xu, W., et al. (2017). SUMO-specific cysteine protease 1 promotes epithelial mesenchymal transition of prostate cancer cells via regulating SMAD4 deSUMOylation. Int. J. Mol. Sci. 18 (4), 808. doi:10.3390/ijms18040808
Zhang, Y., Ma, Y., Wu, G., Xie, M., Luo, C., Huang, X., et al. (2021). SENP1 promotes MCL pathogenesis through regulating JAK-STAT5 pathway and SOCS2 expression. Cell Death Discov. 7 (1), 192. doi:10.1038/s41420-021-00578-x
Zhang, Y., Shen, Y., Wei, W., Wang, W., Jiang, D., Ren, Y., et al. (2022). Dysregulation of SIRT3 SUMOylation confers AML chemoresistance via controlling HES1-dependent fatty acid oxidation. Int. J. Mol. Sci. 23 (15), 8282. doi:10.3390/ijms23158282
Zhao, X. (2018). SUMO-mediated regulation of nuclear functions and signaling processes. Mol. Cell 71 (3), 409–418. doi:10.1016/j.molcel.2018.07.027
Zhao, Y., Wang, Z., Zhang, J., and Zhou, H. (2016). Identification of SENP1 inhibitors through in silico screening and rational drug design. Eur. J. Med. Chem. 122, 178–184. doi:10.1016/j.ejmech.2016.06.018
Zhou, G. Q., Han, F., Shi, Z. L., Yu, L., Li, X. F., Yu, C., et al. (2018a). miR-133a-3p targets SUMO-specific protease 1 to inhibit cell proliferation and cell cycle progress in colorectal cancer. Oncol. Res. 26 (5), 795–800. doi:10.3727/096504017X15004613574679
Zhou, H. J., Xu, Z., Wang, Z., Zhang, H., Zhuang, Z. W., Simons, M., et al. (2018b). SUMOylation of VEGFR2 regulates its intracellular trafficking and pathological angiogenesis. Nat. Commun. 9 (1), 3303. doi:10.1038/s41467-018-05812-2
Zhou, J., and Sun, C. (2020). SENP1/HIF-1α axis works in angiogenesis of human dental pulp stem cells. J. Biochem. Mol. Toxicol. 34 (3), e22436. doi:10.1002/jbt.22436
Zhou, M., Bian, Z., Liu, B., Zhang, Y., Cao, Y., Cui, K., et al. (2021). Long noncoding RNA MCM3AP-AS1 enhances cell proliferation and metastasis in colorectal cancer by regulating miR-193a-5p/SENP1. Cancer Med. 10 (7), 2470–2481. doi:10.1002/cam4.3830
Zhu, S., Hu, J., Cui, Y., Liang, S., Gao, X., Zhang, J., et al. (2021). Knockdown of SENP1 inhibits HIF-1α SUMOylation and suppresses oncogenic CCNE1 in Wilms tumor. Mol. Ther. Oncolytics 23, 355–366. doi:10.1016/j.omto.2021.07.007
Zhu, X., Ding, S., Qiu, C., Shi, Y., Song, L., Wang, Y., et al. (2017). SUMOylation negatively regulates angiogenesis by targeting endothelial NOTCH signaling. Circ. Res. 121 (6), 636–649. doi:10.1161/CIRCRESAHA.117.310696
Keywords: SENP1, deSUMOylation, proliferation and apoptosis, invasion and metastasis, stemness, angiogenesis, metabolism, drug resistance
Citation: Lin M, Zhang M, Yi B, Chen J, Wen S, Chen R, Chen T and Li Z (2024) Emerging role of SENP1 in tumorigenesis and cancer therapy. Front. Pharmacol. 15:1354323. doi: 10.3389/fphar.2024.1354323
Received: 12 December 2023; Accepted: 31 January 2024;
Published: 08 February 2024.
Edited by:
Qingbin Cui, University of Toledo College of Medicine and Life Sciences, United StatesReviewed by:
Jing Pei, St. Jude Children’s Research Hospital, United StatesSong Yizuo, First Affiliated Hospital of Wenzhou Medical University, China
Yang Tian, University of Arkansas, United States
Copyright © 2024 Lin, Zhang, Yi, Chen, Wen, Chen, Chen and Li. This is an open-access article distributed under the terms of the Creative Commons Attribution License (CC BY). The use, distribution or reproduction in other forums is permitted, provided the original author(s) and the copyright owner(s) are credited and that the original publication in this journal is cited, in accordance with accepted academic practice. No use, distribution or reproduction is permitted which does not comply with these terms.
*Correspondence: Zhao Li, emhhb2xpMTk4OTA0MTFAMTYzLmNvbQ==
†These authors share first authorship