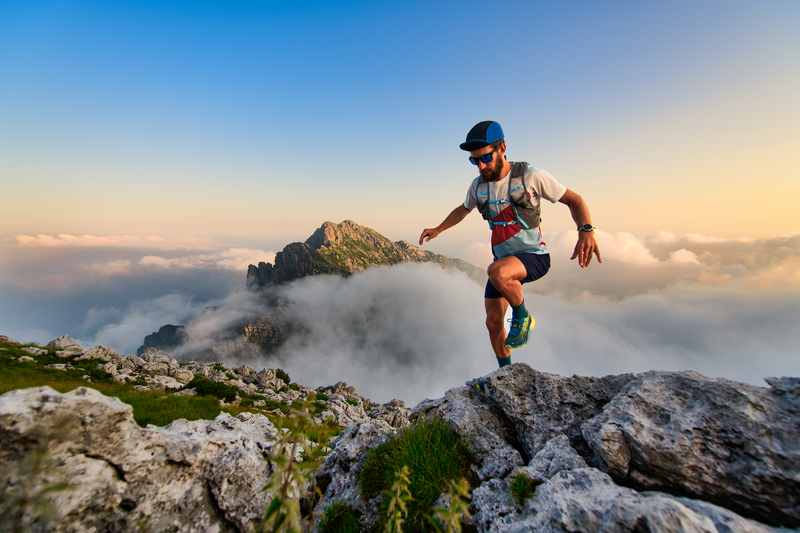
94% of researchers rate our articles as excellent or good
Learn more about the work of our research integrity team to safeguard the quality of each article we publish.
Find out more
REVIEW article
Front. Pharmacol. , 15 February 2024
Sec. Inflammation Pharmacology
Volume 15 - 2024 | https://doi.org/10.3389/fphar.2024.1345779
A wound takes a long time to heal and involves several steps. Following tissue injury, inflammation is the primary cause of tissue regeneration and repair processes. As a result, the pathophysiological processes involving skin damage, healing, and remodeling depend critically on the control of inflammation. The fact that it is a feasible target for improving the prognosis of wound healing has lately become clear. Mesenchymal stem cells (MSCs) are an innovative and effective therapeutic option for wound healing due to their immunomodulatory and paracrine properties. By controlling the inflammatory milieu of wounds through immunomodulation, transplanted MSCs have been shown to speed up the healing process. In addition to other immunomodulatory mechanisms, including handling neutrophil activity and modifying macrophage polarization, there may be modifications to the activation of T cells, natural killer (NK) cells, and dendritic cells (DCs). Furthermore, several studies have shown that pretreating MSCs improves their ability to modulate immunity. In this review, we summarize the existing knowledge about how MSCs influence local inflammation in wounds by influencing immunity to facilitate the healing process. We also provide an overview of MSCs optimizing techniques when used to treat wounds.
The biggest multipurpose organ in the human body, the skin, acts as a barrier to keep out harmful materials and creatures from the external environment, such as toxins and physical assaults (Yang J. et al., 2020). A wound is a physical harm that happens when the skin deforms due to damage to the skin’s internal and exterior components (Ayavoo et al., 2021). Wound healing can be impacted by several variables, including infection, foreign substances, necrosis, and comorbidities, even though the skin can restore itself to its normal condition and function (Falanga, 2005; Han and Ceilley, 2017). Prolonged wound closure raises the possibility of bacterial colonization, invasion, and even sepsis, all of which can be fatal for patients (Lemsanni et al., 2021; Soh, 2021).
Traditional wound treatment options comprise the application of antibiotics, various dressings, debridement, laser therapy, biological scaffolds, topical administration of specific growth factors, skin/flap grafting, gene therapy, and negative-pressure suction. These techniques have certain drawbacks, such as the lengthy treatment duration, high cost, and significant psychological and financial stress placed on patients (Qin et al., 2023). The initiation of tissue regeneration and repair is commonly attributed to the activation of the inflammatory response (Zhu et al., 2023). During the whole healing process, the initial stage is the inflammatory reaction. Inflammation is caused by the invasion of an area by inflammatory cells, which activates both the innate and adaptive immune systems (Gentek and Hoeffel, 2017; Rieckmann et al., 2019). Excessive inflammatory reactions can lead to severe pathological changes, including scarring and impeded wound healing. In contrast, well-controlled inflammation often promotes wound healing (Xu et al., 2012; Ogawa, 2017; Wang Z.-C. et al., 2020; Yu et al., 2021; Zhang et al., 2021). Thus, it is critical for wound healing to identify a treatment regimen that effectively modulates immune-related cells and cytokines at the site of damage to reduce inflammation at the appropriate time point (Hong et al., 2023).
Recent years have seen a notable advancement in the field of study on mesenchymal stem cells’ (MSCs) ability to promote wound healing. A subset of pluripotent stem cells known as MSCs was found in the bone marrow stroma during the 1960s. When transplanted into ectopic areas, these cells may be able to generate new bone (Friedenstein et al., 1970; Bianco et al., 2013). MSCs are thought to be the best seed cells for tissue engineering, according to recent research (Tsiapalis and O’Driscoll, 2020; Khayambashi et al., 2021). They may be extracted from a variety of tissues, such as the skin, bone marrow, adipose, umbilical cord, and dental pulp. In tissue injury, it not only has an extremely strong self-proliferation, multidirectional differentiation ability, and powerful secretion ability, but more importantly, it has an immunomodulatory function that can form a favorable inflammatory microenvironment at the site of tissue injury, release growth factors, and activate endogenous tissue repair (Wang et al., 2014; Wang et al., 2022). Existing evidence suggests that MSCs-based therapies can control and reduce inflammation but not replace damaged cells. MSCs have been used, for example, to alter organ inflammation at different tissue locations in the early stages of treating acute and chronic liver damage. For COVID-19, intravenous infusion of MSCs has also been suggested as a treatment to suppress inflammatory storms in the lungs (Verkhratsky et al., 2020). As a result, the use of MSCs in regenerative medicine must highlight both their limited ability to differentiate and their role in immunomodulation, which entails establishing a supportive immune environment and secreting growth factors to promote endogenous tissue healing (Wang et al., 2014).
However, the relationship between MSCs and inflammation is complex. It has been suggested that the immune regulation of MSCs is not intrinsic but is triggered by inflammation and is dynamically influenced by the type and amount of inflammatory cytokines (Ren et al., 2008; Wang et al., 2014). As an alternative, MSCs might respond to changes in tissue pathophysiological signals in an active manner. This would mobilize many components of the tissue immune microenvironment to achieve inflammation control, which would then aid in tissue regeneration and repair (Wang et al., 2014; Gao et al., 2021; Soliman et al., 2021). Currently, in vitro-amplified MSCs are a hotspot for therapeutic research, but there are still some outstanding problems. It has been discovered that in vitro-amplified MSCs are quickly identified and eliminated by the host’s immune system upon exogenous delivery. In addition, when MSCs are administered to some tissues with low inflammatory status, changes in their inflammatory microenvironment may lead to unstable efficacy of MSCs. These problems are considerable obstacles to its application. Therefore, knowing how to treat in vitro-expanded MSCs before transplantation is significant to improve their clinical efficacy. To increase the therapeutic effects of MSCs, many researchers have recently investigated diverse bioengineering methodologies and approaches for optimizing and pre-treating MSCs (Wang et al., 2022).
We have reviewed recent studies on the mechanisms of the role of MSCs in wound healing. In particular, we focused on the immunomodulatory role mediated by MSCs during the inflammatory phase. This article also presents a review of studies that strive to increase the therapeutic potential of MSCs and analyzes their implications for optimizing MSCs usage for tissue regeneration in clinical settings.
Dynamic and multifaceted interactions between a variety of cells, growth factors, cytokines, chemokines, and other chemicals are necessary for wound healing. Three stages are involved in the recovery of wounds: proliferative, remodeling, and hemostatic/inflammatory (Gurtner et al., 2008; Hong et al., 2023). These stages occur sequentially, although they also overlap. To initiate tissue repair and regeneration, the hemostatic/inflammatory phase is essential (Zhu et al., 2023). The sudden creation of a platelet-fibrin clot at the wound site is known as hemostasis, and it is triggered by severe skin damage. It involves almost immediate platelet aggregation and vasoconstriction (Clark, 2003; Zeng and Liu, 2021). This clot stops blood flow, lessens subsequent blood loss, and acts as a scaffold for the migration of immune and resident skin cells (Reish and Eriksson, 2008). Therefore, the histamine, bradykinin, and leukotrienes produced by nearby mast cells draw immune cells and widen blood vessels (Hong et al., 2023). Neutrophils are activated after skin injury as “first responders” by recruiting “find me” signals from the blood (Zhu et al., 2023). Several signals are produced by the wounded or infected area, including lipid mediators, hydrogen peroxide, damage-associated molecular patterns (DAMPs), calcium waves, and chemokines (Su and Richmond, 2015). Many tactics are used by activated neutrophils to degrade pathogens by proteolytic hydrolysis and oxidative bursts of microbicidal reactive oxygen species (ROS) released. This process is known as neutrophil extracellular traps (NETs) formation (Clark et al., 2007; Kolaczkowska and Kubes, 2013; Lood et al., 2016). Activated neutrophils also use phagocytosis and expel DNA decorated with particles from proteolytic hydrolysis to immobilize and eventually kill pathogens. Macrocytizing macrophages phagocytize neutrophils that have undergone extensive apoptosis in the final stages of inflammation. Inflammatory monocytes are drawn from the peripheral circulation 48–96 h after injury, and when they get to the site of damage, they change into macrophages (Geissmann et al., 2010). Macrophages (also called M1 type) are essential during the early stages of wound healing because they help promote angiogenesis and fibroplasia, synthesize nitric oxide (NO), and move the wound from an inflammatory to a proliferative phase. By producing pro-inflammatory cytokines, including tumor necrosis factor alpha (TNF-α), interleukin-1β (IL-1β), and IL-6, they can also fight off infection. Reducing inflammation and restoring tissue homeostasis is made possible by macrophages’ ability to develop anti-inflammatory qualities and support tissue healing. The anti-inflammatory type M2 phenotype is developed as a result of this change. In response to IL-4/IL-13 or apoptotic neutrophils, type M2 macrophages produce platelet-derived growth factor (PDGF), transforming growth factor-β (TGF-β), insulin-like growth factor-1 (IGF-1), and vascular endothelial growth factor (VEGF), which encourages the proliferation of keratinocytes and fibroblasts (Russell et al., 2019). Dendritic cells (DCs) provide antigens, which stimulate T-cell responses. Helper T-cells 1 (Th1), which have proinflammatory effects during the acute inflammatory phase of the wound, are primarily driven by interferon-γ (IFN-γ). The T cells get more and more polarized throughout the inflammatory phase, which eventually turns into a Th2 response. These cells produce IL-4, IL-5, IL-10, and IL-13, which are anti-inflammatory and also have significant pro-fibrotic effects (Wallace et al., 1994; Ong et al., 1999). Th17 cells induce an immune response that overlaps with Th1 and Th2 immune responses, promoting follicular neogenesis and pro-inflammatory effects through neutrophil recruitment and wound re-epithelialization (Zhu et al., 2023). Moreover, it has been found that regulatory T cells (Tregs) promote tissue homeostasis and regeneration after tissue injury by reducing excessive inflammation (Zhu et al., 2023) Figure 1.
FIGURE 1. Immune cells in wound healing. Neutrophils directly phagocytose pathogens during wound healing, release proteases for hydrolysis, and create neutrophil extracellular traps (NETs) to engulf them–activation of monocytes into macrophages. Early inflammation causes pathogens to be phagocytosed by macrophages. In order to combat infection, M1-type macrophages emit proinflammatory molecules such as IL-6, IL-1β, and TNF-α. They also release monocyte chemokine-1 (MCP-1) to draw in more monocytes. Macrophages phagocytose apoptotic neutrophils later in the inflammatory process. M2-type macrophages stimulate angiogenesis and extracellular matrix deposition by releasing VEGF-γ and TGF-β. Macrophages phagocytose extracellular matrix and remnants of cells during remodeling. Certain DCs activate T cells, which subsequently differentiate into CD4+ and CD8+ T cells, respectively. Additionally, plasmacytoid DCs (PDC) in injured skin are triggered to generate interferon-α (IFN-α), a critical component of the acute inflammatory response. Tryptase and histamine are produced by mast cells.
While an overabundance of inflammation leads to scarring, delayed wound healing, and perhaps severe pathological changes, a moderate amount of inflammation encourages wound repair (Xu et al., 2012; Ogawa, 2017; Wang Z.-C. et al., 2020; Yu et al., 2021; Zhang et al., 2021). Inflammation is a necessary component in the healing of wounds. The length and severity of the inflammatory response affect how well the lesion heals. Consequently, in order to facilitate wound healing, it is crucial to preserve a suitable and timely balance between the inflammatory and reparative phases (Hong et al., 2023). Targeting pathways that either lower inflammation or trigger repair has been the focus of intense research over the past 30 years in an effort to enhance the prognosis of wound healing/. Examples of these efforts include the use of curcumin (Akbik et al., 2014), neurotensin (NT) (Liu et al., 2019), photodynamic therapy (PDT) (Yang T. et al., 2020), silver nanoparticles (AgNPs) (Choudhury et al., 2020), and others.
Curcumin’s ability to lower inflammation is supported by the majority of research (Akbik et al., 2014). By inhibiting the synthesis of TNF-α and IL-1 as well as the activation of the nuclear factor κ light chain enhancer (NF- κ B) in activated B-cells, curcumin controls inflammation in wounds (Joe et al., 2004). As the primary source of inflammation during wound healing activity, Gopinath et al. (2004) discovered that curcumin had a scavenging impact on ROS. Unfortunately, curcumin’s quick metabolism, low solubility, high photosensitivity, and low bioavailability restrict its medicinal usefulness (Akbik et al., 2014).
NT is also a critical immunomodulator that modulates cellular functions in innate and adaptive immunity (Evers et al., 1994; Ramez et al., 2001; Bernatchez et al., 2013). Moura et al. (2014) showed that the expression of IL-1β was reduced in mice treated with NT and NT-loaded collagen, which promoted wound healing. Additional research is required to address this issue as NT therapy alone has little therapeutic potential, as does NT-collagen loading treatment. However, it dramatically accelerates wound healing and leaves more noticeable scars once recovery is complete (Moura et al. (2014).
PDT has drawn a lot of interest as a wound therapy method in recent years. Research has indicated that PDT creates pro-inflammatory cytokines such as IL-1, lipids, and a local acute inflammatory response (Gollnick et al., 2006; Brackett and Gollnick, 2011; Anzengruber et al., 2015). It also dramatically influences neutrophil activation. However, 5-aminolevulinic acid (ALA) -PDT treatment was effective in diminishing inflammatory infiltration with relatively low expression levels of proinflammatory cytokines, such as TNF-α, IL-1β, and IL-23, according to Yang et al. (2021). In the mid-to-late phases of the wound-healing process, PDT therapy can decrease chronic inflammation and increase acute inflammation early in the healing phase (Yang T. et al., 2020). This indicates that PDT therapy can regulate inflammation and facilitate wound healing. However, PDT has some limitations, including pain and discomfort during treatment, long treatment duration, and instability of treatment results (Cottrell et al., 2008; Syed Azhar et al., 2018; Kaw et al., 2020), and its limited optical depth limits its application in deep tissue therapy (Li and Lin, 2022).
The anti-inflammatory activity of AgNPs has also been demonstrated in skin wounds (Tian et al., 2007; Nadworny et al., 2008; Liu X. et al., 2014; Wasef et al., 2020). It has been found that increased expression of type I collagen alpha 1 chain (Col1A1) suppressed inflammatory cytokines (IL-1α and IL-6), and inflammation was maintained at low equilibrium levels after treatment with AgNPs, effectively facilitating the healing process and decreasing the risk of chronicity in wounds (Shahverdi et al., 2007). The keratinocyte growth factor/p38 mitogen-activated protein kinase (KGF-2/p38MAPK) signaling pathway, which AgNPs mediate, controls the inflammatory response by promoting the production of early pro-inflammatory cytokines while inhibiting the early phase of the skin wound anti-inflammatory cytokine, IL-10, following injury. Zhang et al. (2020) showed that while early inflammation is crucial, prolonged inflammation is harmful to wound healing. In fact, giving mice a little late injection of AgNPs to reduce inflammation actually aided in their ability to repair wounds. However, in wounds, cell types are diverse, and the microenvironment is spatially heterogeneous, so targeting specific inflammatory factors or signaling pathways does not achieve great effectiveness (Gurtner et al., 2008; Forrester et al., 2009; Wang et al., 2022).
Therefore, the modulation of the inflammatory microenvironment is crucial in promoting wound healing. MSCs are gradually becoming the focus of promoting wound repair by modulating the inflammatory microenvironment of wounds from multiple mechanisms.
Previous research has indicated that MSCs’ primary capacity to facilitate wound healing may stem from their secretion of pro-regenerative cytokines (Garg et al., 2014). As per the latest research, MSCs could suppress immune responses in the presence of enriched inflammatory cytokines, including infection, trauma, or immune-mediated diseases. These immunomodulatory properties were discovered in preclinical and clinical trials (Margiana et al., 2022). Therefore, when it comes to the use of in vitro-expanded MSCs in regenerative medicine, we shouldn’t overemphasize their limited differentiation potential (“cell replacement”). Instead, we should concentrate on a range of responses that MSCs have to injury signals, such as migration, immunomodulation, growth factor release, tissue remodeling, etc. Growth factors are released to start endogenous tissue healing (“cellular empowerment”) and to create an environment that is favorable for the immune system (Wang et al., 2014). Specifically, MSCs exert their inflammatory modulatory effects mainly relying on direct cell-to-cell communication and paracrine secretion (Keshtkar et al., 2018). Almost all immune cells, including mast cells, natural killer (NK) cells, DCs, T lymphocytes, and so on, can interact with and change the activity of MSCs in order to restore immunological homeostasis and prevent the immune response from spiraling out of control (Wang et al., 2014; Shi et al., 2018a; Han et al., 2022). Paracrine effects are thought to be the primary mechanism of their immunomodulation (Qiu et al., 2018; Qin et al., 2023). By releasing a range of growth factors, inflammatory modulators, and extracellular vesicles (e.g., exosomes (Exos)), the local inflammatory response can be regulated by MSCs after they enter an inflammatory environment (Shi et al., 2018b). MSCs positively impact wound healing, which is supported by their capacity to control the inflammatory response to wounds. The number of inflammatory cells and pro-inflammatory cytokines was dramatically reduced by human umbilical cord-derived MSCs (e.g., IL-1, IL-6, and TNF-α) in a rat model of severe burns, according to Liu L. et al. (2014). Crucially, these wounds also showed the higher speed of healing and shorter recovery periods (Liu L. et al., 2014). Further studies have shown that Exos in the MSCs secretome are increasingly recognized as critical intercellular mediators for transmitting biological signals rather than wasted products initially thought to be processed by cells. Numerous studies have demonstrated that Exos produced from MSCs actively participate in every step of wound healing (Qin et al., 2023) Figure 2
FIGURE 2. Mechanisms of immunomodulatory effects of MSCs in wound healing. Through the synthesis of metabolites, cytokines, growth factors, chemokines, exosomes, etc., as well as T-cell death-mediated immunomodulation, MSCs elicit immunomodulatory effects. MSCs can stimulate the differentiation of primitive CD4+ T cells into Tregs, restrict T-cell proliferation, and teach macrophages to adopt an immunosuppressive phenotype (M2-type). MSCs induce T-cell death, which triggers TGF-β release from macrophages, leading to Tregs differentiation and immunological tolerance. MSCs induce increased T-cell differentiation towards Th2 and decreased differentiation towards Th1 and Th17. MSCs promote mast cell degranulation. These mechanisms modulate the local inflammatory environment of the wound, thereby promoting wound healing.
An increasing amount of evidence points to the possibility that excessive neutrophil infiltration, which results in an overabundance of NETs, may damage the structure of the wound, hinder angiogenesis in the vicinity of the injury, and alter the quantity or quality of cells participating in wound repair, all of which might delay the healing process (Zhu et al., 2021). The neutrophils’ departure shows a decrease in inflammation. On the other hand, chronic wounds include diabetic foot ulcers, pressure sores, and venous leg ulcers, which are caused by persistent inflammation that is brought on by neutrophil persistence (Chen and Rogers, 2007). It was found that treatment with MSCs or MSCs conditioned medium (CM) significantly reduced the number of infiltrating neutrophils and alleviated progression in an acute injury mouse model (Ionescu et al., 2012; Wang et al., 2018). Interestingly, however, lipopolysaccharide (LPS) -activated MSCs present different regulatory effects on neutrophils. In vitro experiments by Brandau et al. (2014) showed enhanced neutrophil phagocytosis and increased survival after CM exposure to LPS-activated MSCs. Munir et al. (2020) found that injecting LPS-induced MSCs into mouse wounds significantly accelerated wound healing compared to uninduced MSCs. This was due to increased neutrophil granulocyte activation, NET formation, and ROS production, resulting in improved wound healing in alloplastic wounds. During the later phases, a large number of apoptotic neutrophils were taken up by macrophages, and there was an increase in TGF-β release. In turn, this promoted neovascularization and sped up the healing process overall by stimulating myofibroblast differentiation and wound contraction. However, in the repair of specialized wounds, such as diabetic wounds, excessive NETs formation is detrimental to wound healing. Chu et al. (2023) found that Exos derived from hypoxia-pretreated MSCs could promote wound healing by blocking excessive NETs formation through the transfer of miR-17-5p to target the Toll-like receptor-4 (TLR-4)/ROS/MAPK pathway. This shows that the role of neutrophils varies from one wound to another and from one period of time to another. Therefore, when applying the property of immunomodulation of MSCs for wound treatment, it is necessary to consider different characteristics of wounds and other periods of inflammation to understand more thoroughly the need for neutrophil activity in wounds and to use MSCs to regulate neutrophils more precisely. Still lacking, however, is a thorough understanding of how MSCs provide adaptive responses to modify neutrophil activity and activation state following tissue damage.
The primary way that MSCs influence macrophages is by controlling the polarization of these cells. Zhang et al. (2010) observed that following co-culturing with MSCs, macrophages exhibited an anti-inflammatory M2 phenotype, indicated by elevated levels of the anti-inflammatory cytokine IL-10 (Fairweather and Cihakova, 2009; Kim and Hematti, 2009; Martinez et al., 2009), a reduction in the pro-inflammatory cytokine TNF-α, and an increase in the expression of CD206, a recognized marker of M2 macrophages. Most importantly, injection of MSCs also led to a decrease in local inflammation, an increase in angiogenesis, and a notable speeding up of the healing process of the mouse wound, indicating that MSCs can support the M2 macrophage phenotype, which helps with cutaneous wound healing. da Costa Manso et al. (2023) found that skin wound healing was accelerated in diabetic mice receiving biotherapeutics containing MSCs and detected increases in TGF-β gene expression and M2 macrophage-related markers. These results confirmed findings from other studies suggesting that M2-type macrophages produced mediators necessary for tissue remodeling and inflammation reduction, thereby promoting wound repair (Tiemessen et al., 2007; Fairweather and Cihakova, 2009). Research has demonstrated that MSCs can release PGE2, which changes the phenotype of macrophages from polarized M1-type macrophages to M2-type (Spaggiari et al., 2009; Chen et al., 2018; Ko et al., 2020). To control macrophage polarization, MSCs release large amounts of the anti-inflammatory cytokine IL-6 (Philipp et al., 2018). Granulocyte-macrophage colony-stimulating factor (GM-CSF) and IL-6 generated by MSCs have been shown to significantly accelerate wound healing by causing macrophages to undergo M2 polarization (Spaggiari et al., 2009; Zhang et al., 2010). In a mouse model of injury, Zhao et al. demonstrated that MSCs-produced TSG6 induced polarization from pro-inflammatory to anti-inflammatory M1 phenotypes and sped up tissue regeneration by inhibiting the NF-κB pathway (Zhao et al., 2021). Interestingly, via binding to CD44, TSG6 can also prevent macrophage migration into areas of inflammation and damage (Day and Milner, 2019). Furthermore, it has been discovered that human MSCs cultivated in hypoxia could produce IGF2, which acts on monocytes during their maturation from monocyte to macrophage, giving them long-lasting anti-inflammatory qualities rather than directly influencing Tregs differentiation or the polarization of mature macrophages. This characteristic shows that MSCs may continually repair aberrant immunological responses and restore immune homeostasis since it lasts long after they mature into macrophages and even after stimulation with LPS or IFN-γ (Du et al., 2019; Wang X. et al., 2020). It is commonly recognized that wound healing requires reestablishing local immunological homeostasis and rectifying aberrant immune responses. A growing body of research suggested that MSCs-derived apoptotic vesicles or exosomes might also accelerate the healing of cutaneous wounds by inducing macrophage polarization toward the M2 phenotype or by changing M2 polarization (He et al., 2019; Luo et al., 2021; Geng et al., 2022). Exos derived from melatonin-stimulated MSCs have been used to target the PTEN/AKT pathway, modulate macrophage M2 polarization, and increase IL-10 and arginase-1 (Arg-1) expression in order to enhance diabetic wound healing (Liu et al., 2020). According to one study, systemic treatment of MSCs produced from bone marrow could cause macrophages to become more polarized toward the M2 phenotype. It facilitates their migration to the site of the lesion. Following investigations have demonstrated that miR-223, which is produced from bone marrow MSC-Exos, targets pknox1 to control macrophage polarization and facilitate the healing of wounds (He et al., 2019). In conclusion, MSCs can control macrophage polarization via a variety of mechanisms, which is critical for wound healing and restoration Table 1.
The final immune cells that penetrate the wound are T cells, which are crucial to the skin’s adaptive immunological response. T cells are drawn to chemokines generated within the wound, including chemokine C-C motif ligand-9 (CCL9) and CCL10 (Balaji et al., 2015). According to research, MSCs primarily control T-cell differentiation and proliferation by inhibiting T-cell proliferation (Hong et al., 2023). By coming into close touch with T cells and having paracrine actions, MSCs can control them. Two crucial molecules on MSCs membranes implicated in T-cell regulation are Fas ligand (FASL) and Programmed cell death protein-L1 (PD-L1). By directing the expression of PD-1 or CD80 on T cells, PD-L1 on MSCs prevents T cell growth by sending out inhibitory signals (Luz-Crawford et al., 2012). Similar to this, FALS on MSC’s membrane interacts with T cell’s Fas to cause T-cell apoptosis (Wang L. et al., 2012). Galactagogue-1, a member of the galactagogue family, is extensively expressed on the surface of MSCs and limits T-cell proliferation via controlling inflammation (Sioud, 2011). In addition to these key cell surface molecules, MSCs can also regulate T cells through paracrine effects. Human umbilical cord MSCs have been demonstrated to inhibit T cell activation and development by generating TGF-β and preventing T cells from entering the G0 phase Vellasamy et al. (2013). More of the catabolic enzyme indoleamine-2,3-dioxygenase (IDO), which changes tryptophan into kynurenine, may be produced by MSCs. Additionally, they can inhibit T-cell proliferation, activate GCN2(a general control non-suppressible factor), promote local tryptophan depletion, and convert naïve CD4+ T cells into Tregs (Munn et al., 2005; Fallarino et al., 2006). In addition, IDO generates soluble compounds like kynurenine and its byproducts that can bind to and activate the aryl hydrocarbon receptor. This increases Tregs development (Mezrich et al., 2010) and causes DCs to take on an immunosuppressive character (Quintana et al., 2010). In addition, it has been demonstrated that MSCs counteract pro-inflammatory and anti-inflammatory responses, prevent T cells from differentiating into the Th1 and Th17, and encourage the development of Tregs (Duffy et al., 2011b; Vellasamy et al., 2013). Furthermore, MSCs modify T-cell polarization and cytokine secretion profiles, transforming pro-inflammatory Th1 cells into anti-inflammatory Th2 cells (Di Nicola et al., 2002; William et al., 2003; Wang et al., 2008). Th1 cytokine production has been shown to be inhibited by MSCs via PGE-2-dependent inhibition, Th17 cytokine production is constrained by MSCs by upregulating PD-1 expression and IL-10 production, and MSCs inhibitory activity is activated through CCL2-dependent inhibition (Darlington et al., 2010; Duffy et al., 2011a; Luz-Crawford et al., 2012; Mohammadzadeh et al., 2014). In addition, it has been found that T-cell apoptosis resulting from inhibition by MSCs enables macrophages to produce TGF-β, which promotes the induction of Tregs (Ren et al., 2008). It has been shown that chemokine receptor 2 (CCR2) overexpressing MSCs speed wound healing and encourage the build-up of Tregs in diabetic wounds Kuang et al. (2021). Studies have indicated that Th17 cell development is suppressed by leukemia inhibitory factor (LIF) released by MSCs Cao et al. (2011), and LIF has also been shown to promote the differentiation of Treg cells Spaggiari et al. (2009). In summary, MSCs can function to promote wound healing by regulating T cells. In summary, MSCs can function to promote wound healing by regulating T cells Table 2.
Further studies are needed on the regulation of B lymphocyte activity by MSCs. It has been suggested that MSCs inhibit the synthesis of immunoglobulins such as IgG, IgM, and IgA by activated B cells, thus preventing these cells from differentiating into plasma cells. These cells also decrease the expression of chemokines and their receptors at the level of B lymphocytes, which may negatively affect their migratory capacity (Fan et al., 2016; Boukani et al., 2023). A growing number of scholars have investigated the potential of using MSCs to modulate mast cells for wound repair based on their biological properties. Throughout the healing process of wounds, mast cells interact with several other immune cells (Artuc et al., 1999). Mast cells have the ability to produce antimicrobial peptides that prevent skin infections in their early stages (Wang Z. et al., 2012). Mast cells have been shown to produce histamine and VEGF, which cause vascular permeability and permit neutrophil inflow, as well as chymotrypsin and trypsin-like enzymes necessary for the breakdown of extracellular matrix (ECM) (Dvorak, 2005; Younan et al., 2010). Additionally, released histamine promotes the remodeling of the epithelium and the proliferation of keratinocytes (Weller et al., 2006). Trypsin-like enzymes and histamine also encourage the synthesis of collagen and the proliferation of fibroblasts, which enhances wound contraction and is necessary for the proliferative and remodeling phases (Abe et al., 2000; Garbuzenko et al., 2002). Studies have indicated that MSCs significantly decreased inflammation and mast cell degranulation by producing PGE-2 and TGF-β (Kashyap et al., 2005; Kim et al., 2015). It was found that media from TNF-α-stimulated MSCs reduced mast cell activation and histamine release and attenuated experimental allergic conjunctivitis through a cyclooxygenase-2 (COX-2)-dependent mechanism (Su et al., 2015). More research is required to determine the specific molecular pathways by which human MSCs govern mast cell activity and its consequences for wound healing, although research on animals has shown that MSCs regulate mast cells. Additionally, MSCs inhibit the conversion of immature dendritic cells to adult dendritic cells and limit the movement of dendritic cells into tissues (Maqbool et al., 2019; Boukani et al., 2023). MSCs also can decrease T-cell activation by inhibiting the growth and activation of DCs (Chiesa et al., 2011; Le Blanc and Mougiakakos, 2012). PGE2, secreted by MSCs, can impede DC maturation and antigen-presentation capabilities. It can also lessen T-cell inflammatory processes (Spaggiari et al., 2009). In response to IL-6, PGE-2, and Jagged-2, it has been demonstrated that MSCs may also generate regulatory and tolerogenic DCs, which will suppress T-cell proliferation and stimulate the growth of Tregs (Spaggiari et al., 2009; Zhang et al., 2009; Deng et al., 2014). We believe that the activities of other immune cells should not be ignored during wound healing as well. More research would be necessary to fully comprehend the mechanism behind the interaction between MSCs and other immune cells Table 3.
The immunomodulatory effects of MSCs are not intrinsic but are triggered by inflammation (Wang et al., 2022). Ren et al. (2008) showed that IFN-γ, especially in conjunction with TNF-α or IL-1α/β combos, initiates the immunosuppression of T cells by MSCs. This suggests a mutually beneficial interaction between MSCs and the milieux that causes inflammation. Using a checkerboard titration of IFN-γ and TNF-α, Li et al. (2012) evaluated the impact of cytokine concentration on immunosuppression in MSCs, providing first-hand evidence for the concept that MSCs are malleable in controlling immunological responses, particularly adaptive immunity. The kind and quantity of inflammatory mediators present in the tissue microenvironment determine this particular trait (Moonen et al., 2012; Wang et al., 2014). For instance, IL-17 in the surrounding environment might intensify MSCs’ ability to inhibit the immune system (Han et al., 2014). In contrast, TGF-β and IL-10 reduce the immunosuppressive capacity of MSCs (Renner et al., 2009; Xu et al., 2014). Another factor affecting MSCs’ capacity to regulate immunity is their capacity to identify microbial molecular patterns through TLRs. For instance, TLR-3 activation results in anti-inflammatory signaling, while TLR-4 activation causes pro-inflammatory reactions. It’s interesting to note that TLRs have also been linked to significant skin damage healing. According to Lin et al., TLR-4-deficient mice’s wounds produced much less IL-1β and IL-6. Mice lacking the TLR-4 gene also showed a substantial decrease in epidermal growth factor at the edges of their wounds (Chen et al., 2013). In order to stimulate the regeneration of hair follicles, Nelson et al. found that TLR-3, together with its downstream effectors, IL-6 and transducer and activator of transcription 3 (STAT3), was activated with the release of dsRNA from wounded skin. In contrast, TLR-3-deficient animals failed to initiate trauma-induced hair regeneration (WIHN), which is detrimental to wound healing (Nelson et al., 2015). Consequently, MSCs can be efficiently enabled by “matching” signals to control inflammatory responses and work in concert with activated MSCs to reestablish tissue homeostasis. Nevertheless, the therapeutic impact of MSCs will be reduced if they come into contact with “mismatched” signals, such as inadequate proinflammatory cytokines and the existence of immunosuppressive substances or factors (Wang et al., 2022). We need to understand the different needs of inflammation regulation in various types of wounds and at other times of wound healing and amplify their “matching signals” to advance the realization of clinical precision therapy. In addition, the potential mechanisms of MSCs’ plasticity in immune regulation will expand the clinical indications of MSCs and guide the development of new therapeutic strategies for MSCs (Wang et al., 2022). This functional flexibility also offers a potential theoretical foundation for optimizing MSCs before transplantation, for example, before therapy, to maximize their ability to promote wound healing.
Several studies have shown that modulation of biological, biochemical, and biophysical factors can influence the fate, lineage-specific differentiation, and function of MSCs and enhance their therapeutic potential (Nava et al., 2012; Huang et al., 2015). To increase MSC activity, survival, and therapeutic efficacy, researchers have proposed and summarized a variety of strategies that hold promise for improving clinical outcomes (Baldari et al., 2017; Huerta et al., 2023). In the following, we will discuss in detail several aspects of cytokines, hypoxia, and drug-chemical pretreatment, as well as biomaterial encapsulation and three-dimensional (3D) culture Table 4.
TABLE 4. Pre-transplantation optimization strategies for MSCs to enhance their immunomodulatory effects.
Numerous studies have shown that the inflammatory state of the receptor should be taken into account before the treatment of MSCs. To increase the efficacy of MSCs-based therapies in clinical settings, it is therefore advised to pretreat MSCs by stimulating them with inflammatory cytokines that mimic the inflammatory microenvironment of tissues before infusion. As a result, MSCs will no longer be able to inhibit the immune system and will secrete more immunomodulatory and anti-inflammatory substances (Song et al., 2020). It has been demonstrated that pretreating MSCs with IFN-γ improves their immunosuppressive capabilities. According to Nelson et al., the release of dsRNA from injured skin activates TLR-3 together with its downstream effectors, IL-6 and STAT3. Research indicates that co-cultivating activated lymphocytes and MSCs sensitized to IFN-γ can lower the frequency of Th17 cells, secrete more IL-6 and IL-10, and produce less IFN-γ and TNF-α (Wang et al., 2016). While inhibiting T cell effector activity, IFN-γ-sensitized MSCs also upregulate PDL-1, which increases the production of HLA molecules (Chinnadurai et al., 2014). According to the research carried out by Vigo et al. (2017), due to early phosphorylation of STAT1/STAT3 signal transducers and activators of transcription and a decrease in rapamycin-targeting (mTOR) activity, MSCs from mice treated with IFN-γ exhibited immunosuppressive characteristics. Genes associated with immunoregulation were upregulated, and genes related to differentiation, proliferation, and stem cells were downregulated as a result of mTOR activity. Inhibiting the mTOR pathway also improves the immunoregulatory potential of human MSCs (Vigo et al., 2017). Chinnadurai et al. (2017) discovered that by activating regulatory molecules (such as IDO), IFN-γ pretreatment might restore the immunosuppressive characteristics of senescent MSCs. TNF-α pretreatment is frequently utilized in cytokine combinations to precondition MSCs since it also increases the upregulation of immunoregulatory factors in MSCs, albeit to a lesser degree than IFN-γ triggering (Prasanna et al., 2010). Co-preconditioning MSCs with IFN-γ and TNF-α, as shown by Francois et al., boosted IDO activity in MSCs and caused monocytes to differentiate into M2-type macrophages, which were implicated in T-cell suppression, thereby augmenting MSCs’ immunosuppressive capabilities (François et al., 2012). Liu C. et al. (2022) demonstrated that high levels of CCL2 and IL-6 were found in MSCs after co-pretreatment with IFN-γ and TNF-α, promoting macrophage migration and polarization. Preclinical research has shown that pretreating MSCs with TNF-α and IFN-γ increased their therapeutic efficiency in the healing of skin wounds (Zhu et al., 2020). Notably, there was a transient expression of human leukocyte antigen DR (HLA-DR) in MSCs when pretreated by combining TNF-α with IFN-γ, which may lead to unpredictable immune responses and tissue damage (Wen et al., 2021). Therefore, it is essential to monitor HLA-DR levels as one of the critical parameters for quality control to avoid harmful therapeutic effects in MSCs. Furthermore, MSCs treated with IL-17 stimulated the development of suppressive Tregs and prevented T cells from secreting Th1 cytokines (TNF-α, IFN-γ, and IL-2) (Sivanathan et al., 2017; Wen et al., 2021). According to Ma et al. (2018), MSCs pretreated with IL-17 significantly extended the survival time of allogeneic grafted skins compared to the non-pretreated group. In transplanted skin grafts, they also noticed elevated tagged MSCs levels, a proportion of the Tregs subpopulation, a notable rise in IL-10 and TGF-β, and a drop in IFN-γ levels. Such preconditioning strategies can mimic the pathological inflammatory environment present in disease states, and the evidence from their practical use points to the significance of inflammation in triggering MSCs’ anti-inflammatory properties (Wang et al., 2014).
MSCs exist in vivo in microenvironments with generally low oxygen tension (i.e.,1%-5% O2). To improve MSCs implantation, survival in ischemic microenvironments, and angiogenic potential in vitro, moderate hypoxia has been utilized to simulate the survival conditions of MSCs in vivo. This has been achieved by significantly changing cellular metabolism during MSCs expansion and enhancing resistance to oxidative stress (Noronha et al., 2019). Numerous investigations have demonstrated that MSCs grown in hypoxic environments release a variety of soluble bioactive substances (De Witte et al., 2016; Saparov et al., 2016) and increase proliferation ability (Kheirandish et al., 2017). HIF-1α produced by hypoxia preconditioned MSCs can impair dendritic cell differentiation, reduce NK cell-mediated cytolysis (Ejtehadifar et al., 2015), and increase resident macrophage recruitment to wounds to accelerate wound healing (Mahjoor et al., 2023). Dong et al. (2021) showed that hypoxia pretreatment of MSCs enhanced their immunomodulatory effects and was able to attenuate inflammation by increasing exosomal miR146a-5p.
Pretreatment of MSCs with drugs or chemical reagents is also a promising strategy to improve the implantation and survival of MSCs in damaged tissues, thus enhancing the therapeutic efficacy (Baldari et al., 2017). The inhibition of the mTOR signaling pathway caused by rapamycin did not affect all MSCs in the same way. Before co-culturing with activated human CD4+ T cells or mice splenocytes, BM-MSCs were briefly exposed to rapamycin. In vitro, this led to an increase in the immunosuppressive effects of COX-2 and PGE-2, independent of inflammatory stimuli, and a decrease in mTOR signaling. However, after extended rapamycin exposure, MSCs showed no such impact (Wang et al., 2017). Nuclear retinoic acid receptors (RARs) are occupied by all-trans retinoic acid (ATRA), which is another starting component that is used. ATRA is essential for immune system function, differentiation, apoptosis, and cell development. Rat bone marrow MSCs were pretreated with ATRA, increasing the expression of COX-2, hypoxia-inducible factor-1 (HIF-1), CCR2, and VEGF genes. ATRA-sensitized MSCs improved the capacity of wound healing in vivo (Wang et al., 2017). Bai et al. (2018) demonstrated that stimulation of adipose MSCs-derived exosomes using low-dose H2O2 promoted flap neovascularization, attenuated flap inflammation, reduced apoptosis, and improved flap survival after ischemia-reperfusion injury. Rhodiola rosea glycoside, the active ingredient in the Tibetan medicine Rhodiola rosea, can regulate the proliferation, migration, and differentiation of MSCs. The pretreatment with Rhodiola rosea glycoside was able to boost the survival rate of MSCs and improve the migratory capacity of MSCs damaged by hyperglycemia. It was also able to successfully reverse the suppression of essential wound healing factors caused by hyperglycemia in bone marrow MSCs. Research conducted in vivo revealed that administering Rhodiola rosea glycosides before transplantation considerably boosted MSCs’ ability to promote wound healing in diabetic mice (Ariyanti et al., 2019).
The development of biocompatible materials has a direct bearing on the use of MSCs in tissue engineering. These materials can enhance MSCs effectiveness by promoting cell survival and directing in vivo cell differentiation (Kusuma et al., 2017). Drug delivery, tissue engineering scaffolds, transplantation treatment, and MSCs cell encapsulation are just a few of the applications for hydrogels that have seen widespread use (Akbari et al., 2020). Because of their capacity to replicate ECM, high biocompatibility and flexibility, noninvasive drug administration, and adjustable physical and chemical characteristics, they are the perfect bioactive scaffolding materials for MSCs tissue creation (Shi et al., 2018b). The viscosity of hydrogels reduces the mechanical forces exerted in syringe-based drug delivery, significantly reducing the loss of MSCs during the procedure (Baldari et al., 2017). In addition, encapsulation/culture of MSCs in hydrogels improved cell differentiation, accelerated routine wound healing, and promoted neovascularization (Rustad et al., 2012; Chen et al., 2015), cell viability, homing, and proliferation (Nava et al., 2012; Ansari et al., 2017). Because of their porous nature, chitin nanofibers are an excellent material for wound dressings since they match the fibrous structure of the extracellular matrix seen in nature. It also encourages cell proliferation, permits the diffusion of nutrients, oxygen, and waste products, and absorbs secretions from wounds to prevent bacterial infections and excessive dehydration. According to Liu Y. et al. (2022), MSCs-loaded β-chitin nanofiber hydrogels caused a significant increase in angiogenesis, which in turn encouraged the healing and repair of damaged tissue. Furthermore, they observed that these hydrogels decreased Smad phosphorylation while increasing the synthesis of VEGF, α-SMA (α-smooth muscle actin), and matrix metalloproteinase tissue inhibitory factor-1 (TIMP-1). Using a decellularization and decalcification procedure, bioactive fish scale scaffolds were produced. This allowed for the preservation of residual bioactive substances within the fish scale scaffolds, and the surface of the decalcified fish scale retained oriented collagen nanofibers, which facilitated the growth of oriented cells in a suitable environment. Lin et al. (2022) found that the fish scale scaffolds loaded with MSCs effectively promoted angiogenesis, regulated macrophage polarization toward the M2 phenotype polarization, and attenuated local inflammatory responses.
The physical microenvironment influences the function of MSCs. Cell polarity and cell-to-cell contacts are crucial, as demonstrated by studies employing cell aggregates Kusuma et al. (2017). By simulating in vivo developmental and signaling activity, 3D cultivation, often called spherical cultivation, increases cell-to-cell and cell-ECM interactions, enhancing the therapeutic potential of human MSCs (Kouroupis and Correa, 2021). The phenotypic of cultured cells, including proliferation, cell-cell interactions, and paracrine factor gene expression, can be changed by 3D culture in comparison to traditional 2D culture (Ryu et al., 2019; Białkowska et al., 2020). Sphere formation increased the survival of MSCs after transplantation (Bhang et al., 2012), enhanced tissue regeneration properties, reduced in vitro senescence, and, more importantly, enhanced angiogenesis and anti-inflammatory effects (Cesarz and Tamama, 2016). Hildebrandt et al. showed improved nutrient delivery and enhanced viability of MSCs based on rotary cultures, suspension-droplet technique, and 3D cultures in non-adherent culture vessels (Hildebrandt et al., 2011). Bartlett et al. showed improved nutrient delivery and enhanced viability of MSCs (Hildebrandt et al., 2011). It was demonstrated by Bartosh et al. (2010) that the high expression of TSG-6 was responsible for the powerful anti-inflammatory effects of 3D-cultured MSCs. It was demonstrated that via increasing PGE-2 and HGF levels, 3D-cultured MSCs also strengthened immunosuppressive effects (Follin et al., 2016). Many techniques, including pellet culture, suspension drops, spinning flasks, magnetic levitation, and space culture, have been used to create spheroids thus far (Blaber et al., 2014; Ryu et al., 2019; Wnorowski et al., 2019; Białkowska et al., 2020). For the most part, sphere creation is a labor-intensive procedure that takes a long time. Human MSCs were cultured by Park et al. (2023) using acoustic levitation to produce spheroids, which took significantly less time, had a denser and more homogeneous morphology, and showed higher levels of metabolic activity, viability, and proliferative capacity. The genes encoding angiogenic, senescent, and proliferative factors were also expressed at elevated levels.
Stem cells exhibit the capacity to repair tissue and heal wounds. Studies in both the clinic and the laboratory have shown that MSCs-based inflammatory regulation treatment is a successful tactic for accelerating wound healing. Nonetheless, the primary issue limiting their practical use is the limited survival and retention of cells following implantation. Current research emphasizes how critical it is to control inflammation early in the healing process and how MSCs can help heal wounds by influencing the local inflammatory milieu through primary immunomodulatory action. Furthermore, the adaptability of MSCs’ immunomodulation is highlighted, and methods to boost MSCs’ therapeutic effectiveness—which has been demonstrated to raise MSCs migration and survival, encourage angiogenic potential, improve immunomodulation, and increase overall efficacy—are further explored. These findings hold potential for the advancement of MSCs-based treatment approaches. In the clinical management of wounds, clinicians tend to administer antimicrobial and anti-inflammatory treatments immediately after trauma to prevent excessive inflammatory responses. In contrast, the need for inflammation may vary during different periods of wound healing. Thus, to advance the realization of precision therapy in the clinic, more thorough research is still needed to understand the molecular mechanisms underlying the interactions between MSCs and each type of immune cell, particularly the distinct requirements for inflammation regulation for different kinds of wounds and varying periods of wound healing. Future research endeavors may expand upon this study and offer valuable perspectives on the practical applications of these results.
MG: Conceptualization, Investigation, Software, Visualization, Writing–original draft, Writing–review and editing. HG: Conceptualization, Investigation, Methodology, Writing–original draft. XD: Conceptualization, Investigation, Writing–original draft. ZW: Conceptualization, Writing–review and editing. ZY: Conceptualization, Writing–review and editing. QS: Writing–review and editing. QW: Conceptualization, Methodology, Writing–original draft, Writing–review and editing.
The author(s) declare financial support was received for the research, authorship, and/or publication of this article. This work was supported by the Henan Provincial Key R and D and Promotion Special Program (Grant No. 222102310420).
The authors declare that the research was conducted in the absence of any commercial or financial relationships that could be construed as a potential conflict of interest.
All claims expressed in this article are solely those of the authors and do not necessarily represent those of their affiliated organizations, or those of the publisher, the editors and the reviewers. Any product that may be evaluated in this article, or claim that may be made by its manufacturer, is not guaranteed or endorsed by the publisher.
Abe, M., Kurosawa, M., Ishikawa, O., and Miyachi, Y. (2000). Effect of mast cell–derived mediators and mast cell–related neutral proteases on human dermal fibroblast proliferation and type i collagen production. J. allergy Clin. Immunol. 106, S78–S84. doi:10.1067/mai.2000.106058
Akbari, A., Jabbari, N., Sharifi, R., Ahmadi, M., Vahhabi, A., Seyedzadeh, S. J., et al. (2020). Free and hydrogel encapsulated exosome-based therapies in regenerative medicine. Life Sci. 249, 117447. doi:10.1016/j.lfs.2020.117447
Akbik, D., Ghadiri, M., Chrzanowski, W., and Rohanizadeh, R. (2014). Curcumin as a wound healing agent. Life Sci. 116, 1–7. doi:10.1016/j.lfs.2014.08.016
Ansari, S., Chen, C., Hasani-Sadrabadi, M. M., Yu, B., Zadeh, H. H., Wu, B. M., et al. (2017). Hydrogel elasticity and microarchitecture regulate dental-derived mesenchymal stem cell-host immune system cross-talk. Acta biomater. 60, 181–189. doi:10.1016/j.actbio.2017.07.017
Anzengruber, F., Avci, P., de Freitas, L. F., and Hamblin, M. R. (2015). T-cell mediated anti-tumor immunity after photodynamic therapy: why does it not always work and how can we improve it? Photochem. Photobiological Sci. 14, 1492–1509. doi:10.1039/c4pp00455h
Ariyanti, A. D., Zhang, J., Marcelina, O., Nugrahaningrum, D. A., Wang, G., Kasim, V., et al. (2019). Salidroside-pretreated mesenchymal stem cells enhance diabetic wound healing by promoting paracrine function and survival of mesenchymal stem cells under hyperglycemia. Stem Cells Transl. Med. 8, 404–414. doi:10.1002/sctm.18-0143
Artuc, M., Hermes, B., Stckelings, U., Grützkau, A., and Henz, B. (1999). Mast cells and their mediators in cutaneous wound healing–active participants or innocent bystanders? Exp. Dermatol. 8, 1–16. doi:10.1111/j.1600-0625.1999.tb00342.x
Ayavoo, T., Murugesan, K., and Gnanasekaran, A. (2021). Roles and mechanisms of stem cell in wound healing. Stem Cell Investig. 8, 4. doi:10.21037/sci-2020-027
Bai, Y., Yan, X.-l., Ren, J., Zeng, Q., Li, X.-d., Pei, X.-t., et al. (2018). Adipose mesenchymal stem cell-derived exosomes stimulated by hydrogen peroxide enhanced skin flap recovery in ischemia-reperfusion injury. Biochem. Biophysical Res. Commun. 500, 310–317. doi:10.1016/j.bbrc.2018.04.065
Balaji, S., Watson, C. L., Ranjan, R., King, A., Bollyky, P. L., and Keswani, S. G. (2015). Chemokine involvement in fetal and adult wound healing. Adv. wound care 4, 660–672. doi:10.1089/wound.2014.0564
Baldari, S., Di Rocco, G., Piccoli, M., Pozzobon, M., Muraca, M., and Toietta, G. (2017). Challenges and strategies for improving the regenerative effects of mesenchymal stromal cell-based therapies. Int. J. Mol. Sci. 18, 2087. doi:10.3390/ijms18102087
Bartosh, T. J., Ylöstalo, J. H., Mohammadipoor, A., Bazhanov, N., Coble, K., Claypool, K., et al. (2010). Aggregation of human mesenchymal stromal cells (mscs) into 3d spheroids enhances their antiinflammatory properties. Proc. Natl. Acad. Sci. 107, 13724–13729. doi:10.1073/pnas.1008117107
Bernatchez, S. F., Menon, V., Stoffel, J., Walters, S.-A. H., Lindroos, W. E., Crossland, M. C., et al. (2013). Nitric oxide levels in wound fluid may reflect the healing trajectory. Wound Repair Regen. 21, 410–417. doi:10.1111/wrr.12048
Bhang, S. H., Lee, S., Shin, J.-Y., Lee, T.-J., and Kim, B.-S. (2012). Transplantation of cord blood mesenchymal stem cells as spheroids enhances vascularization. Tissue Eng. Part A 18, 2138–2147. doi:10.1089/ten.TEA.2011.0640
Białkowska, K., Komorowski, P., Bryszewska, M., and Miłowska, K. (2020). Spheroids as a type of three-dimensional cell cultures—examples of methods of preparation and the most important application. Int. J. Mol. Sci. 21, 6225. doi:10.3390/ijms21176225
Bianco, P., Cao, X., Frenette, P. S., Mao, J. J., Robey, P. G., Simmons, P. J., et al. (2013). The meaning, the sense and the significance: translating the science of mesenchymal stem cells into medicine. Nat. Med. 19, 35–42. doi:10.1038/nm.3028
Blaber, E., Sato, K., and Almeida, E. A. (2014). Stem cell health and tissue regeneration in microgravity. Stem cells Dev. 23, 73–78. doi:10.1089/scd.2014.0408
Boukani, L. M., Khosroshahi, R. F., Kh, S. A., Rashtbar, M., and Khosroshahi, A. F. (2023). Statistical study of clinical trials with stem cells and their function in skin wound. Cell Tissue Res. 1–12. doi:10.1007/s00441-023-03793-3
Brackett, C. M., and Gollnick, S. O. (2011). Photodynamic therapy enhancement of anti-tumor immunity. Photochem. photobiological Sci. 10, 649–652. doi:10.1039/c0pp00354a
Brandau, S., Jakob, M., Bruderek, K., Bootz, F., Giebel, B., Radtke, S., et al. (2014). Mesenchymal stem cells augment the anti-bacterial activity of neutrophil granulocytes. PLoS One 9, e106903. doi:10.1371/journal.pone.0106903
Cao, W., Yang, Y., Wang, Z., Liu, A., Fang, L., Wu, F., et al. (2011). Leukemia inhibitory factor inhibits t helper 17 cell differentiation and confers treatment effects of neural progenitor cell therapy in autoimmune disease. Immunity 35, 273–284. doi:10.1016/j.immuni.2011.06.011
Cesarz, Z., and Tamama, K. (2016). Spheroid culture of mesenchymal stem cells. Stem cells Int. 2016, 9176357. doi:10.1155/2016/9176357
Chen, C.-Y., Rao, S.-S., Ren, L., Hu, X.-K., Tan, Y.-J., Hu, Y., et al. (2018). Exosomal dmbt1 from human urine-derived stem cells facilitates diabetic wound repair by promoting angiogenesis. Theranostics 8, 1607–1623. doi:10.7150/thno.22958
Chen, L., Guo, S., Ranzer, M. J., and DiPietro, L. A. (2013). Toll-like receptor 4 has an essential role in early skin wound healing. J. Investigative Dermatology 133, 258–267. doi:10.1038/jid.2012.267
Chen, S., Shi, J., Zhang, M., Chen, Y., Wang, X., Zhang, L., et al. (2015). Mesenchymal stem cell-laden anti-inflammatory hydrogel enhances diabetic wound healing. Sci. Rep. 5, 18104. doi:10.1038/srep18104
Chen, W. J., and Rogers, A. A. (2007). Recent insights into the causes of chronic leg ulceration in venous diseases and implications on other types of chronic wounds. Wound repair Regen. 15, 434–449. doi:10.1111/j.1524-475X.2007.00250.x
Chiesa, S., Morbelli, S., Morando, S., Massollo, M., Marini, C., Bertoni, A., et al. (2011). Mesenchymal stem cells impair in vivo t-cell priming by dendritic cells. Proc. Natl. Acad. Sci. 108, 17384–17389. doi:10.1073/pnas.1103650108
Chinnadurai, R., Copland, I. B., Patel, S. R., and Galipeau, J. (2014). Ido-independent suppression of t cell effector function by ifn-γ–licensed human mesenchymal stromal cells. J. Immunol. 192, 1491–1501. doi:10.4049/jimmunol.1301828
Chinnadurai, R., Rajan, D., Ng, S., McCullough, K., Arafat, D., Waller, E. K., et al. (2017). Immune dysfunctionality of replicative senescent mesenchymal stromal cells is corrected by ifnγ priming. Blood Adv. 1, 628–643. doi:10.1182/bloodadvances.2017006205
Choudhury, H., Pandey, M., Lim, Y. Q., Low, C. Y., Lee, C. T., Marilyn, T. C. L., et al. (2020). Silver nanoparticles: advanced and promising technology in diabetic wound therapy. Mater. Sci. Eng. C 112, 110925. doi:10.1016/j.msec.2020.110925
Chu, Z., Huang, Q., Ma, K., Liu, X., Zhang, W., Cui, S., et al. (2023). Novel neutrophil extracellular trap-related mechanisms in diabetic wounds inspire a promising treatment strategy with hypoxia-challenged small extracellular vesicles. Bioact. Mater. 27, 257–270. doi:10.1016/j.bioactmat.2023.04.007
Clark, R. (2003). Fibrin is a many splendored thing. J. Investigative Dermatology 121, xxi–xxii. doi:10.1046/j.1523-1747.2003.12575.x
Clark, S. R., Ma, A. C., Tavener, S. A., McDonald, B., Goodarzi, Z., Kelly, M. M., et al. (2007). Platelet tlr4 activates neutrophil extracellular traps to ensnare bacteria in septic blood. Nat. Med. 13, 463–469. doi:10.1038/nm1565
Cottrell, W. J., Paquette, A. D., Keymel, K. R., Foster, T. H., and Oseroff, A. R. (2008). Irradiance-dependent photobleaching and pain in δ-aminolevulinic acid-photodynamic therapy of superficial basal cell carcinomas. Clin. cancer Res. 14, 4475–4483. doi:10.1158/1078-0432.CCR-07-5199
da Costa Manso, G. M., Elias-Oliveira, J., Guimarães, J. B., Pereira, Í. S., Rodrigues, V. F., Burger, B., et al. (2023). Xenogeneic mesenchymal stem cell biocurative improves skin wounds healing in diabetic mice by increasing mast cells and the regenerative profile. Regen. Ther. 22, 79–89. doi:10.1016/j.reth.2022.12.006
Darlington, P. J., Boivin, M.-N., Renoux, C., François, M., Galipeau, J., Freedman, M. S., et al. (2010). Reciprocal th1 and th17 regulation by mesenchymal stem cells: implication for multiple sclerosis. Ann. neurology 68, 540–545. doi:10.1002/ana.22065
Day, A. J., and Milner, C. M. (2019). Tsg-6: a multifunctional protein with anti-inflammatory and tissue-protective properties. Matrix Biol. 78, 60–83. doi:10.1016/j.matbio.2018.01.011
Deng, Y., Yi, S., Wang, G., Cheng, J., Zhang, Y., Chen, W., et al. (2014). Umbilical cord-derived mesenchymal stem cells instruct dendritic cells to acquire tolerogenic phenotypes through the il-6-mediated upregulation of socs1. Stem cells Dev. 23, 2080–2092. doi:10.1089/scd.2013.0559
De Witte, S. F., Franquesa, M., Baan, C. C., and Hoogduijn, M. J. (2016). Toward development of imesenchymal stem cells for immunomodulatory therapy. Front. Immunol. 6, 648. doi:10.3389/fimmu.2015.00648
Di Nicola, M., Carlo-Stella, C., Magni, M., Milanesi, M., Longoni, P. D., Matteucci, P., et al. (2002). Human bone marrow stromal cells suppress t-lymphocyte proliferation induced by cellular or nonspecific mitogenic stimuli. Blood, J. Am. Soc. Hematol. 99, 3838–3843. doi:10.1182/blood.v99.10.3838
Dong, L., Wang, Y., Zheng, T., Pu, Y., Ma, Y., Qi, X., et al. (2021). Hypoxic hucmsc-derived extracellular vesicles attenuate allergic airway inflammation and airway remodeling in chronic asthma mice. Stem Cell Res. Ther. 12, 4–14. doi:10.1186/s13287-020-02072-0
Du, L., Lin, L., Li, Q., Liu, K., Huang, Y., Wang, X., et al. (2019). Igf-2 preprograms maturing macrophages to acquire oxidative phosphorylation-dependent anti-inflammatory properties. Cell metab. 29, 1363–1375.e8. doi:10.1016/j.cmet.2019.01.006
Duffy, M. M., Pindjakova, J., Hanley, S. A., McCarthy, C., Weidhofer, G. A., Sweeney, E. M., et al. (2011a). Mesenchymal stem cell inhibition of t-helper 17 cell-differentiation is triggered by cell–cell contact and mediated by prostaglandin e2 via the ep4 receptor. Eur. J. Immunol. 41, 2840–2851. doi:10.1002/eji.201141499
Duffy, M. M., Ritter, T., Ceredig, R., and Griffin, M. D. (2011b). Mesenchymal stem cell effects on t-cell effector pathways. Stem Cell Res. Ther. 2, 34–39. doi:10.1186/scrt75
Dvorak, A. M. (2005). “Mast cell-derived mediators of enhanced microvascular permeability, vascular permeability factor/vascular endothelial growth factor, histamine, and serotonin, cause leakage of macromolecules through a new endothelial cell permeability organelle, the vesiculo-vacuolar organelle,” in Ultrastructure of mast cells and basophils (Karger Publishers), 185–204.
Ejtehadifar, M., Shamsasenjan, K., Movassaghpour, A., Akbarzadehlaleh, P., Dehdilani, N., Abbasi, P., et al. (2015). The effect of hypoxia on mesenchymal stem cell biology. Adv. Pharm. Bull. 5, 141–149. doi:10.15171/apb.2015.021
Evers, B. M., Bold, R. J., Ehrenfried, J. A., Li, J., Townsend, C., and Klimpel, G. (1994). Characterization of functional neurotensin receptors on human lymphocytes. Surgery 116, 134–140.
Fairweather, D., and Cihakova, D. (2009). Alternatively activated macrophages in infection and autoimmunity. J. Autoimmun. 33, 222–230. doi:10.1016/j.jaut.2009.09.012
Falanga, V. (2005). Wound healing and its impairment in the diabetic foot. Lancet 366, 1736–1743. doi:10.1016/S0140-6736(05)67700-8
Fallarino, F., Grohmann, U., You, S., McGrath, B. C., Cavener, D. R., Vacca, C., et al. (2006). The combined effects of tryptophan starvation and tryptophan catabolites down-regulate t cell receptor ζ-chain and induce a regulatory phenotype in naive t cells. J. Immunol. 176, 6752–6761. doi:10.4049/jimmunol.176.11.6752
Fan, L., Hu, C., Chen, J., Cen, P., Wang, J., and Li, L. (2016). Interaction between mesenchymal stem cells and b-cells. Int. J. Mol. Sci. 17, 650. doi:10.3390/ijms17050650
Follin, B., Juhl, M., Cohen, S., Pedersen, A. E., Kastrup, J., and Ekblond, A. (2016). Increased paracrine immunomodulatory potential of mesenchymal stromal cells in three-dimensional culture. Tissue Eng. Part B Rev. 22, 322–329. doi:10.1089/ten.TEB.2015.0532
Forrester, J. S., Makkar, R. R., and Marbán, E. (2009). Long-term outcome of stem cell therapy for acute myocardial infarction: right results, wrong reasons. J. Am. Coll. Cardiol. 53, 2270–2272. doi:10.1016/j.jacc.2009.03.023
François, M., Romieu-Mourez, R., Li, M., and Galipeau, J. (2012). Human msc suppression correlates with cytokine induction of indoleamine 2, 3-dioxygenase and bystander m2 macrophage differentiation. Mol. Ther. 20, 187–195. doi:10.1038/mt.2011.189
Friedenstein, A., Chailakhjan, R., and Lalykina, K. (1970). The development of fibroblast colonies in monolayer cultures of Guinea-pig bone marrow and spleen cells. Cell Prolif. 3, 393–403. doi:10.1111/j.1365-2184.1970.tb00347.x
Gao, L., Yu, Q., Zhang, H., Wang, Z., Zhang, T., Xiang, J., et al. (2021). A resident stromal cell population actively restrains innate immune response in the propagation phase of colitis pathogenesis in mice. Sci. Transl. Med. 13, eabb5071. doi:10.1126/scitranslmed.abb5071
Garbuzenko, E., Nagler, A., Pickholtz, D., Gillery, P., Reich, R., Maquart, F.-X., et al. (2002). Human mast cells stimulate fibroblast proliferation, collagen synthesis and lattice contraction: a direct role for mast cells in skin fibrosis. Clin. Exp. Allergy 32, 237–246. doi:10.1046/j.1365-2222.2002.01293.x
Garg, R. K., Rennert, R. C., Duscher, D., Sorkin, M., Kosaraju, R., Auerbach, L. J., et al. (2014). Capillary force seeding of hydrogels for adipose-derived stem cell delivery in wounds. Stem cells Transl. Med. 3, 1079–1089. doi:10.5966/sctm.2014-0007
Geissmann, F., Manz, M. G., Jung, S., Sieweke, M. H., Merad, M., and Ley, K. (2010). Development of monocytes, macrophages, and dendritic cells. Science 327, 656–661. doi:10.1126/science.1178331
Geng, X., Qi, Y., Liu, X., Shi, Y., Li, H., and Zhao, L. (2022). A multifunctional antibacterial and self-healing hydrogel laden with bone marrow mesenchymal stem cell-derived exosomes for accelerating diabetic wound healing. Biomater. Adv. 133, 112613. doi:10.1016/j.msec.2021.112613
Gentek, R., and Hoeffel, G. (2017). The innate immune response in myocardial infarction, repair, and regeneration. Immunol. Cardiovasc. Homeost. pathology 1003, 251–272. doi:10.1007/978-3-319-57613-8_12
Gollnick, S. O., Owczarczak, B., and Maier, P. (2006). Photodynamic therapy and anti-tumor immunity. Lasers Surg. Med. 38, 509–515. doi:10.1002/lsm.20362
Gopinath, D., Ahmed, M. R., Gomathi, K., Chitra, K., Sehgal, P., and Jayakumar, R. (2004). Dermal wound healing processes with curcumin incorporated collagen films. Biomaterials 25, 1911–1917. doi:10.1016/s0142-9612(03)00625-2
Gurtner, G. C., Werner, S., Barrandon, Y., and Longaker, M. T. (2008). Wound repair and regeneration. Nature 453, 314–321. doi:10.1038/nature07039
Han, G., and Ceilley, R. (2017). Chronic wound healing: a review of current management and treatments. Adv. Ther. 34, 599–610. doi:10.1007/s12325-017-0478-y
Han, X., Yang, Q., Lin, L., Xu, C., Zheng, C., Chen, X., et al. (2014). Interleukin-17 enhances immunosuppression by mesenchymal stem cells. Cell Death Differ. 21, 1758–1768. doi:10.1038/cdd.2014.85
Han, Y., Yang, J., Fang, J., Zhou, Y., Candi, E., Wang, J., et al. (2022). The secretion profile of mesenchymal stem cells and potential applications in treating human diseases. Signal Transduct. Target. Ther. 7, 92. doi:10.1038/s41392-022-00932-0
He, X., Dong, Z., Cao, Y., Wang, H., Liu, S., Liao, L., et al. (2019). Msc-derived exosome promotes m2 polarization and enhances cutaneous wound healing. Stem cells Int. 2019, 7132708. doi:10.1155/2019/7132708
Hildebrandt, C., Büth, H., and Thielecke, H. (2011). A scaffold-free in vitro model for osteogenesis of human mesenchymal stem cells. Tissue Cell 43, 91–100. doi:10.1016/j.tice.2010.12.004
Hong, Y.-K., Chang, Y.-H., Lin, Y.-C., Chen, B., Guevara, B. E. K., and Hsu, C.-K. (2023). Inflammation in wound healing and pathological scarring. Adv. Wound Care 12, 288–300. doi:10.1089/wound.2021.0161
Huang, C., Dai, J., and Zhang, X. A. (2015). Environmental physical cues determine the lineage specification of mesenchymal stem cells. Biochimica Biophysica Acta (BBA)-General Subj. 1850, 1261–1266. doi:10.1016/j.bbagen.2015.02.011
Huerta, C. T., Ortiz, Y. Y., Liu, Z.-J., and Velazquez, O. C. (2023). Methods and limitations of augmenting mesenchymal stem cells for therapeutic applications. Adv. Wound Care 12, 467–481. doi:10.1089/wound.2022.0107
Ionescu, L., Byrne, R. N., van Haaften, T., Vadivel, A., Alphonse, R. S., Rey-Parra, G. J., et al. (2012). Stem cell conditioned medium improves acute lung injury in mice: in vivo evidence for stem cell paracrine action. Am. J. Physiology-Lung Cell. Mol. Physiology 303, L967–L977. doi:10.1152/ajplung.00144.2011
Joe, B., Vijaykumar, M., and Lokesh, B. (2004). Biological properties of curcumin-cellular and molecular mechanisms of action. Crit. Rev. food Sci. Nutr. 44, 97–111. doi:10.1080/10408690490424702
Kashyap, M., Bailey, D. P., Gomez, G., Rivera, J., Huff, T. F., and Ryan, J. J. (2005). Tgf-β1 inhibits late-stage mast cell maturation. Exp. Hematol. 33, 1281–1291. doi:10.1016/j.exphem.2005.07.001
Kaw, U., Ilyas, M., Bullock, T., Rittwage, L., Riha, M., Vidimos, A., et al. (2020). A regimen to minimize pain during blue light photodynamic therapy of actinic keratoses: bilaterally controlled, randomized trial of simultaneous versus conventional illumination. J. Am. Acad. Dermatology 82, 862–868. doi:10.1016/j.jaad.2019.09.010
Keshtkar, S., Azarpira, N., and Ghahremani, M. H. (2018). Mesenchymal stem cell-derived extracellular vesicles: novel frontiers in regenerative medicine. Stem Cell Res. Ther. 9, 63–69. doi:10.1186/s13287-018-0791-7
Khayambashi, P., Iyer, J., Pillai, S., Upadhyay, A., Zhang, Y., and Tran, S. D. (2021). Hydrogel encapsulation of mesenchymal stem cells and their derived exosomes for tissue engineering. Int. J. Mol. Sci. 22, 684. doi:10.3390/ijms22020684
Kheirandish, M., Gavgani, S. P., and Samiee, S. (2017). The effect of hypoxia preconditioning on the neural and stemness genes expression profiling in human umbilical cord blood mesenchymal stem cells. Transfus. Apher. Sci. 56, 392–399. doi:10.1016/j.transci.2017.03.015
Kim, H.-S., Yun, J.-W., Shin, T.-H., Lee, S.-H., Lee, B.-C., Yu, K.-R., et al. (2015). Human umbilical cord blood mesenchymal stem cell-derived pge2 and tgf-β1 alleviate atopic dermatitis by reducing mast cell degranulation. Stem cells 33, 1254–1266. doi:10.1002/stem.1913
Kim, J., and Hematti, P. (2009). Mesenchymal stem cell–educated macrophages: a novel type of alternatively activated macrophages. Exp. Hematol. 37, 1445–1453. doi:10.1016/j.exphem.2009.09.004
Ko, J. H., Kim, H. J., Jeong, H. J., Lee, H. J., and Oh, J. Y. (2020). Mesenchymal stem and stromal cells harness macrophage-derived amphiregulin to maintain tissue homeostasis. Cell Rep. 30, 3806–3820.e6. doi:10.1016/j.celrep.2020.02.062
Kolaczkowska, E., and Kubes, P. (2013). Neutrophil recruitment and function in health and inflammation. Nat. Rev. Immunol. 13, 159–175. doi:10.1038/nri3399
Kouroupis, D., and Correa, D. (2021). Increased mesenchymal stem cell functionalization in three-dimensional manufacturing settings for enhanced therapeutic applications. Front. Bioeng. Biotechnol. 9, 621748. doi:10.3389/fbioe.2021.621748
Kuang, S., He, F., Liu, G., Sun, X., Dai, J., Chi, A., et al. (2021). Ccr2-engineered mesenchymal stromal cells accelerate diabetic wound healing by restoring immunological homeostasis. Biomaterials 275, 120963. doi:10.1016/j.biomaterials.2021.120963
Kusuma, G. D., Carthew, J., Lim, R., and Frith, J. E. (2017). Effect of the microenvironment on mesenchymal stem cell paracrine signaling: opportunities to engineer the therapeutic effect. Stem cells Dev. 26, 617–631. doi:10.1089/scd.2016.0349
Le Blanc, K., and Mougiakakos, D. (2012). Multipotent mesenchymal stromal cells and the innate immune system. Nat. Rev. Immunol. 12, 383–396. doi:10.1038/nri3209
Lemsanni, M., Najeb, Y., Zoukal, S., Chafik, R., Madhar, M., and Elhaoury, H. (2021). Necrotizing fasciitis of the upper extremity: a retrospective analysis of 19 cases. Hand Surg. Rehabilitation 40, 505–512. doi:10.1016/j.hansur.2021.02.004
Li, B., and Lin, L. (2022). Internal light source for deep photodynamic therapy. Light Sci. Appl. 11, 85. doi:10.1038/s41377-022-00780-1
Li, W., Ren, G., Huang, Y., Su, J., Han, Y., Li, J., et al. (2012). Mesenchymal stem cells: a double-edged sword in regulating immune responses. Cell Death Differ. 19, 1505–1513. doi:10.1038/cdd.2012.26
Lin, X., Kong, B., Zhu, Y., and Zhao, Y. (2022). Bioactive fish scale scaffolds with mscs-loading for skin flap regeneration. Adv. Sci. 9, 2201226. doi:10.1002/advs.202201226
Liu, C., Lu, Y., Du, P., Yang, F., Guo, P., Tang, X., et al. (2022a). Mesenchymal stem cells pretreated with proinflammatory cytokines accelerate skin wound healing by promoting macrophages migration and m2 polarization. Regen. Ther. 21, 192–200. doi:10.1016/j.reth.2022.06.009
Liu, J., Yan, L., Yang, W., Lan, Y., Zhu, Q., Xu, H., et al. (2019). Controlled-release neurotensin-loaded silk fibroin dressings improve wound healing in diabetic rat model. Bioact. Mater. 4, 151–159. doi:10.1016/j.bioactmat.2019.03.001
Liu, L., Yu, Y., Hou, Y., Chai, J., Duan, H., Chu, W., et al. (2014a). Human umbilical cord mesenchymal stem cells transplantation promotes cutaneous wound healing of severe burned rats. PloS one 9, e88348. doi:10.1371/journal.pone.0088348
Liu, W., Yu, M., Xie, D., Wang, L., Ye, C., Zhu, Q., et al. (2020). Melatonin-stimulated msc-derived exosomes improve diabetic wound healing through regulating macrophage m1 and m2 polarization by targeting the pten/akt pathway. Stem Cell Res. Ther. 11, 259. doi:10.1186/s13287-020-01756-x
Liu, X., Hao, W., Lok, C.-N., Wang, Y. C., Zhang, R., and Wong, K. K. (2014b). Dendrimer encapsulation enhances anti-inflammatory efficacy of silver nanoparticles. J. Pediatr. Surg. 49, 1846–1851. doi:10.1016/j.jpedsurg.2014.09.033
Liu, Y., Liu, Y., Wu, M., Zou, R., Mao, S., Cong, P., et al. (2022b). Adipose-derived mesenchymal stem cell-loaded β-chitin nanofiber hydrogel promote wound healing in rats. J. Mater. Sci. Mater. Med. 33, 12. doi:10.1007/s10856-021-06630-7
Lood, C., Blanco, L. P., Purmalek, M. M., Carmona-Rivera, C., De Ravin, S. S., Smith, C. K., et al. (2016). Neutrophil extracellular traps enriched in oxidized mitochondrial dna are interferogenic and contribute to lupus-like disease. Nat. Med. 22, 146–153. doi:10.1038/nm.4027
Luo, Z., Lin, J., Sun, Y., Wang, C., and Chen, J. (2021). Bone marrow stromal cell-derived exosomes promote muscle healing following contusion through macrophage polarization. Stem Cells Dev. 30, 135–148. doi:10.1089/scd.2020.0167
Luz-Crawford, P., Noël, D., Fernandez, X., Khoury, M., Figueroa, F., Carrion, F., et al. (2012). Mesenchymal stem cells repress th17 molecular program through the pd-1 pathway. PLoS One 7, e45272. doi:10.1371/journal.pone.0045272
Ma, T., Wang, X., Jiao, Y., Wang, H., Qi, Y., Gong, H., et al. (2018). Interleukin 17 (il-17)-induced mesenchymal stem cells prolong the survival of allogeneic skin grafts. Ann. Transplant. 23, 615–621. doi:10.12659/AOT.909381
Mahjoor, M., Fakouri, A., Farokhi, S., Nazari, H., Afkhami, H., and Heidari, F. (2023). Regenerative potential of mesenchymal stromal cells in wound healing: unveiling the influence of normoxic and hypoxic environments. Front. Cell Dev. Biol. 11, 1245872. doi:10.3389/fcell.2023.1245872
Maqbool, M., Algraittee, S. J. R., Boroojerdi, M. H., Sarmadi, V. H., John, C. M., Vidyadaran, S., et al. (2019). Human mesenchymal stem cells inhibit the differentiation and effector functions of monocytes. Innate Immun. 26, 424–434. doi:10.1177/1753425919899132
Margiana, R., Markov, A., Zekiy, A. O., Hamza, M. U., Al-Dabbagh, K. A., Al-Zubaidi, S. H., et al. (2022). Clinical application of mesenchymal stem cell in regenerative medicine: a narrative review. Stem Cell Res. Ther. 13, 366. doi:10.1186/s13287-022-03054-0
Martinez, F. O., Helming, L., and Gordon, S. (2009). Alternative activation of macrophages: an immunologic functional perspective. Annu. Rev. Immunol. 27, 451–483. doi:10.1146/annurev.immunol.021908.132532
Mezrich, J. D., Fechner, J. H., Zhang, X., Johnson, B. P., Burlingham, W. J., and Bradfield, C. A. (2010). An interaction between kynurenine and the aryl hydrocarbon receptor can generate regulatory t cells. J. Immunol. 185, 3190–3198. doi:10.4049/jimmunol.0903670
Mohammadzadeh, A., Pourfathollah, A. A., Shahrokhi, S., Hashemi, S. M., Moradi, S. L. A., and Soleimani, M. (2014). Immunomodulatory effects of adipose-derived mesenchymal stem cells on the gene expression of major transcription factors of t cell subsets. Int. Immunopharmacol. 20, 316–321. doi:10.1016/j.intimp.2014.03.003
Moonen, J.-R. A., Harmsen, M. C., and Krenning, G. (2012). Cellular plasticity: the good, the bad, and the ugly? microenvironmental influences on progenitor cell therapy. Can. J. physiology Pharmacol. 90, 275–285. doi:10.1139/y11-107
Moura, L. I., Dias, A. M., Suesca, E., Casadiegos, S., Leal, E. C., Fontanilla, M. R., et al. (2014). Neurotensin-loaded collagen dressings reduce inflammation and improve wound healing in diabetic mice. Biochimica Biophysica Acta (BBA)-Molecular Basis Dis. 1842, 32–43. doi:10.1016/j.bbadis.2013.10.009
Munir, S., Basu, A., Maity, P., Krug, L., Haas, P., Jiang, D., et al. (2020). Tlr4-dependent shaping of the wound site by mscs accelerates wound healing. EMBO Rep. 21, e48777. doi:10.15252/embr.201948777
Munn, D. H., Sharma, M. D., Baban, B., Harding, H. P., Zhang, Y., Ron, D., et al. (2005). Gcn2 kinase in t cells mediates proliferative arrest and anergy induction in response to indoleamine 2, 3-dioxygenase. Immunity 22, 633–642. doi:10.1016/j.immuni.2005.03.013
Nadworny, P. L., Wang, J., Tredget, E. E., and Burrell, R. E. (2008). Anti-inflammatory activity of nanocrystalline silver in a porcine contact dermatitis model. Nanomedicine Nanotechnol. Biol. Med. 4, 241–251. doi:10.1016/j.nano.2008.04.006
Nava, M. M., Raimondi, M. T., and Pietrabissa, R. (2012). Controlling self-renewal and differentiation of stem cells via mechanical cues. BioMed Res. Int. 2012, 797410. doi:10.1155/2012/797410
Nelson, A. M., Reddy, S. K., Ratliff, T. S., Hossain, M. Z., Katseff, A. S., Zhu, A. S., et al. (2015). Dsrna released by tissue damage activates tlr3 to drive skin regeneration. Cell stem Cell 17, 139–151. doi:10.1016/j.stem.2015.07.008
Noronha, N. d. C., Mizukami, A., Caliári-Oliveira, C., Cominal, J. G., Rocha, J. L. M., Covas, D. T., et al. (2019). Priming approaches to improve the efficacy of mesenchymal stromal cell-based therapies. Stem Cell Res. Ther. 10, 131. doi:10.1186/s13287-019-1224-y
Ogawa, R. (2017). Keloid and hypertrophic scars are the result of chronic inflammation in the reticular dermis. Int. J. Mol. Sci. 18, 606. doi:10.3390/ijms18030606
Ong, C. J., Ip, S., Teh, S.-J., Wong, C., Jirik, F. R., Grusby, M. J., et al. (1999). A role for t helper 2 cells in mediating skin fibrosis in tight-skin mice. Cell. Immunol. 196, 60–68. doi:10.1006/cimm.1999.1537
Park, J. H., Lee, J.-R., Park, S., Kim, Y.-J., Yoon, J.-K., Park, H. S., et al. (2023). Subaqueous 3d stem cell spheroid levitation culture using anti-gravity bioreactor based on sound wave superposition. Biomaterials Res. 27, 51. doi:10.1186/s40824-023-00383-w
Philipp, D., Suhr, L., Wahlers, T., Choi, Y.-H., and Paunel-Görgülü, A. (2018). Preconditioning of bone marrow-derived mesenchymal stem cells highly strengthens their potential to promote il-6-dependent m2b polarization. Stem Cell Res. Ther. 9, 286. doi:10.1186/s13287-018-1039-2
Prasanna, S. J., Gopalakrishnan, D., Shankar, S. R., and Vasandan, A. B. (2010). Pro-inflammatory cytokines, ifnγ and tnfα, influence immune properties of human bone marrow and wharton jelly mesenchymal stem cells differentially. PloS one 5, e9016. doi:10.1371/journal.pone.0009016
Qin, X., He, J., Wang, X., Wang, J., Yang, R., and Chen, X. (2023). The functions and clinical application potential of exosomes derived from mesenchymal stem cells on wound repair: a review of recent research advances. Front. Immunol. 14, 1256687. doi:10.3389/fimmu.2023.1256687
Qiu, G., Zheng, G., Ge, M., Wang, J., Huang, R., Shu, Q., et al. (2018). Mesenchymal stem cell-derived extracellular vesicles affect disease outcomes via transfer of micrornas. Stem Cell Res. Ther. 9, 320–329. doi:10.1186/s13287-018-1069-9
Quintana, F. J., Murugaiyan, G., Farez, M. F., Mitsdoerffer, M., Tukpah, A.-M., Burns, E. J., et al. (2010). An endogenous aryl hydrocarbon receptor ligand acts on dendritic cells and t cells to suppress experimental autoimmune encephalomyelitis. Proc. Natl. Acad. Sci. 107, 20768–20773. doi:10.1073/pnas.1009201107
Ramez, M., Bagot, M., Nikolova, M., Boumsell, L., Bensussan, A., Vita, N., et al. (2001). Functional characterization of neurotensin receptors in human cutaneous t cell lymphoma malignant lymphocytes. J. investigative dermatology 117, 687–693. doi:10.1046/j.0022-202x.2001.01439.x
Reish, R. G., and Eriksson, E. (2008). Scars: a review of emerging and currently available therapies. Plastic Reconstr. Surg. 122, 1068–1078. doi:10.1097/PRS.0b013e318185d38f
Ren, G., Zhang, L., Zhao, X., Xu, G., Zhang, Y., Roberts, A. I., et al. (2008). Mesenchymal stem cell-mediated immunosuppression occurs via concerted action of chemokines and nitric oxide. Cell stem Cell 2, 141–150. doi:10.1016/j.stem.2007.11.014
Renner, P., Eggenhofer, E., Rosenauer, A., Popp, F., Steinmann, J., Slowik, P., et al. (2009). Mesenchymal stem cells require a sufficient, ongoing immune response to exert their immunosuppressive function. Transplant. Proc. 41, 2607–2611. doi:10.1016/j.transproceed.2009.06.119
Rieckmann, M., Delgobo, M., Gaal, C., Büchner, L., Steinau, P., Reshef, D., et al. (2019). Myocardial infarction triggers cardioprotective antigen-specific t helper cell responses. J. Clin. investigation 129, 4922–4936. doi:10.1172/JCI123859
Russell, D. G., Huang, L., and VanderVen, B. C. (2019). Immunometabolism at the interface between macrophages and pathogens. Nat. Rev. Immunol. 19, 291–304. doi:10.1038/s41577-019-0124-9
Rustad, K. C., Wong, V. W., Sorkin, M., Glotzbach, J. P., Major, M. R., Rajadas, J., et al. (2012). Enhancement of mesenchymal stem cell angiogenic capacity and stemness by a biomimetic hydrogel scaffold. Biomaterials 33, 80–90. doi:10.1016/j.biomaterials.2011.09.041
Ryu, N.-E., Lee, S.-H., and Park, H. (2019). Spheroid culture system methods and applications for mesenchymal stem cells. Cells 8, 1620. doi:10.3390/cells8121620
Saparov, A., Ogay, V., Nurgozhin, T., Jumabay, M., and Chen, W. C. (2016). Preconditioning of human mesenchymal stem cells to enhance their regulation of the immune response. Stem cells Int. 2016, 3924858. doi:10.1155/2016/3924858
Shahverdi, A. R., Fakhimi, A., Shahverdi, H. R., and Minaian, S. (2007). Synthesis and effect of silver nanoparticles on the antibacterial activity of different antibiotics against staphylococcus aureus and escherichia coli. Nanomedicine Nanotechnol. Biol. Med. 3, 168–171. doi:10.1016/j.nano.2007.02.001
Shi, Y., Wang, Y., Li, Q., Liu, K., Hou, J., Shao, C., et al. (2018a). Immunoregulatory mechanisms of mesenchymal stem and stromal cells in inflammatory diseases. Nat. Rev. Nephrol. 14, 493–507. doi:10.1038/s41581-018-0023-5
Shi, Y., Wang, Y., Li, Q., Liu, K., Hou, J., Shao, C., et al. (2018b). Mesenchymal stem cell-derived extracellular vesicles: novel frontiers in regenerative medicine. Nat. Rev. Nephrol. 14, 493–507. doi:10.1038/s41581-018-0023-5
Sioud, M. (2011). New insights into mesenchymal stromal cell-mediated t-cell suppression through galectins. Scand. J. Immunol. 73, 79–84. doi:10.1111/j.1365-3083.2010.02491.x
Sivanathan, K. N., Rojas-Canales, D., Grey, S. T., Gronthos, S., and Coates, P. T., (2017). Transcriptome profiling of il-17a preactivated mesenchymal stem cells: a comparative study to unmodified and ifn-γ modified mesenchymal stem cells. Stem cells Int. 2017, 1025820. doi:10.1155/2017/1025820
Sivanathan, K. N., Rojas-Canales, D. M., Hope, C. M., Krishnan, R., Carroll, R. P., Gronthos, S., et al. (2015). Interleukin-17a-induced human mesenchymal stem cells are superior modulators of immunological function. Stem Cells 33, 2850–2863. doi:10.1002/stem.2075
Soh, E. Z. F. (2021). Necrotizing soft tissue infection of left shoulder and upper limb following intravenous injection of non-steroidal anti-inflammatory drug. Cureus 13, e18068. doi:10.7759/cureus.18068
Soliman, H., Theret, M., Scott, W., Hill, L., Underhill, T. M., Hinz, B., et al. (2021). Multipotent stromal cells: one name, multiple identities. Cell Stem Cell 28, 1690–1707. doi:10.1016/j.stem.2021.09.001
Song, N., Scholtemeijer, M., and Shah, K. (2020). Mesenchymal stem cell immunomodulation: mechanisms and therapeutic potential. Trends Pharmacol. Sci. 41, 653–664. doi:10.1016/j.tips.2020.06.009
Spaggiari, G. M., Abdelrazik, H., Becchetti, F., and Moretta, L. (2009). Mscs inhibit monocyte-derived dc maturation and function by selectively interfering with the generation of immature dcs: central role of msc-derived prostaglandin e2. Blood, J. Am. Soc. Hematol. 113, 6576–6583. doi:10.1182/blood-2009-02-203943
Su, W., Wan, Q., Huang, J., Han, L., Chen, X., Chen, G., et al. (2015). Culture medium from tnf-α–stimulated mesenchymal stem cells attenuates allergic conjunctivitis through multiple antiallergic mechanisms. J. Allergy Clin. Immunol. 136, 423–432. doi:10.1016/j.jaci.2014.12.1926
Su, Y., and Richmond, A. (2015). Chemokine regulation of neutrophil infiltration of skin wounds. Adv. wound care 4, 631–640. doi:10.1089/wound.2014.0559
Syed Azhar, S. N. A., Ashari, S. E., and Salim, N. (2018). Development of a kojic monooleate-enriched oil-in-water nanoemulsion as a potential carrier for hyperpigmentation treatment. Int. J. nanomedicine 13, 6465–6479. doi:10.2147/IJN.S171532
Tian, J., Wong, K. K., Ho, C.-M., Lok, C.-N., Yu, W.-Y., Che, C.-M., et al. (2007). Topical delivery of silver nanoparticles promotes wound healing. ChemMedChem Chem. Enabling Drug Discov. 2, 129–136. doi:10.1002/cmdc.200600171
Tiemessen, M. M., Jagger, A. L., Evans, H. G., van Herwijnen, M. J., John, S., and Taams, L. S. (2007). Cd4+ cd25+ foxp3+ regulatory t cells induce alternative activation of human monocytes/macrophages. Proc. Natl. Acad. Sci. 104, 19446–19451. doi:10.1073/pnas.0706832104
Tsiapalis, D., and O’Driscoll, L. (2020). Mesenchymal stem cell derived extracellular vesicles for tissue engineering and regenerative medicine applications. Cells 9, 991. doi:10.3390/cells9040991
Vellasamy, S., Sandrasaigaran, P., Vidyadaran, S., Abdullah, M., George, E., and Ramasamy, R. (2013). Mesenchymal stem cells of human placenta and umbilical cord suppress t-cell proliferation at g0 phase of cell cycle. Cell Biol. Int. 37, 250–256. doi:10.1002/cbin.10033
Verkhratsky, A., Li, Q., Melino, S., Melino, G., and Shi, Y. (2020). Can covid-19 pandemic boost the epidemic of neurodegenerative diseases? Biol. direct 15, 28–8. doi:10.1186/s13062-020-00282-3
Vigo, T., Procaccini, C., Ferrara, G., Baranzini, S., Oksenberg, J. R., Matarese, G., et al. (2017). Ifn-γ orchestrates mesenchymal stem cell plasticity through the signal transducer and activator of transcription 1 and 3 and mammalian target of rapamycin pathways. J. Allergy Clin. Immunol. 139, 1667–1676. doi:10.1016/j.jaci.2016.09.004
Wallace, V. A., Kondo, S., Kono, T., Xing, Z., Timms, E., Furlonger, C., et al. (1994). A role for cd4+ t cells in the pathogenesis of skin fibrosis in tight skin mice. Eur. J. Immunol. 24, 1463–1466. doi:10.1002/eji.1830240634
Wang, B., Lin, Y., Hu, Y., Shan, W., Liu, S., Xu, Y., et al. (2017). Mtor inhibition improves the immunomodulatory properties of human bone marrow mesenchymal stem cells by inducing cox-2 and pge2. Stem Cell Res. Ther. 8, 292. doi:10.1186/s13287-017-0744-6
Wang, G., Cao, K., Liu, K., Xue, Y., Roberts, A. I., Li, F., et al. (2018). Kynurenic acid, an Ido metabolite, controls tsg-6-mediated immunosuppression of human mesenchymal stem cells. Cell Death Differ. 25, 1209–1223. doi:10.1038/s41418-017-0006-2
Wang, L., Zhao, Y., and Shi, S. (2012a). Interplay between mesenchymal stem cells and lymphocytes: implications for immunotherapy and tissue regeneration. J. Dent. Res. 91, 1003–1010. doi:10.1177/0022034512460404
Wang, Q., Sun, B., Wang, D., Ji, Y., Kong, Q., Wang, G., et al. (2008). Murine bone marrow mesenchymal stem cells cause mature dendritic cells to promote t-cell tolerance. Scand. J. Immunol. 68, 607–615. doi:10.1111/j.1365-3083.2008.02180.x
Wang, Q., Yang, Q., Wang, Z., Tong, H., Ma, L., Zhang, Y., et al. (2016). Comparative analysis of human mesenchymal stem cells from fetal-bone marrow, adipose tissue, and warton’s jelly as sources of cell immunomodulatory therapy. Hum. Vaccines Immunother. 12, 85–96. doi:10.1080/21645515.2015.1030549
Wang, X., Lin, L., Lan, B., Wang, Y., Du, L., Chen, X., et al. (2020a). Igf2r-initiated proton rechanneling dictates an anti-inflammatory property in macrophages. Sci. Adv. 6, eabb7389. doi:10.1126/sciadv.abb7389
Wang, Y., Chen, X., Cao, W., and Shi, Y. (2014). Plasticity of mesenchymal stem cells in immunomodulation: pathological and therapeutic implications. Nat. Immunol. 15, 1009–1016. doi:10.1038/ni.3002
Wang, Y., Fang, J., Liu, B., Shao, C., and Shi, Y. (2022). Reciprocal regulation of mesenchymal stem cells and immune responses. Cell Stem Cell 29, 1515–1530. doi:10.1016/j.stem.2022.10.001
Wang, Z., Lai, Y., Bernard, J. J., MacLeod, D. T., Cogen, A. L., Moss, B., et al. (2012b). Skin mast cells protect mice against vaccinia virus by triggering mast cell receptor s1pr2 and releasing antimicrobial peptides. J. Immunol. 188, 345–357. doi:10.4049/jimmunol.1101703
Wang, Z.-C., Zhao, W.-Y., Cao, Y., Liu, Y.-Q., Sun, Q., Shi, P., et al. (2020b). The roles of inflammation in keloid and hypertrophic scars. Front. Immunol. 11, 603187. doi:10.3389/fimmu.2020.603187
Wasef, L. G., Shaheen, H. M., El-Sayed, Y. S., Shalaby, T. I., Samak, D. H., Abd El-Hack, M. E., et al. (2020). Effects of silver nanoparticles on burn wound healing in a mouse model. Biol. trace Elem. Res. 193, 456–465. doi:10.1007/s12011-019-01729-z
Weller, K., Foitzik, K., Paus, R., Syska, W., Maurer, M., Weller, K., et al. (2006). Mast cells are required for normal healing of skin wounds in mice. FASEB J. 20, 2366–2368. doi:10.1096/fj.06-5837fje
Wen, Y.-T., Ho, Y.-C., Lee, Y.-C., Ding, D.-C., Liu, P.-K., and Tsai, R.-K. (2021). The benefits and hazards of intravitreal mesenchymal stem cell (msc) based-therapies in the experimental ischemic optic neuropathy. Int. J. Mol. Sci. 22, 2117. doi:10.3390/ijms22042117
William, T. T., Pendleton, J. D., Beyer, W. M., Egalka, M. C., and Guinan, E. C. (2003). Suppression of allogeneic t-cell proliferation by human marrow stromal cells: implications in transplantation. Transplantation 75, 389–397. doi:10.1097/01.TP.0000045055.63901.A9
Wnorowski, A., Sharma, A., Chen, H., Wu, H., Shao, N.-Y., Sayed, N., et al. (2019). Effects of spaceflight on human induced pluripotent stem cell-derived cardiomyocyte structure and function. Stem Cell Rep. 13, 960–969. doi:10.1016/j.stemcr.2019.10.006
Xu, C., Yu, P., Han, X., Du, L., Gan, J., Wang, Y., et al. (2014). Tgf-β promotes immune responses in the presence of mesenchymal stem cells. J. Immunol. 192, 103–109. doi:10.4049/jimmunol.1302164
Xu, J., Wu, W., Zhang, L., Dorset-Martin, W., Morris, M. W., Mitchell, M. E., et al. (2012). The role of microrna-146a in the pathogenesis of the diabetic wound-healing impairment: correction with mesenchymal stem cell treatment. Diabetes 61, 2906–2912. doi:10.2337/db12-0145
Yang, J., Chen, Z., Pan, D., Li, H., and Shen, J. (2020a). Umbilical cord-derived mesenchymal stem cell-derived exosomes combined pluronic f127 hydrogel promote chronic diabetic wound healing and complete skin regeneration. Int. J. Nanomedicine 15, 5911–5926. doi:10.2147/IJN.S249129
Yang, T., Tan, Y., Zhang, W., Yang, W., Luo, J., Chen, L., et al. (2020b). Effects of ala-pdt on the healing of mouse skin wounds infected with pseudomonas aeruginosa and its related mechanisms. Front. Cell Dev. Biol. 8, 585132. doi:10.3389/fcell.2020.585132
Yang, Z., Hu, X., Zhou, L., He, Y., Zhang, X., Yang, J., et al. (2021). Photodynamic therapy accelerates skin wound healing through promoting re-epithelialization. Burns Trauma 9, tkab008. doi:10.1093/burnst/tkab008
Younan, G., Suber, F., Xing, W., Shi, T., Kunori, Y., Åbrink, M., et al. (2010). The inflammatory response after an epidermal burn depends on the activities of mouse mast cell proteases 4 and 5. J. Immunol. 185, 7681–7690. doi:10.4049/jimmunol.1002803
Yu, Y., Wu, H., Zhang, Q., Ogawa, R., and Fu, S. (2021). Emerging insights into the immunological aspects of keloids. J. Dermatology 48, 1817–1826. doi:10.1111/1346-8138.16149
Zeng, Q.-L., and Liu, D.-W. (2021). Mesenchymal stem cell-derived exosomes: an emerging therapeutic strategy for normal and chronic wound healing. World J. Clin. Cases 9, 6218–6233. doi:10.12998/wjcc.v9.i22.6218
Zhang, B., Liu, R., Shi, D., Liu, X., Chen, Y., Dou, X., et al. (2009). Mesenchymal stem cells induce mature dendritic cells into a novel jagged-2–dependent regulatory dendritic cell population. Blood, J. Am. Soc. Hematol. 113, 46–57. doi:10.1182/blood-2008-04-154138
Zhang, D., Li, B., and Zhao, M. (2021). Therapeutic strategies by regulating interleukin family to suppress inflammation in hypertrophic scar and keloid. Front. Pharmacol. 12, 667763. doi:10.3389/fphar.2021.667763
Zhang, K., Lui, V. C., Chen, Y., Lok, C. N., and Wong, K. K. (2020). Delayed application of silver nanoparticles reveals the role of early inflammation in burn wound healing. Sci. Rep. 10, 6338. doi:10.1038/s41598-020-63464-z
Zhang, Q.-Z., Su, W.-R., Shi, S.-H., Wilder-Smith, P., Xiang, A. P., Wong, A., et al. (2010). Human gingiva-derived mesenchymal stem cells elicit polarization of m2 macrophages and enhance cutaneous wound healing. Stem cells 28, 1856–1868. doi:10.1002/stem.503
Zhao, Y., Zhu, X.-Y., Song, T., Zhang, L., Eirin, A., Conley, S., et al. (2021). Mesenchymal stem cells protect renal tubular cells via tsg-6 regulating macrophage function and phenotype switching. Am. J. Physiology-Renal Physiology 320, F454–F463. doi:10.1152/ajprenal.00426.2020
Zhu, M., Cao, L., Melino, S., Candi, E., Wang, Y., Shao, C., et al. (2023). Orchestration of mesenchymal stem/stromal cells and inflammation during wound healing. Stem Cells Transl. Med. 12, 576–587. doi:10.1093/stcltm/szad043
Zhu, M., Chu, Y., Shang, Q., Zheng, Z., Li, Y., Cao, L., et al. (2020). Mesenchymal stromal cells pretreated with pro-inflammatory cytokines promote skin wound healing through vegfc-mediated angiogenesis. Stem cells Transl. Med. 9, 1218–1232. doi:10.1002/sctm.19-0241
Keywords: skin, wound healing, MSCs, inflammation, immune response, pre-implant optimization
Citation: Gao M, Guo H, Dong X, Wang Z, Yang Z, Shang Q and Wang Q (2024) Regulation of inflammation during wound healing: the function of mesenchymal stem cells and strategies for therapeutic enhancement. Front. Pharmacol. 15:1345779. doi: 10.3389/fphar.2024.1345779
Received: 30 November 2023; Accepted: 05 February 2024;
Published: 15 February 2024.
Edited by:
Pier Maria Fornasari, Regen Health Solutions, ItalyReviewed by:
Fei Mao, Jiangsu University, ChinaCopyright © 2024 Gao, Guo, Dong, Wang, Yang, Shang and Wang. This is an open-access article distributed under the terms of the Creative Commons Attribution License (CC BY). The use, distribution or reproduction in other forums is permitted, provided the original author(s) and the copyright owner(s) are credited and that the original publication in this journal is cited, in accordance with accepted academic practice. No use, distribution or reproduction is permitted which does not comply with these terms.
*Correspondence: Qiying Wang, d2FuZ3FpeWluZ0B6enUuZWR1LmNu
Disclaimer: All claims expressed in this article are solely those of the authors and do not necessarily represent those of their affiliated organizations, or those of the publisher, the editors and the reviewers. Any product that may be evaluated in this article or claim that may be made by its manufacturer is not guaranteed or endorsed by the publisher.
Research integrity at Frontiers
Learn more about the work of our research integrity team to safeguard the quality of each article we publish.