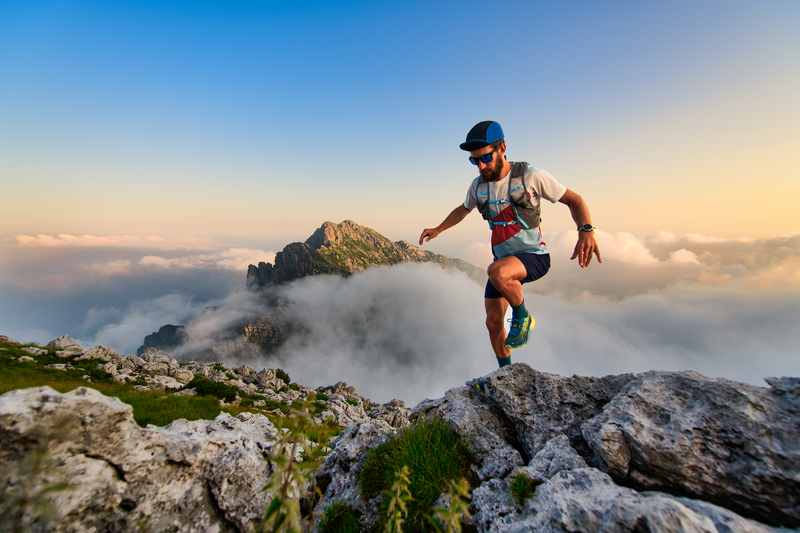
95% of researchers rate our articles as excellent or good
Learn more about the work of our research integrity team to safeguard the quality of each article we publish.
Find out more
ORIGINAL RESEARCH article
Front. Pharmacol. , 31 January 2024
Sec. Experimental Pharmacology and Drug Discovery
Volume 15 - 2024 | https://doi.org/10.3389/fphar.2024.1318797
P66Shc and Rac1 proteins are responsible for tumor-associated inflammation, particularly in brain tumors characterized by elevated oxidative stress and increased reactive oxygen species (ROS) production. Quercetin, a natural polyphenolic flavonoid, is a well-known redox modulator with anticancer properties. It has the capacity to cross the blood–brain barrier and, thus, could be a possible drug against brain tumors. In this study, we explored the effect of quercetin on Rac1/p66Shc-mediated tumor cell inflammation, which is the principal pathway for the generation of ROS in brain cells. Glioma cells transfected with Rac1, p66Shc, or both were treated with varying concentrations of quercetin for different time points. Quercetin significantly reduced the viability and migration of cells in an ROS-dependent manner with the concomitant inhibition of Rac1/p66Shc expression and ROS production in naïve and Rac1/p66Shc-transfected cell lines, suggestive of preventing Rac1 activation. Through molecular docking simulations, we observed that quercetin showed the best binding compared to other known Rac1 inhibitors and specifically blocked the GTP-binding site in the A-loop of Rac1 to prevent GTP binding and, thus, Rac1 activation. We conclude that quercetin exerts its anticancer effects via the modulation of Rac1-p66Shc signaling by specifically inhibiting Rac1 activation, thus restraining the production of ROS and tumor growth.
Gliomas are the most common brain tumors, accounting for almost 30% of primary brain tumors and 80% of all malignant tumors. Gliomas have disturbed redox homeostasis, resulting in the activation of reactive oxygen species (ROS)-mediated cell survival and tumor growth signaling pathways (Olivier et al., 2021). P66Shc is an oxidative stress regulating protein that, along with P52Shc and P46Shc, belongs to the ShcA family of proteins. P66Shc plays a dual role in cell growth, regulating both cell proliferation and cell apoptosis by modulating ROS levels in cells (Mir et al., 2020). In response to oxidative stress conditions, p66Shc promotes apoptotic signals by undergoing ser36 phosphorylation at its CH2 domain (Mir et al., 2022). However, when stimulated by growth factors, p66Shc gets tyrosine phosphorylated and promotes mitogenic signals. P66Shc, in response to epidermal growth (EGF) stimulation, promotes cell proliferation, cell migration, and cell invasion by regulating the activity of small GTPases (Bhat et al., 2015). P66Shc overexpression has been correlated with the metastatic activity of various cancers. In addition, p66Shc, in association with SNTA1 and Grb2, has been reported to enhance the metastatic activity of breast cancers by enhancing Rac1 activity (Ali et al., 2021; Ali et al., 2022).
Rac1, a member of small monomeric GTP-binding proteins of the Rho (Ras homology) family, is a well-established regulator of ROS levels (Liang et al., 2021). To maintain the normal redox state of cells, the activation of Rac1 levels must be regulated precisely. Rac1 has two key functions: it regulates the organization of the actin cytoskeleton and controls the release of superoxides in cells via the modulation of the activity of the NADPH oxidase enzyme (Ma et al., 2023). A direct link between the activation of Rac1 and ROS production has been observed. Rac1 showed a direct correlation with ROS to promote cell migration of head and neck squamous cell carcinoma (Chen Y-H. et al., 2023). Apart from this, Rac1 has a role in diverse cellular processes, for example, membrane trafficking [5], hypoxia-stimulated breast cancer cell migration, cell adhesion, and cell proliferation (Chen M. et al., 2023; Bogucka-Janczi et al., 2023; Yang et al., 2023). Rac1 also has a role in cancer initiation, progression, invasion, and metastasis (Zhang et al., 2023). The overexpression of Rac-1 has been associated to the development of cancers such as testicular cancer, head and neck squamous cell cancer, colorectal, pancreatic, and breast cancer, and leukemia (Ramos et al., 2022; An et al., 2023; Gupta et al., 2023; Nakhaei-Rad et al., 2023).
Immense complexity of the human brain has made the treatment of gliomas an exigent task. Chemotherapeutics, though effective in treating cancer, show undesirable effects and are highly expensive. On the other hand, natural products have played a significant role in cancer drug discovery and have proven advantageous over conventional synthetic molecules. The chemopreventive properties of natural bioactive products have been increasingly important to researchers in the field of medicine in recent years (Harvey, 2008; Shen, 2015). It is believed that regular intake of vegetables and fruits can aid in preventing gliomas (Zhang et al., 2022). One possible reason is the presence of dietary antioxidants, for example, flavonoids in plant products since these are capable of decreasing oxidative stress and related damages (Mahomoodally et al., 2018; Slika et al., 2022; Hassan et al., 2023). The beneficial effects are proclaimed because of their capability to scavenge free radicals and reactive oxygen species. It is believed that free radicals and ROS induce DNA damage, cellular inflammation, and oxidative stress, ultimately leading to malignancies (Devika et al., 2022; Dewanjee et al., 2022). Therefore, it is likely that strengthening the antioxidant defense with an exogenous antioxidant might diminish these processes by decreasing the reactive species. Pronounced effects against these pathologies and cancer can, thus, be expected from treatment with antioxidants.
Quercetin is a dietary polyphenolic compound having high antioxidant activity and is chemically composed of three benzene rings and five hydroxyl groups. The sources of quercetin include apples, buckwheat, onions, and citrus fruits. Quercetin, as an antioxidant, has been suggested to have antidiabetic, anti-inflammatory, antioxidant, antimicrobial, anti-Alzheimer’s, antiarthritic, cardiovascular, and wound-healing effects (Alizadeh and Ebrahimzadeh, 2022; Michala and Pritsa, 2022). In addition, quercetin also shows antitumor properties against different cancers including pancreatic cancer, colon cancer breast cancer, and ovarian cancer (Salehi et al., 2020). The concentration at which quercetin exhibits anticancer activities under in vitro conditions ranges from 10 μM to 200 µM depending on the cancer cell type (Kedhari Sundaram et al., 2019; Salehi et al., 2020). The pharmacokinetic effects of intravenous quercetin injections were also studied in phase I clinical trials in 51 patients at doses of 60–2000 mg/m2. The study showed that a dose of 945 mg/m2 was safe, beyond which vomiting, high blood pressure, nephrotoxicity, and decreased serum potassium were reported (Ferry et al., 1996). Though quercetin has been shown to have anticancer activities, the molecular mechanisms behind are still inconclusive. Considering the facts that p66Shc regulates ROS-mediated tumor formation and quercetin being an antioxidant possessing anticancer properties, we were intrigued to explore its effects on p66Shc/Rac1-mediated ROS production and cell migration in an attempt to elucidate its possible mechanism of action.
Quercetin (3, 3′, 4′, 5, 7-pentahydroxyflavone) was purchased from Sigma (6151-25-3) and was dissolved in dimethyl sulfoxide (DMSO) before use. The final concentration of DMSO did not exceed 0.1% (v/v) throughout the experiments.
Xpress-tagged P66Shc WT cDNA in the PCDNA 3.1/His A and Rac1 vector were gifted by Dr. Shaida Andrabi.
Antibody to Shc (BD BioSciences, 610082), anti-vinculin (Upstate Biotechnology Inc., 05-386), anti-Rac1 (Millipore, 23A8), anti-β-actin (Sigma, A1978), and horseradish peroxidase-conjugated secondary antibodies (Sigma-Aldrich, OR03L) were used.
C6 (CCL-107) rat glioma and IMR-32 (CCL-27) human neuroblastoma cells were procured from the National Centre for Cell Science (NCCS), India, and were grown up to a number of 50 passages. These cells were grown in Dulbecco’s Modified Eagle’s Medium (DMEM) (Sigma) supplemented with 10% fetal bovine serum (Sigma) and antibiotics (100 U/mL penicillin and 100 μg/mL streptomycin). All the cells were maintained at 37°C in a 5% CO2 incubator. C6 and IMR-32 cells were selected for this because of significant endogenous expression levels of the p66Shc protein. However, based on better transfection efficiency, most of the experiments were carried out in C6 cells.
Cells after attaining 70%–80% confluence were treated with various concentrations of quercetin (in DMSO) ranging from 25 μM to 200 µM for different intervals of time (0, 12, 24, 48, and 72 h). Quercetin concentrations were selected based on some previous studies which had reported its antitumor properties. To check for the involvement of ROS, N-acetylcysteine (NAC), which is a known ROS scavenger, was added to the cells either alone or in combination with quercetin. Vehicle controls were cells treated only with DMSO.
For transient transfection, cells were grown overnight in 10 cm dishes (Thermo Fisher Scientific (Carlsbad, CA, United States)) up to 70% confluence. The next day, complete media were removed, and serum-free media were added followed by transient transfection using the cationic lipid-based Lipofectamine 2000 (Invitrogen, United States) transfection reagent. Transfection was performed according to the protocol provided by the manufacturer; two microcentrifuge tubes with an equal volume of serum-free media were taken, and to one, Lipofectamine was added, while to another microcentrifuge tube, DNA along with P2000 was added. DNA mixture was then added dropwise to Lipofectamine tube, mixed, and incubated for 15–20 min at room temperature. The mixture was then dropwise added to cell culture dishes and incubated overnight. After transfection, the cells were analyzed for different time points (discussed in results) and were harvested or assayed as per the protocol.
Cell viability was monitored using MTT (3-(4, 5-dimethylthiazol-2-yl)-2, 5-diphenyltetrazolium bromide) colorimetric assay. C6 and IMR-32 cells were seeded in 96-well plates (104 cells per well) and cultured for different time intervals (12, 24, 48, and 72 h), followed by the treatment with varying concentrations of quercetin (25 μM, 50 μM, 100 μM, and 200 µM). Thereafter, the culture medium was aspirated, and 100 μL of MTT dye (1 mg/mL in PBS) was added into every well and incubated at 37°C for 3–4 h.
Acidified isopropanol (0.1N HCl) or DMSO was added to each well to solubilize the blue formazan crystals (if formed). Absorbance was read at 570 nm using a microplate reader (Epoc Biotech). The index of cell viability was determined by measuring the OD of the color produced by the reduction of MTT dye, compared with that of the untreated control cells [(OD of test compound-treated cells/OD of solvent-treated cells) ×100].
C6 cells were seeded in 100 mm dishes to the density of 2.2 × 106 per dish and transfected with the desired plasmid constructs. Transfected cells were treated with different doses of quercetin for specific time points depending on the experiments. Cells were then collected by trypsinization, washed with PBS, and lysed in lysis buffer [150 mM NaCl, 20 mM Tris-HCl (pH 7.4), 2 mM ethylenediaminetetraacetic acid (EDTA), 1% Nonidet P-40, 20% glycerol, 1 mM phenylmethylsulfonyl fluoride, and 5 mM NaF and containing a protease inhibitor cocktail]. The cell lysates (20 μg–40 μg) were solubilized in sample buffer [50 mM Tris-HCl (pH 6.8), 10% glycerol, 4% SDS, 5% β-mercaptoethanol, and 0.00125% bromophenol blue] and boiled for 5 min. Then, 30 μg of total protein from each cell lysate was resolved by and subjected to 10% sodium dodecyl sulfate-polyacrylamide gel electrophoresis (SDS-PAGE). Resolved proteins were then electro-transferred to the polyvinylidene difluoride (PVDF) membrane (Millipore). All the steps of Western blotting were followed as done previously [40]. Anti-Shc (1:3000), anti-Rac1 (1:1000), anti-vinculin (1:5000), and anti-β-actin (1:500) antibodies were used. Following transfer, immuno-detection was carried out, and the immunoreactive bands were visualized by chemiluminescence using the ECL system (Cell Biosciences).
The amount of Rac1 activation (GTP-bound form) was determined using a commercial assay kit (Upstate Biotechnology, United States) that affinity precipitates GTP-Rac1 in cell lysates using the agarose conjugate-Rac1-binding domain of PAK. An activation assay was performed as per the protocol provided by the manufacturer, and the GTP-bound form of Rac1 associated with GST-PAK1 was detected using a monoclonal antibody against Rac1, also provided with the kit.
Extracellular ROS generation in cells was assessed using the Amplex Ultra Red reagent (10 mM; Molecular Probes, Invitrogen). The Amplex Ultra Red reagent is a highly sensitive assay for detecting ROS levels. The Amplex Ultra Red reagent in the presence of peroxidase reacts with H2O2 in a 1:1 stoichiometry to produce the red-fluorescent oxidation product, resorufin. Cells seeded in six-well plates (0.3 × 106 cells per well) were transfected with either p66Shc or Rac1 or both and then treated with quercetin. To each well, 50 μL of 10 mM Amplex UltraRed reagent and 100 μL of 10 U/mL horseradish peroxidase (HRP) were added. The plates were then kept in a CO2 incubator for 20 min incubation. The ROS generation was quantified by measuring fluorescence intensity (excitation, 568 nm; emission, 581 nm) using a spectrofluorophotometer (Shimadzu, RF-5301) against appropriate assay controls.
The effect of quercetin on the migratory potential of C6 cells was determined using wound-healing assay. In brief, the cells were seeded in a 24-well plate at a density of 1 × 105 cells per well. At 70%–80% confluence, the cells were transfected with either p66Shc or Rac1 or both the plasmids together. A P-10 pipette tip was used to create a clean wound area across the center of the culture plate. The cells were then treated with 100 µM quercetin or 1 mM NAC; however, control cells were left untreated. The movement of the cells in the area of the wound was examined. The images of the distance migrated by the cells were taken under a light microscope (Olympus).
The X-ray-diffracted crystal structure of Rac1 protein was extracted from the Research Collaboratory for Structural Bioinformatics (RSCB) with accession number PDB ID 3TH5. It encompassed six helices and six small beta sheets and possessed a structure weight of 46561.09 A.U. (asymmetric unit), with a resolution of approximately 2.3 Å. The 3D ligand conformations were extracted from the PubChem database: (a) quercetin (Chem ID 5280343), (b) InSolution™ Rac1 Inhibitor (Chem ID: 16760632), (c) NSC 23766 (Chem ID: 16759159), (d) EHT 1864 (Chem ID: 9938202), (e) 6-mercaptopurine (Chem ID: 667490), and (f) ouabain (Chem ID: 439501).
AutoDock 4.2 (Morris et al., 2009) was used to perform molecular docking simulations and to elucidate the binding conformations of the selected compound(s). Polar hydrogen atoms were added to the receptor protein and ligands; all bonds were allowed to rotate and were flexible during docking. Using AutoGrid, grid maps were generated by marking the grids around the whole receptor. Each grid was centered at the structure of the corresponding receptor. The grid dimensions were 30 Å * 30 Å * 30 Å with a spacing of 0.375 Å. The Lamarckian genetic algorithm was used for flexible ligand docking with 100 runs, a population size of 150, 2.5 * 106 evaluations, a maximum number of 27 * 103 iterations, an elitism value of 1, a mutation rate of 0.02, and a crossover rate of 0.80 (Mokhdomi et al., 2016). The binding conformations were then analyzed based on the energy values and the interacting residues. For comparing the molecular interaction of quercetin with Rac1 protein, the top five known Rac1 inhibitors were used: a) InSolution™ Rac1 Inhibitor (Chem ID: 16760632), b) NSC 23766 (Chem ID: 16759159), c) EHT 1864 (Chem ID: 9938202), d) 6-mercaptopurine (Chem ID: 667490), and e) ouabain (Chem ID: 439501). All molecular visualizations were carried out using Accelrys Discovery Studio Client 4.2.
Using Desmond 2020.1 (Shaw et al., 2010), MD simulations were performed on the 3TH5 + quercetin complex. In this system, the OPLS-2005 force field (Bowers et al., 2006; Chow et al., 2008; Shivakumar et al., 2010) and explicit solvent model with the SPC water molecules (Jorgensen et al., 1983) were used in a period boundary salvation box with dimensions of 1.0 Å × 1.0 Å × 1.0 Å. In order to neutralize the electrical charge, sodium ions (Na+) were introduced. The metabolic environment was replicated by introducing a solution containing 0.15 M NaCl into the apparatus. Initially, the system underwent retraining by subjecting the protein–ligand complexes to an NVT ensemble for a duration of 10 nanoseconds. Following the preceding procedure, an NPT ensemble was used to conduct a brief 12-ns run of equilibration and minimization. The NPT ensemble was established using the Nose–Hoover chain coupling method (Martyna et al., 1994). In each of the models, the temperature was varied while maintaining a constant relaxation time of 1.0 picoseconds and a pressure of 1 bar. The temporal increment used in the simulation was 2 femtoseconds. The Martyna–Tuckerman–Klein chain coupling scheme barostat technique (Martyna et al., 1992) was used to regulate the pressure. The period of relaxation was chosen as 2 picoseconds. In the computation of long-range electrostatic interactions, we used the particle mesh Ewald approach. The radius for the coulomb interactions was maintained at a constant value of 9 Å. The bonded forces for each trajectory were calculated using the RESPA integrator with a time step of 2 fs. The most recent manufacturing run was conducted with a temporal duration of one hundred nanoseconds per unit. To assess the stability of the molecular dynamics (MD) simulations, several key parameters were quantified, including the root mean square deviation (RMSD), radius of gyration (Rg), root mean square fluctuation (RMSF), and number of hydrogen bonds (H-bonds). The aforementioned values were used for the purpose of assessing the stability of the MD simulations.
Statistical analyses were carried out using GraphPad Prism 6 (v 6.01). For dose- and time-dependent studies, a statistical analysis was carried out using one-way analysis of variance (ANOVA), with multiple comparisons using Dunnett’s post hoc test, and the level of significance was tested at a p-value ranging from 0.05 to 0.001. In comparative analysis, Student’s t-test (one tailed) was used. The level of significance was tested at 95% CI, p < 0.05. All the data are expressed as the mean ± SD of at least three independent experiments. Asterisks represent statistical significances and are indicated as follows: *p < 0.05, **p < 0.01, and ***p < 0.001.
The effect of quercetin on the viability of C6 and IMR-32 cells was evaluated using MTT assay. Cells were treated with increasing concentrations of quercetin (25 μM, 50 μM, 100 μM, and 200 µM) for different time points (12, 24, 48, or 72 h). We found that quercetin significantly inhibited the growth and proliferation of both C6 and IMR-32 cells in a dose-dependent manner but showed time dependence till 24 h only (doubling time of cells). The inhibition of proliferation beyond 24 h drug treatment showed little or no difference at each tested dose (Figure 1).
FIGURE 1. Quercetin treatment inhibits the proliferation of neuronal cells. Cells were incubated with different concentrations of quercetin (25 μM, 50 μM, 100 μM, and 200 µM) for 12, 24, 48, or 72 h, respectively. Control comprised cells without quercetin treatment. (A) Bar graph showing the effect of quercetin on rat glioma C6 cells in a concentration- and time-dependent manner. (B) Bar graph showing the effect of quercetin on human neuroblastoma IMR-32 cells in a concentration- and time-dependent manner. The data shown represent the mean ± standard deviation of three biological replicates. The statistical analysis was carried out using one-way ANOVA with multiple comparisons using Dunnett’s post hoc test to determine the level of significance. **p < 0.01; *** p < 0.0001.
Both p66Shc and Rac1 share a common activation pattern as both are being induced by cellular stress and show interdependence. The former acts as scaffolding protein to bring about the expression of Rac1, which, in turn, releases stress factors and induces the expression of p66Shc. To elucidate the effect of quercetin on p66Shc and Rac1 expression, C6 cells were transfected with p66Shc and Rac1, incubated with 25 μM, 50 μM, 100 μM, and 200 µM concentrations of quercetin, and harvested after 24 h incubation. After harvesting, cell lysates were titrated against anti-p66Shc and anti-Rac1 antibodies. The results indicate that quercetin inhibited the expression of endogenously and exogenously transected p66Shc/Rac1 expression in a dose-dependent manner (Figures 2A, B), (Figures 3A, B).
FIGURE 2. Quercetin decreases the expression levels of p66Shc. (A) Representative immunoblot showing the dose-dependent effect of quercetin for 24 h on endogenous p66Shc. (B) Immunoblot showing the effect of quercetin for 24 h on exogenous (+) p66Shc (externally transfected) in C6 cells. (C) Bar diagram (densitometric analysis) showing the fold change in the expression levels of endogenous and exogenous p66Shc in response to quercetin treatment. (D) Immunoblot showing the time-dependent effect of quercetin on the endogenous expression of p66Shc. (E) Immunoblot showing the time-dependent effect of quercetin on exogenous (+) p66Shc expression in cells treated with 100 µM quercetin. Also detected in the blot are two other Shc isoforms, i.e., p52Shc and p46Shc. QVC: vehicle control. Q25–Q200: 25 µM–200 µM concentrations of quercetin used. Q12 hr–Q 72 h: 100 µM concentration of quercetin used for 12 h–72 h. Lower panels represent the expression of vinculin protein used as loading control, in respective treatment groups. (F) Bar diagram densitometric analysis showing the fold change in the expression of p66shc in response to quercetin in a time-dependent manner. The results shown were repeated at least three times and are expressed as mean ± SD fold change over the control level set to 1 unit and normalized to total protein. The statistical analysis was carried out using one-way ANOVA with multiple comparisons using Dunnett’s post hoc test. **p < 0.01 represents significant variation compared to respective vehicle controls.
FIGURE 3. Effect of quercetin on the expression of Rac1 protein. (A) Western blot immunoblot showing a dose-dependent effect of quercetin on endogenous Rac1 at different time points in C6 glioma cells. (B) Western blot immunoblot showing a dose-dependent effect of quercetin on exogenous Rac1 at different time points in C6 glioma cells. (C) Bar diagram (densitometric analysis) showing the fold change in the expression levels of endogenous and exogenous Rac1 in response to quercetin treatment in a concentration-dependent manner. (D) Immunoblots showing the time-dependent effect of quercetin on the expression levels of endogenous Rac1 in C6 glioma cells and harvested after 24 h. (E) Immunoblots showing the time-dependent effect of quercetin on the expression levels of exogenous Rac1 in C6 glioma cells harvested after 24 h. QVC: vehicle control. Q25–Q200: 25 µM–200 µM concentrations of quercetin used. Q12 hr–Q72 h: 100 µM concentration of quercetin used for 12 hr–72 h. Lower panels represent the expression of vinculin protein used as loading control, in respective treatment groups. (F) Bar diagram (densitometric analysis) showing the fold change in the expression levels of endogenous and exogenous Rac1 in response to quercetin treatment in a time-dependent manner. The results shown were repeated at least three times and are expressed as mean ± SD fold change over the control level set to 1 unit and normalized to total protein. The statistical analysis was carried out using one-way ANOVA with multiple comparisons using Dunnett’s post hoc test. **p < 0.01 represents significant variation compared to respective vehicle controls.
We also looked for the time dependence of quercetin treatment on the expression of p66Shc and Rac1 proteins. A consistent decrease in Rac1 expression was observed in both transfected and untransfected cells upon quercetin (100 µM) treatment (Figures 2D, E; Figure 3D; Figure 2E); however, the decrease in the expression of p66Shc was less prominent after 24 h.
Both p66Shc and Rac1 are known to be involved in ROS generation and the regulation of cellular oxidative stress; hence, the effect of quercetin on ROS production via the p66Shc–Rac1 pathway was studied. Cells were transiently transfected with (i) p66Shc, (ii) Rac1, and/or (iii) p66Shc and Rac1 together. The Amplex UltraRed reagent was used to monitor extracellular ROS generation in C6 glioma cells, following quercetin treatment. The overexpression of p66Shc and/or Rac1 in cells resulted in a significant increase in ROS generation, which showed a peak when both the vectors were expressed together. However, the treatment of cells with quercetin not only reduced the levels of ROS (Figure 4) but also showed a significant inhibition of p66Shc/Rac1, irrespective of whether both the vectors are co-expressed or not.
FIGURE 4. Quercetin decreases p66Shc/Rac1-mediated ROS production: comparison of ROS production in cells transfected either with p66Shc, Rac1, and/or both the plasmid constructs together following quercetin (100 µM) treatment. Controls represent untransfected cells. The results shown were repeated at least three times and are expressed as mean ± SD. The statistical analysis was carried out using one-way ANOVA with multiple comparisons using Dunnett’s post hoc test. *p < 0.05, **p < 0.01, and ***p < 0.00 represent significant variations compared to respective vehicle controls.
Since a consistent decline in the expression of both p66Shc and Rac1 proteins as well as ROS production was observed upon quercetin treatment, we looked for the effect of quercetin on the activation of Rac1 to validate the specificity of quercetin action. C6 cells were transiently transfected with the Rac1 plasmid and treated with varying concentrations of quercetin. The pull-down experiments showed a 2–2.5-fold decrease in active Rac1 upon quercetin treatment (Figure 5) compared to untreated cells.
FIGURE 5. Quercetin decreases active Rac1 levels in c6 cells. (A) Representative immunoblot showing a decrease in active Rac1 levels upon quercetin treatment in immuno-precipitated lysates from C6 cells transiently transfected with the Rac1 plasmid construct. (B) Western densitometry quantifications showing fold decrease in active Rac1 upon quercetin treatment compared to control (untreated). The results shown were repeated at least three times and are expressed as mean ± SD. The statistical analysis was carried out using one-way ANOVA with multiple comparisons using Dunnett’s post hoc test. *p < 0.05, **p < 0.01, and ***p < 0.00 represent significant variations compared to respective vehicle controls.
Cellular stress induced by ROS has been implicated in stimulating migration in benign tumor cells (Mir et al., 2020). Since ROS generation is primarily regulated by p66Shc-Rac1 signaling, we looked for the effect of quercetin on the migratory potential of gliomas using wound-healing assay by modulating p66Shc, Rac1, or ROS titer. Quercetin effectively decreased the migratory potential of cells, even after transfection with p66Shc or Rac1 (Figure 6), which otherwise increases cell proliferation (data not shown). When both of these proteins, viz., p66Shc and Rac1, were co-expressed in cells, wound healing conspicuously increased after 48 h following the wound. However, there was a noticeable decrease in cell migration in these cells after quercetin treatment. The effect of quercetin on cell migration was comparable to that of NAC (1 mM), a known ROS scavenger which showed a considerable decrease in wound healing at 48 h, thus pointing toward an ROS-dependent inhibition of cell migration by quercetin. The results were further corroborated by combinatorial treatment of quercetin and NAC on wound healing which drastically reduced cell migration across the wound area (Figure 6).
FIGURE 6. Quercetin inhibits cell migration in ROS-dependent manner. Representative photomicrographs depicting the effect of quercetin (100 µM) in the absence and presence of NAC (1 mM) on cell migration in cultures of C6 cells transfected with p66Shc and/or Rac1 plasmid constructs following scratch wound. Representative photographs illustrate the results of three independent experiments.
The activation of Rac1 is dependent on the binding of GTP to its nucleotide-binding pocket, stimulating effector proteins, and regulates cellular processes, viz., cell adhesion, cell proliferation, and ROS production. To evaluate the quercetin-mediated inhibition of Rac1 activation, we performed molecular docking simulation (MDS) of Rac1 with quercetin and the top five Rac1 inhibitors targeting the GTP-binding site in the P-loop of Rac1 (Figure 7). Quercetin showed the best binding (∆G = −9.86 kcal/mol) with the P-loop and specifically blocked the GTP-binding site (KiQuer = 58.82 uM) compared to other Rac1 inhibitors, viz., a) InSolution™ Rac1 Inhibitor (∆G = −4.88, KiInSol = 264.46 uM), b) NSC 23766 (∆G = −5.46; KiNSC = 99.15 uM), c) EHT 1864 (∆G = −5.22; KiEHT = 149.5 uM), d) 6-mercaptopurine (∆G = −5.66; KiMer = 71.38 Um), and e) ouabain (∆G = −4.51; KiOuab = 490.82 uM). The binding interactions of quercetin with the GTP-binding site of Rac1 comprised amino acids GLY12, ALA13, VAL14, GLY15, LYS16, and THR17 in the P-loop and ASP57, THR58, ALA59, GLY60, and GLN61 in the A-loop of Rac1. Quercetin showed the highest H-bonding (n = 5) compared to other Rac1 inhibitors with a bond energy of −11.65 kcal/mol. As docking studies showed significant binding affinity of Rac1 with quercetin, we further utilized molecular dynamics simulation to further evaluate the stability of the Rac1–quercetin complex.
FIGURE 7. Comparative binding modes of Rac1 inhibitors and quercetin. The results of the molecular docking simulation of Rac1 protein with (A) InSolution™ Rac1 Inhibitor, (B) NSC 23766, (C) EHT 1864, (D) 6-mercaptopurine, (E) ouabain, and (F) quercetin showing respective binding modes of inhibitors targeting the GTP-binding site. Ligand interactions at the GTP-binding site of Rac1 are depicted in molecular models.
MD and simulation studies were carried out in order to determine the stability and convergence of Rac1 + quercetin. Simulation of 100 ns displayed almost stable conformation while comparing the RMSD. The RMSD of the Cα-backbone of Rac1 + quercetin up to 60 ns exhibited dangling deviations, which later became more stable up to 100 ns (Figure 8A). A stable RMSD plot during simulation signifies a good convergence and stable conformations. Thus, it can be suggested that Rac1 was quite stable in complex with quercetin due to higher affinity. Moreover, RMSF plots displayed large spikes of fluctuation in Rac1 observed at the 1st to 40th residues, which may be due to the higher flexibility of the residues (Figure 8B), but no significant fluctuations were observed later on. Most of the residues less fluctuating during the entire 100 ns simulation (Figure S1) indicate the rigid amino acid conformations during the simulation time. Therefore, from RMSF plots, it can be suggested that the protein structure is rigid during simulation in ligand-bound conformations. Rg is the measure of compactness of the protein. In this study, the Rac1 + quercetin Cα-backbone displayed the lowering of Rg from 22.3 to 22.2 Å (Figure 8C). The lowering of Rg indicates the highly compact orientation of the protein in the ligand-bound state. The number of hydrogen bonds between the protein and the ligand suggests the significant interaction and stability of the complex. The number of hydrogen bonds between Rac1 and quercetin showed initially up to 65 ns one hydrogen bond observed on average, while later on, an addition of hydrogen bonds was observed up to 100 ns (Figure 8D). Followed by Rg analysis, patterns were also observed in the solvent-accessible surface area (SASA) in both ligand-bound and -unbound states. It is clearly visible from Figure 8E that in the unbound state, Rac1 displayed high surface area accessible to solvent in all the cases. The SASA value lowered as compared to the unbound state while bound with quercetin (Figure 8E). The overall study of Rg signifies that the ligand binding compels the respective proteins to become more compact.
FIGURE 8. MD simulation analysis of the 100 ns trajectories of (A) the Cα backbone RMSD of 3TH5 bound to ligand quercetin. (B) RMSF of the Cα backbone of 3TH5 bound to ligand quercetin. (C) Cα backbone radius of gyration (Rg) of 3TH5 bound to ligand quercetin. (D) Formation of hydrogen bonds in 3TH5 bound to ligand quercetin. (E) Solvent-accessible surface area of 3TH5 bound to ligand quercetin.
Utilizing the MD simulation trajectory, the binding free energy along with other contributing energies in the form of MM-GBSA was determined for complex Rac1 + quercetin. The results (Table 1) suggested that the maximum contribution to ΔGbind in the stability of the simulated complexes was due to ΔGbindCoulomb, ΔGbindvdW, and ΔGbindLipo, while, ΔGbindCovalent and ΔGbindSolvGB contributed to the instability of the corresponding complexes. Furthermore, the Rac1 + quercetin complex has significantly higher binding free energies (Table 1). These results supported the potential of Rac1 + quercetin having high affinity of binding to the protein as well as efficiency in binding to the selected protein and the ability to form stable protein–ligand complexes.
RhoGTPase Rac1 protein, primarily involved in cell adhesion and motility in migrating cells, has been implicated in oxidative stress, enhancing the production of ROS and, in particular, ROS-mediated tumor inflammation (Azarova et al., 2022; Marwaha et al., 2022; Liao et al., 2023). Although the human body has an innate antioxidant defense mechanism to counter oxidative species in cells, it may not function once ROS exceeds a threshold level leading to cellular stress. The overproduction of ROS, particularly peroxides, tends to alter cell cycle phases, eventually leading to cancer (Mehraj et al., 2022a; Mehraj et al., 2022b; Chen J-S. et al., 2023; Liu et al., 2023). The situation becomes more vulnerable in metabolically active tissues such as the brain which, apart from being less equipped with innate antioxidant defense, also tend to resist the delivery of drugs due to the blood–brain barrier (Rinaldi et al., 2016). Augmenting the innate antioxidant defense with external antioxidants having the ability to cross the blood–brain barrier could, thus, be beneficial in the treatment of brain tumors (Mittal et al., 2023).
Quercetin is a powerful antioxidant and effective scavenger for highly reactive species such as hydroxyl radicals, peroxinitrite, and superoxide radicals and has the ability to cross the blood–brain barrier and, therefore, is a potential drug for brain tumors (Gibellini et al., 2011; Zhang et al., 2011; Asgharian et al., 2022). The effect of antioxidants such as quercetin on the regulation of the p66shc-Rac1 pathway which principally drives the generation of ROS apart from regulating apoptosis and/or cell proliferation has not been evaluated so far. Therefore, in this study, we explored the effect of quercetin on Rac1 signaling and tumor cell proliferation.
We show that quercetin effectively inhibited the proliferation of both human neuroblastoma cells (IMR-32 cells) and rat glioma C6 cells (Figure 1). The decline in cell proliferation was consistent with inhibition in the expression of p66Shc protein (Figure 2), pointing toward the modulation of RhoGTPase Rac1 signaling by quercetin. Quercetin not only inhibited the expression of intracellular p66Shc but also prevented the expression of the transfected p66Shc gene. P66Shc has been recognized as a ROS producer since it acts as an oxidoreductase enzyme capable of oxidizing cytochrome C and H2O2 production, resulting in the opening of permeability transition pores (PTPs) and release of cytochrome C (Giorgio et al., 2005). In cytosol, p66Shc acts as a switch in separating Sos1 from the Grb2/Sos1 complex to the Eps8/E3b1 complex, resulting in the activation of Rac1 and ROS production (Baba et al., 2012). The GTPase Rac1, once activated, triggers the production of ROS via a varied number of intracellular processes. The prime target of activated Rac1 is the plasma membrane-NADPH oxidase, which is responsible for oxidative burst. Rac1, in turn, increases phosphorylation, lessens ubiquitination, and increases the stability of p66Shc protein, consequently resulting in further increase in ROS, thus maintaining cytosolic oxidative stress through mechanisms involving reciprocal regulation through Rac1 (Khanday et al., 2006; Mushtaq et al., 2021). Since the decrease in expression levels of p66Shc protein was already observed, the reciprocal regulation between p66Shc and Rac1 intrigued us to study the effect of quercetin on Rac1 expression. Our results showed that quercetin prevented basal expression of Rac1 and inhibited the expression of transfected Rac1 construct (Figure 3).
Since Rac1 expression is favored by the pro-inflammatory microenvironment (Barth et al., 2009), it could be argued that the decrease in expression of Rac1 could be a consequence of decreased ROS production and vice versa. To further look into the mutualistic interdependence between ROS production and Rac1 expression, we monitored the activation of Rac1 following quercetin treatment. Quercetin effectively prevented the activation of Rac1 with a concomitant decrease in ROS production even in transfected Rac1, p66Shc, or Rac1/p66Shc cells (Figures 4, 5). ROS has also been implicated in the metastasis and migration of cancer cells. Although quercetin mediated the prevention of invasion and migration via the downregulation of Rac1 expression and ROS has previously been observed in MCF10A cells (Song et al., 2014), our results gave a direct evidence of quercetin-mediated ROS inhibition in cell migration assays (Figure 6).
All these findings suggest a targeted inhibition of Rac1 by quercetin, which might possibly prevent Rac1 activation and eventually lead to decreased ROS production and prevent tumor cell migration or metastasis. To investigate such a possibility, computational approaches including molecular docking and thermodynamic-based MD simulation were used. These methods are important tools to understand the functional mechanisms of proteins and other biomolecules in deciphering the structural basis for disease and in the design and optimization of small molecules, peptides, and proteins (Hollingsworth and Dror, 2018; Singh et al., 2022; Bender et al., 2023). We performed molecular docking simulation of Rac1 with quercetin to analyze the mode of inhibition, if any exists. Rac1 is a RhoGTPase and, thus, requires GTP binding for its phosphorylation and subsequent activation. We, therefore, targeted the GTP-binding site of Rac1 with quercetin and also compared it with known Rac1 inhibitors to quantify the extent of possible inhibition by quercetin. In consonance with our postulated theory, quercetin not only prevented the activation of Rac1 by binding efficiently to its GTP-binding site but also depicted the highest extent of inhibition compared to other established inhibitors of Rac1 currently in use (Figure 7; Table 2). MDS studies further confirmed the stability of the Rac1–quercetin complex, validating the high binding energies of the Rac1 and quercetin complex observed in docking studies. The simulation studies further revealed that quercetin binding increases the compactness of Rac1, evident by a decreased radius of gyration and SAS area (Figures 8A–E). Together, in silico and in vitro findings establish how quercetin modulates Rac1-p66Shc signaling and participates in the control of ROS generation within cells (Figure 9). The study also suggests the modulation of Rac1-p66Shc signaling and scavenging of ROS by quercetin could be prime mechanisms through which dietary flavonoids exert their antioxidant effects, which may play a critical role in their protective action in cancers. In lieu of the achieved results, additional studies are required to understand the role(s) of quercetin on Rac1-p66Shc signaling to establish its role as a therapeutic drug particularly in brain tumors.
FIGURE 9. Proposed model of quercetin action: Quercetin binds to Rac1 and decreases cell migration and cell proliferation by inhibiting p66Shc mediated activation of Rac1 and ROS production.
Glioma is the most common and aggressive brain tumor having the poorest survival with only 0.05%–4.7% of patients surviving 5 years after diagnosis (Cruz et al., 2022). This tumor is highly invasive and difficult to treat because of blood–brain barrier permeabilization (Cruz et al., 2022). Quercetin not only possesses anticancer activities but can also cross the blood–brain barrier and has potential to treat brain tumors. The present study shows the novel mechanisms exploited by quercetin to target gliomas. We show that quercetin targets the p66Shc/Rac1/ROS pathway, inhibiting tumor growth, and could, thus, be explored in glioma therapeutics. In addition to these findings, there are some limitations associated with this study. One limitation is that this study was carried out only under in vitro conditions and needs to be validated under in vivo conditions. Another limitation is the less-detailed mechanism explored in this study. The p66Shc/Rac1 pathway has been shown to regulate cell survival, apoptosis, and migration in tumors; therefore, further studies are required before establishing Rac1-p66Shc signaling as a therapeutic target of quercetin.
The original contributions presented in the study are included in the article/Supplementary Material, further inquiries can be directed to the corresponding authors.
Ethical approval was not required for the studies on humans in accordance with the local legislation and institutional requirements because only commercially available established cell lines were used. Ethical approval was not required for the studies on animals in accordance with the local legislation and institutional requirements because only commercially available established cell lines were used.
RB: conceptualization, data curation, formal analysis, investigation, methodology, and writing–original draft. HM: conceptualization, data curation, formal analysis, writing–original draft, and writing–review and editing. TM: formal analysis, methodology, and writing–review and editing. HB: formal analysis, investigation, methodology, and writing–review and editing. AA: formal analysis, writing–review and editing, investigation, and validation. FK: conceptualization, data curation, formal analysis, supervision, writing–review and editing, funding acquisition, project administration, resources, and validation.
The authors declare that financial support was received for the research, authorship, and/or publication of this article. This work was supported by financial aid from the Department of Science and Technology, Govt. of India, No. SR/SO/BB-09/2009. APC was supported by RSP2024R350, KSU, Saudi Arabia.
The authors are grateful to the Department of Science and Technology, Govt. of India for supporting this work and also express our gratitude to Sheikh Mansoor Shafi, Postdoctoral Researcher at Jeju National University, for his invaluable feedback and suggestions, which significantly improved the overall quality of our work. The authors would also like to extend their sincere appreciation to the Researchers Supporting Project Number (RSP2024R350), King Saud University, Riyadh, Saudi Arabia.
The authors declare that the research was conducted in the absence of any commercial or financial relationships that could be construed as a potential conflict of interest.
All claims expressed in this article are solely those of the authors and do not necessarily represent those of their affiliated organizations, or those of the publisher, the editors, and the reviewers. Any product that may be evaluated in this article, or claim that may be made by its manufacturer, is not guaranteed or endorsed by the publisher.
The Supplementary Material for this article can be found online at: https://www.frontiersin.org/articles/10.3389/fphar.2024.1318797/full#supplementary-material
Ali, R., Mir, H., Hamid, R., Bhat, B., Shah, R., Khanday, F., et al. (2022). Actin modulation regulates the alpha-1-syntrophin/p66Shc mediated redox signaling contributing to the RhoA GTPase protein activation in breast cancer cells. Front. Oncol. 12, 841303. doi:10.3389/fonc.2022.841303
Ali, R., Mir, H. A., Hamid, R., Shah, R. A., Khanday, F. A., and Bhat, S. S. (2021). Jasplakinolide attenuates cell migration by impeding alpha-1-syntrophin protein phosphorylation in breast cancer cells. protein J. 40 (2), 234–244. doi:10.1007/s10930-021-09963-y
Alizadeh, S. R., and Ebrahimzadeh, M. A. (2022). Quercetin derivatives: drug design, development, and biological activities, a review. Eur. J. Med. Chem. 229, 114068. doi:10.1016/j.ejmech.2021.114068
An, S., Vo, T. T. L., Son, T., Choi, H., Kim, J., Lee, J., et al. (2023). SAMHD1-induced endosomal FAK signaling promotes human renal clear cell carcinoma metastasis by activating Rac1-mediated lamellipodia protrusion. Exp. Mol. Med. 55 (4), 779–793. doi:10.1038/s12276-023-00961-x
Asgharian, P., Tazekand, A. P., Hosseini, K., Forouhandeh, H., Ghasemnejad, T., Ranjbar, M., et al. (2022). Potential mechanisms of quercetin in cancer prevention: focus on cellular and molecular targets. Cancer cell Int. 22 (1), 257. doi:10.1186/s12935-022-02677-w
Azarova, I., Klyosova, E., and Polonikov, A. (2022). Association between RAC1 gene variation, redox homeostasis and type 2 diabetes mellitus. Eur. J. Clin. Investigation 52 (8), e13792. doi:10.1111/eci.13792
Baba, R. A., Bhat, H. F., Wani, L. A., Bashir, M., Wani, M. M., Qadri, S. K., et al. (2012). E3B1/ABI-1 isoforms are down-regulated in cancers of human gastrointestinal tract. Dis. Markers 32 (4), 273–279. doi:10.3233/DMA-2011-0881
Barth, B. M., Stewart-Smeets, S., and Kuhn, T. B. (2009). Proinflammatory cytokines provoke oxidative damage to actin in neuronal cells mediated by Rac1 and NADPH oxidase. Mol. Cell. Neurosci. 41 (2), 274–285. doi:10.1016/j.mcn.2009.03.007
Bender, O., Shoman, M. E., Ali, T. F., Dogan, R., Celik, I., Mollica, A., et al. (2023). Discovery of oxindole-based FLT3 inhibitors as a promising therapeutic lead for acute myeloid leukemia carrying the oncogenic ITD mutation. Arch. Pharm. 356 (2), 2200407. doi:10.1002/ardp.202200407
Bhat, S. S., Anand, D., and Khanday, F. A. (2015). p66Shc as a switch in bringing about contrasting responses in cell growth: implications on cell proliferation and apoptosis. Mol. cancer 14, 76–12. doi:10.1186/s12943-015-0354-9
Bogucka-Janczi, K., Harms, G., Coissieux, M.-M., Bentires-Alj, M., Thiede, B., and Rajalingam, K. (2023). ERK3/MAPK6 dictates CDC42/RAC1 activity and ARP2/3-dependent actin polymerization. Elife 12, e85167. doi:10.7554/eLife.85167
K. J. Bowers, E. Chow, H. Xu, R. O. Dror, M. P. Eastwood, B. A. Gregersenet al. (2006). “Scalable algorithms for molecular dynamics simulations on commodity clusters,” in Proceedings of the 2006 ACM/IEEE Conference on Supercomputing.
Chen, J.-S., Chiu, S.-C., Huang, S.-Y., Chang, S.-F., and Liao, K.-F. (2023). Isolinderalactone induces apoptosis, autophagy, cell cycle arrest and MAPK activation through ROS–mediated signaling in colorectal cancer cell lines. Int. J. Mol. Sci. 24 (18), 14246. doi:10.3390/ijms241814246
Chen, M., Li, H., Xu, X., Bao, X., Xue, L., Ai, X., et al. (2023). Identification of RAC1 in promoting brain metastasis of lung adenocarcinoma using single-cell transcriptome sequencing. Cell Death Dis. 14 (5), 330. doi:10.1038/s41419-023-05823-y
Chen, Y.-H., Hsu, J.-Y., Chu, C.-T., Chang, Y.-W., Fan, J.-R., Yang, M.-H., et al. (2023). Loss of cell–cell adhesion triggers cell migration through Rac1-dependent ROS generation. Life Sci. alliance 6 (2), e202201529. doi:10.26508/lsa.202201529
Chow, E., Rendleman, C. A., Bowers, K. J., Dror, R. O., Hughes, D. H., Gullingsrud, J., et al. Desmond performance on a cluster of multicore processors. DE Shaw Research Technical Report DESRES/TR--2008-01. 2008.
Cruz, J. V. R., Batista, C., Afonso, B. H., Alexandre-Moreira, M. S., Dubois, L. G., Pontes, B., et al. (2022). Obstacles to glioblastoma treatment two decades after temozolomide. Cancers 14 (13), 3203. doi:10.3390/cancers14133203
Devika, M., Vysakh, A., Vijeesh, V., Jisha, N., and Ruby, P. M. (2022). Flavonoid glycoside diosmin induces apoptosis and cell cycle arrest in DLD-1 human colon cancer cell line. J. Biol. Act. Prod. Nat. 12 (3), 232–242. doi:10.1080/22311866.2022.2081608
Dewanjee, S., Chakraborty, P., Bhattacharya, H., Singh, S. K., Dua, K., Dey, A., et al. (2022). Recent advances in flavonoid-based nanocarriers as an emerging drug delivery approach for cancer chemotherapy. Drug Discov. Today 28, 103409. doi:10.1016/j.drudis.2022.103409
Ferry, D. R., Smith, A., Malkhandi, J., Fyfe, D. W., deTakats, P. G., Anderson, D., et al. (1996). Phase I clinical trial of the flavonoid quercetin: pharmacokinetics and evidence for in vivo tyrosine kinase inhibition. Clin. cancer Res. 2 (4), 659–668.
Gibellini, L., Pinti, M., Nasi, M., Montagna, J. P., De Biasi, S., Roat, E., et al. (2011). Quercetin and cancer chemoprevention. Evidence-based complementary Altern. Med. 2011, 591356. doi:10.1093/ecam/neq053
Giorgio, M., Migliaccio, E., Orsini, F., Paolucci, D., Moroni, M., Contursi, C., et al. (2005). Electron transfer between cytochrome c and p66Shc generates reactive oxygen species that trigger mitochondrial apoptosis. Cell 122 (2), 221–233. doi:10.1016/j.cell.2005.05.011
Gupta, J., Ahmed, A. T., Tayyib, N. A., Zabibah, R. S., Shomurodov, Q., Kadheim, M. N., et al. (2023). A state-of-art of underlying molecular mechanisms and pharmacological interventions/nanotherapeutics for cisplatin resistance in gastric cancer. Biomed. Pharmacother. 166, 115337. doi:10.1016/j.biopha.2023.115337
Harvey, A. L. (2008). Natural products in drug discovery. Drug Discov. today 13 (19-20), 894–901. doi:10.1016/j.drudis.2008.07.004
Hassan, F., Mir, H., Shafi, A., Khanday, F. A., Mobarez, S., Salim, I. H., et al. (2023). Dietary zinc-chitosan nanoparticles addition influences on growth performance, apparent total tract digestibility, carcass indices, and immune function in weaned rabbits. Arabian J. Sci. Eng., 1–9. doi:10.1080/10495398.2023.2197467
Hollingsworth, S. A., and Dror, R. O. (2018). Molecular dynamics simulation for all. Neuron 99 (6), 1129–1143. doi:10.1016/j.neuron.2018.08.011
Jorgensen, W. L., Chandrasekhar, J., Madura, J. D., Impey, R. W., and Klein, M. L. (1983). Comparison of simple potential functions for simulating liquid water. J. Chem. Phys. 79 (2), 926–935. doi:10.1063/1.445869
Kedhari Sundaram, M., Raina, R., Afroze, N., Bajbouj, K., Hamad, M., Haque, S., et al. (2019). Quercetin modulates signaling pathways and induces apoptosis in cervical cancer cells. Biosci. Rep. 39 (8), BSR20190720. doi:10.1042/BSR20190720
Khanday, F. A., Santhanam, L., Kasuno, K., Yamamori, T., Naqvi, A., DeRicco, J., et al. (2006). Sos-mediated activation of rac1 by p66shc. J. cell Biol. 172 (6), 817–822. doi:10.1083/jcb.200506001
Liang, J., Oyang, L., Rao, S., Han, Y., Luo, X., Yi, P., et al. (2021). Rac1, a potential target for tumor therapy. Front. Oncol. 11, 674426. doi:10.3389/fonc.2021.674426
Liao, K., Zheng, R., Feng, J., Liu, H., and Xie, G. (2023). Effect of overexpression of Rac1 on radiosensitivity of nasopharyngeal carcinoma xenografts in nude mice, and the underlying mechanism. Trop. J. Pharm. Res. 22 (4), 789–794. doi:10.4314/tjpr.v22i4.11
Liu, H., Zhang, W., Jin, L., Liu, S., Liang, L., and Wei, Y. (2023). Plumbagin exhibits genotoxicity and induces G2/M cell cycle arrest via ROS-mediated oxidative stress and activation of ATM-p53 signaling pathway in hepatocellular cells. Int. J. Mol. Sci. 24 (7), 6279. doi:10.3390/ijms24076279
Ma, N., Xu, E., Luo, Q., and Song, G. (2023). Rac1: a regulator of cell migration and a potential target for cancer therapy. Molecules 28 (7), 2976. doi:10.3390/molecules28072976
Mahomoodally, M. F., Atalay, A., Picot, M. C. N., Bender, O., Celebi, E., Mollica, A., et al. (2018). Chemical, biological and molecular modelling analyses to probe into the pharmacological potential of Antidesma madagascariense Lam.: a multifunctional agent for developing novel therapeutic formulations. J. Pharm. Biomed. Analysis 161, 425–435. doi:10.1016/j.jpba.2018.09.002
Martyna, G. J., Klein, M. L., and Tuckerman, M. (1992). Nosé–Hoover chains: the canonical ensemble via continuous dynamics. J. Chem. Phys. 97 (4), 2635–2643. doi:10.1063/1.463940
Martyna, G. J., Tobias, D. J., and Klein, M. L. (1994). Constant pressure molecular dynamics algorithms. J. Chem. Phys. 101 (5), 4177–4189. doi:10.1063/1.467468
Marwaha, R., Rawal, S., Khuntia, P., Jaiswal, M., and Das, T. (2022). Mechanosensitive dynamics of lysosomes regulates the emergence of leader cells during collective cell migration. bioRxiv. doi:10.1101/2022.08.03.502740
Mehraj, U., Mir, I. A., Alkhanani, M., Wani, N. A., and Mir, M. A. (2022b). Adapalene and doxorubicin synergistically promote apoptosis of TNBC Cells by hyperactivation of the ERK1/2 pathway through ROS induction. Front. Oncol. 12, 938052. doi:10.3389/fonc.2022.938052
Mehraj, U., Wani, N. A., Hamid, A., Alkhanani, M., Almilaibary, A., and Mir, M. A. (2022a). Adapalene inhibits the growth of triple-negative breast cancer cells by S-phase arrest and potentiates the antitumor efficacy of GDC-0941. Front. Pharmacol. 13, 958443. doi:10.3389/fphar.2022.958443
Michala, A.-S., and Pritsa, A. (2022). Quercetin: a molecule of great biochemical and clinical value and its beneficial effect on diabetes and cancer. Diseases 10 (3), 37. doi:10.3390/diseases10030037
Mir, H., Ali, R., Wani, Z., and Khanday, F. (2022). Pro-oxidant vitamin C mechanistically exploits p66Shc/Rac1 GTPase pathway in inducing cytotoxicity. Int. J. Biol. Macromol. 205, 154–168. doi:10.1016/j.ijbiomac.2022.02.046
Mir, H. A., Ali, R., Mushtaq, U., and Khanday, F. A. (2020). Structure-functional implications of longevity protein p66Shc in health and disease. Ageing Res. Rev. 63, 101139. doi:10.1016/j.arr.2020.101139
Mittal, P., Dhankhar, S., Chauhan, S., Garg, N., Bhattacharya, T., Ali, M., et al. (2023). A review on natural antioxidants for their role in the treatment of Parkinson’s disease. Pharmaceuticals 16 (7), 908. doi:10.3390/ph16070908
Mokhdomi, T. A., Bukhari, S., Chikan, N. A., Amin, A., Wafai, A. H., Wani, S. H., et al. (2016). A novel kinase mutation in VEGFR-1 predisposes its αC-helix/activation loop towards allosteric activation: atomic insights from protein simulation. Eur. J. Hum. Genet. 24 (9), 1287–1293. doi:10.1038/ejhg.2016.26
Morris, G. M., Huey, R., Lindstrom, W., Sanner, M. F., Belew, R. K., Goodsell, D. S., et al. (2009). AutoDock4 and AutoDockTools4: automated docking with selective receptor flexibility. J. Comput. Chem. 30 (16), 2785–2791. doi:10.1002/jcc.21256
Mushtaq, U., Bashir, M., Nabi, S., and Khanday, F. A. (2021). Epidermal growth factor receptor and integrins meet redox signaling through P66shc and Rac1. Cytokine 146, 155625. doi:10.1016/j.cyto.2021.155625
Nakhaei-Rad, S., Soleimani, Z., Vahedi, S., and Gorjinia, Z. (2023). Testicular germ cell tumors: genomic alternations and RAS-dependent signaling. Crit. Rev. Oncology/Hematology. 183, 103928. doi:10.1016/j.critrevonc.2023.103928
Olivier, C., Oliver, L., Lalier, L., and Vallette, F. M. (2021). Drug resistance in glioblastoma: the two faces of oxidative stress. Front. Mol. Biosci. 7, 620677. doi:10.3389/fmolb.2020.620677
Ramos, D. F. V., Mancuso, R. I., Contieri, B., Duarte, A., Paiva, L., de Melo Carrilho, J., et al. (2022). Rac GTPases in acute myeloid leukemia cells: expression profile and biological effects of pharmacological inhibition. Toxicol. Appl. Pharmacol. 442, 115990. doi:10.1016/j.taap.2022.115990
Rinaldi, M., Caffo, M., Minutoli, L., Marini, H., Abbritti, R. V., Squadrito, F., et al. (2016). ROS and brain gliomas: an overview of potential and innovative therapeutic strategies. Int. J. Mol. Sci. 17 (6), 984. doi:10.3390/ijms17060984
Salehi, B., Machin, L., Monzote, L., Sharifi-Rad, J., Ezzat, S. M., Salem, M. A., et al. (2020). Therapeutic potential of quercetin: new insights and perspectives for human health. Acs Omega 5 (20), 11849–11872. doi:10.1021/acsomega.0c01818
Shaw, D. E., Maragakis, P., Lindorff-Larsen, K., Piana, S., Dror, R. O., Eastwood, M. P., et al. (2010). Atomic-level characterization of the structural dynamics of proteins. Science 330 (6002), 341–346. doi:10.1126/science.1187409
Shen, B. (2015). A new golden age of natural products drug discovery. Cell 163 (6), 1297–1300. doi:10.1016/j.cell.2015.11.031
Shivakumar, D., Williams, J., Wu, Y., Damm, W., Shelley, J., and Sherman, W. (2010). Prediction of absolute solvation free energies using molecular dynamics free energy perturbation and the OPLS force field. J. Chem. theory Comput. 6 (5), 1509–1519. doi:10.1021/ct900587b
Singh, S., Baker, Q. B., and Singh, D. B. (2022). Molecular docking and molecular dynamics simulation. Bioinformatics: Elsevier, 291–304.
Slika, H., Mansour, H., Wehbe, N., Nasser, S. A., Iratni, R., Nasrallah, G., et al. (2022). Therapeutic potential of flavonoids in cancer: ROS-mediated mechanisms. Biomed. Pharmacother. 146, 112442. doi:10.1016/j.biopha.2021.112442
Song, N. R., Chung, M.-Y., Kang, N. J., Seo, S. G., Jang, T. S., Lee, H. J., et al. (2014). Quercetin suppresses invasion and migration of H-Ras-transformed MCF10A human epithelial cells by inhibiting phosphatidylinositol 3-kinase. Food Chem. 142, 66–71. doi:10.1016/j.foodchem.2013.07.002
Yang, X., Cruz, M. I., Nguyen, E. V., Huang, C., Schittenhelm, R. B., Luu, J., et al. (2023). The pseudokinase NRBP1 activates Rac1/Cdc42 via P-Rex1 to drive oncogenic signalling in triple-negative breast cancer. Oncogene 42 (11), 833–847. doi:10.1038/s41388-023-02594-w
Zhang, F.-f., Jiang, C., Jiang, D.-P., Cui, Y.-Z., Wang, X.-Y., Sun, L.-Z., et al. (2023). ARHGAP15 promotes metastatic colonization in gastric cancer by suppressing RAC1-ROS pathway. PLoS Genet. 19 (2), e1010640. doi:10.1371/journal.pgen.1010640
M. Zhang, S. G. Swarts, L. Yin, C. Liu, Y. Tian, Y. Caoet al. (2011). Antioxidant properties of quercetin. Oxygen transport to tissue XXXII (Springer).
Keywords: quercetin, ROS, cell proliferation, cell migration, P66shc, oxidative stress
Citation: Baba RA, Mir HA, Mokhdomi TA, Bhat HF, Ahmad A and Khanday FA (2024) Quercetin suppresses ROS production and migration by specifically targeting Rac1 activation in gliomas. Front. Pharmacol. 15:1318797. doi: 10.3389/fphar.2024.1318797
Received: 12 October 2023; Accepted: 10 January 2024;
Published: 31 January 2024.
Edited by:
Onur Bender, Ankara University, TürkiyeReviewed by:
Romina Deza-Ponzio, Albert Einstein College of Medicine, United StatesCopyright © 2024 Baba, Mir, Mokhdomi, Bhat, Ahmad and Khanday. This is an open-access article distributed under the terms of the Creative Commons Attribution License (CC BY). The use, distribution or reproduction in other forums is permitted, provided the original author(s) and the copyright owner(s) are credited and that the original publication in this journal is cited, in accordance with accepted academic practice. No use, distribution or reproduction is permitted which does not comply with these terms.
*Correspondence: Hilal A. Mir, aGlsYWxtaXIxNjEzQGdtYWlsLmNvbQ==; Firdous A. Khanday, a2hhbmRheWZAa2FzaG1pcnVuaXZlcnNpdHkuYWMuaW4=
†These authors have contributed equally to this work and share first authorship
Disclaimer: All claims expressed in this article are solely those of the authors and do not necessarily represent those of their affiliated organizations, or those of the publisher, the editors and the reviewers. Any product that may be evaluated in this article or claim that may be made by its manufacturer is not guaranteed or endorsed by the publisher.
Research integrity at Frontiers
Learn more about the work of our research integrity team to safeguard the quality of each article we publish.