- 1Program in Molecular Medicine, SickKids Research Institute, Toronto, ON, Canada
- 2Department of Biochemistry, University of Toronto, Toronto, ON, Canada
- 3Program in Translational Medicine, SickKids Research Institute, Toronto, ON, Canada
- 4Department of Cardiovascular Surgery, The Hospital for Sick Children, Toronto, ON, Canada
- 5Department of Anesthesia and Pain Medicine, Hospital for Sick Children, Toronto, ON, Canada
- 6Department of Anesthesiology and Pain Medicine, University of Toronto, Toronto, ON, Canada
Many challenges remain in the preclinical evaluation, adjudication, and prioritization of novel compounds in therapeutic discovery pipelines. These obstacles are evident by the large number of candidate or lead compounds failing to reach clinical trials, significantly due to a lack of efficacy in the disease paradigm of interest and/or the presence of innate chemical toxicity. The consequential compound attrition in discovery pipelines results in added monetary and time costs, potential danger to patients, and a slowed discovery of true therapeutics. The low rate of successful translation calls for improved models that can recapitulate in vivo function in preclinical testing to ensure the removal of toxic compounds earlier in the discovery process, in particular for the assessment of cardiotoxicity, the leading cause of post-market drug withdrawal. With recent advances in the development of human Inducible pluripotent stem cell derived cardiomyocytes (iPSC-CMs), novel compounds can be assessed with better disease relevance while more accurately assessing human safety. In this review, we discuss the utility of iPSC-CMs in preclinical testing by taking advantage of the inherent ability to mimic CMs in vivo. We explore the similarities and differences in electrophysiology, calcium handling, cellular signaling, contractile machinery, and metabolism between iPSC-CMs and adult CMs as these complex coordinated functions directly relate to toxicity evaluation. We will highlight considerations when using iPSC-CMs, such as maturation protocols, to ensure a more representative phenotype of the adult human CM, and how different populations of CMs can affect results in compound testing.
1 Introduction
The predictive and accurate preclinical evaluation of novel compounds in therapeutic discovery pipelines remains a considerable challenge, illustrated by the high rate of lead compounds failing to reach clinical implementation. A consequential contributor to this high failure rate is the lack of proper assessment of toxicity and off-target effects in highly translational and physiologically relevant pre-clinical models. The resulting attrition of compounds incurs significant monetary and time costs but also poses potential risks to those in need of the therapeutic (Seyhan, 2019). Evidence of post-market withdrawal due to cardiotoxicity has been reported in several studies. In an analysis of 121 withdrawn medicinal products between 1960–1999, 8.7% of the withdraws were due to unanticipated cardiotoxicity (Fung et al., 2001). Another study identified that 63 out of 462 (14%) of withdrawn medicinal products from 1953–2013 were due to unexpected adverse cardiovascular effects (Onakpoya et al., 2016). More recently, an analysis of 78 drugs with known side effects on cardiovascular function yielded that 27 of these compounds were withdrawn from the market due to overt cardiotoxicity (Mamoshina et al., 2021). Examples of post-market withdrawal for cardiotoxicity include dronedarone, an effective therapeutic for treating atrial fibrillation that had proved its disease efficacy in a double blinded, multi-center, randomized trial (Singh et al., 2007), but was later found to increase the mortality in patients predominantly due to the exacerbation of pre-existing heart failure (Køber et al., 2008). More commonly known and notorious cardiotoxic drugs include the anthracycline class of anticancer pharmaceuticals, which are still utilized in oncology (Di Marco et al., 1964) despite known drug-induced cardiac dysfunction (Tan et al., 1967). Unknown and unanticipated cardiotoxic effects extend to numerous therapeutic classes including anti-diabetics (Lee et al., 2022), fluoroquinolone antibiotics (Frothingham, 2001), and selective serotonin reuptake inhibitor antidepressants (Funk and Bostwick, 2013), which all have pro-arrhythmic effects discovered post market release. Pre-clinical models which facilitate evaluation more consistent with in vivo human physiology would provide an opportunity to identify compound cardiotoxicity earlier and more accurately in the development pipeline, reducing post-market drug withdrawal and improving patient safety.
The ability to reprogram end-differentiated human cells into pluripotent stem cells with subsequent re-differentiation back into a target tissue provides a platform for assessing novel compounds in a system that more closely resembles the in vivo human environment (Takahashi et al., 2007). This technology gave rise to the generation of inducible pluripotent stem cell derived cardiomyocytes (iPSC-CMs) as a model for heart function (Kałużna et al., 2022; Yang et al., 2022). This review explores the similarities and differences between iPSC-CMs and in vivo cardiomyocytes (CMs) found in the human heart, and how iPSC-CMs can be used to assess adverse changes to ion channel function, signal transduction, contractile function, and metabolism, as mechanisms for compound toxicity. We review steps to improve model translation, including cell maturation, to more closely mimic the adult human heart. Significantly, clinical cardiotoxicity manifests as changes to heart beating (rate or rhythm) and contractile force (inotropy). While these are inherent phenotypes that can be measured in the in vivo heart itself, in isolated cardiomyocytes or cardiac tissue from model species, or in iPSC-CMs, the underlying etiology is primarily related to altered activity (direct or indirect) of ion channels. The delicate and coordinated function of the multitude of ion channels present in the cardiomyocyte requires the presence (expression) and normal activity of each channel, both considerations that are changed naturally during cardiac development and in model systems. Effects on channels affecting the three primary ions (sodium, potassium, and calcium) are important to consider, including how these are represented in the testing system itself, before knowing if they can be evaluated for pharmacologic modification. The calcium cycling machinery is particularly affected in iPSC-CM compared to the in vivo environment and significant experimental efforts are made to bring the two closer aligned prior to compound evaluation. Through better cardiotoxicity prediction, iPSC-CMs can provide a highly valuable tool to improve discovery pipeline efficiency and candidate drug safety, advancing therapeutic discovery.
2 Functional similarities between iPSC-CMs and the human in vivo cardiomyocytes
To enhance the accuracy of predicting potential cardiotoxicity, the electrical and mechanobiology of the iPSC-CMs must mimic the cellular physiology of a CM within the human heart. Although the iPSC-CMs contains a human proteome, developmental stage/maturation-dependent protein expression (i.e., myosin heavy chain isoform) and the general lack of supporting non-cardiomyocyte cell types (i.e., fibroblast, endothelial cell) can affect these metrics and alter responses when testing compound activity.
2.1 Electrophysiology and ion channels
iPSC-CMs are electrically active cells, recapitulating the complex ionic current profiles observed in the human endogenous cardiomyocyte that constitute the CM action potential (AP). Characterized currents include depolarizing sodium (INa), transient outward potassium (Ito), L-type and T-type calcium (ICa), slow and rapid delayed rectifier (IKs, IKr), pacemaker (If), and the inward rectifying potassium (IK1) currents. The similarities and differences of these currents between in vitro and in vivo environments plays an important role in how iPSC-CMs can be used for detecting the potentially arrhythmogenic effects of compounds.
The APs in iPSC-CMs can vary depending on the cell population of a developed beating monolayer, and are classified as either atrial-, nodal-, or ventricular-like cells (Zhang et al., 2009; Zwi et al., 2009; Burridge et al., 2014). The AP differences between the cell constituents of the potentially heterogenous population in iPSC-CMs were delineated (Ma et al., 2011). The resting membrane potential of iPSC-CMs have been reported as −68.1 mV (Van de Sande et al., 2021), compared to the in vivo human atrial and ventricular resting potentials of −70 mV and −87 mV, respectively (Trautwein et al., 1962). Comparing ventricular-like iPSC-CMs to human mature CMs, the action potential durations (APD) are similar, ranging from 100 to 400 ms depending on the type of CM (Luo and Rudy, 1994; Shih, 1994; Ma et al., 2011). Differences in the AP between iPSC-CMs and human mature CMs arise from changes to upstroke velocity, waveform (lack of phase 1 in iPSC-CMs), and a less pronounced depolarization plateau.
2.1.1 INa
The sodium channel Nav1.5 contributes to the same phase 0 (depolarization) of the AP in both iPSC-CMs and human CMs. The activation and inactivation kinetics and current densities are similar between iPSC-CM and in vivo cardiomyocytes (Ma et al., 2011). Interestingly, recovery from inactivation is faster in iPSC-CMs, with fast (τf) and slow (τs) recovery time constants of 2.58 ± 0.31 ms and 47.17 ± 7.01 ms (Selga et al., 2018), compared to atrial CMs isolated from the adult heart (τf = 7.5 ms, τs = 61.4 ms) (Sakakibara et al., 1992). Recovery time constants reported from human ventricular CMs equally showed slower values than iPSC-CMs (τf = 5 ms, τs = 67.2 ms) (Sakakibara et al., 1993). In contrast, the upstroke velocity of iPSC-CMs was reported to be 50% slower than in cells from human heart tissue, highlighting a critical inherent difference observed between iPSC-CM sources and differentiation/maturation methods (Zhang et al., 2009; Ma et al., 2011; Davis et al., 2012). Other reports show an even slower iPSC-CM upstroke velocity of ∼50 V/s (Lundy et al., 2013) compared to CMs found in the human adult ventricular free wall at ∼250 V/s (Drouin et al., 1995). These findings may partially explain the slower beat rate commonly observed in iPSC-CMs (although other factors are involved, like maturation state).
Despite these differences, iPSC-CMs are very sensitive in detecting compound effects on Nav1.5, and iPSC-CMs sodium channels have similar tetrodotoxin sensitivity compared to adult human CMs (Lemoine et al., 2017) (Figure 1). iPSC-CMs can recapitulate the electrophysiological characteristics of sodium channelopathies allowing for therapeutic testing using patient-derived cells (Davis et al., 2012) or incorporated into platforms that can accurately measure conduction velocity for compound testing (Dou et al., 2020). The robustness of the sodium channels in iPSC-CMs is further exemplified by the measurement of previously unknown cardiotoxic mechanisms of local anesthetics (Plakhotnik et al., 2022).
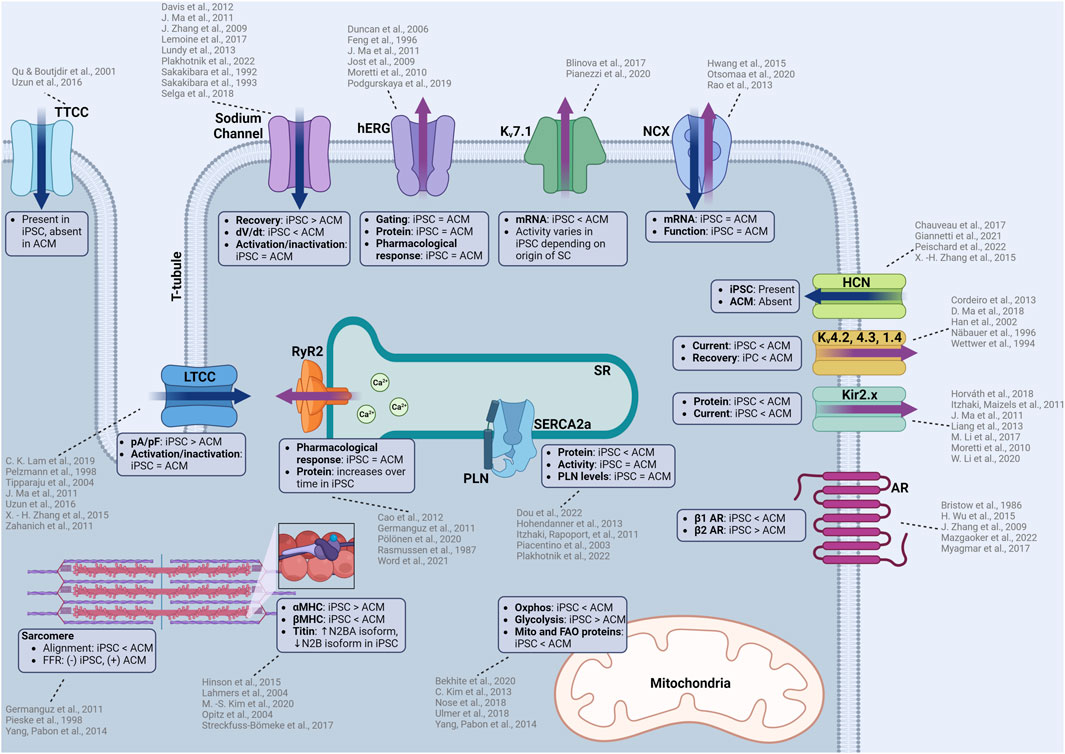
FIGURE 1. Summary of functional similarities and differences between iPSC-CMs and adult human CMs (ACM). iPSC-CMs are similar in many aspects including electrophysiology, calcium handling, contractile machinery which allows the use of iPSC-CMs to predict cellular responses to novel compounds. However, there are some differences that must be considered when considering iPSC-CMs as a model for cardiotoxicity testing. Some of these differences include ion channel kinetics, upstroke velocities, structural differences in the contractile machinery, isoform of proteins, and metabolic function. TTCC: T-type calcium channel, LTCC: L-type calcium channel, hERG: human-ether-a-go-go (rapid delayed rectifier potassium channel), Kv7.1: slow delayed rectifier postassium channel, NCX: sodium calcium exchanger, HCN: hyperpolarization-activated cyclic nucleotide gated channel, Kv4.2, 4.3, 1.4: voltage dependent potassium channels responsible for the transient outward current, AR: adrenergic receptor, RyR2: ryanodine receptor, SERCA2a: sarcoendoplasmic reticulum calcium ATPase, PLN: phospholamban, Oxphos: oxidative phosphorylation, MHC: myosin heavy chain. Created with www.biorender.com.
2.1.2 ICa
Most calcium currents in iPSC-CMs originate from the L-type calcium channel (Cav1.2) but residual currents from T-type calcium channels exist related to the maturation process for the cells.
L-type calcium channels (LTCC) are present in iPSC-CMs and the cells have the expected response to nifedipine, a specific LTCC inhibitor, which causes cessation of spontaneous beating at 200 nM (Zahanich et al., 2011). Another independent study confirmed channel inhibition with a 20% reduction in spontaneous ICa through nifedipine inhibition (Zhang et al., 2015), emulating the pharmacological response of adult heart CMs. The iPSC-CM L-type calcium channels have similar activation and inactivation characteristics to adult human CMs (Ma et al., 2011; Uzun et al., 2016) and have similar or higher transcript or protein levels to the adult human heart (Ivashchenko et al., 2013; Rao et al., 2013; Fernández-Morales et al., 2019). While similarities exist, the ICa density is larger than human CMs (Ma et al., 2011), when compared to atrial CMs (Tipparaju et al., 2004) and CMs obtained from Tetralogy of Fallot hearts (Pelzmann et al., 1998). The patient-specific sensitivity of LTCC inhibition was modeled in iPSC-CMs, including observed reductions in the transcripts for contractility related genes (TNNI3, MYH7) after verapamil treatment (Lam et al., 2019). These findings demonstrate how iPSC-CMs can accurately detect patient-specific functional and transcriptomic effects as a result of calcium channel antagonists.
T-type calcium channels (Cav3.1/2) are present in the embryonic stages of the heart but diminish over time and are absent in the adult heart (Qu and Boutjdir, 2001). Interestingly, iPSC-CMs are reported to have T-type calcium channel expression which contributes to ICa (Uzun et al., 2016). The presence of these channels is a sign of cell immaturity, and their presence should be considered when investigating modulators of the calcium flux as the observed effect could result from a mix of altered L- and T-type currents (Figure 1).
2.1.3 IKr and IKs
Rapid and slow delayed rectifier currents are generated by the Kv11.1 (also known as the human ether-a-go-go channel, or hERG) and Kv7.1 potassium channels, respectively. Both potassium channels are present in iPSC-CMs (Moretti et al., 2010), with similar gating properties compared to adult human CMs (Feng et al., 1996; Jost et al., 2009; Ma et al., 2011). Pharmacological testing revealed that IKr in iPSC-CMs are sensitive to erythromycin (Podgurskaya et al., 2019), a known hERG channel antagonist and arrhythmogen (Duncan et al., 2006). The sensitivity of iPSC-CMs toward hERG channel antagonists make them a valuable model for pharmacological proarrhythmic risk assessment as the hERG channel is the canonical target for arrhythmogenesis from QT-prolongation.
Differences in potassium currents between iPSC-CM and adult cardiomyocytes originate from an alteration in IKs, where lower levels of Kv7.1 (KCNQ1) mRNA in iPSC-CMs was measured compared to adult ventricular CMs (Blinova et al., 2017) (Figure 1). In addition to reduced expression, the activity of Kv7.1 can vary depending on the origin of the stem cell (even when using different source tissue within the same patient). The highest activity of IKs was measured in iPSC-CMs derived from cardiac mesenchymal progenitor cells, followed by cells derived from bone marrow mesenchymal stem cells, with the lowest activity in cells derived from dermal fibroblasts (Pianezzi et al., 2020). The exact etiology for these differences in source tissue is not clear. In adult CMs, IKs is known to be modulated by adrenergic signaling where the activity is increased through PKA dependent phosphorylation (Terrenoire et al., 2005). This finding is illustrated by action potential duration increases in human ventricular tissue when exposed to the β-blocker sotalol (Jost et al., 2005). Similar adrenergic-dependent increases in IKs activity are observed in iPSC-CMs in response to sympathetic stimulation (Moretti et al., 2010; Zhang et al., 2014).
2.1.4 IK1
The inward rectifying potassium current is primarily driven by the Kir2.x subfamily of potassium channels. Very low Kir2.x protein and IK1 current was detected in iPSC-CMs, compared to adult heart tissue (Ma et al., 2011; Li et al., 2020), illustrating that overexpression of Kir2.x channels (Li et al., 2017) may be necessary to increase IK1 current to better assess the risk of QT-prolongation (Figure 1). One report showed that the IK1 current was similar to human right atrial (RA) and left ventricular (LV) tissue (Horváth et al., 2018), and argued that the current differences others have reported can be attributed to the lower resting membrane potential of iPSC-CMs (Moretti et al., 2010; Itzhaki and Maizels, 2011; Liang et al., 2013). While possible, the low levels of Kir2.x protein and IK1 current can also be explained by the typical location of Kir2.x channels in T-tubules, a structure commonly absent in immature iPSC-CMs without maturation protocols (Clark et al., 2001).
2.1.5 If
The If is the pacemaker current generated from the hyperpolarization-activated cyclic-nucleotide gated channel (HCN) commonly found in the sinoatrial node, atrioventricular node, and Purkinje fibers of conductive tissue. The presence of If in iPSC-CMs is a result of its automaticity and a sign of heterogeneity as healthy adult human ventricular CMs do not have HCN channels or If (Peischard et al., 2022). The If in iPSC-CMs is persistent, even with longer cultures and maturation, demonstrating a typical mixed iPSC-CM population (ventricular, atrial, and nodal) (Giannetti et al., 2021), although the spontaneous rate does decrease as culture time increases. As specialized differentiation protocols become more widely available, ventricular-only iPSC-CM populations (or atrial or nodal) allow for HCN expression in the proper end-differentiated cell desired (Figure 1). There are some disagreements regarding the function of the HCN channel. Initially Zhang et al. reported that ivabradine, a specific HCN channel inhibitor, had no effect on the automaticity in iPSC-CMs (Zhang et al., 2015). More recently, Chauveau et al. demonstrated that ivabradine had a concentration-dependent effect on slowing the automaticity of iPSC-CMs until complete cessation (Chauveau et al., 2017). The differences in their observations could be a result of altered differentiation or maturation protocols, illustrating that the If in iPSC-CMs needs to be carefully characterized for relevant studies.
2.1.6 Ito
The Ito is driven by Kv4.2, Kv4.3, and Kv1.4 potassium channels, which contribute to the phase 1 “notch” in the cardiac AP. When measuring Ito in patch clamping experiments with iPSC-CMs, phase 1 of the AP is often subtle or missing, but was exacerbated through increased pacing (Cordeiro et al., 2013). Recovery from activation of the potassium channels responsible for Ito are generally slower (Cordeiro et al., 2013) when compared to human epicardial ventricular CMs (Wettwer et al., 1994) and the current densities of Ito were observed to be ∼50% of those found in human sub-epicardial ventricular CMs (Näbauer et al., 1996; Ma et al., 2018) (Figure 1). Interestingly, there were higher currents observed in 20% of the cells, which points to the heterogenous population of cells in mixed differentiated iPSC-CM cultures. Temperature during patch clamp experiments may additionally be a factor as Kv4.2, Kv4.3, and Kv1.4 potassium channel activity can be temperature sensitive (Han et al., 2002). These channel activity differences demonstrate some of the reasons why the APs are partially inconsistent between iPSC-CMs and adult CMs.
2.2 Calcium cycling
2.2.1 T-tubules
iPSC-CMs have high amounts of calcium flux, indicative of the presence of calcium-induced calcium release (CICR) to facilitate contraction. An important structural component of CICR are the T-tubules, invaginations into CMs for spatial positioning of ion channels for efficient ion conduction. Unmatured iPSC-CMs are known to lack T-tubules, a detriment to efficient CICR (Lieu et al., 2009; Gherghiceanu et al., 2011; Yang et al., 2014). The absence of T-tubules are confirmed through electron microscopy (Gherghiceanu et al., 2011; Lundy et al., 2013). As T-tubules develop over time in the human heart throughout fetal development (Ziman et al., 2010), the general immaturity of iPSC would explain the absence of T-tubules. However, there are many ways to enhance T-tubule formation in iPSC-CMs through maturation protocols, thereby positively contributing to the calcium handling capabilities. The effects of t-tubule formation as iPSC-CMs undergo maturation protocols will be explored in a later section.
2.2.2 Junctophilin-2
Junctophilin-2 (JP2) is a structural protein that tethers the T-tubule to the sarcoplasmic reticulum (SR) to spatially orient LTCC and ryanodine receptors (RyR) for coordinated CICR (Takeshima et al., 2000). iPSC-CMs express fully functional JP2 and there are increases in expression level proportional to culture time as a function of maturation (Pretorius et al., 2021; Weninger et al., 2022). JP2 found in iPSC-CMs is cleavable by calpain (Weninger et al., 2022), which is a known target of proteolysis in human hearts during cardiac injury (Murphy et al., 2013; Guo et al., 2015; Chan et al., 2019). Intrinsic changes in JP2 function in iPSC-CMs has not been explored significantly, but one study did generate patient-specific iPSC-CMs with a JP2 T161K variant and demonstrated an arrhythmogenic phenotype (Valtonen et al., 2023). The functional expression of JP2 in iPSC-CMs and the ability to recapitulate genetic variant phenotype could prove iPSC-CMs as a valuable model for developing therapeutics targeting JP2.
2.2.3 Ryanodine receptors
RyRs facilitate calcium release from the SR upon calcium binding. iPSC-CMs express functional RyR (Germanguz et al., 2011) with its expression increasing as the cells are matured (Cao et al., 2012). The RyR expressed in iPSC-CMs is able to emulate RyR mutations and shows responsiveness to modulators such as carvedilol, which inhibit the phosphorylation of RyR though β-adrenergic inhibition (Pölönen et al., 2020); demonstrating an ability to detect physiologically relevant effects on CICR (Figure 1). While RyR function in iPSC-CMs appears to be identical to Ryr in adult human CMs, iPSC-CM RyR response to caffeine is much lower (Rasmussen et al., 1987; Germanguz et al., 2011), indicative of potential binding kinetic and activity differences. This lower response should be considered if total SR calcium release is to be measured using caffeine stimulation. The efficacy of a novel RyR2 inhibitor, EL20, for the treatment of catecholaminergic polymorphic ventricular tachycardia was assessed using patient-derived iPSC-CMs (Word et al., 2021). The drug was found to have an IC50 of 82 nM and it successfully suppressed arrhythmogenic activity while maintaining homeostatic calcium handling within the iPSC-CM, illustrating a potential for precision therapeutic testing.
2.2.4 Sarco-endoplasmic reticulum calcium ATPase (SERCA-2a) and phospholamban (PLN)
SERCA-2a is the primary channel for the reuptake of calcium into the SR after contraction and is lower in both activity and expression level in heart failure (Figure 1). SERCA-2a activity can be negatively modulated by PLN through allosteric binding. The presence of SERCA-2a was verified by measuring calcium fluxes within iPSC-CMs with activity inhibited by thapsigargin, a channel-specific antagonist (Itzhaki and Rapoport, 2011). Overall, calcium handling characteristics are similar to those found in the human heart and have been extensively characterized (Itzhaki and Rapoport, 2011; Dou et al., 2022). Some minor differences include lower levels of SERCA-2a expression but similar levels of PLN transcripts compared to the adult human heart (Rao et al., 2013). SERCA-2a activity in iPSC-CMs has been quantified, with a time decay constant tau reported as ∼0.1 s (Plakhotnik et al., 2022), comparable to those measured in rabbit heart ∼0.07 s (Wang et al., 2021) but faster than rat hearts (0.3 s) (Mohamed et al., 2013). A similar tau value of 0.209 s was reported in human non-failing ventricular CMs (Piacentino et al., 2003). Interestingly, the tau in healthy human ventricular CMs was reported as 0.51 s (Hohendanner et al., 2013), which indicates lower SERCA-2a activity than iPSC-CMs. However, this phenomenon is likely be due to regional differences in cytosolic calcium release as discussed in the study manuscript.
2.2.5 Sodium calcium exchanger (NCX)
Transcript levels of NCX in iPSC-CMs are comparable to those found in the adult human heart (Rao et al., 2013). NCX antiporters in iPSC-CMs have robust function and even contribute to calcium removal during diastole (Hwang et al., 2015). The utility of iPSC-CMs was demonstrated when an inhibitor of NCX, ORM-11372, was discovered and characterized (Otsomaa et al., 2020). The IC50 for the outward current in iPSC-CMs was 4.8 nM, compared to an IC50 of 11.3 nM in rat primary ventricular CMs, a nearly three-fold change to be considered when observing intracellular calcium or sodium homeostasis.
2.3 Cellular signaling
2.3.1 Calmodulin
Calmodulin is a calcium-responsive modulator of downstream kinases, importantly involved in responses to calcium signaling. Calmodulin signaling is present in iPSC-CMs and can be manipulated by changing calcium levels to emulate human disease phenotypes (Rocchetti et al., 2017; Liu et al., 2021). The similarity of calmodulin signaling between iPSC-CMs and adult human CMs can be utilized to investigate calmodulin modulators as a therapy for heart failure, and assessing any potential cardiotoxic effects associated with alterations in protein activity (Schulman and Anderson, 2010).
2.3.2 Adrenergic signaling
Adrenergic signaling in iPSC-CM is similar to adult human CMs. Isoproterenol, a canonical β-adrenergic agonist, induces a predictable positive chronotropic (two-fold increase in beating rate at 1 μM drug concentration) and inotropic (contractility) response (Zhang et al., 2009; Wang et al., 2018; Dou et al., 2023). However, a major difference between iPSC-CMs and in vivo CM response is that the positive chronotropic and inotropic effect of isoproterenol is primarily modulated through β2 adrenergic receptors in unmatured iPSC-CMs (Wu et al., 2015), compared to β1 receptors in the human heart (Bristow et al., 1986; Myagmar et al., 2017). The difference in adrenergic receptor subtype could contribute to changes in sensitivity to any cardiotoxic compounds that affect the adrenergic system. Downstream effectors of adrenergic signaling, such as the cAMP/PKA pathway, are present in iPSC-CMs and play an important role in modulating calcium release and automaticity (Mazgaoker et al., 2022).
2.4 Contractile machinery
The ability of iPSC-CMs to contract make it possible to quantify compound-induced changes to inotropy (Wang et al., 2020). There are several differences in the contractile machinery between iPSC-CMs and the human heart. Even with maturation, the sarcomere organization of iPSC-CMs is more disorganized, with misaligned actin, and the cells presenting a more spherical (compared to rectangular) form (Yang et al., 2014). However, despite the differences in cellular morphology and actin structure, the average sarcomere length of ∼1.98 μm in iPSC-CMs (Lee et al., 2012) were similar to the measurements of samples acquired from human ventricular biopsies (Herron et al., 2006). A fundamental property of the human heart is the force-frequency relationship, an endogenous mechanism of the heart to match the frequency of beating to the contractile force (Endoh, 2004). iPSC-CMs exhibit a negative force-frequency relationship which is more akin to the level of contractility in heart failure (Pieske et al., 1998; Germanguz et al., 2011), but possess mild post-rest potentiation, a phenomenon where contraction after rest becomes stronger in adult CMs (Germanguz et al., 2011). Contractile forces of iPSC-CMs in 3D constructs were lower (∼0.08 mN/mm2) (Tulloch et al., 2011) compared to human myocardial strips (44 ± 11.7 mN/mm2) (Hasenfuss et al., 1991). Single cell contractile forces of iPSC-CMs were reported to be in the range of 0.1nN–144 nN depending on experimental conditions (Rodriguez et al., 2014), which is lower than measurements made in adult rat CMs (Yasuda et al., 2001; Nishimura et al., 2004) and human ventricular CMs (Van Der Velden et al., 1998).
Cardiac troponin, β-myosin heavy chain, and myosin light chain protein levels are similar to or slightly lower than the levels in the human fetal heart (Rao et al., 2013). There is higher α-myosin heavy chain levels in iPSC-CMs compared to adult CMs, which is converted to β-myosin heavy chain as the iPSC-CMs mature (Kim et al., 2020), thereby emphasizing the importance of maturation protocols to ensure the function of the iPSC-CMs can more closely recapitulate adult CM function. Titin isoforms are also different in iPSC-CMs, as human hearts have a shift from the more compliant larger N2BA isoform to the smaller and stiffer N2B isoform post-natally (Lahmers et al., 2004; Opitz et al., 2004). While iPSC-CMs express titin, it is predominantly in the N2BA isoform (Hinson et al., 2015; Streckfuss-Bömeke et al., 2017). The isoform differences do not negatively affect the ability to create iPSC-CM models that can recapitulate phenotypes associated with pathological titin changes (Hinson et al., 2015), demonstrating that small molecule modulation of titin would likely be detected. Similar to isoform differences in β-myosin heavy chain, adult cardiac troponin I (cTnI) levels in iPSC-CMs were found to be absent or lower in favor of slow skeletal troponin I (ssTnI) (Miki et al., 2021) which is an isoform found in the developing hearts that eventually switches to adult cTnI as the heart matures (Saggin et al., 1989; Hunkeler et al., 1991).
2.5 Metabolism
In the adult human heart, 95% of the ATP is generated through oxidative phosphorylation utilizing fatty acids as a primary carbon source (Lopaschuk et al., 2021). In iPSC-CMs, the baseline metabolic profile is primarily glycolytic, but fatty acid can be utilized for ATP generation, especially with cell maturation and forced utilization of mitochondrial metabolism (Kim et al., 2013; Yang et al., 2014). Quantification of mitochondrial and fatty acid oxidation-related proteins and transcripts reveals lower amounts in iPSC-CM (before maturation) compared to the adult human heart (Nose et al., 2018; Ulmer et al., 2018; Bekhite et al., 2020). Furthermore, iPSC-CMs express higher levels of fetal glucose transporters (GLUT1) opposed to the adult form (GLUT4) (Bowman et al., 2019). After maturation protocols, expression of mitochondrial proteins, oxygen consumption, and fatty acid utilization are all increased, indicative of a successful shift and maturation of the metabolic phenotype towards a more adult-like state (Nose et al., 2018; Ulmer et al., 2018; Bekhite et al., 2020).
3 Considerations when using iPSC-CMs for cardiotoxicity testing
3.1 Maturation
As described, the phenotype exhibited by iPSC-CMs after initial differentiation is closer to a human fetal/neonatal cell, not fully reflective of the physiological characteristics of an adult CM (Van Den Berg et al., 2015). It is important to consider how maturation can improve these aspects of cellular physiology in order to acquire a better representation of potential cardiotoxic effects (Figure 2).
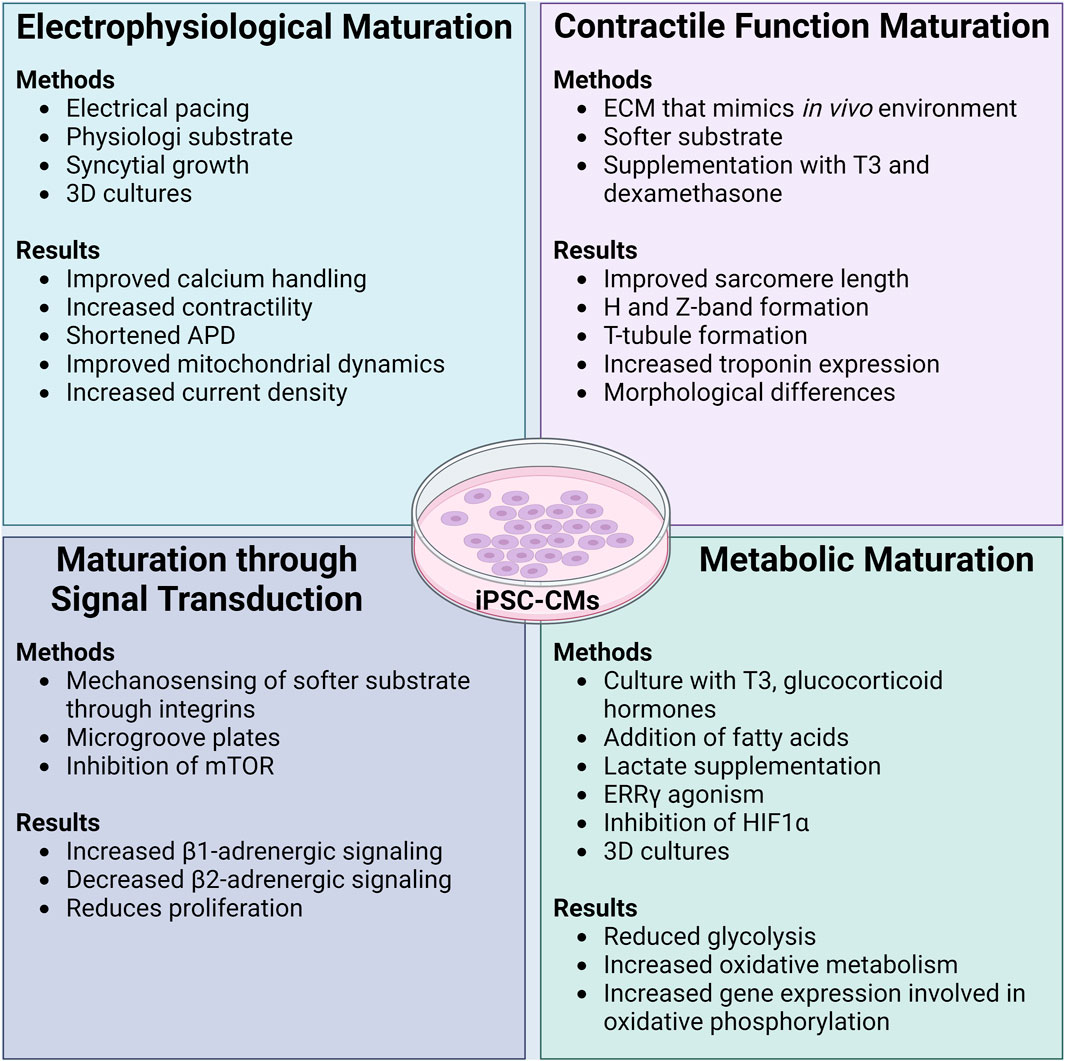
FIGURE 2. Methods and functional results of maturation protocols in iPSC-CMs. Initially differentiated iPSC-CMs are known to have an immature phenotype akin to a neonatal CM. Many different maturation protocols have been developed to ensure that functional parameters and the cellular phenotype of iPSC-CMs can more closely and accurately reflect that of an adult human CM. In general, maturation protocols should aim to improve the electrophysiology, contractility, signal transduction, and metabolism of iPSC-CMs. While the combination of different maturation protocols leads to the best results of functional improvement in iPSC-CMs, it appears that culturing iPSC-CMs on soft substrates with extracellular matrix coating that is similar to the in vivo environment is imperative. ADP: action potential duration, ECM: extracellular matrix, T3: thyroid hormone T3, mTOR: mammalian target of rapamycin, ERRγ: estrogen related receptor γ, HIF1α: hypoxia inducible factor-1α. Created with www.biorender.com.
3.1.1 Electrophysiological maturation
A mature electrophysiological system increases reliability when assessing a candidate compound for arrhythmogenic effects. Several methods improve the electrophysiological function of iPSC-CMs. Currently, the most common method of electrophysiological maturation is by pacing iPSC-CMs with an electrode, improving calcium handling and contractile function (Wu et al., 2021; Shen et al., 2022).
Improvements to (increasing) the AP upstroke velocity in iPSC-CMs was made through maturation by plating the cells on substrates possessing a stiffness more akin to a healthy heart (i.e., softer than normal tissue culture plastic), with a scaffold/extracellular matrix (ECM) composed of polydimethylsiloxane (PDMS) and Matrigel (Herron et al., 2016). In addition to ECM composition, cell density plays a role in the maturity of electrophysiology. Syncytial growth (multinucleate cell formation) of iPSC-CMs with proper cell-cell contact can improve the IK1 current and the expression Kir2.1 channels (Li et al., 2020). Some groups have created three-dimensional constructs for denser cell-cell connections, improving upstroke velocity through increases in INa density, resulting in an AP waveform that more closely resembles those observed in LV tissue (Lemoine et al., 2017). Other 3D microtissue techniques saw improved electrical coupling between the cells and the formation of refined intercalated disks (Giacomelli et al., 2020). Interestingly, overexpression of the Kir2.1 channel improved functional parameters, such as a shortened APD, an increased upstroke velocity, and improvements to calcium signaling and mitochondrial dynamics (Zhou et al., 2023). While this approach is novel and shows signs of promising improvements in electrophysiology, the overexpression could negatively alter the homeostatic balance of (intracellular) ions with unknown consequences (Nerbonne et al., 2001). The endoplasmic reticulum unfolded protein response, mediated through protein kinase-like ER kinase (PERK) and inositol-requiring protein-1 (IRE1), can have negative effects on Nav1.5 and hERG expression in CMs (Liu et al., 2018). The inhibition of these pathways could evoke electrophysiological maturation.
Other novel approaches to electrophysiological maturation include pharmacological additions of SGLT2 inhibitors, which resulted in an increase in INa and IK1 (Dago et al., 2022). Co-culturing iPSC-CMs with Kir2.1 expressing human embryonic kidney (HEK) cells to create an electrical syncytium where the HEK cells acted as a pacer, resulted in decreased spontaneous beating (of the cardiomyocytes) and an increase in contraction amplitude (Costa et al., 2021). Whether this technique confers an advantage over more common maturation methods of pacing is unclear.
3.1.2 Maturation through signal transduction
The importance of signal transduction can be attributed to mechanosensing through integrin signaling. iPSC-CMs plated on PDMS and Matrigel ECM showed improvements in conduction velocity (55 cm/s), similar to adult LV CMs (Herron et al., 2016).
As discussed, iPSC-CM adrenergic function is partially mediated through β2-adrenergic receptors, as opposed to relying solely on β1-adrenergic receptors like in the adult heart. This change can be addressed as the increasing β1-adrenergic signaling and decreasing β2-adrenergic signaling are a function of maturation (Jung et al., 2016). The shift in adrenergic signaling can be achieved through biomechanical stimulation, such as culturing iPSC-CMs on PDMS micro-grooved devices (Jung et al., 2016).
Another signaling pathway that modulate the maturity of iPSC-CMs is the mTOR pathway. Inhibition of mTOR leads to improved contractility and more adult-like metabolic and electrophysiological profiles (Garbern et al., 2020). The exact mechanism for this effect is still unknown but the authors speculate that it can be attributed to the inhibition of proliferative signals and switching the iPSC-CMs to a quiescent state to mimic the low proliferative potential of adult CMs.
3.1.3 Maturation of contractile function
Maturation of iPSC-CMs leads to the development and refinement of the contractile machinery through increased sarcomere length, with distinct H and Z-bands (Lundy et al., 2013). A method commonly used to mature contractile function is to plate iPSC-CMs on a substrate with a Young’s modulus more similar to the in vivo environment (like PDMS), coated with ECM proteins (i.e., gelatin, collagen, fibronectin) (Wang et al., 2018; Knight et al., 2021; Körner et al., 2021; Dhahri et al., 2022). The addition of a softer substrate results in rod-shaped cells and more organized actin filaments (Lemoine et al., 2017). Furthermore, addition of microgrooves within the substrate can enhance sarcomere alignment and improve contractility (Dou et al., 2021; 2023). Increases in mechanical outputs (as measured by sarcomere shortening) were found by utilizing a softer substrate for culture and cell maturation (Ribeiro et al., 2015).
Improvements to the CICR can be made through the maturation of T-tubules and calcium handling proteins. Supplementation with thyroid hormone T3 (100 nM) and dexamethasone (1 mM) on iPSC-CMs plated on a Matrigel coating (3 mL media/well) showed enhanced formation of T-tubules, which are essential for efficient CICR (Parikh et al., 2017). The formation of T-tubules was attributed to the function of bridging integrator-1 (BIN1), which contributes to the co-proximity of LTCC and the RyR (Guo et al., 2022). This raises the possibility of modulating BIN1 activity for further CICR maturation. T-tubule formation can be enhanced through supplementation with an estrogen-related receptor gamma (ERRγ) agonist (e.g., T112 at 3 μM), in conjunction with an S-phase kinase-associated protein inhibitor (e.g., T623 at 40 nM) (Miki et al., 2021). This approach has the added benefit of increasing the expression of adult troponin I (TNNI3) but did not appear to reduce fetal troponin I (TNNI1). Within this study, the iPSC-CMs were still spherical in morphology, a sign of continued immaturity and indicating that the agonist/antagonist alone is not enough for full maturation of the contractile machinery. As maturation progresses, increases in SERCA-2a activity and calcium cycling are observed, although a plateau is reached after 21 days of culture (Hwang et al., 2015).
A softer substrate and ECM are beneficial for maturation to occur, and modulation of specific signaling pathways is beneficial to the maturation of the contractile function in iPSC-CMs. When comparing cells grown on glass (25 GPa) and PDMS (1.5 kPa, 15kPa, 28 kPa) surfaces, iPSC-CMs grown on PDMS had improved contractility and calcium handling, similar to those of an adult CM (Körner et al., 2021). Similarly, culturing iPSC-CMs on softer substrates have yielded improvements in conduction velocity (doubling to 43.6 ± 7 cm/s), structural maturation (gap junction formation and hypertrophic growth), and activation of integrin signaling pathways (Herron et al., 2016). While the signs of maturation as a result of a softer substrate are substantial, the optimal combination of maturation protocols remains to be elucidated and standardized.
Culturing iPSC-CMs in 3D scaffolds is another approach used to mature contractile function through the organization of myofibrils and better spatial positioning of CICR related proteins (Silbernagel et al., 2020; Pinton et al., 2023). These approaches have demonstrated success in the formation of 3D structures more akin to that of heart tissue.
3.1.4 Metabolic maturation
Metabolic maturation of iPSC-CMs is often overlooked and can take up to 200 days for maximal effect (Ebert et al., 2019). Many protocols suggest culturing iPSC-CMs with thyroid and glucocorticoid hormones (Yang et al., 2014; Birket et al., 2015; Parikh et al., 2017) and fatty acids (Rana et al., 2012; Horikoshi et al., 2019; M. Feyen et al., 2020) thereby reducing the glycolytic phenotype and promoting fatty acid uptake/utilization. Lactate supplementation additionally shows an improvement in the metabolic profile, along with an increased expression of genes involved in oxidative phosphorylation (PPARα, CPT-1B, PGC-1α, PDK4) (Bekhite et al., 2020).
In addition to the positive effects ERRγ agonism can have on iPSC-CM contractility, ERRγ is a regulator for the metabolic shift towards oxidative metabolism (Alaynick et al., 2007). Adding an ERRγ agonist (T112 at 3 μM) increased oxygen consumption in iPSC-CMs, indicative of enhanced mitochondrial function and metabolic maturation (Miki et al., 2021). Additionally, inhibition of hypoxia induced factor-1α and lactate dehydrogenase A can cause partial shifts of iPSC-CM metabolism from aerobic glycolysis to fatty acid oxidation (Hu et al., 2018).
Considering structural approaches, three dimensional engineered heart tissue showed robust maturation of metabolism through increases in ATP synthesis and an upregulation of fatty acid oxidation-related proteins (Ulmer et al., 2018). 3D iPSC-CM engineering approaches with iPSC-CMs have gained traction and show promising signs of maturation in many aspects of cellular physiology. The advantages and applications of 3D iPSC-CM cultures have been discussed in other reviews (Zuppinger, 2019; Cho et al., 2021; Liu et al., 2022).
3.2 Sources of iPSC-CMs
iPSC-CMs can be derived from different tissues, resulting in altered responses to tested compounds (even when from the same individual). Commercial sources offer cells that are quality controlled, with pre-defined plating efficiencies, and are delivered already differentiated into CMs with high purity. While commercial sources offer several advantages, there is some evidence showing batch-to-batch variability (Nozaki et al., 2016; Mannhardt et al., 2017). A comparison of the effects of pro-arrhythmic drugs from two different lots of commercial iPSC-CMs showed terfenadine (potent IKr inhibitor) induced early after depolarizations (EADs) in one but not the other cell source (Nozaki et al., 2016). Other drugs tested in this study showed no variability between the different cell lots. Despite the variance in the production of EADs, terfenadine still produced similar changes to beat rate and FPDc, and the study concluded that lot-to-lot variability was within acceptable margins.
Other assessments of iPSC-CMs from 10 different sources (5 commercial, 5 academic) showed major differences in baseline metrics such as beat rate, contractile force, time to peak contraction, and relaxation time (Mannhardt et al., 2020). Despite these differences at baseline, the sarcomere lengths between all sources were similar and tested pharmaceuticals elicited similar responses, as measured by changes to contractile force. Although some cellular physiological differences will exist in cells from different sources, relative changes from baseline and data reproducibility are more important for drug evaluation.
Another source of iPSC-CMs are those derived from a specific patient with a desired phenotype/disease state. The phenotype of the resulting cells can be highly patient tissue specific, a detriment to the generalization of cardiotoxicity predictions. For example, atrial and ventricular fibroblast-derived iPSC-CMs were noted to have different field potential durations and higher action potential duration/conduction velocities (Wang et al., 2023) than iPSC-CM derived from dermal fibroblasts (Lan et al., 2020) or blood (Lam et al., 2020). The tissue sources of iPSC-CMs can also affect how fast and how well the resultant iPSC-CM can mature, as illustrated with iPSC-CMs derived from cardiac sources having more ion channels and calcium handling proteins than those derived from non-cardiac sources with the same duration of maturation (Pianezzi et al., 2020).
3.3 The heterogenous population of iPSC-CMs
Unless differentiation protocols are designed to produce a specific subset of CMs, the resultant population of iPSC-CMs can include a heterogenous mixture of nodal, atrial, ventricular, and Purkinje related cell types (Zhang et al., 2009; Ma et al., 2013). Although the majority of iPSC-CMs tend towards more ventricular-like phenotypes, the composition is important to determine what anatomical or cellular part of the heart a novel compound may be targeting. Maturation protocols can be customized to what type of iPSC-CMs is desired. For instance, a PPARα agonist, dexamethasone, T3, and palmitate in low-glucose media worked well for maturing iPSC-CMs towards a ventricular phenotype (cTNT+, MYL2+) (Funakoshi et al., 2021). For more atrial-like iPSC-CMs, retinoic acid was found to be important, along with lower BMP4 and activin-A concentrations (Goldfracht et al., 2020). Atrial-like iPSC-CMs were delineated with the absence of MLC2v and confirmed with electrophysiological measurements. Modulation of ion channels can be used to push iPSC-CM populations towards a pacemaker-like phenotype (Kleger et al., 2010).
Contractile force and frequency of beating are known to be different in atrial or ventricular iPSC-CMs (Zhao et al., 2019). The advantage of delineating the different populations was demonstrated where a small segment of engineered heart tissue on a “biowire” platform was created with both atrial and ventricular iPSC-CMs, effectively making a “mini heart”.
There are many variations on maturation protocols, and it is difficult to determine which one is objectively the most beneficial. It is evident that often maturing one aspect of iPSC-CM cellular function improves others concurrently, and no one technique can mature all aspects at once. Nonetheless, if functional metrics in electrophysiology, cell signaling, contractile function, calcium handling, and metabolism show maturation, it would support a reliable platform for reproducible and translatable results in cardiotoxicity testing.
4 Examples of drug testing using iPSC-CMs
The utilization of iPSC-CMs for cardiotoxicity testing is a growing area and is one of the main motives for the Comprehensive In vitro Proarrhythmia Assessment assay (CiPA) initiative, established by the Cardiac Safety Research Consortium, the Food and Drug Administration, and the Environmental Sciences Institute (Sager et al., 2014). CiPA was formed to address novel approaches in assessing drug-induced proarrhythmia risk with three major components: 1) assessing the effect of candidate drugs on crucial ventricular ion channel currents, 2) evaluation of potential effects on ion channels in silico for assessment of the cumulative effects on the cardiac AP, and 3) observing and quantifying discrepancies in a systems biology approach through the use of iPSC-CMs and human electrocardiograms (Colatsky et al., 2016).
The initiative has resulted in the advancement of consensus protocols for the use of iPSC-CM in large multisite studies for proarrhythmic analysis with high reproducibility and accurate predictions. A large study enrolled 10 laboratories around the world using two commercial sources of iPSC-CMs and were able to assess the arrhythmic risks in 28 blinded drugs at an 87% predictivity (Blinova et al., 2018). A similar undertaking was performed by the same group in 2017 using two different commercial iPSC-CM lines which demonstrated that iPSC-CMs are sensitive to hERG and LTCC channel blockers, but had variable responses to late INa blockers (Blinova et al., 2017). Another multi-site study included four iPSC-CM cell lines and 18 individual investigation sites, demonstrating the sensitivity of iPSC-CMs towards multi-channel blocking compounds for accurate predictions of proarrhythmic risk (Millard et al., 2018). The testing found that there were consistent concentration-dependent effects for all of the 8 drugs tested, including prolongation of beat period and field potential duration with the IKr blocker E-4031, or the shortening of BP and FPD with nifedipine. The authors did note that 8/18 (44%) of the independent studies failed to detect delayed repolarization with E-4031 and attributed it to inadequate drug exposure, poor culturing techniques, and differences in ion channel densities. These studies illustrate that iPSC-CMs have a great potential for assessing the proarrhythmic risk of compounds and are in line with the goals of the CiPA initiative.
There have not been large scale studies assessing other (non-arrhythmogenic) metrics of cardiotoxicity, but smaller studies have been performed. iPSC-CMs were used to assess the negative effects of the breast cancer drug trastuzumab on CM structure and function (Kitani et al., 2019). Patient-derived iPSC-CMs created from individuals with identified trastuzumab-induced cardiotoxicity were able to recapitulate patient-specific responses, demonstrating the utility of iPSC-CMs in precision medicine-type cardiotoxicity testing. The analysis of iPSC-CM responses to trastuzumab elucidated putative metabolic mechanisms that contribute to trastuzumab cardiotoxicity. In a separate study, a library of iPSC-CMs was generated from patients with various genetic and hereditary disorders. The extent of drug cardiotoxicity effects were quantified utilizing the derived cells, which accurately recapitulated the clinical susceptibilities observed (Liang et al., 2013).
5 Conclusion
iPSC-CMs are powerful tools for assessing the cardiotoxicity of novel or existing compounds due to their similarity in cellular physiology to endogenous CMs. They can be used to predict the effects of candidate compounds in discovery pipelines on the complex electrophysiology, cellular signaling, metabolism, and contractile machinery in the adult CM. However, it is crucial to acknowledge that when considering iPSC-CMs as a model for cardiotoxicity testing, there remains certain disparities that should be considered. The distinctions encompass variances in ion channel kinetics and function, structural differences in the contractile machinery, isoforms of proteins, and metabolic function. These considerations are especially important if the target of the drug is one that is known to be different between adult CM and iPSC-CMs as highlighted in this review. The integration of iPSC-CMs into preclinical testing pipelines holds potential to expedite the discovery and assess the safety of novel therapeutic compounds, resulting in greater bench to bedside efficiency. For wider implementation in pre-clinical drug discovery, the use of iPSC-CMs should be (minimally internally) standardized regarding culture conditions, maturation protocols, and the assessment of cellular subtype, to ensure reproducibility. The incorporation of iPSC-CMs represents a crucial step towards addressing challenges of compound attrition and improving the landscape of drug development.
Author contributions
TL: Conceptualization, Data curation, Investigation, Writing–original draft, Writing–review and editing. JC: Supervision, Writing–review and editing. JM: Conceptualization, Funding acquisition, Supervision, Writing–review and editing.
Funding
The author(s) declare financial support was received for the research, authorship, and/or publication of this article. This work was supported by a Canadian Institutes of Health Research Project Grant, the SickKids Foundation through the Curtis Joseph and Harold Groves Chair in Anesthesia and Pain Medicine (JTM) and the Department of Anesthesiology and Pain Medicine, University of Toronto through a Merit Award (JTM). TYTL is supported by the CIHR Canadian Graduate Scholarship–Doctoral Award.
Acknowledgments
The authors thank the members of the Department of Anesthesia and Pain Medicine at the Hospital for Sick Children (Toronto, Ontario, Canada) for the protection of research time for the study investigators. Dr. Maynes would also like to thank the donors and SickKids Foundation as the holder of the Curtis Joseph and Harold Groves Chair in Anesthesia and Pain Medicine and the Department of Anesthesiology and Pain Medicine at the University of Toronto as the holder of a Merit Award.
Conflict of interest
The authors declare that the research was conducted in the absence of any commercial or financial relationships that could be construed as a potential conflict of interest.
Publisher’s note
All claims expressed in this article are solely those of the authors and do not necessarily represent those of their affiliated organizations, or those of the publisher, the editors and the reviewers. Any product that may be evaluated in this article, or claim that may be made by its manufacturer, is not guaranteed or endorsed by the publisher.
References
Alaynick, W. A., Kondo, R. P., Xie, W., He, W., Dufour, C. R., Downes, M., et al. (2007). ERRgamma directs and maintains the transition to oxidative metabolism in the postnatal heart. Cell Metab. 6 (1), 13–24. doi:10.1016/j.cmet.2007.06.007
Bekhite, M. M., González Delgado, A., Menz, F., Kretzschmar, T., Wu, J. M. F., Bekfani, T., et al. (2020). Longitudinal metabolic profiling of cardiomyocytes derived from human-induced pluripotent stem cells. Basic Res. Cardiol. 115 (4), 37. doi:10.1007/s00395-020-0796-0
Birket, M. J., Ribeiro, M. C., Kosmidis, G., Ward, D., Leitoguinho, A. R., van de Pol, V., et al. (2015). Contractile defect caused by mutation in MYBPC3 revealed under conditions optimized for human PSC-cardiomyocyte function. Cell Rep. 13 (4), 733–745. doi:10.1016/j.celrep.2015.09.025
Blinova, K., Dang, Q., Millard, D., Smith, G., Pierson, J., Guo, L., et al. (2018). International multisite study of human-induced pluripotent stem cell-derived cardiomyocytes for drug proarrhythmic potential assessment. Cell Rep. 24 (13), 3582–3592. doi:10.1016/j.celrep.2018.08.079
Blinova, K., Stohlman, J., Vicente, J., Chan, D., Johannesen, L., Hortigon-Vinagre, M. P., et al. (2017). Comprehensive translational assessment of human-induced pluripotent stem cell derived cardiomyocytes for evaluating drug-induced arrhythmias. Toxicol. Sci. 155 (1), 234–247. doi:10.1093/toxsci/kfw200
Bowman, P. R. T., Smith, G. L., and Gould, G. W. (2019). GLUT4 expression and glucose transport in human induced pluripotent stem cell-derived cardiomyocytes. PLoS ONE 14 (7), e0217885. doi:10.1371/journal.pone.0217885
Bristow, M. R., Ginsburg, R., Umans, V., Fowler, M., Minobe, W., Rasmussen, R., et al. (1986). Beta 1- and beta 2-adrenergic-receptor subpopulations in nonfailing and failing human ventricular myocardium: coupling of both receptor subtypes to muscle contraction and selective beta 1-receptor down-regulation in heart failure. Circulation Res. 59 (3), 297–309. doi:10.1161/01.RES.59.3.297
Burridge, P. W., Matsa, E., Shukla, P., Lin, Z. C., Churko, J. M., Ebert, A. D., et al. (2014). Chemically defined generation of human cardiomyocytes. Nat. Methods 11 (8), 855–860. doi:10.1038/nmeth.2999
Cao, N., Liu, Z., Chen, Z., Wang, J., Chen, T., Zhao, X., et al. (2012). Ascorbic acid enhances the cardiac differentiation of induced pluripotent stem cells through promoting the proliferation of cardiac progenitor cells. Cell Res. 22 (1), 219–236. doi:10.1038/cr.2011.195
Chan, B. Y. H., Roczkowsky, A., Cho, W. J., Poirier, M., Lee, T. Y. T., Mahmud, Z., et al. (2019). Junctophilin-2 is a target of matrix metalloproteinase-2 in myocardial ischemia–reperfusion injury. Basic Res. Cardiol. 114 (6), 42. doi:10.1007/s00395-019-0749-7
Chauveau, S., Anyukhovsky, E. P., Ben-Ari, M., Naor, S., Jiang, Y. P., Danilo, P., et al. (2017). Induced pluripotent stem cell–derived cardiomyocytes provide in vivo biological pacemaker function. Circulation. Arrhythmia Electrophysiol. 10 (5), e004508. doi:10.1161/CIRCEP.116.004508
Cho, S., Lee, C., Skylar-Scott, M. A., Heilshorn, S. C., and Wu, J. C. (2021). Reconstructing the heart using iPSCs: engineering strategies and applications. J. Mol. Cell. Cardiol. 157, 56–65. doi:10.1016/j.yjmcc.2021.04.006
Clark, R. B., Tremblay, A., Melnyk, P., Allen, B. G., Giles, W. R., and Fiset, C. (2001). T-tubule localization of the inward-rectifier K+ channel in mouse ventricular myocytes: a role in K+ accumulation. J. Physiology 537 (3), 979–992. doi:10.1111/j.1469-7793.2001.00979.x
Colatsky, T., Fermini, B., Gintant, G., Pierson, J. B., Sager, P., Sekino, Y., et al. (2016). The comprehensive in vitro proarrhythmia assay (CiPA) initiative — update on progress. J. Pharmacol. Toxicol. Methods 81, 15–20. doi:10.1016/j.vascn.2016.06.002
Cordeiro, J. M., Nesterenko, V. V., Sicouri, S., Goodrow, R. J., Treat, J. A., Desai, M., et al. (2013). Identification and characterization of a transient outward K+ current in human induced pluripotent stem cell-derived cardiomyocytes. J. Mol. Cell. Cardiol. 60, 36–46. doi:10.1016/j.yjmcc.2013.03.014
Costa, A. D. S., Mortensen, P., Hortigon-Vinagre, M. P., van der Heyden, M. A. G., Burton, F. L., Gao, H., et al. (2021). Electrophysiology of hiPSC-cardiomyocytes Co-cultured with HEK cells expressing the inward rectifier channel. Int. J. Mol. Sci. 22 (12), 6621. doi:10.3390/ijms22126621
Dago, M., Crespo-García, T., Cámara-Checa, A., Rapún, J., Rubio-Alarcón, M., Marín, M., et al. (2022). Empagliflozin and dapagliflozin increase Na+ and inward rectifier K+ current densities in human cardiomyocytes derived from induced pluripotent stem cells (hiPSC-CMs). Cells 11 (23), 3707. doi:10.3390/cells11233707
Davis, R. P., Casini, S., van den Berg, C. W., Hoekstra, M., Remme, C. A., Dambrot, C., et al. (2012). Cardiomyocytes derived from pluripotent stem cells recapitulate electrophysiological characteristics of an overlap syndrome of cardiac sodium channel disease. Circulation 125 (25), 3079–3091. doi:10.1161/CIRCULATIONAHA.111.066092
Dhahri, W., Sadikov Valdman, T., Wilkinson, D., Pereira, E., Ceylan, E., Andharia, N., et al. (2022). In vitro matured human pluripotent stem cell–derived cardiomyocytes form grafts with enhanced structure and function in injured hearts. Circulation 145 (18), 1412–1426. doi:10.1161/CIRCULATIONAHA.121.053563
Di Marco, A., Gaetani, M., Orezzi, P., Scarpinato, B. M., Silvestrini, R., Soldati, M., et al. (1964). “Daunomycin”, a new antibiotic of the rhodomycin group. Nature 201 (4920), 706–707. doi:10.1038/201706a0
Dou, W., Daoud, A., Chen, X., Wang, T., Malhi, M., Gong, Z., et al. (2023). Ultrathin and flexible bioelectronic arrays for functional measurement of iPSC-cardiomyocytes under cardiotropic drug administration and controlled microenvironments. Nano Lett. 23 (6), 2321–2331. doi:10.1021/acs.nanolett.3c00017
Dou, W., Malhi, M., Cui, T., Wang, M., Wang, T., Shan, G., et al. (2022). A carbon-based biosensing platform for simultaneously measuring the contraction and electrophysiology of iPSC-cardiomyocyte monolayers. ACS Nano 16 (7), 11278–11290. doi:10.1021/acsnano.2c04676
Dou, W., Wang, L., Malhi, M., Liu, H., Zhao, Q., Plakhotnik, J., et al. (2021). A microdevice platform for characterizing the effect of mechanical strain magnitudes on the maturation of iPSC-Cardiomyocytes. Biosens. Bioelectron. 175, 112875. doi:10.1016/j.bios.2020.112875
Dou, W., Zhao, Q., Malhi, M., Liu, X., Zhang, Z., Wang, L., et al. (2020). Label-free conduction velocity mapping and gap junction assessment of functional iPSC-Cardiomyocyte monolayers. Biosens. Bioelectron. 167, 112468. doi:10.1016/j.bios.2020.112468
Drouin, E., Charpentier, F., Gauthier, C., Laurent, K., and Le Marec, H. (1995). Electrophysiologic characteristics of cells spanning the left ventricular wall of human heart: evidence for presence of M cells. J. Am. Coll. Cardiol. 26 (1), 185–192. doi:10.1016/0735-1097(95)00167-X
Duncan, R. S., Ridley, J. M., Dempsey, C. E., Leishman, D. J., Leaney, J. L., Hancox, J. C., et al. (2006). Erythromycin block of the HERG K+ channel: accessibility to F656 and Y652. Biochem. Biophysical Res. Commun. 341 (2), 500–506. doi:10.1016/j.bbrc.2006.01.008
Ebert, A., Joshi, A. U., Andorf, S., Dai, Y., Sampathkumar, S., Chen, H., et al. (2019). Proteasome-dependent regulation of distinct metabolic states during long-term culture of human iPSC-derived cardiomyocytes. Circulation Res. 125 (1), 90–103. doi:10.1161/CIRCRESAHA.118.313973
Endoh, M. (2004). Force–frequency relationship in intact mammalian ventricular myocardium: physiological and pathophysiological relevance. Eur. J. Pharmacol. 500 (1), 73–86. doi:10.1016/j.ejphar.2004.07.013
Feng, J., Li, G. R., Fermini, B., and Nattel, S. (1996). Properties of sodium and potassium currents of cultured adult human atrial myocytes. Am. J. Physiology-Heart Circulatory Physiology 270 (5), H1676–H1686. doi:10.1152/ajpheart.1996.270.5.H1676
Fernández-Morales, J.-C., Hua, W., Yao, Y., and Morad, M. (2019). Regulation of Ca2+ signaling by acute hypoxia and acidosis in cardiomyocytes derived from human induced pluripotent stem cells. Cell calcium 78, 1–14. doi:10.1016/j.ceca.2018.12.006
Feyen, M. D. A., McKeithan, W. L., Bruyneel, A. A. N., Spiering, S., Hörmann, L., Ulmer, B., et al. (2020). Metabolic maturation media improve physiological function of human iPSC-derived cardiomyocytes. Cell Rep. 32 (3), 107925. doi:10.1016/j.celrep.2020.107925
Frothingham, R. (2001). Rates of torsades de Pointes associated with ciprofloxacin, ofloxacin, levofloxacin, gatifloxacin, and moxifloxacin. Pharmacother. J. Hum. Pharmacol. Drug Ther. 21 (12), 1468–1472. doi:10.1592/phco.21.20.1468.34482
Funakoshi, S., Fernandes, I., Mastikhina, O., Wilkinson, D., Tran, T., Dhahri, W., et al. (2021). Generation of mature compact ventricular cardiomyocytes from human pluripotent stem cells. Nat. Commun. 12, 3155. doi:10.1038/s41467-021-23329-z
Fung, M., Thornton, A., Mybeck, K., Wu, J. H. H., Hornbuckle, K., and Muniz, E. (2001). Evaluation of the characteristics of safety withdrawal of prescription drugs from worldwide pharmaceutical markets-1960 to 1999. Drug Inf. J. DIJ/Drug Inf. Assoc. 35 (1), 293–317. doi:10.1177/009286150103500134
Funk, K. A., and Bostwick, J. R. (2013). A comparison of the risk of QT prolongation among SSRIs. Ann. Pharmacother. 47 (10), 1330–1341. doi:10.1177/1060028013501994
Garbern, J. C., Helman, A., Sereda, R., Sarikhani, M., Ahmed, A., Escalante, G. O., et al. (2020). Inhibition of mTOR signaling enhances maturation of cardiomyocytes derived from human induced pluripotent stem cells via p53-induced quiescence. Circulation 141 (4), 285–300. doi:10.1161/CIRCULATIONAHA.119.044205
Germanguz, I., Sedan, O., Zeevi-Levin, N., Shtrichman, R., Barak, E., Ziskind, A., et al. (2011). Molecular characterization and functional properties of cardiomyocytes derived from human inducible pluripotent stem cells. J. Cell. Mol. Med. 15 (1), 38–51. doi:10.1111/j.1582-4934.2009.00996.x
Gherghiceanu, M., Barad, L., Novak, A., Reiter, I., Itskovitz-Eldor, J., Binah, O., et al. (2011). Cardiomyocytes derived from human embryonic and induced pluripotent stem cells: comparative ultrastructure. J. Cell. Mol. Med. 15 (11), 2539–2551. doi:10.1111/j.1582-4934.2011.01417.x
Giacomelli, E., Meraviglia, V., Campostrini, G., Cochrane, A., Cao, X., van Helden, R. W. J., et al. (2020). Human-iPSC-Derived cardiac stromal cells enhance maturation in 3D cardiac microtissues and reveal non-cardiomyocyte contributions to heart disease. Cell Stem Cell 26 (6), 862–879. doi:10.1016/j.stem.2020.05.004
Giannetti, F., Benzoni, P., Campostrini, G., Milanesi, R., Bucchi, A., Baruscotti, M., et al. (2021). A detailed characterization of the hyperpolarization-activated “funny” current (If) in human-induced pluripotent stem cell (iPSC)–derived cardiomyocytes with pacemaker activity. Pflugers Arch. 473 (7), 1009–1021. doi:10.1007/s00424-021-02571-w
Goldfracht, I., Protze, S., Shiti, A., Setter, N., Gruber, A., Shaheen, N., et al. (2020). Generating ring-shaped engineered heart tissues from ventricular and atrial human pluripotent stem cell-derived cardiomyocytes. Nat. Commun. 11, 75. doi:10.1038/s41467-019-13868-x
Guo, A., Hall, D., Zhang, C., Peng, T., Miller, J. D., Kutschke, W., et al. (2015). Molecular determinants of calpain-dependent cleavage of junctophilin-2 protein in cardiomyocytes. J. Biol. Chem. 290 (29), 17946–17955. doi:10.1074/jbc.M115.652396
Guo, J., Tian, Q., Barth, M., Xian, W., Ruppenthal, S., Schaefers, H. J., et al. (2022). Human BIN1 isoforms grow, maintain, and regenerate excitation–contraction couplons in adult rat and human stem cell-derived cardiomyocytes. Cardiovasc. Res. 118 (6), 1479–1491. doi:10.1093/cvr/cvab195
Han, W., Zhang, L., Schram, G., and Nattel, S. (2002). Properties of potassium currents in Purkinje cells of failing human hearts. Am. J. Physiology-Heart Circulatory Physiology 283 (6), H2495–H2503. doi:10.1152/ajpheart.00389.2002
Hasenfuss, G., Mulieri, L. A., Blanchard, E. M., Holubarsch, C., Leavitt, B. J., Ittleman, F., et al. (1991). Energetics of isometric force development in control and volume-overload human myocardium. Comparison with animal species. Circulation Res. 68 (3), 836–846. doi:10.1161/01.RES.68.3.836
Herron, T. J., Rocha, A. M. D., Campbell, K. F., Ponce-Balbuena, D., Willis, B. C., Guerrero-Serna, G., et al. (2016). Extracellular matrix–mediated maturation of human pluripotent stem cell–derived cardiac monolayer structure and electrophysiological function. Circulation Arrhythmia Electrophysiol. 9 (4), e003638. doi:10.1161/CIRCEP.113.003638
Herron, T. J., Rostkova, E., Kunst, G., Chaturvedi, R., Gautel, M., and Kentish, J. C. (2006). Activation of myocardial contraction by the N-terminal domains of myosin binding protein-C. Circulation Res. 98 (10), 1290–1298. doi:10.1161/01.RES.0000222059.54917.ef
Hinson, J. T., Chopra, A., Nafissi, N., Polacheck, W. J., Benson, C. C., Swist, S., et al. (2015). HEART DISEASE. Titin mutations in iPS cells define sarcomere insufficiency as a cause of dilated cardiomyopathy. Sci. (New York, N.Y.) 349 (6251), 982–986. doi:10.1126/science.aaa5458
Hohendanner, F., Ljubojević, S., MacQuaide, N., Sacherer, M., Sedej, S., Biesmans, L., et al. (2013). Intracellular dyssynchrony of diastolic cytosolic [Ca²⁺] decay in ventricular cardiomyocytes in cardiac remodeling and human heart failure. Circulation Res. 113 (5), 527–538. doi:10.1161/CIRCRESAHA.113.300895
Horikoshi, Y., Yan, Y., Terashvili, M., Wells, C., Horikoshi, H., Fujita, S., et al. (2019). Fatty acid-treated induced pluripotent stem cell-derived human cardiomyocytes exhibit adult cardiomyocyte-like energy metabolism phenotypes. Cells 8 (9), 1095. doi:10.3390/cells8091095
Horváth, A., Lemoine, M. D., Löser, A., Mannhardt, I., Flenner, F., Uzun, A. U., et al. (2018). Low resting membrane potential and low inward rectifier potassium currents are not inherent features of hiPSC-derived cardiomyocytes. Stem Cell Rep. 10 (3), 822–833. doi:10.1016/j.stemcr.2018.01.012
Hu, D., Linders, A., Yamak, A., Correia, C., Kijlstra, J. D., Garakani, A., et al. (2018). Metabolic maturation of human pluripotent stem cell derived cardiomyocytes by inhibition of HIF1α and LDHA. Circulation Res. 123 (9), 1066–1079. doi:10.1161/CIRCRESAHA.118.313249
Hunkeler, N. M., Kullman, J., and Murphy, A. M. (1991). Troponin I isoform expression in human heart. Circulation Res. 69 (5), 1409–1414. doi:10.1161/01.RES.69.5.1409
Hwang, H. S., Kryshtal, D. O., Feaster, T. K., Sánchez-Freire, V., Zhang, J., Kamp, T. J., et al. (2015). Comparable calcium handling of human iPSC-derived cardiomyocytes generated by multiple laboratories. J. Mol. Cell. Cardiol. 85, 79–88. doi:10.1016/j.yjmcc.2015.05.003
Itzhaki, I., Maizels, L., Huber, I., Zwi-Dantsis, L., Caspi, O., Winterstern, A., et al. (2011). Modelling the long QT syndrome with induced pluripotent stem cells. Nature 471 (7337), 225–229. doi:10.1038/nature09747
Itzhaki, I., Rapoport, S., Huber, I., Mizrahi, I., Zwi-Dantsis, L., Arbel, G., et al. (2011). Calcium handling in human induced pluripotent stem cell derived cardiomyocytes. PLOS ONE 6 (4), e18037. doi:10.1371/journal.pone.0018037
Ivashchenko, C. Y., Pipes, G. C., Lozinskaya, I. M., Lin, Z., Xiaoping, X., Needle, S., et al. (2013). Human-induced pluripotent stem cell-derived cardiomyocytes exhibit temporal changes in phenotype. Am. J. Physiology-Heart Circulatory Physiology 305 (6), H913–H922. doi:10.1152/ajpheart.00819.2012
Jost, N., Acsai, K., Horváth, B., Bányász, T., Baczkó, I., Bitay, M., et al. (2009). Contribution of I Kr and I K1 to ventricular repolarization in canine and human myocytes: is there any influence of action potential duration? Basic Res. Cardiol. 104 (1), 33–41. doi:10.1007/s00395-008-0730-3
Jost, N., Virág, L., Bitay, M., Takács, J., Lengyel, C., Biliczki, P., et al. (2005). Restricting excessive cardiac action potential and QT prolongation: a vital role for IKs in human ventricular muscle. Circulation 112 (10), 1392–1399. doi:10.1161/CIRCULATIONAHA.105.550111
Jung, G., Fajardo, G., Ribeiro, A. J. S., Kooiker, K. B., Coronado, M., Zhao, M., et al. (2016). Time-dependent evolution of functional vs. remodeling signaling in induced pluripotent stem cell-derived cardiomyocytes and induced maturation with biomechanical stimulation. FASEB J. 30 (4), 1464–1479. doi:10.1096/fj.15-280982
Kałużna, E., Nadel, A., Zimna, A., Rozwadowska, N., and Kolanowski, T. (2022). Modeling the human heart ex vivo—current possibilities and strive for future applications. J. Tissue Eng. Regen. Med. 16 (10), 853–874. doi:10.1002/term.3335
Kim, C., Wong, J., Wen, J., Wang, S., Wang, C., Spiering, S., et al. (2013). Studying arrhythmogenic right ventricular dysplasia with patient-specific iPSCs. Nature 494 (7435), 105–110. doi:10.1038/nature11799
Kim, M.-S., Fleres, B., Lovett, J., Anfinson, M., Samudrala, S. S. K., Kelly, L. J., et al. (2020). Contractility of induced pluripotent stem cell-cardiomyocytes with an MYH6 head domain variant associated with hypoplastic left heart syndrome. Front. Cell Dev. Biol. 8, 440. doi:10.3389/fcell.2020.00440
Kitani, T., Ong, S. G., Lam, C. K., Rhee, J. W., Zhang, J. Z., Oikonomopoulos, A., et al. (2019). Human-induced pluripotent stem cell model of trastuzumab-induced cardiac dysfunction in patients with breast cancer. Circulation 139 (21), 2451–2465. doi:10.1161/CIRCULATIONAHA.118.037357
Kleger, A., Seufferlein, T., Malan, D., Tischendorf, M., Storch, A., Wolheim, A., et al. (2010). Modulation of calcium-activated potassium channels induces cardiogenesis of pluripotent stem cells and enrichment of pacemaker-like cells. Circulation 122 (18), 1823–1836. doi:10.1161/CIRCULATIONAHA.110.971721
Knight, W. E., Cao, Y., Dillon, P., and Song, K. (2021). A simple protocol to produce mature human-induced pluripotent stem cell-derived cardiomyocytes. Star. Protoc. 2 (4), 100912. doi:10.1016/j.xpro.2021.100912
Køber, L., Torp-Pedersen, C., McMurray, J. J. V., Gøtzsche, O., Lévy, S., Crijns, H., et al. (2008). Increased mortality after dronedarone therapy for severe heart failure. N. Engl. J. Med. 358 (25), 2678–2687. doi:10.1056/NEJMoa0800456
Körner, A., Mosqueira, M., Hecker, M., and Ullrich, N. D. (2021). Substrate stiffness influences structural and functional remodeling in induced pluripotent stem cell-derived cardiomyocytes. Front. Physiology 12, 710619. doi:10.3389/fphys.2021.710619
Lahmers, S., Wu, Y., Call, D. R., Labeit, S., and Granzier, H. (2004). Developmental control of titin isoform expression and passive stiffness in fetal and neonatal myocardium. Circulation Res. 94 (4), 505–513. doi:10.1161/01.RES.0000115522.52554.86
Lam, C. K., Tian, L., Belbachir, N., Wnorowski, A., and Shrestha, R. (2019). Identifying the transcriptome signatures of calcium channel blockers in human induced pluripotent stem cell–derived cardiomyocytes. Circulation Res. 125 (2), 212–222. doi:10.1161/CIRCRESAHA.118.314202
Lam, Y.-Y., Keung, W., Chan, C. H., Geng, L., Wong, N., Brenière-Letuffe, D., et al. (2020). Single-cell transcriptomics of engineered cardiac tissues from patient-specific induced pluripotent stem cell-derived cardiomyocytes reveals abnormal developmental trajectory and intrinsic contractile defects in hypoplastic right heart syndrome. J. Am. Heart Assoc. 9 (20), e016528. doi:10.1161/JAHA.120.016528
Lan, H., Xu, Q., El-Battrawy, I., Zhong, R., Li, X., Lang, S., et al. (2020). Ionic mechanisms of disopyramide prolonging action potential duration in human-induced pluripotent stem cell-derived cardiomyocytes from a patient with short QT syndrome type 1. Front. Pharmacol. 11, 554422. doi:10.3389/fphar.2020.554422
Lee, H. M., Hahn, S. J., and Choi, B. H. (2022). The antidiabetic drug rosiglitazone blocks Kv1.5 potassium channels in an open state. Korean J. Physiology Pharmacol. 26 (2), 135–144. doi:10.4196/kjpp.2022.26.2.135
Lee, P., Klos, M., Bollensdorff, C., Hou, L., Ewart, P., Kamp, T. J., et al. (2012). Simultaneous voltage and calcium mapping of genetically purified human induced pluripotent stem cell–derived cardiac myocyte monolayers. Circulation Res. 110 (12), 1556–1563. doi:10.1161/CIRCRESAHA.111.262535
Lemoine, M. D., Mannhardt, I., Breckwoldt, K., Prondzynski, M., Flenner, F., Ulmer, B., et al. (2017). Human iPSC-derived cardiomyocytes cultured in 3D engineered heart tissue show physiological upstroke velocity and sodium current density. Sci. Rep. 7 (1), 5464. doi:10.1038/s41598-017-05600-w
Li, M., Kanda, Y., Ashihara, T., Sasano, T., Nakai, Y., Kodama, M., et al. (2017). Overexpression of KCNJ2 in induced pluripotent stem cell-derived cardiomyocytes for the assessment of QT-prolonging drugs. J. Pharmacol. Sci. 134 (2), 75–85. doi:10.1016/j.jphs.2017.05.004
Li, W., Han, J. L., and Entcheva, E. (2020). Syncytium cell growth increases Kir2.1 contribution in human iPSC-cardiomyocytes. Am. J. Physiology-Heart Circulatory Physiology 319 (5), H1112. doi:10.1152/ajpheart.00148.2020
Liang, P., Lan, F., Lee, A. S., Gong, T., Sanchez-Freire, V., Wang, Y., et al. (2013). Drug screening using a library of human induced pluripotent stem cell–derived cardiomyocytes reveals disease-specific patterns of cardiotoxicity. Circulation 127 (16), 1677–1691. doi:10.1161/CIRCULATIONAHA.113.001883
Lieu, D. K., Liu, J., Siu, C. W., McNerney, G. P., Tse, H. F., Abu-Khalil, A., et al. (2009). Absence of transverse tubules contributes to non-uniform Ca2+ wavefronts in mouse and human embryonic stem cell–derived cardiomyocytes. Stem Cells Dev. 18 (10), 1493–1500. doi:10.1089/scd.2009.0052
Liu, C., Feng, X., Li, G., Gokulnath, P., and Xiao, J. (2022). Generating 3D human cardiac constructs from pluripotent stem cells. eBioMedicine 76, 103813. doi:10.1016/j.ebiom.2022.103813
Liu, M., Shi, G., Zhou, A., Rupert, C. E., Coulombe, K. L. K., and Dudley, S. C. (2018). Activation of the unfolded protein response downregulates cardiac ion channels in human induced pluripotent stem cell-derived cardiomyocytes. J. Mol. Cell. Cardiol. 117, 62–71. doi:10.1016/j.yjmcc.2018.02.011
Liu, X., Wang, S., Guo, X., Li, Y., Ogurlu, R., Lu, F., et al. (2021). Increased reactive oxygen species–mediated Ca2+/calmodulin-dependent protein kinase II activation contributes to calcium handling abnormalities and impaired contraction in barth syndrome. Circulation 143 (19), 1894–1911. doi:10.1161/CIRCULATIONAHA.120.048698
Lopaschuk, G. D., Karwi, Q. G., Tian, R., Wende, A. R., and Abel, E. D. (2021). Cardiac energy metabolism in heart failure. Circulation Res. 128 (10), 1487–1513. doi:10.1161/CIRCRESAHA.121.318241
Lundy, S. D., Zhu, W. Z., Regnier, M., and Laflamme, M. A. (2013). Structural and functional maturation of cardiomyocytes derived from human pluripotent stem cells. Stem Cells Dev. 22 (14), 1991–2002. doi:10.1089/scd.2012.0490
Luo, C. H., and Rudy, Y. (1994). A dynamic model of the cardiac ventricular action potential. I. Simulations of ionic currents and concentration changes. Circulation Res. 74 (6), 1071–1096. doi:10.1161/01.RES.74.6.1071
Ma, D., Liu, Z., Loh, L. J., Zhao, Y., Li, G., Liew, R., et al. (2018). Identification of an INa-dependent and Ito-mediated proarrhythmic mechanism in cardiomyocytes derived from pluripotent stem cells of a Brugada syndrome patient. Sci. Rep. 8, 11246. doi:10.1038/s41598-018-29574-5
Ma, D., Wei, H., Lu, J., Ho, S., Zhang, G., Sun, X., et al. (2013). Generation of patient-specific induced pluripotent stem cell-derived cardiomyocytes as a cellular model of arrhythmogenic right ventricular cardiomyopathy. Eur. Heart J. 34 (15), 1122–1133. doi:10.1093/eurheartj/ehs226
Ma, J., Guo, L., Fiene, S. J., Anson, B. D., Thomson, J. A., Kamp, T. J., et al. (2011). High purity human-induced pluripotent stem cell-derived cardiomyocytes: electrophysiological properties of action potentials and ionic currents. Am. J. Physiology-Heart Circulatory Physiology 301 (5), H2006–H2017. doi:10.1152/ajpheart.00694.2011
Mamoshina, P., Rodriguez, B., and Bueno-Orovio, A. (2021). Toward a broader view of mechanisms of drug cardiotoxicity. Cell Rep. Med. 2 (3), 100216. doi:10.1016/j.xcrm.2021.100216
Mannhardt, I., Eder, A., Dumotier, B., Prondzynski, M., Krämer, E., Traebert, M., et al. (2017). Blinded contractility analysis in hiPSC-cardiomyocytes in engineered heart tissue format: comparison with human atrial trabeculae. Toxicol. Sci. 158 (1), 164–175. doi:10.1093/toxsci/kfx081
Mannhardt, I., Saleem, U., Mosqueira, D., Loos, M. F., Ulmer, B. M., Lemoine, M. D., et al. (2020). Comparison of 10 control hPSC lines for drug screening in an engineered heart tissue format. Stem Cell Rep. 15 (4), 983–998. doi:10.1016/j.stemcr.2020.09.002
Mazgaoker, S., Weiser-Bitoun, I., Brosh, I., Binah, O., and Yaniv, Y. (2022). cAMP-PKA signaling modulates the automaticity of human iPSC-derived cardiomyocytes. J. General Physiology 155 (1), e202213153. doi:10.1085/jgp.202213153
Miki, K., Deguchi, K., Nakanishi-Koakutsu, M., Lucena-Cacace, A., Kondo, S., Fujiwara, Y., et al. (2021). ERRγ enhances cardiac maturation with T-tubule formation in human iPSC-derived cardiomyocytes. Nat. Commun. 12 (1), 3596. doi:10.1038/s41467-021-23816-3
Millard, D., Dang, Q., Shi, H., Zhang, X., Strock, C., Kraushaar, U., et al. (2018). Cross-site reliability of human induced pluripotent stem cell-derived cardiomyocyte based safety assays using microelectrode arrays: results from a blinded CiPA pilot study. Toxicol. Sci. 164 (2), 550–562. doi:10.1093/toxsci/kfy110
Mohamed, T. M. A., Abou-Leisa, R., Baudoin, F., Stafford, N., Neyses, L., Cartwright, E. J., et al. (2013). Development and characterization of a novel fluorescent indicator protein PMCA4-GCaMP2 in cardiomyocytes. J. Mol. Cell. Cardiol. 63, 57–68. doi:10.1016/j.yjmcc.2013.07.007
Moretti, A., Bellin, M., Welling, A., Jung, C. B., Lam, J. T., Bott-Flügel, L., et al. (2010). Patient-specific induced pluripotent stem-cell models for long-QT syndrome. N. Engl. J. Med. 363 (15), 1397–1409. doi:10.1056/NEJMoa0908679
Murphy, R. M., Dutka, T. L., Horvath, D., Bell, J. R., Delbridge, L. M., and Lamb, G. D. (2013). Ca2+-dependent proteolysis of junctophilin-1 and junctophilin-2 in skeletal and cardiac muscle. J. Physiology 591 (3), 719–729. doi:10.1113/jphysiol.2012.243279
Myagmar, B.-E., Flynn, J. M., Cowley, P. M., Swigart, P. M., Montgomery, M. D., Thai, K., et al. (2017). Adrenergic receptors in individual ventricular myocytes: the beta-1 and alpha-1B are in all cells, the alpha-1A is in a subpopulation, and the beta-2 and beta-3 are mostly absent. Circulation Res. 120 (7), 1103–1115. doi:10.1161/CIRCRESAHA.117.310520
Näbauer, M., Beuckelmann, D. J., Uberfuhr, P., and Steinbeck, G. (1996). Regional differences in current density and rate-dependent properties of the transient outward current in subepicardial and subendocardial myocytes of human left ventricle. Circulation 93 (1), 168–177. doi:10.1161/01.CIR.93.1.168
Nerbonne, J. M., Nichols, C. G., Schwarz, T. L., and Escande, D. (2001). Genetic manipulation of cardiac K+ channel function in mice. Circulation Res. 89 (11), 944–956. doi:10.1161/hh2301.100349
Nishimura, S., Yasuda, S. i., Katoh, M., Yamada, K. P., Yamashita, H., Saeki, Y., et al. (2004). Single cell mechanics of rat cardiomyocytes under isometric, unloaded, and physiologically loaded conditions. Am. J. Physiology-Heart Circulatory Physiology 287 (1), H196–H202. doi:10.1152/ajpheart.00948.2003
Nose, N., Werner, R. A., Ueda, Y., Günther, K., Lapa, C., Javadi, M. S., et al. (2018). Metabolic substrate shift in human induced pluripotent stem cells during cardiac differentiation: functional assessment using in vitro radionuclide uptake assay. Int. J. Cardiol. 269, 229–234. doi:10.1016/j.ijcard.2018.06.089
Nozaki, Y., Honda, Y., Watanabe, H., Saiki, S., Koyabu, K., Itoh, T., et al. (2016). CSAHi study: validation of multi-electrode array systems (MEA60/2100) for prediction of drug-induced proarrhythmia using human iPS cell-derived cardiomyocytes -assessment of inter-facility and cells lot-to-lot-variability-. Regul. Toxicol. Pharmacol. 77, 75–86. doi:10.1016/j.yrtph.2016.02.007
Onakpoya, I. J., Heneghan, C. J., and Aronson, J. K. (2016). Post-marketing withdrawal of 462 medicinal products because of adverse drug reactions: a systematic review of the world literature. BMC Med. 14 (1), 10. doi:10.1186/s12916-016-0553-2
Opitz, C. A., Leake, M. C., Makarenko, I., Benes, V., and Linke, W. A. (2004). Developmentally regulated switching of titin size alters myofibrillar stiffness in the perinatal heart. Circulation Res. 94 (7), 967–975. doi:10.1161/01.RES.0000124301.48193.E1
Otsomaa, L., Levijoki, J., Wohlfahrt, G., Chapman, H., Koivisto, A. P., Syrjänen, K., et al. (2020). Discovery and characterization of ORM-11372, a novel inhibitor of the sodium-calcium exchanger with positive inotropic activity. Br. J. Pharmacol. 177 (24), 5534–5554. doi:10.1111/bph.15257
Parikh, S. S., Blackwell, D. J., Gomez-Hurtado, N., Frisk, M., Wang, L., Kim, K., et al. (2017). Thyroid and glucocorticoid hormones promote functional T-tubule development in human-induced pluripotent stem cell derived cardiomyocytes. Circulation Res. 121 (12), 1323–1330. doi:10.1161/CIRCRESAHA.117.311920
Peischard, S., Möller, M., Disse, P., Ho, H. T., Verkerk, A. O., Strutz-Seebohm, N., et al. (2022). Virus-induced inhibition of cardiac pacemaker channel HCN4 triggers bradycardia in human-induced stem cell system. Cell. Mol. Life Sci. 79 (8), 440. doi:10.1007/s00018-022-04435-7
Pelzmann, B., Schaffer, P., Bernhart, E., Lang, P., Mächler, H., Rigler, B., et al. (1998). L-type calcium current in human ventricular myocytes at a physiological temperature from children with tetralogy of Fallot. Cardiovasc. Res. 38 (2), 424–432. doi:10.1016/S0008-6363(98)00002-9
Piacentino, V., Weber, C. R., Chen, X., Weisser-Thomas, J., Margulies, K. B., Bers, D. M., et al. (2003). Cellular basis of abnormal calcium transients of failing human ventricular myocytes. Circulation Res. 92 (6), 651–658. doi:10.1161/01.RES.0000062469.83985.9B
Pianezzi, E., Altomare, C., Bolis, S., Balbi, C., Torre, T., Rinaldi, A., et al. (2020). Role of somatic cell sources in the maturation degree of human induced pluripotent stem cell-derived cardiomyocytes. Biochimica Biophysica Acta (BBA) - Mol. Cell Res. 1867 (3), 118538. doi:10.1016/j.bbamcr.2019.118538
Pieske, B., Trost, S., Schütt, K., Minami, K., Just, H., and Hasenfuss, G. (1998). Influence of Forskolin on the force-frequency behavior in nonfailing and end-stage failing human myocardium. Basic Res. Cardiol. 93 (1), s066–s075. doi:10.1007/s003950050222
Pinton, L., Khedr, M., Lionello, V. M., Sarcar, S., Maffioletti, S. M., Dastidar, S., et al. (2023). 3D human induced pluripotent stem cell–derived bioengineered skeletal muscles for tissue, disease and therapy modeling. Nat. Protoc. 18 (4), 1337–1376. doi:10.1038/s41596-022-00790-8
Plakhotnik, J., Zhang, L., Estrada, M., Coles, J. G., Lonnqvist, P. A., and Maynes, J. T. (2022). Local anesthetic cardiac toxicity is mediated by cardiomyocyte calcium dynamics. Anesthesiology 137 (6), 687–703. doi:10.1097/ALN.0000000000004389
Podgurskaya, A. D., Tsvelaya, V. A., Slotvitsky, M. M., Dementyeva, E. V., Valetdinova, K. R., and Agladze, K. I. (2019). The use of iPSC-derived cardiomyocytes and optical mapping for erythromycin arrhythmogenicity testing. Cardiovasc. Toxicol. 19 (6), 518–528. doi:10.1007/s12012-019-09532-x
Pölönen, R. P., Swan, H., and Aalto-Setälä, K. (2020). Mutation-specific differences in arrhythmias and drug responses in CPVT patients: simultaneous patch clamp and video imaging of iPSC derived cardiomyocytes. Mol. Biol. Rep. 47 (2), 1067–1077. doi:10.1007/s11033-019-05201-y
Pretorius, D., Kahn-Krell, A. M., Lou, X., Fast, V. G., Berry, J. L., Kamp, T. J., et al. (2021). Layer-by-layer fabrication of large and thick human cardiac muscle patch constructs with superior electrophysiological properties. Front. Cell Dev. Biol. 9, 670504. doi:10.3389/fcell.2021.670504
Qu, Y., and Boutjdir, M. (2001). Gene expression of SERCA2a and L- and T-type Ca channels during human heart development. Pediatr. Res. 50 (5), 569–574. doi:10.1203/00006450-200111000-00006
Rana, P., Anson, B., Engle, S., and Will, Y. (2012). Characterization of human-induced pluripotent stem cell–derived cardiomyocytes: bioenergetics and utilization in safety screening. Toxicol. Sci. 130 (1), 117–131. doi:10.1093/toxsci/kfs233
Rao, C., Prodromakis, T., Kolker, L., Chaudhry, U. A. R., Trantidou, T., Sridhar, A., et al. (2013). The effect of microgrooved culture substrates on calcium cycling of cardiac myocytes derived from human induced pluripotent stem cells. Biomaterials 34 (10), 2399–2411. doi:10.1016/j.biomaterials.2012.11.055
Rasmussen, C. A., Sutko, J. L., and Barry, W. H. (1987). Effects of ryanodine and caffeine on contractility, membrane voltage, and calcium exchange in cultured heart cells. Circulation Res. 60 (4), 495–504. doi:10.1161/01.RES.60.4.495
Ribeiro, A. J. S., Ang, Y. S., Fu, J. D., Rivas, R. N., Mohamed, T. M. A., Higgs, G. C., et al. (2015). Contractility of single cardiomyocytes differentiated from pluripotent stem cells depends on physiological shape and substrate stiffness. Proc. Natl. Acad. Sci. 112 (41), 12705–12710. doi:10.1073/pnas.1508073112
Rocchetti, M., Sala, L., Dreizehnter, L., Crotti, L., Sinnecker, D., Mura, M., et al. (2017). Elucidating arrhythmogenic mechanisms of long-QT syndrome CALM1-F142L mutation in patient-specific induced pluripotent stem cell-derived cardiomyocytes. Cardiovasc. Res. 113 (5), 531–541. doi:10.1093/cvr/cvx006
Rodriguez, M. L., Graham, B. T., Pabon, L. M., Han, S. J., Murry, C. E., and Sniadecki, N. J. (2014). Measuring the contractile forces of human induced pluripotent stem cell-derived cardiomyocytes with arrays of microposts. J. Biomechanical Eng. 136 (5), 051005. doi:10.1115/1.4027145
Sager, P. T., Gintant, G., Turner, J. R., Pettit, S., and Stockbridge, N. (2014). Rechanneling the cardiac proarrhythmia safety paradigm: a meeting report from the Cardiac Safety Research Consortium. Am. Heart J. 167 (3), 292–300. doi:10.1016/j.ahj.2013.11.004
Saggin, L., Gorza, L., Ausoni, S., and Schiaffino, S. (1989). Troponin I switching in the developing heart. J. Biol. Chem. 264 (27), 16299–16302. doi:10.1016/S0021-9258(18)71621-9
Sakakibara, Y., Furukawa, T., Singer, D. H., Jia, H., Backer, C. L., Arentzen, C. E., et al. (1993). Sodium current in isolated human ventricular myocytes. Am. J. Physiology-Heart Circulatory Physiology 265 (4), H1301–H1309. doi:10.1152/ajpheart.1993.265.4.H1301
Sakakibara, Y., Wasserstrom, J. A., Furukawa, T., Jia, H., Arentzen, C. E., Hartz, R. S., et al. (1992). Characterization of the sodium current in single human atrial myocytes. Circulation Res. 71 (3), 535–546. doi:10.1161/01.RES.71.3.535
Schulman, H., and Anderson, M. E. (2010). Ca/Calmodulin-dependent protein kinase II in heart failure. Drug Discov. today. Dis. Mech. 7 (2), e117–e122. doi:10.1016/j.ddmec.2010.07.005
Selga, E., Sendfeld, F., Martinez-Moreno, R., Medine, C. N., Tura-Ceide, O., Wilmut, S. I., et al. (2018). Sodium channel current loss of function in induced pluripotent stem cell-derived cardiomyocytes from a Brugada syndrome patient. J. Mol. Cell. Cardiol. 114, 10–19. doi:10.1016/j.yjmcc.2017.10.002
Seyhan, A. A. (2019). Lost in translation: the valley of death across preclinical and clinical divide – identification of problems and overcoming obstacles. Transl. Med. Commun. 4 (1), 18. doi:10.1186/s41231-019-0050-7
Shen, S., Sewanan, L. R., Shao, S., Halder, S. S., Stankey, P., Li, X., et al. (2022). Physiological calcium combined with electrical pacing accelerates maturation of human engineered heart tissue. Stem Cell Rep. 17 (9), 2037–2049. doi:10.1016/j.stemcr.2022.07.006
Shih, H. T. (1994). Anatomy of the action potential in the heart. Tex. Heart Inst. J. 21 (1), 30–41.
Silbernagel, N., Körner, A., Balitzki, J., Jaggy, M., Bertels, S., Richter, B., et al. (2020). Shaping the heart: structural and functional maturation of iPSC-cardiomyocytes in 3D-micro-scaffolds. Biomaterials 227, 119551. doi:10.1016/j.biomaterials.2019.119551
Singh, B. N., Connolly, S. J., Crijns, H. J. G. M., Roy, D., Kowey, P. R., Capucci, A., et al. (2007). Dronedarone for maintenance of sinus rhythm in atrial fibrillation or flutter. N. Engl. J. Med. 357 (10), 987–999. doi:10.1056/NEJMoa054686
Streckfuss-Bömeke, K., Tiburcy, M., Fomin, A., Luo, X., Li, W., Fischer, C., et al. (2017). Severe DCM phenotype of patient harboring RBM20 mutation S635A can be modeled by patient-specific induced pluripotent stem cell-derived cardiomyocytes. J. Mol. Cell. Cardiol. 113, 9–21. doi:10.1016/j.yjmcc.2017.09.008
Takahashi, K., Tanabe, K., Ohnuki, M., Narita, M., Ichisaka, T., Tomoda, K., et al. (2007). Induction of pluripotent stem cells from adult human fibroblasts by defined factors. Cell 131 (5), 861–872. doi:10.1016/j.cell.2007.11.019
Takeshima, H., Komazaki, S., Nishi, M., Iino, M., and Kangawa, K. (2000). Junctophilins: a novel family of junctional membrane complex proteins. Mol. Cell 6 (1), 11–22. doi:10.1016/s1097-2765(00)00003-4
Tan, C., Tasaka, H., Yu, K. P., Murphy, M. L., and Karnofsky, D. A. (1967). Daunomycin, an antitumor antibiotic, in the treatment of neoplastic disease. Clinical evaluation with special reference to childhood leukemia. Cancer 20 (3), 333–353. doi:10.1002/1097-0142(1967)20:3<333::AID-CNCR2820200302>3.0.CO;2-K
Terrenoire, C., Clancy, C. E., Cormier, J. W., Sampson, K. J., and Kass, R. S. (2005). Autonomic control of cardiac action potentials: role of potassium channel kinetics in response to sympathetic stimulation. Circulation Res. 96 (5), e25–e34. doi:10.1161/01.RES.0000160555.58046.9a
Tipparaju, S. M., Kumar, R., Wang, Y., Joyner, R. W., and Wagner, M. B. (2004). Developmental differences in L-type calcium current of human atrial myocytes. Am. J. Physiology-Heart Circulatory Physiology 286 (5), H1963–H1969. doi:10.1152/ajpheart.01011.2003
Trautwein, W., Kassebaum, D. G., Nelson, R. M., and Hechthh, (1962). Electrophysiological study of human heart muscle. Circulation Res. 10 (3), 306–312. doi:10.1161/01.RES.10.3.306
Tulloch, N. L., Muskheli, V., Razumova, M. V., Korte, F. S., Regnier, M., Hauch, K. D., et al. (2011). Growth of engineered human myocardium with mechanical loading and vascular coculture. Circulation Res. 109 (1), 47–59. doi:10.1161/CIRCRESAHA.110.237206
Ulmer, B. M., Stoehr, A., Schulze, M. L., Patel, S., Gucek, M., Mannhardt, I., et al. (2018). Contractile work contributes to maturation of energy metabolism in hiPSC-derived cardiomyocytes. Stem Cell Rep. 10 (3), 834–847. doi:10.1016/j.stemcr.2018.01.039
Uzun, A. U., Mannhardt, I., Breckwoldt, K., Horváth, A., Johannsen, S. S., Hansen, A., et al. (2016). Ca2+-Currents in human induced pluripotent stem cell-derived cardiomyocytes effects of two different culture conditions. Front. Pharmacol. 7, 300. doi:10.3389/fphar.2016.00300
Valtonen, J., Prajapati, C., Cherian, R. M., Vanninen, S., Ojala, M., Leivo, K., et al. (2023). The junctophilin-2 mutation p.(Thr161Lys) is associated with hypertrophic cardiomyopathy using patient-specific iPS cardiomyocytes and demonstrates prolonged action potential and increased arrhythmogenicity. Biomedicines 11 (6), 1558. doi:10.3390/biomedicines11061558
Van Den Berg, C. W., Okawa, S., Chuva de Sousa Lopes, S. M., van Iperen, L., Passier, R., Braam, S. R., et al. (2015). Transcriptome of human foetal heart compared with cardiomyocytes from pluripotent stem cells. Development 142, 3231–3238. doi:10.1242/dev.123810
Van Der Velden, J., Klein, L. J., van der Bijl, M., Huybregts, M. A., Stooker, W., Witkop, J., et al. (1998). Force production in mechanically isolated cardiac myocytes from human ventricular muscle tissue. Cardiovasc. Res. 38 (2), 414–423. doi:10.1016/S0008-6363(98)00019-4
Van de Sande, D. V., Kopljar, I., Maaike, A., Teisman, A., Gallacher, D. J., Bart, L., et al. (2021). The resting membrane potential of hSC-CM in a syncytium is more hyperpolarised than that of isolated cells. Channels 15 (1), 239–252. doi:10.1080/19336950.2021.1871815
Wang, L., Dou, W., Malhi, M., Zhu, M., Liu, H., Plakhotnik, J., et al. (2018). Microdevice platform for continuous measurement of contractility, beating rate, and beating rhythm of human-induced pluripotent stem cell-cardiomyocytes inside a controlled incubator environment. ACS Appl. Mater. Interfaces 10 (25), 21173–21183. doi:10.1021/acsami.8b05407
Wang, L., Myles, R. C., Lee, I. J., Bers, D. M., and Ripplinger, C. M. (2021). Role of reduced sarco-endoplasmic reticulum Ca2+-ATPase function on sarcoplasmic reticulum Ca2+ alternans in the intact rabbit heart. Front. Physiology 12, 656516. doi:10.3389/fphys.2021.656516
Wang, L., Nguyen, T., Rosa-Garrido, M., Zhou, Y., Cleveland, D. C., and Zhang, J. (2023). Comparative analysis of the cardiomyocyte differentiation potential of induced pluripotent stem cells reprogrammed from human atrial or ventricular fibroblasts. Front. Bioeng. Biotechnol. 11, 1108340. doi:10.3389/fbioe.2023.1108340
Wang, X., Wang, L., Dou, W., Huang, Z., Zhao, Q., Malhi, M., et al. (2020). Electrical impedance-based contractile stress measurement of human iPSC-Cardiomyocytes. Biosens. Bioelectron. 166, 112399. doi:10.1016/j.bios.2020.112399
Weninger, G., Pochechueva, T., El Chami, D., Luo, X., Kohl, T., Brandenburg, S., et al. (2022). Calpain cleavage of Junctophilin-2 generates a spectrum of calcium-dependent cleavage products and DNA-rich NT1-fragment domains in cardiomyocytes. Sci. Rep. 12, 10387. doi:10.1038/s41598-022-14320-9
Wettwer, E., Amos, G. J., Posival, H., and Ravens, U. (1994). Transient outward current in human ventricular myocytes of subepicardial and subendocardial origin. Circulation Res. 75 (3), 473–482. doi:10.1161/01.RES.75.3.473
Word, T. A., Quick, A. P., Miyake, C. Y., Shak, M. K., Pan, X., Kim, J. J., et al. (2021). Efficacy of RyR2 inhibitor EL20 in induced pluripotent stem cell-derived cardiomyocytes from a patient with catecholaminergic polymorphic ventricular tachycardia. J. Cell. Mol. Med. 25 (13), 6115–6124. doi:10.1111/jcmm.16521
Wu, H., Lee, J., Vincent, L. G., Wang, Q., Gu, M., Lan, F., et al. (2015). Epigenetic regulation of phosphodiesterases 2A and 3A underlies compromised β-adrenergic signaling in an iPSC model of dilated cardiomyopathy. Cell Stem Cell 17 (1), 89–100. doi:10.1016/j.stem.2015.04.020
Wu, P., Deng, G., Sai, X., Guo, H., Huang, H., and Zhu, P. (2021). Maturation strategies and limitations of induced pluripotent stem cell-derived cardiomyocytes. Biosci. Rep. 41 (6), BSR20200833. doi:10.1042/BSR20200833
Yang, X., Pabon, L., and Murry, C. E. (2014). Engineering adolescence: maturation of human pluripotent stem cell-derived cardiomyocytes. Circulation Res. 114 (3), 511–523. doi:10.1161/CIRCRESAHA.114.300558
Yang, X., Ribeiro, A. J. S., Pang, L., and Strauss, D. G. (2022). Use of human iPSC-CMs in nonclinical regulatory studies for cardiac safety assessment. Toxicol. Sci. 190 (2), 117–126. doi:10.1093/toxsci/kfac095
Yang, X., Rodriguez, M., Pabon, L., Fischer, K. A., Reinecke, H., Regnier, M., et al. (2014). Tri-iodo-L-thyronine promotes the maturation of human cardiomyocytes-derived from induced pluripotent stem cells. J. Mol. Cell. Cardiol. 72, 296–304. doi:10.1016/j.yjmcc.2014.04.005
Yasuda, S.-I., Sugiura, S., Kobayakawa, N., Fujita, H., Yamashita, H., Katoh, K., et al. (2001). A novel method to study contraction characteristics of a single cardiac myocyte using carbon fibers. Am. J. Physiology-Heart Circulatory Physiology 281 (3), H1442–H1446. doi:10.1152/ajpheart.2001.281.3.H1442
Zahanich, I., Sirenko, S. G., Maltseva, L. A., Tarasova, Y. S., Spurgeon, H. A., Boheler, K. R., et al. (2011). Rhythmic beating of stem cell-derived cardiac cells requires dynamic coupling of electrophysiology and Ca cycling. J. Mol. Cell. Cardiol. 50 (1), 66–76. doi:10.1016/j.yjmcc.2010.09.018
Zhang, J., Wilson, G. F., Soerens, A. G., Koonce, C. H., Yu, J., Palecek, S. P., et al. (2009). Functional cardiomyocytes derived from human induced pluripotent stem cells. Circulation Res. 104 (4), e30–e41. doi:10.1161/CIRCRESAHA.108.192237
Zhang, M., D’Aniello, C., Verkerk, A. O., Wrobel, E., Frank, S., Ward-van Oostwaard, D., et al. (2014). Recessive cardiac phenotypes in induced pluripotent stem cell models of Jervell and Lange-Nielsen syndrome: disease mechanisms and pharmacological rescue. Proc. Natl. Acad. Sci. 111 (50), E5383–E5392. doi:10.1073/pnas.1419553111
Zhang, X.-H., Wei, H., Šarić, T., Hescheler, J., Cleemann, L., and Morad, M. (2015). Regionally diverse mitochondrial calcium signaling regulates spontaneous pacing in developing cardiomyocytes. Cell calcium 57 (0), 321–336. doi:10.1016/j.ceca.2015.02.003
Zhao, Y., Rafatian, N., Feric, N. T., Cox, B. J., Aschar-Sobbi, R., Wang, E. Y., et al. (2019). A platform for generation of chamber-specific cardiac tissues and disease modeling. Cell 176 (4), 913–927.e18. doi:10.1016/j.cell.2018.11.042
Zhou, J., Cui, B., Wang, X., Wang, H., Zheng, J., Guo, F., et al. (2023). Overexpression of KCNJ2 enhances maturation of human-induced pluripotent stem cell-derived cardiomyocytes. Stem Cell Res. Ther. 14, 92. doi:10.1186/s13287-023-03312-9
Ziman, A. P., Gómez-Viquez, N. L., Bloch, R. J., and Lederer, W. J. (2010). Excitation-contraction coupling changes during postnatal cardiac development. J. Mol. Cell. Cardiol. 48 (2), 379–386. doi:10.1016/j.yjmcc.2009.09.016
Zuppinger, C. (2019). 3D cardiac cell culture: a critical review of current technologies and applications. Front. Cardiovasc. Med. 6, 87. doi:10.3389/fcvm.2019.00087
Keywords: ipsc-cm, cardiotoxicity, cardiac, preclinical, pharmaceutical, drug discovery
Citation: Lee TYT, Coles JG and Maynes JT (2024) iPSC-cardiomyocytes in the preclinical prediction of candidate pharmaceutical toxicity. Front. Pharmacol. 15:1308217. doi: 10.3389/fphar.2024.1308217
Received: 06 October 2023; Accepted: 15 February 2024;
Published: 28 February 2024.
Edited by:
Jonathan Satin, University of Kentucky, United StatesReviewed by:
Cheavar Blair, University of Kentucky, United StatesMatthew Perry, Victor Chang Cardiac Research Institute, Australia
Copyright © 2024 Lee, Coles and Maynes. This is an open-access article distributed under the terms of the Creative Commons Attribution License (CC BY). The use, distribution or reproduction in other forums is permitted, provided the original author(s) and the copyright owner(s) are credited and that the original publication in this journal is cited, in accordance with accepted academic practice. No use, distribution or reproduction is permitted which does not comply with these terms.
*Correspondence: Jason T. Maynes, jason.maynes@sickkids.ca