- 1Department of Pediatrics, The Second School of Medicine, Wenzhou Medical University, The Second Affiliated Hospital and Yuying Children’s Hospital of Wenzhou Medical University, Wenzhou, Zhejiang, China
- 2Department of Clinical Medicine, Pingyang County Traditional Chinese Medicine Hospital, Meizhou, Zhejiang, China
- 3Department of Pediatrics, Sichuan Provincial Maternity and Child Health Care Hospital, Chengdu Medical College, Chengdu, Sichuan, China
Ginseng is frequently used in traditional Chinese medicine to treat neurological disorders. The primary active component of ginseng is ginsenoside, which has been classified into more than 110 types based on their chemical structures. Ginsenoside Rb1 (GsRb1)—a protopanaxadiol saponin and a typical ginseng component—exhibits anti-inflammatory, anti-oxidant, anti-apoptotic, and anti-autophagy properties in the nervous system. Neurological disorders remain a leading cause of death and disability globally. GsRb1 effectively treats neurological disorders. To contribute novel insights to the understanding and treatment of neurological disorders, we present a comprehensive review of the pharmacokinetics, actions, mechanisms, and research development of GsRb1 in neurological disorders.
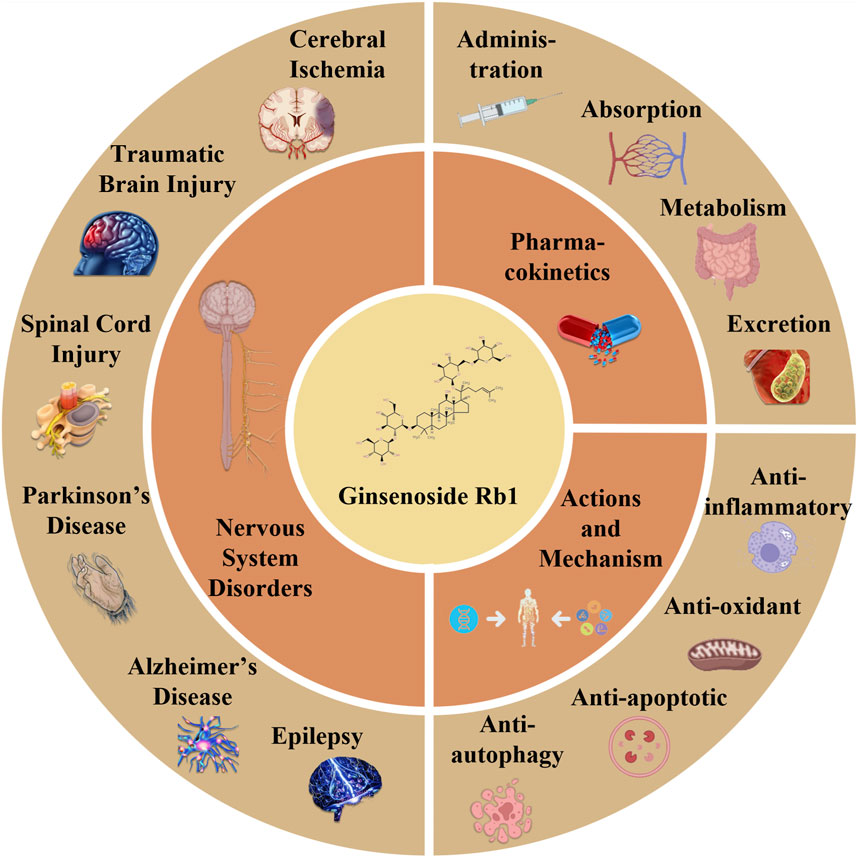
GRAPHICAL ABSTRACT | The article reviews the pharmacokinetics, effects, processes, and investigations in developing GsRb1 research. The four components of GsRb1’s pharmacokinetics are administration route, absorption, metabolism, and excretion. GsRb1 can have anti-inflammatory, anti-oxidant, anti-apoptotic, and anti-autophagy properties in the neurological system. Therapeutic applications of GsRb1 include the treatment of epilepsy, Parkinson’s disease, traumatic brain damage, spinal cord injury, cerebral ischemia, and other neurological disorders.
1 Introduction
The “king of all herbs” is ginseng, a tonic and medicinal herb (Tao et al., 2023). Traditional Chinese medicine attributes ginseng’s efficacy to prolonging life and replenishing vital energy (Im, 2020). Ginseng’s therapeutic benefits on neurological disorders have been backed by extensive preclinical and clinical data (Mancuso and Santangelo, 2017). The active components of ginseng include saponin, polysaccharide, essential oil, and polypeptide (Ha et al., 2007; Ni et al., 2022; Tao et al., 2023). Ginsenosides consist of 20(S)-protopanaxadiol and 20(S)-protopanaxatriol saponins of the dammarane type (Zhou et al., 2019a). Ginsenoside Rb1 (GsRb1) is a highly prevalent ginsenoside and serves as the primary protopanaxadiol saponin (Figure 1) (Kim et al., 2022; Ni et al., 2022).
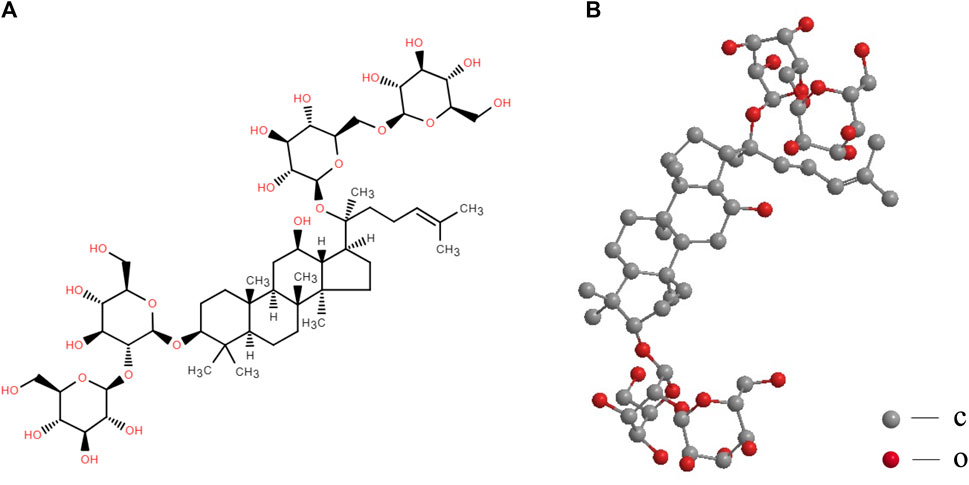
FIGURE 1. Structural formula (A) and molecular stereo structure (B) of ginsenoside Rb1. The structural formula of GsRb1 (C54H92O23) is illustrated in (A), with a dammarane (a tetracyclic triterpenoid) at its center and several R groups connecting its upper and lower ends. In (A), the dashed triangles are typically used to represent wedged bonds, indicating that the chemical bond extends outwards from the plane of the paper towards the observer. Solid triangles represent hashed bonds, signifying that the chemical bond extends inward, away from the observer into the plane of the paper. The stereoscopic structure of this molecule, which conceals the hydrogen atoms, is displayed in (B). The oxygen and carbon atoms are represented by the red and gray balls, respectively.
GsRb1 can be used therapeutically to treat multi-system illnesses affecting the nervous, cardiovascular, and endocrine systems (Zheng et al., 2017; Zhou et al., 2019b; Gong et al., 2022). GsRb1 has been found to exhibit several biological activities, particularly in the nervous system. These activities can penetrate the blood-brain barrier and exert neuroprotective effects such as anti-inflammatory, anti-oxidant, anti-apoptotic, and anti-autophagy (Kim, 2012; Kim et al., 2013; Ong et al., 2015; Zhou et al., 2019b). Recent studies have suggested that GsRb1 can inhibit inflammation, oxidative stress, and excitotoxicity, attenuate neuronal damage, and promote neuronal cell repair to treat neurological diseases (Kiefer and Pantuso, 2003; Yang JE. et al., 2020; Shi et al., 2020). These findings suggest that GsRb1 may be more effective in treating epilepsy, Alzheimer’s disease (AD), and Parkinson’s disease (PD).
Cerebrovascular disease, spinal cord lesions, epilepsy, and neurodegenerative disorders are among the most prevalent neurological conditions in humans (Gooch et al., 2017). Lifelong medication is mandatory for most patients, resulting in substantial adverse effects (Cai et al., 2020). Studies have demonstrated that GsRb1 is a prospective new star in the pharmacological treatment of neurological disorders because it has good pharmacokinetics, numerous neuroprotective properties, and fewer side effects (Gong et al., 2022). The present review provides an overview of the pharmacokinetics, results, mechanisms, and progress of research on GsRb1 in neurological disorders to generate novel ideas for the treatment and study of such conditions.
2 Pharmacokinetics of ginsenoside Rb1
GsRb1 has better pharmacokinetics and can be delivered into the human body through various methods. Its absorption, metabolism, and excretion follow a certain pattern (Figure 2). Following gavage, GsRb1 is marginally absorbed in the stomach (Kim, 2018). Most GsRb1 is then absorbed in the entire intestine, with the jejunum having specific absorption sites and a higher concentration of the drug than in the ileum and duodenum, and the intestines are then passively absorbed into the blood (Li et al., 2009; Kim, 2018). A study demonstrated that the absorption, rate, cumulative percent absorption, and half-life did not significantly correlate with the potential of hydrogen (pH) of the intestinal segment’s circulating fluid (Li et al., 2009). Human intestinal flora can quickly metabolize and degrade GsRb1 after it enters the gastrointestinal system, and the large intestine is the primary metabolic location, followed by the stomach (Bae et al., 2000; Kim, 2018; Zhao et al., 2023). In vivo, GsRb1 was first transformed into ginsenosides Rd (GsRd) and ginsenosides F2 (GsF2) and then into ginsenoside compound K (CK) (Figure 3), also known as 20-O-glucopyranosyl-20 (S) protopanaxadiol (Kim, 2013; Chen et al., 2015). GsRb1 and compound K have neuroprotective properties. After administering 9 g of GsRb1 orally to ten healthy Korean male subjects, the researchers quickly identified GsRb1 in the plasma of the subjects, which reached its Maximum Concentration (Cmax) of (3.94 ± 1.97) ng/mL in approximately 5 h and its half-life (t1/2) of (58.47 ± 14.28) h (Kim, 2013). After oral administration of GsRb1 to fasted Sprague-Dawley rats (weighing 300–320 g, obtained from the Laboratory Animal Center of Fudan University, Shanghai, China) at a dose of 600 mg/kg using a 120 mg/mL panax notoginseng saponins (PNS) aqueous solution, the serum concentration of Rb1 peaked at 1.5 h, reaching 47.13 mg/mL, and remained elevated for 72 h, resulting in an absolute oral bioavailability of 4.35% (Xu et al., 2003; Won et al., 2019). Furthermore, GsRb1 may boost its bioavailability when paired with the P-glycoprotein (P-gp) inhibitor verapamil because it serves as a substrate for P-gp, a significant transporter protein linked to drug transport in cell membranes (Li et al., 2009). GsRb1 has a low bioavailability as it is excreted in the stomach, colon, and liver; however, increasing the permeability of its membranes might improve its absorption (Han et al., 2006). Ginsenosides are excreted through the kidneys and biliary tract, and GsRb1 passes via both sluggish renal excretion and quick biliary excretion (Won et al., 2019). When Sprague-Dawley rats weighing 250–300 g, sourced from the Laboratory Animal Center of Fudan University in Shanghai, China, were intravenously administered a 50 mg/mL PNS physiological saline solution at a dose of 50 mg/kg, bile excretion reached equilibrium and entered a plateau phase at 6 h (Han et al., 2007). At 10 h after administration, GsRb1 exhibited a bile excretion rate of (3.94 ± 1.49)% of the intravenous dose, with an absolute bioavailability of 65.77% following portal vein injection, a mean residence time (MRT) of 24.6 h, and a high (>80%) plasma protein binding rate. In contrast to oral administration, intravenous (IV) administration showed lower bile excretion but higher absolute bioavailability and plasma protein binding rates, indicating that IV administration is the preferred route for GsRb1 for therapeutic purposes (Han et al., 2006; Han et al., 2007). Intratympanic (IT) is a novel mode of administration compared with IV, as it can disseminate GsRb1 to the inner ear and then transport it to the brain (Chen et al., 2007; Long et al., 2014). This delivery approach circumvents both the Blood-Labyrinth Barrier (BLB) and the Blood-Brain Barrier (BBB), allowing the drug to reach its target more directly at lower doses. As a result, it achieves higher drug concentrations in the inner ear and the brain while minimizing systemic side effects (Long et al., 2014). According to the data, Cmax was 1.5 and 0.4 times higher in cerebrospinal fluid and brain tissue, respectively, after IT than after IV, and the area under the curve (AUC) was 0.5 and 1.2 times higher, respectively, after IT than after IV, but Cmax and AUC in plasma were 45.9% and 33.1% lower, respectively, after IT than after IV (Chen et al., 2011). Additionally, intranasal administration is another efficient way to reach the central nervous system and is superior to other approaches because of its ease of usage, quick absorption, and avoidance of the initial excretion. Intranasal delivery of GsRb1 rapidly penetrates the brain within 5 min, and local bioavailability in specific brain areas is likewise significantly higher than that in plasma (Lu et al., 2011).
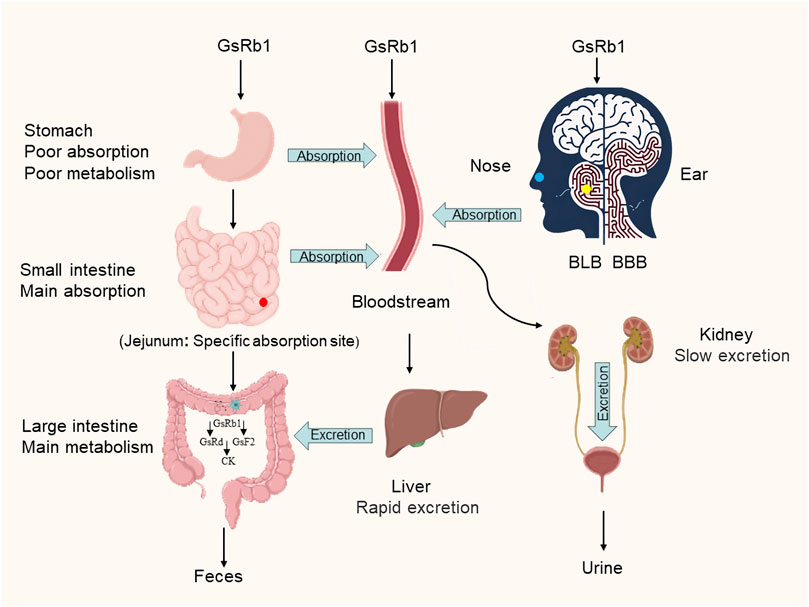
FIGURE 2. Metabolic pathways of ginsenoside Rb1. GsRb1 can enter the body and circulate in the bloodstream through various administration routes, including oral ingestion, intravenous injection, intratympanic administration (indicated by yellow dots representing the location of the middle ear), and intranasal administration (represented by blue dots indicating the nasal administration site). After oral ingestion, GsRb1 is characterized by complete intestinal absorption, with the jejunum showing a specific absorption site (indicated by red dots representing the location of the jejunum). Intratympanic administration allows GsRb1 to bypass the BLB and BBB, resulting in higher drug concentrations in the inner ear and the brain. Once GsRb1 enters the intestines, it undergoes rapid metabolism and breakdown facilitated by gut microbiota, leading to the formation of GsRd, GsF2, and CK. The primary metabolic site is the colon (major metabolic site), followed by the stomach (minor metabolic site). GsRb1 is primarily excreted through rapid biliary excretion and slow renal excretion. Abbreviation: GsRb1, Ginsenoside Rb1; BLB, Blood-Labyrinth Barrier; BBB, the Blood-Brain Barrier; GsRd, Ginsenoside Rd; GsF2, Ginsenoside F2; CK, Compound K.
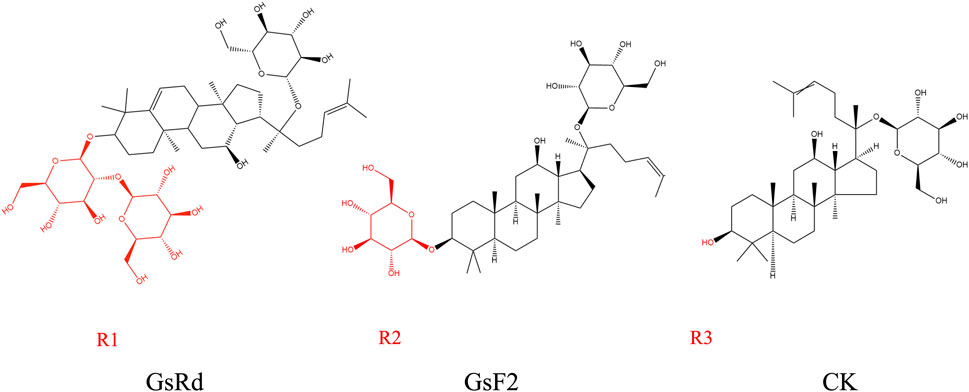
FIGURE 3. Metabolites of ginsenoside Rb1. GsRb1 undergoes primary metabolic conversion into GsRd, GsF2, and CK. These three compounds share structural similarities, with the main distinction lying in their respective R groups, as highlighted in red in each molecular structure diagram. GsRd has an R group denoted as R1, which is a disaccharide structure formed by a glycosidic bond between two monosaccharides. GsF2 possesses an R group known as R2, which is a monosaccharide structure. CK features an R group designated as R3, which consists of a hydroxyl group. Abbreviation: GsRb1, Ginsenoside Rb1; GsRd, Ginsenoside Rd; GsF2, Ginsenoside F2; CK, Compound K.
Furthermore, GsRb1 can be converted into the stable chemical derivative Rb1, dihydroginsenoside Rb1 (dgRb1), and pertinent studies have demonstrated that the effective dose of dgRb1 is ten times lower than that of GsRb1 (Sakanaka et al., 2007). Additionally, IV infusion of an effective dose of dgRb1 does not affect systemic parameters like brain temperature, blood pressure, or cerebral blood flow when repairing damaged neurons (Sakanaka et al., 2007). Panax ginseng Meyer Herbal Preparation HRG80 has garnered widespread recognition both domestically and internationally, with demonstrated significant efficacy, particularly in preventing and alleviating stress-induced cognitive function impairment in healthy individuals (Mariage et al., 2020). Certain GsRb1 class drugs, such as Vitacost Ginseng Extract Complex, have received drug approval numbers and have been proven to enhance energy levels, attention, and memory. These medications boast unique formulations and ingredients, with GsRb1 being acknowledged for its outstanding stress resistance and neuroprotective properties, contributing to memory enhancement, improved attention, and the promotion of cognitive abilities (Ahmed et al., 2016; Jakaria et al., 2018). Nowadays, oral medications predominate; therefore, developing strategies that enhance a drug’s oral bioavailability is crucial. However, GsRb1 has a low oral bioavailability, which significantly reduces its efficacy and hinders the continued exertion of clinical efficacy. GsRb1 possesses pharmacological effects that extend beyond its single-molecule form; it can be converted to dgRb1 to play an important role; accordingly, it has application prospects in preventing and treating neurological disorders. IV, IT, and intranasal administrations currently provide more options for entering GsRb1 into the brain.
3 Effect and mechanism of ginsenoside Rb1
GsRb1 has been shown to possess a diverse array of biological activities, including the capacity to cross the blood-brain barrier and produce neuroprotective effects, such as anti-inflammatory, anti-oxidant, anti-apoptotic, and anti-autophagic effects (Figure 4; Table 1) (Lin et al., 2022; Zhang M. et al., 2023; Hu et al., 2023; Jiang et al., 2023).
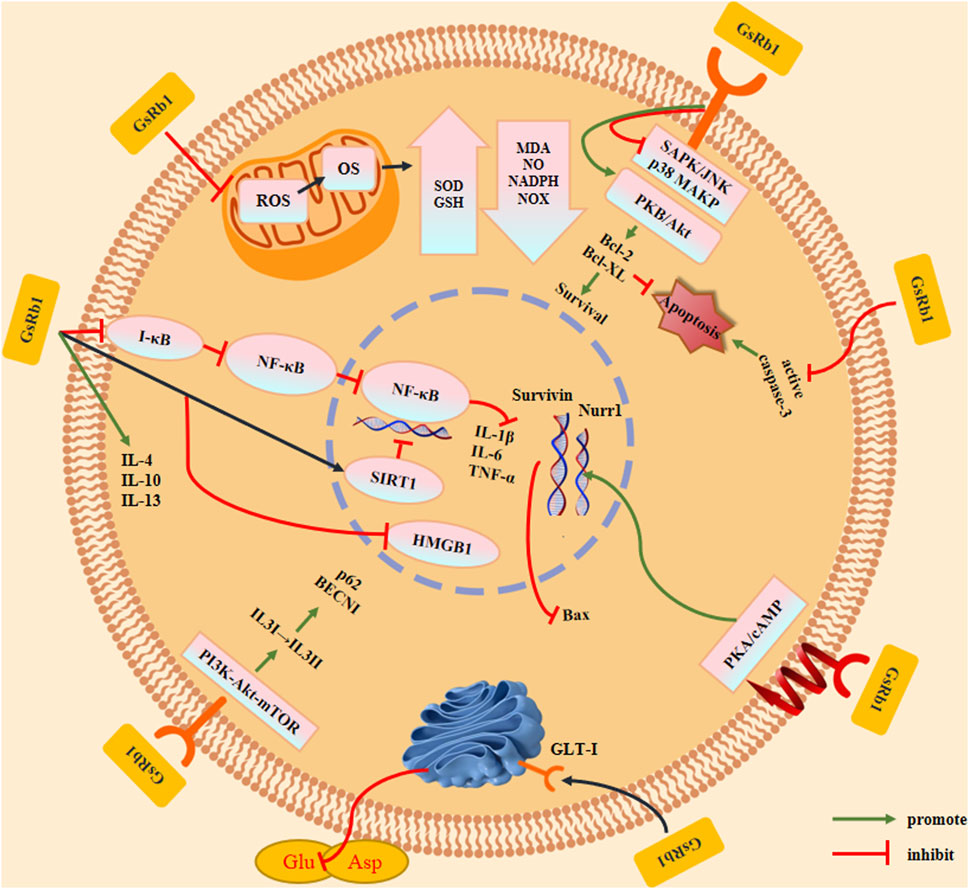
FIGURE 4. Effects and mechanisms of ginsenoside Rb1. GsRb1 can act as an anti-inflammatory, anti-oxidant, anti-apoptotic, and anti-autophagic factor in cells. By controlling IkB-α to prevent the formation of NF-κB dimers and activation of SIRT1, GsRb1 reduces inflammation by inducing the production of anti-inflammatory factors (IL-4, IL-10, and IL-13), thereby inhibiting HMGB1 inflammatory signaling and blocking the synthesis of inflammatory factors (IL-1β, IL-6, and TNF-α). GsRb1 modulates ROS levels in mitochondria that affect OS by upregulating SOD and GSH expression, downregulating MDA, NO, NADPH, and NOX expression, and lowering the neurotoxicity brought on by free radicals and other substances. GsRb1 enhanced cell survival by upregulating the expression of Bcl-2 and Bcl-XL, decreasing caspase-3 activation to limit apoptosis, and decreasing phosphoprotein expression of SAPK/JNK or p38 MAPK. These changes also increased the expression of Bcl-2 and Bcl-XL. Concurrently, GsRb1 controls the PKA/cAMP signaling pathway to reduce Nurr1 levels and increase Survivin expression, which prevents apoptosis. GsRb1 also increases GLT-1 expression on vesicle membranes and decreases the amount of Glu and Asp in tissues to prevent amino acid excitotoxicity. GsRb1 reduces the autophagy marker protein BECN1, downregulates the autophagic breakdown substrate p62, and significantly attenuates hyperactivated autophagy in cells. It also activates PI3K-Akt-mTOR in vivo and changes LC3 from type I to type II. Abbreviation: Akt, protein kinase B; Bcl-2, B-cell lymphoma 2; Bcl-XL, B-cell lymphoma-extra large; BECN1, Beclin 1; cAMP, Cyclic adenosine monophosphate; GLT-1, Glutamate transporter 1; Glu, Glutamate; GSH, Glutathione; IL, Interleukin; HMGB1, High mobility group box protein 1; JNK, c-Jun N-terminal kinase; LC3, Light chain 3; MDA, Malondialdehyde; mTOR, Mammalian target of rapamycin; NADPH, Nicotinamide adenine dinucleotide phosphate; NF-κB, Nuclear factor kappa B; NO, Nitric oxide; NOX, NADPH oxidase; Nurr1, Nuclear receptor-associated protein 1; OS, Oxidative stress; p38 MAPK, p38 mitogen-activated protein kinases; PI3K, Phosphoinositide 3-kinase; PKA, Protein kinase A; ROS, Reactive oxygen species; SAPK, Stress-activated protein kinase; SIRT1, Sirtuin 1; SOD, Superoxide dismutase; TNF-α, Tumor necrosis factor alpha.
3.1 Anti-inflammation
The anti-inflammatory actions of GsRb1 are primarily manifested in the suppression of inflammatory factor production, activation of anti-inflammatory factor synthesis, and inhibition of inflammatory conduction pathways in the inflammatory response. First, GsRb1 prevents the production of inflammatory factors by controlling two key inflammatory regulators, cyclooxygenase-2 (COX-2) and nuclear factor-kappa B (NF-κB) (Wang et al., 2011). According to studies, GsRb1 controls NF-κB in two ways: on the one hand, by controlling Inhibitor of nuclear factor kappa-B Kinase Subunit Alpha (IkB-α), which prevents the synthesis of NF-κB dimers, and on the other hand, by reducing inflammation (Li et al., 2007; Wang et al., 2011). By contrast, GsRb1 downregulates the expression of interleukin (IL)-1β (IL-1β), IL-6, and tumor necrosis factor-α (TNF-α) in ischemic brain injury and decreases the production of inflammatory factors by activating silent information regulator 1 (SIRT1) (Zhou et al., 2018; Cheng et al., 2019; Qi et al., 2020; Zheng et al., 2020). Furthermore, GsRb1 can increase anti-inflammatory M2 macrophage polarization by promoting the expression of the anti-inflammatory cytokines, such as IL-4, IL-10, and IL-13, as well as two conventional M2 macrophage markers, arginase-I (Arg-I), and macrophage mannose receptor (Chen et al., 2015; Zhang X. et al., 2018). Inhibiting inflammatory high mobility group box protein 1 (HMGB1) signaling, a frequently occurring molecule that exacerbates inflammatory damage, may allow GsRb1 to have anti-inflammatory effects (Liu et al., 2018). HMGB1 is a highly conserved non-histone deoxyribonucleic acid (DNA)-binding nucleoprotein (Chen et al., 2004). Inflammation is a common underlying mechanism in various conditions such as brain injury, cerebral ischemia, aging, and neurodegenerative diseases. GsRb1 demonstrates its anti-inflammatory effects through multiple pathways, making it a valuable asset in the treatment of neurological disorders. GsRb1’s actions are governed by a complex molecular regulatory network, and future research can delve deeper into understanding the intricate interactions among these mechanisms, providing a more comprehensive grasp of its anti-inflammatory properties. Moreover, GsRb1 not only suppresses the synthesis of pro-inflammatory cytokines but also facilitates the expression of anti-inflammatory cytokines like IL-4, IL-10, and IL-13. This pivotal role in maintaining the balance within the inflammatory response warrants further investigation. Subsequent studies can explore how GsRb1 precisely modulates the equilibrium between pro-inflammatory and anti-inflammatory factors, thus paving the way for more targeted anti-inflammatory treatments. In summary, GsRb1, with its multifaceted mechanisms and effects as an anti-inflammatory agent, offers promising avenues for future research in diseases associated with inflammation. Researchers can delve deeper into these mechanisms to develop more effective treatment strategies, fully unlocking the medical potential of GsRb1.
3.2 Anti-oxidant
GsRb1 has an anti-oxidant effect on oxidative stress (OS) by controlling the amounts of reactive oxygen species (ROS) in mitochondria, reducing the neurotoxicity induced by free radicals (Ni et al., 2022). ROS, including oxygen-containing radicals, oxygen-free radicals, non-radical derivatives, and hydroperoxides, are produced mainly by oxidative stress in mitochondria (Zhou et al., 2019b). Neurological disorders can develop because of severe OS that can cause brain cell malfunctions, such as proliferative arrest, aging, apoptosis, and necrosis (Rottenberg and Hoek, 2017). GsRb1 can directly reduce ROS levels in mitochondria or promote the production and transformation of mitochondrial energy through related metabolic enzymes, specifically by enhancing anti-oxidant enzyme activity and inhibiting oxidase activity to inhibit ROS accumulation in mitochondria during oxidative stress, such as increasing anti-oxidant enzyme superoxide dismutase (SOD) levels and increasing the expression of ROS scavenger glutathione (GSH), exerting anti-oxidant effects and reducing malondialdehyde (MDA), nitric oxide (NO) and nicotinamide adenine dinucleotide phosphate (NADPH) expression, markers of oxidative stress (Dong et al., 2017; Shi et al., 2018; Zhou et al., 2019b; Liu et al., 2023). GsRb1 can also boost mitochondrial membrane potential, protect the normal oxidative stress function of mitochondria, and maintain the integrity of mitochondrial function, all of which reduce mitochondrial damage in various neurons (Cheng et al., 2019). GsRb1 also inhibits the primary ROS source in vivo and lowers ROS generation by negatively regulating nicotinamide adenine dinucleotide phosphate oxidase (NOX)-1, NOX-2, and NOX-4 activity following ischemia (Dong et al., 2017; Xie et al., 2021).
An increasing body of research indicates that as individuals age, the functionality of the body’s antioxidant systems gradually diminishes. Meanwhile, oxidative stress, driven by ROS, plays a pivotal role in the pathogenesis of various neurological disorders. The protective effects of GsRb1 on mitochondria may constitute a critical aspect of its antioxidant capabilities. Furthermore, GsRb1 is likely to mitigate oxidative stress through multiple pathways, negatively regulating the activity of NADPH oxidases, thereby reducing ROS production. This may represent another key mechanism underlying its antioxidant properties. In the future, a more in-depth exploration into how GsRb1 influences the generation and accumulation of ROS within mitochondria can be pursued. Additionally, investigating the interactions between GsRb1 and various subtypes of NADPH oxidases, as well as its involvement in mitochondrial antioxidant processes, holds promising prospects. Summarizing these insights and perspectives will provide valuable directions for further comprehensive research into GsRb1’s antioxidant effects and its potential applications in the treatment of neurological disorders. Such studies will contribute to a deeper understanding of GsRb1’s mechanisms in neuroprotection.
3.3 Anti-apoptotic
GsRb1 prevents neuronal death by controlling gene and enzyme expression, encouraging neurotrophin release, and blocking the excitotoxic effects of amino acids and calcium overload. In apoptosis caused by 1-methyl-4-phenylpyridine (MPP+), GsRb1 effectively reduced MPP + -induced caspase-3 activation and DNA fragmentation and increased B-cell lymphoma-extra large (Bcl-XL) gene expression in pheochromocytoma cells (PC12) (Undifferentiated rat pheochromocytoma PC12 cells were cultured in 75 cm2 tissue culture flasks. Cells were maintained in Dulbecco’s modified Eagle’s medium containing 5% heat-inactivated Fetal Bovine Serum and 10% heat-inactivated Horse Serum supplemented with 100 U/mL penicillin and 100 μg/mL streptomycin in a water-saturated atmosphere of 5% Carbon Dioxide in air at 37°C.), which significantly improved cell viability (Zhang et al., 2006; Hashimoto et al., 2012; Zhang et al., 2012; Dong et al., 2022). Furthermore, GsRb1 can suppress microglial apoptosis, increase neuronal recovery in vitro, and upregulate the expression of anti-apoptotic proteins, including B-cell lymphoma 2 (Bcl-2) (Lee et al., 2018). By lowering the phosphorylated protein expression of stress-activated protein kinase/c-Jun N-terminal kinase (SAPK/JNK) or p38 mitogen-activated protein kinases (p38 MAPK), GsRb1 can increase the phosphorylated protein expression of protein kinase B (Akt/PKB) and extracellular regulated protein kinase (ERK) 1/2 (ERK1/2) (Hashimoto et al., 2012; Chen et al., 2016). Moreover, GsRb1 reduces the expression of pro-apoptotic Bax protein by regulating the levels of nuclear receptor-associated protein 1 (Nurr1), an essential factor in inhibiting microglial apoptosis, and by regulating the protein kinase A/cyclic adenosine monophosphate (PKA/cAMP) signaling pathway (Lee et al., 2018). GsRb1 can also prevent apoptosis by encouraging the expression of the apoptosis suppressor gene Survivin (Ye et al., 2019). GsRb1 reduces the levels of glutamate (Glu) and aspartate (Asp) in brain tissue by increasing the expression of glutamate transporter 1 (GLT-1) on the vesicle membrane (Wang et al., 2017; Yang F. et al., 2020). Furthermore, GsRb1 prevented neurons from Glu toxicity, inhibited L-type voltage-gated calcium channels in hippocampal neurons in a dose-dependent manner, decreased cell death, and increased the longevity of surviving neurons, exerting a protective effect on cortical neurons and dopaminergic neurons (Lin et al., 2012; Ahmed et al., 2016). In the artificial abnormal microenvironment caused by microperfusion of L-glutamate and calcium ions (Ca2+), the protein kinase B/mammalian target of rapamycin/phosphatase and tensin homolog signaling pathway (Akt/mTOR/PTEN) signaling pathway is involved in cell growth and proliferation and inhibits apoptosis. GsRb1 increases the expression of phosphorylated-Akt (p-Akt) and phosphorylated-mTOR (p-mTOR) and decreases phosphorylated-PTEN (p-PTEN) in vitro and in vivo, thereby alleviating neuronal injury after abnormal microperfusion, proving that GsRb1 inhibits amino acid excitotoxicity and calcium overload (Guo et al., 2018). In the context of preventing neurological disorders, the control of cell apoptosis can be instrumental in reducing the incidence of these diseases and, potentially, in slowing down or preventing their progression. It is imperative to conduct further in-depth research into the influence of GsRb1 on apoptotic signaling pathways, encompassing its regulatory mechanisms involving key molecules such as Akt/mTOR, PKA/cAMP, and the Bcl-2 family. Through comprehensive molecular-level investigations, we can unravel the variations in the effects of GsRb1 across diverse cell types and disease models. This, in turn, will provide a robust scientific basis for the development of more precise therapeutic strategies. GsRb1 manifests its anti-apoptotic effects through multiple pathways, effectively inhibiting neuronal apoptosis and alleviating damage to the nervous system. These actions contribute to the maintenance of normal bodily functions, underscoring its substantial potential value in the prevention and treatment of neurological disorders.
3.4 Anti-autophagy
GsRb1 has anti-autophagy effects and can activate the phosphoinositide 3-pinase/protein pinase B signaling pathway (PI3K/Akt) pathway in vivo (Zhou et al., 2019b; Qin et al., 2021). Excessive cellular activation of autophagy can be significantly decreased in the presence of a PI3K activator, Akt activator, or mTOR activator (Guan et al., 2017). The mechanism underlying this decrease in autophagy is that GsRb1 converts microtubule-associated protein light chain 3 (LC3) from type I to type II, significantly increases the expression ratio of ILC3-II/LC3-I (Zhou et al., 2018; Zou et al., 2022), decreases the autophagy marker protein beclin1 (BECN1) (Chen et al., 2010; Luo et al., 2014), and downregulates the autophagic degradation substrate sequestosome 1 (p62) (Li et al., 2017; Liu et al., 2020), thereby inhibiting excessive autophagy in neurons (He et al., 2017). Further investigation is necessary to uncover the precise mechanisms by which GsRb1 activates the PI3K/Akt pathway, its interactions with key molecules such as PI3K, Akt, mTOR, as well as its mechanisms for diminishing the autophagic marker Beclin1 and reducing the autophagic substrate p62. In the nervous system, although autophagy is generally protective, it accelerates the injurious degeneration of neurons after acute injury to the nervous system (Choi et al., 2022), whereas GsRb1 can resist autophagy, bringing potential novel multi-targeted therapeutic strategies for these diseases.
4 Ginsenoside Rb1 and neurological system disorders
Previous research has demonstrated that GsRb1 has various effects, such as anti-inflammatory, anti-oxidant, anti-apoptotic, and anti-autophagy in the nervous system, and can play an essential role in developing and progressing neurological disorders. Some studies have also demonstrated that GsRb1 is a potential therapeutic agent for various neurological disorders, such as cerebral ischemia, traumatic brain injury, spinal cord injury, PD, AD, and epilepsy (Table 2).
4.1 Ginsenoside Rb1 and cerebral ischemia
Cerebral ischemia is currently associated with the most significant fatality and disability rates among cardiovascular disorders (Yang et al., 2022). It broadly refers to a lack of cerebral blood flow that hinders the support of brain tissue metabolism. Its progression can lead to neurosensory and motor dysfunction, which includes brain damage and aberrant changes in brain function (Zhang et al., 2022). Its causes are numerous and complicated, including smoking, diabetes, hypertension, and atherosclerosis (Sveinsson et al., 2014). Because inflammation can result in subsequent injury following cerebral ischemia, the pathological process of cerebral ischemia is complicated (Zeng et al., 2022). GsRb1 can prevent inflammation, safeguard the tight junctional activity of endothelial cells, and preserve the BBB structural integrity (Chen et al., 2015; Dong et al., 2017). GsRb1 attenuates neurological impairment, lowers the area of cerebral infarction, and enhances anti-inflammatory factors in localized cerebral ischemia (Liu et al., 2013; Zeng et al., 2022). Besides, it lessens inflammation-related redness, swelling, and heat discomfort (Yang F. et al., 2020). Multiple pathways, mostly mitochondria-mediated apoptosis, can be used by cerebral ischemia to cause apoptosis (Zhou et al., 2019b). GsRb1 can protect the structure and function of mitochondria, inhibit neuronal apoptosis and excessive autophagy, and attenuate neurological injury caused by ischemia-reperfusion and autophagic neuronal death induced by ischemic injury (Yang et al., 2008; Luo et al., 2014; Zhao D. et al., 2018). GsRb1 preserved neuronal cells, increased levels of brain-derived neurotrophic factor (BDNF) and glial cell-derived neurotrophic factor (GDNF), and greatly enhanced sensorimotor scores in rats (Adult male Wistar rats weighing 250–300 g were utilized in all experiments. These inbred strain rats were sourced from the Animal House Center at the Third Military Medical University in Chongqing, China. Middle cerebral artery occlusion was induced using the intraluminal vascular occlusion method.) suffering from cerebral ischemic stroke (Yuan et al., 2007; Gao et al., 2010; Jiang et al., 2013). The microenvironment of the central neuron changes when there is cerebral ischemia, which causes neuronal injury (Wang et al., 2017). GsRb1 can influence the phosphorylation of mTOR/PTEN under abnormal microenvironments, reverse pyramidal cell injury in the cornu ammonis (CA) 1 (CA1) region of the hippocampus, attenuate neuronal damage, and lessen cognitive dysfunction following cerebral ischemia (Guo et al., 2018). Regulating oxidative stress is an effective therapeutic method to protect neurons from cerebral ischemia-reperfusion (Wu et al., 2020). GsRb1 can be a protective agent against hypoxia, protecting mitochondrial function, decreasing ROS generation, and enhancing neuronal survival (Park et al., 2005; Xu et al., 2020).
Cheng Z et al. found that GsRb1 is a better ginsenoside to reduce intracellular neuronal apoptosis by comparing the protective effects of six ginsenosides (Rg1, Rb1, Rh2, Rg3, Rg5, and Re) on cerebral ischemia, which can significantly reduce cerebral ischemic injury and neurological deficit and may be used as an effective drug to treat cerebral ischemia (Cheng et al., 2019). Additionally, GsRb1 can be therapeutically valuable up to 24 h post-stroke, and it works well to increase the likelihood of long-term neurological recovery by activating the cyclic adenosine monophosphate/protein kinase A/cAMP response element-binding protein (cAMP/PKA/CREB) signaling pathway in vivo and encouraging axonal regeneration in the event of delayed stroke treatment (Yoshikawa et al., 2008; Gao et al., 2020). In conclusion, because GsRb1 can lessen the severity of ischemia injury, attenuate neuronal damage, and enhance cognitive function, it is anticipated to be a viable therapeutic agent in cerebral ischemic illnesses.
4.2 Ginsenoside Rb1 and traumatic brain injury
Traumatic brain injury (TBI) typically results from a primary injury caused by physical force to the head area, which subsequently develops into secondary injuries that cause neurological abnormalities that may be transient or permanent (Galgano et al., 2017), such as cerebral edema and cerebral hemorrhage (Hackenberg and Unterberg, 2016). Connexin 40 (Cx40) expression and the severity of TBI are firmly connected, and research has demonstrated that GsRb1 can significantly increase ERK1/2 phosphorylation levels, decrease Cx40 expression, and lessen the severity of TBI (Chen et al., 2016). Furthermore, after brain injury in rats (Male Sprague Dawley rats weighing between 250 and 300 g were subjected to subarachnoid hemorrhage induction using the modified double hemorrhage model, followed by intravenous treatment administration.), GsRb1 can relieve brain edema, weaken basilar artery vasospasm, reduce lumen thickness, and enhance neurobehavior (Li et al., 2011). Besides, autophagy is increased post-TBI, and GsRb1 acts as a neuroprotective agent in TBI rats by suppressing excessive autophagy (Guo et al., 2014), reducing neurological dysfunction scores, and ameliorating neurological damage (Zou et al., 2022). Traditional Chinese Medicine (TCM) has been receiving attention as an effective treatment for TBI, but anti-apoptotic and anti-autophagic activities of GsRb1 are also neuroprotective against TBI and could be further investigated as a potential therapeutic agent.
4.3 Ginsenoside Rb1 and spinal cord injury
Spinal cord injury (SCI) causes severe damage to the central nervous system, resulting in sensorimotor dysfunction of the trunk and extremities (Fan et al., 2018). Approximately 70% of SCI patients can develop chronic neuropathic pain (NP), which can manifest as abnormal pressure pain, nociceptive hypersensitivity (increased sensitivity to non-injurious stimuli), nociceptive hypersensitivity (increased sensitivity to injurious stimuli), or spontaneous pain (Finnerup, 2013; Dosenovic et al., 2017). The induction and maintenance of NP are importantly linked to the activation of MAPK (p38MAPK, ERK, and JNK) in glial cells (Hains and Waxman, 2006; Choi et al., 2012; Jendelova, 2018), and GsRb1 can exert an anti-apoptotic effect, inhibit this pathway, and ameliorate the clinical manifestations of NP. Inflammatory cytokines and mediators contributing to SCI-induced NP include IL-6, inducible nitric oxide synthase (iNOS), and COX-2, produced by activated glial cells (Zhang and De Koninck, 2006; Detloff et al., 2008; Jendelova, 2018). Studies have demonstrated that GsRb1 can lessen neuronal damage and enhance neurological function in SCI models by lowering neuronal apoptosis, blocking autophagy, attenuating oxidative stress, and encouraging the restoration of motor function (Huang et al., 2015; Bisicchia et al., 2017; Wang et al., 2018; Wen et al., 2023). Additionally, a study discovered that GsRb1 reduced the amplitude of nerve action potentials to treat cervical spondylosis similar to SCI and heal spinal cord damage (Lee et al., 2021). GsRb1 and its metabolite CK can be used in the clinic as an anti-injury (reduction of mechanical, cold, and thermal nociceptive hypersensitivity) drug to alleviate NP symptoms through the endoplasmic reticulum-mediated expression of estrogen receptor (ER)-α (ER-α) and ER-β expressed in the dorsal horn neurons of the L4-L5 spinal cord (Lee et al., 2021; Shoaib et al., 2023), which provides new insight into the future strategy for the diagnosis and treatment of SCI in humans.
4.4 Ginsenoside Rb1 and Parkinson’s disease
Dyskinesia and non-motor symptoms are the hallmarks of PD—a chronic, progressive neurodegenerative condition (Mahoney-Sánchez et al., 2021). From a pathophysiological perspective, PD is primarily characterized by neurodegeneration coupled with misfolding and aggregation of the neuronal inclusions α-synuclein (α-Syn), which accumulate into protein inclusions within the cell and lead to the formation of Lewy bodies, as well as the loss or degeneration of nigrostriatal dopaminergic neurons (Beitz, 2014; Bridi and Hirth, 2018; Dickson, 2018; Degirmenci et al., 2023). A key etiology of PD is an imbalance between the excitatory Glu and inhibitory γ-aminobutyric acid (GABA) systems, which results in neuro excitotoxicity and dopaminergic cell death in the substantia nigra compacta (Rose, 2016). Gamma-aminobutyric acid A receptor alpha 1 (GABAARα1) may interact with GsRb1 through hydrophobic interactions to upregulate the expression of GABAARα1 in cells, which enhances GABAergic transmission and reduces the dysfunction of GABA-mediated inhibitory transmission (Liu et al., 2019). In mice (Ten-week-old male C57BL/6 mice were obtained from SLAC Laboratory Animal Co., Ltd., Shanghai, China.), GsRb1 showed increased postsynaptic density protein 95 (PSD-95) expression in an α-Syn-dependent manner in vitro and in vivo, slowed memory loss and long-term potentiation (LTP) induced by 1-Methyl-4-phenyl-1,2,3,6-tetrahydropyridine (MPTP) (which induces typical parkinsonism in both humans and primates), whereas GsRb1 alleviated glutamate excitotoxicity by upregulating glutamate transporter expression and treated memory impairment and dyskinesia in PD patients with PD (Zhang YL. et al., 2018; Qu et al., 2019).
Furthermore, Ahmed T et al. discovered that GsRb1 affects the length of neural protrusions and increases the lifespan of neurons, which further supports the idea that GsRb1 has a beneficial therapeutic effect on neurodegenerative illnesses (Ahmed et al., 2016). Memory loss is a non-motor symptom of PD, and the hippocampus of the brain has a memory processing storage function and spatial information processing function, and the protection of hippocampal function is crucial to treating PD (Györfi et al., 2018). Lei Liu et al. and Qu S et al. demonstrated that reduced α-Syn in the hippocampal CA3 region causes memory deficits and that oral administration of GsRb1 significantly increases cell survival in the dentate gyrus and hippocampal CA3 region as one of the mechanisms for improving spatial learning and memory (Liu et al., 2011; Qu et al., 2019). As a novel neurotarget therapy for PD, GsRb1 affects hippocampal neurons in the brain by selectively inhibiting L-type calcium channels (Lin et al., 2012; Liss and Striessnig, 2019). PD is an untreatable condition that develops gradually over time. GsRb1 may be a possible medication to treat PD, owing to its capacity to ameliorate symptoms and enhance patients’ quality of life. Pharmacological treatment is the first option for Parkinson’s patients and is the cornerstone of the total treatment process.
4.5 Ginsenoside Rb1 and Alzheimer’s disease
The pathomechanism of AD—a neurodegenerative condition that impairs cognitive function in the brain—is characterized by the development of amyloid plaques, neurofibrillary tangles (NFTs), and significant neuronal loss (Selkoe, 2001; Town, 2010). According to studies, beta-amyloid peptides can cause tau protein (the microtubule-associated protein) to phosphorylate abnormally, which causes microtubule destabilization, poor axonal transport, and ultimately neuronal death (Zheng et al., 2002; Huang and Jiang, 2009). GsRb1 reduces calpain and p25 expression levels and attenuates β-amyloid peptide (25–35)-induced tau hyperphosphorylation in cortical neurons via the cyclin-dependent kinase 5 (CDK5) pathway, which promotes increased microtubule stability, intracellular calcium homeostasis in neuronal cells, neuronal cell protection and has significant potential to treat AD (Humbert et al., 2000; Chen et al., 2008; Shalaby et al., 2023; Sharari et al., 2023). In a study involving mice (Healthy male Sprague Dawley rats (clean grade) with a weight range of 300–320 g were supplied by the Animal Center of the First Hospital affiliated with Harbin Medical University, located in Harbin, China.) AD models and β-amyloid peptide (25–35), GsRb1 significantly improved axonal atrophy, synaptic loss, and memory impairment (Tohda et al., 2004). By contrast, another study demonstrated that GsRb1 could encourage neural stem cell proliferation and differentiation in a rat (Alzheimer’s disease animal models were induced by injecting Amyloid beta 1–40 in healthy male Sprague Dawley rats, 6 weeks old, from SLC, Shizuoka, Japan.) model of AD (Zhao J. et al., 2018), indicating that GsRb1 may have several therapeutic roles in AD, delaying AD progression and enhancing the patient’s quality of life. Oxidative stress plays a significant role in the pathogenesis of neurological disorders; thus, GsRb1 may offer fresh perspectives on preventing and treating AD by encouraging reverse cholesterol transport and reducing ROS formation (Changhong et al., 2021). Neuroinflammation plays a substantial role in AD etiology (Leng and Edison, 2021), which can activate neurodegenerative signaling pathways and promote plaque aggregation (Zhang W. et al., 2023). GsRb1 exerted anti-neuroinflammatory effects and corrected the loss of learning and memory skills in a rat (Male Wistar rats weighing 250–300 g and aged 3–4 months old were used to create Alzheimer’s disease rat models through intracerebroventricular injection of Amyloid beta 1–42.) model of AD (Wang et al., 2011; Jiang et al., 2023). Currently, GsRb1 can protect the nerves, enhance cognitive function, and be applied in preclinical and clinical studies of AD through several administration methods. Drug delivery to the central nervous system remains a complex process (Passeri et al., 2022), and there has been no improved treatment for AD.
4.6 Ginsenoside Rb1 and epilepsy
Epilepsy is a chronic disease with transient disorders of brain function. A significant cause of seizures is the imbalance between excitatory and inhibitory neurotransmission (Amtul and Aziz, 2017). The onset of the disease is characterized by sudden abnormal discharges of neurons in the brain. The type of the disease onset is linked to the patient’s genetics and the environment. The oxidative stress in the body and the impairment of autophagy can also lead to epileptic seizures (Berkovic et al., 2006; Mcmahon et al., 2012). In a rat (Healthy male Sprague-Dawley rats, with a weight range of 220–240 g, were sourced from the Animal Experimental Center of Zhengzhou University.) study, researchers used the antiepileptic drug γ-aminobutyric acid receptor antagonist pentylenetetrazole to mimic epilepsy and then treated with GsRb1, demonstrating that MDA and Bcl-2 expressions were upregulated during seizures and that the expressions of GSH, iNOS, and LC3 were downregulated, suggesting that GsRb1 acts as an inhibitor of oxidative stress, autophagy, and apoptosis, and attenuates neurological damage during seizures in epileptic rats (Shi et al., 2018; Zeng et al., 2018). GsRb1 also increases the in vivo and in vitro expression of Nrf2 and heme oxygenase-1 (HO-1) and protects the nerves by activating the nuclear factor erythroid 2-related factor 2/antioxidant response element (Nrf2/ARE) signaling pathway, reducing oxidative stress and neuronal apoptosis, shortening seizure duration while prolonging the latency period for seizure reoccurrence, thereby reducing brain damage during seizures, decreasing seizure severity, and improving cognitive dysfunction (Shi et al., 2018; Chen et al., 2019). At the moment, drug-based treatment is the only option available to stop the development or progression of epilepsy (Xu et al., 2013). Identifying effective medications to lower the frequency of seizures is necessary because treating recurrent seizures is currently difficult. GsRb1 can be used as a new antiepileptic medication as it contains anti-inflammatory, anti-apoptotic, and neurogenesis-inducing properties that can prevent neuronal damage, lessen the frequency and severity of seizures, and improve cognitive impairment.
5 Conclusion
This comprehensive review focuses on GsRb1, a natural compound with significant medicinal potential. A thorough analysis of its pharmacokinetic data provides a comprehensive understanding of the metabolic pathways and kinetic characteristics of GsRb1 in the human body, which is crucial for optimizing its clinical applications and drug development. Additionally, we delve into the actions and mechanisms of GsRb1 in the human body, such as its roles in suppressing inflammation, oxidative stress, apoptosis, and autophagy. By elaborating on how GsRb1 modulates multiple biomolecules and signaling pathways, we offer an in-depth insight into the compound’s multifaceted mechanisms at the cellular level. This comprehensive understanding can inspire new research directions and perspectives, including the development of multi-target drugs. Given GsRb1’s involvement in multiple signaling pathways, exploring its potential as a multi-target drug is not only feasible but also particularly promising for diseases that require the simultaneous regulation of various physiological processes, such as neurodegenerative diseases or autoimmune disorders. Furthermore, we can translate these cellular-level findings into clinical research and human trials in the future to evaluate the effectiveness and safety of GsRb1 in practical treatments. These considerations can significantly expand our understanding of GsRb1, providing fresh perspectives and insights for future research and clinical applications.
GsRb1 plays an indispensable role in the nervous system. Its potential for neuroprotection and treatment has garnered widespread attention in the scientific community, offering new hope for research and clinical treatment of neurological diseases. This comprehensive review aims to provide researchers and clinicians with a rich resource for understanding GsRb1, promoting further research and application. It holds profound significance for current scientific research and medical practice. The aforementioned studies have some limitations. There have been few preclinical efficacy assessments of GsRb1, and further research is required to determine whether the drug’s mechanism of action in cellular or animal models is entirely transferable to people. Second, additional research is warranted to determine whether GsRb1 causes other systemic side effects when used to treat neurological disorders because GsRb1 has some targets binding to the cardiovascular and endocrine systems.
Author contributions
GL: Conceptualization, Data curation, Formal Analysis, Investigation, Methodology, Writing–original draft. MZ: Writing–original draft, Data curation, Formal Analysis. CC: Writing–original draft, Data curation. YW: Data curation, Formal Analysis, Writing–original draft. QG: Conceptualization, Data curation, Writing–original draft. JL: Data curation, Writing–original draft. HY: Data curation, Writing–original draft. WJ: Conceptualization, Data curation, Investigation, Writing–original draft. WL: Writing–review and editing, Conceptualization, Data curation, Formal Analysis, Funding acquisition, Writing–original draft. LY: Writing–review and editing, Conceptualization, Data curation, Formal Analysis.
Funding
The author(s) declare financial support was received for the research, authorship, and/or publication of this article. This study was supported by National Natural Science Foundation of China (82201902), Zhejiang Province Medical and Health Science and Technology Program (2023RC048), and Wenzhou Science and Technology Plan Project (Y20210008).
Conflict of interest
The authors declare that the research was conducted in the absence of any commercial or financial relationships that could be construed as a potential conflict of interest.
Publisher’s note
All claims expressed in this article are solely those of the authors and do not necessarily represent those of their affiliated organizations, or those of the publisher, the editors and the reviewers. Any product that may be evaluated in this article, or claim that may be made by its manufacturer, is not guaranteed or endorsed by the publisher.
References
Ahmed, T., Raza, S. H., Maryam, A., Setzer, W. N., Braidy, N., Nabavi, S. F., et al. (2016). Ginsenoside Rb1 as a neuroprotective agent: a review. Brain Res. Bull. 125, 30–43. doi:10.1016/j.brainresbull.2016.04.002
Amtul, Z., and Aziz, A. A. (2017). Microbial proteins as novel industrial biotechnology hosts to treat epilepsy. Mol. Neurobiol. 54 (10), 8211–8224. doi:10.1007/s12035-016-0279-3
Bae, E. A., Park, S. Y., and Kim, D. H. (2000). Constitutive beta-glucosidases hydrolyzing ginsenoside Rb1 and Rb2 from human intestinal bacteria. Biol. Pharm. Bull. 23 (12), 1481–1485. doi:10.1248/bpb.23.1481
Beitz, J. M. (2014). Parkinson's disease: a review. Front. Biosci. Sch. Ed. 6, 65–74. doi:10.2741/s415
Berkovic, S. F., Mulley, J. C., Scheffer, I. E., and Petrou, S. (2006). Human epilepsies: interaction of genetic and acquired factors. Trends Neurosci. 29 (7), 391–397. doi:10.1016/j.tins.2006.05.009
Bisicchia, E., Latini, L., Cavallucci, V., Sasso, V., Nicolin, V., Molinari, M., et al. (2017). Autophagy inhibition favors survival of rubrospinal neurons after spinal cord hemisection. Mol. Neurobiol. 54 (7), 4896–4907. doi:10.1007/s12035-016-0031-z
Bridi, J. C., and Hirth, F. (2018). Mechanisms of α-synuclein induced synaptopathy in Parkinson's disease. Front. Neurosci. 12, 80. doi:10.3389/fnins.2018.00080
Cai, B., Zhang, Y., Wang, Z., Xu, D., Jia, Y., Guan, Y., et al. (2020). Therapeutic potential of diosgenin and its major derivatives against neurological diseases: recent advances. Oxid. Med. Cell Longev. 2020, 3153082. doi:10.1155/2020/3153082
Changhong, K., Peng, Y., Yuan, Z., and Cai, J. (2021). Ginsenoside Rb1 protected PC12 cells from Aβ(25-35)-induced cytotoxicity via PPARγ activation and cholesterol reduction. Eur. J. Pharmacol. 893, 173835. doi:10.1016/j.ejphar.2020.173835
Chen, G., Hou, S. X., Hu, P., Jin, M. z., and Liu, J. (2007). Preliminary study on brain-targeted drug delivery via inner ear. Yao Xue Xue Bao 42 (10), 1102–1106. doi:10.16438/j.0513-4870.2007.10.019
Chen, G., Mu, L., Zhang, X., Hou, S., and Nan, H. (2011). In vivo distribution and pharmacokinetics of multiple effective components contained in Panax notoginseng saponins after intratympanic administration. Zhongguo Zhong Yao Za Zhi 36 (13), 1815–1820. doi:10.4268/cjcmm20111326
Chen, G., Ward, M. F., Sama, A. E., and Wang, H. (2004). Extracellular HMGB1 as a proinflammatory cytokine. J. Interferon Cytokine Res. 24 (6), 329–333. doi:10.1089/107999004323142187
Chen, S., Li, X., Wang, Y., Mu, P., Chen, C., Huang, P., et al. (2019). Ginsenoside Rb1 attenuates intestinal ischemia/reperfusion-induced inflammation and oxidative stress via activation of the PI3K/Akt/Nrf2 signaling pathway. Mol. Med. Rep. 19 (5), 3633–3641. doi:10.3892/mmr.2019.10018
Chen, W., Guo, Y., Yang, W., Zheng, P., Zeng, J., and Tong, W. (2015). Protective effect of ginsenoside Rb1 on integrity of blood-brain barrier following cerebral ischemia. Exp. Brain Res. 233 (10), 2823–2831. doi:10.1007/s00221-015-4352-3
Chen, W., Guo, Y., Yang, W., Zheng, P., Zeng, J., and Tong, W. (2016). Involvement of Connexin40 in the protective effects of ginsenoside Rb1 against traumatic brain injury. Cell Mol. Neurobiol. 36 (7), 1057–1065. doi:10.1007/s10571-015-0299-y
Chen, X., Huang, T., Zhang, J., Song, J., Chen, L., and Zhu, Y. (2008). Involvement of calpain and p25 of CDK5 pathway in ginsenoside Rb1's attenuation of beta-amyloid peptide25-35-induced tau hyperphosphorylation in cortical neurons. Brain Res. 1200, 99–106. doi:10.1016/j.brainres.2007.12.029
Chen, Z., Lu, T., Yue, X., Wei, N., Jiang, Y., Chen, M., et al. (2010). Neuroprotective effect of ginsenoside Rb1 on glutamate-induced neurotoxicity: with emphasis on autophagy. Neurosci. Lett. 482 (3), 264–268. doi:10.1016/j.neulet.2010.07.052
Cheng, Z., Zhang, M., Ling, C., Zhu, Y., Ren, H., Hong, C., et al. (2019). Neuroprotective effects of ginsenosides against cerebral ischemia. Mol. 24 (6), 1102. doi:10.3390/molecules24061102
Choi, D. C., Lee, J. Y., Lim, E. J., Baik, H. H., Oh, T. H., and Yune, T. Y. (2012). Inhibition of ROS-induced p38MAPK and ERK activation in microglia by acupuncture relieves neuropathic pain after spinal cord injury in rats. Exp. Neurol. 236 (2), 268–282. doi:10.1016/j.expneurol.2012.05.014
Choi, H. M. C., Li, Y., Suraj, D., Hsia, R. C., Sarkar, C., Wu, J., et al. (2022). Autophagy protein ULK1 interacts with and regulates SARM1 during axonal injury. Proc. Natl. Acad. Sci. U. S. A. 119 (47), e2203824119. doi:10.1073/pnas.2203824119
Degirmenci, Y., Angelopoulou, E., Georgakopoulou, V. E., and Bougea, A. (2023). Cognitive impairment in Parkinson's disease: an updated overview focusing on emerging pharmaceutical treatment approaches. Med. Kaunas. 59 (10), 1756. doi:10.3390/medicina59101756
Detloff, M. R., Fisher, L. C., Mcgaughy, V., Longbrake, E. E., Popovich, P. G., and Basso, D. M. (2008). Remote activation of microglia and pro-inflammatory cytokines predict the onset and severity of below-level neuropathic pain after spinal cord injury in rats. Exp. Neurol. 212 (2), 337–347. doi:10.1016/j.expneurol.2008.04.009
Dickson, D. W. (2018). Neuropathology of Parkinson disease. Park. Relat. Disord. 46 (1), S30–s33. doi:10.1016/j.parkreldis.2017.07.033
Dong, W., Chen, W., Zou, H., Shen, Z., and Yu, D. (2022). Ginsenoside Rb1 prevents oxidative stress-induced apoptosis and mitochondrial dysfunction in muscle stem cells via NF-κB pathway. Oxid. Med. Cell Longev. 2022, 9159101. doi:10.1155/2022/9159101
Dong, X., Zheng, L., Lu, S., and Yang, Y. (2017). Neuroprotective effects of pretreatment of ginsenoside Rb1 on severe cerebral ischemia-induced injuries in aged mice: involvement of anti-oxidant signaling. Geriatr. Gerontol. Int. 17 (2), 338–345. doi:10.1111/ggi.12699
Dosenovic, S., Jelicic Kadic, A., Miljanovic, M., Biocic, M., Boric, K., Cavar, M., et al. (2017). Interventions for neuropathic pain: an overview of systematic reviews. Anesth. Analg. 125 (2), 643–652. doi:10.1213/ANE.0000000000001998
Fan, B., Wei, Z., Yao, X., Shi, G., Cheng, X., Zhou, X., et al. (2018). Microenvironment imbalance of spinal cord injury. Cell Transpl. 27 (6), 853–866. doi:10.1177/0963689718755778
Finnerup, N. B. (2013). Pain in patients with spinal cord injury. Pain 154 (1), S71–s76. doi:10.1016/j.pain.2012.12.007
Galgano, M., Toshkezi, G., Qiu, X., Russell, T., Chin, L., and Zhao, L. R. (2017). Traumatic brain injury: current treatment strategies and future endeavors. Cell Transpl. 26 (7), 1118–1130. doi:10.1177/0963689717714102
Gao, X., Zhang, X., Cui, L., Chen, R., Zhang, C., Xue, J., et al. (2020). Ginsenoside Rb1 promotes motor functional recovery and axonal regeneration in post-stroke mice through cAMP/PKA/CREB signaling pathway. Brain Res. Bull. 154, 51–60. doi:10.1016/j.brainresbull.2019.10.006
Gao, X. Q., Yang, C. X., Chen, G. J., Wang, G. Y., Chen, B., Tan, S. K., et al. (2010). Ginsenoside Rb1 regulates the expressions of brain-derived neurotrophic factor and caspase-3 and induces neurogenesis in rats with experimental cerebral ischemia. J. Ethnopharmacol. 132 (2), 393–399. doi:10.1016/j.jep.2010.07.033
Gong, L., Yin, J., Zhang, Y., Huang, R., Lou, Y., Jiang, H., et al. (2022). Neuroprotective mechanisms of ginsenoside Rb1 in central nervous system diseases. Front. Pharmacol. 13, 914352. doi:10.3389/fphar.2022.914352
Gooch, C. L., Pracht, E., and Borenstein, A. R. (2017). The burden of neurological disease in the United States: a summary report and call to action. Ann. Neurol. 81 (4), 479–484. doi:10.1002/ana.24897
Guan, H., Piao, H., Qian, Z., Zhou, X., Sun, Y., Gao, C., et al. (2017). 2,5-Hexanedione induces autophagic death of VSC4.1 cells via a PI3K/Akt/mTOR pathway. Mol. Biosyst. 13 (10), 1993–2005. doi:10.1039/c7mb00001d
Guo, Y., Wang, L. P., Li, C., Xiong, Y. X., Yan, Y. T., Zhao, L. Q., et al. (2018). Effects of ginsenoside Rb1 on expressions of phosphorylation akt/phosphorylation mTOR/phosphorylation PTEN in artificial abnormal hippocampal microenvironment in rats. Neurochem. Res. 43 (10), 1927–1937. doi:10.1007/s11064-018-2612-x
Guo, Z., Cao, G., Yang, H., Zhou, H., and Li, L. (2014). A combination of four active compounds alleviates cerebral ischemia-reperfusion injury in correlation with inhibition of autophagy and modulation of AMPK/mTOR and JNK pathways. J. Neurosci. Res. 92 (10), 1295–1306. doi:10.1002/jnr.23400
Györfi, O., Nagy, H., Bokor, M., and Kéri, S. (2018). Insights into the structure and function of the hippocampal formation: relevance to Parkinson's disease. Ideggyogy Sz. 71 (1-02), 15–24. doi:10.18071/isz.71.0015
Ha, Y. W., Lim, S. S., Ha, I. J., Na, Y. C., Seo, J. J., Shin, H., et al. (2007). Preparative isolation of four ginsenosides from Korean red ginseng (steam-treated Panax ginseng C. A. Meyer), by high-speed counter-current chromatography coupled with evaporative light scattering detection. J. Chromatogr. A 1151 (1-2), 37–44. doi:10.1016/j.chroma.2007.01.038
Hackenberg, K., and Unterberg, A. (2016). Traumatic brain injury. Nervenarzt 87 (2), 203–214. ; quiz 215-6. doi:10.1007/s00115-015-0051-3
Hains, B. C., and Waxman, S. G. (2006). Activated microglia contribute to the maintenance of chronic pain after spinal cord injury. J. Neurosci. 26 (16), 4308–4317. doi:10.1523/JNEUROSCI.0003-06.2006
Han, M., Fu, S., and Fang, X. L. (2007). Comparison between the characteristics of absorption and pharmacokinetic behavior of ginsenoside Rg1 and ginsenoside Rb, of Panax notoginseng saponins. Yao Xue Xue Bao 42 (8), 849–853. doi:10.16438/j.0513-4870.2007.08.002
Han, M., Han, L. M., Wang, Q. S., Bai, Z. H., and Fang, X. L. (2006). Mechanism of oral absorption of panaxnotoginseng saponins. Yao Xue Xue Bao 41 (6), 498–505. doi:10.16438/j.0513-4870.2006.06.004
Hashimoto, R., Yu, J., Koizumi, H., Ouchi, Y., and Okabe, T. (2012). Ginsenoside Rb1 prevents MPP(+)-Induced apoptosis in PC12 cells by stimulating estrogen receptors with consequent activation of ERK1/2, Akt and inhibition of SAPK/JNK, p38 MAPK. Evid. Based Complement. Altern. Med. 2012, 693717. doi:10.1155/2012/693717
He, Q., Li, Z., Wang, Y., Hou, Y., Li, L., and Zhao, J. (2017). Resveratrol alleviates cerebral ischemia/reperfusion injury in rats by inhibiting NLRP3 inflammasome activation through Sirt1-dependent autophagy induction. Int. Immunopharmacol. 50, 208–215. doi:10.1016/j.intimp.2017.06.029
Hu, S., Sun, Q., Xu, F., Jiang, N., and Gao, J. (2023). Age-related hearing loss and its potential drug candidates: a systematic review. Chin. Med. 18 (1), 121. doi:10.1186/s13020-023-00825-6
Huang, F., Li, Y. N., Yin, F., Wu, Y. T., and Zhao, D. X. (2015). Ginsenoside Rb1 inhibits neuronal apoptosis and damage, enhances spinal aquaporin 4 expression and improves neurological deficits in rats with spinal cord ischemia-reperfusion injury. Mol. Med. Rep. 11 (5), 3565–3572. doi:10.3892/mmr.2015.3162
Huang, H. C., and Jiang, Z. F. (2009). Accumulated amyloid-beta peptide and hyperphosphorylated tau protein: relationship and links in Alzheimer's disease. J. Alzheimers Dis. 16 (1), 15–27. doi:10.3233/JAD-2009-0960
Humbert, S., Dhavan, R., and Tsai, L. (2000). p39 activates cdk5 in neurons, and is associated with the actin cytoskeleton. J. Cell Sci. 113 (6), 975–983. doi:10.1242/jcs.113.6.975
Im, D. S. (2020). Pro-resolving effect of ginsenosides as an anti-inflammatory mechanism of panax ginseng. Biomolecules 10 (3), 444. doi:10.3390/biom10030444
Jakaria, M., Haque, M. E., Kim, J., Cho, D. Y., Kim, I. S., and Choi, D. K. (2018). Active ginseng components in cognitive impairment: therapeutic potential and prospects for delivery and clinical study. Oncotarget 9 (71), 33601–33620. doi:10.18632/oncotarget.26035
Jendelova, P. (2018). Therapeutic strategies for spinal cord injury. Int. J. Mol. Sci. 19 (10), 3200. doi:10.3390/ijms19103200
Jiang, N., Lv, J., Zhang, Y., Sun, X., Yao, C., Wang, Q., et al. (2023). Protective effects of ginsenosides Rg1 and Rb1 against cognitive impairment induced by simulated microgravity in rats. Front. Pharmacol. 14, 1167398. doi:10.3389/fphar.2023.1167398
Jiang, Z., Wang, Y., Zhang, X., Peng, T., Lu, Y., Leng, J., et al. (2013). Preventive and therapeutic effects of ginsenoside Rb1 for neural injury during cerebral infarction in rats. Am. J. Chin. Med. 41 (2), 341–352. doi:10.1142/S0192415X13500250
Kim, D. H. (2018). Gut microbiota-mediated pharmacokinetics of ginseng saponins. J. Ginseng Res. 42 (3), 255–263. doi:10.1016/j.jgr.2017.04.011
Kim, E. H., Kim, I. H., Ha, J. A., Choi, K. T., Pyo, S., and Rhee, D. K. (2013). Antistress effect of red ginseng in brain cells is mediated by TACE repression via PADI4. J. Ginseng Res. 37 (3), 315–323. doi:10.5142/jgr.2013.37.315
Kim, H. J., Oh, T. K., Kim, Y. H., Lee, J., Moon, J. M., Park, Y. S., et al. (2022). Pharmacokinetics of ginsenoside Rb1, Rg3, Rk1, Rg5, F2, and compound K from red ginseng extract in healthy Korean volunteers. Evid. Based Complement. Altern. Med. 2022, 8427519. doi:10.1155/2022/8427519
Kim, H. K. (2013). Pharmacokinetics of ginsenoside Rb1 and its metabolite compound K after oral administration of Korean Red Ginseng extract. J. Ginseng Res. 37 (4), 451–456. doi:10.5142/jgr.2013.37.451
Kim, J. H. (2012). Cardiovascular diseases and panax ginseng: a review on molecular mechanisms and medical applications. J. Ginseng Res. 36 (1), 16–26. doi:10.5142/jgr.2012.36.1.16
Lee, J. Y., Choi, H. Y., Park, C. S., Kim, D. H., and Yune, T. Y. (2021). Total saponin extract, ginsenoside Rb1, and compound K alleviate peripheral and central neuropathic pain through estrogen receptors on rats. Phytother. Res. 35 (4), 2119–2132. doi:10.1002/ptr.6960
Lee, S., Lee, S. O., Kim, G. L., and Rhee, D. K. (2018). Estrogen receptor-β of microglia underlies sexual differentiation of neuronal protection via ginsenosides in mice brain. CNS Neurosci. Ther. 24 (10), 930–939. doi:10.1111/cns.12842
Leng, F., and Edison, P. (2021). Neuroinflammation and microglial activation in Alzheimer disease: where do we go from here? Nat. Rev. Neurol. 17 (3), 157–172. doi:10.1038/s41582-020-00435-y
Li, L. F., Ma, Z. C., Wang, Y. G., Tang, X. L., Tan, H. L., Xiao, C. R., et al. (2017). Protective effect of ginsenoside Rb₁ on doxorubicin-induced myocardial autophagy. Zhongguo Zhong Yao Za Zhi 42 (7), 1365–1369. doi:10.19540/j.cnki.cjcmm.20170222.009
Li, W., Nan, L., Ji, Y., Sun, X., and Sun, Z. (2009). Studies on influence factors of gnsenoside Rg1 and Rb1 absorption in intestines of rats. Zhongguo Zhong Yao Za Zhi 34 (20), 2627–2632.
Li, Y., Tang, J., Khatibi, N. H., Zhu, M., Chen, D., Tu, L., et al. (2011). Treatment with ginsenoside rb1, a component of panax ginseng, provides neuroprotection in rats subjected to subarachnoid hemorrhage-induced brain injury. Acta Neurochir. Suppl. 110 (2), 75–79. doi:10.1007/978-3-7091-0356-2_14
Li, Y., Zhao, Y., Li, G., Wang, J., Li, T., Li, W., et al. (2007). Regulation of neuronal nitric oxide synthase exon 1f gene expression by nuclear factor-kappaB acetylation in human neuroblastoma cells. J. Neurochem. 101 (5), 1194–1204. doi:10.1111/j.1471-4159.2006.04407.x
Lin, Z., Xie, R., Zhong, C., Huang, J., Shi, P., and Yao, H. (2022). Recent progress (2015-2020) in the investigation of the pharmacological effects and mechanisms of ginsenoside Rb(1), a main active ingredient in Panax ginseng Meyer. J. Ginseng Res. 46 (1), 39–53. doi:10.1016/j.jgr.2021.07.008
Lin, Z. Y., Chen, L. M., Zhang, J., Pan, X. d., Zhu, Y. g., Ye, Q. y., et al. (2012). Ginsenoside Rb1 selectively inhibits the activity of L-type voltage-gated calcium channels in cultured rat hippocampal neurons. Acta Pharmacol. Sin. 33 (4), 438–444. doi:10.1038/aps.2011.181
Liss, B., and Striessnig, J. (2019). The potential of L-type calcium channels as a drug target for neuroprotective therapy in Parkinson's disease. Annu. Rev. Pharmacol. Toxicol. 59, 263–289. doi:10.1146/annurev-pharmtox-010818-021214
Liu, A., Zhu, W., Sun, L., Han, G., Liu, H., Chen, Z., et al. (2018). Ginsenoside Rb1 administration attenuates focal cerebral ischemic reperfusion injury through inhibition of HMGB1 and inflammation signals. Exp. Ther. Med. 16 (4), 3020–3026. doi:10.3892/etm.2018.6523
Liu, J. W., Ren, Y. L., Liu, X. L., Xia, H. L., Zhang, H. L., Jin, S. H., et al. (2013). Effect of ginsenoside Rb1 on cerebral infarction volume and IL-1 beta in the brain tissue and sera of focal cerebral ischemia/reperfusion injury model rats. Zhongguo Zhong Xi Yi Jie He Za Zhi 33 (12), 1696–1700. doi:10.7661/CJIM.2013.12.1696
Liu, L., Hoang-Gia, T., Wu, H., Lee, M. R., Wang, C., et al. (2011). Ginsenoside Rb1 improves spatial learning and memory by regulation of cell genesis in the hippocampal subregions of rats. Brain Res. 1382, 147–154. doi:10.1016/j.brainres.2011.01.051
Liu, X., Chen, J., Sun, N., Zhang, Z., and Zheng, T. (2020). Ginsenoside Rb1 ameliorates autophagy via the AMPK/mTOR pathway in renal tubular epithelial cells in vitro and in vivo. Int. J. Biol. Macromol. 163, 996–1009. doi:10.1016/j.ijbiomac.2020.07.060
Liu, Y., Wang, X., Xie, J., and Tang, M. (2023). Regulation of NAD(+)/NADH redox involves the protective effects of ginsenoside Rb1 against oxygen-glucose deprivation/reoxygenation-induced astrocyte lesions. Int. J. Mol. Sci. 24 (22), 16059. doi:10.3390/ijms242216059
Liu, Y., Zong, X., Huang, J., Guan, Y., Li, Y., Du, T., et al. (2019). Ginsenoside Rb1 regulates prefrontal cortical GABAergic transmission in MPTP-treated mice. Aging (Albany NY) 11 (14), 5008–5034. doi:10.18632/aging.102095
Long, W., Zhang, S. C., Wen, L., Yang, F., and Chen, G. (2014). In vivo distribution and pharmacokinetics of multiple active components from Danshen and Sanqi and their combination via inner ear administration. J. Ethnopharmacol. 156, 199–208. doi:10.1016/j.jep.2014.08.041
Lu, T., Jiang, Y., Zhou, Z., Yue, X., Wei, N., Chen, Z., et al. (2011). Intranasal ginsenoside Rb1 targets the brain and ameliorates cerebral ischemia/reperfusion injury in rats. Biol. Pharm. Bull. 34 (8), 1319–1324. doi:10.1248/bpb.34.1319
Luo, T., Liu, G., Ma, H., Lu, B., Xu, H., Wang, Y., et al. (2014). Inhibition of autophagy via activation of PI3K/Akt pathway contributes to the protection of ginsenoside Rb1 against neuronal death caused by ischemic insults. Int. J. Mol. Sci. 15 (9), 15426–15442. doi:10.3390/ijms150915426
Mahoney-Sánchez, L., Bouchaoui, H., Ayton, S., Devos, D., Duce, J. A., and Devedjian, J. C. (2021). Ferroptosis and its potential role in the physiopathology of Parkinson's Disease. Prog. Neurobiol. 196, 101890. doi:10.1016/j.pneurobio.2020.101890
Mancuso, C., and Santangelo, R. (2017). Panax ginseng and Panax quinquefolius: from pharmacology to toxicology. Food Chem. Toxicol. 107, 362–372. doi:10.1016/j.fct.2017.07.019
Mariage, P. A., Hovhannisyan, A., and Panossian, A. G. (2020). Efficacy of panax ginseng meyer herbal preparation HRG80 in preventing and mitigating stress-induced failure of cognitive functions in healthy subjects: a pilot, randomized, double-blind, placebo-controlled crossover trial. Pharm. (Basel) 13 (4), 57. doi:10.3390/ph13040057
Mcmahon, J., Huang, X., Yang, J., Komatsu, M., Yue, Z., Qian, J., et al. (2012). Impaired autophagy in neurons after disinhibition of mammalian target of rapamycin and its contribution to epileptogenesis. J. Neurosci. 32 (45), 15704–15714. doi:10.1523/JNEUROSCI.2392-12.2012
Ni, X. C., Wang, H. F., Cai, Y. Y., Yang, D., Alolga, R. N., Liu, B., et al. (2022). Ginsenoside Rb1 inhibits astrocyte activation and promotes transfer of astrocytic mitochondria to neurons against ischemic stroke. Redox Biol. 54, 102363. doi:10.1016/j.redox.2022.102363
Ong, W. Y., Farooqui, T., Koh, H. L., Farooqui, A. A., and Ling, E. A. (2015). Protective effects of ginseng on neurological disorders. Front. Aging Neurosci. 7, 129. doi:10.3389/fnagi.2015.00129
Park, J. K., Namgung, U., Lee, C. J., Jin, S. H., and Kwon, O. B., (2005). Calcium-independent CaMKII activity is involved in ginsenoside Rb1-mediated neuronal recovery after hypoxic damage. Life Sci. 76 (9), 1013–1025. doi:10.1016/j.lfs.2004.10.011
Passeri, E., Elkhoury, K., Morsink, M., Broersen, K., Linder, M., Tamayol, A., et al. (2022). Alzheimer's disease: treatment strategies and their limitations. Int. J. Mol. Sci. 23 (22), 13954. doi:10.3390/ijms232213954
Qi, S. H., Xiao, F., Wei, B., and Qin, C. (2020). Value of ginsenoside Rb1 in alleviating coronary artery lesion in a mouse model of Kawasaki disease. Zhongguo Dang Dai Er Ke Za Zhi 22 (9), 1034–1040. doi:10.7499/j.issn.1008-8830.2003147
Qin, G. W., Lu, P., Peng, L., and Jiang, W. (2021). Ginsenoside Rb1 inhibits cardiomyocyte autophagy via PI3K/Akt/mTOR signaling pathway and reduces myocardial ischemia/reperfusion injury. Am. J. Chin. Med. 49 (8), 1913–1927. doi:10.1142/S0192415X21500907
Qu, S., Meng, X., Liu, Y., Zhang, X., and Zhang, Y. (2019). Ginsenoside Rb1 prevents MPTP-induced changes in hippocampal memory via regulation of the α-synuclein/PSD-95 pathway. Aging (Albany NY) 11 (7), 1934–1964. doi:10.18632/aging.101884
Rottenberg, H., and Hoek, J. B. (2017). The path from mitochondrial ROS to aging runs through the mitochondrial permeability transition pore. Aging Cell 16 (5), 943–955. doi:10.1111/acel.12650
Sakanaka, M., Zhu, P., Zhang, B., Wen, T. C., Cao, F., Ma, Y. J., et al. (2007). Intravenous infusion of dihydroginsenoside Rb1 prevents compressive spinal cord injury and ischemic brain damage through upregulation of VEGF and Bcl-XL. J. Neurotrauma 24 (6), 1037–1054. doi:10.1089/neu.2006.0182
Selkoe, D. J. (2001). Alzheimer's disease: genes, proteins, and therapy. Physiol. Rev. 81 (2), 741–766. doi:10.1152/physrev.2001.81.2.741
Shalaby, A. M., Alnasser, S. M., Ahmed Khairy, D., Alabiad, M. A., Alorini, M., Jaber, F. A., et al. (2023). The neuroprotective effect of ginsenoside Rb1 on the cerebral cortex changes induced by aluminium chloride in a mouse model of Alzheimer's disease: a histological, immunohistochemical, and biochemical study. J. Chem. Neuroanat. 129, 102248. doi:10.1016/j.jchemneu.2023.102248
Sharari, S., Vaikath, N. N., Tsakou, M., Ghanem, S. S., and Vekrellis, K. (2023). Screening for novel inhibitors of amyloid beta aggregation and toxicity as potential drugs for Alzheimer's disease. Int. J. Mol. Sci. 24 (14), 11326. doi:10.3390/ijms241411326
Shi, Y., Miao, W., Teng, J., and Zhang, L. (2018). Ginsenoside Rb1 protects the brain from damage induced by epileptic seizure via Nrf2/ARE signaling. Cell Physiol. Biochem. 45 (1), 212–225. doi:10.1159/000486768
Shi, Y. H., Li, Y., Wang, Y., Xu, Z., Fu, H., and Zheng, G. Q. (2020). Ginsenoside-Rb1 for ischemic stroke: a systematic review and meta-analysis of preclinical evidence and possible mechanisms. Front. Pharmacol. 11, 285. doi:10.3389/fphar.2020.00285
Shoaib, R. M., Ahsan, M. Z., Akhtar, U., Ahmad, K. A., Ali, U., Deng, M. Y., et al. (2023). Ginsenoside Rb1, a principal effective ingredient of Panax notoginseng, produces pain antihypersensitivity by spinal microglial dynorphin A expression. Neurosci. Res. 188, 75–87. doi:10.1016/j.neures.2022.11.003
Sveinsson, O. A., Kjartansson, O., and Valdimarsson, E. M. (2014). Cerebral ischemia/infarction - epidemiology, causes and symptoms. Laeknabladid 100 (5), 271–279. doi:10.17992/lbl.2014.05.543
Tao, R., Lu, K., Zong, G., Xia, Y., Han, H., Zhao, Y., et al. (2023). Ginseng polysaccharides: potential antitumor agents. J. Ginseng Res. 47 (1), 9–22. doi:10.1016/j.jgr.2022.07.002
Tohda, C., Matsumoto, N., Zou, K., Meselhy, M. R., and Komatsu, K. (2004). Abeta(25-35)-induced memory impairment, axonal atrophy, and synaptic loss are ameliorated by M1, A metabolite of protopanaxadiol-type saponins. Neuropsychopharmacology 29 (5), 860–868. doi:10.1038/sj.npp.1300388
Town, T. (2010). Inflammation, immunity, and Alzheimer's disease. CNS Neurol. Disord. Drug Targets 9 (2), 129–131. doi:10.2174/187152710791012008
Wang, P., Lin, C., Wu, S., Huang, K., Wang, Y., Bao, X., et al. (2018). Inhibition of autophagy is involved in the protective effects of ginsenoside Rb1 on spinal cord injury. Cell Mol. Neurobiol. 38 (3), 679–690. doi:10.1007/s10571-017-0527-8
Wang, S., Li, M., Guo, Y., Li, C., Wu, L., Zhou, X. F., et al. (2017). Effects of Panax notoginseng ginsenoside Rb1 on abnormal hippocampal microenvironment in rats. J. Ethnopharmacol. 202, 138–146. doi:10.1016/j.jep.2017.01.005
Wang, Y., Liu, J., Zhang, Z., Bi, P., Qi, Z., and Zhang, C. (2011). Anti-neuroinflammation effect of ginsenoside Rbl in a rat model of Alzheimer disease. Neurosci. Lett. 487 (1), 70–72. doi:10.1016/j.neulet.2010.09.076
Wen, S., Zou, Z. R., Cheng, S., Guo, H., Hu, H. S., Zeng, F. Z., et al. (2023). Ginsenoside Rb1 improves energy metabolism after spinal cord injury. Neural Regen. Res. 18 (6), 1332–1338. doi:10.4103/1673-5374.357915
Won, H. J., Kim, H. I., Park, T., Jo, K., and Jeon, H. (2019). Non-clinical pharmacokinetic behavior of ginsenosides. J. Ginseng Res. 43 (3), 354–360. doi:10.1016/j.jgr.2018.06.001
Wu, L., Xiong, X., Wu, X., Ye, Y., Jian, Z., Zhi, Z., et al. (2020). Targeting oxidative stress and inflammation to prevent ischemia-reperfusion injury. Front. Mol. Neurosci. 13, 28. doi:10.3389/fnmol.2020.00028
Xie, W., Wang, X., Xiao, T., Cao, Y., Wu, Y., Yang, D., et al. (2021). Protective effects and network analysis of ginsenoside Rb1 against cerebral ischemia injury: a pharmacological review. Front. Pharmacol. 12, 604811. doi:10.3389/fphar.2021.604811
Xu, D., Miller, S. D., and Koh, S. (2013). Immune mechanisms in epileptogenesis. Front. Cell Neurosci. 7, 195. doi:10.3389/fncel.2013.00195
Xu, Q. F., Fang, X. L., and Chen, D. F. (2003). Pharmacokinetics and bioavailability of ginsenoside Rb1 and Rg1 from Panax notoginseng in rats. J. Ethnopharmacol. 84 (2-3), 187–192. doi:10.1016/s0378-8741(02)00317-3
Xu, Z., Liu, W., and Huang, H. (2020). Astragaloside IV alleviates cerebral ischemia-reperfusion injury by activating the janus kinase 2 and signal transducer and activator of transcription 3 signaling pathway. Pharmacology 105 (3-4), 181–189. doi:10.1159/000503361
Yang, C. X., Liu, J. X., Sun, Z. L., Gao, X. Q., Deng, L., and Yuan, Q. L. (2008). Effects of Ginsenoside RB1 on neural cell apoptosis and expressions of Bcl-2 and Bax in rats following subjected to cerebral ischemia-reperfusion. Sichuan Da Xue Xue Bao Yi Xue Ban. 39 (2), 214–217.
Yang, F., Ma, Q., Matsabisa, M. G., Chabalala, H., Braga, F. C., and Tang, M. (2020b). Panax notoginseng for cerebral ischemia: a systematic review. Am. J. Chin. Med. 48 (6), 1331–1351. doi:10.1142/S0192415X20500652
Yang, J. E., Jia, N., Wang, D., He, Y., Dong, L., and Yang, A. G. (2020a). Ginsenoside Rb1 regulates neuronal injury and Keap1-Nrf2/ARE signaling pathway in cerebral infarction rats. J. Biol. Regul. Homeost. Agents 34 (3), 1091–1095. doi:10.23812/20-143-L-7
Yang, K., Zeng, L., Ge, A., Wang, S., Zeng, J., Yuan, X., et al. (2022). A systematic review of the research progress of non-coding RNA in neuroinflammation and immune regulation in cerebral infarction/ischemia-reperfusion injury. Front. Immunol. 13, 930171. doi:10.3389/fimmu.2022.930171
Ye, J. T., Li, F. T., Huang, S. L., Xue, J. L., Aihaiti, Y., Wu, H., et al. (2019). Effects of ginsenoside Rb1 on spinal cord ischemia-reperfusion injury in rats. J. Orthop. Surg. Res. 14 (1), 259. doi:10.1186/s13018-019-1299-2
Yoshikawa, T., Akiyoshi, Y., Susumu, T., Tokado, H., Fukuzaki, K., Nagata, R., et al. (2008). Ginsenoside Rb1 reduces neurodegeneration in the peri-infarct area of a thromboembolic stroke model in non-human primates. J. Pharmacol. Sci. 107 (1), 32–40. doi:10.1254/jphs.fp0071297
Yuan, Q. L., Yang, C. X., Xu, P., Gao, X. Q., Deng, L., Chen, P., et al. (2007). Neuroprotective effects of ginsenoside Rb1 on transient cerebral ischemia in rats. Brain Res. 1167, 1–12. doi:10.1016/j.brainres.2007.06.024
Zeng, M., Zhang, R., Yang, Q., Guo, L., Zhang, X., Yu, B., et al. (2022). Pharmacological therapy to cerebral ischemia-reperfusion injury: focus on saponins. Biomed. Pharmacother. 155, 113696. doi:10.1016/j.biopha.2022.113696
Zeng, X., Hu, K., Chen, L., Zhou, L., Luo, W., Li, C., et al. (2018). The effects of ginsenoside compound K against epilepsy by enhancing the γ-aminobutyric acid signaling pathway. Front. Pharmacol. 9, 1020. doi:10.3389/fphar.2018.01020
Zhang, B., Hata, R., Zhu, P., Sato, K., Wen, T. C., Yang, L., et al. (2006). Prevention of ischemic neuronal death by intravenous infusion of a ginseng saponin, ginsenoside Rb(1), that upregulates Bcl-x(L) expression. J. Cereb. Blood Flow. Metab. 26 (5), 708–721. doi:10.1038/sj.jcbfm.9600225
Zhang, J., and De Koninck, Y. (2006). Spatial and temporal relationship between monocyte chemoattractant protein-1 expression and spinal glial activation following peripheral nerve injury. J. Neurochem. 97 (3), 772–783. doi:10.1111/j.1471-4159.2006.03746.x
Zhang, L., Ding, W., Sun, H., Zhou, Q., Huang, J., Li, X., et al. (2012). Salidroside protects PC12 cells from MPP⁺-induced apoptosis via activation of the PI3K/Akt pathway. Food Chem. Toxicol. 50 (8), 2591–2597. doi:10.1016/j.fct.2012.05.045
Zhang, M., Lin, W., Tao, X., Zhou, W., Liu, Z., Zhang, Z., et al. (2023a). Ginsenoside Rb1 inhibits ferroptosis to ameliorate hypoxic-ischemic brain damage in neonatal rats. Int. Immunopharmacol. 121, 110503. doi:10.1016/j.intimp.2023.110503
Zhang, W., Xiao, D., Mao, Q., and Xia, H. (2023b). Role of neuroinflammation in neurodegeneration development. Signal Transduct. Target Ther. 8 (1), 267. doi:10.1038/s41392-023-01486-5
Zhang, W. J., Hu, D. X., Lin, S. J., Fang, X. Q., and Ye, Z. F. (2022). Contribution of P2X purinergic receptor in cerebral ischemia injury. Brain Res. Bull. 190, 42–49. doi:10.1016/j.brainresbull.2022.09.009
Zhang, X., Liu, M. H., Qiao, L., and Dong, M. (2018a). Ginsenoside Rb1 enhances atherosclerotic plaque stability by skewing macrophages to the M2 phenotype. J. Cell Mol. Med. 22 (1), 409–416. doi:10.1111/jcmm.13329
Zhang, Y. L., Liu, Y., Kang, X. P., Dou, C. Y., Zhuo, R. G., Huang, S. Q., et al. (2018b). Ginsenoside Rb1 confers neuroprotection via promotion of glutamate transporters in a mouse model of Parkinson's disease. Neuropharmacology 131, 223–237. doi:10.1016/j.neuropharm.2017.12.012
Zhao, D., Zhang, M., Yuan, H., Meng, C., Zhang, B., and Wu, H. (2018a). Ginsenoside Rb1 protects against spinal cord ischemia-reperfusion injury in rats by downregulating the Bax/Bcl-2 ratio and caspase-3 and p-Ask-1 levels. Exp. Mol. Pathol. 105 (3), 229–235. doi:10.1016/j.yexmp.2018.09.001
Zhao, J., Lu, S., Yu, H., and Duan, S. (2018b). Baicalin and ginsenoside Rb1 promote the proliferation and differentiation of neural stem cells in Alzheimer's disease model rats. Brain Res. 1678, 187–194. doi:10.1016/j.brainres.2017.10.003
Zhao, L., Sui, M., Zhang, T., and Zhang, K. (2023). The interaction between ginseng and gut microbiota. Front. Nutr. 10, 1301468. doi:10.3389/fnut.2023.1301468
Zheng, Q., Bao, X. Y., Zhu, P. C., Tong, Q., Zheng, G. Q., and Wang, Y. (2017). Ginsenoside Rb1 for myocardial ischemia/reperfusion injury: preclinical evidence and possible mechanisms. Oxid. Med. Cell Longev. 2017, 6313625. doi:10.1155/2017/6313625
Zheng, W. H., Bastianetto, S., Mennicken, F., Ma, W., and Kar, S. (2002). Amyloid beta peptide induces tau phosphorylation and loss of cholinergic neurons in rat primary septal cultures. Neuroscience 115 (1), 201–211. doi:10.1016/s0306-4522(02)00404-9
Zheng, Z., Wang, M., Cheng, C., Liu, D., Wu, L., Zhu, J., et al. (2020). Ginsenoside Rb1 reduces H2O2-induced HUVEC dysfunction by stimulating the sirtuin-1/AMP-activated protein kinase pathway. Mol. Med. Rep. 22 (1), 247–256. doi:10.3892/mmr.2020.11096
Zhou, P., Xie, W., He, S., Sun, Y., Meng, X., Sun, G., et al. (2019a). Ginsenoside Rb1 as an anti-diabetic agent and its underlying mechanism analysis. Cells 8 (3), 204. doi:10.3390/cells8030204
Zhou, P., Xie, W., Luo, Y., Lu, S., Dai, Z., Wang, R., et al. (2018). Inhibitory effects of ginsenoside Rb1 on early atherosclerosis in ApoE-/- mice via inhibition of apoptosis and enhancing autophagy. Mol. 23 (11), 2912. doi:10.3390/molecules23112912
Zhou, P., Xie, W., Sun, Y., Dai, Z., Li, G., Sun, G., et al. (2019b). Ginsenoside Rb1 and mitochondria: a short review of the literature. Mol. Cell Probes 43, 1–5. doi:10.1016/j.mcp.2018.12.001
Zou, S., Chen, W., Ding, H., Qi, Y., Wang, Z., Fu, J., et al. (2022). Involvement of autophagy in the protective effects of ginsenoside Rb1 in a rat model of traumatic brain injury. Eur. J. Drug Metab. Pharmacokinet. 47 (6), 869–877. doi:10.1007/s13318-022-00799-0
Glossary
Keywords: Ginsenoside Rb1, pharmacokinetics, actions, mechanisms, neurological disorders
Citation: Ling G, Zhang M, Chen C, Wang Y, Gao Q, Li J, Yuan H, Jin W, Lin W and Yang L (2024) Progress of Ginsenoside Rb1 in neurological disorders. Front. Pharmacol. 15:1280792. doi: 10.3389/fphar.2024.1280792
Received: 21 August 2023; Accepted: 11 January 2024;
Published: 24 January 2024.
Edited by:
Ik-Hyun Cho, Kyung Hee University, Republic of KoreaReviewed by:
Guoqi Zhu, Anhui University of Chinese Medicine, ChinaYan Gao, Capital Medical University, China
Copyright © 2024 Ling, Zhang, Chen, Wang, Gao, Li, Yuan, Jin, Lin and Yang. This is an open-access article distributed under the terms of the Creative Commons Attribution License (CC BY). The use, distribution or reproduction in other forums is permitted, provided the original author(s) and the copyright owner(s) are credited and that the original publication in this journal is cited, in accordance with accepted academic practice. No use, distribution or reproduction is permitted which does not comply with these terms.
*Correspondence: Wei Lin, bGlud2VpMTExMEAxNjMuY29t; Lingrong Yang, eWxyZG9jdG9yQDE2My5jb20=