- 1Department of Basic Medical Science, College of Medicine, Prince Sattam Bin Abdulaziz University, Al-Kharj, Saudi Arabia
- 2Centre for Interdisciplinary Research in Basic Sciences, Jamia Millia Islamia, New Delhi, India
- 3Centre of Medical and Bio-Allied Health Sciences Research, Ajman University, Ajman, United Arab Emirates
- 4Respiratory Translational Research Group, Department of Laboratory Medicine, School of Health Sciences, College of Health and Medicine, University of Tasmania, Launceston, TAS, Australia
Traumatic brain injury (TBI) leads to brain damage, comprising both immediate primary damage and a subsequent cascade of secondary injury mechanisms. The primary injury results in localized brain damage, while the secondary damage initiates inflammatory responses, followed by the disruption of the blood-brain barrier, infiltration of peripheral blood cells, brain edema, and the release of various immune mediators, including chemotactic factors and interleukins. TBI disrupts molecular signaling, cell structures, and functions. In addition to physical tissue damage, such as axonal injuries, contusions, and haemorrhages, TBI interferes with brain functioning, impacting cognition, decision-making, memory, attention, and speech capabilities. Despite a deep understanding of the pathophysiology of TBI, an intensive effort to evaluate the underlying mechanisms with effective therapeutic interventions is imperative to manage the repercussions of TBI. Studies have commenced to explore the potential of employing natural compounds as therapeutic interventions for TBI. These compounds are characterized by their low toxicity and limited interactions with conventional drugs. Moreover, many natural compounds demonstrate the capacity to target various aspects of the secondary injury process. While our understanding of the pathophysiology of TBI, there is an urgent need for effective therapeutic interventions to mitigate its consequences. Here, we aimed to summarize the mechanism of action and the role of phytochemicals against TBI progression. This review discusses the therapeutic implications of various phytonutrients and addresses primary and secondary consequences of TBI. In addition, we highlighted the roles of emerging phytochemicals as promising candidates for therapeutic intervention of TBI. The review highlights the neuroprotective roles of phytochemicals against TBI and the mechanistic approach. Furthermore, our efforts focused on the underlying mechanisms, providing a better understanding of the therapeutic potential of phytochemicals in TBI therapeutics.
1 Introduction
A traumatic brain injury (TBI) is an injury that takes place when an external force inflicts harm upon the brain. This force can arise from various situations, including falls, accidents, sports-related incidents, and physical violence (Maas et al., 2022). TBIs can exhibit a range of severities, encompassing mild to severe, and can result in lasting and significant effects on an individual’s physical, cognitive, emotional, and behavioral abilities. TBI can be categorized into two phases: first, the initial injury arising from the mechanical trauma, and second, the TBI-related consequences. The initial injury can result in the direct bruising of cranial tissue or the shearing of axons as the brain is rapidly compelled to change its position (Johnson et al., 2013a). Several secondary injury cascades (SICs) are commenced following the mechanical trauma. If left uncontrolled, these cascades result in further TBI-related pathology, leading to various neurological issues. The clinical symptoms of TBI correlate directly with the severity of the initial injury and the extent and duration of the SICs. The onset and scale of TBI-related SIC are highly intricate processes, resulting in a disturbance of the mechanisms that typically regulate multiple factors for normal brain functioning. These subsequent injuries involve the disturbance of the blood-brain barrier (BBB), excitotoxicity induced by glutamate, oxidative stress, and neuroinflammation, all of which can develop in a time-dependent manner following the initial mechanical injury (Chiu et al., 2016).
One key goal of therapeutic management after the trauma is to manage or reduce the progression of SIC. These events could potentially result in the salvation of nearby regions (known as the penumbra) and improve the chances of a favorable outcome. Researchers have explored the potential of substances possessing antioxidant properties as innovative therapeutic agents to reduce oxidative stress and decelerate cellular harm induced by reactive compounds. Antioxidants can either intervene in the generation of reactive oxygen species (ROS) within mitochondria or function as inhibitors of nicotinamide adenine dinucleotide phosphate (NADP). These mechanisms can potentially reduce secondary injuries and enhance clinical outcomes (Fernández-Gajardo, Matamala, Carrasco, Gutiérrez, Melo and Rodrigo, 2014).
Many naturally occurring substances exhibit antioxidant properties (Alam et al., 2022a; Ali et al., 2022; Alam et al., 2022b; Alam et al., 2022c). Their antioxidant mechanisms are linked to their influence on the pathways and enzymes within mitochondria. Utilizing substances with antioxidant properties can decelerate the degeneration of the brain and influence the outcome or the intensity of the complications. Increasing evidence suggests that neuroinflammation and oxidative stress play a significant role in contributing to neurological impairments and the development of posttraumatic epileptogenesis, in addition to cytotoxicity and damage to neurons and glial cells (Shin et al., 2011; Battaglini et al., 2021; Fatima et al., 2022a; Fatima et al., 2022b; Alam S. et al, 2022d; Anwar et al., 2023).
Over the years, researchers have investigated different facets of TBI using various animal models to gain deeper insights into its pathophysiology and explore potential treatment options. Various TBI models have been used to study the progression of the disease and the role of multiple modulators of TBI. The promising role of phytochemicals in TBI treatment offers an exciting avenue for research and clinical applications. The future holds great potential for personalized, multi-targeted therapies, innovative delivery systems, and a more comprehensive understanding of TBI, ultimately leading to improved outcomes and quality of life for TBI patients.
In the review, we have summarized the pathophysiology of TBI, mechanisms associated with TBI progression, and the role of phytochemicals as neuroprotective agents in TBI. Various mechanisms, such as glutamate-induced excitotoxicity, mitochondrial dysfunction, neurotoxicity, etc., are associated with the consequences of TBI. Phytochemicals have emerged as a neuroprotective agent in TBI by acting against these consequences. This review further highlights the role of different phytochemicals and the mechanical approach associated with the phytochemical to exhibit the neuroprotective effect in TBI.
2 Pathophysiology of traumatic brain injury
In the United States, TBI accounts for around 40% of deaths resulting from acute injuries and stands as the primary cause of death in individuals under the age of 45. Moreover, TBI imposes a significant financial burden, with an estimated total annual cost of $37.6 billion, of which $12.7 billion represents lost income due to premature death (Werner and Engelhard, 2007; Popescu, Anghelescu, Daia and Onose, 2015). TBI results from external mechanical forces that can cause temporary or permanent physical, psychological, or cognitive impairments, often accompanied by an altered state of consciousness (Zhao et al., 2011; Maxwell, 2012). Symptoms of TBI encompass dizziness, headaches, amnesia (forgetfulness), and nausea, which may show improvement over days to weeks following the injury. However, in cases of severe injury, long-term behavioral and cognitive impairments can result (Yamamoto, Levin and Prough, 2018; Thapa, Khan, Singh and Kaur, 2021). There are fragmented pieces of evidence indicating a higher occurrence of neurodegenerative disorders (NDs) like Alzheimer’s disease (AD), Parkinson’s disease (PD), and chronic traumatic encephalopathy because of head trauma (Safinia et al., 2016). TBI treatment includes pharmacotherapy, cognitive therapies and surgeries, which depend on the severity of the condition (Stocchetti et al., 2017).
TBI classification involves several scales and metrics. For instance, the Glasgow Coma Scale (GCS), along with the duration of altered levels of consciousness (LOC) and post-traumatic amnesia (PTA), serve as valuable tools to evaluate the clinical severity of TBI (Mehta and Chinthapalli, 2019; Bodien et al., 2021). Individuals who experience severe TBI face an elevated risk of secondary brain injury, which can result in adverse outcomes such as disability, a vegetative state, or even death. Additional classification is based on the regions of injury: focal injury encompasses mass lesions like contusions, subdural hematoma, epidural hematoma, and intra-parenchymal haemorrhage, while diffuse injury involves axonal, hypoxic-ischemic and micro-vascular injuries (Leitgeb et al., 2012). As a result of brain trauma, TBI can be categorized into primary and secondary brain injuries, depending on the outcome (Mckee and Daneshvar, 2015).
Primary brain injury results in brain damage, reduced oxygen supply, and extensive tissue necrosis, affecting all cellular components and blood vessels within the brain. Neuronal and glial cell death in the necrotic region is linked to impaired blood flow and the disruption of the blood-brain barrier (BBB) integrity, potentially resulting in additional intracerebral haemorrhage and edema due to vascular leakage (Corps, Roth and McGavern, 2015). The astroglial syncytial systems, assisted by aquaporin-4 (AQP-4), facilitate the removal of excessive edema fluid from the brain (Rash, Davidson, Yasumura and Furman, 2004). The intensity of post-injury inflammation assisted with pro-inflammatory cytokines contributes to blood vessel damage (Kwiecien et al., 2020).
TBIs can further be classified as penetrating and closed-head injuries. In penetrating injuries, the object penetrates both the skull and the dura, resulting in direct injury to the brain, while in closed-head injuries, the skull and dura remain intact. TBIs are categorized based on severity as mild, moderate, and severe based on clinical parameters such as loss of consciousness, amnesia, neurological symptoms, and the findings from structural brain imaging, such as CT or MRI scans (Blyth and Bazarian, 2010). Moderate and severe TBIs require neurosurgical interventions and intensive care. In contrast, mild TBI and concussion are synonymous terms to describe the mildest form of TBIs, comprising 80%–90% of cases [4–6]. Mild TBI usually stems from non-penetrating blunt head trauma, leading to temporary symptoms identified through clinical assessments, patient self-reporting, or witness observations (if present). Symptoms can vary widely and encompass physical indications (such as nausea, vomiting, dizziness, and headaches), cognitive manifestations (like difficulties in concentration and memory), behavioral changes (including irritability and emotional instability), and the potential for loss of consciousness [5]. TBI is further associated with secondary injury cascades through various mechanisms. Various factors such as excitotoxicity, oxidative stress, and others play a significant role in the SICs. Some of them are discussed in the following paragraphs. Figure 1 shows the pathophysiology and SICs associated with TBI.
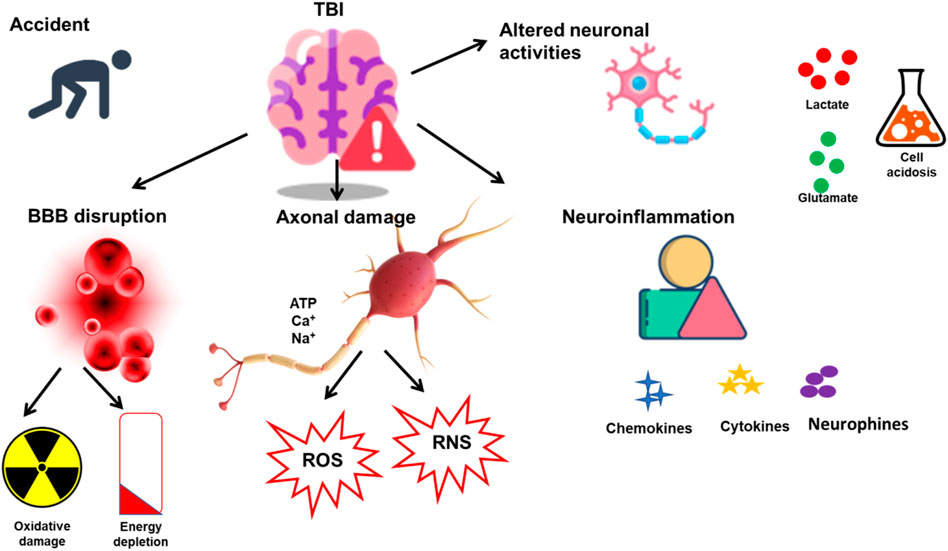
FIGURE 1. SICs associated with TBI. TBI is induced in response to sudden damage to the neurons and brain. Various SICs are related to the onset of TBI, such as BBB disruption, neuronal damage, and neuroinflammation. The SICs further result in alterations in various cellular functions such as oxidative damage, ROS and RNS release, neuroinflammatory agents, and cell acidosis.
3 Excitotoxicity in TBI
The disruption of the BBB resulting from TBI leads to the release of excessive neurotransmitters and a breakdown in the normal glutamate reuptake by transporters (Chamoun, Suki, Gopinath, Goodman and Robertson, 2010). Glutamate and its various byproducts bind and activate the ionotropic (IT) and metabotropic (MT) types of glutamate receptors. IT glutamate receptors, such as NMDA and AMPA receptors, permit the entry of sodium, potassium, and calcium ions, causing membrane depolarization (Brustovetsky, Bolshakov and Brustovetsky, 2010). In TBI, the excessive glutamate release leads to an upregulation of these receptors, disrupting ion balance by allowing extracellular calcium and sodium ions into the cells (Meldrum, 2000). It has been observed that the GluN2B subunit, present in synaptic cytosol, plays a role in mediating the excitotoxic response (Wyllie, Livesey and Hardingham, 2013). Elevated intracellular calcium levels trigger downstream signalling molecules like Ca2+/calmodulin-dependent protein kinase II (CAMK II), protein kinase C (PKC), mitogen-activated protein kinases (MAPK), and protein phosphatases (PPs).
The excessive cytosolic calcium activates apoptotic proteins such as calpain, calcineurin, and caspases, ultimately leading to cell death (Folkerts, Parks, Dedman, Kaetzel, Lyeth and Berman, 2007; Weber, 2012). Excitatory neurotransmitters cause cells to succumb to oxidative stress, resulting in excitotoxic cell death (Chamoun et al., 2010). Shortly after the trauma, the shearing and stretching forces resulting from the head injury induce glutamate-independent excitotoxicity through NMDA receptor activation (LaPlaca and Thibault, 1998). Various studies have identified the GluN2B subunit as a mediator of mechanosensitive responses (P. Singh et al., 2012). Various phytochemicals have shown protective effects against glutamate-induced excitotoxicity and are summarized in Table 1.
4 Mitochondrial dysfunction
Mitochondrial dysfunction is a prominent event of TBI, resulting in disruptions to physiological and metabolic processes and ultimately culminating in cell death. The increased entry of calcium ions (Ca2+) into mitochondria can lead to ROS generation and depolarization of the mitochondrial membrane, all occurring without ATP synthesis (Susin et al., 1998; Xiong et al., 1999). Consequently, the electron transport chain (ETC) and oxidative phosphorylation (OXPHOS) disrupt calcium regulation and metabolic function (Naga et al., 2007). Mitochondrial dysfunction additionally leads to a structural alteration in the adenine nucleotide translocator protein when it interacts with Cyclophilin D. Mitochondrial damage results in oxidative stress, followed by apoptosis and reduced cellular energy production. These changes in brain cells impair neurological functions, evident in individuals suffering from TBI. The intricate mitochondrial dysfunction following TBI necessitates targeted treatment to address the secondary injury. Phytochemicals, naturally occurring compounds found in various plant sources, have gained attention for their potential therapeutic role in treating TBI by targeting mitochondrial dysfunction. Astaxanthin (AXT), a carotenoid compound, is utilized as a dietary supplement (Hussein et al., , 2006). AXT was studied in mouse neuronal cells (HT22) with glutamate toxicity at doses 1.25–5 µM. AXT showed various effects on HT22 cells, including attenuating mitochondrial dysfunction by regulating the Akt/glycogen synthase kinase (GSK)-3β signaling pathway. Barberine has also shown neuroprotective effects via elevating the mitochondrial membrane potential (MMP) (Moneim, 2015).
Curcumin has also shown inhibitory effects on the mitochondrial dysfunction in PC12 cells, reducing ROS production and neurotoxicity (R. Wang et al., 2008). Protopanaxadiol is a natural compound that prevents glutamate-induced toxicity by improving mitochondrial function and enhancing anti-oxidant activity (Bak et al., 2016). Tanshinone IIA also suppresses mitochondrial dysfunction by modulating the MAPK pathways in the neuroblastoma cell line (Li et al., 2017).
5 Oxidative stress
Elevated levels of free radicals, encompassing both reactive nitrogen species (RNS) and reactive oxygen species (ROS), may arise due to secondary cell death and oxidative stress. Excessive production of ROS disturbs mitochondrial function, damaging the mitochondrial membrane through lipid peroxidation (Shohami and Kohen, 2011). In response to the damaged cells after TBI, there is an increased ROS generation from the electron transport chain (ETC). Conversely, after TBI, the accumulation of Ca2+ facilitates nitric oxide (NO) generation by nitric oxide synthases (NOS) (Deng et al., 2007). Moreover, the continuous release of ROS and lipid peroxidation unfavourable influences cerebral blood flow, leading to immunosuppression and brain plasticity (Shohami and Kohen, 2011). Table 2 summarizes some phytochemicals associated with reducing oxidative stress in neurological cell lines and TBI models.
6 Neuroinflammation
TBI triggers a complex array of immune and inflammatory responses in tissues, resembling the reactions observed in ischemia/reperfusion injury. The process activates cellular mediators of inflammation, such as prostaglandins and free radicals (Werner and Engelhard, 2007; Thapa et al., 2021). Analysis of cerebrospinal fluid and post-mortem brain tissue from individuals who suffered TBIs (Frugier et al., 2010; Goodman et al., 2009) and experiments conducted on rodents (Lotocki et al., 2009; Semple et al., 2010) indicated that within 24 h of the trauma, polymorphonuclear leukocytes and cytokines released inflammatory agents like IL-6, IL-1β, and TNF-α. The persistent secretion of cytokines indicates disruptions in the blood-brain barrier (BBB), resulting in edema and neurological impairments. TNF-α, belonging to the Fas family, exhibits a robust interaction with the Fas ligand and triggers caspases, initiating programmed cell death (Bye et al., 2007).
Moreover, endothelial and leukocyte cell adhesion molecules like ICAM-1 and VCAM-1 promote the recruitment of leukocytes and immune cells to the site of injury through their interactions with endothelial cells (Frugier et al., 2010). In cases of prolonged neuroinflammation, macrophages activate microglial cells, leading to an increased release of astrocytes, a phenomenon observed in survivors of TBI for many years following the initial injury (Johnson et al., 2013b). The involvement of GSK-3β in the physiological framework of mild traumatic brain injury (mTBI) has been investigated at both the cellular and behavioral dimensions (Shapira et al., 2007).
The reduction of neuroinflammation in TBI through phytochemicals is a subject of growing interest in neurology and medicine. Research suggests that phytochemicals can modulate the release of proinflammatory cytokines, such as TNF-α and IL-6, and reduce oxidative stress, ultimately contributing to TBI neuroinflammation. Various phytochemicals have been studied for their inhibitory effects on neuroinflammation. Curcumin mitigated the neurotoxic effects induced by glutamate through the inhibition of endoplasmic reticulum stress-associated activation of TXNIP/NLRP3 inflammasome in a manner that relied on 5’ AMP-activated protein kinase, as documented in reference (Feeney et al., 1981). Additionally, Lovastatin was associated with a decrease in the expression of proinflammatory cytokines, specifically TNF-α and IL-1β. A summarized neuro score demonstrated significant improvement during the initial 7 days.
In an experimental study, a pretreatment approach was employed to assess lovastatin’s potential as a neuroprotective agent in the context of a subsequent moderate TBI (CCI-induced TBI), as described in reference (S.-F. Chen et al., 2007). Adult rats received lovastatin injections (4 mg/kg, intraperitoneal) for 5 days preceding a unilateral injury focused on the bregma region. 6 h after the traumatic event, there was a notable decrease in the messenger RNA (mRNA) levels of TNF-α and IL-1β. 4 days after the injury, the researchers observed a significant decrease in the contusion volume, accompanied by a reduction in FJB staining.
The reduction of neuroinflammation in TBI through phytochemicals is a subject of growing interest in neurology and medicine. Research suggests that phytochemicals can modulate the release of proinflammatory cytokines, such as TNF-α and IL-6, and reduce oxidative stress, ultimately contributing to TBI neuroinflammation. Various phytochemicals have been studied for their inhibitory effects on neuroinflammation. Curcumin mitigated the neurotoxic effects induced by glutamate through the inhibition of endoplasmic reticulum stress-associated activation of TXNIP/NLRP3 inflammasome in a manner that relied on 5’ AMP-activated protein kinase, as documented in reference (Feeney et al., 1981).
7 BBB breakdown
While it is technically considered part of the primary injury, the breach of the blood-brain barrier (BBB) plays a role in the development of brain edema, which is a critical early element of secondary injury cascades (SIC) associated with various forms of brain injury (Kelley et al., 2007; Das et al., 2012; Mendes Arent et al., 2014). Cerebral edema is characterized by an expansion in brain tissue volume resulting from the buildup of fluid, and it occurs through two fundamental mechanisms: vasogenic and cytotoxic (Vink and Nimmo, 2009; Hinson et al.,, 2015). Vasogenic edema arises due to heightened blood-brain barrier (BBB) permeability, leading to an imbalance in the oncotic and hydrostatic pressures that control the movement of fluids between the bloodstream and the brain interstitial space (Kimelberg, 1995). An intact blood-brain barrier (BBB) prevents the diffusion of water-soluble molecules exceeding 500 Da. However, once the BBB is compromised, brain-specific proteins can be observed in the bloodstream and cerebrospinal fluid (CSF) (Reiber and Peter, 2001; Stahel et al., 2001; Blyth et al., 2009). Cytotoxic edema is marked by the swelling of brain structural cells within the intracellular space, and this occurs while the blood-brain barrier remains unbroken. This edema primarily manifests in grey matter due to energy depletion and insufficient blood supply. Regarding its mechanism, cytotoxic edema is linked to malfunctioning ATPase-dependent cellular pumps and water buildup within the cells among osmotically active solutes (Unterberg et al., 2004; Stiefel et al., 2005). Elevated glutamate levels can play a role in promoting cytotoxic edema by raising intracellular sodium levels and facilitating the movement of water into the cells, subsequently increasing the volume of intracellular fluid (Duvdevani et al., 1995; Floyd et al, 2005; Yi and Hazell, 2006).
In a recent investigation, the application of Baicalein was examined using a subarachnoid haemorrhage model, a condition frequently observed following TBIs, which may hold significance for advancing innovative therapeutic strategies. Treatment was initiated 30 min after the injury with 30 mg/kg or 100 mg/kg of Baicalein. This treatment regimen led to a reduction in blood-brain barrier (BBB) permeability, edema, and neuronal apoptosis, ultimately resulting in an enhancement of the subjects’ neuroscores (Chen et al., 2008; Kerman et al., 2012; Wang et al., 2015). In a separate investigation, adult male rats were exposed to mild to moderate TBI induced by controlled cortical impact (CCI). These rats were then administered CAPE therapy at 10 mg/kg via intraperitoneal injection, commencing 30 min after the injury.
In some cases, the treatment was extended for an additional 4 days. When assessed 24 h after a single treatment, the rats displayed decreased blood-brain barrier (BBB) permeability and a decrease in the loss of cortical cells (Kerman et al., 2012; Zhao et al., 2012). In a recent study, the potential therapeutic benefits of EA were examined following a mild to moderate diffuse injury, explicitly using the Marmarou model, in young adult rats. These animals received a pretreatment regimen for 7 days through oral administration of EA at a dose of 100 mg/kg. This pre-treatment approach reduced BBB permeability (Marmarou et al., 1994). Figure 2 shows various factors leading to TBI.
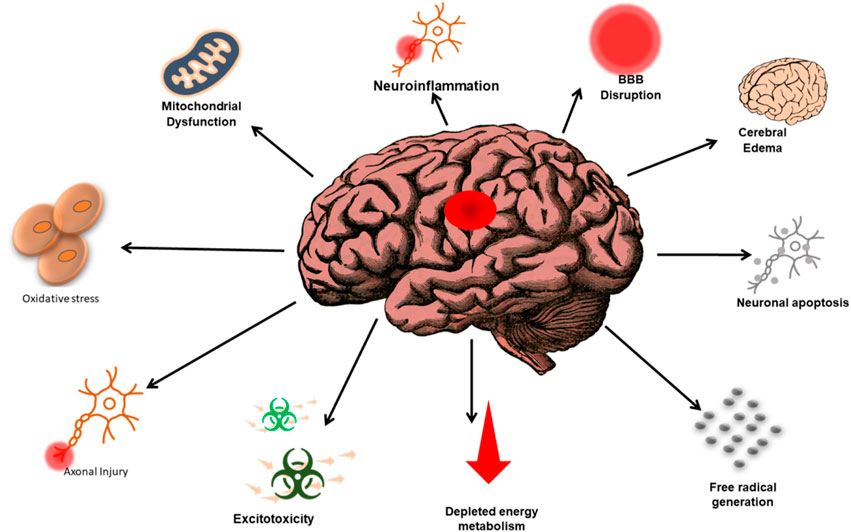
FIGURE 2. Representation of the complex relationship between the various factors that lead to TBI and the subsequent consequences associated with TBI-related events. The annotations aim to enhance understanding and awareness of the multifaceted nature of TBI and its impacts.
8 Role of phytochemicals in TBI
TBI is a significant public health concern, with over 2.8 million new cases annually in the United States alone (Taylor et al., 2017; Horstemeyer et al., 2019). TBI occurs when an external mechanical force impacts the head, leading to temporary or permanent impairments in brain function (Jenkins, 2023). TBI could be of forms, i.e., primary and secondary phases of damages (Ye et al., 2023). The primary injury induced immediately by the external force causes tissue damage through skull fractures, haemorrhages, and vascular injury (Xiong et al., 2013). However, the subsequent secondary injury arising minutes to months after the initial insult exacerbates the neuropathology through various cellular processes, including neuroinflammation, oxidative stress, metabolic dysfunction, and excitotoxicity (Walker and Tesco, 2013). This secondary phase offers a window for therapeutic interventions to mitigate further neuronal damage and improve outcomes. Currently, no FDA-approved drugs target the secondary neurodegenerative cascades induced by TBI (Prins and Matsumoto, 2014).
Therefore, research has focused on identifying neuroprotective agents from natural sources, such as dietary phytochemicals, which we will discuss in the later sections of this literature. Phytochemicals from fruits, vegetables, spices, teas, and herbs demonstrate therapeutic efficacy in preclinical TBI models through their anti-inflammatory, antioxidant, and anti-apoptotic properties. However, most findings arise from in vitro studies, warranting further research through in vivo models and clinical trials to validate their neuroprotective effects and mechanisms. The pathological changes could also include altered brain metabolism, excitotoxicity, free radical generation, neuroinflammation, blood-brain barrier dysfunction, and apoptosis. Phytochemicals derived from dietary sources can mitigate secondary brain injury by activating endogenous neuroprotective mechanisms.
During the secondary TBI phase, impaired mitochondrial function reduces ATP production, disrupting ionic homeostasis and promoting anaerobic metabolism. This results in lactic acid accumulation, cytokine release, and free radical generation (Hakiminia et al., 2022). Excitotoxicity also ensues, whereby excess extracellular glutamate overactivates NMDA receptors, increasing intracellular calcium levels and triggering protease activation and nitric oxide production (Engin and Engin, 2021).
Furthermore, oxidative stress arises from mitochondrial dysfunction and inflammation (Khan et al., 2022). This damages lipids, proteins, and nucleic acids. Lastly, activated microglia release proinflammatory cytokines like TNF-alpha and IL-1beta, inducing leukocyte infiltration, edema, and neurotoxicity (Jarrahi et al., 2020). Phytochemicals can modulate these secondary injury mechanisms through their anti-inflammatory, antioxidant, and anti-apoptotic properties (Al-Haj et al., 2022). For instance, curcumin inhibits TNF-alpha release from glial cells. Resveratrol reduces IL-1beta and other cytokines by inhibiting NF-kappaB signaling. Anthocyanins suppress microglial activation and subsequent neuroinflammation. Curcumin and anthocyanins also attenuate oxidative damage by scavenging free radicals and boosting endogenous antioxidants like glutathione (Pandey et al., 2022). Additionally, ginsenosides inhibit glutamate excitotoxicity by modulating NMDA receptor signaling and calcium fluxes.
By mitigating secondary injury processes, phytochemicals can salvage viable neurons and improve neurological outcomes after TBI. In conclusion, phytochemicals activate cytoprotective mechanisms that can potentially prevent the progression of secondary brain damage following TBI. While no FDA-approved drugs currently target TBI pathogenesis, phytochemicals are promising therapeutic candidates warranting further investigation through rigorous in vivo and clinical studies. Optimizing one’s dietary phytochemical intake may help mitigate secondary neurodegeneration and impairment after a TBI. Figure 3 shows some of the phytochemicals as therapeutic agents in TBI management and their mechanism of action.
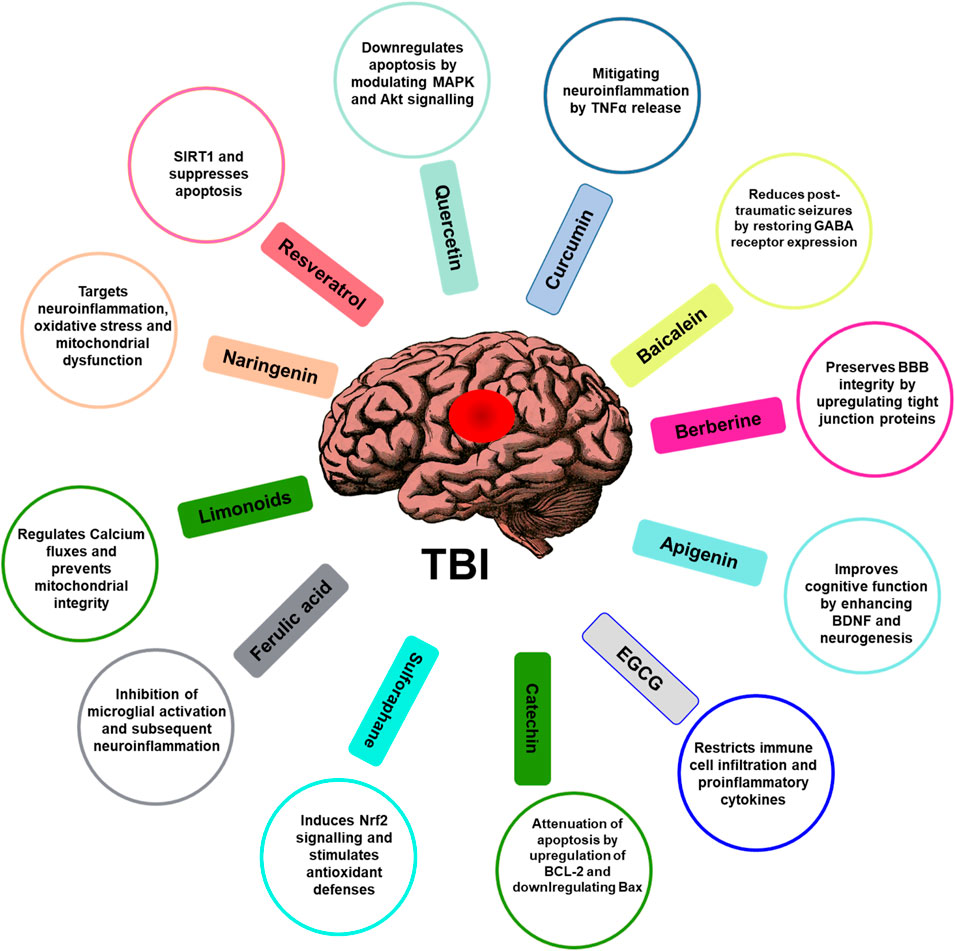
FIGURE 3. Role of phytochemicals in attenuating TBI. Phytochemicals and their mechanism of action associated with the function are illustrated. The figure illustrates phytochemicals such as flavonoids, polyphenols, alkaloids, or other compounds found in plants known for their potential neuroprotective properties in the therapeutic management of TBI and their possible mechanisms of action.
8.1 Resveratrol and its neuroprotective effects in TBI
Resveratrol (3,5,4′-trihydroxy-trans-stilbene) is a natural polyphenol in various plants such as grapes, peanuts, and berries (Ahmed et al., 2017). Several preclinical studies have elucidated the ameliorative effects of resveratrol against TBI-induced neuronal damage and cognitive impairments. Resveratrol remarkably attenuates neuronal apoptosis triggered by TBI. In a mouse model, resveratrol pretreatment conferred antiapoptotic effects by downregulating Bax, upregulating Bcl-2, and inhibiting caspase-3 activation (Xu et al., 2019).
The ability of resveratrol to modulate sirtuin-1 (SIRT1) and suppress acetylation of p53, a pro-apoptotic protein, has also been evidenced (Sin et al., 2014). A recent study showed that resveratrol pretreatment prevented neuronal apoptosis by reducing the expression of miR-21 through SIRT1 activation in a rat model of TBI (Kaminska et al., 2016). Resveratrol exhibits antioxidative properties that aid in combating TBI-induced oxidative stress. Administration of resveratrol suppresses malondialdehyde (MDA) and nitric oxide (NO) levels and restores glutathione (GSH) levels and superoxide dismutase (SOD) activity (Zhang et al., 2019). The Nrf2/HO-1 signaling cascade is also activated by resveratrol to confer neuroprotection against oxidative damage.
Neuroinflammation is another hallmark of secondary injury after TBI. Resveratrol attenuates the expression of proinflammatory mediators like IL-1β, IL-6, TNF-α, iNOS, and COX-2 (Rashad et al., 2018). The NF-κB pathway is also inhibited by resveratrol, thus reducing inflammation. In a mouse model, resveratrol suppressed neutrophil infiltration, microglia activation, and cytokine production induced by TBI (Phillips and Fahimi, 2018). In preclinical studies, resveratrol exhibits pleiotropic effects against TBI-induced neuronal apoptosis, oxidative stress, and neuroinflammation. Given its high bioavailability and ability to cross the blood-brain barrier, resveratrol is a potential adjuvant therapeutic for ameliorating secondary brain damage after TBI. However, further clinical trials are necessitated to establish its efficacy and safety for clinical use.
8.2 Quercetin and its ameliorating effects against TBI
Quercetin (3,3′,4′,5,7-pentahydroxyflavone) is a ubiquitous bioactive plant flavonoid in fruits, vegetables, leaves, seeds, and grains having numerous health benefits (Yousuf et al., 2020; Waseem et al., 2022). Emerging preclinical evidence indicates the neuroprotective effects of quercetin against neuronal damage associated with TBI. Quercetin treatment remarkably attenuates apoptotic cell death after TBI by modulating critical proteins involved in the mitochondrial apoptotic pathway. Studies report downregulation of Bax, cytochrome c, cleaved caspase-3, and upregulation of Bcl-2 levels upon quercetin administration in rodent models.
Quercetin also reduces neuronal apoptosis by inhibiting MAPK signaling and restoring PI3K/Akt pathway (Grewal et al., 2021). Furthermore, quercetin’s antioxidative activity facilitates oxidative stress-mediated secondary damage post-TBI. Quercetin treatment decreases malondialdehyde (MDA) and 8-iso-prostaglandin F2α (8-iso-PGF2α) levels and increases antioxidant enzymes like SOD and glutathione peroxidase (GPx) (Moslemi et al., 2022). Quercetin also exhibited free radical scavenging capacity by elevating glutathione (GSH) levels in the injured rat brain (Ansari et al., 2008). Neuroinflammation is a crucial contributor to TBI-induced neuropathology. Quercetin is evidenced to mitigate glial activation, inhibit production of pro-inflammatory cytokines like TNF-α, IL-1β, and IL-6, and attenuate NF-κB signaling after TBI. Moreover, quercetin reduces leukocyte rolling and adhesion to brain microvessels, indicating its anti-inflammatory effects (Shen et al., 2019). In TBI models, quercetin treatment confers multifaceted neuroprotection against oxidative stress, neuroinflammation, and apoptosis. However, clinical trials are warranted to translate the promising preclinical findings into viable therapeutic approaches for TBI patients.
8.3 Curcumin and its pleiotropic effects against TBI
Curcumin, a polyphenol derived from turmeric, has emerged as a potential neuroprotective phytochemical for TBI owing to its multifaceted effects. Studies demonstrate the potent antioxidant capacity of curcumin by mitigating oxidative damage and restoring the endogenous antioxidant systems after TBI. Curcumin remarkably reduces malondialdehyde (MDA) and nitrotyrosine levels and induces superoxide dismutase (SOD) and glutathione peroxidase (GPx) post-TBI (Hakiminia et al., 2022). Additionally, curcumin exhibits significant anti-inflammatory properties by downregulating microglial activation, reducing proinflammatory cytokines, and attenuating TLR4/MyD88/NF-κB signaling cascade after TBI (Xie et al., 2014; Zhu et al., 2014). Curcumin also reduces BBB disruption by modulating matrix metalloproteinases and tight junction proteins (Yang et al., 2007).
Curcumin shows neuroprotective effects by stabilizing mitochondrial membrane potential, inhibiting cytochrome c release and subsequent caspase-3 activation, leading to reduced apoptosis (Jayaraj et al., 2013). It also stimulates mitochondrial biogenesis in the injured rat brain by upregulating PGC-1α and NRF-1. Autophagic flux disruption is another crucial pathology in TBI mitigated by curcumin treatment via mTOR inhibition and AMPK/ULK1 pathway activation. Enhanced hippocampal neurogenesis is also evidenced upon curcumin administration in TBI models (Kim et al., 2011). Thus, the neuroprotective effects exhibited by curcumin in preclinical TBI models can be attributed to its ability to modulate oxidative stress, neuroinflammation, mitochondrial dysfunction, and autophagy. Further clinical studies substantiating curcumin’s therapeutic potential would pave the way for its clinical translation in TBI management.
8.4 Baicalein mitigates secondary injury after TBI
Baicalein is a bioactive flavone derived from the roots of Scutellaria baicalensis. Emerging evidence from preclinical studies indicates baicalein’s neuroprotective effects against neuronal damage associated with TBI. Post-traumatic seizures are a common occurrence after TBI, which exacerbates neuropathology. Baicalein administration reduces seizure severity, duration, and frequency in rodent models of TBI (Fu et al., 2020). It also restores the decreased expression of γ-aminobutyric acid (GABA) receptors induced by TBI in the rat hippocampus. Additionally, baicalein exhibits antioxidant activity by enhancing superoxide dismutase (SOD) and glutathione peroxidase (GPx) antioxidant enzymes and reducing malondialdehyde (MDA) levels after TBI (Fang et al., 2018). It also mitigates inflammation by downregulating microglial activation, NF-κB signaling, and proinflammatory cytokine production in the injured rat brain (Kong et al., 2019).
Apoptosis of neurons is a crucial contributor to TBI-induced neurologic impairments. Studies demonstrate baicalein remarkably reduces neuronal apoptosis by downregulating the expression of pro-apoptotic proteins like Bax, cleaved caspase-3, and upregulating the anti-apoptotic Bcl-2 protein (Liu et al., 2018). It also activates the PI3k/Akt pathway to inhibit neuronal apoptosis after TBI. Thus, we can conclude that baicalein treatment confers neuroprotection against post-traumatic seizures, oxidative damage, neuroinflammation and neuronal apoptosis in animal models of TBI. Further investigations into baicalein’s therapeutic potential, especially using higher-order species, would help facilitate its clinical translation for TBI management.
8.5 Berberine exhibits neuroprotection in TBI models
Berberine is a natural isoquinoline alkaloid found in various plants such as barberry, goldenseal, and Oregon grape. Studies indicate berberine’s therapeutic potential for ameliorating secondary brain damage following TBI. Berberine administration is evidenced to improve cognitive impairment and neurological deficits and reduce brain edema in rodent models of TBI. It is found to upregulate tight junction proteins like occludin, which helps maintain blood-brain barrier integrity after TBI (Li R. et al., 2022a). Additionally, berberine exhibits anti-inflammatory effects by inhibiting microglial activation, reducing TNF-α, IL-1β, and IL-6 levels, and attenuating the NF-κB pathway in the injured brain (Li et al., 2023a). Berberine also inhibits MMP-9 activity, thereby facilitating neuroinflammation and BBB disruption. Apoptosis of hippocampal neurons is mitigated by berberine through downregulation of ROS, increased Bcl-2/Bax ratio, and prevention of mitochondrial cytochrome c release in models of TBI. Berberine also reduces apoptosis by modulating the PI3K/Akt and p38-MAPK pathways (Han et al., 2023).
Moreover, berberine promotes neurogenesis in the dentate gyrus after TBI by increasing the expression of Brain-derived neurotrophic factor (BDNF) (Suwannakot et al., 2022). Enhanced neurogenesis by berberine leads to improved cognitive recovery following TBI. Berberine treatment confers neuroprotection against oxidative stress, neuroinflammation, and apoptosis. It ameliorates functional outcomes post-TBI. Further preclinical and clinical studies could provide evidence for berberine’s clinical utility in attenuating secondary brain injury after TBI.
8.6 Apigenin ameliorates neuronal damage following TBI
Apigenin is a natural flavone in many fruits and vegetables, such as parsley, onions, and oranges. Emerging evidence indicates apigenin’s therapeutic potential in attenuating secondary brain injury after TBI (Welcome, 2020). Studies demonstrate that apigenin administration improves cognitive and memory deficits in TBI rodent models, as evidenced by enhanced performance in the Morris water maze and Step-down inhibitory avoidance tests (Guo et al., 2023). Apigenin also preserves hippocampal synaptic plasticity and upregulates BDNF, contributing to its nootropic effects post-TBI. Additionally, apigenin exhibits antioxidant activity by increasing superoxide dismutase (SOD), glutathione peroxidase (GPx), and reduced malondialdehyde (MDA) levels in the injured brain (T. Zhang et al., 2015). It also stimulates Nrf2 signaling, which induces endogenous antioxidant enzyme expression after TBI.
Apigenin showed anti-inflammatory effects by suppressing microglial activation, reducing TNF-α, IL-1β and attenuating the NF-κB pathway in TBI models (Li Y. et al, 2022b). It also inhibited neuronal apoptosis by modulating Bax/Bcl-2 expression and caspase-3 activation (K. Kim and Choi, 2021). Moreover, apigenin promotes adult neurogenesis in the hippocampus, further contributing to cognitive recovery following TBI. Overall, apigenin treatment confers multifaceted neuroprotection against oxidative damage, neuroinflammation, and neuronal loss induced by TBI. Further studies could provide pre-clinical evidence supporting apigenin’s translational potential in TBI management.
8.7 Epigallocatechin gallate ameliorates secondary injury following TBI
Epigallocatechin gallate (EGCG) is a natural polyphenol and the most abundant catechin in green tea (M. Alam, S. Ali, et al., 2022). Emerging evidence from preclinical studies indicates therapeutic potential of EGCG in attenuating secondary brain damage after TBI (Sebastiani et al., 2021). EGCG administration significantly reduces brain infarct volume and edema in rodent models of TBI (Yessenkyzy et al., 2020). EGCG also prevents blood-brain barrier disruption by modulating tight junction proteins and restricts the extravasation of blood-derived products into the brain parenchyma. Additionally, EGCG exhibits anti-inflammatory effects by inhibiting microglial activation, reducing TNF-α, IL-1β, and IL-6, and attenuating the NF-κB pathway in the injured rat brain (Li R. et al., 2022a). EGCG also reduces neutrophil infiltration and proinflammatory cytokine production after TBI (Zeng et al., 2023). EGCG remarkably attenuates neuronal apoptosis in the hippocampus induced by TBI by upregulating Bcl-2, downregulating Bax, and cleaved caspase-3 expression (Ye et al., 2023). It also reduces apoptosis by activating the Nrf2 signaling pathway and inducing antioxidant enzymes.
EGCG promotes adult neurogenesis in the dentate gyrus and subventricular zone post-TBI, contributing to cognitive recovery (Salehpour et al., 2023). EGCG treatment confers neurovascular protection and ameliorates neuroinflammation, oxidative damage and neuronal loss induced by TBI. Further preclinical and clinical studies could provide evidence for EGCG’s translational potential in TBI management.
8.8 Catechin attenuates secondary pathology following TBI
Catechin is a natural flavan-3-ol found abundantly in green tea, cocoa, and many fruits. Emerging preclinical evidence indicates the therapeutic potential of catechin in ameliorating secondary brain damage after TBI (Salehpour et al., 2023). Studies demonstrate that catechin administration significantly improves neurological function, as evidenced by reduced Neurological Severity Score (NSS) in rodent models of TBI (Y. Wu and Cui, 2020). Catechin treatment also remarkably improves sensorimotor deficits post-TBI in rats (Wu and Cui, 2020). Additionally, catechin exhibits anti-inflammatory properties by suppressing microglial activation reducing TNF-α, IL-1β, and IL-6 levels. It inhibits the NF-κB pathway in the injured rat brain. Catechin attenuates neuronal apoptosis in the cortex and hippocampus induced by TBI by downregulating Bax, cleaved caspase-3 expression, and upregulating Bcl-2 levels (Gupta et al., 2021). It also reduces neuronal apoptosis by activating Nrf2 signaling and inducing antioxidant enzymes.
Catechin administration increases hippocampal neurogenesis in the subgranular zone, contributing to cognitive recovery following TBI (Z. Xu, Chen et al., 2006). Catechin treatment confers neuroprotection against neuroinflammation and oxidative damage. It improves functional outcomes post-TBI. Further studies could provide pre-clinical evidence for catechin’s translational potential in TBI management.
8.9 Sulforaphane mitigates secondary brain injury after TBI
Sulforaphane is an organosulfur compound derived from cruciferous vegetables like broccoli and Brussels sprouts. Emerging preclinical evidence indicates the neuroprotective effects of sulforaphane in TBI models. Studies demonstrate that sulforaphane administration improves the TBI rodent model’s cognitive function and spatial learning deficits (Gray et al., 2022). It also inhibits neuronal apoptosis in the hippocampus induced after TBI (Uddin et al., 2020). Additionally, sulforaphane exhibits antioxidant effects by increasing superoxide dismutase (SOD), glutathione peroxidase (GPx) activity, reducing malondialdehyde (MDA) and nitric oxide (NO) levels in the injured brain (Chen et al., 2021). It also induces Nrf2 signaling, which stimulates endogenous antioxidant defenses post-TBI.
Sulforaphane exerts anti-neuroinflammatory effects by suppressing microglial activation, reducing TNF-α, IL-1β, IL-6. It inhibits the NF-κB pathway in the injured cortex and hippocampus (Q. Wu et al., 2021). It also restricts the infiltration of peripheral immune cells into the brain. Sulforaphane promotes neurogenesis in the subgranular and subventricular zone post-TBI, thereby aiding cognitive recovery (Thapa et al., 2021). Sulforaphane treatment confers neuroprotection against oxidative stress neuroinflammation and improves functional outcomes in TBI models. Further clinical evaluation could provide evidence for sulforaphane’s translational value in attenuating secondary brain injury after TBI.
8.10 Ferulic acid exhibits neuroprotection in TBI models
Ferulic acid is a natural phenolic compound found abundantly in fruits, vegetables, and grains. Emerging evidence indicates the therapeutic potential of ferulic acid in attenuating secondary brain injury following TBI (Li Y. et al., 2022b). Studies demonstrate that ferulic acid administration dose-dependently improves neurobehavioral function, including motor coordination in mouse TBI models and reduces brain edema and neuronal loss in the injured cortex (Li R. et al., 2022a). Additionally, ferulic acid exhibits antioxidant activity by upregulating nuclear factor erythroid 2–related factor 2 (Nrf2) signaling and inducing superoxide dismutase (SOD), glutathione peroxidase (GPx) antioxidant enzymes in the injured brain (Adeyi et al., 2023). It also suppresses lipid peroxidation and nitric oxide (NO) levels post-TBI (J. Chen et al., 2023). Ferulic acid attenuates neuroinflammation by inhibiting microglial activation, reducing TNF-α, IL-1β, IL-6 levels, and NF-κB activity in the injured rat brain (Sun, Ma, Li et al., 2022). It also restricts the infiltration of peripheral neutrophils and macrophages into the brain parenchyma.
Ferulic acid inhibits the TLR4/MyD88 innate immune signaling pathway, which produces proinflammatory cytokines after TBI (Li Y. et al., 2022b). Overall, ferulic acid treatment confers neuroprotection against oxidative damage and neuroinflammation, improving outcomes in TBI models. Further clinical studies could provide evidence for ferulic acid’s translational potential for TBI management.
8.11 Role of limonoids in TBI
Limonoids are a class of highly oxygenated triterpenoid compounds found abundantly in citrus fruits. Emerging evidence indicates limonoids confer neuroprotection in models of neurodegenerative diseases, including TBI (Mukherjee et al., 2019). TBI pathology involves primary injury directly induced by biomechanical forces, succeeded by secondary injury mediated by excitotoxicity, calcium dyshomeostasis, oxidative stress, neuroinflammation, and mitochondrial dysfunction (Maas et al., 2017). Limonoids may confer neuroprotection by ameliorating these secondary injury factors.
Dyshomeostasis of calcium is another crucial pathological event in TBI. TBI causes excessive glutamate release and overstimulation of NMDA receptors, resulting in cytotoxic calcium influx. Limonoids may confer protection by regulating calcium flux. For instance, limonin demonstrated anti-excitotoxic effects in murine hippocampal neurons exposed to glutamate (Nagini et al., 2023). Limonin attenuated calcium influx, preventing oxidative damage and loss of viability. Mitochondrial dysfunction is both a consequence and driver of secondary injury after TBI (Thapa et al., 2021). Limonoids protect mitochondrial integrity by reducing calcium overload and oxidative damage (Qi and Wang, 2020). Limonoids may also stimulate the biogenesis of healthy mitochondria. In TBI rats, increasing mitochondrial mass, Obacunone upregulated PGC-1α and mitochondrial transcription factors.
Limonoids exhibit versatile neuroprotective mechanisms that target key secondary injury factors like neuroinflammation, oxidative stress, excitotoxicity, and mitochondrial dysfunction. While most evidence involves rodent TBI models, emerging clinical research also reveals therapeutic potential. For instance, a pilot study on TBI patients found daily orange juice consumption improved antioxidant status and reduced lipid peroxidation (Cao et al., 2021). Given the lack of effective TBI pharmacological treatments, further research on limonoids is warranted.
8.12 Neuroprotective role of naringenin in TBI
Naringenin is a flavonoid prevalent in citrus fruits that has exhibited antioxidant properties and shown neuroprotective effects in preclinical models of TBI (Anwar et al., 2021; Yousuf et al., 2022; Li et al., 2023b). The primary mechanical injury in TBI triggers secondary injury cascades involving neuroinflammation, oxidative stress, mitochondrial dysfunction, and excitotoxicity. Naringenin may mitigate these secondary injury factors through its anti-inflammatory, antioxidant, and anti-apoptotic properties. Naringenin reduced hippocampal expression of proinflammatory cytokines, including TNF-α, IL-1β, and IL-6, in a controlled cortical impact model of TBI in mice (Culhuac et al., 2023). This anti-neuroinflammatory effect was accompanied by attenuated neuronal apoptosis, edema, and improved cognitive function.
The anti-inflammatory actions were mediated by inhibiting NF-κB and MAPK signaling. In another study, naringenin ameliorated neurobehavioral deficits and improved long-term potentiation in the rat hippocampus after TBI by suppressing microglial activation (Wang et al., 2023). Naringenin also exhibits antioxidant activity by scavenging ROS and inducing endogenous antioxidant defenses. In a rat model, naringenin treatment following TBI elevated glutathione peroxidase and superoxide dismutase activity while reducing malondialdehyde levels in the hippocampus (Long et al., 2020). This antioxidant effect was associated with reduced neuronal apoptosis and cerebral edema. Naringenin may induce antioxidant defenses by activating the Nrf2 pathway. Mitochondrial dysfunction is a central secondary injury mechanism in TBI mediated by calcium overload and ROS-induced damage (Thapa et al., 2021).
Naringenin attenuates mitochondrial membrane potential dissipation, preserves complex I activity, and inhibits cytochrome c release in TBI rats (Bai et al., 2020). This mitochondrial protection was accompanied by reduced neuronal apoptosis. Excitotoxicity resulting from excessive glutamate release is another secondary injury factor in naringenin targets. In mixed neuronal-glial cultures exposed to glutamate excitotoxicity, naringenin inhibited calcium influx through NMDA receptors and subsequent activation of apoptotic pathways (Socała et al., 2020). Naringenin confers multifaceted protective effects in TBI models by targeting neuroinflammation, oxidative stress, mitochondrial dysfunction, and excitotoxicity. While rodent studies show promising results, clinical research is still lacking. One pilot study found that supplementation with a citrus bioflavonoid blend containing naringenin improved cognitive performance in TBI patients (Atoki et al., 2023). Further clinical trials are warranted to validate the therapeutic potential of naringenin in TBI.
9 Future directions and challenges
TBI has emerged as a significant global health and socioeconomic challenge, placing a substantial healthcare burden on contemporary society and necessitating the development of efficient treatment strategies (Maxwell, 2012). They suppress neuronal cell death mechanisms and restore normal function in non-neuronal cells by employing occupational therapies (Bayley et al., 2023; Wheeler and Acord-Vira, 2023). Many treatments have been devised, encompassing neurorestorative, anti-inflammatory, and neuroprotective agents.
Nevertheless, one of the immediate ramifications of TBI pertains to preserving the BBB, as the impairment of the BBB following TBI contributes to subsequent harm. Peptides and therapeutic proteins are the exclusive substances capable of traversing the endothelial tight junctions and reaching the injury site through the intranasal route. In the context of an animal model of TBI, it is possible to directly administer therapeutic agents to the cerebrospinal fluid (CSF) via an intraventricular pathway. However, in clinical practice, surgical intervention is frequently necessary to alleviate intracranial pressure and oedema, allowing for the direct delivery of drugs. Hence, the development of an efficient drug delivery system has the potential to facilitate a continuous and regulated release of therapeutic substances, thereby fostering recovery from the secondary damage to the brain following TBI (Aqel et al., 2023; Mohammed et al., 2023).
Neuroprotective approaches in treating TBI are designed to address distinct mechanisms within the intricate cascade of secondary injuries. Historically, the primary focus has been on modifying the cellular processes after the injury, constituting an essential aspect of neuroprotection. In contemporary neuroprotection strategies, the emphasis is on therapeutic interventions that initiate the restoration of neurons with optimal functionality by restraining the principal mechanism of cell death. Focusing on precise molecular mechanisms for managing and providing post-injury care in TBI is paramount. There is a critical necessity to progress and innovate new treatments to minimize the repercussions of TBI. Numerous therapies directed at specific targets have been suggested, some of which have yet to undergo clinical assessment. There should be an increased emphasis on formulating outcome-oriented treatments to address the management and treatment of TBI efficiently.
Phytochemicals have emerged as adjuvant and complementary medicines in the treatment of various disorders (Azhar et al., 2023; Majrashi et al., 2023). Phytochemicals possess various beneficial properties, including antioxidant, anti-inflammatory, anti-cancer, neuro-protectant, etc. As mentioned in the review, various phytochemicals have shown positive roles in recovery from TBI. However, various challenges are encountered in the use of polyphenols for the treatment of TBIs, which include: i. Bioavailability: Numerous phytochemicals exhibit limited bioavailability, implying they are inadequately absorbed and processed within the body, thereby constraining their capacity to reach the damaged brain tissue effectively. ii. BBB restrictions: The BBB serves as a barrier that hampers the passage of specific phytochemicals into the brain, posing a challenge for these compounds to exert their neuroprotective effects directly at the injury site. iii. Dosage: Establishing the ideal dosage and timing for administering phytochemicals to realize therapeutic advantages for TBIs is a complex task, given that distinct polyphenols can have differing pharmacokinetics and mechanisms of action. iv. Lack of clinical evidence: Although there is encouraging preclinical research indicating the neuroprotective potential of phytochemicals in TBIs, the absence of comprehensive clinical data hinders the ability to substantiate their effectiveness and safety in human patients. v. Interactions with medications: Phytochemicals can potentially interact with medications frequently employed in the treatment of TBIs, which may result in adverse effects or modifications in drug metabolism. vi. Regulatory approvals: Securing regulatory approval for TBI treatments based on phytochemicals can be a daunting task because of the intricate characteristics of polyphenols and the requirement for rigorous clinical trials.
Understanding the diverse nature of TBIs may pave the way for personalised or precision medicine strategies. Tailoring treatments to an individual’s injury characteristics, genetics, and other factors could optimize therapeutic interventions. Ongoing research into neuroprotective agents aims to identify drugs or compounds that can mitigate the initial and secondary damage caused by TBI, targeting various pathways such as inflammation, excitotoxicity, and oxidative stress. Focusing on the inflammatory response associated with TBI holds promise for future treatments, as modulating the immune response may reduce secondary injury processes and enhance overall recovery. The exploration of stem cells for neural repair and regeneration is an active area of research, offering promise for tissue healing and functional recovery after TBI.
Additionally, ongoing efforts in developing targeted drug delivery systems capable of effectively penetrating the blood-brain barrier and delivering therapeutic agents directly to the injury site represent a crucial research focus. Emerging technologies, including nanotechnology, may contribute to advancements in this field. While these future directions present potential breakthroughs, translating research findings into clinically effective treatments requires sustained dedication, collaboration, and a profound understanding of the intricate nature of traumatic brain injury (Dwyer and Morrison, 2022; Hanna and Pfister, 2023; Johnson et al., 2023; Popa et al., 2023; Rauchman et al., 2023).
10 Conclusion
In summary, the application of phytochemicals in the context of traumatic brain injury (TBI) presents both promising benefits and significant challenges. While preclinical studies offer robust evidence of the neuroprotective and anti-inflammatory properties associated with various phytochemical compounds, confirming their clinical efficacy and safety in human TBI patients is notably lacking, primarily due to the absence of comprehensive clinical data. A major hurdle in realizing the therapeutic potential of phytochemicals lies in their limited bioavailability and the potential for interactions with conventional medications used in TBI treatment. Determining the optimal dosage, timing, and administration methods for phytochemical interventions is complex, given the diverse range of polyphenols and their distinct pharmacokinetic profiles. Unleashing the full potential of phytochemicals in TBI management requires further dedicated research efforts. Despite the potential of phytochemicals as supplementary therapies for TBI, their integration into clinical practice and attainment of regulatory approval necessitates a thorough understanding of their impacts, a meticulous assessment of potential interactions with existing treatments, and the precise design of clinical trials. Despite these challenges, the prospective advantages of phytochemical-based treatments in improving TBI outcomes make them an enticing avenue for ongoing research and development.
Author contributions
GH: Conceptualization, Investigation, Methodology, Project administration, Resources, Supervision, Visualization, Writing–original draft. SA: Conceptualization, Data curation, Formal Analysis, Investigation, Methodology, Project administration, Resources, Visualization, Writing–original draft. AS: Funding acquisition, Investigation, Methodology, Project administration, Software, Supervision, Validation, Visualization, Writing–review and editing. SS: Data curation, Formal Analysis, Investigation, Supervision, Visualization, Writing–review and editing. MH: Data curation, Formal Analysis, Funding acquisition, Investigation, Project administration, Software, Supervision, Visualization, Writing–review and editing.
Funding
The author(s) declare financial support was received for the research, authorship, and/or publication of this article. This work is sponsored by Prince Sattam Bin Abdulaziz University (Grant No. 2023/RV/012).
Acknowledgments
GH thanks the Prince Sattam Bin Abdulaziz University (Grant No. 2023/RV/012).
Conflict of interest
The authors declare that the research was conducted in the absence of any commercial or financial relationships that could be construed as a potential conflict of interest.
The author(s) declared that they were an editorial board member of Frontiers, at the time of submission. This had no impact on the peer review process and the final decision.
Publisher’s note
All claims expressed in this article are solely those of the authors and do not necessarily represent those of their affiliated organizations, or those of the publisher, the editors and the reviewers. Any product that may be evaluated in this article, or claim that may be made by its manufacturer, is not guaranteed or endorsed by the publisher.
Abbreviations
Traumatic brain injury, TBI; Secondary injury cascades, SIC; Blood-brain barrier, BBB; Neurodegenerative Disorders, NDs; Alzheimer’s Disease, AD; Parkinson’s Disease, PD; Altered Levels of Consciousness, LOC; Post-Traumatic Amnesia, PTA; Glasgow Coma Scale, GCS; Computed Tomography, CT; Magnetic Resonance Imaging, MRI.
References
Adeyi, O. E., Somade, O. T., James, A. S., Adeyi, A. O., Ogbonna-Eze, S. N., Salako, O. Q., et al. (2023). Ferulic acid mitigates 2-methoxyethanol-induced testicular oxidative stress via combined downregulation of FoxO1, PTEN, and modulation of Nrf2-Hmox1-NQO1 signaling pathway in rats. Pharmacol. Research-Modern Chin. Med. 7, 100257. doi:10.1016/j.prmcm.2023.100257
Ahmed, T., Javed, S., Javed, S., Tariq, A., Šamec, D., Tejada, S., et al. (2017). Resveratrol and Alzheimer’s disease: mechanistic insights. Mol. Neurobiol. 54, 2622–2635. doi:10.1007/s12035-016-9839-9
Alam, M., Ahmed, S., Elasbali, A. M., Adnan, M., Alam, S., Hassan, M. I., et al. (2022a). Therapeutic implications of caffeic acid in cancer and neurological diseases. Front. Oncol. 12, 860508. doi:10.3389/fonc.2022.860508
Alam, M., Ali, S., Ashraf, G. M., Bilgrami, A. L., Yadav, D. K., and Hassan, M. I. (2022b). Epigallocatechin 3-gallate: from green tea to cancer therapeutics. Food Chem. 379, 132135. doi:10.1016/j.foodchem.2022.132135
Alam, M., Hasan, G. M., Ansari, M. M., Sharma, R., Yadav, D. K., and Hassan, M. I. (2022c). Therapeutic implications and clinical manifestations of thymoquinone. Phytochemistry 200, 113213. doi:10.1016/j.phytochem.2022.113213
Alam, S., Mohammad, T., Padder, R. A., Hassan, M. I., and Husain, M. (2022d). Thymoquinone and quercetin induce enhanced apoptosis in non-small cell lung cancer in combination through the Bax/Bcl2 cascade. J. Cell Biochem. 123 (2), 259–274. doi:10.1002/jcb.30162
Al-Haj, N., Issa, H., Zein, O. E., Ibeh, S., Reslan, M. A., Yehya, Y., et al. (2022). “Phytochemicals as micronutrients: what is their therapeutic promise in the management of traumatic brain injury?,” in Role of micronutrients in brain health (Springer), 245–276.
Ali, S., Alam, M., Khatoon, F., Fatima, U., Elasbali, A. M., Adnan, M., et al. (2022). Natural products can be used in therapeutic management of COVID-19: probable mechanistic insights. Biomed. Pharmacother. 147, 112658. doi:10.1016/j.biopha.2022.112658
Al Mamun, A., Hashimoto, M., Katakura, M., Hossain, S., and Shido, O. (2015). NEUROPROTECTIVE EFFECT OF THYMOQUINONE AGAINST GLUTAMATE-INDUCED TOXICITY IN SH-SY5Y CELLS. Curr. Top. Nutraceutical Res. 13 (3).
Anbai, S., Niazi, F., Siahposht-Khachaki, A., Sefidari, L., and Farzin, D. (2022). Neuroprotective effects of berberine after severe traumatic brain injury in male rats: the role of IL-1β and IL 10. J. Mazandaran Univ. Med. Sci. 31 (204), 1–13.
Ansari, M. A., Roberts, K. N., and Scheff, S. W. (2008). Oxidative stress and modification of synaptic proteins in hippocampus after traumatic brain injury. Free Radic. Biol. Med. 45 (4), 443–452. doi:10.1016/j.freeradbiomed.2008.04.038
Anwar, S., Khan, S., Shamsi, A., Anjum, F., Shafie, A., Islam, A., et al. (2021). Structure-based investigation of MARK4 inhibitory potential of Naringenin for therapeutic management of cancer and neurodegenerative diseases. J. Cell Biochem. 122 (10), 1445–1459. doi:10.1002/jcb.30022
Anwar, S., Mohammad, T., Azhar, M. K., Fatima, H., Alam, A., Hasan, G. M., et al. (2023). Investigating MARK4 inhibitory potential of Bacopaside II: targeting Alzheimer's disease. Int. J. Biol. Macromol. 245, 125364. doi:10.1016/j.ijbiomac.2023.125364
Aqel, S., Al-Thani, N., Haider, M. Z., Abdelhady, S., Al Thani, A. A., Kobeissy, F. H., et al. (2023). Biomaterials in traumatic brain injury: challenges and perspectives.
Atoki, A. V., Aja, P. M., Shinkafi, T. S., Ondari, E. N., and Awuchi, C. G. (2023). Naringenin: its chemistry and roles in neuroprotection. Nutr. Neurosci., 1–30. doi:10.1080/1028415X.2023.2243089
Azhar, M. K., Anwar, S., Hasan, G. M., Shamsi, A., Islam, A., Parvez, S., et al. (2023). Comprehensive insights into biological roles of rosmarinic acid: implications in diabetes, cancer and neurodegenerative diseases. Nutrients 15 (19), 4297. doi:10.3390/nu15194297
Bai, X., Tan, T.-Y., Li, Y.-X., Li, Y., Chen, Y.-F., Ma, R., et al. (2020). The protective effect of cordyceps sinensis extract on cerebral ischemic injury via modulating the mitochondrial respiratory chain and inhibiting the mitochondrial apoptotic pathway. Biomed. Pharmacother. 124, 109834. doi:10.1016/j.biopha.2020.109834
Bak, D.-H., Kim, H. D., Kim, Y. O., Park, C. G., Han, S.-Y., and Kim, J.-J. (2016). Neuroprotective effects of 20 (S)-protopanaxadiol against glutamate-induced mitochondrial dysfunction in PC12 cells. Int. J. Mol. Med. 37 (2), 378–386. doi:10.3892/ijmm.2015.2440
Battaglini, D., Siwicka-Gieroba, D., Rocco, P. R., Cruz, F. F., Silva, P. L., Dabrowski, W., et al. (2021). Novel synthetic and natural therapies for traumatic brain injury. Curr. Neuropharmacol. 19 (10), 1661–1687. doi:10.2174/1570159X19666210225145957
Bayley, M. T., Janzen, S., Harnett, A., Teasell, R., Patsakos, E., Marshall, S., et al. (2023). INCOG 2.0 guidelines for cognitive rehabilitation following traumatic brain injury: methods, overview, and principles. J. Head Trauma Rehabilitation 38 (1), 7–23. doi:10.1097/HTR.0000000000000838
Bie, X.-D., Han, J., and Dai, H.-B. (2010). Effects of hydroxysafflor yellow A on the experimental traumatic brain injury in rats. J. Asian Nat. Prod. Res. 12 (3), 239–247. doi:10.1080/10286020903510636
Blyth, B. J., and Bazarian, J. J. (2010). Traumatic alterations in consciousness: traumatic brain injury. Emerg. Med. Clin. 28 (3), 571–594. doi:10.1016/j.emc.2010.03.003
Blyth, B. J., Farhavar, A., Gee, C., Hawthorn, B., He, H., Nayak, A., et al. (2009). Validation of serum markers for blood-brain barrier disruption in traumatic brain injury. J. neurotrauma 26 (9), 1497–1507. doi:10.1089/neu.2008.0738
Bodien, Y. G., Barra, A., Temkin, N. R., Barber, J., Foreman, B., Vassar, M., et al. (2021). Diagnosing level of consciousness: the limits of the glasgow coma scale total score. J. neurotrauma 38 (23), 3295–3305. doi:10.1089/neu.2021.0199
Brustovetsky, T., Bolshakov, A., and Brustovetsky, N. (2010). Calpain activation and Na+/Ca2+ exchanger degradation occur downstream of calcium deregulation in hippocampal neurons exposed to excitotoxic glutamate. J. Neurosci. Res. 88 (6), 1317–1328. doi:10.1002/jnr.22295
Bye, N., Habgood, M. D., Callaway, J. K., Malakooti, N., Potter, A., Kossmann, T., et al. (2007). Transient neuroprotection by minocycline following traumatic brain injury is associated with attenuated microglial activation but no changes in cell apoptosis or neutrophil infiltration. Exp. Neurol. 204 (1), 220–233. doi:10.1016/j.expneurol.2006.10.013
Cao, Y., Yang, L., Qiao, X., Xue, C., and Xu, J. (2021). Dietary astaxanthin: an excellent carotenoid with multiple health benefits. Crit. Rev. Food Sci. Nutr. 63, 3019–3045. doi:10.1080/10408398.2021.1983766
Chamoun, R., Suki, D., Gopinath, S. P., Goodman, J. C., and Robertson, C. (2010). Role of extracellular glutamate measured by cerebral microdialysis in severe traumatic brain injury. J. Neurosurg. 113 (3), 564–570. doi:10.3171/2009.12.JNS09689
Chang, C.-H., Chen, H.-X., Yü, G., Peng, C.-C., and Peng, R. Y. (2014). Curcumin-protected PC12 cells against glutamate-induced oxidative toxicity. Food Technol. Biotechnol. 52 (4), 468–478. doi:10.17113/ftb.52.04.14.3622
Chen, C.-C., Hung, T.-H., Lee, C. Y., Wang, L.-F., Wu, C.-H., Ke, C.-H., et al. (2014). Berberine protects against neuronal damage via suppression of glia-mediated inflammation in traumatic brain injury. PloS one 9 (12), e115694. doi:10.1371/journal.pone.0115694
Chen, J., Zhu, T., Yu, D., Yan, B., Zhang, Y., Jin, J., et al. (2023). Moderate intensity of treadmill exercise rescues TBI-induced ferroptosis, neurodegeneration, and cognitive impairments via suppressing STING pathway. Mol. Neurobiol. 60, 4872–4896. doi:10.1007/s12035-023-03379-8
Chen, L., Zhang, W.-L., Xie, D.-q., and Jia, W. (2021). Sulforaphane alleviates hepatic ischemia–reperfusion injury through promoting the activation of Nrf-2/HO-1 signaling. Transpl. Immunol. 68, 101439. doi:10.1016/j.trim.2021.101439
Chen, S. F., Hsu, C. W., Huang, W. H., and Wang, J. Y. (2008). Post-injury baicalein improves histological and functional outcomes and reduces inflammatory cytokines after experimental traumatic brain injury. Br. J. Pharmacol. 155 (8), 1279–1296. doi:10.1038/bjp.2008.345
Chen, S.-F., Hung, T.-H., Chen, C.-C., Lin, K.-H., Huang, Y.-N., Tsai, H.-C., et al. (2007). Lovastatin improves histological and functional outcomes and reduces inflammation after experimental traumatic brain injury. Life Sci. 81 (4), 288–298. doi:10.1016/j.lfs.2007.05.023
Chiu, C.-C., Liao, Y.-E., Yang, L.-Y., Wang, J.-Y., Tweedie, D., Karnati, H. K., et al. (2016). Neuroinflammation in animal models of traumatic brain injury. J. Neurosci. methods 272, 38–49. doi:10.1016/j.jneumeth.2016.06.018
Çolak, R., Celik, A., Diniz, G., Alkan Özdemir, S., Yilmaz, O., and Calkavur, S. (2021). Evaluation of the neuroprotective effect of pycnogenol in a hypoxic–ischemic brain injury model in newborn rats. Am. J. Perinatology 40 (06), 612–618. doi:10.1055/s-0041-1730349
Corps, K. N., Roth, T. L., and McGavern, D. B. (2015). Inflammation and neuroprotection in traumatic brain injury. JAMA neurol. 72 (3), 355–362. doi:10.1001/jamaneurol.2014.3558
Culhuac, E. B., Maggiolino, A., Elghandour, M. M., De Palo, P., and Salem, A. Z. (2023). Antioxidant and anti-inflammatory properties of phytochemicals found in the yucca genus. Antioxidants 12 (3), 574. doi:10.3390/antiox12030574
Das, M., Mohapatra, S., and Mohapatra, S. S. (2012). New perspectives on central and peripheral immune responses to acute traumatic brain injury. J. neuroinflammation 9, 236. doi:10.1186/1742-2094-9-236
Deng, H., Xu, Q., Guo, H.-Y., Huang, X., Chen, F., Jin, L., et al. (2022). Application of cinnamic acid in the structural modification of natural products: a review. Phytochemistry, 113532. doi:10.1016/j.phytochem.2022.11353
Deng, Y., Thompson, B. M., Gao, X., and Hall, E. D. (2007). Temporal relationship of peroxynitrite-induced oxidative damage, calpain-mediated cytoskeletal degradation and neurodegeneration after traumatic brain injury. Exp. Neurol. 205 (1), 154–165. doi:10.1016/j.expneurol.2007.01.023
Duvdevani, R., Roof, R. L., Fülöp, Z., Hoffman, S. W., and Stein, D. G. (1995). Blood–brain barrier breakdown and edema formation following frontal cortical contusion: does hormonal status play a role? J. neurotrauma 12 (1), 65–75. doi:10.1089/neu.1995.12.65
Dwyer, M. K. R., and Morrison, B. (2022). Recent advancements in in vitro models of traumatic brain injury. Curr. Opin. Biomed. Eng. 23, 100396. doi:10.1016/j.cobme.2022.100396
Engin, A., and Engin, A. B. (2021). N-Methyl-d-aspartate receptor signaling-protein kinases crosstalk in cerebral ischemia. Protein Kinase-mediated Decis. Between Life Death, 259–283. doi:10.1007/978-3-030-49844-3_10
Fang, J., Wang, H., Zhou, J., Dai, W., Zhu, Y., Zhou, Y., et al. (2018). Baicalin provides neuroprotection in traumatic brain injury mice model through Akt/Nrf2 pathway. Drug Des. Dev. Ther. 12, 2497–2508. doi:10.2147/DDDT.S163951
Farkhondeh, T., Samarghandian, S., Shahri, A. M. P., and Samini, F. (2018). The neuroprotective effects of thymoquinone: a review. Dose-response 16 (2), 1559325818761455. doi:10.1177/1559325818761455
Fatima, U., Roy, S., Ahmad, S., Ali, S., Elkady, W. M., Khan, I., et al. (2022b). Pharmacological attributes of Bacopa monnieri extract: current updates and clinical manifestation. Front. Nutr. 9, 972379. doi:10.3389/fnut.2022.972379
Fatima, U., Roy, S., Ahmad, S., Al-Keridis, L. A., Alshammari, N., Adnan, M., et al. (2022a). Investigating neuroprotective roles of Bacopa monnieri extracts: mechanistic insights and therapeutic implications. Biomed. Pharmacother. 153, 113469. doi:10.1016/j.biopha.2022.113469
Feeney, D. M., Boyeson, M. G., Linn, R. T., Murray, H. M., and Dail, W. G. (1981). Responses to cortical injury: I. Methodology and local effects of contusions in the rat. Brain Res. 211 (1), 67–77. doi:10.1016/0006-8993(81)90067-6
Fernández-Gajardo, R., Matamala, J. M., Carrasco, R., Gutiérrez, R., Melo, R., and Rodrigo, R. (2014). Novel therapeutic strategies for traumatic brain injury: acute antioxidant reinforcement. CNS drugs 28, 229–248. doi:10.1007/s40263-013-0138-y
Ferreira, A. P. O., Rodrigues, F. S., Della-Pace, I. D., Mota, B. C., Oliveira, S. M., Gewehr, C. d. C. V., et al. (2013). The effect of NADPH-oxidase inhibitor apocynin on cognitive impairment induced by moderate lateral fluid percussion injury: role of inflammatory and oxidative brain damage. Neurochem. Int. 63 (6), 583–593. doi:10.1016/j.neuint.2013.09.012
Floyd, C. L., Gorin, F. A., and Lyeth, B. G. (2005). Mechanical strain injury increases intracellular sodium and reverses Na+/Ca2+ exchange in cortical astrocytes. Glia 51 (1), 35–46. doi:10.1002/glia.20183
Folkerts, M. M., Parks, E. A., Dedman, J. R., Kaetzel, M. A., Lyeth, B. G., and Berman, R. F. (2007). Phosphorylation of calcium calmodulin—dependent protein kinase II following lateral fluid percussion brain injury in rats. J. neurotrauma 24 (4), 638–650. doi:10.1089/neu.2006.0188
Fouad, I. A., Sharaf, N. M., Abdelghany, R. M., and El Sayed, N. S. E. D. (2018). Neuromodulatory effect of thymoquinone in attenuating glutamate-mediated neurotoxicity targeting the amyloidogenic and apoptotic pathways. Front. neurology 9, 236. doi:10.3389/fneur.2018.00236
Frugier, T., Morganti-Kossmann, M. C., O'Reilly, D., and McLean, C. A. (2010). In situ detection of inflammatory mediators in post mortem human brain tissue after traumatic injury. J. neurotrauma 27 (3), 497–507. doi:10.1089/neu.2009.1120
Fu, P., Yuan, Q., Sun, Y., Wu, X., Du, Z., Li, Z., et al. (2020). Baicalein ameliorates epilepsy symptoms in a pilocarpine-induced rat model by regulation of IGF1R. Neurochem. Res. 45, 3021–3033. doi:10.1007/s11064-020-03150-8
Goodman, J., Van, M., Gopinath, S., and Robertson, C. (2009). Pro-inflammatory and pro-apoptotic elements of the neuroinflammatory response are activated in traumatic brain injury. Acta Neurochir. Suppl., 437–439. doi:10.1007/978-3-211-85578-2_85
Gray, N. E., Farina, M., Tucci, P., and Saso, L. (2022). The role of the NRF2 pathway in maintaining and improving cognitive function. Biomedicines 10 (8), 2043. doi:10.3390/biomedicines10082043
Grewal, A. K., Singh, T. G., Sharma, D., Sharma, V., Singh, M., Rahman, M. H., et al. (2021). Mechanistic insights and perspectives involved in neuroprotective action of quercetin. Biomed. Pharmacother. 140, 111729. doi:10.1016/j.biopha.2021.111729
Gülşen, İ., Ak, H., Çölçimen, N., Alp, H. H., Akyol, M. E., Demir, I., et al. (2016). Neuroprotective effects of thymoquinone on the hippocampus in a rat model of traumatic brain injury. World Neurosurg. 86, 243–249. doi:10.1016/j.wneu.2015.09.052
Guo, S., Xing, N., Xiang, G., Zhang, Y., and Wang, S. (2023). Eriodictyol: a review of its pharmacological activities and molecular mechanisms related to ischemic stroke. Food & Funct. 14, 1851–1868. doi:10.1039/d2fo03417d
Gupta, R., Ambasta, R. K., and Kumar, P. (2021). Autophagy and apoptosis cascade: which is more prominent in neuronal death? Cell. Mol. Life Sci. 78 (24), 8001–8047. doi:10.1007/s00018-021-04004-4
Hakiminia, B., Alikiaii, B., Khorvash, F., and Mousavi, S. (2022). Oxidative stress and mitochondrial dysfunction following traumatic brain injury: from mechanistic view to targeted therapeutic opportunities. Fundam. Clin. Pharmacol. 36 (4), 612–662. doi:10.1111/fcp.12767
Han, Y., Guo, S., Li, Y., Li, J., Zhu, L., Liu, Y., et al. (2023). Phytomedicine.Berberine ameliorate inflammation and apoptosis via modulating PI3K/AKT/NFκB and MAPK pathway on dry eye155081
Hanna, M. E., and Pfister, B. J. (2023). Advancements in in vitro models of traumatic brain injury. Curr. Opin. Biomed. Eng. 25, 100430. doi:10.1016/j.cobme.2022.100430
Hinson, H. E., Rowell, S., and Schreiber, M. (2015). Clinical evidence of inflammation driving secondary brain injury: a systematic review. J. trauma acute care Surg. 78 (1), 184–191. doi:10.1097/TA.0000000000000468
Horstemeyer, M. F., Panzer, M. B., and Prabhu, R. K. (2019). State-of-the-art modeling and simulation of the brain’s response to mechanical loads. Ann. Biomed. Eng. 47, 1829–1831. doi:10.1007/s10439-019-02351-9
Huang, S.-X., Qiu, G., Cheng, F.-R., Pei, Z., Yang, Z., Deng, X.-H., et al. (2018). Berberine protects secondary injury in mice with traumatic brain injury through anti-oxidative and anti-inflammatory modulation. Neurochem. Res. 43, 1814–1825. doi:10.1007/s11064-018-2597-5
Hussein, G., Sankawa, U., Goto, H., Matsumoto, K., and Watanabe, H. (2006). Astaxanthin, a carotenoid with potential in human health and nutrition. J. Nat. Prod. 69 (3), 443–449. doi:10.1021/np050354+
Jarrahi, A., Braun, M., Ahluwalia, M., Gupta, R. V., Wilson, M., Munie, S., et al. (2020). Revisiting traumatic brain injury: from molecular mechanisms to therapeutic interventions. Biomedicines 8 (10), 389. doi:10.3390/biomedicines8100389
Jayaraj, R. L., Tamilselvam, K., Manivasagam, T., and Elangovan, N. (2013). Neuroprotective effect of CNB-001, a novel pyrazole derivative of curcumin on biochemical and apoptotic markers against rotenone-induced SK-N-SH cellular model of Parkinson’s disease. J. Mol. Neurosci. 51, 863–870. doi:10.1007/s12031-013-0075-8
Jenkins, P. O. (2023). Persistent symptoms after a mild traumatic brain injury: assessment and management. Medicine 51, 581–585. doi:10.1016/j.mpmed.2023.05.009
Johnson, N. H., de Rivero Vaccari, J. P., Bramlett, H. M., Keane, R. W., and Dietrich, W. D. (2023). Inflammasome activation in traumatic brain injury and Alzheimer's disease. Transl. Res. 254, 1–12. doi:10.1016/j.trsl.2022.08.014
Johnson, V. E., Stewart, J. E., Begbie, F. D., Trojanowski, J. Q., Smith, D. H., and Stewart, W. (2013a). Inflammation and white matter degeneration persist for years after a single traumatic brain injury. Brain 136 (1), 28–42. doi:10.1093/brain/aws322
Johnson, V. E., Stewart, W., and Smith, D. H. (2013b). Axonal pathology in traumatic brain injury. Exp. Neurol. 246, 35–43. doi:10.1016/j.expneurol.2012.01.013
Kaminska, B., Mota, M., and Pizzi, M. (2016). Signal transduction and epigenetic mechanisms in the control of microglia activation during neuroinflammation. Biochimica Biophysica Acta (BBA)-Molecular Basis Dis. 1862 (3), 339–351. doi:10.1016/j.bbadis.2015.10.026
Kelley, B. J., Lifshitz, J., and Povlishock, J. T. (2007). Neuroinflammatory responses after experimental diffuse traumatic brain injury. J. Neuropathology Exp. Neurology 66 (11), 989–1001. doi:10.1097/NEN.0b013e3181588245
Kempuraj, D., Thangavel, R., Kempuraj, D. D., Ahmed, M. E., Selvakumar, G. P., Raikwar, S. P., et al. (2021). Neuroprotective effects of flavone luteolin in neuroinflammation and neurotrauma. Biofactors 47 (2), 190–197. doi:10.1002/biof.1687
Kerman, M., Kanter, M., Coşkun, K. K., Erboga, M., and Gurel, A. (2012). Neuroprotective effects of caffeic acid phenethyl ester on experimental traumatic brain injury in rats. J. Mol. histology 43, 49–57. doi:10.1007/s10735-011-9376-9
Khan, T., Waseem, R., Zehra, Z., Aiman, A., Bhardwaj, P., Ansari, J., et al. (2022). Mitochondrial dysfunction: pathophysiology and mitochondria-targeted drug delivery approaches. Pharmaceutics 14 (12), 2657. doi:10.3390/pharmaceutics14122657
Kim, J.-H., Park, S.-H., Nam, S.-W., Kwon, H.-J., Kim, B.-W., Kim, W.-J., et al. (2011). Curcumin stimulates proliferation, stemness acting signals and migration of 3T3-L1 preadipocytes. Int. J. Mol. Med. 28 (3), 429–435. doi:10.3892/ijmm.2011.680
Kim, K., and Choi, S. H. (2021). Cardiovascular safety of SGLT2 inhibitors compared to DPP4 inhibitors and sulfonylureas as the second-line of therapy in T2DM using large, real-world clinical data in Korea. Diabetes Metab. J. 45 (4), 502–504. doi:10.4093/dmj.2021.0158
Kimelberg, H. K. (1995). Current concepts of brain edema: review of laboratory investigations. J. Neurosurg. 83 (6), 1051–1059. doi:10.3171/jns.1995.83.6.1051
Kong, L., Yao, Y., Xia, Y., Liang, X., Ni, Y., and Yang, J. (2019). Osthole alleviates inflammation by down-regulating NF-κB signaling pathway in traumatic brain injury. Immunopharmacol. Immunotoxicol. 41 (2), 349–360. doi:10.1080/08923973.2019.1608560
Kumar, A., Rinwa, P., and Dhar, H. (2014). Microglial inhibitory effect of ginseng ameliorates cognitive deficits and neuroinflammation following traumatic head injury in rats. Inflammopharmacology 22, 155–167. doi:10.1007/s10787-013-0187-3
Kwiecien, J. M., Dabrowski, W., Dąbrowska-Bouta, B., Sulkowski, G., Oakden, W., Kwiecien-Delaney, C. J., et al. (2020). Prolonged inflammation leads to ongoing damage after spinal cord injury. PloS one 15 (3), e0226584. doi:10.1371/journal.pone.0226584
Lai, Z., Li, C., Ma, H., Hua, S., Liu, Z., Huang, S., et al. (2023). Hydroxysafflor yellow a confers neuroprotection against acute traumatic brain injury by modulating neuronal autophagy to inhibit NLRP3 inflammasomes. J. Ethnopharmacol. 308, 116268. doi:10.1016/j.jep.2023.116268
LaPlaca, M. C., and Thibault, L. E. (1998). Dynamic mechanical deformation of neurons triggers an acute calcium response and cell injury involving the N-methyl-D-aspartate glutamate receptor. J. Neurosci. Res. 52 (2), 220–229. doi:10.1002/(SICI)1097-4547(19980415)52:2<220::AID-JNR10>3.0.CO;2-B
Lee, M. Y., Kim, M., Kim, G. R., Lee, H. J., Sayson, L. V., Ortiz, D. M. D., et al. (2023). Korean Red Ginseng extract attenuates alcohol-induced addictive responses and cognitive impairments by alleviating neuroinflammation. J. Ginseng Res. 47, 583–592. doi:10.1016/j.jgr.2023.02.003
Leitgeb, J., Mauritz, W., Brazinova, A., Janciak, I., Majdan, M., Wilbacher, I., et al. (2012). Outcome after severe brain trauma due to acute subdural hematoma. J. Neurosurg. 117 (2), 324–333. doi:10.3171/2012.4.JNS111448
Li, H., Han, W., Wang, H., Ding, F., Xiao, L., Shi, R., et al. (2017). Tanshinone IIA inhibits glutamate-induced oxidative toxicity through prevention of mitochondrial dysfunction and suppression of MAPK activation in SH-SY5Y human neuroblastoma cells. Oxidative Med. Cell. Longev. doi:10.1155/2017/4517486
Li, R., Zhou, Y., Zhang, S., Li, J., Zheng, Y., and Fan, X. (2022a). The natural (poly) phenols as modulators of microglia polarization via TLR4/NF-κB pathway exert anti-inflammatory activity in ischemic stroke. Eur. J. Pharmacol. 914, 174660. doi:10.1016/j.ejphar.2021.174660
Li, X., Huang, W., Tan, R., Xu, C., Chen, X., Li, S., et al. (2023a). The benefits of hesperidin in central nervous system disorders, based on the neuroprotective effect. Biomed. Pharmacother. 159, 114222. doi:10.1016/j.biopha.2023.114222
Li, X., Yang, X., Lu, H., Wang, W., Cai, L., Chen, J., et al. (2023b). Calycosin attenuates the inflammatory damage of microglia induced by oxygen and glucose deprivation through the HMGB1/TLR4/NF-κB signaling pathway: calycosin attenuates OGD/R damage in microglia. Acta Biochimica Biophysica Sinica 55 (9), 1415–1424. doi:10.3724/abbs.2023125
Li, Y., Wei, J.-Y., Liu, H., Wang, K.-J., Jin, S.-N., Su, Z.-K., et al. (2022b). An oxygen-adaptive interaction between SNHG12 and occludin maintains blood-brain barrier integrity. Cell Rep. 39 (2), 110656. doi:10.1016/j.celrep.2022.110656
Li, Z., Dong, X., Zhang, J., Zeng, G., Zhao, H., Liu, Y., et al. (2014). Formononetin protects TBI rats against neurological lesions and the underlying mechanism. J. neurological Sci. 338 (1-2), 112–117. doi:10.1016/j.jns.2013.12.027
Lin, T.-Y., Lin, Y.-W., Lu, C.-W., Huang, S.-K., and Wang, S.-J. (2013). Berberine inhibits the release of glutamate in nerve terminals from rat cerebral cortex. PloS one 8 (6), e67215. doi:10.1371/journal.pone.0067215
Lin, T. Y., Lu, C. W., Wang, C.-C., Wang, Y.-C., and Wang, S.-J. (2011). Curcumin inhibits glutamate release in nerve terminals from rat prefrontal cortex: possible relevance to its antidepressant mechanism. Prog. Neuro-Psychopharmacology Biol. Psychiatry 35 (7), 1785–1793. doi:10.1016/j.pnpbp.2011.06.012
Liu, F., Zhang, J., Qian, J., Wu, G., and Ma, Z. (2018). Baicalin attenuates liver hypoxia/reoxygenation injury by inducing autophagy. Exp. Ther. Med. 16 (2), 657–664. doi:10.3892/etm.2018.6284
Loane, D. J., Stoica, B. A., Byrnes, K. R., Jeong, W., and Faden, A. I. (2013). Activation of mGluR5 and inhibition of NADPH oxidase improves functional recovery after traumatic brain injury. J. neurotrauma 30 (5), 403–412. doi:10.1089/neu.2012.2589
Long, J.-y., Chen, J.-m., Liao, Y.-j., Zhou, Y.-j., Liang, B.-y., and Zhou, Y. (2020). Naringin provides neuroprotection in CCL2-induced cognition impairment by attenuating neuronal apoptosis in the hippocampus. Behav. Brain Funct. 16, 4–13. doi:10.1186/s12993-020-00166-6
Lotocki, G., de Rivero Vaccari, J. P., Perez, E. R., Sanchez-Molano, J., Furones-Alonso, O., Bramlett, H. M., et al. (2009). Alterations in blood-brain barrier permeability to large and small molecules and leukocyte accumulation after traumatic brain injury: effects of post-traumatic hypothermia. J. neurotrauma 26 (7), 1123–1134. doi:10.1089/neu.2008.0802
Lv, C., Yuan, X., Zeng, H.-W., Liu, R.-H., and Zhang, W.-D. (2017). Protective effect of cinnamaldehyde against glutamate-induced oxidative stress and apoptosis in PC12 cells. Eur. J. Pharmacol. 815, 487–494. doi:10.1016/j.ejphar.2017.09.009
Maas, A. I., Menon, D. K., Adelson, P. D., Andelic, N., Bell, M. J., Belli, A., et al. (2017). Traumatic brain injury: integrated approaches to improve prevention, clinical care, and research. Lancet Neurology 16 (12), 987–1048. doi:10.1016/S1474-4422(17)30371-X
Maas, A. I., Menon, D. K., Manley, G. T., Abrams, M., Åkerlund, C., Andelic, N., et al. (2022). Traumatic brain injury: progress and challenges in prevention, clinical care, and research. Lancet Neurology 21, 1004–1060. doi:10.1016/S1474-4422(22)00309-X
Majrashi, T. A., Alshehri, S. A., Alsayari, A., Muhsinah, A. B., Alrouji, M., Alshahrani, A. M., et al. (2023). Insight into the biological roles and mechanisms of phytochemicals in different types of cancer: targeting cancer therapeutics. Nutrients 15 (7), 1704. doi:10.3390/nu15071704
Mao, X.-Y., Zhou, H.-H., Li, X., and Liu, Z.-Q. (2016). Huperzine A alleviates oxidative glutamate toxicity in hippocampal HT22 cells via activating BDNF/TrkB-dependent PI3K/Akt/mTOR signaling pathway. Cell. Mol. Neurobiol. 36, 915–925. doi:10.1007/s10571-015-0276-5
Marmarou, A., Foda, M. A. A.-E., Van Den Brink, W., Campbell, J., Kita, H., and Demetriadou, K. (1994). A new model of diffuse brain injury in rats: Part I: pathophysiology and biomechanics. J. Neurosurg. 80 (2), 291–300. doi:10.3171/jns.1994.80.2.0291
Maxwell, W. L. (2012). Traumatic brain injury in the neonate, child and adolescent human: an overview of pathology. Int. J. Dev. Neurosci. 30 (3), 167–183. doi:10.1016/j.ijdevneu.2011.12.008
Mckee, A. C., and Daneshvar, D. H. (2015). The neuropathology of traumatic brain injury. Handb. Clin. neurology 127, 45–66. doi:10.1016/B978-0-444-52892-6.00004-0
Mehta, R., and Chinthapalli, K.consultant neurologist (2019). Glasgow coma scale explained. BMJ 365, l1296. doi:10.1136/bmj.l1296
Meldrum, B. S. (2000). Glutamate as a neurotransmitter in the brain: review of physiology and pathology. J. Nutr. 130 (4), 1007S–1015S. doi:10.1093/jn/130.4.1007S
Mendes Arent, A., Souza, L. F. d., Walz, R., and Dafre, A. L. (2014). Perspectives on molecular biomarkers of oxidative stress and antioxidant strategies in traumatic brain injury. BioMed Res. Int. 2014, 723060. doi:10.1155/2014/723060
Mohammed, F. S., Omay, S. B., Sheth, K. N., and Zhou, J. (2023). Nanoparticle-based drug delivery for the treatment of traumatic brain injury. Expert Opin. Drug Deliv. 20 (1), 55–73. doi:10.1080/17425247.2023.2152001
Moneim, A. E. A. (2015). Mercury-induced neurotoxicity and neuroprotective effects of berberine. Neural Regen. Res. 10 (6), 881–882. doi:10.4103/1673-5374.158336
Moslemi, E., Dehghan, P., and Khani, M. (2022). The effect of date seed (Phoenix dactylifera) supplementation on inflammation, oxidative stress biomarkers, and performance in active people: a blinded randomized controlled trial protocol. Contemp. Clin. Trials Commun. 28, 100951. doi:10.1016/j.conctc.2022.100951
Mukherjee, S., Tripathi, A. K., Kumar, G., Patnaik, R., Dhanesha, N., and Mishra, D. (2019). Advancement in the pathophysiology of cerebral stroke, 155–175.Neuroprotective potential of small molecule phytochemicals in stroke therapy
Naga, K. K., Sullivan, P. G., and Geddes, J. W. (2007). High cyclophilin D content of synaptic mitochondria results in increased vulnerability to permeability transition. J. Neurosci. 27 (28), 7469–7475. doi:10.1523/JNEUROSCI.0646-07.2007
Nagini, S., Palrasu, M., and Bishayee, A. (2023). Limonoids from neem (Azadirachta Indica A. Juss.) are potential anticancer drug candidates. Med. Res. Rev. doi:10.1002/med.21988
Nouri, Z., Fakhri, S., El-Senduny, F. F., Sanadgol, N., Abd-ElGhani, G. E., Farzaei, M. H., et al. (2019). On the neuroprotective effects of naringenin: pharmacological targets, signaling pathways, molecular mechanisms, and clinical perspective. Biomolecules 9 (11), 690. doi:10.3390/biom9110690
Ozoner, B., Yuceli, S., Aydin, S., Yazici, G. N., Sunar, M., Arslan, Y. K., et al. (2019). Effects of pycnogenol on ischemia/reperfusion-induced inflammatory and oxidative brain injury in rats. Neurosci. Lett. 704, 169–175. doi:10.1016/j.neulet.2019.04.010
Pandey, S. N., Singh, G., Semwal, B. C., Gupta, G., Alharbi, K. S., Almalki, W. H., et al. (2022). Therapeutic approaches of nutraceuticals in the prevention of Alzheimer's disease. J. Food Biochem. 46 (12), e14426. doi:10.1111/jfbc.14426
Pérez, R., Burgos, V., Marín, V., Camins, A., Olloquequi, J., González-Chavarría, I., et al. (2023). Caffeic acid phenethyl ester (CAPE): biosynthesis, derivatives and formulations with neuroprotective activities. Antioxidants 12 (8), 1500. doi:10.3390/antiox12081500
Phillips, C., and Fahimi, A. (2018). Immune and neuroprotective effects of physical activity on the brain in depression. Front. Neurosci. 12, 498. doi:10.3389/fnins.2018.00498
Popa, L. L., Chira, D., Strilciuc, Ș., and Mureșanu, D. F. (2023). Non-invasive systems application in traumatic brain injury rehabilitation. Brain Sci. 13 (11), 1594. doi:10.3390/brainsci13111594
Popescu, C., Anghelescu, A., Daia, C., and Onose, G. (2015). Actual data on epidemiological evolution and prevention endeavours regarding traumatic brain injury. J. Med. life 8 (3), 272–277.
Prins, M. L., and Matsumoto, J. H. (2014). The collective therapeutic potential of cerebral ketone metabolism in traumatic brain injury. J. lipid Res. 55 (12), 2450–2457. doi:10.1194/jlr.R046706
Qi, X., and Wang, J. (2020). Melatonin improves mitochondrial biogenesis through the AMPK/PGC1α pathway to attenuate ischemia/reperfusion-induced myocardial damage. Aging (Albany NY) 12 (8), 7299–7312. doi:10.18632/aging.103078
Rash, J., Davidson, K., Yasumura, T., and Furman, C. (2004). Freeze-fracture and immunogold analysis of aquaporin-4 (AQP4) square arrays, with models of AQP4 lattice assembly. Neuroscience 129 (4), 915–934. doi:10.1016/j.neuroscience.2004.06.076
Rashad, M. M., Galal, M. K., Abou-El-Sherbini, K. S., El-Behairy, A. M., Gouda, E. M., and Moussa, S. Z. (2018). Nano-sized selenium attenuates the developmental testicular toxicity induced by di-n-butyl phthalate in pre-pubertal male rats. Biomed. Pharmacother. 107, 1754–1762. doi:10.1016/j.biopha.2018.09.006
Rauchman, S. H., Zubair, A., Jacob, B., Rauchman, D., Pinkhasov, A., Placantonakis, D. G., et al. (2023). Traumatic brain injury: mechanisms, manifestations, and visual sequelae. Front. Neurosci. 17, 1090672. doi:10.3389/fnins.2023.1090672
Reiber, H., and Peter, J. B. (2001). Cerebrospinal fluid analysis: disease-related data patterns and evaluation programs. J. neurological Sci. 184 (2), 101–122. doi:10.1016/s0022-510x(00)00501-3
Sadeghnia, H. R., Kolangikhah, M., Asadpour, E., Forouzanfar, F., and Hosseinzadeh, H. (2017). Berberine protects against glutamate-induced oxidative stress and apoptosis in PC12 and N2a cells. Iran. J. Basic Med. Sci. 20 (5), 594–603. doi:10.22038/IJBMS.2017.8847
Salehpour, F., Sadigh-Eteghad, S., Mahmoudi, J., Kamari, F., Cassano, P., and Hamblin, M. R. (2023). “Action mechanisms of photobiomodulation in neuronal cells and the brain,” in Photobiomodulation for the brain: photobiomodulation therapy in neurology and neuropsychiatry (Springer), 49–85.
Scheff, S. W., Ansari, M. A., and Roberts, K. N. (2013). Neuroprotective effect of Pycnogenol® following traumatic brain injury. Exp. Neurol. 239, 183–191. doi:10.1016/j.expneurol.2012.09.019
Sebastiani, G., Almeida-Toledano, L., Serra-Delgado, M., Navarro-Tapia, E., Sailer, S., Valverde, O., et al. (2021). Therapeutic effects of catechins in less common neurological and neurodegenerative disorders. Nutrients 13 (7), 2232. doi:10.3390/nu13072232
Semple, B. D., Bye, N., Rancan, M., Ziebell, J. M., and Morganti-Kossmann, M. C. (2010). Role of CCL2 (MCP-1) in traumatic brain injury (TBI): evidence from severe TBI patients and CCL2−/− mice. J. Cereb. Blood Flow Metabolism 30 (4), 769–782. doi:10.1038/jcbfm.2009.262
Shapira, M., Licht, A., Milman, A., Pick, C. G., Shohami, E., and Eldar-Finkelman, H. (2007). Role of glycogen synthase kinase-3beta in early depressive behavior induced by mild traumatic brain injury. Mol. Cell. Neurosci. 34 (4), 571–577. doi:10.1016/j.mcn.2006.12.006
Sharma, S., Ying, Z., and Gomez-Pinilla, F. (2010). A pyrazole curcumin derivative restores membrane homeostasis disrupted after brain trauma. Exp. Neurol. 226 (1), 191–199. doi:10.1016/j.expneurol.2010.08.027
Sharma, S., Zhuang, Y., Ying, Z., Wu, A., and Gomez-Pinilla, F. (2009). Dietary curcumin supplementation counteracts reduction in levels of molecules involved in energy homeostasis after brain trauma. Neuroscience 161 (4), 1037–1044. doi:10.1016/j.neuroscience.2009.04.042
Shen, X., Liu, X., Wan, S., Fan, X., He, H., Wei, R., et al. (2019). Discovery of coumarin as microtubule affinity-regulating kinase 4 inhibitor that sensitize hepatocellular carcinoma to paclitaxel. Front. Chem. 7, 366. doi:10.3389/fchem.2019.00366
Shin, E.-J., Jeong, J. H., Chung, Y. H., Kim, W.-K., Ko, K.-H., Bach, J.-H., et al. (2011). Role of oxidative stress in epileptic seizures. Neurochem. Int. 59 (2), 122–137. doi:10.1016/j.neuint.2011.03.025
Shohami, E., and Kohen, R. (2011). The role of reactive oxygen species in the pathogenesis of traumatic brain injury. Oxidative Stress Free Radic. Damage Neurology, 99–118. doi:10.1007/978-1-60327-514-9_7
Sin, T. K., Yu, A. P., Yung, B. Y., Yip, S. P., Chan, L. W., Wong, C. S., et al. (2014). Modulating effect of SIRT1 activation induced by resveratrol on Foxo1-associated apoptotic signalling in senescent heart. J. physiology 592 (12), 2535–2548. doi:10.1113/jphysiol.2014.271387
Singh, L., Kaur, H., Chandra Arya, G., and Bhatti, R. (2023). Neuroprotective potential of formononetin, a naturally occurring isoflavone phytoestrogen. Chem. Biol. drug Des.
Singh, P., Doshi, S., Spaethling, J. M., Hockenberry, A. J., Patel, T. P., Geddes-Klein, D. M., et al. (2012). N-methyl-D-aspartate receptor mechanosensitivity is governed by C terminus of NR2B subunit. J. Biol. Chem. 287 (6), 4348–4359. doi:10.1074/jbc.M111.253740
Socała, K., Szopa, A., Serefko, A., Poleszak, E., and Wlaź, P. (2020). Neuroprotective effects of coffee bioactive compounds: a review. Int. J. Mol. Sci. 22 (1), 107. doi:10.3390/ijms22010107
Stahel, P. F., Morganti-Kossmann, M. C., Perez, D., Redaelli, C., Gloor, B., Trentz, O., et al. (2001). Intrathecal levels of complement-derived soluble membrane attack complex (sC5b-9) correlate with blood–brain barrier dysfunction in patients with traumatic brain injury. J. neurotrauma 18 (8), 773–781. doi:10.1089/089771501316919139
Stiefel, M. F., Tomita, Y., and Marmarou, A. (2005). Secondary ischemia impairing the restoration of ion homeostasis following traumatic brain injury. J. Neurosurg. 103 (4), 707–714. doi:10.3171/jns.2005.103.4.0707
Stocchetti, N., Carbonara, M., Citerio, G., Ercole, A., Skrifvars, M. B., Smielewski, P., et al. (2017). Severe traumatic brain injury: targeted management in the intensive care unit. Lancet Neurology 16 (6), 452–464. doi:10.1016/S1474-4422(17)30118-7
Sun, X., Ma, L., Li, X., Wang, J., Li, Y., and Huang, Z. (2022). Ferulic acid alleviates retinal neovascularization by modulating microglia/macrophage polarization through the ROS/NF-κB axis. Front. Immunol. 13, 976729. doi:10.3389/fimmu.2022.976729
Susin, S. A., Zamzami, N., and Kroemer, G. (1998). Mitochondria as regulators of apoptosis: doubt no more. Biochimica Biophysica Acta (BBA)-Bioenergetics 1366 (1-2), 151–165. doi:10.1016/s0005-2728(98)00110-8
Suwannakot, K., Sritawan, N., Naewla, S., Aranarochana, A., Sirichoat, A., Pannangrong, W., et al. (2022). Melatonin attenuates methotrexate-induced reduction of antioxidant activity related to decreases of neurogenesis in adult rat hippocampus and prefrontal cortex. Oxidative Med. Cell. Longev., 2022.
Taylor, C. A., Bell, J. M., Breiding, M. J., and Xu, L. (2017). Traumatic brain injury–related emergency department visits, hospitalizations, and deaths—United States, 2007 and 2013. MMWR Surveill. Summ. 66 (9), 1–16. doi:10.15585/mmwr.ss6609a1
Thapa, K., Khan, H., Singh, T. G., and Kaur, A. (2021). Traumatic brain injury: mechanistic insight on pathophysiology and potential therapeutic targets. J. Mol. Neurosci. 71 (9), 1725–1742. doi:10.1007/s12031-021-01841-7
Uddin, M. S., Al Mamun, A., Jakaria, M., Thangapandiyan, S., Ahmad, J., Rahman, M. A., et al. (2020). Emerging promise of sulforaphane-mediated Nrf2 signaling cascade against neurological disorders. Sci. Total Environ. 707, 135624. doi:10.1016/j.scitotenv.2019.135624
Unterberg, A., Stover, J., Kress, B., and Kiening, K. (2004). Edema and brain trauma. Neuroscience 129 (4), 1021–1029. doi:10.1016/j.neuroscience.2004.06.046
Vink, R., and Nimmo, A. J. (2009). Multifunctional drugs for head injury. Neurotherapeutics 6 (1), 28–42. doi:10.1016/j.nurt.2008.10.036
Walker, K. R., and Tesco, G. (2013). Molecular mechanisms of cognitive dysfunction following traumatic brain injury. Front. aging Neurosci. 5, 29. doi:10.3389/fnagi.2013.00029
Wang, C.-x., Xie, G.-b., Zhou, C.-h., Zhang, X.-s., Li, T., Xu, J.-g., et al. (2015). Baincalein alleviates early brain injury after experimental subarachnoid hemorrhage in rats: possible involvement of TLR4/NF-κB-mediated inflammatory pathway. Brain Res. 1594, 245–255. doi:10.1016/j.brainres.2014.10.014
Wang, F., Wan, J., Liao, Y., Liu, S., Wei, Y., and Ouyang, Z. (2023). Dendrobium species regulate energy homeostasis in neurodegenerative diseases: a review. Food Sci. Hum. Wellness 12 (6), 2151–2174. doi:10.1016/j.fshw.2023.03.029
Wang, J., and Zhang, Y. (2018). Neuroprotective effect of berberine agonist against impairment of learning and memory skills in severe traumatic brain injury via Sirt1/p38 MAPK expression. Mol. Med. Rep. 17 (5), 6881–6886. doi:10.3892/mmr.2018.8674
Wang, R., Li, Y.-B., Li, Y.-H., Xu, Y., Wu, H.-l., and Li, X.-J. (2008). Curcumin protects against glutamate excitotoxicity in rat cerebral cortical neurons by increasing brain-derived neurotrophic factor level and activating TrkB. Brain Res. 1210, 84–91. doi:10.1016/j.brainres.2008.01.104
Waseem, M., Kaushik, P., Dutta, S., Chakraborty, R., Hassan, M. I., and Parvez, S. (2022). Modulatory role of quercetin in mitochondrial dysfunction in titanium dioxide nanoparticle-induced hepatotoxicity. ACS Omega 7 (4), 3192–3202. doi:10.1021/acsomega.1c04740
Weber, J. T. (2012). Altered calcium signaling following traumatic brain injury. Front. Pharmacol. 3, 60. doi:10.3389/fphar.2012.00060
Welcome, M. O. (2020). Neuroinflammation in CNS diseases: molecular mechanisms and the therapeutic potential of plant derived bioactive molecules. PharmaNutrition 11, 100176. doi:10.1016/j.phanu.2020.100176
Werner, C., and Engelhard, K. (2007). Pathophysiology of traumatic brain injury. Br. J. Anaesth. 99 (1), 4–9. doi:10.1093/bja/aem131
Wheeler, S., and Acord-Vira, A. (2023). Occupational therapy practice guidelines for adults with traumatic brain injury. Am. J. Occup. Ther. 77 (4), 7704397010. doi:10.5014/ajot.2023.077401
Wu, A., Ying, Z., and Gomez-Pinilla, F. (2006). Dietary curcumin counteracts the outcome of traumatic brain injury on oxidative stress, synaptic plasticity, and cognition. Exp. Neurol. 197 (2), 309–317. doi:10.1016/j.expneurol.2005.09.004
Wu, A., Ying, Z., Schubert, D., and Gomez-Pinilla, F. (2011). Brain and spinal cord interaction: a dietary curcumin derivative counteracts locomotor and cognitive deficits after brain trauma. Neurorehabilitation neural repair 25 (4), 332–342. doi:10.1177/1545968310397706
Wu, Q., Wang, Y., and Li, Q. (2021). Matairesinol exerts anti-inflammatory and antioxidant effects in sepsis-mediated brain injury by repressing the MAPK and NF-κB pathways through up-regulating AMPK. Aging (Albany NY) 13 (20), 23780–23795. doi:10.18632/aging.203649
Wu, Y., and Cui, J. (2020). (-) Epigallocatechin-3-gallate provides neuroprotection via AMPK activation against traumatic brain injury in a mouse model. Naunyn-Schmiedeberg's Archives Pharmacol. 393 (11), 2209–2220. doi:10.1007/s00210-020-01841-1
Wyllie, D., Livesey, M., and Hardingham, G. (2013). Influence of GluN2 subunit identity on NMDA receptor function. Neuropharmacology 74, 4–17. doi:10.1016/j.neuropharm.2013.01.016
Xie, Y., Zhao, Q. Y., Li, H. Y., Zhou, X., Liu, Y., and Zhang, H. (2014). Curcumin ameliorates cognitive deficits heavy ion irradiation-induced learning and memory deficits through enhancing of Nrf2 antioxidant signaling pathways. Pharmacol. Biochem. Behav. 126, 181–186. doi:10.1016/j.pbb.2014.08.005
Xiong, Y., Mahmood, A., and Chopp, M. (2013). Animal models of traumatic brain injury. Nat. Rev. Neurosci. 14 (2), 128–142. doi:10.1038/nrn3407
Xiong, Y., Mahmood, A., and Chopp, M. (2024). Mesenchymal stem cell-derived extracellular vesicles as a cell-free therapy for traumatic brain injury via neuroprotection and neurorestoration. Neural Regen. Res. 19 (1), 49–54. doi:10.4103/1673-5374.374143
Xiong, Y., Peterson, P., and Lee, C. (1999). Effect of N-acetylcysteine on mitochondrial function following traumatic brain injury in rats. J. neurotrauma 16 (11), 1067–1082. doi:10.1089/neu.1999.16.1067
Xu, H., Cheng, J., Wang, X., Liu, H., Wang, S., Wu, J., et al. (2019). Resveratrol pretreatment alleviates myocardial ischemia/reperfusion injury by inhibiting STIM1-mediated intracellular calcium accumulation. J. physiology Biochem. 75, 607–618. doi:10.1007/s13105-019-00704-5
Xu, J., Wang, H., Lu, X., Ding, K., Zhang, L., He, J., et al. (2014). Posttraumatic administration of luteolin protects mice from traumatic brain injury: implication of autophagy and inflammation. Brain Res. 1582, 237–246. doi:10.1016/j.brainres.2014.07.042
Xu, X.-H., Ma, C.-M., Han, Y.-Z., Li, Y., Liu, C., Duan, Z.-H., et al. (2015). Protective effect of naringenin on glutamate-induced neurotoxicity in cultured hippocampal cells. Archives Biol. Sci. 67 (2), 639–646. doi:10.2298/abs140811023x
Xu, Z., Chen, S., Li, X., Luo, G., Li, L., and Le, W. (2006). Neuroprotective effects of (-)-epigallocatechin-3-gallate in a transgenic mouse model of amyotrophic lateral sclerosis. Neurochem. Res. 31, 1263–1269. doi:10.1007/s11064-006-9166-z
Xue, N., Wu, X., Wu, L., Li, L., and Wang, F. (2019). Antinociceptive and anti-inflammatory effect of Naringenin in different nociceptive and inflammatory mice models. Life Sci. 217, 148–154. doi:10.1016/j.lfs.2018.11.013
Yamamoto, S., Levin, H. S., and Prough, D. S. (2018). Mild, moderate and severe: terminology implications for clinical and experimental traumatic brain injury. Curr. Opin. neurology 31 (6), 672–680. doi:10.1097/WCO.0000000000000624
Yang, Y., Estrada, E. Y., Thompson, J. F., Liu, W., and Rosenberg, G. A. (2007). Matrix metalloproteinase-mediated disruption of tight junction proteins in cerebral vessels is reversed by synthetic matrix metalloproteinase inhibitor in focal ischemia in rat. J. Cereb. Blood Flow Metabolism 27 (4), 697–709. doi:10.1038/sj.jcbfm.9600375
Ye, J., Hu, X., Wang, Z., Li, R., Gan, L., Zhang, M., et al. (2023). The role of mtDAMPs in the trauma-induced systemic inflammatory response syndrome. Front. Immunol. 14, 1164187. doi:10.3389/fimmu.2023.1164187
Yessenkyzy, A., Saliev, T., Zhanaliyeva, M., Masoud, A.-R., Umbayev, B., Sergazy, S., et al. (2020). Polyphenols as caloric-restriction mimetics and autophagy inducers in aging research. Nutrients 12 (5), 1344. doi:10.3390/nu12051344
Yi, J.-H., and Hazell, A. S. (2006). Excitotoxic mechanisms and the role of astrocytic glutamate transporters in traumatic brain injury. Neurochem. Int. 48 (5), 394–403. doi:10.1016/j.neuint.2005.12.001
Yousuf, M., Khan, P., Shamsi, A., Shahbaaz, M., Hasan, G. M., Haque, Q. M. R., et al. (2020). Inhibiting CDK6 activity by quercetin is an attractive strategy for cancer therapy. ACS Omega 5 (42), 27480–27491. doi:10.1021/acsomega.0c03975
Yousuf, M., Shamsi, A., Khan, S., Khan, P., Shahwan, M., Elasbali, A. M., et al. (2022). Naringenin as a potential inhibitor of human cyclin-dependent kinase 6: molecular and structural insights into anti-cancer therapeutics. Int. J. Biol. Macromol. 213, 944–954. doi:10.1016/j.ijbiomac.2022.06.013
Zeng, J., Bao, T., Yang, K., Zhu, X., Wang, S., Xiang, W., et al. (2023). The mechanism of microglia-mediated immune inflammation in ischemic stroke and the role of natural botanical components in regulating microglia: a review. Front. Immunol. 13, 1047550.
Zhang, L., Fang, Y., Cheng, X., Lian, Y.-J., and Xu, H.-L. (2019). Silencing of long noncoding RNA SOX21-AS1 relieves neuronal oxidative stress injury in mice with Alzheimer’s disease by upregulating FZD3/5 via the Wnt signaling pathway. Mol. Neurobiol. 56, 3522–3537. doi:10.1007/s12035-018-1299-y
Zhang, T., Su, J., Guo, B., Wang, K., Li, X., and Liang, G. (2015). Apigenin protects blood–brain barrier and ameliorates early brain injury by inhibiting TLR4-mediated inflammatory pathway in subarachnoid hemorrhage rats. Int. Immunopharmacol. 28 (1), 79–87. doi:10.1016/j.intimp.2015.05.024
Zhao, J., Li, G., Zhang, Y., Su, X., and Hang, C. (2011). The potential role of JAK2/STAT3 pathway on the anti-apoptotic effect of recombinant human erythropoietin (rhEPO) after experimental traumatic brain injury of rats. Cytokine 56 (2), 343–350. doi:10.1016/j.cyto.2011.07.018
Zhao, J., Pati, S., Redell, J. B., Zhang, M., Moore, A. N., and Dash, P. K. (2012). Caffeic acid phenethyl ester protects blood–brain barrier integrity and reduces contusion volume in rodent models of traumatic brain injury. J. neurotrauma 29 (6), 1209–1218. doi:10.1089/neu.2011.1858
Keywords: phytonutrients, traumatic brain injury, multi-targeted therapies, therapeutic intervention, secondary injury cascades (SIC), blood-brain barrier (BBB), neurodegenerative disorders (NDs)
Citation: Hasan GM, Anwar S, Shamsi A, Sohal SS and Hassan MI (2024) The neuroprotective potential of phytochemicals in traumatic brain injury: mechanistic insights and pharmacological implications. Front. Pharmacol. 14:1330098. doi: 10.3389/fphar.2023.1330098
Received: 30 October 2023; Accepted: 15 December 2023;
Published: 04 January 2024.
Edited by:
Andleeb Khan, Integral University, IndiaReviewed by:
Md. Khurshid Khan, B. S. Abdur Rahman Crescent Institute of Science and Technology, IndiaTimir Tripathi, North Eastern Hill University, India
Mohammad Amir, The University of Iowa, United States
Khurshid Ahmad, Yeungnam University, Republic of Korea
Amit Kumar Singh, Sharda University, India
Copyright © 2024 Hasan, Anwar, Shamsi, Sohal and Hassan. This is an open-access article distributed under the terms of the Creative Commons Attribution License (CC BY). The use, distribution or reproduction in other forums is permitted, provided the original author(s) and the copyright owner(s) are credited and that the original publication in this journal is cited, in accordance with accepted academic practice. No use, distribution or reproduction is permitted which does not comply with these terms.
*Correspondence: Anas Shamsi, YW5hcy5zaGFtc2kxOEBnbWFpbC5jb20=; Md. Imtaiyaz Hassan, bWloYXNzYW5Aam1pLmFjLmlu