- 1College of Sport and Health, Shandong Sport University, Jinan, China
- 2Department of Pediatric Surgery, Zibo Central Hospital, Zibo, China
- 3Department of Clinical Laboratory, Zibo Central Hospital, Zibo, China
T cells play a pivotal role in the immune system by distinguishing between various harmful pathogens and cancerous cells within the human body and initiating an immune response. Within the tumor microenvironment (TME), immune effector T cells encounter both immunosuppressive cells and factors that hinder their functionality. Additionally, they endure robust and persistent antigenic stimulation, often leading to exhaustion and apoptosis. However, the stemness of T cells, characterized by their ability to survive and self-renew over extended periods, represents a primary target in immune checkpoint therapies such as anti-PD-1 therapy. T cell stemness encompasses specific memory T cell subsets and progenitor-exhausted T cells with stem cell-like properties. Therefore, understanding the impact of the TME on T cell stemness, including factors like K+, lactate, and H+, holds significant importance and can facilitate the mitigation of terminal T-cell depletion, the identification of potential resilient biomarkers or therapeutic targets resistant to immune checkpoint therapies, and ultimately lead to sustained anti-tumor effects. Thus, it offers a novel perspective for advancing tumor immunotherapy.
Introduction
Malignant tumors represent a significant global health challenge, characterized by elevated incidence and mortality rates, posing a severe threat to human well-being. In 2019, the World Health Organization reported that cancer stands as the second leading cause of death among individuals under the age of 70 in 112 of 183 countries worldwide (Ferlay et al., 2021). Recent investigations have revealed a strong association between the emergence and progression of tumors and the tumor microenvironment (TME) (Xiao and Yu, 2021), and alterations in the TME exert profound effects not only on the immune response within tumors but also on the efficacy of tumor treatments (de Visser and Joyce, 2023).
In addition to conventional approaches such as surgery, chemotherapy, and radiotherapy, the field of malignant tumor treatment has witnessed rapid advancements in research areas such as stem cell biology, immunology, molecular technology, and tissue engineering. Immunotherapy, recognized as a safe and effective treatment modality, has found an increasingly prominent role in comprehensive cancer management (Lu et al., 2022). Among the immune cells operating in the TME, T lymphocytes, especially CD8+ T cells, have been identified as pivotal players in the eradication of cancer cells (Reiser and Banerjee, 2016). Nonetheless, many cancer treatments are limited by T cell exhaustion (Yang et al., 2022), and addressing the causes of T cell exhaustion to sustain their stemness has been shown to enhance the efficacy of immunotherapy in tumor treatment, resulting in superior anti-tumor capabilities (Vodnala et al., 2019; Li et al., 2020; Mo et al., 2021). T cell stemness, characterized by the potential for prolonged survival, self-renewal and immune reconstitution, has garnered substantial evidence supporting its therapeutic relevance (Gattinoni et al., 2017; Morrot, 2017). Immunotherapy, which can harness the inherent capacity of T cells, is now considered a highly effective option for the treatment of cancer patients. Herein, this article focuses on investigating the impact of alterations in the TME on T cell stemness.
T cell stemness has been observed to be influenced by a range of factors, including the androgen receptor (Yang et al., 2022), G protein signaling 16 (Weisshaar et al., 2022), and changes in the TME (Vodnala et al., 2019; Feng et al., 2022; Xie et al., 2023). The TME represents a complex, interconnected system comprising components within and surrounding tumor cells. Beyond the tumor cells themselves, the TME can be broadly categorized into the immune microenvironment, primarily composed of immune cells, and the non-immune microenvironment, primarily comprised of fibroblasts. Additionally, ions and microorganisms are also present within the TME (Smetana and Masarik, 2022; de Visser and Joyce, 2023). Notably, a distinctive hallmark of the TME is hypoxia, which compels tumor cells to rely on anaerobic glycolysis for energy metabolism, resulting in lactate accumulation (Vaupel and Multhoff, 2017; Wei et al., 2021). Furthermore, ion-exchange proteins on the tumor cell membrane continuously transport intracellular H+ ions to the extracellular space (Chen and Pagel, 2015; Wei et al., 2021). Moreover, studies have revealed that both in murine and human tumor cells, cell necrosis releases intracellular K+ ions into the extracellular fluid, elevating K+ ion concentrations in the TME (Eil et al., 2016). Elevated levels of K+, lactate, and H+ in the TME have the potential to diminish T cell apoptosis, thereby promoting T cell stemness (Vodnala et al., 2019; Feng et al., 2022; Cheng et al., 2023). Given that T cell stemness can have long-lasting anti-tumor effects in immunotherapy, in this brief review, we summarize the effects of TME changes on T cell stem-cell properties for providing insights into optimizing and enhancing immunotherapy strategies.
T cell status
T cells are highly heterogeneous and can be categorized into distinct subsets based on their activation status, including naive T cells, effector T cells, and memory T cells (Lanzavecchia and Sallusto, 2005). Upon activation, naive T cells differentiate into effector T cells, which do not engage in lymphocyte recirculation but rather migrate to peripheral inflammatory sites or specific organ tissues to execute immune responses, often culminating in exhaustion (Wherry and Kurachi, 2015). On the other hand, another portion of naive T cells differentiate into memory T cells, which represent two key states: T cell stemness and exhaustion. T cell stemness describes the stem cell-like behavior of T cells, including self-renewal, multipotency, and sustained functionality (Vodnala et al., 2019; Li et al., 2020). On the other hand, T cell exhaustion signifies the progressive impairment of effector functions due to prolonged to antigens, and exhausted T cells (TEX) exhibit high expression of various inhibitory receptors and demonstrate severe defects in cell proliferation and cytokine production (Wherry and Kurachi, 2015; Gonzalez et al., 2021). Therefore, it can be confirmed that the TME harbors diverse T cell states including naive T cells, effector T cells, TEX, senescent T cells, and stem-like T cells, each assuming distinct roles (Crespo et al., 2013). Mouse studies have demonstrated that blocking T cell differentiation can stimulate the generation of T cell stemness (Crespo et al., 2013).
T cell exhausted
T cell exhaustion signifies the gradual decline of inflammatory and antigen-responsive T cells during chronic infections and cancer progression. They progressively lose their effector functions and memory T cell characteristics, leading to an inability of the body to sustain a durable and effective immune response (Wherry, 2011), and in this state, the T cells become resistant to restimulation (Crespo et al., 2013). T cell exhaustion is mainly characterized by the loss of interleukin-2 (IL-2) production, diminished proliferative potential, reduced cytolytic activity, and decreased expression of interferon-γ (IFN-γ) and tumor necrosis factor-α (TNF-α), ultimately compromising their protective capabilities (Wherry, 2011). However, exhausted T cell exhibits heterogeneity in both phenotype and function and can be divided into two main subsets: progenitor-exhausted T (TPEX) cells and terminally TEX. TPEX cells possess a stem-like exhausted phenotype characterized by the expression of PD-1, CD127, the chemokine receptor CXCR5, and high levels of TCF-1 (encoded by Tcf7) (McLane et al., 2019). The TCF-1 subset is pivotal for memory formation, as it possesses self-renewal and proliferative capacities and exhibits a favorable response to immunotherapy (Miller et al., 2019). TCF-1 serves as a critical marker for T cell stemness (Wen et al., 2021). Compared to TPEX cells, terminally TEX display impaired proliferative potential and lack TCF-1 expression, rendering them unresponsive to activation (Hudson et al., 2019; Siddiqui et al., 2019). Additionally, these two types of TEX exhibit distinct metabolic characteristics. TPEX cells predominantly engage in catabolic metabolism, characterized by mitochondrial fatty acid oxidation (FAO) and oxidative phosphorylation (OXPHOS) (Adams et al., 2016). On the other hand, terminally TEX primarily relies on glycolytic metabolism. However, prolonged antigen stimulation can induce changes in mitochondrial structure, resulting in impaired glycolysis and OXPHOS (Scharping et al., 2016; Schurich et al., 2016). Notably, an immunotherapy trial in lymphoma patients revealed higher levels of TPEX cells in lymph nodes without cancer metastases (Rahim et al., 2023).
T cell stemness
The concept of T cell stemness was first recognized in 2001 when mouse central memory T cells (TCM) were experimentally inhibited at the pre-differentiation stage using transcriptional inhibitors, which resulted in the preservation of their replicative potential and their ability to generate effector T cells over the long term upon encountering a subsequent antigenic challenge (Fearon et al., 2001; Crespo et al., 2013). Recent investigations have revealed that T cells undergo a programmed sequential process involving activation upon stimulation and differentiation into stem cell-like cells (Gattinoni et al., 2009; Vodnala et al., 2019). Following stimulation of the T cell receptor (TCR), naive T cells become activated and can differentiate into effector T cells and memory T cells. Memory T cells can be further categorized into effector memory T cells (TEM), central memory T cells (TCM), tissue-resident memory T cells, and stem cell memory T cells (TSCM) (Pace, 2021). The differentiation of naive T cells into memory and effector states is primarily influenced by the intensity of the signals they receive, predominantly through the TCR (Vodnala et al., 2019). Studies have indicated that once effector T cells enter the TME, they are not only subjected to continuous antigen stimulation but also to immunosuppression and other limiting factors, which often leads to apoptosis after antigen clearance, with only a small fraction of T cells (5%–10%) surviving. These survivors are referred to as memory precursor T cells or progenitor-depleted T cells, and they subsequently differentiate into various subtypes of memory T cells. Consequently, reducing or altering the immunosuppressive conditions in the TME is crucial for generating T cells with stemness-like properties capable of long-term survival under sustained antigen stimulation, ultimately achieving an effective and enduring anti-tumor response (Wang et al., 2018; Li et al., 2022). In particular, TSCM can maintain self-renewal and memory capacity for up to 25 years (Gao et al., 2021; Li et al., 2021; Fazeli et al., 2023). The differing lifespans of T cells in various states can be attributed to changes in their transcription factor promoters, with methylation imparting self-renewal ability while demethylation does not. Furthermore, TSCM possesses telomeres that are protected from damage (Gao et al., 2021; Wang et al., 2021; Fazeli et al., 2023). T cells exhibiting stemness characteristics display high expression of stemness-associated genes, including TCF-1, Il7r, and Cxcr3, while showing low expression of the genes Ifng, Gzmb, Gzmc, and Gzmf (Feng et al., 2022). Additionally, they exhibit elevated expression of CD62L and CCR7, diminished expression of CD44, and the capacity to rapidly acquire effector functions like memory cells following TCR stimulation (Gattinoni et al., 2011).
Despite T cells with stemness characteristics exhibiting lower secretion of IFN-γ, TNF-α, and granzyme B (GZMB), their capacity for tumor cell destruction is diminished (Gattinoni et al., 2011). Nevertheless, research has demonstrated that these T cells with stemness properties possess enhanced self-renewal, sustained anti-tumor effect and proliferative potential, along with the ability to differentiate into various subtypes of memory cells (Gattinoni et al., 2011; Fuertes Marraco et al., 2015). Compared to naive T cells and effector T cells, stemness in T cells is associated with increased mitochondrial mass and a predominant reliance on FAO and mitochondrial OXPHOS for prolonged survival (Fox et al., 2005). Given their enduring persistence, T cells with stemness properties can be harnessed to enhance T cell-based immunotherapy against tumors. Studies have indicated that T cells with stemness attributes, as well as other memory-like T cell subsets like TPEX, exhibit longer-lasting anti-infection and anti-tumor functions, which prove advantageous for tumor immunotherapy (Golubovskaya and Wu, 2016; Vodnala et al., 2019; Biasco et al., 2021). Environmental factors, particularly those within the TME, exert a pivotal influence on the status of T cells. The TME may either enhance or suppress T cell function, making it a potential target for intervention. Therefore, understanding the factors within the TME that promote T cell stemness introduces a fresh perspective for advancing tumor immunotherapy.
Tumor microenvironment high K+
High K+ induces autophagy in T cells
Autophagy, first discovered in 1962, refers to the cellular process where autophagosomes are formed by cells to degrade organelles, proteins, and other components under the control of specific genes. Ultimately lysosomes are used to break down their own organelles and contents, facilitating cellular metabolism and renewal (Kundu and Thompson, 2008). Autophagy serves to prevent cellular damage and promote cell survival in response to various stressors, such as nutrient deprivation or hypoxia (Lum et al., 2005). One notable characteristic of the TME is the rapid division of cancer cells, which compete for limited local resources (Sitkovsky and Lukashev, 2005). These factors can result in cellular apoptosis and necrosis (Richards et al., 2011). However, cell death can also alter the extracellular environment and lead to the release of intracellular ions. Research has indicated that the concentration of K+ in the interstitial fluid of tumors is higher than that in normal tissue interstitial fluid (Zimmerli and Gallin, 1988). Elevated K+ levels significantly reduce glycolytic intermediates and essential amino acids in T cells, affecting nutrient uptake—a phenomenon termed “caloric restriction” by (Vodnala et al., 2019). Nonetheless, this reduction in nutrient availability does not diminish T cell viability or proliferative potential; rather, it induces autophagy in T cells (Vodnala et al., 2019). Autophagy occurs concurrently with an increase in the methyl donor S-adenosylmethionine (SAM) (DeVorkin et al., 2019). It was found that the increased deposition of H3K27me3 in the high K+ situation of TME suggest a shift towards T cell stemness (Vodnala et al., 2019). The Kennedy pathway is the responsible for synthesizing phosphatidylethanolamine (PE), an early initiator of autophagosome formation and autophagy (Gibellini and Smith, 2010). The autophagy marker protein microtubule-associated protein LC3b-I is converted to LC3b-II through conjugation with PE. LC3b-II is a structural protein of autophagosomes, and its expression reflects the level of autophagic activity (Panda and Singh, 2023). Immunoblot analysis conducted under high K+ conditions confirmed an increase in LC3b-II, demonstrating that despite caloric restriction induced by T cells in a high K+ environment, mitochondrial integrity and oxygen-consuming capacity are preserved, which limits sustained energy consumption under high K+ conditions. Moreover, a decrease in the phosphorylation level of phosphorylation 3-kinase (PI3K)/AKT/mammalian target of the rapamycin (mTOR) signaling induces nutrient protection and a metabolic state resembling functional starvation, thereby prompting autophagy (Vodnala et al., 2019).
High K+ on T cell activation pathways
Previous studies have demonstrated that PI3K-Akt-mTOR signaling promotes the differentiation of CD8+ T cells into effector T cells while concurrently inhibiting the generation of memory T cells (Rao et al., 2010; van der Waart et al., 2014; Crompton et al., 2015; Li et al., 2020). Elevated K+ in the TME also affects the activation process of T cells, which inhibits the functionality of TCR-driven effector proteins by suppressing the PI3K-Akt-mTOR pathway. Sustained strong TCR signaling may induce T cells to become terminal T cells and may predispose them to apoptosis. In contrast, weak TCR signals may not be sufficient to effectively activate T cells but may prevent T cell differentiation during immune initiation and arrest at the TSCM stage (Wang et al., 2018). Thus, T cell activation is reduced, and the inhibition of the TCR by high K+ occurs mainly by reducing TCR activation-induced phosphorylation of AKT-targeted serine/threonine residues (Vodnala et al., 2019), via a mechanism involving serine/threonine phosphatases to regulate the activity of Akt downstream of PI3K (Eil et al., 2016; Vodnala et al., 2019). During increased levels of K+, the use of okadaic acid (OA), an inhibitor of the serine/threonine phosphatase PP2A21, was found to significantly restore T cell functions, and related analyses revealed that OA could reverse the reduced phosphorylation of Akt and S6 induced by elevated K+. Furthermore, disruption of the PP2A gene also reversed T cell functionality, and high K+ inhibits TCR-induced PI3K-Akt-mTOR phosphorylation by depending on PP2A (Eil et al., 2016). Therefore, T cells cannot be activated, thus leading to their stemness.
High K+ leads to T cell stemness
Elevated levels of K+ in the TME initiate functional metabolic restrictions in T cells, prompting a starvation response and modifying cellular metabolism. Under high K+ conditions, it has been observed that there is an increase in the total acetyl coenzyme A (AcCoA), but the levels of AcCoA and its precursor citrate decrease in the cell nucleus (Pietrocola et al., 2015). This alteration subsequently affects gene expression (Eil et al., 2016; Vodnala et al., 2019). High K+ primarily maintains T cells in a stem-like state by influencing TCF-1 expression (Vodnala et al., 2019). In human T lymphocytes, K+ efflux is regulated by two K+ channels: Kv1.3 (a voltage-gated K+ channel activated by membrane depolarization) and KCa3.1 (a K+ channel activated by increased cytoplasmic Ca2+; also known as IK1 or Gardos channel) (Srivastava et al., 2009). Additionally, it has been observed that blocking Kv1.3 and KCa3.1 channels in the TME with high K+ levels can inhibit T cell function, driving T cells toward a stemness state (Feske et al., 2015).
Kir2.1, an inward rectifying potassium channel, plays a significant role in this context. Knockout of the Kir2.1 channel in tumor-associated macrophages (TAMs) results in elevated K+ levels and induces a metabolic shift from OXPHOS to glycolysis (Chen et al., 2022). The reduction in nuclear-cytoplasmic AcCoA levels and its precursor citrate in T cells, along with the depletion of glycolytic metabolites, indicates a preference for AcCoA production and utilization in mitochondria for OXPHOS, aligning with the findings in TAMs (Vodnala et al., 2019). Thus, in conditions of elevated K+, the regulation of AcCoA influences histone acetylation and gene expression and initiates metabolic reprogramming. Studies have demonstrated alterations in the expression of acetyl-CoA synthetase 1 (Acss1), primarily located in mitochondria, under these conditions. These changes lead to metabolic reprogramming, enhanced oxidative capacity, and increased stem cell-like properties in T cells, suggesting that the Acss1 pathway may serve as a potential target for enhancing T cell stem cell-like capabilities (Vodnala et al., 2019).
Moreover, high K+ levels have been shown to inhibit the production of key cytokines such as IFN-γ, GZMB, and IL-2 in T cells (Vodnala et al., 2019). In CD8+ T cells exposed to high K+, an increase in the expression of the lymphoid homing marker CD62L and the co-stimulatory marker CD27 has been observed. Both Tcf7 transcripts and proteins also exhibit an increase under high K+ conditions (Vodnala et al., 2019). Amino acids, including methionine, become depleted in T cells when exposed to elevated K+. Consequently, the metabolites required to support relevant aspects of T cell stemness are depleted (Vodnala et al., 2019). However, T cells subjected to high K+ conditions possess an enhanced capacity for recalling their response to antigens, remain relatively undifferentiated, and demonstrate greater persistence and self-renewal upon re-exposure to antigens (Gattinoni et al., 2012). T cells that regain their stemness can promptly acquire effector functions following stimulation via the TCR (Gattinoni et al., 2011).
Tumor microenvironment high lactic
Lactic on T cell function
Tumor cells undergo metabolic changes characterized by increased glucose uptake and a higher proportion of pyruvate conversion to lactic acid, even in normoxic conditions, and this phenomenon, known as aerobic glycolysis, is commonly referred to as the Warburg effect (Warburg, 1961). Previous studies have suggested that lactate plays a contributing role in tumor development and is detrimental to tumor therapy, There exists a correlation between lactate concentration in tumor tissue and the incidence of metastasis, with higher lactate concentrations associated with shorter survival (Walenta et al., 1997; Walenta et al., 2000; Walenta et al., 2004). Lactate within tumors fuels regulatory T cells and within progression of macrophages toward an M2 phenotype (Ohashi et al., 2017; Watson et al., 2021). Moreover, the rapid proliferation and growth of tumors can result in hypoxic conditions (Petrova et al., 2018), leading to energy metabolism through anaerobic glycolysis and the accumulation of lactic acid. Interventions targeting lactic acid can induce up to 60% cell death in cytotoxic T lymphocytes (CTLs) (Fischer et al., 2007). Lactic acid treatment leads to a decrease in the production of IFN-γ and IL-2 by CTL (Fischer et al., 2007), as well as reduced content of granule enzymes and perforin in T cells and NK cells (Fischer et al., 2007; Husain et al., 2013). The ability of lactic acid-treated CTLs to eliminate target cells is reduced by half compared to the control group. Elevated expression of monocarboxylate transporter 1 (MCT-1) in CTLs from melanoma patients suggests that lactic acid in the tumor environment hinders T cell function (Fischer et al., 2007).
However, T cells exposed to lactic acid exhibit a substantial increase in the expression of genes related to T cell functional and signaling, such as Gzmb, Ifng, and TCF-1, leading to the initiation of changes associated with T cell stemness, ultimately enhancing the long-term anti-tumor immune response in T cells (Feng et al., 2022). Notably, the administration of a sodium lactate solution was found to significantly decrease tumor growth when injected into tumor-bearing mice. Moreover, when sodium lactate was co-administered with either a PD-1 antibody or a tumor vaccine, synergistic anti-tumor effects were achieved (Feng et al., 2022). Thus, while lactate does hinder T cell function, it is plausible that T cells with stem-like characteristics possess enduring anti-tumor capabilities and contribute to the synergy in immune therapy. However, upon the in vivo removal of CD8+ T cells, the anti-tumor effect resulting from lactate intervention diminishes (Feng et al., 2022). Consequently, the anti-tumor efficacy of sodium lactate is mediated through T cells and is an outcome of T cell stemness.
High lactic leads to T cell stemness
Lactic acid treatment was observed to significantly enhance the therapeutic effectiveness of anti-PD-1 treatment across various tumor models through reduced tumor growth rates and extended survival periods. In vitro intervention with lactic acid in T cells demonstrated a notable elevation in the expression of the stemness marker gene TCF-1 (Feng et al., 2022). The primary mechanism through which elevated lactate affects T cell stemness predominantly involves the inhibition of histone deacetylase activity in CD8+ T cells, which leads to the retention of histone acetylation at the TCF-1 locus during the differentiation of CD8+ T cells, with a notable 3.5-fold increase in acetylation levels at the H3K27 locus, thereby resulting in a reduction in the apoptosis and depletion of CD8+ T cells (Feng et al., 2022).
Tumor microenvironment high H+
High H+ on T cell metabolism
During glycolysis, tumor cells continuously transport intracellular H+ ions to the extracellular space through ion exchange proteins on their cell membranes, preventing self-acidosis (Chen and Pagel, 2015; Wei et al., 2021). Acidosis is t a defining feature of solid tumors, characterized by a pH range of 5.7–7 (Ashby, 1966; Vaupel et al., 1989). Extracellular acidosis has the effect of enhancing the endocytosis of dendritic cells (DCs) and influencing macrophage activity, leading to increased expression of MHC class II and CD86 (Vermeulen et al., 2004; Colegio et al., 2014). Under prolonged exposure to high extracellular H+ conditions, T cells experience reduced glycolysis and amino acid metabolism. Conversely, short-term exposure to high H+ levels only inhibits glycolysis without significant effects on amino acids (Cheng et al., 2023). High H+ levels severely impede glucose entry into the tricarboxylic acid cycle in T cells while promoting the breakdown of palmitic acid into AcCoA and citrate, resulting in metabolic reprogramming. Consequently, high H+ levels stimulate FAO in T cells (Cheng et al., 2023). T cells exhibit a higher oxygen consumption rate (OCR) and spare respiratory capacity (SRC) as characteristic trait of long-lived memory T cells (van der Windt et al., 2012; Cheng et al., 2023). This metabolic reprogramming mainly induces inhibition of the PI3K -AKT-mTOR signaling pathway, thereby inhibiting TCR activation and favoring the formation of CD8+ T cells stemness (Pollizzi et al., 2015; Ron-Harel et al., 2016; Cheng et al., 2023).
High H+ triggers metabolic reprogramming of T cells
There is increasing evidence indicating that one-carbon metabolism plays a crucial role in determining cellular fate and influencing their functional states (Xie et al., 2023). Effector T cells characterized by stemness exhibit heightened glycolytic activity and rely on one-carbon metabolism. Conversely, T cell stemness is distinguished by unique metabolic traits, including increased FAO and SRC, which are critical for sustaining effective anti-tumor capabilities (Cheng et al., 2023). Therefore, metabolic reprogramming is crucial for the effective acquisition of stemness in T cells and their long-term survival. High H+ inhibits the expression of enzymes related to the methionine cycle (MTR, AHCY, and BHMT) as well as genes involved in folate metabolism (SHMT1 and SHMT2) in T cells (Cheng et al., 2023). The expression of methionine transporter SLC7A5 on the surface of T cells is dependent on Myc gene (c-Myc) expression (Han et al., 2021). Elevated H+ concentrations can suppress c-Myc expression, further diminishing the expression of SLC7A5 and impeding the uptake of extracellular methionine by T cells, this ultimately hampers the production of SAM (Ron-Harel et al., 2016). As a methyl donor for histone methylation modifications, reduced levels of SAM can lead to alterations in histone modifications within T cells, thereby affecting gene expression. Investigations of various tumor-infiltrating CD8+ T cell subpopulations, revealed decreased expression of both c-Myc and SLC7A in T cell stemness.
High H+ leads to T cell stemness
Under high H+ conditions (10 mM) in vitro culture significantly increased the expression of BACH2, CCR7, LEF1, and TCF7 expression in CD8+ T cells. Additionally, research has demonstrated a H+ concentration-dependent effect on the expression of CCR7 and TCF-1 expression. Under high H+ conditions, both human and murine CD8+ T cells exhibit a substantial increase in TCF-1 expression at the protein level, a key factor associated with stem properties. These T cell stemness also display heightened expression of CCR7 and CD62L, along with a notable reduction in the production of IFN-γ and tumor necrosis TNF-α within the cells (Cheng et al., 2023). Quinn et al revealed demonstrated that exposure to a high-lactate, low-glucose environment induces reductive stress, ultimately resulting in decreased glycolysis and reduced serine production in effector and regulatory T cells (Quinn et al., 2020). High H+ levels lead to a decrease in inhibitory histone marks H3K27 and KLFe3 at memory-related gene loci (such as TCF7, CCR7, ID3, and LEF2) in T cells. Treatment with high H+ treatment significantly inhibits the expression of the methyltransferase EZH2, which governs H3K27me3 modification, ultimately lowering intracellular levels of H3K27me3 (Cheng et al., 2023). The decrease in H3K27me3 induced by extracellular acid treatment promotes the transcriptional activation of stemness-related genes within T cells, ultimately maintaining the stem-like characteristics of T cells; therefore, T cells cultured under high H+ conditions exhibit strong anti-tumor activity in vivo (McLane et al., 2019).
Conclusion
The adoptive transfer of tumor antigen-specific T cells has marked a significant advancement in cancer therapy, often resulting in the complete regression of certain malignant tumors, which depends largely on the persistence and differentiation status of the infused T cells. Preferably, less differentiated memory T cells with lower levels of differentiation are chosen for adoptive T cell transfer (ACT) due to their possession of stem cell-like attributes, including self-renewal and multipotent properties. Thus, T cell stemness plays a pivotal role in tumor immune surveillance and immunotherapy.
In recent years, there has been a growing interest in preclinical trials involving immune cells with stem cell characteristics for use in immunotherapy. The extended lifespan and robust proliferation potential make T cell stemness cells an ideal candidate for immunotherapy. Stem-like T cells can originate directly from naive lymphocytes, and notably, TPEX-phase cells are also regarded as stem-like T cells (Wen et al., 2021).
Recent studies uncovered that the TME exerts a notable influence on the development of T cell stemness. Despite prior findings indicating the suppressive role of lactate and acidic microenvironments in tumor immunity, it has been discovered that elevated levels of K+, lactate, and H+ all can restore and sustain T cell stemness by reshaping the metabolism within the T cells (Figure 1). T cell stemness not only enhances the efficacy of immunotherapy but also endures longer in vivo, leading to a more robust anti-tumor response (Bailey et al., 2017). The primary mechanism by which these three factors in the TME modify T cell stemness involves metabolic reprogramming, subsequently influencing gene expression, with a particular focus on altering TCF-1 gene expression. TCF-1 possesses intrinsic histone deacetylase (HDAC) activity, contributing to the maintenance of T cell stemness by repressing genes inappropriate for the T cell lineage (e.g., Cd4, Foxp3) (Xing et al., 2016). There is growing mounting evidence supporting the notion that metabolic pathways determine the fate of T cells and shape their epigenetic and functional states. Among the three treatment approaches aimed at regulating the metabolic reprogramming of T cells, the control of cellular energy intake or the targeting of molecules such as AcCoA represents a novel therapeutic strategy, to enhance resistance against T cell exhaustion. Furthermore, this paper introduces additional possibilities for improving tumor immunotherapy, suggesting that elevating K+ and lactate levels, along with increasing H+, can augment T cell stemness and, consequently, enhance the effectiveness of immunotherapy. Of immunotherapy.
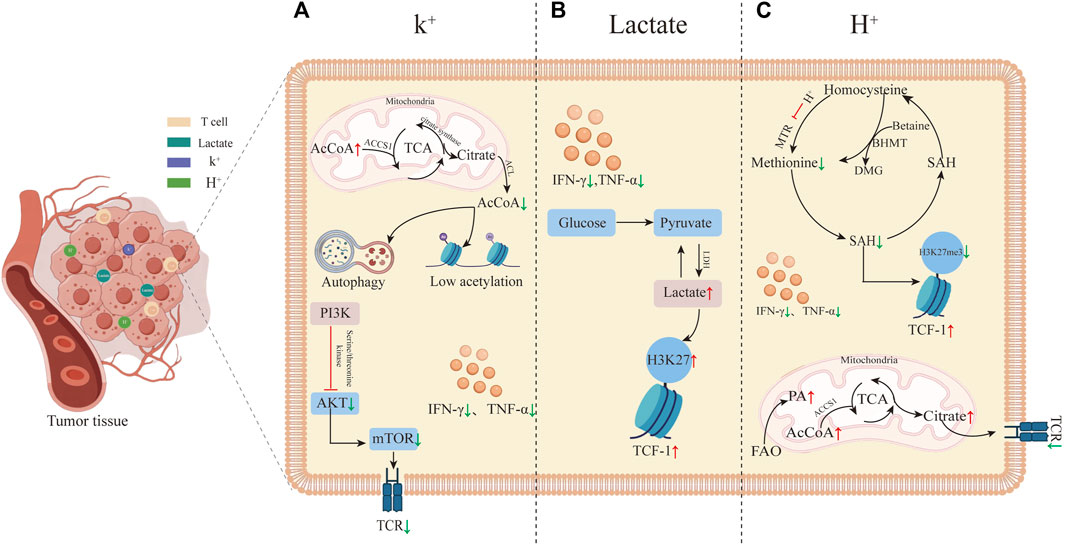
FIGURE 1. Tumor microenvironment-induced T cell stemness. (A) High K+ levels in the tumor microenvironment promote T cell stemness. High K+ leads to an increase in AcCoA within mitochondria while decreasing it in the nucleus, inducing autophagy and histone hypoacetylation. These alterations in gene expression reduce T cell differentiation into effector T cells by inhibiting the TCR signaling pathway. The inhibition of TCR by high K+ primarily occurs through reduced phosphorylation of Akt-targeted serine/threonine residues upon TCR activation. (B) Elevated lactate in the tumor microenvironment induces T cell stemness by increasing acetylation levels at the H3K27 locus within the TCF-1 gene during CD8+ T cell differentiation. This elevated acetylation preserves cellular stemness. (C) T cell stemness induced by high H+ levels in the tumor microenvironment. Elevated FAO results in increased AcCoA levels, leading to TCR inhibition, reduced T cell activation, and inhibition of methionine synthase MTR, thereby decreasing methionine synthesis. This reduction further lowers the synthesis of SAM and S-adenosylhomocysteine (SAH). SAM is a critical methyl donor for DNA and histone methylation reactions. The decreased methylation levels, particularly the histone marker H3K27me3, enhance TCF-1 expression.
Author contributions
YL: Conceptualization, Data curation, Formal Analysis, Investigation, Methodology, Project administration, Software, Supervision, Validation, Writing–original draft. TW: Conceptualization, Writing–review and editing. WM: Writing–review and editing. ZJ: Writing–review and editing. QW: Funding acquisition, Writing–review and editing. MZ: Funding acquisition, Writing–review and editing. YL: Writing–review and editing. HS: Writing–review and editing.
Funding
The author(s) declare financial support was received for the research, authorship, and/or publication of this article. This work was supported by grants from the central government guides local science and technology development funds (YDZX2022091), the National Social Science Fund of China (19BTY114).
Conflict of interest
The authors declare that the research was conducted in the absence of any commercial or financial relationships that could be construed as a potential conflict of interest.
Publisher’s note
All claims expressed in this article are solely those of the authors and do not necessarily represent those of their affiliated organizations, or those of the publisher, the editors and the reviewers. Any product that may be evaluated in this article, or claim that may be made by its manufacturer, is not guaranteed or endorsed by the publisher.
References
Adams, W. C., Chen, Y. H., Kratchmarov, R., Yen, B., Nish, S. A., Lin, W. W., et al. (2016). Anabolism-associated mitochondrial stasis driving lymphocyte differentiation over self-renewal. Cell Rep. 17, 3142–3152. doi:10.1016/j.celrep.2016.11.065
Ashby, B. S. (1966). pH studies in human malignant tumours. Lancet 2, 312–315. doi:10.1016/s0140-6736(66)92598-0
Bailey, S. R., Nelson, M. H., Majchrzak, K., Bowers, J. S., Wyatt, M. M., Smith, A. S., et al. (2017). Human CD26(high) T cells elicit tumor immunity against multiple malignancies via enhanced migration and persistence. Nat. Commun. 8, 1961. doi:10.1038/s41467-017-01867-9
Biasco, L., Izotova, N., Rivat, C., Ghorashian, S., Richardson, R., Guvenel, A., et al. (2021). Clonal expansion of T memory stem cells determines early anti-leukemic responses and long-term CAR T cell persistence in patients. Nat. Cancer 2, 629–642. doi:10.1038/s43018-021-00207-7
Chen, L. Q., and Pagel, M. D. (2015). Evaluating pH in the extracellular tumor microenvironment using CEST MRI and other imaging methods. Adv. Radiol. 2015, 206405. doi:10.1155/2015/206405
Chen, S., Cui, W., Chi, Z., Xiao, Q., Hu, T., Ye, Q., et al. (2022). Tumor-associated macrophages are shaped by intratumoral high potassium via Kir2.1. Cell Metab. 34, 1843–1859 e11. doi:10.1016/j.cmet.2022.08.016
Cheng, H., Qiu, Y., Xu, Y., Chen, L., Ma, K., Tao, M., et al. (2023). Extracellular acidosis restricts one-carbon metabolism and preserves T cell stemness. Nat. Metab. 5, 314–330. doi:10.1038/s42255-022-00730-6
Colegio, O. R., Chu, N. Q., Szabo, A. L., Chu, T., Rhebergen, A. M., Jairam, V., et al. (2014). Functional polarization of tumour-associated macrophages by tumour-derived lactic acid. Nature 513, 559–563. doi:10.1038/nature13490
Crespo, J., Sun, H., Welling, T. H., Tian, Z., and Zou, W. (2013). T cell anergy, exhaustion, senescence, and stemness in the tumor microenvironment. Curr. Opin. Immunol. 25, 214–221. doi:10.1016/j.coi.2012.12.003
Crompton, J. G., Sukumar, M., Roychoudhuri, R., Clever, D., Gros, A., Eil, R. L., et al. (2015). Akt inhibition enhances expansion of potent tumor-specific lymphocytes with memory cell characteristics. Cancer Res. 75, 296–305. doi:10.1158/0008-5472.CAN-14-2277
de Visser, K. E., and Joyce, J. A. (2023). The evolving tumor microenvironment: from cancer initiation to metastatic outgrowth. Cancer Cell 41, 374–403. doi:10.1016/j.ccell.2023.02.016
DeVorkin, L., Pavey, N., Carleton, G., Comber, A., Ho, C., Lim, J., et al. (2019). Autophagy regulation of metabolism is required for CD8(+) T cell anti-tumor immunity. Cell Rep. 27, 502–513. doi:10.1016/j.celrep.2019.03.037
Eil, R., Vodnala, S. K., Clever, D., Klebanoff, C. A., Sukumar, M., Pan, J. H., et al. (2016). Ionic immune suppression within the tumour microenvironment limits T cell effector function. Nature 537, 539–543. doi:10.1038/nature19364
Fazeli, P., Kalani, M., and Hosseini, M. (2023). T memory stem cell characteristics in autoimmune diseases and their promising therapeutic values. Front. Immunol. 14, 1204231. doi:10.3389/fimmu.2023.1204231
Fearon, D. T., Manders, P., and Wagner, S. D. (2001). Arrested differentiation, the self-renewing memory lymphocyte, and vaccination. Science 293, 248–250. doi:10.1126/science.1062589
Feng, Q., Liu, Z., Yu, X., Huang, T., Chen, J., Wang, J., et al. (2022). Lactate increases stemness of CD8 + T cells to augment anti-tumor immunity. Nat. Commun. 13, 4981. doi:10.1038/s41467-022-32521-8
Ferlay, J., Colombet, M., Soerjomataram, I., Parkin, D. M., Pineros, M., Znaor, A., et al. (2021). Estimating the global cancer incidence and mortality in 2018: GLOBOCAN sources and methods. Int. J. Cancer 144, 1941–1953. doi:10.1002/ijc.31937
Feske, S., Wulff, H., and Skolnik, E. Y. (2015). Ion channels in innate and adaptive immunity. Annu. Rev. Immunol. 33, 291–353. doi:10.1146/annurev-immunol-032414-112212
Fischer, K., Hoffmann, P., Voelkl, S., Meidenbauer, N., Ammer, J., Edinger, M., et al. (2007). Inhibitory effect of tumor cell-derived lactic acid on human T cells. Blood 109, 3812–3819. doi:10.1182/blood-2006-07-035972
Fox, C. J., Hammerman, P. S., and Thompson, C. B. (2005). Fuel feeds function: energy metabolism and the T-cell response. Nat. Rev. Immunol. 5, 844–852. doi:10.1038/nri1710
Fuertes Marraco, S. A., Soneson, C., Cagnon, L., Gannon, P. O., Allard, M., Abed Maillard, S., et al. (2015). Long-lasting stem cell-like memory CD8+ T cells with a naive-like profile upon yellow fever vaccination. Sci. Transl. Med. 7, 282ra48. doi:10.1126/scitranslmed.aaa3700
Gao, S., Liang, X., Wang, H., Bao, B., Zhang, K., Zhu, Y., et al. (2021). Stem cell-like memory T cells: a perspective from the dark side. Cell Immunol. 361, 104273. doi:10.1016/j.cellimm.2020.104273
Gattinoni, L., Klebanoff, C. A., and Restifo, N. P. (2012). Paths to stemness: building the ultimate antitumour T cell. Nat. Rev. Cancer 12, 671–684. doi:10.1038/nrc3322
Gattinoni, L., Lugli, E., Ji, Y., Pos, Z., Paulos, C. M., Quigley, M. F., et al. (2011). A human memory T cell subset with stem cell-like properties. Nat. Med. 17, 1290–1297. doi:10.1038/nm.2446
Gattinoni, L., Speiser, D. E., Lichterfeld, M., and Bonini, C. (2017). T memory stem cells in health and disease. Nat. Med. 23, 18–27. doi:10.1038/nm.4241
Gattinoni, L., Zhong, X. S., Palmer, D. C., Ji, Y., Hinrichs, C. S., Yu, Z., et al. (2009). Wnt signaling arrests effector T cell differentiation and generates CD8+ memory stem cells. Nat. Med. 15, 808–813. doi:10.1038/nm.1982
Gibellini, F., and Smith, T. K. (2010). The Kennedy pathway--de novo synthesis of phosphatidylethanolamine and phosphatidylcholine. IUBMB Life 62, 414–428. doi:10.1002/iub.337
Golubovskaya, V., and Wu, L. (2016). Different subsets of T cells, memory, effector functions, and CAR-T immunotherapy. Cancers (Basel) 8, 36. doi:10.3390/cancers8030036
Gonzalez, N. M., Zou, D., Gu, A., and Chen, W. (2021). Schrodinger's T cells: molecular insights into stemness and exhaustion. Front. Immunol. 12, 725618. doi:10.3389/fimmu.2021.725618
Han, C., Ge, M., Ho, P. C., and Zhang, L. (2021). Fueling T-cell antitumor immunity: amino acid metabolism revisited. Cancer Immunol. Res. 9, 1373–1382. doi:10.1158/2326-6066.CIR-21-0459
Hudson, W. H., Gensheimer, J., Hashimoto, M., Wieland, A., Valanparambil, R. M., Li, P., et al. (2019). Proliferating transitory T cells with an effector-like transcriptional signature emerge from PD-1(+) stem-like CD8(+) T cells during chronic infection. Immunity 51, 1043–1058. doi:10.1016/j.immuni.2019.11.002
Husain, Z., Huang, Y., Seth, P., and Sukhatme, V. P. (2013). Tumor-derived lactate modifies antitumor immune response: effect on myeloid-derived suppressor cells and NK cells. J. Immunol. 191, 1486–1495. doi:10.4049/jimmunol.1202702
Kundu, M., and Thompson, C. B. (2008). Autophagy: basic principles and relevance to disease. Annu. Rev. Pathol. 3, 427–455. doi:10.1146/annurev.pathmechdis.2.010506.091842
Lanzavecchia, A., and Sallusto, F. (2005). Understanding the generation and function of memory T cell subsets. Curr Opin Immunol. 17, 326–332. doi:10.1016/j.coi.2005.04.010
Li, F., Liu, H., Zhang, D., Ma, Y., and Zhu, B. (2022). Metabolic plasticity and regulation of T cell exhaustion. Immunology 167 (2022), 482–494. doi:10.1111/imm.13575
Li, W., Lu, L., Lu, J., Wang, X., Yang, C., Jin, J., et al. (2020). cGAS-STING-mediated DNA sensing maintains CD8(+) T cell stemness and promotes antitumor T cell therapy. Sci. Transl. Med. 12, eaay9013. doi:10.1126/scitranslmed.aay9013
Li, Y., Wu, D., Yang, X., and Zhou, S. (2021). Immunotherapeutic potential of T memory stem cells. Front. Oncol. 11, 723888. doi:10.3389/fonc.2021.723888
Lu, C., Liu, Y., Ali, N. M., Zhang, B., and Cui, X. (2022). The role of innate immune cells in the tumor microenvironment and research progress in anti-tumor therapy. Front. Immunol. 13, 1039260. doi:10.3389/fimmu.2022.1039260
Lum, J. J., DeBerardinis, R. J., and Thompson, C. B. (2005). Autophagy in metazoans: cell survival in the land of plenty. Nat. Rev. Mol. Cell Biol. 6, 439–448. doi:10.1038/nrm1660
McLane, L. M., Abdel-Hakeem, M. S., and Wherry, E. J. (2019). CD8 T cell exhaustion during chronic viral infection and cancer. Annu. Rev. Immunol. 37, 457–495. doi:10.1146/annurev-immunol-041015-055318
Miller, B. C., Sen, D. R., Al Abosy, R., Bi, K., Virkud, Y. V., LaFleur, M. W., et al. (2019). Subsets of exhausted CD8(+) T cells differentially mediate tumor control and respond to checkpoint blockade. Nat. Immunol. 20, 326–336. doi:10.1038/s41590-019-0312-6
Mo, F., Yu, Z., Li, P., Oh, J., Spolski, R., Zhao, L., et al. (2021). An engineered IL-2 partial agonist promotes CD8(+) T cell stemness. Nature 597, 544–548. doi:10.1038/s41586-021-03861-0
Morrot, A. (2017). Human stem memory T cells (T(SCM)) as critical players in the long-term persistence of immune responses. Ann. Transl. Med. 5, 120. doi:10.21037/atm.2017.02.28
Ohashi, T., Aoki, M., Tomita, H., Akazawa, T., Sato, K., Kuze, B., et al. (2017). M2-like macrophage polarization in high lactic acid-producing head and neck cancer. Cancer Sci. 108, 1128–1134. doi:10.1111/cas.13244
Pace, L. (2021). Temporal and epigenetic control of plasticity and fate decision during CD8(+) T-cell memory differentiation. Cold Spring Harb. Perspect. Biol. 13, a037754. doi:10.1101/cshperspect.a037754
Panda, S. P., and Singh, V. (2023). The dysregulated MAD in Mad: a neuro-theranostic approach through the induction of autophagic biomarkers LC3B-II and ATG. Mol. Neurobiol. 60, 5214–5236. doi:10.1007/s12035-023-03402-y
Petrova, V., Annicchiarico-Petruzzelli, M., Melino, G., and Amelio, I. (2018). The hypoxic tumour microenvironment. Oncogenesis 7, 10. doi:10.1038/s41389-017-0011-9
Pietrocola, F., Galluzzi, L., Bravo-San Pedro, J. M., Madeo, F., and Kroemer, G. (2015). Acetyl coenzyme A: a central metabolite and second messenger. Cell Metab. 21, 805–821. doi:10.1016/j.cmet.2015.05.014
Pollizzi, K. N., Patel, C. H., Sun, I. H., Oh, M. H., Waickman, A. T., Wen, J., et al. (2015). mTORC1 and mTORC2 selectively regulate CD8⁺ T cell differentiation. J. Clin. Invest. 125, 2090–2108. doi:10.1172/JCI77746
Quinn, W. J., Jiao, J., TeSlaa, T., Stadanlick, J., Wang, Z., Wang, L., et al. (2020). Lactate limits T cell proliferation via the NAD(H) redox state. Cell Rep. 33, 108500. doi:10.1016/j.celrep.2020.108500
Rahim, M. K., Okholm, T. L. H., Jones, K. B., McCarthy, E. E., Liu, C. C., Yee, J. L., et al. (2023). Dynamic CD8(+) T cell responses to cancer immunotherapy in human regional lymph nodes are disrupted in metastatic lymph nodes. Cell 186, 1127–1143 e18. doi:10.1016/j.cell.2023.02.021
Rao, R. R., Li, Q., Odunsi, K., and Shrikant, P. A. (2010). The mTOR kinase determines effector versus memory CD8+ T cell fate by regulating the expression of transcription factors T-bet and Eomesodermin. Immunity 32, 67–78. doi:10.1016/j.immuni.2009.10.010
Reiser, J., and Banerjee, A. (2016). Effector, memory, and dysfunctional CD8(+) T cell fates in the antitumor immune response. J. Immunol. Res. 2016, 8941260. doi:10.1155/2016/8941260
Richards, C. H., Mohammed, Z., Qayyum, T., Horgan, P. G., and McMillan, D. C. (2011). The prognostic value of histological tumor necrosis in solid organ malignant disease: a systematic review. Future Oncol. 7, 1223–1235. doi:10.2217/fon.11.99
Ron-Harel, N., Santos, D., Ghergurovich, J. M., Sage, P. T., Reddy, A., Lovitch, S. B., et al. (2016). Mitochondrial biogenesis and proteome remodeling promote one-carbon metabolism for T cell activation. Cell Metab. 24, 104–117. doi:10.1016/j.cmet.2016.06.007
Scharping, N. E., Menk, A. V., Moreci, R. S., Whetstone, R. D., Dadey, R. E., Watkins, S. C., et al. (2016). The tumor microenvironment represses T cell mitochondrial biogenesis to drive intratumoral T cell metabolic insufficiency and dysfunction. Immunity 45, 374–388. doi:10.1016/j.immuni.2016.07.009
Schurich, A., Pallett, L. J., Jajbhay, D., Wijngaarden, J., Otano, I., Gill, U. S., et al. (2016). Distinct metabolic requirements of exhausted and functional virus-specific CD8 T cells in the same host. Cell Rep. 16, 1243–1252. doi:10.1016/j.celrep.2016.06.078
Siddiqui, I., Schaeuble, K., Chennupati, V., Fuertes Marraco, S. A., Calderon-Copete, S., Pais Ferreira, D., et al. (2019). Intratumoral tcf1(+)pd-1(+)cd8(+) T cells with stem-like properties promote tumor control in response to vaccination and checkpoint blockade immunotherapy. Immunity 50, 195–211. doi:10.1016/j.immuni.2018.12.021
Sitkovsky, M., and Lukashev, D. (2005). Regulation of immune cells by local-tissue oxygen tension: HIF1 alpha and adenosine receptors. Nat. Rev. Immunol. 5, 712–721. doi:10.1038/nri1685
Smetana, K., and Masarik, M. (2022). Advances in cancer metabolism and tumour microenvironment. Int. J. Mol. Sci. 23, 4071. doi:10.3390/ijms23084071
Srivastava, S., Di, L., Zhdanova, O., Li, Z., Vardhana, S., Wan, Q., et al. (2009). The class II phosphatidylinositol 3 kinase C2beta is required for the activation of the K+ channel KCa3.1 and CD4 T-cells. Mol. Biol. Cell 20, 3783–3791. doi:10.1091/mbc.e09-05-0390
van der Waart, A. B., van de Weem, N. M., Maas, F., Kramer, C. S., Kester, M. G., Falkenburg, J. H., et al. (2014). Inhibition of Akt signaling promotes the generation of superior tumor-reactive T cells for adoptive immunotherapy. Blood 124, 3490–3500. doi:10.1182/blood-2014-05-578583
van der Windt, G. J., Everts, B., Chang, C. H., Curtis, J. D., Freitas, T. C., Amiel, E., et al. (2012). Mitochondrial respiratory capacity is a critical regulator of CD8+ T cell memory development. Immunity 36, 68–78. doi:10.1016/j.immuni.2011.12.007
Vaupel, P., Kallinowski, F., and Okunieff, P. (1989). Blood flow, oxygen and nutrient supply, and metabolic microenvironment of human tumors: a review. Cancer Res. 49, 6449–6465.
Vaupel, P., and Multhoff, G. (2017). Accomplices of the hypoxic tumor microenvironment compromising antitumor immunity: adenosine, lactate, acidosis, vascular endothelial growth factor, potassium ions, and phosphatidylserine. Front. Immunol. 8, 1887. doi:10.3389/fimmu.2017.01887
Vermeulen, M., Giordano, M., Trevani, A. S., Sedlik, C., Gamberale, R., Fernandez-Calotti, P., et al. (2004). Acidosis improves uptake of antigens and MHC class I-restricted presentation by dendritic cells. J. Immunol. 172, 3196–3204. doi:10.4049/jimmunol.172.5.3196
Vodnala, S. K., Eil, R., Kishton, R. J., Sukumar, M., Yamamoto, T. N., Ha, N. H., et al. (2019). T cell stemness and dysfunction in tumors are triggered by a common mechanism. Science 363, eaau0135. doi:10.1126/science.aau0135
Walenta, S., Salameh, A., Lyng, H., Evensen, J. F., Mitze, M., Rofstad, E. K., et al. (1997). Correlation of high lactate levels in head and neck tumors with incidence of metastasis. Am. J. Pathol. 150, 409–415.
Walenta, S., Schroeder, T., and Mueller-Klieser, W. (2004). Lactate in solid malignant tumors: potential basis of a metabolic classification in clinical oncology. Curr. Med. Chem. 11, 2195–2204. doi:10.2174/0929867043364711
Walenta, S., Wetterling, M., Lehrke, M., Schwickert, G., Sundfor, K., Rofstad, E. K., et al. (2000). High lactate levels predict likelihood of metastases, tumor recurrence, and restricted patient survival in human cervical cancers. Cancer Res. 60, 916–921.
Wang, W., He, Y., and Wu, S. (2018). Harnessing the CD8+ T-cell subsets with stemness for tumor immunotherapy. Future Oncol. 14, 2433–2436. doi:10.2217/fon-2018-0238
Wang, Y., Qiu, F., Xu, Y., Hou, X., Zhang, Z., Huang, L., et al. (2021). Stem cell-like memory T cells: the generation and application. J. Leukoc. Biol. 110, 1209–1223. doi:10.1002/JLB.5MR0321-145R
Warburg, O. (1961). On the facultative anaerobiosis of cancer cells and its use in chemotherapy. Munch Med. Wochenschr 103, 2504–2506.
Watson, M. J., Vignali, P. D. A., Mullett, S. J., Overacre-Delgoffe, A. E., Peralta, R. M., Grebinoski, S., et al. (2021). Metabolic support of tumour-infiltrating regulatory T cells by lactic acid. Nature 591, 645–651. doi:10.1038/s41586-020-03045-2
Wei, C., Liu, X., Wang, Q., Li, Q., and Xie, M. (2021). Identification of hypoxia signature to assess the tumor immune microenvironment and predict prognosis in patients with ovarian cancer. Int. J. Endocrinol. 2021, 4156187. doi:10.1155/2021/4156187
Weisshaar, N., Wu, J., Ming, Y., Madi, A., Hotz-Wagenblatt, A., Ma, S., et al. (2022). Rgs16 promotes antitumor CD8(+) T cell exhaustion. Sci. Immunol. 7, eabh1873. doi:10.1126/sciimmunol.abh1873
Wen, S., Lu, H., Wang, D., Guo, J., Dai, W., and Wang, Z. (2021). TCF-1 maintains CD8(+) T cell stemness in tumor microenvironment. J. Leukoc. Biol. 110, 585–590. doi:10.1002/JLB.5MR1120-778R
Wherry, E. J., and Kurachi, M. (2015). Molecular and cellular insights into T cell exhaustion. Nat. Rev. Immunol. 15, 486–499. doi:10.1038/nri3862
Xiao, Y., and Yu, D. (2021). Tumor microenvironment as a therapeutic target in cancer. Pharmacol. Ther. 221, 107753. doi:10.1016/j.pharmthera.2020.107753
Xie, J., Kittur, F. S., Hung, C. Y., and Ding, T. T. (2023). Regulation of one-carbon metabolism may open new avenues to slow down the initiation and progression of Huntington's disease. Neural Regen. Res. 18, 2401–2402. doi:10.4103/1673-5374.371363
Xing, S., Li, F., Zeng, Z., Zhao, Y., Yu, S., Shan, Q., et al. (2016). Tcf1 and Lef1 transcription factors establish CD8(+) T cell identity through intrinsic HDAC activity. Nat. Immunol. 17, 695–703. doi:10.1038/ni.3456
Yang, C., Jin, J., Yang, Y., Sun, H., Wu, L., Shen, M., et al. (2022). Androgen receptor-mediated CD8(+) T cell stemness programs drive sex differences in antitumor immunity. Immunity 55, 1747. doi:10.1016/j.immuni.2022.07.016
Keywords: tumor microenvironment, H+, lactate, K+, cancer
Citation: Liu Y, Wang T, Ma W, Jia Z, Wang Q, Zhang M, Luo Y and Sun H (2023) Metabolic reprogramming in the tumor microenvironment: unleashing T cell stemness for enhanced cancer immunotherapy. Front. Pharmacol. 14:1327717. doi: 10.3389/fphar.2023.1327717
Received: 25 October 2023; Accepted: 01 December 2023;
Published: 19 December 2023.
Edited by:
Yi Zhigao, National University of Singapore, SingaporeReviewed by:
Huilan Zhuang, Fujian Normal University, ChinaPanpan Xue, Fujian Normal University, China
Copyright © 2023 Liu, Wang, Ma, Jia, Wang, Zhang, Luo and Sun. This is an open-access article distributed under the terms of the Creative Commons Attribution License (CC BY). The use, distribution or reproduction in other forums is permitted, provided the original author(s) and the copyright owner(s) are credited and that the original publication in this journal is cited, in accordance with accepted academic practice. No use, distribution or reproduction is permitted which does not comply with these terms.
*Correspondence: Ying Luo, bHVveWluZzEyNDJAMTI2LmNvbQ==; Hongmei Sun, NTExNjMzODEwQHFxLmNvbQ==