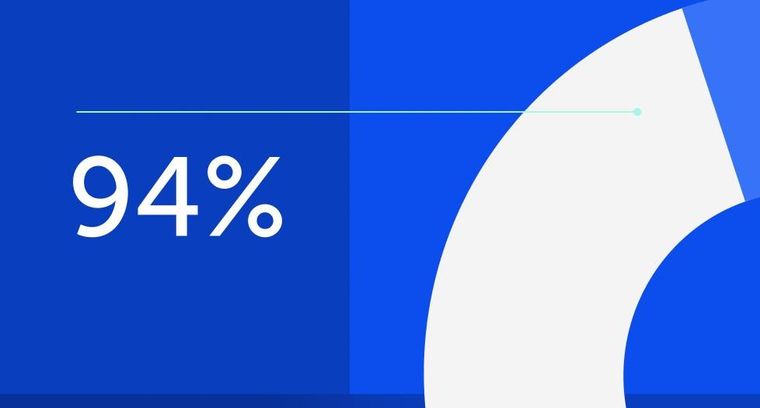
94% of researchers rate our articles as excellent or good
Learn more about the work of our research integrity team to safeguard the quality of each article we publish.
Find out more
REVIEW article
Front. Pharmacol., 09 November 2023
Sec. Gastrointestinal and Hepatic Pharmacology
Volume 14 - 2023 | https://doi.org/10.3389/fphar.2023.1286449
Metabolic dysfunction-associated steatotic liver disease (MASLD) is considered a “multisystem” disease that simultaneously suffers from metabolic diseases and hepatic steatosis. Some may develop into liver fibrosis, cirrhosis, and even hepatocellular carcinoma. Given the close connection between metabolic diseases and fatty liver, it is urgent to identify drugs that can control metabolic diseases and fatty liver as a whole and delay disease progression. Ferroptosis, characterized by iron overload and lipid peroxidation resulting from abnormal iron metabolism, is a programmed cell death mechanism. It is an important pathogenic mechanism in metabolic diseases or fatty liver, and may become a key direction for improving MASLD. In this article, we have summarized the physiological and pathological mechanisms of iron metabolism and ferroptosis, as well as the connections established between metabolic diseases and fatty liver through ferroptosis. We have also summarized MASLD therapeutic drugs and potential active substances targeting ferroptosis, in order to provide readers with new insights. At the same time, in future clinical trials involving subjects with MASLD (especially with the intervention of the therapeutic drugs), the detection of serum iron metabolism levels and ferroptosis markers in patients should be increased to further explore the efficacy of potential drugs on ferroptosis.
Metabolic dysfunction-associated fatty liver disease (MASLD), also referred to as non-alcoholic fatty liver disease (NAFLD) or metabolic dysfunction-associated fatty liver disease (MAFLD), encompasses both simple hepatic steatosis and metabolic dysfunction-associated steatohepatitis (MASH), previously known as non-alcoholic steatohepatitis (NASH). Some cases of MASLD can progress to liver fibrosis, cirrhosis, and even hepatocellular carcinoma. In 2020, an international expert group recommended renaming NAFLD to MAFLD based on previous research and clinical evidence (Eslam et al., 2020). However, in June 2023, NAFLD was renamed MASLD again based on Multi-society Delphi consensus (Rinella et al., 2023). Currently, MASLD is recognized as a “multisystem” disease that coexists with metabolic disorders and hepatic steatosis. Metabolic diseases are characterized by at least one of the following: obesity/overweight, type 2 diabetes mellitus (T2DM), hypertension or dyslipidemia. The diagnosis of fatty liver is based on liver biopsy histology and imaging examinations indicating the presence of hepatic steatosis. The two renames of NAFLD have gradually expanded the diagnostic criteria for metabolic diseases (from including at least two metabolic diseases to including at least one of them), emphasizing the strong connection between fatty liver and metabolic diseases, and highlighting the significance of controlling the progression of metabolic disorders in the treatment and management of MASLD. Currently, a combination of drugs, such as hypoglycemic drugs, and antioxidants, has been recommended for comprehensive control and management in clinical practice (Yin et al., 2023).
Ferroptosis is a newly discovered form of programmed cell death resulting from abnormal intracellular iron metabolism, which leads to iron overload and lipid peroxidation. The main factors contributing to the development of ferroptosis include abnormal iron metabolism or excessive iron intake, oxidation of unsaturated fatty acids, and impairment of antioxidant repair mechanisms (Li J. et al., 2020). Recently, ferroptosis has gained considerable attention in medical research due to its involvement in various conditions such as cancer, diabetes, cardio-cerebrovascular disease, and liver disease (Cheng et al., 2023; Miao et al., 2023; Zhang et al., 2023). Importantly, existing evidence suggests an interrelation between metabolic diseases, fatty liver, and ferroptosis, with the latter playing a crucial role in their pathogenesis. Therefore, targeting ferroptosis may serve as a common therapeutic approach for both metabolic diseases and fatty liver, potentially improving the progression of MASLD. Based on these considerations, we have conducted a comprehensive review of current research on fatty liver, metabolic diseases, and ferroptosis, and explored the potential of relevant drugs as therapeutic interventions, aiming to provide new insights and references for readers in this field.
Ferroptosis, is a novel cellular mechanism of damage, characterized by iron overload and lipid peroxidation resulting from aberrant intracellular iron metabolism. The physiological and pathological processes involved primarily encompass the following four aspects. We have summarized the physiological and pathological mechanisms related to ferroptosis in MASLD (Figure 1) and summarized relevant research evidence.
FIGURE 1. Physiological and pathological mechanisms related to ferroptosis in MASLD. In normal cells, the intake and metabolism of iron, esterification of unsaturated fatty acids, and clearance of peroxides are maintained in a relatively stable state. The main factors contributing to the development of ferroptosis are abnormal iron metabolism (A), lipid peroxidation (B), as well as abnormal antioxidant defense (C and D). The red pathway indicates an abnormality in the process. The figure was drawn by Figdraw.
Iron metabolism is a complex process. Under normal conditions, transferrin (Tf) in plasma binds to transferrin receptor 1 (TfR1), facilitating the entry of Fe3+ into the cell (Yu et al., 2020). The six-transmembrane epithelial antigen of the prostate 3 (STEAP3), a member of the metal reductase family, acts as a ferrous reductase activity and converts Fe3+ to Fe2+. This Fe2+ is transported to the cytoplasm through divalent metal transporter 1 (DMT1) and zinc transporter 8/14 (ZIP8/14), resulting in the formation of a labile iron pool (LIP) (Frazer and Anderson, 2014). Free Fe2+ can be transferred to ferritin, with ferritin heavy chain 1 (FTH1) playing a pivotal role, through poly (rC) binding protein 1 (PCBP1), and it can be converted back to Fe3+ to maintain intracellular iron balance or be transported to the mitochondria for utilization (Protchenko et al., 2021). Ferroportin 1 (FPN1) enables the export of Fe2+ to the extracellular space. Nuclear receptor coactivator 4 (NCOA4) regulates ferritin degradation in lysosomes during this process (Mancias et al., 2014). Disruptions or imbalances in certain metabolic pathways can trigger ferroptosis when iron metabolism is disrupted. Increased ferritin degradation, elevated TfR1 expression, or decreased FPN1 expression can lead to intracellular Fe2+ accumulation, which generates hydroxyl peroxides through the Fenton reaction with peroxides (Haschka et al., 2021). Polyunsaturated fatty acid (PUFA)-phospholipids (PL) become susceptible targets, resulting in the production of a substantial amount of peroxides.
Multiple clinical studies have uncovered anomalies in intestinal iron absorption and iron metabolism levels among patients afflicted with MASLD. Hoki et al. conducted an oral iron absorption test, which demonstrated an augmented uptake of intestinal iron absorption in individuals with NASH. This increase is attributed to the upregulation of DMT1 expression, regulated by iron regulatory proteins (Hoki et al., 2015). The concentration of serum serves as a crucial indicator of iron reserve and in vivo iron metabolism. Real-world studies have consistently revealed a positive correlation between serum ferritin levels and the incidence rate of MASLD (OR:1.725, 95%CI:1.427–2.085, p < 0.001) (Wang J-W. et al., 2022).
Furthermore, the occurrence of MASLD is significantly more prevalent in individuals with hyperproteinemia and those adhering to a high iron intake diet in comparison with normal individuals (p < 0.001) (Yang et al., 2019). Patients with hyperproteinemia exhibit more severe disruptions in lipid and glucose metabolism, alongside higher levels of transaminase levels (p < 0.05). Histopathological analysis reveals a positive correlation between increased serum ferritin levels and the severity of steatosis and iron staining (p < 0.05) (Wang Q. et al., 2022).
Moreover, a double-sample Mendelian randomization study utilizing an open genome-wide association research database substantiates the association between heightened genetic prediction of liver iron and an elevated risk of MAFLD (odds ratio: 1.193, 95% CI: 1.074–1.326, p = 0.001). The study also establishes a significant connection between hereditarily predicted elevated serum ferritin levels predicted by heredity and MAFLD (Dataset 1: β = 0.038, 95% CI: 0.014–0.062, p = 0.002; Dataset 2: β = 0.081, 95% CI: 0.025–0.136, p = 0.004) (He et al., 2022).
Previous preclinical studies have also investigated the potential relationship between iron intake and MASLD. In one study, the progression of liver fat lesions in HFE mice subjected to a high-calorie diet with iron deficiency was compared to those on a normal iron high-calorie diet. The findings revealed that mice with iron deficiency exhibited lower liver weight and diminished expression of iron transporters and iron regulatory genes (Crawford et al., 2021). Additionally, levels of 4-hydroxynonenal (4-HNE) and malondialdehyde (MDA), which are markers of ferroptosis, were significantly elevated in hepatocytes induced by a high iron diet.
Furthermore, another preclinical study utilizing a hepatocyte-specific Trf knockout mice (Trf LKO) model discovered that feeding mice a high iron diet increased the incidence of ferroptosis-induced liver fibrosis (Yu et al., 2020). However, the administration of a ferroptosis inhibitor, ferrostatin-1 (Fer-1), effectively reversed liver fibrosis in this model. In addition, relevant studies have shown that iron overload related ferroptosis is associated with upregulation of TfR1, DMT1 expression, and downregulation of FPN1 expression (Wang et al., 2021a; Liu et al., 2022).
The excessive synthesis of PUFA-PL can serve as a substrate for hydroxide ion (OH−) to facilitate the generation of lipid peroxides, inducing ferroptosis (Gan, 2022). This process primarily involves PUFAs, PL, and crucial enzymes such as long-chain acyl CoA synthase family member 4 (ACSL4), lysophosphatidylcholine acyltransferase 3 (LPCAT3), lipoxygenase (LOXs), etc (Yuan et al., 2016a; Lee et al., 2021).
Previous studies have shown that elevated arachidonic acid metabolism promotes the occurrence of liver ferroptosis in mice (Li X. et al., 2020; Tong et al., 2023), and upregulation of LOX15 (25) was observed in MAFLD mice fed on a high-fat diet. Additionally, gut microbiota metabolites also promoted the expression of ACSL4 and induced ferroptosis. The iron chelating agent effectively controlled this effect (Liu et al., 2022).
The cell possesses antioxidant defense systems that regulate lipid reactive oxygen species (ROS) and lipid peroxides to counteract ferroptosis. These defense mechanisms include.
1. Glutathione peroxidase 4 (GPX4): an endogenous enzyme that removes lipid peroxides. GPX4 is synthesized using glutathione as its precursor. The Xc− System transports cystine into the cell, which undergoes a series of reactions to generate glutathione (GSH), further synthesizing GPX4. The Xc− System-GSH-GPX4 axis is typically inactive during ferroptosis (Chen et al., 2021).
2. Kelch-like epichlorohydrin-associated protein-1 (Keap1)-nuclear factor erythroid 2-related factor 2 (Nrf2) pathway: this intracellular antioxidant pathway regulates the expression of downstream antioxidant factors, including heme oxygenase 1 (HO-1), to mitigate ferroptosis (Song and Long, 2020).
3. Guanosine triphosphate cyclization hydrolase 1 (GCH1)/tetrahydrobiopterin (BH4)/dihydrofolate reductase (DHFR) pathway: this pathway promotes coenzyme Q (CoQ) synthesis, inhibits lipid peroxide accumulation, and provides resistance against ferroptosis (Kraft et al., 2020).
4. Ferroptosis suppressor protein 1 (FSP1): FSP1, as an oxidoreductase, reduces CoQ to CoQH2, an antioxidant that scavenges lipophilic free radicals, thus preventing lipid peroxide accumulation (Bersuker et al., 2019).
5. Mitochondrial defense system: mitochondria have defense mechanisms to against ferroptosis. For example, mitochondrial ferritin reduces iron content by storing iron, GPX4 and dihydroorotate dehydrogenase (DHODH) in the mitochondria eliminate lipid peroxides (Mao et al., 2021), and CDGSH iron sulfur domain 1 (CISD1) and voltage-dependent anion channel (VDAC) regulate iron concentration and respiratory substrate content in mitochondria, respectively (Lemasters, 2017; Lipper et al., 2019).
Two studies evaluated the progression of NAFLD using mice fed a high-fat diet (Qi et al., 2020; Ding et al., 2023) and a methionine-deficient diet (Li X. et al., 2020), indicating that elevated arachidonic acid metabolism promoted the occurrence of liver ferroptosis in mice, with the accumulation of lipid peroxides, an increase in mitochondrial reactive oxygen species, and changes in mitochondrial morphology. Meanwhile, in animal models of fatty liver, ferroptosis leads to a decrease in liver GPX4, an increase in 12/15-LOX (34), and a decrease in Nrf2(35).
Besides these primary physiological and pathological mechanisms, recent studies have identified key factors involved in iron metabolism significantly impact ferroptosis. For instance, the p53 protein has been found to inhibit ferroptosis by suppressing dipeptidyl peptidase 4 (DPP4) and promote ferroptosis by inhibiting the solute carrier family 7—member 11 (SLC7A11) gene. This dual regulatory function of p53 holds great potential for cancer treatment (Kang et al., 2019). Additionally, ROS accumulation induces endoplasmic reticulum stress, affecting the activity of peroxisome proliferator-activated receptor (PPAR) (Yakubov et al., 2023) and influencing iron metabolism through inflammatory signaling pathways (Cheng et al., 2021a; Zhao et al., 2023). Moreover, as research progresses, emerging potential targets and receptors are being identified. The farnesoid X receptor (FXR) (Kim et al., 2022) and AMP-activated protein kinase (AMPK) (Lee et al., 2020; Wang et al., 2022c) have emerged as potential key targets of ferroptosis. For example, it has been suggested that a high-fat diet can impact Nrf2 levels by inhibiting AMPK-mediated mechanistic target of rapamycin (mTOR) activation (Liu et al., 2023). These findings enhance our understanding of the complex mechanisms underlying ferroptosis and provide potential avenues for therapeutic intervention.
T2DM is primarily characterized by insulin resistance. A meta-analysis indicates that fatty liver has a prevalence of 55.5% (95% CI, 47.3–63.7) among T2DM patients (Younossi et al., 2019a). In the liver of T2DM patients, insulin resistance can lead to fat accumulation and accelerate the progression of MASLD. Recent studies have demonstrated the impact of diabetes on the liver by examining ferroptosis. For example, a study observed the pathological changes in the livers of male C57BL/6 mice with diabetes. The findings revealed fibrotic symptoms, increased levels of inflammatory and oxidative stress markers, and weakened activity of the antioxidant system in the livers of diabetic mice. Treatment with Fer-1 effectively reversed and delayed the adverse liver outcomes in diabetic mice, as evidenced by improvements in alanine transaminase (ALT) and triglyceride levels, enhanced liver antioxidant systems such as Nrf2 and GPX4, and reduced levels of interleukin-6 (IL-6) and tumor necrosis factor a (TNF-α) (Younossi et al., 2019a).
In addition to insulin resistance, pancreatic β cell dysfunction and injury are also pathological features of T2DM. Excessive iron deposition in the cells can contribute to pancreatic dysfunction (Coffey and Knutson, 2017). Iron metabolism in the body is associated with the development of T2DM. A meta-analysis summarizing the results of 12 case-control and cohort studies found a significant correlation between elevated serum ferritin levels and the prevalence of T2DM (OR = 1.43, 95% CI: 1.29–1.59) (Liu et al., 2020). Furthermore, conditions like thalassemia and hemochromatosis, which can result in iron overload, are linked to pancreatic β cell damage and insulin resistance (Noetzli et al., 2012; Pelusi et al., 2016). Iron, an essential component of ferrum-sulfur (Fe-S) clusters, plays a crucial role in the mitochondrial oxidative synthesis, processing and secretion of insulin (Marku et al., 2021). Disruption of intracellular iron metabolism can interfere with these metabolic processes and induce ferroptosis, leading to a decrease in insulin secretion (Bruni et al., 2018). A study (Wei et al., 2020) suggests that arsenic can induce ferroptosis by mediating ferritin autophagy in pancreatic β cells . NCOA4, as a selective receptor for ferritin autophagy, mediates intracellular iron transport to autophagosomes by binding to FTH1, ultimately releasing Fe2+, and this damage may depend on an increase in mitochondrial reactive oxygen species (MtROS). The comparative experiment of using streptozotocin (STZ) and Fer-1, the inducer of diabetes, in male C57BL/6 mice, and the comparative experiment of using erastin and Fer-1 in human islet cell clusters also have proved the relationship between ferroptosis and pancreatic β cells (Li and Leung, 2020). Meanwhile, research has found that under high glucose conditions, the expression of GPX4 in pancreatic β cells is inhibited, the synthesis of GSH is reduced, leading to ferroptosis (Li D. et al., 2020; Krümmel et al., 2021). Xc− system is also necessary for insulin synthesis and secretion (de Baat et al., 2023). Based on the above research, Xc− System-GSH-GPX4 axis may be crucial for clearing lipid peroxidation and maintaining normal homeostasis in pancreatic β cells. Additionally, high glucose, hydrogen peroxide, and STZ induced increased intracellular ROS, decreased activity of the Nrf2-GPX4 pathway, and reduced mitochondrial membrane potential in Rin-5F cells (Frey et al., 2020; Stancic et al., 2022).
Obesity, a metabolic disorder characterized by excessive fat accumulation and storage in the body, is closely associated with an increased prevalence of metabolic diseases. MASLD represents a comprehensive manifestation of obesity and metabolic syndrome in the liver. A study in France revealed that over 20% of obese individuals with metabolic syndrome suffered from NASH(55). The expression of inflammatory cytokines in the liver and white adipose tissue may play a crucial role in the molecular signaling pathway that links obesity to fatty liver. Chronic low-level inflammation associated with obesity contributes to the production of inflammatory cytokines including IL-6 and TNF-α(56), which may directly result in liver pathology via endocrine mechanisms. Various adipokines produced by adipose tissue, including leptin, adiponectin, and others (Britton et al., 2016; Jorge et al., 2018), have been shown to influence the development of MASLD. Iron homeostasis is significantly connected to adipose factors, and high iron is an important negative regulator of both leptin and adiponectin (Fernández-Real et al., 2015; Harrison et al., 2023). A study using a mouse model of iron overload induced by an iron-rich diet revealed an upregulation of adipose factor levels associated with insulin resistance (Dongiovanni et al., 2013). Meanwhile, the reduction of FPN in adipocytes can lead to iron load, decreased adiponectin, and insulin resistance, which can further affect the metabolism of other organs (Gabrielsen et al., 2012). It was accompanied by a notable reduction in adipocytes as well as a potential correlation with the proliferation and hypertrophy of visceral adipose tissues. Regarding mitochondrial function, obesity can lead to mitochondrial dysfunction, which mainly occurs in the liver, muscles, and adipose tissue. The morphology and quantity of mitochondria have also changed: mitochondria in skeletal muscles have become smaller and shorter, mitochondria in white adipose tissue are small and slender, and cristae are irregular (Zhang S. et al., 2022). GPX4, a crucial enzyme for the maintenance of lipid peroxidation levels, plays a vital role in the inhibition of ferroptosis. A recent study (Schwärzler et al., 2022) found that mice with specific GPX4 deficiency in adipose tissue exhibited an increase in the number of white adipocytes and the level of serum TNF-α. In isolated adipocytes with impaired GPX4 activity, there was an increase in the level of 4-HNE and the production of inflammatory factors such as TNF-α, IL-1β, and IL-6. Furthermore, obese mice fed a high-fat diet showed a significant decrease in GPX4 expression (Schwärzler et al., 2022). In addition, obese mice with GPX4 deficiency also displayed lipid peroxidation in the liver (Katunga et al., 2015). Based on these findings, it could be inferred that GPX4 may play a role in the inhibition of lipid peroxidation, the prevention of adipose tissue inflammation, as well as the mitigation of low-level systemic inflammation. In addition, ACSL4 may have effects such as promoting the participation of arachidonic acid in phospholipids, causing liver fat accumulation, and triggering inflammation of white adipose tissue (Chen et al., 2023). For example, specific knockout of ACSL4 in mouse adipocytes effectively prevented obesity induced by a high-fat diet and reduced levels of lipid peroxidation product 4-HNE (Killion et al., 2018).
Recently, hypertension and dyslipidemia are also considered as the common metabolic disorder of MASLD. Although there is less connection between the two diseases and liver steatosis, ferroptosis plays a role in some pathogenesis of hypertension and atherosclerosis induced by hyperlipidemia. Targeted therapy for ferroptosis may be a promising new therapy.
Dyslipidemia is closely associated with hepatic steatosis and can serve as an independent predictor (Zou et al., 2021). Daily consumption of a high-fat diet exacerbates the metabolic burden on the liver, leading to the accumulation of hepatic lipids. Lipid-lowering therapy and a diet aimed at reducing fat intake are recommended management measures for MASLD. Conversely, MASLD can contribute to the development of dyslipidemia and is correlated with vascular calcification, significantly increasing the risk of cardiovascular disease. Dyslipidemia impacts the function of vascular endothelial cells and vascular smooth muscle cells, thereby promoting the development of atherosclerosis. Furthermore, iron overload and ferroptosis have been observed in vascular endothelial cells within atherosclerotic lesions.
Hypertension is now recognized as a risk factor for MASLD. Additionally, hypertension represents one of the primary clinical outcomes of MASLD, demonstrating a strong correlation between these two conditions. Recent studies have revealed that angiotensin II, a key factor in the development of hypertension, can induce astrocytes to secrete inflammatory cytokines, promote ferroptosis, and elevate the levels of ferroptosis markers. The involvement of angiotensin II in various pathological processes, including cardiac remodeling, myocardial hypertrophy, and ischemic reperfusion injury, has been observed in hypertensive mice.
MASLD is a complex condition involving multiple systems. Obesity, T2DM, hyperlipidemia, and fatty liver disease can interact with each other, thereby contributing to the development of comorbidities and collectively increasing the risk of cardiovascular disease (Figure 2). Ferroptosis is related with the normal functioning of pancreatic β cells, hepatocytes, vascular endothelial cells, as well as adipocytes. The disruption in various systems further results in the imbalance of glucose and lipid metabolism, with multiple organs involved, promoting the onset and progression of MASLD. It seems that ferroptosis is intricately intertwined with the pathogenic processes of metabolic diseases and MASLD. Metabolic diseases are often accompanied by disturbances in iron metabolism, a condition termed dysmetabolic iron overload syndrome (DIOS). DIOS primarily occurs in overweight individuals, such as those with type 2 diabetes, potentially progressing to MASLD.
FIGURE 2. Risk association between MASLD and metabolic diseases. Evidence of ferroptosis in pancreatic β cells (A); Evidence of ferroptosis in adipocytes (B); The correlation between metabolic diseases and liver steatosis (C). The figure was drawn by Figdraw.
Relevant studies primarily focus on the inhibition of ferroptosis in MASLD. There are three main approaches. Firstly, it involves reducing intracellular iron overload by either decreasing excessive iron intake or regulating iron metabolism. Secondly, it entails enhancing the defense system against lipid peroxidation and increasing the expression of antioxidant factors. Lastly, it involves the regulation of the activity of key enzymes implicated in the lipid oxidation process, accompanied by the modulation of the unsaturated fatty acid metabolism. Key proteins targeted in these approaches include ACSL4, GPX4, and Nrf2, among others. In this article, we provided a summary of the mechanisms of anti-ferroptosis drugs that have demonstrated efficacy in clinical trials for MASLD and other liver diseases. Our goal was to analyze and explore the commonalities and potential therapeutic targets among these drugs in MASLD treatment using different targets (Table 1).
Currently, hypoglycemic drugs, such as glucagon-like peptide-1 receptor agonists (GLP-1RA) and thiazolidinedione (TZDs), have been recommended as treatment options for MASLD in guidelines (Chalasani et al., 2018; Tokushige et al., 2021). Although metformin, sodium-glucose linked transporter 2 (SGLT2) inhibitors, along with DPP4 inhibitors have shown certain efficacy in clinical trials, their strength of evidence has remained insufficient for first-line drugs. TZDs have been identified as inhibitors of ferroptosis (Doll et al., 2017; Kung et al., 2022), predominantly by inhibiting the activity of ACSL4, thereby attenuating the enzymatic conversion of unsaturated fatty acids. Studies have shown that rosiglitazone can improve arsenic-induced ferroptosis in hepatocytes by targeting ACSL4 (72). In neurons, pioglitazone exhibits anti-ferroptosis activity in neurons by increasing the expression of PPAR-γ, downregulating cyclooxygenase-2 (COX2) expression (Liang et al., 2022), and cooperating with Nrf2(74). Furthermore, pioglitazone and mitoglitazone were found to target CISD1 through the stabilizing Fe-S clusters, thereby alleviating mitochondrial ferroptosis (Yuan et al., 2016b; Qi et al., 2023). Liraglutide has also demonstrated a delay in fatty liver progression in db/db mice through various aspects, including the improvement of iron metabolism and GPX4 activity (Song J-X. et al., 2022). Similar anti-ferroptosis mechanisms of liraglutide have been observed in neurons (An et al., 2022) as well. Although the research on the impact of metformin, DPP4 inhibitors, and SGLT2 inhibitors on liver ferroptosis is limited, existing fundamental studies indicate their potential influences on various cellular systems. For instance, metformin has been demonstrated to inhibit ferroptosis in various conditions, including pancreatic β cell injury, cardiac ischemia/reperfusion, colitis, osteoarthritis, polycystic ovary syndrome, as well as hyperlipidemia-associated vascular calcification. The underlying mechanisms involve the activation of AMPK and Nrf2 pathways (Ma et al., 2021; Yan J. et al., 2022; Sun Y. et al., 2023; Sun SP. et al., 2023; Liao et al., 2023; Peng et al., 2023; Wu et al., 2023). Vildagliptin has demonstrated the ability to upregulate the expression of GPX4 and improve ferroptosis in neurons (Zhang Y. et al., 2022), suggesting its possible inhibitory impact on DPP4 similar to p53 (87). SGLT2 inhibitors have been found to play an anti-ferroptosis role in renal tubular cells of diabetic nephropathy mice by combining with FPN1 to reduce its ubiquitination degradation (Huang et al., 2022), as well as in cardiomyocytes of heart failure mice (Ma et al., 2022). Further research is imperative to fully understand the potential roles and mechanisms of these pharmaceutical agents pertaining to the selective targeting of ferroptosis within the context of MASLD.
As reported by the International Lipid Expert Group in 2023, the beneficial clinical evidence of various nutritional supplements in the context of nutritional food therapy for MASLD have been highlighted (Rizzo et al., 2023). These supplements include vitamin D, vitamin E, silymarin, green tea extract, curcumin, fish oil, berberine, and resveratrol, et al. These supplements have shown efficacy in delaying the progression of MASLD and regulating ferroptosis in basic research. Some of these supplements may also provide benefits for individuals with both diabetes and fatty liver (Table 1).
Moreover, several endogenous target active molecules and biological analogues have progressed to clinical phase II-III trials. Examples include fibroblast growth factor 21 analogues (FGF21) (Luo Y. et al., 2022) and the FXR agonist obeticholic acid (Younossi et al., 2019b). Research has demonstrated the involvement of FGF21 and FXR agonist in the regulation of ferroptosis. These emerging therapeutic options hold promise for the management of MASLD.
In the realm of basic research, various active components and endogenous substances found in drugs have demonstrated potential in improving ferroptosis associated with metabolic diseases and fatty liver. These substances hold promise as key drug molecules, acting on relevant targets to ameliorate symptoms of MASLD. Additionally, the continuous discovery of novel targets further expands our understanding in this field. We summarized the effective active ingredients in Table 2.
TABLE 2. The mechanism of ferroptosis targeted by other active substances in the basic research stage.
In summary, it is evident that metabolic diseases and fatty liver share a close relationship and mutually influence each other as risk factors. Both conditions are characterized by iron overload and ferroptosis. Consequently, ferroptosis indirectly contributes to the interplay between metabolic diseases and fatty liver, promoting the onset and progression of MASLD.
Regarding drug treatment, numerous clinical studies emphasize the significance of hypoglycemic drugs as essential therapeutic agents for managing MASLD. These drugs exhibit potential anti-ferroptotic effects in various cell types and align with the previously discussed key targets. As a result, they offer promise in combating ferroptosis. Moreover, apart from their intrinsic hypoglycemic effects, these drugs serve as an ideal option for comprehensive control of MASLD occurrence and progression. Furthermore, ongoing clinical research investigating nutritional supplements and active ingredients derived from traditional Chinese medicine has demonstrated effective anti-ferroptotic properties. These therapeutic approaches present notable advantages and should not be underestimated. Considering the multifaceted nature of MASLD the identification of effective drugs, such as hypoglycemic agents, for both metabolic diseases and MASLD could yield promising therapeutic outcomes in controlling risk factors and providing targeted treatment. Additionally, we recommend an increased assessment of serum iron metabolism markers in future clinical trials involving individuals with MASLD, particularly when evaluating the effects of the aforementioned drugs. This approach will enable a more thorough investigation into the potential effectiveness of these drugs in mitigating ferroptosis.
This article summarizes the possible relationship between MASLD and related metabolic diseases and ferroptosis. Research has shown that ferroptosis affects multiple signaling molecules and pathways in the body, leading to metabolic disorders and inducing MASLD. Ferroptosis inhibitors can alleviate liver steatosis. It can be inferred that ferroptosis may be an important pathogenesis for the occurrence and development of MASLD. The article summarizes the relevant targets or pathways of MASLD therapeutic drugs acting on ferroptosis, as well as the mechanisms and pathways of targeted drugs/active substances inhibiting ferroptosis, in order to explore the process and form of ferroptosis in MASLD. At present, there is still a lack of further research to elaborate on the specific mechanisms by which these drugs or active substances affect the progression of MASLD through ferroptosis. The pathways of ferroptosis-induced diseases are complex and diverse, with different therapeutic drugs targeting different targets. Perhaps multi-drug combinations or ferroptosis multi-pathway inhibitors can more effectively reverse MASLD. In addition, more clinical studies on MASLD drugs are needed to confirm that inhibition of ferroptosis can improve MASLD in clinical practice. We believe that more research will reveal the regulatory mechanisms of ferroptosis in the future, providing strong evidence for targeted ferroptosis prevention and treatment of MASLD.
BZ: Writing–original draft, Data curation, Investigation. YW: Writing–original draft. MZ: Data curation, Investigation, Resources, Writing–original draft. SY: Data curation, Investigation, Resources, Writing–original draft. RT: Funding acquisition, Writing–review and editing. WL: Supervision, Writing–review and editing. EL: Funding acquisition, Supervision, Visualization, Writing–review and editing.
The author(s) declare financial support was received for the research, authorship, and/or publication of this article. This paper was funded by the Sichuan Provincial Department of Science and Technology (2021YFS0197, 2019YFS0514), National key R&D Program of China (2020YFC2005500).
The authors declare that the research was conducted in the absence of any commercial or financial relationships that could be construed as a potential conflict of interest.
All claims expressed in this article are solely those of the authors and do not necessarily represent those of their affiliated organizations, or those of the publisher, the editors and the reviewers. Any product that may be evaluated in this article, or claim that may be made by its manufacturer, is not guaranteed or endorsed by the publisher.
An, J.-R., Su, J.-N., Sun, G.-Y., Wang, Q.-F., Fan, Y.-D., Jiang, N., et al. (2022). Liraglutide alleviates cognitive deficit in db/db mice: involvement in oxidative stress, iron overload, and ferroptosis. Neurochem. Res. 47 (2), 279–294. doi:10.1007/s11064-021-03442-7
Bao, L., Jin, Y., Han, J., Wang, W., Qian, L., and Wu, W. (2023). Berberine regulates GPX4 to inhibit ferroptosis of islet β cells. Planta Med. 89 (3), 254–261. doi:10.1055/a-1939-7417
Bersuker, K., Hendricks, J. M., Li, Z., Magtanong, L., Ford, B., Tang, P. H., et al. (2019). The CoQ oxidoreductase FSP1 acts parallel to GPX4 to inhibit ferroptosis. Nature 575 (7784), 688–692. doi:10.1038/s41586-019-1705-2
Britton, L. J., Subramaniam, V. N., and Crawford, D. H. (2016). Iron and non-alcoholic fatty liver disease. World J. Gastroenterol. 22 (36), 8112–8122. doi:10.3748/wjg.v22.i36.8112
Bruni, A., Pepper, A. R., Pawlick, R. L., Gala-Lopez, B., Gamble, A. F., Kin, T., et al. (2018). Ferroptosis-inducing agents compromise in vitro human islet viability and function. Cell. Death Dis. 9 (6), 595. doi:10.1038/s41419-018-0506-0
Cai, X., Hua, S., Deng, J., Du, Z., Zhang, D., Liu, Z., et al. (2022). Astaxanthin activated the Nrf2/HO-1 pathway to enhance autophagy and inhibit ferroptosis, ameliorating acetaminophen-induced liver injury. ACS Appl. Mater Interfaces 14 (38), 42887–42903. doi:10.1021/acsami.2c10506
Cao, L., Qin, R., and Liu, J. (2023). Farnesoid X receptor protects against lipopolysaccharide-induced endometritis by inhibiting ferroptosis and inflammatory response. Int. Immunopharmacol. 118, 110080. doi:10.1016/j.intimp.2023.110080
Carlson, B. A., Tobe, R., Yefremova, E., Tsuji, P. A., Hoffmann, V. J., Schweizer, U., et al. (2016). Glutathione peroxidase 4 and vitamin E cooperatively prevent hepatocellular degeneration. Redox Biol. 9, 22–31. doi:10.1016/j.redox.2016.05.003
Chalasani, N., Younossi, Z., Lavine, J. E., Charlton, M., Cusi, K., Rinella, M., et al. (2018). The diagnosis and management of nonalcoholic fatty liver disease: practice guidance from the American Association for the Study of Liver Diseases. Hepatology 67 (1), 328–357. doi:10.1002/hep.29367
Chen, F., Kang, R., Liu, J., and Tang, D. (2023). The ACSL4 network regulates cell death and autophagy in diseases. Biology 12 (6), 864. doi:10.3390/biology12060864
Chen, J., Li, X., Ge, C., Min, J., and Wang, F. (2022a). The multifaceted role of ferroptosis in liver disease. Cell. Death Differ. 29 (3), 467–480. doi:10.1038/s41418-022-00941-0
Chen, X., Yu, C., Kang, R., Kroemer, G., and Tang, D. (2021). Cellular degradation systems in ferroptosis. Cell. Death Differ. 28 (4), 1135–1148. doi:10.1038/s41418-020-00728-1
Chen, Y., Zhu, S., Chen, Z., Liu, Y., Pei, C., Huang, H., et al. (2022b). Gingerenone A alleviates ferroptosis in secondary liver injury in colitis mice via activating nrf2-gpx4 signaling pathway. J. Agric. Food Chem. 70 (39), 12525–12534. doi:10.1021/acs.jafc.2c05262
Cheng, K., Huang, Y., and Wang, C. (2021a). 1,25(OH)(2)D(3) inhibited ferroptosis in zebrafish liver cells (ZFL) by regulating keap1-nrf2-GPx4 and NF-κB-hepcidin Axis. Int. J. Mol. Sci. 22 (21), 11334. doi:10.3390/ijms222111334
Cheng, K., Huang, Y., and Wang, C. (2021b). 1,25(OH)2D3 inhibited ferroptosis in zebrafish liver cells (ZFL) by regulating keap1-nrf2-GPx4 and NF-κB-hepcidin Axis. Int. J. Mol. Sci. 22 (21), 11334. doi:10.3390/ijms222111334
Cheng, X., Yu, C., Yang, X., Wang, F., and Min, J. (2023). A panoramic view of ferroptosis in cardiovascular disease. Kidney Dis. (Basel). 9 (3), 173–186. doi:10.1159/000530046
Coffey, R., and Knutson, M. D. (2017). The plasma membrane metal-ion transporter ZIP14 contributes to nontransferrin-bound iron uptake by human β-cells. Am. J. Physiol. Cell. Physiol. 312(2), C169-C175-C75. doi:10.1152/ajpcell.00116.2016
Crawford, D. H. G., Ross, D. G. F., Jaskowski, L.-A., Burke, L. J., Britton, L. J., Musgrave, N., et al. (2021). Iron depletion attenuates steatosis in a mouse model of non-alcoholic fatty liver disease: role of iron-dependent pathways. Biochim. Biophys. Acta Mol. Basis Dis. 1867 (7), 166142. doi:10.1016/j.bbadis.2021.166142
de Baat, A., Meier, D. T., Rachid, L., Fontana, A., Böni-Schnetzler, M., and Donath, M. Y. (2023). Cystine/glutamate antiporter System xc-deficiency impairs insulin secretion in mice. Diabetologia 66 (11), 2062–2074. doi:10.1007/s00125-023-05993-6
Ding, S.-B., Chu, X.-L., Jin, Y.-X., Jiang, J.-J., Zhao, X., and Yu, M. (2023). Epigallocatechin gallate alleviates high-fat diet-induced hepatic lipotoxicity by targeting mitochondrial ROS-mediated ferroptosis. Front. Pharmacol. 14, 1148814. doi:10.3389/fphar.2023.1148814
Doll, S., Proneth, B., Tyurina, Y. Y., Panzilius, E., Kobayashi, S., Ingold, I., et al. (2017). ACSL4 dictates ferroptosis sensitivity by shaping cellular lipid composition. Nat. Chem. Biol. 13 (1), 91–98. doi:10.1038/nchembio.2239
Dongiovanni, P., Ruscica, M., Rametta, R., Recalcati, S., Steffani, L., Gatti, S., et al. (2013). Dietary iron overload induces visceral adipose tissue insulin resistance. Am. J. Pathol. 182 (6), 2254–2263. doi:10.1016/j.ajpath.2013.02.019
Duan, C., Jiao, D., Wang, H., Wu, Q., Men, W., Yan, H., et al. (2022). Activation of the PPARγ prevents ferroptosis-induced neuronal loss in response to intracerebral hemorrhage through synergistic actions with the Nrf2. Front. Pharmacol. 13, 869300. doi:10.3389/fphar.2022.869300
Eslam, M., Newsome, P. N., Sarin, S. K., Anstee, Q. M., Targher, G., Romero-Gomez, M., et al. (2020). A new definition for metabolic dysfunction-associated fatty liver disease: an international expert consensus statement. J. Hepatol. 73 (1), 202–209. doi:10.1016/j.jhep.2020.03.039
Fernández-Real, J. M., McClain, D., and Manco, M. (2015). Mechanisms linking glucose homeostasis and iron metabolism toward the onset and progression of type 2 diabetes. Diabetes Care 38 (11), 2169–2176. doi:10.2337/dc14-3082
Frazer, D. M., and Anderson, G. J. (2014). The regulation of iron transport. BioFactors Oxf. Engl. 40 (2), 206–214. doi:10.1002/biof.1148
Frey, S., Patouraux, S., Debs, T., Gugenheim, J., Anty, R., and Iannelli, A. (2020). Prevalence of NASH/NAFLD in people with obesity who are currently classified as metabolically healthy. Surg. Obes. Relat. Dis. 16 (12), 2050–2057. doi:10.1016/j.soard.2020.07.009
Gabrielsen, J. S., Gao, Y., Simcox, J. A., Huang, J., Thorup, D., Jones, D., et al. (2012). Adipocyte iron regulates adiponectin and insulin sensitivity. J. Clin. Investig. 122 (10), 3529–3540. doi:10.1172/JCI44421
Gan, B. (2022). ACSL4, PUFA, and ferroptosis: new arsenal in anti-tumor immunity. Signal Transduct. Target. Ther. 7 (1), 128. doi:10.1038/s41392-022-01004-z
Gao, G., Xie, Z., Li, E.-W., Yuan, Y., Fu, Y., Wang, P., et al. (2021). Dehydroabietic acid improves nonalcoholic fatty liver disease through activating the Keap1/Nrf2-ARE signaling pathway to reduce ferroptosis. J. Nat. Med. 75 (3), 540–552. doi:10.1007/s11418-021-01491-4
Han, D., Yao, Y., Chen, L., Miao, Z., and Xu, S. (2022). Apigenin ameliorates di(2-ethylhexyl) phthalate-induced ferroptosis: the activation of glutathione peroxidase 4 and suppression of iron intake. Food Chem. Toxicol. 164, 113089. doi:10.1016/j.fct.2022.113089
Harrison, A. V., Lorenzo, F. R., and McClain, D. A. (2023). Iron and the pathophysiology of diabetes. Annu. Rev. Physiol. 85, 339–362. doi:10.1146/annurev-physiol-022522-102832
Haschka, D., Hoffmann, A., and Weiss, G. (2021). Iron in immune cell function and host defense. Seminars Cell. & Dev. Biol. 115, 27–36. doi:10.1016/j.semcdb.2020.12.005
He, H., Liao, S., Zeng, Y., Liang, L., Chen, J., and Tao, C. (2022). Causal relationships between metabolic-associated fatty liver disease and iron status: two-sample Mendelian randomization. Liver Int. 42 (12), 2759–2768. doi:10.1111/liv.15455
Hoki, T., Miyanishi, K., Tanaka, S., Takada, K., Kawano, Y., Sakurada, A., et al. (2015). Increased duodenal iron absorption through up-regulation of divalent metal transporter 1 from enhancement of iron regulatory protein 1 activity in patients with nonalcoholic steatohepatitis. Hepatology 62 (3), 751–761. doi:10.1002/hep.27774
Homma, T., Osaki, T., Kobayashi, S., Sato, H., and Fujii, J. (2022). d-Cysteine supplementation partially protects against ferroptosis induced by xCT dysfunction via increasing the availability of glutathione. J. Clin. Biochem. Nutr. 71 (1), 48–54. doi:10.3164/jcbn.21-143
Huang, B., Wen, W., and Ye, S. (2022). Dapagliflozin ameliorates renal tubular ferroptosis in diabetes via SLC40A1 stabilization. Oxid. Med. Cell. Longev. 2022, 9735555. doi:10.1155/2022/9735555
Jiang, H., Zhang, X., Yang, W., Li, M., Wang, G., and Luo, Q. (2022a). Ferrostatin-1 ameliorates liver dysfunction via reducing iron in thioacetamide-induced acute liver injury in mice. Front. Pharmacol. 13, 869794. doi:10.3389/fphar.2022.869794
Jiang, J.-J., Zhang, G.-F., Zheng, J.-Y., Sun, J.-H., and Ding, S.-B. (2022b). Targeting mitochondrial ROS-mediated ferroptosis by quercetin alleviates high-fat diet-induced hepatic lipotoxicity. Front. Pharmacol. 13, 876550. doi:10.3389/fphar.2022.876550
Jorge, A. S. B., Andrade, J. M. O., Paraíso, A. F., Jorge, G. C. B., Silveira, C. M., de Souza, L. R., et al. (2018). Body mass index and the visceral adipose tissue expression of IL-6 and TNF-alpha are associated with the morphological severity of non-alcoholic fatty liver disease in individuals with class III obesity. Obes. Res. Clin. Pract. 12(2), 1–8. doi:10.1016/j.orcp.2016.03.009
Kang, R., Kroemer, G., and Tang, D. (2019). The tumor suppressor protein p53 and the ferroptosis network. Free Radic. Biol. Med. 133, 162–168. doi:10.1016/j.freeradbiomed.2018.05.074
Katunga, L. A., Gudimella, P., Efird, J. T., Abernathy, S., Mattox, T. A., Beatty, C., et al. (2015). Obesity in a model of gpx4 haploinsufficiency uncovers a causal role for lipid-derived aldehydes in human metabolic disease and cardiomyopathy. Mol. Metab. 4 (6), 493–506. doi:10.1016/j.molmet.2015.04.001
Killion, E. A., Reeves, A. R., El Azzouny, M. A., Yan, Q.-W., Surujon, D., Griffin, J. D., et al. (2018). A role for long-chain acyl-CoA synthetase-4 (ACSL4) in diet-induced phospholipid remodeling and obesity-associated adipocyte dysfunction. Mol. Metab. 9, 43–56. doi:10.1016/j.molmet.2018.01.012
Kim, D.-H., Choi, H.-I., Park, J. S., Kim, C. S., Bae, E. H., Ma, S. K., et al. (2022). Farnesoid X receptor protects against cisplatin-induced acute kidney injury by regulating the transcription of ferroptosis-related genes. Redox Biol. 54, 102382. doi:10.1016/j.redox.2022.102382
Kose, T., Vera-Aviles, M., Sharp, P. A., and Latunde-Dada, G. O. (2019). Curcumin and (-)- epigallocatechin-3-gallate protect murine MIN6 pancreatic beta-cells against iron toxicity and erastin-induced ferroptosis. Pharm. (Basel) 12 (1), 26. doi:10.3390/ph12010026
Kraft, V. A. N., Bezjian, C. T., Pfeiffer, S., Ringelstetter, L., Müller, C., Zandkarimi, F., et al. (2020). GTP Cyclohydrolase 1/Tetrahydrobiopterin Counteract Ferroptosis through Lipid Remodeling. ACS central Sci. 6(1), 41–53. doi:10.1021/acscentsci.9b01063
Krümmel, B., Plötz, T., Jörns, A., Lenzen, S., and Mehmeti, I. (2021). The central role of glutathione peroxidase 4 in the regulation of ferroptosis and its implications for pro-inflammatory cytokine-mediated beta-cell death. Biochim. Biophys. Acta Mol. Basis Dis. 1867 (6), 166114. doi:10.1016/j.bbadis.2021.166114
Kung, Y.-A., Chiang, H.-J., Li, M.-L., Gong, Y.-N., Chiu, H.-P., Hung, C.-T., et al. (2022). Acyl-coenzyme A synthetase long-chain family member 4 is involved in viral replication organelle formation and facilitates virus replication via ferroptosis. mBio 13 (1), e0271721. doi:10.1128/mbio.02717-21
Lee, H., Zandkarimi, F., Zhang, Y., Meena, J. K., Kim, J., Zhuang, L., et al. (2020). Energy-stress-mediated AMPK activation inhibits ferroptosis. Nat. Cell. Biol. 22 (2), 225–234. doi:10.1038/s41556-020-0461-8
Lee, J. Y., Kim, W. K., Bae, K. H., Lee, S. C., and Lee, E. W. (2021). Lipid metabolism and ferroptosis. Biology 10 (3), 184. doi:10.3390/biology10030184
Lemasters, J. J. (2017). Evolution of voltage-dependent anion channel function: from molecular sieve to governator to actuator of ferroptosis. Front. Oncol. 7, 303. doi:10.3389/fonc.2017.00303
Li, D., Jiang, C., Mei, G., Zhao, Y., Chen, L., Liu, J., et al. (2020c). Quercetin alleviates ferroptosis of pancreatic β cells in type 2 diabetes. Nutrients 12 (10), 2954. doi:10.3390/nu12102954
Li, J., Cao, F., Yin, H. L., Huang, Z. J., Lin, Z. T., Mao, N., et al. (2020a). Ferroptosis: past, present and future. Cell. Death Dis. 11 (2), 88. doi:10.1038/s41419-020-2298-2
Li, X., Wang, T.-X., Huang, X., Li, Y., Sun, T., Zang, S., et al. (2020b). Targeting ferroptosis alleviates methionine-choline deficient (MCD)-diet induced NASH by suppressing liver lipotoxicity. Liver Int. 40 (6), 1378–1394. doi:10.1111/liv.14428
Li, X. Y., and Leung, P. S. (2020). Erastin-induced ferroptosis is a regulator for the growth and function of human pancreatic islet-like cell clusters. Cell. Regen. 9 (1), 16. doi:10.1186/s13619-020-00055-3
Li, Y., Jiang, W., Feng, Y., Wu, L., Jia, Y., and Zhao, R. (2022). Betaine alleviates high-fat diet-induced disruptionof hepatic lipid and iron homeostasis in mice. Int. J. Mol. Sci. 23 (11), 6263. doi:10.3390/ijms23116263
Liang, H., Tang, T., Huang, H., Li, T., Gao, C., Han, Y., et al. (2022). Peroxisome proliferator-activated receptor-γ ameliorates neuronal ferroptosis after traumatic brain injury in mice by inhibiting cyclooxygenase-2. Exp. Neurol. 354, 114100. doi:10.1016/j.expneurol.2022.114100
Liao, H.-H., Ding, W., Zhang, N., Zhou, Z.-Y., Ling, Z., Li, W.-J., et al. (2023). Activation of AMPKα2 attenuated doxorubicin-induced cardiotoxicity via inhibiting lipid peroxidation associated ferroptosis. Free Radic. Biol. Med. 205, 275–290. doi:10.1016/j.freeradbiomed.2023.06.004
Lipper, C. H., Stofleth, J. T., Bai, F., Sohn, Y. S., Roy, S., Mittler, R., et al. (2019). Redox-dependent gating of VDAC by mitoNEET. Proc. Natl. Acad. Sci. U. S. A. 116 (40), 19924–19929. doi:10.1073/pnas.1908271116
Liu, J., Li, Q., Yang, Y., and Ma, L. (2020). Iron metabolism and type 2 diabetes mellitus: a meta-analysis and systematic review. J. Diabetes Investig. 11 (4), 946–955. doi:10.1111/jdi.13216
Liu, P., Anandhan, A., Chen, J., Shakya, A., Dodson, M., Ooi, A., et al. (2023). Decreased autophagosome biogenesis, reduced NRF2, and enhanced ferroptotic cell death are underlying molecular mechanisms of non-alcoholic fatty liver disease. Redox Biol. 59, 102570. doi:10.1016/j.redox.2022.102570
Liu, S., Gao, Z., He, W., Wu, Y., Liu, J., Zhang, S., et al. (2022). The gut microbiota metabolite glycochenodeoxycholate activates TFR-ACSL4-mediated ferroptosis to promote the development of environmental toxin-linked MAFLD. Free Radic. Biol. Med. 193 (1), 213–226. doi:10.1016/j.freeradbiomed.2022.10.270
Luo, L., Huang, F., Zhong, S., Ding, R., Su, J., and Li, X. (2022b). Astaxanthin attenuates ferroptosis via Keap1-Nrf2/HO-1 signaling pathways in LPS-induced acute lung injury. Life Sci. 311, 121091. doi:10.1016/j.lfs.2022.121091
Luo, Y., Decato, B. E., Charles, E. D., Shevell, D. E., McNaney, C., Shipkova, P., et al. (2022a). Pegbelfermin selectively reduces secondary bile acid concentrations in patients with non-alcoholic steatohepatitis. JHEP Rep. 4 (1), 100392. doi:10.1016/j.jhepr.2021.100392
Ma, S., He, L.-L., Zhang, G.-R., Zuo, Q.-J., Wang, Z.-L., Zhai, J.-L., et al. (2022). Canagliflozin mitigates ferroptosis and ameliorates heart failure in rats with preserved ejection fraction. Naunyn Schmiedeb. Arch. Pharmacol. 395 (8), 945–962. doi:10.1007/s00210-022-02243-1
Ma, W.-Q., Sun, X.-J., Zhu, Y., and Liu, N.-F. (2021). Metformin attenuates hyperlipidaemia-associated vascular calcification through anti-ferroptotic effects. Free Radic. Biol. Med. 165, 229–242. doi:10.1016/j.freeradbiomed.2021.01.033
Mancias, J. D., Wang, X., Gygi, S. P., Harper, J. W., and Kimmelman, A. C. (2014). Quantitative proteomics identifies NCOA4 as the cargo receptor mediating ferritinophagy. Nature 509 (7498), 105–109. doi:10.1038/nature13148
Mao, C., Liu, X., Zhang, Y., Lei, G., Yan, Y., Lee, H., et al. (2021). DHODH-mediated ferroptosis defence is a targetable vulnerability in cancer. Nature 593 (7860), 586–590. doi:10.1038/s41586-021-03539-7
Marku, A., Galli, A., Marciani, P., Dule, N., Perego, C., and Castagna, M. (2021). Iron metabolism in pancreatic beta-cell function and dysfunction. Cells 10 (11), 2841. doi:10.3390/cells10112841
Miao, R., Fang, X., Zhang, Y., Wei, J., Zhang, Y., and Tian, J. (2023). Iron metabolism and ferroptosis in type 2 diabetes mellitus and complications: mechanisms and therapeutic opportunities. Cell. Death Dis. 14 (3), 186. doi:10.1038/s41419-023-05708-0
Niu, B., Lei, X., Xu, Q., Ju, Y., Xu, D., Mao, L., et al. (2022). Protecting mitochondria via inhibiting VDAC1 oligomerization alleviates ferroptosis in acetaminophen-induced acute liver injury. Cell. Biol. Toxicol. 38 (3), 505–530. doi:10.1007/s10565-021-09624-x
Noetzli, L. J., Mittelman, S. D., Watanabe, R. M., Coates, T. D., and Wood, J. C. (2012). Pancreatic iron and glucose dysregulation in thalassemia major. Am. J. Hematol. 87 (2), 155–160. doi:10.1002/ajh.22223
Ouyang, C., Ma, X., Zhao, J., Li, S., Liu, C., Tang, Y., et al. (2023). Oleanolic acid inhibits mercury chloride induced-liver ferroptosis by regulating ROS/iron overload. Ecotoxicol. Environ. Saf. 258, 114973. doi:10.1016/j.ecoenv.2023.114973
Pelusi, C., Gasparini, D. I., Bianchi, N., and Pasquali, R. (2016). Endocrine dysfunction in hereditary hemochromatosis. J. Endocrinol. Investig. 39 (8), 837–847. doi:10.1007/s40618-016-0451-7
Peng, Q., Chen, X., Liang, X., Ouyang, J., Wang, Q., Ren, S., et al. (2023). Metformin improves polycystic ovary syndrome in mice by inhibiting ovarian ferroptosis. Front. Endocrinol. (Lausanne) 14, 1070264. doi:10.3389/fendo.2023.1070264
Protchenko, O., Baratz, E., Jadhav, S., Li, F., Shakoury-Elizeh, M., Gavrilova, O., et al. (2021). Iron chaperone poly rC binding protein 1 protects mouse liver from lipid peroxidation and steatosis. Hepatology 73 (3), 1176–1193. doi:10.1002/hep.31328
Qi, J., Kim, J.-W., Zhou, Z., Lim, C.-W., and Kim, B. (2020). Ferroptosis affects the progression of nonalcoholic steatohepatitis via the modulation of lipid peroxidation-mediated cell death in mice. Am. J. Pathol. 190 (1), 68–81. doi:10.1016/j.ajpath.2019.09.011
Qi, Y., Hu, M., Qiu, Y., Zhang, L., Yan, Y., Feng, Y., et al. (2023). Mitoglitazone ameliorates renal ischemia/reperfusion injury by inhibiting ferroptosis via targeting mitoNEET. Toxicol. Appl. Pharmacol. 465, 116440. doi:10.1016/j.taap.2023.116440
Rinella, M. E., Lazarus, J. V., Ratziu, V., Francque, S. M., Sanyal, A. J., Kanwal, F., et al. (2023). A multi-society Delphi consensus statement on new fatty liver disease nomenclature. J. Hepatol. doi:10.1016/j.jhep.2023.06.003
Rizzo, M., Colletti, A., Penson, P. E., Katsiki, N., Mikhailidis, D. P., Toth, P. P., et al. (2023). Nutraceutical approaches to non-alcoholic fatty liver disease (NAFLD): a position paper from the International Lipid Expert Panel (ILEP). Pharmacol. Res. 189, 106679. doi:10.1016/j.phrs.2023.106679
Salama, S. A., Abdel-Bakky, M. S., and Mohamed, A. A. (2022). Upregulation of Nrf2 signaling and suppression of ferroptosis and NF-κB pathway by leonurine attenuate iron overload-induced hepatotoxicity. Chem. Biol. Interact. 356, 109875. doi:10.1016/j.cbi.2022.109875
Schwärzler, J., Mayr, L., Radlinger, B., Grabherr, F., Philipp, M., Texler, B., et al. (2022). Adipocyte GPX4 protects against inflammation, hepatic insulin resistance and metabolic dysregulation. Int. J. Obes. (Lond). 46 (5), 951–959. doi:10.1038/s41366-022-01064-9
Shi, H., Yan, Y., Yang, H., Pu, P., and Tang, H. (2022b). Schisandrin B diet inhibits oxidative stress to reduce ferroptosis and lipid peroxidation to prevent pirarubicin-induced hepatotoxicity. Biomed. Res. Int. 2022, 5623555. doi:10.1155/2022/5623555
Shi, P., Song, C., Qi, H., Ren, J., Ren, P., Wu, J., et al. (2022a). Up-regulation of IRF3 is required for docosahexaenoic acid suppressing ferroptosis of cardiac microvascular endothelial cells in cardiac hypertrophy rat. J. Nutr. Biochem. 104, 108972. doi:10.1016/j.jnutbio.2022.108972
Song, C., Li, D., Zhang, J., and Zhao, X. (2023). Berberine hydrochloride alleviates imatinib mesylate - induced cardiotoxicity through the inhibition of Nrf2-dependent ferroptosis. Food Funct. 14 (2), 1087–1098. doi:10.1039/d2fo03331c
Song, J.-X., An, J.-R., Chen, Q., Yang, X.-Y., Jia, C.-L., Xu, S., et al. (2022a). Liraglutide attenuates hepatic iron levels and ferroptosis in db/db mice. Bioengineered 13 (4), 8334–8348. doi:10.1080/21655979.2022.2051858
Song, X., and Long, D. (2020). Nrf2 and ferroptosis: a new research direction for neurodegenerative diseases. Front. Neurosci. 14, 267. doi:10.3389/fnins.2020.00267
Song, X.-Y., Liu, P.-C., Liu, W.-W., Zhou, J., Hayashi, T., Mizuno, K., et al. (2022b). Silibinin inhibits ethanol- or acetaldehyde-induced ferroptosis in liver cell lines. Toxicol Vitro 82, 105388. doi:10.1016/j.tiv.2022.105388
Stancic, A., Saksida, T., Markelic, M., Vucetic, M., Grigorov, I., Martinovic, V., et al. (2022). Ferroptosis as a novel determinant of β-cell death in diabetic conditions. Oxid. Med. Cell. Longev. 2022, 3873420. doi:10.1155/2022/3873420
Sun, S. P., Lu, Y. F., Li, H., Weng, C. Y., Chen, J. J., Lou, Y. J., et al. (2023b). AMPK activation alleviated dextran sulfate sodium-induced colitis by inhibiting ferroptosis. J. Dig. Dis. 24 (3), 213–223. doi:10.1111/1751-2980.13176
Sun, X., Zhang, X., Yan, H., Wu, H., Cao, S., Zhao, W., et al. (2023c). Protective effect of curcumin on hepatolenticular degeneration through copper excretion and inhibition of ferroptosis. Phytomedicine 113, 154539. doi:10.1016/j.phymed.2022.154539
Sun, Y., Guo, L.-Q., Wang, D.-G., Xing, Y.-J., Bai, Y.-P., Zhang, T., et al. (2023a). Metformin alleviates glucolipotoxicity-induced pancreatic β cell ferroptosis through regulation of the GPX4/ACSL4 axis. Eur. J. Pharmacol. 956, 175967. doi:10.1016/j.ejphar.2023.175967
Tang, X., Li, Z., Yu, Z., Li, J., Zhang, J., Wan, N., et al. (2021). Effect of curcumin on lung epithelial injury and ferroptosis induced by cigarette smoke. Hum. Exp. Toxicol. 40, S753–S762. doi:10.1177/09603271211059497
Tokushige, K., Ikejima, K., Ono, M., Eguchi, Y., Kamada, Y., Itoh, Y., et al. (2021). Evidence-based clinical practice guidelines for nonalcoholic fatty liver disease/nonalcoholic steatohepatitis 2020. J. Gastroenterol. 56 (11), 951–963. doi:10.1007/s00535-021-01796-x
Tong, J., Lan, X.-T., Zhang, Z., Liu, Y., Sun, D.-Y., Wang, X.-J., et al. (2023). Ferroptosis inhibitor liproxstatin-1 alleviates metabolic dysfunction-associated fatty liver disease in mice: potential involvement of PANoptosis. Acta Pharmacol. Sin. 44 (5), 1014–1028. doi:10.1038/s41401-022-01010-5
Wang, C., Liu, T., Tong, Y., Cui, R., Qu, K., Liu, C., et al. (2021b). Ulinastatin protects against acetaminophen-induced liver injury by alleviating ferroptosis via the SIRT1/NRF2/HO-1 pathway. Am. J. Transl. Res. 13 (6), 6031–6042.
Wang, C., Wang, X., Song, G., Xing, H., Yang, L., Han, K., et al. (2021a). A high-fructose diet in rats induces systemic iron deficiency and hepatic iron overload by an inflammation mechanism. J. Food Biochem. 45 (1), e13578. doi:10.1111/jfbc.13578
Wang, H., Jiang, C., Yang, Y., Li, J., Wang, Y., Wang, C., et al. (2022e). Resveratrol ameliorates iron overload induced liver fibrosis in mice by regulating iron homeostasis. PeerJ 10, e13592. doi:10.7717/peerj.13592
Wang, J.-W., Jin, C.-H., Ke, J.-F., Ma, Y.-L., Wang, Y.-J., Lu, J.-X., et al. (2022a). Serum iron is closely associated with metabolic dysfunction-associated fatty liver disease in type 2 diabetes: a real-world study. Front. Endocrinol. (Lausanne) 13, 942412. doi:10.3389/fendo.2022.942412
Wang, Q., Zhu, M., Li, H., Chen, P., Wang, M., Gu, L., et al. (2022b). Hyperferritinemia correlates to metabolic dysregulation and steatosis in Chinese biopsy-proven nonalcoholic fatty liver disease patients. Diabetes Metab. Syndr. Obes. 15, 1543–1552. doi:10.2147/DMSO.S361187
Wang, X., Chen, X., Zhou, W., Men, H., Bao, T., Sun, Y., et al. (2022c). Ferroptosis is essential for diabetic cardiomyopathy and is prevented by sulforaphane via AMPK/NRF2 pathways. Acta Pharm. Sin. B 12 (2), 708–722. doi:10.1016/j.apsb.2021.10.005
Wang, X., Xiao, A., Yang, Y., Zhao, Y., Wang, C. C., Wang, Y., et al. (2022d). DHA and EPA prevent seizure and depression-like behavior by inhibiting ferroptosis and neuroinflammation via different mode-of-actions in a pentylenetetrazole-induced kindling model in mice. Mol. Nutr. Food Res. 66 (22), e2200275. doi:10.1002/mnfr.202200275
Wang, Y., Chen, Q., Shi, C., Jiao, F., and Gong, Z. (2019). Mechanism of glycyrrhizin on ferroptosis during acute liver failure by inhibiting oxidative stress. Mol. Med. Rep. 20 (5), 4081–4090. doi:10.3892/mmr.2019.10660
Wei, S., Qiu, T., Wang, N., Yao, X., Jiang, L., Jia, X., et al. (2020). Ferroptosis mediated by the interaction between Mfn2 and IREα promotes arsenic-induced nonalcoholic steatohepatitis. Environ. Res. 188, 109824. doi:10.1016/j.envres.2020.109824
Wei, S., Qiu, T., Yao, X., Wang, N., Jiang, L., Jia, X., et al. (2020). Arsenic induces pancreatic dysfunction and ferroptosis via mitochondrial ROS-autophagy-lysosomal pathway. J. Hazard. Mater. 384, 121390. doi:10.1016/j.jhazmat.2019.121390
Wei, Z., Shaohuan, Q., Pinfang, K., and Chao, S. (2022). Curcumin attenuates ferroptosis-induced myocardial injury in diabetic cardiomyopathy through the Nrf2 pathway. Cardiovasc Ther. 2022, 3159717. doi:10.1155/2022/3159717
Wu, A., Feng, B., Yu, J., Yan, L., Che, L., Zhuo, Y., et al. (2021). Fibroblast growth factor 21 attenuates iron overload-induced liver injury and fibrosis by inhibiting ferroptosis. Redox Biol. 46, 102131. doi:10.1016/j.redox.2021.102131
Wu, Z., Bai, Y., Qi, Y., Chang, C., Jiao, Y., Bai, Y., et al. (2023). Metformin ameliorates ferroptosis in cardiac ischemia and reperfusion by reducing NOX4 expression via promoting AMPKα. Pharm. Biol. 61 (1), 886–896. doi:10.1080/13880209.2023.2212700
Xie, Y., Zhu, S., Song, X., Sun, X., Fan, Y., Liu, J., et al. (2017). The tumor suppressor p53 limits ferroptosis by blocking DPP4 activity. Cell. Rep. 20 (7), 1692–1704. doi:10.1016/j.celrep.2017.07.055
Xue, M., Tian, Y., Sui, Y., Zhao, H., Gao, H., Liang, H., et al. (2022). Protective effect of fucoidan against iron overload and ferroptosis-induced liver injury in rats exposed to alcohol. Biomed. Pharmacother. 153, 113402. doi:10.1016/j.biopha.2022.113402
Yakubov, E., Schmid, S., Hammer, A., Chen, D., Dahlmanns, J. K., Mitrovic, I., et al. (2023). Ferroptosis and PPAR-gamma in the limelight of brain tumors and edema. Front. Oncol. 13, 1176038. doi:10.3389/fonc.2023.1176038
Yan, J., Feng, G., Ma, L., Chen, Z., and Jin, Q. (2022a). Metformin alleviates osteoarthritis in mice by inhibiting chondrocyte ferroptosis and improving subchondral osteosclerosis and angiogenesis. J. Orthop. Surg. Res. 17 (1), 333. doi:10.1186/s13018-022-03225-y
Yan, W., Wang, D., Wan, N., Wang, S., Shao, C., Zhang, H., et al. (2022b). Living cell-target responsive accessibility profiling reveals silibinin targeting ACSL4 for combating ferroptosis. Anal. Chem. 94 (43), 14820–14826. doi:10.1021/acs.analchem.2c03515
Yang, Y., Chen, J., Gao, Q., Shan, X., Wang, J., and Lv, Z. (2020). Study on the attenuated effect of Ginkgolide B on ferroptosis in high fat diet induced nonalcoholic fatty liver disease. Toxicology 445, 152599. doi:10.1016/j.tox.2020.152599
Yang, Z., Wu, J., Li, X., Xie, D., Wang, Y., and Yang, T. (2019). Association between dietary iron intake and the prevalence of nonalcoholic fatty liver disease: a cross-sectional study. Med. Baltim. 98 (43), e17613. doi:10.1097/MD.0000000000017613
Ye, Q., Jiang, Y., Wu, D., Cai, J., Jiang, Z., Zhou, Z., et al. (2023). Atractylodin alleviates nonalcoholic fatty liver disease by regulating Nrf2-mediated ferroptosis. Heliyon 9 (7), e18321. doi:10.1016/j.heliyon.2023.e18321
Yin, X., Guo, X., Liu, Z., and Wang, J. (2023). Advances in the diagnosis and treatment of non-alcoholic fatty liver disease. Int. J. Mol. Sci. 24 (3), 2844. doi:10.3390/ijms24032844
Younossi, Z. M., Golabi, P., de Avila, L., Paik, J. M., Srishord, M., Fukui, N., et al. (2019a). The global epidemiology of NAFLD and NASH in patients with type 2 diabetes: a systematic review and meta-analysis. J. Hepatol. 71 (4), 793–801. doi:10.1016/j.jhep.2019.06.021
Younossi, Z. M., Ratziu, V., Loomba, R., Rinella, M., Anstee, Q. M., Goodman, Z., et al. (2019b). Obeticholic acid for the treatment of non-alcoholic steatohepatitis: interim analysis from a multicentre, randomised, placebo-controlled phase 3 trial. Lancet 394 (10215), 2184–2196. doi:10.1016/S0140-6736(19)33041-7
Yu, Y., Jiang, L., Wang, H., Shen, Z., Cheng, Q., Zhang, P., et al. (2020). Hepatic transferrin plays a role in systemic iron homeostasis and liver ferroptosis. Blood 136 (6), 726–739. doi:10.1182/blood.2019002907
Yuan, H., Li, X., Zhang, X., Kang, R., and Tang, D. (2016a). Identification of ACSL4 as a biomarker and contributor of ferroptosis. Biochem. Biophys. Res. Commun. 478 (3), 1338–1343. doi:10.1016/j.bbrc.2016.08.124
Yuan, H., Li, X., Zhang, X., Kang, R., and Tang, D. (2016b). CISD1 inhibits ferroptosis by protection against mitochondrial lipid peroxidation. Biochem. Biophys. Res. Commun. 478 (2), 838–844. doi:10.1016/j.bbrc.2016.08.034
Zhang, R., Chen, J., Wang, S., Zhang, W., Zheng, Q., and Cai, R. (2023). Ferroptosis in cancer progression. Cells 12 (14), 1820. doi:10.3390/cells12141820
Zhang, S., Sun, Z., Jiang, X., Lu, Z., Ding, L., Li, C., et al. (2022a). Ferroptosis increases obesity: crosstalk between adipocytes and the neuroimmune system. Front. Immunol. 13, 1049936. doi:10.3389/fimmu.2022.1049936
Zhang, X., Jiang, L., Chen, H., Wei, S., Yao, K., Sun, X., et al. (2022d). Resveratrol protected acrolein-induced ferroptosis and insulin secretion dysfunction via ER-stress- related PERK pathway in MIN6 cells. Toxicology 465, 153048. doi:10.1016/j.tox.2021.153048
Zhang, X., Wu, S., Guo, C., Guo, K., Hu, Z., Peng, J., et al. (2022c). Vitamin E exerts neuroprotective effects in pentylenetetrazole kindling epilepsy via suppression of ferroptosis. Neurochem. Res. 47 (3), 739–747. doi:10.1007/s11064-021-03483-y
Zhang, Y., Zhang, X., Wee Yong, V., and Xue, M. (2022b). Vildagliptin improves neurological function by inhibiting apoptosis and ferroptosis following intracerebral hemorrhage in mice. Neurosci. Lett. 776, 136579. doi:10.1016/j.neulet.2022.136579
Zhang, Y., Zhao, S., Fu, Y., Yan, L., Feng, Y., Chen, Y., et al. (2020). Computational repositioning of dimethyl fumarate for treating alcoholic liver disease. Cell. Death Dis. 11 (8), 641. doi:10.1038/s41419-020-02890-3
Zhang, Z., Liu, D., Yi, B., Liao, Z., Tang, L., Yin, D., et al. (2014). Taurine supplementation reduces oxidative stress and protects the liver in an iron-overload murine model. Mol. Med. Rep. 10 (5), 2255–2262. doi:10.3892/mmr.2014.2544
Zhao, C., Xiao, C., Feng, S., and Bai, J. (2023). Artemisitene alters LPS-induced oxidative stress, inflammation and ferroptosis in liver through Nrf2/HO-1 and NF-kB pathway. Front. Pharmacol. 14, 1177542. doi:10.3389/fphar.2023.1177542
Zhao, T., Yu, Z., Zhou, L., Wang, X., Hui, Y., Mao, L., et al. (2022b). Regulating Nrf2-GPx4 axis by bicyclol can prevent ferroptosis in carbon tetrachloride-induced acute liver injury in mice. Cell. Death Discov. 8 (1), 380. doi:10.1038/s41420-022-01173-4
Zhao, Y., Mei, G., Zhou, F., Kong, B., Chen, L., Chen, H., et al. (2022a). Vitamin D decreases pancreatic iron overload in type 2 diabetes through the NF-κB-DMT1 pathway. J. Nutr. Biochem. 99, 108870. doi:10.1016/j.jnutbio.2021.108870
Zhu, Z., Zhang, Y., Huang, X., Can, L., Zhao, X., Wang, Y., et al. (2021). Thymosin beta 4 alleviates non-alcoholic fatty liver by inhibiting ferroptosis via up-regulation of GPX4. Eur. J. Pharmacol. 908, 174351. doi:10.1016/j.ejphar.2021.174351
Zou, Y., Zhong, L., Hu, C., Zhong, M., Peng, N., and Sheng, G. (2021). LDL/HDL cholesterol ratio is associated with new-onset NAFLD in Chinese non-obese people with normal lipids: a 5-year longitudinal cohort study. Lipids Health Dis. 20 (1), 28. doi:10.1186/s12944-021-01457-1
Keywords: metabolic disease, ferroptosis, therapeutic drugs, metabolic dysfunction-associated steatotic liver disease (MASLD), mechanism
Citation: Zhu B, Wei Y, Zhang M, Yang S, Tong R, Li W and Long E (2023) Metabolic dysfunction-associated steatotic liver disease: ferroptosis related mechanisms and potential drugs. Front. Pharmacol. 14:1286449. doi: 10.3389/fphar.2023.1286449
Received: 31 August 2023; Accepted: 24 October 2023;
Published: 09 November 2023.
Edited by:
Chenghai Liu, Shanghai University of Traditional Chinese Medicine, ChinaReviewed by:
Feng Zhang, Nanjing University of Chinese Medicine, ChinaCopyright © 2023 Zhu, Wei, Zhang, Yang, Tong, Li and Long. This is an open-access article distributed under the terms of the Creative Commons Attribution License (CC BY). The use, distribution or reproduction in other forums is permitted, provided the original author(s) and the copyright owner(s) are credited and that the original publication in this journal is cited, in accordance with accepted academic practice. No use, distribution or reproduction is permitted which does not comply with these terms.
*Correspondence: Enwu Long, ZHJhZ29uOTg0MTY5QDEyNi5jb20=; Wenyuan Li, d2VueXVhbmxpNzRAMTYzLmNvbQ==
†These authors have contributed equally to this work
Disclaimer: All claims expressed in this article are solely those of the authors and do not necessarily represent those of their affiliated organizations, or those of the publisher, the editors and the reviewers. Any product that may be evaluated in this article or claim that may be made by its manufacturer is not guaranteed or endorsed by the publisher.
Research integrity at Frontiers
Learn more about the work of our research integrity team to safeguard the quality of each article we publish.