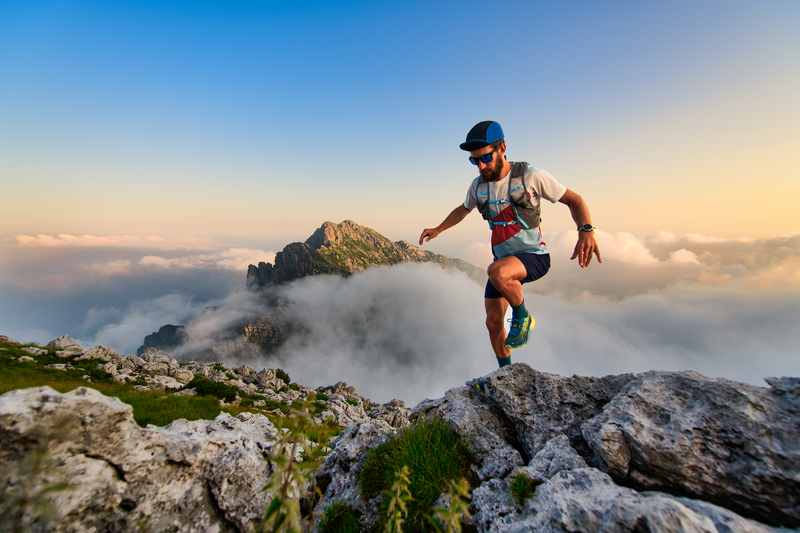
95% of researchers rate our articles as excellent or good
Learn more about the work of our research integrity team to safeguard the quality of each article we publish.
Find out more
REVIEW article
Front. Pharmacol. , 15 November 2023
Sec. Ethnopharmacology
Volume 14 - 2023 | https://doi.org/10.3389/fphar.2023.1283784
Lipid-lowering therapy is an important tool for the treatment of lipid metabolic diseases, which are increasing in prevalence. However, the failure of conventional lipid-lowering drugs to achieve the desired efficacy in some patients, and the side-effects of these drug regimens, highlight the urgent need for novel lipid-lowering drugs. The liver and intestine are important in the production and removal of endogenous and exogenous lipids, respectively, and have an important impact on circulating lipid levels. Elevated circulating lipids predisposes an individual to lipid deposition in the vascular wall, affecting vascular function. Berberine (BBR) modulates liver lipid production and clearance by regulating cellular targets such as cluster of differentiation 36 (CD36), acetyl-CoA carboxylase (ACC), microsomal triglyceride transfer protein (MTTP), scavenger receptor class B type 1 (SR-BI), low-density lipoprotein receptor (LDLR), and ATP-binding cassette transporter A1 (ABCA1). It influences intestinal lipid synthesis and metabolism by modulating gut microbiota composition and metabolism. Finally, BBR maintains vascular function by targeting proteins such as endothelial nitric oxide synthase (eNOS) and lectin-like oxidized low-density lipoprotein receptor-1 (LOX-1). This paper elucidates and summarizes the pharmacological mechanisms of berberine in lipid metabolic diseases from a multi-organ (liver, intestine, and vascular system) and multi-target perspective.
In recent years, with economic development and changes in diet, the incidence of lipid metabolic diseases, such as non-alcoholic fatty liver disease (NAFLD), atherosclerosis (AS), obesity, and hyperlipidemia, has increased, placing a significant burden on human health and the health insurance system (Loomba et al., 2021; Fan and Watanabe, 2022). Elevated lipid levels are a common feature of these diseases, and thus lipid-lowering therapies are essential for their treatment. Commonly used lipid-lowering drugs such as statins, protein convertase subtilisin/kexin type 9 (PCSK9) inhibitors, and ezetimibe have been developed based on critical targets of lipid metabolism. The widespread use of these drugs effectively lowers lipid levels and reduces lipid deposition in tissues such as blood vessels. However, adverse effects of long-term treatment, such as liver damage and rhabdomyolysis, have been widely documented, emphasizing the need to develop new lipid-lowering therapies (Liu et al., 2019).
Berberine (BBR) is widely used in Asian countries because of its good clinical efficacy and safety in reducing lipids (Song et al., 2020). Importantly, in contrast to drugs developed for a single target of lipid metabolism in a single organ, BBR can coordinate multiple key targets of lipid metabolism and intestinal flora in the intestine and liver to regulate lipid levels. It can also act directly on blood vessels to ameliorate endothelial dysfunction, inhibit macrophage foam cell formation, and regulate vascular smooth muscle cell (VSMC) proliferation and migration to prevent and treat AS (Rui et al., 2021) (Figure 1). The guidelines of the European Society of Cardiology and the European Atherosclerosis Society recommend BBR as a dietary supplement and functional food for treating dyslipidemia (Members et al., 2016). This article provides an in-depth review of recent advances in utilizing BBR to prevent and treat lipid metabolic disorders. It examines the progress from the per-spective of the liver, intestine, and blood vessels, and discusses how BBR acts on cellular targets including cluster of differentiation 36 (CD36), microsomal triglyceride transfer protein (MTTP), low-density lipoprotein receptor (LDLR), lectin-like oxidized low-density lipoprotein receptor-1 (LOX-1), acyl-CoA-cholesterol acyltransferase (ACAT), and adenosine monophosphate-activated protein kinase (AMPK), as well as gut microbiota. The aim of this review is to provide a modern scientific perspective for a holistic and systematic understanding of the mechanisms by which BBR regulates lipid lowering in multiple organs and to provide new ideas for treating lipid metabolic diseases.
FIGURE 1. The berberine (BBR) pathway in the prevention and treatment of lipid metabolic diseases. When oral BBR enters the gut, it interacts with intestinal microorganisms. Intestinal flora convert BBR into BBR derivatives such as demethyleneberberine, jatrorrhizine, berberrubine, and thalifendine BBR can also directly regulate intestinal branched-chain amino acid (BCAA), short-chain fatty acid (SCFA), and trimethylamine-N-oxide (TMAO) production. These intestinal metabolites are absorbed into the liver through blood vessels, which reduce liver lipid production and increase circulating lipid removal by inhibiting liver cluster of differentiation 36 (CD36), acetyl-CoA carboxylase (ACC), and microsomal triglyceride transfer protein (MTTP) expression and upregulating ATP-binding cassette transporter A1 (ABCA1), scavenger receptor class B type 1 (SR-BI), bile acid, and low-density lipoprotein receptor (LDLR) expression. In addition, metabolites entering blood vessels also act directly on blood vessel walls to reduce vascular endothelial dysfunction, reduce the formation of macrophage foam cells, inhibit vascular smooth muscle cell proliferation, and, thus, alleviate atherosclerosis (AS). Additional figure abbreviations: apoA-1, apolipoprotein AI; HDL, high-density lipoprotein; LCFA, long-chain fatty acid; LDL, low-density lipoprotein; LOX-1, lectin-like oxidized low-density lipoprotein receptor-1; VLDL, very low-density lipoprotein.
Because intravenous BBR administration can cause serious clinical side effects, oral administration is still the main route of administration of BBR. In an animal study in rats, berberine (400 mg/kg) was administered orally in rats, Cmax was 0.260 μg/mL. AUC0-t was low for the oral route of administration (Yu et al., 2022a). The poor absorption after BBR oral administration is caused by poor permeability, P-glycoprotein mediated efflux, hepatobiliary reexcretion, and self-aggregation. Absorbed BBR is rapidly and widely distributed in a variety of tissues such as the brain, intestine, stomach, pancreas, heart, kidney, and liver with the highest concentration in the liver (Tan et al., 2013). The liver and intestine, as the main sites of BBR metabolism, can metabolize BBR into a variety of derivatives through a variety of mechanisms (specifically, see section “2.2 Metabolism of BBR in the liver and intestine”). BBR is mainly excreted fecally, followed by through the urine and bile. When orally administered, BBR is majorly excreted through the feces and primarily in the original form. In contrast, BBR metabolites are mainly excreted through the urine and bile (Feng et al., 2021). Bile, as one of the pathways of BBR excretion, is also regulated by BBR-related signaling pathways. It has been found that BBR affects bile excretion by regulating the gut microbiota and acting on the farnesoid X receptor (FXR) -CYP7A1/CYP8B1 pathway (Guo et al., 2016; Wolf et al., 2021). However, due to the hepatoenteric circulation of bile acids (BAs), the amount of BBR and its derivatives excreted through bile is small.
In both rats and humans, the liver plays a significant role in BBR metabolism (Kumar et al., 2015). BBR is mainly converted into berberrubine, demethyleneberberine, jatrorrhizine, and thalifendine via hepatic metabolism (Feng et al., 2021). Following oral administration, BBR metabolite levels are highest in the liver (Zhu et al., 2016). Moreover, the half-life of BBR in hepatic tissue is longer than that in other tissues (Wei et al., 2016b), suggesting that the liver may be the main site of BBR metabolism.
Cytochrome P450 enzymes (CYPs) mediate the phase I metabolic pathway of BBR in the liver (Guo et al., 2011a). Clinical studies have shown that CYP2D6 is the primary human CYP that produces BBR metabolites, followed by CYP1A2, CYP3A4, CYP2E1, and CYP2C19 (Guo et al., 2011b). Thus, quinidine and furafylline are important metabolic inhibitors of BBR as they inhibit CYP2D6 and CYP1A2 (Khoshandam et al., 2022), respectively. Li et al. showed that CYP2D6 and CYP1A2 can convert BBR to the BBR metabolite thalifendine. Thalifendine increases LDLR mRNA expression with an activity level of 26% of that of BBR (Li et al., 2011a; Tan et al., 2013). CYP2D6, CYP1A2, and CYP3A4 participate in demethyleneberberine production. In the liver, intracellular demethyleneberberine is 25.14 times more enriched than extracellular demethyleneberberine (Liu et al., 2022). After the formation of phase I metabolites of BBR, phase II metabolites of BBR may be formed by glucuronidation. UDP-glucuronosyltransferases (UGTs), particularly UGT1 and UGT2, are known to mediate glucuronidation. In a human study, UGT1A1, UGT1A3, UGT1A7, UGT1A8, UGT1A9, and UGT1A10 in the liver mediated jatrorrhizine glucuronidation (Zhou et al., 2013). Berberrubine, demethyleneberberine, and their corresponding glucuronides were also be produced via incubation with the liver S9 fraction, but at a slower pace than with liver microsomes (Liu et al., 2009).
Animal studies have shown that a large proportion of BBR (200 mg/kg) is distributed in the liver after oral administration, which may contribute to its low blood concentration. Among BBR, berberrubine, demethyleneberberine, and jatrorrhizine, demethyleneberberine has the highest distribution in the liver and jatrorrhizine has the lowest (Liu et al., 2010). The liver also affects BBR clearance. For example, liver tissue clearance of BBR is higher in normal rats than in rats with acute hepatitis. Therefore, clinical doses of BBR should be carefully controlled when using it to treat acute hepatitis (Dai et al., 2018). In addition, tandem mass spectrometry revealed that the content of BBR metabolites in human liver microsomes is significantly different from that in rat liver microsomes (Li et al., 2017). Therefore, data related to the rat liver metabolism of BBR should be carefully refer-enced when developing BBR drugs.
To evaluate its intestinal first-pass metabolism, BBR was administered to rats via four distinct routes (intragastric, intraduodenal, intraportal, and intravenous). Significant differences are found in circulating BBR concentrations after intraduodenal and intravenous administration compared to intragastric administration, indicating that the intestinal first-pass effect of BBR is substantial (Liu et al., 2010). Moreover, utilizing intestinal post-mitochondrial (S9), cytosolic, and microsomal fractions, researchers have examined the intestinal metabolism of BBR (Ma et al., 2014). Five metabolites (berberrubine, demethyleneberberine, jatrorrhizine, berberrubine glucuronide, and demethyleneberberine glucuronide) were produced in the enterocyte fraction S9 and intestinal perfusates in vitro studies. Among them, berberrubine, demethyleneberberine glucuronide, and jatrorrhizine were the major intestinal metabolites (Liu et al., 2010).
Moreover, the intestinal flora is the largest microecosystem in the body and significantly affects material and energy metabolism. CYP51 in intestinal flora stably binds BBR and facilitates its conversion into demethylated metabolites. Adding a CYP51 inhibitor slows BBR metabolism, preventing the production of demethylated metabolites, including thalifendine and berberrubine (Zhang et al., 2021a). Furthermore, the intestinal flora can also increase circulating BBR levels by converting BBR to a more readily absorbed form, dihydroberine (DHB), via nitroreductase. Additionally, observations of the gut microbial modulation of pharmacokinetics in beagle dogs after oral administration of BBR (50 mg/kg/d) by single or multiple doses for 7 days revealed that their fecal BBR excretion levels were higher on days 3 and 7. Butyrate in the plasma and feces increased 3.1-fold and 2.7-fold, respectively, after 1 day of BBR treatment, and the number of bacteria that produce butyrate and nitroreductase was elevated after 7 days of treatment. The plasma and feces were found to contain eleven metabolites, including eight phase I and three metabolites of phase II (Feng et al., 2018b). These studies suggest that the gut and its flora are essential for BBR metabolism.
The liver is an essential organ for endogenous lipid production, and BBR can regulate hepatic lipid metabolism to prevent and treat lipid metabolic diseases, such as and NAFLD. The mechanisms of action of BBR include inhibiting hepatic lipid production, increasing hepatic lipid clearance, improving insulin resistance, promoting AMPK phosphorylation (p-), influencing apoptosis and autophagy in lipid cells, and regulating epigenetic modifications.
CD36, acetyl-CoA carboxylase (ACC), and MTTP are essential regulators of lipid uptake and synthesis in the liver. BBR reduces circulating lipid levels and hepatic lipid accumulation by inhibiting these regulators.
CD36 belongs to the scavenger receptor family, also known as fatty acid translocases, which facilitates the uptake of long-chain fatty acids (LCFAs) (Zhong et al., 2017). Hepatocyte CD36 is a crucial regulator of de novo hepatic lipogenesis. High fat diet (HFD)-induced liver steatosis and insulin resistance are attenuated in CD36-knockout mice (Zeng et al., 2022). Excessive accumulation of liver fat is a crucial factor in the pathogenesis of NAFLD. Therefore, suppression of CD36 to reduce liver fat uptake and synthesis may have a potential effect in preventing and treating NAFLD. Berberrubine, a main BBR metabolite, alleviates NAFLD by reducing CD36 expression Moreover, berberine maintains glucose homeostasis in HFD-fed mice by upregulating glucose transporter 2 (GLUT-2) and glycogen synthase kinase 3β (GSK3β) protein expression and inhibiting glucose 6 phosphatase (G6Pase) protein expression (Yang et al., 2022c).
Accumulating evidence has indicated that CD36 is an essential regulator of intracellular fatty acid homeostasis (Samovski et al., 2015). CD36 is involved in fatty acid oxidation (Samovski et al., 2015) and lipophagy (Li et al., 2019a) by activating AMPK, thus regulating the storage or use of fatty acids. These studies suggest that promoting fatty acid oxidation and correcting lipophagy defects by inhibiting CD36 expression in hepatocytes may be a novel strategy for treating liver fat accumulation. BBR lowers cholesterol by inhibiting bile salt hydrolase (BSH), increasing conjugated BA levels, and activating the FXR signaling pathway, among other mechanisms; this leads to reduced hepatic CD36 levels, thereby reducing both hepatic LCFA absorption and lipid accumulation (Sun et al., 2017). Therefore, BBR may play an important role in the prevention and protection against NAFLD by regulating CD36 expression.
ACC is a crucial enzyme involved in fatty acid biosynthesis and metabolism. It has two tissue-specific isomers, ACC1 and ACC2, which catalyze the conversion of acetyl coenzyme A to malonyl coenzyme A and exhibit different distributions. A cytosolic enzyme expressed mainly in adipose tissue such as the liver, ACC1 is responsible for the rate-limiting step in LCFA biosynthesis (Munday, 2002). The rate-limiting enzyme ACC carboxylates acetyl coenzyme A into malonyl coenzyme A during adipogenesis, subsequently converting this into LCFAs and then into triglycerides (TGs) via a multi-step reaction with fatty acid synthase (FAS) (Gathercole et al., 2011). Over-synthesis of TG is a major pathogenic factor in NAFLD. Indeed, several studies have shown that ACC1 inhibitors can combat NAFLD (Wu and Huang, 2019). In HFD-induced NAFLD rats, the protein expression levels of sirtuin 3 (SIRT3), p-AMPK, and p-ACC were significantly higher in the livers of rats in the BBR treatment group, and the serum and liver lipid profiles and liver injury status improved. These data suggest that the mechanism by which BBR ameliorates HFD-induced hepatic steatosis may be related to activation of the liver SIRT3/AMPK/ACC pathway (Zhang et al., 2019b). In addition to NAFLD, BBR improves hepatic lipid metabolism in rats with alcohol-induced alcoholic fatty liver disease by reducing hepatic lipid synthesis through the inhibition of ACC, which may be related to the thyroid hormone responsive gene responsible for fatty acid synthesis (Ke et al., 2022). Single-drug therapy for fatty liver disease is typically ineffective, as it has a complex etiology and affects multiple systems (Brown et al., 2021; Zhu et al., 2021). Therefore, combining drugs with different mechanisms may enhance overall efficacy (Dufour et al., 2020; Kessoku et al., 2020). For example, the p62/nuclear factor erythroid 2-related factor 2 (NRF2)/carboxylesterase 2 (CES2) and p62/NRF2/PPARα signaling axes are restored by bicyclol, which enhances lipolysis and β-oxidation, while BBR reduces de novo lipogenesis by suppressing ACC and FAS expression; the combination of the two drugs has already been demonstrated to enhance their overall therapeutic effect in improving NAFLD (Li et al., 2022a).
NAFLD is characterized by considerable accumulation of TGs in the liver, which, under normal circumstances, are excreted from the liver as very low-density lipoprotein (VLDL). Therefore, the timely synthesis and secretion of VLDL are vital for mitigating hepatic TG deposition. The assembly and maturation of VLDL are closely related to MTTP activity (Anastasia et al., 2021). Lipids and apolipoprotein B (ApoB) are essential components of VLDL, and MTTP stabilizes ApoB expression to reduce ApoB degradation and promote lipid transfer to VLDL particles to facilitate VLDL maturation (Demignot et al., 2014). Wang et al. (Wang et al., 1999) found that inhibition of MTTP activity impedes VLDL assembly, leading to increased intrahepatic TG levels. Lomitapide is a drug developed to target the downregulation of MTTP activity. It reduces ApoB secretion but causes side effects such as increased hepatic lipid compensation and exacerbated NAFLD, thus limiting its range of application (Stefanutti, 2020). It is believed that decreased MTTP expression in NAFLD rats may decrease hepatic TG excretion in VLDL, favoring the accumulation of hepatic fat. BBR treatment reverses the abnormal HFD-induced expression of MTTP thus improving fatty liver (Chen et al., 2021). DNA methylation is one of the pathways that leads to gene expression dysregulation via interactions with environmental factors. DNA methylation levels in the MTTP promoter of NAFLD rats are elevated in the liver, and a strong negative correlation has been observed between MTTP expression and DNA methylation. BBR selectively inhibits HFD-induced MTTP methylation to partially counteract HFD-induced MTTP dysregulation, which promotes normal levels of VLDL secretion in the liver, thereby reducing hepatic fat content and alleviating NAFLD (Chang et al., 2010).
Circulating high-density lipoprotein cholesterol (HDL-C) and low-density lipoprotein cholesterol (LDL-C) are taken up and cleared by hepatocytes via hepatic scavenger receptor class B type I (SR-BI) and LDLR, respectively. The liver transfers cholesterol to apolipoprotein AI (ApoAI) via the ATP-binding cassette transporter A1 (ABCA1) to form high-density lipoprotein (HDL), which is a protective factor for blood lipids to a certain extent. BBR reduces circulating and hepatic lipid levels by acting on these targets of lipid metabolism.
SR-BI is a hairpin-looped structure with two short transmembrane domains, two cytoplasmic tails, and a large extracellular loop. A large cavity traverses the entire length of the SR-BI molecule, and mutagenesis of SR-BI showed that the cavity is a lipophilic tunnel through which cholesterol ester is delivered from the bound lipoprotein to the outer leaflet of the plasma membrane (Neculai et al., 2013). SR-BI is highly expressed in human and animal livers and can lower circulating cholesterol levels by taking up cholesterol from circulating HDL, VLDL, and other lipoproteins (Yang et al., 2013). In the SR-BI−/− mouse model, circulating total cholesterol (TC) levels increase 2.5-fold (Cao et al., 2018a). While, overexpression of SR-BI in mouse liver can reduce the level of circulating lipid (Zhang et al., 2005). In addition, SR-BI variants in humans can lead to increased circulating cholesterol levels and an increased risk of cardiovascular disease (CVD) (May et al., 2021). Thus, regulation of SR-BI expression can influence lipid levels and AS progression.
In HFD-fed ApoE−/− mice, circulating lipid levels increased and hepatic SR-BI expression decreased. After addition of BBR, SR-BI expression was restored and the levels of circulating TC, TG, LDL-C and HDL-C decreased, delaying the progression of AS plaques and hepatic steatosis (Ma et al., 2021a). BBR may regulate SR-BI via various mechanisms. Liver X receptors (LXRs) and peroxisome proliferator-activated receptors (PPAR-α, PPAR-β/δ, and PPAR-γ) regulate human SR-BI gene expression by forming a heterodimer with the retinoid X receptor (RXR) and bind to the SR-BI promoter (Shen et al., 2018). Mitogen-activated protein kinase (MAPK) affects hepatic SR-BI expression by targeting PPAR, further altering the ability of cells to export cholesterol (Wood et al., 2011).
The mechanisms related to the regulation of lipid metabolism by BBR via hepatic SR-B1 have not been well studied. Considering that LXR, PPAR, and MAPK are known targets of BBR (Han et al., 2015; Bansod et al., 2021) and that LXR, PPAR, and MAPK have regulatory effects on hepatic SR-B1, whether BBR can affect circulating cholesterol levels by regulating hepatic SR-B1 via LXR, PPAR, and MAPK deserves further exploration.
Plasma LDL is mainly removed from circulation through LDLR, and LDLR deficiency increases circulating cholesterol levels and accelerates AS (Xu and Weng, 2020). Three proteins are known to bind to AU-rich element (ARE): heterologous nuclear ribonucleoprotein D (hnRNP D), hnRNP I, and KH-type splicing regulatory protein (KSRP) (Li et al., 2009a; Pan et al., 2020). These proteins interact with the LDLR 3′UTR, leading to a reduction in LDLR mRNA levels. BBR stabilizes LDLR expression by reducing the affinity between hnRNP I or KSRP and LDLR (Singh et al., 2014; Momtazi et al., 2017). Poly A-binding protein (PABP) maintains mRNA stability by protecting it against nucleophilic attack (Behm-Ansmant et al., 2007). In vitro, BBR indirectly enhances LDLR mRNA stability by promoting binding between PABP and the poly adenine (polyA) tail of LDLR mRNA. BBR also improves LDLR mRNA stability by targeting the polyA tail directly (Yuan et al., 2015). BBR stabilizes LDLR mRNA and enhances plasma LDL-C clearance by activating the AMPK/extracellular signal-regulated kinase (ERK) signaling pathway (Abidi et al., 2005). BBR can directly upregulate LDLR mRNA expression in hepatocytes by activating the Jun N-terminal kinase (JNK)/c-Jun signaling pathway (Fan et al., 2021), resulting in increased LDL uptake, lower circulating LDL-C levels, and alleviation of AS. These studies suggest that BBR plays a role in reducing circulating cholesterol levels by stabilizing or increasing LDLR mRNA expression.
LDLR levels are also regulated by PCSK9, which promotes LDLR degradation and increases circulating LDL-C levels by binding to LDLR (Ataei et al., 2022). BBR reduces serum LDL-C levels and delays aortic plaque formation in ApoE−/− mice by activating ERK1/2 to downregulate PCSK9 (Ma et al., 2021b). Sterol regulatory element-binding protein (SREBP) and hepatocyte nuclear factor 1α (HNF1α) control PCSK9 protein synthesis at the transcriptional level. Through ubiquitin-proteasome degradation, BBR inhibits SREBP2 and HNF1α expression. As a result, blood PCSK9 levels could be lowered via decreased transcription of its mRNA, resulting in increased LDLR mRNA expression (Cao et al., 2018a; Shafabakhsh et al., 2021). Furthermore, when added to hepatocytes with statins, BBR counteracts the statin-inducted transcription of PCSK9 (Li et al., 2009b) and is therefore a potential drug for lipid regulation in combination with statins. In conclusion, BBR can not only increase LDLR expression by stabilizing LDLR mRNA, but also reduce LDLR degradation by down-regulating PCSK9, which indirectly improves LDLR expression and increases circulating cholesterol clearance.
Human ABCA1 gene has been mapped to chromosome 9q31.1, spans 149 kb, and comprises 50 exons and 49 introns (Santamarina-Fojo et al., 2000). The study found that ABCA1 may transport cellular cholesterol and phospholipids to extracellular ApoAI through nine different models (Sun and Li, 2022) to generate nascent HDL particles (Ogura, 2022). Upregulation of ABCA1 promotes intracellular lipid efflux and increases circulating HDL levels to alleviate lipid metabolic diseases (Matsuo, 2022). Relative to wild-type mice, ApoE−/− mice fed an HFD exhibit reduced hepatic ABCA1 expression, whereas BBR treatment enhances the expression of ABCA1 and alleviates atherosclerotic lesions and hepatic steatosis (Ma et al., 2021a). Protein kinase Cδ (PKCδ) increases ABCA1 serine phosphorylation and reduces the rate of ABCA1 protein degradation (Weng et al., 2018). In vitro and in vivo studies demonstrate that BBR decreases hepatic cholesterol and TG levels by increasing ABCA1 protein levels through PKCδ, whereas the PKCδ inhibitor rottlerin and PKCδ siRNA completely abolish the effect of BBR on ABCA1. Furthermore, the inhibition of ABCA1 and its siRNA eliminate the ability of BBR to lower cellular cholesterol levels. These results suggest that BBR increases ABCA1 protein levels via PKCδ, thereby reducing hepatic steatosis (Liang and Wang, 2018). Therefore, ABCA1 may be an important target of BBR for the treatment of lipid metabolic diseases.
AMPK, which plays a key role in energy metabolism, has been the focus of research on lipid-related metabolic diseases (Wang et al., 2018). When AMPK is activated, genes linked to lipogenesis are downregulated, and energy consumption is increased. As a result, lipid buildup is reduced, and liver function is enhanced.
In HFD-fed rats, the BBR- and simvastatin-treated groups showed similar decreases in LDL-C levels (−26.8% and −28.3%, respectively), and the combination of the two drugs resulted in a more pronounced decrease in LDL levels (−46.2%) (Kong et al., 2008) and slowed AS progression, potentially via AMPK activation by BBR (Cao et al., 2013), which inhibit 3-hydroxy-3-methylglutaryl-CoA reductase (HMGCR) and cholesterol biosynthesis (Song et al., 2012). AMPK can modulate lipid metabolism by acting on SREBP, a regulator of hepatic lipogenesis (Ferré et al., 2021). p-AMPK inhibits the expression of SREBP-1c via Ser372 phosphorylation and blocks SREBP-1c nuclear translocation, thus inhibiting the transcription and translation of SREBP-1c target genes such ACC1, FAS, and stearoyl CoA desaturase 1 (SCD1) (Li et al., 2011b). The role AMPK in lipid metabolism is further supported by the discovery that BBR treatment promotes hepatic lipolysis (CPT and LPL) and reduces hepatic lipid deposition in largemouth bass via AMPK/SREBP1 (Gong, 2022). In addition, the mRNA levels of two crucial cholesterol production enzymes, 3-Hydroxy-3-Methylglutaryl-CoA Synthase (HMGCS) and HMGCR, are reduced by the activation of AMPK, which is consistent with decreased levels of SREBP-2 (Li et al., 2011a). Reduced TG and cholesterol biosynthesis, owing to reduced expression of ACC1, FAS, SCD1, HMGCS, and HMGCR, improves hepatic steatosis (Li et al., 2011b). Columbamine, a BBR metabolite, also has the advantage on lowering TG levels via the activation of AMPK (Cao et al., 2013).
BBR and its derivatives can block mitochondrial respiratory chain complex I, thereby affecting levels of intracellular AMP and ADP, and activating AMPK (Sanders et al., 2007; Turner et al., 2008). Activated AMPK promotes the expression of uncoupling protein-1 (UCP1) and −2 (UCP2) in adipose tissue and increases the expression of other thermogenic genes. Fatty acids are burned off in the form of heat (Wang et al., 2011; Zhang et al., 2011; Zhang et al., 2014). At the same time, in HFD-induced NAFLD rats, BBR-induced activation of SIRT3, a crucial gene for fatty acid oxidation, alters the expression of ACC and carnitine palmitoyltransferase-1A (CPT-1A), thereby reducing hepatic steatosis (Zhang et al., 2019a). In AMPKα1−/− HepG2 cells, the stimulating effects of BBR on the activity p-AMPKα1, p-AMPKα and AMPK, and its effects on glucose and lipid metabolism, are completely suppressed (Ren et al., 2020). These studies suggest that BBR may reduce circulating fat levels by inhibiting adipogenesis and promoting fat consumption through AMPK and may be used to treat lipid metabolic diseases.
The mitochondria are an important location for energy (including lipid) metabolism in cells, and are composed of an outer membrane, intermembrane space, inner membrane, and matrix. Studies have shown that excessive lipid accumulation in hepatocytes during NAFLD leads to abnormal fatty acid oxidation, increased mitochondrial reactive oxygen species production, and abnormal mitochondrial membrane lipids and proteins (Prasun et al., 2021). Mitochondria-associated ER membranes (MAMs) are lipid raft-like domains closely associated with mitochondria, which are located between mitochondria and the endoplasmic reticulum. MAMs not only structurally connect the endoplasmic reticulum and mitochondria, but are also rich in a variety of functional proteins related to lipid metabolism such as acyl-coA:cholesterol acyltransferase-1 (ACAT1/SOAT1), acyl-CoA:diacylglycerol acyltransferase 2 (DGAT2) (Ma et al., 2021b), and phosphatidylethanolamine N-methyltransferase (PEMT) (Zhao et al., 2009). Administration of BBR to rats fed a high-fat diet reduced the expression of DGAT in liver tissues and the level of circulating blood lipids (Zhu et al., 2017). In vitro experiments have shown that BBR can inhibit ATP synthesis, enhance glycolysis, and promote glucose metabolism by inhibiting the mitochondrial respiratory chain complex I (Xu et al., 2014). Animal experiments have shown that BBR upregulates the mitochondrial content in brown and white adipocytes in diabetic mice, stimulates UCP1 -mediated thermogenesis, and accelerates fat catabolism. These mechanisms may be related to AMPK signaling (Zhang et al., 2014). In addition, Sirtuin 3 (SIRT3), a deacetylase mainly located in mitochondria, is a key regulator of mitochondrial function. SIRT3 is highly expressed in metabolically active tissues (such as the liver). Mice fed a high-fat diet for sustained periods exhibit decreased Sirt3 expression, resulting in mitochondrial dysfunction and over-acetylation of proteins in the liver. These changes can increase the risk of aging-related diseases such as NAFLD and obesity (Choudhury et al., 2011). Potential mechanisms underlying these findings include the downregulation of ERK-CREB-Bnip3 and inhibition of the mitochondrial autophagy pathway (Li et al., 2018a). BBR also promotes fatty acid β oxidation through SIRT3-LCAD, thereby slowing the progression of NAFLD (Xu et al., 2019). By activating SIRT3, BBR can also improve adipose tissue remodeling, downregulate the expression of TNF-α and NF-kB, which play an anti-hyperlipidemic and anti-hyperglycemic role (Li et al., 2022b). Therefore, by regulating mitochondria, MAMs, and their related proteins is one of the important ways for BBR to exert lipid-lowering effects.
Lipid droplets are separated by autophagy and sent to hepatocyte lysosomes for degradation, enabling the breakdown and excretion of lipids such as TG and cholesterol (Zhang et al., 2018). The Akt/mammalian target of rapamycin (mTOR) axis is the main signaling pathway that regulates autophagy (Jung et al., 2010). Autophagy activation by mTOR inhibitors promotes lipid degradation (Lin et al., 2013). BBR slows NAFLD progression by inhibiting ERK/mTOR-induced autophagy, thereby improving hepatic lipid accumulation in vitro and in vivo (He et al., 2017). BBR enhances autophagy by preventing cyclooxygenase-2 (COX-2)-mediated prostaglandin formation, thereby reducing p-AKT and p-mTOR levels (Sun et al., 2018a; Gendy et al., 2022). In ApoE−/− mice fed a high-fat diet, BBR regulates autophagy, reduces lipid levels, and antagonizes carotid lipid accumulation by modulating the phosphatidylinositol 3-kinase (PI3K)/AKT/mTOR signaling pathway (Song and Chen, 2021). Moreover, SIRT1 is essential for BBR to potentiate autophagy and inhibit lipid storage in the mouse liver in response to fasting. BBR stimulates SIRT1 deacetylation and induces autophagy in an autophagy-related protein 5 (ATG5)-dependent manner (Sun et al., 2018b). Therefore, promoting autophagy is a key method by which BBR reduces circulating lipid levels.
Increasing evidence suggests that insulin resistance and lipid metabolism are tightly connected as tissue lipid accumulation could lead to severe insulin resistance (Yang et al., 2018). In addition, the elevation of insulin levels after a meal signal the liver to adjust lipid metabolism (Titchenell et al., 2017). Moreover, lipid metabolic diseases, such as obesity, hyperlipidemia, and AS, are often associated with insulin resistance (Lee et al., 2022). BBR can improve insulin resistance (Yan et al., 2015) by upregulating insulin receptor substrate-2 (IRS-2) (Xing et al., 2011) and down regulating UCP2 at the mRNA and protein levels along with a decrease in TC, TG, and LDL-C and an increase in HDL-C in HFD-induced NAFLD model rats (Zhao et al., 2017).
Long noncoding RNA (lncRNA) and microRNA (miRNA) are important regulators of lipid homeostasis. BBR therapy reverses the expression of numerous genes, including 881 mRNAs and 538 lncRNAs, in steatotic liver (Yuan et al., 2015). In BBR-treated immortalized hepatocyte cell lines MIHA and HepG2, upregulation of miR-373 levels is observed, which in turn inhibits the AKT/mTOR/ribosomal S6 kinase (S6K) signaling pathway associated with steatosis in hepatocytes, thereby reducing abnormal lipid deposition in the liver (Li et al., 2018b). In addition, BBR therapy prevents type 2 diabetic mice from developing hepatic gluconeogenesis and lipid metabolism abnormalities, by lowering miR122 (Wei et al., 2016a). Moreover, BBR affects the expression of lncRNAs associated with the rapamycin, MAPK, and apoptosis pathways in the plasma of patients with stable coronary artery disease, which may be involved in coronary heart disease prognosis (Han et al., 2022). DNA demethylation and histone acetylation are also involved in BBR-mediated lipid regulation. L-type pyruvate kinase (L-PK) is the third rate-limiting enzyme in glycolysis and is closely associated with NAFLD and diabetes. BBR treatment restores the expression of L-PK by demethylating the L-PK promoter and increasing the acetylation levels of histones H3 and H4 around L-PK and could therefore be used to treat NAFLD (Zhang et al., 2015). BBR treatment reduces hepatic lipid deposition by reversing abnormal epigenetic modifications, such as DNA modification, histone modification, and noncoding RNA regulation, thereby maintaining normal hepatic lipid metabolism.
Like the endogenous lipid-producing organ, the liver, the intestine is an important organ for exogenous lipid production and makes an important contribution to the body’s lipid levels (Figures 2, 3). BBR can prevent and treat lipid metabolic diseases by directly regulating intestinal lipid metabolism and interacting with intestinal flora.
FIGURE 3. Efficacy and underlying mechanisms of berberine against lipid metabolic diseases. The liver and intestine are important organs for endogenous and exogenous lipid production, respectively, and regulate the body’s lipid levels. Cluster of differentiation 36 (CD36), acetyl-CoA carboxylase (ACC), and microsomal triglyceride transfer protein (MTTP) are the liver targets for lipid production. At the same time, ATP-binding cassette transporter A1 (ABCA1), scavenger receptor class B type 1 (SR-BI), and low-density lipoprotein receptor (LDLR) are the liver targets for lipid clearance. Risk factors such as irrational diet and disease promote lipogenesis and inhibit lipid clearance by up-regulating CD36, ACC, and MTTP and down-regulating SR-BI, LDLR, and ABCA1, elevating circulating lipid levels and exacerbating hepatic fat accumulation. Intestinal flora is an important micro-ecosystem of the intestine, which is greatly influenced by diet. High-fat diet aggravates the body’s lipid burden by altering the intestinal flora’s composition and its metabolites’ production. Elevated circulating lipid levels lead to vascular endothelial dysfunction, macrophage foam cell formation, and vascular smooth muscle cell hyperproliferation, accelerating the formation of atherosclerosis. Berberine works against lipid metabolic diseases by synergistically regulating multiple lipid metabolism-related targets in the liver, gut, and blood vessels.
Compared to the many adverse effects of ACAT1 deficiency, such as cholesterol accumulation, macrophage death, and destabilization of cell membrane function, ACAT2 deficiency protects against AS (Rudel et al., 2005). ACAT2 promotes the conversion of cholesterol into cholesteryl esters (CEs), which in turn synthesize lipoproteins and affect lipid levels. Furthermore, ACAT2 affects intestinal cholesterol absorption, which is significantly reduced in mice lacking ACAT2 (Temel et al., 2005).
Free cholesterol is incorporated into mixed micelles in the intestinal lumen after fat digestion and de-esterification, before being delivered to the small intestine’s absorptive epithelium. This step determines the amount of cholesterol available for uptake on the apical side of the enterocytes. ACAT2 converts free cholesterol taken up by intestinal cells into CEs in the intestine and further assembles CEs into chylomicrons (CMs). In HFD-fed rats, BBR treatment reduces the level of circulating total and non-HDL cholesterol. In vitro studies reveal that BBR treatment reduces the monolayer permeability of Caco-2 cells and their uptake and esterification of cholesterol, the mechanism of which might be related to the inhibition of ACAT2 gene and protein expression in Caco-2 cells by BBR (Wang et al., 2014). This study suggests that BBR may affect blood lipid levels by inhibiting intestinal ACAT2 and may be used to combat lipid metabolic disorders. Studies on the relevant targets of BBR in regulating intestinal lipid metabolism are currently scarce and need to be conducted in the future.
Because of the low oral bioavailability of BBR and its direct antibacterial effect (Huang et al., 2020), it can alter intestinal flora composition (Hu et al., 2022b). In HFD-induced atherosclerotic mice, BBR treatment exerts a beneficial effect on reducing circulating lipid levels (Wu et al., 2020) by increasing the abundance of Akkermansia, Firmicutes, and Verrucomicrobia (Zhu et al., 2018) and decreasing the abundance of Bacteroidetes and Proteobacteria in the mouse intestinal flora (Shi et al., 2018). In HFD-induced NAFLD rats, BBR reduces body weight and TC, TG, and LDL-C levels. This improved phenotype is associated with an increase in intestinal Bacteroidetes and Proteobacteria and a decrease in Firmicutes and Cyanobacteria in rats treated with BBR (Wang et al., 2020b).
A clinical study found that circulating cholesterol levels decrease significantly after BBR treatment, with little change in TG levels. Simultaneously, suppression of Blautia in the gut flora eliminates the cholesterol-lowering effect of BBR, suggesting that the lipid-lowering effect of BBR is closely related to its modulation of the gut microbiota. Interestingly, baseline levels of Blautia accurately predict the anti-hypercholesterolemic efficiency of BBR in subsequent treatments (Wu et al., 2022). Microbiome analysis indicates that Blautia is closely associated with BBR lipid-modulating activities and that BBR selectively promotes Blautia producta (Wu et al., 2022). Further studies found that B. producta significantly increases LDLR expression in the liver and contributes to the role of BBR in the production of butyrate and the inhibition of BSH, thereby reducing obesity and alleviating hyperlipidemia in mice (Yang et al., 2022a). The intestinal microbiota, especially B. producta, may contribute to the BBR-directed prevention and treatment of lipid metabolic diseases (Yang et al., 2022b).
In addition to intestinal bacteria, intestinal fungi play an essential role in BBR-mediated regulation of lipid metabolism. Tilletia bornmuelleri and Tilletia bromi content increase after BBR treatment, reducing serum lipids and inhibiting hepatic lipid accumulation in HFD-fed mice (Yang et al., 2022). In addition, intestinal BBR levels are higher in hyperlipidemic rats than in normal rats, and BBR bioavailability is reduced after the administration of antibiotics. This suggests that the higher circulating BBR levels in hyperlipidemic rats may be related to their higher intestinal microbial population (Zhang et al., 2021a). However, another human study found a negative correlation between circulating BBR and gut microbial counts (Alolga et al., 2016). This discrepancy between studies may be due to differences in the study subjects; therefore, more research and clinical trials are needed.
In conclusion, BBR can alleviate HFD-induced lipid metabolic disorders by modulating intestinal flora composition.
BBR interacts with intestinal microorganisms to derive a variety of metabolites, such as short-chain fatty acids (SCFAs), trimethylamine-N-oxide (TMAO), and branched-chain amino acids (BCAAs) (Fang et al., 2022). These derivatives and the signaling pathways they influence have a large impact on lipid levels and, as such, may be used to treat lipid metabolic diseases.
SCFAs are carboxylic acids with two to six carbon atoms (Ohira et al., 2017). The intestinal flora syn-thesizes SCFAs, such as butyric, acetic, and propionic acids, by fermenting undigested dietary fiber (Li et al., 2022). Many studies have suggested that these SCFAs delay AS progression (Hu et al., 2022a). In HFD-fed ApoE−/− mice, the addition of propionic acid increases circulating interleukin (IL)-10 and inhibits the expression of the intestinal cholesterol transporter Niemann-Pick C1-like 1 (NPC1L1), reducing circulating cholesterol levels and alleviating AS (Haghikia et al., 2022). The addition of butyric acid downregulates the expression of Npc1l1 in intestinal cells and upregulates the mRNA levels of ATP-binding cassette transporters G5 and G8 (ABCG5 and ABCG8), thereby reducing lipid intake, increasing lipid efflux in intestinal cells, and protecting ApoE−/− mice from HFD-induced AS (Chen et al., 2018).
Both in vitro and in vivo experiments have shown that BBR increases intestinal butyrate production, thereby reducing circulating lipid levels. Moreover, intraperitoneal BBR administration does not increase butyrate levels but reduces lipid and glucose levels. This suggests that BBR reduces hyperlipidemia in two ways, through the direct effect of circulating BBR and its indirect action through intestinal microbiota (Wang et al., 2017b). BBR modifies the SCFA biosynthesis-related enzymes to control SCFA expression. (Yue et al., 2019a). found that BBR treatment significantly increased intestinal butyric acid levels by promoting the expression and activity of butyryl-CoA:acetate-CoA transferase (BUT). When BBR is present, the expression of BUT and two butyrate synthesis precursors (crotonyl-CoA and butyryl-CoA) is elevated, thereby reducing blood lipid levels (Wang et al., 2017c). BBR also regulates SCFA levels by regulating the abundance of SCFA-producing bacteria (Zhang et al., 2019a). The proportions of SCFA-producing bacteria in the gut microbiota, including of Blautia, Bacteroidales, and Roseburia, are elevated following BBR treatment (Jia et al., 2019b). Another study revealed that the SCFA producers Butyricimonas, Eubacterium, and Clostridium were significantly enriched with BBR treatment, indicating that SCFA-producing bacteria probably play a key role in the effectiveness of BBR (Mukherjee et al., 2020).
Trimethylamine (TMA) is synthesized from undigested choline, carnitine, and betaine obtained from food by enzymes produced by the gut bacteria (Velasquez et al., 2016). Intestinal TMA absorbed into the blood is further transformed into its pro-atherogenic form, TMAO, by flavin monooxygenase family members, such as flavin-containing monooxygenases (FMO3), in the liver. TMAO can promote AS by interfering with reverse cholesterol transport and promoting inflammation and thrombosis, among other mechanisms (Poli, 2020).
Several in vitro and ex vivo experiments have suggested that BBR may act, at least in part, by reducing TMAO during AS treatment. Decreased serum inflammatory factor expression in HFD-induced mouse models after BBR treatment might be related to the downregulation of the FMO3-TMAO pathway (Shi et al., 2018). BBR can also reduce circulating TMA and TMAO levels by downregulating enzymes associated with TMA production (Wu et al., 2020) and genes associated with TMA production (Li et al., 2021a). BBR treatment reduces intestinal TMAO biosynthesis and interrupts hamster vascular plaque formation by interacting with the choline-trimethylamine lyase (CutC) and FMO in the gut microbiota of HFD-fed hamsters. In a subsequent clinical study, patients in the BBR group were observed to have lower plaque scores and reduced circulating and fecal TMA and TMAO levels than those in the rosuvastatin plus aspirin group, suggesting that BBR might treat AS through the choline-TMA-TMAO production pathway (Ma et al., 2022). Furthermore, BBR administration reshapes the structure of rat intestinal microbiota, increasing the levels of Lactobacillus spp. and decreasing the levels of CutC and TMAO, leading to the attenuation of the TMAO-induced phosphorylation of ERK1/2 and JNK in platelets and reduced platelet reactivity to collagen and risk of atherothrombosis (Xie et al., 2021). Wang et al. (Wang et al., 2022) reported that BBR alleviates Ang II-induced vascular dysfunction and pathological remodeling in hypertensive mice by inhibiting FMO3 expression and TMA/TMAO production. In summary, the vasoprotective effect of BBR treatment through the inhibition of TMAO may delay AS progression.
BCAAs are essential amino acids mainly obtained from food. Elevated BCAA levels are strongly connected with lipid metabolic disorders such as hyperlipidemia, obesity, and NAFLD (Cuomo et al., 2022). A case-control study found that dietary BCAAs are positively associated with circulating TC and LDL-C levels, thereby increasing the risk of dyslipidemia (Yu et al., 2022). Numerous bacterial taxa, including Bacteroides vulgatus, the Bacil-lus–Lactobacillus–Streptococcus group, Prevotella copri, and Proteobacteria, participate in BCAA synthesis (Pedersen et al., 2016). BBR regulates BCAA synthesis, probably by inhibiting microbial BCAA production. For example, treatment with BBR reduces serum TC, HDL, TG, fasting plasma glucose (FPG), and insulin resistance levels in obese mice, which may be associated with a reduction in the relative abundance of BCAA-producing bacteria, such as Clostridiaceae and Prevotellaceae, in the gut of these mice (Yue et al., 2019b). Abnormal mTOR activation is linked to a number of illnesses, including ischemic diseases (Hua et al., 2019); BCAAs, particularly leucine, activate the mTOR pathway (Yoon, 2016). The inhibitory effect of BBR on BCAAs may therefore play a role in reducing the risk of developing ischemic diseases via mTOR. In summary, in treating lipid metabolic disorders, it is crucial to restrict the proliferation of BCAA-producing bacteria and lower BCAA levels.
Intestinal flora can convert primary BAs to secondary BAs through decoupling and dehydroxylation reactions (Long et al., 2017). It was found that after the addition of BBR, the abundances of Akkermansia spp., Verrucomicrobia, and Firmicutes increased, while those of Proteobacteria and Bacteroidetes decreased (Tian et al., 2019). Furthermore, the proportion of total BAs and primary BAs (such as cholic acid (CA) and chenodeoxycholic acid (CDCA)) in serum increased, while the proportion of secondary BAs (such as deoxycholic acid (DCA) and lithocholic acid (LCA)) was reduced. These findings were associated with increasing BBR concentrations in a dose-dependent manner (Guo et al., 2016). These changes in the BA pool can affect body function by regulating BA receptors in the gut (Martinot et al., 2017). The BA receptors mainly consist of G protein-coupled BA receptor 1 (TGR5) and nuclear receptors such as FXR (Fiorucci et al., 2018). Various BA receptors bind to different types of BAs with different affinities. For example, DCA and LCA have a high affinity for TGR5, while CDCA and CA have a high affinity for FXR. Upon BBR treatment, increased CA and CDCA activate FXR (Wolf et al., 2021). FXR plays a central role in regulating BA synthesis. FXR regulates the expression of rate-limiting enzymes (such as CYP7A1 and CYP8B1) in BA synthesis by inducing negative nuclear receptor small heterodimer partner (SHP) (Gómez-Ambrosi et al., 2017). At the same time, BBR can increase the excretion of BA and decrease the level of circulating and liver cholesterol by directly up-regulating the expression of CYP7A1 and CYP8B1 in mice (Guo et al., 2016). In addition, activated FXR can downregulate the expression of adipogenic genes, including SREGBP-1C, FAS, and acetyl-CoA carboxylase through the FXR/SHP pathway in mice, thus playing a role in the prevention and treatment of lipid metabolic diseases (Watanabe et al., 2004).
Food and medication can be biotransformed by the gut microbiota into secondary metabolites (Wang et al., 2017a). Modern technologies show that the gut bacteria can convert BBR into DHB, berberrubine, demethyleneberberine, jatrorrhizine, and thalifendine (Habtemariam, 2020). In addition to having pharmacological effects similar to those of BBR, these metabolites also have their own characteristics and advantages and contribute significantly to the hypolipidemic effect.
The intestinal absorption of BBR is aided by the nitroreductase (NR) activity of the intestinal bacteria (Wang et al., 2017b). Intestinal NR catalyzes the conversion of BBR to DHB (Tan et al., 2019). Compared to BBR, DHB has a higher rate of intestinal absorption (Feng et al., 2015; Pan et al., 2019). The gut microbial metabolite DHB, which is produced from BBR, may be critical for explaining the absorption of BBR in the gut. Two procedures are involved in the circulatory sys-tem’s uptake of DHB from the intestine: first, the conversion of BBR to DHB, catalyzed by the enzymes of the intestinal microbiota, is uptaked by the intestinal epithelial cells, and second, the subsequent absorption of DHB by the intestinal epithelial cells is further oxidized to BBR, which is absorbed into the circulatory system (Feng et al., 2015). DHB is converted to BBR via a nonenzymatic process. NR plays an important role in the BBR-DHB-BBR conversion process, with enhanced BBR uptake being the basis for promoting subsequent lipid-regulating effects. In a randomized controlled crossover trial, after oral administration of 500 mg of BBR or 100 and 200 mg DHB to study subjects, the peak concentration was found to be higher in the DHB group than in the oral-administration BBR group (Moon et al., 2021). Liu et al. found that DHB improves the lipid profile of hyperlipidemic rats by increasing LDL-R expression and decreasing PCSK-9 expression in liver tissues (Liu et al., 2015b). Given its good intestinal absorption rate and lipid-lowering efficacy, DHB can potentially be used to treat lipid metabolic diseases.
The gut microbiota can break down the five-membered dioxymethylene ring in BBR to create jatrorrhizine (Wang et al., 2017c). Jatrorrhizine is safer than BBR as a microbial reduction product. The lethal dose 50 (LD50) of jatrorrhizine in mice is approximately 5,500 mg/kg, whereas the LD50 of BBR is 763 mg/kg. Additionally, jatrorrhizine reduces circulating cholesterol levels by upregulating LDLR and CYP7A1 mRNA and protein expression (Wu et al., 2014). Yang et al. found that jatrorrhizine downregulates mRNA expression of SREBP-1c and FAS in the liver of hyperlipidemic mice to inhibit adipogenesis and upregulates PPAR-α and CPT1A mRNA expression to enhance lipid oxidation and improve hyperlipidemia (Yang et al., 2016). In obese mice, jatrorrhizine treatment reduces body weight and serum lipid levels and improves hepatic lipid metabolism via the IRS1/PI3K/AKT signaling pathway (He et al., 2022). In addition, jatrorrhizine treatment increases endothelial nitric oxide (NO) release; normalizes plasma lipid levels in diabetic and obese mice; and improves glucose sensitivity, fatty liver, and morphological changes, possibly related to its ability to inhibit ER and oxidative stress by enhancing the Akt/endothelial nitric oxide synthase (eNOS) pathway and NO bioavailability (Zhou et al., 2022). These studies suggest that jatrorrhizine, an intestinal microbial metabolite of BBR, may improve circulatory and hepatic lipid levels through multiple pathways.
The CYP51 enzyme in the intestinal microbiota converts BBR into demethylated metabolites such as berberrubine (Zhang et al., 2021b). Both BBR and berberrubine treatments significantly upregulate the expression of proteins associated with lipolysis (ALGL) and fatty acid β-oxidation (CPT-1 and PPAR-α) while significantly decreasing the expression of proteins associated with de novo adipogenesis (ACC1 and FAS) and fatty acid translation (CD36). In addition, compared with BBR, berberrubine maintains glucose homeostasis in HFD-fed mice via glucose transporter 2 (GLUT2), glycogen synthase kinase 3 (GSK3), and glucose-6-phosphatase (G6Pase) via a mechanism that may be related to the FXR signaling pathway (Sun et al., 2021). Berberrubine also acts as a cholesterol-lowering agent in human hepatoma HepG2 cells by inhibiting PCSK9 expression and upregulating LDLR expression via the ERK signaling pathway (Cao et al., 2018b). Additionally, berberrubine outperforms BBR in terms of absolute bioavailability and fat solubility (Liu et al., 2016). In summary, berberrubine, a demethylated metabolite of BBR, offers more benefits than BBR, which may explain the lipid-regulating activity of BBR.
The gut microbiota can convert BBR to oxyberberine (OBB) through oxidation, and this transformation is significantly inhibited after oral antibiotics (Li et al., 2020). When BBR was metabolized to its oxidized derivative OBB, the structure of C-8 quaternary ammonium turned to more active lactam ring, and the lipophilicity would be enhanced and easier to be absorbed through biofilm, which was beneficial to enhance its biological activity (Singh et al., 2016). In addition, OBB exhibits more favorable safety profile as compared to BBR, with LD50 value above 5,000 mg/kg in mice (the LD50 value of BBR was 713.57 mg/kg) (Li et al., 2019b). OBB can inhibit inflammation and maintain normal intestinal barrier function by inhibiting TLR4-MyD88-NF-κB signaling (Li et al., 2020). In the streptozotocin (STZ)-induced diabetic rat model, the addition of OBB significantly upregulated the expression of the Nrf2 and PI3K/Akt signaling pathways in the model group. OBB also had good hypoglycemic and protective effects on pancreatic β cells, which was superior to that of BBR at an equivalent dose (Dou et al., 2021). Another study found that OBB significantly inhibited abnormal phosphorylation of IRS in rat models of NAFLD (induced via high-fat diets), and improved hepatic insulin signaling. OBB can also reduce chronic adipose tissue inflammation by inhibiting WAT expansion, reducing macrophage migration, and promoting M2-macrophage phenotypic transformation, thus playing an anti-NAFLD role. These findings may be related to AMPK activation (Li et al., 2021b). In view of the superior effect of OBB in improving glucose and lipid metabolism, it may be a potential therapeutic option for the treatment of lipid metabolic diseases in the future.
Elevated lipid levels can cause endothelial cell (EC) dysfunction, foam cell formation, and VSMC proliferation, ultimately inducing the development of AS. In contrast, BBR alleviates endothelial dysfunction and inhibits macrophage foam cell formation and VSMC over-proliferation by reducing dyslipidemia (Figure 1).
Cardiovascular events can be predicted based on endothelial dysfunction, which also causes AS (Xu et al., 2021). NO is a key regulator of endothelial function (Cyr et al., 2020), and hyperlipidemia not only reduces circulating NO levels by downregulating eNOS expression but also affects related pathways through LOX-1 entry into ECs, leading to endothelial dysfunction. In contrast, BBR improves endothelial function by promoting eNOS and inhibiting LOX-1 expression.
eNOS is the main weapon of ECs against vascular diseases, and the production of NO is essential for maintaining normal endothelial function (Benincasa et al., 2022). Hyperlipidemia can reduce NO production and exacerbate endothelial damage by downregulating eNOS through signaling pathways, such as HMGB1/TLR4/Caveolin-1 (Gliozzi et al., 2019), NRF2 (Abu-Saleh et al., 2021), and AMPK/PI3K/AkteNOS (García-Prieto et al., 2015). In turn, the damaged vascular endothelium reduces NO production and exacerbates endothelial dysfunction (Wu et al., 2019).
A previous study showed that BBR treatment reduces plasma TG levels, increases eNOS mRNA and protein expression and serum NO levels, improves endothelium-dependent vasorelaxation in the aorta, and restores endothelial dysfunction in diabetic rats (Wang et al., 2009b). In a palmitate-induced dysfunction model of human umbilical vein endothelial cells (HUVECs), the addition of BBR raised eNOS and NO levels, ameliorating palmitate-induced endothelial dysfunction, and further experiments revealed that this modulatory effect of BBR might be due to AMPK activation (Zhang et al., 2013). In addition, endothelial dysfunction is a key event linking obesity, diabetes, and CVDs. In a model of hyperglycemia-induced endothelial injury, BBR concentration-dependently enhanced eNOS phosphorylation and promoted eNOS binding to heat shock protein 90 (HSP90). This led to increased NO production, which in turn caused endothelium-dependent vasodilation and alleviated high glucose-mediated endothelial dysfunction (Wang et al., 2009a). Despite current controversies, innovative findings have shown that endothelial progenitor cells (EPCs) may be used in cell therapy for cardiovascular repair (Bianconi et al., 2018). BBR mobilizes EPCs by increasing plasma NO concentrations (Xu et al., 2009), increasing the engagement of EPCs and, consequently, the elasticity of small arteries (Xu et al., 2008). These findings suggest that BBR improves endothelial function by increasing NO production through the upregulation of eNOS expression.
Circulating ox-LDL enters the vascular endothelium via LOX-1. This leads to increased intracellular reactive oxygen species (ROS) production and induces mitochondrial dysfunction by increasing NADPH oxidase (Liang et al., 2018) and myeloperoxidase (Liu et al., 2015a), and decreasing NRF2 (Huang et al., 2013). This promotes EC senescence (Bian et al., 2020) and accelerates AS progression (Jiang et al., 2020). As such, LOX-1−/− mice exhibit slow AS progression (Mehta et al., 2007).
Induction of HUVECs with ox-LDL upregulates LOX-1 expression and thus increases the uptake of ox-LDL by Ecs (Guo et al., 2022), resulting in abnormal proliferation and dysfunction of vascular ECs, possibly associated with the activation of the PI3K/Akt, ERK1/2, and MAPK signaling pathways. The subsequent addition of BBR reduces the expression of pAkt, pERK1/2, and p38MAPK, downregulates LOX-1 expression, and significantly inhibits the excessive proliferation of HUVECs, indicating that BBR is a protective agent against vascular endothelial dysfunction (Xu et al., 2017). BBR has also been shown to improve endothelial function in ApoE−/− mice by modulating mitochondrial dysfunction and targeting ApoAI to reduce plasma ox-LDL (Tan et al., 2020) levels. Further exposure of HUVEC to ox-LDL or tumor necrosis factor-alpha (TNFα) for 24 h signifi-cantly increases LOX-1 expression, which is downregulated by the addition of BBR or lovastatin. However, only treatment with BBR reduces TNFα-induced expression of vascular cell adhesion molecule-1 (VCAM-1) and intercellular adhesion molecule-1 (ICAM-1) associated with endothelial dysfunction, possibly through phosphorylation of MAPK/ERK1/2, compared to treatment with lovastatin (Caliceti et al., 2017). Therefore, it has been suggested that BBR can improve endothelial dysfunction in a multifaceted manner compared with lovastatin.
Macrophage foam cells are abundant in AS plaques. BBR inhibits lipid uptake by macrophages through the downregulation of LOX-1 and promotes intracellular lipid efflux through the upregulation of ABCA1/G1, thereby reducing intracellular lipid deposition and macrophage foam cell formation and slowing AS progression.
LOX-1 is also expressed in macrophages and promotes their conversion into foam cells, thereby accelerating macrophage senescence and AS progression (Akhmedov et al., 2021). Ox-LDL induces macrophage senescence by increasing lipid accumulation in macrophages and upregulating senescence-associated proteins, such as p53, p21, and p16 (Ahmad and Leake, 2019; Jia et al., 2019a). The use of an anti-LOX-1 antibody significantly reduces the uptake of ox-LDL by macro-phages and slows AS progression (Dandapat et al., 2007). In ox-LDL-induced human-derived macrophages, ox-LDL significantly increases LOX-1 expression; in contrast, BBR treatment reduces foam cell formation in a dose- and time-dependent manner and inhibits LOX-1 expression by activating the AMPK/SIRT1/PPARγ pathway (Guan et al., 2010). Chi et al. exposed monocyte-derived macrophages to a combination of BBR and atorvastatin and found that LOX-1 expression decreased progressively with increasing doses of BBR, provided that the atorvastatin dosage was fixed. Furthermore, knockdown of the endothelin-1 (ET-1) receptor prevented the inhibitory effect of the combination of BBR and atorvastatin on LOX-1 expression. Berberine combined with atorvastatin has been suggested to downregulate LOX-1 expression via ET-1 receptors in mono-cytes/macrophages (Chi et al., 2014). In clinical practice, BBR may be therefore combined with statins to enhance their lipid-lowering efficacy.
ABCA1/G1 is an important macrophage receptor that promotes intracellular cholesterol efflux and reduces the formation of macrophage foam cells. The promotion of ABCA1/G1 expression is a promising strategy for AS treatment. BBR increases ABCA1/G1 expression by decreasing the degradation rate of ABCG1 mRNA, whereas the effects of BBR on increasing ABCA1/G1 protein levels and promoting cholesterol efflux are blocked when NRF2 is silenced, suggesting that BBR might exert a protective effect by inhibiting foam formation through the NRF2-mediated ABCA1/G1 signaling pathway (Yang et al., 2020). The regulatory role of LXR in ABCA1/G1 has been reported in many papers (Morin et al., 2020). Knockdown of LXRα mRNA expression by siRNAs abrogates BBR-mediated ABCA1 protein expression and affects macrophage cholesterol efflux. These data suggest that BBR reduces macrophage foam cell formation by enhancing LXRα-ABCA1-dependent cholesterol efflux (Lee et al., 2010). In contrast, another study found that in ox-LDL-induced human macrophage-derived foam cells, BBR treatment has no effect on ABCA1 expression, but rather reduces foam cell formation by inhibiting LOX-1 uptake of ox-LDL (Guan et al., 2010), possibly because of the different culture conditions of the model cells. Further studies are needed to elucidate the effect of BBR on the macrophage cholesterol efflux regulator ABCA1.
The contractile VSMC phenotype is typically observed in healthy vessels. When the vessel is injured, VSMCs are converted to a highly proliferative synthetic phenotype, accelerating VSMC migration and proliferation and the secretion of extracellular matrix molecules, thereby accelerating AS progression (Basatemur et al., 2019). Recent studies have shown that hypercholesterolemia can induce VSMC proliferation through the ERK1/2 (Verdeguer et al., 2007) and Wnt signaling pathways (Zhuang et al., 2016). The role of BBR in preventing VSMC proliferation by reducing circulating lipid levels has been demonstrated in many studies and is closely related to the Wnt (Dong et al., 2022) and ERK1/2 (Qu et al., 2020) pathways. In a murine model of diabetic AS, VSMC proliferation could be induced by hypertension-induced mechanical stretch stress and advanced glycosylation end products (AGEs) alone or in combination. BBR inhibits this progression by decreasing protein disulfide isomerase (PDI) expression to prevent vein graft stenosis (Ping et al., 2017). Endoplasmic reticulum stress (ERS) is essential for VSMC proliferation in CVD (Hetz, 2012). BBR attenuates VSMC proliferation by inhibiting the PDI-ERS system (Wang et al., 2020a). Also, PPARα and NO are involved in the proliferation of VSMCs, and BBR was found to upregulate the PPARα-NO signaling pathway and increase vascular NO production, thereby inhibiting the proliferation of Ang IV-stimulated VSMCs (Qiu et al., 2017). In summary, BBR inhibits the excessive proliferation of VSMC through multiple pathways, thereby maintaining normal vascular function.
In addition to drug efficacy, drug safety is important for patient health (Tan et al., 2016). There have been reports of adverse effects, such as cardiac discomfort, in some individuals after the use of BBR (Feng et al., 2018a). However, the safety of BBR, a drug that has been used for thousands of years, is well established in clinical practice. In a meta-analysis of the efficacy and safety of BBR in the treatment of dyslipidemia, 16 randomized controlled trials (RCTs) were included, of which 11 reported 164 adverse events (45 in the berberine group and 119 in the control group). Further combined analysis of the incidence of adverse events in each group showed no significant differences (Ju et al., 2018). In addition, non-oral formulations developed to improve the bioavailability of BBR, such as transdermal formulations of BBR and dihydroberberine, showed no changes in renal and hepatic biomarkers during treatment, supporting the safe use of transdermal compositions (Buchanan et al., 2018). Meanwhile, the use of nutraceuticals containing BBR has gradually expanded owing to their better lipid-lowering effects, and no adverse events have been reported during their administration (Riccioni et al., 2018). Statins are routinely used in the treatment of hyperlipidemia, and the incidence of adverse events is not statistically significant when comparing BBR treatment to treatment with statin (Yang et al., 2023).
In addition to adverse drug reactions, tolerability is another concern in clinical practice. Statins are first-line drugs for the treatment of hyperlipidemia. However, even with lower statin doses, intolerance still occurs in about 10%–15% of patients (Banach et al., 2015). Nutritional preparations containing BBR have recently been recommended as alternative lipid-lowering strategies for statin-intolerant patients (Banach et al., 2018). For example, the nutritional combination of red yeast rice, lipotriol, and BBR is effective and well tolerated for lipid management in patients with a low to moderate risk of hypercholesterolemia (Gonnelli et al., 2015). In addition, several clinical trials have comprehensively studied the commercially available nutritional drug Armolipid Plus, which contains red yeast rice, cholesterol, BBR, folic acid, astaxanthin, and coenzyme Q10. Armolipid Plus has been shown to reduce TC and LDL-C levels in patients with mild to moderate dyslipidemia, particularly in those intolerant to statins (Barrios et al., 2017). Therefore, this evidence suggests that berberine-containing nutraceuticals may be a reasonable option for patients with mild to moderate dyslipidemia, particularly those who are intolerant to statins.
Lipid metabolic diseases, such as obesity, hyperlipidemia, NAFLD, and AS, are chronic diseases that require long-term management. Many challenges such as drug resistance and adverse drug reactions occur during drug treatment. BBR is a safe and effective natural product and a potential option for long-term treatment and management of lipid metabolic diseases. Emerging research over the past decade has suggested that BBR may synergistically regulate lipid metabolism in the liver, gut, and blood vessels to combat lipid metabolic diseases, with specific targets involving CD36, MTTP, SR-BI, ACAT, LOX-1, ABCA1, and intestinal flora (Figure 3). Many studies have been conducted on CD36, ACAT, ABCA1, and LOX-1 targets, and there is a clear understanding of the role of these targets and the potential regulatory mechanisms of BBR on them, and thus further high-quality and large-scale studies should be conducted on these targets to facilitate clinical drug development.
However, earlier MTTP-targeting drugs caused side effects. Furthermore, the impact of SR-BI expresson on blood lipids varies between tissues. It is therefore crucial to conduct further research to investigate whether the regulatory effects of BBR on these two targets can effectively reduce blood lipids, while minimizing potential drug side effects. MTTP promotes lipid binding to ApoB and stabilizes ApoB expression, and a lack of MTTP decreases the secretion of ApoB-containing lipoproteins in the gut and liver (Takahashi et al., 2021). Meanwhile, an article published in JAMA on lipids and lipoproteins indicated that lowering ApoB levels was key to successful lipid-lowering therapies compared to LDL-C or TG (Ference et al., 2020); therefore, lowering ApoB levels through the downregulation of MTTP may be a promising lipid-lowering strategy. Lomitapide, a drug that targets the downregulation of MTTP, reduces ApoB secretion but causes hepatic side effects, such as increased liver fat accumulation, which limits its clinical application (Stefanutti, 2020). In contrast, BBR can reverse the abnormal expression of MTTP to alleviate hepatic lipid accumulation (Chen et al., 2021) and may be a potential drug for further lipid lowering in synergy with lomitapide.
SR-BI is expressed in the liver, intestines, and vascular macrophages. However, its function is tissue-specific, with SR-BI on vascular macrophages being responsible for transferring intracellular cholesterol to circulating HDL, thereby reducing macrophage foam cell formation (Yan et al., 2022). Circulating HDL-CE is further taken up by liver SR-BI and converted into BA for excretion into the intestine. When this reverse cholesterol transport functions properly, circulating cholesterol levels are reduced. Although the function of intestinal SR-BI is controversial, one study found that it is present in the intestinal parietal membrane and acts as a high-affinity receptor for intestinal cholesterol (Labonté et al., 2007). However, mice lacking SR-BI exhibit normal or enhanced intestinal cho-lesterol absorption (Mardones et al., 2001). Another study showed that circulating triglyceride-rich lipoproteins (TRL) levels and that of their remnants, cholesterol and ApoB48, were lower in SR-BI−/− mice than in wild-type mice, suggesting that SR-BI may be a novel regulator of CM production (Lino et al., 2015). In Caco-2 cells, ApoB48, a component of AS plaques, is secreted more often when SR-B1 is overexpressed (Fuentes et al., 2019). In rats and Caco-2/TC7 cell lines, LXR reduces CM secretion and alleviates postprandial triglyceridemia by inhibiting SR-B1 via a miRNA post-transcriptional mechanism (Briand et al., 2016). BBR is a known LXR agonist that can improve lipid levels by activating SR-BI in hepatocytes and macrophages, suggesting that BBR may reduce circulating TRL and its remnant levels through intestinal SR-BI (Ma et al., 2021b). Meanwhile, the European Atherosclerosis Society consensus states that TRL and its remnants may contribute significantly to residual cardiovascular risk in patients on optimized LDL-lowering therapy (Ginsberg et al., 2021), suggesting that BBR may be a promising target for the further reduction of residual cardiovascular risk. In addition, activation of LXR in the intestine reduces cholesterol absorption by upregulating intestinal ABCG5/G8 and increases cholesterol efflux by upregulating ABCA1, thus delaying AS progression (Sasso et al., 2010). The modulatory effect of BBR on LXR has been demonstrated in many studies, and BBR may act on intestinal ABCG5/8 to promote intestinal lipid excretion and improve blood lipid levels. Few studies have examined how BBR affects intestinal lipid metabolism targets, and further research in this area should be pursued.
Furthermore, the low solubility and bioavailability of BBR limits its clinical use. However, this is also one of its advantages, as safety is relatively assured, even with rapidly increasing oral doses. Even so, numerous studies have focused on improving the structure of BBR drugs or enhancing their penetration with additives to improve its bioavailability (Imenshahidi and Hosseinzadeh, 2019). Various nanocarriers for encapsulating BBR have small particles, high surface reactivity, and high adsorption capacity, which improve its efficacy and bioavailability (Hori et al., 2023). Mixed micelles loaded with BBR using Pluronic P85 and Tween 80 enhance intestinal absorption and plasma concentrations of BBR by inhibiting P-gp- and CYP450-mediated efflux and metabolism of BBR in the intestine (Kwon et al., 2020). In addition, transdermal formulations of BBR may be more effective in treating dyslipidemia or hypercholesterolemia because of the higher concentration of circulating BBR compared with oral administration (Buchanan et al., 2018).
Thus, BBR is a potential natural compound for use in treating lipid metabolic disorders. Considering the important role of TRL and its remnants in dyslipidemia and atherosclerotic cardiovascular disease processes, and the relatively few studies on the modulation of intestinal lipid metabolic targets by BBR, future in-depth studies in this area may be an important direction for further lipid reduction. Further structural modifications to BBR may improve its oral bioavailability and efficacy. Blends of BBR with other nutraceuticals should also be carefully analyzed, as pure BBR was not used in these studies. Moreover, large-scale, high-quality, and multicenter clinical trials must be carefully designed to assess the safety, toxicological profile, and clinical utility of BBR in patients with lipid metabolism disorders.
YC: Writing–original draft. QY: Writing–original draft. YY: Visualization, Writing–review and editing. FY: Visualization, Writing–review and editing. RB: Conceptualization, Writing–review and editing. XF: Conceptualization, Writing–review and editing.
The author(s) declare financial support was received for the research, authorship, and/or publication of this article. This research was funded by the National Natural Science Foundation of China (No. 82174216), the Fundamental Research Funds for the Central public welfare research institutes (Nos ZZ13-YQ-005, ZZ13-YQ-005-C1, and ZZ15-YQ-008), and Scientific and Technological Innovation Project of China Academy of Chinese Medical Sciences (grant number CI2021A04617).
The image is created by biorender.
The authors declare that the research was conducted in the absence of any commercial or financial relationships that could be construed as a potential conflict of interest.
All claims expressed in this article are solely those of the authors and do not necessarily represent those of their affiliated organizations, or those of the publisher, the editors and the reviewers. Any product that may be evaluated in this article, or claim that may be made by its manufacturer, is not guaranteed or endorsed by the publisher.
Abidi, P., Zhou, Y., Jiang, J. D., and Liu, J. (2005). Extracellular signal-regulated kinase-dependent stabilization of hepatic low-density lipoprotein receptor mRNA by herbal medicine berberine. Arter. Throm. Vas. 25, 2170–2176. doi:10.1161/01.ATV.0000181761.16341.2b
Abu-Saleh, N., Yaseen, H., Kinaneh, S., Khamaisi, M., and Abassi, Z. (2021). Combination of hyperglycaemia and hyperlipidaemia induces endothelial dysfunction: role of the endothelin and nitric oxide systems. J. Cell. Mol. Med. 25, 1884–1895. doi:10.1111/jcmm.15787
Ahmad, F., and Leake, D. S. (2019). Lysosomal oxidation of LDL alters lysosomal pH, induces senescence, and increases secretion of pro-inflammatory cytokines in human macrophages. J. Lipid Res. 60, 98–110. doi:10.1194/jlr.M088245
Akhmedov, A., Sawamura, T., Chen, C. H., Kraler, S., Vdovenko, D., and Lüscher, T. F. (2021). Lectin-like oxidized low-density lipoprotein receptor-1 (LOX-1): a crucial driver of atherosclerotic cardiovascular disease. Eur. Heart. J. 42, 1797–1807. doi:10.1093/eurheartj/ehaa770
Alolga, R. N., Fan, Y., Chen, Z., Liu, L. W., Zhao, Y. J., Li, J., et al. (2016). Significant pharmacokinetic differences of berberine are attributable to variations in gut microbiota between Africans and Chinese. Sci. Rep-Uk. 6, 27671. doi:10.1038/srep27671
Anastasia, I., Ilacqua, N., Raimondi, A., Lemieux, P., Ghandehari-Alavijeh, R., Faure, G., et al. (2021). Mitochondria-rough-ER contacts in the liver regulate systemic lipid homeostasis. Cell Rep. 34, 108873. doi:10.1016/j.celrep.2021.108873
Ataei, S., Kesharwani, P., and Sahebkar, A. (2022). Berberine: ins and outs of a nature-made PCSK9 inhibitor. Excli. J. 21, 1099–1110. doi:10.17179/excli2022-5234
Banach, M., Patti, A. M., Giglio, R. V., Cicero, A. F., Atanasov, A. G., Bajraktari, G., et al. (2018). The role of nutraceuticals in statin intolerant patients. J. Am. Coll. Cardiol. 72, 96–118. doi:10.1016/j.jacc.2018.04.040
Banach, M., Rizzo, M., Toth, P. P., Farnier, M., Davidson, M. H., Al-Rasadi, K., et al. (2015). Statin intolerance - an attempt at a unified definition. Position paper from an International Lipid Expert Panel. Arch. Med. Sci. 11, 1–23. doi:10.5114/aoms.2015.49807
Bansod, S., Saifi, M. A., and Godugu, C. (2021). Molecular updates on berberine in liver diseases: bench to bedside. Phytother. Res. 35, 5459–5476. doi:10.1002/ptr.7181
Barrios, V., Escobar, C., Cicero, A. F. G., Burke, D., Fasching, P., Banach, M., et al. (2017). A nutraceutical approach (Armolipid Plus) to reduce total and LDL cholesterol in individuals with mild to moderate dyslipidemia: review of the clinical evidence. Atheroscler. Suppl. 24, 1–15. doi:10.1016/j.atherosclerosissup.2016.10.003
Basatemur, G. L., Jørgensen, H. F., Clarke, M. C., Bennett, M. R., and Mallat, Z. (2019). Vascular smooth muscle cells in atherosclerosis. Nat. Rev. Cardiol. 16, 727–744. doi:10.1038/s41569-019-0227-9
Behm-Ansmant, I., Gatfield, D., Rehwinkel, J., Hilgers, V. E. R., and Izaurralde, E. (2007). A conserved role for cytoplasmic poly (A)-binding protein 1 (PABPC1) in nonsense-mediated mRNA decay. Embo J. 26, 1591–1601. doi:10.1038/sj.emboj.7601588
Benincasa, G., Coscioni, E., and Napoli, C. (2022). Cardiovascular risk factors and molecular routes underlying endothelial dysfunction: novel opportunities for primary prevention. Biochem. Pharmacol. 202, 115108. doi:10.1016/j.bcp.2022.115108
Bian, W., Jing, X., Yang, Z., Shi, Z., Chen, R., Xu, A., et al. (2020). Downregulation of LncRNA NORAD promotes Ox-LDL-induced vascular endothelial cell injury and atherosclerosis. Aging 12, 6385–6400. doi:10.18632/aging.103034
Bianconi, V., Sahebkar, A., Kovanen, P., Bagaglia, F., Ricciuti, B., Calabrò, P., et al. (2018). Endothelial and cardiac progenitor cells for cardiovascular repair: a controversial paradigm in cell therapy. Pharmacol. Ther. 181, 156–168. doi:10.1016/j.pharmthera.2017.08.004
Briand, O., Touche, V., Colin, S., Brufau, G., Davalos, A., Schonewille, M., et al. (2016). Liver X receptor regulates triglyceride absorption through intestinal down-regulation of scavenger receptor class B, type 1. Gastroenterology 150, 650–658. doi:10.1053/j.gastro.2015.11.015
Brown, E., Hydes, T., Hamid, A., and Cuthbertson, D. (2021). Emerging and established therapeutic approaches for nonalcoholic fatty liver disease. Clin. Ther. 43, 1476–1504. doi:10.1016/j.clinthera.2021.07.013
Buchanan, B., Meng, Q., Poulin, M. M., Zuccolo, J., Azike, C. G., Gabriele, J., et al. (2018). Comparative pharmacokinetics and safety assessment of transdermal berberine and dihydroberberine. PloS One 13, 01949799–e195020. doi:10.1371/journal.pone.0194979
Caliceti, C., Rizzo, P., Ferrari, R., Fortini, F., Aquila, G., Leoncini, E., et al. (2017). Novel role of the nutraceutical bioactive compound berberine in lectin-like OxLDL receptor 1-mediated endothelial dysfunction in comparison to lovastatin. Nutr. Metab. Cardiovas. 27, 552–563. doi:10.1016/j.numecd.2017.04.002
Cao, J., Xu, Y., Li, F., Shang, L., Fan, D., Yu, H., et al. (2018a). Protein markers of dysfunctional HDL in scavenger receptor class B type I deficient mice. J. Transl. Med. 16, 155–213. doi:10.1186/s12967-018-1502-y
Cao, S., Xu, P., Yan, J., Liu, H., Liu, L., Cheng, L., et al. (2018b). Berberrubine and its analog, hydroxypropyl-berberrubine, regulate LDLR and PCSK9 expression via the ERK signal pathway to exert cholesterol-lowering effects in human hepatoma HepG2 cells. J. Cell. Biochem. 120, 1340–1349. doi:10.1002/jcb.27102
Cao, S., Zhou, Y., Xu, P., Wang, Y., Yan, J., Bin, W., et al. (2013). Berberine metabolites exhibit triglyceride-lowering effects via activation of AMP-activated protein kinase in Hep G2 cells. J. Ethnopharmacol. 149, 576–582. doi:10.1016/j.jep.2013.07.025
Chang, X., Yan, H., Fei, J., Jiang, M., Zhu, H., Lu, D., et al. (2010). Berberine reduces methylation of the MTTP promoter and alleviates fatty liver induced by a high-fat diet in rats. J. Lipid Res. 51, 2504–2515. doi:10.1194/jlr.M001958
Chen, P., Li, Y., and Xiao, L. (2021). Berberine ameliorates nonalcoholic fatty liver disease by decreasing the liver lipid content via reversing the abnormal expression of MTTP and LDLR. Exp. Ther. Med. 22, 1109–1118. doi:10.3892/etm.2021.10543
Chen, Y., Xu, C., Huang, R., Song, J., Li, D., Xia, M., et al. (2018). Butyrate from pectin fermentation inhibits intestinal cholesterol absorption and attenuates atherosclerosis in apolipoprotein E-deficient mice. J. Nutr. Biochem. 56, 175–182. doi:10.1016/j.jnutbio.2018.02.011
Chi, L., Peng, L., Hu, X., Pan, N., and Zhang, Y. (2014). Berberine combined with atorvastatin downregulates LOX-1 expression through the ET-1 receptor in monocyte/macrophages. Int. J. Mol. Med. 34, 283–290. doi:10.3892/ijmm.2014.1748
Choudhury, M., Jonscher, K. R., and Friedman, J. E. (2011). Reduced mitochondrial function in obesity-associated fatty liver: SIRT3 takes on the fat. Aging 3, 175–178. doi:10.18632/aging.100289
Cuomo, P., Capparelli, R., Iannelli, A., and Iannelli, D. (2022). Role of branched-chain amino acid metabolism in type 2 diabetes, obesity, cardiovascular disease and non-alcoholic fatty liver disease. Int. J. Mol. Sci. 23, 4325–4411. doi:10.3390/ijms23084325
Cyr, A. R., Huckaby, L. V., Shiva, S. S., and Zuckerbraun, B. S. (2020). Nitric oxide and endothelial dysfunction. Crit. Care Clin. 36, 307–321. doi:10.1016/j.ccc.2019.12.009
Dai, G., Sun, B., Wu, L., Gao, X., Song, S., Sun, H., et al. (2018). Comparative pharmacokinetics of three alkaloids in normal and acute hepatitis rats after oral administration of Yanhuanglian total alkaloids extract. Biomed. Chromatogr. 32, e4329. doi:10.1002/bmc.4329
Dandapat, A., Hu, C., Sun, L., and Mehta, J. L. (2007). Small concentrations of oxLDL induce capillary tube formation from endothelial cells via LOX-1-dependent redox-sensitive pathway. Arter. Throm. Vas. 27, 2435–2442. doi:10.1161/ATVBAHA.107.152272
Demignot, S., Beilstein, F., and Morel, E. (2014). Triglyceride-rich lipoproteins and cytosolic lipid droplets in enterocytes: key players in intestinal physiology and metabolic disorders. Biochimie 96, 48–55. doi:10.1016/j.biochi.2013.07.009
Dong, Y., Fan, H., Zhang, Z., Jiang, F., Li, M., Zhou, H., et al. (2022). Berberine ameliorates DSS-induced intestinal mucosal barrier dysfunction through microbiota-dependence and Wnt/β-catenin pathway. Int. J. Biol. Sci. 18, 1381–1397. doi:10.7150/ijbs.65476
Dou, Y., Huang, R., Li, Q., Liu, Y., Li, Y., Chen, H., et al. (2021). Oxyberberine, an absorbed metabolite of berberine, possess superior hypoglycemic effect via regulating the PI3K/Akt and Nrf2 signaling pathways. Biomed. Pharmacother. 137, 111312. doi:10.1016/j.biopha.2021.111312
Dufour, J. F., Caussy, C., and Loomba, R. (2020). Combination therapy for non-alcoholic steatohepatitis: rationale, opportunities and challenges. Gut 69, 1877–1884. doi:10.1136/gutjnl-2019-319104
Fan, J., and Watanabe, T. (2022). Atherosclerosis: known and unknown. Pathol. Int. 72, 151–160. doi:10.1111/pin.13202
Fan, T. Y., Yang, Y. X., Zeng, Q. X., Wang, X. L., Wei, W., Guo, X. X., et al. (2021). Structure-activity relationship and biological evaluation of berberine derivatives as PCSK9 down-regulating agents. Bioorg. Chem. 113, 104994. doi:10.1016/j.bioorg.2021.104994
Fang, X., Wu, H., Wang, X., Lian, F., Li, M., Miao, R., et al. (2022). Modulation of gut microbiota and metabolites by berberine in treating mice with disturbances in glucose and lipid metabolism. Front. Pharmacol. 13, 870407. doi:10.3389/fphar.2022.870407
Feng, P., Zhao, L., Guo, F., Zhang, B., Fang, L., Zhan, G., et al. (2018a). The enhancement of cardiotoxicity that results from inhibiton of CYP 3A4 activity and hERG channel by berberine in combination with statins. Chem-Biol. Interact. 293, 115–123. doi:10.1016/j.cbi.2018.07.022
Feng, R., Shou, J. W., Zhao, Z. X., He, C. Y., Ma, C., Huang, M., et al. (2015). Transforming berberine into its intestine-absorbable form by the gut microbiota. Sci. Rep-Uk. 5, 12155–12215. doi:10.1038/srep12155
Feng, R., Zhao, Z. X., Ma, S. R., Guo, F., Wang, Y., Jiang, J. D., et al. (2018b). Gut microbiota-regulated pharmacokinetics of berberine and active metabolites in beagle dogs after oral administration. Front. Pharmacol. 9, 214. doi:10.3389/fphar.2018.00214
Feng, X., Wang, K., Cao, S., Ding, L., and Qiu, F. (2021). Pharmacokinetics and excretion of berberine and its nine metabolites in rats. Front. Pharmacol. 11, 594852. doi:10.3389/fphar.2020.594852
Ference, B. A., Kastelein, J. J., and Catapano, A. L. (2020). Lipids and lipoproteins in 2020. JAMA 324, 595–596. doi:10.1001/jama.2020.5685
Ferré, P., Phan, F., and Foufelle, F. (2021). SREBP-1c and lipogenesis in the liver: an update. Biochem. J. 478, 3723–3739. doi:10.1042/BCJ20210071
Fiorucci, S., Biagioli, M., Zampella, A., and Distrutti, E. (2018). Bile acids activated receptors regulate innate immunity. Front. Immunol. 9, 1853. doi:10.3389/fimmu.2018.01853
Fuentes, M., Santander, N., and Cortés, V. (2019). Insulin increases cholesterol uptake, lipid droplet content, and apolipoprotein B secretion in CaCo-2 cells by upregulating SR-BI via a PI3K, AKT, and mTOR-dependent pathway. J. Cell. Biochem. 120, 1550–1559. doi:10.1002/jcb.27410
García-Prieto, C. F., Hernández-Nuño, F., Rio, D. D., Ruiz-Hurtado, G., Aránguez, I., Ruiz-Gayo, M., et al. (2015). High-fat diet induces endothelial dysfunction through a down-regulation of the endothelial AMPK-PI3K-Akt-eNOS pathway. Mol. Nutr. Food Res. 59, 520–532. doi:10.1002/mnfr.201400539
Gathercole, L. L., Morgan, S. A., Bujalska, I. J., Hauton, D., Stewart, P. M., Tomlinson, J. W., et al. (2011). Regulation of lipogenesis by glucocorticoids and insulin in human adipose tissue. PloS One 6, e26223. doi:10.1371/journal.pone.0026223
Gendy, A. M., Elnagar, M. R., Allam, M. M., Mousa, M. R., Khodir, A. E., El-Haddad, A. E., et al. (2022). Berberine-loaded nanostructured lipid carriers mitigate warm hepatic ischemia/reperfusion-induced lesion through modulation of HMGB1/TLR4/NF-κB signaling and autophagy. Biomed. Pharmacother. 145, 112122. doi:10.1016/j.biopha.2021.112122
Ginsberg, H. N., Packard, C. J., Chapman, M. J., Borén, J., Aguilar-Salinas, C. A., Averna, M., et al. (2021). Triglyceride-rich lipoproteins and their remnants: metabolic insights, role in atherosclerotic cardiovascular disease, and emerging therapeutic strategies—a consensus statement from the European Atherosclerosis Society. Eur. Heart J. 42, 4791–4806. doi:10.1093/eurheartj/ehab551
Gliozzi, M., Scicchitano, M., Bosco, F., Musolino, V., Carresi, C., Scarano, F., et al. (2019). Modulation of nitric oxide synthases by oxidized LDLs: role in vascular inflammation and atherosclerosis development. Int. J. Mol. Sci. 20, 3294. doi:10.3390/ijms20133294
Gómez-Ambrosi, J., Gallego-Escuredo, J. M., Catalán, V., Rodríguez, A., Domingo, P., Moncada, R., et al. (2017). FGF19 and FGF21 serum concentrations in human obesity and type 2 diabetes behave differently after diet-or surgically-induced weight loss. Clin. Nutr. 36, 861–868. doi:10.1016/j.clnu.2016.04.027
Gong, Y., Lu, Q., Liu, Y., Xi, L., Zhang, Z., Liu, H., et al. (2022). Dietary berberine alleviates high carbohydrate diet-induced intestinal damages and improves lipid metabolism in largemouth bass (Micropterus salmoides). Front. Nutr. 9, 1010859. doi:10.3389/fnut.2022.1010859
Gonnelli, S., Caffarelli, C., Stolakis, K., Cuda, C., Giordano, N., Nuti, R., et al. (2015). Efficacy and tolerability of a nutraceutical combination (red yeast rice, policosanols, and berberine) in patients with low-moderate risk hypercholesterolemia: a double-blind, placebo-controlled study. Curr. Ther. Res. 77, 1–6. doi:10.1016/j.curtheres.2014.07.003
Guan, S., Wang, B., Li, W., Guan, J., and Fang, X. (2010). Effects of berberine on expression of LOX-1 and SR-BI in human macrophage-derived foam cells induced by ox-LDL. Am. J. Chin. Med. 38, 1161–1169. doi:10.1142/S0192415X10008548
Guo, X., Guo, Y., Wang, Z., Cao, B., Zheng, C., Zeng, Z., et al. (2022). Reducing the damage of ox-LDL/LOX-1 pathway to vascular endothelial barrier can inhibit atherosclerosis. Oxid. Med. Cell. Longev. 2022, 7541411–7541416. doi:10.1155/2022/7541411
Guo, Y., Li, F., Ma, X., Cheng, X., Zhou, H., Klaassen, C. D., et al. (2011a). CYP2D plays a major role in berberine metabolism in liver of mice and humans. Xenobiotica 41, 996–1005. doi:10.3109/00498254.2011.597456
Guo, Y., Pope, C., Cheng, X., Zhou, H., and Klaassen, C. D. (2011b). Dose-response of berberine on hepatic cytochromes P450 mRNA expression and activities in mice. J. Ethnopharmacol. 138, 111–118. doi:10.1016/j.jep.2011.08.058
Guo, Y., Zhang, Y., Huang, W., Selwyn, F. P., and Klaassen, C. D. (2016). Dose-response effect of berberine on bile acid profile and gut microbiota in mice. BMC Complem. Altern. Med. 16, 394–412. doi:10.1186/s12906-016-1367-7
Habtemariam, S. (2020). Berberine pharmacology and the gut microbiota: a hidden therapeutic link. Pharmacol. Res. 155, 104722. doi:10.1016/j.phrs.2020.104722
Haghikia, A., Zimmermann, F., Schumann, P., Jasina, A., Roessler, J., Schmidt, D., et al. (2022). Propionate attenuates atherosclerosis by immune-dependent regulation of intestinal cholesterol metabolism. Eur. Heart J. 43, 518–533. doi:10.1093/eurheartj/ehab644
Han, L., Yang, Q. H., Zhang, Y. P., Yan, H. Z., Zhu, X. F., Gong, X. W., et al. (2015). Intervention of berberine on lipid deposition in liver cells of non-alcoholic fatty liver disease rats induced by high fat diet. Zhongguo Zhong Xi Yi Jie He Za Zhi Zhongguo Zhongxiyi Jiehe Zazhi= Chin. J. Integr. Traditional West. Med. 35, 314–319.
Han, Y. C., Xie, H. Z., Lu, B., Xiang, R. L., Li, J. Y., Qian, H., et al. (2022). Effect of berberine on global modulation of lncRNAs and mRNAs expression profiles in patients with stable coronary heart disease. BMC Genomics 23, 400. doi:10.1186/s12864-022-08641-2
He, H., Deng, J., Yang, M., An, L., Ye, X., Li, X., et al. (2022). Jatrorrhizine from Rhizoma Coptidis exerts an anti-obesity effect in db/db mice. J. Ethnopharmacol. 298, 115529. doi:10.1016/j.jep.2022.115529
He, Q., Mei, D., Sha, S., Fan, S., Wang, L., Dong, M., et al. (2017). ERK-dependent mTOR pathway is involved in berberine-induced autophagy in hepatic steatosis. J. Mol. Endocrinol. 59, X1. doi:10.1530/JME-16-0139e
Hetz, C. (2012). The unfolded protein response: controlling cell fate decisions under ER stress and beyond. Nat. Rev. Mol. Cell Bio. 13, 89–102. doi:10.1038/nrm3270
Hori, I., Harashima, H., and Yamada, Y. (2023). Development of a mitochondrial targeting lipid nanoparticle encapsulating berberine. Int. J. Mol. Sci. 24, 903. doi:10.3390/ijms24020903
Hu, H., Xu, K., Wang, K., Zhang, F., and Bai, X. (2022a). Dissecting the effect of berberine on the intestinal microbiome in the weaned piglets by metagenomic sequencing. Front. Microbiol. 13, 862882. doi:10.3389/fmicb.2022.862882
Hu, T., Wu, Q., Yao, Q., Jiang, K., Yu, J., Tang, Q., et al. (2022b). Short-chain fatty acid metabolism and multiple effects on cardiovascular diseases. Ageing Res. Rev. 81, 101706. doi:10.1016/j.arr.2022.101706
Hua, H., Kong, Q., Zhang, H., Wang, J., Luo, T., Jiang, Y., et al. (2019). Targeting mTOR for cancer therapy. J. Hematol. Oncol. 12, 71–19. doi:10.1186/s13045-019-0754-1
Huang, C. S., Lin, A. H., Liu, C. T., Tsai, C. W., Chang, I. S., Chen, H. W., et al. (2013). Isothiocyanates protect against oxidized LDL-induced endothelial dysfunction by upregulating Nrf2-dependent antioxidation and suppressing NFκB activation. Mol. Nutr. Food Res. 57, 1918–1930. doi:10.1002/mnfr.201300063
Huang, X., Zheng, M., Yi, Y., Patel, A., Song, Z., Li, Y., et al. (2020). Inhibition of berberine hydrochloride on Candida albicans biofilm formation. Biotechnol. Lett. 42, 2263–2269. doi:10.1007/s10529-020-02938-6
Imenshahidi, M., and Hosseinzadeh, H. (2019). Berberine and barberry (Berberis vulgaris): a clinical review. Phytother. Res. 33, 504–523. doi:10.1002/ptr.6252
Jia, Q., Cao, H., Shen, D., Yan, L., Chen, C., Xing, S., et al. (2019a). Fisetin, via CKIP-1/REGγ, limits oxidized LDL-induced lipid accumulation and senescence in RAW264. 7 macrophage-derived foam cells. Eur. J. Pharmacol. 865, 172748. doi:10.1016/j.ejphar.2019.172748
Jia, X., Jia, L., Mo, L., Yuan, S., Zheng, X., He, J., et al. (2019b). Berberine ameliorates periodontal bone loss by regulating gut microbiota. J. Dent. Res. 98, 107–116. doi:10.1177/0022034518797275
Jiang, Y. H., Jiang, L. Y., Wang, Y. C., Ma, D. F., and Li, X. (2020). Quercetin attenuates atherosclerosis via modulating oxidized LDL-induced endothelial cellular senescence. Front. Pharmacol. 11, 512–611. doi:10.3389/fphar.2020.00512
Ju, J., Li, J., Lin, Q., and Xu, H. (2018). Efficacy and safety of berberine for dyslipidaemias: a systematic review and meta-analysis of randomized clinical trials. Phytomedicine 50, 25–34. doi:10.1016/j.phymed.2018.09.212
Jung, C. H., Ro, S. H., Cao, J., Otto, N. M., and Kim, D. H. (2010). mTOR regulation of autophagy. Febs Lett. 584, 1287–1295. doi:10.1016/j.febslet.2010.01.017
Ke, X., Zhang, R., Li, P., Zuo, L., Wang, M., Yang, J., et al. (2022). Hydrochloride Berberine ameliorates alcohol-induced liver injury by regulating inflammation and lipid metabolism. Biochem. Biophys. Res. Commun. 610, 49–55. doi:10.1016/j.bbrc.2022.04.009
Kessoku, T., Kobayashi, T., Ozaki, A., Iwaki, M., Honda, Y., Ogawa, Y., et al. (2020). Rationale and design of a randomised, double-blind, placebo-controlled, parallel-group, investigator-initiated phase 2a study to investigate the efficacy and safety of elobixibat in combination with cholestyramine for non-alcoholic fatty liver disease. BMJ Open 10, e037961. doi:10.1136/bmjopen-2020-037961
Khoshandam, A., Imenshahidi, M., and Hosseinzadeh, H. (2022). Pharmacokinetic of berberine, the main constituent of Berberis vulgaris L.: a comprehensive review. Phytother. Res. 36, 4063–4079. doi:10.1002/ptr.7589
Kong, W. J., Wei, J., Zuo, Z. Y., Wang, Y. M., Song, D. Q., You, X. F., et al. (2008). Combination of simvastatin with berberine improves the lipid-lowering efficacy. Metabolism 57, 1029–1037. doi:10.1016/j.metabol.2008.01.037
Kumar, A., Chopra, K., Mukherjee, M., Pottabathini, R., and Dhull, D. K. (2015). Current knowledge and pharmacological profile of berberine: an update. Eur. J. Pharmacol. 761, 288–297. doi:10.1016/j.ejphar.2015.05.068
Kwon, M., Lim, D. Y., Lee, C. H., Jeon, J. H., Choi, M. K., Song, I. S., et al. (2020). Enhanced intestinal absorption and pharmacokinetic modulation of berberine and its metabolites through the inhibition of P-glycoprotein and intestinal metabolism in rats using a berberine mixed micelle formulation. Pharmaceutics 12, 882. doi:10.3390/pharmaceutics12090882
Labonté, E. D., Howles, P. N., Granholm, N. A., Rojas, J. C., Davies, J. P., Ioannou, Y. A., et al. (2007). Class B type I scavenger receptor is responsible for the high affinity cholesterol binding activity of intestinal brush border membrane vesicles. Biochim. Biophys. Acta. 1771, 1132–1139. doi:10.1016/j.bbalip.2007.03.002
Lee, S. H., Park, S. Y., and Choi, C. S. (2022). Insulin resistance: from mechanisms to therapeutic strategies. Diabetes Metab. J. 46, 15–37. doi:10.4093/dmj.2021.0280
Lee, T. S., Pan, C. C., Peng, C. C., Kou, Y. R., Chen, C. Y., Ching, L. C., et al. (2010). Anti-atherogenic effect of berberine on LXRalpha-ABCA1-dependent cholesterol efflux in macrophages. J. Cell. Biochem. 111, 104–110. doi:10.1002/jcb.22667
Li, C., Ai, G., Wang, Y., Lu, Q., Luo, C., Tan, L., et al. (2020). Oxyberberine, a novel gut microbiota-mediated metabolite of berberine, possesses superior anti-colitis effect: impact on intestinal epithelial barrier, gut microbiota profile and TLR4-MyD88-NF-κB pathway. Pharmacol. Res. 152, 104603. doi:10.1016/j.phrs.2019.104603
Li, C. H., Tang, S. C., Wong, C. H., Wang, Y., Jiang, J. D., Chen, Y., et al. (2018a). Berberine induces miR-373 expression in hepatocytes to inactivate hepatic steatosis associated AKT-S6 kinase pathway. Eur. J. Pharmacol. 825, 107–118. doi:10.1016/j.ejphar.2018.02.035
Li, C. L., Tan, L. H., Wang, Y. F., Luo, C. D., Chen, H. B., Lu, Q., et al. (2019a). Comparison of anti-inflammatory effects of berberine, and its natural oxidative and reduced derivatives from Rhizoma Coptidis in vitro and in vivo. Phytomedicine 52, 272–283. doi:10.1016/j.phymed.2018.09.228
Li, D., Yang, C., Zhu, J. Z., Lopez, E., Zhang, T., Tong, Q., et al. (2022). Berberine remodels adipose tissue to attenuate metabolic disorders by activating sirtuin 3. Acta Pharmacol. Sin. 43, 1285–1298. doi:10.1038/s41401-021-00736-y
Li, H., Chen, W., Zhou, Y., Abidi, P., Sharpe, O., Robinson, W. H., et al. (2009a). Identification of mRNA binding proteins that regulate the stability of LDL receptor mRNA through AU-rich elements. J. Lipid Res. 50, 820–831. doi:10.1194/jlr.M800375-JLR200
Li, H., Dong, B., Park, S. W., Lee, H. S., Chen, W., Liu, J., et al. (2009b). Hepatocyte nuclear factor 1alpha plays a critical role in PCSK9 gene transcription and regulation by the natural hypocholesterolemic compound berberine. J. Biol. Chem. 284, 28885–28895. doi:10.1074/jbc.M109.052407
Li, H., Liu, N. N., Li, J. R., Dong, B., Wang, M. X., Tan, J. L., et al. (2022a). Combined use of bicyclol and berberine alleviates mouse nonalcoholic fatty liver disease. Front. Pharmacol. 13, 843872. doi:10.3389/fphar.2022.843872
Li, J., Li, J., Ni, J., Zhang, C., Jia, J., Wu, G., et al. (2022b). Berberine relieves metabolic syndrome in mice by inhibiting liver inflammation caused by a high-fat diet and potential association with gut microbiota. Front. Microbiol. 12, 752512. doi:10.3389/fmicb.2021.752512
Li, Q. P., Dou, Y. X., Huang, Z. W., Chen, H. B., Li, Y. C., Chen, J. N., et al. (2021a). Therapeutic effect of oxyberberine on obese non-alcoholic fatty liver disease rats. Phytomedicine 85, 153550. doi:10.1016/j.phymed.2021.153550
Li, R., Xin, T., Li, D., Wang, C., Zhu, H., and Zhou, H. (2018b). Therapeutic effect of Sirtuin 3 on ameliorating nonalcoholic fatty liver disease: the role of the ERK-CREB pathway and Bnip3-mediated mitophagy. Redox Biol. 18, 229–243. doi:10.1016/j.redox.2018.07.011
Li, X., Su, C., Jiang, Z., Yang, Y., Zhang, Y., Yang, M., et al. (2021b). Berberine attenuates choline-induced atherosclerosis by inhibiting trimethylamine and trimethylamine-N-oxide production via manipulating the gut microbiome. Npj Biofilms Microbi 7, 36–14. doi:10.1038/s41522-021-00205-8
Li, Y., Ren, G., Wang, Y. X., Kong, W. J., Yang, P., Wang, Y. M., et al. (2011a). Bioactivities of berberine metabolites after transformation through CYP450 isoenzymes. J. Transl. Med. 9, 62–10. doi:10.1186/1479-5876-9-62
Li, Y., Xu, S., Mihaylova, M. M., Zheng, B., Hou, X., Jiang, B., et al. (2011b). AMPK phosphorylates and inhibits SREBP activity to attenuate hepatic steatosis and atherosclerosis in diet-induced insulin-resistant mice. Cell. Metab. 13, 376–388. doi:10.1016/j.cmet.2011.03.009
Li, Y., Yang, P., Zhao, L., Chen, Y., Zhang, X., Zeng, S., et al. (2019b). CD36 plays a negative role in the regulation of lipophagy in hepatocytes through an AMPK-dependent pathway. J. Lipid Res. 60, 844–855. doi:10.1194/jlr.M090969
Li, Y., Zhou, Y., Si, N., Han, L., Ren, W., Xin, S., et al. (2017). Comparative metabolism study of five protoberberine alkaloids in liver microsomes from rat, rhesus monkey, and human. Planta Med. 83, 1281–1288. doi:10.1055/s-0043-108249
Liang, C., Wang, Q. S., Yang, X., Niu, N., Hu, Q. Q., Zhang, B. L., et al. (2018). Oxidized low-density lipoprotein stimulates epithelial sodium channels in endothelial cells of mouse thoracic aorta. Br. J. Pharmacol. 175, 1318–1328. doi:10.1111/bph.13853
Liang, H., and Wang, Y. (2018). Berberine alleviates hepatic lipid accumulation by increasing ABCA1 through the protein kinase C δ pathway. Biochem. Bioph. Res. Co. 498, 473–480. doi:10.1016/j.bbrc.2018.03.003
Lin, C. W., Zhang, H., Li, M., Xiong, X., Chen, X., Chen, X., et al. (2013). Pharmacological promotion of autophagy alleviates steatosis and injury in alcoholic and non-alcoholic fatty liver conditions in mice. J. Hepatol. 58, 993–999. doi:10.1016/j.jhep.2013.01.011
Lino, M., Farr, S., Baker, C., Fuller, M., Trigatti, B., Adeli, K., et al. (2015). Intestinal scavenger receptor class B type I as a novel regulator of chylomicron production in healthy and diet-induced obese states. Am. J. Physiol. Gastrointest. Liver Physiol. 309, G350–G359. doi:10.1152/ajpgi.00086.2015
Liu, A., Wu, Q., Guo, J., Ares, I., Rodríguez, J. L., Martínez-Larrañaga, M. R., et al. (2019). Statins: adverse reactions, oxidative stress and metabolic interactions. Pharmacol. Ther. 195, 54–84. doi:10.1016/j.pharmthera.2018.10.004
Liu, C. S., Zheng, Y. R., Zhang, Y. F., and Long, X. Y. (2016). Research progress on berberine with a special focus on its oral bioavailability. Fitoterapia 109, 274–282. doi:10.1016/j.fitote.2016.02.001
Liu, D. L., Xu, L. J., Dong, H., Chen, G., Huang, Z. Y., Zou, X., et al. (2015a). Inhibition of proprotein convertase subtilisin/kexin type 9: a novel mechanism of berberine and 8-hydroxy dihydroberberine against hyperlipidemia. Chin. J. Integr. Med. 21, 132–138. doi:10.1007/s11655-014-1775-1
Liu, W. Q., Zhang, Y. Z., Wu, Y., Zhang, J. J., Li, T. B., Jiang, T., et al. (2015b). Myeloperoxidase-derived hypochlorous acid promotes ox-LDL-induced senescence of endothelial cells through a mechanism involving β-catenin signaling in hyperlipidemia. Biochem. Bioph. Res. Co. 467, 859–865. doi:10.1016/j.bbrc.2015.10.053
Liu, X., Li, W., Zhang, H., Wang, X., Huang, Y., Li, Y., et al. (2022). Biodistribution and pharmacokinetic profile of berberine and its metabolites in hepatocytes. Phytomedicine 104, 154288. doi:10.1016/j.phymed.2022.154288
Liu, Y., Hao, H., Xie, H., Lv, H., Liu, C., Wang, G., et al. (2009). Oxidative demethylenation and subsequent glucuronidation are the major metabolic pathways of berberine in rats. J. Pharm. Sci-Us. 98, 4391–4401. doi:10.1002/jps.21721
Liu, Y. T., Hao, H. P., Xie, H. G., Lai, L., Wang, Q., Liu, C. X., et al. (2010). Extensive intestinal first-pass elimination and predominant hepatic distribution of berberine explain its low plasma levels in rats. Drug Metab. Dispos. 38, 1779–1784. doi:10.1124/dmd.110.033936
Long, S. L., Gahan, C. G., and Joyce, S. A. (2017). Interactions between gut bacteria and bile in health and disease. Mol. Asp. Med. 56, 54–65. doi:10.1016/j.mam.2017.06.002
Loomba, R., Friedman, S. L., and Shulman, G. I. (2021). Mechanisms and disease consequences of nonalcoholic fatty liver disease. Cell 184, 2537–2564. doi:10.1016/j.cell.2021.04.015
Ma, C. Y., Shi, X. Y., Wu, Y. R., Zhang, Y., Yao, Y. H., Qu, H. L., et al. (2021a). Berberine attenuates atherosclerotic lesions and hepatic steatosis in ApoE-/-mice by down-regulating PCSK9 via ERK1/2 pathway. Ann. Transl. Med. 9, 1517. doi:10.21037/atm-20-8106
Ma, S. R., Tong, Q., Lin, Y., Pan, L. B., Fu, J., Peng, R., et al. (2022). Berberine treats atherosclerosis via a vitamine-like effect down-regulating Choline-TMA-TMAO production pathway in gut microbiota. Signal Transduct. Target 7, 207. doi:10.1038/s41392-022-01027-6
Ma, X., Qian, H., Chen, A., Ni, H. M., and Ding, W. X. (2021b). Perspectives on mitochondria–ER and mitochondria–lipid droplet contact in hepatocytes and hepatic lipid metabolism. Cells 10, 2273. doi:10.3390/cells10092273
Ma, Y., Zeng, M., Sun, R., and Hu, M. (2014). Disposition of flavonoids impacts their efficacy and safety. Curr. Drug Metab. 15, 841–864. doi:10.2174/1389200216666150206123719
Mardones, P., Quiñones, V., Amigo, L., Moreno, M., Miquel, J. F., Schwarz, M., et al. (2001). Hepatic cholesterol and bile acid metabolism and intestinal cholesterol absorption in scavenger receptor class B type I-deficient mice. J. Lipid Res. 42, 170–180. doi:10.1016/S0022-2275(20)31676-X
Martinot, E., Sèdes, L., Baptissart, M., Lobaccaro, J. M., Caira, F., Beaudoin, C., et al. (2017). Bile acids and their receptors. Mol. Asp. Med. 56, 2–9. doi:10.1016/j.mam.2017.01.006
Matsuo, M. (2022). ABCA1 and ABCG1 as potential therapeutic targets for the prevention of atherosclerosis. J. Pharmacol. Sci. 148, 197–203. doi:10.1016/j.jphs.2021.11.005
May, S. C., Dron, J. S., Hegele, R. A., and Sahoo, D. (2021). Human variant of scavenger receptor BI (R174C) exhibits impaired cholesterol transport functions. J. Lipid Res. 62, 100045. doi:10.1016/j.jlr.2021.100045
Mehta, J. L., Sanada, N., Hu, C. P., Chen, J. D., Dandapat, A., Sugawara, F., et al. (2007). Deletion of LOX-1 reduces atherogenesis in LDLR knockout mice fed high cholesterol diet. Circ. Res. 100, 1634–1642. doi:10.1161/CIRCRESAHA.107.149724
Members, T. F., Catapano, A. L., Graham, I., De Backer, G., Wiklund, O., Chapman, M. J., et al. (2016). 2016 ESC/EAS guidelines for the management of dyslipidaemias: the task force for the management of dyslipidaemias of the European society of Cardiology (ESC) and European atherosclerosis society (EAS) developed with the special contribution of the European assocciation for cardiovascular prevention and rehabilitation (EACPR). Atherosclerosis 253, 281–344. doi:10.1016/j.atherosclerosis.2016.08.018
Momtazi, A. A., Banach, M., Pirro, M., Katsiki, N., and Sahebkar, A. (2017). Regulation of PCSK9 by nutraceuticals. Pharmacol. Res. 120, 157–169. doi:10.1016/j.phrs.2017.03.023
Moon, J. M., Ratliff, K. M., Hagele, A. M., Stecker, R. A., Mumford, P. W., Kerksick, C. M., et al. (2021). Absorption kinetics of berberine and dihydroberberine and their impact on glycemia: a randomized, controlled, crossover pilot trial. Nutrients 14, 124. doi:10.3390/nu14010124
Morin, E. E., Guo, Y., He, H., Yuan, W., Souery, W. N., Fawaz, M. V., et al. (2020). Synergetic effect of rHDL and LXR agonist on reduction of atherosclerosis in mice. Front. Pharmacol. 11, 513031–513110. doi:10.3389/fphar.2020.513031
Mukherjee, A., Lordan, C., Ross, R. P., and Cotter, P. D. (2020). Gut microbes from the phylogenetically diverse genus Eubacterium and their various contributions to gut health. Gut Microbes 12, 1802866. doi:10.1080/19490976.2020.1802866
Munday, M. R. (2002). Regulation of mammalian acetyl-CoA carboxylase. Biochem. Soc. Trans. 30, 1059–1064. doi:10.1042/bst0301059
Neculai, D., Schwake, M., Ravichandran, M., Zunke, F., Collins, R. f., Peters, J., et al. (2013). Structure of LIMP-2 provides functional insights with implications for SR-BI and CD36. Nature 504, 172–176. doi:10.1038/nature12684
Ogura, M. (2022). HDL, cholesterol efflux, and ABCA1: free from good and evil dualism. J. Pharmacol. Sci. 150, 81–89. doi:10.1016/j.jphs.2022.07.004
Ohira, H., Tsutsui, W., and Fujioka, Y. (2017). Are short chain fatty acids in gut microbiota defensive players for inflammation and atherosclerosis. J. Atheroscler. Thromb. 24, 660–672. doi:10.5551/jat.RV17006
Pan, C., Guo, Q., and Lu, N. (2019). Role of gut microbiota in the pharmacological effects of natural products. Evid-Based. Compl. Alt. Med. 2019, 2682748–2682757. doi:10.1155/2019/2682748
Pan, R., Cai, W., Sun, J., Yu, C., Li, P., Zheng, M., et al. (2020). Inhibition of KHSRP sensitizes colorectal cancer to 5-fluoruracil through miR-501-5p-mediated ERRFI1 mRNA degradation. J. Cell. Physiol. 235, 1576–1587. doi:10.1002/jcp.29076
Pedersen, H. K., Gudmundsdottir, V., Nielsen, H. B., Hyotylainen, T., Nielsen, T., Jensen, B. A., et al. (2016). Human gut microbes impact host serum metabolome and insulin sensitivity. Nature 535, 376–381. doi:10.1038/nature18646
Ping, S., Liu, S., Zhou, Y., Li, Z., Li, Y., Liu, K., et al. (2017). Protein disulfide isomerase-mediated apoptosis and proliferation of vascular smooth muscle cells induced by mechanical stress and advanced glycosylation end products result in diabetic mouse vein graft atherosclerosis. Cell Death Dis. 8, e2818. doi:10.1038/cddis.2017.213
Poli, A. (2020). What connection is there between intestinal microbiota and heart disease. Eur. Heart J. Suppl. 22, L117-L120–L120. doi:10.1093/eurheartj/suaa149
Prasun, P., Ginevic, I., and Oishi, K. (2021). Mitochondrial dysfunction in nonalcoholic fatty liver disease and alcohol related liver disease. Transl. Gastroenterol. Hepatol. 6, 4. doi:10.21037/tgh-20-125
Qiu, H., Wu, Y., Wang, Q., Liu, C., Xue, L., Wang, H., et al. (2017). Effect of berberine on PPARα-NO signalling pathway in vascular smooth muscle cell proliferation induced by angiotensin IV. Pharm. Biol. 55, 227–232. doi:10.1080/13880209.2016.1257642
Qu, H., Song, X., Song, Z., Jiang, X., Gao, X., Bai, L., et al. (2020). Berberine reduces temozolomide resistance by inducing autophagy via the ERK1/2 signaling pathway in glioblastoma. Cancer Cell Int. 20, 592–613. doi:10.1186/s12935-020-01693-y
Ren, G., Guo, J. H., Qian, Y. Z., Kong, W. J., and Jiang, J. D. (2020). Berberine improves glucose and lipid metabolism in HepG2 cells through AMPKα1 activation. Front. Pharmacol. 11, 647. doi:10.3389/fphar.2020.00647
Riccioni, G., Gammone, M. A., Currenti, W., and D’Orazio, N. (2018). Effectiveness and safety of dietetic supplementation of a new nutraceutical on lipid profile and serum inflammation biomarkers in hypercholesterolemic patients. Biomacromolecules 23, 1168. doi:10.3390/molecules23051168
Rudel, L. L., Lee, R. G., and Parini, P. (2005). ACAT2 is a target for treatment of coronary heart disease associated with hypercholesterolemia. Arter. Throm. Vas. 25, 1112–1118. doi:10.1161/01.ATV.0000166548.65753.1e
Rui, R., Yang, H., Liu, Y., Zhou, Y., Xu, X., Li, C., et al. (2021). Effects of berberine on atherosclerosis. Front. Pharmacol. 12, 764175. doi:10.3389/fphar.2021.764175
Samovski, D., Sun, J., Pietka, T., Gross, R. W., Eckel, R. H., Su, X., et al. (2015). Regulation of AMPK activation by CD36 links fatty acid uptake to β-oxidation. Diabetes 64, 353–359. doi:10.2337/db14-0582
Sanders, M. J., Grondin, P. O., Hegarty, B. D., Snowden, M. A., and Carling, D. (2007). Investigating the mechanism for AMP activation of the AMP-activated protein kinase cascade. Biochem. J. 403, 139–148. doi:10.1042/BJ20061520
Santamarina-Fojo, S., Peterson, K., Knapper, C., Qiu, Y., Freeman, L., Cheng, J. F., et al. (2000). Complete genomic sequence of the human ABCA1 gene: analysis of the human and mouse ATP-binding cassette A promoter. Proc. Natl. Acad. Sci. 97, 7987–7992. doi:10.1073/pnas.97.14.7987
Sasso, G. L., Murzilli, S., Salvatore, L., D'Errico, I., Petruzzelli, M., Conca, P., et al. (2010). Intestinal specific LXR activation stimulates reverse cholesterol transport and protects from atherosclerosis. Cell Metab. 12, 187–193. doi:10.1016/j.cmet.2010.07.002
Shafabakhsh, R., Reiner, Ž., Hallajzadeh, J., Mirsafaei, L., and Asemi, Z. (2021). Are anti-inflammatory agents and nutraceuticals-novel inhibitors of PCSK9. Crit. Rev. Food Sci. Nutr. 61, 325–336. doi:10.1080/10408398.2020.1731678
Shen, W. J., Azhar, S., and Kraemer, F. B. (2018). SR-B1: a unique multifunctional receptor for cholesterol influx and efflux. Annu. Rev. Physiol. 80, 95–116. doi:10.1146/annurev-physiol-021317-121550
Shi, Y., Hu, J., Geng, J., Hu, T., Wang, B., Yan, W., et al. (2018). Berberine treatment reduces atherosclerosis by mediating gut microbiota in apoE-/-mice. Biomed. Pharmacother. 107, 1556–1563. doi:10.1016/j.biopha.2018.08.148
Singh, A. B., Li, H., Kan, C. F. K., Dong, B., Nicolls, M. R., Liu, J., et al. (2014). The critical role of mRNA destabilizing protein heterogeneous nuclear ribonucleoprotein D in 3′ untranslated region-mediated decay of low-density lipoprotein receptor mRNA in liver tissue. Arter. Throm. Vas. 34, 8–16. doi:10.1161/ATVBAHA.112.301131
Singh, S., Verma, M., Malhotra, M., Prakash, S., and Singh, T. D. (2016). Cytotoxicity of alkaloids isolated from Argemone mexicana on SW480 human colon cancer cell line. Pharm. Biol. 54, 740–745. doi:10.3109/13880209.2015.1073334
Song, B., Tang, X., Wang, X., Huang, X., Ye, Y., Lu, X., et al. (2012). Bererine induces peripheral lymphocytes immune regulations to realize its neuroprotective effects in the cerebral ischemia/reperfusion mice. Cell. Immunol. 276, 91–100. doi:10.1016/j.cellimm.2012.04.006
Song, T., and Chen, W. D. (2021). Berberine inhibited carotid atherosclerosis through PI3K/AKTmTOR signaling pathway. Bioengineered 12, 8135–8146. doi:10.1080/21655979.2021.1987130
Song, D., Hao, J., and Fan, D. (2020). Biological properties and clinical applications of berberine. Front. Med-Prc. 14, 564–582. doi:10.1007/s11684-019-0724-6
Stefanutti, C. (2020). Lomitapide-a microsomal triglyceride transfer protein inhibitor for homozygous familial hypercholesterolemia. Curr. Atheroscler. Rep. 22, 38–11. doi:10.1007/s11883-020-00858-4
Sun, H., Liu, Q., Hu, H., Jiang, Y., Shao, W., Wang, Q., et al. (2018a). Berberine ameliorates blockade of autophagic flux in the liver by regulating cholesterol metabolism and inhibiting COX2-prostaglandin synthesis. Cell Death Dis. 9, 824. doi:10.1038/s41419-018-0890-5
Sun, R., Kong, B., Yang, N., Cao, B., Feng, D., Yu, X., et al. (2021). The hypoglycemic effect of berberine and berberrubine involves modulation of intestinal farnesoid X receptor signaling pathway and inhibition of hepatic gluconeogenesis. Drug Metab. Dispos. 49, 276–286. doi:10.1124/dmd.120.000215
Sun, R., Yang, N., Kong, B., Cao, B., Feng, D., Yu, X., et al. (2017). Orally administered berberine modulates hepatic lipid metabolism by altering microbial bile acid metabolism and the intestinal FXR signaling pathway. Mol. Pharmacol. 91, 110–122. doi:10.1124/mol.116.106617
Sun, Y., and Li, X. (2022). Cholesterol efflux mechanism revealed by structural analysis of human ABCA1 conformational states. Nat. Cardiovas. Res. 1, 238–245. doi:10.1038/s44161-022-00022-y
Sun, Y., Xia, M., Yan, H., Han, Y., Zhang, F., Hu, Z., et al. (2018b). Berberine attenuates hepatic steatosis and enhances energy expenditure in mice by inducing autophagy and fibroblast growth factor 21. Brit. J. Pharmacol. 175, 374–387. doi:10.1111/bph.14079
Takahashi, M., Okazaki, H., Ohashi, K., Ogura, M., Ishibashi, S., Okazaki, S., et al. (2021). Current diagnosis and management of abetalipoproteinemia. J. Atheroscler. Thromb. 28, 1009–1019. doi:10.5551/jat.RV17056
Tan, L., Wang, Y., Ai, G., Luo, C., Chen, H., Li, C., et al. (2019). Dihydroberberine, a hydrogenated derivative of berberine firstly identified in Phellodendri Chinese Cortex, exerts anti-inflammatory effect via dual modulation of NF-κB and MAPK signaling pathways. Int. Immunopharmacol. 75, 105802. doi:10.1016/j.intimp.2019.105802
Tan, W., Wang, Y., Wang, K., Wang, S., Liu, J., Qin, X., et al. (2020). Improvement of endothelial dysfunction of berberine in atherosclerotic mice and mechanism exploring through TMT-based proteomics. Oxid. Med. Cell. Longev. 2020, 8683404–8683422. doi:10.1155/2020/8683404
Tan, X. S., Ma, J. Y., Feng, R., Ma, C., Chen, W. J., Sun, Y. P., et al. (2013). Tissue distribution of berberine and its metabolites after oral administration in rats. PloS One 8, e77969. doi:10.1371/journal.pone.0077969
Tan, Y., Hu, Y., Liu, X., Yin, Z., Chen, X. W., Liu, M., et al. (2016). Improving drug safety: from adverse drug reaction knowledge discovery to clinical implementation. Methods 110, 14–25. doi:10.1016/j.ymeth.2016.07.023
Temel, R. E., Lee, R. G., Kelley, K. L., Davis, M. A., Shah, R., Sawyer, J. K., et al. (2005). Intestinal cholesterol absorption is substantially reduced in mice deficient in both ABCA1 and ACAT2. J. Lipid Res. 46, 2423–2431. doi:10.1194/jlr.M500232-JLR200
Tian, Y., Cai, J., Gui, W., Nichols, R. G., Koo, I., Zhang, J., et al. (2019). Berberine directly affects the gut microbiota to promote intestinal farnesoid X receptor activation. Drug Metab. Dispos. 47, 86–93. doi:10.1124/dmd.118.083691
Titchenell, P. M., Lazar, M. A., and Birnbaum, M. J. (2017). Unraveling the regulation of hepatic metabolism by insulin. Trends. endocrin. Mater. 28, 497–505. doi:10.1016/j.tem.2017.03.003
Turner, N., Li, J. Y., Gosby, A., To, S. W., Cheng, Z., Miyoshi, H., et al. (2008). Berberine and its more biologically available derivative, dihydroberberine, inhibit mitochondrial respiratory complex I: a mechanism for the action of berberine to activate AMP-activated protein kinase and improve insulin action. Diabetes 57, 1414–1418. doi:10.2337/db07-1552
Velasquez, M. T., Ramezani, A., Manal, A., and Raj, D. S. (2016). Trimethylamine N-oxide: the good, the bad and the unknown. Toxins 8, 326–411. doi:10.3390/toxins8110326
Verdeguer, F., Castro, C., Kubicek, M., Pla, D., Vila-Caballer, M., Vinué, Á., et al. (2007). Complement regulation in murine and human hypercholesterolemia and role in the control of macrophage and smooth muscle cell proliferation. Cardiovasc. Res. 76, 340–350. doi:10.1016/j.cardiores.2007.06.028
Wang, C., Li, J., Lv, X., Zhang, M., Song, Y., Chen, L., et al. (2009a). Ameliorative effect of berberine on endothelial dysfunction in diabetic rats induced by high-fat diet and streptozotocin. Eur. J. Pharmacol. 620, 131–137. doi:10.1016/j.ejphar.2009.07.027
Wang, K., Feng, X., Chai, L., Cao, S., and Qiu, F. (2017a). The metabolism of berberine and its contribution to the pharmacological effects. Drug Metab. Rev. 49, 139–157. doi:10.1080/03602532.2017.1306544
Wang, L., Deng, L., Lin, N., Shi, Y., Chen, J., Zhou, Y., et al. (2020a). Berberine inhibits proliferation and apoptosis of vascular smooth muscle cells induced by mechanical stretch via the PDI/ERS and MAPK pathways. Life Sci. 259, 118253. doi:10.1016/j.lfs.2020.118253
Wang, Q., Liu, S., Zhai, A., Zhang, B., and Tian, G. (2018). AMPK-mediated regulation of lipid metabolism by phosphorylation. Biol. Pharm. Bull. 41, 985–993. doi:10.1248/bpb.b17-00724
Wang, Q., Zhang, M., Liang, B., Shirwany, N., Zhu, Y., Zou, M. H., et al. (2011). Activation of AMP-activated protein kinase is required for berberine-induced reduction of atherosclerosis in mice: the role of uncoupling protein 2. PloS One 6, e25436. doi:10.1371/journal.pone.0025436
Wang, Y., Huang, Y., Lam, K. S., Li, Y., Wong, W. T., Ye, H., et al. (2009b). Berberine prevents hyperglycemia-induced endothelial injury and enhances vasodilatation via adenosine monophosphate-activated protein kinase and endothelial nitric oxide synthase. Cardiovasc. Res. 82, 484–492. doi:10.1093/cvr/cvp078
Wang, Y., Shou, J. W., Li, X. Y., Zhao, Z. X., Fu, J., He, C. Y., et al. (2017b). Berberine-induced bioactive metabolites of the gut microbiota improve energy metabolism. Metabolism 70, 72–84. doi:10.1016/j.metabol.2017.02.003
Wang, Y., Tong, Q., Shou, J. W., Zhao, Z. X., Li, X. Y., Zhang, X. F., et al. (2017c). Gut microbiota-mediated personalized treatment of hyperlipidemia using berberine. Theranostics 7, 2443–2451. doi:10.7150/thno.18290
Wang, Y., Tran, K., and Yao, Z. (1999). The activity of microsomal triglyceride transfer protein is essential for accumulation of triglyceride within microsomes in McA-RH7777 cells: a unified model for the assembly of very low density lipoproteins. J. Biol. Chem. 274, 27793–27800. doi:10.1074/jbc.274.39.27793
Wang, Y., Yi, X., Ghanam, K., Zhang, S., Zhao, T., Zhu, X., et al. (2014). Berberine decreases cholesterol levels in rats through multiple mechanisms, including inhibition of cholesterol absorption. Metabolism 63, 1167–1177. doi:10.1016/j.metabol.2014.05.013
Wang, Y., Zheng, J., Hou, H., Zhang, J., Qi, S., Li, Y., et al. (2020b). Effects of berberine on intestinal flora of non-alcoholic fatty liver induced by high-fat diet through 16S rRNA gene segmentation. J. King Saud. Univ. Sci. 32, 2603–2609. doi:10.1016/j.jksus.2020.04.020
Wang, Z., Wu, F., Zhou, Q., Qiu, Y., Zhang, J., Tu, Q., et al. (2022). Berberine improves vascular dysfunction by inhibiting trimethylamine-N-oxide via regulating the gut microbiota in angiotensin II-induced hypertensive mice. Front. Microbiol. 13, 814855–814912. doi:10.3389/fmicb.2022.814855
Watanabe, M., Houten, S. M., Wang, L., Moschetta, A., Mangelsdorf, D. J., Heyman, R. A., et al. (2004). Bile acids lower triglyceride levels via a pathway involving FXR, SHP, and SREBP-1c. J. Clin. Invest. 113, 1408–1418. doi:10.1172/JCI21025
Wei, S., Zhang, M., Yu, Y., Lan, X., Yao, F., Yan, X., et al. (2016a). Berberine attenuates development of the hepatic gluconeogenesis and lipid metabolism disorder in type 2 diabetic mice and in palmitate-incubated HepG2 cells through suppression of the HNF-4α miR122 pathway. PloS One 11, e0152097. doi:10.1371/journal.pone.0152097
Wei, X., Wang, C., Hao, S., Song, H., and Yang, L. (2016b). The therapeutic effect of berberine in the treatment of nonalcoholic fatty liver disease: a meta-analysis. Evid-Based. Compl. Alt. Med. 2016, 3593951. doi:10.1155/2016/3593951
Weng, Y., Mizuno, N., Dong, J., Segawa, R., Yonezawa, T., Cha, B. Y., et al. (2018). Induction of thymic stromal lymphopoietin by a steroid alkaloid derivative in mouse keratinocytes. Int. Immunopharmacol. 55, 28–37. doi:10.1016/j.intimp.2017.11.045
Wolf, P. G., Devendran, S., Doden, H. L., Ly, L. K., Moore, T., Takei, H., et al. (2021). Berberine alters gut microbial function through modulation of bile acids. Bmc. Microbiol. 21, 24–15. doi:10.1186/s12866-020-02020-1
Wood, P., Mulay, V., Darabi, M., Chan, K. C., Heeren, J., Pol, A., et al. (2011). Ras/mitogen-activated protein kinase (MAPK) signaling modulates protein stability and cell surface expression of scavenger receptor SR-BI. J. Biol. Chem. 286, 23077–23092. doi:10.1074/jbc.M111.236398
Wu, C., Zhao, Y., Zhang, Y., Yang, Y., Su, W., Yang, Y., et al. (2022). Gut microbiota specifically mediates the anti-hypercholesterolemic effect of berberine (BBR) and facilitates to predict BBR’s cholesterol-decreasing efficacy in patients. J. Adv. Res. 37, 197–208. doi:10.1016/j.jare.2021.07.011
Wu, H., He, K., Wang, Y., Xue, D., Ning, N., Zou, Z., et al. (2014). The antihypercholesterolemic effect of jatrorrhizine isolated from Rhizoma Coptidis. Phytomedicine 21, 1373–1381. doi:10.1016/j.phymed.2014.05.002
Wu, M., Yang, S., Wang, S., Cao, Y., Zhao, R., Li, X., et al. (2020). Effect of berberine on atherosclerosis and gut microbiota modulation and their correlation in high-fat diet-fed ApoE-/- mice. Front. Pharmacol. 11, 223. doi:10.3389/fphar.2020.00223
Wu, W. Z., Hu, D. J., Wang, Z. Y., Liao, L. S., and Li, C. C. (2019). Endothelial progenitor cell impairment mediated vasodilation dysfunction via diminishing nitric oxide production in postmenopausal females. Mol. Med. Rep. 19, 2449–2457. doi:10.3892/mmr.2019.9888
Wu, X., and Huang, T. (2019). Recent development in acetyl-CoA carboxylase inhibitors and their potential as novel drugs. Future Med. Chem. 12, 533–561. doi:10.4155/fmc-2019-0312
Xie, Z., Liu, X., Huang, X., Liu, Q., Yang, M., Huang, D., et al. (2021). Remodelling of gut microbiota by Berberine attenuates trimethylamine N-oxide-induced platelet hyperreaction and thrombus formation. Eur. J. Pharmacol. 911, 174526. doi:10.1016/j.ejphar.2021.174526
Xing, L. J., Zhang, L., Liu, T., Hua, Y. Q., Zheng, P. Y., Ji, G., et al. (2011). Berberine reducing insulin resistance by up-regulating IRS-2 mRNA expression in nonalcoholic fatty liver disease (NAFLD) rat liver. Eur. J. Pharmacol. 668, 467–471. doi:10.1016/j.ejphar.2011.07.036
Xu, M., Wang, J., Chen, L., Wang, Y., Yang, Z., Tao, J., et al. (2008). Berberine-induced mobilization of circulating endothelial progenitor cells improves human small artery elasticity. J. Hum. Hypertens. 22, 389–393. doi:10.1038/sj.jhh.1002311
Xu, M., Xiao, Y., Yin, J., Hou, W., Yu, X., Shen, L., et al. (2014). Berberine promotes glucose consumption independently of AMP-activated protein kinase activation. PLoS One 9, e103702. doi:10.1371/journal.pone.0103702
Xu, M. G., Wang, J. M., Chen, L., Wang, Y., Yang, Z., Tao, J., et al. (2009). Berberine-induced upregulation of circulating endothelial progenitor cells is related to nitric oxide production in healthy subjects. Cardiology 112, 279–286. doi:10.1159/000157336
Xu, R. X., Sun, X. C., Ma, C. Y., Yao, Y. H., Li, X. L., Guo, Y. L., et al. (2017). Impacts of berberine on oxidized LDL-induced proliferation of human umbilical vein endothelial cells. Am. J. Transl. Res. 9, 4375–4389. doi:10.1016/j.apradiso.2017.05.025
Xu, S., Ilyas, I., Little, P. J., Li, H., Kamato, D., Zheng, X., et al. (2021). Endothelial dysfunction in atherosclerotic cardiovascular diseases and beyond: from mechanism to pharmacotherapies. Pharmacol. Rev. 73, 924–967. doi:10.1124/pharmrev.120.000096
Xu, S., and Weng, J. (2020). Familial hypercholesterolemia and atherosclerosis: animal models and therapeutic advances. Trends endocrin. Mater. 31, 331–333. doi:10.1016/j.tem.2020.02.007
Xu, X., Zhu, X. P., Bai, J. Y., Xia, P., Li, Y., Lu, Y., et al. (2019). Berberine alleviates nonalcoholic fatty liver induced by a high-fat diet in mice by activating SIRT3. FASEB J. 33, 7289–7300. doi:10.1096/fj.201802316R
Yan, H. M., Xia, M. F., Wang, Y., Chang, X. X., Yao, X. Z., Rao, S. X., et al. (2015). Efficacy of berberine in patients with non-alcoholic fatty liver disease. PloS One 10, e0134172. doi:10.1371/journal.pone.0134172
Yan, L., Guo, J., and Gao, X. (2022). Expression of SR-BI and LXRα in macrophage origin foam cells of mouse RAW264. 7 up-regulated by Astragalus polysaccharide. Contrast Media Mol. Imaging 2022, 3920584–3920585. doi:10.1155/2022/3920584
Yang, L., Zhu, W., Zhang, X., Zhou, X., Wu, W., Shen, T., et al. (2023). Efficacy and safety of berberine for several cardiovascular diseases: a systematic review and meta-analysis of randomized controlled trials. Phytomedicine 112, 154716. doi:10.1016/j.phymed.2023.154716
Yang, Q., Vijayakumar, A., and Kahn, B. B. (2018). Metabolites as regulators of insulin sensitivity and metabolism. Nat. Rev. Mol. Cell Bio. 19, 654–672. doi:10.1038/s41580-018-0044-8
Yang, S., Cao, S., Li, C., Zhang, J., Liu, C., Qiu, F., et al. (2022a). Berberrubine, a main metabolite of berberine, alleviates non-alcoholic fatty liver disease via modulating glucose and lipid metabolism and restoring gut microbiota. Front. Pharmacol. 13, 913378. doi:10.3389/fphar.2022.913378
Yang, W., She, L., Yu, K., Yan, S., Zhang, X., Tian, X., et al. (2016). Jatrorrhizine hydrochloride attenuates hyperlipidemia in a high-fat diet-induced obesity mouse model. Mol. Med. Rep. 14, 3277–3284. doi:10.3892/mmr.2016.5634
Yang, X. J., Liu, F., Feng, N., Ding, X. S., Chen, Y., Zhu, S. X., et al. (2020). Berberine attenuates cholesterol accumulation in macrophage foam cells by suppressing AP-1 activity and activation of the Nrf2/HO-1 pathway. J. Cardiovasc. Pharm. 75, 45–53. doi:10.1097/FJC.0000000000000769
Yang, X. P., Amar, M. J., Vaisman, B., Bocharov, A. V., Vishnyakova, T. G., Freeman, L. A., et al. (2013). Scavenger receptor-BI is a receptor for lipoprotein (a). J. Lipid Res. 54, 2450–2457. doi:10.1194/jlr.M038877
Yang, Y., Cao, S., Xu, W., Zang, C., Zhang, F., Xie, Y., et al. (2022c). Dual modulation of gut bacteria and fungi manifests the gut-based anti-hyperlipidemic effect of Coptidis Rhizoma. Biomed. Pharmacother. 153, 113542. doi:10.1016/j.biopha.2022.113542
Yang, Y. N., Wang, Q. C., Xu, W., Yu, J., Zhang, H., Wu, C., et al. (2022b). The berberine-enriched gut commensal Blautia producta ameliorates high-fat diet (HFD)-induced hyperlipidemia and stimulates liver LDLR expression. Biomed. Pharmacother. 155, 113749. doi:10.1016/j.biopha.2022.113749
Yoon, M. S. (2016). The emerging role of branched-chain amino acids in insulin resistance and metabolism. Nutrients 8, 405. doi:10.3390/nu8070405
Yu, L., Zhu, Q., Li, Y., Song, P., and Zhang, J. (2022a). Dietary branched-chain amino acids (BCAAs) and risk of dyslipidemia in a Chinese population. Nutrients 14, 1824–1914. doi:10.3390/nu14091824
Yu, Q., Li, M., Chen, H., Xu, L., Cheng, J., Lin, G., et al. (2022b). The discovery of berberine erythrocyte-hemoglobin self-assembly delivery system: a neglected carrier underlying its pharmacokinetics. Drug Deliv. 29, 856–870. doi:10.1080/10717544.2022.2036870
Yuan, X., Wang, J., Tang, X., Li, Y., Xia, P., Gao, X., et al. (2015a). Berberine ameliorates nonalcoholic fatty liver disease by a global modulation of hepatic mRNA and lncRNA expression profiles. J. Transl. Med. 13, 24–11. doi:10.1186/s12967-015-0383-6
Yuan, Z. Y., Lu, X., Lei, F., Chai, Y. S., Wang, Y. G., Jiang, J. F., et al. (2015b). TATA boxes in gene transcription and poly (A) tails in mRNA stability: new perspective on the effects of berberine. Sci. Rep-Uk. 5, 18326. doi:10.1038/srep18326
Yue, M., Tao, Y., Fang, Y., Lian, X., Zhang, Q., Xia, Y., et al. (2019a). The gut microbiota modulator berberine ameliorates collagen-induced arthritis in rats by facilitating the generation of butyrate and adjusting the intestinal hypoxia and nitrate supply. Faseb J. 33, 12311–12323. doi:10.1096/fj.201900425RR
Yue, S. J., Liu, J., Wang, A. T., Meng, X. T., Yang, Z. R., Peng, C., et al. (2019b). Berberine alleviates insulin resistance by reducing peripheral branched-chain amino acids. Am. J. Physiol-Endoc. M. 316, E73-E85–E85. doi:10.1152/ajpendo.00256.2018
Zeng, H., Qin, H., Liao, M., Zheng, E., Luo, X., Xiao, A., et al. (2022). CD36 promotes de novo lipogenesis in hepatocytes through INSIG2-dependent SREBP1 processing. Mol. Metab. 57, 101428. doi:10.1016/j.molmet.2021.101428
Zhang, L., Wu, X., Yang, R., Chen, F., Liao, Y., Zhu, Z., et al. (2021a). Effects of berberine on the gastrointestinal microbiota. Front. Cell. Infect. Mi. 10, 588517. doi:10.3389/fcimb.2020.588517
Zhang, M., Wang, C. M., Li, J., Meng, Z. J., Wei, S. N., Li, J., et al. (2013). Berberine protects against palmitate-induced endothelial dysfunction: involvements of upregulation of AMPK and eNOS and downregulation of NOX4. Mediat. Inflamm. 2013, 260464–260468. doi:10.1155/2013/260464
Zhang, Q., Xiao, X., Feng, K., Wang, T., Li, W., Yuan, T., et al. (2011). Berberine moderates glucose and lipid metabolism through multipathway mechanism. Evid-Based. Compl. Alt. 2011, 924851. doi:10.1155/2011/924851
Zhang, W., Xu, J. H., Yu, T., and Chen, Q. K. (2019a). Effects of berberine and metformin on intestinal inflammation and gut microbiome composition in db/db mice. Biomed. Pharmacother. 118, 109131. doi:10.1016/j.biopha.2019.109131
Zhang, X., Evans, T. D., Jeong, S. J., and Razani, B. (2018). Classical and alternative roles for autophagy in lipid metabolism. Curr. Opin. Lipidol. 29, 203–211. doi:10.1097/MOL.0000000000000509
Zhang, Y., Chang, X., Song, X., Chen, C., Chen, H., Lu, Z., et al. (2015). Berberine reverses abnormal expression of L-type pyruvate kinase by DNA demethylation and histone acetylation in the livers of the non-alcoholic fatty disease rat. Int. J. Clin. Exp. Med. 8, 7535–7543.
Zhang, Y., Da Silva, J. R., Reilly, M., Billheimer, J. T., Rothblat, G. H., and Rader, D. J. (2005). Hepatic expression of scavenger receptor class B type I (SR-BI) is a positive regulator of macrophage reverse cholesterol transport in vivo. J. Clin. Invest. 115, 2870–2874. doi:10.1172/JCI25327
Zhang, Y. P., Deng, Y. J., Tang, K. R., Chen, R. S., Liang, S., Liang, Y. J., et al. (2019b). Berberine ameliorates high-fat diet-induced non-alcoholic fatty liver disease in rats via activation of SIRT3/AMPK/ACC pathway. Curr. Med. Sci. 39, 37–43. doi:10.1007/s11596-019-1997-3
Zhang, Z., Zhang, H., Li, B., Meng, X., Wang, J., Zhang, Y., et al. (2014). Berberine activates thermogenesis in white and brown adipose tissue. Nat. Commun. 5, 5493. doi:10.1038/ncomms6493
Zhang, Z. W., Cong, L., Peng, R., Han, P., Ma, S. R., Pan, L. B., et al. (2021b). Transformation of berberine to its demethylated metabolites by the CYP51 enzyme in the gut microbiota. J. Pharm. Anal. 11, 628–637. doi:10.1016/j.jpha.2020.10.001
Zhao, L., Cang, Z., Sun, H., Nie, X., Wang, N., Lu, Y., et al. (2017). Berberine improves glucogenesis and lipid metabolism in nonalcoholic fatty liver disease. BMC Endocr. Disord. 17, 13–18. doi:10.1186/s12902-017-0165-7
Zhao, Y., Su, B., Jacobs, R. E. L., Kennedy, B., Francis, G. A., Waddington, E., et al. (2009). Lack of phosphatidylethanolamine N-methyltransferase alters plasma VLDL phospholipids and attenuates atherosclerosis in mice. Arterioscler. Thromb. Vasc. Biol. 29, 1349–1355. doi:10.1161/ATVBAHA.109.188672
Zhong, S., Zhao, L., Wang, Y., Zhang, C., Liu, J., Wang, P., et al. (2017). Cluster of differentiation 36 deficiency aggravates macrophage infiltration and hepatic inflammation by upregulating monocyte chemotactic protein-1 expression of hepatocytes through histone deacetylase 2-dependent pathway. Antioxid. Redox Sign. 27, 201–214. doi:10.1089/ars.2016.6808
Zhou, H., Shi, R., Ma, B., Ma, Y., Wang, C., Wu, D., et al. (2013). CYP450 1A2 and multiple UGT1A isoforms are responsible for jatrorrhizine metabolism in human liver microsomes. Biopharm. Drug Dispos. 34, 176–185. doi:10.1002/bdd.1835
Zhou, Y., Wang, Y., Vong, C. T., Zhu, Y., Xu, B., Ruan, C. C., et al. (2022). Jatrorrhizine improves endothelial function in diabetes and obesity through suppression of endoplasmic reticulum stress. Int. J. Mol. Sci. 23, 12064. doi:10.3390/ijms232012064
Zhu, B., Chan, S. L., Li, J., Li, K., Wu, H., Cui, K., et al. (2021). Non-alcoholic steatohepatitis pathogenesis, diagnosis, and treatment. Front. Cardiovasc. Med. 8, 742382. doi:10.3389/fcvm.2021.742382
Zhu, L., Zhang, D., Zhu, H., Zhu, J., Weng, S., Dong, L., et al. (2018). Berberine treatment increases Akkermansia in the gut and improves high-fat diet-induced atherosclerosis in Apoe-/- mice. Atherosclerosis 268, 117–126. doi:10.1016/j.atherosclerosis.2017.11.023
Zhu, T. L., Yang, B., Guo, Y. S., Ji, Y. S., and Li, X. Y. (2017). Effects of the traditional Chinese medicine berberine on antiatheroscloresis and antioxidant activities in hyperlipoidemic model rats. Chin. J. Appl. Physiol. 33, 369–372. doi:10.12047/j.cjap.5546.2017.089
Zhu, X., Bian, H., and Gao, X. (2016). The potential mechanisms of berberine in the treatment of nonalcoholic fatty liver disease. Molecules 21, 1336. doi:10.3390/molecules21101336
Keywords: berberine, berberine metabolite, intestinal-hepatic-vascular tissue, pharmacological mechanism, lipid metabolic disease
Citation: Cai Y, Yang Q, Yu Y, Yang F, Bai R and Fan X (2023) Efficacy and underlying mechanisms of berberine against lipid metabolic diseases: a review. Front. Pharmacol. 14:1283784. doi: 10.3389/fphar.2023.1283784
Received: 27 August 2023; Accepted: 03 November 2023;
Published: 15 November 2023.
Edited by:
Ruiwen Zhang, University of Houston, United StatesReviewed by:
Zhongwen Yuan, Third Affiliated Hospital of Guangzhou Medical University, ChinaCopyright © 2023 Cai, Yang, Yu, Yang, Bai and Fan. This is an open-access article distributed under the terms of the Creative Commons Attribution License (CC BY). The use, distribution or reproduction in other forums is permitted, provided the original author(s) and the copyright owner(s) are credited and that the original publication in this journal is cited, in accordance with accepted academic practice. No use, distribution or reproduction is permitted which does not comply with these terms.
*Correspondence: Ruina Bai, YnJudGNsQDEyNi5jb20=; Xiaodi Fan, eGlhb2RpLjEwMThAMTYzLmNvbQ==
†These authors have contributed equally to this work and share first authorship
Disclaimer: All claims expressed in this article are solely those of the authors and do not necessarily represent those of their affiliated organizations, or those of the publisher, the editors and the reviewers. Any product that may be evaluated in this article or claim that may be made by its manufacturer is not guaranteed or endorsed by the publisher.
Research integrity at Frontiers
Learn more about the work of our research integrity team to safeguard the quality of each article we publish.