- Biomedical Research Centre, School of Science, Engineering and Environment, University of Salford, Manchester, United Kingdom
Background: Leukemias are a common cancer in adults and children. While existing treatments are effective, they are associated with severe side-effects compounded by the emergence of drug resistance. This necessitates the need to develop new drugs and phytopharmaceuticals offer a largely untapped source. Oleoresins produced by plants in the genus Boswellia have been used for centuries in traditional medicine and recent work suggests they may exhibit anti-cancer activity. However, the underlying mechanisms remain unclear and most existing research focusses on Boswellia serrata; just one of many species in the Boswellia genus. To address these limitations, we elucidated the anti-cancer potential and associated mechanisms of action of Boswellia carterii.
Methods: A methanolic solvent extraction method was optimised. The effect of methanolic extracts of B. carterii on leukaemia (K562, MOLT-4 and CCRF-CEM) and normal (PBMC) cell line viability was assessed using MTT assay and flow cytometry. Cell morphology, apoptosis (Annexin-V/propidium iodide), mitochondrial membrane potential (Rhodamine-123) and the cell cycle (propidium iodide) were evaluated using flow cytometry. Regulatory protein expression was quantified using Western Blot.
Results: Methanolic extracts of B. carterii oleoresin reduced the viability of K562, MOLT-4 and CCRF-CEM cell lines with selectivity indexes of between 1.75 and 2.68. Extracts increased the proportion of cells in late apoptosis by 285.4% ± 51.6%. Mitochondrial membrane potential was decreased by 41% ± 2% and the expression of cleaved caspase-3, -7, and -9 was increased by 5.7, 3.3, and 1.5-fold respectively. Extracts increased the proportion of cells in subG1 and G1 phase by 867.8% ± 122.9% and 14.0 ± 5.5 and decreased those in S phase and G2/M by 63.4% ± 2.0% and 57.6% ± 5.3%. Expression of CDK2, CDK6, cyclin D1, and cyclin D3 were decreased by 2.8, 4.9, 3.9, and 2.5-fold.
Conclusion: We are the first to report that methanolic extracts of B. carterii are selectively cytotoxic against three leukemia cell lines. Cytotoxic mechanisms likely include activation of the intrinsic apoptotic pathway and cell cycle arrest through downregulation of CDK2, CDK6, cyclin D1, and cyclin D3. Our findings suggest that B. carterii may be an important source of novel chemotherapeutic drugs and justifies further investigation.
1 Introduction
Leukaemia is a common cancer that presents as increased blood leucocyte count and remains a considerable global health burden (Du et al., 2022). In children and young adults, the incidence of leukaemia increases each year and now accounts for one-third of all cancer diagnoses (Hao et al., 2019; CRUK, 2021). Despite this, improvements in chemotherapeutics—which remain at the forefront of treatment regimens—have produced net 5-year survival rates of between 37% and 89% dependent on leukaemia subtype (Bhayat et al., 2009; Chang et al., 2021; Kantarjian et al., 2021; Sekeres and Taylor, 2022). Drugs commonly used to treat leukaemia include anthracyclines, topoisomerase inhibitors, mitotic inhibitors, alkylating agents and antimetabolites (Estey, 2018) which produce cell cycle arrest and apoptosis via various mechanisms (Ralhan and Kaur, 2007; Luengo et al., 2017; Martins-Teixeira and Carvalho, 2020). However, despite their effectivity against leukaemia, many produce severe short and long-term off-target effects in multiple organ systems (Oeffinger et al., 2006; Armstrong et al., 2014) which are particularly severe in developing children and adolescents (Ikonomidou, 2018). Therefore, there remains a need to develop novel effective chemotherapeutics with fewer off-target effects.
Many anti-cancer drugs are derivatives of plant-based compounds (Demain and Vaishnav, 2011). Yet, phytopharmaceuticals offer a largely untapped source for the development of novel anti-cancer drugs (Mtewa et al., 2021) despite a tendency to exhibit fewer and less severe side-effects (Iten et al., 2002). Plants in the genus Boswellia provide a notable example (Lim et al., 2019).
Boswellia species are shrub-like trees, widely distributed from the Horn of Africa, throughout the Middle East and into India and China (Abdel-Tawab et al., 2011). These plants produce oleoresins—commonly known as Frankincense—which have been used for centuries as a part of Ayurvedic and traditional medicine practices (Siddiqui, 2011). However, recent evidence suggests that Boswellia oleoresins and oleoresin-derived compounds may be effective in the treatment of a variety of diseases. For example, previous work highlights that extracts of Boswellia oleoresins show anti-inflammatory (Koeberle et al., 2018) and anti-microbial (Al-Dosary, 2018) activity. Furthermore, several studies show that extracts of the Boswellia oleoresins exhibit anti-cancer activity against multiple cancer cell types in vitro (Ahmed et al., 2015; Zhang et al., 2016; Mazzio et al., 2017; Ranjbarnejad et al., 2017; Schmiech et al., 2019) through mechanisms such as apoptosis (Moussaieff and Mechoulam, 2009; Ahmed et al., 2015; Ranjbarnejad et al., 2017), and cell cycle arrest (Yazdanpanahi et al., 2014).
However, studies that seek to elucidate the mechanistic actions of Boswellia are limited, use a variety of cancer cell types and tend to focus on one particular mechanistic element. As such, a cohesive description of the mechanistic effects of Boswellia remains unreported. Furthermore, the majority of existing research focusses on Boswellia serrata which is one of many species in the Boswellia genus (Moussaieff and Mechoulam, 2009; Abdel-Tawab et al., 2011; Efferth and Oesch, 2022). As a result, much less is known about the biological activity of other accessible Boswellia species such as Boswellia carterii.
To address these limitations, we sought to investigate the anti-cancer effects of B. carterii oleoresin extracts against leukemia cell lines. We then took an integrative methodological approach to reveal the associated mechanisms of action thus producing a unified description of the anti-cancer activity of B. carterii.
2 Materials and methods
2.1 Chemicals and reagents
All chemicals and reagents were purchased from Fisher scientific, United States, unless otherwise stated.
2.2 Phytochemical extraction
The B. carterii Birdw oleoresin samples used for this study was provided by United Kingdom Essential oils and authenticated by Steven Holmes as a part of industrial procurement quality control. Voucher specimens are stored at The University of Salford, United Kingdom.
B. carterii oleoresin was macerated to a fine powder and then extracted using either acetonitrile (99.5%) (AcN), distilled water (dH2O), ethanol (99%) (EtOH), methanol (99.5%) (MeOH) or propan-2-ol (99.5%) (PrOH) at a final concentration of 100 mg/mL. The mixture was then stirred continuously for 2 h at room temperature. After 2 h, the supernatant was removed, and fresh extraction solvent added. This process was repeated three times. The supernatants were pooled then filtered through Whatman no 1 filter paper, before being dried to a powder using a rotary evaporator (Eppendorf, United States) and stored at −20°C. All extracts used for further experimentation were dissolved in dimethyl sulphoxide (DMSO) to a stock concentration of 50 mg/mL.
Each of the extracts is characterized by gradient high-performance liquid chromatography with diode-array detection (HPLC-DAD) as described in Supplementary Data S1.1 with spectra for each extraction method shown in Supplementary Figures S1–S4.
2.3 Cell culture
Three human leukaemia cell lines were used; K562, a chronic myeloid leukaemia (CML) and MOLT-4 and CCRF-CEM, which are both acute lymphoblastic leukaemias (ALL). Cell lines were provided by the Kidscan Children’s cancer research laboratories at The University of Salford, United Kingdom and originally sourced from American Type Culture Collection (ATCC, United States). All 3 cell lines were cultured using Roswell Park Memorial Institute (RPMI) 1,640 cell culture medium (Gibco, United Kingdom) supplemented with 10% v/v foetal bovine serum (FBS) (Gibco, United Kingdom) and 1% v/v penicillin-streptomycin (Penicillin: 10,000 units/mL, Streptomycin: 10,000 μg/mL) (Gibco, United Kingdom) as described in Gaigneaux et al. (2002) and Deesrisak et al. (2021). All cells were maintained at 37°C in a 5% CO2 atmosphere.
2.4 Peripheral blood mononuclear cell isolation
Peripheral blood mononuclear cells (PBMC’s) were isolated, to serve as a normal cell type, from Type O positive whole human blood (National Health Service Blood & Transplant, Manchester, United Kingdom) as described by Chen et al. (2020). Following isolation, PBMC’s were maintained in RPMI 1640 supplemented with 10% FBS and 1% penicillin-streptomycin at 37°C in a 5% CO2 atmosphere.
2.5 Measurement of cytotoxicity and calculation of anti-cancer selectivity
The cytotoxic effects of the B. carterii oleoresin extracts were determined using 3-(4,5-dimethylthiazol-2-yl)-2,5-diphenyltetrazolium bromide (MTT) assays, as described by Carreño et al. (2021) against K562, MOLT-4, CEM-CCRF and PBMC’s. To evaluate the time dependent effects of the B. carterii oleoresin extracts, MTT assays were performed at 24, 72, and 120 h. For all experiments, 50 µM doxorubicin hydrochloride was used as a positive control. IC50 values were calculated using SigmaPlot 12.3 (Systat Software, Inc., United States). The selectivity of the B. carterii oleoresin methanolic extract was calculated using the equation shown below.
2.6 Evaluation of cellular morphology
Cellular morphology was measured at a constant temperature of 37°C in a 5% CO2 atmosphere by time-lapse microscopy using a Cytation 3 cell Imaging multi-mode reader (BioTek Instruments, United States). Leukaemia cells were imaged hourly for 72 h, at ×100 magnification, following exposure to 25–100 μg/ml B. carterii oleoresin methanolic extract. Key morphological changes were examined and annotated offline using ImageJ (National Institutes of Health, United States) (Schneider et al., 2012).
2.7 Evaluation of cell size and granularity
Cell densities of 50,000 cells were treated for 24–72 h with 25–100 μg/ml B. carterii oleoresin methanolic extract then washed with 1x PBS. A minimum of 10,000 cells were measured using flow cytometry (FACSverse flow cytometer & FACSuite software, Becton, Dickinson and Company, United States) to determine relative cell size (using forward scatter) and granularity (side scatter) as described in Haynes et al. (2009). All changes were normalised to treatment with the vehicle (0.5% DMSO v/v) alone.
2.8 Evaluation of apoptosis by annexin-V and propidium iodide co-staining
Cell densities of 100,000 cells were incubated for 48 h with 25–100 μg/ml B. carterii oleoresin methanolic extract or a vehicle control (0.5% v/v DMSO) then washed with 1x PBS. Cells were then co-stained with annexin-V conjugated to FITC (to measure apoptosis) and propidium iodide (to measure necrosis) using a proprietary kit (Invitrogen, United States) as per manufacturers instructions. Annexin-V (Excitation: 490, Emission: 525 nm) and propidium iodide (Excitation: 535, Emission: 617 nm) fluorescence in a minimum of 10,000 cells was measured using flow cytometry (FACSverse flow cytometer & FACSuite software).
2.9 Qualitative assessment of mitochondrial membrane potential
The effect of the B. carterii oleoresin methanolic extract on leukaemia mitochondrial membrane potential (Δψm) was evaluated by flow cytometry as previously described by Panwar et al. (2020). Cell densities of 100,000 cells were incubated for 24–72 h with 25–100 μg/ml B. carterii oleoresin extract, a vehicle control (0.5% v/v DMSO) or a positive control (the oxidative phosphorylation uncoupler carbonyl cyanide 3-chlorophenylhydrazone (CCCP) (Sigma Aldrich, United States). Cells were then washed with 1x PBS and loaded with 200 nM rhodamine-123. Rhodamine-123 was excited at 508 nm and fluorescence measured at 528 nm using a FACSverse flow cytometer & FACSuite software.
2.10 Cell cycle analysis
To assess the effect of the B. carterii methanolic extract on the cell cycle, was conducted as previously described by Pozarowski and Darzynkiewicz (2004). Cell densities of 200,000 cells were incubated for 24 or 48 h with 25–100 μg/ml B. carterii oleoresin extract, a vehicle control (0.5% v/v DMSO). Cells were then washed with 1x PBS and fixed with ice-cold 70% v/v ethanol. Following fixation cells were treated with 100 μg/mL RNase A (ThermoFisher Scientific, United States) and stained with 50 μg/mL propidium iodide (Excitation: 535, Emission: 617 nm) [(Acros Organics, United States)]. A minimum of 10,000 cells were then immediately analysed using a FACSverse flow cytometer & FACSuite software.
2.11 Quantification of regulatory proteins using Western blotting
Protein was extracted from 1 × 106 cells pre-treated with varying concentrations of the B. carterii oleoresin methanolic extract for 48 h using radioimmunoprecipitation assay lysis buffer (Thermo Fisher Scientific, United States). Protein was quantified using bicinchoninic acid protein assays. A concentration of 50 µg of whole cell protein lysate for each condition was separated using sodium dodecyl sulphate (SDS) PAGE over a gradient of 4%–20% (Merck, United States) and transferred to 0.45 µm Polyvinylidene fluoride (PVDF) membranes (Immobilon, United States) as described in Mahmood and Yang (2012). The primary and secondary antibodies utilised are described within the Supplementary Section S1.2. Protein expression was then visualised by chemiluminescence using Crescendo Western horseradish peroxidase (HRP) substrate (Immobilon, United States). Chemiluminescent Images were taken using a G:Box Chemi-XX6 imager and the GeneSys V1.5.2.0 software (both Syngene, United States). Protein abundance was quantified using the software package ImageJ (National Institutes of Health, United States).
2.12 Statistical analysis
Appropriate statistical tests (as indicated in figure legends) were performed using SigmaPlot 12.3 (Systat Software, Inc., United States). Statistical significance was accepted when p < 0.05. A minimum of three independent repeats were conducted for all experiments.
2.13 Ethical approval
Ethical approval was granted (STR1718-35) by the University of Salford Research, Innovation and Academic Engagement Ethical Approval Panel.
3 Results
3.1 The effects of extraction solvent on B. carterii oleoresin extract yield
Supplementary Figure S5A shows the extraction yields produced by acetonitrile, distilled water, ethanol, methanol, and propanol. Statistical indicators have been removed for clarity, though Supplementary Figure S5B provides p-values for all pairwise comparisons. The yields produced by ethanol, methanol and propanol were 50 ± 7, 55% ± 3% and 50% ± 8% respectively (n = 3) and significantly greater than those produced by acetonitrile and water; 14% ± 5% and 8% ± 2% respectively (n = 3, p < 0.001). The yield produced between each alcoholic and non-alcoholic solvent did not differ significantly.
3.2 The concentration-dependent effects B. carterii oleoresin extract on cytotoxicity
Figures 1A–C shows that with the exception of distilled water, all solvents produced a concentration-dependent cytotoxic effect against CML (K562) and ALL (MOLT-4 & CCRF-CEM) cell lines. Figures 1D–F show the derived IC50 values. In K562 cells (Figure 1D), the IC50 of methanol (52.2 ± 5.5 μg/mL) was significantly less than those of acetonitrile, ethanol, and propanol (97.5 ± 8.6 μg/mL, 90.3 ± 9.3 μg/mL, 95.0 ± 6.5 μg/mL respectively, n = 12, p < 0.001). In MOLT-4 cells (Figure 1E), the IC50 of acetonitrile, methanol, and propanol (75.5 ± 2.7, 51.1 ± 7.7 and 86.3 ± 4.8 μg/mL respectively) was significantly less than that of ethanol (126.8 ± 6.2 μg/mL) though the IC50 of methanol was significantly lowest (n = 12, p < 0.001). In CCRF-CEM cells (Figure 1F) IC50 values for acetonitrile (38.3 ± 2.5 μg/mL), ethanol (48.3 ± 2.9 μg/mL), methanol (41.6 ± 1.9 μg/mL) and propanol (48.6 ± 3.5 μg/mL) were similar with the only significant difference being that acetonitrile produced a lower IC50 than propanol (n = 12, p = 0.032).
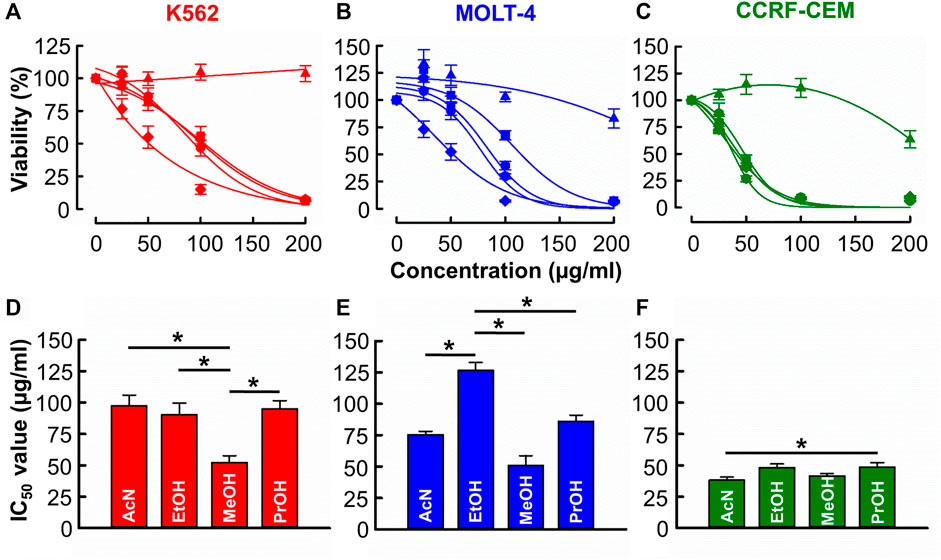
FIGURE 1. The of effects B. carterii oleoresin solvent extracts on leukemia cytotoxicity. (A–C) Dose response curves comparing the cytotoxicity of B. carterii oleoresin solvent extracts [Acetonitrile (), distilled water (
), Ethanol (
), Methanol (
), Propan-2-ol (
)] in K562, MOLT-4 and CCRF-CCM. In all examples, cells were treated for 72 h and all data points are normalised to vehicle alone (0.5% v/v DMSO). (D–F) Corresponding normalsied mean ± SEM IC50 values from 12 repeats. Statistically significant (p < 0.05) pairwise comparisons are indicated by asterisks (*).
3.3 The time-dependence and specificity of B. carterii oleoresin methanolic extract cytotoxicity
Given the relative yield and potency values, methanolic extracts were used for all subsequent experiments (see Section 4.1 for detailed rationale). The independent experiment shown in Figure 2A was designed to evaluate the time-dependent effect of B. carterii in K562 cells. The effect of each concentration tested on viability increases with incubation time. This is quantified in Figure 2B which compares the IC50 values of equivalent dose response curves following 24-, 72- and 120-h incubation periods. This reveals a significant time-dependent effect (24 h; 92.8 ± 10.6, 72 h; 54.2 ± 5.7, 120 h; 27.9 ± 4.6 μg/mL, n = 12, p < 0.001). This pattern of effect was also observed in MOLT-4 and CCRF-CEM cells (Supplementary Figure S6A, D).
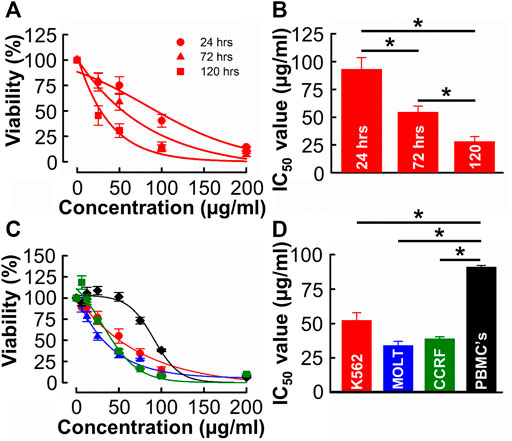
FIGURE 2. The time and concentration dependent effects of B. carterii oleoresin methanolic extract on leukemia and peripheral blood mononuclear cell cytotoxicity (A) Dose response curves comparing the cytotoxicity of B. carterii methanolic extract following treatment for 24, 72 and 120 h. In all examples, K562 cells were used. All data points are normalised to vehicle alone (0.5% v/v DMSO). (B) Corresponding normalised mean ± SEM IC50 values from 12 repeats. (C) Dose response curves comparing concentration dependent cytotoxicity of the B. carterii oleoresin methanolic extract against K562 (red), MOLT-4 (blue) and CCRF-CEM (green) leukaemia cell lines and the non-cancerous PBMC’s (black). In all examples, cells were treated for 72 h, and all data points are normalised to vehicle alone (0.5% v/v DMSO). (D) Corresponding normalized mean ± SEM IC50 values from 12 repeats. Statistically significant (p < 0.05) pairwise comparisons are indicated by asterisks (*).
We next evaluated the specificity of effect of B. carterii by comparing dose response curves in leukemia cells to that in an equivalent normal cell-line (PBMCs, Figure 2C). The average IC50 value (Figure 2D) in PBMC cells (90.8 ± 1.3 μg/mL) was significantly greater than those in K562, MOLT-4 and CCRF cells (52.08 ± 5.9, 33.8 ± 3.2, 38.7 ± 1.7 μg/mL respectively, n = 12, p < 0.001). The calculated selectivity indices towards K562, MOLT 4 and CCRF-CEM were 1.75, 2.68, and 2.39 respectively.
3.4 The effect of B. carterii oleoresin methanolic extract on cell morphology
Given the anti-leukemic activity of the B. carterii oleoresin, its effect on cell morphology was evaluated. A time and concentration-dependent decrease in cell size and increase in cell granularity is apparent upon visual inspection of the live-cell, time-lapse micrographs shown in Figure 3A. These phenomena were quantified using flow cytometry. Figures 3B, C show that following a 24-h incubation, 100 μg/ml B. carterii oleoresin produced a modest 3.6% ± 1.1% decrease (n = 6, p = 0.03) and 9.6% ± 3.2% decrease (n = 6, p = 0.007) in cell size and granularity respectively. For both, a time-dependence of effect was observed whereby following 48 and 72-h incubation periods, 50 μg/ml B. carterii oleoresin also significantly altered cell morphology by 1) decreasing cell size while 2) increasing granularity. For example, following a 72-h incubation, B. carterii oleoresin produced a 20.1% ± 7.1% decrease (n = 6, p = 0.005) and 20.6% ± 6.6% increase (n = 6, p = 0.003) in cell size and granularity respectively.
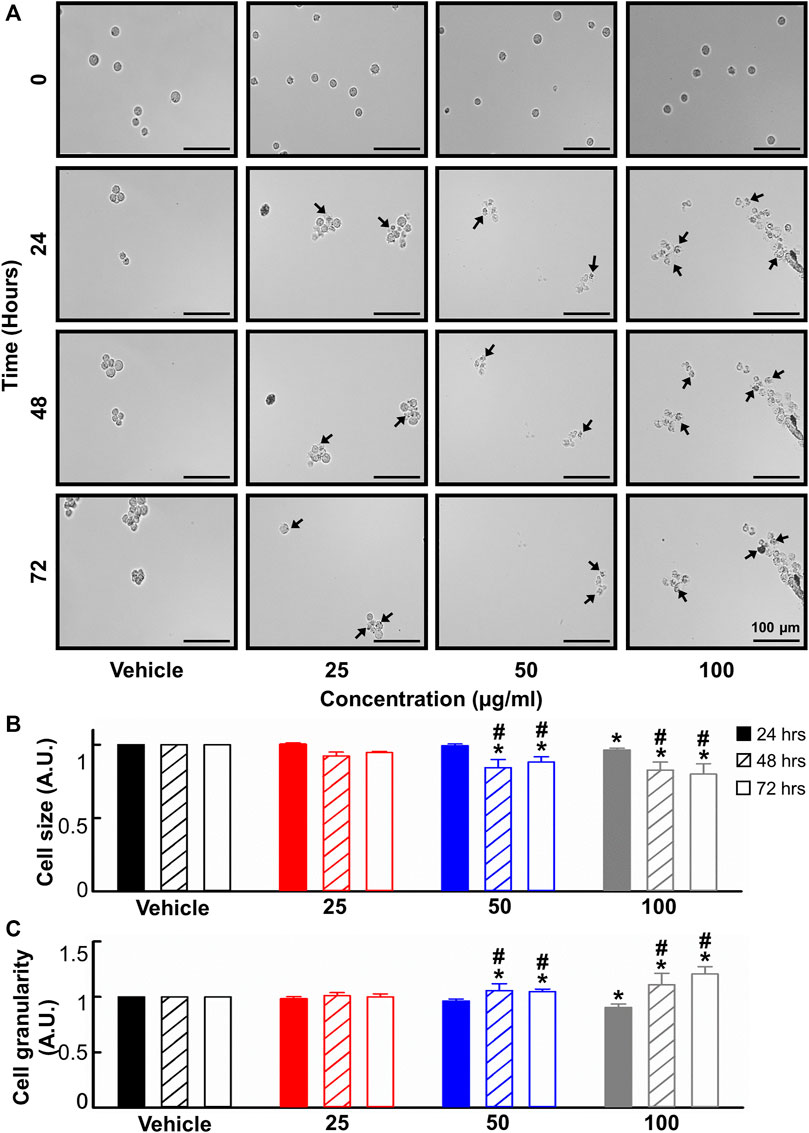
FIGURE 3. The effects of B. carterii oleoresin methanolic extract on cell morphology. (A) Specimen micrographs showing K562 morphology following treatment with B. carterii oleoresin methanolic extract for 24, 48, and 72 h. Arrows indicate examples cells with key morphological changes. Scale bars represent 100 µm. (B) Normalised mean ± SEM cell size. (C) Normalised mean ± SEM cell granularity. Means are calculated from 6 repeats. Asterisks (*) indicate concentration means that are statistically different to vehicle alone (0.5% v/v DMSO). Hashes (#) indicate incubation-time means that are statistically different to 24 h for a given concentration.
3.5 The effects of B. carterii oleoresin methanolic extract on apoptosis and necrosis
To determine whether the changes reported in 3.4 were associated with apoptosis, we next used flow cytometry with Annexin V and propidium iodide co-staining. Figures 4A, B show that B. carterii oleoresin produced a concentration dependent decrease in cell viability and increase in late apoptosis. For example, at the highest concentration tested (100 μg/mL) B. carterii oleoresin decreased the proportion of viable cells by 95.1% ± 3.8% (Vehicle; 69.4% ± 1.2%, 100 μg/mL; 3.3% ± 2.5%, n = 4, p < 0.001) and increased the proportion of cells in late apoptosis by 285.4% ± 51.6% (Vehicle; 21.1% ± 2.8%, 100 μg/mL; 81.7% ± 13.8%, n = 6, p < 0.001). Over the time-course of our experiments, B. carterii oleoresin did not induce early apoptosis or necrosis.
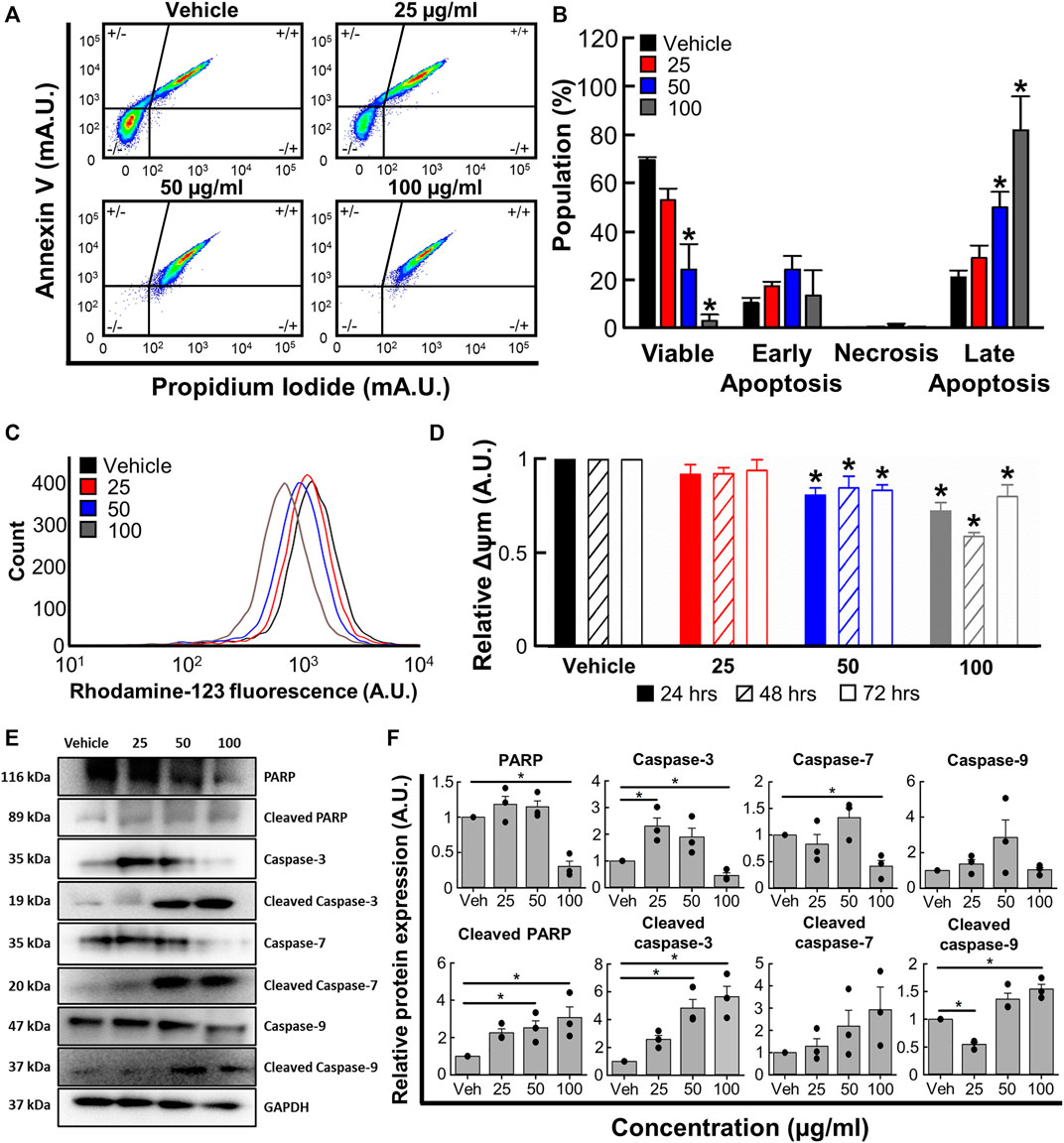
FIGURE 4. The effects of B. carterii oleoresin methanolic extract on apoptosis. (A) Specimen flow cytometry plots showing K562 annexin V/propidium iodide co-staining following treatment with B. carterii oleoresin methanolic extract for 48 h (B) Normalised mean ± SEM cell state proportion from 4 repeats. (C) Specimen flow cytometry plot showing the effect of B. carterii oleoresin methanolic extract on rhodamine-123 fluorescence in K562 cells. (D) Normalised mean ± SEM mitochondrial membrane potential (Δψm) following treatment for 24, 48, and 72 h. Mean calculated from 6 repeats. (E) Specimen Western blots for key apoptosis regulatory proteins following treatment for 48 h. GAPDH served as a loading control. (F) Relative mean ± SEM protein expression of caspases-3, -7, -9, PARP and their associated cleavage fragments in K562 cells following treatment for 48 h. All means calculated from 3 repeats. Asterisks (*) indicate concentration means that are statistically different to vehicle alone (0.5% v/v DMSO).
We next evaluated the effect of B. carterii oleoresin on mitochondrial membrane potential (Δψm) by measuring rhodamine-123 fluorescence with flow cytometry. The specimen flow plot (Figure 4C) shows a leftward shift in rhodamine-123 fluorescence indicating a decrease in mitochondrial membrane potential. The mean data shown in Figure 4D demonstrates this effect is concentration, but not incubation time dependent. For example, incubation with the highest concentration tested (100 μg/mL) for an intermediate period (48 h) decreased relative mitochondrial membrane potential by 41% ± 2% (n = 6, p < 0.001).
Western blotting revealed significant modifications to pro-apoptotic protein expression following treatment for 48 h (Figures 4E, F). For example, 100 μg/ml B. carterii oleoresin decreased relative PARP expression by 3.3-fold (n = 3, p = 0.003) while the 89 kDa cleavage fragment of PARP was increased 3.1-fold (n = 3, p = 0.023). The concentration-dependent effect of B. carterii oleoresin on caspase-3 and 7 expression was complex, though 100 μg/mL reduced expression by 2.2 (n = 3, p = 0.01) and 2.4 (n = 3, p = 0.01) fold respectively. Caspase 9 expression was unaltered. B. carterii oleoresin produced a concentration-dependent increase in cleaved caspase-3, cleaved caspase-7 and cleaved caspase-9 expression with 100 μg/mL producing a 5.7 (n = 3, p = 0.003), 3.3 (n = 3, p = 0.03) and 1.5 (n = 3, p = 0.01) fold increase respectively.
3.6 The effects of B. carterii oleoresin methanolic extract on cell cycle regulation
Next, we used flow cytometry to determine if B. carterii oleoresin altered cell cycle regulation. Figure 5A show specimen cell cycle plots. Associated mean data (Figure 5B) reveals a concentration-dependent effect on the proportion of cells in sub G1, G1, S phase and G2. 100 μg/mL increased the proportion of cells in sub G1 and G1 by 867.8% ± 122.9% (n = 4, p < 0.001) and 14.0% ± 5.5% (n = 4, p = 0.013) respectively. The proportion of cells in S phase and G2 were decreased by 63.4% ± 2.0% (n = 4, p < 0.001) and 57.6% ± 5.3% (n = 4, p = 0.001) respectively.
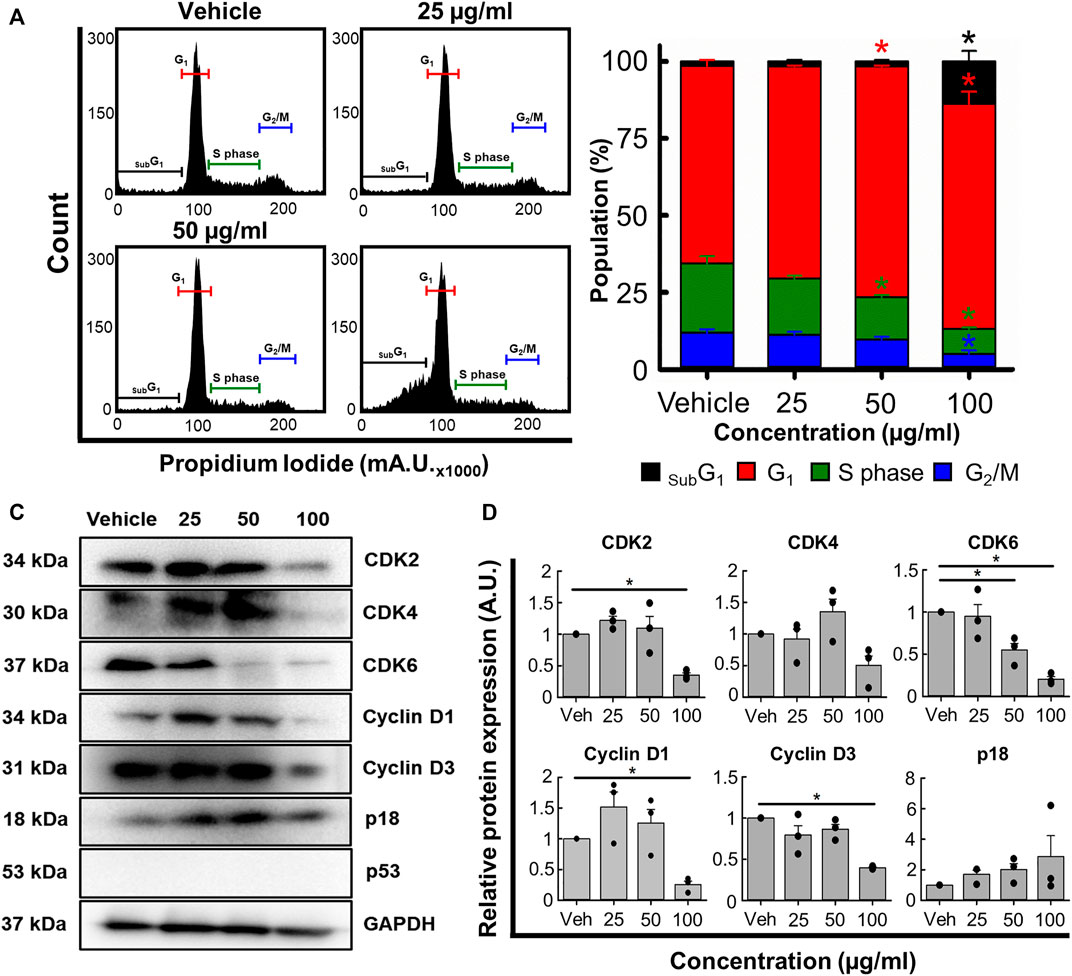
FIGURE 5. The effects of the B. carterii oleoresin methanolic extract on cell cycle regulation. (A) Specimen flow cytometry plots showing the effect of B. carterii oleoresin methanolic extract on cell cycle regulation following treatment for 48 h. (B) Normalised mean ± SEM cell cycle phase proportionality. Means calculated from 4 repeats. (C) Specimen Western blots for key cell cycle regulatory proteins following treatment for 48 h. GAPDH served as a loading control. (D) Relative mean ± SEM protein expression of CDK2, CDK4, CDK6, cyclin D1, cyclin D3 and p18 following treatment for 48 h. All means calculated from 3 repeats. Asterisks (*) indicate concentration means that are statistically different (p < 0.05) to vehicle alone (0.5% v/v DMSO).
To explain these changes, we next examined expression of key cell cycle regulatory proteins (Figures 5C, D). 100 μg/ml B. carterii oleoresin significantly decreased CDK2, CDK6, cyclin D1 and cyclin D3 expression by 2.8-fold (n = 3, p = 0.025), 4.9-fold (n = 3, p = 0.002), 3.9-fold (n = 3, p < 0.001) and 2.5-fold (n = 3, p = 0.003) respectively. CDK 4 was decreased by 2-fold but did not reach significance (n = 3, p = 0.059). The expression of p18 was unaltered.
4 Discussion
Over the last 40 years, the development of effective and specific treatments means the prognosis and long-term survival rate for leukaemia has improved considerably. However, these trends may be compromised by the emergence and increased incidence of chemotherapeutic resistant leukaemia’s (Zhang et al., 2019; Niu et al., 2022; Poudel et al., 2022). Furthermore, it remains the case that many existing chemotherapeutic strategies are associated and the severe side-effects, which is especially the case in children. For both reasons, the discovery and development of novel chemotherapeutics remains a priority.
Natural products are an important source given 1) the richness and diversity of potentially therapeutic species, many of which remain unexplored and 2) that compounds isolated from natural sources frequently produce fewer or attenuated side-effects (Cragg and Newman, 2005). One plant genus of interest is Boswellia, which has been used in traditional Chinese and Indian medicine for centuries. More recently, several studies show B. serrata to be an effective anti-cancer chemotherapeutic in vitro (Jaafari-Ashkavandi et al., 2017; Ranjbarnejad et al., 2017; Alipanah and Zareian, 2018; Schmiech et al., 2019). However, a cohesive description of the mechanistic effects of Boswellia remains unreported. Furthermore, the majority of existing research focusses on B. serrata which is one of many species in the Boswellia genus. To address these limitations, we used an integrated approach to elucidate the anti-leukemic potential and mechanisms of effect of B. carterii.
4.1 Which extraction solvent produces the greatest yield and cytotoxic potential?
Previous investigators predominantly use alcoholic extractions to evaluate the biological activity of Boswellia oleoresins (Uma Mahesh et al., 2013; Ahmed et al., 2015; Zhang et al., 2016; Alipanah and Zareian, 2018). However, it wasn’t clear if alcoholic solvents are optimal given that no previous studies have directly compared the extraction yield and biological activity of alternative solvent extracts. Our findings revealed that alcohol-based solvents 1) produce the highest yield (Supplementary Figure S5) and 2) appear to effectively isolate potentially biologically active compounds (Figure 1). Given the high proportion of alcohol soluble material within Boswellia oleoresins (Abdel-Tawab et al., 2011), this observation may not surprise. However, we deemed it important to rule out the potential superiority of non-alcoholic solvents.
Integrating the data of Supplementary Figure S5 and 1 reveals that of the alcohols tested, methanol produces the highest yield and extracts with the greatest potency and diversity of effect against leukemia cell lines. For this reason, methanolic extracts were used in all further experiments. Methanol is a commonly used as a Boswellia extraction solvent (Yazdanpanahi et al., 2014; Ranjbarnejad et al., 2017), though our study is the first to empirically demonstrate it is indeed most effective.
4.2 The cytotoxic profile of B. carterii
B. carterii oleoresin methanolic extract was cytotoxic against CML (K562) and ALL (MOLT-4 and CCRF-CEM) leukaemia in a time (Figures 2A, B) and concentration (Figures 2C, D) manner. This contributes to a more complete understanding of the B. carterii cytotoxic profile, and we are the first to report cytotoxic effects in leukemia.
Clinically used chemotherapeutic agents are associated with severe and long-term side effects (Pearce et al., 2017; Nurgali et al., 2018). While it is encouraging that clinical trials using other Boswellia species report few off target effects (Sengupta et al., 2008), similar evidence does not exist for B. carterii specifically. As such, we also began to investigate the anti-cancer selectivity of the B. carterii oleoresin methanolic extract. Figures 2C, D show minimal cytotoxic activity against human PBMC’s over anti-leukemic concentration ranges. Here, the IC50 value of 90.8 ± 1.3 μg/mL was around 2–3 times that against K562, MOLT-4 and CCRF cell lines. Measured IC50 values were used to calculate selectivity indexes. This is important, as selectivity indexes calculated during in vitro studies predict the likelihood of side effects when compounds are administered in vivo (Peña-Morán et al., 2016). The selectivity indexes for K562, MOLT-4 and CCRF-CEM were 1.75, 2.68 and 2.39 respectively which are considered mildly selective according to the recognised standard scale (Peña-Morán et al., 2016). However, it should be noted that the methanolic extract of B. carterii oleoresin is more selective than clinically employed chemotherapeutic agents such as anthracyclines, which have selectivity indexes in the region of 0.2–1.5 (Badmus et al., 2019).
4.3 Does the methanolic extract of B. carterii produce apoptosis or necrosis?
Previous studies show oleoresin extracts from other Boswellia species induce apoptotic cell death in colorectal (Ahmed et al., 2015; Ranjbarnejad et al., 2017) breast (Yazdanpanahi et al., 2014) and oral squamous cell (Jaafari-Ashkavandi et al., 2017) carcinomas. However, the precise pathways and molecular mechanisms remain ambiguous.
Visual review (Figure 3A) of cells revealed concentration and time-dependent morphological changes. These included increased granularity (including formation for apoptotic blebs), decreased cell size and evidence of pyknosis The effects on granularity and cells size were confirmed quantitively using flow cytometry (Figures 3B, C) and are consistent with apoptosis (Elmore, 2007; Jiang et al., 2016). Further evidence for a pro-apoptotic action is provided by annexin V/propidium iodide co-staining (Figure 4A) thus molecular investigation of flippase phosphatidylserine translocation; a phenomenon commonly associated with apoptosis (Segawa and Nagata, 2015). The mean data shown in Figure 4B 1) confirms the effect on cell viability reported in Figure 2 and 2) reveals that apoptosis but not necrosis is responsible.
Our next experiments sought to establish whether apoptosis resulted from activation of the intrinsic or extrinsic pathway. Figures 4C, D show that B. carterii oleoresin methanolic extract decreases mitochondrial membrane potential. In apoptotic cells, mitochondrial membrane potential is decreased by the BCL-2 family of proteins (Bid, Bax, and Bak), which form oligomers on mitochondrial outer membrane. This process results in membrane permeabilisation so the release of intramitochondrial factors that include cytochrome C (Wang and Youle, 2009; Jin et al., 2010). This mitochondrial deplolarisation is a key factor in the development of the apoptosome in conjunction with caspase 9 and more strongly associated with the intrinsic pathway (Peña-Blanco and García-Sáez, 2018; Al-Aamri et al., 2021).
Western blotting revealed increased cleavage of the DNA repair enzyme PARP, the loss of which is associated with caspase activation to prevent depletion of adenosine triphosphate pools (Chaitanya et al., 2010). Furthermore, we observed increased cleavage of the cysteinyl proteases caspases-3, -7 and 9 (Figures 4E, F). Cleavage of caspase-3 and -7 coverts them to their active isoforms; a process vital for the effector pathways of apoptosis (Li and Yuan, 2008; Li et al., 2017). That we see an upregulation of cleaved caspase-9 strongly suggests it is activation of the intrinsic pathway that leads to activation of effector pathway (Li et al., 2017).
The induction of apoptosis without necrosis is an important consideration for prospective cytotoxic agents. Cells killed via apoptosis can be removed by the immune system (Nagata, 2018). This prevents leakage of intracellular components into the extracellular space thus reduces inflammation (Elmore, 2007; Yee and Li, 2021) further decreasing the likelihood of side effects if translated to an in vivo model.
4.4 Does the methanolic extract of B. carterii produce cell cycle dysregulation?
Many cancer cells overexpress cell division promoting proteins (Nenclares and Harrington, 2020). As such, another target of chemotherapeutic agents is the cell cycle thus prevention of uncontrolled, indefinite cancer cell division (Hanahan and Weinberg, 2011; Bai et al., 2017; Jhaveri et al., 2021). Previous work suggests that B. serrata extracts can induce subG1 and G1 cell cycle arrests in vitro (Ranjbarnejad et al., 2017; Trivedi et al., 2023) though the underlying mechanisms remain unclear. Therefore, we determined whether B. carterii oleoresin methanolic extract impacted cell cycle regulation. Figures 5A, B show that extracts increase the proportion of cells in subG1 which is indicative of DNA damage (Plesca et al., 2008; Bergandi et al., 2018). Furthermore, the accumulation of cells in G1 and decrease of cells in S phase suggests inhibition of transition which ought to prevent, or at least slow division (Bertoli et al., 2013).
To better understand the molecular mechanisms underlying cell cycle dysregulation we measured the expression of key regulatory proteins (Figures 5C, D). B. carterii oleoresin methanolic extract did not significantly alter the expression of p18; a protein that inhibits transition from G1 to S phase of the cell cycle. However, the extract reduced the expression of cyclins D1 and D3, and CDK2 and CDK6; proteins that facilitate the transition from G1 to S phase of the cell cycle (Nenclares and Harrington, 2020).
Our data suggests it is the downregulation of transition-promoting proteins, rather than upregulation of inhibitory proteins that is primarily responsible for the proportional changes reported in Figure 5B. However, we cannot rule out a role for other inhibitory proteins such as p21 and p27 (Foster et al., 2010; Bai et al., 2017). For this, further work is required.
4.5 The wider therapeutic potential of B. carterii
There remains a global need to develop new anti-cancer drugs given the ever present off-target effects and increasing incidence of drug resistance associated with existing compounds. Our data suggests that B. carterii may be a promising source of novel anti-cancer agents. The cytotoxic effects were specific and produced by the controlled mechanisms of apoptosis and cell-cycle arrest; common targets for other chemotherapeutics. Furthermore, we know that oleresins from other Bosweillia species are well tolerated in animal models and clinical trials (Gupta et al., 1998; Kimmatkar et al., 2003; Sengupta et al., 2008). The therapeutic potential of B. carterii may be of value in low-income and developing countries. Here, mainstream chemotherapeutics are frequently inaccessible so plant-based and traditional medicines are used widely (Khan, 2014). However, further research into the pharmacodynamics and pharmacokinetics of B. carterii oleoresin methanolic extracts is required.
4.6 Summary
We are the first to report that methanolic extracts of B. carterii are selectively cytotoxic against three leukemia cell lines. Cytotoxicity results from 1) activation of the intrinsic apoptotic pathway and 2) cell cycle arrest through downregulation of CDK2, CDK6, cyclin D1 and cyclin D3. These data suggest that B. carterii may be an important source of potential novel chemotherapeutic drugs. This warrants further investigation to elucidate and purify the precise compounds responsible for the reported effects.
Data availability statement
The raw data supporting the conclusion of this article will be made available by the authors, without undue reservation.
Ethics statement
Ethical approval was not required for the studies on humans in accordance with the local legislation and institutional requirements because only commercially available established cell lines were used.
Author contributions
MJ: Data curation, Formal Analysis, Investigation, Methodology, Software, Visualization, Writing–original draft, Writing–review and editing. AB: Investigation, Methodology, Writing–review and editing. DG: Conceptualization, Data curation, Funding acquisition, Project administration, Resources, Supervision, Visualization, Writing–original draft, Writing–review and editing.
Funding
The author(s) declare financial support was received for the research, authorship, and/or publication of this article. MJ’s iPhD studentship was co-funded by United Kingdom Essential oils Ltd. and The University of Salford AB’s undergraduate research placement was funded by Kidscan children’s cancer research.
Acknowledgments
The authors would like to thank Steven Holmes and Peter Cobain of United Kingdom Essential oils for their funding and for supplying the B. carterii oleoresins used during this study.
Conflict of interest
The authors declare that the research was conducted in the absence of any commercial or financial relationships that could be construed as a potential conflict of interest.
Publisher’s note
All claims expressed in this article are solely those of the authors and do not necessarily represent those of their affiliated organizations, or those of the publisher, the editors and the reviewers. Any product that may be evaluated in this article, or claim that may be made by its manufacturer, is not guaranteed or endorsed by the publisher.
Supplementary material
The Supplementary Material for this article can be found online at: https://www.frontiersin.org/articles/10.3389/fphar.2023.1282239/full#supplementary-material
References
Abdel-Tawab, M., Werz, O., and Schubert-Zsilavecz, M. (2011). Boswellia serrata: an overall assessment of in vitro, preclinical, pharmacokinetic and clinical data. Clin. Pharmacokinet. 50 (6), 349–369. doi:10.2165/11586800-000000000-00000
Ahmed, H. H., Abd-Rabou, A. A., Hassan, A. Z., and Kotob, S. E. (2015). Phytochemical analysis and anti-cancer investigation of boswellia serrata bioactive constituents in vitro. Asian Pac J. Cancer Prev. 16 (16), 7179–7188. doi:10.7314/apjcp.2015.16.16.7179
Al-Aamri, H. M., Irving, H. R., Bradley, C., and Meehan-Andrews, T. (2021). Intrinsic and extrinsic apoptosis responses in leukaemia cells following daunorubicin treatment. BMC Cancer 21 (1), 438. doi:10.1186/s12885-021-08167-y
Al-Dosary, S. K. (2018). Antibacterial effect of Thymus sp. and Boswellia sp. extracts on Streptococcus pneumoniae and Klebsiella pneumoniae isolates. Afr. J. Biotechnol. 17 (5), 133–138. doi:10.5897/AJB2017.16051
Alipanah, H., and Zareian, P. (2018). Anti-cancer properties of the methanol extract of Boswellia serrata gum resin: cell proliferation arrest and inhibition of angiogenesis and metastasis in BALB/c mice breast cancer model. Physiology Pharmacol. 22 (3), 183–194.
Armstrong, G. T., Kawashima, T., Leisenring, W., Stratton, K., Stovall, M., Hudson, M. M., et al. (2014). Aging and risk of severe, disabling, life-threatening, and fatal events in the childhood cancer survivor study. J. Clin. Oncol. 32 (12), 1218–1227. doi:10.1200/JCO.2013.51.1055
Badmus, J. A., Ekpo, O. E., Hussein, A. A., Meyer, M., and Hiss, D. C. (2019). Cytotoxic and cell cycle arrest properties of two steroidal alkaloids isolated from Holarrhena floribunda (G. Don) T. Durand & Schinz leaves. BMC Complement. Altern. Med. 19 (1), 112. doi:10.1186/s12906-019-2521-9
Bai, J., Li, Y., and Zhang, G. (2017). Cell cycle regulation and anticancer drug discovery. Cancer Biol. Med. 14 (4), 348–362. doi:10.20892/j.issn.2095-3941.2017.0033
Bergandi, L., Mungo, E., Morone, R., Bosco, O., Rolando, B., and Doublier, S. (2018). Hyperglycemia promotes chemoresistance through the reduction of the mitochondrial DNA damage, the bax/bcl-2 and bax/bcl-XL ratio, and the cells in sub-G1 phase due to antitumoral drugs induced-cytotoxicity in human colon adenocarcinoma cells. Front. Pharmacol. 9, 866. doi:10.3389/fphar.2018.00866
Bertoli, C., Skotheim, J. M., and de Bruin, R. A. (2013). Control of cell cycle transcription during G1 and S phases. Nat. Rev. Mol. Cell Biol. 14 (8), 518–528. doi:10.1038/nrm3629
Bhayat, F., Das-Gupta, E., Smith, C., McKeever, T., and Hubbard, R. (2009). The incidence of and mortality from leukaemias in the UK: a general population-based study. BMC Cancer 9, 252. doi:10.1186/1471-2407-9-252
Carreño, E. A., Alberto, A. V. P., de Souza, C. A. M., de Mello, H. L., Henriques-Pons, A., and Anastacio Alves, L. (2021). Considerations and technical pitfalls in the employment of the MTT assay to evaluate photosensitizers for photodynamic therapy. Appl. Sci. 11 (6), 2603. doi:10.3390/app11062603
Chaitanya, G. V., Steven, A. J., and Babu, P. P. (2010). PARP-1 cleavage fragments: signatures of cell-death proteases in neurodegeneration. Cell Commun. Signal 8, 31. doi:10.1186/1478-811x-8-31
Chang, J. H., Poppe, M. M., Hua, C. H., Marcus, K. J., and Esiashvili, N. (2021). Acute lymphoblastic leukemia. Pediatr. Blood Cancer 68 (Suppl. 2), e28371. doi:10.1002/pbc.28371
Chen, H., Schürch, C. M., Noble, K., Kim, K., Krutzik, P. O., O’Donnell, E., et al. (2020). Functional comparison of PBMCs isolated by cell preparation tubes (CPT) vs. Lymphoprep tubes. BMC Immunol. 21 (1), 15–13. doi:10.1186/s12865-020-00345-0
Cragg, G. M., and Newman, D. J. (2005). Plants as a source of anti-cancer agents. J. Ethnopharmacol. 100 (1-2), 72–79. doi:10.1016/j.jep.2005.05.011
Deesrisak, K., Chatupheeraphat, C., Roytrakul, S., Anurathapan, U., and Tanyong, D. (2021). Autophagy and apoptosis induction by sesamin in MOLT-4 and NB4 leukemia cells. Oncol. Lett. 21 (1), 32. doi:10.3892/ol.2020.12293
Demain, A. L., and Vaishnav, P. (2011). Natural products for cancer chemotherapy. Microb. Biotechnol. 4 (6), 687–699. doi:10.1111/j.1751-7915.2010.00221.x
Du, M., Chen, W., Liu, K., Wang, L., Hu, Y., Mao, Y., et al. (2022). The global burden of leukemia and its attributable factors in 204 countries and territories: findings from the global burden of disease 2019 study and projections to 2030. J. Oncol. 2022, 1612702. doi:10.1155/2022/1612702
Efferth, T., and Oesch, F. (2022). Anti-inflammatory and anti-cancer activities of frankincense: targets, treatments and toxicities. Semin. Cancer Biol. 80, 39–57. doi:10.1016/j.semcancer.2020.01.015
Elmore, S. (2007). Apoptosis: a review of programmed cell death. Toxicol. Pathol. 35 (4), 495–516. doi:10.1080/01926230701320337
Estey, E. H. (2018). Acute myeloid leukemia: 2019 update on risk-stratification and management. Am. J. Hematol. 93 (10), 1267–1291. doi:10.1002/ajh.25214
Foster, D. A., Yellen, P., Xu, L., and Saqcena, M. (2010). Regulation of G1 cell cycle progression: distinguishing the restriction point from a nutrient-sensing cell growth checkpoint(s). Genes Cancer 1 (11), 1124–1131. doi:10.1177/1947601910392989
Gaigneaux, A., Ruysschaert, J. M., and Goormaghtigh, E. (2002). Infrared spectroscopy as a tool for discrimination between sensitive and multiresistant K562 cells. Eur. J. Biochem. 269 (7), 1968–1973. doi:10.1046/j.1432-1033.2002.02841.x
Gupta, I., Gupta, V., Parihar, A., Gupta, S., Lüdtke, R., Safayhi, H., et al. (1998). Effects of Boswellia serrata gum resin in patients with bronchial asthma: results of a double-blind, placebo-controlled, 6-week clinical study. Eur. J. Med. Res. 3 (11), 511–514.
Hanahan, D., and Weinberg, R. A. (2011). Hallmarks of cancer: the next generation. Cell 144 (5), 646–674. doi:10.1016/j.cell.2011.02.013
Hao, T., Li-Talley, M., Buck, A., and Chen, W. (2019). An emerging trend of rapid increase of leukemia but not all cancers in the aging population in the United States. Sci. Rep. 9 (1), 12070. doi:10.1038/s41598-019-48445-1
Haynes, M. K., Strouse, J. J., Waller, A., Leitao, A., Curpan, R. F., Bologa, C., et al. (2009). Detection of intracellular granularity induction in prostate cancer cell lines by small molecules using the HyperCyt high-throughput flow cytometry system. J. Biomol. Screen. 14 (6), 596–609. doi:10.1177/1087057109335671
Ikonomidou, C. (2018). Chemotherapy and the pediatric brain. Mol. Cell Pediatr. 5 (1), 8. doi:10.1186/s40348-018-0087-0
Iten, , Reichling, , and Saller, (2002). Adverse effects and interactions of phytotherapeutic drugs. Ther. Umsch. 59 (6), 283–291. doi:10.1024/0040-5930.59.6.283
Jaafari-Ashkavandi, Z., Hamedi, A., Assar, S., and Ebrahimpour, A. (2017). The effects of frankincense on oral squamous cell carcinoma cell line. Int. J. Cancer Manag. 10 (5). doi:10.5812/ijcm.6416
Jhaveri, K., Burris Rd, H. A., Yap, T. A., Hamilton, E., Rugo, H. S., Goldman, J. W., et al. (2021). The evolution of cyclin dependent kinase inhibitors in the treatment of cancer. Expert Rev. Anticancer Ther. 21 (10), 1105–1124. doi:10.1080/14737140.2021.1944109
Jiang, L., Tixeira, R., Caruso, S., Atkin-Smith, G. K., Baxter, A. A., Paone, S., et al. (2016). Monitoring the progression of cell death and the disassembly of dying cells by flow cytometry. Nat. Protoc. 11 (4), 655–663. doi:10.1038/nprot.2016.028
Jin, S. M., Lazarou, M., Wang, C., Kane, L. A., Narendra, D. P., and Youle, R. J. (2010). Mitochondrial membrane potential regulates PINK1 import and proteolytic destabilization by PARL. J. Cell Biol. 191 (5), 933–942. doi:10.1083/jcb.201008084
Kantarjian, H. M., Kadia, T. M., DiNardo, C. D., Welch, M. A., and Ravandi, F. (2021). Acute myeloid leukemia: treatment and research outlook for 2021 and the MD Anderson approach. Cancer 127 (8), 1186–1207. doi:10.1002/cncr.33477
Khan, H. (2014). Medicinal plants in light of history: recognized therapeutic modality. J. Evid. Based Complement. Altern. Med. 19 (3), 216–219. doi:10.1177/2156587214533346
Kimmatkar, N., Thawani, V., Hingorani, L., and Khiyani, R. (2003). Efficacy and tolerability of Boswellia serrata extract in treatment of osteoarthritis of knee--a randomized double blind placebo controlled trial. Phytomedicine 10 (1), 3–7. doi:10.1078/094471103321648593
Koeberle, A., Henkel, A., Verhoff, M., Tausch, L., König, S., Fischer, D., et al. (2018). Triterpene acids from frankincense and semi-synthetic derivatives that inhibit 5-lipoxygenase and cathepsin G. Molecules 23 (2), 506. doi:10.3390/molecules23020506
Li, J., and Yuan, J. (2008). Caspases in apoptosis and beyond. Oncogene 27 (48), 6194–6206. doi:10.1038/onc.2008.297
Li, P., Zhou, L., Zhao, T., Liu, X., Zhang, P., Liu, Y., et al. (2017). Caspase-9: structure, mechanisms and clinical application. Oncotarget 8 (14), 23996–24008. doi:10.18632/oncotarget.15098
Lim, Y. Z., Hussain, S. M., Cicuttini, F. M., and Wang, Y. (2019). “Chapter 6 - nutrients and dietary supplements for osteoarthritis,” in Bioactive food as dietary interventions for arthritis and related inflammatory diseases Editors R R Watson, and V R Preedy. Second Edition (Cambridge, Massachusetts, United States: Academic Press), 97–137. doi:10.1016/B978-0-12-813820-5.00006-4
Luengo, A., Gui, D. Y., and Vander Heiden, M. G. (2017). Targeting metabolism for cancer therapy. Cell Chem. Biol. 24 (9), 1161–1180. doi:10.1016/j.chembiol.2017.08.028
Mahmood, T., and Yang, P.-C. (2012). Western blot: technique, theory, and trouble shooting. North Am. J. Med. Sci. 4 (9), 429–434. doi:10.4103/1947-2714.100998
Martins-Teixeira, M. B., and Carvalho, I. (2020). Antitumour anthracyclines: progress and perspectives. ChemMedChem 15 (11), 933–948. doi:10.1002/cmdc.202000131
Mazzio, E. A., Lewis, C. A., and Soliman, K. F. A. (2017). Transcriptomic profiling of MDA-MB-231 cells exposed to boswellia serrata and 3-O-acetyl-B-boswellic acid; ER/UPR mediated programmed cell death. Cancer Genomics Proteomics 14 (6), 409–425. doi:10.21873/cgp.20051
Moussaieff, A., and Mechoulam, R. (2009). Boswellia resin: from religious ceremonies to medical uses; a review of in-vitro, in-vivo and clinical trials. J. Pharm. Pharmacol. 61 (10), 1281–1293. doi:10.1211/jpp/61.10.0003
Mtewa, A. G., Egbuna, C., Beressa, T. B., Ngwira, K. J., and Lampiao, F. (2021). “Chapter 2 - phytopharmaceuticals: efficacy, safety, and regulation,” in Preparation of phytopharmaceuticals for the management of disorders. Editors C. Egbuna, A. P. Mishra, and M. R. Goyal (Cambridge, Massachusetts, United States: Academic Press), 25–38. doi:10.1016/B978-0-12-820284-5.00010-1
Nagata, S. (2018). Apoptosis and clearance of apoptotic cells. Annu. Rev. Immunol. 36, 489–517. doi:10.1146/annurev-immunol-042617-053010
Nenclares, P., and Harrington, K. (2020). The biology of cancer. Medicine 48 (2), 67–72. doi:10.1016/j.mpmed.2019.11.001
Niu, J., Peng, D., and Liu, L. (2022). Drug resistance mechanisms of acute myeloid leukemia stem cells. Front. Oncol. 12, 896426. doi:10.3389/fonc.2022.896426
Nurgali, K., Jagoe, R. T., and Abalo, R. (2018). Editorial: adverse effects of cancer chemotherapy: anything new to improve tolerance and reduce sequelae? Front. Pharmacol. 9, 245. doi:10.3389/fphar.2018.00245
Oeffinger, K. C., Mertens, A. C., Sklar, C. A., Kawashima, T., Hudson, M. M., Meadows, A. T., et al. (2006). Chronic health conditions in adult survivors of childhood cancer. N. Engl. J. Med. 355 (15), 1572–1582. doi:10.1056/NEJMsa060185
Panwar, P., Burusco, K. K., Abubaker, M., Matthews, H., Gutnov, A., Fernández-Álvaro, E., et al. (2020). Lead optimization of dehydroemetine for repositioned use in malaria. Antimicrob. agents Chemother. 64 (4), e01444–e01419. doi:10.1128/AAC.01444-19
Pearce, A., Haas, M., Viney, R., Pearson, S. A., Haywood, P., Brown, C., et al. (2017). Incidence and severity of self-reported chemotherapy side effects in routine care: a prospective cohort study. PLoS One 12 (10), e0184360. doi:10.1371/journal.pone.0184360
Peña-Blanco, A., and García-Sáez, A. J. (2018). Bax, Bak and beyond - mitochondrial performance in apoptosis. Febs J. 285 (3), 416–431. doi:10.1111/febs.14186
Peña-Morán, O. A., Villarreal, M. L., Álvarez-Berber, L., Meneses-Acosta, A., and Rodríguez-López, V. (2016). Cytotoxicity, post-treatment recovery, and selectivity analysis of naturally occurring podophyllotoxins from Bursera fagaroides var. fagaroides on breast cancer cell lines. Molecules 21 (8), 1013. doi:10.3390/molecules21081013
Plesca, D., Mazumder, S., and Almasan, A. (2008). DNA damage response and apoptosis. Methods Enzymol. 446, 107–122. doi:10.1016/s0076-6879(08)01606-6
Poudel, G., Tolland, M. G., Hughes, T. P., and Pagani, I. S. (2022). Mechanisms of resistance and implications for treatment strategies in chronic myeloid leukaemia. Cancers (Basel) 14 (14), 3300. doi:10.3390/cancers14143300
Pozarowski, P., and Darzynkiewicz, Z. (2004). Analysis of cell cycle by flow cytometry. Methods Mol. Biol. 281, 301–311. doi:10.1385/1-59259-811-0:301
Ralhan, R., and Kaur, J. (2007). Alkylating agents and cancer therapy. Expert Opin. Ther. Pat. 17 (9), 1061–1075. doi:10.1517/13543776.17.9.1061
Ranjbarnejad, T., Saidijam, M., Moradkhani, S., and Najafi, R. (2017). Methanolic extract of Boswellia serrata exhibits anti-cancer activities by targeting microsomal prostaglandin E synthase-1 in human colon cancer cells. Prostagl. other lipid Mediat. 131, 1–8. doi:10.1016/j.prostaglandins.2017.05.003
Schmiech, M., Lang, S. J., Werner, K., Rashan, L. J., Syrovets, T., and Simmet, T. (2019). Comparative analysis of pentacyclic triterpenic acid compositions in oleogum resins of different Boswellia species and their in vitro cytotoxicity against treatment-resistant human breast cancer cells. Molecules 24 (11), 2153. doi:10.3390/molecules24112153
Schneider, C. A., Rasband, W. S., and Eliceiri, K. W. (2012). NIH Image to ImageJ: 25 years of image analysis. Nat. Methods 9 (7), 671–675. doi:10.1038/nmeth.2089
Segawa, K., and Nagata, S. (2015). An apoptotic 'eat me' signal: phosphatidylserine exposure. Trends Cell Biol. 25 (11), 639–650. doi:10.1016/j.tcb.2015.08.003
Sekeres, M. A., and Taylor, J. (2022). Diagnosis and treatment of myelodysplastic syndromes: a review. Jama 328 (9), 872–880. doi:10.1001/jama.2022.14578
Sengupta, K., Alluri, K. V., Satish, A. R., Mishra, S., Golakoti, T., Sarma, K. V., et al. (2008). A double blind, randomized, placebo controlled study of the efficacy and safety of 5-Loxin® for treatment of osteoarthritis of the knee. Arthritis Res. Ther. 10 (4), 1–11. doi:10.1186/ar2461
Siddiqui, M. Z. (2011). Boswellia serrata, A potential antiinflammatory agent: an overview. Indian J. Pharm. Sci. 73 (3), 255–261. doi:10.4103/0250-474X.93507
Trivedi, V. L., Soni, R., Dhyani, P., Sati, P., Tejada, S., Sureda, A., et al. (2023). Anti-cancer properties of boswellic acids: mechanism of action as anti-cancerous agent. Front. Pharmacol. 14, 1187181. doi:10.3389/fphar.2023.1187181
Uma Mahesh, B., Shrivastava, S., Kuncha, M., Sahu, B. D., Swamy, C. V., Pragada, R. R., et al. (2013). Ethanolic extract of Boswellia ovalifoliolata bark and leaf attenuates doxorubicin-induced cardiotoxicity in mice. Environ. Toxicol. Pharmacol. 36 (3), 840–849. doi:10.1016/j.etap.2013.07.016
Wang, C., and Youle, R. J. (2009). The role of mitochondria in apoptosis. Annu. Rev. Genet. 43, 95–118. doi:10.1146/annurev-genet-102108-134850
Yazdanpanahi, N., Behbahani, M., and Yektaeian, A. (2014). Effect of boswellia thurifera gum methanol extract on cytotoxicity and p53 gene expression in human breast cancer cell line. Iran. J. Pharm. Res. 13 (2), 719–724.
Yee, P. P., and Li, W. (2021). Tumor necrosis: a synergistic consequence of metabolic stress and inflammation. Bioessays 43 (7), e2100029. doi:10.1002/bies.202100029
Zhang, J., Biggs, I., Sirdaarta, J., White, A., and Cock, I. E. (2016). Antibacterial and anticancer properties of Boswellia carteri Birdw. and Commiphora molmol Engl. oleo-resin solvent extractions. Pharmacogn. Commun. 8 (3), 120–136. doi:10.5530/pc.2016.3.2
Keywords: Boswellia, phytomedicine, cancer, leukaemia, apoptosis, cell cycle
Citation: Jones MA, Borun A and Greensmith DJ (2023) Boswellia carterii oleoresin extracts induce caspase-mediated apoptosis and G1 cell cycle arrest in human leukaemia subtypes. Front. Pharmacol. 14:1282239. doi: 10.3389/fphar.2023.1282239
Received: 23 August 2023; Accepted: 04 December 2023;
Published: 14 December 2023.
Edited by:
Mona Abdel Tawab, Central Laboratory of German Pharmacists, GermanyReviewed by:
Daniel Gideon, Bishop Heber College, IndiaEbtesam Abdullah Al-Suhaimi, King Abdulaziz and His Companions Foundation for Giftedness and Creativity, Saudi Arabia
Copyright © 2023 Jones, Borun and Greensmith. This is an open-access article distributed under the terms of the Creative Commons Attribution License (CC BY). The use, distribution or reproduction in other forums is permitted, provided the original author(s) and the copyright owner(s) are credited and that the original publication in this journal is cited, in accordance with accepted academic practice. No use, distribution or reproduction is permitted which does not comply with these terms.
*Correspondence: David James Greensmith, ZC5qLmdyZWVuc21pdGhAc2FsZm9yZC5hYy51aw==