- 1The Medical College, Shandong University of Traditional Chinese Medicine, Jinan, China
- 2School of Chinese Medicine, Shandong University of Traditional Chinese Medicine, Jinan, China
- 3Department of Histology and Embryology, Shandong University of Traditional Chinese Medicine, Jinan, China
Tanshinone is a lipophilic compound that is present in traditional Chinese medicine and is derived from the roots of Salvia miltiorrhiza (Danshen). It has been proven to be highly effective in combating tumors in various parts of the body, including liver carcinoma, gastric cancer, ovarian cancer, cervix carcinoma, breast cancer, colon cancer, and prostate cancer. Tanshinone can efficiently prevent the reproduction of cancerous cells, induce cell death, and inhibit the spread of cancerous cells, which are mainly involved in the PI3K/Akt signaling pathway, NF-κB pathway, Bcl-2 family, Caspase cascades, MicroRNA, MAPK signaling pathway, p21, STAT3 pathway, miR30b-P53-PTPN11/SHP2 axis, β-catenin, and Skp2. However, the properties and mechanisms of tanshinone’s anti-tumor effects remain unclear currently. Thus, this study aims to review the research progress on tumor prevention and mechanisms of tanshinone to gain new perspectives for further development and clinical application of tanshinone.
1 Introduction
Malignant tumors have had a crushing impact on human health and are identified as a main reason for death (Zuo et al., 2022). The global figures from 2020 revealed that there were around 19.30 million new cancer cases reported, resulting in approximately 10 million deaths. It is a matter of great concern that cancer incidence is increasing steadily, and by 2040, it is estimated to affect around 28.40 million people (Sung et al., 2021). Given this condition, significant focus has been placed on available treatments for malignant tumors. As a result, identifying the effective medical approach to treat malignant tumors has been of utmost importance to numerous researchers and clinical doctors.
Danshen has been extensively researched as a natural active pharmaceutical ingredient since the 1930s (Li et al., 2009). According to the Chinese Pharmacopoeia’s 2020 Edition, Salvia miltiorrhiza is known for its ability to alleviate pain and eliminate blood stasis, as well as promote blood circulation, clear the heart, and reduce irritation. It contains both fat-soluble and water-soluble components that are effective. The fat-soluble components consist of tanshinone IIA, cryptotanshinone, tanshinone I, and dihydrotanshinone (Figure 1). While, the components that dissolve in water are salvianolic acid A, salvianolic acid B, purple oxalic acid, and rosmarinic acid. Among these two categories, the fat-soluble component, tanshinone, is highly valued due to its minimal toxicity, high effectiveness, safety, and ability to produce various pharmacological actions that are against tumors (Jin et al., 2021), inflammation (Ye et al., 2020), myocardial ischemia (Zhu et al., 2023), oxidative stress (Wu et al., 2021) and thrombosis (Feng et al., 2021), inhibit left ventricular hypertrophy (Pang et al., 2014), dilate blood vessels (Lu et al., 2021), resist atherosclerosis (Wen et al., 2020), protect brain tissue (Wang et al., 2022), improve microcirculation (Qin et al., 2023), significantly inhibit pulmonary fibrosis (Feng et al., 2020), and activate immunity (Chen et al., 2022).
Tanshinone has been shown to have anti-tumor properties among various common malignant tumors, including gastric (Ni et al., 2022), lung (Li et al., 2021), liver (Lee et al., 2010), breast (Wang et al., 2005) and colorectal (Jieensinue et al., 2018) cancers, which is achieved through various molecular mechanisms. Notably, the molecular mechanism behind the anti-tumor effect of continuous research has garnered significant attention, making it a research hotspot for numerous researchers. However, presently, there exists a requirement for a thorough review of the molecular mechanisms underlying tanshinone’s anti-tumor effects. Researchers have discovered that tanshinone primarily acts as an anti-tumor agent by promoting tumor cell death, inhibiting their proliferation, preventing migration and incursion, hindering immune evasion, and increasing the sensitivity of radiation and chemotherapy (Table 1). Thus, this article outlines the molecular mechanism of tanshinone in malignancies based on the five aforementioned aspects, in order to furnish scientific researchers and clinical workers with novel perspectives.
2 Tanshinone induces tumor cell death
2.1 Tanshinone induces tumor cell apoptosis
2.1.1 PI3K/Akt signaling pathway
PI3K is imperative in activating Akt, which is essential for enhancing cellular survival and proliferation (Liu et al., 2009; Santarpia et al., 2012). The PI3K/Akt axis is a commonly dysregulated kinase cascade in human cancer and is activated by growth factor receptors (McCubrey et al., 2011). Research on non-small-cell lung cancer revealed that when the quantity of tanshinone IIA increased, the viability of A549 cells was reduced (Liao et al., 2019). Meanwhile, tanshinone IIA intervention had a concentration-dependent impact on the suppression of PI3K and downstream Akt protein phosphorylation. Nevertheless, the expression of Caspase 3, a protein that aids in cell apoptosis, significantly increased. Combining these findings, tanshinone IIA stimulated cell apoptosis by modulating the PI3K/Akt pathway and influencing Caspase 3 activity.
Schaf et al. (2023) reported the tanshinone IIA’s role in glioblastomas and the induction of apoptosis on GBM59 cells in a manner dependent on concentration. During that study, TSA treatment resulted in a reduction in the phosphorylated PI3K levels within the nucleus of glioblastoma cells, as well as a decrease in levels of AKT and mTOR regulated by PI3K. This suggested that tanshinone IIA effectively inhibited the multiplication of GBM59 cells and triggered their cell death, possibly due to the reduction of the PI3K/AKT/mTOR pathway. Another bioactive component tanshinone IA significantly induced apoptosis and promoted autophagy in vitro and in vivo by inactivating the PI3K/AKT/mTOR pathway in ovarian cancer (Zhou et al., 2020a). Consistent with the study above, Tan IIA appears to suppress the PI3K/AKT survival pathway, which aids in apoptosis induction signaling. According to a study conducted by Won et al. (2010), the levels of PI3K p85 subunit expression, AKT phosphorylation, and mTOR in LNCaP cells were decreased following Tan IIA treatment. Additionally, Tan IIA treatment triggered the mitochondria to release cytochrome c into the cytoplasm, while suppressing the expression of Mcl-1L and increasing levels of caspases-9 and 3, as well as PARP. Taken together, this study indicated that Tan IIA’s stimulation of apoptosis involved the activation of caspase cascades within the mitochondria and the suppression of the PI3K/AKT/mTOR axis.
Additional research into the mechanism revealed that tanshinone IIA hindered the PI3K/AKT/mTOR signaling pathway by diminishing levels of EGFR, IGFR, and VEGFR (Su, 2018). It was the initial study to show that Tan IIA hindered the growth of MiaPaCa-2 pancreatic cancer cells by reducing levels of EGFR, IGFR, and VEGFR, while also suppressing the PI3K/Akt/mTOR axis. Consistently, tan IIA in gastric carcinoma could substantially and concentration-dependently reduce EGFR, IGFR, PI3K, AKT, and mTOR protein expression both in vitro and in vivo (Su and Chiu, 2016). This report first unveiled tan IIA’s potential to inhibit gastric cancer via blocking the PI3K/Akt/mTOR pathway and lowering the EGFR and IGFR protein levels in AGS cell xenograft tumors.
JNK is activated by phosphorylation and triggered by cytokines, growth factors, or stress (Wagner and Nebreda, 2009). In a study on ovarian cancer, it was discovered that Tan-IIA deactivated the PI3K/AKT/JNK pathway to activate caspase-3, caspase-8, caspases-9 and downregulate Bcl-w, Mcl-1L, which led to cell apoptosis (Zhang et al., 2019b). It was also revealed that PI3K overexpression canceled out the influence of Tan IIA on AKT and JNK expression, and prevented ovarian cancer cells from apoptosis that Tan IIA induced. This suggested that Tan IIA’s anti-tumor activity might be linked to its suppression of the PI3K/AKT/JNK axis.
2.1.2 NF-κB signaling pathway
The NF-κB transcription factor is a well-established regulatory protein that serves a crucial role in preventing cell apoptosis (Verzella et al., 2020). Research on breast cancer has revealed the viability of MDA-MB-231 was reduced with an increased concentration of tanshinone IIA (Su et al., 2012). Meanwhile, intervention with tanshinone IIA suppressed NF-κB p65 and increased Caspase 3 level concentration-dependently. After analyzing this study, tanshinone IIA possessed the capacity to trigger cellular apoptosis through the modulation of the NF-κB pathway and the activation of Caspase cascades.
Another study reported tanshinone IIA’s function in colon cancer and found it suppressed the growth of HCT116/COLO205 cells in a manner dependent on dose (Bai et al., 2016). In that study, the use of TSA reduced the NF-κB regulated gene expression levels, including COX-2, c-Myc, and Bcl-2, as well as the amount of phosphorylated NF-κB p65 in the nucleus of colon cancer cells. Additionally, another bioactive component cryptotanshinone was found to significantly induce apoptosis of HCCC-9810 and RBE cells by inactivation of the NF-κB pathway in cholangiocarcinoma (Ke et al., 2017). During this study, it was discovered that decreasing PI3K and Akt activity resulted in the deactivation of the NF-κB pathway and the alteration of the Bcl-2/Bax ratio. These indicated that tanshinone can significantly trigger the apoptosis of cancerous cells, which might be related to the decrease of anti-apoptotic proteins mediated by the NF-κB signaling pathway.
2.1.3 Bcl-2 family
Bcl-2 is a molecule that can lessen caspase cascade reactions triggered by cytochrome c and prevent mitochondria from releasing pro-apoptotic proteins, ultimately serving as an anti-apoptotic agent (Straten and Andersen, 2010). Bcl-2, therefore, is crucial for the treatment of tumors. The research found that tanshinone IIA could promote MDA-MB-231 cell death in breast cancer via elevating the pro-apoptotic protein Bax and lowering the anti-apoptotic protein Bcl-2 levels (Su and Lin, 2008).
TCTP possesses an anti-apoptotic property that can be associated with the binding of MCL-1 and Bcl-xL (Zhang et al., 2002; Graidist et al., 2004; Liu et al., 2005), and the antagonization of Bax (Yang et al., 2005). In a pancreatic carcinoma study, it was found that the increased concentration of Tan IIA led to an increase in levels of Bax and Caspase-3, while the decrease of TCTP, Mcl-1, and Bcl-xL, thus inducing BxPC-3 cells cell apoptosis (Huang et al., 2013). During this process, the decrement of BXPC-3 cell activity and the increase of toxicity exhibited a direct correlation with time and dosage. Overall, Tan IIA has the potential to trigger apoptosis in pancreatic carcinoma by inhibiting the anti-apoptotic proteins of TCTP, Mcl-1, Bcl-xL and initiating the Caspase pathway’s activation.
The mutation or deactivation of p53 and the bcl-2 overexpression are frequently present in malignant tumors (Vousden and Lane, 2007). A study has reported Tan IIA promoted apoptosis in breast cancer when 4T1 cells were exposed to it at varying concentrations for 24, 48, and 72 h (Liu et al., 2023). It was found that Tan IIA could significantly suppress proliferation and facilitate apoptosis, concurrently resulting in the reduction of Bcl-2 expression and upregulation of phosphorylated p53 and Bax. To conclude, this shows that Tan IIA serves as an anti-neoplastic agent in breast cancer by controlling the levels of suppressor gene p53 and apoptotic factor Bcl-2.
2.1.4 Caspase pathway
Caspases, endoprotease family, control cell death and inflammation. After signaling events, enzymes activate substrates and create a signaling cascade to induce apoptosis. A study in colon cancer discovered that dihydrotanshinone I-induced AIF increase significantly upregulated mitochondrial cytochrome c release in HCT116 cells (Wang et al., 2015a). At the same time, cytochrome c release stimulated caspase cascades that contributed to dihydrotanshinone I’s anti-colon cancer efficacy in vitro and in vivo. Additionally, Chiu et al. found that tan IIA increased ER stress by raising PERK, IRE1α, caspase-12, and ATF6 expression in BxPC3-derived xenograft tumors. These proteins caused eIF2α, and CHOP overexpression, leading to lowered Bcl-2 expression and enhanced caspase-3-mediated apoptosis in vivo (Chiu and Su, 2017). This finding indicated that tan IIA stimulated caspase cascades via ER stress, becoming a promising candidate for pancreatic cancer treatment.
A study in ovarian carcinoma revealed that tan IIA concentration-dependently increased caspase-9, caspase-3, and PARP cleavage due to survivin suppression (Lin et al., 2015). In this process, the effect of TRAIL was enhanced by tanshinone IIA through the downregulation of survivin in OVCAR3 and SKOV3 cells. Furthermore, Tan I, according to Liu et al. (2010), promoted apoptosis of myeloid leukemia cells via activating the caspase-3 cascade which might be associated with inactivation of the PI3K/Akt/survivin pathway. Similarly, during chronic myelogenous leukemia, tanshinone IIA decreased mitochondrial membrane potential (MMP), released cytochrome c, and triggered caspase-3 and 9, confirming mitochondria-dependent apoptosis (Yun et al., 2013). The Caspase cascade involved the increase of JNK and p38 phosphorylation expression in KBM 5 cells. In addition, tan IIA decreased hepatocellular carcinoma growth in a J5 xenograft animal model by boosting Bax and caspase 3 and lowering CD31 expression in vivo (Chien et al., 2012). Overall, tanshinone, according to different signaling events, modulates caspase expression to enhance the therapy of cancerous diseases.
2.1.5 miRNA
MicroRNA (miRNA) encompasses a group of non-coding, single-stranded RNA molecules that exert a pivotal part in the initiation and advancement of tumors (Zhang et al., 2017; Tang et al., 2018). According to research, the upregulation of miR-125 expression may potentially augment the stemness of breast cancer cells. Nevertheless, tanshinone IIA therapy reduced cell viability and raised the propensity for apoptosis in MCF-7 cells (Li et al., 2022a). Meanwhile, miR-125b expression was downregulated whereas STARD13 expression was upregulated. Additionally, study found that tanshinone IIA effectively impeded the growth of breast cells by modulating STARD13 expression, which was achieved through the decrease of miR-125b. This study is the first to propose that STARD13 contributes to the development of breast cells, in conjunction with the miR-125b/STARD13 axis.
Similarly, Ge et al. conducted research to determine if tanshinone IIA’s effects on colorectal cancer were due to the miRNA/AVEN axis (Ge and Zhang, 2022). Apoptosis and caspase activation inhibitor (AVEN) exerts a cancer-facilitating part in tumors (Choi et al., 2006; Eissmann et al., 2013; Han et al., 2015a; Baranski et al., 2015). An in vitro study showed that tanshinone IIA could concurrently suppress SW480/R cell proliferation by targeting miR-30b-5p and enhance apoptosis by targeting AVEN in colorectal cancer. The study showed that tanshinone IIA treatment significantly increased miR-30b-5p expression in SW480/R cells, and miR-30b-5p suppression fully negated the inhibition of SW480/R cells’ malignant behaviors using tan IIA. While this was happening, AVEN expression decreased whereas activities of cleaved-caspase 3, Bax enhanced concurrently. These results indicated that tanshinone IIA induced apoptosis in colorectal cancer by increasing miR-30b-5p and decreasing AVEN.
In non-small-cell lung cancer, apoptosis of A549 and H292 cells was facilitated by tanshinone IIA through the circ_0020123/miR-1299/HMGB3 axis, which raised miR-1299 expression while decreased circ_0020123 and HMGB3 expression (Sun et al., 2023). Similarly, in osteosarcoma, tanshinone I induced apoptosis of U2OS and MG63 cells targeting the circ_0000376/miR-432-5p/Bcl-2 axis, lowering circ_0000376 and Bcl-2 and elevating miR-432-5p (Ye et al., 2022). This was the first study to reveal the connection between miR-432-5p and circ_0000376 or Bcl-2. Additionally, Nie et al. (2020) found that tanshinone IIA affected acute leukemia cell viability via the miR-497-5p/Akt3 axis, elevating miR-497-5p and lowering Akt3. This study was the first to reveal that tan IIA controlled AML growth by upregulating miR-497-5p. Overall, circRNAs and miRNAs function as mediators in the progression of malignancies, providing novel avenues for cancer therapy. The mechanism by which it induces apoptosis can be viewed in Figure 2.
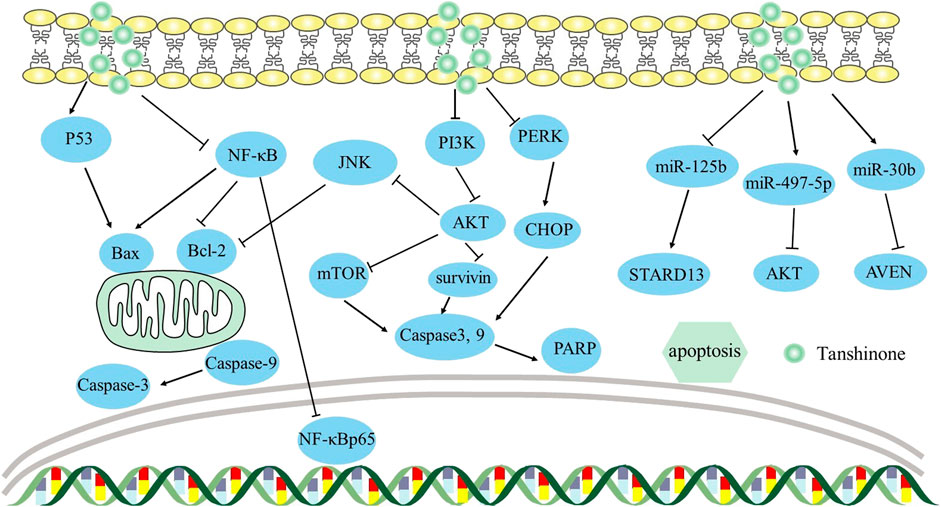
FIGURE 2. Mechanism of Tanshinone inducing apoptosis in tumor cells. Contents are as follows. Bcl-2 family: PI3K/AKT/JNK axis decreases Bcl-2 levels; NF-κB elevates Bax and decreases Bcl-2 levels; P53 elevates Bax. Caspase cascades: PI3K/Akt/survivin elevates Caspase-3 and Caspase-9 levels; PI3K/AKT/mTOR elevates Caspase-3 and Caspase-9 levels; PERK causes CHOP overexpression, leading to caspase-3-mediated apoptosis by ER stress. miRNA: miR-125b/STARD13 axis; miR-30b/AVEN; miR-497-5p/AKT.
2.2 Tanshinone induces tumor cell autophagy
In various cancer models, autophagy has two opposing effects. Autophagy has a cytoprotective impact in several cancer models but it could also convert from cytoprotective to cytotoxic autophagy in specific circumstances (Levy et al., 2017). Recently, Li et al. (2017) discovered that Tanshinone IIA reduced melanoma growth by disrupting the PI3K-Akt-mTOR-p70S6K1 axis and activating autophagocytosis in A375 cells. Similarly, Ding et al. (2017) found that tan IIA blocked the PI3K/Akt/mTOR pathway and lowered p-PI3K and p-Akt levels in U251 glioma cells, inducing autophagy and inhibiting cell viability. In acute promyelocytic leukemia, tan IIA blocked the PI3K/Akt/mTOR axis and promoted autophagy of NB4 cells, decreasing PI3K, Akt, and mTOR protein levels while raising p-ULK-1 and LC3B levels (Pan et al., 2021). Similar to these results, tan IIA inhibited PI3K/Akt/mTOR to trigger acute myeloid leukemia U937 cell death and autophagy in vitro and in vivo (Zhang et al., 2019c). In conclusion, these findings suggested that tan IIA could block the PI3K/Akt/mTOR axis to induce autophagy in cancerous cells. Additionally, Kim et al. (2022) found that tanshinone IIA blocked β-catenin translocation into the nucleus, leading to increased levels of autophagy-related genes (LC3B, beclin-1, Atg7) and the formation of autophagosomes in 786-O and Caki-1 cells, further inducing renal cell carcinoma cells autophagic death. Conversely, a study in hepatocellular carcinoma found that inhibition of p53/DRAM-mediated autophagy resulted in lower expression of LC3B and beclin-1, ultimately inducing cell death (Liu and Liu, 2020). Furthermore, in comparison to Tanshinone I alone, the autophagy inhibitor 3-MA dramatically reduced cell proliferation and viability in HepG2 and Huh7 cells. Taken together, autophagy has a contradictory effect on distinct cancer cell types, suggesting it might be a cancer therapy target.
Furthermore, according to many studies, tanshinone-induced cell autophagy involves ROS accumulation in many malignancies. Li et al. (2016a) observed that tanshinone IIA induced PC-3 cell autophagy by increasing beclin1 and LC3B expression in prostate cancer. In this process, tanshinone IIA-induced ROS was vital for autophagy start, while ROS scavenger NAC decreased beclin1, LC3B, and cleaved caspase-3 expression. Similarly, tanshinone I triggered ROS-dependent autophagy in glioblastoma, elevating LC3B and beclin-1 expression significantly in U87 MG cells (Jian et al., 2020). Taken together, these results showed that tanshinone-induced ROS was essential for autophagy initiation.
2.3 Tanshinone induces tumor cell ferroptosis
Ferroptosis is a non-apoptotic cell death that involves iron-dependent lipid peroxidation (Mou et al., 2019). At present, tan IIA’s ferroptosis mechanism against cancer has been identified, providing significant insight into its application in cancer intervention. In gastric cancer, tanshinone IIA caused BGC-823 and NCI-H87 cells ferroptosis via the p53/SLC7A11 pathway (Guan et al., 2020). It concentration-dependently upregulated p53 and inhibited xCT, lowering intracellular GSH and cysteine and increasing ROS levels to inhibit the growth of gastric cancer. Similarly, tanshinone IIA suppressed gastric cancer cell stemness via SLC7A11-mediated ferroptosis (Ni et al., 2022). The proportion of subpopulation CD44+ cells, a tumor stemness marker, was inhibited during this process. As a consequence, ferroptosis has been regarded as a prospective candidate for therapeutic intervention in cancer stem cells.
Additionally, tan IIA treatment significantly lowered the survival and invasiveness of FaDu cells by decreasing the ferroptosis gene FTH1, which is widely expressed in head and neck squamous cell carcinoma (Mao et al., 2022). This study also revealed that due to cancer cells’ high iron levels and higher sensitivity to ferroptosis, ferroptosis may be a potential cancer therapy.
2.4 Tanshinone induces tumor cell pyroptosis
Pyroptosis is a type of programmed cell death characterized by inflammation, which is correlated with the NLRP3 inflammasome (NLRP3, ASC, and caspase-1) and the release of pro-inflammatory proteins (IL-18, IL-1β) (Li et al., 2022b). Notably, gasdermin D (GSDMD) serves as the principal mediator of pyroptotic cell death. Tong et al. (2020) observed that tanshinone IIA exhibited the capacity to enhance pyroptosis in HeLa cells by regulating the miR-145/GSDMD cascade, causing a decrease in cell proliferation. This study was the first to link miR-145 to pyroptosis and consider miR-145 as a therapy target for cervical cancer. In addition, Wang et al. (2021) discovered that pyroptotic levels were raised in nasopharyngeal carcinoma by modifying the miR- 125b/foxp3/caspase-1 cascade. Tanshinone IIA increased GSDMD and caspase-1 expression, raised ROS and LDH levels, and upregulated IL-18 and IL-1β, thus triggering the pyroptosis of HK1 cells. Collectively, studies of pyroptosis might provide vital insights on the utilization of tan IIA in cancer therapy. The mechanism by which it induces pyroptosis can be viewed in Figure 3.
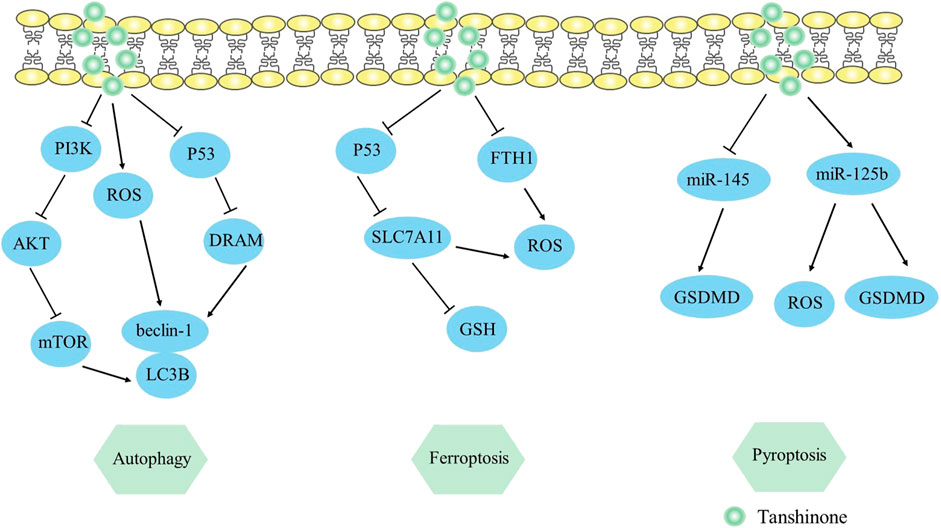
FIGURE 3. Mechanism of Tanshinone inducing autophagy, ferroptosis, pyroptosis in tumor cells. Contents are as follows. Autophagy: PI3K/AKT/mTOR elevates LC3B, beclin-1 levels; ROS elevates LC3B, beclin-1 levels; P53/DRAM mediates autophagy. Ferroptosis: P53/SLC7A11 pathway elevates ROS and decreases GSH expression; Decrease the ferroptosis gene FTH1. Pyroptosis: Regulate the miR-145/GSDMD cascade; miR-125b elevates GSDMD and ROS levels.
3 Tanshinone prevents tumor cells from proliferating
Malignant tumors often exhibit aberrant cell proliferation as one of their defining characteristics (Hanahan and Weinberg, 2011). In clinical practice, preventing the growth of tumor cells has proven to be an effective strategy in treating tumors.
3.1 MAPK signaling pathway
MAPK is classified as a serine/threonine kinase and is activated by various signals to enhance cell survival, proliferation, and apoptosis (Molina and Adjei, 2006). The MAPK pathway contains ERK, p38, and JNK. The p38 MAPK and the JNK MAPK pathways have been associated with anti-proliferative functions, but the ERK MAPK pathway appears to exert opposing effects (Nordstrom et al., 2009). According to the report, tan IIA boosted the production of p-p38 and p-JNK, while p-ERK expression was decreased. Meanwhile, the activation of p53 led to a rise in p21 level, which in turn decreased CDC2 and cyclin B1 expression, and triggered AGS cells to be arrested in the G2/M phase (Su, 2014). This indicated that Tan IIA likely caused the cell cycle to stall in gastric cancer by upregulating p-p38, p-JNK, p53, and p21, downregulating CDC2 and cyclin B1 levels. Consistently, Yan et al. (2012) observed that tanshinone IIA inhibited BT-20 human breast cancer cells’ ability to proliferate by activating ER stress (upregulating caspase-12, CHOP levels and downregulating Bcl-2 expression) and MAPK pathway (upregulating p-p38, p-JNK protein expression and downregulating p-ERK expression).
Another study on prostate cancer revealed that tanshinone analog treatment led to a reduction in survival and proliferation, possibly due to the increase in p38 and p53 proteins (Wang et al., 2019a). Subsequently, p38 and p53 respective downstream pathways such as cyclin b1/CDC2 and GADD45A/PLK1 were inactivated, inducing G2/M arrest in the two PCa cells. This research indicates that p38/cyclin b1/CDC2 and p53-dependent GADD45A/PLK1 pathways are two promising therapeutic strategies for inhibiting proliferation.
3.2 p21 protein
P21, a CDK inhibitor, modifies the G1 or S phases of the cell cycle (Roskoski, 2019). It plays a crucial role as a downstream target of p53, facilitating the growth-inhibitory consequences of p53 in neoplastic formations (el-Deiry et al., 1993). Given the important function of p53 in regulating cell cycle progression and the impact of p21/WAF1 proteins as CDK inhibitors, research regarding pertinent mechanisms has been conducted. Won et al. (2012) discovered that tanshinone IIA induced G1 stagnation by activating p53/p21 signaling and inhibiting androgen receptors in LNCaP cells. Furthermore, tanshinone IIA induced the cell cycle in prostate cancer to stall at the G1 phase by lowering cyclin D1, CDK2, and CDK4. Another research found that treatment with cryptotanshinone could potentially enhance P21 and P53 expression in melanoma (A375 cells), and reduce Rb phosphorylation, CDK2 activation, and cyclin A2 expression (Zanre et al., 2022). This report first reveals that cryptotanshinone inhibits human melanoma cell growth by reducing P21 and P53 protein expression.
The FOXM1 gene, which belongs to the FOX family, has been correlated with a negative prognosis in patients with cancerous tumors when it is overexpressed (Gormally et al., 2014). A study has shown that tanshinone IIA can reduce the expression of FOXM1 in gastric tumors, resulting in a rise in p21 and a decrease in PCNA and Ki-67 proteins, ultimately inhibiting the proliferation of SGC-7901 cells (Yu et al., 2017). Overall, tanshinone IIA could suppress the growth of gastric tumor cells by acting as a mediator of FOXM1.
3.3 STAT3 signaling pathway
The oncogene STAT3 has been extensively researched for the activation of its signal transduction pathway in tumorigenesis (Choudhari et al., 2007; Timofeeva et al., 2013). Given the important role of the JAK/STAT3 signaling pathway in tumor growth when IL-6 is activated in the microenvironment (Guo et al., 2012), studies on pertinent mechanisms have been undertaken. Wang et al. (2019b) found that tan I intervention remarkably prevented IL-6-induced JAK1/2 and STAT3 activation and concurrently suppressed the phosphorylation of JAK1/2 and STAT3. Additionally, this report implied that tan I could inhibit osteosarcoma cell growth and metastasis by restraining the interactions between STAT3 and its target genes Bcl-2, Cyclin B1, and MMP2. Additionally, another study revealed STAT3 may regulate SIRT3 production at the transcript stage, which further impacted levels of glycolysis-related proteins, including GLUT1, LDHA, and HK2. Cryptotanshinone was found to inhibit cell reproduction of ovarian cancer induced by glycolysis through suppression of the STAT3/SIRT3 pathway (Yang et al., 2018).
According to a study on gastric cancer, tan IIA reduced the survival of SNU-638, MKN1, and AGS cells in a manner dependent on dose and caused apoptosis by activating Bax and caspase 3 (Zhang et al., 2018). In the meantime, the level of phosphorylation of STAT3 was significantly inhibited and the same outcome was observed in the xenograft model. Notably, when SNU-638 cells were stimulated to overexpress STAT3, tan IIA was unable to inhibit cell reproduction. That is because STAT3 overexpression could counteract the influence of tan IIA on cell proliferation.
3.4 miR30b-P53-PTPN11/SHP2 axis
P53 was the sole transcription factor for the PTPN11 and the SHP2 protein was encoded by PTPN11 (Bentires-Alj et al., 2004; Han et al., 2015b). The research on hepatocellular carcinoma revealed that tanshinone IIA decreased the viability of HepG2 cells dose-dependently and inhibited proliferation with the inactivation of miR30b (Ren et al., 2017). Meanwhile, levels of P53 and P21 were notably increased, while Cyclin D1 and CDK6 were reduced in HepG2 cells. Notably, suppression of miR30b could trigger the expression of P53-PTPN11/SHP2 in HepG2 cells, and downregulation of cell cycle-related proteins, thereby inducing HepG2 cell cycle stagnation at G1/G0 checkpoints. Overall, Tanshinone IIA hindered the growth of liver carcinoma cells by blocking miR30b expression and stimulating the P53-PTPN11/SHP2 axis. The mechanism by which it inhibits proliferation is depicted in Figure 4.
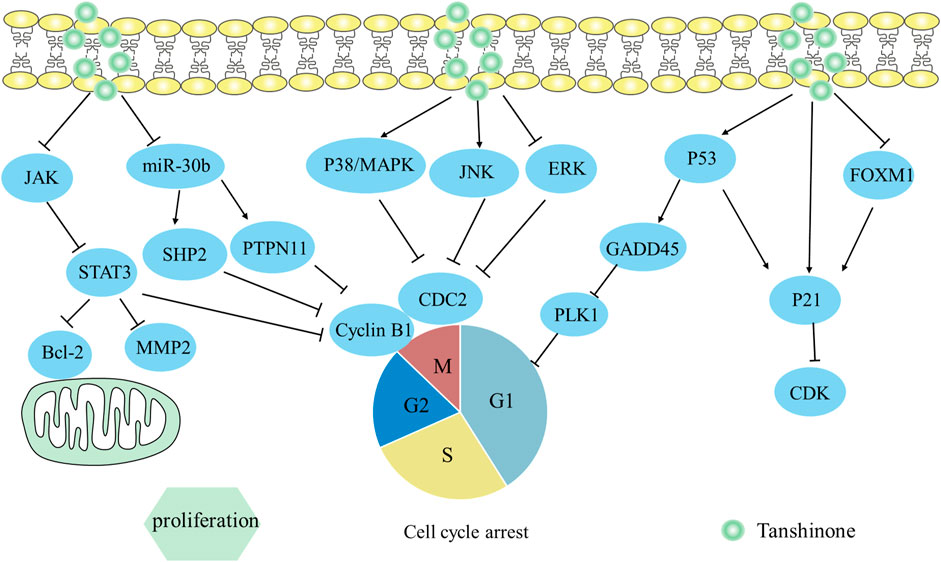
FIGURE 4. Mechanism of Tanshinone inhibiting proliferation in tumor cells. Contents are as follows. JAK/STAT3 restrains its target genes Bcl-2, Cyclin B1; miR30b-P53-PTPN11/SHP2 downregulate cell cycle-related proteins; Activate p38 MAPK and JNK MAPK pathways, but inactivate ERK MAPK pathway to downregulate CDC2 and cyclin B1; Activate p53-dependent GADD45A/PLK1 pathway; Activate p53/p21; Activate FOXM1/p21.
4 Tanshinone prevents tumor cells from migrating and invading
Tumor cells possess the capacity for migration and incursion (Friedl and Wolf, 2003), which are the hallmarks of tumor malignancy (Zeng et al., 2019). Incursion requires active migration to occur beforehand (Entschladen et al., 2004), thus it is effective in impeding the migration and incursion of cancerous cells when treating tumors.
4.1 Tanshinone hinders tumor cells migration and invasion
4.1.1 EMT
The activation of EMT enables cancerous cells to migrate and invade, assuming a pivotal role in cancer’s progression (Mani et al., 2008). EMT is a biological process where cells shift from epithelium to mesenchymal, and gain increased motility and invasive abilities by secreting MMPs. And it also can be induced by EGF and TGF-β1 (Puisieux et al., 2014). In accordance with a study, the level of EMT indicator E-cadherin was substantially decreased when induced by EGF and TGF-β1, while levels of Vimentin, N-cadherin, MMPs, and transcription factors (Snail, Slug, Twist) were significantly elevated in HepG2 cells (Zhang et al., 2019a). In that study, tan IIA can reverse EMT biomarker protein (MMP-2, E-cadherin, N-cadherin, vimentin, and Snail) changes in HepG2 cells, counteracting EMT triggered by EGF and TGF-β1. Additionally, elevated p-PI3K, p-Akt, and p-ERK expression were successfully reversed. This showed that Tan IIA hindered the process of EMT on liver cancer triggered by EGF and TGF-β1 by regulating the PI3K/Akt/ERK axis.
In colorectal cancer, the vitro study proved that tan IIA might hinder EMT in SW480 cells via reducing MMP-9 and Vimentin while raising E-cadherin, hence preventing cell migration and incursion (Zhang et al., 2016). Similarly, tanshinone derivatives inhibited prostate cancer cell metastasis by reducing VEGF-1 and MMP-9 protein expression (Wang et al., 2019a).
Additionally, Wang et al. (2023) observed that tanshinone derivative reduced Cavin-1 expression in NSCLC cells and suppressed the EMT process by decreasing levels of EMT indicators (N-cadherin, Vimentin, snail, slug, MMP2/7/9). In the meantime, tanshinone derivative efficiently reduced the ERK/Smad2 axis’s activation in normal cells and Cavin-1 transfected cell lines. This indicated tanshinone derivative impeded migration and incursion of NSCLC cells by suppressing the Cavin-1-mediated ERK/Smad2 axis.
4.1.2 β-catenin
The molecule β-catenin is crucial for cancer cell motility, invasion, and angiogenesis, which is part of the Wnt/β-catenin pathway (Sui et al., 2015). β-catenin is liberated from the Wnt/β-catenin protein complex when activated by its upstream genes like TGF-β1 and HIF-1α (Jeong and Park, 2013). Subsequently, β-catenin can be combined with TCF/LEF and trigger the production of VEGF synergistically (Wu et al., 2015), leading to the promotion of angiogenesis and migration of tumors. Sui et al. (2017) discovered that tanIIA hindered VEGF-mediated angiogenesis under normoxic and hypoxic conditions. It worked by blocking different signaling pathways in each condition: TGF-β1/β-catenin/TCF3/LEF1 in normoxic conditions and HIF-1α/β-catenin/TCF3/LEF1 in hypoxic conditions. This implied that tan IIA potentially impeded colorectal cancer’s spread by blocking the β-catenin/TCF3/LEF1 signaling pathway via the suppression of TGF-β1, HIF-1α.
Another study in colon cancer revealed that tanshinone IIA impeded the incursion and migration of HC8693 cells by suppressing angiogenesis (Ma et al., 2018). In the meantime, the Wnt/β-catenin axis was inactivated, together with reductions in COX-2 and VEGF. Notably, the reduction of β-catenin can result in a decrease in VEGF expression and lead to block angiogenesis in tumor cells, thus affecting tumor cell growth and migration (Kim et al., 2016; Fu et al., 2017). Overall, tanshinone IIA suppresses the migration of colorectal carcinoma cells by blocking the COX-2-Wnt/β-catenin axis.
Yuan et al. (2020) discovered that tan IIA exerted an anti-invasion impact on gastric cancer, in a way that depended on time and concentration. During the study, levels of HMGB2, β-catenin, and various downstream molecules including c-myc, cyclin D1, N-card, and Vimentin were reduced simultaneously through the use of tan IIA intervention. Additionally, miR-874 overexpression had a negative effect on the HMGB2/β-catenin pathway, which suggested targeting miR-874 could be an important strategy for treating gastric cancer. This result indicated that tan IIA inhibited the spread and incursion of AGS, MGC-803 cells by the miR-874/HMGB2/β-catenin pathway.
4.1.3 Hippo signaling pathway
The dysregulation of the Hippo pathway has the potential to cause tumor metastasis and oncogenic effects (Qiao et al., 2018). It is comprised of a principal kinase cascade that includes Mst1/2, and LATS1/2, as well as downstream transcription co-activators YAP or TAZ. Qian et al. (2018) discovered that tan IIA/IL-2 activated the Mst1-Hippo pathway and elicited INF2-related mitochondrial fission of SW480 cells, subsequently promoting apoptosis and inhibiting migration in colorectal cancer. This finding suggested a strong relationship between the Mst1-Hippo pathway and mitochondria.
Furthermore, YAP is a crucial component of the Hippo pathway. A study has been discovered regarding the relationship between SMAD7 and the Hippo/YAP axis in liver carcinoma (Ma et al., 2019). SMAD7 negatively regulates the Hippo/YAP pathway which could promote E3 ligase βTrcp expression to advance the degradation of YAP protein. Ma et al. (2019) observed that Tan IIA could boost E-cadherin and SMAD7 expression and reduce N-cadherin and YAP expression, which inhibited the metastasis and invasion of Bel-7404, SMMC-7721, and Bel-7402 cells. This indicated that tan IIA could impede the spread and incursion of liver cancer via inhibiting the Hippo/YAP pathway.
Qin et al. (2018) discovered that tan IIA therapy inhibited HuR translocation from the nucleus’s interior, causing a substantial downregulation of YAP which was highly expressed in cervix carcinoma. Meanwhile, the downstream target protein E-cadherin increased, while N-cadherin decreased, which weakened the capacity of HeLa and C33A cells to metastasize and invade. This indicated that tan IIA was capable of hindering cervical carcinoma cells from spreading and invading via decreasing the production of YAP.
4.1.4 Skp2 signaling pathway
Skp2 is elevated in human cancer and may be crucial for the progression of cancer (Lin et al., 2017). Skp2 could accelerate the spread of cancer by regulating the proteins involved in EMT, such as MMP-9 and snail1 (Hung et al., 2010; Wei et al., 2013; Yang et al., 2014). Consistently, the Skp2 complex controls RhoA transcription, which is crucial for cancer migration (Chan et al., 2010). According to research, dihydrotanshinone I could reduce the expression of Skp2 in HCT 116 and HT29 cells, leading to lower levels of RhoA and snail1, ultimately inhibiting the spread of colon cancer (Lin et al., 2017). In addition, dihydrotanshinone I also exerted the prostate cancer’s ability to forestall metastasis by mediating Skp2 in vitro. Meanwhile, Skp2 and its downstream EMT genes’ expression, including RhoA and snail1, were inhibited following dihydrotanshinone I treatment in a manner dependent on concentration, which resulted in reduced invasiveness of PC-3 cells (Wu et al., 2017). These studies showed that inhibiting Skp2 could be a promising strategy for cancer treatment. Its particular mechanism for preventing migration and incursion is depicted in Figure 5.
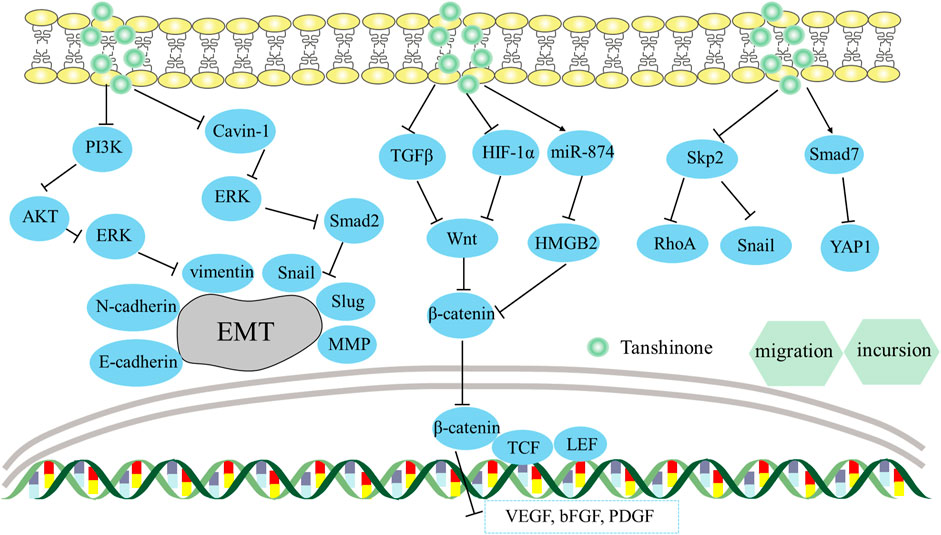
FIGURE 5. Mechanism of Tanshinone preventing migration and incursion in tumor cells. Contents are as follows. Hinder EMT via regulating the PI3K/Akt/ERK axis; Suppress the Cavin-1-mediated ERK/Smad2 axis; Block the β-catenin/TCF3/LEF1 axis via the suppression of TGF-β1, HIF-1α; Regulate miR-874/HMGB2/β-catenin pathway; Inhibit Skp2 and its downstream EMT genes’ expression, including RhoA and Snail; Boost SMAD7 levels and reduce YAP levels.
4.2 Tanshinone inhibits tumor angiogenesis and permeability
Tumour angiogenesis helps tumors develop, invade, and metastasize. A recent study showed that the hypoxic microenvironment is the main mechanism that shifts tumors from avascular to angiogenesis (Huang et al., 2016). The hypoxic microenvironment stimulates HIF-1α in tumor cells, leading to higher expression of angiogenic factors like VEGF and bFGF, which promote tumor formation and vascularization. Conversely, Angiostatin and Endostatin hinder angiogenesis. Study data in lung cancer showed VEGF expression reduced while Angiostatin and Endostatin expressions elevated, implying that tanshinone IIA treatment decreased angiogenesis of tumor cells. In addition, COX-2 overexpression also triggers increased VEGF production, exerting a distinctly encouraging effect on angiogenesis. Zhou et al. (2012) found that tan II dramatically reduced COX-2 and VEGF levels in HCT-116 cells, blocking the angiogenesis of colorectal cancer.
Furthermore, HIF-1α expression is still a major marker for detecting cancer neovascularization (Kasai et al., 2018). Research revealed that tanshinone I could attenuate hypoxia-induced HIF-1α accumulation and phosphorylation of Tyr705-STAT3 in MCF-7 cells (Wang et al., 2015b). Subsequently, a decrease in VEGF secretion could prevent endothelial cell activation, exerting tanshinone I’s antiangiogenesis effects. Additionally, Zhou et al. (2020b) discovered that tan IIA reduced HUVEC proliferation, tube formation, and metastasis by decreasing HIF-1α, VEGF, and bFGF expression dose-dependently in HCT-116 cells, preventing angiogenesis in colorectal cancer. Taken together, these findings demonstrate that tanshinone-mediated HIF-1α emerges as an alternative cancer treatment.
Tumour blood vessels have abnormal morphology, resulting in permeable, immature, weak, and poorly perfused vessels. Tumour blood vessels’ permeable profile impedes the transportation of oxygen, immune cells, and therapeutic drugs, resulting in an acidic and hypoxic milieu, impaired medication delivery, and immune cell infiltration (Viallard and Larrivée, 2017). Zou et al. (2021) found that tan IIA lowered blood vessel permeability and improved colon cancer’s vascular integrity by targeting the Ang2-Tie2-AKT-MLCK pathway in transplanted HT-29 tumors. Overall, reverting premature and permeable blood vessels to normal blood vessels could become an efficient tumor treatment method.
4.3 Tanshinone suppresses the formation of metastases
Surgery is necessary for most solid tumors, however, it might lead to recurrent and metastatic lesions, hence it is vital to avoid metastases after surgery. Wang et al. (2012) found that tanshinone IIA can reduce hepatocellular carcinoma metastasis after palliative resection via normalizing VEGFR1/PDGFR-related vascular function. It did significantly raise microvessel integrity throughout this procedure. This finding suggested that proangiogenic “vessel normalizing” treatments may block the formation of metastases and improve patients’ survival. Besides, in colon carcinoma, tanshinone IIA reduced uPA, MMP-2, and MMP-9 and increased TIMP-1, and TIMP-2 dose-dependently to limit the formation of metastases in vitro and in vivo (Shan et al., 2009). Furthermore, tanshinone lowered HMGB1, a metastasis-related gene, limiting the metastases of gastric cancer (Nishiguchi et al., 2019). Therefore, tanshione may be an option for blocking the formation of metastases.
5 Tanshinone increases the sensitivity of radiation and chemotherapy
Chemotherapy and radiotherapy are known to cause negative effects and increase drug resistance (Sekeres et al., 2021). A relevant study on lung cancer found that H358-IR and H157-IR cells of radio-resistance had notably lower levels of Phosphoribosyl pyrophosphate aminotransferase (PPAT) after being treated with tanshinone I. This treatment also led to raised Caspase 3 and Caspase 8 levels, more DNA damage, and a notable increase in apoptosis (Yan et al., 2018). This finding suggests that tanshinone I has the potential to improve cell sensitivity to radiotherapy by reducing the levels of PPAT, which is known to promote tumor growth in cells.
The obstacles of chemotherapy are closely related to multidrug resistance (MDR). The primary cause of MDR is the increased levels of ABC transporter proteins, such as MRP1, P-gp, and BCRP, expressed in cancerous cells (Amawi et al., 2019). Cancer cells that are resistant to chemotherapy may overexpress export transporters, which can transfer chemotherapeutics away from cancer cells, and decrease their cellular storage and effectiveness for treatment. According to a study, tan IIA can reduce the expression of MRP1 in gastric carcinoma cells, which causes SNU-719R cell cycle stagnation and apoptosis (Xu et al., 2018). Co-treatment of tanshinone IIA and doxorubicin promoted apoptosis by modulating the expression of Bcl-2, Bax, and P53, and triggered G2/M stage cell cycle stagnation by modulating levels of cyclin B1 and CDK1. There was also a study reporting that cryptotanshinone and dihydrotanshinone reversed doxorubicin and irinotecan resistance in SW620 cells of colon cancer by decreasing the level of P-gp mRNA and blocking P-gp ATPase activity (Hu et al., 2014). Taken together, reductions of MDR expression and function have a significant impact on chemotherapy.
It was also reported that tanshinone IIA may be effective in overcoming doxorubicin resistance in MCF-7 cells of breast cancer, which was achieved by blocking the PI3K/AKT axis and reducing levels of ABC transporter proteins, including MRP1, P-gp, and BCRP (Li et al., 2019). Moreover, Tan IIA’s intervention prevented AKT activation due to the increase of PTEN expression. In vivo study also revealed that tan IIA improved the effectiveness of doxorubicin in treating AGS cell xenograft tumors of nude mice (Li et al., 2019), while simultaneously mitigating negative adverse effects, such as loss of weight, myelosuppression, and renal toxicity. The mechanism by which it enhances the body’s response to chemotherapy is viewed in Figure 6.
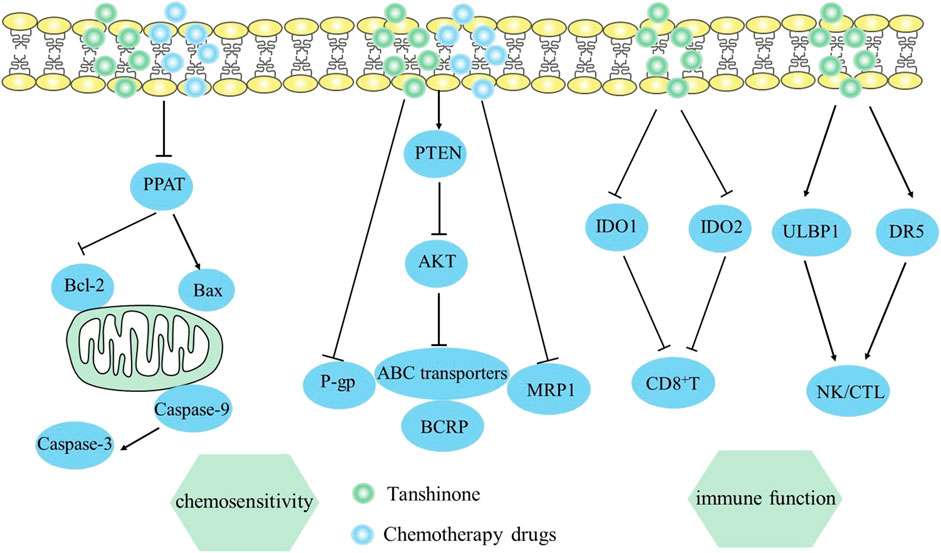
FIGURE 6. Mechanism of Tanshinone increasing sensitivity of chemotherapy and hindering immune evasion in tumor cells. Contents are as follows. Sensitivity of chemotherapy: Increase PTEN and block the PI3K/AKT axis expression to reduce ABC transporter proteins. Immune evasion: Increase ULBP1 and DR5 to enhance NK/CTL activities; Suppress IDO1 and TDO2 to elevate CD8+ T cells.
6 Tanshinone hinders tumor immune evasion
Multiple immune evasion mechanisms in cancer cell proliferation contribute to their ongoing occurrence and development, making immune response crucial for tumor treatment (Li et al., 2016b). The study in lung cancer found that tanshinone IIA treatment significantly increased CD4+ and CD4+/CD8+ levels in vivo, as well as NK cell activity, further improving immune function and strengthen anti-tumor effect (Li et al., 2016b). Similarly, Sun et al. (2020) found that tanshinone IIA might improve NSCLC cell sensitivity to NK cell-mediated lysis by increasing ULBP1 and DR5, demonstrating tanshinone IIA’s potential in NK cell-based cancer immunotherapy. Additionally, mice treated with tanshinone and radix astragali enhanced CD4+ and CD8+ percentages and NK/CTL activities in vivo, affecting immune system functions in melanoma (Wu et al., 2016). Zhang et al. (2022) indicated that tanshinone IIA sulfonate might be used as an immunotherapy for colorectal cancer by suppressing IDO1 and TDO2 that promote tumor immune evasion. Ultimately, tanshinone IIA sulfonate enhanced tumor immunotherapy by decreasing Tregs and elevating CD8+ T cells respectively. Overall, tanshinone enhances the cytotoxicity of CD8+ T cells or NK cells, avoiding tumor immune escapes. The mechanism by which it hinders tumor immune evasion is viewed in Figure 6.
7 Summary and perspective
Tanshinone has garnered significant focus due to its various pharmacological effects. Several studies have demonstrated its ability to fight tumors through diverse molecular mechanisms such as apoptosis, ferroptosis, pyroptosis, reproduction, migration, incursion, chemosensitivity, and immune evasion. Regardless of multiple studies, further research is still required to fully comprehend the tumor-fighting mechanism of tanshinone. There are techniques such as network pharmacology and molecular docking that can be used to investigate potential mechanisms. However, it is also vital to conduct experimental validation in order to provide more reliable references for clinical workers.
Moreover, tanshinone is a crucial component in chemotherapy, and its impact on a patient’s prognosis is significant. When used alongside other chemotherapy medications, tanshinone can enhance their effectiveness and improve patient outcomes. However, one limitation of tanshinone is its limited bioavailability when taken orally. Thus, researchers are working on developing new formulations to improve its pharmacokinetic properties. To conclude, more research is needed to fully comprehend the potential benefits of tanshinone for clinical use in the future.
Author contributions
PZ: Writing–original draft, Writing–review and editing. WL: Formal Analysis, Writing–review and editing. YW: Writing–review and editing.
Funding
The author(s) declare financial support was received for the research, authorship, and/or publication of this article. This study was supported by the Natural Science Foundation of China (Grant No. 81703839), Scientific Innovation Team of Shandong University of Traditional Chinese Medicine (2020-54-17), Major Basic Research Projects of Natural Science Foundation of Shandong Province (No. ZR2019ZD23), Jinan Higher Education Institution Innovation Team Project (2020GXRC012), Team Project of “Qingchuang Science and Technology Plan” in Shandong Higher Education Institutions (No. 2019KJK013).
Conflict of interest
The authors declare that the research was conducted in the absence of any commercial or financial relationships that could be construed as a potential conflict of interest.
Publisher’s note
All claims expressed in this article are solely those of the authors and do not necessarily represent those of their affiliated organizations, or those of the publisher, the editors and the reviewers. Any product that may be evaluated in this article, or claim that may be made by its manufacturer, is not guaranteed or endorsed by the publisher.
References
Amawi, H., Sim, H. M., Tiwari, A. K., Ambudkar, S. V., and Shukla, S. (2019). ABC transporter-mediated multidrug-resistant cancer. Adv. Exp. Med. Biol. 114 (1), 549–580. doi:10.1007/978-981-13-7647-4_12
Bai, Y., Zhang, L., Fang, X., and Yang, Y. (2016). Tanshinone IIA enhances chemosensitivity of colon cancer cells by suppressing nuclear factor-κB. Exp. Ther. Med. 11 (3), 1085–1089. doi:10.3892/etm.2016.2984
Baranski, Z., Booij, T. H., Cleton-Jansen, A. M., Price, L. S., van de Water, B., Bovee, J. V., et al. (2015). Aven-mediated checkpoint kinase control regulates proliferation and resistance to chemotherapy in conventional osteosarcoma. J. Pathol. 236 (3), 348–359. doi:10.1002/path.4528
Bentires-Alj, M., Paez, J. G., David, F. S., Keilhack, H., Halmos, B., Naoki, K., et al. (2004). Activating mutations of the noonan syndrome-associated SHP2/PTPN11 gene in human solid tumors and adult acute myelogenous leukemia. Cancer Res. 64 (25), 8816–8820. doi:10.1158/0008-5472.CAN-04-1923
Chan, C. H., Lee, S. W., Li, C. F., Wang, J., Yang, W. L., Wu, C. Y., et al. (2010). Deciphering the transcriptional complex critical for RhoA gene expression and cancer metastasis. Nat. Cell Biol. 12 (5), 457–467. doi:10.1038/ncb2047
Chen, H., Shu, H., Su, W., Li, B., Zhang, H., Li, L., et al. (2022). Tanshinone IIA has a potential therapeutic effect on kawasaki disease and suppresses megakaryocytes in rabbits with immune vasculitis. Front. Cardiovasc Med. 9, 873851–055X. doi:10.3389/fcvm.2022.873851
Chien, S. Y., Kuo, S. J., Chen, Y. L., Chen, D. R., Cheng, C. Y., and Su, C. C. (2012). Tanshinone IIA inhibits human hepatocellular carcinoma J5 cell growth by increasing Bax and caspase 3 and decreasing CD31 expression in vivo. Mol. Med. Rep. 5 (1), 282–286. doi:10.3892/mmr.2011.631
Chiu, T. L., and Su, C. C. (2017). Tanshinone IIA increases protein expression levels of PERK, ATF6, IRE1α, CHOP, caspase‑3 and caspase‑12 in pancreatic cancer BxPC‑3 cell‑derived xenograft tumors. Mol. Med. Rep. 15 (5), 3259–3263. doi:10.3892/mmr.2017.6359
Choi, J., Hwang, Y. K., Sung, K. W., Kim, D. H., Yoo, K. H., Jung, H. L., et al. (2006). Aven overexpression: association with poor prognosis in childhood acute lymphoblastic leukemia. Leuk. Res. 30 (8), 1019–1025. doi:10.1016/j.leukres.2005.11.001
Choudhari, S. R., Khan, M. A., Harris, G., Picker, D., Jacob, G. S., Block, T., et al. (2007). Deactivation of Akt and STAT3 signaling promotes apoptosis, inhibits proliferation, and enhances the sensitivity of hepatocellular carcinoma cells to an anticancer agent, Atiprimod. Atiprimod. Mol. Cancer Ther. 6 (1), 112–121. doi:10.1158/1535-7163.MCT-06-0561
Ding, L., Ding, L., Wang, S., Wang, S., Wang, W., Wang, W., et al. (2017). Tanshinone IIA affects autophagy and apoptosis of glioma cells by inhibiting phosphatidylinositol 3-kinase/akt/mammalian target of rapamycin signaling pathway. Pharmacology 99 (3-4), 188–195. doi:10.1159/000452340
Eissmann, M., Melzer, I. M., Fernandez, S. B., Michel, G., Hrabe de Angelis, M., Hoefler, G., et al. (2013). Overexpression of the anti-apoptotic protein AVEN contributes to increased malignancy in hematopoietic neoplasms. Oncogene 32 (20), 2586–2591. doi:10.1038/onc.2012.263
el-Deiry, W. S., Tokino, T., Velculescu, V. E., Levy, D. B., Parsons, R., Trent, J. M., et al. (1993). WAF1, a potential mediator of p53 tumor suppression. Cell 75 (4), 817–825. doi:10.1016/0092-8674(93)90500-p
Entschladen, F., Drell, T. L. t., Lang, K., Joseph, J., and Zaenker, K. S. (2004). Tumour-cell migration, invasion, and metastasis: navigation by neurotransmitters. Lancet Oncol. 5 (4), 254–258. doi:10.1016/S1470-2045(04)01431-7
Feng, F., Li, N., Cheng, P., Zhang, H., Wang, H., Wang, Y., et al. (2020). Tanshinone IIA attenuates silica-induced pulmonary fibrosis via inhibition of TGF-β1-Smad signaling pathway. Biomed. Pharmacother. 121, 109586. doi:10.1016/j.biopha.2019.109586
Feng, J., Liu, L., Yao, F., Zhou, D., He, Y., and Wang, J. (2021). The protective effect of tanshinone IIa on endothelial cells: a generalist among clinical therapeutics. Expert Rev. Clin. Pharmacol. 14 (2), 239–248. doi:10.1080/17512433.2021.1878877
Friedl, P., and Wolf, K. (2003). Tumour-cell invasion and migration: diversity and escape mechanisms. Nat. Rev. Cancer 3 (5), 362–374. doi:10.1038/nrc1075
Fu, Q. R., Song, W., Deng, Y. T., Li, H. L., Mao, X. M., Lin, C. L., et al. (2017). ESC-3 induces apoptosis of human ovarian carcinomas through Wnt/β-catenin and Notch signaling in vitro and in vivo. Int. J. Oncol. 50 (1), 241–251. doi:10.3892/ijo.2016.3773
Ge, T., and Zhang, Y. (2022). Tanshinone IIA reverses oxaliplatin resistance in colorectal cancer through microRNA-30b-5p/AVEN axis. Open Med. (Wars) 17 (1), 1228–1240. doi:10.1515/med-2022-0512
Gormally, M. V., Dexheimer, T. S., Marsico, G., Sanders, D. A., Lowe, C., Matak-Vinkovic, D., et al. (2014). Suppression of the FOXM1 transcriptional programme via novel small molecule inhibition. Nat. Commun. 5, 5165. doi:10.1038/ncomms6165
Graidist, P., Phongdara, A., and Fujise, K. (2004). Antiapoptotic protein partners fortilin and MCL1 independently protect cells from 5-fluorouracil-induced cytotoxicity. J. Biol. Chem. 279 (39), 40868–40875. doi:10.1074/jbc.M401454200
Guan, Z., Chen, J., Li, X., and Dong, N. (2020). Tanshinone IIA induces ferroptosis in gastric cancer cells through p53-mediated SLC7A11 down-regulation. Biosci. Rep. 40 (8). doi:10.1042/BSR20201807
Guo, Y., Xu, F., Lu, T., Duan, Z., and Zhang, Z. (2012). Interleukin-6 signaling pathway in targeted therapy for cancer. Cancer Treat. Rev. 38 (7), 904–910. doi:10.1016/j.ctrv.2012.04.007
Han, K. Y., Hwang, J. W., Bae, G. U., Kim, S. N., and Kim, Y. K. (2015a). Akt regulation of Aven contributes to the sensitivity of cancer cells to chemotherapeutic agents. Mol. Med. Rep. 11 (5), 3866–3871. doi:10.3892/mmr.2015.3158
Han, T., Xiang, D. M., Sun, W., Liu, N., Sun, H. L., Wen, W., et al. (2015b). PTPN11/Shp2 overexpression enhances liver cancer progression and predicts poor prognosis of patients. J. Hepatol. 63 (3), 651–660. doi:10.1016/j.jhep.2015.03.036
Hanahan, D., and Weinberg, R. A. (2011). Hallmarks of cancer: the next generation. Cell 144 (5), 646–674. doi:10.1016/j.cell.2011.02.013
Hu, T., To, K. K., Wang, L., Zhang, L., Lu, L., Shen, J., et al. (2014). Reversal of P-glycoprotein (P-gp) mediated multidrug resistance in colon cancer cells by cryptotanshinone and dihydrotanshinone of Salvia miltiorrhiza. Phytomedicine 21 (11), 1264–1272. doi:10.1016/j.phymed.2014.06.013
Huang, C. Y., Chiu, T. L., Kuo, S. J., Chien, S. Y., Chen, D. R., and Su, C. C. (2013). Tanshinone IIA inhibits the growth of pancreatic cancer BxPC-3 cells by decreasing protein expression of TCTP, MCL-1 and Bcl-xL. Mol. Med. Rep. 7 (3), 1045–1049. doi:10.3892/mmr.2013.1290
Huang, W. C., Chen, S. H., Chiang, W. H., Huang, C. W., Lo, C. L., Chern, C. S., et al. (2016). Tumor microenvironment-responsive nanoparticle delivery of chemotherapy for enhanced selective cellular uptake and transportation within tumor. Biomacromolecules 17 (12), 3883–3892. doi:10.1021/acs.biomac.6b00956
Hung, W. C., Tseng, W. L., Shiea, J., and Chang, H. C. (2010). Skp2 overexpression increases the expression of MMP-2 and MMP-9 and invasion of lung cancer cells. Cancer Lett. 288 (2), 156–161. doi:10.1016/j.canlet.2009.06.032
Jeong, J. K., and Park, S. Y. (2013). HIF-1α-induced β-catenin activation prevents prion-mediated neurotoxicity. Int. J. Mol. Med. 32 (4), 931–937. doi:10.3892/ijmm.2013.1457
Jian, S., Chen, L., Minxue, L., Hongmin, C., Ronghua, T., Xiaoxuan, F., et al. (2020). Tanshinone I induces apoptosis and protective autophagy in human glioblastoma cells via a reactive oxygen species-dependent pathway. Int. J. Mol. Med. 45 (4), 983–992. doi:10.3892/ijmm.2020.4499
Jieensinue, S., Zhu, H., Li, G., Dong, K., Liang, M., and Li, Y. (2018). Tanshinone IIA reduces SW837 colorectal cancer cell viability via the promotion of mitochondrial fission by activating JNK-Mff signaling pathways. BMC Cell Biol. 19 (1), 21. doi:10.1186/s12860-018-0174-z
Jin, Z., Chenghao, Y., and Cheng, P. (2021). Anticancer effect of tanshinones on female breast cancer and gynecological cancer. Front. Pharmacol. 12, 824531. doi:10.3389/fphar.2021.824531
Kasai, M., Van Damme, N., Berardi, G., Geboes, K., Laurent, S., and Troisi, R. I. (2018). The inflammatory response to stress and angiogenesis in liver resection for colorectal liver metastases: a randomized controlled trial comparing open versus laparoscopic approach. Acta Chir. Belg 118 (3), 172–180. doi:10.1080/00015458.2017.1407118
Ke, F., Wang, Z., Song, X., Ma, Q., Hu, Y., Jiang, L., et al. (2017). Cryptotanshinone induces cell cycle arrest and apoptosis through the JAK2/STAT3 and PI3K/Akt/NFκB pathways in cholangiocarcinoma cells. Drug Des. Devel Ther. 11, 1753–1766. doi:10.2147/DDDT.S132488
Kim, N. Y., Jung, Y. Y., Yang, M. H., Chinnathambi, A., Govindasamy, C., Narula, A. S., et al. (2022). Tanshinone IIA exerts autophagic cell death through down-regulation of β-catenin in renal cell carcinoma cells. Biochimie 200, 119–130. doi:10.1016/j.biochi.2022.05.018
Kim, Y. E., Choi, H. C., Lee, I. C., Yuk, D. Y., Lee, H., and Choi, B. Y. (2016). 3-Deoxysappanchalcone promotes proliferation of human hair follicle dermal papilla cells and hair growth in C57bl/6 mice by modulating WNT/β-Catenin and STAT signaling. Biomol. Ther. Seoul. 24 (6), 572–580. doi:10.4062/biomolther.2016.183
Lee, W. Y., Cheung, C. C., Liu, K. W., Fung, K. P., Wong, J., Lai, P. B. S., Yeung, J. H. K., et al. (2010). Cytotoxic effects of tanshinones from Salvia miltiorrhiza on doxorubicin-resistant human liver cancer cells. J. Nat. Prod. 73 (5), 854–859. doi:10.1021/np900792p
Levy, J. M. M., Towers, C. G., and Thorburn, A. (2017). Targeting autophagy in cancer. Nat. Rev. Cancer 17 (9), 528–542. doi:10.1038/nrc.2017.53
Li, C., Han, X., Zhang, H., Wu, J., and Li, B. (2016a). The interplay between autophagy and apoptosis induced by tanshinone IIA in prostate cancer cells. Tumour Biol. 37 (6), 7667–7674. doi:10.1007/s13277-015-4602-9
Li, K., Liu, W., Zhao, Q., Wu, C., Fan, C., Lai, H., et al. (2019). Combination of tanshinone IIA and doxorubicin possesses synergism and attenuation effects on doxorubicin in the treatment of breast cancer. Phytother. Res. 33 (6), 1658–1669. doi:10.1002/ptr.6353
Li, Q., Hu, K., Tang, S., Xu, L. F., and Luo, Y. C. (2016b). Anti-tumor activity of tanshinone IIA in combined with cyclophosphamide against Lewis mice with lung cancer. Asian Pac J. Trop. Med. 9 (11), 1084–1088. doi:10.1016/j.apjtm.2016.09.003
Li, X., Jia, Q., Zhou, Y., Jiang, X., Song, L., Wu, Y., et al. (2022a). Tanshinone IIA attenuates the stemness of breast cancer cells via targeting the miR-125b/STARD13 axis. Exp. Hematol. Oncol. 11 (1), 2. doi:10.1186/s40164-022-00255-4
Li, X., Li, Z., Li, X., Liu, B., and Liu, Z. (2017). Mechanisms of Tanshinone II a inhibits malignant melanoma development through blocking autophagy signal transduction in A375 cell. BMC Cancer 17 (1), 357. doi:10.1186/s12885-017-3329-y
Li, Y., Fu, Y., Sun, J., Shen, J., Liu, F., Ning, B., et al. (2022b). Tanshinone IIA alleviates NLRP3 inflammasome-mediated pyroptosis in Mycobacterium tuberculosis-(H37Ra-) infected macrophages by inhibiting endoplasmic reticulum stress. J. Ethnopharmacol. 10 (6), 114595. doi:10.1016/j.jep.2021.114595
Li, Y. G., Song, L., Liu, M., Hu, Z. B., and Wang, Z. T. (2009). Advancement in analysis of Salviae miltiorrhizae Radix et Rhizoma (Danshen). J. Chromatogr. A 1216 (11), 1941–1953. doi:10.1016/j.chroma.2008.12.032
Li, Z., Zhang, Y., Zhou, Y., Wang, F., Yin, C., Ding, L., et al. (2021). Tanshinone IIA suppresses the progression of lung adenocarcinoma through regulating CCNA2-CDK2 complex and AURKA/PLK1 pathway. Sci. Rep. 11 (1), 23681. doi:10.1038/s41598-021-03166-2
Liao, X. Z., Gao, Y., Huang, S., Chen, Z. Z., Sun, L. L., Liu, J. H., et al. (2019). Tanshinone IIA combined with cisplatin synergistically inhibits non-small-cell lung cancer in vitro and in vivo via down-regulating the phosphatidylinositol 3-kinase/Akt signalling pathway. Phytother. Res. 33 (9), 2298–2309. doi:10.1002/ptr.6392
Lin, J. Y., Ke, Y. M., Lai, J. S., and Ho, T. F. (2015). Tanshinone IIA enhances the effects of TRAIL by downregulating survivin in human ovarian carcinoma cells. Phytomedicine 22 (10), 929–938. doi:10.1016/j.phymed.2015.06.012
Lin, Y. Y., Lee, I. Y., Huang, W. S., Lin, Y. S., Kuan, F. C., Shu, L. H., et al. (2017). Danshen improves survival of patients with colon cancer and dihydroisotanshinone I inhibit the proliferation of colon cancer cells via apoptosis and skp2 signaling pathway. J. Ethnopharmacol. 209, 305–316. doi:10.1016/j.jep.2017.08.011
Liu, H., Peng, H. W., Cheng, Y. S., Yuan, H. S., and Yang-Yen, H. F. (2005). Stabilization and enhancement of the antiapoptotic activity of mcl-1 by TCTP. Mol. Cell Biol. 25 (8), 3117–3126. doi:10.1128/MCB.25.8.3117-3126.2005
Liu, J., Zhang, C., Liu, S., Wang, X., Wu, X., and Hao, J. (2023). Tanshinone IIA promotes apoptosis by downregulating BCL2 and upregulating TP53 in triple-negative breast cancer. Naunyn Schmiedeb. Arch. Pharmacol. 396 (2), 365–374. doi:10.1007/s00210-022-02316-1
Liu, J. J., Liu, W. D., Yang, H.-Z., Zhang, Y., Fang, Z.-G., Liu, P.-Q., Lin, D.-J., et al. (2010). Inactivation of PI3k/Akt signaling pathway and activation of caspase-3 are involved in tanshinone I-induced apoptosis in myeloid leukemia cells in vitro. Ann. Hematol. 89 (11), 1089–1097. doi:10.1007/s00277-010-0996-z
Liu, P., Cheng, H., Roberts, T. M., and Zhao, J. J. (2009). Targeting the phosphoinositide 3-kinase pathway in cancer. Nat. Rev. Drug Discov. 8 (8), 627–644. doi:10.1038/nrd2926
Liu, X., and Liu, J. (2020). Tanshinone I induces cell apoptosis by reactive oxygen species-mediated endoplasmic reticulum stress and by suppressing p53/DRAM-mediated autophagy in human hepatocellular carcinoma. Artif. Cells Nanomed Biotechnol. 48 (1), 488–497. doi:10.1080/21691401.2019.1709862
Lu, Y., Yan, Y., and Liu, X. (2021). Effects of alprostadil combined with tanshinone IIa injection on microcirculation disorder, outcomes, and cardiac function in AMI patients after PCI. Ann. Palliat. Med. 10 (1), 97–103. doi:10.21037/apm-20-2147
Ma, L., Jiang, H., Xu, X., Zhang, C., Niu, Y., Wang, Z., et al. (2019). Tanshinone IIA mediates SMAD7-YAP interaction to inhibit liver cancer growth by inactivating the transforming growth factor beta signaling pathway. Aging (Albany NY) 11 (21), 9719–9737. doi:10.18632/aging.102420
Ma, S., Lei, Y., Zhang, L., Zhang, L., Wang, J., and Wang, J. (2018). Research on the inhibiting effect of tanshinone IIA on colon cancer cell growth via COX-2-Wnt/β-catenin signaling pathway. J. BUON 23 (5), 1337–1342.
Mani, S. A., Guo, W., Liao, M. J., Eaton, E. N., Ayyanan, A., Zhou, A. Y., et al. (2008). The epithelial-mesenchymal transition generates cells with properties of stem cells. Cell 133 (4), 704–715. doi:10.1016/j.cell.2008.03.027
Mao, W., Ding, J., Li, Y., Huang, R., and Wang, B. (2022). Inhibition of cell survival and invasion by Tanshinone IIA via FTH1: a key therapeutic target and biomarker in head and neck squamous cell carcinoma. Exp. Ther. Med. 24 (2), 521. doi:10.3892/etm.2022.11449
McCubrey, J. A., Steelman, L. S., Kempf, C. R., Chappell, W. H., Abrams, S. L., Stivala, F., et al. (2011). Therapeutic resistance resulting from mutations in Raf/MEK/ERK and PI3K/PTEN/Akt/mTOR signaling pathways. J. Cell Physiol. 226 (11), 2762–2781. doi:10.1002/jcp.22647
Molina, J. R., and Adjei, A. A. (2006). The Ras/Raf/MAPK pathway. J. Thorac. Oncol. 1 (1), 7–9. doi:10.1097/01243894-200601000-00004
Mou, Y., Wang, J., Wu, J., He, D., Zhang, C., Duan, C., et al. (2019). Ferroptosis, a new form of cell death: opportunities and challenges in cancer. J. Hematol. Oncol. 12 (1), 34. doi:10.1186/s13045-019-0720-y
Ni, H., Ruan, G., Sun, C., Yang, X., Miao, Z., Li, J., et al. (2022). Tanshinone IIA inhibits gastric cancer cell stemness through inducing ferroptosis. Environ. Toxicol. 37 (2), 192–200. doi:10.1002/tox.23388
Nie, Z. Y., Zhao, M. H., Cheng, B. Q., Pan, R. F., Wang, T. R., Qin, Y., et al. (2020). Tanshinone IIA regulates human AML cell proliferation, cell cycle, and apoptosis through miR-497-5p/AKT3 axis. Cancer Cell Int. 20, 379. doi:10.1186/s12935-020-01468-5
Nishiguchi, Y., Oue, N., Fujiwara-Tani, R., Sasaki, T., Ohmori, H., Kishi, S., et al. (2019). Role of metastasis-related genes in cisplatin chemoresistance in gastric cancer. Int. J. Mol. Sci. 21 (1), 254. doi:10.3390/ijms21010254
Nordstrom, E., Fisone, G., and Kristensson, K. (2009). Opposing effects of ERK and p38-JNK MAP kinase pathways on formation of prions in GT1-1 cells. FASEB J. 23 (2), 613–622. doi:10.1096/fj.08-115360
Pan, Y., Chen, L., Li, R., Liu, Y., Nan, M., and Hou, L. (2021). Tanshinone IIa induces autophagy and apoptosis via PI3K/Akt/mTOR Axis in acute promyelocytic leukemia NB4 cells. Evid. Based Complement. Altern. Med. 2021, 3372403. doi:10.1155/2021/3372403
Pang, H., Han, B., Yu, T., and Peng, Z. (2014). The complex regulation of tanshinone IIA in rats with hypertension-induced left ventricular hypertrophy. PLoS One 9 (3), e92216. doi:10.1371/journal.pone.0092216
Puisieux, A., Brabletz, T., and Caramel, J. (2014). Oncogenic roles of EMT-inducing transcription factors. Nat. Cell Biol. 16 (6), 488–494. doi:10.1038/ncb2976
Qian, J., Fang, D., Lu, H., Cao, Y., Zhang, J., Ding, R., et al. (2018). Tanshinone IIA promotes IL2-mediated SW480 colorectal cancer cell apoptosis by triggering INF2-related mitochondrial fission and activating the Mst1-Hippo pathway. Biomed. Pharmacother. 108, 1658–1669. doi:10.1016/j.biopha.2018.09.170
Qiao, Y., Li, T., Zheng, S., and Wang, H. (2018). The Hippo pathway as a drug target in gastric cancer. Cancer Lett. 420, 14–25. doi:10.1016/j.canlet.2018.01.062
Qin, C., Liu, S., Zhou, S., Xia, X., Hu, J., Yu, Y., et al. (2023). Tanshinone IIA promotes vascular normalization and boosts Sorafenib's anti-hepatoma activity via modulating the PI3K-AKT pathway. Front. Pharmacol. 14, 1189532. doi:10.3389/fphar.2023.1189532
Qin, J., Shi, H., Xu, Y., Zhao, F., and Wang, Q. (2018). Tanshinone IIA inhibits cervix carcinoma stem cells migration and invasion via inhibiting YAP transcriptional activity. Biomed. Pharmacother. 105, 758–765. doi:10.1016/j.biopha.2018.06.028
Ren, X., Wang, C., Xie, B., Hu, L., Chai, H., Ding, L., et al. (2017). Tanshinone IIA induced cell death via miR30b-p53-PTPN11/SHP2 signaling pathway in human hepatocellular carcinoma cells. Eur. J. Pharmacol. 796, 233–241. doi:10.1016/j.ejphar.2016.11.046
Roskoski, R. (2019). Cyclin-dependent protein serine/threonine kinase inhibitors as anticancer drugs. Pharmacol. Res. 139, 471–488. doi:10.1016/j.phrs.2018.11.035
Santarpia, L., Lippman, S. M., and El-Naggar, A. K. (2012). Targeting the MAPK-RAS-RAF signaling pathway in cancer therapy. Expert Opin. Ther. Targets 16 (1), 103–119. doi:10.1517/14728222.2011.645805
Schaf, J., Shinhmar, S., Zeng, Q., Pardo, O. E., Beesley, P., Syed, N., et al. (2023). Enhanced Sestrin expression through Tanshinone 2A treatment improves PI3K-dependent inhibition of glioma growth. Cell Death Discov. 9 (1), 172. doi:10.1038/s41420-023-01462-6
Sekeres, M. J., Bradley-Garcia, M., Martinez-Canabal, A., and Winocur, G. (2021). Chemotherapy-induced cognitive impairment and hippocampal neurogenesis: a review of physiological mechanisms and interventions. Int. J. Mol. Sci. 22 (23), 12697. doi:10.3390/ijms222312697
Shan, Y. F., Shen, X., Xie, Y.-k., Chen, J.-C., Shi, H.-Q., Yu, Z.-p., et al. (2009). Inhibitory effects of tanshinone II-A on invasion and metastasis of human colon carcinoma cells. Acta Pharmacol. Sin. 30 (11), 1537–1542. doi:10.1038/aps.2009.139
Straten, P., and Andersen, M. H. (2010). The anti-apoptotic members of the Bcl-2 family are attractive tumor-associated antigens. Oncotarget 1 (4), 239–245. doi:10.18632/oncotarget.100804
Su, C. C. (2014). Tanshinone IIA inhibits gastric carcinoma AGS cells through increasing p-p38, p-JNK and p53 but reducing p-ERK, CDC2 and cyclin B1 expression. Anticancer Res. 34 (12), 7097–7110.
Su, C. C. (2018). Tanshinone IIA can inhibit MiaPaCa-2 human pancreatic cancer cells by dual blockade of the Ras/Raf/MEK/ERK and PI3K/AKT/mTOR pathways. Oncol. Rep. 40 (5), 3102–3111. doi:10.3892/or.2018.6670
Su, C. C., Chien, S. Y., Kuo, S. J., Chen, Y. L., Cheng, C. Y., and Chen, D. R. (2012). Tanshinone IIA inhibits human breast cancer MDA-MB-231 cells by decreasing LC3-II, Erb-B2 and NF-κBp65. Mol. Med. Rep. 5 (4), 1019–1022. doi:10.3892/mmr.2012.756
Su, C. C., and Chiu, T. L. (2016). Tanshinone IIA decreases the protein expression of EGFR, and IGFR blocking the PI3K/Akt/mTOR pathway in gastric carcinoma AGS cells both in vitro and in vivo. Oncol. Rep. 36 (2), 1173–1179. doi:10.3892/or.2016.4857
Su, C. C., and Lin, Y. H. (2008). Tanshinone IIA inhibits human breast cancer cells through increased Bax to Bcl-xL ratios. Int. J. Mol. Med. 22 (3), 357–361. doi:10.3892/ijmm_00000030
Sui, H., Xu, H., Ji, Q., Liu, X., Zhou, L., Song, H., et al. (2015). 5-hydroxytryptamine receptor (5-HT1DR) promotes colorectal cancer metastasis by regulating Axin1/β-catenin/MMP-7 signaling pathway. Oncotarget 6 (28), 25975–25987. doi:10.18632/oncotarget.4543
Sui, H., Zhao, J., Zhou, L., Wen, H., Deng, W., Li, C., et al. (2017). Tanshinone IIA inhibits β-catenin/VEGF-mediated angiogenesis by targeting TGF-β1 in normoxic and HIF-1α in hypoxic microenvironments in human colorectal cancer. Cancer Lett. 403, 86–97. doi:10.1016/j.canlet.2017.05.013
Sun, F., Yang, X., Song, W., Yu, N., and Lin, Q. (2023). Tanshinone IIA (TSIIA) represses the progression of non-small cell lung cancer by the circ_0020123/miR-1299/HMGB3 pathway. Mol. Cell Biochem. 478 (9), 1973–1986. doi:10.1007/s11010-022-04646-3
Sun, Y., Gong, C., Ni, Z., Hu, D., Ng, W., Zhu, X., et al. (2020). Tanshinone IIA enhances susceptibility of non-small cell lung cancer cells to NK cell-mediated lysis by up-regulating ULBP1 and DR5. J. Leukoc. Biol. 110 (2), 315–325. doi:10.1002/JLB.5MA1120-776RR
Sung, H., Ferlay, J., Siegel, R. L., Laversanne, M., Soerjomataram, I., Jemal, A., et al. (2021). Global cancer statistics 2020: GLOBOCAN estimates of incidence and mortality worldwide for 36 cancers in 185 countries. CA A Cancer J. Clin. 71 (3), 209–249. doi:10.3322/caac.21660
Tang, T., Yang, Z., Zhu, Q., Wu, Y., Sun, K., Alahdal, M., et al. (2018). Up-regulation of miR-210 induced by a hypoxic microenvironment promotes breast cancer stem cell metastasis, proliferation, and self-renewal by targeting E-cadherin. FASEB J. 32, 6965–6981. doi:10.1096/fj.201801013R
Timofeeva, O. A., Tarasova, N. I., Zhang, X., Chasovskikh, S., Cheema, A. K., Wang, H., et al. (2013). STAT3 suppresses transcription of proapoptotic genes in cancer cells with the involvement of its N-terminal domain. Proc. Natl. Acad. Sci. U. S. A. 110 (4), 1267–1272. doi:10.1073/pnas.1211805110
Tong, W., Guo, J., and Yang, C. (2020). Tanshinone II A enhances pyroptosis and represses cell proliferation of HeLa cells by regulating miR-145/GSDMD signaling pathway. Biosci. Rep. 40 (4), BSR20200259. doi:10.1042/BSR20200259
Verzella, D., Pescatore, A., Capece, D., Vecchiotti, D., Ursini, M. V., Franzoso, G., et al. (2020). Life, death, and autophagy in cancer: NF-κB turns up everywhere. Cell Death Dis. 11 (3), 210. doi:10.1038/s41419-020-2399-y
Viallard, C., and Larrivée, B. (2017). Tumor angiogenesis and vascular normalization: alternative therapeutic targets. Angiogenesis 20 (4), 409–426. doi:10.1007/s10456-017-9562-9
Vousden, K. H., and Lane, D. P. (2007). p53 in health and disease. Nat. Rev. Mol. Cell Biol. 8 (4), 275–283. doi:10.1038/nrm2147
Wagner, E. F., and Nebreda, A. R. (2009). Signal integration by JNK and p38 MAPK pathways in cancer development. Nat. Rev. Cancer 9 (8), 537–549. doi:10.1038/nrc2694
Wang, L., Hu, T., Shen, J., Zhang, L., Chan, R. L., Lu, L., et al. (2015a). Dihydrotanshinone I induced apoptosis and autophagy through caspase dependent pathway in colon cancer. Phytomedicine 22 (12), 1079–1087. doi:10.1016/j.phymed.2015.08.009
Wang, L., Jiang, G., and Li, X. (2023). Diterpenoid tanshinone attenuates the metastasis of non-small-cell lung cancer (NSCLC) cells by inhibiting the Cavin-1-mediated ERK/Smad2 signaling pathway. Anticancer Agents Med. Chem. 23, 1618–1625. doi:10.2174/1871520623666230417090504
Wang, M., Zeng, X., Li, S., Sun, Z., Yu, J., Chen, C., et al. (2019a). A novel tanshinone analog exerts anti-cancer effects in prostate cancer by inducing cell apoptosis, arresting cell cycle at G2 phase and blocking metastatic ability. Int. J. Mol. Sci. 20 (18), 4459. doi:10.3390/ijms20184459
Wang, W., Li, J., Ding, Z., Li, Y., Wang, J., Chen, S., et al. (2019b). Tanshinone I inhibits the growth and metastasis of osteosarcoma via suppressing JAK/STAT3 signalling pathway. J. Cell Mol. Med. 23 (9), 6454–6465. doi:10.1111/jcmm.14539
Wang, W. Q., Liu, L., Sun, H.-C., Fu, Y.-L., Xu, H.-X., Chai, Z.-T., et al. (2012). Tanshinone IIA inhibits metastasis after palliative resection of hepatocellular carcinoma and prolongs survival in part via vascular normalization. J. Hematol. Oncol. 5, 69. doi:10.1186/1756-8722-5-69
Wang, X., Wang, W. M., Han, H., Zhang, Y., Liu, J. L., Yu, J. Y., et al. (2022). Tanshinone IIA protected against lipopolysaccharide-induced brain injury through the protective effect of the blood-brain barrier and the suppression of oxidant stress and inflammatory response. Food Funct. 13 (15), 8304–8312. doi:10.1039/d2fo00710j
Wang, X., Wei, Y., Yuan, S., Liu, G., Lu, Y., Zhang, J., et al. (2005). Potential anticancer activity of tanshinone IIA against human breast cancer. Int. J. Cancer 116 (5), 799–807. doi:10.1002/ijc.20880
Wang, Y., Jin, W., and Wang, J. (2021). Tanshinone IIA regulates microRNA-125b/foxp3/caspase-1 signaling and inhibits cell viability of nasopharyngeal carcinoma. Mol. Med. Rep. 23 (5), 371. doi:10.3892/mmr.2021.12010
Wang, Y., Li, J. X., Wang, Y. Q., and Miao, Z. H. (2015b). Tanshinone I inhibits tumor angiogenesis by reducing Stat3 phosphorylation at Tyr705 and hypoxia-induced HIF-1α accumulation in both endothelial and tumor cells. Oncotarget 6 (18), 16031–16042. doi:10.18632/oncotarget.3648
Wei, Z., Jiang, X., Qiao, H., Zhai, B., Zhang, L., Zhang, Q., et al. (2013). STAT3 interacts with Skp2/p27/p21 pathway to regulate the motility and invasion of gastric cancer cells. Cell Signal 25 (4), 931–938. doi:10.1016/j.cellsig.2013.01.011
Wen, J., Chang, Y., Huo, S., Li, W., Huang, H., Gao, Y., et al. (2020). Tanshinone IIA attenuates atherosclerosis via inhibiting NLRP3 inflammasome activation. Aging (Albany NY) 13 (1), 910–932. doi:10.18632/aging.202202
Won, S. H., Lee, H. J., Jeong, S. J., Lu, J., and Kim, S. H. (2012). Activation of p53 signaling and inhibition of androgen receptor mediate tanshinone IIA induced G1 arrest in LNCaP prostate cancer cells. Phytother. Res. 26 (5), 669–674. doi:10.1002/ptr.3616
Won, S. H., Lee, H. J., Jeong, S.-J., Lee, H.-J., Lee, E.-O., Jung, D.-B., Shin, J.-M., et al. (2010). Tanshinone IIA induces mitochondria dependent apoptosis in prostate cancer cells in association with an inhibition of phosphoinositide 3-kinase/AKT pathway. Biol. Pharm. Bull. 33 (11), 1828–1834. doi:10.1248/bpb.33.1828
Wu, C., Chen, J., Chen, C., Wang, W., Wen, L., Gao, K., et al. (2015). Wnt/β-catenin coupled with HIF-1α/VEGF signaling pathways involved in galangin neurovascular unit protection from focal cerebral ischemia. Sci. Rep. 5, 16151. doi:10.1038/srep16151
Wu, C. Y., Yang, Y. H., Lin, Y. Y., Kuan, F. C., Lin, Y. S., Lin, W. Y., et al. (2017). Anti-cancer effect of danshen and dihydroisotanshinone I on prostate cancer: targeting the crosstalk between macrophages and cancer cells via inhibition of the STAT3/CCL2 signaling pathway. Oncotarget 8 (25), 40246–40263. doi:10.18632/oncotarget.14958
Wu, J., Xu, H., Zhang, L., and Zhang, X. (2016). Radix astragali and tanshinone help carboplatin inhibit B16 tumor cell growth. Technol. Cancer Res. Treat. 15 (4), 583–588. doi:10.1177/1533034615588682
Wu, Y. T., Xie, L. P., Hua, Y., Xu, H. L., Chen, G. H., Han, X., et al. (2021). Tanshinone I inhibits oxidative stress-induced cardiomyocyte injury by modulating Nrf2 signaling. Front. Pharmacol. 12, 644116. doi:10.3389/fphar.2021.644116
Xu, Z., Chen, L., Xiao, Z., Zhu, Y., Jiang, H., Jin, Y., et al. (2018). Potentiation of the anticancer effect of doxorubicinin drug-resistant gastric cancer cells by tanshinone IIA. Phytomedicine 51, 58–67. doi:10.1016/j.phymed.2018.05.012
Yan, M. Y., Chien, S. Y., Kuo, S. J., Chen, D. R., and Su, C. C. (2012). Tanshinone IIA inhibits BT-20 human breast cancer cell proliferation through increasing caspase 12, GADD153 and phospho-p38 protein expression. Int. J. Mol. Med. 29 (5), 855–863. doi:10.3892/ijmm.2012.908
Yan, Y. L., Su, W. P., Zeng, S. S., Qian, L., Chen, X., Wei, J., et al. (2018). Effect and mechanism of tanshinone I on the radiosensitivity of lung cancer cells. Mol. Pharm. 15 (11), 4843–4853. doi:10.1021/acs.molpharmaceut.8b00489
Yang, Q., Huang, J., Wu, Q., Cai, Y., Zhu, L., Lu, X., et al. (2014). Acquisition of epithelial-mesenchymal transition is associated with Skp2 expression in paclitaxel-resistant breast cancer cells. Br. J. Cancer 110 (8), 1958–1967. doi:10.1038/bjc.2014.136
Yang, Y., Cao, Y., Chen, L., Liu, F., Qi, Z., Cheng, X., et al. (2018). Cryptotanshinone suppresses cell proliferation and glucose metabolism via STAT3/SIRT3 signaling pathway in ovarian cancer cells. Cancer Med. 7 (9), 4610–4618. doi:10.1002/cam4.1691
Yang, Y., Yang, F., Xiong, Z., Yan, Y., Wang, X., Nishino, M., et al. (2005). An N-terminal region of translationally controlled tumor protein is required for its antiapoptotic activity. Oncogene 24 (30), 4778–4788. doi:10.1038/sj.onc.1208666
Ye, B., Qiao, K., Zhao, Q., Jiang, Z., Hu, N., and Wang, F. (2022). Tanshinone I restrains osteosarcoma progression by regulating circ_0000376/miR-432-5p/BCL2 axis. Mol. Cell Biochem. 477 (1), 1–13. doi:10.1007/s11010-021-04257-4
Ye, T., Xiong, D., Chen, L., Li, Y., Gong, S., Zhang, L., et al. (2020). Effect of Danshen on TLR2-triggered inflammation in macrophages. Phytomedicine 70, 153228. doi:10.1016/j.phymed.2020.153228
Yu, J., Wang, X., Li, Y., and Tang, B. (2017). Tanshinone IIA suppresses gastric cancer cell proliferation and migration by downregulation of FOXM1. Oncol. Rep. 37 (3), 1394–1400. doi:10.3892/or.2017.5408
Yuan, F., Zhao, Z. T., Jia, B., Wang, Y. P., and Lei, W. (2020). TSN inhibits cell proliferation, migration, invasion, and EMT through regulating miR-874/HMGB2/β-catenin pathway in gastric cancer. Neoplasma 67 (5), 1012–1021. doi:10.4149/neo_2020_190919N931
Yun, S. M., Jeong, S. J., Kim, J.-H., Jung, J. H., Lee, H.-J., Sohn, E. J., Lee, M.-H., et al. (2013). Activation of c-Jun N-terminal kinase mediates tanshinone IIA-induced apoptosis in KBM-5 chronic myeloid leukemia cells. Biol. Pharm. Bull. 36 (2), 208–214. doi:10.1248/bpb.b12-00537
Zanre, V., Campagnari, R., Cerulli, A., Masullo, M., Cardile, A., Piacente, S., et al. (2022). Salviolone from Salvia miltiorrhiza roots impairs cell cycle progression, colony formation, and metalloproteinase-2 activity in A375 melanoma cells: involvement of P21(cip1/waf1) expression and STAT3 phosphorylation. Int. J. Mol. Sci. 23 (3), 1121. doi:10.3390/ijms23031121
Zeng, A., Hua, H., Liu, L., and Zhao, J. (2019). Betulinic acid induces apoptosis and inhibits metastasis of human colorectal cancer cells in vitro and in vivo. Bioorg Med. Chem. 27 (12), 2546–2552. doi:10.1016/j.bmc.2019.03.033
Zhang, D., Li, F., Weidner, D., Mnjoyan, Z. H., and Fujise, K. (2002). Physical and functional interaction between myeloid cell leukemia 1 protein (MCL1) and Fortilin. The potential role of MCL1 as a fortilin chaperone. J. Biol. Chem. 277 (40), 37430–37438. doi:10.1074/jbc.M207413200
Zhang, L., Lin, W., Chen, X., Wei, G., Zhu, H., and Xing, S. (2019a). Tanshinone IIA reverses EGF- and TGF-β1-mediated epithelial-mesenchymal transition in HepG2 cells via the PI3K/Akt/ERK signaling pathway. Oncol. Lett. 18 (6), 6554–6562. doi:10.3892/ol.2019.11032
Zhang, R., Wang, Y., Liu, D., Luo, Q., Du, P., Zhang, H., et al. (2022). Sodium tanshinone IIA sulfonate as a potent Ido1/TDO2 dual inhibitor enhances anti-PD1 therapy for colorectal cancer in mice. Front. Pharmacol. 13, 870848. doi:10.3389/fphar.2022.870848
Zhang, R. W., Liu, Z. G., Xie, Y., Wang, L. X., Li, M. C., and Sun, X. (2016). In vitro inhibition of invasion and metastasis in colon cancer cells by TanIIA. Genet. Mol. Res. 15 (3). doi:10.4238/gmr.15039008
Zhang, X., Zhou, Y., and Gu, Y. E. (2019b). Tanshinone IIA induces apoptosis of ovarian cancer cells in vitro and in vivo through attenuation of PI3K/AKT/JNK signaling pathways. Oncol. Lett. 17 (2), 1896–1902. doi:10.3892/ol.2018.9744
Zhang, Y., Geng, Y., He, J., Wu, D., Zhang, T., Xue, L., et al. (2019c). Tanshinone IIA induces apoptosis and autophagy in acute monocytic leukemia via downregulation of PI3K/Akt pathway. Am. J. Transl. Res. 11 (5), 2995–3006.
Zhang, Y., Guo, S., Fang, J., Peng, B., Zhang, Y., and Cao, T. (2018). Tanshinone IIA inhibits cell proliferation and tumor growth by downregulating STAT3 in human gastric cancer. Exp. Ther. Med. 16 (4), 2931–2937. doi:10.3892/etm.2018.6562
Zhang, Y., Meng, X., Li, C., Tan, Z., Guo, X., Zhang, Z., et al. (2017). MiR-9 enhances the sensitivity of A549 cells to cisplatin by inhibiting autophagy. Biotech. Lett. 39 (7), 959–966. doi:10.1007/s10529-017-2325-2
Zhou, J., Jiang, Y. Y., Chen, H., Wu, Y. C., and Zhang, L. (2020a). Tanshinone I attenuates the malignant biological properties of ovarian cancer by inducing apoptosis and autophagy via the inactivation of PI3K/AKT/mTOR pathway. Cell Prolif. 53 (2), e12739. doi:10.1111/cpr.12739
Zhou, L., Sui, H., Wang, T., Jia, R., Zhang, Z., Fu, J., et al. (2020b). Tanshinone IIA reduces secretion of pro-angiogenic factors and inhibits angiogenesis in human colorectal cancer. Oncol. Rep. 43 (4), 1159–1168. doi:10.3892/or.2020.7498
Zhou, L. H., Hu, Q., Sui, H., Ci, S.-J., Ci, S., Wang, Y., et al. (2012). Tanshinone II--a inhibits angiogenesis through down regulation of COX-2 in human colorectal cancer. Asian Pac J. Cancer Prev. 13 (9), 4453–4458. doi:10.7314/apjcp.2012.13.9.4453
Zhu, P. C., Shen, J., Qian, R. Y., Xu, J., Liu, C., Hu, W. M., et al. (2023). Effect of tanshinone IIA for myocardial ischemia/reperfusion injury in animal model: preclinical evidence and possible mechanisms. Front. Pharmacol. 14, 1165212. doi:10.3389/fphar.2023.1165212
Zou, W., Qian, C., Zhang, S., Wan, X., Wei, Z., Li, X., et al. (2021). Targeting the ang2/tie2 Axis with tanshinone IIA elicits vascular normalization in ischemic injury and colon cancer. Oxid. Med. Cell Longev. 2021, 7037786. doi:10.1155/2021/7037786
Keywords: tanshinone, cancer, molecular mechanism, traditional Chinese medicine, anti-tumor
Citation: Zhang P, Liu W and Wang Y (2023) The mechanisms of tanshinone in the treatment of tumors. Front. Pharmacol. 14:1282203. doi: 10.3389/fphar.2023.1282203
Received: 23 August 2023; Accepted: 18 October 2023;
Published: 26 October 2023.
Edited by:
Onur Bender, Ankara University, TürkiyeReviewed by:
Abdullah Aslan, Firat University, TürkiyeMing Xu, Shimonoseki City University, Japan
Saheed Benson, Liverpool John Moores University, United Kingdom
Copyright © 2023 Zhang, Liu and Wang. This is an open-access article distributed under the terms of the Creative Commons Attribution License (CC BY). The use, distribution or reproduction in other forums is permitted, provided the original author(s) and the copyright owner(s) are credited and that the original publication in this journal is cited, in accordance with accepted academic practice. No use, distribution or reproduction is permitted which does not comply with these terms.
*Correspondence: Yuan Wang, ZGVtaTA1MzFAMTYzLmNvbQ==