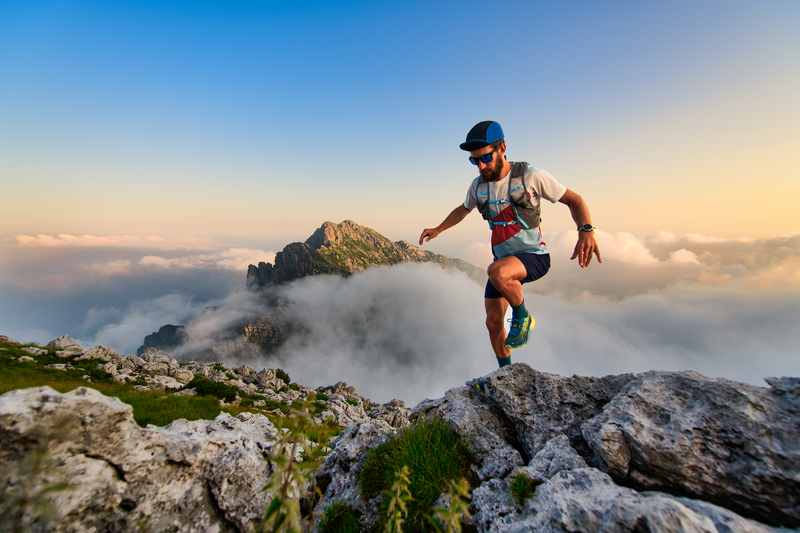
94% of researchers rate our articles as excellent or good
Learn more about the work of our research integrity team to safeguard the quality of each article we publish.
Find out more
REVIEW article
Front. Pharmacol. , 01 December 2023
Sec. Ethnopharmacology
Volume 14 - 2023 | https://doi.org/10.3389/fphar.2023.1272241
This article is part of the Research Topic Reviews in Ethnopharmacology: 2023 View all 30 articles
Medicinal plants are the primary sources for the discovery of novel medicines and the basis of ethnopharmacological research. While existing studies mainly focus on the chemical compounds, there is little research about the functions of other contents in medicinal plants. Extracellular vesicles (EVs) are functionally active, nanoscale, membrane-bound vesicles secreted by almost all eukaryotic cells. Intriguingly, plant-derived extracellular vesicles (PDEVs) also have been implicated to play an important role in therapeutic application. PDEVs were reported to have physical and chemical properties similar to mammalian EVs, which are rich in lipids, proteins, nucleic acids, and pharmacologically active compounds. Besides these properties, PDEVs also exhibit unique advantages, especially intrinsic bioactivity, high stability, and easy absorption. PDEVs were found to be transferred into recipient cells and significantly affect their biological process involved in many diseases, such as inflammation and tumors. PDEVs also could offer unique morphological and compositional characteristics as natural nanocarriers by innately shuttling bioactive lipids, RNA, proteins, and other pharmacologically active substances. In addition, PDEVs could effectively encapsulate hydrophobic and hydrophilic chemicals, remain stable, and cross stringent biological barriers. Thus, this study focuses on the pharmacological action and mechanisms of PDEVs in therapeutic applications. We also systemically deal with facets of PDEVs, ranging from their isolation to composition, biological functions, and biotherapeutic roles. Efforts are also made to elucidate recent advances in re-engineering PDEVs applied as stable, effective, and non-immunogenic therapeutic applications to meet the ever-stringent demands. Considering its unique advantages, these studies not only provide relevant scientific evidence on therapeutic applications but could also replenish and inherit precious cultural heritage.
Extracellular vesicles (EVs), which are biological membrane-bound vesicles of 40–150 nm, are secreted by all mammalian cells (Colombo et al., 2014; Peng et al., 2020). Despite being initially considered as cellular debris and therefore underestimated, EVs are now recognized as significant factors in controlling numerous physiological and pathological mechanisms in multicellular organisms. Further investigation has revealed that these EVs are surrounded by a double layer of fats and proteins, containing various functional nucleic acids, lipids, proteins, RNAs, and other elements. Although most EVs share analogical structures, the uptake and signal transduction capacity primarily rely on the heterogeneity of their source and components. Due to these distinctive features, EVs play a vital role in facilitating intercellular communication and the transfer of information and exchange of materials in both prokaryotes and higher eukaryotes. Since their first discovery in the 1980s, mammalian extracellular vesicles (MEVs) have been extensively researched, and their biogenesis mechanisms and functions of mediating intercellular transmission have been gradually elucidated (Colombo et al., 2014; Abels and Breakefield, 2016; Isaac et al., 2021). For example, the arrangement of lipids in a bilayer enables MEVs to trap both water-loving and water-fearing molecular substances. Moreover, MEVs have also been reported to have remarkable specific targeting capabilities (Shao et al., 2020; Wu et al., 2022; Zhang et al., 2022). Intriguingly, EVs possess physiological activity and have the ability to cross bio-membrane barriers, evade phagocytosis by the reticuloendothelial system, and extend the lifespan of drugs (Duan et al., 2021; Nguyen and Fuhrmann, 2022; Xie et al., 2023). Although they exist in the nanodomain, EVs are considered low toxicity and capable of decomposing. As a result, extensive research has been conducted on EVs in diverse scientific fields, such as drug delivery systems, nutrition, disease treatment, and clinical diagnosis. Furthermore, EVs have proven effective in treating cardiovascular diseases, cancer, and neurodegenerative diseases and facilitating tissue repair (Cheng and Hill, 2022; Zhou et al., 2022). For instance, Qiao et al. developed a composite EVs based on HT1080 cells, which enclosed doxorubicin, leading to a substantial increase in the concentration of doxorubicin in the tumor tissue and augmenting its effectiveness against tumor cells (Qiao et al., 2020). In addition, MEVs are also applied to deliver vulnerable reagents, such as nucleic acid and lipids. According to recent research, it has been proved that the EVs obtained from dendritic cells contained siRNA, effectively reducing the expression of beta-site amyloid precursor protein cleaving enzyme-1 (Alvarez-Erviti et al., 2011). Moreover, EVs originating from brain endothelial cells have also been demonstrated to cross blood-brain bio-membrane barriers and transport the anti-tumor medications doxorubicin and paclitaxel to the brains of mice (Khan et al., 2021; Fang and Liu, 2022b). Based on these characteristics, the MEVs have been regarded as promising drug delivery strategies in previous studies.
Despite the therapeutic potential of MEVs for drug delivery, their clinical application is still plagued by several challenges, including quick blood clearance, potential cancer stimulation, low production, and high expenses (Imai et al., 2015; Yamashita et al., 2016; Charoenviriyakul et al., 2017; Baldini et al., 2018). Accordingly, exploring safe, high-yield, and readily available sources of EVs has become a talking point for all researchers. According to the history of plant-derived extracellular vesicles (PDEVs), the first observed PDEVs were in 1967 and first isolated in 2009 (Figure 1). Since then, the research on PDEVs has been deepening and bringing the field into a period of diversified development. Interestingly, extracellular vesicles derived from plants (PDEVs) have been discovered in different plant species and possess exceptional qualities, making them a subject of increased interest in recent times. For example, PDEVs do not contain human or zoonotic pathogens and pose reduced immune risks in vivo. Hence, the PDEVs bestow non-immunogenic characteristics absent in MEVs and other artificial drug delivery methods (Zhuang et al., 2015; Baldini et al., 2018; Cao et al., 2019). PDEVs containing proteins, DNA, RNA, lipids, and other substances can acquire specific bioactive properties from the original plants, such as antioxidant, anti-inflammatory, and anti-cancer effects (Ju et al., 2013; Zhuang et al., 2015; Liu B. et al., 2020; Han et al., 2021). Furthermore, PDEVs, which have biological capabilities, can be utilized as carriers for molecules to enhance the therapeutic effects of drugs through synergistic effects. The transfer of these PDEV-loaded bioactive compounds can significantly impact the biological behaviors of recipient cells, particularly those related to various diseases like tumors and inflammation. Recent research has demonstrated that multiple PDEVs possess the ability to withstand challenging conditions in the gastrointestinal tract and overcome biological obstacles (Liu et al., 2023). It suggests that PDEVs may have great promise as platforms for delivering drugs. Various separate teams have utilized biochemical technologies to show the significant functions of PDEVs as potential intermediaries in intercellular communication and the exchange of bio-information among different species (Feng et al., 2023). For instance, it has been demonstrated that Helianthus annuu derived PDEVs containing lectins play a role in inflammation, cancer, and autoimmunity disease (Regente et al., 2009). Phosphoric acid can be transported by PDEVs derived from Zingiber officinale Roscoe to enhance chronic periodontitis while regulating pro-inflammatory cytokines can help alleviate colitis symptoms and preventing microglia activation can moderate encephalitis (Sundaram et al., 2019; Liu and Liu, 2022; Sundaram et al., 2022). Furthermore, various PDEVs from Aloe ferox, Acrotome angustifolia, Beta vulgaris, Anthemis arvensis, and Vaccinium myrsinites are being developed for disease therapy (De Robertis et al., 2020; Zeng et al., 2021; Chen et al., 2022; Mahdipour, 2022). Although growing evidence has also revealed that PDEVs could be absorbed by animal cells, some differences still exist between PDEVs and MEVs. For example, an apparent difference between PDEVs and MEVs is that PDEVs are abundant in phospholipids, such as phosphatidylethanolamines (PE), phosphatidic acids (PA) and phosphatidylcholine (PC). In contrast, MEVs mainly comprise sphingomyelins and cholesterol (Zhang et al., 2016b). Here, we summarized the advantages and disadvantages of PDEVs and MEVs in Table 1.
Accordingly, the magnitude of PDEVs in the field of biomedicine has witnessed a growing appreciation. Although PDEVs were discovered approximately a decade before MEVs, there is still limited understanding of the research conducted on drug delivery systems associated with PDEVs (Duan et al., 2021; Xu et al., 2021; Nguyen and Fuhrmann, 2022; Zhou et al., 2022). Therefore, this review aims to offer a brief summary of the PDEVs investigation, including the generation, formulation, thorough analysis, and loading methods in detail. Specifically, the focus is on revealing the capacity of PDEVs as therapies for diseases and as effective systems for delivering drugs based on ongoing research. We also tried to thoroughly evaluate the role of PDEVs as a lucrative mechanism for delivering drugs. In conclusion, we present our distinct perspectives on pharmacological significance, toxicity and immunogenicity, clinical utilization, possibilities, and obstacles of PDEVs.
The presence of cell walls implies that plant cells possess more intricate communication methods than MEVs, making it difficult to investigate the forming process of PDEVs. According to recent research, the potential development of PDEVs may occur in various ways, such as through multivesicular bodies (MVBs), exocyst-positive-organelles (EXPOs), autophagosomes, and vacuolar pathways (Cui et al., 2020; Fang and Liu, 2022a). The primary types of PDEVs consist of the endosome (50–150 nm), microvesicles generated by the protrusion of the cell membrane (150–1,000 nm), and apoptotic bodies (1–1.5 μm), categorized by their origin within the cell, biological purpose, and physical characteristics (Fang and Liu, 2022a; Cheng and Hill, 2022).
The combination of MVBs and the plasma membrane (PM) results in the liberation of the intraluminal vesicle (ILV) and is controlled by the endosomal sorting complex required for transport (ESCRT), which is the predominant route for EVs creation in mammals. MVB pathway in plant cells bears a resemblance to that of mammalian cells (van Niel et al., 2022). The first observation of the MVBs occurred in the 1960s through a transmission electron microscope (TEM) (Ly et al., 2023). Numerous studies on different subjects, including unconventional protein secretion (UPS) and interactions between plants and pathogens, have validated the presence of PDEVs and their biogenesis mechanisms (An et al., 2006; Regente et al., 2012). For example, MVBs could discharge vesicles by merging with the PM or releasing them to particular infection locations while the fungus invades plant tissue (An et al., 2006). Multiple studies have additionally indicated that these MVBs, originating from the leaf tissues of Arabidopsis thaliana at the site of fungal infection, could be marked with Rab5-like GTPase ARA-6 and TETRASPANIN(TET)8-GFP (Cai et al., 2018; Cai et al., 2019). Specifically, the ARA-6 is situated at the site of infection and persists on the PM for subsequent transportation. Simultaneously, the Tetraspanin-8 is located on the released vesicles, resembling the CD63 marker of MEVs, and is anticipated to function as a marker for plant EVs (Cai et al., 2018). In addition, studies also proved that the fusion between MVBs and PM was also promoted by plant-microbe symbiosis, and these EVs were released into the periarbuscular space between plants and fungi.
There is also supporting evidence for alternative routes, including secretion through EXPO, autophagosomes, and fusion between vacuoles and the plasma membrane (Ly et al., 2023). Autophagosomes and EXPO are spherical double-membrane organelles. In spite of their comparable structure, EXPOs do not coexist with autophagosomes, nor do they experience any impact from nutrient deprivation. As a result, these two cellular components are regarded as separate entities. EXPO, possessing a dual-layered composition, has the capability to merge with the plasma membrane and discharge its internal vesicles into the extracellular space (Wang et al., 2010). However, EXPO exhibits morphological differences compared to MVBs and operates separately from endosomes and autophagosomes. Following fusion with the PM, EXPO secrete vesicles into the extracellular environment, which are larger by 200–500 nm (Cai et al., 2021). The recent discovery that glycosyltransferases and EXPO markers are found together in tobacco indicates that PDEVs derived from EXPO might play a role in releasing arabinogalactan proteins in plants. In addition to the MVBs and EXPO pathways discussed earlier, vacuoles may also play a role in forming PDEVs. Vacuoles containing hydrolytic enzymes are crucial organelles in plant defense (Pérez-Bermúdez et al., 2017; Wang et al., 2017). To combat pathogen infection, plants regulate the merging of vacuoles and plasma membranes, releasing substances like hydrolases and defense proteins into the external environment (Hatsugai et al., 2009). According to recent research, it has been discovered that central vacuoles in Arabidopsis originated from the transition of MVBs to small vacuoles (SVs) and the subsequent fusion of SVs (Cui et al., 2019). The fusion of autophagosomes and MVBs in yeast cells results in the formation of secondary vesicles, indicating a potential co-secretion of PDEVs through these secretory pathways (Manjithaya and Subramani, 2010).
Consequently, PDEVs have been discovered to have a crucial role in protecting the immune system and facilitating communication between cells. Nevertheless, the precise mechanisms of biogenesis for PDEVs remain uncertain, and PDEVs from various plant origins might be released in intricate ways. Hence, it is imperative to conduct additional research on the biogenesis process of PDEVs, as this could potentially aid in the systematic regulation of their secretion in the future.
Utilizing PDEVs as naturally sustainable raw resources could be a promising approach in the medical field (Hernández-Díaz et al., 2021). MEVs have demonstrated significant promise in the biomedical domain, and PDEVs have also garnered considerable interest. In comparison to the MEVs, PDEVs offer a more comprehensive range of choices and enhanced safety (De Palma et al., 2020; Ly et al., 2023). However, achieving successful isolation of these PDEVs may be essential for practical applications. PDEVs are typically obtained directly from the extracellular surroundings, like apoplastic fluids or root exudate. These involve immersing plant raw samples in an infiltration buffer and isolating PDEVs by multiple methods, including ultracentrifugation, precipitation with polyethylene glycol, immunoaffinity, and size exclusion chromatography (Wang et al., 2021; Ali et al., 2022; Lai et al., 2022; Yin et al., 2022). Actually, the proper separation technique should be applied according to the final purpose of the PDEVs, among which basic research and clinical application are the bases for the selection of methods (Ströhle et al., 2022). However, there are currently limited separation methods for PDEVs, most of which refer to MEVs (Jackson et al., 2023). Due to the differences between animal cells and plant cells, such as starch and cellulose, the same isolation technique may be less effective in the extraction of PDEVs (Gardiner et al., 2016). As a result, we summarized the advantages and disadvantages of different isolation methods in Table 2 and provided a brief overview of the typical preparation and characterization of PDEV. It is predictable that an appropriate separation method could be more conducive to the acquisition of PDEVs with high purity, high yield, and high activity.
The preparation of PDEVs follows similar methods established for MEVs. The extraction and purification technique used could be vital for the functioning of PDEVs since they are frequently present in a highly intricate environment. In existing research, numerous studies have aimed to enhance both extraction specificity and efficiency (Yang et al., 2021). Every method has its unique benefits and limitations. For example, a more precise strategy, like immunoaffinity, usually yields a pure sample but lacks efficiency and recovery. On the other hand, several methods, like membrane filtration, which have high efficiencies, may lack specificity and purity. However, it is important to mention that the functioning of PDEVs relies on the undamaged bilayer membrane (Ju et al., 2013; Wen et al., 2023). Therefore, it is crucial to exercise caution when extracting and storing it to avoid any deterioration. It can be achieved by employing protease inhibitors, keeping them at low temperatures, avoiding the freeze-thaw process, and maintaining a neutral pH (Li et al., 2017; Cheng et al., 2019). Extraction methods such as ultracentrifugation, ultrafiltration, immunoaffinity capture, precipitation, or microfluidics often rely on their distinct properties, such as their density, size, shape, and protein composition (Suharta et al., 2021). For instance, some studies suggested that blending is a superior choice for acquiring increased amounts of phytochemicals from grapefruits compared to juicing or manually squeezing (Uckoo et al., 2012). Wang and others determined that out of blending, high-speed centrifugal juicing, and low-speed juicing, the latter two preserved the most excellent phytochemical profiles and antioxidant activities of 19 vegetables, such as Brassica oleracea, Raphanus raphanistrum, and Beta vulgaris (Wang et al., 2021). This section summarized the typical techniques frequently employed for isolating PDEVs (Table 2).
Among the techniques mentioned above, ultracentrifugation is often utilized as the primary method for EVs isolation due to its straightforwardness, user-friendliness, cost-effectiveness over time, reasonable time requirement, and lack of complex sample preparation (Li et al., 2017). Accordingly, ultracentrifugation usually divides into a differential or a density gradient centrifugation (Figure 2A). Differential centrifugation relies on the separation of particles by their density, size, and shape through a sequence of high-speed centrifugation in a uniform medium (Li et al., 2017; Qiao et al., 2020). Actually, PDEVs are commonly extracted using the method of differential centrifugation (Gardiner et al., 2016). Various sizes of particles in the medium can separate different components with different rates of sedimentation. Generally, the intact cells could be eliminated at a low speed through centrifugation. At the same time, high-speed centrifugation can separate the sizable pieces and undamaged organelles. Ultimately, PDEVs are separated and enhanced through ultracentrifugation. It should be emphasized that prolonged and repeated ultracentrifugation can potentially disrupt the structure of the PDEVs. However, an additional cushion was incorporated at the base of the centrifuge tube, demonstrating its efficacy in enhancing the condition (Li et al., 2017; Yang D. et al., 2020; Lai et al., 2022). Differential centrifugation is the most common technology to extract PDEVs now. Despite the drawbacks of time-consuming processes and expensive costs, this technology offers the benefits of easy operation and a plentiful yield of PDEVs.
FIGURE 2. The typical method for the isolation of PDEVs. (A) Ultracentrifugation is often utilized as the primary method for extracellular vesicle isolation and usually divides into a differential or a density gradient centrifugation. (B) Precipitation isolates PDEVs by regulating the solubility and dispersion of PDEVs. For the separation of PDEVs, polyethylene glycol (PEG) is commonly employed as a coprecipitate.
The density gradient centrifugation principle is based on the varying sedimentation coefficients of particles. Different particles settle in the area with varying density gradients under the influence of centrifugal force (Lai et al., 2022). Sucrose density gradient centrifugation is commonly used for purifying PDEVs that are acquired through differential centrifugation. The suspension of PDEVs was included in the solution with sucrose gradients. Following ultracentrifugation, the majority of PDEVs were typically concentrated within the density range of 1.13–1.19 g/mL. The primary origin of PDEVs is the sucrose gradient found in the sucrose layer, ranging from 30% to 45% (Stanly et al., 2019; Yang D. et al., 2020). Density gradient centrifugation yields PDEVs with excellent purity and a well-maintained structure, although the process is intricate and time-intensive. Accordingly, the most mature technique for preparing PDEVs is the combination of density gradient centrifugation and differential centrifugation.
By regulating the solubility and dispersion of PDEVs, it is possible to induce the precipitation of PDEVs from the solution (Figure 2B). For the separation of PDEVs, polyethylene glycol (PEG) is commonly employed as a coprecipitate (Fang and Liu, 2022b). Hence, a less expensive technique for extracting EVs was developed, involving a sequence of centrifugation steps and purification with polyethylene glycol-6000 (PEG6000). The presence of this overcrowding agent has the potential to form a mesh-like arrangement capable of ensnaring PDEVs prior to their precipitation (Ali et al., 2022). The utilization of this technique can be extended to cause the precipitation of tiny particles and even viruses (Adams, 1973; Lewis and Metcalf, 1988). By modifying the PEG-6000 concentration employed during purification, this technique successfully yielded diverse average nanoparticles, measuring 365 nm, 304 nm, 256 nm, and 252 nm at PEG-6000 concentrations of 8%, 10%, 12%, and 15%, respectively, in contrast to the 403 nm achieved through ultracentrifugation. Despite having a lower yield and reduced bioactive polyphenol compounds, the PDEVs acquired through this technique exhibited comparable efficiency in macrophage cell uptake and profiles of small RNAs, proteins, and lipids compared to those obtained through ultracentrifugation (Kalarikkal et al., 2020). In addition, the precipitation technique tends to co-precipitate additional impurities that are not EVs, such as different vesicles, aggregates, protein complexes, and proteins of significant size (Zarovni et al., 2015; Castillo et al., 2018). For instance, Sreeram and others designed a technique for purifying PDEVs extracted from ginger roots utilizing PEG6000. These PDEVs precipitated by PEG6000 possess identical dimensions, capabilities, and biochemical makeup to PDEVs obtained through ultracentrifugation (Kalarikkal et al., 2020). Due to the straightforward procedure and excellent productivity, these techniques have also been employed in extraction kits (Umezu et al., 2021). While coprecipitation methods to isolate PDEVs exclusively have been limited to a few researchers, resulting from the tendency of coprecipitation techniques to cause EVs aggregation and coprecipitation of contaminants like lipoproteins (Gardiner et al., 2016).
The immunoaffinity technique is also frequently used to extract PDEVs (Figure 3A). The success of this method relies on the interactions between identifying particular antibodies and distinct proteins located on the surface of EVs. The protein target must be exclusively present or abundant within the EVs, bound within the membrane, and not present as a soluble component in the sample (Zarovni et al., 2015). The immunoaffinity technique can coat magnetic beads with specific antibodies that target proteins found in the membrane of PDEVs (Gardiner et al., 2016). This technique exhibits a high level of specificity and rapid separation rate. For instance, recent research successfully utilized the interaction between the phosphatidylserine on the surface of EVs and the T-cell immunoglobulin and mucin domain-containing protein 4 (Tim4) based on Ca2+. Therefore, it allowed the liberation of the EVs from the beads through the application of Ca2+ chelators (Yang M. et al., 2020). Furthermore, Field-flow fractionation (AF4) is an innovative method of isolating PDEVs based on their particle dimensions (Sitar et al., 2015). The Field-flow fractionation, in combination with light scattering detection, is appropriate for separating and characterizing a diverse range of analyses due to the vast array of mobile eluents available. For example, the AF4 method effectively isolated EVs from B16F10 cells (Zhang et al., 2018). Furthermore, PDEVs were effectively isolated from citrus and bitter gourd through dialysis and electrophoresis. The morphology and quantity of PDEVs separated using this technique were comparable to ultracentrifugation, as revealed by nanoparticle tracking analysis (NTA) or transmission electron microscopy (TEM) analysis (Yang M. et al., 2020; Yang et al., 2021). Additionally, aqueous biphasic systems were employed to extract PDEVs from garlic, with consistent particle size and a diverse range of labeled proteins (Özkan et al., 2021). However, the immunoaffinity technique has a limitation due to the heterogeneity of PDEVs. And only a fraction of EVs in a sample would contain the desired antigen. In contrast, the remaining subpopulation lacking this antigen would not be trapped by the antibody employed in the laboratory. Consequently, resulting from the absent study for the surface composition of PDEVs, the immunoaffinity method has not been studied extensively for extraction.
FIGURE 3. The typical method for the isolation of PDEVs. (A) The immunoaffinity technique is also frequently used to extract PDEV based on the interactions between identifying particular antibodies and distinct proteins located on the surface of extracellular vesicles; (B) Another method for preparing PDEVs is based on size exclusion chromatography (SEC) through the correlation between the hydrodynamic radius of the particles present in the sample and the pore size of the resin beads within the column.
Another method for preparing PDEVs is based on size exclusion chromatography (SEC) (Figure 3B). By considering the correlation between the hydrodynamic radius of the particles present in the sample and the pore size of the resin beads within the column, this technique also effectively separates EVs (Hajj et al., 2013; Fang and Liu, 2022b). Due to the larger hydrodynamic radii, EVs were unable to pass through the pores, leading to their early elution. In contrast, components with smaller hydrodynamic radii, capable of entering the pores, experienced late elution. Some studies evaluated the efficacy of ultracentrifugation, precipitation with polyethylene glycol, and SEC for isolating PDEVs from cabbage. The SEC technique was employed to isolate PDEVs, which exhibited greater yields and more comprehensive functional properties compared to ultracentrifugation (Monguió-Tortajada et al., 2019; Martínez-Greene et al., 2021). In regards to purity, SEC produced outcomes that were over 20 times greater than the two techniques mentioned before (Nordin et al., 2015). These EVs also could preserve biological functionality and structure intact. Jae et al. successfully applied SEC to isolate PDEVs from carrots and cabbage, resulting from the SEC not exerting more changes in their physicochemical properties (You et al., 2021). The SEC method is recognized as the primary technique for extracting PDEVs due to its simplicity, scalability, affordability, and rapid separation rate. It has been applied to extract PDEVs from cucumbers, tomatoes, and peppers (Sidhom et al., 2020a; You et al., 2021). As SEC depends on gravity, it has the potential to maintain the original bioactivity and structures of PDEVs. Although obtaining PDEVs with higher purity can also be achieved by utilizing HPLC, challenges may arise in terms of scaling up and the availability of equipment (Yang D. et al., 2020).
As the most potential candidate for drug delivery platforms, biochemical and physical characterization of PDEVs may be crucial. For example, accurate analysis of their physicochemical properties is significant for drug delivery applications. The description of the characterization and structure of particles also can verify the effective extraction of PDEVs. PDEVs have been reported to transfer multiple cargoes to recipient cells and transform their phenotype, containing the surface morphology, lipids, proteins, RNA, and small molecular substances of PDEVs (Wang et al., 2022). For instance, PDEVs packaged with secondary metabolites could facilitate them to pass the membrane barrier (Woith et al., 2021). Accordingly, these bioavailable molecules encapsulated on PDEVs make them unique and powerful tools for health-beneficial purposes. However, an extensive database of the physicochemical properties related to PDEVs is still unavailable due to the diversity of plants. Therefore, we summarized the currently typical characterization of PDEVs to display their natural clinical and pharmaceutical benefits.
The assessment of morphology is essential in the study of PDEVs since it offers researchers direct insights into the characteristics. In most studies, the features, including shape, size, and surface charge, have been the primary focus of physical investigations of PDEVs. For instance, the majority of studies have adopted TEM and revealed that the morphology of PDEVs is typically round or saucer-shaped and characterized by lipid bilayers with lipid bilayers, which is regarded as the classic extracellular vesicle morphology. The size distributions of PDEVs separated through the disruption process range from 18–1,000 nm and exhibit negative surface charges. Recent studies suggested that the alteration of environmental pH or temperature may modify the size and charge of PDEVs. Up to now, PDEVs have been characterized by several approaches, including dynamic light scattering (DLS), nanoparticle tracking analysis (NTA), and electron microscopy (EM). Dynamic light scattering (DLS) has the capability to evaluate the hydrodynamic size of PDEVs through the fluctuation of light scattering. However, the size of PDEVs may not be accurately analyzed by dynamic light scattering, resulting from their polydispersity and potentially low scattering contrast (Sokolova et al., 2011; Hadisurya et al., 2023). The size may differ depending on the testing technique, as each method operates based on distinct principles and preparations. Moreover, nanoparticle tracking analysis (NTA) is the most common method to assess the size distribution and provides information on the number of PDEVs (McIlvenna et al., 2023). Despite the absence of standardized configurations and the limited scattering index of PDEVs, NTA is still the prevailing method for characterizing PDEVs.
In addition, the stability and optimal preservation of PDEVs have not been extensively elucidated compared with MEVs or synthetic liposomes. However, it is crucial to clarify the capability of PDEVs for biological management and scalability through these necessary measures. Therefore, various experiments have been carried out to examine the durability of PDEVs in diverse environments by modifying variables such as pH ranges, temperature, freeze-thaw cycles, and external manipulation. For example, numerous studies implicated that PDEVs derived from Z. officinale, Daucus carota, and Aframomum sulcatum could remain stable across a broad pH range under physiological temperatures (Mu et al., 2014). Moreover, the pH level may affect the size and surface charge of PDEVs. For example, PDEVs derived from Citrus paradisi maintained their size and surface charge in neutral and alkaline pH conditions while increasing in acidic conditions (Wang et al., 2014). Similarly, the PDEVs of Z. officinale exhibited an increased size and a transition in surface charge from negative to positive in an acidic solution (Zhuang et al., 2015; Zhang et al., 2016a). PDEVs derived from Citrus paradisi and Citrus limon also showed significant resistance to the digestive environment in both simulated conditions and mouse models. In addition, several studies indicated that PDEVs might be unstable under boiling or sonication. The antiproliferative function of PDEVs extracted from citrus fruits was destroyed under sonication or boiling conditions (Alzahrani et al., 2023). Consistently, the PDEVs obtained from apples were susceptible after boiling and sonication (Fujita et al., 2018). PDEVs derived from Ginseng quinquefolium could promote the polarization of macrophages compared with un-sonicated samples (Cao et al., 2019). It is crucial for researchers to understand that sonication is a commonly employed technique for drug incorporation (Wang et al., 2013; Zhang et al., 2016c; Zhuang et al., 2016; Ly et al., 2023; Raguraman et al., 2023). Therefore, the morphology of PDEVs should be evaluated during drug loading. PDEVs have been extensively applied as drug carriers, resulting from their adjustable dimensions and excellent stability. For example, it is possible to improve the stability and bioavailability of the delivery platform by combining hydrophobic agents with Z. officinale isolated from PDEVs. Without affecting biological activities, Citrus paradisi-derived PDEVs could be modified with biomolecules such as zymosan A, folic acid, or curcumin. Moreover, these lipid particles exhibited more excellent stability compared to cationic liposomes when exposed to a 10% bovine serum solution at 37°C (Wang et al., 2013).
The description of the distinctive protein composition and function in PDEVs is crucial for further classification of PDEVs. According to the International Society for Extracellular Vesicles (ISEV), it is recommended that researchers should document a minimum of three proteins in each EVs sample, such as transmembrane and cytosolic proteins (Lötvall et al., 2014). Interestingly, PDEVs usually comprise a low concentration of proteins, including cytosolic proteins like actin and proteases, and membrane proteins acting as channels and transporters, like aquaporins and chloride channels (Zhang et al., 2016a; Alzahrani et al., 2023; Ipinmoroti et al., 2023; Wang et al., 2023). Cao and others detected 3,129 proteins in PDEVs derived from G. quinquefolium by mass spectrometry. According to Gene Ontology, these proteins were categorized into three groups, which are biological processes, cellular compartments, and molecular functions (Cao et al., 2019; Ou et al., 2023). Other groups analyzed the protein composition of PDEVs derived from Citrus limon and identified 162 proteins related to transportation (Stanly et al., 2019). Surprisingly, 56.7% of these proteins matched with proteins extracted from MEVs, according to the ExoCarta protein database (Fang and Liu, 2022b). However, extracellular vesicles derived from mammals comprise over 1,000 proteins, whereas PDEVs, like those obtained from G. quinquefolium, only have 28 proteins (Ju et al., 2013; Zhang et al., 2016a). Interestingly, some studies reported that the surface protein of PDEVs derived from Allium sativum may control their absorption by HepG2 (Song et al., 2020). Subsequently, they found a significant decrease in the absorption of PDEVs after inhibiting CD98 receptors on HepG2. In detail, Song et al. indicated that the interaction of lectin-like protein from PDEVs with the glycoprotein CD98 on the cell surface facilitated the uptake of A. sativum-PDEVs. This discovery provided a deeper comprehension of how cells take up PDEVs. On the other hand, the PDEVs contain proteins that help the plant to be resistant to stress (Rutter and Innes, 2017). For example, Rutter et al. detected the proteomics of the apoplastic PDEVs from Arabidopsis leaves and revealed that they contained a high concentration of stress response-related proteins (De Vallée et al., 2023). In a recent study, researchers compared the proteomics between apoplastic and whole-leaf PDEVs from A. thaliana. As expected, PDEVs obtained through tissue rupture methods contained more composition of proteins than those obtained exclusively from extracellular fluid (Liu Y. et al., 2020). Furthermore, several proteins present in MEVs have also been identified in PDEVs, including GPI-anchored proteins, annexins, heat-shock protein 70 (HSP70), and aquaporin proteins (Ju et al., 2013). In summary, complete profiling of proteins inside PDEVs is quite tricky due to the varying protein databases needed for matching in different plants.
Lipids have been considered the main composition of PDEVs, as the lipid bilayer composition influences their efficiency taken up by recipient cells and used for therapeutic purposes. Moreover, the proportion of lipids has a crucial role in the drug encapsulation and transportation capacity of PDEVs. The diversity in lipid composition determines the physical distribution and transport function of PDEVs. For instance, the primary lipid in PDEVs of Citrus paradisi and A. sativum was phosphatidylcholine (PC). In contrast, PDEVs derived from Z. officinale mainly contained phosphatidic acid (PA) as their main lipid component (Wang et al., 2014; Teng et al., 2018). However, PDEVs have been found to lack cholesterol and sphingomyelin, unlike MEVs and synthetic liposomes (Teng et al., 2018). In addition, this peculiarity in lipid compositions impacted the distribution of PDEVs. For instance, the lipid composition and structure of PDEVs played a crucial role in their distinctive absorption by the cells in the intestine. PDEVs derived from Vitis amurensis were plentiful in PA (53.2%), primarily found in the intestinal tract, and rarely absorbed by intestinal stem cells and macrophages (Ju et al., 2013; Mu et al., 2014; Zhuang et al., 2015; Zhang et al., 2016a). By contrast, the enrichment of PC lipids by PDEVs determined the migration from the gut to the liver (Teng et al., 2018). The presence of phosphatidylcholine (PC) was observed to promote the movement of PDEVs into liver cells, as demonstrated by analyzing images of mouse intestinal cells and liver cells at 1–6 h after consuming fluorescence-labeled PDEVs (Teng et al., 2018). PDEVs derived from Z. officinale migrated from the gut to the liver via the bloodstream and were absorbed by hepatocytes and Kupffer cells, resulting in significant accumulation in the liver (Zhuang et al., 2015; Kilasoniya et al., 2023). In the same way, Z. officinale-PDEVs produced using the thin-layer dispersion technique were absorbed by RAW264.7 and colon-26 cells and persisted in the stomach, ileum, and colon, indicating a focus on the intestines (Zhang et al., 2017). Mouse intestinal stem cells rarely absorbed PDEVs derived from V. amurensis, whereas they could absorb recombinant liposomes, which then accumulated in the intestinal tract (Ju et al., 2013; Record, 2013). Therefore, it is crucial to lucubrate the lipid role of PDEVs to elucidate the mechanism of PDEVs and utilize them in drug delivery systems.
miRNAs, which are small RNA molecules approximately 22 nt in size, do not possess coding properties. Their primary roles include regulating the cleavage or translation of mRNAs and inducing the expression of specific target genes (Bartel, 2004; Olmi et al., 2023). Numerous studies have confirmed the existence of abundant miRNAs in PDEVs, typically thought to regulate gene expression in mammals (Chin et al., 2016). Besides, PDEVs are also proposed to protect miRNAs against degradation during transportation. Some studies also implicated that PDEVs have the potential to facilitate the interaction between the gut microbiota and the host immune systems by impacting the composition of gut microbes due to their possession of diverse miRNAs (Teng et al., 2018). Previous studies have detected 418 miRNAs in different species of PDEVs, suggesting that the over-expressed miRNAs might participate in inflammatory responses and signaling pathways associated with cancer (Xiao et al., 2018). Moreover, various methods, including gel electrophoresis and mass spectrometry, have been applied to validate miRNAs in PDEVs derived from V. amurensis (Ju et al., 2013). For instance, Zhang et al. identified 125 different miRNAs in Z. officinale-PDEVs, mediating immunomodulatory or metabolic regulation (Zhang et al., 2016a; Yin et al., 2022). Recent studies have demonstrated the ability of miRNA from PDEVs to regulate the composition of the gut microbiota. For example, miR167a from Z. officinale-PDEVs may activate the aryl hydrocarbon receptor (AHR) signaling pathway to alleviate ulcerative colitis by promoting IL-22 expression (Teng et al., 2018). Zhou and others demonstrated that miRNA-2911 derived from Lonicera sempervirens has the ability to suppress Influenza Virus A (IVA) (Zhou et al., 2015). Hence, the miRNA derived from PDEVs holds promise for therapeutic implications. Accordingly, due to the ability of miRNA to engage in intercellular communication, further study of PDEVs may be considered as one of the most potential therapeutic strategies in the future, especially as drug delivery systems.
PDEVs contained compounds inherit the bioactive properties of their source plants, and at the same time, their distinct structures also suggest the potential in the field of biomolecule transportation. For example, PDEVs, consisting of a double layer of lipids, enable the incorporation of hydrophobic medications, enhance the effectiveness of medicines, and efficiently accommodate hydrophilic chemicals to prevent adverse effects resulting from drug leakage (Nemati et al., 2022). Recent studies have implicated that the application of PDEVs has been shown to enhance the bioavailability, solubility, and stability of biomolecules (Kalani et al., 2016). For example, the PDEVs derived from V. amurensis were applied to encapsulate the methotrexate (GDN-MTX), preserving the anti-inflammatory properties of GDN-MTX and enhancing the uptake of GDN-MTX by intestinal macrophages (Wang et al., 2014). Moreover, the GDN-MTX effectively alleviated symptoms of ulcerative colitis, reduced the secretion of pro-inflammatory cytokines by intestinal macrophages, and mitigated the adverse effects of MTX while playing a more substantial anti-inflammatory impact in the mouse colitis model (Wang et al., 2014). Recent studies also suggest that PDEVs from V. amurensis coated with white cell membranes on their surface have the ability to improve target and accumulate at the site of inflammation in various models. Furthermore, these membrane fusion techniques also were proven to improve the targeting of PDEVs, prolong the drug circulation time, and enhance the accumulation of the medicine in the target tissue (Wang et al., 2015). In addition, several researchers also found that combining PDEVs with ligands, such as folic acid (FA), could enhance their targeting ability. For example, FA-modified PDEVs derived from Z. officinale could encapsulate the doxorubicin without size change, delay the doxorubicin release, produce sustained cytotoxicity, and improve the tumor targeting ability (Zhang et al., 2016c). Similarly, functional heparin (HR)-modified PDEVs from Citrus limon could load doxorubicin with a slight size variation, which effectively improved the internalization efficiency of HR-PDEVs (Xiao et al., 2022). At the same time, the diverse endocytosis capacity of HR-PDEVs efficiently dissipated intracellular energy, decreasing drug efflux (Xiao et al., 2022).
Accordingly, we summarized various PDEVs that have been extensively used to develop novel drug delivery systems for treating particular diseases or maintaining normal functions (Figure 4). Different edible and medical plants are applied to isolate therapeutic PDEVs with diverse functionalities. This review also lists the main types of PDEVs for their therapeutic applications (Table 3).
FIGURE 4. Multiple sources of PDEVs have been extensively used to develop novel drug delivery systems for treating particular diseases or maintaining normal functions. PDEVs and engineered PDEVs both are directly applied as therapeutic agents or delivery platforms, which could precisely target the different cells by administration to the body.
Despite being a relative innovation, PDEVs have already been employed to alleviate various diseases resulting from their advantageous healing properties and precise targeting of specific tissues (Wang et al., 2014). Especially, PDEVs were identified to bind to receptors and serve as recognition elements for remote locations due to the biomolecules composed in PDEVs, including proteins, lipids, and miRNAs (Wang et al., 2014; Teng et al., 2018). Therefore, these PDEVs may be directly applied as therapeutic agents. For instance, PDEVs from V. amurensis have been proven to relieve dextran sulfate sodium (DSS)-induced colitis in mice (Ju et al., 2013). Interestingly, these PDEVs also enhanced the growth of intestinal stem cells, which could potentially generate enduring intestinal organoid structures in vitro, possibly being the sole source of such stem cells (Deng et al., 2017). Furthermore, these PDEVs dramatically improve the regeneration of mucosal epithelium and successfully revive the structural integrity of the entire intestinal tract (Deng et al., 2017). Similarly, some studies found that PDEVs derived from B. oleracea displayed significant preventative and curative properties in relation to three forms of colitis (Wang et al., 2014). In detail, these PDEVs exhibit strong preventive properties against both acute and chronic colitis in vivo experiments. These were supported by evidence of heightened levels of anti-inflammatory cytokines and increased histoscore, as indicated by a decrease in colon shortening, loss of weight, and mucosa inflammation (Wang et al., 2014). Additionally, these B. oleracea-PDEVs were found to preserve the immune environment of the intestines while causing minimal negative responses by activating the Adenosine monophosphate-activated protein kinase (AMPK) pathway (Wang et al., 2014). Furthermore, PDEVs also have been reported to alleviate some liver diseases (Zhuang et al., 2015). Zingiber officinale has been applied in traditional medicine for centuries, providing numerous health advantages due to its inherent chemical components like shogaol and gingerol (Brahmbhatt et al., 2013; Kandemir et al., 2019). The expression level of a set of detoxifying/antioxidant genes, including glutamate-cysteine ligase modifier subunit (GCLM), heme oxygenase-1 (HO-1), glutamate-cysteine ligase catalytic subunit (GCLC), and NADPH quinone oxidoreductase-1 (NQO1), was increased by Z. officinale-derived PDEVs, leading to alleviating liver damage. In addition, the analysis of tissue showed that mice administered Z. officinale-PDEVs experienced a significant decrease in fat deposits within the liver. Moreover, Z. officinale-PDEVs markedly reduced levels of triglycerides and the weight of the liver. The mice that were given Z. officinale-PDEVs also exhibited histopathological alterations in the liver, indicating that PDEVs safeguard the liver against the toxicity of alcohol.
Interestingly, PDEVs also have dramatic anti-tumor activity. Raimondo et al. have reported that PDEVs derived from Citrus limon significantly suppressed the growth of tumors and reduced the multiple angiogenesis-related cytokines secretion in chronic myeloid leukemia (CML) through the Tumor necrosis-related apoptosis-inducing ligand (TRAIL) pathway (Raimondo et al., 2015). At the same time, these PDEVs targeted the tumor site within 15 min, exhibiting enduring effects lasting over 24 h in vivo. Actually, these PDEVs participate in cellular interaction and contribute to eliminating cancer cells via activation of TRAIL signaling, displaying their potential as chemotherapy agents (Raimondo et al., 2015). Similarly, PDEVs derived from citrus fruits have demonstrated the ability to suppress the survival of cancer cells, such as LAMA84, SW480, and A549, dependent on both the dosage and duration, compared to normal cell lines (HS5, HUVEC, and PBMC). Additional analysis indicated that these PDEVs induced apoptotic cell death in tumor cells by activating the TRAIL pathway, which was further validated in the xenograft model (Raimondo et al., 2018). Specifically, it was shown that the proteomics analysis revealed a correlation between the suppression of acetyl-CoA carboxylase (ACC) and the anti-cancer impact of these PDEVs, specifically in the case of colorectal cancer (Raimondo et al., 2018).
Numerous research studies have focused on the development of drug-delivery systems that possess the ability to target particular cells, tissues, or organs specifically. PDEVs exhibited a promising potential due to their inherent capacity to target desired tissues specifically. Zingiber officinale-PDEV exhibited distinct transport characteristics in a study focused on devising strategies to protect liver cells against the toxicity of alcohol. Zingiber officinale-PDEVs labeled with tetramethylindotricarbocyanine iodide (DiR) were observed to accumulate primarily in the liver in vivo assay. Using confocal microscopy to image immunostaining provided evidence that Z. officinale-PDEVs could enter and shift the hepatic vascular system. Caco-2 cells were found to internalize PDEVs derived from apples following co-incubation (Fujita et al., 2018). Therefore, the inherent targeting capabilities of PDEVs are expected to enhance their application as natural drug delivery systems.
PDEVs have exhibited some inherent advantages, containing stability, firmness, and an appropriate structure, enabling the inclusion of drugs in their lipid bilayer and precise targeting of specific tissues (Wang et al., 2013; Wang et al., 2015; Teng et al., 2018). Recent studies also suggested that PDEVs could transport biomolecules safely and maintain long-term circulation in the blood when administered systemically. Besides inherent biological activities, PDEVs could be engineered as desired drug delivery platforms for the precise delivery of poorly soluble agents or therapeutic compounds, such as surface functionalization and content modification. Recent studies have focused on modification techniques to improve the drug delivery precision and efficiency of PDEVs (Chen et al., 2023).
Numerous studies have also indicated that PDEVs inherently possess the capability to target cells specifically. The surface of PDEVs, consisting of different lipids, exhibited distinct advantages like stability and precise targeting (Wang et al., 2013; Huang et al., 2023). The lack of studies compared with MEVs has resulted in limited knowledge about the fundamental mechanisms responsible for their natural targeting capabilities. However, recent studies implicated that the specificity of PDEVs is conferred by a specific alignment of proteins and lipids. According to research on the source plants, it has also been proposed that the selective absorption of PDEVs by particular cells is attributed to specific genes (that encode miRNAs and siRNAs) and small compounds, which act as external ligands for the targeting components on the cells (Wang et al., 2013; Teng et al., 2018). PDEVs exhibit excellent inherent targeting ability, minimal off-target effects, and rare toxicity compared to synthetic nanoparticles. It excludes the necessity for extra alterations to enhance biocompatibility, internal stability, and properties related to pharmacokinetics, which would otherwise be necessary to increase their effectiveness as treatments when they are modified with particular ligands. Furthermore, PDEVs are regarded as optimal nanocarriers due to their inclusion of typical proteins involved in transfer and trafficking (Ju et al., 2013; Wang et al., 2014). Although they possess inherent targeting characteristics, numerous endeavors have been made to refine their targeting effects and enhance the efficacy of their intracellular delivery (Teng et al., 2018). PDEVs could be developed to precisely and consistently target organs or cells by modifications using targeting ligands, like chemicals and genetic drugs on their membrane, and merging with cell membranes (Wang et al., 2013). These biomolecular modifications on the surface of PDEVs have proved to improve the stability and uniformity of the nanostructures (Li et al., 2018).
Several modification techniques focus on the surface functionalization of PDEVs to incorporate cell membranes or ligands. For instance, some studies focused on improving the precision of PDEVs, and the lipid-thin film is formed by adding small molecules like folic acid (FA) to the extracted lipids from PDEVs, which are applied to fabricate nanovesicles. It also demonstrated the targeting effect of the ligands exhibited on the membrane surface via the assay of biodistribution in vitro and in vivo (Wang et al., 2013; Yáñez-Mó et al., 2015). Following intravenous administration, some studies demonstrated that FA-PDEVs remained detectable even after 48 h of circulation in vivo, thereby enhancing their potential to infiltrate tumors. Thus, FA-PDEVs might serve as a perfect carrier for delivering drugs against tumors without causing the adverse impact of freely circulating medications. Some studies developed a drug delivery system utilizing Z. officinale-PDEVs combined with siRNA as targeting ligands (Adams, 1973; Lewis and Metcalf, 1988). Different physical configurations and angles, referred to as arrow-tail and arrow-head, were achieved by manipulating the RNA three-way junction (3WJ) architecture. The analysis of their absorption by cancer cells revealed varying degrees of cellular internalization based on the orientation of the 3WJ nanostructure. In order to selectively focus on cancer cells that have an overexpression of folate receptors, arrow-tail siRNA was conjugated with FA, which served as a targeting ligand and attached to the outer surface of the ginger-PDEVs. The decreased levels of survivin mRNA levels confirmed the therapeutic potential of FA-3WJ-PDEVs. These vectors led to a significantly enhanced distribution of tumor tissues compared to unmodified PDEVs (Wang et al., 2013). Recent studies also designed an intelligent delivery platform by utilizing PDEVs covered with a plasma membrane derived from activated leukocytes, aiming to improve their therapeutic capabilities and targeting precision (Wang et al., 2015). Moreover, using the extrusion technique, PDEVs were ready to create a personalized transport mechanism by merging with the cell membrane derived from leukocytes. Zhuang et al. also designed biomimetic nanoplatforms that integrated electrodynamic Pd-Pt nanosheets and PDEVs from Z. officinale (Qiao et al., 2022). These engineered PDEVs endow great anti-infection with prolonged blood circulation and accumulation at infection sites. In this process, the engineered PDEVs could specifically target inflamed tissues and diminish the off-side impacts while transporting drugs (Wang et al., 2015).
The technology of encapsulation in the delivery system could transport biomolecules safely and maintain long-term circulation in the blood when administered systemically. As a result, PDEVs with content modification may be regarded as promising vectors for targeted delivery in tumorigenic disorders and chronic illnesses (Teng et al., 2018; Wang et al., 2019). Multiple techniques have been devised for loading diverse shipments into PDEVs. PDEVs can be loaded with desired cargo molecules using passive loading techniques, such as co-incubating with drugs (Vashisht et al., 2017). However, passive loading methods might not succeed in attaining a substantial encapsulation rate. Thus, subjecting PDEVs to sonication, freeze-thaw cycling, and similar mechanical treatments can destroy the integrity of their membranes and improve the efficiency of loading cargo. In order to prevent potential internal toxicity caused by impurities and achieve nanocarriers with consistent size, PDEVs can be designed using lipids and combined with cargo while preparing a lipid thin film (Li et al., 2018; Uddin et al., 2023). The method efficiently loads various shipments, including lipophilic drugs (DOX) and biomacromolecules like antibodies, siRNA, as well as DNA expression vectors (Wang et al., 2013; Zhang et al., 2016c). The encapsulation efficiency of doxorubicin through this method has resulted in high rates (95.9% ± 0.3%) (Zhang et al., 2016c). Moreover, conventional drug delivery systems loaded with some gene medicines, including siRNA and miRNA, have been lucubrated. However, the limited loading efficiencies of substances result in suboptimal therapeutic effects, and adverse consequences appear inevitable (Amreddy et al., 2017; Mainini and Eccles, 2020). In contrast, recent studies implicated that PDEVs could address these disadvantages (Zhu et al., 2022). For example, some studies suggested PDEVs from Z. officinale were used to carry specific siRNA targeting CD98 for the treatment of ulcerative colitis (Zhang et al., 2017). In comparison to a commercially accessible cholesterol and dioleoylphosphatidylethanolamine liposome product (DC-Chol/DOPE), it was identified that these PDEVs exhibited excellent biocompatibility, causing reduced toxicity and apoptosis in macrophage and eliminating colon-26 cells during in vitro assay (Zhang et al., 2017; Li et al., 2023). The PDEVs from Z. officinale were transfected with siRNA against CD98 using sonication to assess the mRNA expression of CD98. In detail, after being orally administered, the complexes of siRNA-CD98/PDEVs were successfully retained in the gastrointestinal tract and exhibited a notable decrease in CD98 expression in the gastrointestinal system compared to scrambled siRNA/PDEVs (Zhang et al., 2017). PDEVs derived from Z. officinale, which have low toxicity and high biocompatibility in contrast to synthetic liposomes, were discovered to be appropriate for transporting divalent metal-ion transporter-1 (Dmt1)-siRNA blunts to intestinal epithelial cells via reducing iron accumulation in hereditary hemochromatosis (Wang et al., 2019). Moreover, these PDEVs incorporating folic acid (FA) can enhance their capacity to specifically target the duodenum and seamlessly integrate into the intestinal tract through the proton-coupled folate transporter. In detail, they found that these PDEVs may decrease ferritin, transferrin saturation (TSAT), and non-heme Ferrum in multiple organs, such as the pancreas, heart, kidney, and liver, by reducing Dmt1-mRNA expression and iron loading (Wang et al., 2019).
Engineered PDEVs offer several unusual properties that render them highly appropriate as drug delivery systems for future medical purposes, such as transporting hydrophobic medications, improving the target precision, evading enzymatic decomposition, and eluding immune response. For example, some content modification of PDEVs has ensured excellent stability and biocompatibility in diverse physiological environments, including the bloodstream and different pH. All of these stellar features, coupled with the discovery of their intrinsic therapeutic actions, make engineered PDEVs desired for their advent into the arena of bioactive molecule delivery applications. Here, we shed light on engineered PDEVs as the therapeutic system and drug delivery systems.
Several studies have proved that the surface functionalization of PDEVs can prevent the harmful effects of chemotherapy by transporting a higher amount of medication to tumors rather than normal organs. For instance, FA-modified PDEVs are predominantly gathered in tumor tissues, whereas unmodified PDEVs are primarily localized to the spleen and liver. Interestingly, Zhang et al. also found that FA- PDEVs loading doxorubicin (DOX) has a dramatic effect on colon cancer (Zhang et al., 2016c). FA-PDEVs-DOX or FA-PDEVs were fabricated using a conventional approach that relies on the hydration of lipid films, which is similar to the technique employed for producing liposomes. Compared with unmodified PDEVs, FA-PDEVs showed an enhanced capacity to target colorectal tumor cells via active FA and folate receptor (FR) interactions. While the FA-PDEVs exhibited dramatic reduction levels within the spleen and liver compared to PDEVs. It suggests that utilizing FA-PDEVs as the delivery platform may diminish the systemic toxicity of drugs toward healthy tissues. Following intravenous administration, some studies demonstrated that FA-PDEVs remained detectable even after 48 h of circulation in vivo, thereby enhancing their potential to infiltrate tumors. Thus, FA-PDEVs might serve as a perfect carrier for delivering drugs against tumors without causing the adverse impact of freely circulating medications. In addition, recent studies also designed an intelligent delivery platform by utilizing PDEVs covered with a plasma membrane derived from activated leukocytes, aiming to improve their therapeutic capabilities and targeting precision (Wang et al., 2015). By modifying the membrane-coated PDEVs, specialized inflammatory receptor-enriched plasma membrane-coated PDEVs (IPDEVs) were obtained, which possess distinct cellular targeting capabilities. To evaluate the inflammatory potential of IPDEVs or PDEVs, they were introduced to the upper layer of a transwell plate containing a cultured monolayer of human umbilical vein endothelial cells (HUVECs), which served as a blood-brain barrier model. The study yielded more significant quantities of IPDEVs migrating through HUVECs within 48 h compared to PDEVs, and the inclusion of chemokines further improved the efficiency of migration. The impact of IPDEVs on peripheral circulation was further validated in models of inflammation and chronic inflammatory cancer models. Compared to PDEVs, the inflammatory sites exhibited a notable rise in the accumulation of IPDEVs, and in vivo, IPDEVs and DOX were consistently administered for tumor treatment. Compared to free-DOX and PDEVs-DOX, IPDEVs demonstrated an improvement in tumor permeability and a decrease in tumor volume. As the result of inflammation is a prevalent occurrence in numerous affected tissues, the exceptional capacity of these engineered PDEVs to specifically target sites of inflammation renders them highly effective nanocarriers.
In addition, the technology of encapsulation in the delivery system could transport biomolecules safely and maintain long-term circulation in the blood when administered systemically. Multiple studies suggested that PDEVs with content modification may be regarded as promising vectors for targeted delivery in tumorigenic disorders and chronic illnesses. As a result, they have the capability to transport medications with accurate localization to specific tissues instead of causing widespread effects throughout the body, resulting in enhanced therapeutic results and reduced side effects. To evaluate the drug-carrying capability of PDEVs, multiple groups assess the effectiveness of ginger-PDEVs in loading and releasing doxorubicin through a pre-loading strategy (Zhang et al., 2016c). Furthermore, the drug release kinetics of PDEVs containing doxorubicin and DC-Chol/DOPE liposomes loaded with doxorubicin were analyzed at different pH levels (pH 5.5, 6.0, 6.5, 7.0, and 7.5). PDEVs from Z. officinale carrying doxorubicin could effectively secret DOX in an acidic tumor microenvironment, and these PDEVs diffused more rapidly compared to the liposomes (Zhang et al., 2016c). The capacity of PDEVs derived from Citrus paradisi to offer precise drug delivery to intestinal macrophages was also assessed (Wang et al., 2013). According to their findings, the primary immune cells of the intestine, known as intestinal macrophages, took up the majority of PDEVs from Citrus paradisi in the lamina propria of both the small and large intestines (Wang et al., 2013). However, liposomes that are readily accessible in the market exhibited significantly lower efficacy in directing their action toward intestinal macrophages. Moreover, PDEVs from Citrus paradisi were developed to conjugate with methotrexate (MTX), a chemical drug that suppresses the immune response and reduces inflammation (Zhuang et al., 2016; Niu et al., 2021). In a mouse model of ulcerative colitis induced by DSS, PDEVs from Citrus paradisi loading MTX could alleviate the loss of body weight and the shortening of the colon. These findings indicate alleviated colon tissue damage and infiltration of inflammatory cells in mice treated with grape-PDEVs-MTX (Niu et al., 2021). Accordingly, the PDEVs-MTX were effective in targeting intestinal macrophages for treating inflammatory-related diseases in the intestines (Nishad et al., 2021; Niu et al., 2021).
Ever since the identification of PDEVs, they have demonstrated remarkable characteristics in the fields of biotherapy, delivering medication, and traversing physiological obstacles. PDEVs, derived from plant sources, are believed to hold immense promise in the field of biomedicine, particularly as drug delivery systems. PDEVs present excellent benefits compared to artificial carriers and MEVs in terms of drug encapsulation, resistance to gastrointestinal fluid, cellular absorption, efficiency, and safety. However, the technological limitations currently constrain the utilization of PDEVs as drug delivery systems.
Firstly, one of the primary challenges encountered by drug delivery applications of PDEVs is the absence of a universal and standardized approach to separation techniques. In the laboratory, various techniques like ultracentrifugation, density gradient centrifugation, ultrafiltration, and size exclusion chromatography find extensive application for the isolation and analysis of PDEVs. In comparison, the application of these techniques in the process of industrial transformation is hindered by the time-consuming and limited productivity. Researchers using ultracentrifugation may experience inconsistent results due to variations in centrifugation speed, force, and rotor type, as reported (Kameli et al., 2021b). Thus, it is imperative to investigate and develop a perfect isolation procedure to acquire consistent, steady, and productive PDEVs. Furthermore, it is also crucial to precisely analyze and regulate the quality and origin of raw materials owing to variations in plant types, growth phases, and geographical locations.
Further exploring the biological properties and transportation mechanisms of PDEVs remains one of the primary obstacles in utilizing them for drug delivery. PDEVs demonstrate exceptional tissue specificity and gastrointestinal resilience, yet this specific characteristic remains insufficiently elucidated. Further elucidating the tissue-specific targeting mechanism of PDEVs has the potential to overcome the challenge encountered in drug delivery applications. The ordered structure or lipid composition of PDEVs may enhance these capabilities of PDEVs. To understand these mechanisms of PDEVs, gaining a comprehensive comprehension of their physical characteristics might be the appropriate direction. The regulation of information in PDEVs appears to involve proteins, lipids, and miRNA, and the source of PDEVs may contribute to the variation in regulation. While articulating a comprehensive regulatory mechanism appears to be challenging, it may be more efficient to concentrate on regulating PDEVs in particular plants or species rather than across all plants. Simultaneously, the differentiation between regulatory mode and targeting mechanism facilitates the administration of diverse medications, enabling researchers to select from a range of PDEV origins based on their suitability for a particular ailment. Furthermore, the unpredictable element of the natural active component transported by PDEVs is not only capable of enhancing the effects of medications but also influencing their effectiveness (Nemati et al., 2022). Therefore, it is imperative to conduct comprehensive and profound research to elucidate the makeup of natural active constituents in PDEVs and the interaction mechanism between these constituents and medications to achieve efficient incorporation or collaborative therapeutic outcomes.
Insufficient targeting ability poses a challenge for the drug delivery application of PDEVs. On the other hand, proteins may have a critical role in receptor recognition. Several studies have indicated that PDEVs exhibit a lower protein count in comparison to MEVs, implying that PDEVs possess relatively limited selectivity (Pocsfalvi et al., 2018). The application of engineering was extensively utilized in the examination of MEVs, resulting in enhanced flexibility of MEVs (van der Koog et al., 2022). Thus, engineering PDEVs is expected to play a crucial role in the application of PDEVs as drug delivery platforms. PDEVs could also be modified using ligands like FA or peptides to enhance cargo targeting capability. The utilization of membrane fusion technology has the potential to combine PDEVs with synthesized liposomes, as well as mammalian EVs and bacterial EVs. The resulting hybrid PDEVs could potentially provide enhanced biological attributes. This strategy could improve the targeting precision and immune evasion capabilities of PDEVs.
In particular, several clinical studies on the therapeutic activities of PDEVs for clinical management have been registered (Alfieri et al., 2021). For example, the derivants of PDEVs as a delivery system to increase the bioavailability of oral curcumin are being tested on colorectal cancer patients (NCT01294072) (Wu et al., 2017). In a subsequent study, PDEVs derived from grapes and their derivates were proven to alleviate adverse reactions induced by chemoradiation in head and neck cancer patients, such as inflammatory and oral mucositis pain (NCT01668849) (Wu et al., 2017). Another clinical trial confirmed the prospects against inflammation of PDEVs from Z. officinale for effectively alleviating irritable bowel disease (NCT04879810) (Zhang et al., 2016a). Thus, considering these promising advantages, the researchers should carry out more preclinical and clinical trials to evaluate the bioactivities of PDEVs and engineered PDEVs in patients and define their minimum dosage. However, the application of PDEVs in clinical management is still limited by technology.
Firstly, one of the main problems of PDEVs translated from lab to clinical state is the lack of unified and standardized separation methods. These isolation methods have the problems of time-consuming and low yields in the industrial transformation (Kameli et al., 2021b). For example, ultracentrifugation may be a cost-effective and simple method, but it has been reported to disrupt PDEVs due to the centrifugation force and speed (Ramirez et al., 2018). Moreover, there have reportedly been inconsistencies in the separation of EVs by ultracentrifugation due to the rotor (Furi et al., 2017). Additionally, ultracentrifugation and other methods also have the risk of co-contamination with toxic proteins (Momen-Heravi et al., 2013). Further research of PDEVs to understand their biological role and transport mechanism in physiological or pathological processes may be one of the major challenges in the transformation from lab to clinical application (Cloet et al., 2021). For instance, components in PDEVs seem to be involved in the transport or regulation mechanism, and their different sources may be the primary reason (Kameli et al., 2021a). However, the current general regulation may be complicated to depict clearly. Therefore, it could be more effective to focus on the regulation of PDEVs in specific species rather than all plants.
Overall, drug delivery systems based on PDEVs demonstrated dramatic benefits in terms of biocompatibility, stability, distribution, and cellular absorption. Furthermore, employing PDEVs for the administration of biomolecules can decrease the adverse reactions of medications while also exhibiting the inherent capabilities of PDEVs. The unique attributes of PDEVs allow for the development of an innovative approach to drug delivery platforms. Since biomedical research on PDEVs is still in the infancy stages, there may be numerous challenges in utilizing PDEVs as drug carriers. Accordingly, further exploration of PDEVs in drug delivery is worthwhile, as these challenges can be overcome through additional research in the future.
PW: Conceptualization, Writing–original draft, Writing–review and editing. WW: Writing–original draft. SZ: Writing–original draft. JH: Writing–review and editing. CL: Visualization, Writing–review and editing. HY: Visualization, Writing–review and editing. XpC: Conceptualization, Supervision, Writing–original draft. XaC: Conceptualization, Writing–original draft.
The author(s) declare financial support was received for the research, authorship, and/or publication of this article. This work was supported by grants from the Sichuan Science and Technology Program (Grant Nos 2022JDRC0131 and 2023NSFSC1759), the Sichuan Administration of Traditional Chinese Medicine (Gran No. 2023MS333), the China Postdoctoral Science Foundation (Grant Nos 2021M693784 and 2022T150078), the National Natural Science Foundation of China (No. 82004097), Potential Postdoctoral Program of Chengdu University of Traditional Chinese Medicine Grant No. BSH2019019.
We would like to acknowledge Yifan Bao (University of Vienna) for the construction of the figures, which are graphics created with BioRender.com. We also acknowledge the cited publications in this review, yet we were unable to mention them due to space limitations.
The authors declare that the research was conducted in the absence of any commercial or financial relationships that could be construed as a potential conflict of interest.
All claims expressed in this article are solely those of the authors and do not necessarily represent those of their affiliated organizations, or those of the publisher, the editors and the reviewers. Any product that may be evaluated in this article, or claim that may be made by its manufacturer, is not guaranteed or endorsed by the publisher.
Abels, E. R., and Breakefield, X. O. (2016). Introduction to extracellular vesicles: biogenesis, RNA cargo selection, content, release, and uptake. Cell Mol. Neurobiol. 36 (3), 301–312. doi:10.1007/s10571-016-0366-z
Adams, A. (1973). Concentration of Epstein-Barr virus from cell culture fluids with polyethylene glycol. J. Gen. Virol. 20 (3), 391–394. doi:10.1099/0022-1317-20-3-391
Alfieri, M., Leone, A., and Ambrosone, A. (2021). Plant-derived nano and microvesicles for human health and therapeutic potential in nanomedicine. Pharmaceutics 13 (4), 498. doi:10.3390/pharmaceutics13040498
Ali, N. B., Abdull Razis, A. F., Ooi, J., Chan, K. W., Ismail, N., and Foo, J. B. (2022). Theragnostic applications of mammal and plant-derived extracellular vesicles: latest findings, current technologies, and prospects. Molecules 27 (12), 3941. doi:10.3390/molecules27123941
Alvarez-Erviti, L., Seow, Y., Yin, H., Betts, C., Lakhal, S., and Wood, M. J. (2011). Delivery of siRNA to the mouse brain by systemic injection of targeted exosomes. Nat. Biotechnol. 29 (4), 341–345. doi:10.1038/nbt.1807
Alzahrani, F. A., Khan, M. I., Kameli, N., Alsahafi, E., and Riza, Y. M. (2023). Plant-derived extracellular vesicles and their exciting potential as the future of next-generation drug delivery. Biomolecules 13 (5), 839. doi:10.3390/biom13050839
Amreddy, N., Babu, A., Muralidharan, R., Munshi, A., and Ramesh, R. (2017). Polymeric nanoparticle-mediated gene delivery for lung cancer treatment. Top. Curr. Chem. (Cham) 375 (2), 35. doi:10.1007/s41061-017-0128-5
An, Q., Ehlers, K., Kogel, K. H., van Bel, A. J., and Hückelhoven, R. (2006). Multivesicular compartments proliferate in susceptible and resistant MLA12-barley leaves in response to infection by the biotrophic powdery mildew fungus. New Phytol. 172 (3), 563–576. doi:10.1111/j.1469-8137.2006.01844.x
Baldini, N., Torreggiani, E., Roncuzzi, L., Perut, F., Zini, N., and Avnet, S. (2018). Exosome-like nanovesicles isolated from citrus limon L. Exert antioxidative effect. Curr. Pharm. Biotechnol. 19 (11), 877–885. doi:10.2174/1389201019666181017115755
Bartel, D. P. (2004). MicroRNAs: genomics, biogenesis, mechanism, and function. Cell 116 (2), 281–297. doi:10.1016/s0092-8674(04)00045-5
Brahmbhatt, M., Gundala, S. R., Asif, G., Shamsi, S. A., and Aneja, R. (2013). Ginger phytochemicals exhibit synergy to inhibit prostate cancer cell proliferation. Nutr. Cancer 65 (2), 263–272. doi:10.1080/01635581.2013.749925
Cai, Q., He, B., and Jin, H. (2019). A safe ride in extracellular vesicles – small RNA trafficking between plant hosts and pathogens. Curr. Opin. Plant Biol. 52, 140–148. doi:10.1016/j.pbi.2019.09.001
Cai, Q., He, B., Wang, S., Fletcher, S., Niu, D., Mitter, N., et al. (2021). Message in a bubble: shuttling small RNAs and proteins between cells and interacting organisms using extracellular vesicles. Annu. Rev. Plant Biol. 72 (1), 497–524. doi:10.1146/annurev-arplant-081720-010616
Cai, Q., Qiao, L., Wang, M., He, B., Lin, F.-M., Palmquist, J., et al. (2018). Plants send small RNAs in extracellular vesicles to fungal pathogen to silence virulence genes. Science 360 (6393), 1126–1129. doi:10.1126/science.aar4142
Cao, M., Yan, H., Han, X., Weng, L., Wei, Q., Sun, X., et al. (2019). Ginseng-derived nanoparticles alter macrophage polarization to inhibit melanoma growth. J. Immunother. Cancer 7 (1), 326. doi:10.1186/s40425-019-0817-4
Castillo, J., Bernard, V., San Lucas, F. A., Allenson, K., Capello, M., Kim, D. U., et al. (2018). Surfaceome profiling enables isolation of cancer-specific exosomal cargo in liquid biopsies from pancreatic cancer patients. Ann. Oncol. 29 (1), 223–229. doi:10.1093/annonc/mdx542
Charoenviriyakul, C., Takahashi, Y., Morishita, M., Matsumoto, A., Nishikawa, M., and Takakura, Y. (2017). Cell type-specific and common characteristics of exosomes derived from mouse cell lines: yield, physicochemical properties, and pharmacokinetics. Eur. J. Pharm. Sci. 96, 316–322. doi:10.1016/j.ejps.2016.10.009
Chen, J., Pan, J., Liu, S., Zhang, Y., Sha, S., Guo, H., et al. (2023). Fruit-derived extracellular-vesicle-engineered structural droplet drugs for enhanced glioblastoma chemotherapy. Adv. Mater 35, e2304187. doi:10.1002/adma.202304187
Chen, Q., Li, Q., Liang, Y., Zu, M., Chen, N., Canup, B. S. B., et al. (2022). Natural exosome-like nanovesicles from edible tea flowers suppress metastatic breast cancer via ROS generation and microbiota modulation. Acta Pharm. Sin. B 12 (2), 907–923. doi:10.1016/j.apsb.2021.08.016
Cheng, L., and Hill, A. F. (2022). Therapeutically harnessing extracellular vesicles. Nat. Rev. Drug Discov. 21 (5), 379–399. doi:10.1038/s41573-022-00410-w
Cheng, Y., Zeng, Q., Han, Q., and Xia, W. (2019). Effect of pH, temperature and freezing-thawing on quantity changes and cellular uptake of exosomes. Protein Cell 10 (4), 295–299. doi:10.1007/s13238-018-0529-4
Chin, A. R., Fong, M. Y., Somlo, G., Wu, J., Swiderski, P., Wu, X., et al. (2016). Cross-kingdom inhibition of breast cancer growth by plant miR159. Cell Res. 26 (2), 217–228. doi:10.1038/cr.2016.13
Cloet, T., Momenbeitollahi, N., and Li, H. (2021). Recent advances on protein-based quantification of extracellular vesicles. Anal. Biochem. 622, 114168. doi:10.1016/j.ab.2021.114168
Colombo, M., Raposo, G., and Théry, C. (2014). Biogenesis, secretion, and intercellular interactions of exosomes and other extracellular vesicles. Annu. Rev. Cell Dev. Biol. 30, 255–289. doi:10.1146/annurev-cellbio-101512-122326
Cong, M., Tan, S., Li, S., Gao, L., Huang, L., Zhang, H. G., et al. (2022). Technology insight: plant-derived vesicles-How far from the clinical biotherapeutics and therapeutic drug carriers? Adv. Drug Deliv. Rev. 182, 114108. doi:10.1016/j.addr.2021.114108
Cui, Y., Cao, W., He, Y., Zhao, Q., Wakazaki, M., Zhuang, X., et al. (2019). A whole-cell electron tomography model of vacuole biogenesis in Arabidopsis root cells. Nat. Plants 5 (1), 95–105. doi:10.1038/s41477-018-0328-1
Cui, Y., Gao, J., He, Y., and Jiang, L. (2020). Plant extracellular vesicles. Protoplasma 257 (1), 3–12. doi:10.1007/s00709-019-01435-6
Deng, Z., Rong, Y., Teng, Y., Mu, J., Zhuang, X., Tseng, M., et al. (2017). Broccoli-derived nanoparticle inhibits mouse colitis by activating dendritic cell AMP-activated protein kinase. Mol. Ther. 25 (7), 1641–1654. doi:10.1016/j.ymthe.2017.01.025
De Palma, M., Ambrosone, A., Leone, A., Del Gaudio, P., Ruocco, M., Turiák, L., et al. (2020). Plant roots release small extracellular vesicles with antifungal activity. Plants (Basel) 9 (12), 1777. doi:10.3390/plants9121777
De Robertis, M., Sarra, A., D'Oria, V., Mura, F., Bordi, F., Postorino, P., et al. (2020). Blueberry-derived exosome-like nanoparticles counter the response to TNF-α-induced change on gene expression in EA.hy926 cells. Biomolecules 10 (5), 742. doi:10.3390/biom10050742
De Vallée, A., Dupuy, J. W., Moriscot, C., Gallet, B., Vanderperre, S., Guignard, G., et al. (2023). Extracellular vesicles of the plant pathogen botrytis cinerea. J. Fungi (Basel) 9 (4), 495. doi:10.3390/jof9040495
Duan, L., Xu, L., Xu, X., Qin, Z., Zhou, X., Xiao, Y., et al. (2021). Exosome-mediated delivery of gene vectors for gene therapy. Nanoscale 13 (3), 1387–1397. doi:10.1039/D0NR07622H
Fang, Z., and Liu, K. (2022a). Plant-derived extracellular vesicles as oral drug delivery carriers. J. Control. Release 350, 389–400. doi:10.1016/j.jconrel.2022.08.046
Fang, Z., and Liu, K. (2022b). Plant-derived extracellular vesicles as oral drug delivery carriers. J. Control Release 350, 389–400. doi:10.1016/j.jconrel.2022.08.046
Feng, J., Xiu, Q., Huang, Y., Troyer, Z., Li, B., and Zheng, L. (2023). Plant-derived vesicle-like nanoparticles as promising biotherapeutic tools: present and future. Adv. Mater 35 (24), e2207826. doi:10.1002/adma.202207826
Fujita, D., Arai, T., Komori, H., Shirasaki, Y., Wakayama, T., Nakanishi, T., et al. (2018). Apple-derived nanoparticles modulate expression of organic-anion-transporting polypeptide (OATP) 2B1 in caco-2 cells. Mol. Pharm. 15 (12), 5772–5780. doi:10.1021/acs.molpharmaceut.8b00921
Furi, I., Momen-Heravi, F., and Szabo, G. (2017). Extracellular vesicle isolation: present and future. Ann. Transl. Med. 5 (12), 263. doi:10.21037/atm.2017.03.95
Gardiner, C., Di Vizio, D., Sahoo, S., Théry, C., Witwer, K. W., Wauben, M., et al. (2016). Techniques used for the isolation and characterization of extracellular vesicles: results of a worldwide survey. J. Extracell. Vesicles 5, 32945. doi:10.3402/jev.v5.32945
Hadisurya, M., Lee, Z. C., Luo, Z., Zhang, G., Ding, Y., Zhang, H., et al. (2023). Data-independent acquisition phosphoproteomics of urinary extracellular vesicles enables renal cell carcinoma grade differentiation. Mol. Cell Proteomics 22 (5), 100536. doi:10.1016/j.mcpro.2023.100536
Hajj, G. N., Arantes, C. P., Dias, M. V., Roffé, M., Costa-Silva, B., Lopes, M. H., et al. (2013). The unconventional secretion of stress-inducible protein 1 by a heterogeneous population of extracellular vesicles. Cell Mol. Life Sci. 70 (17), 3211–3227. doi:10.1007/s00018-013-1328-y
Han, J. M., Song, H. Y., Lim, S. T., Kim, K. I., Seo, H. S., and Byun, E. B. (2021). Immunostimulatory potential of extracellular vesicles isolated from an edible plant, petasites japonicus, via the induction of murine dendritic cell maturation. Int. J. Mol. Sci. 22 (19), 10634. doi:10.3390/ijms221910634
Hatsugai, N., Iwasaki, S., Tamura, K., Kondo, M., Fuji, K., Ogasawara, K., et al. (2009). A novel membrane fusion-mediated plant immunity against bacterial pathogens. Genes Dev. 23 (21), 2496–2506. doi:10.1101/gad.1825209
Hernández-Díaz, J. A., Garza-García, J. J., Zamudio-Ojeda, A., León-Morales, J. M., López-Velázquez, J. C., and García-Morales, S. (2021). Plant-mediated synthesis of nanoparticles and their antimicrobial activity against phytopathogens. J. Sci. Food Agric. 101 (4), 1270–1287. doi:10.1002/jsfa.10767
Huang, H., Yi, X., Wei, Q., Li, M., Cai, X., Lv, Y., et al. (2023). Edible and cation-free kiwi fruit derived vesicles mediated EGFR-targeted siRNA delivery to inhibit multidrug resistant lung cancer. J. Nanobiotechnology 21 (1), 41. doi:10.1186/s12951-023-01766-w
Imai, T., Takahashi, Y., Nishikawa, M., Kato, K., Morishita, M., Yamashita, T., et al. (2015). Macrophage-dependent clearance of systemically administered B16BL6-derived exosomes from the blood circulation in mice. J. Extracell. Vesicles 4, 26238. doi:10.3402/jev.v4.26238
Ipinmoroti, A. O., Turner, J., Bellenger, E. J., Crenshaw, B. J., Xu, J., Reeves, C., et al. (2023). Characterization of cannabis strain-plant-derived extracellular vesicles as potential biomarkers. Protoplasma 260 (6), 1603–1606. doi:10.1007/s00709-023-01870-6
Isaac, R., Reis, F. C. G., Ying, W., and Olefsky, J. M. (2021). Exosomes as mediators of intercellular crosstalk in metabolism. Cell Metab. 33 (9), 1744–1762. doi:10.1016/j.cmet.2021.08.006
Jackson, K. K., Mata, C., and Marcus, R. K. (2023). A rapid capillary-channeled polymer (C-CP) fiber spin-down tip approach for the isolation of plant-derived extracellular vesicles (PDEVs) from 20 common fruit and vegetable sources. Talanta 252, 123779. doi:10.1016/j.talanta.2022.123779
Jia, L., Li, B., Fang, C., Liang, X., Xie, Y., Sun, X., et al. (2022). Extracellular vesicles of mesenchymal stem cells are more effectively accessed through polyethylene glycol-based precipitation than by ultracentrifugation. Stem Cells Int. 2022, 3577015. doi:10.1155/2022/3577015
Ju, S., Mu, J., Dokland, T., Zhuang, X., Wang, Q., Jiang, H., et al. (2013). Grape exosome-like nanoparticles induce intestinal stem cells and protect mice from DSS-induced colitis. Mol. Ther. 21 (7), 1345–1357. doi:10.1038/mt.2013.64
Kalani, A., Chaturvedi, P., Kamat, P. K., Maldonado, C., Bauer, P., Joshua, I. G., et al. (2016). Curcumin-loaded embryonic stem cell exosomes restored neurovascular unit following ischemia-reperfusion injury. Int. J. Biochem. Cell Biol. 79, 360–369. doi:10.1016/j.biocel.2016.09.002
Kalarikkal, S. P., Prasad, D., Kasiappan, R., Chaudhari, S. R., and Sundaram, G. M. (2020). A cost-effective polyethylene glycol-based method for the isolation of functional edible nanoparticles from ginger rhizomes. Sci. Rep. 10 (1), 4456. doi:10.1038/s41598-020-61358-8
Kameli, N., Borman, R., Lpez-Iglesias, C., Savelkoul, P., and Stassen, F. R. M. (2021a). Characterization of feces-derived bacterial membrane vesicles and the impact of their origin on the inflammatory response. Front. Cell Infect. Microbiol. 11, 667987. doi:10.3389/fcimb.2021.667987
Kameli, N., Dragojlovic-Kerkache, A., Savelkoul, P., and Stassen, F. R. (2021b). Plant-derived extracellular vesicles: current findings, challenges, and future applications. Membr. (Basel) 11 (6), 411. doi:10.3390/membranes11060411
Kandemir, F. M., Yildirim, S., Caglayan, C., Kucukler, S., and Eser, G. (2019). Protective effects of zingerone on cisplatin-induced nephrotoxicity in female rats. Environ. Sci. Pollut. Res. Int. 26 (22), 22562–22574. doi:10.1007/s11356-019-05505-3
Khan, H., Pan, J. J., Li, Y., Zhang, Z., and Yang, G. Y. (2021). Native and bioengineered exosomes for ischemic stroke therapy. Front. Cell Dev. Biol. 9, 619565. doi:10.3389/fcell.2021.619565
Kilasoniya, A., Garaeva, L., Shtam, T., Spitsyna, A., Putevich, E., Moreno-Chamba, B., et al. (2023). Potential of plant exosome vesicles from grapefruit (citrus × paradisi) and tomato (Solanum lycopersicum) juices as functional ingredients and targeted drug delivery vehicles. Antioxidants (Basel) 12 (4), 943. doi:10.3390/antiox12040943
Lai, J. J., Chau, Z. L., Chen, S. Y., Hill, J. J., Korpany, K. V., Liang, N. W., et al. (2022). Exosome processing and characterization approaches for research and technology development. Adv. Sci. (Weinh) 9 (15), e2103222. doi:10.1002/advs.202103222
Lewis, G. D., and Metcalf, T. G. (1988). Polyethylene glycol precipitation for recovery of pathogenic viruses, including hepatitis A virus and human rotavirus, from oyster, water, and sediment samples. Appl. Environ. Microbiol. 54 (8), 1983–1988. doi:10.1128/aem.54.8.1983-1988.1988
Li, N., Wang, M., Lyu, Z., Shan, K., Chen, Z., Chen, B., et al. (2023). Medicinal plant-based drug delivery system for inflammatory bowel disease. Front. Pharmacol. 14, 1158945. doi:10.3389/fphar.2023.1158945
Li, P., Kaslan, M., Lee, S. H., Yao, J., and Gao, Z. (2017). Progress in exosome isolation techniques. Theranostics 7 (3), 789–804. doi:10.7150/thno.18133
Li, Z., Wang, H., Yin, H., Bennett, C., Zhang, H. G., and Guo, P. (2018). Arrowtail RNA for ligand display on ginger exosome-like nanovesicles to systemic deliver siRNA for cancer suppression. Sci. Rep. 8 (1), 14644. doi:10.1038/s41598-018-32953-7
Liu, B., Lu, Y., Chen, X., Muthuraj, P. G., Li, X., Pattabiraman, M., et al. (2020a). Protective role of shiitake mushroom-derived exosome-like nanoparticles in D-galactosamine and lipopolysaccharide-induced acute liver injury in mice. Nutrients 12 (2), 477. doi:10.3390/nu12020477
Liu, C., and Liu, B. (2022). Boundedness in a quasilinear two-species chemotaxis system with nonlinear sensitivity and nonlinear signal secretion. J. Differ. Equations 320, 206–246. doi:10.1016/j.jde.2022.03.004
Liu, H., Deng, S., Han, L., Ren, Y., Gu, J., He, L., et al. (2022). Mesenchymal stem cells, exosomes and exosome-mimics as smart drug carriers for targeted cancer therapy. Colloids Surf. B Biointerfaces 209 (1), 112163. doi:10.1016/j.colsurfb.2021.112163
Liu, Y., Ahumada, A. L., Bayraktar, E., Schwartz, P., Chowdhury, M., Shi, S., et al. (2023). Enhancing oral delivery of plant-derived vesicles for colitis. J. Control Release 357, 472–483. doi:10.1016/j.jconrel.2023.03.056
Liu, Y., Wu, S., Koo, Y., Yang, A., Dai, Y., Khant, H., et al. (2020b). Characterization of and isolation methods for plant leaf nanovesicles and small extracellular vesicles. Nanomedicine 29, 102271. doi:10.1016/j.nano.2020.102271
Logozzi, M., Di Raimo, R., Mizzoni, D., and Fais, S. (2022). The potentiality of plant-derived nanovesicles in human health-A comparison with human exosomes and artificial nanoparticles. Int. J. Mol. Sci. 23 (9), 4919. doi:10.3390/ijms23094919
Lötvall, J., Hill, A. F., Hochberg, F., Buzás, E. I., Di Vizio, D., Gardiner, C., et al. (2014). Minimal experimental requirements for definition of extracellular vesicles and their functions: a position statement from the International Society for Extracellular Vesicles. J. Extracell. Vesicles 3, 26913. doi:10.3402/jev.v3.26913
Ly, N. P., Han, H. S., Kim, M., Park, J. H., and Choi, K. Y. (2023). Plant-derived nanovesicles: current understanding and applications for cancer therapy. Bioact. Mater 22, 365–383. doi:10.1016/j.bioactmat.2022.10.005
Mahdipour, E. (2022). Beta vulgaris juice contains biologically active exosome-like nanoparticles. Tissue Cell 76, 101800. doi:10.1016/j.tice.2022.101800
Mainini, F., and Eccles, M. R. (2020). Lipid and polymer-based nanoparticle siRNA delivery systems for cancer therapy. Molecules 25 (11), 2692. doi:10.3390/molecules25112692
Manjithaya, R., and Subramani, S. (2010). Role of autophagy in unconventional protein secretion. Autophagy 6 (5), 650–651. doi:10.4161/auto.6.5.12066
Martínez-Greene, J. A., Hernández-Ortega, K., Quiroz-Baez, R., Resendis-Antonio, O., Pichardo-Casas, I., Sinclair, D. A., et al. (2021). Quantitative proteomic analysis of extracellular vesicle subgroups isolated by an optimized method combining polymer-based precipitation and size exclusion chromatography. J. Extracell. Vesicles 10 (6), e12087. doi:10.1002/jev2.12087
McIlvenna, L. C., Parker, H. J., Seabright, A. P., Sale, B., Anghileri, G., Weaver, S. R. C., et al. (2023). Single vesicle analysis reveals the release of tetraspanin positive extracellular vesicles into circulation with high intensity intermittent exercise. J. Physiol. 601, 5093–5106. doi:10.1113/jp284047
Momen-Heravi, F., Balaj, L., Alian, S., Mantel, P.-Y., Halleck, A. E., Trachtenberg, A. J., et al. (2013). Current methods for the isolation of extracellular vesicles. Biol. Chem. 394 (10), 1253–1262. doi:10.1515/hsz-2013-0141
Monguió-Tortajada, M., Gálvez-Montón, C., Bayes-Genis, A., Roura, S., and Borràs, F. E. (2019). Extracellular vesicle isolation methods: rising impact of size-exclusion chromatography. Cell. Mol. Life Sci. 76 (12), 2369–2382. doi:10.1007/s00018-019-03071-y
Mu, J., Zhuang, X., Wang, Q., Jiang, H., Deng, Z. B., Wang, B., et al. (2014). Interspecies communication between plant and mouse gut host cells through edible plant derived exosome-like nanoparticles. Mol. Nutr. Food Res. 58 (7), 1561–1573. doi:10.1002/mnfr.201300729
Muraoka, S., Jedrychowski, M. P., Yanamandra, K., Ikezu, S., Gygi, S. P., and Ikezu, T. (2020). Proteomic profiling of extracellular vesicles derived from cerebrospinal fluid of alzheimer's disease patients: a pilot study. Cells 9 (9), 1959. doi:10.3390/cells9091959
Nemati, M., Singh, B., Mir, R. A., Nemati, M., Babaei, A., Ahmadi, M., et al. (2022). Plant-derived extracellular vesicles: a novel nanomedicine approach with advantages and challenges. Cell Commun. Signal 20 (1), 69. doi:10.1186/s12964-022-00889-1
Nguyen, J., and Fuhrmann, G. (2022). Extracellular vesicles - a versatile biomaterial. Adv. Healthc. Mater 11 (5), e2200192. doi:10.1002/adhm.202200192
Nishad, J., Dutta, A., Saha, S., Rudra, S. G., Varghese, E., Sharma, R. R., et al. (2021). Ultrasound-assisted development of stable grapefruit peel polyphenolic nano-emulsion: optimization and application in improving oxidative stability of mustard oil. Food Chem. 334, 127561. doi:10.1016/j.foodchem.2020.127561
Niu, W., Xiao, Q., Wang, X., Zhu, J., Li, J., Liang, X., et al. (2021). A biomimetic drug delivery system by integrating grapefruit extracellular vesicles and doxorubicin-loaded heparin-based nanoparticles for glioma therapy. Nano Lett. 21 (3), 1484–1492. doi:10.1021/acs.nanolett.0c04753
Nordin, J. Z., Lee, Y., Vader, P., Mäger, I., Johansson, H. J., Heusermann, W., et al. (2015). Ultrafiltration with size-exclusion liquid chromatography for high yield isolation of extracellular vesicles preserving intact biophysical and functional properties. Nanomedicine Nanotechnol. Biol. Med. 11 (4), 879–883. doi:10.1016/j.nano.2015.01.003
Olmi, L., Pepe, G., Helmer-Citterich, M., Canini, A., and Gismondi, A. (2023). Looking for plant microRNAs in human blood samples: bioinformatics evidence and perspectives. Plant Foods Hum. Nutr. 78, 399–406. doi:10.1007/s11130-023-01063-9
Ou, X., Wang, H., Tie, H., Liao, J., Luo, Y., Huang, W., et al. (2023). Novel plant-derived exosome-like nanovesicles from Catharanthus roseus: preparation, characterization, and immunostimulatory effect via TNF-α/NF-κB/PU.1 axis. J. Nanobiotechnology 21 (1), 160. doi:10.1186/s12951-023-01919-x
Özkan, İ., Koçak, P., Yıldırım, M., Ünsal, N., Yılmaz, H., Telci, D., et al. (2021). Garlic (Allium sativum)-derived SEVs inhibit cancer cell proliferation and induce caspase mediated apoptosis. Sci. Rep. 11 (1), 14773. doi:10.1038/s41598-021-93876-4
Peng, L. H., Wang, M. Z., Chu, Y., Zhang, L., Niu, J., Shao, H. T., et al. (2020). Engineering bacterial outer membrane vesicles as transdermal nanoplatforms for photo-TRAIL-programmed therapy against melanoma. Sci. Adv. 6 (27), eaba2735. doi:10.1126/sciadv.aba2735
Pérez-Bermúdez, P., Blesa, J., Soriano, J. M., and Marcilla, A. (2017). Extracellular vesicles in food: experimental evidence of their secretion in grape fruits. Eur. J. Pharm. Sci. 98, 40–50. doi:10.1016/j.ejps.2016.09.022
Pocsfalvi, G., Turiák, L., Ambrosone, A., Del Gaudio, P., Puska, G., Fiume, I., et al. (2018). Protein biocargo of citrus fruit-derived vesicles reveals heterogeneous transport and extracellular vesicle populations. J. Plant Physiol. 229, 111–121. doi:10.1016/j.jplph.2018.07.006
Qiao, L., Hu, S., Huang, K., Su, T., Li, Z., Vandergriff, A., et al. (2020). Tumor cell-derived exosomes home to their cells of origin and can be used as Trojan horses to deliver cancer drugs. Theranostics 10 (8), 3474–3487. doi:10.7150/thno.39434
Qiao, Z., Zhang, K., Liu, J., Cheng, D., Yu, B., Zhao, N., et al. (2022). Biomimetic electrodynamic nanoparticles comprising ginger-derived extracellular vesicles for synergistic anti-infective therapy. Nat. Commun. 13 (1), 7164. doi:10.1038/s41467-022-34883-5
Qin, X., Wang, X., Xu, K., Zhang, Y., Ren, X., Qi, B., et al. (2022). Digestion of plant dietary miRNAs starts in the mouth under the protection of coingested food components and plant-derived exosome-like nanoparticles. J. Agric. Food Chem. 70 (14), 4316–4327. doi:10.1021/acs.jafc.1c07730
Raguraman, R., Bhavsar, D., Kim, D., Ren, X., Sikavitsas, V., Munshi, A., et al. (2023). Tumor-targeted exosomes for delivery of anticancer drugs. Cancer Lett. 558, 216093. doi:10.1016/j.canlet.2023.216093
Raimondo, S., Naselli, F., Fontana, S., Monteleone, F., Lo Dico, A., Saieva, L., et al. (2015). Citrus limon-derived nanovesicles inhibit cancer cell proliferation and suppress CML xenograft growth by inducing TRAIL-mediated cell death. Oncotarget 6 (23), 19514–19527. doi:10.18632/oncotarget.4004
Raimondo, S., Saieva, L., Cristaldi, M., Monteleone, F., Fontana, S., and Alessandro, R. (2018). Label-free quantitative proteomic profiling of colon cancer cells identifies acetyl-CoA carboxylase alpha as antitumor target of Citrus limon-derived nanovesicles. J. Proteomics 173, 1–11. doi:10.1016/j.jprot.2017.11.017
Ramirez, M. I., Amorim, M. G., Gadelha, C., Milic, I., Welsh, J. A., Freitas, V. M., et al. (2018). Technical challenges of working with extracellular vesicles. Nanoscale 10 (3), 881–906. doi:10.1039/c7nr08360b
Ratnadewi, D., Widjaja, C. H., Barlian, A., Amsar, R. M., Ana, I. D., Hidajah, A. C., et al. (2022). Isolation of native plant-derived exosome-like nanoparticles and their uptake by human cells. HAYATI J. Biosci. 30 (1), 182–192. doi:10.4308/hjb.30.1.182-192
Record, M. (2013). Exosome-like nanoparticles from food: protective nanoshuttles for bioactive cargo. Mol. Ther. 21 (7), 1294–1296. doi:10.1038/mt.2013.130
Regente, M., Corti-Monzón, G., Maldonado, A. M., Pinedo, M., Jorrín, J., and de la Canal, L. (2009). Vesicular fractions of sunflower apoplastic fluids are associated with potential exosome marker proteins. FEBS Lett. 583 (20), 3363–3366. doi:10.1016/j.febslet.2009.09.041
Regente, M., Pinedo, M., Elizalde, M., and de la Canal, L. (2012). Apoplastic exosome-like vesicles: a new way of protein secretion in plants? Plant Signal Behav. 7 (5), 544–546. doi:10.4161/psb.19675
Rutter, B. D., and Innes, R. W. (2017). Extracellular vesicles isolated from the leaf apoplast carry stress-response proteins. Plant Physiol. 173 (1), 728–741. doi:10.1104/pp.16.01253
Shao, J., Zaro, J., and Shen, Y. (2020). Advances in exosome-based drug delivery and tumor targeting: from tissue distribution to intracellular fate. Int. J. Nanomedicine 15, 9355–9371. doi:10.2147/ijn.S281890
Shinge, S., Xiao, Y., Xia, J., Liang, Y., and Duan, L. (2022). Extracellular Vesicles and Circulating Nucleic Acids New insights of engineering plant exosome-like nanovesicles as a nanoplatform for therapeutics and drug delivery. Extracell. Vesicles Circulating Nucleic Acids 3, 150–162. doi:10.20517/evcna.2021.25
Sidhom, K., Obi, P. O., and Saleem, A. (2020a). A review of exosomal isolation methods: is size exclusion chromatography the best option? Int. J. Mol. Sci. 21 (18), 6466. doi:10.3390/ijms21186466
Sidhom, K., Obi, P. O., and Saleem, A. (2020b). A review of exosomal isolation methods: is size exclusion chromatography the best option? Int. J. Mol. Sci. 21 (18), 6466. doi:10.3390/ijms21186466
Singh, A., Sharma, B., and Deswal, R. (2018). Green silver nanoparticles from novel Brassicaceae cultivars with enhanced antimicrobial potential than earlier reported Brassicaceae members. J. Trace Elem. Med. Biol. 47, 1–11. doi:10.1016/j.jtemb.2018.01.001
Sitar, S., Kejžar, A., Pahovnik, D., Kogej, K., Tušek-Žnidarič, M., Lenassi, M., et al. (2015). Size characterization and quantification of exosomes by asymmetrical-flow field-flow fractionation. Anal. Chem. 87 (18), 9225–9233. doi:10.1021/acs.analchem.5b01636
Sokolova, V., Ludwig, A. K., Hornung, S., Rotan, O., Horn, P. A., Epple, M., et al. (2011). Characterisation of exosomes derived from human cells by nanoparticle tracking analysis and scanning electron microscopy. Colloids Surf. B Biointerfaces 87 (1), 146–150. doi:10.1016/j.colsurfb.2011.05.013
Song, H., Canup, B. S. B., Ngo, V. L., Denning, T. L., Garg, P., and Laroui, H. (2020). Internalization of garlic-derived nanovesicles on liver cells is triggered by interaction with CD98. ACS Omega 5 (36), 23118–23128. doi:10.1021/acsomega.0c02893
Stanly, C., Moubarak, M., Fiume, I., Turiák, L., and Pocsfalvi, G. (2019). Membrane transporters in citrus clementina fruit juice-derived nanovesicles. Int. J. Mol. Sci. 20 (24), 6205. doi:10.3390/ijms20246205
Ströhle, G., Gan, J., and Li, H. (2022). Affinity-based isolation of extracellular vesicles and the effects on downstream molecular analysis. Anal. Bioanal. Chem. 414 (24), 7051–7067. doi:10.1007/s00216-022-04178-1
Suharta, S., Barlian, A., Hidajah, A. C., Notobroto, H. B., Ana, I. D., Indariani, S., et al. (2021). Plant-derived exosome-like nanoparticles: a concise review on its extraction methods, content, bioactivities, and potential as functional food ingredient. J. Food Sci. 86 (7), 2838–2850. doi:10.1111/1750-3841.15787
Sundaram, K., Miller, D. P., Kumar, A., Teng, Y., Sayed, M., Mu, J., et al. (2019). Plant-derived exosomal nanoparticles inhibit pathogenicity of porphyromonas gingivalis. iScience 21, 308–327. doi:10.1016/j.isci.2019.10.032
Sundaram, K., Mu, J., Kumar, A., Behera, J., Lei, C., Sriwastva, M. K., et al. (2022). Garlic exosome-like nanoparticles reverse high-fat diet induced obesity via the gut/brain axis. Theranostics 12 (3), 1220–1246. doi:10.7150/thno.65427
Sundaram, P. M., Casadei, L., Lopez, G., Braggio, D., Balakirsky, G., Pollock, R., et al. (2020). Multi-layer micro-nanofluidic device for isolation and capture of extracellular vesicles derived from liposarcoma cell conditioned media. J. Microelectromech Syst. 29 (5), 776–782. doi:10.1109/jmems.2020.3006786
Teng, Y., Ren, Y., Sayed, M., Hu, X., Lei, C., Kumar, A., et al. (2018). Plant-derived exosomal MicroRNAs shape the gut microbiota. Cell Host Microbe 24 (5), 637–652. doi:10.1016/j.chom.2018.10.001
Uckoo, R. M., Jayaprakasha, G. K., Balasubramaniam, V. M., and Patil, B. S. (2012). Grapefruit (Citrus paradisi Macfad) phytochemicals composition is modulated by household processing techniques. J. Food Sci. 77 (9), C921–C926. doi:10.1111/j.1750-3841.2012.02865.x
Uddin, N., Binzel, D. W., Shu, D., Fu, T. M., and Guo, P. (2023). Targeted delivery of RNAi to cancer cells using RNA-ligand displaying exosome. Acta Pharm. Sin. B 13 (4), 1383–1399. doi:10.1016/j.apsb.2022.11.019
Umezu, T., Takanashi, M., Murakami, Y., Ohno, S. I., Kanekura, K., Sudo, K., et al. (2021). Acerola exosome-like nanovesicles to systemically deliver nucleic acid medicine via oral administration. Mol. Ther. Methods Clin. Dev. 21, 199–208. doi:10.1016/j.omtm.2021.03.006
van der Koog, L., Gandek, T. B., and Nagelkerke, A. (2022). Liposomes and extracellular vesicles as drug delivery systems: a comparison of composition, pharmacokinetics, and functionalization. Adv. Healthc. Mater 11 (5), e2100639. doi:10.1002/adhm.202100639
van Niel, G., Carter, D. R. F., Clayton, A., Lambert, D. W., Raposo, G., and Vader, P. (2022). Challenges and directions in studying cell–cell communication by extracellular vesicles. Nat. Rev. Mol. Cell Biol. 23 (5), 369–382. doi:10.1038/s41580-022-00460-3
Vashisht, M., Rani, P., Onteru, S. K., and Singh, D. (2017). Curcumin encapsulated in milk exosomes resists human digestion and possesses enhanced intestinal permeability in vitro. Appl. Biochem. Biotechnol. 183 (3), 993–1007. doi:10.1007/s12010-017-2478-4
Wang, B., Zhuang, X., Deng, Z. B., Jiang, H., Mu, J., Wang, Q., et al. (2014). Targeted drug delivery to intestinal macrophages by bioactive nanovesicles released from grapefruit. Mol. Ther. 22 (3), 522–534. doi:10.1038/mt.2013.190
Wang, J., Ding, Y., Wang, J., Hillmer, S., Miao, Y., Lo, S. W., et al. (2010). EXPO, an exocyst-positive organelle distinct from multivesicular endosomes and autophagosomes, mediates cytosol to cell wall exocytosis in Arabidopsis and tobacco cells. Plant Cell 22 (12), 4009–4030. doi:10.1105/tpc.110.080697
Wang, J., Jayaprakasha, G. K., Patil, B. S., and Son, C. G. (2021). Total antioxidant capacity in HBV carriers, a promising biomarker for evaluating hepatic fibrosis: a pilot study. ACS Food Sci. Technol. 1 (1), 77–87. doi:10.3390/antiox10010077
Wang, L., Wang, D., Ye, Z., and Xu, J. (2023). Engineering extracellular vesicles as delivery systems in therapeutic applications. Adv. Sci. (Weinh) 10 (17), e2300552. doi:10.1002/advs.202300552
Wang, Q., Ren, Y., Mu, J., Egilmez, N. K., Zhuang, X., Deng, Z., et al. (2015). Grapefruit-derived nanovectors use an activated leukocyte trafficking pathway to deliver therapeutic agents to inflammatory tumor sites. Cancer Res. 75 (12), 2520–2529. doi:10.1158/0008-5472.Can-14-3095
Wang, Q., Zhuang, X., Mu, J., Deng, Z. B., Jiang, H., Zhang, L., et al. (2013). Delivery of therapeutic agents by nanoparticles made of grapefruit-derived lipids. Nat. Commun. 4, 1867. doi:10.1038/ncomms2886
Wang, X., Chung, K. P., Lin, W., and Jiang, L. (2017). Protein secretion in plants: conventional and unconventional pathways and new techniques. J. Exp. Bot. 69 (1), 21–37. doi:10.1093/jxb/erx262
Wang, X., Zhang, M., Flores, S. R. L., Woloshun, R. R., Yang, C., Yin, L., et al. (2019). Oral gavage of ginger nanoparticle-derived lipid vectors carrying Dmt1 siRNA blunts iron loading in murine hereditary hemochromatosis. Mol. Ther. 27 (3), 493–506. doi:10.1016/j.ymthe.2019.01.003
Wang, Y., Wang, J., Ma, J., Zhou, Y., and Lu, R. (2022). Focusing on future applications and current challenges of plant derived extracellular vesicles. Pharm. (Basel) 15 (6), 708. doi:10.3390/ph15060708
Wei, X., Li, X., Zhang, Y., Wang, J., and Shen, S. (2023). Advances in the therapeutic applications of plant-derived exosomes in the treatment of inflammatory diseases. Biomedicines 11 (6), 1554. doi:10.3390/biomedicines11061554
Wen, Z., Yu, J., Jeong, H., Kim, D. U., Yang, J. Y., Hyun, K. A., et al. (2023). An all-in-one platform to deplete pathogenic bacteria for rapid and safe enrichment of plant-derived extracellular vesicles. Lab. Chip 23 (20), 4483–4492. doi:10.1039/d3lc00585b
Woith, E., Guerriero, G., Hausman, J. F., Renaut, J., Leclercq, C. C., Weise, C., et al. (2021). Plant extracellular vesicles and nanovesicles: focus on secondary metabolites, proteins and lipids with perspectives on their potential and sources. Int. J. Mol. Sci. 22 (7), 3719. doi:10.3390/ijms22073719
Wu, K., Xing, F., Wu, S. Y., and Watabe, K. (2017). Extracellular vesicles as emerging targets in cancer: recent development from bench to bedside. Biochim. Biophys. Acta Rev. Cancer 1868 (2), 538–563. doi:10.1016/j.bbcan.2017.10.001
Wu, P., Han, J., Gong, Y., Liu, C., Yu, H., and Xie, N. (2022). Nanoparticle-based drug delivery systems targeting tumor microenvironment for cancer immunotherapy resistance: current advances and applications. Pharmaceutics 14 (10), 1990. doi:10.3390/pharmaceutics14101990
Xiao, J., Feng, S., Wang, X., Long, K., Luo, Y., Wang, Y., et al. (2018). Identification of exosome-like nanoparticle-derived microRNAs from 11 edible fruits and vegetables. PeerJ 6, e5186. doi:10.7717/peerj.5186
Xiao, Q., Zhao, W., Wu, C., Wang, X., Chen, J., Shi, X., et al. (2022). Lemon-derived extracellular vesicles nanodrugs enable to efficiently overcome cancer multidrug resistance by endocytosis-triggered energy dissipation and energy production reduction. Adv. Sci. (Weinh) 9 (20), e2105274. doi:10.1002/advs.202105274
Xie, N., Shen, G., Gao, W., Huang, Z., Huang, C., and Fu, L. (2023). Neoantigens: promising targets for cancer therapy. Signal Transduct. Target Ther. 8 (1), 9. doi:10.1038/s41392-022-01270-x
Xu, X.-H., Yuan, T.-J., Dad, H. A., Shi, M.-Y., Huang, Y.-Y., Jiang, Z.-H., et al. (2021). Plant exosomes as novel nanoplatforms for MicroRNA transfer stimulate neural differentiation of stem cells in vitro and in vivo. Nano Lett. 21 (19), 8151–8159. doi:10.1021/acs.nanolett.1c02530
Yamashita, T., Takahashi, Y., Nishikawa, M., and Takakura, Y. (2016). Effect of exosome isolation methods on physicochemical properties of exosomes and clearance of exosomes from the blood circulation. Eur. J. Pharm. Biopharm. 98, 1–8. doi:10.1016/j.ejpb.2015.10.017
Yáñez-Mó, M., Siljander, P. R., Andreu, Z., Zavec, A. B., Borràs, F. E., Buzas, E. I., et al. (2015). Biological properties of extracellular vesicles and their physiological functions. J. Extracell. Vesicles 4, 27066. doi:10.3402/jev.v4.27066
Yang, D., Zhang, W., Zhang, H., Zhang, F., Chen, L., Ma, L., et al. (2020a). Progress, opportunity, and perspective on exosome isolation - efforts for efficient exosome-based theranostics. Theranostics 10 (8), 3684–3707. doi:10.7150/thno.41580
Yang, M., Liu, X., Luo, Q., Xu, L., and Chen, F. (2020b). An efficient method to isolate lemon derived extracellular vesicles for gastric cancer therapy. J. Nanobiotechnology 18 (1), 100. doi:10.1186/s12951-020-00656-9
Yang, M., Luo, Q., Chen, X., and Chen, F. (2021). Bitter melon derived extracellular vesicles enhance the therapeutic effects and reduce the drug resistance of 5-fluorouracil on oral squamous cell carcinoma. J. Nanobiotechnology 19 (1), 259. doi:10.1186/s12951-021-00995-1
Yin, L., Yan, L., Yu, Q., Wang, J., Liu, C., Wang, L., et al. (2022). Characterization of the MicroRNA profile of ginger exosome-like nanoparticles and their anti-inflammatory effects in intestinal caco-2 cells. J. Agric. Food Chem. 70 (15), 4725–4734. doi:10.1021/acs.jafc.1c07306
You, J. Y., Kang, S. J., and Rhee, W. J. (2021). Isolation of cabbage exosome-like nanovesicles and investigation of their biological activities in human cells. Bioact. Mater. 6 (12), 4321–4332. doi:10.1016/j.bioactmat.2021.04.023
Zarovni, N., Corrado, A., Guazzi, P., Zocco, D., Lari, E., Radano, G., et al. (2015). Integrated isolation and quantitative analysis of exosome shuttled proteins and nucleic acids using immunocapture approaches. Methods 87, 46–58. doi:10.1016/j.ymeth.2015.05.028
Zeng, L., Wang, H., Shi, W., Chen, L., Chen, T., Chen, G., et al. (2021). Aloe derived nanovesicle as a functional carrier for indocyanine green encapsulation and phototherapy. J. Nanobiotechnology 19 (1), 439. doi:10.1186/s12951-021-01195-7
Zhang, H., Freitas, D., Kim, H. S., Fabijanic, K., Li, Z., Chen, H., et al. (2018). Identification of distinct nanoparticles and subsets of extracellular vesicles by asymmetric flow field-flow fractionation. Nat. Cell Biol. 20 (3), 332–343. doi:10.1038/s41556-018-0040-4
Zhang, J., Nguyen, L. T. H., Hickey, R., Walters, N., Wang, X., Kwak, K. J., et al. (2021). Immunomagnetic sequential ultrafiltration (iSUF) platform for enrichment and purification of extracellular vesicles from biofluids. Sci. Rep. 11 (1), 8034. doi:10.1038/s41598-021-86910-y
Zhang, M., Viennois, E., Prasad, M., Zhang, Y., Wang, L., Zhang, Z., et al. (2016a). Edible ginger-derived nanoparticles: a novel therapeutic approach for the prevention and treatment of inflammatory bowel disease and colitis-associated cancer. Biomaterials 101, 321–340. doi:10.1016/j.biomaterials.2016.06.018
Zhang, M., Viennois, E., Xu, C., and Merlin, D. (2016b). Plant derived edible nanoparticles as a new therapeutic approach against diseases. Tissue Barriers 4 (2), e1134415. doi:10.1080/21688370.2015.1134415
Zhang, M., Wang, X., Han, M. K., Collins, J. F., and Merlin, D. (2017). Oral administration of ginger-derived nanolipids loaded with siRNA as a novel approach for efficient siRNA drug delivery to treat ulcerative colitis. Nanomedicine (Lond) 12 (16), 1927–1943. doi:10.2217/nnm-2017-0196
Zhang, M., Xiao, B., Wang, H., Han, M. K., Zhang, Z., Viennois, E., et al. (2016c). Edible ginger-derived nano-lipids loaded with doxorubicin as a novel drug-delivery approach for colon cancer therapy. Mol. Ther. 24 (10), 1783–1796. doi:10.1038/mt.2016.159
Zhang, Y., Li, J., Gao, W., and Xie, N. (2022). Exosomes as anticancer drug delivery vehicles: prospects and challenges. Front. Biosci. (Landmark Ed. 27 (10), 293. doi:10.31083/j.fbl2710293
Zhou, Q., Ma, K., Hu, H., Xing, X., Huang, X., and Gao, H. (2022). Extracellular vesicles: their functions in plant-pathogen interactions. Mol. Plant Pathol. 23 (6), 760–771. doi:10.1111/mpp.13170
Zhou, Z., Li, X., Liu, J., Dong, L., Chen, Q., Liu, J., et al. (2015). Honeysuckle-encoded atypical microRNA2911 directly targets influenza A viruses. Cell Res. 25 (1), 39–49. doi:10.1038/cr.2014.130
Zhu, W., Dong, Y., Xu, P., Pan, Q., Jia, K., Jin, P., et al. (2022). A composite hydrogel containing resveratrol-laden nanoparticles and platelet-derived extracellular vesicles promotes wound healing in diabetic mice. Acta Biomater. 154, 212–230. doi:10.1016/j.actbio.2022.10.038
Zhuang, X., Deng, Z. B., Mu, J., Zhang, L., Yan, J., Miller, D., et al. (2015). Ginger-derived nanoparticles protect against alcohol-induced liver damage. J. Extracell. Vesicles 4, 28713. doi:10.3402/jev.v4.28713
Keywords: traditional medicinal plants, extracellular vesicles, lipids, pharmacology, therapy, tumor, inflammation
Citation: Wu P, Wu W, Zhang S, Han J, Liu C, Yu H, Chen X and Chen X (2023) Therapeutic potential and pharmacological significance of extracellular vesicles derived from traditional medicinal plants. Front. Pharmacol. 14:1272241. doi: 10.3389/fphar.2023.1272241
Received: 03 August 2023; Accepted: 20 November 2023;
Published: 01 December 2023.
Edited by:
Rajeev K. Singla, Sichuan University, ChinaReviewed by:
Haroon Khan, Shanghai Jiao Tong University, ChinaCopyright © 2023 Wu, Wu, Zhang, Han, Liu, Yu, Chen and Chen. This is an open-access article distributed under the terms of the Creative Commons Attribution License (CC BY). The use, distribution or reproduction in other forums is permitted, provided the original author(s) and the copyright owner(s) are credited and that the original publication in this journal is cited, in accordance with accepted academic practice. No use, distribution or reproduction is permitted which does not comply with these terms.
*Correspondence: Xiaofeng Chen, eGlhb2ZlbmdjaGVuQGNkdXRjbS5lZHUuY24=; Xiping Chen, eGlwaW5nY2hlbmNkdXRjbUAxNjMuY29t
†These authors have contributed equally to this work
Disclaimer: All claims expressed in this article are solely those of the authors and do not necessarily represent those of their affiliated organizations, or those of the publisher, the editors and the reviewers. Any product that may be evaluated in this article or claim that may be made by its manufacturer is not guaranteed or endorsed by the publisher.
Research integrity at Frontiers
Learn more about the work of our research integrity team to safeguard the quality of each article we publish.