- Department of Pharmacy, The First Affiliated Hospital of Zhengzhou University, Zhengzhou, China
The burden of liver diseases such as metabolic-associated fatty liver diseases and hepatocellular carcinoma has increased rapidly worldwide over the past decades. However, pharmacological therapies for these liver diseases are insufficient. Sulforaphane (SFN), an isothiocyanate that is mainly found in cruciferous vegetables, has been found to have a broad spectrum of activities like antioxidation, anti-inflammation, anti-diabetic, and anticancer effects. Recently, a growing number of studies have reported that SFN could significantly ameliorate hepatic steatosis and prevent the development of fatty liver, improve insulin sensitivity, attenuate oxidative damage and liver injury, induce apoptosis, and inhibit the proliferation of hepatoma cells through multiple signaling pathways. Moreover, many clinical studies have demonstrated that SFN is harmless to the human body and well-tolerated by individuals. This emerging evidence suggests SFN to be a promising drug candidate in the treatment of liver diseases. Nevertheless, limitations exist in the development of SFN as a hepatoprotective drug due to its special properties, including instability, water insolubility, and high inter-individual variation of bioavailability when used from broccoli sprout extracts. Herein, we comprehensively review the recent progress of SFN in the treatment of common liver diseases and the underlying mechanisms, with the aim to provide a better understanding of the therapeutic potential of SFN in liver diseases.
1 Introduction
Over the past decades, liver diseases have become one of the major diseases threatening human health globally, with the burden of liver diseases increasing rapidly (Pimpin et al., 2018; Sarin et al., 2020). Although plenty of studies have been published focusing on the pathogenesis and regulatory mechanisms of different kinds of liver diseases, effective strategies for the prevention and treatment of liver diseases are still insufficient. Recently, a number of natural products have been shown to be effective in the treatment of liver diseases, such as fatty liver disease and drug-induced liver injury, which provides a new direction for the development of drugs to treat liver diseases (Chen et al., 2017; Tarantino et al., 2021; Sun et al., 2022). Sulforaphane (SFN) is an aliphatic isothiocyanate derived from the hydrolysis of glucoraphanin catalyzed by myrosinase in plants, which is abundant in cruciferous vegetables like broccoli, cabbage, and brussels sprouts (Kaiser et al., 2021). Since it was discovered, the beneficial effects of SFN on health have been widely reported, such as antioxidative, anti-inflammatory, antidiabetic, anticancer, neuroprotective, and cardiovascular protective activities, along with having low toxicity to the human body (Greaney et al., 2016; Feng et al., 2017; Patel et al., 2018; Houghton, 2019; Calabrese and Kozumbo, 2021). In recent years, clinical trials have been performed to assess the therapeutic efficacy of SFN in the treatment of several diseases, such as different types of cancer, neuro-degenerative diseases, autism spectrum disorder, and non-alcoholic fatty liver disease (NAFLD) (Singh et al., 2014; Schepici et al., 2020; Mangla et al., 2021). The effects of SFN on chronic and acute liver diseases were investigated more than a decade by studies using in vitro or in vivo models (Kim et al., 2006; Zhao et al., 2010; Xu et al., 2019). Previous studies have indicated that the antioxidative activity and biological functions of SFN are partially dependent on the activation of nuclear factor-erythroid 2-related factor 2 (Nrf2), which is a master regulator of cellular homeostasis that is ubiquitously expressed in many organs (Houghton et al., 2016; Cuadrado et al., 2018; Zhou et al., 2022). The beneficial effects of SFN on lipid metabolism and NAFLD through the activation of Nrf2 have been reviewed previously (Xu et al., 2019; Du et al., 2021). However, with a growing number of studies focusing on the role of SFN in liver diseases, increasing pathways and targets that are mediated by SFN have been discovered. In this review, the efficacy and related mechanisms of SFN in the treatment of liver diseases, including fatty liver disease, hepatic insulin resistance, xenobiotic-induced liver injury, hepatic ischemia-reperfusion injury, and hepatocellular carcinoma (HCC), were summarized. Additionally, strategies for solving problems of SFN like instability and insolubility, as well as a selection of formulation and dose schedules in clinical trials, were discussed, with the aim to provide insights into the future studies of SFN in the treatment of liver diseases.
2 The bioavailability, pharmacokinetics, and safety of SFN
SFN is a sulfur-containing compound that is insoluble in water, but glucoraphanin, its precursor and storage form in plants, is water-soluble and is used more frequently in clinical research either in its pure form or in the form of broccoli seed and sprout extracts. On average, the bioavailability of pure SFN is about seven times that of glucoraphanin (Shapiro et al., 2006; Egner et al., 2011; Fahey et al., 2012). However, the source materials that were used in clinical studies showed significant influence on the bioavailability of SFN in human body. For instance, the peak plasma concentration of SFN in subjects who received fresh broccoli sprouts was 7-fold higher than that of broccoli supplements, and the urinary excretion was 5-fold higher, which indicated a much higher bioavailability of SFN in humans when administered from a whole food source (Clarke et al., 2011a). The reason was thought to be due to the presence of myrosinase in fresh broccoli sprouts (Fahey et al., 2015). Thus, a dietary supplement containing myrosinase could dramatically increase the bioavailability of SFN in human subjects receiving cooked broccoli (Okunade et al., 2018). Moreover, different cooking methods were also reported to affect the bioavailability of SFN (Orlando et al., 2022). The bioavailability of SFN was found to be affected by gastric acidity when broccoli seeds were co-delivered, which might be associated with altered activity of myrosinase as it could be improved by the use of omeprazole or enteric coating of myrosinase (Fahey et al., 2019). Additionally, the bioavailability of SFN was also reported to be influenced by gut microflora because of its ability to secrete myrosinase and convert glucoraphanin to SFN (Tian et al., 2018b).
SFN is absorbed and metabolized quickly in the human body after ingestion. The plasma concentration of SFN peaks within 3 h and is cleared from the body in 24 h, with a half-life of about 2 h, which might be slightly affected by the formulation used in different studies (Al Janobi et al., 2006; Egner et al., 2008; Atwell et al., 2015). Once entering systemic circulation, SFN is distributed to several organs, like the small intestine, prostate, kidney, and lung (Clarke et al., 2011b; Livingstone et al., 2022). In the human body, SFN is primarily metabolized via the mercapturic acid pathway. Under the catalysis of glutathione transferases, SFN is conjugated with glutathione (GSH) to form SFN-GSH, then metabolized to SFN-cysteinylglycine, SFN-cysteine, and SFN-N-acetylcysteine under the catalyzation of γ-glutamyltranspeptidase, cysteinylglycinase, and N-acetyltransferase, respectively (Kolm et al., 1995; Egner et al., 2008). Subsequently, these metabolites are excreted in urine (Conaway et al., 2000; Ye et al., 2002). Some SFN could be reversibly converted to erucin by oxidation in vivo, which also demonstrated similar biological activities (Clarke et al., 2011a).
Although SFN showed anticancer activity and cytotoxicity to cancer cells, it is relatively safe for normal cells. For instance, SFN inhibited the viability of about 70% of HepG2 cells after exposure for 48 h at 20 μM, but no significant cytotoxicity was observed in cultured human hepatocytes when exposed to 50 μM of SFN for 48 h (Park et al., 2007; Gross-Steinmeyer et al., 2010). Moreover, the safety of SFN was also confirmed in clinical studies as no severe adverse effects were observed in participants who were administrated SFN either for a short period or for several weeks (Kensler et al., 2005; Shapiro et al., 2006; Alumkal et al., 2015). Occasionally, a few mild adverse effects of SFN were observed in clinical studies, like grade 2 constipation, nausea, headache, and bloating (Alumkal et al., 2015; Tahata et al., 2018; Zhang et al., 2020). But SFN treatment did not increase the risk of side effects in patients (Ghazizadeh-Hashemi et al., 2021). In a 15-week randomized parallel double-blind placebo-controlled clinical trial in children with autism spectrum disorder, SFN was found to be associated with insomnia, irritability, and intolerance of taste and smell, but no severe adverse effects were observed and serum chemistry profile, urinalysis, and complete blood count of patients were normal (Zimmerman et al., 2021). Collectively, SFN is regarded as safe to the human body when used within limited doses.
3 The efficacy of SFN in the treatment of liver diseases
SFN has multiple effects on the liver. Initially, it was shown to induce detoxifying and antioxidant enzymes in the liver, which consequently mediates the detoxification and clearance of carcinogens and reactive oxygen species to elevate cell defense (Hu et al., 2004; Hu et al., 2006). As well as chemopreventive and anti-cancer activities, many studies have reported the protective role of SFN in oxidative stress-induced liver injury and hepatic inflammation caused by drugs, alcohols, toxins, and so forth. In recent years, emerging studies have found that SFN affects the metabolism of glucose and lipids in the liver, and could improve insulin resistance and reduce hepatic lipid accumulation. In this part, the efficacy of SFN in the treatment of several liver diseases, including fatty liver disease, xenobiotic-induced liver injury, hepatic insulin resistance, hepatic ischemia-reperfusion injury, and HCC, as well as related mechanisms, will be discussed. Research data from preclinical and clinical studies about the effects of SFN on liver diseases were collected from PubMed, Web of Science, and the scientific Databases of Science Direct. Keywords included sulforaphane, steatosis, fatty liver, insulin resistance, hepatotoxicity, liver injury, ischemia reperfusion, and hepatocellular carcinoma and were used to retrieve literature published before 31 January 2023.
3.1 SFN and fatty liver disease
Fatty liver disease (FLD) is a common liver disease around the world with a broad spectrum from simple steatosis to steatohepatitis, fibrosis, and cirrhosis, and is characterized by excessive lipid accumulation in the liver (Louvet and Mathurin, 2015; Cotter and Rinella, 2020). Patients with hepatic steatosis are usually diagnosed with alcoholic fatty liver disease (AFLD) or nonalcoholic fatty liver disease (NAFLD) according to the consumption of alcohol or not. Heterogeneity in the pathogenesis of FLD has been recognized by clinicians and scientists (Eslam et al., 2020). However, this disease has been found to be accompanied by several physiological characters like inflammation, endoplasmic reticulum (ER) stress, oxidative stress, and mitochondrial dysfunction (Bessone et al., 2019; Arroyave-Ospina et al., 2021). The usage of natural compounds from herbs in the treatment of liver diseases has been applied for a long time. Recently, the beneficial effects of phytochemical active compounds like SFN, silybin, and curcumin on FLD have been proven by both experimental and clinical studies, mostly due to the antioxidative and anti-inflammation activities (Bagherniya et al., 2018; Cheng et al., 2019; Du et al., 2021).
SFN was first reported to activate Nrf2 and prevent liver X receptor-α (LXRα) agonist-induced hepatic lipogenesis and steatosis by activating farnesoid X receptor (FXR) and inducting small heterodimer partner in mice in 2011 (Kay et al., 2011). The following year, a study reported that coadministration of SFN with a methionine- and choline-deficient diet significantly decreased inflammatory cell infiltration and suppressed fibrosis and oxidative stress in the livers of mice with steatohepatitis (Okada et al., 2012). More recently, SFN was found to enhance lipid droplet degradation and ameliorate steatosis in hepatocytes and rat liver through Nrf2-mediated lipophagy (Lei et al., 2022). The efficacy of SFN in preventing alcohol-induced hepatic steatosis has also been reported: the intake of SFN showed protective effects against ethanol-induced liver injury and alleviated steatosis in mice, which were mainly associated with the upregulation of antioxidant capacity and suppression of ER stress through Nrf2 pathway (Zhou et al., 2014; Lei et al., 2018; Wang and Zhou, 2020). Apart from activation of Nrf2, SFN was also shown to prevent high-fat diet (HFD)-induced NAFLD in mice by inhibiting NOD-like receptor family pyrin domain containing 3 (NLRP3) inflammasome in liver through AMP-activated protein kinase (AMPK)-autophagy axis when administered with a daily dose of 30 mg/kg for 9 weeks along with HFD (Yang et al., 2016). In recent years, the number of new mechanisms and molecular targets of SFN in the treatment of fatty liver reported by experimental studies has increased rapidly (Figure 1), such as inhibition of lipogenic enzymes via ER stress-dependent decrease of X-box binding protein 1 (XBP1) expression and ER stress-independent blocking of sterol regulatory element binding protein-1c (SREBP1c) pathways (Tian et al., 2018a), alleviated ER stress through the upregulation of AMPK and peroxisome proliferators-activated receptor α (PPARα) (Mansour et al., 2022), enhanced mitochondrial function via Nrf2 activation or promotion of mitochondrial biogenesis by peroxisome proliferator-activated receptor alpha co-activator pathway (Lei et al., 2019), and regulation of FXR-mediated bile acid metabolism and LXRα-mediated fatty acid synthesis pathways (Ma et al., 2022). SFN was also found to alleviate HFD-induced lipid deposition and suppress apoptosis by regulating the AMPK/SREBP1c/FAS signaling pathway both in vitro and in vivo (Li et al., 2021). In addition, fibroblast growth factor 21 (FGF21), which is expected to be a promising therapeutic target for the treatment of hepatic steatosis due to its beneficial effects on lipid metabolism in liver (Fisher and Maratos-Flier, 2016), was newly reported to be a target of SFN (Tian et al., 2021b). A continuous administration of SFN for 6 weeks significantly increased hepatic expression of FGF21 and facilitated fatty acid metabolism through preserving FGF receptor 1 protein and preventing the phosphorylation of p38MAPK in HFD-induced NAFLD mice (Wu et al., 2022). In addition, gut microbiota and gut microbiome-derived metabolite, indole-3-acetic acid, were also reported to contribute to the efficacy of SFN in improving HFD-induced hepatic steatosis along with Aryl hydrocarbon receptor (AHR)/SREBP1c pathway-mediated lipid metabolism (Xu et al., 2021). Together, these experimental studies suggest the great potential of SFN in the treatment of FLD.
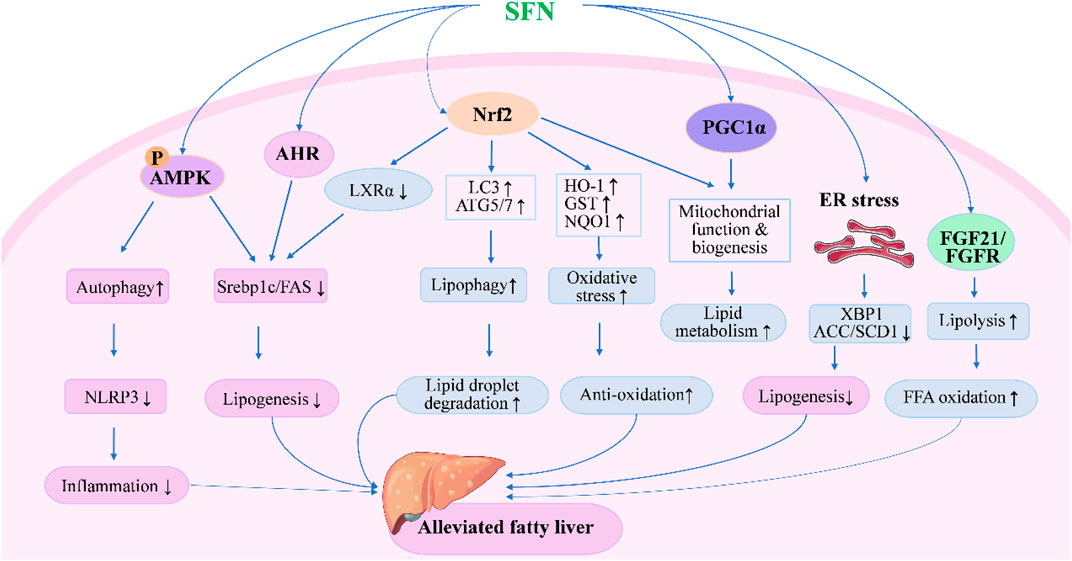
FIGURE 1. Molecular mechanisms under the efficacy of SFN in the treatment of fatty liver disease. SFN could alleviate fatty liver either through Nrf2-dependent or independent pathways. Upon activation of Nrf2, SFN inhibits lipogenesis and oxidative stress while enhancing lipid droplet degradation through modulating the expression of genes involved in lipid synthesis, metabolism, and oxidation. Moreover, SFN modulates autophagy, lipolysis, mitochondrial function, and ER stress to alleviate fatty liver through AMPK-, AHR-, PGC1α-, and FGF21-mediated pathways.
Although clinical studies for SFN have been performed for many years, there has been little focus on the efficacy in treatment of fatty liver disease. Until now, only one published clinical study has reported the effect of SFN-containing broccoli sprout extract on fatty liver in humans. In that randomized, placebo-controlled, and double-blind trial, daily dietary supplementation with broccoli sprout extract containing glucoraphanin, a precursor of SFN, showed a positive effect on improving liver function in male subjects with fatty liver. The levels of serum biomarkers of liver function, including alanine aminotransferases (ALT), γ-glutamyl transpeptidase (γ-GT), alkali phosphatase (ALP), and urinary level of 8-OHdG, an oxidative stress marker, were significantly decreased in subjects after a daily supplementation of broccoli sprout extract containing 30 mg of glucoraphanin for 2 months (Kikuchi et al., 2015). Although only a small group of subjects were enrolled and broccoli sprout extract was used instead of SFN, the findings in this study are still valuable. A clinical trial focusing on the efficacy of dietary supplementation of SFN in treatment of NAFLD, obesity, and metabolic syndrome is still ongoing (Retrieved from https://clinicaltrials.gov/ct2/show/NCT04364360). Obviously, more clinical studies about the efficacy of SFN in the treatment of FLD are meaningful and anticipated.
3.2 SFN and hepatic insulin resistance
Under normal conditions, insulin regulates the homeostasis of glucose and lipid metabolism in liver by direct or indirect signal pathways (Titchenell et al., 2017). But hepatic insulin resistance occurs in some pathologic states, such as obesity and type 2 diabetes, which leads to an increase of hepatic glucose production and lipid synthesis (Santoleri and Titchenell, 2019). Thus, hepatic insulin resistance is closely linked to NAFLD. Several therapies have been applied in clinical practice to treat hepatic insulin resistance, like weight loss with diet or bariatric surgery, aerobic exercise, and pharmacological strategies (Samuel and Shulman, 2018). Antioxidants are considered as a new therapy for the treatment of insulin resistance and diabetes because of their association with oxidative stress. In a parallel, randomized, double-blind, and placebo-controlled clinical study, broccoli sprout powder containing high amounts of SFN was found to decrease serum concentration of insulin and a homeostasis model assessment of insulin resistance indices in patients with type 2 diabetes (Bahadoran et al., 2012). This suggests the therapeutic potential of SFN in the treatment of insulin resistance.
In high-fructose-fed rats, treatment of SFN showed comparable improvements of insulin resistance and hepatoprotective effect when compared to the standard insulin sensitizer pioglitazone (Shawky et al., 2019). Experimentally, both pre- and post-treatment with SFN significantly elevated insulin sensitivity and hepatic glycogen concentration in streptozotocin-induced diabetes in rats but did not impact antioxidant response in the liver (de Souza et al., 2012; de Souza et al., 2016). Long-term coadministration of glucoraphanin with HFD in mice showed benefits for ameliorating insulin resistance and hepatic steatosis in a Nrf2-dependent manner, and these benefits might be attributed to the inhibition of metabolic endotoxemia-related chronic inflammation and oxidative stress by glucoraphanin (Nagata et al., 2017). Except for alleviating hepatic steatosis, SFN showed antidiabetic effects and ameliorated insulin resistance either by enhancing antioxidant capacity and improving FGF21 resistance in HFD and streptozotocin-induced type 2 diabetes or through activating AMPK/Nrf2-mediated antioxidative effect via inactivation of glutathione peroxidase 4 in HFD-induced insulin resistance in mice (Tian et al., 2021a; Zhang et al., 2022). Through the activation of Nrf2, SFN inhibited hepatic glucose production and decreased the expression of key enzymes in gluconeogenesis in HFD-induced diabetic rats, and reversed the gene expression profiles of the hepatic disease signature of diabetes. Moreover, clinical data suggested that the antidiabetic effect of SFN was found to be most effective in obese patients with dysregulated type 2 diabetes without causing severe adverse effects (Axelsson et al., 2017).
Additionally, new targets of SFN in the treatment of hepatic insulin resistance have been identified by researchers in recent years (Figure 2). The mitochondria-associated ER membranes are considered as hubs for hepatic metabolism and are tightly related with gluconeogenesis in hepatocytes. Disruption of mitochondria-ER interactions occurs in the early stage of hepatic insulin resistance, and targeting mitochondria-ER interactions has been proven to be effective in improving insulin resistance in diabetic mice (Tubbs et al., 2014; Beaulant et al., 2022). In a recent study, SFN showed a significant effect on restoring mitochondria-ER interactions along with alleviating hepatic insulin resistance and glucose intolerance both in vitro and in vivo, with a disruption of mitochondria-ER interactions in hepatocytes or mice induced by HFD and high-sucrose diet counteracted by coadministration of SFN (Tubbs et al., 2018). Apart from that, ceramides, which have been shown to correlate with lipid-induced hepatic insulin resistance, were also reported to be a target of SFN (Petersen and Shulman, 2017; Chaurasia et al., 2019; Teng et al., 2019). In insulin-resistant HepG2 cells, SFN treatment counteracted palmitic acid-induced increase of ceramides, and alleviated insulin resistance through blocking ceramide biosynthesis by reducing the expression of serine palmitoyltransferase 3. Moreover, long-term treatment of SFN completely normalized hepatic ceramide levels and improved insulin sensitivity in HFD-induced mice (Teng et al., 2019).
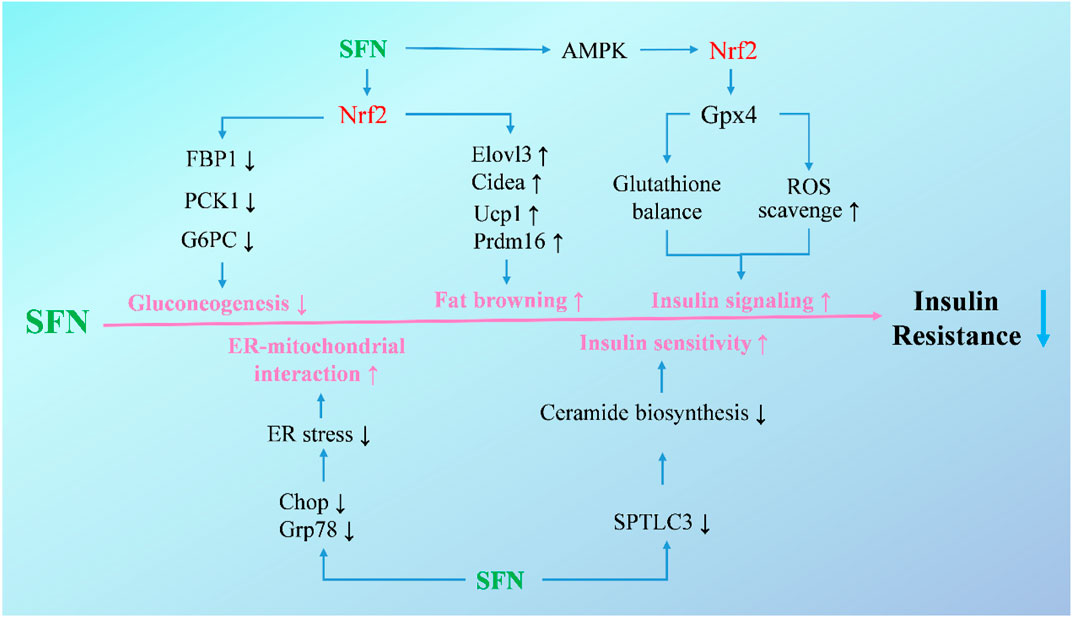
FIGURE 2. Molecular mechanisms under the efficacy of SFN in the treatment of hepatic insulin resistance. Upon activation of Nrf2, SFN inhibits gluconeogenetic enzymes and decreases gluconeogenesis to attenuate exaggerated glucose production and glucose intolerance, increases brown fat-selective genes, and promotes adipose tissue browning to mitigate obesity and insulin resistance. Additionally, SFN increases insulin signaling in hepatocytes and ameliorates HFD-induced insulin resistance through activating the AMPK-Nrf2-Gpx4 pathway. SFN also alleviates ER stress to enhance ER-mitochondrial interaction and blocks ceramide biosynthesis to improve insulin sensitivity.
3.3 SFN and xenobiotic-induced liver injury
Liver injury induced by xenobiotics, such as drugs, endocrine-disrupting chemicals, pollutants, and herbs, is an important part of liver diseases that could lead to liver failure or even death (Fontana et al., 2014; Andrade et al., 2019). Prevention and treatment of xenobiotic-induced liver injury are rather challenging due to the difficulties in diagnosis and incomplete understanding of pathogenesis, especially of idiosyncratic liver injuries (Fontana, 2014; Hassan and Fontana, 2019). However, several studies have reported the protective role and possible mechanisms of SFN in xenobiotic-induced liver injury by using in vivo models (Figure 3). In acetaminophen-induced acute liver damage in mice, pretreatment with SFN showed a protective effect against severe liver injury and oxidative stress by inhibition of reactive oxygen species (ROS) formation and induction of heme oxygenase-1 (HO-1) expression (Noh et al., 2015). The hepatic expression of genes related to detoxification and GSH synthesis were induced by broccoli sprout extract treatment, which might contribute to the liver-protective effect of SFN in acetaminophen-induced injury (Yoshida et al., 2015). The antioxidant activity of SFN and its effect on GSH synthesis are similar to that of N-acetyl cysteine, an accomplished antioxidant that has been widely used clinically in the treatment of acetaminophen-induced injury (Ntamo et al., 2021; Schwalfenberg, 2021). The hepatic protective effect of SFN was also reported in cisplatin-induced liver injury. Pretreatment with SFN in rats could prevent hepatic damage induced by cisplatin and the decrease of antioxidant enzyme activity as well as mitochondrial alterations in oxygen consumption were also attenuated (Gaona-Gaona et al., 2011). Further, coadministration of SFN showed a protective effect in sodium valproate-induced liver injury in rats, and reduced the content of malondialdehyde (MDA) and tumor necrosis factor α (TNFα), while the concentration of GSH and HO-1 were increased (Nazmy et al., 2017). Olanzapine is a widely used antipsychotic drug, but side effects such as weight gain, dyslipidemia, and liver injury are common. In a HFD plus olanzapine-induced chronic liver injury model in mice, SFN was found to effectively prevent the exacerbated liver damage caused by the interaction of olanzapine and HFD; moreover, fat accumulation and inflammation were significantly decreased along with 4-HNE, a biomarker of oxidative/nitrosative stress (Isaacson et al., 2020).
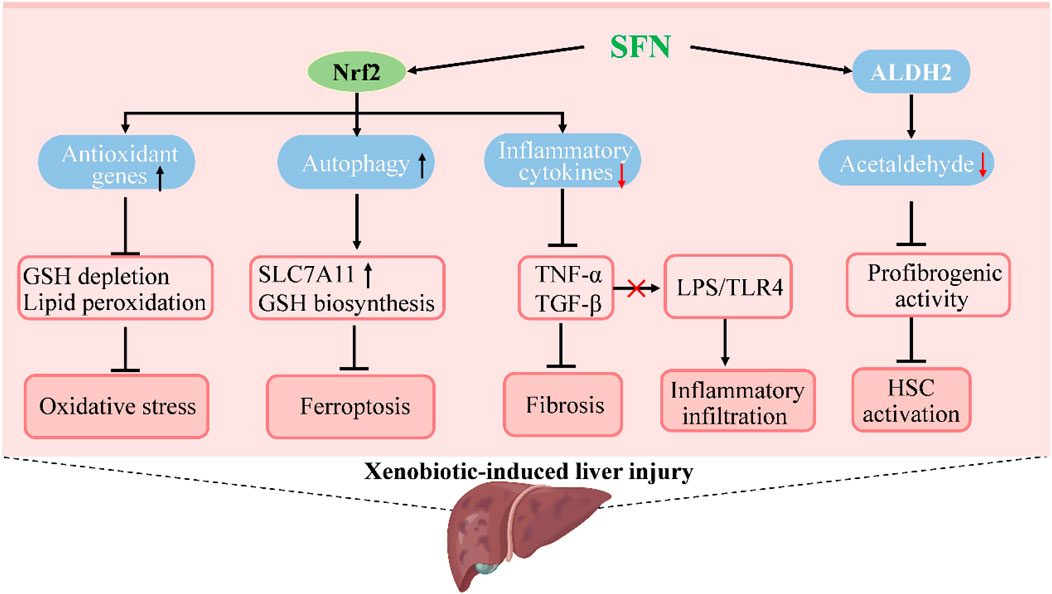
FIGURE 3. Molecular mechanisms under the efficacy of SFN in the treatment of xenobiotic-induced liver injury. SFN activates Nrf2 to increase the expression of genes in antioxidation and autophagy to suppress oxidative stress and ferroptosis, which prevents or attenuates xenobiotic-induced liver injury. The activation of Nrf2 by SFN also decreases inflammatory cytokines to inhibit inflammatory infiltration and hepatic fibrosis that occurs in liver injury. Moreover, SFN induces the activity of acetaldehyde-metabolizing enzyme ALDH2 to promote acetaldehyde metabolism and suppresses acetaldehyde-induced proliferation and profibrogenic activity of hepatic stellate cells to ameliorate alcoholic liver injury.
Apart from drug-induced liver injury, the role of SFN in acute liver injuries induced by other substances has been studied, like carbon tetrachloride (CCl4), D-galactose, lipopolysaccharide (LPS), nanoparticles, and environmental toxicants. Oral administration of SFN after CCl4 significantly decreased serum ALT in mice and reduced necrotic zones and lipid peroxidation in the liver (Baek et al., 2008). Liver fibrosis caused by ethanol/CCl4 in mice was also ameliorated by SFN treatment, possibly through Nrf2-mediated antioxidation and enhanced acetaldehyde metabolism (Ishida et al., 2021). Recently, SFN was found to reduce ferroptosis in acute liver injury in an Nrf2-dependent manner. Upon activating Nrf2, SFN induced autophagy and upregulated the expression of solute carrier family 7 member 11 and promoted its membrane transfer, which finally suppressed ferroptosis to alleviate acute liver injury both in vitro and in vivo (Liu et al., 2023). Because of its antioxidant and anti-inflammatory capacity, SFN showed benefits not only in alleviating liver damage and reducing mortality in D-galactose/LPS-induced fulminant hepatic failure, but also in prevention of hepatic fibrosis and injury caused by D-galactose in rats (Sayed et al., 2014; Saleh et al., 2019). However, although anti-inflammatory effects were obvious in the liver, no improvement of LPS-induced sickness behavior in mice was observed by a short pretreatment with SFN (Townsend and Johnson, 2017). In the study of cadmium selenide quantum dots-induced hepatotoxicity, treatment with SFN significantly reduced cell death in human hepatocytes and decreased liver damage in mice through the induction of Nrf2 pathway and autophagy (Wang et al., 2015). In addition, SFN was found to prevent arsenic-induced hepatotoxicity in mice by PI3K/Akt mediated Nrf2 signaling pathway, which suggests a protective effect of SFN on toxicant-induced liver injury (Thangapandiyan et al., 2019). Though the efficacy of SFN in preventing liver injury or in therapy of xenobiotic-induced liver injuries has been demonstrated by various experimental studies, clinical data from patients are lacking. In view of the therapeutic potential, evaluating the effect of SFN on xenobiotic-induced liver injuries in patients in the future is meaningful.
3.4 SFN and hepatic ischemia-reperfusion injury
Hepatic ischemia-reperfusion injury (IRI) is a major complication of hemorrhagic shock and transplantation and is the most difficult problem in liver transplantation, often leading to liver dysfunction or failure of transplantation. Hepatic IRI is considered as a local proinflammatory response mediated by the innate immune system (Zhai et al., 2013). Although the mechanisms of hepatic IRI are largely unknown, several targets have been revealed to be associated with its pathogenesis by preclinical studies such as proliferators-activated receptor γ, family with sequence similarity 3A, and some noncoding RNAs (Yang et al., 2018). Targeting Nrf2 through SFN was found to be effective in handling hepatic IRI due to its antioxidative activity. This suggests that SFN could be useful in the treatment of hepatic IRI, since clinical trials have reported that antioxidants like N-acetyl cysteine could improve liver function of patients with liver transplantation by inhibiting oxidative stress and inflammation (Ntamo et al., 2022). In hepatic IRI rats, pretreatment with SFN could alleviate liver injury by inhibiting oxidative stress and improving antioxidative activity as well as mitochondrial function in liver tissues, which was accompanied with the activation of Nrf2 pathway (Zhao et al., 2010; Chi et al., 2015; Oguz et al., 2015). Upregulation of carbonyl reductase 1, an enzyme that protects cells against oxidative stress and cell death by inhibiting the formation of lipid peroxides, was observed in SFN-pretreated hepatic IRI mice in an Nrf2-dependent manner (Kwon et al., 2019). Apart from antioxidation, an anti-inflammatory effect was also involved in the protection of hepatic IRI by SFN. The upregulation of inflammatory cytokines in hepatic IRI rats, like TNFα, Interleukin 6, and Monocyte chemoattractant protein-1, were inhibited by SFN treatment which was dependent on the activation of Nrf2 (Chen et al., 2021). More recently, immunomodulatory effects of SFN in alleviating hepatic IRI were further identified in a murine model. In male C57BL/6 mice with hemorrhagic shock followed by resuscitation, SFN treatment reduced inflammatory cytokine secretion of Kupffer cells, decreased the infiltration of neutrophils in the liver, and alleviated liver damage through the Nrf2-mediated pathway (Liang et al., 2022). Together, these results indicate that antioxidative and anti-inflammatory effects are indispensable for SFN in the treatment of hepatic IRI.
3.5 SFN and hepatocellular carcinoma
The antineoplastic activity of SFN has been widely studied since its discovery in the 1990s, and multiple molecular pathways have been revealed, including HCC (Kaiser et al., 2021). SFN exhibited antiproliferative activity in HepG2 cells by inducing apoptosis through the activation of caspase-3 and upregulation of Bcl-2-associated X protein, as well as downregulation of Bcl-2 and Bcl-XL expression (Park et al., 2007). In another study, SFN-mediated apoptosis in Huh7 cells was found to be associated with the inhibition of 6-phosphofructo-2-kinase/fructose-2,6-biphosphatase 4 expression and HIF-1α pathway, and the inhibition was even higher under hypoxic conditions (Jeon et al., 2011). Moreover, SFN suppressed angiogenesis and tumor growth by inhibiting STAT3/HIF-1α/VEGF pathway in HepG2 cells and tumor tissues as the expression of HIF-1α, STAT3, and VEGF were decreased (Liu et al., 2017). In addition, the biomarkers of ER stress, like C/EBP homologous protein and XBP-1, were upregulated in apoptotic HepG2 cells induced by SFN treatment, suggesting the involvement of ER stress in SFN-induced apoptosis (Zou et al., 2017). Apart from apoptosis, SFN treatment inhibited the formation of fibroblast-like mesenchymal cells and expression of vimentin in HepG2 cells, while it increased the expression of E-cadherin, which indicates that SFN suppresses epithelial-mesenchymal transition in HCC (Wu et al., 2016). In Hep3B cells, SFN not only decreased cell viability but also inhibited telomerase activity via reducing the expression of telomerase reverse transcriptase in a ROS-dependent manner, suggesting a novel mechanism of the antineoplastic activity of SFN (Moon et al., 2010). Recently, active metabolites of SFN, such as SFN-GSH, SFN-cysteine, and SFN-N-acetylcysteine, were found to have similar chemopreventive activities to SFN in HepG2 cells (Liu et al., 2018). Furthermore, SFN was found to be a potent sensitizer for tumor necrosis factor-related apoptosis-inducing ligand-induced apoptosis in hepatoma cells through the generation of ROS and subsequent upregulation of death receptor 5 (Kim et al., 2006). Together, these in vitro findings suggest an effective role of SFN in the treatment of HCC.
However, in vivo studies on the chemopreventive activity of SFN in HCC are very limited. In a HepG2 cell-derived xenograft tumor model in Balb/c athymic nude mice, treatment with SFN for 13 days significantly inhibited tumor growth and reduced the volume of tumors (Wu et al., 2016). But in another in vivo study, an SFN-containing diet did not prevent the hepatic tumorigenesis induced by the diethylnitrosamine in C57BL/6J mice (Chen et al., 2016). In this study, broccoli powder was used instead of SFN and administered along with a western diet during the study period. Although SFN content was 4 mmol/kg broccoli powder in the present study, the source, dose, and route of administration of SFN may affect its final efficacy as it is metabolized quickly in the body with a relatively short half-life (Yagishita et al., 2019).
4 Future perspectives
SFN has become a promising phytochemical in the treatment of several diseases in the last few years, including various cancers, autism spectrum disorder, schizophrenia, obesity, and fatty liver disease. Although few clinical trials have focused on the efficacy of SFN in the treatment of liver diseases, previous findings from preclinical studies are exciting, especially in fatty liver disease. Thus, advanced research and clinical trials that focus on the efficacy of SFN in the treatment of liver diseases are necessary in the future. Nevertheless, there are some problems that need to be solved by future research.
Firstly, the development of SFN is limited by its water-insoluble and unstable properties. SFN is sensitive to temperature and easy to degrade in aqueous and protic solvents and polar aprotic environments (Franklin et al., 2014). Developing strategies to improve the stability of SFN in formulation is an important direction for future research. Novel drug delivery systems are expected to be suitable candidates in future studies. Previously, a commercially available product of stabilized SFN was developed, named Prostaphane®, but its storage condition is limited to between 4°C–8°C. Besides, novel delivery systems have been reported to successfully increase the stability and solubility of SFN in recent years, such as coated microparticles, microencapsulation, and nanoparticles, and these strategies for improving the stabilization of SFN have been discussed in a recent review (Yuanfeng et al., 2021). Nevertheless, it is worth noting that most of these formulations remain to be tested on the human body to evaluate their safety, as some of these materials may cause unwanted effects. For instance, a high incidence of mild stomach upset has been observed in a clinical trial of α-cyclodextrin inclusion of SFN (Fahey et al., 2017). Further in vivo studies as well as safety evaluations of these delivery systems will greatly promote the translational research of SFN from bench to bedside.
Secondly, the formulation is an important factor that affects the bioavailability and efficacy of SFN in humans. In most of the published clinical studies, broccoli sprout extracts or glucoraphanin-rich preparations were used instead of SFN. But administration of these formulations showed significant inter-individual variation of the bioavailability of SFN. Among which, the myrosinase-catalyzed conversion of glucoraphanin has demonstrated large differences in effect among individuals and is considered as an important factor that affects the bioavailability of SFN. Additionally, the precise dosages of SFN that subjects received from these formulations are difficult to quantify, and the efficacy of SFN in clinical trials may be influenced (Alumkal et al., 2015; Cipolla et al., 2015). The use of formulation containing stable and pure SFN is recommended for future studies due to its advantage of dose control and relative high bioavailability.
Thirdly, the selection of dose and dose schedule of SFN needs to be optimized in future studies. In previous studies, SFN or SFN-containing formulations were usually administered daily with a single dose. However, the half-life of SFN is very short due to its rapid metabolism in the human body. To date, there are few dose-response studies on SFN that have been reported and the range of its effective doses is unclear. Doses used in most animal studies have exceeded the highest dose of SFN used in humans. Thus, high quality dose-response research of SFN in the treatment of liver diseases are needed, which will provide valuable data for developing reasonable dose schedules of SFN in future clinical studies.
5 Conclusion
In this review, the therapeutic potential of SFN, a phytochemical derived from broccoli and other cruciferous vegetables, in the treatment of several liver diseases and related mechanisms were summarized and future research directions were discussed. In the past decades, both in vitro and in vivo studies have demonstrated significant benefits of SFN in the treatment of several liver diseases, including fatty liver disease, hepatic insulin resistance, liver injuries, and hepatocellular carcinoma. In terms of mechanism, Nrf2-mediated pathways play important roles in the therapeutic effects of SFN, such as Nrf2-mediated inhibition of lipogenesis and oxidative stress, anti-inflammation, and suppression of ER stress, Nrf2-mediated lipophagy, and mitochondrial function. Moreover, new regulatory pathways like FGF21-regulated enhancement of fatty acid metabolism, restoration of mitochondrial-ER stress interaction, and blocking of ceramide biosynthesis were found to be mediated by SFN. However, there is still a gap between the basic research and clinical application of SFN. More efficient delivery systems and precise dose schedules of SFN are expected to be developed in future studies, which would improve its solubility, stability, and bioavailability and reduce inter-individual variations in humans. And these future studies will greatly facilitate the translational research of SFN in the treatment of liver diseases and ultimately promote its clinical application (Figure 4).
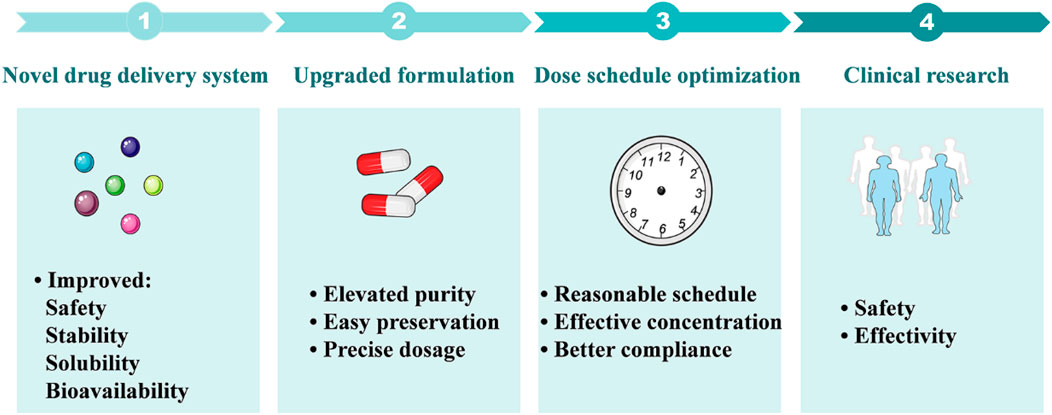
FIGURE 4. Future research perspectives of SFN in the treatment of liver diseases. 1: Novel drug delivery systems including nanoparticles, microencapsulation, and coated microparticles are expected to improve the solubility and stability of SFN, as well as its bioavailability and safety. 2: Advanced formulations will provide pure and stable SFN that is suitable for preservation and dose control. 3: Optimizing the dose schedule of SFN will provide reasonable dosing intervals, benefit the maintenance of effective blood concentration, and improve drug compliance. 4: Future clinical research focusing on the safety and efficacy of SFN in the treatment of liver diseases will facilitate its clinical transformation.
Author contributions
LY: Conceptualization, Funding acquisition, Investigation, Visualization, Writing–original draft, Writing–review and editing. YY: Investigation, Visualization, Writing–original draft.
Funding
The authors declare financial support was received for the research, authorship, and/or publication of this article. This work was financially supported by the National Natural Science Foundation of China (grant No. 81803645 to LY).
Conflict of interest
The authors declare that the research was conducted in the absence of any commercial or financial relationships that could be construed as a potential conflict of interest.
Publisher’s note
All claims expressed in this article are solely those of the authors and do not necessarily represent those of their affiliated organizations, or those of the publisher, the editors and the reviewers. Any product that may be evaluated in this article, or claim that may be made by its manufacturer, is not guaranteed or endorsed by the publisher.
References
Al Janobi, A. A., Mithen, R. F., Gasper, A. V., Shaw, P. N., Middleton, R. J., Ortori, C. A., et al. (2006). Quantitative measurement of sulforaphane, iberin and their mercapturic acid pathway metabolites in human plasma and urine using liquid chromatography-tandem electrospray ionisation mass spectrometry. J. Chromatogr. B Anal. Technol. Biomed. Life Sci. 844 (2), 223–234. doi:10.1016/j.jchromb.2006.07.007
Alumkal, J. J., Slottke, R., Schwartzman, J., Cherala, G., Munar, M., Graff, J. N., et al. (2015). A phase II study of sulforaphane-rich broccoli sprout extracts in men with recurrent prostate cancer. Invest. New Drugs 33 (2), 480–489. doi:10.1007/s10637-014-0189-z
Andrade, R. J., Chalasani, N., Bjornsson, E. S., Suzuki, A., Kullak-Ublick, G. A., Watkins, P. B., et al. (2019). Drug-induced liver injury. Nat. Rev. Dis. Prim. 5 (1), 58. doi:10.1038/s41572-019-0105-0
Arroyave-Ospina, J. C., Wu, Z., Geng, Y., and Moshage, H. (2021). Role of oxidative stress in the pathogenesis of non-alcoholic fatty liver disease: implications for prevention and therapy. Antioxidants (Basel) 10 (2), 174. doi:10.3390/antiox10020174
Atwell, L. L., Hsu, A., Wong, C. P., Stevens, J. F., Bella, D., Yu, T. W., et al. (2015). Absorption and chemopreventive targets of sulforaphane in humans following consumption of broccoli sprouts or a myrosinase-treated broccoli sprout extract. Mol. Nutr. Food Res. 59 (3), 424–433. doi:10.1002/mnfr.201400674
Axelsson, A. S., Tubbs, E., Mecham, B., Chacko, S., Nenonen, H. A., Tang, Y., et al. (2017). Sulforaphane reduces hepatic glucose production and improves glucose control in patients with type 2 diabetes. Sci. Transl. Med. 9 (394), eaah4477. doi:10.1126/scitranslmed.aah4477
Baek, S. H., Park, M., Suh, J. H., and Choi, H. S. (2008). Protective effects of an extract of young radish (Raphanus sativus L) cultivated with sulfur (sulfur-radish extract) and of sulforaphane on carbon tetrachloride-induced hepatotoxicity. Biosci. Biotechnol. Biochem. 72 (5), 1176–1182. doi:10.1271/bbb.70545
Bagherniya, M., Nobili, V., Blesso, C. N., and Sahebkar, A. (2018). Medicinal plants and bioactive natural compounds in the treatment of non-alcoholic fatty liver disease: a clinical review. Pharmacol. Res. 130, 213–240. doi:10.1016/j.phrs.2017.12.020
Bahadoran, Z., Tohidi, M., Nazeri, P., Mehran, M., Azizi, F., and Mirmiran, P. (2012). Effect of broccoli sprouts on insulin resistance in type 2 diabetic patients: a randomized double-blind clinical trial. Int. J. Food Sci. Nutr. 63 (7), 767–771. doi:10.3109/09637486.2012.665043
Beaulant, A., Dia, M., Pillot, B., Chauvin, M. A., Ji-Cao, J., Durand, C., et al. (2022). Endoplasmic reticulum-mitochondria miscommunication is an early and causal trigger of hepatic insulin resistance and steatosis. J. Hepatol. 77 (3), 710–722. doi:10.1016/j.jhep.2022.03.017
Bessone, F., Razori, M. V., and Roma, M. G. (2019). Molecular pathways of nonalcoholic fatty liver disease development and progression. Cell Mol. Life Sci. 76 (1), 99–128. doi:10.1007/s00018-018-2947-0
Calabrese, E. J., and Kozumbo, W. J. (2021). The phytoprotective agent sulforaphane prevents inflammatory degenerative diseases and age-related pathologies via Nrf2-mediated hormesis. Pharmacol. Res. 163, 105283. doi:10.1016/j.phrs.2020.105283
Chaurasia, B., Tippetts, T. S., Mayoral Monibas, R., Liu, J., Li, Y., Wang, L., et al. (2019). Targeting a ceramide double bond improves insulin resistance and hepatic steatosis. Science 365 (6451), 386–392. doi:10.1126/science.aav3722
Chen, L., Zhang, W. L., Xie, D. Q., and Jia, W. (2021). Sulforaphane alleviates hepatic ischemia-reperfusion injury through promoting the activation of Nrf-2/HO-1 signaling. Transpl. Immunol. 68, 101439. doi:10.1016/j.trim.2021.101439
Chen, Q., Wang, T., Li, J., Wang, S., Qiu, F., Yu, H., et al. (2017). Effects of natural products on fructose-induced nonalcoholic fatty liver disease (NAFLD). Nutrients 9 (2), 96. doi:10.3390/nu9020096
Chen, Y. J., Myracle, A. D., Wallig, M. A., and Jeffery, E. H. (2016). Dietary broccoli protects against fatty liver development but not against progression of liver cancer in mice pretreated with diethylnitrosamine. J. Funct. Foods 24, 57–62. doi:10.1016/j.jff.2016.03.028
Cheng, C., Zhuo, S., Zhang, B., Zhao, X., Liu, Y., Liao, C., et al. (2019). Treatment implications of natural compounds targeting lipid metabolism in nonalcoholic fatty liver disease, obesity and cancer. Int. J. Biol. Sci. 15 (8), 1654–1663. doi:10.7150/ijbs.33837
Chi, X., Zhang, R., Shen, N., Jin, Y., Alina, A., Yang, S., et al. (2015). Sulforaphane reduces apoptosis and oncosis along with protecting liver injury-induced ischemic reperfusion by activating the Nrf2/ARE pathway. Hepatol. Int. 9 (2), 321–329. doi:10.1007/s12072-014-9604-y
Cipolla, B. G., Mandron, E., Lefort, J. M., Coadou, Y., Della Negra, E., Corbel, L., et al. (2015). Effect of sulforaphane in men with biochemical recurrence after radical prostatectomy. Cancer Prev. Res. (Phila) 8 (8), 712–719. doi:10.1158/1940-6207.CAPR-14-0459
Clarke, J. D., Hsu, A., Riedl, K., Bella, D., Schwartz, S. J., Stevens, J. F., et al. (2011a). Bioavailability and inter-conversion of sulforaphane and erucin in human subjects consuming broccoli sprouts or broccoli supplement in a cross-over study design. Pharmacol. Res. 64 (5), 456–463. doi:10.1016/j.phrs.2011.07.005
Clarke, J. D., Hsu, A., Williams, D. E., Dashwood, R. H., Stevens, J. F., Yamamoto, M., et al. (2011b). Metabolism and tissue distribution of sulforaphane in Nrf2 knockout and wild-type mice. Pharm. Res. 28 (12), 3171–3179. doi:10.1007/s11095-011-0500-z
Conaway, C. C., Getahun, S. M., Liebes, L. L., Pusateri, D. J., Topham, D. K., Botero-Omary, M., et al. (2000). Disposition of glucosinolates and sulforaphane in humans after ingestion of steamed and fresh broccoli. Nutr. Cancer 38 (2), 168–178. doi:10.1207/S15327914NC382_5
Cotter, T. G., and Rinella, M. (2020). Nonalcoholic fatty liver disease 2020: the state of the disease. Gastroenterology 158 (7), 1851–1864. doi:10.1053/j.gastro.2020.01.052
Cuadrado, A., Manda, G., Hassan, A., Alcaraz, M. J., Barbas, C., Daiber, A., et al. (2018). Transcription factor NRF2 as a therapeutic target for chronic diseases: a systems medicine approach. Pharmacol. Rev. 70 (2), 348–383. doi:10.1124/pr.117.014753
de Souza, C. G., da Motta, L. L., de Assis, A. M., Rech, A., Bruch, R., Klamt, F., et al. (2016). Sulforaphane ameliorates the insulin responsiveness and the lipid profile but does not alter the antioxidant response in diabetic rats. Food Funct. 7 (4), 2060–2065. doi:10.1039/c5fo01620g
de Souza, C. G., Sattler, J. A., de Assis, A. M., Rech, A., Perry, M. L., and Souza, D. O. (2012). Metabolic effects of sulforaphane oral treatment in streptozotocin-diabetic rats. J. Med. Food 15 (9), 795–801. doi:10.1089/jmf.2012.0016
Du, K., Fan, Y., and Li, D. (2021). Sulforaphane ameliorates lipid profile in rodents: an updated systematic review and meta-analysis. Sci. Rep. 11 (1), 7804. doi:10.1038/s41598-021-87367-9
Egner, P. A., Chen, J. G., Wang, J. B., Wu, Y., Sun, Y., Lu, J. H., et al. (2011). Bioavailability of sulforaphane from two broccoli sprout beverages: results of a short-term, cross-over clinical trial in qidong, China. Cancer Prev. Res. (Phila) 4 (3), 384–395. doi:10.1158/1940-6207.CAPR-10-0296
Egner, P. A., Kensler, T. W., Chen, J. G., Gange, S. J., Groopman, J. D., and Friesen, M. D. (2008). Quantification of sulforaphane mercapturic acid pathway conjugates in human urine by high-performance liquid chromatography and isotope-dilution tandem mass spectrometry. Chem. Res. Toxicol. 21 (10), 1991–1996. doi:10.1021/tx800210k
Eslam, M., Sanyal, A. J., George, J., and International Consensus, P. (2020). Mafld: a consensus-driven proposed nomenclature for metabolic associated fatty liver disease. Gastroenterology 158 (7), 1999–2014.e1. doi:10.1053/j.gastro.2019.11.312
Fahey, J. W., Holtzclaw, W. D., Wehage, S. L., Wade, K. L., Stephenson, K. K., and Talalay, P. (2015). Sulforaphane bioavailability from glucoraphanin-rich broccoli: control by active endogenous myrosinase. PLoS One 10 (11), e0140963. doi:10.1371/journal.pone.0140963
Fahey, J. W., Wade, K. L., Stephenson, K. K., Panjwani, A. A., Liu, H., Cornblatt, G., et al. (2019). Bioavailability of sulforaphane following ingestion of glucoraphanin-rich broccoli sprout and seed extracts with active myrosinase: a pilot study of the effects of proton pump inhibitor administration. Nutrients 11 (7), 1489. doi:10.3390/nu11071489
Fahey, J. W., Wade, K. L., Wehage, S. L., Holtzclaw, W. D., Liu, H., Talalay, P., et al. (2017). Stabilized sulforaphane for clinical use: phytochemical delivery efficiency. Mol. Nutr. Food Res. 61 (4), 1600766. doi:10.1002/mnfr.201600766
Fahey, J. W., Wehage, S. L., Holtzclaw, W. D., Kensler, T. W., Egner, P. A., Shapiro, T. A., et al. (2012). Protection of humans by plant glucosinolates: efficiency of conversion of glucosinolates to isothiocyanates by the gastrointestinal microflora. Cancer Prev. Res. (Phila) 5 (4), 603–611. doi:10.1158/1940-6207.CAPR-11-0538
Feng, S., Xu, Z., Wang, F., Yang, T., Liu, W., Deng, Y., et al. (2017). Sulforaphane prevents methylmercury-induced oxidative damage and excitotoxicity through activation of the nrf2-ARE pathway. Mol. Neurobiol. 54 (1), 375–391. doi:10.1007/s12035-015-9643-y
Fisher, F. M., and Maratos-Flier, E. (2016). Understanding the physiology of FGF21. Annu. Rev. Physiol. 78, 223–241. doi:10.1146/annurev-physiol-021115-105339
Fontana, R. J., Hayashi, P. H., Gu, J., Reddy, K. R., Barnhart, H., Watkins, P. B., et al. (2014). Idiosyncratic drug-induced liver injury is associated with substantial morbidity and mortality within 6 months from onset. Gastroenterology 147 (1), 96–108.e4. doi:10.1053/j.gastro.2014.03.045
Fontana, R. J. (2014). Pathogenesis of idiosyncratic drug-induced liver injury and clinical perspectives. Gastroenterology 146 (4), 914–928. doi:10.1053/j.gastro.2013.12.032
Franklin, S. J., Dickinson, S. E., Karlage, K. L., Bowden, G. T., and Myrdal, P. B. (2014). Stability of sulforaphane for topical formulation. Drug Dev. Ind. Pharm. 40 (4), 494–502. doi:10.3109/03639045.2013.768634
Gaona-Gaona, L., Molina-Jijon, E., Tapia, E., Zazueta, C., Hernandez-Pando, R., Calderon-Oliver, M., et al. (2011). Protective effect of sulforaphane pretreatment against cisplatin-induced liver and mitochondrial oxidant damage in rats. Toxicology 286 (1-3), 20–27. doi:10.1016/j.tox.2011.04.014
Ghazizadeh-Hashemi, F., Bagheri, S., Ashraf-Ganjouei, A., Moradi, K., Shahmansouri, N., Mehrpooya, M., et al. (2021). Efficacy and safety of sulforaphane for treatment of mild to moderate depression in patients with history of cardiac interventions: a randomized, double-blind, placebo-controlled clinical trial. Psychiatry Clin. Neurosci. 75 (8), 250–255. doi:10.1111/pcn.13276
Greaney, A. J., Maier, N. K., Leppla, S. H., and Moayeri, M. (2016). Sulforaphane inhibits multiple inflammasomes through an Nrf2-independent mechanism. J. Leukoc. Biol. 99 (1), 189–199. doi:10.1189/jlb.3A0415-155RR
Gross-Steinmeyer, K., Stapleton, P. L., Tracy, J. H., Bammler, T. K., Strom, S. C., and Eaton, D. L. (2010). Sulforaphane- and phenethyl isothiocyanate-induced inhibition of aflatoxin B1-mediated genotoxicity in human hepatocytes: role of GSTM1 genotype and CYP3A4 gene expression. Toxicol. Sci. 116 (2), 422–432. doi:10.1093/toxsci/kfq135
Hassan, A., and Fontana, R. J. (2019). The diagnosis and management of idiosyncratic drug-induced liver injury. Liver Int. 39 (1), 31–41. doi:10.1111/liv.13931
Houghton, C. A., Fassett, R. G., and Coombes, J. S. (2016). Sulforaphane and other nutrigenomic Nrf2 activators: can the clinician's expectation Be matched by the reality? Oxid. Med. Cell Longev. 2016, 7857186. doi:10.1155/2016/7857186
Houghton, C. A. (2019). Sulforaphane: its "coming of age" as a clinically relevant nutraceutical in the prevention and treatment of chronic disease. Oxid. Med. Cell Longev. 2019, 2716870. doi:10.1155/2019/2716870
Hu, R., Hebbar, V., Kim, B. R., Chen, C., Winnik, B., Buckley, B., et al. (2004). In vivo pharmacokinetics and regulation of gene expression profiles by isothiocyanate sulforaphane in the rat. J. Pharmacol. Exp. Ther. 310 (1), 263–271. doi:10.1124/jpet.103.064261
Hu, R., Xu, C., Shen, G., Jain, M. R., Khor, T. O., Gopalkrishnan, A., et al. (2006). Gene expression profiles induced by cancer chemopreventive isothiocyanate sulforaphane in the liver of C57BL/6J mice and C57BL/6J/Nrf2 (-/-) mice. Cancer Lett. 243 (2), 170–192. doi:10.1016/j.canlet.2005.11.050
Isaacson, R. H., Beier, J. I., Khoo, N. K., Freeman, B. A., Freyberg, Z., and Arteel, G. E. (2020). Olanzapine-induced liver injury in mice: aggravation by high-fat diet and protection with sulforaphane. J. Nutr. Biochem. 81, 108399. doi:10.1016/j.jnutbio.2020.108399
Ishida, K., Kaji, K., Sato, S., Ogawa, H., Takagi, H., Takaya, H., et al. (2021). Sulforaphane ameliorates ethanol plus carbon tetrachloride-induced liver fibrosis in mice through the Nrf2-mediated antioxidant response and acetaldehyde metabolization with inhibition of the LPS/TLR4 signaling pathway. J. Nutr. Biochem. 89, 108573. doi:10.1016/j.jnutbio.2020.108573
Jeon, Y. K., Yoo, D. R., Jang, Y. H., Jang, S. Y., and Nam, M. J. (2011). Sulforaphane induces apoptosis in human hepatic cancer cells through inhibition of 6-phosphofructo-2-kinase/fructose-2,6-biphosphatase4, mediated by hypoxia inducible factor-1-dependent pathway. Biochim. Biophys. Acta 1814 (10), 1340–1348. doi:10.1016/j.bbapap.2011.05.015
Kaiser, A. E., Baniasadi, M., Giansiracusa, D., Giansiracusa, M., Garcia, M., Fryda, Z., et al. (2021). Sulforaphane: a broccoli bioactive phytocompound with cancer preventive potential. Cancers (Basel) 13 (19), 4796. doi:10.3390/cancers13194796
Kay, H. Y., Kim, W. D., Hwang, S. J., Choi, H. S., Gilroy, R. K., Wan, Y. J., et al. (2011). Nrf2 inhibits LXRα-dependent hepatic lipogenesis by competing with FXR for acetylase binding. Antioxid. Redox Signal 15 (8), 2135–2146. doi:10.1089/ars.2010.3834
Kensler, T. W., Chen, J. G., Egner, P. A., Fahey, J. W., Jacobson, L. P., Stephenson, K. K., et al. (2005). Effects of glucosinolate-rich broccoli sprouts on urinary levels of aflatoxin-DNA adducts and phenanthrene tetraols in a randomized clinical trial in He Zuo township, Qidong, People's Republic of China. Cancer Epidemiol. Biomarkers Prev. 14 (11 Pt 1), 2605–2613. doi:10.1158/1055-9965.EPI-05-0368
Kikuchi, M., Ushida, Y., Shiozawa, H., Umeda, R., Tsuruya, K., Aoki, Y., et al. (2015). Sulforaphane-rich broccoli sprout extract improves hepatic abnormalities in male subjects. World J. Gastroenterol. 21 (43), 12457–12467. doi:10.3748/wjg.v21.i43.12457
Kim, H., Kim, E. H., Eom, Y. W., Kim, W. H., Kwon, T. K., Lee, S. J., et al. (2006). Sulforaphane sensitizes tumor necrosis factor-related apoptosis-inducing ligand (TRAIL)-resistant hepatoma cells to TRAIL-induced apoptosis through reactive oxygen species-mediated up-regulation of DR5. Cancer Res. 66 (3), 1740–1750. doi:10.1158/0008-5472.CAN-05-1568
Kolm, R. H., Danielson, U. H., Zhang, Y., Talalay, P., and Mannervik, B. (1995). Isothiocyanates as substrates for human glutathione transferases: structure-activity studies. Biochem. J. 311 ( Pt 2)(Pt 2), 453–459. doi:10.1042/bj3110453
Kwon, J. H., Lee, J., Kim, J., Kirchner, V. A., Jo, Y. H., Miura, T., et al. (2019). Upregulation of carbonyl reductase 1 by Nrf2 as a potential therapeutic intervention for ischemia/reperfusion injury during liver transplantation. Mol. Cells 42 (9), 672–685. doi:10.14348/molcells.2019.0003
Lei, P., Hu, Y., Gao, P., Ding, Q., Yan, J., Zhao, J., et al. (2022). Sulforaphane ameliorates hepatic lipid metabolism via modulating lipophagy in vivo and in vitro. J. Agric. Food Chem. 70 (48), 15126–15133. doi:10.1021/acs.jafc.2c06311
Lei, P., Tian, S., Teng, C., Huang, L., Liu, X., Wang, J., et al. (2019). Sulforaphane improves lipid metabolism by enhancing mitochondrial function and biogenesis in vivo and in vitro. Mol. Nutr. Food Res. 63 (4), e1800795. doi:10.1002/mnfr.201800795
Lei, P., Zhao, W., Pang, B., Yang, X., Li, B. L., Ren, M., et al. (2018). Broccoli sprout extract alleviates alcohol-induced oxidative stress and endoplasmic reticulum stress in C57bl/6 mice. J. Agric. Food Chem. 66 (22), 5574–5580. doi:10.1021/acs.jafc.8b01653
Li, J., Xie, S., and Teng, W. (2021). Sulforaphane attenuates nonalcoholic fatty liver disease by inhibiting hepatic steatosis and apoptosis. Nutrients 14 (1), 76. doi:10.3390/nu14010076
Liang, W., Greven, J., Qin, K., Fragoulis, A., Horst, K., Blasius, F., et al. (2022). Sulforaphane exerts beneficial immunomodulatory effects on liver tissue via a Nrf2 pathway-related mechanism in a murine model of hemorrhagic shock and resuscitation. Front. Immunol. 13, 822895. doi:10.3389/fimmu.2022.822895
Liu, J., Huang, C., Liu, J., Meng, C., Gu, Q., Du, X., et al. (2023). Nrf2 and its dependent autophagy activation cooperatively counteract ferroptosis to alleviate acute liver injury. Pharmacol. Res. 187, 106563. doi:10.1016/j.phrs.2022.106563
Liu, P., Atkinson, S. J., Akbareian, S. E., Zhou, Z., Munsterberg, A., Robinson, S. D., et al. (2017). Sulforaphane exerts anti-angiogenesis effects against hepatocellular carcinoma through inhibition of STAT3/HIF-1α/VEGF signalling. Sci. Rep. 7 (1), 12651. doi:10.1038/s41598-017-12855-w
Liu, P., Wang, W., Zhou, Z., Smith, A. J. O., Bowater, R. P., Wormstone, I. M., et al. (2018). Chemopreventive activities of sulforaphane and its metabolites in human hepatoma HepG2 cells. Nutrients 10 (5), 585. doi:10.3390/nu10050585
Livingstone, T. L., Saha, S., Bernuzzi, F., Savva, G. M., Troncoso-Rey, P., Traka, M. H., et al. (2022). Accumulation of sulforaphane and alliin in human prostate tissue. Nutrients 14 (16), 3263. doi:10.3390/nu14163263
Louvet, A., and Mathurin, P. (2015). Alcoholic liver disease: mechanisms of injury and targeted treatment. Nat. Rev. Gastroenterol. Hepatol. 12 (4), 231–242. doi:10.1038/nrgastro.2015.35
Ma, S., Pang, X., Tian, S., Sun, J., Hu, Q., Li, X., et al. (2022). The protective effects of sulforaphane on high-fat diet-induced metabolic associated fatty liver disease in mice via mediating the FXR/LXRα pathway. Food Funct. 13 (24), 12966–12982. doi:10.1039/d2fo02341e
Mangla, B., Javed, S., Sultan, M. H., Kumar, P., Kohli, K., Najmi, A., et al. (2021). Sulforaphane: a review of its therapeutic potentials, advances in its nanodelivery, recent patents, and clinical trials. Phytother. Res. 35 (10), 5440–5458. doi:10.1002/ptr.7176
Mansour, S. Z., Moustafa, E. M., and Moawed, F. S. M. (2022). Modulation of endoplasmic reticulum stress via sulforaphane-mediated AMPK upregulation against nonalcoholic fatty liver disease in rats. Cell Stress Chaperones 27 (5), 499–511. doi:10.1007/s12192-022-01286-w
Moon, D. O., Kang, S. H., Kim, K. C., Kim, M. O., Choi, Y. H., and Kim, G. Y. (2010). Sulforaphane decreases viability and telomerase activity in hepatocellular carcinoma Hep3B cells through the reactive oxygen species-dependent pathway. Cancer Lett. 295 (2), 260–266. doi:10.1016/j.canlet.2010.03.009
Nagata, N., Xu, L., Kohno, S., Ushida, Y., Aoki, Y., Umeda, R., et al. (2017). Glucoraphanin ameliorates obesity and insulin resistance through adipose tissue browning and reduction of metabolic endotoxemia in mice. Diabetes 66 (5), 1222–1236. doi:10.2337/db16-0662
Nazmy, E. A., El-Khouly, O. A., Atef, H., and Said, E. (2017). Sulforaphane protects against sodium valproate-induced acute liver injury. Can. J. Physiol. Pharmacol. 95 (4), 420–426. doi:10.1139/cjpp-2016-0447
Noh, J. R., Kim, Y. H., Hwang, J. H., Choi, D. H., Kim, K. S., Oh, W. K., et al. (2015). Sulforaphane protects against acetaminophen-induced hepatotoxicity. Food Chem. Toxicol. 80, 193–200. doi:10.1016/j.fct.2015.03.020
Ntamo, Y., Ziqubu, K., Chellan, N., Nkambule, B. B., Nyambuya, T. M., Mazibuko-Mbeje, S. E., et al. (2022). Clinical use of N-acetyl cysteine during liver transplantation: implications of oxidative stress and inflammation as therapeutic targets. Biomed. Pharmacother. 147, 112638. doi:10.1016/j.biopha.2022.112638
Ntamo, Y., Ziqubu, K., Chellan, N., Nkambule, B. B., Nyambuya, T. M., Mazibuko-Mbeje, S. E., et al. (2021). Drug-induced liver injury: clinical evidence of N-acetyl cysteine protective effects. Oxid. Med. Cell Longev. 2021, 3320325. doi:10.1155/2021/3320325
Oguz, A., Kapan, M., Kaplan, I., Alabalik, U., Ulger, B. V., Uslukaya, O., et al. (2015). The effects of sulforaphane on the liver and remote organ damage in hepatic ischemia-reperfusion model formed with pringle maneuver in rats. Int. J. Surg. 18, 163–168. doi:10.1016/j.ijsu.2015.04.049
Okada, K., Warabi, E., Sugimoto, H., Horie, M., Tokushige, K., Ueda, T., et al. (2012). Nrf2 inhibits hepatic iron accumulation and counteracts oxidative stress-induced liver injury in nutritional steatohepatitis. J. Gastroenterol. 47 (8), 924–935. doi:10.1007/s00535-012-0552-9
Okunade, O., Niranjan, K., Ghawi, S. K., Kuhnle, G., and Methven, L. (2018). Supplementation of the diet by exogenous myrosinase via mustard seeds to increase the bioavailability of sulforaphane in healthy human subjects after the consumption of cooked broccoli. Mol. Nutr. Food Res. 62 (18), e1700980. doi:10.1002/mnfr.201700980
Orlando, P., Nartea, A., Silvestri, S., Marcheggiani, F., Cirilli, I., Dludla, P. V., et al. (2022). Bioavailability study of isothiocyanates and other bioactive compounds of Brassica oleracea L. Var. Italica boiled or steamed: functional food or dietary supplement? Antioxidants (Basel) 11 (2), 209. doi:10.3390/antiox11020209
Park, S. Y., Kim, G. Y., Bae, S. J., Yoo, Y. H., and Choi, Y. H. (2007). Induction of apoptosis by isothiocyanate sulforaphane in human cervical carcinoma HeLa and hepatocarcinoma HepG2 cells through activation of caspase-3. Oncol. Rep. 18 (1), 181–187. doi:10.3892/or.18.1.181
Patel, B., Mann, G. E., and Chapple, S. J. (2018). Concerted redox modulation by sulforaphane alleviates diabetes and cardiometabolic syndrome. Free Radic. Biol. Med. 122, 150–160. doi:10.1016/j.freeradbiomed.2018.02.004
Petersen, M. C., and Shulman, G. I. (2017). Roles of diacylglycerols and ceramides in hepatic insulin resistance. Trends Pharmacol. Sci. 38 (7), 649–665. doi:10.1016/j.tips.2017.04.004
Pimpin, L., Cortez-Pinto, H., Negro, F., Corbould, E., Lazarus, J. V., Webber, L., et al. (2018). Burden of liver disease in Europe: epidemiology and analysis of risk factors to identify prevention policies. J. Hepatol. 69 (3), 718–735. doi:10.1016/j.jhep.2018.05.011
Saleh, D. O., Mansour, D. F., Hashad, I. M., and Bakeer, R. M. (2019). Effects of sulforaphane on D-galactose-induced liver aging in rats: role of keap-1/nrf-2 pathway. Eur. J. Pharmacol. 855, 40–49. doi:10.1016/j.ejphar.2019.04.043
Samuel, V. T., and Shulman, G. I. (2018). Nonalcoholic fatty liver disease as a nexus of metabolic and hepatic diseases. Cell Metab. 27 (1), 22–41. doi:10.1016/j.cmet.2017.08.002
Santoleri, D., and Titchenell, P. M. (2019). Resolving the paradox of hepatic insulin resistance. Cell Mol. Gastroenterol. Hepatol. 7 (2), 447–456. doi:10.1016/j.jcmgh.2018.10.016
Sarin, S. K., Kumar, M., Eslam, M., George, J., Al Mahtab, M., Akbar, S. M. F., et al. (2020). Liver diseases in the asia-pacific region: a lancet gastroenterology & hepatology commission. Lancet Gastroenterol. Hepatol. 5 (2), 167–228. doi:10.1016/S2468-1253(19)30342-5
Sayed, R. H., Khalil, W. K., Salem, H. A., Kenawy, S. A., and El-Sayeh, B. M. (2014). Sulforaphane increases the survival rate in rats with fulminant hepatic failure induced by D-galactosamine and lipopolysaccharide. Nutr. Res. 34 (11), 982–989. doi:10.1016/j.nutres.2014.10.003
Schepici, G., Bramanti, P., and Mazzon, E. (2020). Efficacy of sulforaphane in neurodegenerative diseases. Int. J. Mol. Sci. 21 (22), 8637. doi:10.3390/ijms21228637
Schwalfenberg, G. K. (2021). N-acetylcysteine: A review of clinical usefulness (an old drug with new tricks). J. Nutr. Metab. 2021, 9949453. doi:10.1155/2021/9949453
Shapiro, T. A., Fahey, J. W., Dinkova-Kostova, A. T., Holtzclaw, W. D., Stephenson, K. K., Wade, K. L., et al. (2006). Safety, tolerance, and metabolism of broccoli sprout glucosinolates and isothiocyanates: a clinical phase I study. Nutr. Cancer 55 (1), 53–62. doi:10.1207/s15327914nc5501_7
Shawky, N. M., Shehatou, G. S. G., Suddek, G. M., and Gameil, N. M. (2019). Comparison of the effects of sulforaphane and pioglitazone on insulin resistance and associated dyslipidemia, hepatosteatosis, and endothelial dysfunction in fructose-fed rats. Environ. Toxicol. Pharmacol. 66, 43–54. doi:10.1016/j.etap.2018.12.008
Singh, K., Connors, S. L., Macklin, E. A., Smith, K. D., Fahey, J. W., Talalay, P., et al. (2014). Sulforaphane treatment of autism spectrum disorder (ASD). Proc. Natl. Acad. Sci. U. S. A. 111 (43), 15550–15555. doi:10.1073/pnas.1416940111
Sun, Y. K., Zhang, Y. F., Xie, L., Rong, F., Zhu, X. Y., Xie, J., et al. (2022). Progress in the treatment of drug-induced liver injury with natural products. Pharmacol. Res. 183, 106361. doi:10.1016/j.phrs.2022.106361
Tahata, S., Singh, S. V., Lin, Y., Hahm, E. R., Beumer, J. H., Christner, S. M., et al. (2018). Evaluation of biodistribution of sulforaphane after administration of oral broccoli sprout extract in melanoma patients with multiple atypical nevi. Cancer Prev. Res. (Phila) 11 (7), 429–438. doi:10.1158/1940-6207.CAPR-17-0268
Tarantino, G., Balsano, C., Santini, S. J., Brienza, G., Clemente, I., Cosimini, B., et al. (2021). It is high time physicians thought of natural products for alleviating NAFLD. Is there sufficient evidence to use them? Int. J. Mol. Sci. 22 (24), 13424. doi:10.3390/ijms222413424
Teng, W., Li, Y., Du, M., Lei, X., Xie, S., and Ren, F. (2019). Sulforaphane prevents hepatic insulin resistance by blocking serine palmitoyltransferase 3-mediated ceramide biosynthesis. Nutrients 11 (5), 1185. doi:10.3390/nu11051185
Thangapandiyan, S., Ramesh, M., Hema, T., Miltonprabu, S., Uddin, M. S., Nandhini, V., et al. (2019). Sulforaphane potentially ameliorates arsenic induced hepatotoxicity in albino wistar rats: implication of PI3K/Akt/Nrf2 signaling pathway. Cell Physiol. Biochem. 52 (5), 1203–1222. doi:10.33594/000000082
Tian, S., Li, B., Lei, P., Yang, X., Zhang, X., Bao, Y., et al. (2018a). Sulforaphane improves abnormal lipid metabolism via both ERS-dependent XBP1/ACC &SCD1 and ERS-independent SREBP/FAS pathways. Mol. Nutr. Food Res. 62 (6), e1700737. doi:10.1002/mnfr.201700737
Tian, S., Li, X., Wang, Y., and Lu, Y. (2021a). The protective effect of sulforaphane on type II diabetes induced by high-fat diet and low-dosage streptozotocin. Food Sci. Nutr. 9 (2), 747–756. doi:10.1002/fsn3.2040
Tian, S., Liu, X., Lei, P., Zhang, X., and Shan, Y. (2018b). Microbiota: A mediator to transform glucosinolate precursors in cruciferous vegetables to the active isothiocyanates. J. Sci. Food Agric. 98 (4), 1255–1260. doi:10.1002/jsfa.8654
Tian, S., Wang, Y., Li, X., Liu, J., Wang, J., and Lu, Y. (2021b). Sulforaphane regulates glucose and lipid metabolisms in obese mice by restraining JNK and activating insulin and FGF21 signal pathways. J. Agric. Food Chem. 69 (44), 13066–13079. doi:10.1021/acs.jafc.1c04933
Titchenell, P. M., Lazar, M. A., and Birnbaum, M. J. (2017). Unraveling the regulation of hepatic metabolism by insulin. Trends Endocrinol. Metab. 28 (7), 497–505. doi:10.1016/j.tem.2017.03.003
Townsend, B. E., and Johnson, R. W. (2017). Sulforaphane reduces lipopolysaccharide-induced proinflammatory markers in hippocampus and liver but does not improve sickness behavior. Nutr. Neurosci. 20 (3), 195–202. doi:10.1080/1028415X.2015.1103463
Tubbs, E., Axelsson, A. S., Vial, G., Wollheim, C. B., Rieusset, J., and Rosengren, A. H. (2018). Sulforaphane improves disrupted ER-mitochondria interactions and suppresses exaggerated hepatic glucose production. Mol. Cell Endocrinol. 461, 205–214. doi:10.1016/j.mce.2017.09.016
Tubbs, E., Theurey, P., Vial, G., Bendridi, N., Bravard, A., Chauvin, M. A., et al. (2014). Mitochondria-associated endoplasmic reticulum membrane (MAM) integrity is required for insulin signaling and is implicated in hepatic insulin resistance. Diabetes 63 (10), 3279–3294. doi:10.2337/db13-1751
Wang, J., and Zhou, H. (2020). Protective effects of sulforaphane and aerobic exercise on acute alcoholic hepatic injury in mice. Saudi J. Biol. Sci. 27 (11), 3145–3149. doi:10.1016/j.sjbs.2020.07.014
Wang, W., He, Y., Yu, G., Li, B., Sexton, D. W., Wileman, T., et al. (2015). Sulforaphane protects the liver against CdSe quantum dot-induced cytotoxicity. PLoS One 10 (9), e0138771. doi:10.1371/journal.pone.0138771
Wu, J., Han, J., Hou, B., Deng, C., Wu, H., and Shen, L. (2016). Sulforaphane inhibits TGF-beta-induced epithelial-mesenchymal transition of hepatocellular carcinoma cells via the reactive oxygen species-dependent pathway. Oncol. Rep. 35 (5), 2977–2983. doi:10.3892/or.2016.4638
Wu, Y. K., Ren, Z. N., Zhu, S. L., Wu, Y. Z., Wang, G., Zhang, H., et al. (2022). Sulforaphane ameliorates non-alcoholic fatty liver disease in mice by promoting FGF21/FGFR1 signaling pathway. Acta Pharmacol. Sin. 43 (6), 1473–1483. doi:10.1038/s41401-021-00786-2
Xu, L., Nagata, N., and Ota, T. (2019). Impact of glucoraphanin-mediated activation of Nrf2 on non-alcoholic fatty liver disease with a focus on mitochondrial dysfunction. Int. J. Mol. Sci. 20 (23), 5920. doi:10.3390/ijms20235920
Xu, X., Sun, S., Liang, L., Lou, C., He, Q., Ran, M., et al. (2021). Role of the Aryl hydrocarbon receptor and gut microbiota-derived metabolites indole-3-acetic acid in sulforaphane alleviates hepatic steatosis in mice. Front. Nutr. 8, 756565. doi:10.3389/fnut.2021.756565
Yagishita, Y., Fahey, J. W., Dinkova-Kostova, A. T., and Kensler, T. W. (2019). Broccoli or sulforaphane: is it the source or dose that matters? Molecules 24 (19), 3593. doi:10.3390/molecules24193593
Yang, G., Lee, H. E., and Lee, J. Y. (2016). A pharmacological inhibitor of NLRP3 inflammasome prevents non-alcoholic fatty liver disease in a mouse model induced by high fat diet. Sci. Rep. 6, 24399. doi:10.1038/srep24399
Yang, W., Chen, J., Meng, Y., Chen, Z., and Yang, J. (2018). Novel targets for treating ischemia-reperfusion injury in the liver. Int. J. Mol. Sci. 19 (5), 1302. doi:10.3390/ijms19051302
Ye, L., Dinkova-Kostova, A. T., Wade, K. L., Zhang, Y., Shapiro, T. A., and Talalay, P. (2002). Quantitative determination of dithiocarbamates in human plasma, serum, erythrocytes and urine: pharmacokinetics of broccoli sprout isothiocyanates in humans. Clin. Chim. Acta 316 (1-2), 43–53. doi:10.1016/s0009-8981(01)00727-6
Yoshida, K., Ushida, Y., Ishijima, T., Suganuma, H., Inakuma, T., Yajima, N., et al. (2015). Broccoli sprout extract induces detoxification-related gene expression and attenuates acute liver injury. World J. Gastroenterol. 21 (35), 10091–10103. doi:10.3748/wjg.v21.i35.10091
Yuanfeng, W., Chengzhi, L., Ligen, Z., Juan, S., Xinjie, S., Yao, Z., et al. (2021). Approaches for enhancing the stability and formation of sulforaphane. Food Chem. 345, 128771. doi:10.1016/j.foodchem.2020.128771
Zhai, Y., Petrowsky, H., Hong, J. C., Busuttil, R. W., and Kupiec-Weglinski, J. W. (2013). Ischaemia-reperfusion injury in liver transplantation-from bench to bedside. Nat. Rev. Gastroenterol. Hepatol. 10 (2), 79–89. doi:10.1038/nrgastro.2012.225
Zhang, Y., Wu, Q., Liu, J., Zhang, Z., Ma, X., Zhang, Y., et al. (2022). Sulforaphane alleviates high fat diet-induced insulin resistance via AMPK/Nrf2/GPx4 axis. Biomed. Pharmacother. 152, 113273. doi:10.1016/j.biopha.2022.113273
Zhang, Z., Garzotto, M., Davis, E. W., Mori, M., Stoller, W. A., Farris, P. E., et al. (2020). Sulforaphane bioavailability and chemopreventive activity in men presenting for biopsy of the prostate gland: a randomized controlled trial. Nutr. Cancer 72 (1), 74–87. doi:10.1080/01635581.2019.1619783
Zhao, H. D., Zhang, F., Shen, G., Li, Y. B., Li, Y. H., Jing, H. R., et al. (2010). Sulforaphane protects liver injury induced by intestinal ischemia reperfusion through Nrf2-ARE pathway. World J. Gastroenterol. 16 (24), 3002–3010. doi:10.3748/wjg.v16.i24.3002
Zhou, J., Zheng, Q., and Chen, Z. (2022). The Nrf2 pathway in liver diseases. Front. Cell Dev. Biol. 10, 826204. doi:10.3389/fcell.2022.826204
Zhou, R., Lin, J., and Wu, D. (2014). Sulforaphane induces Nrf2 and protects against CYP2E1-dependent binge alcohol-induced liver steatosis. Biochim. Biophys. Acta 1840 (1), 209–218. doi:10.1016/j.bbagen.2013.09.018
Zimmerman, A. W., Singh, K., Connors, S. L., Liu, H., Panjwani, A. A., Lee, L. C., et al. (2021). Randomized controlled trial of sulforaphane and metabolite discovery in children with Autism Spectrum Disorder. Mol. Autism 12 (1), 38. doi:10.1186/s13229-021-00447-5
Keywords: sulforaphane, Nrf2, metabolic liver disease, antioxidant, autophagy
Citation: Yan L and Yan Y (2023) Therapeutic potential of sulforaphane in liver diseases: a review. Front. Pharmacol. 14:1256029. doi: 10.3389/fphar.2023.1256029
Received: 10 July 2023; Accepted: 11 August 2023;
Published: 29 August 2023.
Edited by:
Sílvio Terra Stefanello, Westfälischen Wilhelms-Universität Münster, GermanyReviewed by:
Phiwayinkosi V. Dludla, South African Medical Research Council, South AfricaMilton Prabu, Annamalai University, India
Copyright © 2023 Yan and Yan. This is an open-access article distributed under the terms of the Creative Commons Attribution License (CC BY). The use, distribution or reproduction in other forums is permitted, provided the original author(s) and the copyright owner(s) are credited and that the original publication in this journal is cited, in accordance with accepted academic practice. No use, distribution or reproduction is permitted which does not comply with these terms.
*Correspondence: Liang Yan, ZmNjeWFubGlhbmdAenp1LmVkdS5jbg==