- 1Centro Interdisciplinario de Neurociencia de Valparaíso, Facultad de Ciencias, Universidad de Valparaíso, Valparaíso, Chile
- 2Departamento de Fisiología y Comportamiento Animal, Facultad de Ciencias Naturales, Exactas y Tecnología, Universidad de Panamá, Ciudad de Panamá, Panamá
- 3Centro de Investigación de Estudios Avanzados del Maule (CIEAM), Vicerrectoría de Investigación y Postgrado Universidad Católica del Maule, Talca, Chile
- 4Laboratorio de Bioinformática y Química Computacional, Departamento de Medicina Traslacional, Facultad de Medicina, Universidad Católica del Maule, Talca, Chile
- 5Núcleo de Investigación en Data Science, Facultad de Ingeniería y Negocios, Universidad de las Américas, Santiago, Chile
The heat and capsaicin receptor TRPV1 channel is widely expressed in nerve terminals of dorsal root ganglia (DRGs) and trigeminal ganglia innervating the body and face, respectively, as well as in other tissues and organs including central nervous system. The TRPV1 channel is a versatile receptor that detects harmful heat, pain, and various internal and external ligands. Hence, it operates as a polymodal sensory channel. Many pathological conditions including neuroinflammation, cancer, psychiatric disorders, and pathological pain, are linked to the abnormal functioning of the TRPV1 in peripheral tissues. Intense biomedical research is underway to discover compounds that can modulate the channel and provide pain relief. The molecular mechanisms underlying temperature sensing remain largely unknown, although they are closely linked to pain transduction. Prolonged exposure to capsaicin generates analgesia, hence numerous capsaicin analogs have been developed to discover efficient analgesics for pain relief. The emergence of in silico tools offered significant techniques for molecular modeling and machine learning algorithms to indentify druggable sites in the channel and for repositioning of current drugs aimed at TRPV1. Here we recapitulate the physiological and pathophysiological functions of the TRPV1 channel, including structural models obtained through cryo-EM, pharmacological compounds tested on TRPV1, and the in silico tools for drug discovery and repositioning.
1 Introduction
Throughout evolution, different groups of organisms have developed highly conserved proteins. These proteins enable them to perceive and integrate fluctuations in environmental cues, including temperature. Temperature is a pervasive physical signal that varies in time and space, significantly affecting the metabolism, physiology, and behavior of organisms. Animals and other organisms have developed complex proteins that allow them to perceive changes in temperature and respond physiologically to adapt, survive, and reproduce. This process is crucial for their survival and evolution. The perception of temperature changes and other stimuli is facilitated by the expression of specialized receptors in the cell membrane. These receptors aid in the perception, interpretation, and differentiation of varying temperature ranges, allowing organisms to appropriately respond and adapt to external conditions. However, sensing errors can have detrimental and potentially fatal consequences for survival.
Temperature sensing is made possible through the expression of a subset of channel receptors that belong to the transient receptor potential (TRP) ion channel superfamily. These receptors are known as thermoTRP channels. The origin of the name TRP comes from the discoveries made by Cosens and Manning, (1969), with a mutant strain of Drosophila melanogaster that shows an abnormal transient response to light instead of a sustained receptor potential during light exposure that is normally observed in the wild phenotype, then the mutant was named trp (transient receptor potential). In 1989 the gene responsible for this trp phenotype was cloned in D. melanogaster and was suggested that may function as a channel or receptor (Montell and Rubin, 1989). Homologues of these channels were subsequently identified in several taxa, and currently 28 members of the TRP channel superfamily have been identified, grouped into eight subfamilies: TRPC (“canonical”), TRPA (“ankyrin”), TRPV (“vanilloid”), TRPM (“melastatin”), TRPS (“soromelastatin”), TRPML (“mucolipin”), TRPP (“polycystin”) and TRPN (“Non-mecano-potential or NOMP-C”) (Himmel et al., 2021). Most TRP channels function as polymodal receptors that detect and transduce physical and chemical signals such as pressure, pH, temperature, lipids, toxins, chemical ligands, and other stimuli. Functional TRP channels are tetramers formed by subunits with a topology of six transmembrane segments with the pore domain between the S5-S6 segments, and the N- and C-terminal domains facing intracellularly (Gees et al., 2012). A subset of TRP channels belonging to TRPV (1–4), TRPM (2, 3, 4, 5, 8), TRPA1 and TRPC5, are thermally gated and respond to temperatures ranging from noxious cold (<15°C) to noxious heat (>42°C) and are classified as “thermoTRPs” (Zimmermann et al., 2011; Ferrandiz-Huertas et al., 2014; Song et al., 2016). The TRPV1 known for its involvement in nociception and pain, is a highly-researched member of thermoTRP channels. Biomedical studies aim to identify suitable molecules for pain relief therapeutic intervention, with particular focus on this ion channel.
1.1 Transient receptor potential vanilloid type 1 channel
The TRPV1 channel, initially named the capsaicin and heat receptor (VR1) (Montell et al., 2002), was the first thermoTRP channel to be identified and characterized from the dorsal root ganglion (DRG) in 1997. It can be activated by temperature >42°C, protons, and the pungent compound found in chili peppers called capsaicin (Caterina et al., 1997). TRPV1 channels are non-selective cationic channels that preferentially allow the passage of Ca2+ ions. They can be modulated by a multitude of stimuli, including changes in voltage or pH, as well as the presence of lipids, vanilloids, phyto- and endocannabinoids, PI (4,5)P2, a variety of chemical compounds and pharmacological agents. These channels have the potential to be useful in biomedical applications (Figure 1) (Szallasi et al., 2007). At the sensory nerve endings, the activation of TRPV1 channels by temperature causes an influx Ca2+ ions and membrane depolarization. This initiates the generation of action potentials that synapse at the dorsal root ganglion and propagate to the CNS, generating thermosensation and thermoregulatory processes, as well as the perception of pain (Julius, 2013). The perception of pain involves numerous signaling pathways and relays within the central nervous system, leading to complex processing. ThermoTRP channels expressed in free nerve endings play a critical role in various physiological and pathophysiological pain types, including but not limited to neuropathic, mechanical, inflammatory, dental, migraine, and visceral pain (Mickle et al., 2016). Numerous inflammatory agents, such as protons, prostaglandins, bradykinin, reactive oxygen species, interleukins, histamines, and others, have the capability to modulate and sensitize thermoTRP channels. As a result, these ion channels act as the molecular basis for noxious peripheral, physical, thermal and chemical stimuli. Electrical signal conversion from the detected stimuli commence in the peripheral tissues upon the activation of thermoTRP channels. The Ca2+ influx depolarize C or Aδ fibers increasing action potential firing rate. The electric signal travels to the dorsal root of the spinal cord, where it can produce the stimulation of a motor neuron, resulting in a rapid withdrawal reflex to a noxious stimulus. Alternatively, it can ascend through pathways of the brainstem, synapse in the thalamus, and ultimately reach the somatosensory cortex or preoptic area. These regions are responsible for the perception of temperature and thermoregulation, respectively (Figure 2).
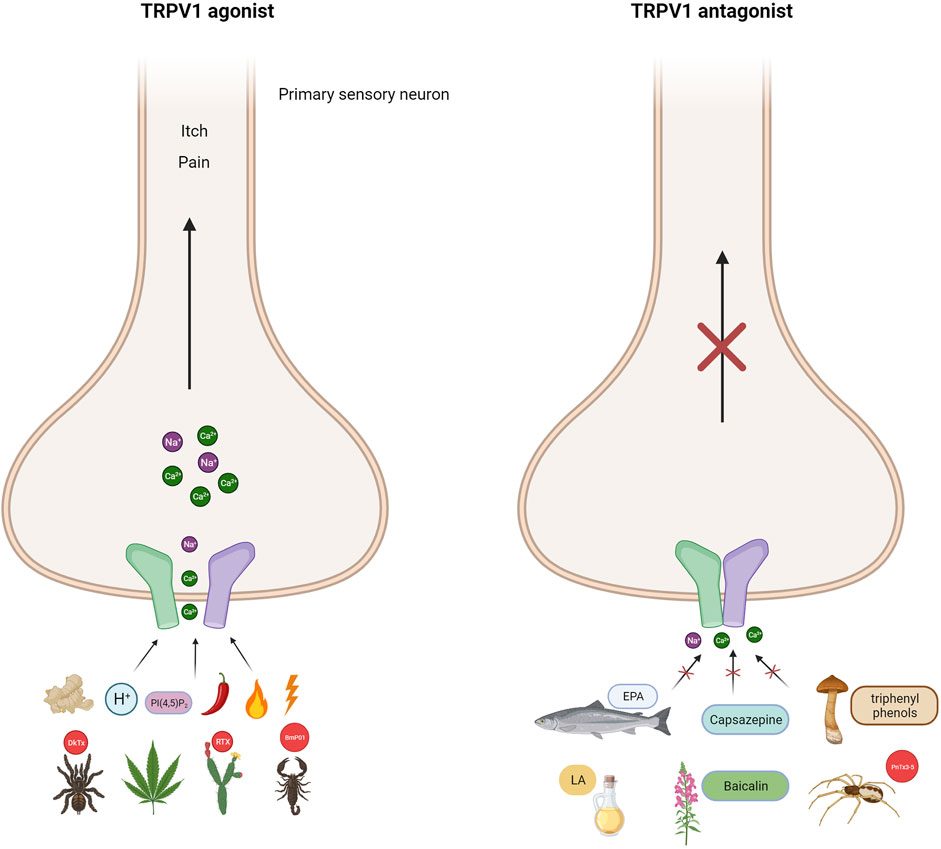
FIGURE 1. TRPV1 channel as polymodal receptor. TRPV1 channels in mammals can be activated by a range of ligands including heat above 42°C, capsaicin (the compound responsible for spicy in peppers), voltage, endogenous stimuli such as protons, lipids PI(4,5)P2, ginger (gingerol), Cannabis sativa (endocannabinoids), and plant and animal toxins. Indeed, resiniferatoxin (RTX) from the native Moroccan species Euphorbia resinifera, the native Nigerian species Einhorbia possonii, DkTx peptide toxins from the terrestrial tiger tarantula (Ornithoctonus huwena), and BmP01 from the Chinese scorpion (Mesobuthus martensii). This activation makes the flow of cations more permeable, causing pain and itching. Antagonists of the TRPV1 channel on the other hand, cause the channel to close, blocking its activity. Capsazepine is a synthetic compound structurally similar to capsaicin and is a classic antagonist of TRPV1 channels. Fascinating antagonists of natural origin have also been discovered, such as eicosapentaenoic acid (EPA), derived from fish, and linoleic acid (LA), mainly present in oils, both of which are fatty acids. The group of flavonoids includes baicalin from the plant Scutellaria baicalensis and triprenyl phenols (albaconol, grifolina, neogrifolina) from the fungal genus Albatrellus spp. Additionally, the peptide toxin PnTx3-5 from the banana spider Phoneutria nigrivente is also a member of this group. The broad range of TRPV1 modulators allows for the development of new analgesics which can act as either TRPV1 agonists or antagonists (Created with BioRender.com)
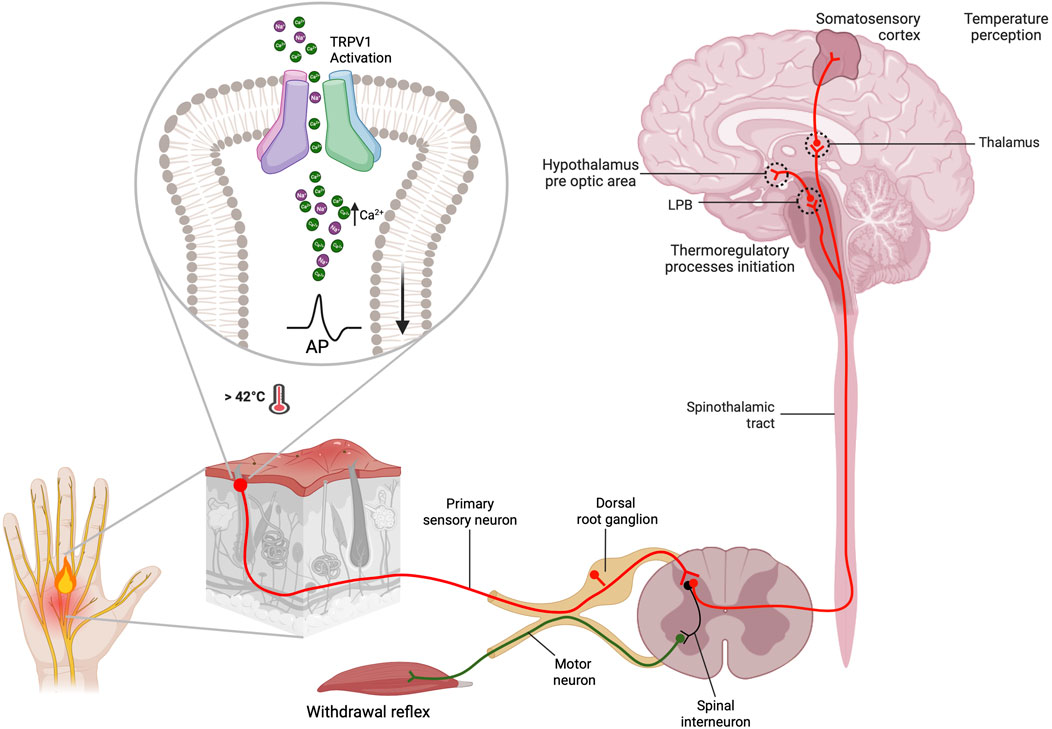
FIGURE 2. Thermosensation and thermoregulation processes occur from the periphery to the central nervous system. TRPV1 channels are mainly located in the primary afferent neurons (C and Aδ fibers) of the somatosensory system. They function as the primary integrator of noxious stimuli, leading to thermosensation and pain perception. After being activated by a noxious stimulus, such as a temperature above 42°C, nociceptors allow Ca2+ influx. This leads to local membrane depolarization by opening voltage-dependent sodium channels, which consequently generates afferent action potentials. These action potentials allow information to propagate to the central nervous system. The action potentials generated in the periphery travel to the dorsal horn. There, they form two types of synapses: one with motoneurons via spinal interneurons to give rise to the withdrawal reflex, and another with sensory neurons of the spinothalamic tract. The information transmitted at this synapse gives rise to two important processes: thermosensation and thermoregulation. The perception of temperature originates in the primary somatosensory cortex after the information is transmitted from the thalamus through thalamocortical radiations, where. Additionally, the information is transmitted to the hypothalamus, specifically to the preoptic area (POAI, via lateral parabrachial neurons (LPB), to initiate thermoregulatory processes (Created with BioRender.com)
The sustained exposure of TRPV1 to agonists, such as capsaicin, causes its desensitization, stopping its function, which produces analgesia, thus the search for capsaicin analogs for pain relief has been the focus of intense biomedical research. The associated mechanisms for TRPV1 desensitization appear to occur by pore closure, or by tachyphylaxis (Touska et al., 2011; Luo et al., 2019; Tian et al., 2019). In the former a reduction in pore diameter prevents ion permeation through the channel, whereas in the latter, channel internalization occurs with recycling to the plasma membrane with a temporal recovery depending on stimulus intensity. It has been suggested that long-term desensitization of TRPV1 may involve its removal from the plasma membrane by a clathrin-independent endocytic mechanism (Sanz-Salvador et al., 2012). The discovery of the ion channels acting as temperature receptors, and touch receptors, ion channels leads to David Julius and Ardem Patapoutian being awarded the Nobel Prize in Physiology or Medicine in 2021 (Latorre and Díaz-Franulic, 2022).
TRPV1 channels are expressed in excitable and non-excitable cells and tissues, where is associated with several physiological and pathophysiological conditions, including nociception and pain. The expression of TRPV1 is found throughout the peripheral nervous system and is predominant in small myelinated (Aδ) and unmyelinated (C) fibers from sensory ganglia of the dorsal root ganglion (DRG) in the spinal cord, the trigeminal ganglia, and nodose ganglia neurons (Caterina et al., 1997; Hwang and Valtschanoff, 2003). A role in memory and learning in the CNS has also been proposed. Although there is no certainty about the precise location of TRPV1 at central synapses, it is known that the receptor can modulate both neurotransmitter release at presynaptic terminals, as well as the synaptic efficacy in postsynaptic compartments (Matta et al., 2010; Meza et al., 2022). In fact, it has been observed that TRPV1 activity suppresses the excitatory transmission in the dentate gyrus of the hippocampus, and that its synaptic activation induces a form of long-term depression, mediated by endogenous anandamide (Chávez et al., 2010). TRPV1 is also expressed in corneal afferent neurons and in the cortical areas, and in the lamina I and II of the dorsal horn of the spinal cord (Fernandes et al., 2012; Mickle et al., 2015; Morales-Lázaro and Rosenbaum, 2015). Its subcellular expression has been also been found at dendritic spines and synaptic vesicles (Ho et al., 2012), as well as in various neurons of the brain, including all cortical areas, hippocampus, and dentate gyrus, fimbrial, dorsal and lateral septal nuclei, central amygdala, medial and lateral habenula, mampillary and interpeduncular nuclei, stria terminalis, suprachiasmatic nucleus, inferior olive, and others (Mezey et al., 2000), human sympathetic ganglia (Kokubun et al., 2015), smooth muscle cells (Kark et al., 2008), endothelial cells (Golech et al., 2004), liver (Li et al., 2012), colon (Matsumoto et al., 2009), pancreas (Akiba et al., 2004), lung (Johansen et al., 2006), bladder and male genital tract (Stein et al., 2004), uterus (Contassot et al., 2004), stomach (Faussone-Pellegrini et al., 2005), cornea (Okada et al., 2011), spleen (Bertin et al., 2014) and kidney (Cortright et al., 2001).
Pain perception is a natural physiological warning mechanism that enables individuals to prevent harm and safeguard the tissue that has experienced trauma. Prolonged pain beyond the anticipated healing period may lead to an impaired state referred to as chronic pain, which affects a multitude of individuals globally. This condition can inflict long-term and incapacitating consequences, resulting in substantial disability and imposing significant health and socioeconomic burden.
Chronic pain is characterized by spontaneous pain such as burning or aching sensations, as well as pain that is induced by noxious (hyperalgesia) or non-noxious (allodynia) stimuli. The plasticity of neurons in pain pathways and circuits consisting of primary sensory neurons within the dorsal root ganglion (DRG) and trigeminal ganglia (leading to peripheral sensitization), as well as in pain-processing neurons located in the spinal cord and brain (resulting in central sensitization), may contribute to the development of chronic pain (Ji et al., 2003; Latremoliere and Woolf, 2009).
If well we are focused on TRPV1 channel in this contribution, it is important to note that peripheral sensitization, which is characterized by hypersensitivity and hyperexcitability of nociceptors due to tissue injury and inflammation, can be triggered by a diverse array of ion channels. These include thermoTRPs, sodium channels such a Nav1.7, Nav1.8, and Nav1.9 (Waxman et al., 1999; Amaya et al., 2000), and mechanosensitive piezo ion channels (Eijkelkamp et al., 2013).
1.2 TRPV1 channel sensitization
TRPV1 integrates of exogenous and endogenous signals, including pro-inflammatory mediators. A a polymodal receptor, TRPV1 senses multiple physical and chemical signals. Such sensations include burning, thermal pain, irritants, toxins derived from plants and animals (e.g., vanillotoxins, capsaicin, resiniferatoxin, and double-knot toxin). TRPV1 serves as a major receptor for painful stimuli from molecules created through metabolic activity or by receptors internally. This occurs during inflammation, nerve damage, chemotherapy, diabetes, or other pain-producing disorders, specifically via ligands such as lipoxygenase, endocannabinoids, and derivatives of arachidonic acid, linoleic acid, and lysophosphatidic acid. Many of the signals can lead to the sensitization of TRPV1 channels, which results in an increased response to low-intensity stimuli due to the channels’ greater plasma membrane availability and phosphorylation. Different signaling cascades such as bradykinin, prostanoids, nerve growth factor (NGF), and ATP can initiate this sensitization process. Furthermore, TRPV1 gating can be enhanced by protons, heat, and depolarization. Therefore, comprehending the regulatory mechanisms behind TRPV1 activation is vital for advancing new objectives for pain management and other medical treatments.
Injury or inflammation releases inflammatory mediators that lower the pain threshold. At least three pathways sensitize the TRPV1 channel, including cellular pathways related to G protein-coupled receptors (GPCRs) and several protein kinases such as PKC, PKA, CaMKII and Src that phosphorylate the channel (Figure 3). This produces a deep sensitization that triggers nociception, hyperalgesia and allodynia (Jung et al., 2004; Rosenbaum and Simon, 2007; Studer and McNaughton, 2010). The Gq-coupled B1 and B2 receptors activation by bradykinin decreases the threshold for temperature activation by promoting the PKCε (Cesare and Mcnaughton, 1996; Cesare et al., 1999; Numazaki et al., 2002; Bhave et al., 2003) phosphorylation and an increase in channel open probability under several activators. The metabolism of PIP2 by PLCβ could potentially impact the channel sensitization; however, this is a contentious issue because some reports suggest that PIP2 acts as a channel inhibitor (Chuang et al., 2001), while others indicate that it works as a channel activator (Stein et al., 2006; Lishko et al., 2007; Poblete et al., 2015). On the other hand, in sensory neurons, PGE2 activates the Gs coupled EP4 receptor leading to sensitization by triggering the phosphorylation of TRPV1 by PKA (Bhave et al., 2002; Distler et al., 2003). NGF sensitizes TRPV1 through PI3 kinase and the tyrosine kinase Src. This result in the phosphorylation of the channel and enhances its trafficking to the plasma membrane (Zhang et al., 2005; Stein et al., 2006).
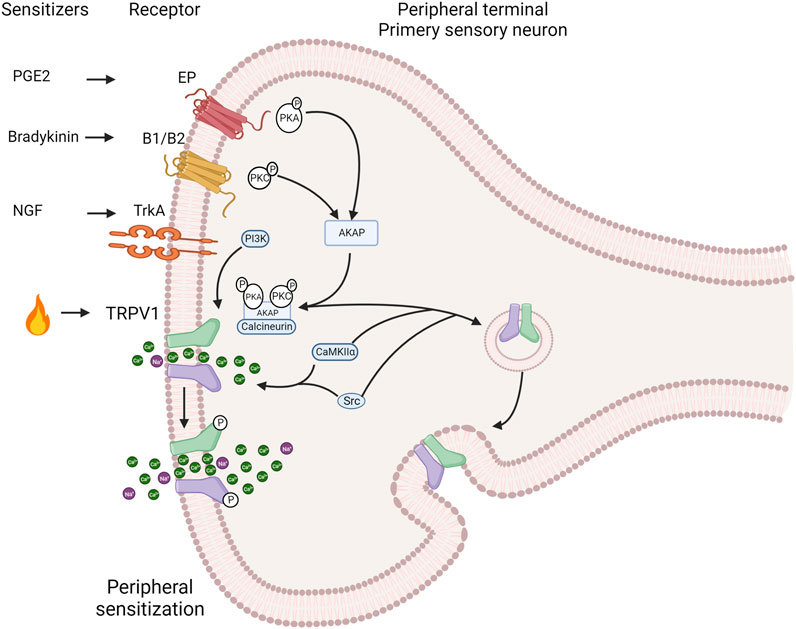
FIGURE 3. Induction of the TRPV1 channel deep sensitization by exogenous and endogenous signals. The activation of intracellular pathways related to G protein-coupled receptors (GPCRs) and protein kinases such as protein kinase C PKC), protein kinase A (PKA), calcium/calmdulin-dependent protein kinase II (CaMKII) and Src directly affects TRPV1 channel sensitization. During the inflammatory process nerve growth factor (NGF) is secreted, which binds to tropomyosin receptor kinase A (TrkA), leading to receptor dimerization and autophosphorylation. This, in turn, sensitizes TRPV1 through phosphorylation by phosphatidylinositol 3-kinase (PI3K) and the tyrosine kinase Src. Bradykinin activates B1 and B2 Gq-coupled receptors. This activation promotes PKCe phosphorylation and subsequent TRPV1 phosphorylation, resulting in channel sensitization. Similarly, PGE2 activates the Gs-coupled EP4 receptor, leading to sensitization through phosphorylation of TRPV1 by PKA. The scaffold protein AKAP 79/150 facilitates specific sensitization by phosphorylation by bringing macromolecular complexes, including PKA, PKC, and calcineurin, close to TRPV1. AKAP 79/150 bindsto the C-terminal region, specifically at residues D738, R740, C742 and V745. Inhibition of TRPV1 sensitization occurs when AKAP79/150-TRPV1 binding is disrupted. AKAP79/150 is involved in TRPV1 trafficking to the plasma membrane via PKC. The major site involved in trafficking control is S502. The tyrosine kinase Src can promote membrane trafficking by phosphorylating Y200, but does so independently of AKAP79/150 (Created with BioRender.com).
The specificity and speed of channel sensitization via phosphorylation is facilitated by scaffolding proteins such as AKAP (A-kinase anchoring protein) that promote the encounter between kinases and the channel. AKAP 79/150 is indispensable in bringing macromolecular complexes, including PKA, PKC and calcineurin, near TRPV1 for their modulatory influence. In rodents (Zhang et al., 2008a; Brandao et al., 2012), AKAP79 and TRPV1 are co-expressed in small nociceptive sensory neurons. The sensitization of heat pain modulation, also referred as hyperalgesia, depends on the formation of a macromolecular signaling complex including the scaffold protein AKAP 79/150, TRPV1 and the enzymes PKC, PKA, and calcineurin enzymes (Zhang et al., 2008b; Jeske et al., 2009). AKAP79/150 binds to the C-terminal region of TRPV1, which facilitates channel phosphorylation and sensitization by bradykinin and PGE2. In vitro disruption of AKAP79/150 binding to TRPV1 or its binding mechanisms abolished TRPV1 sensitization, and a cell-permeant peptide that impedes the interaction of both proteins abolishes inflammatory hyperalgesia in vivo (Fischer et al., 2013). This anchoring protein is involved in trafficking TRPV1 to the plasma membrane through promotion of phosphorylation either by PKC and Src kinase (Morenilla-Palao et al., 2004; Zhang et al., 2005). Src has independent effects on TRPV1, compared to PKC or PKA (Numazaki et al., 2002; Bhave et al., 2003), and key residues in the C-terminal domain of TRPV1 identified for AKAP binding correspond to D738, R740, C742 and V745 (Fischer et al., 2013).
AKAP is capable to organizing various kinases and phosphatases into macromolecular complexes with their respective targets (Leiser et al., 1986; Bregman et al., 1989; Smith et al., 2006), such as PKA, PKC, and calcineurin (Coghlan et al., 1995; Klauck et al., 1996). Inhibiting pain sensitization through endogenous signaling presents a significant challenge due to complex macromolecular interactions of protein kinases and phosphatases, which regulate the phosphorylation status of the TRPV1 channel, as well as other ion channels like potassium and calcium channels and glutamate receptors. These protein complexes are also involved in various cellular functions and transduction pathways (Gao et al., 1997; Colledge et al., 2000; Altierab et al., 2002; Hoshi et al., 2003; Hoshi et al., 2005; Sandoz et al., 2006; Chai et al., 2007; Lu et al., 2007; Oliveria et al., 2007; Navedo et al., 2008; Fabbro et al., 2015), and the cessation of their activity could result in unintended consequences.
Heat hyperalgesia, a condition where injury or inflammation is lowers the threshold of heat-induced pain, is relieved in the absence of TRPV1 (Caterina et al., 2000; Davis et al., 2000). In rodent models the disruption of interaction between TRPV1 and AKAP 79/150 eradicates the inflammatory hyperalgesia (Fischer et al., 2013).
It has been reported that the activity of TRPV1 and Nav1.8 increases when phosphorylated by p38 MAP kinase. This results in peripheral sensitization when exposed to TNF and IL-1β in DRG neurons (Ji et al., 2002; Obata et al., 2004; Binshtok et al., 2008; Constantin et al., 2008; Matsuda et al., 2017). The elevated expression of TRPV1 prolongs the state of peripheral sensitization causing the transition from acute to chronic pain (Ji et al., 2002; Amaya et al., 2003, 2004). Additionally, the activation of p38 MAP kinase in C- and Aδ-fibers present in DRG neurons contributes to hypersensitivity to pain (Mizukoshi et al., 2013).
Phosphorylation has two distinct effects of on TRPV1 in whole-cell recordings: first it lowers the thermal threshold needed to activate TRPV1 and, second, it enhances the sensitivity of TRPV1 to other activating factors such as capsaicin, protons, and anandamide (Vellani et al., 2001). In addition, the TRPV1 channel is highly trafficked to the surface membrane following phosphorylation (Morenilla-Palao et al., 2004; Zhang et al., 2005), which plays a role in the development of heat hyperalgesia.
TRPV1 single-channel activity is significantly enhanced by PKC activation, in response to capsaicin. TRPV1 phosphorylation does not alter single-channel conductance and the increase in current that flowed through a single channel could be attributed to a higher probability to find the channel in the open state. The effect on the probability of the channel opening resembled that produced by an increase in capsaicin concentration (Studer and McNaughton, 2010), that is consistent with the observation that PKC activation produces an approximately 2-fold displacement in the capsaicin-induced TRPV1 dose-response curve in the whole-cell measurements (Vellani et al., 2001). PKC activation has two main effects on TRPV1. It shortens the time constant, decreases the occupancy of a closed state, and increases the occupancy of an open state. These changes ocurr with no significant difference in the time constant activation. PKC phosphorylates serine residues at positions 502 and 801 at C-terminus region. The first one is situated in a loop between transmembrane segments 2 and 3, which is close to the binding site for capsaicin, while the latter is in the C-terminal region. Phosphorylation boosts TRPV1 activity, by capsaicin and protons, whose binding sites are situated intracellularly and extracellularly, respectively (Jung et al., 1999; Jordt et al., 2000; Vellani et al., 2001; Jordt and Julius, 2002; Ryu et al., 2003). Phosphorylation of TRPV1 also enhances heat activation, but the location of the sensor for thermal stimulus is unknown.
Several neuropeptides are released from primary sensory nerve terminals into the peripheral terminals when C- or Aδ-fibers dorsal root ganglion (DRG) neurons are activated (Holzer and Maggi, 1998). These mediators directly or indirectly act on TRPV1, leading to nociceptors, immunocytes and endothelial cells activation and sensitization, strongly suggesting a significant of cross-talk between the nervous and immune systems. Indeed, these peptide mediators act on nociceptors, mast cells, immune cells, and vascular smooth muscle cells triggering inflammatory signaling cascades, edema and pain (Richardson and Vasko, 2002). This occurs because ATP and acidosis harm cells during injury. This, along with cytokines generated by immune cells, activate and sensitize sensory neurons, a condition known as neurogenic inflammation (Richardson and Vasko, 2002). Neurogenic inflammation is initiated by nerve stimulation, resulting in the release of neuropeptides and rapid plasma extravasation and edema, contributing to various pain conditions such as migraines, irritable bowel syndrome, vulvodynia, fibromyalgia, multiple sclerosis, painful bladder syndromes, arthrosis, airway inflammation conditions, and others (Richardson and Vasko, 2002; Birklein and Schmelz, 2008; Chiu et al., 2012). Neurogenic inflammation has been identified as prominently associated with inflammatory ailments such as asthma and psoriasis (Ji et al., 2018) in various clinical conditions. Even though eliminating nociceptors reduces neurogenic inflammation, it can have adverse effects because they can provide a favorable modulatory role to regulate inflammation in bacterial infections (Chiu et al., 2013; Chiu, 2018). Neurogenic inflammation is not solely provoked by the activation of peripheral C-fibers; it can also be initiated by local inflammation events or even through the activation of CNS primary afferents (Xanthos and Sandkühler, 2013; Ji et al., 2018). In fact, the CNS can experience neurogenic inflammation in response to neuroinflammatory events in the brain or spinal cord.
Neuroinflammation involves the activation of glial cells in dorsal root ganglia, spinal cord, and brain which leads to the production of proinflammatory cytokines and chemokines that drives peripheral and central sensitization (Ransohoff and Brown, 2012). On the other hand, neuroinflammation is a form of localized inflammation that can manifest in both the peripheral and CNS (Ji et al., 2014). It is characterized by heightened vascular permeability, infiltration of leukocytes, activation of glial cell, and exaggerated production of inflammatory mediators like cytokines and chemokines (Ji et al., 2014). The elevated permeability of the blood-brain barrier permits the infiltration of peripheral immune cells into the CNS. Neuroinflammation is linked chronic pain conditions, including postsurgical pain following major procedures such as amputation, thoracotomy, and mastectomy, as well as postoperative complications like delirium (Ji et al., 2018). Chronic pain persists beyond the resolution of clinical signs and inflammation symptoms, and neuroinflammation is closely associated with chronic pain states, potentially mediating and prolonging pain in human patients (Shi et al., 2012). Investigating the effects of various neuroinflammatory mediators on pain sensitivity in the pain neurocircuitry presents an intriguing research opportunity.
2 The molecular structure of TRPV1 channel
2.1 The TRPV1 channel: a close view into its architecture, agonist, and antagonist binding sites
The structure of rat TRPV1 obtained by single-particle cryogenic electron microscopy (cryo-EM) (Liao et al., 2013), confirmed the homotetrameric arrangement of the channel, in which each subunit consists of a protein containing 838 amino acids, composed of six transmembrane segments, intracellular ankyrin repeat domains ARD), and the amino- and carboxy-terminal domains, both facing the cytoplasm (Figure 4). Currently, the Protein Data Bank (PDB), contains 51 TRPV1 channel structures in different conformations at different temperatures and with different ligands, which have contributed to the understanding of channel gating since 2013 (Cao et al., 2013a; Liao et al., 2013; Gao et al., 2016; Kwon et al., 2021; Zhang et al., 2021; Kwon et al., 2022; Neuberger et al., 2023).
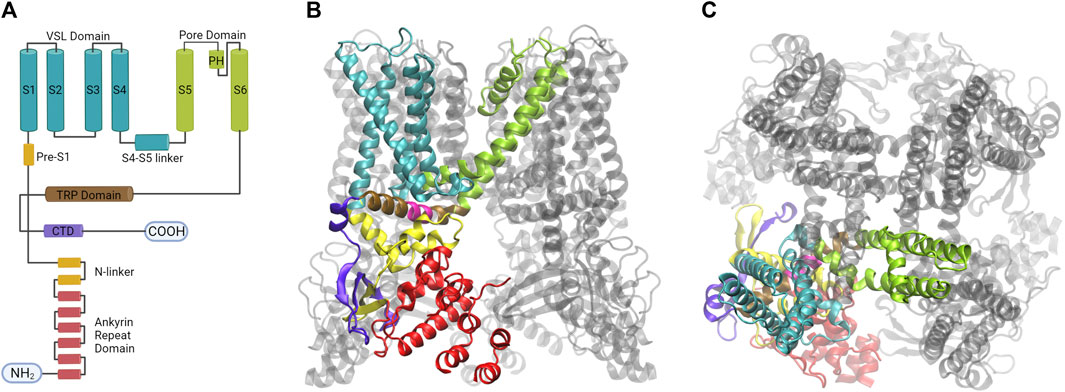
FIGURE 4. The homotetramer of the TRPV1 channel is organized by a quadruple-symmetric architecture. (A) Diagram illustrating the topology of the major structural domains found in a subunit of the TRPV1 channel. Each domain is color-coded: the voltage sensing like domain (cyan), the pore domain (lime), the TRP domain (brown), the ankyrin repeat domains (red), and the CTD domain (violet). These colors correspond to the colors of the ribbon diagrams shown to the right. (B) The image displays the tetrameric structure of the TRPV1 channel with one subunit highlighted from a lateral view (C) The architecture of the fourfold symmetry Is shown from a top view, revealing the interaction between S1- S4 and PD in a domain-swapped arrangement (Created with BioRender.com, Protein Data Bank: 7LPD).
Segments S1 to S6 form the transmembrane domain (TMD), the TMD and ARD are connected by the coupling domain (CD), which includes a helix-loop-helix motif (HLHCD), a β-sheet (βCD), the pre-S1 helix (pre-S1CD), and a C-terminal domain (CTD) (Kwon et al., 2021). The N-terminal segment connects the sixth ankyrin repeat domain (ARD) to S1. The S1-S4 segments are considered to be the voltage sensor-like domain (VSLD), but the molecular nature of the voltage sensor has not been demonstrated, whereas the S5-S6 segments form the pore domain (PD). The pore helix acts as a pore radius regulator in the selectivity filter. In addition, the S1-S4 and PD interact with each other in a domain-swapped arrangement (Myers et al., 2008; Gao et al., 2016). The TRPV1 permeation pathway has two constrictions, one located at position G643 in the selectivity filter and the other located at residue I679 in the lower part of the S6 (Jara-Oseguera et al., 2019). The selectivity filter (SF) is located in the upper part of the pore between residues 643–646 and is composed of the residues glycine (G), methionine (M), glycine (G) and aspartate (D) (GMGD) whose backbone carbonyls or side chains point into the central pathway. The upper restriction does not act as a gate with defined open and closed states, but as a highly dynamic filter that allows permeation of small and large cations while contributing to the modulation of the lower gate (Jara-Oseguera et al., 2019; Zhang et al., 2021). In the C-terminal domain, the TRP box domain is oriented almost parallel to the membrane plane and is located below S6 and interacts with the S4–S5 linker, which facilitates coupling between different channel domains (Cao et al., 2013b; Liao et al., 2013) (Figure 5).
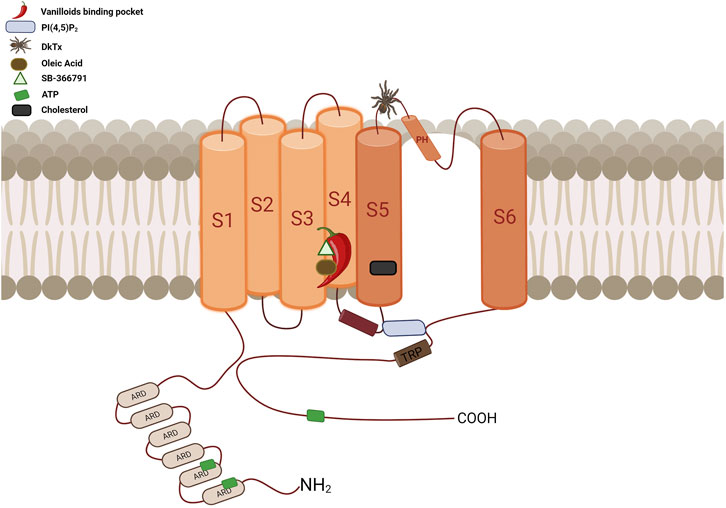
FIGURE 5. Diagrammatic overview of the TRPV1 channel’s topological arrangement and key binding sites location. The vanilloid binding site, represented by a chili pepper, is located between segments S3, S4, the S4-S5 linker, and the PD of the adjacent subunit. This site accommodates a variety of ligands such as CAP, RTX, CPZ, OA, PI, among others, all of which interact with at least one of these residues: Y512, S513, E571, T550, T551, and R557. The cholesterol interaction site is located between residues 579 to 586 at S5, specifically at the CRAC motif. ATP has three interaction sites: ANKR1, ANKR2 and the distal C-terminus. The binding site for PI(4,5)P2 is located between S3 and the S4-S5 linker, above the TRP domain. It interacts with residues R557 in the S4 segment, E570, R575, and R579 in the S4-S5 linker, and residue K694 of the TRP domain of an adjacent subunit. The DkTx site, represented by a spider, binds to the channel on the extracellular side, specifically in the outer pore region. It contacts residues at the top of the pore helix from one subunit and the outer pore loop of the adjacent subunit, interacting with residues I599, F649, A657, and F659 (Created with BioRender.com).
2.2 The vanilloid binding pocket: a heterogeneous binding site in the transmembrane domain
The vanilloid pocket, located in the TMD and facing the cytoplasmic leaflet of the membrane, consists of segments S3, S4 and the S4-S5 linker of one subunit and the pore domain (S5-S6) of the adjacent subunit. It owes its name to the favorable accommodation of compounds possessing the vanillyl group, such as capsaicin, a classical activator of the TRPV1 channel, and resiniferatoxin (RTX), the most potent activator described to date, both compounds of natural origin (Cao et al., 2013a). These vanilloid compounds appear to interact directly with the S4–S5 linker pulling it away from the central pore and consequently facilitating the opening of the lower gate (Gao et al., 2016). Indeed, the distance between the side chains is 5.3 Å in the unliganded state, whereas upon vanilloid binding, I679 rotates away from the central axis causing an expansion to 7.6 Å (Liao et al., 2013). Capsazepine, a synthetic vanilloid derived from capsaicin (Bevan and Geppetti, 1992), binds to this site and acts as a competitive inhibitor. In addition, several structures obtained by cryo-EM have shown that in the apo state the vanilloid pocket is occupied by a resident lipid, although the exact identity of the lipid has not been determined, which could be phosphatidylcholine, PI, PI(4,5)P2, and the displacement of these lipid molecules could lead to channel activation (Gao et al., 2016; Zhang et al., 2021; Neuberger et al., 2023). Although these ligands differ in structure and function, binding to the vanilloid pocket is favored by the caveolae spacing of the site and the rearrangement of the side chains of some key constituent residues, one of the most important being T511 (in the rat channel).
2.3 Capsaicin and RTX
In the vanilloid binding pocket, capsaicin adopts a “tail-up, head-down” configuration, where the tail interacts with the channel through Van der Waals forces (VDW) that contribute to binding affinity, while the interaction between the head and the channel is given by the hydrogen bonds between its vanillyl structure and residues Y512, S513, E571, T550, T551, and R557 within the TRPV1 channel (Yang et al., 2015a; Gao et al., 2016). After capsaicin binding, structural rearrangements occur to stabilize the S4-S5 linker in its outward (activated) conformation by “pulling” on E571 through hydrogen bonding and VDW contact with the linker. As the S4-S5 linker moves outward, S6 follows to open the activation gate. (Yang et al., 2015a). Φ-analysis has revealed that upon capsaicin binding, a conformational wave is initiated from the vanilloid binding pocket, propagates through the S4-S5 linker into the S6 bundle, and finally reaches the selectivity filter region, which opens the pore (Yang et al., 2018).
RTX is stabilized in the pocket by hydrophobic interactions between the diterpene ring of RTX and several residues including L515, V518 in S3 segment, M547 in S4, I573 in the S4-S5 linker and the L669 in S6 segment from the adjacent subunit. These interactions may be the reason for the high affinity binding of RTX. Also, RTX binding facilitates the interaction between R557 and E570, this interaction takes place in the space previously occupied by that of the PIP2 head group, thus moving the S4-S5 linker away from the central shaft, facilitating the opening of the lower gate (Gao et al., 2016; Zhang et al., 2021). As in the case of capsaicin binding, it has been proposed that upon RTX binding, TRPV1 undergoes a wave-like conformational propagation initiated in the vanilloid-binding pocket, first by opening of the S6 gate, then by opening of the SF, followed by reorganization of the PL and external pore (with further opening of the S6 gate and SF). This conformational wave is apparently due to additive and concerted conformational changes in many subdomains (Kwon et al., 2022). A recent study shows that RTX enhances structural fluctuations of TRPV1, but it has not been determined whether structural fluctuations are involved in channel gating (Sumino et al., 2023).
2.4 Lipids
Lipids play a crucial role in regulating TRPV1 activity. Specifically, phospholipids, fats, and steroids can modulateTRPV1 activity as agonists or antagonists (Rohacs, 2014; Zhai et al., 2020; Zhang et al., 2020; Manchanda et al., 2021; Rosenbaum and Islas, 2023).
It has been postulated that the activation of TRPV1 by annular lipids might facilitate allosteric communication between the vanilloid site and the peripheral cavities. The opening of TRPV1 necessitates the rotation of a conserved residue on the S6 segment from the S4-S5 linker towards the pore. This motion is linked to dehydration of the peripheral cavities between S6 and the S4-S5 linker. Hydration studies indicate that vanilloids and spider venom toxins bind to cause the ring lipids to adopt a distinctive “buried” conformation, which projects their hydrophobic tails into the peripheral cavities, encouraging dehydration and in consequence the opening of the channel (Gianti et al., 2019). This mechanism, which entails alterations in hydration, can explicate the lodging of molecules that serve as agonists or antagonists of the channel in the same pocket. These molecules may have varying associations with the pore, ultimately impacting the gating of the channel.
Phosphatidylinositol (PI), a resident lipid found within TRPV1, may have a significant influence on the allosteric activation of TRPV1 by vanilloid compounds and analogs. Research indicates that PI and capsaicin or resiniferatoxin all ocupy the same inter-subunit binding pocket in TRPV1, located between the voltage sensor-like domain and the pore domain. Capsaicin binding in the vanilloid pocket is potentially reliant on lipids through a sequential cooperativity mechanism.
In silico analysis suggests an initial, transient binding site with low affinity collaborates sequentially with a subsequent, dominant binding site with strong affinity. This cooperation may enable the release of the enclosed resident lipid (i.e., PI) for allosteric activation of TRPV1 by vanilloids or analogs through non-covalent interactions (Wang, 2021). High-resolution structures obtained through cryo-EM reveal a continued competition occurring within the vanilloid binding pocket (VBP) between vanilloid agonists and the resident regulatory PI lipid, utilizing a two-step process. Vanilloid agonists displace the regulatory PI lipid and compete for the aliphatic tail of PI. This is followed by the displacement of the inositol head group. It is worth noting that for the agonist to induce sufficient movement of S5, which is necessary for S6 movement and subsequently opening the lower gate of TRPV1, it must fully displace endogenous PI lipids in all subunits (Zhang et al., 2021). It has been hypothesized that heat destabilizes a hydrophobic cluster in the C-terminal domain, facilitating interactions between residues R739 and R743, and the corresponding adjacent linker domains. This leads to the breaking of hydrogen bonds between the linker domain and S2-S3 linker, which results in the formation of the vanilloid pocket. Then, the lipid in the VBP is displaced, and a new hydrogen bond is created between S4 and S5. This leads to S6 moving away from the ion channel, ultimately opening up the conduction pathway (Melnick and Kaviany, 2018).
The nature of TRPV1 interactions with membrane phosphoinositide molecules and whether these interactions facilitate or inhibit channel opening are controversial issues (Gunthorpe et al., 2004; Cao et al., 2013b; Gao et al., 2016; Zhang et al., 2021; Neuberger et al., 2023).
Several TRPV1 structures show a low density in the vanilloid binding pocket, identified as membrane phosphoinositide (PI) lipids. An allosteric mechanism has been proposed in which PI is released or displaced from the vanilloid binding pocket upon arrival of agonist at the site (Gunthorpe et al., 2004; Cao et al., 2013a; Gao et al., 2016; Zhang et al., 2021).
As mentioned above, it has been suggested that the TRPV1 channel may be negatively regulated by PI lipids as they inhibit TRPV1 by reducing sensitivity to chemical and thermal stimuli to prevent uncontrolled activation of TRPV1 in resting cells (Cao et al., 2013b). In the binding site where resident PI was identified, its branched acyl chains extend upward between S4 of one subunit and between S5 and S6 of an adjacent subunit, within a cleft that faces the interior of the membrane. The inositol ring is bounded on either side by S3 and the S4-S5 linker elbow, with the TRP domain below. The amino acid residue R557 (S4) can form polar interactions between the phosphate hydroxyl group at position 1 and E570 (S4-S5 linker) and a hydroxyl group at position 6 of the inositol ring; these interactions increase the stability of the PI at this site (Gao et al., 2016).
For PI(4,5)P2, a binding pocket has been proposed where the inositol ring is located between the S3 segment and the S4-S5 linker and above the TRP domain, where the presence of PI(4,5)P2 activates the channel (Poblete et al., 2015). The phosphate groups of PI(4,5)P2 interact with residues R557 in the S4 segment, E570, R575 and R579 in the S4-S5 linker, and residue K694 of the TRP domain of an adjacent subunit. Residue R701 may help stabilize the binding pocket once formed (Poblete et al., 2015). Several configurations of PI(4,5)P2 are in the vanilloid pocket and partially or fully displaced from it, suggesting that vanilloid binding displaces PIP2 from the pocket. Displacement of PI(4,5)P2 from the vanilloid pocket is essential for RTX-mediated activation, because if it is not fully displaced, the RTX-induced conformational change of S5 cannot be transmitted via the TRP helix to the lower gate in the S6 segment for TRPV1 channel opening (Zhang et al., 2021; Neuberger et al., 2023). It has been proposed that PI(4,5)P2 activates the channel through direct interaction with the proximal C-terminus (Ufret-Vincenty et al., 2011), and experiments with chimeras between TRPM8 and TRPV1 show that in both cases key residues in the TRP domain are involved in determining the apparent PI(4,5)P2 affinity of the channel (Brauchi et al., 2007).
2.5 Oleic acid (OA)
Oleic acid is a fatty acid found naturally in animals and vegetables and belongs to the omega-9 fatty acids. OA has been shown to be an allosteric inhibitor of the TRPV1 channel, and consistent with this, electrophysiological recordings showed that incubation of cleaved patches in OA 5 µM together with capsaicin 4 µM showed an 85% reduction in macroscopic current amplitude compared to those obtained in the presence of capsaicin 4 µM alone. In addition, 5 µM OA inhibited the current generated by noxious heat by 72% and by endogenous TRPV1 activators such as lysophosphatidic acid (LPA) by 95%, cPA by 79% and protons by 98%. Using competition assays, the researchers found that OA may compete with capsaicin for the same binding site, the vanilloid binding pocket. Molecular docking revealed that residue T550 forms hydrogen bonds with the carbonyl group of OA, as well as the residues Y511 and S512 is also important for OA binding. Mutagenic analysis using the Y511A-S512A-T550A triple mutant produces TRPV1 channels that are only 26% inhibited compared to 85% of wild-type rat TRPV1 channels (Morales-Lázaro et al., 2016).
2.6 Capsazepine
Capsazepine (CZP) is a synthetic compound derived from capsaicin that acts as a competitive inhibitor of the TRPV1 channel (Bevan et al., 1992) by exclusively occupying the vanilloid binding site. Binding of capsazepine to the channel promotes stabilization of the closed state removing the interaction between the side chains of residues R557 and E570, thus preventing channel opening. In addition, mutagenic analysis showed that the T551V mutation affects capsazepine-mediated inhibition (Yang et al., 2015a; Gao et al., 2016). Contrary to the effect of RTX, CZP decreases the structural fluctuation of TRPV1, but as mentioned above, it has not been established whether structural fluctuation is involved in channel opening (Sumino et al., 2023).
2.7 SB-366791
In 2004, the pharmaceutical company GlaxoSmithKline identified and characterized the compound SB-366791 (N-(3-methoxyphenyl)-4-chlorocinnamide) as a potent TRPV1 channel antagonist, as it inhibits the activation induced by various agonists such as capsaicin, protons, and noxious heat (Gunthorpe et al., 2004). Recently, the structure of the human TRPV1 channel in the presence of this compound was obtained, identifying four densities, one in each channel subunit, whose binding site corresponds to the vanilloid pocket. In the binding site, SB-366791 forms a hydrogen bond with residue Y511, an essential residue for capsaicin-mediated activation, and also forms hydrophobic interactions with residues L515 in the S3 segment and L547, T550, and L553 in the S4 segment. Mutagenic analysis showed that elimination of the hydrophobic interaction with residues L515, L547, and T550 renders the channel insensitive to SB-366791, and elimination of the hydrogen bond with residue Y511 decreases sensitivity to the compound, demonstrating that the vanilloid pocket corresponds to the binding site of the antagonist SB-366791 which acts as a competitive inhibitor (Neuberger et al., 2023).
2.8 Cholesterol
Cholesterol, a major component of plasma membranes, has been described to inhibit TRPV1 channel activity by binding to a cholesterol binding motif (CRAC) located between residues 579 to 586 in S5, specifically interacting with residue L585 in rTRPV1. The L585I substitution in rTRPV1 renders the channel sensitive to cholesterol (Picazo-Juárez et al., 2011).
2.9 Extracellular and intracellular binding sites
2.9.1 DkTx
The Double Knot Toxin (DkTx), a toxin found in the venom of the Chinese Bird spider species, selectively and irreversibly activates TRPV1 channel. It is composed of 75 amino acids showing two inhibitory cysteine knot (ICK) motifs named knot1 (K1) and knot2 (K2) domains connected by a short linker (Bohlen et al., 2010; Bae et al., 2012). DkTx act as a bivalent ligand that engages the outer region of TRPV1, with each toxin moieties sitting at the subunit interfaces, contacting residues at the top of the pore helix from one subunit and the outer pore loop proximal to the S6 segment from the neighboring subunit, interacting with residues I599, F649, A657, F659 (Cao et al., 2013b). Mutations of these residues to alanine turned out TRPV1 to be DkTx insensitive (Bohlen et al., 2010). A comparative analysis of the DkTx bound and unbound structures indicates that binding of DkTx promotes changes in the VSLD of each subunit, affecting their relative arrangements. Furthermore, when DkTx is fully coupled to the channel in the open state, the VSLD is tilted and twisted, acting as a major module that allosterically couples changes in the outer pore to the lower gate. As a result, all these changes open the constrictions observed in the apo structure, both in the SF region and in the intracellular gate (Bae et al., 2016; Zhang et al., 2021).
2.9.2 ATP
ATP acts as a positive allosteric modulator that directly prevents tachyphylaxis to repeated applications of capsaicin. The binding site for ATP was found in the ARD specifically located in ankyrin repeats 1-3, where the triphosphate interacts with residues R115 in inner helix 1 and K155 and K160 in inner helix 2, while residues L163 also in inner helix 2 and Y199 in finger 2 were found to be intercalated with the adenine base. The N6 amine of adenine also interacts with Q202 in finger 2 and E210 in inner helix 3. Mutagenic analysis of residues K155A, K160A, or Y199A/Q202A showed little tachyphylaxis even in the absence of ATP (Lishko et al., 2007). In addition, mutation of K735, in the distal C- terminal, resulted in a strong inhibition of ATP binding (Grycova et al., 2007).
3 Temperature sensing
The physical fundaments of thermosensitivity in thermoTRP channels is unknown, although several theoretical frameworks have been proposed to explain this remarkable feature of these transmembrane proteins. The characterization of the activity of thermoTRP channels at different temperature ranges has been accurately determined by electrophysiology under temperature-controlled conditions.
Due to the polymodal nature of these ion channels and species-specific differences in thermal sensitivities, the search for the temperature sensor in thermoTRP channels has been challenging. Reconstitued ThermoTRP channels in artificial membrane systems, confirmed their intrinsic temperature sensitivities (Tominaga et al., 1998; Saito and Shingai, 2006; Zakharian et al., 2010; Yao et al., 2011; Sardar et al., 2012; Saito and Tominaga, 2015; Saito et al., 2016; Saito and Tominaga, 2017; Hori and Saito, 2023). The existence of a temperature sensor has implicit the presence of a domain or residues containing the structural elements for temperature detection, in analogue manner as the voltage sensor in voltage-gated K+ channels. Evidence of the existence of structurally defined modules in thermoTRP channels harboring temperature sensors comes from experiments that exchanged entire modules between channels with different thermal sensitivities (Brauchi et al., 2006; Cordero-Morales et al., 2011; Gracheva et al., 2011; Yao et al., 2011; Zhong et al., 2012).
Four members of the TRPV subclass are activated by temperature. TRPV1 is activated by temperatures above 42°C (Caterina et al., 1997, 1999; Smith et al., 2002; Watanabe et al., 2002; Xu et al., 2002; Patapoutian et al., 2003a; Moqrich et al., 2005; Voets et al., 2005; Togashi et al., 2006; Song et al., 2017; Vandewauw et al., 2018; Paricio-Montesinos et al., 2020). In fact, several publications demonstrate that TRPA1 is a noxious cold receptor (Bandell et al., 2004; Sawada et al., 2007; Karashima et al., 2009; Kremeyer et al., 2010), but others indicate that this channel is not activated by cold (Jordt et al., 2004; Bautista et al., 2006; Zurborg et al., 2007; Cordero-Morales et al., 2011) temperature (Moparthi et al., 2016).
Structural modules involved in temperature sensing are thought to be located in the N- and C-termini (Brauchi et al., 2006; Yao et al., 2011), pore turret, and pore (Grandl et al., 2010; Yang et al., 2010) of the TRPV1 channel. However, it was shown that deleting the entire pore turret of the channel (residues 604–626), it remains activated by heat (Liao et al., 2013). The thermal sensitivities of TRPV1 (heat-activated) and TRPM8 (cold-activated), are exchanged by swapping the C-terminal domains between them (Brauchi et al., 2006). In addition, a region between amino acids Q727 and W752 of TRPV1 could convert TRPM8 into a warm-activated channel (Brauchi et al., 2007). In both TRPV1 and TRPV2 channels, is thought to be that the membrane proximal domain (MPD) is a key component of the temperature sensing mechanism (Yao et al., 2011). Ground squirrels and camels exhibit high tolerance to environmental heat, enabled by the expression of TRPV1 channels with a deeply reduced temperature sensitivity (Laursen et al., 2016). This is caused by a single mutation of N190 in ground squirrels and camels, which is a serine residue in rat, in the first ankyrin repeat domain (ARD). In fact, heat sensitivity is completely restored by replacing this amino acid in the insensitive clones (Laursen et al., 2016).
Temperature dependence in chemical systems, including ion channels, can be quantified by Q10, a dimensionless factor that represents the change in reaction rate for a 10° change in temperature (Ito et al., 2015). In thermodynamics, a high value of Q10 indicates the presence of a large activation energy (i.e., large enthalpy) over a short period of time during the transition process between the closed and open states of the channels (Ito et al., 2015). In ion channels Q10 and thermodynamic state functions can be estimated from voltage-activated currents obtained at different temperatures as described before (Baez et al., 2014; Feng, 2014; Castillo et al., 2018). An increase in temperature speeds up the activation of most proteins. In ion channels that are not temperature-sensitive, the gating processes exhibit a Q10 of ∼3 (Hille, 1987). It was suggested that a thermoTRP channel must possess a Q10 ≥ 5 to be classified as a temperature-sensitive TRP channel (Voets, 2012). Accordingly, temperature changes trigger significantly high Q10 values in cold and heat receptors, which have Q10s ≥ 25 (Liu et al., 2003; Brauchi et al., 2004; Yao et al., 2010; Raddatz et al., 2014).
The temperature the channel deactivation kinetics of temperature-gated currents displays the temperature dependence in cold receptors, whilethe temperature-dependent process in heat receptors is reflected in the channel activation kinetics (Brauchi et al., 2004; Voets et al., 2004; Yao et al., 2010; Baez et al., 2014; Raddatz et al., 2014). In the case of the TRPV1 channel, the enthalpy change related to channel activation is ∼100 kcal/mol, with a corresponding Q10 of ∼50 for its activation (Yao et al., 2010). Compared to that, deactivation of TRPM8 channels results in a total enthalpy change of ∼46 kcal/mol, which corresponds to a Q10 of ∼33 (Raddatz et al., 2014). These large enthalpy changes are counteracted by significant entropy changes, enabling the closed-open equilibrium to be reversible.
Electrophysiological recordings have revealed details of the mechanisms of activation of TRPV1 by capsaicin, heat and low pH (Premkumar et al., 2002; Hui et al., 2003; Liu et al., 2003; Ryu et al., 2003). Single-channel measurements and analysis have yielded significant insights into the behavior and energetics of TRPV1 activity as temperature increases, facilitating a more comprehensive of channel functions. Since thermoTRP channel activity involves substantial entropic and enthalpic changes, the assessment of opening energy has proven essential. However, it is experimentally challenging to measure this energy when a high temperature (≥40°C) is necessary for full channel activation (Nagy and Rang, 1999; Vyklický et al., 1999; Welch et al., 2000; Liu et al., 2003; Brauchi et al., 2004; Voets et al., 2004). Single-channel analysis has revealed that the gating process based gating on temperature involves complex kinetics with several closed and open states (Liu et al., 2003). The effect of temperature is mainly noticeable in longer closures, while short closures and openings exhibit little dependence on temperature. The observed kinetic characteristics and voltage gating modeled at varying temperatures align with the notion that channel opening is triggered by temperature and that the single-channel long closures are the microscopic origin of the activation time course (Voets et al., 2004). The duration of bursts in single-channel activity is significantly temperature-dependent. Nevetherless, the open and closed components of the bursts are impervious to temperature. This implies that the apparent temperature sensitivity of the bursts is a secondary effect. It emerges from a shift in the occupancy of populations rather than fluctuations in opening and closing rates (Liu et al., 2003).
To date, at least three different mechanisms have been proposed to explain the exquisite temperature sensitivity of thermoTRP channels: i) if ΔH and ΔS are temperature independent, the balance between large changes in enthalpy and entropy drives the cold or heat sensitivity (Liu et al., 2003; Brauchi et al., 2004; Voets et al., 2004); ii) another potential mechanism is based on changes in heat capacity between the closed and open states (Clapham and Miller, 2011), which requires that a thermoTRP channel can be activated by heat and cold and exhibits an inverted bell-shaped curve (dual thermosensitivity) in the Po-temperature curve (Feng, 2014), a situation that has only been tested experimentally in hTRPA1 (Moparthi et al., 2016); and iii) allosteric thermoTRP channel models with intrinsically temperature-dependent coupling constants can also generate heat- and cold-activated channels (Jara-Oseguera and Islas, 2013).
Despite the numerous cryo-EM structures acquired for TRP channels, information regarding how temperature induces rearrangements in thermoTRP channels is lacking. As a result, the molecular foundation for the gating by temperature remains elusive.
4 Pharmacological compounds targeting the TRPV1 channel
The role of TRPV1 channels as pain receptors in physiological and pathophysiological processes represents a promising alternative for the therapeutic development of new compounds with analgesic functions. Although narcotics (opioids) have been used effectively as analgesics for several decades, their use is associated with addiction and brain damage, which creates several work and social problems (Hser et al., 2015). The identification of the TRPV1 channel as a pain receptor has led to intense biomedical research over the last 20 years in the search for new TRPV1 receptor agonists and antagonists to be used as analgesics without the undesirable side effects of opioids. Currently, a variety of exogenous ligands have been developed and characterized for targeting TRPV1 (Table 1). This include various natural compounds, synthesized molecules, and toxins derived animal, fungal, plant, and bacterial venoms. In particular, a classic agonist of TRPV1 is capsaicin, whose therapeutic effect has led to the development of drugs for pain relief. The prolonged exposure of TRPV1 channel to capsaicin activates TRPV1 channel and then produce channel desensitization, by Ca2+−dependent mechanisms, which reduce the channel activity, producing the analgesic effect. This has led to the development of the capsaicin transdermal patch (8%), indicated for moderate musculoskeletal and peripheral neuropathic pain, which is applied directly to the side of the pain. However, when TRPV1 channels are sensitized in inflammatory pain conditions the use of agonists is limited due to the side effects caused by activation of the channel, such as increased purgency and neurotoxic effects. In this context, the use of antagonists in the development of pain medications has been considered to have greater therapeutic advantages than TRPV1 agonists (Caterina, 2008; Anand and Bley, 2011).
The effects of capsaicin on the activation of TRPV1 can be potentiated by acidic pH (Caterina et al., 1997), consistent with the synergism of protons and the heat receptor in the excitation of pain pathways (Bevan and Geppetti, 1994). Subcutaneous, oral, and central administration of TRPV1 agonists induces hypothermia (Jancsó-Gábor et al., 1970; Hori, 1984; Szelényi et al., 2004; Gavva et al., 2007a; Yoshida et al., 2016; Inagaki et al., 2019), suggesting a role in thermal homeostasis. Administration of agonists of capsaicin rinvanil induces hypothermia (Jancsó-Gábor et al., 1970; Muzzi et al., 2012), whereas administration antagonist blocks agonists-induced hypothermia and also causes an increase in temperature (hyperthermia) (Gavva et al., 2007b; Gavva, 2008). Consistently, the TRPV1-deficient mouse confirmed its involvement in painful thermal sensation and hyperalgesia (Caterina et al., 1997). In addition, TRPV1 KO mice undergo hyperthermia and heat loss when exposed to warm, suggesting that central expression of TRPV1 is relevant in thermosensation and thermoregulation (Yonghak et al., 2020).
Therefore, the search for specific TRPV1 channel antagonists has been a major focus of research in recent years. However, the use of blockers or inhibitors of TRPV1 present some concerns that emerged from clinical trials such as hyperthermia caused by the peripheral block of the channel and an increase in the threshold for temperature sensitivity, which can cause burnings (Gavva et al., 2007a; Papakosta et al., 2011; Vay et al., 2012). These has slow down the develop of TRPV1 blockers as analgesics.
5 Physiology and pathophysiology related to TRPV1
As described above, TRPV1 is predominantly distributed in the primary afferent neurons of the somatosensory system and are known to be the primary input molecular substrate leading to pain perception (Caterina et al., 1997). Although the first processes identified for TRPV1 were nociception and thermosensation, research has been conducted to elucidate the molecular mechanisms underlying other physiological and pathophysiological processes. Involved in nociceptive, inflammatory, and neuropathic pain, TRPV1s are activated by noxious stimuli (heat or chemical stimulation) and allow Ca2+ influx, allowing local depolarization by opening voltage-dependent sodium channels and generating afferent action potentials that allow information to propagate to central nervous system (Patapoutian et al., 2009b). In this context, a human genetic variant of TRPV1 has recently been identified that implicates residue K710 as a critical site in the control of TRPV1 nociception (He et al., 2023).
Activation of TRPV1 in the organ of Corti and spiral ganglion cells suggests hearing loss due to increased generation of reactive oxygen species (ROS) in the cochlea (Zheng et al., 2003; Mukherjea et al., 2008). Similarly, salicylate has been described to induce tinnitus via TRPV1 (Kizawa et al., 2010). The distribution of TRPV1 in the brain affects neuronal activity by playing a fundamental role in synaptic transmission, neurotransmitter release, and plasticity (Li et al., 2008; Maione et al., 2009). In fact, increased expression of TRPV1 channels has been found in the hippocampus in epileptic conditions, suggesting an important role in this pathophysiology (Bhaskaran and Smith, 2010; Saffarzadeh et al., 2016). TRPV1, located in the cardiovascular system, has been shown to modulate vasoconstriction, regulate blood flow, and increase systemic blood pressure (Phan et al., 2020). TRPV1 activity reduces lipid storage, which is a promising therapeutic tool for atherosclerosis, as the use of copper sulfide (CuS) nanoparticles targeting TRPV1 channels in vascular smooth muscle cells helps to attenuate fat accumulation (Gao et al., 2018). TRPV1, expressed in islet β cells and nerve fibers innervating the pancreas, promotes insulin release by increasing Ca2+ concentration, which regulates appetite and body weight (Razavi et al., 2006; Waluk et al., 2013; Jeong et al., 2018), and modulates type 1 and type 2 diabetes by participating in adiponectin and leptin signaling (Derbenev and Zsombok, 2016; Liu et al., 2023). In the bladder, TRPV1 channel expression is distributed throughout the lower urinary tract structures and plays an important role in the micturition reflex (Birder et al., 2002; Daly et al., 2007; Mistretta et al., 2014). Similarly, TRPV1 expression in the respiratory tract is involved in the cough reflex (Adcock, 2009; Lee et al., 2011). In the male reproductive system, TRPV1 has been implicated in sperm calcium homeostasis (Xiao and Chen, 2022).
TRPV1 channels have recently been found to play an important role in cancer development and tumorigenesis, in various types of cancer. Recently, it has been reported that expression of TRPV1 in gastric cancer suppresses tumor development (Gao et al., 2020). In addition, capsaicin activation in breast cancer inhibits cell growth and induces apoptosis, thereby improving prognosis (Weber et al., 2016). Similar cases have been reported in thyroid cancer (Xu et al., 2018), prostate cancer (Czifra et al., 2009), pancreatic cancer (Huang et al., 2020), skin cancer (Bode et al., 2009), and bladder cancer (Mistretta et al., 2014). The regulation of TRPV1 in metastasis has recently been suggested to play a possible inhibitory role in migration and proliferation through Ca2+- activated pathways triggered by ion flux across the channel (Li et al., 2021).
6 In silico tools for TRP channel drug discovery/repositioning
The foregoing strongly supports the search for compounds capable of regulating TRPV1 channels to treat various pathophysiological and pathological conditions. Although clinical trials are the most expensive phase of drug development, significant opportunities for time and cost savings lie in the earlier stages of discovery, also known as Computer-Aided Drug Discovery (CADD). These early stages include identifying potential drug targets, designing, and synthesizing compound libraries, conducting in vitro and in vivo experiments, and optimizing drug candidates for further evaluation. By prioritizing efficient strategies in these early stages, pharmaceutical companies can streamline the drug development process, reduce costs, and accelerate the availability of safe and effective therapies (Sadybekov and Katritch, 2023).
6.1 Leverage the wealth of 3D protein structures for targeted CADD
The exponential growth of protein data enabled by cryo-electron microscopy (cryo-EM) technology has provided remarkable insights into the 3D structures of ion channels, including the transient receptor potential (TRP) family (Fernandez-Leiro and Scheres, 2016; Nannenga and Gonen, 2019). While Cryo-EM has successfully resolved the majority of Homo sapiens TRP channels, offering detailed insights into their structural peculiarities, such as modulation by various biologically relevant ligands and the effects of different physicochemical conditions on their activity, it’ essential to note that cryo-EM data provides 3D snapshots of a channel’s conformations. In most cases, these snapshots represent a single physiologically important conformation. Recent advances in artificial intelligence, exemplified by the groundbreacking AI system AlphaFold2 (AF2) during CASP14, have transformed the field of protein structure prediction (Pahari et al., 2019; Jumper et al., 2021; Pereira et al., 2021; Marcu et al., 2022; Varadi and Velankar, 2022). The ability of AF2 to predict protein structures has profound implications for target identification and validation tasks in the CADD process, even for targets such as the TRP superfamily with limited structural information for some members or physiologically relevant conformations.
The functional relevance of membrane proteins, such as the TRP superfamily, in all domains of life lies in their ability to adopt multiple interconverting conformational states by crossing kinetic barriers (Liu and Montell, 2015; López-Romero et al., 2019). In general, these multiple states are intricately linked to the specific function of the protein and are crucial for understanding the gating mechanism (Boehr et al., 2009; Cournia et al., 2015; Stein and McHaourab, 2022). Recognizing the importance of these conformational states is particularly important in CADD. By targeting specific conformations, researchers can develop drugs that selectively modulate the activity of the protein to promote desired therapeutic outcomes. In this context, Saldaño T. et al. report that AF2 predominantly predicts the holo form in about 70% of cases, showing limitations in capturing the observed conformational diversity equally for holo and apo conformers, suggesting the potential use of predicted local model quality scores to infer ligand-binding induced conformational changes.
Nevertheless, the integration of stochastic subsampling into multiple sequence alignment within AF2 has shown potential in aiding the creation of models that aim to approximate the multiple conformations of membrane proteins (Del Alamo et al., 2022). These conformations are posited to bear resemblance to native structures, acknowledging that our current methodologies, including cryo-EM and crystallography, may not provide a definitive picture of native states, as alluded to by recent discussions in the field (Shoemaker and Ando, 2018). In addition, it has been tested that the ability of AF2 to predict multiple conformations for a given protein sequence holds potential for engineering protein and mutant induced activity (Cummins et al., 2022). Another success derived from AF2 is the recent update of the Membranome Database 3.0, which incorporates models generated by AF2 and validated with experimental information (Lomize et al., 2022).
6.2 Exploring binding sites in TRP channels with CADD
Identification of primary ligand-binding sites is crucial in CADD for targeting proteins. These sites are where ligands directly interact with enzymes, competing with substrates or cofactors to alter or change protein activity. For TRP channels, these pockets/sites are where direct modulation of channel gating occurs exemplified by the vanilloid site in TRPV1 that bind capsaicin (Yang et al., 2015b; Darré and Domene, 2015). Understanding the structural and functional characteristics of these ligand-binding sites is essential for the rational design of therapeutic molecules with improved potency, selectivity and efficacy to treat diseases and disorders associated with TRP channel dysfunction. One of the main challenges in designing in silico modulator that target these sites is to achieve higher affinity than natural ligands or control ligand, which have been finely honed by evolution for tight and specific interactions with their corresponding sites. Consequently, creating modulators that can compete with these natural ligands involves innovative strategies and deep knowledge of the interaction site (Clifton and Jackson, 2016).
The identification of allosteric sites has important implications for the development of drugs that specifically target ion channels belonging to the TRP superfamily and represents another promising avenue for drug discovery and the development of innovative therapeutic interventions, as allosteric and competitive modulators act through different mechanisms (Nussinov and Tsai, 2012; Nussinov and Tsai, 2014). Allosteric drugs alter the distribution of interchangeable conformational states, resulting in increased affinity (stronger protein-ligand interactions) and specificity (selectivity for a particular protein) for the target. Binding at these sites often leads to structural changes that can influence the binding characteristics at the primary site without direct competition (Guarnera and Berezovsky, 2019, 2020; Egbert et al., 2022; Xiao et al., 2022).
However, the shortcoming of computational protocols for predicting allosteric drugs lies in their inability to find their binding sites without prior knowledge, consequently the predictive accuracy and reliability of these methods are compromised, posing a challenge in CADD of allosteric drugs (Nussinov et al., 2014). Cryptic binding sites, on the other hand, are hidden or inaccessible pockets under normal conditions, even with conventional simulations, due to the slow motions of their components (Meller and Ward, 2023). Noteworthy, advancements have been made in developing algorithms to uncover these cryptic pockets, including using Markov state models (MSM) (Konovalov et al., 2021; Cruz et al., 2022) and enhanced sampling strategies (Comitani and Gervasio, 2018) or adaptive sampling approaches (Zimmerman and Bowman, 2015), as well as a combination of these methods such as classical Molecular Dynamics simulations (MDs), MSM, and Deep Learning (DL) with the gold of detecting cryptic pockets and identifying allosteric inhibitors in a pharmacological target related to cancer (Meller and de Oliveira, 2023). In this sense, the potential of AF2 to accelerate the discovery of cryptic pockets was investigated by generating ensembles of structures. They report that AF2 successfully samples open states in six out of ten known cryptic pocket examples. Simulations based on AF2-generated structures effectively sample cryptic pocket openings, overcoming the limitations of simulations using ligand-free experimental structures. MSM constructed from AF2-seeded simulations provide a reliable free energy landscape of cryptic pocket openings, demonstrating the utility of AF2 in this area (Hart et al., 2017; Meller and Bhakat, 2023).
To enhance the success rate in the design and discovery of new modulators, strategies must be employed to address the challenges posed by the low success rates of traditional docking methods. One of these problems is an unbalanced distribution between few active and many inactive states in proteins, resulting in docking runs with starting points from mostly inactive states. Some authors suggest that the modeling of proteins in the following iterations of AF2 be intentionally biased towards active states as a solution to increase the hit rate in ensemble-based docking protocols (Nussinov et al., 2023). Another factor in the low hit rate of docking is related to poorly parameterized energy terms in the scoring function used. The SF is typically derived from physics-based force fields, empirical functions, or knowledge-based terms, but lacks proper electronic treatment of the atoms involved in the protein-ligand complex. In addition, the use of fixed dielectric charges in the docking SF contributes to an increase in both false-positive and false-negative results (Khodarahmi et al., 2015). It is important to note that the electronic component of the potential energy plays a critical role in non-bonding interactions. To improve the accuracy of predicting the binding energy of a protein-ligand complex, it is necessary to accurately calculate the charge transfer and polarization, which can be achieved using quantum mechanics (QM) methods. However, applying QM methods to large biological macromolecules is computationally demanding. As a solution, an intermediate approach called QM/MM (quantum mechanics/molecular mechanics) has been developed. In the QM/MM method, only the atoms involved in the interatomic interactions within the protein-ligand complex are handled at the QM level, while the remaining parts of the system are handled using conventional molecular mechanics (MM) calculations. This combination allows for more efficient and accurate calculations of the complex (Ryde and Söderhjelm, 2016; Kollar and Frecer, 2018).
6.3 The rapid expansion of chemical space: challenges and opportunities in CADD
With the advent of the Internet, the change and storage of data has become possible, leading to the development of several databases containing chemical compounds or metabolites that play a critical role in research in various fields. These databases are essential for CADD, investigating studying protein-ligand interactions, exploring controlled drug release mechanisms, developing new organic materials, and conducting data-driven studies using machine learning, among other applications.
Currently, numerous open access databases of chemical compounds or bioactive molecules are available, including PubChem (Kim et al., 2023), ChEMBL (Mendez et al., 2019), ZINC (Irwin et al., 2020), BindingDB (Gilson et al., 2016), ChEBI (Hastings et al., 2016), eMolecules (https://www.emolecules.com, consulting May 2023), DrugBank (Wishart et al., 2018), HMDB (Wishart et al., 2022), IUPHAR/BPS (Harding et al., 2022), COCONUT (Sorokina et al., 2021), Drug Repurposing Hub (Corsello et al., 2017), e-Drug3D (Douguet, 2018), Promiscuous (Gallo et al., 2021) and the Natural Products Atlas (Van Santen et al., 2022). These databases are just a few examples of the resources available for research purposes and to obtain specific information.
Furthermore, many of these databases of molecular compounds are freely downloadable, while others provide diverse access methods to facilitate the work of researchers, including the use of an Application Programming Interface (API) or the implementation of web scraping techniques (Hanwell et al., 2017). An example of this is the ChEMBL database, which provides a web interface (ChEMBL Database) for data retrieval, a RESTful API (REST = REpresentational State Transfer) which means that it responds to a variety of programming languages such as Java, Python, JavaScript, and works with XML (Extensible Markup Language), JSON (JavaScript Object Notation) or YAML formats (Davies et al., 2015; Nowotka et al., 2017). In addition, for Python users, there is a library called the “Python client” that provides seamless access to the ChEMBL API, simplifying data retrieval (GitHub - chembl/chembl_webresource_client: Official Python client for accessing ChEMBL API, 2023). These tools become indispensable when dealing with complex queries or filtering specific datasets (data cleaning) for subsequent in-depth analysis or training models using machine learning techniques.
For example, using a Python client, we performed a search to determine the number of reported molecules in the ChEMBL database (2023) for each member of the TRP superfamily. We specifically considered molecules reporting IC50 or EC50 values from the STANDARD_TYPE column and restricted our analysis to those reported for the TRP superfamily in humans (Papadatos et al., 2015). To date, the TRPV family accounts for 4,477 reported molecules, representing 66.06% of the total reported molecules within the TRP superfamily. The TRPM and TRPA families follow with 927 and 808 reported molecules, respectively, representing 13.68% and 11.92% of the total. The TRPML and TRPC families have the lowest number of reported molecules with 4.6% and 3.73% respectively, and the TRPP family has no molecules with reported IC50 or EC50 values (Figure 6A). Analyzing the data for TRPV family members in detail (Figure 6B), TRPV1 has the highest number of reported molecules, with 3,479, representing 77.71% of the total 4,477 molecules. The reported molecules in TRPV4 and TRPV3 represent 11.68% and 9.36% respectively, and the TRPV2/5/6 families together represent only 1.25% of the total molecules.
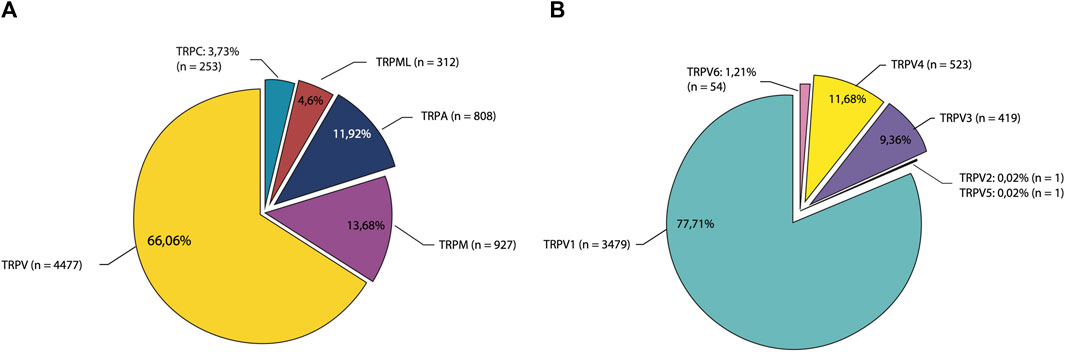
FIGURE 6. Exploring molecule dispersal rates across TRP superfamily and TRPV family members in the ChEMBL database. The pie chart shows the distribution of reported molecules in the ChEMBL database, categorized by (A) the entire TRP superfamily, with the TRPV family being the most represented, and (B) individual members within the TRPV family, with TRPV1 having the highest number of reported molecules.
This significant number of molecules allows for exploratory data analysis (EDA) and the potential application of machine learning models to train predictive classifiers. This has been demonstrated in previous research, such as the development of classifiers to predict cyclooxygenase-2 (COX-2) inhibitors by categorizing molecules from the literature as highly or weakly active inhibitors (Brown et al., 2019). Additionally, Tinivella et al. (2021) described a study in which they used machine learning models to predict the activity and selectivity of inhibitors against human carbonic anhydrase (hCA), which are potential therapeutic targets. In their research, they used curated data extracted from ChEMBL, which enabled them to train their models and make accurate predictions, highlighting the value of leveraging the extensive data set to build robust predictive models.
With the rapidly expanding chemical space, researchers face the challenge of dealing with a vast number of molecules. This requires the use of efficient tools and methods. One such tool is the open-source software KNIME Analytics Platform (Berthold et al., 2009) which allows the visual creation of interconnected workflows with nodes containing custom algorithms. Within this platform, there is a GET Request node that facilitates interaction with databases that support RESTful web services, such as ChEMBL, PubChem or other biological databases. There are numerous research studies that use this tool in their workflows (Sydow et al., 2019; Kojima et al., 2020; Alegría-Arcos et al., 2022; Smajić et al., 2022), because it provides seamless integration with programming languages such as Python or R and libraries like RDkit (RDKit, 2023) and Scikit-learn (Pedregosa et al., 2012). Additionally, it facilitates integration with other commercial software programs such as the Schrödinger suite (Maestro, 2021).
In addition, Jupyter notebooks have gained significant popularity as a tool for documenting and ensuring the reproducibility of pipelines and reports in exploratory chemical data analysis and other research methodologies that use computational workflows for specific purposes. These notebooks are often hosted in repositories such as GitHub, Zenodo (Zenodo, 2023), Bitbucket (Git solution for teams using Jira, 2023), and similar platforms.
It is also worth noting that explosion of the chemical space has been driven by the need to discover new drugs, leading to the generation of combinatorial chemical libraries (Saldívar-González et al., 2020). Ruddigkeit et al. (2012) have generated a database called GDB-17 which houses more than 166 billion synthetic molecules, including various drug analogs and lead compounds for their applications in CADD techniques such as virtual screening. CHIPMUNK is another database of 95 million virtual small molecules that can be synthesized for subsequent analysis (Humbeck et al., 2018). These chemical libraries were generated using the combinatorial enumeration approach. In the enumeration approach, chemical structures are systemically generated by exploring various combinations and modifications of molecular fragments within a given scaffold. This approach allows the exploration of a vast chemical space, enabling the identification of diverse compound libraries for screening and lead optimization. In a recent study applying the enumeration approach to capsaicin, resulted in the generation of 2174 analogs. Using this approach, it was discovered four highly selective modulators of TRPV1, that exhibited greater selectivity compared to other members of the TRPV ion channel family (Bustos et al., 2022). Bioisosteres, on the other hand, involve the strategic replacement of one group or atom within a molecule with another group or atom that has similar physical or chemical properties. Bioisosteres aim to optimize the pharmacological properties of a compound while preserving its overall molecular framework. It was found a TRPV1 inhibitor through a bioisosteres protocol in the Lys-Trp (Nps) peptide scaffold (Zaccaro et al., 2011). Both techniques provide valuable tools for drug developers to enhance the potency, selectivity, pharmacokinetic properties, and overall drug-like characteristics of potential modulators for TRPV1 channels. They contribute to the generation of novel compounds with improved activity profiles and increased chances of successful drug development.
On another note, the growth of chemical space has expanded the capability to synthesize and select from a larger number of compounds than was previously possible. This has increased the synthetic feasibility of molecules or compounds in recent years, with suppliers and libraries offering readily available or custom-made molecules. These advances facilitate the acceleration of scientific research.
7 Conclusion
Although the TRPV1 channel has been extensively studied and its molecular intricacies are known, the discovery of specific and efficient drugs that target the channel in pathological conditions remains a challenge. Similarly, the existence of molecular elements for temperature sensing is a matter of debate in the field of ion channels. While capsaicin analogs are well suited to relieve peripheral pain when administered topically, they can cause unpleasant pain amplification in pathological pain conditions such as inflammatory or neuropathic pain. Furthermore, central administration of capsaicin or its analogs induces thermosensory and thermoregulatory distortions. In addition, although several published structures elucidate many aspects of channel function, they provide information on static states, thus limiting knowledge of important aspects of the dynamics of TRPV1 channel gating by different types of ligands. In silico tools and predictive algorithms can aid in the challenge of finding drugs that can be experimentally tested and have no unwanted side effects for the treatment of various pain and other TRPV1-related disorders.
Author contributions
KC and MA-A: conceptualization, writing-original draft. CA-R, KC-Z, DB: write the draft, and prepare figures. All authors contributed to the article and approved the submitted version.
Funding
The author(s) declare financial support was received for the research, authorship, and/or publication of this article. OAS Academic Scholarship Program Graduate Studies or Graduate Research (0000165325, CA-R), Beca de Doctorado Nacional ANID Chile (2139/2023, KC-Z), thanks to ANID FONDECYT Initiation Project No. 11220444 (DB), thanks to regular research project UDLA 2023 DI-31/23 (MA-A). To the student Vannessa Martinez Acevedo for her help in obtaining the data.
Conflict of interest
The authors declare that the research was conducted in the absence of any commercial or financial relationships that could be construed as a potential conflict of interest.
Publisher’s note
All claims expressed in this article are solely those of the authors and do not necessarily represent those of their affiliated organizations, or those of the publisher, the editors and the reviewers. Any product that may be evaluated in this article, or claim that may be made by its manufacturer, is not guaranteed or endorsed by the publisher.
References
Adcock, J. J. (2009). TRPV1 receptors in sensitisation of cough and pain reflexes. Pulm. Pharmacol. Ther. 22 (2), 65–70. doi:10.1016/J.PUPT.2008.12.014
Akiba, Y., Kato, S., Katsube, K. i., Nakamura, M., Takeuchi, K., Ishii, H., et al. (2004). Transient receptor potential vanilloid subfamily 1 expressed in pancreatic islet β cells modulates insulin secretion in rats. Biochem. Biophysical Res. Commun. 321 (1), 219–225. doi:10.1016/J.BBRC.2004.06.149
Alegría-Arcos, M., Barbosa, T., Sepúlveda, F., Combariza, G., González, J., Gil, C., et al. (2022). Network pharmacology reveals multitarget mechanism of action of drugs to be repurposed for COVID-19. Front. Pharmacol. 13, 2921. doi:10.3389/fphar.2022.952192
Altierab, C., Dubel, S. J., Barrère, C., Jarvis, S. E., Stotz, S. C., Spaetgens, R. L., et al. (2002). Trafficking of L-type calcium channels mediated by the postsynaptic scaffolding protein AKAP79. J. Biol. Chem. 277 (37), 33598–33603. doi:10.1074/jbc.M202476200
Amaya, F., Decosterd, I., Samad, T. A., Plumpton, C., Tate, S., Mannion, R. J., et al. (2000). Diversity of expression of the sensory neuron-specific TTX-resistant voltage-gated sodium ion channels SNS and SNS2. Mol. Cell. Neurosci. 15 (4), 331–342. doi:10.1006/MCNE.1999.0828
Amaya, F., Oh-hashi, K., Naruse, Y., Iijima, N., Ueda, M., Shimosato, G., et al. (2003). Local inflammation increases vanilloid receptor 1 expression within distinct subgroups of DRG neurons. Brain Res. 963 (1–2), 190–196. doi:10.1016/S0006-8993(02)03972-0
Amaya, F., Shimosato, G., Nagano, M., Ueda, M., Hashimoto, S., Tanaka, Y., et al. (2004). NGF and GDNF differentially regulate TRPV1 expression that contributes to development of inflammatory thermal hyperalgesia. Eur. J. Neurosci. 20 (9), 2303–2310. doi:10.1111/J.1460-9568.2004.03701.X
Anand, P., and Bley, K. (2011). Topical capsaicin for pain management: therapeutic potential and mechanisms of action of the new high-concentration capsaicin 8% patch. BJA Br. J. Anaesth. 107 (4), 490–502. doi:10.1093/BJA/AER260
Bae, C., Anselmi, C., Kalia, J., Jara-Oseguera, A., Schwieters, C. D., Krepkiy, D., et al. (2016). Structural insights into the mechanism of activation of the TRPV1 channel by a membrane-bound tarantula toxin. eLife 5, 1–30. doi:10.7554/elife.11273
Bae, C., Kalia, J., Song, I., Yu, J., Kim, H. H., Swartz, K. J., et al. (2012). High yield production and refolding of the double-knot toxin, an activator of TRPV1 channels. PLoS ONE 7 (12), e51516. doi:10.1371/journal.pone.0051516
Baez, D., Raddatz, N., Ferreira, G., Gonzalez, C., and Latorre, R. (2014). Gating of thermally activated channels. Curr. Top. Membr. 74 (1), 51–87. doi:10.1016/B978-0-12-800181-3.00003-8
Bandell, M., Story, G. M., Hwang, S. W., Viswanath, V., Eid, S. R., Petrus, M. J., et al. (2004). Noxious cold ion channel TRPA1 is activated by pungent compounds and bradykinin. Neuron 41 (6), 849–857. doi:10.1016/S0896-6273(04)00150-3
Bautista, D. M., Jordt, S. E., Nikai, T., Tsuruda, P. R., Read, A. J., Poblete, J., et al. (2006). TRPA1 mediates the inflammatory actions of environmental irritants and proalgesic agents. Cell 124 (6), 1269–1282. doi:10.1016/J.CELL.2006.02.023
Berthold, M. R., Cebron, N., Dill, F., Gabriel, T. R., Kötter, T., Meinl, T., et al. (2009). KNIME - the Konstanz information miner. ACM SIGKDD Explor. Newsl. 11, 26–31. doi:10.1145/1656274.1656280
Bertin, S., Aoki-Nonaka, Y., de Jong, P. R., Nohara, L. L., Xu, H., Stanwood, S. R., et al. (2014) ‘The ion channel TRPV1 regulates the activation and proinflammatory properties of CD4⁺ T cells’, Nat. Immunol. 15(11), pp. 1055–1063. doi:10.1038/ni.3009
Bevan, S., and Geppetti, P. (1994). Protons: small stimulants of capsaicin-sensitive sensory nerves. Trends Neurosci. 17 (12), 509–512. doi:10.1016/0166-2236(94)90149-X
Bevan, S., Hothi, S., Hughes, G., James, I. F., Rang, H. P., Shah, K., et al. (1992). Capsazepine: a competitive antagonist of the sensory neurone excitant capsaicin. Br. J. Pharmacol. 107 (2), 544–552. doi:10.1111/J.1476-5381.1992.TB12781.X
Bhaskaran, M. D., and Smith, B. N. (2010). Effects of TRPV1 activation on synaptic excitation in the dentate gyrus of a mouse model of temporal lobe epilepsy. Exp. Neurol. 223 (2), 529–536. doi:10.1016/J.EXPNEUROL.2010.01.021
Bhave, G., Hu, H. J., Glauner, K. S., Zhu, W., Wang, H., Brasier, D. J., et al. (2003). Protein kinase C phosphorylation sensitizes but does not activate the capsaicin receptor transient receptor potential vanilloid 1 (TRPV1). Proc. Natl. Acad. Sci. 100 (21), 12480–12485. doi:10.1073/PNAS.2032100100
Bhave, G., Zhu, W., Wang, H., Brasier, D. J., Oxford, G. S., and Gereau, R. W. (2002). cAMP-dependent protein kinase regulates desensitization of the capsaicin receptor (VR1) by direct phosphorylation. Neuron 35 (4), 721–731. doi:10.1016/S0896-6273(02)00802-4
Binshtok, A. M., Wang, H., Zimmermann, K., Amaya, F., Vardeh, D., Shi, L., et al. (2008). Nociceptors are interleukin-1beta sensors. J. Neurosci. official J. Soc. Neurosci. 28 (52), 14062–14073. doi:10.1523/JNEUROSCI.3795-08.2008
Birder, L. A., Nakamura, Y., Kiss, S., Nealen, M. L., Barrick, S., Kanai, A. J., et al. (2002). Altered urinary bladder function in mice lacking the vanilloid receptor TRPV1. Nat. Neurosci. 5 (9), 856–860. doi:10.1038/nn902
Birklein, F., and Schmelz, M. (2008). Neuropeptides, neurogenic inflammation and complex regional pain syndrome (CRPS). Neurosci. Lett. 437 (3), 199–202. doi:10.1016/J.NEULET.2008.03.081
Bode, A. M., Cho, Y. Y., Zheng, D., Zhu, F., Ericson, M. E., Ma, W. Y., et al. (2009). Transient receptor potential type vanilloid 1 suppresses skin carcinogenesis. Cancer Res. 69 (3), 905–913. doi:10.1158/0008-5472.CAN-08-3263
Boehr, D. D., Nussinov, R., and Wright, P. E. (2009). The role of dynamic conformational ensembles in biomolecular recognition. Nat. Chem. Biol. 5 (11), 789–796. doi:10.1038/nchembio.232
Bohlen, C. J., Priel, A., Zhou, S., King, D., Siemens, J., and Julius, D. (2010). A bivalent tarantula toxin activates the capsaicin receptor, TRPV1, by targeting the outer pore domain. Cell 141 (5), 834–845. doi:10.1016/j.cell.2010.03.052
Brandao, K. E., Dell’Acqua, M. L., and Levinson, S. R. (2012). A-kinase anchoring protein 150 expression in a specific subset of TRPV1- and CaV 1.2-positive nociceptive rat dorsal root ganglion neurons. J. Comp. neurology 520 (1), 81–99. doi:10.1002/CNE.22692
Brauchi, S., Orio, P., and Latorre, R. (2004). Clues to understanding cold sensation: thermodynamics and electrophysiological analysis of the cold receptor TRPM8. Proc. Natl. Acad. Sci. U. S. A. 101 (43), 15494–15499. doi:10.1073/pnas.0406773101
Brauchi, S., Orta, G., Mascayano, C., Salazar, M., Raddatz, N., Urbina, H., et al. (2007). Dissection of the components for PIP2 activation and thermosensation in TRP channels. Proc. Natl. Acad. Sci. 104 (24), 10246–10251. doi:10.1073/PNAS.0703420104
Brauchi, S., Orta, G., Salazar, M., Rosenmann, E., and Latorre, R. (2006). A hot-sensing cold receptor: C-terminal domain determines thermosensation in transient receptor potential channels. J. Neurosci. 26 (18), 4835–4840. doi:10.1523/JNEUROSCI.5080-05.2006
Bregman, D. B., Bhattacharyya, N., and Rubin, C. S. (1989). High affinity binding protein for the regulatory subunit of cAMP-dependent protein kinase II-B. J. Biol. Chem. 264 (8), 4648–4656. doi:10.1016/s0021-9258(18)83792-9
Brown, N., Fiscato, M., Segler, M. H. S., and Vaucher, A. C. (2019). GuacaMol: benchmarking models for de novo molecular design. J. Chem. Inf. Model. 59 (3), 1096–1108. doi:10.1021/acs.jcim.8b00839
Bustos, D., Galarza, C., Ordoñez, W., Brauchi, S., and Benso, B. (2022). Cost-effective pipeline for a rational design and selection of capsaicin analogues targeting TRPV1 channels. ACS Omega 8, 11736–11749. doi:10.1021/ACSOMEGA.2C05672
Cao, E., Cordero-Morales, J. F., Liu, B., Qin, F., and Julius, D. (2013b). TRPV1 channels are intrinsically heat sensitive and negatively regulated by phosphoinositide lipids. Neuron 77 (4), 667–679. doi:10.1016/j.neuron.2012.12.016
Cao, E., Liao, M., Cheng, Y., and Julius, D. (2013a). TRPV1 structures in distinct conformations reveal activation mechanisms. Nature 504 (7478), 113–118. doi:10.1038/nature12823
Castillo, K., Diaz-Franulic, I., Canan, J., Gonzalez-Nilo, F., and Latorre, R. (2018). Thermally activated TRP channels: molecular sensors for temperature detection. Phys. Biol. Inst. Phys. Publ. 15, 021001. doi:10.1088/1478-3975/aa9a6f
Caterina, M. J. (2008). On the thermoregulatory perils of TRPV1 antagonism. Pain 136 (1–2), 3–4. doi:10.1016/J.PAIN.2008.02.033
Caterina, M. J., Leffler, A., Malmberg, A. B., Martin, W. J., Trafton, J., Petersen-Zeitz, K. R., et al. (2000). Impaired nociception and pain sensation in mice lacking the capsaicin receptor. Science 288 (5464), 306–313. doi:10.1126/science.288.5464.306
Caterina, M. J., Rosen, T. A., Tominaga, M., Brake, A. J., and Julius, D. (1999) ‘A capsaicin-receptor homologue with a high threshold for noxious heat’, Nature 398(6726), pp. 436–441. doi:10.1038/18906
Caterina, M. J., Schumacher, M. A., Tominaga, M., Rosen, T. A., Levine, J. D., and Julius, D. (1997). The capsaicin receptor: a heat-activated ion channel in the pain pathway. Natue 389, 816–824. doi:10.1038/39807
Cesare, P., Dekker, L. V., Sardini, A., Parker, P. J., and McNaughton, P. A. (1999). Specific involvement of PKC-ε in sensitization of the neuronal response to painful heat. Neuron 23 (3), 617–624. doi:10.1016/S0896-6273(00)80813-2
Cesare, P., and Mcnaughton, P. (1996). A novel heat-activated current in nociceptive neurons and its sensitization by bradykinin. Proc. Natl. Acad. Sci. 93 (26), 15435–15439. doi:10.1073/PNAS.93.26.15435
Chai, S., Li, M., Lan, J., Xiong, Z. G., Saugstad, J. A., and Simon, R. P. (2007). A kinase-anchoring protein 150 and calcineurin are involved in regulation of acid-sensing ion channels ASIC1a and ASIC2a. J. Biol. Chem. 282 (31), 22668–22677. doi:10.1074/JBC.M703624200
Chávez, A. E., Chiu, C. Q., and Castillo, P. E. (2010) ‘TRPV1 activation by endogenous anandamide triggers postsynaptic long-term depression in dentate gyrus’, Nat. Neurosci. 13(12), 1511–1518. doi:10.1038/nn.2684
ChEMBL Database (2023). ) ebi. Available at: https://www.ebi.ac.uk/chembl/(Accessed: October 16, 2023).
Chiu, I. M. (2018). Infection, pain, and itch. Neurosci. Bull. 34 (1), 109–119. doi:10.1007/s12264-017-0098-1
Chiu, I. M., Heesters, B. A., Ghasemlou, N., Von Hehn, C. A., Zhao, F., Tran, J., et al. (2013) ‘Bacteria activate sensory neurons that modulate pain and inflammation’, Nature 501 (7465), 52–57. doi:10.1038/nature12479
Chiu, I. M., Von Hehn, C. A., and Woolf, C. J. (2012) ‘Neurogenic inflammation and the peripheral nervous system in host defense and immunopathology’, Nat. Neurosci. 15(8), 1063–1067. doi:10.1038/nn.3144
Chuang, H. H., Prescott, E. D., Kong, H., Shields, S., Jordt, S. E., Basbaum, A. I., et al. (2001) ‘Bradykinin and nerve growth factor release the capsaicin receptor from PtdIns(4,5)P2-mediated inhibition’, Nature 411 (6840), pp. 957–962. doi:10.1038/35082088
Clapham, D. E., and Miller, C. (2011). A thermodynamic framework for understanding temperature sensing by transient receptor potential (TRP) channels. Proc. Natl. Acad. Sci. 108 (49), 19492–19497. doi:10.1073/PNAS.1117485108
Clifton, B. E., and Jackson, C. J. (2016). Ancestral protein reconstruction yields insights into adaptive evolution of binding specificity in solute-binding proteins. Cell Chem. Biol. 23 (2), 236–245. doi:10.1016/J.CHEMBIOL.2015.12.010
Coghlan, V. M., Perrino, B. A., Howard, M., Langeberg, L. K., Hicks, J. B., Gallatin, W. M., et al. (1995). Association of protein kinase A and protein phosphatase 2B with a common anchoring protein. Science 267 (5194), 108–111. doi:10.1126/SCIENCE.7528941
Colledge, M., Dean, R. A., Scott, G. K., Langeberg, L. K., Huganir, R. L., and Scott, J. D. (2000). Targeting of PKA to glutamate receptors through a MAGUK-AKAP complex. Neuron 27 (1), 107–119. doi:10.1016/S0896-6273(00)00013-1
Comitani, F., and Gervasio, F. L. (2018). Exploring cryptic pockets formation in targets of pharmaceutical interest with SWISH. J. Chem. Theory Comput. 14 (6), 3321–3331. doi:10.1021/acs.jctc.8b00263
Constantin, C. E., Mair, N., Sailer, C. A., Andratsch, M., Xu, Z. Z., Blumer, M. J. F., et al. (2008). Endogenous tumor necrosis factor alpha (TNFalpha) requires TNF receptor type 2 to generate heat hyperalgesia in a mouse cancer model. J. Neurosci. 28 (19), 5072–5081. doi:10.1523/JNEUROSCI.4476-07.2008
Contassot, E., Tenan, M., Schnüriger, V., Pelte, M. F., and Dietrich, P. Y. (2004). Arachidonyl ethanolamide induces apoptosis of uterine cervix cancer cells via aberrantly expressed vanilloid receptor-1. Gynecol. Oncol. 93 (1), 182–188. doi:10.1016/J.YGYNO.2003.12.040
Cordero-Morales, J. F., Gracheva, E. O., and Julius, D. (2011). Cytoplasmic ankyrin repeats of transient receptor potential A1 (TRPA1) dictate sensitivity to thermal and chemical stimuli. Proc. Natl. Acad. Sci. U. S. A. 108 (46), E1184–E1191. doi:10.1073/pnas.1114124108
Corsello, S. M., Bittker, J. A., Liu, Z., Gould, J., McCarren, P., Hirschman, J. E., et al. (2017) ‘The Drug Repurposing Hub: a next-generation drug library and information resource’, Nat. Med. 23(4), 405–408. doi:10.1038/nm.4306
Cortright, D. N., Crandall, M., Sanchez, J. F., Zou, T., Krause, J. E., and White, G. (2001). The tissue distribution and functional characterization of human VR1. Biochem. Biophysical Res. Commun. 281 (5), 1183–1189. doi:10.1006/BBRC.2001.4482
Cosens, D. J., and Manning, A. (1969). Abnormal electroretinogram from a Drosophila mutant. Nature 224 (5216), 285–287. doi:10.1038/224285a0
Cournia, Z., Allen, T. W., Andricioaei, I., Antonny, B., Baum, D., Brannigan, G., et al. (2015). Membrane protein structure, function, and dynamics: a perspective from experiments and theory. J. Membr. Biol. 248 (4), 611–640. doi:10.1007/S00232-015-9802-0
Cruz, M. A., Frederick, T. E., Mallimadugula, U. L., Singh, S., Vithani, N., Zimmerman, M. I., et al. (2022). A cryptic pocket in Ebola VP35 allosterically controls RNA binding. Nat. Commun. 13 (1), 2269–2310. doi:10.1038/s41467-022-29927-9
Cummins, M. C., Jacobs, T. M., Teets, F. D., DiMaio, F., Tripathy, A., and Kuhlman, B. (2022). AlphaFold accurately predicts distinct conformations based on the oligomeric state of a de novo designed protein. Protein Sci. 31 (7), e4368. doi:10.1002/PRO.4368
Czifra, G., Varga, A., Nyeste, K., Marincsák, R., Tóth, B. I., Kovács, I., et al. (2009). Increased expressions of cannabinoid receptor-1 and transient receptor potential vanilloid-1 in human prostate carcinoma. J. Cancer Res. Clin. Oncol. 135 (4), 507–514. doi:10.1007/s00432-008-0482-3
Daly, D., Rong, W., Chess-Williams, R., Chapple, C., and Grundy, D. (2007). Bladder afferent sensitivity in wild-type and TRPV1 knockout mice. J. Physiology 583 (2), 663–674. doi:10.1113/JPHYSIOL.2007.139147
Darré, L., and Domene, C. (2015). Binding of capsaicin to the TRPV1 ion channel. Mol. Pharm. 12 (12), 4454–4465. doi:10.1021/acs.molpharmaceut.5b00641
Davies, M., Nowotka, M., Papadatos, G., Dedman, N., Gaulton, A., Atkinson, F., et al. (2015). ChEMBL web services: streamlining access to drug discovery data and utilities. Nucleic acids Res. 43 (W1), W612–W620. doi:10.1093/NAR/GKV352
Davis, J. B., Gray, J., Gunthorpe, M. J., Hatcher, J. P., Davey, P. T., Overend, P., et al. (2000) ‘Vanilloid receptor-1 is essential for inflammatory thermal hyperalgesia’, Nature 405 (6783), 183–187. doi:10.1038/35012076
Dedov, V. N., Tran, V. H., Duke, C. C., Connor, M., Christie, M. J., Mandadi, S., et al. (2002). Gingerols: a novel class of vanilloid receptor (VR1) agonists. Br. J. Pharmacol. 137 (6), 793–798. doi:10.1038/SJ.BJP.0704925
Del Alamo, D., Sala, D., Mchaourab, H. S., and Meiler, J. (2022). Sampling alternative conformational states of transporters and receptors with AlphaFold2. eLife 11, e75751. doi:10.7554/eLife.75751
De Petrocellis, L., Ligresti, A., Moriello, A. S., Allarà, M., Bisogno, T., Petrosino, S., et al. (2011). Effects of cannabinoids and cannabinoid-enriched Cannabis extracts on TRP channels and endocannabinoid metabolic enzymes. Br. J. Pharmacol. 163 (7), 1479–1494. doi:10.1111/J.1476-5381.2010.01166.X
Derbenev, A. V., and Zsombok, A. (2016). Potential therapeutic value of TRPV1 and TRPA1 in diabetes mellitus and obesity. Seminars Immunopathol. 38 (3), 397–406. doi:10.1007/s00281-015-0529-x
Distler, C., Rathee, P. K., Lips, K. S., Obreja, O., Neuhuber, W., and Kress, M. (2003). Fast Ca2+-induced potentiation of heat-activated ionic currents requires cAMP/PKA signaling and functional AKAP anchoring. J. Neurophysiology 89 (5), 2499–2505. doi:10.1152/jn.00713.2002
Douguet, D. (2018). Data sets representative of the structures and experimental properties of FDA-approved drugs. ACS Med. Chem. Lett. 9 (3), 204–209. doi:10.1021/acsmedchemlett.7b00462
Egbert, M., Jones, G., Collins, M. R., Kozakov, D., and Vajda, S. (2022). FTMove: a web server for detection and analysis of cryptic and allosteric binding sites by mapping multiple protein structures. J. Mol. Biol. 434 (11), 167587. doi:10.1016/J.JMB.2022.167587
Eijkelkamp, N., Linley, J. E., Torres, J. M., Bee, L., Dickenson, A. H., Gringhuis, M., et al. (2013) ‘A role for Piezo2 in EPAC1-dependent mechanical allodynia’, Nat. Commun. 4(1), 1682–1713. doi:10.1038/ncomms2673
Fabbro, D., Cowan-Jacob, S. W., and Moebitz, H. (2015). Ten things you should know about protein kinases: IUPHAR Review 14. Br. J. Pharmacol. 172 (11), 2675–2700. doi:10.1111/BPH.13096
Fajrin, F. A., Nugroho, A. E., Nurrochmad, A., and Susilowati, R. (2020). Ginger extract and its compound, 6-shogaol, attenuates painful diabetic neuropathy in mice via reducing TRPV1 and NMDAR2B expressions in the spinal cord, J. Ethnopharmacol. 249. doi:10.1016/J.JEP.2019.112396
Faussone-Pellegrini, M. S., Taddei, A., Bizzoco, E., Lazzeri, M., Vannucchi, M. G., and Bechi, P. (2005). Distribution of the vanilloid (capsaicin) receptor type 1 in the human stomach. Histochem. Cell Biol. 124 (1), 61–68. doi:10.1007/s00418-005-0025-9
Feng, Q. (2014). Temperature sensing by thermal TRP channels: thermodynamic basis and molecular insights. Curr. Top. Membr. 74 (1), 19–50. doi:10.1016/B978-0-12-800181-3.00002-6
Fernandes, E. S., Fernandes, M. A., and Keeble, J. E. (2012). The functions of TRPA1 and TRPV1: moving away from sensory nerves. Br. J. Pharmacol. 166 (2), 510–521. doi:10.1111/J.1476-5381.2012.01851.X
Fernandez-Leiro, R., and Scheres, S. H. W. (2016) ‘Unravelling biological macromolecules with cryo-electron microscopy’, Nature 537(7620), 339–346. doi:10.1038/nature19948
Ferrandiz-Huertas, C., Mathivanan, S., Wolf, C. J., Devesa, I., and Ferrer-Montiel, A. (2014). Trafficking of thermo TRP channels. Membranes 4, 525–564. MDPI AG. doi:10.3390/membranes4030525
Fischer, M. J. M., Btesh, J., and McNaughton, P. A. (2013). Disrupting sensitization of transient receptor potential vanilloid subtype 1 inhibits inflammatory hyperalgesia. J. Neurosci. 33 (17), 7407–7414. doi:10.1523/JNEUROSCI.3721-12.2013
Gallo, K., Goede, A., Eckert, A., Moahamed, B., Preissner, R., and Gohlke, B. O. (2021). PROMISCUOUS 2.0: a resource for drug-repositioning. Nucleic Acids Res. 49 (D1), D1373–D1380. doi:10.1093/NAR/GKAA1061
Gao, N., Yang, F., Chen, S., Wan, H., Zhao, X., and Dong, H. (2020). The role of TRPV1 ion channels in the suppression of gastric cancer development. J. Exp. Clin. Cancer Res. 39 (1), 206–217. doi:10.1186/s13046-020-01707-7
Gao, T., Yatani, A., Dell'Acqua, M. L., Sako, H., Green, S. A., Dascal, N., et al. (1997). cAMP-dependent regulation of cardiac L-type Ca2+ channels requires membrane targeting of PKA and phosphorylation of channel subunits. Neuron 19 (1), 185–196. doi:10.1016/S0896-6273(00)80358-X
Gao, W., Sun, Y., Cai, M., Zhao, Y., Cao, W., Liu, Z., et al. (2018). Copper sulfide nanoparticles as a photothermal switch for TRPV1 signaling to attenuate atherosclerosis. Nat. Commun. 9 (1), 231–310. doi:10.1038/s41467-017-02657-z
Gao, Y., Cao, E., Julius, D., and Cheng, Y. (2016) ‘TRPV1 structures in nanodiscs reveal mechanisms of ligand and lipid action’, Nature 534 (7607), 347–351. doi:10.1038/nature17964
Gavva, N. R. (2008). Body-temperature maintenance as the predominant function of the vanilloid receptor TRPV1. Trends Pharmacol. Sci. 29 (11), 550–557. doi:10.1016/J.TIPS.2008.08.003
Gavva, N. R., Bannon, A. W., Hovland, D. N., Lehto, S. G., Klionsky, L., Surapaneni, S., et al. (2007a). Repeated administration of vanilloid receptor TRPV1 antagonists attenuates hyperthermia elicited by TRPV1 blockade. J. Pharmacol. Exp. Ther. 323 (1), 128–137. doi:10.1124/JPET.107.125674
Gavva, N. R., Bannon, A. W., Surapaneni, S., Hovland, D. N., Lehto, S. G., Gore, A., et al. (2007b). The vanilloid receptor TRPV1 is tonically activated in vivo and involved in body temperature regulation. J. Neurosci. 27 (13), 3366–3374. doi:10.1523/JNEUROSCI.4833-06.2007
Gees, M., Owsianik, G., Nilius, B., and Voets, T. (2012). TRP channels. Compr. Physiol. 2, 563–608. doi:10.1002/cphy.c110026
Geng, S., Zheng, Y., Meng, M., Guo, Z., Cao, N., Ma, X., et al. (2016). Gingerol reverses the cancer-promoting effect of capsaicin by increased TRPV1 level in a urethane-induced lung carcinogenic model. J. Agric. Food Chem. 64 (31), 6203–6211. doi:10.1021/acs.jafc.6b02480
Gianti, E., Klein, M. L., Rohacs, T., and Carnevale, V. (2019). Activation of TRPV1 by lipids: can lipid tails bridge the gap between the vanilloid binding site and the peripheral cavities? BpJ 116 (3), 536a. doi:10.1016/J.BPJ.2018.11.2885
Gilson, M. K., Liu, T., Baitaluk, M., Nicola, G., Hwang, L., and Chong, J. (2016). BindingDB in 2015: a public database for medicinal chemistry, computational chemistry and systems pharmacology. Nucleic acids Res. 44 (D1), D1045–D1053. doi:10.1093/NAR/GKV1072
GitHub - chembl/chembl_webresource_client: Official Python client for accessing ChEMBL API (2023). ) github. Available at: https://github.com/chembl/chembl_webresource_client (Accessed: October 16, 2023).
Git solution for teams using Jira (2023). Bitbucket. Available at: https://bitbucket.org/(Accessed: October 16, 2023).
Golech, S. A., McCarron, R. M., Chen, Y., Bembry, J., Lenz, F., Mechoulam, R., et al. (2004). Human brain endothelium: coexpression and function of vanilloid and endocannabinoid receptors. Brain Res. Mol. Brain Res. 132 (1), 87–92. doi:10.1016/J.MOLBRAINRES.2004.08.025
Gonçalves, J. C. R., Silveira, A. L., de Souza, H. D. N., Nery, A. A., Prado, V. F., Prado, M. A. M., et al. (2013). The monoterpene (–)-carvone: a novel agonist of TRPV1 channels. Cytom. Part A 83A (2), 212–219. doi:10.1002/CYTO.A.22236
Gracheva, E. O., Cordero-Morales, J. F., González-Carcacía, J. A., Ingolia, N. T., Manno, C., Aranguren, C. I., et al. (2011) Ganglion-specific splicing of TRPV1 underlies infrared sensation in vampire bats, Nature 476 (7358), 88–91. doi:10.1038/nature10245
Grandl, J., Kim, S. E., Uzzell, V., Bursulaya, B., Petrus, M., Bandell, M., et al. (2010) ‘Temperature-induced opening of TRPV1 ion channel is stabilized by the pore domain’, Nat. Neurosci. 13 (6), 708–714. doi:10.1038/nn.2552
Grycova, L., Lansky, Z., Friedlova, E., Vlachova, V., Kubala, M., Obsilova, V., et al. (2007). ATP binding site on the C-terminus of the vanilloid receptor. Archives Biochem. Biophysics 465 (2), 389–398. doi:10.1016/j.abb.2007.06.035
Guarnera, E., and Berezovsky, I. N. (2019). On the perturbation nature of allostery: sites, mutations, and signal modulation. Curr. Opin. Struct. Biol. 56, 18–27. doi:10.1016/J.SBI.2018.10.008
Guarnera, E., and Berezovsky, I. N. (2020). Allosteric drugs and mutations: chances, challenges, and necessity. Curr. Opin. Struct. Biol. 62, 149–157. doi:10.1016/J.SBI.2020.01.010
Gunthorpe, M. J., Rami, H. K., Jerman, J. C., Smart, D., Gill, C. H., Soffin, E. M., et al. (2004). Identification and characterisation of SB-366791, a potent and selective vanilloid receptor (VR1/TRPV1) antagonist. Neuropharmacology 46 (1), 133–149. doi:10.1016/S0028-3908(03)00305-8
Hanwell, M. D., De Jong, W. A., and Harris, C. J. (2017). Open chemistry: RESTful web APIs, JSON, NWChem and the modern web application. J. Cheminformatics 9 (1), 55–10. doi:10.1186/s13321-017-0241-z
Harding, S. D., Armstrong, J. F., Faccenda, E., Southan, C., Alexander, S. P. H., Davenport, A. P., et al. (2022). The IUPHAR/BPS guide to PHARMACOLOGY in 2022: curating pharmacology for COVID-19, malaria and antibacterials. Nucleic Acids Res. 50 (D1), D1282–D1294. doi:10.1093/NAR/GKAB1010
Hart, K. M., Moeder, K. E., Ho, C. M. W., Zimmerman, M. I., Frederick, T. E., and Bowman, G. R. (2017). Designing small molecules to target cryptic pockets yields both positive and negative allosteric modulators. PLOS ONE 12 (6), e0178678. doi:10.1371/JOURNAL.PONE.0178678
Hastings, J., Owen, G., Dekker, A., Ennis, M., Kale, N., Muthukrishnan, V., et al. (2016). ChEBI in 2016: improved services and an expanding collection of metabolites. Nucleic acids Res. 44 (D1), D1214–D1219. doi:10.1093/NAR/GKV1031
He, S., Zambelli, V. O., Sinharoy, P., Brabenec, L., Bian, Y., Rwere, F., et al. (2023). A human TRPV1 genetic variant within the channel gating domain regulates pain sensitivity in rodents. J. Clin. Investigation 133 (3), e163735. doi:10.1172/JCI163735
Hellwig, V., Nopper, R., Mauler, F., Freitag, J., Ji-Kai, L., Zhi-Hui, D., et al. (2003). Activities of prenylphenol derivatives from fruitbodies of Albatrellus spp. on the human and rat vanilloid receptor 1 (VR1) and characterisation of the novel natural product, confluentin. Arch. Pharm. 336 (2), 119–126. doi:10.1002/ARDP.200390008
Hille, B. (1987). Ion channels in excitable membranes as short circuits between electrical double layers. J. Electrochem. Soc. 134 (2), 343–346. doi:10.1149/1.2100457
Himmel, N. J., Gray, T. R., and Cox, D. N. (2021). Corrigendum to: phylogenetics identifies two eumetazoan TRPM clades and an eighth TRP family, TRP soromelastatin (TRPS). Mol. Biol. Evol. 38 (1), 329. doi:10.1093/molbev/msaa254
Ho, K. W., Ward, N. J., and Calkins, D. J. (2012). TRPV1: a stress response protein in the central nervous system. Am. J. Neurodegener. Dis. 1 (1), 1–14.
Holzer, P., and Maggi, C. A. (1998). Dissociation of dorsal root ganglion neurons into afferent and efferent-like neurons. Neuroscience 86 (2), 389–398. doi:10.1016/S0306-4522(98)00047-5
Hori, S., and Saitoh, O. (2023). Decreased heat sensitivity of lungfish TRPV1 revealed by the heterologous expression system. Biochem. Biophysical Res. Commun. 647, 16–22. doi:10.1016/J.BBRC.2023.01.060
Hori, T. (1984). Capsaicin and central control of thermoregulation. Pharmacol. Ther. 26 (3), 389–416. doi:10.1016/0163-7258(84)90041-X
Hoshi, N., Langeberg, L. K., and Scott, J. D. (2005) ‘Distinct enzyme combinations in AKAP signalling complexes permit functional diversity’, Nat. Cell Biol. 7(11), 1066–1073. doi:10.1038/ncb1315
Hoshi, N., Zhang, J. S., Omaki, M., Takeuchi, T., Yokoyama, S., Wanaverbecq, N., et al. (2003). AKAP150 signaling complex promotes suppression of the M-current by muscarinic agonists. Nat. Neurosci. 6 (6), 564–571. doi:10.1038/nn1062
Hser, Y. I., Evans, E., Grella, C., Ling, W., and Anglin, D. (2015). Long-term course of opioid addiction. Harv. Rev. Psychiatry 23 (2), 76–89. doi:10.1097/HRP.0000000000000052
Huang, J., Liu, J., and Qiu, L. (2020). Transient receptor potential vanilloid 1 promotes EGFR ubiquitination and modulates EGFR/MAPK signalling in pancreatic cancer cells. Cell Biochem. Funct. 38 (4), 401–408. doi:10.1002/CBF.3483
Hui, K., Liu, B., and Qin, F. (2003). Capsaicin activation of the pain receptor, VR1: multiple open states from both partial and full binding. Biophysical J. 84 (5), 2957–2968. doi:10.1016/S0006-3495(03)70022-8
Humbeck, L., Weigang, S., Schäfer, T., Mutzel, P., and Koch, O. (2018). CHIPMUNK: a virtual synthesizable small-molecule library for medicinal chemistry, exploitable for protein-protein interaction modulators. ChemMedChem 13 (6), 532–539. doi:10.1002/CMDC.201700689
Hwang, S. J., and Valtschanoff, J. G. (2003). Vanilloid receptor VR1-positive afferents are distributed differently at different levels of the rat lumbar spinal cord. Neurosci. Lett. 349 (1), 41–44. doi:10.1016/S0304-3940(03)00750-X
Inagaki, H., Kurganov, E., Park, Y., Furube, E., and Miyata, S. (2019). Oral gavage of capsaicin causes TRPV1-dependent acute hypothermia and TRPV1-independent long-lasting increase of locomotor activity in the mouse. Physiology Behav. 206, 213–224. doi:10.1016/J.PHYSBEH.2019.04.015
Irwin, J. J., Tang, K. G., Young, J., Dandarchuluun, C., Wong, B. R., Khurelbaatar, M., et al. (2020). ZINC20 - a free ultralarge-scale chemical database for ligand discovery. J. Chem. Inf. Model. 60 (12), 6065–6073. doi:10.1021/acs.jcim.0c00675
Ito, E., Ikemoto, Y., and Yoshioka, T. (2015). Thermodynamic implications of high Q10 of thermoTRP channels in living cells. BIOPHYSICS 11 (0), 33–38. doi:10.2142/BIOPHYSICS.11.33
Iwasaki, Y., Morita, A., Iwasawa, T., Kobata, K., Sekiwa, Y., Morimitsu, Y., et al. (2013). A nonpungent component of steamed ginger—[10]-shogaol—increases adrenaline secretion via the activation of TRPV1. Nutr. Neurosci. 9(3–4), 169–178. doi:10.1080/110284150600955164
Iwasaki, Y., Tanabe, M., Kayama, Y., Abe, M., Kashio, M., Koizumi, K., et al. (2009). Miogadial and miogatrial with alpha,beta-unsaturated 1,4-dialdehyde moieties--novel and potent TRPA1 agonists. Life Sci. 85 (1–2), 60–69. doi:10.1016/J.LFS.2009.04.017
Jancsó-Gábor, A., Szolcsányi, J., and Jancsó, N. (1970). Irreversible impairment of thermoregulation induced by capsaicin and similar pungent substances in rats and Guinea-pigs. J. Physiology 206 (3), 495–507. doi:10.1113/JPHYSIOL.1970.SP009027
Jara-Oseguera, A., Huffer, K. E., and Swartz, K. J. (2019). The ion selectivity filter is not an activation gate in TRPV1-3 channels. eLife 8, e51212–e51227. doi:10.7554/eLife.51212
Jara-Oseguera, A., and Islas, L. D. (2013). The role of allosteric coupling on thermal activation of thermo-TRP channels. Biophysical J. 104 (10), 2160–2169. doi:10.1016/J.BPJ.2013.03.055
Jeong, J. H., Lee, D. K., Liu, S. M., Chua, S. C., Schwartz, G. J., and Jo, Y. H. (2018). Activation of temperature-sensitive TRPV1-like receptors in ARC POMC neurons reduces food intake. PLOS Biol. 16 (4), e2004399. doi:10.1371/JOURNAL.PBIO.2004399
Jeske, N. A., Patwardhan, A. M., Ruparel, N. B., Akopian, A. N., Shapiro, M. S., and Henry, M. A. (2009). A-kinase anchoring protein 150 controls protein kinase C-mediated phosphorylation and sensitization of TRPV1. PAIN® 146 (3), 301–307. doi:10.1016/J.PAIN.2009.08.002
Ji, R. R., Kohno, T., Moore, K. A., and Woolf, C. J. (2003). Central sensitization and LTP: do pain and memory share similar mechanisms? Trends Neurosci. 26 (12), 696–705. doi:10.1016/J.TINS.2003.09.017
Ji, R. R., Nackley, A., Huh, Y., Terrando, N., and Maixner, W. (2018). Neuroinflammation and central sensitization in chronic and widespread pain. Anesthesiology 129 (2), 343–366. doi:10.1097/ALN.0000000000002130
Ji, R. R., Samad, T. A., Jin, S. X., Schmoll, R., and Woolf, C. J. (2002). p38 MAPK activation by NGF in primary sensory neurons after inflammation increases TRPV1 levels and maintains heat hyperalgesia. Neuron 36 (1), 57–68. doi:10.1016/S0896-6273(02)00908-X
Ji, R. R., Xu, Z. Z., and Gao, Y. J. (2014). Emerging targets in neuroinflammation-driven chronic pain. Nat. Rev. Drug Discov. 13 (7), 533–548. doi:10.1038/nrd4334
Johansen, M. E., Reilly, C. A., and Yost, G. S. (2006). TRPV1 antagonists elevate cell surface populations of receptor protein and exacerbate TRPV1-mediated toxicities in human lung epithelial cells. Toxicol. Sci. 89 (1), 278–286. doi:10.1093/TOXSCI/KFI292
Jordt, S. E., Bautista, D. M., Chuang, H. H., McKemy, D. D., Zygmunt, P. M., Högestätt, E. D., et al. (2004). Mustard oils and cannabinoids excite sensory nerve fibres through the TRP channel ANKTM1. Nature 427(6971), 260–265. doi:10.1038/nature02282
Jordt, S. E., and Julius, D. (2002). Molecular basis for species-specific sensitivity to “hot” chili peppers. Cell 108 (3), 421–430. doi:10.1016/S0092-8674(02)00637-2
Jordt, S. E., Tominaga, M., and Julius, D. (2000). Acid potentiation of the capsaicin receptor determined by a key extracellular site. Proc. Natl. Acad. Sci. 97 (14), 8134–8139. doi:10.1073/PNAS.100129497
Julius, D. (2013). TRP channels and pain. Annu. Rev. Cell Dev. Biol. 29, 355–384. doi:10.1146/annurev-cellbio-101011-155833
Jumper, J., Evans, R., Pritzel, A., Green, T., Figurnov, M., Ronneberger, O., et al. (2021). Applying and improving AlphaFold at CASP14. Proteins Struct. Funct. Bioinforma. 89 (12), 1711–1721. doi:10.1002/PROT.26257
Jung, J., Hwang, S. W., Kwak, J., Lee, S. Y., Kang, C. J., Kim, W. B., et al. (1999). Capsaicin binds to the intracellular domain of the capsaicin-activated ion channel. J. Neurosci. 19 (2), 529–538. doi:10.1523/JNEUROSCI.19-02-00529.1999
Jung, J., Shin, J. S., Lee, S. Y., Hwang, S. W., Koo, J., Cho, H., et al. (2004). Phosphorylation of vanilloid receptor 1 by Ca 2+/Calmodulin-dependent kinase II regulates its vanilloid binding. J. Biol. Chem. 279 (8), 7048–7054. doi:10.1074/jbc.M311448200
Karashima, Y., Talavera, K., Everaerts, W., Janssens, A., Kwan, K. Y., Vennekens, R., et al. (2009). TRPA1 acts as a cold sensor in vitro and in vivo. Proc. Natl. Acad. Sci. U. S. America, 106. MP4, 1273–1278. doi:10.1073/pnas.0808487106
Kark, T., Bagi, Z., Lizanecz, E., Pásztor, E. T., Erdei, N., Czikora, A., et al. (2008). Tissue-specific regulation of microvascular diameter: opposite functional roles of neuronal and smooth muscle located vanilloid receptor-1. Mol. Pharmacol. 73 (5), 1405–1412. doi:10.1124/MOL.107.043323
Khodarahmi, G., Asadi, P., Farrokhpour, H., Hassanzadeh, F., and Dinari, M. (2015). Design of novel potential aromatase inhibitors via hybrid pharmacophore approach: docking improvement using the QM/MM method. RSC Adv. 5 (71), 58055–58064. doi:10.1039/c5ra10097f
Kim, S., Chen, J., Cheng, T., Gindulyte, A., He, J., He, S., et al. (2023). PubChem 2023 update. Nucleic Acids Res. 51 (D1), D1373–D1380. doi:10.1093/NAR/GKAC956
Kizawa, K., Kitahara, T., Horii, A., Maekawa, C., Kuramasu, T., Kawashima, T., et al. (2010). Behavioral assessment and identification of a molecular marker in a salicylate-induced tinnitus in rats. Neuroscience 165 (4), 1323–1332. doi:10.1016/J.NEUROSCIENCE.2009.11.048
Klauck, T. M., Faux, M. C., Labudda, K., Langeberg, L. K., Jaken, S., and Scott, J. D. (1996). Coordination of three signaling enzymes by AKAP79, a mammalian scaffold protein. Science 271 (5255), 1589–1592. doi:10.1126/SCIENCE.271.5255.1589
Kobata, K., Tate, H., Iwasaki, Y., Tanaka, Y., Ohtsu, K., Yazawa, S., et al. (2008). Isolation of coniferyl esters from Capsicum baccatum L., and their enzymatic preparation and agonist activity for TRPV1. Phytochemistry 69 (5), 1179–1184. doi:10.1016/J.PHYTOCHEM.2007.11.017
Kojima, R., Ishida, S., Ohta, M., Iwata, H., Honma, T., and Okuno, Y. (2020). KGCN: a graph-based deep learning framework for chemical structures. J. Cheminformatics 12 (1), 32–10. doi:10.1186/s13321-020-00435-6
Kokubun, S., Sato, T., Ogawa, C., Kudo, K., Goto, K., Fujii, Y., et al. (2015). Distribution of TRPV1 and TRPV2 in the human stellate ganglion and spinal cord. Neurosci. Lett. 590, 6–11. doi:10.1016/J.NEULET.2015.01.074
Kollar, J., and Frecer, V. (2018). How accurate is the description of ligand–protein interactions by a hybrid QM/MM approach? J. Mol. Model. 24 (1), 11–20. doi:10.1007/s00894-017-3537-z
Konovalov, K. A., Unarta, I. C., Cao, S., Goonetilleke, E. C., and Huang, X. (2021). Markov state models to study the functional dynamics of proteins in the wake of machine learning. JACS Au 1 (9), 1330–1341. doi:10.1021/jacsau.1c00254
Kremeyer, B., Lopera, F., Cox, J. J., Momin, A., Rugiero, F., Marsh, S., et al. (2010). A gain-of-function mutation in TRPA1 causes familial episodic pain syndrome. Neuron 66 (5), 671–680. doi:10.1016/J.NEURON.2010.04.030
Kwon, D. H., Zhang, F., Fedor, J. G., Suo, Y., and Lee, S. Y. (2022). Vanilloid-dependent TRPV1 opening trajectory from cryoEM ensemble analysis. Nat. Commun. 13 (1), 2874–2912. doi:10.1038/s41467-022-30602-2
Kwon, D. H., Zhang, F., Suo, Y., Bouvette, J., Borgnia, M. J., and Lee, S. Y. (2021). Heat-dependent opening of TRPV1 in the presence of capsaicin. Nat. Struct. Mol. Biol. 28 (7), 554–563. doi:10.1038/s41594-021-00616-3
Latorre, R., and Díaz-Franulic, I. (2022). Profile of David Julius and Ardem patapoutian: 2021 Nobel laureates in physiology or medicine. Proc. Natl. Acad. Sci. U. S. A. 119 (1), e2121015119. doi:10.1073/pnas.2121015119
Latremoliere, A., and Woolf, C. J. (2009). Central sensitization: a generator of pain hypersensitivity by central neural plasticity. J. Pain 10 (9), 895–926. doi:10.1016/J.JPAIN.2009.06.012
Laursen, W. J., Schneider, E. R., Merriman, D. K., Bagriantsev, S. N., and Gracheva, E. O. (2016). Low-cost functional plasticity of TRPV1 supports heat tolerance in squirrels and camels. Proc. Natl. Acad. Sci. U. S. A. 113 (40), 11342–11347. doi:10.1073/pnas.1604269113
Lee, L. Y., Ni, D., Hayes, D., and Lin, R. L. (2011). TRPV1 as a cough sensor and its temperature-sensitive properties. Pulm. Pharmacol. Ther. 24 (3), 280–285. doi:10.1016/J.PUPT.2010.12.003
Leiser, M., Rubins, C. S., and Erlichman, J. (1986). Differential binding of the regulatory subunits (RII) of cAMP-dependent protein kinase II from bovine brain and muscle to RII-binding proteins. J. Biol. Chem. 261 (4), 1904–1908. doi:10.1016/s0021-9258(17)36027-1
Li, H. B., Mao, R. R., Zhang, J. C., Yang, Y., Cao, J., and Xu, L. (2008). Antistress effect of TRPV1 channel on synaptic plasticity and spatial memory. Biol. Psychiatry 64 (4), 286–292. doi:10.1016/J.BIOPSYCH.2008.02.020
Li, L., Chen, C., Chiang, C., Xiao, T., Chen, Y., Zhao, Y., et al. (2021). The impact of TRPV1 on cancer pathogenesis and therapy: a systematic review. Int. J. Biol. Sci. 17 (8), 2034–2049. doi:10.7150/IJBS.59918
Li, L., Chen, J., Ni, Y., Feng, X., Zhao, Z., Wang, P., et al. (2012). TRPV1 activation prevents nonalcoholic fatty liver through UCP2 upregulation in mice. Pflugers Archiv Eur. J. Physiology 463 (5), 727–732. doi:10.1007/s00424-012-1078-y
Liao, M., Cao, E., Julius, D., and Cheng, Y. (2013). Structure of the TRPV1 ion channel determined by electron cryo-microscopy. Nature 504 (7478), 107–112. doi:10.1038/NATURE12822
Lishko, P. V., Procko, E., Jin, X., Phelps, C. B., and Gaudet, R. (2007). The ankyrin repeats of TRPV1 bind multiple ligands and modulate channel sensitivity. Neuron 54 (6), 905–918. doi:10.1016/j.neuron.2007.05.027
Liu, B., Hui, K., and Qin, F. (2003). Thermodynamics of heat activation of single capsaicin ion channels VR1. Biophysical J. 85 (5), 2988–3006. doi:10.1016/S0006-3495(03)74719-5
Liu, C., Miao, R., Raza, F., Qian, H., and Tian, X. (2023). Research progress and challenges of TRPV1 channel modulators as a prospective therapy for diabetic neuropathic pain. Eur. J. Med. Chem. 245, 114893. doi:10.1016/J.EJMECH.2022.114893
Liu, C., and Montell, C. (2015). Forcing open TRP channels: mechanical gating as a unifying activation mechanism. Biochem. Biophysical Res. Commun. 460 (1), 22–25. doi:10.1016/J.BBRC.2015.02.067
Lomize, A. L., Schnitzer, K. A., Todd, S. C., Cherepanov, S., Outeiral, C., Deane, C. M., et al. (2022). Membranome 3.0: database of single-pass membrane proteins with AlphaFold models. Protein Sci. 31 (5), e4318. doi:10.1002/PRO.4318
López-Romero, A. E., Hernández-Araiza, I., Torres-Quiroz, F., Tovar-Y-Romo, L. B., Islas, L. D., and Rosenbaum, T. (2019). TRP ion channels: proteins with conformational flexibility. Proteins conformational Flex. 13(1), 207–226. doi:10.1080/19336950.2019.1626793
Lu, Y., Allen, M., Halt, A. R., Weisenhaus, M., Dallapiazza, R. F., Hall, D. D., et al. (2007). Age-dependent requirement of AKAP150-anchored PKA and GluR2-lacking AMPA receptors in LTP. EMBO J. 26 (23), 4879–4890. doi:10.1038/SJ.EMBOJ.7601884
Luo, L., Wang, Y., Li, B., Xu, L., Kamau, P. M., Zheng, J., et al. (2019). Molecular basis for heat desensitization of TRPV1 ion channels. Nat. Commun. 10 (1), 2134. doi:10.1038/s41467-019-09965-6
Maione, S., Cristino, L., Migliozzi, A. L., Georgiou, A. L., Starowicz, K., Salt, T. E., et al. (2009). TRPV1 channels control synaptic plasticity in the developing superior colliculus. J. Physiology 587 (11), 2521–2535. doi:10.1113/JPHYSIOL.2009.171900
Manchanda, M., Leishman, E., Sangani, K., Alamri, A., and Bradshaw, H. B. (2021). Activation of TRPV1 by capsaicin or heat drives changes in 2-acyl glycerols and N-acyl ethanolamines in a time, dose, and temperature dependent manner. Front. Cell Dev. Biol. 9, 611952. doi:10.3389/fcell.2021.611952
Marcu, Ş. B., Tăbîrcă, S., and Tangney, M. (2022). An overview of alphafold’s breakthrough. Front. Artif. Intell. 5, 875587. doi:10.3389/FRAI.2022.875587
Matsuda, M., Oh-Hashi, K., Yokota, I., Sawa, T., and Amaya, F. (2017). Acquired exchange protein directly activated by cyclic adenosine monophosphate activity induced by p38 mitogen-activated protein kinase in primary afferent neurons contributes to sustaining postincisional nociception. Anesthesiology 126 (1), 150–162. doi:10.1097/ALN.0000000000001401
Matsumoto, K., Kurosawa, E., Terui, H., Hosoya, T., Tashima, K., Murayama, T., et al. (2009). Localization of TRPV1 and contractile effect of capsaicin in mouse large intestine: high abundance and sensitivity in rectum and distal colon. Am. J. Physiology - Gastrointest. Liver Physiology 297 (2), 348–360. doi:10.1152/ajpgi.90578.2008
Matta, J., Ahern, P., and Gerard, P. A. (2010). TRPV1 and synaptic transmission. Curr. Pharm. Biotechnol. 12 (1), 95–101. doi:10.2174/138920111793937925
Matta, J. A., Miyares, R. L., and Ahern, G. P. (2007). TRPV1 is a novel target for omega-3 polyunsaturated fatty acids. J. Physiology 578 (2), 397–411. doi:10.1113/JPHYSIOL.2006.121988
Meller, A., Bhakat, S., Solieva, S., and Bowman, G. R. (2023). Accelerating cryptic pocket discovery using AlphaFold. J. Chem. theory Comput. 19, 4355–4363. [Preprint]. doi:10.1021/ACS.JCTC.2C01189
Meller, A., de Oliveira, S., Davtyan, A., Abramyan, T., Bowman, G. R., and van den Bedem, H. (2023). ‘Discovery of a cryptic pocket in the AI-predicted structure of PPM1D phosphatase explains the binding site and potency of its allosteric inhibitors. bioRxiv Prepr. Serv. Biol., 2023.03.22.533829. [Preprint]. doi:10.1101/2023.03.22.533829
Meller, A., Ward, M., Borowsky, J., Kshirsagar, M., Lotthammer, J. M., Oviedo, F., et al. (2023). Predicting locations of cryptic pockets from single protein structures using the PocketMiner graph neural network. Nat. Commun. 14 (1), pp. 1177–1215. doi:10.1038/s41467-023-36699-3
Melnick, C., and Kaviany, M. (2018). Thermal actuation in TRPV1: role of embedded lipids and intracellular domains. J. Theor. Biol. 444, 38–49. doi:10.1016/J.JTBI.2018.02.004
Melo Júnior, J., Damasceno, M. D. B. M. V., Santos, S. A. A. R., Barbosa, T. M., Araújo, J. R. C., Vieira-Neto, A. E., et al. (2017). Acute and neuropathic orofacial antinociceptive effect of eucalyptol. Inflammopharmacology 25 (2), 247–254. doi:10.1007/s10787-017-0324-5
Mendez, D., Gaulton, A., Bento, A. P., Chambers, J., De Veij, M., Félix, E., et al. (2019). ChEMBL: towards direct deposition of bioassay data. Nucleic Acids Res. 47 (D1), D940. doi:10.1093/NAR/GKY1075
Meza, R. C., Ancatén-González, C., Chiu, C. Q., and Chávez, A. E. (2022). Transient receptor potential vanilloid 1 function at central synapses in health and disease. Front. Cell. Neurosci. 16, 864828. doi:10.3389/fncel.2022.864828
Mezey, É., Tóth, Z. E., Cortright, D. N., Arzubi, M. K., Krause, J. E., Elde, R., et al. (2000). Distribution of mRNA for vanilloid receptor subtype 1 (VR1), and VR1-like immunoreactivity, in the central nervous system of the rat and human. Proc. Natl. Acad. Sci. U. S. A. 97 (7), 3655–3660. doi:10.1073/pnas.060496197
Mickle, A. D., Shepherd, A. J., and Mohapatra, D. P. (2015). Sensory TRP channels: the key transducers of nociception and pain. Prog. Mol. Biol. Transl. Sci. 131, 73–118. doi:10.1016/BS.PMBTS.2015.01.002
Mickle, A. D., Shepherd, A. J., and Mohapatra, D. P. (2016). Nociceptive TRP channels: sensory detectors and transducers in multiple pain pathologies. Pharmaceuticals 9 (4), 72. doi:10.3390/PH9040072
Mistretta, F., Buffi, N. M., Lughezzani, G., Lista, G., Larcher, A., Fossati, N., et al. (2014). Bladder cancer and urothelial impairment: the role of TRPV1 as potential drug target. BioMed Res. Int. 2014, 987149. doi:10.1155/2014/987149
Mizukoshi, K., Sasaki, M., Izumi, Y., Miura, M., Watanabe, M., and Amaya, F. (2013). Activation of p38 mitogen-activated protein kinase in the dorsal root ganglion contributes to pain hypersensitivity after plantar incision. Neuroscience 234, 77–87. doi:10.1016/J.NEUROSCIENCE.2013.01.001
Montell, C., Birnbaumer, L., Flockerzi, V., Bindels, R. J., Bruford, E. A., Caterina, M. J., et al. (2002). A unified nomenclature for the superfamily of TRP cation channels. Mol. Cell 9, 229–231. doi:10.1016/s1097-2765(02)00448-3
Montell, C., and Rubin, G. M. (1989). Molecular characterization of the Drosophila trp locus: a putative integral membrane protein required for phototransduction. Neuron 2, 1313–1323. doi:10.1016/0896-6273(89)90069-x
Moparthi, L., Kichko, T. I., Eberhardt, M., Högestätt, E. D., Kjellbom, P., Johanson, U., et al. (2016). Human TRPA1 is a heat sensor displaying intrinsic U-shaped thermosensitivity. Sci. Rep. 6 (1), 28763–28810. doi:10.1038/srep28763
Moqrich, A., Hwang, S. W., Earley, T. J., Petrus, M. J., Murray, A. N., Spencer, K. S. R., et al. (2005). Impaired thermosensation in mice lacking TRPV3, a heat and camphor sensor in the skin. Science 307 (5714), 1468–1472. doi:10.1126/science.1108609
Morales-Lázaro, S. L., Llorente, I., Sierra-Ramírez, F., López-Romero, A. E., Ortíz-Rentería, M., Serrano-Flores, B., et al. (2016). Inhibition of TRPV1 channels by a naturally occurring omega-9 fatty acid reduces pain and itch. Nat. Commun. 7, 13092. doi:10.1038/ncomms13092
Morales-Lázaro, S. L., and Rosenbaum, T. (2015). A painful link between the TRPV1 channel and lysophosphatidic acid. Life Sci. 125, 15–24. doi:10.1016/J.LFS.2014.10.004
Morenilla-Palao, C., Planells-Cases, R., García-Sanz, N., and Ferrer-Montiel, A. (2004). Regulated exocytosis contributes to protein kinase C potentiation of vanilloid receptor activity. J. Biol. Chem. 279 (24), 25665–25672. doi:10.1074/JBC.M311515200
Mukherjea, D., Jajoo, S., Whitworth, C., Bunch, J. R., Turner, J. G., Rybak, L. P., et al. (2008). Short interfering RNA against transient receptor potential vanilloid 1 attenuates cisplatin-induced hearing loss in the rat. J. Neurosci. 28 (49), 13056–13065. doi:10.1523/JNEUROSCI.1307-08.2008
Muzzi, M., Felici, R., Cavone, L., Gerace, E., Minassi, A., Appendino, G., et al. (2012). Ischemic neuroprotection by TRPV1 receptor-induced hypothermia. J. Cereb. Blood Flow Metabolism 32 (6), 978–982. doi:10.1038/jcbfm.2012.36
Myers, B. R., Bohlen, C. J., and Julius, D. (2008). A yeast genetic screen reveals a critical role for the pore helix domain in TRP channel gating. Neuron 58 (3), 362–373. doi:10.1016/j.neuron.2008.04.012
Nagy, I., and Rang, H. P. (1999). Similarities and differences between the responses of rat sensory neurons to noxious heat and capsaicin. J. Neurosci. 19 (24), 10647–10655. doi:10.1523/JNEUROSCI.19-24-10647.1999
Nannenga, B. L., and Gonen, T. (2019) ‘The cryo-EM method microcrystal electron diffraction (MicroED)’, Nat. Methods 16(5), 369–379. doi:10.1038/s41592-019-0395-x
Navedo, M. F., Nieves-Cintrón, M., Amberg, G. C., Yuan, C., Votaw, V. S., Lederer, W. J., et al. (2008). AKAP150 is required for stuttering persistent Ca2+ sparklets and angiotensin II–induced hypertension. Circulation Res. 102 (2), e1–e11. doi:10.1161/CIRCRESAHA.107.167809
Neuberger, A., Oda, M., Nikolaev, Y. A., Nadezhdin, K. D., Gracheva, E. O., Bagriantsev, S. N., et al. (2023) ‘Human TRPV1 structure and inhibition by the analgesic SB-366791’, Nat. Commun. 14(1), 2451–2510. doi:10.1038/s41467-023-38162-9
Nowotka, M. M., Gaulton, A., Mendez, D., Bento, A. P., Hersey, A., and Leach, A. (2017). Using ChEMBL web services for building applications and data processing workflows relevant to drug discovery. Expert Opin. Drug Discov. 12 (8), 757–767. doi:10.1080/17460441.2017.1339032
Numazaki, M., Tominaga, T., Toyooka, H., and Tominaga, M. (2002). Direct phosphorylation of capsaicin receptor VR1 by protein kinase Cepsilon and identification of two target serine residues. J. Biol. Chem. 277 (16), 13375–13378. doi:10.1074/jbc.C200104200
Nussinov, R., and Tsai, C.-J. (2012). The different ways through which specificity works in orthosteric and allosteric drugs. Curr. Pharm. Des. 18 (9), 1311–1316. doi:10.2174/138161212799436377
Nussinov, R., and Tsai, C. J. (2014). Unraveling structural mechanisms of allosteric drug action. Trends Pharmacol. Sci. 35 (5), 256–264. doi:10.1016/J.TIPS.2014.03.006
Nussinov, R., Tsai, C. J., and Liu, J. (2014). Principles of allosteric interactions in cell signaling. J. Am. Chem. Soc. 136 (51), 17692–17701. doi:10.1021/ja510028c
Nussinov, R., Zhang, M., Liu, Y., and Jang, H. (2023). AlphaFold, allosteric, and orthosteric drug discovery: ways forward. Drug Discov. Today 28, 103551. doi:10.1016/j.drudis.2023.103551
Obata, K., Yamanaka, H., Kobayashi, K., Dai, Y., Mizushima, T., Katsura, H., et al. (2004). Role of mitogen-activated protein kinase activation in injured and intact primary afferent neurons for mechanical and heat hypersensitivity after spinal nerve ligation. J. Neurosci. 24 (45), 10211–10222. doi:10.1523/JNEUROSCI.3388-04.2004
Okada, Y., Reinach, P. S., Shirai, K., Kitano, A., Kao, W. W. Y., Flanders, K. C., et al. (2011). TRPV1 involvement in inflammatory tissue fibrosis in mice. Am. J. Pathology 178 (6), 2654–2664. doi:10.1016/J.AJPATH.2011.02.043
Oláh, Z., Rédei, D., Pecze, L., Vizler, C., Jósvay, K., Forgó, P., et al. (2017). Pellitorine, an extract of Tetradium daniellii, is an antagonist of the ion channel TRPV1. Phytomedicine 34, 44–49. doi:10.1016/J.PHYMED.2017.06.006
Oliveria, S. F., Dell’Acqua, M. L., and Sather, W. A. (2007). AKAP79/150 anchoring of calcineurin controls neuronal L-type Ca2+ channel activity and nuclear signaling. Neuron 55 (2), 261–275. doi:10.1016/J.NEURON.2007.06.032
Pahari, S., Sun, L., and Alexov, E. (2019). ‘PKAD: a database of experimentally measured pKa values of ionizable groups in proteins. Database 2019 (1), baz024. doi:10.1093/DATABASE/BAZ024
Papadatos, G., Gaulton, A., Hersey, A., and Overington, J. P. (2015). Activity, assay and target data curation and quality in the ChEMBL database. J. Computer-Aided Mol. Des. 29 (9), 885–896. doi:10.1007/s10822-015-9860-5
Papakosta, M., Dalle, C., Haythornthwaite, A., Cao, L., Stevens, E. B., Burgess, G., et al. (2011). The chimeric approach reveals that differences in the TRPV1 pore domain determine species-specific sensitivity to block of heat activation. J. Biol. Chem. 286 (45), 39663–39672. doi:10.1074/jbc.M111.273581
Paricio-Montesinos, R., Schwaller, F., Udhayachandran, A., Rau, F., Walcher, J., Evangelista, R., et al. (2020). The sensory coding of warm perception. Neuron 106 (5), 830–841. doi:10.1016/J.NEURON.2020.02.035
Patapoutian, A., Peier, A. M., Story, G. M., and Viswanath, V. (2003a). ThermoTRP channels and beyond: mechanisms of temperature sensation. Nat. Rev. Neurosci. 4 (7), 529–539. doi:10.1038/nrn1141
Patapoutian, A., Tate, S., and Woolf, C. J. (2009b). Transient receptor potential channels: targeting pain at the source. Nat. Rev. Drug Discov. 8 (1), 55–68. doi:10.1038/nrd2757
Pedregosa, F., Varoquaux, G., Gramfort, A., Michel, V., Thirion, B., Grisel, O., et al. (2012). Scikit-learn: machine learning in Python. J. Mach. Learn. Res. 12, 2825–2830. doi:10.48550/arXiv.1201.0490
Pereira, J., Simpkin, A. J., Hartmann, M. D., Rigden, D. J., Keegan, R. M., and Lupas, A. N. (2021). High-accuracy protein structure prediction in CASP14. Proteins Struct. Funct. Bioinforma. 89 (12), 1687–1699. doi:10.1002/PROT.26171
Phan, T. X., Ton, H. T., Gulyás, H., Pórszász, R., Tóth, A., Russo, R., et al. (2020). TRPV1 expressed throughout the arterial circulation regulates vasoconstriction and blood pressure. J. Physiology 598 (24), 5639–5659. doi:10.1113/JP279909
Picazo-Juárez, G., Romero-Suárez, S., Nieto-Posadas, A., Llorente, I., Jara-Oseguera, A., Briggs, M., et al. (2011). Identification of a binding motif in the S5 helix that confers cholesterol sensitivity to the TRPV1 ion channel. J. Biol. Chem. 286 (28), 24966–24976. doi:10.1074/jbc.M111.237537
Poblete, H., Oyarzún, I., Olivero, P., Comer, J., Zuñiga, M., Sepulveda, R. V., et al. (2015). Molecular determinants of phosphatidylinositol 4,5-bisphosphate (PI(4,5)P2) binding to transient receptor potential V1 (TRPV1) channels. J. Biol. Chem. 290 (4), 2086–2098. doi:10.1074/jbc.M114.613620
Premkumar, L. S., Agarwal, S., and Steffen, D. (2002). Single-channel properties of native and cloned rat vanilloid receptors. J. Physiology 545 (1), 107–117. doi:10.1113/JPHYSIOL.2002.016352
Raddatz, N., Castillo, J. P., Gonzalez, C., Alvarez, O., and Latorre, R. (2014). Temperature and voltage coupling to channel opening in transient receptor potential melastatin 8 (TRPM8). J. Biol. Chem. 289 (51), 35438–35454. doi:10.1074/jbc.M114.612713
Ransohoff, R. M., and Brown, M. A. (2012). Innate immunity in the central nervous system. J. Clin. Investigation 122 (4), 1164–1171. doi:10.1172/JCI58644
Razavi, R., Chan, Y., Afifiyan, F. N., Liu, X. J., Wan, X., Yantha, J., et al. (2006). TRPV1+ sensory neurons control β cell stress and islet inflammation in autoimmune diabetes. Cell 127 (6), 1123–1135. doi:10.1016/J.CELL.2006.10.038
RDKit (2023). RDKit. Available at: https://www.rdkit.org/ (Accessed: October 16, 2023).
Richardson, J. D., and Vasko, M. R. (2002). Cellular mechanisms of neurogenic inflammation. J. Pharmacol. Exp. Ther. 302 (3), 839–845. Available at:. doi:10.1124/JPET.102.032797
Riera, C. E., Menozzi-Smarrito, C., Affolter, M., Michlig, S., Munari, C., Robert, F., et al. (2009). Compounds from Sichuan and Melegueta peppers activate, covalently and non-covalently, TRPA1 and TRPV1 channels. Br. J. Pharmacol. 157 (8), 1398–1409. doi:10.1111/J.1476-5381.2009.00307.X
Rita Pereira, E. M., Souza, J. M., Carobin, N. V., Silva, J. F., Santos, D. C., Silva Júnior, C. A., et al. (2020). Phoneutria toxin PnTx3-5 inhibits TRPV1 channel with antinociceptive action in an orofacial pain model. Neuropharmacology 162, 107826. doi:10.1016/J.NEUROPHARM.2019.107826
Rohacs, T. (2014). Phosphoinositide regulation of TRP channels. Handb. Exp. Pharmacol. 223, 1143–1176. doi:10.1007/978-3-319-05161-1_18
Rosenbaum, T., and Islas, L. D. (2023). Molecular physiology of TRPV channels: controversies and future challenges. Annu. Rev. Physiology 85, 293–316. doi:10.1146/annurev-physiol-030222-012349
Rosenbaum, T., and Morales-Lázaro, S. L. (2023). Regulation of ThermoTRP channels by PIP2 and cholesterol. Adv. Exp. Med. Biol. 1422, 245–277. doi:10.1007/978-3-031-21547-6_9
Rosenbaum, T., and Simon, S. A. (2007). TRPV1 receptors and signal transduction. TRP Ion Channel Funct. Sens. Transduct. Cell. Signal. Cascades, 91–106. doi:10.1201/9781420005844-11
Rossato, M. F., Trevisan, G., Walker, C. I. B., Klafke, J. Z., de Oliveira, A. P., Villarinho, J. G., et al. (2011). Eriodictyol: a flavonoid antagonist of the TRPV1 receptor with antioxidant activity. Biochem. Pharmacol. 81 (4), 544–551. doi:10.1016/J.BCP.2010.11.004
Ruddigkeit, L., van Deursen, R., Blum, L. C., and Reymond, J. L. (2012). Enumeration of 166 billion organic small molecules in the chemical universe database GDB-17. J. Chem. Inf. Model. 52 (11), 2864–2875. doi:10.1021/ci300415d
Ryde, U., and Söderhjelm, P. (2016). Ligand-binding affinity estimates supported by quantum-mechanical methods. Chem. Rev. 116 (9), 5520–5566. doi:10.1021/acs.chemrev.5b00630
Ryu, S., Liu, B., and Qin, F. (2003). Low pH potentiates both capsaicin binding and channel gating of VR1 receptors. J. General Physiology 122 (1), 45–61. doi:10.1085/JGP.200308847
Sadybekov, A. V., and Katritch, V. (2023). Computational approaches streamlining drug discovery. Nature 616 (7958), 673–685. doi:10.1038/s41586-023-05905-z
Saffarzadeh, F., Eslamizade, M. J., Mousavi, S. M. M., Abraki, S. B., Hadjighassem, M. R., and Gorji, A. (2016). TRPV1 receptors augment basal synaptic transmission in CA1 and CA3 pyramidal neurons in epilepsy. Neuroscience 314, 170–178. doi:10.1016/J.NEUROSCIENCE.2015.11.045
Saito, S., Ohkita, M., Saito, C. T., Takahashi, K., Tominaga, M., and Ohta, T. (2016). Evolution of heat sensors drove shifts in thermosensation between Xenopus species adapted to different thermal niches. J. Biol. Chem. 291 (21), 11446–11459. doi:10.1074/jbc.M115.702498
Saito, S., and Shingai, R. (2006). Evolution of thermoTRP ion channel homologs in vertebrates. Physiol. genomics 27 (3), 219–230. doi:10.1152/PHYSIOLGENOMICS.00322.2005
Saito, S., and Tominaga, M. (2015). Functional diversity and evolutionary dynamics of thermoTRP channels. Cell Calcium 57 (3), 214–221. doi:10.1016/J.CECA.2014.12.001
Saito, S., and Tominaga, M. (2017) ‘Evolutionary tuning of TRPA1 and TRPV1 thermal and chemical sensitivity in vertebrates. Temp. (Austin). 4(2), 141–152. doi:10.1080/23328940.2017.1315478
Saldívar-González, F. I., Huerta-García, C. S., and Medina-Franco, J. L. (2020). Chemoinformatics-based enumeration of chemical libraries: a tutorial. J. Cheminformatics 12 (1), 64. doi:10.1186/s13321-020-00466-z
Sandoz, G., Thümmler, S., Duprat, F., Feliciangeli, S., Vinh, J., Escoubas, P., et al. (2006). AKAP150, a switch to convert mechano-pH- and arachidonic acid-sensitive TREK K+ channels into open leak channels. EMBO J. 25 (24), 5864–5872. doi:10.1038/SJ.EMBOJ.7601437
Sanz-Salvador, L., Andrés-Borderia, A., Ferrer-Montiel, A., and Planells-Cases, R. (2012). Agonist- and Ca2+-dependent desensitization of TRPV1 channel targets the receptor to lysosomes for degradation. J. Biol. Chem. 287 (23), 19462–19471. doi:10.1074/jbc.M111.289751
Sardar, P., Kumar, A., Bhandari, A., and Goswami, C. (2012). Conservation of tubulin-binding sequences in TRPV1 throughout evolution. PLoS ONE 7 (4), e31448. doi:10.1371/journal.pone.0031448
Sawada, Y., Hosokawa, H., Hori, A., Matsumura, K., and Kobayashi, S. (2007). Cold sensitivity of recombinant TRPA1 channels. Brain Res. 1160 (1), 39–46. doi:10.1016/J.BRAINRES.2007.05.047
Shi, Y., Gelman, B. B., Lisinicchia, J. G., and Tang, S. J. (2012). Chronic-pain-associated astrocytic reaction in the spinal cord dorsal horn of human immunodeficiency virus-infected patients. J. Neurosci. 32 (32), 10833–10840. doi:10.1523/JNEUROSCI.5628-11.2012
Shoemaker, S. C., and Ando, N. (2018). X-Rays in the cryo-electron microscopy era: structural biology's dynamic future. Biochemistry 57, 277–285. doi:10.1021/ACS.BIOCHEM.7B01031
Smajić, A., Grandits, M., and Ecker, G. F. (2022). Using Jupyter Notebooks for re-training machine learning models. J. Cheminformatics 14 (1), 1–9. doi:10.1186/S13321-022-00635-2/TABLES/4
Smith, G. D., Gunthorpe, M. J., Kelsell, R. E., Hayes, P. D., Reilly, P., Facer, P., et al. (2002). TRPV3 is a temperature-sensitive vanilloid receptor-like protein, Nature 418 (6894), 186–190. doi:10.1038/nature00894
Smith, K. E., Gibson, E. S., and Dell’Acqua, M. L. (2006). cAMP-dependent protein kinase postsynaptic localization regulated by NMDA receptor activation through translocation of an A-kinase anchoring protein scaffold protein. J. Neurosci. 26 (9), 2391–2402. doi:10.1523/JNEUROSCI.3092-05.2006
Song, K., Wang, H., Kamm, G. B., Pohle, J., Reis, F. d. C., Heppenstall, P., et al. (2016). The TRPM2 channel is a hypothalamic heat sensor that limits fever and can drive hypothermia. Science 353 (6306), 1393–1398. doi:10.1126/science.aaf7537
Song, S., Ayon, R. J., Yamamura, A., Yamamura, H., Dash, S., Babicheva, A., et al. (2017). Capsaicin-induced Ca2+ signaling is enhanced via upregulated TRPV1 channels in pulmonary artery smooth muscle cells from patients with idiopathic PAH. Am. J. Physiology - Lung Cell. Mol. Physiology 312 (3), L309–L325. doi:10.1152/ajplung.00357.2016
Sorokina, M., Merseburger, P., Rajan, K., Yirik, M. A., and Steinbeck, C. (2021). COCONUT online: collection of open natural products database. J. Cheminformatics 13 (1), 2–13. doi:10.1186/s13321-020-00478-9
Stein, A. T., Ufret-Vincenty, C. A., Hua, L., Santana, L. F., and Gordon, S. E. (2006). Phosphoinositide 3-kinase binds to TRPV1 and mediates NGF-stimulated TRPV1 trafficking to the plasma membrane. J. General Physiology 128 (5), 509–522. doi:10.1085/JGP.200609576
Stein, R. A., and McHaourab, H. S. (2022). SPEACH_AF: sampling protein ensembles and conformational heterogeneity with Alphafold2. PLOS Comput. Biol. 18 (8), e1010483. doi:10.1371/JOURNAL.PCBI.1010483
Stein, R. J., Santos, S., Nagatomi, J., Hayashi, Y., Minnery, B. S., Xavier, M., et al. (2004). COOL (TRPM8) AND HOT (TRPV1) RECEPTORS IN THE BLADDER AND MALE GENITAL TRACT. J. Urology 172 (3), 1175–1178. doi:10.1097/01.JU.0000134880.55119.CF
Studer, M., and McNaughton, P. A. (2010). Modulation of single-channel properties of TRPV1 by phosphorylation. J. Physiology 588 (19), 3743–3756. doi:10.1113/JPHYSIOL.2010.190611
Sui, F., Zhang, C. B., Yang, N., Li, L. F., Guo, S. Y., Huo, H. R., et al. (2010). Anti-nociceptive mechanism of baicalin involved in intervention of TRPV1 in DRG neurons in vitro. J. Ethnopharmacol. 129 (3), 361–366. doi:10.1016/J.JEP.2010.03.039
Sumino, A., Zhao, Y., Mukai, D., Sumikama, T., Puppulin, L., Hattori, M., et al. (2023). Antithetic effects of agonists and antagonists on the structural fluctuations of TRPV1 channel. Proc. Natl. Acad. Sci. 120 (20). doi:10.1073/pnas.2301013120
Sydow, D., Wichmann, M., Rodríguez-Guerra, J., Goldmann, D., Landrum, G., and Volkamer, A. (2019). TeachOpenCADD-KNIME: a teaching platform for computer-aided drug design using KNIME workflows. J. Chem. Inf. Model. 59, 4083–4086. [Preprint]. doi:10.1021/acs.jcim.9b00662
Szallasi, A., and Blumberg, P. M. (1989). Resiniferatoxin, a phorbol-related diterpene, acts as an ultrapotent analog of capsaicin, the irritant constituent in red pepper. Neuroscience 30 (2), 515–520. doi:10.1016/0306-4522(89)90269-8
Szallasi, A., Cortright, D. N., Blum, C. A., and Eid, S. R. (2007). The vanilloid receptor TRPV1: 10 years from channel cloning to antagonist proof-of-concept. Nat. Rev. Drug Discov. 6 (5), 357–372. doi:10.1038/nrd2280
Szelényi, Z., Hummel, Z., Szolcsányi, J., and Davis, J. B. (2004). Daily body temperature rhythm and heat tolerance in TRPV1 knockout and capsaicin pretreated mice. Eur. J. Neurosci. 19 (5), 1421–1424. doi:10.1111/J.1460-9568.2004.03221.X
Terada, Y., Masuda, H., and Watanabe, T. (2015). Structure-activity relationship study on isothiocyanates: comparison of TRPA1-activating ability between allyl isothiocyanate and specific flavor components of wasabi, horseradish, and white mustard. J. Nat. Prod. 78 (8), 1937–1941. doi:10.1021/acs.jnatprod.5b00272
Tian, Q., Hu, J., Xie, C., Mei, K., Pham, C., Mo, X., et al. (2019). Recovery from tachyphylaxis of TRPV1 coincides with recycling to the surface membrane. Proc. Natl. Acad. Sci. U. S. A. 116 (11), 5170–5175. doi:10.1073/pnas.1819635116
Tinivella, A., Pinzi, L., and Rastelli, G. (2021). Prediction of activity and selectivity profiles of human Carbonic Anhydrase inhibitors using machine learning classification models. J. Cheminformatics 13 (1), 18–15. doi:10.1186/s13321-021-00499-y
Togashi, K., Hara, Y., Tominaga, T., Higashi, T., Konishi, Y., Mori, Y., et al. (2006). TRPM2 activation by cyclic ADP-ribose at body temperature is involved in insulin secretion. EMBO J. 25 (9), 1804–1815. doi:10.1038/SJ.EMBOJ.7601083
Tominaga, M., Caterina, M. J., Malmberg, A. B., Rosen, T. A., Gilbert, H., Skinner, K., et al. (1998). The cloned capsaicin receptor integrates multiple pain-producing stimuli. Neuron 21 (3), 531–543. doi:10.1016/s0896-6273(00)80564-4
Touska, F., Marsakova, L., Teisinger, J., and Vlachova, V. (2011). A “cute” desensitization of TRPV1. Curr. Pharm. Biotechnol. 12 (1), 122–129. doi:10.2174/138920111793937826
Ufret-Vincenty, C. A., Klein, R. M., Hua, L., Angueyra, J., and Gordon, S. E. (2011). Localization of the PIP2 sensor of TRPV1 ion channels. J. Biol. Chem. 286 (11), 9688–9698. doi:10.1074/jbc.M110.192526
Vandewauw, I., De Clercq, K., Mulier, M., Held, K., Pinto, S., Van Ranst, N., et al. (2018). A TRP channel trio mediates acute noxious heat sensing. Nature 555 (7698), 662–666. doi:10.1038/nature26137
Van Santen, J. A., Poynton, E. F., Iskakova, D., McMann, E., Alsup, T. A., Clark, T. N., et al. (2022). The Natural Products Atlas 2.0: a database of microbially-derived natural products. Nucleic Acids Res. 50 (D1), D1317–D1323. doi:10.1093/NAR/GKAB941
Varadi, M., and Velankar, S. (2022). The impact of AlphaFold Protein Structure Database on the fields of life sciences. PROTEOMICS. 2200128. doi:10.1002/PMIC.202200128
Vay, L., Gu, C., and McNaughton, P. A. (2012). The thermo-TRP ion channel family: properties and therapeutic implications. Br. J. Pharmacol. 165 (4), 787–801. doi:10.1111/J.1476-5381.2011.01601.X
Vellani, V., Mapplebeck, S., Moriondo, A., Davis, J. B., and McNaughton, P. A. (2001). Protein kinase C activation potentiates gating of the vanilloid receptor VR1 by capsaicin, protons, heat and anandamide. J. Physiology 534 (3), 813–825. doi:10.1111/J.1469-7793.2001.00813.X
Voets, T. (2012). Quantifying and modeling the temperature-dependent gating of TRP channels. Rev. Physiology, Biochem. Pharmacol. 162, 91–119. doi:10.1007/112_2011_5
Voets, T., Droogmans, G., Wissenbach, U., Janssens, A., Flockerzi, V., and Nilius, B. (2004). The principle of temperature-dependent gating in cold- and heat-sensitive TRP channels. Nature 430:(7001). 748–754. doi:10.1038/nature02732
Voets, T., Talavera, K., Owsianik, G., and Nilius, B. (2005). Sensing with TRP channels. Nat. Chem. Biol. 1 (2), 85–92. doi:10.1038/nchembio0705-85
Vyklický, L., Vlachová, V., Vitásková, Z., Dittert, I., Kabát, M., and Orkand, R. K. (1999). Temperature coefficient of membrane currents induced by noxious heat in sensory neurones in the rat. J. Physiology 517 (1), 181–192. doi:10.1111/J.1469-7793.1999.0181Z.X
Waluk, D. P., Vielfort, K., Derakhshan, S., Aro, H., and Hunt, M. C. (2013). N-Acyl taurines trigger insulin secretion by increasing calcium flux in pancreatic β-cells. Biochem. Biophysical Res. Commun. 430 (1), 54–59. doi:10.1016/J.BBRC.2012.11.026
Wang, G. (2021). Lipid-dependent sequential allosteric activation of heat-sensing TRPV1 channels by anchor-stereoselective “hot” vanilloid compounds and analogs. Biochem. Biophysics Rep. 28, 101109. doi:10.1016/J.BBREP.2021.101109
Wang, S., Yamamoto, S., Kogure, Y., Zhang, W., Noguchi, K., and Dai, Y. (2016). Partial activation and inhibition of TRPV1 channels by evodiamine and rutaecarpine, two major components of the fruits of evodia rutaecarpa. J. Nat. Prod. 79 (5), 1225–1230. doi:10.1021/acs.jnatprod.5b00599
Watanabe, H., Vriens, J., Suh, S. H., Benham, C. D., Droogmans, G., and Nilius, B. (2002). Heat-evoked activation of TRPV4 channels in a HEK293 cell expression system and in native mouse aorta endothelial cells. J. Biol. Chem. 277 (49), 47044–47051. doi:10.1074/jbc.M208277200
Waxman, S. G., Dib-Hajj, S., Cummins, T. R., and Black, J. A. (1999). Sodium channels and pain. Proc. Natl. Acad. Sci. U. S. A. 96 (14), 7635–7639. doi:10.1073/pnas.96.14.7635
Weber, L. V., Al-Refae, K., Wölk, G., Bonatz, G., Altmüller, J., Becker, C., et al. (2016). Expression and functionality of TRPV1 in breast cancer cells. Breast Cancer Targets Ther. 8, 243–252. doi:10.2147/BCTT.S121610
Wei, L. S., Chen, S., Huang, X. J., Yao, J., and Liu, X. M. (2013). Material basis for inhibition of dragon’s blood on capsaicin-induced TRPV1 receptor currents in rat dorsal root ganglion neurons. Eur. J. Pharmacol. 702 (1–3), 275–284. doi:10.1016/J.EJPHAR.2013.01.052
Welch, J. M., Simon, S. A., and Reinhart, P. H. (2000). The activation mechanism of rat vanilloid receptor 1 by capsaicin involves the pore domain and differs from the activation by either acid or heat. Proc. Natl. Acad. Sci. 97 (25), 13889–13894. doi:10.1073/PNAS.230146497
Winter, J., Dray, A., Wood, J. N., Yeats, J. C., and Bevan, S. (1990). Cellular mechanism of action of resiniferatoxin: a potent sensory neuron excitotoxin. Brain Res. 520 (1–2), 131–140. doi:10.1016/0006-8993(90)91698-G
Wishart, D. S., Feunang, Y. D., Guo, A. C., Lo, E. J., Marcu, A., Grant, J. R., et al. (2018). DrugBank 5.0: a major update to the DrugBank database for 2018. Nucleic Acids Res. 46 (D1), D1074–D1082. doi:10.1093/NAR/GKX1037
Wishart, D. S., Guo, A., Oler, E., Wang, F., Anjum, A., Peters, H., et al. (2022). HMDB 5.0: the human metabolome database for 2022. Nucleic Acids Res. 50 (D1), D622–D631. doi:10.1093/NAR/GKAB1062
Xanthos, D. N., and Sandkühler, J. (2013). Neurogenic neuroinflammation: inflammatory CNS reactions in response to neuronal activity. Nat. Rev. Neurosci. 15 (1), 43–53. doi:10.1038/nrn3617
Xiao, S., Tian, H., and Tao, P. (2022). PASSer2.0: accurate prediction of protein allosteric sites through automated machine learning. Front. Mol. Biosci. 9, 879251. doi:10.3389/fmolb.2022.879251
Xiao, W., and Chen, Y. (2022). TRPV1 in male reproductive system: focus on sperm function. Mol. Cell. Biochem. 477 (11), 2567–2579. doi:10.1007/S11010-022-04469-2
Xu, H., Ramsey, I. S., Kotecha, S. A., Moran, M. M., Chong, J. A., Lawson, D., et al. (2002). TRPV3 is a calcium-permeable temperature-sensitive cation channel. Nature 418 (6894), 181–186. doi:10.1038/nature00882
Xu, S., Zhang, L., Cheng, X., Yu, H., Bao, J., and Lu, R. (2018). Capsaicin inhibits the metastasis of human papillary thyroid carcinoma BCPAP cells through the modulation of the TRPV1 channel. Food & Funct. 9 (1), 344–354. doi:10.1039/C7FO01295K
Yang, F., Cui, Y., Wang, K., and Zheng, J. (2010). Thermosensitive TRP channel pore turret is part of the temperature activation pathway. Proc. Natl. Acad. Sci. U. S. A. 107 (15), 7083–7088. doi:10.1073/pnas.1000357107
Yang, F., Xiao, X., Cheng, W., Yang, W., Yu, P., Song, Z., et al. (2015a). Structural mechanism underlying capsaicin binding and activation of the TRPV1 ion channel. Nat. Chem. Biol. 11 (7), 518–524. doi:10.1038/nchembio.1835
Yang, F., Xiao, X., Lee, B. H., Vu, S., Yang, W., Yarov-Yarovoy, V., et al. (2018). The conformational wave in capsaicin activation of transient receptor potential vanilloid 1 ion channel. Nat. Commun. 9 (1), 2879. doi:10.1038/s41467-018-05339-6
Yang, S., Yang, F., Wei, N., Hong, J., Li, B., Luo, L., et al. (2015b). A pain-inducing centipede toxin targets the heat activation machinery of nociceptor TRPV1. Nat. Commun. 6 (1), 8297–8311. doi:10.1038/ncomms9297
Yang, S., Yang, F., Zhang, B., Lee, B. H., Li, B., Luo, L., et al. (2017). A bimodal activation mechanism underlies scorpion toxin-induced pain. Sci. Adv. 3 (8), e1700810. doi:10.1126/sciadv.1700810
Yao, J., Liu, B., and Qin, F. (2010). Kinetic and energetic analysis of thermally activated TRPV1 channels. Biophysical J. 99 (6), 1743–1753. doi:10.1016/J.BPJ.2010.07.022
Yao, J., Liu, B., and Qin, F. (2011). Modular thermal sensors in temperature-gated transient receptor potential (TRP) channels. Proc. Natl. Acad. Sci. U. S. A. 108 (27), 11109–11114. doi:10.1073/pnas.1105196108
Yeon, K. Y., Kim, S. A., Kim, Y. H., Lee, M. K., Ahn, D. K., Kim, H. J., et al. (2009). Curcumin produces an antihyperalgesic effect via antagonism of TRPV1. J. Dent. Res. 89 (2), 170–174. doi:10.1177/0022034509356169
Yin, Y., Dong, Y., Vu, S., Yang, F., Yarov-Yarovoy, V., Tian, Y., et al. (2019). Structural mechanisms underlying activation of TRPV1 channels by pungent compounds in gingers, British journal of pharmacology 176 (17), 3364–3377. doi:10.1111/BPH.14766
Yonghak, P., Miyata, S., and Kurganov, E. (2020). TRPV1 is crucial for thermal homeostasis in the mouse by heat loss behaviors under warm ambient temperature, Sci. Rep. 10(1). 8799–8812. doi:10.1038/s41598-020-65703-9
Yoshida, A., Furube, E., Mannari, T., Takayama, Y., Kittaka, H., Tominaga, M., et al. (2016). TRPV1 is crucial for proinflammatory STAT3 signaling and thermoregulation-associated pathways in the brain during inflammation. Nat. Publ. Group 6, 26088. [Preprint]. doi:10.1038/srep26088
Zaccaro, L., García-López, M. T., González-Muñiz, R., García-Martínez, C., Ferrer-Montiel, A., Albericio, F., et al. (2011). TRPV1 modulators: structure–activity relationships using a rational combinatorial approach. Bioorg. Med. Chem. Lett. 21 (12), 3541–3545. doi:10.1016/J.BMCL.2011.04.141
Zakharian, E., Cao, C., and Rohacs, T. (2010). Gating of transient receptor potential melastatin 8 (TRPM8) channels activated by cold and chemical agonists in planar lipid bilayers. J. Neurosci. 30 (37), 12526–12534. doi:10.1523/JNEUROSCI.3189-10.2010
Zenodo (2023). ) Zenodo. Available at: https://zenodo.org/(Accessed: October 16, 2023).
Zhai, K., Liskova, A., Kubatka, P., and Büsselberg, D. (2020). Calcium entry through TRPV1: a potential target for the regulation of proliferation and apoptosis in cancerous and healthy cells. Int. J. Mol. Sci. 21 (11), 4177. doi:10.3390/IJMS21114177
Zhang, C., Ye, L., Zhang, Q., Wu, F., and Wang, L. (2020). The role of TRPV1 channels in atherosclerosis. Channels 14 (1), 141–150. doi:10.1080/19336950.2020.1747803
Zhang, G., Lin, R. L., Wiggers, M., Snow, D. M., and Lee, L. Y. (2008a). Altered expression of TRPV1 and sensitivity to capsaicin in pulmonary myelinated afferents following chronic airway inflammation in the rat. J. Physiology 586 (23), 5771–5786. doi:10.1113/JPHYSIOL.2008.161042
Zhang, K., Julius, D., and Cheng, Y. (2021). Structural snapshots of TRPV1 reveal mechanism of polymodal functionality. Cell 184 (20), 5138–5150. doi:10.1016/J.CELL.2021.08.012
Zhang, X., Huang, J., and McNaughton, P. A. (2005). NGF rapidly increases membrane expression of TRPV1 heat-gated ion channels. EMBO J. 24 (24), 4211–4223. doi:10.1038/SJ.EMBOJ.7600893
Zhang, X., Li, L., and McNaughton, P. A. (2008b). Proinflammatory mediators modulate the heat-activated ion channel TRPV1 via the scaffolding protein AKAP79/150. Neuron 59 (3), 450–461. doi:10.1016/j.neuron.2008.05.015
Zheng, J., Dai, C., Steyger, P. S., Kim, Y., Vass, Z., Ren, T., et al. (2003). Vanilloid receptors in hearing: altered cochlear sensitivity by vanilloids and expression of TRPV1 in the organ of Corti. J. Neurophysiology 90 (1), 444–455. doi:10.1152/jn.00919.2002
Zhong, L., Bellemer, A., Yan, H., Ken, H., Jessica, R., Hwang, R. Y., et al. (2012). Thermosensory and nonthermosensory isoforms of Drosophila melanogaster TRPA1 reveal heat-sensor domains of a ThermoTRP channel. Cell Rep. 1 (1), 43–55. doi:10.1016/J.CELREP.2011.11.002
Zimmerman, M. I., and Bowman, G. R. (2015). FAST conformational searches by balancing exploration/exploitation trade-offs. J. Chem. Theory Comput. 11 (12), 5747–5757. doi:10.1021/acs.jctc.5b00737
Zimmermann, K., Lennerz, J. K., Hein, A., Link, A. S., Kaczmarek, J. S., Delling, M., et al. (2011). Transient receptor potential cation channel, subfamily C, member 5 (TRPC5) is a cold-transducer in the peripheral nervous system. Proc. Natl. Acad. Sci. U. S. A. 108 (44), 18114–18119. doi:10.1073/pnas.1115387108
Keywords: temperature, TRP channels, drug discovery, pain, structure, transient receptor potential vanilloid type 1 (TRPV1) channel
Citation: Amaya-Rodriguez CA, Carvajal-Zamorano K, Bustos D, Alegría-Arcos M and Castillo K (2024) A journey from molecule to physiology and in silico tools for drug discovery targeting the transient receptor potential vanilloid type 1 (TRPV1) channel. Front. Pharmacol. 14:1251061. doi: 10.3389/fphar.2023.1251061
Received: 30 June 2023; Accepted: 14 December 2023;
Published: 24 January 2024.
Edited by:
Domenico Tricarico, University of Bari Aldo Moro, ItalyReviewed by:
Alexander V. Zholos, Taras Shevchenko National University of Kyiv, UkraineArthur Neuberger, University of Cambridge, United Kingdom
Copyright © 2024 Amaya-Rodriguez, Carvajal-Zamorano, Bustos, Alegría-Arcos and Castillo. This is an open-access article distributed under the terms of the Creative Commons Attribution License (CC BY). The use, distribution or reproduction in other forums is permitted, provided the original author(s) and the copyright owner(s) are credited and that the original publication in this journal is cited, in accordance with accepted academic practice. No use, distribution or reproduction is permitted which does not comply with these terms.
*Correspondence: Melissa Alegría-Arcos, bWFsZWdyaWFhQHVkbGEuY2w=; Karen Castillo, a2Nhc3RpbGxvQHVjbS5jbA==, a2FyZW4uY2FzdGlsbG9AY2ludi5jbA==