- 1Department of Chemistry, Durham University, Durham, United Kingdom
- 2School of Health and Life Sciences, Teesside University, Middlesbrough, United Kingdom
- 3National Horizons Centre, Darlington, United Kingdom
Chagas disease is a vector-borne illness caused by the protozoan parasite Trypanosoma cruzi (T. cruzi). It poses a significant public health burden, particularly in the poorest regions of Latin America. Currently, there is no available vaccine, and chemotherapy has been the traditional treatment for Chagas disease. However, the treatment options are limited to just two outdated medicines, nifurtimox and benznidazole, which have serious side effects and low efficacy, especially during the chronic phase of the disease. Collectively, this has led the World Health Organization to classify it as a neglected disease. To address this problem, new drug regimens are urgently needed. Drug repurposing, which involves the use of existing drugs already approved for the treatment of other diseases, represents an increasingly important option. This approach offers potential cost reduction in new drug discovery processes and can address pharmaceutical bottlenecks in the development of drugs for Chagas disease. In this review, we discuss the state-of-the-art of drug repurposing approaches, including combination therapy with existing drugs, to overcome the formidable challenges associated with treating Chagas disease. Organized by original therapeutic area, we describe significant recent advances, as well as the challenges in this field. In particular, we identify candidates that exhibit potential for heightened efficacy and reduced toxicity profiles with the ultimate objective of accelerating the development of new, safe, and effective treatments for Chagas disease.
1 Introduction
Chagas disease (CD), also known as American trypanosomiasis, is a potentially life-threatening Neglected Tropical Disease (NTD) caused by the protozoan parasite Trypanosoma cruzi (T. cruzi). An estimated 6 to 7 million people worldwide are infected with T. cruzi (WHO, 2023). The disease is found mainly in endemic areas of 21 Latin American countries but with climate change and population movement the impact is spreading to other regions of the globe (Figure 1). CD places a significant direct financial burden on healthcare system and society that has been estimated to exceed $625 million in healthcare costs and 806,170 DALYs (Gómez-Ochoa et al., 2022). However, the full economic cost to endemic communities is much more significant, resulting in a loss of 752,000 workdays annually due to premature deaths, which carries an average financial burden of 1.2 billion dollars per year in the southern countries of Latin America (Ramsey et al., 2014). Additionally, it is currently estimated that <1% of those infected with CD have access to care and treatment (Cucunubá et al., 2017).
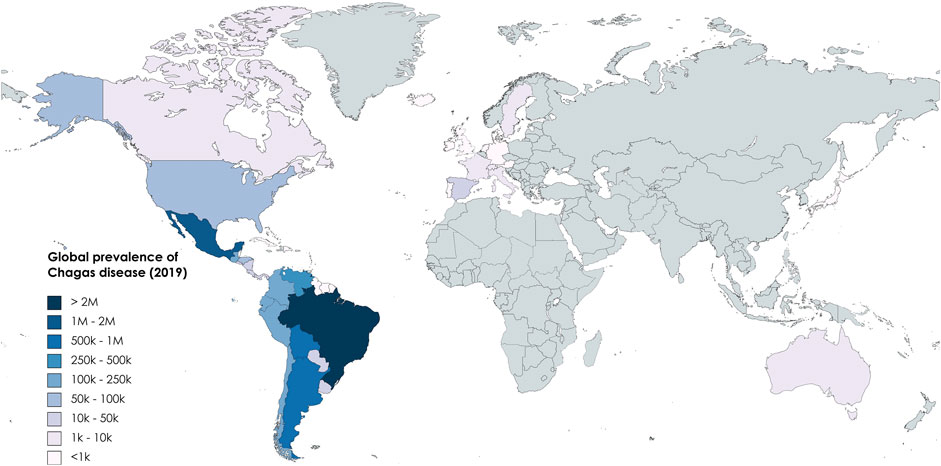
FIGURE 1. Global prevalence of CD based on data from Global Burden of Disease (2019).
There is no vaccine for CD and there are only two drugs’ treatments approved for this illness. These are benznidazole 1 (BZN), in use since 1971, and nifurtimox 2 (NFX), which was first approved in 1965 (Figure 2). While these old medications remain effective in preventing or curbing disease progression in infected adults, especially those with no symptoms and during the acute infection, they are not without challenges (Crespillo-Andújar et al., 2022). The duration of treatment is long (up to 2 months) and possible adverse reactions can occur in up to 40% of treated adult patients (Jackson et al., 2020), potentially requiring additional treatment for cardiac, digestive, or neurological manifestations. The drugs are contraindicated for certain populations, such as pregnant women and people with kidney or liver failure (Meymandi et al., 2018). Collectively, these factors can contribute to poor patient adherence, which may result in relapse and the development of resistance.
Given this, there is an urgent need for the development of new cost-effective, efficacious drugs. This is challenged by the global resources dedicated to ameliorating the burden of the NTDs. Moreover, even within this limited space, research into CD received only 0.67% of the overall funding allocated to all NTDs over a span of 10 years (2010–2020) (Miranda-Arboleda et al., 2021) reinforcing its reputation as the “most neglected of the neglected tropical diseases” (Zaidel and Sosa Liprandi, 2021).
Drug repurposing (the process of finding new indications for existing drugs) presents a promising approach to addressing this challenge. In this review, we discuss the use of drug repositioning as a cost-effective strategy for the development of new solutions to this devastating disease. We not only explore the latest advances in this approach but also shed light on the challenges that remain for T. cruzi chemotherapies.
2 Chagas disease
Identifying and developing new drugs against T. cruzi is complicated by the parasite’s complex life cycle (Figure 3). The life cycle has four developmental stages, involving, metacyclic trypomastigotes, epimastigotes, blood-form trypomastigotes, and intracellular amastigotes, which span two host organisms; a triatomine insect, commonly known as a kissing bug, and the mammalian host (Nagajyothi et al., 2012). In humans and other mammals, transmission primarily occurs through insect vectors. This happens when an infected triatomine insect feeds on the blood of a host. Trypomastigotes are released in its faeces near the site of the bite wound. The trypomastigotes can then enter the host’s body through the wound or through intact mucosal membranes, such as the conjunctiva. Alternative modes of transmission can occur through ingestion of contaminated food, mother-to-child transmission and infected blood transfusions and organ transplantation.
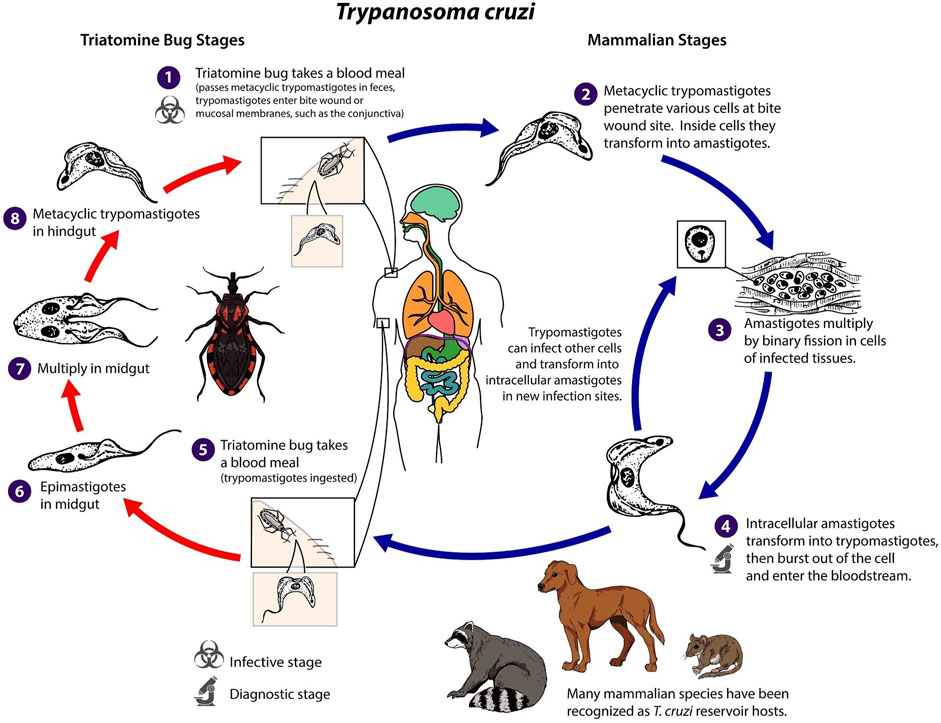
FIGURE 3. Trypanosoma cruzi life cycle adapted from Centers for Diseases Control and Prevention (CDC, 2023).
Once inside the host, the trypomastigotes invade nearby cells and differentiate into intracellular amastigotes. These amastigotes multiply by binary fission and differentiate back into trypomastigotes, which are then released into the bloodstream. The trypomastigotes can infect cells from various tissues and transform into intracellular amastigotes in new infection sites. Unlike African trypanosomes (T. brucei), bloodstream trypomastigotes of T. cruzi do not replicate. Replication only resumes when the parasites enter another cell or are ingested by another vector. The cycle is propagated when an uninfected kissing bug feeds on human or animal blood that contains circulating parasites. The ingested trypomastigotes transform into epimastigotes in the vector’s midgut, where they multiply and differentiate. The parasites then differentiate into infective metacyclic trypomastigotes in the hindgut, ready to be transmitted to another host. This life cycle leads to a disease with two distinct phases (PAHO, 2023). Following first infection there is an initial acute phase, which can last for several months, during which a high number of parasites circulate in the blood. This can lead to a range of visible signs, initially a skin lesion or swelling of the eye lids but may involve fever, headache, enlarged lymph glands, muscle pain, breathing difficulties, and abdominal and chest discomfort. However, challenging early diagnosis for the majority of individuals, these symptoms are often mild and not easily attributed to a T. cruzi infection. During the chronic phase, the blood population of the parasite falls dramatically, and the parasite reside mainly in the heart and digestive muscles. One to three decades later, up to a third of patients suffer from cardiac disorders (CD is the second leading cause of chronic heart failure in Latin America) and up to 1 in 10 suffer from digestive (typically enlargement of the esophagus or colon), neurological or mixed alterations. Then, the infection can lead to progressive damage to the nervous system and heart muscle, resulting in cardiac arrhythmias, heart failure, and sudden death. This unusually slow progression, often without noticeable symptoms has led CD to be labelled as a “silent and silenced disease” and challenges diagnosis and a recognition of the severity of the problem (WHO, 2023).
3 Drug discovery vs. drug repurposing
De novo drug discovery, spanning from biochemical concept to the clinic, is an intricate, time-consuming, and expensive journey encompassing several crucial stages. These stages include compound identification, formulation, verification of efficacy and safety in animal models and human volunteers, numerous rounds of pre-clinical and clinical trials, and, ultimately, registration and regulatory approval. Collectively, this is estimated to take between 12–18 years and cost $1–2B to develop a new drug (Figure 4A). Moreover, the probability of failures, most commonly at later more expensive clinical trial stages, are high and this is reflected in the high cost of patented drugs. Consequently, the discovery of new drugs for CD, and other NTDs, for which the average patient exists on an income of less than $2 per day (Clark et al., 2014), is simply not viable for pharmaceutical companies without significant public cross-subsidy.
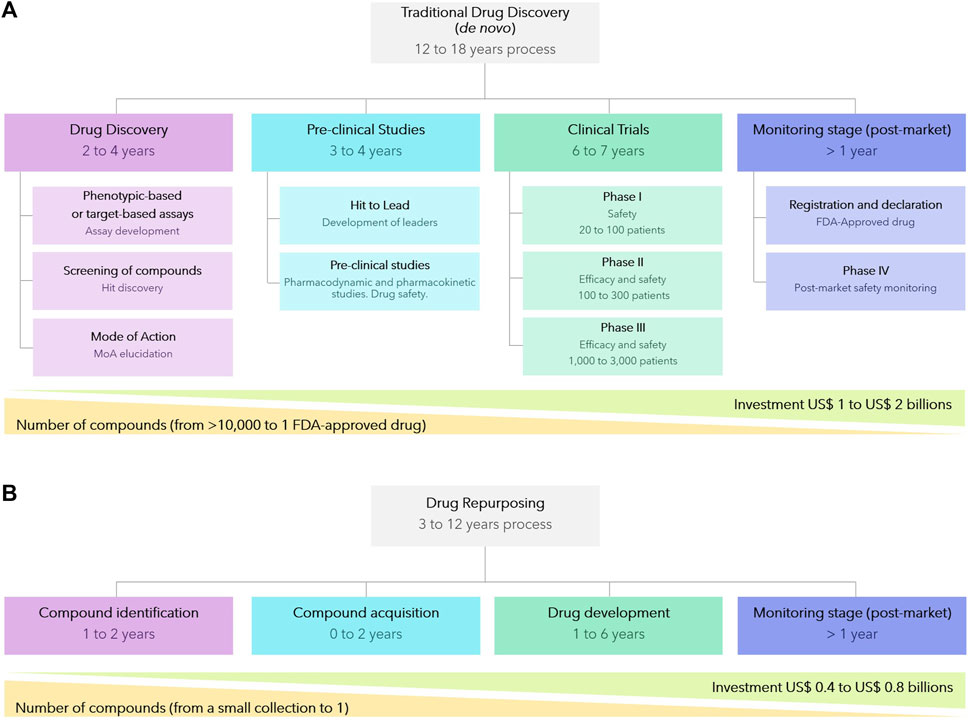
FIGURE 4. Comparison between de novo traditional drug discovery (A) and drug repurposing strategies (B).
Drug repurposing, also known as drug repositioning or drug reprofiling, in which compounds developed for one indication are then utilized to provide solutions to an alternative disease, offers various advantages over developing an entirely new drug for a given indication (Pushpakom et al., 2019; Jourdan et al., 2020). Firstly, the risk of failure is lower because the repurposed drug has, if early-stage trials have been completed, already been found to be sufficiently safe in preclinical models and humans. Secondly, the drug development time frame is commonly both shorter and cheaper (Figure 4B) since some preclinical testing, safety assessments, and sometimes even formulation development, will have been completed. Given the reduced costs, drug repurposing is a particularly attractive approach for NTD (Krishnamurthy et al., 2022). Whilst repurposing approaches has been successful applied to afford approved drugs for other diseases, including NTDs such as Human African Trypanosomiasis and Leishmaniasis (where the majority of the current chemotherapy are repurposed drug (Charlton et al., 2018; Braga, 2019), there has yet not been a successful outcome for CD.
4 Approaches and techniques for drug repurposing
Strategies for drug repurposing follow traditional models for other drug discovery processes and encompass a combination of molecular and empirical approaches to enhance effectiveness and efficiency. Molecular approaches, commonly known as target-based strategies, primarily rely on hypothesis-driven methods at the single protein level. On the other hand, empirical approaches, known as phenotypic strategies, are centered around evaluating observable measures of response in a whole cell, organism, or system (Schenone et al., 2013; Swinney and Lee, 2020). Both strategies have their advantages and disadvantages that have been discussed in numerous review articles (Swinney, 2013; Moffat et al., 2017).
The process of discovering drugs through phenotypic screening is also referred to as “forward (or classical) pharmacology” or “forward chemical biology”. For instance, a sensitive assay to identify compound activity against T. cruzi was developed by Sykes and Avery (2015). This used an image-based assay to estimate the effect of compound treatment on T. cruzi amastigotes in 3T3 fibroblasts and host cells. This assay identified active compounds from an in-house FDA-approved drug library and the MMV Malaria Box collection. Prominent compounds include camptothecin 3, clemastine 4, crystal violet 5, and clotrimazole 6 (Figure 5A), all with sub-micromolar activities against this protozoan. In another phenotypic-based assay using a library of 100 registered drugs with drug repositioning potential for NTDs, two compounds with in vitro activity against T. cruzi were discovered (Kaiser et al., 2015). These compounds were tadalafil 7, a phosphodiesterase type 5 inhibitor used to treat erectile dysfunction, with an EC50 of 8.6 μM and a selectivity index >26 (selectivity index or SI is defined as the ratio of the EC50 of host cell versus the EC50 of T. cruzi), and the antispasmodic mebeverine 8 with an EC50 of 3.89 μM and a SI = 18.
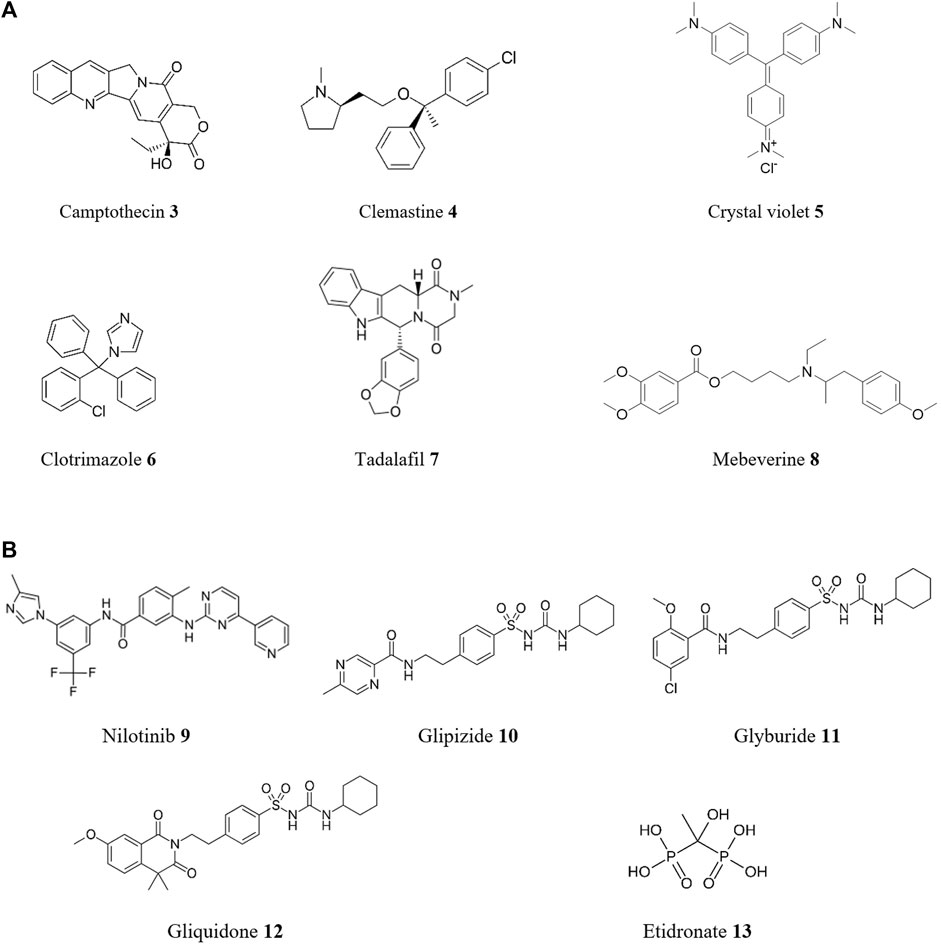
FIGURE 5. Structures of reported repurposed drugs for CD that have been identified using phenotypic-based approaches [(A), compounds 3 to 8] or by in silico target-based approaches [(B), compounds 9 to 13].
In contrast, target-based drug discovery, also known as ‘reverse pharmacology,’ begins with the formulation of a hypothesis that modulation of a specific protein target, believed to be crucial in disease modification, will yield favorable therapeutic effects (Swinney, 2013). The initial step involves the identification and validation of a molecular target, such as an enzyme or receptor, that plays a significant role in the disease process. Subsequently, a range of strategies is employed to discover and develop drugs capable of modulating the target’s activity. These strategies encompass diverse techniques including simple biochemical and biophysical assays, in both single and high-throughput formats (vide infra), as well as virtual screening and other in silico methods. In the spirit of reducing cost, in silico methods which can screen large numbers of drug candidate compounds in a virtual manner using computer generated models of targets or known ligands are ideally suited to repurposing previously reported structures. By employing these techniques, researchers strive to identify innovative therapeutic interventions for CD, aiming to tackle the disease at its molecular roots. Over the past decade, numerous successful cases of drug repurposing for the treatment of CD have been reported using in silico approaches (Figure 5B). As an example, a novel virtual screening approach was developed by Juárez-Saldivar et al. (2020) to identify drug repositioning opportunities against T. cruzi infection by targeting the bifunctional enzyme dihydrofolate reductase-thymidylate synthase (DHFR-TS). Ten putative TcDHFR-TS inhibitors were identified, including nilotinib 9, glipizide 10, glyburide 11, and gliquidone 12. These compounds showed growth inhibitory activity against T. cruzi epimastigotes. In other study (Valera-Vera et al., 2020), a virtual screening strategy was employed to identify potential inhibitors of enolase, a key enzyme and potential drug target for CD. This in silico study proposed etidronate 13, a bisphosphonate inhibitor of bone resorption, as a potential inhibitor of this molecular target. Due to its safety profile, etidronate 13 provides valuable insights for the development of new drugs targeting T. cruzi enolase (TcENO). However, further exploration of its potential through in vitro and in vivo studies is necessary.
An important technique in both approaches is high-throughput screening (HTS), which allows researchers to rapidly screen thousands to millions of compounds in a relatively short period of time, greatly accelerating the drug discovery process. In a typical HTS experiment, a library of compounds is screened against a particular molecular target (target-based) or whole cell (phenotypic-based) assay (Blay et al., 2020). For instance, in the search for drug targets against T. cruzi, a HTS campaign focused on TcGlcK, a glucokinase enzyme was performed by Mercaldi et al. (2019). Glucokinase and hexokinase are crucial in T. cruzi’s metabolic pathways, making their inhibition a promising strategy for drug discovery. Out of 13,040 compounds screened, the HTS campaign found 25 enzyme inhibitors from nine chemical classes. Thirteen compounds displayed low micromolar IC50 values for enzyme inhibition, with four showing low toxicity to NIH-3T3 murine host cells and notable in vitro trypanocidal activity. These four compounds, belonging to three chemical classes (3-nitro-2-phenyl-2H-chromene, N-phenyl-benzenesulfonamide, and gossypol scaffolds), include two potential hit-to-lead candidates (Figure 6A) from the 3-nitro-2-phenyl-2H-chromene class (namely, GLK2-003 14 and GLK2-004 15), holding promise for further exploration in drug discovery.
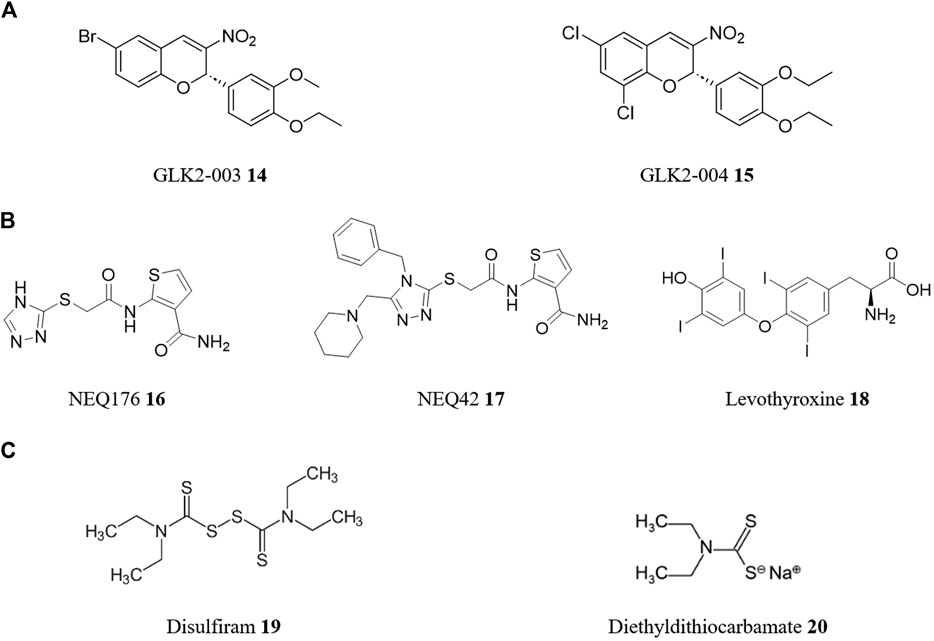
FIGURE 6. Structures of reported repurposed drugs for CD that have been identified using HTS [(A), compounds 14 and 15] or by a combination of both target-based and phenotypic-based approaches [(B), compounds 16 to 18]. Structures of repurposed drugs for combinatory therapy for CD treatment [(C), compounds 19 and 20].
However, it is important to note that drug repurposing strategies often incorporate both molecular and empirical approaches in complementary ways. This integrative approach maximizes the chances of success by leveraging the strengths of each strategy. By combining target-based and phenotypic methods, researchers can gain a more comprehensive understanding of the underlying biology, discover new targets or pathways, and optimize drug candidates for efficacy and safety. For instance, cysteine proteases are a class of enzymes that have been validated as drug targets for the development of safe and effective pharmacological agents for CD. One such cysteine protease is cruzain, the major cysteine protease of T. cruzi. It has been shown to be essential in various stages of the T. cruzi life cycle and is a target of rational drug design for chemotherapy of CD (Ferreira et al., 2022). A study screened the ZINC database for compounds with lead-like properties to find new inhibitors of the cruzain protease (Wiggers et al., 2013). Using consensus scoring, target-based molecular docking, and ligand-based similarity searching, 8,600 lead structures were identified from an initial library of 8.5 million compounds. These were then screened through Glide XP and HQSAR, and the top 5% were visually inspected. 23 compounds were selected for in vitro phenotypic-based testing against T. cruzi-infected cells, with the top two hits (NEQ176 16 with an EC50 of 108 μM and NEQ42 17 with an EC50 of 10.6 μM—Figure 6B) being structurally characterized by X-ray crystallography to understand their binding modes with cruzain. In a second virtual screening campaign, 163 FDA-approved and investigational drugs identified from the DrugBank database, were screened against cruzipain, another major cysteine protease and validated molecular target of T. cruzi (Bellera et al., 2014). In this case, using a combination of target-based and phenotypic-based approaches, levothyroxine 18 (Figure 6B), a drug used in hormone replacement therapy for hypothyroidism, had dose-dependent inhibition of cruzipain (IC50 of 38.43 μM) and antiproliferative activity on the T. cruzi epimastigotes (EC50 of 121 mM).
An intriguing strategy for drug repositioning involves the use of combination drug therapy. Combination therapy or polytherapy is a therapeutic intervention in which more than one medication or modality is used. This approach has become increasingly common in medicine, particularly in the treatment of cancer (Bayat Mokhtari et al., 2017) and infectious diseases (Shyr et al., 2021), as it can offer several benefits over single-drug therapies including enhanced efficacy, commonly greater than simple additive effects (synergistic effect), delaying the development of drug resistance, and reducing the risk of side effects and toxicity. Combining two or more drugs with different mechanisms of action can increase the success rate of drug repositioning by providing an alternative approach to treatment (Sun et al., 2016). For example, disulfiram 19, a medicine used to treat chronic alcoholism, is currently undergoing Phase I/II clinical trials as combined chemotherapy with BZN 1 for CD (Saraiva et al., 2021). In vitro and in vivo experiments of BZN 1 with disulfiram 19 and/or its metabolite diethyldithiocarbamate 20 (Figure 6C) demonstrated synergistic effects, inhibiting parasite proliferation in infected macrophages and improved survival rates in infected mice compared to BZN 1 alone (Almeida-Silva et al., 2022). Microscopic analysis revealed structural alterations in the parasites, whilst diethyldithiocarbamate 20 treatment increased reactive oxygen species production.
5 Advances on drug repurposing for CD
Given the pressing need for new treatments for CD, significant progress has been made over the past years through drug repositioning efforts. In this review, we present an overview of these works based on the therapeutic origin of the drugs used (for a comprehensive list of the compounds discussed and their biological activities, please refer to Supplementary Table S1; Supplementary Figures S1–S3, in Supplementary Material).
Many natural products exhibit significant therapeutic potential and possess a diverse array of biological properties, including antibacterial, antiprotozoal, antimycobacterial, antileishmanial, antitumor, and anti-human immunodeficiency virus (HIV) activities and represent a valuable and fruitful resource for the discovery and development of potent drugs against a variety of diseases (Álvarez-Bardón et al., 2020; Tempone et al., 2021; Lazarin-Bidóia et al., 2022). As such, there are numerous review studies focusing on the potential use of natural products with effects on T. cruzi, providing valuable insights for further investigation (Scotti et al., 2016; Nekoei et al., 2022; Barbosa et al., 2023). For instance, antibiotics such as echinomycin derived from Streptomyces echinatus, exhibit notable anti-T. cruzi activity at nanomolar concentrations (EC50 of 1.1 nM) (Annang et al., 2015). However, reflecting the complexities associated with the use of natural products as repurposing therapies, notably the lack of comprehensive in vivo studies and limited progress through clinical phases that hinder their immediate application, they will not be further explored in this review.
In these next sections, we delve into the exciting frontier of FDA-approved drug repositioning towards CD, investigating the rationale behind this approach, the challenges encountered, and the successful examples that have paved the way for the identification of novel therapeutic options. Although the majority of drugs discussed in this review are FDA-approved, we have also included, in certain instances, noteworthy molecules that are currently or have been undergoing clinical assessments, expanding the scope of potential therapeutic options for CD. The ultimate target product profile for CD is a multifactorial issue, but for simplicity in this review is described in terms of enhance efficacy and selectivity, safety and tolerability, and is realized by comparison to BZN 1 (DNDi, 2023). Additionally, we classified the selected examples of drug repurposing for CD in two ways: by approach (target-based or phenotypic-based strategies) and by technique. Within the technique category, we further subcategorize our findings into single drug trials (or small libraries), high-throughput screening, virtual screening, and combination drug therapy.
5.1 Repurposing anticancer drugs
Anticancer agents, targeting a wide range of proteins such as kinases, epigenetic regulators, DNA repair enzymes, and proteasomes (Zhong et al., 2021), have emerged as a compelling avenue for repurposing towards CD, attracting considerable attention due to their diverse mechanisms of action and potential for multi-targeted effects. For these reasons, there are many instances of anticancer drugs being repurposed to other diseases, including Alzheimer’s disease (Ancidoni et al., 2021), malaria (Porta et al., 2019), COVID-19 (El Bairi et al., 2020), among others.
The tyrosine kinase inhibitor imatinib 21 (Figure 7), the first small-molecule tyrosine kinase inhibitor (TKI) approved for clinical use by the US Food and Drug Administration (FDA) (Savage and Antman, 2002), was screened for activity against T. cruzi (Simões-Silva et al., 2019a). In this phenotypic-based approach, imatinib 21 showed an in vitro trypanocidal activity (EC50) of 24.8 μM against the intracellular forms of the parasite (SI = 1.5, Y strain) and an EC50 of 30.0 μM against the extracellular forms. An additive effect was observed in the combination of imatinib 21 + BZN 1, in fixed-ratio proportions. Similarly, by using this drug as a pharmacophore in a hit-to-lead strategy, a promising synthetic analogue was developed: LS2/89 22 (Nesic de Freitas et al., 2023). Although not a repurposed compound, this analogue demonstrated significantly improved potency, with antiparasitic activities more than 100-times lower against the intracellular form (EC50 of 0.19 μM) and 10-times lower against the trypomastigote form (EC50 of 2.67 μM), compared to imatinib 21.
In another phenotypic assay, this time in HTS format, screening 7680 compounds from the Repurposing, Focused Rescue, and Accelerated Medchem (ReFRAME) library against T. cruzi (CA-I/72) identified seven compounds with potent in vitro activity (Bernatchez et al., 2020). These included the DNA topoisomerase inhibitor NSC-706744 23 with a EC50 of 0.44 nM (SI = 214) and the EGFR inhibitor ASP-8273 24 (naquotinib) with a EC50 of 2.7 nM (SI = 191).
Miltefosine 25, an anti-breast cancer drug already repositioned for leishmaniasis, was evaluated as a monotherapy and in combination with BZN 1 against T. cruzi (Gulin et al., 2022). Miltefosine 25 alone showed efficacy against the parasite (VD strain, DTU TcVI) in both in vitro (amastigotes and trypomastigotes) and in vivo models, with no observed cytotoxic effects on host cells. When combined with BZN 1, miltefosine 25 exhibited enhanced effectiveness, preventing parasitemia rebound. Given that miltefosine 25 is currently the sole approved oral antileishmanial drug and has demonstrated success in its repositioning for leishmaniasis treatment, it emerges as a highly promising and attractive candidate for further exploration in clinical phases to address CD.
Many drug repositioning studies for CD have shown promising in vitro activity profiles. However, there is often a lack of data on subsequent in vivo studies, which is crucial as many repositioned drugs fail during this stage. Tamoxifen 26 is an example of this. This antineoplastic drug was tested for its activity against T. cruzi and showed promising results in vitro against the epimastigote, trypomastigote, and amastigote forms of the CL14, Y, and Y benznidazole-resistant T. cruzi strains (Miguel et al., 2010). Tamoxifen 26 demonstrated activity against all life-cycle stages of the parasite, with EC50 ranging from 0.7 to 17.9 µM across all strains. However, in vivo tests using two experimental models of acute CD showed no significant differences in parasitemia or mortality between tamoxifen-treated and control mice.
5.2 Repurposing antihistaminic compounds
De Rycker et al. (2016) has described a screening cascade for the identification of compounds with anti-T. cruzi activity from 963 clinically tested compounds (from NIH Clinical Collection and the SelleckChem FDA-approved drug library). The cascade includes a primary assay that allows the determination of 14,080 single point measurements (for library screening) or up to 1,280 potency determinations in a single run, and secondary assays to assess static-cidal, rate-of-kill, and cytochrome P450 CYP51 inhibition. A number of promising targets were identified including the first-generation H1 histamine antagonists clemastine 4 and azelastine 27 (Figure 8). Although further optimization is needed due to limitations in their pharmacokinetic and toxicity profiles, these compounds could offer valuable starting points for the development of effective treatments for CD.
The dye crystal violet 5 is known for its ability to eliminate T. cruzi in blood banks (Docampo et al., 1983) by inhibiting proline uptake through the proline permease TcAAAP069. However, due to its high cytotoxicity and low selectivity, it is not considered a suitable candidate for drug repositioning. Using ligand based in silico drug repurposing, the FDA-approved antihistamines loratadine 28 and cyproheptadine 29 were identified as structurally related compounds to crystal violet 5 (Sayé et al., 2020). These drugs inhibited TcAAAP069 activity and displayed trypanocidal action against all T. cruzi life stages in different strains. When combined with BZN 1, a synergistic effect was observed with loratadine 28 or cyproheptadine 29.
Alberca et al. (2018) reported a combined ligand- and structure-based virtual screening campaign to find inhibitors of putrescine (a polyamine) uptake in T. cruzi. Using an ensemble of linear ligand-based classifiers as an initial screening filter and then docking the results into a homology model of the putrescine permease TcPAT12, the DrugBank and Sweetlead databases were screened for drug repositioning opportunities. As a result, cinnarizine 30, an antihistamine drug for motion sickness and balance disorder, was selected and tested against T. cruzi. Its trypanocidal effects and inhibitory effects on putrescine uptake were confirmed by in vitro studies.
5.3 Repurposing CNS drugs
CNS drugs are medications that affect the central nervous system (CNS). There are many different types of drugs that work on the CNS, including anesthetics, anticonvulsants, antiemetics, antiparkinsonian agents, CNS stimulants, muscle relaxants, narcotic analgesics (pain relievers), nonnarcotic analgesics (such as acetaminophen and NSAIDs), and sedatives. Additionally, pathological studies in CD have confirmed that nodular encephalitis in multiple foci is a key finding in the acute nervous form of the disease (Pittella, 2009). CNS involvement is uncommon in mild cases but can occur in immunosuppressed patients. Ischemic cerebral changes associated with chronic Chagas cardiomyopathy are also common. Given these findings, it is not surprising that the repurposing of CNS medications for the treatment of CD shows promise. For example, ifenprodil 31 (Figure 9), an inhibitor of the NMDA receptor that is currently undergoing clinical trials as a potential repurposed treatment for COVID-19 (Hashimoto, 2021), and ziprasidone 32, an atypical antipsychotic drug, were identified as promising candidates for drug repurposing through the high content phenotypic screening described above (De Rycker et al., 2016).
In a second example, sertraline 33, an antidepressant drug, displayed effectiveness against T. cruzi strains in vitro, with EC50 values of 1.4 μM for intracellular amastigotes (Y strain, SI = 17.8) and 14 μM for bloodstream trypomastigotes (Ferreira et al., 2018). Sertraline 33 affected the mitochondrial integrity of T. cruzi, decreasing ATP levels. In silico approaches using chemogenomic target fishing, homology modeling, and molecular docking suggested TcIDH2 (isocitrate dehydrogenase 2) as a potential target for this drug.
It is well-established that T. cruzi relies on the uptake of polyamines from the extracellular medium for survival, therefore, polyamine transporters are promising targets for trypanosomatids (Talevi et al., 2019), as well as other protozoan parasites (Panozzo-Zénere et al., 2018). A ligand-based virtual screening using Ant4, an anthracene-putrescine conjugate inhibitor of the polyamine transport system, as a reference molecule identified three CNS drugs as possible inhibitors of polyamine transport (Reigada et al., 2019a). The antipsychotics promazine 34 and chlorpromazine 35, and the antidepressant clomipramine 36 were effective inhibitors of putrescine uptake and showed good trypanocidal activity against T. cruzi (EC50 < 10 μM in all cases). Molecular docking simulations suggest good interactions between the T. cruzi polyamine transporter TcPAT12 and these drugs. Additionally, these phenothiazine derivatives have been reported as specific inhibitors of parasite-trypanothione reductase (Iribarne et al., 2009). A study explored combining clomipramine 36 with BZN 1 (García et al., 2016). In vitro and in vivo tests showed a synergistic effect against T. cruzi. During the acute phase, BZN 1 reduced parasitemia, but combining it with clomipramine 36 completely suppressed it. Importantly in the chronic phase, mice treated with both drugs had lower heart damage and inflammation when compared to BZN 1 alone suggesting that this combination shows potential to enable lower BZN 1 doses and concomitant improved safety.
In another case, a structure-based drug repositioning approach was conducted over a set of 20 T. cruzi targets to find new treatments for CD (Adasme et al., 2020). The screening yielded over 500 molecules as hits, out of which 38 drugs were prioritized. Compounds showing growth inhibitory activity (<100 μM) when tested on T. cruzi trypomastigotes and epimastigotes were selected for further in vivo investigation. In mice, a single dose of 100 mg/kg body weight of the nonsteroidal anti-inflammatory naproxen 37 demonstrated the highest inhibition of parasitemia (85.8%) of this collection. For reference, a single dose of at 100 mg/kg body weight of NFX 2 exhibited parasitemia inhibition of 77.8%. Another non-steroidal anti-inflammatory drug nimesulide 38 was identified by Trindade et al. (2021) as a potential candidate for repositioning as a treatment for CD. Treatment of T. cruzi epimastigotes with nimesulide 38 resulted in dose-dependent cell death. Nimesulide 38 also inhibited the replication of intracellular amastigotes in T. cruzi-infected macrophages. The study suggested that nimesulide 38 affects the parasite’s cell redox balance, leading to cell death primarily through oxidative stress. Ultrastructural changes and a mixed mechanism of cell death involving both apoptosis and necrosis were observed in nimesulide-treated epimastigotes.
5.4 Repurposing cholesterol-lowering medicines
Trypanosoma cruzi has a high affinity for host lipoproteins and uses the low-density lipoprotein receptor to invade cells. Moreover, Johndrow et al. (2014) has shown that T. cruzi infection is associated with an accumulation of low-density lipoprotein and cholesterol in tissues during both the acute and chronic stages of murine CD. This has led to the suggestion that drugs that help lower cholesterol levels in the blood, may have positive effects for CD. The most common anti-cholesterol drugs are the statins, for which the primary target is HMG-CoA reductase. Interestingly, this enzyme is also relevant in the case of T. cruzi (Peña-Diaz et al., 2004). However, statins also been shown to possess additional roles including antioxidative, anti-inflammatory, antiatherogenic, and chemotherapeutic activities (Liao and Laufs, 2005; Zhang et al., 2020). Considering these factors together, there have been numerous reports on the repurposing of statins in the context of T. cruzi. In this respect, it is noteworthy that the antiparasitic effect of the antifungal ketoconazole (vide infra) was improved by lovastatin in a murine model of CD (Urbina et al., 1993). This is proposed to arise through statin mediated reduction of heart inflammation that is commonly observed in chronic T. cruzi infection (Guzmán-Rivera et al., 2020).
The activity and selectivity of atorvastatin 39 (Figure 10), an inhibitor of cholesterol synthesis, against T. cruzi were evaluated by Araujo-Lima et al. (2018). Atorvastatin 39 showed activity against different strains (Y and Tulahuen) and forms of the parasite (amastigotes and trypomastigotes) with good selectivity in all cases (SI > 20). Combinatory approaches using atorvastatin 39 and BZN 1 in fixed ratio gave synergistic interactions against both trypomastigotes and intracellular forms. Recently, a phase II, multicenter trial to evaluate the impact of atorvastatin 39 on inflammation and cardiac function in patients with chronic CD has been described (Campos-Estrada et al., 2023).
Clofibrate 40, a lipid-lowering agent commonly used to control high cholesterol and triglyceride levels in the blood, belongs to the class of fibrates. Two groups independently explored its therapeutic potential using an initial virtual screening approach. On one hand, a computer-guided drug repositioning method was employed to identify potential FDA-approved drugs as inhibitors of cruzain, the major cysteine protease of T. cruzi (Palos et al., 2017). Through virtual screening of 3180 FDA drugs, clofibrate 40 was selected for in vitro and in vivo testing. Interestingly, clofibrate 40 emerged as one of the highlighted and selected drugs in the cascade screening conducted by De Rycker et al. (2016), as discussed previously. However, whilst clofibrate 40 exhibited superior activity profiles compared to commercially available drugs (EC50 in amastigotes of 6.31 μM), BZN 1 and NFX 2, in in vitro studies, it demonstrated inferior results in short-term in vivo studies to reduce parasitemia in infected mice, using a single dose of 100 mg/kg of each drug (reduction to 50% of parasitemia at 6 h for BZN 1, and a range of 60%–90% for clofibrate 40).
5.5 Repurposing medicines for heart conditions
Given that CD frequently manifest itself in cardiac failure it is not surprising that repurposing drugs targeted toward other heart conditions has been a popular avenue to explore. The combination of BZN 1 with amiodarone 41 (Figure 11), an antiarrhythmic drug used to treat chronic cardiac CD and also previously recognized as a trypanocidal agent (Adesse et al., 2011), has been investigated (Barbosa et al., 2022). The combined treatment did not improve the direct trypanocidal effect of amiodarone 41 but attenuated the infection-induced cytoskeleton damage of host cells and cytotoxic effects of amiodarone 41. Therefore, this combination treatment may favor parasite control and limit tissue damage. An alternative coupling of amiodarone 41 with the antifungal itraconazole 51 (vide infra) provides an effective treatment of T. cruzi-infected dogs and supports the potential therapeutic application of this combination against trypanosomatid infections in humans (Benaim et al., 2021).
Carvedilol 42, a beta-blocker, selected through virtual screening on the cysteine protease cruzipain of the SWEETLEAD library of approved drugs, promotes the accumulation of immature autophagosomes with decreased acidity and hydrolytic properties by inhibiting the autophagy flux (Rivero et al., 2021). As a result, the viability of trypomastigotes is compromised, and the replication of epimastigotes and amastigotes at 10 μM is hindered, leading to a significant reduction in infection and parasite load. Significantly this effect is maintained in in vivo studies with carvedilol diminishing the peak whole-body parasite burden in infected mice.
The antihypertensive drug manidipine 43 was evaluated in vitro against T. cruzi (Correa et al., 2021) showing potent antiparasitic activity against multiple life cycle stages (EC50 of 0.1 μM in amastigotes and 3 μM in trypomastigotes), with promising selectivity against intracellular amastigotes versus the host cell (SI > 1459). Fluorometric analysis showed that manidipine 43 caused depolarization of the plasma membrane and decreased ATP levels, suggesting mitochondrial bioenergetic alteration of the parasite as a potential mode of action.
Finally, benidipine 44, a calcium channel blocker, has emerged as another promising candidate for repurposing as a trypanocidal drug (Bellera et al., 2015). Discovered by computer-aided screening as a cruzipain inhibitor, this compound underwent comprehensive evaluation, including biochemical and cellular studies, as well as biopharmaceutical, toxicological, physiopathological, and preclinical experiments utilizing an acute model of infection. Remarkably, benidipine 44 demonstrated potent efficacy in reducing parasitemia in an experimental preclinical acute murine infection model at significantly lower doses compared to the positive control, BZN 1 (10 mg/kg/day versus 100 mg/kg/day, respectively). The same research group later found that chronically infected mice treated with this compound showed a reduction in both quantitative and qualitative measures of inflammation compared to untreated mice (Sbaraglini et al., 2016). This was particularly significant in cardiac and skeletal muscle. The reduction in tissue damage can be attributed to the parasiticidal properties of cruzipain inhibitors.
5.6 Repurposing antifungal drugs
Unlike mammalian cells, trypanosomatids primarily produce ergosterol rather than cholesterol, which is similar to the sterol metabolism found in fungi. As such, the sterol biosynthesis pathway presents promising targets for the development of new drugs to treat kinetoplastid infections (Porta et al., 2014; Porta et al., 2023). These targets are sensitive to azoles (Leaver, 2018). These well-known antifungal drugs are already recognized for their activity against T. cruzi and act, generally, via inhibition of 14-alpha-sterol demethylase (Buckner and Urbina, 2012). For instance, using a phenotypic-based repurposing strategy, the trypanocidal activity of terconazole 45 (Figure 12), a triazole antifungal drug, was evaluated (Reigada et al., 2019b), revealing trypanocidal activity in vitro against epimastigotes of different parasite strains and clinically relevant life-stages of T. cruzi (EC50 between 4 and 25 μM). Consistent with the above premise, molecular docking simulations suggested that terconazole inhibits T. cruzi cytochrome P450 14-alpha-demethylase.
In another case, a collection of 100 registered drugs with repositioning potential for NTDs was gathered and assessed in vitro (Kaiser et al., 2015). From a dose-response phenotypic-based screen seven azoles and triazoles were identified as the most potent class of inhibitors from this set of compounds. These active compounds displayed EC50 values in the range of 0.003–0.3 μM and SI > 100, and include the imidazoles bifonazole 46, clotrimazole 6, econazole nitrate 47, miconazole 48 and tioconazole 49, and the triazoles itraconazole 50 and ketoconazole 51.
However, the failure of two triazole antifungals, posaconazole 52 and E1224 53, to demonstrate sustained clearance of T. cruzi parasitemia in chronically infected patients in phase II clinical trials (Molina et al., 2014) has challenged the sole use of azoles as a therapeutic class for the treatment of CD. Given this, coupled with the limited efficacy of treatment for CD in the chronic phase, a study was conducted in dogs infected with a benznidazole-resistant strain of T. cruzi to test the effectiveness of combining BZN 1 with the antifungal azole itraconazole 50 (Cunha et al., 2022). The dogs were divided into four groups and treated with BZN 1, itraconazole 50, a combination of BZN 1 and itraconazole 50, or left untreated. Over a period of 24 months, the BZN 1 and BZN 1 + itraconazole 50 groups showed negative results in PCR and hemoculture tests, while BZN 1 alone showed partial success. Reactive immunoassay results persisted in all treated animals. Echocardiography and histopathological analysis indicated improved cardiac conditions in the BZN 1 + itraconazole 50 group compared to itraconazole 50 alone. Inflammation and fibrosis were significantly reduced in the BZN 1 + itraconazole 50 group, suggesting an improvement or stabilization of the dogs’ clinical condition.
5.7 Repurposing antibacterial and antiviral drugs
Metronidazole 54 (Figure 13) is a broad-spectrum nitroimidazole antibiotic with activity against various parasites including trichomonas and giardia. In in vitro repurposing studies, as a monotherapy, metronidazole 54 had low potency against T. cruzi, but in combination with BZN 1, it increased BZN’s efficacy. In vivo, metronidazole 54 did not suppress parasitemia but improved survival at certain doses, and the combination therapy with BZN 1 prevented mortality and protected against electric cardiac alterations caused by the parasite (Simões-Silva et al., 2017).
A number of other antibiotics have emerged from various screening campaigns. For example, in the structure-based screening conducted by Adasme et al. (2020), the most effective in vitro trypanocidal compound was the fluoroquinolone antibiotic ciprofloxacin 55. Further evaluation in an in vivo experiment, showed that ciprofloxacin 55 demonstrated good levels of inhibition of parasitemia in mice (66.7% in a single dose of ciprofloxacin 55 at 100 mg/kg body weight). Similarly, piperacillin 56, a β-lactam antibiotic, emerged as the lead candidate from a virtual screening of 3180 FDA-approved drugs conducted by Palos et al. (2017) for potential inhibition of the T. cruzi cysteine protease cruzain. Piperacillin 56 exhibited superior in vitro trypanocidal activity profiles and comparable results in reducing parasitemia in infected mice during in short-term in vivo studies, when compared to the standard drug BZN 1. A similar target-based virtual screening study identified the antibiotic clofazimine 57, which finds current use for the treatment of leprosy, as a promising lead structure for the inhibition of proline uptake through the proline permease TcAAAP069 (Sayé et al., 2020).
A number of antiviral drugs have been evaluated for their potential against CD, with mixed results. Saquinavir 58, an antiretroviral drug, showed potential as a trypanocidal compound in in vitro assays (Bellera et al., 2015). However, poor solubility prevented it from advancing to in vivo studies in mice. In contrast, the antiherpetic compound 348U87 59 has demonstrated considerable promise against T. cruzi CA-I/72 strain showing a sub-nanomolar EC50 (0.63 nM) and a SI of 1294 (Bernatchez et al., 2020).
5.8 Repurposing antiparasitic drugs
It is only logical that existing antiparasitic agents are candidates for drug repositioning for treatments for CD. One resource is the open-access Pathogen Box collection provided by the Medicines for Malaria Venture (MMV), (MMV, 2023). Pathogen Box is a collection of 400 compounds, including 200 drug-like and 200 probe-like compounds. These were selected from over 20,000 antimalarial hits from corporate and academic libraries and represent a structurally diverse set. Testing the complete Pathogen Box identified many molecules with good activity (micromolar and sub-micromolar) and SI (>5) in T. cruzi phenotypic-based assays (Duffy et al., 2017). Among them, 7 compounds stood out (Figure 14): MMV687776 60, MMV637229 61, MMV689028 62, MMV689029 63, MMV688796 64, MMV688371 65, and MMV689709 66. Whilst not formally repurposing, all these structures represent strong starting points for the search for new chemical entities against CD.
In a true repurposing study, the broad-spectrum antiparasitic ivermectin 67 was investigated as a trypanocidal agent against T. cruzi (Fraccaroli et al., 2022). In this case, ivermectin 67 affected the proliferation of T. cruzi epimastigotes and amastigotes, and the viability of trypomastigotes in a dose-dependent manner, with a SI of 12 for the amastigote stage. However, drug combinations of ivermectin 67 with BZN 1 or NFX 2 showed mainly additive effects. In contrast, a combination of BZN 1 and the anti-malarial drug chloroquine 68 significantly reduced T. cruzi infection in vitro and was eight times more effective in reducing T. cruzi infection in vivo than BZN 1 monotherapy. This could enable higher treatment efficacy while mitigating the adverse effects of high doses of BZN 1 (Pandey et al., 2022).
Co-administration of the anthelminthic drug levamisole 69, with BZN 1 partially reduced parasitemia and slightly promoted animal survival (Simões-Silva et al., 2019b). As levamisole 69 has immunomodulatory activity, and when tested alone did not decrease parasitemia or mortality rates in a murine infection model, this suggest that these effects are related to Th1-response modulation. Similarly, resveratrol 70, an activator of type III KDACs, which has been shown to exhibit a range of anti-parasitic properties including anti-T. cruzi effects (Campo, 2017), also has a host cell response. For example, a resveratrol 70 dosage partially protected mammal cells from infection without activating apoptosis. However, these were in vitro experiments and further in vivo studies are needed to determine if this therapy could be used as a pre-exposure prophylactic drug (Rodriguez et al., 2022).
Finally, a notable example of drug repositioning is the translational research program by DNDi (Drugs for Neglected Diseases initiative) that repositioned fexinidazole 71 for treatment of CD. Originally developed in the late 1970s as a broad-spectrum anti-infective agent, fexinidazole 71 is a pro-drug of 5-nitroimidazole activated by NTR-1. It was later selected, among other 700 nitroheterocyclic compounds, for development by the DNDi as a treatment for sleeping sickness (Torreele et al., 2010). It has shown superior efficacy in curing experimental T. cruzi infections compared to BZN 1 and NFX 2. It also has been reported for its oral efficacy in acute and chronic experimental models of benznidazole-susceptible, partially resistant, or resistant T. cruzi isolates (Bahia et al., 2012). A Phase II clinical trial evaluating its effectiveness, safety, and tolerability in patients with asymptomatic chronic infections was completed at the end of 2022 (Torrico et al., 2023).
6 Perspective, challenges, and future directions
In line with the WHO’s roadmap 2021–2030 for eradicating NTDs, and especially in the context of CD, there is an urgent need to expand the range of chemotherapeutic options (Casulli, 2021). The current therapy for this condition is limited, inefficient, and insufficient, consisting of only two outdated drugs. As such, finding a rapid solution is crucial. As evidenced by successes in treating other diseases (Charlton et al., 2018; Hua et al., 2022), coupled with the encouraging results achieved over the past years, summarized in this article, to develop to develop new and improved therapies for CD, drug repurposing offers hope for making progress in the fight against one of the most neglected NTDs.
Despite its promise, drug repositioning (and drug discovery in general) in T. cruzi faces several challenges, both technical and applied. One major challenge is the lack of comprehensive knowledge of T. cruzi biology and drug targets (Jansen et al., 2020). This is exemplified by the extensive morphological and metabolic transformations that occur throughout the various developmental stages of T. cruzi, which challenge efforts to develop effective drugs against this parasite (Teixeira et al., 2012). Most notably, the intracellular amastigote can enter in a metabolically quiescent or dormant state which can be non-responsive to otherwise effective trypanocidal drugs (Sánchez-Valdéz et al., 2018; Bhattacharya et al., 2020). As such, there is a pressing need for compounds that can address this chronic state or even restore the sensitivity of the dormant parasite. In this respect, a very recent study by Rial et al. (2023) suggest that isotretinoin 72 (Figure 15), an FDA-approved drug used for severe acne that has previously shown reduction of the trypomastigote burst from infected mammal cells at nanomolar concentrations (Reigada et al., 2017), can also reduce blood parasitemia, prevent negative chronotropic effects and lead to reduced anti-T. cruzi antibody levels in murine models of chronic CD.
The success of drug repurposing remains reliant on the availability of existing drugs with well-established safety profiles, which can limit the pool of potential drug candidates. Moreover, this limited pool, and the very nature of repurposing, can mean that innovation and new modes of action are less likely to be revealed. Despite this, there are multitude of libraries available for testing and it is possible to initiate large-scale screening programs that can effectively harness these resources and unlock new treatment options. However, HTS is not cheap and for repurposing it is expected that in silico drug-target evaluation will have a growing role. By leveraging computational tools, researchers can predict the activity of existing drugs against specific targets in T. cruzi, allowing for the prioritization of compounds for further testing (Trevisan et al., 2020). While this approach is well-established in other diseases, alternative rational methods such as network-based and signature-based approaches have yet to be applied in the context of CD (Bellera et al., 2020). The future application of artificial intelligence (AI) and machine learning to this field holds great promise (Villalta and Rachakonda, 2019).
CD chemotherapy is further challenged by the extensive tissue distribution of T. cruzi which requires a similarly wide drug distribution profile in order to clear all parasites (De Rycker et al., 2023). Furthermore, the pharmacological management of this disease is further complicated by the resistance of different parasite strains to currently available drugs, potentially leading to treatment failures (García-Huertas and Cardona-Castro, 2021). One increasingly popular solution to these issues is the use of combination drug therapy, which involves administering multiple drugs with different profiles and modes of action. This can also help address challenges due to drug toxicity. Reflecting this, most combinations that have been reported involve BZN 1 or NFX 2 as the currently approved therapies. However, other repurposing combinations have been explored (Machado et al., 2020; Rocha-Hasler et al., 2021). For instance, combinations of Posaconazole 52 with either amlodipine 73 (Figure 15) or clemastine 4 demonstrated synergistic activity and greater effectiveness in reducing parasitemia levels in mice (Planer et al., 2014). Although attractive for these reasons, developing drug combinations does have hurdles that may not exist for a single repurposed drug, particularly in the assessment of pharmacokinetic and pharmacodynamic parameters as well as efficacy and safety within patients.
In conclusion, repurposing approved drugs presents a fast and appealing approach to address the challenge of CD (Supplementary Table S1, Supplementary Material), with a number of advantages compared to conventional bottom-up drug discovery. Firstly, it significantly reduces the time and cost associated with drug development by leveraging the known safety profiles and pharmacokinetic properties of these drugs, streamlining the regulatory approval process. Secondly, repositioning can rapidly expand the limited treatment options currently available for CD, potentially uncovering additional effective therapies. By exploring diverse mechanisms of action, repurposed drugs may address drug resistance and target different stages of the disease, leading to improved treatment outcomes. Moreover, combining repurposed drugs with existing treatments can result in synergistic effects, enhancing their efficacy in a safer manner. Lastly, utilizing existing drug supply chains facilitates broader access to medications for CD, particularly in resource-constrained regions. Despite these advantages, rigorous research and clinical trials remain essential to confirm the safety, efficacy, and optimal usage of repurposed drugs for CD. Furthermore, the regulatory landscape for drug repurposing is complex, with challenges related to intellectual property, regulatory approval, and commercialization (Halabi, 2018). Despite these minor limitations, whilst not the sole solution, drug repositioning represents a valuable, relatively fast, and cost-effective strategy for developing essential new therapies, particularly for NTDs such as CD.
Author contributions
All authors conceptualized the overall structure of the review article and contributed to writing the article. EP prepared all the figures. All listed authors have made a substantial, direct, and intellectual contribution to the article and have approved the submitted version.
Funding
We thank The Royal Society (The Royal Society International Collaboration Awards for Research Professors 2016: IC160044), UKRI for the MRC impact acceleration accounts (grant code MR/X502947/1) and UKRI Grand Challenges Research Fund (“A Global Network for Neglected Tropical Diseases” grant number MR/P027989/1) for financial support. KK acknowledges funding from The Royal Society of Chemistry (Research Fund R21-3545544506) and The Royal Society (Research Grant RGS\R2\222343).
Conflict of interest
The authors declare that the research was conducted in the absence of any commercial or financial relationships that could be construed as a potential conflict of interest.
Publisher’s note
All claims expressed in this article are solely those of the authors and do not necessarily represent those of their affiliated organizations, or those of the publisher, the editors and the reviewers. Any product that may be evaluated in this article, or claim that may be made by its manufacturer, is not guaranteed or endorsed by the publisher.
Supplementary material
The Supplementary Material for this article can be found online at: https://www.frontiersin.org/articles/10.3389/fphar.2023.1233253/full#supplementary-material
References
Adasme, M. F., Bolz, S. N., Adelmann, L., Salentin, S., Haupt, V. J., Moreno-Rodríguez, A., et al. (2020). Repositioned drugs for Chagas disease unveiled via structure-based drug repositioning. Int. J. Mol. Sci. 21 (22), 8809. doi:10.3390/ijms21228809
Adesse, D., Azzam, E. M., Meirelles, M. N., Urbina, J. A., and Garzoni, L. R. (2011). Amiodarone inhibits Trypanosoma cruzi infection and promotes cardiac cell recovery with gap junction and cytoskeleton reassembly in vitro. Antimicrob. Agents Chemother. 55 (1), 203–210. doi:10.1128/AAC.01129-10
Alberca, L. N., Sbaraglini, M. L., Morales, J. F., Dietrich, R., Ruiz, M. D., Pino Martínez, A. M., et al. (2018). Cascade ligand- and structure-based virtual screening to identify new trypanocidal compounds inhibiting putrescine uptake. Front. Cell. Infect. Microbiol. 8, 173. doi:10.3389/fcimb.2018.00173
Almeida-Silva, J., Menezes, D. S., Fernandes, J. M. P., Almeida, M. C., Vasco-Dos-Santos, D. R., Saraiva, R. M., et al. (2022). The repositioned drugs disulfiram/diethyldithiocarbamate combined to benznidazole: Searching for Chagas disease selective therapy, preventing toxicity and drug resistance. Front. Cell. Infect. Microbiol. 12, 926699. doi:10.3389/fcimb.2022.926699
Álvarez-Bardón, M., Pérez-Pertejo, Y., Ordóñez, C., Sepúlveda-Crespo, D., Carballeira, N. M., Tekwani, B. L., et al. (2020). Screening marine natural products for new drug leads against trypanosomatids and malaria. Mar. Drugs. 18 (4), 187. doi:10.3390/md18040187
Ancidoni, A., Bacigalupo, I., Remoli, G., Lacorte, E., Piscopo, P., Sarti, G., et al. (2021). Anticancer drugs repurposed for alzheimer's disease: A systematic review. Alzheimers Res. Ther. 13 (1), 96. doi:10.1186/s13195-021-00831-6
Annang, F., Pérez-Moreno, G., García-Hernández, R., Cordon-Obras, C., Martín, J., Tormo, J. R., et al. (2015). High-throughput screening platform for natural product-based drug discovery against 3 neglected tropical diseases: Human african trypanosomiasis, leishmaniasis, and Chagas disease. J. Biomol. Screen. 20 (1), 82–91. doi:10.1177/1087057114555846
Araujo-Lima, C. F., Peres, R. B., Silva, P. B., Batista, M. M., Aiub, C. A. F., Felzenszwalb, I., et al. (2018). Repurposing strategy of atorvastatin against trypanosoma cruzi: In vitro monotherapy and combined therapy with benznidazole exhibit synergistic trypanocidal activity. Antimicrob. Agents Chemother. 62 (9), 009799–e1018. doi:10.1128/AAC.00979-18
Bahia, M. T., de Andrade, I. M., Martins, T. A., do Nascimento, Á. F., Diniz, L. de F., Caldas, I. S., et al. (2012). Fexinidazole: A potential new drug candidate for Chagas disease. PLoS Negl. Trop. Dis. 6 (11), e1870. doi:10.1371/journal.pntd.0001870
Barbosa, H., Thevenard, F., Quero Reimão, J., Tempone, A. G., Honorio, K. M., and Lago, J. H. G. (2023). The potential of secondary metabolites from plants as drugs or leads against trypanosoma cruzi-an update from 2012 to 2021. Curr. Top. Med. Chem. 23 (3), 159–213. doi:10.2174/1568026623666221212111514
Barbosa, J. M. C., Pedra-Rezende, Y., Pereira, L. D., de Melo, T. G., Barbosa, H. S., Lannes-Vieira, J., et al. (2022). Benznidazole and amiodarone combined treatment attenuates cytoskeletal damage in Trypanosoma cruzi-infected cardiac cells. Front. Cell Infect. Microbiol. 12, 975931. doi:10.3389/fcimb.2022.975931
Bayat Mokhtari, R., Homayouni, T. S., Baluch, N., Morgatskaya, E., Kumar, S., Das, B., et al. (2017). Combination therapy in combating cancer. Oncotarget 8 (23), 38022–38043. doi:10.18632/oncotarget.16723
Bellera, C. L., Alberca, L. N., Sbaraglini, M. L., and Talevi, A. (2020). In silico drug repositioning for Chagas disease. Curr. Med. Chem. 27 (5), 662–675. doi:10.2174/0929867326666191016114839
Bellera, C. L., Balcazar, D. E., Alberca, L., Labriola, C. A., Talevi, A., and Carrillo, C. (2014). Identification of levothyroxine antichagasic activity through computer-aided drug repurposing. ScientificWorldJournal 2014, 279618. doi:10.1155/2014/279618
Bellera, C. L., Balcazar, D. E., Vanrell, M. C., Casassa, A. F., Palestro, P. H., Gavernet, L., et al. (2015). Computer-guided drug repurposing: Identification of trypanocidal activity of clofazimine, benidipine and saquinavir. Eur. J. Med. Chem. 93, 338–348. doi:10.1016/j.ejmech.2015.01.065
Benaim, G., Paniz-Mondolfi, A. E., and Sordillo, E. M. (2021). The rationale for use of amiodarone and its derivatives for the treatment of Chagas' disease and leishmaniasis. Curr. Pharm. Des. 27 (15), 1825–1833. doi:10.2174/1381612826666200928161403
Bernatchez, J. A., Chen, E., Hull, M. V., McNamara, C. W., McKerrow, J. H., and Siqueira-Neto, J. L. (2020). High-throughput screening of the ReFRAME library identifies potential drug repurposing candidates for trypanosoma cruzi. Microorganisms 8 (4), 472. doi:10.3390/microorganisms8040472
Bhattacharya, A., Corbeil, A., do Monte-Neto, R. L., and Fernandez-Prada, C. (2020). Of drugs and trypanosomatids: New tools and knowledge to reduce bottlenecks in drug discovery. Genes (Basel) 11 (7), 722. doi:10.3390/genes11070722
Blay, V., Tolani, B., Ho, S. P., and Arkin, M. R. (2020). High-throughput screening: today's biochemical and cell-based approaches. Drug. Discov. Today. 25 (10), 1807–1821. doi:10.1016/j.drudis.2020.07.024
Braga, S. S. (2019). Multi-target drugs active against leishmaniasis: A paradigm of drug repurposing. Eur. J. Med. Chem. 183, 111660. doi:10.1016/j.ejmech.2019.111660
Buckner, F. S., and Urbina, J. A. (2012). Recent developments in sterol 14-demethylase inhibitors for Chagas disease. Int. J. Parasitol. Drugs Drug Resist 2, 236–242. doi:10.1016/j.ijpddr.2011.12.002
Campo, V. A. (2017). Comparative effects of histone deacetylases inhibitors and resveratrol on Trypanosoma cruzi replication, differentiation, infectivity and gene expression. Int. J. Parasitol. Drugs Drug Resist. 7 (1), 23–33. doi:10.1016/j.ijpddr.2016.12.003
Campos-Estrada, C., Urarte, E., Denegri, M., Villalón, L., González-Herrera, F., Kemmerling, U., et al. (2023). Effect of statins on inflammation and cardiac function in patients with chronic Chagas disease: A protocol for pathophysiological studies in a multicenter, placebo-controlled, proof-of-concept phase II trial. PLoS One 18 (1), e0280335. doi:10.1371/journal.pone.0280335
Casulli, A. (2021). New global targets for NTDs in the WHO roadmap 2021-2030. PLoS Negl. Trop. Dis. 15 (5), e0009373. doi:10.1371/journal.pntd.0009373
CDC (2023). Centers for diseases control and prevention. Available at: https://www.cdc.gov/parasites/chagas/ (Accessed May, 2023).
Charlton, R. L., Rossi-Bergmann, B., Denny, P. W., and Steel, P. G. (2018). Repurposing as a strategy for the discovery of new anti-leishmanials: The-state-of-the-art. Parasitology 145 (2), 219–236. doi:10.1017/S0031182017000993
Clark, E. H., Sherbuk, J., Okamoto, E., Jois, M., Galdos-Cardenas, G., Vela-Guerra, J., et al. (2014). Hyperendemic Chagas disease and the unmet need for pacemakers in the Bolivian Chaco. PLoS Negl. Trop. Dis. 8 (6), e2801. doi:10.1371/journal.pntd.0002801
Correa, I. T. S., da Costa-Silva, T. A., and Tempone, A. G. (2021). Bioenergetics impairment of trypanosoma cruzi by the antihypertensive manidipine: A drug repurposing strategy. Acta Trop. 214, 105768. doi:10.1016/j.actatropica.2020.105768
Crespillo-Andújar, C., Comeche, B., Hamer, D. H., Arevalo-Rodriguez, I., Alvarez-Díaz, N., Zamora, J., et al. (2022). Use of benznidazole to treat chronic Chagas disease: An updated systematic review with a meta-analysis. PLoS Negl. Trop. Dis. 16 (5), e0010386. doi:10.1371/journal.pntd.0010386
Cucunubá, Z. M., Nouvellet, P., Conteh, L., Vera, M. J., Angulo, V. M., Dib, J. C., et al. (2017). Modelling historical changes in the force-of-infection of Chagas disease to inform control and elimination programmes: Application in Colombia. BMJ Glob. Health 2 (3), e000345. doi:10.1136/bmjgh-2017-000345
Cunha, E. L. A., Torchelsen, F. K. V. D. S., Fonseca, K. D. S., Sousa, L. R. D., Vieira, P. M. A., Carneiro, C. M., et al. (2022). Benznidazole, itraconazole, and their combination for the treatment of chronic experimental Chagas disease in dogs. Exp. Parasitol. 238, 108266. doi:10.1016/j.exppara.2022.108266
De Rycker, M., Thomas, J., Riley, J., Brough, S. J., Miles, T. J., and Gray, D. W. (2016). Identification of trypanocidal activity for known clinical compounds using a new trypanosoma cruzi hit-discovery screening cascade. PLoS Negl. Trop. Dis. 10 (4), e0004584. doi:10.1371/journal.pntd.0004584
De Rycker, M., Wyllie, S., Horn, D., Read, K. D., and Gilbert, I. H. (2023). Anti-trypanosomatid drug discovery: Progress and challenges. Nat. Rev. Microbiol. 21 (1), 35–50. doi:10.1038/s41579-022-00777-y
DNDi (2023). Drugs for neglected diseases initiative. Available at: https://dndi.org/diseases/chagas/target-product-profile/ (Accessed July, 2023).
Docampo, R., Moreno, S. N., Muniz, R. P., Cruz, F. S., and Mason, R. P. (1983). Light-enhanced free radical formation and trypanocidal action of gentian violet (crystal violet). Science 220 (4603), 1292–1295. doi:10.1126/science.6304876
Duffy, S., Sykes, M. L., Jones, A. J., Shelper, T. B., Simpson, M., Lang, R., et al. (2017). Screening the medicines for malaria venture pathogen Box across multiple pathogens reclassifies starting points for open-source drug discovery. Antimicrob. Agents Chemother. 61 (9), 003799–e417. doi:10.1128/AAC.00379-17
El Bairi, K., Trapani, D., Petrillo, A., Le Page, C., Zbakh, H., Daniele, B., et al. (2020). Repurposing anticancer drugs for the management of COVID-19. Eur. J. Cancer. 141, 40–61. doi:10.1016/j.ejca.2020.09.014
Ferreira, D. D., Mesquita, J. T., da Costa Silva, T. A., Romanelli, M. M., da Gama Jaen Batista, D., da Silva, C. F., et al. (2018). Efficacy of sertraline against trypanosoma cruzi: An in vitro and in silico study. J. Venom. Anim. Toxins Incl. Trop. Dis. 24, 30. doi:10.1186/s40409-018-0165-8
Ferreira, L. L. G., de Moraes, J., and Andricopulo, A. D. (2022). Approaches to advance drug discovery for neglected tropical diseases. Drug. Discov. Today. 27 (8), 2278–2287. doi:10.1016/j.drudis.2022.04.004
Fraccaroli, L., Ruiz, M. D., Perdomo, V. G., Clausi, A. N., Balcazar, D. E., Larocca, L., et al. (2022). Broadening the spectrum of ivermectin: Its effect on Trypanosoma cruzi and related trypanosomatids. Front. Cell. Infect. Microbiol. 12, 885268. doi:10.3389/fcimb.2022.885268
García, M. C., Ponce, N. E., Sanmarco, L. M., Manzo, R. H., Jimenez-Kairuz, A. F., and Aoki, M. P. (2016). Clomipramine and benznidazole act synergistically and ameliorate the outcome of experimental Chagas disease. Antimicrob. Agents Chemother. 60 (6), 3700–3708. doi:10.1128/AAC.00404-16
García-Huertas, P., and Cardona-Castro, N. (2021). Advances in the treatment of Chagas disease: Promising new drugs, plants and targets. Biomed. Pharmacother. 142, 112020. doi:10.1016/j.biopha.2021.112020
Global Burden of Disease (2019). Global burden of disease. Available at: http://ghdx.healthdata.org/ (Accessed July, 2023).
Gómez-Ochoa, S. A., Rojas, L. Z., Echeverría, L. E., Muka, T., and Franco, O. H. (2022). Global, regional, and national trends of Chagas disease from 1990 to 2019: Comprehensive analysis of the global burden of disease study. Glob. Heart 17 (1), 59. doi:10.5334/gh.1150
Gulin, J. E. N., Bisio, M. M. C., Rocco, D., Altcheh, J., Solana, M. E., and García-Bournissen, F. (2022). Miltefosine and benznidazole combination improve anti-trypanosoma cruzi in vitro and in vivo efficacy. Front. Cell. Infect. Microbiol. 12, 855119. doi:10.3389/fcimb.2022.855119
Guzmán-Rivera, D., Liempi, A., González-Herrera, F., Fuentes-Retamal, S., Carrillo, I., Abarca, P., et al. (2020). Simvastatin improves cardiac function through notch 1 activation in BALB/c mice with chronic Chagas cardiomyopathy. Antimicrob. Agents Chemother. 64 (8), 021411–e2219. doi:10.1128/AAC.02141-19
Halabi, S. F. (2018). The drug repurposing ecosystem: Intellectual property incentives, market exclusivity, and the future of "new" medicines 20 yale journal of law and technology. Available at: https://scholarship.law.missouri.edu/facpubs/717.
Hashimoto, K. (2021). Repurposing of CNS drugs to treat COVID-19 infection: Targeting the sigma-1 receptor. Eur. Arch. Psychiatry Clin. Neurosci. 271 (2), 249–258. doi:10.1007/s00406-020-01231-x
Hua, Y., Dai, X., Xu, Y., Xing, G., Liu, H., Lu, T., et al. (2022). Drug repositioning: Progress and challenges in drug discovery for various diseases. Eur. J. Med. Chem. 234, 114239. doi:10.1016/j.ejmech.2022.114239
Iribarne, F., Paulino, M., Aguilera, S., and Tapia, O. (2009). Assaying phenothiazine derivatives as trypanothione reductase and glutathione reductase inhibitors by theoretical docking and molecular dynamics studies. J. Mol. Graph. Model. 28 (4), 371–381. doi:10.1016/j.jmgm.2009.09.003
Jackson, Y., Wyssa, B., and Chappuis, F. (2020). Tolerance to nifurtimox and benznidazole in adult patients with chronic Chagas' disease. J. Antimicrob. Chemother. 75 (3), 690–696. doi:10.1093/jac/dkz473
Jansen, A. M., Xavier, S. C. D. C., and Roque, A. L. R. (2020). Landmarks of the knowledge and trypanosoma cruzi biology in the wild environment. Front. Cell. Infect. Microbiol. 10, 10. doi:10.3389/fcimb.2020.00010
Johndrow, C., Nelson, R., Tanowitz, H., Weiss, L. M., and Nagajyothi, F. (2014). Trypanosoma cruzi infection results in an increase in intracellular cholesterol. Microbes Infect. 16 (4), 337–344. doi:10.1016/j.micinf.2014.01.001
Jourdan, J. P., Bureau, R., Rochais, C., and Dallemagne, P. (2020). Drug repositioning: A brief overview. J. Pharm. Pharmacol. 72 (9), 1145–1151. doi:10.1111/jphp.13273
Juárez-Saldivar, A., Schroeder, M., Salentin, S., Haupt, V. J., Saavedra, E., Vázquez, C., et al. (2020). Computational drug repositioning for Chagas disease using protein-ligand interaction profiling. Int. J. Mol. Sci. 21 (12), 4270. doi:10.3390/ijms21124270
Kaiser, M., Mäser, P., Tadoori, L. P., Ioset, J. R., and Brun, R. (2015). Antiprotozoal activity profiling of approved drugs: A starting point toward drug repositioning. PLoS One 10 (8), e0135556. doi:10.1371/journal.pone.0135556
Krishnamurthy, N., Grimshaw, A. A., Axson, S. A., Choe, S. H., and Miller, J. E. (2022). Drug repurposing: A systematic review on root causes, barriers and facilitators. BMC Health. Serv. Res. 22 (1), 970. doi:10.1186/s12913-022-08272-z
Lazarin-Bidóia, D., Garcia, F. P., Ueda-Nakamura, T., Silva, S. O., and Nakamura, C. V. (2022). Natural compounds based chemotherapeutic against Chagas disease and leishmaniasis: Mitochondrion as a strategic target. Mem. Inst. Oswaldo Cruz. 117, e220396. doi:10.1590/0074-02760220396
Leaver, D. J. (2018). Synthesis and biological activity of sterol 14α-demethylase and sterol C24-methyltransferase inhibitors. Molecules 23 (7), 1753. doi:10.3390/molecules23071753
Liao, J. K., and Laufs, U. (2005). Pleiotropic effects of statins. Annu. Rev. Pharmacol. Toxicol. 45, 89–118. doi:10.1146/annurev.pharmtox.45.120403.095748
Machado, Y. A., Bahia, M. T., Caldas, I. S., Mazzeti, A. L., Novaes, R. D., Vilas Boas, B. R., et al. (2020). Amlodipine increases the therapeutic potential of ravuconazole upon trypanosoma cruzi infection. Antimicrob. Agents Chemother. 64 (8), 024977–e2519. doi:10.1128/AAC.02497-19
Mercaldi, G. F., D'Antonio, E. L., Aguessi, A., Rodriguez, A., and Cordeiro, A. T. (2019). Discovery of antichagasic inhibitors by high-throughput screening with Trypanosoma cruzi glucokinase. Bioorg. Med. Chem. Lett. 29 (15), 1948–1953. doi:10.1016/j.bmcl.2019.05.037
Meymandi, S., Hernandez, S., Park, S., Sanchez, D. R., and Forsyth, C. (2018). Treatment of Chagas disease in the United States. Curr. Treat. Options Infect. Dis. 10 (3), 373–388. doi:10.1007/s40506-018-0170-z
Miguel, D. C., Ferraz, M. L., Alves, R. O., Yokoyama-Yasunaka, J. K., Torrecilhas, A. C., Romanha, A. J., et al. (2010). The anticancer drug tamoxifen is active against Trypanosoma cruzi in vitro but ineffective in the treatment of the acute phase of Chagas disease in mice. Mem. Inst. Oswaldo Cruz. 105 (7), 945–948. doi:10.1590/s0074-02762010000700021
Miranda-Arboleda, A. F., Zaidel, E. J., Marcus, R., Pinazo, M. J., Echeverría, L. E., Saldarriaga, C., et al. (2021). Roadblocks in Chagas disease care in endemic and nonendemic countries: Argentina, Colombia, Spain, and the United States. The NET-heart project. PLoS. Negl. Trop. Dis. 15 (12), e0009954. doi:10.1371/journal.pntd.0009954
MMV (2023). Medicines for malaria venture. Available at: https://www.mmv.org/mmv-open/pathogen-box/about-pathogen-box (Accessed May, 2023).
Moffat, J. G., Vincent, F., Lee, J. A., Eder, J., and Prunotto, M. (2017). Opportunities and challenges in phenotypic drug discovery: An industry perspective. Nat. Rev. Drug. Discov. 16 (8), 531–543. doi:10.1038/nrd.2017.111
Molina, I., Gómez i Prat, J., Salvador, F., Treviño, B., SulleiroSerre, N. E., et al. (2014). Randomized trial of posaconazole and benznidazole for chronic Chagas' disease. N. Engl. J. Med. 370 (20), 1899–1908. doi:10.1056/NEJMoa1313122
Nagajyothi, F., Machado, F. S., Burleigh, B. A., Jelicks, L. A., Scherer, P. E., Mukherjee, S., et al. (2012). Mechanisms of Trypanosoma cruzi persistence in Chagas disease. Cell Microbiol. 14 (5), 634–643. doi:10.1111/j.1462-5822.2012.01764.x
Nekoei, S., Khamesipour, F., Habtemariam, S., de Souza, W., Mohammadi Pour, P., and Hosseini, S. R. (2022). The anti-trypanosoma activities of medicinal plants: A systematic review of the literature. Vet. Med. Sci. 8 (6), 2738–2772. doi:10.1002/vms3.912
Nesic de Freitas, L. S. F., da Silva, C. F., Intagliata, S., Amata, E., Salerno, L., and Soeiro, M. N. C. (2023). In vitro and in silico analysis of imatinib analogues as anti-Trypanosoma cruzi drug candidates. Parasitology 150 (4), 1–18. doi:10.1017/S0031182023000057
PAHO (2023). Pan American health organization. Available at: https://www.paho.org/en/topics/chagas-disease (Accessed May, 2023).
Palos, I., Lara-Ramirez, E. E., Lopez-Cedillo, J. C., Garcia-Perez, C., Kashif, M., Bocanegra-Garcia, V., et al. (2017). Repositioning FDA drugs as potential cruzain inhibitors from trypanosoma cruzi: Virtual screening, in vitro and in vivo studies. Molecules 22 (6), 1015. doi:10.3390/molecules22061015
Pandey, R. P., Nascimento, M. S., Franco, C. H., Bortoluci, K., Silva, M. N., Zingales, B., et al. (2022). Drug repurposing in Chagas disease: Chloroquine potentiates benznidazole activity against trypanosoma cruzi in vitro and in vivo. Antimicrob. Agents Chemother. 66 (11), e0028422. doi:10.1128/aac.00284-22
Panozzo-Zénere, E. A., Porta, E. O. J., Arrizabalaga, G., Fargnoli, L., Khan, S. I., Tekwani, B. L., et al. (2018). A minimalistic approach to develop new anti-apicomplexa polyamines analogs. Eur. J. Med. Chem. 2143, 866–880. doi:10.1016/j.ejmech.2017.11.069
Peña-Diaz, J., Montalvetti, A., Flores, C. L., Constán, A., Hurtado-Guerrero, R., De Souza, W., et al. (2004). Mitochondrial localization of the mevalonate pathway enzyme 3-Hydroxy-3-methyl-glutaryl-CoA reductase in the Trypanosomatidae. Mol. Biol. Cell. 15 (3), 1356–1363. doi:10.1091/mbc.e03-10-0720
Pittella, J. E. (2009). Central nervous system involvement in Chagas disease: A hundred-year-old history. Trans. R. Soc. Trop. Med. Hyg. 103 (10), 973–978. doi:10.1016/j.trstmh.2009.04.012
Planer, J. D., Hulverson, M. A., Arif, J. A., Ranade, R. M., Don, R., and Buckner, F. S. (2014). Synergy testing of FDA-approved drugs identifies potent drug combinations against Trypanosoma cruzi. PLoS Negl. Trop. Dis. 8 (7), e2977. doi:10.1371/journal.pntd.0002977
Porta, E. O., Carvalho, P. B., Avery, M. A., Tekwani, B. L., and Labadie, G. R. (2014). Click chemistry decoration of amino sterols as promising strategy to developed new leishmanicidal drugs. Steroids 79, 28–36. doi:10.1016/j.steroids.2013.10.010
Porta, E. O. J., Ballari, M. S., Carlucci, R., Wilkinson, S., Ma, G., Tekwani, B. L., et al. (2023). Systematic study of 1,2,3-triazolyl sterols for the development of new drugs against parasitic Neglected Tropical Diseases. Eur. J. Med. Chem. 254, 115378. doi:10.1016/j.ejmech.2023.115378
Porta, E. O. J., Bofill Verdaguer, I., Perez, C., Banchio, C., Ferreira de Azevedo, M., Katzin, A. M., et al. (2019). Repositioning Salirasib as a new antimalarial agent. Medchemcomm 10 (9), 1599–1605. doi:10.1039/c9md00298g
Pushpakom, S., Iorio, F., Eyers, P. A., Escott, K. J., Hopper, S., Wells, A., et al. (2019). Drug repurposing: Progress, challenges and recommendations. Nat. Rev. Drug. Discov. 18 (1), 41–58. doi:10.1038/nrd.2018.168
Ramsey, J. M., Elizondo-Cano, M., Sanchez-González, G., Peña-Nieves, A., and Figueroa-Lara, A. (2014). Opportunity cost for early treatment of Chagas disease in Mexico. PLoS Negl. Trop. Dis. 8 (4), e2776. doi:10.1371/journal.pntd.0002776
Reigada, C., Sayé, M., Phanstiel, O., Valera-Vera, E., Miranda, M. R., and Pereira, C. A. (2019a). Identification of trypanosoma cruzi polyamine transport inhibitors by computational drug repurposing. Front. Med. (Lausanne) 6, 256. doi:10.3389/fmed.2019.00256
Reigada, C., Sayé, M., Valera-Vera, E., Miranda, M. R., and Pereira, C. A. (2019b). Repurposing of terconazole as an anti Trypanosoma cruzi agent. Heliyon 25 (6), e01947. doi:10.1016/j.heliyon.2019.e01947
Reigada, C., Valera-Vera, E. A., Sayé, M., Errasti, A. E., Avila, C. C., Miranda, M. R., et al. (2017). Trypanocidal effect of isotretinoin through the inhibition of polyamine and amino acid transporters in trypanosoma cruzi. PLoS Negl. Trop. Dis. 11 (3), e0005472. doi:10.1371/journal.pntd.0005472
Rial, M. S., Reigada, C., Prado, N., Bua, J., Esteva, M., Pereira, C. A., et al. (2023). Effectiveness of the repurposed drug isotretinoin in an experimental murine model of Chagas disease. Acta Trop. 242, 106920. doi:10.1016/j.actatropica.2023.106920
Rivero, C. V., Martínez, S. J., Novick, P., Cueto, J. A., Salassa, B. N., Vanrell, M. C., et al. (2021). Repurposing carvedilol as a novel inhibitor of the trypanosoma cruzi autophagy flux that affects parasite replication and survival. Front. Cell Infect. Microbiol. 11, 657257. doi:10.3389/fcimb.2021.657257
Rocha-Hasler, M., de Oliveira, G. M., da Gama, A. N., Fiuza, L. F. A., Fesser, A. F., Cal, M., et al. (2021). Combination with tomatidine improves the potency of posaconazole against trypanosoma cruzi. Front. Cell Infect. Microbiol. 11, 617917. doi:10.3389/fcimb.2021.617917
Rodriguez, M. E., Tekiel, V., and Campo, V. A. (2022). In vitro evaluation of Resveratrol as a potential pre-exposure prophylactic drug against Trypanosoma cruzi infection. Int. J. Parasitol. Drugs Drug Resist. 20, 54–64. doi:10.1016/j.ijpddr.2022.08.003
Sánchez-Valdéz, F. J., Padilla, A., Wang, W., Orr, D., and Tarleton, R. L. (2018). Spontaneous dormancy protects Trypanosoma cruzi during extended drug exposure. Elife 7, e34039. doi:10.7554/eLife.34039
Saraiva, R. M., Portela, L. F., Silveira, G. P. E., Gomes, N. L. S., Pinto, D. P., Silva, A. C. d. A. d., et al. (2021). Disulfiram repurposing in the combined chemotherapy of Chagas disease: A protocol for phase I/II clinical trial. Med. Case Rep. Study Protoc. 2 (7), e0110. doi:10.1097/MD9.0000000000000110
Savage, D. G., and Antman, K. H. (2002). Imatinib mesylate--a new oral targeted therapy. N. Engl. J. Med. 346 (9), 683–693. doi:10.1056/NEJMra013339
Sayé, M., Gauna, L., Valera-Vera, E., Reigada, C., Miranda, M. R., and Pereira, C. A. (2020). Crystal violet structural analogues identified by in silico drug repositioning present anti-Trypanosoma cruzi activity through inhibition of proline transporter TcAAAP069. PLoS Negl. Trop. Dis. 14 (1), e0007481. doi:10.1371/journal.pntd.0007481
Sbaraglini, M. L., Bellera, C. L., Fraccaroli, L., Larocca, L., Carrillo, C., Talevi, A., et al. (2016). Novel cruzipain inhibitors for the chemotherapy of chronic Chagas disease. Int. J. Antimicrob. Agents. 48 (1), 91–95. doi:10.1016/j.ijantimicag.2016.02.018
Schenone, M., Dančík, V., Wagner, B. K., and Clemons, P. A. (2013). Target identification and mechanism of action in chemical biology and drug discovery. Nat. Chem. Biol. 9 (4), 232–240. doi:10.1038/nchembio.1199
Scotti, M. T., Scotti, L., Ishiki, H., Ribeiro, F. F., Cruz, R. M., Oliveira, M. P., et al. (2016). Natural products as a source for antileishmanial and antitrypanosomal agents. Comb. Chem. High. Throughput Screen. 19 (7), 537–553. doi:10.2174/1386207319666160506123921
Shyr, Z. A., Cheng, Y. S., Lo, D. C., and Zheng, W. (2021). Drug combination therapy for emerging viral diseases. Drug. Discov. Today. 26 (10), 2367–2376. doi:10.1016/j.drudis.2021.05.008
Simões-Silva, M. R., Brandão Peres, R., Britto, C., Machado Cascabulho, C., de Melo Oliveira, G., Nefertiti da Gama, A., et al. (2019b). Impact of levamisole in co-administration with benznidazole on experimental Chagas disease. Parasitology 146 (8), 1055–1062. doi:10.1017/S0031182019000374
Simões-Silva, M. R., De Araújo, J. S., Oliveira, G. M., Demarque, K. C., Peres, R. B., D'Almeida-Melo, I., et al. (2017). Drug repurposing strategy against trypanosoma cruzi infection: In vitro and in vivo assessment of the activity of metronidazole in mono- and combined therapy. Biochem. Pharmacol. 145, 46–53. doi:10.1016/j.bcp.2017.08.025
Simões-Silva, M. R., De Araújo, J. S., Peres, R. B., Da Silva, P. B., Batista, M. M., De Azevedo, L. D., et al. (2019a). Repurposing strategies for Chagas disease therapy: The effect of imatinib and derivatives against trypanosoma cruzi. Parasitology 146 (8), 1006–1012. doi:10.1017/S0031182019000234
Sun, W., Sanderson, P. E., and Zheng, W. (2016). Drug combination therapy increases successful drug repositioning. Drug. Discov. Today. 21 (7), 1189–1195. doi:10.1016/j.drudis.2016.05.015
Swinney, D. C., and Lee, J. A. (2020). Recent advances in phenotypic drug discovery. F1000Res 9, F1000 Faculty Rev-944. doi:10.12688/f1000research.25813.1
Swinney, D. C. (2013). Phenotypic vs. target-based drug discovery for first-in-class medicines. Clin. Pharmacol. Ther. 93 (4), 299–301. doi:10.1038/clpt.2012.236
Sykes, M. L., and Avery, V. M. (2015). Development and application of a sensitive, phenotypic, high-throughput image-based assay to identify compound activity against Trypanosoma cruzi amastigotes. Int. J. Parasitol. Drugs Drug Resist. 5 (3), 215–228. doi:10.1016/j.ijpddr.2015.10.001
Talevi, A., Carrillo, C., and Comini, M. (2019). The thiol-polyamine metabolism of trypanosoma cruzi: Molecular targets and drug repurposing strategies. Curr. Med. Chem. 26 (36), 6614–6635. doi:10.2174/0929867325666180926151059
Teixeira, D. E., Benchimol, M., Crepaldi, P. H., and de Souza, W. (2012). Interactive multimedia to teach the life cycle of Trypanosoma cruzi, the causative agent of Chagas disease. PLoS Negl. Trop. Dis. 6 (8), e1749. doi:10.1371/journal.pntd.0001749
Tempone, A. G., Pieper, P., Borborema, S. E. T., Thevenard, F., Lago, J. H. G., Croft, S. L., et al. (2021). Marine alkaloids as bioactive agents against protozoal neglected tropical diseases and malaria. Nat. Prod. Rep. 38 (12), 2214–2235. doi:10.1039/d0np00078g
Torreele, E., Bourdin Trunz, B., Tweats, D., Kaiser, M., Brun, R., Mazué, G., et al. (2010). Fexinidazole--a new oral nitroimidazole drug candidate entering clinical development for the treatment of sleeping sickness. PLoS Negl. Trop. Dis. 4 (12), e923. doi:10.1371/journal.pntd.0000923
Torrico, F., Gascón, J., Ortiz, L., Pinto, J., Rojas, G., Palacios, A., et al. (2023). A phase 2, randomized, multicenter, placebo-controlled, proof-of-concept trial of oral fexinidazole in adults with chronic indeterminate Chagas disease. Clin. Infect. Dis. 76 (3), e1186–e1194. doi:10.1093/cid/ciac579
Trevisan, R. O., Santos, M. M., Desidério, C. S., Alves, L. G., de Jesus Sousa, T., de Castro Oliveira, L., et al. (2020). In silico identification of new targets for diagnosis, vaccine, and drug candidates against Trypanosoma cruzi. Dis. Markers 2020, 9130719. doi:10.1155/2020/9130719
Trindade, J. D. S., Freire-de-Lima, C. G., Côrte-Real, S., Decote-Ricardo, D., and Freire de Lima, M. E. (2021). Drug repurposing for Chagas disease: In vitro assessment of nimesulide against trypanosoma cruzi and insights on its mechanisms of action. PLoS One 16 (10), e0258292. doi:10.1371/journal.pone.0258292
Urbina, J. A., Lazardi, K., Marchan, E., Visbal, G., Aguirre, T., Piras, M. M., et al. (1993). Mevinolin (lovastatin) potentiates the antiproliferative effects of ketoconazole and terbinafine against trypanosoma (schizotrypanum) cruzi: In vitro and in vivo studies. Antimicrob. Agents Chemother. 37 (3), 580–591. doi:10.1128/AAC.37.3.580
Valera-Vera, E. A., Sayé, M., Reigada, C., Miranda, M. R., and Pereira, C. A. (2020). In silico repositioning of etidronate as a potential inhibitor of the Trypanosoma cruzi enolase. J. Mol. Graph. Model. 95, 107506. doi:10.1016/j.jmgm.2019.107506
Villalta, F., and Rachakonda, G. (2019). Advances in preclinical approaches to Chagas disease drug discovery. Expert Opin. Drug Discov. 14 (11), 1161–1174. doi:10.1080/17460441.2019.1652593
WHO (2023). World health organization. Available at: https://www.who.int/health-topics/chagas-disease (Accessed May, 2023).
Wiggers, H. J., Rocha, J. R., Fernandes, W. B., Sesti-Costa, R., Carneiro, Z. A., Cheleski, J., et al. (2013). Non-peptidic cruzain inhibitors with trypanocidal activity discovered by virtual screening and in vitro assay. PLoS Negl. Trop. Dis. 7 (8), e2370. doi:10.1371/journal.pntd.0002370
Zaidel, E. J., and Sosa Liprandi, Á. (2021). Neglected of the neglected: Crude reality of Chagas disease. Med. (B Aires) 81 (4), 675–676.
Zhang, Q., Dong, J., and Yu, Z. (2020). Pleiotropic use of Statins as non-lipid-lowering drugs. Int. J. Biol. Sci. 16 (14), 2704–2711. doi:10.7150/ijbs.42965
Keywords: Chagas disease, combination therapy, drug discovery, drug repositioning, drug repurposing, neglected tropical diseases, parasitic disease, Trypanosoma cruzi
Citation: Porta EOJ, Kalesh K and Steel PG (2023) Navigating drug repurposing for Chagas disease: advances, challenges, and opportunities. Front. Pharmacol. 14:1233253. doi: 10.3389/fphar.2023.1233253
Received: 01 June 2023; Accepted: 18 July 2023;
Published: 27 July 2023.
Edited by:
Alan Talevi, National University of La Plata, ArgentinaReviewed by:
Edezio Ferreira Cunha-Junior, Federal University of Rio de Janeiro, BrazilJaume Bastida, University of Barcelona, Spain
Copyright © 2023 Porta, Kalesh and Steel. This is an open-access article distributed under the terms of the Creative Commons Attribution License (CC BY). The use, distribution or reproduction in other forums is permitted, provided the original author(s) and the copyright owner(s) are credited and that the original publication in this journal is cited, in accordance with accepted academic practice. No use, distribution or reproduction is permitted which does not comply with these terms.
*Correspondence: Exequiel O. J. Porta, ZXhlcXVpZWwuby5wb3J0YUBkdXJoYW0uYWMudWs=; Patrick G. Steel, cC5nLnN0ZWVsQGR1cmhhbS5hYy51aw==