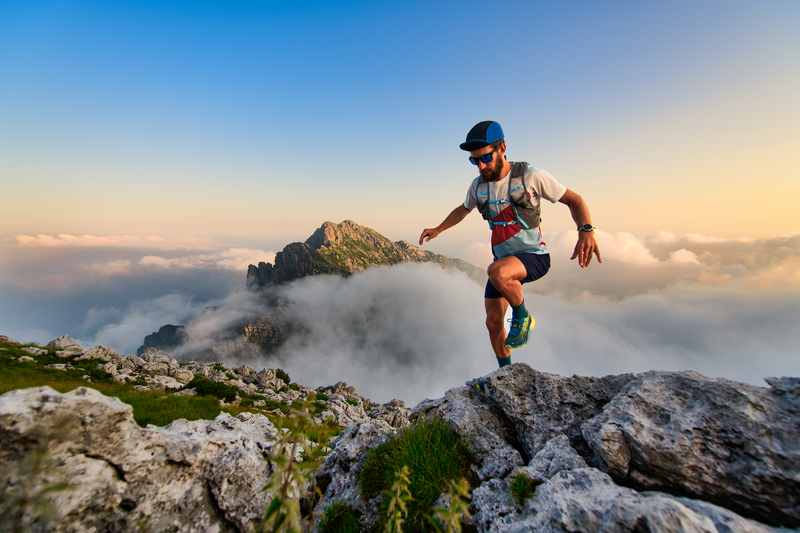
95% of researchers rate our articles as excellent or good
Learn more about the work of our research integrity team to safeguard the quality of each article we publish.
Find out more
REVIEW article
Front. Pharmacol. , 15 June 2023
Sec. Cardiovascular and Smooth Muscle Pharmacology
Volume 14 - 2023 | https://doi.org/10.3389/fphar.2023.1209890
This article is part of the Research Topic Emerging Mechanisms in Cardiovascular Disease View all 8 articles
Hypertension generally causes target organ damage (TOD) in the heart, brain, kidney, and blood vessels. This can result in atherosclerosis, plaque formation, cardiovascular and cerebrovascular events, and renal failure. Recent studies have indicated that mitochondrial dysfunction is crucial in hypertensive target organ damage. Consequently, mitochondria-targeted therapies attract increasing attention. Natural compounds are valuable resources for drug discovery and development. Many studies have demonstrated that natural compounds can ameliorate mitochondrial dysfunction in hypertensive target organ damage. This review examines the contribution of mitochondrial dysfunction to the development of target organ damage in hypertension. Moreover, it summarizes therapeutic strategies based on natural compounds that target mitochondrial dysfunction, which may be beneficial for preventing and treating hypertensive target organ damage.
Over 1.2 billion individuals suffer from hypertension worldwide, and its prevalence is projected to rise (Benjamin et al., 2018). It can damage essential organs, such as the heart, kidney, brain, and blood vessels, resulting in severe complications, such as stroke, heart attack, and kidney failure. These complications are associated with high disability and mortality rates and significant social and economic costs (Zhou et al., 2021). Therefore, preventing and treating hypertensive target organ damage (TOD) are crucial global challenges. Novel therapeutic techniques are necessary to prevent hypertensive TOD and enhance cardiovascular outcomes.
Mitochondria are essential organelles with two membranes that serve as the primary source of cellular energy. They are necessary for cardiovascular function because they generate adenosine triphosphate (ATP), control intracellular calcium ions (Ca2+), and metabolize amino acids and fatty acids (Paggio et al., 2019; Abdel-Rahman et al., 2021). Over the past two decades, experimental research has demonstrated that hypertension can cause aberrant mitochondrial function in various tissues, including the heart, brain, kidneys, and blood vessels. In hypertensive TOD, mitochondrial dysfunction is characterized by mitochondrial energy dysregulation, impairment of biogenesis and dynamics, and oxidative damage to DNA, proteins, and lipids in the cell caused by excess generation of reactive oxygen species (ROS). Accordingly, hypertensive TOD pathogenesis and progression depend greatly on mitochondrial function (Shirakabe et al., 2016; Dikalova et al., 2020; Forte et al., 2020).
Several studies have explored mitochondrial dysfunction as a potential therapeutic target (Vaka et al., 2018; Li et al., 2019a). Some anti-hypertensive drugs can reduce mitochondrial damage caused by hypertension, however, therapies that directly target mitochondria may be more effective in reducing hypertensive mitochondrial malfunction or organ damage (de Cavanagh et al., 2006). Natural compounds affecting mitochondrial function have emerged as promising candidates for treating hypertensive TOD. However, there is a lack of comprehensive research regarding the efficacy of natural compounds targeting mitochondrial dysfunction for treating and managing hypertensive TOD. Here, we summarize the mechanism of mitochondrial dysfunction in hypertensive TOD and some recent natural compound-based treatments targeting mitochondrial dysfunction.
Mitochondria are double-membrane organelles between 0.5 and 1 µm in diameter that hold their genetic material (Shpilka et al., 2021). However, their genomes are limited, and most proteins are derived from the nuclear genome (Münch and Harper, 2016). Mitochondria are the powerhouses of cells as they produce the majority of ATP by aerobic cellular respiration. Mitochondria are also involved in various other cellular functions, including cell differentiation, signal transduction, apoptosis, cell proliferation, and cell cycle regulation (Lampert et al., 2019). Therefore, mitochondrial activity is essential for cellular homeostasis and survival.
Mitochondrial dysfunction may be caused by abnormalities in mitochondrial morphology and structure, poor ATP synthesis, excessive ROS production, dysregulated Ca2+ handling, dynamics imbalance, and modulating mitochondrial DNA (mtDNA) damage (Zhou et al., 2019; Xue et al., 2020). These modifications can affect cellular energy metabolism, redox balance, and signaling pathways. The pathogenesis and progression of various human diseases have been linked to mitochondrial malfunction. Consequently, mitochondrial targeting is essential for disease treatment.
Mitochondrial dysfunction can result from abnormalities in mitochondrial morphology and structure, impaired ATP synthesis, excessive ROS production, dysregulated Ca2+ handling, dynamics imbalance, and mtDNA damage (Zhou et al., 2019; Xue et al., 2020). These alterations can affect cellular energy metabolism, redox balance, and signaling pathways. Mitochondrial malfunction has been associated with the pathogenesis and progression of various human diseases. Therefore, targeting mitochondria is crucial for disease treatment.
Hypertension affects mitochondrial adaptations and degrades mitochondrial function, resulting in decreased ATP production, increased oxidative stress, and loss of mitochondrial integrity (Eirin et al., 2014). Changes like these can damage many organs and cause a steady reduction in mitochondrial function. Hypertension harms to the kidneys, brain, heart, and vasculature through mitochondrial dysfunction-related processes.
Mitochondrial dysfunction is a key factor in the pathogenesis of cardiomyocyte death and cardiac impairment caused by various cardiovascular diseases (Nargesi et al., 2021). Hypertension-induced cardiac hypertrophy, a common complication that leads to heart failure, can be modeled by spontaneously hypertensive rats (SHRs) and pressure overload rats induced by transverse aortic constriction (TAC) (Musumeci et al., 2011). Heart failure is characterized by reduced energy production due to mitochondrial dysfunction, which affects complex I activity, ATP levels, and mitochondrial ROS (mtROS) generation (Shen et al., 1999; Dai et al., 2011a). Cardiomyocytes have substantial energy demands, with mitochondria occupying 30% of their intracellular volume and producing 6–30 kg of ATP daily (Weiss et al., 2005). Heart failure could alters mitochondrial dynamics, shifting toward fission and mitochondrial disintegration (Wai et al., 2015). Mitochondrial malfunction also contributes to diverse forms of myocyte death in heart failure (Li et al., 2019b). Consequently, mitochondria have a significant role in the progression of hypertensive heart disease structurally and functionally.
Research has demonstrated that hypertension induces mitochondrial dysfunction in vascular endothelial and smooth muscle cells, elevating reactive oxygen species generation (Dikalova et al., 2010). Dynamics-related protein 1 (DRP1) activation and optic atrophy protein 1 (OPA1) downregulation impairs mitochondrial dynamics in the small mesenteric arteries of SHRs, leading to an increase in mtROS emission, inflammation, and growth factor signaling, which causes medial thickening and luminal constriction (Li et al., 2022a). Vascular mitochondrial dysfunction can stimulate proliferation and phenotypic switching from contractile to proliferative in vascular smooth muscle cells (VSMCs), resulting in vascular remodeling and stiffness (Lu et al., 2021; Ye et al., 2021). Mitochondrial dynamics is essential for VSMCs proliferation (Marsboom et al., 2012). Mitochondrial fission restriction can inhibit VSMCs migration by modifying mitochondrial energy and ROS levels (Wang et al., 2015). Endothelial mitochondria are signaling variables that control endothelial function and ROS generation (Shukla et al., 2020). Mitochondria influence endothelial pathophysiology through Ca2+ signaling (Yamamoto et al., 2018), nitric oxide (NO) production (Dedkova et al., 2004), apoptosis and autophagy (Gao et al., 2020), thereby contributing to end-organ damage. Furthermore, numerous studies have demonstrated that hyperacetylation of mitochondrial proteins (SIRT3, SOD2, or CyPD) can impair mitochondrial metabolism and oxidative stress, inducing hypertensive vascular dysfunction (Dikalova et al., 2017; Dikalov and Dikalova, 2019; Dikalova et al., 2020).
Hypertension causes glomerular capillary damage and tubulointerstitial inflammation in kidneys. The glomerular filtration rate decreases due to a diminished filtration surface area and nephron count (Lee et al., 2019). The commonly used models are animals with bilateral renal artery stenosis and high salt-induced renal injury model. Hypertension increases the risk of kidney failure by inducing kidney hypoxia (Friederich-Persson et al., 2013). The main contributors to hypoxia in the hypertensive kidney are oxidative stress-mediated excess of ROS and a NO deficiency, which inhibits mitochondrial oxygen utilization (Adler and Huang, 2002). Extensive research indicates that renal medullary hypoxia contributes to the advancement of kidney damage in chronic kidney disease and hypertension (Li et al., 2008). Recent research has demonstrated that mitochondrial malfunction due to a deficiency of apoptosis-inducing factor (AIF) (Coughlan et al., 2016), mitochondrial complex I activity (Forbes et al., 2013), or faulty fatty acid oxidation (Kang et al., 2015) may contribute to the development of chronic kidney disease. Moreover, urine mtDNA is related to renal impairment and dysfunction markers in hypertensive patients, demonstrating mitochondrial damage in kidney impairment in hypertension (Eirin et al., 2016a).
Hypertension alters cerebral blood flow control and blood-brain barrier permeability. Spontaneously hypertensive stroke rats (SHRSP) exhibit cerebrovascular alterations similar to human disease; they are frequently employed as a model to examine hypertensive brain injury (Fredriksson et al., 1988). Mitochondrial respiratory chain dysfunction and high mtROS levels in SHRSP neurons result in cell death and cognitive impairment (Rubattu et al., 2016). Mitochondria in the brain are essential for sustaining cerebral energy metabolism, antioxidant defense, anti-inflammatory response, and survival and function of neurons. Their dysfunction can precipitate neurodegeneration and increases the risk of cognitive impairment and stroke (Lopez-Campistrous et al., 2008; Sumbalová et al., 2010). Several mitochondrial proteins, including complexes I, II, and IV, adenine dinucleotide translocase (ANT), ATP-sensitive potassium channel (mitoKATP), and mitochondrial permeability transition pore (mPTP), which are involved in energy production, ion transport, and cell death regulation, have been identified as potential neuroprotective targets in hypertensive stroke (Jin et al., 2016). Moreover, brain damage caused by ischemia or trauma can disrupt mitochondrial structure and function in neurons, influencing their neurotransmission and plasticity (Kislin et al., 2017).
Mitochondrial dynamics is a process that regulates the size, shape, number, and function of mitochondria in response to the metabolic demands and stress conditions of the cells, which involves four key events: fission, fusion, biogenesis, and mitophagy (Archer, 2013). During fission, DRP1 and mitochondrial fission 1 protein (FIS1) are responsible, while during fusion, mitofusin 1 and 2 (MFN1 and MFN2) and OPA1 are responsible (Archer, 2013). In hypertensive TOD, mitochondrial dynamics are disturbed, contributing to its pathogenesis. Therefore, treatments promoting mitochondrial fusion or preventing fission may reduce hypertensive TOD. Mitochondrial biogenesis, which produces new mitochondria, requires approximately 150 proteins, including transcription factors, enzymes, and receptors. Three key synergistic components are peroxisome proliferator-activated receptor gamma co-activator 1α (PGC-1α), adenylate-activated protein kinase (AMPK), and sirtuin-1 (SIRT1) in mitochondrial biosynthesis (Cantó et al., 2009; Luo et al., 2012). During mitochondrial biogenesis, the cell’s mitochondrial mass and number increase. Mitochondrial autophagy, a quality control program regulated by the PTEN-induced kinase 1 (PINK1)-Parkin pathway, is also essential for hypertensive TOD (Shirakabe et al., 2016) Taken together, the modulation of mitochondrial homeostasis can improve hypertensive TOD.
Mitochondrial bioenergetics involve energy production and conversion in mitochondria by enzymatic and metabolic pathways. Mitochondria in cardiomyocytes and renal tubular cells generate substantial ATP (Suzuki et al., 2016). Mitochondrial respiration involves a series of processes in the inner mitochondrial membrane, including the generation of a proton gradient, movement of electrons by the electron transport chain (ETC.), and phosphorylation of adenosine diphosphate (ADP) to form ATP (Cogliati et al., 2013). Cardiolipin, a critical component of the inner mitochondrial membrane, is necessary for the construction and function of, ETC. Cardiolipin content and composition are reduced in hypertensive animals’ cardiac and renal tissues, indicating impaired mitochondrial bioenergetics (Zachman et al., 2010; Eirin et al., 2016b). Consequently, addressing mitochondrial bioenergetics and increasing ATP production may be crucial in treating hypertensive TOD.
Mitochondria dysfunction is caused by oxidative stress, an imbalance between ROS and intracellular homeostasis. ROS can be generated by xanthine oxidase, NADPH oxidase, and uncoupled NO synthase, among others (Beswick et al., 2001). However, mitochondria are the primary source and target of ROS in cells. Abnormal mitochondrial respiration can impede oxidative phosphorylation and increase ROS generation, resulting in oxidative damage to cellular and mitochondrial proteins, lipids, and DNA, exacerbating mitochondrial dysfunction, and forming a vicious cycle of damage (Satoh et al., 2021).
The pathophysiology of hypertensive TOD is linked to mitochondrial oxidative stress. Several studies have depicted that elevated mtROS production is associated with cardiac hypertrophy (Dai et al., 2011a; Dai et al., 2011b), renal dysfunction (Gu et al., 2015), arterial endothelial dysfunction (Doughan et al., 2008; Dikalov et al., 2014), and cerebrovascular remodeling (Fang et al., 2021) in various hypertension models. Modulating mitochondrial oxidative stress is a potential therapeutic strategy to reduce TOD in hypertension.
Another mechanism connecting mitochondrial dysfunction with hypertensive TOD is mitochondria-mediated apoptosis. Mitochondria are crucial regulators of apoptosis, the process of programmed cell death process that preserves cellular homeostasis and viability. Apoptosis can be triggered by intrinsic or extrinsic pathways (Ivanova et al., 2019). In response to intracellular damage, such as oxidative stress, Ca2+ excess, or DNA damage, the intrinsic pathway is initiated by the permeabilization of outer mitochondrial membrane (Cheng et al., 2016). A key step in the intrinsic route, the opening of the mPTP, results in the release of pro-apoptotic proteins, such as cytochrome c and the activation of caspase-9 and caspase-3. These caspases can cleave diverse substrates and induce cell death (Volkova et al., 2011).
It is believed that mitochondria-mediated apoptosis contributes to the pathogenesis of hypertensive TOD. Inhibition of mPTP opening can limit the release of pro-apoptotic proteins and protect against mitochondrial malfunction and cell death. This is possible using mitochondrial antioxidants or cardiolipin-protective agents. Mitochondrial antioxidants such as mitoQ can scavenge mtROS and reduce oxidative damage to mitochondria and cells (Pak et al., 2018; Goh et al., 2019). Cardiolipin-protective agents can maintain cardiolipin content and function and stabilize the inner mitochondrial membrane (Mulligan et al., 2012; Eirin et al., 2016b).
Natural compounds are small molecules derived from natural sources, such as plants, animals, fungi, or bacteria. Due to their low side effects and toxicity, they have been a significant source of novel drugs for human diseases. More than 30% of medications approved by the Food and Drug Administration (FDA) contain natural components or their derivatives (Enghiad et al., 2021). In recent years, natural compounds that modulate mitochondrial activity have emerged as a promising area of therapeutic development. Therefore, natural compounds that affect mitochondrial function may have beneficial effects on TOD in hypertensive. This section reviews some natural compounds that modulate mitochondrial activity to treat hypertensive TOD.
Flavonoids are a large class of low molecular weight compounds widely distributed in foods and medicinal plants. They have various health benefits, including antioxidant, anti-inflammatory, antitumor, and chemopreventive activities (Sayed et al., 2020). Epidemiological evidence suggests that consuming of foods or beverages rich in flavonoids can reduce the incidence of cardiovascular diseases (Mahmoud et al., 2019).
Acacetin (ACT) is a naturally occurring flavonoid isolated from the traditional Chinese medicine snow lotus. It has several pharmacological actions, including antimicrobial, anti-inflammatory, anti-proliferative, and anticancer (Singh et al., 2020). Recent in vivo and in vitro studies have demonstrated that ACT protects against hypertensive TOD. For example, Li et al. reported that ACT protects against vascular endothelial dysfunction in hypertension by activating the AKT/eNOS pathway and targeting mitochondrial mPTP and DRP1/OPA1-dependent regulation of mitochondrial dynamics (Li et al., 2022b). Yuan et al. found that ACT improves cardiac mitochondrial dysfunction by regulating PI3K/AKT signaling pathway-mediated mitochondrial apoptosis, oxidative stress, and mitochondrial fission (MFF, DRP1) and fusion (MFN2) in SHRs with insulin resistance, thus suggesting a beneficial role for ACT in the treatment of mitochondrial dysfunction (Yuan et al., 2022). These results suggested that ACT, which modulates mitochondrial activity, may be a natural remedy for hypertensive TOD.
Naringin (NRG) is one of the most important flavonoids found mainly in the peel and pulp of Citrus fruits (Rutacea). NRG possesses diverse pharmacological actions, including antioxidant, anti-inflammatory, and anti-hypertensive activities (Ikemura et al., 2012). NRG (about 100 mg/kg/day) can reduce systolic blood pressure and improve vascular and ventricular dysfunction in rats fed with a high-carbohydrate, high-fat diet. These beneficial effects of NRG on cardiovascular health are associated with maintaining the structural and functional integrity of mitochondrial preparations. The high respiratory control ratio suggests that NRG can enhance mitochondrial respiratory chain function and lipid metabolism (Alam et al., 2013).
Icariin (ICA) is a flavonol glycoside derived from the Chinese medicinal herb Epimedium. It has been used to treat hypertension, Alzheimer’s disease, cerebral ischemia, and depression (Li et al., 2022a). Qian et al. observed that ICA had a protective effect on cardiac remodeling in SHRs and that oral administration of ICA (20 and 40 mg/kg/day) prevented apoptosis of cardiomyocytes and improved left ventricular remodeling and mitochondrial abnormalities. Further mechanistic studies suggested ICA therapy upregulated Bcl-2 and downregulated p53, Bok, Bax and cleaved caspase 3, indicating a possible mechanism of blocking the mitochondrial apoptotic pathway (Qian et al., 2017). Similarly, intragastric administration of Icariside II (ICA II), a form of ICA metabolite, has been demonstrated to reduce blood pressure, promote cardiac function recovery, and improve ventricular remodeling in SHRs. These cardioprotective effects of ICA II were related to downregulating the activation of oxidative stress-associated proteins ASK1, p38 and JNK, inhibiting the expression of p53, Bax and cleaved caspase 3, and upregulation of the expression of Bcl-2 expression in the mitochondrial apoptotic pathway. This process may involve the prevention of mitochondrial apoptosis mediated by the ASK1-JNK/p38 signaling pathway (Wu et al., 2018).
Epigallocatechin-3-gallate (EGCG), a flavonoid found in green tea, has beneficial effects on hypertension and its complications. EGCG (200 mg/kg, i.g. for 12 weeks) protects neuronal against apoptosis by regulating mitochondria-mediated apoptotic pathways, including decreased Bax/Bcl-2, Bak/Bcl-xL, cytochrome C release, caspase-9 activation, and increase SIRT1/PI3K/AKT-related pro-survival pathway. This suggests a therapeutic potential of EGCG for hypertension-induced brain damage (Hsieh et al., 2021). EGCG (50 mg/kg/day, i.p., for 21 days) reduced hypertension-induced ventricular hypertrophy in rats by modulating mtDNA copy number and respiratory chain complexes I, III, and IV (Chen et al., 2009). EGCG may be a promising natural compound for treating hypertensive brain and heart damage by altering mitochondrial function.
Quercetin (QEC) is a prevalent flavonoid found in numerous plants. It has antioxidative properties due to ortho-diphenolic hydroxyl groups at B-rings and double bonds at C-rings (Sekher Pannala et al., 2001). Cui et al. (2017) reported that QEC prevents mitochondrial dysfunction, including increases the mitochondrial potential and ATP production and inhibits phosphate-induced apoptosis and calcification of VSMCs by reducing oxidative stress and mitochondrial fission by downregulating DRP1 expression and phosphorylation. Similarly, recent research has demonstrated that QEC can also improve cardiac function by reducing mitochondrial superoxide and preserving mitochondrial structure in vivo. It also attenuated angiotensin II (Ang II) -induced cardiac hypertrophy in vitro. Notably, mitochondrial protection and PARP-1 suppression by QEC were partially abolished by SIRT3 knockdown. These findings imply that QEC suppresses cardiac hypertrophy by regulating the SIRT3/PARP-1 pathway to preserve mitochondrial function (Chen et al., 2021a).
Dihydromyricetin (DHY), a flavonoid derived from Garcinia cambogia, possesses anticancer, antioxidant, anti-inflammatory, and neuroprotective properties (Zhang et al., 2018a). DHY pretreatment (250 mg/kg/day, i.g, for 4 weeks) could significantly improve cardiac function and reduce cardiac index after TAC-induced hypertrophy. DHY inhibited oxidative stress by decreasing ROS generation and malondialdehyde levels, and increasing total antioxidant capacity and superoxide dismutase activity in the myocardium. Importantly, DHY enhanced the expression and activity of SIRT3, a key regulator of mitochondrial function and oxidative stress resistance, as well as its downstream targets, forkhead-box-protein 3a (FOXO3a) and SOD2, in the myocardium. These findings imply that DHY ameliorates pressure overload-induced myocardial hypertrophy in mice by activating the SIRT3 pathway and decreasing oxidative stress (Chen et al., 2018).
Baicalein (BCL) is a naturally occurring flavonoid found in the roots of Scutellaria baicalensis that exhibits several pharmacological activities, including antibacterial, antioxidation, anticancer, and antiviral (Liao et al., 2019). Cai et al. demonstrated that BCL is a promising treatment for cardiac hypertrophy in rats with abdominal aortic constriction (AAC). These benefic effects of BCL were related to modulating the SIRT3/LKB1/AMPK signaling pathway. Further mechanism research indicates that BCL inhibits proteasome degradation and activates the 20S proteasome subunit beta Type 5 (PSMB5) to promote the production of SIRT3 protein, a key mitochondrial deacetylation modifying enzyme that affects mitochondrial structure and function. These results demonstrated that BCL inhibits cardiac hypertrophy by a SIRT3-dependent mechanism, indicating its potential use in treating cardiac hypertrophy and heart failure (Cai et al., 2023).
Phenolics are natural organic compounds with phenolic hydroxyl groups. Polyphenols, a subgroup of phenolics, have garnered popular interest due to their oxidative coupling capacity. By controlling oxidative stress or associated signaling pathways, notably by limiting mitochondrial damage, they can protect against hypertensive TOD (Table 1).
Resveratrol (RSV) is a polyphenol found in Vaccinium berries and other plants. It reduces blood pressure by modulating mitochondrial biogenesis signaling. Zhang et al. discovered that RSV promoted the expression of mitochondrial fusion-related MFN2 and OPA1 in a model of chronic ocular hypertension (Zhang et al., 2018b). Moreover, RSV ameliorates Ang II-induced cardiac remodeling in double transgenic rats (dTGR) with human renin and angiotensinogen genes by increasing the expression of mitochondrial biogenesis markers, including PGC-1α, mitochondrial transcription factor A (TFAM), and nuclear respiratory factor (NRF). These results indicate that the beneficial effects of RSV are mediated by a blood pressure-dependent pathway and are associated with an increase in mitochondrial biogenesis (Biala et al., 2010). Similarly, RSV (18 mg/kg/day, for 8 weeks) maintains mitochondrial mass and biogenesis in Dahl salt-sensitive rats with hypertension-induced heart failure via preserving mitochondrial fatty acid oxidation and PPARα expression (Rimbaud et al., 2011). Banday and Lokhandwala (2020) reported that dopamine oxidation in high salt-fed rats impairs lysosomal and mitochondrial function and induces renal inflammation. RSV (50 mg/L in drinking water for 6 weeks) did not affect basal mitochondrial oxidation or respiration but protected mitochondrial function, preserved renal function and reduced hypertension.
Curcumin (CCM), a diarylheptanoid compound derived from turmeric, possesses diverse pharmacological properties, including anti-inflammatory, antioxidant, anti-liver fibrosis, anti-tumor, and anti-atherosclerosis (Tapia et al., 2012). Due to its low toxicity and negligible side effects, CCM is one of the world’s most utilized natural food pigments. Several studies have depicted that CCM has a possible protective effect against mitochondrial abnormalities in several disease types (Cox et al., 2022). The beneficial effects of CCM (100 mg/kg/day, gavage, 10 weeks) on NG-nitro-L-arginine methyl ester (L-NAME)-induced hypertensive kidney injury were mediated by its antioxidant capacity and downregulation of AT1R, including reduction of apoptosis and preservation of mtDNA (Greish et al., 2020). Lan et al. (2018) observed that CCM administration (100 mg/kg/day, gavage, for 10 weeks) delayed stroke onset and increased SHRSP survival by decreasing ROS and improving endothelial-dependent carotid artery relaxation. Further mechanism studies demonstrated that the mechanism of CCM reducing oxidative stress and increasing NO production to improve vascular endothelial function to prevent hypertensive stroke was related to the activation of uncoupling protein 2 (UCP2) signal, a physiological regulator of mtROS generation.
Apocynin (APO), a natural organic compound derived from the roots of Apocynum cannabinum, is frequently used as an NADPH oxidase inhibitor (Kovacevic et al., 2020). APO possesses anti-hypertensive properties by suppressing the release of superoxide ions and inhibiting the transport of p47phox to the mitochondrial membrane (Babior, 2004). APO also inhibits the rise in mitochondrial H2O2 generation that Ang II induces in bovine aortic endothelial cells (BAECs). Knocking down the p22phox subunit of NADPH oxidase with small interfering RNA diminished Ang II-induced mtROS production. Moreover, Ang II reduced BAECs’ mitochondrial glutathione levels, disrupted mitochondrial respiration states 3 and 4, and lowered the mitochondrial respiratory control ratio. However, apocynin reversed these effects (Doughan et al., 2008).
Punicalagin (PUN) is a polyphenolic compound isolated from pomegranate (PunicagranatumL.) leaves that exhibit antioxidant effects (Shao et al., 2016). Pomegranate fruit and juice have traditionally been employed to treat and promote health. Punicalagin-containing pomegranate extract can reduce blood pressure and prevent heart hypertrophy, probably by increasing mitochondrial function in the paraventricular nucleus of hypertensive rats. This could involve increasing mitochondrial biogenesis, enhancing mitochondrial dynamics and clearance, and decreasing mitochondrial superoxide anion levels. The AMPK- NRF2 pathway is a potential mechanism (Sun et al., 2016).
Salvianolic acid D (Sal-D) is a natural compound isolated from a natural herbal Salvia miltiorrhiza Bunge with cardiovascular benefits. Sal-D is an important antioxidant from Danshen and has attracted increasing research interest (Lu et al., 2022). Chen et al. (2023) demonstrated that Sal-D can treat hypertension-induced heart failure (HF) by reducing blood pressure, attenuating cardiac remodeling, and enhancing cardiac function. These beneficial effects on the heart may entail the protection of mitochondria by reducing mitochondrial ultrastructure damage and energy charge depletion. Further investigations revealed that Sal-D could improve heart function in SHRs by concurrently blocking the Ras pathway and activating the PI3K/AKT pathway.
Alkaloids are nitrogen organic compounds widespread in plants, animals, and microorganisms. Certain alkaloids can regulate mitochondrial function and have favorable effects on cardiovascular diseases.
Tetrandrine (TET) is a bisbenzylisoquinoline alkaloid that is obtained from the root of Stephania tetrandra S Moore, a plant that can treat cardiovascular diseases. Previous research reported that hypertensive rats with left ventricular hypertrophy induced by DOCA-salt had greater mitochondrial Ca2+ levels than normal rats. However, TET administration (50 mg/kg/day, gastric, for 9 weeks) decreased heart mass mitochondrial Ca2+ levels in hypertensive rats, indicating that TET alleviates cardiac pressure overload by regulating intracellular Ca2+ of myocardial mitochondria (Xu and Rao, 1995).
Chelerythrine (CHE) is a naturally occurring benzo[c] phenanthridine alkaloid present in many plant species, including Chelidonium majus, Sanguinaria Canadensis, and Macleaya cordata (Tang et al., 2017a). It inhibits proteinase C selectively and has a vasodilatory effect on the vasculature. CHE suppressed the increase in mitochondrial H2O2 generation caused by Ang II in bovine aortic endothelial cells (BAECs), indicating that CHE decreases the effects of Ang II-induced mtROS (Doughan et al., 2008). Liu et al. (2013) reported that chelerythrine improved hypertension-induced nonischemic cardiomyopathy, which was involved in improving mitochondrial ROS overproduction and restoring Na+ current in myocardial tissue and myocytes.
Spermidine (SPE) is a natural polyamine ubiquitous in organisms that plays an important role in cell transcription, growth, and differentiation (Maki et al., 2017). Previous research has demonstrated that supplementation with spermidine can reduce blood pressure and enhance heart function by improving cardiac mitochondrial autophagy (mitophagy) and respiration in vivo (Eisenberg et al., 2016). SPE protects against mitochondrial dysfunction in cardiovascular disease, indicating its potential as a therapeutic agent against mitochondrial malfunction.
Melatonin (MEL) is a tryptophan-derived natural substance with multiple therapeutic benefits, including antihypertensive properties (Ding et al., 2018). The importance of employing MEL as a therapeutic tool to target mitochondria was emphasized in a previous review covering the role of melatonin in modulating mitochondrial physiology in hypertensive TOD (Baltatu et al., 2017). Wang et al. (2023) discovered that MEL decreased mammalian STE20-like kinase 1 (Mst1) expression, increased autophagy, and decreased apoptosis in myocardial microvascular endothelial cells (MMECs) under hypertension conditions. MEL also increased the mitochondrial membrane potential and autophagosome formation in MMECs, thereby providing cardioprotection effects.
Terpenoids are a wide group of natural products with a molecular skeleton composed of isoprene units. They are diverse and abundant in nature, especially in plants. They have various physiological functions, including antimicrobial, antihypertensive, antitumor, anti-inflammatory, antioxidant, and immunomodulatory activities (Chen et al., 2022; Hu et al., 2022; Xu et al., 2022). In addition, the significance of terpenoids in alleviating mitochondrial dysfunction cannot be overlooked.
Astaxanthin (ATX) is a red carotenoid nutrient found in numerous living organisms and commonly consumed by humans through food. Due to its potent ability to scavenge free radicals, ATX possesses antioxidant and anti-inflammatory effects that influence biological and pharmacological processes (Hatabu et al., 2020). In SHRs, ATX has been reported to reduce blood pressure and improve vascular remodeling (Monroy-Ruiz et al., 2011). A mechanism study has revealed that ATX can improve hypertensive vascular remodeling by ameliorating mitochondrial structural damage, inhibiting mitochondrial fission (DRP1), increasing mitophagy (Parkin and PINK1) and mitochondrial biosynthesis (PGC-1α), and decreasing mtROS (Chen et al., 2020).
Corosolic acid (CRA) is a pentacyclic triterpenoid with antioxidative, anti-inflammatory, and antihypertension properties (Yamaguchi et al., 2006). CRA protects vascular endothelial homeostasis by regulating DRP1 phosphorylation (Ser637) in an AMPK-dependent manner to prevent mitochondrial fission and suppress NLRP3 inflammasome activation in endothelial cells (Li et al., 2016).
Astragaloside IV (ASIV) is a cycloalkane-type triterpenoid saponin derived from Astragalus membranaceus (Fisch.) Bge., a traditional Chinese medicine widely used for cardiovascular diseases (Jing et al., 2021). ASIV reverses Ang II-induced ROS production, NADPH oxidase, xanthine oxidase activities, ΔΨm reduction, and Mn-SOD activity decrease in rat aortic VSMCs. Moreover, ASIV treatment enhances mRNA expression of TFAM and PGC-1α and protein expression of PGC-1α, Parkin, and DRP1 in aortic VSMCs. These findings indicate that ASIV protects aortic VSMCs by suppressing mitochondrial fission and ROS overproduction and increasing mitochondrial autophagy and biogenesis (Lu et al., 2015a; Lu et al., 2015b).
Diallyl trisulfide (DATS) is a major antioxidant component in garlic and its usefulness in cardiovascular illnesses, particularly hypertension, has been studied (Chen et al., 2021a). Besides its antioxidant activity, DATS may also protect mitochondrial function and exert beneficial effects (Liu et al., 2014). A recent study by Lu et al. (2020) found that DRP1-mediated mitochondrial fission enhanced mtROS generation in primary mouse VSMCs, contributing to Ang II-induced VSMC responses. Additionally, Ang II increased the activity of the protein kinase ROCK1, which controls VSMC phenotypic switching and mitochondrial fission by phosphorylating DRP1. However, DATS abrogated the biological effect of Ang II. In an animal model of Ang II-induced vascular remodeling, DATS also significantly alleviated mitochondrial fission, VSMCs differentiation, and vessel wall thickening, which are regulated by the ROCK1/DRP1 pathway. These findings indicate that DATS attenuates Ang II-induced vascular remodeling by suppressing DRP1-mediated mitochondrial fission in a ROCK1-dependent manner.
Trehalose (TRE) is a non-reducing disaccharide that lower organisms including yeasts and tardigrades synthesize. It possesses antioxidant effects and promotes autophagy (Sarkar et al., 2007; Tang et al., 2017b). In an SHRSP model, TRE attenuated renal damage and stroke incidence. These results were linked to a significant improvement in mitochondrial health, including the restoration of cerebral autophagy/mitophagy and mitochondrial mass. Further mechanisms indicate that these effects are linked to increased nuclear translocation of transcription factor EB (TFEB), a critical regulator of autophagy (Forte et al., 2021). Moreover, TRE is a natural substance without side effects and a perfect medicinal molecule. Therefore, it may be a viable dietary supplement to augment pharmaceutical therapy for stroke patients with hypertension.
In summary, numerous studies have demonstrated that natural compounds can improve mitochondrial function in animal and cellular models of hypertensive TOD. Most of these compounds are flavonoids, polyphenols, terpenoids, and alkaloids originating from plants. Thus, they may have therapeutic potential in regulating mitochondrial diseases associated with hypertensive TOD.
Hypertension is a significant global public health issue and the leading risk factor for mortality and cardiovascular, cerebrovascular, and renal diseases. However, its pathogenesis remains unclear. Mitochondrial dysfunction is a prevalent pathobiological mechanism in many diseases (Rubattu et al., 2014; Kućmierz et al., 2021). Targeting mitochondria with small-molecule drugs may be an effective method for preventing and treating hypertensive TOD. This review covers numerous natural substances, such as QEC, RSV, CCM, and MEL, which affect mitochondrial activity in hypertensive TOD.
Most natural products improve mitochondrial function and reduce mitochondrial oxidative stress to prevent TOD in hypertension (Table 1). The balance between antioxidants and oxidants is critical for maintaining mitochondrial homeostasis in hypertensive TOD. Consequently, natural compounds that increase mitochondrial biogenesis and reduce oxidative stress are essential for maintaining mitochondrial function. These compounds have been found to reduce mitochondrial dysfunction and promote cell survival in various hypertensive TOD models. Moreover, as displayed in Figure 1, some compounds, such as RSV, QEC, ACT, and ATX, can modulate multiple aspects of mitochondrial function (biogenesis, dynamics, bioenergetics, or apoptosis) as well as regulate mitochondrial oxidative stress-induced damage. Interestingly, most of these compounds are phenolic compounds, which suggests a protective effect of these natural compounds on hypertensive TOD by targeting mitochondria. This may be due to their antioxidant activity that prevents and attenuates the cytotoxic by-products of cellular respiration and ATP production in damaged mitochondria. Moreover, natural antioxidants can affect mitochondrial biogenesis, dynamics, membrane potential, and apoptosis (Table 1; Figure 1). However, some of the biological activities of phenolic compounds, such as mitochondrial biogenesis and dynamics, are not directly related to their antioxidant activity.
FIGURE 1. Natural compounds improve TOD in hypertension by modulating mitochondrial dysfunction. Mitochondrial dysfunction includes many aspects, such as reduced mitochondrial biogenesis, reduced ATP production, impaired mitophagy and imbalance fission/fusion, increased ROS production, and increased mitochondrial apoptosis. Natural products, including phenols, terpenoids, alkaloids, and flavonoids, can significantly amend one or several facets associated with mitochondrial dysfunction and thus subsequent amelioration of TOD in hypertension (Figure was created with https://biorender.com).
There is experimental evidence that mitochondrial damage contributes to the initiation and progression of hypertensive TOD. Nevertheless, mitochondrial damage’s cause and therapeutic significance in hypertensive TOD remain unclear. Natural compounds that enhance the morphology and function of mitochondria have demonstrated beneficial effects in experimental hypertension. However, their efficacy and safety in alleviating hypertensive TOD need further experimental and clinical validation. Therefore, mitochondria-targeted natural compounds may be effective as adjuvant therapy to standard antihypertensive drugs in treating hypertensive TOD. Future clinical trials in hypertensive patients will require more experimental studies to elucidate their mechanisms of action.
XL searched the literature and drafted the manuscript. JL and YW conceived and designed the review. YuH and YiH examined the literature and made the figures. JL and YW made a critical revision of the review. All authors contributed to the article and approved the submitted version.
This work was supported by the National Natural Science Foundation of China (81874464 and 82174357), and the Education Department Scientific Research Foundation of Hunan Province of China (18A228).
The authors declare that the research was conducted in the absence of any commercial or financial relationships that could be construed as a potential conflict of interest.
All claims expressed in this article are solely those of the authors and do not necessarily represent those of their affiliated organizations, or those of the publisher, the editors and the reviewers. Any product that may be evaluated in this article, or claim that may be made by its manufacturer, is not guaranteed or endorsed by the publisher.
Abdel-Rahman, E. A., Hosseiny, S., Aaliya, A., Adel, M., Yasseen, B., Al-Okda, A., et al. (2021). Sleep/wake calcium dynamics, respiratory function, and ROS production in cardiac mitochondria. J. Adv. Res. 31, 35–47. doi:10.1016/j.jare.2021.01.006
Adler, S., and Huang, H. (2002). Impaired regulation of renal oxygen consumption in spontaneously hypertensive rats. J. Am. Soc. Nephrol. 13 (7), 1788–1794. doi:10.1097/01.asn.0000019781.90630.0f
Alam, M. A., Kauter, K., and Brown, L. (2013). Naringin improves diet-induced cardiovascular dysfunction and obesity in high carbohydrate, high fat diet-fed rats. Nutrients 5 (3), 637–650. doi:10.3390/nu5030637
Archer, S. L. (2013). Mitochondrial dynamics-mitochondrial fission and fusion in human diseases. N. Engl. J. Med. 369 (23), 2236–2251. doi:10.1056/NEJMra1215233
Babior, B. M. (2004). NADPH oxidase. Curr. Opin. Immunol. 16 (1), 42–47. doi:10.1016/j.coi.2003.12.001
Baltatu, O. C., Amaral, F. G., Campos, L. A., and Cipolla-Neto, J. (2017). Melatonin, mitochondria and hypertension. Cell. Mol. Life Sci. 74 (21), 3955–3964. doi:10.1007/s00018-017-2613-y
Banday, A. A., and Lokhandwala, M. F. (2020). Renal dopamine oxidation and inflammation in high salt fed rats. J. Am. Heart Assoc. 9 (1), e014977. doi:10.1161/JAHA.119.014977
Benjamin, E. J., Virani, S. S., Callaway, C. W., Chamberlain, A. M., Chang, A. R., Cheng, S., et al. (2018). Heart disease and stroke statistics-2018 update: A report from the American heart association. Circulation 137 (12), e67–e492. doi:10.1161/CIR.0000000000000558
Beswick, R. A., Dorrance, A. M., Leite, R., and Webb, R. C. (2001). NADH/NADPH oxidase and enhanced superoxide production in the mineralocorticoid hypertensive rat. Hypertension 38 (5), 1107–1111. doi:10.1161/hy1101.093423
Biala, A., Tauriainen, E., Siltanen, A., Shi, J., Merasto, S., Louhelainen, M., et al. (2010). Resveratrol induces mitochondrial biogenesis and ameliorates Ang II-induced cardiac remodeling in transgenic rats harboring human renin and angiotensinogen genes. Blood Press 19 (3), 196–205. doi:10.3109/08037051.2010.481808
Cai, Y., Jiang, S., Huang, C., Shen, A., Zhang, X., Yang, W., et al. (2023). Baicalin inhibits pressure overload-induced cardiac hypertrophy by regulating the SIRT3-dependent signaling pathway. Phytomedicine 114, 154747. doi:10.1016/j.phymed.2023.154747
Cantó, C., Gerhart-Hines, Z., Feige, J. N., Lagouge, M., Noriega, L., Milne, J. C., et al. (2009). AMPK regulates energy expenditure by modulating NAD+ metabolism and SIRT1 activity. Nature 458 (7241), 1056–1060. doi:10.1038/nature07813
Chen, C. Y., Tsai, T. Y., and Chen, B. H. (2021b). Effects of black garlic extract and nanoemulsion on the deoxy corticosterone acetate-salt induced hypertension and its associated mild cognitive impairment in rats. Antioxidants (Basel) 10 (10), 1611. doi:10.3390/antiox10101611
Chen, D. D., Dong, Y. G., Liu, D., and He, J. G. (2009). Epigallocatechin-3-gallate attenuates cardiac hypertrophy in hypertensive rats in part by modulation of mitogen-activated protein kinase signals. Clin. Exp. Pharmacol. Physiol. 36 (9), 925–932. doi:10.1111/j.1440-1681.2009.05173.x
Chen, K., Guan, Y., Wu, S., Quan, D., Yang, D., Wu, H., et al. (2023). Salvianolic acid D: A potent molecule that protects against heart failure induced by hypertension via Ras signalling pathway and PI3K/akt signalling pathway. Heliyon 9 (2), e12337. doi:10.1016/j.heliyon.2022.e12337
Chen, C. Y., Cheng, Y., Li, W., Dong, X. K., Wei, J. L., Yang, C. H., et al. (2021a). Quercetin attenuates cardiac hypertrophy by inhibiting mitochondrial dysfunction through SIRT3/PARP-1 pathway. Front. Pharmacol. 12, 739615. doi:10.3389/fphar.2021.739615
Chen, X. L., Geng, Y. J., Li, F., Hu, W. Y., and Zhang, R. P. (2022). Cytotoxic terpenoids from Tripterygium hypoglaucum against human pancreatic cancer cells SW1990 by increasing the expression of Bax protein. J. Ethnopharmacol. 289, 115010. doi:10.1016/j.jep.2022.115010
Chen, Y., Li, S., Guo, Y., Yu, H., Bao, Y., Xin, X., et al. (2020). Astaxanthin attenuates hypertensive vascular remodeling by protecting vascular smooth muscle cells from oxidative stress-induced mitochondrial dysfunction. Oxid. Med. Cell. Longev. 2020, 4629189. doi:10.1155/2020/4629189
Chen, Y., Luo, H. Q., Sun, L. L., Xu, M. T., Yu, J., Liu, L. L., et al. (2018). Dihydromyricetin attenuates myocardial hypertrophy induced by transverse aortic constriction via oxidative stress inhibition and SIRT3 pathway enhancement. Int. J. Mol. Sci. 19 (9), 2592. doi:10.3390/ijms19092592
Cheng, A., Yang, Y., Zhou, Y., Maharana, C., Lu, D., Peng, W., et al. (2016). Mitochondrial SIRT3 mediates adaptive responses of neurons to exercise and metabolic and excitatory challenges. Cell. Metab. 23 (1), 128–142. doi:10.1016/j.cmet.2015.10.013
Cogliati, S., Frezza, C., Soriano, M. E., Varanita, T., Quintana-Cabrera, R., Corrado, M., et al. (2013). Mitochondrial cristae shape determines respiratory chain supercomplexes assembly and respiratory efficiency. Cell. 155 (1), 160–171. doi:10.1016/j.cell.2013.08.032
Coughlan, M. T., Higgins, G. C., Nguyen, T. V., Penfold, S. A., Thallas-Bonke, V., Tan, S. M., et al. (2016). Deficiency in apoptosis-inducing factor recapitulates chronic kidney disease via aberrant mitochondrial homeostasis. Diabetes 65 (4), 1085–1098. doi:10.2337/db15-0864
Cox, F. F., Misiou, A., Vierkant, A., Ale-Agha, N., Grandoch, M., Haendeler, J., et al. (2022). Protective effects of curcumin in cardiovascular diseases-impact on oxidative stress and mitochondria. Cells 11 (3), 342. doi:10.3390/cells11030342
Cui, L., Li, Z., Chang, X., Cong, G., and Hao, L. (2017). Quercetin attenuates vascular calcification by inhibiting oxidative stress and mitochondrial fission. Vasc. Pharmacol. 88, 21–29. doi:10.1016/j.vph.2016.11.006
Dai, D. F., Chen, T., Szeto, H., Nieves-Cintrón, M., Kutyavin, V., Santana, L. F., et al. (2011a). Mitochondrial targeted antioxidant Peptide ameliorates hypertensive cardiomyopathy. J. Am. Coll. Cardiol. 58 (1), 73–82. doi:10.1016/j.jacc.2010.12.044
Dai, D. F., Johnson, S. C., Villarin, J. J., Chin, M. T., Nieves-Cintrón, M., Chen, T., et al. (2011b). Mitochondrial oxidative stress mediates angiotensin II-induced cardiac hypertrophy and Galphaq overexpression-induced heart failure. Circ. Res. 108 (7), 837–846. doi:10.1161/CIRCRESAHA.110.232306
de Cavanagh, E. M., Toblli, J. E., Ferder, L., Piotrkowski, B., Stella, I., and Inserra, F. (2006). Renal mitochondrial dysfunction in spontaneously hypertensive rats is attenuated by losartan but not by amlodipine. Am. J. Physiol. Regul. Integr. Comp. Physiol. 290 (6), R1616–R1625. doi:10.1152/ajpregu.00615.2005
Dedkova, E. N., Ji, X., Lipsius, S. L., and Blatter, L. A. (2004). Mitochondrial calcium uptake stimulates nitric oxide production in mitochondria of bovine vascular endothelial cells. Am. J. Physiol. Cell. Physiol. 286 (2), C406–C415. doi:10.1152/ajpcell.00155.2003
Dikalov, S. I., and Dikalova, A. E. (2019). Crosstalk between mitochondrial hyperacetylation and oxidative stress in vascular dysfunction and hypertension. Antioxid. Redox Signal 31 (10), 710–721. doi:10.1089/ars.2018.7632
Dikalov, S. I., Nazarewicz, R. R., Bikineyeva, A., Hilenski, L., Lassègue, B., Griendling, K. K., et al. (2014). Nox2-induced production of mitochondrial superoxide in angiotensin II-mediated endothelial oxidative stress and hypertension. Antioxid. Redox Signal 20 (2), 281–294. doi:10.1089/ars.2012.4918
Dikalova, A. E., Bikineyeva, A. T., Budzyn, K., Nazarewicz, R. R., McCann, L., Lewis, W., et al. (2010). Therapeutic targeting of mitochondrial superoxide in hypertension. Circ. Res. 107 (1), 106–116. doi:10.1161/CIRCRESAHA.109.214601
Dikalova, A. E., Itani, H. A., Nazarewicz, R. R., McMaster, W. G., Flynn, C. R., Uzhachenko, R., et al. (2017). Sirt3 impairment and SOD2 hyperacetylation in vascular oxidative stress and hypertension. Circ. Res. 121 (5), 564–574. doi:10.1161/CIRCRESAHA.117.310933
Dikalova, A. E., Pandey, A., Xiao, L., Arslanbaeva, L., Sidorova, T., Lopez, M. G., et al. (2020). Mitochondrial deacetylase Sirt3 reduces vascular dysfunction and hypertension while Sirt3 depletion in essential hypertension is linked to vascular inflammation and oxidative stress. Circ. Res. 126 (4), 439–452. doi:10.1161/CIRCRESAHA.119.315767
Ding, F., Wang, G., and Zhang, S. (2018). Exogenous melatonin mitigates methyl viologen-triggered oxidative stress in poplar leaf. Molecules 23 (11), 2852. doi:10.3390/molecules23112852
Doughan, A. K., Harrison, D. G., and Dikalov, S. I. (2008). Molecular mechanisms of angiotensin II-mediated mitochondrial dysfunction: Linking mitochondrial oxidative damage and vascular endothelial dysfunction. Circ. Res. 102 (4), 488–496. doi:10.1161/CIRCRESAHA.107.162800
Eirin, A., Ebrahimi, B., Kwon, S. H., Fiala, J. A., Williams, B. J., Woollard, J. R., et al. (2016b). Restoration of mitochondrial cardiolipin attenuates cardiac damage in swine renovascular hypertension. J. Am. Heart Assoc. 5 (6), e003118. doi:10.1161/JAHA.115.003118
Eirin, A., Lerman, A., and Lerman, L. O. (2014). Mitochondrial injury and dysfunction in hypertension-induced cardiac damage. Eur. Heart J. 35 (46), 3258–3266. doi:10.1093/eurheartj/ehu436
Eirin, A., Saad, A., Tang, H., Herrmann, S. M., Woollard, J. R., Lerman, A., et al. (2016a). Urinary mitochondrial DNA copy number identifies chronic renal injury in hypertensive patients. Hypertension 68 (2), 401–410. doi:10.1161/HYPERTENSIONAHA.116.07849
Eisenberg, T., Abdellatif, M., Schroeder, S., Primessnig, U., Stekovic, S., Pendl, T., et al. (2016). Cardioprotection and lifespan extension by the natural polyamine spermidine. Nat. Med. 22 (12), 1428–1438. doi:10.1038/nm.4222
Enghiad, B., Huang, C., Guo, F., Jiang, G., Wang, B., Tabatabaei, S. K., et al. (2021). Cas12a-assisted precise targeted cloning using in vivo Cre-lox recombination. Nat. Commun. 12 (1), 1171. doi:10.1038/s41467-021-21275-4
Fang, S., Cheng, Y., Deng, F., and Zhang, B. (2021). RNF34 ablation promotes cerebrovascular remodeling and hypertension by increasing NADPH-derived ROS generation. Neurobiol. Dis. 156, 105396. doi:10.1016/j.nbd.2021.105396
Forbes, J. M., Ke, B. X., Nguyen, T. V., Henstridge, D. C., Penfold, S. A., Laskowski, A., et al. (2013). Deficiency in mitochondrial complex I activity due to Ndufs6 gene trap insertion induces renal disease. Antioxid. Redox Signal 19 (4), 331–343. doi:10.1089/ars.2012.4719
Forte, M., Bianchi, F., Cotugno, M., Marchitti, S., De Falco, E., Raffa, S., et al. (2020). Pharmacological restoration of autophagy reduces hypertension-related stroke occurrence. Autophagy 16 (8), 1468–1481. doi:10.1080/15548627.2019.1687215
Forte, M., Marchitti, S., Cotugno, M., Di Nonno, F., Stanzione, R., Bianchi, F., et al. (2021). Trehalose, a natural disaccharide, reduces stroke occurrence in the stroke-prone spontaneously hypertensive rat. Pharmacol. Res. 173, 105875. doi:10.1016/j.phrs.2021.105875
Fredriksson, K., Kalimo, H., Nordborg, C., Johansson, B. B., and Olsson, Y. (1988). Nerve cell injury in the brain of stroke-prone spontaneously hypertensive rats. Acta Neuropathol. 76 (3), 227–237. doi:10.1007/BF00687769
Friederich-Persson, M., Thörn, E., Hansell, P., Nangaku, M., Levin, M., and Palm, F. (2013). Kidney hypoxia, attributable to increased oxygen consumption, induces nephropathy independently of hyperglycemia and oxidative stress. Hypertension 62 (5), 914–919. doi:10.1161/HYPERTENSIONAHA.113.01425
Gao, J., Wei, T., Huang, C., Sun, M., and Shen, W. (2020). Sirtuin 3 governs autophagy-dependent glycolysis during Angiotensin II-induced endothelial-to-mesenchymal transition. Faseb J. 34 (12), 16645–16661. doi:10.1096/fj.202001494R
Goh, K. Y., He, L., Song, J., Jinno, M., Rogers, A. J., Sethu, P., et al. (2019). Mitoquinone ameliorates pressure overload-induced cardiac fibrosis and left ventricular dysfunction in mice. Redox Biol. 21, 101100. doi:10.1016/j.redox.2019.101100
Greish, S. M., Abdel-Hady, Z., Mohammed, S. S., Abdel-Hamed, A. R., Masoud, R. E., Eltamany, D. A., et al. (2020). Protective potential of curcumin in L-NAME-induced hypertensive rat model: AT1R, mitochondrial DNA synergy. Int. J. Physiol. Pathophysiol. Pharmacol. 12 (5), 134–146.
Gu, Q., Zhao, L., Ma, Y. P., and Liu, J. D. (2015). Contribution of mitochondrial function to exercise-induced attenuation of renal dysfunction in spontaneously hypertensive rats. Mol. Cell. Biochem. 406 (1-2), 217–225. doi:10.1007/s11010-015-2439-6
Hatabu, T., Harada, T., Takao, Y., Thi, D. H., Yamasato, A., Horiuchi, T., et al. (2020). Daily meal supplemented with astaxanthin-enriched yolk has mitigative effects against hypertension in spontaneously hypertensive rats. Biol. Pharm. Bull. 43 (3), 404–408. doi:10.1248/bpb.b19-01013
Hsieh, M. H., Cui, Z. Y., Yang, A. L., Nhu, N. T., Ting, S. Y., Yu, S. H., et al. (2021). Cerebral cortex apoptosis in early aged hypertension: Effects of epigallocatechin-3-gallate. Front. Aging Neurosci. 13, 705304. doi:10.3389/fnagi.2021.705304
Hu, Y. J., Chen, M. L., and Liang, D. (2022). Lignans and terpenoids from Gaultheria leucocarpa var. yunnanensis and their anti-inflammatory and antioxidant activities. Fitoterapia 162, 105293. doi:10.1016/j.fitote.2022.105293
Ikemura, M., Sasaki, Y., Giddings, J. C., and Yamamoto, J. (2012). Preventive effects of hesperidin, glucosyl hesperidin and naringin on hypertension and cerebral thrombosis in stroke-prone spontaneously hypertensive rats. Phytother. Res. 26 (9), 1272–1277. doi:10.1002/ptr.3724
Ivanova, S., Polajnar, M., Narbona-Perez, A. J., Hernandez-Alvarez, M. I., Frager, P., Slobodnyuk, K., et al. (2019). Regulation of death receptor signaling by the autophagy protein TP53INP2. Embo J. 38 (10), e99300. doi:10.15252/embj.201899300
Jin, Z., Wu, J., and Yan, L. J. (2016). Chemical conditioning as an approach to ischemic stroke tolerance: Mitochondria as the target. Int. J. Mol. Sci. 17 (3), 351. doi:10.3390/ijms17030351
Jing, H., Xie, R., Bai, Y., Duan, Y., Sun, C., Wang, Y., et al. (2021). The mechanism actions of astragaloside IV prevents the progression of hypertensive heart disease based on network Pharmacology and experimental Pharmacology. Front. Pharmacol. 12, 755653. doi:10.3389/fphar.2021.755653
Kang, H. M., Ahn, S. H., Choi, P., Ko, Y. A., Han, S. H., Chinga, F., et al. (2015). Defective fatty acid oxidation in renal tubular epithelial cells has a key role in kidney fibrosis development. Nat. Med. 21 (1), 37–46. doi:10.1038/nm.3762
Kislin, M., Sword, J., Fomitcheva, I. V., Croom, D., Pryazhnikov, E., Lihavainen, E., et al. (2017). Reversible disruption of neuronal mitochondria by ischemic and traumatic injury revealed by quantitative two-photon imaging in the neocortex of anesthetized mice. J. Neurosci. 37 (2), 333–348. doi:10.1523/JNEUROSCI.1510-16.2016
Kovacevic, S., Ivanov, M., Miloradovic, Z., Brkic, P., Vajic, U. J., Zivotic, M., et al. (2020). Hyperbaric oxygen preconditioning and the role of NADPH oxidase inhibition in postischemic acute kidney injury induced in spontaneously hypertensive rats. PLoS One 15 (1), e0226974. doi:10.1371/journal.pone.0226974
Kućmierz, J., Frąk, W., Młynarska, E., Franczyk, B., and Rysz, J. (2021). Molecular interactions of arterial hypertension in its target organs. Int. J. Mol. Sci. 22 (18), 9669. doi:10.3390/ijms22189669
Lampert, M. A., Orogo, A. M., Najor, R. H., Hammerling, B. C., Leon, L. J., Wang, B. J., et al. (2019). BNIP3L/NIX and FUNDC1-mediated mitophagy is required for mitochondrial network remodeling during cardiac progenitor cell differentiation. Autophagy 15 (7), 1182–1198. doi:10.1080/15548627.2019.1580095Å,
Lan, C., Chen, X., Zhang, Y., Wang, W., Wang, W. E., Liu, Y., et al. (2018). Curcumin prevents strokes in stroke-prone spontaneously hypertensive rats by improving vascular endothelial function. BMC Cardiovasc Disord. 18 (1), 43. doi:10.1186/s12872-018-0768-6
Lee, Y. Q., Lumbers, E. R., Oldmeadow, C., Collins, C. E., Johnson, V., Keogh, L., et al. (2019). The relationship between maternal adiposity during pregnancy and fetal kidney development and kidney function in infants: The gomeroi gaaynggal study. Physiol. Rep. 7 (17), e14227. doi:10.14814/phy2.14227
Li, L. R., Sethi, G., Zhang, X., Liu, C. L., Huang, Y., Liu, Q., et al. (2022b). The neuroprotective effects of icariin on ageing, various neurological, neuropsychiatric disorders, and brain injury induced by radiation exposure. Aging (Albany NY) 14 (3), 1562–1588. doi:10.18632/aging.203893
Li, N., Chen, L., Yi, F., Xia, M., and Li, P. L. (2008). Salt-sensitive hypertension induced by decoy of transcription factor hypoxia-inducible factor-1alpha in the renal medulla. Circ. Res. 102 (9), 1101–1108. doi:10.1161/CIRCRESAHA.107.169201
Li, Q., Youn, J. Y., Siu, K. L., Murugesan, P., Zhang, Y., and Cai, H. (2019a). Knockout of dihydrofolate reductase in mice induces hypertension and abdominal aortic aneurysm via mitochondrial dysfunction. Redox Biol. 24, 101185. doi:10.1016/j.redox.2019.101185
Li, Q., Chen, B., Yang, X., Zhang, C., Jiao, Y., Li, P., et al. (2019b). S100a8/a9 signaling causes mitochondrial dysfunction and cardiomyocyte death in response to ischemic/reperfusion injury. Circulation 140 (9), 751–764. doi:10.1161/CIRCULATIONAHA.118.039262
Li, L. R., Dang, Q., Li, Z., Han, C., Yang, Y., Li, M., et al. (2022a). Restoration of mitochondrial function is essential in the endothelium-dependent vasodilation induced by acacetin in hypertensive rats. Int. J. Mol. Sci. 23 (19), 11350. doi:10.3390/ijms231911350
Li, Y., Zhou, Z. H., Chen, M. H., Yang, J., Leng, J., Cao, G. S., et al. (2016). Inhibition of mitochondrial fission and NOX2 expression prevent NLRP3 inflammasome activation in the endothelium: The role of corosolic acid action in the amelioration of endothelial dysfunction. Antioxid. Redox Signal 24 (16), 893–908. doi:10.1089/ars.2015.6479
Liao, H., Gao, Y., Lian, C., Zhang, Y., Wang, B., Yang, Y., et al. (2019). Oral absorption and lymphatic transport of baicalein following drug-phospholipid complex incorporation in self-microemulsifying drug delivery systems. Int. J. Nanomedicine 14, 7291–7306. doi:10.2147/IJN.S214883
Liu, L. L., Yan, L., Chen, Y. H., Zeng, G. H., Zhou, Y., Chen, H. P., et al. (2014). A role for diallyl trisulfide in mitochondrial antioxidative stress contributes to its protective effects against vascular endothelial impairment. Eur. J. Pharmacol. 725, 23–31. doi:10.1016/j.ejphar.2014.01.010
Liu, M., Gu, L., Sulkin, M. S., Liu, H., Jeong, E. M., Greener, I., et al. (2013). Mitochondrial dysfunction causing cardiac sodium channel downregulation in cardiomyopathy. J. Mol. Cell. Cardiol. 54, 25–34. doi:10.1016/j.yjmcc.2012.10.011
Lopez-Campistrous, A., Hao, L., Xiang, W., Ton, D., Semchuk, P., Sander, J., et al. (2008). Mitochondrial dysfunction in the hypertensive rat brain: Respiratory complexes exhibit assembly defects in hypertension. Hypertension 51 (2), 412–419. doi:10.1161/HYPERTENSIONAHA.107.102285
Lu, J. L., Zeng, X. S., Zhou, X., Yang, J. L., Ren, L. L., Long, X. Y., et al. (2022). Molecular basis underlying hepatobiliary and renal excretion of phenolic acids of Salvia miltiorrhiza roots (danshen). Front. Pharmacol. 13, 911982. doi:10.3389/fphar.2022.911982
Lu, X., Zhang, J., Liu, H., Ma, W., Yu, L., Tan, X., et al. (2021). Cannabidiol attenuates pulmonary arterial hypertension by improving vascular smooth muscle cells mitochondrial function. Theranostics 11 (11), 5267–5278. doi:10.7150/thno.55571
Lu, Y., Li, S., Wu, H., Bian, Z., Xu, J., Gu, C., et al. (2015a). Beneficial effects of astragaloside IV against angiotensin II-induced mitochondrial dysfunction in rat vascular smooth muscle cells. Int. J. Mol. Med. 36 (5), 1223–1232. doi:10.3892/ijmm.2015.2345
Lu, Y., Xu, J., Bian, Z., Wu, H., Gu, C., Chen, X., et al. (2015b). Astragaloside IV ameliorates mitochondrial dysfunction of vascular smooth muscle cells induced by angiotensin II in rats. J. Nanjing Med. Univ. Nat. Sci. 35 (07), 975–980.
Lu, Z. Y., Qi, J., Yang, B., Cao, H. L., Wang, R. Y., Wang, X., et al. (2020). Diallyl trisulfide suppresses angiotensin II-induced vascular remodeling via inhibition of mitochondrial fission. Cardiovasc Drugs Ther. 34 (5), 605–618. doi:10.1007/s10557-020-07000-1
Luo, Z., Ma, L., Zhao, Z., He, H., Yang, D., Feng, X., et al. (2012). TRPV1 activation improves exercise endurance and energy metabolism through PGC-1α upregulation in mice. Cell. Res. 22 (3), 551–564. doi:10.1038/cr.2011.205
Mahmoud, A. M., Hernández Bautista, R. J., Sandhu, M. A., and Hussein, O. E. (2019). Beneficial effects of Citrus flavonoids on cardiovascular and metabolic health. Oxid. Med. Cell. Longev. 2019, 5484138. doi:10.1155/2019/5484138
Maki, K., Shibata, T., and Kawabata, S. I. (2017). Transglutaminase-catalyzed incorporation of polyamines masks the DNA-binding region of the transcription factor Relish. J. Biol. Chem. 292 (15), 6369–6380. doi:10.1074/jbc.M117.779579
Marsboom, G., Toth, P. T., Ryan, J. J., Hong, Z., Wu, X., Fang, Y. H., et al. (2012). Dynamin-related protein 1-mediated mitochondrial mitotic fission permits hyperproliferation of vascular smooth muscle cells and offers a novel therapeutic target in pulmonary hypertension. Circ. Res. 110 (11), 1484–1497. doi:10.1161/CIRCRESAHA.111.263848
Monroy-Ruiz, J., Sevilla, M., Carrón, R., and Montero, M. J. (2011). Astaxanthin-enriched-diet reduces blood pressure and improves cardiovascular parameters in spontaneously hypertensive rats. Pharmacol. Res. 63 (1), 44–50. doi:10.1016/j.phrs.2010.09.003
Mulligan, C. M., Sparagna, G. C., Le, C. H., De Mooy, A. B., Routh, M. A., Holmes, M. G., et al. (2012). Dietary linoleate preserves cardiolipin and attenuates mitochondrial dysfunction in the failing rat heart. Cardiovasc Res. 94 (3), 460–468. doi:10.1093/cvr/cvs118
Münch, C., and Harper, J. W. (2016). Mitochondrial unfolded protein response controls matrix pre-RNA processing and translation. Nature 534 (7609), 710–713. doi:10.1038/nature18302
Musumeci, M., Maccari, S., Sestili, P., Signore, M., Molinari, P., Ambrosio, C., et al. (2011). Propranolol enhances cell cycle-related gene expression in pressure overloaded hearts. Br. J. Pharmacol. 164 (8), 1917–1928. doi:10.1111/j.1476-5381.2011.01504.x
Nargesi, A. A., Farah, M. C., Zhu, X. Y., Zhang, L., Tang, H., Jordan, K. L., et al. (2021). Renovascular hypertension induces myocardial mitochondrial damage, contributing to cardiac injury and dysfunction in pigs with metabolic syndrome. Am. J. Hypertens. 34 (2), 172–182. doi:10.1093/ajh/hpaa202
Paggio, A., Checchetto, V., Campo, A., Menabò, R., Di Marco, G., Di Lisa, F., et al. (2019). Identification of an ATP-sensitive potassium channel in mitochondria. Nature 572 (7771), 609–613. doi:10.1038/s41586-019-1498-3
Pak, O., Scheibe, S., Esfandiary, A., Gierhardt, M., Sydykov, A., Logan, A., et al. (2018). Impact of the mitochondria-targeted antioxidant MitoQ on hypoxia-induced pulmonary hypertension. Eur. Respir. J. 51, 1701024. doi:10.1183/13993003.01024-2017
Qian, Z. Q., Wang, Y. W., Li, Y. L., Li, Y. Q., Ling, Z., and Yang, D. L. (2017). Icariin prevents hypertension-induced cardiomyocyte apoptosis through the mitochondrial apoptotic pathway. Biomed. Pharmacother. 88, 823–831. doi:10.1016/j.biopha.2017.01.147
Rimbaud, S., Ruiz, M., Piquereau, J., Mateo, P., Fortin, D., Veksler, V., et al. (2011). Resveratrol improves survival, hemodynamics and energetics in a rat model of hypertension leading to heart failure. PLoS One 6 (10), e26391. doi:10.1371/journal.pone.0026391
Rubattu, S., Di Castro, S., Schulz, H., Geurts, A. M., Cotugno, M., Bianchi, F., et al. (2016). Ndufc2 gene inhibition is associated with mitochondrial dysfunction and increased stroke susceptibility in an animal model of complex human disease. J. Am. Heart Assoc. 5 (2), e002701. doi:10.1161/JAHA.115.002701
Rubattu, S., Pagliaro, B., Pierelli, G., Santolamazza, C., Castro, S. D., Mennuni, S., et al. (2014). Pathogenesis of target organ damage in hypertension: Role of mitochondrial oxidative stress. Int. J. Mol. Sci. 16 (1), 823–839. doi:10.3390/ijms16010823
Sarkar, S., Davies, J. E., Huang, Z., Tunnacliffe, A., and Rubinsztein, D. C. (2007). Trehalose, a novel mTOR-independent autophagy enhancer, accelerates the clearance of mutant huntingtin and alpha-synuclein. J. Biol. Chem. 282 (8), 5641–5652. doi:10.1074/jbc.M609532200
Satoh, T., Wang, L., Espinosa-Diez, C., Wang, B., Hahn, S. A., Noda, K., et al. (2021). Metabolic syndrome mediates ROS-miR-193b-NFYA-dependent downregulation of soluble guanylate cyclase and contributes to exercise-induced pulmonary hypertension in heart failure with preserved ejection fraction. Circulation 144 (8), 615–637. doi:10.1161/CIRCULATIONAHA.121.053889
Sayed, A. M., Hassanein, E. H. M., Salem, S. H., Hussein, O. E., and Mahmoud, A. M. (2020). Flavonoids-mediated SIRT1 signaling activation in hepatic disorders. Life Sci. 259, 118173. doi:10.1016/j.lfs.2020.118173
Sekher Pannala, A., Chan, T. S., O'Brien, P. J., and Rice-Evans, C. A. (2001). Flavonoid B-ring chemistry and antioxidant activity: Fast reaction kinetics. Biochem. Biophys. Res. Commun. 282 (5), 1161–1168. doi:10.1006/bbrc.2001.4705
Shao, J., Wang, P., Liu, A., Du, X., Bai, J., and Chen, M. (2016). Punicalagin prevents hypoxic pulmonary hypertension via anti-oxidant effects in rats. Am. J. Chin. Med. 44 (4), 785–801. doi:10.1142/S0192415X16500439
Shen, W., Asai, K., Uechi, M., Mathier, M. A., Shannon, R. P., Vatner, S. F., et al. (1999). Progressive loss of myocardial ATP due to a loss of total purines during the development of heart failure in dogs: A compensatory role for the parallel loss of creatine. Circulation 100 (20), 2113–2118. doi:10.1161/01.cir.100.20.2113
Shirakabe, A., Zhai, P., Ikeda, Y., Saito, T., Maejima, Y., Hsu, C. P., et al. (2016). Drp1-Dependent mitochondrial autophagy plays a protective role against pressure overload-induced mitochondrial dysfunction and heart failure. Circulation 133 (13), 1249–1263. doi:10.1161/CIRCULATIONAHA.115.020502
Shpilka, T., Du, Y., Yang, Q., Melber, A., Uma Naresh, N., Lavelle, J., et al. (2021). UPR(mt) scales mitochondrial network expansion with protein synthesis via mitochondrial import in Caenorhabditis elegans. Nat. Commun. 12 (1), 479. doi:10.1038/s41467-020-20784-y
Shukla, H., Chitrakar, R., Bibi, H. A., Gaje, G., Koucheki, A., Trush, M. A., et al. (2020). Reactive oxygen species production by BP-1,6-quinone and its effects on the endothelial dysfunction: Involvement of the mitochondria. Toxicol. Lett. 322, 120–130. doi:10.1016/j.toxlet.2020.01.011
Singh, S., Gupta, P., Meena, A., and Luqman, S. (2020). Acacetin, a flavone with diverse therapeutic potential in cancer, inflammation, infections and other metabolic disorders. Food Chem. Toxicol. 145, 111708. doi:10.1016/j.fct.2020.111708
Sumbalová, Z., Kucharská, J., and Kristek, F. (2010). Losartan improved respiratory function and coenzyme Q content in brain mitochondria of young spontaneously hypertensive rats. Cell. Mol. Neurobiol. 30 (5), 751–758. doi:10.1007/s10571-010-9501-4
Sun, W., Yan, C., Frost, B., Wang, X., Hou, C., Zeng, M., et al. (2016). Pomegranate extract decreases oxidative stress and alleviates mitochondrial impairment by activating AMPK-Nrf2 in hypothalamic paraventricular nucleus of spontaneously hypertensive rats. Sci. Rep. 6, 34246. doi:10.1038/srep34246
Suzuki, T., Yamaguchi, H., Kikusato, M., Hashizume, O., Nagatoishi, S., Matsuo, A., et al. (2016). Mitochonic acid 5 binds mitochondria and ameliorates renal tubular and cardiac myocyte damage. J. Am. Soc. Nephrol. 27 (7), 1925–1932. doi:10.1681/ASN.2015060623
Tang, Z. H., Zheng, G., Feng, Z., Chen, Y., Lou, Y., Wang, C., et al. (2017b). Trehalose ameliorates oxidative stress-mediated mitochondrial dysfunction and ER stress via selective autophagy stimulation and autophagic flux restoration in osteoarthritis development. Cell. Death Dis. 8 (10), e3081. doi:10.1038/cddis.2017.453
Tang, Z. H., Cao, W. X., Wang, Z. Y., Lu, J. H., Liu, B., Chen, X., et al. (2017a). Induction of reactive oxygen species-stimulated distinctive autophagy by chelerythrine in non-small cell lung cancer cells. Redox Biol. 12, 367–376. doi:10.1016/j.redox.2017.03.009
Tapia, E., Soto, V., Ortiz-Vega, K. M., Zarco-Márquez, G., Molina-Jijón, E., Cristóbal-García, M., et al. (2012). Curcumin induces Nrf2 nuclear translocation and prevents glomerular hypertension, hyperfiltration, oxidant stress, and the decrease in antioxidant enzymes in 5/6 nephrectomized rats. Oxid. Med. Cell. Longev. 2012, 269039. doi:10.1155/2012/269039
Vaka, V. R., McMaster, K. M., Cunningham, M. W., Ibrahim, T., Hazlewood, R., Usry, N., et al. (2018). Role of mitochondrial dysfunction and reactive oxygen species in mediating hypertension in the reduced uterine perfusion pressure rat model of preeclampsia. Hypertension 72 (3), 703–711. doi:10.1161/HYPERTENSIONAHA.118.11290
Volkova, M., Palmeri, M., Russell, K. S., and Russell, R. R. (2011). Activation of the aryl hydrocarbon receptor by doxorubicin mediates cytoprotective effects in the heart. Cardiovasc Res. 90 (2), 305–314. doi:10.1093/cvr/cvr007
Wai, T., García-Prieto, J., Baker, M. J., Merkwirth, C., Benit, P., Rustin, P., et al. (2015). Imbalanced OPA1 processing and mitochondrial fragmentation cause heart failure in mice. Science 350 (6265), aad0116. doi:10.1126/science.aad0116
Wang, L., Wang, W., Han, R., Liu, Y., Wu, B., and Luo, J. (2023). Protective effects of melatonin on myocardial microvascular endothelial cell injury under hypertensive state by regulating Mst1. BMC Cardiovasc Disord. 23 (1), 179. doi:10.1186/s12872-023-03159-1
Wang, L., Yu, T., Lee, H., O'Brien, D. K., Sesaki, H., and Yoon, Y. (2015). Decreasing mitochondrial fission diminishes vascular smooth muscle cell migration and ameliorates intimal hyperplasia. Cardiovasc Res. 106 (2), 272–283. doi:10.1093/cvr/cvv005
Weiss, R. G., Gerstenblith, G., and Bottomley, P. A. (2005). ATP flux through creatine kinase in the normal, stressed, and failing human heart. Proc. Natl. Acad. Sci. U. S. A. 102 (3), 808–813. doi:10.1073/pnas.0408962102
Wu, Y., Qian, Z., Fu, S., Yue, Y., Li, Y., Sun, R., et al. (2018). IcarisideII improves left ventricular remodeling in spontaneously hypertensive rats by inhibiting the ASK1-JNK/p38 signaling pathway. Eur. J. Pharmacol. 819, 68–79. doi:10.1016/j.ejphar.2017.11.035
Xu, M. L., Peng, B., Bai, J., Li, L., Du, Y., Wang, Z. Q., et al. (2022). Diosgenin exerts an antihypertensive effect in spontaneously hypertensive rats via gut-brain communication. Food Funct. 13 (18), 9532–9543. doi:10.1039/d2fo00946c
Xu, Y., and Rao, M. R. (1995). Effects of tetrandrine on left ventricle hypertrophy in deoxycorticosterone acetate-salt hypertensive rats. Eur. J. Pharmacol. 278 (1), 1–7. doi:10.1016/0014-2999(95)00055-p
Xue, W., Wang, X., Tang, H., Sun, F., Zhu, H., Huang, D., et al. (2020). Vitexin attenuates myocardial ischemia/reperfusion injury in rats by regulating mitochondrial dysfunction induced by mitochondrial dynamics imbalance. Biomed. Pharmacother. 124, 109849. doi:10.1016/j.biopha.2020.109849
Yamaguchi, Y., Yamada, K., Yoshikawa, N., Nakamura, K., Haginaka, J., and Kunitomo, M. (2006). Corosolic acid prevents oxidative stress, inflammation and hypertension in SHR/NDmcr-cp rats, a model of metabolic syndrome. Life Sci. 79 (26), 2474–2479. doi:10.1016/j.lfs.2006.08.007
Yamamoto, K., Imamura, H., and Ando, J. (2018). Shear stress augments mitochondrial ATP generation that triggers ATP release and Ca(2+) signaling in vascular endothelial cells. Am. J. Physiol. Heart Circ. Physiol. 315 (5), H1477–H1485. doi:10.1152/ajpheart.00204.2018
Ye, C., Tong, Y., Wu, N., Wan, G. W., Zheng, F., Chen, J. Y., et al. (2021). Inhibition of miR-135a-5p attenuates vascular smooth muscle cell proliferation and vascular remodeling in hypertensive rats. Acta Pharmacol. Sin. 42 (11), 1798–1807. doi:10.1038/s41401-020-00608-x
Yuan, P., Zhang, Q., Fu, Y., Hou, Y., Gao, L., Wei, Y., et al. (2022). Acacetin inhibits myocardial mitochondrial dysfunction by activating PI3K/AKT in SHR rats fed with fructose. J. Nat. Med. 77, 262–275. doi:10.1007/s11418-022-01666-7
Zachman, D. K., Chicco, A. J., McCune, S. A., Murphy, R. C., Moore, R. L., and Sparagna, G. C. (2010). The role of calcium-independent phospholipase A2 in cardiolipin remodeling in the spontaneously hypertensive heart failure rat heart. J. Lipid Res. 51 (3), 525–534. doi:10.1194/jlr.M000646
Zhang, J., Chen, Y., Luo, H., Sun, L., Xu, M., Yu, J., et al. (2018a). Recent update on the pharmacological effects and mechanisms of dihydromyricetin. Front. Pharmacol. 9, 1204. doi:10.3389/fphar.2018.01204
Zhang, J., Feng, Y., Wang, Y., Wang, J., Xiang, D., Niu, W., et al. (2018b). Resveratrol ameliorates disorders of mitochondrial biogenesis and dynamics in a rat chronic ocular hypertension model. Life Sci. 207, 234–245. doi:10.1016/j.lfs.2018.06.010
Zhou, B., Perel, P., Mensah, G. A., and Ezzati, M. (2021). Global epidemiology, health burden and effective interventions for elevated blood pressure and hypertension. Nat. Rev. Cardiol. 18 (11), 785–802. doi:10.1038/s41569-021-00559-8
Keywords: natural compounds, mitochondrial dysfunction, target organ damage, hypertension, flavonoids
Citation: Liao X, Han Y, He Y, Liu J and Wang Y (2023) Natural compounds targeting mitochondrial dysfunction: emerging therapeutics for target organ damage in hypertension. Front. Pharmacol. 14:1209890. doi: 10.3389/fphar.2023.1209890
Received: 21 April 2023; Accepted: 08 June 2023;
Published: 15 June 2023.
Edited by:
Ali H. Eid, Qatar University, QatarReviewed by:
Yongnan Li, Lanzhou University, ChinaCopyright © 2023 Liao, Han, He, Liu and Wang. This is an open-access article distributed under the terms of the Creative Commons Attribution License (CC BY). The use, distribution or reproduction in other forums is permitted, provided the original author(s) and the copyright owner(s) are credited and that the original publication in this journal is cited, in accordance with accepted academic practice. No use, distribution or reproduction is permitted which does not comply with these terms.
*Correspondence: Jianjun Liu, ODAyMjg2NjFAcXEuY29t; Yuhong Wang, d3loMTA3QGhudWNtLmVkdS5jbg==
Disclaimer: All claims expressed in this article are solely those of the authors and do not necessarily represent those of their affiliated organizations, or those of the publisher, the editors and the reviewers. Any product that may be evaluated in this article or claim that may be made by its manufacturer is not guaranteed or endorsed by the publisher.
Research integrity at Frontiers
Learn more about the work of our research integrity team to safeguard the quality of each article we publish.