- 1School of Food and Biomedicine, Zaozhuang University, Zaozhuang, Shandong, China
- 2School of Public Health, Weifang Medical University, Weifang, Shandong, China
Background: Since its discovery, poly (ADP-ribose) polymerase 1 (PARP-1) has been extensively studied due to its regulatory role in numerous biologically crucial pathways. PARP inhibitors have opened new therapeutic avenues for cancer patients and have gained approval as standalone treatments for certain types of cancer. With continued advancements in the research of PARP inhibitors, we can fully realize their potential as therapeutic targets for various diseases.
Purpose: To assess the current understanding of PARP-1 mechanisms in radioprotection and radiotherapy based on the literature.
Methods: We searched the PubMed database and summarized information on PARP inhibitors, the interaction of PARP-1 with DNA, and the relationships between PARP-1 and p53/ROS, NF-κB/DNA-PK, and caspase3/AIF, respectively.
Results: The enzyme PARP-1 plays a crucial role in repairing DNA damage and modifying proteins. Cells exposed to radiation can experience DNA damage, such as single-, intra-, or inter-strand damage. This damage, associated with replication fork stagnation, triggers DNA repair mechanisms, including those involving PARP-1. The activity of PARP-1 increases 500-fold on DNA binding. Studies on PARP-1-knockdown mice have shown that the protein regulates the response to radiation. A lack of PARP-1 also increases the organism’s sensitivity to radiation injury. PARP-1 has been found positively or negatively regulate the expression of specific genes through its modulation of key transcription factors and other molecules, including NF-κB, p53, Caspase 3, reactive oxygen species (ROS), and apoptosis-inducing factor (AIF).
Conclusion: This review provides a comprehensive analysis of the physiological and pathological roles of PARP-1 and examines the impact of PARP-1 inhibitors under conditions of ionizing radiation exposure. The review also emphasizes the challenges and opportunities for developing PARP-1 inhibitors to improve the clinical outcomes of ionizing radiation damage.
1 Introduction
The PARP-1 nucleoprotein is expressed at high levels in eukaryotic cells, where it serves as an essential component of the DNA base excision-repair (BER) system (Demin et al., 2021). Recent evidence has also shown that PARP-1 plays roles in nucleotide excision repair (NER) (Pines et al., 2012; Robu et al., 2013), classical non-homologous end joining (cNHEJ) (Ruscetti et al., 1998; Spagnolo et al., 2012; Luijsterburg et al., 2016), alternative NHEJ (aNHEJ) (Wang et al., 2006; Fattah et al., 2010; Mansour et al., 2010; Cheng et al., 2011; Sfeir and de Lange., 2012; Ceccaldi et al., 2015; Mateos-Gomez et al., 2015), microhomology-mediated end-joining (MMEJ) (Sfeir and Symington, 2015; Dutta et al., 2017), homologous recombination (HR) (Hochegger et al., 2006; Hu et al., 2014), DNA mismatch repair (MMR) (Liu et al., 2011), and maintenance of replication fork stability (Yang et al., 2004; Bryant et al., 2009; Ray Chaudhuri et al., 2012; Berti et al., 2013; Ronson et al., 2018) pathways. Two recent reviews have explored how PARP-1 functions in these different pathways (Martin-Hernandez et al., 2017; Ray Chaudhuri and Nussenzweig, 2017). In addition to its role as a mediator of DNA repair, PARP-1 also functions as a regulator of transcription, post-transcriptional gene expression, cell death, and inflammatory activity (Rajawat et al., 2017). PARP-1 belongs to a family of enzymes that catalyze the transfer of ADP-ribose from the substrate, nicotinamide adenine dinucleotide (NAD), to particular nucleoprotein targets (Figure 1). Functionally, PARP-1 acts as a sensor for gaps in DNA and can interact with various DNA repair factors in the BER pathway (Muiras, 2003). PARP-1 contains several conserved and functionally distinct domains, including a self-modifying domain, a 55-kDa catalytic domain, and two zinc finger domains that bind DNA (Kotova et al., 2010). PARP-1 is mobilized to the locations of DNA damage. This mobilization results in the two homologous N-terminal PARP-1 zinc finger domains binding to the compromised DNA, consequently leading to a 500-fold enhancement in the enzymatic activity of PARP-1 (Langelier et al., 2008; Tao et al., 2008). A third zinc finger domain is specific to PARP-1 and essential for its DNA-dependent activity (Gamsjaeger et al., 2007). The structure of PARP-1 is shown in Figure 2.
PARP-1 inhibitors can be used in combination with ionizing radiation or chemotherapy to enhance the sensitivity of tumor cells to these treatments. They can also serve as standalone treatments that are both effective and relatively non-toxic for cancers with DNA repair gene defects, including those with BRCA-1/2 mutations, such as some triple-negative breast cancers. Most PARP inhibitors developed to date are not specific for PARP-1 and function by interacting with the catalytic NAD-binding domain and thus competing with NAD for PARP-1 binding by simulating the substrate-enzyme interaction and interfering with double-stranded break (DSB) repair (Langelier et al., 2018). Others inhibit PARP-1 allosterically by binding to the catalytic domain, impairing the DNA-binding ability and, thus, the DSB repair response (Ogden et al., 2021; Rouleau-Turcotte et al., 2022).
The transcription factor NF-κB, associated with inflammation, is typically found in the cytosol bound to the inhibitory protein IκB. When stimulated by intracellular or extracellular stimuli, IκB is degraded, leading to the nuclear translocation of NF-κB, which binds to the promoter regions of specific target genes, upregulating their expression (Perkins, 2000; Negi and Sharma, 2015). PARP-1 can form a complex with NF-κB to promote NF-κB target gene expression (Ullrich et al., 2001). Both NF-κB DNA-binding activity and induced nitric oxide synthase (iNOS) expression in macrophages were found to be reduced by PARP-1 inhibitors (Chiarugi, 2002). PARP-knockdown mice exposed to endotoxin-induced shock also exhibited impaired NF-κB activation and iNOS upregulation (Oliver et al., 1999). Indeed, PARP-1 and NF-κB are closely associated with one another, with synthesized PAR facilitating the trans-stimulation of NF-κB (Smith, 2001), enabling PARP-1 to regulate NF-κB activity (Hunter et al., 2012). NF-κB activation is important for p53 expression and activity, and PARP can thus potentially shape cell fate outcomes by regulating p53, NF-κB, and a range of different effector proteins (Qin et al., 1999; Ryan et al., 2000). PAR-binding sequence motifs exist in many proteins, including NF-κB, p53, and DNA-dependent protein kinase (DNA-PK) (Pleschke et al., 2000). The PAR-binding zinc finger (PBZ) motif in several eukaryotic proteins is involved in the DNA damage response and checkpoint regulation (Ahel et al., 2008; Eustermann et al., 2010).
Cell death facilitated by PARP-1 shares many features with typical apoptosis, necessitating the relocation of the mitochondrial AIF-1 to the nuclear compartment (Lautier et al., 1993; Alano et al., 2004). Sphingosine and radiation can enhance caspase-independent apoptotic death in radioresistant Jurkat T cell clones, demonstrating a role for PARP-1 activation in AIF translocation to the nucleus (Park et al., 2005). PARP-1 activation-induced cell death is a key mechanism associated with cell death mediated by AIF, with AIF being central to this cytotoxic death pathway (Yu et al., 2002). Activation of PARP-1 contributes to the loss of mitochondrial membrane potential, nuclear translocation of AIF, nuclear agglutination, and, ultimately, cell death. The translocation of AIF occurs after the activation of PARP-1 but before the release of cytochrome C from the mitochondria or the activation of caspase activity such that AIF-neutralizing antibodies can partially disrupt the PARP-1-mediated induction of cell death (Hong et al., 2004). In severe, extensive DNA damage, PARP-1 overactivation can consume available NAD and ATP energy reserves, thus spurring AIF nuclear translocation and facilitating non-caspase-dependent apoptosis (Koh et al., 2005).
Exposure to ionizing radiation increases PARP-1 activity levels significantly within one minute. Inhibiting PARP-1 activity can significantly slow the rate of DNA damage repair (Ryabokon et al., 2008; Ryabokon et al., 2009). PARP-1 inhibitors can increase the degree of single-stranded break (SSB) formation in the DNA, unlike DNA-PK inhibitors, which only affect DSB repair (Zou et al., 2015). Given PARP-1’s critical role in DNA damage repair, using PARP-1 inhibitors to enhance the efficacy of anti-cancer treatments could increase tumor cell sensitivity to radiotherapy or chemotherapy (Underhill et al., 2011). PARP-1 inhibitors have been shown to interfere with PARP-1-mediated SSB/DSB repair. This inhibition of PARP-1 activity can induce apoptosis after exposure to ionizing radiation due to the lack of DSB repair while also promoting necrotic death due to energy deficiencies resulting from reduced PAR production after DNA damage, thus depleting the NAD reservoir (Barth et al., 2006). In most therapeutic settings, PARP-1 inhibitors are combined with chemo- or radiotherapy (Haince et al., 2005; De Soto and Deng., 2006). More detailed reference examples are in the “1. PARP inhibitors” section. PARP-1 activity in active and inactive cells at 24 and 48 h following irradiation was associated with increased ROS production (Chiu et al., 2021), and the inhibition of PARP-1-related ROS production may provide an additional opportunity to increase tumor cell sensitivity to other therapeutic agents (Doroshow, 2006).
2 PARP inhibitors
PARP inhibitors disrupt DNA repair, providing ample opportunities for radiosensitization, adjuvant treatment, or chemical synergism. Many such inhibitors have been evaluated in various preclinical and clinical studies, demonstrating their primary function of interfering with PARP-1 activities in DNA repair and transcriptional regulation. The PARP substrate 3-aminobenzamide (3-AB) is a first-generation PARP inhibitor lacking in specificity, although it can inhibit a reported 96% of PARP-1 activity in cells, adversely affecting DNA synthesis, glucose metabolism, and overall cellular viability (Rajawat et al., 2017). 3-AB can promote apoptotic death in HeLa cells by interfering with PARP expression and blocking G2 phase arrest following X-ray irradiation (Masutani et al., 1995). Pairing the PARP inhibitor Veliparib with ionizing radiation can swiftly trigger senescence in tumor cells (Zhang et al., 2016). The PARP-1 inhibitor amplifies ionizing radiation-induced cytotoxicity by suppressing NF-κB activation (Veuger et al., 2009). In K562 cells exposed to ionizing radiation, PARP-1 inhibition can promote ROS production while suppressing PAR production and dramatically reducing the rate of DNA-break repair (Cieślar-Pobuda et al., 2012). Ionizing radiation-induced cytotoxicity sensitivity was observed in both PLC-PRF-5 and HepG2 cell lines one-hour post-treatment with ABT-888 (Guillot et al., 2014). γ-radiation exposure can trigger an overexpression of nuclear PARP, leading to the depletion of both NAD and ATP stores and, subsequently, the induction of oxidative stress. Studies indicate that 3-AB can facilitate wound healing, typically delayed in mice undergoing whole-body γ-irradiation, by modulating the inflammatory and oxidative responses to such irradiation (El-Hamoly et al., 2015). Functionally, 3-AB disrupts the interaction between NAD and the PARP-1 active site. This obstruction inhibits its activation and DNA binding, impairing its ability to recognize DNA sequence breaks (Garon and Dubinett., 2010). Radiosensitive OPSCC cells positive for HPV infection showed upregulation of proteins involved in the SSB and BER repair processes, including PARP1, XRCC1, and PNKP. Treating HPV-negative OPSCCs with the PARP inhibitor Olaparib enhanced their sensitivity to radiation, emphasizing the potential value of combining PARP inhibition and radiotherapy when treating both types of OPSCC (Nickson et al., 2017). Overexpression of the PARP-1 DNA-binding region significantly reduces DSB repair in irradiated cells, indicating that PARP inhibitors have pronounced radiosensitizing activity. Mechanistically, inhibiting PARP-1 may raise the odds of repair failures due to the loss or rapid-to-slow reconnection transitions from PAR-induced DSB due to the expression of a dominant negative form of PARP-1 (Rudat et al., 2001). SSBs can result in rapid PARP-1 activation, and low-dose radiosensitization can be achieved by treating cells with chemical PARP inhibitors in CHO, V79, and T89G cells in the exponential growth phase. Rapidly dividing cells are most sensitive to PARP inhibition, resulting in sensitivity to radiation doses as low as 0.05–0.3 Gy (Chalmers et al., 2004). All of these tumors exhibited increased radiosensitivity when treated with the PARP-1 inhibitor MK-48287 at 50 mg/kg/d (treatment once per hour) to lower intratumoral PAR levels, providing a valuable tool to increase therapeutic efficacy (Wang et al., 2012).
The use of Olaparib to inhibit BER pathway activity resulted in SSB accumulation due to a lack of genomic repair, resulting in the collapse of the replication fork during the S phase of the cell cycle and ultimately contributing to deleterious DSB formation (Bochum et al., 2018). Niraparib activates the cGAS/STING signaling pathway and can thus alter immune responses, and this activity can be further enhanced when combined with PD-L1 blockade, providing a valuable opportunity for clinical intervention (Meng et al., 2021). Rucaparib, a small molecule PARP inhibitor, has been administered to patients with specific resistant and advanced stages of ovarian carcinoma. This treatment resulted in moderate and transient elevations in serum aminotransferase activity in the treated patients. Nevertheless, these changes have not been associated with clinically significant hepatic injury (LiverTox, 2012). The oral PARP inhibitor, Talazoparib, has been used to treat certain breast cancer patients, but the moderate increases in serum aminotransferase levels observed in some cases suggest the possibility of clinically detectable liver damage (Guney, 2019). In clinical trials, Veliparib has demonstrated promising efficacy in treating breast cancers that harbor BRCA mutations and HR pathway deficiencies (HRD). However, additional work is needed to fully explore its optimal dosing and compatibility with other antineoplastic agents (George et al., 2022). In a randomized phase II study of triple-negative breast cancer patients, the PARP inhibitor Iniparib reportedly exhibited promising activity (Mateo et al., 2013). The selective and potent PARP-1 inhibitor AZD5305 also has PARP-1-DNA trapper activity, shown in a preclinical in vivo patient-derived xenograft (PDX) model of BRCA mutant breast cancer (HBCx-17) (Johannes et al., 2021). The investigational PARP-1 and PARP-2 inhibitor Pamiparib has also shown promising antitumor efficacy, suppressing the proliferation of tumor cell lines harboring BRCA1/2 mutations or HRD (Wang et al., 2020). When treating platinum-sensitive relapse ovarian cancer cells with germline BRCA1/2 mutations, Fluzoparib also exhibited good safety and antitumor activity profiles (Li et al., 2021). BGP-15 reportedly reduced the ischemia-reperfusion-induced self-ADP-ribosylation of nuclear PARP and the mono-ADP-ribosylation of GRP78, an ER chaperone (Szabados et al., 2000). E7449 can inhibit the enzymatic activity of PARP, trapping PARP-1 in association with damaged DNA in a manner that contributes to higher rates of cytotoxic cell death. Consistently, cells with defects in DNA repair pathways showed high levels of E7449 sensitivity, which exhibited single-agent activity in BRCA-derived xenograft models, had its activity enhanced in combination with chemotherapeutic agents, and suppressed Wnt/β-catenin pathway signaling in colon cancer cells, likely via inhibition of TNKS (McGonigle et al., 2015). BYK204165 is an isoquinolindione that reportedly exhibits 100-fold greater selectivity for PARP-1, making it a promising tool for studies specifically focused on the effects of inhibiting PARP-1 (Eltze et al., 2008). The PARP inhibitor A-966492 can increase the radiosensitivity of U87MG spheroids, while TPT increases such radiosensitivity by interfering with DNA damage repair and resulting in S-phase cell accumulation. Combining the topoisomerase I inhibitor TPT and A-966492 can enhance tumor cell radiosensitivity in an additive manner (Koosha et al., 2017). DPQ and PJ-34 can suppress inflammation and other deleterious effects associated with the overexpression of PARP-1 while increasing cell viability, underscoring their potential clinical utility (Scalia et al., 2013). It has been suggested that 3-AB can significantly augment acetylcholine-induced, endothelium-dependent, nitric oxide-mediated vasorelaxation, normalizing the function of endothelial tissue following exposure to hydrogen peroxide (400 μM) (Radovits et al., 2007). Details of the mechanisms of the inhibitors are listed in Table 1.
The potency of PARP inhibitors was shown to be associated with both the recommended therapeutic dosage and the severity of adverse effects. In 2018, the FDA authorized Talazoparib to treat HER2-negative and BRCA-mutated breast cancer. Cases with breast cancer who have the BRCA mutation had a 21%–37% survival rate when given 1 mg/day of Talazoparib (Turner et al., 2019). Cases with advanced breast cancer with BRCA mutations who received Talazoparib had a higher median survival rate (62.6% vs. 27.2%) than those who received conventional treatment (Litton et al., 2018). Talazoparib, the most potent PARP inhibitor, has been associated with the highest incidence of anemia. The lowest effective dosage is 1 mg orally once daily (de Bono et al., 2017; Litton et al., 2018; Pilie et al., 2019). Talazoparib has been shown to enhance the efficacy of in vivo radiotherapy and chemotherapy in various cancers, including lung cancer, colorectal cancer, glioblastoma, and serous ovarian cancer xenotransplantation (Calabrese et al., 2004; Donawho et al., 2007; Bi et al., 2018). Specifically for glioblastoma, a study found that Talazoparib has a greater radiosensitization effect on stem cells compared to other PARP inhibitors (including Olaparib and AG14361), even at lower concentrations (Lesueur et al., 2018). Talazoparib has been shown to enhance the efficacy of in vivo radiotherapy and chemotherapy in various cancers, including lung cancer, colorectal cancer, glioblastoma, and serous ovarian cancer xenotransplantation (Calabrese et al., 2004; Donawho et al., 2007; Bi et al., 2018). Specifically for glioblastoma, a study found that Talazoparib has a greater radiosensitization effect on stem cells compared to other PARP inhibitors (including Olaparib and AG14361), even at lower concentrations (Lesueur et al., 2018). The European Medicines Agency (EMA) authorized Olaparib in 2014 as a maintenance therapy for BRCA mutant ovarian malignancies when platinum chemotherapy can be tolerated following three or more lines of chemotherapy. Currently, the standard dosage is 300 mg twice/day. Patients with advanced BRCA1/2-mutated ovarian cancer who have progressed or relapsed following platinum-based chemotherapy do not benefit more from treatment with Olaparib than those who get pegylated liposome doxorubicin (PLD) (Kaye et al., 2012). The use of Olaparib as a radiation sensitizer during breast radiation therapy may not be associated with an increased risk of late complications. Therefore, we suggest that in future clinical trials, when Olaparib is combined with breast radiation therapy, the radiation sensitization dose should be 200 mg twice a day to evaluate the antitumor effect of the combination (Loap et al., 2022). In 2016, the FDA authorized Rucaparib to treat advanced ovarian cancer cases with somatic BRCA1/2 or germline mutations. The median survival was extended from roughly 5 months with a placebo to 16.6 or 21 months with Niraparib (300 mg once/day) and Rucaparib (600 mg twice/day), according to phase 3 studies (Coleman et al., 2017; Mirza et al., 2016). Single-agent administration of Veliparib did not improve the clinical outcomes for patients with pancreatic and ovarian cancers (Coleman et al., 2015; Lowery et al., 2018). Nevertheless, combining Veliparib with Paclitaxel and Carboplatin enhanced survival after maintenance treatment more than Paclitaxel and Carboplatin alone in a recent phase 3 study of advanced serous ovarian malignancy (Coleman et al., 2019). Veliparib stands out as the most promising PARP inhibitor with therapeutic implications, capable of being administered alongside standard chemotherapy without eliciting undesirable toxicity. A Phase I multicenter study was initiated with 30 participants diagnosed with either inflammatory or recurrent breast cancer localized to the surgical resection area. This trial involved the administration of Veliparib concomitant with radiotherapy targeting the chest wall and regional lymph nodes. Despite the highest experimental dose, severe acute toxicity did not surpass 30%. However, nearly half of the surviving patients exhibited grade 3 adverse events after 3 years, underscoring the critical necessity of long-term toxicity monitoring in trials involving radiation sensitizers (Jagsi et al., 2018).
3 The role of PARP-1 in DSB and SSB repair
PARP-1, the most abundantly expressed member of the PARP family, acts as a key component in the BER pathway (Anwar et al., 2015). In addition to its direct effects as a mediator of DNA repair, PARP-1 is also associated with inflammation, post-transcriptional gene regulation, and cell death (Langelier et al., 2008; Tao et al., 2008; Rajawat et al., 2017). PARP-1, when drawn to sites of DNA damage, plays an instrumental role in maintaining or regaining genomic stability, thereby aiding in cell survival (Veuger et al., 2003; Chalmers et al., 2004; Haince et al., 2008). Upon the incidence of DNA damage, PARP-1 is activated, catalyzing the splitting of NAD + into nicotinamide and ADP-ribose. This reaction leads to the formation of elongated branches of ADP-ribose polymers capable of modifying target proteins (Aguilar-Quesada et al., 2007). Overactivation of PARP, however, may result in NAD+ and ATP depletion, compromising the energy supply at a cellular level and potentially provoking necrotic cell death (Sodhi et al., 2010; Underhill et al., 2011).
High glucose levels have been associated with the activation of PARP1 in endothelial cells (Garcia Soriano et al., 2001; Du et al., 2003; Piconi et al., 2004). This activation is partly due to DNA damage and oxidative stress induction. Thus, PARP-1 expression may be associated with ROS production in cells. If the resultant oxidative stress is not adequately controlled, it can cause DNA breaks that contribute to PARP-1 overactivation and potential necrotic or apoptotic cell death (Zakaria et al., 2016). Inhibition of PARP can protect against ROS-mediated cell death and preserve the mitochondrial membrane potential in an MKP-1 and ATF4-dependent manner (Hocsak et al., 2017). Recent work has demonstrated that PARP-1 activation is directly triggered by ROS-induced damage to the DNA, ultimately contributing to a loss of cellular viability (Cole and Perez-Polo., 2002). Although research has frequently explored PARP-1 regulation of DNA in tumor cells, less is known about PARP-1-mediated regulation in normal cells.
Tumor cell resistance to radiotherapy is primarily mediated by robust DNA repair functionality. Therefore, actions such as silencing, inhibiting, or knocking out PARP-1 can significantly enhance the efficacy of radiotherapy by impairing DNA repair. PARP-1 is an important SSB and DSB repair mediator, orchestrating appropriate cellular responses after radiation exposure. (Veuger et al., 2003; Chalmers et al., 2004; Chen et al., 2018; Sefer et al., 2022). By targeting these DNA repair mechanisms, PARP inhibitors can increase the cytotoxic effects of radiotherapy and chemotherapy (Curtin, 2012; Steffen et al., 2013). Exposure to ionizing radiation can induce both SSB and DSB in DNA, which can be repaired by activating appropriate DNA repair enzymes, thus preserving cell and tissue function. Several clinical trials have validated the potential of PARP inhibitors for use as a radiosensitizing treatment, while experimental studies indicate an increase in radiosensitivity following the silencing of PARP-1. This enhancement in radiosensitivity is somewhat distinct from the effects observed under PARP-1 inhibition, which predominantly impacts cells in the S-phase (Godon et al., 2008). Olaparib, which inhibits PARP-1/2/3, modifies SSB repair and displays significant radiosensitizing effectiveness when administered for treating Lewis lung cancer cells and tumor xenografts. This effectiveness might be associated with an elevation in DNA DSB formation and an upregulation of the pro-apoptotic Bax/Bcl-2 upon irradiation (Wang et al., 2016). Observations of increased PARP-1 expression in response to adaptive radiation imply a potential role for this enzyme in cellular adaptation to exposure to low-dose radiation (Cheng et al., 2010). This radiation-induced PARP-1 activation can also drive the migratory and invasive activity of CNE-2 nasopharyngeal carcinoma cells (Lu et al., 2018). One study found that high linear energy transfer (LET) ionizing radiation was only sufficient to inhibit the Ku (XRCC5)-dependent NHEJ pathway but not the PARP-1-dependent NHEJ or homologous recombination repair (HRR) pathways. Exposure to high LET, facilitated by highly charged particles, can lead to impaired DNA repair and more DSB fragments that are less than 40 base pairs in size. This prevents the Ku protein from binding to the ends of these DSB fragments more effectively than exposure to a dose of low LET ionizing radiation from X-rays, which delays Ku-dependent repair (Zhang, 2008). PARP can be activated in a dose-dependent manner in response to synchrotron radiation X (SRX)-associated tissue damage. At the same time, this is inhibited by treatment with the antioxidant n-acetylcysteine (NAC), highlighting the role of oxidative stress in the mediation of SRX-induced PARP activation (Ying, 2013).
PARP-1 plays diverse and complex roles in repairing DNA damage and preserving genomic integrity (Martin-Hernandez et al., 2017; Ray Chaudhuri and Nussenzweig., 2017). In the context of DNA repair, the three main steps are detecting DNA damage, the PAR-mediated recruitment of repair-related factors, and the PAR-mediated regulation of key biochemical processes. A multitude of studies elaborates on the involvement of PARP-1 in the SSB repair and BER pathways (Masson et al., 1998; Trucco et al., 1998; Dantzer et al., 2000; Lavrik et al., 2001; Prasad et al., 2001; El-Khamisy et al., 2003; Leppard et al., 2003; Fisher et al., 2007), with SSB damage detection being followed by the PAR-mediated recruitment of XRCC1 to these sites of DNA damage.
PARP-1 is a highly conserved nuclear protein that binds rapidly to SSB and DSB sites in the genome and exhibits PAR catalytic activity (Herceg and Wang., 2001). The binding of PARP-1 to DNA damage sites and its PAR catalytic activity triggers its enzymatic activation. This activation leads to the modification of various nuclear proteins via the covalent attachment of branching PAR chains (Figure 3) (D'Amours et al., 1999). Cells and animals deficient in PARP-1 present marked genomic instability and diminished BER activity, highlighting this enzyme’s critical role in cellular responses to low doses of ionizing radiation (Shall and de Murcia., 2000).
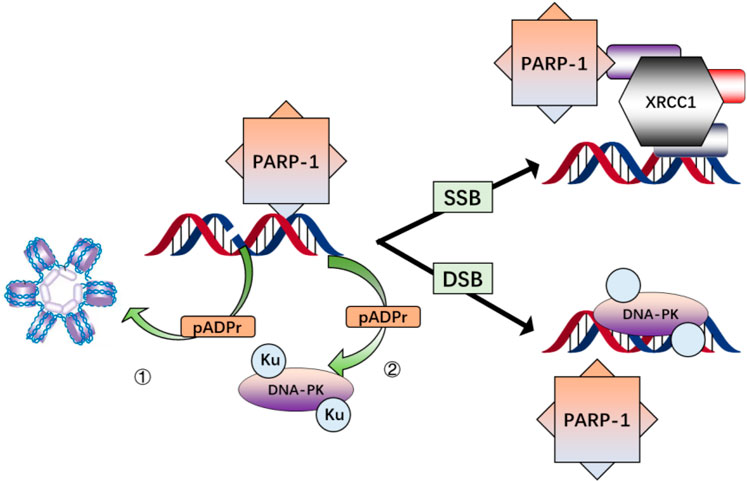
FIGURE 3. Simplified schema illustrating the major interactions of PARP-1 after DNA damage. PARP-1 is activated by binding to single-or double-strand breaks in DNA, resulting in the modification of nuclear proteins via poly(ADP-ribosylation) (pADPr) and direct interaction while histone modification contributes to chromatin relaxation 1); pADPr of DNA-PKcs and physical associations with Ku proteins enhance DNA-PK recruitment and activation 2); pADPr of components of the base excision repair pathway and direct interaction of PARP-1 with XRCC1 facilitates assembly and activation of the base excision repair complex.
4 Interactions between PARP-1 and p53/ROS
ROS and p53 mutually regulate one another in a feedback cycle (Maillet and Pervaiz, 2012), with p53 serving to protect the genome from ROS-induced DNA damage. At baseline conditions, minimal p53 expression is necessary to stimulate the expression of antioxidant genes sufficient for mitigating oxidative stress (Sablina et al., 2005). In addition to regulating DNA damage, apoptotic death, and senescence, p53 can modulate autophagy. Consequently, depletion or inhibition of p53 can promote autophagy in mice, humans, and nematode cells (Lakin and Jackson., 1999; Tavernarakis et al., 2008). PARP does not subject DNA ligase I to PAR modification but regulates DNA repair by altering chromosomal structures to ensure that the damaged sites are accessible to the appropriate repair enzymes (Bhat et al., 2006). Evidence shows that p53 can modulate glioblastoma cell sensitivity to combined TPT, radiotherapy, and PARP inhibitor treatment. Specifically, cells with activated p53 have greater sensitivity to a combination of radiotherapy and PARP inhibitor treatment, while cells with inactive p53 are more sensitive to combinations of TPT and PARP inhibitors (Sabbatino et al., 2014). The combination of radiation and emodin can induce PARP-1 cleavage and the downregulation of epigenetic signaling mediators, including JMJD1A and JMJD2B, thus contributing to apoptotic death. Accordingly, emodin overcame HepG2 cell radioresistance by promoting the upregulation of apoptotic signaling molecules and downregulation of pro-proliferation signaling factors (Hwang et al., 2015). Trans-dominant inhibition of PARP-1, resulting from the overexpression of the PARP-1 DNA-binding domain in MCF-7 cells, can lead to a reduction in p21 induction and G1 cell cycle arrest instigated by p53 following exposure to ionizing radiation. Inhibiting the functionality of endogenous PARP-1 can thus suppress p53 transactivating activity following radiation exposure. PARP-1 is thus a key regulator of p53-mediated responses to DNA damage (Wieler et al., 2003). The p53 status of BRCA1 and HR-proficient tumors can determine the degree to which PARP inhibitor treatment sensitizes them to ionizing radiation, providing an opportunity to identify patients with the greatest chance of benefiting from the combination treatment (Sizemore et al., 2018). Indeed, capitalizing on the mutational status of p53 in human tumors, in conjunction with the inactivation of PARP-1, could potentially render otherwise resistant cells susceptible to radiotherapy.
Various strategies have been used to inhibit PAR synthesis. Studies in cells lacking PARP activity have emphasized the critical role of poly(ADP-ribosyl)ation in regulating DNA repair and DNA metabolism (D'Amours et al., 1999). Investigations using PARP knockout mice revealed beneficial roles of PARP in maintaining genomic integrity and survival after exposure to whole-body γ-radiation. The capacity of PARP to hinder recombination at sites of DNA strand breaks was clearly shown in studies involving PARP and another key DNA strand break-binding protein, the DNA-dependent protein kinase (D'Amours et al., 1999; Le Rhun et al., 1998). Decreases in the covalent PAR modification of p53 also coincided with increases in the expression levels of p53-responsive genes, including Fas and Bax, indicating a possible role for poly-(ADP-ribosyl)ation as a regulator of the function of p53 while highlighting the importance of PARP and PAR modification during the early stages of apoptotic cell death (Prives and Hall., 1999; Simbulan-Rosenthal et al., 1999). The activation of p53 and its upregulation occurs within minutes to hours following DNA damage (Prives and Hall., 1999), while PARP-1 can respond to DNA damage in seconds to minutes (D'Amours et al., 1999), indicating a potential upstream role for poly(ADP-ribosylation) in the regulation of the expression and function of p53 following genotoxic stress exposure.
The simultaneous mutation of both PARP-1 and Wrn results in a more pronounced shift in gene expression profiles than those observed when either gene is mutated in isolation. Moreover, cultured cells with mutations in both genes exhibit extensive dysregulation of signal transduction, cell cycle progression, metabolism, embryonic development, and apoptosis-related genes. In vivo studies using dual-gene mutant mice have demonstrated higher rates of embryonic developmental defects, apoptosis, and elevated levels of oxidative damage and intracellular phosphorylation in adult tissue samples (Deschênes et al., 2005) (Figure 4).
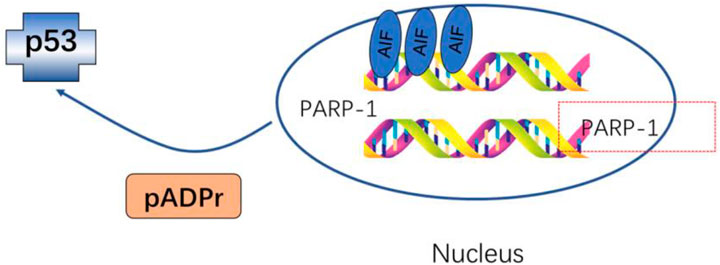
FIGURE 4. pADPr of p53 and transcription factors influence cell cycle progression and reduce overall levels of gene transcription.
Oncogenesis is a complex process driven by exogenous and endogenous stimuli, including endogenous ROS-mediated damage to the deoxyribosyl DNA backbone (Valko et al., 2004; Guo et al., 2016. The p53 protein is capable of regulating ROS-related stress and genomic damage. Correspondingly, the loss of p53 function enhances the radiosensitizing activity of Olaparib in both isogenic and non-isogenic cell line models. This coincides with increased levels of PARP-1 expression in bladder cancer cell lines and associated tumors. The simultaneous loss of p53 and impairment of ataxia-telangiectasia mutated (ATM) activity can produce more robust radiosensitivity (Liu et al., 2018). Investigations into the impact of PARP-1 inhibition on ROS generation and DNA repair post-exposure to ionizing radiation revealed no significant variances in DNA strand breaks between cells treated with PARP inhibitors and those that were untreated after a period of 3 h. However, at 12 and 48 h following irradiation, cells treated with PARP inhibitors displayed markedly higher surges in ROS levels compared to their untreated counterparts (Cieślar-Pobuda et al., 2012). Interfering with PARP-1 activity can also increase ROS levels in ovarian cancer cells, leading to DSB in the DNA. NAC is an antioxidant that inhibits PARP-1-mediated dysregulation of cell proliferation, highlighting a protective role for PARP-1 in augmenting the antioxidant capacity of ovarian cancer cells (Hou, 2015). A combined treatment approach using radiation and flavonoids from Rosa roxburghii Tratt (FRT) can enhance radioresistance. This improvement is mediated by PARP-1-dependent cell death pathways, which involve PARP-1 activation and ROS generation inhibition. The combination of FRT treatment and PARP-1 knockout significantly reduces ROS levels in cells and prevents the mitochondrial translocation of AIF (Figure 5) (Xu et al., 2020).
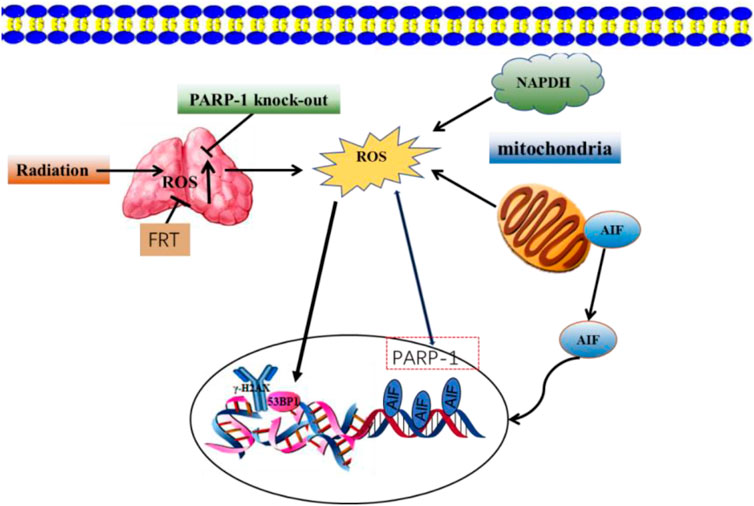
FIGURE 5. FRT protects against radiation damage by regulating the PARP-1/ROS/DNA pathway and by inhibiting the activation of PARP-1/AIF.
5 Interactions between PARP-1 and NF-κB/DNA-PK
5.1 Interactions between PARP-1 and DNA-PK
In vitro and in vivo analyses have demonstrated that the loss or inhibition of PARP-1 function can compromise DNA repair, making tumors more sensitive to chemotherapy and radiotherapy (Huang and Huang, 2006). In nasopharyngeal carcinoma cells, PARP-1 can activate autophagy through the AMPK/mTOR pathway such that interfering with PARP-1 or AMPK activity can suppress autophagic activity and increase CNE-2 nasopharyngeal carcinoma cell sensitivity to radiation treatment (Chen et al., 2015; Chen, 2016). DSBs that develop in the genome following radiation exposure are generally repaired by the NHEJ pathway, which relies on DNA-PK activity. PARP-1, while best known for its role in SSB repair, can also mediate DSB repair. DNA-PK and PARP-1 can promote the rapid repair of DSBs caused by ionizing radiation, and inactivating these enzymes does not yield additive effects, indicating that they cooperate to promote the same DSB repair response pathway (Mitchell et al., 2009). DNA-PK and PARP-1 are also involved in rapid changes in radiosensitivity in cells exposed to short pulses of ionizing radiation. The inactivation of DNA-PK through Wortmannin can intensify the cytotoxicity induced by irradiation without modifying the oscillatory character of early radiation responses. On the contrary, 3-AB mediated inhibition of PARP disrupts this oscillatory response, a disruption also observed in 3T3 fibroblasts with silenced PARP-1 (Fernet et al., 2000). The function of nuclear receptor subfamily 4 group A (NR4A) proteins is to directly target DNA-PK catalytic subunit (DNA-PKcs) that have been poly-ADP-ribosylated, facilitating its autophosphorylation, which promotes DNA-PK kinase assembly at DNA damage sites. Selective targeting of the PAR-binding pocket of NR4A presents an opportunity for cancer therapy (Munnur et al., 2019). Replication forks may be better protected, repaired, and restarted if XRCC1 is recruited in a PARP and DNA-PK-dependent approach (Ying et al., 2016). Using ATM-deficient cells, it was found that the combined use of AZD7648, a DNA-PK inhibitor, and Olaparib, a PARP inhibitor, increased genomic instability in cells lacking ATM, leading to reduced cell growth and the promotion of apoptosis (Fok et al., 2019). Treatment with PARP inhibitors increased the phosphorylation of DNA-PK substrates and stimulated NHEJ in cells lacking HR, leading to potential errors. Notably, inhibition of DNA-PK activity could reverse the genomic instability induced by inhibiting PARP (Patel et al., 2011). The PARP inhibitor Olaparib was observed to induce both phosphorylation and stabilization of p53 in a DNA-PK-dependent manner in mantle cell lymphoma cells lacking ATM and promote the expression of p53-responsive factors responsible for regulating cell-cycle checkpoints. At the same time, Olaparib toxicity could be reduced by inhibiting DNA-PK in these cells (Williamson et al., 2012). Animal investigations indicate that using the PARP inhibitor Olaparib and the DNA-PK inhibitor NU7441 in conjunction with ionizing radiation boosts the suppression of HPV-negative head and neck squamous cell cancer (HNSCC), indicating that this may be a useful and novel approach to treating cases with HPV-negative HNSCC (Zeng et al., 2020).
5.2 Interactions between PARP-1 and NF-κB
The transcription factor NF-κB is an essential regulator of inflammatory signaling activity and oncogenic processes. Constitutive NF-κB expression is seen in tumors, promoting the upregulation of proliferation, invasion, metastasis, and angiogenesis-related genes. Several tumors exhibit altered or elevated basal NF-κB activity levels that coincide with increased resistance to radiotherapy and chemotherapy (Martin-Oliva et al., 2006; Sorriento et al., 2012). Exposure to ionizing radiation can induce complex, multifaceted responses in mammalian cells. These responses include activating pro-survival pathways that lead to the transient activation of specific transcription factors. These factors include NF-κB and members of the signal transducers and activators of the transcription (STAT) family, which serve as core mediators of pro-inflammatory and oncogenic signaling activity. These factors ultimately drive the expression of a diverse range of anti-apoptotic and pro-inflammatory genes that contribute to enhanced cell survival, invasivity, and angiogenesis, rendering tumors more resistant to radiation exposure. The upregulation of inflammatory cytokines, including IL-1β, IL-6, and TNF-α in response to radiation can also cause symptoms such as fatigue, localized inflammation, and pain in patients undergoing cancer treatment (Deorukhkar and Krishnan, 2010). LRP16 can interact with IκB kinase (IKK) and PARP-1, thereby facilitating the lesion-specific recruitment of these factors and the recruitment of PIASy to IKK following DSB formation (Wu et al., 2015). In the ovaries, irradiation can enhance the expression of PARP-1 and NF-κB, thereby promoting the upregulation of inflammatory factor mRNAs, including IL-6, IL-8, and visfatin, together with reduced IL-10 and increased iNOS and COX-2 protein expression. Resveratrol treatment can reportedly restore function to the ovaries by increasing levels of AMH and reducing inflammatory activity through mechanisms that largely center around PPAR-γ and SIRT1 upregulation and the consequent inhibition of NF-κB-induced inflammatory cytokine signaling (Said et al., 2016). Emerging evidence indicates a role of PARP-1 in the activation of NF-kB. Research has shown that the application of PARP-1 inhibitor AG14361 on breast cancer cell lines, which constitutively express NF-kB, successfully suppressed the expression of a luciferase reporter gene activated by ionizing radiation. This observation was mirrored in mouse embryonic fibroblasts, irrespective of their PARP-1 or NF-kB p65 expression. PARP-1 is thus a key mediator of radiation-driven NF-kB activation, with the potentiation of radiation-mediated cytotoxicity by AG14361 treatment resulting from NF-kB inhibition (Veuger et al., 2009). The shRNA-mediated silencing of EWS-FL1 ablated the impact of inhibiting PARP-1 on Ewing sarcoma cell radiation responsivity. Combining 4 Gy radiation with PARP-1 inhibition reduced the growth of SK-N-MC xenograft tumors, demonstrating that inhibiting PARP-1 in Ewing sarcomas can synergistically prolong and exacerbate radiation-induced DNA damage-promoting apoptotic death in an EWS-FLI1-dependent manner (Lee et al., 2013).
Onjisaponin B (OB) is an inhibitor of NF-κB signaling that can protect against endotoxin-induced liver damage (Fu et al., 2016). OB can also prevent the proliferation of osteoclasts and protect against bone loss by downregulating NF-κB (Yang et al., 2015). OB may also control NF-κB signaling through PI3K/AKT pathways, and this action is independent of radiation. However, the mechanism by which OB confers radioprotection to cells by inhibiting p65 remains unclear. Both caspase-3 and NF-κB become activated following radiation exposure. Notably, in a neonatal retinal hypoxia model, NF-κB is reported to promote caspase-3 activation, which contributes to retinal ganglion cell death.
Inhibitors of NF-κB can also suppress caspase-3-dependent apoptotic cell death (Li et al., 2020). Activating NF-κB leads to additional caspase-3 activation, while suppressing NF-κB activity suppresses the activity of caspase-3 (Cao et al., 2013). Given the ability of NF-κB to promote caspase-3 activation, OB may inhibit this activation and thereby shield cells from radiation-induced damage (Wang et al., 2022).
6 Interactions between PARP-1 and Caspase-3/AIF
Caspase-3 is a key effector caspase that triggers apoptotic cell death, partly through its ability to cleave substrate proteins, including PARP-1, DNA fragmentation factor (DFF45), and Lamin B (Boulares et al., 2001). Severe DNA damage and the consequent overactivation of PARP-1 can result in the excessive consumption of NAD+ and ATP energy stores. Caspase-3 activation can help preserve these energy supplies by cleaving the Asp-Glu-Val-Asp sequence in the PARP-1 nuclear localization signal, yielding two PARP-1 fragments (p89 and p24). The PARP-1 DNA binding domain is thus separated from its catalytic domain, inactivating its enzymatic activity. These p89 and p24 fragments can also serve as inhibitors of PARP-1 activity through monomeric poly aggregation and DNA binding, ultimately contributing to internucleosomal DNA degradation and eventual apoptotic death (Li and Darzynkiewicz, 2000). The positive feedback function of caspase-mediated PARP-1 inhibition underscores the critical role PARP-1 plays in apoptotic cell death (Figure 6).
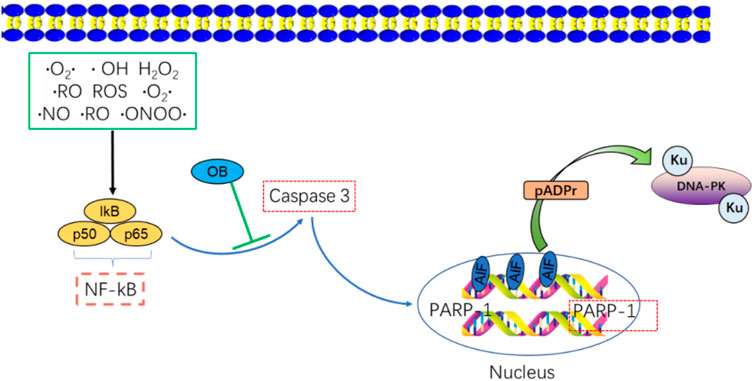
FIGURE 6. NF-κB activates the apoptosis-related molecule, caspase-3, OB inhibits caspase-3 activation by NF-κB/Caspase-3 activation resulting in the cleavage of PARP-1 and increased apoptosis. FRT may inhibit Bcl-2(Ca2+)/Caspase-3/PARP-1 signaling to reduce apoptosis in thymus cells.
Caspase-3 is a key protease that can cleave PARP and associated substrates, thereby facilitating the repair of DNA SSBs (Xu et al., 2016). High radiation levels can contribute to PARP-1 overactivation and consequent AIF release (Yu et al., 2006; Doti et al., 2014). Knocking down ATRX in the H460 lung cancer cell line promoted radiation-induced cell death, potentially through mechanisms linked to this PARP-1/Caspase-3 axis (Wieler et al., 2003). 5-azacyclobutane can enhance the expression of caspase-3 and the cleavage of PARP induced by ionizing radiation, thereby increasing the radiosensitivity of C666-1 nasopharyngeal carcinoma cells (Cai et al., 2017). HMGB1 silencing was associated with higher levels of Caspase-3, PARP, p-p38, and p-JNK proteins compared with radiation alone in vitro, suggesting that HMGB1 knockdown can promote caspase-3 upregulation and the cleavage of PARP. Increases in ROS production can promote DNA damage, increasing cell radiosensitivity (Han, 2016). Severe DNA damage can potentially trigger PARP-1 overactivation, which leads to the depletion of energy reservoirs NAD and ATP, and subsequently induces the translocation of AIF to the nucleus to facilitate caspase-independent apoptosis (Srinivasan, 1997). According to reports, the accumulation of PAR can trigger AIF-dependent cell death upon exposure to carbon ion radiation (Park et al., 2020). Poly (ADP- ribose) glycohydrolase (PARg) is a key enzyme important for PAR degradation, and the effects of PARg deficiencies on the sensitivity of murine embryonic stem (ES) cells to low and high LET radiation have been analyzed in prior reports. Increases in PARg-deficient ES cell sensitivity to γ-radiation are related to defective DSB repair and coincide with sensitivity to many other forms of radiation (Shirai et al., 2013). Combining plant-derived sphingosine and γ-radiation can enhance the death of radioresistant tumor cell lines through the nuclear translocation of AIF in response to ROS-mediated Bax relocation and the activation of PARP-1 independent of ROS. NAC is an antioxidant that can prevent AIF translocation by suppressing Bax relocation, although it does not suppress PARP-1 activation. AIF translocation is also reduced by pretreatment with the PARP-1 inhibitor DPQ(3, 4-dihydro-5)-[4-(1-pilon-butanoxy) -butanoxy]-1(2H)-isopentone or by PARP-1 knockdown (Park et al., 2005).
FRT can significantly suppress the expression of pro-apoptotic factors such as caspase-3/8/9/10 and PARP-1/AIF-related signaling activity in thymus cells following radiation exposure, thereby enhancing radioprotective activity at least in part through the regulation of the PARP-1 and caspase-mediated pathways. FRT can also decrease the mRNA expression of several inflammatory factors, such as ICAM-1, VCAM-1, TNF-α, and NF-κB, without impacting the expression levels of IL-6 or IL-1α. It can also reduce the protein levels of ICAM-1, IL-1α, IL-6, TNF-α, and NF-κB after radiation exposure without affecting those of VCAM-1. As such, FRT may exert radioprotective efficacy by targeting these inflammatory pathways to reduce the inflammatory response (Figure 7) (Xu et al., 2018).
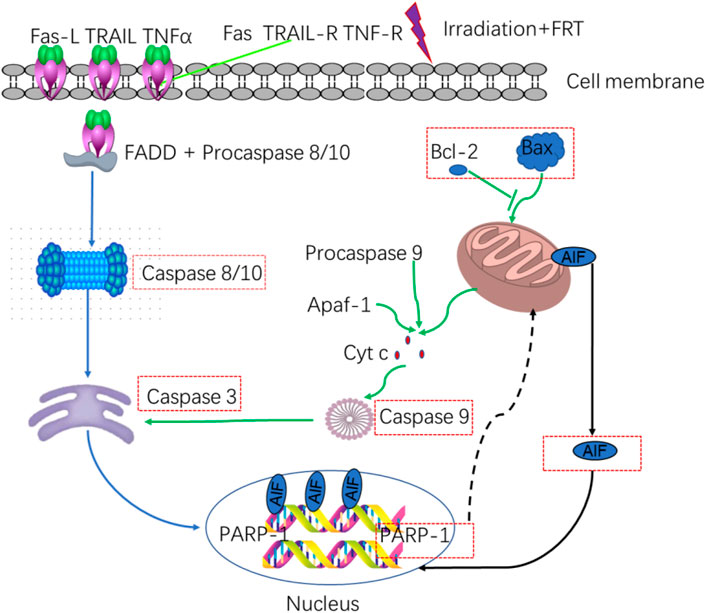
FIGURE 7. Relationship between apoptotic and inflammatory biomarkers. Biomarkers of apoptosis: caspases 3/8–10 and PARP-1/AIF.
In summary, efforts to protect individuals from the damage associated with ionizing radiation exposure and to promote recovery following irradiation remain an area of active research interest. PARP-1 is a critical regulator of genomic integrity that facilitates DNA damage repair. Extensive DNA damage can result in PARP-1 overactivation, which can deplete cellular energy stores, culminating in cell death. Identifying mutations in genes involved in DNA repair may help guide the selection of appropriate candidates for PARP inhibitor treatment, informing clinicians as to whether these inhibitors should be provided as single-agent therapies or components of combination therapeutic regimens also incorporating inhibitors targeting other components of the DNA repair pathway, radiotherapy, or DNA damaging drugs. While many studies have explored PARP-1 as a target for radiosensitization in cancer treatment, considerably fewer have investigated FRT on normal healthy tissue. PARP-1 can function as a key modulator of cell fate and survival through its ability to control the activity of NF-κB, p53, and caspase-3/AIF (Figure 3). Although considerable progress has been made in understanding the relationship between PARP-1 and radiation sensitivity and in developing several PARP-1 inhibitors, the precise mechanisms connecting PARP-1 and radioprotection remain to be fully clarified. Therefore, further investigations are required to inform the design and selection of suitable radioprotective agents interacting with this pathway.
7 Opportunities and challenges of PARP inhibitor
Since its discovery, PARP-1 has been extensively characterized as a regulator of numerous biologically important pathways. PARP inhibitors provide new therapeutic opportunities to cancer patients, leading to their approval as single-agent treatments for certain cancer types. While the next-generation of these small-molecule inhibitors is in active development, the current versions continue to be applied as radiosensitizing drugs in clinical trials in combination with radiotherapy and chemotherapy. Further advancement in PARP inhibitor research could fully underscore the value of targeting this pathway for treating various diseases, thus providing novel therapeutic options to numerous patients, including those currently without effective treatments.
(1) Determining an optimal low-intensity dose regimen
While early experiments showed that single doses of Olaparib, Rucaparib, Niraparib, and Talazoparib were the maximum doses tolerated, it is unclear whether the maximum tolerated dose is appropriate for a tumor-selective drug that is not expected to damage normal tissues. Therefore, opting for the optimal biological dose instead of the maximum tolerated dose might be preferable to improve patients’ quality of life. Further research is needed to determine whether the low-intensity dose regimen, which may be less toxic and more affordable, is as effective as the approved single-dose regimen.
Toxicity issues and the need for meticulous titration of the cytotoxic agents and PARP inhibitors still constrain the combined use of PARP inhibitors with genotoxic chemotherapy and radiotherapy. A well-designed dose plan will be necessary to optimize the therapeutic index, which may have to be considered on an individual basis. The preclinical and clinical data show that when PARP inhibitors are combined with cytotoxic drugs, curative effects, and tolerance can be achieved with shorter treatment courses and lower dosages. We posit that it is necessary to reclassify PARP inhibitors based on their dual molecular mechanisms to accurately evaluate suitable combination strategies (Murai et al., 2012; Murai et al., 2014; Fojo and Bates, 2013).
(2) Identifying predictive biomarkers
A crucial objective, alongside determining the optimum tolerance for combined PARP inhibitor therapy, is the discovery of predictive biomarkers for patient segregation in conjunction with the therapy and understanding the impact of this combination on the resistance mechanisms against PARP inhibitors to achieve a more sustained clinical response. A critical query is whether a combined treatment regimen could alleviate the resistance to PARP inhibitor monotherapy seen in patients with BRCA mutations. It remains uncertain how much the combined administration of PARP inhibitors and other drugs can delay or prevent the onset of PARP inhibitor resistance in these patients and potentially lead to longer-lasting responses. Furthermore, the sequence and timing of administering the therapeutic agents demand careful consideration.
(3) Understanding the clinical mechanisms that drive resistance to PARP inhibitors in BRCA-mutant cancers
The prospect of mitigating resistance to PARP inhibitor monotherapy in patients with BRCA mutations through combination therapy presents an intriguing question. It is unclear whether the simultaneous use of PARP inhibitors and auxiliary agents could impede or prevent the emergence of resistance to PARP inhibitors in these patients, potentially resulting in more enduring responses. Furthermore, the arrangement and timing of administering these therapeutic agents call for meticulous planning and examination.
The presence of BRCA mutations does not universally predict the sensitivity of tumors to PARP inhibitors. In fact, PARP inhibitors display a spectrum of sensitivity in BRCA-mutant cancers. For instance, ovarian cancers with BRCA mutations show greater sensitivity than breast, prostate, or pancreatic cancers with the same mutations. Furthermore, cancers with BRCA mutations that are usually unrelated to BRCA carriers are even less sensitive to PARP inhibitors (Curtin et al., 2019; Jonsson et al., 2019). Genome screening techniques, like Myriad myChoice, may help identify tumors with defective homologous recombination but might produce false positives. These methods also detect genomic changes resulting from diminished HRR, which can persist even after HRR recovery (a phenomenon known as genomic scarring). This could result in failing to identify tumors where the HRD phenotype has been reversed and are resistant to PARP inhibitors. The most reliable predictive biomarker of HRD may be functional, such as the ability of tumor cells to form RAD51 lesions, which has been used to show that approximately one-third of abdominal tumors and high-frequency lung tumors contain homologous recombination defects (Patterson et al., 2014; Gentles et al., 2019).
(4) Leveraging biological insights into the response to PARP inhibitors to guide the design of combination therapy
Enhancing our understanding of the biological responses to PARP inhibitors could significantly contribute to designing effective combination therapies involving PARP inhibitors. Given that PARP inhibitors trigger extreme genomic instability, resulting in cancer cell death, one potential strategy could involve leveraging this phenotype. This could be achieved by pairing PARP inhibitors with agents that amplify cell death processes, such as apoptosis or necrosis, in the context of enhanced genomic instability (Yuan et al., 2013).
The research conducted over the past few decades indicates that the combined use of PARPi holds immense promise for cancer treatment, provided several significant hurdles can be overcome.
Author contributions
W-HL: writing, reviewing and editing; FW: data curation, writing, original draft preparation; G-YS: validation, writing; Q-HY and R-PD: consult the literature and summarize; PX: supervision. All authors contributed to the article and approved the submitted version.
Funding
The Natural Science Foundation of China (NSFC) provided financial support for this investigation (Nos 81773358, 11705158, and U1504824).
Conflict of interest
The authors declare that the research was conducted in the absence of any commercial or financial relationships that could be construed as a potential conflict of interest.
Publisher’s note
All claims expressed in this article are solely those of the authors and do not necessarily represent those of their affiliated organizations, or those of the publisher, the editors and the reviewers. Any product that may be evaluated in this article, or claim that may be made by its manufacturer, is not guaranteed or endorsed by the publisher.
References
Aguilar-Quesada, R., Muñoz-Gámez, J. A., Martín-Oliva, D., Peralta-Leal, A., Quiles-Pérez, R., Rodríguez-Vargas, J. M., et al. (2007). Modulation of transcription by PARP-1: Consequences in carcinogenesis and inflammation. Curr. Med. Chem. 14, 1179–1187. doi:10.2174/092986707780597998
Ahel, I., Ahel, D., Matsusaka, T., Clark, A. J., Pines, J., Boulton, S. J., et al. (2008). Poly(ADP-ribose)-binding zinc finger motifs in DNA repair/checkpoint proteins. Nature 451, 81–85. doi:10.1038/nature06420
Alano, C. C., Ying, W., and Swanson, R. A. (2004). Poly(ADP-ribose) polymerase-1-mediated cell death in astrocytes requires NAD+ depletion and mitochondrial permeability transition. J. Biol. Chem. 279, 18895–18902. doi:10.1074/jbc.M313329200
Alhosaini, K., Ansari, M. A., Nadeem, A., Bakheet, S. A., Attia, S. M., Alhazzani, K., et al. (2021). 5-Aminoisoquinolinone, a PARP-1 inhibitor, ameliorates immune abnormalities through upregulation of anti-inflammatory and downregulation of inflammatory parameters in T cells of BTBR mouse model of autism. Brain Sci. 11, 249. doi:10.3390/brainsci11020249
Anwar, M., Aslam, H. M., and Anwar, S. (2015). PARP inhibitors. Hered. Cancer Clin. Pract. 13, 4. doi:10.1186/s13053-014-0024-8
Barth, E., Radermacher, P., and Szabó, C. (2006). The world according to poly(ADP-ribose) polymerase (PARP)--update 2006. Intensive Care Med. 32, 1470–1474. doi:10.1007/s00134-006-0336-x
Berti, M., Ray Chaudhuri, A., Thangavel, S., Gomathinayagam, S., Kenig, S., Vujanovic, M., et al. (2013). Human RECQ1 promotes restart of replication forks reversed by DNA topoisomerase I inhibition. Nat. Struct. Mol. Biol. 20, 347–354. doi:10.1038/nsmb.2501
Bhat, K. R., Benton, B. J., and Ray, R. (2006). Poly (ADP-ribose) polymerase (PARP) is essential for sulfur mustard-induced DNA damage repair, but has no role in DNA ligase activation. J. Appl. Toxicol. 26, 452–457. doi:10.1002/jat.1161
Bi, Y., Verginadis, I. I., Dey, S., Lin, L., Guo, L., Zheng, Y., et al. (2018). Radiosensitization by the PARP inhibitor olaparib in BRCA1-proficient and deficient high-grade serous ovarian carcinomas. Gynecol. Oncol. 150 (3), 534–544. doi:10.1016/j.ygyno.2018.07.002
Bochum, S., Berger, S., and Martens, U. M. (2018). Olaparib. Recent Results Cancer Res. 211, 217–233. doi:10.1007/978-3-319-91442-8_15
Boulares, A. H., Zoltoski, A. J., Yakovlev, A., Xu, M., and Smulson, M. E. (2001). Roles of DNA fragmentation factor and poly(ADP-ribose) polymerase in an amplification phase of tumor necrosis factor-induced apoptosis. J. Biol. Chem. 276, 38185–38192. doi:10.1074/jbc.M100629200
Bridges, K. A., Toniatti, C., Buser, C. A., Liu, H., Buchholz, T. A., and Meyn, R. E. (2014). Niraparib (MK-4827), a novel poly(ADP-Ribose) polymerase inhibitor, radiosensitizes human lung and breast cancer cells. Oncotarget 5, 5076–5086. doi:10.18632/oncotarget.2083
Brock, W. A., Milas, L., Bergh, S., Lo, R., Szabó, C., and Mason, K. A. (2004). Radiosensitization of human and rodent cell lines by INO-1001, a novel inhibitor of poly(ADP-ribose) polymerase. Cancer Lett. 205, 155–160. doi:10.1016/j.canlet.2003.10.029
Bryant, H. E., Petermann, E., Schultz, N., Jemth, A. S., Loseva, O., Issaeva, N., et al. (2009). PARP is activated at stalled forks to mediate Mre11-dependent replication restart and recombination. EMBO J. 28, 2601–2615. doi:10.1038/emboj.2009.206
Cai, R., Chen, Q. Q., and Jiang, W. (2017). Experiment of 5-azacytidine increasing radiosensitivity of nasopharyngeal carcinoma cell line C666-1. Cancer Prev. Res. (Phila) 44, 94–97.
Calabrese, C. R., Almassy, R., Barton, S., Batey, M. A., Calvert, A. H., Canan-Koch, S., et al. (2004). Anticancer chemosensitization and radiosensitization by the novel poly(ADP-ribose) polymerase-1 inhibitor AG14361. J. Natl. Cancer Inst. 96 (1), 56–67. doi:10.1093/jnci/djh005
Cao, Z. H., Yin, W. D., Zheng, Q. Y., Feng, S. L., Xu, G. L., and Zhang, K. Q. (2013). Caspase-3 is involved in IFN-γ- and TNF-α-mediated MIN6 cells apoptosis via NF-κB/Bcl-2 pathway. Cell biochem. Biophys. 67, 1239–1248. doi:10.1007/s12013-013-9642-4
Ceccaldi, R., Liu, J. C., Amunugama, R., Hajdu, I., Primack, B., Petalcorin, M. I., et al. (2015). Homologous-recombination-deficient tumours are dependent on Polθ-mediated repair. Nature 518, 258–262. doi:10.1038/nature14184
Chalmers, A., Johnston, P., Woodcock, M., Joiner, M., and Marples, B. (2004). PARP-1, PARP-2, and the cellular response to low doses of ionizing radiation. Int. J. Radiat. Oncol. Biol. Phys. 58, 410–419. doi:10.1016/j.ijrobp.2003.09.053
Chen, J. K., Lin, W. L., Chen, Z., and Liu, H. W. (2018). PARP-1-dependent recruitment of cold-inducible RNA-binding protein promotes double-strand break repair and genome stability. Proc. Natl. Acad. Sci. U. S. A. 115, E1759–E1768. doi:10.1073/pnas.1713912115
Chen, Z. T. (2016). The relationship between PARP-1 and LKB1-AMPK-mTOR pathway in autophagy of nasopharyngeal carcinoma cells induced by radiation and the effect of interfering with this pathway on radioresistance. Nanning, Qingxiu: Guangxi Medical University.
Chen, Z. T., Zhao, W., Qu, S., Li, L., Lu, X. D., Su, F., et al. (2015). PARP-1 promotes autophagy via the AMPK/mTOR pathway in CNE-2 human nasopharyngeal carcinoma cells following ionizing radiation, while inhibition of autophagy contributes to the radiation sensitization of CNE-2 cells. Mol. Med. Rep. 12, 1868–1876. doi:10.3892/mmr.2015.3604
Cheng, G. H., Wu, N., Jiang, D. F., Zhao, H. G., Zhang, Q., Wang, J. F., et al. (2010). Increased levels of p53 and PARP-1 in EL-4 cells probably related with the immune adaptive response induced by low dose ionizing radiation in vitro. Biomed. Environ. Sci. 23, 487–495. doi:10.1016/S0895-3988(11)60012-3
Cheng, Q., Barboule, N., Frit, P., Gomez, D., Bombarde, O., Couderc, B., et al. (2011). Ku counteracts mobilization of PARP1 and MRN in chromatin damaged with DNA double-strand breaks. Nucleic Acids Res. 39, 9605–9619. doi:10.1093/nar/gkr656
Chiarugi, A. (2002). Poly(ADP-ribose) polymerase: Killer or conspirator? The 'suicide hypothesis' revisited. Trends Pharmacol. Sci. 23, 122–129. doi:10.1016/S0165-6147(00)01902-7
Chiu, L. Y., Wu, N. L., Hung, C. F., Bai, P., Dai, Y. S., and Lin, W. W. (2021). PARP-1 involves in UVB-induced inflammatory response in keratinocytes and skin injury via regulation of ROS-dependent EGFR transactivation and p38 signaling. FASEB J. 35, e21393. doi:10.1096/fj.202002285RR
Cieślar-Pobuda, A., Saenko, Y., and Rzeszowska-Wolny, J. (2012). PARP-1 inhibition induces a late increase in the level of reactive oxygen species in cells after ionizing radiation. Mutat. Res. 732, 9–15. doi:10.1016/j.mrfmmm.2012.01.005
Cole, K. K., and Perez-Polo, J. R. (2002). Poly(ADP-ribose) polymerase inhibition prevents both apoptotic-like delayed neuronal death and necrosis after H(2)O(2) injury. J. Neurochem. 82, 19–29. doi:10.1046/j.1471-4159.2002.00935.x
Coleman, R. L., Fleming, G. F., Brady, M. F., Swisher, E. M., Steffensen, K. D., Friedlander, M., et al. (2019). Veliparib with first-line chemotherapy and as maintenance therapy in ovarian cancer. N. Engl. J. Med. 381, 2403–2415. doi:10.1056/NEJMoa1909707
Coleman, R. L., Oza, A. M., Lorusso, D., Aghajanian, C., Oaknin, A., Dean, A., et al. (2017). Rucaparib maintenance treatment for recurrent ovarian carci-noma after response to platinum therapy (ARIEL3): A randomised, double-blind, placebo-controlled, phase 3 trial. Lancet 390, 1949–1961. doi:10.1016/S0140-6736(17)32440-6
Coleman, R. L., Sill, M. W., Bell-McGuinn, K., Aghajanian, C., Gray, H. J., Tewari, K. S., et al. (2015). A phase II evaluation of the potent, highly selective PARP inhibitor veliparib in the treatment of persistent or recurrent epithelial ovarian, fallopian tube, or primary peritoneal cancer in patients who carry a germline BRCA1 or BRCA2 mutation—An NRG oncology/gynecologic oncology group study. Gynecol. Oncol. 137, 386–391. doi:10.1016/j.ygyno.2015.03.042
Curtin, N. J. (2012). DNA repair dysregulation from cancer driver to therapeutic target. Nat. Rev. Cancer. 12, 801–817. doi:10.1038/nrc3399
Curtin, N. J., Drew, Y., and Sharma-Saha, S. (2019). Why BRCA mutations are not tumour-agnostic biomarkers for PARP inhibitor therapy. Nat. Rev. Clin. Oncol. 16, 725–726. doi:10.1038/s41571-019-0285-2
D'Amours, D., Desnoyers, S., D'Silva, I., and Poirier, G. G. (1999). Poly(ADP-ribosyl)ation reactions in the regulation of nuclear functions. Biochem. J. 342, 249–268. doi:10.1042/0264-6021:3420249
Dal Piaz, F., Ferro, P., Vassallo, A., Vasaturo, M., Forte, G., Chini, M. G., et al. (2015). Identification and mechanism of action analysis of the new PARP-1 inhibitor 2″-hydroxygenkwanol A. Biochim. Biophys. Acta. 1850, 1806–1814. doi:10.1016/j.bbagen.2015.05.014
Dallavalle, S., Musso, L., Artali, R., Aviñó, A., Scaglioni, L., Eritja, R., et al. (2021). G-quadruplex binding properties of a potent PARP-1 inhibitor derived from 7-azaindole-1-carboxamide. Sci. Rep. 11, 3869. doi:10.1038/s41598-021-83474-9
Dantzer, F., de La Rubia, G., Ménissier-De Murcia, J., Hostomsky, Z., de Murcia, G., and Schreiber, V. (2000). Base excision repair is impaired in mammalian cells lacking Poly(ADP-ribose) polymerase-1. Biochem. 39, 7559–7569. doi:10.1021/bi0003442
de Bono, J., Ramanathan, R. K., Mina, L., Chugh, R., Glaspy, J., Rafii, S., et al. (2017). Phase I, dose-Escalation, two-part trial of the PARP inhibitor talazoparib in patients with advanced germline BRCA1/2 mutations and selected sporadic cancers. Cancer Discov. 7, 620–629. doi:10.1158/2159-8290.CD-16-1250
De Soto, J. A., and Deng, C. X. (2006). PARP-1 inhibitors: Are they the long-sought genetically specific drugs for BRCA1/2-associated breast cancers? Int. J. Med. Sci. 3, 117–123. doi:10.7150/ijms.3.117
Demin, A. A., Hirota, K., Tsuda, M., Adamowicz, M., Hailstone, R., Brazina, J., et al. (2021). XRCC1 prevents toxic PARP1 trapping during DNA base excision repair. Mol. Cell. 81, 3018–3030. doi:10.1016/j.molcel.2021.05.009
Deorukhkar, A., and Krishnan, S. (2010). Targeting inflammatory pathways for tumor radiosensitization. Biochem. Pharmacol. 80, 1904–1914. doi:10.1016/j.bcp.2010.06.039
Deschênes, F., Massip, L., Garand, C., and Lebel, M. (2005). In vivo misregulation of genes involved in apoptosis, development and oxidative stress in mice lacking both functional Werner syndrome protein and poly(ADP-ribose) polymerase-1. Hum. Mol. Genet. 14, 3293–3308. doi:10.1093/hmg/ddi362
Donawho, C. K., Luo, Y., Luo, Y., Penning, T. D., Bauch, J. L., Bouska, J. J., et al. (2007). ABT-888, an orally active poly(ADP-ribose) polymerase inhibitor that potentiates DNA-damaging agents in preclinical tumor models. Clin. Cancer Res. 13, 2728–2737. doi:10.1158/1078-0432.CCR-06-3039
Doroshow, J. H. (2006). Redox modulation of chemotherapy-induced tumor cell killing and normal tissue toxicity. J. Natl. Cancer Inst. 98, 223–225. doi:10.1093/jnci/djj065
Doti, N., Reuther, C., Scognamiglio, P. L., Dolga, A. M., Plesnila, N., Ruvo, M., et al. (2014). Inhibition of the AIF/CypA complex protects against intrinsic death pathways induced by oxidative stress. Cell Death Dis. 5, e993. doi:10.1038/cddis.2013.518
Du, X., Matsumura, T., Edelstein, D., Rossetti, L., Zsengellér, Z., Szabó, C., et al. (2003). Inhibition of GAPDH activity by poly(ADP-ribose) polymerase activates three major pathways of hyperglycemic damage in endothelial cells. J. Clin. Invest. 112, 1049–1057. doi:10.1172/JCI18127
Dutta, A., Eckelmann, B., Adhikari, S., Ahmed, K. M., Sengupta, S., Pandey, A., et al. (2017). Microhomology-mediated end joining is activated in irradiated human cells due to phosphorylation-dependent formation of the XRCC1 repair complex. Nucleic Acids Res. 45, 2585–2599. doi:10.1093/nar/gkw1262
El-Hamoly, T., El-Denshary, E. S., Saad, S. M., and El-Ghazaly, M. A. (2015). 3-aminobenzamide, a poly (ADP ribose) polymerase inhibitor, enhances wound healing in whole body gamma irradiated model. Wound Repair Regen. 23, 672–684. doi:10.1111/wrr.12330
El-Khamisy, S. F., Masutani, M., Suzuki, H., and Caldecott, K. W. (2003). A requirement for PARP-1 for the assembly or stability of XRCC1 nuclear foci at sites of oxidative DNA damage. Nucleic Acids Res. 31, 5526–5533. doi:10.1093/nar/gkg761
Eltze, T., Boer, R., Wagner, T., Weinbrenner, S., McDonald, M. C., Thiemermann, C., et al. (2008). Imidazoquinolinone, imidazopyridine, and isoquinolindione derivatives as novel and potent inhibitors of the poly(ADP-ribose) polymerase (PARP): A comparison with standard PARP inhibitors. Mol. Pharmacol. 74, 1587–1598. doi:10.1124/mol.108.048751
Eustermann, S., Brockmann, C., Mehrotra, P. V., Yang, J. C., Loakes, D., West, S. C., et al. (2010). Solution structures of the two PBZ domains from human APLF and their interaction with poly(ADP-ribose). Nat. Struct. Mol. Biol. 17, 241–243. doi:10.1038/nsmb.1747
Fatima, S., Jatavath, M. B., Bathini, R., Sivan, S. K., and Manga, V. (2014). Multiple receptor conformation docking, dock pose clustering and 3D QSAR studies on human poly(ADP-ribose) polymerase-1 (PARP-1) inhibitors. J. Recept. Signal Transduct. Res. 34, 417–430. doi:10.3109/10799893.2014.917323
Fattah, F., Lee, E. H., Weisensel, N., Wang, Y., Lichter, N., and Hendrickson, E. A. (2010). Ku regulates the non-homologous end joining pathway choice of DNA double-strand break repair in human somatic cells. PLoS Genet. 6, e1000855. doi:10.1371/journal.pgen.1000855
Fernet, M., Ponette, V., Deniaud-Alexandre, E., Ménissier-De Murcia, J., De Murcia, G., Giocanti, N., et al. (2000). Poly(ADP-ribose) polymerase, a major determinant of early cell response to ionizing radiation. Int. J. Radiat. Biol. 76, 1621–1629. doi:10.1080/09553000050201118
Fisher, A. E., Hochegger, H., Takeda, S., and Caldecott, K. W. (2007). Poly(ADP-ribose) polymerase 1 accelerates single-strand break repair in concert with poly(ADP-ribose) glycohydrolase. Mol. Cell Biol. 27, 5597–5605. doi:10.1128/MCB.02248-06
Fojo, T., and Bates, S. (2013). Mechanisms of resistance to PARP inhibitors-three and counting. Cancer Discov. 3 (1), 20–23. doi:10.1158/2159-8290.CD-12-0514
Fok, J. H. L., Ramos-Montoya, A., Vazquez-Chantada, M., Wijnhoven, P. W. G., Follia, V., James, N., et al. (2019). AZD7648 is a potent and selective DNA-PK inhibitor that enhances radiation, chemotherapy and olaparib activity. Nat. Commun. 10, 5065. doi:10.1038/s41467-019-12836-9
Friedlander, M., Meniawy, T., Markman, B., Mileshkin, L., Harnett, P., Millward, M., et al. (2019). Pamiparib in combination with tislelizumab in patients with advanced solid tumours: Results from the dose-escalation stage of a multicentre, open-label, phase 1a/b trial. Lancet Oncol. 20, 1306–1315. doi:10.1016/S1470-2045(19)30396-1
Fu, H., Hu, Z., Di, X., Zhang, Q., Zhou, R., and Du, H. (2016). Tenuigenin exhibits protective effects against LPS-induced acute kidney injury via inhibiting TLR4/NF-κB signaling pathway. Eur. J. Pharmacol. 791, 229–234. doi:10.1016/j.ejphar.2016.08.013
Gamsjaeger, R., Liew, C. K., Loughlin, F. E., Crossley, M., and Mackay, J. P. (2007). Sticky fingers: Zinc-fingers as protein-recognition motifs. Trends biochem. Sci. 32, 63–70. doi:10.1016/j.tibs.2006.12.007
Garcia Soriano, F., Virág, L., Jagtap, P., Szabó, E., Mabley, J. G., Liaudet, L., et al. (2001). Diabetic endothelial dysfunction: The role of poly(ADP-ribose) polymerase activation. Nat. Med. 7, 108–113. doi:10.1038/83241
Garon, E. B., and Dubinett, S. M. (2010). Poly(ADP-ribose) polymerase inhibitors. J. Thorac. Oncol. 5 (12), S455–S456. doi:10.1097/01.JTO.0000391363.10517.56
Gentles, L., Goranov, B., Matheson, E., Herriott, A., Kaufmann, A., Hall, S., et al. (2019). Exploring the frequency of homologous recombination DNA repair dysfunction in multiple cancer types. Cancers 11 (3), 354. doi:10.3390/cancers11030354
George, R. R., Thomas, R., Davice, A., and Mathew, M. S. (2022). Veliparib for the treatment of solid malignancies. J. Oncol. Pharm. Pract. 28, 924–934. doi:10.1177/10781552221073990
Godon, C., Cordelières, F. P., Biard, D., Giocanti, N., Mégnin-Chanet, F., Hall, J., et al. (2008). PARP inhibition versus PARP-1 silencing: Different outcomes in terms of single-strand break repair and radiation susceptibility. Nucleic Acids Res. 36, 4454–4464. doi:10.1093/nar/gkn403
Guillot, C., Favaudon, V., Herceg, Z., Sagne, C., Sauvaigo, S., Merle, P., et al. (2014). PARP inhibition and the radiosensitizing effects of the PARP inhibitor ABT-888 in in vitro hepatocellular carcinoma models. BMC Cancer 14, 603. doi:10.1186/1471-2407-14-603
Guo, C., Zhang, F., Wu, X., Yu, X., Wu, X., Shi, D., et al. (2020). BTH-8, a novel poly (ADP-ribose) polymerase-1 (PARP-1) inhibitor, causes DNA double-strand breaks and exhibits anticancer activities in vitro and in vivo. Int. J. Biol. Macromol. 150, 238–245. doi:10.1016/j.ijbiomac.2020.02.069
Guo, X., Li, Q., Shi, J., Shi, L., Li, B., Xu, A., et al. (2016). Perfluorooctane sulfonate exposure causes gonadal developmental toxicity in Caenorhabditis elegans through ROS-induced DNA damage. Chemosphere 155, 115–126. doi:10.1016/j.chemosphere.2016.04.046
Haikarainen, T., Narwal, M., Joensuu, P., and Lehtiö, L. (2013). Evaluation and structural basis for the inhibition of tankyrases by PARP inhibitors. ACS Med. Chem. Lett. 5 (1), 18–22. doi:10.1021/ml400292s
Haince, J. F., McDonald, D., Rodrigue, A., Déry, U., Masson, J. Y., Hendzel, M. J., et al. (2008). PARP1-dependent kinetics of recruitment of MRE11 and NBS1 proteins to multiple DNA damage sites. J. Biol. Chem. 283, 1197–1208. doi:10.1074/jbc.M706734200
Haince, J. F., Rouleau, M., Hendzel, M. J., Masson, J. Y., and Poirier, G. G. (2005). Targeting poly(ADP-ribosyl)ation: A promising approach in cancer therapy. Trends Mol. Med. 11, 456–463. doi:10.1016/j.molmed.2005.08.003
Han, G. H. (2016). Effect of down-regulation of HMGB1 expression on radiosensitivity of esophageal squamous cell carcinoma TE-1 cells and its mechanism. Zhenjiang, Jingkou: jiangsu university.
Hauser, B., Gröger, M., Ehrmann, U., Albicini, M., Brückner, U. B., Schelzig, H., et al. (2006). The parp-1 inhibitor ino-1001 facilitates hemodynamic stabilization without affecting DNA repair in porcine thoracic aortic cross-clamping-induced ischemia/reperfusion. Shock 25, 633–640. doi:10.1097/01.shk.0000209561.61951.2e
Herceg, Z., and Wang, Z. Q. (2001). Functions of poly(ADP-ribose) polymerase (PARP) in DNA repair, genomic integrity and cell death. Mutat. Res. 477, 97–110. doi:10.1016/s0027-5107(01)00111-7
Hochegger, H., Dejsuphong, D., Fukushima, T., Morrison, C., Sonoda, E., Schreiber, V., et al. (2006). Parp-1 protects homologous recombination from interference by Ku and Ligase IV in vertebrate cells. EMBO J. 25, 1305–1314. doi:10.1038/sj.emboj.7601015
Hocsak, E., Szabo, V., Kalman, N., Antus, C., Cseh, A., Sumegi, K., et al. (2017). PARP inhibition protects mitochondria and reduces ROS production via PARP-1-ATF4-MKP-1-MAPK retrograde pathway. Free Radic. Biol. Med. 108, 770–784. doi:10.1016/j.freeradbiomed.2017.04.018
Hong, S. J., Dawson, T. M., and Dawson, V. L. (2004). Nuclear and mitochondrial conversations in cell death: PARP-1 and AIF signaling. Trends Pharmacol. Sci. 25, 259–264. doi:10.1016/j.tips.2004.03.005
Hou, D. (2015). Expression of PARP1 in ovarian cancer and its antioxidant effect. Jinan, Licheng: Shandong University.
Hu, Y., Petit, S. A., Ficarro, S. B., Toomire, K. J., Xie, A., Lim, E., et al. (2014). PARP1-driven poly-ADP-ribosylation regulates BRCA1 function in homologous recombination-mediated DNA repair. Cancer Discov. 4, 1430–1447. doi:10.1158/2159-8290.CD-13-0891
Huang, S. H., and Huang, Z. Y. (2006). PARP-1: A new target for tumor therapy. Shi Jie Hua Ren. Xiao Hua Za Zhi 14, 841–847.
Hunter, J. E., Willmore, E., Irving, J. A., Hostomsky, Z., Veuger, S. J., and Durkacz, B. W. (2012). NF-κB mediates radio-sensitization by the PARP-1 inhibitor, AG-014699. Oncogene 31, 251–264. doi:10.1038/onc.2011.229
Hwang, S. Y., Heo, K., Kim, J. S., Im, J. W., Lee, S. M., Cho, M., et al. (2015). Emodin attenuates radioresistance induced by hypoxia in HepG2 cells via the enhancement of PARP1 cleavage and inhibition of JMJD2B. Oncol. Rep. 33, 1691–1698. doi:10.3892/or.2015.3744
Iwashita, A., Tojo, N., Matsuura, S., Yamazaki, S., Kamijo, K., Ishida, J., et al. (2004). A novel and potent poly(ADP-ribose) polymerase-1 inhibitor, FR247304 (5-chloro-2-[3-(4-phenyl-3,6-dihydro-1(2H)-pyridinyl)propyl]-4(3H)-quinazolinone), attenuates neuronal damage in in vitro and in vivo models of cerebral ischemia. J. Pharmacol. Exp. Ther. 310, 425–436. doi:10.1124/jpet.104.066944
Jacewicz, M., Czapski, G. A., Katkowska, I., and Strosznajder, R. P. (2009). Systemic administration of lipopolysaccharide impairs glutathione redox state and object recognition in male mice. The effect of PARP-1 inhibitor. Folia Neuropathol. 47, 321–328.
Jagsi, R., Griffith, K. A., Bellon, J. R., Woodward, W. A., Horton, J. K., Ho, A., et al. (2018). Concurrent veliparib with chest wall and nodal radiotherapy in patients with inflammatory or locoregionally recurrent breast cancer: The TBCRC 024 phase I multicenter study. J. Clin. Oncol. 36 (13), 1317–1322. doi:10.1200/JCO.2017.77.2665
Johannes, J. W., Balazs, A., Barratt, D., Bista, M., Chuba, M. D., Cosulich, S., et al. (2021). Discovery of 5-{4-[(7-Ethyl-6-oxo-5,6-dihydro-1,5-naphthyridin-3-yl)methyl]piperazin-1-yl}-N-methylpyridine-2-carboxamide (AZD5305): A PARP1-DNA trapper with high selectivity for PARP1 over PARP2 and other PARPs. J. Med. Chem. 64, 14498–14512. doi:10.1021/acs.jmedchem.1c01012
Jones, P., Altamura, S., Boueres, J., Ferrigno, F., Fonsi, M., Giomini, C., et al. (2009). Discovery of 2-{4-[(3S)-piperidin-3-yl]phenyl}-2H-indazole-7-carboxamide (MK-4827): A novel oral poly(ADP-ribose)polymerase (PARP) inhibitor efficacious in BRCA-1 and -2 mutant tumors. J. Med. Chem. 52, 7170–7185. doi:10.1021/jm901188v
Jonsson, P., Bandlamudi, C., Cheng, M. L., Srinivasan, P., Chavan, S. S., Friedman, N. D., et al. (2019). Tumour lineage shapes BRCA-mediated phenotypes. Nature 571 (7766), 576–579. doi:10.1038/s41586-019-1382-1
Kaye, S. B., Lubinski, J., Matulonis, U., Ang, J. E., Gourley, C., Karlan, B. Y., et al. (2012). Phase II, open-label, randomized, multicenter study comparing the efficacy and safety of olaparib, a poly (ADP-ribose) polymerase inhibitor, and pegylated liposomal doxorubicin in patients with BRCA1 or BRCA2 mutations and recurrent ovarian cancer. J. Clin. Oncol. 30, 372–379. doi:10.1200/JCO.2011.36.9215
Koh, D. W., Dawson, T. M., and Dawson, V. L. (2005). Mediation of cell death by poly(ADP-ribose) polymerase-1. Pharmacol. Res. 52, 5–14. doi:10.1016/j.phrs.2005.02.011
Koosha, F., Neshasteh-Riz, A., Takavar, A., Eyvazzadeh, N., Mazaheri, Z., Eynali, S., et al. (2017). The combination of A-966492 and Topotecan for effective radiosensitization on glioblastoma spheroids. Biochem. Biophys. Res. Commun. 491, 1092–1097. doi:10.1016/j.bbrc.2017.08.018
Kotova, E., Jarnik, M., and Tulin, A. V. (2010). Uncoupling of the transactivation and transrepression functions of PARP1 protein. Proc. Natl. Acad. Sci. U. S. A. 107, 6406–6411. doi:10.1073/pnas.0914152107
Lai, W. G., Farah, N., Moniz, G. A., and Wong, Y. N. (2011). A Baeyer-Villiger oxidation specifically catalyzed by human flavin-containing monooxygenase 5. Drug Metab. Dispos. 39, 61–70. doi:10.1124/dmd.110.035360
Lakin, N. D., and Jackson, S. P. (1999). Regulation of p53 in response to DNA damage. Oncogene 18, 7644–7655. doi:10.1038/sj.onc.1203015
Langelier, M. F., Servent, K. M., Rogers, E. E., and Pascal, J. M. (2008). A third zinc-binding domain of human poly(ADP-ribose) polymerase-1 coordinates DNA-dependent enzyme activation. J. Biol. Chem. 283, 4105–4114. doi:10.1074/jbc.M708558200
Langelier, M. F., Zandarashvili, L., Aguiar, P. M., Black, B. E., and Pascal, J. M. (2018). NAD+ analog reveals PARP-1 substrate-blocking mechanism and allosteric communication from catalytic center to DNA-binding domains. Nat. Commun. 9, 844. doi:10.1038/s41467-018-03234-8
Lautier, D., Lagueux, J., Thibodeau, J., Ménard, L., and Poirier, G. G. (1993). Molecular and biochemical features of poly (ADP-ribose) metabolism. Mol. Cell Biochem. 122, 171–193. doi:10.1007/BF01076101
Lavrik, O. I., Prasad, R., Sobol, R. W., Horton, J. K., Ackerman, E. J., and Wilson, S. H. (2001). Photoaffinity labeling of mouse fibroblast enzymes by a base excision repair intermediate. Evidence for the role of poly(ADP-ribose) polymerase-1 in DNA repair. J. Biol. Chem. 276, 25541–25548. doi:10.1074/jbc.M102125200
Le Rhun, Y., Kirkland, J. B., and Shah, G. M. (1998). Cellular responses to DNA damage in the absence of Poly(ADP-ribose) polymerase. Biochem. Biophys. Res. Commun. 245, 1–10. doi:10.1006/bbrc.1998.8257
Lee, H. J., Yoon, C., Schmidt, B., Park, D. J., Zhang, A. Y., Erkizan, H. V., et al. (2013). Combining PARP-1 inhibition and radiation in Ewing sarcoma results in lethal DNA damage. Mol. Cancer Ther. 12, 2591–2600. doi:10.1158/1535-7163.MCT-13-0338
Leppard, J. B., Dong, Z., Mackey, Z. B., and Tomkinson, A. E. (2003). Physical and functional interaction between DNA ligase IIIalpha and poly(ADP-Ribose) polymerase 1 in DNA single-strand break repair. Mol. Cell Biol. 23, 5919–5927. doi:10.1128/mcb.23.16.5919-5927.2003
Lesueur, P., Chevalier, F., El-Habr, E. A., Junier, M. P., Chneiweiss, H., Castera, L., et al. (2018). Radiosensitization effect of talazoparib, a parp inhibitor, on glioblastoma stem cells exposed to low and high linear energy transfer radiation. Sci. Rep. 8 (1), 3664. doi:10.1038/s41598-018-22022-4
Li, N., Bu, H., Liu, J., Zhu, J., Zhou, Q., Wang, L., et al. (2021). An open-label, multicenter, single-arm, phase II study of fluzoparib in patients with germline BRCA1/2 mutation and platinum-sensitive recurrent ovarian cancer. Clin. cancer Res. 27, 2452–2458. doi:10.1158/1078-0432.CCR-20-3546
Li, W. H., Yang, Y. L., Cheng, X., Liu, M., Zhang, S. S., Wang, Y. H., et al. (2020). Baicalein attenuates caspase-independent cells death via inhibiting PARP-1 activation and AIF nuclear translocation in cerebral ischemia/reperfusion rats. Apoptosis 25, 354–369. doi:10.1007/s10495-020-01600-w
Li, X., and Darzynkiewicz, Z. (2000). Cleavage of poly(ADP-ribose) polymerase measured in situ in individual cells: Relationship to DNA fragmentation and cell cycle position during apoptosis. Exp. Cell Res. 255, 125–132. doi:10.1006/excr.1999.4796
Litton, J. K., Rugo, H. S., Ettl, J., Hurvitz, S. A., Gonçalves, A., Lee, K. H., et al. (2018). Talazoparib in patients with advanced breast cancer and a germline BRCA mutation BRCA mutation. N. Engl. J. Med. 379, 753–763. doi:10.1056/NEJMoa1802905
Liu, M., Li, Z., Chen, G. W., Li, Z. M., Wang, L. P., Ye, J. T., et al. (2014). AG-690/11026014, a novel PARP-1 inhibitor, protects cardiomyocytes from AngII-induced hypertrophy. Mol. Cell Endocrinol. 392, 14–22. doi:10.1016/j.mce.2014.05.010
Liu, Q., Gheorghiu, L., Drumm, M., Clayman, R., Eidelman, A., Wszolek, M. F., et al. (2018). PARP-1 inhibition with or without ionizing radiation confers reactive oxygen species-mediated cytotoxicity preferentially to cancer cells with mutant TP53. Oncogene 37, 2793–2805. doi:10.1038/s41388-018-0130-6
Liu, Y., Kadyrov, F. A., and Modrich, P. (2012). PARP-1 enhances the mismatch-dependence of 5'-directed excision in human mismatch repair in vitro. DNA repair 10, 1145–1153. doi:10.1016/j.dnarep.2011.08.012
LiverTox (2012). Clinical and Research Information on Drug-Induced Liver Injury. National Institute of Diabetes and Digestive and Kidney Diseases.
Loap, P., Loirat, D., Berger, F., Rodrigues, M., Bazire, L., Pierga, J. Y., et al. (2022). Concurrent olaparib and radiotherapy in patients with triple-negative breast cancer: The phase 1 olaparib and radiation therapy for triple-negative breast cancer trial. JAMA Oncol. 8 (12), 1802–1808. doi:10.1001/jamaoncol.2022.5074
Lowery, M. A., Kelsen, D. P., Capanu, M., Smith, S. C., Lee, J. W., Stadler, Z. K., et al. (2018). Phase II trial of veliparib in patients with previously treated BRCA-mutated pancreas ductal adenocarcinoma. Eur. J. Cancer 89, 19–26. doi:10.1016/j.ejca.2017.11.004
Lu, Q. T., Qu, S., and Li, L. (2018). Effect of PARP-1 on migration and invasion of nasopharyngeal carcinoma CNE-2 cells. Chin. J. Cancer Prev. Treat. 5, 10–14.
Lucarini, L., Durante, M., Lanzi, C., Pini, A., Boccalini, G., Calosi, L., et al. (2017). HYDAMTIQ, a selective PARP-1 inhibitor, improves bleomycin-induced lung fibrosis by dampening the TGF-β/SMAD signalling pathway. J. Cell Mol. Med. 21, 324–335. doi:10.1111/jcmm.12967
Luijsterburg, M. S., de Krijger, I., Wiegant, W. W., Shah, R. G., Smeenk, G., de Groot, A. J. L., et al. (2016). PARP1 links CHD2-mediated chromatin expansion and H3.3 deposition to DNA repair by non-homologous end-joining. Mol. Cell. 61, 547–562. doi:10.1016/j.molcel.2016.01.019
Luo, Q., Li, Y., Deng, J., and Zhang, Z. (2015). PARP-1 inhibitor sensitizes arsenic trioxide in hepatocellular carcinoma cells via abrogation of G2/M checkpoint and suppression of DNA damage repair. Chem. Biol. Interact. 226, 12–22. doi:10.1016/j.cbi.2014.12.007
Maillet, A., and Pervaiz, S. (2012). Redox regulation of p53, redox effectors regulated by p53: A subtle balance. Antioxid. Redox Signal 16, 1285–1294. doi:10.1089/ars.2011.4434
Mansour, W. Y., Rhein, T., and Dahm-Daphi, J. (2010). The alternative end-joining pathway for repair of DNA double-strand breaks requires PARP1 but is not dependent upon microhomologies. Nucleic Acids Res. 38, 6065–6077. doi:10.1093/nar/gkq387
Martin-Hernandez, K., Rodriguez-Vargas, J. M., Schreiber, V., and Dantzer, F. (2017). Expanding functions of ADP-ribosylation in the maintenance of genome integrity. Semin. Cell Dev. Biol. 63, 92–101. doi:10.1016/j.semcdb.2016.09.009
Martin-Oliva, D., Aguilar-Quesada, R., O'valle, F., Muñoz-Gámez, J. A., Martínez-Romero, R., García Del Moral, R., et al. (2006). Inhibition of poly(ADP-ribose) polymerase modulates tumor-related gene expression, including hypoxia-inducible factor-1 activation, during skin carcinogenesis. Cancer Res. 66, 5744–5756. doi:10.1158/0008-5472.CAN-05-3050
Masson, M., Niedergang, C., Schreiber, V., Muller, S., Menissier-de Murcia, J., and de Murcia, G. (1998). XRCC1 is specifically associated with poly(ADP-ribose) polymerase and negatively regulates its activity following DNA damage. Mol. Cell Biol. 18, 3563–3571. doi:10.1128/mcb.18.6.3563
Masutani, M., Nozaki, T., Wakabayashi, K., and Sugimura, T. (1995). Role of poly(ADP-ribose) polymerase in cell-cycle checkpoint mechanisms following gamma-irradiation. Biochimie 77, 462–465. doi:10.1016/0300-9084(96)88161-2
Mateo, J., Ong, M., Tan, D. S., Gonzalez, M. A., and de Bono, J. S. (2013). Appraising iniparib, the PARP inhibitor that never was--what must we learn? Nat. Rev. Clin. Oncol. 10, 688–696. doi:10.1038/nrclinonc.2013.177
Mateos-Gomez, P. A., Gong, F., Nair, N., Miller, K. M., Lazzerini-Denchi, E., and Sfeir, A. (2015). Mammalian polymerase θ promotes alternative NHEJ and suppresses recombination. Nature 518, 254–257. doi:10.1038/nature14157
McGonigle, S., Chen, Z., Wu, J., Chang, P., Kolber-Simonds, D., Ackermann, K., et al. (2015). E7449: A dual inhibitor of PARP1/2 and tankyrase1/2 inhibits growth of DNA repair deficient tumors and antagonizes Wnt signaling. Oncotarget 6, 41307–41323. doi:10.18632/oncotarget.5846
Meli, E., Pangallo, M., Picca, R., Baronti, R., Moroni, F., and Pellegrini-Giampietro, D. E. (2004). Differential role of poly(ADP-ribose) polymerase-1in apoptotic and necrotic neuronal death induced by mild or intense NMDA exposure in vitro. Mol. Cell Neurosci. 25, 172–180. doi:10.1016/j.mcn.2003.09.016
Menear, K. A., Adcock, C., Boulter, R., Cockcroft, X. L., Copsey, L., Cranston, A., et al. (2008). 4-[3-(4-cyclopropanecarbonylpiperazine-1-carbonyl)-4-fluorobenzyl]-2H-phthalazin-1-one: A novel bioavailable inhibitor of poly(ADP-ribose) polymerase-1. J. Med. Chem. 51 (20), 6581–6591. doi:10.1021/jm8001263
Meng, J., Peng, J., Feng, J., Maurer, J., Li, X., Li, Y., et al. (2021). Niraparib exhibits a synergistic anti-tumor effect with PD-L1 blockade by inducing an immune response in ovarian cancer. J. Transl. Med. 19, 415. doi:10.1186/s12967-021-03073-0
Mitchell, J., Smith, G. C., and Curtin, N. J. (2009). Poly(ADP-Ribose) polymerase-1 and DNA-dependent protein kinase have equivalent roles in double strand break repair following ionizing radiation. Int. J. Radiat. Oncol. Biol. Phys. 75, 1520–1527. doi:10.1016/j.ijrobp.2009.07.1722
Mirza, M. R., Monk, B. J., Herrstedt, J., Oza, A. M., Mahner, S., Redondo, A., et al. (2016). Niraparib Maintenance Therapy in Platinum-Sensitive, Recurrent Ovarian Cancer. N. Engl. J. Med. 375, 2154–2164. doi:10.1056/NEJMoa1611310
Morretta, E., Tosco, A., Festa, C., Mozzicafreddo, M., Monti, M. C., and Casapullo, A. (2020). Crellastatin A, a PARP-1 inhibitor discovered by complementary proteomic approaches. ChemMedChem 15, 317–323. doi:10.1002/cmdc.201900634
Mosca, L., Rotili, D., Tempera, I., Masci, A., Fontana, M., Chiaraluce, R., et al. (2011). Biological effects of MC2050, a quinazoline-based PARP-1 inhibitor, in human neuroblastoma and EBV-positive Burkitt's lymphoma cells. ChemMedChem 6, 606–611. doi:10.1002/cmdc.201000536
Muiras, M. L. (2003). Mammalian longevity under the protection of PARP-1's multi-facets. Ageing Res. Rev. 2, 129–148. doi:10.1016/s1568-1637(02)00062-4
Munnur, D., Somers, J., Skalka, G., Weston, R., Jukes-Jones, R., Bhogadia, M., et al. (2019). NR4A nuclear receptors target poly-ADP-ribosylated DNA-PKcs protein to promote DNA repair. Cell Rep. 26 (8), 2028–2036. doi:10.1016/j.celrep.2019.01.083
Murai, J., Huang, S. y. N., Das, B. B., Renaud, A., Zhang, Y., Doroshow, J. H., et al. (2012). Trapping of PARP1 and PARP2 by clinical PARP inhibitors. Cancer Res. 72 (21), 5588–5599. doi:10.1158/0008-5472.CAN-12-2753
Murai, J., Zhang, Y., Morris, J., Ji, J., Takeda, S., Doroshow, J. H., et al. (2014). Rationale for poly(ADP-ribose) polymerase (PARP) inhibitors in combination therapy with camptothecins or temozolomide based on PARP trapping versus catalytic inhibition. J. Pharmacol. Exp. Ther. 349 (3), 408–416. doi:10.1124/jpet.113.210146
Myongjae, L., Park, J. Tae., Yang, J. H., Kim, D., Je, I. G., Lee, Y. S., et al. (2018). Abstract A106: Development of IDX-1197, a novel, selective, and highly potent PARP inhibitor. Pennsylvania, United States: American Association for Cancer Research.
Negi, G., and Sharma, S. S. (2015). Inhibition of IκB kinase (IKK) protects against peripheral nerve dysfunction of experimental diabetes. Mol. Neurobiol. 51, 591–598. doi:10.1007/s12035-014-8784-8
Nickson, C. M., Moori, P., Carter, R. J., Rubbi, C. P., and Parsons, J. L. (2017). Misregulation of DNA damage repair pathways in HPV-positive head and neck squamous cell carcinoma contributes to cellular radiosensitivity. Oncotarget 8, 29963–29975. doi:10.18632/oncotarget.16265
Nilov, D., Maluchenko, N., Kurgina, T., Pushkarev, S., Lys, A., Kutuzov, M., et al. (2020). Molecular mechanisms of PARP-1 inhibitor 7-methylguanine. Int. J. Mol. Sci. 21, 2159. doi:10.3390/ijms21062159
O'Connor, L. O., Rulten, S. L., Cranston, A. N., Odedra, R., Brown, H., Jaspers, J. E., et al. (2016). The PARP inhibitor AZD2461 provides insights into the role of PARP3 inhibition for both synthetic lethality and tolerability with chemotherapy in preclinical models. Cancer Res. 76, 6084–6094. doi:10.1158/0008-5472.CAN-15-3240
Ogden, T. E. H., Yang, J. C., Schimpl, M., Easton, L. E., Underwood, E., Rawlins, P. B., et al. (2021). Dynamics of the HD regulatory subdomain of PARP-1; substrate access and allostery in PARP activation and inhibition. Nucleic Acids Res. 49, 2266–2288. doi:10.1093/nar/gkab020
Oliver, F. J., Ménissier-de Murcia, J., Nacci, C., Decker, P., Andriantsitohaina, R., Muller, S., et al. (1999). Resistance to endotoxic shock as a consequence of defective NF-kappaB activation in poly (ADP-ribose) polymerase-1 deficient mice. EMBO J. 18, 4446–4454. doi:10.1093/emboj/18.16.4446
Papeo, G., Orsini, P., Avanzi, N. R., Borghi, D., Casale, E., Ciomei, M., et al. (2019). Discovery of stereospecific PARP-1 inhibitor isoindolinone NMS-P515. ACS Med. Chem. Lett. 10, 534–538. doi:10.1021/acsmedchemlett.8b00569
Papeo, G., Posteri, H., Borghi, D., Busel, A. A., Caprera, F., Casale, E., et al. (2015). Discovery of 2-[1-(4,4-Difluorocyclohexyl)piperidin-4-yl]-6-fluoro-3-oxo-2,3-dihydro-1H-isoindole-4-carboxamide (nms-P118): A potent, orally available, and highly selective PARP-1 inhibitor for cancer therapy. J. Med. Chem. 58, 6875–6898. doi:10.1021/acs.jmedchem.5b00680
Park, H., Kam, T. I., Dawson, T. M., and Dawson, V. L. (2020). Poly (ADP-ribose) (PAR)-dependent cell death in neurodegenerative diseases. Int. Rev. Cell Mol. Biol. 353, 1–29. doi:10.1016/bs.ircmb.2019.12.009
Park, M. T., Kim, M. J., Kang, Y. H., Choi, S. Y., Lee, J. H., Choi, J. A., et al. (2005). Phytosphingosine in combination with ionizing radiation enhances apoptotic cell death in radiation-resistant cancer cells through ROS-dependent and -independent AIF release. Blood 105, 1724–1733. doi:10.1182/blood-2004-07-2938
Patel, A. G., Sarkaria, J. N., and Kaufmann, S. H. (2011). Nonhomologous end joining drives poly(ADP-ribose) polymerase (PARP) inhibitor lethality in homologous recombination-deficient cells. Proc. Natl. Acad. Sci. U. S. A. 108, 3406–3411. doi:10.1073/pnas.1013715108
Patterson, M. J., Sutton, R. E., Forrest, I., Sharrock, R., Lane, M., Kaufmann, A., et al. (2014). Assessing the function of homologous recombination DNA repair in malignant pleural effusion (MPE) samples. Br. J. Cancer. 111, 94–100. doi:10.1038/bjc.2014.261
Penning, T. D., Zhu, G. D., Gong, J., Thomas, S., Gandhi, V. B., Liu, X., et al. (2010). Optimization of phenyl-substituted benzimidazole carboxamide poly(ADP-ribose) polymerase inhibitors: Identification of (S)-2-(2-fluoro-4-(pyrrolidin-2-yl)phenyl)-1H-benzimidazole-4-carboxamide (A-966492), a highly potent and efficacious inhibitor. J. Med. Chem. 53, 3142–3153. doi:10.1021/jm901775y
Perkins, N. D. (2000). The rel/NF-kappa B family: Friend and foe. Trends Biochem. Sci. 25, 434–440. doi:10.1016/s0968-0004(00)01617-0
Piconi, L., Quagliaro, L., Da Ros, R., Assaloni, R., Giugliano, D., Esposito, K., et al. (2004). Intermittent high glucose enhances ICAM-1, VCAM-1, E-selectin and interleukin-6 expression in human umbilical endothelial cells in culture: The role of poly(ADP-ribose) polymerase. J. Thromb. Haemost. 2, 1453–1459. doi:10.1111/j.1538-7836.2004.00835.x
Pilié, P. G., Gay, C. M., Byers, L. A., O’Connor, M. J., and Yap, T. A. (2019). PARP inhibitors: Extending benefit beyond BRCA-mutant cancers. Clin. Cancer Res. 25, 3759–3771. doi:10.1158/1078-0432.CCR-18-0968
Pines, A., Vrouwe, M. G., Marteijn, J. A., Typas, D., Luijsterburg, M. S., Cansoy, M., et al. (2012). PARP1 promotes nucleotide excision repair through DDB2 stabilization and recruitment of ALC1. J. Cell Biol. 199, 235–249. doi:10.1083/jcb.201112132
Pleschke, J. M., Kleczkowska, H. E., Strohm, M., and Althaus, F. R. (2000). Poly(ADP-ribose) binds to specific domains in DNA damage checkpoint proteins. J. Biol. Chem. 275, 40974–40980. doi:10.1074/jbc.M006520200
Prasad, R., Lavrik, O. I., Kim, S. J., Kedar, P., Yang, X. P., Vande Berg, B. J., et al. (2001). DNA polymerase beta -mediated long patch base excision repair. Poly(ADP-ribose)polymerase-1 stimulates strand displacement DNA synthesis. J. Biol. Chem. 276, 32411–32414. doi:10.1074/jbc.C100292200
Prives, C., and Hall, P. A. (1999). The p53 pathway. J. Pathol. 187, 112–126. doi:10.1002/(SICI)1096-9896(199901)187:1<112::AID-PATH250>3.0.CO;2-3
Qin, Z. H., Chen, R. W., Wang, Y., Nakai, M., Chuang, D. M., and Chase, T. N. (1999). Nuclear factor kappaB nuclear translocation upregulates c-Myc and p53 expression during NMDA receptor-mediated apoptosis in rat striatum. J. Neurosci. 19, 4023–4033. doi:10.1523/JNEUROSCI.19-10-04023.1999
Radovits, T., Lin, L. N., Zotkina, J., Gero, D., Szabó, C., Karck, M., et al. (2007). Poly(ADP-ribose) polymerase inhibition improves endothelial dysfunction induced by reactive oxidant hydrogen peroxide in vitro. Eur. J. Pharmacol. 564, 158–166. doi:10.1016/j.ejphar.2007.02.060
Rajawat, J., Shukla, N., and Mishra, D. P. (2017). Therapeutic targeting of poly(ADP-ribose) polymerase-1 (PARP1) in cancer: Current developments, therapeutic strategies, and future opportunities. Med. Res. Rev. 37, 1461–1491. doi:10.1002/med.21442
Ray Chaudhuri, A., Hashimoto, Y., Herrador, R., Neelsen, K. J., Fachinetti, D., Bermejo, R., et al. (2012). Topoisomerase I poisoning results in PARP-mediated replication fork reversal. Nat. Struct. Mol. Biol. 19, 417–423. doi:10.1038/nsmb.2258
Ray Chaudhuri, A., and Nussenzweig, A. (2017). The multifaceted roles of PARP1 in DNA repair and chromatin remodelling. Nat. Rev. Nat. Rev. Mol. Cell Biol. 18, 610–621. doi:10.1038/nrm.2017.53
Robu, M., Shah, R. G., Petitclerc, N., Brind'Amour, J., Kandan-Kulangara, F., and Shah, G. M. (2013). Role of poly(ADP-ribose) polymerase-1 in the removal of UV-induced DNA lesions by nucleotide excision repair. Proc. Natl. Acad. Sci. U. S. A. 110, 1658–1663. doi:10.1073/pnas.1209507110
Ronson, G. E., Piberger, A. L., Higgs, M. R., Olsen, A. L., Stewart, G. S., McHugh, P. J., et al. (2018). PARP1 and PARP2 stabilise replication forks at base excision repair intermediates through Fbh1-dependent Rad51 regulation. Nat. Commun. 9, 746. doi:10.1038/s41467-018-03159-2
Rouleau-Turcotte, É., Krastev, D. B., Pettitt, S. J., Lord, C. J., and Pascal, J. M. (2022). Captured snapshots of PARP1 in the active state reveal the mechanics of PARP1 allostery. Mol. Cell. 82, 2939–2951. doi:10.1016/j.molcel.2022.06.011
Rudat, V., Bachmann, N., Küpper, J. H., and Weber, K. J. (2001). Overexpression of the DNA-binding domain of poly(ADP-ribose) polymerase inhibits rejoining of ionizing radiation-induced DNA double-strand breaks. Int. J. Radiat. Biol. 77, 303–307. doi:10.1080/09553000010009026
Ruscetti, T., Lehnert, B. E., Halbrook, J., Le Trong, H., Hoekstra, M. F., Chen, D. J., et al. (1998). Stimulation of the DNA-dependent protein kinase by poly(ADP-ribose) polymerase. J. Biol. Chem. 273, 14461–14467. doi:10.1074/jbc.273.23.14461
Russo, A. L., Kwon, H. C., Burgan, W. E., Carter, D., Beam, K., Weizheng, X., et al. (2009). In vitro and in vivo radiosensitization of glioblastoma cells by the poly (ADP-ribose) polymerase inhibitor E7016. Clin. Cancer Res. 15, 607–612. doi:10.1158/1078-0432.CCR-08-2079
Rutkowski, B., Slominska, E., Szolkiewicz, M., Smolenski, R. T., Striley, C., Rutkowski, P., et al. (2003). N-methyl-2-pyridone-5-carboxamide: A novel uremic toxin? Kidney Int. Suppl. 63, S19–S21. doi:10.1046/j.1523-1755.63.s84.36.x
Ryabokon, N. I., Cieślar-Pobuda, A., and Rzeszowska-Wolny, J. (2009). Inhibition of poly(ADP-ribose) polymerase activity affects its subcellular localization and DNA strand break rejoining. Acta biochem. Pol. 56, 243–248. doi:10.18388/abp.2009_2455
Ryabokon, N. I., Goncharova, R. I., Duburs, G., Hancock, R., and Rzeszowska-Wolny, J. (2008). Changes in poly(ADP-ribose) level modulate the kinetics of DNA strand break rejoining. Mutat. Res. 637, 173–181. doi:10.1016/j.mrfmmm.2007.08.005
Ryan, K. M., Ernst, M. K., Rice, N. R., and Vousden, K. H. (2000). Role of NF-kappaB in p53-mediated programmed cell death. Nature 404, 892–897. doi:10.1038/35009130
Sabbatino, F., Fusciello, C., Somma, D., Pacelli, R., Poudel, R., Pepin, D., et al. (2014). Effect of p53 activity on the sensitivity of human glioblastoma cells to PARP-1 inhibitor in combination with topoisomerase I inhibitor or radiation. Cytom. A 85, 953–961. doi:10.1002/cyto.a.22563
Sablina, A. A., Budanov, A. V., Ilyinskaya, G. V., Agapova, L. S., Kravchenko, J. E., and Chumakov, P. M. (2005). The antioxidant function of the p53 tumor suppressor. Nat. Med. 11, 1306–1313. doi:10.1038/nm1320
Said, R. S., El-Demerdash, E., Nada, A. S., and Kamal, M. M. (2016). Resveratrol inhibits inflammatory signaling implicated in ionizing radiation-induced premature ovarian failure through antagonistic crosstalk between silencing information regulator 1 (SIRT1) and poly(ADP-ribose) polymerase 1 (PARP-1). Biochem. Pharmacol. 103, 140–150. doi:10.1016/j.bcp.2016.01.019
Salech, F., Ponce, D. P., Paula-Lima, A. C., SanMartin, C. D., and Behrens, M. I. (2020). Nicotinamide, a poly [ADP-Ribose] polymerase 1 (PARP-1) inhibitor, as an adjunctive therapy for the treatment of alzheimer's disease. Front. Aging Neurosci. 12, 255. doi:10.3389/fnagi.2020.00255
Scalia, M., Satriano, C., Greca, R., Stella, A. M., Rizzarelli, E., and Spina-Purrello, V. (2013). PARP-1 inhibitors DPQ and PJ-34 negatively modulate proinflammatory commitment of human glioblastoma cells. Neurochem. Res. 38, 50–58. doi:10.1007/s11064-012-0887-x
Sefer, A., Kallis, E., Eilert, T., Röcker, C., Kolesnikova, O., Neuhaus, D., et al. (2022). Structural dynamics of DNA strand break sensing by PARP-1 at a single-molecule level. Nat. Commun. 13, 6569. doi:10.1038/s41467-022-34148-1
Sfeir, A., and de Lange, T. (2012). Removal of shelterin reveals the telomere end-protection problem. Science 336, 593–597. doi:10.1126/science.1218498
Sfeir, A., and Symington, L. S. (2015). Microhomology-mediated end joining: A back-up survival mechanism or dedicated pathway? Trends biochem. Sci. 40, 701–714. doi:10.1016/j.tibs.2015.08.006
Shall, S., and de Murcia, G. (2000). Poly(ADP-ribose) polymerase-1: What have we learned from the deficient mouse model? Mutat. Res. 460, 1–15. doi:10.1016/s0921-8777(00)00016-1
Shi, Y., Maag, D. X., Palma, J. P., Patterson, M. J., Ellis, P. A., Surber, B. W., et al. (2012). Iniparib nonselectively modifies cysteine-containing proteins in tumor cells and is not a bona fide PARP inhibitor. Clin. Cancer Res. 18, 510–523. doi:10.1158/1078-0432.CCR-11-1973
Shirai, H., Fujimori, H., Gunji, A., Maeda, D., Hirai, T., Poetsch, A. R., et al. (2013). Parg deficiency confers radio-sensitization through enhanced cell death in mouse ES cells exposed to various forms of ionizing radiation. Biochem. Biophys. Res. Commun. 435, 100–106. doi:10.1016/j.bbrc.2013.04.048
Shirley, M. (2019). Rucaparib: A review in ovarian cancer. Target Oncol. 14, 237–246. doi:10.1007/s11523-019-00629-5
Simbulan-Rosenthal, C. M., Rosenthal, D. S., Luo, R., and Smulson, M. E. (1999). Poly(ADP-ribosyl)ation of p53 during apoptosis in human osteosarcoma cells. Cancer Res. 59, 2190–2194.
Sizemore, S. T., Mohammad, R., Sizemore, G. M., Nowsheen, S., Yu, H., Ostrowski, M. C., et al. (2018). Synthetic lethality of PARP inhibition and ionizing radiation is p53-dependent. Mol. Cancer Res. 16, 1092–1102. doi:10.1158/1541-7786.MCR-18-0106
Smith, L. M., Willmore, E., Austin, C. A., and Curtin, N. J. (2005). The novel poly(ADP-Ribose) polymerase inhibitor, AG14361, sensitizes cells to topoisomerase I poisons by increasing the persistence of DNA strand breaks. Clin. Cancer Res. 11, 8449–8457. doi:10.1158/1078-0432.CCR-05-1224
Smith, S. (2001). The world according to PARP. Trends Biochem. Sci. 26, 174–179. doi:10.1016/s0968-0004(00)01780-1
Sodhi, R. K., Singh, N., and Jaggi, A. S. (2010). Poly(ADP-ribose) polymerase-1 (PARP-1) and its therapeutic implications. Vasc. Pharmacol. 53, 77–87. doi:10.1016/j.vph.2010.06.003
Sorriento, D., Illario, M., Finelli, R., and Iaccarino, G. (2012). To NFκB or not to NFκB: The dilemma on how to inhibit a cancer cell fate regulator. Transl. Med. UniSa. 4, 73–85.
Spagnolo, L., Barbeau, J., Curtin, N. J., Morris, E. P., and Pearl, L. H. (2012). Visualization of a DNA-PK/PARP1 complex. Nucleic Acids Res. 40, 4168–4177. doi:10.1093/nar/gkr1231
Srinivasan, S. (1997). The design and synthesis of novel heterocyclic inhibitors of the DNA-repair enzyme, poly(ADP-ribose) polymerase, as potential resistance-modifying agents. United Kingdom: Newcastle University.
Steffen, J. D., Brody, J. R., Armen, R. S., and Pascal, J. M. (2013). Structural implications for selective targeting of PARPs. Front. Oncol. 3, 301. doi:10.3389/fonc.2013.00301
Szabados, E., Literati-Nagy, P., Farkas, B., and Sumegi, B. (2000). BGP-15, a nicotinic amidoxime derivate protecting heart from ischemia reperfusion injury through modulation of poly(ADP-ribose) polymerase. Biochem. Pharmacol. 59, 937–945. doi:10.1016/s0006-2952(99)00418-9
Tao, Z., Gao, P., Hoffman, D. W., and Liu, H. W. (2008). Domain C of human poly(ADP-ribose) polymerase-1 is important for enzyme activity and contains a novel zinc-ribbon motif. Biochem. 47, 5804–5813. doi:10.1021/bi800018a
Tavernarakis, N., Pasparaki, A., Tasdemir, E., Maiuri, M. C., and Kroemer, G. (2008). The effects of p53 on whole organism longevity are mediated by autophagy. Autophagy 4, 870–873. doi:10.4161/auto.6730
Tentori, L., Leonetti, C., Scarsella, M., D'Amati, G., Vergati, M., Portarena, I., et al. (2003). Systemic administration of GPI 15427, a novel poly(ADP-ribose) polymerase-1 inhibitor, increases the antitumor activity of temozolomide against intracranial melanoma, glioma, lymphoma. Clin. Cancer Res. 9, 5370–5379.
Tentori, L., Leonetti, C., Scarsella, M., Vergati, M., Xu, W., Calvin, D., et al. (2005). Brain distribution and efficacy as chemosensitizer of an oral formulation of PARP-1 inhibitor GPI 15427 in experimental models of CNS tumors. Int. J. Oncol. 26, 415–422. doi:10.3892/ijo.26.2.415
Trucco, C., Oliver, F. J., de Murcia, G., and Ménissier-de Murcia, J. (1998). DNA repair defect in poly(ADP-ribose) polymerase-deficient cell lines. Nucleic Acids Res. 26, 2644–2649. doi:10.1093/nar/26.11.2644
Turner, N. C., Telli, M. L., Rugo, H. S., Mailliez, A., Ettl, J., Grischke, E. M., et al. (2019). A phase II study of talazoparib after platinum or cytotoxic nonplatinum regimens in patients with advanced breast cancer and germline BRCA1/2 mutations (ABRAZO). Clin. Cancer Res. 25, 2717–2724. doi:10.1158/1078-0432.CCR-18-1891
Ullrich, O., Diestel, A., Eyüpoglu, I. Y., and Nitsch, R. (2001). Regulation of microglial expression of integrins by poly(ADP-ribose) polymerase-1. Nat. Cell Biol. 3, 1035–1042. doi:10.1038/ncb1201-1035
Underhill, C., Toulmonde, M., and Bonnefoi, H. (2011). A review of PARP inhibitors: From bench to bedside. Ann. Oncol. 22, 268–279. doi:10.1093/annonc/mdq322
Valko, M., Izakovic, M., Mazur, M., Rhodes, C. J., and Telser, J. (2004). Role of oxygen radicals in DNA damage and cancer incidence. Mol. Cell Biochem. 266, 37–56. doi:10.1023/b:mcbi.0000049134.69131.89
Vazquez-Ortiz, G., Chisholm, C., Xu, X., Lahusen, T. J., Li, C., Sakamuru, S., et al. (2014). Drug repurposing screen identifies lestaurtinib amplifies the ability of the poly (ADP-ribose) polymerase 1 inhibitor AG14361 to kill breast cancer associated gene-1 mutant and wild type breast cancer cells. Breast Cancer Res. 16, R67. doi:10.1186/bcr3682
Veuger, S. J., Curtin, N. J., Richardson, C. J., Smith, G. C., and Durkacz, B. W. (2003). Radiosensitization and DNA repair inhibition by the combined use of novel inhibitors of DNA-dependent protein kinase and poly(ADP-ribose) polymerase-1. Cancer Res. 63, 6008–6015.
Veuger, S. J., Hunter, J. E., and Durkacz, B. W. (2009). Ionizing radiation-induced NF-kappaB activation requires PARP-1 function to confer radioresistance. Oncogene 28, 832–842. doi:10.1038/onc.2008.439
Wang B, B., Chu, D., Feng, Y., Shen, Y., Aoyagi-Scharber, M., and Post, L. E. (2016). Discovery and characterization of (8S,9R)-5-Fluoro-8-(4-fluorophenyl)-9-(1-methyl-1H-1,2,4-triazol-5-yl)-2,7,8,9-tetrahydro-3H-pyrido[4,3,2-de]phthalazin-3-one (BMN 673, talazoparib), a novel, highly potent, and orally efficacious poly(ADP-ribose) polymerase-1/2 inhibitor, as an anticancer agent. J. Med. Chem. 59, 335–357. doi:10.1021/acs.jmedchem.5b01498
Wang, H., Ren, B., Liu, Y., Jiang, B., Guo, Y., Wei, M., et al. (2020). Discovery of Pamiparib (BGB-290), a potent and selective poly (ADP-ribose) polymerase (PARP) inhibitor in clinical development. J. Med. Chem. 63, 15541–15563. doi:10.1021/acs.jmedchem.0c01346
Wang, L., Mason, K. A., Ang, K. K., Buchholz, T., Valdecanas, D., Mathur, A., et al. (2012). MK-4827, a PARP-1/-2 inhibitor, strongly enhances response of human lung and breast cancer xenografts to radiation. Invest. New Drugs 30, 2113–2120. doi:10.1007/s10637-011-9770-x
Wang, L., Yang, C., Xie, C., Jiang, J., Gao, M., Fu, L., et al. (2019). Pharmacologic characterization of fluzoparib, a novel poly(ADP-ribose) polymerase inhibitor undergoing clinical trials. Cancer Sci. 110, 1064–1075. doi:10.1111/cas.13947
Wang, M., Wu, W., Wu, W., Rosidi, B., Zhang, L., Wang, H., et al. (2006). PARP-1 and Ku compete for repair of DNA double strand breaks by distinct NHEJ pathways. Nucleic Acids Res. 34, 6170–6182. doi:10.1093/nar/gkl840
Wang, T. Y., Hu, Y. J., Wang, X., Li, Y. F., Zhang, F., Yan, Y. D., et al. (2022). Targeting p65 to inhibit Cas3 transcription by Onjisaponin B for radiation damage therapy in p65+/- mice. Phytomedicine 104, 154317. doi:10.1016/j.phymed.2022.154317
Wang W, W., Duan, B., and Zeng, L. (2016). Effect and mechanism of radiosensitization of poly (ADP-Ribose) polymerase inhibitor n Lewis cells and xenografts. Chin. J. Lung Cancer 19, 16–23. doi:10.3779/j.issn.1009-3419.2016.01.02
Węsierska-Gądek, J., Mauritz, M., Mitulovic, G., and Cupo, M. (2015). Differential potential of pharmacological PARP inhibitors for inhibiting cell proliferation and inducing apoptosis in human breast cancer cells. J. Cell Biochem. 116, 2824–2839. doi:10.1002/jcb.25229
Wieler, S., Gagné, J. P., Vaziri, H., Poirier, G. G., and Benchimol, S. (2003). Poly(ADP-ribose) polymerase-1 is a positive regulator of the p53-mediated G1 arrest response following ionizing radiation. J. Biol. Chem. 278, 18914–18921. doi:10.1074/jbc.M211641200
Williamson, C. T., Kubota, E., Hamill, J. D., Klimowicz, A., Ye, R., Muzik, H., et al. (2012). Enhanced cytotoxicity of PARP inhibition in mantle cell lymphoma harbouring mutations in both ATM and p53. EMBO Mol. Med. 4, 515–527. doi:10.1002/emmm.201200229
Wu, Z., Wang, C., Bai, M., Li, X., Mei, Q., Li, X., et al. (2015). An LRP16-containing preassembly complex contributes to NF-κB activation induced by DNA double-strand breaks. Nucleic Acids Res. 43, 3167–3179. doi:10.1093/nar/gkv161
Xu, P., Cai, X., Zhang, W., Li, Y., Qiu, P., Lu, D., et al. (2016). Flavonoids of Rosa roxburghii Tratt exhibit radioprotection and anti-apoptosis properties via the Bcl-2(Ca(2+))/Caspase-3/PARP-1 pathway. Apoptosis 21, 1125–1143. doi:10.1007/s10495-016-1270-1
Xu, S. J., Wang, X., Wang, T. Y., Lin, Z. Z., Hu, Y. J., Huang, Z. L., et al. (2020). Flavonoids from Rosaroxburghii Tratt prevent reactive oxygen species-mediated DNA damage in thymus cells both combined with and without PARP-1 expression after exposure to radiation in vivo. Aging 12, 16368–16389. doi:10.18632/aging.103688
Xu, S. J., Zhang, F., Wang, L. J., Hao, M. H., Yang, X. J., Li, N. N, et al. (2018). Flavonoids of Rosa roxburghii Tratt offers protection against radiation induced apoptosis and inflammation in mouse thymus. Apoptosis 23, 470–483. doi:10.1007/s10495-018-1466-7
Yamamura, E., Muto, S., Yamada, K., Sato, Y., Iwase, Y., and Uno, Y. (2015). Chromosomal damage and micronucleus induction by MP-124, a novel poly(ADP-ribose) polymerase-1 (PARP-1) inhibitor: Evidence for a non-DNA-reactive mode of action. Mutat. Res. Genet. Toxicol. Environ. Mutagen. 782, 1–8. doi:10.1016/j.mrgentox.2015.02.006
Yang, S., Li, X., Cheng, L., Wu, H., Zhang, C., and Li, K. (2015). Tenuigenin inhibits RANKL-induced osteoclastogenesis by down-regulating NF-κB activation and suppresses bone loss in vivo. Biochem. Biophys. Res. Commun. 466, 615–621. doi:10.1016/j.bbrc.2015.09.093
Yang, Y. G., Cortes, U., Patnaik, S., Jasin, M., and Wang, Z. Q. (2004). Ablation of PARP-1 does not interfere with the repair of DNA double-strand breaks, but compromises the reactivation of stalled replication forks. Oncogene 23, 3872–3882. doi:10.1038/sj.onc.1207491
Yin, S., Cheryan, V. T., Xu, L., Rishi, A. K., and Reddy, K. B. (2017). Myc mediates cancer stem-like cells and EMT changes in triple negative breast cancers cells. PLoS One 12, e0183578. doi:10.1371/journal.pone.0183578
Ying, S., Chen, Z., Medhurst, A. L., Neal, J. A., Bao, Z., Mortusewicz, O., et al. (2016). DNA-PKcs and PARP1 bind to unresected stalled DNA replication forks where they recruit XRCC1 to mediate repair. Cancer Res. 76 (5), 1078–1088. doi:10.1158/0008-5472.CAN-15-0608
Ying, W. (2013). Roles of NAD (+), PARP-1, and sirtuins in cell death, ischemic brain injury, and synchrotron radiation X-ray-induced tissue injury. Sci. (Cairo) 2013, 691251. doi:10.1155/2013/691251
Yong, M. K., Kyun-Seop, B., Nam, S. B., Kyungun, K., Lee, W. S., and Eun-Jihn, R. (2021). First-in-human dose-finding study of venadaparib (IDX-1197), a potent and selective PARP inhibitor, in patients with advanced solid tumors. J. Clin. Oncol. 39, 3107.
Yu, S. W., Andrabi, S. A., Wang, H., Kim, N. S., Poirier, G. G., Dawson, T. M., et al. (2006). Apoptosis-inducing factor mediates poly(ADP-ribose) (PAR) polymer-induced cell death. Proc. Natl. Acad. Sci. U. S. A. 103, 18314–18319. doi:10.1073/pnas.0606528103
Yu, S. W., Wang, H., Poitras, M. F., Coombs, C., Bowers, W. J., Federoff, H. J., et al. (2002). Mediation of poly(ADP-ribose) polymerase-1-dependent cell death by apoptosis-inducing factor. Science 297, 259–263. doi:10.1126/science.1072221
Yuan, K., Sun, Y., Zhou, T., McDonald, J., and Chen, Y. (2013). PARP-1 regulates resistance of pancreatic cancer to TRAIL therapy. Clin. Cancer Res. 19 (17), 4750–4759. doi:10.1158/1078-0432.CCR-13-0516
Zakaria, E. M., El-Bassossy, H. M., El-Maraghy, N. N., Ahmed, A. F., and Ali, A. A. (2016). PARP-1 inhibition alleviates diabetic cardiac complications in experimental animals. Eur. J. Pharmacol. 791, 444–454. doi:10.1016/j.ejphar.2016.09.008
Zeng, L., Boggs, D. H., Xing, C., Zhang, Z., Anderson, J. C., Wajapeyee, N., et al. (2020). Combining PARP and DNA-PK inhibitors with irradiation inhibits HPV-negative head and neck cancer squamous carcinoma growth. Front. Genet. 11, 1036. doi:10.3389/fgene.2020.01036
Zhang, J. H., Hu, K., and Yang, Z. K. (2016). Study on aging of mouse breast cancer cells induced by X-ray radiation combined with Veliparib. Chin. J. Cancer. 23, 1141–1148.
Zhang, P., Wang, X., and Wang, Y. (2008). The Ku-dependent non-homologous end-joining but not other repair pathway is inhibited by high linear energy transfer ionizing radiation. DNA Repair 7, 725–733. doi:10.1016/j.dnarep.2008.01.010
Zou, L. H., Shang, Z. F., Tan, W., Liu, X. D., Xu, Q. Z., Song, M., et al. (2015). TNKS1BP1 functions in DNA double-strand break repair though facilitating DNA-PKcs autophosphorylation dependent on PARP-1. Oncotarget 6, 7011–7022. doi:10.18632/oncotarget.3137
Glossary
Keywords: ionizing radiation, PARP inhibitor, p53/ROS, NF-κB/DNA-PK, Caspese-3/AIF
Citation: Li W-H, Wang F, Song G-Y, Yu Q-H, Du R-P and Xu P (2023) PARP-1: a critical regulator in radioprotection and radiotherapy-mechanisms, challenges, and therapeutic opportunities. Front. Pharmacol. 14:1198948. doi: 10.3389/fphar.2023.1198948
Received: 02 April 2023; Accepted: 22 May 2023;
Published: 06 June 2023.
Edited by:
Ali H. Eid, Qatar University, QatarReviewed by:
Yoshihisa Matsumoto, Tokyo Institute of Technology, JapanJulio Morales, University of Oklahoma, United States
Copyright © 2023 Li, Wang, Song, Yu, Du and Xu. This is an open-access article distributed under the terms of the Creative Commons Attribution License (CC BY). The use, distribution or reproduction in other forums is permitted, provided the original author(s) and the copyright owner(s) are credited and that the original publication in this journal is cited, in accordance with accepted academic practice. No use, distribution or reproduction is permitted which does not comply with these terms.
*Correspondence: Ping Xu, MTMyNzM3MzAyNzFAMTYzLmNvbQ==