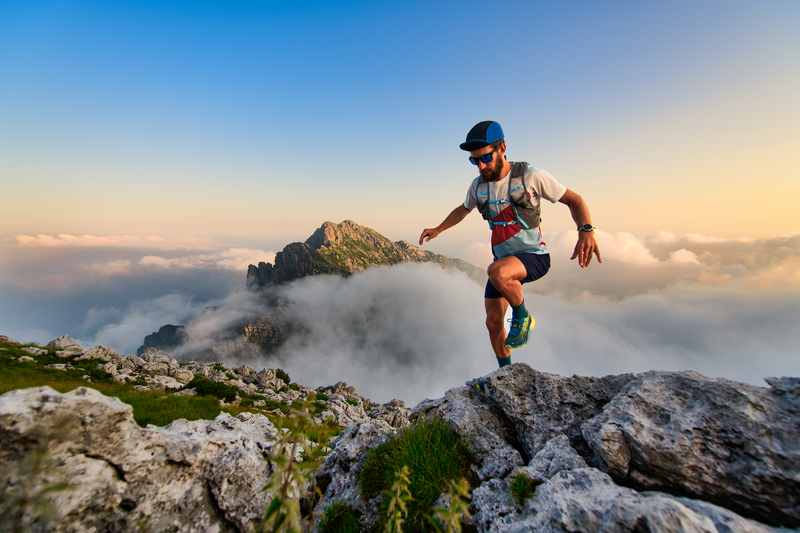
94% of researchers rate our articles as excellent or good
Learn more about the work of our research integrity team to safeguard the quality of each article we publish.
Find out more
ORIGINAL RESEARCH article
Front. Pharmacol. , 25 May 2023
Sec. Neuropharmacology
Volume 14 - 2023 | https://doi.org/10.3389/fphar.2023.1183019
The pharmacological profile of cannabigerol (CBG), which acid form constitutes the main precursor of the most abundant cannabinoids, has been scarcely studied. It has been reported to target α2-adrenoceptor and 5-HT1A receptor. The locus coeruleus (LC) and the dorsal raphe nucleus (DRN) are the main serotonergic (5-HT) and noradrenergic (NA) areas in the rat brain, respectively. We aimed to study the effect of CBG on the firing rate of LC NA cells and DRN 5-HT cells and on α2-adrenergic and 5-HT1A autoreceptors by electrophysiological techniques in male Sprague-Dawley rat brain slices. The effect of CBG on the novelty-suppressed feeding test (NSFT) and the elevated plus maze test (EPMT) and the involvement of the 5-HT1A receptor was also studied. CBG (30 μM, 10 min) slightly changed the firing rate of NA cells but failed to alter the inhibitory effect of NA (1–100 µM). However, in the presence of CBG the inhibitory effect of the selective α2-adrenoceptor agonist UK14304 (10 nM) was decreased. Perfusion with CBG (30 μM, 10 min) did not change the firing rate of DRN 5-HT cells or the inhibitory effect of 5-HT (100 μM, 1 min) but it reduced the inhibitory effect of ipsapirone (100 nM). CBG failed to reverse ipsapirone-induced inhibition whereas perfusion with the 5-HT1A receptor antagonist WAY100635 (30 nM) completely restored the firing rate of DRN 5-HT cells. In the EPMT, CBG (10 mg/kg, i.p.) significantly increased the percentage of time the rats spent on the open arms and the number of head-dipping but it reduced the anxiety index. In the NSFT, CBG decreased the time latency to eat in the novel environment but it did not alter home-cage consumption. The effect of CBG on the reduction of latency to feed was prevented by pretreatment with WAY100635 (1 mg/kg, i.p.). In conclusion, CBG hinders the inhibitory effect produced by selective α2-adrenoceptor and 5-HT1A receptor agonists on the firing rate of NA-LC and 5-HT-DRN neurons by a yet unknown indirect mechanism in rat brain slices and produces anxiolytic-like effects through 5-HT1A receptor.
Cannabis sativa plant contains more than 120 phytocannabinoids including psychoactive and non-psychoactive compounds (Turner et al., 2017). Several studies have been performed to characterize the pharmacological effects of the non-psychoactive cannabinoids. The best characterized one is cannabidiol (CBD), which has been shown to target the Gi/o protein-coupled 5-HT1A receptor to mediate its principal therapeutical effects, such as anxiolytic or antiepileptic effects (De Gregorio et al., 2019; Silvestro et al., 2020). Another non-psychoactive phytocannabinoid is cannabigerol (CBG), which acid form has been identified as the main precursor of the most abundant cannabinoids. CBG has been suggested to constitute a potential drug for disease treatment since it stimulates appetite (Brierley et al., 2016) and shows analgesic, anti-inflammatory (di Giacomo et al., 2020), antiemetic (Rock et al., 2011) or anxiolytic (Zagzoog et al., 2020) effects in rodents. However, to date little is known about its pharmacological profile, although it has been postulated to be between that of Δ9-THC and CBD (Nachnani et al., 2021).
The main nucleus in the central nervous system (CNS) enriched with noradrenergic (NA) cells is the locus coeruleus (LC), which activity is regulated, among others, by the Gi/o protein-coupled α2-adrenoceptor (Aghajanian and Wang, 1987). The dorsal raphe nucleus (DRN) is the principal 5-HT nucleus in the rat brain. 5-HT cells of the DRN expresses the Gi/o protein-coupled 5-HT1A autoreceptor and its activation results in the inhibition of the firing activity of 5-HT cells via G protein-coupled inwardly rectifying potassium channels (GIRKs) (Courtney and Ford, 2016). Both monoaminergic nuclei play a pivotal role in the regulation of physiological processes and pathological conditions including sleep-wake phase, arousal, pain, anxiety and depression (Aston-Jones et al., 1999; Michelsen et al., 2007; Lowry et al., 2008; Monti, 2010; Takahashi et al., 2010; Felippotti et al., 2011; Lin et al., 2011; Campion et al., 2016; Morris et al., 2020; Suárez-Pereira et al., 2022).
Several studies have demonstrated that psychoactive and non-psychoactive cannabinoids regulate monoaminergic systems (Mendiguren et al., 2018). On the one hand, CB1 receptor agonists and/or antagonists modulate the neuronal activity of DRN 5-HT cells or LC NA cells in vivo and in vitro (Gobbi et al., 2005; Muntoni et al., 2006; Mendiguren and Pineda, 2009; Bambico et al., 2012; Mendiguren et al., 2018). On the other hand, CBD reduces the firing rate of DRN 5-HT cells in vivo (De Gregorio et al., 2019) and modulates 5-HT1A receptor-mediated effects on the firing rate of DRN 5-HT cells in vitro (Mendiguren et al., 2022). Furthermore, several in vivo studies have reported that the 5-HT or NA systems are involved in the anxiolytic (De Gregorio et al., 2019; Warren et al., 2022) and antidepressant effects of cannabinoids (Gobbi et al., 2005; Zanelati et al., 2010; Kirilly et al., 2013; Linge et al., 2016; Sartim et al., 2016; Sales et al., 2018). However, the in vivo and in vitro effects of the non-psychoactive cannabinoid CBG have been scarcely studied. In vitro data have shown that CBG targets α2-adrenoceptor and 5-HT1A receptor (Cascio et al., 2010; Rock et al., 2011). Thus, a single study performed by binding techniques in mouse brain membranes revealed that CBG behaves as potent α2-adrenoceptor agonist and moderately potent 5-HT1A receptor antagonist (Cascio et al., 2010). In addition, few data exist on the pharmacological effects (i.e., anxiolytic effect) of CBG in rodent. Moreover, the involvement of 5-HT1A receptor in the in vivo effects of CBG have not been investigated yet, even though it has been reported that CBG exerts 5-HT1A receptor-mediated neuroprotective effects in vitro (Echeverry et al., 2021).
Therefore, considering that the α2-adrenoceptor is abundant in the LC and that the 5-HT1A receptor is widely distributed in the DRN, the aim of our work was to characterize the effect of CBG on the firing rate of NA and 5-HT cells and on the 5-HT1A and α2- autoreceptors activation by electrophysiological techniques in rat brain slices from the LC and DRN. Furthermore, we studied the effect of CBG on anxiety-like behavior by the elevated plus maze test (EPMT) and the novelty-suppressed feeding test (NSFT) and the putative involvement of the 5-HT1A receptor.
Male Sprague-Dawley (200–300 g) rats (total, n = 176; behavioral tests, n = 118; and electrophysiological assays, n = 58) were kept under controlled environmental conditions (22 °C, 12L:12D schedule, 65–70% humidity, food and water ad libitum). The experiments were conducted following the European Directive on the protection of animals for scientific purposes (2010/63/EU). All the procedures were accepted by the Institutional Ethical Committee for Research and Teaching of the University of the Basque Country (UPV/EHU, Spain) and the Department of Sustainability and Natural Environment of Provincial Council from Bizkaia (ref. CEEA M20-2018-025 and CEEA M20-2018-262). A minimum number of animals was used and we made an effort to avoid animal suffering.
The rat was anaesthetized with chloral hydrate (400 mg/kg i.p.) and then brain was extracted after decapitation. The tissue was transferred to an ice-cold artificial cerebrospinal fluid (ACSF), in which NaCl was replaced by sucrose to enhance neuronal survival. Coronal slices containing the LC or the DRN of 500–600 µm thickness were cut by a vibratome and they were allowed to recover from the slicing for 2 h. Then, the brainstem sections were placed in a custom-made modified Haas-type interface chamber. The slice was continuously perfused with ACSF (flow rate: 1.5 mL/min, 33 °C), which was composed of NaCl 130 mM, KCl 3 mM, NaH2PO4 1.25 mM, MgSO4 2 mM, CaCl2 2 mM, NaHCO3 20 mM and D-glucose 10 mM bubbled with 95% O2/5% CO2 (pH = 7.34) (Mendiguren and Pineda, 2009).
Single-unit extracellular recordings of LC NA neurons and DRN 5-HT neurons were made as previously described (Mendiguren and Pineda, 2009; Medrano et al., 2017; Mendiguren et al., 2022). An Omegadot glass micropipette was prepared with a horizontal pipette puller and filled with NaCl (0.05 M). Then, the tip was broken to a diameter of 2–5 µm for a final resistance of 3–5 MΩ. The electrode was placed under binocular microscope in the recording area (LC or DRN). The LC nucleus was visually identified in the rostral pons as a dark oval area on the lateral borders of the central gray and the fourth ventricle, anterior to the genu of the facial nerve. The DRN was localized visually as a dark area in the ventromedial part of the periaqueductal gray. The extracellular signal was filtered and amplied through a high-input impedance amplifier. Then, an oscilloscope and audio analyzer were used to monitor the signal. Individual (single-unit) neuronal spikes were discriminated from the background noise with a window discriminator. The firing rate was analyzed by a PC-based custom-made software, which generated consecutive histogram bars representing the accumulated number of spikes in 10 s. NA LC cells were identified by their spontaneous and regular discharge activity, slow firing rate, and long-lasting biphasic positive-negative waveforms (Andrade and Aghajanian, 1984; Medrano et al., 2017). The effect of GABA (1 mM) was used as a control for the perfusion system and to normalize the inhibitory effects of NA. We only selected the cells that were initially inhibited by GABA (1 mM, 1 min). One animal was used for each experiment and only one cell from each slice was recorded. DRN 5-HT cells were selected based on electrophysiological and pharmacological criteria. The electrophysiological features to identify 5-HT cells were the following: a regular discharging pattern, a slow firing rate and a long-lasting biphasic positive-negative waveform (2 ms). As a pharmacological criteria, the response to short perfusion of 5-HT (50–100 μM, 1 min) was used (Aghajanian and Lakoski, 1984; Hajós et al., 1996; Mendiguren and Pineda, 2009; Mendiguren et al., 2022). Only the neurons that showed the mentioned electrophysiological features and were inhibited by 5-HT were selected for the study. In all cases, the firing rate was driven by perfusion with the α1-adrenoceptor agonist phenylephrine (PE, 15 μM) because in slices from the DRN the NA excitatory afferents are cut and 5-HT cells fail to discharge spontaneously (Mendiguren and Pineda, 2009; Mendiguren et al., 2022).
To study the effect of CBG (30 µM) on the firing rate of LC NA cells and on the PE-driven firing activity of DRN 5-HT cells, we first perfused the vehicle of the drug (DMSO ≤0.1%) and then the cannabinoid for 10 min. The effect of CBG on α2-adrenoceptor mediated inhibition of the firing rate was studied by perfusing increasing concentrations of NA (1–100 μM, x3, 1 min each) or the more selective α2-adrenoceptor agonist UK14304 (1 nM and 10 nM) in the absence and in the continuous presence of CBG. UK14304 was applied until a maximal inhibition plateau was reached within 10 min of drug perfusion. Unlike NA, which is easily washed out from the slice, experiments of UK14304 in the absence or the presence of CBG were performed in NA cells from different slices. To avoid the influence that changes of the firing rate produced by CBG may have on the quantification of the effects of NA on the firing rate, GABA (1 mM, 1 min) was administrated before performing the concentration-effect curves in the absence and the presence of CBG to normalize the inhibitory effects of NA.
The effect of CBG (30 µM) on the inhibition of the firing rate elicited by 5-HT1A receptor agonists was investigated by studying and comparing the inhibitory responses to application of the endogenous ligand 5-HT (100 μM, 1 min) or the more selective 5-HT1A receptor agonist ipsapirone (100 nM, 10 min) in the absence or the presence of the cannabinoid in DRN 5-HT cells. We also studied whether CBG (30 μM) could restore the firing rate of previously inhibited 5-HT cells and mimick the effect of a competitive 5-HT1A receptor antagonist by perfusing the cannabinoid for 10 min. Finally, after administration of CBG the selective 5-HT1A receptor antagonist WAY100635 (30 nM) was perfused in the same 5-HT cell to restore the firing activity.
The EPM consisted of a cross-shaped, elevated platform with two open (50 cm long x 10 cm wide) and two closed arms (50 cm long x 10 cm wide). Each rat was placed in the central platform facing to the open arm and its behavior was recorded for 5 min. Arm entries were considered as introduction of four paws into the arm. The number of entries and the time spent in the open arms were measured. The frequency of the following ethological parameters was also observed: head-dipping (animal sticking the head toward the floor from the open arm) and rearing (vertical standing of rodent on two hind legs) (Walf and Frye, 2007). Increases of number of entries, time spent in the open arms and the number of head-dipping have been shown to indicate an anxiolytic-like effect (Griebel et al., 1997) while changes in rearing have been suggested to reflect alteration of motor activity (Cruz et al., 1994).
NSFT assesses the ability of the animal to resolve a conflict between a novel context that induces heightened anxiety and a drive to approach an appetitive stimulus. Thereby it can be used for evaluating the potential anxiolytic effect of drugs. Novel environment consisted of a clean 100 cm × 100 cm × 40 cm box with open anxiogenic arena. The ground was covered with wooden bedding, which was changed after each single animal experiment. A small piece of rat chow was positioned in the center of the arena onto a circular filter paper, which was illuminated (1000 lm, peripheral intensity 800 lm). The surrounding environment remained lightless. The rat underwent a food restriction period of 24 h with free access to water. After habituation to the room (at least 90 min), the animal was introduced in a corner of the open field and the time it took to eat from the highly-illuminated food was recorded (latency to eat). Longer latency to eat indicates higher level of anxiety-like-behavior. Anxiolytic drugs decrease the latency to feed in food-deprived rats exposed to a novel environment (Shephard and Broadhurst, 1982). An experimental cut off time of 10 min was set. Immediately after, the animal was returned back to its home-cage, where it was provided with a weighed amount of food. After 10 min the amount of food ingested by each rat was determined by weighing the remaining rat chow (home-cage food consumption). Both latency to eat in the home-cage and home-cage food consumption were measured to assure that the tested drugs did not alter food intake since cannabinoids could have hyperphagic effects (Williams and Kirkham, 2002; Nachnani et al., 2021).
CBG (3–10 mg/kg, i.p.) was administrated 60 min prior to carry out the test, according to previous behavioral and pharmacokinetics studies (Deiana et al., 2012; Zagzoog et al., 2020) (CBG group). The control group was injected with the corresponding volume of the vehicle of CBG (3 or 10 mL/kg of 5% cremophor, 5% ethanol, 90% saline) 60 min prior to the test. As no differences were observed in the tested effects among the groups injected with different volumes of the vehicle, all the data from the vehicle-injected animals were gathered in the same group for further comparison analysis. Finally, to study the receptor involved in these effects, some animals received an injection of the 5-HT1A receptor antagonist WAY100635 (1 mg/kg, i.p.) 30 min before administration of CBG (10 mg/kg, i.p.) (WAY100635 + CBG group) or the vehicle of CBG (WAY100635 group).
γ-Aminobutyric acid (GABA), 5-Bromo-6-(2-imidazolin-2-ylamino)quinoxaline tartrate (UK14304), (−) Cannabigerol (CBG), 2-[4-[4-(2-pyrimidinyl)-1-piperazinyl]butyl]-1,2-benzisothiazol-3(2H)-one-1,1-dioxide (ipsapirone) and phenylephrine (PE) hydrochloride were purchased from Tocris (Bristol, United Kingdom). 5-hydroxytryptamine (5-HT), noradrenaline (NA) and N-[2-[4-(2-Methoxyphenyl)-1-piperazinyl]ethyl]-N-2-pyridinylcyclohexanecarboxamide (WAY100635) were purchased from Sigma (St Louis, MO, United States).
For electrophysiological assays, stock solutions of ipsapirone, UK14304 and CBG were prepared in dimethylsulphoxide (DMSO), and those of GABA, 5-HT, NA, PE, and WAY100635 in milliQ water. Final solutions were freshly prepared and diluted in ACSF for the desired concentration. Equivalent maximal concentrations of the vehicles in which the drugs were dissolved were applied as a control. The maximal concentration of DMSO in the ACSF was ≤0.1%.
For behavioral studies, CBG was dissolved in a mixture of 5% cremophor, 5% ethanol and 90% saline (0.9% NaCl). WAY100635 was dissolved in 0.9% NaCl (saline).
In electrophysiological experiments, the effect of CBG on the firing rate of NA LC cells and on 5-HT DRN cells was measured at the time of the maximal change in the firing rate after administration of the cannabinoid, which was quantified as the percentage change from the basal firing rate. The inhibitory effects of increasing concentrations of NA on the firing rate of LC cells were normalized to GABA (1 mM, 1 min)-induced inhibition. To construct concentration-effect curves for NA, fitting analysis was performed to obtain the best simple non-linear fit to the following three-parameter logistic equation: E = Emax/1 + (EC50/A)n x 100, where [A] is the concentration of NA, E is the effect on the firing rate induced by NA, Emax is the maximal inhibitory effect, EC50 is the concentration of the agonist required to promote the 50% of the Emax and n represents the slope factor of the curve. From this analysis EC50, Emax and n values were calculated. For comparison purposes, EC50 values were converted and expressed as the negative logarithm values (pEC50, M), which adjusted the variable to a Gaussian distribution.
The effect of 5-HT was calculated by integrating the firing rate values (spikes/10 s, 60 s) after 5-HT application in the absence and in the presence of CBG while the effect of the selective 5-HT1A receptor or α2-adrenoceptor agonists was measured by calculating the maximal change in the firing rate within 10 min of drug perfusion. These values were subtracted to the firing rate value (spikes/10 s) before application of the 5-HT1A receptor agonists and then quantified as the percentage change from the basal firing rate.
In behavioral studies, the percentage of time the rats spent on the open arms ([seconds on the open arms]/[300 s] × 100), the percentage of open arm entries ([open entries]/[total entries] × 100) and the anxiety index were calculated in the EPMT for comparisons between CBG and vehicle-treated groups. Anxiety index was expressed as follows: AI = 1 − ([time spent on the open arms/test duration] + [entries into the open arms/total number of entries]/2). Values range from 0 to 1, where an increase in the index expresses higher anxiety-like behavior (Cohen et al., 2013). To study the effect of CBG on the NSFT, the time latency to feed in the novel environment (s), home-cage consumption (g) and latency to eat in the housing cage (s) were measured both in the CBG-treated group and in the matched vehicle-treated group. To characterize the influence of WAY100635 administration on CBG-induced effects, changes in the latency to feed, home-cage consumption and latency to eat in the housing cage produced by the cannabinoid were studied both in the absence (vehicle) and the presence of the 5-HT1A receptor antagonist. The effects of CBG in the absence of WAY100635 were calculated as the percentages of latency to eat, home-cage consumption and latency to feed in the housing cage after CBG administration with respect to the mean value in the control group (vehicle-treated group). The effects of CBG in the presence of WAY100635 were estimated as the percentages of the latency to eat, home-cage consumption and latency to feed in the housing cage after CBG and WAY100635 administration with respect to the mean value in its control group (WAY100635-treated group).
Data are given as mean ± standard error of the mean (SEM). Analysis of the results were done by Graph Pad Prism. For statistical analysis, paired Student’s t-test was performed when the effects before and after drug application were compared within the same cell, and by a two-sample Student’s t-test when two independent experimental conditions were compared. One-way analysis of variance (ANOVA) followed by the Bonferroni’s multiple comparison poshoc test was used to compare more than two independent groups. The non-parametric Kruskal–Wallis test was used when the criteria for the parametric statistics were not met (frequency of head-dipping and rearing). Subsequently, appropriate paired comparisons were performed using Mann-Whitney U test. The level of significance was set at p < 0.05.
CBG has been shown to be a potent agonist at the α2-adrenoceptor in binding assays performed in brain mouse membranes (Cascio et al., 2010). Therefore, we first studied the effect of CBG on the firing rate of NA cells in the LC. Administration of CBG (30 μM, 10 min) slightly decreased the firing rate of LC cells (FR before CBG = 0.88 ± 0.08 Hz vs. FR after CBG = 0.77 ± 0.08 Hz, n = 16, p < 0.005) (Figures 1A, B). The inhibitory effect of CBG was 12.8 ± 3.8%, suggesting that CBG did not behave as a full agonist at the α2-adrenoceptor in LC neurons. Perfusion with the vehicle of CBG (DMSO 0.1%, 10 min), which was administrated before the cannabinoid, failed to alter the firing rate of NA cells (FR before: 0.85 ± 0.09 Hz vs. FR after: 0.88 ± 0.08 Hz, n = 16) (Figure 1B). In order to study whether CBG (30 µM) changed the effect of α2-adrenoceptor agonists on the neuronal activity of LC neurons, we tested the effect of the cannabinoid on NA and UK14304-induced inhibition of the firing rate of NA cells. Increasing concentrations of NA (1–100 μM, x3, 1 min each) inhibited the neuronal activity of LC cells in a concentration-dependent manner with an EC50 value of 8.93 µM (n = 6), which was consistent with that previously reported in LC brain slices (Grandoso et al., 2005). Complete inhibition of the firing rate of NA cells was achieved at the highest concentration of NA (Emax = 97.4 ± 1.9%, n = 6) (Figure 1C). Continuous perfusion with CBG (30 µM) failed to change the inhibitory effect of NA (1–100 μM, 1 min each). Thus, in the presence of CBG, the EC50 value of the concentration-effect curve for NA was 8.70 µM and the Emax = 96.8 ± 2.2% (n = 6) (Figure 1C). However, administration of CBG (30 µM) reduced the maximal inhibitory effect of UK14304 (10 nM, 5–10 min) (Emax in the absence of CBG = 100%, n = 6 vs. Emax in the presence of CBG = 85.9 ± 7.6%, n = 8). Thus, the decrease of the firing rate induced by UK14304 (10 nM) in the absence of CBG was significantly higher than that in the presence of CBG (decrease of the FR in the absence of CBG: 0.85 ± 0.09 Hz, n = 6 vs. decrease of the FR in the presence of CBG: 0.56 ± 0.09 Hz, n = 8, p < 0.05) (Figure 1D). However, CBG did not change the inhibitory effect of a lower concentration of UK14304 (1 nM, 5–10 min) (0.15 ± 0.04 Hz, n = 6 vs. 0.11 ± 0.03 Hz, n = 9) (Figure 1D).
FIGURE 1. Effect of CBG on the firing rate of LC NA cells and on α2-adrenoceptor agonist-induced inhibition (A) Representative example of the firing rate recording of LC neuron, which represents the effect of CBG (30 μM) on the basal firing rate. The vertical line indicates the number of spikes recorded every 10 s and the horizontal line shows the period of drug application. (B) Bar histograms showing the firing rate (mean ± SEM) in the absence and the presence of CBG (30 μM) (n = 16) (C) Concentration-effect curves for NA in the absence (non-filled circles, n = 6) and the presence of CBG (30 μM) (filled circles, n = 6). The horizontal axis shows the concentration of NA in a semi-logarithmic scale. The vertical axis indicates the reduction in the firing rate of LC neurons as the percentage of the inhibitory effect of GABA (1 mM). Data points are the mean ± SEM at each NA concentration obtained from n cells. The line through the data is the theoretical curve constructed from the mean of the individual concentration-effect curve parameters, as estimated by nonlinear regression. The parameters of the concentration-effect curve for NA in the absence (control) and the presence of CBG (30 μM) were the following: Emax (97.4 ± 1.9 vs. 96.8 ± 2.2%), pEC50 (5.05 ± 0.07 M vs. 5.06 ± 0.07 M) and EC50 (8.93 µM vs. 8.70 µM) (n = 6). (D) Bar histograms showing the inhibitory effect (decrease in spikes/10 s; mean ± SEM) of UK14304 in the absence (control) (1 nM, n = 6 and 10 nM, n = 6) and the presence of CBG (30 μM) (1 nM, n = 9 and 10 nM, n = 8). *p < 0.005 compared to the firing rate before CBG (30 μM) administration by a paired Student’s t-test. †p < 0.05 compared to the inhibitory effect induced by UK14304 (10 nM) in the absence of CBG (30 μM) by an unpaired Student’s t-test.
The non-psychoactive cannabinoid CBG has been shown to be a moderate 5-HT1A receptor antagonist in binding assays by blocking 8-OH-DPAT effects in brain mouse membranes (Cascio et al., 2010). In a recent work, we have demonstrated that the best-studied non-psychoactive cannabinoid CBD hinders the effects of 5-HT1A receptor agonists without altering the firing rate of DRN 5-HT cells in rat brain slices (Mendiguren et al., 2022). Therefore, in order to study the effect of CBG on the neuronal activity of 5-HT cells and on the 5-HT1A receptor in the DRN we followed the same pharmacological procedure. Perfusion with CBG (30 μM, 10 min) failed to change the basal firing rate of DRN 5-HT cells (FR before CBG: 1.01 ± 0.13 Hz vs. FR after CBG: 0.96 ± 0.12 Hz, n = 9) (Figures 2A, C). To test whether the cannabinoid regulated 5-HT1A receptor-mediated effects in the DRN we administrated 5-HT (100 µM) in the absence and in the continuous presence of CBG (30 µM). As expected, administration of 5-HT (100 μM, 1 min) significantly inhibited the firing rate of DRN 5-HT cells (Figures 2B, D). Perfusion with CBG (30 μM, 10 min) did not change the inhibitory effect of 5-HT (100 μM, 1 min) (Figures 2B, D). Thus, in the presence of CBG (30 μM, 10 min) administration of 5-HT (100 μM, 1 min) significantly inhibited the firing rate of DRN 5-HT cells (FR before 5-HT: 0.96 ± 0.12 Hz vs. FR after 5-HT: 0.36 ± 0.17 Hz, n = 9, p < 0.005) and the inhibition was not different from that induced by 5-HT in the absence of CBG (FR before 5-HT: 0.99 ± 0.1 Hz vs. FR after 5-HT: 0.21 ± 0.05 Hz, n = 9, p < 0.005). Administration of the vehicle of CBG (DMSO 0.1%) did not affect the firing rate of 5-HT cells (FR before DMSO: 0.95 ± 0.13 Hz vs. FR after DMSO: 1.01 ± 0.13 Hz, n = 9) (Figure 2C).
FIGURE 2. Effect of CBG on the firing rate of 5-HT cells and on 5-HT-induced inhibition of the firing activity of DRN 5-HT cells (A,B) Representative examples of firing rate recordings from DRN cells, which show the effect of CBG (30 μM) on the firing activity of 5-HT neurons (A) and the effect of CBG (30 μM) on 5-HT (100 μM)-induced inhibition (B). The vertical lines refer to the integrated firing rate values (spikes per 10 s) and the horizontal lines represent the time scale. Drugs were perfused at the concentration and for the time indicated by the horizontal bars. (C) Bar histograms showing the firing rate of 5-HT cells (mean ± SEM) before (n = 9) and after perfusion with CBG (30 μM) (n = 9). (D) Bar histograms showing the inhibitory effect (decrease in spikes/10 s; mean ± SEM) induced by 5-HT (100 µM) in the absence (n = 9) and the presence of CBG (30 μM) (n = 9).
5-HT activates different 5-HT receptors including the 5-HT1A, 5-HT1B or 5-HT7 receptors. To avoid the action of 5-HT on non-5-HT1A receptor, we used the more selective 5-HT1A receptor agonist ipsapirone (100 nM), which has been studied due to its anxiolytic effects in several clinical trials. Administration of ipsapirone (100 nM, 10 min) completely inhibited the firing rate of DRN 5-HT cells (inhibitory effect: 98.4 ± 0.9%, n = 12) (Figures 3A, C–E). Thus, the firing rate after perfusion with ipsapirone was significantly lower than the firing rate before 5-HT1A receptor agonist perfusion (FR before ipsapirone: 0.89 ± 0.11 Hz vs. FR after ipsapirone: 0.01 ± 0.01 Hz, n = 12, p < 0.005). In the presence of CBG (30 μM, 10 min), the inhibitory effect of ipsapirone (100 nM) on the firing rate of DRN 5-HT cells was reduced to 49.1 ± 13.8% (n = 9) (Figures 3B, D). The decrease of the firing rate produced by ipsapirone in the absence of CBG was significantly higher than that in the presence of the cannabinoid (decrease of the FR in the absence of CBG: 0.88 ± 0.11 Hz, n = 12 vs. decrease of the FR in the presence of CBG: 0.45 ± 0.14 Hz, n = 9, p < 0.05) (Figure 3D). It is known that a competitive 5-HT1A receptor antagonist would restore the firing rate of a 5-HT neuron that has been previously inhibited by a 5-HT1A receptor agonist. In order to study whether CBG mimicked the effect of a competitive 5-HT1A receptor antagonist in the DRN, the cannabinoid was perfused for 10 min in 5-HT cells that were completely inhibited by ipsapirone. In these experiments, CBG (30 μM) did not reverse the inhibition induced by ipsapirone whereas perfusion with the 5-HT1A receptor antagonist WAY100635 (30 nM) completely restored the firing rate of 5-HT cells to the initial firing rate value (Basal FR: 0.91 ± 0.11 Hz vs. FR after WAY100635: 0.98 ± 0.22 Hz, n = 5) (Figures 3C, E).
FIGURE 3. Effect of CBG on ipsapirone-induced inhibition of the firing activity of DRN 5-HT cells (A–C) Representative examples of firing rate recordings from DRN 5-HT cells, which show the inhibition of the firing activity of 5-HT neurons by ipsapirone (100 nM) (A), the blockade of the inhibitory effect of ipsapirone (100 nM) by CBG (B) and the effect of CBG and WAY100635 on a neuron completely inhibited by ipsapirone (C). The vertical lines refer to the integrated firing rate values (spikes per 10 s) and the horizontal lines represent the time scale. Drugs were perfused at the concentration and for the time indicated by the horizontal bars. (D) Bar histograms showing the inhibitory effect (decrease in spikes/10 s; mean ± SEM) induced by ipsapirone (100 nM) in the absence (n = 12) and the presence of CBG (30 μM, n = 9) (E) Time course of the firing rate of DRN 5-HT cells (mean ± SEM) in the presence of ipsapirone (n = 5), CBG (n = 5) or WAY100635 (n = 5). *p < 0.05 compared to the inhibitory effect induced by ipsapirone (100 nM) in the absence of CBG (30 μM) by an unpaired Student’s t-test.
The non-psychoactive CBD has been reported to produce anxiolytic effects through 5-HT1A receptor-mediated mechanism in rodents (Resstel et al., 2009; Marinho et al., 2015; De Gregorio et al., 2019) and it has been suggested that modulation 5-HT1A receptor of the DRN could be involved in its pharmacological effects (Rock et al., 2012; De Gregorio et al., 2019). The present study reveals that CBG shows similar pharmacological profile to that previously described for CBD at DRN 5-HT1A receptor in rat brain slices (Mendiguren et al., 2022). Therefore, we studied whether CBG produced anxiolytic-like effects by different behavioral tests and then tested the putative involvement of the 5-HT1A receptor.
First, the effect of acute administration of CBG (3 and 10 mg/kg, i.p.) was characterized in the EPMT. Our data revealed a significant increase in the percentage of time the rats spent on the open arms after administration of CBG (p < 0.05) and a trend towards an increase in the percentage of open arm entries (Figures 4A, B). Post hoc analysis showed that the significant effect was due to the dose of 10 mg/kg (vehicle group: 1.4 ± 0.5%, n = 28 vs. CBG 10 mg/kg group: 8.2 ± 2.7%, n = 11, p < 0.05). Furthermore, a significant lower anxiety index in the CBG-treated group was observed (p < 0.05). Post hoc analysis revealed that the reduction of anxiety index also occurs at the dose of 10 mg/kg (vehicle group: 0.97 ± 0.01, n = 28 vs. CBG 10 mg/kg group: 0.84 ± 0.05, n = 11, p < 0.01). These data suggest that acute injection of CBG (10 mg/kg, i.p.) produces an anxiolytic effect in rats. Injection of lower dose of CBG (3 mg/kg, i.p., n = 21) did not significantly change the percentage of time spent in the open arms, the open arms entries or the anxiety index compared to the vehicle-treated group (Figures 4A–C). No statistical differences were observed between CBG (3 mg/kg)-treated and CBG (10 mg/kg)-treated groups in the mentioned variables.
FIGURE 4. Effect of CBG on the anxiety-like behavior in rats by the EPMT (A) Percentage of time spent by the rats in the open arms, (B) percentage of open arms entries, (C) the anxiety index,(D) the frequency of head-dipping and (E) the frequency of rearing in rats injected with the vehicle (up to 10 mL/kg, i.p., n = 28), CBG (3 mg/kg, i.p., n = 21) and CBG (10 mg/kg, i.p., n = 11). Each bar represents the mean ± SEM of n animals. *p < 0.05 compared to percentage of time spent by the rats in the open arms in the vehicle-treated group and **p < 0.01 compared to the anxiety index value in the vehicle-treated group by the one-way ANOVA followed by Bonferroni’s Multiple Comparison Test. †p < 0.05 compared to the number of head-dipping in the vehicle-treated group by the Kruskal–Wallis test followed by Mann–Whitney U test.
In addition, administration of CBG (10 mg/kg, i.p.), but not CBG (3 mg/kg, i.p.), significantly increased the frequency of head-dipping compared to the vehicle-treated group (vehicle group: 0.82 ± 0.39, n = 28 vs. CBG 10 mg/kg group: 2.82 ± 0.88, n = 11, p < 0.05), which is indicative of a reduction of anxiety-like behavior in cannabinoid-treated group (Figure 4D). CBG (3 and 10 mg/kg i.p.) did not change the frequency of rearing with respect to the control group (vehicle group: 11.89 ± 0.96, n = 28 vs. CBG 3 mg/kg group: 11.48 ± 1.23, n = 21; CBG 10 mg/kg group: 12.18 ± 1.54, n = 11) (Figure 4E).
After observing the anxiolytic-like effect produced by CBG (10 mg/kg, i.p.) on the EPMT, we performed the NSFT to characterize the action of the cannabinoid both in the novel environment and home-cage consumption. Acute administration of CBG (10 mg/kg, i.p.) significantly reduced the time until the first feeding event in the novel environment compared to the vehicle group (n = 13, p < 0.05) (Figure 5A), suggesting an anxiolytic-like effect. In fact, the rats treated with CBG took significantly less time to eat the food placed in the center of the field (vehicle group: 208.90 ± 20.32 s, n = 20; CBG group: 146 ± 20.83 s, n = 13; p < 0.05) (Figure 5A). Putative interaction of CBG with central targets could lead to stimulation of feeding behavior and yield confounding results in the NSFT. Therefore, to rule out differences in food intake between groups we measured the amount of food ingested by the animals when they were returned back to the home-cage. In the housing cage, consumption of food in CBG (10 mg/kg, i.p.)-treated group did not significantly differ from that of the vehicle-treated group (n = 13 and n = 20, respectively) (Figure 5B). Furthermore, no differences were observed in the time latency to eat in the CBG-treated group (98.15 ± 18.50 s) compared to that in the vehicle-treated group (87.94 ± 19.4 s). This suggests that the reduction of latency time until the first feeding event produced by CBG in the novel environment did not result from stimulation of feeding behavior.
FIGURE 5. Effect of CBG on the anxiety-like behavior in rats by the NSFT and the involvement of 5-HT1A receptor (A) Time latency to feed in the novel environment (s) and (B) home-cage consumption (g) in rats treated with the vehicle (up to 10 mL/kg, i.p., n = 20) or CBG (10 mg/kg, i.p., n = 13). Values are the mean ± SEM of n animals (C) Effect of CBG (10 mg/kg, i.p.) on the latency to feed and on home-cage consumption (D) in the absence of WAY100635 (1 mg/kg, i.p., n = 13) and in rats pretreated with WAY100635 (1 mg/kg, i.p., n = 12). Values are the mean ± SEM of the effect of CBG on the latency to feed or home-cage consumption in n animals, calculated as the percentage of the value of their corresponding control groups without CBG. *p < 0.05 compared to the time latency to feed in the vehicle-treated group by the unpaired Student’s t-test. †p < 0.01 compared to the effect of CBG in the absence of WAY100635 by the unpaired Student’s t-test.
In order to study if the effect of CBG was mediated by the 5-HT1A receptor, the 5-HT1A receptor antagonist WAY100635 (1 mg/kg, i.p.) was injected 30 min before administration of CBG (10 mg/kg, i.p.) and NSFT was performed. Treatment with WAY100635 (1 mg/kg, i.p.) by itself did not affect the time latency to feed (vehicle group: 208.90 ± 20.32 s, n = 20 vs. WAY100635 group: 225.80 ± 50.28 s, n = 13) or the home-cage consumption (vehicle group: 1.23 ± 0.15 g, n = 20 vs. WAY100635 group: 1.03 ± 0.23 g, n = 13). However, it increased the latency to eat in the home-cage (vehicle group: 87.94 ± 19.41 s vs. WAY100635 group: 225.10 ± 66.33 s). Pretreatment with WAY100635 (1 mg/kg, i.p.) significantly reduced the effect of CBG (10 mg/kg, i.p.) on the latency to feed in the novel environment Thus, in the absence of WAY100635, the effect of CBG (10 mg/kg, i.p.) on the latency to feed, which was estimated as the percentage of time latency to feed after CBG with respect to its corresponding control group (vehicle-treated group), was 69.91 ± 9.97% while in the presence of the 5-HT1A receptor antagonist, the effect of CBG (10 mg/kg, i.p.) was 120.14 ± 21.90% of that in its control group (WAY100635 treated group) (n = 12, p < 0.05) (Figure 5C). No significant change in home-cage consumption was found after CBG (10 mg/kg, i.p.) injection in rats pretreated with WAY100635 (1 mg/kg, i.p.) (n = 12) with respect to that in the vehicle-treated group (n = 13) (Figure 5D). Similarly, the effect of CBG on the lactency to feed in the home-cage in rats pretreated with WAY100635 was not different from that in the group that was not injected with the 5-HT1A receptor antagonist. Thus, in the absence of WAY100635, the effect of CBG on the latency to feed in the home-cage, which was estimated as the percentage of time latency to feed after CBG with respect to its corresponding control group (vehicle-treated group), was 111.60 ± 21.03%, whereas in the presence of WAY100635 the effect of CBG was 95.71 ± 30.43% of that in its control group (WAY100635-treated group). Our data suggest that the anxiolytic-like effect of CBG on NSFT was mediated by activation of 5-HT1A receptor.
The present work was carried out to characterize the effect of CBG on the neuronal activity of NA and 5-HT cells and on the anxiety-like behavior in rats. Our results show that CBG slightly reduces the basal firing rate of LC NA cells but fails to change the firing rate of DRN 5-HT cells. CBG reduces the inhibitory effect of the selective α2-adrenoceptor agonist UK14304 on LC NA cells and of the selective 5-HT1A receptor agonist ipsapirone on DRN 5-HT cells, but fails to alter the inhibitory effect of the endogenous neurotransmitters NA and 5-HT. In addition, CBG does not reverse the inhibitory effect of ipsapirone on the firing rate of DRN 5-HT cells. Besides, our data reveal that CBG produces anxiolytic-like effects in rat by a 5-HT1A receptor-mediated mechanism.
In this study, single-unit extracellular recording techniques in brain slices were used to study the regulation by CBG of 5-HT1A and α2-adrenoreceptors-mediated effects on the firing rate of LC NA and DRN 5-HT cells in rats. In vitro techniques of cell recording allow to isolate somatodendritic responses of neurons to bath application of drugs. The concentrations and time of application of cannabinoids and 5-HT1A and α2-adrenoreceptor agonists or antagonist were selected based on the reported Ki values for the receptors and their use in previous electrophysiological assays performed in monoaminergic nuclei from rat brain slices (Haj-Dahmane et al., 1991; Nörenberg et al., 1997; Corradetti et al., 1998; Mendiguren and Pineda, 2004; Grandoso et al., 2005; Jones et al., 2010; Mendiguren et al., 2022). For behavioral assays, the EPMT and NSFT were used to elucidate the effect of CBG on both the anxiolytic-like behavior and/or food consumption. CBG was administrated at the dose previously reported to produce an anxiolytic-like effect by the open-field test (Zagzoog et al., 2020) and at the time range in which the maximal concentration was achieved after i.p. injection in rodent (Deiana et al., 2012). Accordingly, the 5-HT1A receptor antagonist WAY100635 was administrated at the dose and time point reported to block 5-HT1A receptor-mediated anxiolytic effects in rodent (Bektas et al., 2020).
We observed that CBG induces a slight reduction of the firing rate of LC NA cells. A previous binding assay performed in mouse whole brain membranes has revealed that CBG behaves as a highly potent α2-adrenoceptor agonist (EC50 = 0.2 nM) (Cascio et al., 2010). In fact, stimulation of [35S]GTPɣS binding induced by CBG was blocked by the α2-adrenoceptor antagonist yohimbine. Additionally, electrically-evoked contractions of mouse vas deferens have been observed to be inhibited by CBG, further pointing to agonistic properties of the cannabinoid at the α2-adrenoceptor (Cascio et al., 2010).
However, in previous electrophysiological assays performed in slices from the LC, α2-adrenoceptor agonists have been shown to elicit complete inhibition of the neuronal activity of NA cells at rather low concentrations (nM) (Chiu et al., 1995), which would have been expected to occur after CBG perfusion. Moreover, binding assays showing potent agonist action of CBG at the α2-adrenoceptor were performed in brainstem membranes preparation, which contain several brain regions with different type of cells that may account for the overall effect of the cannabinoid. Therefore, although an α2-adrenoceptor antagonist was not tested in our study, one could speculate that the slight reduction of the firing rate of LC cells produced by CBG (30 µM) could rather result from a non-α2-adrenoceptor-mediated mechanism.
Interestingly, we observed that CBG regulates α2-adrenoceptor-mediated inhibitory responses on the neuronal activity of LC cells since the effect of the selective α2-adrenoceptor agonist UK14304 was reduced, although not that of the endogenous adrenergic neurotransmitter NA. This fact could be due to the lack of selectivity of NA onto α-adrenoreceptors since activation of α1-adrenoreceptors could have masked α2-adrenoreceptor-mediated inhibitory responses. Additionally, the endogenous adrenergic neurotransmitter NA could have been rapidly reuptake from the synaptic cleft while the structurally different UK14304 could remain longer in the slice preparation. One possible hypothesis that would explain the regulation of selective α2-adrenoceptor agonist-induced inhibition of the firing rate by CBG would be an indirect (i.e., allosteric) interaction with the α2-adrenoceptor.
In our study CBG fails to affect the firing rate of DRN 5-HT cells but reduces the inhibitory effect of the 5-HT1A receptor agonist ipsapirone in the DRN suggesting an antagonist action at the serotonergic receptor. Two approaches were used to test the effect of CBG on 5-HT1A receptor-mediated responses in the DRN cells. On the one hand, we perfused ipsapirone, which has been shown to cause a complete inhibition of the firing rate of DRN neurons at the concentration used (Haj-Dahmane et al., 1991; Mendiguren et al., 2022), both in the absence and the presence of CBG. As previously stated, we observed that CBG partially prevented the inhibitory effect of ipsapirone. On the other hand, the same concentration of CBG was used to investigate if it could mimick the effect of a classical competitive antagonist of the 5-HT1A receptor (i.e., WAY100635). Indeed, a potent competitive antagonist at 5-HT1A receptor in the DRN (Corradetti et al., 1998) would reverse the inhibition produced by a selective 5-HT1A receptor agonist in electrophysiological studies. Although few data exist on the interaction of CBG with the 5-HT1A receptor, our results are partially in line with the reported blockade of 5-HT1A receptor-mediated responses described by other authors in vitro (Cascio et al., 2010; Nachnani et al., 2021). Thus, CBG antagonized the effect of 5-HT1A receptor agonist 8-OH-DPAT on [35S]GTPɣS binding to mouse brain membranes (Cascio et al., 2010). However, Cascio et al. reported that CBG behaved as a neutral antagonist at 5-HT1A receptor while our study rather points to a non-competitive antagonist at the 5-HT1A receptor since CBG fails to reverse the inhibitory effect of ipsapirone on the firing activity of DRN 5-HT cells but the previously characterized competitive antagonist WAY100635 completely restores it. In fact, according to our results the effect of CBG on ipsapirone-induced inhibition of the firing rate of DRN 5-HT cells would not derive from its action onto the orthosteric site of the 5-HT1A receptor but would rather result from a negative allosteric modulation of the receptor. An indirect modulation of the 5-HT1A receptor has also been previously described for CBD (Rock et al., 2012; Galaj and Xi, 2021), one of the best studied non-psychoactive cannabinoid that shares pharmacological features with CBG (Nachnani et al., 2021). Thus, in brain slices from the DRN, CBD reduced the effect of 5-HT1A receptor agonist by an indirect mechanism (Mendiguren et al., 2022). As previously mentioned, the reasons behind the discrepancy between binding data (competitive antagonist) and electrophysiological assays (non-competitive antagonist) could rely on the differences in the preparations used (brain membranes vs. slices), techniques (binding vs. electrophysiology) or species (rat vs. mouse).
Our data show that CBG fails to modify the inhibition induced by 5-HT on the DRN. This is in line with previously reported lack of effect of the non-psychoactive CBD on 5-HT-induced inhibition (Mendiguren et al., 2022), which could result from the ability of 5-HT to activate non-5-HT1A receptors. Thus, ipsapirone has been shown to be selective for 5-HT1A receptor where as 5-HT has been described to activate 5-HT7 receptors (Roberts et al., 2004; Albert and Vahid-Ansari, 2019) and the presynaptic 5-HT1B receptors (Morikawa et al., 2000; Adell et al., 2001). 5-HT7 receptors modulate GABAergic neurons while some presynaptic 5-HT1B receptors have been shown to behave as heteroreceptors to inhibit glutamatergic neurotransmission in the DRN (Lemos et al., 2006; Geddes et al., 2016). In addition, several evidence shows that 5-HT and selective 5-HT1A receptor agonists may activate differently the 5-HT1A receptor. On the one hand, there are neurons that are completely inhibited by ipsapirone but are not equally responsive to 5-HT. On the other hand, 5-HT and selective 5-HT1A receptor agonist could also differ in coupling to adenylate cyclase (Varrault and Bockaert, 1992; Polter and Li, 2010).
In our study, CBG (10 mg/kg, i.p.) increases the time spent by the rats in the open arms or the number of head-dipping and reduces the anxiety index in the EPMT. In the NSFT, CBG decreases the time latency to feed in the novel environment. These are all indicative of an anxiolytic-like effect of the cannabinoid. Few studies have tested the anxiolytic effects of CBG in rodents and the reported results have been controversial. Thus, in agreement with our results, Zagzoog et al. demonstrated that acute administration of CBG produced anxiolytic-like effects in the open-field test in mice at the same dose, via and time point herein used (Zagzoog et al., 2020). In contrast, a very recent study has reported that injection of a single dose of CBG failed to produce anxiolytic effects in the light-dark test in mice (Zhou et al., 2022). However, in the latter study a mouse model of post-traumatic stress disorder was used, which could have accounted for a higher basal anxiety level of the animals and subsequent lack of effect of the cannabinoid.
With regard to previous data published in rats, it was reported that acute low doses of CBG (2.5 mg/kg, i.p.) failed to produce anxiolytic-like effects in the light-dark immersion test (O’Brien et al., 2013), which is in line with the lack of effect observed in our study at similar doses of CBG (3 mg/kg). In contrast to our results, other authors have reported that CBG did not produce anxiolytic effects even when given at higher doses in the open field test (Brierley et al., 2016). The discrepancy between the latter and our data could arise from the differences in the strain of rats (Lister Hooded vs. Sprague-Dawley) or route of administration (p.o vs. i.p.) used. In fact, Lister Hooded rats are known to be less responsive to anxiolytic drugs (McDermott and Kelly, 2008). Moreover, the amount of CBG reaching the site of action could have been substantially reduced after oral administration due to degradation.
However, at this point we cannot completely exclude other effects of CBG that could have contributed to the reduction of latency time to feed in the NSFT or to the increase in the time spent in the open arms in the EPMT observed in our study, such as alteration of motor activity. In view of previous authors data this seems unlikely to occur because no changes of locomotor activity have been reported at similar doses, via or administration time herein used in rodents (Brierley et al., 2016; Zagzoog et al., 2020). Furthermore, in our experiments CBG does not change the number of rearings in the EPMT, which have been related to alteration of motor activity (Cruz et al., 1994). Interestingly, we observed that CBG fails to alter food intake at the same dose that reduces the latency to feed in the novel environment, which is also in line with the lack of effect on food intake previously reported (Farrimond et al., 2012). In fact, both alteration of motor performance and hyperphagic effects of cannabinoids have been shown to occur mainly by activation of CB1 receptor (Farrimond et al., 2012; Polissidis et al., 2013) and low affinity at the cannabinoid receptor has been reported for CBG (Nachnani et al., 2021). Therefore, our findings further support an anxiolytic-like effect of CBG at the tested dose. At this stage, the putative involvement of the CB2 receptor in the effects of CBG could not be ruled out since the cannabinoid has been shown to target CB2 receptor (Navarro et al., 2018). Thus, previous evidence has reported the expression of CB2 receptor in several brain areas regulating emotional states (Kibret et al., 2022) and has described its role in anxiety-related behavior (García-Gutiérrez and Manzanares, 2011). Our study reveals that CBG decreases the time of first feeding event in the NSFT by a 5-HT1A receptor-mediated mechanism. Involvement of the 5-HT1A receptor in the in vivo effects of CBG has not been reported yet although 5-HT1A receptor-mediated neuroprotective effect of CBG has been recently observed in vitro (Echeverry et al., 2021). Thus, in previous studies showing anti-inflammatory (Carrillo-Salinas et al., 2014; Valdeolivas et al., 2015; Burgaz et al., 2021), analgesic or anxiolytic properties of CBG (Zagzoog et al., 2020) the effect of a 5-HT1A receptor antagonist was not tested. Given the fact that CBG shows similar pharmacological profile to CBD onto DRN 5-HT1A receptors in vitro and that CBD produces anxiolytic (Resstel et al., 2009) and other effects (analgesic, antiepileptic, antidepressant) via 5-HT1A receptor in vivo (Zanelati et al., 2010; Linge et al., 2016; Sartim et al., 2016; De Gregorio et al., 2019; Peng et al., 2022) we cannot rule out that CBG may regulate DRN 5-HT1A receptor to mediate some in vivo effect (i.e., anxiolytic). Additionally, in view of our results revealing modulation of α2-adrenoceptor-mediated inhibitory effects by CBG in the LC, the involvement of this receptor in the anxiolytic effects of the cannabinoid should not be discarded. Considering that anxiolytic effects have been attributed to some drugs that regulate the α2-adrenoceptor (Garakani et al., 2020) it would be of interest to investigate the role of the α2-adrenoceptor in the in vivo effects of CBG.
In conclusion, CBG slightly affects the firing rate of LC NA cells but not that of DRN 5-HT cells and hinders both the inhibitory effect produced by selective α2-adrenoceptor agonist on the NA-LC cells and by 5-HT1A receptor agonist on 5-HT-DRN cells in vitro. One possible hypothesis is an allosteric modulation of LC α2-adrenoceptor and DRN 5-HT1A receptor by CBG. Furthermore, the data suggest that CBG produces anxiolytic effect in rat through a 5-HT1A receptor-mediated mechanism. In the future, it would be interesting to study the possible contribution of the modulation of 5-HT1A receptors located in the DRN to the in vivo effects of the non-psychoactive cannabinoids.
To our knowledge this is the first study in which the effect of CBG on the neuronal activity of 5-HT and NA cells in vitro was tested and the involvement of 5-HT1A receptor in an in vivo effect induced by CBG was reported. To characterize the effect of CBG on the neuronal activity of LC and DRN could be interesting in view of the role of these nuclei in the modulation of emotional state, arousal or control of pain, functions that could be altered by cannabinoids (Lowry et al., 2008; McDevitt and Neumaier, 2011; Campion et al., 2016). Our study provides new insights into the effects of CBG on monoaminergic systems in the rat. However, more studies are needed to elucidate the effects of CBG on NA and 5-HT systems both in vitro and in vivo and to delve into the mechanisms by which the cannabinoid regulate 5-HT1A and α2-adrenoceptors.
The raw data supporting the conclusion of this article will be made available by the authors, without undue reservation.
The experiments were conducted following the European Directive on the protection of animals for scientific purposes (2010/63/EU). All the procedures were accepted by the Institutional Ethical Committee for Research and Teaching of the University of the Basque Country (UPV/EHU, Spain) and the Department of Sustainability and Natural Environment of Provincial Council from Bizkaia (ref. CEEA M20-2018-025 and CEEA M20-2018-262).
AM, EA, and IR performed the electrophysiological assays. AM, IP, and EN performed behavioral studies. AM and JP conceived and designed the study and wrote the manuscript. All authors contributed to the article and approved the submitted version.
This work was supported by the Ministerio de Sanidad, Consumo y Bienestar Social. Delegación del Gobierno para el Plan Nacional Sobre Drogas, PND 2018I025, (PND18/04) and University of the Basque Country (UPV/EHU) (Grant GIU19/076). EA and IR were supported by a predoctoral fellowship from the Basque and Spanish Government, respectively.
The authors declare that the research was conducted in the absence of any commercial or financial relationships that could be construed as a potential conflict of interest.
All claims expressed in this article are solely those of the authors and do not necessarily represent those of their affiliated organizations, or those of the publisher, the editors and the reviewers. Any product that may be evaluated in this article, or claim that may be made by its manufacturer, is not guaranteed or endorsed by the publisher.
ACSF, artificial cerebrospinal fluid; CBD, cannabidiol; CBG, cannabigerol; CNS, central nervous system; DMSO, dimethylsulphoxide; DRN, dorsal raphe nucleus; EPMT, elevated plus maze test; GABA, γ-aminobutyric acid; GIRKs, G protein-coupled inwardly rectifying potassium channels; 5-HT, serotonin; ipsapirone, 2-[4-[4-(2-pyrimidinyl)-1-piperazinyl]butyl]-1,2-benzisothiazol-3(2H)-one-1,1-dioxide; LC, locus coeruleus; NA, noradrenaline; NSFT, novelty-suppressed feeding test; PE, Phenylephrine; UK14304, 5-Bromo-6-(2-imidazolin-2-ylamino)quinoxaline tartrate; WAY100635, N-[2-[4-(2-Methoxyphenyl)-1-piperazinyl]ethyl]-N-2-yridinylcyclohexanecarboxamide.
Adell, A., Celada, P., and Artigas, F. (2001). The role of 5-HT1B receptors in the regulation of serotonin cell firing and release in the rat brain. J. Neurochem. 79, 172–182. doi:10.1046/j.1471-4159.2001.00550.x
Aghajanian, G. K., and Lakoski, J. M. (1984). Hyperpolarization of serotonergic neurons by serotonin and LSD: Studies in brain slices showing increased K+ conductance. Brain Res. 305, 181–185. doi:10.1016/0006-8993(84)91137-5
Aghajanian, G. K., and Wang, Y. Y. (1987). Common alpha 2- and opiate effector mechanisms in the locus coeruleus: Intracellular studies in brain slices. Neuropharmacology 26, 793–799. doi:10.1016/0028-3908(87)90054-2
Albert, P. R., and Vahid-Ansari, F. (2019). The 5-HT1A receptor: Signaling to behavior. Biochimie 161, 34–45. doi:10.1016/j.biochi.2018.10.015
Andrade, R., and Aghajanian, G. K. (1984). Locus coeruleus activity in vitro: Intrinsic regulation by a calcium-dependent potassium conductance but not alpha 2-adrenoceptors. J. Neurosci. 4, 161–170. doi:10.1523/JNEUROSCI.04-01-00161.1984
Aston-Jones, G., Rajkowski, J., and Cohen, J. (1999). Role of locus coeruleus in attention and behavioral flexibility. Biol. Psychiatry 46, 1309–1320. doi:10.1016/S0006-3223(99)00140-7
Bambico, F. R., Hattan, P. R., Garant, J. P., and Gobbi, G. (2012). Effect of delta-9-tetrahydrocannabinol on behavioral despair and on pre- and postsynaptic serotonergic transmission. Prog. Neuro-Psychopharmacology Biol. Psychiatry 38, 88–96. doi:10.1016/j.pnpbp.2012.02.006
Bektas, N., Arslan, R., and Alyu, F. (2020). The anxiolytic effect of perampanel and possible mechanisms mediating its anxiolytic effect in mice. Life Sci. 261, 118359. doi:10.1016/j.lfs.2020.118359
Brierley, D. I., Samuels, J., Duncan, M., Whalley, B. J., and Williams, C. M. (2016). Cannabigerol is a novel, well-tolerated appetite stimulant in pre-satiated rats. Psychopharmacol. Berl. 233, 3603–3613. doi:10.1007/s00213-016-4397-4
Burgaz, S., García, C., Gómez-Cañas, M., Navarrete, C., García-Martín, A., Rolland, A., et al. (2021). Neuroprotection with the cannabigerol quinone derivative VCE-003.2 and its analogs CBGA-Q and CBGA-Q-Salt in Parkinson’s disease using 6-hydroxydopamine-lesioned mice. Mol. Cell. Neurosci. 110, 103583. doi:10.1016/j.mcn.2020.103583
Campion, K. N., Saville, K. A., and Morgan, M. M. (2016). Relative contribution of the dorsal raphe nucleus and ventrolateral periaqueductal gray to morphine antinociception and tolerance in the rat. Eur. J. Neurosci. 44, 2667–2672. doi:10.1111/ejn.13378
Carrillo-Salinas, F. J., Navarrete, C., Mecha, M., Feliú, A., Collado, J. A., Cantarero, I., et al. (2014). A cannabigerol derivative suppresses immune responses and protects mice from experimental autoimmune encephalomyelitis. PLoS One 9, 947333–e94812. doi:10.1371/journal.pone.0094733
Cascio, M. G., Gauson, L. A., Stevenson, L. A., Ross, R. A., and Pertwee, R. G. (2010). Evidence that the plant cannabinoid cannabigerol is a highly potent alpha2-adrenoceptor agonist and moderately potent 5HT1A receptor antagonist. Br. J. Pharmacol. 159, 129–141. doi:10.1111/j.1476-5381.2009.00515.x
Chiu, T., Chen, M., Yang, Y., Yang, J., and Tang, F. (1995). Action of dexmedetomidine on rat locus coeruleus neurones: Intracellular recording in vitro. Eur. J. Pharmacol. 285, 261–268. doi:10.1016/0014-2999(95)00417-j
Cohen, H., Matar, M. A., and Joseph, Z. (2013). Animal models of post-traumatic stress disorder. Curr. Protoc. Neurosci. 1, 5–18. doi:10.1002/0471142301.ns0945s64
Corradetti, R., Laaris, N., Hanoun, N., Laporte, A. M., Le Poul, E., Hamon, M., et al. (1998). Antagonist properties of (-)-pindolol and WAY 100635 at somatodendritic and postsynaptic 5-HT1A receptors in the rat brain. Br. J. Pharmacol. 123 (3), 449–462. doi:10.1038/sj.bjp.0701632
Courtney, N. A., and Ford, C. P. (2016). Mechanisms of 5-HT1A receptor-mediated transmission in dorsal raphe serotonin neurons. J. Physiol. 594, 953–965. doi:10.1113/JP271716
Cruz, A. P. M., Frei, F., and Graeff, F. G. (1994). Ethopharmacological analysis of rat behavior on the elevated plus-maze. Pharmacol. Biochem. Behav. 49, 171–176. doi:10.1016/0091-3057(94)90472-3
De Gregorio, D., McLaughlin, R. J., Posa, L., Ochoa-Sanchez, R., Enns, J., Lopez-Canul, M., et al. (2019). Cannabidiol modulates serotonergic transmission and reverses both allodynia and anxiety-like behavior in a model of neuropathic pain. Pain 160, 136–150. doi:10.1097/j.pain.0000000000001386
Deiana, S., Watanabe, A., Yamasaki, Y., Amada, N., Arthur, M., Fleming, S., et al. (2012). Plasma and brain pharmacokinetic profile of cannabidiol (CBD), cannabidivarine (CBDV), Δ⁹-tetrahydrocannabivarin (THCV) and cannabigerol (CBG) in rats and mice following oral and intraperitoneal administration and CBD action on obsessive-compulsive behaviour. Psychopharmacol. Berl. 219, 859–873. doi:10.1007/s00213-011-2415-0
di Giacomo, V., Chiavaroli, A., Orlando, G., Cataldi, A., Rapino, M., di Valerio, V., et al. (2020). Neuroprotective and neuromodulatory effects induced by cannabidiol and cannabigerol in rat hypo-E22 cells and isolated hypothalamus. Antioxidants 9. doi:10.3390/antiox9010071
Echeverry, C., Prunell, G., Narbondo, C., de Medina, V. S., Nadal, X., Reyes-Parada, M., et al. (2021). A comparative in vitro study of the neuroprotective effect induced by cannabidiol, cannabigerol, and their respective acid forms: Relevance of the 5-ht1a receptors. Neurotox. Res. 39, 335–348. doi:10.1007/s12640-020-00277-y
Farrimond, J. A., Whalley, B. J., and Williams, C. M. (2012). Cannabinol and cannabidiol exert opposing effects on rat feeding patterns. Psychopharmacol. Berl. 223, 117–129. doi:10.1007/s00213-012-2697-x
Felippotti, T. T., Dos Reis Ferreira, C. M., De Freitas, R. L., De Oliveira, R. C., De Oliveira, R., Paschoalin-Maurin, T., et al. (2011). Paradoxical effect of noradrenaline-mediated neurotransmission in the antinociceptive phenomenon that accompanies tonic-clonic seizures: Role of locus coeruleus neurons and α(2)- and β-noradrenergic receptors. Epilepsy Behav. 22, 165–177. doi:10.1016/j.yebeh.2011.06.028
Galaj, E., and Xi, Z. X. (2021). Possible receptor mechanisms underlying cannabidiol effects on addictive-like behaviors in experimental animals. Int. J. Mol. Sci. 22, 134–214. doi:10.3390/ijms22010134
Garakani, A., Murrough, J. W., Freire, R. C., Thom, R. P., Larkin, K., Buono, F. D., et al. (2020). Pharmacotherapy of anxiety disorders: Current and emerging treatment options. Front. Psychiatry 11, 595584–595621. doi:10.3389/fpsyt.2020.595584
García-Gutiérrez, M. S., and Manzanares, J. (2011). Overexpression of CB2 cannabinoid receptors decreased vulnerability to anxiety and impaired anxiolytic action of alprazolam in mice. J. Psychopharmacol. 25, 111–120. doi:10.1177/0269881110379507
Geddes, S. D., Assadzada, S., Lemelin, D., Sokolovski, A., Bergeron, R., Haj-Dahmane, S., et al. (2016). Target-specific modulation of the descending prefrontal cortex inputs to the dorsal raphe nucleus by cannabinoids. Proc. Natl. Acad. Sci. U. S. A. 113, 5429–5434. doi:10.1073/pnas.1522754113
Gobbi, G., Bambico, F. R., Mangieri, R., Bortolato, M., Campolongo, P., Solinas, M., et al. (2005). Antidepressant-like activity and modulation of brain monoaminergic transmission by blockade of anandamide hydrolysis. Proc. Natl. Acad. Sci. U. S. A. 102, 18620–18625. doi:10.1073/pnas.0509591102
Grandoso, L., Torrecilla, M., Pineda, J., and Ugedo, L. (2005). alpha(2)-Adrenoceptor involvement in the in vitro inhibitory effect of citalopram on a subpopulation of rat locus coeruleus neurons. Eur. J. Pharmacol. 517, 51–58. doi:10.1016/j.ejphar.2005.05.033
Griebel, G., Rodgers, R. J., Perrault, G., and Sanger, D. J. (1997). Risk assessment behaviour: Evaluation of utility in the study of 5-HT-related drugs in the rat elevated plus-maze test. Pharmacol. Biochem. Behav. 57, 817–827. doi:10.1016/S0091-3057(96)00402-9
Haj-Dahmane, S., Hamon, M., and Lanfumey, L. (1991). K+ channel and 5-hydroxytryptamine1A autoreceptor interactions in the rat dorsal raphe nucleus: An in vitro electrophysiological study. Neuroscience 41, 495–505. doi:10.1016/0306-4522(91)90344-N
Hajós, M., Sharp, T., and Newberry, N. R. (1996). Intracellular recordings from burst-firing presumed serotonergic neurones in the rat dorsal raphe nucleus in vivo. Brain Res. 737, 308–312. doi:10.1016/0006-8993(96)00936-5
Jones, N. A., Hill, A. J., Smith, I., Bevan, S. A., Williams, C. M., Whalley, B. J., et al. (2010). Cannabidiol displays antiepileptiform and antiseizure properties in vitro and in vivo. J. Pharmacol. Exp. Ther. 332, 569–577. doi:10.1124/jpet.109.159145
Kibret, B. G., Ishiguro, H., Horiuchi, Y., and Onaivi, E. S. (2022). New insights and potential therapeutic targeting of CB2 cannabinoid receptors in CNS disorders. Int. J. Mol. Sci. 23, 975. doi:10.3390/ijms23020975
Kirilly, E., Hunyady, L., and Bagdy, G. (2013). Opposing local effects of endocannabinoids on the activity of noradrenergic neurons and release of noradrenaline: Relevance for their role in depression and in the actions of CB1 receptor antagonists. J. Neural Transm. 120, 177–186. doi:10.1007/s00702-012-0900-1
Lemos, J. C., Pan, Y. Z., Ma, X., Lamy, C., Akanwa, A. C., and Beck, S. G. (2006). Selective 5-HT receptor inhibition of glutamatergic and GABAergic synaptic activity in the rat dorsal and median raphe. Eur. J. Neurosci. 24, 3415–3430. doi:10.1111/j.1460-9568.2006.05222.x
Lin, Y., Sarfraz, Y., Jensen, A., Dunn, A. J., and Stone, E. A. (2011). Participation of brainstem monoaminergic nuclei in behavioral depression. Pharmacol. Biochem. Behav. 100, 330–339. doi:10.1016/j.pbb.2011.08.021
Linge, R., Jiménez-Sánchez, L., Campa, L., Pilar-Cuéllar, F., Vidal, R., Pazos, A., et al. (2016). Cannabidiol induces rapid-acting antidepressant-like effects and enhances cortical 5-HT/glutamate neurotransmission: Role of 5-HT1A receptors. Neuropharmacology 103, 16–26. doi:10.1016/j.neuropharm.2015.12.017
Lowry, C. A., Hale, M. W., Evans, A. K., Heerkens, J., Staub, D. R., Gasser, P. J., et al. (2008). Serotonergic systems, anxiety, and affective disorder: Focus on the dorsomedial part of the dorsal raphe nucleus. Ann. N. Y. Acad. Sci. 1148, 86–94. doi:10.1196/annals.1410.004
Marinho, A. L. Z., Vila-Verde, C., Fogaça, M. V., and Guimarães, F. S. (2015). Effects of intra-infralimbic prefrontal cortex injections of cannabidiol in the modulation of emotional behaviors in rats: Contribution of 5HT₁A receptors and stressful experiences. Behav. Brain Res. 286, 49–56. doi:10.1016/j.bbr.2015.02.023
McDermott, C., and Kelly, J. P. (2008). Comparison of the behavioural pharmacology of the Lister-Hooded with 2 commonly utilised albino rat strains. Prog. Neuro-Psychopharmacology Biol. Psychiatry 32, 1816–1823. doi:10.1016/j.pnpbp.2008.08.004
McDevitt, R. A., and Neumaier, J. F. (2011). Regulation of dorsal raphe nucleus function by serotonin autoreceptors: A behavioral perspective. J. Chem. Neuroanat. 41, 234–246. doi:10.1016/j.jchemneu.2011.05.001
Medrano, M. C., Santamarta, M. T., Pablos, P., Aira, Z., Buesa, I., Azkue, J. J., et al. (2017). Characterization of functional μ opioid receptor turnover in rat locus coeruleus: An electrophysiological and immunocytochemical study. Br. J. Pharmacol. 174, 2758–2772. doi:10.1111/bph.13901
Mendiguren, A., Aostri, E., Alberdi, E., Pérez-Samartín, A., and Pineda, J. (2022). Functional characterization of cannabidiol effect on the serotonergic neurons of the dorsal raphe nucleus in rat brain slices. Front. Pharmacol. 13, 956886–956913. doi:10.3389/fphar.2022.956886
Mendiguren, A., Aostri, E., and Pineda, J. (2018). Regulation of noradrenergic and serotonergic systems by cannabinoids: Relevance to cannabinoid-induced effects. Life Sci. 192, 115–127. doi:10.1016/j.lfs.2017.11.029
Mendiguren, A., and Pineda, J. (2004). Cannabinoids enhance N-methyl-D-aspartate-induced excitation of locus coeruleus neurons by CB1 receptors in rat brain slices. Neurosci. Lett. 363, 1–5. doi:10.1016/j.neulet.2004.02.073
Mendiguren, A., and Pineda, J. (2009). Effect of the CB 1 receptor antagonists rimonabant and AM251 on the firing rate of dorsal raphe nucleus neurons in rat brain slices. Br. J. Pharmacol. 158, 1579–1587. doi:10.1111/j.1476-5381.2009.00434.x
Michelsen, K. A., Schmitz, C., and Steinbusch, H. W. M. (2007). The dorsal raphe nucleus-From silver stainings to a role in depression. Brain Res. Rev. 55, 329–342. doi:10.1016/j.brainresrev.2007.01.002
Monti, J. M. (2010). The structure of the dorsal raphe nucleus and its relevance to the regulation of sleep and wakefulness. Sleep. Med. Rev. 14, 307–317. doi:10.1016/j.smrv.2009.11.004
Morikawa, H., Manzoni, O. J., Crabbe, J. C., and Williams, J. T. (2000). Regulation of central synaptic transmission by 5-HT(1B) auto- and Heteroreceptors. Mol. Pharmacol. 58, 1271–1278. doi:10.1124/mol.58.6.1271
Morris, L. S., McCall, J. G., Charney, D. S., and Murrough, J. W. (2020). The role of the locus coeruleus in the generation of pathological anxiety. Brain Neurosci. Adv. 4, 2398212820930321. doi:10.1177/2398212820930321
Muntoni, A. L., Pillolla, G., Melis, M., Perra, S., Gessa, G. L., and Pistis, M. (2006). Cannabinoids modulate spontaneous neuronal activity and evoked inhibition of locus coeruleus noradrenergic neurons. Eur. J. Neurosci. 23, 2385–2394. doi:10.1111/j.1460-9568.2006.04759.x
Nachnani, R., Raup-Konsavage, W. M., and Vrana, K. E. (2021). The pharmacological case for cannabigerol. J. Pharmacol. Exp. Ther. 376, 204–212. doi:10.1124/jpet.120.000340
Navarro, G., Varani, K., Reyes-Resina, I., de Medina, V. S., Rivas-Santisteban, R., Callado, C. S. C., et al. (2018). Cannabigerol action at cannabinoid CB1 and CB2 receptors and at CB1-CB2 heteroreceptor complexes. Front. Pharmacol. 9, 632–714. doi:10.3389/fphar.2018.00632
Nörenberg, W., Schöffel, E., Szabo, B., and Starke, K. (1997). Subtype determination of soma-dendritic alpha2-autoreceptors in slices of rat locus coeruleus. Naunyn. Schmiedeb. Arch. Pharmacol. 356, 159–165. doi:10.1007/PL00005036
O’Brien, L. D., Wills, K. L., Segsworth, B., Dashney, B., Rock, E. M., Limebeer, C. L., et al. (2013). Effect of chronic exposure to rimonabant and phytocannabinoids on anxiety-like behavior and saccharin palatability. Pharmacol. Biochem. Behav. 103, 597–602. doi:10.1016/j.pbb.2012.10.008
Peng, J., Fan, M., An, C., Ni, F., Huang, W., and Luo, J. (2022). A narrative review of molecular mechanism and therapeutic effect of cannabidiol (CBD). Basic Clin. Pharmacol. Toxicol. 130, 439–456. doi:10.1111/bcpt.13710
Polissidis, A., Galanopoulos, A., Naxakis, G., Papahatjis, D., Papadopoulou-Daifoti, Z., and Antoniou, K. (2013). The cannabinoid CB1 receptor biphasically modulates motor activity and regulates dopamine and glutamate release region dependently. Int. J. Neuropsychopharmacol. 16, 393–403. doi:10.1017/S1461145712000156
Polter, A. M., and Li, X. (2010). 5-HT1A receptor-regulated signal transduction pathways in brain. Cell. Signal. 22, 1406–1412. doi:10.1016/j.cellsig.2010.03.019
Resstel, L. B. M., Tavares, R. F., Lisboa, S. F. S., Joca, S. R. L., Corrêa, F. M. A., and Guimarães, F. S. (2009). 5-HT 1A receptors are involved in the cannabidiol-induced attenuation of behavioural and cardiovascular responses to acute restraint stress in rats. Br. J. Pharmacol. 156, 181–188. doi:10.1111/j.1476-5381.2008.00046.x
Roberts, C., Thomas, D. R., Bate, S. T., and Kew, J. N. C. (2004). GABAergic modulation of 5-HT7 receptor-mediated effects on 5-HT efflux in the Guinea-pig dorsal raphe nucleus. Neuropharmacology 46, 935–941. doi:10.1016/j.neuropharm.2004.01.010
Rock, E. M., Bolognini, D., Limebeer, C. L., Cascio, M. G., Anavi-Goffer, S., Fletcher, P. J., et al. (2012). Cannabidiol, a nonpsychotropic component of cannabis, attenuates vomiting and nausea-like behaviour via indirect agonism of 5-HT 1A somatodendritic autoreceptors in the dorsal raphe nucleus. Br. J. Pharmacol. 165, 2620–2634. doi:10.1111/j.1476-5381.2011.01621.x
Rock, E. M., Goodwin, J. M., Limebeer, C. L., Breuer, A., Pertwee, R. G., Mechoulam, R., et al. (2011). Interaction between non-psychotropic cannabinoids in marihuana: Effect of cannabigerol (CBG) on the anti-nausea or anti-emetic effects of cannabidiol (CBD) in rats and shrews. Psychopharmacol. Berl. 215, 505–512. doi:10.1007/s00213-010-2157-4
Sales, A. J., Crestani, C. C., Guimarães, F. S., and Joca, S. R. L. (2018). Antidepressant-like effect induced by Cannabidiol is dependent on brain serotonin levels. Prog. Neuro-Psychopharmacology Biol. Psychiatry 86, 255–261. doi:10.1016/j.pnpbp.2018.06.002
Sartim, A. G., Guimarães, F. S., and Joca, S. R. L. (2016). Antidepressant-like effect of cannabidiol injection into the ventral medial prefrontal cortex-Possible involvement of 5-HT1A and CB1 receptors. Behav. Brain Res. 303, 218–227. doi:10.1016/j.bbr.2016.01.033
Shephard, R. A., and Broadhurst, P. L. (1982). Hyponeophagia and arousal in rats: Effects of diazepam, 5-methoxy-N,N-dimethyltryptamine, d-amphetamine and food deprivation. Psychopharmacol. Berl. 78, 368–372. doi:10.1007/BF00433744
Silvestro, S., Schepici, G., Bramanti, P., and Mazzon, E. (2020). Molecular targets of cannabidiol in experimental models of neurological disease. Molecules 25, 5186–5215. doi:10.3390/molecules25215186
Suárez-Pereira, I., Llorca-Torralba, M., Bravo, L., Camarena-Delgado, C., Soriano-Mas, C., and Berrocoso, E. (2022). The role of the locus coeruleus in pain and associated stress-related disorders. Biol. Psychiatry 91, 786–797. doi:10.1016/j.biopsych.2021.11.023
Takahashi, K., Kayama, Y., Lin, J. S., and Sakai, K. (2010). Locus coeruleus neuronal activity during the sleep-waking cycle in mice. Neuroscience 169, 1115–1126. doi:10.1016/j.neuroscience.2010.06.009
Turner, S. E., Williams, C. M., Iversen, L., and Whalley, B. J. (2017). “Molecular pharmacology of phytocannabinoids,” in Phytocannabinoids: Unraveling the complex chemistry and Pharmacology of cannabis sativa. Editors A. D. Kinghorn, H. Falk, S. Gibbons, and J. Kobayashi (Berlin, Germany: Springer), 61–101. doi:10.1007/978-3-319-45541-9_3
Valdeolivas, S., Navarrete, C., Cantarero, I., Bellido, M. L., Muñoz, E., and Sagredo, O. (2015). Neuroprotective properties of cannabigerol in huntington’s disease: Studies in R6/2 mice and 3-Nitropropionate-lesioned mice. Neurotherapeutics 12, 185–199. doi:10.1007/s13311-014-0304-z
Varrault, A., and Bockaert, J. (1992). Differential coupling of 5-HT1A receptors occupied by 5-HT or 8-OH-DPAT to adenylyl cyclase. Naunyn. Schmiedeb. Arch. Pharmacol. 346, 367–374. doi:10.1007/BF00171076
Walf, A. A., and Frye, C. A. (2007). The use of the elevated plus maze as an assay of anxiety-related behavior in rodents. Nat. Protoc. 2, 322–328. doi:10.1038/nprot.2007.44
Warren, W. G., Papagianni, E. P., Stevenson, C. W., and Stubbendorff, C. (2022). In it together? The case for endocannabinoid–noradrenergic interactions in fear extinction. Eur. J. Neurosci. 55, 952–970. doi:10.1111/ejn.15200
Williams, C. M., and Kirkham, T. C. (2002). Observational analysis of feeding induced by Delta9-THC and anandamide. Physiol. Behav. 76, 241–250. doi:10.1016/S0031-9384(02)00725-4
Zagzoog, A., Mohamed, K. A., Kim, H. J. J., Kim, E. D., Frank, C. S., Black, T., et al. (2020). In vitro and in vivo pharmacological activity of minor cannabinoids isolated from Cannabis sativa. Sci. Rep. 10, 20405–20413. doi:10.1038/s41598-020-77175-y
Zanelati, T. V., Biojone, C., Moreira, F. A., Guimarães, F. S., and Joca, S. R. L. (2010). Antidepressant-like effects of cannabidiol in mice: Possible involvement of 5-HT 1A receptors. Br. J. Pharmacol. 159, 122–128. doi:10.1111/j.1476-5381.2009.00521.x
Keywords: dorsal raphe nucleus, slice, firing, noradrenaline, locus coeruleus, cannabigerol, anxiety, serotonin
Citation: Mendiguren A, Aostri E, Rodilla I, Pujana I, Noskova E and Pineda J (2023) Cannabigerol modulates α2-adrenoceptor and 5-HT1A receptor-mediated electrophysiological effects on dorsal raphe nucleus and locus coeruleus neurons and anxiety behavior in rat. Front. Pharmacol. 14:1183019. doi: 10.3389/fphar.2023.1183019
Received: 09 March 2023; Accepted: 12 May 2023;
Published: 25 May 2023.
Edited by:
Francisco Navarrete Rueda, Miguel Hernández University of Elche, SpainReviewed by:
Alline C. Campos, University of São Paulo, BrazilCopyright © 2023 Mendiguren, Aostri, Rodilla, Pujana, Noskova and Pineda. This is an open-access article distributed under the terms of the Creative Commons Attribution License (CC BY). The use, distribution or reproduction in other forums is permitted, provided the original author(s) and the copyright owner(s) are credited and that the original publication in this journal is cited, in accordance with accepted academic practice. No use, distribution or reproduction is permitted which does not comply with these terms.
*Correspondence: Aitziber Mendiguren, YWl0emliZXIubWVuZGlndXJlbkBlaHUuZXVz
Disclaimer: All claims expressed in this article are solely those of the authors and do not necessarily represent those of their affiliated organizations, or those of the publisher, the editors and the reviewers. Any product that may be evaluated in this article or claim that may be made by its manufacturer is not guaranteed or endorsed by the publisher.
Research integrity at Frontiers
Learn more about the work of our research integrity team to safeguard the quality of each article we publish.