- 1Chongqing Municipal Key Laboratory of Oral Biomedical Engineering of Higher Education, Chongqing Medical University, Chongqing, China
- 2Chongqing Key Laboratory of Oral Diseases and Biomedical Sciences, Chongqing Medical University, Chongqing, China
- 3State Key Laboratory of Ultrasound in Medicine and Engineering, College of Biomedical Engineering, Chongqing Medical University, Chongqing, China
- 4Department of Oral and Maxillofacial Surgery, Stomatological Hospital of Chongqing Medical University, Chongqing, China
- 5Department of Oral Sciences, Sir John Walsh Research Institute, Faculty of Dentistry, University of Otago, Dunedin, New Zealand
In the last decade, immune checkpoint blockade (ICB) has revolutionized the standard of treatment for solid tumors. Despite success in several immunogenic tumor types evidenced by improved survival, ICB remains largely unresponsive, especially in “cold tumors” with poor lymphocyte infiltration. In addition, side effects such as immune-related adverse events (irAEs) are also obstacles for the clinical translation of ICB. Recent studies have shown that focused ultrasound (FUS), a non-invasive technology proven to be effective and safe for tumor treatment in clinical settings, could boost the therapeutic effect of ICB while alleviating the potential side effects. Most importantly, the application of FUS to ultrasound-sensitive small particles, such as microbubbles (MBs) or nanoparticles (NPs), allows for precise delivery and release of genetic materials, catalysts and chemotherapeutic agents to tumor sites, thus enhancing the anti-tumor effects of ICB while minimizing toxicity. In this review, we provide an updated overview of the progress made in recent years concerning ICB therapy assisted by FUS-controlled small-molecule delivery systems. We highlight the value of different FUS-augmented small-molecules delivery systems to ICB and describe the synergetic effects and underlying mechanisms of these combination strategies. Furthermore, we discuss the limitations of the current strategies and the possible ways that FUS-mediated small-molecule delivery systems could boost novel personalized ICB treatments for solid tumors.
1 Introduction
Cancers are devastating life-threatening diseases. Globally, an estimated 19.3 million new cancer cases, and nearly 10 million cancer deaths, occurred in 2020. By 2040, the global cancer burden is expected to reach 28.4 million cases (Sung et al., 2021). Surgery, chemotherapy and radiotherapy are the major therapies for malignant tumors (Wan et al., 2016), yet frequently they fail to cure a range of cancers, especially solid tumors because of post-surgical tumor residue, resistance to radiotherapy and chemotherapy, or the broad spectrum functional status of the tumor microenvironment (TME) (Xiao and Yu, 2021; Zhang C. et al., 2022). In recent years, the emergence and development of immunotherapy has changed the paradigm of cancer therapy (Mellman et al., 2011; Rosenberg, 2014; Riley et al., 2019). Immunotherapy activates and enhances the anti-tumor immune response, as well as alleviating tumor escape by manipulating the patient’s autoimmune system (Abbott and Ustoyev, 2019; Riley et al., 2019; Kennedy and Salama, 2020). In a variety of experiments and clinical studies, it has been found that immunotherapy has advantages that traditional anti-tumor therapy cannot match. These advantages include: 1) high accuracy, specificity and targeting (Salgia et al., 2003; Schanda et al., 2021); 2) wide adaptability that can control and kill multiple types of tumors (Schmid et al., 2018; Zhang and Zhang, 2020); 3) thorough removal of residual tumor cells (Tan S. et al., 2020); 4) sustained clinical responses to the immunotherapy (Forde et al., 2018; Zhang and Zhang, 2020); and 5) fewer side effects than the traditional treatments (Tan S. et al., 2020). At present, there are approximately a dozen different kinds of immunotherapies for cancer treatment, including immune checkpoint inhibitors (ICIs) that cause immune checkpoint blockade (ICB), lymphocyte-activating cytokines, chimeric antigen receptor (CAR)-T cells or other cellular therapies, agonistic antibodies against co-stimulatory receptors, bispecific antibodies, tumor vaccines and oncolytic viruses (Dillman, 2011; Riley et al., 2019; Ou et al., 2022). Among these strategies, ICB is one of the most well-studied and promising immunotherapies (Webb et al., 2018) because it often has a broader applicability across cancer types and results in a more durable clinical response than other strategies when treatment is effective (Ribas and Wolchok, 2018; He and Xu, 2020). Despite the significant advances achieved, the clinical application of ICB has been impeded by multiple challenges, both in terms of effectiveness (Maleki Vareki et al., 2017) and safety (June et al., 2017). The physical and chemical barriers in solid tumors greatly hinder the entry of therapeutic agents, and this may be responsible for the low response rate in some patients (Kruger et al., 2019). Additionally, the autoimmune side effects caused by systemic delivery of ICB could be dangerous and difficult to overcome (Morad et al., 2021). These problems require novel approaches to administer ICB in a more effective and controllable manner. Advances in delivery technologies, in particular, could improve the curative potential of ICB and could also reduce ICB-related toxicities (Kubli et al., 2021). Multiple delivery modes, including photons (Cheng H. et al., 2022; Chen et al., 2022), ultrasound (Lee H. et al., 2022), alternating magnetic fields (Ge et al., 2023), and radiofrequencies (Shou et al., 2022), can increase the accumulation of small-molecule drugs within the TME, enable more efficient and precise targeting of the specific tumor and/or immune cells, re-shape the TME and reduce off-target adverse effects (Izci et al., 2021). Therefore, combining ICB with various delivery platforms has been one of the promising strategies to improve ICB for solid tumors (Gong et al., 2021).
Among these combination strategies, the recently much-discussed technology, focused ultrasound (FUS), has been found to enhance the anti-tumor immune response in humans (Quaia et al., 2006; Mahnken et al., 2013). Sonodynamic therapy (SDT), which is an application of FUS (Mess et al., 2023), refers to sonochemical or sonophotochemical events in the acoustic field that result in cytotoxicity dependent on the presence of a sensitizer (Xu et al., 2023). It can promote the killing of tumor cells by various mechanisms, including ROS generation and ultrasonic cavitation (Guo et al., 2022). However, such therapy is usually associated with inertial cavitation and unnecessary healthy tissue damage due to the insufficient sensitivity of current sonosensitizers to ultrasound (Fan et al., 2023). In addition to its use in SDT, FUS also excels in the field of treatment through its thermal and mechanical effects (Sivaramakrishnan and Incharoensakdi, 2018; Baek et al., 2021). FUS has the potential to enhance the therapeutic effect of ICB while simultaneously alleviating the potential side effects in solid tumors (Wu et al., 2022). More importantly, FUS-assisted drug deliver is a promising application in solid tumors because it can deliver small-molecule drugs to targeted deep areas with a high degree of precision and minimize the adverse effects on healthy tissues. Small molecules could also be directly delivered into cells by FUS through a variety of mechanisms (Armulik et al., 2010). Furthermore, the FUS-mediated delivery system can also activate the anti-tumor immune response by delivering genes and antigens into the cancer cells or tissues (Unga and Hashida, 2014) or through other ways.
Due to the great potential of FUS-mediated therapy in boosting immunotherapy especially ICB, in this review we summarize the recent research progress of FUS-controlled small-molecule delivery systems in ICB therapy. In addition, we discuss the possible mechanisms by which FUS-mediated small-molecule delivery systems could facilitate personalized ICB therapy for solid tumors.
2 ICB treatment and small-molecule drugs for solid tumors
2.1 ICB treatment for solid tumors
Physiologically, immune checkpoints are points in cellular pathways at which key molecules maintain the immune homeostasis and protect healthy tissues from immune attack (Mahoney et al., 2015). To date, multiple immune checkpoint molecules have been discovered, including programmed cell death protein 1 (PD-1), cytotoxic T lymphocyte-associated protein 4 (CTLA-4), lymphocyte activation gene-3 (LAG-3 or CD223), T Cell immunoglobulin-3 (TIM-3), B7-H3 (CD276) and B7-H4 (B7S1, B7x, or Vtcn1) and natural killer group protein 2A (NKG2A) (Marin-Acevedo et al., 2021). The two major classes of immune checkpoint molecules are PD-1/PD-L1 and CTLA-4 (Pardoll, 2012; Granier et al., 2017). PD-1 is an inhibitor of both adaptive and innate immune responses (Ahmadzadeh et al., 2009) that plays an important role in reducing ineffective or harmful immune responses and maintaining immune tolerance (Han et al., 2020; Ma et al., 2021). However, some tumor cells can evade recognition and elimination by T Cells through expressing PD-L1, which binds to PD-1 on T Cells to inhibit their activation (Alsaab et al., 2017; Zhou et al., 2017). Blocking the interaction between PD-1 and PD-L1, therefore, enables T Cell-mediated tumor cell death. Likewise, by blocking the interaction between CTLA-4 and its ligands, T Cells remain active and can recognize and kill tumor cells. ICB has been proved clinically efficient for a range of tumors, including melanoma (Maibach et al., 2020), non-small cell lung cancer (Sul et al., 2016), head and neck cancers (Vos et al., 2021), renal cell cancer (Motzer et al., 2015), Merkel cell cancer (Maniar et al., 2020), Hodgkin’s lymphoma (Tobin et al., 2021), gastric cancer (Zeng et al., 2021), urothelial cancer (Ning et al., 2017), cervical cancer (Peng et al., 2022) and mismatch repair deficient (dMMR)/microsatellite instability high (MSI-H) tumors (Heinhuis et al., 2019). The combination of anti-PD-1 and anti-CTLA-4 monoclonal antibodies was approved as a first-line therapy for patients with unresectable or metastatic melanoma (Larkin et al., 2015; Postow et al., 2015), achieving an objective response rate (ORR) of 59% (Johnson et al., 2022).
The clinical impact of ICB has grown considerably over recent decades yet several challenges remained to be overcome. Notably, the response rate to ICB varies for different types of cancers (Table 1). Results from various clinical studies have shown that the clinical response rates to ICB in melanoma, lung cancer, and renal cell carcinoma can reach as high as 20–30% (Topalian et al., 2012; Brahmer et al., 2015); about one-third of advanced metastatic melanoma patients responded to ICB using anti-PD-1 (Postow et al., 2015; Weber et al., 2015). Other types of cancers, such as breast and bladder cancers, have been reported to respond to ICB monotherapies at a rate of approximately 10%–20% (Tabana et al., 2021; van der Heijden et al., 2021). The underlying factors determining the low response rate or non-responsiveness to ICB are being investigated intensely (Maleki Vareki et al., 2017). It is currently believed that immunological phenotypes in which immune cells are either absent (immune desert) or immune cells fail to properly infiltrate the tumor (immune-excluded tumors) (Ji et al., 2012; Hegde et al., 2016) rarely respond to ICB monotherapy, which may explain the heterogeneity of therapeutic efficacy (Herbst et al., 2014). The hostile TME, a highly complex and dynamic milieu, plays the key role in the formation of these phenotypes, which can result in the lack of tumor-infiltrating T Cells, the abnormal regulation of immune checkpoints on tumor cells or T Cells, and adapted immune resistance (AIR) to ICB (Restifo et al., 2016; Dillman, 2017; Zhang J. et al., 2022; Kim et al., 2022).
Another difficult problem is that systemic administration of ICB can lead to severe irAEs in numerous organs (Friedman et al., 2016; Naidoo et al., 2017; Elia et al., 2020). Early toxicity (between 1 and 12 weeks after treatment initiation) from both CTLA-4 and PD-1 inhibitors most commonly presents as dermatological effects (Sandigursky and Mor, 2018). Other common types of irAEs include gastrointestinal, hepatic and renal toxicities, vitiligo, rash, arthralgia, pneumonitis, hypothyroidism, and hypophysitis (Khoja et al., 2017; Xu et al., 2018; Ramos-Casals et al., 2020). The severity of irAEs varies, but can be serious, or even deadly in some cases (Helmink et al., 2020). Moreover, the onset of irAE usually does not follow a predictable time-course, with some starting days to weeks after therapy and others months later (Postow et al., 2018; Pauken et al., 2019). In light of the above, novel approaches to increase the response rate and reduce the irAEs of ICB are urgently needed. Combination with other therapies, such as small-molecule drugs, may provide a way to overcome these critical problems.
2.2 Small-molecule drugs for solid tumors
Small molecule compounds have been studied as drugs for solid tumors for decades and significant progress has been made in recent years. Over 60 small-molecule drugs were FDA-approved for treating solid tumors from 2011 to 2021, including various kinase or enzyme inhibitors, receptor antagonists, transcription inhibitors and therapeutic radiopharmaceuticals (Sochacka-Cwikla et al., 2022). These agents could control the progression of tumors by dampening the proliferation of cancer cells, suppressing angiogenesis or reconstructing the TME (Belgiovine et al., 2017; Gan et al., 2018) when used alone or in combination with other therapeutic agents. Importantly, the additional effects of small-molecule drugs on immunotherapies, especially ICB, are being evaluated in ongoing clinical trials (Yamaguchi et al., 2022). Small-molecule drugs can directly, or indirectly, modulate immune checkpoints and strengthen the anti-tumor effects of ICIs through disrupting the immune checkpoint interactions (CA-170) (Sasikumar et al., 2021), epigenetic regulation (DNA hypomethylating agents, HDAC inhibitors) (Yang et al., 2014; Gao et al., 2020), transcriptional regulation (EGFR inhibitors (Jiang et al., 2017), PI3K-AKT-mTOR pathway inhibition (Janse van Rensburg et al., 2018; Du et al., 2020), JAK-STAT pathway inhibition (Shen M. et al., 2019) or protein stability modification (poly (ADP-ribose) polymerase (PARP) inhibitor (Wu Z. et al., 2021), or through proteolysis-targeting chimeras (PROTACs)) of the immune checkpoint molecules (Cotton et al., 2021). Besides targeting immune checkpoints on T Cells, small-molecule agents can also target the innate immune system. For example, the stimulator of interferon genes (STING) agonists could activate the antigen-presenting cells (APCs), especially CD8+ DCs, which promote T Cell infiltration through cross-presentation (Li A. et al., 2019; Ding et al., 2020; Jiang et al., 2020). In addition, these agents increase the expression of antigen-presenting related molecules, including Tap1, Tap2 and MHC-I, with type-I IFN upregulation, which may improve the tumor immune surveillance (Grabosch et al., 2019). Combination with small-molecule drugs and anti-CTLA4 antibody or anti-PD-L1 antibody have shown successful tumor control both in preclinical models and clinical trials (Hellmann et al., 2017). Additionally, small-molecule immunotherapeutics play a role in a TME-directed approach. The tryptophan-metabolizing enzyme, indoleamine 2,3-dioxygenase 1 (IDO1), is expressed in the TME of multiple tumor types, and mediates the depletion of tryptophan and the increase of kynurenine and other metabolites. IDO1 was shown to promote Treg cells and reduce effector T Cell number and functions (Fujiwara et al., 2022). The selective IDO1 inhibitor, epacadostat, displayed a good overall response rate in a phase 1–2 clinical trial in combination with pembrolizumab (Brochez et al., 2017). Unfortunately, the phase 3 trial did not show clinical benefit when compared with pembrolizumab monotherapy in patients with advanced malignant melanoma (Long et al., 2019). This unsuccessful outcome indicated that the combination strategies are not suitable in at least some tumor types, which may benefit from better delivery systems.
Delivery systems offer numerous benefits over the use of small-molecule drugs alone (Toy and Roy, 2016; Oliva et al., 2017). On the one hand, they can be designed to protect therapeutic agents until they are delivered to the targeted tissue (Aikins et al., 2020). On the other hand, delivery systems can make spatial-, temporal-, and dosage-controlled delivery of therapeutics possible if they are responsive to stimuli such as pH (Knight et al., 2019), light (Tao et al., 2020) or ultrasound, thereby keeping the therapeutic agents inactive until they accumulate within targets (Pan et al., 2018). In this review, we focus on ultrasound-based delivery systems, and discuss their safety and efficacy when combined with ICB treatment of solid tumors.
3 Focused ultrasound technology
The exploration of the application of ultrasound in medicine began in the early 20th century in an attempt to detect the presence of tumors in brain tissue based on tissue density (White, 1988). In 1950, Wild (1950) reported the difference in density between cancerous and healthy tissues and the diagnostic values of these findings (Wild and Reid, 1956). Since then, the medical applications of ultrasound have been greatly expanded (Newman and Rozycki, 1998), and it remains one of the major imaging approaches, including for the assessment of cancers. Diagnostic ultrasound is usually delivered at a power of 0.1 W/cm2, while higher energy administration is classified as either high intensity (1,000–10,000 W/cm2), medium intensity, or low intensity (<3 W/cm2) (Xin et al., 2016). High-intensity focused ultrasound (HIFU) was first proposed for therapeutic use in 1942, when Lynn et al. found it caused the destruction of liver tissue and changes in brain structure and behavior in living mice (Lynn et al., 1942). It was not until 1999 that Gelet et al. reported the first clinical study of the use of HIFU for the treatment of local prostate cancer (Gelet et al., 1999). Since then, the application of FUS has expanded to treat a wide variety of diseases (Phenix et al., 2014; Zhang et al., 2014; Wu et al., 2017). Basically, FUS can destroy the targeted tissues (Ter Haar, 2016), increase membrane permeability to enhance the uptake and cytotoxic effects of drugs, resulting in tumor cell death in cancerous tissues (Sasaki et al., 2017; Chowdhury et al., 2020; Sasaki et al., 2022).
3.1 Thermal and mechanical effects of focused ultrasound
FUS can induce biological effects within deep tissue in a minimally or non-invasive manner (Lafond et al., 2022). The specific actions of FUS can be divided into two main effects, the thermal and mechanical effects. The thermal effects are generated by the large amplitude, high-duty cycle of the HIFU regime, and occur when the local tissue temperature rises higher than the level causing thermal necrosis (>56°C) (Izadifar et al., 2020). Heating effects will lead to protein denaturation and tissue damage as tissue temperatures rise above 43°C (Pahk, 2021). Usually, temperatures at or above 60°C for 1 s will cause irreversible cell death in most tissues (Zhou, 2011). Coagulative thermal necrosis is the main mechanism of tissue destruction in HIFU thermal therapy (Izadifar et al., 2020). HIFU has the ability to focus acoustic energy into a small volume and generate rapid and spatially confined heating of the target while minimizing the impact on surrounding tissues (Tempany et al., 2011). Combined with diagnostic ultrasound (USgFUS), or preferably MR imaging (MRgFUS) to map the lesioned area (Bachu et al., 2021), HIFU can be precisely applied from the minimal distance. It has been reported that this distance was limited to approximately 10 cells (250–300 μm) when ablating hepatocytes (Ter Haar and Robertson, 1993). The other essential mechanisms of action of FUS are the mechanical effects, including acoustic flow, radiative force and, most importantly, cavitation (stable and inertial). These effects are most often generated by high-pressure and short-duration ultrasound pulses, which achieve high instantaneous intensity without accumulating thermal energy or producing thermal effects (Izadifar et al., 2020). Ultrasound can induce shear stress on nearby tissues, or form jet streams and shock waves, depending largely on its intensity (Yang et al., 2021). Various ultrasound-based therapies rely on mechanical effects, including ultrasound microbubble targeted destruction (UMTD) (Tian et al., 2020), histotripsy (Khokhlova et al., 2015; Bader et al., 2016), lithotripsy (Xu Z. et al., 2021), sonodynamic therapy (Lafond et al., 2019), sonothrombolysis (Kooiman et al., 2020), and blood–brain barrier opening (Beccaria et al., 2020; Bunevicius et al., 2020).
3.2 Applications of focused ultrasound
Due to the thermal effects of HIFU, the most commonly explored application is thermal ablation (Ter Haar, 2016). Monitoring the formation of lesions during ablative pulses is extremely important from the perspective of efficacy and safety. MRI- and thermometry-guided FUS are more popular than diagnostic ultrasound-guided FUS because they enable real-time monitoring of lesion formation and tissue temperature (Zhang and Wang, 2010). Since its first application, thermal ablation has become a popular treatment for cancers of the bone, liver, pancreas, breast, and kidney (Shehata, 2012). As a non-invasive method, MR-HIFU treatment is characterized as patient-friendly, with low complication rates, few side effects and is repeatable when necessary (Anneveldt et al., 2021). Integration with MRI makes real-time spatial guidance possible, further improving the safety and therapeutic efficacy (Schmitt et al., 2014).
FUS techniques can also be applied in neuromodulation therapies. Low-intensity focused ultrasound (LIFU) plays a useful role in neuromodulation compared with the ablative effect caused by HIFU (Darrow, 2019). Indeed, LIFU produces a non-thermal mechanical disturbance in voltage-gated ion channels that affects electrical signaling across membranes and therefore regulates the neuronal activity. Furthermore, the neuromodulation effects are not limited to the duration of the LIFU treatment but can last for hours to even days (Harary et al., 2018). Both preclinical and clinical studies have reported the relative safety and efficacy of LIFU for neuromodulation. For example, LIFU was reported to inhibit specific thalamic nuclei and regulate the swine sensory thalamus without affecting nearby nuclei, nor creating any tissue injury or inducing any thermal effects to regulate the swine sensory thalamus (Dallapiazza et al., 2018). Some studies have investigated the application of FUS for neuromodulation in patients. Sanguinetti et al. found that FUS could improve mood and affect the connectivity of neural networks related to emotional regulation (Sanguinetti et al., 2020). In addition, FUS has the potential to treat patients with epilepsy and decrease the seizure frequency (Lee C. C. et al., 2022).
Recently, FUS has been shown to improve the immune response against tumors (Hu et al., 2007; Poon et al., 2021). The underlying mechanisms remain unclear, but it is widely accepted that subcellular debris generated in situ by FUS are taken up by dendritic cells (Hu et al., 2005) which then triggers cytotoxic T Cell activation (Xing et al., 2008). Pulsed FUS, or low-intensity FUS, have been shown to drive Th1 inflammation, and stimulate production of localized cell recruitment factors and tumor cell surface immunogenic proteins which increase CD8+/T regulatory cell proportions (Maloney et al., 2017). Furthermore, reshaping the hypoxic TME by FUS can enhance the endogenous immune response and also improve the response to radiotherapy and chemotherapy (Wang and Sun, 2002; Barsoum et al., 2014; Noman et al., 2014). LIFU-activated adoptive transfer immune cells also generate good anti-tumor effects (Rezayat and Toostani, 2016). Recent research demonstrated that short-pulsed FUS stimulation can activate the CAR-T Cells at the desired time and location with an inducible CAR cassette under the control of a promoter for the heat-shock protein, which suppressed the tumor growth in vivo with satisfying safety (Wu Y. et al., 2021).
More importantly, the potential of FUS to assist drug delivery due to its impacts on membrane permeability has recently been explored (Tian et al., 2020). FUS is able to spatiotemporally activate specific drug delivery particles via thermal and/or mechanical effects. To respond to the thermal effects of FUS, the particles that carry small-molecule agents to the specific sites are designed to be temperature-sensitive, with a certain heat or energy threshold for the drug to be released (Dromi et al., 2007; Luo et al., 2017). Futhermore, the particles can act as additional nucleation sites and reduce the cavitation threshold during microbubble (MB) formation, which enhances the mechanical effects of FUS and increases drug unloading through acoustic manipulation at the specific positions (Airan et al., 2017; Khirallah et al., 2019; Chen et al., 2021). These particles can be either organic, such as lipid- or polymer-based, or inorganic, for example, metallic, or a combination of both types (Mai et al., 2021). The application of FUS as delivery technology to boost ICB has been a major novel and promising combination strategy and is discussed further below.
4 FUS-mediated delivery systems enhance the efficacy of ICB for solid tumors
FUS has been proven as a strategy to improve tumor responsiveness to ICIs (Sheybani and Price, 2019) (Table 2). Studies have shown that HIFU treatment led to an upregulation of immune checkpoint molecules, such as PD-1, PD-L1 or CD80/86 (ligand of CTLA-4) on tumor cells (Eranki et al., 2020; Abe et al., 2022), dendritic cells (DC) (Hu et al., 2005) or T Cells (Garg et al., 2015), resulting in better control of the tumors. More importantly, FUS has also been applied in drug delivery. In this case, FUS is used to improve the local delivery of therapeutic reagents carried by ultrasound-responsive materials and enhance the anti-tumor efficacy (Timbie et al., 2015; Yildirim et al., 2019). These studies indicated the potential of FUS-mediated combinatorial strategies in solid tumors to improve the efficacy of ICB in anti-tumor immunity and outcome.
4.1 FUS enhances the efficacy of ICB for solid tumors
To date, the feasibility of FUS and ICB combinatorial regimens in solid tumors is under preclinical investigation. The study of Abe et al. showed that mechanical-HIFU (M-HIFU) elicited stronger systemic cellular antitumor immunity and suppression of tumor growth than thermal-HIFU (T-HIFU) in murine breast cancer models because M-HIFU induced an inflammatory, M1-biased, macrophage population which contributed to enhanced antitumor immunity in M-HIFU-treated tumors (Abe et al., 2022). However, many M-HIFU-treated mice eventually developed progressive disease and the upregulation of PD-L1 rather than PD-1 and TIM3 on neutrophils, DCs, and macrophages was found in M-HIFU-treated tumors. Concurrent anti-PD-L1 antibody administration activated CD8 T Cells markedly and increased CD8 and NK cell-mediated systemic antitumor immunity, which resulted in superior systemic antitumor immune responses and distant tumor growth suppression. In another study, murine orthotopic pancreatic KPC tumors were treated with both pulsed-HIFU (pHIFU) and anti-CTLA-4/anti-PD-1 antibodies (ICI-treated) (Mouratidis et al., 2021). Increased infiltration of CD8+ T Cells in the tumors of both pHIFU and pHIFU + ICI-treated groups was observed compared with the sham-exposed group. Survival of the mice in the pHIFU + ICI-treated group was prolonged compared to both control untreated group and pHIFU or ICI alone groups. The study showed that the balance within the tumor was skewed towards a pro-inflammatory phenotype in pHIFU + ICI-treated mice caused by an acute infiltration of CD8+ TILs and CD8+ IFNγ+ TILs, raising the ratio of CD8+ IFNγ+ TILs to regulatory T Cells, MDSCs and CD4+ T Cells while retaining the levels of regulatory T Cells and MDSCs at the levels seen in control mice. A similar protocol was also carried out in a refractory murine neuroblastoma model. M-HIFU increased the expression of PD-L1 on most tumor cells significantly and induced a systemic immune activation of DCs, tumor-infiltrating T Cells, proinflammatory cytokine changes, and damage-associated molecular pattern (DAMP) changes, as well as concurrent downregulation of protumor regulators such as regulatory T Cells, IL10, TGFb, and VEGF-A (Eranki et al., 2020). In this study, M-HIFU combined with αCTLA-4 + αPD-L1 significantly prolonged survival from 0% to 62.5% in a refractory unilateral and bilateral neuroblastoma tumor model. This combination treatment of HIFU and ICB was also found to be adoptively transferable, and this could induce long-term memory responses and slow subsequent de novo tumor engraftment (Eranki et al., 2020). Another FUS therapy, histotripsy, was used to treat immunocompetent C57BL/6 mice implanted with poorly immunogenic melanoma or hepatocellular carcinoma tumors (Qu et al., 2020). It was found that histotripsy ablation of tumors significantly inhibited primary tumor growth and abscopal pulmonary metastasis by induction of systemic inflammatory changes and stimulation of potent local intratumoral infiltration of innate and adaptive immune cell populations. When used together with anti-CTLA-4 antibody, histotripsy augmented the efficacy of checkpoint inhibition immunotherapy (Qu et al., 2020).
Overall, the combination of FUS and ICB has been shown to exert a superior anti-tumor effect by inducing a local or systemic inflammatory immune activation, and enhancing the intratumoral infiltration and maturity of innate and adaptive immune cells, as well as concurrently downregulating protumor regulators in different types of solid tumors.
4.2 FUS-mediated small-molecule delivery synergizes with ICB in solid tumors
Accumulative evidence suggests that FUS has potential in small-molecule drug delivery (Mullick Chowdhury et al., 2017; Al Sawaftah and Husseini, 2020) because of its non-invasiveness, clinical convenience, and spatial-, temporal-, and dosage-controlled delivery with a high degree of precision (Tzu-Yin et al., 2013; Mullick Chowdhury et al., 2016). This is especially beneficial for cancer treatment as it could minimize the adverse effects (Al Sawaftah and Husseini, 2020). Various inorganic or organic materials have been used for targeted drug delivery including inorganic nanoparticles (NPs) (Li et al., 2016), liposomes (Zhao et al., 2015), polymer micelles (Shi et al., 2014), and polymer NPs(Shen X. et al., 2019) for superior anti-tumor effect. We have summarized the research progress for FUS-mediated small-molecule delivery combined with ICB in the treatment of solid tumors treatment in Figure 1.
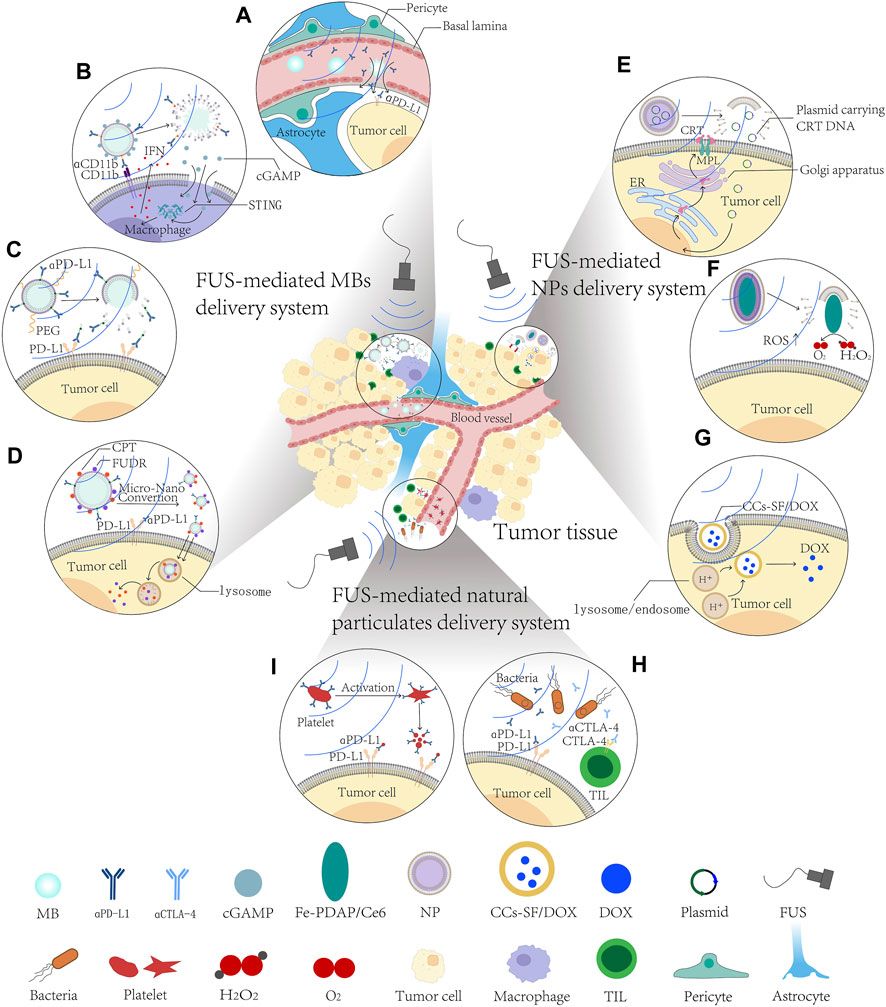
FIGURE 1. Schematic diagram of FUS-mediated small-molecule delivery systems in solid tumor treatment. (A–D) FUS-mediated microbubble delivery system improves the therapeutic effects and diminishes side effects of ICB. (A) FUS-MBs delivery system disrupt BBB/BTB to enhance penetration and distribution of αPD1 antibody. (B) FUS-mediated cGAMP loading ncMBs targeting macrophages specifically activate cGAS-STING pathway. (C) FUS-mediated IMC delivery to alleviate their immunogenicity. (D) FUS-mediated αPFC MBs to reach higher tumor cell uptake and deeper tumor penetration. (E–G) FUS-mediated nanoparticles delivery system synergizes with ICB in solid tumors. (E) FUS-mediated CRT-NPs upregulate CRT expression and induce ICD in tumor cells. (F) FUS-mediated MFC targeting TME to stimulate reactive oxygen species (ROS) production. (G) FUS-mediated CCs-SF/DOX NPs prevent premature release and increase intracellular nflux of drug. (H, I) FUS-mediated natural particulate delivery targets ICs in solid tumors. (H) FUS-mediated engineered platelets effectively target incompletely ablated tumor cells and release αPD-L1 antibody. (I) FUS-mediated therapeutic bacteria home to, and engraft in, tumors to chronically release αPD-L1 and αCTLA-4. αCTLA-4, anti-CTLA-4 antibody; αPD-L1, anti-PD-L1 antibody; CCs-SF/DOX, silk fibroin-modified doxorubicin preloaded calcium carbonates; cGAMP, cyclic GMP-AMP; CPT, camptothecin; CRT, calreticulin; ER, endoplasmic reticulum; FUDR, floxuridine; FUS, focused ultrasound; IFN, interferon; MBs, microbubbles; MPL, thrombopoietin receptor; NPs, nanoparticles; PEG, polyethylene glycol; ROS, reactive oxygen species; STING, stimulator of interferon genes; TIL, tumor infiltrating lymphocyte.
4.2.1 FUS-mediated microbubble delivery system improves the therapeutic effects, and diminishes the side effects, of ICB
MBs are stabilized gas bubbles with diameters typically 1–4.5 μm (Blomley et al., 2001), that were originally used as vascular contrast agents to improve the acoustic contrast in diagnostic imaging. However, when subjected to incident ultrasound bursts, these stable tiny gas-filled bubbles undergo high frequency vibrations and exert circumferential or shear stress to the surrounding cells (Sheikov et al., 2008; Alonso et al., 2010). Cellular and molecular evidence suggests that these physical effects can promote paracellular transport through temporary reorganization of the tight junctions (Jalali et al., 2010; Shang et al., 2011) and facilitate transcellular passage by vesicle/caveolae transport (Deng et al., 2012; Xia et al., 2012). When the ultrasound beam is focused, these physical changes and functions are localized in the focal region and can lead to a local increase in the vascular permeability for up to 24 h post sonication (Hynynen et al., 2001; Aryal et al., 2014; Meairs, 2015), providing a window for spatio-temporal targeted drug delivery (Aryal et al., 2014). These findings indicate the feasibility of US-responsive MBs to deliver various types of anti-cancer agents, including chemotherapeutics, antibodies, nanoparticle drug conjugates, and viruses (Curley et al., 2017; Schoen et al., 2022). Recently, the impacts of FUS-MB delivery on the immune system and TME in solid tumors are under investigation. Numerous studies have revealed that FUS-MB, delivery systems could remodel the TME (Alkins et al., 2016; Song et al., 2021), including affecting the immune cells and tumor vasculatures (Liu et al., 2022), to enhance the effect of immunotherapy. Sheybani et al. investigated the impact of blood brain/tumor barrier disruption (BBB/BTB-D) by FUS and MBs on innate and adaptive immune responses in an orthotopic murine glioblastoma model (Sheybani et al., 2022) (Figure 1A). They found that FUS did not alter the frequency of CD8+ T Cells across evaluated organs significantly, but upregulated checkpoint molecules (PD-1 and TIM-3) on these cells were detected at 1-week post-FUS, suggesting the increased activation of the T Cells. Similarly, the study of Lee et al. indicated that a closed-loop controlled MB-FUS system could lead to a better penetration and distribution of the anti-PD1 antibody in the GL261 glioma mouse model (Lee H. et al., 2022). Their proposed delivery system increased the interaction of proinflammatory macrophages within the PD-1-expressing TME without promoting global inflammation in non-tumor tissue. When compared to anti-PD1-treated mice with no sonication, closed-loop controlled FUS-MBs in combination with anti-PD-1 elicited antitumor immunity and conferred a significant improvement in survival. These results indicated that FUS-MB delivery system in combination with adjunct therapeutic strategies targeting immune checkpoints (such as PD-1, CTLA-4, and TIM-3) may improve the outcomes of anti-tumor therapy.
Depending on the surface-modified antibody, the US-responsive MBs can target different types of cells. Li et al. synthesized cyclic GMP-AMP (cGAMP) loaded nanocomplex-conjugated microbubbles (ncMBs) that target antigen-presenting cells (Li et al., 2022) (APCs, Figure 1B). cGAMP can activate the ‘cyclic GMP-AMP synthase-stimulator of interferon genes’ (cGAS-STING) pathway which plays an essential role in priming tumor-specific cytotoxic T Cells (Cousin et al., 2019; Ilovitsh et al., 2020; Xu N. et al., 2021). However, non-specific activation of the cGAS-STING pathway may cause widespread inflammatory responses (Li Y. et al., 2019; Decout et al., 2021), impeding their clinical application. Li et al. found that the ncMBs targeted APCs and efficiently delivered cGAMP into the macrophages’ cytosol when exposed to ultrasound, resulting in the activation of the cGAS-STING pathway and downstream proinflammatory pathways that efficiently prime antigen-specific T Cells (Li et al., 2022). This bridging of innate and adaptive immunity inhibited tumor growth and prolonged survival in both localized and metastatic murine breast cancer models. Furthermore, the addition of anti-PD-1 antibody exhibited not only enhanced primary tumor control, but also a superior survival benefit with a 76% increase in median survival and approximately 60% decrease in pulmonary metastatic nodules.
Although the concurrent application of FUS and ICB has shown some preclinical promise (Eranki et al., 2020; Mouratidis et al., 2021; Abe et al., 2022), few studies indicate that FUS can directly alleviate the adverse effects of ICB. As mentioned above, the irAEs that are caused by off-target binding and overactivation of the immune system (Michot et al., 2016; Moslehi et al., 2018; Postow et al., 2018) are usually diverse and unpredictable, potentially affecting almost every organ system (Wang et al., 2018). The transportation of active pharmaceutical ingredients in carriers such as MBs has become a common way to enhance the pharmacokinetic/dynamic profiles in vivo (Ma and Mumper, 2013). Use of ultrasound-sensitive immune-engineered complexes targeting ICs is an emerging strategy that may improve the tumor response rate while reducing the potential side effects. Kim et al. designed an immune-microbubble complex (IMC), in which PD-L1 antibodies were conjugated with MBs to alleviate their immunogenicity because the polyethylene glycol chains on the MBs surface prevented the macrophages from recognizing and activating an immune response against the PD-L1 antibodies (Kim et al., 2020) (Figure 1C). It turned out that the toxicity was markedly decreased when the IMCs were administered intravenously in the CT26 colon carcinoma mice group compared to that of the group receiving the unmodified PD-L1 antibodies at the same concentrations. When combined with FUS, IMCs exhibited higher efficiency in suppressing the tumor growth than the combination of the PD-L1 antibody with FUS, IMC only, PD-L1 antibody only, and FUS only. Enhanced localization of the PD-L1 antibody was also observed in the IMC + FUS group. These results suggested that upon FUS treatment, the IMCs underwent cavitation to increase extravasation, and eventually broke down to expose the individual antibodies which then bound to the surface of PD-L1-expressing tumors. Apart from ICIs, US-responsive MBs could also deliver other anti-cancer agents, such as chemotherapeutics. Sun et al. fabricated US-responsive MBs consisting of camptothecin (CPT)−floxuridine (FUDR) (CF) conjugate and anti-PD-L1 (αPFC MBs) in order to achieve higher tumor cell uptake and deeper tumor penetration (Sun et al., 2022) (Figure 1D). In the TME, αPFC MBs were hydrolyzed, then CPT and FUDR were further released at a fixed 1:1 molar ratio to induce immunogenic cell death (ICD) to enhance anti-tumor efficacy, while the anti-PD-L1 antibody could block the PD-L1 receptor on the tumor cells to promote cytotoxic T Cell (CTL) infiltration, and thus achieve robust therapeutic effects and reduce the irAEs. These findings highlighted the potential of FUS-MBs delivery targeting ICs to boost the therapeutic efficacy and avoid the side effects of ICB.
4.2.2 FUS-mediated nanoparticle delivery systems synergize with ICB in solid tumors
NPs, which are typically less than 100 nm in at least one dimension, have been found to take advantage of the enhanced permeability and retention (EPR) effect to sustainably deliver small-molecule drugs to solid tumors after intravenous administration (Hewakuruppu et al., 2013; Baetke et al., 2015). However, malformed and highly permeable tumor blood vessels, malfunctioning lymphatics and dense extracellular matrix (ECM) in tumors elevate interstitial fluid pressure (IFP) and create a solid physical barrier for drug delivery, leading to a gathering of NPs in the perivascular space of tumors (Viallard and Larrivee, 2017; Zhan et al., 2018; Jin, 2021). Hence, the delivery of NPs from the circulatory system to the particular cells and intracellular targets of the tumor remains inefficient and can be ineffective. Recent progress in materials science and drug delivery makes the application of spatial-, temporal-, and dosage-controlled nanoparticle drug delivery systems possible, and the requisite characteristics of NPs can be achieved by using stimuli-responsive materials during their synthesis (Amreddy et al., 2018). Ultrasound interaction with NPs induces enhanced drug delivery and can also be used in image-guided delivery. Here we describe the strategies using FUS and NPs to deliver anticancer agents to tumors, and improve the synergistic antitumor efficacy of ICB.
It has been extensively reported that the release of DAMPs such as calreticulin (CRT) in solid tumors can lead to local activation of ICD (Obeid et al., 2007; Panaretakis et al., 2008; Galluzzi et al., 2017). CRT enhances the phagocytosis and immunogenic recognition of dying cancer cells by APCs and improves interactions with tumor-infiltrating leukocytes (Wang et al., 2012; Wang et al., 2017). When tumor cells experience ICD, the immune stimulatory effects of ICD increase the populations of tumor antigen-presenting and CTL cells, enhancing antitumor immune responses (Green et al., 2009; Fucikova et al., 2016; Cui, 2017). However, CRT activation is often inconsistent in most solid tumors and generally is insufficient to generate an effective antitumor immune response (Garg et al., 2015). To find a clinically translatable method for reproducibly inducing CRT expression, a novel liposome-based calreticulin-nanoparticle (CRT-NP), in which DNA encoding full-length calreticulin was encapsulated, was developed (Sethuraman et al., 2020) (Figure 1E). The CRT-NP was intratumorally injected into B16F10 melanoma mice followed by FUS treatment. It was found that CRT-NP plus FUS (CFUS) upregulated the CRT expression, expanded the population of melanoma TRP-2 specific functional CD4+ and CD8+ T Cells and tumor-suppressing M1 phenotype macrophages, and increased the expression of PD-1 and PD-L1 in T Cells. Moreover, CFUS markedly inhibited primary B16 melanoma growth and prevented tumor growth in distal untreated sites. These results suggested that the potential combination of CFUS and PD-1/PD-L1 blockade may provide a better anti-tumor effect.
Depending on the practical needs, NPs can be designed to target the receptors on the cell or the intracellular target elements, but also specific factors in the TME (Yu et al., 2012). Several studies show that the highly hypoxic TME makes it difficult to generate robust reactive oxygen species (ROS) during cancer treatment, leading to insufficient tumor-associated antigen (TAA) generation and a limited anti-tumor immune effect (Phua et al., 2019; Yu et al., 2019; Yang et al., 2020). As a sonosensitizer, chlorin e6 (Ce6) can stimulate ROS production by the ultrasonic cavitation effect, which directly kills the tumor cells (Rosenthal et al., 2004). Hence, membrane-coated Fe-PDAP/Ce6 (MFC), an H2O2 economizer was developed for on-demand O2 supply and sonosensitizer-mediated ROS production during ultrasound activation was developed. Both in vitro and in vivo experiments demonstrated that MFC could be effectively accumulated in tumor cells and tumor tissue via homologous targeting mechanisms, and ultrasound irradiation promoted MFC dissociation and the exposure of Fe-PDAP for a more robust O2 supply (Jiang et al., 2022). After combination with anti-PD-1 antibody (αPD-1) administration, MFC-reinforced ultrasound suppressed both primary and distant tumors more effectively. Survival analysis showed that 80% percent of mice treated with MFC-reinforced ultrasound + αPD-1 survived to day 45, a survival rate more than 2-fold higher than that of other groups. Further exploration of the underlying mechanism of the tumor-specific immunological effect evoked by MFC + US+αPD-1 showed an increased number of mature DCs in the tumor-draining lymph nodes (TDLNs) (Jiang et al., 2022). Moreover, the upregulation and infiltration of CD8+ T Cells as well as the downregulation of Tregs in distant tumors caused by MFC + US+αPD-1 also contributed to a strong anti-tumor immunity against both primary tumors and distant tumors (Jiang et al., 2022) (Figure 1F). On the basis of this work, Wu et al. constructed a focused acoustic vortex (FAV) system with a hollow cylindrical focal region, which exhibited a larger focal region compared to conventional FUS of the same frequency (Wu et al., 2022). Then a synergistic therapy based on integrated FAV double combination sequence-regulated phase-transformation nanodroplets containing ce6 (CPDA@PFH) with checkpoint blockade immunotherapy was applied to metastatic murine breast cancer in a mouse model. Their study found that FAV + CPDA@PFH resulted in significantly higher ROS levels than the other groups both in vitro and in vivo. Treatment with FAV + CPDA@PFH led to higher inhibition of tumor growth than that with traditional FUS + CPDA@PFH(Wu et al., 2022). Moreover, FAV + CPDA@PFH combined with anti-PD-L1 antibodies induced a systemic immune response that not only inhibited the primary and distal 4T1 tumor growth but also suppressed lung metastasis (Wu et al., 2022). In addition, FAV + CPDA@PFH + anti-PD-L1 induced long-term immune memory that effectively inhibited tumor growth and prolonged the survival of mice (Wu et al., 2022).
Although significant progress has been made in the use of a large number of chemotherapeutic agents with good anti-tumor effects in preclinical trials, their efficacy in clinical phase trials remains frustrating (Dias et al., 2021; Leon-Ferre et al., 2021). Therefore, new technology is urgently needed to break through the bottleneck of existing chemotherapy. The combination of chemotherapy and immunotherapy has been regarded as redefining cancer therapy (Song et al., 2018). Tan et al. constructed silk fibroin-modified doxorubicin preloaded calcium carbonates (CCs-SF/DOX) that could prevent premature drug release (Tan M. et al., 2020) (Figure 1G). When accompanied with LIFU, the CCs-SF/DOX nanocomposites showed a markedly increased intracellular influx through acoustic pertubation-facilitated delivery or endocytic uptake, which aided the chemosensitization of the cancer. After targeting to TME, the acidic endosomes (pH 5.0–6.0) or lysosomes (pH 4.0–5.0) in the cancer cells promoted the decomposition of CCs and the release of DOX for efficient cytotoxicity, which suppressed tumor growth in both the 4T1 tumor-bearing nude mouse model and the primary breast tumor-bearing immunogenic Balb/c mouse model. Residual CCs-SF/DOX or uploaded DOX from dead or dying cells were then encapsulated in vesicles which fused with the plasma membrane of cells and was excreted into the extracellular space, leading to the formation of extracellular vesicles (EVs). Then the EVs responded to the acidic environment in the TME and infected neighboring cancer cells repeatedly, thus resulting in deep penetration of the drug-based EV therapy. Furthermore, administration of αPD-1 combined with CCs-SF/DOX plus LIFU showed improved synergistic antitumor efficacy (Tan M. et al., 2020).
Synthetic and biological NPs are multifunctional and able to carry different kinds of immunotherapy reagents, DNA, proteins, cytokines, adjuvants and antigens (Gorbet et al., 2020). They can be used in combination with FUS and ICB to promote the clinical therapeutical efforts. However, combining FUS-NP delivery with ICB treatment is still in its early stages, systems and methods to track the NPs and ICB biodistribution, kinetics and their cargo during FUS need to be further explored.
4.2.3 FUS-mediated natural particulate delivery to target ICs in solid tumors
In addition to synthetic drug vehicles, such as MBs and NPs mentioned above, natural particulates also have the potential to be applied in drug delivery (Yoo et al., 2011). They have characteristics of evasion from the host immune system, a natural tropism that engenders highly selective and efficient entry into target cells, longevity in the host, and varied therapeutic payloads (Yeo et al., 2013). Natural particulate-based delivery systems, such as erythrocytes (Han et al., 2018), immune cells (Chu et al., 2018), stem cells (Yeo et al., 2013), viruses (Yeo et al., 2013) and exosomes (Lakhal and Wood, 2011; van den Boorn et al., 2011) have generated increased attention as biocompatible drug delivery systems.
Inspired by the excellent inflammation targeting (Morrell et al., 2014) and immunomodulator releasing ability of platelets (Ruggeri and Mendolicchio, 2007), Han et al. synthesized αPD-L1 antibody-coupled engineered platelets, which could effectively target incompletely ablated tumors and inhibit the residual tumor growth and increase the survival rate in triple-negative breast carcinoma (4T1) mouse models with thermal ablation (Han et al., 2019) (TA, Figure 1H). Moreover, upon their activation and dissociation, the engineered platelets could release anti-PD-L1 antibodies as well as pro-inflammatory cytokines. Based on this result, they further tested the targeting ability of the engineered platelets after other physical ablation treatments including HIFU, photodynamic therapy (PDT) and irradiotherapy (RT), and observed a large recruitment of engineered platelets in all ablated areas with potential residual tumors. This result indicated that the platelet-based delivery strategy could be extended to IC-targeted treatment and improve therapeutic effects. In addition, the impaired immune activity of some tumor cores provides a favorable microenvironment for the colonization and growth of certain bacteria, which can reach the tumors after systemic administration (Dang et al., 2001; Leschner et al., 2009; Kang et al., 2020). Meanwhile, a recent study demonstrated that FUS can control the expression of bacterial genes when used with temperature-responsive repressors (Piraner et al., 2017). Capitalizing on these tumor-infiltrating and FUS-stimulated gene expression properties, Abedi et al. engineered FUS-activated therapeutic bacteria (Escherichia coli Nissle 1917) the genome of which was optimized to express anti-CTLA-4 and anti-PD-L1 (Abedi et al., 2022) (Figure 1I). In an A20 tumor mouse model, the systemically administrated engineered bacteria were able to home to, and engraft in, tumors and be reliably and chronically activated by a brief non-invasive FUS treatment to release anti-CTLA-4 and anti-PD-L1 and significantly suppress tumor growth.
Since systemic administration of foreign proteins (such as therapeutic bacteria) may increase the risk of inducing a strong immune response (Chinol et al., 1998), and modification of foreign molecules on the membrane of platelets may to some degree induce alloimmunization (Han et al., 2018), future research should focus on solving these problems. It is evident that FUS-mediated natural particulate delivery targeting ICs could act as a new platform to address a wide array of indications in the foreseeable future.
4.3 Combination of FUS, ICB and other treatments for solid tumors
In general, “immunologically cold” tumors such as B16F10 melanoma exhibit limited APC functions, leading to an inability to accumulate cytotoxic infiltrating lymphocytes, upregulated PD-L1 expression on tumor cells, and poor response to ICIs in advanced stages, thereby evading antitumor immunity (Lechner et al., 2013; Twyman-Saint Victor et al., 2015). Studies show that anti-CD40 agonist antibody (αCD40) attaches to the CD40 receptor on APCs, activating CD40 signaling pathway as well as the expression of CD80, IL-12, and CCR7. These effects cause efficient APC activity and T cell-based cytotoxic responses (Beatty et al., 2011; Thompson et al., 2015; Long et al., 2016). Resent research has shown that both thermal (Singh et al., 2019; Sethuraman et al., 2020) and mechanical (Qu et al., 2020) FUS can play a role in immune-modulation. Hence, Singh et al. investigated the effect of FUS-based boiling histotripsy (HT) and insitu αCD40 combinatorial therapy on ICB refractory murine melanoma (Singh et al., 2021). They found that HT + αCD40 (HT40) upregulated a variety of inflammatory markers associated with phagocytosis, cell adhesion, cytokines, and antigen processing and presentation, and the proliferation rates of the melanoma-specific memory T Cell population in tumors. Consistent with T Cell activation, the checkpoint marker genes (CTLA-4, PD-L1, PD-1, TIM3, and LAG3) were upregulated in αCD40 and HT40 treatment. The following combination of HT40 and ICB therapy (anti-CTLA-4 and anti-PD-L1) exhibited superior inhibition of distant untreated tumors, and prolonged survival rates compared to the control group. These data indicated that HT and insitu αCD40 combinatorial therapy reprogrammed immunologically cold tumors and sensitized them to ICB. Thus this approach may be clinically useful for treating “immunologically cold” tumors.
Not all ICB and FUS combination therapies, however, achieve significant results. Sheybani et al. applied thermally ablative FUS in combination with gemcitabine (GEM), a chemotherapy, in murine metastatic TNBC models (Sheybani et al., 2020). The treatment restricted primary tumor outgrowth significantly, improved survival and enhanced immunogenicity by inhibiting myeloid-derived suppressor cell (MDSC) - driven immunosuppression. The addition of anti-PD-1 antibodies did not markedly enhance growth restriction on a FUS + GEM background. The limited effect of PD-1 blockade on this background may be explained partly by the result that a major source of PD-L1 in the TME is MDSC, which has been inhibited by GEM, and the possibility that other mechanisms of resistance to tumor immunity may play a role in the current model system. Future studies could also focus on targeting PD-L1 or other immune checkpoint molecules such as CTLA-4, Tim3, LAG3, or OX-40.
These studies provide timely and provocative insights into the immunogenicity of FUS and the role of FUS-ICB-based combinatorial strategies in solid tumors. To this end, future studies are expected to optimize treatment frequency, and drug dosage in larger animal models and clinical trials.
5 Challenges and limitations
Although the field of drug delivery systems as a whole is advancing at a rapid pace, the design of FUS-mediated delivery technologies for this field is still in its infancy, especially when in combination with ICB for human solid tumors. The major challenges still remain in the TME, with high interstitial fluid pressure, compressed vasculature and dense fibrotic tissue surrounding solid tumors that hinders T Cell infiltration and dampens the efficacy of ICB. Small molecule agents that change the physical conditions of TME could be the future targets of the FUS-mediated delivery systems. Losartan (an angiotensin inhibitor), which is under investigation in patients with pancreatic cancer in a phase II clinical trial (NCT01821729), was shown to potentiate the therapeutic delivery of chemotherapeutic agents to several solid tumor types (Chauhan et al., 2013). Treatment with angiotensin inhibitors was reported to normalize the extracellular matrix, reduce the progression of pancreatic cancer, and increase the T Cell and APC activity (Liu et al., 2017), which indicates the possibility of reshaping the TME with the assistance of FUS. In addition, the cellular components in the TME could be another type of fundamental target exploited to deliver immunotherapy. For example, the tumor-associated macrophages (TAMs) could be engineered to directly attack tumor cells by inhibiting SHP substrate 1 (Alvey et al., 2017), or spare the T Cell from exhaustion by losing TAM antigen presentation in an IRF8-dependent manner (Nixon et al., 2022). It is noteworthy that the immune checkpoint molecules, such as PD-1 and Tim-3, are also the markers of exhausted T Cells. The upregulated expression of these markers post-FUS indicated that FUS may affect the T Cell exhaustion program, but the underlying mechanism remains unclear (Garg et al., 2015; Sheybani et al., 2022). Further understanding of the molecular regulation of T Cell exhaustion could improve the designs or technology of FUS-mediated delivery, and provide novel targets to relieve or even reverse the dysfunctional status of cytotoxic immune cells.
Despite its potential, several limitations of FUS-assisted drug delivery remain to be solved, including the short duration of the effect and erratic drug uptake (Sun et al., 2021). In clinical practice, larger tumors may require longer and more complex treatment regimes (Payne et al., 2010), which raises safety concerns. A better understanding of ICB penetration into the tumor and uptake from the circulatory system following different ultrasound schemes and conditions in different kinds of tumor models is needed to optimize treatment protocols. Future work could focus on developing novel technologies to prolong the controllable duration of drug delivery, and more preclinical models and clinical trials are needed to examine their safety.
6 Conclusion
ICB has been extensively explored as one of the most promising cancer immunotherapies, yet it has failed to acquire satisfactory clinical outcomes for solid tumors. Small-molecule drugs could assist ICB through different mechanisms, but need precise and safe delivery. In this review, we summarized the current frontline studies of FUS-assisted small-molecule drug delivery in combination with ICB to treat solid tumors. This research provides a proof-of-concept for FUS-mediated delivery serving as a pioneer and effective combinatorial regimen for ICB. By further understanding the underlying mechanisms of the TME and developing novel technologies, FUS-mediated small-molecule delivery could definitely boost the efficacy and the safety of ICB in solid tumors.
Author contributions
QW, ZO, and PJ designed the review. QW, YX, and XX conducted the literature searching. XD, XP, FZ, ST, JS, SW and XX collected the related references and participated in the discussion. QW and YX performed the visualization. QW, YX, and ZO wrote the manuscript. LM, RC, ZO, and PJ reviewed and revised the manuscript. All authors read and approved the final manuscript.
Funding
This study was supported by grants from the National Natural Science Foundation of China (31871464 and 82071115).
Conflict of interest
The authors declare that the research was conducted in the absence of any commercial or financial relationships that could be construed as a potential conflict of interest.
Publisher’s note
All claims expressed in this article are solely those of the authors and do not necessarily represent those of their affiliated organizations, or those of the publisher, the editors and the reviewers. Any product that may be evaluated in this article, or claim that may be made by its manufacturer, is not guaranteed or endorsed by the publisher.
References
Abbott, M., and Ustoyev, Y. (2019). Cancer and the immune system: The history and background of immunotherapy. Semin. Oncol. Nurs. 35 (5), 150923. doi:10.1016/j.soncn.2019.08.002
Abe, S., Nagata, H., Crosby, E. J., Inoue, Y., Kaneko, K., Liu, C. X., et al. (2022). Combination of ultrasound-based mechanical disruption of tumor with immune checkpoint blockade modifies tumor microenvironment and augments systemic antitumor immunity. J. Immunother. Cancer 10 (1), e003717. doi:10.1136/jitc-2021-003717
Abedi, M. H., Yao, M. S., Mittelstein, D. R., Bar-Zion, A., Swift, M. B., Lee-Gosselin, A., et al. (2022). Ultrasound-controllable engineered bacteria for cancer immunotherapy. Nat. Commun. 13 (1), 1585. doi:10.1038/s41467-022-29065-2
Adams, S., Othus, M., Patel, S. P., Miller, K. D., Chugh, R., Schuetze, S. M., et al. (2022). A multicenter phase II trial of ipilimumab and nivolumab in unresectable or metastatic metaplastic breast cancer: Cohort 36 of dual anti-CTLA-4 and anti-PD-1 blockade in rare tumors (DART, SWOG S1609). Clin. Cancer Res. 28 (2), 271–278. doi:10.1158/1078-0432.CCR-21-2182
Ahmadzadeh, M., Johnson, L. A., Heemskerk, B., Wunderlich, J. R., Dudley, M. E., White, D. E., et al. (2009). Tumor antigen-specific CD8 T cells infiltrating the tumor express high levels of PD-1 and are functionally impaired. Blood 114 (8), 1537–1544. doi:10.1182/blood-2008-12-195792
Aikins, M. E., Xu, C., and Moon, J. J. (2020). Engineered nanoparticles for cancer vaccination and immunotherapy. Acc. Chem. Res. 53 (10), 2094–2105. doi:10.1021/acs.accounts.0c00456
Airan, R. D., Meyer, R. A., Ellens, N. P., Rhodes, K. R., Farahani, K., Pomper, M. G., et al. (2017). Noninvasive targeted transcranial neuromodulation via focused ultrasound gated drug release from nanoemulsions. Nano Lett. 17 (2), 652–659. doi:10.1021/acs.nanolett.6b03517
Al Sawaftah, N. M., and Husseini, G. A. (2020). Ultrasound-mediated drug delivery in cancer therapy: A review. J. Nanosci. Nanotechnol. 20 (12), 7211–7230. doi:10.1166/jnn.2020.18877
Alkins, R., Burgess, A., Kerbel, R., Wels, W. S., and Hynynen, K. (2016). Early treatment of HER2-amplified brain tumors with targeted NK-92 cells and focused ultrasound improves survival. Neuro Oncol. 18 (7), 974–981. doi:10.1093/neuonc/nov318
Alley, E. W., Lopez, J., Santoro, A., Morosky, A., Saraf, S., Piperdi, B., et al. (2017). Clinical safety and activity of pembrolizumab in patients with malignant pleural mesothelioma (KEYNOTE-028): Preliminary results from a non-randomised, open-label, phase 1b trial. Lancet Oncol. 18 (5), 623–630. doi:10.1016/S1470-2045(17)30169-9
Alonso, A., Reinz, E., Jenne, J. W., Fatar, M., Schmidt-Glenewinkel, H., Hennerici, M. G., et al. (2010). Reorganization of gap junctions after focused ultrasound blood-brain barrier opening in the rat brain. J. Cereb. Blood Flow. Metab. 30 (7), 1394–1402. doi:10.1038/jcbfm.2010.41
Alsaab, H. O., Sau, S., Alzhrani, R., Tatiparti, K., Bhise, K., Kashaw, S. K., et al. (2017). PD-1 and PD-L1 checkpoint signaling inhibition for cancer immunotherapy: Mechanism, combinations, and clinical outcome. Front. Pharmacol. 8, 561. doi:10.3389/fphar.2017.00561
Alvey, C. M., Spinler, K. R., Irianto, J., Pfeifer, C. R., Hayes, B., Xia, Y., et al. (2017). SIRPA-inhibited, marrow-derived macrophages engorge, accumulate, and differentiate in antibody-targeted regression of solid tumors. Curr. Biol. 27 (14), 2065–2077.e6. doi:10.1016/j.cub.2017.06.005
Amreddy, N., Babu, A., Muralidharan, R., Panneerselvam, J., Srivastava, A., Ahmed, R., et al. (2018). Recent advances in nanoparticle-based cancer drug and gene delivery. Adv. Cancer Res. 137, 115–170. doi:10.1016/bs.acr.2017.11.003
Andre, T., Shiu, K. K., Kim, T. W., Jensen, B. V., Jensen, L. H., Punt, C., et al. (2020). Pembrolizumab in microsatellite-instability-high advanced colorectal cancer. N. Engl. J. Med. 383 (23), 2207–2218. doi:10.1056/NEJMoa2017699
Anneveldt, K. J., van 't Oever, H. J., Nijholt, I. M., Dijkstra, J. R., Hehenkamp, W. J., Veersema, S., et al. (2021). Systematic review of reproductive outcomes after High Intensity Focused Ultrasound treatment of uterine fibroids. Eur. J. Radiol. 141, 109801. doi:10.1016/j.ejrad.2021.109801
Ansell, S. M., Lesokhin, A. M., Borrello, I., Halwani, A., Scott, E. C., Gutierrez, M., et al. (2015). PD-1 blockade with nivolumab in relapsed or refractory Hodgkin's lymphoma. N. Engl. J. Med. 372 (4), 311–319. doi:10.1056/NEJMoa1411087
Antonia, S. J., Villegas, A., Daniel, D., Vicente, D., Murakami, S., Hui, R., et al. (2017). Durvalumab after chemoradiotherapy in stage III non-small-cell lung cancer. N. Engl. J. Med. 377 (20), 1919–1929. doi:10.1056/NEJMoa1709937
Armand, P., Engert, A., Younes, A., Fanale, M., Santoro, A., Zinzani, P. L., et al. (2018). Nivolumab for relapsed/refractory classic Hodgkin lymphoma after failure of autologous hematopoietic cell transplantation: Extended follow-up of the multicohort single-arm phase II CheckMate 205 trial. J. Clin. Oncol. 36 (14), 1428–1439. doi:10.1200/JCO.2017.76.0793
Armulik, A., Genove, G., Mae, M., Nisancioglu, M. H., Wallgard, E., Niaudet, C., et al. (2010). Pericytes regulate the blood-brain barrier. Nature 468 (7323), 557–561. doi:10.1038/nature09522
Aryal, M., Arvanitis, C. D., Alexander, P. M., and McDannold, N. (2014). Ultrasound-mediated blood-brain barrier disruption for targeted drug delivery in the central nervous system. Adv. Drug Deliv. Rev. 72, 94–109. doi:10.1016/j.addr.2014.01.008
Bachu, V. S., Kedda, J., Suk, I., Green, J. J., and Tyler, B. (2021). High-intensity focused ultrasound: A review of mechanisms and clinical applications. Ann. Biomed. Eng. 49 (9), 1975–1991. doi:10.1007/s10439-021-02833-9
Bader, K. B., Haworth, K. J., Shekhar, H., Maxwell, A. D., Peng, T., McPherson, D. D., et al. (2016). Efficacy of histotripsy combined with rt-PA in vitro. Phys. Med. Biol. 61 (14), 5253–5274. doi:10.1088/0031-9155/61/14/5253
Baek, H., Yang, Y., Pacia, C. P., Xu, L., Yue, Y., Bruchas, M. R., et al. (2021). Mechanical and mechanothermal effects of focused ultrasound elicited distinct electromyographic responses in mice. Phys. Med. Biol. 66 (13), 135005. doi:10.1088/1361-6560/ac08b1
Baetke, S. C., Lammers, T., and Kiessling, F. (2015). Applications of nanoparticles for diagnosis and therapy of cancer. Br. J. Radiol. 88 (1054), 20150207. doi:10.1259/bjr.20150207
Balar, A. V., Galsky, M. D., Rosenberg, J. E., Powles, T., Petrylak, D. P., Bellmunt, J., et al. (2017). Atezolizumab as first-line treatment in cisplatin-ineligible patients with locally advanced and metastatic urothelial carcinoma: A single-arm, multicentre, phase 2 trial. Lancet 389 (10064), 67–76. doi:10.1016/S0140-6736(16)32455-2
Barsoum, I. B., Smallwood, C. A., Siemens, D. R., and Graham, C. H. (2014). A mechanism of hypoxia-mediated escape from adaptive immunity in cancer cells. Cancer Res. 74 (3), 665–674. doi:10.1158/0008-5472.CAN-13-0992
Beatty, G. L., Chiorean, E. G., Fishman, M. P., Saboury, B., Teitelbaum, U. R., Sun, W., et al. (2011). CD40 agonists alter tumor stroma and show efficacy against pancreatic carcinoma in mice and humans. Science 331 (6024), 1612–1616. doi:10.1126/science.1198443
Beccaria, K., Canney, M., Bouchoux, G., Puget, S., Grill, J., and Carpentier, A. (2020). Blood-brain barrier disruption with low-intensity pulsed ultrasound for the treatment of pediatric brain tumors: A review and perspectives. Neurosurg. Focus 48 (1), E10. doi:10.3171/2019.10.FOCUS19726
Belgiovine, C., Bello, E., Liguori, M., Craparotta, I., Mannarino, L., Paracchini, L., et al. (2017). Lurbinectedin reduces tumour-associated macrophages and the inflammatory tumour microenvironment in preclinical models. Br. J. Cancer 117 (5), 628–638. doi:10.1038/bjc.2017.205
Blomley, M. J., Cooke, J. C., Unger, E. C., Monaghan, M. J., and Cosgrove, D. O. (2001). Microbubble contrast agents: A new era in ultrasound. BMJ 322 (7296), 1222–1225. doi:10.1136/bmj.322.7296.1222
Brahmer, J., Reckamp, K. L., Baas, P., Crino, L., Eberhardt, W. E., Poddubskaya, E., et al. (2015). Nivolumab versus docetaxel in advanced squamous-cell non-small-cell lung cancer. N. Engl. J. Med. 373 (2), 123–135. doi:10.1056/NEJMoa1504627
Brochez, L., Chevolet, I., and Kruse, V. (2017). The rationale of indoleamine 2,3-dioxygenase inhibition for cancer therapy. Eur. J. Cancer 76, 167–182. doi:10.1016/j.ejca.2017.01.011
Bunevicius, A., McDannold, N. J., and Golby, A. J. (2020). Focused ultrasound strategies for brain tumor therapy. Oper. Neurosurg. Hagerst. 19 (1), 9–18. doi:10.1093/ons/opz374
Burtness, B., Harrington, K. J., Greil, R., Soulieres, D., Tahara, M., de Castro, G., et al. (2019). Pembrolizumab alone or with chemotherapy versus cetuximab with chemotherapy for recurrent or metastatic squamous cell carcinoma of the head and neck (KEYNOTE-048): A randomised, open-label, phase 3 study. Lancet 394 (10212), 1915–1928. doi:10.1016/S0140-6736(19)32591-7
Chauhan, V. P., Martin, J. D., Liu, H., Lacorre, D. A., Jain, S. R., Kozin, S. V., et al. (2013). Angiotensin inhibition enhances drug delivery and potentiates chemotherapy by decompressing tumour blood vessels. Nat. Commun. 4, 2516. doi:10.1038/ncomms3516
Chen, H., Zhang, P., Shi, Y., Liu, C., Zhou, Q., Zeng, Y., et al. (2022). Functional nanovesicles displaying anti-PD-L1 antibodies for programmed photoimmunotherapy. J. Nanobiotechnology 20 (1), 61. doi:10.1186/s12951-022-01266-3
Chen, J., Nan, Z., Zhao, Y., Zhang, L., Zhu, H., Wu, D., et al. (2021). Enhanced HIFU theranostics with dual-frequency-ring focused ultrasound and activatable perfluoropentane-loaded polymer nanoparticles. Micromachines (Basel) 12 (11), 1324. doi:10.3390/mi12111324
Cheng, A. L., Qin, S., Ikeda, M., Galle, P. R., Ducreux, M., Kim, T. Y., et al. (2022). Updated efficacy and safety data from IMbrave150: Atezolizumab plus bevacizumab vs. sorafenib for unresectable hepatocellular carcinoma. J. Hepatol. 76 (4), 862–873. doi:10.1016/j.jhep.2021.11.030
Cheng, H., Fan, X., Ye, E., Chen, H., Yang, J., Ke, L., et al. (2022). Dual tumor microenvironment remodeling by glucose-contained radical copolymer for MRI-guided photoimmunotherapy. Adv. Mater 34 (25), e2107674. doi:10.1002/adma.202107674
Cheon, J., Yoo, C., Hong, J. Y., Kim, H. S., Lee, D. W., Lee, M. A., et al. (2022). Efficacy and safety of atezolizumab plus bevacizumab in Korean patients with advanced hepatocellular carcinoma. Liver Int. 42 (3), 674–681. doi:10.1111/liv.15102
Chinol, M., Casalini, P., Maggiolo, M., Canevari, S., Omodeo, E. S., Caliceti, P., et al. (1998). Biochemical modifications of avidin improve pharmacokinetics and biodistribution, and reduce immunogenicity. Br. J. Cancer 78 (2), 189–197. doi:10.1038/bjc.1998.463
Chowdhury, S. M., Abou-Elkacem, L., Lee, T., Dahl, J., and Lutz, A. M. (2020). Ultrasound and microbubble mediated therapeutic delivery: Underlying mechanisms and future outlook. J. Control Release 326, 75–90. doi:10.1016/j.jconrel.2020.06.008
Chu, D., Dong, X., Shi, X., Zhang, C., and Wang, Z. (2018). Neutrophil-based drug delivery systems. Adv. Mater 30 (22), e1706245. doi:10.1002/adma.201706245
Cohen, E. E. W., Soulieres, D., Le Tourneau, C., Dinis, J., Licitra, L., Ahn, M. J., et al. (2019). Pembrolizumab versus methotrexate, docetaxel, or cetuximab for recurrent or metastatic head-and-neck squamous cell carcinoma (KEYNOTE-040): A randomised, open-label, phase 3 study. Lancet 393 (10167), 156–167. doi:10.1016/S0140-6736(18)31999-8
Cotton, A. D., Nguyen, D. P., Gramespacher, J. A., Seiple, I. B., and Wells, J. A. (2021). Development of antibody-based PROTACs for the degradation of the cell-surface immune checkpoint protein PD-L1. J. Am. Chem. Soc. 143 (2), 593–598. doi:10.1021/jacs.0c10008
Cousin, C., Oberkampf, M., Felix, T., Rosenbaum, P., Weil, R., Fabrega, S., et al. (2019). Persistence of integrase-deficient lentiviral vectors correlates with the induction of STING-independent CD8(+) T cell responses. Cell. Rep. 26 (5), 1242–1257.e7. doi:10.1016/j.celrep.2019.01.025
Cui, S. (2017). Immunogenic chemotherapy sensitizes renal cancer to immune checkpoint blockade therapy in preclinical models. Med. Sci. Monit. 23, 3360–3366. doi:10.12659/msm.902426
Curley, C. T., Sheybani, N. D., Bullock, T. N., and Price, R. J. (2017). Focused ultrasound immunotherapy for central nervous system pathologies: Challenges and opportunities. Theranostics 7 (15), 3608–3623. doi:10.7150/thno.21225
Dallapiazza, R. F., Timbie, K. F., Holmberg, S., Gatesman, J., Lopes, M. B., Price, R. J., et al. (2018). Noninvasive neuromodulation and thalamic mapping with low-intensity focused ultrasound. J. Neurosurg. 128 (3), 875–884. doi:10.3171/2016.11.JNS16976
Dang, L. H., Bettegowda, C., Huso, D. L., Kinzler, K. W., and Vogelstein, B. (2001). Combination bacteriolytic therapy for the treatment of experimental tumors. Proc. Natl. Acad. Sci. U. S. A. 98 (26), 15155–15160. doi:10.1073/pnas.251543698
Darrow, D. P. (2019). Focused ultrasound for neuromodulation. Neurotherapeutics 16 (1), 88–99. doi:10.1007/s13311-018-00691-3
Decout, A., Katz, J. D., Venkatraman, S., and Ablasser, A. (2021). The cGAS-STING pathway as a therapeutic target in inflammatory diseases. Nat. Rev. Immunol. 21 (9), 548–569. doi:10.1038/s41577-021-00524-z
Deng, J., Huang, Q., Wang, F., Liu, Y., Wang, Z., Wang, Z., et al. (2012). The role of caveolin-1 in blood-brain barrier disruption induced by focused ultrasound combined with microbubbles. J. Mol. Neurosci. 46 (3), 677–687. doi:10.1007/s12031-011-9629-9
Dias, M. P., Moser, S. C., Ganesan, S., and Jonkers, J. (2021). Understanding and overcoming resistance to PARP inhibitors in cancer therapy. Nat. Rev. Clin. Oncol. 18 (12), 773–791. doi:10.1038/s41571-021-00532-x
Dillman, R. O. (2011). Cancer immunotherapy. Cancer Biother Radiopharm. 26 (1), 1–64. doi:10.1089/cbr.2010.0902
Dillman, R. O. (2017). Is there a role for therapeutic cancer vaccines in the age of checkpoint inhibitors? Hum. Vaccin Immunother. 13 (3), 528–532. doi:10.1080/21645515.2016.1244149
Ding, C., Song, Z., Shen, A., Chen, T., and Zhang, A. (2020). Small molecules targeting the innate immune cGAS‒STING‒TBK1 signaling pathway. Acta Pharm. Sin. B 10 (12), 2272–2298. doi:10.1016/j.apsb.2020.03.001
Dromi, S., Frenkel, V., Luk, A., Traughber, B., Angstadt, M., Bur, M., et al. (2007). Pulsed-high intensity focused ultrasound and low temperature-sensitive liposomes for enhanced targeted drug delivery and antitumor effect. Clin. Cancer Res. 13 (9), 2722–2727. doi:10.1158/1078-0432.CCR-06-2443
Du, L., Lee, J. H., Jiang, H., Wang, C., Wang, S., Zheng, Z., et al. (2020). β-Catenin induces transcriptional expression of PD-L1 to promote glioblastoma immune evasion. J. Exp. Med. 217 (11), e20191115. doi:10.1084/jem.20191115
El-Khoueiry, A. B., Sangro, B., Yau, T., Crocenzi, T. S., Kudo, M., Hsu, C., et al. (2017). Nivolumab in patients with advanced hepatocellular carcinoma (CheckMate 040): An open-label, non-comparative, phase 1/2 dose escalation and expansion trial. Lancet 389 (10088), 2492–2502. doi:10.1016/S0140-6736(17)31046-2
Elia, G., Ferrari, S. M., Galdiero, M. R., Ragusa, F., Paparo, S. R., Ruffilli, I., et al. (2020). New insight in endocrine-related adverse events associated to immune checkpoint blockade. Best. Pract. Res. Clin. Endocrinol. Metab. 34 (1), 101370. doi:10.1016/j.beem.2019.101370
Emens, L. A., Cruz, C., Eder, J. P., Braiteh, F., Chung, C., Tolaney, S. M., et al. (2019). Long-term clinical outcomes and biomarker analyses of atezolizumab therapy for patients with metastatic triple-negative breast cancer: A phase 1 study. JAMA Oncol. 5 (1), 74–82. doi:10.1001/jamaoncol.2018.4224
Eranki, A., Srinivasan, P., Ries, M., Kim, A., Lazarski, C. A., Rossi, C. T., et al. (2020). High-intensity focused ultrasound (HIFU) triggers immune sensitization of refractory murine neuroblastoma to checkpoint inhibitor therapy. Clin. Cancer Res. 26 (5), 1152–1161. doi:10.1158/1078-0432.CCR-19-1604
Fan, C. H., Wu, N., and Yeh, C. K. (2023). Enhanced sonodynamic therapy by carbon dots-shelled microbubbles with focused ultrasound. Ultrason. Sonochem 94, 106342. doi:10.1016/j.ultsonch.2023.106342
Fedorova, L. V., Lepik, K. V., Volkov, N. P., Kotselyabina, P. V., Borzenkova, E. S., Popova, M. O., et al. (2022). Efficacy and safety of nivolumab combined with brentuximab vedotin after nivolumab monotherapy failure in patients with relapsed and refractory classic Hodgkin lymphoma. Int. J. Clin. Oncol. 27 (3), 626–632. doi:10.1007/s10147-021-02085-6
Fehrenbacher, L., Spira, A., Ballinger, M., Kowanetz, M., Vansteenkiste, J., Mazieres, J., et al. (2016). Atezolizumab versus docetaxel for patients with previously treated non-small-cell lung cancer (POPLAR): A multicentre, open-label, phase 2 randomised controlled trial. Lancet 387 (10030), 1837–1846. doi:10.1016/S0140-6736(16)00587-0
Fennell, D. A., Ewings, S., Ottensmeier, C., Califano, R., Hanna, G. G., Hill, K., et al. (2021). Nivolumab versus placebo in patients with relapsed malignant mesothelioma (CONFIRM): A multicentre, double-blind, randomised, phase 3 trial. Lancet Oncol. 22 (11), 1530–1540. doi:10.1016/S1470-2045(21)00471-X
Finn, R. S., Qin, S., Ikeda, M., Galle, P. R., Ducreux, M., Kim, T. Y., et al. (2020a). Atezolizumab plus bevacizumab in unresectable hepatocellular carcinoma. N. Engl. J. Med. 382 (20), 1894–1905. doi:10.1056/NEJMoa1915745
Finn, R. S., Ryoo, B. Y., Merle, P., Kudo, M., Bouattour, M., Lim, H. Y., et al. (2020b). Pembrolizumab as second-line therapy in patients with advanced hepatocellular carcinoma in KEYNOTE-240: A randomized, double-blind, phase III trial. J. Clin. Oncol. 38 (3), 193–202. doi:10.1200/JCO.19.01307
Forde, P. M., Chaft, J. E., and Pardoll, D. M. (2018). Neoadjuvant PD-1 blockade in resectable lung cancer. N. Engl. J. Med. 379 (9), e14. doi:10.1056/NEJMc1808251
Friedman, C. F., Proverbs-Singh, T. A., and Postow, M. A. (2016). Treatment of the immune-related adverse effects of immune checkpoint inhibitors: A review. JAMA Oncol. 2 (10), 1346–1353. doi:10.1001/jamaoncol.2016.1051
Fuchs, C. S., Doi, T., Jang, R. W., Muro, K., Satoh, T., Machado, M., et al. (2018). Safety and efficacy of pembrolizumab monotherapy in patients with previously treated advanced gastric and gastroesophageal junction cancer: Phase 2 clinical KEYNOTE-059 trial. JAMA Oncol. 4 (5), e180013. doi:10.1001/jamaoncol.2018.0013
Fucikova, J., Becht, E., Iribarren, K., Goc, J., Remark, R., Damotte, D., et al. (2016). Calreticulin expression in human non-small cell lung cancers correlates with increased accumulation of antitumor immune cells and favorable prognosis. Cancer Res. 76 (7), 1746–1756. doi:10.1158/0008-5472.CAN-15-1142
Fujiwara, Y., Kato, S., Nesline, M. K., Conroy, J. M., DePietro, P., Pabla, S., et al. (2022). Indoleamine 2,3-dioxygenase (Ido) inhibitors and cancer immunotherapy. Cancer Treat. Rev. 110, 102461. doi:10.1016/j.ctrv.2022.102461
Galluzzi, L., Buque, A., Kepp, O., Zitvogel, L., and Kroemer, G. (2017). Immunogenic cell death in cancer and infectious disease. Nat. Rev. Immunol. 17 (2), 97–111. doi:10.1038/nri.2016.107
Galsky, M. D., Arija, J. A. A., Bamias, A., Davis, I. D., De Santis, M., Kikuchi, E., et al. (2020). Atezolizumab with or without chemotherapy in metastatic urothelial cancer (IMvigor130): A multicentre, randomised, placebo-controlled phase 3 trial. Lancet 395 (10236), 1547–1557. doi:10.1016/S0140-6736(20)30230-0
Gan, L., Yang, Y., Li, Q., Feng, Y., Liu, T., and Guo, W. (2018). Epigenetic regulation of cancer progression by EZH2: From biological insights to therapeutic potential. Biomark. Res. 6, 10. doi:10.1186/s40364-018-0122-2
Gao, Y., Nihira, N. T., Bu, X., Chu, C., Zhang, J., Kolodziejczyk, A., et al. (2020). Acetylation-dependent regulation of PD-L1 nuclear translocation dictates the efficacy of anti-PD-1 immunotherapy. Nat. Cell. Biol. 22 (9), 1064–1075. doi:10.1038/s41556-020-0562-4
Garg, A. D., Elsen, S., Krysko, D. V., Vandenabeele, P., de Witte, P., and Agostinis, P. (2015). Resistance to anticancer vaccination effect is controlled by a cancer cell-autonomous phenotype that disrupts immunogenic phagocytic removal. Oncotarget 6 (29), 26841–26860. doi:10.18632/oncotarget.4754
Ge, J., Yang, N., Yang, Y., Yu, H., Yang, X., Wang, Y., et al. (2023). The combination of eddy thermal effect of biodegradable magnesium with immune checkpoint blockade shows enhanced efficacy against osteosarcoma. Bioact. Mater 25, 73–85. doi:10.1016/j.bioactmat.2023.01.008
Gelet, A., Chapelon, J. Y., Bouvier, R., Pangaud, C., and Lasne, Y. (1999). Local control of prostate cancer by transrectal high intensity focused ultrasound therapy: Preliminary results. J. Urol. 161 (1), 156–162. doi:10.1016/s0022-5347(01)62087-1
Gong, N., Sheppard, N. C., Billingsley, M. M., June, C. H., and Mitchell, M. J. (2021). Nanomaterials for T-cell cancer immunotherapy. Nat. Nanotechnol. 16 (1), 25–36. doi:10.1038/s41565-020-00822-y
Gorbet, M. J., Singh, A., Mao, C., Fiering, S., and Ranjan, A. (2020). Using nanoparticles for in situ vaccination against cancer: Mechanisms and immunotherapy benefits. Int. J. Hyperth. 37 (3), 18–33. doi:10.1080/02656736.2020.1802519
Grabosch, S., Bulatovic, M., Zeng, F., Ma, T., Zhang, L., Ross, M., et al. (2019). Cisplatin-induced immune modulation in ovarian cancer mouse models with distinct inflammation profiles. Oncogene 38 (13), 2380–2393. doi:10.1038/s41388-018-0581-9
Granier, C., De Guillebon, E., Blanc, C., Roussel, H., Badoual, C., Colin, E., et al. (2017). Mechanisms of action and rationale for the use of checkpoint inhibitors in cancer. ESMO Open 2 (2), e000213. doi:10.1136/esmoopen-2017-000213
Green, D. R., Ferguson, T., Zitvogel, L., and Kroemer, G. (2009). Immunogenic and tolerogenic cell death. Nat. Rev. Immunol. 9 (5), 353–363. doi:10.1038/nri2545
Guo, Q. L., Dai, X. L., Yin, M. Y., Cheng, H. W., Qian, H. S., Wang, H., et al. (2022). Nanosensitizers for sonodynamic therapy for glioblastoma multiforme: Current progress and future perspectives. Mil. Med. Res. 9 (1), 26. doi:10.1186/s40779-022-00386-z
Han, X., Chen, J., Chu, J., Liang, C., Ma, Q., Fan, Q., et al. (2019). Platelets as platforms for inhibition of tumor recurrence post-physical therapy by delivery of anti-PD-L1 checkpoint antibody. J. Control Release 304, 233–241. doi:10.1016/j.jconrel.2019.05.008
Han, X., Wang, C., and Liu, Z. (2018). Red blood cells as smart delivery systems. Bioconjug Chem. 29 (4), 852–860. doi:10.1021/acs.bioconjchem.7b00758
Han, Y., Liu, D., and Li, L. (2020). PD-1/PD-L1 pathway: Current researches in cancer. Am. J. Cancer Res. 10 (3), 727–742.
Harary, M., Segar, D. J., Huang, K. T., Tafel, I. J., Valdes, P. A., and Cosgrove, G. R. (2018). Focused ultrasound in neurosurgery: A historical perspective. Neurosurg. Focus 44 (2), E2. doi:10.3171/2017.11.FOCUS17586
He, X., and Xu, C. (2020). Immune checkpoint signaling and cancer immunotherapy. Cell. Res. 30 (8), 660–669. doi:10.1038/s41422-020-0343-4
Hegde, P. S., Karanikas, V., and Evers, S. (2016). The where, the when, and the how of immune monitoring for cancer immunotherapies in the era of checkpoint inhibition. Clin. Cancer Res. 22 (8), 1865–1874. doi:10.1158/1078-0432.CCR-15-1507
Heinhuis, K. M., Ros, W., Kok, M., Steeghs, N., Beijnen, J. H., and Schellens, J. H. M. (2019). Enhancing antitumor response by combining immune checkpoint inhibitors with chemotherapy in solid tumors. Ann. Oncol. 30 (2), 219–235. doi:10.1093/annonc/mdy551
Hellmann, M. D., Rizvi, N. A., Goldman, J. W., Gettinger, S. N., Borghaei, H., Brahmer, J. R., et al. (2017). Nivolumab plus ipilimumab as first-line treatment for advanced non-small-cell lung cancer (CheckMate 012): Results of an open-label, phase 1, multicohort study. Lancet Oncol. 18 (1), 31–41. doi:10.1016/S1470-2045(16)30624-6
Helmink, B. A., Reddy, S. M., Gao, J., Zhang, S., Basar, R., Thakur, R., et al. (2020). B cells and tertiary lymphoid structures promote immunotherapy response. Nature 577 (7791), 549–555. doi:10.1038/s41586-019-1922-8
Herbst, R. S., Soria, J. C., Kowanetz, M., Fine, G. D., Hamid, O., Gordon, M. S., et al. (2014). Predictive correlates of response to the anti-PD-L1 antibody MPDL3280A in cancer patients. Nature 515 (7528), 563–567. doi:10.1038/nature14011
Hewakuruppu, Y. L., Dombrovsky, L. A., Chen, C., Timchenko, V., Jiang, X., Baek, S., et al. (2013). Plasmonic "pump-probe" method to study semi-transparent nanofluids. Appl. Opt. 52 (24), 6041–6050. doi:10.1364/AO.52.006041
Horn, L., Mansfield, A. S., Szczesna, A., Havel, L., Krzakowski, M., Hochmair, M. J., et al. (2018). First-line atezolizumab plus chemotherapy in extensive-stage small-cell lung cancer. N. Engl. J. Med. 379 (23), 2220–2229. doi:10.1056/NEJMoa1809064
Hu, H., Yu, J., Zhang, L., Zhang, A., Li, Y., Jiang, Y., et al. (2007). Pulse source based on directly modulated laser and phase modulator. Opt. Express 15 (14), 8931–8937. doi:10.1364/oe.15.008931
Hu, Z., Yang, X. Y., Liu, Y., Morse, M. A., Lyerly, H. K., Clay, T. M., et al. (2005). Release of endogenous danger signals from HIFU-treated tumor cells and their stimulatory effects on APCs. Biochem. Biophys. Res. Commun. 335 (1), 124–131. doi:10.1016/j.bbrc.2005.07.071
Hynynen, K., McDannold, N., Vykhodtseva, N., and Jolesz, F. A. (2001). Noninvasive MR imaging-guided focal opening of the blood-brain barrier in rabbits. Radiology 220 (3), 640–646. doi:10.1148/radiol.2202001804
Ilovitsh, T., Feng, Y., Foiret, J., Kheirolomoom, A., Zhang, H., Ingham, E. S., et al. (2020). Low-frequency ultrasound-mediated cytokine transfection enhances T cell recruitment at local and distant tumor sites. Proc. Natl. Acad. Sci. U. S. A. 117 (23), 12674–12685. doi:10.1073/pnas.1914906117
Izadifar, Z., Izadifar, Z., Chapman, D., and Babyn, P. (2020). An introduction to high intensity focused ultrasound: Systematic review on principles, devices, and clinical applications. J. Clin. Med. 9 (2), 460. doi:10.3390/jcm9020460
Izci, M., Maksoudian, C., Manshian, B. B., and Soenen, S. J. (2021). The use of alternative strategies for enhanced nanoparticle delivery to solid tumors. Chem. Rev. 121 (3), 1746–1803. doi:10.1021/acs.chemrev.0c00779
Jalali, S., Huang, Y., Dumont, D. J., and Hynynen, K. (2010). Focused ultrasound-mediated bbb disruption is associated with an increase in activation of AKT: Experimental study in rats. BMC Neurol. 10, 114. doi:10.1186/1471-2377-10-114
Janse van Rensburg, H. J., Azad, T., Ling, M., Hao, Y., Snetsinger, B., Khanal, P., et al. (2018). The hippo pathway component TAZ promotes immune evasion in human cancer through PD-L1. Cancer Res. 78 (6), 1457–1470. doi:10.1158/0008-5472.CAN-17-3139
Ji, R. R., Chasalow, S. D., Wang, L., Hamid, O., Schmidt, H., Cogswell, J., et al. (2012). An immune-active tumor microenvironment favors clinical response to ipilimumab. Cancer Immunol. Immunother. 61 (7), 1019–1031. doi:10.1007/s00262-011-1172-6
Jiang, M., Chen, P., Wang, L., Li, W., Chen, B., Liu, Y., et al. (2020). cGAS-STING, an important pathway in cancer immunotherapy. J. Hematol. Oncol. 13 (1), 81. doi:10.1186/s13045-020-00916-z
Jiang, Q., Qiao, B., Lin, X., Cao, J., Zhang, N., Guo, H., et al. (2022). A hydrogen peroxide economizer for on-demand oxygen production-assisted robust sonodynamic immunotherapy. Theranostics 12 (1), 59–75. doi:10.7150/thno.64862
Jiang, X. M., Xu, Y. L., Huang, M. Y., Zhang, L. L., Su, M. X., Chen, X., et al. (2017). Osimertinib (AZD9291) decreases programmed death ligand-1 in EGFR-mutated non-small cell lung cancer cells. Acta Pharmacol. Sin. 38 (11), 1512–1520. doi:10.1038/aps.2017.123
Jin, Z. H. (2021). Oscillatory interstitial fluid pressure and velocity in a solid tumor with partial surface fluid leakage. Microvasc. Res. 133, 104097. doi:10.1016/j.mvr.2020.104097
Johnson, D. B., Nebhan, C. A., Moslehi, J. J., and Balko, J. M. (2022). Immune-checkpoint inhibitors: Long-term implications of toxicity. Nat. Rev. Clin. Oncol. 19 (4), 254–267. doi:10.1038/s41571-022-00600-w
June, C. H., Warshauer, J. T., and Bluestone, J. A. (2017). Is autoimmunity the Achilles' heel of cancer immunotherapy? Nat. Med. 23 (5), 540–547. doi:10.1038/nm.4321
Kang, S. R., Jo, E. J., Nguyen, V. H., Zhang, Y., Yoon, H. S., Pyo, A., et al. (2020). Imaging of tumor colonization by Escherichia coli using (18)F-FDS PET. Theranostics 10 (11), 4958–4966. doi:10.7150/thno.42121
Kaseb, A. O., Hasanov, E., Cao, H. S. T., Xiao, L., Vauthey, J. N., Lee, S. S., et al. (2022). Perioperative nivolumab monotherapy versus nivolumab plus ipilimumab in resectable hepatocellular carcinoma: A randomised, open-label, phase 2 trial. Lancet Gastroenterol. Hepatol. 7 (3), 208–218. doi:10.1016/S2468-1253(21)00427-1
Kato, K., Cho, B. C., Takahashi, M., Okada, M., Lin, C. Y., Chin, K., et al. (2019). Nivolumab versus chemotherapy in patients with advanced oesophageal squamous cell carcinoma refractory or intolerant to previous chemotherapy (ATTRACTION-3): A multicentre, randomised, open-label, phase 3 trial. Lancet Oncol. 20 (11), 1506–1517. doi:10.1016/S1470-2045(19)30626-6
Kennedy, L. B., and Salama, A. K. S. (2020). A review of cancer immunotherapy toxicity. CA Cancer J. Clin. 70 (2), 86–104. doi:10.3322/caac.21596
Khirallah, J., Schmieley, R., Demirel, E., Rehman, T. U., Howell, J., Durmaz, Y. Y., et al. (2019). Nanoparticle-mediated histotripsy (NMH) using perfluorohexane 'nanocones. Phys. Med. Biol. 64 (12), 125018. doi:10.1088/1361-6560/ab207e
Khoja, L., Day, D., Wei-Wu Chen, T., Siu, L. L., and Hansen, A. R. (2017). Tumour- and class-specific patterns of immune-related adverse events of immune checkpoint inhibitors: A systematic review. Ann. Oncol. 28 (10), 2377–2385. doi:10.1093/annonc/mdx286
Khokhlova, V. A., Fowlkes, J. B., Roberts, W. W., Schade, G. R., Xu, Z., Khokhlova, T. D., et al. (2015). Histotripsy methods in mechanical disintegration of tissue: Towards clinical applications. Int. J. Hyperth. 31 (2), 145–162. doi:10.3109/02656736.2015.1007538
Kim, D., Lee, S. S., Moon, H., Park, S. Y., and Lee, H. J. (2020). PD-L1 targeting immune-microbubble complex enhances therapeutic index in murine colon cancer models. Pharm. (Basel) 14 (1), 6. doi:10.3390/ph14010006
Kim, T. K., Vandsemb, E. N., Herbst, R. S., and Chen, L. (2022). Adaptive immune resistance at the tumour site: Mechanisms and therapeutic opportunities. Nat. Rev. Drug Discov. 21 (7), 529–540. doi:10.1038/s41573-022-00493-5
Knight, F. C., Gilchuk, P., Kumar, A., Becker, K. W., Sevimli, S., Jacobson, M. E., et al. (2019). Mucosal immunization with a pH-responsive nanoparticle vaccine induces protective CD8(+) lung-resident memory T cells. ACS Nano 13 (10), 10939–10960. doi:10.1021/acsnano.9b00326
Kojima, T., Shah, M. A., Muro, K., Francois, E., Adenis, A., Hsu, C. H., et al. (2020). Randomized phase III KEYNOTE-181 study of pembrolizumab versus chemotherapy in advanced esophageal cancer. J. Clin. Oncol. 38 (35), 4138–4148. doi:10.1200/JCO.20.01888
Kooiman, K., Roovers, S., Langeveld, S. A. G., Kleven, R. T., Dewitte, H., O'Reilly, M. A., et al. (2020). Ultrasound-responsive cavitation nuclei for therapy and drug delivery. Ultrasound Med. Biol. 46 (6), 1296–1325. doi:10.1016/j.ultrasmedbio.2020.01.002
Kruger, S., Ilmer, M., Kobold, S., Cadilha, B. L., Endres, S., Ormanns, S., et al. (2019). Advances in cancer immunotherapy 2019 - latest trends. J. Exp. Clin. Cancer Res. 38 (1), 268. doi:10.1186/s13046-019-1266-0
Kubli, S. P., Berger, T., Araujo, D. V., Siu, L. L., and Mak, T. W. (2021). Beyond immune checkpoint blockade: Emerging immunological strategies. Nat. Rev. Drug Discov. 20 (12), 899–919. doi:10.1038/s41573-021-00155-y
Kudo, M., Finn, R. S., Edeline, J., Cattan, S., Ogasawara, S., Palmer, D. H., et al. (2022). Updated efficacy and safety of KEYNOTE-224: A phase II study of pembrolizumab in patients with advanced hepatocellular carcinoma previously treated with sorafenib. Eur. J. Cancer 167, 1–12. doi:10.1016/j.ejca.2022.02.009
Lafond, M., Lambin, T., Drainville, R. A., Dupre, A., Pioche, M., Melodelima, D., et al. (2022). Pancreatic ductal adenocarcinoma: Current and emerging therapeutic uses of focused ultrasound. Cancers (Basel) 14 (11), 2577. doi:10.3390/cancers14112577
Lafond, M., Yoshizawa, S., and Umemura, S. I. (2019). Sonodynamic therapy: Advances and challenges in clinical translation. J. Ultrasound Med. 38 (3), 567–580. doi:10.1002/jum.14733
Lakhal, S., and Wood, M. J. (2011). Exosome nanotechnology: An emerging paradigm shift in drug delivery: Exploitation of exosome nanovesicles for systemic in vivo delivery of RNAi heralds new horizons for drug delivery across biological barriers. Bioessays 33 (10), 737–741. doi:10.1002/bies.201100076
Larkin, J., Chiarion-Sileni, V., Gonzalez, R., Grob, J. J., Cowey, C. L., Lao, C. D., et al. (2015). Combined nivolumab and ipilimumab or monotherapy in untreated melanoma. N. Engl. J. Med. 373 (1), 23–34. doi:10.1056/NEJMoa1504030
Larkin, J., Chiarion-Sileni, V., Gonzalez, R., Grob, J. J., Rutkowski, P., Lao, C. D., et al. (2019). Five-year survival with combined nivolumab and ipilimumab in advanced melanoma. N. Engl. J. Med. 381 (16), 1535–1546. doi:10.1056/NEJMoa1910836
Le, D. T., Kim, T. W., Van Cutsem, E., Geva, R., Jager, D., Hara, H., et al. (2020). Phase II open-label study of pembrolizumab in treatment-refractory, microsatellite instability-high/mismatch repair-deficient metastatic colorectal cancer: KEYNOTE-164. J. Clin. Oncol. 38 (1), 11–19. doi:10.1200/JCO.19.02107
Lechner, M. G., Karimi, S. S., Barry-Holson, K., Angell, T. E., Murphy, K. A., Church, C. H., et al. (2013). Immunogenicity of murine solid tumor models as a defining feature of in vivo behavior and response to immunotherapy. J. Immunother. 36 (9), 477–489. doi:10.1097/01.cji.0000436722.46675.4a
Lee, C. C., Chou, C. C., Hsiao, F. J., Chen, Y. H., Lin, C. F., Chen, C. J., et al. (2022). Pilot study of focused ultrasound for drug-resistant epilepsy. Epilepsia 63 (1), 162–175. doi:10.1111/epi.17105
Lee, H., Guo, Y., Ross, J. L., Schoen, S., Degertekin, F. L., and Arvanitis, C. (2022). Spatially targeted brain cancer immunotherapy with closed-loop controlled focused ultrasound and immune checkpoint blockade. Sci. Adv. 8 (46), eadd2288. doi:10.1126/sciadv.add2288
Lee, M. S., Ryoo, B. Y., Hsu, C. H., Numata, K., Stein, S., Verret, W., et al. (2020). Atezolizumab with or without bevacizumab in unresectable hepatocellular carcinoma (GO30140): An open-label, multicentre, phase 1b study. Lancet Oncol. 21 (6), 808–820. doi:10.1016/S1470-2045(20)30156-X
Leon-Ferre, R. A., Hieken, T. J., and Boughey, J. C. (2021). The landmark series: Neoadjuvant chemotherapy for triple-negative and HER2-positive breast cancer. Ann. Surg. Oncol. 28 (4), 2111–2119. doi:10.1245/s10434-020-09480-9
Leschner, S., Westphal, K., Dietrich, N., Viegas, N., Jablonska, J., Lyszkiewicz, M., et al. (2009). Tumor invasion of Salmonella enterica serovar Typhimurium is accompanied by strong hemorrhage promoted by TNF-alpha. PLoS One 4 (8), e6692. doi:10.1371/journal.pone.0006692
Li, A., Yi, M., Qin, S., Song, Y., Chu, Q., and Wu, K. (2019). Activating cGAS-STING pathway for the optimal effect of cancer immunotherapy. J. Hematol. Oncol. 12 (1), 35. doi:10.1186/s13045-019-0721-x
Li, T., Shen, X., Geng, Y., Chen, Z., Li, L., Li, S., et al. (2016). Folate-functionalized magnetic-mesoporous silica nanoparticles for drug/gene codelivery to potentiate the antitumor efficacy. ACS Appl. Mater Interfaces 8 (22), 13748–13758. doi:10.1021/acsami.6b02963
Li, X., Khorsandi, S., Wang, Y., Santelli, J., Huntoon, K., Nguyen, N., et al. (2022). Cancer immunotherapy based on image-guided STING activation by nucleotide nanocomplex-decorated ultrasound microbubbles. Nat. Nanotechnol. 17 (8), 891–899. doi:10.1038/s41565-022-01134-z
Li, Y., James, S. J., Wyllie, D. H., Wynne, C., Czibula, A., Bukhari, A., et al. (2019). TMEM203 is a binding partner and regulator of STING-mediated inflammatory signaling in macrophages. Proc. Natl. Acad. Sci. U. S. A. 116 (33), 16479–16488. doi:10.1073/pnas.1901090116
Liu, H., Naxerova, K., Pinter, M., Incio, J., Lee, H., Shigeta, K., et al. (2017). Use of angiotensin system inhibitors is associated with immune activation and longer survival in nonmetastatic pancreatic ductal adenocarcinoma. Clin. Cancer Res. 23 (19), 5959–5969. doi:10.1158/1078-0432.CCR-17-0256
Liu, S., Zhang, Y., Liu, Y., Wang, W., Gao, S., Yuan, W., et al. (2022). Ultrasound-targeted microbubble destruction remodels tumour microenvironment to improve immunotherapeutic effect. Br. J. Cancer 128, 715–725. doi:10.1038/s41416-022-02076-y
Long, G. V., Dummer, R., Hamid, O., Gajewski, T. F., Caglevic, C., Dalle, S., et al. (2019). Epacadostat plus pembrolizumab versus placebo plus pembrolizumab in patients with unresectable or metastatic melanoma (ECHO-301/KEYNOTE-252): A phase 3, randomised, double-blind study. Lancet Oncol. 20 (8), 1083–1097. doi:10.1016/S1470-2045(19)30274-8
Long, K. B., Gladney, W. L., Tooker, G. M., Graham, K., Fraietta, J. A., and Beatty, G. L. (2016). IFNγ and CCL2 cooperate to redirect tumor-infiltrating monocytes to degrade fibrosis and enhance chemotherapy efficacy in pancreatic carcinoma. Cancer Discov. 6 (4), 400–413. doi:10.1158/2159-8290.CD-15-1032
Luo, Z., Jin, K., Pang, Q., Shen, S., Yan, Z., Jiang, T., et al. (2017). On-demand drug release from dual-targeting small nanoparticles triggered by high-intensity focused ultrasound enhanced glioblastoma-targeting therapy. ACS Appl. Mater Interfaces 9 (37), 31612–31625. doi:10.1021/acsami.7b10866
Lynn, J. G., Zwemer, R. L., Chick, A. J., and Miller, A. E. (1942). A new method for the generation and use of focused ultrasound in experimental biology. J. Gen. Physiol. 26 (2), 179–193. doi:10.1085/jgp.26.2.179
Ma, F., Vayalil, J., Lee, G., Wang, Y., and Peng, G. (2021). Emerging role of tumor-derived extracellular vesicles in T cell suppression and dysfunction in the tumor microenvironment. J. Immunother. Cancer 9 (10), e003217. doi:10.1136/jitc-2021-003217
Ma, P., and Mumper, R. J. (2013). Paclitaxel nano-delivery systems: A comprehensive review. J. Nanomed Nanotechnol. 4 (2), 1000164. doi:10.4172/2157-7439.1000164
Mahnken, A. H., Pereira, P. L., and de Baere, T. (2013). Interventional oncologic approaches to liver metastases. Radiology 266 (2), 407–430. doi:10.1148/radiol.12112544
Mahoney, K. M., Rennert, P. D., and Freeman, G. J. (2015). Combination cancer immunotherapy and new immunomodulatory targets. Nat. Rev. Drug Discov. 14 (8), 561–584. doi:10.1038/nrd4591
Mai, X., Chang, Y., You, Y., He, L., and Chen, T. (2021). Designing intelligent nano-bomb with on-demand site-specific drug burst release to synergize with high-intensity focused ultrasound cancer ablation. J. Control Release 331, 270–281. doi:10.1016/j.jconrel.2020.09.051
Maibach, F., Sadozai, H., Seyed Jafari, S. M., Hunger, R. E., and Schenk, M. (2020). Tumor-infiltrating lymphocytes and their prognostic value in cutaneous melanoma. Front. Immunol. 11, 2105. doi:10.3389/fimmu.2020.02105
Maleki Vareki, S., Garrigos, C., and Duran, I. (2017). Biomarkers of response to PD-1/PD-L1 inhibition. Crit. Rev. Oncol. Hematol. 116, 116–124. doi:10.1016/j.critrevonc.2017.06.001
Maloney, E., Khokhlova, T., Pillarisetty, V. G., Schade, G. R., Repasky, E. A., Wang, Y. N., et al. (2017). Focused ultrasound for immuno-adjuvant treatment of pancreatic cancer: An emerging clinical paradigm in the era of personalized oncotherapy. Int. Rev. Immunol. 36 (6), 338–351. doi:10.1080/08830185.2017.1363199
Maniar, R., Anderson, M., Taback, B., Khan, S., McDonnell, D., Saqi, A., et al. (2020). Case of Merkel cell carcinoma in a patient with pre-existing ILD. J. Immunother. Cancer 8 (2), e001672. doi:10.1136/jitc-2020-001672
Marin-Acevedo, J. A., Kimbrough, E. O., and Lou, Y. (2021). Next generation of immune checkpoint inhibitors and beyond. J. Hematol. Oncol. 14 (1), 45. doi:10.1186/s13045-021-01056-8
Meairs, S. (2015). Facilitation of drug transport across the blood-brain barrier with ultrasound and microbubbles. Pharmaceutics 7 (3), 275–293. doi:10.3390/pharmaceutics7030275
Mellman, I., Coukos, G., and Dranoff, G. (2011). Cancer immunotherapy comes of age. Nature 480 (7378), 480–489. doi:10.1038/nature10673
Mess, G., Anderson, T., Kapoor, S., Thombre, R., Liang, R., Derin, E., et al. (2023). Sonodynamic therapy for the treatment of glioblastoma multiforme in a mouse model using a portable benchtop focused ultrasound system. J. Vis. Exp. 192. doi:10.3791/65114
Michot, J. M., Bigenwald, C., Champiat, S., Collins, M., Carbonnel, F., Postel-Vinay, S., et al. (2016). Immune-related adverse events with immune checkpoint blockade: A comprehensive review. Eur. J. Cancer 54, 139–148. doi:10.1016/j.ejca.2015.11.016
Miles, D., Gligorov, J., Andre, F., Cameron, D., Schneeweiss, A., Barrios, C., et al. (2021). Primary results from IMpassion131, a double-blind, placebo-controlled, randomised phase III trial of first-line paclitaxel with or without atezolizumab for unresectable locally advanced/metastatic triple-negative breast cancer. Ann. Oncol. 32 (8), 994–1004. doi:10.1016/j.annonc.2021.05.801
Morad, G., Helmink, B. A., Sharma, P., and Wargo, J. A. (2021). Hallmarks of response, resistance, and toxicity to immune checkpoint blockade. Cell. 184 (21), 5309–5337. doi:10.1016/j.cell.2021.09.020
Morrell, C. N., Aggrey, A. A., Chapman, L. M., and Modjeski, K. L. (2014). Emerging roles for platelets as immune and inflammatory cells. Blood 123 (18), 2759–2767. doi:10.1182/blood-2013-11-462432
Moslehi, J. J., Salem, J. E., Sosman, J. A., Lebrun-Vignes, B., and Johnson, D. B. (2018). Increased reporting of fatal immune checkpoint inhibitor-associated myocarditis. Lancet 391 (10124), 933. doi:10.1016/S0140-6736(18)30533-6
Motzer, R. J., Escudier, B., McDermott, D. F., George, S., Hammers, H. J., Srinivas, S., et al. (2015). Nivolumab versus everolimus in advanced renal-cell carcinoma. N. Engl. J. Med. 373 (19), 1803–1813. doi:10.1056/NEJMoa1510665
Motzer, R. J., Penkov, K., Haanen, J., Rini, B., Albiges, L., Campbell, M. T., et al. (2019). Avelumab plus axitinib versus sunitinib for advanced renal-cell carcinoma. N. Engl. J. Med. 380 (12), 1103–1115. doi:10.1056/NEJMoa1816047
Mouratidis, P. X. E., Costa, M., Rivens, I., Repasky, E. E., and Ter Haar, G. (2021). Pulsed focused ultrasound can improve the anti-cancer effects of immune checkpoint inhibitors in murine pancreatic cancer. J. R. Soc. Interface 18 (180), 20210266. doi:10.1098/rsif.2021.0266
Mullick Chowdhury, S., Lee, T., and Willmann, J. K. (2017). Ultrasound-guided drug delivery in cancer. Ultrasonography 36 (3), 171–184. doi:10.14366/usg.17021
Mullick Chowdhury, S., Wang, T. Y., Bachawal, S., Devulapally, R., Choe, J. W., Abou Elkacem, L., et al. (2016). Ultrasound-guided therapeutic modulation of hepatocellular carcinoma using complementary microRNAs. J. Control Release 238, 272–280. doi:10.1016/j.jconrel.2016.08.005
Naidoo, J., Wang, X., Woo, K. M., Iyriboz, T., Halpenny, D., Cunningham, J., et al. (2017). Pneumonitis in patients treated with anti-programmed death-1/programmed death ligand 1 therapy. J. Clin. Oncol. 35 (7), 709–717. doi:10.1200/JCO.2016.68.2005
Nanda, R., Chow, L. Q., Dees, E. C., Berger, R., Gupta, S., Geva, R., et al. (2016). Pembrolizumab in patients with advanced triple-negative breast cancer: Phase ib KEYNOTE-012 study. J. Clin. Oncol. 34 (21), 2460–2467. doi:10.1200/JCO.2015.64.8931
Newman, P. G., and Rozycki, G. S. (1998). The history of ultrasound. Surg. Clin. North Am. 78 (2), 179–195. doi:10.1016/s0039-6109(05)70308-x
Ning, Y. M., Suzman, D., Maher, V. E., Zhang, L., Tang, S., Ricks, T., et al. (2017). FDA approval summary: Atezolizumab for the treatment of patients with progressive advanced urothelial carcinoma after platinum-containing chemotherapy. Oncologist 22 (6), 743–749. doi:10.1634/theoncologist.2017-0087
Nixon, B. G., Kuo, F., Ji, L., Liu, M., Capistrano, K., Do, M., et al. (2022). Tumor-associated macrophages expressing the transcription factor IRF8 promote T cell exhaustion in cancer. Immunity 55 (11), 2044–2058.e5. doi:10.1016/j.immuni.2022.10.002
Noman, M. Z., Desantis, G., Janji, B., Hasmim, M., Karray, S., Dessen, P., et al. (2014). PD-L1 is a novel direct target of HIF-1α, and its blockade under hypoxia enhanced MDSC-mediated T cell activation. J. Exp. Med. 211 (5), 781–790. doi:10.1084/jem.20131916
O'Malley, D. M., Bariani, G. M., Cassier, P. A., Marabelle, A., Hansen, A. R., De Jesus Acosta, A., et al. (2022). Pembrolizumab in patients with microsatellite instability-high advanced endometrial cancer: Results from the KEYNOTE-158 study. J. Clin. Oncol. 40 (7), 752–761. doi:10.1200/JCO.21.01874
Obeid, M., Tesniere, A., Ghiringhelli, F., Fimia, G. M., Apetoh, L., Perfettini, J. L., et al. (2007). Calreticulin exposure dictates the immunogenicity of cancer cell death. Nat. Med. 13 (1), 54–61. doi:10.1038/nm1523
Oliva, N., Conde, J., Wang, K., and Artzi, N. (2017). Designing hydrogels for on-demand therapy. Acc. Chem. Res. 50 (4), 669–679. doi:10.1021/acs.accounts.6b00536
Ou, Z., Qiu, L., Rong, H., Li, B., Ren, S., Kuang, S., et al. (2022). Bibliometric analysis of chimeric antigen receptor-based immunotherapy in cancers from 2001 to 2021. Front. Immunol. 13, 822004. doi:10.3389/fimmu.2022.822004
Overman, M. J., McDermott, R., Leach, J. L., Lonardi, S., Lenz, H. J., Morse, M. A., et al. (2017). Nivolumab in patients with metastatic DNA mismatch repair-deficient or microsatellite instability-high colorectal cancer (CheckMate 142): An open-label, multicentre, phase 2 study. Lancet Oncol. 18 (9), 1182–1191. doi:10.1016/S1470-2045(17)30422-9
Pahk, K. J. (2021). Control of the dynamics of a boiling vapour bubble using pressure-modulated high intensity focused ultrasound without the shock scattering effect: A first proof-of-concept study. Ultrason. Sonochem 77, 105699. doi:10.1016/j.ultsonch.2021.105699
Pan, Y., Yoon, S., Sun, J., Huang, Z., Lee, C., Allen, M., et al. (2018). Mechanogenetics for the remote and noninvasive control of cancer immunotherapy. Proc. Natl. Acad. Sci. U. S. A. 115 (5), 992–997. doi:10.1073/pnas.1714900115
Panaretakis, T., Joza, N., Modjtahedi, N., Tesniere, A., Vitale, I., Durchschlag, M., et al. (2008). The co-translocation of ERp57 and calreticulin determines the immunogenicity of cell death. Cell. Death Differ. 15 (9), 1499–1509. doi:10.1038/cdd.2008.67
Pardoll, D. M. (2012). The blockade of immune checkpoints in cancer immunotherapy. Nat. Rev. Cancer 12 (4), 252–264. doi:10.1038/nrc3239
Pauken, K. E., Dougan, M., Rose, N. R., Lichtman, A. H., and Sharpe, A. H. (2019). Adverse events following cancer immunotherapy: Obstacles and opportunities. Trends Immunol. 40 (6), 511–523. doi:10.1016/j.it.2019.04.002
Payne, A., Vyas, U., Blankespoor, A., Christensen, D., and Roemer, R. (2010). Minimisation of HIFU pulse heating and interpulse cooling times. Int. J. Hyperth. 26 (2), 198–208. doi:10.3109/02656730903436459
Paz-Ares, L., Dvorkin, M., Chen, Y., Reinmuth, N., Hotta, K., Trukhin, D., et al. (2019). Durvalumab plus platinum-etoposide versus platinum-etoposide in first-line treatment of extensive-stage small-cell lung cancer (CASPIAN): A randomised, controlled, open-label, phase 3 trial. Lancet 394 (10212), 1929–1939. doi:10.1016/S0140-6736(19)32222-6
Peng, H., He, X., and Wang, Q. (2022). Immune checkpoint blockades in gynecological cancers: A review of clinical trials. Acta Obstet. Gynecol. Scand. 101 (9), 941–951. doi:10.1111/aogs.14412
Phenix, C. P., Togtema, M., Pichardo, S., Zehbe, I., and Curiel, L. (2014). High intensity focused ultrasound technology, its scope and applications in therapy and drug delivery. J. Pharm. Pharm. Sci. 17 (1), 136–153. doi:10.18433/j3zp5f
Phua, S. Z. F., Yang, G., Lim, W. Q., Verma, A., Chen, H., Thanabalu, T., et al. (2019). Catalase-integrated hyaluronic acid as nanocarriers for enhanced photodynamic therapy in solid tumor. ACS Nano 13 (4), 4742–4751. doi:10.1021/acsnano.9b01087
Piraner, D. I., Abedi, M. H., Moser, B. A., Lee-Gosselin, A., and Shapiro, M. G. (2017). Tunable thermal bioswitches for in vivo control of microbial therapeutics. Nat. Chem. Biol. 13 (1), 75–80. doi:10.1038/nchembio.2233
Poon, C., Pellow, C., and Hynynen, K. (2021). Neutrophil recruitment and leukocyte response following focused ultrasound and microbubble mediated blood-brain barrier treatments. Theranostics 11 (4), 1655–1671. doi:10.7150/thno.52710
Postow, M. A., Chesney, J., Pavlick, A. C., Robert, C., Grossmann, K., McDermott, D., et al. (2015). Nivolumab and ipilimumab versus ipilimumab in untreated melanoma. N. Engl. J. Med. 372 (21), 2006–2017. doi:10.1056/NEJMoa1414428
Postow, M. A., Sidlow, R., and Hellmann, M. D. (2018). Immune-related adverse events associated with immune checkpoint blockade. N. Engl. J. Med. 378 (2), 158–168. doi:10.1056/NEJMra1703481
Qu, S., Worlikar, T., Felsted, A. E., Ganguly, A., Beems, M. V., Hubbard, R., et al. (2020). Non-thermal histotripsy tumor ablation promotes abscopal immune responses that enhance cancer immunotherapy. J. Immunother. Cancer 8 (1), e000200. doi:10.1136/jitc-2019-000200
Quaia, E., D'Onofrio, M., Palumbo, A., Rossi, S., Bruni, S., and Cova, M. (2006). Comparison of contrast-enhanced ultrasonography versus baseline ultrasound and contrast-enhanced computed tomography in metastatic disease of the liver: Diagnostic performance and confidence. Eur. Radiol. 16 (7), 1599–1609. doi:10.1007/s00330-006-0192-7
Quispel-Janssen, J., van der Noort, V., de Vries, J. F., Zimmerman, M., Lalezari, F., Thunnissen, E., et al. (2018). Programmed death 1 blockade with nivolumab in patients with recurrent malignant pleural mesothelioma. J. Thorac. Oncol. 13 (10), 1569–1576. doi:10.1016/j.jtho.2018.05.038
Ramos-Casals, M., Brahmer, J. R., Callahan, M. K., Flores-Chavez, A., Keegan, N., Khamashta, M. A., et al. (2020). Immune-related adverse events of checkpoint inhibitors. Nat. Rev. Dis. Prim. 6 (1), 38. doi:10.1038/s41572-020-0160-6
Restifo, N. P., Smyth, M. J., and Snyder, A. (2016). Acquired resistance to immunotherapy and future challenges. Nat. Rev. Cancer 16 (2), 121–126. doi:10.1038/nrc.2016.2
Rezayat, E., and Toostani, I. G. (2016). A review on brain stimulation using low intensity focused ultrasound. Basic Clin. Neurosci. 7 (3), 187–194. doi:10.15412/J.BCN.03070303
Ribas, A., Hamid, O., Daud, A., Hodi, F. S., Wolchok, J. D., Kefford, R., et al. (2016). Association of pembrolizumab with tumor response and survival among patients with advanced melanoma. JAMA 315 (15), 1600–1609. doi:10.1001/jama.2016.4059
Ribas, A., and Wolchok, J. D. (2018). Cancer immunotherapy using checkpoint blockade. Science 359 (6382), 1350–1355. doi:10.1126/science.aar4060
Riley, R. S., June, C. H., Langer, R., and Mitchell, M. J. (2019). Delivery technologies for cancer immunotherapy. Nat. Rev. Drug Discov. 18 (3), 175–196. doi:10.1038/s41573-018-0006-z
Rini, B. I., Plimack, E. R., Stus, V., Gafanov, R., Hawkins, R., Nosov, D., et al. (2019). Pembrolizumab plus axitinib versus sunitinib for advanced renal-cell carcinoma. N. Engl. J. Med. 380 (12), 1116–1127. doi:10.1056/NEJMoa1816714
Rizvi, N. A., Mazieres, J., Planchard, D., Stinchcombe, T. E., Dy, G. K., Antonia, S. J., et al. (2015). Activity and safety of nivolumab, an anti-PD-1 immune checkpoint inhibitor, for patients with advanced, refractory squamous non-small-cell lung cancer (CheckMate 063): A phase 2, single-arm trial. Lancet Oncol. 16 (3), 257–265. doi:10.1016/S1470-2045(15)70054-9
Rosenberg, J. E., Hoffman-Censits, J., Powles, T., van der Heijden, M. S., Balar, A. V., Necchi, A., et al. (2016). Atezolizumab in patients with locally advanced and metastatic urothelial carcinoma who have progressed following treatment with platinum-based chemotherapy: A single-arm, multicentre, phase 2 trial. Lancet 387 (10031), 1909–1920. doi:10.1016/S0140-6736(16)00561-4
Rosenberg, S. A. (2014). IL-2: The first effective immunotherapy for human cancer. J. Immunol. 192 (12), 5451–5458. doi:10.4049/jimmunol.1490019
Rosenthal, I., Sostaric, J. Z., and Riesz, P. (2004). Sonodynamic therapy-a review of the synergistic effects of drugs and ultrasound. Ultrason. Sonochem 11 (6), 349–363. doi:10.1016/j.ultsonch.2004.03.004
Ruggeri, Z. M., and Mendolicchio, G. L. (2007). Adhesion mechanisms in platelet function. Circ. Res. 100 (12), 1673–1685. doi:10.1161/01.RES.0000267878.97021.ab
Salgia, R., Lynch, T., Skarin, A., Lucca, J., Lynch, C., Jung, K., et al. (2003). Vaccination with irradiated autologous tumor cells engineered to secrete granulocyte-macrophage colony-stimulating factor augments antitumor immunity in some patients with metastatic non-small-cell lung carcinoma. J. Clin. Oncol. 21 (4), 624–630. doi:10.1200/JCO.2003.03.091
Sandigursky, S., and Mor, A. (2018). Immune-related adverse events in cancer patients treated with immune checkpoint inhibitors. Curr. Rheumatol. Rep. 20 (10), 65. doi:10.1007/s11926-018-0770-0
Sanguinetti, J. L., Hameroff, S., Smith, E. E., Sato, T., Daft, C. M. W., Tyler, W. J., et al. (2020). Transcranial focused ultrasound to the right prefrontal cortex improves mood and alters functional connectivity in humans. Front. Hum. Neurosci. 14, 52. doi:10.3389/fnhum.2020.00052
Sasaki, N., Ikenaka, Y., Aoshima, K., Aoyagi, T., Kudo, N., Nakamura, K., et al. (2022). Safety assessment of ultrasound-assisted intravesical chemotherapy in normal dogs: A pilot study. Front. Pharmacol. 13, 837754. doi:10.3389/fphar.2022.837754
Sasaki, N., Ishi, K., Kudo, N., Nakayama, S. M. M., Nakamura, K., Morishita, K., et al. (2017). Spatial and temporal profile of cisplatin delivery by ultrasound-assisted intravesical chemotherapy in a bladder cancer model. PLoS One 12 (11), e0188093. doi:10.1371/journal.pone.0188093
Sasikumar, P. G., Sudarshan, N. S., Adurthi, S., Ramachandra, R. K., Samiulla, D. S., Lakshminarasimhan, A., et al. (2021). PD-1 derived CA-170 is an oral immune checkpoint inhibitor that exhibits preclinical anti-tumor efficacy. Commun. Biol. 4 (1), 699. doi:10.1038/s42003-021-02191-1
Schanda, N., Sauer, T., Kunz, A., Huckelhoven-Krauss, A., Neuber, B., Wang, L., et al. (2021). Sensitivity and specificity of CD19.CAR-T cell detection by flow cytometry and PCR. Cells 10 (11), 3208. doi:10.3390/cells10113208
Schmid, P., Adams, S., Rugo, H. S., Schneeweiss, A., Barrios, C. H., Iwata, H., et al. (2018). Atezolizumab and nab-paclitaxel in advanced triple-negative breast cancer. N. Engl. J. Med. 379 (22), 2108–2121. doi:10.1056/NEJMoa1809615
Schmid, P., Rugo, H. S., Adams, S., Schneeweiss, A., Barrios, C. H., Iwata, H., et al. (2020). Atezolizumab plus nab-paclitaxel as first-line treatment for unresectable, locally advanced or metastatic triple-negative breast cancer (IMpassion130): Updated efficacy results from a randomised, double-blind, placebo-controlled, phase 3 trial. Lancet Oncol. 21 (1), 44–59. doi:10.1016/S1470-2045(19)30689-8
Schmitt, A., Mougenot, C., and Chopra, R. (2014). Spatiotemporal filtering of MR-temperature artifacts arising from bowel motion during transurethral MR-HIFU. Med. Phys. 41 (11), 113302. doi:10.1118/1.4897382
Schoen, S., Kilinc, M. S., Lee, H., Guo, Y., Degertekin, F. L., Woodworth, G. F., et al. (2022). Towards controlled drug delivery in brain tumors with microbubble-enhanced focused ultrasound. Adv. Drug Deliv. Rev. 180, 114043. doi:10.1016/j.addr.2021.114043
Sethuraman, S. N., Singh, M. P., Patil, G., Li, S., Fiering, S., Hoopes, P. J., et al. (2020). Novel calreticulin-nanoparticle in combination with focused ultrasound induces immunogenic cell death in melanoma to enhance antitumor immunity. Theranostics 10 (8), 3397–3412. doi:10.7150/thno.42243
Shah, M. A., Kojima, T., Hochhauser, D., Enzinger, P., Raimbourg, J., Hollebecque, A., et al. (2019). Efficacy and safety of pembrolizumab for heavily pretreated patients with advanced, metastatic adenocarcinoma or squamous cell carcinoma of the esophagus: The phase 2 KEYNOTE-180 study. JAMA Oncol. 5 (4), 546–550. doi:10.1001/jamaoncol.2018.5441
Shang, X., Wang, P., Liu, Y., Zhang, Z., and Xue, Y. (2011). Mechanism of low-frequency ultrasound in opening blood-tumor barrier by tight junction. J. Mol. Neurosci. 43 (3), 364–369. doi:10.1007/s12031-010-9451-9
Shehata, I. A. (2012). Treatment with high intensity focused ultrasound: Secrets revealed. Eur. J. Radiol. 81 (3), 534–541. doi:10.1016/j.ejrad.2011.01.047
Sheikov, N., McDannold, N., Sharma, S., and Hynynen, K. (2008). Effect of focused ultrasound applied with an ultrasound contrast agent on the tight junctional integrity of the brain microvascular endothelium. Ultrasound Med. Biol. 34 (7), 1093–1104. doi:10.1016/j.ultrasmedbio.2007.12.015
Shen, M., Xu, Z., Xu, W., Jiang, K., Zhang, F., Ding, Q., et al. (2019). Inhibition of ATM reverses EMT and decreases metastatic potential of cisplatin-resistant lung cancer cells through JAK/STAT3/PD-L1 pathway. J. Exp. Clin. Cancer Res. 38 (1), 149. doi:10.1186/s13046-019-1161-8
Shen, X., Li, T., Chen, Z., Xie, X., Zhang, H., Feng, Y., et al. (2019). NIR-Light-Triggered anticancer strategy for dual-modality imaging-guided combination therapy via a bioinspired hybrid PLGA nanoplatform. Mol. Pharm. 16 (3), 1367–1384. doi:10.1021/acs.molpharmaceut.8b01321
Sheybani, N. D., and Price, R. J. (2019). Perspectives on recent progress in focused ultrasound immunotherapy. Theranostics 9 (25), 7749–7758. doi:10.7150/thno.37131
Sheybani, N. D., Witter, A. R., Garrison, W. J., Miller, G. W., Price, R. J., and Bullock, T. N. J. (2022). Profiling of the immune landscape in murine glioblastoma following blood brain/tumor barrier disruption with MR image-guided focused ultrasound. J. Neurooncol 156 (1), 109–122. doi:10.1007/s11060-021-03887-4
Sheybani, N. D., Witter, A. R., Thim, E. A., Yagita, H., Bullock, T. N. J., and Price, R. J. (2020). Combination of thermally ablative focused ultrasound with gemcitabine controls breast cancer via adaptive immunity. J. Immunother. Cancer 8 (2), e001008. doi:10.1136/jitc-2020-001008
Shi, S., Shi, K., Tan, L., Qu, Y., Shen, G., Chu, B., et al. (2014). The use of cationic MPEG-PCL-g-PEI micelles for co-delivery of Msurvivin T34A gene and doxorubicin. Biomaterials 35 (15), 4536–4547. doi:10.1016/j.biomaterials.2014.02.010
Shou, J., Mo, F., Zhang, S., Lu, L., Han, N., Liu, L., et al. (2022). Combination treatment of radiofrequency ablation and peptide neoantigen vaccination: Promising modality for future cancer immunotherapy. Front. Immunol. 13, 1000681. doi:10.3389/fimmu.2022.1000681
Singh, M. P., Sethuraman, S. N., Miller, C., Malayer, J., and Ranjan, A. (2021). Boiling histotripsy and in-situ CD40 stimulation improve the checkpoint blockade therapy of poorly immunogenic tumors. Theranostics 11 (2), 540–554. doi:10.7150/thno.49517
Singh, M. P., Sethuraman, S. N., Ritchey, J., Fiering, S., Guha, C., Malayer, J., et al. (2019). In-situ vaccination using focused ultrasound heating and anti-CD-40 agonistic antibody enhances T-cell mediated local and abscopal effects in murine melanoma. Int. J. Hyperth. 36, 64–73. doi:10.1080/02656736.2019.1663280
Sivaramakrishnan, R., and Incharoensakdi, A. (2018). Microalgae as feedstock for biodiesel production under ultrasound treatment - a review. Bioresour. Technol. 250, 877–887. doi:10.1016/j.biortech.2017.11.095
Sochacka-Cwikla, A., Maczynski, M., and Regiec, A. (2022). FDA-approved small molecule compounds as drugs for solid cancers from early 2011 to the end of 2021. Molecules 27 (7), 2259. doi:10.3390/molecules27072259
Socinski, M. A., Jotte, R. M., Cappuzzo, F., Orlandi, F., Stroyakovskiy, D., Nogami, N., et al. (2018). Atezolizumab for first-line treatment of metastatic nonsquamous NSCLC. N. Engl. J. Med. 378 (24), 2288–2301. doi:10.1056/NEJMoa1716948
Song, H. W., Lee, H. S., Kim, S. J., Kim, H. Y., Choi, Y. H., Kang, B., et al. (2021). Sonazoid-conjugated natural killer cells for tumor therapy and real-time visualization by ultrasound imaging. Pharmaceutics 13 (10), 1689. doi:10.3390/pharmaceutics13101689
Song, W., Shen, L., Wang, Y., Liu, Q., Goodwin, T. J., Li, J., et al. (2018). Synergistic and low adverse effect cancer immunotherapy by immunogenic chemotherapy and locally expressed PD-L1 trap. Nat. Commun. 9 (1), 2237. doi:10.1038/s41467-018-04605-x
Sul, J., Blumenthal, G. M., Jiang, X., He, K., Keegan, P., and Pazdur, R. (2016). FDA approval summary: Pembrolizumab for the treatment of patients with metastatic non-small cell lung cancer whose tumors express programmed death-ligand 1. Oncologist 21 (5), 643–650. doi:10.1634/theoncologist.2015-0498
Sun, S., Tang, Q., Wang, Y., Zhang, L., Chen, J., Xu, M., et al. (2022). In situ micro-nano conversion augmented tumor-localized immunochemotherapy. ACS Appl. Mater Interfaces 14, 27013–27027. doi:10.1021/acsami.2c02490
Sun, S., Wang, P., Sun, S., and Liang, X. (2021). Applications of micro/nanotechnology in ultrasound-based drug delivery and therapy for tumor. Curr. Med. Chem. 28 (3), 525–547. doi:10.2174/0929867327666200212100257
Sung, H., Ferlay, J., Siegel, R. L., Laversanne, M., Soerjomataram, I., Jemal, A., et al. (2021). Global cancer statistics 2020: GLOBOCAN estimates of incidence and mortality worldwide for 36 cancers in 185 countries. CA Cancer J. Clin. 71 (3), 209–249. doi:10.3322/caac.21660
Tabana, Y., Okoye, I. S., Siraki, A., Elahi, S., and Barakat, K. H. (2021). Tackling immune targets for breast cancer: Beyond PD-1/PD-L1 Axis. Front. Oncol. 11, 628138. doi:10.3389/fonc.2021.628138
Tan, M., Chen, Y., Guo, Y., Yang, C., Liu, M., Guo, D., et al. (2020). A low-intensity focused ultrasound-assisted nanocomposite for advanced triple cancer therapy: Local chemotherapy, therapeutic extracellular vesicles and combined immunotherapy. Biomater. Sci. 8 (23), 6703–6717. doi:10.1039/d0bm00804d
Tan, S., Li, D., and Zhu, X. (2020). Cancer immunotherapy: Pros, cons and beyond. Biomed. Pharmacother. 124, 109821. doi:10.1016/j.biopha.2020.109821
Tao, Y., Chan, H. F., Shi, B., Li, M., and Leong, K. W. (2020). Light: A magical tool for controlled drug delivery. Adv. Funct. Mater 30 (49), 2005029. doi:10.1002/adfm.202005029
Tempany, C. M., McDannold, N. J., Hynynen, K., and Jolesz, F. A. (2011). Focused ultrasound surgery in oncology: Overview and principles. Radiology 259 (1), 39–56. doi:10.1148/radiol.11100155
Ter Haar, G. (2016). HIFU tissue ablation: Concept and devices. Adv. Exp. Med. Biol. 880, 3–20. doi:10.1007/978-3-319-22536-4_1
Ter Haar, G. R., and Robertson, D. (1993). Tissue destruction with focused ultrasound in vivo. Eur. Urol. 23, 8–11. doi:10.1159/000474672
Thompson, E. A., Liang, F., Lindgren, G., Sandgren, K. J., Quinn, K. M., Darrah, P. A., et al. (2015). Human anti-CD40 antibody and poly IC:LC adjuvant combination induces potent T cell responses in the lung of nonhuman primates. J. Immunol. 195 (3), 1015–1024. doi:10.4049/jimmunol.1500078
Tian, Y., Liu, Z., Tan, H., Hou, J., Wen, X., Yang, F., et al. (2020). New aspects of ultrasound-mediated targeted delivery and therapy for cancer. Int. J. Nanomedicine 15, 401–418. doi:10.2147/IJN.S201208
Timbie, K. F., Mead, B. P., and Price, R. J. (2015). Drug and gene delivery across the blood-brain barrier with focused ultrasound. J. Control Release 219, 61–75. doi:10.1016/j.jconrel.2015.08.059
Tobin, J. W. D., Bednarska, K., Campbell, A., and Keane, C. (2021). PD-1 and LAG-3 checkpoint blockade: Potential avenues for therapy in B-cell lymphoma. Cells 10 (5), 1152. doi:10.3390/cells10051152
Tomita, Y., Motzer, R. J., Choueiri, T. K., Rini, B. I., Miyake, H., Uemura, H., et al. (2022). Efficacy and safety of avelumab plus axitinib in elderly patients with advanced renal cell carcinoma: Extended follow-up results from JAVELIN renal 101. ESMO Open 7 (2), 100450. doi:10.1016/j.esmoop.2022.100450
Topalian, S. L., Hodi, F. S., Brahmer, J. R., Gettinger, S. N., Smith, D. C., McDermott, D. F., et al. (2012). Safety, activity, and immune correlates of anti-PD-1 antibody in cancer. N. Engl. J. Med. 366 (26), 2443–2454. doi:10.1056/NEJMoa1200690
Toy, R., and Roy, K. (2016). Engineering nanoparticles to overcome barriers to immunotherapy. Bioeng. Transl. Med. 1 (1), 47–62. doi:10.1002/btm2.10005
Twyman-Saint Victor, C., Rech, A. J., Maity, A., Rengan, R., Pauken, K. E., Stelekati, E., et al. (2015). Radiation and dual checkpoint blockade activate non-redundant immune mechanisms in cancer. Nature 520 (7547), 373–377. doi:10.1038/nature14292
Tzu-Yin, W., Wilson, K. E., Machtaler, S., and Willmann, J. K. (2013). Ultrasound and microbubble guided drug delivery: Mechanistic understanding and clinical implications. Curr. Pharm. Biotechnol. 14 (8), 743–752. doi:10.2174/1389201014666131226114611
Unga, J., and Hashida, M. (2014). Ultrasound induced cancer immunotherapy. Adv. Drug Deliv. Rev. 72, 144–153. doi:10.1016/j.addr.2014.03.004
van den Boorn, J. G., Schlee, M., Coch, C., and Hartmann, G. (2011). SiRNA delivery with exosome nanoparticles. Nat. Biotechnol. 29 (4), 325–326. doi:10.1038/nbt.1830
van der Heijden, M. S., Loriot, Y., Duran, I., Ravaud, A., Retz, M., Vogelzang, N. J., et al. (2021). Atezolizumab versus chemotherapy in patients with platinum-treated locally advanced or metastatic urothelial carcinoma: A long-term overall survival and safety update from the phase 3 IMvigor211 clinical trial. Eur. Urol. 80 (1), 7–11. doi:10.1016/j.eururo.2021.03.024
Viallard, C., and Larrivee, B. (2017). Tumor angiogenesis and vascular normalization: Alternative therapeutic targets. Angiogenesis 20 (4), 409–426. doi:10.1007/s10456-017-9562-9
Vos, J. L., Elbers, J. B. W., Krijgsman, O., Traets, J. J. H., Qiao, X., van der Leun, A. M., et al. (2021). Neoadjuvant immunotherapy with nivolumab and ipilimumab induces major pathological responses in patients with head and neck squamous cell carcinoma. Nat. Commun. 12 (1), 7348. doi:10.1038/s41467-021-26472-9
Wan, G. Y., Liu, Y., Chen, B. W., Liu, Y. Y., Wang, Y. S., and Zhang, N. (2016). Recent advances of sonodynamic therapy in cancer treatment. Cancer Biol. Med. 13 (3), 325–338. doi:10.20892/j.issn.2095-3941.2016.0068
Wang, D. Y., Salem, J. E., Cohen, J. V., Chandra, S., Menzer, C., Ye, F., et al. (2018). Fatal toxic effects associated with immune checkpoint inhibitors: A systematic review and meta-analysis. JAMA Oncol. 4 (12), 1721–1728. doi:10.1001/jamaoncol.2018.3923
Wang, H. T., Lee, H. I., Guo, J. H., Chen, S. H., Liao, Z. K., Huang, K. W., et al. (2012). Calreticulin promotes tumor lymphocyte infiltration and enhances the antitumor effects of immunotherapy by up-regulating the endothelial expression of adhesion molecules. Int. J. Cancer 130 (12), 2892–2902. doi:10.1002/ijc.26339
Wang, J., Gao, Z. P., Qin, S., Liu, C. B., and Zou, L. L. (2017). Calreticulin is an effective immunologic adjuvant to tumor-associated antigens. Exp. Ther. Med. 14 (4), 3399–3406. doi:10.3892/etm.2017.4989
Wang, X., and Sun, J. (2002). High-intensity focused ultrasound in patients with late-stage pancreatic carcinoma. Chin. Med. J. Engl. 115 (9), 1332–1335.
Webb, E. S., Liu, P., Baleeiro, R., Lemoine, N. R., Yuan, M., and Wang, Y. H. (2018). Immune checkpoint inhibitors in cancer therapy. J. Biomed. Res. 32 (5), 317–326. doi:10.7555/JBR.31.20160168
Weber, J. S., D'Angelo, S. P., Minor, D., Hodi, F. S., Gutzmer, R., Neyns, B., et al. (2015). Nivolumab versus chemotherapy in patients with advanced melanoma who progressed after anti-CTLA-4 treatment (CheckMate 037): A randomised, controlled, open-label, phase 3 trial. Lancet Oncol. 16 (4), 375–384. doi:10.1016/S1470-2045(15)70076-8
West, H., McCleod, M., Hussein, M., Morabito, A., Rittmeyer, A., Conter, H. J., et al. (2019). Atezolizumab in combination with carboplatin plus nab-paclitaxel chemotherapy compared with chemotherapy alone as first-line treatment for metastatic non-squamous non-small-cell lung cancer (IMpower130): A multicentre, randomised, open-label, phase 3 trial. Lancet Oncol. 20 (7), 924–937. doi:10.1016/S1470-2045(19)30167-6
White, D. N. (1988). Neurosonology pioneers. Ultrasound Med. Biol. 14 (7), 541–561. doi:10.1016/0301-5629(88)90121-4
Wild, J. J., and Reid, J. M. (1956). Diagnostic use of ultrasound. Br. J. Phys. Med. 19 (11), 248–257.
Wild, J. J. (1950). The use of ultrasonic pulses for the measurement of biologic tissues and the detection of tissue density changes. Surgery 27 (2), 183–188.
Wolchok, J. D., Chiarion-Sileni, V., Gonzalez, R., Grob, J. J., Rutkowski, P., Lao, C. D., et al. (2022). Long-term outcomes with nivolumab plus ipilimumab or nivolumab alone versus ipilimumab in patients with advanced melanoma. J. Clin. Oncol. 40 (2), 127–137. doi:10.1200/JCO.21.02229
Wu, M., Wang, Y., Wang, Y., Zhang, M., Luo, Y., Tang, J., et al. (2017). Paclitaxel-loaded and A10-3.2 aptamer-targeted poly(lactide-co-glycolic acid) nanobubbles for ultrasound imaging and therapy of prostate cancer. Int. J. Nanomedicine 12, 5313–5330. doi:10.2147/IJN.S136032
Wu, P., Ya, Z., Li, Y., Zhu, M., Zhang, L., Zong, Y., et al. (2022). Focused acoustic vortex-regulated composite nanodroplets combined with checkpoint blockade for high-performance tumor synergistic therapy. ACS Appl. Mater Interfaces 14 (27), 30466–30479. doi:10.1021/acsami.2c02137
Wu, Y., Liu, Y., Huang, Z., Wang, X., Jin, Z., Li, J., et al. (2021). Control of the activity of CAR-T cells within tumours via focused ultrasound. Nat. Biomed. Eng. 5 (11), 1336–1347. doi:10.1038/s41551-021-00779-w
Wu, Z., Cui, P., Tao, H., Zhang, S., Ma, J., Liu, Z., et al. (2021). The synergistic effect of PARP inhibitors and immune checkpoint inhibitors. Clin. Med. Insights Oncol. 15, 1179554921996288. doi:10.1177/1179554921996288
Xia, C. Y., Liu, Y. H., Wang, P., and Xue, Y. X. (2012). Low-frequency ultrasound irradiation increases blood-tumor barrier permeability by transcellular pathway in a rat glioma model. J. Mol. Neurosci. 48 (1), 281–290. doi:10.1007/s12031-012-9770-0
Xiao, Y., and Yu, D. (2021). Tumor microenvironment as a therapeutic target in cancer. Pharmacol. Ther. 221, 107753. doi:10.1016/j.pharmthera.2020.107753
Xin, Z., Lin, G., Lei, H., Lue, T. F., and Guo, Y. (2016). Clinical applications of low-intensity pulsed ultrasound and its potential role in urology. Transl. Androl. Urol. 5 (2), 255–266. doi:10.21037/tau.2016.02.04
Xing, Y., Lu, X., Pua, E. C., and Zhong, P. (2008). The effect of high intensity focused ultrasound treatment on metastases in a murine melanoma model. Biochem. Biophys. Res. Commun. 375 (4), 645–650. doi:10.1016/j.bbrc.2008.08.072
Xu, C., Chen, Y. P., Du, X. J., Liu, J. Q., Huang, C. L., Chen, L., et al. (2018). Comparative safety of immune checkpoint inhibitors in cancer: Systematic review and network meta-analysis. BMJ 363, k4226. doi:10.1136/bmj.k4226
Xu, N., Palmer, D. C., Robeson, A. C., Shou, P., Bommiasamy, H., Laurie, S. J., et al. (2021). STING agonist promotes CAR T cell trafficking and persistence in breast cancer. J. Exp. Med. 218 (2), e20200844. doi:10.1084/jem.20200844
Xu, Q., Xiu, W., Li, Q., Zhang, Y., Li, X., Ding, M., et al. (2023). Emerging nanosonosensitizers augment sonodynamic-mediated antimicrobial therapies. Mater Today Bio 19, 100559. doi:10.1016/j.mtbio.2023.100559
Xu, Z., Hall, T. L., Vlaisavljevich, E., and Lee, F. T. (2021). Histotripsy: The first noninvasive, non-ionizing, non-thermal ablation technique based on ultrasound. Int. J. Hyperth. 38 (1), 561–575. doi:10.1080/02656736.2021.1905189
Yamaguchi, H., Hsu, J. M., Yang, W. H., and Hung, M. C. (2022). Mechanisms regulating PD-L1 expression in cancers and associated opportunities for novel small-molecule therapeutics. Nat. Rev. Clin. Oncol. 19 (5), 287–305. doi:10.1038/s41571-022-00601-9
Yang, H., Bueso-Ramos, C., DiNardo, C., Estecio, M. R., Davanlou, M., Geng, Q. R., et al. (2014). Expression of PD-L1, PD-L2, PD-1 and CTLA4 in myelodysplastic syndromes is enhanced by treatment with hypomethylating agents. Leukemia 28 (6), 1280–1288. doi:10.1038/leu.2013.355
Yang, J., Hou, M., Sun, W., Wu, Q., Xu, J., Xiong, L., et al. (2020). Sequential PDT and PTT using dual-modal single-walled carbon nanohorns synergistically promote systemic immune responses against tumor metastasis and relapse. Adv. Sci. (Weinh) 7 (16), 2001088. doi:10.1002/advs.202001088
Yang, Q., Zhou, Y., Chen, J., Huang, N., Wang, Z., and Cheng, Y. (2021). Gene therapy for drug-resistant glioblastoma via lipid-polymer hybrid nanoparticles combined with focused ultrasound. Int. J. Nanomedicine 16, 185–199. doi:10.2147/IJN.S286221
Yap, T. A., Nakagawa, K., Fujimoto, N., Kuribayashi, K., Guren, T. K., Calabro, L., et al. (2021). Efficacy and safety of pembrolizumab in patients with advanced mesothelioma in the open-label, single-arm, phase 2 KEYNOTE-158 study. Lancet Respir. Med. 9 (6), 613–621. doi:10.1016/S2213-2600(20)30515-4
Yeo, R. W., Lai, R. C., Zhang, B., Tan, S. S., Yin, Y., Teh, B. J., et al. (2013). Mesenchymal stem cell: An efficient mass producer of exosomes for drug delivery. Adv. Drug Deliv. Rev. 65 (3), 336–341. doi:10.1016/j.addr.2012.07.001
Yildirim, A., Blum, N. T., and Goodwin, A. P. (2019). Colloids, nanoparticles, and materials for imaging, delivery, ablation, and theranostics by focused ultrasound (FUS). Theranostics 9 (9), 2572–2594. doi:10.7150/thno.32424
Yoo, J. W., Irvine, D. J., Discher, D. E., and Mitragotri, S. (2011). Bio-inspired, bioengineered and biomimetic drug delivery carriers. Nat. Rev. Drug Discov. 10 (7), 521–535. doi:10.1038/nrd3499
Younes, A., Santoro, A., Shipp, M., Zinzani, P. L., Timmerman, J. M., Ansell, S., et al. (2016). Nivolumab for classical hodgkin's lymphoma after failure of both autologous stem-cell transplantation and brentuximab vedotin: A multicentre, multicohort, single-arm phase 2 trial. Lancet Oncol. 17 (9), 1283–1294. doi:10.1016/S1470-2045(16)30167-X
Yu, M. K., Park, J., and Jon, S. (2012). Targeting strategies for multifunctional nanoparticles in cancer imaging and therapy. Theranostics 2 (1), 3–44. doi:10.7150/thno.3463
Yu, W., Liu, T., Zhang, M., Wang, Z., Ye, J., Li, C. X., et al. (2019). O(2) economizer for inhibiting cell respiration to combat the hypoxia obstacle in tumor treatments. ACS Nano 13 (2), 1784–1794. doi:10.1021/acsnano.8b07852
Zeng, D., Wu, J., Luo, H., Li, Y., Xiao, J., Peng, J., et al. (2021). Tumor microenvironment evaluation promotes precise checkpoint immunotherapy of advanced gastric cancer. J. Immunother. Cancer 9 (8), e002467. doi:10.1136/jitc-2021-002467
Zhan, W., Alamer, M., and Xu, X. Y. (2018). Computational modelling of drug delivery to solid tumour: Understanding the interplay between chemotherapeutics and biological system for optimised delivery systems. Adv. Drug Deliv. Rev. 132, 81–103. doi:10.1016/j.addr.2018.07.013
Zhang, C., Liu, X., Jin, S., Chen, Y., and Guo, R. (2022). Ferroptosis in cancer therapy: A novel approach to reversing drug resistance. Mol. Cancer 21 (1), 47. doi:10.1186/s12943-022-01530-y
Zhang, J., Huang, D., Saw, P. E., and Song, E. (2022). Turning cold tumors hot: From molecular mechanisms to clinical applications. Trends Immunol. 43 (7), 523–545. doi:10.1016/j.it.2022.04.010
Zhang, L., and Wang, Z. B. (2010). High-intensity focused ultrasound tumor ablation: Review of ten years of clinical experience. Front. Med. China 4 (3), 294–302. doi:10.1007/s11684-010-0092-8
Zhang, X., Zheng, Y., Wang, Z., Huang, S., Chen, Y., Jiang, W., et al. (2014). Methotrexate-loaded PLGA nanobubbles for ultrasound imaging and Synergistic Targeted therapy of residual tumor during HIFU ablation. Biomaterials 35 (19), 5148–5161. doi:10.1016/j.biomaterials.2014.02.036
Zhang, Y., and Zhang, Z. (2020). The history and advances in cancer immunotherapy: Understanding the characteristics of tumor-infiltrating immune cells and their therapeutic implications. Cell. Mol. Immunol. 17 (8), 807–821. doi:10.1038/s41423-020-0488-6
Zhao, X., Li, F., Li, Y., Wang, H., Ren, H., Chen, J., et al. (2015). Co-delivery of HIF1α siRNA and gemcitabine via biocompatible lipid-polymer hybrid nanoparticles for effective treatment of pancreatic cancer. Biomaterials 46, 13–25. doi:10.1016/j.biomaterials.2014.12.028
Zhou, G., Sprengers, D., Boor, P. P. C., Doukas, M., Schutz, H., Mancham, S., et al. (2017). Antibodies against immune checkpoint molecules restore functions of tumor-infiltrating T cells in hepatocellular carcinomas. Gastroenterology 153 (4), 1107–1119.e10. doi:10.1053/j.gastro.2017.06.017
Zhou, Y. F. (2011). High intensity focused ultrasound in clinical tumor ablation. World J. Clin. Oncol. 2 (1), 8–27. doi:10.5306/wjco.v2.i1.8
Zhu, A. X., Finn, R. S., Edeline, J., Cattan, S., Ogasawara, S., Palmer, D., et al. (2018). Pembrolizumab in patients with advanced hepatocellular carcinoma previously treated with sorafenib (KEYNOTE-224): A non-randomised, open-label phase 2 trial. Lancet Oncol. 19 (7), 940–952. doi:10.1016/S1470-2045(18)30351-6
Glossary
Keywords: focused ultrasound, small-molecule delivery systems, microbubbles, nanoparticles, immune checkpoint blockade, solid tumors
Citation: Wu Q, Xia Y, Xiong X, Duan X, Pang X, Zhang F, Tang S, Su J, Wen S, Mei L, Cannon RD, Ji P and Ou Z (2023) Focused ultrasound-mediated small-molecule delivery to potentiate immune checkpoint blockade in solid tumors. Front. Pharmacol. 14:1169608. doi: 10.3389/fphar.2023.1169608
Received: 19 February 2023; Accepted: 03 April 2023;
Published: 26 April 2023.
Edited by:
Zhong Zheng, University of California, Los Angeles, United StatesCopyright © 2023 Wu, Xia, Xiong, Duan, Pang, Zhang, Tang, Su, Wen, Mei, Cannon, Ji and Ou. This is an open-access article distributed under the terms of the Creative Commons Attribution License (CC BY). The use, distribution or reproduction in other forums is permitted, provided the original author(s) and the copyright owner(s) are credited and that the original publication in this journal is cited, in accordance with accepted academic practice. No use, distribution or reproduction is permitted which does not comply with these terms.
*Correspondence: Ping Ji, SmlwaW5nQGhvc3BpdGFsLmNxbXUuZWR1LmNu Zhanpeng Ou, NTAxNTE4QGhvc3BpdGFsLmNxbXUuZWR1LmNu
†These authors have contributed equally to this work
‡ORCID: Richard D. Cannon, http://orcid.org/0000-0002-5398-2066; Ping Ji, http://orcid.org/0000-0002-2608-1035