- Department of Physiology and Cell Biology, University of Arkansas for Medical Sciences, Little Rock, AR, United States
This review summarizes the current understanding of the role of plasma membrane transporters in regulating intracellular inorganic phosphate ([Pi]In) in mammals. Pi influx is mediated by SLC34 and SLC20 Na+-Pi cotransporters. In non-epithelial cells other than erythrocytes, Pi influx via SLC20 transporters PiT1 and/or PiT2 is balanced by efflux through XPR1 (xenotropic and polytropic retrovirus receptor 1). Two new pathways for mammalian Pi transport regulation have been described recently: 1) in the presence of adequate Pi, cells continuously internalize and degrade PiT1. Pi starvation causes recycling of PiT1 from early endosomes to the plasma membrane and thereby increases the capacity for Pi influx; and 2) binding of inositol pyrophosphate InsP8 to the SPX domain of XPR1 increases Pi efflux. InsP8 is degraded by a phosphatase that is strongly inhibited by Pi. Therefore, an increase in [Pi]In decreases InsP8 degradation, increases InsP8 binding to SPX, and increases Pi efflux, completing a feedback loop for [Pi]In homeostasis. Published data on [Pi]In by magnetic resonance spectroscopy indicate that the steady state [Pi]In of skeletal muscle, heart, and brain is normally in the range of 1–5 mM, but it is not yet known whether PiT1 recycling or XPR1 activation by InsP8 contributes to Pi homeostasis in these organs. Data on [Pi]In in cultured cells are variable and suggest that some cells can regulate [Pi] better than others, following a change in [Pi]Ex. More measurements of [Pi]In, influx, and efflux are needed to determine how closely, and how rapidly, mammalian [Pi]In is regulated during either hyper- or hypophosphatemia.
1 Introduction
Inorganic orthophosphate (H2PO4−/HPO4=; Pi) plays numerous metabolic roles as a reactant (glycolysis, oxidative phosphorylation, glycogen phosphorolysis, and mineralization; Figure 1A) and product (nucleic acid synthesis, ATPases, GTPases, and phosphatases; Figure 1B). In addition, Pi is now recognized as a signaling molecule (Khoshniat et al., 2011; Chande and Bergwitz, 2018; Michigami et al., 2018; Abbasian et al., 2020a; Kritmetapak and Kumar, 2021). As expected for a metabolite of such central importance, plasma [Pi] is normally regulated within the relatively narrow range of 0.8–1.5 mM in adult humans by the combined actions of the kidney, intestine, bone, and parathyroid gland (Bergwitz and Jüppner, 2010; Komaba and Fukagawa, 2016; Beck and Beck-Cormier, 2020; Sun et al., 2020; Figueres et al., 2021). Less is known about intracellular Pi ([Pi]In) regulation in mammals.
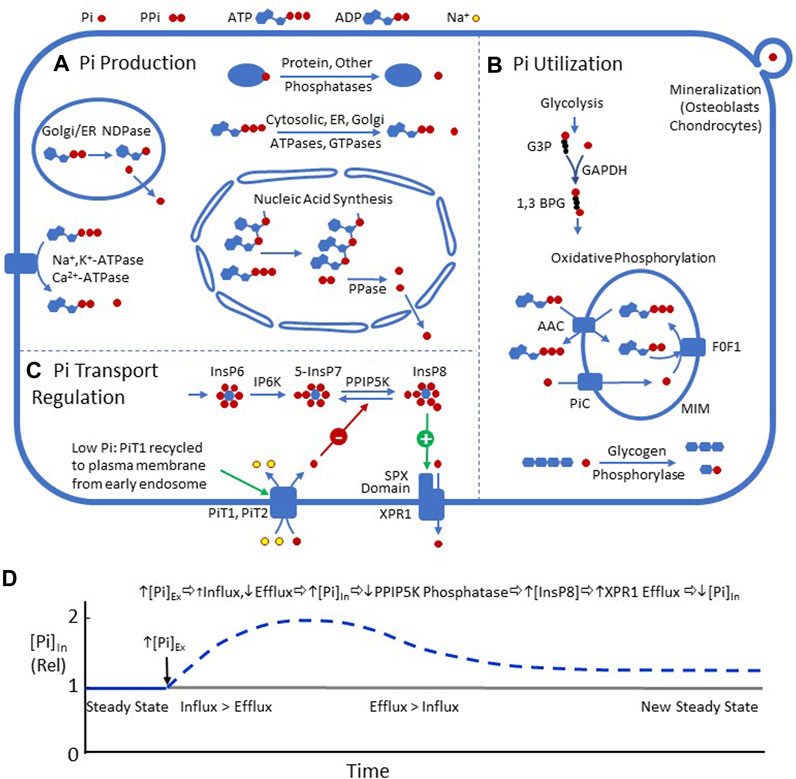
FIGURE 1. (A) Metabolic processes resulting in intracellular Pi production, including nucleic acid synthesis, NTPases, NDPases, and phosphatases. (B) Processes utilizing Pi in mammalian cells, including glycolysis (GAPDH), oxidative phosphorylation, glycogen phosphorolysis, and the formation of matrix vesicles in mineralizing tissues. (C) Two recently described pathways for regulating plasma membrane Pi transport. 1) PiT1 is recycled to the plasma membrane during Pi depletion (Zechner et al., 2022). 2) Efflux mediated by XPR1 is stimulated by InsP8 (Wild et al., 2016) (Gu et al., 2017) (Wilson et al., 2013) (López-Sánchez et al., 2020) (Li et al., 2020). (D) Hypothetical time course of [Pi]In, following a step increase in [Pi]Ex, driven by InsP8 regulation of XPR1. The increase in [Pi]Ex, by mass action, should decrease efflux through XPR1 and slightly increase PiT1/PiT2-mediated influx. The imbalance between influx and efflux should increase [Pi]In. Increased [Pi]In should inhibit the phosphatase activity of PPIP5K and increase [InsP8], which binds to the SPX domain of XPR1 and stimulates efflux, resulting in a decrease in [Pi]In. Eventually, a new steady state should be reached with [Pi]In higher than the previous state.
The goal of this review is to summarize the current knowledge of the roles of plasma membrane transporters in regulating mammalian [Pi]In. The emphasis is on non-epithelial cells because many reviews of renal and intestinal Pi transport have been published in the past several years. This review is focused on transport and does not include several topics that are related to Pi: normal and abnormal mineralization, muscle metabolism and fatigue, polyphosphate, tumor microenvironment, nutrition, Pi toxicity, intestinal Pi transport, and non-mammalian Pi homeostasis.
2 Pi influx: Mammalian Na+-Pi cotransporters
SLC17 proteins (originally known as Type I Na+-Pi cotransporters) function primarily as organic anion transporters (Reimer and Edwards, 2004) and are not included in this review.
2.1 SLC34
Pi entry into mammalian cells is through SLC34 (Type II) and SLC20 (Type III) Na+-Pi cotransporters. SLC34 transporters are expressed mainly in epithelia and play a critical role in regulating plasma [Pi]. Readers are referred to several excellent reviews for discussion of the structural, functional, and pharmacological properties of SLC34 transporters (Virkki et al., 2007; Forster et al., 2013; Wagner et al., 2014; Levi et al., 2019; Hernando et al., 2021; Wagner, 2023).
2.2 SLC20 (PiT1 and PiT2) general properties
Type III Na+-Pi cotransporters PiT1 (SLC20A1) and PiT2 (SLC20A2) are widely expressed and were first identified as retrovirus receptors (Kavanaugh et al., 1994). PiT1 and PiT2 catalyze electrogenic 2 Na+: 1 H2PO4−cotransport (Virkki et al., 2007; Hernando et al., 2021). The half-maximal [Pi]Ex for influx via PiT1 and PiT2 is ∼0.1–0.5 mM (Kavanaugh et al., 1994; Tenenhouse et al., 1998; Zoidis et al., 2004; Bøttger et al., 2006; Ravera et al., 2007; Villa-Bellosta et al., 2007). Therefore, raising [Pi]Ex above 1 mM should cause only a slight increase in Pi influx through PiT1 or PiT2.
2.3 SLC20 pharmacology
SLC20 transporters are less sensitive than SLC34 transporters to inhibition by phosphonoformic acid (Foscarnet) (Ravera et al., 2007). Recently, the first high-affinity SLC20 inhibitor, EOS789, has been described (Tsuboi et al., 2020). Human and rat PiT1 and PiT2 expressed in CHO cells are inhibited half-maximally by 1–2 μM EOS789. SLC34 transporters are also inhibited by EOS789 but at slightly higher concentrations. The properties of EOS789 indicate that it has therapeutic potential for hyperphosphatemia associated with chronic kidney disease (Tsuboi et al., 2020; Hill Gallant et al., 2021; Doshi and Wish, 2022; Tsuboi et al., 2022). For a recent review of Na+-Pi cotransporter pharmacology, see Wagner, 2023.
2.4 PiT1 pathophysiology
Mouse PiT1 is essential for liver development, and global Slc20A1 deletion is embryonic lethal (Festing et al., 2009; Beck et al., 2010). PiT1 knockdown decreases proliferation of HeLa and HepG2 cells, independent of PiT1 transport activity (Beck et al., 2009). High expression of PiT1 is associated with a poor prognosis in some breast cancers (Sato and Akimoto, 2017; Onaga et al., 2021). PiT1 is the main Pi transporter in vascular smooth muscle cells (Jono et al., 2000), and PiT1-mediated Pi influx is required for vascular smooth muscle cell calcification in response to elevated [Pi]Ex (Li et al., 2006).
2.5 PiT1 regulation
Pi depletion increases PiT1 mRNA in mammalian cells by variable amounts (Kavanaugh et al., 1994; Zoidis et al., 2004; Guillén et al., 2019) but causes a large increase in plasma membrane PiT1 protein levels in HEK 293 cells (Zechner et al., 2022). With adequate Pi, PiT1 is continuously internalized and degraded; during Pi depletion, PiT1 is recycled from early endosomes to the plasma membrane by a pathway that depends on the sorting nexin SNX17 (Zechner et al., 2022). If graded recycling of PiT1 takes place under physiological conditions, it would constitute a mechanism for up-regulating Pi influx in response to hypophosphatemia.
2.6 PiT2 pathophysiology
Deletion of mouse Slc20a2 results in placental calcifications and sub-viable pups (Wallingford et al., 2016), indicating a role for PiT2 in Pi transport from maternal to fetal blood. Mouse PiT2 deficiency also causes brain calcifications (Jensen et al., 2013; Ren et al., 2021), and in humans, SLC20A2 loss-of-function mutations are associated with familial brain calcification (Wang et al., 2012; Peters et al., 2020). The effect of PiT2 deficiency on brain calcification likely results from elevated CSF [Pi], which is normally lower than plasma [Pi] because of the PiT2-dependent transport through the choroid plexus from CSF to blood (Guerreiro et al., 2014; Jensen et al., 2016).
2.7 PiT2 regulation
Incubation of mammalian cells in low-Pi medium causes a major increase in PiT2 mRNA and protein (Chien et al., 1997), and a low-Pi diet increases the amount of PiT2 protein in the apical membrane of the rat proximal tubule (Villa-Bellosta et al., 2009). It is not known whether PiT2 is regulated by the recycling mechanism recently described for PiT1 (Zechner et al., 2022).
2.8 Redundant roles of PiT1 and PiT2 in skeletal and vascular smooth muscles
In skeletal muscle, PiT2 is more highly expressed than PiT1 (Kavanaugh et al., 1994). Deletion of Pit2 alone does not affect the muscle, but knockout of both Pit1 and Pit2 in the mouse skeletal muscle leads to energy stress and myofiber necrosis resulting from low [Pi]In (Chande et al., 2020). Therefore, Pit1 can compensate for the lack of Pit2, but either one or the other is necessary in skeletal muscle. Pit1 and Pit2 also play redundant roles in vascular smooth muscle calcification resulting from hyperphosphatemia (Crouthamel et al., 2013).
2.9 PiT1/PiT2 sensing of extracellular Pi
Pit2-knockout mice have a much smaller drop in serum FGF23 in response to a low-Pi diet than wild-type mice, indicating a role for Pit2 in sensing [Pi]Ex (Bon et al., 2018a) by a mechanism involving Pit1/Pit2 heterodimerization, without the need for Pi influx (Bon et al., 2018b). Earlier work showed that Pit2 dimerization is enhanced by low [Pi]Ex (Salaün et al., 2002; Salaün et al., 2004). [Pi]Ex sensing by Pit1/Pit2 does not have a known role in regulating [Pi]In.
3 Pi efflux transporter XPR1 (SLC53A1)
With the normal mammalian cell Na+ gradient and membrane potential, the driving force for transport through PiT1 and PiT2 is inward, even if [Pi]In/[Pi]Ex >100 (Virkki et al., 2007). [Pi]In is much lower than 100 mM; therefore, in the steady state, Na+-coupled Pi influx is balanced by efflux. In mammalian cells other than erythrocytes, the only known Pi efflux transporter is XPR1 (SLC53A1) (Giovannini et al., 2013). XPR1 is the receptor for murine gammaretrovirus X-MLV (Battini et al., 1999), and XPR1-mediated Pi efflux is inhibited by a peptide (XRBD) derived from X-MLV (Giovannini et al., 2013).
3.1 Functions of XPR1
In keeping with a critical role for XPR1 in Pi efflux, mouse Xpr1−/− pups are not viable (Ansermet et al., 2017). Conditional knockout of mouse kidney Xpr1 results in reduced proximal tubule Pi efflux, hypophosphatemia, and other abnormalities similar to Fanconi syndrome (Ansermet et al., 2017), which is consistent with XPR1 being the basolateral exit pathway for Pi in the proximal tubule.
Loss-of-function mutations in human XPR1 are associated with familial brain calcification (Legati et al., 2015; Anheim et al., 2016). The fact that brain calcification is caused by LOF mutations in either SLC20A2 (Pi influx) or XPR1 (Pi efflux) could be explained if PiT2 is the apical and XPR1 is the basolateral Pi transporter in the choroid plexus (Wallingford et al., 2017). A defect in either transporter would reduce Pi transport from the CSF to blood and raise CSF [Pi]. However, XPR1 has not been shown definitively to be the basolateral Pi transporter in any mammalian epithelium (Wagner et al., 2019).
XPR1 is highly expressed in the placental spongiotrophoblast layer (Xu et al., 2020). In Xpr1 ± and Xpr1 −/− fetuses, there is reduced [Pi] in amniotic fluid and severe placental calcifications, indicating a role for Xpr1 in maternal to fetal [Pi] transport (Xu et al., 2020), as shown previously for PiT2 (Wallingford et al., 2016).
Recent work has implicated XPR1 in the tumorigenicity of ovarian cancer cells (Akasu-Nagayoshi et al., 2022; Bondeson et al., 2022), platelet polyphosphate metabolism (Mailer et al., 2021), glucose-induced Pi efflux from pancreatic β cells (Barker et al., 2021), and protection from uremic vascular calcification (Arase et al., 2022). The relationships between these processes and [Pi]In homeostasis are not clear.
3.2 Regulation of XPR1 by inositol pyrophosphate InsP8
XPR1 has a cytoplasmic N-terminal SPX domain (Battini et al., 1999), but this is not required for Pi transport (Giovannini et al., 2013). SPX domains bind to Pi with low affinity, but they bind to inositol pyrophosphates much more tightly (Wild et al., 2016). IP6 kinases (IP6K1-2) produce 5-InsP7 from InsP6 (Wilson et al., 2013), and PPIP5-kinase (PPIP5K) produces InsP8 from 5-InsP7 (Gu et al., 2017). Knockdown of IP6K1-2 results in decreased XPR1-mediated Pi efflux and undetectable levels of 5-InsP7 and InsP8 (Wilson et al., 2019). In addition, PiT2 overexpression increases XPR1-mediated Pi efflux by a mechanism dependent on IP6K1-2 (López-Sánchez et al., 2020). These results indicate that 5-InsP7 and/or InsP8 stimulate the XPR1 transport.
PPIP5K is a dual-function enzyme with a phosphatase domain that converts InsP8 back to 5-InsP7; this phosphatase activity is strongly inhibited by Pi (Gu et al., 2017). Therefore, an increase in [Pi]In inhibits the conversion of InsP8 to 5-InsP7 and increases [InsP8]. Using a combination of approaches, Shears and coworkers recently provided strong evidence that InsP8 is the dominant regulator of XPR1 (Li et al., 2020). SPX is an autoinhibitory domain in yeast low-affinity Pi transporters (Hürlimann et al., 2009; Secco et al., 2012), and it is possible that InsP8 binding stimulates transport by relieving SPX inhibition of XPR1.
These recent studies provide a new mechanistic framework for homeostasis of mammalian [Pi]In (Figures 1C, D): An increase in [Pi]In should lower PPIP5K phosphatase activity, increase [InsP8], and stimulate Pi efflux via XPR1, resulting in reduction in [Pi]In toward the original level. The same pathways should also stabilize [Pi]In, following a decrease in [Pi]Ex. It is not known how many cell types share the Pi transport regulatory mechanisms, as shown in Figure 1. It is also not known how uniform [Pi]In is among different mammalian cells.
4 Steady-state mammalian cell [Pi]In
4.1 Erythrocytes have a lower [Pi]In than other mammalian cells
The erythrocyte has a Pi efflux pathway that is distinct from XPR1. Like other cells, human erythrocytes have Na+-coupled Pi influx (Shoemaker et al., 1988; Timmer and Gunn, 2000). Unlike other cells, red blood cell membranes have extremely high levels of the Cl−/HCO3− exchanger AE1 (SLC4A1). AE1 transports Pi much more slowly than Cl− (Jennings, 2021), but there is so much AE1 in red blood cells that the AE1-mediated Cl−-Pi exchange drives [Pi]In toward the Donnan equilibrium ([Pi]In/[Pi]Ex ∼0.6 for H2PO4− and ∼0.36 for HPO4=). The balance between Na+-coupled influx and AE1-mediated efflux results in [Pi]In lower than [Pi]Ex but not as low as that expected for the Donnan equilibrium (Challa et al., 1985; Bevington et al., 1986).
4.2 31P magnetic resonance spectroscopy
31P magnetic resonance spectroscopy (MRS) is a powerful method for measuring concentrations of Pi, ATP, and other metabolites in vivo (Meyerspeer et al., 2020). MRS is also used to measure intracellular pH (Moon and Richards, 1973; Gillies et al., 1982) and metabolic fluxes (Pesta et al., 2016). The major 31Pi peak in intact muscle and brain results mainly from cytosolic Pi, but a minor peak from extracellular and/or mitochondrial Pi is resolved in some studies (Kan et al., 2010). A partial list of published MRS data on [Pi]In (Table 1, upper) shows that in mammalian cells other than erythrocytes, [Pi]In is generally equal to or higher than [Pi]Ex. The MRS data indicate that [Pi]In under resting conditions is ∼4 mM in skeletal muscle (highest in slow-twitch fibers) and ∼1 mM in the heart and brain.
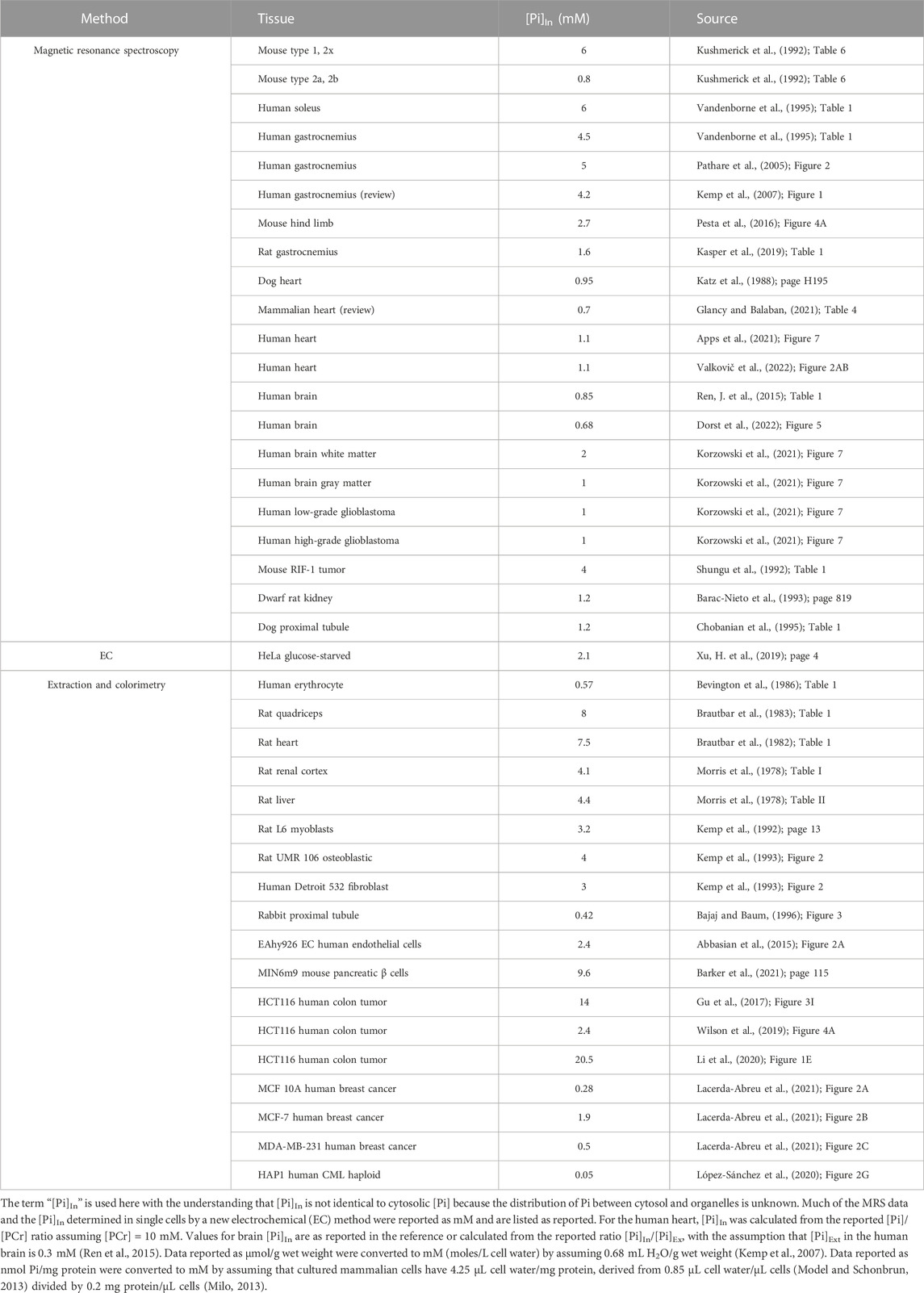
TABLE 1. Partial list of published estimates of mammalian [Pi]In under resting physiological conditions.
4.3 [Pi]In determined by extraction and colorimetry
[Pi]In data from cultured mammalian cells obtained by extraction and colorimetry are considerably more variable (Table 1, lower), but many of the values are in the 1–5 mM range. Hydrolysis of phosphoesters during extraction, even in carefully freeze-clamped samples, likely causes overestimates of [Pi] in muscle (Meyer et al., 1985). The labile phosphoester concentrations are lower in other tissues, but ester hydrolysis is still a potential source of overestimation of [Pi] in extracts of tissues and cultured cells. In summary, physiological [Pi]In, in many but not all mammalian cells, is about 1–5 mM.
5 Ongoing questions
5.1 How does [Pi]In change, following an increase in [Pi]Ex?
Although [Pi]Ex is normally regulated, hyperphosphatemia is not uncommon and can result from kidney disease, high P intake, hypoparathyroidism, or tumor lysis syndrome (Davidson et al., 2004; Hruska et al., 2008; Howard et al., 2011). Elevated [Pi]Ex has many adverse effects:
• Vascular calcification (Jono et al., 2000; Giachelli et al., 2005)
• Release of procoagulant microvesicles from endothelial cells (Abbasian et al., 2020b; Abbasian and Harper, 2021)
• Increased release of reactive oxygen species in a variety of cells (Lacerda-Abreu and Meyer-Fernandes, 2021)
• Premature aging (Ohnishi and Razzaque, 2010)
• Enhanced tumorigenesis (Camalier et al., 2010; Brown and Razzaque, 2018; Arnst and Beck, 2021
• Increased risk of cardiovascular events in people with coronary disease (Tonelli et al., 2005)
• Increased risk of death in critically ill patients (Zheng et al., 2022)
These effects of hyperphosphatemia are caused by a combination of [Pi]Ex and [Pi]In. Despite a sizable body of information on [Pi]In and Pi transporters, it is not known how much (and how rapidly) [Pi]In changes after an increase in [Pi]Ex. Published data on the effects of increased [Pi]Ex on mammalian or avian [Pi]In show varying degrees of homeostasis:
• In rat skeletal muscle, a two-fold increase in plasma [Pi] causes a ∼33% increase in skeletal muscle [Pi] (Bevington et al., 1986).
• In UMR106 cells or Detroit 532 fibroblasts, raising [Pi]Ex from 1 to 3 mM for 1 h has little effect on [Pi]In (Kemp et al., 1993).
• In HCT116 cells, raising [Pi]Ex from 1 mM to 5 mM for 1 hour has little effect on [Pi]In (Gu et al., 2017).
• In chick tibial chondrocytes, raising [Pi]Ex from 3 mM to 5 mM for 24 h causes a nearly 10-fold increase in [Pi]In (Mansfield et al., 2001).
• In EAhy926 vascular endothelial cells, raising [Pi]Ex from 1 to 2.5 mM for 48 h nearly doubles [Pi]In (Abbasian et al., 2015).
• Raising [Pi]Ex from 1 mM to 2 mM for 24 h causes a ∼2-fold increase in [Pi]In in MCF10-A but a much smaller change in MCF-7 breast cancer cells (Lacerda-Abreu et al., 2021).
These data indicate that at least some mammalian cells do not maintain near-constant [Pi]In when [Pi]Ex is increased. Now that the InsP8 mechanism for up-regulating the XPR1 activity has been described, it will be important to determine how broadly this mechanism applies and how closely [Pi]In is regulated during hyperphosphatemia in various cell types.
5.2 How does [Pi]In change, following a decrease in [Pi]Ex?
Hypophosphatemia has several possible causes (genetics, malnutrition, sepsis, and dialysis) and can result in muscle weakness (Pesta et al., 2016; Romagnoli et al., 2022), erythrocyte abnormalities (Travis et al., 1971; Worley et al., 1998), and other symptoms (Gaasbeek and Meinders, 2005; Amanzadeh and Reilly, 2006). Erythrocyte vulnerability to low [Pi]Ex is likely the result of Pi efflux via AE1 (see Section 4.1). In other mammalian cells, there are very limited data on the effect of a moderate decrease in [Pi]Ex on [Pi]In. A 50% decrease in plasma [Pi] causes human and rat skeletal muscle [Pi]In to decrease ∼20–35% (Brautbar et al., 1983; Bevington et al., 1986).
During hemodialysis, there is Pi efflux from skeletal muscle to blood (Lemoine et al., 2016; Chazot et al., 2021). XPR1 expression is relatively low in skeletal muscle (https://www.ncbi.nlm.nih.gov/gene/9213), and it is not known whether skeletal muscle uses the XPR1/InsP8 pathway for [Pi]In homeostasis or if XPR1 mediates muscle Pi efflux during dialysis.
5.3 How do changes in [Pi]In driven by metabolism affect transport?
Changes in [Pi]In can be driven by metabolism. One example is the drop in proximal tubule [Pi]In associated with Fanconi syndrome of cystinosis, probably resulting from a deficiency in apical Na+-Pi cotransporters (Baum, 1998). Other metabolic changes in [Pi]In have unknown effects on transporters:
• Exercise causes an increase in skeletal muscle [Pi]In (Westerblad et al., 2002).
• Fructose loading causes a large drop in [Pi]In in the kidney cortex and liver (Morris et al., 1978).
• In the perfused rat heart, insulin causes a >50% decrease in [Pi]In (Polgreen et al., 1994).
• Glucose starvation causes a two-fold increase in pancreatic β cell or insulinoma cell [Pi]In (Papas et al., 1997; Barker et al., 2021).
5.4 What is the driving force for XPR1-mediated [Pi] transport?
XPR1 expressed in tobacco leaves mediates Pi efflux (Wege and Poirier, 2014), but the transporter is difficult to express in Xenopus oocytes, and the nature of XPR1-mediated Pi transport is not known. Possibilities include the following:
• H+- Pi cotransport. The XPR1 homologs in yeast (Pho 87 and Pho90) (Hürlimann et al., 2009; Ghillebert et al., 2011) and Arabidopsis (PHO1) (Stefanovic et al., 2011; Chiou, 2020) are not known to be H+-Pi cotransporters, but an SPX-containing vacuolar Pi transporter in rice, OsSPX-MFS3, carries out the H+-Pi cotransport (Wang, C. et al., 2015). There is indirect evidence that XPR1 catalyzes the H+-Pi cotransport: stimulation of osteoclastic differentiation by RANK-L causes an increase in both XPR1 expression (Sharma et al., 2010) and H+-stimulated Pi transport (Ito et al., 2005; Ito et al., 2007).
• Pi-X or Pi-Pi exchange. Many transporters have partial reactions, resulting in exchange (Rosenberg and Wilbrandt, 1957; Schroers et al., 1997; Lytle et al., 1998; Russell, 2000; Glynn, 2002); the same could be true of XPR1. Exchange could be with another anion or with Pi itself. XPR1-mediated tracer efflux is stimulated by [Pi]Ex (Giovannini et al., 2013; Li et al., 2020; López-Sánchez et al., 2020); trans-stimulation could represent the Pi–Pi exchange rather than regulatory processes. The Pi–Pi exchange could also explain the XPR1-dependent tracer Pi influx found under some conditions (Li et al., 2020). In addition, the 2:1 H2PO4− -HPO4= exchange has been proposed for proximal tubular basolateral Pi transport (Barac-Nieto et al., 2002), which may be mediated by XPR1 (Ansermet et al., 2017; Kritmetapak and Kumar, 2021).
• Pi uniporter. The mitochondrial H+-Pi cotransporter can be converted reversibly into a uniporter (Schroers et al., 1997), and an uncoupled Pi uniport via XPR1 has not been ruled out.
5.5 What is the basis for Na+-independent Pi transport that is not mediated by XPR1?
XPR1 is the only identified transporter with Pi efflux as the primary function, but there are many examples of Na+-independent Pi transport processes that are not known to be mediated by XPR1 in non-erythroid mammalian cells, including Caco2BBE (Candeal et al., 2014), vascular smooth muscle (Villa-Bellosta et al., 2007; Hortells et al., 2020), articular chondrocytes (Solomon et al., 2007), brain endothelial cells (Dallaire and Béliveau, 1992), and breast cancer cells (Lacerda-Abreu et al., 2019; Lacerda-Abreu et al., 2020). The genes and proteins associated with these processes are unknown. Other evidence for XPR1-independent Pi efflux is that, when XPR1 is inhibited with an XBRD peptide or expression is knocked down, Pi efflux is certainly reduced, but 25% or more of the Pi efflux does not seem dependent on XPR1 (Giovannini et al., 2013; Li et al., 2020; López-Sánchez et al., 2020). The XPR1-independent efflux could conceivably be via a diffusional “leak,” but it could also be mediated by a transporter that has yet to be identified.
6 Conclusion and perspective
In the past several years, there have been important advances in understanding the role of transporters in mammalian [Pi]In homeostasis, but there is still much to learn. More data are needed on steady-state [Pi]In and the time courses of changes in [Pi]In in response to changes in conditions. The MRS data on skeletal muscle, heart, and brain [Pi]In are reasonably consistent, but the colorimetry data on cultured cells are more variable, possibly because somewhat different procedures (timing, temperature, detergents, phosphatase inhibitors, etc.) were used for extraction.
Quantitative understanding of Pi transport regulation will require measurement of tracer influx and efflux in the same units under the same conditions. Tracer Pi efflux (in units convertible to moles per cell per minute) is difficult to measure because neither the intracellular Pi specific activity after tracer loading is generally unknown nor is the proportion of tracer that has been converted into organic phosphate during loading. Despite these complexities, it is possible to determine intracellular [Pi]-specific activity, following tracer loading (Kemp et al., 1992; Kemp et al., 1993); the specific activity, [Pi]In, and the initial rate of tracer loss could then be used to determine efflux. Measurement of both tracer influx and efflux, in the same units under the same conditions, would make it possible to determine whether net flux, following a change in [Pi]Ex, is caused by a change in influx, efflux, or both. This kind of experiment would make it possible to test hypotheses about how the newly described feedback mechanisms (Figure 1) regulate [Pi]In in various cells under normal and pathophysiological conditions.
Author contributions
MJ is the sole author and prepared the text, figure, table, and references.
Funding
The preparation of this review was supported by salary from the Department of Physiology and Cell Biology, College of Medicine, University of Arkansas for Medical Sciences.
Conflict of interest
The author declares that the research was conducted in the absence of any commercial or financial relationships that could be construed as a potential conflict of interest.
Publisher’s note
All claims expressed in this article are solely those of the authors and do not necessarily represent those of their affiliated organizations, or those of the publisher, the editors, and the reviewers. Any product that may be evaluated in this article, or claim that may be made by its manufacturer, is not guaranteed or endorsed by the publisher.
Abbreviations
Pi, inorganic orthophosphate; [Pi]Ex, extracellular concentration of inorganic orthophosphate; [Pi]In, intracellular concentration of inorganic orthophosphate; PFA, phosphonoformic acid; PiT1, Na+-Pi cotransporter SLC20A1; PiT2, Na+-Pi cotransporter SLC20A2; XPR1, xenotropic and polytropic retrovirus receptor 1; SPX, suppressor of yeast gpa1 (Syg1), yeast phosphatase 81 (Pho81), and XPR1; X-MLV, xenotropic murine leukemia virus; XRBD, X-MLV receptor-binding domain; InsP6, inositol hexakisphosphate; 5-InsP7, 5-diphosphoinositol 1,2,3,4,6 pentakisphosphate; IP6K1-2, IP6 kinases 1 and -2; InsP8, 1,5 bisdiphosphoinositol 2,3,4,6 tetrakisphosphate; PPIP5K, 5-diphosphoinositol 1,2,3,4,6 pentakisphosphate kinase; PPase, pyrophosphataseSNX17: Sorting nexin 17; AAC, mitochondrial ATP/ADP exchanger; PiC, mitochondrial Pi transporter; GAPDH, glyceraldehyde-3-phosphate dehydrogenase; G3P, glyceraldehyde-3-phosphate; 1,3-BPG, 1,3-bisphosphoglycerage; MIM, mitochondrial inner membrane; F0F1, mitochondrial ATPase/ATP synthase; ER, endoplasmic reticulum; SLC, solute carrier family; MFS, major facilitator superfamily; MRS, magnetic resonance spectroscopy; EC, electrochemical.
References
Abbasian, N., Bevington, A., Burton, J. O., Herbert, K. E., Goodall, A. H., and Brunskill, N. J. (2020a). Inorganic phosphate (Pi) signaling in endothelial cells: A molecular basis for generation of endothelial microvesicles in uraemic cardiovascular disease. Int. J. Mol. Sci. 21, 6993. doi:10.3390/ijms21196993
Abbasian, N., Burton, J. O., Herbert, K. E., Tregunna, B. E., Brown, J. R., Ghaderi-Najafabadi, M., et al. (2015). Hyperphosphatemia, phosphoprotein phosphatases, and microparticle release in vascular endothelial cells. J. Am. Soc. Nephrol. 26, 2152–2162. [doi]. doi:10.1681/ASN.2014070642
Abbasian, N., Goodall, A. H., Burton, J. O., Bursnall, D., Bevington, A., and Brunskill, N. J. (2020b). Hyperphosphatemia drives procoagulant microvesicle generation in the rat partial nephrectomy model of CKD. J. Clin. Med. 9, 3534. doi:10.3390/jcm9113534
Abbasian, N., and Harper, M. T. (2021). High extracellular phosphate increases platelet polyphosphate content 32, 992–994. doi:10.1080/09537104.2020.1817358
Akasu-Nagayoshi, Y., Hayashi, T., Kawabata, A., Shimizu, N., Yamada, A., Yokota, N., et al. (2022). Phosphate exporter XPR1/SLC53A1 is required for the tumorigenicity of epithelial ovarian cancer. Cancer. Sci. 113, 2034–2043. [doi]. doi:10.1111/cas.15358
Amanzadeh, J., and Reilly, R. F. (2006). Hypophosphatemia: An evidence-based approach to its clinical consequences and management. Nat. Clin. Pract. Nephrol. 2, 136–148. doi: ncpneph0124 [pii]. doi:10.1038/ncpneph0124
Anheim, M., López-Sánchez, U., Giovannini, D., Richard, A. C., Touhami, J., N'Guyen, L., et al. (2016). XPR1 mutations are a rare cause of primary familial brain calcification. J. Neurol. 263, 1559–1564. [doi]. doi:10.1007/s00415-016-8166-4
Ansermet, C., Moor, M. B., Centeno, G., Auberson, M., Hu, D. Z., Baron, R., et al. (2017). Renal Fanconi syndrome and hypophosphatemic rickets in the absence of xenotropic and polytropic retroviral receptor in the nephron. J. Am. Soc. Nephrol. 28, 1073–1078. [doi]. doi:10.1681/ASN.2016070726
Apps, A., Valkovič, L., Peterzan, M., Lau, J. Y. C., Hundertmark, M., Clarke, W., et al. (2021). Quantifying the effect of dobutamine stress on myocardial Pi and pH in healthy volunteers: A (31) P MRS study at 7T. Magn. Reson. Med. 85, 1147–1159. doi:10.1002/mrm.28494
Arase, H., Yamada, S., Torisu, K., Tokumoto, M., Taniguchi, M., Tsuruya, K., et al. (2022). Protective roles of xenotropic and polytropic retrovirus receptor 1 (XPR1) in uremic vascular calcification. Calcif. Tissue Int. 110, 685–697. [doi]. doi:10.1007/s00223-022-00947-3
Arnst, J. L., and Beck, G. R. (2021). Modulating phosphate consumption, a novel therapeutic approach for the control of cancer cell proliferation and tumorigenesis. Biochem. Pharmacol. 183 (20), 114305. [pii]. doi:10.1016/j.bcp.2020.114305
Bajaj, G., and Baum, M. (1996). Proximal tubule dysfunction in cystine-loaded tubules: Effect of phosphate and metabolic substrates. Am. J. Physiol. 271, 717–F722. [doi]. doi:10.1152/ajprenal.1996.271.3.F717
Barac-Nieto, M., Alfred, M., and Spitzer, A. (2002). Basolateral phosphate transport in renal proximal-tubule-like OK cells. Exp. Biol. Med. (Maywood) 227, 626–631. [doi]. doi:10.1177/153537020222700811
Barac-Nieto, M., Corey, H., Liu, S. M., and Spitzer, A. (1993). Role of intracellular phosphate in the regulation of renal phosphate transport during development. Pediatr. Nephrol. 7, 819–822. doi:10.1007/BF01213367
Barker, C. J., Tessaro, F. H. G., Ferreira, S. S., Simas, R., Ayala, T. S., Köhler, M., et al. (2021). XPR1 mediates the pancreatic β-cell phosphate flush 70, 111–118. doi:10.2337/db19-0633
Battini, J. L., Rasko, J. E., and Miller, A. D. (1999). A human cell-surface receptor for xenotropic and polytropic murine leukemia viruses: Possible role in G protein-coupled signal transduction. Proc. Natl. Acad. Sci. U. S. A. 96, 1385–1390. doi:10.1073/pnas.96.4.1385
Baum, M. (1998). The Fanconi syndrome of cystinosis: Insights into the pathophysiology. Pediatr. Nephrol. 12, 492–497. doi:10.1007/s004670050495
Beck, L., and Beck-Cormier, S. (2020). Extracellular phosphate sensing in mammals: What do we know? J. Mol. Endocrinol. 65, R53–R63. JME-20-0121 [pii]. doi:10.1530/JME-20-0121
Beck, L., Leroy, C., Beck-Cormier, S., Forand, A., Salaün, C., Paris, N., et al. (2010). The phosphate transporter PiT1 (Slc20a1) revealed as a new essential gene for mouse liver development. PLoS One 5, e9148. doi:10.1371/journal.pone.0009148
Beck, L., Leroy, C., Salaün, C., Margall-Ducos, G., Desdouets, C., and Friedlander, G. (2009). Identification of a novel function of PiT1 critical for cell proliferation and independent of its phosphate transport activity. J. Biol. Chem. 284, 31363–31374. [doi]. doi:10.1074/jbc.M109.053132
Bergwitz, C., and Jüppner, H. (2010). Regulation of phosphate homeostasis by PTH, vitamin D, and FGF23. Annu. Rev. Med. 61, 91–104. doi:10.1146/annurev.med.051308.111339
Bevington, A., Mundy, K. I., Yates, A. J., Kanis, J. A., Russell, R. G., Taylor, D. J., et al. (1986). A study of intracellular orthophosphate concentration in human muscle and erythrocytes by 31P nuclear magnetic resonance spectroscopy and selective chemical assay. Clin. Sci. (Lond) 71, 729–735. [doi]. doi:10.1042/cs0710729
Bon, N., Couasnay, G., Bourgine, A., Sourice, S., Beck-Cormier, S., Guicheux, J., et al. (2018a). Phosphate (P(i))-regulated heterodimerization of the high-affinity sodium-dependent P(i) transporters PiT1/Slc20a1 and PiT2/Slc20a2 underlies extracellular P(i) sensing independently of P(i) uptake. J. Biol. Chem. 293, 2102–2114. [doi]. doi:10.1074/jbc.M117.807339
Bon, N., Frangi, G., Sourice, S., Guicheux, J., Beck-Cormier, S., and Beck, L. (2018b). Phosphate-dependent FGF23 secretion is modulated by PiT2/Slc20a2. Mol. Metab. 11, 197–204. doi:10.1016/j.molmet.2018.02.007
Bondeson, D. P., Paolella, B. R., Asfaw, A., Rothberg, M. V., Skipper, T. A., Langan, C., et al. (2022). Phosphate dysregulation via the XPR1-KIDINS220 protein complex is a therapeutic vulnerability in ovarian cancer. Nat. Cancer 3, 681–695. [doi]. doi:10.1038/s43018-022-00360-7
Bøttger, P., Hede, S. E., Grunnet, M., Høyer, B., Klaerke, D. A., and Pedersen, L. (2006). Characterization of transport mechanisms and determinants critical for Na+-dependent Pi symport of the PiT family paralogs human PiT1 and PiT2. Am. J. Physiol. Cell. Physiol. 291, 1377–C1387. doi:10.1152/ajpcell.00015.2006
Brautbar, N., Baczynski, R., Carpenter, C., Moser, S., Geiger, P., Finander, P., et al. (1982). Impaired energy metabolism in rat myocardium during phosphate depletion. Am. J. Physiol. 242, 699–F704. doi:10.1152/ajprenal.1982.242.6.F699
Brautbar, N., Carpenter, C., Baczynski, R., Kohan, R., and Massry, S. G. (1983). Impaired energy metabolism in skeletal muscle during phosphate depletion. Kidney Int. 24, 53–57. S0085-2538(15)32995-1 [pii]. doi:10.1038/ki.1983.125
Brown, R. B., and Razzaque, M. S. (2018). Phosphate toxicity and tumorigenesis. Biochim. Biophys. Acta Rev. Cancer. 1869 (18), 303–309. 30042-8 [pii]. doi:10.1016/j.bbcan.2018.04.007
Camalier, C. E., Young, M. R., Bobe, G., Perella, C. M., Colburn, N. H., and Beck, G. R. J. (2010). Elevated phosphate activates N-ras and promotes cell transformation and skin tumorigenesis. Cancer. Prev. Res. (Phila) 3, 359–370. doi:10.1158/1940-6207.CAPR-09-0068
Candeal, E., Caldas, Y. A., Guillén, N., Levi, M., and Sorribas, V. (2014). Na+-independent phosphate transport in Caco2BBE cells. Am. J. Physiol. Cell. Physiol. 307, 1113–C1122. [doi]. doi:10.1152/ajpcell.00251.2014
Challa, A., Bevington, A., Angier, C. M., Asbury, A. J., Preston, C. J., and Russell, R. G. (1985). A technique for the measurement of orthophosphate in human erythrocytes, and some studies of its determinants. Clin. Sci. (Lond) 69, 429–434. [doi]. doi:10.1042/cs0690429
Chande, S., and Bergwitz, C. (2018). Role of phosphate sensing in bone and mineral metabolism. Nat. Rev. Endocrinol. 14, 637–655. doi:10.1038/s41574-018-0076-3
Chande, S., Caballero, D., Ho, B. B., Fetene, J., Serna, J., Pesta, D., et al. (2020). Slc20a1/Pit1 and Slc20a2/Pit2 are essential for normal skeletal myofiber function and survival. Sci. Rep. 10, 3069. doi:10.1038/s41598-020-59430-4
Chazot, G., Lemoine, S., Kocevar, G., Kalbacher, E., Sappey-Marinier, D., Rouvière, O., et al. (2021). Intracellular phosphate and ATP depletion measured by magnetic resonance spectroscopy in patients receiving maintenance hemodialysis. J. Am. Soc. Nephrol. 32, 229–237. [doi]. doi:10.1681/ASN.2020050716
Chien, M. L., Foster, J. L., Douglas, J. L., and Garcia, J. V. (1997). The amphotropic murine leukemia virus receptor gene encodes a 71-kilodalton protein that is induced by phosphate depletion. J. Virol. 71, 4564–4570. doi:10.1128/JVI.71.6.4564-4570.1997
Chiou, T. (2020). The diverse roles of rice PHO1 in phosphate transport: From root to node to grain. Plant Cell Physiol. 61, 1384–1386. doi:10.1093/pcp/pcaa097
Chobanian, M. C., Anderson, M. E., and Brazy, P. C. (1995). An NMR study of cellular phosphates and membrane transport in renal proximal tubules. Am. J. Physiol. 268, 375–F384. doi:10.1152/ajprenal.1995.268.3.F375
Crouthamel, M. H., Lau, W. L., Leaf, E. M., Chavkin, N. W., Wallingford, M. C., Peterson, D. F., et al. (2013). Sodium-dependent phosphate cotransporters and phosphate-induced calcification of vascular smooth muscle cells: Redundant roles for PiT-1 and PiT-2. Arterioscler. Thromb. Vasc. Biol. 33, 2625–2632. doi:10.1161/ATVBAHA.113.302249
Dallaire, L., and Béliveau, R. (1992). Phosphate transport by capillaries of the blood-brain barrier. J. Biol. Chem. 267, 22323–22327. doi:10.1016/s0021-9258(18)41673-0
Davidson, M. B., Thakkar, S., Hix, J. K., Bhandarkar, N. D., Wong, A., and Schreiber, M. J. (2004). Pathophysiology, clinical consequences, and treatment of tumor lysis syndrome. Am. J. Med. 116, 546–554. S0002934303007253 [pii]. doi:10.1016/j.amjmed.2003.09.045
Dorst, J., Borbath, T., Ruhm, L., and Henning, A. (2022). Phosphorus transversal relaxation times and metabolite concentrations in the human brain at 9.4 T. NMR Biomed. 35, e4776. [doi]. doi:10.1002/nbm.4776
Doshi, S. M., and Wish, J. B. (2022). Past, present, and future of phosphate management. Kidney Int. Rep. 7, 688–698. doi:10.1016/j.ekir.2022.01.1055
Festing, M. H., Speer, M. Y., Yang, H., and Giachelli, C. M. (2009). Generation of mouse conditional and null alleles of the type III sodium-dependent phosphate cotransporter PiT-1. Genesis 47, 858–863. doi:10.1002/dvg.20577
Figueres, L., Beck-Cormier, S., Beck, L., and Marks, J. (2021). The complexities of organ crosstalk in phosphate homeostasis: Time to put phosphate sensing back in the limelight. Int. J. Mol. Sci. 22, 5701. doi:10.3390/ijms22115701
Forster, I. C., Hernando, N., Biber, J., and Murer, H. (2013). Phosphate transporters of the SLC20 and SLC34 families. Mol. Asp. Med. 34, 386–395. doi:10.1016/j.mam.2012.07.007
Gaasbeek, A., and Meinders, A. E. (2005). Hypophosphatemia: An update on its etiology and treatment. Am. J. Med. 118, 1094–1101. doi:10.1016/j.amjmed.2005.02.014
Ghillebert, R., Swinnen, E., De Snijder, P., Smets, B., and Winderickx, J. (2011). Differential roles for the low-affinity phosphate transporters Pho87 and Pho90 in Saccharomyces cerevisiae. Biochem. J. 434, 243–251. doi:10.1042/BJ20101118
Giachelli, C. M., Speer, M. Y., Li, X., Rajachar, R. M., and Yang, H. (2005). Regulation of vascular calcification: Roles of phosphate and osteopontin. Circ. Res. 96, 717–722. [pii]. doi:10.1161/01.RES.0000161997.24797.c0
Gillies, R. J., Ogino, T., Shulman, R. G., and Ward, D. C. (1982). 31P nuclear magnetic resonance evidence for the regulation of intracellular pH by Ehrlich ascites tumor cells. J. Cell Biol. 95, 24–28. doi:10.1083/jcb.95.1.24
Giovannini, D., Touhami, J., Charnet, P., Sitbon, M., and Battini, J. L. (2013). Inorganic phosphate export by the retrovirus receptor XPR1 in metazoans. Cell. Rep. 3, 1866–1873. doi:10.1016/j.celrep.2013.05.035
Glancy, B., and Balaban, R. S. (2021). Energy metabolism design of the striated muscle cell. Physiol. Rev. 101, 1561–1607. doi:10.1152/physrev.00040.2020
Glynn, I. M. (2002). A hundred years of sodium pumping. Annu. Rev. Physiol. 64, 1–18. doi:10.1146/annurev.physiol.64.081501.130716
Gu, C., Nguyen, H. N., Hofer, A., Jessen, H. J., Dai, X., Wang, H., et al. (2017). The significance of the bifunctional kinase/phosphatase activities of diphosphoinositol pentakisphosphate kinases (PPIP5Ks) for coupling inositol pyrophosphate cell signaling to cellular phosphate homeostasis. J. Biol. Chem. 292, 4544–4555. [doi]. doi:10.1074/jbc.M116.765743
Guerreiro, P. M., Bataille, A. M., Parker, S. L., and Renfro, J. L. (2014). Active removal of inorganic phosphate from cerebrospinal fluid by the choroid plexus. Am. J. Physiol. Ren. Physiol. 306, 1275–F1284. [doi]. doi:10.1152/ajprenal.00458.2013
Guillén, N., Caldas, Y. A., Levi, M., and Sorribas, V. (2019). Identification and expression analysis of type II and type III P(i) transporters in the opossum kidney cell line. Exp. Physiol. 104, 149–161. doi:10.1113/EP087217
Hernando, N., Gagnon, K., and Lederer, E. (2021). Phosphate transport in epithelial and nonepithelial tissue. Physiol. Rev. 101, 1–35. doi:10.1152/physrev.00008.2019
Hill Gallant, K. M., Stremke, E. R., Trevino, L. L., Moorthi, R. N., Doshi, S., Wastney, M. E., et al. (2021). EOS789, a broad-spectrum inhibitor of phosphate transport, is safe with an indication of efficacy in a phase 1b randomized crossover trial in hemodialysis patients. Kidney Int. 99, 1225–1233. doi:10.1016/j.kint.2020.09.035
Hortells, L., Guillén, N., Sosa, C., and Sorribas, V. (2020). Several phosphate transport processes are present in vascular smooth muscle cells. Am. J. Physiol. Heart Circ. Physiol. 318, H448–H460. [doi]. doi:10.1152/ajpheart.00433.2019
Howard, S. C., Jones, D. P., and Pui, C. (2011). The tumor lysis syndrome. N. Engl. J. Med. 364, 1844–1854. doi:10.1056/NEJMra0904569
Hruska, K. A., Mathew, S., Lund, R., Qiu, P., and Pratt, R. (2008). Hyperphosphatemia of chronic kidney disease. Kidney Int. 74, 148–157. doi:10.1038/ki.2008.130
Hürlimann, H. C., Pinson, B., Stadler-Waibel, M., Zeeman, S. C., and Freimoser, F. M. (2009). The SPX domain of the yeast low-affinity phosphate transporter Pho90 regulates transport activity. EMBO Rep. 10, 1003–1008. doi:10.1038/embor.2009.105
Ito, M., Haito, S., Furumoto, M., Uehata, Y., Sakurai, A., Segawa, H., et al. (2007). Unique uptake and efflux systems of inorganic phosphate in osteoclast-like cells. Am. J. Physiol. Cell. Physiol. 292, C526. C534. doi:10.1152/ajpcell.00357.2006
Ito, M., Matsuka, N., Izuka, M., Haito, S., Sakai, Y., Nakamura, R., et al. (2005). Characterization of inorganic phosphate transport in osteoclast-like cells. Am. J. Physiol. Cell. Physiol. 288, C921. C931. doi:10.1152/ajpcell.00412.2004
Jennings, M. L. (2021). Cell physiology and molecular mechanism of anion transport by erythrocyte band 3/AE1. Am. J. Physiol. Cell. Physiol. 321, C1028–C1059. doi:10.1152/ajpcell.00275.2021
Jensen, N., Autzen, J. K., and Pedersen, L. (2016). Slc20a2 is critical for maintaining a physiologic inorganic phosphate level in cerebrospinal fluid, 17, 125–130. doi:10.1007/s10048-015-0469-6
Jensen, N., Schrøder, H. D., Hejbøl, E. K., Füchtbauer, E., de Oliveira, J. R. M., and Pedersen, L. (2013). Loss of function of Slc20a2 associated with familial idiopathic Basal Ganglia calcification in humans causes brain calcifications in mice. J. Mol. Neurosci. 51, 994–999. doi:10.1007/s12031-013-0085-6
Jono, S., McKee, M. D., Murry, C. E., Shioi, A., Nishizawa, Y., Mori, K., et al. (2000). Phosphate regulation of vascular smooth muscle cell calcification. Circ. Res. 87, 10–E17. doi:10.1161/01.res.87.7.e10
Kan, H. E., Klomp, D. W. J., Wong, C. S., Boer, V. O., Webb, A. G., Luijten, P. R., et al. (2010). In vivo31P MRS detection of an alkaline inorganic phosphate pool with short T1 in human resting skeletal muscle. NMR Biomed. 23, 995–1000. doi:10.1002/nbm.1517
Kasper, J. D., Meyer, R. A., Beard, D. A., and Wiseman, R. W. (2019). Effects of altered pyruvate dehydrogenase activity on contracting skeletal muscle bioenergetics. Am. J. Physiol. Regul. Integr. Comp. Physiol. 316, R76–R86. doi:10.1152/ajpregu.00321.2018
Katz, L. A., Swain, J. A., Portman, M. A., and Balaban, R. S. (1988). Intracellular pH and inorganic phosphate content of heart in vivo: A 31P-nmr study. Am. J. Physiol. 255, 189–H196. [doi]. doi:10.1152/ajpheart.1988.255.1.H189
Kavanaugh, M. P., Miller, D. G., Zhang, W., Law, W., Kozak, S. L., Kabat, D., et al. (1994). Cell-surface receptors for gibbon ape leukemia virus and amphotropic murine retrovirus are inducible sodium-dependent phosphate symporters. Proc. Natl. Acad. Sci. U. S. A. 91, 7071–7075. [doi]. doi:10.1073/pnas.91.15.7071
Kemp, G. J., Khouja, H. I., Ahmado, A., Graham, R., Russell, G., and Bevington, A. (1993). Regulation of the phosphate (Pi) concentration in UMR 106 osteoblast-like cells: Effect of Pi, Na+ and K+. Cell biochem. Funct. 11, 13–23. doi:10.1002/cbf.290110103
Kemp, G. J., Meyerspeer, M., and Moser, E. (2007). Absolute quantification of phosphorus metabolite concentrations in human muscle in vivo by 31P MRS: A quantitative review. NMR Biomed. 20, 555–565. doi:10.1002/nbm.1192
Kemp, G. J., Polgreen, K. E., and Radda, G. K. (1992). Skeletal muscle Pi transport and cellular [Pi] studied in L6 myoblasts and rabbit muscle-membrane vesicles. Biochim. Biophys. Acta Mol. Cell Res. 1137, 10–18. doi:10.1016/0167/4889(92)90093-Q
Khoshniat, S., Bourgine, A., Julien, M., Weiss, P., Guicheux, J., and Beck, L. (2011). The emergence of phosphate as a specific signaling molecule in bone and other cell types in mammals. Cell Mol. Life Sci. 68, 205–218. doi:10.1007/s00018-010-0527-z
Komaba, H., and Fukagawa, M. (2016). Phosphate-a poison for humans? Kidney Int. 90, 753–763. doi:10.1016/j.kint.2016.03.039
Korzowski, A., Weckesser, N., Franke, V. L., Breitling, J., Goerke, S., Schlemmer, H., et al. (2021). Mapping an extended metabolic profile of gliomas using high-resolution (31)P MRSI at 7T. Front. Neurol. 12, 735071. doi:10.3389/fneur.2021.735071
Kritmetapak, K., and Kumar, R. (2021). Phosphate as a signaling molecule. Calcif. Tissue Int. 108, 16–31. [doi]. doi:10.1007/s00223-019-00636-8
Kushmerick, M. J., Moerland, T. S., and Wiseman, R. W. (1992). Mammalian skeletal muscle fibers distinguished by contents of phosphocreatine, ATP, and Pi. Proc. Natl. Acad. Sci. U. S. A. 89, 7521–7525. doi:10.1073/pnas.89.16.7521
Lacerda-Abreu, M. A., and Meyer-Fernandes, J. R. (2021). Extracellular inorganic phosphate-induced release of reactive oxygen species: Roles in physiological processes and disease development. Int. J. Mol. Sci. 22, 7768. doi:10.3390/ijms22157768
Lacerda-Abreu, M. A., Russo-Abrahão, T., Cosentino-Gomes, D., Nascimento, M. T. C., Carvalho-Kelly, L. F., Gomes, T., et al. (2019). H(+)-dependent inorganic phosphate transporter in breast cancer cells: Possible functions in the tumor microenvironment. Biochim. Biophys. Acta Mol. Basis Dis. 1865, 2180–2188. doi:10.1016/j.bbadis.2019.04.015
Lacerda-Abreu, M. A., Russo-Abrahão, T., and Meyer-Fernandes, J. R. (2020). The roles of sodium-independent inorganic phosphate transporters in inorganic phosphate homeostasis and in cancer and other diseases. Int. J. Mol. Sci. 21, 9298. doi:10.3390/ijms21239298
Lacerda-Abreu, M. A., Russo-Abrahão, T., Rocco-Machado, N., Cosentino-Gomes, D., Dick, C. F., Carvalho-Kelly, L. F., et al. (2021). Hydrogen peroxide generation as an underlying response to high extracellular inorganic phosphate (Pi) in breast cancer cells. Int. J. Mol. Sci. 22, 10096. doi:10.3390/ijms221810096
Legati, A., Giovannini, D., Nicolas, G., López-Sánchez, U., Quintáns, B., Oliveira, J. R., et al. (2015). Mutations in XPR1 cause primary familial brain calcification associated with altered phosphate export. Nat. Genet. 47, 579–581. [doi]. doi:10.1038/ng.3289
Lemoine, S., Fournier, T., Kocevar, G., Belloi, A., Normand, G., Ibarrola, D., et al. (2016). Intracellular phosphate dynamics in muscle measured by magnetic resonance spectroscopy during hemodialysis. J. Am. Soc. Nephrol. 27, 2062–2068. doi:10.1681/ASN.2015050546
Levi, M., Gratton, E., Forster, I. C., Hernando, N., Wagner, C. A., Biber, J., et al. (2019). Mechanisms of phosphate transport. Nat. Rev. Nephrol. 15, 482–500. [doi]. doi:10.1038/s41581-019-0159-y
Li, X., Gu, C., Hostachy, S., Sahu, S., Wittwer, C., Jessen, H. J., et al. (2020). Control of XPR1-dependent cellular phosphate efflux by InsP8 is an exemplar for functionally-exclusive inositol pyrophosphate signaling 117, 3568–3574. doi:10.1073/pnas.1908830117
Li, X., Yang, H., and Giachelli, C. M. (2006). Role of the sodium-dependent phosphate cotransporter, Pit-1, in vascular smooth muscle cell calcification. Circ. Res. 98, 905–912. doi:10.1161/01.RES.0000216409.20863.e7
López-Sánchez, U., Tury, S., Nicolas, G., Wilson, M. S., Jurici, S., Ayrignac, X., et al. (2020). Interplay between primary familial brain calcification-associated SLC20A2 and XPR1 phosphate transporters requires inositol polyphosphates for control of cellular phosphate homeostasis. J. Biol. Chem. 295, 9366–9378. [doi]. doi:10.1074/jbc.RA119.011376
Lytle, C., McManus, T. J., and Haas, M. (1998). A model of Na-K-2Cl cotransport based on ordered ion binding and glide symmetry. Am. J. Physiol. 274, 299–C309. doi:10.1152/ajpcell.1998.274.2.C299
Mailer, R. K., Allende, M., Heestermans, M., Schweizer, M., Deppermann, C., Frye, M., et al. (2021). Xenotropic and polytropic retrovirus receptor 1 regulates procoagulant platelet polyphosphate, 137, 1392–1405. [doi]. doi:10.1182/blood.2019004617
Mansfield, K., Teixeira, C. C., Adams, C. S., and Shapiro, I. M. (2001). Phosphate ions mediate chondrocyte apoptosis through a plasma membrane transporter mechanism. through a plasma Membr. Transp. Mech. 28, 1–8. (00)00409-9 [pii]. doi:10.1016/s8756-3282(00)00409-9
Meyer, R. A., Brown, T. R., and Kushmerick, M. J. (1985). Phosphorus nuclear magnetic resonance of fast- and slow-twitch muscle. Am. J. Physiol. 248, 279–C287. doi:10.1152/ajpcell.1985.248.3.C279
Meyerspeer, M., Boesch, C., Cameron, D., Dezortová, M., Forbes, S. C., Heerschap, A., et al. (2020). (31) P magnetic resonance spectroscopy in skeletal muscle: Experts' consensus recommendations. NMR Biomed. 34, e4246. [doi]. doi:10.1002/nbm.4246
Michigami, T., Kawai, M., Yamazaki, M., and Ozono, K. (2018). Phosphate as a signaling molecule and its sensing mechanism. Physiol. Rev. 98, 2317–2348. doi:10.1152/physrev.00022.2017
Milo, R. (2013). What is the total number of protein molecules per cell volume? A call to rethink some published values. Bioessays 35, 1050–1055. [doi]. doi:10.1002/bies.201300066
Model, M. A., and Schonbrun, E. (2013). Optical determination of intracellular water in apoptotic cells. J. Physiol. 591, 5843–5849. doi:10.1113/jphysiol.2013.263228
Moon, R. B., and Richards, J. H. (1973). Determination of intracellular pH by 31P magnetic resonance. J. Biol. Chem. 248, 7276–7278. S0021-9258(19)43389-9 [pii]. doi:10.1016/s0021-9258(19)43389-9
Morris, R. C. J., Nigon, K., and Reed, E. B. (1978). Evidence that the severity of depletion of inorganic phosphate determines the severity of the disturbance of adenine nucleotide metabolism in the liver and renal cortex of the fructose-loaded rat. J. Clin. Invest. 61, 209–220. doi:10.1172/JCI108920
Ohnishi, M., and Razzaque, M. S. (2010). Dietary and genetic evidence for phosphate toxicity accelerating mammalian aging. FASEB J. 24, 3562–3571. doi:10.1096/fj.09-152488
Onaga, C., Tamori, S., Motomura, H., Ozaki, A., Matsuda, C., Matsuoka, I., et al. (2021). High SLC20A1 expression is associated with poor prognoses in claudin-low and basal-like breast cancers. Anticancer Res. 41, 43–54. doi:10.21873/anticanres.14750
Papas, K. K., Long, R. C. J., Constantinidis, I., and Sambanis, A. (1997). Role of ATP and Pi in the mechanism of insulin secretion in the mouse insulinoma betaTC3 cell line. Biochem. J. 326 (3), 807–814. doi:10.1042/bj3260807
Pathare, N., Walter, G. A., Stevens, J. E., Yang, Z., Okerke, E., Gibbs, J. D., et al. (2005). Changes in inorganic phosphate and force production in human skeletal muscle after cast immobilization. J. Appl. Physiol. 98, 307–314. doi:10.1152/japplphysiol.00612.2004
Pesta, D. H., Tsirigotis, D. N., Befroy, D. E., Caballero, D., Jurczak, M. J., Rahimi, Y., et al. (2016). Hypophosphatemia promotes lower rates of muscle ATP synthesis. FASEB J. 30, 3378–3387. doi:10.1096/fj.201600473R
Peters, M. E. M., de Brouwer, E. J. M., Bartstra, J. W., Mali, W. P. T. M., Koek, H. L., Rozemuller, A. J. M., et al. (2020). Mechanisms of calcification in Fahr disease and exposure of potential therapeutic targets. Neurol. Clin. Pract. 10, 449–457. doi:10.1212/CPJ.0000000000000782
Polgreen, K. E., Kemp, G. J., Clarke, K., and Radda, G. K. (1994). Transsarcolemmal movement of inorganic phosphate in glucose-perfused rat heart: A 31P nuclear magnetic resonance spectroscopic study. J. Mol. Cell. Cardiol. 26, 219–228. doi:10.1006/jmcc.1994.1025
Ravera, S., Virkki, L. V., Murer, H., and Forster, I. C. (2007). Deciphering PiT transport kinetics and substrate specificity using electrophysiology and flux measurements. Am. J. Physiol. Cell. Physiol. 293, C606. C620. doi:10.1152/ajpcell.00064.2007
Reimer, R. J., and Edwards, R. H. (2004). Organic anion transport is the primary function of the SLC17/type I phosphate transporter family. Pflugers Arch. 447, 629–635. doi:10.1007/s00424-003-1087-y
Ren, J., Sherry, A. D., and Malloy, C. R. (2015). (31)P-MRS of healthy human brain: ATP synthesis, metabolite concentrations, pH, and T1 relaxation times. NMR Biomed. 28, 1455–1462. [doi]. doi:10.1002/nbm.3384
Ren, Y., Shen, Y., Si, N., Fan, S., Zhang, Y., Xu, W., et al. (2021). Slc20a2-deficient mice exhibit multisystem abnormalities and impaired spatial learning memory and sensorimotor gating but normal motor coordination abilities. Front. Genet. 12, 639935. doi:10.3389/fgene.2021.639935
Romagnoli, C., Iantomasi, T., and Brandi, M. L. (2022). Impact of X-linked hypophosphatemia on muscle symptoms. Genes (Basel) 13, 2415. doi:10.3390/genes13122415
Rosenberg, T., and Wilbrandt, W. (1957). Uphill transport induced by counterflow. J. Gen. Physiol. 41, 289–296. doi:10.1085/jgp.41.2.289
Russell, J. M. (2000). Sodium-potassium-chloride cotransport. Physiol. Rev. 80, 211–276. doi:10.1152/physrev.2000.80.1.211
Salaün, C., Gyan, E., Rodrigues, P., and Heard, J. M. (2002). Pit2 assemblies at the cell surface are modulated by extracellular inorganic phosphate concentration. J. Virol. 76, 4304–4311. doi:10.1128/jvi.76.9.4304-4311.2002
Salaün, C., Maréchal, V., and Heard, J. M. (2004). Transport-deficient Pit2 phosphate transporters still modify cell surface oligomers structure in response to inorganic phosphate. J. Mol. Biol. 340, 39–47. doi:10.1016/j.jmb.2004.04.050
Sato, K., and Akimoto, K. (2017). Expression levels of KMT2C and SLC20A1 identified by information-theoretical analysis are powerful prognostic biomarkers in estrogen receptor-positive breast cancer. Clin. Breast Cancer 17, e135–e142. doi:10.1016/j.clbc.2016.11.005
Schroers, A., Krämer, R., and Wohlrab, H. (1997). The reversible antiport-uniport conversion of the phosphate carrier from yeast mitochondria depends on the presence of a single cysteine. J. Biol. Chem. 272, 10558–10564. doi:10.1074/jbc.272.16.10558
Secco, D., Wang, C., Arpat, B. A., Wang, Z., Poirier, Y., Tyerman, S. D., et al. (2012). The emerging importance of the SPX domain-containing proteins in phosphate homeostasis. New Phytol. 193, 842–851. doi:10.1111/j.1469-8137.2011.04002.x
Sharma, P., Patntirapong, S., Hann, S., and Hauschka, P. V. (2010). RANKL-RANK signaling regulates expression of xenotropic and polytropic virus receptor (XPR1) in osteoclasts. Biochem. Biophys. Res. Commun. 399, 129–132. [doi]. doi:10.1016/j.bbrc.2010.07.022
Shoemaker, D. G., Bender, C. A., and Gunn, R. B. (1988). Sodium-phosphate cotransport in human red blood cells. Kinetics and role in membrane metabolism. J. Gen. Physiol. 92, 449–474. doi:10.1085/jgp.92.4.449
Shungu, D. C., Bhujwalla, Z. M., Li, S. J., Rose, L. M., Wehrle, J. P., and Glickson, J. D. (1992). Determination of absolute phosphate metabolite concentrations in RIF-1 tumors in vivo by 31P-1H-2H NMR spectroscopy using water as an internal intensity reference. Magn. Reson. Med. 28, 105–121. doi:10.1002/mrm.1910280111
Solomon, D. H., Wilkins, R. J., Meredith, D., and Browning, J. A. (2007). Characterisation of inorganic phosphate transport in bovine articular chondrocytes. Cell. Physiol. biochem. 20, 99–108. doi:10.1159/000104158
Stefanovic, A., Arpat, A. B., Bligny, R., Gout, E., Vidoudez, C., Bensimon, M., et al. (2011). Over-expression of PHO1 in Arabidopsis leaves reveals its role in mediating phosphate efflux. Plant J. 66, 689–699. doi:10.1111/j.1365-313X.2011.04532.x
Sun, M., Wu, X., Yu, Y., Wang, L., Xie, D., Zhang, Z., et al. (2020). Disorders of calcium and phosphorus metabolism and the proteomics/metabolomics-based research. Front. Cell. Dev. Biol. 8, 576110. doi:10.3389/fcell.2020.576110
Tenenhouse, H. S., Gauthier, C., Martel, J., Gesek, F. A., Coutermarsh, B. A., and Friedman, P. A. (1998). Na+ -phosphate cotransport in mouse distal convoluted tubule cells: Evidence for glvr-1 and ram-1 gene expression. J. Bone Min. Res. 13, 590–597. doi:10.1359/jbmr.1998.13.4.590
Timmer, R. T., and Gunn, R. B. (2000). The molecular basis for Na-dependent phosphate transport in human erythrocytes and K562 cells. J. Gen. Physiol. 116, 363–378. doi:10.1085/jgp.116.3.363
Tonelli, M., Sacks, F., Pfeffer, M., Gao, Z., and Curhan, G. (2005). Relation between serum phosphate level and cardiovascular event rate in people with coronary disease 112, 2627–2633. doi:10.1161/CIRCULATIONAHA.105.553198
Travis, S. F., Sugerman, H. J., Ruberg, R. L., Dudrick, S. J., Delivoria-Papadopoulos, M., Miller, L. D., et al. (1971). Alterations of red-cell glycolytic intermediates and oxygen transport as a consequence of hypophosphatemia in patients receiving intravenous hyperalimentation. N. Engl. J. Med. 285, 763–768. doi:10.1056/NEJM197109302851402
Tsuboi, Y., Ichida, Y., Murai, A., Maeda, A., Iida, M., Kato, A., et al. (2022). EOS789, pan-phosphate transporter inhibitor, ameliorates the progression of kidney injury in anti-GBM-induced glomerulonephritis rats. Pharmacol. Res. Perspect. 2022 10, e00973. doi:10.1002/prp2.973
Tsuboi, Y., Ohtomo, S., Ichida, Y., Hagita, H., Ozawa, K., Iida, M., et al. (2020). EOS789, a novel pan-phosphate transporter inhibitor, is effective for the treatment of chronic kidney disease–mineral bone disorder. Kidney Int. 98, 343–354. doi:10.1016/j.kint.2020.02.040
Valkovič, L., Apps, A., Ellis, J., Neubauer, S., Tyler, D. J., Schmid, A. I., et al. (2022). Increased cardiac Pi/PCr in the diabetic heart observed using phosphorus magnetic resonance spectroscopy at 7T. PLoS One 17, e0269957. doi:10.1371/journal.pone.0269957
Vandenborne, K., Walter, G., Ploutz-Snyder, L., Staron, R., Fry, A., De Meirleir, K., et al. (1995). Energy-rich phosphates in slow and fast human skeletal muscle. Am. J. Physiol. 268, 869–C876. doi:10.1152/ajpcell.1995.268.4.C869
Villa-Bellosta, R., Bogaert, Y. E., Levi, M., and Sorribas, V. (2007). Characterization of phosphate transport in rat vascular smooth muscle cells: Implications for vascular calcification. Arterioscler. Thromb. Vasc. Biol. 27, 1030–1036. doi:10.1161/ATVBAHA.106.132266
Villa-Bellosta, R., Ravera, S., Sorribas, V., Stange, G., Levi, M., Murer, H., et al. (2009). The Na+-Picotransporter PiT-2 (SLC20A2) is expressed in the apical membrane of rat renal proximal tubules and regulated by dietary Pi. Am. J. Physiol. Ren. Physiol. 296, 691–F699. doi:10.1152/ajprenal.90623.2008
Virkki, L. V., Biber, J., Murer, H., and Forster, I. C. (2007). Phosphate transporters: A tale of two solute carrier families. Am. J. Physiol. Ren. Physiol. 293, F643. F654. doi:10.1152/ajprenal.00228.2007
Wagner, C. A., Hernando, N., Forster, I. C., and Biber, J. (2014). The SLC34 family of sodium-dependent phosphate transporters. Pflugers Arch. 466, 139–153. doi:10.1007/s00424-013-1418-6
Wagner, C. A. (2023). Pharmacology of mammalian Na+- dependent transporters of inorganic phosphate. Handb. Exp. Pharmacol. doi:10.1007/164_2022_633
Wagner, C. A., Rubio-Aliaga, I., and Hernando, N. (2019). Renal phosphate handling and inherited disorders of phosphate reabsorption: An update. Pediatr. Nephrol. 34, 549–559. doi:10.1007/s00467-017-3873-3
Wallingford, M. C., Chia, J. J., Leaf, E. M., Borgeia, S., Chavkin, N. W., Sawangmake, C., et al. (2017). SLC20A2 deficiency in mice leads to elevated phosphate levels in cerbrospinal fluid and glymphatic pathway-associated arteriolar calcification, and recapitulates human idiopathic basal ganglia calcification. Brain Pathol. 27, 64–76. doi:10.1111/bpa.12362
Wallingford, M. C., Gammill, H. S., and Giachelli, C. M. (2016). Slc20a2 deficiency results in fetal growth restriction and placental calcification associated with thickened basement membranes and novel CD13 and lamininα1 expressing cells. Reprod. Biol. 16, 13–26. doi:10.1016/j.repbio.2015.12.004
Wang, C., Li, Y., Shi, L., Ren, J., Patti, M., Wang, T., et al. (2012). Mutations in SLC20A2 link familial idiopathic basal ganglia calcification with phosphate homeostasis. Nat. Genet. 44, 254–256. doi:10.1038/ng.1077
Wang, C., Yue, W., Ying, Y., Wang, S., Secco, D., Liu, Y., et al. (2015). Rice SPX-major facility Superfamily3, a vacuolar phosphate efflux transporter, is involved in maintaining phosphate homeostasis in rice. Plant Physiol. 169, 2822–2831. [doi]. doi:10.1104/pp.15.01005
Wege, S., and Poirier, Y. (2014). Expression of the mammalian Xenotropic Polytropic Virus Receptor 1 (XPR1) in tobacco leaves leads to phosphate export. FEBS Lett. 588, 482–489. doi:10.1016/j.febslet.2013.12.013
Westerblad, H., Allen, D. G., and Lännergren, J. (2002). Muscle fatigue: Lactic acid or inorganic phosphate the major cause? News Physiol. Sci. 17, 17–21. doi:10.1152/physiologyonline.2002.17.1.17
Wild, R., Gerasimaite, R., Jung, J. -., Truffault, V., Pavlovic, I., Schmidt, A., et al. (2016). Control of eukaryotic phosphate homeostasis by inositol polyphosphate sensor domains. Science 352, 986–990. doi:10.1126/science.aad9858
Wilson, M. S., Jessen, H. J., and Saiardi, A. (2019). The inositol hexakisphosphate kinases IP6K1 and -2 regulate human cellular phosphate homeostasis, including XPR1-mediated phosphate export. J. Biol. Chem. 294, 11597–11608. [doi]. doi:10.1074/jbc.RA119.007848
Wilson, M. S., Livermore, T. M., and Saiardi, A. (2013). Inositol pyrophosphates: Between signalling and metabolism. Biochem. J. 452, 369–379. doi:10.1042/BJ20130118
Worley, G., Claerhout, S. J., and Combs, S. P. (1998). Hypophosphatemia in malnourished children during refeeding. Clin. Pediatr. (Phila) 37, 347–352. doi:10.1177/000992289803700603
Xu, H., Yang, D., Jiang, D., and Chen, H. (2019). Phosphate assay kit in one cell for electrochemical detection of intracellular phosphate Ions at single cells. Front. Chem. 7, 360. doi:10.3389/fchem.2019.00360
Xu, X., Li, X., Sun, H., Cao, Z., Gao, R., Niu, T., et al. (2020). Murine placental-fetal phosphate dyshomeostasis caused by an Xpr1 deficiency accelerates placental calcification and restricts fetal growth in late gestation. J. Bone Min. Res. 35, 116–129. [doi]. doi:10.1002/jbmr.3866
Zechner, C., Henne, W. M., Sathe, A. A., Xing, C., Hernandez, G., Sun, S., et al. (2022). Cellular abundance of sodium phosphate cotransporter SLC20A1/PiT1 and phosphate uptake are controlled post-transcriptionally by ESCRT. J. Biol. Chem. 298, 101945. doi:10.1016/j.jbc.2022.101945
Zheng, W., Yao, Y., Zhou, H., Xu, Y., and Huang, H. (2022). Hyperphosphatemia and outcomes in critically ill patients: A systematic review and meta-analysis. Front. Med. (Lausanne) 9, 870637. doi:10.3389/fmed.2022.870637
Keywords: inorganic phosphate, transport, regulation, SLC20, XPR1, inositol pyrophosphates
Citation: Jennings ML (2023) Role of transporters in regulating mammalian intracellular inorganic phosphate. Front. Pharmacol. 14:1163442. doi: 10.3389/fphar.2023.1163442
Received: 10 February 2023; Accepted: 17 March 2023;
Published: 30 March 2023.
Edited by:
Stefan Oswald, Rostock University Medical Center, GermanyReviewed by:
Amr Zaineldin, Animal Health Research Institute, EgyptRosalinde (Roos) Masereeuw, Utrecht University, Netherlands
Hewang Lee, George Washington University, United States
Copyright © 2023 Jennings. This is an open-access article distributed under the terms of the Creative Commons Attribution License (CC BY). The use, distribution or reproduction in other forums is permitted, provided the original author(s) and the copyright owner(s) are credited and that the original publication in this journal is cited, in accordance with accepted academic practice. No use, distribution or reproduction is permitted which does not comply with these terms.
*Correspondence: Michael L. Jennings, SmVubmluZ3NNaWNoYWVsTEB1YW1zLmVkdQ==