- 1Department of Pharmaceutics, KIET Group of Institutions (KIET School of Pharmacy), Delhi NCR, Ghaziabad, India
- 2Dr. A.P.J. Abdul Kalam Technical University, Lucknow, Uttar Pradesh, India
- 3Department of Biochemistry, IMS, Management and Science University, University Drive, Shah Alam, Selangor, Malaysia
- 4Department of Anantomy, IMS, Management and Science University, University Drive, Shah Alam, Selangor, Malaysia
- 5Department of Pathology, IMS, Management and Science University, University Drive, Shah Alam, Selangor, Malaysia
- 6Department of Pharmaceutical Sciences, Hemvati Nandan Bahuguna Garhwal University (A Central University), Chauras Campus, Tehri Garhwal, Uttarakhand, India
- 7School of Pharmacy, YBN University, Ranchi, Jharkhand, India
- 8School of Pharmacy, Management and Science University, Shah Alam, Selangor, Malaysia
- 9Department of Pharmaceutical Sciences, Assam University (A Central University), Silchar, Assam, India
A brain tumor is an uncontrolled cell proliferation, a mass of tissue composed of cells that grow and divide abnormally and appear to be uncontrollable by the processes that normally control normal cells. Approximately 25,690 primary malignant brain tumors are discovered each year, 70% of which originate in glial cells. It has been observed that the blood-brain barrier (BBB) limits the distribution of drugs into the tumour environment, which complicates the oncological therapy of malignant brain tumours. Numerous studies have found that nanocarriers have demonstrated significant therapeutic efficacy in brain diseases. This review, based on a non-systematic search of the existing literature, provides an update on the existing knowledge of the types of dendrimers, synthesis methods, and mechanisms of action in relation to brain tumours. It also discusses the use of dendrimers in the diagnosis and treatment of brain tumours and the future possibilities of dendrimers. Dendrimers are of particular interest in the diagnosis and treatment of brain tumours because they can transport biochemical agents across the BBB to the tumour and into the brain after systemic administration. Dendrimers are being used to develop novel therapeutics such as prolonged release of drugs, immunotherapy, and antineoplastic effects. The use of PAMAM, PPI, PLL and surface engineered dendrimers has proven revolutionary in the effective diagnosis and treatment of brain tumours.
1 Introduction
Cancer is currently associated with extremely high mortality and morbidity rates worldwide. The World Health Organization (WHO) predicts that early deaths will increase by 70% in the next 20 years (Cancer, 2022). In 2015, cancer was the cause of death for 8.8 million inhabitants around the world, and this number is expected to increase to 12 million by 2030. Although cancer incidence is lower in low- and middle-income countries (LIMICs), overall cancer mortality is much higher in LMICs, especially among those under 65 years of age. For this reason, human development and wellbeing are affected by cancer (Kesharwani et al., 2015; Thakur et al., 2015; Dwivedi et al., 2016).
A high mortality rate is associated with this type of disease when the metastatic growth is uncontrolled in the central nervous systems (CNS) (brain, spinal cord) (Sacks and Rahman, 2020). Approximately 25,690 cases of primary malignant brain tumors are diagnosed annually, 70% of which originate primarily in glial cells (Ostrom et al., 2021). World health organization (WHO) has classified brain tumors into four grades, ranging from I to IV depending on severity. Glioblastoma (GB), referred as type IV glioma, which is severe and invasive carcinoma with a survival rate of 5.1% (Tadros and Ray-Chaudhury, 2020; Katano and Yamashita, 2022). The radiation therapy, surgery and systemically administered chemotherapy are the three main techniques used in the conventional treatment of cancer. According to them, a median survival of 9 months is possible, with a survival rate of about 10% of 2 years. However, systemic chemotherapy has limited efficacy in brain tumors due to minimal drug uptake into the tumor cells, drug metabolism within tumor cells and intrinsic sensitivity of tumor cells (Zaal and Berkers, 2018; Tan et al., 2020; Stine et al., 2022).
Patients with multiple drug-resistant gliomas are more likely to have poor prognosis as they cannot be completely removed surgically and there is a possibility for a new primary tumor to form after surgery. Major challenges include the complexity and heterogeneity of glioblastoma (GB) molecular biology. As a result, the prognosis could differ significantly for every patient receiving the same treatment. Adjuvant chemotherapy and radiotherapy (RT) are primarily utilized to treat GB whereby both therapies can cause genotoxicity (Thakur et al., 2015).
Blood Brain Barrier (BBB) poses a challenge to the efficient treatment of brain tumours by preventing the transfer of drugs to the affected area. The BBB is a very important part of how drug molecules move from the bloodstream to the brain. It also prevents toxins and large and higher concentrations of therapeutic drugs from entering the brain microenvironment (Thakur et al., 2015). The main physiological barriers at the brain surface include the choroid plexus, arachnoid plexus and blood vessels. Other challenges include non-specific binding, bioavailability of drugs and imaging agents (Kesharwani et al., 2015).
Numerous studies have found that nanocarriers are effective in treating brain diseases. Drug distribution in the brain can be divided into two categories: First, bypassing and evading the BBB due to the different architectures, and second, targeting the drug across BBB vai utilization of characteristics of polymeric nanocarriers. Nanocarriers offer special benefits for drug delivery. The optimal physicochemical characteristics, such as solubility, particle size, potential, and shape, help to increase pharmacokinetics and biodistribution. Moreover, surface modification may boost medication accumulation in the target tissue to enhance the therapeutic effect. Additionally, the nanocarriers have a particular drug release characteristic that enhances drug concentration in the target site and decreases drug concentration in the non-target site, minimising the likelihood of unfavourable reactions. Moreover, it is simple to mix treatments with nanocarriers to generate synergistic effects. Hence, nanocarriers offer a good platform for the investigation of medications that target brain tumours. Few most commonly utilized nanocarriers are nanoparticles, liposomes, quantom dots, magnetic nanoparticles, dendrimers, micelles, carbon nanotubes, nanoemulsions, solid lipid nanoparticles, etc., (Sahu, et al., 2021; Yeini et al., 2021; Bhatt et al., 2022; Grover et al., 2022; Setia et al., 2022).
Dendrimers consist of either chemical conjugation of terminal functional groups on the drug surface or physical encapsulation of drug molecules in the internal cavities of the dendrimer (Kesharwani et al., 2015). The efficient passage of therapeutic molecules through the BBB can be attributed to their lipophilicity. Based on past studies, dendrimers are excellent solubilizers, particularly for macromolecules. Positively charged dendrimers, which consist of enmeshed Nanoparticles (NPs) with mucus are known to have increased cellular uptake when they are associated with mucus. In a recent study, the drug bortezomib was found to be less toxic when loaded into dendrimers that are acid and pH resistant and have the ability to altered the drug release. Therefore, this treatment shows great promise for the treatment of malignancies (Singh et al., 2019). Modifying the external features of dendrimers can be considered as a cost-effective way to acquire new capabilities. Modifying the surface of dendrimers would alter their biopharmaceutical properties, such as improved biocompatibility, release kinetics, targeting to the BBB or brain tumor, and delivery of bioactive and imaging agents through the BBB (Zhu et al., 2019).
This review summarizes the current status of brain tumors, available treatments, major challenges in the application of diagnostic and therapeutic nanocarrier systems and aspirations for future research. The architecture, species, physicochemical and biological properties of dendrimers, and synthesis methods are described in great detail. In addition, the pharmaceutical delivery and brain tumor imaging using dendrimer-based nanovesicles are highlighted as well. The article aims to provide information on the potential and problems associated with effective brain tumor targeting for therapy and diagnosis in a clear manner that can be understood by all. Finally, the limitations that dendrimers face in clinical applications and ways to overcome them will be addressed. The focus of this review is on recent therapeutic developments using dendrimers for brain tumor targeting and imaging.
2 Dendrimers and their characteristics
The current circumstances and obstacles related to brain tumors make it difficult for researchers to develop an efficient technique to detect and treat brain tumors. For this reason, it is highly recommended to develop a safe and effective delivery system that protects the payload and increases the effectiveness of the drug. Furthermore, this developed system must have improved drug release and offers reduced toxicity in the non-targeted organs due to aggregation of the charged cytotoxic materials. Although various nano-delivery systems have been utilized in recent decades, dendrimers have emerged as the leading system in brain tumor treatment and imaging due to their immense potential alongside existing nanocarriers (Akhter et al., 2013). Dendrimers are polymeric structures range of 1 nm–10 nm size that are firmly established, spherical, macromolecular, hyperbranched, three-dimensional, and multivalent., which give them better flexibility and monodispersibility (Chenthamara et al., 2019). With a large number of hydrophobic pockets, dendrimers are able to encapsulate a variety of bioactive substances, allowing for regulated and sustained release of drugs (Noriega-Luna et al., 2014; Choudhary et al., 2017; Rahman et al., 2020).
3 Structure of dendrimers
Dendrimers are composed of a central core, branches (dendrons), and terminal functional groups (Figure 1). The attachment of the branches is made possible by the central nucleus, which consists of a single atom accompanied by at least one functional group. The branches (dendrons) arise from the atomic units of the core and bridge among themselves repeatedly at least one branching junction, resulting in an organized, radially concentric, layer-based geometric structure known as generations. Thus, the radially concentric layer is created by bridging between the branching units, which are referred as “generation (G)” (Birdhariya et al., 2015). As the generations are repeated, dendrimers of higher generations with spherical shape are formed, namely, the first generation (G1), the second generation (G2), the third generation (G3) and so on. The defined area formed between the dendrons is protected by a surface decorated with a multivalent end group. This empty area is used to encapsulate various bioactive materials and bio-imaging agents (Klimova et al., 2018; Tomalia et al., 2020). The loading efficiency of dendrimers would increase with every generation level. For example, cationic amino groups on the surface of higher generation cationic dendrimers would contribute to better DNA binding and cellular uptake by transforming the complex into nanoscale polyplexes (Noriega-Luna et al., 2014; Kesharwani et al., 2015; Tomalia et al., 2020). Therefore, the three main domains of the dendrimer architecture can be used for drug delivery, molecular sensors, genetic materials, enzymes, and bioimaging applications (Figure 2).
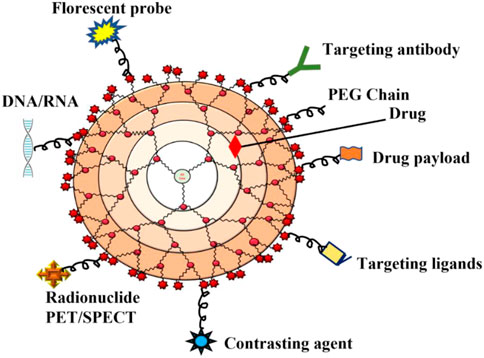
FIGURE 2. Schematic diagram that indicates the application of dendrimers in various biomedical fields.
4 Methods of dendrimer synthesis
Dendrimers are compact spherical structures formed by high degree branching of polymers (Shi et al., 2006). In most cases, their synthesis involves the repetitive attachment of monomers to a central core that has multiple functional groups. Several different functional groups form the core of the structure. The addition of monomers to each functional group produces the next dendrimer and forms end groups for the next reactions (Walter and Malkoch, 2012; Mittal et al., 2021). The size of the dendrimers increases with packing after each generation and finally reaches its densely packed spherical structure with maximum size.
Depending on the goals of the study, either convergent or divergent strategies can be used to synthesis dendrimers (Šebestík et al., 2012; Kalhapure et al., 2015; Malkoch and García-Gallego, 2020). In the divergent method, the activation of the functional groups of the multivalent surface occurs in the first step and the addition of monomer units for continuous branch elongation begins (Jain et al., 2012; Kesharwani et al., 2015; Chauhan, 2018; Sherje et al., 2018; Janaszewska et al., 2019; Kumbhar et al., 2021; Sheikh and Kesharwani, 2021). The advantages of divergent methods are the possibility of surface modification and the ability to construct dendrimers with different physiochemical properties. Meanwhile, the convergent technique for the synthesis of dendrimers begins with the attachment of functionalized monomers to the interface where the dendrons are to be joined together to produce the finalized dendrimer architecture. In this process, the dendrons are linked together to form the dendrimer. Compared to the divergent method, which often results in incomplete branching, the main advantage of this method is the low error rate in the final structure (Cammidge, 2003; Svenson and Tomalia, 2005).
5 Dendrimer physical and biological properties
Dendrimers are spherical macromolecular structures at the nanoscale connected to various branched structures. They are best used for drug delivery and imaging. Conventional polymeric carriers are disperse, while dendrimers are mmonodisperse and have well-defined chemical structures. Furthermore, dendrimers’ unique framework enables them to be loaded with therapeutic agents via covalent conjugation or electrostatic adsorption (Du et al., 2013). Dendrimers normally resemble a set of biological structures in terms of size. For example, the fifth-generation polyamidoamine dendrimers (PAMAM) resemble hemoglobin in size and shape (5.5 nm) (Esfand and Tomalia, 2001).
The surface group of dendrimers can be either positive, negative, or neutral, which affects which group is best suited for drug transport. Compared to neutral or anionic-charged dendrimers, negatively charged dendrimers do not cause as much cell loss or hemolysis as positively charged dendrimers do (Malkoch and García-Gallego, 2020). In lieu of this, PEGylation would alter zeta-potential, plasma attainment and even distribution in vivo to overcome this issue. It is extremely difficult for free gene molecules to reach the targeted cells in vivo which is a major obstacle in gene therapy (Vannucci et al., 2013). Electrostatically, the positively charged dendrimers would be attracted to the negatively charged polynucleotides, leading to the formation of stable dendriplexes. After being taken up by the cell, these dendriplexes are unloaded into endosomes due to the sponge effect, which stimulates gene transcription in the cell (Sonawane et al., 2003; Perumal et al., 2008). Dendrimers can protect nucleotide substances from degradation, allowing the penetration of nucleic acids into the cells and maintaining the biological activity of gene molecules when they are utilized as gene delivery carriers.
6 Types of dendrimers
Developing an optimal delivery method for the treatment of brain tumours is challenging for researchers and clinical investigators, as there are currently many obstacles in this area (Dande et al., 2006). These limitations further necessitate the use of a safer and more effective carrier that protects the payload from degradation, penetrates the targeted area and increases the efficacy of the drug molecules. In addition, the carrier must also be able to regulate or optimize the release of the drug. Dendrimers, which have emerged in recent decades with numerous nanocarriers, are being touted as the stars on the current horizon because they are multitasking and flexible (Chis et al., 2020). Dendrimers, which possess unique properties such as nanosize, defined composition, and programmable surface functions, have been extensively explored for brain tumor therapy. Table 1 explains the basic properties of the different types of dendrimers and their advantages and disadvantages.
6.1 PAMAM dendrimers
Polyamidoamine dendrimers essentially have a central ethylenediamine core with ascending divisions with amide groups which form a wall of the spherical structure and at the surface of each terminal with amine functional groups (Figure 3A). PAMAM dendrimers are good candidates for carrying drugs and peptides because they are biocompatible and have well-defined spherical nanoparticles with bifunctional modifying parts. Dendrimers PAM are used in the nanomedicine platform to maximize the bioavailability of drugs and minimize the number of doses required (Pedziwiatr-Werbicka et al., 2019). However, there are some limitations associated with the use of PAMAM, particularly those with positively charged groups. It leads to the accumulation produces cytotoxicity. PAMAM dendrimers of G5 are mostly considered as a non-toxic (Beezer et al., 2003). To address the aforementioned limitation, the surface is reconfigured with polyethylene glycol (PEG). Compared to “other dendrimers,” PAMAM has the broadest range of applications in therapeutics such as antibacterial, antiviral, antioxidant, and diagnostic agents.
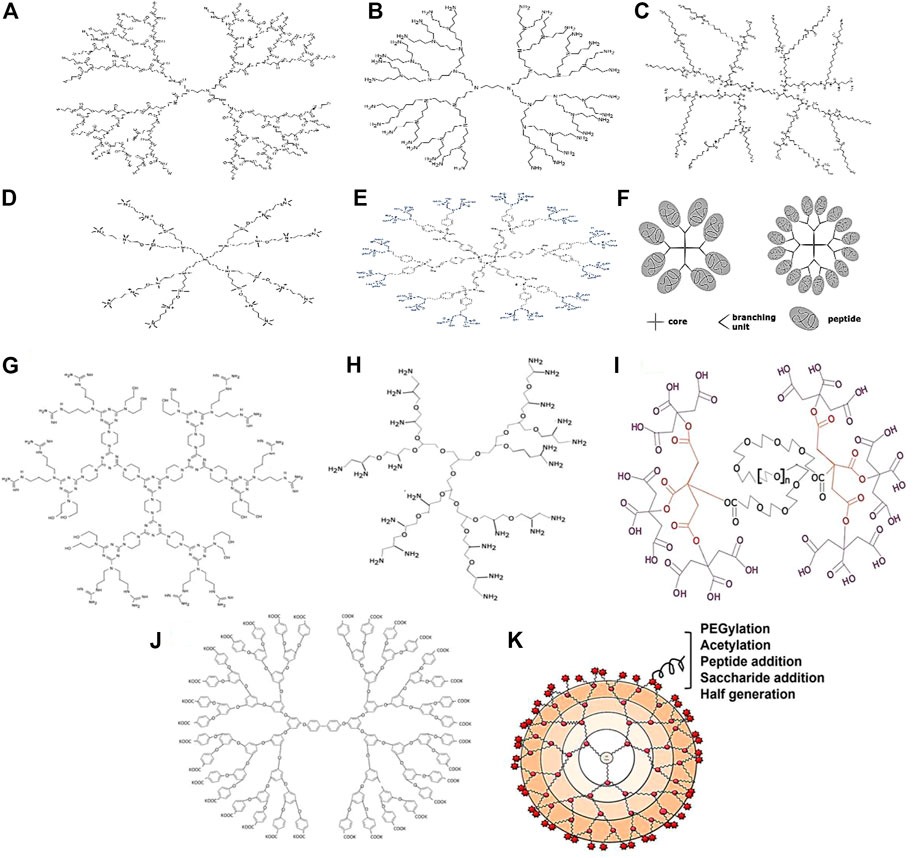
FIGURE 3. Types of dendrimers: (A) PAMAM Dendrimers; (B) PPI Dendrimers; (C) PLL Dendrimers; (D) Carbosilane Dendrimers; (E) Phosphorus Dendrimers; (F) Peptide dendrimers. Types of dendrimers: (G) Triazine dendrimers; (H) Polyglycerol dendrimers; (I) Citric acid dendrimer; (J) Polyether dendrimers; (K) Surface engineered dendrimers.
6.2 Polypropylenimine dendrimers
The dendrimer polypropylenimine (PPI) is the first known dendrimer used industrially as a therapeutic agent. There are also other names used for PPI dendrimers which are astramol or butylenediamine (BDA) or “polypropyleneamine” (POPAM) (Noske et al., 2020). These dendrimers mainly consist of butylenediamine as the core and repeat propylenimine branching units via sequential Figure 3B is the reaction of Michael’s addition of acrylonitrile to a primary amino group, followed by hydrogenation of the nitrile groups to form primary amino groups (Sherje et al., 2018). The amino-terminal groups contribute to its solubility in water. PPI Dendrimers are characterized by their ability to improve the water solubility of hydrophobic drugs entrapped in the hydrophobic inner crevices of PPI due to this property. Cell membranes can be destabilized by positively charged PPIs, leading to cell lysis. As a result, PPIs have a lower ability to load drugs than PAMAMs. Furthermore, the PPI/drug complex has lower stability than PPI alone. PEGylation and acetylation of the surface groups are selected. Acetylation is preferred as it is extremely efficient and able to penetrate deeply. Moreover, the steric hindrance of the PEG chain can affect the interaction of the entrapped drug molecules with the surface functional groups (Fant et al., 2010). Diaminoethane or diamino-propane would form the central functional groups in Poly (ethyleneimine) (PEI) dendrimers, a subclass of PPI dendrimers.
6.3 Poly-l-lysine dendrimers
Dendrimers containing poly-l-lysine residues such as poly-l-lysine dendrimers (PLL) or dendrigraft poly-l-lysine dendrimers (DGL) also possess lysine residues (Figure 3C). These dendrimers have higher cytocompatibility, lower cytotoxicity, convenient enzymatic degradation and resultant efflux of low molecular products (Ryan et al., 2013). Furthermore, DGL dendrimers have been researched for the use of delivery vectors for siRNAs. “The ability to respond to stimuli on demand by incorporating specific protein sequences into PLL dendrimers is quite tantalizing”. Higher-generation PLL dendrimers have exhibited improved gene transfection efficiency whereas conventional linear poly (lysine) dendrimers have exhibited lower genome transfection efficiency and higher cytotoxicity compared to PLL dendrimers (Byrne et al., 2013).
6.4 Carbosilane dendrimers
Carbosilane dendrimers consist of carbon and silicon molecules as building blocks. With the widespread use “of silicon chemistry, a new class of carbosilane dendrimers with hydrophobic scaffolds and superior thermal stability have emerged (Figure 3D) (Uchida et al., 1990). The chemical properties of silicon are utilized in dendrimers preparation because they allow the “nucleophilic molecules to reach the electrophilic silicon (Si+) easily” (Zhou and Roovers, 1993). However, the presence of the C-Si bond in carbosilane dendrimers provides low polarity and high energy which makes them more hydrophobic compared to other dendrimers (Sepúlveda-Crespo et al., 2015). Although they have a hydrophobic endoskeleton, carbosilane dendrimers can be modified to polar molecules by surface chemistry with polar moieties such as Si-H, Si-Cl, Si- CH = CH2, and Si-CH2CH = CH2, allowing the introduction of various other intriguing inorganic, organic, and organometallic substituents. This would lead to increased applications in the pharmaceutical fields. The ability of the generation 2 ammonium-terminating carbosilane dendrimer “has been tested on a variety of cell types including the glial cells, progenitor cells, leukocytes, granulocytes and human peripheral blood mononuclear cells, general pluripotent stem cells, primary cells as well as suspension cells” (Liu et al., 2012).
6.5 Phosphorus dendrimers
Cationic dendrimers are dendrimers with cationic phosphorus as the core and decorated surface groups that have been studied in a variety of biological and theragnostic applications due to their special properties (Figure 3E). In phosphorus dendrimers, the “presence of phosphorus at each branch point” and reactive end groups provide a hydrophilic shell and hydrophobic backbone that can affect their internalization into cells. Moreover, they have effects on the growth of cells as well such as neurons, immune and cancer cells (Caminade and Majoral, 2013). Rolland et al. (2008) investigated on phosphorus-terminated dendrimers that could be explored for immunotherapy to target and activate monocytes. Based on the study conducted, such dendritic materials have been shown to be able to affect the aggregation of amyloid oligomers and tau protein in neurodegenerative diseases (Wasiak et al., 2012). Polyphosphorhydrazone (PPH) dendrimers have also shown the ability to deliver antisense siRNAs to target cells (Dzmitruk et al., 2015) and treat HIV infection with gene therapy (Briz et al., 2012).
6.6 Peptide dendrimers
Peptide “dendrimers are macromolecules which comprise either branched polypeptide core” or radically arranged peripheral polypeptide chain or both (Figure 3F) (Sadler and Tam, 2002). These dendrimers are divided into three categories. The first category is grafted dendrimers with amino acids chain only at their surface. The second category is grafted dendrimers composed entirely of amino acids, while the third category consists of amino acids that branch both in the core and on the surface and have non-peptide branching units. Divergent and convergent techniques are often used to synthesize peptide dendrimers, and with the advent of solid-phase peptide synthesis methods, extensive “libraries of peptide dendrimers can be prepared and tested for desired properties.” Peptide dendrimers are used as surfactants as well as multiple antigen peptides (MAP) (Bruckdorfer et al., 2004), protein analogs (Thompson and Scholz, 2021), drugs, and gene transporters in the biomedical field. They are also used “as contrast agents for magnetic resonance imaging and angiography, fluorescence imaging” and in serum analysis (Choi et al., 2000).
6.7 Glycodendrimers
Dendrimers that comprise carbohydrates “moieties such as monosaccharides (glucose, mannose, galactose) (Woller and Cloninger, 2001) and disaccharides (chondroitin sulfate) (Roy and Baek, 2002) ”into their structure are referred as glycodendrimers. Although most of “the researched glycodendrimers have sugar residues on their exterior surfaces, glycodendrimers” with a sugar unit as the central core in which all branches would emerge have been discovered as well. In general, glycodendrimers are classified into three types: “Carbohydrate-centered, carbohydrate-based and carbohydrate-coated dendrimers (Oliveira et al., 2010). There is a potential application for these dendrimers which is site-specific delivery to lectin-rich” tissues. These dendrimers are predicted to have a stronger affinity for lectin-anchored systems than monosaccharide-anchored systems (Mousavifar and Roy, 2021).
6.8 Triazine dendrimers
Triazine dendrimers are aptly named as they “consist of 1,3,5-triazine rings as branches with amine groups at both ends” (Figure 3G). A study was conducted recently on the siRNA transport ability of a series of “triazine dendrimers with different core structures, generation numbers and surface functions”. Dendrimers with inflexible structures and “arginine-like or hydrophobic terminals had the most efficient siRNA gene repression effects” on Hela cells in an experimental luciferase model (Simanek, 2021). Triazine dendrimers are deemed suitable for various biomedical applications due to their liquid crystalline structure and non-linear optical properties. In one study, triazine dendrimers were evaluated for their potential for drug delivery. The results showed that they are suitable for hydrophobic drugs as solvents and carrier systems and are not toxic to organs at a dose of up to 10 mg/kg via the intraperitoneal route in an animal model. However, these dendrimers should be explored further for various applications with or without surface functionalization by appropriate moieties (Merkel et al., 2010).
6.9 Polyglycerol dendrimers
Dendritic polyglycerols are hyperbranched structures that are made of glycerol with a wide range of sizes (at the nanoscale) and functional groups at their ends which allow them to interact with different biological receptors (Maršić et al., 2021). Sheikhi Mehrabadi et al. (2015) have developed polyglycerol dendrimers (PG) with a variety of cationic amine end groups, including a star-shaped oligoamine shell (Figure 3H). These dendrimers have a neutral biocompatible aliphatic polyether core, numerous siRNA-binding and complexing amine end groups. PG-PEHA (Polyglycerol-pentaethylene hexamine) was the most efficient at silencing genes, whereas polyglycerol-amine (PG-NH2) was the least effective. “The favorable primary amines at the 1,2-position of PG-NH2” might be the reason for the reported high efficiency in the transfer of siRNA (Sheikhi Mehrabadi et al., 2015). Moreover, PG-NH2 could be used to deliver siRNA in animal models by intravenous injection, resulting in efficient gene silencing with low toxicity. This further indicates the potential utility of the dendrimer for siRNA therapy delivery (Sheikhi Mehrabadi et al., 2015).
6.10 Citric acid dendrimers
Citric acid dendrimers can be good candidates for an efficient drug delivery system as they are relatively stable in water with good drug deposition and release properties (Figure 3I). Namazi et al. prepared β-cyclodextrin (β-CD)-modified citric acid dendrimers with -cyclodextrin (CD) to increase the loading capacity and encapsulation properties of the dendrimers. The results showed that by increasing the number of branches, it had further increased the internal cavity, hence responsible for the resulting loading potential (Namazi and Hamrahloo, 2011). Similarly, Namazi and his colleagues prepared dendrimers with PEG as the central core and repeating citric acid units as surface functionalized groups (Namazi et al., 2011). In the viability assay, the viability of HT1080 cells which were exposed to the PEG-citric acid dendrimer was found to be more than 80% up to 2 days at a high concentration of 1 mg mL-1. In other toxicity tests, the dendrimers were shown to be extremely safe. The tests done included hemolysis assay, lactate dehydrogenase assay and prothrombin time assay (Naeini et al., 2010). The biocompatible dendrimer exhibited high drug loading and prolonged drug release, indicating its potential application as a vehicle for cancer treatment (Adeli et al., 2013).
6.11 Polyether dendrimers
Polyether dendrimers are functional dendrimers that are spherical and highly branched with cationic ether groups on the outer surface. Polyether dendrimers were synthesized for the first time by Hawker and Frechet in 1990 via the convergent approach (Figure 3J) (Hawker and Frechet, 1990). This polyether group is composed of the core material “1,1,1-tris(4′-hydroxyphenyl) ethane and the branching material benzyl bromide and 3,5-dihydroxybenzyl alcohol”. Jayaraman et al. described the synthesis of dendrimers with a backbone that consists of aliphatic polyethers. Due to the presence of “2-hydroxymethyl-1,3-propanediol moieties”, the prepared dendrimers emerged as good prospects for drug delivery with improved solubility (Kumbhar et al., 2021).
In another study, Malik et al. performed in vitro tests on the biocompatibility of these convergently synthesized polyether dendrimers. The findings indicated that such dendrimers with carboxylate and malonate interfacial conjugates were more biocompatible and hemolytic than cationic dendrimers. However, they were not hemolytic up to 1 h but were as hemolytic as anionic dendrimers after 24 h. It seems that these biodegradable polymers may less hazardous than standard dendrimers and their usage for drug delivery could be extended (Duncan, 2014).
6.12 Surface tailored dendrimers
It seems that making changes to the surface is one of the most effective ways to make dendrimers less harmful (Figure 3K). In this approach, the exposure of cationic groups such as amino groups to the surface of the dendrimer is minimized by modification or decoration with natural or anionic molecules to prevent their electrostatic interaction with cell membrane molecules, thus avoiding cytotoxicity mediated by cationic groups. Apart from reduced toxicity, surface-engineered dendrimers also can improve drug loading, biodistribution, pharmacokinetic profile, solubility, site-specific targeting stability, antimicrobial activity and gene delivery efficiency (Satija et al., 2007). There are several surface engineering approaches to modify surface groups on dendrimers such as PEGylation through the addition of PEG, which increases drug loading and decreases hemolytic toxicity, as seen in PAMAM dendrimers (Santos et al., 2019), saccharide addition via maltose, which decreases hemolytic toxicity, as seen in PPI dendrimers (Bhadra et al., 2003), acetylation by adding an acetyl group lessening toxicity effects and increase absorptivity of PAMAM dendrimers (Klajnert et al., 2008), bisection by adding carboxylic acid to minimize cytotoxicity (Kolhatkar et al., 2007) and peptide conjugation by tripeptide (arginine-glycerol aspartate) to minimize toxicity of cationic dendrimers (Jevprasesphant et al., 2003).
7 Brain tumor drug targeting approaches
The main obstacles in the detection and treatment of cancer are the development of drug/gene delivery systems that selectively target cancer cells while leaving normal healthy cells/tissues unaffected. This could be achieved by effective delivery of anticancer drugs into tumor cells (Khizar et al., 2021). Nanotechnology-based delivery systems, namely, phytosomes, liposomes, nanoparticles, carbon nanotubes, neosomes, and dendrimers, are believed to be the most practical choices. The nanocarriers produced must overcome a number of physiological and biological obstacles. Their use as delivery systems requires that their size, biocompatibility, and surface functionality are suitable to promote the binding of specific drugs and diagnostics to their target sites and avoid unwanted interactions (Truskewycz et al., 2022).
Nanocarrier systems can be employed to transport therapeutic agents or genetic material into cancer cells or tissues. This can be done either actively or passively (Attia et al., 2019). Passive targeting enhances the amount of drug or the delivery of a drug to a specific region due to its physicochemical characteristics (particle size, circulation time), pathophysiological conditions (hypoxia, inflammation) and tumor biology (leakiness, vascularity) (Iyer et al., 2014). Active targeting, on the other hand, requires specific adjustments to the delivery system that would result in the coupling of active agonists with significant selectivity for a specific cell or organ in the body (Figure 4).
7.1 Passive targeting approach
The use of polymeric delivery systems takes advantage of a drug’s pharmacokinetic properties, such as its solubility, half-life, and prolongation of plasma circulation time, to achieve optimal passive targeting. This ensures that the drug matrix is passively transported to the solid tumour (Ekladious et al., 2019). Furthermore, passive targeting certainly depends strongly on two parameters: The tumour endothelial permeability to macromolecules and the presence of reduced lymphatic outflow. These two factors determine how well passive targeting works. When these factors are improved, the likelihood of passive targeting would increase. The enhanced effect of permeation and retention, often called as the EPR effect, is a phenomenon unique to tumours. It was first discovered and stated by Matsumura and Maeda in 1986. Because tumours have defective blood vessels, they form vascular permeability factors. These factors ensure that the tumour tissue receives adequate nutrients and oxygen, allowing the tumour to develop rapidly. Aliphatic polyester dendrimers containing dimethylolpropionic acid have been identified as a promising possibility for the preparation of therapeutic anticancer conjugates. The results of in vitro and in vivo evaluation showed that the water-soluble polyester dendrimers were biocompatible. The delayed aggregation of dendrimers in important organs results in a prolonged period of dendrimer-mediated drug administration, which appears to be beneficial for the EPR effect of passively targeting tumours (Kheraldine et al., 2021). In addition, tumour cells would stimulate dilatation of blood channels through excessive release of permeability mediators (Greish et al., 2003; Gillies and Fréchet, 2005).
Polymeric drug conjugates and drug loaded polymeric micelles, dendrimers, polymeric nanoparticles and carbon nanotubes would selectively accumulate inside solid tumor owing to the EPR effect (Puri et al., 2009; Shcharbina et al., 2013). Constructions with hydrophilic surfaces and molecular weighing over 25 kDa–30 kDa would provide enhanced chances of targeting tumors by virtue of EPR, which is accompanied by a longer retention time during circulation (Kesharwani et al., 2015). Etrych et al. (2008) developed a biodegradable PAMAM dendrimer with a semi-telechelic Hydroxypropyl Methyl Cellulose (HPMA) polymer embedded with doxorubicin as the core for passive tumor targeting. The findings revealed that the dendronized nanoparticles with diameters ranging from 10 to several hundred nanometers were able to achieve passive targeting through the EPR effect (Etrych et al., 2011). The size range of nanocarriers will determine whether or not they can be retained and localised in target areas. There is general agreement that dendrimers up to 10 nm–20 nm in diameter are most appropriate for passive tumour targeting (She et al., 2013).
Massive membrane proteins and other macromolecules which are coupled with dendrimers would decrease their plasma clearance, resulting in increased half-life in the bloodstream. As a result, these conjugation systems provide a prolonged and precise delivery to tumor targets. Furthermore, a cisplatin-loaded 3.5 G PAMAM dendrimer demonstrated a 50-times rise in cisplatin accumulation at the tumor position when compared to free drugs (Kaminskas et al., 2011).
7.2 Active targeting approaches
Chemotherapy is an essential procedure for cancer treatment in modern times but there is a lack of antineoplastic drugs that can act against the tumor mass. To find solutions to these problems, researchers and scientists have focused their efforts on developing novel anticancer drugs and drug delivery systems. Some examples of these innovations include tailored drug delivery methods that have high therapeutic efficacy and low toxicity. Active targeting is a strategy that can decrease the absorption of a drug in normal tissue and increase its concentration in malignant tissue (Janaszewska et al., 2019). It was found that the most efficient method for drug accumulation in solid tumours is known as EPR, which is achieved by passive tumour targeting. Although the majority of the pharmaceuticals are notoriously non-diffusible, intracellular targeting of cancer cells via passive diffusion of these substances is notoriously hard. In addition, administration of a low dose of drugs to certain cancer cells can lead to ineffectiveness of chemotherapy or other negative outcomes, such as the growth of cancers resistant to multiple drugs (also known as MDR malignancies) (Basile et al., 2012). Passive targeting and the EPR are therefore only able to deliver drugs to solid tumours that are porous and permeable. On the other hand, the EPR effect does not occur in a number of malignant tumours that are resistant to radiation because these cancers are impermeable (Gottesman et al., 2002). Active targeting overcomes some of these limitations by attaching precisely targeted ligands to the outside of nanostructures that exert an attractive force on specific receptors on cancer cells (Gottesman et al., 2002).
Dendrimers have several polar functional groups which might attached to a diversity of ligands on their surface to target tumors in active targeting. In one study, polymethacrylate (PMA)-crosslinked PAMAM dendrimers were prepared so that medications could more easily targeted the acidic microenvironment of the tumour. Folate-PEGylated PMA-PAMAM had a significant effect on drug accumulation and tumor regression at the tumor site (Shen et al., 2012).
The adhesion molecule integrin αvβ3 is commonly expressed in prostate, breast, ovarian, glioblastoma and melanoma cells. Peptides that include the amino acid sequence Arg-Gly-Asp (RGD) show a better affinity for the integrin αvβ3 protein (Li and Xu, 2005). PEGylated PAMAM dendrimers coated with RGD embedded with Doxorubicin (DOX) prolonged plasma circulation time, drug accumulation and bioavailability in brain tumors compared to DOX solution alone (Zhang et al., 2011).
According to the findings of Gupta and colleagues, a dendrimer loaded with DOX and folate-conjugated PPI was the improved option for targeting cancer. The novel dosage showed improved drug stability, release profile and toxicity, as well as better drug uptake in the cancer cell MCF -7 (Gupta et al., 2010). There are several ligands for targeting purposes in the brain and other body tissues such as thiamine, glucose, choline, serum albumin, folate, lactoferrin, L-glutamate, L-aspartate, folic acid, nucleoside, biotin, oligopeptide and aptamers. Nanoparticles that can be formed on the surface of dendrimers efficiently controlled the tumor progression (Lockman et al., 2003; Huang et al., 2008; Ulbrich et al., 2011).
Across the brain many transporters such as glucose transporter 1 (GLUT1), vitamin C transporter 2 (SVCT2), Na + -dependent vitamin transporter (SMVT), L-amino acid transporter 1 (LAT1), mono-carboxylic acid transporter 1 (MCT1) etc., works as carrier mediated transporters to transport nutrient through the BBB. Modification of surface characteristics of Dendrimers with the substrates or their analoues can promotes drug into brain via mentioned transporters ((Zhao et al., 2015; Jiang et al., 2021; Zhao et al., 2021).
Another most widely used way to internalized large drug moieties and growth factors in brain through brain tumor targeted delivery systems is receptor mediated transport. Most commonly expressed receptor on brain are low density lipoprotein receptor (LDL-R), apolipoprotein E (ApoE) receptor, EGFR, transferrin receptor (TfR), insulin receptor (IR) and integrin receptor (αvβ3) and can be employed efficiently to promote drugs and diagnostic agents into the brain for brain tumor treatment and imaging respectively (Yeini et al., 2021).
8 Application of dendrimers as drug delivery systems for the brain
With the help of dendrimers, nucleic acids and drugs can be sent to the brain and cancer cells without using a virus. This is due to the high-branched structure and the available internal cavities of these polymers which make them excellent delivery systems for genes and drugs.
8.1 PAMAM dendrimers as brain drug delivery
The BBB functions as a filter to control molecules that have entered the brain from the blood (Li et al., 2020). A series of specialized cells and transporters have been developed to manage the chemical environment of the CNS. It consists of endothelial cells that form specialized capillaries and extravascular components such as astrocytes, pericytes, and interneurons that make up the BBB (Xu et al., 2014). CNS nutrients and waste are regulated by these components which constitute as a physical barrier. The extracellular nucleases and peptidases, intracellular monoamine oxidase and cytochrome P450 enzymes are some of the proteins and enzymes that prevent toxicity of the central nervous system (Pisoschi et al., 2021).
The potent anticancer drug methotrexate is an antimetabolite of folate that has been shown to be effective in the treatment of various cancers. PAMAM dendrimers do possess capability of good drug carriers owing to precise description of their structures and a variety of different types of groups (De et al., 2022). Wu and colleagues utilized methotrexate to develop a drug carrier that targeted the epidermal growth factor receptor (EGFR) as well as their mutant isoform EG-FRvIII. This was done by binding an average of 12.6 methotrexate molecules to every fifth PAMAM dendrimer molecule, which was then conjugated to cetuximab. According to the researchers, particular molecular targeting is just one of several properties that an antibody-drug bioconjugate must meet to be therapeutically useful. However, no therapeutic benefit was observed when tumor mice were administered the bio-conjugate via CED instead of free methotrexate or cetuximab (Wu et al., 2006).
Table 2 presents an overview of the numerous PAMAM dendrimer types that have been utilized.
8.2 PAMAM dendrimers in small molecules brain delivery
PAMAM dendrimers could be used to carry peptides and drugs to specific sites in a way that is effective and efficient (Abedi-Gaballu, et al., 2018). This is because they can enhance the biocompatibility of active compounds and decrease the number of times they need to be taken. The fact that PAMAM dendrimers produced natural podophyllotoxine and estramustine more bioavailable showed that they could be used as carriers. Along with stopping and killing more cells, PAMAM dendrimers also modified the manner in which these antimitotic agents were released. It also made these antimitotic agents work better at stopping tubulin polymerization, which is important for glioma cell survival (Tarach P & Janaszewska, 2021; Dixit & Sen, 2013).
In one work, PEGylated dendrimers, wheat germ agglutinin, and transferring ligands were used to encapsulate doxorubicin in G4 PAMAM dendrimers. The ability of the dendrimers to cross the BBB was enhanced by targeting wheat germ agglutinin (WGA) and transferrin (Tf). In addition, microscopic study showed that the nanoparticles had a size of about 20 nm. Compared with free drugs and dendrimers without Tf and WGA, the dendrimers containing Tf and WGA delivered a higher payload of doxorubicin to brain tumour sites. In another study, Swami and colleagues utilized PAMAM dendrimers to combine docetaxel (DTX) with p-hydroxylbenzoic acid (pHBA). The findings suggested that pHBA has a strong affinity for beta receptors which are found primarily in the CNS. Compared to free Docetaxel (DTX), dendrimers containing G4-pHBA-DTX delivered more drugs to the brain (Swami et al., 2015; Florendo et al., 2018; Igartúa et al., 2018; Vasconcelos-Ferreira et al., 2022).
8.3 PAMAM dendrimers in brain delivery of genes
When it comes to how they interact with nucleic acids, small molecule drugs are the complete opposite of dendrimers. Nucleic acids (DNA or RNA) can be delivered using dendrimers with surface amines. During physiological conditions, dendrimers are formed when amines interact with nucleic acids to produce dendrimer complexes. In terms of their physicochemical properties, hydroxyl and carboxyl groups are considered neutral under physiological conditions, whereas nucleic acids are considered to be anionic. Therefore, dendrimers containing these surface functional molecules cannot bind nucleic acids. The dendrimers and nucleic acids that form on amine-terminated dendrimers can exhibit considerable variation in size and structure due to the multiple charges in both (Dey et al., 2022). In addition to individual characterizing dendrimers (generations), the sizes are affected by the nucleic acid, the N/P ratio (also known as the charge ratio) between dendrimers and nucleic acids and the solvent properties (Sahu et al., 2023). As the dendrimers are being generated, their DNA binding affinity would increase. At charge ratios close to 1, the complexes would typically aggregate and fail. Therefore, dendrimer complexes with a higher density tend to have a smaller size distribution and better transfection efficiency. Apart from that, complexes with an increased dendrimer generation ratio have an increased charge ratio as well (two times more amines than phosphates in a dendrimer complex). Generally, nanometer-sized dendriplexes are formed when the generations and the N/P ratio are higher (Demeule et al., 2008; Huang et al., 2008). The efficiency of transfection varies greatly between dendrimer-nucleic acid complexes. In addition to depending on the structure of the complex, it also varies based on the type of cell and the density of the cell population. Dendriplexes have shown promising results in the field of biology. Therefore, it is an excellent opportunity for researchers to apply a range of analytical techniques to accurately describe the complexes formed by dendriplex formation.
According to a study done by Huang and his team, transferrin that is attached to dendrimer DNA dendriplexes of PAMAM increased the number of genes that were expressed in the brain twice. The same study also revealed “that conjugating lactoferrin to PAMAM dendrimers” using PEG spacers boosted dendrimer brain absorption by “4.6-fold compared to non-conjugated PAMAM dendrimers and by 2.2-fold compared to dendrimers conjugated to transferrin” in BALB/c mice (Huang et al., 2008). Low density lipoprotein (LRP) receptors are extensively produced in mammalian neural cells. Angiopep has demonstrated selectivity in targeting LRP receptors. “Angiopep-PEG-PAMAM loaded with DNA” caused higher gene expression “in the cortex, caudate putamen, hippocampus and substantia nigra of BALB/c mice than unconjugated PAMAM loaded with DNA (Ke et al., 2009).
8.4 Carbosilane dendrimers as a brain drug delivery
Researchers have addressed the possibility that dendrimers may treat or ward off neurological and degenerative diseases of the brain. Because of the BBB, treating and/or preventing diseases that affect the central nervous system can be extremely difficult. One of the factors contributing to the ineffectiveness of this barrier is inadequate drug penetration. This particular limitation, however, can be overcome through the usage of dendrimers as nanocarriers (Rabiee et al., 2020).
A previous study by Serrama et al. (2015) used carbosilane dendrimers with targeted siRNA to prevent gene expression of a specific protein production in primary astrocytes and cytotoxicity. This study sheds light on the potential of carbosilane dendrimers for drug targeting in the brain.
8.5 PLL dendrimers as a potential therapeutic strategy for brain tumors
Poly-l-lysine (PLL) dendrimers are an alternative option to PAMAM dendrimers. Their natural antiangiogenic properties and lower vascularization make them less likely to cause side effects. PLL inhibits tumor development by destroying necrosis and stimulating apoptosis. This contributes to the non-toxic effect PLL dendrimers have on healthy cells, bringing their therapeutic potential to the level of marketed anti-angiogenic drugs (Ryan et al., 2013; Singh et al., 2022). A DOX-loaded PLL dendrimer coupled mainly with folate was synthesised by Jain and colleagues. This dendrimer responded to pH and exhibited antiangiogenic and anticancer activities developed by the researchers (Gauro et al., 2021). There is also the possibility that PLL dendrimers could serve as vehicles for the delivery of anticancer drugs (Gao et al., 2016). Scientists have developed PLL dendrimers that can deliver anticancer drugs to specific tumor sites by binding DOX to the acid-labile bond HSBA (4-hydrazinosulfonylbenzoic acid). Due to the acid-labile binding, only 10% of the drug could be released at a pH of 7.4, while the entire amount of drug could be released at a pH of 5. The results also showed that a decrease in metabolic lability led to an increase in cellular absorption in vivo, suggesting that mechanistic targeting of PLL dendrimers may be possible (Kaminskas et al., 2011). Niidome and colleagues developed a G6 PLL dendrimer with PEG-linked penta-alanine or pentaphenylalanine, which made it possible to administer DOX in a targeted manner. The DOX was encased in either a penta-alanine or penta-phenylalanine core, both of which are hydrophobic. Penta-phenylalanine was found to have higher encapsulation efficiency than penta-alanine. DOX was released from the hydrophobic cavity over time as a function of pH. The created dendrimer accumulated more in cancer cells and significantly suppressed tumor development without causing weight loss, suggesting that PLL dendrimers can be targeted in the brain (Malik et al., 2018). Table 2 (Gao et al., 2016; Sharma et al., 2017; Malik et al., 2018; Nh et al., 2016) lists several types of PLL dendrimers.
8.6 Poly (propylene imine) (PPI) dendrimers as a brain drug delivery
A new synthesis approach is utilized to develop PPI dendrimers which are hyperbranched macromolecules (Kwan and Leung, 2020). The presence of amino terminal functional groups allows them to be conjugated with ligands such as folate and antibodies to deliver anticancer drugs to specific sites. Dendrimers are deemed as dangerous due to their cationic nature. Wang et al. (2012) utilized a method to circumvent this problem by preparing acetylated PPI dendrimers with 14.2% acylation and 95.3% Doxorubicin/methotrexate (DOX/MTX) loading. Dendrimers with more than 80% acylation were found to be protective against A549 and MCF -7 cells when injected and released over an extended period of time. Another study found that preparation of a folate-free diaminobutane G4 PPI dendrimer ligand would result in lower cytotoxicity. The results underscored that an ETP-loaded dendrimer had long-lasting activity and enhanced site-specific drug delivery (Sideratou et al., 2001). Gajbhiye and Jain conducted research to determine the effectiveness of a polysorbate 80 (P80)-anchored PPI dendrimer (P80-PPI) in transporting DTX (Docetaxel) to the cells in the brain. The study found that DTX-P80-PPI was more cytotoxic than the combination DTX-PPI and the free drug in a human glioma cell line U87MG. Within one week, DTX-P80-PPI demonstrated a possibility of declination in the size of brain tumors (by 50%) in which the significant permeation of P80 through the BBB was linked to this particular finding (Gajbhiye and Jain, 2011). Patel et al. developed a thiamine PPI dendrimer combination that was loaded with Paclitaxel (PTX) so that it could be transported to the brain. In this way, paclitaxel (PTX) could be transported into the brain (PTX-TM-PPI). According to the results of the study, the molecule could prolong the duration of action of drugs while reducing their harmful effects on the body. The PTX-loaded dendrimers and free drug were compared with PTX-TM-PPI, which significantly suppressed tumor formation in a human IMR-32 neuroblastoma cell line. In a separate research study, Patel et al. (2012) examined how effectively PPI dendrimers combined with other ligands, such as concanavalin A (Con A), sialic acid and glucosamine, transported PTX in the brain. According to the cytotoxicity data, the IC50 value of the ligand-anchored PPI dendrimer was three to six times lower than that of the free drug. As the biodistribution was studied, it was found that the ligand-anchored PPI dendrimers showed a higher concentration of the medication in the brain when compared to the free PTX. Not only that, the sialic acid also had better target efficacy than Con A and glucosamine. Table 2 has listed a few examples of PPI dendrimers.
9 Dendrimers in brain tumor imaging
Limited accessibility to the BBB is a hurdle that prevents successful identification and treatment of brain tumors. Metastases often occur in advanced stages of cancer and require difficult surgical intervention; therefore, early detection is critical for optimal treatment. The efficacy of nanodiagnostics depends on a well-established imaging technique that can accurately assess the pharmacokinetic profile, bioavailability, tumor neovascularization, uptake by tumor cells and drug and imaging agent release kinetics (Saluja et al., 2021).
A wide range of non-invasive imaging modalities known as molecular imaging have been utilized to visualize, interpret and assess the physiological changes at the molecular/cellular/tissue level to gain insight into the mechanisms of oncogenesis (Zukotynski et al., 2020). Therefore, molecular imaging techniques would be useful for forecasting therapy response, prudently segmenting patients, measuring biodistribution, and determining the drug release profile (Bernsen et al., 2013; Li et al., 2021).
Magnetic resonance imaging (MRI) technique was the first one utilised to detect and diagnose brain tumour lesions (Khan et al., 2020; Almalki et al., 2022; Wu et al., 2022; Yazdan et al., 2022). Meanwhile, gadolinium (Gd)-chelated diagnostic agents are extensively utilized as contrast agents for tumor imaging (Rodríguez-Galván et al., 2020). Currently, there are seven Gd contrast agents approved for clinical use by US-FDA (Heshmatzadeh Behzadi & McDonald, 2022). In the twenty years, ferromagnetic Gd contrast agents coupled with dendrimers have been utilised for the purposes of image intensification, better elimination qualities, and possible targeting while MRI monitoring is being performed (Wu et al., 2022). Dendrimers have been described as nanomedicines in the past. These nanomedicines have the ability to tolerate bigger Gd charges and improve the signal contrast of an MRI contrast agent with nearby tissue in vivo (Longmire et al., 2014; McMahon and Bulte, 2018).
In 2011, Han and his co-workers developed a contrast agent for MRI that could be used in the diagnosis of glioma. They came up with the idea of developing PEG-linked PAMAM dendrimers that were loaded with the tumor-selective peptide HAIYPRH (T7). These dendrimers were then connected with diethylenetriaminepentaacetic acid (DTPA) and Gd chelates, which resulted in GdDTPA-PAMAM-PEG-T7. To evaluate the use of dendrimers in the diagnosis of brain tumours, an early brain glioma model was chosen. According to the results, these dendrimers were not able to detect early gliomas. This is because it is difficult to accurately determine the location of a brain tumour as well as its growth due to the challenges posed by the BBB and various pathophysiological conditions (Han et al., 2011).
Recently, Rasouli et al. (2021) investigated the potential of 99 mTc-labelled dendrimer-phenylalanine conjugates in C6 glioma cell lines for brain tumour diagnosis using single photon emission computed tomography (SPECT). The results showed that these dendrimers did not exhibit toxicity in the brain, whereas phenylalanine increased the accumulation and deposition of 99 mTc-labelled dendrimer in brain tumours.
According to the results of a study conducted by Zhao, the resulting G5-NH2 terminal PAMAM dendrimer nanoplatform was further conjugated with PEG, chlorotoxin (CTX) and 3-(4'-hydroxyphenyl)propionic acid-Osu (HPAO). After this step, the remaining terminal amine groups were acetylated and finally labelled with radioactive 131I. In vivo studies using the mouse glioma model showed an increase in signal intensity and an anti-cancer effect in SPECT imaging by radionuclides (Zhao et al., 2015).
Subbarayan et al. (2001) prepared two types of soluble dendrimer-based porphyrins (P1 and P2) with a central core of 5,10,15,20-tetrakis [4-(carboxymethyleneoxy)-phenyl]porphyrin (T4CPP) radiolabeled with 99mTc for glioma imaging and diagnosis. The results showed that these dendrimers had an efficient imaging potential with a satisfactory diagnostic level in the C6 glioma tumor model. Table 3 consists of a list of dendrimers that can be employed in cancer as contrast agents for MRI, PET and SPECT scans (Han et al., 2011; Huang et al., 2011; Yang et al., 2015; Zhao et al., 2015; Sun et al., 2017; Xu et al., 2019; Rasouli et al., 2021).
10 Conclusion
According to the results of various animal studies, dendrimers are an interesting candidate for systemic drug delivery for the treatment of brain tumours. Dendrimers and nanotechnology have proven to be the best solution for the treatment of brain tumours. This is because they are an excellent alternative to the conventional chemotherapy available today. Due to their spherical architecture and multivalent periphery, dendrimers can also be used for simultaneous treatment and diagnosis. By using dendrimers, it is possible to reliably target the brain tumour with sustained drug release. In recent years, researchers have become more interested in treating and monitoring brain tumours by taking advantage of the multifunctional properties of BBB, which could improve patient survival. Nanomedicines based on dendrimers, which are highly targeted, effective, biocompatible, and cost-effective, are becoming more popular in the clinic with advances in oncology research. However, dendrimers need further accurate evidence on efficacy, targeting, safety, and mortality concerns to validate them as suitable and viable nanoarchitectures for brain tumour imaging and treatment.
The global outlook for dendrimers in the coming years appears favourable, raising hopes for more successful clinical translation in brain tumours and other malignancies. The ability to provide treatment at the appropriate time depends on rapid diagnosis. This goal may one day be achieved through the use of personalised treatment planning for brain tumours, which aims to improve tumour diagnosis, and drugs that have predictable side effects. Medical advances are shown to have increased patient satisfaction with their life expectancy. Dendrimers are expected to be produced and moved into clinical trials in the next few years.
Author contributions
Authors MK, RS, SR, AJ, DR, AS, and HA-G drafted the paper by collecting material from different sources. While review and editing of the draft has been done by MK, JK, RS, and AA. All authors have given consent for the publication of this manuscript.
Conflict of interest
The authors declare that the research was conducted in the absence of any commercial or financial relationships that could be construed as a potential conflict of interest.
Publisher’s note
All claims expressed in this article are solely those of the authors and do not necessarily represent those of their affiliated organizations, or those of the publisher, the editors and the reviewers. Any product that may be evaluated in this article, or claim that may be made by its manufacturer, is not guaranteed or endorsed by the publisher.
References
Abedi-Gaballu, F., Dehghan, G., Ghaffari, M., Yekta, R., Abbaspour-Ravasjani, S., Baradaran, B., et al. (2018). PAMAM dendrimers as efficient drug and gene delivery nanosystems for cancer therapy. Appl. Mater. today 12, 177–190. doi:10.1016/j.apmt.2018.05.002
Adeli, M., Rasoulian, B., Saadatmehr, F., and Zabihi, F. (2013). Hyperbranched poly (citric acid) and its application as anticancer drug delivery system. J. Appl. Polym. Sci. 129 (6), 3665–3671. doi:10.1002/app.39028
Akhter, S., Ahmad, I., Ahmad, M. Z., Ramazani, F., Singh, A., Rahman, Z., et al. (2013). Nanomedicines as cancer therapeutics: Current status. Curr. cancer drug targets 13 (4), 362–378. doi:10.2174/1568009611313040002
Almalki, Y. E., Ali, M. U., Kallu, K. D., Masud, M., Zafar, A., Alduraibi, S. K., et al. (2022). Isolated convolutional-neural-network-based deep-feature extraction for brain tumor classification using shallow classifier. Diagn. (Basel, Switz. 12 (8), 1793. doi:10.3390/diagnostics12081793
Apartsin, E. K., Knauer, N., Kahlert, U. D., and Caminade, A. M. (2022). Amphiphilic triazine-phosphorus metallodendrons possessing anti-cancer stem cell activity. Pharmaceutics 14 (2), 393. doi:10.3390/pharmaceutics14020393
Attia, M. F., Anton, N., Wallyn, J., Omran, Z., and Vandamme, T. F. (2019). An overview of active and passive targeting strategies to improve the nanocarriers efficiency to tumour sites. J. Pharm. Pharmacol. 71 (8), 1185–1198. doi:10.1111/jphp.13098
Bae, Y., Lee, J., Kho, C., Choi, J. S., and Han, J. (2021). Apoptin gene delivery by a PAMAM dendrimer modified with a nuclear localization signal peptide as a gene carrier for brain cancer therapy. Korean J. physiology Pharmacol. official J. Korean Physiological Soc. Korean Soc. Pharmacol. 25 (5), 467–478. doi:10.4196/kjpp.2021.25.5.467
Bae, Y., Thuy, L. T., Lee, Y. H., Ko, K. S., Han, J., and Choi, J. S. (2019). Polyplexes of functional PAMAM dendrimer/apoptin gene induce apoptosis of human primary glioma cells in vitro. Polymers 11 (2), 296. doi:10.3390/polym11020296
Ban, J., Li, S., Zhan, Q., Li, X., Xing, H., Chen, N., et al. (2021). PMPC modified PAMAM dendrimer enhances brain tumor-targeted drug delivery. Macromol. Biosci. 21 (4), e2000392. doi:10.1002/mabi.202000392
Basile, L., Pignatello, R., and Passirani, C. (2012). Active targeting strategies for anticancer drug nanocarriers. Curr. drug Deliv. 9 (3), 255–268. doi:10.2174/156720112800389089
Beezer, A. E., King, A. S. H., Martin, I. K., Mitchel, J. C., Twyman, L. J., and Wain, C. F. (2003). Dendrimers as potential drug carriers; encapsulation of acidic hydrophobes within water soluble PAMAM derivatives. Tetrahedron 59 (22), 3873–3880. doi:10.1016/s0040-4020(03)00437-x
Bernsen, M. R., Ruggiero, A., van Straten, M., Kotek, G., Haeck, J. C., Wielopolski, P. A., et al. (2013). Computed tomography and magnetic resonance imaging. Progres dans les Rech. cancer 187, 3–63. doi:10.1007/978-3-642-10853-2_1
Bhadra, D., Bhadra, S., Jain, S., and Jain, N. K. (2003). A PEGylated dendritic nanoparticulate carrier of fluorouracil. Int. J. Pharm. 257 (1-2), 111–124. doi:10.1016/s0378-5173(03)00132-7
Bhatt, S., Pathak, A., Grover, P., Bharadwaj, A., Bhatia, D., Tomar, R., et al. (2022). Different aspects of polymers - A review article. Materials Today: Proceedings 64 (3), 1490–1495.
Birdhariya, B., Kesharwani, P., and Jain, N. K. (2015). Effect of surface capping on targeting potential of folate decorated poly (propylene imine) dendrimers. Drug Dev. industrial Pharm. 41 (8), 1393–1399. doi:10.3109/03639045.2014.954584
Biswas, S., Deshpande, P. P., Navarro, G., Dodwadkar, N. S., and Torchilin, V. P. (2013). Lipid modified triblock PAMAM-based nanocarriers for siRNA drug co-delivery. Biomaterials 34 (4), 1289–1301. doi:10.1016/j.biomaterials.2012.10.024
Briz, V., Serramía, M. J., Madrid, R., Hameau, A., Caminade, A. M., Majoral, J. P., et al. (2012). Validation of a generation 4 phosphorus-containing polycationic dendrimer for gene delivery against HIV-1. Curr. Med. Chem. 19 (29), 5044–5051. doi:10.2174/0929867311209025044
Bruckdorfer, T., Marder, O., and Albericio, F. (2004). From production of peptides in milligram amounts for research to multi-tons quantities for drugs of the future. Curr. Pharm. Biotechnol. 5 (1), 29–43. doi:10.2174/1389201043489620
Byrne, M., Victory, D., Hibbitts, A., Lanigan, M., Heise, A., and Cryan, S. A. (2013). Molecular weight and architectural dependence of well-defined star-shaped poly(lysine) as a gene delivery vector. Biomaterials Sci. 1 (12), 1223–1234. doi:10.1039/c3bm60123d
Caminade, A. M., and Majoral, J. P. (2013). Positively charged phosphorus dendrimers. An overview of their properties. New J. Chem. 37 (11), 3358–3373. doi:10.1039/c3nj00583f
Cammidge, A. (2003). Dendrimers and other dendritic polymers jean mj Fréchet and donald A Tomalia (Eds). Chichester, UK; John Wiley and Sons, 647, price£ 195.00€ 321.80 ISBN 0-471-63850-1.
Carnahan, M. A., and Grinstaff, M. W. (2001). Synthesis and characterization of polyether-ester dendrimers from glycerol and lactic acid. J. Am. Chem. Soc. 123 (12), 2905–2906. doi:10.1021/ja005726+
Chauhan, A. S. (2018). Dendrimers for drug delivery. Mol. (Basel, Switz. 23 (4), 938. doi:10.3390/molecules23040938
Chenthamara, D., Subramaniam, S., Ramakrishnan, S. G., Krishnaswamy, S., Essa, M. M., Lin, F. H., et al. (2019). Therapeutic efficacy of nanoparticles and routes of administration. Biomaterials Res. 23, 20. doi:10.1186/s40824-019-0166-x
Chis, A. A., Dobrea, C., Morgovan, C., Arseniu, A. M., Rus, L. L., Butuca, A., et al. (2020). Applications and limitations of dendrimers in biomedicine. Mol. (Basel, Switz. 25 (17), 3982. doi:10.3390/molecules25173982
Choi, J. S., Joo, D. K., Kim, C. H., Kim, K., and Park, J. S. (2000). Synthesis of a barbell-like triblock copolymer, poly (L-lysine) dendrimer-block-poly (ethylene glycol)-block-poly (L-lysine) dendrimer, and its self-assembly with plasmid DNA. J. Am. Chem. Soc. 122 (3), 474–480. doi:10.1021/ja9931473
Choudhary, S., Gupta, L., Rani, S., Dave, K., and Gupta, U. (2017). Impact of dendrimers on solubility of hydrophobic drug molecules. Front. Pharmacol. 8, 261. doi:10.3389/fphar.2017.00261
Cieślak, M., Ryszawy, D., Pudełek, M., Urbanowicz, M., Morawiak, M., Staszewska-Krajewska, O., et al. (2020). Bioinspired bola-type peptide dendrimers inhibit proliferation and invasiveness of glioblastoma cells in a manner dependent on their structure and amphipathic properties. Pharmaceutics 12 (11), 1106. doi:10.3390/pharmaceutics12111106
Dande, P., Prakash, T. P., Sioufi, N., Gaus, H., Jarres, R., Berdeja, A., et al. (2006). Improving RNA interference in mammalian cells by 4'-thio-modified small interfering RNA (siRNA): Effect on siRNA activity and nuclease stability when used in combination with 2'-O-alkyl modifications. J. Med. Chem. 49 (5), 1624–1634. doi:10.1021/jm050822c
De, R., Mahata, M. K., and Kim, K. T. (2022). Structure-based varieties of polymeric nanocarriers and influences of their physicochemical properties on drug delivery profiles. Adv. Sci. (Weinheim, Baden-Wurttemberg, Ger. 9 (10), e2105373. doi:10.1002/advs.202105373
Demeule, M., Currie, J. C., Bertrand, Y., Ché, C., Nguyen, T., Régina, A., et al. (2008). Involvement of the low-density lipoprotein receptor-related protein in the transcytosis of the brain delivery vector angiopep-2. J. Neurochem. 106 (4), 1534–1544. doi:10.1111/j.1471-4159.2008.05492.x
Denora, N., Laquintana, V., Lopalco, A., Iacobazzi, R. M., Lopedota, A., Cutrignelli, A., et al. (2013). In vitro targeting and imaging the translocator protein TSPO 18-kDa through G(4)-PAMAM-FITC labeled dendrimer. J. Control. release official J. Control. Release Soc. 172 (3), 1111–1125. doi:10.1016/j.jconrel.2013.09.024
Dey, A. D., Bigham, A., Esmaeili, Y., Ashrafizadeh, M., Moghaddam, F. D., Tan, S. C., et al. (2022). Dendrimers as nanoscale vectors: Unlocking the bars of cancer therapy. Seminars cancer Biol. 86 (2), 396–419. doi:10.1016/j.semcancer.2022.06.003
Dhanikula, R. S., Argaw, A., Bouchard, J. F., and Hildgen, P. (2008). Methotrexate loaded polyether-copolyester dendrimers for the treatment of gliomas: Enhanced efficacy and intratumoral transport capability. Mol. Pharm. 5 (1), 105–116. doi:10.1021/mp700086j
Du, X., Shi, B., Liang, J., Bi, J., Dai, S., and Qiao, S. Z. (2013). Developing functionalized dendrimer-like silica nanoparticles with hierarchical pores as advanced delivery nanocarriers. Adv. Mater. Deerf. Beach, Fla.) 25 (41), 5981–5985. doi:10.1002/adma.201302189
Duncan, R. (2014). 2. Commentary on dendrimers: Why dendrimers?: Original research article: Relationship between structure and biocompatibility in vitro preliminary studies on the biodistribution of 125I-labelled polyamidoamine dendrimers (2000). J. Control. release official J. Control. Release Soc. 190, 32–34.
Dwivedi, N., Shah, J., Mishra, V., Mohd Amin, M. C., Iyer, A. K., Tekade, R. K., et al. (2016). Dendrimer-mediated approaches for the treatment of brain tumor. J. biomaterials Sci. Polym. Ed. 27 (7), 557–580. doi:10.1080/09205063.2015.1133155
Dzmitruk, V., Szulc, A., Shcharbin, D., Janaszewska, A., Shcharbina, N., Lazniewska, J., et al. (2015). Anticancer siRNA cocktails as a novel tool to treat cancer cells. Part (B). Efficiency of pharmacological action. Int. J. Pharm. 485 (1-2), 288–294. doi:10.1016/j.ijpharm.2015.03.034
Ekladious, I., Colson, Y. L., and Grinstaff, M. W. (2019). Polymer-drug conjugate therapeutics: Advances, insights and prospects. Nat. Rev. Drug Discov. 18 (4), 273–294. doi:10.1038/s41573-018-0005-0
Esfand, R., and Tomalia, D. A. (2001). Poly(amidoamine) (PAMAM) dendrimers: From biomimicry to drug delivery and biomedical applications. Drug Discov. today 6 (8), 427–436. doi:10.1016/s1359-6446(01)01757-3
Etrych, T., Chytil, P., Mrkvan, T., Sírová, M., Ríhová, B., and Ulbrich, K. (2008). Conjugates of doxorubicin with graft HPMA copolymers for passive tumor targeting. J. Control. release official J. Control. Release Soc. 132 (3), 184–192. doi:10.1016/j.jconrel.2008.04.017
Etrych, T., Strohalm, J., Chytil, P., Černoch, P., Starovoytova, L., Pechar, M., et al. (2011). Biodegradable star HPMA polymer conjugates of doxorubicin for passive tumor targeting. Eur. J. Pharm. Sci. official J. Eur. Fed. Pharm. Sci. 42 (5), 527–539. doi:10.1016/j.ejps.2011.03.001
Fana, M., Gallien, J., Srinageshwar, B., Dunbar, G. L., and Rossignol, J. (2020). PAMAM dendrimer nanomolecules utilized as drug delivery systems for potential treatment of glioblastoma: A systematic review. Int. J. nanomedicine 15, 2789–2808. doi:10.2147/IJN.S243155
Fant, K., Esbjörner, E. K., Jenkins, A., Grossel, M. C., Lincoln, P., and Nordén, B. (2010). Effects of PEGylation and acetylation of PAMAM dendrimers on DNA binding, cytotoxicity and in vitro transfection efficiency. Mol. Pharm. 7 (5), 1734–1746. doi:10.1021/mp1001312
Farabi, K., Manabe, Y., Ichikawa, H., Miyake, S., Tsutsui, M., Kabayama, K., et al. (2020). Concise and reliable syntheses of glycodendrimers via self-activating click chemistry: A robust strategy for mimicking multivalent glycan-pathogen interactions. J. Org. Chem. 85 (24), 16014–16023. doi:10.1021/acs.joc.0c01547
Florendo, M., Figacz, A., Srinageshwar, B., Sharma, A., Swanson, D., Dunbar, G. L., et al. (2018). Use of polyamidoamine dendrimers in brain diseases. Mol. (Basel, Switz. 23 (9), 2238. doi:10.3390/molecules23092238
Frechet, J. M., and Tomalia, D. A. (2001). Dendrimers and other dendritic polymers. Hoboken, New Jersey, U.S: Wiley Publisher.
Gajbhiye, V., and Jain, N. K. (2011). The treatment of Glioblastoma Xenografts by surfactant conjugated dendritic nanoconjugates. Biomaterials 32 (26), 6213–6225. doi:10.1016/j.biomaterials.2011.04.057
Gamage, N. H., Jing, L., Worsham, M. J., and Ali, M. M. (2016). Targeted theranostic approach for glioma using dendrimer-based curcumin nanoparticle. J. nanomedicine Nanotechnol. 7 (4), 393. doi:10.4172/2157-7439.1000393
Gao, S., Tian, H., Xing, Z., Zhang, D., Guo, Y., Guo, Z., et al. (2016). A non-viral suicide gene delivery system traversing the blood brain barrier for non-invasive glioma targeting treatment. J. Control. release official J. Control. Release Soc. 243, 357–369. doi:10.1016/j.jconrel.2016.10.027
Gasparri, R., Guaglio, A., and Spaggiari, L. (2022). Early diagnosis of lung cancer: The urgent need of a clinical test. J. Clin. Med. 11 (15), 4398. doi:10.3390/jcm11154398
Gauro, R., Nandave, M., Jain, V. K., and Jain, K. (2021). Advances in dendrimer-mediated targeted drug delivery to the brain. J. Nanoparticle Res. 23, 76–20. doi:10.1007/s11051-021-05175-8
Gillies, E. R., and Fréchet, J. M. (2005). Dendrimers and dendritic polymers in drug delivery. Drug Discov. today 10 (1), 35–43. doi:10.1016/S1359-6446(04)03276-3
Gillies, E. R. (2011). Glycodendrimers and their biological applications. Engineered carbohydrate-based materials for biomedical applications: Polymers, surfaces, dendrimers, nanoparticles, and hydrogels, 261–305.
Gorzkiewicz, M., Kopeć, O., Janaszewska, A., Konopka, M., Pędziwiatr-Werbicka, E., Tarasenko, I. I., et al. (2020). Poly(lysine) dendrimers form complexes with siRNA and provide its efficient uptake by myeloid cells: Model studies for therapeutic nucleic acid delivery. Int. J. Mol. Sci. 21 (9), 3138. doi:10.3390/ijms21093138
Gottesman, M. M., Fojo, T., and Bates, S. E. (2002). Multidrug resistance in cancer: Role of ATP-dependent transporters. Nat. Rev. Cancer 2 (1), 48–58. doi:10.1038/nrc706
Greish, K., Fang, J., Inutsuka, T., Nagamitsu, A., and Maeda, H. (2003). Macromolecular therapeutics: Advantages and prospects with special emphasis on solid tumour targeting. Clin. Pharmacokinet. 42 (13), 1089–1105. doi:10.2165/00003088-200342130-00002
Grover, P., Mehta, L., Nagarajan, K., Kumar, P., Pathak, A., and Sharma, K. (2022). Targeted delivery approaches for synthesis of plant based nanoparticles as anticancer chemotherapeutics. Materials Today: Proceedings 64 (3), 1193–1196. doi:10.1016/j.matpr.2022.03.483
Gupta, U., Dwivedi, S. K., Bid, H. K., Konwar, R., and Jain, N. K. (2010). Ligand anchored dendrimers based nanoconstructs for effective targeting to cancer cells. Int. J. Pharm. 393 (1-2), 185–196. doi:10.1016/j.ijpharm.2010.04.002
Haiba, N. S., Khalil, H. H., Bergas, A., Abu-Serie, M. M., Khattab, S. N., and Teleb, M. (2022). First-in-Class star-shaped triazine dendrimers endowed with MMP-9 inhibition and VEGF suppression capacity: Design, synthesis, and anticancer evaluation. ACS omega 7 (24), 21131–21144. doi:10.1021/acsomega.2c01949
Han, L., Li, J., Huang, S., Huang, R., Liu, S., Hu, X., et al. (2011). Peptide-conjugated polyamidoamine dendrimer as a nanoscale tumor-targeted T1 magnetic resonance imaging contrast agent. Biomaterials 32 (11), 2989–2998. doi:10.1016/j.biomaterials.2011.01.005
Hawker, C. J., and Frechet, J. M. (1990). Preparation of polymers with controlled molecular architecture. A new convergent approach to dendritic macromolecules. J. Am. Chem. Soc. 112 (21), 7638–7647. doi:10.1021/ja00177a027
Hegde, N., Velingkar, V., and Prabhakar, B. (2019). An update on design and pharmacology of dendritic poly (l-lysine). Int. J. Peptide Res. Ther. 25, 1539–1562. doi:10.1007/s10989-018-9798-2
Heshmatzadeh Behzadi, A., and McDonald, J. (2022). Gadolinium-based contrast agents for imaging of the central nervous system: A multicenter European prospective study. Medicine 101 (34), e30163. doi:10.1097/MD.0000000000030163
Huang, R., Han, L., Li, J., Liu, S., Shao, K., Kuang, Y., et al. (2011). Chlorotoxin-modified macromolecular contrast agent for MRI tumor diagnosis. Biomaterials 32 (22), 5177–5186. doi:10.1016/j.biomaterials.2011.03.075
Huang, R., Ke, W., Liu, Y., Jiang, C., and Pei, Y. (2008). The use of lactoferrin as a ligand for targeting the polyamidoamine-based gene delivery system to the brain. Biomaterials 29 (2), 238–246. doi:10.1016/j.biomaterials.2007.09.024
Igartúa, D. E., Martinez, C. S., Temprana, C. F., Alonso, S. D. V., and Prieto, M. J. (2018). PAMAM dendrimers as a carbamazepine delivery system for neurodegenerative diseases: A biophysical and nanotoxicological characterization. Int. J. Pharm. 544 (1), 191–202. doi:10.1016/j.ijpharm.2018.04.032
Iyer, A. K., Duan, Z., and Amiji, M. M. (2014). Nanodelivery systems for nucleic acid therapeutics in drug resistant tumors. Mol. Pharm. 11 (8), 2511–2526. doi:10.1021/mp500024p
Jain, K., Kesharwani, P., Gupta, U., and Jain, N. K. (2012). A review of glycosylated carriers for drug delivery. Biomaterials 33 (16), 4166–4186. doi:10.1016/j.biomaterials.2012.02.033
Janaszewska, A., Lazniewska, J., Trzepiński, P., Marcinkowska, M., and Klajnert-Maculewicz, B. (2019). Cytotoxicity of dendrimers. Biomolecules 9 (8), 330. doi:10.3390/biom9080330
Janiszewska, J., Posadas, I., Játiva, P., Bugaj-Zarebska, M., Urbanczyk-Lipkowska, Z., and Ceña, V. (2016). Second generation amphiphilic poly-lysine dendrons inhibit glioblastoma cell proliferation without toxicity for neurons or astrocytes. PloS one 11 (11), e0165704. doi:10.1371/journal.pone.0165704
Jevprasesphant, R., Penny, J., Jalal, R., Attwood, D., McKeown, N. B., and D'Emanuele, A. (2003). The influence of surface modification on the cytotoxicity of PAMAM dendrimers. Int. J. Pharm. 252 (1-2), 263–266. doi:10.1016/s0378-5173(02)00623-3
Jiang, B., Zhao, Y., Cao, J., Yang, Y., Kuang, T., Zhang, Q., et al. (2021). Synthesis and preliminary biological evaluation of naproxen-probenecid conjugate for central nervous system (CNS) delivery. Pak. J. Pharm. Sci. 34 (6), 2197–2203.
Jiang, Y., Lv, L., Shi, H., Hua, Y., Lv, W., Wang, X., et al. (2016). PEGylated Polyamidoamine dendrimer conjugated with tumor homing peptide as a potential targeted delivery system for glioma. Colloids surfaces. B, Biointerfaces 147, 242–249. doi:10.1016/j.colsurfb.2016.08.002
Kalhapure, R. S., Kathiravan, M. K., Akamanchi, K. G., and Govender, T. (2015). Dendrimers - from organic synthesis to pharmaceutical applications: An update. Pharm. Dev. Technol. 20 (1), 22–40. doi:10.3109/10837450.2013.862264
Kaminskas, L. M., Boyd, B. J., and Porter, C. J. (2011). Dendrimer pharmacokinetics: The effect of size, structure and surface characteristics on ADME properties. Nanomedicine Lond. Engl. 6 (6), 1063–1084. doi:10.2217/nnm.11.67
Kaminskas, L. M., Kelly, B. D., McLeod, V. M., Sberna, G., Owen, D. J., Boyd, B. J., et al. (2011). Characterisation and tumour targeting of PEGylated polylysine dendrimers bearing doxorubicin via a pH labile linker. J. Control. release official J. Control. Release Soc. 152 (2), 241–248. doi:10.1016/j.jconrel.2011.02.005
Katano, A., and Yamashita, H. (2022). Brain metastasis: Recent treatment modalities and future-perspectives. Oncol. Lett. 23 (6), 191. doi:10.3892/ol.2022.13311
Ke, W., Shao, K., Huang, R., Han, L., Liu, Y., Li, J., et al. (2009). Gene delivery targeted to the brain using an Angiopep-conjugated polyethyleneglycol-modified polyamidoamine dendrimer. Biomaterials 30 (36), 6976–6985. doi:10.1016/j.biomaterials.2009.08.049
Kesharwani, P., Banerjee, S., Padhye, S., Sarkar, F. H., and Iyer, A. K. (2015). Parenterally administrable nano-micelles of 3,4-difluorobenzylidene curcumin for treating pancreatic cancer. Colloids surfaces. B, Biointerfaces 132, 138–145. doi:10.1016/j.colsurfb.2015.05.007
Kesharwani, P., Mishra, V., and Jain, N. K. (2015). Validating the anticancer potential of carbon nanotube-based therapeutics through cell line testing. Drug Discov. today 20 (9), 1049–1060. doi:10.1016/j.drudis.2015.05.004
Kesharwani, P., Tekade, R. K., and Jain, N. K. (2015). Generation dependent safety and efficacy of folic acid conjugated dendrimer based anticancer drug formulations. Pharm. Res. 32 (4), 1438–1450. doi:10.1007/s11095-014-1549-2
Khan, H. A., Jue, W., Mushtaq, M., and Mushtaq, M. U. (2020). Brain tumor classification in MRI image using convolutional neural network. Math. Biosci. Eng. MBE 17 (5), 6203–6216. doi:10.3934/mbe.2020328
Kheraldine, H., Rachid, O., Habib, A. M., Al Moustafa, A. E., Benter, I. F., and Akhtar, S. (2021). Emerging innate biological properties of nano-drug delivery systems: A focus on PAMAM dendrimers and their clinical potential. Adv. drug Deliv. Rev. 178, 113908. doi:10.1016/j.addr.2021.113908
Khizar, S., Ahmad, N. M., Zine, N., Jaffrezic-Renault, N., Errachid-el-salhi, A., and Elaissari, A. (2021). Magnetic nanoparticles: From synthesis to theranostic applications. ACS Appl. Nano Mater. 4 (5), 4284–4306. doi:10.1021/acsanm.1c00852
Klajnert, B., Appelhans, D., Komber, H., Morgner, N., Schwarz, S., Richter, S., et al. (2008). The influence of densely organized maltose shells on the biological properties of poly(propylene imine) dendrimers: New effects dependent on hydrogen bonding. Chem. (Weinheim der Bergstrasse, Ger. 14 (23), 7030–7041. doi:10.1002/chem.200800342
Klimova, B., Maresova, P., Novotny, M., and Kuca, K. (2018). A global view on narcolepsy - a review study. Mini Rev. Med. Chem. 18 (5), 458–464. doi:10.2174/1389557516666160801095630
Knauer, N., Arkhipova, V., Li, G., Hewera, M., Pashkina, E., Nguyen, P. H., et al. (2022). In vitro validation of the therapeutic potential of dendrimer-based nanoformulations against tumor stem cells. Int. J. Mol. Sci. 23 (10), 5691. doi:10.3390/ijms23105691
Kolhatkar, R. B., Kitchens, K. M., Swaan, P. W., and Ghandehari, H. (2007). Surface acetylation of polyamidoamine (PAMAM) dendrimers decreases cytotoxicity while maintaining membrane permeability. Bioconjugate Chem. 18 (6), 2054–2060. doi:10.1021/bc0603889
Kumbhar, S. A., Gorain, B., Choudhury, H., and Kesharwani, P. (2021). “Safety and toxicity issues of dendrimers,” in Dendrimer-based nanotherapeutics (Academic Press), 143–162.
Kwan, C. S., and Leung, K. C. (2020). Development and advancement of rotaxane dendrimers as switchable macromolecular machines. Mater. Chem. Front. 4 (10), 2825–2844. doi:10.1039/d0qm00368a
Lalatsa, A., Schatzlein, A. G., and Uchegbu, I. F. (2014). Strategies to deliver peptide drugs to the brain. Mol. Pharm. 11 (4), 1081–1093. doi:10.1021/mp400680d
Lamy, C. M., Sallin, O., Loussert, C., and Chatton, J. Y. (2012). Sodium sensing in neurons with a dendrimer-based nanoprobe. ACS Nano 6 (2), 1176–1187. doi:10.1021/nn203822t
Li, B., Zhao, M., Feng, L., Dou, C., Ding, S., Zhou, G., et al. (2020). Organic NIR-II molecule with long blood half-life for in vivo dynamic vascular imaging. Nat. Commun. 11 (1), 3102. doi:10.1038/s41467-020-16924-z
Li, H., Sun, J., Zhu, H., Wu, H., Zhang, H., Gu, Z., et al. (2021). Recent advances in development of dendritic polymer-based nanomedicines for cancer diagnosis. Nanomedicine nanobiotechnology 13 (2), e1670. doi:10.1002/wnan.1670
Li, Q., and Xu, W. (2005). Novel anticancer targets and drug discovery in post genomic age. Anti-cancer agents 5 (1), 53–63. doi:10.2174/1568011053352631
Li, X., Ta, W., Hua, R., Song, J., and Lu, W. (2022). A review on increasing the targeting of PAMAM as carriers in glioma therapy. Biomedicines 10 (10), 2455. doi:10.3390/biomedicines10102455
Lim, J., Guan, B., Nham, K., Hao, G., Sun, X., and Simanek, E. E. (2019). Tumor uptake of triazine dendrimers decorated with four, sixteen, and sixty-four PSMA-targeted ligands: Passive versus active tumor targeting. Biomolecules 9 (9), 421. doi:10.3390/biom9090421
Lim, J., and Simanek, E. E. (2012). Triazine dendrimers as drug delivery systems: From synthesis to therapy. Adv. drug Deliv. Rev. 64 (9), 826–835. doi:10.1016/j.addr.2012.03.008
Liu, X., Rocchi, P., and Peng, L. (2012). Dendrimers as non-viral vectors for siRNA delivery. New J. Chem. 36 (2), 256–263. doi:10.1039/c1nj20408d
Lockman, P. R., Oyewumi, M. O., Koziara, J. M., Roder, K. E., Mumper, R. J., and Allen, D. D. (2003). Brain uptake of thiamine-coated nanoparticles. J. Control. release official J. Control. Release Soc. 93 (3), 271–282. doi:10.1016/j.jconrel.2003.08.006
Longmire, M. R., Ogawa, M., Choyke, P. L., and Kobayashi, H. (2014). Dendrimers as high relaxivity MR contrast agents. Wiley Interdiscip. Rev. Nanomedicine nanobiotechnology 6 (2), 155–162. doi:10.1002/wnan.1250
Malik, Y. S., Sheikh, M. A., Xing, Z., Guo, Z., Zhu, X., Tian, H., et al. (2018). Polylysine-modified polyethylenimine polymer can generate genetically engineered mesenchymal stem cells for combinational suicidal gene therapy in glioblastoma. Acta biomater. 80, 144–153. doi:10.1016/j.actbio.2018.09.015
M. Malkoch, and S. G. Gallego (Editors) (2020). Dendrimer Chemistry: Synthetic approaches towards complex architectures (Burlington House, Piccadilly, London, UK: Royal Society of Chemistry).
Maysinger, D., Zhang, Q., and Kakkar, A. (2020). Dendrimers as modulators of brain cells. Mol. (Basel, Switz. 25 (19), 4489. doi:10.3390/molecules25194489
McMahon, M. T., and Bulte, J. W. M. (2018). Two decades of dendrimers as versatile MRI agents: A tale with and without metals. Wiley Interdiscip. Rev. Nanomedicine nanobiotechnology 10 (3), e1496. doi:10.1002/wnan.1496
Merkel, O. M., Mintzer, M. A., Librizzi, D., Samsonova, O., Dicke, T., Sproat, B., et al. (2010). Triazine dendrimers as nonviral vectors for in vitro and in vivo RNAi: The effects of peripheral groups and core structure on biological activity. Mol. Pharm. 7 (4), 969–983. doi:10.1021/mp100101s
Mignani, S., Bignon, J., Shi, X., and Majoral, J. P. (2021). First-in-Class phosphorus dendritic framework, a wide surface functional group palette bringing noteworthy anti-cancer and anti-tuberculosis activities: What lessons to learn? Mol. (Basel, Switz. 26 (12), 3708. doi:10.3390/molecules26123708
Mignani, S., Shi, X., Bryszewska, M., Shcharbin, D., and Majoral, J. P. (2022). Engineered phosphorus dendrimers as powerful non-viral nanoplatforms for gene delivery: A great hope for the future of cancer therapeutics. Explor. Target. anti-tumor Ther. 3 (1), 50–61. doi:10.37349/etat.2022.00071
Mignani, S., Shi, X., Ceña, V., Shcharbin, D., Bryszewska, M., and Majoral, J. P. (2021). In vivo therapeutic applications of phosphorus dendrimers: State of the art. Drug Discov. today 26 (3), 677–689. doi:10.1016/j.drudis.2020.11.034
Mittal, P., Saharan, A., Verma, R., Altalbawy, F. M. A., Alfaidi, M. A., Batiha, G. E., et al. (2021). Dendrimers: A new race of pharmaceutical nanocarriers. BioMed Res. Int. 2021, 8844030. doi:10.1155/2021/8844030
Mousavifar, L., and Roy, R. (2021). Design, synthetic strategies, and therapeutic applications of heterofunctional glycodendrimers. Mol. (Basel, Switz. 26 (9), 2428. doi:10.3390/molecules26092428
Naeini, A. T., Adeli, M., and Vossoughi, M. (2010). Poly(citric acid)-block-poly(ethylene glycol) copolymers--new biocompatible hybrid materials for nanomedicine. Nanomedicine Nanotechnol. Biol. Med. 6 (4), 556–562. doi:10.1016/j.nano.2009.11.008
Namazi, H., Hashemipour, S. S., and Toomari, Y. (2017). Synthesis of citric-acid-based dendrimers decorated with ferrocenyl groups and investigation of their electroactivity. Polym. Bull. 74, 3783–3796. doi:10.1007/s00289-017-1930-5
Namazi, H., Motamedi, S., and Namvari, M. (2011). Synthesis of new functionalized citric acid-based dendrimers as nanocarrier agents for drug delivery. BioImpacts BI 1 (1), 63–69. doi:10.5681/bi.2011.009
Namazi, H., and Toomari Hamrahloo, Y. (2011). Novel PH sensitive nanocarrier agents based on citric acid dendrimers containing conjugated β-cyclodextrins. Adv. Pharm. Bull. 1 (1), 40–47. doi:10.5681/apb.2011.006
Nangare, S., Vispute, Y., Tade, R., Dugam, S., and Patil, P. (2021). Pharmaceutical applications of citric acid. Future J. Pharm. Sci. 7 (1), 54–23. doi:10.1186/s43094-021-00203-9
Noriega-Luna, B., Godínez, L. A., Rodríguez, F. J., Rodríguez, A., Larrea, G. Z. L. D., Sosa-Ferreyra, C. F., et al. (2014). Applications of dendrimers in drug delivery agents, diagnosis, therapy, and detection. J. Nanomater., 2014, 1–19. doi:10.1155/2014/507273
Noske, S., Karimov, M., Aigner, A., and Ewe, A. (2020). Tyrosine-modification of polypropylenimine (PPI) and polyethylenimine (PEI) strongly improves efficacy of siRNA-mediated gene knockdown. Nanomater. (Basel, Switz. 10 (9), 1809. doi:10.3390/nano10091809
Oliveira, J. M., Salgado, A. J., Sousa, N., Mano, J. F., and Reis, R. L. (2010). Dendrimers and derivatives as a potential therapeutic tool in regenerative medicine strategies—a review. Prog. Polym. Sci. 35 (9), 1163–1194. doi:10.1016/j.progpolymsci.2010.04.006
Ooya, T., and Lee, J. (2022). Hydrotropic hydrogels prepared from polyglycerol dendrimers: Enhanced solubilization and release of paclitaxel. Gels (Basel, Switz. 8 (10), 614. doi:10.3390/gels8100614
Ostrom, Q. T., Cioffi, G., Waite, K., Kruchko, C., and Barnholtz-Sloan, J. S. (2021). CBTRUS statistical report: Primary brain and other central nervous system tumors diagnosed in the United States in 2014-2018. Neuro-oncology 23 (2), iii1–iii105. doi:10.1093/neuonc/noab200
Patel, H. K., Gajbhiye, V., Kesharwani, P., and Jain, N. K. (2016). Ligand anchored poly(propyleneimine) dendrimers for brain targeting: Comparative in vitro and in vivo assessment. J. colloid interface Sci. 482, 142–150. doi:10.1016/j.jcis.2016.07.047
Patel, S. K., Gajbhiye, V., and Jain, N. K. (2012). Synthesis, characterization and brain targeting potential of paclitaxel loaded thiamine-PPI nanoconjugates. J. drug Target. 20 (10), 841–849. doi:10.3109/1061186X.2012.719231
Pedziwiatr-Werbicka, E., Milowska, K., Dzmitruk, V., Ionov, M., Shcharbin, D., and Bryszewska, M. (2019). Dendrimers and hyperbranched structures for biomedical applications. Eur. Polym. J. 119, 61–73. doi:10.1016/j.eurpolymj.2019.07.013
Perisé-Barrios, A. J., Gómez, R., Corbí, A. L., de la Mata, J., Domínguez-Soto, A., and Muñoz-Fernandez, M. A. (2015). Use of carbosilane dendrimer to switch macrophage polarization for the acquisition of antitumor functions. Nanoscale 7 (9), 3857–3866. doi:10.1039/c4nr04038d
Perumal, O. P., Inapagolla, R., Kannan, S., and Kannan, R. M. (2008). The effect of surface functionality on cellular trafficking of dendrimers. Biomaterials 29 (24-25), 3469–3476. doi:10.1016/j.biomaterials.2008.04.038
Pisoschi, A. M., Pop, A., Iordache, F., Stanca, L., Predoi, G., and Serban, A. I. (2021). Oxidative stress mitigation by antioxidants - an overview on their chemistry and influences on health status. Eur. J. Med. Chem. 209, 112891. doi:10.1016/j.ejmech.2020.112891
Posadas, I., Romero-Castillo, L., Ronca, R. A., Karpus, A., Mignani, S., Majoral, J. P., et al. (2022). Engineered neutral phosphorous dendrimers protect mouse cortical neurons and brain organoids from excitotoxic death. Int. J. Mol. Sci. 23 (8), 4391. doi:10.3390/ijms23084391
Puri, A., Loomis, K., Smith, B., Lee, J. H., Yavlovich, A., Heldman, E., et al. (2009). Lipid-based nanoparticles as pharmaceutical drug carriers: From concepts to clinic. Crit. Rev. Ther. drug Carr. Syst. 26 (6), 523–580. doi:10.1615/critrevtherdrugcarriersyst.v26.i6.10
Qiu, J., Chen, L., Zhan, M., Laurent, R., Bignon, J., Mignani, S., et al. (2021). Facile synthesis of amphiphilic fluorescent phosphorus dendron-based micelles as antiproliferative agents: First investigations. Bioconjugate Chem. 32 (2), 339–349. doi:10.1021/acs.bioconjchem.0c00716
Rabiee, N., Ahmadvand, S., Ahmadi, S., Fatahi, Y., Dinarvand, R., Bagherzadeh, M., et al. (2020). Carbosilane dendrimers: Drug and gene delivery applications. J. Drug Deliv. Sci. Technol. 59, 101879. doi:10.1016/j.jddst.2020.101879
Rabiee, N., Ahmadvand, S., Ahmadi, S., Fatahi, Y., Dinarvand, R., Bagherzadeh, M., et al. (2020). Carbosilane dendrimers: Drug and gene delivery applications. J. Drug Deliv. Sci. Technol. 59, 101879. doi:10.1016/j.jddst.2020.101879
Rabiee, N., Ahmadvand, S., Ahmadi, S., Fatahi, Y., Dinarvand, R., Bagherzadeh, M., et al. (2020). Carbosilane dendrimers: Drug and gene delivery applications. J. Drug Deliv. Sci. Technol. 59, 101879. doi:10.1016/j.jddst.2020.101879
Rahman, H. S., Othman, H. H., Hammadi, N. I., Yeap, S. K., Amin, K. M., Abdul Samad, N., et al. (2020). Novel drug delivery systems for loading of natural plant extracts and their biomedical applications. Int. J. nanomedicine 15, 2439–2483. doi:10.2147/IJN.S227805
Rasouli, R., Zaaeri, F., Rajabi, A. B., Darbandi-Azar, A., Faridi-Majidi, R., and Ardestani, M. S. (2021). 99mTc-anionic linear globular dendrimer-G2-phenylalanine conjugate: Novel brain tumor SPECT imaging. Biointerface Res. Appl. Chem. 11 (4), 11244–11255.
Rodríguez-Galván, A., Rivera, M., García-López, P., Medina, L. A., and Basiuk, V. A. (2020). Gadolinium-containing carbon nanomaterials for magnetic resonance imaging: Trends and challenges. J. Cell. Mol. Med. 24 (7), 3779–3794. doi:10.1111/jcmm.15065
Rodríguez-Prieto, T., Barrios-Gumiel, A., de la Mata, F. J., Sanchez-Nieves, J., and Gomez, R. (2016). Synthesis of degradable cationic carbosilane dendrimers based on Si–O or ester bonds. Tetrahedron 72 (39), 5825–5830. doi:10.1016/j.tet.2016.07.084
Rolland, O., Griffe, L., Poupot, M., Maraval, A., Ouali, A., Coppel, Y., et al. (2008). Tailored control and optimisation of the number of phosphonic acid termini on phosphorus-containing dendrimers for the ex-vivo activation of human monocytes. Chem. (Weinheim der Bergstrasse, Ger. 14 (16), 4836–4850. doi:10.1002/chem.200701063
Roy, R., and Baek, M. G. (2002). Glycodendrimers: Novel glycotope isosteres unmasking sugar coding. Case study with T-antigen markers from breast cancer MUC1 glycoprotein. J. Biotechnol. 90 (3-4), 291–309. doi:10.1016/s1389-0352(01)00065-4
Roy, R., Shiao, T. C., and Rittenhouse-Olson, K. (2013). Glycodendrimers: Versatile tools for nanotechnology. Braz. J. Pharm. Sci. 49, 85–108. doi:10.1590/s1984-82502013000700008
Ryan, G. M., Kaminskas, L. M., Bulitta, J. B., McIntosh, M. P., Owen, D. J., and Porter, C. J. H. (2013). PEGylated polylysine dendrimers increase lymphatic exposure to doxorubicin when compared to PEGylated liposomal and solution formulations of doxorubicin. J. Control. release official J. Control. Release Soc. 172 (1), 128–136. doi:10.1016/j.jconrel.2013.08.004
Sacks, P., and Rahman, M. (2020). Epidemiology of brain metastases. Neurosurg. Clin. N. Am. 31 (4), 481–488. doi:10.1016/j.nec.2020.06.001
Sadler, K., and Tam, J. P. (2002). Peptide dendrimers: Applications and synthesis. J. Biotechnol. 90 (3-4), 195–229. doi:10.1016/s1389-0352(01)00061-7
Sahu, R. K., Aboulthana, W. M., and Mehta, D. K. (2021). Phyto-phospholipid complexation as a novel drug delivery dystem for management of cancer with better bioavailability: current perspectives and future prospects. Anticancer Agents Med. Chem. 21 (11), 1403–1412. doi:10.2174/1871520620999201110191741
Saluja, V., Mishra, Y., Mishra, V., Giri, N., and Nayak, P. (2021). Dendrimers based cancer nanotheranostics: An overview. Int. J. Pharm. 600, 120485. doi:10.1016/j.ijpharm.2021.120485
Sanader Maršić, Ž., Maysinger, D., and Bonačić-Kouteckỳ, V. (2021). Insights into interactions between interleukin-6 and dendritic polyglycerols. Int. J. Mol. Sci. 22 (5), 2415. doi:10.3390/ijms22052415
Santos, A., Veiga, F., and Figueiras, A. (2019). Dendrimers as pharmaceutical excipients: Synthesis, properties, toxicity and biomedical applications. Mater. (Basel, Switz. 13 (1), 65. doi:10.3390/ma13010065
Satija, J., Gupta, U., and Jain, N. K. (2007). Pharmaceutical and biomedical potential of surface engineered dendrimers. Crit. Rev. Ther. drug Carr. Syst. 24 (3), 257–306. doi:10.1615/critrevtherdrugcarriersyst.v24.i3.20
Sebestik, J., Reinis, M., and Jezek, J. (2012). Biomedical applications of peptide-, glyco-and glycopeptide dendrimers, and analogous dendrimeric structures. Springer Science and Business Media.
Sepúlveda-Crespo, D., Gómez, R., De La Mata, F. J., Jiménez, J. L., and Muñoz-Fernández, M. Á. (2015). Polyanionic carbosilane dendrimer-conjugated antiviral drugs as efficient microbicides: Recent trends and developments in HIV treatment/therapy. Nanomedicine Nanotechnol. Biol. Med. 11 (6), 1481–1498. doi:10.1016/j.nano.2015.03.008
Serramía, M. J., Álvarez, S., Fuentes-Paniagua, E., Clemente, M. I., Sánchez-Nieves, J., Gómez, R., et al. (2015). In vivo delivery of siRNA to the brain by carbosilane dendrimer. J. Control. release official J. Control. Release Soc. 200, 60–70. doi:10.1016/j.jconrel.2014.12.042
Setia, A., Sahu, R. K., Ray, S., Widyowati, R., Ekasari, W., and Saraf, S. (2022). Advances in hybrid vesicular-based drug delivery systems: improved biocompatibility, targeting, therapeutic efficacy and pharmacokinetics of anticancer drugs. Current drug metabolism 23 (9), 757–780. doi:10.2174/1389200223666220627110049
Sharma, A. K., Gupta, L., Sahu, H., Qayum, A., Singh, S. K., Nakhate, K. T., et al. (2018). Chitosan engineered PAMAM dendrimers as nanoconstructs for the enhanced anti-cancer potential and improved in vivo brain pharmacokinetics of temozolomide. Pharm. Res. 35 (1), 9. doi:10.1007/s11095-017-2324-y
Sharma, A., Sharma, R., Zhang, Z., Liaw, K., Kambhampati, S. P., Porterfield, J. E., et al. (2020). Dense hydroxyl polyethylene glycol dendrimer targets activated glia in multiple CNS disorders. Sci. Adv. 6 (4), eaay8514. doi:10.1126/sciadv.aay8514
Sharma, R., Kim, S. Y., Sharma, A., Zhang, Z., Kambhampati, S. P., Kannan, S., et al. (2017). Activated microglia targeting dendrimer-minocycline conjugate as therapeutics for neuroinflammation. Bioconjugate Chem. 28 (11), 2874–2886. doi:10.1021/acs.bioconjchem.7b00569
Sharma, R., Liaw, K., Sharma, A., Jimenez, A., Chang, M., Salazar, S., et al. (2021). Glycosylation of PAMAM dendrimers significantly improves tumor macrophage targeting and specificity in glioblastoma. J. Control. release official J. Control. Release Soc. 337, 179–192. doi:10.1016/j.jconrel.2021.07.018
Shcharbin, D., Shcharbina, N., Dzmitruk, V., Pedziwiatr-Werbicka, E., Ionov, M., Mignani, S., et al. (2017). Dendrimer-protein interactions versus dendrimer-based nanomedicine. Colloids surfaces. B, Biointerfaces 152, 414–422. doi:10.1016/j.colsurfb.2017.01.041
Shcharbina, N., Shcharbin, D., and Bryszewska, M. (2013). Nanomaterials in stroke treatment: Perspectives. Stroke 44 (8), 2351–2355. doi:10.1161/STROKEAHA.113.001298
She, W., Li, N., Luo, K., Guo, C., Wang, G., Geng, Y., et al. (2013). Dendronized heparin-doxorubicin conjugate based nanoparticle as pH-responsive drug delivery system for cancer therapy. Biomaterials 34 (9), 2252–2264. doi:10.1016/j.biomaterials.2012.12.017
Sheikh, A., and Kesharwani, P. (2021). An insight into aptamer engineered dendrimer for cancer therapy. Eur. Polym. J. 159, 110746. doi:10.1016/j.eurpolymj.2021.110746
Sheikhi Mehrabadi, F., Zeng, H., Johnson, M., Schlesener, C., Guan, Z., and Haag, R. (2015). Multivalent dendritic polyglycerolamine with arginine and histidine end groups for efficient siRNA transfection. Beilstein J. Org. Chem. 11, 763–772. doi:10.3762/bjoc.11.86
Shen, M., Huang, Y., Han, L., Qin, J., Fang, X., Wang, J., et al. (2012). Multifunctional drug delivery system for targeting tumor and its acidic microenvironment. J. Control. release official J. Control. Release Soc. 161 (3), 884–892. doi:10.1016/j.jconrel.2012.05.013
Sherje, A. P., Jadhav, M., Dravyakar, B. R., and Kadam, D. (2018). Dendrimers: A versatile nanocarrier for drug delivery and targeting. Int. J. Pharm. 548 (1), 707–720. doi:10.1016/j.ijpharm.2018.07.030
Shi, X., Bányai, I., Rodriguez, K., Islam, M. T., Lesniak, W., Balogh, P., et al. (2006). Electrophoretic mobility and molecular distribution studies of poly(amidoamine) dendrimers of defined charges. Electrophoresis 27 (9), 1758–1767. doi:10.1002/elps.200500818
Shi, X., Ma, R., Lu, Y., Cheng, Y., Fan, X., Zou, J., et al. (2020). iRGD and TGN co-modified PAMAM for multi-targeted delivery of ATO to gliomas. Biochem. biophysical Res. Commun. 527 (1), 117–123. doi:10.1016/j.bbrc.2020.04.064
Sideratou, Z., Tsiourvas, D., and Paleos, C. M. (2001). Solubilization and release properties of PEGylated diaminobutane poly (propylene imine) dendrimers. J. colloid interface Sci. 242 (1), 272–276. doi:10.1006/jcis.2001.7787
Simanek, E. E. (2021). Two decades of triazine dendrimers. Mol. (Basel, Switz. 26 (16), 4774. doi:10.3390/molecules26164774
Singh, A., Ansari, V. A., Mahmood, T., Ahsan, F., and Wasim, R. (2022). Dendrimers: A neuroprotective lead in alzheimer disease: A review on its synthetic approach and applications. Drug Res. 72 (8), 417–423. doi:10.1055/a-1886-3208
Singh, A. K., Gothwal, A., Rani, S., Rana, M., Sharma, A. K., Yadav, A. K., et al. (2019). Dendrimer donepezil conjugates for improved brain delivery and better in vivo pharmacokinetics. ACS Omega 4 (3), 4519–4529. doi:10.1021/acsomega.8b03445
Singh, M. K., Kuncha, M., Nayak, V. L., Sarma, A. V. S., Kumar, M. J. M., Chauhan, A. S., et al. (2019). An innovative in situ method of creating hybrid dendrimer nano-assembly: An efficient next generation dendritic platform for drug delivery. Nanomedicine Nanotechnol. Biol. Med. 21, 102043. doi:10.1016/j.nano.2019.102043
Sk, U. H., Dixit, D., and Sen, E. (2013). Comparative study of microtubule inhibitors--estramustine and natural podophyllotoxin conjugated PAMAM dendrimer on glioma cell proliferation. Eur. J. Med. Chem. 68, 47–57. doi:10.1016/j.ejmech.2013.07.007
Somani, S., Blatchford, D. R., Millington, O., Stevenson, M. L., and Dufès, C. (2014). Transferrin-bearing polypropylenimine dendrimer for targeted gene delivery to the brain. J. Control. release official J. Control. Release Soc., 188, 78–86. doi:10.1016/j.jconrel.2014.06.006
Sonawane, N. D., Szoka, F. C., and Verkman, A. S. (2003). Chloride accumulation and swelling in endosomes enhances DNA transfer by polyamine-DNA polyplexes. J. Biol. Chem. 278 (45), 44826–44831. doi:10.1074/jbc.M308643200
Song, N., Zhao, L., Xu, X., Zhu, M., Liu, C., Sun, N., et al. (2020). LyP-1-Modified multifunctional dendrimers for targeted antitumor and antimetastasis therapy. ACS Appl. Mater. interfaces 12 (11), 12395–12406. doi:10.1021/acsami.9b18881
Sowińska, M., Szeliga, M., Morawiak, M., Zabłocka, B., and Urbanczyk-Lipkowska, Z. (2022). Design, synthesis and activity of new N1-alkyl tryptophan functionalized dendrimeric peptides against glioblastoma. Biomolecules 12 (8), 1116. doi:10.3390/biom12081116
Stalmans, S., Wynendaele, E., Bracke, N., Knappe, D., Hoffmann, R., Peremans, K., et al. (2014). Blood-brain barrier transport of short proline-rich antimicrobial peptides. Protein peptide Lett. 21 (4), 399–406. doi:10.2174/09298665113206660110
Stine, Z. E., Schug, Z. T., Salvino, J. M., and Dang, C. V. (2022). Targeting cancer metabolism in the era of precision oncology. Nat. Rev. Drug Discov. 21 (2), 141–162. doi:10.1038/s41573-021-00339-6
Strašák, T., Malý, J., Wróbel, D., Malý, M., Herma, R., Čermák, J., et al. (2017). Phosphonium carbosilane dendrimers for biomedical applications–synthesis, characterization and cytotoxicity evaluation. Rsc Adv. 7 (30), 18724–18744. doi:10.1039/c7ra01845b
Subbarayan, M., Shetty, S. J., Srivastava, T. S., Noronha, O. P., Samuel, A. M., and Mukhtar, H. (2001). Water-soluble 99mTc-labeled dendritic novel porphyrins tumor imaging and diagnosis. Biochem. biophysical Res. Commun. 281 (1), 32–36. doi:10.1006/bbrc.2001.4289
Sun, T., Jiang, X., Wang, Q., Chen, Q., Lu, Y., Liu, L., et al. (2017). Substance P mediated DGLs complexing with DACHPt for targeting therapy of glioma. ACS Appl. Mater. interfaces 9 (40), 34603–34617. doi:10.1021/acsami.7b05997
Svenson, S., and Tomalia, D. A. (2005). Dendrimers in biomedical applications--reflections on the field. Adv. drug Deliv. Rev. 57 (15), 2106–2129. doi:10.1016/j.addr.2005.09.018
Swami, R., Singh, I., Kulhari, H., Jeengar, M. K., Khan, W., and Sistla, R. (2015). p-Hydroxy benzoic acid-conjugated dendrimer nanotherapeutics as potential carriers for targeted drug delivery to brain: an in vitro and in vivo evaluation. J. Nanoparticle Res. 17, 1–11. doi:10.1007/s11051-015-3063-9
Tadros, S., and Ray-Chaudhury, A. (2020). Pathological features of brain metastases. Neurosurg. Clin. N. Am. 31 (4), 549–564. doi:10.1016/j.nec.2020.06.005
Tan, A. C., Ashley, D. M., López, G. Y., Malinzak, M., Friedman, H. S., and Khasraw, M. (2020). Management of glioblastoma: State of the art and future directions. CA a cancer J. Clin. 70 (4), 299–312. doi:10.3322/caac.21613
Tarach, P., and Janaszewska, A. (2021). Recent advances in preclinical research using PAMAM dendrimers for cancer gene therapy. Int. J. Mol. Sci. 22 (6), 2912. doi:10.3390/ijms22062912
Teow, H. M., Zhou, Z., Najlah, M., Yusof, S. R., Abbott, N. J., and D'Emanuele, A. (2013). Delivery of paclitaxel across cellular barriers using a dendrimer-based nanocarrier. Int. J. Pharm. 441 (1-2), 701–711. doi:10.1016/j.ijpharm.2012.10.024
Thakur, S., Kesharwani, P., Tekade, R. K., and Jain, N. K. (2015). Impact of pegylation on biopharmaceutical properties of dendrimers. Polymer 59, 67–92. doi:10.1016/j.polymer.2014.12.051
Thompson, M., and Scholz, C. (2021). Highly branched polymers based on poly(amino acid)s for biomedical application. Nanomater. (Basel, Switz. 11 (5), 1119. doi:10.3390/nano11051119
Tomalia, D. A., Nixon, L. S., and Hedstrand, D. M. (2020). The role of branch cell symmetry and other critical nanoscale design parameters in the determination of dendrimer encapsulation properties. Biomolecules 10 (4), 642. doi:10.3390/biom10040642
Truskewycz, A., Yin, H., Halberg, N., Lai, D. T. H., Ball, A. S., Truong, V. K., et al. (2022). Carbon dot therapeutic platforms: Administration, distribution, metabolism, excretion, toxicity, and therapeutic potential. Small (Weinheim der Bergstrasse, Ger. 18 (16), e2106342. doi:10.1002/smll.202106342
Uchida, H., Kabe, Y., Yoshino, K., Kawamata, A., Tsumuraya, T., and Masamune, S. (1990). General strategy for the systematic synthesis of oligosiloxanes. Silicone dendrimers. J. Am. Chem. Soc. 112 (19), 7077–7079. doi:10.1021/ja00175a062
Ulbrich, K., Knobloch, T., and Kreuter, J. (2011). Targeting the insulin receptor: Nanoparticles for drug delivery across the blood-brain barrier (BBB). J. drug Target. 19 (2), 125–132. doi:10.3109/10611861003734001
Vannucci, L., Lai, M., Chiuppesi, F., Ceccherini-Nelli, L., and Pistello, M. (2013). Viral vectors: A look back and ahead on gene transfer technology. new Microbiol. 36 (1), 1–22.
Vasconcelos-Ferreira, A., Carmo-Silva, S., Codêsso, J. M., Silva, P., Martinez, A. R. M., França, M. C., et al. (2022). The autophagy-enhancing drug carbamazepine improves neuropathology and motor impairment in mouse models of Machado-Joseph disease. Neuropathology Appl. Neurobiol. 48 (1), e12763. doi:10.1111/nan.12763
Walter, M. V., and Malkoch, M. (2012). Simplifying the synthesis of dendrimers: Accelerated approaches. Chem. Soc. Rev. 41 (13), 4593–4609. doi:10.1039/c2cs35062a
Wang, F., Cai, X., Su, Y., Hu, J., Wu, Q., Zhang, H., et al. (2012). Reducing cytotoxicity while improving anti-cancer drug loading capacity of polypropylenimine dendrimers by surface acetylation. Acta biomater. 8 (12), 4304–4313. doi:10.1016/j.actbio.2012.07.031
Wang, K., Zhang, X., Liu, Y., Liu, C., Jiang, B., and Jiang, Y. (2014). Tumor penetrability and anti-angiogenesis using iRGD-mediated delivery of doxorubicin-polymer conjugates. Biomaterials 35 (30), 8735–8747. doi:10.1016/j.biomaterials.2014.06.042
Wasiak, T., Ionov, M., Nieznanski, K., Nieznanska, H., Klementieva, O., Granell, M., et al. (2012). Phosphorus dendrimers affect Alzheimer's (Aβ1-28) peptide and MAP-Tau protein aggregation. Mol. Pharm. 9 (3), 458–469. doi:10.1021/mp2005627
Woller, E. K., and Cloninger, M. J. (2001). Mannose functionalization of a sixth generation dendrimer. Biomacromolecules 2 (3), 1052–1054. doi:10.1021/bm015560k
Wu, G., Barth, R. F., Yang, W., Kawabata, S., Zhang, L., and Green-Church, K. (2006). Targeted delivery of methotrexate to epidermal growth factor receptor-positive brain tumors by means of cetuximab (IMC-C225) dendrimer bioconjugates. Mol. cancer Ther. 5 (1), 52–59. doi:10.1158/1535-7163.MCT-05-0325
Wu, M., Liu, Q., Yan, C., and Sen, G. (2022). Multi-Classification of brain tumors on magnetic resonance images using an ensemble of pre-trained convolutional neural networks. Curr. Med. imaging. Advance online publication. doi:10.2174/1573405618666220415122843
Xie, F., Li, R., Shu, W., Zhao, L., and Wan, J. (2022). Self-assembly of Peptide dendrimers and their bio-applications in theranostics. Bio 14, 100239. doi:10.1016/j.mtbio.2022.100239
Xu, L., Zhang, H., and Wu, Y. (2014). Dendrimer advances for the central nervous system delivery of therapeutics. ACS Chem. Neurosci. 5 (1), 2–13. doi:10.1021/cn400182z
Xu, X., Li, J., Han, S., Tao, C., Fang, L., Sun, Y., et al. (2016). A novel doxorubicin loaded folic acid conjugated PAMAM modified with borneol, a nature dual-functional product of reducing PAMAM toxicity and boosting BBB penetration. Eur. J. Pharm. Sci. official J. Eur. Fed. Pharm. Sci. 88, 178–190. doi:10.1016/j.ejps.2016.02.015
Xu, X., Liu, K., Wang, Y., Zhang, C., Shi, M., Wang, P., et al. (2019). A multifunctional low-generation dendrimer-based nanoprobe for the targeted dual mode MR/CT imaging of orthotopic brain gliomas. J. Mater. Chem. B 7 (23), 3639–3643. doi:10.1039/c9tb00416e
Yang, J., Luo, Y., Xu, Y., Li, J., Zhang, Z., Wang, H., et al. (2015). Conjugation of iron oxide nanoparticles with RGD-modified dendrimers for targeted tumor MR imaging. ACS Appl. Mater. interfaces 7 (9), 5420–5428. doi:10.1021/am508983n
Yang, S. K., Shi, X., Park, S., Ha, T., and Zimmerman, S. C. (2013). A dendritic single-molecule fluorescent probe that is monovalent, photostable and minimally blinking. Nat. Chem. 5 (8), 692–697. doi:10.1038/nchem.1706
Yazdan, S. A., Ahmad, R., Iqbal, N., Rizwan, A., Khan, A. N., and Kim, D. H. (2022). An efficient multi-scale convolutional neural network based multi-class brain MRI classification for SaMD. Tomogr. Ann. Arbor. Mich.) 8 (4), 1905–1927. doi:10.3390/tomography8040161
Yeini, E., Ofek, P., Albeck, N., Rodriguez Ajamil, D., Neufeld, L., Eldar-Boock, A., et al. (2021). Targeting glioblastoma: Advances in drug delivery and novel therapeutic approaches. Adv. Ther. 4 (1), 2000124. doi:10.1002/adtp.202000124
Zaal, E. A., and Berkers, C. R. (2018). The influence of metabolism on drug response in cancer. Front. Oncol. 8, 500. doi:10.3389/fonc.2018.00500
Zarebkohan, A., Najafi, F., Moghimi, H. R., Hemmati, M., Deevband, M. R., and Kazemi, B. (2016). SRL-coated PAMAM dendrimer nano-carrier for targeted gene delivery to the glioma cells and competitive inhibition by lactoferrin. Iran. J. Pharm. Res. IJPR 15 (4), 629–640.
Zhang, L., Zhu, S., Qian, L., Pei, Y., Qiu, Y., and Jiang, Y. (2011). RGD-Modified PEG-PAMAM-DOX conjugates: In vitro and in vivo studies for glioma. Eur. J. Pharm. Biopharm. official J. Arbeitsgemeinschaft fur Pharmazeutische Verfahrenstechnik e.V 79 (2), 232–240. doi:10.1016/j.ejpb.2011.03.025
Zhang, W., Dhumal, D., Zhu, X., Ralahy, B., Ellert-Miklaszewska, A., Wu, J., et al. (2022). Bola-amphiphilic glycodendrimers: New carbohydrate-mimicking scaffolds to target carbohydrate-binding proteins. Chem. (Weinheim der Bergstrasse, Ger. 28 (58), e202201400. doi:10.1002/chem.202201400
Zhao, J., Zhang, B., Shen, S., Chen, J., Zhang, Q., Jiang, X., et al. (2015). CREKA peptide-conjugated dendrimer nanoparticles for glioblastoma multiforme delivery. J. colloid interface Sci. 450, 396–403. doi:10.1016/j.jcis.2015.03.019
Zhao, L., Zhu, J., Cheng, Y., Xiong, Z., Tang, Y., Guo, L., et al. (2015). Chlorotoxin-conjugated multifunctional dendrimers labeled with radionuclide 131I for single photon emission computed tomography imaging and radiotherapy of gliomas. ACS Appl. Mater. interfaces 7 (35), 19798–19808. doi:10.1021/acsami.5b05836
Zhao, Y., Yue, P., Peng, Y., Sun, Y., Chen, X., Zhao, Z., et al. (2023). Recent advances in drug delivery systems for targeting brain tumors. Drug Deliv. 30 (1), 1–18. doi:10.1080/10717544.2022.2154409
Zhao, Y., Zhao, Z., Cui, Y., Chen, X., Chen, C., Xie, C., et al. (2021). Redox-responsive glycosylated combretastatin A-4 derivative as novel tubulin polymerization inhibitor for glioma and drug delivery. Drug Dev. Res. 82 (7), 1063–1072. doi:10.1002/ddr.21889
Zhou, L. L., and Roovers, J. (1993). Synthesis of novel carbosilane dendritic macromolecules. Macromolecules 26 (5), 963–968. doi:10.1021/ma00057a013
Zhu, Y., Liu, C., and Pang, Z. (2019). Dendrimer-based drug delivery systems for brain targeting. Biomolecules 9 (12), 790. doi:10.3390/biom9120790
Keywords: dendrimers, brain tumor, brain tumor imaging, brain targeting, theranostics
Citation: Kaurav M, Ruhi S, Al-Goshae HA, Jeppu AK, Ramachandran D, Sahu RK, Sarkar AK, Khan J and Ashif Ikbal AM (2023) Dendrimer: An update on recent developments and future opportunities for the brain tumors diagnosis and treatment. Front. Pharmacol. 14:1159131. doi: 10.3389/fphar.2023.1159131
Received: 05 February 2023; Accepted: 27 February 2023;
Published: 16 March 2023.
Edited by:
Sankha Bhattacharya, SVKM’s Narsee Moonjee Institute of Management and Studies (NMIMS), IndiaReviewed by:
Yihenew Simegniew Birhan, Debre Markos University, EthiopiaPriya Singh, Babasaheb Bhimrao Ambedkar University, India
Copyright © 2023 Kaurav, Ruhi, Al-Goshae, Jeppu, Ramachandran, Sahu, Sarkar, Khan and Ashif Ikbal. This is an open-access article distributed under the terms of the Creative Commons Attribution License (CC BY). The use, distribution or reproduction in other forums is permitted, provided the original author(s) and the copyright owner(s) are credited and that the original publication in this journal is cited, in accordance with accepted academic practice. No use, distribution or reproduction is permitted which does not comply with these terms.
*Correspondence: Ram Kumar Sahu, cmFtc2FodTc5QGdtYWlsLmNvbQ==