- Dongfang Hospital of Beijing University of Chinese Medicine, Beijing, China
Alzheimer’s Disease (AD) is a global chronic disease in adults with beta-amyloid (Aβ) deposits and hyperphosphorylated tau protein as the pathologic characteristics. Although the exact etiology of AD is still not fully elucidated, aberrant metabolism including insulin signaling and mitochondria dysfunction plays an important role in the development of AD. Binding to insulin receptor substrates, insulin can transport through the blood-brain barrier (BBB), thus mediating insulin signaling pathways to regulate physiological functions. Impaired insulin signaling pathways, including PI3K/Akt/GSK3β and MAPK pathways, could cause damage to the brain in the pathogenesis of AD. Mitochondrial dysfunction and overexpression of TXNIP could also be causative links between AD and DM. Some antidiabetic medicines may have benefits in the treatment of AD. Metformin can be beneficial for cognition improvement in AD patients, although results from clinical trials were inconsistent. Exendin-4 may affect AD in animal models but there is a lack of clinical trials. Liraglutide and dulaglutide could also benefit AD patients in adequate clinical studies but not semaglutide. Dipeptidyl peptidase IV inhibitors (DPP4is) such as saxagliptin, vildagliptin, linagliptin, and sitagliptin could boost cognitive function in animal models. And SGLT2 inhibitors such as empagliflozin and dapagliflozin were also considerably protective against new-onset dementia in T2DM patients. Insulin therapy is a promising therapy but some studies indicated that it may increase the risk of AD. Herbal medicines are helpful for cognitive function and neuroprotection in the brain. For example, polyphenols, alkaloids, glycosides, and flavonoids have protective benefits in cognition function and glucose metabolism. Focusing on glucose metabolism, we summarized the pharmacological mechanism of hypoglycemic drugs and herbal medicines. New treatment approaches including antidiabetic synthesized drugs and herbal medicines would be provided to patients with AD. More clinical trials are needed to produce definite evidence for the effectiveness of hypoglycemic medications.
1 Introduction
Alzheimer’s disease (AD) is clinically manifested as cognitive decline and memory impairment (Reitz and Mayeux, 2014; Liu et al., 2019). It is one of the leading causes of global mortality and the most common type of dementia worldwide (Chornenkyy et al., 2019; Kumar et al., 2022b). And the prevalence of AD has been increasing steadily over the past decades (Nichols et al., 2019). The major pathological characteristics of AD are beta-amyloid (Aβ) deposits and intracellular neurofibrillary tangles (NFTs) formed by hyperphosphorylated tau protein (Grundke-Iqbal et al., 1986; Selkoe and Schenk, 2003). However, a full understanding of the mechanism is still lacking (Kumar et al., 2022a; Kumar et al., 2022b). Currently, there are still no specific drugs, despite the increasing incidence of AD and the social problems it poses (Moran et al., 2019; Srivastava et al., 2021; Michailidis et al., 2022).
Studies have demonstrated that multiple factors are associated with the onset of AD (Exalto et al., 2013; Norton et al., 2014; Andrews et al., 2021), which can be broadly classified into two categories: non-metabolic and metabolic. The non-metabolic ones include age, gender (Rahman et al., 2020), depression (Barnes and Yaffe, 2011; Feinkohl et al., 2015; Biessels and Whitmer, 2020; Srikanth et al., 2020), smoking (Barnes and Yaffe, 2011; Baumgart et al., 2015), low level of education (Exalto et al., 2013), physical inactivity (Rolandi et al., 2020), stress (Dye et al., 2017), sleep disorders (Flolingue et al., 2018), and environmental factors (Paul et al., 2018). A large amount of literature has indicated that metabolic factors such as obesity (Gendron et al., 2013; Dye et al., 2017), hyperglycemia, hypertension, and dyslipidemia (Launer, 2002; Biessels and Despa, 2018) are associated with cognitive decline.
Hyperglycemia, the main characteristic of Diabetes Mellitus (DM), is a critical metabolic risk factor in the development of AD. Researches show that DM is correlated with the development of AD (Ott et al., 1999; Butterfield et al., 2014; Natunen et al., 2020; Chae et al., 2022). T2DM and AD share common pathogenesis associated with aberrant glucose metabolism, including insulin resistance, oxidative stress, and inflammation (De Felice and Ferreira, 2014; Rosales-Corral et al., 2015; Baglietto-Vargas et al., 2016; Pugazhenthi et al., 2017; Barone et al., 2021). DM could be responsible for the progression of not only peripheral nervous system diseases but central nervous system (CNS) diseases. Abnormal metabolism in the brain is regarded as one of the main features of AD, including impaired glucose metabolism (Jack et al., 2013; Kumar et al., 2022b; González et al., 2022) and the imbalance of energy metabolism (Akhtar et al., 2016; Carvalho and Cardoso, 2021; González et al., 2022). This may eventually lead to brain lesions through a series of impaired metabolic pathways (Kellar and Craft, 2020; Michailidis et al., 2022). Dysfunction of metabolism in DM may result in neurodegeneration in the brain (Patrone et al., 2014), taking the form of Aβ accumulation and tau hyperphosphorylation as the hallmarks of AD (Grundke-Iqbal et al., 1986; Selkoe and Schenk, 2003) which is accompanied by synaptic dysfunction, neurogenesis, and neuronal disorders (Michailidis et al., 2022).
New strategies should be explored to treat AD based on its pathogenesis. Some hypoglycemic drugs that correct glucose metabolism disorders may promote brain metabolism and regeneration, and significantly improve memory and cognition (Patrone et al., 2014). Studies find that hypoglycemic drugs like Metformin, Dipeptidyl peptidase IV inhibitors (DPP4is) and glucagon-like peptide-1 (GLP-1) receptor agonists (Ras), sodium-glucose cotransporter 2 inhibitors (SGLT2is) are effective in treating Alzheimer’s disease by regulating insulin pathways and energy metabolism. Botanical medicines (Thota et al., 2020) also play an important role in regulating blood sugar and improving cognition in the treatment of both AD and DM (Ebrahimpour et al., 2020; Thota et al., 2020).
This article summarized the pathogenic relationship of AD and abnormal glucose metabolism to explain the pharmacological effects of hypoglycemic drugs and herbal medicine on AD, and aimed to provide new ideas for the treatment of AD.
2 Glucose metabolism and AD
Glucose homeostasis is critical to the prevention of AD. One of the tipping points for late-onset AD risk in midlife aging may be metabolic shifts (Mishra et al., 2022). The decreased glucose level in the cerebrospinal fluid (CSF) and brain can cause synaptic inactivity, which increases the chance of cognitive impairment and AD (He et al., 2022). In preclinical AD, decreased aerobic glycolysis is correlated with tau deposition (Vlassenko et al., 2018). Reduced glucose metabolism is often shown in the brains of AD patients, particularly in hippocampal and cortical regions (Wang W. et al., 2020). In post-mortem tissues of AD, the increased Blood-Brain Barrier (BBB) permeability and impaired glucose transport are observed (Sweeney et al., 2018b), and cerebral blood flow shortfall and dysfunction of the BBB may be responsible for AD (Sweeney et al., 2018a). In diabetes, high glucose levels in the plasma and other metabolic abnormalities such as hyperinsulinemia can damage the BBB and then cause hyperglycemia in CSF (Nigrovic et al., 2012; Bogush et al., 2017; Arnold et al., 2018), which can subsequently contribute to impaired insulin signaling, mitochondria dysfunction, oxidative stress, and inflammation in the brain (Szendroedi et al., 2011).
2.1 Insulin signaling in AD
The brain is an insulin-sensitive organ (Milstein and Ferris, 2021). Insulin plays an important physiological role in the brain except for glucose homeostasis (Duarte et al., 2012). Although the idea of synthesis of insulin in the brain is controversial (Arnold et al., 2018), it is now widely accepted that peripheral insulin can cross the BBB (Banks, 2004; Heneka and Nicotera, 2016) and bind to insulin receptors which are commonly distributed in the brain (Coll et al., 2007). Insulin signaling is involved in neuronal growth, altering synaptic plasticity (Kleinridders et al., 2014), and regulating neurotransmission (Cai W. et al., 2018), thus participating in learning, memory, and emotional functions of the brain (Roden and Shulman, 2019) by triggering the two canonical signaling cascades, the Mitogen-Activated Protein Kinase (MAPK) pathway and Akt signaling pathway (Sims-Robinson et al., 2010; Stanley et al., 2016; Arnold et al., 2018).
The abnormalities of insulin levels and insulin signaling can be significant for the onset of AD (de la Monte, 2017). In a genome-wide association study, AD was found to be correlated with insulin-related genes (Fanelli et al., 2022). Emerging evidence has demonstrated that peripheral insulin resistance is closely related to declined cerebral glucose metabolism and is a risk factor for AD in middle-aged adults (Willette et al., 2015; Femminella et al., 2021). Besides hyperinsulinemia, insulin deficiency in the plasma is involved in AD development (van der Harg et al., 2017; Imamura et al., 2020). Furthermore, disturbed insulin signaling pathways, including the Akt signaling pathway and MAPK pathway, also play an important role in the production of Aβ and phosphorylation of tau regardless of insulin levels (Goncalves et al., 2019). Starting at dysfunction of insulin receptors substrate-1 (IRS-1) (Sims-Robinson et al., 2010; Talbot et al., 2012; Gupta et al., 2018), the dysregulation of Phosphatidylinositol 3-kinase (PI3K)/Akt pathways, results in the activation of glycogen synthase kinase 3β (GSK3β) to enhance tau phosphorylation and the generation of Aβ (Phiel et al., 2003; Avila et al., 2012; Stanley et al., 2016; Dey et al., 2017; Gupta et al., 2018). The deposition of Aβ plays a role in disrupted insulin signaling, and it can compete with insulin for binding to the insulin receptor thus downregulating PI3K/Akt activation in return (Townsend et al., 2007; Kim and Feldman, 2015). Amyloid beta oligomers (AβO), a synaptic toxic formation of Aβ accumulated in the AD brain (Ferreira and Klein, 2011), can also lead to loss of insulin receptors on the cell surface, thus inhibiting the PI3K/Akt pathway (De Felice et al., 2014). What’s more, MAPK signaling participates in the phosphorylation of tau (Sims-Robinson et al., 2010), which can be proximately divided into the two subtypes, c-Jun N-Terminal Kinase (JNK) and extracellular signal-regulated kinases (ERK) (Burillo et al., 2021).
Thioredoxin-interacting protein (TXNIP) is a negative regulator of the thioredoxin (TRX) system and a modulator of oxidative stress and inflammation (Tsubaki et al., 2020). TXNIP plays an important role in AD development and may be a novel target for AD treatment. Overexpression of TXNIP in the hippocampus and cortex of AD indicated that TXNIP is related to tau and Aβ accumulation (Li et al., 2019; Ismael et al., 2021). High glucose concentration can lead to TXNIP overexpression (Nasoohi et al., 2018a). TXNIP binds to glucose transporter-1 (GLUT-1) and GLUT-4 and suppresses the glucose uptake in response to glucose evaluation. And it can mediate cerebral insulin resistance and inhibit Akt/GSK3β (Nasoohi et al., 2018b). Aβ deposition may increase TXNIP levels (Wang et al., 2019), thus promoting tau and p38 MAPK phosphorylation (Melone et al., 2018). TXNIP can also be induced by AMP-activated protein kinase (AMPK), which phosphorylates to balance energy status (Mihaylova and Shaw, 2011; Wu et al., 2013).
2.2 Mitochondria dysfunction
Mitochondria are essential for glucose homeostasis. Glucose metabolism is determined by mitochondria, which is regarded as a “powerhouse” (Ding et al., 2021). The tricarboxylic acid (TCA) cycle of glycolysis occurs in the mitochondria and is accompanied by energy generation. Hyperglycemia state can lead to mitochondrial calcium ion imbalance, dysregulation of the electron transport chain, and disturbed membrane potential, leading to a state of oxidative stress in mitochondria (Paul et al., 2021b). It is also revealed that blood glucose fluctuations, either hyperglycemic or hypoglycemic, can lead to oxidative stress and mitochondrial dysfunction, which is an overall concept that encompasses abnormalities in morphology and physiological function (Carvalho and Cardoso, 2021).
Mitochondria dysfunction is also a pathological link between diabetes and AD (Pugazhenthi et al., 2017). It can also interact with the pathological products of AD. Defects in mitochondrial quality control, also known as mitochondrial dysfunction could lead to cognitive dysfunction in DM (Ng et al., 2021; Luo et al., 2022). Decreased mitochondrial respiration, disrupted membrane potential, and reduced energy synthesis, which is associated with the progression of AD, cause synaptic injury in diabetic AD mice (Carvalho et al., 2015). Further studies have revealed that in transgenic AD mice, mitochondria dysfunction is related to human amyloid precursor protein (APP) which is the source of the Aβ (Ronnback et al., 2016). Aβ can also induce mitochondria dysfunction and cause metabolic disorders including impaired TCA cycle (Teo et al., 2019). An integrated metabolomics and transcriptomics study with computational models has already demonstrated that Aβ may contribute to mitochondria dysfunction and neuroinflammation by mediating oxidative stress (Butterfield and Boyd-Kimball, 2019). Moreover, tau hyperphosphorylation can trigger neurodegeneration in the diabetic retina by disrupting mitochondria function (Zhu et al., 2018).
Mitochondria dysfunction is corelative with oxidative stress and inflammation, both of which are the characteristics of AD. Disruption of calcium ion homeostasis in mitochondria and mitophagy can promote the deposition of Aβ and tau (Fang et al., 2019; Jadiya et al., 2019), both of which could increase oxidative stress and energy deficiency (Kerr et al., 2017; Fertan et al., 2019). In the brain of the AD patient, oxidative stress as a result of reduced glucose metabolism could cause mitochondrial dysfunction and neuronal death (Butterfield and Halliwell, 2019). The receptor for advanced glycation end products (RAGE) is a proinflammatory ligand for advanced glycation end products (AGEs), which is also contributed to AD. RAGE/TXNIP/Nod-like receptor protein 3 (NLRP3) inflammasome is related to mitochondria dysfunction with Aβ (Figure 1).
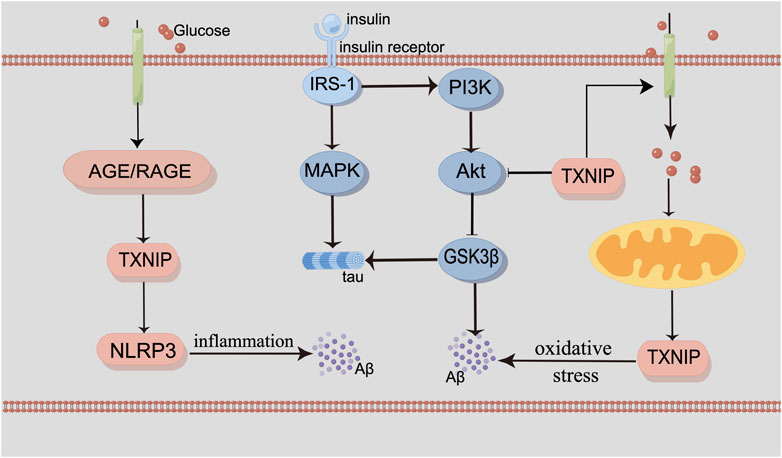
FIGURE 1. By Figdraw. The interaction of glucose metabolism and the pathogenesis of AD. Insulin binds to the insulin receptor, recruiting the IRS-1 proteins and triggering two signaling cascades, including the MAPK pathway and the PI3K/Akt/GSK3β pathway. Inhibition of PI3K and downregulation of the phosphorylated Akt protein subsequently activate GSK3β. TXNIP is a modulator of glucose metabolism and oxidative stress. It can also be induced by AMPK. RAGE/TXNIP/NLRP3 inflammasome can lead to neuroinflammation. Abnormal mitochondrial function can interact with glucose metabolism. MAPK signaling participates in the phosphorylation of tau. And the activation of GSK3β can also contribute to Aβ production and tau phosphorylation, as well as neuroinflammation and oxidative stress.
3 Antidiabetic drugs
Studies have shown that some antidiabetic drugs, in addition to regulating the metabolism of peripheral tissues, are also able to modulate the metabolism of the brain, reduce inflammation and have a direct protective effect on nerves. Antidiabetic drugs may improve cognition and memory and be a viable option for AD treatment. This section of the review enumerates the possible therapeutic mechanisms for AD of metformin, GLP-1 receptor agonists, DPP4 inhibitors, insulin, and SGLT2 inhibitors, all of which are widely applied to patients with diabetes according to official Clinical Guidelines.
3.1 Metformin
Metformin, the most important medicine for treating people with type 2 diabetes, can lower blood glucose levels by inhibiting hepatic gluconeogenesis and increasing glucose absorption in peripheral tissues (Patrone et al., 2014). It quickly penetrates the BBB (Łabuzek et al., 2010; Ying et al., 2014), and is disseminated to several brain regions (Łabuzek et al., 2010). Metformin enhanced hippocampal-dependent and non-dependent cognitive function in prediabetic rats by lowering brain inflammation, microglia hyperactivity, brain mitochondrial dysfunction, necrosis, and tau hyperphosphorylation (Jinawong et al., 2020). Metformin also decreases brain mitochondrial dysfunction, apoptosis, Aβ aggregation, and inflammation during ischemia in non-diabetic rats following cardiac ischemia/reperfusion (Benjanuwattra et al., 2020). Metformin, aspirin, simvastatin, and angiotensin-converting enzyme inhibitors (ACEI) were called antidiabetic polypill (PP). They could ameliorate learning and memory deficits in AD or AD-T2DM mice. PP treatment improved metabolic parameters, ameliorated brain atrophy and neuronal loss, rescued synaptic loss, reduced Aβ levels, and tau phosphorylation (Infante-Garcia et al., 2018). Metformin improved cognitive deficits in the hippocampus of the APP/PS1 mice model of AD via promoting Chaperone-mediated autophagy (CMA)-activated degradation of Aβ, which suggested that it might be a possible therapy for AD (Xu et al., 2021).
Metformin not only regulates metabolism in peripheral tissues but also prevents the onset and development of AD by alleviating metabolic abnormalities. In high glucose-incubated HT22 cells and brains of db/db mice with cognitive dysfunction, metformin stimulated AMPK to enhance autophagic activity. It reduced hyperphosphorylated tau and improved cognitive dysfunction (Chen et al., 2019). By triggering the mammalian target of rapamycin (mTOR) signaling in elderly ApoE3-target replacement (TR) mice, metformin increased the expression of postsynaptic proteins, thus enhancing cognition (Zhang et al., 2019). Intranasal metformin produced higher metformin concentrations in the hippocampus and lowered plasma concentrations, which enhanced insulin sensitivity more than oral treatment in mice with intracerebroventricular-streptozotocin (ICV-STZ)-induced AD (Kazkayasi et al., 2022). In SH-SY5Y cells and Transgenic mice (Tg6799), metformin exacerbated the pathogenesis of AD by promoting β-APP processing in autophagosomes to boost the production of Aβ (Son et al., 2016). In transgenic Caenorhabditis elegans with low levels of constitutive pan-neuronal expression of human Aβ1-42 (GRU102), metformin reversed Aβ-induced metabolic defects, normalized mitochondrial function, reduced protein aggregation, and repaired defects that affect lifespan. Changes in the TCA cycle metabolism were identified even at low levels of Aβ expression by combining metabolomics, transcriptomics, and computational modeling (Teo et al., 2019).
Clinical studies have also revealed the relationship between metformin and cognitive decline. In a retrospective investigation, metformin was connected to better memory function in older people who were cognitively healthy, which suggested that metformin was connected to a lower risk of dementia (Wu et al., 2020). Metformin improved cognitive performance and decreased the risk of dementia in individuals with T2D compared to those who did not use oral antidiabetic medications, according to a population-based investigation of seniors (65 years or older) (Cheng et al., 2014). A paired study revealed that metformin might reduce hippocampus and cortical atrophy to cognitive improvement. Higher levels of Aβ and lower levels of tau and phosphorylated tau (p-Tau) in CSF were observed in patients with metformin (Pomilio et al., 2022). Results from a randomized controlled trial suggested that metformin improved cognitive function in patients with non-dementia vascular cognitive impairment (NDVCI) and abnormal glucose metabolism. Possible mechanisms were the improvement in IR and attenuation of intima-media thickness (IMT) progression (Lin et al., 2018). A prospective open-cohort study showed that metformin use was associated with a slower decline in Mini-Mental State Examination (MMSE) scores over time. The findings suggest that metformin might have a direct neuroprotective effect on participants (Secnik et al., 2021).
However, some studies suggested metformin, as a side effect, was found to possibly expedite the development of AD. A population-based nested case-control study revealed a link between the use of metformin and a higher risk of AD in newly diagnosed diabetic patients (Ha et al., 2021). A prospective research showed that metformin might be a factor in cognitive impairment, while calcium and vitamin B12 supplements helped relieve the metformin-induced vitamin B12 deficit and improved cognitive results (Moore et al., 2013). According to a study on experimental animals, metformin elevated APP and Aβ in mice, impairing the mitochondrial function of brain neurons. Additionally, metformin might directly interact with Aβ, causing Aβ aggregates to accumulate, primarily in the cortex region. Apoptotic neurons were seen in the cortical area where metformin-induced Aβ aggregates were concentrated (Picone et al., 2016). Furthermore, in APP/PS1 mice with tau seeding, metformin was found to ameliorate autophagic damage in microglia and promote phagocytosis of NP tau. This suggested that metformin could reduce Aβ load and NP tau aggregation by enhancing autophagic activity in microglia (Chen et al., 2021). But in aged APOE TR mice, metformin also enhanced tau phosphorylation, suggesting that there might be adverse implications in humans (Zhang et al., 2019). Metformin activated signaling pathways that at least partially included AMPK to influence the transcription of β-secretase (BACE1), which then catalyzed APP cleavage and significantly increased the production of Aβ (Chen et al., 2009). In LAN5 neuroblastoma cells, metformin increased APP and presenilin levels, which raised APP cleavage and intracellular accumulation of Aβ (Picone et al., 2015). It remains controversial whether metformin is a promising agent for AD. Further research should be undertaken to elucidate.
3.2 GLP-1 receptor agonists
The hypoglycemic effects of the hormone GLP-1 are increasing insulin secretion, decreasing glucagon secretion, delaying stomach emptying, increasing satiety, and increasing insulin sensitivity in a range of tissues (Amer Diabet, 2013). GLP-1 Ras play a crucial role in maintaining glucose homeostasis in the body (Nuamnaichati et al., 2020). Currently, some animal studies provide evidence that GLP-1 Ras improve cognitive impairment, but clinical evidence is still lacking. We have reviewed the pharmacological effects of several GLP-1 Ras agonists on AD, including short-acting GLP-1 Ras, long-acting GLP-1 Ras, a GLP-1/Glucose-dependent insulinotropic polypeptide (GIP) Dual-Agonist, and a triple GLP-1/GIP/glucagon receptor agonist (TA).
3.2.1 Exendin-4/exenatide
Exendin-4 and exenatide are short-acting GLP-1 Ras. Exenatide is a synthetic product of exendin-4 (Nielsen et al., 2004). In a rat model of AD, exenatide might inhibit the inflammation response and increase cholinergic activity to protect hippocampus neurons and improve cognitive impairment (Solmaz et al., 2015). Exendin-4 can cross the BBB (Holscher, 2014; Salameh et al., 2020) and protect neurons against apoptosis induced by AβO in differentiated human neural progenitor cells (Velmurugan et al., 2012). In presenilin-1 knock-in (PS1-KI) mice, exenatide might improve cognition by increasing anaerobic glycolysis in the brain (Bomba et al., 2013). Exendin-4 treatment might prevent AD-related tau hyperphosphorylation by regulating the insulin signaling pathway and enhance memory and cognition in the brain of rats (Xu et al., 2015). Exendin-4 intervention increased PI3K/Akt activity and decreased GSK3β activity in rats (Chen et al., 2012). In the brains of type 2 diabetic rats, exendin-4 also increased protein kinase A (PKA), increased cyclic guanosine monophosphate (cGMP), increased AMPK levels, and decreased JNK activation. Furthermore, exendin-4 protected neural cells against apoptosis in the rat brain by regulating several autophagic markers (Candeias et al., 2018). In transgenic (Tg) mice, exendin-4 prevented the impaired insulin signaling in the hippocampus and enhanced cognition, as demonstrated by a decline in hippocampal serine phosphorylation of IRS-1 (IRS-1pSer) and activation of JNK. It could inhibit AβO-induced neuronal pathologies observed in vitro (Bomfim et al., 2012). Exenatide enhanced the long-term memory ability of adult wild-type mice, stimulated the Brain-Derived Neurotrophic Factor (BDNF)-tropomyosin-related kinase B receptor (TrkB) neurotrophic axis, and prevented apoptosis by reducing p75-neurotrophin-receptor-mediated signaling (Bomba et al., 2018). Exenatide treatment altered cognitive deficits in a triple transgenic mice model of AD (3xTg-AD) undergoing a high-fat diet by improving BDNF signaling and reducing inflammation (Bomba et al., 2019). However, the outcomes of a pilot study showed no trends in improvement in cognition, but a reduction of Aβ42 in extracellular vesicles (EV) was observed (Mullins et al., 2019).
3.2.2 Liraglutide
Liraglutide, a short-acting GLP-1 Ras, can cross BBB (Hunter and Hoelscher, 2012; Hoelscher, 2014). Recent experiments have proven the direct neuroprotective effect of liraglutide on cognitive function and neuron growth. Liraglutide exerted a neuroprotective role by triggering cytoprotective growth factors on the human neuroblastoma cell line SH-SY5Y during methylglyoxal stress (Sharma et al., 2014). The application of liraglutide enhanced late-phase long-term potentiation (L-LTP), synaptic plasticity (LTP) and spatial memory in rats (McClean et al., 2010; Han et al., 2013). Liraglutide also reduced overall Aβ plaques and inflammatory responses and increased the number of young neurons in the dentate gyrus (McClean et al., 2011). In addition, liraglutide has neuroprotective properties through the regulation of pathways related to glucose metabolism. It was demonstrated that liraglutide protected against diabetes-induced neuronal loss and synaptic ultrastructure degradation in the hippocampus of DM mice with cognitive impairment and might promote autophagy via the AMPK/mTOR pathway in primary cultured mice hippocampal neurons (Kong et al., 2018). Peripherally injected liraglutide prevented tau phosphorylation by promoting Akt activity and decreased GSK3β activity in db/db mice (Ma et al., 2015). Liraglutide greatly decreased insulin receptor aberrations, amyloid plaque load, and glial cell activation in APP(SWE)/PS1d9E mice (Long-Smith et al., 2013). Intravenous liraglutide improved AD-like learning and memory deficits, lowered the level of tau protein hyperphosphorylation, and reduced neurodegeneration, by strengthening JNK and ERK signaling pathways and boosting o-glycosylation of neuronal cytoskeletal proteins in STZ mice (Xiong et al., 2013). Intraperitoneally administered liraglutide relieved brain chronic inflammatory responses in mice by lowering the number of activated microglia and activated astrocytes (Parthsarathy and Hoelscher, 2013). Besides, female 3xTg-AD mice given chronic liraglutide treatment, reduced the cortical Aβ1-42, partially decreased inflammatory and oxidative stress in both plasma and brain, and partially normalized brain estradiol, GLP-1 content, and PKA levels (Duarte et al., 2020).
A randomized study also demonstrated that liraglutide restored blood-brain glucose transfer capacity at BBB (Gejl et al., 2017). In another randomized, placebo-controlled double-blinded clinical trial, liraglutide blocked the decline of glucose metabolism in the brain of AD, although no significant differences were presented in Aβ load and cognition measures to the placebo with PET of [18F]fluoro-2-deoxyglucose ([18F]FDG) metabolism (Gejl et al., 2016). In 12 weeks of a placebo-controlled study, liraglutide significantly improved the results of functional magnetic resonance imaging (fMRI). Intrinsic connectivity within the default mode network (DMN) was improved in the liraglutide group relative to placebo (Watson et al., 2019).
3.2.3 Other GLP-1 receptor agonists
Both lixisenatide and Val(8) GLP-1 could permeate the BBB (Gengler et al., 2012; Hunter and Hoelscher, 2012; Hoelscher, 2014), and protect LTP (Gengler et al., 2012; McClean and Hoelscher, 2014). They helped reduce the amount of amyloid plaque and tau hyperphosphorylation in AD mice (Li et al., 2012; Cai H.-Y. et al., 2018). The short-acting GLP-1 Ras lixisenatide significantly decreased neuroinflammation (McClean and Hoelscher, 2014) via activating PKA-CREB signaling and inhibiting p38-MAPK pathway. In a randomized, double-blind placebo-controlled trial, dulaglutide treatment in long term may improve cognition in patients with type 2 diabetes (Cukierman-Yaffe et al., 2020). Semaglutide, a long-acting GLP-1 receptor agonist, had a protective role against Aβ in AD by enhancing autophagy and inhibiting apoptosis (Chang et al., 2020).
GLP-1/GIP Dual-Agonist-5 (DA5-CH) can enhance memory function in AD mice by metabolism pathways. In the hippocampus of APP/PS1 mice, DA5-CH decreased hippocampal amyloid senile plaques and phosphorylated tau protein, increased levels of p-PI3K and p-Akt growth factor kinase, and prevented excessive activation of p-GSK3β (Cao et al., 2018). TA therapy could significantly correct the memory deficits in APP/PS1 mice, which elevated BDNF levels and further boosted neurogenesis in the dentate gyrus. TA decreased the overall level of Aβ as well as oxidative stress and neuroinflammation in the cortex and hippocampus (Kim et al., 2018).
3.3 DPP4 inhibitors
Diabetes treatment with DPP4 is linked to higher GLP-1 levels (Lambeir et al., 2008). Many studies have proven that DPP4is could benefit cognitive function such as saxagliptin, vildagliptin, linagliptin, and sitagliptin.
Saxagliptin and vildagliptin not only boosted GLP-1 levels in the hippocampus but corrected cognitive impairments, as well as decreased levels of Aβ42 and p-tau in the animal model. Moreover, TNF-α and IL-1β, two important cytokines produced by activated microglia, were decreased to reduce inflammation after the treatment of the two DPP4is (Kosaraju et al., 2013a; Kosaraju et al., 2013b).
Linagliptin and sitagliptin also alleviated cognitive impairments and amyloid load in AD model besides raising GLP-1 levels in the brain. Linagliptin raised GIP incretin levels and decreased tau phosphorylation caused by the downregulation of GSK3β in the brain of AD mice. It also ameliorated neuroinflammation by significantly reducing glial fibrillary acidic protein (GFAP) (Kosaraju et al., 2017). In APP/PS1 animals, sitagliptin therapy protected synaptic plasticity in AD mice by markedly activating GLP-1 and BDNF-TrkB signaling (Dong et al., 2019). It might restore damaged insulin signaling in cultured human neuronal cells, thus preventing tau hyperphosphorylation and GSK3β activation. The formation of intracellular reactive oxygen species (ROS) and mitochondria dysfunction brought on by Aβ were mitigated by sitagliptin, which may be related to the activation of 5′AMPK-Sirt1 signaling (Kornelius et al., 2015). But a conflicting view held that Sitagliptin was ineffective on tau phosphorylation in OLETF type 2 diabetic rats and instead exacerbated it, possibly through the activation of GSK3β. Additionally, sitagliptin raised ser-616 phosphorylation of IRS-1, which suggested elevated insulin resistance in the brain (Kim et al., 2012).
Clinical studies supported the findings of some experimental studies. A cross-sectional study revealed that enhanced dipeptidyl peptidase IV (DPP4) activity and lower BDNF activity were linked to a higher risk of moderate cognitive impairment (MCI) in older persons. However, it remained uncertain whether DPP4 could reduce BDNF ratio (DBR) by targeting increased plasma DPP4 activity, thereby improving MCI in older patients with type 2 diabetes (Zheng et al., 2018). In a prospective and observational study, older diabetic individuals who took sitagliptin for 6 months showed enhanced cognitive performance (Isik et al., 2017). A retrospective investigation revealed that DPP4i usage was linked to a low amyloid load and improved long-term cognitive outcomes in diabetic patients with Alzheimer’s disease-related cognitive impairment (Jeong et al., 2021). DPP4is showed a protective effect against the risk of dementia in Alzheimer’s disease, according to a meta-analysis (Zhou et al., 2020). In individuals with diabetes and dementia, DPP4is improved global cognition as measured by the MMSE, according to a prospective open-cohort study (Secnik et al., 2021). In a retrospective investigation, DPP4i usage was linked to slower rates of memory loss in AD patients. Additionally, DPP4is were more advantageous for APOE4 carriers (Wu et al., 2020).
3.4 SGLT2 inhibitors
SGLT2 inhibitors prevent the kidneys from reabsorbing glucose, which lowers blood glucose levels (Vallon and Thomson, 2017). Empagliflozin, a selective SGLT2 inhibitor, significantly prevented cognitive decline in db/db mice. This protection of cognitive abilities was linked to a reduction in brain oxidative stress and an increase in brain-derived neurotrophic factors (Lin et al., 2014). Dapagliflozin inhibited ROS-dependent neuronal apoptosis, increased the expression of PI3K/Akt/GSK-3β pathway, and suppressed neuroinflammation by reducing the activation of nuclear factor-kappa B (NF-κB) pathway and TNF-α levels to exert neuroprotective effects (Arab et al., 2021).
A retrospective study showed that when compared to DPP4is, the use of SGLT2 inhibitors was considerably more protective in T2DM patients against new-onset dementia, but not against new-onset AD (Mui et al., 2021). T2DM individuals who used SGLT2 inhibitors had a lower chance of developing dementia according to a retrospective study. The lowest risk of dementia was shown in dapagliflozin, followed by empagliflozin, while canagliflozin had no association (Wu et al., 2022).
3.5 Insulin therapy
The effects of insulin on cognitive impairment are debatable. Aβ-induced loss of spatial memory was prevented by intrahippocampal insulin injections (Ghasemi et al., 2014). A single insulin injection reversed additional brain Aβ accumulation and cognitive deficits in a mice model of AD induced by a high-fat diet (Vandal et al., 2014). By shifting APP processing to non-amyloidogenic pathways, intranasal insulin improved cognitive impairments and decreased brain Aβ levels and Aβ plaque deposition in APP/PS1 mice (Mao et al., 2016). Intranasal insulin improved verbal memory and acutely increased plasma Aβ42 levels. However, further research has to be done to determine the clinical implications of changed plasma Aβ42 levels (Reger et al., 2008). Intravenous insulin infusion decreased Aβ42 clearance but increased Aβ42 accumulation in plasma, which has been demonstrated in the past to cause peripheral insulin resistance (Swaminathan et al., 2018). A meta-analysis also revealed that patients with T2DM under treatment with insulin presented the lowest risk of dementia compared with other antidiabetic drugs (Zhou et al., 2020). (Table 1)
4 Herbal medicines
Plants can be used as dietary supplements or as drug composition for the prevention and treatment of diseases. Phytochemicals possess abundant biological properties, and the active components of some plants are also very useful in the treatment of diseases. Numerous studies have found that phytoconstituents including plant extracts and active components have antioxidant, anti-inflammatory, and hypoglycemic properties, which can target abnormal enzyme activities and thus have a therapeutic effect on diseases. This section of the review enumerates the therapeutic value of plants, their extracts, and active metabolites for both AD and DM. Considering the link between abnormal glucose metabolism and AD, phytomedicines can be used as a promising drug treatment for AD by modulating glucose metabolism.
4.1 Curcumin
Curcumin is a polyphenol derived from the rhizomes of Curcuma longa (Thiruvengadam et al., 2021). It is an antioxidant and has anti-inflammatory effect. (Bradford, 2013). It has been reported that curcumin may be adopted as a potential neuroprotective agent against cognitive decline or AD (Caruso et al., 2022). Moreover, curcumin can also reverse aberrant glucose metabolism to alleviate memory decline. In AD mice, curcumin can upregulate PI3K/Akt pathways while decreasing the expression of insulin receptors and insulin receptor substrates in the hippocampus (Feng et al., 2016; Wang et al., 2017). Daily dietary supplementation with curcumin may also improve abnormal glucose metabolism and reduce the risk of diabetes and AD. Curcumin can also decrease the activation of the TXNIP/NLRP3 inflammasome to prevent cell death (Zhang M. et al., 2021). Using the micro-positron emission tomography (PET) technique in AD mice, it has been revealed that curcumin can improve glucose metabolism (Wang et al., 2017). In a high-fat diet rat model, curcumin nanoparticle (CurNP) can improve Aβ accumulation and tau hyperphosphorylation in the hippocampus through alleviating redox and inflammation and downregulating MAPK/ERK pathway (Abdulmalek et al., 2021). In a 12-week randomized controlled trial, participants with prediabetes who consumed 180 mg of curcumin daily had significantly reduced GSK3β and IAPP in serum samples after 12 weeks compared with placebo groups, reducing insulin resistance and the risk of developing AD and DM (Thota et al., 2020). In a randomized, double-blind, placebo-controlled trial, healthy older people who took solid lipid curcumin had significantly better memory performance and mood (Cox et al., 2015). A randomized, placebo-controlled, double-blind, pilot clinical trial in 6 months has shown that curcumin can help patients with AD decrease the accumulation of Aβ in the plasma (Baum et al., 2008).
4.2 Resveratrol
Resveratrol is a polyphenolic phytocompound, derived from various plants, including fruits (e.g., blueberries, blackberries), and herbal medicine (e.g., Polygonum cuspidatum herb) (Gambini et al., 2015). It has benefits in both lifespan and health span (Bhullar and Hubbard, 2015). Previous studies have shown that resveratrol can act as an anti-diabetic drug to decrease plasma glucose levels, and increase insulin sensitivity (Berman et al., 2017). Resveratrol is used in treating age-related diseases such as AD because of its anti-inflammatory and antioxidant propertities (Li et al., 2018). Moreover, resveratrol also can permeate the BBB and be used as a potential neuroprotective agent (Wicinski et al., 2020). A clinical study of older adults has shown that a supplement of resveratrol could improve memory function and increase hippocampal functional connectivity and glucose metabolism (Albani et al., 2010; Witte et al., 2014). It has been demonstrated that resveratrol can regulate glucose metabolism and inhibit tau phosphorylation through the PI3K/Akt pathway, respectively (Ghafouri-Fard et al., 2022). The mechanism of resveratrol in the treatment of AD and DM is the same in the SIRT1 and AMPK pathways (Meng et al., 2020). But there is insufficient evidence that resveratrol can treat AD merely by modulating glucose metabolism (Ribeiro et al., 2022). Resveratrol also had the ability to inhibit the inflammatory factors and the TXNIP/TRX/NLRP3 signaling pathway, both of which were simulated by Aβ (Feng and Zhang, 2019; Zhang M. et al., 2021). Resveratrol consumption could protect mitochondrial function, which reduces the risk of developing AD and DM. Carrasco-Pozo etc. al have found that resveratrol could affect mitochondria dysfunction and oxidative stress induced by indomethacin in vitro, although quercetin but not resveratrol is the most effective one (Carrasco-Pozo et al., 2012). In addition, resveratrol can promote autophagy and treat AD and DM in vivo and in vitro (Shakeri et al., 2020).
The treatment with resveratrol, however, has its drawbacks. A recent study has shown that Resveratrol could upregulate autophagy in the prefrontal cortex, but BDNF and synaptophysin decreased after the treatment of resveratrol in mice (Yang et al., 2019). Although dietary supplementation with resveratrol reduced the risk of developing AD, the bioavailability of resveratrol and the level of resveratrol in the brain were reduced in diabetic rats. This may be related to diabetic-induced osmotic diuresis (Chen T.-Y. et al., 2017). A randomized, double-blind, placebo-controlled trial has shown that the treatment of resveratrol had no benefits in biomarkers of AD and glucose metabolism. And brain volume loss was increased in the resveratrol group. This further prevents resveratrol from being a potential therapeutic agent to regulate both peripheral and central glucose metabolism.
New technological applications for resveratrol can provide broader prospects for drug formulations of resveratrol. Because of its limited bioavailability, micronized resveratrol formulations can effectively solve this problem (Singh et al., 2019). Besides, both resveratrol and its analog pterostilbene have the common stilbene backbone, as well as pleiotropic actions. Therefore, stilbene-based drug development is also an emerging direction for the treatment of AD (Giacomini et al., 2016).
4.3 Berberine (BBR)
BBR is an isoquinoline alkaloid extracted mainly from Berberis aristata (shrub). It has a broad spectrum of treatments such as anti-inflammatory, antitumor, lipid-lowering properties, etc (Yao et al., 2015). BBR can be used as an anti-diabetic agent by regulating peripheral glucose metabolism (Lan et al., 2015; Yao et al., 2015). Since the BBB is favorably permeable to BBR, it can also be used in the treatment of central nervous system disorders (Behl et al., 2022). In recent years, it has also been shown that BBR can improve central glucose metabolism and insulin signaling to ameliorate AD-related pathological lesions. In vitro, BBR induced increased glucose utilization in neurons and inhibited the production of oligomer Aβ by activating the PI3K/PGC epsilon pathway, thereby reducing AD-related neuronal damage (Wu et al., 2021). BBR can modulate mitochondria dysfunction to regulate energy metabolism in the AD cell model. It also reduces pro-inflammatory cytokines in activated microglial cells (Wong et al., 2021). In a high-fat diet diabetic rat model, BBR enhanced cognitive function by improving both peripheral and central IR, and reduced hippocampal phosphorylation of tau as well as neuronal apoptosis (Zhang J.-H. et al., 2021). BBR also inhibited insulin resistance in the medial prefrontal cortex and downregulated PI3K/Akt/mTOR and MAPK signaling pathways in diabetic rats (Chen Q. et al., 2017). In addition, in a combined rat model of AD and T2DM, BBR played a neuroprotective role by suppressing endoplasmic reticulum (ER) stress, and also attenuated memory deficits (Xuan et al., 2020). Nevertheless, due to the limited pharmacokinetic characteristics, the combination of nanotechnology and BBR is still a promising therapeutic approach (Behl et al., 2022).
Some other alkaloids can also modulate glucose metabolism for the treatment of AD. Peganum harmala (P. harmala) is an Egyptian herbal medicine, whose extract can also decrease hippocampal Aβ, phosphorylated tau, and GSK-3β (Saleh et al., 2021). Another study (Li et al., 2017) has shown that the alkaloids of P. Harmala, including harmine and harmaline (Saleh et al., 2021), were the most active components. Huperzine A (Hup A) is a Lycopodium alkaloid extracted from the moss Huperzia serrata. A recent study has shown that Hup A treatment can improve metabolism and cognition by upregulating the insulin and phosphorylated Akt levels in the cortex of high fat-induced mice, although the same results were not seen in ob/ob mice (Wang H.-y. et al., 2020).
4.4 Glycosides
Glycosides, especially saponins, are an important group of phytochemicals due to their diverse pharmacological activities. The therapeutic effects of these active components have received much attention in recent years. Geniposide is extracted from the fruit of Gardenia jasminoides Ellis and has various pharmacological properties (Ma et al., 2019). Primary studies have suggested that geniposide had a promising ability to treat AD by reducing amyloid plaques, inhibiting tau phosphorylation, and preventing memory impairment (Liu et al., 2015). It can also be used in managing the pathological alterations of AD by targeting glucose metabolism-related pathways such as GLP-1 receptors and insulin signaling. Geniposide can stimulate the GLP-1 receptor, which not only has an effect on diabetes but promotes neurogenesis and cognitive function via correcting metabolism (Sun et al., 2021). Two studies have revealed that geniposide enhanced the effect of insulin signaling on AD-related changes (Zhang et al., 2015; Zhang et al., 2016). In the diabetic AD mouse model, geniposide significantly decreased the phosphorylated level of tau directly. What’s more, the phosphorylation of GSK-3β was decelerated in vivo, and the phosphorylation of Akt was increased in vitro cells (Zhang et al., 2016). Geniposide also upregulated the levels of BACE1 and insulin-degrading enzyme (IDE), both in vivo and in vitro (Zhang et al., 2015).
Some favorable results of sapogenins have been shown in recent studies. Sarsasapogenin is extracted from the Anemarrhena asphodeloides Bunge (rhizome). It could inhibit Aβ overproduction and tau hyperphosphorylation by upregulating BACE1 and inactivating of Akt/GSK-3β cascade respectively in vivo and in vitro (Zhang Y.-M. et al., 2021). Asiaticoside, found in Centella asiatica (L.) Urban, also could remarkedly improve the cognitive deficits in diabetic rats by modulating oxidative stress, PI3K/Akt/NF-kappa B pathway, and synaptic function (Yin et al., 2015). Ginsenoside is an active compound of Panax ginseng C. A. Meyer or Panax quinquefolium L. (American Ginseng). In the STZ-injected mice model, ginsenoside Rb1 can enhance insulin sensitivity. At the same time, this study also revealed that it improved memory and cognition (Yang et al., 2020), which could have benefits in diabetic encephalopathy, as well as AD. Ginsenoside Rg2, steroid glycoside extracted from Panax ginseng could induce autophagy in the AD mice model, thus improving cognitive behaviors and metabolism (Fan et al., 2017).
4.5 Quercetin
Quercetin is a natural flavonoid from a variety of plants, including fruits and vegetable (Miltonprabu, 2019). Moreover, quercetin has beneficial effects on many diseases including diabetes and AD (Costa et al., 2016). Quercetin may protect cells from mitochondria dysfunction and oxidative stress in vitro, which was the most efficient compared with rutin, resveratrol, and epigallocatechin gallate (Carrasco-Pozo et al., 2012). This may reveal a joint role of quercetin in the treatment of abnormal glucose metabolism and AD-related cognitive impairment by targeting mitochondria. Subsequent studies found that quercetin improved cognitive function and aberrant peripheral glucose metabolism in db/db mice. What’s more, quercetin increased the expression of brain-derived BDNF and SIRT1 protein, which indicated a neuroprotective role of quercetin (Hu et al., 2020). A recent study aimed to identify the potential target of quercetin suggested that quercetin may have a synergic effect on DM and AD by targeting MAPK pathways (Zu et al., 2021).
Although quercetin has pleiotropic effects and a few negligible adverse effects, its low bioavailability may be a roadblock preventing it from being a therapeutic agent. Nanoparticles with quercetin may solve this problem, which has been already applied in cancer treatment (Ebrahimpour et al., 2020). Future studies could focus on improving the bioavailability of quercetin through nanotechnology and other pharmaceutical technologies.
Other flavonoid components also have great therapeutic value. Emerging studies have shown that luteolin and its derivatives (Choi et al., 2014b; Liu et al., 2014), scutellarin (Chledzik et al., 2018), and apigenin as well as its derivatives (Choi et al., 2014a), had properties of anti-diabetes and anti-AD. Luteolin treatment in high-fat diet mice, significantly improved the central insulin resistance and peripheral glucose metabolism, to alleviate cognitive decline for AD and diabetes induced by obesity (Liu et al., 2014). Some flavonoids can also reduce the overexpression of TXNIP and activation of NLRP3 inflammasome (Zhang M. et al., 2021). Notably, regarding the structure-activity relationships of flavonoids, isomers of flavonoids also play an influential role in the treatment of AD and DM, such as isomeric C-glycosylated derivatives of luteolin and apigenin (Choi et al., 2014a; Choi et al., 2014b). This may indicate that future phytochemical investigations could focus on the structure-activity relationships to clarify the pharmacological effects.
4.6 Herbal plants and extracts
Many natural products have inhibitive effects on both AD and diabetes. Herbal medicine has been used for thousands of years since ancient times in many countries such as China, India, and some Africa countries. Modern pharmaceutical research has also provided evidence for the application of these botanicals in the treatment of diabetes and AD. Non-etheless, plenty of research indicated that the effects of herbal plants or their extracts on diabetes and AD were associated with the inhibition of enzymes instead of modulating pathological processes. For example, many plants and extracts showed a high capacity to inhibit acetylcholinesterases (AChE) and butyrylcholinesterase (BuChE), alpha-glucosidase, and alpha-amylase (Ali et al., 2015; Zengin et al., 2015; Trampetti et al., 2019; Mahomoodally et al., 2020; Floris et al., 2021). However, most herbal plants and their extracts may belong to the restorative category, so the treatment may not delay the progress of AD (Brimson et al., 2020). But several studies have revealed the therapeutic role of herbal plants in rodent models of diabetic cognitive decline or diabetes-related AD. On the one hand, herbal plants and exacts can improve cognitive impairment in diabetic rats. Piperine can improve the cognition of diabetes rats and reduce the expression of AD-associated genes (Kumar et al., 2021). Artemisinin reduced the formation of Aβ plaques in the hippocampus of rats with central and peripheral STZ injections (Poorgholam et al., 2021). Mangifera indica Linn extracts reduced central inflammation and atrophy in the hippocampus and cortex. The metabolic parameters (body weight, glucose, and insulin levels) were reduced after the treatment of Mangifera indica Linn extracts in the diabetes mice model (Infante-Garcia et al., 2017). Withania somnifera (L.) Dunal (Solanaceae) can enhance peripheral glucose metabolisms such as insulin sensitivity and glucose uptake. It can also have properties against Aβ induced toxicity and APP (Paul et al., 2021a). Rhinacanthus nasutus (L.) Kurz (Acanthaceae) or its compound could be beneficial for diabetes and neurodegenerative diseases like AD (Brimson et al., 2020). Propolis and its extracts can ameliorate diabetic symptoms and improve glucose metabolism in the animal model. Propolis also prevented neurons from oxidative stress (Zulhendri et al., 2021).
Recent studies have further identified the therapeutic effects of some herbal plants on AD through modulating central glucose metabolism. Lychee seed extract decreased the level of Aβ and tau formation, as well as insulin resistance and AGE in the hippocampus of diabetes rats (Tang et al., 2018). Yuzu (Citrus junos Tanaka) extract also had a neuroprotective role in the hippocampus of AD rats and also attenuated hippocampal insulin signaling (Yang et al., 2013). (Table 2 and Table 3).
5 Discussion
The incidence and prevalence of Alzheimer’s disease, the most common form of dementia, have been rising rapidly over the past few decades. Although the pathology of AD is not fully understood, some studies focused on central metabolism including insulin signaling and mitochondria dysfunction have demonstrated the relationship between aberrant glucose metabolism and AD. Hyperglycemia is a major risk factor in the development of AD, and the central nervous system can also respond to glucose metabolism except for peripheral systems. Insulin can bind to insulin substrate through the BBB, thus mediating insulin signaling pathways to regulate energy metabolism and protect neurons. Impaired insulin signaling pathways, including PI3K/Akt/GSK3β and MAPK pathways, could cause damage to the brain in the pathogenesis of AD. Mitochondrial dysfunction and overexpression of TXNIP could also be causative links between AD and DM. These pathological processes do not exist independently, along with Aβ and phosphorylated tau. They can influence and interfere with each other to form a vicious circle.
Anti-diabetic medicines can also affect protein aggregation metabolism and neuroinflammation in the brain, which can dramatically enhance memory and cognition. Metformin has been shown to ameliorate AD, but results from multiple studies were inconsistent in rodent models. Some indicated that metformin is beneficial for cognitive decline and pathology of AD, but others argued that metformin might contribute to them because of vitamin B12 deficiency. The effect of Exendin-4 on AD is prominent in animal experiments, but more clinical trials are still lacking. Liraglutide as a promising drug for current treatment has confirmed its effect on AD in animal models and restored the decline of glucose metabolism in the brain in clinical trials. Semaglutide was proven to be effective in AD treatment with animal models and yet there is a lack of clinical trials. DPP4is such as saxagliptin, vildagliptin, linagliptin, and sitagliptin were effective in animal models. And only sitagliptin can enhance cognitive performance in current clinical trials. Compared to DPP4is, SGLT2is were considerably more protective in T2DM patients against new-onset dementia according to a retrospective study. In the existing studies of SGLT2is, empagliflozin, and dapagliflozin have neuroprotective effects on AD in animal models, and animals with dapagliflozin had the lowest chance of developing dementia in a retrospective study. The effects of DPP4is and SGLT2is still need more clinical trials to clarify. Insulin therapy is a promising therapy but some studies indicated that it may increase the risk of AD. To fully explore their potentials for treating AD, more research on the impact of antidiabetics on dementia risk is required, particularly in the clinical environment.
Moreover, the evidence in the text showed that herbal medicine also has effects on AD and diabetes. Plants and their extracts can improve cognition, and ameliorate the pathological changes associated with AD. Polyphenols like curcumin and resveratrol, BBR, glycosides such as geniposide and sarsasapogenin, and quercetin can correct metabolic abnormalities through PI3K/Akt/GSK3β and MAPK signaling pathways in AD models. Active metabolites of plants are the most promising component to apply for medicine formation, but their low bioavailability remains to be solved. Nanotechnology may offer broader prospects for the adoption of bioactive ingredients. More clinical trials are needed to verify the effectiveness of herbal medicines for AD based on animal studies.
Author contributions
Preparing the original manuscripts, YW, HH, and XL; writing and revising manuscripts, YW, HH, XL, and XG. All authors have read and agreed to the published version of the manuscript.
Funding
This study was supported by grants from the National Natural Science Foundation of China (Grant No. 82174351 and 81303130).
Conflict of interest
The authors declare that the research was conducted in the absence of any commercial or financial relationships that could be construed as a potential conflict of interest.
Publisher’s note
All claims expressed in this article are solely those of the authors and do not necessarily represent those of their affiliated organizations, or those of the publisher, the editors and the reviewers. Any product that may be evaluated in this article, or claim that may be made by its manufacturer, is not guaranteed or endorsed by the publisher.
References
Abdulmalek, S., Nasef, M., Awad, D., and Balbaa, M. (2021). Protective effect of natural antioxidant, curcumin nanoparticles, and zinc oxide nanoparticles against type 2 diabetes-promoted hippocampal neurotoxicity in rats. Pharmaceutics 13 (11), 1937. doi:10.3390/pharmaceutics13111937
Akhtar, M. W., Sanz-Blasco, S., Dolatabadi, N., Parker, J., Chon, K., Lee, M. S., et al. (2016). Elevated glucose and oligomeric beta-amyloid disrupt synapses via a common pathway of aberrant protein S-nitrosylation. Nat. Commun. 7, 10242. doi:10.1038/ncomms10242
Albani, D., Polito, L., Signorini, A., and Forloni, G. (2010). Neuroprotective properties of resveratrol in different neurodegenerative disorders. Biofactors 36 (5), 370–376. doi:10.1002/biof.118
Ali, M. Y., Jung, H. A., and Choi, J. S. (2015). Anti-diabetic and anti-Alzheimer's disease activities of Angelica decursiva. Archives Pharmacal Res. 38 (12), 2216–2227. doi:10.1007/s12272-015-0629-0
Amer Diabet, A. (2013). Standards of medical care in diabetes-2013. Diabetes Care 36, S11–S66. doi:10.2337/dc13-S011
Andrews, S. J., Fulton-Howard, B., O'Reilly, P., Marcora, E., Goate, A. M., and Alzheimer's Dis, G. (2021). Causal associations between modifiable risk factors and the alzheimer's phenome. Ann. Neurology 89 (1), 54–65. doi:10.1002/ana.25918
Arab, H. H., Safar, M. M., and Shahin, N. N. (2021). Targeting ROS-dependent AKT/GSK-3β/NF-κB and DJ-1/nrf2 pathways by dapagliflozin attenuates neuronal injury and motor dysfunction in rotenone-induced Parkinson's disease rat model. ACS Chem. Neurosci. 12 (4), 689–703. doi:10.1021/acschemneuro.0c00722
Arnold, S. E., Arvanitakis, Z., Macauley-Rambach, S. L., Koenig, A. M., Wang, H.-Y., Ahima, R. S., et al. (2018). Brain insulin resistance in type 2 diabetes and alzheimer disease: Concepts and conundrums. Nat. Rev. Neurol. 14 (3), 168–181. doi:10.1038/nrneurol.2017.185
Avila, J., León-Espinosa, G., García, E., García-Escudero, V., Hernández, F., and DeFelipe, J. (2012). Tau phosphorylation by GSK3 in different conditions. Int. J. Alzheimer’s Dis. 2012, 578373. doi:10.1155/2012/578373
Baglietto-Vargas, D., Shi, J., Yaeger, D. M., Ager, R., and LaFerla, F. M. (2016). Diabetes and Alzheimer's disease crosstalk. Neurosci. Biobehav. Rev. 64, 272–287. doi:10.1016/j.neubiorev.2016.03.005
Banks, W. A. (2004). The source of cerebral insulin. Eur. J. Pharmacol. 490 (1-3), 5–12. doi:10.1016/j.ejphar.2004.02.040
Barnes, D. E., and Yaffe, K. (2011). The projected effect of risk factor reduction on Alzheimer's disease prevalence. Lancet Neurol. 10 (9), 819–828. doi:10.1016/S1474-4422(11)70072-2
Barone, E., Di Domenico, F., Perluigi, M., and Butterfield, D. A. (2021). The interplay among oxidative stress, brain insulin resistance and AMPK dysfunction contribute to neurodegeneration in type 2 diabetes and Alzheimer disease. Free Radic. Biol. Med. 176, 16–33. doi:10.1016/j.freeradbiomed.2021.09.006
Baum, L., Lam, C. W. K., Cheung, S. K.-K., Kwok, T., Lui, V., Tsoh, J., et al. (2008). Six-month randomized, placebo-controlled, double-blind, pilot clinical trial of curcumin in patients with alzheimer disease. J. Clin. Psychopharmacol. 28 (1), 110–113. doi:10.1097/jcp.0b013e318160862c
Baumgart, M., Snyder, H. M., Carrillo, M. C., Fazio, S., Kim, H., and Johns, H. (2015). Summary of the evidence on modifiable risk factors for cognitive decline and dementia: A population-based perspective. Alzheimers Dementia 11 (6), 718–726. doi:10.1016/j.jalz.2015.05.016
Behl, T., Singh, S., Sharma, N., Zahoor, I., Albarrati, A., Albratty, M., et al. (2022). Expatiating the pharmacological and nanotechnological aspects of the alkaloidal drug berberine: Current and future trends. Molecules 27 (12), 3705. doi:10.3390/molecules27123705
Benjanuwattra, J., Apaijai, N., Chunchai, T., Kerdphoo, S., Jaiwongkam, T., Arunsak, B., et al. (2020). Metformin preferentially provides neuroprotection following cardiac ischemia/reperfusion in non-diabetic rats. Biochimica Biophysica Acta-Molecular Basis Dis. 1866 (10), 165893. doi:10.1016/j.bbadis.2020.165893
Berman, A. Y., Motechin, R. A., Wiesenfeld, M. Y., and Holz, M. K. (2017). The therapeutic potential of resveratrol: A review of clinical trials. NPJ Precis. Oncol. 1, 35. doi:10.1038/s41698-017-0038-6
Bhullar, K. S., and Hubbard, B. P. (2015). Lifespan and healthspan extension by resveratrol. Biochimica Biophysica Acta-Molecular Basis Dis. 1852 (6), 1209–1218. doi:10.1016/j.bbadis.2015.01.012
Biessels, G. J., and Despa, F. (2018). Cognitive decline and dementia in diabetes mellitus: Mechanisms and clinical implications. Nat. Rev. Endocrinol. 14 (10), 591–604. doi:10.1038/s41574-018-0048-7
Biessels, G. J., and Whitmer, R. A. (2020). Cognitive dysfunction in diabetes: How to implement emerging guidelines. Diabetologia 63 (1), 3–9. doi:10.1007/s00125-019-04977-9
Bogush, M., Heldt, N. A., and Persidsky, Y. (2017). Blood brain barrier injury in diabetes: Unrecognized effects on brain and cognition. J. Neuroimmune Pharmacol. 12 (4), 593–601. doi:10.1007/s11481-017-9752-7
Bomba, M., Ciavardelli, D., Silvestri, E., Canzoniero, L. M. T., Lattanzio, R., Chiappini, P., et al. (2013). Exenatide promotes cognitive enhancement and positive brain metabolic changes in PS1-KI mice but has no effects in 3xTg-AD animals. Cell Death Dis. 4, e612. doi:10.1038/cddis.2013.139
Bomba, M., Granzotto, A., Castelli, V., Massetti, N., Silvestri, E., Canzoniero, L. M. T., et al. (2018). Exenatide exerts cognitive effects by modulating the BDNF-TrkB neurotrophic axis in adult mice. Neurobiol. Aging 64, 33–43. doi:10.1016/j.neurobiolaging.2017.12.009
Bomba, M., Granzotto, A., Castelli, V., Onofrj, M., Lattanzio, R., Cimini, A., et al. (2019). Exenatide reverts the high-fat-diet-induced impairment of BDNF signaling and inflammatory response in an animal model of alzheimer's disease. J. Alzheimers Dis. 70 (3), 793–810. doi:10.3233/JAD-190237
Bomfim, T. R., Forny-Germano, L., Sathler, L. B., Brito-Moreira, J., Houzel, J.-C., Decker, H., et al. (2012). An anti-diabetes agent protects the mouse brain from defective insulin signaling caused by Alzheimer's disease-associated A beta oligomers. J. Clin. Investigation 122 (4), 1339–1353. doi:10.1172/JCI57256
Brimson, J. M., Prasanth, M. I., Malar, D. S., Brimson, S., and Tencomnao, T. (2020). Rhinacanthus nasutus "tea" infusions and the medicinal benefits of the constituent phytochemicals. Nutrients 12 (12), 3776. doi:10.3390/nu12123776
Burillo, J., Marques, P., Jimenez, B., Gonzalez-Blanco, C., Benito, M., and Guillen, C. (2021). Insulin resistance and diabetes mellitus in alzheimer's disease. Cells 10 (5), 1236. doi:10.3390/cells10051236
Butterfield, D. A., and Boyd-Kimball, D. (2019). Redox proteomics and amyloid beta-peptide: Insights into alzheimer disease. J. Neurochem. 151 (4), 459–487. doi:10.1111/jnc.14589
Butterfield, D. A., Di Domenico, F., and Barone, E. (2014). Elevated risk of type 2 diabetes for development of alzheimer disease: A key role for oxidative stress in brain. Biochimica Biophysica Acta-Molecular Basis Dis. 1842 (9), 1693–1706. doi:10.1016/j.bbadis.2014.06.010
Butterfield, D. A., and Halliwell, B. (2019). Oxidative stress, dysfunctional glucose metabolism and Alzheimer disease. Nat. Rev. Neurosci. 20 (3), 148–160. doi:10.1038/s41583-019-0132-6
Cai, H.-Y., Yang, J.-T., Wang, Z.-J., Zhang, J., Yang, W., Wu, M.-N., et al. (2018a). Lixisenatide reduces amyloid plaques, neurofibrillary tangles and neuroinflammation in an APP/PS1/tau mouse model of Alzheimer's disease. Biochem. Biophysical Res. Commun. 495 (1), 1034–1040. doi:10.1016/j.bbrc.2017.11.114
Cai, W., Xue, C., Sakaguchi, M., Konishi, M., Shirazian, A., Ferris, H. A., et al. (2018b). Insulin regulates astrocyte gliotransmission and modulates behavior. J. Clin. Investigation 128 (7), 2914–2926. doi:10.1172/JCI99366
Candeias, E., Sebastiao, I., Cardoso, S., Carvalho, C., Santos, M. S., Oliveira, C. R., et al. (2018). Brain GLP-1/IGF-1 signaling and autophagy mediate exendin-4 protection against apoptosis in type 2 diabetic rats. Mol. Neurobiol. 55 (5), 4030–4050. doi:10.1007/s12035-017-0622-3
Cao, Y., Holscher, C., Hu, M.-M., Wang, T., Zhao, F., Bai, Y., et al. (2018). DA5-CH, a novel GLP-1/GIP dual agonist, effectively ameliorates the cognitive impairments and pathology in the APP/PS1 mouse model of Alzheimer's disease. Eur. J. Pharmacol. 827, 215–226. doi:10.1016/j.ejphar.2018.03.024
Carrasco-Pozo, C., Luisa Mizgier, M., Speisky, H., and Gotteland, M. (2012). Differential protective effects of quercetin, resveratrol, rutin and epigallocatechin gallate against mitochondrial dysfunction induced by indomethacin in Caco-2 cells. Chemico-Biological Interact. 195 (3), 199–205. doi:10.1016/j.cbi.2011.12.007
Caruso, G., Torrisi, S. A., Mogavero, M. P., Currenti, W., Castellano, S., Godos, J., et al. (2022). Polyphenols and neuroprotection: Therapeutic implications for cognitive decline. Pharmacol. Ther. 232, 108013. doi:10.1016/j.pharmthera.2021.108013
Carvalho, C., and Cardoso, S. (2021). Diabetes-Alzheimer's disease link: Targeting mitochondrial dysfunction and redox imbalance. Antioxidants Redox Signal. 34 (8), 631–649. doi:10.1089/ars.2020.8056
Carvalho, C., Santos, M. S., Oliveira, C. R., and Moreira, P. I. (2015). Alzheimer's disease and type 2 diabetes-related alterations in brain mitochondria, autophagy and synaptic markers. Biochimica Biophysica Acta-Molecular Basis Dis. 1852 (8), 1665–1675. doi:10.1016/j.bbadis.2015.05.001
Chae, C. W., Choi, G. E., Jung, Y. H., Lim, J. R., Cho, J. H., Yoon, J. H., et al. (2022). High glucose-mediated VPS26a down-regulation dysregulates neuronal amyloid precursor protein processing and tau phosphorylation. Br. J. Pharmacol. 179 (15), 3934–3950. doi:10.1111/bph.15836
Chang, Y. F., Zhang, D., Hu, W. M., Liu, D. X., and Li, L. (2020). Semaglutide-mediated protection against A beta correlated with enhancement of autophagy and inhibition of apotosis. J. Clin. Neurosci. 81, 234–239. doi:10.1016/j.jocn.2020.09.054
Chen, J.-L., Luo, C., Pu, D., Zhang, G.-Q., Zhao, Y.-X., Sun, Y., et al. (2019). Metformin attenuates diabetes-induced tau hyperphosphorylation in vitro and in vivo by enhancing autophagic clearance. Exp. Neurol. 311, 44–56. doi:10.1016/j.expneurol.2018.09.008
Chen, Q., Mo, R., Wu, N., Zou, X., Shi, C., Gong, J., et al. (2017a). Berberine ameliorates diabetes-associated cognitive decline through modulation of aberrant inflammation response and insulin signaling pathway in DM rats. Front. Pharmacol. 8, 334. doi:10.3389/fphar.2017.00334
Chen, S., Liu, A.-r., An, F.-m., Yao, W.-b., and Gao, X.-d. (2012). Amelioration of neurodegenerative changes in cellular and rat models of diabetes-related Alzheimer's disease by exendin-4. Age 34 (5), 1211–1224. doi:10.1007/s11357-011-9303-8
Chen, T.-Y., Ferruzzi, M. G., Wu, Q.-L., Simon, J. E., Talcott, S. T., Wang, J., et al. (2017b). Influence of diabetes on plasma pharmacokinetics and brain bioavailability of grape polyphenols and their phase II metabolites in the Zucker diabetic fatty rat. Mol. Nutr. Food Res. 61 (10), 1700111. doi:10.1002/mnfr.201700111
Chen, Y., Zhao, S., Fan, Z., Li, Z., Zhu, Y., Shen, T., et al. (2021). Metformin attenuates plaque-associated tau pathology and reduces amyloid-beta burden in APP/PS1 mice. Alzheimers Res. Ther. 13 (1), 40. doi:10.1186/s13195-020-00761-9
Chen, Y., Zhou, K., Wang, R., Liu, Y., Kwak, Y.-D., Ma, T., et al. (2009). Antidiabetic drug metformin (Glucophage(R)) increases biogenesis of Alzheimer's amyloid peptides via up-regulating BACE1 transcription. Proc. Natl. Acad. Sci. U. S. A. 106 (10), 3907–3912. doi:10.1073/pnas.0807991106
Cheng, C., Lin, C.-H., Tsai, Y.-W., Tsai, C.-J., Chou, P.-H., and Lan, T.-H. (2014). Type 2 diabetes and antidiabetic medications in relation to dementia diagnosis. Journals Gerontology Ser. a-Biological Sci. Med. Sci. 69 (10), 1299–1305. doi:10.1093/gerona/glu073
Chledzik, S., Strawa, J., Matuszek, K., and Nazaruk, J. (2018). Pharmacological effects of scutellarin, an active component of genus scutellaria and Erigeron: A systematic review. Am. J. Chin. Med. 46 (2), 319–337. doi:10.1142/s0192415x18500167
Choi, J. S., Islam, M. N., Ali, M. Y., Kim, E. J., Kim, Y. M., and Jung, H. A. (2014a). Effects of C-glycosylation on anti-diabetic, anti-Alzheimer's disease and anti-inflammatory potential of apigenin. Food Chem. Toxicol. 64, 27–33. doi:10.1016/j.fct.2013.11.020
Choi, J. S., Islam, M. N., Ali, M. Y., Kim, Y. M., Park, H. J., Sohn, H. S., et al. (2014b). The effects of C-glycosylation of luteolin on its antioxidant, anti-Alzheimer's disease, anti-diabetic, and anti-inflammatory activities. Archives Pharmacal Res. 37 (10), 1354–1363. doi:10.1007/s12272-014-0351-3
Chornenkyy, Y., Wang, W. X., Wei, A., and Nelson, P. T. (2019). Alzheimer's disease and type 2 diabetes mellitus are distinct diseases with potential overlapping metabolic dysfunction upstream of observed cognitive decline. Brain Pathol. 29 (1), 3–17. doi:10.1111/bpa.12655
Coll, A. P., Farooqi, I. S., and O'Rahilly, S. (2007). The hormonal control of food intake. Cell 129 (2), 251–262. doi:10.1016/j.cell.2007.04.001
Costa, L. G., Garrick, J. M., Roque, P. J., and Pellacani, C. (2016). Mechanisms of neuroprotection by quercetin: Counteracting oxidative stress and more. Oxidative Med. Cell. Longev. 2016, 2986796. doi:10.1155/2016/2986796
Cox, K. H. M., Pipingas, A., and Scholey, A. B. (2015). Investigation of the effects of solid lipid curcumin on cognition and mood in a healthy older population. J. Psychopharmacol. 29 (5), 642–651. doi:10.1177/0269881114552744
Cukierman-Yaffe, T., Gerstein, H. C., Colhoun, H. M., Diaz, R., Garcia-Perez, L. E., Lakshmanan, M., et al. (2020). Effect of dulaglutide on cognitive impairment in type 2 diabetes: An exploratory analysis of the REWIND trial. Lancet Neurol. 19 (7), 582–590. doi:10.1016/s1474-4422(20)30173-3
De Felice, F. G., and Ferreira, S. T. (2014). Inflammation, defective insulin signaling, and mitochondrial dysfunction as common molecular denominators connecting type 2 diabetes to alzheimer disease. Diabetes 63 (7), 2262–2272. doi:10.2337/db13-1954
De Felice, F. G., Lourenco, M. V., and Ferreira, S. T. (2014). How does brain insulin resistance develop in Alzheimer's disease? Alzheimers Dement. 10 (1), S26–S32. doi:10.1016/j.jalz.2013.12.004
de la Monte, S. M. (2017). Insulin resistance and neurodegeneration: Progress towards the development of new therapeutics for alzheimer's disease. Drugs 77 (1), 47–65. doi:10.1007/s40265-016-0674-0
Dey, A., Hao, S., Wosiski-Kuhn, M., and Stranahan, A. M. (2017). Glucocorticoid-mediated activation of GSK3 beta promotes tau phosphorylation and impairs memory in type 2 diabetes. Neurobiol. Aging 57, 75–83. doi:10.1016/j.neurobiolaging.2017.05.010
Ding, X.-W., Robinson, M., Li, R., Aldhowayan, H., Geetha, T., and Babu, J. R. (2021). Mitochondrial dysfunction and beneficial effects of mitochondria-targeted small peptide SS-31 in Diabetes Mellitus and Alzheimer's disease. Pharmacol. Res. 171, 105783. doi:10.1016/j.phrs.2021.105783
Dong, Q., Teng, S.-W., Wang, Y., Qin, F., Li, Y., Ai, L.-L., et al. (2019). Sitagliptin protects the cognition function of the Alzheimer's disease mice through activating glucagon-like peptide-1 and BDNF-TrkB signalings. Neurosci. Lett. 696, 184–190. doi:10.1016/j.neulet.2018.12.041
Duarte, A. I., Candeias, E., Alves, I. N., Mena, D., Silva, D. F., Machado, N. J., et al. (2020). Liraglutide protects against brain amyloid-β1-42 accumulation in female mice with early alzheimer's disease-like pathology by partially rescuing oxidative/nitrosative stress and inflammation. Int. J. Mol. Sci. 21 (5), 1746. doi:10.3390/ijms21051746
Duarte, A. I., Moreira, P. I., and Oliveira, C. R. (2012). Insulin in central nervous system: More than just a peripheral hormone. J. Aging Res. 2012, 384017. doi:10.1155/2012/384017
Dye, L., Boyle, N. B., Champ, C., and Lawton, C. (2017). The relationship between obesity and cognitive health and decline. Proc. Nutr. Soc. 76 (4), 443–454. doi:10.1017/s0029665117002014
Ebrahimpour, S., Zakeri, M., and Esmaeili, A. (2020). Crosstalk between obesity, diabetes, and alzheimer's disease: Introducing quercetin as an effective triple herbal medicine. Ageing Res. Rev. 62, 101095. doi:10.1016/j.arr.2020.101095
Exalto, L. G., Biessels, G. J., Karter, A. J., Huang, E. S., Katon, W. J., Minkoff, J. R., et al. (2013). Risk score for prediction of 10 year dementia risk in individuals with type 2 diabetes: A cohort study. Lancet Diabetes & Endocrinol. 1 (3), 183–190. doi:10.1016/s2213-8587(13)70048-2
Fan, Y., Wang, N., Rocchi, A., Zhang, W., Vassar, R., Zhou, Y., et al. (2017). Identification of natural products with neuronal and metabolic benefits through autophagy induction. Autophagy 13 (1), 41–56. doi:10.1080/15548627.2016.1240855
Fanelli, G., Franke, B., De Witte, W., Ruisch, I. H., Haavik, J., van Gils, V., et al. (2022). Insulinopathies of the brain? Genetic overlap between somatic insulin-related and neuropsychiatric disorders. Transl. Psychiatry 12 (1), 59. doi:10.1038/s41398-022-01817-0
Fang, E. F., Hou, Y. J., Palikaras, K., Adriaanse, B. A., Kerr, J. S., Yang, B. M., et al. (2019). Mitophagy inhibits amyloid-beta and tau pathology and reverses cognitive deficits in models of Alzheimer's disease. Nat. Neurosci. 22(3), 401-412. doi:10.1038/s41593-018-0332-9
Feinkohl, I., Price, J. F., Strachan, M. W., and Frier, B. M. (2015). The impact of diabetes on cognitive decline: Potential vascular, metabolic, and psychosocial risk factors. Alzheimers Res. Ther. 7 (1), 46. doi:10.1186/s13195-015-0130-5
Femminella, G. D., Livingston, N. R., Raza, S., van der Doef, T., Frangou, E., Love, S., et al. (2021). Does insulin resistance influence neurodegeneration in non-diabetic Alzheimer's subjects? Alzheimers Res. Ther. 13 (1), 47. doi:10.1186/s13195-021-00784-w
Feng, H.-l., Dang, H.-z., Fan, H., Chen, X.-p., Rao, Y.-x., Ren, Y., et al. (2016). Curcumin ameliorates insulin signalling pathway in brain of Alzheimer's disease transgenic mice. Int. J. Immunopathol. Pharmacol. 29 (4), 734–741. doi:10.1177/0394632016659494
Feng, L., and Zhang, L. (2019). Resveratrol suppresses aβ-induced microglial activation through the TXNIP/TRX/NLRP3 signaling pathway. DNA Cell Biol. 38 (8), 874–879. doi:10.1089/dna.2018.4308
Ferreira, S. T., and Klein, W. L. (2011). The Aβ oligomer hypothesis for synapse failure and memory loss in Alzheimer’s disease. Neurobiol. Learn. Mem. 96 (4), 529–543. doi:10.1016/j.nlm.2011.08.003
Fertan, E., Rodrigues, G. J., Wheeler, R. V., Goguen, D., Wong, A. A., James, H., et al. (2019). Cognitive decline, cerebral-spleen tryptophan metabolism, oxidative stress, cytokine production, and regulation of the txnip gene in a triple transgenic mouse model of alzheimer disease. Am. J. Pathol. 189 (7), 1435–1450. doi:10.1016/j.ajpath.2019.03.006
Flolingue, C., Wennberg, A., Berger, S., Polotsky, V. Y., and Spira, A. P. (2018). Disturbed sleep and diabetes: A potential nexus of dementia risk. Metabolism-Clinical Exp. 84, 85–93. doi:10.1016/j.metabol.2018.01.021
Floris, S., Fais, A., Medda, R., Pintus, F., Piras, A., Kumar, A., et al. (2021). Washingtonia filifera seed extracts inhibit the islet amyloid polypeptide fibrils formations and alpha-amylase and alpha-glucosidase activity. J. Enzyme Inhibition Med. Chem. 36 (1), 517–524. doi:10.1080/14756366.2021.1874945
Gambini, J., Ingles, M., Olaso, G., Lopez-Grueso, R., Bonet-Costa, V., Gimeno-Mallench, L., et al. (2015). Properties of resveratrol: In vitro and in vivo studies about metabolism, bioavailability, and biological effects in animal models and humans. Oxid. Med. Cell Longev. 2015, 837042. doi:10.1155/2015/837042
Gejl, M., Brock, B., Egefjord, L., Vang, K., Rungby, J., and Gjedde, A. (2017). Blood-brain glucose transfer in alzheimer's disease: Effect of GLP-1 analog treatment. Sci. Rep. 7, 17490. doi:10.1038/s41598-017-17718-y
Gejl, M., Gjedde, A., Egefjord, L., Moller, A., Hansen, S. B., Vang, K., et al. (2016). In alzheimer's disease, 6-month treatment with GLP-1 analog prevents decline of brain glucose metabolism: Randomized, placebo-controlled, double-blind clinical trial. Front. Aging Neurosci. 8, 108. doi:10.3389/fnagi.2016.00108
Gendron, T. F., Zhang, Y. J., and Petrucelli, L. (2013). Does obesity-induced tau phosphorylation tip the scale toward dementia? Diabetes 62 (5), 1365–1366. doi:10.2337/db12-1784
Gengler, S., McClean, P. L., McCurtin, R., Gault, V. A., and Hoelscher, C. (2012). Val(8)GLP-1 rescues synaptic plasticity and reduces dense core plaques in APP/PS1 mice. Neurobiol. Aging 33 (2), 265–276. doi:10.1016/j.neurobiolaging.2010.02.014
Ghafouri-Fard, S., Bahroudi, Z., Shoorei, H., Hussen, B. M., Talebi, S. F., Baig, S. G., et al. (2022). Disease-associated regulation of gene expression by resveratrol: Special focus on the PI3K/AKT signaling pathway. Cancer Cell Int. 22 (1), 298. doi:10.1186/s12935-022-02719-3
Ghasemi, R., Zarifkar, A., Rastegar, K., Maghsoudi, N., and Moosavi, M. (2014). Insulin protects against A beta-induced spatial memory impairment, hippocampal apoptosis and MAPKs signaling disruption. Neuropharmacology 85, 113–120. doi:10.1016/j.neuropharm.2014.01.036
Giacomini, E., Rupiani, S., Guidotti, L., Recanatini, M., and Roberti, M. (2016). The use of stilbene scaffold in medicinal chemistry and multi-target drug design. Curr. Med. Chem. 23 (23), 2439–2489. doi:10.2174/0929867323666160517121629
Goncalves, R. A., Wijesekara, N., Fraser, P. E., and De Felice, F. G. (2019). The link between tau and insulin signaling: Implications for alzheimer's disease and other tauopathies. Front. Cell Neurosci. 13, 17. doi:10.3389/fncel.2019.00017
González, A., Calfío, C., Churruca, M., and Maccioni, R. B. (2022). Glucose metabolism and AD: Evidence for a potential diabetes type 3. Alzheimers Res. Ther. 14 (1), 56. doi:10.1186/s13195-022-00996-8
Grundke-Iqbal, I., Iqbal, K., Tung, Y. C., Quinlan, M., Wisniewski, H. M., and Binder, L. I. (1986). Abnormal phosphorylation of the microtubule-associated protein tau (tau) in Alzheimer cytoskeletal pathology. Proc. Natl. Acad. Sci. U. S. A. 83 (13), 4913–4917. doi:10.1073/pnas.83.13.4913
Gupta, S., Yadav, K., Mantri, S. S., Singhal, N. K., Ganesh, S., and Sandhir, R. (2018). Evidence for compromised insulin signaling and neuronal vulnerability in experimental model of sporadic alzheimer's disease. Mol. Neurobiol. 55 (12), 8916–8935. doi:10.1007/s12035-018-0985-0
Ha, J., Choi, D. W., Kim, K. J., Cho, S. Y., Kim, H., Kim, K. Y., et al. (2021). Association of metformin use with alzheimer's disease in patients with newly diagnosed type 2 diabetes: A population-based nested case-control study. Sci. Rep. 11 (1), 24069. doi:10.1038/s41598-021-03406-5
Han, W.-N., Hoelscher, C., Yuan, L., Yang, W., Wang, X.-H., Wu, M.-N., et al. (2013). Liraglutide protects against amyloid-beta protein-induced impairment of spatial learning and memory in rats. Neurobiol. Aging 34 (2), 576–588. doi:10.1016/j.neurobiolaging.2012.04.009
He, C., Li, Q., Cui, Y., Gao, P., Shu, W., Zhou, Q., et al. (2022). Recurrent moderate hypoglycemia accelerates the progression of Alzheimer's disease through impairment of the TRPC6/GLUT3 pathway. Jci Insight 7 (5), e154595. doi:10.1172/jci.insight.154595
Heneka, M. T., and Nicotera, P. (2016). Thoughts on obesity and brain glucose. Cell 165 (4), 773–775. doi:10.1016/j.cell.2016.04.057
Hoelscher, C. (2014). The incretin hormones glucagonlike peptide 1 and glucose-dependent insulinotropic polypeptide are neuroprotective in mouse models of Alzheimer's disease. Alzheimers Dementia 10, S47–S54. doi:10.1016/j.jalz.2013.12.009
Holscher, C. (2014). Central effects of GLP-1: New opportunities for treatments of neurodegenerative diseases. J. Endocrinol. 221 (1), T31–T41. doi:10.1530/joe-13-0221
Hu, T., Lu, X.-Y., Shi, J.-J., Liu, X.-Q., Chen, Q.-B., Wang, Q., et al. (2020). Quercetin protects against diabetic encephalopathy via SIRT1/NLRP3 pathway in db/db mice. J. Cell. Mol. Med. 24 (6), 3449–3459. doi:10.1111/jcmm.15026
Hunter, K., and Hoelscher, C. (2012). Drugs developed to treat diabetes, liraglutide and lixisenatide, cross the blood brain barrier and enhance neurogenesis. Bmc Neurosci. 13, 33. doi:10.1186/1471-2202-13-33
Imamura, T., Yanagihara, Y. T., Ohyagi, Y., Nakamura, N., Iinuma, K. M., Yamasaki, R., et al. (2020). Insulin deficiency promotes formation of toxic amyloid-beta 42 conformer co-aggregating with hyper-phosphorylated tau oligomer in an Alzheimer's disease model. Neurobiol. Dis. 137, 104739. doi:10.1016/j.nbd.2020.104739
Infante-Garcia, C., Jose Ramos-Rodriguez, J., Hierro-Bujalance, C., Ortegon, E., Pickett, E., Jackson, R., et al. (2018). Antidiabetic polypill improves central pathology and cognitive impairment in a mixed model of alzheimer's disease and type 2 diabetes. Mol. Neurobiol. 55 (7), 6130–6144. doi:10.1007/s12035-017-0825-7
Infante-Garcia, C., Jose Ramos-Rodriguez, J., Marin-Zambrana, Y., Teresa Fernandez-Ponce, M., Casas, L., Mantell, C., et al. (2017). Mango leaf extract improves central pathology and cognitive impairment in a type 2 diabetes mouse model. Brain Pathol. 27 (4), 499–507. doi:10.1111/bpa.12433
Isik, A. T., Soysal, P., Yay, A., and Usarel, C. (2017). The effects of sitagliptin, a DPP-4 inhibitor, on cognitive functions in elderly diabetic patients with or without Alzheimer's disease. Diabetes Res. Clin. Pract. 123, 192–198. doi:10.1016/j.diabres.2016.12.010
Ismael, S., WajidunnisaSakata, K., McDonald, M. P., Liao, F. F., and Ishrat, T. (2021). ER stress associated TXNIP-NLRP3 inflammasome activation in hippocampus of human Alzheimer's disease. Neurochem. Int. 148, 105104. doi:10.1016/j.neuint.2021.105104
Jack, C. R., Knopman, D. S., Jagust, W. J., Petersen, R. C., Weiner, M. W., Aisen, P. S., et al. (2013). Tracking pathophysiological processes in alzheimer's disease: An updated hypothetical model of dynamic biomarkers. Lancet Neurol. 12 (2), 207–216. doi:10.1016/s1474-4422(12)70291-0
Jadiya, P., Kolmetzky, D. W., Tomar, D., Di Meco, A., Lombardi, A. A., Lambert, J. P., et al. (2019). Impaired mitochondrial calcium efflux contributes to disease progression in models of Alzheimer's disease. Nat. Commun. 10, 3885. doi:10.1038/s41467-019-11813-6
Jeong, S. H., Kim, H. R., Kim, J., Kim, H., Hong, N., Jung, J. H., et al. (2021). Association of dipeptidyl peptidase-4 inhibitor use and amyloid burden in patients with diabetes and AD-related cognitive impairment. Neurology 97 (11), e1110–e1122. doi:10.1212/WNL.0000000000012534
Jinawong, K., Apaijai, N., Wongsuchai, S., Pratchayasakul, W., Chattipakorn, N., and Chattipakorn, S. C. (2020). Necrostatin-1 mitigates cognitive dysfunction in prediabetic rats with No alteration in insulin sensitivity. Diabetes 69 (7), 1411–1423. doi:10.2337/db19-1128
Kazkayasi, I., Telli, G., Nemutlu, E., and Uma, S. (2022). Intranasal metformin treatment ameliorates cognitive functions via insulin signaling pathway in ICV-STZ-induced mice model of Alzheimer's disease. Life Sci. 299, 120538. doi:10.1016/j.lfs.2022.120538
Kellar, D., and Craft, S. (2020). Brain insulin resistance in alzheimer's disease and related disorders: Mechanisms and therapeutic approaches. Lancet Neurol. 19 (9), 758–766. doi:10.1016/s1474-4422(20)30231-3
Kerr, J. S., Adriaanse, B. A., Greig, N. H., Mattson, M. P., Cader, M. Z., Bohr, V. A., et al. (2017). Mitophagy and alzheimer's disease: Cellular and molecular mechanisms. Trends Neurosci. 40 (3), 151–166. doi:10.1016/j.tins.2017.01.002
Kim, B., and Feldman, E. L. (2015). Insulin resistance as a key link for the increased risk of cognitive impairment in the metabolic syndrome. Exp. Mol. Med. 47, e149. doi:10.1038/emm.2015.3
Kim, D.-H., Huh, J.-W., Jang, M., Suh, J.-H., Kim, T.-W., Park, J.-S., et al. (2012). Sitagliptin increases tau phosphorylation in the hippocampus of rats with type 2 diabetes and in primary neuron cultures. Neurobiol. Dis. 46 (1), 52–58. doi:10.1016/j.nbd.2011.12.043
Kim, J., Lee, S., Lee, S. H., Jung, S., Kim, Y., Choi, I., et al. (2018). Neuroprotective effects of HM15211, a novel long-acting GLP-1/GIP/glucagon triple agonist in the neurodegenerative disease models. Diabetologia 61, S84–S85.
Kleinridders, A., Ferris, H. A., Cai, W., and Kahn, C. R. (2014). Insulin action in brain regulates systemic metabolism and brain function. Diabetes 63 (7), 2232–2243. doi:10.2337/db14-0568
Kong, F.-J., Wu, J.-H., Sun, S.-Y., Ma, L.-L., and Zhou, J.-Q. (2018). Liraglutide ameliorates cognitive decline by promoting autophagy via the AMP-activated protein kinase/mammalian target of rapamycin pathway in a streptozotocin-induced mouse model of diabetes. Neuropharmacology 131, 316–325. doi:10.1016/j.neuropharm.2018.01.001
Kornelius, E., Lin, C.-L., Chang, H.-H., Li, H.-H., Huang, W.-N., Yang, Y.-S., et al. (2015). DPP-4 inhibitor linagliptin attenuates A beta-induced cytotoxicity through activation of AMPK in neuronal cells. Cns Neurosci. Ther. 21 (7), 549–557. doi:10.1111/cns.12404
Kosaraju, J., Gali, C. C., Khatwal, R. B., Dubala, A., Chinni, S., Holsinger, R. M. D., et al. (2013a). Saxagliptin: A dipeptidyl peptidase-4 inhibitor ameliorates streptozotocin induced alzheimer's disease. Neuropharmacology 72, 291–300. doi:10.1016/j.neuropharm.2013.04.008
Kosaraju, J., Holsinger, R. M. D., Guo, L., and Tam, K. Y. (2017). Linagliptin, a dipeptidyl peptidase-4 inhibitor, mitigates cognitive deficits and pathology in the 3xTg-AD mouse model of alzheimer's disease. Mol. Neurobiol. 54 (8), 6074–6084. doi:10.1007/s12035-016-0125-7
Kosaraju, J., Murthy, V., Khatwal, R. B., Dubala, A., Chinni, S., Nataraj, S. K. M., et al. (2013b). Vildagliptin: An anti-diabetes agent ameliorates cognitive deficits and pathology observed in streptozotocin-induced alzheimer's disease. J. Pharm. Pharmacol. 65 (12), 1773–1784. doi:10.1111/jphp.12148
Kumar, S., Chowdhury, S., Razdan, A., Kumari, D., Purty, R. S., Ram, H., et al. (2021). Downregulation of candidate gene expression and neuroprotection by piperine in streptozotocin-induced hyperglycemia and memory impairment in rats. Front. Pharmacol. 11, 595471. doi:10.3389/fphar.2020.595471
Kumar, V., Kim, S.-H., and Bishayee, K. (2022a). Dysfunctional glucose metabolism in alzheimer's disease onset and potential pharmacological interventions. Int. J. Mol. Sci. 23 (17), 9540. doi:10.3390/ijms23179540
Kumar, V., Kim, S. H., and Bishayee, K. (2022b). Dysfunctional glucose metabolism in alzheimer's disease onset and potential pharmacological interventions. Int. J. Mol. Sci. 23 (17), 9540. doi:10.3390/ijms23179540
Łabuzek, K., Suchy, D., Gabryel, B., Bielecka, A., Liber, S., and Okopień, B. (2010). Quantification of metformin by the HPLC method in brain regions, cerebrospinal fluid and plasma of rats treated with lipopolysaccharide. Pharmacol. Rep. 62 (5), 956–965. doi:10.1016/s1734-1140(10)70357-1
Lambeir, A. M., Scharpé, S., and De Meester, I. (2008). DPP4 inhibitors for diabetes--what next? Biochem. Pharmacol. 76 (12), 1637–1643. doi:10.1016/j.bcp.2008.07.029
Lan, J. R., Zhao, Y. Y., Dong, F. X., Yan, Z. Y., Zheng, W. J., Fan, J. P., et al. (2015). Meta-analysis of the effect and safety of berberine in the treatment of type 2 diabetes mellitus, hyperlipemia and hypertension. J. Ethnopharmacol. 161, 69–81. doi:10.1016/j.jep.2014.09.049
Launer, L. J. (2002). Demonstrating the case that AD is a vascular disease: Epidemiologic evidence. Ageing Res. Rev. 1 (1), 61–77. doi:10.1016/s0047-6374(01)00364-5
Li, L., Ismael, S., Nasoohi, S., Sakata, K., Liao, F. F., McDonald, M. P., et al. (2019). Thioredoxin-interacting protein (TXNIP) associated NLRP3 inflammasome activation in human alzheimer's disease brain. J. Alzheimers Dis. 68 (1), 255–265. doi:10.3233/JAD-180814
Li, L., Zhang, Z.-F., Holscher, C., Gao, C., Jiang, Y.-H., and Liu, Y.-Z. (2012). (Val⁸) glucagon-like peptide-1 prevents tau hyperphosphorylation, impairment of spatial learning and ultra-structural cellular damage induced by streptozotocin in rat brains. Eur. J. Pharmacol. 674 (2-3), 280–286. doi:10.1016/j.ejphar.2011.11.005
Li, S., Cheng, X., and Wang, C. (2017). A review on traditional uses, phytochemistry, pharmacology, pharmacokinetics and toxicology of the genus Peganum. J. Ethnopharmacol. 203, 127–162. doi:10.1016/j.jep.2017.03.049
Li, Y.-R., Li, S., and Lin, C.-C. (2018). Effect of resveratrol and pterostilbene on aging and longevity. Biofactors 44 (1), 69–82. doi:10.1002/biof.1400
Lin, B., Koibuchi, N., Hasegawa, Y., Sueta, D., Toyama, K., Uekawa, K., et al. (2014). Glycemic control with empagliflozin, a novel selective SGLT2 inhibitor, ameliorates cardiovascular injury and cognitive dysfunction in obese and type 2 diabetic mice. Cardiovasc. Diabetol. 13, 148. doi:10.1186/s12933-014-0148-1
Lin, Y., Wang, K., Ma, C., Wang, X., Gong, Z., Zhang, R., et al. (2018). Evaluation of metformin on cognitive improvement in patients with non-dementia vascular cognitive impairment and abnormal glucose metabolism. Front. Aging Neurosci. 10, 227. doi:10.3389/fnagi.2018.00227
Liu, P. P., Xie, Y., Meng, X. Y., and Kang, J. S. (2019). History and progress of hypotheses and clinical trials for Alzheimer's disease. Signal Transduct. Target Ther. 4, 29. doi:10.1038/s41392-019-0063-8
Liu, W., Li, G., Hoelscher, C., and Li, L. (2015). Neuroprotective effects of geniposide on Alzheimer's disease pathology. Rev. Neurosci. 26 (4), 371–383. doi:10.1515/revneuro-2015-0005
Liu, Y., Fu, X., Lan, N., Li, S., Zhang, J., Wang, S., et al. (2014). Luteolin protects against high fat diet-induced cognitive deficits in obesity mice. Behav. Brain Res. 267, 178–188. doi:10.1016/j.bbr.2014.02.040
Long-Smith, C. M., Manning, S., McClean, P. L., Coakley, M. F., O'Halloran, D. J., Holscher, C., et al. (2013). The diabetes drug liraglutide ameliorates aberrant insulin receptor localisation and signalling in parallel with decreasing both amyloid-beta plaque and glial pathology in a mouse model of alzheimer's disease. Neuromolecular Med. 15 (1), 102–114. doi:10.1007/s12017-012-8199-5
Luo, J.-S., Ning, J.-Q., Chen, Z.-Y., Li, W.-J., Zhou, R.-L., Yan, R.-Y., et al. (2022). The role of mitochondrial quality control in cognitive dysfunction in diabetes. Neurochem. Res. 47 (8), 2158–2172. doi:10.1007/s11064-022-03631-y
Ma, D.-L., Chen, F.-Q., Xu, W.-J., Yue, W.-Z., Yuan, G., and Yang, Y. (2015). Early intervention with glucagon-like peptide 1 analog liraglutide prevents tau hyperphosphorylation in diabetic db/db mice. J. Neurochem. 135 (2), 301–308. doi:10.1111/jnc.13248
Ma, S. L., Zhang, C., Zhang, Z. Y., Dai, Y. X., Gu, R., and Jiang, R. (2019). Geniposide protects PC12 cells from lipopolysaccharide-evoked inflammatory injury via up-regulation of miR-145-5p. Artif. Cells Nanomedicine Biotechnol. 47 (1), 2875–2881. doi:10.1080/21691401.2019.1626406
Mahomoodally, M. F., Picot-Allain, M. C. N., Zengin, G., Llorent-Martinez, E. J., Abdullah, H. H., Ak, G., et al. (2020). Phytochemical analysis, network pharmacology and in silico investigations on anacamptis pyramidalis tuber extracts. Molecules 25 (10), 2422. doi:10.3390/molecules25102422
Mao, Y. F., Guo, Z. Y., Zheng, T. T., Jiang, Y. S., Yan, Y. P., Yin, X. Z., et al. (2016). Intranasal insulin alleviates cognitive deficits and amyloid pathology in young adult APPswe/PS1dE9 mice. Aging Cell 15 (5), 893–902. doi:10.1111/acel.12498
McClean, P. L., Gault, V. A., Harriott, P., and Hoelscher, C. (2010). Glucagon-like peptide-1 analogues enhance synaptic plasticity in the brain: A link between diabetes and alzheimer's disease. Eur. J. Pharmacol. 630 (1-3), 158–162. doi:10.1016/j.ejphar.2009.12.023
McClean, P. L., and Hoelscher, C. (2014). Lixisenatide, a drug developed to treat type 2 diabetes, shows neuroprotective effects in a mouse model of Alzheimer's disease. Neuropharmacology 86, 241–258. doi:10.1016/j.neuropharm.2014.07.015
McClean, P. L., Parthsarathy, V., Faivre, E., and Hoelscher, C. (2011). The diabetes drug liraglutide prevents degenerative processes in a mouse model of alzheimer's disease. J. Neurosci. 31 (17), 6587–6594. doi:10.1523/jneurosci.0529-11.2011
Melone, M. A. B., Dato, C., Paladino, S., Coppola, C., Trebini, C., Giordana, M. T., et al. (2018). Verapamil inhibits ser202/thr205 phosphorylation of tau by blocking TXNIP/ROS/p38 MAPK pathway. Pharm. Res. 35 (2), 44. doi:10.1007/s11095-017-2276-2
Meng, X., Zhou, J., Zhao, C. N., Gan, R. Y., and Li, H. B. (2020). Health benefits and molecular mechanisms of resveratrol: A narrative review. Foods 9 (3), 340. doi:10.3390/foods9030340
Michailidis, M., Moraitou, D., Tata, D. A., Kalinderi, K., Papamitsou, T., and Papaliagkas, V. (2022). Alzheimer's disease as type 3 diabetes: Common pathophysiological mechanisms between alzheimer's disease and type 2 diabetes. Int. J. Mol. Sci. 23 (5), 2687. doi:10.3390/ijms23052687
Mihaylova, M. M., and Shaw, R. J. (2011). The AMPK signalling pathway coordinates cell growth, autophagy and metabolism. Nat. Cell Biol. 13 (9), 1016–1023. doi:10.1038/ncb2329
Milstein, J. L., and Ferris, H. A. (2021). The brain as an insulin-sensitive metabolic organ. Mol. Metab. 52, 101234. doi:10.1016/j.molmet.2021.101234
Miltonprabu, S. (2019). “Chapter 2.9 - quercetin: A flavonol with versatile therapeutic applications and its interactions with other drugs,” in Nonvitamin and nonmineral nutritional supplements. Editors S. M. Nabavi, and A. S. Silva (Academic Press), 75–83.
Mishra, A., Wang, Y., Yin, F., Vitali, F., Rodgers, K. E., Soto, M., et al. (2022). A tale of two systems: Lessons learned from female mid-life aging with implications for Alzheimer's prevention & treatment. Ageing Res. Rev. 74, 101542. doi:10.1016/j.arr.2021.101542
Moore, E. M., Mander, A. G., Ames, D., Kotowicz, M. A., Carne, R. P., Brodaty, H., et al. (2013). Increased risk of cognitive impairment in patients with diabetes is associated with metformin. Diabetes Care 36 (10), 2981–2987. doi:10.2337/dc13-0229
Moran, C., Callisaya, M. L., Srikanth, V., and Arvanitakis, Z. (2019). Diabetes therapies for dementia. Curr. Neurology Neurosci. Rep. 19 (8), 58. doi:10.1007/s11910-019-0973-4
Mui, J. V., Zhou, J., Lee, S., Leung, K. S. K., Lee, T. T. L., Chou, O. H. I., et al. (2021). Sodium-glucose cotransporter 2 (SGLT2) inhibitors vs. Dipeptidyl peptidase-4 (DPP4) inhibitors for new-onset dementia: A propensity score-matched population-based study with competing risk analysis. Front. Cardiovasc. Med. 8, 747620. doi:10.3389/fcvm.2021.747620
Mullins, R. J., Mustapic, M., Chia, C. W., Carlson, O., Gulyani, S., Tran, J., et al. (2019). A pilot study of exenatide actions in alzheimer's disease. Curr. Alzheimer Res. 16 (8), 741–752. doi:10.2174/1567205016666190913155950
Nasoohi, S., Ismael, S., and Ishrat, T. (2018a). Thioredoxin-interacting protein (TXNIP) in cerebrovascular and neurodegenerative diseases: Regulation and implication. Mol. Neurobiol. 55 (10), 7900–7920. doi:10.1007/s12035-018-0917-z
Nasoohi, S., Parveen, K., and Ishrat, T. (2018b). Metabolic syndrome, brain insulin resistance, and alzheimer's disease: Thioredoxin interacting protein (TXNIP) and inflammasome as core amplifiers. J. Alzheimers Dis. 66 (3), 857–885. doi:10.3233/JAD-180735
Natunen, T., Martiskainen, H., Marttinen, M., Gabbouj, S., Koivisto, H., Kemppainen, S., et al. (2020). Diabetic phenotype in mouse and humans reduces the number of microglia around β-amyloid plaques. Mol. Neurodegener. 15 (1), 66. doi:10.1186/s13024-020-00415-2
Ng, M. Y. W., Wai, T., and Simonsen, A. (2021). Quality control of the mitochondrion. Dev. Cell 56 (7), 881–905. doi:10.1016/j.devcel.2021.02.009
Nichols, E., Szoeke, C. E. I., Vollset, S. E., Abbasi, N., Abd-Allah, F., Abdela, J., et al. (2019). Global, regional, and national burden of alzheimer's disease and other dementias, 1990-2016: A systematic analysis for the global burden of disease study 2016. Lancet Neurol. 18 (1), 88–106. doi:10.1016/s1474-4422(18)30403-4
Nielsen, L. L., Young, A. A., and Parkes, D. G. (2004). Pharmacology of exenatide (synthetic exendin-4): A potential therapeutic for improved glycemic control of type 2 diabetes. Regul. Pept. 117 (2), 77–88. doi:10.1016/j.regpep.2003.10.028
Nigrovic, L. E., Kimia, A. A., Shah, S. S., and Neuman, M. I. (2012). Relationship between cerebrospinal fluid glucose and serum glucose. N. Engl. J. Med. 366 (6), 576–578. doi:10.1056/NEJMc1111080
Norton, S., Matthews, F. E., Barnes, D. E., Yaffe, K., and Brayne, C. (2014). Potential for primary prevention of alzheimer's disease: An analysis of population-based data. Lancet Neurol. 13 (8), 788–794. doi:10.1016/S1474-4422(14)70136-X
Nuamnaichati, N., Mangmool, S., Chattipakorn, N., and Parichatikanond, W. (2020). Stimulation of GLP-1 receptor inhibits methylglyoxal-induced mitochondrial dysfunctions in H9c2 cardiomyoblasts: Potential role of epac/PI3K/Akt pathway. Front. Pharmacol. 11, 805. doi:10.3389/fphar.2020.00805
Ott, A., Stolk, R. P., van Harskamp, F., Pols, H. A., Hofman, A., and Breteler, M. M. (1999). Diabetes mellitus and the risk of dementia: The Rotterdam Study. Neurology 53 (9), 1937–1942. doi:10.1212/wnl.53.9.1937
Parthsarathy, V., and Hoelscher, C. (2013). The type 2 diabetes drug liraglutide reduces chronic inflammation induced by irradiation in the mouse brain. Eur. J. Pharmacol. 700 (1-3), 42–50. doi:10.1016/j.ejphar.2012.12.012
Patrone, C., Eriksson, O., and Lindholm, D. (2014). Diabetes drugs and neurological disorders: New views and therapeutic possibilities. Lancet Diabetes Endocrinol. 2 (3), 256–262. doi:10.1016/s2213-8587(13)70125-6
Paul, K. C., Jerrett, M., and Ritz, B. (2018). Type 2 diabetes mellitus and alzheimer's disease: Overlapping biologic mechanisms and environmental risk factors. Curr. Environ. Health Rep. 5 (1), 44–58. doi:10.1007/s40572-018-0176-1
Paul, S., Chakraborty, S., Anand, U., Dey, S., Nandy, S., Ghorai, M., et al. (2021a). Withania somnifera (L.) dunal (ashwagandha): A comprehensive review on ethnopharmacology, pharmacotherapeutics, biomedicinal and toxicological aspects. Biomed. Pharmacother. 143, 112175. doi:10.1016/j.biopha.2021.112175
Paul, S., Saha, D., and Bk, B. (2021b). Mitochondrial dysfunction and mitophagy closely cooperate in neurological deficits associated with alzheimer's disease and type 2 diabetes. Mol. Neurobiol. 58 (8), 3677–3691. doi:10.1007/s12035-021-02365-2
Phiel, C. J., Wilson, C. A., Lee, V. M., and Klein, P. S. (2003). GSK-3alpha regulates production of Alzheimer's disease amyloid-beta peptides. Nature 423 (6938), 435–439. doi:10.1038/nature01640
Picone, P., Nuzzo, D., Caruana, L., Messina, E., Barera, A., Vasto, S., et al. (2015). Metformin increases APP expression and processing via oxidative stress, mitochondrial dysfunction and NF-kappa B activation: Use of insulin to attenuate metformin's effect. Biochimica Biophysica Acta-Molecular Cell Res. 1853 (5), 1046–1059. doi:10.1016/j.bbamcr.2015.01.017
Picone, P., Vilasi, S., Librizzi, F., Contardi, M., Nuzzo, D., Caruana, L., et al. (2016). Biological and biophysics aspects of metformin-induced effects: Cortex mitochondrial dysfunction and promotion of toxic amyloid pre-fibrillar aggregates. Aging-Us 8 (8), 1718–1734. doi:10.18632/aging.101004
Pomilio, C., Gonzalez Perez, N., Calandri, I., Crivelli, L., Allegri, R., Sevlever, G., et al. (2022). Diabetic patients treated with metformin during early stages of alzheimer's disease show a better integral performance: Data from ADNI study. Geroscience 44 (3), 1791–1805. doi:10.1007/s11357-022-00568-6
Poorgholam, P., Yaghmaei, P., Noureddini, M., and Hajebrahimi, Z. (2021). Effects of artemisinin and TSP-1-human endometrial-derived stem cells on a streptozocin-induced model of Alzheimer's disease and diabetes in Wistar rats. Acta Neurobiol. Exp. 81 (2), 141–150. doi:10.21307/ane-2021-013
Pugazhenthi, S., Qin, L. M., and Reddy, P. H. (2017). Common neurodegenerative pathways in obesity, diabetes, and Alzheimer's disease. Biochimica Biophysica Acta-Molecular Basis Dis. 1863 (5), 1037–1045. doi:10.1016/j.bbadis.2016.04.017
Rahman, A., Schelbaum, E., Hoffman, K., Diaz, I., Hristov, H., Andrews, R., et al. (2020). Sex-driven modifiers of alzheimer risk: A multimodality brain imaging study. Neurology 95 (2), e166–e178. doi:10.1212/wnl.0000000000009781
Reger, M. A., Watson, G. S., Green, P. S., Baker, L. D., Cholerton, B., Fishel, M. A., et al. (2008). Intranasal insulin administration dose-dependently modulates verbal memory and plasma amyloid-beta in memory-impaired older adults. J. Alzheimers Dis. 13 (3), 323–331. doi:10.3233/jad-2008-13309
Reitz, C., and Mayeux, R. (2014). Alzheimer disease: Epidemiology, diagnostic criteria, risk factors and biomarkers. Biochem. Pharmacol. 88 (4), 640–651. doi:10.1016/j.bcp.2013.12.024
Ribeiro, R., Santos, A. C., Calazans, M. O., De Oliveira, A. C. P., and Vieira, L. B. (2022). Is resveratrol a prospective therapeutic strategy in the co-association of glucose metabolism disorders and neurodegenerative diseases? Nutr. Neurosci. 25 (11), 2442–2457. doi:10.1080/1028415x.2021.1972514
Roden, M., and Shulman, G. I. (2019). The integrative biology of type 2 diabetes. Nature 576 (7785), 51–60. doi:10.1038/s41586-019-1797-8
Rolandi, E., Zaccaria, D., Vaccaro, R., Abbondanza, S., Pettinato, L., Davin, A., et al. (2020). Estimating the potential for dementia prevention through modifiable risk factors elimination in the real-world setting: A population-based study. Alzheimers Res. Ther. 12 (1), 94. doi:10.1186/s13195-020-00661-y
Ronnback, A., Pavlov, P. F., Mansory, M., Gonze, P., Marliere, N., Winblad, B., et al. (2016). Mitochondrial dysfunction in a transgenic mouse model expressing human amyloid precursor protein (APP) with the Arctic mutation. J. Neurochem. 136 (3), 497–502. doi:10.1111/jnc.13410
Rosales-Corral, S., Tan, D.-X., Manchester, L., and Reiter, R. J. (2015). Diabetes and alzheimer disease, two overlapping pathologies with the same background: Oxidative stress. Oxidative Med. Cell. Longev. 2015, 985845. doi:10.1155/2015/985845
Salameh, T. S., Rhea, E. M., Talbot, K., and Banks, W. A. (2020). Brain uptake pharmacokinetics of incretin receptor agonists showing promise as Alzheimer's and Parkinson's disease therapeutics. Biochem. Pharmacol. 180, 114187. doi:10.1016/j.bcp.2020.114187
Saleh, R. A., Eissa, T. F., Abdallah, D. M., Saad, M. A., and El-Abhar, H. S. (2021). Peganum harmala enhanced GLP-1 and restored insulin signaling to alleviate-AlCl3-induced Alzheimer-like pathology model. Sci. Rep. 11 (1), 12040. doi:10.1038/s41598-021-90545-4
Secnik, J., Xu, H., Schwertner, E., Hammar, N., Alvarsson, M., Winblad, B., et al. (2021). The association of antidiabetic medications and Mini-Mental State Examination scores in patients with diabetes and dementia. Alzheimers Res. Ther. 13 (1), 197. doi:10.1186/s13195-021-00934-0
Selkoe, D. J., and Schenk, D. (2003). Alzheimer's disease: Molecular understanding predicts amyloid-based therapeutics. Annu. Rev. Pharmacol. Toxicol. 43, 545–584. doi:10.1146/annurev.pharmtox.43.100901.140248
Shakeri, F., Bianconi, V., Pirro, M., and Sahebkar, A. (2020). Effects of plant and animal natural products on mitophagy. Oxidative Med. Cell. Longev. 2020, 6969402. doi:10.1155/2020/6969402
Sharma, M. K., Jalewa, J., and Hoelscher, C. (2014). Neuroprotective and anti-apoptotic effects of liraglutide on SH-SY5Y cells exposed to methylglyoxal stress. J. Neurochem. 128 (3), 459–471. doi:10.1111/jnc.12469
Sims-Robinson, C., Kim, B., Rosko, A., and Feldman, E. L. (2010). How does diabetes accelerate Alzheimer disease pathology? Nat. Rev. Neurol. 6 (10), 551–559. doi:10.1038/nrneurol.2010.130
Singh, A. P., Singh, R., Verma, S. S., Rai, V., Kaschula, C. H., Maiti, P., et al. (2019). Health benefits of resveratrol: Evidence from clinical studies. Med. Res. Rev. 39 (5), 1851–1891. doi:10.1002/med.21565
Solmaz, V., Cinar, B. P., Yigitturk, G., Cavusoglu, T., Taskiran, D., and Erbas, O. (2015). Exenatide reduces TNF-alpha expression and improves hippocampal neuron numbers and memory in streptozotocin treated rats. Eur. J. Pharmacol. 765, 482–487. doi:10.1016/j.ejphar.2015.09.024
Son, S. M., Shin, H.-J., Byun, J., Kook, S. Y., Moon, M., Chang, Y. J., et al. (2016). Metformin facilitates amyloid-beta generation by beta- and gamma-secretases via autophagy activation. J. Alzheimers Dis. 51 (4), 1197–1208. doi:10.3233/jad-151200
Srikanth, V., Sinclair, A. J., Hill-Briggs, F., Moran, C., and Biessels, G. J. (2020). Type 2 diabetes and cognitive dysfunction-towards effective management of both comorbidities. Lancet Diabetes & Endocrinol. 8 (6), 535–545. doi:10.1016/S2213-8587(20)30118-2
Srivastava, S., Ahmad, R., and Khare, S. K. (2021). Alzheimer's disease and its treatment by different approaches: A review. Eur. J. Med. Chem. 216, 113320. doi:10.1016/j.ejmech.2021.113320
Stanley, M., Macauley, S. L., and Holtzman, D. M. (2016). Changes in insulin and insulin signaling in Alzheimer’s disease: Cause or consequence? J. Exp. Med. 213 (8), 1375–1385. doi:10.1084/jem.20160493
Sun, B., Jia, X., Yang, F., Ren, G., and Wu, X. (2021). CREB-mediated generation and neuronal growth regulates the behavioral improvement of geniposide in diabetes-associated depression mouse model. Neurosci. Res. 165, 38–44. doi:10.1016/j.neures.2020.05.003
Swaminathan, S. K., Ahlschwede, K. M., Sarma, V., Curran, G. L., Omtri, R. S., Decklever, T., et al. (2018). Insulin differentially affects the distribution kinetics of amyloid beta 40 and 42 in plasma and brain. J. Cereb. Blood Flow Metabolism 38 (5), 904–918. doi:10.1177/0271678X17709709
Sweeney, M. D., Kisler, K., Montagne, A., Toga, A. W., and Zlokovic, B. V. (2018a). The role of brain vasculature in neurodegenerative disorders. Nat. Neurosci. 21 (10), 1318–1331. doi:10.1038/s41593-018-0234-x
Sweeney, M. D., Sagare, A. P., and Zlokovic, B. V. (2018b). Blood–brain barrier breakdown in Alzheimer disease and other neurodegenerative disorders. Nat. Rev. Neurol. 14 (3), 133–150. doi:10.1038/nrneurol.2017.188
Szendroedi, J., Phielix, E., and Roden, M. (2011). The role of mitochondria in insulin resistance and type 2 diabetes mellitus. Nat. Rev. Endocrinol. 8 (2), 92–103. doi:10.1038/nrendo.2011.138
Talbot, K., Wang, H.-Y., Kazi, H., Han, L.-Y., Bakshi, K. P., Stucky, A., et al. (2012). Demonstrated brain insulin resistance in Alzheimer’s disease patients is associated with IGF-1 resistance, IRS-1 dysregulation, and cognitive decline. J. Clin. Investigation 122 (4), 1316–1338. doi:10.1172/jci59903
Tang, Y., Yu, C., Wu, J., Chen, H., Zeng, Y., Wang, X., et al. (2018). Lychee seed extract protects against neuronal injury and improves cognitive function in rats with type II diabetes mellitus with cognitive impairment. Int. J. Mol. Med. 41 (1), 251–263. doi:10.3892/ijmm.2017.3245
Teo, E., Ravi, S., Barardo, D., Kim, H.-S., Fong, S., Cazenave-Gassiot, A., et al. (2019). Metabolic stress is a primary pathogenic event in transgenic Caenorhabditis elegans expressing pan-neuronal human amyloid beta. Elife 8, e50069. doi:10.7554/eLife.50069
Thiruvengadam, M., Venkidasamy, B., Subramanian, U., Samynathan, R., Ali Shariati, M., Rebezov, M., et al. (2021). Bioactive compounds in oxidative stress-mediated diseases: Targeting the NRF2/ARE signaling pathway and epigenetic regulation. Antioxidants 10 (12), 1859. doi:10.3390/antiox10121859
Thota, R. N., Rosato, J. I., Dias, C. B., Burrows, T. L., Martins, R. N., and Garg, M. L. (2020). Dietary supplementation with curcumin reduce circulating levels of glycogen synthase kinase-3 beta and islet amyloid polypeptide in adults with high risk of type 2 diabetes and alzheimer's disease. Nutrients 12 (4), 1032. doi:10.3390/nu12041032
Townsend, M., Mehta, T., and Selkoe, D. J. (2007). Soluble Abeta inhibits specific signal transduction cascades common to the insulin receptor pathway. J. Biol. Chem. 282 (46), 33305–33312. doi:10.1074/jbc.M610390200
Trampetti, F., Pereira, C., Rodrigues, M. J., Celaj, O., D'Abrosca, B., Zengin, G., et al. (2019). Exploring the halophyte cistanche phelypaea (L.) cout as a source of health promoting products: In vitro antioxidant and enzyme inhibitory properties, metabolomic profile and computational studies. J. Pharm. Biomed. Analysis 165, 119–128. doi:10.1016/j.jpba.2018.11.053
Tsubaki, H., Tooyama, I., and Walker, D. G. (2020). Thioredoxin-interacting protein (TXNIP) with focus on brain and neurodegenerative diseases. Int. J. Mol. Sci. 21 (24), 9357. doi:10.3390/ijms21249357
Vallon, V., and Thomson, S. C. (2017). Targeting renal glucose reabsorption to treat hyperglycaemia: The pleiotropic effects of SGLT2 inhibition. Diabetologia 60 (2), 215–225. doi:10.1007/s00125-016-4157-3
van der Harg, J. M., Eggels, L., Bangel, F. N., Ruigrok, S. R., Zwart, R., Hoozemans, J. J. M., et al. (2017). Insulin deficiency results in reversible protein kinase A activation and tau phosphorylation. Neurobiol. Dis. 103, 163–173. doi:10.1016/j.nbd.2017.04.005
Vandal, M., White, P. J., Tremblay, C., St-Amour, I., Chevrier, G., Emond, V., et al. (2014). Insulin reverses the high-fat diet-induced increase in brain A beta and improves memory in an animal model of alzheimer disease. Diabetes 63 (12), 4291–4301. doi:10.2337/db14-0375
Velmurugan, K., Bouchard, R., Mahaffey, G., and Pugazhenthi, S. (2012). Neuroprotective actions of Glucagon-like peptide-1 in differentiated human neuroprogenitor cells. J. Neurochem. 123 (6), 919–931. doi:10.1111/jnc.12036
Vlassenko, A. G., Gordon, B. A., Goyal, M. S., Su, Y., Blazey, T. M., Durbin, T. J., et al. (2018). Aerobic glycolysis and tau deposition in preclinical Alzheimer's disease. Neurobiol. Aging 67, 95–98. doi:10.1016/j.neurobiolaging.2018.03.014
Wang, H.-y., Wu, M., Diao, J.-l., Li, J.-b., Sun, Y.-x., and Xiao, X.-q. (2020a). Huperzine A ameliorates obesity-related cognitive performance impairments involving neuronal insulin signaling pathway in mice. Acta Pharmacol. Sin. 41 (2), 145–153. doi:10.1038/s41401-019-0257-1
Wang, P., Su, C., Feng, H., Chen, X., Dong, Y., Rao, Y., et al. (2017). Curcumin regulates insulin pathways and glucose metabolism in the brains of APPswe/PS1dE9 mice. Int. J. Immunopathol. Pharmacol. 30 (1), 25–43. doi:10.1177/0394632016688025
Wang, W., Zhao, F., Ma, X., Perry, G., and Zhu, X. (2020b). Mitochondria dysfunction in the pathogenesis of alzheimer's disease: Recent advances. Mol. Neurodegener. 15 (1), 30. doi:10.1186/s13024-020-00376-6
Wang, Y., Wang, Y., Bharti, V., Zhou, H., Hoi, V., Tan, H., et al. (2019). Upregulation of thioredoxin-interacting protein in brain of amyloid-beta protein precursor/presenilin 1 transgenic mice and amyloid-beta treated neuronal cells. J. Alzheimers Dis. 72 (1), 139–150. doi:10.3233/JAD-190223
Watson, K. T., Wroolie, T. E., Tong, G., Foland-Ross, L. C., Frangou, S., Singh, M., et al. (2019). Neural correlates of liraglutide effects in persons at risk for Alzheimer's disease. Behav. Brain Res. 356, 271–278. doi:10.1016/j.bbr.2018.08.006
Wicinski, M., Domanowska, A., Wodkiewicz, E., and Malinowski, B. (2020). Neuroprotective properties of resveratrol and its derivatives-influence on potential mechanisms leading to the development of alzheimer's disease. Int. J. Mol. Sci. 21 (8), 2749. doi:10.3390/ijms21082749
Willette, A. A., Bendlin, B. B., Starks, E. J., Birdsill, A. C., Johnson, S. C., Christian, B. T., et al. (2015). Association of insulin resistance with cerebral glucose uptake in late middle-aged adults at risk for alzheimer disease. Jama Neurol. 72 (9), 1013–1020. doi:10.1001/jamaneurol.2015.0613
Witte, A. V., Kerti, L., Margulies, D. S., and Floel, A. (2014). Effects of resveratrol on memory performance, hippocampal functional connectivity, and glucose metabolism in healthy older adults. J. Neurosci. 34 (23), 7862–7870. doi:10.1523/jneurosci.0385-14.2014
Wong, L. R., Tan, E. A., Lim, M. E. J., Shen, W., Lian, X. L., Wang, Y., et al. (2021). Functional effects of berberine in modulating mitochondrial dysfunction and inflammatory response in the respective amyloidogenic cells and activated microglial cells - in vitro models simulating Alzheimer's disease pathology. Life Sci. 282, 119824. doi:10.1016/j.lfs.2021.119824
Wu, C.-Y., Ouk, M., Wong, Y. Y., Anita, N. Z., Edwards, J. D., Yang, P., et al. (2020). Relationships between memory decline and the use of metformin or DPP4 inhibitors in people with type 2 diabetes with normal cognition or Alzheimer's disease, and the role APOE carrier status. Alzheimers Dementia 16 (12), 1663–1673. doi:10.1002/alz.12161
Wu, C. Y., Iskander, C., Wang, C., Xiong, L. Y., Shah, B. R., Edwards, J. D., et al. (2022). Association of sodium-glucose cotransporter-2 inhibitors with time to dementia: A population-based cohort study. Diabetes Care 46, 297–304. doi:10.2337/dc22-1705
Wu, N., Liu, W., Wang, J., Han, Y., Ye, Y., Liu, X., et al. (2021). Berberine ameliorates neuronal AD-like change via activating Pi3k/PGC epsilon pathway. Biofactors 47 (4), 587–599. doi:10.1002/biof.1725
Wu, N., Zheng, B., Shaywitz, A., Dagon, Y., Tower, C., Bellinger, G., et al. (2013). AMPK-dependent degradation of TXNIP upon energy stress leads to enhanced glucose uptake via GLUT1. Mol. Cell 49 (6), 1167–1175. doi:10.1016/j.molcel.2013.01.035
Xiong, H., Zheng, C., Wang, J., Song, J., Zhao, G., Shen, H., et al. (2013). The neuroprotection of liraglutide on alzheimer-like learning and memory impairment by modulating the hyperphosphorylation of tau and neurofilament proteins and insulin signaling pathways in mice. J. Alzheimers Dis. 37 (3), 623–635. doi:10.3233/JAD-130584
Xu, W., Yang, Y., Yuan, G., Zhu, W., Ma, D., and Hu, S. (2015). Exendin-4, a glucagon-like peptide-1 receptor agonist, reduces alzheimer disease-associated tau hyperphosphorylation in the Hippocampus of rats with type 2 diabetes. J. Investigative Med. 63 (2), 267–272. doi:10.1097/JIM.0000000000000129
Xu, X., Sun, Y., Cen, X., Shan, B., Zhao, Q., Xie, T., et al. (2021). Metformin activates chaperone-mediated autophagy and improves disease pathologies in an Alzheimer disease mouse model. Protein & Cell 12 (10), 769–787. doi:10.1007/s13238-021-00858-3
Xuan, W.-t., Wang, H., Zhou, P., Ye, T., Gao, H.-w., Ye, S., et al. (2020). Berberine ameliorates rats model of combined Alzheimer's disease and type 2 diabetes mellitus via the suppression of endoplasmic reticulum stress. 3 Biotech. 10 (8), 359. doi:10.1007/s13205-020-02354-7
Yang, A. J. T., Frendo-Cumbo, S., and MacPherson, R. E. K. (2019). Resveratrol and metformin recover prefrontal cortex AMPK activation in diet-induced obese mice but reduce BDNF and synaptophysin protein content. J. Alzheimers Dis. 71 (3), 945–956. doi:10.3233/JAD-190123
Yang, H. J., Hwang, J. T., Kwon, D. Y., Kim, M. J., Kang, S., Moon, N. R., et al. (2013). Yuzu extract prevents cognitive decline and impaired glucose homeostasis in beta-amyloid-infused rats. J. Nutr. 143 (7), 1093–1099. doi:10.3945/jn.112.173401
Yang, R., Jiang, X., He, X., Liang, D., Sun, S., and Zhou, G. (2020). Ginsenoside Rb1 improves cognitive impairment induced by insulin resistance through cdk5/p35-NMDAR-IDE pathway. Biomed Res. Int. 2020, 3905719. doi:10.1155/2020/3905719
Yao, J., Kong, W., and Jiang, J. (2015). Learning from berberine: Treating chronic diseases through multiple targets. Sci. China-Life Sci. 58 (9), 854–859. doi:10.1007/s11427-013-4568-z
Yin, Z., Yu, H., Chen, S., Ma, C., Ma, X., Xu, L., et al. (2015). Asiaticoside attenuates diabetes-induced cognition deficits by regulating PI3K/Akt/NF-kappa B pathway. Behav. Brain Res. 292, 288–299. doi:10.1016/j.bbr.2015.06.024
Ying, M. A., Maruschak, N., Mansur, R., Carvalho, A. F., Cha, D. S., and McIntyre, R. S. (2014). Metformin: Repurposing opportunities for cognitive and mood dysfunction. CNS Neurol. Disord. Drug Targets 13 (10), 1836–1845. doi:10.2174/1871527313666141130205514
Zengin, G., Sarikurkcu, C., Gunes, E., Uysal, A., Ceylan, R., Uysal, S., et al. (2015). Two ganoderma species: Profiling of phenolic compounds by HPLC-DAD, antioxidant, antimicrobial and inhibitory activities on key enzymes linked to diabetes mellitus, alzheimer's disease and skin disorders. Food & Funct. 6 (8), 2794–2802. doi:10.1039/c5fo00665a
Zhang, J.-H., Zhang, J.-F., Song, J., Bai, Y., Deng, L., Feng, C.-P., et al. (2021a). Effects of berberine on diabetes and cognitive impairment in an animal model: The mechanisms of action. Am. J. Chin. Med. 49 (06), 1399–1415. doi:10.1142/S0192415X21500658
Zhang, J., Lin, Y., Dai, X., Fang, W., Wu, X., and Chen, X. (2019). Metformin treatment improves the spatial memory of aged mice in an APOE genotype-dependent manner. Faseb J. 33 (6), 7748–7757. doi:10.1096/fj.201802718R
Zhang, M., Hu, G., Shao, N., Qin, Y., Chen, Q., Wang, Y., et al. (2021b). Thioredoxin-interacting protein (TXNIP) as a target for alzheimer's disease: Flavonoids and phenols. Inflammopharmacology 29 (5), 1317–1329. doi:10.1007/s10787-021-00861-4
Zhang, Y.-M., Zheng, T., Huang, T.-T., Gu, P.-P., Gou, L.-S., Ma, T.-F., et al. (2021c). Sarsasapogenin attenuates Alzheimer-like encephalopathy in diabetes. Phytomedicine 91, 153686. doi:10.1016/j.phymed.2021.153686
Zhang, Y., Yin, F., Liu, J., and Liu, Z. (2016). Geniposide attenuates the phosphorylation of tau protein in cellular and insulin-deficient APP/PS1 transgenic mouse model of alzheimer's disease. Chem. Biol. Drug Des. 87 (3), 409–418. doi:10.1111/cbdd.12673
Zhang, Y., Yin, F., Liu, J., Liu, Z., Guo, L., Xia, Z., et al. (2015). Geniposide attenuates insulin-deficiency-induced acceleration of beta-amyloidosis in an APP/PS1 transgenic model of Alzheimer's disease. Neurochem. Int. 89, 7–16. doi:10.1016/j.neuint.2015.04.002
Zheng, T., Liu, H., Qin, L., Chen, B., Zhang, X., Hu, X., et al. (2018). Oxidative stress-mediated influence of plasma DPP4 activity to BDNF ratio on mild cognitive impairment in elderly type 2 diabetic patients: Results from the GDMD study in China. Metabolism-Clinical Exp. 87, 105–112. doi:10.1016/j.metabol.2018.03.014
Zhou, J.-B., Tang, X., Han, M., Yang, J., and Simo, R. (2020). Impact of antidiabetic agents on dementia risk: A bayesian network meta-analysis. Metabolism-Clinical Exp. 109, 154265. doi:10.1016/j.metabol.2020.154265
Zhu, H., Zhang, W., Zhao, Y., Shu, X., Wang, W., Wang, D., et al. (2018). GSK3-mediated tau hyperphosphorylation triggers diabetic retinal neurodegeneration by disrupting synaptic and mitochondrial functions. Mol. Neurodegener. 13, 62. doi:10.1186/s13024-018-0295-z
Zu, G., Sun, K., Li, L., Zu, X., Han, T., and Huang, H. (2021). Mechanism of quercetin therapeutic targets for Alzheimer disease and type 2 diabetes mellitus. Sci. Rep. 11 (1), 22959. doi:10.1038/s41598-021-02248-5
Zulhendri, F., Ravalia, M., Kripal, K., Chandrasekaran, K., Fearnley, J., and Perera, C. O. (2021). Propolis in metabolic syndrome and its associated chronic diseases: A narrative review. Antioxidants 10 (3), 348. doi:10.3390/antiox10030348
Glossary
3xTg-AD: triple transgenic mice model of AD
ACEI: angiotensin-converting enzyme inhibitors
AChE: acetylcholinesterases
AD: Alzheimer’s Disease
AGEs: advanced glycation end products
AMPK: AMP-activated protein kinase
APP: amyloid precursor protein
Aβ: β-amyloid
AβO: Amyloid beta oligomers
BACE1: beta-site APP cleaving enzyme
BBB: Blood-Brain Barrier
BBR: Berberine
BDNF: Brain-Derived Neurotrophic Factor
BuChE: butyrylcholinesterase
cGMP: cyclic guanosine monophosphate
CMA: Chaperone-mediated autophagy
CNS: central nervous system
CSF: cerebrospinal fluid
CurNP: curcumin nanoparticle
DA5-CH: GLP-1/GIP Dual-Agonist-5
DBR: BDNF ratio
DM: Diabetes Mellitus
DMN: default mode network
DPP4: dipeptidyl peptidase IV
DPP4is: dipeptidyl peptidase IV inhibitors
ER: endoplasmic reticulum
ERK: extracellular signal-regulated kinase
EV: extracellular vesicles
fMRI: functional magnetic resonance imaging
GFAP: glial fibrillary acidic protein
GLP-1: Glucagon-like peptide-1
GLUT-1: glucose transporter-1
GIP: Glucose-dependent insulinotropic polypeptide
GRU102: transgenic Caenorhabditis elegans with low levels of constitutive pan-neuronal expression of human Aβ1-42
GSK3β: glycogen synthase kinase 3β
Hup A: Huperzine A
IAPP: islet amyloid polypeptide
ICV-STZ: intracerebroventricular-streptozotocin
IDE: insulin-degrading enzyme
IMT: intima-media thickness
IRS-1: insulin receptors
IRS-1pSer: serine phosphorylation of IRS-1
JNK: c-Jun N-terminal kinase
L-LTP: late-phase long-term potentiation
LTP: long-term potentiation
MAPK: Mitogen-Activated Protein Kinase
MCI: moderate cognitive impairment
MMSE: Mini-Mental State Examination
mTOR: mammalian target of rapamycin
NDVCI: non-dementia vascular cognitive impairment
NFTs: intracellular neurofibrillary tangles
NF-κB: nuclear factor-kappa B
NLRP3: Nod-like receptor protein 3
PET: micro-positron emission tomography
PI3K: Phosphatidylinositol 3-kinase
PKA: protein kinase A
PP: antidiabetic polypill
PS1-KI: presenilin-1 knock-in
P-tau: phosphorylated tau
P. harmala: Peganum harmala
Ras: receptor agonists
RAGE: receptor for advanced glycation end products
ROS: reactive oxygen species
SGLT2is: sodium-glucose cotransporter 2 inhibitors
TA: triple GLP-1/GIP/glucagon receptor agonist
TCA: ricarboxylic acid cycle
Tg: transgenic
Tg6799: Transgenic mice
TR: target replacement
TrkB: tropomyosin-related kinase B receptor
TRX: thioredoxin
TXNIP: Thioredoxin-interacting protein
Keywords: Alzheimer’s disease, glucose metabolism, insulin signaling, mitochondria dysfunction, hypoglycemic medicines, herbal medicines
Citation: Wang Y, Hu H, Liu X and Guo X (2023) Hypoglycemic medicines in the treatment of Alzheimer’s disease: Pathophysiological links between AD and glucose metabolism. Front. Pharmacol. 14:1138499. doi: 10.3389/fphar.2023.1138499
Received: 05 January 2023; Accepted: 13 February 2023;
Published: 23 February 2023.
Edited by:
Weidong Cheng, Southern Medical University, ChinaReviewed by:
Lorena Perrone, University of Campania Luigi Vanvitelli, ItalyBruno Araujo Serra Pinto, Federal University of Maranhão, Brazil
Copyright © 2023 Wang, Hu, Liu and Guo. This is an open-access article distributed under the terms of the Creative Commons Attribution License (CC BY). The use, distribution or reproduction in other forums is permitted, provided the original author(s) and the copyright owner(s) are credited and that the original publication in this journal is cited, in accordance with accepted academic practice. No use, distribution or reproduction is permitted which does not comply with these terms.
*Correspondence: Xiangyu Guo, Z3h5YzEwMDNAMTYzLmNvbQ==
†These authors have contributed equally to this work