- 1Department of Pharmacy, The Second Xiangya Hospital, Institute of Clinical Pharmacy, Central South University, Changsha, China
- 2Department of Pharmacy, The First Hospital of Lanzhou University, Lanzhou, China
- 3Department of Pharmacy, Xiangya Hospital, Central South University, Changsha, China
- 4National Clinical Research Center for Geriatric Disorders, Xiangya Hospital, Central South University, Changsha, China
- 5Hunan Key Laboratory of the Research and Development of Novel Pharmaceutical Preparations, Changsha Medical University, Changsha, China
Objectives: Augmented renal clearance (ARC) is a state of enhanced renal function commonly observed in 30%–65% of critically ill patients despite normal serum creatinine levels. Using unadjusted standard dosing regimens of renally eliminated drugs in ARC patients often leads to subtherapeutic concentrations, poor clinical outcomes, and the emergence of multidrug-resistant bacteria. We summarized pharmaceutical, pharmacokinetic, and pharmacodynamic research on the definition, underlying mechanisms, and risk factors of ARC to guide individualized dosing of antibiotics and various strategies for optimizing outcomes.
Methods: We searched for articles between 2010 and 2022 in the MEDLINE database about ARC patients and antibiotics and further provided individualized antibiotic dosage regimens for patients with ARC.
Results: 25 antibiotic dosage regimens for patients with ARC and various strategies for optimization of outcomes, such as extended infusion time, continuous infusion, increased dosage, and combination regimens, were summarized according to previous research.
Conclusion: ARC patients, especially critically ill patients, need to make individualized adjustments to antibiotics, including dose, frequency, and method of administration. Further comprehensive research is required to determine ARC staging, expand the range of recommended antibiotics, and establish individualized dosing guidelines for ARC patients.
1 Introduction
The concept of augmented renal clearance (ARC) was proposed by Udy et al. (Udy et al., 2010) in 2010, who defined a phenomenon in critically ill patients characterized by enhanced creatinine clearance (CrCl) and removal of renally eliminated circulating solutes (Udy et al., 2010; Udy et al., 2011). Using conventional dosing regimens for ARC patients may lead to suboptimal drug exposure and therapeutic failure (Udy et al., 2010; Udy et al., 2011; Baptista et al., 2019; Luo et al., 2021). This review discusses the characteristics of ARC, its influence on antibiotic therapy, and dose optimization strategies to improve outcomes.
2 Definition of ARC
Augmented renal clearance (ARC) is clinically defined as creatinine clearance (CrCl) of more than 130 mL/min/1.73m2 (Udy et al., 2011; Mahmoud and Shen, 2017). Other threshold values of 120, 150, and 160 mL/min/1.73m2 have additionally been suggested (Udy et al., 2010; Campassi et al., 2014; Carrie et al., 2019). CrCl is measured through urine collection followed by evaluation of the creatinine concentration using the formula: creatinine clearance = [urine creatinine concentration × urine volume]/[serum creatinine concentration × time], abbreviated as CrCl = [UCr×Uvol]/[SCr×Tmin](Cherry et al., 2002; Claus et al., 2013; Baptista et al., 2014). Measurements of CrCl at 8, 12, or 24 h are more accurate in critically ill patients with normal serum creatinine (sCr) levels since glomerular filtration rate (GFR) values obtained using Cockroft-Gault (CG), Chronic Kidney Disease Epidemiology Collaboration (CKD-EPI) and Modification of Diet in Renal Disease Study (MDRD) formulae underestimate renal function in ARC (Baptista et al., 2014; Morbitzer et al., 2019). Several scoring systems have additionally been developed to predict ARC (Udy et al., 2013; Barletta et al., 2017; Gijsen et al., 2020; Saito et al., 2020). However, validation of the accuracy of the models and unification of standards for definitive diagnosis of ARC remains an urgent clinical need.
3 Mechanism of ARC
The mechanism of ARC in patients has not been clearly defined and needs to be studied more. Generally, the underlying mechanisms of ARC are characterized by a hyperkinetic state, elevated cardiac output, and greater blood flow to major organs, which may lead to increased kidney perfusion (Bilbao-Meseguer et al., 2018). Three main possible mechanisms are proposed based on the comprehensive analysis of previous studies (shown in Figure 1). One possible mechanism is systemic inflammatory response syndrome (SIRS), which may arise in patients with several conditions, including sepsis, burns, major surgery, and severe trauma, with or without infection (Balk, 2014; Chakraborty and Burns, 2022). Dysregulated cytokines and pro-inflammatory mediators may lead to decreased vascular resistance, increased cardiac output, and increased capillary permeability. As a result, these critically ill patients have increased renal vascular flow, leading to better clearance of hydrophilic medications when used with intensive fluid therapy and inotropic drugs (Fuster-Lluch et al., 2008; Sime et al., 2015).
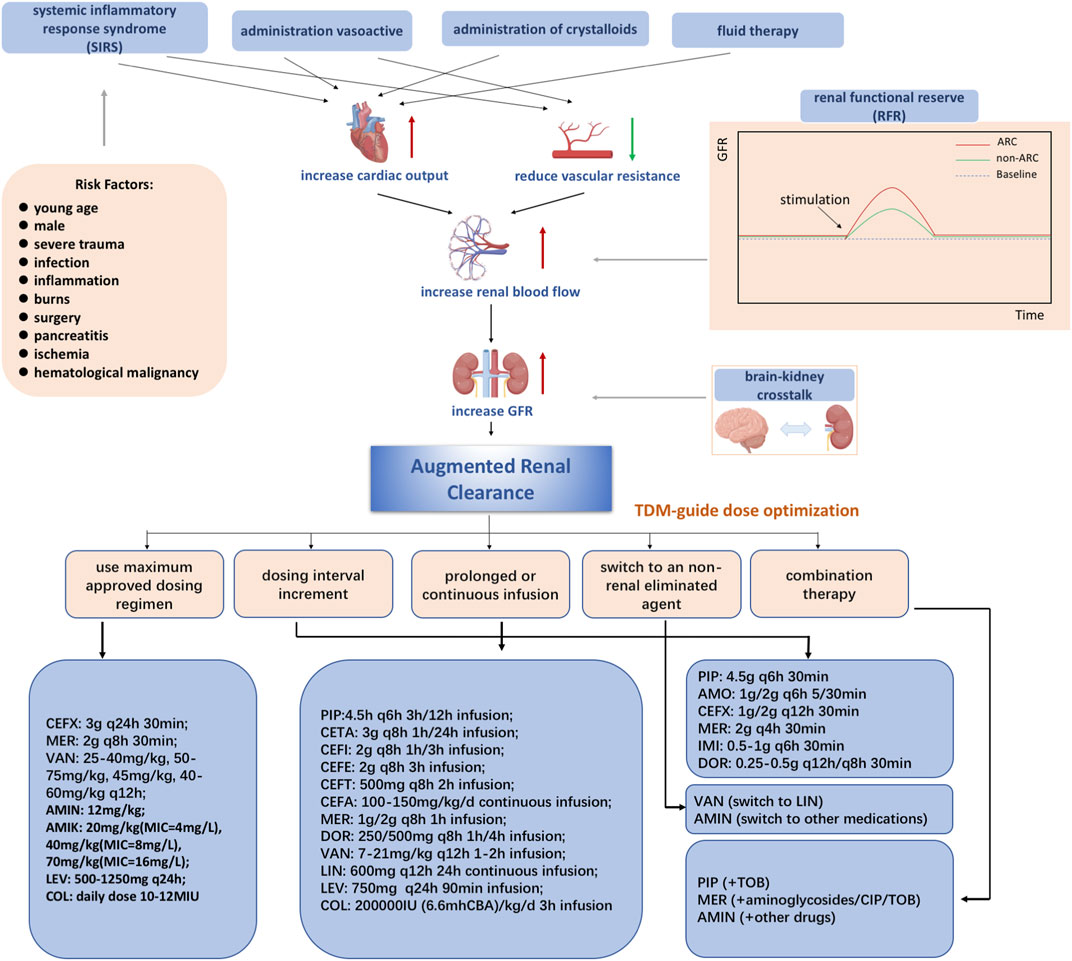
FIGURE 1. Mechanisms of augmented renal clearance Abbreviations: CEFX, ceftriaxone; MER, meropenem; VAN, vancomycin; AMIN, aminoglycoside; AMIK, amikacin; LEV, levofloxacin; COL, colistin; PIP, piperacillin-tazobactam; AMO, amoxicillin/clavulanic acid; IMI, imipenem; DOR, doripenem; CETA, ceftolozane/tazobactam; CEFI, cefiderocol; CEFE, cefepime; CEFT, ceftobiprole; CIP, ciprofloxacin; CEFA, cefazolin; LIN, linezolid; TOB, tobramycin.
Another possible mechanism is renal function reserve (RFR), which represents the kidney’s ability to increase glomerular filtration rate (GFR) under certain physiological and pathological conditions, including hyperfiltration states, cardiorenal patient conditions, and so on (Hoffmann et al., 2018). The renal stress test is executed to estimate the renal filtration rate based on the baseline and stress glomerular filtration rates, which cause the release of prostaglandins and relaxation factors that increase RFR (Sharma et al., 2014; Kindgen-Milles et al., 2020). RFR and ARC have overlapping populations and mechanisms (Baptista et al., 2020). In addition, some studies have shown that integrating SIRS and RFR may be a comprehensive mechanism in ARC (Bilbao-Meseguer et al., 2018).
Recently, it has been suggested that the concept proposed by Nongnuch is closely related to ARC, defined as “brain-kidney crosstalk” (Nongnuch et al., 2014). Both brain and kidney function can be preserved through autoregulation, according to Dias et al. They found that both PRx (cranial perfusion pressure, intracranial pressure) and CPP (cerebral perfusion pressure) can also be preserved (Dias et al., 2015). According to Udy et al., ARC is observed in traumatic brain-injured patients with normal plasma creatinine levels and may be caused by a hyperdynamic vascular state and increased intracranial pressure (Udy et al., 2015).
4 Antibacterial therapy in ARC patients
Accumulating studies have explored the impact of ARC status on antimicrobial exposure and antibiotic dosage recommendations. According to recent research, we provided dosage recommendations for 25 antibiotics (Supplementary Table S1).
4.1 Penicillins
4.1.1 Piperacillin-tazobactam
Piperacillin-tazobactam (PIP-TAZ) is an 8:1 β-lactam/β-lactamase inhibitor commonly used as a hydrophilic antibiotic against Gram-positive and Gram-negative aerobic and anaerobic bacteria in critically ill patients presenting signs of infection (Landersdorfer et al., 2012). The kidney eliminates ∼50–60% of piperacillin-tazobactam without much metabolic involvement (Bryson and Brogden, 1994; Hayashi et al., 2010). Like other β-lactams, PIP-TAZ has a time-dependent antibacterial activity, defined by the duration (T) during which the unbound drug concentration must be above the minimum inhibitory concentration (MIC). Clinical efficacy requires a
However, the therapeutic concentrations are clearly inadequate in ARC patients treated with normal dosing regimens, resulting in poor clinical outcomes. Earlier studies showed that a higher ARC rate led to higher free piperacillin clearance, with failure to achieve 100%
Optimizing PIP-TAZ therapy is becoming attractive in populations such as the critically ill. Enhancing exposure times via prolonged or continuous infusion administration for time-dependent antibiotics could present a satisfactory strategy to attain the therapeutic target. For patients at increased risk for augmented renal function, dose increments or continuous infusion may be necessary to increase the probability of achieving therapeutic plasma concentrations (Obrink-Hansen et al., 2015; Beranger et al., 2019). Enhanced dosage of PIP-TAZ could also be considered effective to some extent. For example, higher than licensed doses of PIP-TAZ attained the therapeutic target in patients with CrCl ≥150 mL/min (Besnard et al., 2019). Data obtained with a dynamic hollow-fiber in vitro infection model showed that dose increments improved bacterial killing and minimized the development of resistance in patients with normal renal function (Bergen et al., 2016). Extended infusion delivery and continuous infusion regimens were considered sufficient owing to cost savings of ∼50.0–66.7% (Akers et al., 2014). Continuous infusion is most effective in cases of elevated MIC for a bacterial pathogen. However, prolonged infusion requires considering some practical aspects, such as the loading dose and drug stability (Lodise et al., 2007). PIP-TAZ is stable at 37°C for at least 24 h, supporting its suitability for continuous infusion (De Waele et al., 2015). However, continuous PIP-TAZ (16 g/2 g/day) infusion is inadequate for ARC patients, especially those with high CrCl ≥170 ml/min. Monte Carlo simulation (MCS) has been proposed frequently as simulated pharmacokinetic profiles to evaluate the probability of target attainment (PTA) of experimental dosage regimens in attaining prespecified pharmacodynamic targets against specific pathogens{Kuti et al., 2003 #4}{Matsu et al., 2019 #5}. It is widely used in studies on antibiotic dosing regimens, and some cases are reviewed below. MCS indicated that higher doses (20 g/2.5 g/24 h) should be necessary without the additional risk of excessive dosing and neurotoxicity (Carrie et al., 2018a). Continual infusions may not be effective in increasing penetration in target tissues with high MICs, since higher blood concentrations are needed (Roberts et al., 2009; Dahyot-Fizelier et al., 2010).
4.1.2 Amoxicillin/clavulanic acid
Amoxicillin/clavulanic acid is a β-lactam/β-lactamase inhibitor oral or intravenous antibiotic against Gram-positive and Gram-negative bacteria commonly used in critically ill patients presenting signs of infection. Amoxicillin/clavulanic acid is commonly used in typical indications such as community-acquired pneumonia, skin, soft tissue, and abdominal infections (Ceelie et al., 2011). Amoxicillin/clavulanic acid is most readily eliminated in urine (∼60–80% for amoxicillin and 30%–50% for clavulanic acid) after oral administration. Amoxicillin is excreted mainly by tubular secretion, and clavulanic acid is primarily eliminated by glomerular filtration (Todd and Benfield, 1990). Like other β-lactams, amoxicillin/clavulanic acid displays time-dependent antibacterial activity. A pharmacokinetic/pharmacodynamic (PK/PD) index of 40%
Limited published studies are available on recommended amoxicillin/clavulanic acid dosing in critically ill patients with a risk of clinical failure due to ARC. Renal function may be a significant covariate for amoxicillin and clavulanic acid clearance. Underdosing of amoxicillin/clavulanic acid could lead to clinical failure. Enhancing exposure times via prolonged or continuous infusion administration could present a strategy to attain the therapeutic target. A minimum dosing regimen of 25 mg/kg amoxicillin every 4 h (as a 1-h infusion) is recommended for infections in this patient group. To maximize tissue penetration and prevent issues with drug stability, longer infusion times are avoided (De Cock et al., 2015). However, in another study, prolongation of infusion time from 30 min to 2 h achieved favorable target attainment (Anne Fournier et al., 2018; Carrie et al., 2019). Another strategy is to maximize the dosing regimen as much as possible. Population pharmacokinetic (PPK) analysis of amoxicillin in critically ill burn patients showed that increased antibiotic doses were required for ARC patients or cases with higher MIC.
4.2 Cephalosporins
4.2.1 Ceftolozane/tazobactam
Ceftolozane/tazobactam (C/T), a combination of a potent antipseudomonal cephalosporin (ceftolozane) with a β-lactamase inhibitor (tazobactam), has activity against many Gram-negative pathogens for complicated intra-abdominal infections, complicated urinary tract infections and hospital-acquired pneumonia (HAP) or ventilator-associated pneumonia (VAP) (López Montesinos et al., 2021). It displays time-dependent antibacterial activity. Ceftolozane/tazobactam (C/T) is mainly excreted via the kidney, thus requiring dose adjustments in patients with varying renal function (van Duin and Bonomo, 2016). The probability of target attainment (PTA) for C/T has been benchmarked at 40% free concentration time above the MIC (
Although the concentration of C/T is affected by different renal functional parameters, including ARC, PTA of C/T has little been failed to achieve with 60 min infusion. According to PPK analysis, ARC patients do not require dose adjustments of C/T. However, following patient exposure, steady-state volumes of ceftolozane and tazobactam in plasma and pulmonary epithelial lining fluid were significantly decreased. Ceftolozane and tazobactam also displayed high PTA in simulated plasma and epithelial lining fluid (ELF), irrespective of renal function category, including that of patients with severe ARC (Shorr et al., 2021). Another prospective multicenter study reported higher plasma clearance and lower exposure levels of ceftolozane and tazobactam in ARC patients than in healthy subjects (Nicolau et al., 2021).
The dosing regimen adjusts the administration time into prolonged or continuous infusions. Continuous intravenous infusion of C/Z was successfully administered over 24 h in patients with cystic fibrosis and estimated glomerular filtration rates of 215 mL/min (Elizabeth Davis et al., 2019). Extension of the infusion time was sufficient for therapeutic benefit with an MCS (Natesan et al., 2017). However, with another MCS in patients with different renal functions, including ARC, the approved dosing regimens attained target levels for bactericidal activity at MIC of 4 mg/L (Xiao et al., 2017), which could be attributed to different MIC values. The approved dosing regimens with standard intervals are sufficient to obtain PTA at a low MIC value. However, at higher MIC values, prolonged infusion time may improve efficacy.
4.2.2 Cefiderocol
Cefiderocol, a novel parenteral siderophore cephalosporin, exhibits potent efficacy against most Gram-negative bacteria, including carbapenem-resistant strains of Pseudomonas aeruginosa, Acinetobacter baumannii, and Enterobacteriaceae (Ito et al., 2016; Kohira et al., 2016). It is being developed as a therapeutic drug for CR-GNB infections, including bloodstream infections, nosocomial pneumonia, and complicated urinary tract infection. It displays time-dependent antibacterial activity. About 60%–70% of cefiderocol is excreted unchanged via the kidney, supporting the importance of renal function for clearance of this drug. A bacteriostatic effect at 40%–70%
4.2.3 Cefepime
Cefepime is an extended-spectrum cephalosporin against Gram-positive and Gram-negative pathogens for treating pneumonia, soft tissue, and complicated urinary tract infections (Suttels et al., 2022). It displays time-dependent antibacterial activity. Cefepime is primarily eliminated through the renal pathway (∼80%) as an unchanged drug (Barradell and Bryson, 1994). In general, cefepime is safe and efficacious, with a goal exposure target of 70%
Prolonged or continuous infusions are the referenced strategy to optimize the dosing regimen. Daily doses of 2 g in MCS for cases with 140 mL/min CrCl resulted in relatively low PTA. However, intermittent infusion under conditions of renal failure may improve clinical outcomes than intravenous infusion (Liu et al., 2020). For febrile neutropenic patients with normal renal function or ARC, cefepime 2 g t.i.d. with 3 h of infusion was effective in several cases (Yamashita et al., 2016). A study on pediatric patients recommended a regimen of 100 mgkg-1d-1 administered as a continuous infusion to attain higher target values (de Cacqueray et al., 2022).
4.2.4 Ceftazidime/avibactam
Ceftazidime/avibactam (CZA-AVI) is a β-lactam/β-lactamase inhibitor antimicrobial agent to combat increasing antimicrobial resistance among Gram-negative pathogens, predominantly used in serious bacterial infections. It displays time-dependent antibacterial activity. Ceftazidime is not metabolized and is mainly eliminated through urine (Richards and Brogden, 1985), and avibactam is removed unchanged by renal excretion (Lagace-Wiens et al., 2014).
Limited studies to date have focused on the effect of ARC on CZA-AVI therapeutic outcomes. MCS showed that CZA-AVI of 2.5 g achieved PTA (50%
4.2.5 Other cephalosporins
Cefathiamidine is commonly used to treat children with ARC with empirical antimicrobial therapy. It is excreted primarily in an unchanged form by the kidney (>90%) within 12 h after intravenous administration (Tze-ying et al., 1979). Consequently, kidney function plays a significant role in cefathiamidine’s pharmacokinetics. A 70%
Ceftazidime, a broad-spectrum cephalosporins with a spectrum of activity, is usually given as 2 g i.v. every 8 h. It is primarily eliminated by glomerular filtration, with elimination half-lives of 1.8–2.3 h in patients with normal renal function. Higher than licensed dosing regimens of β-lactams may be effective and safe in reducing the rate of recurrence and therapeutic failure in critically ill ARC patients (Carrie et al., 2019). In patients with late-onset hospital-acquired pneumonia (HAP), MCS indicated that prolonged infusion of ceftazidime could achieve a high probability of target attainment (Kim et al., 2009). However, this study did not include ARC patients (Kim et al., 2009).
Ceftobiprole, the active moiety of ceftobiprole medocaril, is recommended as a 500 mg 2-h intravenous infusion every 8 h in adults with normal renal function (Murthy and Schmitt-Hoffmann, 2008). As an antimicrobial, it is effective against Gram-positive and Gram-negative pathogens (Farrell et al., 2014). Dose adjustments of ceftobiprole are recommended according to renal function. When treating critically ill patients with ARC, ceftobiprole should be instilled over 4 h for optimal drug exposure (Torres et al., 2016).
Ceftriaxone, one of the most commonly used antibiotics for community-acquired infections, has pharmacokinetic properties, including high binding to albumin, mixed biliary and renal elimination, and long elimination half-life. In earlier studies, trough concentrations were lower in ARC patients (Joynt et al., 2001; Schleibinger et al., 2015). Increasing the dosing regimen may therefore be necessary to obtain PTA of 99% in patients with CrCl ≥150 mL/min (Ollivier et al., 2019). Another multicenter PPK study supported the recommendation of 3–4 g per day, administered in divided doses or as a continuous infusion (Heffernan et al., 2022).
Cefuroxime, a second-generation cephalosporin, is a time-dependent antibiotic frequently used to treat critically ill patients (Vree and Hekster, 1990).In patients with normal renal function, 95% of cefuroxime is excreted unchanged in the urine (Foord, 1976). Continuous infusion of higher doses after the loading dose of cefuroxime has been proposed as a better strategy to achieve therapeutic targets in critically ill patients (Carlier et al., 2014). However, this dosage regimen was insufficient for patients with CrCl ≥300 mL/min (Carlier et al., 2014).
Cefazolin is a narrow-spectrum cephalosporin active against methicillin-sensitive Staphylococcus aureus (MSSA) infection. The hydrophilic drug is highly bound to human serum albumin, and eliminated by the kidney (Weis et al., 2019). Among critically ill children with normal and augmented renal function infected with methicillin-susceptible S. aureus (MSSA), continuous infusion of cefazolin served as the most feasible scheme to reach the PK target of 100%
4.3 Carbapenems
4.3.1 Meropenem
Wide-spectrum carbapenem antibiotic meropenem is commonly used in ICU therapy to combat life-threatening infections caused by Gram-positive and Gram-negatives (Mattoes et al., 2004). It is a time-dependent bactericidal antibiotic and unbound concentrations of 100%
The first dosing regimen strategy is to use prolonged or continuous infusions to take advantage of the nature of time-dependent antibiotics. After 180 min of infusion, PTA ≥90% remained within the range of 100–300 mL/min CrCl with dosing regimens of 1 g every 8 h and 2 g every 8 h. A 2 g q8h dosing regimen with 180 min infusion was established as the empirical therapy in ARC patients (Tamatsukuri et al., 2018). Prolongation of infusion time (usually at least 3 h) is one of the most efficient ways to achieve the therapeutic target (Kim et al., 2018). However, even when the dose was administered as an extended infusion over a 3 h period, up to 37% of ARC patients were potentially at risk for treatment failure, as this minimum PK/PD target without dose uptitration was not achieved in critically ill patients treated with meropenem (Carlier et al., 2013). Among patients with ventilator-associated pneumonia (VAP), prolonged meropenem infusion was confirmed as a more appropriate strategy than dose elevation in those with ARC (Liebchen et al., 2020; Razzazzadeh et al., 2022).
Maximizing the dosing regimen as much as possible is another strategy to attain the PTA. The meropenem regimen was increased to 2 g q8h to improve clinical outcomes. Therapeutic drug monitoring showed the significant utility of increased meropenem doses in preventing failure to receive sufficient antibiotics (Troger et al., 2012; Selig et al., 2022). However, toxicity should be considered when increasing the antibiotic dose. No data is defined in the literature on toxic levels for meropenem since β-lactam antibiotics were considered safe. Limited studies report the neurotoxicity related to plasmatic levels of β-lactams, with Cmin concentrations of 64.2 mg/L and 44.45 mg/L increased by 50%, the risk of developing neurotoxicity and nephrotoxicity{Imani, 2017 #1}. Therefore, therapeutic drug monitoring-guided meropenem treatment may be necessary to ensure adequate drug exposure, suggesting a Cmin therapeutic target for empiric therapy of 8–32 mg/L to enhance efficacy and reduce toxicity{Steffens et al., 2021 #2}.
Combination therapy protocols may be utilized as the third optional optimal dosing regimen. A combination of aminoglycosides with β-lactams demonstrated good bactericidal activity and suppression of resistance in critically ill patients with ARC, supporting its therapeutic potential. Meropenem and ciprofloxacin regimens were administered intermittently due to monotherapy’s failure against P. aeruginosa in patients with ARC. In vitro, the combination regimens were generally effective at suppressing the resistance of isolates susceptible to one or both antibiotics and promoting synergistic killing. In conjunction with ciprofloxacin, higher than approved daily doses of meropenem may benefit cases infected with more resistant clinical isolates (Agyeman et al., 2021). Another study reported that continuous infusion of meropenem combined with a high dose of daily tobramycin effectively suppressed the regrowth and amplification of less resistant isolates (Yadav et al., 2019).
4.3.2 Other carbapenems
A carbapenem antibiotic, doripenem, is effective against many Gram-positive and Gram-negative pathogens. PTA of
A PK/PD target of 40%
The utility of relebactam as a fixed-dose combination with imipenem/cilastatin has been documented. An earlier PPK analysis of imipenem/relebactam included ARC patients with >90% PTA(Bhagunde et al., 2019).
4.4 Glycopeptides
4.4.1 Vancomycin
The glycopeptide antibiotic vancomycin (VCM) is used in the treatment of Gram-positive bacteria like methicillin-resistant S. aureus (MRSA) and Methicillin-resistant coagulase-negative staphylococci (MRCNS) (Liu et al., 2011). Most of the drug is excreted through the renal route, and >80%–90% is eliminated primarily through urine within 24 h (Rubinstein and Keynan, 2014). Therefore, renal function greatly influences vancomycin pharmacokinetics (da Silva Alves et al., 2017). Therapeutic drug monitoring (TDM) is necessary to maintain a trough concentration between 10 and 20 g/mL to ensure safe and effective dosing. The ratio of the area under the concentration-time curve to the minimum inhibitory concentration (AUC0–24h/MIC) was used as the goal for vancomycin treatment optimization, and the target was to achieve a target of AUC0–24h/MIC ≥400. If a patient has a severe MRSA infection with an assumed AUC/MIC ratio of 400–600 (MIC of 1 mg/L), trough monitoring should not be performed (Rybak et al., 2020). Thus, performing TDM with AUC0–24h/MIC guide dosing is important to ensure effective and safe therapy.
Vancomycin clearance by the kidney is accelerated in ARC patients owing to an elevated creatinine clearance rate, resulting in reduced effectiveness (Bakke et al., 2017; Bilbao-Meseguer et al., 2018; Izumisawa et al., 2019; Chu et al., 2020; Zhao et al., 2021; Scully et al., 2022; Yu et al., 2022). ARC risk is significantly increased by febrile neutropenia or neurosurgery, and clearance of VCM is augmented, resulting in subtherapeutic levels (Hirai et al., 2016; Kim et al., 2016).
Higher doses of VCM may be necessary to achieve sufficient therapeutic effects (Mikami et al., 2022). Data from PK/PD modeling and MCS in children with hematological malignancies showed that at MIC of 0.5 or 1 mg/L, the recommended doses to achieve target AUC0–24h/MIC ≥400 were 25–40 and 50–75 mg/kg/d, respectively (Lv et al., 2020). Even at the maximum recommended dose of VCM of 161.9 mL/min/1.73m2 provided to a patient with eGFR, therapeutic efficacy could not be achieved, with consequent treatment failure and a fatal outcome, clearly highlighting the need for early dose adjustment of VCM based on urinary CrCl (Pata et al., 2021). The typical VCM dosage was insufficient and higher doses were also required to achieve adequate exposure in critically ill infants with ARC (Huang et al., 2022). However, patients with higher exposure to vancomycin are more likely to experience toxicity, such as acute kidney injury{Kim et al., 2023 #3}. AUC0–24h/MIC ≥400 based on TDM was used to ensure therapeutic effectiveness and safety.
A few studies currently recommend a continuous infusion of vancomycin to improve outcomes. In these reports, loading and continuous infusion were conducted to avoid subtherapeutic serum VCM concentrations in ARC patients (Curth et al., 2015; Villanueva et al., 2019).
A therapeutic switch from VCM to linezolid could improve outcomes since linezolid is mainly eliminated through a non-renal pathway. However, elevated linezolid clearance was recently reported in ARC patients (Barrasa et al., 2020).
4.4.2 Teicoplanin
Teicoplanin is a glycopeptide antibiotic that is active against methicillin-resistant S. aureus infections. Approximately 2%–3% of a teicoplanin dose administered intravenously is metabolized, and most of its elimination mainly occurs via the kidney. This drug was approved for three loading doses of 12 mg/kg/d q12 h followed by one maintenance dose of 12 mg/kg/d qd. Based on the TDM, 15–30 mg/L is the probability of achieving the target concentration range. AUC/MIC is the pharmacokinetic/pharmacodynamic (PK/PD) index used to establish teicoplanin efficacy, but the specific PK/PD target for teicoplanin remains to be defined (Byrne et al., 2017). In two previous small-scale clinical studies, patients with MRSA infections had AUC of 750–800 mg h/L (MIC of 1 mg/L) (Craig, 2003; Kanazawa et al., 2011). Patients with neutropenia displayed a 25% increase in clearance compared with non-neutropenia cases. Regimen A was recommended for patients with neutropenia and 600 mg loading and 400 mg maintenance doses were used for ARC. Regimen B (600 mg–400 mg on day 3) was proposed for patients without neutropenia simulated in a PPK model study (Sako et al., 2021).
Few reports to date have focused on dose adjustment of teicoplanin. A high dose might be one of the optional regimens. A loading dose of 800 mg q12h three times with a maintenance dose of 800 mg (severe infection) or 600/400 mg (mild infection) was employed for ARC patients (Hu et al., 2022). The optimal dosing regimens for children with different renal functions were stimulated with the PK model and those with normal or augmented renal function recommended three loading doses of 12 mg/kg q12h, followed by a maintenance dose of 10 mg/kg quarter in die (Gao et al., 2020).
4.5 Oxazolidinones
Linezolid, the first member of oxazolidinone antibiotics, has activity against various Gram-positive microorganisms, including methicillin-resistant S. aureus (Hashemian et al., 2018). Regardless of a patient’s liver or renal function, the recommended dose of linezolid is 600 mg every 12 h. It is an antibiotic with concentration- and time-dependent activity. Moderate linezolid binding to plasma proteins (31%) has been demonstrated, with ∼65% clearance via a non-renal pathway (Dryden, 2011). Plasma concentrations above MIC (%T > MIC) and AUC24/MIC ratio are the PK/PD parameters that best predict clinical efficacy. The recommended PTA is reported as AUC24/MIC >80 or %T > MIC >85% (Minichmayr et al., 2017; Barrasa et al., 2019). The correlation between linezolid clearance and creatinine clearance is currently a subject of controversy. A few researchers suggest no relationship between renal function and linezolid pharmacokinetics in critically ill patients, while others believe that renal function affects linezolid pharmacokinetics (Barrasa et al., 2019). Thus, the standard dose regimen may not be the most suitable for all patients.
The status of ARC in critically ill patients significantly affects the pharmacokinetics of linezolid. While only 30%–35% of linezolid is normally excreted in the urine, a significant increase in linezolid clearance and a high risk of underexposure in patients is associated with ARC (Cojutti et al., 2018). PTA (AUC24/MIC >80 or %T > MIC >85%) has not been reached in ARC patients. Based on MCS, a regimen of 600 mg Q8h with 30 min infusions did not improve the probability of successful treatment, highlighting the necessity to modify the dosing strategy for ARC patients with increased CL from linezolid. Continuous infusion may thus present a useful strategy (Barrasa et al., 2020). For instance, a 24 h continuous infusion (2,400 mg/day) regimen for ARC patients was reported to achieve sufficient efficacy (Wang et al., 2021). Thus, ARC patients may benefit from continuous infusion and dose increase strategies for the delivery of linezolid, and TDM has potential utility in optimizing PTA (Richards and Brink, 2014).
4.6 Aminoglycosides
As part of the empirical treatment of sepsis in critically ill pediatric patients, aminoglycosides, commonly available for Gram-negative bacterial infections, are frequently used in conjunction with β-lactams (Weis et al., 2019). Aminoglycosides are concentration-dependent bactericidal agents with a long-term post-antibiotic effect. Therefore, enhancing the Cmax/MIC or AUC/MIC value is expected to increase the PTA (LeBras et al., 2016). A well-known fact is that critically ill patients exhibit altered organ function and volume of distribution, which significantly reduces their response to aminoglycoside therapy. Given the narrow therapeutic window of aminoglycosides and relatively different degrees of renal elimination, optimizing the aminoglycoside dosage in ARC patients is a considerable challenge (Roberts and Lipman, 2009; Yu et al., 2015).
The incidence of ARC is at least 20% among pediatric intensive care unit (PICU) patients receiving aminoglycosides (Avedissian et al., 2020a). A population-based pharmacokinetic model in the pediatric ICU showed a significantly higher clearance rate (CL) and volume of distribution (Vd) and significantly lower AUC24h in ARC than non-ARC patients (Avedissian et al., 2021). An increase in empirical aminoglycoside dosing in ARC patients is a possible strategy. However, the risk of toxicity should be carefully considered in the younger patient population. Patients with ARC and MIC >1 g/mL should consider combination therapy or drugs other than aminoglycosides (Avedissian et al., 2021). PK/PD values are unlikely optimal when these drugs are administered at standard doses (Avedissian et al., 2021).
Cmax/MIC ratio ≥8 for amikacin is a parameter to predict treatment efficacy. The kidney almost wholly eliminates the drug by glomerular filtration (Marsot et al., 2017). Based on Bayesian algorithms, optimal dosage regimens for this drug could minimize the development of bacterial resistance and maximize the efficacy of antimicrobial therapy limited by underdosing or adverse events from overdosing in patients with different renal function levels (Arechiga-Alvarado et al., 2020).
4.7 Fluoroquinolones
The implications of ARC in dosing this class of antibacterial are poorly understood. Levofloxacin, a fluoroquinolone antimicrobial, is commonly used to treat Gram-positive and Gram-negative microorganisms’ infections (Anderson and Perry, 2008). Levofloxacin is a lipophilic drug with almost complete renal elimination (Hurst et al., 2002). PTA has been achieved at AUC24h of 50–150 mg h/L for levofloxacin.
Levofloxacin doses higher than the standard values may be needed to achieve therapeutic effects. Patients with morbid obesity often have augmented renal function caused by glomerular hyperfiltration. Among 15 obese patients intravenously administered 750 mg levofloxacin, three with ARC (measured CrCl of 184.3 mL/min) had lower AUC than the remaining 12 patients with normal renal function (Cook et al., 2011). According to data from a study conducted on severely obese subjects (BMI 40 kg/m2), higher doses based on weight are not necessarily effective (Pai et al., 2014).
Ciprofloxacin, an antimicrobial drug used for many infections, is mainly excreted by glomerular filtration and tubular secretion (50%–60%) (Campoli-Richards et al., 1988). For critically ill patients with MIC ≥0.5 mg/L and eGFR >100 mL/min, doses up to 600 mg q6h or greater were estimated. Higher doses than the standard licensed dose was necessary to achieve target attainment for patients with ARC or those infected with less susceptible pathogens (Roberts et al., 2019; Gieling et al., 2020).
4.8 Colistin
Colistin is administered intravenously as a cationic lipopeptide antibiotic known as colistimethate sodium (CMS) hydrolyzed in vivo into its active component, colistin (Orwa et al., 2001). The drug is a last resort for treating critically ill patients suffering from multidrug-resistant (MDR) systemic Gram-negative infections (Li et al., 2006). Colistin is mainly disposed of via non-renal pathways, while CMS is excreted by the kidney (including renal tubular secretion) (Li et al., 2004; Kassamali et al., 2013). The bactericidal action of colistin is concentration-rather than time-dependent (Michalopoulos and Karatza, 2010). For colistin, the area under the plasma concentration-time curve across 24 h at steady state (AUCss, 24 h) of ∼50 mg h/L is required that equates to a target average steady-state plasma concentration (Css,avg) of ∼2 mg/L for total drug (Tsuji et al., 2019).
Limited studies are available regarding the effects of ARC on colistin therapy. A study on colistin use recommended that colistin-induced acute kidney (AKI) is less common in ARC patients (Aitullina et al., 2019). Dalfino et al. (Dalfino et al., 2015) suggested that ARC patients should be administered increased colistin doses, such as 12 MU daily, in cases where CrCl is >130 mL/min. The apparent clearance of colistin decreased with declining kidney function since a higher amount of CMS was converted to colistin with each dose (Li et al., 2006; Ooi et al., 2019). Owing to the influence of creatinine clearance on the PK of formed colistin, it may be necessary to increase the CMS dose in ARC patients and decrease the dose in patients with renal impairment. Furthermore, in cases where the dose is increased, the possibility of nephrotoxicity or other adverse effects should be considered. Therefore, TDM of the plasma colistin concentration may serve as a useful strategy for dose adjustment.
4.9 Fosfomycin
Fosfomycin is a broad-spectrum antibiotic that is active against Gram-positive and Gram-negative bacteria. It is used to treat critically ill patients with multidrug-resistant bacteria. Since fosfomycin is hydrophilic with little protein binding, it is almost entirely excreted via glomerular filtration and is subject to a patient’s renal function. The increase in renal clearance rate in critically ill patients with ARC may lead to low blood concentrations and predispose patients to the risk of treatment failure (Parker et al., 2013). To our knowledge, no clinical reports on the effect of ARC on fosfomycin clearance are documented. In two earlier studies, Cmax was reduced by up to 35% compared with healthy patients, suggesting that critically ill patients are likely to have higher Vd of fosfomycin, resulting in lower Cmax (Frossard et al., 2000; Pfausler et al., 2004).
4.10 Daptomycin
Daptomycin, the first of the cyclic lipopeptide class of antibiotics, has a wide range of Gram-positive bacteria, especially MRSA and VRE. About 50% of daptomycin was eliminated by renal excretion (Dvorchik et al., 2003). Earlier PPK analysis of daptomycin disclosed a decrease in t1/2 in critically ill patients with CrCl ≥80 mL/min (Gregoire et al., 2019). Other optimized dose regimens were not proposed in this study.
5 Limitations and perspectives
There were some limitations in our study. Most of the included studies are observational single centers studies in a specific population or with different definitions and diagnostic techniques of ARC. Furthermore, there are few or no studies on some drugs excreted mainly through the kidney. Hence, larger samples or multicenter prospective studies are needed to explore these related issues to provide more evidence for optimal dosing regimens and conduct a safe and effective therapy. For those patients with confirmed ARC, individualized strategies are needed for all renally cleared medications.
Most of these studies presented the dosing adjustment regimens based on the in-silicon simulations, lacking clinical research support. A unified solution for dose adjustment guidelines was also not reached an agreement. In the future, more accurate population PK/PD modeling or physiologically based pharmacokinetic (PBPK) modeling will need to be developed, which is confirmed by clinical trials. As an approach to accurately determining the target concentration of drugs, TDM technologies show their advantages in drug dose adjustment. More rapid, convenient, and accurate technologies, such as point-of-care testing (Irving and Gecse, 2022), and semi-automated TDM(Jayanti et al., 2022), are being vigorously developed. Finally, another limitation in this review was the English-language restriction. Studies in other languages were not included. More studies are needed to guide the individualized medication of clinical ARC patients.
6 Conclusion
In critically ill patients, ARC is a prevalent phenomenon that significantly affects optimal therapeutic exposure of antibiotics predominantly eliminated by renal excretion. Increased elimination of antibiotics in patients with ARC often results in a high risk of subtherapeutic concentrations and clinical failure. Therefore, optimizing dosing regimens for individual patients should be considered to overcome these limitations. Any delay in achieving therapeutic concentrations will lead to increased morbidity and mortality.
Accurate assessment of renal function is essential to identify patients with ARC. Urinary CrCl measurement is more recommended because the GFR estimated by equations leads to the underdiagnosis of ARC. Regular therapeutic drug monitoring may be necessary to optimize treatment efficacy and minimize the risk of treatment failure and/or the emergence of antibiotic resistance. In this review, 25 antibiotic dosage regimens for patients with ARC and various strategies for optimization of outcomes were summarized, including extended infusion time, continuous infusion, increased dosage, and combination regimens. ARC patients, especially critically ill patients, need to make individualized adjustments to antibiotics, including dose, frequency, and method of administration. Further comprehensive research is required to determine ARC staging, expand the range of recommended antibiotics, and establish individualized dosing guidelines for ARC patients.
Author contributions
JQ took responsibility for concept formation. A-XS and QQ did literature selection and screening, supervision, visualization, data curation. A-XS drafted the manuscript. H-HZ and X-QT collected data to summarize figure and tables. All authors contributed to the article and approved the submitted version.
Acknowledgments
We thank the Figdraw platform for providing drawing assistance.
Conflict of interest
The authors declare that the research was conducted in the absence of any commercial or financial relationships that could be construed as a potential conflict of interest.
Publisher’s note
All claims expressed in this article are solely those of the authors and do not necessarily represent those of their affiliated organizations, or those of the publisher, the editors and the reviewers. Any product that may be evaluated in this article, or claim that may be made by its manufacturer, is not guaranteed or endorsed by the publisher.
Supplementary material
The Supplementary Material for this article can be found online at: https://www.frontiersin.org/articles/10.3389/fphar.2023.1137975/full#supplementary-material
Abbreviations
ARC, augmented renal clearance; CrCl, creatinine clearance; ICU, intensive care unit; sCr, serum creatinine; SIRS, systemic inflammatory response syndrome; RFR, renal function reserve; GFR, glomerular filtration rate; MIC, minimum inhibitory concentration; CVVH, continuous veno-venous hemofiltration; TDM, therapeutic drug monitoring; VCM, vancomycin; PICUs, pediatric intensive care units; PIP-TAZ, piperacillin-tazobactam; C/T, ceftolozane/tazobactam; HFIM, hollow-fiber in vitro infection model; MSSA, methicillin-sensitive Staphylococcus aureus; MRSA, methicillin-resistant Staphylococcus aureus; MRCNS, methicillin-resistant coagulase-negative staphylococci; AUC, area under the curve; CMS, colistimethate sodium; MDR, multidrug-resistant; PPK, population pharmacokinetic.
References
Abdul-Aziz, M. H., Alffenaar, J. C., Bassetti, M., Bracht, H., Dimopoulos, G., Marriott, D., et al. (2020). Antimicrobial therapeutic drug monitoring in critically ill adult patients: A position Paper<sub/>. Intensive Care Med. 46 (6), 1127–1153. doi:10.1007/s00134-020-06050-1
Agyeman, A. A., Rogers, K. E., Tait, J. R., Bergen, P. J., Kirkpatrick, C. M., Wallis, S. C., et al. (2021). Evaluation of meropenem-ciprofloxacin combination dosage regimens for the pharmacokinetics of critically ill patients with augmented renal clearance. Clin. Pharmacol. Ther. 109 (4), 1104–1115. doi:10.1002/cpt.2191
Aitullina, A., Krumina, A., Svirskis, S., and Purvina, S. (2019). Colistin use in patients with extreme renal function: From dialysis to augmented clearance. Med. Kaunas. 55 (2), 33. doi:10.3390/medicina55020033
Akers, K. S., Niece, K. L., Chung, K. K., Cannon, J. W., Cota, J. M., and Murray, C. K. (2014). Modified Augmented Renal Clearance score predicts rapid piperacillin and tazobactam clearance in critically ill surgery and trauma patients. J. Trauma Acute Care Surg. 77 (32), S163–S170. doi:10.1097/TA.0000000000000191
Anderson, V. R., and Perry, C. M. (2008). Levofloxacin: A review of its use as a high-dose, short-course treatment for bacterial infection. Drugs 68 (4), 535–565. doi:10.2165/00003495-200868040-00011
Anne Fournier, S. G., Que, Y-A., Eggimann, P., Pantet, O., Sadeghipour, F., Voirol, P., et al. (2018). Population pharmacokinetic study of amoxicillin-treated burn patients hospitalized at a tertiary Swiss centre. Antimicrob. Agents Chemother. 62 (9), 00505–00518. doi:10.1128/AAC.00505-18
Arechiga-Alvarado, N. A., Medellin-Garibay, S. E., Milan-Segovia, R. D. C., Ortiz-Alvarez, A., Magana-Aquino, M., and Romano-Moreno, S. (2020). Population pharmacokinetics of amikacin administered once daily in patients with different renal functions. Antimicrob. Agents Chemother. 64 (5), 021788–e2219. doi:10.1128/AAC.02178-19
Avedissian, S. N., Rhodes, N. J., Kim, Y., Bradley, J., Valdez, J. L., and Le, J. (2020a). Augmented renal clearance of aminoglycosides using population-based pharmacokinetic modelling with Bayesian estimation in the paediatric ICU. J. Antimicrob. Chemother. 75 (1), 162–169. doi:10.1093/jac/dkz408
Avedissian, S. N., Rohani, R., Bradley, J., Le, J., and Rhodes, N. J. (2021). Optimizing aminoglycoside dosing regimens for critically ill pediatric patients with augmented renal clearance: A convergence of parametric and nonparametric population approaches. Antimicrob. Agents Chemother. 65 (4), 026299–e2720. doi:10.1128/AAC.02629-20
Avedissian, S. N., Skochko, S. M., Le, J., Hingtgen, S., Harvey, H., Capparelli, E. V., et al. (2020b). Use of simulation strategies to predict subtherapeutic meropenem exposure caused by augmented renal clearance in critically ill pediatric patients with sepsis. J. Pediatr. Pharmacol. Ther. 25 (5), 413–422. doi:10.5863/1551-6776-25.5.413
Bakke, V., Sporsem, H., Von der Lippe, E., Nordoy, I., Lao, Y., Nyrerod, H. C., et al. (2017). Vancomycin levels are frequently subtherapeutic in critically ill patients: A prospective observational study. Acta Anaesthesiol. Scand. 61 (6), 627–635. doi:10.1111/aas.12897
Balk, R. A. (2014). Systemic inflammatory response syndrome (SIRS): Where did it come from and is it still relevant today? Virulence 5 (1), 20–26. doi:10.4161/viru.27135
Baptista, J. P., Martins, P. J., Marques, M., and Pimentel, J. M. (2020). Prevalence and risk factors for augmented renal clearance in a population of critically ill patients. J. Intensive Care Med. 35 (10), 1044–1052. doi:10.1177/0885066618809688
Baptista, J. P., Neves, M., Rodrigues, L., Teixeira, L., Pinho, J., and Pimentel, J. (2014). Accuracy of the estimation of glomerular filtration rate within a population of critically ill patients. J. Nephrol. 27 (4), 403–410. doi:10.1007/s40620-013-0036-x
Baptista, J. P., Roberts, J. A., and Udy, A. A. (2019). Augmented renal clearance: A real phenomenon with an uncertain cause. Anaesth. Crit. Care Pain Med. 38 (4), 335–336. doi:10.1016/j.accpm.2019.03.002
Barletta, J. F., Mangram, A. J., Byrne, M., Sucher, J. F., Hollingworth, A. K., Ali-Osman, F. R., et al. (2017). Identifying augmented renal clearance in trauma patients: Validation of the augmented renal clearance in trauma intensive care scoring system. J. Trauma Acute Care Surg. 82 (4), 665–671. doi:10.1097/TA.0000000000001387
Barradell, L. B., and Bryson, H. M. (1994). Cefepime. A review of its antibacterial activity, pharmacokinetic properties and therapeutic use. Drugs 47 (3), 471–505. doi:10.2165/00003495-199447030-00007
Barrasa, H., Soraluce, A., Isla, A., Martin, A., Maynar, J., Canut, A., et al. (2019). Pharmacokinetics of linezolid in critically ill patients on continuous renal replacement therapy: Influence of residual renal function on PK/PD target attainment. J. Crit. Care 50, 69–76. doi:10.1016/j.jcrc.2018.11.016
Barrasa, H., Soraluce, A., Uson, E., Sainz, J., Martin, A., Sanchez-Izquierdo, J. A., et al. (2020). Impact of augmented renal clearance on the pharmacokinetics of linezolid: Advantages of continuous infusion from a pharmacokinetic/pharmacodynamic perspective. Int. J. Infect. Dis. 93, 329–338. doi:10.1016/j.ijid.2020.02.044
Beranger, A., Benaboud, S., Urien, S., Moulin, F., Bille, E., Lesage, F., et al. (2019). Piperacillin population pharmacokinetics and dosing regimen optimization in critically ill children with normal and augmented renal clearance. Clin. Pharmacokinet. 58 (2), 223–233. doi:10.1007/s40262-018-0682-1
Bergen, P. J., Bulitta, J. B., Kirkpatrick, C. M., Rogers, K. E., McGregor, M. J., Wallis, S. C., et al. (2016). Effect of different renal function on antibacterial effects of piperacillin against Pseudomonas aeruginosa evaluated via the hollow-fibre infection model and mechanism-based modelling. J. Antimicrob. Chemother. 71 (9), 2509–2520. doi:10.1093/jac/dkw153
Besnard, T., Carrie, C., Petit, L., and Biais, M. (2019). Increased dosing regimens of piperacillin-tazobactam are needed to avoid subtherapeutic exposure in critically ill patients with augmented renal clearance. Crit. Care 23 (1), 13. doi:10.1186/s13054-019-2308-x
Bhagunde, P., Patel, P., Lala, M., Watson, K., Copalu, W., Xu, M., et al. (2019). Population pharmacokinetic analysis for imipenem-relebactam in healthy volunteers and patients with bacterial infections. CPT Pharmacometrics Syst. Pharmacol. 8 (10), 748–758. doi:10.1002/psp4.12462
Bilbao-Meseguer, I., Rodriguez-Gascon, A., Barrasa, H., Isla, A., and Solinis, M. A. (2018). Augmented renal clearance in critically ill patients: A systematic review. Clin. Pharmacokinet. 57 (9), 1107–1121. doi:10.1007/s40262-018-0636-7
Bryson, H. M., and Brogden, R. N. (1994). Piperacillin/tazobactam. A review of its antibacterial activity, pharmacokinetic properties and therapeutic potential. Drugs 47 (3), 506–535. doi:10.2165/00003495-199447030-00008
Byrne, C. J., Roberts, J. A., McWhinney, B., Ryder, S. A., Fennell, J. P., O'Byrne, P., et al. (2017). Population pharmacokinetics of teicoplanin and attainment of pharmacokinetic/pharmacodynamic targets in adult patients with haematological malignancy. Clin. Microbiol. Infect. 23 (9), 674.e7–674674. doi:10.1016/j.cmi.2017.02.032
Campassi, M. L., Gonzalez, M. C., Masevicius, F. D., Vazquez, A. R., Moseinco, M., Navarro, N. C., et al. (2014). Augmented renal clearance in critically ill patients: Incidence, associated factors and effects on vancomycin treatment. Rev. Bras. Ter. Intensiva 26 (1), 13–20. doi:10.5935/0103-507x.20140003
Campoli-Richards, D. M., Monk, J. P., Price, A., Benfield, P., Todd, P. A., and Ward, A. (1988). Ciprofloxacin. A review of its antibacterial activity, pharmacokinetic properties and therapeutic use. Drugs 35 (4), 373–447. doi:10.2165/00003495-198835040-00003
Carlier, M., Carrette, S., Roberts, J. A., Stove, V., Verstraete, A., Hoste, E., et al. (2013). Meropenem and piperacillin/tazobactam prescribing in critically ill patients: Does augmented renal clearance affect pharmacokinetic/pharmacodynamic target attainment when extended infusions are used? Crit. Care 17 (3), R84. doi:10.1186/cc12705
Carlier, M., Noe, M., Roberts, J. A., Stove, V., Verstraete, A. G., Lipman, J., et al. (2014). Population pharmacokinetics and dosing simulations of cefuroxime in critically ill patients: Non-standard dosing approaches are required to achieve therapeutic exposures. J. Antimicrob. Chemother. 69 (10), 2797–2803. doi:10.1093/jac/dku195
Carrie, C., Chadefaux, G., Sauvage, N., de Courson, H., Petit, L., Nouette-Gaulain, K., et al. (2019). Increased beta-lactams dosing regimens improve clinical outcome in critically ill patients with augmented renal clearance treated for a first episode of hospital or ventilator-acquired pneumonia: A before and after study. Crit. Care 23 (1), 379. doi:10.1186/s13054-019-2621-4
Carrie, C., Legeron, R., Petit, L., Ollivier, J., Cottenceau, V., d'Houdain, N., et al. (2018a). Higher than standard dosing regimen are needed to achieve optimal antibiotic exposure in critically ill patients with augmented renal clearance receiving piperacillin-tazobactam administered by continuous infusion. J. Crit. Care 48, 66–71. doi:10.1016/j.jcrc.2018.08.026
Carrie, C., Rubin, S., Sioniac, P., Breilh, D., and Biais, M. (2018b). The kinetic glomerular filtration rate is not interchangeable with measured creatinine clearance for prediction of piperacillin underexposure in critically ill patients with augmented renal clearance. Crit. Care 22 (1), 177. doi:10.1186/s13054-018-2117-7
Ceelie, I., van der Starre, C., Tibboel, D., Stol, K., Koren, G., and de Wildt, S. N. (2011). Evaluation of drug formularies for pediatric intensive care. Pediatr. Crit. Care Med. 12 (1), e14–e19. doi:10.1097/PCC.0b013e3181d90228
Chakraborty, R. K., and Burns, B. (2022). “Systemic inflammatory response syndrome,” in StatPearls (Treasure Island: StatPearls Publishing LLC).
Cherry, R. A., Eachempati, S. R., Hydo, L., and Barie, P. S. (2002). Accuracy of short-duration creatinine clearance determinations in predicting 24-hour creatinine clearance in critically ill and injured patients. J. Trauma 53 (2), 267–271. doi:10.1097/00005373-200208000-00013
Chu, Y., Luo, Y., Jiang, M., and Zhou, B. (2020). Application of vancomycin in patients with augmented renal clearance. Eur. J. Hosp. Pharm. 27 (5), 276–279. doi:10.1136/ejhpharm-2018-001781
Claus, B. O., Hoste, E. A., Colpaert, K., Robays, H., Decruyenaere, J., and De Waele, J. J. (2013). Augmented renal clearance is a common finding with worse clinical outcome in critically ill patients receiving antimicrobial therapy. J. Crit. Care 28 (5), 695–700. doi:10.1016/j.jcrc.2013.03.003
Cojutti, P. G., Barbarino, C., De Monte, A., Hope, W., and Pea, F. (2018). Higher than standard meropenem and linezolid dosages needed for appropriate treatment of an intracerebral hemorrhage patient with augmented renal clearance. Eur. J. Clin. Pharmacol. 74 (8), 1091–1092. doi:10.1007/s00228-018-2465-x
Cook, A. M., Martin, C., Adams, V. R., and Morehead, R. S. (2011). Pharmacokinetics of intravenous levofloxacin administered at 750 milligrams in obese adults. Antimicrob. Agents Chemother. 55 (7), 3240–3243. doi:10.1128/AAC.01680-10
Craig, W. A., and Andes, D. R. (2013). In vivo activities of ceftolozane, a new cephalosporin, with and without tazobactam against Pseudomonas aeruginosa and Enterobacteriaceae, including strains with extended-spectrum beta-lactamases, in the thighs of neutropenic mice. Antimicrob. Agents Chemother. 57 (4), 1577–1582. doi:10.1128/AAC.01590-12
Craig, W. A. (2003). Basic pharmacodynamics of antibacterials with clinical applications to the use of beta-lactams, glycopeptides, and linezolid. Infect. Dis. Clin. North Am. 17 (3), 479–501. doi:10.1016/s0891-5520(03)00065-5
Curth, H. M., Pelc, A., Kutting, F., and Steffen, H. M. (2015). Augmented renal vancomycin clearance in cancer patients: A case report and review of the literature. Oncol. Res. Treat. 38 (4), 182–184. doi:10.1159/000377652
Dahyot-Fizelier, C., Lefeuvre, S., Laksiri, L., Marchand, S., Sawchuk, R. J., Couet, W., et al. (2010). Kinetics of imipenem distribution into the peritoneal fluid of patients with severe peritonitis studied by microdialysis. Clin. Pharmacokinet. 49 (5), 323–334. doi:10.2165/11319370-000000000-00000
Dalfino, L., Puntillo, F., Ondok, M. J., Mosca, A., Monno, R., Coppolecchia, S., et al. (2015). Colistin-associated acute kidney injury in severely ill patients: A step toward a better renal care? A prospective cohort study. Clin. Infect. Dis. 61 (12), 1771–1777. doi:10.1093/cid/civ717
da Silva Alves, G. C., da Silva, S. D., Frade, V. P., Rodrigues, D., Baldoni, A. O., de Castro, W. V., et al. (2017). Determining the optimal vancomycin daily dose for pediatrics: A meta-analysis. Eur. J. Clin. Pharmacol. 73 (11), 1341–1353. doi:10.1007/s00228-017-2306-3
de Cacqueray, N., Hirt, D., Zheng, Y., Bille, E., Leger, P. L., Rambaud, J., et al. (2022). Cefepime population pharmacokinetics and dosing regimen optimization in critically ill children with different renal function. Clin. Microbiol. Infect. 28, 1389–1381389. doi:10.1016/j.cmi.2022.05.007
De Cock, P. A., Standing, J. F., Barker, C. I., de Jaeger, A., Dhont, E., Carlier, M., et al. (2015). Augmented renal clearance implies a need for increased amoxicillin-clavulanic acid dosing in critically ill children. Antimicrob. Agents Chemother. 59 (11), 7027–7035. doi:10.1128/AAC.01368-15
De Waele, J. J., Lipman, J., Carlier, M., and Roberts, J. A. (2015). Subtleties in practical application of prolonged infusion of beta-lactam antibiotics. Int. J. Antimicrob. Agents 45 (5), 461–463. doi:10.1016/j.ijantimicag.2015.01.007
Dias, C., Gaio, A. R., Monteiro, E., Barbosa, S., Cerejo, A., Donnelly, J., et al. (2015). Kidney-brain link in traumatic brain injury patients? A preliminary report. Neurocrit Care 22 (2), 192–201. doi:10.1007/s12028-014-0045-1
Dryden, M. S. (2011). Linezolid pharmacokinetics and pharmacodynamics in clinical treatment. J. Antimicrob. Chemother. 66 (4), iv7–iv15. doi:10.1093/jac/dkr072
Du, B., Zhou, Y., Tang, B. H., Wu, Y. E., Yang, X. M., Shi, H. Y., et al. (2021). Population pharmacokinetic study of cefathiamidine in infants with augmented renal clearance. Front. Pharmacol. 12, 630047. doi:10.3389/fphar.2021.630047
Dvorchik, B. H., Brazier, D., DeBruin, M. F., and Arbeit, R. D. (2003). Daptomycin pharmacokinetics and safety following administration of escalating doses once daily to healthy subjects. Antimicrob. Agents Chemother. 47 (4), 1318–1323. doi:10.1128/AAC.47.4.1318-1323.2003
El-Haffaf, I., Caissy, J. A., and Marsot, A. (2021). Piperacillin-tazobactam in intensive care units: A review of population pharmacokinetic analyses. Clin. Pharmacokinet. 60 (7), 855–875. doi:10.1007/s40262-021-01013-1
Elizabeth Davis, S., Ham, J., Hucks, J., Gould, A., Foster, R., Ann Justo, J., et al. (2019). Use of continuous infusion ceftolozane-tazobactam with therapeutic drug monitoring in a patient with cystic fibrosis. Am. J. Health Syst. Pharm. 76 (8), 501–504. doi:10.1093/ajhp/zxz011
Farrell, D. J., Flamm, R. K., Sader, H. S., and Jones, R. N. (2014). Ceftobiprole activity against over 60,000 clinical bacterial pathogens isolated in Europe, Turkey, and Israel from 2005 to 2010. Antimicrob. Agents Chemother. 58 (7), 3882–3888. doi:10.1128/AAC.02465-14
Foord, R. D. (1976). Cefuroxime: Human pharmacokinetics. Antimicrob. Agents Chemother. 9 (5), 741–747. doi:10.1128/AAC.9.5.741
Frossard, M., Joukhadar, C., Erovic, B. M., Dittrich, P., Mrass, P. E., Van Houte, M., et al. (2000). Distribution and antimicrobial activity of fosfomycin in the interstitial fluid of human soft tissues. Antimicrob. Agents Chemother. 44 (10), 2728–2732. doi:10.1128/AAC.44.10.2728-2732.2000
Fuster-Lluch, O., Geronimo-Pardo, M., Peyro-Garcia, R., and Lizan-Garcia, M. (2008). Glomerular hyperfiltration and albuminuria in critically ill patients. Anaesth. Intensive Care 36 (5), 674–680. doi:10.1177/0310057X0803600507
Gao, L., Xu, H., Ye, Q., Li, S., Wang, J., Mei, Y., et al. (2020). Population pharmacokinetics and dosage optimization of teicoplanin in children with different renal functions. Front. Pharmacol. 11, 552. doi:10.3389/fphar.2020.00552
Gieling, E. M., Wallenburg, E., Frenzel, T., de Lange, D. W., Schouten, J. A., Ten Oever, J., et al. (2020). Higher dosage of ciprofloxacin necessary in critically ill patients: A new dosing algorithm based on renal function and pathogen susceptibility. Clin. Pharmacol. Ther. 108 (4), 770–774. doi:10.1002/cpt.1855
Gijsen, M., Elkayal, O., Annaert, P., Van Daele, R., Meersseman, P., Debaveye, Y., et al. (2022). Meropenem target attainment and population pharmacokinetics in critically ill septic patients with preserved or increased renal function. Infect. Drug Resist 15, 53–62. doi:10.2147/IDR.S343264
Gijsen, M., Huang, C. Y., Flechet, M., Van Daele, R., Declercq, P., Debaveye, Y., et al. (2020). Development and external validation of an online clinical prediction model for augmented renal clearance in adult mixed critically ill patients: The augmented renal clearance predictor. Crit. Care Med. 48 (12), e1260–e1268. doi:10.1097/CCM.0000000000004667
Gregoire, N., Marchand, S., Ferrandiere, M., Lasocki, S., Seguin, P., Vourc'h, M., et al. (2019). Population pharmacokinetics of daptomycin in critically ill patients with various degrees of renal impairment. J. Antimicrob. Chemother. 74 (1), 117–125. doi:10.1093/jac/dky374
Guilhaumou, R., Benaboud, S., Bennis, Y., Dahyot-Fizelier, C., Dailly, E., Gandia, P., et al. (2019). Optimization of the treatment with beta-lactam antibiotics in critically ill patients-guidelines from the French Society of Pharmacology and Therapeutics (Societe Francaise de Pharmacologie et Therapeutique-SFPT) and the French Society of Anaesthesia and Intensive Care Medicine (Societe Francaise d'Anesthesie et Reanimation-SFAR). Crit. Care 23 (1), 104. doi:10.1186/s13054-019-2378-9
Haeseker, M., Havenith, T., Stolk, L., Neef, C., Bruggeman, C., and Verbon, A. (2014). Is the standard dose of amoxicillin-clavulanic acid sufficient? BMC Pharmacol. Toxicol. 15, 38. doi:10.1186/2050-6511-15-38
Hashemian, S. M. R., Farhadi, T., and Ganjparvar, M. (2018). Linezolid: A review of its properties, function, and use in critical care. Drug Des. Devel Ther. 12, 1759–1767. doi:10.2147/DDDT.S164515
Hayashi, Y., Roberts, J. A., Paterson, D. L., and Lipman, J. (2010). Pharmacokinetic evaluation of piperacillin-tazobactam. Expert Opin. Drug Metab. Toxicol. 6 (8), 1017–1031. doi:10.1517/17425255.2010.506187
Heffernan, A. J., Sime, F. B., Kumta, N., Wallis, S. C., McWhinney, B., Ungerer, J., et al. (2022). Multicenter population pharmacokinetic study of unbound ceftriaxone in critically ill patients. Antimicrob. Agents Chemother. 66 (6), e0218921. doi:10.1128/aac.02189-21
Hirai, K., Ihara, S., Kinae, A., Ikegaya, K., Suzuki, M., Hirano, K., et al. (2016). Augmented renal clearance in pediatric patients with febrile neutropenia associated with vancomycin clearance. Ther. Drug Monit. 38 (3), 393–397. doi:10.1097/FTD.0000000000000270
Hoffmann, C., Zbinden, S., and Bourquin, V. (2018). Comprendre la réserve fonctionnelle rénale. Rev. Med. Suisse 14 (592), 276–278. doi:10.53738/revmed.2018.14.592.0276
Hu, S., Wang, T., You, H., Chen, S., Zhang, T., and Dong, Y. (2022). Plasma trough concentration distribution and safety of high-dose teicoplanin for patients with augmented renal clearance. J. Clin. Pharm. Ther. 47, 1548–1555. doi:10.1111/jcpt.13700
Huang, G. M., Qiu, Y., Liu, T. T., and Lu, J. J. (2022). Comparison of vancomycin clearance between augmented renal clearance and normal renal function in critically ill infants: A population pharmacokinetics study. J. Clin. Pharmacol. 62 (7), 863–872. doi:10.1002/jcph.2029
Hurst, M., Lamb, H. M., Scott, L. J., and Figgitt, D. P. (2002). Levofloxacin: An updated review of its use in the treatment of bacterial infections. Drugs 62 (14), 2127–2167. doi:10.2165/00003495-200262140-00013
Imani, S., Buscher, H., Marriott, D., Gentili, S., and Sandaradura, I. (2017). Too much of a good thing: A retrospective study of β-lactam concentration-toxicity relationships. J. Antimicrob. Chemother. 72 (10), 2891–2897. doi:10.1093/jac/dkx209
Irving, P. M., and Gecse, K. B. (2022). Optimizing therapies using therapeutic drug monitoring: Current strategies and future perspectives. Gastroenterology 162 (5), 1512–1524. doi:10.1053/j.gastro.2022.02.014
Ito, A., Kohira, N., Bouchillon, S. K., West, J., Rittenhouse, S., Sader, H. S., et al. (2016). In vitro antimicrobial activity of S-649266, a catechol-substituted siderophore cephalosporin, when tested against non-fermenting Gram-negative bacteria. J. Antimicrob. Chemother. 71 (3), 670–677. doi:10.1093/jac/dkv402
Izumisawa, T., Kaneko, T., Soma, M., Imai, M., Wakui, N., Hasegawa, H., et al. (2019). Augmented renal clearance of vancomycin in hematologic malignancy patients. Biol. Pharm. Bull. 42 (12), 2089–2094. doi:10.1248/bpb.b19-00652
Jayanti, R. P., Long, N. P., Phat, N. K., Cho, Y. S., and Shin, J. G. (2022). Semi-automated therapeutic drug monitoring as a pillar toward personalized medicine for tuberculosis management. Pharmaceutics 14 (5), 990. doi:10.3390/pharmaceutics14050990
Joynt, G. M., Lipman, J., Gomersall, C. D., Young, R. J., Wong, E. L., and Gin, T. (2001). The pharmacokinetics of once-daily dosing of ceftriaxone in critically ill patients. J. Antimicrob. Chemother. 47 (4), 421–429. doi:10.1093/jac/47.4.421
Kanazawa, N., Matsumoto, K., Ikawa, K., Fukamizu, T., Shigemi, A., Yaji, K., et al. (2011). An initial dosing method for teicoplanin based on the area under the serum concentration time curve required for MRSA eradication. J. Infect. Chemother. 17 (2), 297–300. doi:10.1007/s10156-010-0105-1
Kassamali, Z., Rotschafer, J. C., Jones, R. N., Prince, R. A., and Danziger, L. H. (2013). Polymyxins: Wisdom does not always come with age. Clin. Infect. Dis. 57 (6), 877–883. doi:10.1093/cid/cit367
Kawaguchi, N., Katsube, T., Echols, R., and Wajima, T. (2018). Population pharmacokinetic analysis of cefiderocol, a parenteral siderophore cephalosporin, in healthy subjects, subjects with various degrees of renal function, and patients with complicated urinary tract infection or acute uncomplicated pyelonephritis. Antimicrob. Agents Chemother. 62 (2), 013911–e1417. doi:10.1128/AAC.01391-17
Kawaguchi, N., Katsube, T., Echols, R., and Wajima, T. (2021). Population pharmacokinetic and pharmacokinetic/pharmacodynamic analyses of cefiderocol, a parenteral siderophore cephalosporin, in patients with pneumonia, bloodstream infection/sepsis, or complicated urinary tract infection. Antimicrob. Agents Chemother. 65 (3), 014377–e1520. doi:10.1128/AAC.01437-20
Kim, A. J., Lee, J. Y., Choi, S. A., and Shin, W. G. (2016). Comparison of the pharmacokinetics of vancomycin in neurosurgical and non-neurosurgical patients. Int. J. Antimicrob. Agents 48 (4), 381–387. doi:10.1016/j.ijantimicag.2016.06.022
Kim, A., Kuti, J. L., and Nicolau, D. P. (2009). Probability of pharmacodynamic target attainment with standard and prolonged-infusion antibiotic regimens for empiric therapy in adults with hospital-acquired pneumonia. Clin. Ther. 31 (11), 2765–2778. doi:10.1016/j.clinthera.2009.11.026
Kim, Y. K., Lee, D. H., Jeon, J., Jang, H. J., Kim, H. K., Jin, K., et al. (2018). Population pharmacokinetic analysis of meropenem after intravenous infusion in Korean patients with acute infections. Clin. Ther. 40 (8), 1384–1395. doi:10.1016/j.clinthera.2018.07.001
Kim, B., Hwang, S., Heo, E., Kim, H. S., Jung, J., Kim, E. S., et al. (2023). Evaluation of vancomycin TDM strategies: Prediction and prevention of kidney injuries based on vancomycin TDM results. J. Korean Med. Sci. 38 (14), e101. doi:10.3346/jkms.2023.38.e101
Kindgen-Milles, D., Slowinski, T., and Dimski, T. (2020). New kidney function tests: Renal functional reserve and furosemide stress test. Med. Klin. Intensivmed. Notfmed 115 (1), 37–42. doi:10.1007/s00063-017-0400-z
Kohira, N., West, J., Ito, A., Ito-Horiyama, T., Nakamura, R., Sato, T., et al. (2015). In vitro antimicrobial activity of a siderophore cephalosporin, S-649266, against enterobacteriaceae clinical isolates, including carbapenem-resistant strains. Antimicrob. Agents Chemother. 60 (2), 729–734. doi:10.1128/AAC.01695-15
Kuti, J. L., Dandekar, P. K., Nightingale, C. H., and Nicolau, D. P. (2003). Use of Monte Carlo simulation to design an optimized pharmacodynamic dosing strategy for meropenem. J. Clin. Pharmacol. 43 (10), 1116–1123. doi:10.1177/0091270003257225
Lagace-Wiens, P., Walkty, A., and Karlowsky, J. A. (2014). Ceftazidime-avibactam: An evidence-based review of its pharmacology and potential use in the treatment of gram-negative bacterial infections. Core Evid. 9, 13–25. doi:10.2147/CE.S40698
Landersdorfer, C. B., Bulitta, J. B., Kirkpatrick, C. M., Kinzig, M., Holzgrabe, U., Drusano, G. L., et al. (2012). Population pharmacokinetics of piperacillin at two dose levels: Influence of nonlinear pharmacokinetics on the pharmacodynamic profile. Antimicrob. Agents Chemother. 56 (11), 5715–5723. doi:10.1128/AAC.00937-12
LeBras, M., Chow, I., Mabasa, V. H., and Ensom, M. H. (2016). Systematic review of efficacy, pharmacokinetics, and administration of intraventricular aminoglycosides in adults. Neurocrit Care 25 (3), 492–507. doi:10.1007/s12028-016-0269-3
Lee, D. H., Kim, Y. K., Jin, K., Kang, M. J., Joo, Y. D., Kim, Y. W., et al. (2017). Population pharmacokinetic analysis of doripenem after intravenous infusion in Korean patients with acute infections. Antimicrob. Agents Chemother. 61 (5), 021855–e2216. doi:10.1128/AAC.02185-16
Lepak, A. J., Reda, A., Marchillo, K., Van Hecker, J., Craig, W. A., and Andes, D. (2014). Impact of MIC range for Pseudomonas aeruginosa and Streptococcus pneumoniae on the ceftolozane in vivo pharmacokinetic/pharmacodynamic target. Antimicrob. Agents Chemother. 58 (10), 6311–6314. doi:10.1128/AAC.03572-14
Li, J., Lovern, M., Green, M. L., Chiu, J., Zhou, D., Comisar, C., et al. (2019). Ceftazidime-avibactam population pharmacokinetic modeling and pharmacodynamic target attainment across adult indications and patient subgroups. Clin. Transl. Sci. 12 (2), 151–163. doi:10.1111/cts.12585
Li, J., Milne, R. W., Nation, R. L., Turnidge, J. D., Smeaton, T. C., and Coulthard, K. (2004). Pharmacokinetics of colistin methanesulphonate and colistin in rats following an intravenous dose of colistin methanesulphonate. J. Antimicrob. Chemother. 53 (5), 837–840. doi:10.1093/jac/dkh167
Li, J., Nation, R. L., Turnidge, J. D., Milne, R. W., Coulthard, K., Rayner, C. R., et al. (2006). Colistin: The re-emerging antibiotic for multidrug-resistant gram-negative bacterial infections. Lancet Infect. Dis. 6 (9), 589–601. doi:10.1016/S1473-3099(06)70580-1
Liebchen, U., Paal, M., Jung, J., Schroeder, I., Frey, L., Zoller, M., et al. (2020). Therapeutic drug monitoring-guided high dose meropenem therapy of a multidrug resistant Acinetobacter baumannii - a case report. Respir. Med. Case Rep. 29, 100966. doi:10.1016/j.rmcr.2019.100966
Liu, C., Bayer, A., Cosgrove, S. E., Daum, R. S., Fridkin, S. K., Gorwitz, R. J., et al. (2011). Clinical practice guidelines by the infectious diseases society of America for the treatment of methicillin-resistant Staphylococcus aureus infections in adults and children: Executive summary. Clin. Infect. Dis. 52 (3), 285–292. doi:10.1093/cid/cir034
Liu, J., Rhodes, N. J., Roberts, J. A., Pais, G. M., Turner, B., Kiel, P. J., et al. (2020). β-lactam dosing strategies: Think before you push. Int. J. Antimicrob. Agents 56 (5), 106151. doi:10.1016/j.ijantimicag.2020.106151
Lodise, T. P., Lomaestro, B., and Drusano, G. L. (2007). Piperacillin-tazobactam for Pseudomonas aeruginosa infection: Clinical implications of an extended-infusion dosing strategy. Clin. Infect. Dis. 44 (3), 357–363. doi:10.1086/510590
Lodise, T. P., Lomaestro, B., Rodvold, K. A., Danziger, L. H., and Drusano, G. L. (2004). Pharmacodynamic profiling of piperacillin in the presence of tazobactam in patients through the use of population pharmacokinetic models and Monte Carlo simulation. Antimicrob. Agents Chemother. 48 (12), 4718–4724. doi:10.1128/AAC.48.12.4718-4724.2004
López Montesinos, I., Montero, M., Sorlí, L., and Horcajada, J. P. (2021). Ceftolozane-tazobactam: When, how and why using it? Rev. Esp. Quimioter. 34 (Suppl. 1), 35–37. Suppl1). doi:10.37201/req/s01.10.2021
Luo, Y., Wang, Y., Ma, Y., Wang, P., Zhong, J., and Chu, Y. (2021). Augmented renal clearance: What have we known and what will we do? Front. Pharmacol. 12, 723731. doi:10.3389/fphar.2021.723731
Lv, C. L., Lu, J. J., Chen, M., Zhang, R., Li, Q. C., Chen, Y. Y., et al. (2020). Vancomycin population pharmacokinetics and dosing recommendations in haematologic malignancy with augmented renal clearance children. J. Clin. Pharm. Ther. 45 (6), 1278–1287. doi:10.1111/jcpt.13206
Mahmoud, S. H., and Shen, C. (2017). Augmented renal clearance in critical illness: An important consideration in drug dosing. Pharmaceutics 9 (3), 36. doi:10.3390/pharmaceutics9030036
Marsot, A., Guilhaumou, R., Riff, C., and Blin, O. (2017). Amikacin in critically ill patients: A review of population pharmacokinetic studies. Clin. Pharmacokinet. 56 (2), 127–138. doi:10.1007/s40262-016-0428-x
Matsuo, Y., Ishibashi, T., Matsumoto, S., Katsube, T., and Wajima, T. (2019). Population pharmacokinetics of doripenem in pediatric patients and monte-carlo pharmacokinetic-pharmacodynamic simulations for dosing regimen assessment. J. Pharm. Sci. 108 (9), 3099–3105. doi:10.1016/j.xphs.2019.04.002
Mattoes, H. M., Kuti, J. L., Drusano, G. L., and Nicolau, D. P. (2004). Optimizing antimicrobial pharmacodynamics: Dosage strategies for meropenem. Clin. Ther. 26 (8), 1187–1198. doi:10.1016/s0149-2918(04)80001-8
Melchers, M. J., Mavridou, E., van Mil, A. C., Lagarde, C., and Mouton, J. W. (2016). Pharmacodynamics of ceftolozane combined with tazobactam against Enterobacteriaceae in a neutropenic mouse thigh model. Antimicrob. Agents Chemother. 60 (12), 7272–7279. doi:10.1128/AAC.01580-16
Michalopoulos, A. S., and Karatza, D. C. (2010). Multidrug-resistant gram-negative infections: The use of colistin. Expert Rev. Anti Infect. Ther. 8 (9), 1009–1017. doi:10.1586/eri.10.88
Mikami, R., Imai, S., Hayakawa, M., Sugawara, M., and Takekuma, Y. (2022). Clinical applicability of urinary creatinine clearance for determining the initial dose of vancomycin in critically ill patients. J. Infect. Chemother. 28 (2), 199–205. doi:10.1016/j.jiac.2021.10.008
Minichmayr, I. K., Schaeftlein, A., Kuti, J. L., Zeitlinger, M., and Kloft, C. (2017). Clinical determinants of target non-attainment of linezolid in plasma and interstitial space fluid: A pooled population pharmacokinetic analysis with focus on critically ill patients. Clin. Pharmacokinet. 56 (6), 617–633. doi:10.1007/s40262-016-0463-7
Morbitzer, K. A., Rhoney, D. H., Dehne, K. A., and Jordan, J. D. (2019). Enhanced renal clearance and impact on vancomycin pharmacokinetic parameters in patients with hemorrhagic stroke. J. Intensive Care 7, 51. doi:10.1186/s40560-019-0408-y
Mouton, J. W., Touzw, D. J., Horrevorts, A. M., and Vinks, A. A. (2000). Comparative pharmacokinetics of the carbapenems: Clinical implications. Clin. Pharmacokinet. 39 (3), 185–201. doi:10.2165/00003088-200039030-00002
Murthy, B., and Schmitt-Hoffmann, A. (2008). Pharmacokinetics and pharmacodynamics of ceftobiprole, an anti-MRSA cephalosporin with broad-spectrum activity. Clin. Pharmacokinet. 47 (1), 21–33. doi:10.2165/00003088-200847010-00003
Natesan, S., Pai, M. P., and Lodise, T. P. (2017). Determination of alternative ceftolozane/tazobactam dosing regimens for patients with infections due to Pseudomonas aeruginosa with MIC values between 4 and 32 mg/L. J. Antimicrob. Chemother. 72 (10), 2813–2816. doi:10.1093/jac/dkx221
Nicolau, D. P., De Waele, J., Kuti, J. L., Caro, L., Larson, K. B., Yu, B., et al. (2021). Pharmacokinetics and pharmacodynamics of ceftolozane/tazobactam in critically ill patients with augmented renal clearance. Int. J. Antimicrob. Agents 57 (4), 106299. doi:10.1016/j.ijantimicag.2021.106299
Nongnuch, A., Panorchan, K., and Davenport, A. (2014). Brain-kidney crosstalk. Crit. Care 18 (3), 225. doi:10.1186/cc13907
Obrink-Hansen, K., Juul, R. V., Storgaard, M., Thomsen, M. K., Hardlei, T. F., Brock, B., et al. (2015). Population pharmacokinetics of piperacillin in the early phase of septic shock: Does standard dosing result in therapeutic plasma concentrations? Antimicrob. Agents Chemother. 59 (11), 7018–7026. doi:10.1128/AAC.01347-15
Ollivier, J., Carrie, C., d'Houdain, N., Djabarouti, S., Petit, L., Xuereb, F., et al. (2019). Are standard dosing regimens of ceftriaxone adapted for critically ill patients with augmented creatinine clearance? Antimicrob. Agents Chemother. 63 (3), 021344–e2218. doi:10.1128/AAC.02134-18
Ooi, M. H., Ngu, S. J., Chor, Y. K., Li, J., Landersdorfer, C. B., and Nation, R. L. (2019). Population pharmacokinetics of intravenous colistin in pediatric patients: Implications for the selection of dosage regimens. Clin. Infect. Dis. 69 (11), 1962–1968. doi:10.1093/cid/ciz067
Orwa, J. A., Govaerts, C., Busson, R., Roets, E., Van Schepdael, A., and Hoogmartens, J. (2001). Isolation and structural characterization of colistin components. J. Antibiot. (Tokyo) 54 (7), 595–599. doi:10.7164/antibiotics.54.595
Pai, M. P., Cojutti, P., and Pea, F. (2014). Levofloxacin dosing regimen in severely morbidly obese patients (BMI ≥40 kg/m(2)) should be guided by creatinine clearance estimates based on ideal body weight and optimized by therapeutic drug monitoring. Clin. Pharmacokinet. 53 (8), 753–762. doi:10.1007/s40262-014-0154-1
Parker, S., Lipman, J., Koulenti, D., Dimopoulos, G., and Roberts, J. A. (2013). What is the relevance of fosfomycin pharmacokinetics in the treatment of serious infections in critically ill patients? A systematic review. Int. J. Antimicrob. Agents 42 (4), 289–293. doi:10.1016/j.ijantimicag.2013.05.018
Pata, R. K., Bastola, C., Nway, N., Patel, M. J., and Adhikari, S. (2021). Augmented renal clearance in a case of sepsis leading to vancomycin failure despite increasing dose as per the estimated glomerular filtration rate. Cureus 13 (3), e14183. doi:10.7759/cureus.14183
Pfausler, B., Spiss, H., Dittrich, P., Zeitlinger, M., Schmutzhard, E., and Joukhadar, C. (2004). Concentrations of fosfomycin in the cerebrospinal fluid of neurointensive care patients with ventriculostomy-associated ventriculitis. J. Antimicrob. Chemother. 53 (5), 848–852. doi:10.1093/jac/dkh158
Por, E. D., Akers, K. S., Chung, K. K., Livezey, J. R., and Selig, D. J. (2021). Population pharmacokinetic modeling and simulations of imipenem in burn patients with and without continuous venovenous hemofiltration in the military health system. J. Clin. Pharmacol. 61 (9), 1182–1194. doi:10.1002/jcph.1865
Razzazzadeh, S., Darazam, I. A., Hajiesmaeili, M., Salamzadeh, J., Mahboubi, A., Sadeghnezhad, E., et al. (2022). Investigation of pharmacokinetic and clinical outcomes of various meropenem regimens in patients with ventilator-associated pneumonia and augmented renal clearance. Eur. J. Clin. Pharmacol. 78 (5), 823–829. doi:10.1007/s00228-022-03291-5
Richards, D. M., and Brogden, R. N. (1985). Ceftazidime. A review of its antibacterial activity, pharmacokinetic properties and therapeutic use. Drugs 29 (2), 105–161. doi:10.2165/00003495-198529020-00002
Richards, G. A., and Brink, A. J. (2014). Therapeutic drug monitoring: Linezolid too? Crit. Care 18 (5), 525. doi:10.1186/s13054-014-0525-x
Roberts, J. A., Alobaid, A. S., Wallis, S. C., Perner, A., Lipman, J., and Sjovall, F. (2019). Defining optimal dosing of ciprofloxacin in patients with septic shock. J. Antimicrob. Chemother. 74 (6), 1662–1669. doi:10.1093/jac/dkz069
Roberts, J. A., and Lipman, J. (2013). Optimal doripenem dosing simulations in critically ill nosocomial pneumonia patients with obesity, augmented renal clearance, and decreased bacterial susceptibility. Crit. Care Med. 41 (2), 489–495. doi:10.1097/CCM.0b013e31826ab4c4
Roberts, J. A., and Lipman, J. (2009). Pharmacokinetic issues for antibiotics in the critically ill patient. Crit. Care Med. 37 (3), 840–851. doi:10.1097/CCM.0b013e3181961bff
Roberts, J. A., Paul, S. K., Akova, M., Bassetti, M., De Waele, J. J., Dimopoulos, G., et al. (2014). Dali: Defining antibiotic levels in intensive care unit patients: Are current beta-lactam antibiotic doses sufficient for critically ill patients? Clin. Infect. Dis. 58 (8), 1072–1083. doi:10.1093/cid/ciu027
Roberts, J. A., Roberts, M. S., Robertson, T. A., Dalley, A. J., and Lipman, J. (2009). Piperacillin penetration into tissue of critically ill patients with sepsis--bolus versus continuous administration? Crit. Care Med. 37 (3), 926–933. doi:10.1097/CCM.0b013e3181968e44
Rubinstein, E., and Keynan, Y. (2014). Vancomycin revisited - 60 years later. Front. Public Health 2, 217. doi:10.3389/fpubh.2014.00217
Rybak, M. J., Le, J., Lodise, T. P., Levine, D. P., Bradley, J. S., Liu, C., et al. (2020). Therapeutic monitoring of vancomycin for serious methicillin-resistant Staphylococcus aureus infections: A revised consensus guideline and review by the American society of health-system pharmacists, the infectious diseases society of America, the pediatric infectious diseases society, and the society of infectious diseases pharmacists. Clin. Infect. Dis. 71 (6), 1361–1364. doi:10.1093/cid/ciaa303
Sader, H. S., Castanheira, M., Flamm, R. K., Farrell, D. J., and Jones, R. N. (2014). Antimicrobial activity of ceftazidime-avibactam against Gram-negative organisms collected from U.S. medical centers in 2012. Antimicrob. Agents Chemother. 58 (3), 1684–1692. doi:10.1128/AAC.02429-13
Saito, K., Kamio, S., Ito, K., Suzuki, N., Abe, K., and Goto, T. (2020). A simple scoring method to predict augmented renal clearance in haematologic malignancies. J. Clin. Pharm. Ther. 45 (5), 1120–1126. doi:10.1111/jcpt.13193
Sako, K. I., Nakamaru, Y., Ikawa, K., Maeda, T., Goto, S., Ishihara, Y., et al. (2021). Population pharmacokinetics of teicoplanin and its dosing recommendations for neutropenic patients with augmented renal clearance for hematological malignancies. Ther. Drug Monit. 43 (4), 519–526. doi:10.1097/FTD.0000000000000906
Salvador, E., Oualha, M., Bille, E., Beranger, A., Moulin, F., Benaboud, S., et al. (2021). Population pharmacokinetics of cefazolin in critically ill children infected with methicillin-sensitive Staphylococcus aureus. Clin. Microbiol. Infect. 27 (3), 413–419. doi:10.1016/j.cmi.2020.04.022
Schleibinger, M., Steinbach, C. L., Topper, C., Kratzer, A., Liebchen, U., Kees, F., et al. (2015). Protein binding characteristics and pharmacokinetics of ceftriaxone in intensive care unit patients. Br. J. Clin. Pharmacol. 80 (3), 525–533. doi:10.1111/bcp.12636
Scully, P. T., Lam, W. M., Coronado Munoz, A. J., and Modem, V. M. (2022). Augmented renal clearance of vancomycin in suspected sepsis: Single-center, retrospective pediatric cohort. Pediatr. Crit. Care Med. 23 (6), 444–452. doi:10.1097/PCC.0000000000002918
Selig, D. J., Akers, K. S., Chung, K. K., Pruskowski, K. A., Livezey, J. R., and Por, E. D. (2022). Meropenem pharmacokinetics in critically ill patients with or without burn treated with or without continuous veno-venous haemofiltration. Br. J. Clin. Pharmacol. 88 (5), 2156–2168. doi:10.1111/bcp.15138
Sharma, A., Mucino, M. J., and Ronco, C. (2014). Renal functional reserve and renal recovery after acute kidney injury. Nephron Clin. Pract. 127 (1-4), 94–100. doi:10.1159/000363721
Shorr, A. F., Bruno, C. J., Zhang, Z., Jensen, E., Gao, W., Feng, H. P., et al. (2021). Ceftolozane/tazobactam probability of target attainment and outcomes in participants with augmented renal clearance from the randomized phase 3 ASPECT-NP trial. Crit. Care 25 (1), 354. doi:10.1186/s13054-021-03773-5
Sime, F. B., Udy, A. A., and Roberts, J. A. (2015). Augmented renal clearance in critically ill patients: Etiology, definition and implications for beta-lactam dose optimization. Curr. Opin. Pharmacol. 24, 1–6. doi:10.1016/j.coph.2015.06.002
Steffens, N. A., Zimmermann, E. S., Nichelle, S. M., and Brucker, N. (2021). Meropenem use and therapeutic drug monitoring in clinical practice: A literature review. J. Clin. Pharm. Ther. 46 (3), 610–621. doi:10.1111/jcpt.13369
Stein, G. E., Smith, C. L., Scharmen, A., Kidd, J. M., Cooper, C., Kuti, J., et al. (2019). Pharmacokinetic and pharmacodynamic analysis of ceftazidime/avibactam in critically ill patients. Surg. Infect. (Larchmt) 20 (1), 55–61. doi:10.1089/sur.2018.141
Strayer, A. H., Gilbert, D. H., Pivarnik, P., Medeiros, A. A., Zinner, S. H., and Dudley, M. N. (1994). Pharmacodynamics of piperacillin alone and in combination with tazobactam against piperacillin-resistant and -susceptible organisms in an in vitro model of infection. Antimicrob. Agents Chemother. 38 (10), 2351–2356. doi:10.1128/AAC.38.10.2351
Suttels, V., André, P., Thoma, Y., Veuve, F., Decosterd, L., Guery, B., et al. (2022). Therapeutic drug monitoring of cefepime in a non-critically ill population: Retrospective assessment and potential role for model-based dosing. JAC Antimicrob. Resist 4 (2), dlac043. doi:10.1093/jacamr/dlac043
Tamatsukuri, T., Ohbayashi, M., Kohyama, N., Kobayashi, Y., Yamamoto, T., Fukuda, K., et al. (2018). The exploration of population pharmacokinetic model for meropenem in augmented renal clearance and investigation of optimum setting of dose. J. Infect. Chemother. 24 (10), 834–840. doi:10.1016/j.jiac.2018.07.007
Teng, X. Q., Qu, Q., Luo, Y., Long, W. M., Zhuang, H. H., Xu, J. H., et al. (2022). Therapeutic drug monitoring of ceftazidime-avibactam concentrations in carbapenem-resistant K. Pneumoniae-infected patients with different kidney statuses. Front. Pharmacol. 13, 780991. doi:10.3389/fphar.2022.780991
Todd, P. A., and Benfield, P. (1990). Amoxicillin/clavulanic acid. An update of its antibacterial activity, pharmacokinetic properties and therapeutic use. Drugs 39 (2), 264–307. doi:10.2165/00003495-199039020-00008
Torres, A., Mouton, J. W., and Pea, F. (2016). Pharmacokinetics and dosing of ceftobiprole medocaril for the treatment of hospital- and community-acquired pneumonia in different patient populations. Clin. Pharmacokinet. 55 (12), 1507–1520. doi:10.1007/s40262-016-0418-z
Troger, U., Drust, A., Martens-Lobenhoffer, J., Tanev, I., Braun-Dullaeus, R. C., and Bode-Boger, S. M. (2012). Decreased meropenem levels in intensive care unit patients with augmented renal clearance: Benefit of therapeutic drug monitoring. Int. J. Antimicrob. Agents 40 (4), 370–372. doi:10.1016/j.ijantimicag.2012.05.010
Tsuji, B. T., Pogue, J. M., Zavascki, A. P., Paul, M., Daikos, G. L., Forrest, A., et al. (2019). International consensus guidelines for the optimal use of the polymyxins: Endorsed by the American college of clinical pharmacy (ACCP), European society of clinical microbiology and infectious Diseases (ESCMID), infectious Diseases society of America (IDSA), international society for anti-infective pharmacology (ISAP), society of critical care medicine (SCCM), and society of infectious Diseases pharmacists (SIDP). Pharmacotherapy 39 (1), 10–39. doi:10.1002/phar.2209
Tze-ying, T., Fu, W., Chih-lin, C., Mei-fang, C., Ching-teh, C., Ying-yuan, C., et al. (1979). Clinical and laboratory studies of a new cephalosporin derivative--cefathiamidine. Chin. Med. J. Engl. 92 (1), 26–36.
Udy, A. A., Lipman, J., Jarrett, P., Klein, K., Wallis, S. C., Patel, K., et al. (2015). Are standard doses of piperacillin sufficient for critically ill patients with augmented creatinine clearance? Crit. Care 19, 28. doi:10.1186/s13054-015-0750-y
Udy, A. A., Roberts, J. A., Boots, R. J., Paterson, D. L., and Lipman, J. (2010). Augmented renal clearance: Implications for antibacterial dosing in the critically ill. Clin. Pharmacokinet. 49 (1), 1–16. doi:10.2165/11318140-000000000-00000
Udy, A. A., Roberts, J. A., and Lipman, J. (2011). Implications of augmented renal clearance in critically ill patients. Nat. Rev. Nephrol. 7 (9), 539–543. doi:10.1038/nrneph.2011.92
Udy, A. A., Roberts, J. A., Shorr, A. F., Boots, R. J., and Lipman, J. (2013). Augmented renal clearance in septic and traumatized patients with normal plasma creatinine concentrations: Identifying at-risk patients. Crit. Care 17 (1), R35. doi:10.1186/cc12544
van Duin, D., and Bonomo, R. A. (2016). Ceftazidime/avibactam and ceftolozane/tazobactam: Second-generation β-Lactam/β-Lactamase inhibitor combinations. Clin. Infect. Dis. 63 (2), 234–241. doi:10.1093/cid/ciw243
Villanueva, R. D., Talledo, O., Neely, S., White, B., Celii, A., Cross, A., et al. (2019). Vancomycin dosing in critically ill trauma patients: The VANCTIC Study. J. Trauma Acute Care Surg. 87 (5), 1164–1171. doi:10.1097/TA.0000000000002492
Vree, T. B., and Hekster, Y. A. (1990). Pharmacokinetics and tissue concentrations of cefuroxime. Pharm. Weekbl. Sci. 12 (6A), 262–266; discussion 267. doi:10.1007/BF01967830
Wang, X., Wang, Y., Yao, F., Chen, S., Hou, Y., Zheng, Z., et al. (2021). Pharmacokinetics of linezolid dose adjustment for creatinine clearance in critically ill patients: A multicenter, prospective, open-label, observational study. Drug Des. Devel Ther. 15, 2129–2141. doi:10.2147/DDDT.S303497
Weis, S., Kesselmeier, M., Davis, J. S., Morris, A. M., Lee, S., Scherag, A., et al. (2019). Cefazolin versus anti-staphylococcal penicillins for the treatment of patients with Staphylococcus aureus bacteraemia. Clin. Microbiol. Infect. 25 (7), 818–827. doi:10.1016/j.cmi.2019.03.010
Xiao, A. J., Caro, L., Popejoy, M. W., Huntington, J. A., and Kullar, R. (2017). PK/PD target attainment with ceftolozane/tazobactam using Monte Carlo simulation in patients with various degrees of renal function, including augmented renal clearance and end-stage renal Disease. Infect. Dis. Ther. 6 (1), 137–148. doi:10.1007/s40121-016-0143-9
Yadav, R., Bergen, P. J., Rogers, K. E., Kirkpatrick, C. M. J., Wallis, S. C., Huang, Y., et al. (2019). Meropenem-tobramycin combination regimens combat carbapenem-resistant Pseudomonas aeruginosa in the hollow-fiber infection model simulating augmented renal clearance in critically ill patients. Antimicrob. Agents Chemother. 64 (1), 016799–e1719. doi:10.1128/AAC.01679-19
Yadav, R., Rogers, K. E., Bergen, P. J., Bulitta, J. B., Kirkpatrick, C. M. J., Wallis, S. C., et al. (2018). Optimization and evaluation of piperacillin-tobramycin combination dosage regimens against Pseudomonas aeruginosa for patients with altered pharmacokinetics via the hollow-fiber infection model and mechanism-based modeling. Antimicrob. Agents Chemother. 62 (5), 000788–e118. doi:10.1128/AAC.00078-18
Yamashita, Y., Kamiyama, H., Yamamoto, A., Kanoh, H., Yuhki, Y., Ueda, A., et al. (2016). Relationship between PK/PD of cefepime and clinical outcome in febrile neutropenic patients with normal renal function. Yakugaku Zasshi 136 (12), 1641–1649. doi:10.1248/yakushi.16-00168
Yu, T., Stockmann, C., Healy, D. P., Olson, J., Wead, S., Neely, A. N., et al. (2015). Determination of optimal amikacin dosing regimens for pediatric patients with burn wound sepsis. J. Burn Care Res. 36 (4), e244–e252. doi:10.1097/BCR.0000000000000159
Yu, Y. X., Lu, J., Lu, H. D., Li, L., Li, J. J., Shi, L., et al. (2022). Predictive performance of reported vancomycin population pharmacokinetic model in patients with different renal function status, especially those with augmented renal clearance. Eur. J. Hosp. Pharm. 29 (1), e6–e14. doi:10.1136/ejhpharm-2020-002477
Keywords: augmented renal clearance, antibiotic, pharmacokinetics, pharmacodynamics, individualized
Citation: Shi A-X, Qu Q, Zhuang H-H, Teng X-Q, Xu W-X, Liu Y-P, Xiao Y-W and Qu J (2023) Individualized antibiotic dosage regimens for patients with augmented renal clearance. Front. Pharmacol. 14:1137975. doi: 10.3389/fphar.2023.1137975
Received: 05 January 2023; Accepted: 12 July 2023;
Published: 26 July 2023.
Edited by:
Ying Chen, Renmin Hospital of Wuhan University, ChinaCopyright © 2023 Shi, Qu, Zhuang, Teng, Xu, Liu, Xiao and Qu. This is an open-access article distributed under the terms of the Creative Commons Attribution License (CC BY). The use, distribution or reproduction in other forums is permitted, provided the original author(s) and the copyright owner(s) are credited and that the original publication in this journal is cited, in accordance with accepted academic practice. No use, distribution or reproduction is permitted which does not comply with these terms.
*Correspondence: Jian Qu, cXVqaWFuc3RhbmxleUBjc3UuZWR1LmNu
†These authors have contributed equally to this work