- 1The Brain Science Center, Beijing Institute of Basic Medical Sciences, Beijing, China
- 2Hengyang Medical College, University of South China, Hengyang, Hunan, China
- 3School of Basic Medical Sciences, Anhui Medical University, Hefei, Anhui, China
- 4Center on Translational Neuroscience, College of Life and Environmental Science, Minzu University of China, Beijing, China
Background: Major depression is one of the most common psychiatric disorders worldwide, inflicting suffering, significant reduction in life span, and financial burdens on families and society. Mounting evidence implicates that exposure to chronic stress can induce the dysregulation of the immune system, and the activation of brain-resident innate immune cells, microglia, leading to depression-like symptoms. However, the specific mechanisms need to be further elucidated.
Method: Animal models of depression were established by chronic restraint stress (CRS), and depression-like behavior was assessed by sucrose preference test (SPT), open field test (OFT), tail suspension test (TST) and forced swimming test (FST). Microglial activation was visualized by immunofluorescent and immunohistochemical staining, and microglial morphological changes were further analyzed by skeleton analysis. The levels of inflammatory cytokines were detected by western blotting and qPCR.
Result: Microglial Dlg1 knockout ameliorates CRS-induced mice depression-like behavior. In contrast to the effect of Dlg1 in the LPS-induced mouse model, Dlg1 knockout had little effect on microglial density, but significantly decreased the number of activated microglia and reversed microglia morphological changes in mice challenged with CRS. Moreover, the upregulation of inflammatory cytokines following CRS exposure was partially reversed by Dlg1 deletion.
Conclusion: Our study provides the evidence that Dlg1 ablation in microglia remarkedly reverses microglial activation and depression-like behavior in mice exposed to CRS, implicating a potential target for the treatment of clinical depression.
Introduction
Major depression, a common psychiatric disorder throughout the world, is a leading cause of disability, with an estimated 5.0% of the adults suffering, according to the WHO. As a major contributor to suicide, especially in adolescent, major depression also increases susceptibility to other illnesses with much poorer prognosis (Benros et al., 2013). Despite the recent decades witnessed advances in the understanding of major depression, the underlining mechanism remains to be elucidated. Cumulative evidence shows that depression and neuroinflammation have reciprocal connections (Wohleb et al., 2016; Beurel et al., 2020). Inflammation is involved in depression, and depression can be induced by immune challenge, the way between depression and immune-activation is bidirectional (Licinio and Wong, 1999; Dowlati et al., 2010; Wong et al., 2016; Colasanto et al., 2020; Cao et al., 2021). Accordingly, studies aiming at neuroinflammation might provide valuable insights into the understanding of major depression.
Neuroinflammation, termed as pathological immune processes within the central nervous system (CNS), is mainly mediated by microglia. Evidence demonstrates that microglial activation can be induced by stress exposure, which has been recognized as a trigger for depression in human (McLaughlin et al., 2010; Park et al., 2015) or depression-like behavior in animals (Yang et al., 2015; Hao et al., 2019; Song et al., 2020). As the innate immune cells in the CNS parenchyma, microglia not only play vital roles in immune surveillance and defense, but also in brain development, homeostatic maintenance, as well as disease initiation and progression (Sierra et al., 2019; Wright-Jin and Gutmann, 2019; Lukens and Eyo, 2022). The delicate ramified processes and highly motile identity allow microglia to sense and react to the local environment (Umpierre and Wu, 2021). During stress exposure, microglia response to these neuronal changes as well as neurotransmitters and hormone alterations, subsequently contribute to stress-induced neuroinflammation, thus shaping neuronal function and associated behavior (Wohleb et al., 2016; Schramm and Waisman, 2022). However, the complexity of stress conditions and communication between microglia and other cells make the exact mechanism of microglial activation remains mostly unclear.
Discs large homolog 1 (Dlg1), a member of the membrane-associated guanylate kinase family, which has been reported to be involved in the peripheral immune response and to be a risk factor for neuropsychiatric disorders (Oliva et al., 2012; Zanin-Zhorov et al., 2012; Gupta et al., 2018). In our previous study, we demonstrated that microglial Dlg1 is a regulator of NF-κB signaling, and Dlg1 deletion inhibits microglial activation and inflammatory cytokine production, thereby alleviating LPS-induced depression behavior (Peng et al., 2021). Despite being considered an acute model of depression, pain, lethargy, hypersensitivity, and reduced social interaction induced by LPS challenge are often considered sickness behaviors, which are hard to differentiate from depression-like behaviors (Dantzer et al., 2008; Wohleb et al., 2016). We therefore employed chronic restraint stress (CRS) model to induce depressive like behavior in mice in this study, and found that microglia activated upon CRS exposure, and Dlg1 deletion in microglia significantly reverses this activation and thus ameliorates depressive like behavior induced by CRS. Collectively, our studies elucidate that Dlg1 microglia-specific knockout not only mitigates inflammation-initiated depression, but also stress-induced depression as well as associated microglial activation.
Materials and methods
Mice
CX3CR1CreER mice were purchased from the Jackson Laboratory (Sacramento, CA, United States). Dlg1flox/flox mice were gifted by Dr. Wanli Liu (Tsinghua University, China) as previously described (Peng et al., 2021). To induce Dlg1 microglia-specific knockout, tamoxifen (S1238, Selleck) was given to mice at the age of 6-week by gavage, and each mouse was administrated a total dose of 18 mg of tamoxifen for three consecutive days. CRS was performed 6 weeks after tamoxifen administration followed by behavioral and pathological analysis. All mice were housed in the Animal Care Facility at the Institute of Basic Medical Sciences with free access to standard rodent chow and clean water. All animal experiments were approved by the Institutional Animal Care and Use Committee of the Beijing Institute of Basic Medical Sciences.
CRS procedures
Chronic restraint stress (CRS) was modified from the procedure previously described (Li et al., 2018). Briefly, the mice were placed in a 50 mL conical tube, with 0.5 cm air holes for breathing, for 3 h per day for 14 consecutive days.
RNA isolation and qPCR
Total RNA from brain tissue was extracted using TRIzol™ reagent (# 15596018, Invitrogen). cDNA was synthesized with a One-step First-strand cDNA synthesis kit (AE311-03, TransGen Biotech, Beijing, China). qPCR was performed using 2 × SYBR green master mix to measure the expression of genes. The primer sequences for qPCR are as follows:
GAPDH forward: 5′-AGGTCGGTGTGAACGGATTTG-3′;
GAPDH reverse: 5′-TGTAGACCATGTAGTTGAGGTCA-3′;
TNFα forward: 5′-CCCTCACACTCAGATCATCTTCT-3′;
TNFα reverse: 5′-GCTACGACGTGGGCTACAG-3′;
Il-6 forward: 5′-CCAAGAGGTGAGTGCCTTCCC-3′;
Il-6 reverse: 5′-CTGTTGTTCAGACTCTCTCCCT-3′;
Il-1β forward: 5′-GCAACTGTTCCTGAACTCAACT-3′;
Il-1β reverse: 5′-ATCTTTTGGGGTCCGTCAACT-3′.
Western blotting
The hippocampal tissue was lysed on ice using RIPA lysis buffer containing a cocktail of protease and phosphatase inhibitors. The lysates were centrifuged at ×15,000 g for 15 min at 4°C, then supernatants were collected and protein concentration was determined by BCA assays and was adjusted to the same final concentration. After heat denaturation, samples were separated by SDS-PAGE and transferred to Nitrocellulose membranes which were blocked in 5% non-fat milk and subsequently incubated overnight with the primary antibody. Relevant Horseradish Peroxidase (HRP)-conjugated secondary antibodies were incubated, and the immunoreactive proteins were then detected using an enhanced chemiluminescent (ECL) substrate. The protein signal was visualized using an X-Ray Film Processor (Optimax, New York, United States). The following antibodies were used: anti-iNOS/NOS Type II (# 610332, BD Biosciences, San Jose, CA, United States), anti- Iba1 (019-19741, Wako, Richmond, VA, United States), anti-Gapdh (#CW0266A, CWBiotech, Beijing, China).
Immunohistochemistry and immunofluorescence
Mice were anesthetized, perfused and sacrificed. Brains were then removed, fixed in 4% paraformaldehyde for 48 h, and dehydrated with gradient sucrose in phosphate buffered saline (PBS). The brains were embedded in optimal cutting temperature compound (OCT) and sectioned on a freezing microtome (CM3050S, Leica, Wetzlar, Germany). The coronal sections (40 μm in thickness) were incubated overnight with anti-goat Iba1 antibody (1:400, 019-19741, Wako, Richmond, VA, United States) and anti-mouse Gfap antibody (1:400; MAB360, Millipore, Darmstadt, Germany).
For immunohistochemical staining, the slices were incubated with biotinylated immunoglobulin G (IgG), followed by streptavidin-conjugated horseradish peroxidase (VECTASTAIN ABC Kit, Zhongshan Jinqiao, Beijing, China), and finally was visualized by reacting with hydrogen peroxide (DAB Kit, Zhongshan Jinqiao). Images were captured using a Leica SCN400 scanner.
For immunofluorescent staining, the sections were labeled with TRITC AffiniPure goat anti-rabbit IgG (111-025-003, Jackson ImmunoResearch) fluorescent secondary antibody, and the images were acquired by Nikon confocal microscope (Nikon, Melville, NY, United Stats). For activated microglia quantification, we determined the activated microglia by larger soma area, shorter and bolder processes.
Behavioral tests
For all behavioral tests, mice, in their home cages, were habituated for 2 h in the quiet and dimly lit test room before testing.
Open field test (OFT)
During the test, spontaneous activity of mice was monitored for a 5-min period as they moved in the apparatus (50 × 50 × 20 cm), time spent in the central area and total distances traveled were analyzed by ANY-maze software.
Tail suspension test (TST)
Mice were suspended by the tails on the instrument with a clip (50 cm above the floor) using a medical tape placed 1 cm from the tip of the tail. The test was recorded for 6 min and the immobility time was measured in the last 4 min of the test.
Forced swimming test (FST)
Mice were allowed to swim in water for 2 min the day before the test for habituation. During the test, mice were placed in a clear glass cylinder (diameter 25 cm), which was filled with water (21°C–23°C) up to a height of 25 cm from the bottom. The test was recorded for 6 min and the immobility time was measured in the last 4 min of the test.
Sucrose preference test (SPT)
The sucrose preference test is performed to evaluate anhedonia in mice. Briefly, mice were given two identical standard drinking bottles and habituated to two bottles filled with water (1 day) and with 1% sucrose water (1 day) for 2 days. After being deprived of food and water for 12 h, mice had free access to two drinking bottles, one containing 1% sucrose and the other with normal drinking water for 5 days. The positions of these two bottles were switched every 12 h to avoid side preferences. The overall sucrose and water intakes were measured, and the sucrose preference rate was calculated as 100% × (sucrose intake/sucrose intake + water intake).
Microglial skeleton analysis
Skeleton analysis of microglia was performed as described in our previous study (Cheng et al., 2021). Briefly, microglial images were obtained on Nikon A1 Confocal Microscope with ×20 objective at ×5 zoom. Skeletonize [two-dimensional (2D)/3D] plugin and Analyze Skeleton plugin of the software ImageJ were used for the skeleton analysis.
Statistical analysis
Data are expressed as mean ± SEM of at least two independent experiments. Statistical differences between the two groups were analyzed using Student’s t-test. Statistical differences for multiple comparisons were analyzed by application of ordinary two-way ANOVA (Tukey’s multiple comparison test). Statistical significance was determined as follows: *p < 0.05, **p < 0.01, and ***p < 0.001. GraphPad Prism software (version 9.0, GraphPad, San Diego, United States) was used for statistical analysis.
Results
Dlg1 depletion in microglia alleviates CRS induced mice depression-like behavior
To evaluate the role of microglial Dlg1 on depression, we established chronic restraint stress (CRS) model, which has higher etiological similarities to depression. Specifically, Dlg1 cKO mice (Dlg1f/f; CX3CR1creER) and their control littermates (Dlg1f/f, term as Dlg1 f/f) were subjected to tamoxifen at 6 weeks of age to induce microglia-specific knockout of Dlg1. These mice were then randomly assigned to either the control or CRS group. 6 weeks after tamoxifen administration, mice in the CRS group underwent chronic restraint, whereas mice in the control group were handled in a separate home cage at an equivalent length of time. After 2 weeks, behavioral tests, namely sucrose preference test (SPT), open field test (OFT), tail suspension test (TST), and forced swimming test (FST) were carried out (Figure 1A). We found that CRS mice exhibited no overt anxiety-like behavior (Figure 1B), but they displayed more prominent depression-like behaviors than non-stressed controls (Figures 1C–F). Stressed Dlg1 f/f mice showed reduced locomotion in OFT (Figure 1C), less sucrose consumption in SPT (Figure 1D), and more immobility time in TST and FST (Figures 1E, F), indicating that the CRS model was established successfully. However, these depression-like behaviors induced by CRS were significantly alleviated in microglial Dlg1 deleted mice. These results suggest that ablation of Dlg1 in microglia ameliorates CRS-induced depression-like behavior.
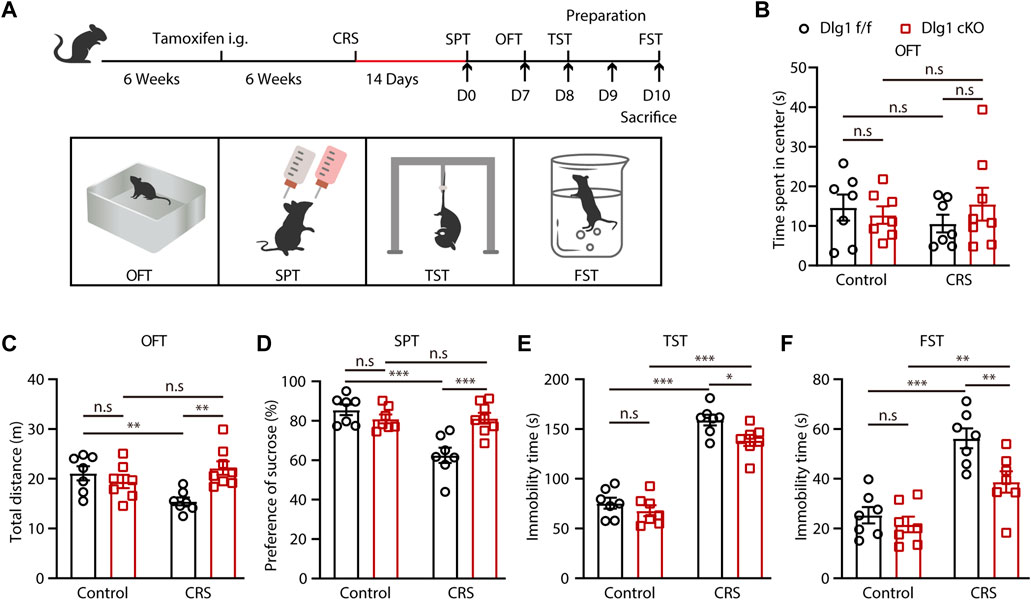
FIGURE 1. Microglial Dlg1 knockout alleviates CRS-induced depression-like behavior in mice. (A) The scheme of tamoxifen administration, stress stimuli and behavioral tests. (B–C) Travel-time spent in the central area and total travel-distance in the OFT of Dlg1 f/f and Dlg1 cKO mice with and without CRS exposure. (D) Sucrose preference of Dlg1 f/f and Dlg1 cKO mice with and without CRS exposure. (E–F) Immobility time of Dlg1 f/f and Dlg1 cKO mice with and without CRS exposure in the TST and FST. Data are presented as the mean ± SEM; *p < 0.05; **p < 0.01, ***p < 0.001, two-way ANOVA with Turkey’s multiple comparisons test.
Dlg1 ablation or CRS exposure has little effect on microglial density
After characterizing that specific knockout of Dlg1 in microglia alleviates depression-like behavior, we next sought to investigate the effects of deleting Dlg1 on microglia. To address this, we performed immunohistochemical staining of brain slices from Dlg1 cKO and their control littermates in both control and CRS group (Figure 2A). The number of microglia in different brain regions, including the cortex (CTX), dentate gyrus (DG), and CA1 of the hippocampus, was nearly unchanged in Dlg1 f/f mice subjected to CRS compared to Dlg1 f/f mice in the control group. Deletion of Dlg1 also showed no effect on microglia density in either the control or CRS groups (Figure 2B). Considering that astrocytes are known to communicate with microglia in a variety of disease states, in addition, we performed immunostaining for Gfap, a commonly used marker of astrocytes, and found that the number of astrocytes were not affected by CRS or deletion of Dlg1 from microglia (Figures 2C, D).
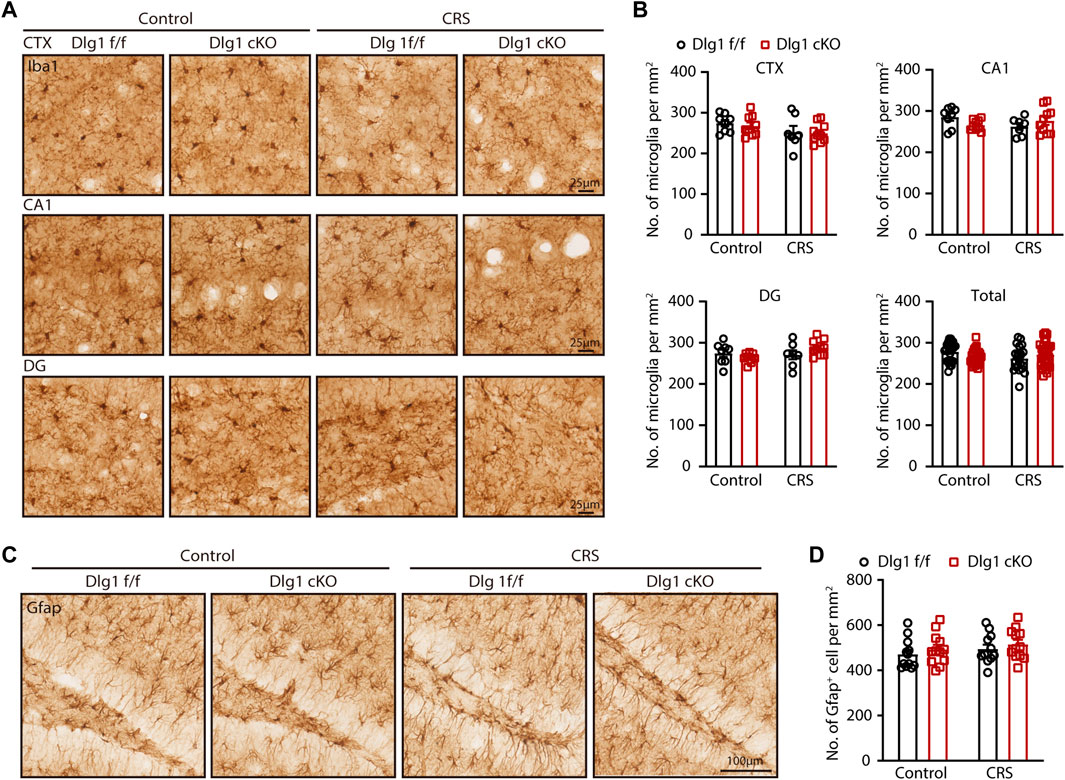
FIGURE 2. Microglial Dlg1 knockout or CRS exposure has little effect on microglial density. (A) Immunohistochemical staining for Iba1 (microglia) of Dlg1 f/f and cKO mice brain slices with and without CRS exposure in indicated brain regions. (Scale bars, 25 μm) (B) Quantifications of microglia density in indicated brain regions. (C) Immunohistochemical staining of Gfap (astrocytes) of Dlg1 f/f and cKO mice brain slices with and without CRS exposure in DG (hippocampus). (Scale bars, 100 μm) (D) Quantification of astrocyte density in DG region. Data are presented as the mean ± SEM; *p < 0.05, **p < 0.01, ***p < 0.001, two-way ANOVA with Turkey’s multiple comparisons test.
Dlg1 deletion reverses microglial activated state found in mice with CRS exposure
All four groups had compatible microglial densities, but some microglia in the CRS group exhibited alterations in morphology. In light of this, we performed immunofluorescence staining for Iba1, a microglia-specific marker, and found that the number of activated microglia, which possessed a larger volume of soma as well as shorter and bolder processes, were markedly increased in the Dlg1 f/f mice subjected to CRS (Figures 3A–C). It is interesting to note that this increase was significantly reversed by microglia-specific knockout of Dlg1 (Figure 3C). In light of the fact that microglia morphology and function are closely linked, we further analyzed the detail changes in microglia morphology (Figure 3D). We found that the soma area of microglia was notably increased while branch number and total branch length were markedly reduced in stressed Dlg1 f/f mice, As expected, these activated morphology changes were significantly absent in Dlg1 deleted microglia (Figures 3E–H). These results demonstrate that CRS provokes microglial activation, and knockout of Dlg1 prominently reverses this effect.
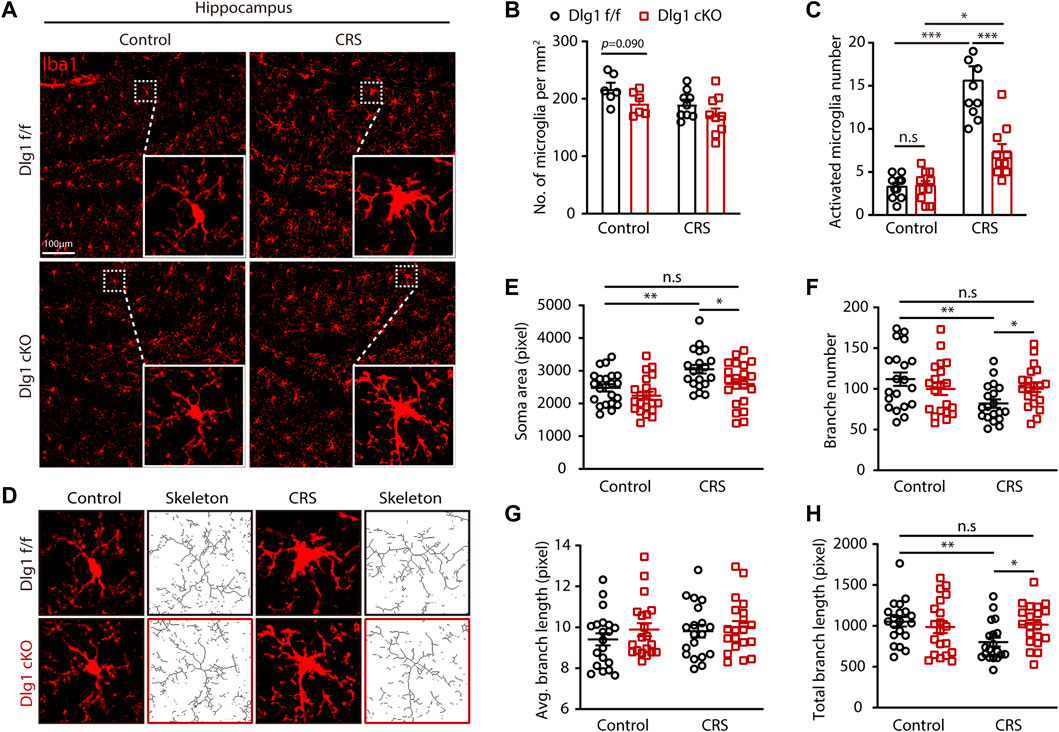
FIGURE 3. Microglial Dlg1 deletion reverses microglia overactive state found in chronic restraint stressed mice. (A) Immunofluorescence staining for Iba1 of Dlg1 f/f and cKO mice brain slices with and without CRS exposure. (B) Quantification of microglia density in indicated four groups. (C) Quantification of the number of activated microglia in a single field of view. (D) Representative images of microglia (Iba1 immunostaining, red) and skeletonized microglia of Dlg1 f/f and cKO mice brain slices with and without CRS exposure. (E,F) Quantitative analysis of microglial soma area (E), branch number (F), average branch length (G), and total branch length (H). Data are presented as the mean ± SEM; *p < 0.05, **p < 0.01, ***p < 0.001, two-way ANOVA with Turkey’s multiple comparisons test.
Stress-induced microglial inflammation was diminished by Dlg1 deletion
We next determined microglial status by examining the inflammatory cytokines. The protein level of inducible nitric oxide synthase (iNOS), termed as a marker of inflammation, was significantly upregulated in Dlg1 f/f mice of CRS group, and Dlg1 knockout reversed this upregulation (Figures 4A, B). In contrast, other cytokines like tumor necrosis factor α (TNFα), interleukin 6 (IL-6) and interleukin 1β (IL-1β) were found to have similar levels among four groups (Figures 3D–F). These results indicate that microglia display a mild activated state in CRS model, and deletion of Dlg1 in microglia recruits its normal state.
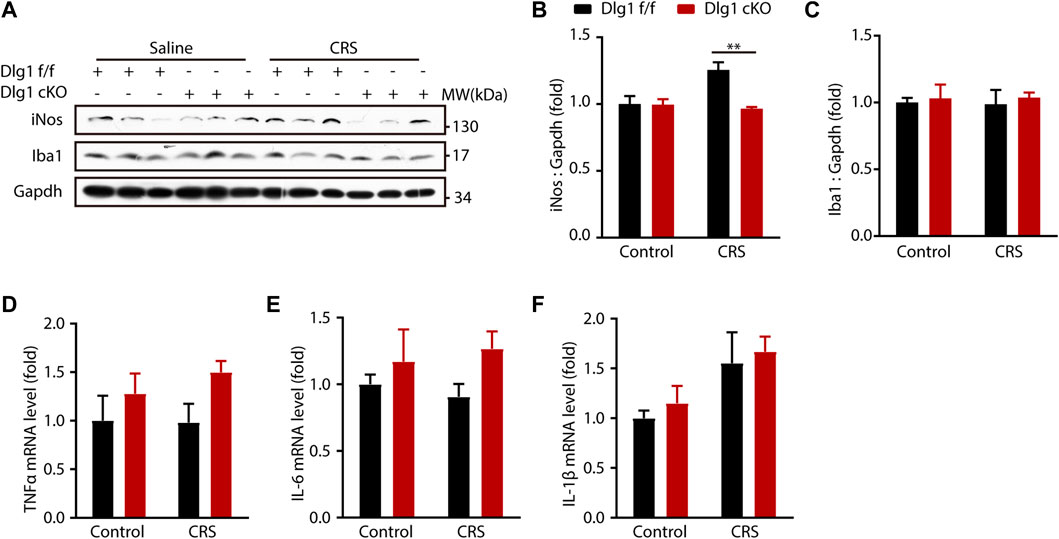
FIGURE 4. Microglial Dlg1 ablation partially decreases inflammation state in the mouse brain. (A) Immunoblots of iNOS, Iba1, and Gapdh in the hippocampus tissue of Dlg1 f/f and cKO mice brain slices with and without CRS exposure. (B,C) Quantitative analysis of iNOS and Iba1 protein levels normalized to Gapdh. (D–F) RT-PCR analysis of TNF-α, IL-6, and IL-1β mRNA levels in the hippocampus tissues of Dlg1 f/f and cKO mice brain slices with and without CRS exposure. Data are presented as the mean ± SEM; *p < 0.05, **p < 0.01, ***p < 0.001, two-way ANOVA with Turkey’s multiple comparisons test.
Discussion
In this study, we demonstrated that Dlg1 ablation in microglia remarkedly reversed microglial activation and depression-like behavior in mice induced by exposure to CRS, indicating a potential target to suppress microglial activation, limit neuroinflammation, and thereby ameliorate depression.
Clinical researches demonstrate that perturbation of microglia homeostasis, like bacterial and viral infections [e.g., coronavirus (Mazza et al., 2020; Rogers et al., 2020), Ebola (Karafillakis et al., 2016)], and inflammation conditions in disease, such as stroke (Medeiros et al., 2020), neurodegenerative diseases (Galts et al., 2019), could contribute to major depression. These immune challenges induce microglial activation and cytokine production. Moreover, the cytokine levels in plasma are highly correlated with the severity of major depression and antidepressant responses (Miller et al., 2009; Black and Miller, 2015; Yirmiya et al., 2015). However, not all patients with depression display an overt inflammation. There are pathophysiological differences among each subtype of major depression (Lamers et al., 2013). In animal model of depression, an often-used immune challenge to establish depression model is lipopolysaccharide (LPS) (O'Connor et al., 2009), which provokes robust microglial activation and cytokine secretion and induces depression-like symptoms. Nevertheless, LPS administration does not cause prolonged anhedonia, lethargy or despair (DellaGioia and Hannestad, 2010). Given the pathophysiological features of different subtypes of depression, stress-induced depression model is needed to study the role of immune dysregulation in depression.
Our previous study adopted the LPS-induced model of depression, and we observed that microglia are activated, with a larger soma volume and shorter, bolder processes, and that microglial density is markedly increased (Peng et al., 2021). In contrast, in the current study, CRS-induced depression model, microglia activated in a much milder manner, without alterations in density, and morphological changes occur in only a fraction of microglia. Furthermore, cytokine levels in the brain that were significantly elevated in mice exposed to LPS showed comparable levels in mice with and without CRS exposure. Interestingly, however, deletion of Dlg1 in microglia inhibits microglial activation and ameliorates depression-like behavior in both the LPS- and CRS-induced models of depression, prompting us to hypothesize that Dlg1 deletion protects the animal from depression more than by targeting NF-κB signaling but restores microglia to homeostasis from a state of stress-induced dysregulation. Numerous studies have revealed that voltage-gated potassium channels are necessary for the activation of microglia, and Kv1.3 is particularly crucial among them (Pike et al., 2022; Zhang et al., 2022; Yuan et al., 2023). Kv1.3 potassium channel regulates microglial activation and is highly expressed in neuroinflammatory diseases (Rangaraju et al., 2017; Pike et al., 2022; Zhang et al., 2022; Yuan et al., 2023). Interestingly, Dlg1 interacts with the Kv1.3 through the PDZ binding domain (Tejedor et al., 1997). Moreover, Kv1.3 expression is downregulated upon Dlg1 deletion in dendritic cells (Dong et al., 2019). These studies provide an insight into the potential mechanism by which Dlg1 regulates microglial activation. Dlg1 knockout might maintain the microglial resting state by downregulating Kv1.3, which requires further study.
Emerging evidence demonstrates that exposed to chronic stress induces alterations in microglial density (Tynan et al., 2010; de Pablos et al., 2014; Kreisel et al., 2014). According to Tynan et al.’s study, the number of microglia was significantly elevated after CRS exposure. In our study, however, we found almost no alterations in microglia density between stressed and non-stressed Dlg1 f/f mice. Interestingly, according to previous studies, the effects of chronic stress on microglial activation are heterogeneous (Yirmiya et al., 2015). In addition to the proliferation of microglia, which is peaked at 2–4 days after stress, chronic stress also induces the apoptosis of microglia (Kreisel et al., 2014; Tong et al., 2017; Schramm and Waisman, 2022). After exposure to stress for several weeks, the microglia density even decreases in some specific brain region (e.g., DG of hippocampus) (Tong et al., 2017; Schramm and Waisman, 2022). In the present study, we sacrificed the mice after performing behavioral tests, and that was 10 days after mice were stressed, while animals were euthanized 24 h after the final stress session in Tynan et al. (2010)’s study. It is possible that the different time points had different effect on the numbers of microglia that were undergoing apoptosis, leading to the different results in microglial density. Additionally, the differences in the animal species, the exact brain regions, the CRS protocol, and the method that the number of microglia was determined may also account for the different outcomes.
Microglia are especially sensitive to the local environment. They react rapidly to what they encountered, thus making them not only a therapeutic target, but also a suitable indicator for monitoring the disease progression and therapeutic effect. Studies aiming at microglia inhibition have achieved promising results in alleviating depression. By blocking microglial activation with minocycline, depression-like behavior can be attenuated (Henry et al., 2008; Wang et al., 2020; Bassett et al., 2021). Clinical study provides more direct evidence in patients with unipolar psychotic depression by using minocycline as an adjunctive therapy (Miyaoka et al., 2012). Other anti-inflammatory drugs, like non-steroidal anti-inflammatory drugs (NSAIDs) also exhibit anti-depressive properties (Lehrer and Rheinstein, 2019). Our studies demonstrate that deletion of Dlg1 attenuates depression-like behavior in both LPS- and CRS- induced mouse models, providing a novel target for the development of treatment strategies.
Data availability statement
The original contributions presented in the study are included in the article/Supplementary Material, further inquiries can be directed to the corresponding authors.
Ethics statement
The animal study was reviewed and approved by the Institutional Animal Care and Use Committee of the Beijing Institute of Basic Medical Sciences.
Author contributions
JC and ZY conceived and directed the project. XL designed and performed most of the experiments, analyzed the data, and wrote the manuscript. ZP and LJ performed mice genotyping and CRS model establishment. PZ and PY helped with data analysis. All authors discussed and commented on the manuscript.
Funding
This work was supported by grants from the National Nature Science Foundation of China (Grant No 82271237, 82071218, 81930029).
Acknowledgments
We sincerely thank Dr. Wanli Liu (Tsinghua University) for providing Dlg1flox/flox mice.
Conflict of interest
The authors declare that the research was conducted in the absence of any commercial or financial relationships that could be construed as a potential conflict of interest.
Publisher’s note
All claims expressed in this article are solely those of the authors and do not necessarily represent those of their affiliated organizations, or those of the publisher, the editors and the reviewers. Any product that may be evaluated in this article, or claim that may be made by its manufacturer, is not guaranteed or endorsed by the publisher.
References
Bassett, B., Subramaniyam, S., Fan, Y., Varney, S., Pan, H., Carneiro, A. M. D., et al. (2021). Minocycline alleviates depression-like symptoms by rescuing decrease in neurogenesis in dorsal hippocampus via blocking microglia activation/phagocytosis. Brain Behav. Immun. 91, 519–530. doi:10.1016/j.bbi.2020.11.009
Benros, M. E., Waltoft, B. L., Nordentoft, M., Ostergaard, S. D., Eaton, W. W., Krogh, J., et al. (2013). Autoimmune diseases and severe infections as risk factors for mood disorders: A nationwide study. JAMA Psychiatry 70 (8), 812–820. doi:10.1001/jamapsychiatry.2013.1111
Beurel, E., Toups, M., and Nemeroff, C. B. (2020). The bidirectional relationship of depression and inflammation: Double trouble. Neuron 107 (2), 234–256. doi:10.1016/j.neuron.2020.06.002
Black, C., and Miller, B. J. (2015). Meta-analysis of cytokines and chemokines in suicidality: Distinguishing suicidal versus nonsuicidal patients. Biol. Psychiatry 78 (1), 28–37. doi:10.1016/j.biopsych.2014.10.014
Cao, P., Chen, C., Liu, A., Shan, Q., Zhu, X., Jia, C., et al. (2021). Early-life inflammation promotes depressive symptoms in adolescence via microglial engulfment of dendritic spines. Neuron 109 (16), 2573–2589.e9. doi:10.1016/j.neuron.2021.06.012
Cheng, J., Dong, Y., Ma, J., Pan, R., Liao, Y., Kong, X., et al. (2021). Microglial Calhm2 regulates neuroinflammation and contributes to Alzheimer's disease pathology. Sci. Adv. 7 (35), eabe3600. doi:10.1126/sciadv.abe3600
Colasanto, M., Madigan, S., and Korczak, D. J. (2020). Depression and inflammation among children and adolescents: A meta-analysis. J. Affect Disord. 277, 940–948. doi:10.1016/j.jad.2020.09.025
Dantzer, R., O'Connor, J. C., Freund, G. G., Johnson, R. W., and Kelley, K. W. (2008). From inflammation to sickness and depression: When the immune system subjugates the brain. Nat. Rev. Neurosci. 9 (1), 46–56. doi:10.1038/nrn2297
de Pablos, R. M., Herrera, A. J., Espinosa-Oliva, A. M., Sarmiento, M., Muñoz, M. F., Machado, A., et al. (2014). Chronic stress enhances microglia activation and exacerbates death of nigral dopaminergic neurons under conditions of inflammation. J. Neuroinflammation 11, 34. doi:10.1186/1742-2094-11-34
DellaGioia, N., and Hannestad, J. (2010). A critical review of human endotoxin administration as an experimental paradigm of depression. Neurosci. Biobehav Rev. 34 (1), 130–143. doi:10.1016/j.neubiorev.2009.07.014
Dong, X., Wei, L., Guo, X., Yang, Z., Wu, C., Li, P., et al. (2019). Dlg1 maintains dendritic cell function by securing voltage-gated K(+) channel integrity. J. Immunol. 202 (11), 3187–3197. doi:10.4049/jimmunol.1900089
Dowlati, Y., Herrmann, N., Swardfager, W., Liu, H., Sham, L., Reim, E. K., et al. (2010). A meta-analysis of cytokines in major depression. Biol. Psychiatry 67 (5), 446–457. doi:10.1016/j.biopsych.2009.09.033
Galts, C. P. C., Bettio, L. E. B., Jewett, D. C., Yang, C. C., Brocardo, P. S., Rodrigues, A. L. S., et al. (2019). Depression in neurodegenerative diseases: Common mechanisms and current treatment options. Neurosci. Biobehav Rev. 102, 56–84. doi:10.1016/j.neubiorev.2019.04.002
Gupta, P., Uner, O. E., Nayak, S., Grant, G. R., and Kalb, R. G. (2018). SAP97 regulates behavior and expression of schizophrenia risk enriched gene sets in mouse hippocampus. PLoS One 13 (7), e0200477. doi:10.1371/journal.pone.0200477
Hao, Y., Ge, H., Sun, M., and Gao, Y. (2019). Selecting an appropriate animal model of depression. Int. J. Mol. Sci. 20 (19), 4827. doi:10.3390/ijms20194827
Henry, C. J., Huang, Y., Wynne, A., Hanke, M., Himler, J., Bailey, M. T., et al. (2008). Minocycline attenuates lipopolysaccharide (LPS)-induced neuroinflammation, sickness behavior, and anhedonia. J. Neuroinflammation 5, 15. doi:10.1186/1742-2094-5-15
Karafillakis, E., Jalloh, M. F., Nuriddin, A., Larson, H. J., Whitworth, J., Lees, S., et al. (2016). Once there is life, there is hope' Ebola survivors' experiences, behaviours and attitudes in Sierra Leone, 2015. BMJ Glob. Health 1 (3), e000108. doi:10.1136/bmjgh-2016-000108
Kreisel, T., Frank, M. G., Licht, T., Reshef, R., Ben-Menachem-Zidon, O., Baratta, M. V., et al. (2014). Dynamic microglial alterations underlie stress-induced depressive-like behavior and suppressed neurogenesis. Mol. Psychiatry 19 (6), 699–709. doi:10.1038/mp.2013.155
Lamers, F., Vogelzangs, N., Merikangas, K. R., de Jonge, P., Beekman, A. T., and Penninx, B. W. (2013). Evidence for a differential role of HPA-axis function, inflammation and metabolic syndrome in melancholic versus atypical depression. Mol. Psychiatry 18 (6), 692–699. doi:10.1038/mp.2012.144
Lehrer, S., and Rheinstein, P. H. (2019). Nonsteroidal anti-inflammatory drugs (NSAIDs) reduce suicidal ideation and depression. Discov. Med. 28 (154), 205–212.
Li, M. X., Zheng, H. L., Luo, Y., He, J. G., Wang, W., Han, J., et al. (2018). Gene deficiency and pharmacological inhibition of caspase-1 confers resilience to chronic social defeat stress via regulating the stability of surface AMPARs. Mol. Psychiatry 23 (3), 556–568. doi:10.1038/mp.2017.76
Licinio, J., and Wong, M. L. (1999). The role of inflammatory mediators in the biology of major depression: Central nervous system cytokines modulate the biological substrate of depressive symptoms, regulate stress-responsive systems, and contribute to neurotoxicity and neuroprotection. Mol. Psychiatry 4 (4), 317–327. doi:10.1038/sj.mp.4000586
Lukens, J. R., and Eyo, U. B. (2022). Microglia and neurodevelopmental disorders. Annu. Rev. Neurosci. 45, 425–445. doi:10.1146/annurev-neuro-110920-023056
Mazza, M. G., De Lorenzo, R., Conte, C., Poletti, S., Vai, B., Bollettini, I., et al. (2020). Anxiety and depression in COVID-19 survivors: Role of inflammatory and clinical predictors. Brain Behav. Immun. 89, 594–600. doi:10.1016/j.bbi.2020.07.037
McLaughlin, K. A., Conron, K. J., Koenen, K. C., and Gilman, S. E. (2010). Childhood adversity, adult stressful life events, and risk of past-year psychiatric disorder: A test of the stress sensitization hypothesis in a population-based sample of adults. Psychol. Med. 40 (10), 1647–1658. doi:10.1017/s0033291709992121
Medeiros, G. C., Roy, D., Kontos, N., and Beach, S. R. (2020). Post-stroke depression: A 2020 updated review. Gen. Hosp. Psychiatry 66, 70–80. doi:10.1016/j.genhosppsych.2020.06.011
Miller, A. H., Maletic, V., and Raison, C. L. (2009). Inflammation and its discontents: The role of cytokines in the pathophysiology of major depression. Biol. Psychiatry 65 (9), 732–741. doi:10.1016/j.biopsych.2008.11.029
Miyaoka, T., Wake, R., Furuya, M., Liaury, K., Ieda, M., Kawakami, K., et al. (2012). Minocycline as adjunctive therapy for patients with unipolar psychotic depression: An open-label study. Prog. Neuropsychopharmacol. Biol. Psychiatry 37 (2), 222–226. doi:10.1016/j.pnpbp.2012.02.002
O'Connor, J. C., Lawson, M. A., André, C., Moreau, M., Lestage, J., Castanon, N., et al. (2009). Lipopolysaccharide-induced depressive-like behavior is mediated by indoleamine 2,3-dioxygenase activation in mice. Mol. Psychiatry 14 (5), 511–522. doi:10.1038/sj.mp.4002148
Oliva, C., Escobedo, P., Astorga, C., Molina, C., and Sierralta, J. (2012). Role of the MAGUK protein family in synapse formation and function. Dev. Neurobiol. 72 (1), 57–72. doi:10.1002/dneu.20949
Park, S., Hatim, A., Si, T. M., Jeon, H. J., Srisurapanont, M., Bautista, D., et al. (2015). Stressful life events preceding the onset of depression in Asian patients with major depressive disorder. Int. J. Soc. Psychiatry 61 (8), 735–742. doi:10.1177/0020764015577842
Peng, Z., Li, X., Li, J., Dong, Y., Gao, Y., Liao, Y., et al. (2021). Dlg1 knockout inhibits microglial activation and alleviates lipopolysaccharide-induced depression-like behavior in mice. Neurosci. Bull. 37 (12), 1671–1682. doi:10.1007/s12264-021-00765-x
Pike, A. F., Szabò, I., Veerhuis, R., and Bubacco, L. (2022). The potential convergence of NLRP3 inflammasome, potassium, and dopamine mechanisms in Parkinson's disease. NPJ Park. Dis. 8 (1), 32. doi:10.1038/s41531-022-00293-z
Rangaraju, S., Raza, S. A., Pennati, A., Deng, Q., Dammer, E. B., Duong, D., et al. (2017). A systems pharmacology-based approach to identify novel Kv1.3 channel-dependent mechanisms in microglial activation. J. Neuroinflammation 14 (1), 128. doi:10.1186/s12974-017-0906-6
Rogers, J. P., Chesney, E., Oliver, D., Pollak, T. A., McGuire, P., Fusar-Poli, P., et al. (2020). Psychiatric and neuropsychiatric presentations associated with severe coronavirus infections: A systematic review and meta-analysis with comparison to the COVID-19 pandemic. Lancet Psychiatry 7 (7), 611–627. doi:10.1016/s2215-0366(20)30203-0
Schramm, E., and Waisman, A. (2022). Microglia as central protagonists in the chronic stress response. Neurol. Neuroimmunol. Neuroinflamm 9 (6), e200023. doi:10.1212/NXI.0000000000200023
Sierra, A., Paolicelli, R. C., and Kettenmann, H. (2019). Cien anos de Microglia: Milestones in a century of microglial research. Trends Neurosci. 42 (11), 778–792. doi:10.1016/j.tins.2019.09.004
Song, A. Q., Gao, B., Fan, J. J., Zhu, Y. J., Zhou, J., Wang, Y. L., et al. (2020). NLRP1 inflammasome contributes to chronic stress-induced depressive-like behaviors in mice. J. Neuroinflammation 17 (1), 178. doi:10.1186/s12974-020-01848-8
Tejedor, F. J., Bokhari, A., Rogero, O., Gorczyca, M., Zhang, J., Kim, E., et al. (1997). Essential role for dlg in synaptic clustering of Shaker K+ channels in vivo. J. Neurosci. 17 (1), 152–159. doi:10.1523/JNEUROSCI.17-01-00152.1997
Tong, L., Gong, Y., Wang, P., Hu, W., Wang, J., Chen, Z., et al. (2017). Microglia loss contributes to the development of major depression induced by different types of chronic stresses. Neurochem. Res. 42 (10), 2698–2711. doi:10.1007/s11064-017-2270-4
Tynan, R. J., Naicker, S., Hinwood, M., Nalivaiko, E., Buller, K. M., Pow, D. V., et al. (2010). Chronic stress alters the density and morphology of microglia in a subset of stress-responsive brain regions. Brain Behav. Immun. 24 (7), 1058–1068. doi:10.1016/j.bbi.2010.02.001
Umpierre, A. D., and Wu, L. J. (2021). How microglia sense and regulate neuronal activity. Glia 69 (7), 1637–1653. doi:10.1002/glia.23961
Wang, B., Huang, X., Pan, X., Zhang, T., Hou, C., Su, W. J., et al. (2020). Minocycline prevents the depressive-like behavior through inhibiting the release of HMGB1 from microglia and neurons. Brain Behav. Immun. 88, 132–143. doi:10.1016/j.bbi.2020.06.019
Wohleb, E. S., Franklin, T., Iwata, M., and Duman, R. S. (2016). Integrating neuroimmune systems in the neurobiology of depression. Nat. Rev. Neurosci. 17 (8), 497–511. doi:10.1038/nrn.2016.69
Wong, M. L., Inserra, A., Lewis, M. D., Mastronardi, C. A., Leong, L., Choo, J., et al. (2016). Inflammasome signaling affects anxiety- and depressive-like behavior and gut microbiome composition. Mol. Psychiatry 21 (6), 797–805. doi:10.1038/mp.2016.46
Wright-Jin, E. C., and Gutmann, D. H. (2019). Microglia as dynamic cellular mediators of brain function. Trends Mol. Med. 25 (11), 967–979. doi:10.1016/j.molmed.2019.08.013
Yang, L., Zhao, Y., Wang, Y., Liu, L., Zhang, X., Li, B., et al. (2015). The effects of psychological stress on depression. Curr. Neuropharmacol. 13 (4), 494–504. doi:10.2174/1570159x1304150831150507
Yirmiya, R., Rimmerman, N., and Reshef, R. (2015). Depression as a microglial disease. Trends Neurosci. 38 (10), 637–658. doi:10.1016/j.tins.2015.08.001
Yuan, X., Han, S., Manyande, A., Gao, F., Wang, J., Zhang, W., et al. (2023). Spinal voltage-gated potassium channel Kv1.3 contributes to neuropathic pain via the promotion of microglial M1 polarization and activation of the NLRP3 inflammasome. Eur. J. Pain 27 (2), 289–302. doi:10.1002/ejp.2059
Zanin-Zhorov, A., Lin, J., Scher, J., Kumari, S., Blair, D., Hippen, K. L., et al. (2012). Scaffold protein Disc large homolog 1 is required for T-cell receptor-induced activation of regulatory T-cell function. Proc. Natl. Acad. Sci. U. S. A. 109 (5), 1625–1630. doi:10.1073/pnas.1110120109
Keywords: Dlg1, microglia, neuroinflammation, chronic restraint stress, depression
Citation: Li X, Peng Z, Jiang L, Zhang P, Yang P, Yuan Z and Cheng J (2023) Dlg1 deletion in microglia ameliorates chronic restraint stress induced mice depression-like behavior. Front. Pharmacol. 14:1124845. doi: 10.3389/fphar.2023.1124845
Received: 15 December 2022; Accepted: 13 February 2023;
Published: 22 February 2023.
Edited by:
Zhuorong Li, Chinese Academy of Medical Sciences, ChinaReviewed by:
Hongyan Li, Xuanwu Hospital, Capital Medical University, ChinaWenting Wang, Air Force Medical University, China
Copyright © 2023 Li, Peng, Jiang, Zhang, Yang, Yuan and Cheng. This is an open-access article distributed under the terms of the Creative Commons Attribution License (CC BY). The use, distribution or reproduction in other forums is permitted, provided the original author(s) and the copyright owner(s) are credited and that the original publication in this journal is cited, in accordance with accepted academic practice. No use, distribution or reproduction is permitted which does not comply with these terms.
*Correspondence: Zengqiang Yuan, enF5dWFuQGJtaS5hYy5jbg==; Jinbo Cheng, Y2hlbmdfamluYm9AMTI2LmNvbQ==