- 1Clinic of Obstetrics and Gynecology, Jessenius Faculty of Medicine, Comenius University in Bratislava, Martin, Slovakia
- 2Cancer Research Institute, Department of Tumor Immunology, Biomedical Research Center, Slovak Academy of Sciences, Bratislava, Slovakia
- 3Centre for Advanced Material Application, Slovak Academy of Sciences, Bratislava, Slovakia
- 4Department of Pathological Physiology, Jessenius Faculty of Medicine, Comenius University in Bratislava, Martin, Slovakia
- 5Department of Medical Biology, Jessenius Faculty of Medicine, Comenius University in Bratislava, Martin, Slovakia
- 6Museum of Literature in Moravia, Rajhrad, Czech Republic
- 7Department of Applied Ecology, Faculty of Environmental Sciences, Czech University of Life Sciences Prague, Prague, Czech Republic
- 8Department of Physiology and Biophysics, Weill Cornell Medicine-Qatar, Education City, Qatar Foundation, Doha, Qatar
- 9Department of Dermatology, Comenius University in Bratislava, Jessenius Faculty of Medicine in Martin and University Hospital Martin, Martin, Slovakia
- 10Predictive, Preventive, Personalised (3P) Medicine, Department of Radiation Oncology, University Hospital Bonn, Rheinische Friedrich-Wilhelms-Universität Bonn, Bonn, Germany
Cancer causes many deaths worldwide each year, especially due to tumor heterogeneity leading to disease progression and treatment failure. Targeted treatment of heterogeneous population of cells - cancer stem cells is still an issue in protecting affected individuals against associated multidrug resistance and disease progression. Nanotherapeutic agents have the potential to go beyond state-of-the-art approaches in overall cancer management. Specially assembled nanoparticles act as carriers for targeted drug delivery. Several nanodrugs have already been approved by the US Food and Drug Administration (FDA) for treating different cancer types. Phytochemicals isolated from plants demonstrate considerable potential for nanomedical applications in oncology thanks to their antioxidant, anti-inflammatory, anti-proliferative, and other health benefits. Phytochemical-based NPs can enhance anticancer therapeutic effects, improve cellular uptake of therapeutic agents, and mitigate the side effects of toxic anticancer treatments. Per evidence, phytochemical-based NPs can specifically target CSCs decreasing risks of tumor relapse and metastatic disease manifestation. Therefore, this review focuses on current outlook of phytochemical-based NPs and their potential targeting CSCs in cancer research studies and their consideration in the framework of predictive, preventive, and personalized medicine (3PM).
1 Introduction
Cancer is a leading cause of death worldwide. According to GLOBOCAN 2020, cancer estimated 19.3 million new cancer cases and almost 10.0 million cancer deaths in 2020 (Sung et al., 2021). In cancer, the genome instability and mutations are the reason of various changes in organism, including avoiding immune destruction, deregulation of cellular energetics, promotion of inflammation, sustaining proliferative signaling, evading growth suppressors, resisting cell death, enabling replicative immortality, activating angiogenesis, invasion and metastasis that consequently lead to disease progression (Hanahan and Weinberg, 2011). Moreover, disease progression and treatment failure are also commonly caused by tumor heterogeneity. There are two types of tumor heterogeneity: inter-tumor heterogeneity (between cancers from different patients) and intra-tumor heterogeneity (within a single tumor). The second one is characterized by phenotypic diversity through alterations in genetic or epigenetic abnormalities, apoptosis, tumor growth, and other hallmarks of cancer (Prasetyanti and Medema, 2017). Furthermore, many tumors contain a heterogeneous population of cells, including cancer stem cells (CSCs) that differentiate into cells to initiate tumor formation (Dick, 2008). CSCs also exert self-renewal and differentiation properties that often lead to the ineffectiveness of conventional therapy to eliminate CSCs. Consequently, the failure in therapy due to treatment resistance often causes tumor relapse and metastases (Lathia et al., 2020; Babaei et al., 2021). Treatment resistance or multi-drug resistance (MDR) describe the resistance to various unrelated therapies, including radiotherapy, chemotherapy, hypoxia, and immunotherapy. Besides, MDR occurs in up to 70% of cancers at the time of diagnosis (Riganti and Contino, 2019; Li et al., 2021a). Moreover, treatment resistance is not associated only with CSCs but MDR exerts multi-factorial character caused by epithelial-mesenchymal transition (EMT), acquired mutations, drug efflux through ABC transporters, drug efflux mediated by extracellular vesicles, drug-loaded lysosomes undergoing exocytosis, deregulation of key signaling pathways, deregulation of cell death mechanisms, activation of DNA damage response, and epigenetic alterations (Assaraf et al., 2019; Li et al., 2021a).Therefore, developing novel potential drugs to overcome the MDR of CSCs is crucial.
Plant-based foods are rich in various phytochemicals that exert many anticancer activities, including proapoptotic, anti-angiogenic, anti-metastatic, anti-inflammatory, antioxidant, or anti-genotoxic effects. However, the therapeutic efficacy can be low due to their low oral bioavailability and poor aqueous solubility (Koklesova et al., 2020a). On the other hand, an encapsulation of phytochemicals into nanocarriers can represent a potential drug delivery system in cancer management. Specific drug delivery into cancer cells and their release at the targeted site can enhance their antineoplastic properties (Kumar et al., 2022). Increased anticancer efficacy can be also achieved by combining various phytochemicals with conventional therapy or other NPs that can be activated through hyperthermia or photothermia (Sun et al., 2015; Li et al., 2019; Jose et al., 2020). Additionally, specifically designed phytochemical-based nanodrug can target CSCs and eliminate them, potentially reversing resistance to therapy or preventing migration and metastasis (Kuo et al., 2019; Yang et al., 2020; Gu et al., 2021).
Nanotechnology is widely used in different areas, including electronics, cosmetics, and diagnostic and therapeutic medical applications (Najahi-Missaoui et al., 2020). The field of nanotechnology in medicine, known as nanomedicine, has multiplied during the last few decades. Nanomedicine includes the use of nano-sized (1–1,000 nm) particles (NPs) as potential therapeutic drugs for various diseases (Missaoui et al., 2018; Tabassum et al., 2018). In cancer research, specifically designed NPs with various sizes and properties represent a new way of delivery systems to targeted delivery into tumor sites without harming the surrounding healthy tissues (Patra et al., 2018). Therefore, this review focuses on the current outlook on phytochemical-based nanodrugs and their potential targeting CSCs in cancer research studies.
2 Nanoparticles
Nanomedicine is represented by small-sized (nanoscale, 1–1,000 nm) drug delivery systems that specifically deliver drug molecules to pathologic sites and accumulate at the target site (Gwinn and Vallyathan, 2006; Tabassum et al., 2018). NPs can also have various shapes, including spherical, rod, oval, cubic, triangular, star, needle, octahedral, flower, cluster, cylinder, branched, platelets, hexagonal, pentagonal, and others (Hamida et al., 2020). NPs can be divided into six groups according to their composition of inner and outer core (Najahi-Missaoui et al., 2020). Moreover, the surface of NPs consists of various ligands with the ability to target damaged (e.g., cancer) cells thanks to their specific selective binding to the overexpressed receptors (Sun et al., 2014). Furthermore, NPs are commonly coated by various agents for better biocompatibility and biodegradability (Singh, 2010). Figure 1 illustrates the schematic structure of NP consisting of an inner core, outer core, and ligands on the outer surface.
NPs can be synthesized in two ways: by bottom-up strategy or top-down strategies, as illustrated in Figure 2. A bottom-up strategy is based on nucleating atomic-sized materials into the eventual NPs. The top-down strategy represents physical degradation of bulk material producing smaller molecules and NPs (Nagarajan, 2008).
3 Tumor cells targeting by nanoparticles
Nanosize of NPs can overcome various biological barriers within the body, such as entering the cell and various cellular compartments (nucleus) (De Jong and Borm, 2008). Therefore, several NPs exhibit the potential for their clinical application; however, their usability depends on various factors such as size, shape, surface functionality, low or no toxicity of the nanocarrier, physical and chemical properties, solubility, stability, drug loading efficiency, drug release, and potential distribution to different organs (Gwinn and Vallyathan, 2006; Puri et al., 2009; Singh, 2010). NPs can act as tumor detector that detects a diseased/cancer site where it accumulates and specifically triggers the therapeutic activity of a circulating drug carrier. Specific targeting of NPs into cancer cells is determined predominantly by ligands on their surface (Zwicke et al., 2012; Yang et al., 2022). At cancer sites, several nanodrugs act as DNA-damaging, immunostimulant, microtubule-inhibiting, or hormone agonist agents that trigger various anticancer pathways (FramptonMifamurtide, 2010; Barenholz, 2012; Gawde et al., 2018; Fu et al., 2020). In hyperthermia events (to 40°C–45°C), cells are susceptible to various forms of damage. Hyperthermia activates various immunological responses, enhances tumor blood flow and oxygenation through higher permeability and vascular perfusion, decreases oxygen consumption, and increases tissue oxygenation by a shift toward anaerobic metabolism. Every mentioned mechanism leads to the alteration of the extracellular microenvironment (Chatterjee et al., 2011). Cancer cells are more thermosensitive than normal healthy cells. Various types of nanostructure can be used for hyperthermia activity, including silica-gold and gold nanoshells, gold nanorods, core-shell gold NPs, solid gold NPs, and carbon nanotubes (Cherukuri et al., 2010; Kaur et al., 2016). One of other hyperthermia event, magnetic hyperthermia can convert the magnetic energy of magnetic NPs into heat energy in the magnetic field. Therefore, magnetic NPs (e.g., metal NPs) can target and kill cancer cells with low toxicity to normal cells. Moreover, a combination of NPs-based magnetic hyperthermia therapy and radiotherapy or chemotherapy can achieve higher thermosensitivity of cancer cells (Maeda, 2001; Jose et al., 2020). Photothermal therapy represents a minimally invasive procedure for cancer treatment. Photo-induced hyperthermia that converts light to heat can be achieved by pulsed and continuous waves or pulsed near-infrared laser irradiation in appropriate dosage (Sahu et al., 2018). For example, gold nanostars presented by star-shaped geometry show therapeutic potential in cancer. Their shape increases light absorption leading to high photon/light-to-heat conversion efficiency through the plasmonic effect. Subsequently, increased temperature causes cell damage at the tumor site (Liu et al., 2018a).
3.1 Nanodrugs in cancer therapy
The US Food and Drug Administration (FDA) approved several nanodrugs for treating various cancer types. FDA-approved nanodrugs used in cancer therapy have different specific targets (e.g., DNA damage, immunostimulation, microtubule, protein synthesis, or hormone inhibition) or formulations. Some of them consist of metallic NPs (Aurimmune®, AuNPs®), polymer-drug conjugates (Eligard®, SMANCS), lipid-based nanoformulations (Marqibo®, Doxil®), recombinant virus (Gendicine®), drug targeted antibody (Kadcyla®), or herbal NPs (nanoformulated curcumin) (Alphandéry et al., 2015). In 1995, the first FDA-approved nanodrug was Doxil®, polyethylene glycol (PEG)ylated liposomal doxorubicin, indicated for the treatment of metastatic ovarian cancer and AIDS-related Kaposi’s sarcoma (Barenholz, 2012). Table 1 represents an overview of some FDA-approved nanodrugs used in cancer therapies.
In addition to the above-mentioned mechanisms of action, the accumulation of NPs at the diseased site causes mitochondria damage and dysfunction, upregulation of apoptotic factors, DNA fragmentation, membrane damage of cancer cells, oxidation of enzymes and proteins, protein denaturation, disassembly of ribosomes, generation of reactive oxygen species (ROS), interruption of electron transport (Roy et al., 2019; Barabadi et al., 2020; Chaudhary et al., 2020). Moreover, the accumulation of NPs at the diseased site demonstrates the diagnostic potential because NPs can act as potential contrast agents for X-ray (gold NPs), magnetic resonance imaging (MRI) (magnetic NPs), computed tomography (CT) and MRI (hybrid NPs from iron oxide and gold) (Smith et al., 2012). Various NPs have been evaluated as potential contrast agents in cancer diagnostics; however, their clinical applications are limited, especially due to their insufficient assessment of biodegradation, elimination and toxicity (Baetke et al., 2015).
Furthermore, thanks to recent FDA approvals of lipid NP-loaded mRNA vaccines for the prevention of COVID-19, the lipid NP-based mRNA vaccines could represent promising way also in cancer therapy in near future (Miao et al., 2021). For example, lipid NP-based mRNA vaccine known as BI1361849 (CV9202) combined with local radiation evaluated in Ib clinical trial (NCT01915524) in patients (n = 26) with stage IV of non-small cell lung cancer. In the majority of patients, the vaccine increased CD4+ and/or CD8+ T cells and BI1361849 antigen-specific immune responses (Papachristofilou et al., 2019). Similarly, enhanced immune responses in patients with stage IIIB/IV non-small cell lung cancer were observed after vaccine BI1361849 in combination with a checkpoint inhibitor, anti-CTLA-4 (tremlimumab) and anti-PD-L1 (duvalumab) in phase I/II study (NCT03164772) (Sebastian et al., 2019). In this way, other mRNA vaccines based on lipid NPs revealed potential in cancer immunotherapy of solid tumors (Huang et al., 2022).
3.2 Phytochemical-based nanodrugs
Phytochemicals are biologically active compounds commonly found in plant-based food such as fruits, vegetables, grains, or nuts, exerting anticancer, antioxidant, anti-inflammatory, immunomodulatory, and other beneficial properties (Cencic and Chingwaru, 2010; Koklesova et al., 2020b). Phytochemicals are classified into five basic groups: phenolics, carotenoids, alkaloids, organosulfur, and nitrogen-containing compounds (Liu, 2004). Figure 3 describes the classification of phytochemicals into basic groups and subgroups.
Several preclinical and clinical studies demonstrate the anticancer potential of phytochemicals alone or their combination or combination with other drugs in preventive and therapeutic cancer management (Abotaleb et al., 2018; Koklesova et al., 2020b; Samec et al., 2020). Therefore, phytochemicals are suitable for nanomedicine, specifically for conjugating with various NPs or for encapsulation into nanocarriers. These nanophyto-formulations demonstrate various potential health benefits in infectious, cardiovascular, and neurodegenerative diseases as well as cancer (Nazer et al., 2020; Hesari et al., 2021; Bhattacharya et al., 2022; Melim et al., 2022).
Despite several FDA-approved nanodrugs for cancer therapy, for medical progress is still important to develop novel drugs or their alterations that could be more sensitive and effective with less side effects or specifically stratified for patients. After all, the aim of nanotechnology is enhancing the bioavailability, solubility, absorption, and controlled-release of drugs (Patra et al., 2018). Natural products represent the low cost, low resistance, less toxic, and effective compounds (Dhupal and Chowdhury, 2020). Moreover, phytochemical-based nanodrugs can overcome the chemotherapeutic resistance of CSCs or can resensitize them to therapy (Chan et al., 2018; Shen et al., 2021).
3.3 Phytochemical-based nanodrugs in cancer research
Various preclinical and clinical studies focused on the phytochemicals conjugated NPs, especially in cancer research.
3.3.1 Gold NPs
Resveratrol-conjugated gold nanoparticles (Res-AuNPs) exerted synergistic anti-tumor effects in human breast, pancreatic, and prostate cancer cells. 3× Res-AuNPs and 3× Res-GA-AuNPs revealed cytotoxic effects, enhanced bioavalability and cellular uptake when compared with 1× Res-AuNPs and 1× Res-GA-AuNPs. In conclusion, Res-AuNPs enhanced phytochemical drug carrier capabilities as a potential application for cancer therapy (Thipe et al., 2019). Furthermore, Res-AuNPs and Resveratrol-nanoemulsion inhibited the growth of BxPC-3pancreatic cancer cells and altered cell cycle regulation and apoptotic events (Inbaraj et al., 2021).
Multifunctional and spherical 20 nm AuNPs conjugated with withanolide-A, a phytocompound from Withania somnifera, demonstrated higher antiproliferative effects when compared with withanolide-A alone in the SKBR-3 breast cancer cell line (Tabassam et al., 2020).
Mango peel phytochemicals coated AuNPs and mangiferin, the most abundant phytochemical in mango peel, conjugated AuNPs were combined with plant phytochemicals from Amalaki (Emblica officinalis), Amra (Mangifera indica), Haridra (Curcumin longa), Babbula (Acacia nilotica), Yashtimadhu (Glycyrrhiza glabra) to create Nano Swarna Bhasma (NSB) drug. NSB drug revealed selective toxicity to MDA-MB-231 cancer cells, reduced tumor volume in MDA-MB-231 mice xenografts. Moreover, in a pilot clinical study, breast cancer patients demonstrated a partial response to the treatment without any disease progression. In summary, NSB therapy in patients with metastatic breast cancer exerted clinical benefits (Khoobchandani et al., 2020).
Silibinin-conjugated gold nanoparticles (Sb-AuNPs) effectively induced in vitro cell death against A549 lung cancer cells with long-term stability. The results showed that the efficacy of Sb improved 4–5 times in inhibiting the cancer cells after the conjugation with AuNPs (Ravi et al., 2022).
3.3.2 Solid lipid NPs
The combination of curcumin and resveratrol solid lipid nanoparticles (Cur-Res-SLNs) inhibited cell migration of B16F10 melanoma cells. Moreover, Cur-Res-SLNs or Cur-Res solution (3:1) revealed strong synergism through the cell proliferation inhibition of SK-MEL-28 melanoma cells (Palliyage et al., 2021).
Moreover, erlotinib and quercetin-loaded solid lipid NPs (EQNPs) showed anticancer effects through increased cellular uptake of NPs.Moreover, EQNPs sensitized and enhanced the induction of apoptosis in Ertb-resistant A549/ER cells (Ganthala et al., 2022).
3.3.3 Chitosan NPs
Quercetin encapsulated chitosan functionalized copper oxide nanoparticle (CuO-ChNPs-Q) demonstrated potent anticancer activity in vitro and in vivo. CuO-ChNPs-Q demonstrated cytotoxic effect against liver, breast, and colorectal cancer cells but safety of CuO-ChNPs-Q on WI38 human normal lung fibroblasts. In vivo study, CuO-ChNPs-Q reduced the breast tumor volume and proliferation, arrested the cell cycle, and induced apoptosis in DMBA-induced female rats (Elsayed et al., 2021).
In another investigation, a hydrogel nanocomposite of chitosan, halloysite, and graphitic-carbon nitride (Ch-HNT-gC3N4) was prepared and loaded by quercetin using an emulsification process to achieve quercetin sustained-release. The prepared drug-loaded delivery system exhibited excellent encapsulation and loading effectiveness, cytotoxic effect, and enhanced apoptotic activity in MCF-7 breast cancer cells (Sabzini et al., 2022).
In a combined in vitro and in vivo experiment, Zhou and others (2022) developed a new nanocarrier called chitosan-gelatin-epigallocatechin-3-gallate (Ch-G-EGCG) for systemic si-TMEM44-AS1 delivery that can silence TMEM44-AS1 gene expression in gastric cancer cells and boost 5-FU sensitivity in gastric cancer cells (Zhou et al., 2022).
3.3.4 Poly (lactic-co-glycolic acid) NPs
In A549 and H1299 lung cancer cells, poly (lactic-co-glycolic acid) NPs loaded with epigallocatechin-3-gallate (PLGA-EGCG) demonstrated antiproliferative andapoptotic events. Furthermore, PLGA-EGCG-NPs decreased tumor volume and weight in the patient-derived xenograft model (Zhang et al., 2020).
Another study revealed that galactose-tailored poly (lactic-co-glycolic acid) NPs loaded with apigenin (API-GAL-NPs) exerted higher cellular internalization, cytotoxic and apoptotic effects in HepG2 human liver hepatocellular carcinoma cancer cells. In the diethylnitrosamine-induced hepatocellular carcinoma rat model, API-GAL-NPs reduced nodule formation and expression of matrix metalloproteinases and triggered apoptosis in the liver (Ganguly et al., 2021).
3.3.5 Iron NPs
Another research group fabricated quercetin-ferrum nanoparticles (Q-F NPs) to improve photothermal therapy (PTT) by modulating the tumor immunosuppressive microenvironment. The prepared nano-photosensitizer induced cancer cell destruction and tumor antigen release, which in turn, stimulated dendritic cell maturation and T-cell activation. Furthermore, the Q-F NPs-PTT-treated mice displayed notably extended survival time and potent anti-tumor immune memory to control tumor metastasis and recurrence (Li et al., 2022).
3.3.6 Folic acid and bovine serum albumin NPs
Difluorinated curcumin (CDF), a synthetic curcumin analog, encapsulated in folic acid and bovine serum albumin NPs (FA-BSA-CDF) and paclitaxel (PTX) encapsulated in folic acid and bovine serum albumin (FA-BSA-PTX) showed anticancer effect through targeting folate receptor and induction of apoptosis in folate overexpressing ovarian and cervical cancers. Separately treatment with either FA-BSA-PTX or FA-BSA-CDF decreased cell viability of SKOV-3 ovarian cancer and HeLa cervical cancer cells Furthermore, the combination of FA-BSA-PTX and FA-BSA-CDF revealed synergism and enhanced cancer cell-killing effect (Gawde et al., 2018).
3.3.7 Zinc oxide NPs
Another in vitro investigation presented quercetin-functionalized wurtzite-type zinc oxide (ZnO-Q) NPs with potent anticancer action against human ovarian cancer cells by inducing intercellular oxidative stress and depolarization of the mitochondrial membrane. Besides, the prepared formulation generated late apoptosis via activating the intrinsic apoptosis signaling pathway in PA-1 cells (Ramalingam et al., 2022).
3.3.8 Silica NPs
Resveratrol encapsulation into mesoporous silica nanoparticles (Res-MSNs) promoted its amorphization and enhanced drug release. Moreover, Res-MSNs reduced cell viability of human A375 and MNT-1 melanoma cells; however, with higher sensitivity in the amelanotic A375 cell line (Marinheiro et al., 2021).
3.3.9 Poly (Glycerol Sebacate) NPs
In vitro study, curcumin-loaded nanoparticles of Poly (Glycerol Sebacate) (Cur-PGS-NPs) demonstrated cytotoxicity, altered cell cycle, and triggered apoptosis in human cervical cancer cells (Massironi et al., 2022).
3.3.10 Micelles
Curcumin encapsulated into monomethyl PEG-polylactide (Cur-MPEG-PLA) micelles demonstrated anticancer potential for melanoma treatment in vitro and in vivo. Cur-MPEG-PLA micelles inhibited proliferation, induced apoptosis, and enhanced cellular uptake in B16 and A375 melanoma cells. Moreover, in mice bearing B16 or A375 subcutaneous melanoma, treatment by Cur-MPEG-PLA micelles decreased tumor volumes and inhibited neovascularization in tumor tissues (Wang et al., 2017).
At the nanoscale, dual-targeted diosmin and berberine hydrochloride-loaded casein micelles (DSN/BRB-CAS MCs) revealed cytotoxicity in HepG2 cells and hepatocellular carcinoma-bearing mice. These micelles decreased cell necrosis, inhibited tumor proliferation, angiogenesis, inflammation, and induced apoptosis (Abdelmoneem et al., 2018).
3.3.11 Quantum dots
A phytochemical from some cruciferous vegetables called allyl isothiocyanate conjugated with silicon quantum dots (AITC- SiQDs) decreased cell viability in Caco-2 cells Moreover, AITC-SiQDs treatment caused a significant increase in ROS, induced DNA damage, and inhibited cell migration and tube formation in the 3D (HUVECs and MII perivascular cells) co-culture model (Liu et al., 2018b).
3.3.12 Green-synthetized NPs and carrier-free NPs
The green-synthesized selenium NPs using apigenin (SeNPs-API) reduced cell proliferation and viability in MCF-7 breast cancer cells. Moreover, the treatment with SeNPs-API increased oxidative stress and ROS production, and triggered apoptosis through modulation of pro-apoptotic and anti-apoptotic markers (Al-Otaibi et al., 2022).
Carrier-free nanodrug (ASP-UA NPs) based on hydrophobic interactions consisting of ursolic acid, a pentacyclic triterpenoid, and aspirin, a non-steroidal anti-inflammatory drug, demonstrated anticancer effects. ASP-UA NPs significantly decreased cell viability in melanoma, cervical, liver, and breast cancer cells. In vivo metastasis assay revealed that ASP-UA NPs inhibited lung metastasis in mice injected with H22 hepatocellular carcinoma mouse cells (Li et al., 2018).
Table 2 describes the detailed anticancer effects of above-mentioned phytochemical-based nanodrugs. Interestingly, more than 300 clinical studies focused on nanotherapy in cancer research (clinicaltrial.gov); however, there is a lack of studies explicitly focused on phytochemical-based nanodrugs.
3.4 Phytochemical-based nanodrugs targeting CSCs
CSCs, a subgroup of cells within the tumor, often cause tumors to recur and progress, consequently contributing to cancer cells’ migration and metastasis. CSCs are also associated with heterogeneously demonstrated resistance (Lathia et al., 2020; Babaei et al., 2021). To overcome the drug resistance of CSCs, combining two or more chemotherapeutic agents or multiple treatment modalities represents the potential anticancer strategy. One of the main strategies for overcoming or eliminating the resistance of CSCs to several drugs is represented by NPs-based drugs (Wang et al., 2015; Wang and He, 2018). The below studies focused on specifically designed phytochemical-based nanodrugs as promising tools against CSCs.
ALDH enzyme that converts aldehydes into carboxylic acids is highly expressed in hematopoietic stem and progenitor cells. Curcumin-loaded chitosan-PLGA-NPs modified with sialic acid and with anti-aldehyde dehydrogenase (Cur-Ch-PLGA-SA-anti-ALDH NPs) revealed anticancer potential against the proliferation of glioblastoma cells and brain CSCs. Interestingly, sialic acid on the surface of NPs helped permeate the blood-brain barrier using N-acetylglucosamine in human brain CSCs and U87MG glioblastoma cells (Kuo et al., 2019). Furthermore, CD123 is expressed explicitly in leukemic CSCs. Specifically designed NPs anti-CD123-Curcumin NPs (anti-CD123-Cur-NPs) increased cellular uptake and induced higher apoptosis in KG-1a human acute myeloid leukemia cells when compared with Cur-NPs, suggesting that anti-CD123-Cur-NPs successfully targeted leukemic CSCs (Nirachonkul et al., 2021). Impressively, a co-delivery system consisting of hyaluronic acid lipoid on the surface of hydrophobic PLGA NPs with paclitaxel as a chemotherapy agent and curcumin as the selective inhibitor of CSCs (HA-PLGA-PTX-Cur NPs) targeted breast CSCs through the interaction between hyaluronic acid lipid and the CD44 receptor on the membrane of breast CSCs leading to anticancer effects via reduced breast CSC population and inhibited their mammosphere formation and migration. Moreover, treatment with mentioned co-delivery system reduced the expression of ALDH1 in MCF7 mammospheres. In MCF7 mice xenografts, the co-delivery system enhanced anticancer efficacy through synergistic inhibition of the growth of non-breast CSCs and breast CSCs (Yang et al., 2017). Another study showed that curcumin combined with glucose nanogold particles (Cur-Glu-AuNPs) reduced radiotherapy resistance in targeted breast CSCs. In MCF-7 and MDA-MB-231 mammospheres, treatment with Cur-Glu-AuNPs was also associated with induced apoptosis followed by G0/G1 phase cell cycle arrest, increased ROS level, and reduced hypoxia-inducible factor-1 alpha (HIF-1α) and heat shock protein 90 (HSP90) expressions (Yang et al., 2020). Furthermore, 3,4-difluorobenzylidene curcumin loaded hyaluronic acid-copoly (styrene maleic acid) (DFBCur-HA-SMA) nanomicelles demonstrated anticancer properties against MiaPaCa-2 and AsPC-1 human pancreatic cancer cells. Treatment improved cellular internalization of nanomicelles in CD44+/CD133+/EpCAM + pancreatic CSCs compared to CD44-/CD133-/EpCAM- CSCs. Moreover, DFBCur-HA-SMA nanomicelles reduced the expression of CD44 and NF-κB, leading to anti-proliferative and anti-invasive effects (Kesharwani et al., 2015). Moreover, curcumin combined with naringenin loaded dextran-coated magnetic nanoparticles (Cur-Nar-D-MNPs) inhibited cell proliferation and induced apoptosis through ROS production, increased P53 and P21, and decreased TNFα and CD44 in MCF-7 human breast cancer cells. Furthermore, CUR-NAR-D-MNPs reduced the tumor volume and caused the cell cycle arrest in DMBA-induced mammary tumor in rats (Askar et al., 2021). Additionally, GANT61, a hexahydro pyrimidine derivative, can target CSCs of different types of human cancers through the GLI1 protein of the Hedgehog pathway. Encapsulated GANT61 and curcumin in PLGA NPs (GANT1-Cur-PLGA NPs) reduced cell viability, proliferation, induced autophagy by the formation of autophagosomes and autophagic flux, and triggered apoptosis in MCF-7 breast adenocarcinoma cell line. Treatment also reduced the nuclear expression of GLI1 and EGFR expression on the cellular membrane, cytoplasm, and the nucleus. Moreover, GANT1-Cur-PLGA NPs inhibited the downstream target proteins Bmi-1 and PI3K of Hedgehog and EGFR pathways (Borah et al., 2020).
Resveratrol NPs decreased metastatic markers CD133, ALDH1, CXCR4 in CSCs-enriched oral cancer cells leading to a reduction in the invasion, proliferation, and growth of CSCs. Moreover, a detailed study on fertilized chick embryos and mice xenografts confirmed that Resveratrol NPs depleted nitric oxide production and decreased angiogenesis and metastasis (Pradhan et al., 2021).
The combination of docetaxel- and sulforaphane-loaded PLGA-hyaluronic acid based NPs (DTX-SFN-PLGA-HA NPs) inhibited breast CSCs through decreased expression of cyclin D1 and β-catenin in MCF-7 breast cancer cells but was less effective in MCF-7 mammospheres with an epithelial-specific antigen (ESA)+CD44+CD24− phenotype. Moreover, the treatment exerted substantial anticancer effects by inhibiting the self-renewal ability of breast CSCs in MCF-7 mice xenografts (Huang et al., 2016). Another study showed that sulforaphane-loaded the mineralized hyaluronic acid-SS-tetradecyl NPs (SFN/M-HA-SS-TA) inhibited breast CSCs through their specific CD44+ targeting and reduced CD44 and CD133 expression, expression of polycomb complex protein involved in the self-renewal of breast CSCs (Bmi1), and breast CSC-like properties, including tumor growth, invasiveness, and self-renewal in MDA-MB-231, Hs578t, and MCF7 cells and MDA-MB-231 mice xenografts (Gu et al., 2021).
Nanoliposomal quercetin combined with CD133 antiserum targeted CD44 and CD133 and decreased expression of NF-κBp65, histone deacetylase 1 (HDAC1), and cyclin D1, increased the expression of caspase-3 and E-cadherin in Eca109/9706 esophageal carcinoma cells (Zheng et al., 2014).
Encapsulated icariin and curcumin in polymer oligomeric hyaluronic acid-hydrazone bond-folic acid-biotin micelles targeted MCF-7 cells and breast CSCs through CD44, folic acid, and/or biotin and inhibited cancer cell invasion (Liu et al., 2019).
Sun et al. (2015) showed that all-trans-retinoic acid and doxorubicin NPs (ATRA-DOX-NPs) could simultaneously deliver the drug to both non-CSCs and breast CSCs to differentiate. ATRA NPs caused CSCs to differentiate into non-CSCs through reduced self-renewal capacity. Treatment also increased sensitivity to chemotherapy (DOX NPs). Therefore, combination therapy consisting of ATRA-DOX-NPs enhanced anticancer properties. NPs increased ATRA and DOX cellular uptake in ALDHhi population MDA-MB-231 mammosphere cells and inhibited the cancer-initiating activity of CSCs. Moreover, ATRA-DOX-NPs decreased the expression of stemness-associated genes Nanog, Sox2, and Oct4.
Table 3 summarizes the preclinical evidence of phytochemical-based nanodrugs with potential targeting CSCs. Several studies revealedthe anticancer properties of phytochemical-based nanodrugs; however, only limited studies described their potential in the specific targeting of CSCs. Therefore, a more detailed molecular analysis of their anticancer effects, including CSCs, should be used that can reverse resistance to therapy or prevent migration and metastasis. Figure 4 illustrated the role of phytochemical-based nanodrugs in targeting CSCs.
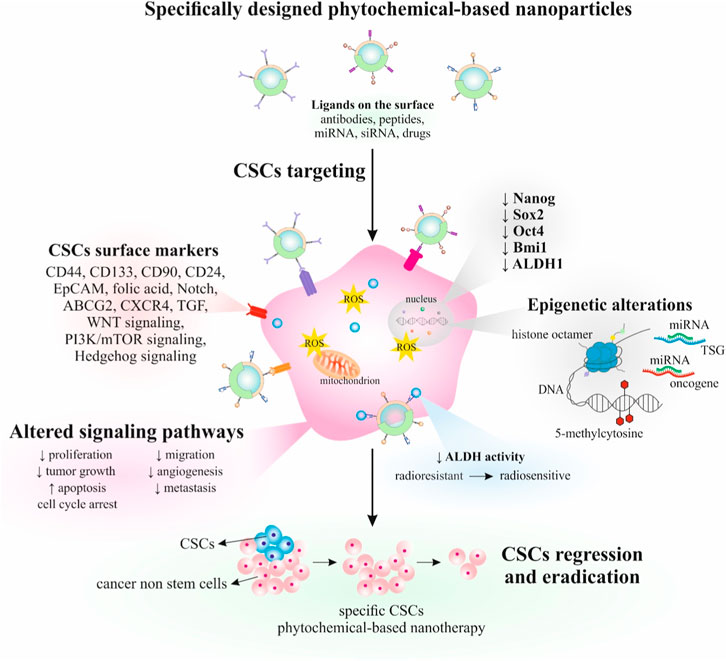
FIGURE 4. Targeting CSCs by phytochemical-based nanodrugs. Abbreviations: CSCs, cancer stem cells, TSG, tumor suppressor gene, ALDH1, aldehyde dehydrogenase 1, ↓ decreased/reduced, ↑, increased/enhanced.
4 Benefits or risks of nanomedicine
In cancer therapies by various NPs, it is crucial to evaluate their safety, potential accumulation in non-targeted sites, clearance, excretion from the body, and others that can potentially lead to life-threatening complications (De Jong and Borm, 2008; Vinluan and Zheng, 2015).
Nanotechnology influences pharmacokinetics that can improve phytochemicals’ stability and solubility and enhance their cellular uptake at the targeted site (Vimala and Kannan, 2021). Nanotechnology also offers specific drug delivery in cancer treatment that can help overcome limitations or side effects of current cancer therapies and reduce multidrug resistance, consequently improving patients’ quality of life and survival (Gavas et al., 2021). Furthermore, phytochemical-based nanodrugs can overcome the chemotherapeutic resistance of CSCs or have the ability to resensitize them to therapy (Shen et al., 2021). Specifically designed NPs deliver drugs into cancer cells and release them only at targeted sites without damaging healthy tissues around the tumor. Moreover, this precise targeting can enhance the therapeutic efficacy of drugs in cancer cells (Mitchell et al., 2021). Furthermore, the enhanced therapeutic efficacy of NPs in cancer treatment can be achieved by hyperthermia, magnetic hyperthermia, or light-mediated phototermia (Chatterjee et al., 2011; Li et al., 2019; Vilas-Boas et al., 2020). Additionally, green nanotechnology (phytoformulations), also known as green or eco-friendly technology, can help reduce energy and fuel use to contribute to environmental sustainability without harming the environment or human health (Verma et al., 2019).
Despite mentioned beneficial properties of NPs, the carrier systems can impose risks to the patients (De Jong and Borm, 2008). Due to the ability to pass some biological barriers, NPs can exert life-threatening toxic effects, especially on essential organs, including the brain, liver, kidney, and others. For example, the accumulation of NPs in reproductive organs can damage the testis, epididymis, ovary, and uterus cells, subsequently leading to reproductive organ dysfunction (Wang et al., 2018). Among other limitations of NPs is clearance by the immune systems or impaired diffusion in the tissue microenvironment (Busatto et al., 2019). Another limitation can be the excretion of NPs from the body. Some NPs cannot be excreted and remain in the organism; however, it usually remains unclear how long NPs remain in the body and what can cause their long-term action (Fischer and ChanNanotoxicity, 2007).
In conclusion, it is crucial to evaluate and realize whether the beneficial properties or risks of using NPs predominate and which are more beneficial to the patient’s treatment.
5 Conclusion and outlook in the framework of 3P medicine
Phytochemicals isolated from plants demonstrate the huge potential for nanomedical applications in oncology thanks to their antioxidant, anti-inflammatory, anti-proliferative, and other health benefits. Phytochemical-based NPs can enhance anticancer therapeutic effects, improve cellular uptake of therapeutic agents, and mitigate the side effects of toxic anticancer treatments. Per evidence, phytochemical-based NPs can specifically target CSCs decreasing risks of tumor relapse and metastatic disease manifestation.
A particular value of phytochemical-based nanodrugs’ implementation is considered in the framework 3PM. The authors have presented related concepts in a series of publications (Kubatka et al., 2021; Link et al., 2021; Liskova et al., 2021; Mazurakova et al., 2022a). Personalized medicine could represent a promising way in cancer therapy through the achievement of the most effective treatment to the individual patient (Yaari et al., 2016; Mitchell et al., 2021). NPs are predicted to be the future of cancer diagnostics, medical imaging, and precise drug delivery; however, it is still important to improve their efficacy or minimize their toxicity and side effects (Li et al., 2021b). Furthermore, several studies also focused on nanotechnological cancer research aimed to cancer prevention (Zhang et al., 2016; Khan et al., 2021; Neelakandan et al., 2022), prediction (Gobbo et al., 2015; Norouzi, 2020; Jeon et al., 2022; Sousa-Junior et al., 2022), or personalized medicine (Benedetto et al., 2015; Yaari et al., 2016). The key points of 3PM are healthcare cost-efficacy and affected individuals’ life quality (Ellinger et al., 2022). Targeted treatment has to be adapted to the individualized patient profile in primary (protection against initial cancer development), secondary (protection against potential metastatic disease development), and tertiary care (towards cascading complications) (Golubnitschaja et al., 2021; Ellinger et al., 2022). To this end, advanced primary care of sub-optimal health conditions plays a pivotal role in protecting affected individuals from the heath-to-disease transition (Wang et al., 2021); principles of 3PM medicine have been recognized by WHO as an advanced approach in the area (Wang et al., 2021). Therefore, specific designed NPs tailored to patients can represent preventive and therapeutic potential in cancer management.
Except for nuclear gene mutations, mitochondria can play also a pivotal role in cancer development and progression. Mitochondria control wide range of cellular functions, including proliferation, apoptosis, signaling events, and cell homeostasis. Alterations in mtDNA copy number, mitochondrial enzymatic activities, or bioenergetic pathways are connected to worse mitochondrial health (Koklesova et al., 2022). Phytochemical-based nanodrugs can be promising agents for the maintenance of mitochondrial health and mitigation of mitochondrial impairments in innovative biomedical research and healthcare (Milane et al., 2015; Tan et al., 2019; Ashrafizadeh et al., 2020; Koklesova et al., 2021; Mazurakova et al., 2022b; Chen et al., 2022; Koklesova et al., 2022).
Author contributions
The manuscript was drafted by LK, AM, MS, KB, SH, MŠ, TH and critically revised by PK, JJ, DB, and DC Figures were prepared by LK and the tables were created by AM and MS Connection with 3PM was prepared by OG Skilled assistance and supervised overall preparation of the manuscript was provided by PK and MP All authors have read and approved final version of the manuscript.
Funding
The present study was supported by the Scientific Grant Agency of the Ministry of Education, Science, Research and Sport of the Slovak Republic (Bratislava, Slovak Republic; grant no. VEGA 1/0045/23) and by the LISPER project (grant Nr. 313011V446) in bilateral agreement with the European Association for Predictive, Preventive and Personalised Medicine, EPMA, Brussels.
Conflict of interest
The authors declare that the research was conducted in the absence of any commercial or financial relationships that could be construed as a potential conflict of interest.
Publisher’s note
All claims expressed in this article are solely those of the authors and do not necessarily represent those of their affiliated organizations, or those of the publisher, the editors and the reviewers. Any product that may be evaluated in this article, or claim that may be made by its manufacturer, is not guaranteed or endorsed by the publisher.
References
Abdelmoneem, M. A., Mahmoud, M., Zaky, A., Helmy, M. W., Sallam, M., Fang, J.-Y., et al. (2018). Dual-targeted casein micelles as green nanomedicine for synergistic phytotherapy of hepatocellular carcinoma. J. Control Release 287, 78–93. doi:10.1016/j.jconrel.2018.08.026
Abe, S., and Otsuki, M. (2002). Styrene maleic acid neocarzinostatin treatment for hepatocellular carcinoma. Curr. Med. Chem. Anticancer Agents 2, 715–726. doi:10.2174/1568011023353679
Abotaleb, M., Samuel, S., Varghese, E., Varghese, S., Kubatka, P., Liskova, A., et al. (2018). Flavonoids in cancer and apoptosis. Cancers 11, 28. doi:10.3390/cancers11010028
Al-Otaibi, A. M., Al-Gebaly, A. S., Almeer, R., Albasher, G., Al-Qahtani, W. S., and Abdel Moneim, A. E. (2022). Potential of green-synthesized selenium nanoparticles using apigenin in human breast cancer MCF-7 cells. Environ. Sci. Pollut. Res. Int. 29, 47539–47548. doi:10.1007/s11356-022-19166-2
Alphandéry, E., Grand-Dewyse, P., Lefèvre, R., Mandawala, C., and Durand-Dubief, M. (2015). Cancer therapy using nanoformulated substances: Scientific, regulatory and financial aspects. Expert Rev. Anticancer Ther. 15, 1233–1255. doi:10.1586/14737140.2015.1086647
Ashrafizadeh, M., Javanmardi, S., Moradi-Ozarlou, M., Mohammadinejad, R., Farkhondeh, T., Samarghandian, S., et al. (2020). Natural products and phytochemical nanoformulations targeting mitochondria in oncotherapy: An updated review on resveratrol. Biosci. Rep. 40. doi:10.1042/BSR20200257
Askar, M. A., El Shawi, O. E., Abou zaid, O. A. R., Mansour, N. A., and Hanafy, A. M. (2021). Breast cancer suppression by curcumin-naringenin-magnetic-nano-particles: In vitro and in vivo studies. Tumor Biol. 43, 225–247. doi:10.3233/TUB-211506
Assaraf, Y. G., Brozovic, A., Gonçalves, A. C., Jurkovicova, D., Linē, A., Machuqueiro, M., et al. (2019). The multi-factorial nature of clinical multidrug resistance in cancer. Drug Resist Updat 46, 100645. doi:10.1016/j.drup.2019.100645
Babaei, G., Aziz, S. G.-G., and Jaghi, N. Z. Z. (2021). EMT, cancer stem cells and autophagy; the three main axes of metastasis. Biomed. Pharmacother. 133, 110909. doi:10.1016/j.biopha.2020.110909
Baetke, S. C., Lammers, T., and Kiessling, F. (2015). Applications of nanoparticles for diagnosis and therapy of cancer. Br. J. Radiol. 88, 20150207. doi:10.1259/bjr.20150207
Barabadi, H., Vahidi, H., Mahjoub, M., Kosar, K., Kamali, K., Ponmurugan, K., et al. (2020). Emerging antineoplastic gold nanomaterials for cervical cancer therapeutics: A systematic review. J. Clust. Sci. 31, 1173–1184. doi:10.1007/s10876-019-01733-2
Barenholz, Y. (2012). Doxil®-the first FDA-approved nano-drug: Lessons learned. J. Control Release 160, 117–134. doi:10.1016/j.jconrel.2012.03.020
Below, J., and M Das, J. (2022). “Vincristine,” in StatPearls (Treasure Island (FL): StatPearls Publishing).
Benedetto, G., Vestal, C. G., and Richardson, C. (2015). Aptamer-functionalized nanoparticles as “smart bombs”: The unrealized potential for personalized medicine and targeted cancer treatment. Target Oncol. 10, 467–485. doi:10.1007/s11523-015-0371-z
Bhattacharya, T., Soares, G. A. B., Chopra, H., Rahman, M. M., Hasan, Z., Swain, S. S., et al. (2022). Applications of phyto-nanotechnology for the treatment of neurodegenerative disorders. Mater. (Basel) 15, 804. doi:10.3390/ma15030804
Borah, A., Pillai, S. C., Rochani, A. K., Palaninathan, V., Nakajima, Y., Maekawa, T., et al. (2020). GANT61 and curcumin-loaded PLGA nanoparticles for GLI1 and PI3K/Akt-Mediated inhibition in breast adenocarcinoma. Nanotechnology 31, 185102. doi:10.1088/1361-6528/ab6d20
Busatto, S., Pham, A., Suh, A., Shapiro, S., and Wolfram, J. (2019). Organotropic drug delivery: Synthetic nanoparticles and extracellular vesicles. Biomed. Microdevices 21, 46. doi:10.1007/s10544-019-0396-7
Cencic, A., and Chingwaru, W. (2010). The role of functional foods, nutraceuticals, and food supplements in intestinal health. Nutrients 2, 611–625. doi:10.3390/nu2060611
Chan, M. M., Chen, R., and Fong, D. (2018). Targeting cancer stem cells with dietary phytochemical - repositioned drug combinations. Cancer Lett. 433, 53–64. doi:10.1016/j.canlet.2018.06.034
Chatterjee, D. K., Diagaradjane, P., and Krishnan, S. (2011). Nanoparticle-mediated hyperthermia in cancer therapy. Ther. Deliv. 2, 1001–1014. doi:10.4155/tde.11.72
Chaudhary, K., and Masram, D. T. (2020). “Biological activities of nanoparticles and mechanism of action,” in Model organisms to study biological activities and toxicity of nanoparticles. Editors B. Siddhardha, M. Dyavaiah, and K. Kasinathan (Singapore: Springer), 19–34.
Chen, Q., Li, N., Wang, X., Yang, Y., Xiang, Y., Long, X., et al. (2022). Mitochondria-targeting chemodynamic therapy nanodrugs for cancer treatment. Front. Pharmacol. 13, 847048. doi:10.3389/fphar.2022.847048
Cherukuri, P., Glazer, E. S., and Curley, S. A. (2010). Targeted hyperthermia using metal nanoparticles. Adv. Drug Deliv. Rev. 62, 339–345. doi:10.1016/j.addr.2009.11.006
De Jong, W. H., and Borm, P. J. A. (2008). Drug delivery and nanoparticles:applications and hazards. Int. J. Nanomedicine 3, 133–149. doi:10.2147/ijn.s596
Dhupal, M., and Chowdhury, D. (2020). Phytochemical-based nanomedicine for advanced cancer theranostics: Perspectives on clinical trials to clinical use. IJN 15, 9125–9157. doi:10.2147/IJN.S259628<
Dick, J. E. (2008). Stem cell concepts renew cancer research. Blood 112, 4793–4807. doi:10.1182/blood-2008-08-077941
Ellinger, J., Alajati, A., Kubatka, P., Giordano, F. A., Ritter, M., Costigliola, V., et al. (2022). Prostate cancer treatment costs increase more rapidly than for any other cancer-how to reverse the trend? EPMA J. 13, 1–7. doi:10.1007/s13167-022-00276-3
Elsayed, A. M., Sherif, N. M., Hassan, N. S., Althobaiti, F., Hanafy, N. A. N., and Sahyon, H. A. (2021). Novel quercetin encapsulated chitosan functionalized copper oxide nanoparticles as anti-breast cancer agent via regulating P53 in rat model. Int. J. Biol. Macromol. 185, 134–152. doi:10.1016/j.ijbiomac.2021.06.085
Fischer, H. C., and ChanNanotoxicity, W. C. W. (2007). Nanotoxicity: The growing need for in vivo study. Curr. Opin. Biotechnol. 18, 565–571. doi:10.1016/j.copbio.2007.11.008
Frampton, J. E. (2020). Liposomal irinotecan: A review in metastatic pancreatic adenocarcinoma. Drugs 80, 1007–1018. doi:10.1007/s40265-020-01336-6
FramptonMifamurtide, J. E. (2010). Mifamurtide: A review of its use in the treatment of osteosarcoma. Paediatr. Drugs 12, 141–153. doi:10.2165/11204910-000000000-00000
Fu, M., Zhuang, X., Zhang, T., Guan, Y., Meng, Q., and Zhang, Y. (2020). PEGylated leuprolide with improved pharmacokinetic properties. Bioorg Med. Chem. 28, 115306. doi:10.1016/j.bmc.2020.115306
Ganguly, S., Dewanjee, S., Sen, R., Chattopadhyay, D., Ganguly, S., Gaonkar, R., et al. (2021). Apigenin-loaded galactose tailored PLGA nanoparticles: A possible strategy for liver targeting to treat hepatocellular carcinoma. Colloids Surf. B Biointerfaces 204, 111778. doi:10.1016/j.colsurfb.2021.111778
Ganthala, P. D., Alavala, S., Chella, N., Andugulapati, S. B., Bathini, N. B., and Sistla, R. (2022). Co-encapsulated nanoparticles of erlotinib and quercetin for targeting lung cancer through nuclear EGFR and PI3K/AKT inhibition. Colloids Surf. B Biointerfaces 211, 112305. doi:10.1016/j.colsurfb.2021.112305
Gavas, S., Quazi, S., and Karpiński, T. M. (2021). Nanoparticles for cancer therapy: Current progress and challenges. Nanoscale Res. Lett. 16, 173. doi:10.1186/s11671-021-03628-6
Gawde, K. A., Sau, S., Tatiparti, K., Kashaw, S. K., Mehrmohammadi, M., Azmi, A. S., et al. (2018). Paclitaxel and di-fluorinated curcumin loaded in albumin nanoparticles for targeted synergistic combination therapy of ovarian and cervical cancers. Colloids Surf. B Biointerfaces 167, 8–19. doi:10.1016/j.colsurfb.2018.03.046
Gobbo, O. L., Sjaastad, K., Radomski, M. W., Volkov, Y., and Prina-Mello, A. (2015). Magnetic nanoparticles in cancer theranostics. Theranostics 5, 1249–1263. doi:10.7150/thno.11544
Golubnitschaja, O., Liskova, A., Koklesova, L., Samec, M., Biringer, K., Büsselberg, D., et al. (2021). Caution, “normal” BMI: Health risks associated with potentially masked individual underweight-EPMA position paper 2021. EPMA J. 12, 243–264. doi:10.1007/s13167-021-00251-4
Gu, H.-F., Ren, F., Mao, X.-Y., and Du, M. (2021). Mineralized and GSH-responsive hyaluronic acid based nano-carriers for potentiating repressive effects of sulforaphane on breast cancer stem cells-like properties. Carbohydr. Polym. 269, 118294. doi:10.1016/j.carbpol.2021.118294
Gwinn, M. R., and Vallyathan, V. (2006). Nanoparticles: Health effects-pros and cons. Environ. Health Perspect. 114, 1818–1825. doi:10.1289/ehp.8871
Hamida, R. S., Ali, M. A., Redhwan, A., and Bin-Meferij, M. M. (2020). Cyanobacteria - a promising platform in green nanotechnology: A review on nanoparticles fabrication and their prospective applications. Int. J. Nanomedicine 15, 6033–6066. doi:10.2147/IJN.S256134
Hanahan, D., and Weinberg, R. A. (2011). Hallmarks of cancer: The next generation. Cell 144, 646–674. doi:10.1016/j.cell.2011.02.013
Hesari, M., Mohammadi, P., Khademi, F., Shackebaei, D., Momtaz, S., Moasefi, N., et al. (2021). Current advances in the use of nanophytomedicine therapies for human cardiovascular diseases. IJN 16, 3293–3315. doi:10.2147/IJN.S295508<
Huang, J., Tao, C., Yu, Y., Yu, F., Zhang, H., Gao, J., et al. (2016). Simultaneous targeting of differentiated breast cancer cells and breast cancer stem cells by combination of docetaxel- and sulforaphane-loaded self-assembled poly(D, L-lactide-Co-Glycolide)/Hyaluronic acid block copolymer-based nanoparticles. J. Biomed. Nanotechnol. 12, 1463–1477. doi:10.1166/jbn.2016.2234
Huang, T., Peng, L., Han, Y., Wang, D., He, X., Wang, J., et al. (2022). Lipid nanoparticle-based MRNA vaccines in cancers: Current advances and future prospects. Front. Immunol. 13, 922301. doi:10.3389/fimmu.2022.922301
Inbaraj, B. S., Hua, L.-H., and Chen, B.-H. (2021). Comparative study on inhibition of pancreatic cancer cells by resveratrol gold nanoparticles and a resveratrol nanoemulsion prepared from grape skin. Pharmaceutics 13, 1871. doi:10.3390/pharmaceutics13111871
Jeon, S., Jun, E., Chang, H., Yhee, J. Y., Koh, E.-Y., Kim, Y., et al. (2022). Prediction the clinical EPR effect of nanoparticles in patient-derived xenograft models. J. Control Release 351, 37–49. doi:10.1016/j.jconrel.2022.09.007
Jose, J., Kumar, R., Harilal, S., Mathew, G. E., Parambi, D. G. T., Prabhu, A., et al. (2020). Magnetic nanoparticles for hyperthermia in cancer treatment: An emerging tool. Environ. Sci. Pollut. Res. 27, 19214–19225. doi:10.1007/s11356-019-07231-2
Kaur, P., Aliru, M. L., Chadha, A. S., Asea, A., and Krishnan, S. (2016). Hyperthermia using nanoparticles – promises and pitfalls. Int. J. Hyperth. 32, 76–88. doi:10.3109/02656736.2015.1120889
Kesharwani, P., Banerjee, S., Padhye, S., Sarkar, F. H., and Iyer, A. K. (2015). Hyaluronic acid engineered nanomicelles loaded with 3,4-difluorobenzylidene curcumin for targeted killing of CD44+ stem-like pancreatic cancer cells. Biomacromolecules 16, 3042–3053. doi:10.1021/acs.biomac.5b00941
Khan, H., Ullah, H., Martorell, M., Valdes, S. E., Belwal, T., Tejada, S., et al. (2021). Flavonoids nanoparticles in cancer: Treatment, prevention and clinical prospects. Semin. Cancer Biol. 69, 200–211. doi:10.1016/j.semcancer.2019.07.023
Khoobchandani, M., Katti, K. K., Karikachery, A. R., Thipe, V. C., Srisrimal, D., Dhurvas Mohandoss, D. K., et al. (2020). New approaches in breast cancer therapy through green nanotechnology and nano-ayurvedic medicine - pre-clinical and pilot human clinical investigations. Int. J. Nanomedicine 15, 181–197. doi:10.2147/IJN.S219042
Koklesova, L., Liskova, A., Samec, M., Buhrmann, C., Samuel, S. M., Varghese, E., et al. (2020). Carotenoids in cancer apoptosis-the road from bench to bedside and back. Cancers (Basel) 12, 2425. doi:10.3390/cancers12092425
Koklesova, L., Liskova, A., Samec, M., Qaradakhi, T., Zulli, A., Smejkal, K., et al. (2020). Genoprotective activities of plant natural substances in cancer and chemopreventive strategies in the context of 3P medicine. EPMA J. 11, 261–287. doi:10.1007/s13167-020-00210-5
Koklesova, L., Mazurakova, A., Samec, M., Kudela, E., Biringer, K., Kubatka, P., et al. (2022). Mitochondrial health quality control: Measurements and interpretation in the framework of predictive, preventive, and personalized medicine. EPMA J. 13, 177–193. doi:10.1007/s13167-022-00281-6
Koklesova, L., Samec, M., Liskova, A., Zhai, K., Büsselberg, D., Giordano, F. A., et al. (2021). Mitochondrial impairments in aetiopathology of multifactorial diseases: Common origin but individual outcomes in context of 3P medicine. EPMA J. 12, 27–40. doi:10.1007/s13167-021-00237-2
Kubatka, P., Mazurakova, A., Samec, M., Koklesova, L., Zhai, K., Al-Ishaq, R., et al. (2021). Flavonoids against non-physiologic inflammation attributed to cancer initiation, development, and progression—3PM pathways. EPMA J. 12, 559–587. doi:10.1007/s13167-021-00257-y
Kumar, P., Yadav, N., Chaudhary, B., Jain, V., Balaramnavar, V. M., Alharbi, K. S., et al. (2022). Promises of phytochemical based nano drug delivery systems in the management of cancer. Chem. Biol. Interact. 351, 109745. doi:10.1016/j.cbi.2021.109745
Kuo, Y.-C., Wang, L.-J., and Rajesh, R. (2019). Targeting human brain cancer stem cells by curcumin-loaded nanoparticles grafted with anti-aldehyde dehydrogenase and sialic acid: Colocalization of ALDH and CD44. Mater Sci. Eng. C Mater Biol. Appl. 102, 362–372. doi:10.1016/j.msec.2019.04.065
Lathia, J., Liu, H., and Matei, D. (2020). The clinical impact of cancer stem cells. Oncologist 25, 123–131. doi:10.1634/theoncologist.2019-0517
Li, C., Lin, J., Wu, P., Zhao, R., Zou, J., Zhou, M., et al. (2018). Small molecule nanodrug assembled of dual-anticancer drug conjugate for synergetic cancer metastasis therapy. Bioconjug Chem. 29, 3495–3502. doi:10.1021/acs.bioconjchem.8b00657
Li, L., Zhang, M., Liu, T., Li, J., Sun, S., Chen, J., et al. Quercetin-ferrum nanoparticles enhance photothermal therapy by modulating the tumor immunosuppressive microenvironment. Acta Biomater. 154, 2022, 454–466. doi:10.1016/j.actbio.2022.10.008
Li, X., Li, W., Wang, M., and Liao, Z. (2021). Magnetic nanoparticles for cancer theranostics: Advances and prospects. J. Control Release 335, 437–448. doi:10.1016/j.jconrel.2021.05.042
Li, Y., Wang, Z., Ajani, J. A., and Song, S. (2021). Drug resistance and cancer stem cells. Cell Commun. Signal 19, 19. doi:10.1186/s12964-020-00627-5
Li, Z., Chen, Y., Yang, Y., Yu, Y., Zhang, Y., Zhu, D., et al. (2019). Recent advances in nanomaterials-based chemo-photothermal combination therapy for improving cancer treatment. Front. Bioeng. Biotechnol. 7, 293. doi:10.3389/fbioe.2019.00293
Libutti, S. K., Paciotti, G. F., Byrnes, A. A., Alexander, H. R., Gannon, W. E., Walker, M., et al. (2010). Phase I and pharmacokinetic studies of CYT-6091, a novel PEGylated colloidal gold-RhTNF nanomedicine. Clin. Cancer Res. 16, 6139–6149. doi:10.1158/1078-0432.CCR-10-0978
Link, B., Torres Crigna, A., Hölzel, M., Giordano, F. A., and Golubnitschaja, O. (2021). Abscopal effects in metastatic cancer: Is a predictive approach possible to improve individual outcomes? J. Clin. Med. 10, 5124. doi:10.3390/jcm10215124
Liskova, A., Samec, M., Koklesova, L., Brockmueller, A., Zhai, K., Abdellatif, B., et al. (2021). Flavonoids as an effective sensitizer for anti-cancer therapy: Insights into multi-faceted mechanisms and applicability towards individualized patient profiles. EPMA J. 12, 155–176. doi:10.1007/s13167-021-00242-5
Liszbinski, R. B., Romagnoli, G. G., Gorgulho, C. M., Basso, C. R., Pedrosa, V. A., and Kaneno, R. (2020). Anti-EGFR-coated gold nanoparticles in vitro carry 5-fluorouracil to colorectal cancer cells. Mater. (Basel) 13, 375. doi:10.3390/ma13020375
Liu, M., Wang, B., Guo, C., Hou, X., Cheng, Z., and Chen, D. (2019). Novel multifunctional triple folic acid, biotin and CD44 targeting PH-sensitive nano-actiniaes for breast cancer combinational therapy. Drug Deliv. 26, 1002–1016. doi:10.1080/10717544.2019.1669734
Liu, P., Behray, M., Wang, Q., Wang, W., Zhou, Z., Chao, Y., et al. (2018). Anti-cancer activities of allyl isothiocyanate and its conjugated silicon quantum dots. Sci. Rep. 8, 1084. doi:10.1038/s41598-018-19353-7
Liu, R. H. (2004). Potential synergy of phytochemicals in cancer prevention: Mechanism of action. J. Nutr. 134, 3479S–3485S. doi:10.1093/jn/134.12.3479S
Liu, Y., Crawford, B. M., and Vo-Dinh, T. (2018). Gold nanoparticles-mediated photothermal therapy and immunotherapy. Immunotherapy 10, 1175–1188. doi:10.2217/imt-2018-0029
Maeda, H. (2001). The enhanced permeability and retention (EPR) effect in tumor vasculature: The key role of tumor-selective macromolecular drug targeting. Adv. Enzyme Regul. 41, 189–207. doi:10.1016/s0065-2571(00)00013-3
Mahmoudi, K., Bouras, A., Bozec, D., Ivkov, R., and Hadjipanayis, C. (2018). Magnetic hyperthermia therapy for the treatment of glioblastoma: A review of the therapy’s history, efficacy and application in humans. Int. J. Hyperth. 34, 1316–1328. doi:10.1080/02656736.2018.1430867
Marinheiro, D., Ferreira, B. J. M. L., Oskoei, P., Oliveira, H., and Daniel-da-Silva, A. L. (2021). Encapsulation and enhanced release of resveratrol from mesoporous silica nanoparticles for melanoma therapy. Mater. (Basel) 14, 1382. doi:10.3390/ma14061382
Martin, M., and López-Tarruella, S. (2016). Emerging therapeutic options for HER2-positive breast cancer. Am. Soc. Clin. Oncol. Educ. Book 35, e64–e70. doi:10.1200/EDBK_159167
Massironi, A., Marzorati, S., Marinelli, A., Toccaceli, M., Gazzotti, S., Ortenzi, M. A., et al. (2022). Synthesis and characterization of curcumin-loaded nanoparticles of poly(glycerol sebacate): A novel highly stable anticancer system. Molecules 27, 6997. doi:10.3390/molecules27206997
Mazurakova, A., Koklesova, L., Samec, M., Kudela, E., Kajo, K., Skuciova, V., et al. (2022). Anti-breast cancer effects of phytochemicals: Primary, secondary, and tertiary care. EPMA J. 13, 315–334. doi:10.1007/s13167-022-00277-2
Mazurakova, A., Samec, M., Koklesova, L., Biringer, K., Kudela, E., Al-Ishaq, R. K., et al. (2022). Anti-prostate cancer protection and therapy in the framework of predictive, preventive and personalised medicine - comprehensive effects of phytochemicals in primary, secondary and tertiary care. EPMA J. 13, 461–486. doi:10.1007/s13167-022-00288-z
Melim, C., Magalhães, M., Santos, A. C., Campos, E. J., and Cabral, C. (2022). Nanoparticles as phytochemical carriers for cancer treatment: News of the last decade. Expert Opin. Drug Deliv. 19, 179–197. doi:10.1080/17425247.2022.2041599
Miao, L., Zhang, Y., and Huang, L. (2021). MRNA vaccine for cancer immunotherapy. Mol. Cancer 20, 41. doi:10.1186/s12943-021-01335-5
Milane, L., Trivedi, M., Singh, A., Talekar, M., and Amiji, M. (2015). Mitochondrial biology, targets, and drug delivery. J. Control Release 207, 40–58. doi:10.1016/j.jconrel.2015.03.036
Missaoui, W. N., Arnold, R. D., and Cummings, B. S. (2018). Toxicological status of nanoparticles: What we know and what we don’t know. Chem. Biol. Interact. 295, 1–12. doi:10.1016/j.cbi.2018.07.015
Mitchell, M. J., Billingsley, M. M., Haley, R. M., Wechsler, M. E., Peppas, N. A., and Langer, R. (2021). Engineering precision nanoparticles for drug delivery. Nat. Rev. Drug Discov. 20, 101–124. doi:10.1038/s41573-020-0090-8
Nagarajan, R. (2008). Building blocks for nanotechnology nanoparticles: Synthesis, stabilization, passivation, and functionalization ACS symposium series. Am. Chem. Soc. 996, ch001. doi:10.1021/bk-2008-0996.ch001
Najahi-Missaoui, W., Arnold, R. D., and CummingsNanoparticles, B. S. S. (2020). Are we there yet? Int. J. Mol. Sci. 22, 385. doi:10.3390/ijms22010385
Nazer, S., Andleeb, S., Ali, S., Gulzar, N., Iqbal, T., Khan, M. A. R., et al. (2020). Synergistic antibacterial efficacy of biogenic synthesized silver nanoparticles using ajuga bractosa with standard antibiotics: A study against bacterial pathogens. Curr. Pharm. Biotechnol. 21, 206–218. doi:10.2174/1389201020666191001123219
Neelakandan, M., Manoharan, S., Muralinaidu, R., and Thara, J. M. (2022). Tumor preventive and antioxidant efficacy of chlorogenic acid-loaded chitosan nanoparticles in experimental skin carcinogenesis. Naunyn Schmiedeb. Arch. Pharmacol. 396, 533–546. doi:10.1007/s00210-022-02330-3
Nirachonkul, W., Ogonoki, S., Thumvijit, T., Chiampanichayakul, S., Panyajai, P., Anuchapreeda, S., et al. (2021). CD123-Targeted nano-curcumin molecule enhances cytotoxic efficacy in leukemic stem cells. Nanomater. (Basel) 11, 2974. doi:10.3390/nano11112974
Norouzi, M. (2020). Gold nanoparticles in glioma theranostics. Pharmacol. Res. 156, 104753. doi:10.1016/j.phrs.2020.104753
Palliyage, G. H., Hussein, N., Mimlitz, M., Weeder, C., Alnasser, M. H. A., Singh, S., et al. (2021). Novel curcumin-resveratrol solid nanoparticles synergistically inhibit proliferation of melanoma cells. Pharm. Res. 38, 851–871. doi:10.1007/s11095-021-03043-7
Papachristofilou, A., Hipp, M. M., Klinkhardt, U., Früh, M., Sebastian, M., Weiss, C., et al. (2019). Phase Ib evaluation of a self-adjuvanted protamine formulated MRNA-based active cancer immunotherapy, BI1361849 (CV9202), combined with local radiation treatment in patients with stage IV non-small cell lung cancer. J. Immunother. Cancer 7, 38. doi:10.1186/s40425-019-0520-5
Patra, J. K., Das, G., Fraceto, L. F., Campos, E. V. R., Rodriguez-Torres, M., del, P., et al. (2018). Nano based drug delivery systems: Recent developments and future prospects. J. Nanobiotechnology 16, 71. doi:10.1186/s12951-018-0392-8
Peng, Z. (2005). Current status of gendicine in China: Recombinant human ad-P53 agent for treatment of cancers. Hum. Gene Ther. 16, 1016–1027. doi:10.1089/hum.2005.16.1016
Pradhan, R., Chatterjee, S., Hembram, K. C., Sethy, C., Mandal, M., and Kundu, C. N. (2021). Nano formulated resveratrol inhibits metastasis and angiogenesis by reducing inflammatory cytokines in oral cancer cells by targeting tumor associated macrophages. J. Nutr. Biochem. 92, 108624. doi:10.1016/j.jnutbio.2021.108624
Prasetyanti, P. R., and Medema, J. P. (2017). Intra-tumor heterogeneity from a cancer stem cell perspective. Mol. Cancer 16, 41. doi:10.1186/s12943-017-0600-4
Puri, A., Loomis, K., Smith, B., Lee, J.-H., Yavlovich, A., Heldman, E., et al. (2009). Lipid-based nanoparticles as pharmaceutical drug carriers: From concepts to clinic. Crit. Rev. Ther. Drug Carr. Syst. 26, 523–580. doi:10.1615/critrevtherdrugcarriersyst.v26.i6.10
Ramalingam, V., Muthukumar Sathya, P., Srivalli, T., and Mohan, H. (2022). Synthesis of quercetin functionalized wurtzite type zinc oxide nanoparticles and their potential to regulate intrinsic apoptosis signaling pathway in human metastatic ovarian cancer. Life Sci. 309, 121022. doi:10.1016/j.lfs.2022.121022
Ravi, R., Zeyaullah, M., Ghosh, S., Khan Warsi, M., Baweja, R., AlShahrani, A. M., et al. (2022). Use of gold nanoparticle-silibinin conjugates: A novel approach against lung cancer cells. Front. Chem. 10, 1018759. doi:10.3389/fchem.2022.1018759
Riganti, C., and Contino, M. (2019). New strategies to overcome resistance to chemotherapy and immune system in cancer. Int. J. Mol. Sci. 20, 4783. doi:10.3390/ijms20194783
Roy, A., Bulut, O., Some, S., Kumar Mandal, A., and Deniz Yilmaz, M. (2019). Green synthesis of silver nanoparticles: Biomolecule-nanoparticle organizations targeting antimicrobial activity. RSC Adv. 9, 2673–2702. doi:10.1039/C8RA08982E
Sabzini, M., Pourmadadi, M., Yazdian, F., Khadiv-Parsi, P., and Rashedi, H. (2022). Development of chitosan/halloysite/graphitic-carbon nitride nanovehicle for targeted delivery of quercetin to enhance its limitation in cancer therapy: An in vitro cytotoxicity against MCF-7 cells. Int. J. Biol. Macromol. 226, 159–171. doi:10.1016/j.ijbiomac.2022.11.189
Sahu, A., Kim, M., Ryu, J., Son, J.-G., Lee, E., Noh, D. Y., et al. (2018). Nanographene oxide as a switch for CW/pulsed NIR laser triggered drug release from liposomes. Mater. Sci. Eng. C 82, 19–24. doi:10.1016/j.msec.2017.08.057
Samec, M., Liskova, A., Koklesova, L., Samuel, S. M., Zhai, K., Buhrmann, C., et al. (2020). Flavonoids against the warburg phenotype-concepts of predictive, preventive and personalised medicine to cut the gordian knot of cancer cell metabolism. EPMA J. 11, 377–398. doi:10.1007/s13167-020-00217-y
Sebastian, M., Schröder, A., Scheel, B., Hong, H. S., Muth, A., von Boehmer, L., et al. (2019). A phase I/IIa study of the MRNA-based cancer immunotherapy CV9201 in patients with stage IIIB/IV non-small cell lung cancer. Cancer Immunol. Immunother. 68, 799–812. doi:10.1007/s00262-019-02315-x
Shen, S., Xu, X., Lin, S., Zhang, Y., Liu, H., Zhang, C., et al. (2021). A nanotherapeutic strategy to overcome chemotherapeutic resistance of cancer stem-like cells. Nat. Nanotechnol. 16, 104–113. doi:10.1038/s41565-020-00793-0
Singh, S. (2010). Nanomedicine-nanoscale drugs and delivery systems. J. Nanosci. Nanotechnol. 10, 7906–7918. doi:10.1166/jnn.2010.3617
Smith, L., Kuncic, Z., Ostrikov, K., Ken), , and Kumar, S. (2012). Nanoparticles in cancer imaging and therapy. J. Nanomater. 2012, 1–7. doi:10.1155/2012/891318
Sousa-Junior, A., Yang, C.-T., Korangath, P., Ivkov, R., and Bakuzis, A. (2022). A predictive pharmacokinetic model for immune cell-mediated uptake and retention of nanoparticles in tumors. Int. J. Mol. Sci. 23, 15664. doi:10.3390/ijms232415664
Sun, R., Liu, Y., Li, S.-Y., Shen, S., Du, X.-J., Xu, C.-F., et al. (2015). Co-delivery of all-trans-retinoic acid and doxorubicin for cancer therapy with synergistic inhibition of cancer stem cells. Biomaterials 37, 405–414. doi:10.1016/j.biomaterials.2014.10.018
Sun, T., Zhang, Y. S., Pang, B., Hyun, D. C., Yang, M., and Xia, Y. (2014). Engineered nanoparticles for drug delivery in cancer therapy. Angew. Chem. Int. Ed. Engl. 53, 12320–12364. doi:10.1002/anie.201403036
Sung, H., Ferlay, J., Siegel, R. L., Laversanne, M., Soerjomataram, I., Jemal, A., et al. (2021). Global cancer statistics 2020: GLOBOCAN estimates of incidence and mortality worldwide for 36 cancers in 185 countries. CA A Cancer J. Clin. 71, 209–249. doi:10.3322/caac.21660
Tabassam, Q., Mehmood, T., Raza, A. R., Ullah, A., Saeed, F., and Anjum, F. M. (2020). Synthesis, characterization and anti-cancer therapeutic potential of withanolide-A with 20nm SAuNPs conjugates against SKBR3 breast cancer cell line. Int. J. Nanomedicine 15, 6649–6658. doi:10.2147/IJN.S258528
Tabassum, N., Verma, V., Kumar, M., Kumar, A., and Singh, B. (2018). Nanomedicine in cancer stem cell therapy: From fringe to forefront. Cell Tissue Res. 374, 427–438. doi:10.1007/s00441-018-2928-5
Tan, X., Zhou, Y., Shen, L., Jia, H., and Tan, X. (2019). A mitochondria-targeted delivery system of doxorubicin and evodiamine for the treatment of metastatic breast cancer. RSC Adv. 9, 37067–37078. doi:10.1039/C9RA07096F
Thipe, V. C., Panjtan Amiri, K., Bloebaum, P., Raphael Karikachery, A., Khoobchandani, M., Katti, K. K., et al. (2019). Development of resveratrol-conjugated gold nanoparticles: Interrelationship of increased resveratrol corona on anti-tumor efficacy against breast, pancreatic and prostate cancers. Int. J. Nanomedicine 14, 4413–4428. doi:10.2147/IJN.S204443
Verma, A., Gautam, S. P., Bansal, K. K., Prabhakar, N., and Rosenholm, J. M. (2019). Green nanotechnology: Advancement in phytoformulation research. Medicines 6, 39. doi:10.3390/medicines6010039
Vilas-Boas, V., Carvalho, F., and Espiña, B. (2020). Magnetic hyperthermia for cancer treatment: Main parameters affecting the outcome of in vitro and in vivo studies. Molecules 25, 2874. doi:10.3390/molecules25122874
Vimala, K., and Kannan, S. (2021). Phyto-drug conjugated nanomaterials enhance apoptotic activity in cancer. Adv. Protein Chem. Struct. Biol. 125, 275–305. doi:10.1016/bs.apcsb.2020.12.003
Vinluan, R. D., and Zheng, J. (2015). Serum protein adsorption and excretion pathways of metal nanoparticles. Nanomedicine (Lond) 10, 2781–2794. doi:10.2217/nnm.15.97
von Minckwitz, G., Huang, C.-S., Mano, M. S., Loibl, S., Mamounas, E. P., Untch, M., et al. (2019). Trastuzumab emtansine for residual invasive HER2-positive breast cancer. N. Engl. J. Med. 380, 617–628. doi:10.1056/NEJMoa1814017
Wang, B., Liu, X., Teng, Y., Yu, T., Chen, J., Hu, Y., et al. (2017). Improving anti-melanoma effect of curcumin by biodegradable nanoparticles. Oncotarget 8, 108624–108642. doi:10.18632/oncotarget.20585
Wang, H., Agarwal, P., Zhao, S., Xu, R. X., Yu, J., Lu, X., et al. (2015). Hyaluronic acid-decorated dual responsive nanoparticles of pluronic F127, PLGA, and chitosan for targeted Co-delivery of doxorubicin and irinotecan to eliminate cancer stem-like cells. Biomaterials 72, 74–89. doi:10.1016/j.biomaterials.2015.08.048
Wang, H., and He, X. (2018). Nanoparticles for targeted drug delivery to cancer stem cells and tumor. Methods Mol. Biol. 1831, 59–67. doi:10.1007/978-1-4939-8661-3_6
Wang, R., Song, B., Wu, J., Zhang, Y., Chen, A., and Shao, L. (2018). Potential adverse effects of nanoparticles on the reproductive system. Int. J. Nanomedicine 13, 8487–8506. doi:10.2147/IJN.S170723
Wang, W., Yan, Y., Guo, Z., Hou, H., Garcia, M., Tan, X., et al. (2021). All around suboptimal health - a joint position paper of the suboptimal health study consortium and European association for predictive, preventive and personalised medicine. EPMA J. 12, 403–433. doi:10.1007/s13167-021-00253-2
Yaari, Z., da Silva, D., Zinger, A., Goldman, E., Kajal, A., Tshuva, R., et al. (2016). Theranostic barcoded nanoparticles for personalized cancer medicine. Nat. Commun. 7, 13325. doi:10.1038/ncomms13325
Yang, K., Liao, Z., Wu, Y., Li, M., Guo, T., Lin, J., et al. (2020). Curcumin and glu-GNPs induce radiosensitivity against breast cancer stem-like cells. Biomed. Res. Int. 2020, 3189217. doi:10.1155/2020/3189217
Yang, Z., Sun, N., Cheng, R., Zhao, C., Liu, J., and Tian, Z. (2017). Hybrid nanoparticles coated with hyaluronic acid lipoid for targeted Co-delivery of paclitaxel and curcumin to synergistically eliminate breast cancer stem cells. J. Mater Chem. B 5, 6762–6775. doi:10.1039/c7tb01510k
Yang, Z., Wang, D., Zhang, C., Liu, H., Hao, M., Kan, S., et al. (2022). The applications of gold nanoparticles in the diagnosis and treatment of gastrointestinal cancer. Front. Oncol. 11, 11. doi:10.3389/fonc.2021.819329
Zhang, L., Chen, W., Tu, G., Chen, X., Lu, Y., Wu, L., et al. (2020). Enhanced chemotherapeutic efficacy of PLGA-encapsulated epigallocatechin gallate (EGCG) against human lung cancer. Int. J. Nanomedicine 15, 4417–4429. doi:10.2147/IJN.S243657
Zhang, M., Kim, H. S., Jin, T., and Moon, W. K. (2017). Near-infrared photothermal therapy using EGFR-targeted gold nanoparticles increases autophagic cell death in breast cancer. J. Photochem. Photobiol. B Biol. 170, 58–64. doi:10.1016/j.jphotobiol.2017.03.025
Zhang, M., Viennois, E., Prasad, M., Zhang, Y., Wang, L., Zhang, Z., et al. (2016). Edible ginger-derived nanoparticles: A novel therapeutic approach for the prevention and treatment of inflammatory bowel disease and colitis-associated cancer. Biomaterials 101, 321–340. doi:10.1016/j.biomaterials.2016.06.018
Zheng, N.-G., Mo, S.-J., Li, J.-P., and Wu, J.-L. (2014). Anti-CSC effects in human esophageal squamous cell carcinomas and eca109/9706 cells induced by nanoliposomal quercetin alone or combined with CD 133 antiserum. Asian Pac J. Cancer Prev. 15, 8679–8684. doi:10.7314/apjcp.2014.15.20.8679
Zhou, M., Dong, J., Huang, J., Ye, W., Zheng, Z., Huang, K., et al. (2022). Chitosan-gelatin-EGCG nanoparticle-meditated LncRNA TMEM44-AS1 silencing to activate the P53 signaling pathway for the synergistic reversal of 5-FU resistance in gastric cancer. Adv. Sci. (Weinh) 9, e2105077. doi:10.1002/advs.202105077
Keywords: nanomedicine, nanoparticles, phytochemicals, plant-derived foods, cancer stem cells therapy, predictive preventive personalized medicine, primary secondary tertiary care
Citation: Koklesova L, Jakubikova J, Cholujova D, Samec M, Mazurakova A, Šudomová M, Pec M, Hassan STS, Biringer K, Büsselberg D, Hurtova T, Golubnitschaja O and Kubatka P (2023) Phytochemical-based nanodrugs going beyond the state-of-the-art in cancer management—Targeting cancer stem cells in the framework of predictive, preventive, personalized medicine. Front. Pharmacol. 14:1121950. doi: 10.3389/fphar.2023.1121950
Received: 12 December 2022; Accepted: 13 March 2023;
Published: 23 March 2023.
Edited by:
Vijayasteltar B. Liju, Ben-Gurion University of the Negev, IsraelReviewed by:
Arunaksharan Narayanankutty, St. Joseph’s College (Autonomous), Devagiri, IndiaVinitha Richard, University of Galway, Ireland
Archana Retnakumary, Rajiv Gandhi Centre for Biotechnology, India
Sankar Jagadeeshan, Ben-Gurion University of the Negev, Israel
Copyright © 2023 Koklesova, Jakubikova, Cholujova, Samec, Mazurakova, Šudomová, Pec, Hassan, Biringer, Büsselberg, Hurtova, Golubnitschaja and Kubatka. This is an open-access article distributed under the terms of the Creative Commons Attribution License (CC BY). The use, distribution or reproduction in other forums is permitted, provided the original author(s) and the copyright owner(s) are credited and that the original publication in this journal is cited, in accordance with accepted academic practice. No use, distribution or reproduction is permitted which does not comply with these terms.
*Correspondence: Tatiana Hurtova, tatiana.hurtova@unm.sk; Olga Golubnitschaja, Olga.Golubnitschaja@ukbonn.de; Peter Kubatka, peter.kubatka@uniba.sk