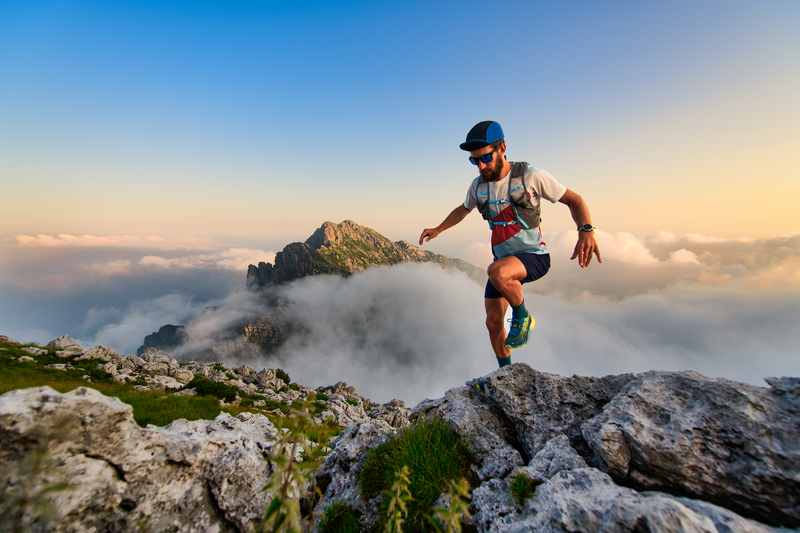
94% of researchers rate our articles as excellent or good
Learn more about the work of our research integrity team to safeguard the quality of each article we publish.
Find out more
MINI REVIEW article
Front. Pharmacol. , 20 January 2023
Sec. Inflammation Pharmacology
Volume 14 - 2023 | https://doi.org/10.3389/fphar.2023.1121819
This article is part of the Research Topic Insights in Inflammation Pharmacology: 2022 View all 8 articles
The importance of biologically active lipid mediators, such as prostanoids, leukotrienes, and specialized pro-resolving mediators, in the regulation of inflammation is well established. While the relevance of cholesterol in the context of atherosclerosis is also widely accepted, the role of cholesterol and its biosynthetic precursors on inflammatory processes is less comprehensively described. In the present mini-review, we summarize the current understanding of the inflammation-regulatory properties of cholesterol and relevant biosynthetic intermediates taking into account the implications of different subcellular distributions. Finally, we discuss the inflammation-regulatory effect of cholesterol homeostasis in the context of SARS-CoV-2 infections.
The high incidence of hypercholesteremia, i.e., pathologically elevated plasma cholesterol levels, remains a matter of great concern, as the concomitantly elevated levels of low-density lipoprotein (LDL) cholesterol pose a major risk factor for the development of cardiovascular diseases such as atherosclerosis (Tsao et al., 2022). In atherosclerosis, LDL-cholesterol is deposited in the arterial intima. Upon uptake of excessive amounts of modified, e.g., oxidized, LDL-cholesterol by macrophages, the latter develop a highly activated foam cell phenotype and, thus, contribute to the inflammatory character of atherosclerosis (Choudhury et al., 2005). Consequently, the use of cholesterol-lowering therapeutics, especially statins, i.e., 3-hydroxy-3-methylglutaryl-coenzyme A (HMG-CoA) reductase inhibitors, increased massively (Salami et al., 2017). The fact that upon inhibition of intracellular cholesterol production cells are able to cover their cholesterol demand by taking up cholesterol from the plasma (Gui et al., 2022), underscores the importance of maintaining intracellular cholesterol levels in a narrow, physiological range to facilitate the crucial functions of cholesterol in cellular membranes and as a precursor for various products (e.g., steroids, bile acids, vitamin D) (Tabas, 2002). Equally important, cells evolved efficient means to export cholesterol to avoid toxicity induced by excessive cholesterol levels (Song et al., 2021). As a side note, elevated cholesterol concentrations also play a pivotal role in the tumor microenvironment, where they not only support proliferation of tumor cells, but also attenuate anti-tumor immune responses. For details on the role of cholesterol homeostasis in tumor immunity see (Halimi and Farjadian, 2022).
With the recent advent of the concept of immunometabolism (Mathis and Shoelson, 2011), the tight integration of metabolic processes with immune responses gained increasing attention and led to propose that intracellular cholesterol dynamics and, similarly important, the cholesterol biosynthetic flux bear immune-regulatory properties (Fessler, 2016; O’Hagan et al., 2022). In this review, we provide a brief overview of the inflammation-regulatory functions of various cholesterol biosynthesis intermediates and discuss implications in the context of SARS-CoV-2.
All mammalian cells share essentially the same mechanisms to regulate their cholesterol content by a dynamic interplay between uptake, de novo synthesis, storage, and export. Cells take up cholesterol from the plasma, where it is transported predominantly packaged in LDL particles, via LDL receptor (LDLR)-mediated endocytosis (Brown and Goldstein, 1986). Upon release from the LDL particles within the endo-lysosomal compartment, cholesterol is distributed intracellularly to endoplasmic reticulum (ER) or plasma membrane by Niemann-Pick type C (NPC) 1 and 2 proteins (Pfeffer, 2019). In addition, cells can de novo synthesize cholesterol in a tightly regulated cascade involving more than 20 enzymes within three subcellular compartments (cytoplasm, ER, and peroxisomes) to satisfy their cholesterol demands (Charles et al., 2020). Due to its toxic properties, excess free intracellular cholesterol is esterified by acyl coenzyme A:cholesterol acyltransferase (ACAT) and either stored in lipid droplets or exported via ATP-binding cassette A1 (ABCA1) or ABCG1 and loaded onto high-density lipoprotein (HDL) (Yu and Tang, 2022).
The delicate cholesterol balance is largely controlled by two opposing transcription factors: Sterol response element-binding protein 2 (SREBP2), which, in response to cholesterol depletion at the ER, is escorted by SREBP cleavage-activating protein (SCAP) from ER to Golgi, where it is cleaved by site 1 protease (S1P) and S2P. The N-terminal transcription factor domain then localizes to the nucleus, and facilitates the expression of target genes, which are important for cholesterol biosynthesis and import (Sakai et al., 1996). In contrast, under elevated cholesterol conditions SREBP2 is retained at the ER, while oxysterols or desmosterol, a direct precursor of cholesterol, activate liver X receptors (LXRα/β) with subsequent induction of genes that reduce cholesterol uptake and promote cholesterol export (Janowski et al., 1996; Yang et al., 2006) (Figure 1). For further details, we refer the readers to the following comprehensive review (Luo et al., 2020).
FIGURE 1. Cholesterol homeostasis and inflammatory regulation. 1. Uptake: Cholesterol is taken up bound to low-density lipoprotein (LDL) particles via LDL-receptor (LDL-R)-associated endocytosis, released from LDL upon fusion of endosomes with lysosomes, and distributed by Niemann-Pick type C (NPC) proteins to either the endoplasmic reticulum (ER) or the plasma membrane. 2. Biosynthesis: Cholesterol is synthesized starting from acetyl-CoA in the cytoplasm, converted via 3-hydroxy-3-methylglutaryl-coenzyme A (HMG-CoA) to mevalonate in the ER, further processed in peroxisomes to the isoprenoids farnesyl pyrophosphate (FPP) and geranylgeranyl pyrophosphate (GGPP), which can also be produced from the GGPP-precursor geranylgeraniol (GGOH). In the ER, two FPP molecules condensate to squalene, which in a multi-step process is processed to lanosterol, which is further metabolized in the parallel Bloch and Kandutsch-Russel (K-R) pathways to desmosterol and 7-dehydrocholesterol (7-DHC), respectively, both of which are direct precursors of cholesterol. ER cholesterol levels are sensed by sterol response element-binding protein 2 (SREBP2), which is bound by SREBP cleavage-activating protein (SCAP) and insulin-induced gene (INSIG) in the ER. Low ER cholesterol levels induce translocation of SCAP-bound SREBP2 to the Golgi, where it undergoes cleavage-dependent activation. The N-terminal SREBP2 fragment acts as transcription factor among others for cholesterol biosynthesis enzymes and LDL-R. 3. Efflux: Excess cholesterol in the ER is esterified by acyl coenzyme A:cholesterol acyltransferase (ACAT) and subsequently either stored in lipid droplets or exported via ATP-binding cassette A1 (ABCA1) or ABCG1 and loaded onto high-density lipoprotein (HDL) and apolipoprotein A-I (apoA-I) for retrograde transport to the liver. Elevated oxysterol and desmosterol levels further activate the transcription factor liver X receptor (LXR) to enhance the expression of cholesterol exporters.
Cholesterol homeostasis and immune responses are tightly intertwined in a bidirectional manner. Since the present mini-review focuses on the effects of cholesterol (and cholesterol precursors) on inflammatory responses in innate immune cells, we refer the readers to excellent reviews for details on the reprogramming of cholesterol metabolism upon infections (Robertson and Ghazal, 2016; Lee and Bensinger, 2022).
A general reduction in the cholesterol biosynthetic flux was described to spontaneously induce interferon-stimulated genes (ISGs) (York et al., 2015), and to enhance anti-viral responses upon infections (Xiao et al., 2020). Moreover, SREBP2 was shown to directly bind and transcriptionally activate ISGs and further pro-inflammatory genes (Kusnadi et al., 2019). Independent of its SREBP2-activating function, the cholesterol sensor SCAP was also observed to connect cholesterol homeostasis to inflammation by shuttling interferon-regulatory factor 3 (IRF3) from ER to the golgi-localized stimulator of interferon genes (STING), thereby facilitating ISG induction upon infection (Chen et al., 2016), and by activation of the NLR family pyrin domain containing 3 (NLRP3) inflammasome (Guo et al., 2018). In the following sections, we will summarize the current understanding of the immunoregulatory functions of cholesterol and associated biosynthetic intermediates.
As exemplified by the pro-inflammatory character of foam cells laden with modified LDL-cholesterol in atherosclerosis (Tall and Yvan-Charvet, 2015), high cholesterol levels in innate immune cells are generally associated with pro-inflammatory functions. Along these lines, cholesterol crystals are recognized as NLRP3 inflammasome inducers in macrophages in atherosclerosis (Duewell et al., 2010).
In addition to total cellular cholesterol levels, its intracellular distribution appears critical for the inflammatory functions. For example, undisturbed cholesterol trafficking to the ER was required to enable activation of nuclear factor ‘kappa-light-chain-enhancer’ of activated B cells (NF-κB) and mitogen-activated protein kinase (MAPK) signaling, and consequently enhanced expression of the pro-inflammatory cytokines interleukin-6 (IL-6) and tumor necrosis factor-α (TNF-α) in response to excess amounts of free cholesterol (Li et al., 2005). Similarly, reducing ER cholesterol levels by either NPC1 inhibition or statin treatment abolished NLRP3 inflammasome activation, resulting in markedly lower IL-1β and IL-18 secretion. While activation of the NLRP3 inflammasome demanded intact shuttling of cholesterol to the ER, assembly and activation of the DNA sensing absent in melanoma 2 (AIM2) inflammasome by poly(deoxyadenylic-deoxythymidylic) acid (poly(dA:dT)) appeared insensitive to disturbances in cholesterol distribution (de la Roche et al., 2018). Interestingly, enhanced accumulation of cholesterol at the ER, brought about by experimental depletion of cholesterol-25-hydroxylase (Ch25h), resulted in mitochondrial (mt) dysfunction and mtDNA release and, thus, induced activation of the AIM2 inflammasome in response to lipopolysaccharide (LPS)-stimulation (Dang et al., 2017).
Furthermore, cholesterol, like other membrane lipids, affects the localization of various pattern-recognition receptors (PRRs) to specific organelles and/or membrane microdomains known as lipid rafts, which again is critical for the regulation of their activity. For further details, readers are referred to comprehensive reviews (Ruysschaert and Lonez, 2015; Köberlin et al., 2016). Therefore, it is not surprising that changes in intracellular cholesterol distribution were associated with altered toll-like receptor (TLR) sensitivity. For instance, increasing cholesterol loading in plasma membranes, by supplementation of methyl-β-cyclodextrin (CD)-complexed cholesterol, sufficed to initiate spontaneous TLR4 signaling in murine macrophages. In contrast, enhanced endosomal cholesterol accumulation, achieved by combined supplementation of acetylated LDL-cholesterol and inhibition of NPC1, induced TLR3 responses (Sun et al., 2009). A recent study further revealed that accumulation of free cholesterol in endosomes and lysosomes upon TLR4 activation is necessary for effective myeloid differentiation primary response 88 (MyD88)-dependent pro-inflammatory signaling in macrophages (Hayakawa et al., 2022).
In addition to uptake and de novo synthesis, cellular export of cholesterol by ABCA1/G1 transporters controls total and subcellular cholesterol levels, and consequently influences inflammatory responses. For example, intracellular cholesterol accumulation in ABCA1-deficient macrophages increased the expression of a broad range of inflammatory mediators upon LPS stimulation (Zhu et al., 2008). Mechanistically, elevated lipid raft cholesterol levels in ABCA1-deficient cells led to enhanced recruitment of TLRs to these membrane domains, thereby increasing the responsiveness to TLR agonists (Yvan-Charvet et al., 2008; Zhu et al., 2010). Cholesterol accumulation due to ABCA1/G1-deficiency was further shown to activate the NLRP3 inflammasome in dendritic cells, resulting in a systemic lupus erythematosus-like autoimmune phenotype in mice (Westerterp et al., 2017). This corroborates recent observations that miltefosine, an FDA-approved drug for the treatment of leishmaniasis, dampens NLRP3 inflammasome assembly and IL-1β release in macrophages by increasing ABCA1-mediated cholesterol efflux (Iacano et al., 2019).
Although cellular cholesterol export is largely associated with anti-inflammatory responses, the role of HDL remains controversial. In line with its function as a main plasma acceptor for cellular cholesterol, HDL was reported to provoke cholesterol export, thereby reducing cholesterol in lipid rafts and consequently attenuating TLR signaling (Cheng et al., 2012). HDL further induced the expression of activating transcription factor 3 (ATF3), a key transcriptional repressor of innate immune response genes, and, thus, downregulated the expression of TLR-induced pro-inflammatory cytokines (De Nardo et al., 2014). In contrast, HDL-associated apolipoprotein A-I (apoA-I) was shown to stimulate MyD88-dependent pro-inflammatory cytokine production in mouse macrophages (Smoak et al., 2010). Interestingly, while exporter-independent, lipid raft-disturbing depletion of cholesterol (e.g., in the presence of high HDL-apoA-I levels) was associated with pro-inflammatory effects, active, exporter-mediated efflux likely accounts for anti-inflammatory HDL effects (van der Vorst et al., 2017). Nevertheless, the majority of information, especially available in vivo findings, rather support the anti-inflammatory aspects of HDL (Fotakis et al., 2019).
Not surprisingly, LXR as the major regulator of ABCA1/G1 was also comprehensively analyzed in the context of inflammation. While the majority of reports described anti-inflammatory properties of LXR (Bilotta et al., 2020), others proposed potential pro-inflammatory functions of LXR as well. For instance, LXR was shown to activate hypoxia-inducible factor-1α (HIF-1α) and subsequent IL-1β secretion in human macrophages independent of its effects on ABCA1/G1 (Ménégaut et al., 2020). Furthermore, long-term activation of LXR was shown to potentiate subsequent LPS responses in human macrophages by increasing TLR4 signaling (Fontaine et al., 2007). Yet, considering the wide-ranging functions of LXR in lipid signaling, exceeding its mere role in cholesterol homeostasis (Wang and Tontonoz, 2018), contradictory findings regarding its impact on inflammatory responses are not entirely unexpected.
While the importance of cholesterol for innate immunity has been extensively studied, the role of specific intermediates generated during cholesterol biosynthesis for inflammatory reactions just emerges. In the following section, we will therefore briefly summarize the current understanding of the role of cholesterol precursors and oxysterols in the context of inflammation (Figure 1).
Mevalonate, the direct product of HMG-CoA reductase, is considered to have pro-inflammatory properties. In line, mevalonate accumulation enhanced a trained immunity phenotype induced by β-Glucan, and, consequently, contributed to elevated TNF-α and IL-6 responses after restimulation with LPS in human monocytes. Mevalonate-driven constitutive trained immunity was further proposed to contribute to recurrent episodes of inflammatory symptoms in patients suffering from the hyper immunoglobulin D syndrome (HIDS), which is characterized by mevalonate kinase deficiency (MKD) accompanied by elevated mevalonate levels (Bekkering et al., 2018). Interestingly, others proposed that the decrease in isoprenoids rather than the increase in mevalonate underlies the hyper-inflammatory syndrome in HIDS (Politiek and Waterham, 2021).
The hydrophobicity-increasing prenylation of members of the Ras superfamily of small GTPases by the peroxisomally produced GGPP or FPP is essential for their functionality and localization, and, thus, directly affects cytoskeletal organization, receptor trafficking, and, consequently, numerous communication processes (Wang and Casey, 2016). Nevertheless, the role of isoprenoids in inflammation remains rather obscure. Some studies observed pro-inflammatory effects, due to enhanced type I or type II IFN signaling in response to GGPP (Veillard et al., 2006; Koike et al., 2021). In contrast, supplementation of the GGPP-precursor geranylgeraniol (GGOH) reduced pro-inflammatory cytokines after LPS stimulation in vitro and in vivo (Giriwono et al., 2013; Giriwono et al., 2019), suggestive for anti-inflammatory properties of GGPP. Accordingly, pharmacological inhibition of FPP synthase reduced FFP and GGPP levels, and concomitantly increased inflammatory cytokine expression in LPS-stimulated murine macrophages, which again could be reversed by the addition of GGOH (Tricarico et al., 2014). These findings substantiate the above-mentioned notion that the lack of isoprenoids might be accountable for hyper-inflammatory episodes in HIDS (Politiek and Waterham, 2021).
Cholesterol biosynthesis intermediates downstream of the isoprenoid pathway for the most part bear anti-inflammatory characteristics. In line, squalene, derived from the condensation of two FPP molecules, dampened NF-κB signaling and pro-inflammatory cytokine expression after LPS stimulation in monocytes and neutrophils as demonstrated by supplementation studies (Cárdeno et al., 2015).
Similarly, lanosterol accumulation, induced by pharmacological inhibition or knockdown of its metabolizing enzyme cytochrome P450 family 51 subfamily A member 1 (CYP51A1), reduced the expression of ISGs and pro-inflammatory cytokines after LPS treatment of murine macrophages (Araldi et al., 2017). Reduced lanosterol levels in mice transgenically overexpressing 3β-hydroxysterol Δ24-reductase (DHCR24), further correlated with progression of atherosclerosis, as well as with enhanced type I IFN responses and NLRP3-dependent inflammasome activation (Zhang et al., 2021). Of note, the authors characterized this pro-inflammatory phenotype mostly with respect to equally reduced desmosterol levels, yet, indicated that reduced lanosterol levels might also be involved.
Desmosterol is the direct precursor of cholesterol in the Bloch pathway. It was described to exert mostly anti-inflammatory functions by activating LXR. Accordingly, the above-described atherosclerosis-promoting phenotype in mice overexpressing DHCR24 in myeloid cells was proposed to result from the depletion of desmosterol, leading to impaired LXR activation and mitochondrial reactive oxygen species formation (Zhang et al., 2021). Along the same lines, DHCR24 inhibition and concomitant desmosterol accumulation, led to LXR-mediated synthesis of polyunsaturated fatty acids, which supported an anti-inflammatory and pro-resolving macrophage phenotype in a murine peritonitis model (Körner et al., 2019). Similarly, desmosterol-induced LXR activation in microglia contributed to inflammation resolution in multiple sclerosis (Berghoff et al., 2021). Interestingly, desmosterol accumulation was also observed in atherosclerotic foam cells, where it unexpectedly also suppressed inflammatory gene expression (Spann et al., 2012).
In contrast, 7-DHC, the direct precursor of cholesterol in the Kandutsch-Russell pathway, was shown to exert pro-inflammatory functions. Specifically, elevated cellular 7-DHC levels, due to deficiency of 7-DHC reductase (DHCR7) or exogenous supplementation of 7-DHC, activated PI3K/AKT3, which contributed to increased IRF3 phosphorylation and type I IFN responses upon viral infections (Xiao et al., 2020).
Oxysterols are well-known for their anti-viral properties by influencing viral entry and replication via alterations of membrane compositions (Lembo et al., 2016; Foo et al., 2022). In addition, oxysterols were shown to induce pro- but also anti-inflammatory immune responses. Most of the anti-inflammatory effects of oxysterols, e.g., 25-hydroxycholesterol (25-HC), are mediated by activation of LXR (Ma and Nelson, 2019), but further LXR-independent functions were described. For instance, 25-HC accumulation restricted AIM2 inflammasome activation and IL-1β secretion in macrophages by reducing ER cholesterol levels and associated mitochondrial damage (Dang et al., 2017). In contrast, increased 25-HC levels enhanced pro-inflammatory responses via an activator protein-1 (AP-1)-dependent increase in TLR expression in macrophages in the context of viral infections (Gold et al., 2014). As the present mini-review aims to provide a quick overview of the role of various cholesterol biosynthesis intermediates in inflammation, readers specifically interested in the role of the more extensively studied oxysterols are referred to comprehensive reviews (Bah et al., 2017; de Freitas et al., 2022).
Conclusively, due to the complexity and highly dynamic regulation of cholesterol biosynthesis, which encompasses numerous feedback loops (Chen et al., 2019), characterizing the exact role of individual sterol precursors remains challenging as supplementation or interference with specific enzymes commonly affects a broad spectrum of other intermediates.
Infections with severe acute respiratory syndrome coronavirus 2 (SARS-CoV-2) induce massive inflammatory responses. In severe cases of the resulting coronavirus disease 2019 (COVID-19), inflammatory events progress to a systemic inflammatory disease syndrome (SIRS), which correlates with poor disease prognosis (Jin et al., 2021). Importantly, while anti-viral, inflammatory responses are generally beneficial in early phases of the infection, development of overshooting systemic inflammatory responses, i.e., a cytokine storm, associated with excess production of pro-inflammatory cytokines, including type I and II IFNs, IL-1β, IL-6, IL-12, IL-18, and TNF-α, and chemokines, including C-X-C motif chemokine ligand 8 (CXCL8), CXCL9, CXCL10, CXCL11, C-C motif chemokine ligand 2 (CCL2), and CCL5, is rather detrimental (Coperchini et al., 2021). Interestingly, enhanced SREBP2 activity, indicative for elevated cellular cholesterol synthesis and biosynthetic flux, was found to correlate with disease severity in COVID-19 patients, and further directly associated with the development of a systemic cytokine storm (Lee et al., 2020). Paradoxically, lipoprotein-bound as well as total cholesterol levels in plasma appear to be reduced in COVID-19 patients, and low LDL- and HDL-cholesterol concentrations predict enhanced disease severity as well as mortality (Chidambaram et al., 2022). The apparent discrepancy between plasma cholesterol levels and SREBP2 activity in severe cases of COVID-19, might serve as an indicator for a generally disturbed cholesterol homeostasis. Presumably, this would result in an intracellular cholesterol overload, predicted to fuel pro-inflammatory responses (Dang and Cyster, 2019). This concept is corroborated by the recent finding that all classes of sterols (oxysterols and intermediates) were increased in extracellular vesicles (EVs) of COVID-19 patients during the hyper-inflammatory phase of the disease (Lam et al., 2021). Since EVs are not only important for intercellular communication (Grieco et al., 2021), but supposedly provide an accessible window into intracellular changes on a systemic level, these observations indeed hint towards increased SREBP2 activity. Considering the elevated levels of SREBP2-inhibitory 25-HC previously shown in response to viral infections (Reboldi et al., 2014), an increased cholesterol biosynthetic flux in the hyper-inflammatory phase of viral infections appears rather surprising. Nevertheless, the observation that the intermediates declined again in the EVs during the early resolution phase, agree with a dynamic response to increased 25-HC levels during the hyper-inflammatory phase (Lam et al., 2021). Taking the highly intertwined cholesterol dynamics into account, the success of therapeutic approaches interfering with cholesterol homeostasis in COVID-19 patients might be highly dependent on the exact disease stage. In fact, plasma EVs and selected cholesterol intermediates therein might be considered as an option to guide the appropriate timing of cholesterol-targeted therapeutic interventions. Of note, the HMG-CoA reductase inhibitor simvastatin was recently shown to reduce viral entry, but also to attenuate the expression of pro-inflammatory mediators in cells already infected by a virus (Teixeira et al., 2022). Furthermore, administration of 25-HC-containing nanovesicles effectively inhibited SREBP2 activity and reduced pro-inflammatory cytokines in COVID-19 patient derived immune cells (Kim et al., 2021).
Conclusively, we speculate that the cholesterol biosynthetic flux and/or levels of certain intermediates or cholesterol itself, as well as their intracellular distribution, might be directly involved in the systemic inflammatory progress of severe COVID-19 cases. This might provide novel opportunities for the development of molecular-targeted, disease stage-tailored interventions for COVID-19 patients. Specifically, while general cholesterol lowering measures bear protective potential during early stages of the disease by attenuating viral entry, the multi-facetted, partially contradictory effects of cholesterol and its biosynthetic intermediates likely require more sophisticated interventions strategies to prevent the detrimental hyper-inflammatory systemic course of the disease.
RB, BB, and TS wrote and edited the manuscript. All authors contributed to the article and approved the submitted version.
This work was supported by the grants of the DFG (GRK 2336 TP6, SCHM2663/7, SFB1039 B04, BR999/25-1) and the Goethe Corona Fonds.
The authors declare that the research was conducted in the absence of any commercial or financial relationships that could be construed as a potential conflict of interest.
All claims expressed in this article are solely those of the authors and do not necessarily represent those of their affiliated organizations, or those of the publisher, the editors and the reviewers. Any product that may be evaluated in this article, or claim that may be made by its manufacturer, is not guaranteed or endorsed by the publisher.
Araldi, E., Fernández-Fuertes, M., Canfrán-Duque, A., Tang, W., Cline, G. W., Madrigal-Matute, J., et al. (2017). Lanosterol modulates TLR4-mediated innate immune responses in macrophages. Cell Rep. 19, 2743–2755. doi:10.1016/j.celrep.2017.05.093
Bah, S. Y., Dickinson, P., Forster, T., Kampmann, B., and Ghazal, P. (2017). Immune oxysterols: Role in mycobacterial infection and inflammation. J. Steroid Biochem. Mol. Biol. 169, 152–163. doi:10.1016/j.jsbmb.2016.04.015
Bekkering, S., Arts, R. J. W., Novakovic, B., Kourtzelis, I., van der Heijden, C. D. C. C., Li, Y., et al. (2018). Metabolic induction of trained immunity through the mevalonate pathway. Cell 172, 135–146.e9. doi:10.1016/j.cell.2017.11.025
Berghoff, S. A., Spieth, L., Sun, T., Hosang, L., Schlaphoff, L., Depp, C., et al. (2021). Microglia facilitate repair of demyelinated lesions via post-squalene sterol synthesis. Nat. Neurosci. 24, 47–60. doi:10.1038/s41593-020-00757-6
Bilotta, M. T., Petillo, S., Santoni, A., and Cippitelli, M. (2020). Liver X receptors: Regulators of cholesterol metabolism, inflammation, autoimmunity, and cancer. Front. Immunol. 11, 584303. doi:10.3389/fimmu.2020.584303
Brown, M. S., and Goldstein, J. L. (1986). A receptor-mediated pathway for cholesterol homeostasis. Science 232, 34–47. doi:10.1126/science.3513311
Cárdeno, A., Aparicio-Soto, M., Montserrat-de la Paz, S., Bermudez, B., Muriana, F. J. G., and Alarcón-de-la-Lastra, C. (2015). Squalene targets pro- and anti-inflammatory mediators and pathways to modulate over-activation of neutrophils, monocytes and macrophages. J. Funct. Foods 14, 779–790. doi:10.1016/j.jff.2015.03.009
Charles, K. N., Shackelford, J. E., Faust, P. L., Fliesler, S. J., Stangl, H., and Kovacs, W. J. (2020). Functional peroxisomes are essential for efficient cholesterol sensing and synthesis. Front. Cell Dev. Biol. 8, 560266. doi:10.3389/fcell.2020.560266
Chen, W., Li, S., Yu, H., Liu, X., Huang, L., Wang, Q., et al. (2016). ER adaptor SCAP translocates and recruits IRF3 to perinuclear microsome induced by cytosolic microbial DNAs. PLoS Pathog. 12, e1005462. doi:10.1371/journal.ppat.1005462
Chen, L., Ma, M.-Y., Sun, M., Jiang, L.-Y., Zhao, X.-T., Fang, X.-X., et al. (2019). Endogenous sterol intermediates of the mevalonate pathway regulate HMGCR degradation and SREBP-2 processing. J. Lipid Res. 60, 1765–1775. doi:10.1194/jlr.RA119000201
Cheng, A. M., Handa, P., Tateya, S., Schwartz, J., Tang, C., Mitra, P., et al. (2012). Apolipoprotein A-I attenuates palmitate-mediated NF-κB activation by reducing toll-like receptor-4 recruitment into lipid rafts. PLoS ONE 7, e33917. doi:10.1371/journal.pone.0033917
Chidambaram, V., Shanmugavel Geetha, H., Kumar, A., Majella, M. G., Sivakumar, R. K., Voruganti, D., et al. (2022). Association of lipid levels with COVID-19 infection, disease severity and mortality: A systematic review and meta-analysis. Front. Cardiovasc. Med. 9, 862999. doi:10.3389/fcvm.2022.862999
Choudhury, R. P., Lee, J. M., and Greaves, D. R. (2005). Mechanisms of disease: Macrophage-derived foam cells emerging as therapeutic targets in atherosclerosis. Nat. Rev. Cardiol. 2, 309–315. doi:10.1038/ncpcardio0195
Coperchini, F., Chiovato, L., Ricci, G., Croce, L., Magri, F., and Rotondi, M. (2021). The cytokine storm in COVID-19: Further advances in our understanding the role of specific chemokines involved. Cytokine & Growth Factor Rev. 58, 82–91. doi:10.1016/j.cytogfr.2020.12.005
Dang, E. V., and Cyster, J. G. (2019). Loss of sterol metabolic homeostasis triggers inflammasomes — how and why. Curr. Opin. Immunol. 56, 1–9. doi:10.1016/j.coi.2018.08.001
Dang, E. V., McDonald, J. G., Russell, D. W., and Cyster, J. G. (2017). Oxysterol restraint of cholesterol synthesis prevents AIM2 inflammasome activation. Cell 171, 1057–1071.e11. doi:10.1016/j.cell.2017.09.029
de Freitas, F. A., Levy, D., Reichert, C. O., Cunha-Neto, E., Kalil, J., and Bydlowski, S. P. (2022). Effects of oxysterols on immune cells and related diseases. Cells 11, 1251. doi:10.3390/cells11081251
de la Roche, M., Hamilton, C., Mortensen, R., Jeyaprakash, A. A., Ghosh, S., and Anand, P. K. (2018). Trafficking of cholesterol to the ER is required for NLRP3 inflammasome activation. J. Cell Biol. 217, 3560–3576. doi:10.1083/jcb.201709057
De Nardo, D., Labzin, L. I., Kono, H., Seki, R., Schmidt, S. V., Beyer, M., et al. (2014). High-density lipoprotein mediates anti-inflammatory reprogramming of macrophages via the transcriptional regulator ATF3. Nat. Immunol. 15, 152–160. doi:10.1038/ni.2784
Duewell, P., Kono, H., Rayner, K. J., Sirois, C. M., Vladimer, G., Bauernfeind, F. G., et al. (2010). NLRP3 inflammasomes are required for atherogenesis and activated by cholesterol crystals. Nature 464, 1357–1361. doi:10.1038/nature08938
Fessler, M. B. (2016). The intracellular cholesterol landscape: Dynamic integrator of the immune response. Trends Immunol. 37, 819–830. doi:10.1016/j.it.2016.09.001
Fontaine, C., Rigamonti, E., Nohara, A., Gervois, P., Teissier, E., Fruchart, J.-C., et al. (2007). Liver X receptor activation potentiates the lipopolysaccharide response in human macrophages. Circ. Res. 101, 40–49. doi:10.1161/CIRCRESAHA.106.135814
Foo, C. X., Bartlett, S., and Ronacher, K. (2022). Oxysterols in the immune response to bacterial and viral infections. Cells 11, 201. doi:10.3390/cells11020201
Fotakis, P., Kothari, V., Thomas, D. G., Westerterp, M., Molusky, M. M., Altin, E., et al. (2019). Anti-inflammatory effects of HDL (High-Density lipoprotein) in macrophages predominate over proinflammatory effects in atherosclerotic plaques. Arterioscler. Thromb. Vasc. Biol. 39, e253–e272. doi:10.1161/ATVBAHA.119.313253
Giriwono, P. E., Shirakawa, H., Ohsaki, Y., Hata, S., Kuriyama, H., Sato, S., et al. (2013). Dietary supplementation with geranylgeraniol suppresses lipopolysaccharide-induced inflammation via inhibition of nuclear factor-κB activation in rats. Eur. J. Nutr. 52, 1191–1199. doi:10.1007/s00394-012-0429-y
Giriwono, P. E., Shirakawa, H., Ohsaki, Y., Sato, S., Aoyama, Y., Ho, H.-J., et al. (2019). Geranylgeraniol suppresses the expression of IRAK1 and TRAF6 to inhibit NFκB activation in lipopolysaccharide-induced inflammatory responses in human macrophage-like cells. Int. J. Mol. Sci. 20, E2320. doi:10.3390/ijms20092320
Gold, E. S., Diercks, A. H., Podolsky, I., Podyminogin, R. L., Askovich, P. S., Treuting, P. M., et al. (2014). 25-Hydroxycholesterol acts as an amplifier of inflammatory signaling. Proc. Natl. Acad. Sci. U. S. A. 111, 10666–10671. doi:10.1073/pnas.1404271111
Grieco, G. E., Fignani, D., Formichi, C., Nigi, L., Licata, G., Maccora, C., et al. (2021). Extracellular vesicles in immune system regulation and type 1 diabetes: Cell-to-Cell communication mediators, disease biomarkers, and promising therapeutic tools. Front. Immunol. 12, 682948. doi:10.3389/fimmu.2021.682948
Gui, Y., Zheng, H., and Cao, R. Y. (2022). Foam cells in atherosclerosis: Novel insights into its origins, consequences, and molecular mechanisms. Front. Cardiovasc. Med. 9, 845942. doi:10.3389/fcvm.2022.845942
Guo, C., Chi, Z., Jiang, D., Xu, T., Yu, W., Wang, Z., et al. (2018). Cholesterol homeostatic regulator SCAP-SREBP2 integrates NLRP3 inflammasome activation and cholesterol biosynthetic signaling in macrophages. Immunity 49, 842–856.e7. doi:10.1016/j.immuni.2018.08.021
Halimi, H., and Farjadian, S. (2022). Cholesterol: An important actor on the cancer immune scene. Front. Immunol. 13, 1057546. doi:10.3389/fimmu.2022.1057546
Hayakawa, S., Tamura, A., Nikiforov, N., Koike, H., Kudo, F., Cheng, Y., et al. (2022). Activated cholesterol metabolism is integral for innate macrophage responses by amplifying Myd88 signaling. JCI Insight 7, e138539. doi:10.1172/jci.insight.138539
Iacano, A. J., Lewis, H., Hazen, J. E., Andro, H., Smith, J. D., and Gulshan, K. (2019). Miltefosine increases macrophage cholesterol release and inhibits NLRP3-inflammasome assembly and IL-1β release. Sci. Rep. 9, 11128. doi:10.1038/s41598-019-47610-w
Janowski, B. A., Willy, P. J., Devi, T. R., Falck, J. R., and Mangelsdorf, D. J. (1996). An oxysterol signalling pathway mediated by the nuclear receptor LXR alpha. Nature 383, 728–731. doi:10.1038/383728a0
Jin, J., Qian, H., Wan, B., Zhou, L., Chen, C., Lv, Y., et al. (2021). Geranylgeranyl diphosphate synthase deficiency hyperactivates macrophages and aggravates lipopolysaccharide-induced acute lung injury. Am. J. Physiol.-Lung Cell. Mol. Physiol. 320, L1011–L1024. doi:10.1152/ajplung.00281.2020
Kim, H., Lee, H. S., Ahn, J. H., Hong, K. S., Jang, J. G., An, J., et al. (2021). Lung-selective 25-hydroxycholesterol nanotherapeutics as a suppressor of COVID-19-associated cytokine storm. Nano Today 38, 101149. doi:10.1016/j.nantod.2021.101149
Köberlin, M. S., Heinz, L. X., and Superti-Furga, G. (2016). Functional crosstalk between membrane lipids and TLR biology. Curr. Opin. Cell Biol. 39, 28–36. doi:10.1016/j.ceb.2016.01.010
Koike, A., Tsujinaka, K., and Fujimori, K. (2021). Statins attenuate antiviral IFN-β and ISG expression via inhibition of IRF3 and JAK/STAT signaling in poly(I:C)-treated hyperlipidemic mice and macrophages. FEBS J. 288, 4249–4266. doi:10.1111/febs.15712
Körner, A., Zhou, E., Müller, C., Mohammed, Y., Herceg, S., Bracher, F., et al. (2019). Inhibition of Δ24-dehydrocholesterol reductase activates pro-resolving lipid mediator biosynthesis and inflammation resolution. Proc. Natl. Acad. Sci. U. S. A. 116, 20623–20634. doi:10.1073/pnas.1911992116
Kusnadi, A., Park, S. H., Yuan, R., Pannellini, T., Giannopoulou, E., Oliver, D., et al. (2019). The cytokine TNF promotes transcription factor SREBP activity and binding to inflammatory genes to activate macrophages and limit tissue repair. Immunity 51, 241–257.e9. doi:10.1016/j.immuni.2019.06.005
Lam, S. M., Zhang, C., Wang, Z., Ni, Z., Zhang, S., Yang, S., et al. (2021). A multi-omics investigation of the composition and function of extracellular vesicles along the temporal trajectory of COVID-19. Nat. Metab. 3, 909–922. doi:10.1038/s42255-021-00425-4
Lee, M.-S., and Bensinger, S. J. (2022). Reprogramming cholesterol metabolism in macrophages and its role in host defense against cholesterol-dependent cytolysins. Cell Mol. Immunol. 19, 327–336. doi:10.1038/s41423-021-00827-0
Lee, W., Ahn, J. H., Park, H. H., Kim, H. N., Kim, H., Yoo, Y., et al. (2020). COVID-19-activated SREBP2 disturbs cholesterol biosynthesis and leads to cytokine storm. Signal Transduct. Target Ther. 5, 186. doi:10.1038/s41392-020-00292-7
Lembo, D., Cagno, V., Civra, A., and Poli, G. (2016). Oxysterols: An emerging class of broad spectrum antiviral effectors. Mol. Aspects Med. 49, 23–30. doi:10.1016/j.mam.2016.04.003
Li, Y., Schwabe, R. F., DeVries-Seimon, T., Yao, P. M., Gerbod-Giannone, M.-C., Tall, A. R., et al. (2005). Free cholesterol-loaded macrophages are an abundant source of tumor necrosis factor-alpha and interleukin-6: Model of NF-kappaB- and map kinase-dependent inflammation in advanced atherosclerosis. J. Biol. Chem. 280, 21763–21772. doi:10.1074/jbc.M501759200
Luo, J., Yang, H., and Song, B.-L. (2020). Mechanisms and regulation of cholesterol homeostasis. Nat. Rev. Mol. Cell Biol. 21, 225–245. doi:10.1038/s41580-019-0190-7
Ma, L., and Nelson, E. R. (2019). Oxysterols and nuclear receptors. Mol. Cell. Endocrinol. 484, 42–51. doi:10.1016/j.mce.2019.01.016
Mathis, D., and Shoelson, S. E. (2011). Immunometabolism: an emerging frontier. Nat. Rev. Immunol. 11, 81. doi:10.1038/nri2922
Ménégaut, L., Thomas, C., Jalil, A., Julla, J. B., Magnani, C., Ceroi, A., et al. (2020). Interplay between liver X receptor and hypoxia inducible factor 1α potentiates interleukin-1β production in human macrophages. Cell Rep. 31, 107665. doi:10.1016/j.celrep.2020.107665
O’Hagan, R., Berg, A. R., Hong, C. G., Parel, P. M., Mehta, N. N., and Teague, H. L. (2022). Systemic consequences of abnormal cholesterol handling: Interdependent pathways of inflammation and dyslipidemia. Front. Immunol. 13, 972140. doi:10.3389/fimmu.2022.972140
Pfeffer, S. R. (2019). NPC intracellular cholesterol transporter 1 (NPC1)-mediated cholesterol export from lysosomes. J. Biol. Chem. 294, 1706–1709. doi:10.1074/jbc.TM118.004165
Politiek, F. A., and Waterham, H. R. (2021). Compromised protein prenylation as pathogenic mechanism in mevalonate kinase deficiency. Front. Immunol. 12, 724991. doi:10.3389/fimmu.2021.724991
Reboldi, A., Dang, E. V., McDonald, J. G., Liang, G., Russell, D. W., and Cyster, J. G. (2014). Inflammation. 25-Hydroxycholesterol suppresses interleukin-1-driven inflammation downstream of type I interferon. Science 345, 679–684. doi:10.1126/science.1254790
Robertson, K. A., and Ghazal, P. (2016). Interferon control of the sterol metabolic network: Bidirectional molecular circuitry-mediating host protection. Front. Immunol. 7, 634. doi:10.3389/fimmu.2016.00634
Ruysschaert, J.-M., and Lonez, C. (2015). Role of lipid microdomains in TLR-mediated signalling. Biochim. Biophys. Acta (BBA) - Biomembr. 1848, 1860–1867. doi:10.1016/j.bbamem.2015.03.014
Sakai, J., Duncan, E. A., Rawson, R. B., Hua, X., Brown, M. S., and Goldstein, J. L. (1996). Sterol-regulated release of SREBP-2 from cell membranes requires two sequential cleavages, one within a transmembrane segment. Cell 85, 1037–1046. doi:10.1016/s0092-8674(00)81304-5
Salami, J. A., Warraich, H., Valero-Elizondo, J., Spatz, E. S., Desai, N. R., Rana, J. S., et al. (2017). National trends in statin use and expenditures in the US adult population from 2002 to 2013: Insights from the medical expenditure panel survey. JAMA Cardiol. 2, 56–65. doi:10.1001/jamacardio.2016.4700
Smoak, K. A., Aloor, J. J., Madenspacher, J., Merrick, B. A., Collins, J. B., Zhu, X., et al. (2010). Myeloid differentiation primary response protein 88 couples reverse cholesterol transport to inflammation. Cell Metab. 11, 493–502. doi:10.1016/j.cmet.2010.04.006
Song, Y., Liu, J., Zhao, K., Gao, L., and Zhao, J. (2021). Cholesterol-induced toxicity: An integrated view of the role of cholesterol in multiple diseases. Cell Metab. 33, 1911–1925. doi:10.1016/j.cmet.2021.09.001
Spann, N. J., Garmire, L. X., McDonald, J. G., Myers, D. S., Milne, S. B., Shibata, N., et al. (2012). Regulated accumulation of desmosterol integrates macrophage lipid metabolism and inflammatory responses. Cell 151, 138–152. doi:10.1016/j.cell.2012.06.054
Sun, Y., Ishibashi, M., Seimon, T., Lee, M., Sharma, S. M., Fitzgerald, K. A., et al. (2009). Free cholesterol accumulation in macrophage membranes activates Toll-like receptors and p38 mitogen-activated protein kinase and induces cathepsin K. Circ. Res. 104, 455–465. doi:10.1161/CIRCRESAHA.108.182568
Tabas, I. (2002). Cholesterol in health and disease. J. Clin. Invest. 110, 583–590. doi:10.1172/JCI16381
Tall, A. R., and Yvan-Charvet, L. (2015). Cholesterol, inflammation and innate immunity. Nat. Rev. Immunol. 15, 104–116. doi:10.1038/nri3793
Teixeira, L., Temerozo, J. R., Pereira-Dutra, F. S., Ferreira, A. C., Mattos, M., Gonçalves, B. S., et al. (2022). Simvastatin downregulates the SARS-CoV-2-induced inflammatory response and impairs viral infection through disruption of lipid rafts. Front. Immunol. 13, 820131. doi:10.3389/fimmu.2022.820131
Tricarico, P. M., Kleiner, G., Valencic, E., Campisciano, G., Girardelli, M., Crovella, S., et al. (2014). Block of the mevalonate pathway triggers oxidative and inflammatory molecular mechanisms modulated by exogenous isoprenoid compounds. Int. J. Mol. Sci. 15, 6843–6856. doi:10.3390/ijms15046843
Tsao, C. W., Aday, A. W., Almarzooq, Z. I., Alonso, A., Beaton, A. Z., Bittencourt, M. S., et al. (2022). Heart disease and stroke statistics—2022 update: A report from the American heart association. Circulation 145, e153–e639. doi:10.1161/CIR.0000000000001052
van der Vorst, E. P. C., Theodorou, K., Wu, Y., Hoeksema, M. A., Goossens, P., Bursill, C. A., et al. (2017). High-density lipoproteins exert pro-inflammatory effects on macrophages via passive cholesterol depletion and PKC-NF-κB/STAT1-IRF1 signaling. Cell Metab. 25, 197–207. doi:10.1016/j.cmet.2016.10.013
Veillard, N. R., Braunersreuther, V., Arnaud, C., Burger, F., Pelli, G., Steffens, S., et al. (2006). Simvastatin modulates chemokine and chemokine receptor expression by geranylgeranyl isoprenoid pathway in human endothelial cells and macrophages. Atherosclerosis 188, 51–58. doi:10.1016/j.atherosclerosis.2005.10.015
Wang, M., and Casey, P. J. (2016). Protein prenylation: unique fats make their mark on biology. Nat. Rev. Mol. Cell Biol. 17, 110–122. doi:10.1038/nrm.2015.11
Wang, B., and Tontonoz, P. (2018). Liver X receptors in lipid signalling and membrane homeostasis. Nat. Rev. Endocrinol. 14, 452–463. doi:10.1038/s41574-018-0037-x
Westerterp, M., Gautier, E. L., Ganda, A., Molusky, M. M., Wang, W., Fotakis, P., et al. (2017). Cholesterol accumulation in dendritic cells links the inflammasome to acquired immunity. Cell Metab. 25, 1294–1304.e6. doi:10.1016/j.cmet.2017.04.005
Xiao, J., Li, W., Zheng, X., Qi, L., Wang, H., Zhang, C., et al. (2020). Targeting 7-dehydrocholesterol reductase integrates cholesterol metabolism and IRF3 activation to eliminate infection. Immunity 52, 109–122.e6. doi:10.1016/j.immuni.2019.11.015
Yang, C., McDonald, J. G., Patel, A., Zhang, Y., Umetani, M., Xu, F., et al. (2006). Sterol intermediates from cholesterol biosynthetic pathway as liver X receptor ligands. J. Biol. Chem. 281, 27816–27826. doi:10.1074/jbc.M603781200
York, A. G., Williams, K. J., Argus, J. P., Zhou, Q. D., Brar, G., Vergnes, L., et al. (2015). Limiting cholesterol biosynthetic flux spontaneously engages type I IFN signaling. Cell 163, 1716–1729. doi:10.1016/j.cell.2015.11.045
Yu, X.-H., and Tang, C.-K. (2022). “ABCA1, ABCG1, and cholesterol homeostasis,” in HDL metabolism and diseases. Advances in experimental medicine and biology. Editor L. Zheng (Singapore: Springer Nature Singapore), 95–107. doi:10.1007/978-981-19-1592-5_7
Yvan-Charvet, L., Welch, C., Pagler, T. A., Ranalletta, M., Lamkanfi, M., Han, S., et al. (2008). Increased inflammatory gene expression in ABC transporter-deficient macrophages: free cholesterol accumulation, increased signaling via toll-like receptors, and neutrophil infiltration of atherosclerotic lesions. Circulation 118, 1837–1847. doi:10.1161/CIRCULATIONAHA.108.793869
Zhang, X., McDonald, J. G., Aryal, B., Canfrán-Duque, A., Goldberg, E. L., Araldi, E., et al. (2021). Desmosterol suppresses macrophage inflammasome activation and protects against vascular inflammation and atherosclerosis. Proc. Natl. Acad. Sci. U.S.A. 118, e2107682118. doi:10.1073/pnas.2107682118
Zhu, X., Lee, J.-Y., Timmins, J. M., Brown, J. M., Boudyguina, E., Mulya, A., et al. (2008). Increased cellular free cholesterol in macrophage-specific Abca1 knock-out mice enhances pro-inflammatory response of macrophages. J. Biol. Chem. 283, 22930–22941. doi:10.1074/jbc.M801408200
Keywords: cholesterol, inflammation, immunometabolism, SARS-CoV-2, COVID-19
Citation: Bauer R, Brüne B and Schmid T (2023) Cholesterol metabolism in the regulation of inflammatory responses. Front. Pharmacol. 14:1121819. doi: 10.3389/fphar.2023.1121819
Received: 12 December 2022; Accepted: 11 January 2023;
Published: 20 January 2023.
Edited by:
Paola Patrignani, University of Studies G. d'Annunzio Chieti and Pescara, ItalyReviewed by:
Annalisa Contursi, University of Studies G. d'Annunzio Chieti and Pescara, ItalyCopyright © 2023 Bauer, Brüne and Schmid. This is an open-access article distributed under the terms of the Creative Commons Attribution License (CC BY). The use, distribution or reproduction in other forums is permitted, provided the original author(s) and the copyright owner(s) are credited and that the original publication in this journal is cited, in accordance with accepted academic practice. No use, distribution or reproduction is permitted which does not comply with these terms.
*Correspondence: Tobias Schmid, dC5zY2htaWRAYmlvY2hlbS51bmktZnJhbmtmdXJ0LmRl
Disclaimer: All claims expressed in this article are solely those of the authors and do not necessarily represent those of their affiliated organizations, or those of the publisher, the editors and the reviewers. Any product that may be evaluated in this article or claim that may be made by its manufacturer is not guaranteed or endorsed by the publisher.
Research integrity at Frontiers
Learn more about the work of our research integrity team to safeguard the quality of each article we publish.