- 1State Key Laboratory of Medical Neurobiology, MOE Frontiers Center for Brain Science, Department of Pharmacology, School of Basic Medical Sciences, Institutes of Brain Science, Fudan University, Shanghai, China
- 2Anhui Provincial Engineering Research Center for Polysaccharide Drugs, Provincial Engineering Laboratory for Screening and Re-evaluation of Active Compounds of Herbal Medicines in Southern Anhui, School of Pharmacy, Wannan Medical College, Wuhu, China
- 3International Institute for Integrative Sleep Medicine (WPI-IIIS) and Faculty of Medicine, University of Tsukuba, Tsukuba, Ibaraki, Japan
Sleep, torpor, and hibernation are three distinct hypometabolic states. However, they have some similar physiological features, such as decreased core body temperature and slowing heart rate. In addition, the accumulation of adenosine seems to be a common feature before entry into these three states, suggesting that adenosine and its receptors, also known as P1 receptors, may mediate the initiation and maintenance of these states. This review, therefore, summarizes the current research on the roles and possible neurobiological mechanisms of adenosine and P1 receptors in sleep, torpor, and hibernation. Understanding these aspects will give us better prospects in sleep disorders, therapeutic hypothermia, and aerospace medicine.
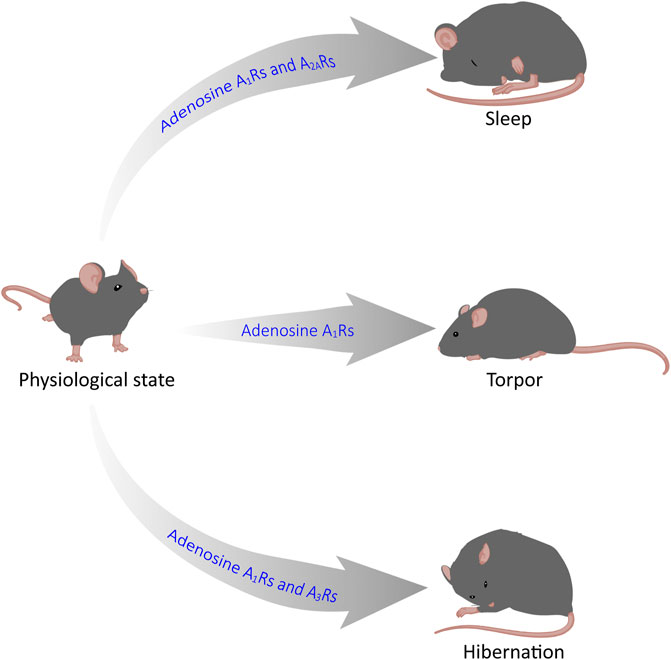
GRAPHICAL ABSTRACT | Adenosine mediates sleep, torpor and hibernation through P1 receptors. Recent reasearch has shown that P1 receptors play a vital role in the regulation of sleep-wake, torpor and hibernation-like states. In this review, we focus on the roles and neurobiological mechanisms of the CNS adenosine and P1 receptors in these three states. Among them, A1 and A2A receptors are key targets for sleep-wake regulation, A1Rs and A3Rs are very important for torpor induction, and activation of A1Rs is sufficient for hibernation-like state.
1 Introduction
Sleep, torpor, and hibernation are three distinct states which can reduce energy expenditure. Sleep, which takes up nearly one-third lifetime of most mammals and birds, is divided into rapid eye movement (REM) sleep and non-REM (NREM) sleep. REM sleep is characterized by phasic changes in various autonomic functions and an elevation in metabolic rate. However, NREM sleep is characterized by the organism’s active contact with the environment and by a decrease in metabolism, body temperature (Tb), and energy expenditure (Silvani and Dampney, 2013; Schmidt, 2014; Silvani et al., 2018). Torpor is an energy-saving strategy in most mammals and birds, sometimes lasting only for a few hours, that helps organisms cope with the stress of an adverse environment (Ruf and Geiser, 2015). Just like NREM sleep, torpor state occurs with a reduction in Tb and metabolic rate (Ruf and Geiser, 2015). Hibernation, also called multi-day torpor, is a seasonal energy conservation strategy that reduces Tb, energy expenditure, and water loss (Geiser, 2013; Ruf and Geiser, 2015). Most hibernators generally remain in hibernation for a winter, which helps them effectively withstand the cold environment.
Adenosine is a ubiquitous endogenous cell signal transducer and regulator, which mainly acts by activating 4 G protein-coupled receptors (GPCRs), namely, adenosine A1, A2A, A2B, and A3 receptors, as known as P1 receptors (Kazemzadeh-Narbat et al., 2015). Activation of A1 and A3 receptors exert inhibitory effects, however A2A and A2B exert excitatory. The four P1 receptors can reduce and increase the intracellular cyclic adenosine-3, 5 monophosphate (cAMP) concentration via inhibiting or activating adenylate cyclase (AC), which makes adenosine and P1 receptors essential for the regulation of energy balance (Chiu and Freund, 2014).
Sleep, torpor, and hibernation are integral to energy balance. At the same time, adenosine which is a homeostatic bioenergetic network regulator appears to accumulate before entry into the three states, suggesting that adenosine and P1 receptors, may mediate sleep, torpor and hibernation (Drew and Jinka, 2013; Silvani et al., 2018). Much evidence suggests that activation or inhibition of the central nervous system (CNS) adenosine receptors by genetic or pharmacological means can alter the states of sleep, torpor, and hibernation. In this review, we focus on the role of adenosine in the CNS and summarize the current research on the roles and possible biological mechanisms of adenosine and P1 receptors in sleep, torpor, and hibernation. This may help us solve many problems in the future, such as treating sleep disorders and using artificial hibernation for medical applications and space exploration.
2 Physiological characteristics during sleep, torpor, and hibernation
Sleep, torpor, and hibernation appear shallow to deep states of diminished body temperature and metabolic rate. Sleep is a relatively rapid and reversible state. However, the animals in a torpor state are more difficult to awaken than sleepers. They may not respond immediately to stimuli, while hibernators typically take an hour or more from hibernation to awakening (Siegel, 2009). Animals control the duration of torpor based on the circadian system, typically remaining dormant for only part of the day and returning to a physiological state when Tb rises to a consistently high level.
In contrast to torpor, hibernation lasts for days or weeks, and hibernators generally do not forage, relying mainly on early food storage or fat storage (Ruf and Geiser, 2015). Hibernation is not as common as daily sleep and torpor; only one-third of mammalian species are hibernators (Berger, 1984). Sleep, torpor, and hibernation are both energy-saving strategies for animals that share similar physiological characteristics and have their own characteristics (Table 1). An interesting commonality between sleep, torpor, and hibernation is the involvement of adenosine receptors. Adenosine is a purine nucleoside involved in many signaling pathways of energy homeostasis. One of the functions of sleep is to restore brain energy homeostasis, while the primary function of hibernation and torpor is to restore or protect body energy homeostasis (Drew and Jinka, 2013). According to many previous studies, adenosine A1 receptors and A2A receptors (A1Rs and A2ARs) play an essential role in inducing NREM, the activation of A1R and A3 receptors (A3Rs) may induce torpor (Silvani et al., 2018), and the onset of hibernation may be due to the activation of A1Rs (Jinka et al., 2011; Frare and Drew, 2021). In the following, we will briefly introduce the physiological characteristics of the three states and expand our review based on this.
2.1 Sleep
Most mammals and birds spend about one-third of their lives asleep, a quiet state in which humans or animals are less sensitive to their environment. Sleep is regulated by biological rhythms and neural loops and plays a vital role in the human body’s functional recovery, learning and memory, and growth and development. It is characterized by loss of consciousness, decreased Tb, metabolism, and a decrease in heart rate (HR) and blood pressure (BP). According to the characteristic electroencephalographic (EEG) patterns, sleep can be divided into NREM and REM sleep.
NREM and REM sleep occur alternately throughout sleep time, with NREM accounting for the majority of the sleep time (Silvani and Dampney, 2013; Schmidt, 2014; Silvani et al., 2018). NREM sleep shows decreased systemic function, regular breathing, HR, reduced energy consumption, an EEG that consisted mainly of slow waves, reduced muscle tension, but still a definite posture, with no noticeable eye changes. NREM sleep is divided into four stages. Stages Ⅰ and Ⅱ are light sleep, and stages Ⅲ and Ⅳ are deep sleep. During deep sleep, cellular metabolism can be promoted throughout the body, immunity can be strengthened, and energy depleted during the wake period can be restored (Silvani et al., 2018). REM sleep is characterized by rapid eye movement, loss of thermoregulation, EEG activity similar to waking, marked decrease or disappearance of muscle tension, muscle relaxation, but active neurons in most brain regions, increased cerebral blood flow, irregular breathing, and increased HR. During REM sleep, humans or animals maintain a relatively high level of vigilance, which is essential for animals to survive in nature (Roth, 2004; Schmidt, 2014).
2.2 Torpor
Torpor, a behavior that saves energy by reducing metabolic rate (MR), is often identical to sleep, which occurs daily or lasts for days, transitions into sleep (also called daily torpor), and is regulated by circadian rhythms (Berger, 1984). A drastic reduction of MR associated with a decrease in Tb results in the occurrence of torpor (Giroud et al., 2020). In addition, the autonomic nervous system is intimately involved in all stages of torpor. During an episode of torpor, the respiratory rate decreased, the HR related to ventilation increased periodically, and the decrease in ventilation was more significant than the MR, resulting in mild respiratory acidosis (Silvani et al., 2018).
A decrease in brain temperature usually accompanies the onset of torpor. If the brain temperature is above 25°C, EEG morphology and frequency during torpor are closest to the characteristics of NREM sleep. Then, both EEG amplitude and power decrease with decreasing Tb. When the brain temperature falls below 25°C, REM sleep gradually disappears, and when the temperature is between 10°C and 20°C, the animals alternate between long NREM sleep and short wakefulness. EEG becomes equipotential when the brain temperature is below 10°C, and it is impossible to determine alertness by electrophysiological methods (Ruf and Geiser, 2015; Ambler et al., 2021; Huang et al., 2021). When electromyography (EMG) was examined, EMG activity was found to decrease significantly with the inhibition of shivering thermogenesis, and a decrease of Tb when entering the state of torpor was observed (Huang et al., 2021). Daily torpor appears independent of ambient temperature (Ta), season, and nutritional status, as it can last only a few hours and is frequently interrupted by activity and foraging. Torpor can occur throughout the year, although it is more frequent in winter. However, in some species that live in warm climates, summer torpor is more common than winter torpor. Compared with waking, the metabolic rate drops to an average of about 30% of the basal metabolic rate (BMR) during torpor. The energy consumption is usually reduced by 10% to 80%, depending on the time and depth of torpor (Geiser, 2013).
2.3 Hibernation
Hibernation is a physiological adaptation that allows endothermic animals to cope with periodic limitations in their energy supply by lowering Tb and metabolism and improve their freezing tolerance, which may enable them to survive seasonal changes in the food supply and temperature reduction (Geiser, 2013; Storey and Storey, 2013; Storey and Storey, 2017). When the metabolic rate decreases during hibernation, ventilation decreases, and prolonged apnea occurs (Milsom and Jackson, 2011). During deep hibernation, the Tb of most mammals is near Ta. However, as Tb approaches the freezing, MR rises sharply, preventing tissue damage from increased heat production (Milsom and Jackson, 2011; Geiser, 2013). Hibernating species include facultative hibernators (hamsters, bats) and obligatory hibernators (ground squirrels, bears, and lemurs). Facultative hibernators are animals that go into hibernation only when they sense cold, lack of food, or photoperiodic changes. Obligatory hibernators are animals that go into hibernation spontaneously and punctually at a specific time of year, regardless of food availability or temperature (Xu et al., 2013; Mohr et al., 2020).
Hibernation is not an uninterrupted process over several months. With the rise of Ta and the accumulation of metabolites, spontaneous periodic awakening may occur and interrupt dormancy. After a brief awakening, the animal returns to dormancy and repeats the cycle of dormancy-awakening until the end of hibernation. This periodic awakening consumes most of the energy during hibernation. The onset of hibernation is highly dependent on temperature. When Ta is between 20°C and 30°C, some species still hibernate, but the duration is usually only a few hours, similar to daily torpor (Geiser, 2013; Ruf and Geiser, 2015; Mohr et al., 2020; Ambler et al., 2021). Gene transcription and translation are significantly inhibited during hibernation, and many other physiological parameters are significantly reduced and recover after awakening, such as HR, respiration, metabolic rate, and so on (Xu et al., 2013).
3 Sources and metabolic pathways of adenosine in the central nervous system
3.1 Source of adenosine
Intracellular adenosine is mainly produced through five pathways (Figure 1): 1) Adenosine triphosphate (ATP) loses two phosphate groups under the action of ATPase to become adenosine monophosphate (AMP), and AMP continues to lose the phosphate group under the action of an internal 5′-nucleotidase (5′-NT) to produce adenosine (Lopes et al., 2011). 2) Adenine reacts with 1-phosphate ribose to form adenosine (Hall and Frenguelli, 2018). 3) S-adenosylmethionine (SAM) and L-homocysteine produce S-adenosylhomocysteine (SAH) and further produce adenosine under the action of S-adenosylhomocysteine hydrolase (SAHH), but this pathway is not common in the CNS (Deussen et al., 1989; Latini and Pedata, 2001). 4) Extracellular adenosine is transported into the cell by the balanced nucleoside transporter in the cell membrane (Liu Y. J. et al., 2019). 5) cAMP is generated from ATP under the action of AC, which is regulated by GPCRs, and then converted through phosphodiesterases (PDEs) to AMP, which is eventually used to generate adenosine (Dos Santos-Rodrigues et al., 2015).
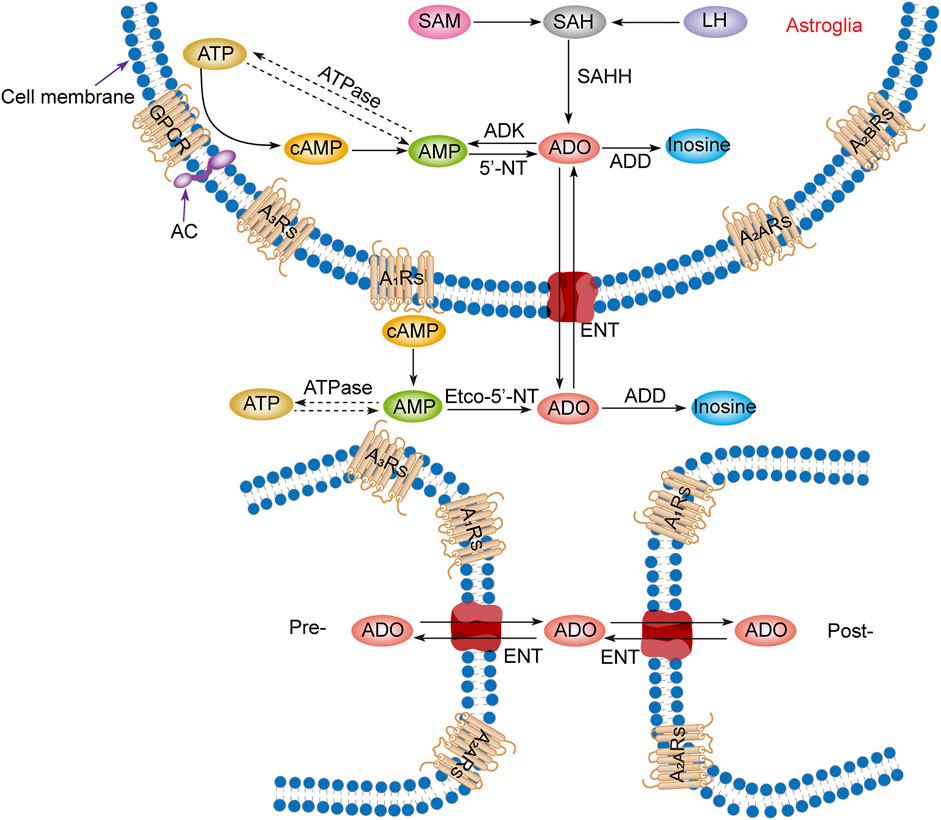
FIGURE 1. Adenosine metabolism and P1 receptors in the central nervous system. Adenosine metabolism occurs mainly in neuronal synapses and astrocytes. In cells, adenosine is formed from ATP, cAMP, or SAH. Extracellular adenosine is produced by ATP and cAMP metabolism but mainly by the balance of nucleoside transporters to regulate the concentration level inside and outside the membrane. SAM, S-adenosylmethionine; SAH, S-adenosyl homocysteine; LH, L-homocysteine; SAHH, S-adenosyl homocysteine hydrolase; ATP, adenosine triphosphate; ADP, adenosine diphosphate; AMP, adenosine monophosphate; cAMP, cyclic adenosine monophosphate; ADO, adenosine; ADD, adenosine deaminase; ADK, adenosine kinase; 5′-NT, 5′-nucleotidase; etco-5′-NT, etco-5′-nucleotidase; AC, adenylate cyclase; GPCR, G protein-coupled receptors; ENT, equilibrating nucleoside transporter; A1Rs, adenosine A1 receptors; A2ARs, adenosine A2A receptors; A2BRs, adenosine A2B receptors; A3Rs, adenosine A3 receptors; Pre-, presynaptic membrane; Post-, postsynaptic membrane.
Production of extracellular adenosine occurs mainly by two pathways (Figure 1): 1) intracellular adenosine is transported to the extracellular space by the balanced nucleoside transporter located in the cell membrane (Sala-Newby et al., 1999). 2) Extracellular ATP and adenosine diphosphate (ADP) are converted to AMP by the enzyme ecto-nucleoside triphosphate diphosphohydrolase (E-NTPDase), also known as CD39. Subsequently, adenosine is generated by ecto-5′-nucleotidase (ecto-5′-NT), also known as CD73, which is mainly expressed on astrocytes, oligodendrocytes and microglia (Lazarus et al., 2019a).
In the equilibrium state, the intracellular adenosine level is 100 nM, and the extracellular adenosine level is 140–200 nM (Dunwiddie and Diao, 1994), but in the pathological state, such as ischemia and hypoxia, extracellular adenosine level increases three- to 10-fold (Andiné et al., 1990; Dux et al., 1990). It is worth noting that although adenosine can be produced from the synaptic terminals of neurons and enter the synaptic space, it is not secreted through vesicles but transported through nucleoside transporters, which has nothing to do with neural activities. Thus, adenosine is not a neurotransmitter but a regulatory factor (Huang et al., 2011; Lopes et al., 2011; Huang et al., 2014).
3.2 Adenosine metabolism
Adenosine has three main metabolic pathways (Figure 1): 1) It becomes inosine under the action of adenosine deaminase [8], and then generates hypoxanthine and hypoxanthine nucleotides by nucleoside phosphorylase, and finally becomes uric acid (Fredholm et al., 2005). 2) Adenosine is transported intracellular and extracellular domain through two-way balanced nucleoside transporter to regulate intracellular and extracellular adenosine levels (Liu Y. J. et al., 2019). 3) Adenosine kinase (ADK), which is mainly found in astrocytes, generates AMP and ADP by phosphorylating adenosine in the presence of ATP. This metabolic pathway can only occur in cells, so extracellular adenosine must enter cells to complete the cycle (Huang et al., 2011; Huang et al., 2014; Garcia-Gil et al., 2021).
4 Excitatory and inhibitory effects of various adenosine receptors
The physiological functions of adenosine is mediated by four purinergic type 1 receptors, known as A1, A2A, A2B, and A3 receptors, which belong to GPCR family. A1Rs and A3Rs belong to the inhibitory adenylate cyclase G protein (Gi) family, whereas A2ARs and A2BRs belong to the stimulatory adenylate cyclase G protein (Gs) family (Wall and Dale, 2008; Lopes et al., 2011).
4.1 A1 receptors
A1Rs have the highest affinity for adenosine and can be activated when the concentration of adenosine is in the pM range. They are the most prominent adenosine receptor in the CNS, distributed mainly in the cerebral cortex, hippocampus, and thalamus. A1Rs are located primarily in the excitatory nerve terminals (Kashfi et al., 2017). Activation of A1Rs can inhibit the activity of adenylate cyclase (AC), decrease the cAMP content, and regulate the activity of cAMP-dependent protein kinase. A1R activation can increase the release of intracellular Ca2+, inhibit N-, Q- and P-type calcium channels, decrease the influx of extracellular Ca2+, block the release of neurotransmitters, and reduce neuronal discharge to regulate neuronal activity (Wall and Dale, 2008). In the postsynaptic membrane, A1Rs are activated to open K+ channels and increase K+ outflow, resulting in membrane hyperpolarization, which reduces excitability and protects neurons. When activated, A1Rs can also open the ATP-sensitive potassium channel (KATP) of substantia nigra neurons, increasing outward currents and decreasing membrane excitability (Stockwell et al., 2017).
4.2 A2A receptors
The affinity of A2ARs for adenosine is lower than that of A1Rs, and the activation concentration of adenosine is in the nM range. A2ARs are mainly distributed in dopaminergic areas, such as striatum, nucleus accumbens (NAc), olfactory nodules and so on (Fang et al., 2017; Dong et al., 2022). When A2ARs are activated, they are coupled with Gs protein in the brain to increase the activity of AC and cAMP in striatal cells. In the hippocampus, A2ARs appear to be coupled with Gi/Go protein (Diógenes et al., 2004). A2ARs are mainly expressed in D2 dopamine receptor cells and are particularly abundant in the plasma membrane of dendrites and dendritic spines, but less so in axons, axon terminals, and glial cells, and has an antagonistic effect with dopamine D2 receptors (D2Rs) (Ferre et al., 1991; Strömberg et al., 2000). Presynaptic A2ARs can regulate the inhibition of A1Rs. In contrast to A1Rs, adenosine promotes the release of excitatory transmitters by activating A2ARs. In astrocytes, A2ARs are involved in the regulation of glutamate release and γ-aminobutyric acid (GABA) uptake (Cristóvão-Ferreira et al., 2009). The balance between A1 and A2ARs is crucial to the adenosine response, and this close interaction between them can produce a response that is different from the sum of the two (Chiu and Freund, 2014).
4.3 A2B receptors
A2BRs have a low affinity for adenosine, and the activation concentration of adenosine should reach μM, suggesting that A2BRs mainly play a role under pathological conditions with increased extracellular adenosine concentration. A2BRs are primarily distributed in hippocampal neurons and glial cells, and a small amount is also found in the thalamus, lateral ventricle, and striatum. A2BRs can activate AC via Gs or phospholipase C (PLC) via Gq. Activation of A2BRs can increase intracellular cAMP, promote glycogen decomposition, and increase the energy supply of neurons to resist the pathological state of ischemia and hypoxia (van Calker et al., 1979; Hösli and Hösli, 1988; Dos Santos-Rodrigues et al., 2015).
4.4 A3 receptors
A3Rs have the lowest sensitivity compared to other adenosine receptors, but activation of A3Rs has neuroprotective and neurotrophic effects. Although A3Rs are distributed throughout the brain, their content varies greatly in different brain regions, especially in the hippocampus and cerebellum. A3Rs act through Gi-mediated AC inhibition and Gq-mediated PLC activation. A3Rs can regulate hippocampal synaptic plasticity and decrease adenylate cyclase activity. In short, A3Rs activation is closely related to inflammation inhibition and cell protection (Lopes et al., 2003; Vlajkovic et al., 2007; Lopes et al., 2011).
5 The roles and neurobiological mechanisms of adenosine and P1 receptors in sleep, torpor, and hibernation
5.1 Increased levels of extracellular adenosine lead to drowsiness
Thanks to neurobiology and molecular biology advances, we are beginning to understand how sleep is initiated and maintained. Sustained wakefulness causes the body to produce and accumulate one or more endogenous somnogenic factors that induce sleep after reaching a certain threshold. The hypnotic effect of adenosine, an endogenous somnogenic factor, was discovered in 1954 (Feldberg and Sherwood, 1954). Typically, extracellular adenosine concentrations in the cerebral cortex and basal forebrain (BF) gradually increase during prolonged arousal, reaching a certain threshold that leads to drowsiness, while slowly decreasing during recovery sleep (Porkka-Heiskanen et al., 1997; Clasadonte et al., 2014; Huang et al., 2014; Tartar et al., 2021; Omond et al., 2022). Extracellular adenosine levels may be partially regulated by glutamatergic neurons (Peng et al., 2020; Sun and Tang, 2020). This is because activation of the glutamatergic BF neurons causes a large increase in extracellular adenosine, and specific ablation of glutamatergic BF neurons reduces the level of extracellular adenosine and significantly impairs sleep homeostasis regulation (Peng et al., 2020). Although adenosine is known to act on four evolutionarily conserved receptors, it is currently thought to regulate sleep-wake states by acting on the A1Rs and A2ARs (Huang et al., 2014; Lazarus et al., 2019b).
5.2 Regulation of sleep homeostasis by A1Rs is brain region-dependent
A1Rs are required for normal sleep homeostasis because the conditional knockout of A1Rs in the CNS during sleep restriction results in a reduced rebound slow-wave activity response (Bjorness et al., 2009). Mainstream research suggests that activation of A1Rs promotes sleep, as A1Rs agonists increase sleep (Radulovacki et al., 1984; Benington et al., 1995), whereas A1Rs antagonists decrease sleep (Methippara et al., 2005; Thakkar et al., 2008). For example, when Oishi et al. (2008) injected the A1Rs-selective agonist N6-cyclopentyladenosine (CPA) into the rat tuberomammillary nucleus (TMN), this significantly increased NREM sleep. A1Rs may mediate sleep through three pathways (Lazarus et al., 2019b): 1) A1Rs promote sleep by inhibiting wake-promoting neurons. A1Rs are expressed in hypocretin/orexin neurons of the lateral hypothalamus (LH) and histaminergic neurons of the TMN, which are typical arousal centers. Activation of A1Rs inhibits excitatory neurotransmission, including cholinergic arousal systems in the brainstem (Rainnie et al., 1994) and BF (Alam et al., 1999; Thakkar et al., 2003), the hypocretin/orexin neurons in the LH (Thakkar et al., 2002; Liu and Gao, 2007), and histaminergic systems in the TMN (Oishi et al., 2008). 2) A1Rs promote sleep by disinhibiting sleep-active neurons in the ventrolateral preoptic nucleus (VLPO) and anterior hypothalamic area (Chamberlin et al., 2003; Morairty et al., 2004). 3) A1Rs mediate homeostatic sleep pressure based on astrocytic gliotransmission (Halassa et al., 2009).
Moreover, A1Rs do not appear to fully promote sleep because A1R knockout mice did not differ from wide-type mice in basal sleep amount and sleep-wake behavior after sleep deprivation (Stenberg et al., 2003). Infusion of CPA into the lateral ventricle of mice did not significantly alter NREM and REM sleep (Urade et al., 2003). However, microdialysis of the adenosine transporter inhibitor nitrobenzyl-thio-inosine (NBTIs) or A1R agonists into the lateral preoptic area (LPO) increased the amount of wakefulness in rats (Methippara et al., 2005). Thus, A1Rs may exert different sleep-wake effects by acting on different brain regions.
5.3 A2ARs are important receptors that mediate the sleep-promoting effect of adenosine
A2ARs are important targets in the regulation of sleep. A2ARs mediate the effects of many sleep-promoting substances, such as ethanol and sake yeast (El Yacoubi et al., 2003; Nakamura et al., 2016; Fang et al., 2017; Nishimon et al., 2021). The selective A2AR agonist CGS21680 injected into the subarachnoid space adjacent to the BF and LPO of rats or the lateral ventricle of mice significantly increased NREM and REM sleep (Satoh et al., 1998; Scammell et al., 2001; Urade et al., 2003; Methippara et al., 2005). Immediately after the cessation of CGS21680 perfusion, there is a strong rebound in wakefulness (Gerashchenko et al., 2000). However, the sleep-promoting effect induced by CGS21680 was abolished entirely in A2AR knockout mice.
In addition, intraperitoneal administration of a positive A2AR allosteric modulator {3, 4-difluoro-2-[(2-fluoro-4-iodophenyl) amino] benzoic acid} in WT mice but not A2AR knockout mice enhanced A2AR signaling and promoted NREM sleep in a dose-dependent manner (Korkutata et al., 2019). Several studies suggested that A2ARs mediated the sleep-regulating effects of prostaglandin D2 (PGD2). After administration of PGD2 or CGS21680 into the rostral BF, c-fos-positive cells were significantly increased in the VLPO, a sleep center, resulting in enhanced induction of NREM sleep, and in contrast, c-fos-positive neurons significantly decreased in the TMN of the posterior hypothalamus, a wake center (Satoh et al., 1999; Scammell et al., 2001). In in-vivo microdialysis experiments, infusion of CGS21680 into the BF dose-dependently decreased histamine release in the frontal cortex and medial preoptic area and increased GABA release in the TMN, but not in the frontal cortex (Hong et al., 2005). Furthermore, VLPO neurons have been divided into two types according to their different responses to serotonin and adenosine: Type-1 neurons were inhibited by serotonin, and type-2 neurons were excited. A2AR agonists excited postsynaptic type-2 neurons in the VLPO but not type-1 neurons. Type-2 neurons were involved in sleep initiation, whereas type-1 neurons may contribute to sleep consolidation because type-1 neurons were activated only when the inhibitory effects of the arousal system were absent (Gallopin et al., 2005). In addition to the VLPO, injection of CGS21680 into the rostral BF also increased c-fos expression in the shell of the NAc and the medial portion of the olfactory tubercle (OT) (Satoh et al., 1999; Scammell et al., 2001). Microinjection of CGS21680 into the NAc shell also induced sleep-promoting effects (Satoh et al., 1999). A2ARs are highly expressed in the caudate putamen, NAc, and OT. Our recent series of studies have shown that activation of A2AR neurons in these nuclei can strongly promote sleep (Oishi et al., 2017; Yuan et al., 2017; Li et al., 2020). Activation of the A2AR neurons of the NAc core projecting to the ventral pallidum (VP) strongly induced NREM sleep. Conversely, inhibiting these neurons reduced sleep but did not affect the sleep homeostasis rebound (Oishi et al., 2017). Yuan et al. demonstrated the important role of the striatal A2AR neurons projecting to the external globus pallidus (GPe) parvalbumin (PV) neurons in sleep control. Chemogenetic inhibition of striatal A2AR neurons significantly decreased NREM sleep in the active period, which was mediated by the formation of inhibitory circuits between striatal A2AR neurons and GPe PV neurons (Yuan et al., 2017). The OT A2AR neurons project to the VP and LH via inhibitory innervations, and pharmacological or chemogenetic activation of OT A2AR neurons resulted in increased NREM sleep in mice (Li et al., 2020). Moreover, A2ARs are co-localized with dopamine D2Rs in these nuclei (Missale et al., 1998). Our studies demonstrated that D2R-expressing neurons are essential for the induction and maintenance of wakefulness (Qu et al., 2008; Qiu et al., 2009; Qu et al., 2010; Liu Y. Y. et al., 2019; Yang et al., 2021). Thus, A2ARs and D2Rs may jointly influence the sleep-wake cycle by balancing their activity.
Caffeine, unlike adenosine, is a wake-promoting substance abundant in refreshing beverages such as coffee and tea. Caffeine is an antagonist of A1Rs and A2ARs, with similar affinity for both at low doses (Fredholm et al., 2001). Using A1R knockout and A2AR knockout mice, Huang et al. demonstrated that caffeine-induced wakefulness is dependent on A2ARs, as caffeine dose-dependently increased wakefulness in both wild-type and A1R knockout but not A2AR knockout mice (Huang et al., 2005). Similarly, selective silencing of A2ARs in the NAc shell inhibited caffeine-induced wakefulness (Lazarus et al., 2011).
In conclusion, the regulatory effect of A1Rs on sleep-wake regulation is brain region-dependent. The excitation of A1Rs in wake-promoting nuclei induces sleep and, conversely, causes arousal on sleep-promoting neurons. The A2ARs are the major sleep-regulating receptors that mediate the wake-promoting effects of caffeine, and activation of A2ARs promotes sleep by inhibiting major arousal systems (Figure 2).
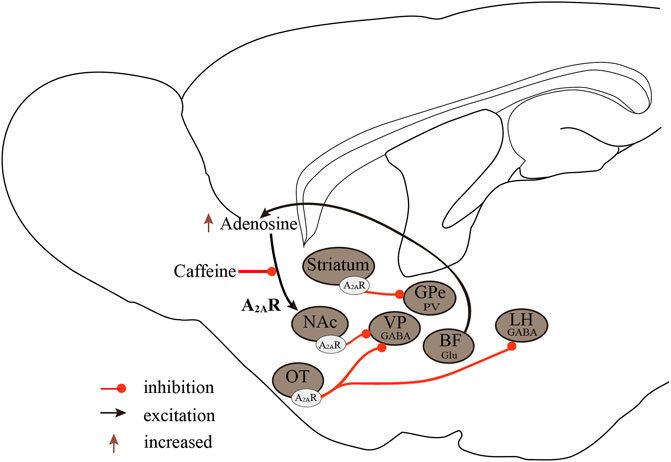
FIGURE 2. Neurobiological mechanisms of the A2ARs regulate sleep-wake states. A2ARs are important targets in sleep regulation, promoting sleep by inhibiting major arousal systems. Activation of A2AR neurons in the NAc core, striatum, and OT promotes sleep, with A2ARs neurons in the NAc core projecting to the VP, striatal A2AR neurons, and GPe PV neurons forming inhibitory circuits, and OT A2AR neurons projecting to the VP and LH. Furthermore, BF glutamatergic neurons may regulate extracellular adenosine levels, and A2ARs rather than A1Rs mediate the wake-promoting effects of caffeine. A2ARs, adenosine A2A receptors; A1Rs, adenosine A1 receptors; NAc, nucleus accumbens; VP, ventral pallidum; GPe, external globus pallidus; OT, olfactory tubercle; LH, lateral hypothalamus; Glu, glutamic acid; GABA, γ-aminobutyric acid.
5.4 Adenosine A1Rs and A3Rs play important roles in torpor
Adenosine may play a key role in torpor, as pyruvate induces torpor in obese mice based on adenosine signaling (Soto et al., 2018). In mice lacking all four adenosine receptors, adenosine does not cause hypothermia, bradycardia, or hypotension typical of the torpor state (Xiao et al., 2019). Peripheral or central infusion of adenosine or AMP results in a decrease in metabolic rate and body temperature similar to that observed in natural torpor, even in rats that do not naturally enter torpor (Swoap et al., 2007; Jinka et al., 2011; Iliff and Swoap, 2012; Olson et al., 2013; Tupone et al., 2013; Carlin et al., 2017; Vicent et al., 2017). Furthermore, the administration of A1R or A3R agonists to mice induces several features of daily torpor, including hypothermia (Anderson et al., 1994; Iliff and Swoap, 2012; Carlin et al., 2017; Swoap, 2017; Vicent et al., 2017), whereas A2ARs and A2BRs agonists do not (Anderson et al., 1994).
Currently, there are three ways to mimic the induction of torpor: 1) inhibition of the raphe pallidus (rPA) neurons in the brainstem (Cerri et al., 2021); 2) activation of A1Rs or A3Rs in the brain; 3) activation of glutamatergic Adcyap1+ neurons in the hypothalamus (Hrvatin et al., 2020). Here, we will discuss the induction of synthetic torpor by controlling A1Rs and A3Rs through pharmacological experiments. Although neither A1Rs nor A3ARs are required for fasting-induced torpor (Carlin et al., 2017), administration of A1R or A3R agonists such as N6-cyclohexyladenosine (CHA) induces torpor-like states in some animals (Jinka et al., 2011; Olson et al., 2013; Tupone et al., 2013; Vicent et al., 2017; Frare et al., 2018), while antagonist administration prevents torpor or causes arousal from torpor during torpor phases (Jinka et al., 2011; Iliff and Swoap, 2012; Tamura et al., 2012). It is not yet certain whether adenosine action triggers the occurrence of natural torpor, but adenosine mediates at least some of the physiological features during torpor. For example, A3R stimulation leads to hypothermia via peripheral mast cell degranulation, histamine release, and activation of central histamine H1 receptors. However, A1R agonist-induced hypothermia occurs via central sites, and the rPA, nucleus of the solitary tract (NTS) and the hypothalamic-pituitary-thyroid axis gate appear to play a pivotal role (Tupone et al., 2013; Carlin et al., 2017; Frare et al., 2018).
In the future, further efforts should be made to confirm the role of adenosine in torpor and its possible neurobiological and molecular mechanisms. First, microdialysis experiments, adenosine probes, and chemogenetic and optogenetic techniques should be used to confirm whether there is an accumulation and dynamic change of adenosine concentration during the initiation and maintenance of torpor and to reveal the possible mechanisms.
5.5 Central activation of A1Rs is sufficient to induce and maintain a hibernation-like state
Seasonal changes in brain adenosine levels may contribute to an increase in A1R sensitivity leading to the onset of hibernation (Frare and Drew, 2021). Although the mechanisms controlling hibernation are currently unclear, activation of A1Rs signaling in the CNS appears to be required for the onset of this phenomenon, as activation of the A1Rs in the CNS can induce hibernation or some hibernation-like states in obligate, facultative, or non-hibernating animals (Drew et al., 2017; Shimaoka et al., 2018; Frare and Drew, 2021). In addition, Shimaoka et al. (2018) activated central A1Rs in rats, a non-hibernating animal, which induced a hypothermia response similar to hibernation.
It is worth noting that activation of A1Rs maintains core body temperature at a low level. In hibernators, core body temperature and metabolic rate reduction occur before hibernation, which may be the key to the A1R-mediated hibernation (Barros et al., 2006). A1Rs are highly expressed throughout the CNS, including the NTS. The NTS is the center that controls cardiovascular, respiratory, and metabolic functions, and the NTS neurons are responsible for the integration of central and peripheral signals related to energy expenditure-related (Barros et al., 2006). A1Rs act as inhibitory receptors whose activation prevents the release of GABA to the NTS neurons that inhibit thermogenesis (Cao et al., 2010). Furthermore, the administration of CHA to the arctic ground squirrel increased c-fos expression in the NTS in both summer and winter (Frare et al., 2019). After the microinjection of CHA into the NTS, it inhibited brown adipose tissue (BAT) thermogenesis and shivering responses. In contrast, inhibition of A1Rs counteracted BAT thermogenesis induced by intracerebroventricular injection of CHA (Tupone et al., 2013). In addition to inhibiting BAT thermogenesis, activation of A1Rs in the NTS increases vasopressin secretion, which constricts blood vessels, including skin vessels, thereby increasing arterial blood pressure (McClure et al., 2005; McClure et al., 2011) and causing bradycardia, one of the initial physiological features of natural hibernation (Jinka, 2012). The rPA, the median preoptic area (MnPO) and the supraoptic nucleus (SON) also appear to mediate the effect of A1Rs in BAT thermogenic, as the rPA and MnPO c-fos expression is lower in winter than in summer after CHA administration, and inhibition of rPA neurons produces hypothermia, however the SON is related to the seasonal increase in vasoconstriction (Cerri et al., 2013; Frare et al., 2019). Therefore, A1Rs could mediate hypothermia similar to hibernation by inhibiting BAT thermogenesis via the NTS and rPA or by inhibiting cardiovascular function. In addition, as previously mentioned, in contrast to sleep, EEG amplitudes are significantly reduced during hibernation (Golanov and Reis, 2001; Magdaleno-Madrigal et al., 2010). Central activation of A1Rs synchronized the EEG, whereas activation in the thalamus significantly reduced EEG amplitude (Saper et al., 2005). After central administration of CHA in rats, the EEG amplitude was greatly reduced, the delta wave amplitude was significantly reduced, and the theta wave almost disappeared (Tupone et al., 2013). Thus, the change in EEG amplitude may be another way A1Rs mediate hibernation.
As with torpor, it is currently unclear whether adenosine accumulation is necessary for the initiation of hibernation, so further efforts are needed to address these scientific questions.
6 Conclusion and future perspective
In this review, we summarize the roles and neurobiological mechanisms of adenosine and its receptors in sleep-wake regulation, torpor, and hibernation (Table 2, Figure 3). The first step toward translating adenosine and P1 receptors into targets for medical applications is to understand their roles and mechanisms underlying these states of diminished metabolism and body temperature. We now know that A1Rs and A2ARs jointly mediate sleep-wake regulation (Huang et al., 2014; Lazarus et al., 2019b), that activation of A1Rs and A3Rs is important for torpor (Carlin et al., 2017) and that hibernation requires A1Rs rather than other adenosine receptors (Shimaoka et al., 2018; Frare and Drew, 2021).
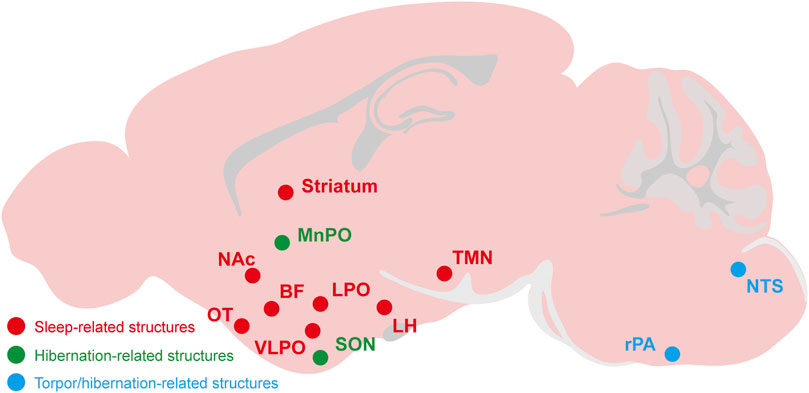
FIGURE 3. The relevant brain regions about adenosine and P1 receptors mediate sleep, torpor, and hibernation. The CNS adenosine and P1 receptors are important for the regulation of sleep-wake, torpor and hibernation. The roles and mechanisms of several brain regions and nuclei have been gradually revealed, such as the A2ARs-expressing neurons in the NAc, striatum, OT and other structures have a significant effect on sleep-wake regulation. The NTS and rPA may be the key brain regions of adenosine and P1 receptors mediating torpor and hibernation. NAc, nucleus accumbens; OT, olfactory tubercle; LH, lateral hypothalamus; BF, basal forebrainvlpo; VLPO, ventrolateral preoptic nucleus; LPO, lateral preoptic area; MnPO, median preoptic area; SON, supraoptic nucleus; TMN, tuberomammillary nucleus; rPA, raphe pallidus; NTS, nucleus tractus solitarius.
It is worth noting that the adenosine system is also altered in various sleep disorders, for example, sleeping sickness and chronic insomnia disorder (Rijo-Ferreira et al., 2020; Ren et al., 2021). Some agonists, antagonists, or allosteric modulators targeting adenosine receptors have the potential to be used for treating sleep disorders (Jenner et al., 2020; Korkutata et al., 2022) or inducing synthetic torpor or hibernation for therapeutic hypothermia, organ preservation, space exploration or longevity promotion (Jinka et al., 2015; Cerri, 2017; Sisa et al., 2017; Hadj-Moussa and Storey, 2019; Al-Attar and Storey, 2020; Cerri et al., 2021), showing that the pharmacological importance of targeting adenosine receptors in the future. However, much work remains to be done because small-molecule drugs targeting adenosine receptors have side effects (Korkutata et al., 2022) and can only mimic some physiological properties of torpor or hibernation by activating adenosine receptors, which is different from natural torpor or hibernation (Swoap, 2017; Vicent et al., 2017). Therefore, it is necessary to explore further the roles and mechanisms of adenosine and its receptors in sleep, torpor, and hibernation and gain more adenosine receptor modulators by structure- and function-based drug discovery. It is important to investigate the neural networks and molecular mechanisms that sleep torpor and hibernation have in common. The first step in conducting these studies is to confirm adenosine accumulation before torpor or hibernation and the dynamic changes in adenosine concentrations during torpor or hibernation using available technologies such as microdialysis, adenosine probes, and chemogenetic and optogenetic methods. Subsequently, several key technologies, from conditional knockout mice based on Cre/lox technology and RNA interference to modulation of neuronal activity with genetic or pharmacological techniques, can be used to confirm neuronal networks of sleep, torpor, and hibernation.
Author contributions
W-XM, P-CY, and HZ wrote the manuscript. L-XK provided some advices and drew figures. Z-LH, W-MQ, Y-QW, and ML edited and revised the manuscript. All the authors have read and agreed to the content of the manuscript.
Funding
This study was supported by the STI 2030-major project (2021ZD0203400 to Z-LH), the China National Key R&D Program; National Key Research and Development Program (2022YFA1604504 to Y-QW), the National Natural Science Foundation of China (82171479, 81871037 to Y-QW; 82020108014 and 32070984 to Z-LH), the Shanghai Science and Technology Innovation Action Plan Laboratory Animal Research Project (201409001800 to Z-LH), Program for Shanghai Outstanding Academic Leaders (to Z-LH), the Shanghai Municipal Science and Technology Major Project, and ZJ Lab, and Shanghai Center for Brain Science and Brain-inspired Technology (2018SHZDZX01 to Z-LH), Japan Society for the Promotion of Science [Grants-in-Aid for Scientific Research B (grant number 21H02802) and RECONNECT Initiative (grant number JP22K21351) to ML]; the Japan Science and Technology Agency [CREST (grant number JPMJCR1655) to ML]; Japan Agency for Medical Research and Development (AMED) [Moonshot Program (grant number JP21zf0127005) to ML]; the project “Social Application of Mobility Innovation and Future Social Engineering Research Phase IV (grant number CRI04006),” a joint research project between Toyota Motor Corporation and the University of Tsukuba to ML; and the World Premier International Research Center Initiative (WPI) from MEXT to ML.
Conflict of interest
The authors declare that the research was conducted in the absence of any commercial or financial relationships that could be construed as a potential conflict of interest.
Publisher’s note
All claims expressed in this article are solely those of the authors and do not necessarily represent those of their affiliated organizations, or those of the publisher, the editors and the reviewers. Any product that may be evaluated in this article, or claim that may be made by its manufacturer, is not guaranteed or endorsed by the publisher.
References
Al-Attar, R., and Storey, K. B. (2020). Suspended in time: Molecular responses to hibernation also promote longevity. Exp. Gerontol. 134, 110889. doi:10.1016/j.exger.2020.110889
Alam, M. N., Szymusiak, R., Gong, H., King, J., and McGinty, D. (1999). Adenosinergic modulation of rat basal forebrain neurons during sleep and waking: Neuronal recording with microdialysis. J. Physiol. 521 Pt 3, 679–690. doi:10.1111/j.1469-7793.1999.00679.x
Ambler, M., Hitrec, T., and Pickering, A. (2021). Turn it off and on again: Characteristics and control of torpor. Wellcome Open Res. 6, 313. doi:10.12688/wellcomeopenres.17379.2
Anderson, R., Sheehan, M. J., and Strong, P. (1994). Characterization of the adenosine receptors mediating hypothermia in the conscious mouse. Br. J. Pharmacol. 113, 1386–1390. doi:10.1111/j.1476-5381.1994.tb17151.x
Andiné, P., Thordstein, M., Kjellmer, I., Nordborg, C., Thiringer, K., Wennberg, E., et al. (1990). Evaluation of brain damage in a rat model of neonatal hypoxic-ischemia. J. Neurosci. Methods 35, 253–260. doi:10.1016/0165-0270(90)90131-x
Barros, R. C., Branco, L. G., and Cárnio, E. C. (2006). Respiratory and body temperature modulation by adenosine A1 receptors in the anteroventral preoptic region during normoxia and hypoxia. Respir. Physiol. Neurobiol. 153, 115–125. doi:10.1016/j.resp.2005.09.013
Benington, J. H., Kodali, S. K., and Heller, H. C. (1995). Stimulation of A1 adenosine receptors mimics the electroencephalographic effects of sleep deprivation. Brain Res. 692, 79–85. doi:10.1016/0006-8993(95)00590-m
Berger, R. J. (1984). Slow wave sleep, shallow torpor and hibernation: Homologous states of diminished metabolism and body temperature. Biol. Psychol. 19, 305–326. doi:10.1016/0301-0511(84)90045-0
Bjorness, T. E., Kelly, C. L., Gao, T., Poffenberger, V., and Greene, R. W. (2009). Control and function of the homeostatic sleep response by adenosine A1 receptors. J. Neurosci. 29, 1267–1276. doi:10.1523/JNEUROSCI.2942-08.2009
Cao, W. H., Madden, C. J., and Morrison, S. F. (2010). Inhibition of Brown adipose tissue thermogenesis by neurons in the ventrolateral medulla and in the nucleus tractus solitarius. Am. J. Physiol. Regul. Integr. Comp. Physiol. 299, R277–R290. doi:10.1152/ajpregu.00039.2010
Carlin, J. L., Jain, S., Gizewski, E., Wan, T. C., Tosh, D. K., Xiao, C., et al. (2017). Hypothermia in mouse is caused by adenosine A(1) and A(3) receptor agonists and AMP via three distinct mechanisms. Neuropharmacology 114, 101–113. doi:10.1016/j.neuropharm.2016.11.026
Cerri, M. (2017). The central control of energy expenditure: Exploiting torpor for medical applications. Annu. Rev. Physiol. 79, 167–186. doi:10.1146/annurev-physiol-022516-034133
Cerri, M., Hitrec, T., Luppi, M., and Amici, R. (2021). Be cool to be far: Exploiting hibernation for space exploration. Neurosci. Biobehav Rev. 128, 218–232. doi:10.1016/j.neubiorev.2021.03.037
Cerri, M., Mastrotto, M., Tupone, D., Martelli, D., Luppi, M., Perez, E., et al. (2013). The inhibition of neurons in the central nervous pathways for thermoregulatory cold defense induces a suspended animation state in the rat. J. Neurosci. 33, 2984–2993. doi:10.1523/JNEUROSCI.3596-12.2013
Chamberlin, N. L., Arrigoni, E., Chou, T. C., Scammell, T. E., Greene, R. W., and Saper, C. B. (2003). Effects of adenosine on gabaergic synaptic inputs to identified ventrolateral preoptic neurons. Neuroscience 119, 913–918. doi:10.1016/s0306-4522(03)00246-x
Chiu, G. S., and Freund, G. G. (2014). Modulation of neuroimmunity by adenosine and its receptors: Metabolism to mental illness. Metabolism 63, 1491–1498. doi:10.1016/j.metabol.2014.09.003
Clasadonte, J., McIver, S. R., Schmitt, L. I., Halassa, M. M., and Haydon, P. G. (2014). Chronic sleep restriction disrupts sleep homeostasis and behavioral sensitivity to alcohol by reducing the extracellular accumulation of adenosine. J. Neurosci. 34, 1879–1891. doi:10.1523/JNEUROSCI.2870-12.2014
Cristóvão-Ferreira, S., Vaz, S. H., Ribeiro, J. A., and Sebastião, A. M. (2009). Adenosine A2A receptors enhance GABA transport into nerve terminals by restraining PKC inhibition of GAT-1. J. Neurochem. 109, 336–347. doi:10.1111/j.1471-4159.2009.05963.x
Deussen, A., Lloyd, H. G., and Schrader, J. (1989). Contribution of S-adenosylhomocysteine to cardiac adenosine formation. J. Mol. Cell. Cardiol. 21, 773–782. doi:10.1016/0022-2828(89)90716-5
Diógenes, M. J., Fernandes, C. C., Sebastião, A. M., and Ribeiro, J. A. (2004). Activation of adenosine A2A receptor facilitates brain-derived neurotrophic factor modulation of synaptic transmission in hippocampal slices. J. Neurosci. 24, 2905–2913. doi:10.1523/JNEUROSCI.4454-03.2004
Dong, H., Chen, Z. K., Guo, H., Yuan, X. S., Liu, C. W., Qu, W. M., et al. (2022). Striatal neurons expressing dopamine D(1) receptor promote wakefulness in mice. Curr. Biol. 32, 600–613.e4. doi:10.1016/j.cub.2021.12.026
Dos Santos-Rodrigues, A., Pereira, M. R., Brito, R., de Oliveira, N. A., and Paes-de-Carvalho, R. (2015). Adenosine transporters and receptors: Key elements for retinal function and neuroprotection. Vitam. Horm. 98, 487–523. doi:10.1016/bs.vh.2014.12.014
Drew, K. L., Frare, C., and Rice, S. A. (2017). Neural signaling metabolites may modulate energy use in hibernation. Neurochem. Res. 42, 141–150. doi:10.1007/s11064-016-2109-4
Drew, K. L., and Jinka, T. R. (2013). The bioenergetic network of adenosine in hibernation, sleep, and thermoregulation, adenosine. Berlin, Germany: Springer, 253–272.
Dunwiddie, T. V., and Diao, L. (1994). Extracellular adenosine concentrations in hippocampal brain slices and the tonic inhibitory modulation of evoked excitatory responses. J. Pharmacol. Exp. Ther. 268, 537–545.
Dux, E., Fastbom, J., Ungerstedt, U., Rudolphi, K., and Fredholm, B. B. (1990). Protective effect of adenosine and a novel xanthine derivative propentofylline on the cell damage after bilateral carotid occlusion in the gerbil hippocampus. Brain Res. 516, 248–256. doi:10.1016/0006-8993(90)90925-2
El Yacoubi, M., Ledent, C., Parmentier, M., Costentin, J., and Vaugeois, J. M. (2003). Caffeine reduces hypnotic effects of alcohol through adenosine A2A receptor blockade. Neuropharmacology 45, 977–985. doi:10.1016/s0028-3908(03)00254-5
Fang, T., Dong, H., Xu, X. H., Yuan, X. S., Chen, Z. K., Chen, J. F., et al. (2017). Adenosine A(2A) receptor mediates hypnotic effects of ethanol in mice. Sci. Rep. 7, 12678. doi:10.1038/s41598-017-12689-6
Feldberg, W., and Sherwood, S. L. (1954). Injections of drugs into the lateral ventricle of the cat. J. Physiol. 123, 148–167.
Ferre, S., von Euler, G., Johansson, B., Fredholm, B. B., and Fuxe, K. (1991). Stimulation of high-affinity adenosine A2 receptors decreases the affinity of dopamine D2 receptors in rat striatal membranes. Proc. Natl. Acad. Sci. U. S. A. 88, 7238–7241. doi:10.1073/pnas.88.16.7238
Frare, C., and Drew, K. L. (2021). Seasonal changes in adenosine kinase in tanycytes of the Arctic ground squirrel (Urocitellus parryii). J. Chem. Neuroanat. 113, 101920. doi:10.1016/j.jchemneu.2021.101920
Frare, C., Jenkins, M. E., Soldin, S. J., and Drew, K. L. (2018). The raphe pallidus and the hypothalamic-pituitary-thyroid Axis gate seasonal changes in thermoregulation in the hibernating arctic ground squirrel (urocitellus parryii). Front. Physiol. 9, 1747. doi:10.3389/fphys.2018.01747
Frare, C., Jenkins, M. E., McClure, K. M., and Drew, K. L. (2019). Seasonal decrease in thermogenesis and increase in vasoconstriction explain seasonal response to N(6) -cyclohexyladenosine-induced hibernation in the Arctic ground squirrel (Urocitellus parryii). J. Neurochem. 151, 316–335. doi:10.1111/jnc.14814
Fredholm, B. B., IJzerman, A. P. I. J., Jacobson, K. A., Klotz, K. N., and Linden, J. (2001). . Pharmacol. Rev. 53, 527–552.
Fredholm, B. B., Chen, J. F., Cunha, R. A., Svenningsson, P., and Vaugeois, J. M. (2005). Adenosine and brain function. Int. Rev. Neurobiol. 63, 191–270. doi:10.1016/S0074-7742(05)63007-3
Gallopin, T., Luppi, P. H., Cauli, B., Urade, Y., Rossier, J., Hayaishi, O., et al. (2005). The endogenous somnogen adenosine excites a subset of sleep-promoting neurons via A2A receptors in the ventrolateral preoptic nucleus. Neuroscience 134, 1377–1390. doi:10.1016/j.neuroscience.2005.05.045
Garcia-Gil, M., Camici, M., Allegrini, S., Pesi, R., and Tozzi, M. G. (2021). Metabolic aspects of adenosine functions in the brain. Front. Pharmacol. 12, 672182. doi:10.3389/fphar.2021.672182
Gerashchenko, D., Okano, Y., Urade, Y., Inoué, S., and Hayaishi, O. (2000). Strong rebound of wakefulness follows prostaglandin D2-or adenosine A2a receptor agonist-induced sleep. J. Sleep. Res. 9, 81–87. doi:10.1046/j.1365-2869.2000.00175.x
Giroud, S., Habold, C., Nespolo, R. F., Mejías, C., Terrien, J., Logan, S. M., et al. (2020). The torpid state: Recent advances in metabolic adaptations and protective mechanisms(†). Front. Physiol. 11, 623665. doi:10.3389/fphys.2020.623665
Golanov, E. V., and Reis, D. J. (2001). Neurons of nucleus of the solitary tract synchronize the EEG and elevate cerebral blood flow via a novel medullary area. Brain Res. 892, 1–12. doi:10.1016/s0006-8993(00)02949-8
Halassa, M. M., Florian, C., Fellin, T., Munoz, J. R., Lee, S. Y., Abel, T., et al. (2009). Astrocytic modulation of sleep homeostasis and cognitive consequences of sleep loss. Neuron 61, 213–219. doi:10.1016/j.neuron.2008.11.024
Hadj-Moussa, H., and Storey, K. B. (2019). Bringing nature back: Using hibernation to reboot organ preservation. Febs J. 286, 1094–1100. doi:10.1111/febs.14683
Hall, J., and Frenguelli, B. G. (2018). The combination of ribose and adenine promotes adenosine release and attenuates the intensity and frequency of epileptiform activity in hippocampal slices: Evidence for the rapid depletion of cellular ATP during electrographic seizures. J. Neurochem. 147, 178–189. doi:10.1111/jnc.14543
Hong, Z. Y., Huang, Z. L., Qu, W. M., Eguchi, N., Urade, Y., and Hayaishi, O. (2005). An adenosine A receptor agonist induces sleep by increasing GABA release in the tuberomammillary nucleus to inhibit histaminergic systems in rats. J. Neurochem. 92, 1542–1549. doi:10.1111/j.1471-4159.2004.02991.x
Hösli, E., and Hösli, L. (1988). Autoradiographic studies on the uptake of adenosine and on binding of adenosine analogues in neurons and astrocytes of cultured rat cerebellum and spinal cord. Neuroscience 24, 621–628. doi:10.1016/0306-4522(88)90355-7
Hrvatin, S., Sun, S., Wilcox, O. F., Yao, H., Lavin-Peter, A. J., Cicconet, M., et al. (2020). Neurons that regulate mouse torpor. Nature 583, 115–121. doi:10.1038/s41586-020-2387-5
Huang, Y. G., Flaherty, S. J., Pothecary, C. A., Foster, R. G., Peirson, S. N., and Vyazovskiy, V. V. (2021). The relationship between fasting-induced torpor, sleep, and wakefulness in laboratory mice. Sleep 44, zsab093. doi:10.1093/sleep/zsab093
Huang, Z. L., Qu, W. M., Eguchi, N., Chen, J. F., Schwarzschild, M. A., Fredholm, B. B., et al. (2005). Adenosine A2A, but not A1, receptors mediate the arousal effect of caffeine. Nat. Neurosci. 8, 858–859. doi:10.1038/nn1491
Huang, Z. L., Urade, Y., and Hayaishi, O. (2011). The role of adenosine in the regulation of sleep. Curr. Top. Med. Chem. 11, 1047–1057. doi:10.2174/156802611795347654
Huang, Z. L., Zhang, Z., and Qu, W. M. (2014). Roles of adenosine and its receptors in sleep-wake regulation. Int. Rev. Neurobiol. 119, 349–371. doi:10.1016/B978-0-12-801022-8.00014-3
Iliff, B. W., and Swoap, S. J. (2012). Central adenosine receptor signaling is necessary for daily torpor in mice. Am. J. Physiol. Regul. Integr. Comp. Physiol. 303, R477–R484. doi:10.1152/ajpregu.00081.2012
Jenner, P., Mori, A., and Kanda, T. (2020). Can adenosine A(2A) receptor antagonists be used to treat cognitive impairment, depression or excessive sleepiness in Parkinson's disease? Park. Relat. Disord. 80, S28–s36. doi:10.1016/j.parkreldis.2020.09.022
Jinka, T. R., Combs, V. M., and Drew, K. L. (2015). Translating drug-induced hibernation to therapeutic hypothermia. ACS Chem. Neurosci. 6, 899–904. doi:10.1021/acschemneuro.5b00056
Jinka, T. R. (2012). “Natural protection against cardiac arrhythmias during hibernation: Significance of adenosine,” in Cardiac Arrhythmias-New Considerations, 151–166.
Jinka, T. R., Tøien, Ø., and Drew, K. L. (2011). Season primes the brain in an arctic hibernator to facilitate entrance into torpor mediated by adenosine A(1) receptors. J. Neurosci. 31, 10752–10758. doi:10.1523/JNEUROSCI.1240-11.2011
Kashfi, S., Ghaedi, K., Baharvand, H., Nasr-Esfahani, M. H., and Javan, M. (2017). A(1) adenosine receptor activation modulates central nervous system development and repair. Mol. Neurobiol. 54, 8128–8139. doi:10.1007/s12035-016-0292-6
Kazemzadeh-Narbat, M., Annabi, N., Tamayol, A., Oklu, R., Ghanem, A., and Khademhosseini, A. (2015). Adenosine-associated delivery systems. J. Drug Target 23, 580–596. doi:10.3109/1061186X.2015.1058803
Korkutata, M., Agrawal, L., and Lazarus, M. (2022). Allosteric modulation of adenosine A(2A) receptors as a new therapeutic avenue. Int. J. Mol. Sci. 23, 2101. doi:10.3390/ijms23042101
Korkutata, M., Saitoh, T., Cherasse, Y., Ioka, S., Duo, F., Qin, R., et al. (2019). Enhancing endogenous adenosine A(2A) receptor signaling induces slow-wave sleep without affecting body temperature and cardiovascular function. Neuropharmacology 144, 122–132. doi:10.1016/j.neuropharm.2018.10.022
Latini, S., and Pedata, F. (2001). Adenosine in the central nervous system: Release mechanisms and extracellular concentrations. J. Neurochem. 79, 463–484. doi:10.1046/j.1471-4159.2001.00607.x
Lazarus, M., Chen, J. F., Huang, Z. L., Urade, Y., and Fredholm, B. B. (2019a). Adenosine and sleep. Handb. Exp. Pharmacol. 253, 359–381. doi:10.1007/164_2017_36
Lazarus, M., Oishi, Y., Bjorness, T. E., and Greene, R. W. (2019b). Gating and the need for sleep: Dissociable effects of adenosine A(1) and A(2A) receptors. Front. Neurosci. 13, 740. doi:10.3389/fnins.2019.00740
Lazarus, M., Shen, H. Y., Cherasse, Y., Qu, W. M., Huang, Z. L., Bass, C. E., et al. (2011). Arousal effect of caffeine depends on adenosine A2A receptors in the shell of the nucleus accumbens. J. Neurosci. 31, 10067–10075. doi:10.1523/JNEUROSCI.6730-10.2011
Li, R., Wang, Y. Q., Liu, W. Y., Zhang, M. Q., Li, L., Cherasse, Y., et al. (2020). Activation of adenosine A(2A) receptors in the olfactory tubercle promotes sleep in rodents. Neuropharmacology 168, 107923. doi:10.1016/j.neuropharm.2019.107923
Liu, Y. J., Chen, J., Li, X., Zhou, X., Hu, Y. M., Chu, S. F., et al. (2019). Research progress on adenosine in central nervous system diseases. CNS Neurosci. Ther. 25, 899–910. doi:10.1111/cns.13190
Liu, Y. Y., Wang, T. X., Zhou, J. C., Qu, W. M., and Huang, Z. L. (2019). . Psychopharmacol. Berl. 236, 3169–3182. doi:10.1007/s00213-019-05275-3
Liu, Z. W., and Gao, X. B. (2007). Adenosine inhibits activity of hypocretin/orexin neurons by the A1 receptor in the lateral hypothalamus: A possible sleep-promoting effect. J. Neurophysiol. 97, 837–848. doi:10.1152/jn.00873.2006
Lopes, L. V., Rebola, N., Pinheiro, P. C., Richardson, P. J., Oliveira, C. R., and Cunha, R. A. (2003). Adenosine A3 receptors are located in neurons of the rat hippocampus. Neuroreport 14, 1645–1648. doi:10.1097/00001756-200308260-00021
Lopes, L. V., Sebastião, A. M., and Ribeiro, J. A. (2011). Adenosine and related drugs in brain diseases: Present and future in clinical trials. Curr. Top. Med. Chem. 11, 1087–1101. doi:10.2174/156802611795347591
Magdaleno-Madrigal, V. M., Martínez-Vargas, D., Valdés-Cruz, A., Almazán-Alvarado, S., and Fernández-Mas, R. (2010). Preemptive effect of nucleus of the solitary tract stimulation on amygdaloid kindling in freely moving cats. Epilepsia 51, 438–444. doi:10.1111/j.1528-1167.2009.02337.x
McClure, J. M., O'Leary, D. S., and Scislo, T. J. (2011). Neural and humoral control of regional vascular beds via A1 adenosine receptors located in the nucleus tractus solitarii. Am. J. Physiol. Regul. Integr. Comp. Physiol. 300, R744–R755. doi:10.1152/ajpregu.00565.2010
McClure, J. M., O'Leary, D. S., and Scislo, T. J. (2005). Stimulation of NTS A1 adenosine receptors evokes counteracting effects on hindlimb vasculature. Am. J. Physiol. Heart Circ. Physiol. 289, H2536–H2542. doi:10.1152/ajpheart.00723.2005
Methippara, M. M., Kumar, S., Alam, M. N., Szymusiak, R., and McGinty, D. (2005). Effects on sleep of microdialysis of adenosine A1 and A2a receptor analogs into the lateral preoptic area of rats. Am. J. Physiol. Regul. Integr. Comp. Physiol. 289, R1715–R1723. doi:10.1152/ajpregu.00247.2005
Milsom, W. K., and Jackson, D. C. (2011). Hibernation and gas exchange. Compr. Physiol. 1, 397–420. doi:10.1002/cphy.c090018
Missale, C., Nash, S. R., Robinson, S. W., Jaber, M., and Caron, M. G. (1998). Dopamine receptors: From structure to function. Physiol. Rev. 78, 189–225. doi:10.1152/physrev.1998.78.1.189
Mohr, S. M., Bagriantsev, S. N., and Gracheva, E. O. (2020). Cellular, molecular, and physiological adaptations of hibernation: The solution to environmental challenges. Annu. Rev. Cell. Dev. Biol. 36, 315–338. doi:10.1146/annurev-cellbio-012820-095945
Morairty, S., Rainnie, D., McCarley, R., and Greene, R. (2004). Disinhibition of ventrolateral preoptic area sleep-active neurons by adenosine: A new mechanism for sleep promotion. Neuroscience 123, 451–457. doi:10.1016/j.neuroscience.2003.08.066
Nakamura, Y., Midorikawa, T., Monoi, N., Kimura, E., Murata-Matsuno, A., Sano, T., et al. (2016). Oral administration of Japanese sake yeast (Saccharomyces cerevisiae sake) promotes non-rapid eye movement sleep in mice via adenosine A(2A) receptors. J. Sleep. Res. 25, 746–753. doi:10.1111/jsr.12434
Nishimon, S., Sakai, N., and Nishino, S. (2021). Sake yeast induces the sleep-promoting effects under the stress-induced acute insomnia in mice. Sci. Rep. 11, 20816. doi:10.1038/s41598-021-00271-0
Oishi, Y., Huang, Z. L., Fredholm, B. B., Urade, Y., and Hayaishi, O. (2008). Adenosine in the tuberomammillary nucleus inhibits the histaminergic system via A1 receptors and promotes non-rapid eye movement sleep. Proc. Natl. Acad. Sci. U. S. A. 105, 19992–19997. doi:10.1073/pnas.0810926105
Oishi, Y., Xu, Q., Wang, L., Zhang, B. J., Takahashi, K., Takata, Y., et al. (2017). Slow-wave sleep is controlled by a subset of nucleus accumbens core neurons in mice. Nat. Commun. 8, 734. doi:10.1038/s41467-017-00781-4
Olson, J. M., Jinka, T. R., Larson, L. K., Danielson, J. J., Moore, J. T., Carpluck, J., et al. (2013). Circannual rhythm in body temperature, torpor, and sensitivity to A₁ adenosine receptor agonist in arctic ground squirrels. J. Biol. Rhythms 28, 201–207. doi:10.1177/0748730413490667
Omond, S. E. T., Hale, M. W., and Lesku, J. A. (2022). Neurotransmitters of sleep and wakefulness in flatworms. Sleep 45 (5), zsac053. doi:10.1093/sleep/zsac053
Peng, W., Wu, Z., Song, K., Zhang, S., Li, Y., and Xu, M. (2020). Regulation of sleep homeostasis mediator adenosine by basal forebrain glutamatergic neurons. Science 369, eabb0556. doi:10.1126/science.abb0556
Porkka-Heiskanen, T., Strecker, R. E., Thakkar, M., Bjorkum, A. A., Greene, R. W., and McCarley, R. W. (1997). Adenosine: A mediator of the sleep-inducing effects of prolonged wakefulness. Science 276, 1265–1268. doi:10.1126/science.276.5316.1265
Qiu, M. H., Qu, W. M., Xu, X. H., Yan, M. M., Urade, Y., and Huang, Z. L. (2009). D(1)/D(2) receptor-targeting L-stepholidine, an active ingredient of the Chinese herb Stephonia, induces non-rapid eye movement sleep in mice. Pharmacol. Biochem. Behav. 94, 16–23. doi:10.1016/j.pbb.2009.06.018
Qu, W. M., Huang, Z. L., Xu, X. H., Matsumoto, N., and Urade, Y. (2008). Dopaminergic D1 and D2 receptors are essential for the arousal effect of modafinil. J. Neurosci. 28, 8462–8469. doi:10.1523/JNEUROSCI.1819-08.2008
Qu, W. M., Xu, X. H., Yan, M. M., Wang, Y. Q., Urade, Y., and Huang, Z. L. (2010). Essential role of dopamine D2 receptor in the maintenance of wakefulness, but not in homeostatic regulation of sleep, in mice. J. Neurosci. 30, 4382–4389. doi:10.1523/JNEUROSCI.4936-09.2010
Radulovacki, M., Virus, R. M., Djuricic-Nedelson, M., and Green, R. D. (1984). Adenosine analogs and sleep in rats. J. Pharmacol. Exp. Ther. 228, 268–274.
Rainnie, D. G., Grunze, H. C., McCarley, R. W., and Greene, R. W. (1994). Adenosine inhibition of mesopontine cholinergic neurons: Implications for EEG arousal. Science 263, 689–692. doi:10.1126/science.8303279
Ren, C. Y., Rao, J. X., Zhang, X. X., Zhang, M., Xia, L., and Chen, G. H. (2021). Changed signals of blood adenosine and cytokines are associated with parameters of sleep and/or cognition in the patients with chronic insomnia disorder. Sleep. Med. 81, 42–51. doi:10.1016/j.sleep.2021.02.005
Rijo-Ferreira, F., Bjorness, T. E., Cox, K. H., Sonneborn, A., Greene, R. W., and Takahashi, J. S. (2020). Sleeping sickness disrupts the sleep-regulating adenosine system. J. Neurosci. 40, 9306–9316. doi:10.1523/JNEUROSCI.1046-20.2020
Ruf, T., and Geiser, F. (2015). Daily torpor and hibernation in birds and mammals. Biol. Rev. Camb Philos. Soc. 90, 891–926. doi:10.1111/brv.12137
Sala-Newby, G. B., Skladanowski, A. C., and Newby, A. C. (1999). The mechanism of adenosine formation in cells. Cloning of cytosolic 5'-nucleotidase-I. J. Biol. Chem. 274, 17789–17793. doi:10.1074/jbc.274.25.17789
Saper, C. B., Scammell, T. E., and Lu, J. (2005). Hypothalamic regulation of sleep and circadian rhythms. Nature 437, 1257–1263. doi:10.1038/nature04284
Satoh, S., Matsumura, H., and Hayaishi, O. (1998). Involvement of adenosine A2A receptor in sleep promotion. Eur. J. Pharmacol. 351, 155–162. doi:10.1016/s0014-2999(98)00302-1
Satoh, S., Matsumura, H., Koike, N., Tokunaga, Y., Maeda, T., and Hayaishi, O. (1999). Region-dependent difference in the sleep-promoting potency of an adenosine A2A receptor agonist. Eur. J. Neurosci. 11, 1587–1597. doi:10.1046/j.1460-9568.1999.00569.x
Scammell, T. E., Gerashchenko, D. Y., Mochizuki, T., McCarthy, M. T., Estabrooke, I. V., Sears, C. A., et al. (2001). An adenosine A2a agonist increases sleep and induces Fos in ventrolateral preoptic neurons. Neuroscience 107, 653–663. doi:10.1016/s0306-4522(01)00383-9
Schmidt, M. H. (2014). The energy allocation function of sleep: A unifying theory of sleep, torpor, and continuous wakefulness. Neurosci. Biobehav Rev. 47, 122–153. doi:10.1016/j.neubiorev.2014.08.001
Shimaoka, H., Kawaguchi, T., Morikawa, K., Sano, Y., Naitou, K., Nakamori, H., et al. (2018). Induction of hibernation-like hypothermia by central activation of the A1 adenosine receptor in a non-hibernator, the rat. J. Physiol. Sci. 68, 425–430. doi:10.1007/s12576-017-0543-y
Siegel, J. M. (2009). Sleep viewed as a state of adaptive inactivity. Nat. Rev. Neurosci. 10, 747–753. doi:10.1038/nrn2697
Silvani, A., Cerri, M., Zoccoli, G., and Swoap, S. J. (2018). Is adenosine action common ground for NREM sleep, torpor, and other hypometabolic states? Physiol. (Bethesda) 33, 182–196. doi:10.1152/physiol.00007.2018
Silvani, A., and Dampney, R. A. (2013). Central control of cardiovascular function during sleep. Am. J. Physiol. Heart Circ. Physiol. 305, H1683–H1692. doi:10.1152/ajpheart.00554.2013
Sisa, C., Turroni, S., Amici, R., Brigidi, P., Candela, M., and Cerri, M. (2017). Potential role of the gut microbiota in synthetic torpor and therapeutic hypothermia. World J. Gastroenterol. 23, 406–413. doi:10.3748/wjg.v23.i3.406
Soto, M., Orliaguet, L., Reyzer, M. L., Manier, M. L., Caprioli, R. M., and Kahn, C. R. (2018). Pyruvate induces torpor in obese mice. Proc. Natl. Acad. Sci. U. S. A. 115, 810–815. doi:10.1073/pnas.1717507115
Stenberg, D., Litonius, E., Halldner, L., Johansson, B., Fredholm, B. B., and Porkka-Heiskanen, T. (2003). Sleep and its homeostatic regulation in mice lacking the adenosine A1 receptor. J. Sleep. Res. 12, 283–290. doi:10.1046/j.0962-1105.2003.00367.x
Stockwell, J., Jakova, E., and Cayabyab, F. S. (2017). Adenosine A1 and A2A receptors in the brain: Current research and their role in neurodegeneration. Molecules 22, 676. doi:10.3390/molecules22040676
Storey, K. B., and Storey, J. M. (2013). Molecular biology of freezing tolerance. Compr. Physiol. 3, 1283–1308. doi:10.1002/cphy.c130007
Storey, K. B., and Storey, J. M. (2017). Molecular physiology of freeze tolerance in vertebrates. Physiol. Rev. 97, 623–665. doi:10.1152/physrev.00016.2016
Strömberg, I., Popoli, P., Müller, C. E., Ferré, S., and Fuxe, K. (2000). Electrophysiological and behavioural evidence for an antagonistic modulatory role of adenosine A2A receptors in dopamine D2 receptor regulation in the rat dopamine-denervated striatum. Eur. J. Neurosci. 12, 4033–4037. doi:10.1046/j.1460-9568.2000.00288.x
Sun, M. J., and Tang, Y. (2020). Extracellular levels of the sleep homeostasis mediator, adenosine, are regulated by glutamatergic neurons during wakefulness and sleep. Purinergic Signal 16, 475–476. doi:10.1007/s11302-020-09758-3
Swoap, S. J. (2017). Central adenosine and daily torpor in mice. Temp. (Austin) 4, 350–352. doi:10.1080/23328940.2017.1345713
Swoap, S. J., Körtner, G., and Geiser, F. (2017). Heart rate dynamics in a marsupial hibernator. J. Exp. Biol. 220, 2939–2946. doi:10.1242/jeb.155879
Swoap, S. J., Rathvon, M., and Gutilla, M. (2007). AMP does not induce torpor. Am. J. Physiol. Regul. Integr. Comp. Physiol. 293, R468–R473. doi:10.1152/ajpregu.00888.2006
Tamura, Y., Shintani, M., Inoue, H., Monden, M., and Shiomi, H. (2012). Regulatory mechanism of body temperature in the central nervous system during the maintenance phase of hibernation in Syrian hamsters: Involvement of β-endorphin. Brain Res. 1448, 63–70. doi:10.1016/j.brainres.2012.02.004
Tartar, J. L., Hiffernan, F. S., Freitas, K. E., Fins, A. I., and Banks, J. B. (2021). A functional adenosine deaminase polymorphism associates with evening melatonin levels and sleep quality. J. Circadian Rhythms 19, 5. doi:10.5334/jcr.209
Thakkar, M. M., Engemann, S. C., Walsh, K. M., and Sahota, P. K. (2008). Adenosine and the homeostatic control of sleep: Effects of A1 receptor blockade in the perifornical lateral hypothalamus on sleep-wakefulness. Neuroscience 153, 875–880. doi:10.1016/j.neuroscience.2008.01.017
Thakkar, M. M., Winston, S., and McCarley, R. W. (2003). A1 receptor and adenosinergic homeostatic regulation of sleep-wakefulness: Effects of antisense to the A1 receptor in the cholinergic basal forebrain. J. Neurosci. 23, 4278–4287. doi:10.1523/JNEUROSCI.23-10-04278.2003
Thakkar, M. M., Winston, S., and McCarley, R. W. (2002). Orexin neurons of the hypothalamus express adenosine A1 receptors. Brain Res. 944, 190–194. doi:10.1016/s0006-8993(02)02873-1
Tupone, D., Madden, C. J., and Morrison, S. F. (2013). Central activation of the A1 adenosine receptor (A1AR) induces a hypothermic, torpor-like state in the rat. J. Neurosci. 33, 14512–14525. doi:10.1523/JNEUROSCI.1980-13.2013
Urade, Y., Eguchi, N., Qu, W. M., Sakata, M., Huang, Z. L., Chen, J. F., et al. (2003). Sleep regulation in adenosine A2A receptor-deficient mice. Neurology 61, S94–S96. doi:10.1212/01.wnl.0000095222.41066.5e
van Calker, D., Müller, M., and Hamprecht, B. (1979). Adenosine regulates via two different types of receptors, the accumulation of cyclic AMP in cultured brain cells. J. Neurochem. 33, 999–1005.
Vicent, M. A., Borre, E. D., and Swoap, S. J. (2017). Central activation of the A(1) adenosine receptor in fed mice recapitulates only some of the attributes of daily torpor. J. Comp. Physiol. B 187, 835–845. doi:10.1007/s00360-017-1084-7
Vlajkovic, S. M., Abi, S., Wang, C. J., Housley, G. D., and Thorne, P. R. (2007). Differential distribution of adenosine receptors in rat cochlea. Cell. Tissue Res. 328, 461–471. doi:10.1007/s00441-006-0374-2
Wall, M., and Dale, N. (2008). Activity-dependent release of adenosine: A critical re-evaluation of mechanism. Curr. Neuropharmacol. 6, 329–337. doi:10.2174/157015908787386087
Xiao, C., Liu, N., Jacobson, K. A., Gavrilova, O., and Reitman, M. L. (2019). Physiology and effects of nucleosides in mice lacking all four adenosine receptors. PLoS Biol. 17, e3000161. doi:10.1371/journal.pbio.3000161
Xu, Y., Shao, C., Fedorov, V. B., Goropashnaya, A. V., Barnes, B. M., and Yan, J. (2013). Molecular signatures of mammalian hibernation: Comparisons with alternative phenotypes. BMC Genomics 14, 567. doi:10.1186/1471-2164-14-567
Yang, Y. F., Dong, H., Shen, Y., Li, L., Lazarus, M., Qu, W. M., et al. (2021). Mesencephalic dopamine neurons are essential for modafinil-induced arousal. Br. J. Pharmacol. 178, 4808–4825. doi:10.1111/bph.15660
Yuan, X. S., Wang, L., Dong, H., Qu, W. M., Yang, S. R., Cherasse, Y., et al. (2017). Striatal adenosine A(2A) receptor neurons control active-period sleep via parvalbumin neurons in external globus pallidus. Elife 6, e29055. doi:10.7554/eLife.29055
Glossary
A1Rs adenosine A1 receptors
A2ARs adenosine A2A receptors
A2BRs adenosine A2B receptors
A3Rs adenosine A3 receptors
AC adenylate cyclase
ADK adenosine kinase
ADP adenosine diphosphate
AMP adenosine monophosphate
ATP adenosine triphosphate
BAT brown adipose tissue
BF basal forebrain
BMR basal metabolic rate
BP blood pressure
cAMP cyclic adenosine-3,5 monophosphate
CHA N6-cyclohexyladenosine
CNS central nervous system
CPA N6-cyclopentyladenosine
EEG electroencephalographic
EMG electromyography
E-NTPDase ecto-nucleoside triphosphate diphosphohydrolase
Gi inhibitory adenylate cyclase G protein
GPCR G protein coupled receptor
GPe external globus pallidus
Gs stimulating adenylate cyclase G protein
HP heart period
HR heart rate
KATP ATP sensitive potassium channel
LH lateral hypothalamus
MR metabolic rates
NAc nucleus accumbens
NBTIs nitrobenzyl-thio-inosine
NREM non-rapid eye movement
NTS nucleus tractus solitarius
OT olfactory tubercle
PAM positive allosteric modulator
PLC phospholipase C
PV parvalbumin
REM rapid eye movement
rPA raphe pallidus
MnPO median preoptic
SON supraoptic
SAH S-adenosylhomocysteine
SAHH S-adenosylhomocysteine hydrolase
SAM S-adenosylmethionine
SWS slow-wave sleep
Ta ambient temperature
Tb body temperature
TMN tuberomammillary nucleus
VLPO ventrolateral preoptic nucleus lateral preoptic area
LPO lateral preoptic
VP ventral pallidum γ-aminobutyric acid
GABA γ-aminobutyric acid
5′-NT 5′-nucleotidase
Keywords: adenosine, P1 receptors, hibernation, sleep, torpor
Citation: Ma W-X, Yuan P-C, Zhang H, Kong L-X, Lazarus M, Qu W-M, Wang Y-Q and Huang Z-L (2023) Adenosine and P1 receptors: Key targets in the regulation of sleep, torpor, and hibernation. Front. Pharmacol. 14:1098976. doi: 10.3389/fphar.2023.1098976
Received: 15 November 2022; Accepted: 27 February 2023;
Published: 10 March 2023.
Edited by:
Yong Tang, Chengdu University of Traditional Chinese Medicine, ChinaCopyright © 2023 Ma, Yuan, Zhang, Kong, Lazarus, Qu, Wang and Huang. This is an open-access article distributed under the terms of the Creative Commons Attribution License (CC BY). The use, distribution or reproduction in other forums is permitted, provided the original author(s) and the copyright owner(s) are credited and that the original publication in this journal is cited, in accordance with accepted academic practice. No use, distribution or reproduction is permitted which does not comply with these terms.
*Correspondence: Wei-Min Qu, cXV3ZWltaW5AZnVkYW4uZWR1LmNu; Yi-Qun Wang, eWlxdW53YW5nQGZ1ZGFuLmVkdS5jbg==; Zhi-Li Huang, aHVhbmd6bEBmdWRhbi5lZHUuY24=
†These authors have contributed equally to this work