- Department of Pharmacology, Faculty of Pharmaceutical Sciences, Government College University of Faisalabad, Faisalabad, Pakistan
Caryopteris odorata (D. Don) B.L. Robinson (Verbenaceae family) is an aromaric shrub traditionally used to treat diabetes and related pathologies (diabetic foot ulcer), cancer/tumors, wound healing, and inflammation. It is enriched with flavonoids and phenolics like coumarins, quercetin, gallic acid, coumaric acid, stigmasterol, α-tocopherol, and iridoids. C. odorata has been reported as having α-glucosidase, anti-inflammatory, and anti-oxidant properties. Its effectiveness in preventing cardiometabolic syndrome has not yet been assessed. This study aims to investigate the potential efficacy of C. odorata and coumarin for characteristic features of cardiometabolic syndrome (CMS), including obesity, dyslipidemia, hyperglycemia, insulin resistance, and hypertension by using high-refined carbohydrate-high fat-cholesterol (HRCHFC)-loaded feed-fed rats. Chronic administration of C. odorata and coumarin for 6 weeks revealed a marked attenuation in body and organ weights, with a consistent decline in feed intake compared to HRCHFC diet fed rats. The test materials also caused a significant reduction in the blood pressure (systolic, diastolic, and mean) and heart rate of HRCHFC-diet fed rats. Improved glucose tolerance and insulin sensitivity tests were also observed in test material administered rats compare to only HRCHFC-diet fed rats. C. odorata and coumarin-treated animals produced a marked decline in serum FBG, TC, TG, LFTs, and RFTs, while an increase in serum HDL-C levels was noticed. C. odorata and coumarin also significantly modulated inflammatory biomarkers (TNFα, IL-6), adipokines (leptin, adiponectin, and chemerin), and HMG-CoA reductase levels, indicating prominent anti-inflammatory, cholesterol-lowering, and anti-hyperglycemic potential. Administration of C. odorata and coumarin exhibited a marked improvement in oxidative stress markers (CAT, SOD, and MDA). Histopathological analysis of liver, heart, kidney, pancreas, aorta, and fat tissues showed a revival of normal tissue architecture in C. odorata and coumarin-treated rats compared to only HRCHFC-diet fed rats. These results suggest that C. odorata and coumarin possess beneficial effects against the characteristic features of CMS (obesity, insulin resistance, hypertension, and dyslipidemia) in HRCHFC feed-administered rats. These effects were possibly mediated through improved adipokines, glucose tolerance, and insulin sensitivity, the attenuation of HMG-CoA reductase and inflammatory biomarkers, and modulated oxidative stress biomarkers. This study thus demonstrates a rationale for the therapeutic potential of C. odorata and coumarin in CMS.
Introduction
Cardiometabolic syndrome (CMS) is a consolidation of metabolic anomalies characterized by central obesity, insulin resistance, hyperglycemia, hyperlipidemia, and hypertension (Ojetola et al., 2021). It is reaching pandemic levels and hence presents a serious global health concern (Agrawal et al., 2018). CMS prevalence varies and depends greatly upon population, gender, age, and race. It is directly proportional to the increased frequency of obesity, which is considered an underlying cause of CMS (Ojetola et al., 2021). It affects approximately 25% of the world’s adult population. According to a survey conducted by the Dow University of Health Sciences, Karachi, Pakistan, ageing and unhealthy lifestyle practices have led to an upsurge in CMS risk factors which affect more than 51.8% adults—of these, 39.7% had hypertension, 29.7% had obesity, 23.1% diabetes, and 11.9% had dyslipidemia (Inam and Shah, 2019). Increased oxidative stress and inflammation are the key factors in obesity which lead to a sequential progression in insulin resistance (IR), type 2 diabetes, dyslipidemia, hepatic steatosis, and hypertension (Sharma et al., 2012), resulting in the development of CMS. Metabolic syndrome and chronic inflammation have an established association with increased adipocytokine (TNFα, IL-6, leptin, and chemerin) and decreased adiponectin levels (Nwakiban-Atchan et al., 2022).
Dietary habits and lifestyle modifications are known to ameliorate the risk factors of CMS for a limited duration. Similarly, weight reduction also attenuates cardiometabolic abnormalities; however, these modifications remain unsuccessful when practiced intermittently in established CMS. Pharmacological treatment involves the use of multiple therapeutic agents, but with increased cost, patient non-compliance, and adverse effects (Gutierrez-Salmean et al., 2014). Hence, there is an urgent need to develop multifactorial approaches to the treatment of CMS to probe its underlying causes. Due to the high prevalence of CMS, WHO recommends an increased use of medicinal herbs/plants to combat its characteristic features. Multiple evidence also indicates that medicinal plants and naturally derived compounds have great potential to combat CMS and related pathologies (Agrawal et al., 2018; De-Oliveira et al., 2021; Ojetola et al., 2021).
Caryopteris odorata (D. Don) B.L. Robinson, belonging to family Verbenaceae, mostly exists in tropical and subtropical climatic regions. The Verbenaceae family comprises of numerous important medicinal plants with a wide range of biological activities and potent phytochemical constituents. In Pakistan, around 35 species and 17 genera of this plant have been found (Shahzadi et al., 2013). It is a shrub which is found mostly in the subtropics or outer Himalayas of Pakistan, Bhutan, India, and Bangladesh. In Pakistan’s traditional system of medicine, its powdered leaves and flowers are used to treat diabetes and associated pathologies like diabetic foot ulcer, tumors/cancer, wound healing, and general body aches (Abbasi et al., 2017; Joshi et al., 2019). It is used as fuel and fodder in northern areas of Pakistan. It has also been claimed to have antiulcer, antitumor, antidiarrhea, anti-inflammatory, antirheumatic, antihemorrhagic, and antitussive effects in Pakistan, China, and Mongolia (Frezza et al., 2019). It has been used for allergic reactions in Nepal (Bhattarai and Tamang, 2017).
The pharmacological effects of C. odorata, its derived iridoids, and the essential oils of its leaf, stem, and flower feature superodixe dismutase (SOD) and nitric oxide (NO) scavenging (Joshi et al., 2019), and antioxidant (Shahzadi et al., 2011; Shahzadi et al., 2012; Shahzadi et al., 2013; Abbasi M. A. et al., 2014; Singh et al., 2014; Joshi et al., 2019) and lipid peroxidation inhibitory activities (Shahzadi et al., 2011). It has also been reported to possess anti-inflammatory (Joshi et al., 2019), antimicrobial, antifungal, hemolytic (Abbasi M. et al., 2014; Singh et al., 2014), anti-urease, and anti-tyrosinase properties (Shahzadi et al., 2012). Furthermore, C. odorata has also shown enzyme inhibition potential against α-glucosidase, acetylcholinesterase, butylcholinestrase, and lipo-oxygenase (Shahzadi et al., 2013; Abbasi M. et al., 2014), thus providing indirect evidence for its use in diabetes, obesity, dyslipidemia, and hypertension. C. odorata has also been reported to be enriched with diverse phytoconstituents, including quercetin, gallic acid, cinnamic acid, vanillic acid, coumaric acid, ursolic acid, coumarins, and their derivative furanocoumarin (psoralen, methoxsalen, oxypeucedanin, isoimperatorin, and bergamottin) (Abbasi et al., 2017), stigmasterol, α-tocopherol, β-caryophyllene, α-and β-longipinene, α-humulene, caryophyllene oxide, germacrene B and D, and α-bisabolol. Various iridoid glycosides (8-O-trans-cinnamoyl caryoptoside, 8-O-trans-cinnamoyl shanzhiside methyl ester, 8-O-trans-cinnamoyl mussaenoside, 8-O-caffeoyl massenoside) have also been isolated from C. odorata. These possess widespread cardiovascular protective, hepatoprotective, cholagogues, antihyperglycemic, analgesic, anti-inflammatory, anti-cancer, spasmolytic, antitumor, antiviral, immune modulator, and laxative (Shahzadi et al., 2013; Joshi et al., 2019) properties, thus providing support for the use of C. odorata in treating CMS. Despite the available precious pharmacological profile of C. odorata, no study has explored its pharmacological effects on CMS.
Coumarins owe their class name from coumarou, the vernacular name of the tonka bean (Dipteryx odorata). Coumarins were first isolated from the tonka bean by Vogel in 1820 (Bruneton, 1999). They are also referred as “plant-derived secondary metabolites”. Coumarins (1,2-benzopyrone or o-hydroxycinamic acid-8-lactone) constitute a large class of phenolic substances; they are abundantly present in many plants like woodruff, tonka beans, cinnamon, green tea, honey, fruits (cloudberry, bilberry), celery, carrots, and chicory (Lake, 1999). Coumarin possesses anti-oxidant properties, which is evident from its ability to protect cells from oxidative damage and stress (Basile et al., 2009). It has also shown anti-inflammatory properties through stimulation of phagocytosis, enzyme production, and proteolysis to removes protein and edematous fluid from injured tissue sites (Piller, 1975). Coumarin inhibits COX and LOX enzymes, produces SOD (Fylaktakidou et al., 2004), and inhibits protein expression of NO synthase and COX-2 enzyme (Huang et al., 2012). It possesses promising therapeutic potential as an anticoagulant (Abdelhafez et al., 2010) and antithrombotic (Jain et al., 2013) agent for cardiovascular disorders. It has also been considered a vitamin K antagonist because of its potential to interfere with vitamin K cycle conversion (Hirsh et al., 2001). It displays an antihypertensive effect (Nguelefack-Mbuyo et al., 2008) through its smooth muscle relaxant actions (Mead et al., 1958). Coumarin is also known for its effectiveness in reducing left ventricular hypertrophy (Najmanova et al., 2015); it has peripheral and coronary vasodilatory efficacy (Gantimur et al., 1986) through platelet aggregation and NO-mediated system and has thus also been used to treat angina pectoris (Najmanova et al., 2015). Coumarin has also shown an antihyperglycemic effect (Murali et al., 2013). It has exhibited antihyperlipidemic action—possibly by activating the AMPK phosphorylation pathway and downregulating FAS and HMGR protein expression (Iwase et al., 2017; Li et al., 2017). Coumarin also possesses antiadipogenic effects. It is known to inhibit lipid accumulation and lipogenic-related gene expressions in 3T3-L1 adipocytes cells, possibly through the PPARγ pathway (Shin E. et al., 2010; Taira et al., 2017). Although coumarin has been studied in isolation and, in part, for its pharmacological effect in hypertension, diabetes, and dyslipidemia, no study has comprehensively ascertained its pharmacological effects against characteristic features of CMS using a rat model.
This study has been designed to provide a pharmacological basis for the protective potential of C. odorata and coumarin in CMS, including obesity, hyperglycemia, insulin resistance, hypertension, and dyslipidemia using HRCHFC-loaded diet-fed rats. This study also explains the possible mechanism(s) of C. odorata and coumarin for anti-oxidant, anti-hypertensive, anti-inflammatory, and HMG-CoA reductase inhibitory pathways. It thus presents sound evidence for the use of C. odorata in CMS. Furthermore, anti-obesity and insulin sensitizing effects have also been endorsed by the modulating potential of C. odorata and coumarin on adipokinin levels (leptin, adiponectin, and chemerin), and inflammatory (TNFα, IL-6) and oxidative stress biomarkers (CAT, SOD, MDA).
Novelty
• This is a pioneer study to show the effectiveness of C. odorata and coumarin in CMS induced by a HRCHFC-loaded diet.
• The quantification of obesity-related candid parameters including chemerin, leptin, adiponectin, and the inhibition of HMG-CoA reductase for the efficacy of C. odorata and coumarin in CMS.
• C. odorata and coumarin exhibit anti-inflammatory and antioxidant potential, which might reflect their effectiveness in CMS.
Materials and methods
Chemicals and drug
Coumarin ≥99% (HPLC), metformin, rosuvastatin, formaldehyde, and cholic acid were purchased from Sigma Aldrich (St. Louis, MA, United States). Cholesterol was bought from PanReac AppliChem (Ottoweg, Darmstadt, Germany). Additional ingredients, including sodium chloride (NaCl), dry powdered milk (Nido/Everyday, Nestle Ltd, Lahore, Pakistan), vegetable oil (Dalda oil, Unilever, Lahore, Pakistan), desi ghee (Pak pure, United Dairy Pharms, Lahore, Pakistan), and multivitamins (Metavit-Super, Batch# PM5483, Prix Pharmaceuticals (Pvt.) Ltd. Lahore, Pakistan), were purchased from respective commercial suppliers. Choker, highly refined wheat flour, fishmeal, molasses, and potassium metabisulfite were obtained from the local market in Faisalabad, Pakistan. All drug solutions and dilutions of the aqueous methanolic extract of C. odorata were prepared freshly on daily basis in distilled water. Stock solution of coumarin was prepared in 1% (v/v) dimethyl sulfoxide (DMSO) and 1% Tween-80 (w/v). All the chemicals and drugs used in this study were of analytical grade purity.
Plant collection, identification and preparation of extract
Whole plant material was obtained from Poonch, Azad Jammu Kashmir (AJK), Pakistan, in May 2020. Dr. Sardar Irfan Mehmood, Department of Botany, Govt. Boy’s Degree College Abbasapur, Poonch AJK, Pakistan, identified and authenticated the plant material. The crude specimen was also submitted to the AJK Medicinal Plants Herbarium (AJKMPH) with issued Voucher no. AJKH: 437 for future reference. Whole plant of C. odorata was shade dried and ground into a fine powder in a mechanical grinder. Approximately 1.5 kg of fine ground powder was soaked in methanol and distilled water (80:20) for 7 days at 25°C. The first filtrate was collected by passing soaked solution through muslin cloth followed by Whatman filter paper No.1. The maceration process was repeated thrice to obtain a sufficient quantity of extract. A rotary evaporator (Model: RE300 Stuart ® United Kingdom) was used to evaporate all of the filtrates. After air drying the final filtrate, the yield of C. odorata aqueous methanolic extract was 9% wt/wt.
Animals
Wistar albino rats weighing 180–220 g were used in this experiment. These were housed in an animal house at standard conditions of 12 h light and dark cycle, 55% relative humidity, and 22 ± 3°C temperature with free access to food and water. All the experiments were performed according to standard housing conditions and laboratory animal protocols as approved by the animal ethical review committee of GCU, Faisalabad No. IRB: 879 (Ref. No. GCUF/ERC/2279).
Quantitative analysis of C. odorata
Estimation of total flavonoid contents (TFC)
C. odorata extract (500 µL) was mixed with 2 ml of distilled water and 0.15 ml of NaNo2 solution (5%). Next, 150 µL of 10% aluminum chloride (AlCl3) solution with 4% sodium hydroxide solution were added into it. Total volume was made up to 5 ml with methanol. After incubation for 15 min, absorbance was measured at 510 nm. The findings were displayed as (mg/g QE) of catechin equivalent of the concentration of plant extract (Kumar and Roy, 2018).
Estimation of total phenolic contents (TPC)
C. odorata extract was prepared at 0.1 g/ml concentration, and 200 µL (two replicate) was poured in a test tube. Folin Ciocalteu reagent (6%) 1 ml and 0.8 ml of sodium carbonate (Na2CO3) solution (7.5% or 0.6 M) were added to the mixture. The test tube mixture was thoroughly mixed and incubated for 30 min. Absorbance was recorded at a wavelength of 765 nm or 725 nm. Total phenolic contents (TPC) were expressed as mg of gallic acid equivalent in milligram GAE/g of dry plant extract. Gallic acid was used as a reference control (Fetni et al., 2020).
DPPH (1,1-diphenyl-2picryl-hydrazyl) radical scavenging activity
The anti-oxidant potential of C. odorata extract was accessed using DPPH by following an earlier practiced method with slight modification (Fetni et al., 2020). To prepare fresh stock solution, 4 mg of DPPH was mixed with 100 ml methanol. A 3 ml aliquot was prepared with 2,800 µL of DPPH and 200 µL from different concentrations of C. odorata extract (500–6.25 μg/ml). This mixture was stored for 30 min in the dark at 25°C. OD (optical density) was noted at 517 nm. Methanol and DPPH were used as negative controls while, methanol was used as blank control. The antioxidant activity of quercetin (standard) was also evaluated. The % age inhibition of the DPPH radical scavenging capacity of the test samples was computed as: scavenging activity/DPPH % age inhibition = absorbance of negative control − absorbance of test sample/absorbance of negative control × 100.
Estimation of reducing power
A 10 µL test sample and 25 µL each of 1% potassium ferrocyanate (K₄ [Fe (CN)₆] ·3H₂O), 0.2 mM (pH = 6.6) phosphate buffer, and (10%) trichloroacetic acid were added to the test tube. It was then centrifuged to separate the supinated layer. Then, 8.5 µL of ferric chloride and 85 µL of distilled water were added. The mixture was incubated for 30 min and absorbance was recorded at a wavelength of 700 nm. The ferric reducing power of the sample was evaluated using following formula:
% age reducing power = Ao-At/Ao × 100 where Ao = negative control absorbance, At = test sample absorbance (Sharma et al., 2016).
Determination of H2O2 scavenging activity
Hydrogen peroxide (H2O2) (40 mM) solution was freshly prepared in 50 mM phosphate buffer (PBS) (pH = 7.4). Different concentrations of C. odorata extract and standard 10–200 μg/ml (50–100 µL) were added to the H2O2 solution (0.6 ml). After 10 min of incubation, the change in absorbance was measured from 30 s to 3 min at a wavelength of 230 nm. The % age of H2O2 scavenged by the sample was calculated using following formula:
% age of H2O2 scavenging activity = Ao -At/Ao × 100, where Ao = negative control absorbance, At = test sample absorbance (Sharma et al., 2016).
Finger print analysis by Fourier transform infrared (FTIR) spectroscopy
An ATR-FTIR spectrophotometer (Alpha-Bruker, Germany) was used for FTIR analysis of C. odorata extract. The sample was supplied with the projected ATR (Attenuated Total Reflectance) accessory system. ATR potassium bromide diamond crystal was thoroughly cleaned with alcohol during procedure. Around 10 mg of C. odorata extract was prepared and cautiously placed on the surface of the FTIR diamond crystal center. The spectrum was analyzed at an absorbance range of 400–4,000 cm–1 with a resolution of 4 cm–1 (Kumar and Roy, 2018).
High performance liquid chromatographic (HPLC) analysis
The quantifiable determination of flavonoids and phenolics present in C. odorata was conducted using the HPLC technique. A sample of C. odorata extract (50 mg) was prepared with methanol (24 ml), distilled water (16 ml), and hydrochloric acid (10 ml, 6 M). It was then incubated at 95°C for 2 h. The prepared solution was purified by membrane filter paper (nylon, 0.45 μm). A gradient reverse phase HPLC system (Shimadzu, Japan Model = SPD-10AV) was utilized to isolate the phenolics and flavonoids from C. odorata. The system was provided with C18 (shim-pack-CLC-ODS) 5 μm column (25 cm × 4.6 mm) attached with an UV-visible spectrophotometer detector system and automatic injector for sampling. Separation of the valuable constituents was achieved through a gradient mobile phase (A: acetic acid and water; B: acetonitrile, 1 ml/min flow rate). Solvent B was used at a different gradient from time to time, like 15% for 0–15 min, 45% for 15–30 min, and 100% for 35–45 min. The compounds were detected at a preset wavelength of 280 nm and temperature of 25°C. Results were recorded by comparing (Rt) retention time with UV-visible peaks previously gained from the standard compound(s). Quantification was achieved by external standardization (Fetni et al., 2020).
Similarly, the standardization of coumarin was carried out in the isocratic mode using acetonitrile (40%)/water (60%); v/v. An injection volume of 20 μL with a flow rate of 0.5 ml/min was used at 274 nm UV detection. Quantification of coumarin in the plant sample was performed by an external standard method by comparison with coumarin (Sigma-Aldrich) as a standard. The stock solution of plant sample was prepared by mixing 224 mg of the dry extract in a 50 ml solution of methanol/water (80:20). The HPLC chromatogram was obtained using the same mobile phase and detection wavelength as for coumarin (Celeghini et al., 2001).
Preventive effect of C. odorata and coumarin on HRCHFC diet induced CMS
A high-refined carbohydrate-high fat-cholesterol loaded diet was used to induce CMS in animals (Agrawal et al., 2018).
Animal diet preparation
Two different types of diets were prepared for the study.
Standard diet (SD)
SD used the following composition for a 15 kg diet: chokar (5 kg), high-refined wheat flour (5 kg), dried powdered milk (2 kg), molasses (150 g), powdered fishmeal (2.25 kg), sodium chloride (75 g), potassium metabisulfite (15 g), vegetable oil (500 g), desi ghee (1 kg), and multivitamin (33 g). All the solid ingredients were ground and mixed to form palatable biscuits for the animals. Water was added in small quantities to make a soft composition (Aziz et al., 2013).
High-refined carbohydrate-high fat-cholesterol loaded diet (HRCHFC)
An earlier protocol was followed with minor modifications (Aziz et al., 2013). The HRCHFC diet comprised 20% protein, 35% carbohydrate, and 50% fat combined with cholesterol (2%w/w) and cholic acid (0.5% w/w).
Experimental design
Wistar albino rats were randomly allocated into eight different groups of six animals prior to dietary manipulation. The animals in Group 1 were considered the negative control and received a normal or standard diet, while the remaining groups, II–VIII, were administered the HRCHFC diet for 12–14 weeks. Group II was considered the disease control, receiving the HRCHFC diet only. The animals in Groups III–VI were further divided into different treatment groups. Group III was given metformin at 300 mg/kg/d, while group IV was administered metformin + rosuvastatin at (200 mg/kg/d + 1.5 mg/kg/d); these were considered the positive controls. Coumarin at 30 and 70 mg/kg was administered to Groups V and VI, serving as treatment. An aqueous methanolic extract of C. odorata (150 and 300 mg/kg) was administered to Groups VII and VIII as treatment. The treatment schedule was started orally from the eighth week of HRCHFC diet intake and continued until the study was terminated (Agrawal et al., 2018).
The doses of coumarin were selected from the results of our preliminary pilot experiment performed on few animals at given doses (appreciable effects were observed against developed parameters of CMS; data not shown) and the dose range of coumarin used in previous animal studies (Pari and Rajarajeswari, 2009) and doses exhibiting hepatic toxicity (Tasdemir et al., 2017).
Considering that the traditional dose of C. odorata plant used in human is 1 teaspoon thrice daily (Yaseen et al., 2015), the doses of C. odorata extract were selected on the basis of converting human doses to animal doses (Shin J. W. et al., 2010), and the effective dose range of similar species used in animal models (Ullah et al., 2019).
Body weight, feed intake and weight of different body organs
Body weights (g) were recorded at Weeks 0, 2, 4, 6, 8, 10, 12, and 14 to assess weight variation. Feed intake (g) was measured daily. Different organ weights (g) of the animals were calculated at the completion of study, such as the liver, heart, and kidney (Sasso et al., 2019).
Non-invasive measurement of blood pressure (NIBP) in conscious rats
Blood pressure was assessed by the tail cuff method in conscious rats. The systolic pressure of the conscious rats was recorded non-invasively using a tail cuff attached with a PowerLab data acquisition system (AD Instruments, Sydney, Australia) at an interval of 2 weeks. For estimation of blood pressure, the cuff sensor was attached to their tails by keeping the rat in a NIBP restrainer of appropriate size. The expected pressure (SBP, 200 mm Hg) was applied to the tail cuff by inflation followed by its gradual deflation. The intensified pulses were noted by PowerLab on Lab chart 7.0 running on a computer during inflation and deflation. Various hemodynamic parameters like systolic blood pressure (SBP), mean blood pressure (MBP), and heart rate (HR) were directly monitored by pulse tracing. Diastolic blood pressure (DBP) was measured by the formula DBP= (3MBP-SBP)/2. Blood pressure parameters were monitored at Weeks 0, 4, 8, 10, 12, and 14 of the diet in conscious rats. For each measurement, an average of 3–5 pressure readings was recorded (Senaphan et al., 2015).
Determination of fasting blood glucose (FBG), oral glucose tolerance test (OGTT), and insulin sensitivity/tolerance test (ITT)
Fasting blood glucose levels (FBG) were measured from overnight-fasted rats using a digital glucometer (EVOCHECK GM700S) at the end of treatment. During the 14th week of treatment, the animals were subjected to OGTT to measure the effect of C. odorata and coumarin administration on glucose metabolism. All rats had been fasted for 12 h. Afterwards, a glucose load (2 g/kg, p.o.) was administered to all test animals. Blood was drawn from their tail veins to assess the glucose levels in the test samples at 0, 30, 60, 90, and 120 min after glucose load through a digital glucometer (EVOCHECK GM700S). Insulin tolerance was tested at Day 5 before the animals were euthanized. ITT was conducted after 6 h of food deprivation. Blood glucose levels were verified in the animals at a fed state (0 min). Thence, 0.75 U/kg/animal weight of insulin (100 U/ml) was administered through intraperitoneal injection. Blood samples were drawn to assess glucose levels at 15, 30, 60, and 120 min by digital glucometer (EVOCHECK GM700S). Area under curves (AUC) was determined for each animal to calculate the mean for the whole study group (Sasso et al., 2019).
Biochemical analysis
After the 14th week of the experiment, the animals were starved for 18 h before being euthanized after deep anesthesia with isoflurane (5–10% v/wt) through inhalation in a closed chamber. Blood was drawn by cardiac puncture from each rat. The collected blood samples were then centrifuged at 4,000 rpm for 10 min to obtain the serum and stored at −80°C. Serum samples were preserved for further biochemical analysis (Javaid et al., 2021).
Assessment of lipid, liver, and kidney profile indices
The lipid profile including triglycerides (TG), high density lipoprotein (HDL), total cholesterol (TC), liver profile indices (aspartate aminotransferase (AST), alanine aminotransferase (ALT), and renal profile indices (urea and creatinine) were measured from isolated serum samples using commercial biochemical assay kits (Germany) as per the manufacturer’s protocol. The results were shown in mg/dl and U/L (Sasso et al., 2019).
Determination of leptin, adiponectin, chemerin, and HMG-CoA reductase levels
Serum biomarkers for obesity, hyperlipidemia and insulin resistance, leptin (E-EL-R0582), adiponectin (E-EL-H6122), HMG-CoA reductase (E-EL-H2472) were sourced from Elabscience, United States, and chemerin (E-0864Ra) from BT LAB, Birmingham, United Kingdom; levels were analyzed by ELISA Kits as per manufacturer’s instructions. 100 µL of serum (reaction mixture) in pre-coated wells with specific rat antibodies of LEP, ADP, HMG-CoA reductase, and CHEM, maintained at 37°C in an ELISA plate reader (DIA source, Germany), were taken. Responses were checked at 450 nm wavelength. 10 µL of serum leptin was taken and diluted 50-fold. The dilution factor was multiplied with sample OD. Serum adiponectin and chemerin levels were shown as pg/ml and ng/ml, respectively, while leptin and HMG-CoA reductase levels were expressed as ng/mL and pmol/ml, respectively (Della-Vedova et al., 2016).
Determination of inflammatory biomarkers (TNF-α and IL-6)
Serum concentration of TNF-α (E-EL-H0109) and IL-6 (E-EL-H0102) were determined using ELISA Kits (Elabscience Biotechnology CO. Ltd., United States) with sensitivity in the 7.6 pg/ml – 46.88 pg/ml range. Assays were performed as per manufacturer's protocol. The reaction mixture was provided 100 μL of serum in previously coated wells in the ELISA plate reader (DIA source, Germany) and kept at 37°C. The reactions were measured at an absorbance of 450 nm. The findings are presented as pg/ml or pg/mg (Adedapo et al., 2020).
Determination of oxidative stress biomarkers
The anti-oxidant potential of C. odorata and coumarin was analyzed by measuring the amount of catalase, superoxide dismutase, and malondialdehyde peroxidase in tissue homogenate. After 14 weeks, animals were starved for 18 h before being euthanized through deep anesthesia with isoflurane (5–10% v/wt) by inhalation in a closed chamber. Organs (liver, heart, kidney, and aorta) were removed and washed with ice-cold normal saline. These were stored at −80°C for further analysis. 100 mg of each organ was homogenized with 5 ml of tris-base/phosphate buffer solution (7.4 pH) and centrifuged at 2000 rpm for 15–20 min at 4°C. The supernatant layer was removed and stored at -80°C for further analysis. For catalase activity (CAT), 50 µL of different organ homogenates were mixed with freshly prepared H2O2 (30 mM, 1 ml) and 50 mM phosphate buffer (7.0 pH, 1.95 ml). Absorbance was measured at 240 nm. Catalase activity was estimated as units/g tissue. Superoxide dismutase (SOD) was estimated by following an earlier practiced method (Younis et al., 2018) where chromogen intensity was measured at an absorbance of 560 nm against blank. It was equated with the known SOD standard curve as units/mg. Lipid peroxidation/malondialdehyde peroxidase (MDA) levels were evaluated using the methodology of Adedapo et al. (2020).
Histological examination
The isolated tissues of heart (5 mm), liver, kidney, aorta, pancreas, and fat (5 μm) were dissected and fixed with 17% formalin. Different organ tissue sections were stained with hematoxylin and eosin. A microtome (Leica, Germany) was used to collect thin sections of organs to assess histopathological changes under a light microscope (ACCU-SCOPE 3001-LED Digital Microscope, United States) (Sasso et al., 2019).
Statistical analysis
The values were presented as mean ± SEM. The significance among results was tested using one-way analysis of variance (ANOVA) followed by Dunnett‘s test, and two-way ANOVA followed by Dunnett‘s test/Bonferroni post doc test and unpaired t-test. Non-linear regression analysis was applied to compare concentration response curves. GraphPad Software 8.4.3 (Diego California United States) was used for statistical analysis and calculation to convert data into graphs.
Results
TFC and TPC
The equivalent contents of TF and TP were quantified using standard regression lines of catechin and gallic acid, respectively. TFC of C. odorata extract were 67.34 mg catechin/g DE and TPC were 86.82 mg GAE/g DE weight.
Antioxidant activities
Aqueous methanolic extract of C. odorata was found to be a promising reducing agent with an IC50 value of 78.38 ± 0.79 mg/ml. C. odorata extract showed 20.86 ± 0.87 U/mg protein of H2O2% inhibition. The DPPH % age activity of C. odorata extract is shown in Figure 1. C. odorata extract showed % age DPPH inhibition with maximum effect of 84.61% at 200 μg/ml concentration. The IC50 value was 29.78 μg/ml, similar to the effect of quercetin (IC50 value = 107.3 μg/ml).
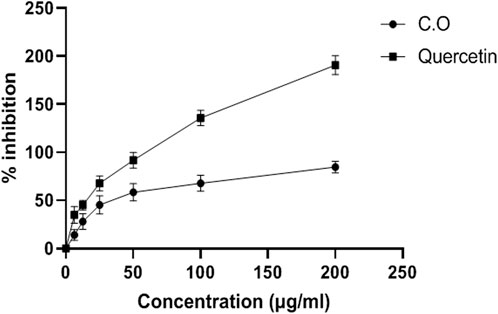
FIGURE 1. Antioxidant DPPH radical scavenging activity of aqueous methanolic extract of C. odorata (in vitro). Values are stated as mean ± SEM (n = 6).
FTIR analysis
Figure 2 depicts the spectrum of the FTIR analysis of C. odorata extract. The ATR-FTIR spectrum was interpreted in comparison with previous reported studies (Table 1). An infrared spectrum represents a fingerprint of a sample with absorption peaks which correspond to the frequencies of vibrations between the bonds of the atoms comprising the material. The absorption signals of 1–11 wave numbers in C. odorata FTIR-spectrum showed first peak at 3,245.75 cm−1, which lies in the reference range of 3,000–3,600. This range represents possible stretching of C–H, O–H and N–H bands of alcohol, phenol, amine, or amide. The second peak of 2,938.41 cm−1 found in the 2,800–2,900 reference range indicates a C–H stretch of alkanes. The peak at 2,366.59 cm−1 and 2059.31 cm−1 at a 2000–2,500 reference range represents C≡N and C≡C bonds of alkynes and isothiocyanate. Peak 5 at 1,607.74 cm−1 of the 1,600–1706 range revealed the presence of amino acids. The peak of 1,521.24 at the 1,500–1,600 range finds N–H bonds of either carboxylic acid salt, amide of amino acids, or nitro compounds. The remaining peak at 1,442.22, 1,358.66, 1,245.68, 1,014.86, and 926.32 wavenumber (cm−1) indicates the aromatic or phenyl group, amide I, phenol, alcohol or nitro, alkyl halide, acyl, or phenyl, ether or aliphatic phosphate, and the methylene (CH2) group of isoprenoids as functional groups, respectively, as detailed in Figure 2 and Table 1.
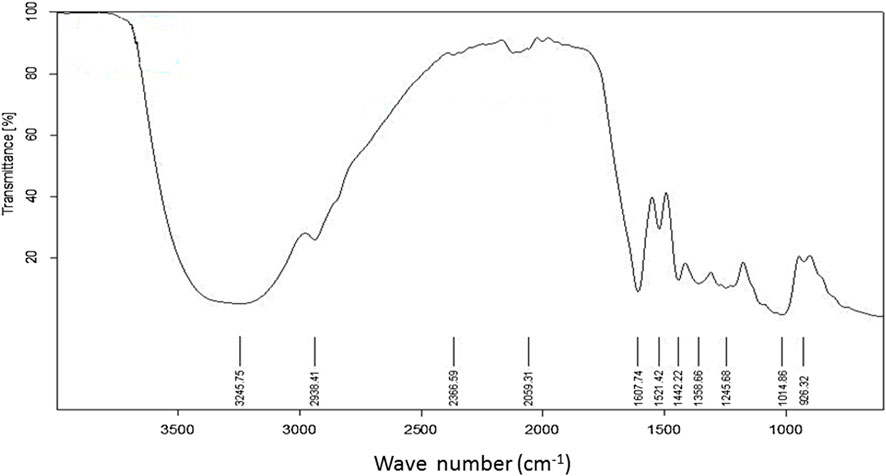
FIGURE 2. FTIR spectrum of aqueous methanolic extract of C. odorata studied at 400–4,000 cm−1 range.
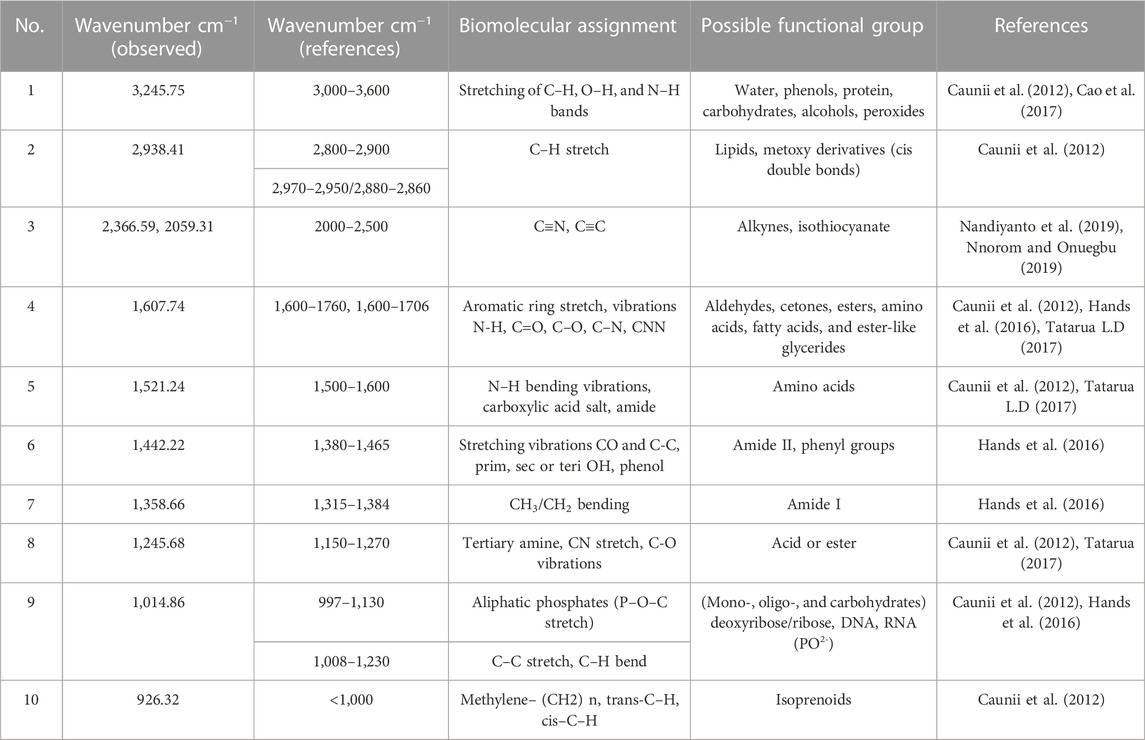
TABLE 1. FTIR frequency range with peaks and functional groups present in aqueous methanolic extract of C. odorata.
HPLC analysis
The achieved chromatograms of C. odorata extract (Figures 3A, B) showed identified compounds as plant constituents. The HPLC profiles of the test materials were related to the standards. The quantitative results were presented in ppm, where quercetin (7.53), catechin (0.34), gallic acid (9.42), caffeic acid (8.75), ferulic acid (176.6), chologenic acid (3.23), syringic acid (0.58), p-coumaric acid (0.84), sinapic acid (0.39) (Figure 3A), and coumarins (416.24) (Figure 3B) were found as active ingredients of C. odorata. The observed retention times (rt) of these standards were 3.09, 3.56, 4.88, 12.06, 13.79, 15.14, 16.22, 17.43, and 26.59 min, respectively. Standard coumarin appeared with 5.63 min (rt) (Figure 3C), while it appeared in plant extract at a retention time of 5.58 min.
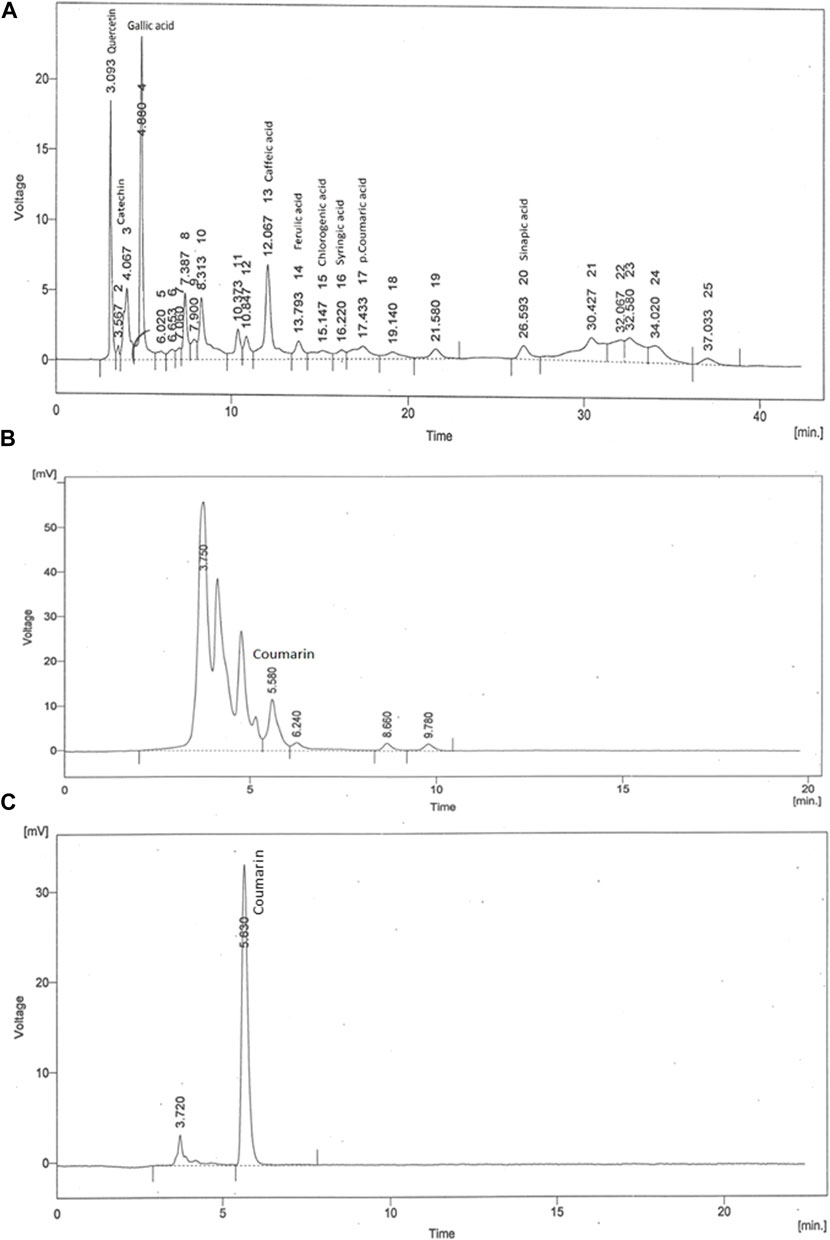
FIGURE 3. HPLC fingerprint of (A) aqueous methanolic extract of C. odorata in gradient mode, (B) aqueous methanolic extract of C. odorata in isocratic mode, and (C) standard coumarin.
Effect of C. odorata and coumarin on body weight, feed intake, and weight of different body organs
Initially, rats in all groups did not show a significant (p > 0.05) difference in their body weight. However, HRCHFC-fed rats showed a significant (p < 0.001) rise at Weeks 10–14. Chronic administration of C. odorata, coumarin or positive controls to HRCHFC-fed rats markedly (p < 0.001) reduced weight gain at Weeks 12–14 of the study (Table 2). Similarly, C. odorata treatment caused a marked (p < 0.001) reduction in feed intake pattern at Weeks 11–14 compared to HRCHFC-fed rat data. Coumarin or positive controls groups showed a consistent pattern of feed intake at the start of experiment and showed significant (p < 0.001) reduction in daily diet intake at Weeks 12–14 of the study period compared to HRCHFC group data (Figure 4). Table 3 shows that HRCHFC-fed rats displayed a marked (p < 0.001) increase in liver and heart weight with no effect on kidney weight at end of the study. Significant (p < 0.001) reductions in liver and heart weight were seen in all treatment groups.
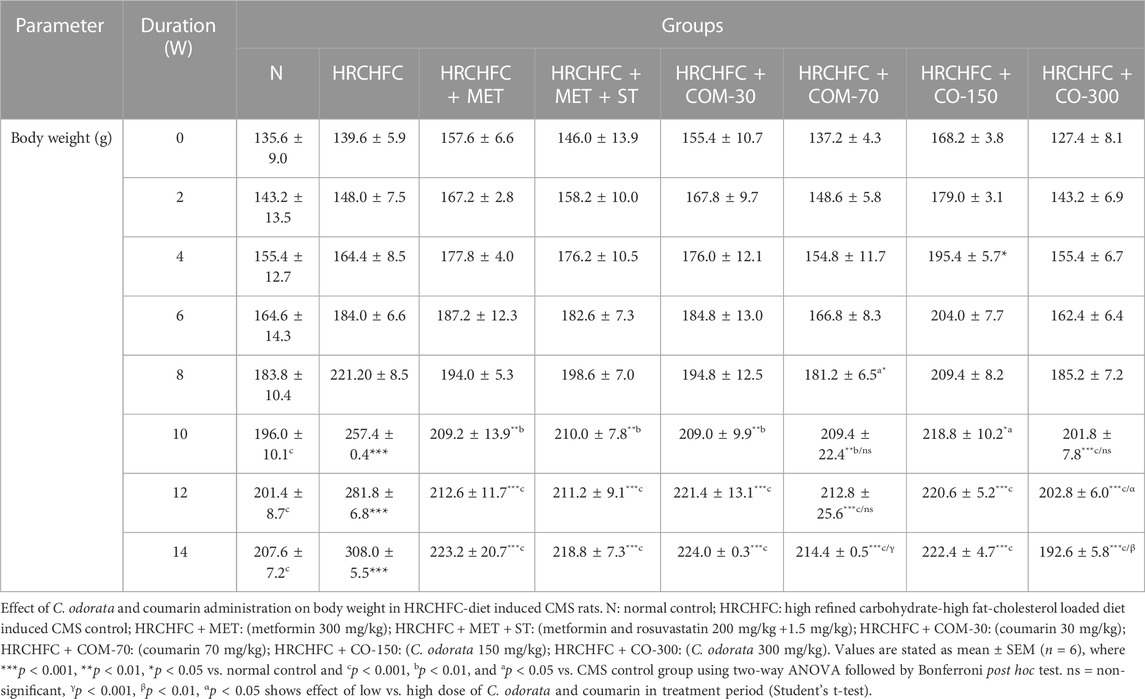
TABLE 2. Effect of C. odorata and coumarin administration on body weight in HRCHFC- diet induced CMS rats.
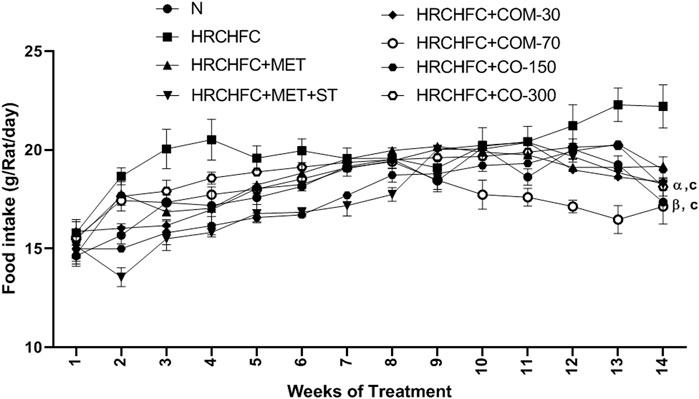
FIGURE 4. Effect of C. odorata and coumarin administration on feed intake in HRCHFC- diet induced CMS rats. N: normal control; HRCHFC: high refined carbohydrate-high fat-cholesterol loaded diet induced CMS control; HRCHFC + MET: (metformin 300 mg/kg); HRCHFC + MET + ST: (metformin and rosuvastatin 200 mg/kg + 1.5 mg/kg); HRCHFC + COM-30: (coumarin 30 mg/kg); HRCHFC + COM-70: (coumarin 70 mg/kg); HRCHFC + CO-150: (C. odorata 150 mg/kg); HRCHFC + CO-300: (C. odorata 300 mg/kg). Values are expressed as mean ± SEM (n = 6), where ***p < 0.001, **p < 0.01, *p < 0.05 vs. normal control and cp < 0.001, bp < 0.01 and ap < 0.05 vs. CMS control group using two-way ANOVA followed by Bonferroni post hoc test. ns = non-significant, γp < 0.001, βp < 0.01, αp < 0.05 shows effect of low vs. high dose of C. odorata and coumarin (Student’s t-test).
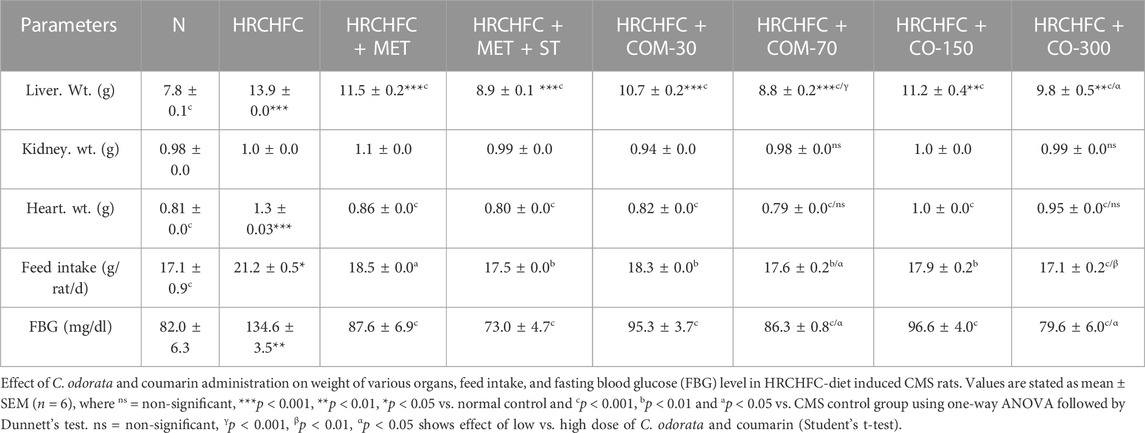
TABLE 3. Effect of C. odorata and coumarin administration on weight of various organs, feed intake, and fasting blood glucose level in HRCHFC-diet induced CMS rats.
Hypotensive effect of C. odorata and coumarin
The hypotensive effect of chronically-administered C. odorata and coumarin on systolic blood pressure, mean arterial pressure, diastolic blood pressure, and heart rate in HRCHFC-fed rats is evident in Tables 4 and 5. The results clearly showed that HRCHFC diet administration revealed a noticeable (p < 0.001) rise in SBP, MAP, and DBP, along with HR, at Weeks 12–14 of the study period compared to the control rats. Subsequently, after 6 weeks of the treatment schedule, all treated rats manifested a significant (p < 0.001) decline in blood pressure (SBP, DBP, MBP) and HR at Weeks 10–14 of the study period compared to HRCHFC-fed rats. The hypotensive effect of C. odorata and coumarin were observed dose-dependent in SBP, MBP (10–14 weeks), and HR (12–14 weeks).
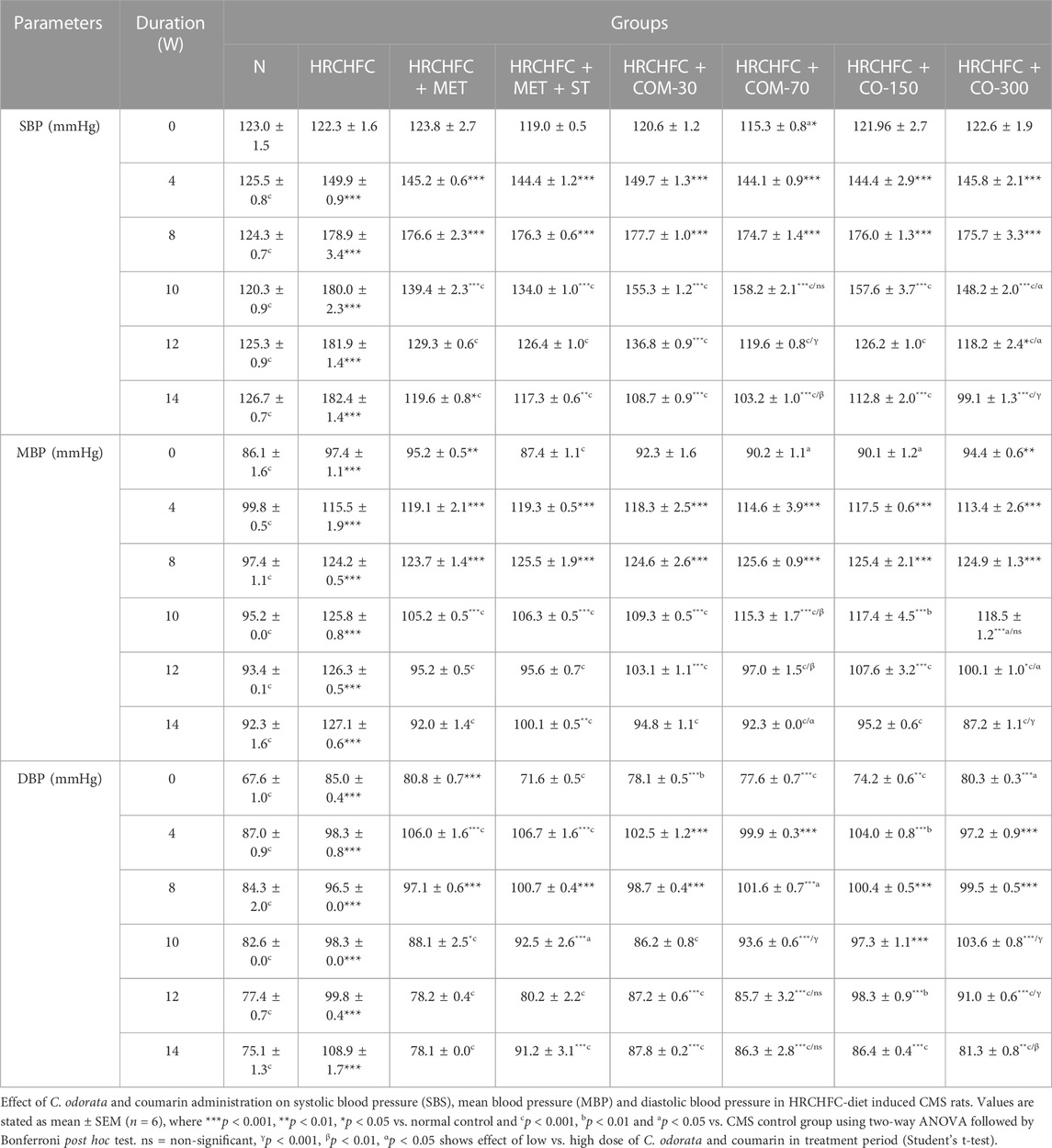
TABLE 4. Effect of C. odorata and coumarin administration on systolic blood pressure (SBP), mean blood pressure (MBP), and diastolic blood pressure (DBP) in HRCHFC-diet induced CMS rats.
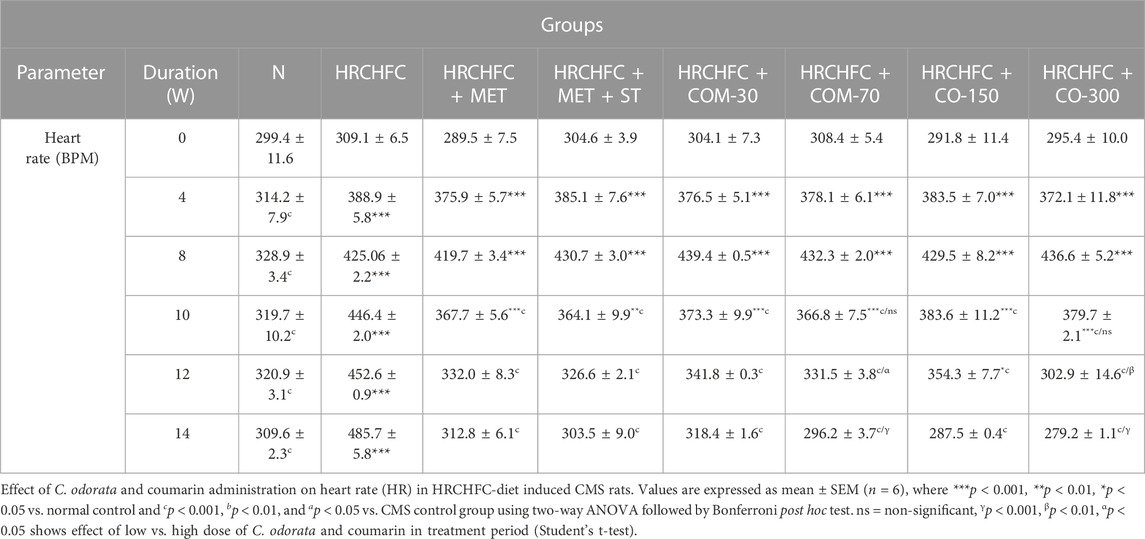
TABLE 5. Effect of C. odorata and coumarin administration on heart rate in HRCHFC-diet induced CMS rats.
Effect of C. odorata and coumarin on fasting blood glucose (FBG), oral glucose tolerance (OGT), and insulin sensitivity tests (ITT)
Administration of C. odorata and coumarin significantly (p < 0.001) showed a dose-dependent reduction in elevated fasting blood glucose in HRCHFC-fed rats (Table 3). These effects were comparable to standards. HRCHFC-fed rats exhibited notably (p < 0.05) impaired glucose tolerance against normal control rats. A remarkably (p < 0.001) faster disposal of glucose levels was noticed from circulation at 60, 90, and 120 min in C. odorata and coumarin-treated rats compared to HRCHFC-fed rats (Figure 5A). The AUC study revealed a significant difference in HRCHFC-fed rats compared to normal control rats (Figure 5B). The ITT results showed that, after 6 weeks of treatment with C. odorata and coumarin, significant (p < 0.001) differences in blood glucose levels were analyzed following insulin administration. ITT showed notably (p < 0.01) lower responsiveness to insulin in HRCHFC-fed rats compared to normal rats. C. odorata and coumarin-treated rats showed marked (p < 0.001) insulin sensitivity compared to HRCHFC-fed rats. These results were verified by AUC analysis between treated groups during the experiment period (Figures 5C, D).
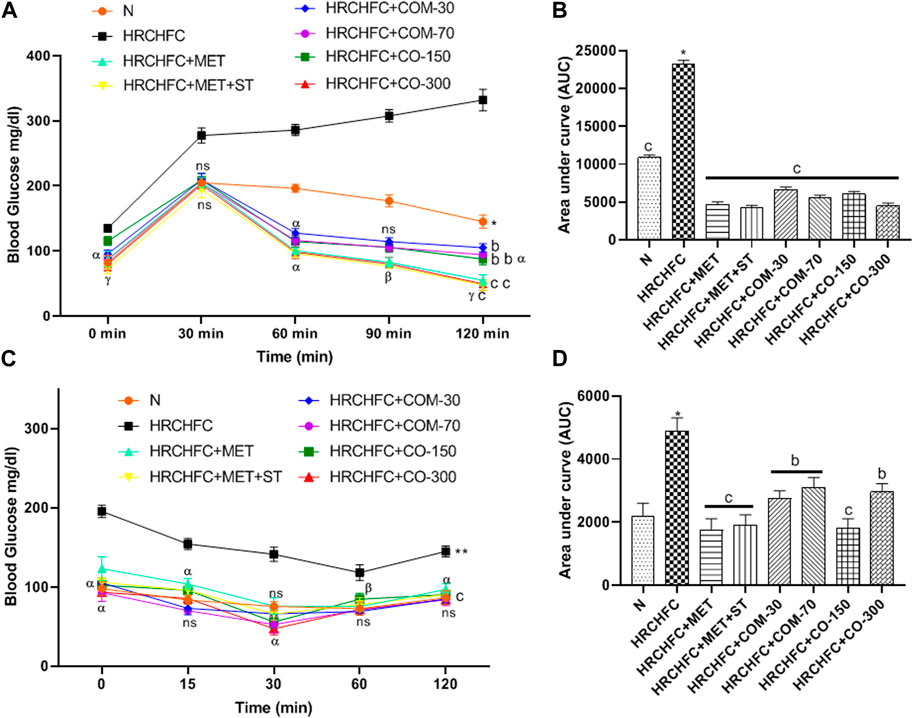
FIGURE 5. Effect of C. odorata and coumarin administration on (A) oral glucose tolerance test during 14th week of study, (B) AUC of blood glucose level of animals measured at end of treatment, (C) insulin tolerance test, and (D) AUC of blood glucose level of animals measured after insulin injection in HRCHFC diet induced CMS rats. N: normal control; HRCHFC: high refined carbohydrate-high fat-cholesterol loaded diet induced CMS control; HRCHFC + MET: (metformin 300 mg/kg); HRCHFC + MET + ST: (metformin and rosuvastatin 200 mg/kg +1.5 mg/kg); HRCHFC + COM-30: (coumarin 30 mg/kg); HRCHFC + COM-70: (coumarin 70 mg/kg); HRCHFC + CO-150: (C. odorata 150 mg/kg); HRCHFC + CO-300: (C. odorata 300 mg/kg). Values are expressed as mean ± SEM (n = 6), where ***p < 0.001, **p < 0.01, *p < 0.05 shows normal control vs. CMS control group (Student’s t-test); cp < 0.001, bp < 0.01 and ap < 0.05 show a comparison of treatment vs. CMS control group using one-way ANOVA followed by Dennett’s test. ns = non-significant, γp < 0.001, βp < 0.01, αp < 0.05 shows effect of low vs. high dose of C. odorata and coumarin (Student’s t-test).
Effect of C. odorata and coumarin on serum biochemical markers
HRCHFC-fed rats showed a significant (p < 0.001) increase in serum triglycerides and total cholesterol (lipid biomarkers), aspartate transaminase, alanine transaminase (liver biomarker enzyme), urea, and creatinine (renal biomarkers) levels, while showing a notable reduction in serum high density lipoprotein cholesterol levels compared to the normal control group. However, concurrent treatment with C. odorata, coumarin, and positive controls produced a notable (p < 0.001) decline in serum TC, TG, ALT, AST, urea, and creatinine levels, while elevated HDL-C levels were observed compared to only HRCHFC-fed rats (Table 6).
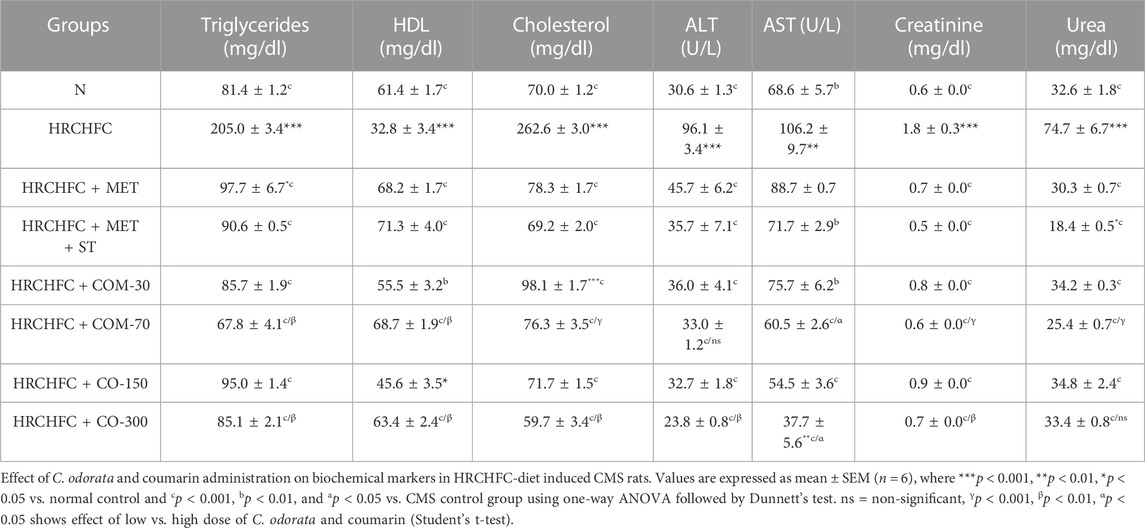
TABLE 6. Effect of C. odorata and coumarin administration on biochemical markers in HRCHFC-diet induced CMS rats.
Effect of C. odorata and coumarin on serum biomarkers of leptin, adiponectin, chemerin, and HMG-CoA reductase levels
The animals on the HRCHFC diet showed a marked (p < 0.001) elevation in serum leptin and chemerin levels and HMG-CoAreductase activity with serum leptin, chemerin and HMG-CoA reductase levels, while adiponectin levels were found to be decreased in diseased animals compared to the normal control group. After the sixth week of treatment with C. odorata, coumarin, and positive controls, there was a marked (p < 0.001) suppression in leptin and chemerin levels and HMG-CoAreductase activity with leptin, chemerin and HMG-CoA reductase levels, while a significant (p < 0.001) rise of adiponectin levels was observed in treated rats compared with data from only HRCHFC-fed rats (Figure 6).
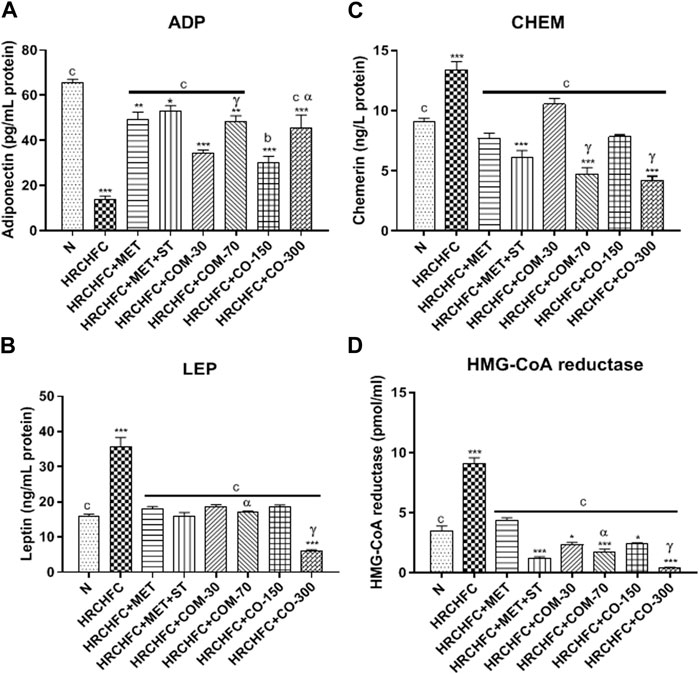
FIGURE 6. Effect of C. odorata and coumarin administration on serum (A) ADP, (B) LEP, (C) CHEM, (D) HMG-CoA reductase in HRCHFC-diet induced CMS rats. N: normal control; HRCHFC: high refined carbohydrate-high fat-cholesterol loaded diet induced CMS control; HRCHFC + MET: (metformin 300 mg/kg); HRCHFC + MET + ST: (metformin and rosuvastatin 200 mg/kg +1.5 mg/kg); HRCHFC + COM-30: (coumarin 30 mg/kg); HRCHFC + COM-70: (coumarin 70 mg/kg); HRCHFC + CO-150: (C. odorata 150 mg/kg); HRCHFC + CO-300: (C. odorata 300 mg/kg). Values are expressed as mean ± SEM (n = 6), where ***p < 0.001, **p < 0.01, *p < 0.05 vs. normal control, and cp < 0.001, bp < 0.01 and ap < 0.05 vs. CMS control group using one-way ANOVA followed by Dunnett’s test. ns = non-significant, γp < 0.001, βp < 0.01, αp < 0.05 shows effect of low vs. high dose of C. odorata and coumarin (Student’s t-test).
Effect of C. odorata and coumarin on inflammatory biomarkers (TNF-α and IL-6)
HRCHFC-fed rats displayed a significant (p < 0.001) elevation in TNF-α and IL-6 levels versus the normal control group. However, oral treatment of C. odorata and coumarin to diseased animals produced a significant (p < 0.001) fall in serum TNF-α and IL-6 levels to normal values, comparable to positive controls (Figure 7).
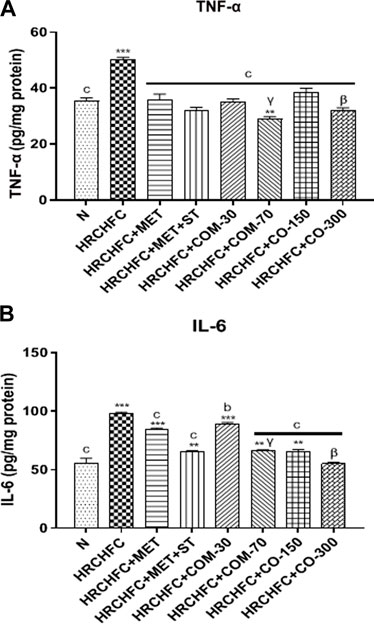
FIGURE 7. Effect of C. odorata and coumarin administration on serum (A) TNF-α, (B) IL-6 in HRCHFC diet induced CMS rats. Values are expressed as mean ± SEM (n = 6), where ***p < 0.001, **p < 0.01, *p < 0.05 vs. normal control and cp < 0.001, bp < 0.01 and ap < 0.05 vs. CMS control group using one-way ANOVA followed by Dunnett’s test. ns = non-significant, γp < 0.001, βp < 0.01, αp < 0.05 shows effect of low vs. high dose of C. odorata and coumarin (Student’s t-test).
Effect of C. odorata and coumarin on oxidative stress markers
Figure 8 shows the effect of C. odorata and coumarin supplementation on oxidative stress markers in liver, heart, kidney, and aorta tissue homogenates. C. odorata and coumarin-treated rats significantly (p < 0.001) raised catalase and superoxide dismutase enzymes in their livers, heart, kidneys, and aortas, which were found depleted in the organs of HRCHFC-fed rats. The detrimental effect of lipid peroxidation in HRCHFC-fed rats markedly (p < 0.001) decreased, representing an attenuation in MDA levels when treated with C. odorata and coumarin.
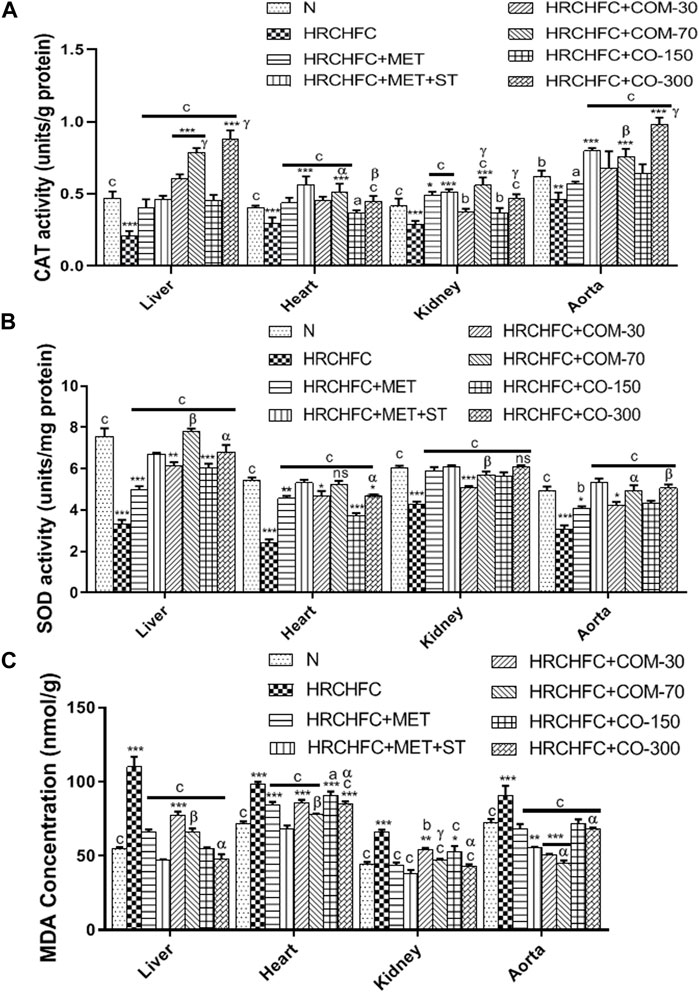
FIGURE 8. Effect of C. odorata and coumarin administration on (A) CAT, (B) SOD, and (C) MDA enzyme in various organs of HRCHFC- diet induced CMS rats. Values are expressed as mean ± SEM (n = 6), where ***p < 0.001, **p < 0.01, *p < 0.05 vs. normal control and cp < 0.001, bp < 0.01 and ap < 0.05 vs. CMS control group using one-way ANOVA followed by Dunnett’s test. ns = non-significant, γp < 0.001, βp < 0.01, αp < 0.05 shows effect of low vs. high dose of C. odorata and coumarin (Student’s t-test).
Effect on histopathology of organs
The histopathological examination of C. odorata and coumarin-treated liver tissues revealed a restored normal or intact texture of hepatocyte with a lesser appearance of fat deposition, reduced hepatic lesions, and necrosis with diminished inflammatory cells. A normal portal vein and dilation of sinusoids were observed in treated groups, while HRCHFC-fed rats caused degenerative and vacuolized hepatocytes, damaged hyaline with fat deposition, inflammatory cell infiltration, and congested sinusoids. The heart tissues of treated rats revealed non-infracted architecture or intact branches of myocardium, lesser cell infiltration, necrosis, and inflammation, and reduced edema with a restoration of myofibril integrity. Restored normal glomerulus with no hyperemia, normal basement membrane and capillaries, no shrinkage of the renal cortex, and absence of inflammatory cells was observed in the kidney tissues of treated rats, whereas the aortic tissues of treated groups presented significant progression of foam cells, repair of elastic fibers, and lessened fat deposition in the tunica media layer of the aorta. An organized pattern, normal architecture, and the regeneration and development of islets of Langerhans and β-cells were evident in the pancreatic tissue of C. odorata and coumarin-treated rats. The dilation of the intra-lobular duct and reduced inflammation of pancreatic tissue were also observed in treated groups. Treated rats reversed the size and number of adipocytes with less inflammation in the fat pad of the epididymal tissues (Figure 9).
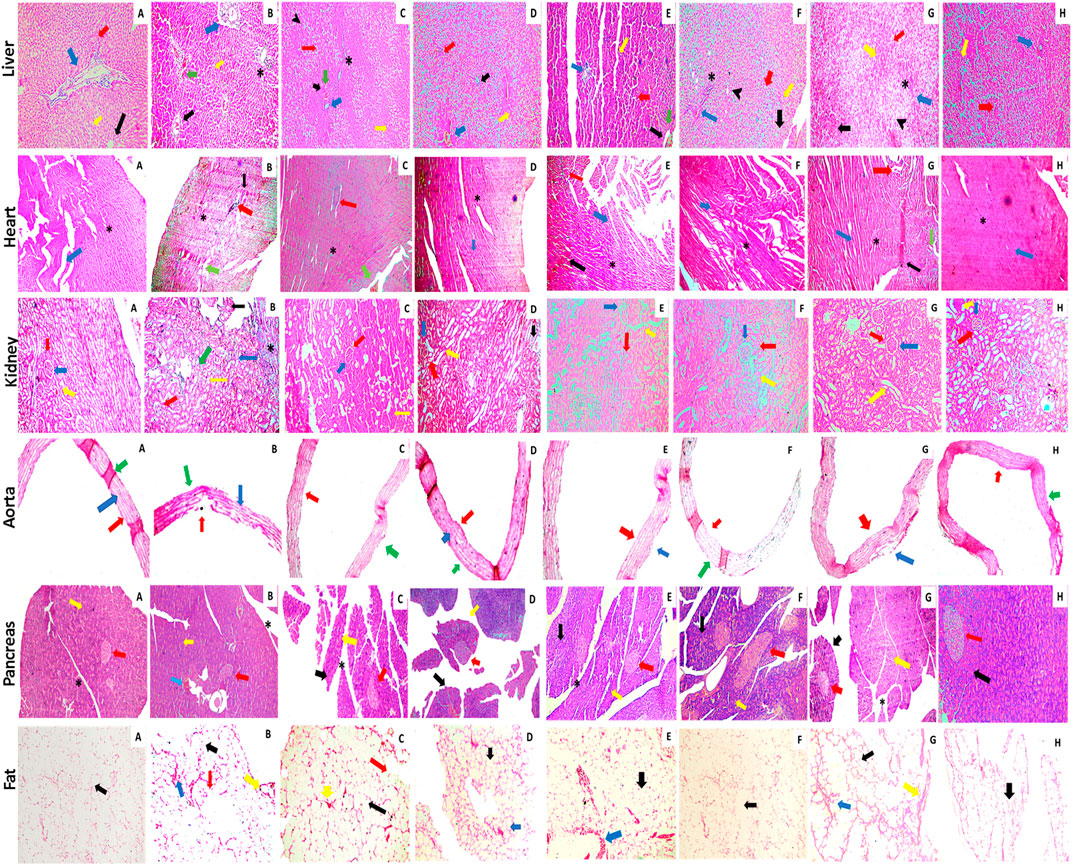
FIGURE 9. Photomicrographic illustration of liver, heart, kidney, aorta, and fat tissue sections stained with H&E dye showing effect of administration of C. odorata: aqueous methanolic extract of C. odorata (150 and 300 mg/kg) and coumarin: (30 and 70 mg/kg) in HRCHFC diet-induced CMS and normal rats. Liver: black, yellow, green, red, blue arrows, stearic, and arrow head, respectively, show central vein, sinusoids, necrosis, hepatocyte, branch of portal triad, cell infiltration, and fat collection. Heart: black, green, red, blue arrows, and stearic, respectively, show inflammation, necrosis, cell infiltration, myofibrils, and cardiomyocytes myofibrils. Kidney: red, blue, yellow, black arrows, and stearic, respectively, show Bowman’s capsule, glomerulus, distal convoluted tubules, shrinkage of cortex, and cell infiltration. Pancreas: yellow, black, blue, red arrows, and stearic, respectively, show exocrine pancreas, pancreatic acinar cells, cell infiltration, islets of Langerhans, and intra lobular duct. Aorta: red, blue, and green arrows show tunica adventitia, media, and intima layers of blood vessel, respectively. Fat: yellow, green, red, and blue arrows, respectively, show collagen fiber, adipose cell, fat deposit, and inflammation.
Discussion
Due to a high mortality and morbidity rate, CMS presents a serious global health risk, thus demanding effective therapeutic alternatives (Agrawal et al., 2018). The results of the present study clearly demonstrated that C. odorata and coumarin improved the characteristic features of CMS by attenuating obesity, dyslipidemia, oxidative stress, insulin resistance, glucose intolerance, and hypertension in HRCHFC-fed rats. A chronic administration of a HRCHFC diet with additional cholic acid induces oxidative stress, and hemodynamic and metabolic alteration (Prasatthong et al., 2021) that mitigate the cellular metabolism of dietary ingredients, resulting in the development of obesity and related comorbidities such as hyperglycemia, glucose intolerance, dyslipidemia, arterial hypertension, insulin resistance, systemic inflammation, and hepatic steatosis (Senaphan et al., 2015).
The traditional use of C. odorata in the treatment of diabetes and its α-glucosidase-inhibitory activity (Abbasi M. et al., 2014) provide a sound basis to its potential use as an anti-diabetic. Furthermore, C. odorata has high amount of coumarin as phenolic content (Joshi et al., 2019). Thus, the beneficial effects of C. odorata and coumarin are attributed to its increased antioxidant activity and attenuation of excessive adipocytokine production, including TNF-α, IL-6, leptin, chemerin, and adiponectin with the regulation of body weight and feed intake. A notable improvement in hepatic steatosis and dyslipidemia is strongly related to the modulation of HMG-CoA reductase and inflammatory cytokines. C. odorata and coumarin reversed HRCHFC- diet induced hypertension, possibly through a NO mediated pathway.
Phytochemical analysis and the oxidative potential of plant extract were examined by HPLC, TFC, TPC, and radical scavenging activities (DPPH, H2O2, reducing power). The conventional FTIR spectrum of C. odorata showed a narrow peak at 3,245.75 cm–1 (O–H and N–H), while a peak at 2,938.41 cm–1 identifies a C–H stretch. Sharp peaks at 2,366.59, 2059.31 cm–1 (C≡N, C≡C) indicate the presence of a CH2 group. The peaks present at 1,607.74, 1,521.24 cm–1 (C=O), 1,442.22 cm–1 (aromatic ring), 1,358.66 (C–H), and 1,245.68 (O–H) indicate that C. odorata contains flavonoids, alkaloids, and phenolics. Interval peaks at 1,014.86 and 926.32 cm–1 show a C–O–C group of saponins. The HPLC analysis revealed a quantitative amount of a variety of polyphenols, mainly phenolic acids, flavonoids, and their derivatives in aqueous methanolic extract of C. odorata: quercetin, catechin, gallic acid, caffeic acid, ferulic acid, chologenic acid, syringic acid, p-coumaric acid, sinapic acid, and a higher expression of coumarins. Many of these polyphenols are reported to have an ameliorating effect on obesity and other features of metabolic syndrome, including glucose intolerance, dyslipidemia, hypertension, and oxidative stress (Prince et al., 2021). Thus, the results obtained from this study that indicate the ability of C. odorata to inhibit obesity and associated metabolic alterations are attributed in part to the presence of these polyphenolic compounds—amongst these, coumarin contributes prominently to the overall effectiveness of C. odorata in CMS.
After the eighth week of induction with the HRCHFC diet, significant weight gain and food consumption were observed due to the addition of added vegetable oil (fat), fish meal (protein), and refined carbohydrate. This may intricate hyperplasia and hypertrophy of adipocytes (BrahmaNaidu et al., 2014).
As a result of high caloric diet intake, the energy metabolism is compromised in the liver (Gutierrez-Salmean et al., 2014). Low energy levels stimulate the hunger center, increasing food intake and resulting in the development of CMS in animals. This suggests that low hepatic energy influences feeding behavior and ultimately causes an increase in body weight (Zhang et al., 2021). This study’s results clearly showed that weight gain is consistent with feed intake. The administration of C. odorata and coumarin significantly and in a dose-dependent manner reduced weight gain and feed intake during the experimental period. Treated groups probably increased energy consumption and enhanced fat oxidation, thus causing weight loss in HRCHFC-fed rats (Mabrouki et al., 2020). Organ weight increase, particularly of the liver and heart, in diseased animals might be due to inflammation, hypertrophy, and steatosis (Nwakiban-Atchan et al., 2022), while a marked decrease was observed in treated groups. These findings indicate the anti-obesity effect of C. odorata and coumarin. This was also confirmed by morphological examination of the adipocyte of fat pads, where a significant increase in the size and number of adipocytes were reversed to normal in the treatment groups.
It is known that metabolic complications related to obesity are prevented by inhibiting elevated adipokine levels. HRCHFC diet-induced obesity and related diseases like diabetes, insulin resistance, and increased fat mass are associated with the dysregulation of adipocytokines. These are secreted from adipocyte, in which adiponectin has anti-diabetic, anti-atherogenic, and anti-inflammatory properties (Sasso et al., 2019). Leptin is appetite regulator hormone which plays a major role in balanced feed intake and body weight control. Leptin secretion is positively correlated with the degree of triglycerides stored in adipose tissues (BrahmaNaidu et al., 2014). Elevated chemerin levels show the interlinking of metabolic syndrome and obesity by producing pathophysiological complications such as increased fat, glucose, lipid metabolism, inflammation, and elevated blood pressure that lead to hyperlipidemia and insulin resistance. Chemerin also stimulates insulin-mediated glucose uptake and improves insulin action in 3T3-L1 adipocyte to treat obesity and insulin resistance (Takahashi et al., 2008). The present study highlights C. odorata and coumarin treated rats as presenting high levels of adiponectin and reduced levels of leptin and chemerin. Reduced leptin may be due to decreased body fat mass and increased leptin sensitivity (BrahmaNaidu et al., 2014). These observations indicate that the regulation of adipokines in treated groups may be due to reduced lipid buildup in adipocytes, which is also consistent with the findings on the lipid profile in treated rats.
Insulin resistance is considered a hallmark for metabolic syndrome and diabetes (Senaphan et al., 2015). It causes elevated adipokine secretion, which develops hyperglycemia or glucose intolerance for metabolic adaptation. A HRCHFC diet produces hepatic insulin resistance, possibly by elevating the circulation of free fatty acid (FFA) levels (Prince et al., 2021). Elevated FFA in HRCHFC-fed rats leads to increased serum glucose levels that manifest obesity-induced glucose intolerance and inhibit muscle glucose uptake (Lasker et al., 2019). Excessive FFA levels also damage the pancreas to produce insulin to combat hyperglycemia (BrahmaNaidu et al., 2014). C. odorata and coumarin alleviate hyperglycemia by reducing FBG levels to prevent insulin resistance. The observed effects might be achieved due to the presence of a diverse nature of phytoconstituents in the plant. This effect is in line with previously reported in vitro α-glucosidase activity of C. odorata (Abbasi M. et al., 2014), which also supports the anti-diabetic action of C. odorata. C. odorata and coumarin administration caused an improvement in HRCHFC diet-induced impaired glucose tolerance in a dose-dependent manner, evident by its positive impact in the oral glucose tolerance test and assessed FBG levels. Treatment groups markedly reduced their blood glucose levels following by insulin administration, thus showing an improvement in insulin sensitivity. This effect possibly occurs due to improvement in one or more defects viz. insulin receptors, insulin receptor substrate, glucose transporters, or glycosylated enzymes. The liberation of inflammatory cytokines is also a strong inducer of insulin resistance (Senaphan et al., 2015). Improved insulin resistance was also shown in the pancreatic tissue of C. odorata and coumarin treated rats, where damaged islets of Langerhans, atrophic β-cell, reduced β-cell mass, and congested intra-lobular duct were revived to normalcy.
Atherogenic dyslipidemia is an intricate disorder related to obesity, diabetes, and metabolic syndrome that enhances the progression of CVD risk (Prince et al., 2021). HRCHFC diet-induced hypertriglyceridemia causes endothelial dysfunction, atherosclerosis, and increased oxidative stress which can be prevented by antioxidants by altering the lipid metabolism (Aziz et al., 2013). In our study, a HRCHFC diet with cholesterol and cholic acid increased TG and TC levels while decreasing HDL-C levels, possibly by increasing FFA in the liver due to insulin deficiency and/or resistance. Hence, more TGs are absorbed into adipose tissues (Nwakiban-Atchan et al., 2022). The reduced liberation of FFA results in elevated levels of VLDL (Ojetola et al., 2021). These changes were endorsed by the observed decreased HMG-CoA reductase activity (Nepal et al., 2011) and TG uptake by peripheral tissues (Sharma et al., 2012). However, reduced HDL-C levels are due to activated lipoprotein lipase and lecithin cholesterol-acyl transferase (LCAT) with reduced cholesterol catabolism (BrahmaNaidu et al., 2014). Treatment with C. odorata and coumarin noticeably improved the lipid profile by causing a decrease in HMG-CoA reductase activity, and TG and TC levels, while increasing HDL-C levels—possibly by inhibiting lipid synthesis—and reducing cholesterol absorption and secretion from the intestine. HMGR (3-hydroxy-3-methylglutaryl-coenzyme A reductase) limits the endogenous enzyme for cholesterol synthesis and is also used to catalyze HMG-CoA reductase conversion to mevalonate (BrahmaNaidu et al., 2014). HMG-CoA reductase inhibitors (lipid-lowering agents) are used as first line agents in treating hyperlipidemia (Javaid et al., 2021). Our study also showed increased HMGR levels in HRCHFC-fed rats, which is an established fact of obesity related to CMS (Kalaivani et al., 2019). Most therapeutic agents that decrease TC levels may also potentially block HMGR enzymes (Istvan, 2002). These findings suggest that C. odorata and coumarin possess anti-dyslipidemic effects.
The elevated hepatic enzymes released in HRCHFC-fed rats may be due to the modified plasma membrane permeability of hepatocytes and biliary obstruction, thus increasing the risk of developing non-alcoholic fatty liver disease (NASH) (Suman et al., 2016). Hepatocyte damage followed by oxidative damage leads to liver injury. Furthermore, higher oxidative stress, dysregulated adipocytokine production, and mitochondrial dysfunction are causative factors of NASH (Sharma et al., 2012). Administration of C. odorata and coumarin to rats caused a remarkable dose-dependent reduction in serum ALT and AST levels to relieve hepatic steatosis through protecting hepatic dysfunction. Increased urea and creatinine levels in CMS-developed animals were found to be strongly associated with renal damage (Bamanikar et al., 2016). Treatment with C. odorata and coumarin offered a significant attenuation in serum urea and creatinine levels to prevent nephropathy. Damaged renal tissues in HRCHFC-fed rats were produced by ROS production (El-Alfy et al., 2005). Intake of C. odorata and coumarin in CMS animals restored renal architecture in HRCHFC-fed rats, possibly by neutralizing free radicals in these tissues. Our results are in accordance with previously published data (Bhatti et al., 2022). The observed findings might be attributed to the presence of abundant phytochemicals in C. odorata, their antioxidant potential, and anti-inflammatory properties.
Manifested features of hepatic steatosis were found to be reduced in hepatic tissues of treated groups, which was evident in revived hepatocyte texture, reduced vacuolization, and congestion in the central vein as well as minimized fat deposition. Similarly, the kidney tissues of treated groups also revived congested glomerular blood vessels, necrotic tubules, inflammation, and cloudy deteriorative parts to a more normal architecture.
The administration of HRCHFC diet for 8 weeks initiated the development of obesity and low-grade chronic inflammation mediated by the liberation of pro-inflammatory cytokines like IL-6 and TNF-α (Sasso et al., 2019). The literature also showed that elevated inflammatory biomarkers and free radicals cause insulin resistance commonly associated with endothelial dysfunction, dyslipidemia, and hyperglycemia (Senaphan et al., 2015). Elevated FFAs in HRCHFC-fed rats directly activate the macrophages to secret pro-inflammatory cytokines (TNF-α primarily stimulate others) that render insulin resistant in peripheral tissues (Jung et al., 2011). This study showed that treatment with C. odorata and coumarin exhibited a significant dose-dependent decrease in serum TNF-α and IL-6 levels. Thus, increased antioxidant enzyme activity and the suppression of inflammatory markers show potential in treating hypertension, insulin resistance, diabetes, obesity, and hepatic steatosis.
C. odorata and coumarin-treated groups displayed significantly elevated catalase and superoxide dismutase enzyme levels compared to HRCHFC-fed rats. SOD and CAT are considered first-line defense anti-oxidant enzymes against ROS (Nepal et al., 2011). The upregulation of CAT levels prevents atherosclerosis and blocks angiotensin-II mediated aortic wall hypertrophy. Elevated SOD levels prevent the inactivation of NO and protect the liver from oxidative damage by scavenging molecular oxygen (Sharma et al., 2012). Thus, the reduction offered in CAT and SOD levels might demonstrate the cardio and hepatoprotective effects of C. odorata and coumarin. A HRCHFC diet administered to animals causes oxidative stress which is either enzymatically or non-enzymatically reflected as higher level of TBARS or reduced levels of SOD, CAT, GPx, GSTs, and GSH (Aziz et al., 2013). Earlier studies reported that increased oxidative stress in HRCHFC-fed rats is usually an outcome of inflammation, insulin resistance, hypertension, dysregulated adipocytokines, and NASH (Kabelova et al., 2021). Oxidative stress develops with increased ROS and reduced antioxidant enzymes, resulting in excessive molecular oxygen and hydrogen peroxide that lead to initiate lipid peroxidation (Sharma et al., 2012). C. odorata and coumarin administration attenuated lipid peroxidation levels, which was evident by reduced MDA levels in treated rats, and thus offers protection against hypertension. Theses finding on C. odorata and coumarin may highlight their therapeutic potential in treating CMS.
Hypertension is a characteristic feature of metabolic syndrome related to obesity (Suman et al., 2016). HRCHFC-fed rats show multiple underlying mechanism(s) that mediate hypertension, including endothelium dysfunction, oxidative degradation of NO, and diminished eNOS activity (Ghibu et al., 2019). Previous studies have reported that decreased NO production in vascular endothelium accounts for impaired vascular function that can result in vascular diseases including hypertension. Endothelial cells also form active oxygen radicals in response to hyperlipidemia and inflammation, which causes destruction of NO and vasoconstriction. A growing body of evidence suggests that HRCHFC diet leads to increased levels of LDL-c and oxidative stress, which was also observed in our study. This increases the levels of oxidized LDL which has a suppressive action on endothelial NO synthase. ROS also increases cytosolic Ca2+ in smooth muscle as well as sensitizing the muscle contractile apparatus in vascular smooth muscle, further augmenting vasoconstriction (Roberts et al., 2000). Elevated ROS, mainly superoxide anions, can strongly inactivate NO, leading to the development of vascular damage (Sharma et al., 2012). Moreover, superoxide anions fuses to NO and tyrosine to make peroxynitrite and nitrotyrosine, respectively, which ultimately attack proteins, lipids, and DNA to cause cellular damage. It has also been reported that hyperlipidemia, insulin resistance, and inflammatory contributors in CMS have repressive effects on eNOS. These increase the wall thickness of conduit vessels, leading to deceased NO synthesis for the development of hypertension (Ojetola et al., 2021). Vasomotor tone modulation and vascular remodeling can also be managed by rendering oxidative stress biomarkers, which are known to mediate cardiovascular complications (Senaphan et al., 2015). Several studies have shown that the intake of natural agents enriched with antioxidants has proven beneficial effects against oxidative stress-augmented pathophysiological anomalies. (Nepal et al., 2011). In our study, the observed anti-hypertensive effect on the part of C. odorata and coumarin by reducing systolic blood pressure (SBP), diastolic blood pressure (DBP), mean blood pressure (MBP), and heart rate (HR) might be associated with its anti-oxidant, antihyperlipidemic, and/or anti-inflammatory activities.
Furthermore, treated rats showed a marked improvement in the morphological features of aortic and cardiac tissues by exhibiting restored myocardial fiber texture, reduced inflamed necrotic areas, and a repaired tunica media layer with less fat deposition, indicating the cardiovascular beneficial potential of the test materials.
Many studies have shown that the phytoconstituents of C. odorata account for its multiple therapeutic properties (Shahzadi et al., 2012; Abbasi M. et al., 2014; Abbasi M. A. et al., 2014; Abbasi et al., 2017; Joshi et al., 2019). Flavonoids and phenolic compounds have much biological potential due to their antioxidant and free radical scavenging properties (Prince et al., 2021). Furthermore, it is unclear which constituent is exactly responsible for attenuating the characteristic features of CMS. Hence, further investigation is needed to determine the biologically active constituent responsible for the actions of C. odorata against CMS.
Conclusion
C. odorata and coumarin improved obesity and dyslipidemia through the modulation of adipocytokines (leptin, adiponectin, chemerin), inhibition of HMG-CoA reductase, and attenuation of the lipid profile. Treatment with C. odorata and coumarin offered an anti-hypertensive effect and caused modulation in insulin resistance, possibly through its effect on amended oxidative stress biomarkers (SOD, CAT, and MDA), inflammatory mediators (TNF-α and IL-6) and improved insulin sensitivity. Its protective effect for hepatic steatosis was evident by its positive influence on hepatic function markers (LFTs). Thus, this study highlights the therapeutic potential of C. odorata and coumarin for treating cardiometabolic syndrome.
Data availability statement
The original contributions presented in the study are included in the article/Supplementary Material; further inquiries can be directed to the corresponding author.
Ethics statement
The animal study design was reviewed and approved by the Ethical Review committee of GCU Faisalabad [IRB:879 (Ref. No. GCUF/ ERC/2279]. Laboratory animals were used in this study as per approved protocol.
Author contributions
MHM proposed the idea and design layout of the research. He supervised this project entirely and was also involved in guidance, data acquisition and analysis, and writing, reviewing and submission of the manuscript. MGA played a role in concept design of the study, experimental performance, data acquisition and analysis, and manuscript writing. SM was involved in data analysis and manuscript writing. MF was involved in data collection and interpretation.
Funding
We acknowledge the Higher Education Commission (HEC) of Pakistan for partial financial support for publication under the NRPU project (Project# 20-9195/NRPU/R&D/HEC/2017-2018) awarded to PI, MHM, supervisor of PhD scholar MGA, HEC-PhD Indigenous Scholar [pin no: 518-77854-2MD5-014 (50043678)].
Acknowledgments
We are very obliged to HEC, Pakistan, for providing partial financial support to complete this research with the help of the indigenous fellowship program and the NRPU project. We are also grateful for the assistance of the helpers in the animal house and faculty members in the labs of the Department of Pharmacology, Faculty of Pharmaceutical Sciences, GCUF.
Conflict of interest
The authors declare that the research was conducted in the absence of any commercial or financial relationships that could be construed as a potential conflict of interest.
Publisher’s note
All claims expressed in this article are solely those of the authors and do not necessarily represent those of their affiliated organizations, or those of the publisher, the editors, and the reviewers. Any product that may be evaluated in this article, or claim that may be made by its manufacturer, is not guaranteed or endorsed by the publisher.
Abbreviations
ALT, alanine transaminase; AST, aspartate transaminase; CAT, catalase; CMS, cardiometabolic syndrome; CRP, c reactive protein; CVD, cardiovascular diseases; DBP, diastolic blood pressure; ELISA, enzyme-linked immunosorbent assay; HDL, high density lipoprotein; HMG-CoA reductase, (3-hydroxy-3-methyl-glutaryl-coenzyme A) reductase; HR, heart rate; HRCHFC, high refined-carbohydrate-high fat-cholesterol; IL-6, interlukin-6; IR, insulin resistance; LFTs, liver function tests; MBP, mean blood pressure; MDA, Malondialdehyde; NIBP, non-invasive blood pressure; RFTs, renal function tests; ROS, reactive oxygen species; SBP, systolic blood pressure; SOD, superoxide dismutase; TC, total cholesterol; TG, total triglycerides; TNF-α, tumor necrosis factor.
References
Abbasi, M. A., Hussain, G., Siddiqui, S. Z., Ahmed, V. U., and Ahmad, V. U. (2017). In silico study of furocoumarins from Caryopteris odorata: Moderate inhibitors of butyryl cholinesterase and lipoxygenase. Asian J. Chem. 29 (4), 758–762. doi:10.14233/ajchem.2017.20241
Abbasi, M. A., Shahzadi, T., and Ahmad, V. U. (2014b). New iridoid glucosides from Caryopteris odorata with suitable antioxidant potential. Chem. Nat. Compd. 50 (5), 836–841. doi:10.1007/s10600-014-1095-5
Abbasi, M., Aziz-ur-Rehman, , Ahmad, V., Riaz, T., and Khlaid, F. (2014a). Evaluation of antibacterial, antifungal, enzyme inhibition and hemolytic activities of Caryopteris odorata fractions. Int. Res. J. Pharm. 4, 9–15. doi:10.7897/2230-8407.041203
Abdelhafez, O. M., Amin, K. M., Batran, R. Z., Maher, T. J., Nada, S. A., and Sethumadhavan, S. (2010). Synthesis, anticoagulant and pivka-ii induced by new 4-hydroxycoumarin derivatives. Bioorg. Amp; Med. Chem. 18 (10), 3371–3378. doi:10.1016/j.bmc.2010.04.009
Adedapo, A. D. A., Ajayi, A. M., Ekwunife, N. L., Falayi, O. O., Oyagbemi, A., Omobowale, T. O., et al. (2020). Antihypertensive effect of phragmanthera incana (schum) balle on NG-nitro-L-arginine methyl ester (L-NAME) induced hypertensive rats. J. Ethanopharmacology 257, 112888. doi:10.1016/j.jep.2020.112888
Agrawal, R., Nath, V., Kumar, H., and Kumar, V. (2018). Deciphering activation in cardiometabolic syndrome: Studies by in silico and in vivo experimental assessment. J. Recept. Signal Transduct. 38 (2), 122–132. doi:10.1080/10799893.2018.1436560
Aziz, N., Mehmood, M. H., and Gilani, A.-H. (2013). Studies on two polyherbal formulations (ZPTO and ZTO) for comparison of their antidyslipidemic, antihypertensive and endothelial modulating activities. BMC Complementary Altern. Med. 13 (1), 371–379. doi:10.1186/1472-6882-13-371
Bamanikar, S., Bamanikar, A., and Arora, A. (2016). Study of Serum urea and Creatinine in Diabetic and non-diabetic patients in in a tertiary teaching hospital. J. Med. Res. 2 (1), 12–15. doi:10.31254/jmr.2016.2104
Basile, A., Sorbo, S., Spadaro, V., Bruno, M., Maggio, A., Faraone, N., et al. (2009). Antimicrobial and antioxidant activities of coumarins from the roots of ferulago campestris (apiaceae). Molecules 14 (3), 939–952. doi:10.3390/molecules14030939
Bhattarai, S. B. S., and Tamang, R. T. R. (2017). Medicinal and aromatic plants: A synopsis of makawanpur district, Central Nepal. Int. J. Indig. Herbs Drugs, 6–15.
Bhatti, J. S., Sehrawat, A., Mishra, J., Sidhu, I. S., Navik, U., Khullar, N., et al. (2022). Oxidative stress in the pathophysiology of type 2 diabetes and related complications: Current therapeutics strategies and future perspectives. Free Radic. Biol. Med. 184, 114–134. doi:10.1016/j.freeradbiomed.2022.03.019
BrahmaNaidu, P., Nemani, H., Meriga, B., Mehar, S. K., Potana, S., and Ramgopalrao, S. (2014). Mitigating efficacy of piperine in the physiological derangements of high fat diet induced obesity in sprague dawley rats. Chemico-biological Interact. 221, 42–51. doi:10.1016/j.cbi.2014.07.008
Bruneton, J. (1999). Immunotoxicity of epicutaneously applied anti-coagulant rodenticide warfarin. Hampshire, UK: Intercept Ltd., 245–263.
Cao, Z., Wang, Z., Shang, Z., and Zhao, J. (2017). Classification and identification of rhodobryum roseum limpr. And its adulterants based on fourier-transform infrared spectroscopy (FTIR) and chemometrics. PLoS One 12 (2), e0172359. doi:10.1371/journal.pone.0172359
Caunii, A., Pribac, G., Grozea, I., Gaitin, D., and Samfira, I. (2012). Design of optimal solvent for extraction of bio–active ingredients from six varieties of medicago sativa. Chem. Central J. 6 (1), 123–128. doi:10.1186/1752-153X-6-123
CeleghinI, R., Yariwake, J., and Lancas, F. (2001). Extraction and quantitative HPLC analysis of coumarin in hydroalcoholic extracts of mikania glomerata spreng: ("guaco") leaves. J. Braz. Chem. Soc. 12, 706–709. doi:10.1590/s0103-50532001000600003
De-Oliveira, A. M., De-Freitas, A. F. S., Costa, M. D. D. S., Torres, M. K. D. S., Castro, Y. A. D. A., Almeida, A. M. R., et al. (2021). Pilosocereus gounellei (cactaceae) stem extract decreases insulin resistance, inflammation, oxidative stress, and cardio-metabolic risk in diet-induced obese mice. J. Ethnopharmacol. 265, 113327. doi:10.1016/j.jep.2020.113327
Della-Vedova, M. C., Muñoz, M. D., Santillan, L. D., Plateo-Pignatari, M. G., Germano, M. J., Rinaldi Tosi, M. E., et al. (2016). A mouse model of diet-induced obesity resembling most features of human metabolic syndrome. Nutr. Metabolic Insights 9, 93–102. NMI-S32907. doi:10.4137/NMI.S32907
El-Alfy, A. T., Ahmed, A. A., and Fatani, A. J. (2005). Protective effect of red grape seeds proanthocyanidins against induction of diabetes by alloxan in rats. Pharmacol. Res. 52 (3), 264–270. doi:10.1016/j.phrs.2005.04.003
Fetni, S., Bertella, N., Ouahab, A., Zapater, J. M. M., and Fernandez, S. D. P.-T. (2020). Composition and biological activity of the Algerian plant rosa caninal. By HPLC-UV-MS. Arabian J. Chem. 13 (1), 1105–1119. doi:10.1016/j.arabjc.2017.09.013
Frezza, C., Venditti, A., Serafini, M., and Bianco, A. (2019). Phytochemistry, chemotaxonomy, Ethnopharmacology, and nutraceutics of lamiaceae. Stud. Nat. Prod. Chem. 62, 125–178. doi:10.1016/B978-0-444-64185-4.00004-6
Fylaktakidou, K. C., Hadjipavlou-Litina, D. J., Litinas, K. E., and Nicolaides, D. N. (2004). Natural and synthetic coumarin derivatives with anti-inflammatory/antioxidant activities. Curr. Pharm. Des. 10 (30), 3813–3833. doi:10.2174/1381612043382710
Gantimur, D., Syrchina, A. I., and Semenov, A. A. (1986). Khellactone derivatives from phlojodicarpus sibiricus. Chem. Nat. Compd. 22 (1), 103–104. doi:10.1007/BF00574597
Ghibu, S., Craciun, C. E., Rusu, R., Morgovan, C., Mogosan, C., Rochette, L., et al. (2019). Impact of alpha-lipoic acid chronic discontinuous treatment in cardiometabolic disorders and oxidative stress induced by fructose intake in rats. Antioxidants 8 (12), 636. doi:10.3390/antiox8120636
Gutierrez-Salmean, G., Pilar, O.-V., Maria, V., Leticia, G.-S., German, C.-C., Meaney, E., et al. (2014). Effects of (-)-Epicatechin on A diet-induced rat model of cardiometabolic risk factors. Eur. J. Pharmacol. 728, 24–30. doi:10.1016/j.ejphar.2014.01.053
Hands, J. R., Clemens, G., Stables, R., Ashton, K. M., Brodbelt, A. R., Davis, C., et al. (2016). Brain tumour differentiation: Rapid stratified serum diagnostics via attenuated total reflection fourier-transform infrared spectroscopy. J. Neuro-Oncology 127 (3), 463–472. doi:10.1007/s11060-016-2060-x
Hirsh, J., Dalen, J., Anderson, D. R., Poller, L., Bussey, H., Ansell, J., et al. (2001). Oral anticoagulants: Mechanism of action, clinical effectiveness, and optimal therapeutic range. Chest 119 (1), 8S–21s. doi:10.1378/chest.119.1_suppl.8s
Huang, G. J., Deng, J. S., Liao, J. C., Hou, W. C., Wang, S. Y., Sung, P. J., et al. (2012). Inducible nitric oxide synthase and cyclooxygenase-2 participate in anti-inflammatory activity of imperatorin from glehnia littoralis. J. Agric. Food Chem. 60 (7), 1673–1681. doi:10.1021/jf204297e
Inam, S., and Shah, N. (2019). Aging, obesity and unhealthy lifestyle behaviors; risk factors for the emergence of cardiometabolic diseases in Pakistani adults (P01-019-19). Curr. Dev. Nutr. 3 (1), nzz028. doi:10.1093/cdn/nzz028.P01-019-19
Iwase, M., Yamamoto, T., Nishimura, K., Takahashi, H., Mohri, S., Li, Y., et al. (2017). Suksdorfin promotes adipocyte differentiation and improves abnormalities in glucose metabolism via PPARγ activation. Lipids 52 (7), 657–664. doi:10.1007/s11745-017-4269-7
Jain, M., Surin, W. R., Misra, A., Prakash, P., Singh, V., Khanna, V., et al. (2013). Antithrombotic activity of a newly synthesized coumarin derivative 3-(5-hydroxy-2,2-dimethyl-chroman-6-yl)-N-{2-[3-(5-hydroxy-2,2-dimethyl-chroman-6-yl)-propionylamino]-ethyl}-propionamide. Chem. Biol. Drug Des. 81 (4), 499–508. doi:10.1111/cbdd.12000
Javaid, F., Mehmood, M. H., and Shaukat, B. (2021). Hydroethanolic Extract of A. officinarum hance ameliorates hypertension and causes diuresis in obesogenic feed-fed rat model. Front. inPharmacology 12, 670433. doi:10.3389/fphar.2021.670433
Joshi, A., Pant, A. K., Prakash, O., Stocki, M., and Isidorov, V. A. (2019). Phytochemical analysis, phenolic content and antioxidant activity of methanolic extract of Caryopteris odorata D. Don. Robin. J. Med. Herbs 9 (4), 189–196.
Jung, J. Y., Lim, Y., Moon, M. S., Kim, J. Y., Kwon, O., and Kwon, O. (2011). Onion peel extracts ameliorate hyperglycemia and insulin resistance in high fat diet/streptozotocin-induced diabetic rats. Nutr. Metabolism 8 (1), 18–8. doi:10.1186/1743-7075-8-18
Kabelova, A., Malinska, H., Markova, I., Oliyarnyk, O., Chylikova, B., and Seda, O. (2021). Ellagic acid affects metabolic and transcriptomic profiles and attenuates features of metabolic syndrome in adult male rats. Nutrients 13 (3), 804. doi:10.3390/nu13030804
Kumar, V., and Roy, B. K. (2018). Population authentication of the traditional medicinal plant Cassia tora l. Based on ISSR markers and FTIR analysis. Sci. Rep. 8 (1), 10714. doi:10.1038/s41598-018-29114-1
Lake, B. G. (1999). Coumarin metabolism, toxicity and carcinogenicity: Relevance for human risk assessment. Food Chem. Toxicol. 37 (4), 423–453. doi:10.1016/s0278-6915(99)00010-1
Lasker, S., Rahman, M. M., Parvez, F., Zamila, M., Miah, P., Nahar, K., et al. (2019). High-fat diet-induced metabolic syndrome and oxidative stress in obese rats are ameliorated by yogurt supplementation. Sci. Rep. 9 (1), 20026. doi:10.1038/s41598-019-56538-0
Li, J., Li, X., Li, Z., Zhang, L., Liu, Y., Ding, H., et al. (2017). Isofraxidin, A coumarin component improves high-fat diet induced hepatic lipid homeostasis disorder and macrophage inflammation in mice. Food Funct. 8 (8), 2886–2896. doi:10.1039/C7FO00290D
Mabrouki, L., Rjeibi, I., Taleb, J., and Zourgui, L. (2020). Cardiac ameliorative effect of moringa oleifera leaf extract in high-fat diet-induced obesity in rat model. BioMed Res. Int. 2020, 6583603. doi:10.1155/2020/6583603
Mead, J. A., Smith, J. N., and Williams, R. T. (1958). Studies in detoxication. 72. The metabolism of coumarin and of o-coumaric acid. Biochem. J. 68 (1), 67–74. doi:10.1042/bj0680067
Murali, R., Srinivasan, S., and Ashokkumar, N. (2013). Antihyperglycemic effect of fraxetin on hepatic key enzymes of carbohydrate metabolism in streptozotocin-induced diabetic rats. Biochimie 95 (10), 1848–1854. doi:10.1016/j.biochi.2013.06.013
Najmanova, I., Dosedel, M., Hrdina, R., Anzenbacher, P., Filipsky, T., Riha, M., et al. (2015). Cardiovascular effects of coumarins besides their antioxidant activity. Curr. Top. Med. Chem. 15 (9), 830–849. doi:10.2174/1568026615666150220112437
Nandiyanto, A. B. D., Oktiani, R., and Ragadhita, R. (2019). How to read and interpret FTIR spectroscope of organic material. Indonesian J. Sci. Technol. 4 (1), 97–118. doi:10.17509/ijost.v4i1.15806
Nepal, S., Malik, S., Sharma, A., Bharti, S., Kumar, N., Siddiqui, K., et al. (2011). Abresham ameliorates dyslipidemia, hepatic steatosis and hypertension in high-fat diet fed rats by repressing oxidative stress, TNF-alpha and normalizing NO production. Exp. Toxicol. Pathology 64 (7-8), 705–712. doi:10.1016/j.etp.2011.01.003
Nguelefack-Mbuyo, P. E., Nguelefack, T. B., Dongmo, A. B., Afkir, S., Azebaze, A. G. B., Dimo, T., et al. (2008). Anti-hypertensive effects of the methanol/methylene chloride stem bark extract of mammea africana in L-name-induced hypertensive rats. J. Ethnopharmacol. 117 (3), 446–450. doi:10.1016/j.jep.2008.02.028
Nnorom, O., and Onuegbu, G. (2019). Authentication of <i&gt;Rothmannia whitfieldii&lt;/i&gt; Dye Extract with FTIR Spectroscopy. J. Text. Sci. Technol. 5 (02), 38–47. doi:10.4236/jtst.2019.52004
Nwakiban-Atchan, A. P., Shivashankara, S. T., Piazza, S., Tchamgoue, A. D., Beretta, G., Dell’Agli, M., et al. (2022). Polyphenol-rich extracts of xylopia and aframomum species show metabolic benefits by lowering hepatic lipid accumulation in diet-induced obese mice. ACS Omega 7 (14), 11914–11928. doi:10.1021/acsomega.2c00050
Ojetola, A. A., Adeyemi, W. J., David, U. E., Ajibade, T. O., Adejumobi, O. A., Omobowale, T. O., et al. (2021). D-ribose-L-cysteine prevents oxidative stress and cardiometabolic syndrome in high fructose high fat diet fed rats. Biomed. Pharmacother. 142, 112017. doi:10.1016/j.biopha.2021.112017
Pari, L., and Rajarajeswari, N. (2009). Efficacy of coumarin on hepatic key enzymes of glucose metabolism in chemical induced type 2 diabetic rats. Chemico-Biological Interact. 181 (3), 292–296. doi:10.1016/j.cbi.2009.07.018
Piller, N. B. (1975). A comparison of the effectiveness of some anti-inflammatory drugs on thermal oedema. Br. J. Exp. Pathology 56 (6), 554–560.
Prasatthong, P., Meephat, S., Rattanakanokchai, S., Bunbupha, S., Prachaney, P., Maneesai, P., et al. (2021). Hesperidin ameliorates signs of the metabolic syndrome and cardiac dysfunction via IRS/Akt/GLUT4 signaling pathway in a rat model of diet-induced metabolic syndrome. Eur. J. Nutr. 60 (2), 833–848. doi:10.1007/s00394-020-02291-4
Prince, M. R. U., Zihad, S., Ghosh, P., Sifat, N., Rouf, R., Al Shajib, G. M., et al. (2021). Amaranthus spinosus attenuated obesity-induced metabolic disorders in high-carbohydrate-high-fat diet-fed obese rats. Front. Nutr. 8, 653918. doi:10.3389/fnut.2021.653918
Roberts, C., Vaziri, N., Wang, X., and Barnard, R. (2000). Enhanced NO inactivation and hypertension induced by a high-fat, refined-carbohydrate diet. Hypertension 36 (3), 423–429. doi:10.1161/01.hyp.36.3.423
Sasso, S., Sampaio, E. S. P. C., Santana, L. F., Cardoso, C. A. L., Alves, F. M., Portugal, L. C., et al. (2019). Use of an extract of annona muricata linn to prevent high-fat diet induced metabolic disorders in C57bl/6 mice. Nutrients 11 (7), 1509. doi:10.3390/nu11071509
Senaphan, K., Kukongviriyapan, U., Sangartit, W., Pakdeechote, P., Pannangpetch, P., Prachaney, P., et al. (2015). Ferulic acid alleviates changes in a rat model of metabolic syndrome induced by high-carbohydrate, high-fat diet. Nutrients 7 (8), 6446–6464. doi:10.3390/nu7085283
Shahzadi, T., Abbasi, M. A., Riaz, T., Rehman, A.-U., Siddiqui, S. Z., and Ajaib, M. (2011). Caryopteris odorata: A rich source of antioxidants for protection against chronic diseases and food products. J. Chil. Chem. Soc. 56 (2), 678–681. doi:10.4067/s0717-97072011000200012
Shahzadi, T., Abbasi, M. A., Ur-Rehman, A., Riaz, T., Khan, K. M., Ashraf, M., et al. (2013). Antioxidant and lipoxygenase inhibiting new iridoid glucosides from Caryopteris odorata. Nat. Prod. Res. 27 (4-5), 302–313. doi:10.1080/14786419.2012.668692
Shahzadi, T., Abbasi, M., Aziz-ur-Rehman, , Riaz, T., Khan, K., Ahmad, V., et al. (2012). Characterization of chemical isolates of Caryopteris odorata. J. Chem. Soc. Pak. 34 (2), 442–447.
Sharma, A., Bharti, S., Bhatia, J., Nepal, S., Malik, S., Ray, R., et al. (2012). Sesamol alleviates diet-induced cardiometabolic syndrome in rats via up-regulating PPARγ, PPARα and e-NOS. J. Nutr. Biochem. 23 (11), 1482–1489. doi:10.1016/j.jnutbio.2011.09.011
Sharma, A., Goyal, R., and Sharma, L. (2016). Potential biological efficacy of pinus plant species against oxidative, inflammatory and microbial disorders. BMC Complementary Altern. Med. 16 (1), 35–11. doi:10.1186/s12906-016-1011-6
Shin, E., Choi, K. M., Yoo, H. S., Lee, C. K., Hwang, B. Y., and Lee, M. K. (2010a). Inhibitory effects of coumarins from the stem barks of fraxinus rhynchophylla on adipocyte differentiation in 3T3-L1 cells. Biol. Pharm. Bull. 33 (9), 1610–1614. doi:10.1248/bpb.33.1610
Shin, J. W., Seol, I. C., and Son, C. G. (2010b). Interpretation of animal dose and human equivalent dose for drug development. J. Korean Med. 31 (3), 1–7.
Singh, D., Mathela, C. S., Panwar, A., and Pande, V. (2014). Sesquiterpene hydrocarbon rich essential oils of Caryopteris odorata (D. Don) robin.: Chemical composition, antioxidant and antimicrobial activity. J. Essent. Oil Res. 26 (4), 274–281. doi:10.1080/10412905.2014.922507
Suman, R. K., Ray Mohanty, I., Borde, M. K., Maheshwari, U., and Deshmukh, Y. A. (2016). Development of an experimental model of diabetes Co-existing with metabolic syndrome in rats. Adv. Pharmacol. Sci. 2016, 9463476. doi:10.1155/2016/9463476
Taira, N., Nugara, R. N., Inafuku, M., Takara, K., Ogi, T., Ichiba, T., et al. (2017). In vivo and in vitro anti-obesity activities of dihydropyranocoumarins derivatives from peucedanum japonicum thunb. J. Funct. Foods 29, 19–28. doi:10.1016/j.jff.2016.11.030
Takahashi, M., Takahashi, Y., Takahashi, K., Zolotaryov, F. N., Hong, K. S., Kitazawa, R., et al. (2008). Chemerin enhances insulin signaling and potentiates insulin-stimulated glucose uptake in 3T3-L1 adipocytes. FEBS Lett. 582 (5), 573–578. 0014-5793 (Print)). doi:10.1016/j.febslet.2008.01.023
Tasdemir, E., Atmaca, M., Yldrm, Y., Bilgin, H. M., Demirtaş, B., Obay, B. D., et al. (2017). Influence of coumarin and some coumarin derivatives on serum lipid profiles in carbontetrachloride-exposed rats. Hum. Exp. Toxicol. 36 (3), 295–301. doi:10.1177/0960327116649675
Tatarua, L. D. (2017). ATR-FTIR spectra fingerprinting of medicinal herbs extracts prepared using microwave extraction. Arabian J. Med. Aromatic Plants 3 (1), 9. doi:10.48347/IMIST.PRSM/ajmap-v3i1.7985
Ullah, K., Jahan, S., Aziz, F., Khan, M. S., Rahman, K. U., Shah, Z. A., et al. (2019). Ameliorative effect of Caryopteris grata benth. Against arsenic-induced enzymatic alterations in testis of albino BALB/c mice. Pol. J. Environ. Stud. 28 (2), 861–866. doi:10.15244/pjoes/84831
Yaseen, G., Ahmad, M., Ahmad, M., Sultana, S., Kayani, S., Andrade Cetto, A., et al. (2015). Traditional management of diabetes in Pakistan: Ethnobotanical investigation from traditional health practitioners. J. Ethnopharmacol. 174, 91–117. doi:10.1016/j.jep.2015.07.041
Younis, W., AlamgeerSchini-Kerth, V. B., Junior, A. G., and Majid, M. (2018). Cardioprotective effect of Asphodelus tenuifolius cav. On blood pressure and metabolic alterations in glucose-induced metabolic syndrome rats–an ethnopharmacological approach. J. Ethnopharmacol. 214, 168–178. doi:10.1016/j.jep.2017.12.005
Keywords: Caryopteris odorata, leptin, adiponectin, chemerin, diet induced cardiometabolic syndrome
Citation: Ahmed MG, Mehmood MH, Mehdi S and Farrukh M (2023) Caryopteris odorata and its metabolite coumarin attenuate characteristic features of cardiometabolic syndrome in high-refined carbohydrate-high fat-cholesterol-loaded feed-fed diet rats. Front. Pharmacol. 14:1097407. doi: 10.3389/fphar.2023.1097407
Received: 13 November 2022; Accepted: 23 February 2023;
Published: 23 March 2023.
Edited by:
Mingxia Gu, Cincinnati Children’s Hospital Medical Center, United StatesReviewed by:
Feihua Wu, China Pharmaceutical University, ChinaAchuthan Raghavamenon, Amala Cancer Research Centre, India
Copyright © 2023 Ahmed, Mehmood, Mehdi and Farrukh. This is an open-access article distributed under the terms of the Creative Commons Attribution License (CC BY). The use, distribution or reproduction in other forums is permitted, provided the original author(s) and the copyright owner(s) are credited and that the original publication in this journal is cited, in accordance with accepted academic practice. No use, distribution or reproduction is permitted which does not comply with these terms.
*Correspondence: Malik Hassan Mehmood, bWFsaWtoYXNzYW4ubWVobW9vZEBnbWFpbC5jb20=, bWFsaWtoYXNzYW5tZWhtb29kQGdjdWYuZWR1LnBr