- 1Department of Neurology, Affiliated Hospital of Zunyi Medical University, Zunyi, China
- 2Department of Nursing, Affiliated Hospital of Zunyi Medical University, Zunyi, China
- 3The Collaborative Innovation Center of Tissue Damage Repair and Regeneration Medicine of Zunyi Medical University, Zunyi, China
Medicines from natural products can not only treat neurodegenerative diseases but also improve the cognitive dysfunction caused by treatments with western medicines. This study reviews the literature related to the regulation of mitochondrial participation in cognitive function by natural products. In this study, we focused on English articles in PubMed, Web of Science, and Google Scholar, from 15 October 2017, to 15 October 2022. Fourteen studies that followed the inclusion criteria were integrated, analyzed, and summarized. Several studies have shown that natural products can improve or reduce cognitive dysfunction by ameliorating mitochondrial dysfunction. These results suggest that natural products may serve as new therapeutic targets for neurodegenerative diseases.
1 Introduction
Natural products contain a wide range of biologically active metabolites (Park et al., 2021). These compounds usually originate from complex biosynthetic systems and exhibit various morphological characteristics (Nair and Jez, 2020). They can be used in pharmaceutical research and development and drug design, as well as in dietary supplements, natural foods without artificial ingredients, and cosmetics (Newman and Cragg, 2012; Nair and Jez, 2020; Park et al., 2021). In this study, we focused on botanical drugs derived from natural products. Natural products are characterized by their chemical diversity and complexity. Many metabolites contain a large number of stereo-specific carbon centers. Thus, an increasing number of scientists are interested in the stereo-complexity of these molecules (Katz and Baltz, 2016). With the continuous development of natural product research, which began with the discovery and medical application of antibiotics in the 1950s, the average lifetime of the population increased significantly over the following 10–15 years (Bérdy, 2012). Katz and Baltz divided the history of natural product discovery into three overlapping periods, from the 1940s–1970s, 1970s–2000s, and beyond 2000 according to their impact on the discovery of new natural products. The first 30 years, the phenotypic screening stage, mainly focused on the discovery of antibacterial and antifungal metabolites; the second 30 years encompassed a huge expansion of screening methods and strategies; the third 30 years incorporated genomics-based approaches (Katz and Baltz, 2016). With the rapid development of science and technology, machine learning models have been used to predict the biochemical and physiological effects of natural products (Jeon et al., 2021). Hence, machine learning models are expected to have a significant impact on the future development and research of the molecular structures of natural products.
Mitochondria are multifunctional organelles (Nunnari and Suomalainen, 2012; Chandel, 2014) that have multiple functions, from bioenergy to cell signaling, and are signaling centers that induce transcription, proteomic, and posttranslational regulation mechanisms (Khacho and Slack, 2017). Mitochondrial function and mitochondrial dynamics play important roles in the process of neural development. Mitochondria play a central role in determining the fate of neural stem cells (NSCs) (Khacho et al., 2019). In the central nervous system, mitochondrial diseases are characterized by cognitive dysfunction, which leads to behavioral abnormalities (Fattal et al., 2006). Metabolic disorders caused by mutations in mitochondrial or nuclear DNA often affect many organs and systems. Among them, nervous system symptoms are the most common and most serious (McFarland et al., 2010), and they manifest as epilepsy, cognitive dysfunction, progressive dementia, and lactic acidosis (Kartsounis et al., 1992; Turconi et al., 1999). Mitochondria maintain their total number and morphology mainly through the regulation of the counterbalance between fission and fusion, namely mitochondrial dynamics. Therefore, changes in mitochondrial morphology and function are indispensable in the process of neurogenesis.
The mitochondria facilitate adenosine triphosphate (ATP) production, calcium regulation, and redox maintenance, hence their dysfunction can lead to various neurodegenerative diseases, including Alzheimer’s disease (AD) (Xia et al., 2018). Mitochondrial and synaptic dysfunction has been proven to be an early symptom of AD (Pérez et al., 2018). To date, there is no standard treatment for mitochondrial disorders. With the development of medical treatments, multiple pharmacological properties of botanical drugs have been applied to study neurodegenerative diseases, such as salidroside and astragalus polysaccharides, which have a protective effect on cognitive-related changes (Zhang et al., 2019; Xie et al., 2020). Asiatic acid can prevent and alleviate seizures (Lu et al., 2021).
Currently, an increasing number of natural product extracts involved in mitochondrial regulation have attracted attention, including their effects on mitochondrial dynamics and autophagy. Therefore, this study reviews the literature on natural products’ regulation of mitochondrial function in cognitive dysfunction diseases to clarify the possible mechanism underlying mitochondrial function as influenced by natural products, identify new therapeutic targets for the treatment of neurodegenerative diseases, and provide an insight into the development of natural products for the treatment of cognitive impairment.
2 Methods
2.1 Search strategy
In this study, we used various keywords to search a range of English databases. We used “natural products,” “mitochondrial,” “mitochondrial function,” “nerve cell,” “neurocyte,” “cognitive dysfunction,” “Alzheimer,” and “dementia” as keywords for search and retrieval. We focused on English articles in PubMed, Web of Science, and Google Scholar from 15 October 2017, to 15 October 2022. The details of this information are shown in Figure 1. Studies that followed the inclusion criteria of the present study were integrated, analyzed, and summarized.
2.2 Data extraction
In the present study, the articles were independently screened and identified by two authors (JT and YL). At the same time, according to the data collection sheet (Supplementary File) designed by the two authors, the first author independently extracted the data that included the author, year, country, type of study, name of natural products, mechanism of regulating mitochondrial function in cognitive dysfunction, and main results (Colquhoun et al., 2014). All authors checked the accuracy of the extracted data.
2.3 Inclusion and exclusion criteria
The inclusion criteria of the literature were determined before searching by JT and ZX to include only studies related to our research questions. The inclusion criteria were as follows: 1) English language studies, 2) articles published in scientific journals, 3) articles containing natural products, 4) studies on the impact of mitochondria on cognitive function, and 5) studies discussing the possible mechanism of correlation with neurological disorders. Articles that did not meet the inclusion criteria were excluded.
2.4 Botanical drugs used in the present study
Erigeron (Dengzhanxixin) [Asteraceae; Erigeron breviscapus (Vaniot) Hand.-Mazz.] injections (DZXI) (specifications: 5.32 mg scutellarin, 2.26 mg 3,4-O-dicaffeoylquinic acid, 1.10 mg 3,5-O-dicaffeoylquinic acid, 1.79 mg erigoster B, 2.70 mg 4,5-O-dicaffeoylquinic acid, and 11.26 mg erigeroster, 10 mL/ampoule, Lot No. 20180137) were provided by Yunnan Biovalley Pharmaceutical Co., Ltd. (Kunming, China), erigeroster (Lot No, 20190701, purity 93.3%) were provided by Yunnan Biovalley Pharmaceutical Co., Ltd. (Kunming, China) and Chengdu Pusi Biotechnology Co., Ltd. (Chengdu, China) (An et al., 2021).
Dried leaves of Centella [Apiaceae; Centella asiatica (L.) Urban] (Oregon’s Wild Harvest, GOT-03193c-OHQ01) was used. CAW (Centella asiatica) was prepared by refluxing CAW (160g) with water (2,000 mL) for 2 h, filtering the solution, and freeze drying to yield a powder (∼16–21 g) (Gray et al., 2018).
Asiatic acid is a triterpene derived from the medicinal botanical drug Centella[Apiaceae; Centella asiatica (L.) Urban]. It included Asiatic acid (purity >99%, Aktin, A98688, Chengdu, China) and kainic acid (Sigma-Aldrich, K0250, St. Louis, MO, USA) (Lu et al., 2021).
Actinidia [Actinidiaceae; Actinidia arguta (Siebold & Zucc.) Planch. ex Miq.] was obtained a hardy kiwi from the National Institute of Forest Science (Suwon, Korea) in September 2013, and it was extracted in 40% ethanol at 40°C for 2 h. It was obtained by filtration, evaporation, freezing, and drying (Ha et al., 2020).
Tetramethylpyrazine (TMP) was extracted from the rhizome of Ligusticum chuanxiong [Apiaceae; Conioselinum anthriscoides “Chuanxiong”] (Huang et al., 2021). The extraction method was not clearly explained.
Schisandra [Schisandraceae; Schisandra chinensis (Turcz.) Baill.] was purchased in Seoul, Korea in September 2017. It was ground and powdered, extracted with 70% EtOH two times at room temperature, then extracted by Diaion HP-20 chromatography and preparative high-performance liquid chromatography (HPLC) (column: YMC-Pack ODS-A, 5 μm, 250 × 20 mm I.D., Japan, 8 mL/min, 10%–35% MeCN, 40 min) (Jang et al., 2020).
(−) – Epicatechin ≥90% (HPLC, EC, Sigma, USA, E1753) is a metabolite (Ling et al., 2022).
Ginkgolide is a natural product from Ginkgo [Ginkgoaceae; Ginkgo biloba L.] leaves. Ginkgolide K (GK) is a metabolite from ginkgolide and diterpene lactone (Liu et al., 2022a).
Capsaicin is metabolite from Capsicum [Solanaceae; Capsicum annuum L.] (Ouyang et al., 2022). The extraction method was not clearly explained (Ouyang et al., 2022).
Trans-cinnamaldehyde (CIN) is a natural product from Cinnamomum [Lauraceae; Cinnamomum verum J.Presl]. Ellagic acid (ELA) is a metabolite. The extraction method was not clearly explained (Pan et al., 2020).
Red ginseng (RG) is a therapeutic material that Panax ginseng [Araliaceae; Panax ginseng C.A.Mey.] (PG) Meyer obtained by steaming and drying. The extraction method was not clearly explained (Shin et al., 2019).
ShenmaYizhi decoction (SMYZD) (composition: Panax ginseng [Araliaceae; Panax ginseng C.A.Mey.], Gastrodia elata [Orchidaceae; Gastrodia elata Blume], Asplenium [Asteraceae, Asplenium thunbergii Kunze], and Ligusticum chuanxiong [Apiaceae; Conioselinum anthriscoides ‘Chuanxiong'] (Chuanxiong) were prepared in a ratio of 3:3:3:2 at Xiyuan Hospital of China, Academy of Chinese Medical Sciences, Beijing Hospital preparation (Beijing Medicine preparation: Z20200005000) (Sun et al., 2021).
Rhein is a metabolite from Rheum [Polygonaceae; Rheum officinale Baill.] that was purchased from Shanghai Aladdin Biochemical Technology Co., Ltd. (Shanghai, China) (Yin et al., 2021; Yin et al., 2022).
2.5 Analysis
We searched the literature library, and 14 articles were finally included in our analysis according to our inclusion criteria. We summarized and sorted the data of the 14 articles and analyzed and discussed what is known about the effect of natural products on mitochondria in cognitive impairment.
3 Results
In the present study, 408 articles were initially retrieved from the database. An additional article related to the purpose of the present study was found through a manual search. A total of 108 repetitive articles were excluded (96 duplicate articles automatically removed by EndNote software, and 12 articles were excluded manually), 274 articles were excluded through article titles and abstract screening, and 13 articles were dropped after full-text screening as they did not meet the purpose of this study. Fourteen articles were included in the final analysis (Figure 1).
3.1 Characteristics of the included studies
The characteristics of the included studies are summarized in the Supplementary File. Of the 14 articles, one article was from the USA (Gray et al., 2018), nine from China (Pan et al., 2020; An et al., 2021; Huang et al., 2021; Sun et al., 2021; Yin et al., 2021; Liu et al., 2022b; Ling et al., 2022; Ouyang et al., 2022; Yin et al., 2022), two from Korea (Shin et al., 2019; Jang et al., 2020), and one from Taiwan (Lu et al., 2021).
All articles used animal experiments except one, which was a mixed clinical trial (An et al., 2021). Among the natural products investigated were DZXI (An et al., 2021), CAW (Gray et al., 2018; Lu et al., 2021), Chloroform fraction Actinidia arguta (CFAA) (Ha et al., 2020), TMP from Ligusticum chuanxiong (Huang et al., 2021), Schisandra chinensis extract (SCE), ascorbic acid (AA) (Jang et al., 2020), (−)-Epicatechin (Epi) from Green tea (Ling et al., 2022), GK from Ginkgo biloba (Liu et al., 2022a), Capsaicin from Capsicum (Ouyang et al., 2022), CIN and ELA from Cinnamomum, Metabolite (Pan et al., 2020), Red ginseng extract (RGE) (Shin et al., 2019), SMYZD from Panax ginseng, Gastrodia elata (Sun et al., 2021), Rheum (Yin et al., 2022), and Rhein, emodin, aloe-emodin, chrysophanol, and physcion from Rheum (Yin et al., 2021).
3.2 Association between natural products acting on mitochondria and cognitive function
The summary results in the Supplementary File show that there is a close relationship between mitochondria and nervous system diseases, and that the natural products of Chinese botanical drugs have protective effects on mitochondria.
Among them, DZXI (Erigeron) treatment can protect mitochondrial functions and increase the resistance of neurons to neurodegeneration (An et al., 2021). Asiatic acid (Centella) can not only prevent damage to the hippocampal synaptic mitochondrial structure in epileptic rats but can also affect mitochondrial proteins related to energy generation (Gray et al., 2018; Lu et al., 2021). CFAA (Actinidia) can effectively protect against high glucose (HG)-induced neurotoxicity, thereby improving cognitive function (Ha et al., 2020). TMP (Ligusticum chuanxiong) treatment reduces amyloid β (Aβ) accumulation in an AD model, promotes the recovery of mitochondrial function in mice, and improves synaptic dysfunction (Huang et al., 2021). The combination of SCE and AA (Schisandra) resulted in higher GluR1 levels in the hippocampus of eight-week-old male C57BL/6 mice than in that of mice injected with SCE or AA alone and enhanced mitochondrial respiration of hippocampal neurons (Jang et al., 2020). Epi upregulated the expression of phosphorylation of adenosine 5′-monophosphate-activated protein kinase (pAMPK) and lost its protective effect in cells blocked by adenosine 5′-monophosphate-activated protein kinase (AMPK). Epi had a protective effect in lipopolysaccharide-induced cells and mouse models (Ling et al., 2022). GK (Ginkgo) promotes neuronal cell survival and prevents apoptosis (Liu et al., 2022b). Capsaicin (Capsicum) exerts a neuroprotective effect on Chronic cerebral hypoperfusion-induced cognitive impairment (Ouyang et al., 2022). The combination of ELA and CIN (Cinnamomum, Metabolite) had a strong and consistent impact on cognitive function in rats (Pan et al., 2020). RGE (Panax ginseng) can restore the respiratory capacity of damaged mitochondria and prevent mitochondrial dysfunction (Shin et al., 2019). SMYZD (Panax ginseng, Gastrodia elata, Asplenium, Ligusticum chuanxiong) can effectively improve the structure and energy metabolism of mitochondria, thereby improving cerebral perfusion insufficiency (Sun et al., 2021). Rhein (Rheum) improved mitochondrial biogenesis, exhibited good antioxidant activity, and significantly increased superoxide dismutase activity (Yin et al., 2021, 2022).
3.3 Mechanism by which natural products regulate mitochondrial function in cognitive dysfunction
DZXI (Erigeron) treatment improved the level of cytochrome C oxidase subunit 2 by regulating the mitochondrial electron transport chain, thus protecting the stability of mitochondria in neural cells (An et al., 2021). CAW (Centella) increased synaptic vesicle exocytosis by kainic acid-induced presynaptic mitochondria and increased ATP production of hippocampal neurons (Gray et al., 2018). It can inhibit glutamate release, increase AKT activation, inhibit protease activation, protect synaptic and mitochondrial functions, and prevent cognitive deficits in kainic acid-induced epilepsy (Lu et al., 2021). CFAA (Actinidia) can effectively improve high-fat diet-induced mitochondrial dysfunction, thereby improving cognitive dysfunction (Ha et al., 2020). TMP (Ligusticum chuanxiong) treatment can significantly change mitochondrial protein levels, increase ATP levels, and achieve neuroprotection by inhibiting the activity of BACE1 (Huang et al., 2021). An SCE-AA (Schisandra) mixture can improve cognitive ability by enhancing mitochondrial respiration, and its short-term treatment can reduce the expression of PSD95 (Jang et al., 2020). Epi protects mitochondria by activating AMPK signaling in sepsis-associated encephalopathy (Ling et al., 2022). Treatment with GK (Ginkgo) can reduce the mitochondrial Ca2+ uniporter (MCU) induced by Aβ in vitro, reduce the level of Ca2+ in the mitochondria, inhibit cell apoptosis, and improve cognitive dysfunction (Liu et al., 2022a). Capsaicin (Capsicum) has a neuroprotective effect on cognitive dysfunction caused by chronic cerebral hypoperfusion (Ouyang et al., 2022). RGE (Panax ginseng) enhances mitochondrial respiratory function, mediates the recovery of mitochondrial function, and prevents Aβ deposition and related pathologies (Shin et al., 2019). SMYZD (Panax ginseng, Gastrodia elata, Asplenium, Ligusticum chuanxiong) plays a therapeutic role by activating AMPK/PPARα to restore and improve the structure and function of mitochondria by inhibiting the oxidation reaction (Sun et al., 2021). Rhein (Rheum) plays an active role in regulating enzymes and antioxidant enzymes in the respiratory chain complex to improve mitochondrial biogenesis, reduce the release of cytochrome C, inhibit the apoptosis cascade, and protect neurons from apoptosis (Yin et al., 2021; Yin et al., 2022) (Table 1).
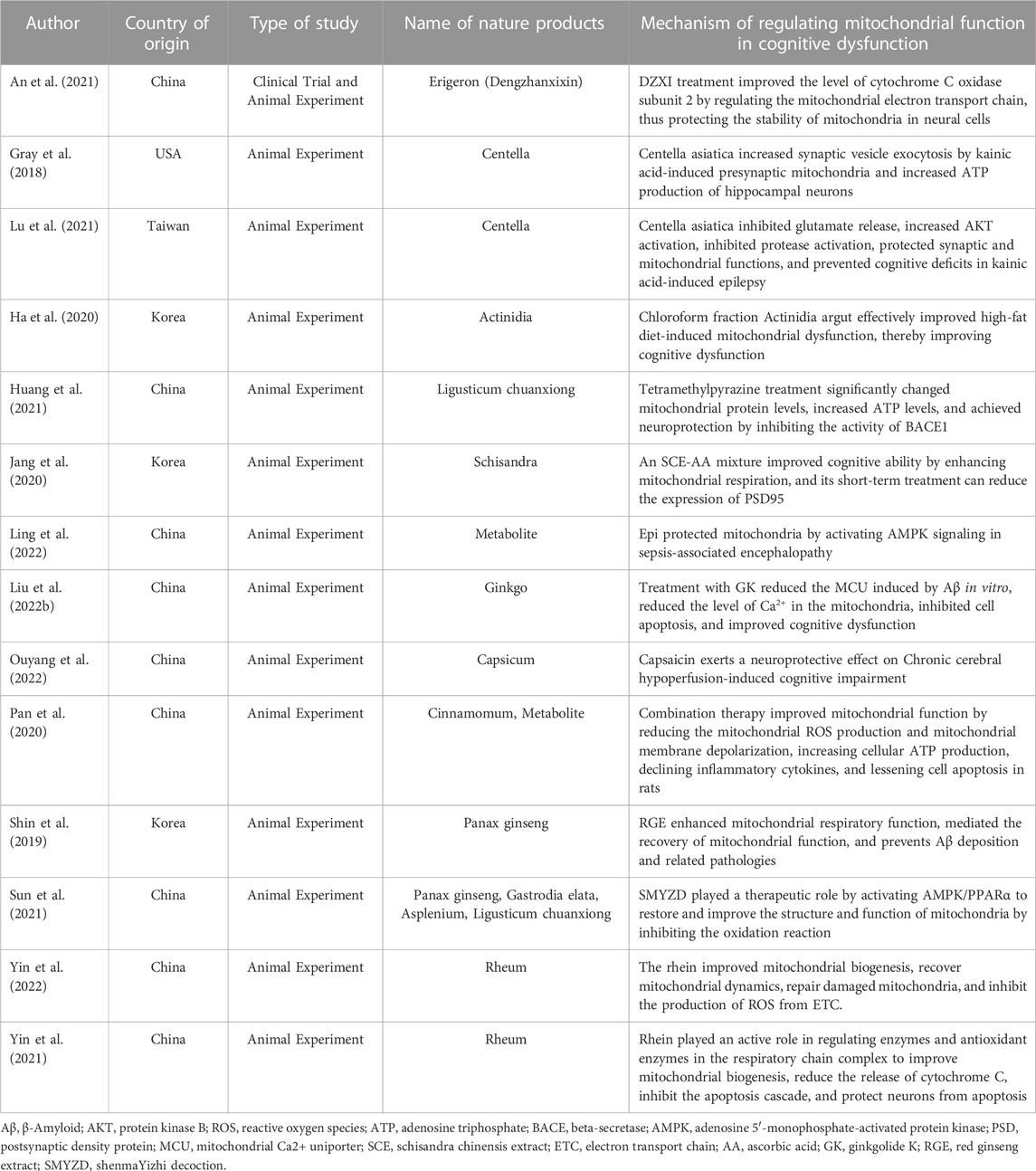
TABLE 1. Mechanisms underlying natural product regulation of mitochondrial function in cognitive dysfunction.
4 Discussion
Mitochondria are multifunctional organelles with an independent genome, and mutations to their genes and structural damage are key factors underlying cognitive impairment in neurodegenerative diseases (Khacho et al., 2019) (Figure 2). Neurons are highly dependent on mitochondria; 20% of the body’s oxygen is consumed by ATP produced by mitochondrial respiration in the brain (Attwell and Laughlin, 2001). Furthermore, the brain consumes 20% of the body’s total glucose intake, especially the nerve cells (Sokoloff, 1999). Although glycolysis can provide some ATP, most ATP is produced by mitochondrial respiration (Rangaraju et al., 2014). Mitochondria play an important role in the maintenance of physiological functions (Misgeld and Schwarz, 2017). Through continuous fission and fusion, a network structure with inner and outer membranes and multiple nucleoli containing mitochondrial DNA is formed (Braschi and McBride, 2010; Nabi et al., 2022). However, errors in mitochondrial fission or fusion can lead to nervous system diseases of varying degrees, and cognitive dysfunction is one of them. Pérez et al. (2018) reported that mitochondrial dysfunction is mediated by abnormal microtubule-associated proteins.
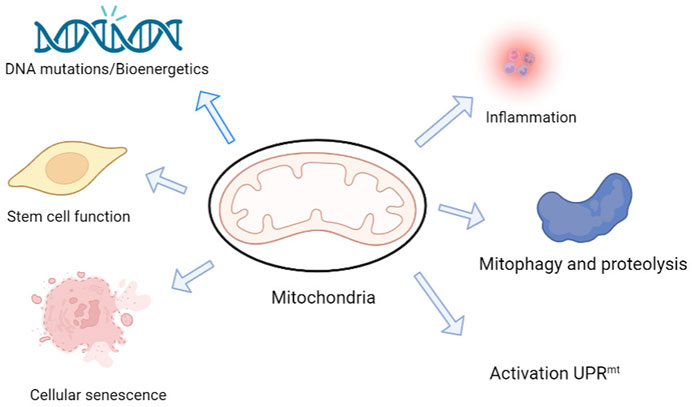
FIGURE 2. Mitochondria are possibly involved in physiological and pathological mechanisms underlying cognition changes in neurodegeneration and aging. Mitochondria are multifunctional organelles with multiple functions, from bioenergy to cell signaling, and are signaling centers that induce transcription, proteomic, and posttranslational regulation mechanisms. The mitochondria play a central role in determining the fate of neural stem cells (NSCs). Mitochondria are also involved in protein assembly and regulation and mediate cell proliferation, differentiation, and death. Mitochondria can initiate a protective program called the mitochondrial unfolded protein response (UPRmt) and use this protective mechanism to maintain normal mitochondrial function (Liu et al., 2022b).
At present, the pathogenesis of neurodegenerative diseases, such as AD, remains incomplete. Many recent studies have discussed the relationship between mitochondrial dysfunction and AD (Klein et al., 2021; Takeda et al., 2021). Tau is a key protein involved in the pathogenesis of AD. Pérez et al. (2018) showed that Tau pathology impaired mitochondrial transport affected mitochondrial dynamics and bioenergetics in AD. Multiple studies have shown that mitochondrial dysfunction is an early symptom of AD (Gibson and Shi, 2010; Cabezas-Opazo et al., 2015) and a driving factor of cognitive impairment in AD. Since mitochondria are easily affected by age, mutations, and metal toxins, dysfunction caused by mitochondrial DNA (mtDNA) damage, mutation, and impairment of metabolism and protein transport may lead to accumulation of Aβ oligomers or fibrils and phosphorylated Tau. Furthermore, the accumulation of damaged mtDNA and other macromolecules during aging leads to metabolic disorders (Abyadeh et al., 2021). The accumulation of damaged mitochondria further accelerates the progression of cognitive dysfunction (Swerdlow, 2018). The dysfunction of mitochondria further leads to the lack of bioenergy, the imbalance of intracellular calcium regulation, and the generation of free radicals. This causes oxidative stress and consequently to aggravation of Aβ oligomeric and Tau pathology, which, in turn, aggravates further synaptic dysfunction, mitochondrial damage, memory loss, and cognitive impairment (Figure 3).
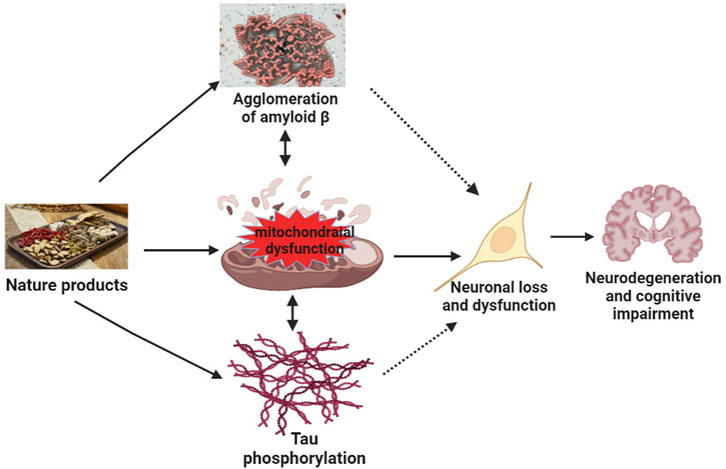
FIGURE 3. Mechanisms of natural products target mitochondrial function to improve neurodegeneration and cognitive impairment. Mitochondrial dysfunction results in the agglomeration of Aβ, tau phosphorylation, and agglomeration of Aβ and tau phosphorylation, further aggravating mitochondrial dysfunction, leading to neuronal loss or dysfunction, and ultimately inducing neurodegeneration and cognitive impairment. Natural products act on the mitochondria to improve tau phosphorylation and the agglomeration of amyloid β, neuronal loss, and dysfunction, thereby improving neurodegeneration and cognitive impairment.
Multiple studies have identified mitochondrial dysfunction as the central mechanism underlying many neurodegenerative diseases (Macdonald et al., 2018; Sharma et al., 2021). Significant progress has been made in understanding mitochondrial diseases, and there are now many treatments available for neurodegenerative diseases, including antioxidants, mitochondrial inducers, energy buffers, and gene therapy. However, satisfactory treatments are still lacking. Natural products have been used in various fields since the emergence of early antibiotics. Penicillin from mold, morphine from poppy, and paclitaxel, an anti-cancer drug, are typical examples of natural product drugs (Hunter, 2008). Natural product preparations may be an important breakthrough in the treatment of neurodegenerative diseases. Most natural products are extracted from microorganisms and exhibit a certain degree of cytotoxicity (Hunter, 2008). Therefore, when used clinically, they should be scientifically verified through clinical drug research at different levels.
The natural products discussed here mainly refer to the natural ingredients extracted from botanical drugs. An et al. (2021) reported that when DZXI (extracted from botanical drugs (Chai et al., 2013)) was administered to patients with acute ischemic stroke (AIS), the neurological and cognitive impairment of these patients was improved by regulating the mitochondrial respiratory chain and retaining the mitochondrial structure in brain tissue. Clinical studies have shown that DZXI treatment is beneficial for patients with AIS (Li et al., 2017). This study showed that the gray matter volume of the right anterior central gyrus, right central sulcus, right superior frontal gyrus, right middle frontal gyrus, and right inferior frontal gyrus in patients with AIS increased after DZXI treatment. Infarctions in these regions are associated with neurological and cognitive dysfunction (Galovic et al., 2013; Cheng et al., 2014; Wu et al., 2015; Henri-Bhargava et al., 2018; Parnaudeau et al., 2018; Zanto and Gazzaley, 2019; Zhu et al., 2019). Furthermore, DZXI treatment restored or reduced the volume of gray matter in specific areas such as the frontal cortex, which is a key area involved in cognitive dysfunction. In addition, DZXI treatment regulated the mitochondrial electron transport process, and the level of cytochrome C oxidase subunit 2 was significantly increased and protected the stability of neuronal mitochondria.
Among the studies retrieved in the present study, there are two articles about Centella asiatica (CAW), which is a plant that used in traditional Chinese medicine to enhance memory and improve cognitive function (Kapoor, 1990; Wattanathorn et al., 2008; Dev et al., 2009; Shinomol et al., 2011; Sirichoat et al., 2015; Gray et al., 2016; Umka Welbat et al., 2016; Chaisawang et al., 2017). In patients with epilepsy, long-term use of the current antiepileptic drugs cannot improve cognitive function (Ortinski and Meador, 2004; 77; Santulli et al., 2016). When CAW (Centella asiatica) is used to treat epileptic patients, it can not only alleviate seizures, but it also improves related memory disorders. The use of CAW before seizures has antiepileptic activity, which can restore the level of synaptophysin and mitochondrial function, reduce neuronal damage, and improve cognitive dysfunction. Therefore, natural medications are effective in treating cognitive dysfunction related to epilepsy. In conclusion, the natural product CAW may be beneficial for the treatment of epilepsy and related cognitive dysfunction.
In a mouse model of high-fat diet (HFD), the antioxidant activity of Actinidia arguta (CFAA) protected against HG-induced neurotoxicity and improved insulin resistance. At the same time, it was observed that the cognitive impairment was also improved through the reduction of oxidative stress and the enhancement of mitochondrial activity. CFAA can be used to treat neurodegenerative diseases caused by HFD.
Many studies on AD treatment have focused on natural products (Jeon et al., 2019). The present study collected five articles on the treatment of AD in patients with neurodegenerative dementia. Tetramethylpyrazine (TMP) from Ligusticum chuanxiong is a calcium antagonist with a strong neuroprotective effect in cerebral ischemia models (Chang et al., 2007). In rat models of Parkinson’s disease, TMP also has neuroprotective effects on dopaminergic neurons (Lu et al., 2014). In the AD mouse model of this study, after TMP treatment, mitochondrial proteins changed significantly, electron transport chain function improved, ATP levels increased, and synaptic dysfunction also improved.
The Ca2+ homeostasis in mitochondria is necessary to maintain normal neuronal function (Moreira et al., 2010). The protective effect of Ginkgolide K (GK) from Ginkgo biloba (a natural metabolite) on neuronal cells can promote neuronal cell survival (Liu et al., 2018), regulate mitochondrial function to exert a prosurvival effect (Ma et al., 2014; Zhou et al., 2017), inhibit MCU expression, reduce the Ca2+ level in the mitochondria, and prevent apoptosis.
Accumulation of Aβ in the brain is another important pathological feature of AD (Moon et al., 2012; Selkoe, 1994; Mancuso et al., 2009). Enhancement of mitochondrial homeostasis can offset Aβ protein toxicity and aggregation (Sorrentino et al., 2017), and Red ginseng (RGE) treatment can significantly restore impaired mitochondrial respiratory capacity and prevent mitochondrial fusion and division imbalance during Aβ-induced mitochondrial dysfunction. Simultaneously, RGE improved mitochondrial dysfunction and adult hippocampal neurogenesis, effectively preventing cognitive dysfunction in AD. The protective effect of RGE on mitochondrial function can reduce neuroinflammation. Therefore, RGE can promote AD therapy by protecting the neurons.
Rhein can improve mitochondrial function (Shin et al., 2019). Rhein activates SIRT1/PGC-1α, and mitochondrial biogenesis may be the key mechanism triggering the mitochondrial antioxidant defense system. After treating with Rhein, the expression level of SIRT1 and PGC-1α in the hippocampus of mice was significantly upregulated together with that of NRF1, which demonstrated that Rhein improved the biogenesis of mitochondria in AD mice, restored mitochondrial dynamics, repaired damaged mitochondria, and inhibited the electron transport chain to produce reactive oxygen species, thereby synergistically relieving neuronal oxidative stress (Yin et al., 2021). Thus, Rhein may have a potential therapeutic effect in AD.
The mixture of Schisandra chinensis extract (SCE) and ascorbic acid (AA) can improve cognitive function by enhancing mitochondrial respiration and inducing the activity of synaptic plasticity regulatory proteins, thereby improving mitochondrial function and memory and alleviating AD and age-related memory decline (Jang et al., 2020).
Sepsis, a common neurological complication, is associated with multiorgan failure, high mortality (Cecconi et al., 2018), and short-term or long-term neurological dysfunction (Silva et al., 2020). To date, there is no clear treatment. (−)-Epicatechin (Epi) from Green tea can be used as a new adjuvant treatment to improve the neurological prognosis of patients with sepsis. Epi is a natural polyphenol substance with an effective neuroprotective effect that has been shown to cross the blood-brain barrier and directly act on neurons (Mandel et al., 2008; Cruz-González et al., 2016; Bernatova, 2018). No obvious side effects have been observed (Borges et al., 2017). Epi controls the quality of mitochondria by activating AMPK signaling in the SAE. Epi treatment can reduce the decline of cognitive function, prevent the loss of neuronal dendritic spines, and reduce neuronal damage, which are related to the improvement of mitochondrial quality and reduction of neuroinflammation.
Cognitive dysfunction in the normal physiological process of aging is accompanied by various physiological phenomena (Beard et al., 2016; d'Avila et al., 2018). In the process of aging, there is dysfunction of organelles, such as mitochondrial enlargement or breakage; dysfunction of the electron transport chain; oxidative damage of mitochondrial DNA (Santos et al., 2013; Lores-Arnaiz et al., 2016). These processes may be important cell targets to protect against the cognitive dysfunction caused by aging. Trans-cinnamaldehyde (CIN) and ellagic acid (ELA) have been shown to inhibit oxidative stress, inflammation, apoptosis, and necrosis in many pathological models (Rajamani et al., 2015; Qi et al., 2016; Absalan et al., 2017; Firdaus et al., 2018; Rahimi et al., 2018). In this study, the combined treatment with ELA and CIN had a stronger and consistent effect on cognitive dysfunction in rats. Combined treatment can improve cognitive dysfunction induced by aging by inhibiting inflammation, protecting the mitochondrial function of the prefrontal cortex, and inhibiting the apoptosis signaling axis.
The main pathogenesis of vascular dementia (VD) includes chronic cerebral hypoperfusion (Damodaran et al., 2019), synaptic disorders (Sun, 2018), axonal abnormalities (Kalaria, 2018), hippocampal neuron loss (Jiang et al., 2019), and white matter vascular changes (Hase et al., 2019), which ultimately lead to cognitive impairment and dementia. The mitochondria are the main organelles involved in ischemia (Lv et al., 2017). Mitochondrial dysfunction in the brain plays an important role in the pathogenesis of VD. The SMYZD (which includes many Chinese botanical drug ingredients), can improve mitochondrial function, energy metabolism, and chronic cerebral perfusion insufficiency by activating the AMPK/PPARα/PGC-1α/UCP2 signaling pathway, which can improve mitochondrial structure and alleviate pathological damage in the rat brain. Anti-apoptotic and antioxidant properties, neurogenesis, and inhibition of mitochondrial dysfunction could exert these effects (Rajabian et al., 2019).
A previous study found that depletion of neuronal specific protein cell-cycle exit and neuronal differentiation 1 (CEND1) could lead to mitochondrial dysfunction, thereby causing cognitive dysfunction, and overexpression of CEND1 could improve cognitive dysfunction (Xie et al., 2022). It is unclear how natural products improve mitochondrial function under conditions of cognitive dysfunction, warranting further research to benefit the clinical treatment of cognitive dysfunction. Mounting evidence shows that the abnormality of mitochondrial dynamics generally precedes the hallmarks of pathology (Wang et al., 2021). Therefore, proper regulation of mitochondrial dynamics may be beneficial to AD patients.
The present study has limitations. The effect of natural products on mitochondria under conditions of cognitive dysfunction may not have been discussed comprehensively because we included few studies. Therefore, in the future, the inclusion criteria should be reconsidered and the mechanism of mitochondrial dysfunction in cognitive dysfunction should be discussed more comprehensively.
Overall, the mechanism underlying the effects of natural products on mitochondria is multileveled. The natural products used in clinical treatment are not only single botanical drugs but have multiple forms of composite applications. Whether there is drug interaction when they are used in combination remains to be determined. Therefore, metabolite botanical drugs, single botanical drugs, formulas, and combination of Chinese and Western medicine remain to be fully investigated to facilitate future clinical composite applications.
5 Conclusion
It is difficult for existing treatments to prevent the occurrence of cognitive dysfunction in neurodegenerative diseases; natural products may be a new therapeutic option. However, as natural botanical drugs are used for treatment, their mechanisms remain to be verified. The clinical use and in-depth research of natural products, especially the unique advantages in the field of cognitive impairment related to mitochondrial dysfunction, may create a new emphasis for drug research and the development of treatments for cognitive dysfunction-related diseases in the future.
Author contributions
JT: Writing the original draft, design, and methodology. YP: Conceptualization and investigation. YL: Supervision and conceptualization. SH: Conceptualization. ZX: Design, methodology, and supervision. All authors contributed to the article and approved the submitted version.
Funding
This work was supported by grants from the Guizhou epilepsy basic and clinical research scientific and technological innovation talent team project (No: CXTD [2022]013), the Collaborative Innovation Center of Chinese Ministry of Education (No: 2020-39), the Guizhou provincial “hundred” level innovative talents funds (No: GCC-2022-038-1), the Guizhou Provincial Science and Technology Foundation (No: ZK2022-656), and the Zunyi City Science and Technology Foundation (No: 2019-71 and 2021-30).
Acknowledgments
We would like to thank Affiliated Hospital of Zunyi Medical University for their valuable help with data collection and analysis.
Conflict of interest
The authors declare that the research was conducted in the absence of any commercial or financial relationships that could be construed as a potential conflict of interest.
Publisher’s note
All claims expressed in this article are solely those of the authors and do not necessarily represent those of their affiliated organizations, or those of the publisher, the editors and the reviewers. Any product that may be evaluated in this article, or claim that may be made by its manufacturer, is not guaranteed or endorsed by the publisher.
Supplementary material
The Supplementary Material for this article can be found online at: https://www.frontiersin.org/articles/10.3389/fphar.2023.1091879/full#supplementary-material
References
Absalan, A., Mesbah-Namin, S. A., Tiraihi, T., and Taheri, T. (2017). Cinnamaldehyde and eugenol change the expression folds of AKT1 and DKC1 genes and decrease the telomere length of human adipose-derived stem cells (hASCs): An experimental and in silico study. Basic Med. Sci. 20, 316–326. doi:10.22038/IJBMS.2017.8362
Abyadeh, M., Gupta, V., Chitranshi, N., Gupta, V., Wu, Y., Saks, D., et al. (2021). Mitochondrial dysfunction in Alzheimer's disease - a proteomics perspective. Expert Rev. Proteomics 18, 295–304. doi:10.1080/14789450.2021.1918550
An, H., Tao, W., Liang, Y., Li, P., Li, M., Zhang, X., et al. (2021). Dengzhanxixin injection ameliorates cognitive impairment through a neuroprotective mechanism based on mitochondrial preservation in patients with acute ischemic stroke. Front. Pharmacol. 12, 712436. doi:10.3389/fphar.2021.712436
Annesley, S. J., and Fisher, P. R. (2019). Mitochondria in health and disease. Cells 8, 680. doi:10.3390/cells8070680
Attwell, D., and Laughlin, S. B. (2001). An energy budget for signaling in the grey matter of the brain. J. Cereb. Blood Flow. Metab. 21, 1133–1145. doi:10.1097/00004647-200110000-00001
Beard, J. R., Officer, A., de Carvalho, I. A., Sadana, R., Pot, A. M., Michel, J. P., et al. (2016). The world report on ageing and health: A policy framework for healthy ageing. Lancet 387, 2145–2154. doi:10.1016/S0140-6736(15)00516-4
Bérdy, J. (2012). Thoughts and facts about antibiotics: Where we are now and where we are heading. J. Antibiot. (Tokyo) 65, 385–395. doi:10.1038/ja.2012.27
Bernatova, I. (2018). Biological activities of (-)-epicatechin and (-)-epicatechin-containing foods: Focus on cardiovascular and neuropsychological health. Biotechnol. Adv. 36, 666–681. doi:10.1016/j.biotechadv.2018.01.009
Borges, G., Ottaviani, J. I., van der Hooft, J. J. J., Schroeter, H., and Crozier, A. (2017). Absorption, metabolism, distribution and excretion of (−)-epicatechin: A review of recent findings. Mol. Asp. Med. 61, 18–30. doi:10.1016/j.mam.2017.11.002
Braschi, E., and McBride, H. M. (2010). Mitochondria and the culture of the borg: Understanding the integration of mitochondrial function within the reticulum, the cell, and the organism. Bioessays 32, 958–966. doi:10.1002/bies.201000073
Cabezas-Opazo, F. A., Vergara-Pulgar, K., Pérez, M. J., Jara, C., Osorio-Fuentealba, C., and Quintanilla, R. A. (2015). Mitochondrial dysfunction contributes to the pathogenesis of Alzheimer’s disease. Oxid. Med. Cell Longev. 2015, 509654. doi:10.1155/2015/509654
Cecconi, M., Evans, L., Levy, M., and Rhodes, A. (2018). Sepsis and septic shock. Lancet 392, 75–87. doi:10.1016/S0140-6736(18)30696-2
Chai, L., Guo, H., Li, H., Wang, S., Wang, Y. L., Shi, F., et al. (2013). Scutellarin and caffeic acid ester fraction, active components of Dengzhanxixin injection, upregulate neurotrophins synthesis and release in hypoxia/reoxygenation rat astrocytes. J. Ethnopharmacol. 150, 100–107. doi:10.1016/j.jep.2013.08.011
Chaisawang, P., Sirichoat, A., Chaijaroonkhanarak, W., Pannangrong, W., Sripanidkulchai, B., Wigmore, P., et al. (2017). Asiatic acid protects against cognitive deficits and reductions in cell proliferation and survival in the rat hippocampus caused by 5-fluorouracil chemotherapy. PLoS One 12, e0180650. doi:10.1371/journal.pone.0180650
Chandel, N. S. (2014). Mitochondria as signaling organelles. BMC Biol. 12, 34. doi:10.1186/1741-7007-12-34
Chang, Y., Hsiao, G., Chen, S. H., Chen, Y. C., Lin, J. H., Lin, K. H., et al. (2007). Tetramethylpyrazine suppresses HIF-1alpha, TNF-alpha, and activated caspase-3 expression in middle cerebral artery occlusion-induced brain ischemia in rats. Acta Pharmacol. Sin. 28, 327–333. doi:10.1111/j.1745-7254.2007.00514.x
Cheng, B., Forkert, N. D., Zavaglia, M., Hilgetag, C. C., Golsari, A., Siemonsen, S., et al. (2014). Influence of stroke infarct location on functional outcome measured by the modified rankin scale. Stroke 45, 1695–1702. doi:10.1161/STROKEAHA.114.005152
Chung, H. Y., Wickel, J., Brunkhorst, F. M., and Geis, C. (2020). Sepsis-associated encephalopathy: From delirium to dementia. J. Clin. Med. 9, 703. doi:10.3390/jcm9030703
Colquhoun, H. L., Levac, D., O'Brien, K. K., Straus, S., Tricco, A. C., Perrier, L., et al. (2014). Scoping reviews: Time for clarity in definition, methods, and reporting. J. Clin. Epidemiol. 67, 1291–1294. doi:10.1016/j.jclinepi.2014.03.013
Cruz-González, T., Cortez-Torres, E., Perez-Severiano, F., Espinosa, B., Guevara, J., Perez-Benitez, A., et al. (2016). Antioxidative stress effect of epicatechin and catechin induced by Aβ25-35 in rats and use of the electrostatic potential and the Fukui function as a tool to elucidate specific sites of interaction. Neuropeptides 59, 89–95. doi:10.1016/j.npep.2016.04.001
d'Avila, J. C., Siqueira, L. D., Mazeraud, A., Azevedo, E. P., Foguel, D., Castro-Faria-Neto, H. C., et al. (2018). Age-related cognitive impairment is associated with long-term neuroinflammation and oxidative stress in a mouse model of episodic systemic inflammation. J. Neuroinflammation 15, 28. doi:10.1186/s12974-018-1059-y
Damodaran, T., Müller, C. P., and Hassan, Z. (2019). Chronic cerebral hypoperfusion-induced memory impairment and hippocampal long-term potentiation deficits are improved by cholinergic stimulation in rats. Pharmacol. Rep. 71, 443–448. doi:10.1016/j.pharep.2019.01.012
Dev, R., Hambali, Z., and Samah, B. A. (2009). Comparison on cognitive effects of Centella asiatica in healthy middle aged female and male volunteers. Eur. J. Sci. Res. 31, 553–565.
Fattal, O., Budur, K., Vaughan, A. J., and Franco, K. (2006). Review of the literature on major mental disorders in adult patients with mitochondrial diseases. Psychosomatics 47, 1–7. doi:10.1176/appi.psy.47.1.1
Firdaus, F., Zafeer, M. F., Anis, E., Ahmad, M., and Afzal, M. (2018). Ellagic acid attenuates arsenic induced neuro-inflammation and mitochondrial dysfunction associated apoptosis. Toxicol. Rep. 5, 411–417. doi:10.1016/j.toxrep.2018.02.017
Galovic, M., Leisi, N., Müller, M., Weber, J., Abela, E., Kägi, G., et al. (2013). Lesion location predicts transient and extended risk of aspiration after supratentorial ischemic stroke. Stroke 44, 2760–2767. doi:10.1161/STROKEAHA.113.001690
Gibson, G. E., and Shi, Q. (2010). A mitocentric view of Alzheimer's disease suggests multi-faceted treatments. J. Alzheimers Dis. 2, S591–S607. doi:10.3233/JAD-2010-100336
Gray, N. E., Harris, C. J., Quinn, J. F., and Soumyanath, A. (2016). Centella asiatica modulates antioxidant and mitochondrial pathways and improves cognitive function in mice. J. Ethnopharmacol. 180, 78–86. doi:10.1016/j.jep.2016.01.013
Gray, N. E., Zweig, J. A., Caruso, M., Martin, M. D., Zhu, J. Y., Quinn, J. F., et al. (2018). Centella asiatica increases hippocampal synaptic density and improves memory and executive function in aged mice. Brain Behav. 8, e01024. doi:10.1002/brb3.1024
Ha, J. S., Kang, J. Y., Kang, J. E., Park, S. K., Kim, J. M., Kim, C. W., et al. (2020). Pentacyclic triterpenoid-rich fraction of the Hardy kiwi (Actinidia arguta) improves brain dysfunction in high fat diet-induced obese mice. Sci. Rep. 10, 5788. doi:10.1038/s41598-020-62810-5
Hase, Y., Ding, R., Harrison, G., Hawthorne, E., King, A., Gettings, S., et al. (2019). White matter capillaries in vascular and neurodegenerative dementias. Acta Neuropathol. Commun. 7, 16. doi:10.1186/s40478-019-0666-x
Henri-Bhargava, A., Stuss, D. T., and Freedman, M. (2018). Clinical assessment of prefrontal lobe functions. Contin. (Minneap Minn) 24, 704–726. doi:10.1212/CON.0000000000000609
Huang, X., Yang, J., Huang, X., Zhang, Z., Liu, J., Zou, L., et al. (2021). Tetramethylpyrazine improves cognitive impairment and modifies the hippocampal proteome in two mouse models of Alzheimer's disease. Front. Cell Dev. Biol. 9, 632843. doi:10.3389/fcell.2021.632843
Hunter, P. (2008). Harnessing nature's wisdom. Turning to nature for inspiration and avoiding her follies. EMBO Rep. 9, 838–840. doi:10.1038/embor.2008.160
Jang, Y., Lee, J. H., Lee, M. J., Kim, S. J., Ju, X., Cui, J., et al. (2020). Schisandra extract and ascorbic acid synergistically enhance cognition in mice through modulation of mitochondrial respiration. Nutrients 12, 897. doi:10.3390/nu12040897
Jeon, J., Kang, S., and Kim, H. U. (2021). Predicting biochemical and physiological effects of natural products from molecular structures using machine learning. Nat. Prod. Rep. 38, 1954–1966. doi:10.1039/d1np00016k
Jeon, S. G., Song, E. J., Lee, D., Park, J., Nam, Y., Kim, J. I., et al. (2019). Traditional oriental medicines and Alzheimer’s disease. Aging Dis. 10, 307–328. doi:10.14336/AD.2018.0328
Jiang, P., Chen, L., Sun, J., Li, J., Xu, J., Liu, W., et al. (2019). Chotosan ameliorates cognitive impairment and hippocampus neuronal loss in experimental vascular dementia via activating the Nrf2-mediated antioxidant pathway. J. Pharmacol. Sci. 139, 105–111. doi:10.1016/j.jphs.2018.12.003
Kalaria, R. N. (2018). The pathology and pathophysiology of vascular dementia. Neuropharmacology 134, 226–239. doi:10.1016/j.neuropharm.2017.12.030
Kapoor, L. (1990). Handbook of Ayurvedic medicinal plants. Boca Raton: CRC Press. doi:10.1201/9781351070997
Kartsounis, L. D., Troung, D. D., Morgan-Hughes, J. A., and Harding, A. E. (1992). The neuropsychological features of mitochondrial myopathies and encephalomyopathies. Arch. Neurol. 49, 158–160. doi:10.1001/archneur.1992.00530260058020
Katz, L., and Baltz, R. H. (2016). Natural product discovery: Past, present, and future. J. Ind. Microbiol. Biotechnol. 43, 155–176. doi:10.1007/s10295-015-1723-5
Khacho, M., Harris, R., and Slack, R. S. (2019). Mitochondria as central regulators of neural stem cell fate and cognitive function. Nat. Rev. Neurosci. 20, 34–48. doi:10.1038/s41583-018-0091-3
Khacho, M., and Slack, R. S. (2017). Mitochondrial activity in the regulation of stem cell self-renewal and differentiation. Curr. Opin. Cell Biol. 49, 1–8. doi:10.1016/j.ceb.2017.11.003
Klein, H. U., Trumpff, C., Yang, H. S., Lee, A. J., Picard, M., Bennett, D. A., et al. (2021). Characterization of mitochondrial DNA quantity and quality in the human aged and Alzheimer's disease brain. Mol. Neurodegener. 16, 75. doi:10.1186/s13024-021-00495-8
Li, J. G., Wang, L. Q., Yang, X. Y., Chen, Z., Lai, L. Y. W., Xu, H., et al. (2017). Chinese herbal medicine dengzhan xixin injection for acute ischemic stroke: A systematic review and meta-analysis of randomised controlled trials. Complement. Ther. Med. 34, 74–85. doi:10.1016/j.ctim.2017.08.004
Ling, J., Wu, Y., Zou, X., Chang, Y., Li, G., and Fang, M. (2022). (-)-Epicatechin reduces neuroinflammation, protects mitochondria function, and prevents cognitive impairment in sepsis-associated encephalopathy. Oxid. Med. Cell Longev. 2022, 2657713. doi:10.1155/2022/2657713
Liu, H., Zhou, J., Zhang, N., Wu, X., Zhang, Q., Zhang, W., et al. (2022b). Two sensory neurons coordinate the systemic mitochondrial stress response via GPCR signaling in C. elegans. Dev. Cell. 57, 2469–2482.e5. doi:10.1016/j.devcel.2022.10.001
Liu, H., Li, Q., Zhang, X., Shi, Y., and Li, J. (2022a). Effect of ginkgolide K on calcium channel activity in Alzheimer's disease. Exp. Ther. Med. 23, 426. doi:10.3892/etm.2022.11353
Liu, Q., Li, X., Li, L., Xu, Z., Zhou, J., and Xiao, W. (2018). Ginkgolide K protects SHSY5Y cells against oxygen-glucose deprivation-induced injury by inhibiting the p38 and JNK signaling pathways. Mol. Med. Rep. 18, 3185–3192. doi:10.3892/mmr.2018.9305
Lores-Arnaiz, S., Lombardi, P., Karadayian, A. G., Orgambide, F., Cicerchia, D., and Bustamante, J. (2016). Brain cortex mitochondrial bioenergetics in synaptosomes and non-synaptic mitochondria during aging. Neurochem. Res. 41, 353–363. doi:10.1007/s11064-015-1817-5
Lu, C. W., Lin, T. Y., Pan, T. L., Wang, P. W., Chiu, K. M., Lee, M. Y., et al. (2021). Asiatic acid prevents cognitive deficits by inhibiting calpain activation and preserving synaptic and mitochondrial function in rats with kainic acid-induced seizure. Biomedicines 9, 284. doi:10.3390/biomedicines9030284
Lu, C., Zhang, J., Shi, X., Miao, S., Bi, L., Zhang, S., et al. (2014). Neuroprotective effects of tetramethylpyrazine against dopaminergic neuron injury in a rat model of Parkinson's disease induced by MPTP. Int. J. Biol. Sci. 10, 350–357. doi:10.7150/ijbs.8366
Lv, J., Bhatia, M., and Wang, X. (2017). Roles of mitochondrial DNA in energy metabolism. Adv. Exp. Med. Biol. 1038, 71–83. doi:10.1007/978-981-10-6674-0_6
Ma, S., Liu, X., Xun, Q., and Zhang, X. (2014). Neuroprotective effect of Ginkgolide K against H2O2-induced PC12 cell cytotoxicity by ameliorating mitochondrial dysfunction and oxidative stress. Biol. Pharm. Bull. 37, 217–225. doi:10.1248/bpb.b13-00378
Macdonald, R., Barnes, K., Hastings, C., and Mortiboys, H. (2018). Mitochondrial abnormalities in Parkinson's disease and Alzheimer's disease: Can mitochondria be targeted therapeutically. Biochem. Soc. Trans. 46, 891–909. doi:10.1042/BST20170501
Mancuso, M., Calsolaro, V., Orsucci, D., Carlesi, C., Choub, A., Piazza, S., et al. (2009). Mitochondria, cognitive impairment, and Alzheimer's disease. Int. J. Alzheimers Dis. 2009, 951548. doi:10.4061/2009/951548
Mandel, S. A., Amit, T., Weinreb, O., Reznichenko, L., and Youdim, M. B. (2008). Simultaneous manipulation of multiple brain targets by green tea catechins: A potential neuroprotective strategy for alzheimer and Parkinson diseases. CNS Neurosci. Ther. 14, 352–365. doi:10.1111/j.1755-5949.2008.00060.x
McFarland, R., Taylor, R. W., and Turnbull, D. M. (2010). A neurological perspective on mitochondrial disease. Lancet Neurol. 9, 829–840. doi:10.1016/S1474-4422(10)70116-2
Misgeld, T., and Schwarz, T. L. (2017). Mitostasis in neurons: Maintaining mitochondria in an extended cellular architecture. Neuron 96, 651–666. doi:10.1016/j.neuron.2017.09.055
Moon, M., Hong, H. S., Nam, D. W., Baik, S. H., Song, H., Kook, S. Y., et al. (2012). Intracellular amyloid-β accumulation in calcium-binding protein-deficient neurons leads to amyloid-β plaque formation in animal model of Alzheimer's disease. J. Alzheimers Dis. 29, 615–628. doi:10.3233/JAD-2011-111778
Moreira, P. I., Carvalho, C., Zhu, X., Smith, M. A., and Perry, G. (2010). Mitochondrial dysfunction is a trigger of Alzheimer's disease pathophysiology. Biochim. Biophys. Acta 1802, 2–10. doi:10.1016/j.bbadis.2009.10.006
Nabi, S. U., Khan, A., Siddiqui, E. M., Rehman, M. U., Alshahrani, S., Arafah, A., et al. (2022). Mechanisms of mitochondrial malfunction in alzheimer’s disease: New therapeutic hope. Oxid. Med. Cell Longev. 2022, 4759963. doi:10.1155/2022/4759963
Nair, S. K., and Jez, J. M. (2020). Natural product biosynthesis: What's next? An introduction to the JBC reviews thematic series. J. Biol. Chem. 295, 335–336. doi:10.1074/jbc.REV119.011586
Newman, D. J., and Cragg, G. M. (2012). Natural products as sources of new drugs over the 30 years from 1981 to 2010. J. Nat. Prod. 75, 311–335. doi:10.1021/np200906s
Nunnari, J., and Suomalainen, A. (2012). Mitochondria: In sickness and in health. Cell. 148, 1145–1159. doi:10.1016/j.cell.2012.02.035
Ortinski, P., and Meador, K. J. (2004). Cognitive side effects of antiepileptic drugs. Epilepsy Behav. 1, S60–S65. doi:10.1016/j.yebeh.2003.11.008
Ouyang, M., Zhang, Q., Shu, J., Wang, Z., Fan, J., Yu, K., et al. (2022). Capsaicin ameliorates the loosening of mitochondria-associated endoplasmic reticulum membranes and improves cognitive function in rats with chronic cerebral hypoperfusion. Front. Cell Neurosci. 16, 822702. doi:10.3389/fncel.2022.822702
Pan, Z., He, X., Zhou, X., Li, X., Rong, B., and Wang, F. (2020). Combination of ellagic acid and trans-cinnamaldehyde alleviates aging-induced cognitive impairment via modulation of mitochondrial function and inflammatory and apoptotic mediators in the prefrontal cortex of aged rats. Chin. J. Physiol. 63, 218–226. doi:10.4103/CJP.CJP_55_20
Park, D., Swayambhu, G., Lyga, T., and Pfeifer, B. A. (2021). Complex natural product production methods and options. Synth. Syst. Biotechnol. 6, 1–11. doi:10.1016/j.synbio.2020.12.001
Parnaudeau, S., Bolkan, S. S., and Kellendonk, C. (2018). The mediodorsal thalamus: An essential partner of the prefrontal cortex for cognition. Biol. Psychiatry 83, 648–656. doi:10.1016/j.biopsych.2017.11.008
Pérez, M. J., Jara, C., and Quintanilla, R. A. (2018). Contribution of tau pathology to mitochondrial impairment in neurodegeneration. Front. Neurosci. 12, 441. doi:10.3389/fnins.2018.00441
Qi, X., Zhou, R., Liu, Y., Wang, J., Zhang, W. N., Tan, H. R., et al. (2016). Trans-cinnamaldehyde protected PC12 cells against oxygen and glucose deprivation/reperfusion (OGD/R)-induced injury via anti-apoptosis and anti-oxidative stress. Mol. Cell Biochem. 421, 67–74. doi:10.1007/s11010-016-2785-z
Rahimi, V. B., Askari, V. R., and Mousavi, S. H. (2018). Ellagic acid reveals promising anti-aging effects against d-galactose-induced aging on human neuroblastoma cell line, SH-SY5Y: A mechanistic study. Biomed. Pharmacother. 108, 1712–1724. doi:10.1016/j.biopha.2018.10.024
Rajabian, A., Rameshrad, M., and Hosseinzadeh, H. (2019). Therapeutic potential of Panax ginseng and its constituents, ginsenosides and gintonin, in neurological and neurodegenerative disorders: A patent review. Expert Opin. Ther. Pat. 29, 55–72. doi:10.1080/13543776.2019.1556258
Rajamani, K., Lin, Y. C., Wen, T. C., Hsieh, J., Subeq, Y. M., Liu, J. W., et al. (2015). The antisenescence effect of trans-cinnamaldehyde on adipose-derived stem cells. Cell Transpl. 24, 493–507. doi:10.3727/096368915X686959
Rangaraju, V., Calloway, N., and Ryan, T. A. (2014). Activity-driven local ATP synthesis is required for synaptic function. Cell. 156, 825–835. doi:10.1016/j.cell.2013.12.042
Santos, R. X., Correia, S. C., Zhu, X., Smith, M. A., Moreira, P. I., Castellani, R. J., et al. (2013). Mitochondrial DNA oxidative damage and repair in aging and Alzheimer's disease. Antioxid. Redox Signal 18, 2444–2457. doi:10.1089/ars.2012.5039
Santulli, L., Coppola, A., Balestrini, S., and Striano, S. (2016). The challenges of treating epilepsy with 25 antiepileptic drugs. Pharmacol. Res. 107, 211–219. doi:10.1016/j.phrs.2016.03.016
Selkoe, D. J. (1994). Alzheimer's disease: A central role for amyloid. J. Neuropathol. Exp. Neurol. 53, 438–447. doi:10.1097/00005072-199409000-00003
Sharma, C., Kim, S., Nam, Y., Jung, U. J., and Kim, S. R. (2021). Mitochondrial dysfunction as a driver of cognitive impairment in Alzheimer’s disease. Int. J. Mol. Sci. 22, 4850. doi:10.3390/ijms22094850
Shin, S. J., Jeon, S. G., Kim, J. I., Jeong, Y. O., Kim, S., Park, Y. H., et al. (2019). Red Ginseng Attenuates Aβ-induced mitochondrial dysfunction and Aβ-mediated pathology in an animal model of Alzheimer’s disease. Int. J. Mol. Sci. 20, 3030. doi:10.3390/ijms20123030
Shinomol, G., Muralidhara, , and Bharath, M. M. (2011). Exploring the role of “brahmi” (bacopa monnieri and Centella asiatica) in brain function and therapy. Recent Pat. Endocr. Metab. Immune Drug Discov. 5, 33–49. doi:10.2174/187221411794351833
Silva, A. Y. O., Amorim, É. A., Barbosa-Silva, M. C., Lima, M. N., Oliveira, H. A., Granja, M. G., et al. (2020). Mesenchymal stromal cells protect the blood-brain barrier, reduce astrogliosis, and prevent cognitive and behavioral alterations in surviving septic mice. Crit. Care Med. 48, e290–e298. doi:10.1097/CCM.0000000000004219
Sirichoat, A., Chaijaroonkhanarak, W., Prachaney, P., Pannangrong, W., Leksomboon, R., Chaichun, A., et al. (2015). Effects of asiatic acid on spatial working memory and cell proliferation in the adult rat hippocampus. Nutrients 7, 8413–8423. doi:10.3390/nu7105401
Sokoloff, L. (1999). Energetics of functional activation in neural tissues. Neurochem. Res. 24, 321–329. doi:10.1023/a:1022534709672
Sorrentino, V., Romani, M., Mouchiroud, L., Beck, J. S., Zhang, H., D'Amico, D., et al. (2017). Enhancing mitochondrial proteostasis reduces amyloid-β proteotoxicity. Nature 552, 187–193. doi:10.1038/nature25143
Sun, C., Liu, M., Liu, J., Zhang, T., Zhang, L., Li, H., et al. (2021). ShenmaYizhi decoction improves the mitochondrial structure in the brain and ameliorates cognitive impairment in VCI rats via the AMPK/UCP2 signaling pathway. Neuropsychiatr. Dis. Treat. 17, 1937–1951. doi:10.2147/NDT.S302355
Sun, M. K. (2018). Potential Therapeutics for vascular cognitive impairment and dementia. Curr. Neuropharmacol. 16, 1036–1044. doi:10.2174/1570159X15666171016164734
Swerdlow, R. H. (2018). Mitochondria and mitochondrial cascades in Alzheimer’s disease. J. Alzheimers Dis. 62, 1403–1416. doi:10.3233/JAD-170585
Takeda, K., Uda, A., Mitsubori, M., Nagashima, S., Iwasaki, H., Ito, N., et al. (2021). Mitochondrial ubiquitin ligase alleviates Alzheimer's disease pathology via blocking the toxic amyloid-β oligomer generation. Commun. Biol. 4, 192. doi:10.1038/s42003-021-01720-2
Turconi, A. C., Benti, R., Castelli, E., Pochintesta, S., Felisari, G., Comi, G., et al. (1999). Focal cognitive impairment in mitochondrial encephalomyopathies: A neuropsychological and neuroimaging study. J. Neurol. Sci. 170, 57–63. doi:10.1016/s0022-510x(99)00199-9
Umka Welbat, J., Sirichoat, A., Chaijaroonkhanarak, W., Prachaney, P., Pannangrong, W., Pakdeechote, P., et al. (2016). Asiatic acid prevents the deleterious effects of valproic acid on cognition and hippocampal cell proliferation and survival. Nutrients 8, 303. doi:10.3390/nu8050303
Wang, S., Ichinomiya, T., Terada, Y., Wang, D., Patel, H. H., and Head, B. P. (2021). Synapsin-promoted Caveolin-1 overexpression maintains mitochondrial morphology and function in PSAPP Alzheimer's disease mice. Cells 10, 2487. doi:10.3390/cells10092487
Wattanathorn, J., Mator, L., Muchimapura, S., Tongun, T., Pasuriwong, O., Piyawatkul, N., et al. (2008). Positive modulation of cognition and mood in the healthy elderly volunteer following the administration of Centella asiatica. J. Ethnopharmacol. 116, 325–332. doi:10.1016/j.jep.2007.11.038
Wu, O., Cloonan, L., Mocking, S. J., Bouts, M. J., Copen, W. A., Cougo-Pinto, P. T., et al. (2015). Role of acute lesion topography in initial ischemic stroke severity and long-term functional outcomes. Stroke 2015 (46), 2438–2444. doi:10.1161/STROKEAHA.115.009643
Xia, X., Jiang, Q., McDermott, J., and Han, J. J. (2018). Aging and Alzheimer's disease: Comparison and associations from molecular to system level. Aging Cell. 17, e12802. doi:10.1111/acel.12802
Xie, W., Guo, D., Li, J., Yue, L., Kang, Q., Chen, G., et al. (2022). CEND1 deficiency induces mitochondrial dysfunction and cognitive impairment in Alzheimer's disease. Cell Death Differ. 29, 2417–2428. doi:10.1038/s41418-022-01027-7
Xie, Z., Lu, H., Yang, S., Zeng, Y., Li, W., Wang, L., et al. (2020). Salidroside attenuates cognitive dysfunction in senescence-accelerated mouse prone 8 (SAMP8) mice and modulates inflammation of the gut-brain axis. Front. Pharmacol. 11, 568423. doi:10.3389/fphar.2020.568423
Yin, Z., Gao, D., Du, K., Han, C., Liu, Y., Wang, Y., et al. (2022). Rhein ameliorates cognitive impairment in an APP/PS1 transgenic mouse model of Alzheimer’s disease by relieving oxidative stress through activating the SIRT1/PGC-1α pathway. Oxid. Med. Cell Longev. 2022, 2524832. doi:10.1155/2022/2524832
Yin, Z., Geng, X., Zhang, Z., Wang, Y., and Gao, X. (2021). Rhein relieves oxidative stress in an Aβ1-42 oligomer-burdened neuron model by activating the SIRT1/PGC-1α-regulated mitochondrial biogenesis. Front. Pharmacol. 12, 746711. doi:10.3389/fphar.2021.746711
Zanto, T. P., and Gazzaley, A. (2019). Aging of the frontal lobe. Handb. Clin. Neurol. 163, 369–389. doi:10.1016/B978-0-12-804281-6.00020-3
Zhang, G., Fang, H., Li, Y., Xu, J., Zhang, D., Sun, Y., et al. (2019). Neuroprotective effect of astragalus Polysacharin on streptozotocin (STZ)-induced diabetic rats. Med. Sci. Monit. 25, 135–141. doi:10.12659/MSM.912213
Zhou, X., Wang, H. Y., Wu, B., Cheng, C. Y., Xiao, W., Wang, Z. Z., et al. (2017). Ginkgolide K attenuates neuronal injury after ischemic stroke by inhibiting mitochondrial fission and GSK-3β-dependent increases in mitochondrial membrane permeability. Oncotarget 8, 44682–44693. doi:10.18632/oncotarget.17967
Keywords: natural products, mitochondrial function, cognitive dysfunction, scoping review, neurodegenerative diseases
Citation: Tuo J, Peng Y, Linghu Y, Tao M, Huang S and Xu Z (2023) Natural products regulate mitochondrial function in cognitive dysfunction—A scoping review. Front. Pharmacol. 14:1091879. doi: 10.3389/fphar.2023.1091879
Received: 07 November 2022; Accepted: 21 February 2023;
Published: 07 March 2023.
Edited by:
Caifeng Xie, Nanchang University, ChinaReviewed by:
Shi Jing Shan, Zunyi Medical University, ChinaTao Ma, Dongfang Hospital, China
Run-Lan Wan, The Affiliated Hospital of Southwest Medical University, China
Copyright © 2023 Tuo, Peng, Linghu, Tao, Huang and Xu. This is an open-access article distributed under the terms of the Creative Commons Attribution License (CC BY). The use, distribution or reproduction in other forums is permitted, provided the original author(s) and the copyright owner(s) are credited and that the original publication in this journal is cited, in accordance with accepted academic practice. No use, distribution or reproduction is permitted which does not comply with these terms.
*Correspondence: Shiming Huang, MTgzMDk1NDQ3OUBxcS5jb20=; Zucai Xu, ZG9jeHpjQDEyNi5jb20=
†These authors have contributed equally to this work and share first authorship