- 1Guang’an Men Hospital, China Academy of Chinese Medical Sciences, Beijing, China
- 2Beijing University of Chinese Medicine, Beijing, China
Atherosclerosis (AS) is the main cause of cardiovascular disease (CVD) and is characterized by endothelial damage, lipid deposition, and chronic inflammation. Gut microbiota plays an important role in the occurrence and development of AS by regulating host metabolism and immunity. As human mitochondria evolved from primordial bacteria have homologous characteristics, they are attacked by microbial pathogens as target organelles, thus contributing to energy metabolism disorders, oxidative stress, and apoptosis. Therefore, mitochondria may be a key mediator of intestinal microbiota disorders and AS aggravation. Microbial metabolites, such as short-chain fatty acids, trimethylamine, hydrogen sulfide, and bile acids, also affect mitochondrial function, including mtDNA mutation, oxidative stress, and mitophagy, promoting low-grade inflammation. This further damages cellular homeostasis and the balance of innate immunity, aggravating AS. Herbal medicines and their monomers can effectively ameliorate the intestinal flora and their metabolites, improve mitochondrial function, and inhibit atherosclerotic plaques. This review focuses on the interaction between gut microbiota and mitochondria in AS and explores a therapeutic strategy for restoring mitochondrial function and intestinal microbiota disorders using herbal medicines, aiming to provide new insights for the prevention and treatment of AS.
1 Introduction
Atherosclerosis (AS) is an inflammatory disease characterized by endothelial cell damage, lipid deposition, and smooth muscle cell proliferation. It is one of the leading causes of cardiovascular disease (CVD) and is responsible for morbidity and mortality worldwide (Cai et al., 2020; Dagenais et al., 2020). Recent studies have focused on the intestinal flora and mitochondrial dysfunction as risk factors for AS (Witkowski et al., 2020). In the distal gastrointestinal tract, gut flora plays an important role in host physiology by establishing bacterial–host symbiosis, whereas its imbalance regulates host inflammation and metabolic and immune disorders, triggering the development of AS (Barrington and Lusis, 2017; Jonsson and Bäckhed, 2017). Moreover, bacterial translocation and metabolites entering circulation aggravate atherosclerotic plaque severity by directly invading arteries, increasing lipid deposition and insulin resistance, and activating the innate immune system (Witkowski et al., 2020). The predominant intestinal flora metabolites include short-chain fatty acids (SCFAs), trimethylamine (TMA), amino acids and their derivatives, secondary bile acids, and vitamins, all of which are associated with AS (Koeth et al., 2013). Although the mechanism and relationship between the gut flora and AS are complex, mitochondria could be an intermediate link, which might explain why AS occurs locally. As the center of cellular aerobic respiration, mitochondria synthesize 90% adenosine triphosphate (ATP) to provide the energy required by cells (Rossmann et al., 2021). Owing to an endosymbiotic relationship with ancient proteobacteria (Roger et al., 2017), mitochondria are also responsible for the signal transduction of innate immunity, oxidative stress, and cell death (Forrester et al., 2018). Thus, mitochondria are often targeted for bacterial attack and show functional abnormalities, including mitochondrial reactive oxygen species (mtROS)-induced oxidative stress, mitochondrial DNA (mtDNA) mutation and release, and imbalance of mitochondrial dynamics, further contributing to arterial wall cell damage, inflammation, and AS (Yu and Bennett, 2014; Oliveira and Vercesi, 2020).
Current treatments for AS mainly aim at improving known risk factors. Despite significant developments in disease control and prevention, there is no clear, effective treatment for these novel mechanisms (Pursnani et al., 2017; Lawton et al., 2022). Herbal medicines have been widely used to treat AS, and a possible therapeutic target is to regulate gut microbiota homeostasis, which is regarded as prebiotics (Anlu et al., 2019). Herbal medicines and their natural compounds can effectively improve mitochondrial function to protect vascular cell homeostasis and survival (Zhou et al., 2022). In this review, we discussed the emerging mechanisms of mitochondria as the key mediator linking gut microbiota to AS and systematically reviewed the role of herbal medicine and their natural compounds in improving AS through the microbiota–mitochondrial axis.
2 Vital role of mitochondria in AS
2.1 Mitochondrial dysfunction
During AS progression, mitochondrial dysfunction is associated with progressive deleterious changes, including 1) the accumulation of mtDNA mutations, 2) excessive production of mtROS and diminished antioxidant defense, leading to increased oxidative damage, and 3) mitophagy inhibition, leading to a decline in mitochondrial renewal.
2.1.1 mtDNA mutation
The genetic mechanisms of atherogenesis depend on the nuclear and mitochondrial genomes. In contrast, mtDNA is more vulnerable to damage from mtROS owing to its lack of introns and histones, leaving DNA exposed, and lack of DNA polymerase, leading to a high error rate and poor stability during mtDNA replication proximate to the site of ROS production (Valente et al., 2016; Stewart and Chinnery, 2021). mtDNA encodes protein subunits necessary for oxidative phosphorylation (Fontana and Gahlon, 2020), resulting in abnormal complex expression, subsequently leading to the generation of excessive mtROS vicious cycle between mtDNA mutation and mtROS (Yang et al., 2020). The other accepted reason for the occurrence of mtDNA mutations is replication errors resulting from de novo events and clonal expansion, and the two have an additive effect and are detrimental to overall health (Ross et al., 2014). Many somatic mtDNA mutations originate from early adulthood to clonally expand preexisting mutations (Greaves et al., 2014) and increase dramatically with age through spontaneous replication errors of mtDNA polymerase γ (Pol γ), accelerate mtDNA turnover, and direct repeats (Khrapko, 2011). The frequency of de novo mtDNA mutation is elevated in mouse female germ cells, being associated with age and parity or sexual maturity (Arbeithuber et al., 2020). All of these factors lead to a high mutation rate and heteroplasmy of mtDNA.
mtDNA mutations occur in the local disturbance of plaques (Sazonova et al., 2015), and the number of mtDNA mutations in the arterial wall cells increases as the lesions become more severe (Ballinger et al., 2002). mtDNA mutations, such as m.1555A>G, m.14459G>A, m.12315G>A, and m.13513G>A occur in plasma leukocytes or aortic plaques, (Sazonova et al., 2015; Sazonova et al., 2017), which may uncouple oxidative phosphorylation (OXPHOS), inactivate ATP synthase, and enhance the basal rate of oxygen consumption (Gilkerson et al., 2012; Orekhov et al., 2020b). High levels of mtDNA4977 deletion are independent risk factors for adverse cardiovascular events and cause mortality in patients with stable coronary heart disease (CHD) (Vecoli et al., 2018; Vecoli et al., 2019). In three cohort studies, mtDNA copy number (mtDNA-CN), which reflects normal mtDNA expression, is negatively correlated with CHD (Ashar et al., 2017; Zhang Y et al., 2017). PolG−/−/ApoE−/− mice lacking mtDNA polymerase γ exhibit extensive mtDNA damage, OXPHOS defects, and severe AS (Yu et al., 2013). ApoE−/− mice overexpressing the mitochondrial helicase Twinkle (Tw+/ApoE−/−) showed increased mtDNA-CN, respiratory complex abundance and respiration, decreased plaque necrotic core, and increased fibrous cap area (Yu et al., 2017). mtDNA mutation contribute to induction of proton leakage, transmembrane potential loss, reduced mitochondrial Ca2+ uptake (Granatiero et al., 2016), and activation of the adenosine monophosphate-activated protein kinase (AMPK) signaling pathway (Orekhov et al., 2020b), promoting arterial wall cell apoptosis and vascular inflammation. Consequently, mtDNA mutations contribute significantly to AS and CHD by disrupting the intracellular environment.
2.1.2 Excessive production of mtROS
Mitochondrial complexes are the main producers of mtROS (Yin et al., 2021), and the excessive production of mtROS and inhibition of antioxidase cause an imbalance in redox reactions (Springo et al., 2015), which promotes mtDNA mutations (Fontana and Gahlon, 2020), aggravates NF-κB activation (Csiszar et al., 2014), excites NAD(P)H oxidase (NOX) in the cytoplasm (Canugovi et al., 2019), and develops vascular oxidative stress and inflammation. Cho et al. (Cho et al., 2013) determined that mtROS significantly increased and augmented superoxide dismutase 2 (SOD2) ubiquitination in coronary endothelial cells (ECs) from type 2 diabetic mice, leading to abnormal vascular relaxation and EC damage. mtROS attenuates nitric oxide (NO) bioavailability, impairs endothelium-dependent dilation, and uncouples nitric oxide synthase (eNOS), contributing to vasodilation inhibition (Robert et al., 2021). Apoe−/−/SOD2+/− mice treated with MitoTEMPO targeting mtROS showed reduced smooth muscle cell apoptosis, necrotic core expansion, and matrix degradation to stabilize plaques (Vendrov et al., 2017). Mitochondria-derived H2O2 induces NF-κB activation in aged endothelial cells, which elevates low-grade vascular inflammation, such as increased levels of proinflammatory cytokines and adhesion molecules (Li Z et al., 2021). Mitochondrial H2O2 also induces endothelial-to-mesenchymal transition (EndoMT) via the p38 MAPK or NF-κB signaling pathways to cause vascular dysfunction and AS (Ko et al., 2019; Xu et al., 2020). EndoMT is a dynamic process that loses characteristic endothelial tight junctions and markers, including CD31, VE-cadherin, and eNOS, and acquires the mesenchymal phenotype (Xu et al., 2020). Administration of natural compounds, such as Baicalein (Shi R et al., 2018) and schizandrin B (You et al., 2019), attenuates EndoMT by suppressing the NF-κB pathway. Mitochondria-targeted antioxidants, including mitoquinone (MitoQ), MitoTEMPO, and coenzyme Q 10 (CoQ10) effectively ameliorate AS (Rossman et al., 2018). MitoQ intervention inhibits nitrotyrosine concentration and improves mitochondrial and endothelial functions (Braakhuis et al., 2018). MitoQ also decreases aortic stiffness in old mice by weakening the aortic pulse wave velocity and restoring the elastin region elastic modulus and elastin expression (Gioscia-Ryan et al., 2018). CoQ10 treatment of AS attenuates mtROS generation and activates the AMPK–YAP–OPA1 pathway to improve mitochondrial function (Xie et al., 2020). Therefore, mitochondria-derived ROS are key risk factors for cell damage, inflammation, and AS. Decreased mtROS production effectively mitigates atherosclerotic lesions.
2.1.3 Inhibition of mitophagy
Mitophagy is a selective type of autophagy that acts by removing unnecessary or aberrant mitochondria and its harmful metabolites, such as mtROS, ensuring mitochondrial quality control and stabilizing cell homeostasis (Kubli and Gustafsson, 2012). Autophagy can be divided into ubiquitin-dependent mitophagy and receptor-regulated mitophagy (Song et al., 2021). Mitophagy is inhibited in plaque lesions. Under high-glucose conditions, putative kinase 1 (PINK)/Parkin acetylation is defective in aortas regulated by sirtuin 3 (SIRT3) or uncoupling protein 2 (UCP2) (Wei et al., 2017), triggering impaired endothelial cells and aggravating AS by excessive mtROS, loss of mitochondrial membrane potential (MMP), and ATP reduction (Zhu W et al., 2018; Forte et al., 2021). Deficiencies in PINK1 or Parkin proteins result in vascular smooth muscle cell (VSMC) apoptosis by inhibiting mitophagy (Swiader et al., 2016) and increasing the occurrence of myocardial infarction by the accumulation of swollen and dysfunctional mitochondria (Kubli et al., 2013). Activation of the PINK1/Parkin pathway maintains mitochondrial integrity and avoids damage to the endothelial cells of obese mice (Wu W et al., 2015), leading to reduced apoptosis by mitigating B Cell lymphoma-associated X (BAX). Apolipoprotein A–I binding protein (AIBP) located at the inner mitochondrial membrane (IMM) is expressed in human and mouse atherosclerotic plaques (Choi et al., 2021). AIBP regulates PINK/parkin-related mitophagy and stimulates mitofusin 1 (MFN1) and MFN2 ubiquitination, improving mitophagy and fission to prevent further cell damage in AS. Therefore, enhanced mitophagy is beneficial for diminishing apoptosis, restoring endothelial function, and protecting the vasculature from sclerosis. In conclusion, the effects of mitochondrial dysfunction on AS have gradually become a focus of research. Injury to mtDNA, mtROS, and mitophagy are all involved in inflammation, disruption of cell homeostasis, and the development of AS.
2.2 Mitochondrial regulation of innate immunity and inflammation
Mitochondria are mostly known for immunity, inflammation, and apoptosis. For example, mitochondrial energy supply depends on the tricarboxylic acid cycle, and its disruption generates metabolites involved in the inflammatory response and modulates the innate immune response in immune cells (Banoth and Cassel, 2018). Downregulation of isocitrate dehydrogenase leads to the conversion of citrate to itaconate (Lampropoulou et al., 2016), which has direct antimicrobial characteristics that stimulate the innate immune response and enhance the proinflammatory properties of M1 (Peace and O'Neill, 2022). Succinate is also proinflammatory and induces mtROS production and IL-1β expression (Banoth and Cassel, 2018).
2.2.1 Mitochondrial damage-associated molecular patterns
As mitochondria evolved from ancient eubacteria, mtDNA contains hypomethylated cytosine-phosphate-guanine sequences similar to those in bacterial DNA (Fang et al., 2016). In addition to mtDNA, mitochondria-derived DAMPs (mtDAMPs) also include other mitochondrial metabolites, such as mtROS, phospholipid cardiolipin, and formyl peptides, which can be recognized as pathogens, elicit inflammation, and induce an innate immune response (Zhang et al., 2010). They are released from impaired mitochondria and activate formyl peptide receptor or pattern recognition proteins (PRPs), which respond to multiple inflammatory signaling pathways, including the nod-like receptor family pyrin domain containing 3 (NLRP3) inflammasome, NF-κB activation, and stimulator of interferon genes (STING) pathways (Misawa et al., 2017). Therefore, mitochondria are crucial elements in sterile inflammation, leading to chronic inflammation in AS.
Mitochondrial permeability transition pores (mPTP) and voltage-dependent anion channel (VDAC) oligomers are responsible for mtDAMP release (Sprenger et al., 2021; Picca et al., 2022). mPTP opening occurs in cells subjected to disrupted calcium levels or oxidative stress (Kim et al., 2019). Binding of mtDNA with charged residues in the N-terminal domain of VDAC1 facilitates VDAC1 oligomerization, which is instrumental in the release of mtDNA fragments over the outer membrane and increases outer membrane permeabilization mediated by BAX and B Cell lymphoma antagonist/killer (BAK) (McArthur et al., 2018). In addition to releasing large molecules from the mitochondria, VDAC also affects ROS production. When VDAC is inhibited by hexokinase, mtROS expression and NLRP3 activation are downregulated (Kim et al., 2019; Xian et al., 2022). Ultimately, mtDAMP, in the form of mitochondrial-derived extracellular vesicles, crosses the cytomembrane (Zhao et al., 2021).
2.2.2 mtDAMPs activate NLRP3 inflammasome
NLRP3 inflammasome is a protein complex of the NLR family. Activated NLRP3 is regulated by the adaptors of damaged mitochondria and endoplasmic reticulum (ER), further inducing caspase-1 expression and promoting interleukin (IL)-1β and IL-18 secretion, further causing inflammation and pyroptosis (Zhou et al., 2011; Song et al., 2020), which can be demonstrated by the colocalization of mitochondria with NLRP3–ASC–caspase–1 (Mohanty et al., 2019). Oxidative stress is a proinflammatory characteristic of impaired mitochondria (Heid et al., 2013). Oxidized mtDNA and mtROS interact with NLRP3 on being released into the cytoplasm (Xian et al., 2022). Depletion of mtDNA using ethidium bromide or knockout of mitochondrial transcription factor A (TFAM) suppresses NLRP3 activation and IL-1β expression (Salnikova et al., 2021).
Mitochondria also provide other key signals to recruit and activate the NLRP3 inflammasome, including translocation of cardiolipin, mitochondrial antiviral signaling proteins (MAVS), and cytosolic potassium ion (K+) efflux (Bird, 2012). Cardiolipin plays a key role in triggering NLRP3 owing to its role as a relic of the prokaryotic cell membrane (Iyer et al., 2013). Cardiolipin is located in the IMM and is transferred to the outer mitochondrial membrane (OMM) after depolarization to recruit NLRP3 (Gkikas et al., 2018). After knockout of cardiolipin synthase to inhibit cardiolipin, the NLRP3 inflammasome is effectively downregulated, and the inflammatory response is attenuated, indicating that cardiolipin triggers NLRP3 (Toksoy et al., 2017). MAVS, located in the OMM, is a critical signal for antiviral infection. MAVS is also involved in NLRP3 activation (Subramanian et al., 2013). Specifically, it recruits NLRP3 to the OMM and mediates its oligomerization and activation by targeting mtROS (Park et al., 2013). MAVS is a key sensor of mtROS, contributing to the production of type I interferon (IFN) and activation of caspase-1, and is responsible for host immunity and inflammation (Buskiewicz et al., 2016). Furthermore, ATP and K+ efflux are regarded as two key NLRP3 activators caused by impaired mitochondria and induce Ca2+ influx and direct damage to mitochondrial Ca2+ homeostasis (Yaron et al., 2015; Katsnelson et al., 2016), further affecting Krebs cycle enzyme activity, leading to a loss of MMP and mitochondrial network fragmentation (Hawkins et al., 2007; Liu et al., 2018). Mitochondrial Ca2+ homeostasis plays a crucial role in plaque calcification and unstable lesions in AS (Forrester et al., 2018).
Mitophagy mediates innate immunity and prevents hyperstimulation of NLRP3 and superfluous inflammation (Orekhov et al., 2020b). As defective mitophagy constantly emits inflammatory signals, cells cannot bear the ongoing stress and undergo apoptosis (Jin et al., 2022). Melatonin inhibits mtROS expression and suppresses NLRP3 inflammasome activation by enhancing mitophagy in lipopolysaccharide-treated (LPS)-treated macrophages, thereby preventing AS progression (Ma S et al., 2018). Accordingly, NLRP3 may be sensitive to mitochondrial dysfunction, explaining the correlation between mitochondrial dysfunction and chronic inflammatory diseases. Furthermore, NLRP3 exacerbates mitochondrial dysfunction. For example, caspase-1 induced by NLRP3 enhances mitochondrial membrane permeabilization and mtROS generation, and NLRP3 disturbs mitochondrial Ca2+ homeostasis (Yu et al., 2014). These suggest a positive feedback loop between NLRP3 and mitochondria (Orekhov et al., 2020a).
2.2.3 mtDAMPs induce other inflammatory pathways
Cyclic guanosine monophosphate–adenosine monophosphate synthase (cGAS) effectively senses and recognizes viral and bacterial DNA in the cytoplasm. Released mtDNA is also an endogenous cGAS ligand that binds to cGAS and catalyzes the generation of cyclic guanosine monophosphate–adenosine monophosphate (cGAMP) (Chowdhury et al., 2022). cGAMP is a secondary messenger that recruits STING (Yu et al., 2020), which stimulates tank-binding kinase in perinuclear endosomes to mediate the phosphorylation of the transcription factor interferon regulatory factor 3 (IRF-3) and transposition into the nucleus (West et al., 2015), promoting type I and III IFN responses and interferon-stimulated gene activation (Puhm et al., 2019). cGAMP expression is elevated in the arteries of ApoE−/− mice, stimulating the accumulation of lipids and macrophages and the secretion of inflammatory cytokines (Pham et al., 2021). However, STING deficiency prevents the progression of atherosclerotic plaques by inhibiting inflammation (Pham et al., 2021). Thus, the cGAS–STING pathway enhances vascular inflammation induced by mtDNA to aggravate AS.
Mitochondria engage in antimicrobial processes of toll-like receptor (TLR) signaling cascades. Activated TLR send signals to the mitochondria by translocating tumor necrosis factor receptor associated factor 6 (TRAF6) and ubiquitinate the evolutionarily conserved mitochondrial signaling intermediate in toll pathways (ECSIT) to stimulate mtROS production and move mitochondria to phagosomes, enhancing antimicrobial activity (West et al., 2011). Depletion of ECSIT and TRAF6 decreases the expression of mtROS and attenuates antimicrobial ability (Banoth and Cassel, 2018). TLR9 is an innate immune receptor that recognizes CpG motifs in bacterial or viral DNA and releases mtDNA (Hotz et al., 2018). Extracellular mtDNA triggers NF-κB nuclearization, induces proinflammatory cytokines, augments type I IFN responses, and provokes a sterile systemic inflammatory response syndrome mediated by TLR9 activation (Zhang et al., 2014; Bliksøen et al., 2016). Circulating oxidative mtDNA triggers TLR9 activation and upregulates proinflammatory cytokines in macrophages of ApoE−/− mice undergoing e-cigarette intervention, which leads to AS. Inhibition of the inflammatory cascade depends on normal mitophagy (Li J et al., 2021). Mitophagy is suppressed via PINK degradation, promoting macrophage polarization of the proinflammatory phenotype M1 and the development of AS (Hung et al., 2021). Consequently, TLRs are recognized and stimulated by mitochondria-derived DAMPs, such as mtDNA, and further trigger amplification of the inflammatory cascade to exacerbate AS progression.
2.3 Mitochondrial regulation in vascular cells
Various cells play different roles in AS progression, including barrier protection function of ECs, vasoconstriction and remodeling function of VSMCs, phagocytosis of lipids and immune function in macrophages, with mitochondria participating in all.
2.3.1 Cholesterol metabolism in macrophages
Mitochondria play a crucial role in lipid metabolism during AS progression. Modified low-density lipoprotein (LDL) is phagocytosed by macrophages and focally stimulates vascular innate immunity, where the mitochondria-associated immune barrier is compromised, contributing to lipid deposition, accumulation of proinflammatory mediators, and transformation of lipid-rich foam cells (Duan et al., 2022). In ECs, intervention with oxidized LDL (ox-LDL) causes mtROS significantly increase, reduction in complex I and III enzyme activity, mPTP opening, cytosolic Ca2+ influx, promoting mitochondrial damage and cell apoptosis, triggering vascular inflammation, and the occurrence of AS (Orekhov et al., 2020b). Mitochondrial respiratory dysfunction negatively influences cholesterol efflux in macrophages. Reverse cholesterol transport (RCT) is mediated by ATP-binding cassette cholesterol transporter proteins (ABCA1) and apolipoprotein A–I (ApoAI) in macrophages, leading to the accumulation of cholesterol and an increase in foam cells (Dumont et al., 2021; Dorighello et al., 2022). ATP is the energy provider for ABCA1-dependent cholesterol efflux. miR-33 also controls cholesterol efflux by limiting ATP production (Karunakaran et al., 2015). Excessive accumulation of cholesterol stimulates mPTP to open and enter the mitochondria, subsequently generating multiple oxysterols via CYP27A1 (a cytochrome p450 oxidase), such as 25- and 27-hydroxycholesterol (25-/27-OH) in the mitochondria, and constantly esterifies oxysterol in atherosclerotic plaques (Korytowski et al., 2015). Cholesterol oxides inhibit ABCA1 and ABCG1 expression and diminish 27-OH efflux inside the mitochondria of macrophages, leading to RCT impairment and peroxidation damage in the mitochondria (Karunakaran et al., 2015). AIBP binds to ApoAI and induces mitophagy and NADPH, whereas a deficiency in AIBP leads to the accumulation of damaged mitochondria and polarization in M1 macrophages to promote inflammation. Furthermore, interventions targeting the mitochondria can recover cholesterol trafficking in AS. Mitochondrial antioxidant Mito-Tempol attenuates cholesteryl accumulation by activating ABCA1 expression to export cholesterol (Ma Y et al., 2018). Pravastatin is involved in mitochondrial fusion and the formation of mitochondria-associated membranes to strengthen the correlation between mitochondria and the ER, further decreasing foam cell generation in a high-lipid environment (Assis et al., 2022). Therefore, recovering mitochondrial respiratory function and providing efficient energy ameliorates cholesterol trafficking, decreases foam cell numbers, and reverses AS progression.
2.3.2 VSMC proliferation, migration, and phenotype switching
VSMC proliferation, migration, and phenotype switching are critical features of AS and contribute to intimal hyperplasia and restenosis in the arteries. VSMC exhibit a contractile phenotype that modulates vascular dilation and contraction under physiological conditions. After vascular injury from various stresses, VSMC convert to a synthetic phenotype, minimizing contractile function and migrating and proliferating from the medial layer to the arterial lumen (Chen et al., 2022). VSMC phenotype switching is closely associated with mitochondrial functions, including fission, oxidative stress, mitophagy, and Ca2+ influx. Mitochondrial fission regulates the quantity and quality of mitochondria in cells and is mediated by dynamin-related GTPases, such as dynamin-related protein 1 (Drp1) (Maimaitijiang et al., 2016). Drp1 activated by AngII, PGDF-BB, or H2O promotes VSMC proliferation by accelerating the cell cycle, whereas mitochondrial division inhibitor-1 (Mdivi-1) stops the cell cycle at the G0 (quiescent)/G1 (long growth) phase by inhibiting Drp1 (Lim et al., 2015), attenuating VSMC proliferation and migration (Zhang X et al., 2018). High glucose induces mitochondrial fragmentation, leading to mtROS production, oxidative stress, and VSMC proliferation, which was reversed by reducing Drp1 expression via Mdivi (Maimaitijiang et al., 2016) or H2S (Sun et al., 2016). Meanwhile, knockdown of the dominant-negative mutant Drp1-K38A or Drp1 also inhibits PDGF-induced mitochondrial fission and mtROS levels, resulting in the suppression of VSMC migration. Mitochondrial Ca2+ uptake by activating Ca2+-dependent kinase II and mitochondrial Ca2+ uniporter also affects mitochondrial translocation to the leading edge of migrating VSMC (Nguyen et al., 2018). Apelin-13 triggers mitochondrial Ca2+ influx and facilitates mtROS expression and mitophagy mediated by the PINK1/Parkin pathway, leading to the proliferation of VSMCs, whereas using mito-TEMPO, Mdivi-1, siRNA-PINK1, or siRNA-Parkin can ameliorate VSMC proliferation (Chen et al., 2022). Thus, VSMC proliferation and migration are mediated by excessive mitochondrial fission, mtROS, Ca2+ influx, and mitophagy, which aggravate vascular intimal remodeling and AS progression.
2.3.3 Cell death
Mitochondria are strongly associated with programmed cell death, and their related mechanisms include apoptosis and pyroptosis (Bock and Tait, 2020), which is a cascade of amplified inflammatory reactions mediated by caspase-1 and inflammasome (Yu et al., 2014). Mitochondria are crucial promoters of inflammasome activation and pyroptosis, and the inhibition of mitophagy exacerbates pyroptosis. NIP3-like protein X (NIX) engages in mitophagy with microtubule-associated protein 1 light chain 3 (LC3) (Peng et al., 2020), suppresses mtROS and NLRP3 expression, inhibits caspase-1 and IL-1β activation, and induces macrophage pyroptosis in AS progression (Peng et al., 2022; Tian et al., 2022).
The main mechanism of apoptosis is the enhancement of OMM permeabilization mediated by the accumulation of Ca2+ and activation of the proapoptotic proteins BAX and BAK (Jeong and Seol, 2008). BAX and BAK oligomerize at the OMM and for a transmembrane pore, resulting in mPTP opening, loss of MMP, and release of mitochondrial intermembrane space proteins, such as cytochrome c, apoptosis-inducing factor, and endonuclease G (Schellenberg et al., 2013). Water moves into the mitochondrial matrix via the opening of the mPTP, causing mitochondrial matrix swelling and OMM rupture, releasing more intermembrane space proteins into the cytoplasm and inducing apoptosis (Han et al., 2019; Liang et al., 2022). After exporting into the cytosol, cytochrome C combines with apoptotic protease-activating factor-1 and procaspase-9, composing the apoptosome structure and initiating an irreversible apoptosis cascade (Zhao Y et al., 2020). Furthermore, nitrosylation of Drp1 regulates fission and triggers the release of proapoptotic factors into the cytoplasm (Kleele et al., 2021; Jenner et al., 2022). Expression of the fusion protein optic atrophy protein 1(OPA1) suppresses apoptosis by limiting cristae remodeling, and OPA1 knockout exacerbates VSMC apoptosis and aggravates AS (Peng et al., 2020). Therefore, owing to their unique structure and function, mitochondria are responsible for the activity and death of vascular cells through the regulation of inflammation, permeability, dynamics, and activation of proapoptotic cytokines (Figure 1). However, the gut microbiota and its metabolites may be potential predisposing factors for these results.
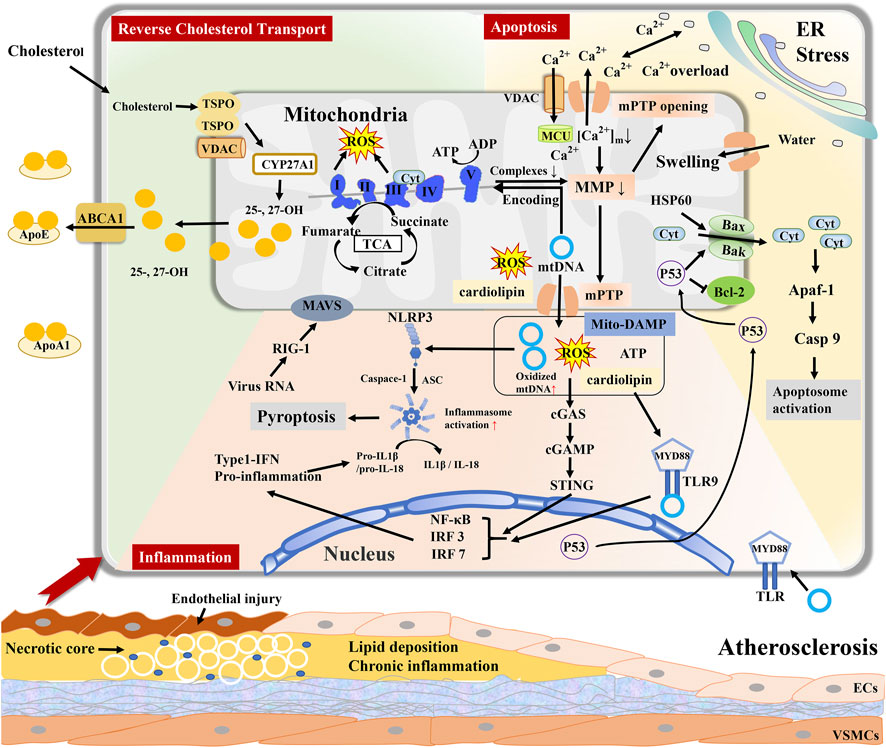
FIGURE 1. Mitochondria regulate reverse cholesterol transport (RCT), cell apoptosis, and inflammatory response during atherosclerosis. Excessive accumulation of cholesterol entry into mitochondria generate and esterify 25- and 27-hydroxycholesterol ((25-/27-OH)) by CYP27A1. Inhibition of ABCA1 results in RCT impairment and peroxidation damage in the mitochondria of macrophages. Mitochondria-DAMPs include mtDNA, mtROS, ATP, and cardiolipin, and export via mPTP. Mito-DAMPs are recognized as a pathogen to elicit inflammation, such as TLR9 signaling, cGAS–cGAMP–STING pathway, and inducing NLRP3 inflammasome to stimulate inflammatory cascade response. mPTP opening causes Ca2+ and cytochrome C (Cyt) efflux and mitochondrial swelling, leading to cellular apoptosis. Mitochondrial dysfunction contributes to lipid deposition, inflammation, and cellular apoptosis in the vascular wall, jointly promoting atherosclerosis progression.
3 Gut microbiota disorders target mitochondria and are closely correlated with AS
There are 100 trillion microbes in the human gastrointestinal tract, including 1000 different bacterial species comprising five phyla: Bacteroidetes, Firmicutes, Proteobacteria, Actinobacteria, and Verrucomicrobia, collectively regarded as “gut microbiota” (Li et al., 2014; Yoo et al., 2022). Gut microbiota and host symbiotic interactions commonly form an intestinal microenvironment to digest indigestible substances and secrete biologically active metabolites, and together maintain host energy metabolism, promote immune homeostasis, and sustain the normal function of distal organs (Gregory et al., 2015; Lian W S et al., 2022). When the intestinal flora and some of its metabolites, such as LPS, enter the bloodstream, they act as PAMP to bind to PRPs in host cells, activate immune cells, induce the production of inflammatory cytokines and chemokines necessary for protective immune responses, and present antigens to antigen-presenting cells in lymphoid tissues, resulting in systemic inflammation and excessive immune response (Verhaar et al., 2020). Meanwhile, the proliferation of opportunistic pathogens or facultative anaerobic bacteria in the intestinal tract causes gut dysbiosis and secretion of numerous abnormal metabolites, which facilitate host fat mass gain, insulin resistance, and various metabolic diseases such as obesity, diabetes, metabolic syndrome, and AS (Le Roy et al., 2022). An imbalance in the proportion of microbiota species is closely correlated with the host’s age, dietary habits, emotional stress, and antibiotic application (Boulangé et al., 2016). Thus, the gut microbiota is not only a digestive apparatus but also a dynamic endocrine organ that regulates the physiological and pathological conditions of the host (Vezza et al., 2020).
3.1 Gut microbiota-mediated damage to mitochondria and AS
3.1.1 Flora translocation in the atherosclerotic plaque
Gut dysbiosis is strongly associated with AS and CVD, mainly through microbiota translocation into atherosclerotic plaques and microbial metabolites from the gut, which act on distal arteries to affect AS progression (Lindskog Jonsson et al., 2017). DNA from Bacteroides forsythus, Prevotella intermedia, Porphyromonas gingivalis, genus Curvibacter, and Actinobacillus actinomycetem comitans have been detected in human endarterectomy plaques (Koren et al., 2011; Ziganshina et al., 2016). Burkholderiales and Propionibacterium are the most abundant taxa in atherosclerotic plaques (Ziganshina et al., 2016). Several structural components of the gut microbiota directly stimulate the expression of proinflammatory cytokines and chemokines, such as LPS, peptidoglycan, flagellin, and bacterial DNA. These bacteria that invade arteries not only contribute to local infections and inflammation but also aggravate the total cholesterol and fibrinogen levels to exacerbate atherosclerotic plaque ruptures (Brown and Hazen, 2018).
Gut bacterial abundance and diversity are altered in ApoE−/−mice with AS, including decreased α-diversity (Kappel et al., 2020) and increased abundance of Verrucomicrobia, Bacteroidaceae, Bacteroides, Akkermansia, and phylum Actinobacteria, which positively correlate with serum cholesterol, triglycerides, and LDL levels, inducing TLR signaling and cytokine receptor interactions (Liu Q et al., 2020). Similar alterations in the gastrointestinal tract of human atherosclerotic patients have also been found, such as enrichment in Collinsella or phylum Actinobacteria, which increase gut permeability and triglyceride synthesis, recruit neutrophils, and trigger the NF-κB pathway (Chen J et al., 2016; Gomez-Arango et al., 2018). In contrast, probiotics are involved in multiple key processes of AS development by attenuating intestinal flora disorders and the risk of infection (Zhai et al., 2022). Lactobacillus strains, such as Lactobacillus rhamnosus JL1 (Yang et al., 2021), Lactobacillus pentosus (Huang et al., 2014), and Lactobacillus acidophilus (Michael et al., 2017), decrease total cholesterol, triglyceride, and LDL levels in mice fed a high-fat diet. Lactobacillus cfermentum effectively protects endothelial function and prevents oxidative stress by maintaining intestinal homeostasis and inhibiting eNOS uncoupling and NOX2 activation (Toral et al., 2018). Moreover, Bifidobacterium ameliorates inflammatory factors, such as TNF-α and IL-6, resulting in the reversal of AS progression (Primec et al., 2019; Zaharuddin et al., 2019).
3.1.2 Mitochondrial injury from gut microbiota
The correlation between the gut microbiota and mitochondria can be traced back to α-Proteobacteria as the origin of the mitochondria (Roger et al., 2017). Mitochondria have homologous characteristics with bacteria; therefore, bacterial and mitochondrial protein-targeting sequences exhibit similarities and close lineage, leading to mitochondria becoming a target for gut microbiota (Degli Esposti et al., 2014). Similar autophagic methods eliminate both mitochondria and bacterial membranes, whereas abnormal autophagy contributes to chronic inflammation by inducing cytokine and inflammasome expression in the host (Kalghatgi et al., 2013). Consequently, mitochondria-related innate immunity activation is an important target of the gut microbiota (Jackson and Theiss, 2020). Clostridium difficile toxin A triggers mtROS production and ATP dissipation, which are associated with upregulation of NF-κB and IL-8 (He et al., 2002). Salmonella enterica serovar Typhimurium stimulates macrophage activation and induces an innate immune response by releasing mtDNA into the cytosol to induce a type I IFN response and the cGAS–STING pathway (Xu L et al., 2022). During these processes, gut bacterial effector proteins transit to the mitochondrial subcompartments via complex import systems and are precisely located in the mitochondria by targeting the sequence domain (Mahmud et al., 2021). Specifically, bacterial effector proteins are recognized and translocated by the translocase of the OMM complex and transported to the OMM, mediated by the sorting and assembly machinery complex. The effector proteins are subsequently transferred into the IMM and matrix by the translocase of the inner membrane 22 (TIM22) and TIM23 complexes, resulting in mitochondrial dysfunction and risk of bacterial survival and infectivity (Mahmud et al., 2021).
Furthermore, as mitochondria are organelles that regulate apoptosis, the consequences of infection from the gut microbiota usually target mitochondria-mediated apoptosis to enhance its virulence. enteropathogenic E coli produces the cytotoxins Maps EspZ and EspF, precisely targeting the mitochondria. EspF induces apoptosis via the release of cytochrome c from the mitochondria, whereas EspZ inhibits apoptosis to sustain MMP (Shames et al., 2011). Maps are also transported to the mitochondria via the import system, further stimulating Drp1 to promote fission, depleting MMP to cause Ca2+ efflux, cytochrome c release, endogenous apoptosis, and triggering p38 MAPK cascades to increase the inflammatory response (Ramachandran et al., 2020). VacA generated by Helicobacter pylori exacerbates apoptosis through similar stimulation, including the formation of anion-selective channels at the OMM to release cytochrome c into the cytosol and activate caspase 3 (Rassow, 2011). Host cell survival or death depends on mechanisms that are conducive to bacterial colonization. FimA, an effector protein produced by Salmonella enterica, maintains the relationship between VDAC1 and the glycolytic enzyme hexokinase to inhibit cytochrome c release by binding to VDAC1 at the OMM (Sukumaran et al., 2010). Another effector protein, SipB, facilitates autophagy-dependent cell death by disrupting the mitochondrial cristae morphology and imbalanced dynamics (Hernandez et al., 2003). In contrast, mitochondrial function also improves gut health and microbiota stability. In mice with increased mitochondrial activity, the relative abundance of Dorea and Oscillospira genera is enhanced and expression of SCFA, NAD, and sirtuin is elevated, leading to facilitation of fatty acid oxidation and reduction of metabolic disorders (Juárez-Fernández et al., 2022). Mitochondrial dysfunction in intestinal epithelial cells aggravates inflammation and oxygenation in the gut barrier and increases the abundance of facultative anaerobes, which are further involved in metabolic disorders (Zhang Y et al., 2022). Moreover, mitochondria also participate in the amelioration of lipid metabolism in the liver and reverse HFD-induced type 2 diabetes (Rodrigues et al., 2021). These data indicate that mitochondria outside vascular cells also respond to AS progression by regulating the gut microbiota. Therefore, gut bacteria not only regulate cell death by secreting toxins targeting mitochondria to promote bacterial colonization and reproduction but also activate the host’s innate immunity mediated by mitochondria to resist gut bacterial infections, subsequently leading to chronic inflammation and systemic diseases, including AS.
3.2 SCFA effects on mitochondria during AS
SCFAs are the most abundant type of gut microbiota metabolites derived from the colon and cecum of non-digestible carbohydrates, including acetate, butyrate, and propionate, which not only serve as ingredients for mitochondrial energy metabolism but are also involved in improving mitochondrial function (den Besten et al., 2013). Most SCFAs are absorbed by the host mainly through active transport mediated by translocated proteins in colonocytes, and the effects of SCFAs on mitochondria may depend on the SCFA concentration. Zhao et al. (Zhao T et al., 2020) found that butyrate mediates 54 genes associated with mitochondrial energy metabolism and exerts protective effects by increasing mtDNA and ATP contents under high insulin conditions. Butyrate also defends islet β cell activity via the depletion of mtROS and activation of antioxidant enzymes to inhibit oxidative and nitrosative stresses, lipogenesis, and loss of excess weight by stimulating GPR43 and beta-arrestin2 activity to favor mitochondrial biogenesis and energy expenditure, including triggering AMPK and the expression of PGC-1α and OXPHOS pathways, reducing the risk factors for AS (Tang et al., 2020). Administration of 3-hydroxybutyrate (3-HT) is beneficial and ameliorates atherogenesis (Zhang et al., 2021). Possibly, 3-HT binds to G-protein-coupled receptor 109a in macrophages to functionally facilitate cholesterol efflux and extracellular Ca2+ influx, leading to a decrease in the proportion of M1 macrophages via the suppression of NLRP3 and inactivation of ER stress via the inhibition of Ca2+ depletion (Zhang et al., 2021). The antioxidant response of butyrate significantly contributes to the induction of mitochondrial manganese–superoxide dismutase (MnSOD) and glutathione peroxidase expression by downregulating glycogen synthase kinase-3 beta and upregulating the nuclear translocation of nuclear factor erythroid 2-related factor 2 (Nrf2) (Tang et al., 2020). Propionic acid also regulates the redox state of the microenvironment through the mtROS content to influence chronic inflammatory diseases. Butyrate and its derivative butyramide attenuate insulin resistance and fat accumulation in the liver cells of obese mice by promoting mitochondrial fusion and the AMPK–acetyl–coenzyme A (CoA) carboxylase pathway to increase the utilization of fatty acids, glucose homeostasis, and mitochondrial respiratory rate (Mollica et al., 2017). SCFAs also suppress blood vessel cell death and chromatin modification by inactivating histone deacetylase, decreasing CD4+ and CD8+ T cell proliferation to inhibit vascular inflammation by delaying the maturation of dendritic cells, ultimately controlling AS progression (Andrade-Oliveira et al., 2015); therefore, SCFAs, especially butyrate, exert protective effects on mitochondria in vascular cells at a certain concentration (Table 1).
3.3 Trimethylamine-N-oxide effects on mitochondria during AS
TMAO is a harmful bacterial metabolite associated with AS. After consuming dietary components containing carnitine, choline, and betaine, TMA derived from the gut microbiota is subsequently oxidized to TMAO via flavin-containing monooxygenases in the liver (Wang et al., 2011). After transplanting the cecal microbiota of C57BL/6J mice with a choline diet and high TMAO levels into germ-free ApoE−/− mice, the recipients bore a greater atherosclerotic plaque burden and a positive association between TMAO levels in the plasma and atherosclerotic lesion area (Gregory et al., 2015). This indicates that choline diet-dependent TMAO levels may enhance atherosclerotic susceptibility. In a 3-year follow-up of 4,007 healthy participants with phosphatidylcholine challenges, the plasma levels of TMAO decreased with oral broad-spectrum antibiotics but increased after the withdrawal of antibiotics, which elevated the risk of major adverse cardiovascular events (MACE) (Tang et al., 2013), demonstrating the crucial role of gut microbiota in AS.
Mitochondria can be a target mediating TMAO effects on AS (Table 1). TMAO enhances aortic valve thickness and osteogenic differentiation by facilitating mitochondrial stress, including stimulation of mitochondrial swelling and NF-κB signaling (Li et al., 2022). TMAO also participates in the inhibition of mitochondrial respiration and OXPHOS. TMAO-treated cardiac fibers contribute to the decline in substrate-dependent respiration with pyruvate by 30% and negatively to β-oxidation, with declines in palmitoyl-CoA-related respiration and energy production (Makrecka-Kuka et al., 2017). TMAO also inhibits phosphocreatine and ATP levels by downregulating complex IV, further aggravating cardiac dysfunction and left ventricular pressure overload (Yoshida et al., 2022). Additionally, TMAO promote vascular endothelial injury by mitochondrial mediation. In ECs, TMAO administration increases succinate dehydrogenase complex subunit B overexpression, which promotes mtROS production and mitochondrial dysfunction, resulting in pyroptosis (Wu et al., 2020). TMAO upregulates mtROS levels and activates NLRP3 expression, likely by inhibiting SOD2 and SIRT3, further aggravating the inflammatory cascade in the blood vessels (Chen et al., 2017). TMAO mediates vascular cell adhesion molecule-1 expression in ECs to enhance monocyte adhesion, trigger scavenger receptors in macrophages, and recruit macrophages to promote the secretion of the inflammatory cytokines TNF-α and IL-6 (Mohammadi et al., 2016; Al-Obaide et al., 2017). In addition to increasing mtROS levels, TMAO-induced endothelial damage is strongly associated with other mitochondrial functions, such as the loss of MMP and Ca2+ influx, which affects NO release and eNOS phosphorylation (Querio et al., 2022). TMAO also induces Ca2+ and mtDNA release from platelets, causing dose-dependent platelet hyperreactivity and thrombus formation, which may be induced by ADP in the mitochondria (Querio et al., 2019). Moreover, TMAO might be reduced by the mitochondrial hmARC1.(Schneider et al., 2018). hmARC1 is an effective constituent of the mitochondrial amidoxime-reducing component protein that can translate TMAO into precursor TMA (Schneider et al., 2018). Overexpression of hmARC1 is correlated with the decline in TMAO in the liver as well as the enrichment of N-reductive activity in OMM. Collectively, mitochondria play a crucial role in mediating the effects of TMAO on AS progression, expanding the negative effects of TMAO on energy metabolism, oxidative stress, inflammatory cascade reactions, EC injury and death, and thrombus formation.
3.4 Hydrogen sulfide effects on mitochondria during AS
The bacterial metabolite H2S, which is generated by the gut microbiota or host cells and is the third physiologic signaling gas after CO and NO, shows dose-dependent effects on vessels, such as facilitating vasodilation and angiogenesis, decreasing monocyte adhesion, and attenuating apoptosis (Paul et al., 2021). The aorta has the highest H2S bioavailability and most H2S bioequivalents (Shen et al., 2013). H2S triggers cGMP synthesis, inhibits the degradation of cGMP and cAMP to promote NO release and vasodilatation, and activates NAD+ in ECs to delay endothelial senescence. Moreover, H2S has a pro-angiogenic effect partly through the regulation of electron transport, OXPHOS, and sulfhydration with mitochondria to elevate glucose uptake and glycolysis (Longchamp et al., 2018).
As H2S is sensitive to oxygen, the metabolic site of H2S is mainly in the mitochondria. The anaerobic microbiota generates a non-negligible amount of H2S in the colon, which is oxidized into detoxicated thiosulfate and sulfate mediated by mitochondria and excreted. The released electrons can be transferred to the electron transport chain (ETC), further improving ATP production during this metabolic process (Libiad et al., 2019). H2S reduces oxidative stress and mitochondrial swelling while enhancing mitophagy and mitochondrial biogenesis and mediates the sulfidation of the mitophagy protein parkin, further activating E3 ubiquitin ligase and inducing mitophagy (Sun et al., 2020). It also improves mitochondrial biogenesis in myocardial cells following ischemia/reperfusion (I/R) injury by downregulating protein phosphatase 2A and upregulating peroxisome PGC-1α (Calvert et al., 2010; Untereiner et al., 2016). In H2O2-treated cardiomyocytes, NaHS effectively attenuates mtROS production, reduces damage from oxidative stress, and enhances cardiomyocyte viability and activity via a SIRT1-dependent pathway (Wu D et al., 2015). SIRT1 is a significant element in mediating the effects of NaHS on reversing oxidative stress and depleting ATP. These effects can be eliminated by SIRT1 silencing (Ma et al., 2019). Furthermore, NaHS ameliorates EC senescence by upregulating SIRT1 and antioxidant defenses (Zheng et al., 2014). Low doses of H2S are beneficial to cells as they promote mitochondrial biological function and ameliorate apoptosis, whereas high concentrations inactivate complex III at the ETC and even lead to apoptosis of aortic SMCs (Murphy et al., 2019). Excess H2S is cytotoxic and harmful to cellular energy metabolism and causes DNA injury through the inhibition of mitochondrial respiration and ATP synthesis (Borisov and Forte, 2021). High concentrations of H2S bind to the copper center of cytochrome c oxidase to inhibit its activation and suppress mitochondrial activity (Módis et al., 2014). This further confirms that mitochondria play a crucial role in regulating the interaction between H2S from the gut microbiota and vascular damage (Table 1).
3.5 Other intestinal flora metabolites
Other metabolites from the gut microbiota also correlate with the development of AS (Table 1). Small metabolites are transported into the bloodstream via transcellular or paracellular routes, whereas large metabolites disrupt the gut barrier and are released into the body (Kirichenko et al., 2020). Indole derivatives, such as indoxyl sulfate, indole ethanol, and indole acrylic acid, are produced by the gut microbiota from tryptophan and induce proinflammatory activity after entering the bloodstream (Xu H et al., 2022). In addition, indolepropionic acid (IPA), which is metabolized from tryptophan, is substantially reduced in patients with coronary artery disease. Oral IPA administration mitigates the extent of atherosclerotic lesions by activating ABCA1 and promoting RCT (Xue et al., 2022). Another amino acid derivative metabolized by the gut microbiota is phenylacetylglutamine, which induces platelet hyper-responsiveness and thrombotic action (Liu et al., 2021) and is positively associated with MACE (Bogiatzi et al., 2018). Furthermore, secondary bile acids (BAs) and LPS are microbiota-dependent proatherogenic molecules. BAs are converted by the gut microbiota from primary BAs and further mediate lipid and glucose metabolic pathways by binding to receptor farnesoid X and membrane receptor Takeda G-protein-coupled receptor 5, resulting in the development of vascular inflammation, lipid deposition, and AS (Pols et al., 2011; Kuipers et al., 2014). Indole-3-carboxylic acid and BAs contribute to mitochondrial swelling and increase mitochondrial permeability by changing the Ca2+ concentration threshold for mPTP opening (Fedotcheva et al., 2021). Binding of secondary BAs to the G-coupled membrane protein 5 and farnesoid X receptor triggers mitochondrial fission via the ERK/DRP1 pathway and mediates fatty acid uptake and β-oxidation, further causing energy metabolism disorders and apoptosis (Mikó et al., 2018). LPS, a large molecule derived from Gram-negative bacteria, translocates into the bloodstream by compromising the intestinal mucosa, leading to metabolic endotoxemia (Giusti et al., 2017). LPS subsequently stimulates the TLR 4 and TLR4/MyD88/TRIF signaling pathways to trigger macrophages and systemic inflammation (Caesar et al., 2015). Additionally, some Gram-negative bacteria can translocate to the arterial intima and directly release LPS, leading to AS progression (Verhaar et al., 2020). Consequently, the gut microbiota–mitochondria axis acts as a significant endocrine metabolic pathway to regulate the host’s systemic chronic diseases, especially AS. The specific mechanism might also be affected by the dominant bacteria in the gut, concentration of metabolites, and other atherosclerotic risk factors. Further studies are necessary to determine the long-distance interactions between the gut microbiota, mitochondria, and AS completely and accurately (Figure 2).
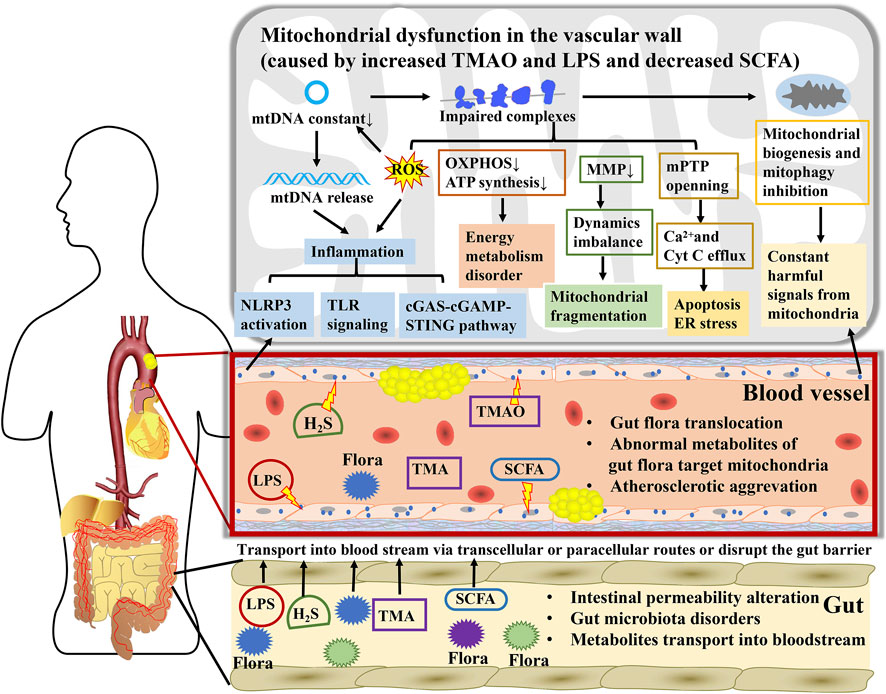
FIGURE 2. A key mediator linking gut microbiota to atherosclerosis, mitochondria participate in the interaction between numerous metabolites from gut microbiota and atherosclerosis. During atherosclerosis, gut microbiota disorders and intestinal permeability alteration contribute to direct transposition of flora to arteries. Microbial metabolites are transported into the bloodstream via transcellular or paracellular routes or disrupting the gut barrier and subsequently affect mitochondrial function in blood vessels cells. Mitochondria expand the influence of TMAO, LPS, H2S, and SCFA on energy metabolism, oxidative stress, inflammatory cascade reaction, and endothelial cell injury through regulating mtDNA release, ATP synthesis, MMP, mPTP, mitochondrial biogenesis, and mitophagy, further promoting or opposing the atherogenic process.
4 Natural compounds in herbal medicine regulating intestinal flora and mitochondria to treat AS
Intervention of gut microbiota and mitochondria is a novel therapeutic target for AS. Lifestyle adjustments may simultaneously regulate gut microbiota and mitochondria (Najjar and Feresin, 2019). Endurance exercise maintains the stability of intestinal flora and reverses host mitochondrial functions by inhibiting enteric inflammation and diminishing the reproduction of pathobionts in the intestinal tract (Clark and Mach, 2017). Plant-based diets or consumption of unsaturated fatty acids can improve gut microbiota diversity and symbiotic homeostasis and recover mitochondrial respiration and fatty acid oxidation (López-Salazar et al., 2021), resulting in enhanced satiety, increased energy expenditure, and elevated insulin sensitivity, ultimately ameliorating AS by reducing body fat and maintaining glycemic stability. However, the therapeutic effects of exercise and dietary changes are limited. Herbal medicine has been used to treat CVDs in China for many years because of the advantages of multi-target, synergistic, and systemic therapy. Herbs are closely related to gut microbiota and mitochondria (Zhou et al., 2022). Gut microbiota converts herbs into active metabolites with higher bioavailability, and herbs regulate gut microbiota biodiversity to ameliorate CVD in the host, similar to prebiotics (Table 2) (Ma et al., 2022; Qi et al., 2022).
4.1 Berberine
Berberine (BBR) is an isoquinoline alkaloid found mainly in Coptis chinensis and Berberis pruinosa var. pruinosa (Habtemariam, 2020). BBR has low oral bioavailability and absorption owing to its poor solubility. Modulating the gut microbiota to some extent increases the efficiency of BBR in CVDs. BBR effectively mitigates AS in high-fat diet-fed ApoE−/− mice by upregulating the abundance of intestinal Akkermansia (Zhu L et al., 2018), Verrucomicrobia, Firmicutes (Shi Y et al., 2018), Alistipes, and Roseburia (Wu et al., 2020), while downregulating that of Lachnospiraceae, Bacteroidales, and Eubacterium. Changes in the intestinal microbiota recover gut barrier integrity, affect serum TMAO (Shi Y et al., 2018), secondary BAs, and SCFA levels, and attenuate proinflammatory cytokines, chemokines, total cholesterol, and very low-density lipoprotein (Wu et al., 2020), thereby reversing atherosclerotic lesions. BBR is metabolized to dihydroberberine by the gut microbiota (Ma et al., 2022), and dihydroberberine further inactivates the enzymes choline-trimethylamine lyase and flavin monooxygenase, enhancing the diversity of the microbiome to suppress TMAO production and decreasing atherosclerotic lesion (Li X et al., 2021). Moreover, BBR ameliorates the risk factors for AS, including obesity (Hu et al., 2012), hyperglycemia (Li M et al., 2020), and hyperlipidemia (Yang et al., 2022), by maintaining the balance of intestinal flora.
BBR might target mitochondria to exert a protective effect on AS mediated by the gut microbiota. BBR protects ECs from oxidative stress by inhibiting Nox4 protein and ROS expression to improve vasodilation (Cheng et al., 2013). Nox4 in vessels is mainly distributed in the mitochondrial membrane and is involved in stimulating mtROS production. A decline in mtROS caused by BBR further elicits a lower expression of the NLRP3 inflammasome and increases MMP. BBR greatly improves mitophagy by preserving myocardial function and suppressing apoptosis. For example, BBR improves cardiac hypertrophy and cardiomyocyte apoptosis via the PINK1/parkin pathway (Abudureyimu et al., 2020), induces mitophagy to inhibit NLRP3 activation by reducing P62 and recruiting LC3 to mitochondria (Liu H et al., 2020), restores cardiomyocyte senescence by activating prohibitin 2 (Wang L et al., 2022), and defends the myocardium from I/R injury via the hypoxia-induced factor (HIF)-1α/BCL2-interacting protein 3(BNIP3) pathway (Zhu et al., 2020). Furthermore, BBR also decreases basal oxygen consumption rates and facilitates fatty acid oxidation by diminishing mitochondrial swelling and upregulating mitochondrial biogenesis mediated by the AMPK/PGC-1α pathway to attenuate lipid deposition and weight in obese mice (Yao et al., 2020; Yu et al., 2021). BBR exerts a protective effect on fatty acid oxidation enzymes, mitochondrial dynamics balance, and ATP synthesis in cells induced by high glucose (Rong et al., 2021). Collectively, BBR remarkably recovered the gut flora and metabolic balance and preserved mitochondrial function, contributing to the inhibition of AS progression.
4.2 Resveratrol
Resveratrol (RSV) is a plant-derived polyphenol extracted from grapes, peanuts, blueberries, and mulberries and has been used as a potential prebiotic in the intervention of AS. RSV attenuates atherosclerotic plaques by enriching Bacteroides, Lactobacillus, Parabacteroides, and Bifidobacterium (Chen M L et al., 2016; Sung et al., 2017), reducing TMAO levels and increasing BA excretion. However, a combination of antibiotics and RSV cannot suppress TMAO or mitigate AS. These results revealed the important role of the gut microbiota in the anti-atherosclerosis treatment of RSV (Chen M L et al., 2016). RSV also mitigates insulin resistance and triglyceride deposition in the liver to suppress the effects of metabolic syndrome on AS by increasing the proportion of Akkermansia spp. in the intestine (Anhê et al., 2015). Fecal transplantation following resveratrol treatment in mice is beneficial for improving glucose metabolism and low-grade inflammation in obese mice (Sung et al., 2017). In turn, the gut microbiota converts resveratrol into its derivatives to enhance bioavailability, such as dihydroresveratrol, 3,4′-dihydroxy-trans-stilbene and 3,4′-dihydroxybibenzyl (Bode et al., 2013), which is the predominant form in the plasma and effectively protects ECs and VSMCs.
RSV induces endothelial relaxation by activating eNOS and NO synthesis, diminishing ET-1, and inhibits VSMCs proliferation and senescence by decreasing β-galactosidase and pro-fibrotic proteins to ameliorate vascular remodeling and sclerosis (Kim et al., 2018). Possible mechanisms are closely related to mitochondrial function. In human coronary arterial ECs, RSV exerts protective effects on mitochondrial biogenesis by upregulating PGC-1α, Nrf-1, and TFAM, and triggering eNOS expression and NO release by inducing SIRT1 (Csiszar et al., 2009; Davinelli et al., 2013). RSV also improves complex I and III expression in the ETC and BNIP3-related mitophagy by triggering HIF1 and AMPK, leading to mitochondrial redox balance and mitochondrial renewal in ox-LDL-induced ECs (Li C et al., 2020). RSV is well-known for its antioxidant properties. It ameliorates mitochondrial fragmentation to diminish mtROS levels by enhancing Mfn1/2 and OPA1 activation (Yang et al., 2019). RSV significantly decreases mtROS levels by activating AMPK-PGC-1α-ERRα-SIRT3 signaling (Zhou et al., 2014) and inhibiting antioxidant enzymes to defend EC activity from H2O2 and deplete apoptosis by attenuating cytochrome c efflux from the mitochondria and Bcl-2 expression (Liu et al., 2013; Liu et al., 2017). SIRT1 is also involved in the antioxidant activity of RSV. RSV is a well-known activator of SIRT1 (Csiszar et al., 2008; Wellman et al., 2017) that can stimulate MnSOD and glutathione (GSH) to reduce the levels of O2•−and H2O2 in the endothelial mitochondria (Ungvari et al., 2009). In clinical trials, RSV-treated older adults with glucose intolerance exhibited symptoms of an enhanced fasting reactive hyperemia index, showing a restoration of endothelial function, probably caused by increasing mitochondrial content and facilitating OXPHOS (Pollack et al., 2017). Consequently, RSV can simultaneously improve endothelial injury, vascular thickening, and fibrosis by modulating intestinal microbiota homeostasis and the mitochondrial redox balance.
4.3 Allicin
Allicin is a thioester of sulfenic acid isolated from Allium sativum L. [Amaryllidaceae] and effectively crosses cell membranes to play antibacterial and antioxidant roles owing to its hydrophobic characteristics. Garlic is a recognized prebiotic; thus, allicin can be metabolized into diallyl disulfide (DAD) and diallyl trisulfide (DAT) to regulate gut microbiota composition and disrupt TMA production in ApoE−/− mice with high concentrations of carnitine (Panyod et al., 2022). Following allicin treatment, the proportions of Bifidobacterium, Lactobacillus, Bacteroidales, and Clostridiales significantly increased in the gut (Shi et al., 2019), while those in some pathogenic bacteria, such as Proteobacteria, Escherichia–Shigella, and Streptococcus, drastically reduced (Hu et al., 2021). Moreover, allicin also regulates the metabolites of gut microbiota, such as upregulating SCFA, while downregulating secondary BAs, TMAO, and indoles, stimulating brown adipose tissue activation, browning in white adipose tissues (Zhang et al., 2020), decline in lipid accumulation in the liver, and weight loss in obese mice (Shi et al., 2019).
Allicin can recover mitochondrial function in ECs and ameliorate AS. DAT contributes to protecting ECs from oxidative stress under high-glucose conditions by facilitating mitochondrial respiration, triggering MnSOD and glutathione peroxidase (GSH-Px) expression, and suppressing lipid peroxidation (Liu et al., 2014). Moreover, allicin is involved in elevating MMP and inhibiting cytochrome c release and ATP export as a DAMP by inactivating antioxidant enzymes and stimulating Nrf2 activation, resulting in the reversal of endothelial apoptosis and vascular inflammation (Zhang M et al., 2017). DAD can prevent cardiac hypertrophy by improving mitochondrial biogenesis, including activation of the mitochondrial complex and the eNOS–Nrf2–TFAM signaling pathway (Khatua et al., 2016). This demonstrated the antibacterial and antioxidant properties of allicin. Allicin, DAD, and DAT are the main probiotic compounds that preserve cardiovascular diastolic function and cell activity by resisting mitochondria-related oxidative stress.
4.4 Quercetin
Quercetin (Que) is a flavonoid naturally found in various vegetables, fruits, nuts, tea, and wine. Que has become an increasingly popular natural dietary supplement owing to its association with CVDs. The anti-atherosclerotic effects of Que in maintaining intestinal homeostasis have been widely reported. Oral administration of Que reduces lipid metabolites, such as atherogenic lysophosphatidyl choline, in the plasma and artery wall, which are negatively correlated with the abundance of Actinobacteria, Firmicutes, and Cyanobacteria in the gut (Nie et al., 2019). Que notably diminishes plasma lipid levels (TC, TG, HDL, and LDL) and plasma inflammation (TNF-α and IL-6) through the BA biosynthesis pathway metabolized by the gut microbiota with a KEGG analysis (Wu et al., 2019). Que also prevents lipid accumulation in vascular macrophages and elevates foam cell survival rates by inducing autophagy and oxidative resistance (Cao et al., 2019). Que is involved in many aspects of lipid metabolism, from the gut to vessels, to attenuate AS.
Que plays a protective role by increasing mitochondria-related cellular viability. Que opens the mitochondrial large-conductance Ca2+-regulated potassium channel, leading to MMP depolarization and maintenance of EC environmental homeostasis (Kampa et al., 2021). Alterations in MMP and permeability caused by oxidative damage could contribute to apoptosis and direct damage to NO content, while Que could reverse these phenomena to preserve EC activity induced by iron or angiotensin II (Lu et al., 2016; Chen et al., 2020). Que also has potential benefits against mitochondrial fragmentation mediated by Drp1 overexpression, which enhances mtROS levels and exacerbates VSMCs apoptosis via cytochrome c release and caspase-3 activation, further causing vascular calcification (Cui et al., 2017). The mitochondrial protective effects have also been confirmed in vivo. Que remarkably mitigated the extent of atherosclerotic plaque area and decreased lipid deposition in the aorta and soluble intercellular adhesion molecule-1 in ApoE−/− mice, probably because of increased MMP and decreased mtROS levels to resist endothelial senescence and apoptosis (Jiang et al., 2020). Briefly, the cardiovascular targets of Que treatment have been established along the gut microbiota–mitochondrial axis.
4.5 Curcumin
Curcumin, a bioactive polyphenolic of Curcuma longa L. [Zingiberaceae], is involved in anti-atherosclerosis by remodeling gut microbiota dysbiosis, altering the level of metabolites, and cytoprotection targeting mitochondria. Curcumin can reduce TMAO levels to diminish atherosclerotic lesions induced by cadmium exposure (Zhang J et al., 2022), probably by increasing bacterial richness (McFadden et al., 2015) and improving the defense of the intestinal barrier (Wang et al., 2017). Effective regulation of intestinal microbiota using curcumin can further inhibit the absorption of intestinal cholesterol (Hong et al., 2022) and mitigate low-grade systemic inflammation (Cox et al., 2022), including a 56% reduction in cholesterol deposition in the aorta (Zou et al., 2018) and a decline in monocyte chemoattractant protein 1 (Zhou et al., 2021), lipocalin 2 (Wan et al., 2016), TNFα, NFκB, and C-reactive protein expression. The therapeutic effect of curcumin on AS also depends on improving bioavailability mediated by the gut microbiota (Lopresti, 2018), further strengthening its positive influence on the distal mitochondria. Curcumin protects ECs and cardiomyocytes from mitochondrial oxidative stress and MMP loss (Gupta et al., 2016; Chen et al., 2021). Specifically, curcumin can upregulate UCP2 to reduce the generation of downstream mtROS, leading to enhanced eNOS and AMPK phosphorylation and recovery of endothelial functions (Gupta et al., 2016). Curcumin inhibits cardiomyocyte autophagy and apoptosis induced by hypoxia and reoxygenation injury by facilitating the expression of antioxidant enzymes, increasing MMP, and suppressing mitochondria-related apoptosis (Chen et al., 2021). Therefore, curcumin acts as an anti-atherogenic agent by ameliorating the intestinal flora and mitochondria to prevent lipid accumulation, inflammation, and oxidative stress.
There are multiple other bioactive compounds extracted from herbal medicines, including ginkgolide B (Lv et al., 2021), pterostilbene (Zhang et al., 2012), and catalpol (Zhang Y et al., 2018), which are beneficial for maintaining the connection between the bacterial flora, metabolites, and mitochondria and contribute to the recovery of vascular stability and inhibition of AS progression. Thus, herbal medicines and their natural compounds can sustain the balance between the microbiota and the host. However, further research is necessary to reveal the therapeutic effects of herbal medicines on the immune and metabolic networks between gut microbiota and mitochondria.
5 Conclusion
Although the risk factors and therapeutic methods for AS have been constantly improving, atherosclerotic CVD remains the leading cause of death worldwide. This review focuses on the close association between gut microbiota and mitochondria during AS progression and simultaneously enumerates several common herbal medicines and their natural compounds to prevent AS by adjusting the intestinal flora and sustaining mitochondrial function. Derived from the endosymbiotic α-proteobacterium, mitochondria possess characteristics similar to those of bacteria, such as exporting DAMP to activate innate immunity, which makes mitochondria a target for the gut microbiota and its metabolites, including SCFA, TMAO, and H2S. Mitochondria not only participate in providing energy but also act as activators of cholesterol metabolism, inflammation, and apoptosis, facilitating the development of AS. Herbal medicines are widely used in China owing to their advantages of multi-target and systemic treatments, which enable the simultaneous regulation of the intestinal flora and vascular mitochondria. Gut microbiota have not only been shown to be endocrine organs that regulate metabolism but are also significantly responsible for reversing the poor bioavailability of natural compounds. Importantly, the interactions between the gut microbiota and mitochondria collectively establish an immune and metabolic network that influences AS progression, which could be a potential target of herbal medicine to prevent AS. However, the causal relationships among microbiota, mitochondria, and AS remain unclear. The safety of herbs, including safe dosage, treatment duration, and potential side effects, has not been investigated in detail. Subsequent analyses are required to systematically elucidate a complete and accurate picture of gut microbiota, metabolites, mitochondria, and local atherosclerotic changes via metagenomic, metabolomic, and cytological methods.
Author contributions
YL and MW designed and directed the manuscript. YL wrote the manuscript. SY: revised the manuscript. JX and DL searched for relevant literature. JL and XW edited the figures and tables. All authors have read and approved the final manuscript.
Funding
The work was supported by the National Natural Science Foundation of China (No. 81202805, 82074254), the Beijing Natural Science Foundation (No.7172185), and Science and Technology Innovation Project of China Academy of Chinese Medical Sciences (No. C12021A01413).
Conflict of interest
The authors declare that the research was conducted in the absence of any commercial or financial relationships that could be construed as a potential conflict of interest.
Publisher’s note
All claims expressed in this article are solely those of the authors and do not necessarily represent those of their affiliated organizations, or those of the publisher, the editors and the reviewers. Any product that may be evaluated in this article, or claim that may be made by its manufacturer, is not guaranteed or endorsed by the publisher.
References
Abudureyimu, M., Yu, W., Cao, R. Y., Zhang, Y., Liu, H., and Zheng, H. (2020). Berberine promotes cardiac function by upregulating PINK1/parkin-mediated mitophagy in heart failure. Front. Physiol. 11, 565751. doi:10.3389/fphys.2020.565751
Al-Obaide, M. A. I., Singh, R., Datta, P., Rewers-Felkins, K. A., Salguero, M. V., Al-Obaidi, I., et al. (2017). Gut microbiota-dependent trimethylamine-N-oxide and serum biomarkers in patients with T2DM and advanced CKD. J. Clin. Med. 6 (9), 86. doi:10.3390/jcm6090086
Andrade-Oliveira, V., Amano, M. T., Correa-Costa, M., Castoldi, A., Felizardo, R. J., de Almeida, D. C., et al. (2015). Gut bacteria products prevent AKI induced by ischemia-reperfusion. J. Am. Soc. Nephrol. 26 (8), 1877–1888. doi:10.1681/asn.2014030288
Anhê, F. F., Roy, D., Pilon, G., Dudonné, S., Matamoros, S., Varin, T. V., et al. (2015). A polyphenol-rich cranberry extract protects from diet-induced obesity, insulin resistance and intestinal inflammation in association with increased Akkermansia spp. population in the gut microbiota of mice. Gut 64 (6), 872–883. doi:10.1136/gutjnl-2014-307142
Anlu, W., Dongcheng, C., He, Z., Qiuyi, L., Yan, Z., Yu, Q., et al. (2019). Using herbal medicine to target the "microbiota-metabolism-immunity" axis as possible therapy for cardiovascular disease. Pharmacol. Res. 142, 205–222. doi:10.1016/j.phrs.2019.02.018
Arbeithuber, B., Hester, J., Cremona, M. A., Stoler, N., Zaidi, A., Higgins, B., et al. (2020). Age-related accumulation of de novo mitochondrial mutations in mammalian oocytes and somatic tissues. PLoS Biol. 18 (7), e3000745. doi:10.1371/journal.pbio.3000745
Ashar, F. N., Zhang, Y., Longchamps, R. J., Lane, J., Moes, A., Grove, M. L., et al. (2017). Association of mitochondrial DNA copy number with cardiovascular disease. JAMA Cardiol. 2 (11), 1247–1255. doi:10.1001/jamacardio.2017.3683
Assis, L. H. P., Dorighello, G. G., Rentz, T., de Souza, J. C., Vercesi, A. E., and de Oliveira, H. C. F. (2022). In vivo pravastatin treatment reverses hypercholesterolemia induced mitochondria-associated membranes contact sites, foam cell formation, and phagocytosis in macrophages. Front. Mol. Biosci. 9, 839428. doi:10.3389/fmolb.2022.839428
Ballinger, S. W., Patterson, C., Knight-Lozano, C. A., Burow, D. L., Conklin, C. A., Hu, Z., et al. (2002). Mitochondrial integrity and function in atherogenesis. Circulation 106 (5), 544–549. doi:10.1161/01.cir.0000023921.93743.89
Banoth, B., and Cassel, S. L. (2018). Mitochondria in innate immune signaling. Transl. Res. 202, 52–68. doi:10.1016/j.trsl.2018.07.014
Barrington, W. T., and Lusis, A. J. (2017). Atherosclerosis: Association between the gut microbiome and atherosclerosis. Nat. Rev. Cardiol. 14 (12), 699–700. doi:10.1038/nrcardio.2017.169
Bird, L. (2012). Innate immunity: Linking mitochondria and microbes to inflammasomes. Nat. Rev. Immunol. 12 (4), 229. doi:10.1038/nri3195
Bliksøen, M., Mariero, L. H., Torp, M. K., Baysa, A., Ytrehus, K., Haugen, F., et al. (2016). Extracellular mtDNA activates NF-κB via toll-like receptor 9 and induces cell death in cardiomyocytes. Basic Res. Cardiol. 111 (4), 42. doi:10.1007/s00395-016-0553-6
Bock, F. J., and Tait, S. W. G. (2020). Mitochondria as multifaceted regulators of cell death. Nat. Rev. Mol. Cell Biol. 21 (2), 85–100. doi:10.1038/s41580-019-0173-8
Bode, L. M., Bunzel, D., Huch, M., Cho, G. S., Ruhland, D., Bunzel, M., et al. (2013). In vivo and in vitro metabolism of trans-resveratrol by human gut microbiota. Am. J. Clin. Nutr. 97 (2), 295–309. doi:10.3945/ajcn.112.049379
Bogiatzi, C., Gloor, G., Allen-Vercoe, E., Reid, G., Wong, R. G., Urquhart, B. L., et al. (2018). Metabolic products of the intestinal microbiome and extremes of atherosclerosis. Atherosclerosis 273, 91–97. doi:10.1016/j.atherosclerosis.2018.04.015
Borisov, V. B., and Forte, E. (2021). Terminal oxidase cytochrome bd protects bacteria against hydrogen sulfide toxicity. Biochem. Mosc. 86 (1), 22–32. doi:10.1134/S000629792101003X
Boulangé, C. L., Neves, A. L., Chilloux, J., Nicholson, J. K., and Dumas, M. E. (2016). Impact of the gut microbiota on inflammation, obesity, and metabolic disease. Genome Med. 8 (1), 42. doi:10.1186/s13073-016-0303-2
Braakhuis, A. J., Nagulan, R., and Somerville, V. (2018). The effect of MitoQ on aging-related biomarkers: A systematic review and meta-analysis. Oxid. Med. Cell Longev. 2018, 8575263. doi:10.1155/2018/8575263
Brown, J. M., and Hazen, S. L. (2018). Microbial modulation of cardiovascular disease. Nat. Rev. Microbiol. 16 (3), 171–181. doi:10.1038/nrmicro.2017.149
Buskiewicz, I. A., Montgomery, T., Yasewicz, E. C., Huber, S. A., Murphy, M. P., Hartley, R. C., et al. (2016). Reactive oxygen species induce virus-independent MAVS oligomerization in systemic lupus erythematosus. Sci. Signal 9 (456), ra115. doi:10.1126/scisignal.aaf1933
Caesar, R., Tremaroli, V., Kovatcheva-Datchary, P., Cani, P. D., and Bäckhed, F. (2015). Crosstalk between gut microbiota and dietary lipids aggravates WAT inflammation through TLR signaling. Cell Metab. 22 (4), 658–668. doi:10.1016/j.cmet.2015.07.026
Cai, X., Zhang, Y., Li, M., Wu, J. H., Mai, L., Li, J., et al. (2020). Association between prediabetes and risk of all cause mortality and cardiovascular disease: Updated meta-analysis. Bmj 370, m2297. doi:10.1136/bmj.m2297
Calvert, J. W., Elston, M., Nicholson, C. K., Gundewar, S., Jha, S., Elrod, J. W., et al. (2010). Genetic and pharmacologic hydrogen sulfide therapy attenuates ischemia-induced heart failure in mice. Circulation 122 (1), 11–19. doi:10.1161/circulationaha.109.920991
Canugovi, C., Stevenson, M. D., Vendrov, A. E., Hayami, T., Robidoux, J., Xiao, H., et al. (2019). Increased mitochondrial NADPH oxidase 4 (NOX4) expression in aging is a causative factor in aortic stiffening. Redox Biol. 26, 101288. doi:10.1016/j.redox.2019.101288
Cao, H., Jia, Q., Yan, L., Chen, C., Xing, S., and Shen, D. (2019). Quercetin suppresses the progression of atherosclerosis by regulating MST1-mediated autophagy in ox-LDL-induced RAW264.7 macrophage foam cells. Int. J. Mol. Sci. 20 (23), 6093. doi:10.3390/ijms20236093
Chen, J., Wright, K., Davis, J. M., Jeraldo, P., Marietta, E. V., Murray, J., et al. (2016). An expansion of rare lineage intestinal microbes characterizes rheumatoid arthritis. Genome Med. 8 (1), 43. doi:10.1186/s13073-016-0299-7
Chen, M. L., Yi, L., Zhang, Y., Zhou, X., Ran, L., Yang, J., et al. (2016). Resveratrol attenuates trimethylamine-N-oxide (TMAO)-Induced atherosclerosis by regulating TMAO synthesis and bile acid metabolism via remodeling of the gut microbiota. mBio 7 (2), e02210–e02215. doi:10.1128/mBio.02210-15
Chen, M. L., Zhu, X. H., Ran, L., Lang, H. D., Yi, L., and Mi, M. T. (2017). Trimethylamine-N-Oxide induces vascular inflammation by activating the NLRP3 inflammasome through the SIRT3-SOD2-mtROS signaling pathway. J. Am. Heart Assoc. 6 (9), e006347. doi:10.1161/jaha.117.006347
Chen, X., Li, H., Wang, Z., Zhou, Q., Chen, S., Yang, B., et al. (2020). Quercetin protects the vascular endothelium against iron overload damages via ROS/ADMA/DDAHⅡ/eNOS/NO pathway. Eur. J. Pharmacol. 868, 172885. doi:10.1016/j.ejphar.2019.172885
Chen, X., Xie, Q., Zhu, Y., Xu, J., Lin, G., Liu, S., et al. (2021). Cardio-protective effect of tetrahydrocurcumin, the primary hydrogenated metabolite of curcumin in vivo and in vitro: Induction of apoptosis and autophagy via PI3K/AKT/mTOR pathways. Eur. J. Pharmacol. 911, 174495. doi:10.1016/j.ejphar.2021.174495
Chen, Z., Zhou, Q., Chen, J., Yang, Y., Chen, W., Mao, H., et al. (2022). MCU-dependent mitochondrial calcium uptake-induced mitophagy contributes to apelin-13-stimulated VSMCs proliferation. Vasc. Pharmacol. 144, 106979. doi:10.1016/j.vph.2022.106979
Cheng, F., Wang, Y., Li, J., Su, C., Wu, F., Xia, W. H., et al. (2013). Berberine improves endothelial function by reducing endothelial microparticles-mediated oxidative stress in humans. Int. J. Cardiol. 167 (3), 936–942. doi:10.1016/j.ijcard.2012.03.090
Cho, Y. E., Basu, A., Dai, A., Heldak, M., and Makino, A. (2013). Coronary endothelial dysfunction and mitochondrial reactive oxygen species in type 2 diabetic mice. Am. J. Physiol. Cell Physiol. 305 (10), C1033–C1040. doi:10.1152/ajpcell.00234.2013
Choi, S. H., Agatisa-Boyle, C., Gonen, A., Kim, A., Kim, J., Alekseeva, E., et al. (2021). Intracellular AIBP (apolipoprotein A-I binding protein) regulates oxidized LDL (Low-Density lipoprotein)-induced mitophagy in macrophages. Arterioscler. Thromb. Vasc. Biol. 41 (2), e82–e96. doi:10.1161/atvbaha.120.315485
Chowdhury, A., Witte, S., and Aich, A. (2022). Role of mitochondrial nucleic acid sensing pathways in health and patho-physiology. Front. Cell Dev. Biol. 10, 796066. doi:10.3389/fcell.2022.796066
Clark, A., and Mach, N. (2017). The crosstalk between the gut microbiota and mitochondria during exercise. Front. Physiol. 8, 319. doi:10.3389/fphys.2017.00319
Cox, F. F., Misiou, A., Vierkant, A., Ale-Agha, N., Grandoch, M., Haendeler, J., et al. (2022). Protective effects of curcumin in cardiovascular diseases-impact on oxidative stress and mitochondria. Cells 11 (3), 342. doi:10.3390/cells11030342
Csiszar, A., Gautam, T., Sosnowska, D., Tarantini, S., Banki, E., Tucsek, Z., et al. (2014). Caloric restriction confers persistent anti-oxidative, pro-angiogenic, and anti-inflammatory effects and promotes anti-aging miRNA expression profile in cerebromicrovascular endothelial cells of aged rats. Am. J. Physiol. Heart Circ. Physiol. 307 (3), H292–H306. doi:10.1152/ajpheart.00307.2014
Csiszar, A., Labinskyy, N., Pinto, J. T., Ballabh, P., Zhang, H., Losonczy, G., et al. (2009). Resveratrol induces mitochondrial biogenesis in endothelial cells. Am. J. Physiol. Heart Circ. Physiol. 297 (1), H13–H20. doi:10.1152/ajpheart.00368.2009
Csiszar, A., Labinskyy, N., Podlutsky, A., Kaminski, P. M., Wolin, M. S., Zhang, C., et al. (2008). Vasoprotective effects of resveratrol and SIRT1: Attenuation of cigarette smoke-induced oxidative stress and proinflammatory phenotypic alterations. Am. J. Physiol. Heart Circ. Physiol. 294 (6), H2721–H2735. doi:10.1152/ajpheart.00235.2008
Cui, J., Li, Z., Zhuang, S., Qi, S., Li, L., Zhou, J., et al. (2018). Melatonin alleviates inflammation-induced apoptosis in human umbilical vein endothelial cells via suppression of Ca(2+)-XO-ROS-Drp1-mitochondrial fission axis by activation of AMPK/SERCA2a pathway. Cell Stress Chaperones 23 (2), 281–293. doi:10.1007/s12192-017-0841-6
Cui, L., Li, Z., Chang, X., Cong, G., and Hao, L. (2017). Quercetin attenuates vascular calcification by inhibiting oxidative stress and mitochondrial fission. Vasc. Pharmacol. 88, 21–29. doi:10.1016/j.vph.2016.11.006
Dagenais, G. R., Leong, D. P., Rangarajan, S., Lanas, F., Lopez-Jaramillo, P., Gupta, R., et al. (2020). Variations in common diseases, hospital admissions, and deaths in middle-aged adults in 21 countries from five continents (PURE): A prospective cohort study. Lancet 395 (10226), 785–794. doi:10.1016/s0140-6736(19)32007-0
Davinelli, S., Sapere, N., Visentin, M., Zella, D., and Scapagnini, G. (2013). Enhancement of mitochondrial biogenesis with polyphenols: Combined effects of resveratrol and equol in human endothelial cells. Immun. Ageing 10 (1), 28. doi:10.1186/1742-4933-10-28
Degli Esposti, M., Chouaia, B., Comandatore, F., Crotti, E., Sassera, D., Lievens, P. M., et al. (2014). Evolution of mitochondria reconstructed from the energy metabolism of living bacteria. PLoS One 9 (5), e96566. doi:10.1371/journal.pone.0096566
den Besten, G., van Eunen, K., Groen, A. K., Venema, K., Reijngoud, D. J., and Bakker, B. M. (2013). The role of short-chain fatty acids in the interplay between diet, gut microbiota, and host energy metabolism. J. Lipid Res. 54 (9), 2325–2340. doi:10.1194/jlr.R036012
Ding, Z., Liu, S., Wang, X., Mathur, P., Dai, Y., Theus, S., et al. (2016). Cross-talk between PCSK9 and damaged mtDNA in vascular smooth muscle cells: Role in apoptosis. Antioxid. Redox Signal 25 (18), 997–1008. doi:10.1089/ars.2016.6631
Dorighello, G. G., Assis, L. H. P., Rentz, T., Morari, J., Santana, M. F. M., Passarelli, M., et al. (2022). Novel role of CETP in macrophages: Reduction of mitochondrial oxidants production and modulation of cell immune-metabolic profile. Antioxidants (Basel) 11 (9), 1734. doi:10.3390/antiox11091734
Duan, M., Chen, H., Yin, L., Zhu, X., Novák, P., Lv, Y., et al. (2022). Mitochondrial apolipoprotein A-I binding protein alleviates atherosclerosis by regulating mitophagy and macrophage polarization. Cell Commun. Signal 20 (1), 60. doi:10.1186/s12964-022-00858-8
Dumont, A., Lee, M., Barouillet, T., Murphy, A., and Yvan-Charvet, L. (2021). Mitochondria orchestrate macrophage effector functions in atherosclerosis. Mol. Asp. Med. 77, 100922. doi:10.1016/j.mam.2020.100922
Fang, C., Wei, X., and Wei, Y. (2016). Mitochondrial DNA in the regulation of innate immune responses. Protein Cell 7 (1), 11–16. doi:10.1007/s13238-015-0222-9
Fedotcheva, N., Olenin, A., and Beloborodova, N. (2021). Influence of microbial metabolites on the nonspecific permeability of mitochondrial membranes under conditions of acidosis and loading with calcium and iron ions. Biomedicines 9 (5), 558. doi:10.3390/biomedicines9050558
Fontana, G. A., and Gahlon, H. L. (2020). Mechanisms of replication and repair in mitochondrial DNA deletion formation. Nucleic Acids Res. 48 (20), 11244–11258. doi:10.1093/nar/gkaa804
Forrester, S. J., Kikuchi, D. S., Hernandes, M. S., Xu, Q., and Griendling, K. K. (2018). Reactive oxygen species in metabolic and inflammatory signaling. Circ. Res. 122 (6), 877–902. doi:10.1161/circresaha.117.311401
Forte, M., Bianchi, F., Cotugno, M., Marchitti, S., Stanzione, R., Maglione, V., et al. (2021). An interplay between UCP2 and ROS protects cells from high-salt-induced injury through autophagy stimulation. Cell Death Dis. 12 (10), 919. doi:10.1038/s41419-021-04188-4
Gilkerson, R. W., De Vries, R. L., Lebot, P., Wikstrom, J. D., Torgyekes, E., Shirihai, O. S., et al. (2012). Mitochondrial autophagy in cells with mtDNA mutations results from synergistic loss of transmembrane potential and mTORC1 inhibition. Hum. Mol. Genet. 21 (5), 978–990. doi:10.1093/hmg/ddr529
Gioscia-Ryan, R. A., Battson, M. L., Cuevas, L. M., Eng, J. S., Murphy, M. P., and Seals, D. R. (2018). Mitochondria-targeted antioxidant therapy with MitoQ ameliorates aortic stiffening in old mice. J. Appl. Physiol. 124 (5), 1194–1202. doi:10.1152/japplphysiol.00670.2017
Giusti, L., Gabriele, M., Penno, G., Garofolo, M., Longo, V., Del Prato, S., et al. (2017). A fermented whole grain prevents lipopolysaccharides-induced dysfunction in human endothelial progenitor cells. Oxid. Med. Cell Longev. 2017, 1026268. doi:10.1155/2017/1026268
Gkikas, I., Palikaras, K., and Tavernarakis, N. (2018). The role of mitophagy in innate immunity. Front. Immunol. 9, 1283. doi:10.3389/fimmu.2018.01283
Gomez-Arango, L. F., Barrett, H. L., Wilkinson, S. A., Callaway, L. K., McIntyre, H. D., Morrison, M., et al. (2018). Low dietary fiber intake increases Collinsella abundance in the gut microbiota of overweight and obese pregnant women. Gut Microbes 9 (3), 189–201. doi:10.1080/19490976.2017.1406584
Granatiero, V., Giorgio, V., Calì, T., Patron, M., Brini, M., Bernardi, P., et al. (2016). Reduced mitochondrial Ca(2+) transients stimulate autophagy in human fibroblasts carrying the 13514A>G mutation of the ND5 subunit of NADH dehydrogenase. Cell Death Differ. 23 (2), 231–241. doi:10.1038/cdd.2015.84
Greaves, L. C., Nooteboom, M., Elson, J. L., Tuppen, H. A., Taylor, G. A., Commane, D. M., et al. (2014). Clonal expansion of early to mid-life mitochondrial DNA point mutations drives mitochondrial dysfunction during human ageing. PLoS Genet. 10 (9), e1004620. doi:10.1371/journal.pgen.1004620
Gregory, J. C., Buffa, J. A., Org, E., Wang, Z., Levison, B. S., Zhu, W., et al. (2015). Transmission of atherosclerosis susceptibility with gut microbial transplantation. J. Biol. Chem. 290 (9), 5647–5660. doi:10.1074/jbc.M114.618249
Gupta, P., Jordan, C. T., Mitov, M. I., Butterfield, D. A., Hilt, J. Z., and Dziubla, T. D. (2016). Controlled curcumin release via conjugation into PBAE nanogels enhances mitochondrial protection against oxidative stress. Int. J. Pharm. 511 (2), 1012–1021. doi:10.1016/j.ijpharm.2016.07.071
Habtemariam, S. (2020). Berberine pharmacology and the gut microbiota: A hidden therapeutic link. Pharmacol. Res. 155, 104722. doi:10.1016/j.phrs.2020.104722
Han, J. H., Park, J., Myung, S. H., Lee, S. H., Kim, H. Y., Kim, K. S., et al. (2019). Noxa mitochondrial targeting domain induces necrosis via VDAC2 and mitochondrial catastrophe. Cell Death Dis. 10 (7), 519. doi:10.1038/s41419-019-1753-4
Hawkins, B. J., Solt, L. A., Chowdhury, I., Kazi, A. S., Abid, M. R., Aird, W. C., et al. (2007). G protein-coupled receptor Ca2+-linked mitochondrial reactive oxygen species are essential for endothelial/leukocyte adherence. Mol. Cell Biol. 27 (21), 7582–7593. doi:10.1128/mcb.00493-07
He, D., Sougioultzis, S., Hagen, S., Liu, J., Keates, S., Keates, A. C., et al. (2002). Clostridium difficile toxin A triggers human colonocyte IL-8 release via mitochondrial oxygen radical generation. Gastroenterology 122 (4), 1048–1057. doi:10.1053/gast.2002.32386
Heid, M. E., Keyel, P. A., Kamga, C., Shiva, S., Watkins, S. C., and Salter, R. D. (2013). Mitochondrial reactive oxygen species induces NLRP3-dependent lysosomal damage and inflammasome activation. J. Immunol. 191 (10), 5230–5238. doi:10.4049/jimmunol.1301490
Hernandez, L. D., Pypaert, M., Flavell, R. A., and Galán, J. E. (2003). A Salmonella protein causes macrophage cell death by inducing autophagy. J. Cell Biol. 163 (5), 1123–1131. doi:10.1083/jcb.200309161
Hong, T., Zou, J., Jiang, X., Yang, J., Cao, Z., He, Y., et al. (2022). Curcumin supplementation ameliorates bile cholesterol supersaturation in hamsters by modulating gut microbiota and cholesterol absorption. Nutrients 14 (9), 1828. doi:10.3390/nu14091828
Hotz, M. J., Qing, D., Shashaty, M. G. S., Zhang, P., Faust, H., Sondheimer, N., et al. (2018). Red blood cells homeostatically bind mitochondrial DNA through TLR9 to maintain quiescence and to prevent lung injury. Am. J. Respir. Crit. Care Med. 197 (4), 470–480. doi:10.1164/rccm.201706-1161OC
Hu, W., Huang, L., Zhou, Z., Yin, L., and Tang, J. (2021). Diallyl disulfide (DADS) ameliorates intestinal Candida albicans infection by modulating the gut microbiota and metabolites and providing intestinal protection in mice. Front. Cell Infect. Microbiol. 11, 743454. doi:10.3389/fcimb.2021.743454
Hu, Y., Ehli, E. A., Kittelsrud, J., Ronan, P. J., Munger, K., Downey, T., et al. (2012). Lipid-lowering effect of berberine in human subjects and rats. Phytomedicine 19 (10), 861–867. doi:10.1016/j.phymed.2012.05.009
Huang, Y., Wang, J., Quan, G., Wang, X., Yang, L., and Zhong, L. (2014). Lactobacillus acidophilus ATCC 4356 prevents atherosclerosis via inhibition of intestinal cholesterol absorption in apolipoprotein E-knockout mice. Appl. Environ. Microbiol. 80 (24), 7496–7504. doi:10.1128/aem.02926-14
Hung, C. H., Lin, Y. C., Tsai, Y. G., Lin, Y. C., Kuo, C. H., Tsai, M. L., et al. (2021). Acrylamide induces mitophagy and alters macrophage phenotype via reactive oxygen species generation. Int. J. Mol. Sci. 22 (4), 1683. doi:10.3390/ijms22041683
Iyer, S. S., He, Q., Janczy, J. R., Elliott, E. I., Zhong, Z., Olivier, A. K., et al. (2013). Mitochondrial cardiolipin is required for Nlrp3 inflammasome activation. Immunity 39 (2), 311–323. doi:10.1016/j.immuni.2013.08.001
Jackson, D. N., and Theiss, A. L. (2020). Gut bacteria signaling to mitochondria in intestinal inflammation and cancer. Gut Microbes 11 (3), 285–304. doi:10.1080/19490976.2019.1592421
Jenner, A., Peña-Blanco, A., Salvador-Gallego, R., Ugarte-Uribe, B., Zollo, C., Ganief, T., et al. (2022). DRP1 interacts directly with BAX to induce its activation and apoptosis. Embo J. 41 (8), e108587. doi:10.15252/embj.2021108587
Jeong, S. Y., and Seol, D. W. (2008). The role of mitochondria in apoptosis. BMB Rep. 41 (1), 11–22. doi:10.5483/bmbrep.2008.41.1.011
Jiang, Y. H., Jiang, L. Y., Wang, Y. C., Ma, D. F., and Li, X. (2020). Quercetin attenuates atherosclerosis via modulating oxidized LDL-induced endothelial cellular senescence. Front. Pharmacol. 11, 512. doi:10.3389/fphar.2020.00512
Jin, Y., Liu, Y., Xu, L., Xu, J., Xiong, Y., Peng, Y., et al. (2022). Novel role for caspase 1 inhibitor VX765 in suppressing NLRP3 inflammasome assembly and atherosclerosis via promoting mitophagy and efferocytosis. Cell Death Dis. 13 (5), 512. doi:10.1038/s41419-022-04966-8
Jonsson, A. L., and Bäckhed, F. (2017). Role of gut microbiota in atherosclerosis. Nat. Rev. Cardiol. 14 (2), 79–87. doi:10.1038/nrcardio.2016.183
Juárez-Fernández, M., Goikoetxea-Usandizaga, N., Porras, D., García-Mediavilla, M. V., Bravo, M., Serrano-Maciá, M., et al. (2022). Enhanced mitochondrial activity reshapes a gut microbiota profile that delays NASH progression. Hepatology. doi:10.1002/hep.32705
Kalghatgi, S., Spina, C. S., Costello, J. C., Liesa, M., Morones-Ramirez, J. R., Slomovic, S., et al. (2013). Bactericidal antibiotics induce mitochondrial dysfunction and oxidative damage in Mammalian cells. Sci. Transl. Med. 5 (192), 192ra85. doi:10.1126/scitranslmed.3006055
Kampa, R. P., Sęk, A., Szewczyk, A., and Bednarczyk, P. (2021). Cytoprotective effects of the flavonoid quercetin by activating mitochondrial BK(Ca) channels in endothelial cells. Biomed. Pharmacother. 142, 112039. doi:10.1016/j.biopha.2021.112039
Kappel, B. A., De Angelis, L., Heiser, M., Ballanti, M., Stoehr, R., Goettsch, C., et al. (2020). Cross-omics analysis revealed gut microbiome-related metabolic pathways underlying atherosclerosis development after antibiotics treatment. Mol. Metab. 36, 100976. doi:10.1016/j.molmet.2020.100976
Karunakaran, D., Thrush, A. B., Nguyen, M. A., Richards, L., Geoffrion, M., Singaravelu, R., et al. (2015). Macrophage mitochondrial energy status regulates cholesterol efflux and is enhanced by anti-miR33 in atherosclerosis. Circ. Res. 117 (3), 266–278. doi:10.1161/circresaha.117.305624
Katsnelson, M. A., Lozada-Soto, K. M., Russo, H. M., Miller, B. A., and Dubyak, G. R. (2016). NLRP3 inflammasome signaling is activated by low-level lysosome disruption but inhibited by extensive lysosome disruption: Roles for K+ efflux and Ca2+ influx. Am. J. Physiol. Cell Physiol. 311 (1), C83–c100. doi:10.1152/ajpcell.00298.2015
Khatua, T. N., Dinda, A. K., Putcha, U. K., and Banerjee, S. K. (2016). Diallyl disulfide ameliorates isoproterenol induced cardiac hypertrophy activating mitochondrial biogenesis via eNOS-Nrf2-Tfam pathway in rats. Biochem. Biophys. Rep. 5, 77–88. doi:10.1016/j.bbrep.2015.11.008
Khrapko, K. (2011). The timing of mitochondrial DNA mutations in aging. Nat. Genet. 43 (8), 726–727. doi:10.1038/ng.895
Kim, E. N., Kim, M. Y., Lim, J. H., Kim, Y., Shin, S. J., Park, C. W., et al. (2018). The protective effect of resveratrol on vascular aging by modulation of the renin-angiotensin system. Atherosclerosis 270, 123–131. doi:10.1016/j.atherosclerosis.2018.01.043
Kim, J., Gupta, R., Blanco, L. P., Yang, S., Shteinfer-Kuzmine, A., Wang, K., et al. (2019). VDAC oligomers form mitochondrial pores to release mtDNA fragments and promote lupus-like disease. Science 366 (6472), 1531–1536. doi:10.1126/science.aav4011
Kirichenko, T. V., Markina, Y. V., Sukhorukov, V. N., Khotina, V. A., Wu, W. K., and Orekhov, A. N. (2020). A novel insight at atherogenesis: The role of microbiome. Front. Cell Dev. Biol. 8, 586189. doi:10.3389/fcell.2020.586189
Kleele, T., Rey, T., Winter, J., Zaganelli, S., Mahecic, D., Perreten Lambert, H., et al. (2021). Distinct fission signatures predict mitochondrial degradation or biogenesis. Nature 593 (7859), 435–439. doi:10.1038/s41586-021-03510-6
Ko, J., Kang, H. J., Kim, D. A., Kim, M. J., Ryu, E. S., Lee, S., et al. (2019). Uric acid induced the phenotype transition of vascular endothelial cells via induction of oxidative stress and glycocalyx shedding. Faseb J. 33 (12), 13334–13345. doi:10.1096/fj.201901148R
Koeth, R. A., Wang, Z., Levison, B. S., Buffa, J. A., Org, E., Sheehy, B. T., et al. (2013). Intestinal microbiota metabolism of L-carnitine, a nutrient in red meat, promotes atherosclerosis. Nat. Med. 19 (5), 576–585. doi:10.1038/nm.3145
Koren, O., Spor, A., Felin, J., Fåk, F., Stombaugh, J., Tremaroli, V., et al. (2011). Human oral, gut, and plaque microbiota in patients with atherosclerosis. Proc. Natl. Acad. Sci. U. S. A. 108 (1), 4592–4598. doi:10.1073/pnas.1011383107
Korytowski, W., Wawak, K., Pabisz, P., Schmitt, J. C., Chadwick, A. C., Sahoo, D., et al. (2015). Impairment of macrophage cholesterol efflux by cholesterol hydroperoxide trafficking: Implications for atherogenesis under oxidative stress. Arterioscler. Thromb. Vasc. Biol. 35 (10), 2104–2113. doi:10.1161/atvbaha.115.306210
Kubli, D. A., and Gustafsson, A. B. (2012). Mitochondria and mitophagy: The yin and yang of cell death control. Circ. Res. 111 (9), 1208–1221. doi:10.1161/circresaha.112.265819
Kubli, D. A., Zhang, X., Lee, Y., Hanna, R. A., Quinsay, M. N., Nguyen, C. K., et al. (2013). Parkin protein deficiency exacerbates cardiac injury and reduces survival following myocardial infarction. J. Biol. Chem. 288 (2), 915–926. doi:10.1074/jbc.M112.411363
Kuipers, F., Bloks, V. W., and Groen, A. K. (2014). Beyond intestinal soap-bile acids in metabolic control. Nat. Rev. Endocrinol. 10 (8), 488–498. doi:10.1038/nrendo.2014.60
Lampropoulou, V., Sergushichev, A., Bambouskova, M., Nair, S., Vincent, E. E., Loginicheva, E., et al. (2016). Itaconate links inhibition of succinate dehydrogenase with macrophage metabolic remodeling and regulation of inflammation. Cell Metab. 24 (1), 158–166. doi:10.1016/j.cmet.2016.06.004
Lawton, J. S., Tamis-Holland, J. E., Bangalore, S., Bates, E. R., Beckie, T. M., Bischoff, J. M., et al. (2022). 2021 ACC/AHA/SCAI guideline for coronary artery revascularization: Executive summary: A report of the American college of cardiology/American heart association joint committee on clinical practice guidelines. Circulation 145 (3), e4–e17. doi:10.1161/cir.0000000000001039
Le Roy, T., Moens de Hase, E., Van Hul, M., Paquot, A., Pelicaen, R., Régnier, M., et al. (2022). Dysosmobacter welbionis is a newly isolated human commensal bacterium preventing diet-induced obesity and metabolic disorders in mice. Gut 71 (3), 534–543. doi:10.1136/gutjnl-2020-323778
Li, C., Tan, Y., Wu, J., Ma, Q., Bai, S., Xia, Z., et al. (2020). Resveratrol improves bnip3-related mitophagy and attenuates high-fat-induced endothelial dysfunction. Front. Cell Dev. Biol. 8, 796. doi:10.3389/fcell.2020.00796
Li, J., Huynh, L., Cornwell, W. D., Tang, M. S., Simborio, H., Huang, J., et al. (2021). Electronic cigarettes induce mitochondrial DNA damage and trigger TLR9 (Toll-Like receptor 9)-mediated atherosclerosis. Arterioscler. Thromb. Vasc. Biol. 41 (2), 839–853. doi:10.1161/atvbaha.120.315556
Li, J., Jia, H., Cai, X., Zhong, H., Feng, Q., Sunagawa, S., et al. (2014). An integrated catalog of reference genes in the human gut microbiome. Nat. Biotechnol. 32 (8), 834–841. doi:10.1038/nbt.2942
Li, J., Zeng, Q., Xiong, Z., Xian, G., Liu, Z., Zhan, Q., et al. (2022). Trimethylamine N-oxide induces osteogenic responses in human aortic valve interstitial cells in vitro and aggravates aortic valve lesions in mice. Cardiovasc Res. 118 (8), 2018–2030. doi:10.1093/cvr/cvab243
Li, M., Zhou, W., Dang, Y., Li, C., Ji, G., and Zhang, L. (2020). Berberine compounds improves hyperglycemia via microbiome mediated colonic TGR5-GLP pathway in db/db mice. Biomed. Pharmacother. 132, 110953. doi:10.1016/j.biopha.2020.110953
Li, S., and Yang, G. (2015). Hydrogen sulfide maintains mitochondrial DNA replication via demethylation of TFAM. Antioxid. Redox Signal 23 (7), 630–642. doi:10.1089/ars.2014.6186
Li, X., Su, C., Jiang, Z., Yang, Y., Zhang, Y., Yang, M., et al. (2021). Berberine attenuates choline-induced atherosclerosis by inhibiting trimethylamine and trimethylamine-N-oxide production via manipulating the gut microbiome. NPJ Biofilms Microbiomes 7 (1), 36. doi:10.1038/s41522-021-00205-8
Li, Z., Li, Q., Wang, L., Li, C., Xu, M., Duan, Y., et al. (2021). Targeting mitochondria-inflammation circle by renal denervation reduces atheroprone endothelial phenotypes and atherosclerosis. Redox Biol. 47, 102156. doi:10.1016/j.redox.2021.102156
Lian N, N., Mao, X., Su, Y., Wang, Y., Wang, Y., Wang, Y., et al. (2022). Hydrogen-rich medium ameliorates lipopolysaccharides-induced mitochondrial fission and dysfunction in human umbilical vein endothelial cells (HUVECs) via up-regulating HO-1 expression. Int. Immunopharmacol. 110, 108936. doi:10.1016/j.intimp.2022.108936
Lian, W. S., Wang, F. S., Chen, Y. S., Tsai, M. H., Chao, H. R., Jahr, H., et al. (2022). Gut microbiota ecosystem governance of host inflammation, mitochondrial respiration and skeletal homeostasis. Biomedicines 10 (4), 860. doi:10.3390/biomedicines10040860
Liang, Y., Chu, P. H., Tian, L., Ho, K. F., Ip, M. S. M., and Mak, J. C. W. (2022). Targeting mitochondrial permeability transition pore ameliorates PM(2.5)-induced mitochondrial dysfunction in airway epithelial cells. Environ. Pollut. 295, 118720. doi:10.1016/j.envpol.2021.118720
Libiad, M., Vitvitsky, V., Bostelaar, T., Bak, D. W., Lee, H. J., Sakamoto, N., et al. (2019). Hydrogen sulfide perturbs mitochondrial bioenergetics and triggers metabolic reprogramming in colon cells. J. Biol. Chem. 294 (32), 12077–12090. doi:10.1074/jbc.RA119.009442
Lim, J. H., Woo, J. S., and Shin, Y. W. (2009). Cilostazol protects endothelial cells against lipopolysaccharide-induced apoptosis through ERK1/2- and P38 MAPK-dependent pathways. Korean J. Intern Med. 24 (2), 113–122. doi:10.3904/kjim.2009.24.2.113
Lim, S., Lee, S. Y., Seo, H. H., Ham, O., Lee, C., Park, J. H., et al. (2015). Regulation of mitochondrial morphology by positive feedback interaction between PKCδ and Drp1 in vascular smooth muscle cell. J. Cell Biochem. 116 (4), 648–660. doi:10.1002/jcb.25016
Lindskog Jonsson, A., Hållenius, F. F., Akrami, R., Johansson, E., Wester, P., Arnerlöv, C., et al. (2017). Bacterial profile in human atherosclerotic plaques. Atherosclerosis 263, 177–183. doi:10.1016/j.atherosclerosis.2017.06.016
Liu, H., You, L., Wu, J., Zhao, M., Guo, R., Zhang, H., et al. (2020). Berberine suppresses influenza virus-triggered NLRP3 inflammasome activation in macrophages by inducing mitophagy and decreasing mitochondrial ROS. J. Leukoc. Biol. 108 (1), 253–266. doi:10.1002/JLB.3MA0320-358RR
Liu, L., Gu, L., Ma, Q., Zhu, D., and Huang, X. (2013). Resveratrol attenuates hydrogen peroxide-induced apoptosis in human umbilical vein endothelial cells. Eur. Rev. Med. Pharmacol. Sci. 17 (1), 88–94.
Liu, L. L., Yan, L., Chen, Y. H., Zeng, G. H., Zhou, Y., Chen, H. P., et al. (2014). A role for diallyl trisulfide in mitochondrial antioxidative stress contributes to its protective effects against vascular endothelial impairment. Eur. J. Pharmacol. 725, 23–31. doi:10.1016/j.ejphar.2014.01.010
Liu, Q., Li, Y., Song, X., Wang, J., He, Z., Zhu, J., et al. (2020). Both gut microbiota and cytokines act to atherosclerosis in ApoE-/- mice. Microb. Pathog. 138, 103827. doi:10.1016/j.micpath.2019.103827
Liu, Q., Zhang, D., Hu, D., Zhou, X., and Zhou, Y. (2018). The role of mitochondria in NLRP3 inflammasome activation. Mol. Immunol. 103, 115–124. doi:10.1016/j.molimm.2018.09.010
Liu, Y., Chen, X., and Li, J. (2017). Resveratrol protects against oxidized low-density lipoprotein-induced human umbilical vein endothelial cell apoptosis via inhibition of mitochondrial-derived oxidative stress. Mol. Med. Rep. 15 (5), 2457–2464. doi:10.3892/mmr.2017.6304
Liu, Y., Liu, S., Zhao, Z., Song, X., Qu, H., and Liu, H. (2021). Phenylacetylglutamine is associated with the degree of coronary atherosclerotic severity assessed by coronary computed tomographic angiography in patients with suspected coronary artery disease. Atherosclerosis 333, 75–82. doi:10.1016/j.atherosclerosis.2021.08.029
Longchamp, A., Mirabella, T., Arduini, A., MacArthur, M. R., Das, A., Treviño-Villarreal, J. H., et al. (2018). Amino acid restriction triggers angiogenesis via GCN2/ATF4 regulation of VEGF and H(2)S production. Cell 173 (1), 117–129. e114. doi:10.1016/j.cell.2018.03.001
López-Salazar, V., Tapia, M. S., Tobón-Cornejo, S., Díaz, D., Alemán-Escondrillas, G., Granados-Portillo, O., et al. (2021). Consumption of soybean or olive oil at recommended concentrations increased the intestinal microbiota diversity and insulin sensitivity and prevented fatty liver compared to the effects of coconut oil. J. Nutr. Biochem. 94, 108751. doi:10.1016/j.jnutbio.2021.108751
Lopresti, A. L. (2018). The problem of curcumin and its bioavailability: Could its gastrointestinal influence contribute to its overall health-enhancing effects? Adv. Nutr. 9 (1), 41–50. doi:10.1093/advances/nmx011
Lu, Y., Wang, R. H., Guo, B. B., and Jia, Y. P. (2016). Quercetin inhibits angiotensin II induced apoptosis via mitochondrial pathway in human umbilical vein endothelial cells. Eur. Rev. Med. Pharmacol. Sci. 20 (8), 1609–1616.
Lv, Z., Shan, X., Tu, Q., Wang, J., Chen, J., and Yang, Y. (2021). Ginkgolide B treatment regulated intestinal flora to improve high-fat diet induced atherosclerosis in ApoE(-/-) mice. Biomed. Pharmacother. 134, 111100. doi:10.1016/j.biopha.2020.111100
Ma, J., Fu, Q., Wang, Z., Zhou, P., Qiao, S., Wang, B., et al. (2019). Sodium hydrosulfide mitigates dexamethasone-induced osteoblast dysfunction by interfering with mitochondrial function. Biotechnol. Appl. Biochem. 66 (4), 690–697. doi:10.1002/bab.1786
Ma, S., Chen, J., Feng, J., Zhang, R., Fan, M., Han, D., et al. (2018). Melatonin ameliorates the progression of atherosclerosis via mitophagy activation and NLRP3 inflammasome inhibition. Oxid. Med. Cell Longev. 2018, 9286458. doi:10.1155/2018/9286458
Ma, S. R., Tong, Q., Lin, Y., Pan, L. B., Fu, J., Peng, R., et al. (2022). Berberine treats atherosclerosis via a vitamine-like effect down-regulating Choline-TMA-TMAO production pathway in gut microbiota. Signal Transduct. Target Ther. 7 (1), 207. doi:10.1038/s41392-022-01027-6
Ma, Y., Huang, Z., Zhou, Z., He, X., Wang, Y., Meng, C., et al. (2018). A novel antioxidant Mito-Tempol inhibits ox-LDL-induced foam cell formation through restoration of autophagy flux. Free Radic. Biol. Med. 129, 463–472. doi:10.1016/j.freeradbiomed.2018.10.412
Mahmud, S. A., Qureshi, M. A., and Pellegrino, M. W. (2021). On the offense and defense: Mitochondrial recovery programs amidst targeted pathogenic assault. FEBS J. doi:10.1111/febs.16126
Maimaitijiang, A., Zhuang, X., Jiang, X., and Li, Y. (2016). Dynamin-related protein inhibitor downregulates reactive oxygen species levels to indirectly suppress high glucose-induced hyperproliferation of vascular smooth muscle cells. Biochem. Biophys. Res. Commun. 471 (4), 474–478. doi:10.1016/j.bbrc.2016.02.051
Makrecka-Kuka, M., Volska, K., Antone, U., Vilskersts, R., Grinberga, S., Bandere, D., et al. (2017). Trimethylamine N-oxide impairs pyruvate and fatty acid oxidation in cardiac mitochondria. Toxicol. Lett. 267, 32–38. doi:10.1016/j.toxlet.2016.12.017
McArthur, K., Whitehead, L. W., Heddleston, J. M., Li, L., Padman, B. S., Oorschot, V., et al. (2018). BAK/BAX macropores facilitate mitochondrial herniation and mtDNA efflux during apoptosis. Science 359 (6378), eaao6047. doi:10.1126/science.aao6047
McFadden, R. M., Larmonier, C. B., Shehab, K. W., Midura-Kiela, M., Ramalingam, R., Harrison, C. A., et al. (2015). The role of curcumin in modulating colonic microbiota during colitis and colon cancer prevention. Inflamm. Bowel Dis. 21 (11), 2483–2494. doi:10.1097/mib.0000000000000522
Michael, D. R., Davies, T. S., Moss, J. W. E., Calvente, D. L., Ramji, D. P., Marchesi, J. R., et al. (2017). The anti-cholesterolaemic effect of a consortium of probiotics: An acute study in C57BL/6J mice. Sci. Rep. 7 (1), 2883. doi:10.1038/s41598-017-02889-5
Mikó, E., Vida, A., Kovács, T., Ujlaki, G., Trencsényi, G., Márton, J., et al. (2018). Lithocholic acid, a bacterial metabolite reduces breast cancer cell proliferation and aggressiveness. Biochimica Biophysica Acta (BBA) - Bioenergetics 1859 (9), 958–974. doi:10.1016/j.bbabio.2018.04.002
Misawa, T., Takahama, M., and Saitoh, T. (2017). “Mitochondria–endoplasmic reticulum contact sites mediate innate immune responses,” in Organelle contact sites: From molecular mechanism to disease. Editors M. Tagaya, and T. Simmen (Singapore: Springer Singapore), 187–197.
Módis, K., Bos, E. M., Calzia, E., van Goor, H., Coletta, C., Papapetropoulos, A., et al. (2014). Regulation of mitochondrial bioenergetic function by hydrogen sulfide. Part II. Pathophysiological and therapeutic aspects. Br. J. Pharmacol. 171 (8), 2123–2146. doi:10.1111/bph.12368
Mohammadi, A., Najar, A. G., Yaghoobi, M. M., Jahani, Y., and Vahabzadeh, Z. (2016). Trimethylamine-N-Oxide treatment induces changes in the ATP-binding cassette transporter A1 and scavenger receptor A1 in murine macrophage J774A.1 cells. Inflammation 39 (1), 393–404. doi:10.1007/s10753-015-0261-7
Mohanty, A., Tiwari-Pandey, R., and Pandey, N. R. (2019). Mitochondria: The indispensable players in innate immunity and guardians of the inflammatory response. J. Cell Commun. Signal 13 (3), 303–318. doi:10.1007/s12079-019-00507-9
Mollica, M. P., Mattace Raso, G., Cavaliere, G., Trinchese, G., De Filippo, C., Aceto, S., et al. (2017). Butyrate regulates liver mitochondrial function, efficiency, and dynamics in insulin-resistant obese mice. Diabetes 66 (5), 1405–1418. doi:10.2337/db16-0924
Murphy, B., Bhattacharya, R., and Mukherjee, P. (2019). Hydrogen sulfide signaling in mitochondria and disease. Faseb J. 33 (12), 13098–13125. doi:10.1096/fj.201901304R
Najjar, R. S., and Feresin, R. G. (2019). Plant-based diets in the reduction of body fat: Physiological effects and biochemical insights. Nutrients 11 (11), 2712. doi:10.3390/nu11112712
Nguyen, E. K., Koval, O. M., Noble, P., Broadhurst, K., Allamargot, C., Wu, M., et al. (2018). CaMKII (Ca(2+)/calmodulin-dependent kinase II) in mitochondria of smooth muscle cells controls mitochondrial mobility, migration, and neointima formation. Arterioscler. Thromb. Vasc. Biol. 38 (6), 1333–1345. doi:10.1161/atvbaha.118.310951
Nie, J., Zhang, L., Zhao, G., and Du, X. (2019). Quercetin reduces atherosclerotic lesions by altering the gut microbiota and reducing atherogenic lipid metabolites. J. Appl. Microbiol. 127 (6), 1824–1834. doi:10.1111/jam.14441
Oliveira, H. C. F., and Vercesi, A. E. (2020). Mitochondrial bioenergetics and redox dysfunctions in hypercholesterolemia and atherosclerosis. Mol. Asp. Med. 71, 100840. doi:10.1016/j.mam.2019.100840
Orekhov, A. N., Nikiforov, N. N., Ivanova, E. A., and Sobenin, I. A. (2020a). Possible role of mitochondrial DNA mutations in chronification of inflammation: Focus on atherosclerosis. J. Clin. Med. 9 (4), 978. doi:10.3390/jcm9040978
Orekhov, A. N., Poznyak, A. V., Sobenin, I. A., Nikifirov, N. N., and Ivanova, E. A. (2020b). Mitochondrion as a selective target for the treatment of atherosclerosis: Role of mitochondrial DNA mutations and defective mitophagy in the pathogenesis of atherosclerosis and chronic inflammation. Curr. Neuropharmacol. 18 (11), 1064–1075. doi:10.2174/1570159x17666191118125018
Panyod, S., Wu, W. K., Chen, P. C., Chong, K. V., Yang, Y. T., Chuang, H. L., et al. (2022). Atherosclerosis amelioration by allicin in raw garlic through gut microbiota and trimethylamine-N-oxide modulation. NPJ Biofilms Microbiomes 8 (1), 4. doi:10.1038/s41522-022-00266-3
Park, S., Juliana, C., Hong, S., Datta, P., Hwang, I., Fernandes-Alnemri, T., et al. (2013). The mitochondrial antiviral protein MAVS associates with NLRP3 and regulates its inflammasome activity. J. Immunol. 191 (8), 4358–4366. doi:10.4049/jimmunol.1301170
Paul, B. D., Snyder, S. H., and Kashfi, K. (2021). Effects of hydrogen sulfide on mitochondrial function and cellular bioenergetics. Redox Biol. 38, 101772. doi:10.1016/j.redox.2020.101772
Peace, C. G., and O'Neill, L. A. (2022). The role of itaconate in host defense and inflammation. J. Clin. Invest. 132 (2), e148548. doi:10.1172/jci148548
Peng, X., Chen, H., Li, Y., Huang, D., Huang, B., and Sun, D. (2020). Effects of NIX-mediated mitophagy on ox-LDL-induced macrophage pyroptosis in atherosclerosis. Cell Biol. Int. 44 (7), 1481–1490. doi:10.1002/cbin.11343
Peng, X., Zhang, C., Zhou, Z. M., Wang, K., Gao, J. W., Qian, Z. Y., et al. (2022). A20 attenuates pyroptosis and apoptosis in nucleus pulposus cells via promoting mitophagy and stabilizing mitochondrial dynamics. Inflamm. Res. 71 (5-6), 695–710. doi:10.1007/s00011-022-01570-6
Pham, P. T., Fukuda, D., Nishimoto, S., Kim-Kaneyama, J. R., Lei, X. F., Takahashi, Y., et al. (2021). STING, a cytosolic DNA sensor, plays a critical role in atherogenesis: A link between innate immunity and chronic inflammation caused by lifestyle-related diseases. Eur. Heart J. 42 (42), 4336–4348. doi:10.1093/eurheartj/ehab249
Picca, A., Guerra, F., Calvani, R., Romano, R., Coelho-Junior, H. J., Damiano, F. P., et al. (2022). Circulating mitochondrial DNA and inter-organelle contact sites in aging and associated conditions. Cells 11 (4), 675. doi:10.3390/cells11040675
Pollack, R. M., Barzilai, N., Anghel, V., Kulkarni, A. S., Golden, A., O'Broin, P., et al. (2017). Resveratrol improves vascular function and mitochondrial number but not glucose metabolism in older adults. J. Gerontol. A Biol. Sci. Med. Sci. 72 (12), 1703–1709. doi:10.1093/gerona/glx041
Pols, T. W., Nomura, M., Harach, T., Lo Sasso, G., Oosterveer, M. H., Thomas, C., et al. (2011). TGR5 activation inhibits atherosclerosis by reducing macrophage inflammation and lipid loading. Cell Metab. 14 (6), 747–757. doi:10.1016/j.cmet.2011.11.006
Primec, M., Klemenak, M., Di Gioia, D., Aloisio, I., Bozzi Cionci, N., Quagliariello, A., et al. (2019). Clinical intervention using Bifidobacterium strains in celiac disease children reveals novel microbial modulators of TNF-α and short-chain fatty acids. Clin. Nutr. 38 (3), 1373–1381. doi:10.1016/j.clnu.2018.06.931
Pu, Y., Zhang, H., Wang, P., Zhao, Y., Li, Q., Wei, X., et al. (2013). Dietary curcumin ameliorates aging-related cerebrovascular dysfunction through the AMPK/uncoupling protein 2 pathway. Cell Physiol. Biochem. 32 (5), 1167–1177. doi:10.1159/000354516
Puhm, F., Afonyushkin, T., Resch, U., Obermayer, G., Rohde, M., Penz, T., et al. (2019). Mitochondria are a subset of extracellular vesicles released by activated monocytes and induce type I IFN and TNF responses in endothelial cells. Circ. Res. 125 (1), 43–52. doi:10.1161/circresaha.118.314601
Pursnani, A., Massaro, J. M., D'Agostino, R. B., Sr, , O'Donnell, C. J., and Hoffmann, U. (2017). Guideline-based statin eligibility, cancer events, and noncardiovascular mortality in the framingham heart study. J. Clin. Oncol. 35 (25), 2927–2933. doi:10.1200/jco.2016.71.3594
Qi, Y., Liu, W., Yan, X., Zhang, C., Zhang, C., Liu, L., et al. (2022). Tongxinluo may alleviate inflammation and improve the stability of atherosclerotic plaques by changing the intestinal flora. Front. Pharmacol. 13, 805266. doi:10.3389/fphar.2022.805266
Querio, G., Antoniotti, S., Geddo, F., Levi, R., and Gallo, M. P. (2022). Trimethylamine N-oxide does not impact viability, ROS production, and mitochondrial membrane potential of adult rat cardiomyocytes. Int. J. Mol. Sci. 23 (7), 3045. doi:10.3390/ijms20123045
Querio, G., Antoniotti, S., Levi, R., and Gallo, M. P. (2019). Trimethylamine N-oxide does not impact viability, ROS production, and mitochondrial membrane potential of adult rat cardiomyocytes. Int. J. Mol. Sci. 20 (12), 3045. doi:10.3390/ijms20123045
Ramachandran, R. P., Spiegel, C., Keren, Y., Danieli, T., Melamed-Book, N., Pal, R. R., et al. (2020). Mitochondrial targeting of the enteropathogenic Escherichia coli map triggers calcium mobilization, ADAM10-MAP kinase signaling, and host cell apoptosis. mBio 11 (5), 013977–e1420. doi:10.1128/mBio.01397-20
Rassow, J. (2011). Helicobacter pylori vacuolating toxin A and apoptosis. Cell Commun. Signal 9, 26. doi:10.1186/1478-811x-9-26
Robert, P., Nguyen, P. M. C., Richard, A., Grenier, C., Chevrollier, A., Munier, M., et al. (2021). Protective role of the mitochondrial fusion protein OPA1 in hypertension. Faseb J. 35 (7), e21678. doi:10.1096/fj.202000238RRR
Rodrigues, R. R., Gurung, M., Li, Z., García-Jaramillo, M., Greer, R., Gaulke, C., et al. (2021). Transkingdom interactions between Lactobacilli and hepatic mitochondria attenuate Western diet-induced diabetes. Nat. Commun. 12 (1), 101. doi:10.1038/s41467-020-20313-x
Roger, A. J., Muñoz-Gómez, S. A., and Kamikawa, R. (2017). The origin and diversification of mitochondria. Curr. Biol. 27 (21), R1177–r1192. doi:10.1016/j.cub.2017.09.015
Rong, Q., Han, B., Li, Y., Yin, H., Li, J., and Hou, Y. (2021). Berberine reduces lipid accumulation by promoting fatty acid oxidation in renal tubular epithelial cells of the diabetic kidney. Front. Pharmacol. 12, 729384. doi:10.3389/fphar.2021.729384
Ross, J. M., Coppotelli, G., Hoffer, B. J., and Olson, L. (2014). Maternally transmitted mitochondrial DNA mutations can reduce lifespan. Sci. Rep. 4, 6569. doi:10.1038/srep06569
Rossman, M. J., Santos-Parker, J. R., Steward, C. A. C., Bispham, N. Z., Cuevas, L. M., Rosenberg, H. L., et al. (2018). Chronic supplementation with a mitochondrial antioxidant (MitoQ) improves vascular function in healthy older adults. Hypertension 71 (6), 1056–1063. doi:10.1161/hypertensionaha.117.10787
Rossmann, M. P., Dubois, S. M., Agarwal, S., and Zon, L. I. (2021). Mitochondrial function in development and disease. Dis. Model Mech. 14 (6), dmm048912. doi:10.1242/dmm.048912
Salnikova, D., Orekhova, V., Grechko, A., Starodubova, A., Bezsonov, E., Popkova, T., et al. (2021). Mitochondrial dysfunction in vascular wall cells and its role in atherosclerosis. Int. J. Mol. Sci. 22 (16), 8990. doi:10.3390/ijms22168990
Sazonova, M. A., Sinyov, V. V., Barinova, V. A., Ryzhkova, A. I., Zhelankin, A. V., Postnov, A. Y., et al. (2015). Mosaicism of mitochondrial genetic variation in atherosclerotic lesions of the human aorta. Biomed. Res. Int. 2015, 825468. doi:10.1155/2015/825468
Sazonova, M. A., Sinyov, V. V., Ryzhkova, A. I., Galitsyna, E. V., Khasanova, Z. B., Postnov, A. Y., et al. (2017). Role of mitochondrial genome mutations in pathogenesis of carotid atherosclerosis. Oxid. Med. Cell Longev. 2017, 6934394. doi:10.1155/2017/6934394
Schellenberg, B., Wang, P., Keeble, J. A., Rodriguez-Enriquez, R., Walker, S., Owens, T. W., et al. (2013). Bax exists in a dynamic equilibrium between the cytosol and mitochondria to control apoptotic priming. Mol. Cell 49 (5), 959–971. doi:10.1016/j.molcel.2012.12.022
Schneider, J., Girreser, U., Havemeyer, A., Bittner, F., and Clement, B. (2018). Detoxification of trimethylamine N-oxide by the mitochondrial amidoxime reducing component mARC. Chem. Res. Toxicol. 31 (6), 447–453. doi:10.1021/acs.chemrestox.7b00329
Shames, S. R., Croxen, M. A., Deng, W., and Finlay, B. B. (2011). The type III system-secreted effector EspZ localizes to host mitochondria and interacts with the translocase of inner mitochondrial membrane 17b. Infect. Immun. 79 (12), 4784–4790. doi:10.1128/iai.05761-11
Shen, X., Carlström, M., Borniquel, S., Jädert, C., Kevil, C. G., and Lundberg, J. O. (2013). Microbial regulation of host hydrogen sulfide bioavailability and metabolism. Free Radic. Biol. Med. 60, 195–200. doi:10.1016/j.freeradbiomed.2013.02.024
Shi, R., Zhu, D., Wei, Z., Fu, N., Wang, C., Liu, L., et al. (2018). Baicalein attenuates monocrotaline-induced pulmonary arterial hypertension by inhibiting endothelial-to-mesenchymal transition. Life Sci. 207, 442–450. doi:10.1016/j.lfs.2018.06.033
Shi, X., Zhou, X., Chu, X., Wang, J., Xie, B., Ge, J., et al. (2019). Allicin improves metabolism in high-fat diet-induced obese mice by modulating the gut microbiota. Nutrients 11 (12), 2909. doi:10.3390/nu11122909
Shi, Y., Hu, J., Geng, J., Hu, T., Wang, B., Yan, W., et al. (2018). Berberine treatment reduces atherosclerosis by mediating gut microbiota in apoE-/- mice. Biomed. Pharmacother. 107, 1556–1563. doi:10.1016/j.biopha.2018.08.148
Song, Y., Xu, Y., Liu, Y., Gao, J., Feng, L., Zhang, Y., et al. (2021). Mitochondrial quality control in the maintenance of cardiovascular homeostasis: The roles and interregulation of UPS, mitochondrial dynamics and mitophagy. Oxid. Med. Cell Longev. 2021, 3960773. doi:10.1155/2021/3960773
Song, Y., Zhou, Y., and Zhou, X. (2020). The role of mitophagy in innate immune responses triggered by mitochondrial stress. Cell Commun. Signal 18 (1), 186. doi:10.1186/s12964-020-00659-x
Sprenger, H. G., MacVicar, T., Bahat, A., Fiedler, K. U., Hermans, S., Ehrentraut, D., et al. (2021). Cellular pyrimidine imbalance triggers mitochondrial DNA-dependent innate immunity. Nat. Metab. 3 (5), 636–650. doi:10.1038/s42255-021-00385-9
Springo, Z., Tarantini, S., Toth, P., Tucsek, Z., Koller, A., Sonntag, W. E., et al. (2015). Aging exacerbates pressure-induced mitochondrial oxidative stress in mouse cerebral arteries. J. Gerontol. A Biol. Sci. Med. Sci. 70 (11), 1355–1359. doi:10.1093/gerona/glu244
Stewart, J. B., and Chinnery, P. F. (2021). Extreme heterogeneity of human mitochondrial DNA from organelles to populations. Nat. Rev. Genet. 22 (2), 106–118. doi:10.1038/s41576-020-00284-x
Subramanian, N., Natarajan, K., Clatworthy, M. R., Wang, Z., and Germain, R. N. (2013). The adaptor MAVS promotes NLRP3 mitochondrial localization and inflammasome activation. Cell 153 (2), 348–361. doi:10.1016/j.cell.2013.02.054
Sukumaran, S. K., Fu, N. Y., Tin, C. B., Wan, K. F., Lee, S. S., and Yu, V. C. (2010). A soluble form of the pilus protein FimA targets the VDAC-hexokinase complex at mitochondria to suppress host cell apoptosis. Mol. Cell 37 (6), 768–783. doi:10.1016/j.molcel.2010.02.015
Sun, A., Wang, Y., Liu, J., Yu, X., Sun, Y., Yang, F., et al. (2016). Exogenous H2S modulates mitochondrial fusion-fission to inhibit vascular smooth muscle cell proliferation in a hyperglycemic state. Cell Biosci. 6, 36. doi:10.1186/s13578-016-0102-x
Sun, Y., Lu, F., Yu, X., Wang, B., Chen, J., Lu, F., et al. (2020). Exogenous H(2)S promoted USP8 sulfhydration to regulate mitophagy in the hearts of db/db mice. Aging Dis. 11 (2), 269–285. doi:10.14336/ad.2019.0524
Sung, M. M., Kim, T. T., Denou, E., Soltys, C. M., Hamza, S. M., Byrne, N. J., et al. (2017). Improved glucose homeostasis in obese mice treated with resveratrol is associated with alterations in the gut microbiome. Diabetes 66 (2), 418–425. doi:10.2337/db16-0680
Swiader, A., Nahapetyan, H., Faccini, J., D'Angelo, R., Mucher, E., Elbaz, M., et al. (2016). Mitophagy acts as a safeguard mechanism against human vascular smooth muscle cell apoptosis induced by atherogenic lipids. Oncotarget 7 (20), 28821–28835. doi:10.18632/oncotarget.8936
Tang, W. H., Wang, Z., Levison, B. S., Koeth, R. A., Britt, E. B., Fu, X., et al. (2013). Intestinal microbial metabolism of phosphatidylcholine and cardiovascular risk. N. Engl. J. Med. 368 (17), 1575–1584. doi:10.1056/NEJMoa1109400
Tang, X., Ma, S., Li, Y., Sun, Y., Zhang, K., Zhou, Q., et al. (2020). Evaluating the activity of sodium butyrate to prevent osteoporosis in rats by promoting osteal GSK-3β/nrf2 signaling and mitochondrial function. J. Agric. Food Chem. 68 (24), 6588–6603. doi:10.1021/acs.jafc.0c01820
Tian, L., Li, N., Li, K., Tan, Y., Han, J., Lin, B., et al. (2022). Ambient ozone exposure induces ROS related-mitophagy and pyroptosis via NLRP3 inflammasome activation in rat lung cells. Ecotoxicol. Environ. Saf. 240, 113663. doi:10.1016/j.ecoenv.2022.113663
Toksoy, A., Sennefelder, H., Adam, C., Hofmann, S., Trautmann, A., Goebeler, M., et al. (2017). Potent NLRP3 inflammasome activation by the HIV reverse transcriptase inhibitor abacavir. J. Biol. Chem. 292 (7), 2805–2814. doi:10.1074/jbc.M116.749473
Toral, M., Romero, M., Rodríguez-Nogales, A., Jiménez, R., Robles-Vera, I., Algieri, F., et al. (2018). Lactobacillus fermentum improves tacrolimus-induced hypertension by restoring vascular redox state and improving eNOS coupling. Mol. Nutr. Food Res. 62, e1800033. doi:10.1002/mnfr.201800033
Ungvari, Z., Labinskyy, N., Mukhopadhyay, P., Pinto, J. T., Bagi, Z., Ballabh, P., et al. (2009). Resveratrol attenuates mitochondrial oxidative stress in coronary arterial endothelial cells. Am. J. Physiol. Heart Circ. Physiol. 297 (5), H1876–H1881. doi:10.1152/ajpheart.00375.2009
Untereiner, A. A., Wang, R., Ju, Y., and Wu, L. (2016). Decreased gluconeogenesis in the absence of cystathionine gamma-lyase and the underlying mechanisms. Antioxid. Redox Signal 24 (3), 129–140. doi:10.1089/ars.2015.6369
Valente, W. J., Ericson, N. G., Long, A. S., White, P. A., Marchetti, F., and Bielas, J. H. (2016). Mitochondrial DNA exhibits resistance to induced point and deletion mutations. Nucleic Acids Res. 44 (18), 8513–8524. doi:10.1093/nar/gkw716
Vecoli, C., Borghini, A., Pulignani, S., Mercuri, A., Turchi, S., Carpeggiani, C., et al. (2018). Prognostic value of mitochondrial DNA(4977) deletion and mitochondrial DNA copy number in patients with stable coronary artery disease. Atherosclerosis 276, 91–97. doi:10.1016/j.atherosclerosis.2018.07.015
Vecoli, C., Borghini, A., Pulignani, S., Mercuri, A., Turchi, S., Picano, E., et al. (2019). Independent and combined effects of telomere shortening and mtDNA(4977) deletion on long-term outcomes of patients with coronary artery disease. Int. J. Mol. Sci. 20 (21), 5508. doi:10.3390/ijms20215508
Vendrov, A. E., Stevenson, M. D., Alahari, S., Pan, H., Wickline, S. A., Madamanchi, N. R., et al. (2017). Attenuated superoxide dismutase 2 activity induces atherosclerotic plaque instability during aging in hyperlipidemic mice. J. Am. Heart Assoc. 6 (11), e006775. doi:10.1161/jaha.117.006775
Verhaar, B. J. H., Prodan, A., Nieuwdorp, M., and Muller, M. (2020). Gut microbiota in hypertension and atherosclerosis: A review. Nutrients 12 (10), 2982. doi:10.3390/nu12102982
Vezza, T., Abad-Jiménez, Z., Marti-Cabrera, M., Rocha, M., and Víctor, V. M. (2020). Microbiota-mitochondria inter-talk: A potential therapeutic strategy in obesity and type 2 diabetes. Antioxidants (Basel) 9 (9), 848. doi:10.3390/antiox9090848
Videja, M., Vilskersts, R., Korzh, S., Cirule, H., Sevostjanovs, E., Dambrova, M., et al. (2020). Microbiota-derived metabolite trimethylamine N-oxide protects mitochondrial energy metabolism and cardiac functionality in a rat model of right ventricle heart failure. Front. Cell Dev. Biol. 8, 622741. doi:10.3389/fcell.2020.622741
Wan, Q., Liu, Z. Y., Yang, Y. P., and Liu, S. M. (2016). Effect of curcumin on inhibiting atherogenesis by down-regulating lipocalin-2 expression in apolipoprotein E knockout mice. Biomed. Mater Eng. 27 (6), 577–587. doi:10.3233/bme-161610
Wang, J., Ghosh, S. S., and Ghosh, S. (2017). Curcumin improves intestinal barrier function: Modulation of intracellular signaling, and organization of tight junctions. Am. J. Physiol. Cell Physiol. 312 (4), C438–c445. doi:10.1152/ajpcell.00235.2016
Wang, L., Tang, X. Q., Shi, Y., Li, H. M., Meng, Z. Y., Chen, H., et al. (2022). Tetrahydroberberrubine retards heart aging in mice by promoting PHB2-mediated mitophagy. Acta Pharmacol. Sin. doi:10.1038/s41401-022-00956-w
Wang, Y., Christopher, B. A., Wilson, K. A., Muoio, D., McGarrah, R. W., Brunengraber, H., et al. (2018). Propionate-induced changes in cardiac metabolism, notably CoA trapping, are not altered by l-carnitine. Am. J. Physiol. Endocrinol. Metab. 315 (4), E622–e633. doi:10.1152/ajpendo.00081.2018
Wang, Z., Klipfell, E., Bennett, B. J., Koeth, R., Levison, B. S., Dugar, B., et al. (2011). Gut flora metabolism of phosphatidylcholine promotes cardiovascular disease. Nature 472 (7341), 57–63. doi:10.1038/nature09922
Wang, Z., Wu, F., Zhou, Q., Qiu, Y., Zhang, J., Tu, Q., et al. (2022). Berberine improves vascular dysfunction by inhibiting trimethylamine-N-oxide via regulating the gut microbiota in angiotensin II-induced hypertensive mice. Front. Microbiol. 13, 814855. doi:10.3389/fmicb.2022.814855
Wei, T., Huang, G., Gao, J., Huang, C., Sun, M., Wu, J., et al. (2017). Sirtuin 3 deficiency accelerates hypertensive cardiac remodeling by impairing angiogenesis. J. Am. Heart Assoc. 6 (8), e006114. doi:10.1161/jaha.117.006114
Wellman, A. S., Metukuri, M. R., Kazgan, N., Xu, X., Xu, Q., Ren, N. S. X., et al. (2017). Intestinal epithelial sirtuin 1 regulates intestinal inflammation during aging in mice by altering the intestinal microbiota. Gastroenterology 153 (3), 772–786. doi:10.1053/j.gastro.2017.05.022
West, A. P., Brodsky, I. E., Rahner, C., Woo, D. K., Erdjument-Bromage, H., Tempst, P., et al. (2011). TLR signalling augments macrophage bactericidal activity through mitochondrial ROS. Nature 472 (7344), 476–480. doi:10.1038/nature09973
West, A. P., Khoury-Hanold, W., Staron, M., Tal, M. C., Pineda, C. M., Lang, S. M., et al. (2015). Mitochondrial DNA stress primes the antiviral innate immune response. Nature 520 (7548), 553–557. doi:10.1038/nature14156
Witkowski, M., Weeks, T. L., and Hazen, S. L. (2020). Gut microbiota and cardiovascular disease. Circ. Res. 127 (4), 553–570. doi:10.1161/circresaha.120.316242
Wu, D., Hu, Q., Liu, X., Pan, L., Xiong, Q., and Zhu, Y. Z. (2015). Hydrogen sulfide protects against apoptosis under oxidative stress through SIRT1 pathway in H9c2 cardiomyocytes. Nitric Oxide 46, 204–212. doi:10.1016/j.niox.2014.11.006
Wu, D. N., Guan, L., Jiang, Y. X., Ma, S. H., Sun, Y. N., Lei, H. T., et al. (2019). Microbiome and metabonomics study of quercetin for the treatment of atherosclerosis. Cardiovasc Diagn Ther. 9 (6), 545–560. doi:10.21037/cdt.2019.12.04
Wu, M., Yang, S., Wang, S., Cao, Y., Zhao, R., Li, X., et al. (2020). Effect of berberine on atherosclerosis and gut microbiota modulation and their correlation in high-fat diet-fed ApoE-/- mice. Front. Pharmacol. 11, 223. doi:10.3389/fphar.2020.00223
Wu, W., Xu, H., Wang, Z., Mao, Y., Yuan, L., Luo, W., et al. (2015). PINK1-Parkin-Mediated mitophagy protects mitochondrial integrity and prevents metabolic stress-induced endothelial injury. PLoS One 10 (7), e0132499. doi:10.1371/journal.pone.0132499
Xian, H., Watari, K., Sanchez-Lopez, E., Offenberger, J., Onyuru, J., Sampath, H., et al. (2022). Oxidized DNA fragments exit mitochondria via mPTP- and VDAC-dependent channels to activate NLRP3 inflammasome and interferon signaling. Immunity 55 (8), 1370–1385.e8. e1378. doi:10.1016/j.immuni.2022.06.007
Xie, T., Wang, C., Jin, Y., Meng, Q., Liu, Q., Wu, J., et al. (2020). CoenzymeQ10-Induced activation of AMPK-YAP-OPA1 pathway alleviates atherosclerosis by improving mitochondrial function, inhibiting oxidative stress and promoting energy metabolism. Front. Pharmacol. 11, 1034. doi:10.3389/fphar.2020.01034
Xu, A., Deng, F., Chen, Y., Kong, Y., Pan, L., Liao, Q., et al. (2020). NF-κB pathway activation during endothelial-to-mesenchymal transition in a rat model of doxorubicin-induced cardiotoxicity. Biomed. Pharmacother. 130, 110525. doi:10.1016/j.biopha.2020.110525
Xu, H., Pan, L. B., Yu, H., Han, P., Fu, J., Zhang, Z. W., et al. (2022). Gut microbiota-derived metabolites in inflammatory diseases based on targeted metabolomics. Front. Pharmacol. 13, 919181. doi:10.3389/fphar.2022.919181
Xu, L., Li, M., Yang, Y., Zhang, C., Xie, Z., Tang, J., et al. (2022). Salmonella induces the cGAS-STING-dependent type I interferon response in murine macrophages by triggering mtDNA release. mBio 13 (3), e0363221. doi:10.1128/mbio.03632-21
Xue, H., Chen, X., Yu, C., Deng, Y., Zhang, Y., Chen, S., et al. (2022). Gut microbially produced indole-3-propionic acid inhibits atherosclerosis by promoting reverse cholesterol transport and its deficiency is causally related to atherosclerotic cardiovascular disease. Circ. Res. 131 (5), 404–420. doi:10.1161/circresaha.122.321253
Yang, J., Zhou, X., Zeng, X., Hu, O., Yi, L., and Mi, M. (2019). Resveratrol attenuates oxidative injury in human umbilical vein endothelial cells through regulating mitochondrial fusion via TyrRS-PARP1 pathway. Nutr. Metab. (Lond) 16, 9. doi:10.1186/s12986-019-0338-7
Yang, L., Lin, X., Tang, H., Fan, Y., Zeng, S., Jia, L., et al. (2020). Mitochondrial DNA mutation exacerbates female reproductive aging via impairment of the NADH/NAD(+) redox. Aging Cell 19 (9), e13206. doi:10.1111/acel.13206
Yang, M., Zheng, J., Zong, X., Yang, X., Zhang, Y., Man, C., et al. (2021). Preventive effect and molecular mechanism of Lactobacillus rhamnosus JL1 on food-borne obesity in mice. Nutrients 13 (11), 3989. doi:10.3390/nu13113989
Yang, Y. N., Wang, Q. C., Xu, W., Yu, J., Zhang, H., and Wu, C. (2022). The berberine-enriched gut commensal Blautia producta ameliorates high-fat diet (HFD)-induced hyperlipidemia and stimulates liver LDLR expression. Biomed. Pharmacother. 155, 113749. doi:10.1016/j.biopha.2022.113749
Yao, S., Yuan, Y., Zhang, H., Meng, X., Jin, L., Yang, J., et al. (2020). Berberine attenuates the abnormal ectopic lipid deposition in skeletal muscle. Free Radic. Biol. Med. 159, 66–75. doi:10.1016/j.freeradbiomed.2020.07.028
Yaron, J. R., Gangaraju, S., Rao, M. Y., Kong, X., Zhang, L., Su, F., et al. (2015). K(+) regulates Ca(2+) to drive inflammasome signaling: Dynamic visualization of ion flux in live cells. Cell Death Dis. 6 (10), e1954. doi:10.1038/cddis.2015.277
Yin, Z., Burger, N., Kula-Alwar, D., Aksentijević, D., Bridges, H. R., Prag, H. A., et al. (2021). Structural basis for a complex I mutation that blocks pathological ROS production. Nat. Commun. 12 (1), 707. doi:10.1038/s41467-021-20942-w
Yoo, J. Y., Sniffen, S., McGill Percy, K. C., Pallaval, V. B., and Chidipi, B. (2022). Gut dysbiosis and immune system in atherosclerotic cardiovascular disease (ACVD). Microorganisms 10 (1), 108. doi:10.3390/microorganisms10010108
Yoshida, Y., Shimizu, I., Shimada, A., Nakahara, K., Yanagisawa, S., Kubo, M., et al. (2022). Brown adipose tissue dysfunction promotes heart failure via a trimethylamine N-oxide-dependent mechanism. Sci. Rep. 12 (1), 14883. doi:10.1038/s41598-022-19245-x
You, S., Qian, J., Wu, G., Qian, Y., Wang, Z., Chen, T., et al. (2019). Schizandrin B attenuates angiotensin II induced endothelial to mesenchymal transition in vascular endothelium by suppressing NF-κB activation. Phytomedicine 62, 152955. doi:10.1016/j.phymed.2019.152955
Yu, C. H., Davidson, S., Harapas, C. R., Hilton, J. B., Mlodzianoski, M. J., Laohamonthonkul, P., et al. (2020). TDP-43 triggers mitochondrial DNA release via mPTP to activate cGAS/STING in ALS. Cell 183 (3), 636–649. e618. doi:10.1016/j.cell.2020.09.020
Yu, E., Calvert, P. A., Mercer, J. R., Harrison, J., Baker, L., Figg, N. L., et al. (2013). Mitochondrial DNA damage can promote atherosclerosis independently of reactive oxygen species through effects on smooth muscle cells and monocytes and correlates with higher-risk plaques in humans. Circulation 128 (7), 702–712. doi:10.1161/circulationaha.113.002271
Yu, E. P., and Bennett, M. R. (2014). Mitochondrial DNA damage and atherosclerosis. Trends Endocrinol. Metab. 25 (9), 481–487. doi:10.1016/j.tem.2014.06.008
Yu, E. P. K., Reinhold, J., Yu, H., Starks, L., Uryga, A. K., Foote, K., et al. (2017). Mitochondrial respiration is reduced in atherosclerosis, promoting necrotic core formation and reducing relative fibrous cap thickness. Arterioscler. Thromb. Vasc. Biol. 37 (12), 2322–2332. doi:10.1161/atvbaha.117.310042
Yu, J., Nagasu, H., Murakami, T., Hoang, H., Broderick, L., Hoffman, H. M., et al. (2014). Inflammasome activation leads to Caspase-1-dependent mitochondrial damage and block of mitophagy. Proc. Natl. Acad. Sci. U. S. A. 111 (43), 15514–15519. doi:10.1073/pnas.1414859111
Yu, M., Alimujiang, M., Hu, L., Liu, F., Bao, Y., and Yin, J. (2021). Berberine alleviates lipid metabolism disorders via inhibition of mitochondrial complex I in gut and liver. Int. J. Biol. Sci. 17 (7), 1693–1707. doi:10.7150/ijbs.54604
Zaharuddin, L., Mokhtar, N. M., Muhammad Nawawi, K. N., and Raja Ali, R. A. (2019). A randomized double-blind placebo-controlled trial of probiotics in post-surgical colorectal cancer. BMC Gastroenterol. 19 (1), 131. doi:10.1186/s12876-019-1047-4
Zhai, T., Wang, P., Hu, X., and Zheng, L. (2022). Probiotics bring new hope for atherosclerosis prevention and treatment. Oxid. Med. Cell Longev. 2022, 3900835. doi:10.1155/2022/3900835
Zhang, C., He, X., Sheng, Y., Yang, C., Xu, J., Zheng, S., et al. (2020). Allicin-induced host-gut microbe interactions improves energy homeostasis. Faseb J. 34 (8), 10682–10698. doi:10.1096/fj.202001007R
Zhang, J., Ou, C., and Chen, M. (2022). Curcumin attenuates cadmium-induced atherosclerosis by regulating trimethylamine-N-oxide synthesis and macrophage polarization through remodeling the gut microbiota. Ecotoxicol. Environ. Saf. 244, 114057. doi:10.1016/j.ecoenv.2022.114057
Zhang, J. Z., Liu, Z., Liu, J., Ren, J. X., and Sun, T. S. (2014). Mitochondrial DNA induces inflammation and increases TLR9/NF-κB expression in lung tissue. Int. J. Mol. Med. 33 (4), 817–824. doi:10.3892/ijmm.2014.1650
Zhang, L., Zhou, G., Song, W., Tan, X., Guo, Y., Zhou, B., et al. (2012). Pterostilbene protects vascular endothelial cells against oxidized low-density lipoprotein-induced apoptosis in vitro and in vivo. Apoptosis 17 (1), 25–36. doi:10.1007/s10495-011-0653-6
Zhang, M., Pan, H., Xu, Y., Wang, X., Qiu, Z., and Jiang, L. (2017). Allicin decreases lipopolysaccharide-induced oxidative stress and inflammation in human umbilical vein endothelial cells through suppression of mitochondrial dysfunction and activation of Nrf2. Cell Physiol. Biochem. 41 (6), 2255–2267. doi:10.1159/000475640
Zhang, Q., Raoof, M., Chen, Y., Sumi, Y., Sursal, T., Junger, W., et al. (2010). Circulating mitochondrial DAMPs cause inflammatory responses to injury. Nature 464 (7285), 104–107. doi:10.1038/nature08780
Zhang, S. J., Li, Z. H., Zhang, Y. D., Chen, J., Li, Y., Wu, F. Q., et al. (2021). Ketone body 3-hydroxybutyrate ameliorates atherosclerosis via receptor gpr109a-mediated calcium influx. Adv. Sci. (Weinh) 8 (9), 2003410. doi:10.1002/advs.202003410
Zhang, X., Chen, W., Li, J., Qi, S., Hong, S., Wang, Y., et al. (2018). Involvement of mitochondrial fission in calcium sensing receptor-mediated vascular smooth muscle cells proliferation during hypertension. Biochem. Biophys. Res. Commun. 495 (1), 454–460. doi:10.1016/j.bbrc.2017.11.048
Zhang, Y., Guallar, E., Ashar, F. N., Longchamps, R. J., Castellani, C. A., Lane, J., et al. (2017). Association between mitochondrial DNA copy number and sudden cardiac death: Findings from the atherosclerosis risk in communities study (ARIC). Eur. Heart J. 38 (46), 3443–3448. doi:10.1093/eurheartj/ehx354
Zhang, Y., Wang, C., Jin, Y., Yang, Q., Meng, Q., Liu, Q., et al. (2018). Activating the PGC-1α/TERT pathway by catalpol ameliorates atherosclerosis via modulating ROS production, DNA damage, and telomere function: Implications on mitochondria and telomere link. Oxid. Med. Cell Longev. 2018, 2876350. doi:10.1155/2018/2876350
Zhang, Y., Zhang, J., and Duan, L. (2022). The role of microbiota-mitochondria crosstalk in pathogenesis and therapy of intestinal diseases. Pharmacol. Res. 186, 106530. doi:10.1016/j.phrs.2022.106530
Zhao, M., Liu, S., Wang, C., Wang, Y., Wan, M., Liu, F., et al. (2021). Mesenchymal stem cell-derived extracellular vesicles attenuate mitochondrial damage and inflammation by stabilizing mitochondrial DNA. ACS Nano 15 (1), 1519–1538. doi:10.1021/acsnano.0c08947
Zhao, T., Gu, J., Zhang, H., Wang, Z., Zhang, W., Zhao, Y., et al. (2020). Sodium butyrate-modulated mitochondrial function in high-insulin induced HepG2 cell dysfunction. Oxid. Med. Cell Longev. 2020, 1904609. doi:10.1155/2020/1904609
Zhao, Y., Guo, Y., Chen, Y., Liu, S., Wu, N., and Jia, D. (2020). Curculigoside attenuates myocardial ischemia-reperfusion injury by inhibiting the opening of the mitochondrial permeability transition pore. Int. J. Mol. Med. 45 (5), 1514–1524. doi:10.3892/ijmm.2020.4513
Zheng, M., Qiao, W., Cui, J., Liu, L., Liu, H., Wang, Z., et al. (2014). Hydrogen sulfide delays nicotinamide-induced premature senescence via upregulation of SIRT1 in human umbilical vein endothelial cells. Mol. Cell Biochem. 393 (1-2), 59–67. doi:10.1007/s11010-014-2046-y
Zhou, P., Zhao, X. N., Ma, Y. Y., Tang, T. J., Wang, S. S., Wang, L., et al. (2022). Virtual screening analysis of natural flavonoids as trimethylamine (TMA)-lyase inhibitors for coronary heart disease. J. Food Biochem. 46, e14376. doi:10.1111/jfbc.14376
Zhou, R., Yazdi, A. S., Menu, P., and Tschopp, J. (2011). A role for mitochondria in NLRP3 inflammasome activation. Nature 469 (7329), 221–225. doi:10.1038/nature09663
Zhou, X., Chen, M., Zeng, X., Yang, J., Deng, H., Yi, L., et al. (2014). Resveratrol regulates mitochondrial reactive oxygen species homeostasis through Sirt3 signaling pathway in human vascular endothelial cells. Cell Death Dis. 5 (12), e1576. doi:10.1038/cddis.2014.530
Zhou, Y., Little, P. J., Xu, S., and Kamato, D. (2021). Curcumin inhibits lysophosphatidic acid mediated MCP-1 expression via blocking ROCK signalling. Molecules 26 (8), 2320. doi:10.3390/molecules26082320
Zhu, L., Zhang, D., Zhu, H., Zhu, J., Weng, S., Dong, L., et al. (2018). Berberine treatment increases Akkermansia in the gut and improves high-fat diet-induced atherosclerosis in Apoe(-/-) mice. Atherosclerosis 268, 117–126. doi:10.1016/j.atherosclerosis.2017.11.023
Zhu, N., Li, J., Li, Y., Zhang, Y., Du, Q., Hao, P., et al. (2020). Berberine protects against simulated ischemia/reperfusion injury-induced H9C2 cardiomyocytes apoptosis in vitro and myocardial ischemia/reperfusion-induced apoptosis in vivo by regulating the mitophagy-mediated HIF-1α/BNIP3 pathway. Front. Pharmacol. 11, 367. doi:10.3389/fphar.2020.00367
Zhu, W., Yuan, Y., Liao, G., Li, L., Liu, J., Chen, Y., et al. (2018). Mesenchymal stem cells ameliorate hyperglycemia-induced endothelial injury through modulation of mitophagy. Cell Death Dis. 9 (8), 837. doi:10.1038/s41419-018-0861-x
Ziganshina, E. E., Sharifullina, D. M., Lozhkin, A. P., Khayrullin, R. N., Ignatyev, I. M., and Ziganshin, A. M. (2016). Bacterial communities associated with atherosclerotic plaques from Russian individuals with atherosclerosis. PLoS One 11 (10), e0164836. doi:10.1371/journal.pone.0164836
Keywords: atherosclerosis, mitochondria, gut microbiota, metabolism, herbal medicine
Citation: Li Y, Yang S, Jin X, Li D, Lu J, Wang X and Wu M (2023) Mitochondria as novel mediators linking gut microbiota to atherosclerosis that is ameliorated by herbal medicine: A review. Front. Pharmacol. 14:1082817. doi: 10.3389/fphar.2023.1082817
Received: 28 October 2022; Accepted: 06 January 2023;
Published: 17 January 2023.
Edited by:
Jian Zhang, Tianjin Medical University, ChinaReviewed by:
Yuanli Chen, Hefei University of Technology, ChinaShiyu Song, Nanjing University, China
Chuanbin Liu, People’s Liberation Army General Hospital, China
Copyright © 2023 Li, Yang, Jin, Li, Lu, Wang and Wu. This is an open-access article distributed under the terms of the Creative Commons Attribution License (CC BY). The use, distribution or reproduction in other forums is permitted, provided the original author(s) and the copyright owner(s) are credited and that the original publication in this journal is cited, in accordance with accepted academic practice. No use, distribution or reproduction is permitted which does not comply with these terms.
*Correspondence: Min Wu, d3VtaW4xOTc2MjAwMEAxMjYuY29t