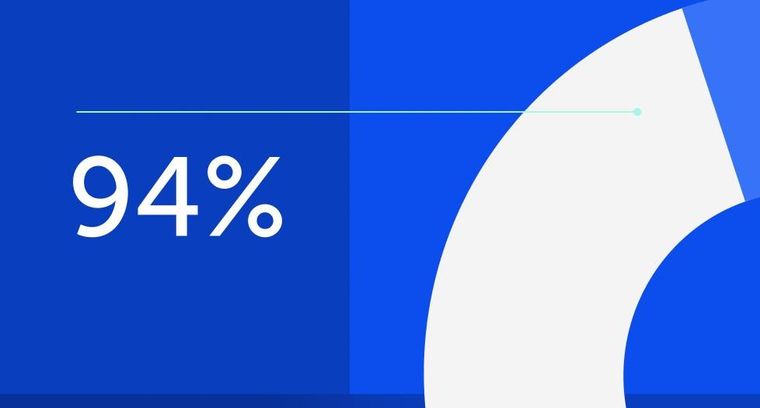
94% of researchers rate our articles as excellent or good
Learn more about the work of our research integrity team to safeguard the quality of each article we publish.
Find out more
ORIGINAL RESEARCH article
Front. Pharmacol., 09 May 2023
Sec. Pharmacology of Anti-Cancer Drugs
Volume 14 - 2023 | https://doi.org/10.3389/fphar.2023.1076815
This article is part of the Research TopicOncogenic PI3KT/Akt/mTOR Pathway Alterations, ROS Homeostasis, Targeted Cancer therapy and drug resistanceView all 10 articles
A retraction of this article was approved in:
Retraction: Mechanism of action of asparagus against multiple myeloma using network pharmacology and molecular docking
Citation: Li Y, Yang X, Wang F, Zhao J, Zhang C, Wu D, Yang B, Gao R, Zhao P, Zan Y, Su M, He Z, Liu Y, Wang J and Tang D (2023) Mechanism of action of Asparagus officinalis extract against multiple myeloma using bioinformatics tools, in silico and in vitro study. Front. Pharmacol. 14:1076815. doi: 10.3389/fphar.2023.1076815
Received: 22 October 2022; Accepted: 28 April 2023;
Published: 09 May 2023; Retracted: 27 January 2025.
Edited by:
Alaa El-Din Bekhit, University of Otago, New ZealandReviewed by:
Rozangela Curi Pedrosa, Federal University of Santa Catarina, BrazilDisclaimer: All claims expressed in this article are solely those of the authors and do not necessarily represent those of their affiliated organizations, or those of the publisher, the editors and the reviewers. Any product that may be evaluated in this article or claim that may be made by its manufacturer is not guaranteed or endorsed by the publisher.
Research integrity at Frontiers
Learn more about the work of our research integrity team to safeguard the quality of each article we publish.